- 1Departamento de Plancton y Ecología Marina, Centro Interdisciplinario de Ciencias Marinas, Instituto Politécnico Nacional, La Paz, Mexico
- 2Centro de Investigaciones Biológicas del Noroeste (CIBNOR), La Paz, Mexico
- 3Centro Interdisciplinario de Ciencias Marinas, Consejo Nacional de Ciencia, Humanidades y Tecnología (CONAHCyT)-Instituto Politécnico Nacional, La Paz, Mexico
Allelopathy refers to biochemical interactions among competing microalgae, it involves a donor species that produces metabolites which can cause inhibitory effects on susceptible species. This phenomenon can participate in the regulation of harmful algal blooms. The dinoflagellate Gymnodinium catenatum is negatively affected by allelopathic interactions with co-occurring microalgae species, like Chattonella marina var. marina, which has been suggested to produce reactive oxygen species (ROS) and free fatty acids (FFA) as nocive and allelopathic agents. This study explored the effect of hydrogen peroxide (H2O2) and the main fatty acids produced by C. marina. An analysis of fatty acids content of C. marina in exponential phase detected 16:0 (12.5 ± 0.01%), 18:4ω-3 (15.4 ± 0.36%) and 20:5ω-3 (35.4 ± 0.71%) as the most abundant. These fatty acids along with H2O2 were used in dose-response bioassays with cultures of G. catenatum in exponential phase. Results suggest that these substances affect cell morphology, including the loss of motility and signs of chlorosis, as well as the chain forming qualities of G. catenatum. Toxicity among these substances varied, suggesting that the polyunsaturated fatty acid 18:4ω-3 can potentially act as a more effective allelochemical (LD50 = 1.7 ± 0.19 mg L-1 at 24 h), followed by 20:5ω-3 (LD50 = 3.6 ± 0.17 mg L-1 at 24 h) and the saturated fatty acid 16:0 (LD50 = 6.2 ± 1.05 at 48 h). Our results suggest these substances can act, at least partially, as allelochemicals, with PUFA being the most effective metabolites. These results contribute in elucidating the potential role of ROS and FFA in allelopathy in marine phytoplankton communities.
1 Introduction
Harmful algae blooms (HABs) are natural phenomena regulated by diverse factors, including biological interactions, such as predation, competition, and parasitism (Solé et al., 2006; Mazzillo et al., 2011; Gleason et al., 2015). Allelopathy is a biochemical interaction in which metabolites are released to the surrounding environment by donor species, in this context called allelochemicals, that influence the growth and development of target species (Rizvi et al., 1992). This phenomenon has been described as concerning only chemical interactions among competing microalgae species in which one or various allelochemicals can cause inhibitory effects on susceptible species; it has been hypothesized that allelopathy encompasses adaptations that confer competitive advantages to some species over others (Keating, 1977; Legrand et al., 2003). In microalgae, the injurious effects of allelopathy on target species include the promotion of oxidative stress, by raising the endogenous production of reactive oxygen species (ROS) and inducing changes in the activity of antioxidant enzymes (Campos et al., 2013; Lu et al., 2017), photosystem II interference, electron transport chain blockage and subsequent photosynthesis inhibition (Weir et al., 2004; Qian et al., 2009; Zhu et al., 2010), disruption of cell membranes and nucleic acids which lead to high mortality in susceptible cells (Granéli et al., 2008).
The allelopathic potential of marine toxins has been explored. It has been reported that okadaic acid produced by Prorocentrum lima can contribute partially to allelopathy with co-occurring species (Windust et al., 1996; Sugg and VanDolah, 1999). Also, karlotoxins produced by dinoflagellates of the genus Karlodinium have been reported as allelochemicals capable of negatively affecting competitors and prey (Adolf et al., 2006; Wang et al., 2020). Continuous efforts to describe the mechanisms of these interactions has demonstrated that allelopathy is mediated by a great diversity of metabolites (Chaïb et al., 2021). The mechanisms of allelopathy are diverse and remain largely unknown (Śliwińska-Wilczewska et al., 2021). Nonetheless, research efforts have demonstrated that various types of secondary metabolites such as alkaloids like fischerellin (Hagmann and Jüttner, 1996; Gantar et al., 2008) and cyclical peptides like microcystins and portoamide (Kearns and Hunter, 2001; Leão et al., 2010) are associated with allelopathy in microalgae. Particularly, fatty acids in their pure form, also known as free fatty acids (FFA) have been suggested as allelochemicals produced by freshwater phytoplankton species like Botryococcus braunii, Chlorella vulgaris and Peridinium bipes (Uchida et al., 1988; Chiang et al., 2004; Song et al., 2017). In marine microalgae, the potential herbicidal qualities of fatty acids produced by the cyanophyte Lyngbya aestuarii have been explored (Entzeroth et al., 1985). Wang et al. (2023) studied the inhibitory effects of linoleic acid (18:2ω-3, LA) on the marine dinoflagellate Karenia mikimotoi which caused the reduction of photosynthetic pigments and an increase in ROS in the organism.
Laboratory studies have suggested that the chain-forming paralytic shellfish toxin (PST) producing dinoflagellate Gymnodinium catenatum can be negatively affected and dominated by the dinoflagellates Gymnodinium impudicum, Margalefidinium polykrikoides, and the raphidophyte Chattonella marina var. marina (Fernández-Herrera et al., 2016; Band-Schmidt et al., 2020; Fernández-Herrera et al., 2021; Fernández-Herrera et al., 2022). The co-occurrence and the dominance of C. marina over G. catenatum was previously reported in the Gulf of California during a HAB event (López-Cortés et al., 2011). Although the identity of the allelochemicals involved in these interactions remains unknown, C. marina and M. polykrikoides, both competitors of G. catenatum via allelopathy, have been hypothesized to produce compounds, including FFA and ROS that can act as allelochemicals (Marshall et al., 2003; Marshall et al., 2005; Tang and Gobler, 2010). The toxicity of FFA and ROS has been previously explored in microalgae, suggesting that susceptibility can vary among species, type of substance and laboratory conditions (Wu et al., 2006; Vale, 2018).
With the aim to document the effects of FFA and ROS towards G. catenatum, a strain of C. marina from Bahía de La Paz, Gulf of California, was isolated and its fatty acid profile was determined to pinpoint the FFA that can potentially act as allelochemicals towards G. catenatum. The response to the most abundant fatty acids in C. marina in their pure form was evaluated, as well as H2O2 under laboratory conditions. Changes in cell density, chain length and morphology were evaluated.
2 Materials and methods
2.1 Strains and culture conditions
Monoalgal strains of G. catenatum and C. marina isolated from Bahía de La Paz, Mexico, were cultured in modified GSe medium (Bustillos-Guzmán et al., 2015). Strain details are shown in Table 1. Culture conditions remained constant throughout this study at 24 ± 1°C, salinity of 34, 12/12 h light/dark cycle and an irradiance of ~150 µmol photons m−1 s−1.
