- 1Climate Change Cluster, Faculty of Science, University of Technology, Sydney, NSW, Australia
- 2School of Life Sciences, Faculty of Science, University of Technology, Sydney, NSW, Australia
- 3School of Life and Environmental Sciences, University of Sydney, Sydney, NSW, Australia
Lipid-based survival mechanisms allow microalgae to occupy wide geographical ranges and survive abiotic stress. The protist Symbiodiniaceae are globally distributed from temperate to tropical environments, and establish mutualisms with numerous hosts, including cnidarians. The ability for these dinoflagellates to maintain cellular function under wide ranging environmental conditions will influence the survival and geographic distribution of their hosts. One mechanism that microalgae utilize to adapt to environmental changes is lipid remodeling, such as increased saturation of membranes to maintain the structural integrity under temperature changes, and lipid accumulation when nutrient availability decreases. Whether Symbiodiniaceae utilize lipid remodeling to adapt to sublethal environmental change is yet to be resolved. This study examines the effects of temperature (16°C to 31°C), and nitrogen (N) and phosphorus (P) availability, on the lipid composition and physiology of cultured Symbiodiniaceae (from genera Breviolum, Cladocopium and Durusdinium) isolated from temperate or tropical environments. Glycerolipids, particularly triacyclglycerols, increased while cell size decreased under N- and NP-nutrient limited cultures, across all Symbiodiniaceae species. P-limitation caused a decrease in phosphatidylcholine, an important membrane lipid, and saw an increase in isoprenol lipids. This suggests a diversion of phosphorus from phospholipid membranes to the biosynthesis of membrane-stabilizing isoprenes. Reduced photophysiology under P-limitation in all Symbiodiniaceae further supports evidence that P-limitation induced stress in these Symbiodiniaceae cells. As expected, growth rate was reduced in all Symbiodiniaceae at temperature extremes (31°C). Significant increases in oxidized lipids, particularly oxidized phosphatidylinositol, and a reduction in ether-linked phospholipids in cultures grown at 31°C, suggests increased reactive oxygen species (ROS) abundance in these cells. In addition, at 31 °C, D. trenchii and both C. goreaui spp. cell size increased, a common sign of ROS accumulation, cell cycle arrest and necrosis. The observed increases in lipid energy storage (triacylglycerols and isoprenoids) under nutrient stress, as well as ROS-mitigation via lipid remodeling leading to increases in saturated fatty acids and oxidized lipids under temperatures stress, suggest Symbiodiniaceae can remodel their lipids to adapt to environmental shifts. If similar mechanisms occur in hospite, this could be an adaptive strategy for coral holobionts under a changing climate.
Introduction
Marine microalgae possess remarkable plasticity and adaptability to abiotic stressors and have evolved a variety of physiological strategies allowing them to survive in a wide range of habitats, including regulation of lipid biosynthesis (Fakhry and El Maghraby, 2015; Holm et al., 2022). Lipids are found within all cells, encompassing a range of functions including energy storage, cell signaling and structure (van Meer et al., 2008; Santos and Preta, 2018). The accumulation of lipids involved in energy storage, such as triacyclglycerols, has been observed in many microalgae species under varied environmental conditions, such as nitrogen and phosphate starvation (Jiang et al., 2014; Yang et al., 2018), light wavelength and intensity, temperature (Dickinson et al., 2017), carbon dioxide levels (Ortiz Montoya et al., 2014), and increased salinity (Zhu et al., 2016). Some microalga taxa can cope with decreased phosphate by remodelling polar-lipid membranes from phospholipids to phosphate-free lipids, such as betaine lipids (Cañavate et al., 2017). Temperature stress induced changes within the saturation of membrane glycolipids and fatty acids in order to maintain cell and thylakoid membrane stability (Tian et al., 2022).
Symbiodiniaceae, a family of dinoflagellate microalgae, associate with numerous hosts across large geographic ranges, including other protists and cnidarians such as reef building corals (LaJeunesse et al., 2018). Symbiodiniaceae are genetically and functionally diverse, with different functional types based on thermal tolerance, and photo physiological performance most frequently studied (Sampayo et al., 2008). Within studies to resolve functional differences between species of Symbiodiniaceae across environmental gradients, it has been found Symbiodiniaceae lipid composition can differ between species (Sikorskaya et al., 2021), and strong evidence suggests that Symbiodiniaceae might modulate the lipid composition in order to maintain cell stability and adapt to environmental alterations (Tchernov et al., 2004; Bachok et al., 2006). Indeed, studies have observed the effects of heat shock (34 °C) on the lipid profile of cultured Symbiodiniaceae from the genera Durusdinium, Breviolum and Cladocopium, which presented increased saturation of fatty acids and higher abundances of energy-rich triacylglycerols, supporting lipid-remodeling survival strategies under stress conditions (Botana et al., 2022). It is clear Symbiodiniaceae can change their lipid profiles, but how this occurs across broader environmental conditions and stress remains unresolved. Membranes rich in unsaturated fatty acids (UFAs) are more susceptible to oxidation by reactive oxygen species (ROS) (Su et al., 2019). Under abiotic stress induced by temperature or light, accumulation of ROS causes oxidative stress and damage to Symbiodiniaceae cells, evidenced by disruptions to the saturated:unsaturated ratio of membranes, increased oxidation of lipids (Koch et al., 2017; Rezayian et al., 2019) and reduced concentrations of unsaturated fatty acids (UFAs) (Ayala et al., 2014). Increase in the biosynthesis of saturated fatty acids (SFAs) and lipogenesis intermediates was observed in endosymbiotic Breviolum minutum after 6 days of heat stress (Hillyer et al., 2017), which may reflect alterations to structural lipids in order to maintain chloroplast structure and function. Symbiodiniaceae species-specific lipid compositions and alterations to the lipid profile under stress have been recorded, with total recomposition of lipids observed in more thermosensitive species (Cladocopium) and higher digalactosyldiacylglycerol:Monogalactosyldiacylglycerol (DGDG : MGDG) ratios, indicating high photosphyiological functioning in thermally tolerent Dusudinium under heat stress (Rosset et al., 2019; Sikorskaya et al., 2021). Studies to date have investigated lipid shifts beyond inhabitable ranges, but their ability to adapt to environmental shifts via lipid remodelling is still unknown. It is therefore important to understand how lipid remodeling strategies differ between Symbiodiniaceae representing different genera, stress tolerances, and geographic distributions (e.g., isolated from temperate versus tropical regions). Nevertheless, Symbiodiniaceae lipid remodeling under wide ranging temperatures and nutrient concentrations remains under-researched despite being an important metric in other algae.
Mutual exchange of nutrients of functional significance between Symbiodiniaceae and corals plays a vital role in governing overall coral performance and survival, including fatty acids and lipids (Matthews et al., 2018, 2017). Lipids are critical for both coral and Symbiodiniaceae energy stores, growth and reproduction, the ability to minimize cellular stress, as well as acting as essential interpartner signaling molecules in this symbiosis (Chen et al., 2017). Corals are able to synthesize lipids, however, benefit from lipids provided by the resident Symbiodiniaceae, particularly those they cannot synthesize themselves (such as some polyunsaturated fatty acids) (Treignier et al., 2008). Therefore, species specific symbiont lipid composition and adaptability may also play a key role in coral energetics and resilience to environmental change (Sampayo et al., 2008; Boulotte et al., 2016). For example, nutrient limitation, alterations to the N:P ratio, and temperature extremes are known to disrupt the stability of some coral-Symbiodiniaceae symbioses, ultimately increasing susceptibility to coral bleaching and mortality (Ezzat et al., 2016; Rosset et al., 2017; Blanckaert et al., 2023). As nutrient concentrations and temperature can affect microalgae lipid composition (Holm et al., 2022; Gao et al., 2023), the plasticity of Symbiodiniaceae lipid composition, and thus what is translocated to the coral hosts, could determine the susceptibility of coral-Symbiodiniaceae associations to climate change and potential for the poleward range shifts of corals (Nielsen and Petrou, 2023).
Studying Symbiodiniaceae in vitro is commonly used to assess differences in performance under both thermal and nutrient stress (Wong et al., 2021; Dilernia et al., 2023). In this study we tested cultured Symbiodiniaceae of the genera Cladocopium, Breviolum and Durusdinium that originated from corals found in both tropical and subtropical regions in eastern Australia. We exposed these Symbiodiniaceae to a variety of temperatures (16°C to 31°C) and nutrient regimes (N and P limitation) (Khan et al., 2018) in order to determine whether Symbiodiniaceae are able to adapt to sublethal environmental shifts via lipid remodeling. This could provide an adaptive strategy for corals as climate pressures continue to change environmental conditions.
Methods and materials
Symbiodiniaceae cultures
Four Symbiodiniaceae cultures originally isolated from cnidarians ranging from tropical to temperate distributions were selected from existing stocks at the University of Technology Sydney. These were Durusdinium trenchii (ITS2 D1a, culture ID: SCF082, isolated from Acropora muricata and previously recorded to be thermally tolerant (LaJeunesse et al., 2018)), two Cladocopium goreaui isolates, Hetero -W (ITS2 C1, culture ID: AIMS-aten-C1-WSY, also isolated from Acropora tenuis, and previously recorded to be more thermally tolerant), and Hetero-M (ITS2 C1, culture ID: SCF055, isolated from Acropora tenuis, and previously recorded to be more thermally sensitive (Levin et al., 2016; Ros et al., 2020), and Breviolum psygmophilum (ITS2: B2, culture ID: PVB18B, isolated from the temperate coral Plesiastrea versipora) (Camp et al., 2022). Each Symbiodiniaceae species was sub-cultured (N = 2 per Symbiodiniaceae species, one allocated to the temperature experiment and one to the nutrient limitation experiment) by adding 5 mL of original cultures in 45 mL of autoclaved and filter sterilized (0.22 µm) artificial seawater (ASW) and F/2 media. Cultures were grown for one month (to achieve a minimum cell density of at least 106 cells/mL) at 26˚C, with an irradiance of 85 ± 15 µmol photons m-2 s-1 (Philips TLD 18W/54 fluorescent tubes, 10 000 K) on a 12h:12h light:dark cycle.
Symbiodiniaceae cell density and size
An aliquot of 184 µL was collected from each culture, photophysiological performance analyzed (see Symbiodiniaceae Photophysiology below) and fixed with 16 µL of 25% glutaraldehyde for direct use in flow cytometry analysis (CytoFLEX S, Beckman Coulter, CA, United States). Symbiodiniaceae cell concentration was assessed by relative cell chlorophyll fluorescence (650 nm) using the flow cytometer gating strategy shown in Supplementary Figure 1. The gating strategy was formed based on the control of each experiment, accounting for differences in gates for each experiment. Several blanks were run prior to sampling to blank correct. Relative cell size was determined from the same data using a calibration curve generated using Forward Scatter Width (FSC-HW) of unstained polystyrene microspheres of known sizes (1-15µm diameter) (Molecular Probes Calibration Kit F-13838), run parallel to the experimental samples.
Nutrient experiment
Each Symbiodiniaceae species (N = 4 replicates per species per nutrient treatment, total N = 64) was exposed to four nutrient treatments; the control (standard ASW F/2) and three nutrient-limited treatments. Nutrient limitation was achieved by reducing the amount of nitrates and phosphates added to 25% of the original concentration as per CSIRO F/2 (Guillard, 1975) (N-limited (ASW F/2 media, N at 25%), P-Limited (ASW F/2 media, P at 25%) and both N:P Limited (ASW F/2 media, N and P at 25%)).ASW + F/2 media, in which the stock cultures were reared, is nutrient (N&P) enriched well above what is found in seawater (Berges et al., 2001). For the purposes of this study, nutrient limitation refers to a rapid reduction in N and P relative to the stock ASW + F/2 media concentrations. Media nutrient (N and P) concentrations were confirmed prior to culture mixing via a Gallery Discrete Analyzer (ThermoFisher, Supplementary Table 1).
From each subculture allocated to the nutrient treatment (generated as above), 2 × 106 cells were collected per replicate (N = 4), centrifuged at 2,000 × g for 5 minutes at 26°C and rinsed twice with ASW to remove residual media solution. Cells were resuspended in 100 mL ASW + F/2 media in sterile culture flasks and placed in Eppendorf Innova Stackable Shaking Incubators with an irradiance of 85 ± 15 µmol photons m-2 s-1 (photosynthetic LED lightbank on a 12h:12h light:dark cycle) and manually shaken once per day to prevent sticking. Temperature was maintained at 26 °C (+/- 0.9°C) for the 14-day period, flasks were randomly distributed within the incubators and rearranged twice per week to account for any slight differences in temperature or light. Flow-cytometric cell abundance was conducted for each Symbiodiniaceae culture every 3-4 days over the 14-day period.
Temperature experiment
From each subculture allocated to the temperature experiment, 106 cells were collected based on flow cytometry cell density results, centrifuged at 2,000 × g for 5 minutes, and then resuspended in 100 mL ASW + F/2 media in sterile culture flasks. Replicates of each Symbiodiniaceae species (N = 4 per species per temperature treatment, total N = 64) were placed directly into four Eppendorf Innova Stackable Shaking Incubators representing each temperature treatment (16°C, 21°C, 26°C and 31°C) and maintained (+/- 0.9°C) for the entire experimental duration (Supplementary Table 27). Temperatures were chosen to ensure Symbiodiniaceae remained viable after the 14-day period yet pushed to the extents of their thermal optima based on results from a pilot experiment (12-33°C). Each incubator had an irradiance of 85 ± 15 µmol photons m-2 s-1 (photosynthetic LED lightbank on a 12h:12h light:dark cycle) and cultures were manually shaken once per day to prevent sticking. Replicates were randomly distributed within the incubators and rearranged twice per week. Flow-cytometric cell abundance was conducted for each Symbiodiniaceae culture every 3-4 days over the 14-day period.
Symbiodiniaceae growth
Specific growth rates (μ) were calculated from the linear regression of the natural log of the flow cytometry cell densities versus time during the exponential growth phase of cultures. Standard error of μ was calculated from μ values from biological replicates (N = 4 per treatment per Symbiodiniaceae species) over the exponential growth period determined to be between 7-11 days in nutrient-treated cultures, and between 4-11 days in temperature treatments. Percentage change in growth was calculated as the difference between μtreatment and μcontrol divided by μcontrol. PERMANOVA (Permutational Multivariate Analysis of Variance, 999 permutations) tests were used to assess variation in exponential growth rates between treatments across each Symbiodiniaceae species in both temperature and nutrient experimentation. All data was transformed using Log(X+1). Due to the small sample size, Montecarlo p-values were used. Pair-wise tests were then undertaken to determine where significance occurred.
Symbiodiniaceae photophysiology
Photophysiological performance of Symbiodiniaceae was assessed using a Closed FluorCam FC 800-C. Photophysiological analyses occurred every 3-4 days. 184 µL of each sample was pipetted into a 96 U-bottom well plate and low light (ca. 5-10 µmol photons m-2 s-1) acclimated for at least 30 minutes prior to measurements. The saturating pulse was delivered using a blue LED excitation source (450 nm) with an intensity of 2930 µE for a duration of 800 ms. All fluorescence yields were adjusted for baseline fluorescence using ASW + F/2. Light response protocols were used to derive the maximum dark-adapted yield of PSII photochemical efficiency (Fv/Fm, dimensionless), which served as a proxy for tracking cellular health. Fv/Fm was compared across treatments over time, and data analyzed using Repeated Measures ANOVA (RMANOVA). Significance was determined using pairwise tests undergoing Bonferroni corrections to account for multiple tests on the same dataset. A one-way ANOVA was utilized to determine whether cell size differed significantly by the final timepoint of this study, with Tukeys post hoc test employed to test for differences between treatments. Where data did not meet assumptions of normality, Kruskal-Wallis One-Way ANOVA was utilized to test for variation in size, followed by a Wilcoxon rank-sum test with p-value adjustment to determine where significant variance occurred. Graphical plots of physiological and photophysiological data were generated using RStudio (2023.06.0 + 421).