For fatty acid analysis, strains were grown in triplicate 1 L cultures in 2 L Erlenmeyer flasks. Each strain was harvested by centrifugation (1000 rpm, 5 min, 4°C) during mid exponential growth phase. For the dose-response bioassays, sterile, polystyrene flat bottom six welled (5 mL) culture plates were employed.
2.2 Growth curves
To determine the exponential growth rate, strains were grown in triplicate in batch cultures in 150 mL of modified GSe medium (Bustillos-Guzmán et al., 2015) within 300 mL Erlenmeyer flasks. Samples of each strain were taken every 48 h. Samples of G. catenatum were fixed in Lugol’s iodine solution and samples of C. marina were fixed with hepes buffered paraformaldehyde (Katano et al., 2009). Cell densities were obtained by counting in a 1 mL Sedgewick-Rafter chamber with an optic microscope (Primo Star, Carl Zeiss). Specific growth rates were calculated using the following equation: k = ln (N2/N1)/(t2 - t1); considering the ratio of the cell counts (N2/N1) at times t2 and t1 (Guillard and Ryther, 1962).
For fatty acid analysis, strains were grown in triplicate 1 L cultures in 2 L Erlenmeyer flasks. Each strain was harvested by centrifugation (1000 rpm, 5 min, 4°C) in the mid exponential growth phase. For the dose-response bioassays, sterile, polystyrene flat bottom six welled (5 mL) culture plates were employed.
2.3 PCR, DNA sequencing and phylogenetic analysis
A 2 mL sample of the raphidophyte C. marina was harvested in exponential growth phase. The supernatant was removed by gentle aspiration. DNA extraction was carried out using the Quick-DNA Miniprep Plus Kit (Zymo Research). The genomic DNA samples were amplified by polymerase chain reaction (PCR) using a thermal cycler (MJ Mini, Bio-Rad, Hercules, CA). The reaction was carried out in 50 μL, employing PCR master mix 2X solution (ThermoFisher) and primers 28S F (5′-TATGCTTAAATTCAGCGGGT-3′) and 28S R (5′-GTGAACCTGCAGAAGGATCA-3′) (Hosoi-Tanabe et al., 2006). PCR conditions are the following: denaturation at 94°C for 1 min, 35 cycles at 94°C for 30 s, annealing at 55°C for 2 min, extension at 72°C for 3 min, and final extension at 72°C for 10 min.
The PCR products were purified using the Zymoclean™ Gel DNA Recovery Kit (Thermo Fisher). Sequencing was carried out by MCLAB (San Francisco, CA) using an automated DNA sequencer ABI 3730XL (Thermo Fisher). The sequence was analyzed using the software BioEdit (ver. 7.2.) and the software Molecular Evolutionary Genetics Analysis (MEGA, ver. 10.1.7.). Existing Chattonella sequences (Supplementary Table S1) were obtained using NCBI Blast (https://blast.ncbi.nlm.nih.gov/Blast.cgi). Sequence alignment was performed with the MUSCLE algorithm. To select the best nucleotide substitution model, a comparison between 24 models was carried out using the Bayesian Information Criterion. Consequently, a phylogenetic analysis with Maximum Likelihood Algorithm was carried out. A sequence of Heterosigma akashiwo was used as an outgroup. The sequence of the strain of Chattonella from Bahía de La Paz was submitted to Genbank (Accession number: OR642744).
2.4 Fatty acids extraction and analysis
Lipid extraction and analysis was carried out using ~100 mg of biomass of each strain, samples were placed in thick-walled glass tubes, 6 mL of Folch’s solution (chloroform/methanol, 2:1 v/v), 10 µL of the antioxidant butylated hydroxytoluene (BHT) and 10 µL of the internal standard fatty acid 23:0 were added to each sample. Samples were sonicated during 15 min and kept at -20°C for 24 h.
Samples were dried under nitrogen gas (N2), 1000 µL of Boron trifluoride-methanol (BF3 10%) were added to the samples and were incubated at 85-95°C during 5 min. After cooling for 5 min at room temperature, 1000 µL of hexane was added to each sample to extract fatty acid methyl esters (FAME). Samples were centrifuged at 2000 rpm for 5 min at 5°C. The hexane phase was washed with deionized water.
Sample analysis was performed in a gas chromatograph (6890 N, Agilent Technologies, Santa Clara, CA) employing a DB-23 silica column (30 m × 0.25 mm internal diameter × 0.25 μm film thickness, 50% Cyanopropyl; 50% Methylpolysiloxane, Agilent J&W, USA), using helium as the carrier gas and a temperature ramp from 110 to 220°C. Identification of FAME was carried out by comparing retention times from the samples and the known standards (47885-U, Supelco, Bellefonte, USA). The concentration of each fatty acid was corrected by the response of the corresponding known standard and the internal standard (23:0). Results were presented as the proportion of each fatty acid out of the total fatty acids.
2.5 Dose-response bioassays
The three most abundant fatty acids from C. marina were acquired as pure analytic standards (Sigma Aldrich). Preliminary bioassays were carried out to determine the range of final concentrations where the inhibitory effect could be observed for each fatty acid. A range of final concentrations was prepared for each fatty acid analyzed by diluting with dimethyl sulfoxide (DMSO): from 0 to 32 mg L-1 for palmitic acid (PA), from 0 to 10 mg L-1 for stearidonic acid (SDA), and from 0 to 15 mg L-1 for eicosapentaenoic acid (EPA). For H2O2, a range of concentrations from 0 to 200 µM was prepared by diluting a 30% w/v solution. For bioassays with fatty acids, DMSO was used as carrier solvent (0.4% of the total volume). Treatments were done in triplicate with an initial cell density of 500 cells mL-1 of G. catenatum. Cultures of G. catenatum in GSe medium and of G. catenatum in GSe medium with the addition of DMSO (0.4%) were used as negative controls. In addition, mixed cultures of G. catenatum and C. marina in a 1:2 cell density proportion were used to compare the effects on G. catenatum.
2.6 Statistical analyses
All statistical analyses were carried out in R software (ver. 4.2.1, R Core Team, 2021). Normality and homogeneity of the data was tested by Shapiro-Wilk and Levene tests, respectively. When parametric conditions were met, Welch’s t test was used in the comparison of two treatments and one-way ANOVA was used for comparisons between three or more treatments followed by a post-hoc Tukey’s test. Data on the proportions of chain lengths did not meet the parametric criteria; therefore, non-parametric Kruskal-Wallis tests followed by Conover-Iman tests were performed. For lethal dose estimation, the drm function from the “dose response curve” package (Ritz et al., 2015) was employed to model the effect of the potential allelochemicals on the cell density of G. catenatum. Several fitted models were compared and the most adequate were chosen based on the Akaike’s information criterion.