Sampling for lipidomics analysis
At 14 days, after cell density, size counts and photophysiology measurements were taken, and 106 (temperature experiment) or 107 (nutrient experiment). Symbiodiniaceae cells were then concentrated by centrifugation at 2,000 × g for 5 minutes at 26°C, the media discarded, and pellets resuspended in 500 µL of ASW before snap freezing in liquid nitrogen. Samples were stored at -80°C prior to lipid extraction. Cell densities differed due to insufficient growth of the higher temperature cultures to reach 107 cells per culture.
Lipid extraction and analysis via RPLC-MS/MS
All steps were performed at 4°C and using LC-MS grade glassware. Lipid extraction was based on the methyl-tert-butyl ether (MTBE) extraction and phase separation protocol for high-throughput lipidomics (Matyash et al., 2008). Symbiodiniaceae samples were thawed on ice, centrifuged at 3000 × g for 5 minutes at 4°C, and the ASW supernatant discarded. To each pellet, 300 µL of 100% methanol (at -20°C) and 5 µL of EquiSPLASH LIPIDOMIX mass spec standard (Avanti Polar Lipid 330707), and ~25 µg acid-washed glass beads were added. Cells were lysed using a bead mill for 3-minutes at 30 hz. Samples were incubated on ice for 10-minutes, vortexed twice during this time. 1,000 µL of MTBE was added, samples were vortexed for 30 s and shaken for 1-hour at 1000 x rmp at 4°C in a rotisserie shaker. To prepare for phase separation, 250 µL of ultra-pure water was added, samples incubated on ice for 10-minutes, and centrifuged at 1,000 × g for 10 minutes at 4°C. 900 µL of the organic layer was collected, dried under a nitrogen stream, and stored at -80°C until analysis. Sample blanks (N = 8) were undertaken alongside each run.
Prior to analysis, samples were resuspended in 100 µL 2:1 isopropanol methanol (IPA : MeOH) and transferred to autosampler glass vials with a 125 µL glass insert. A pooled sample was created to assist with compound analysis, by combining 5 µL of each sample into a single tube and 100 µL of this pooled sample transferred to an autosampler vial with a 125 µL glass insert. 5 µL injections of each sample was processed via Liquid Chromatography Mas-Spectrometry (Themo Orbitrap LC-MS) in positive and negative ion mode. Each sample was run as per methodology developed by Violi, 2022, in positive mode under acidic chromatographic conditions, and in negative mode with neutral chromatographic conditions using an Agilent 1290 UPLC system and Waters ACQUITY UPLC CSH C18 Column (130Å, 1.7 µm, 2.1 mm X 150 mm). The column oven was set to 65°C for both methods. The positive mode method had a flow rate of 0.4 mL/min with mobile phase A consisting of 60:40 ACN : Water + 10 mM Ammonium formate + 0.1% Formic acid and B consisting of 90:10 IPA : ACN + 10mM Ammonium formate + 0.1% formic acid. For Heated electrospray ionization (H-ESI) and DNA acquisition parameters see Supplementary Tables 2, 3. The negative mode method had a flow rate of 0.4 mL/min with mobile phase A consisting of 60:40 ACN : Water + 10 mM Ammonium acetate and B consisting of 90:10 IPA : ACN + 10mM Ammonium acetate. Both methods used the same gradient of the following solvent B: 0.00 min 30%, 2.00 min 30%, 2.50 min 50%, 13.00 min 85%, 13.50 min 99%, 15.00 min 99%, 15.10 min 30%, 18.00 30%. Separated lipids were then ionized into the source of a Thermo Q-Exactive Plus mass spectrometer. Data was acquired via DDA topN and scan range was set to 200 – 1200 m/z, with the only difference between the methods being the polarity (positive or negative mode). Every 9 samples a blank and a QC was injected. When analyzing negative mode temperature data within the LCMS, a system error occurred, compromising several of the samples. These replicates were removed from both negative and positive datasheets, creating an N of 3 for some treatments.
Raw lipid spectral data was exported to.raw files and processed in MS-Dial (version 5.2) (Tsugawa et al., 2015), for peak alignment, blank correction and lipid identification against the LipidMaps LipidFinder (V2) and Structure Database (LMSD). Peak areas for each lipid were exported and normalized to the relative peak area of the internal standards. Data was arranged into all lipids and lipid sub-class (using LipidMaps ontology) data for further analysis. Samples within temperature treatments underwent further normalization to cell density, as some cultures within 31 °C and 16 °C treatments did not reach the cell volume required. All lipid data underwent cube root transformation and scaling through mean centering in MetaboAnalyst (v5.0). Variability in lipid abundance and composition within Symbiodiniaceae across all treatments were analyzed using Principal Component Analysis (PCA) to determine relationships and clustering between the treatments. PERMANOVA permutational MANOVA (999-permuations) was employed using Montecarlo p.value (<0.05) and pairwise testing to highlight variance between treatments in the PRIMER software (V7.0). Variability between individual lipids was tested using an ANOVA with Fischer’s post hoc tests, using a false discovery rate (FDR) of< 0.05. A heatmap was created to visualize all significant lipids and sub-classes, created in MetaboAnalyst using Euclidean distance and ward clustering.
Results
Symbiodiniaceae physiology
Nutrient experiment
The exponential growth rates of D. trenchii and C. goreaui (HW) did not vary significantly between treatments (Figures 1A, B). However, the exponential growth rates of N and NP-Limited treatments of B. psygmophilum were approximately 2% lower than the control group (Supplementary Table 5, (=0.037, (=0.001), but P-limited cultures did not differ significantly. Within C. goreaui (HM), both P- and NP-Limited culture growth rates decreased by 2% on average compared to controls (Figure 1; Supplementary Table 5, (=0.033, (=0.048).
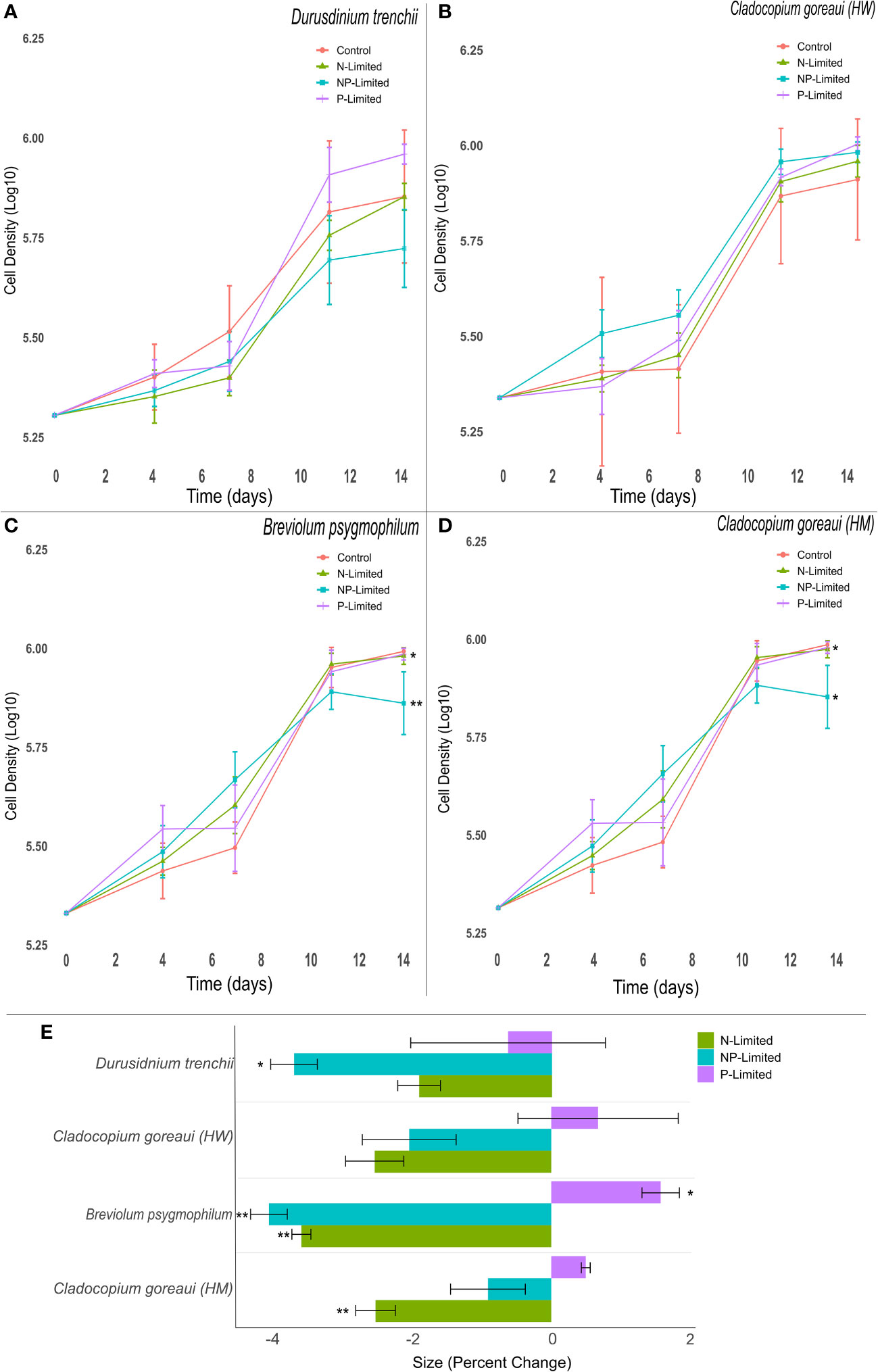
Figure 1 Symbiodiniaceae cell density and size under different nitrogen and phosphate concentrations. Symbiodiniaceae cell densities (ln) for Durusdinium trenchii (A), Cladocopium goreaui HW (B), Breviolum psygmophilum (C), and Cladocopium goreaui HM (D) across the sample period for each nutrient treatment (control nutrient concentrations, and in N- (green), P- (purple), and NP-limited (blue) media). PERMANOVA permutational MANOVA (PERMANOVA) (Pmc) significance shown using *(Pmc =<0.05) and **(Pmc =<0.001). (E): Variation in cell size for each Symbiodiniaceae across nutrient treatments. One-way ANOVA and Tukeys post hoc significance shown using *(P=<0.05) and **(P=<0.001). Values are means and error bars are SEM. N=4 per treatment, per species.
Nutrient concentration affected cell size across the 14-day period, with nutrient treated cultures exhibiting, at the final sampling point, changes in sizes compared to controls across most Symbiodiniaceae species (Figure 1E). NP-Limited cultures of D. trenchii were close to 4% smaller than control cultures (Figure 1E; Supplementary Table 7, ANOVA p=0.028). A similar trend occurred in C. goreaui (HM) cultures grown in N-Limited media, with cell size approximately 3% smaller compared to controls (Figure 1E; Supplementary Table 7, ANOVA p=0.001), and within B. psygmophilum which exhibited approximately 4% lower average cell size in both N and N-P limited treatments compared against the control (Figure 1E; Supplementary Table 7, ANOVA p=<0.001). Meanwhile, B. psygmophilum P-limited cells increased in size relative to controls (Figure 1E; Supplementary Table 7, ANOVA p=0.003). In C. goreaui (HW), none of the treatments changed significantly relative to the control (Figure 1E; Supplementary Table 7).
Across all species, P-Limited cultures had the lowest effective quantum yield of PSII (Fv/Fm) by the end of the 14-day period, dropping below 0.55 across all Symbiodiniaceae species (Figure 2; Supplementary Table 9). The photophysiology of D. trenchii, B. psygmophilum, and C. goreaui (HM) N and NP-Limited cultures remained stable relative to the control, while P-Limited cultures declined significantly by 23%, 20% and 12%, respectively (Figures 2A, C, D; Supplementary Table 9, ANOVA p<0.001). The photosynthetic performance of N-Limited C. goreaui (HW) cultures resembled the control, remaining stable throughout the study period, while P-Limited and NP- Limited C. goreaui (HW) cultures dropped on average by 24% and 7% by the 14th day compared to control cultures (Figure 2B; Supplementary Table 9, ANOVA p<0.01).
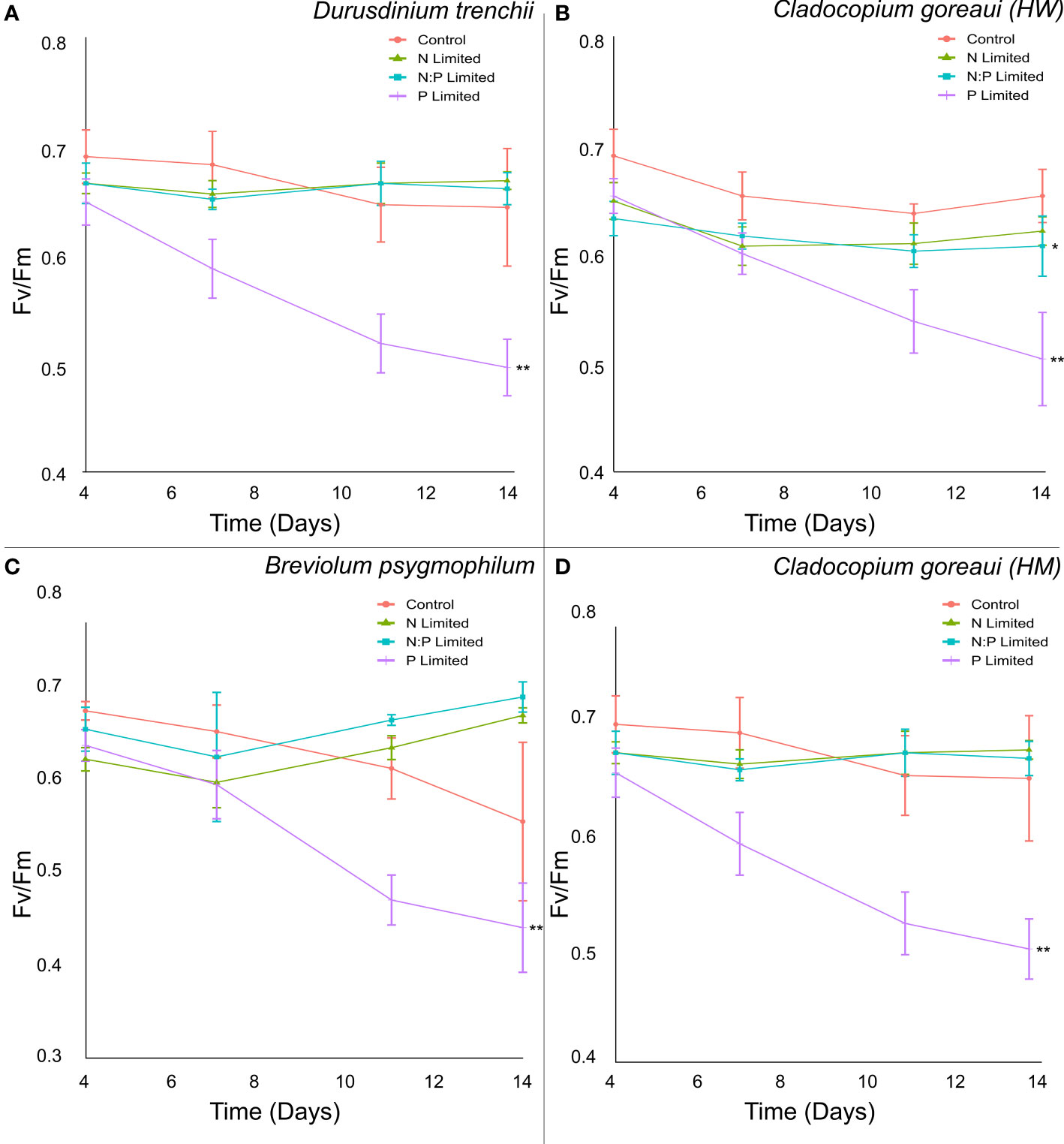
Figure 2 Symbiodiniaceae effective quantum yield of photosystem II under different nitrogen and phosphate concentrations. Fv/Fm (dimensionless) measurements of Durusdinium trenchii (A), Cladocopium goreaui HW (B), Breviolum psygmophilum (C), and Cladocopium goreaui HM (D) cultures grown in control nutrient concentrations, and in N- (green), P- (purple), and NP-limited (blue) media, taken every 3-4 days. Values are means and error bars are SEM, N=4 per treatment, per species. Repeated Measures ANOVA (RMANOVA) significance shown using *(P =<0.05) and **(P =<0.001). N=4 per treatment, per species.