3 Results
3.1 Phylogenetic analysis of the raphidophyte strain
The amplification of a partial sequence of the 28S region in Chattonella sp. strain resulted in a product of approximately 625 bp. A phylogenetic tree was constructed from sequences of 28S region of rDNA from 11 isolates of the Chattonella genus (Figure 1). Phylogenetic analysis placed Chattonella sp. strain within the C. marina cluster with a bootstrap support of 100%. Sequences of C. subsalsa were placed in a separated clade at bootstrap support of 97%, both clades were separated from the H. akashiwo sequence used as an outgroup.
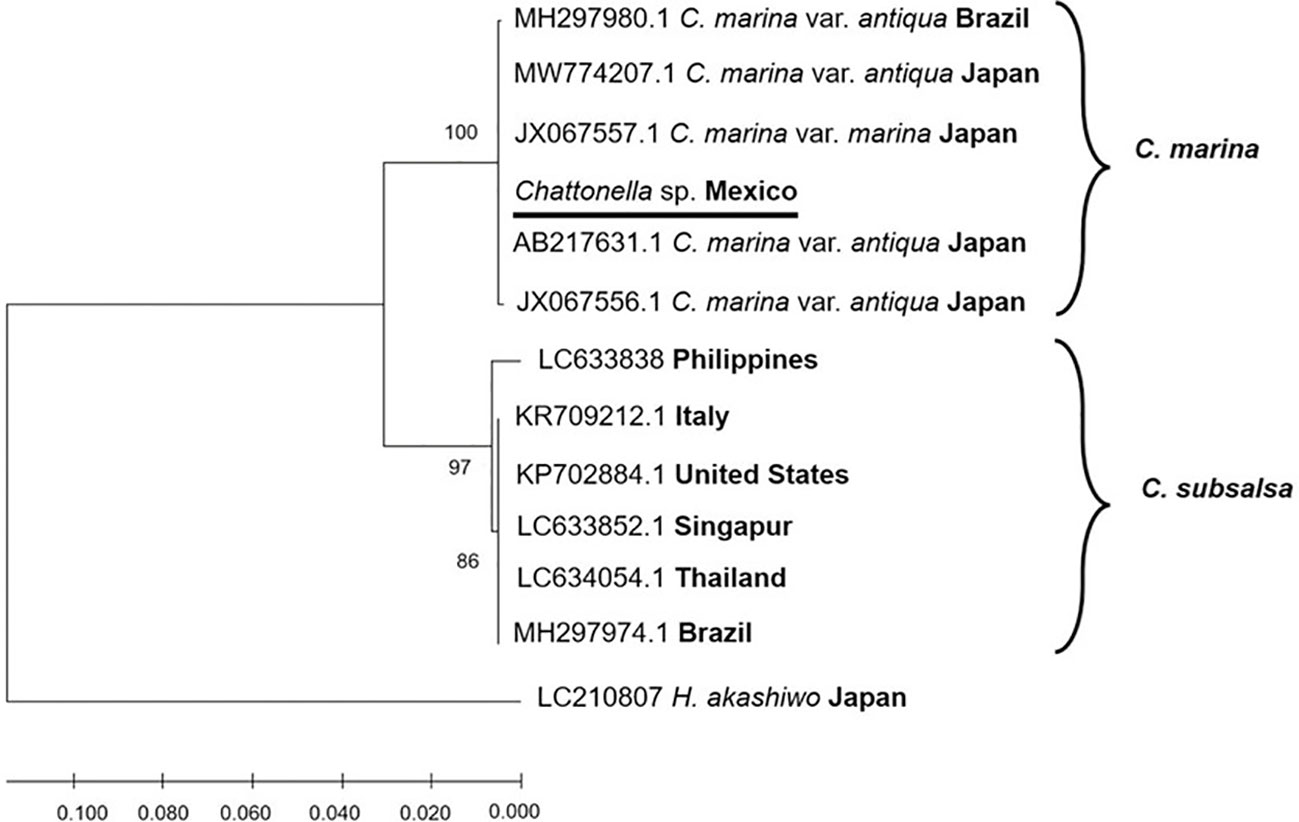
Figure 1 Phylogenetic tree of 28S rDNA sequences of Chattonella strains, using maximum likelihood method with the Kimura two-parameter (K2P) model. The strain used in the present study is underlined.
3.2 Growth
The maximum cell density for G. catenatum in monoalgal cultures was 6,289 ± 375 cells mL-1 which was reached at day 14, while for C. marina 39,209 ± 2,489 cells mL-1 were reached at day 16 (Figure 2). The specific growth rates were 0.26 and 0.15 for G. catenatum and C. marina, respectively; doubling times were 0.21 div day-1 and 0.38 div day-1 for G. catenatum and C. marina, respectively.
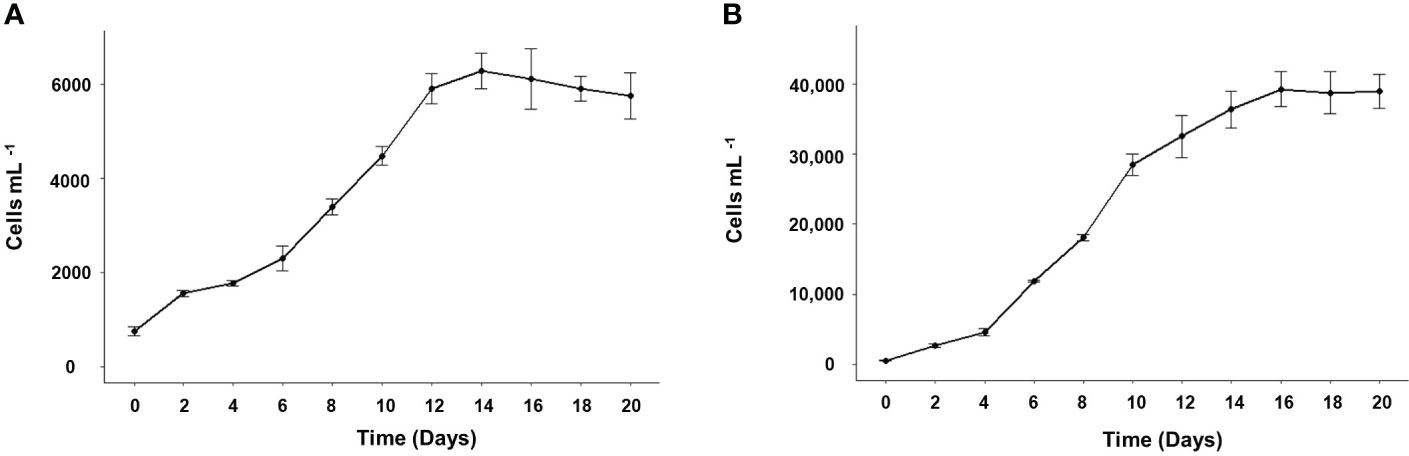
Figure 2 Growth curves of Gymnodinium catenatum (A) and Chattonella marina (B) in modified GSe media at 24°C and salinity of 34. Data are shown as mean ± standard deviation (n = 3).