Temperature experiment
All Symbiodiniaceae species exhibited similar trends in growth, with cultures grown at 16°C and 31°C displaying a reduced growth rate compared against cultures grown at 21°F and 26 °C (Figure 3). At the lowest (16°C) and highest (31°C) temperatures, the exponential growth rate of D. trenchii cultures were on average 10% and 16% lower, respectively, compared to controls (Figure 3A; Supplementary Table 11, (= 0.001). This trend was also observed at 16°C and 31°C in B. psygmophilum with exponential growth rates 10% and 17% lower than control cultures (Figure 3C; Supplementary Table 11, (=0.002, (=0.001), and C. goreaui (HW), with exponential growth rates 6% and 10% lower than control cultures (Figure 3B; Supplementary Table 11, (=0.03, (=0.012). The growth rate of C. goreaui (HM) cultures grown at 31°C was 11% lower than that of control samples (Figure 3D; Supplementary Table 11, (=0.043), but those grown at 16˚C were not significantly different to controls. Meanwhile, C. goreaui (HW) grown at 21°C had, on average, an exponential growth rate 8% higher than the control (Figure 3B; Supplementary Table 5, (=0.007).
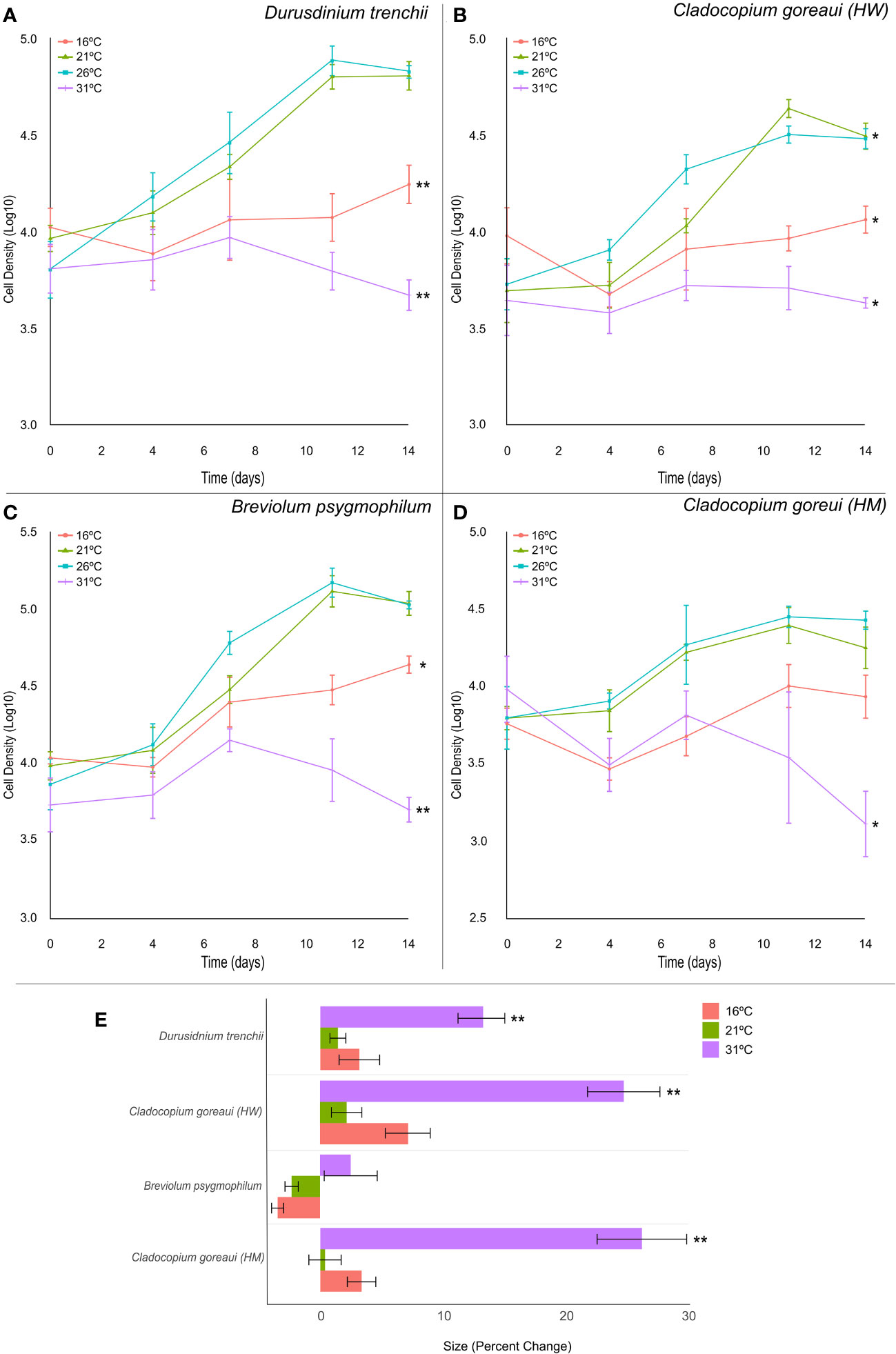
Figure 3 Symbiodiniaceae cell density and size under different temperatures. Symbiodiniaceae cell densities (ln) for Durusdinium trenchii (A), Cladocopium goreaui HW (B), Breviolum psygmophilum (C), and Cladocopium goreaui HM (D) across the sample period for each temperature treatment (16°C (red), 21°C (green), Controls; 26°C (purple), 31°C (blue). PERMANOVA permutational MANOVA (PERMANOVA) (Pmc) significance shown using *(Pmc =<0.05) and **(Pmc =<0.001). (E) Variation in cell size for each Symbiodiniaceae across temperature treatments. One-way ANOVA and Tukeys post hoc significance shown using *(P=<0.05) and **(P=<0.001). Values are means and error bars are SEM. N=4 per treatment, per species.
Symbiodiniaceae cell size was also affected by high temperature (Figure 3E), with relative cell size in cultures grown at 31°C approximately 13%, 25% and 26% larger, respectively, than control samples at the final timepoint in D. trenchii, C. goreaui (HW) and C. goreaui (HM) (Figure 3E; Supplementary Table 13, p-Tukey<0.001). C. goreaui (HM) at 26°C also increased in size, although by only 0.45% (Figure 3E; Supplementary Table 13). The cell size of B. psygmophilum did not vary significantly across treatments, and there was no other significant difference in size across other treatments (Figure 3E; Supplementary Table 13). Effective quantum yield of PSII (Fv/Fm) appeared to be unaffected by high temperature after 14 days in all species except C. goreaui (HM), which had significantly elevated Fv/Fm relative to the control for the 16°C, 21°C and 31°C treatments at the final timepoint (Supplementary Figure 2D, Supplementary Table 14; ANOVA p=0.02, p<0.001, p=0.002). Although RMANOVA revealed large variances in Fv/Fm across the sample period for each culture, all species maintained effective quantum yield values within what is considered a healthy range of Symbiodiniaceae (Supplementary Figure 2; Supplementary Table 15 (Carballo-Bolaños et al., 2019; McRae et al., 2023).
Lipid diversity and abundance
Nutrient experiment
Principal component analysis (PCA) revealed patterns in lipid abundance when Symbiodiniaceae were exposed to changes in nutrient concentration (Figure 4; Supplementary Table 16). For D. trenchii, the majority of the separation occurred across PC1, explaining 55.6% of the variance, and the greatest separation can be seen between P-Limited and N-Limited treatments, mainly driven by fatty acids (FA). N and NP-Limited samples clearly overlapped across both PC1 and PC2 (13.8%), with separation driven mainly by triacylglycerols (TG) (Figure 4A). Within C. goreaui (HW), there was distinct separation on PC1 (35.4%), primarily occurring between control and NP-Limited treatments, whereas P and N-Limited cultures overlapped (Figure 4B; Supplementary Table 16). P- and N-Limited samples were also separated from C and NP-Limited cultures along PC2 (19.2%), with main drivers seemingly FA and hexosylceramides (HexCer) (Figure 4B; Supplementary Table 16). B. psygmophilum also followed a similar pattern to C. goreaui (HW) along PC1 (31.1%), with complete separation between control and NP-Limited cultures. P and N-Limited samples were also completely separated across PC2 (22%) (Figure 4C; Supplementary Table 16). Most of the separation in C. goreaui (HM) occurred between control and all nutrient treated samples across PC1 (39.2%), mainly driven by TG (Figure 4D; Supplementary Table 16). There was some overlap between N and NP-Limited samples, yet both showed distinct separation from P-Limited samples across PC1 (39.2%). Along PC2 (23.2%), there was separation between N and NP-Limited C. goreaui (HM) cultures (Figure 4D; Supplementary Table 16). In both B. psygmophilum and C. goreaui (HM) NP-Limited cultures, cirsiliol (4’,5-dihydroxy-3’,6,7-trimethoxy-flavone) was also a driver of separation from controls (Figures 4C, D; Supplementary Table 16).
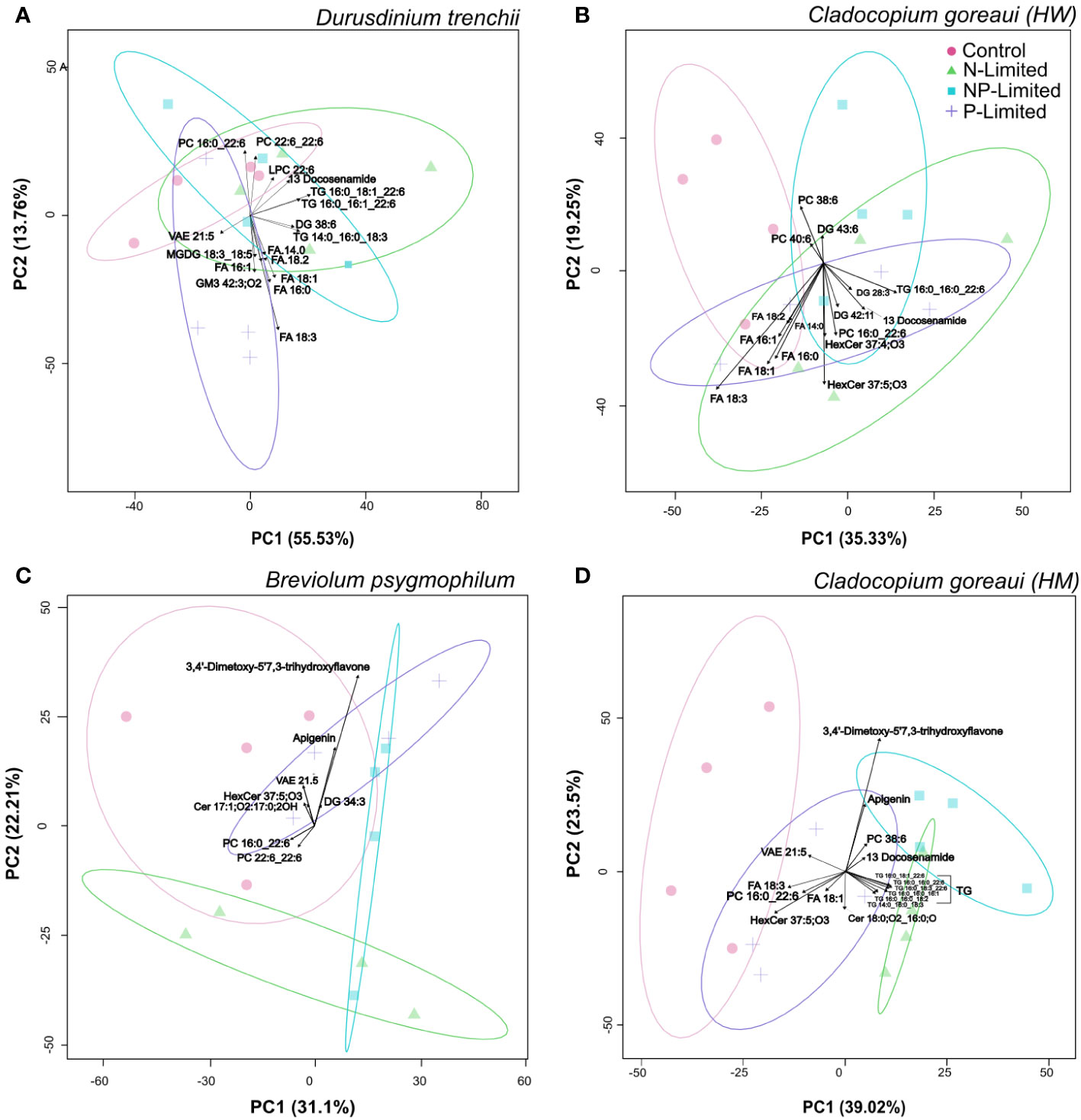
Figure 4 Principal Component Analysis with lipid sub-class biplot of lipid abundance under different nitrogen and phosphate concentrations. Separation of treatments based on lipid composition in Durusdinium trenchii (A), Cladocopium goreaui HW (B), Breviolum psygmophilum (C), and Cladocopium goreaui HM (D) across the sample period for each nutrient treatment (control nutrient concentrations, and in N- (green), P- (purple), and NP-limited (blue) media). N=4 per treatment per species.
For B. psygmophilum, 4 lipid subclasses were significantly changed under N and NP-Limited conditions (vitamin-a fatty esters (VAE), digalactosyldiacylglycerol (DGDG), Ceramide and Hexosylceramide hydroxy fatty acid-sphingosine (Cer_HS, HerCer_HS), which decreased in relative abundance from control conditions (Figure 5A; Supplementary Table 20, ANOVA FDR<0.01). Under P and NP-Limited conditions PC decreased in relative abundance (Supplementary Table 20, ANOVA FDR<0.001) and n-acyl ornithine (NAOrn) changed significantly under nitrogen limited conditions (Figure 5A; Supplementary Table 20, ANOVA FDR=0.018). Within C. goreaui (HM), 2 lipid subclasses changed significantly under N and NP-Limited treatments (DGDG, VAE) (Figure 5B; Supplementary Table 20, ANOVA FDR=0.01). Under NP and P-Limited cultures, phosphatidylcholine (PC) relative abundance decreased significantly (Supplementary Table 20, ANOVA FDR=0.009), and under all nutrient treated cultures, triacylglycerols (TG) relative abundance increased significantly against controls, but especially under N and NP-Limitation (Figure 5B; Supplementary Table 20, ANOVA FDR=0.01). There was no overall change in lipid subclasses between nutrient treatments in either D. trenchii or C. goreaui (HW).