3.3 Fatty acids
The fatty acid composition obtained for G. catenatum and C. marina is shown in Table 2. The most abundant saturated fatty acid was PA (16:0) in both species; C. marina in a proportion of 12.5 ± 0.01%, and in G. catenatum of 27.4 ± 0.93%. The proportion of total saturated fatty acids was 21.9 ± 1.02% for C. marina and 36.2 ± 2.64% for G. catenatum. The most abundant monounsaturated fatty acids were palmitoleic acid (16:1ω-7, 4.2 ± 0.13) in C. marina and oleic acid (18:1ω-9, 2.3 ± 0.45%) in G. catenatum. Both strains had a low percentage of monounsaturated fatty acids (12.4 ± 1.4% in C. marina and 7.1 ± 0.28% in G. catenatum).
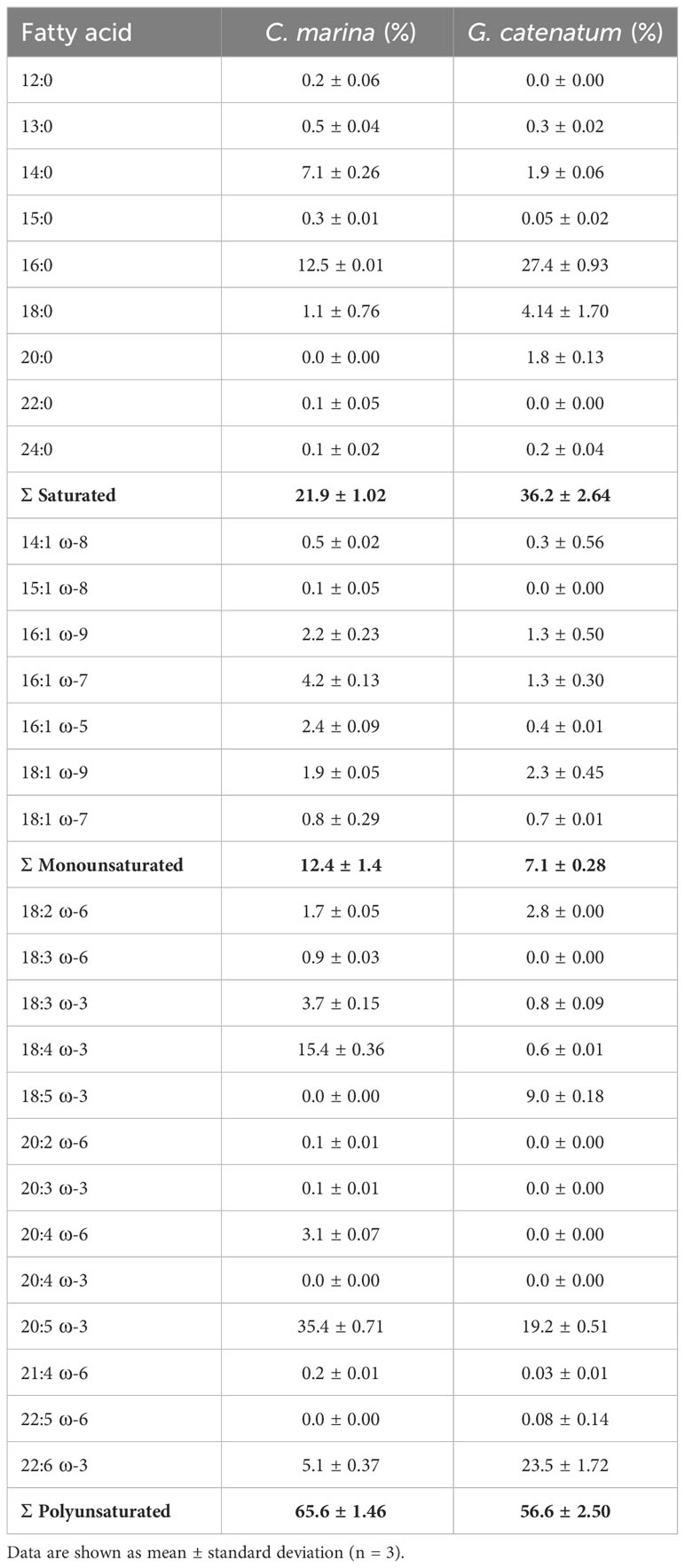
Table 2 Fatty acid composition of Chattonella marina and Gymnodinium catenatum strains from Bahía de La Paz, Gulf of California, Mexico.
The most abundant polyunsaturated fatty acids (PUFA) in C. marina were EPA (20:5ω-3, 35.4 ± 0.71%) and SDA (18:4ω-3, 15.4 ± 0.36%). In contrast, the most abundant PUFA in G. catenatum were docosahexaenoic acid (22:6ω-3, 23.5 ± 1.72%) and EPA (19.22 ± 0.51%). Additionally, octadecapentaenoic acid (18:5ω-3) was detected in G. catenatum as the third most abundant PUFA (9.0 ± 0.18%). The total proportion of PUFA was 65.62 ± 1.46% for C. marina and 56.6 ± 2.50% for G. catenatum.
3.4 Effect of free fatty acids on the cell density and chain length of G. catenatum
Addition of FFA in the culture medium caused mortality in G. catenatum (Figure 3A). A mortality higher than 90% was observed with 32 mg L-1 of PA starting from 48 h and continued increasing at 72 h (> 95%). The lowest PA concentration (1 mg L-1) at 48 h showed a cell density of G. catenatum higher than the mixed culture with C. marina (ANOVA F3,8 = 5.32, p < 0.05). In contrast, there were no significant differences in cell density between the control, the control with DMSO, and the mixed culture with C. marina.