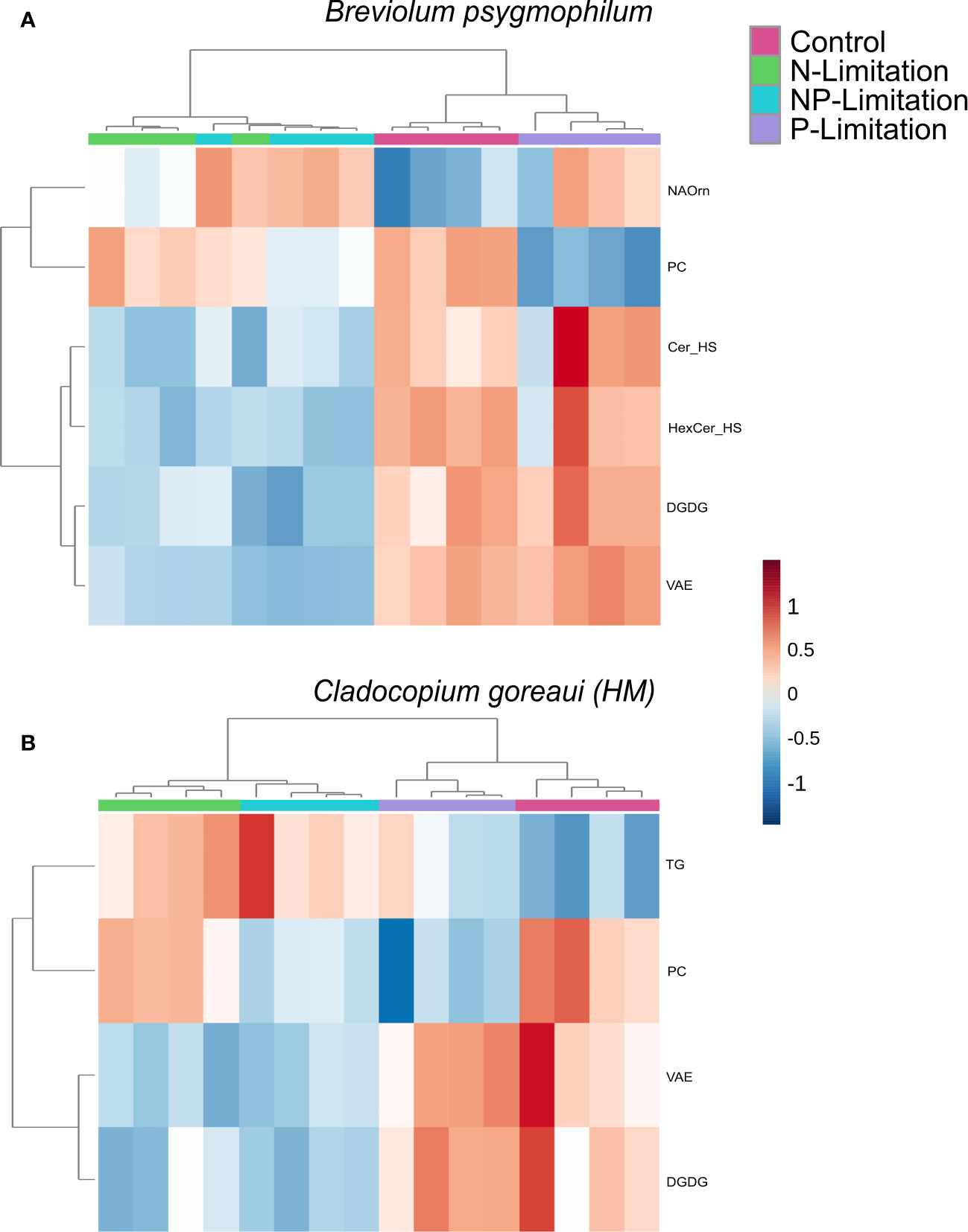
Figure 5 Symbiodiniaceae lipid sub-class relative abundance heatmap under different nutrient concentrations as determined by an ANOVA FDR with Fishers pos hoc in Breviolum psygmophilum (A) and Cladocopium goreaui HM (B) across the sample period for each nutrient treatment (control nutrient concentrations, and in N- (green), P- (purple), and NP-limited (blue) media). N=4 per treatment per species. Significant lipid sub-classes inclusive of ceramide hydroxy fatty acid-sphingosine, (Cer), hexosylceramide hydroxyfatty acid-sphingosine (HexCer), vitamin A fatty acid ester (VAE), digalactosyldiacylglycerol (DGDG), phosphatidylcholine (PC), n-acyl ornithine (NAOrn) and triacylglycerol (TG).
Individual lipid profiles revealed 11 specific lipids significantly changed in D. trenchii across nutrient treatments relative to controls, belonging to five sub-classes in total (Figure 6A; Supplementary Table 18). Across nutrient treatments, C. goreaui (HW) had 57 significant lipids from 10 different sub-classes (Figure 6B; Supplementary Table 18) and B. psygmophilum had 75 significant lipids from 14 differing sub-classes. C. goreaui (HM) had the highest variation in lipid composition when grown under changes in nutrient composition with a total of 142 significant lipids from 21 different sub-classes (Figures 6C, D; Supplementary Table 9). Of these, 39% [22/57; C. goreaui (HW)], 42% [60/142; C. goreaui (HM)] and 28% (21/75; B. psygmophilum) belong to the TG subclass of lipids, all of which were higher in relative abundance under N and NP-limited treatments (Supplementary Table 18). Meanwhile, lipids belonging to the diacylglycerol (DG) subclass showed the opposite, with a decrease in relative abundance across all N- and NP- limited Symbiodiniaceae cultures relative to controls and represented 18% [10/57; C. goreaui (HW)], 17% [24/142; C. goreaui (HM)] and 15% (11/75; B. psygmophilum) of significantly different lipids. No TG or DG lipids were significantly different in the nutrient limited D. trenchii cultures. VAE lipids appeared in all four Symbiodiniaceae species and were commonly higher in relative abundance in P-limited cultures, relative to controls. There was no clear trend in the saturated to unsaturated fatty acid ratio (SFA/UFA) within D. trenchii and B. psygmophilum, but all nutrient treatments within both C. goreaui spp. exhibited slightly higher SFA/UFA, indicating a higher proportion of SFA (Supplementary Table 17). In addition, SFA relative abundance was increased in NP-Limited treatments of C. goreaui (HM), and P-Limited B. psygmophilum treatments (Supplementary Table 17). Of all significant lipids, VAEs accounted for 36% (4/11; D. trenchii), 21% [12/57; C. goreaui (HW)], 11% [16/142; C. goreaui (HM)] and 21% (16/75; B. psygmophilum) of significantly different lipids. A total of 9 lipids (DGDG39:10, DGDG19:5_20:5, DGDG 40:9|DGDG18:3_22:6, DGDG42:11|DGDG20:5_22:6, PC36:6, PC44:12|PC22:6_22:6, VAE18:5, VAE20:4, VAE22:4 and VAE24:6) from 3 sub-classes (DGDG, PC and VAE) were found to be significantly different between treatments across all four Symbiodiniaceae species (Figure 5; Supplementary Table 18, FDR<0.05).
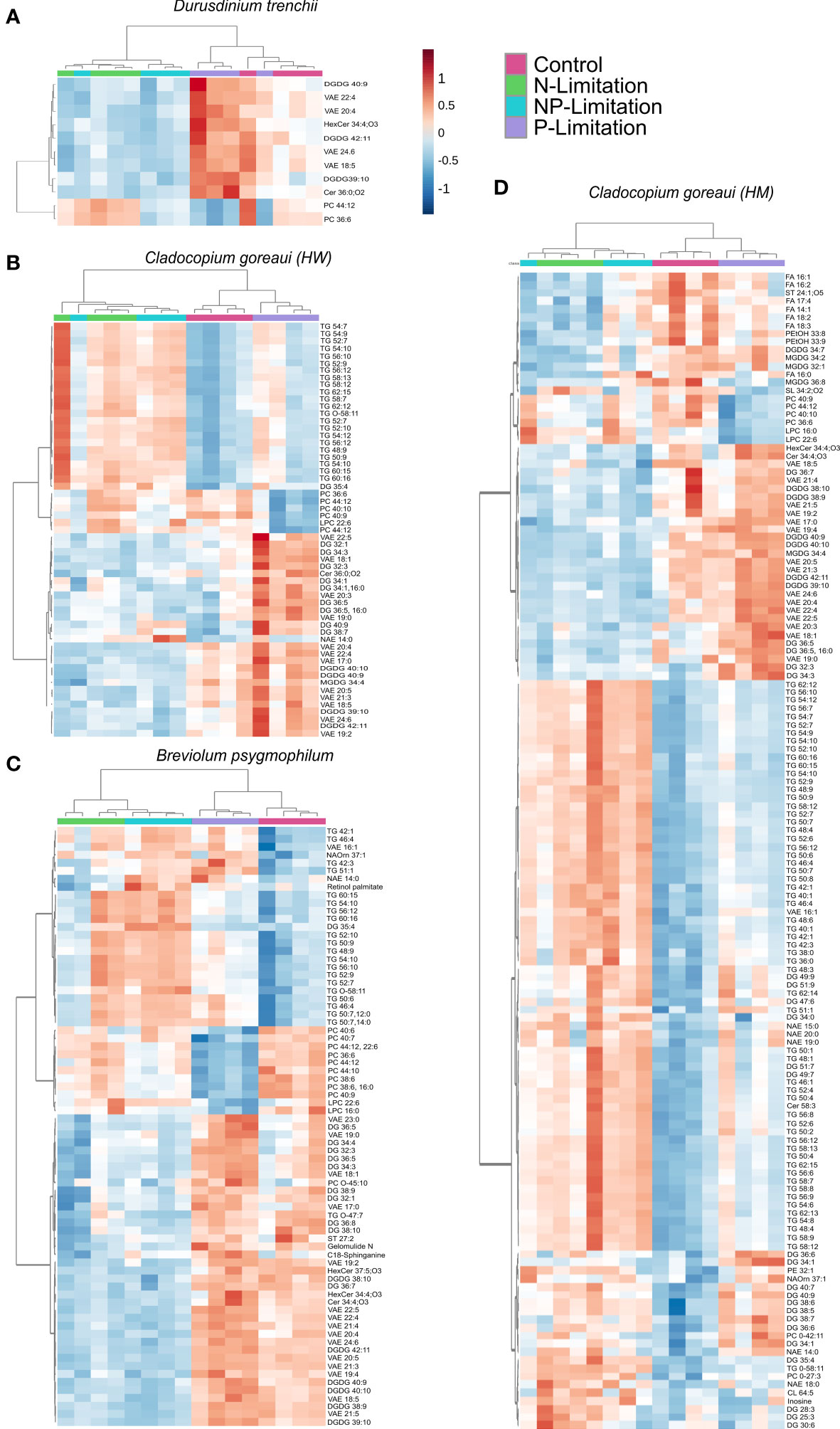
Figure 6 Symbiodiniaceae lipid accumulation and relative abundance heatmap under different nutrient concentrations as determined by an ANOVA FDR with Fishers post hoc in Durusdinium trenchii (A), Cladocopium goreaui HW (B), Breviolum psygmophilum (C), and Cladocopium goreaui HM (D) across the sample period for each nutrient treatment (control nutrient concentrations, and in N- (green), P- (purple), and NP-limited (blue) media). N = 4 per treatment per species.
Temperature experiment
For D. trenchii, the majority of individual lipid separation occurred on PC1, with 71.8% between the control (26°C), and both temperature extremes (31°C and 16°C). Separation in 31°C samples were mainly driven by sphingomyelin (SM), cholesterol (ST) and n-arachidonoyl taurine (NatAU), whereas the main driver in separation for 16°C samples was ceramide hydroxy fatty acid-sphingosine (Cer-HS). There was strong overlap between samples grown under control temperatures and 21°C (Figure 7A; Supplementary Table 21). For C. goreaui (HW), separation was more obvious along PC2 (24.7%), where samples grown at 31°C were distinctly separated from all other cultures, seen to be similarly driven by ST, and NATau, but also phosphatidylcholine (PC) and the fatty amide; docosenamide. There was also slight separation between the 26°C and 21°C samples, and the 16°C samples across PC1 (60.5%) (Figure 7B; Supplementary Table 21). There was clear separation (PC1; 74.1%), between B. psygmophilum cultures grown at 31°C versus 21°C and 26°C, again mainly driven by SM, docosenamide and NATau, and cultures grown at 16°C overlapping all treatments. There was also distinct separation between 21°C and 26°C (PC2; 16.7%) for B. psygmophilum, which was not observed in the other Symbiodiniaceae species (Figure 7C; Supplementary Table 21). C. goreaui (HM) revealed separation between 31°C and all other cultures along PC1 (75.8%), with SM, ST, docosenamide and NATau again driving this change, while 16°C was also separated from 26°C and 21°C along PC2 (14%) (Figure 7D; Supplementary Table 21). Separation of 16°C treatments across all species seemed to be driven by the lack of any specific lipid abundance, grouping closely with control and 21°C treatments.
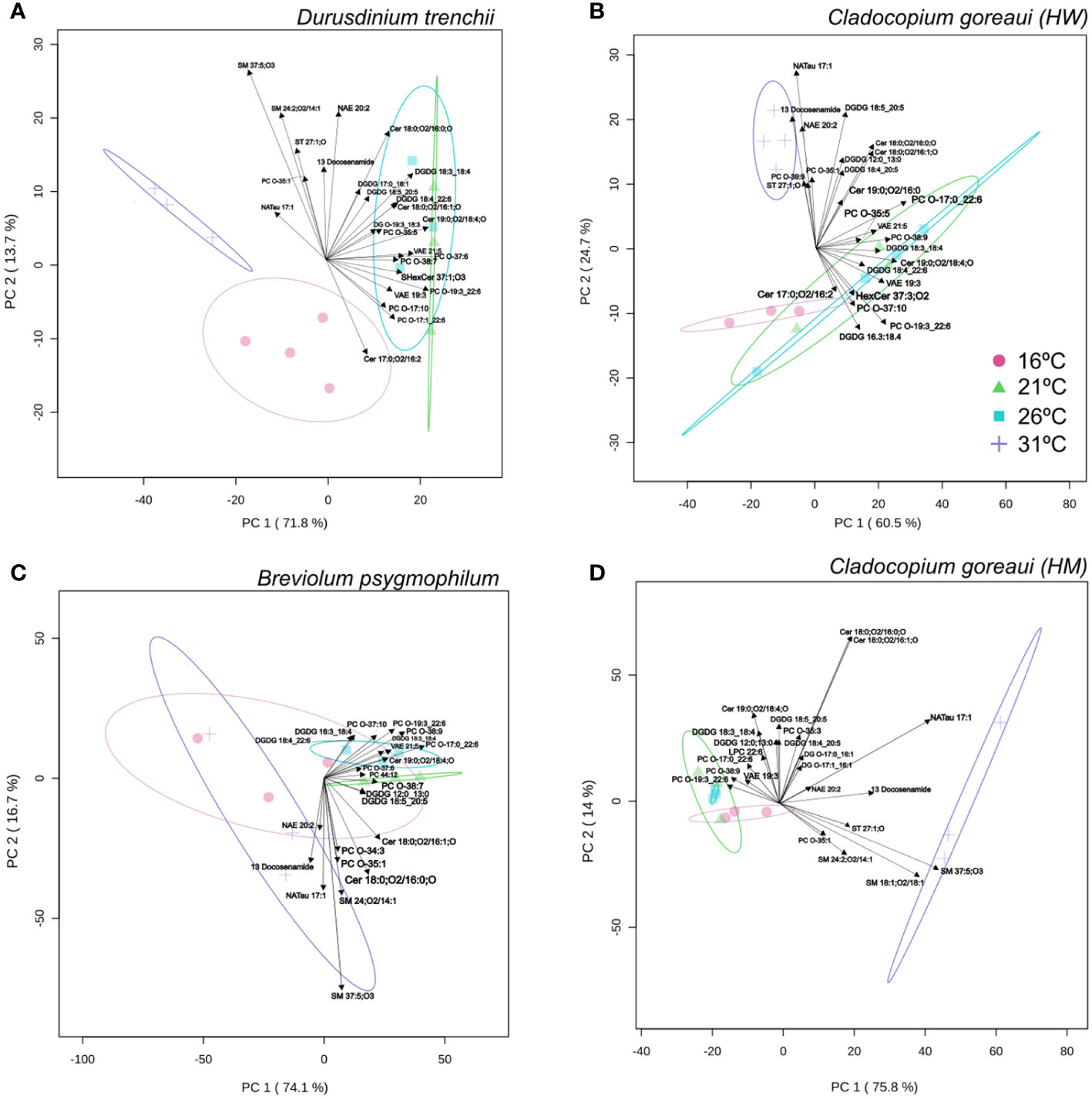
Figure 7 Principal Component Analysis with lipid sub-class biplot of lipid abundance under different temperatures. Separation of treatments based on lipid composition in Durusdinium trenchii (A), Cladocopium goreaui HW (B), Breviolum psygmophilum (C), and Cladocopium goreaui HM (D) across the sample period for each temperature treatment (control temperature [26°C; purple), 16°C (red), 21°C (green), and 31°C (blue)]. N varied between 3-4 per treatment per species.
Within D. trenchii, 56 individual lipids were significantly differently from control temperatures from 23 different subclasses (Figure 8A; Supplementary Table 24). C. goreaui (HW) had 63 significant lipids from 21 different subclasses, B. psygmophilum had 36 significant lipids from 17 subclasses, and C. goreaui (HM) had a total of 55 lipids differing significantly from control samples from a total of 21 sub-classes (Figure 8; Supplementary Table 24). Across all four Symbiodiniaceae, there were 6 lipids across 5 subclasses that significantly differed between treatments (CL 74:6|CL15:0:24:0_16:1_19:5, LPE O-20:5, NAGly 21:0;O, PC O-37:6, PC. O-41:9|PC O19:3:22:6, VAE22:4). Cardiolipins (CL) increased in relative abundance in all 31°C treatments, as did lysophosphatidylethanolamine (LPE), and n-acyl glycine (NAGly) (Supplementary Table 11, ANOVA FDR=<0.025). Ether-linked phosphatidylcholine (Ether-PC) and VAE decreased in relative abundance in both 16°C and 31°C treatments (Supplementary Table 24). There was also an increased presence of oxidized lipids, such as oxidized phosphatidylinositol (OxPI) and oxidized fatty acids (OxFA) across all 31°C treatments, and, within both C. goreaui species, an increased relative abundance of ether-linked phospholipids (Ether-PC) (Supplementary Table 24). The SFA/UFA ratio increased from controls within all Symbiodiniaceae species grown under 31°C as well as 16°C, whereas controls and 21°C were mostly similar (Supplementary Table 22). Additionally, there was a 61%, 69%, 74%, and 23% decrease in ether-linked lipids within D. trenchii, C. goreaui (HW), B. psygmophilum, and C. goreaui (HM) treatments 16°C, respectively, and a 77%, 55%, 83% and 18% decrease within the same Symbiodiniaceae under 31°C treatments (Supplementary Table 23). Both C. goreaui spp. saw a slight increase in ether-linked lipids at 21°C, whereas D. trenchii and B. psygmophilum exhibited the inverse (Supplementary Table 23).
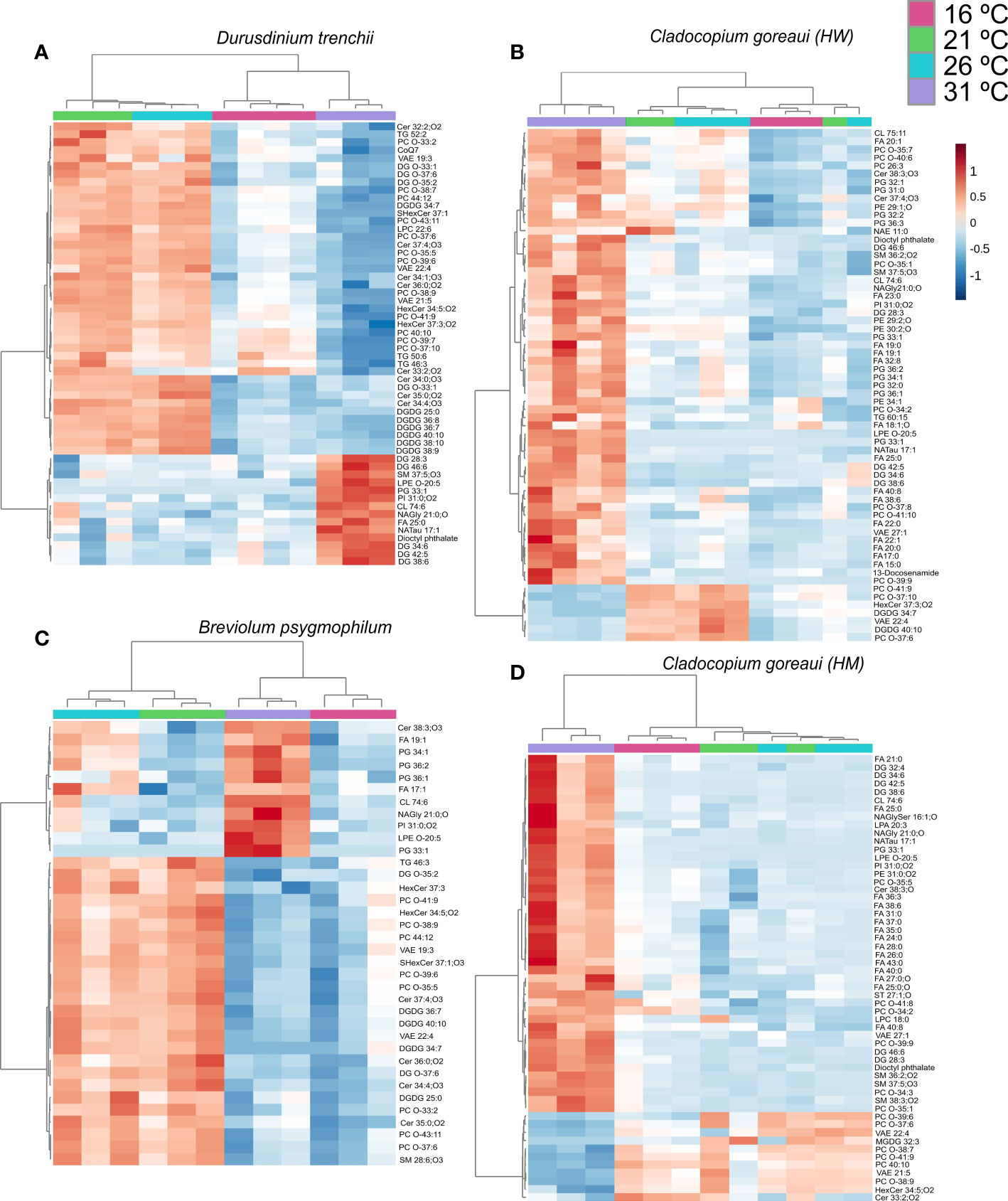
Figure 8 Symbiodiniaceae lipid accumulation and relative abundance heatmap under different temperatures as determined by an ANOVA FDR with Fishers post hoc in Durusdinium trenchii (A), Cladocopium goreaui HW (B), Breviolum psygmophilum (C), and Cladocopium goreaui HM (D) across the sample period for each temperature treatment [control temperature (26°C; purple), 16°C (red), 21°C (green), and 31°C (blue)]. N varied between 3-4 per treatment per species.
When grouped into sub-classes, D. trenchii showed significant differences in 21 lipid subclasses, C. goreaui (HW) and (HM) showed 10, and B. psygmophilum 12 (Figure 9; Supplementary Table 26). Most lipid subclasses followed similar patterns across all Symbiodiniaceae, including NAGly and PG which were relatively more abundant in 31°C treatments (Figure 9; Supplementary Table 26). In all species except B. psygmophilum, DG relative abundance was also significantly increased in 31°C treatments, whereas uniquely, B. psygmophilum, exhibited an increase in ceramide beta-hydroxy fatty acid-sphingosine (Cer-BS) as temperatures increased, with a low concentration in 16°C and 21°C samples, and increased in controlled (26°C) and 31°C treatments (Figure 9; Supplementary Table 26, FDR< 0.01). C. goreaui (HM) also exhibited a unique pattern, with highest relative abundances of PC at 16 °C, which showed an inverse relationship with temperature (Figure 9; Supplementary Table 26, FDR< 0.01).
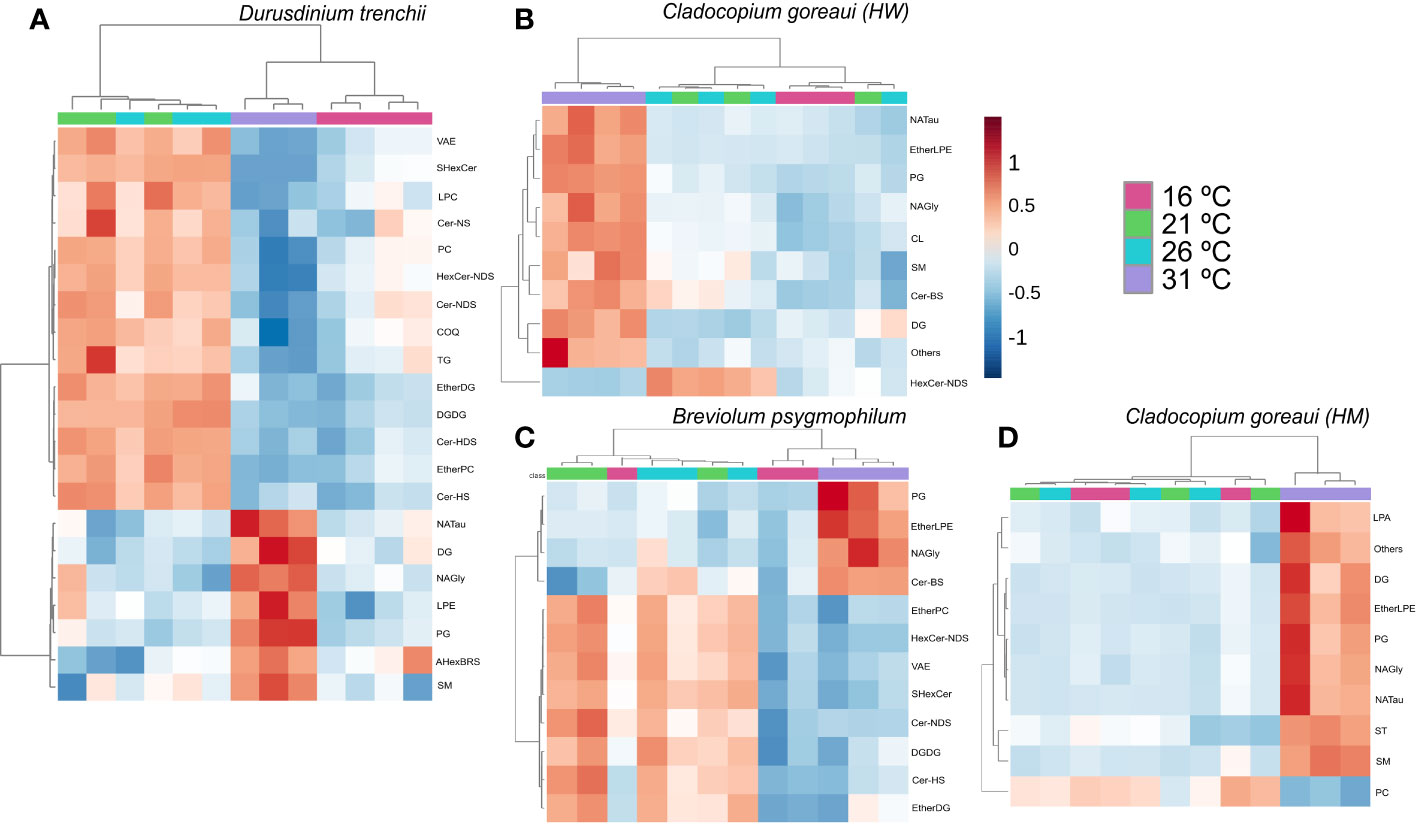
Figure 9 Symbiodiniaceae lipid sub-class abundance heatmap under different temperatures as determined by an ANOVA FDR with Fishers post hoc in Durusdinium trenchii (A), Cladocopium goreaui HW (B), Breviolum psygmophilum (C), and Cladocopium goreaui HM (D) across the sample period for each temperature treatment (control temperature [26°C; purple), 16°C (red), 21°C (green), and 31°C (blue)]. N varied between 3-4 per treatment per species.
Discussion
Microalgae can remodel their lipid composition in response to changes in temperature and nutrient concentration (Jiang et al., 2014; Fakhry and El Maghraby, 2015). The results here suggest Symbiodiniaceae are similarly able to remodel their lipids, including shifting from the biosynthesis of phospholipids to cell membrane-stabilizing isoprenoids in phosphorus limited conditions, increased storage of energy-rich triacylglycerols under nitrogen limitation, and an increase in saturated fatty acid abundance and phosphatidylinositol oxidation under high temperatures. This raises an intriguing possibility that Symbiodiniaceae lipid plasticity, and in turn what is being translocated to the coral host when in hospite, could determine the resilience of coral-Symbiodiniaceae associations.
Phosphorus limitation induced a significant reduction in the relative abundance of phosphatidylcholine (PC) lipids exhibited by all Symbiodiniaceae cultures grown in P-limited and NP-limited media (Figure 6; Supplementary Table 18). PC are the most abundant lipid class of cell membranes, and as such, a reduction in relative abundance can lead to a change in the composition and structure of cellular membranes (Kanno et al., 2007). It has been well established that phosphorus is a limiting nutrient in marine environments (Correll, 1999). For instance, in other microalgae, when phosphorus becomes less available for uptake, membranes biosynthesis shifts from phospholipid headgroups to non-phosphorus headgroups, such as diacylglycerolcarboxyhydroxymethylcholine, (DGCC); and sulfoquinovosyldiacylglycerol (SQDG) (Lowenstein et al., 2021) and betaine lipids (Van Mooy et al., 2009; Martin et al., 2011). By reducing the biosynthesis of phosphorus-containing lipids in membranes, remaining nutrients are able to be conserved for essential cellular functions (Dörmann and Benning, 2002). Phosphorus is a major component of DNA, and thus essential for reproduction, the transmission of chemical energy (ATP) within the cell, and photosynthesis (Li et al., 2016; Brembu et al., 2017). Phosphorus limitation can affect photosynthetic capacity by suppressing photosynthetic efficiency in PSII, as reported for diatoms [e.g., Thalassirosira weissflogii (Liu et al., 2011)], and green algae (e.g., Dunaliella tertiolecta (Geider et al., 1998). While cell density remained unchanged across all Symbiodiniaceae cultures in this study, photosynthetic capacity declined, suggesting a possible diversion of phosphorus from photosynthesis to central metabolism and cell replication, as evidenced by the stable growth rates.
Coinciding with the decrease in PC concentrations in P-Limited cultures, vitamin-a fatty esters (VAE), a type of isoprenol lipid, increased under phosphorus limitation in all Symbiodiniaceae (Figure 6; Supplementary Table 18), and as a class significantly increased in B. psygmophilum and C. goreaui (HM) cultures (Figure 6; Supplementary Table 20). Isoprenoids have been shown to be important components of membrane biosynthesis within algae and cyanobacteria (Lohr et al., 2012; Pattanaik and Lindberg, 2015) and are involved in human cell membrane structure and function (Dingle and Lucy, 1965). Isoprenes are derived from isoprenoids and are thought to possess antioxidant capabilities (Loreto et al., 2001) and stabilize plant cell membranes under stress (Fares et al., 2008). Isoprenoid biosynthesis requires high amounts of phosphorylated intermediates, but decreasing extracellular phosphate has been linked to the diversion of phosphate to isoprenoid synthesis to stabilize membranes (Wadhwa et al., 2018; Jordan et al., 2019). The increase of VAE coinciding with the decrease in PC may therefore indicate the diversion of phosphorus from phospholipid to isoprenoid synthesis to support cell viability under phosphorus deprivation in Symbiodiniaceae. However, whether such potential redirection in pathway activity is involved in either maintaining cell homeostasis, or is a physiological consequence to phosphorous stress, is unclear. Additionally, within B. psygmophilum and C. goreaui (HM) cultures, the polyphenol, cirsiliol, was shown to be a driver of separation in NP-Limited cultures (Figures 4C, D). Cirsiliol has been found to have several biological functions, notably including antioxidative capabilities within plants (Carlini et al., 2022). However, due to high sample variability within treatments, cirsiliol relative abundance was not detected as significant.