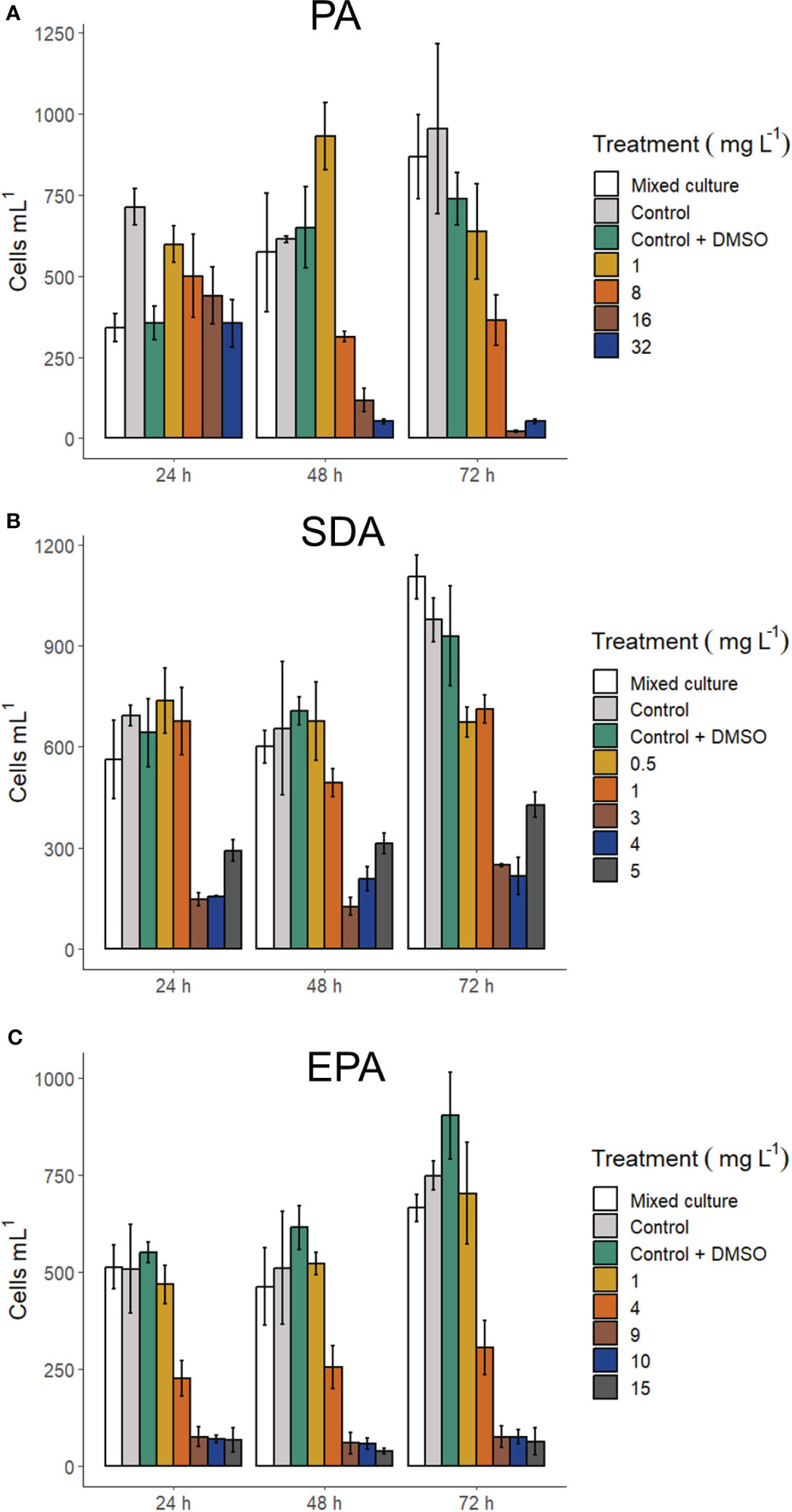
Figure 3 Cell density of Gymnodinium catenatum exposed to fatty acids: Palmitic acid (A), stearidonic acid (B), and eicosapentaenoic acid (C). Mixed culture (G. catenatum and Chattonella marina, 1:2), control (G. catenatum culture in GSe medium), and control with DMSO (0.4%). Data are shown as mean ± standard deviation (n = 3).
Exposure to the free polyunsaturated fatty acids SDA and EPA caused mortalities above 90% at 24 h (Figures 3B, C) with the highest concentrations analyzed (15 mg L-1 and 5 mg L-1, respectively). Exposure to SDA at 24 h caused a decrease in cell density at concentrations above 1 mg L-1; an apparent increase in cell density was observed at concentrations from 3 to 5 mg L-1 SDA at 48 h and 72 h. Mortalities were also higher than 90% at 48 h and 72 h in experiments with EPA with the highest concentrations used (9 to 15 mg L-1).
Changes in chain length of G. catenatum after exposure to the fatty acids is shown in Figure 4. When exposed to the highest concentration of PA (32 mg L-1) at 48 h, the proportion of individual cells (91.9 ± 10.80%) was significantly higher than in the control (56.7 ± 3.16%), the control with DMSO (62.9 ± 1.00%), and the mixed culture with C. marina (58.7 ± 1.58%, Kruskal-Wallis χ2 = 22.54, p < 0.005). The proportion of individual cells of G. catenatum at 72 h was also higher with 32 mg L-1 of PA (91.9 ± 10.80%) than in the control (44.4 ± 6.10%), the control with DMSO (47.1 ± 3.86%), and the mixed culture with C. marina (39.2 ± 3.06%, Kruskal-Wallis χ2 = 22.99, p < 0.005).