This is therefore a potential lipid remodeling strategy to maintain Symbiodiniaceae populations despite low phosphate levels. Furthermore, phosphorus reduction has been shown to inhibit cell division, but not cell growth for the phytoplankton, Emiliania huxleyi (Romano et al., 2015) and dinoflagellate Amphidinium carterae (Li et al., 2016). This is further reflected in the increase in cell size and stability in growth rate observed for P-limited Symbiodiniaceae cultures tested here. As a subclass, isoprenoids (VAE) significantly increased for NP- and P-limited B. psygmophilum and C. goreaui (HM) cultures (Figure 6; Supplementary Table 20), but not for D. trenchii or C. goreaui (HW) cultures. Although individual VAEs were significantly increased in these cultures, encompassing 43% of significant P-limited lipids in D. trenchii (Figure 6A), 20% in C. goreaui (HW) (Figure 6B), 21% of significant P-limited lipids in B. psygmophilum (Figure 6C), and 11% significant P-limited lipids in C. goreaui (HM) (Figure 5; Supplementary Table 18). To date, comparative analysis of different Symbiodiniaceae species under phosphorus stress is not well understood, but as Breviolum sp. and C. goreaui (HM) are known to be more susceptible to thermal stress than D. trenchii or C. goreaui (HW) (Dang et al., 2019; Russnak et al., 2021), temperature stress tolerance mechanisms may also extend to nutrient stress. It is possible that the increase in the isoprenoid class of lipids in stress sensitive species is a mechanism to stabilize cell membranes under the increased physiological stress in these cultures - a cellular response not experienced by D. trenchii or C. goreaui (HW). Indeed, cultures of the relatively ‘stress tolerant’ species D. trenchii had the highest growth rate under phosphorus limitation and cell sizes similar to the control cultures, suggesting no morphological stress response, however, lipid remodeling was observed indicating a response to phosphorous stress (Figure 1A; Supplementary Table 5, Supplementary Table 7).
Glycerolipids were found to be increasingly abundant in all nutrient limited cultures. Glycerolipids encompass a range of lipids, including monoacylglycerol (MG), DG, and TG, and serve essential roles in cellular signaling and as precursors to other biological active molecules (mainly MG and DG) and energy storage (mainly TG). Except for. D. trenchii, TGs encompassed 28-42% of significant lipids within each Symbiodiniaceae species (Figure 6; Supplementary Table 18), in which they were consistently more abundant in N-limited cultures. An increase in TG response under N limitation has been well documented in microalgae (Fakhry and El Maghraby, 2015), and is hypothesized to provide increased energy stores to support cells experiencing nutrient stress (Hu et al., 2008). Indeed, Symbiodiniaceae are thought to be well adapted to N starvation and can divert resources from growth to maintaining functional photophysiology (Rosset et al., 2017). Research within other organisms have also suggested that accumulation of TG may serve an important function in the stress response, aiding in the prevention of lipotoxicity from increased abundances of specific lipids, such as DG, observed within plants (Lu et al., 2020) and yeast (Kurat et al., 2006). These results suggest a similar function may occur within Symbiodiniaceae. Furthermore, increases in TG abundance has been linked to the capacity to maintain photosynthetic activity under nitrogen limitation (Fattore et al., 2021). Indeed, photophysiology in N-Limited cultures remained stable throughout the 14-day period across all Symbiodiniaceae species whereas P-Limited cultures exhibited greatly reduced Fv/Fm. Conversely, P-limitation increased the abundance of DGs relative to the controls (Figure 6; Supplementary Table 18). Under phosphorus deprivation, increases in DG accumulation within plant cells have been shown to coincide with a decrease in PC, hypothesized to be due to hydrolysis of PC within the phosphatidylcholine-specific phospholipase C (PC-PLC) pathway under nutrient stress (Jouhet et al., 2003; Cocco et al., 2015). The same could be occurring within Symbiodiniaceae.
Glycerolipids in the thylakoid membranes are characterized by the presence of monogalactosyldiacylglycerol (MGDG), digalactosyldiacylglycerol (DGDG), sulfoquinovosyldiacylglycerol (SQDG) and phosphatidylglycerol (PG), and the absence of PC (Tchernov et al., 2004; Reue and Brindley, 2008). Nitrogen limitation resulted in a decrease in the relative abundance of specific DGDGs in all Symbiodiniaceae cultures, total DGDGs in C. goreaui (HM) and B. psygmophilum, and specific MGDGs in C. goreaui (HW) and (HM) (Figures 5, 6, Supplementary Table 18, S20). Glyceroglycolipids are also important in cell membranes (Kalisch et al., 2016), and may therefore reflect the reduced cell size observed across N and NP-limited samples across D. trenchii, B. psygmophilum and C. goreaui (HM)(Figure 1; Supplementary Table 7, p<0.028). Similar decreases in cell size were observed within the diatom Stephanodiscus minutulus (Lynn et al., 2000) and other microalgal species (Jia et al., 2015; Latsos et al., 2020). Meanwhile, the relative abundance of DGDG and MGDG lipids increased under P limitation (Figure 6). High concentrations of DGDG and MGDG can support photosynthetic growth under phosphorus limitation (Awai et al., 2007), which corroborates our results as growth rates of P-limited cultures were not reduced in all cultures bar C. goreaui (HM) (Figure 1, Supplementary Table 5).
Alterations to temperature was also shown to induce lipid remodeling within Symbiodiniaceae. Such remodeling may have assisted culture survival over the 14 days, despite an increase in the abundance of oxidized lipids, and significantly reduced growth rate across all Symbiodiniaceae cultures. Across all Symbiodiniaceae, fatty acids (FA) increased in cultures grown at 31°C, of which a higher proportion of SFA : UFA compared to controls were found in all Symbiodiniaceae species (Figure 8; Supplementary Table 22), supporting previous findings for D. trenchii and Cladocopium sp (Rosset et al., 2019). Temperature increase leads to elevated abundance of reactive oxygen species (ROS) in microalgae, which, if it exceeds the antioxidant capacity, can induce oxidative stress, lipotoxicity and cell mortality (Verma et al., 2021). Unsaturated fatty acids are more susceptible to ROS attack than saturated fatty acids (Bacellar and Baptista, 2019), therefore, increased saturation of membrane-bound fatty acids can serve to maintain microalgal cell stability during heat stress (Los and Murata, 2004). ROS accumulation has been shown with increased temperature in Cladocopium sp (McGinty et al., 2012) and D. trenchii (Scharfenstein et al., 2023), and higher abundance of ROS has also been linked to increases in cell size (Lima et al., 2022; Amario et al., 2023). Indeed, D. trenchii and C. goreaui (HM & HW) cell sizes increased when grown at 31°C (Figure 3) in this study, indicating potential cellular swelling prior to cell cycle arrest and necrosis as a result of heat stress (Dunn et al., 2004). N-acyl amino acids (NAAA) increased in all cultures grown at 31°C (Figure 9; Supplementary Table 24). In addition, NAAA, inclusive of NAE, NAGly, n-acyl glycine (NAGlyser) and N-arachidonoyl taurine (NATau) are long chained fatty acids linked to an amino acid through an amide bond, and are important within signaling pathways (Battista et al., 2019). There have been no studies on NAAA within microalgae, however these molecules have been suggested to function within the immune response, and potentially induce apoptosis within murine macrophage cells (Takenouchi et al., 2012). The increased relative abundance of NAAA exhibited by cultures grown at 31°C, in addition to increase in cell size (Figure 3; Supplementary Table 13) suggests these lipids may serve a similar role within Symbiodiniaceae, yet further studies are essential to confirm. Despite the cell size increases, cell growth and photophysiology remained stable, suggesting the increased SFA : UFA ratio served to support cell stability under the higher temperature ranges.
Unsaturation of fatty acids within microalgae actively occurs under decreasing temperatures, and is known to increase membrane permeability, and is considered important in maintaining photosynthetic capacity in thylakoid membranes under cold temperatures (Holm et al., 2022; Ferrer-Ledo et al., 2023). An increased abundance of unsaturated fatty acids under cold stress has been previously recorded in Cladocopium sp. exposed to 16˚C for 4 days (Oakley et al., 2022). When temperatures were decreased to 21˚C, we observed an increase in the abundance of unsaturated fatty acids (and a decrease in the SFA : UFA ratio) across all species, supporting previous physiological responses in microalgae and Symbiodiniaceae (Oakley et al., 2022). However, at the coldest temperature (16˚C), the SFA : UFA ratio increased compared to control cultures across all species (Supplementary Table 22). Longer term exposure (i.e., entire growth cycle) to cold temperatures could increase the level of ROS due to the increased solubility of O2 (Ermilova, 2020), which may have resulted in the relative increase in saturated fatty acids in order to maintain cell stability (Ayala et al., 2014; Su et al., 2019). Betaine lipids are a family of glycerolipids linked to DGs and are considered crucial to low temperature adaptation in other microalgae (Murakami et al., 2018). While the analysis of betaine lipids requires an alternative extraction process to that used here due to their chemical composition, DGs are components, and their abundance could be linked to betaine lipid abundance. DGs were more abundant at 16°C versus the control in all Symbiodiniaceae, suggesting betaine lipids may also provide a lipid remodelling mechanisms of protection against cold temperatures in Symbiodiniaceae. Symbiodiniaceae specific production of betaine lipids has been previously recorded (Sikorskaya et al., 2023), and thus may explain why DGs were significantly higher in relatively stress tolerant strains D. trenchii and C. goreaui (HW) and the temperate Symbiodiniaceae B. minutum, but not the tropical, stress sensitive C. goreaui (HW). Previous studies on D. trenchii and B. psygmophilum noted a distinct lack of change in Fv/Fm when grown under conditions colder than optimum (Dilernia et al., 2023), which may imply that the slightly slowed growth rate exhibited by these cultures in comparison to controls may not indicate stress, but slowed metabolic processes as seen in bacteria (Zhang and Gross, 2021). In order to resolve this current knowledge gap, future studies should be conducted on Symbiodiniaceae physiology and photophysiological response as temperatures are reduced below 16°C, as well as specifically targeting betaine lipid composition.
Over-abundance of ROS under temperature stress can result in photosystem damage and alterations to thylakoid membranes, lipid peroxidation, and an increase in the abundance of oxidized lipids generated by free radicals (Botana et al., 2022; Xing et al., 2022; Amario et al., 2023). Here, there was an increased relative abundance of oxidized phosphatidylinositol (OxPI) across all Symbiodiniaceae species grown at 31°C (Figure 8; Supplementary Table 24). Phosphatidylinositol (PI) is an important secondary messenger in eukaryotic cells, inducing cellular changes (Berridge and Irvine, 1989), and PI signaling pathways (Capelluto, 2013), as found in the slime mold Dictystelium (Stephens and Irvine, 1990). Thermal stress has caused large increases in the relative abundance of inositols in cnidarians (Hillyer et al., 2017; González-Pech et al., 2022), and cultured Symbiodiniaceae (Klueter et al., 2015). The PI pathway, activated through the phosphorylation of inositol, has been observed in Symbiodiniaceae from genera Symbiodinium, Breviolum, Cladocopium and Durusdinium (Rosic et al., 2015). This resembles pathways found in the parasitic taxon Apicomplexa (Ashley et al., 2023), which use PI to impair host cell signaling, preventing host immune responses and allowing for infection (Lüder et al., 2009). This has led to inositol having a predicted function as an important signaling molecule in the cnidarian-Symbiodiniaceae symbiosis (Matthews et al., 2018; González-Pech et al., 2022, 2017). Oxidized derivatives of phosphatidylinositol, OxPI, are less well understood, with studies identifying the presence of OxPI in plants (Nilsson et al., 2014) but not yet able to determine specific function. The presence of oxidized phospholipids has been linked to oxidative damage and increases in ROS (Fellows et al., 2023), potentially impacting membrane structure and permeability, and leading to eventual cell apoptosis (Gaschler and Stockwell, 2017). OxPI and other oxidized phospholipids have not been well explored in algae but could indicate increases in ROS and oxidative stress due to temperature increase. ROS have been shown to leak from Symbiodiniaceae cells, implying that when in hospite would transfer to the coral host (Nielsen and Petrou, 2023), and corals are known to bleach under increased oxidative stress (Lesser, 1997). Therefore, it is possible that increases in relative abundances of OxPI as a result of temperature stress could potentially disrupt signaling between Symbiodiniaceae and coral host when in hospite and contribute to the breakdown of the symbiosis.
This study demonstrates the homeoviscous adaptation of Symbiodiniaceae species under variation to temperature and nutrient concentration. Reef building corals acquire some (mainly PUFAs and fatty acid precursors) of their lipids from endosymbiotic Symbiodiniaceae (Imbs et al., 2010; Matthews et al., 2018), which serve as vital energy sources and key inter-partner signaling molecules (Matthews et al., 2017). Therefore, Symbiodiniaceae lipid remodelling can have significant influence on their physiology that in turn cascades to their host. This is especially important as warming temperatures are causing the increased occurrence of coral bleaching (Hughes et al., 2017) and poleward shift of coral species (Yamano et al., 2011). As bleached corals have been found to consume greater amounts of lipid stores for longer term survival under stress (Liu et al., 2022), it is important to develop upon this knowledge. Future studies may also want to consider further studying homeoviscous adaptation of Symbiodiniaceae under cold stress and how this might mediate poleward range shifts to cooler regions.
Data availability statement
The original contributions presented in the study are included in the article/Supplementary Material. Further inquiries can be directed to the corresponding authors.
Ethics statement
The manuscript presents research on animals that do not require ethical approval for their study.
Author contributions
LL: Conceptualization, Formal Analysis, Investigation, Methodology, Writing – original draft, Writing – review & editing. JM: Conceptualization, Funding acquisition, Methodology, Supervision, Writing – review & editing. EC: Conceptualization, Funding acquisition, Supervision, Writing – review & editing, Methodology. BS: Conceptualization, Supervision, Writing – review & editing. MP: Data curation, Methodology, Resources, Writing – review & editing.
Funding
The author(s) declare financial support was received for the research, authorship, and/or publication of this article. This research was supported by an Australian Government Research Training Program (RTP) Fee Offset Scholarship, Climate Change Cluster (C3) funding, and Future Reefs lab group funding. This work was supported by University of Technology Sydney Chancellor’s Research and Zimmermann Biodiversity Fellowships (awarded to JM).
Acknowledgments
The authors thank the tech staff at the University of Technology, Sydney, for their support during this experimentation.
Conflict of interest
The authors declare that the research was conducted in the absence of any commercial or financial relationships that could be construed as a potential conflict of interest.
Publisher’s note
All claims expressed in this article are solely those of the authors and do not necessarily represent those of their affiliated organizations, or those of the publisher, the editors and the reviewers. Any product that may be evaluated in this article, or claim that may be made by its manufacturer, is not guaranteed or endorsed by the publisher.
Supplementary material
The Supplementary Material for this article can be found online at: https://www.frontiersin.org/articles/10.3389/frpro.2024.1320353/full#supplementary-material
References
Amario M., Villela L. B., Jardim-Messeder D., Silva-Lima A. W., Rosado P. M., De Moura R. L., et al. (2023). Physiological response of Symbiodiniaceae to thermal stress: Reactive oxygen species, photosynthesis, and relative cell size. PloS One 18, e0284717. doi: 10.1371/journal.pone.0284717
Ashley I. A., Kitchen S. A., Gorman L. M., Grossman A. R., Oakley C. A., Suggett D. J., et al. (2023). Genomic conservation and putative downstream functionality of the phosphatidylinositol signalling pathway in the cnidarian-dinoflagellate symbiosis. Front. Microbiol. 13. doi: 10.3389/fmicb.2022.1094255
Awai K., Watanabe H., Benning C., Nishida I. (2007). Digalactosyldiacylglycerol is required for better photosynthetic growth of synechocystis sp. PCC6803 under phosphate limitation. Plant Cell Physiol. 48, 1517–1523. doi: 10.1093/pcp/pcm134
Ayala A., Muñoz M. F., Argüelles S. (2014). Lipid peroxidation: production, metabolism, and signaling mechanisms of malondialdehyde and 4-hydroxy-2-nonenal. Oxid. Med. Cell. Longevity 2014, 1–31. doi: 10.1155/2014/360438
Bacellar I. O. L., Baptista M. S. (2019). Mechanisms of photosensitized lipid oxidation and membrane permeabilization. ACS Omega 4, 21636–21646. doi: 10.1021/acsomega.9b03244
Bachok Z., Mfilinge P., Tsuchiya M. (2006). Characterization of fatty acid composition in healthy and bleached corals from Okinawa, Japan. Coral Reefs 25, 545–554. doi: 10.1007/s00338-006-0130-9
Battista N., Bari M., Bisogno T. (2019). N-acyl amino acids: metabolism, molecular targets, and role in biological processes. Biomolecules 9, 822. doi: 10.3390/biom9120822
Berges J. A., Franklin D. J., Harrison P. J. (2001). EVOLUTION OF AN ARTIFICIAL SEAWATER MEDIUM: IMPROVEMENTS IN ENRICHED SEAWATER, ARTIFICIAL WATER OVER THE LAST TWO DECADES. J. Phycology 37, 1138–1145. doi: 10.1046/j.1529-8817.2001.01052.x
Berridge M. J., Irvine R. F. (1989). Inositol phosphates and cell signalling. Nature 341, 197–205. doi: 10.1038/341197a0
Blanckaert A. C. A., Biscéré T., Grover R., Ferrier-Pagès C. (2023). Species-specific response of corals to imbalanced ratios of inorganic nutrients. IJMS 24, 3119. doi: 10.3390/ijms24043119
Botana M. T., Chaves-Filho A. B., Inague A., Z. Güth A., Saldanha-Corrêa F., Müller M. N., et al. (2022). Thermal plasticity of coral reef symbionts is linked to major alterations in their lipidome composition. Limnology Oceanography 67, 1456–1469. doi: 10.1002/lno.12094
Boulotte N. M., Dalton S. J., Carroll A. G., Harrison P. L., Putnam H. M., Peplow L. M., et al. (2016). Exploring the Symbiodinium rare biosphere provides evidence for symbiont switching in reef-building corals. ISME J. 10, 2693–2701. doi: 10.1038/ismej.2016.54
Brembu T., Mühlroth A., Alipanah L., Bones A. M. (2017). The effects of phosphorus limitation on carbon metabolism in diatoms. Phil. Trans. R. Soc B 372, 20160406. doi: 10.1098/rstb.2016.0406
Camp E. F., Kahlke T., Signal B., Oakley C. A., Lutz A., Davy S. K., et al. (2022). Proteome metabolome and transcriptome data for three Symbiodiniaceae under ambient and heat stress conditions. Sci. Data 9, 153. doi: 10.1038/s41597-022-01258-w
Cañavate J. P., Armada I., Hachero-Cruzado I. (2017). Interspecific variability in phosphorus-induced lipid remodelling among marine eukaryotic phytoplankton. New Phytol. 213, 700–713. doi: 10.1111/nph.14179
Capelluto D. G. S. (2013). Lipid-mediated protein signaling, advances in experimental medicine and biology (Springer Netherlands: Dordrecht). doi: 10.1007/978-94-007-6331-9
Carballo-Bolaños R., Denis V., Huang Y.-Y., Keshavmurthy S., Chen C. A. (2019). Temporal variation and photochemical efficiency of species in Symbiodinaceae associated with coral Leptoria phrygia (Scleractinia; Merulinidae) exposed to contrasting temperature regimes. PloS One 14, e0218801. doi: 10.1371/journal.pone.0218801
Carlini L., Tancreda G., Iobbi V., Caicci F., Bruno S., Esposito A., et al. (2022). The Flavone Cirsiliol from Salvia x jamensis Binds the F1 Moiety of ATP Synthase, Modulating Free Radical Production. Cells 11, 3169. doi: 10.3390/cells11193169
Chen H.-K., Wang L.-H., Chen W.-N. U., Mayfield A. B., Levy O., Lin C.-S., et al. (2017). Coral lipid bodies as the relay center interconnecting diel-dependent lipidomic changes in different cellular compartments. Sci. Rep. 7, 3244. doi: 10.1038/s41598-017-02722-z
Cocco L., Follo M. Y., Manzoli L., Suh P.-G. (2015). Phosphoinositide-specific phospholipase C in health and disease. J. Lipid Res. 56, 1853–1860. doi: 10.1194/jlr.R057984
Correll D. (1999). Phosphorus: a rate limiting nutrient in surface waters. Poultry Sci. 78, 674–682. doi: 10.1093/ps/78.5.674
Dang K. V., Pierangelini M., Roberty S., Cardol P. (2019). Alternative photosynthetic electron transfers and bleaching phenotypes upon acute heat stress in symbiodinium and breviolum spp. (Symbiodiniaceae) Culture. Front. Mar. Sci. 6. doi: 10.3389/fmars.2019.00656
Dickinson S., Mientus M., Frey D., Amini-Hajibashi A., Ozturk S., Shaikh F., et al. (2017). A review of biodiesel production from microalgae. Clean Techn Environ. Policy 19, 637–668. doi: 10.1007/s10098-016-1309-6
Dilernia N. J., Camp E. F., Bartels N., Suggett D. J. (2023). Contrasting the thermal performance of cultured coral endosymbiont photo-physiology. J. Exp. Mar. Biol. Ecol. 561, 151865. doi: 10.1016/j.jembe.2022.151865
Dingle J. T., Lucy J. A. (1965). Membrane phenomenons in relation to vitamin A. Proc. Nutr. Soc 24, 170–172. doi: 10.1079/PNS19650031
Dörmann P., Benning C. (2002). Galactolipids rule in seed plants. Trends Plant Sci. 7, 112–118. doi: 10.1016/S1360-1385(01)02216-6
Dunn S. R., Thomason J. C., Le Tissier M. D. A., Bythell J. C. (2004). Heat stress induces different forms of cell death in sea anemones and their endosymbiotic algae depending on temperature and duration. Cell Death Differ 11, 1213–1222. doi: 10.1038/sj.cdd.4401484
Ermilova E. (2020). Cold stress response: an overview in chlamydomonas. Front. Plant Sci. 11. doi: 10.3389/fpls.2020.569437
Ezzat L., Maguer J.-F., Grover R., Ferrier-Pagès C. (2016). Limited phosphorus availability is the Achilles heel of tropical reef corals in a warming ocean. Sci. Rep. 6, 31768. doi: 10.1038/srep31768
Fakhry E. M., El Maghraby D. M. (2015). Lipid accumulation in response to nitrogen limitation and variation of temperature in Nannochloropsis salina. Bot. Stud. 56, 6. doi: 10.1186/s40529-015-0085-7
Fares S., Brilli F., Noguès I., Velikova V., Tsonev T., Dagli S., et al. (2008). Isoprene emission and primary metabolism in Phragmites australis grown under different phosphorus levels: Isoprene emission and primary metabolism. Plant Biol. 10, 38–43. doi: 10.1055/s-2007-965429
Fattore N., Bellan A., Pedroletti L., Vitulo N., Morosinotto T. (2021). Acclimation of photosynthesis and lipids biosynthesis to prolonged nitrogen and phosphorus limitation in Nannochloropsis gaditana. Algal Res. 58, 102368. doi: 10.1016/j.algal.2021.102368
Fellows A. P., Casford M. T. L., Davies P. B. (2023). In situ investigation of the oxidation of a phospholipid monolayer by reactive oxygen species. Biophys. J. 122, 2007–2022. doi: 10.1016/j.bpj.2022.10.040
Ferrer-Ledo N., Stegemüller L., Janssen M., Wijffels R. H., Barbosa M. J. (2023). Growth and fatty acid distribution over lipid classes in Nannochloropsis oceanica acclimated to different temperatures. Front. Plant Sci. 14. doi: 10.3389/fpls.2023.1078998
Gao B., Hong J., Chen J., Zhang H., Hu R., Zhang C. (2023). The growth, lipid accumulation and adaptation mechanism in response to variation of temperature and nitrogen supply in psychrotrophic filamentous microalga Xanthonema hormidioides (Xanthophyceae). Biotechnol. Biofuels 16, 12. doi: 10.1186/s13068-022-02249-0
Gaschler M. M., Stockwell B. R. (2017). Lipid peroxidation in cell death. Biochem. Biophys. Res. Commun. 482, 419–425. doi: 10.1016/j.bbrc.2016.10.086
Geider R., Graziano L., McKay R. M. (1998). Responses of the photosynthetic apparatus of Dunaliella tertiolecta (Chlorophyceae) to nitrogen and phosphorus limitation. Eur. J. Phycology 33, 315–332. doi: 10.1080/09670269810001736813
González-Pech R. A., Hughes D. J., Strudwick P., Lewis B. M., Booth D. J., Figueira W. F., et al. (2022). Physiological factors facilitating the persistence of Pocillopora aliciae and Plesiastrea versipora in temperate reefs of south-eastern Australia under ocean warming. Coral Reefs 41, 1239–1253. doi: 10.1007/s00338-022-02277-0
Guillard R. R. L. (1975). Culture of phytoplankton for feeding marine invertebrates, in: Culture of Marine Invertebrate Animals Vol. pp (New York, NY: Plenum Publishing Corporation), 29–60.
Hillyer K. E., Dias D. A., Lutz A., Wilkinson S. P., Roessner U., Davy S. K. (2017). Metabolite profiling of symbiont and host during thermal stress and bleaching in the coral Acropora aspera. Coral Reefs 36, 105–118. doi: 10.1007/s00338-016-1508-y
Holm H. C., Fredricks H. F., Bent S. M., Lowenstein D. P., Ossolinski J. E., Becker K. W., et al. (2022). Global ocean lipidomes show a universal relationship between temperature and lipid unsaturation. Science 376, 1487–1491. doi: 10.1126/science.abn7455
Hu Q., Sommerfeld M., Jarvis E., Ghirardi M., Posewitz M., Seibert M., et al. (2008). Microalgal triacylglycerols as feedstocks for biofuel production: perspectives and advances. Plant J. 54, 621–639. doi: 10.1111/j.1365-313X.2008.03492.x
Hughes T. P., Barnes M. L., Bellwood D. R., Cinner J. E., Cumming G. S., Jackson J. B. C., et al. (2017). Coral reefs in the anthropocene. Nature 546, 82–90. doi: 10.1038/nature22901
Imbs A., Latyshev N., Dautova T., Latypov Y. (2010). Distribution of lipids and fatty acids in corals by their taxonomic position and presence of zooxanthellae. Mar. Ecol. Prog. Ser. 409, 65–75. doi: 10.3354/meps08622
Jia J., Han D., Gerken H. G., Li Y., Sommerfeld M., Hu Q., et al. (2015). Molecular mechanisms for photosynthetic carbon partitioning into storage neutral lipids in Nannochloropsis oceanica under nitrogen-depletion conditions. Algal Res. 7, 66–77. doi: 10.1016/j.algal.2014.11.005
Jiang P.-L., Pasaribu B., Chen C.-S. (2014). Nitrogen-deprivation elevates lipid levels in symbiodinium spp. by lipid droplet accumulation: morphological and compositional analyses. PloS One 9, e87416. doi: 10.1371/journal.pone.0087416
Jordan S. F., Nee E., Lane N. (2019). Isoprenoids enhance the stability of fatty acid membranes at the emergence of life potentially leading to an early lipid divide. Interface Focus. 9, 20190067. doi: 10.1098/rsfs.2019.0067
Jouhet J., Maréchal E., Bligny R., Joyard J., Block M. A. (2003). Transient increase of phosphatidylcholine in plant cells in response to phosphate deprivation. FEBS Lett. 544, 63–68. doi: 10.1016/S0014-5793(03)00477-0
Kalisch B., Dörmann P., Hölzl G. (2016). “DGDG and glycolipids in plants and algae,” in Lipids in plant and algae development, subcellular biochemistry, vol. pp . Eds. Nakamura Y., Li-Beisson Y. (Cham, Switzerland: Springer International Publishing), 51–83. doi: 10.1007/978-3-319-25979-6_3
Kanno K., Wu M. K., Scapa E. F., Roderick S. L., Cohen D. E. (2007). Structure and function of phosphatidylcholine transfer protein (PC-TP)/StarD2. Biochim. Biophys. Acta (BBA) - Mol. Cell Biol. Lipids 1771, 654–662. doi: 10.1016/j.bbalip.2007.04.003
Khan M. I., Shin J. H., Kim J. D. (2018). The promising future of microalgae: current status, challenges, and optimization of a sustainable and renewable industry for biofuels, feed, and other products. Microb. Cell Fact 17, 36. doi: 10.1186/s12934-018-0879-x
Klueter A., Crandall J., Archer F., Teece M., Coffroth M. (2015). Taxonomic and environmental variation of metabolite profiles in marine dinoflagellates of the genus symbiodinium. Metabolites 5, 74–99. doi: 10.3390/metabo5010074
Koch K., Hagen W., Graeve M., Bischof K. (2017). Fatty acid compositions associated with high-light tolerance in the intertidal rhodophytes Mastocarpus stellatus and Chondrus crispus. Helgol Mar. Res. 71, 15. doi: 10.1186/s10152-017-0495-x
Kurat C. F., Natter K., Petschnigg J., Wolinski H., Scheuringer K., Scholz H., et al. (2006). Obese yeast: triglyceride lipolysis is functionally conserved from mammals to yeast. J. Biol. Chem. 281, 491–500. doi: 10.1074/jbc.M508414200
LaJeunesse T. C., Parkinson J. E., Gabrielson P. W., Jeong H. J., Reimer J. D., Voolstra C. R., et al. (2018). Systematic revision of symbiodiniaceae highlights the antiquity and diversity of coral endosymbionts. Curr. Biol. 28, 2570–2580.e6. doi: 10.1016/j.cub.2018.07.008
Latsos C., Van Houcke J., Timmermans K. R. (2020). The effect of nitrogen starvation on biomass yield and biochemical constituents of rhodomonas sp. Front. Mar. Sci. 7. doi: 10.3389/fmars.2020.563333
Lesser M. P. (1997). Oxidative stress causes coral bleaching during exposure to elevated temperatures. Coral Reefs 16, 187–192. doi: 10.1007/s003380050073
Levin R. A., Beltran V. H., Hill R., Kjelleberg S., McDougald D., Steinberg P. D., et al. (2016). Sex, scavengers, and chaperones: transcriptome secrets of divergent symbiodinium thermal tolerances. Mol. Biol. Evol. 33, 2201–2215. doi: 10.1093/molbev/msw119
Li M., Shi X., Guo C., Lin S. (2016). Phosphorus deficiency inhibits cell division but not growth in the dinoflagellate amphidinium carterae. Front. Microbiol. 7. doi: 10.3389/fmicb.2016.00826