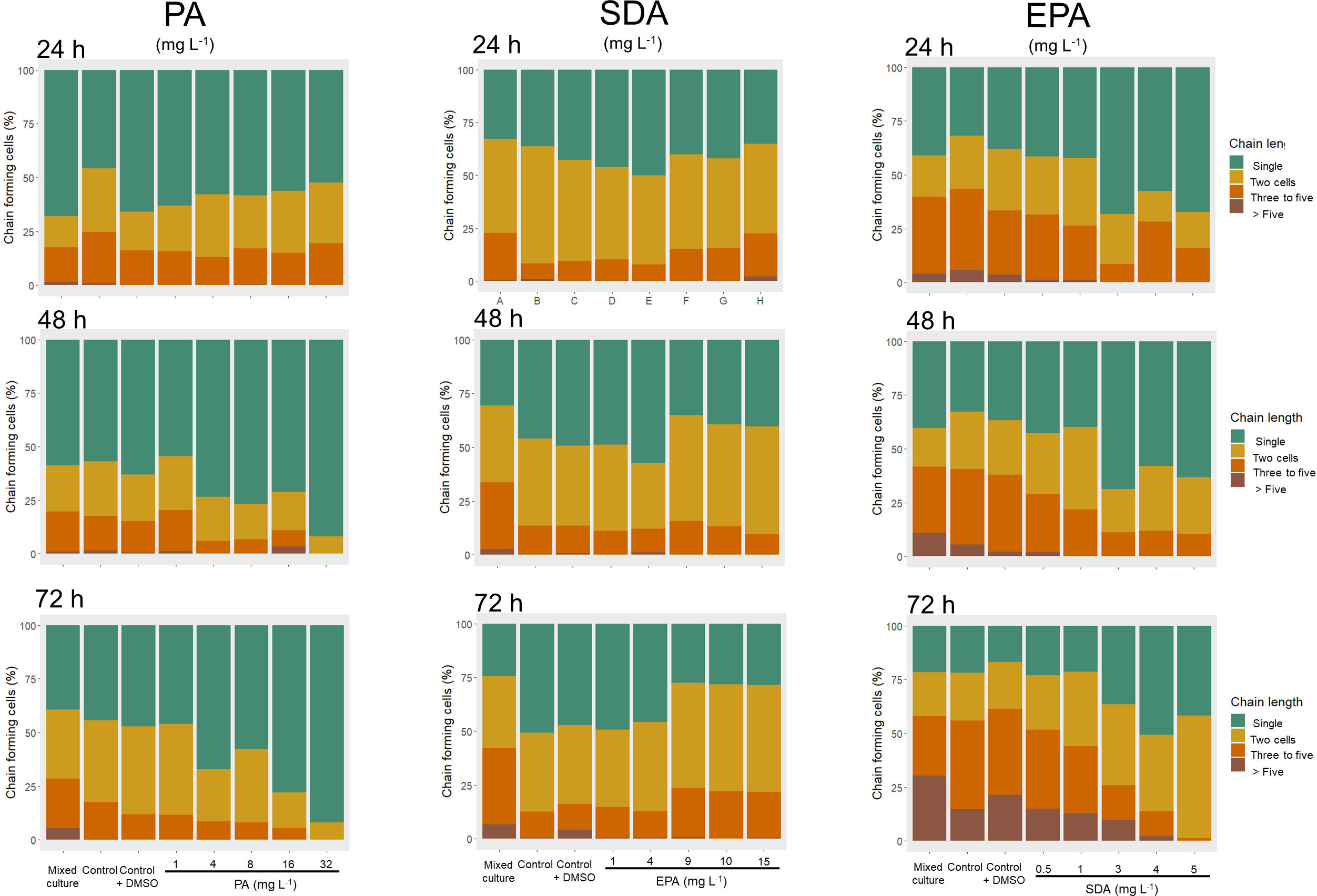
Figure 4 Chain length of Gymnodinium catenatum exposed to free fatty acids: Palmitic acid (16:0, PA), stearidonic acid (18:4ω-3, SDA), and eicosapentaenoic acid (20:5ω-3, EPA). Mixed culture (G. catenatum and Chattonella marina, 1:2), control (G. catenatum culture in GSe medium), and control with DMSO (0.4%). Data are shown as mean ± standard deviation (n = 3).
Exposure to 2 mg L-1 of SDA at 24 h (Figure 4) resulted in a higher proportion of individual cells of G. catenatum (55.7 ± 5.10%) than in the control (36.1 ± 4.82%), the control with DMSO (42.6 ± 3.01%), and the mixed culture with C. marina (32.7 ± 2.78%), as well as with treatments with the highest concentrations of SDA (3 mg L-1, 40.1 ± 2.50%; 4 mg L-1, 41.9 ± 3.04%; 5 mg L-1, 35.2 ± 2.34%; (Kruskal-Wallis χ2 = 22.81, p < 0.005). In contrast, at 72 h after exposure to SDA, individual cells of G. catenatum were higher in the control (50.7 ± 7.87%) than in the treatments with the highest concentrations of SDA (3 mg L-1, 27.4 ± 1.48%; 4 mg L-1, 28.2 ± 1.57%; 5 mg L-1, 28.4 ± 2.74%), (Kruskal-Wallis χ2 = 20.37, p < 0.01). At 48 h of exposure, the proportion of two-cell chains was higher in the treatment with 5 mg L-1 SDA (50.06 ± 0.38%) than in the control with DMSO (37.28 ± 1.53), the mixed culture (35.61 ± 6.45) and the treatment with 1 mg L-1 (30.44 ± 4.5, Kruskal-Wallis χ2 = 19.926, p < 0.05).
When G. catenatum was exposed to the highest concentration of EPA (15 mg L-1) at 24 h the proportion of individual cells (67.2 ± 24.88%) was significantly higher than the control (31.8 ± 2.30%), the control with DMSO (38.0 ± 3.59%) and the mixed culture (41.0 ± 1.27%) with C. marina (Kruskal-Wallis χ2 = 32.87, p < 0.05). Furthermore, single cells of G. catenatum were also higher with 15 mg L-1 EPA at 72 h (41.7 ± 5.01%) than in the control with DMSO (16.8 ± 0.64%, Kruskal-Wallis χ2 = 28.78, p < 0.01). At 72 h the proportion of 2-cell chains was significantly higher in treatments with 9 to 15 mg L-1 (> 30%) than in the control (22.06 ± 2.01%), the control with DMSO (21.80 ± 2.49%) and the mixed culture (20.28 ± 1.36%, Kruskal-Wallis χ2 = 33.662, p < 0.05). chains of 5 or more cells disappeared from the treatment with 15 mg L-1 EPA from 24 to 72 h.
The toxicity of FFA to G. catenatum is shown in Table 3. The toxicity of the three FFA tested varied throughout the sampling times. The LD50 of the free saturated fatty acid PA was calculated after 48 h to be 6.2 ± 1.05 mg L-1. The LD50 of the two free PUFA tested, EPA and SDA, was calculated to be 3.6 ± 0.17 mg L-1 and 1.7 ± 0.19 mg L-1, respectively at 24 h. In addition, the Cedergreen-Ritz-Streibig model (Supplementary Figure S1) suggests a stimulatory response in the surviving cells of G. catenatum exposed to concentrations between 3 to 5 mg L-1 SDA at 48 h (p < 0.05).
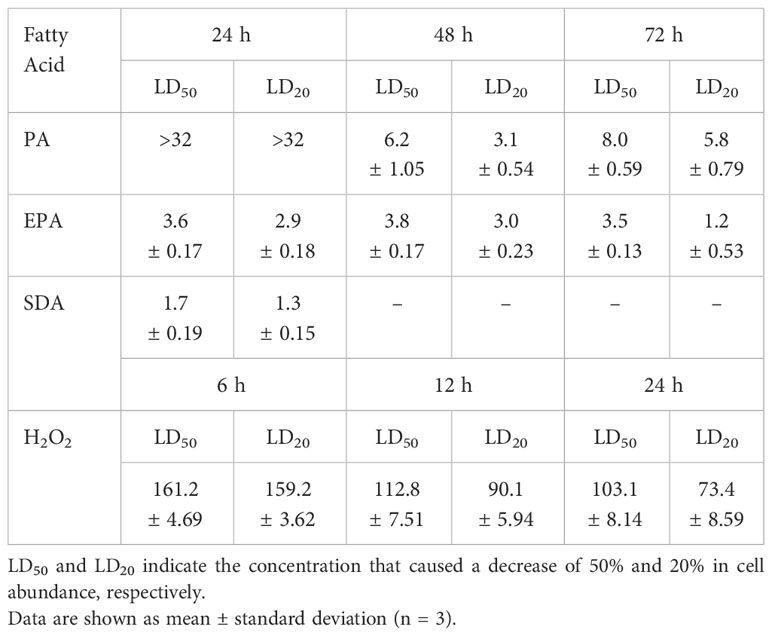
Table 3 Toxicity of fatty acids and hydrogen peroxide towards Gymnodinium catenatum in modified GSe medium.