Lima M. S., Hamerski L., Silva T. A., da Cruz M. L. R., Varasteh T., Tschoeke D. A., et al. (2022). Insights on the biochemical and cellular changes induced by heat stress in the Cladocopium isolated from coral Mussismilia Braziliensis. Front. Microbiol. 13. doi: 10.3389/fmicb.2022.973980
Liu S., Guo Z., Li T., Huang H., Lin S. (2011). Photosynthetic efficiency, cell volume, and elemental stoichiometric ratios in Thalassirosira weissflogii under phosphorus limitation. Chin. J. Ocean. Limnol. 29, 1048–1056. doi: 10.1007/s00343-011-0224-2
Liu C., Zhang Y., Huang L., Yu X., Luo Y., Jiang L., et al. (2022). Differences in fatty acids and lipids of massive and branching reef-building corals and response to environmental changes. Front. Mar. Sci. 9. doi: 10.3389/fmars.2022.882663
Lohr M., Schwender J., Polle J. E. W. (2012). Isoprenoid biosynthesis in eukaryotic phototrophs: A spotlight on algae. Plant Sci. 185–186, 9–22. doi: 10.1016/j.plantsci.2011.07.018
Loreto F., Mannozzi M., Maris C., Nascetti P., Ferranti F., Pasqualini S. (2001). Ozone quenching properties of isoprene and its antioxidant role in leaves. Plant Physiol. 126, 993–1000. doi: 10.1104/pp.126.3.993
Los D. A., Murata N. (2004). Membrane fluidity and its roles in the perception of environmental signals. Biochim. Biophys. Acta (BBA) - Biomembranes 1666, 142–157. doi: 10.1016/j.bbamem.2004.08.002
Lowenstein D. P., Mayers K., Fredricks H. F., Van Mooy B. A. S. (2021). Targeted and untargeted lipidomic analysis of haptophyte cultures reveals novel and divergent nutrient-stress adaptations. Organic Geochemistry 161, 104315. doi: 10.1016/j.orggeochem.2021.104315
Lu J., Xu Y., Wang J., Singer S. D., Chen G. (2020). The role of triacylglycerol in plant stress response. Plants 9, 472. doi: 10.3390/plants9040472
Lüder C. G. K., Stanway R. R., Chaussepied M., Langsley G., Heussler V. T. (2009). Intracellular survival of apicomplexan parasites and host cell modification. Int. J. Parasitol. 39, 163–173. doi: 10.1016/j.ijpara.2008.09.013
Lynn S. G., Kilham S. S., Kreeger D. A., Interlandi S. J. (2000). EFFECT OF NUTRIENT AVAILABILITY ON THE BIOCHEMICAL AND ELEMENTAL STOICHIOMETRY IN THE FRESHWATER DIATOM STEPHANODISCUS MINUTULUS (BACILLARIOPHYCEAE)*. J. Phycology 36, 510–522. doi: 10.1046/j.1529-8817.2000.98251.x
Martin P., Van Mooy B. A., Heithoff A., Dyhrman S. T. (2011). Phosphorus supply drives rapid turnover of membrane phospholipids in the diatom Thalassiosira pseudonana. ISME J. 5, 1057–1060. doi: 10.1038/ismej.2010.192
Matthews J. L., Crowder C. M., Oakley C. A., Lutz A., Roessner U., Meyer E., et al. (2017). Optimal nutrient exchange and immune responses operate in partner specificity in the cnidarian-dinoflagellate symbiosis. Proc. Natl. Acad. Sci. U.S.A. 114, 13194–13199. doi: 10.1073/pnas.1710733114
Matthews J. L., Oakley C. A., Lutz A., Hillyer K. E., Roessner U., Grossman A. R., et al. (2018). Partner switching and metabolic flux in a model cnidarian–dinoflagellate symbiosis. Proc. R. Soc B. 285, 20182336. doi: 10.1098/rspb.2018.2336
Matyash V., Liebisch G., Kurzchalia T. V., Shevchenko A., Schwudke D. (2008). Lipid extraction by methyl-tert-butyl ether for high-throughput lipidomics. J. Lipid Res. 49, 1137–1146. doi: 10.1194/jlr.D700041-JLR200
McGinty E. S., Pieczonka J., Mydlarz L. D. (2012). Variations in reactive oxygen release and antioxidant activity in multiple symbiodinium types in response to elevated temperature. Microb. Ecol. 64, 1000–1007. doi: 10.1007/s00248-012-0085-z
McRae C. J., Keshavmurthy S., Chen H.-K., Ye Z.-M., Meng P.-J., Rosset S. L., et al. (2023). Baseline dynamics of Symbiodiniaceae genera and photochemical efficiency in corals from reefs with different thermal histories. PeerJ 11, e15421. doi: 10.7717/peerj.15421
Murakami H., Nobusawa T., Hori K., Shimojima M., Ohta H. (2018). Betaine lipid is crucial for adapting to low temperature and phosphate deficiency in nannochloropsis. Plant Physiol. 177, 181–193. doi: 10.1104/pp.17.01573
Nielsen D. A., Petrou K. (2023). Lipid stores reveal the state of the coral-algae symbiosis at the single-cell level. ISME Commun. 3, 29. doi: 10.1038/s43705-023-00234-8
Nilsson A. K., Johansson O. N., Fahlberg P., Steinhart F., Gustavsson M. B., Ellerström M., et al. (2014). Formation of oxidized phosphatidylinositol and 12-oxo-phytodienoic acid containing acylated phosphatidylglycerol during the hypersensitive response in Arabidopsis. Phytochemistry 101, 65–75. doi: 10.1016/j.phytochem.2014.01.020
Oakley C. A., Pontasch S., Fisher P. L., Wilkinson S. P., Keyzers R. A., Krueger T., et al. (2022). Thylakoid fatty acid composition and response to short-term cold and heat stress in high-latitude Symbiodiniaceae. Coral Reefs 41, 343–353. doi: 10.1007/s00338-022-02221-2
Ortiz Montoya E. Y., Casazza A. A., Aliakbarian B., Perego P., Converti A., De Carvalho J. C. M. (2014). Production of Chlorella vulgaris as a source of essential fatty acids in a tubular photobioreactor continuously fed with air enriched with CO 2 at different concentrations. Biotechnol. Prog. 30, 916–922. doi: 10.1002/btpr.1885
Pattanaik B., Lindberg P. (2015). Terpenoids and their biosynthesis in cyanobacteria. Life 5, 269–293. doi: 10.3390/life5010269
Reue K., Brindley D. N. (2008). Thematic Review Series: Glycerolipids. Multiple roles for lipins/phosphatidate phosphatase enzymes in lipid metabolism. J. Lipid Res. 49, 2493–2503. doi: 10.1194/jlr.R800019-JLR200
Rezayian M., Niknam V., Ebrahimzadeh H. (2019). Oxidative damage and antioxidative system in algae. Toxicol. Rep. 6, 1309–1313. doi: 10.1016/j.toxrep.2019.10.001
Romano S., Schulz-Vogt H. N., González J. M., Bondarev V. (2015). Phosphate limitation induces drastic physiological changes, virulence-related gene expression, and secondary metabolite production in pseudovibrio sp. Strain FO-BEG1. Appl. Environ. Microbiol. 81, 3518–3528. doi: 10.1128/AEM.04167-14
Ros M., Camp E. F., Hughes D. J., Crosswell J. R., Warner M. E., Leggat W. P., et al. (2020). Unlocking the black-box of inorganic carbon-uptake and utilization strategies among coral endosymbionts (Symbiodiniaceae). Limnol Oceanogr 65, 1747–1763. doi: 10.1002/lno.11416
Rosic N., Ling E. Y. S., Chan C.-K. K., Lee H. C., Kaniewska P., Edwards D., et al. (2015). Unfolding the secrets of coral–algal symbiosis. ISME J. 9, 844–856. doi: 10.1038/ismej.2014.182
Rosset S., Koster G., Brandsma J., Hunt A. N., Postle A. D., D’Angelo C. (2019). Lipidome analysis of Symbiodiniaceae reveals possible mechanisms of heat stress tolerance in reef coral symbionts. Coral Reefs 38, 1241–1253. doi: 10.1007/s00338-019-01865-x
Rosset S., Wiedenmann J., Reed A. J., D’Angelo C. (2017). Phosphate deficiency promotes coral bleaching and is reflected by the ultrastructure of symbiotic dinoflagellates. Mar. pollut. Bull. 118, 180–187. doi: 10.1016/j.marpolbul.2017.02.044
Russnak V., Rodriguez-Lanetty M., Karsten U. (2021). Photophysiological tolerance and thermal plasticity of genetically different symbiodiniaceae endosymbiont species of cnidaria. Front. Mar. Sci. 8. doi: 10.3389/fmars.2021.657348
Sampayo E. M., Ridgway T., Bongaerts P., Hoegh-Guldberg O. (2008). Bleaching susceptibility and mortality of corals are determined by fine-scale differences in symbiont type. Proc. Natl. Acad. Sci. U.S.A. 105, 10444–10449. doi: 10.1073/pnas.0708049105
Santos A. L., Preta G. (2018). Lipids in the cell: organisation regulates function. Cell. Mol. Life Sci. 75, 1909–1927. doi: 10.1007/s00018-018-2765-4
Scharfenstein H. J., Alvarez-Roa C., Peplow L. M., Buerger P., Chan W. Y., Van Oppen M. J. H. (2023). Chemical mutagenesis and thermal selection of coral photosymbionts induce adaptation to heat stress with trait trade-offs. Evolutionary Appl. 16, 1549–1567. doi: 10.1111/eva.13586
Sikorskaya T. V., Efimova K. V., Imbs A. B. (2021). Lipidomes of phylogenetically different symbiotic dinoflagellates of corals. Phytochemistry 181, 112579. doi: 10.1016/j.phytochem.2020.112579
Sikorskaya T. V., Ermolenko E. V., Long P. Q. (2023). Betaine lipids of Symbiodiniaceae hosted by Indo-Pacific corals. Phycological Res. 71, 193–199. doi: 10.1111/pre.12528
Stephens L. R., Irvine R. F. (1990). Stepwise phosphorylation of myo-inositol leading to myo-inositol hexakisphosphate in Dictyostelium. Nature 346, 580–583. doi: 10.1038/346580a0
Su L.-J., Zhang J.-H., Gomez H., Murugan R., Hong X., Xu D., et al. (2019). Reactive oxygen species-induced lipid peroxidation in apoptosis, autophagy, and ferroptosis. Oxid. Med. Cell. Longevity. doi: 10.1155/2019/5080843.
Takenouchi R., Inoue K., Kambe Y., Miyata A. (2012). N-arachidonoyl glycine induces macrophage apoptosis via GPR18. Biochem. Biophys. Res. Commun. 418, 366–371. doi: 10.1016/j.bbrc.2012.01.027
Tchernov D., Gorbunov M. Y., de Vargas C., Narayan Yadav S., Milligan A. J., Häggblom M., et al. (2004). Membrane lipids of symbiotic algae are diagnostic of sensitivity to thermal bleaching in corals. Proc. Natl. Acad. Sci. U.S.A. 101, 13531–13535. doi: 10.1073/pnas.0402907101
Tian J., Tian L., Chen M., Chen Y., Wei A. (2022). Low temperature affects fatty acids profiling and key synthesis genes expression patterns in zanthoxylum bungeanum maxim. IJMS 23, 2319. doi: 10.3390/ijms23042319
Treignier C., Grover R., Ferrier-Pagés C., Tolosa I. (2008). Effect of light and feeding on the fatty acid and sterol composition of zooxanthellae and host tissue isolated from the scleractinian coral Turbinaria reniformis. Limnol. Oceanogr. 53, 2702–2710. doi: 10.4319/lo.2008.53.6.2702
Tsugawa H., Cajka T., Kind T., Ma Y., Higgins B., Ikeda K., et al. (2015). MS-DIAL: data-independent MS/MS deconvolution for comprehensive metabolome analysis. Nat. Methods 12, 523–526. doi: 10.1038/nmeth.3393
van Meer G., Voelker D. R., Feigenson G. W. (2008). Membrane lipids: where they are and how they behave. Nat. Rev. Mol. Cell Biol. 9, 112–124. doi: 10.1038/nrm2330
Van Mooy B. A. S., Fredricks H. F., Pedler B. E., Dyhrman S. T., Karl D. M., Lomas M. W., et al. (2009). Phytoplankton in the ocean use non-phosphorus lipids in response to phosphorus scarcity. Nature 458, 69–72. doi: 10.1038/nature07659
Verma L., Kohli P. S., Maurya K., Thakur J. K., Giri J. (2021). Specific galactolipids species correlate with rice genotypic variability for phosphate utilization efficiency. Plant Physiol. Biochem. 168, 105–115. doi: 10.1016/j.plaphy.2021.10.008
Violi J. P. (2022). Analysis of protein and non-protein amino acids via liquid chromatography-tandem mass spectrometry (Sydney: University of Technology).
Wadhwa M., Srinivasan S., Bachhawat A. K., Venkatesh K. V. (2018). Role of phosphate limitation and pyruvate decarboxylase in rewiring of the metabolic network for increasing flux towards isoprenoid pathway in a TATA binding protein mutant of Saccharomyces cerevisiae. Microb. Cell Fact 17, 152. doi: 10.1186/s12934-018-1000-1
Wong J. C. Y., Enríquez S., Baker D. M. (2021). Towards a trait-based understanding of Symbiodiniaceae nutrient acquisition strategies. Coral Reefs 40, 625–639. doi: 10.1007/s00338-020-02034-1
Xing C., Li J., Yuan H., Yang J. (2022). Physiological and transcription level responses of microalgae Auxenochlorella protothecoides to cold and heat induced oxidative stress. Environ. Res. 211, 113023. doi: 10.1016/j.envres.2022.113023
Yamano H., Sugihara K., Nomura K. (2011). Rapid poleward range expansion of tropical reef corals in response to rising sea surface temperatures: POLEWARD RANGE EXPANSION OF CORALS. Geophys. Res. Lett. 38. doi: 10.1029/2010GL046474
Yang F., Xiang W., Li T., Long L. (2018). Transcriptome analysis for phosphorus starvation-induced lipid accumulation in Scenedesmus sp. Sci. Rep. 8, 16420. doi: 10.1038/s41598-018-34650-x
Zhang Y., Gross C. A. (2021). Cold shock response in bacteria. Annu. Rev. Genet. 55, 377–400. doi: 10.1146/annurev-genet-071819-031654
Keywords: Symbiodiniaceae, lipid remodeling, nutrient limitation, temperature stress, microalgae
Citation: La Motta LM, Padula MP, Sommer B, Camp EF and Matthews JL (2024) Diversity of lipid profiles of Symbiodiniaceae under temperature and nutrient stress. Front. Protistol. 2:1320353. doi: 10.3389/frpro.2024.1320353
Received: 12 October 2023; Accepted: 12 February 2024;
Published: 27 February 2024.
Edited by:
Timothy G. Stephens, Rutgers, The State University of New Jersey, United StatesReviewed by:
Patricia Elena Thome, Institute of Marine Science and Limnology, MexicoXiaoyang Su of senior RE, Rutgers, The State University of New Jersey, United States
Eric N. Chiles of junior RE, Rutgers, The State University of New Jersey, United States, in collaboration with reviewer XS
Copyright © 2024 La Motta, Padula, Sommer, Camp and Matthews. This is an open-access article distributed under the terms of the Creative Commons Attribution License (CC BY). The use, distribution or reproduction in other forums is permitted, provided the original author(s) and the copyright owner(s) are credited and that the original publication in this journal is cited, in accordance with accepted academic practice. No use, distribution or reproduction is permitted which does not comply with these terms.
*Correspondence: Laura M. La Motta, TGF1cmEuTGFtb3R0YUBzdHVkZW50LnV0cy5ldS5hdQ==; Emma F. Camp, RW1tYS5DYW1wQHV0cy5lZHUuYXU=; Jennifer L. Matthews, SmVubmlmZXIuTWF0dGhld3NAdXRzLmVkdS5hdQ==