3.5 Effect of hydrogen peroxide on the cell density and chain length of G. catenatum
Exposure to H2O2 in the culture medium caused mortality in G. catenatum (Figure 5A). A mortality higher than 80% was observed with the highest concentrations of H2O2 used (180 μM and 200 μM) at 12 h of exposure. At 24 h of exposure to 180 μM and 200 μM of H2O2 a mortality higher than 90% was observed. Moreover, at 12 h of exposure, the cell density of G. catenatum was significantly lower in the mixed culture with C. marina than in the control (ANOVA, F (7,16) = 29.95, p < 0.05). However, at 24 h of exposure no differences between cultures were observed. Additionally, a higher cell density of G. catenatum compared to the control was observed at 6 h of exposure to 60 μM H2O2 (t (2.44) = -4.8654, p < 0.05).
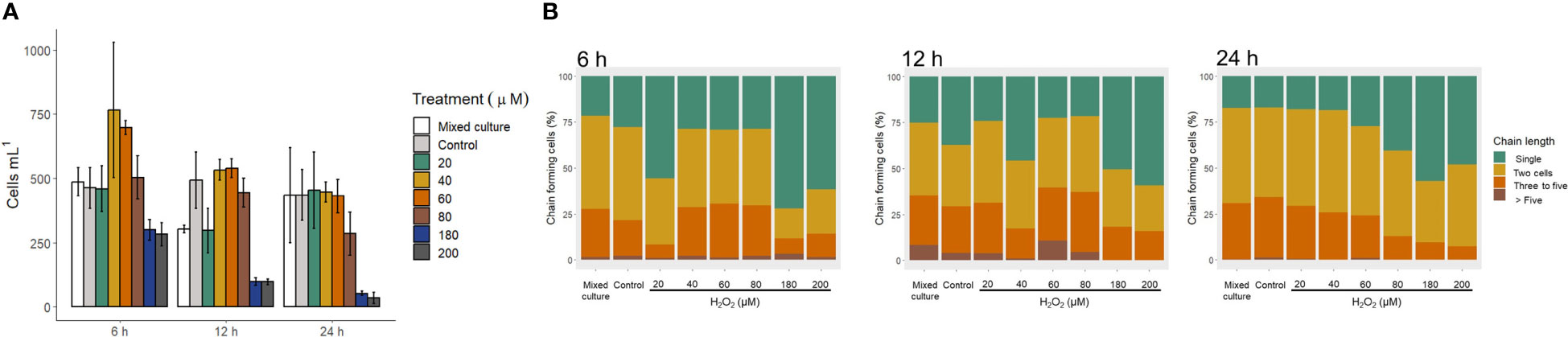
Figure 5 Gymnodinium catenatum exposed to hydrogen peroxide (H2O2). Changes in cell density (A) and chain length (B). Mixed culture (G. catenatum and Chattonella marina, 1:2), and control (G. catenatum culture in modified GSe medium).
Changes in chain length of G. catenatum after exposure to H2O2 is shown in Figure 5B.When G. catenatum was exposed to H2O2 for 6 h, the proportion of individual cells was significantly higher in the treatments with 20 μM (55.6 ± 10.65%), 120 μM (39.4 ± 13.48%), 180 μM (71.9 ± 0.56%), and 200 μM H2O2 (61.6 ± 2.24%) than in the control (27.7 ± 0.91%, Kruskal-Wallis χ2 = 29.565, p < 0.05). After 24 h, the proportion of individual cells was higher in the treatments with 180 μM (57.0 ± 7.43%) and 200 μM H2O2 (48.0 ± 14.72%) than in the treatment with 20 μM H2O2 (17.9 ± 5.06%), the control (17.0 ± 3.17%) and the mixed culture (17.2 ± 6.49%, Kruskal-Wallis χ2 = 26.482, p < 0.05). The toxicity of H2O2 to G. catenatum is shown in Table 3. The LD50 of H2O2 decreased from 161.2 ± 4.69 μM at 6 h to 103.1 ± 8.14 μM at 24 h.
3.6 Changes in the morphology of G. catenatum after exposure to FFA and H2O2
Morphological changes were observed in G. catenatum after exposure to the FFA and H2O2 (Figures 6–9). Evident changes were observed at 48 and 72 h exposure at concentrations above 4 mg L-1 PA, which included swelling, loss of motility and flagella, detachment of the external membrane, lysis, and apparent chlorosis at the highest concentrations (32 mg L-1 PA, Figure 6).
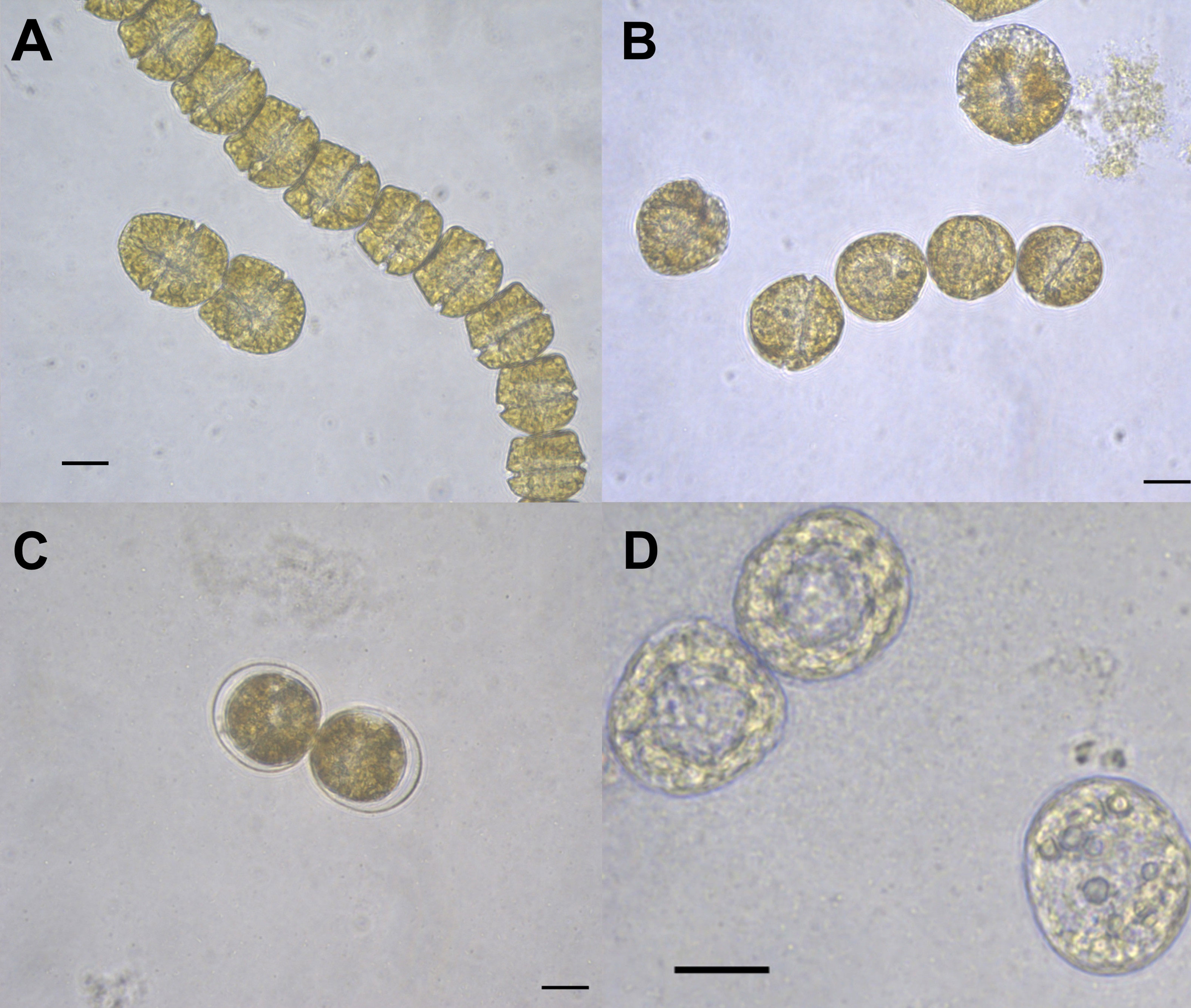
Figure 6 Gymnodinium catenatum cells exposed to palmitic acid (PA) at 72 h. Cell swelling (A, B), cells surrounded by detached external membrane (C), dead cells (D). Scale bar = 20 μm.
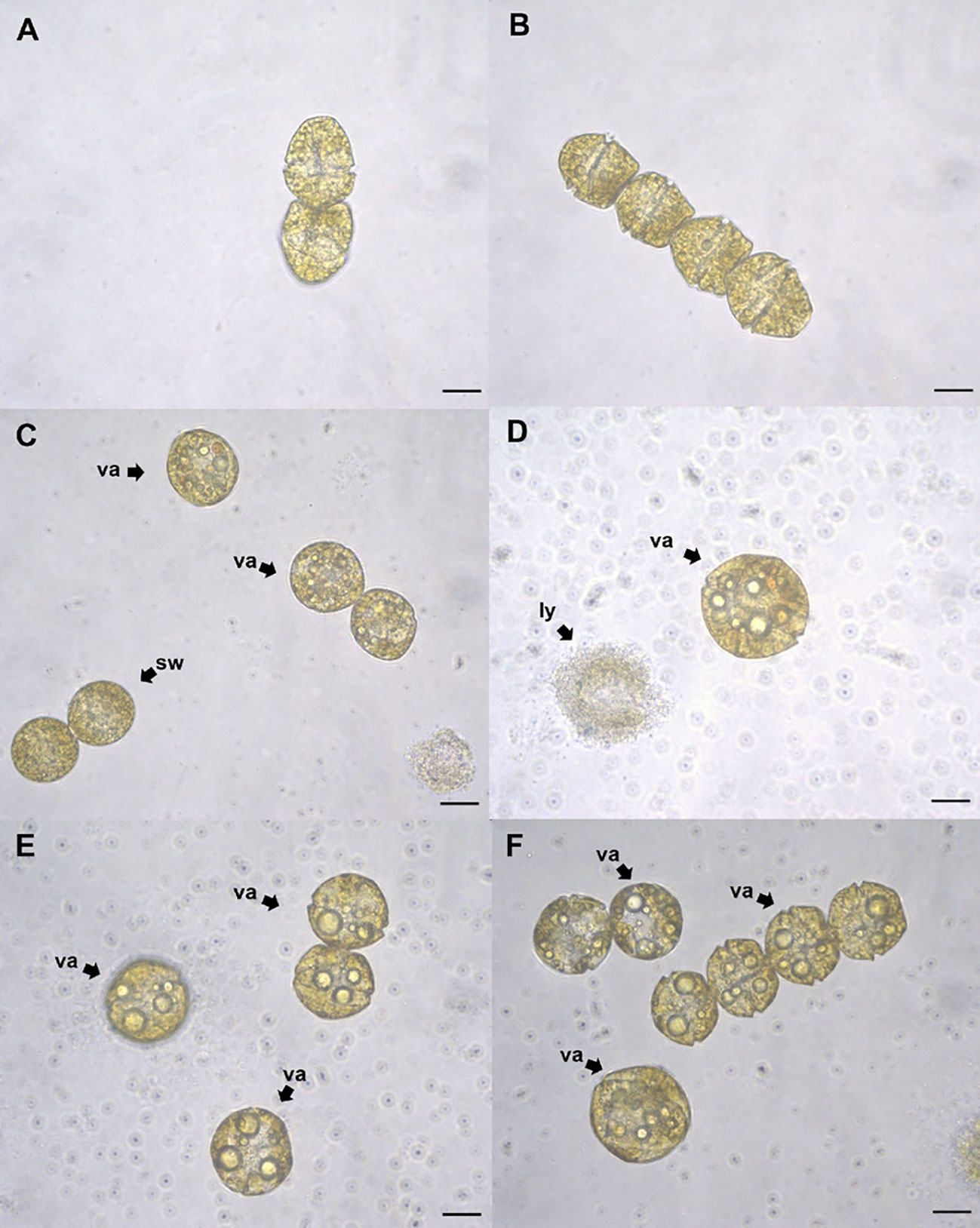
Figure 7 Gymnodinium catenatum cells exposed to eicosapentaenoic acid (EPA) at 24 (h) Control with dimethyl sulfoxide (DMSO) (A), cells exposed to 1 mg L-1 (B), 3 mg L-1 (C), 6 mg L-1 (D), 8 mg L-1 (E), and 10 mg L-1 (F). Swollen cells (sw), vacuolization (va), lysis (ly). Scale bar = 20 μm.
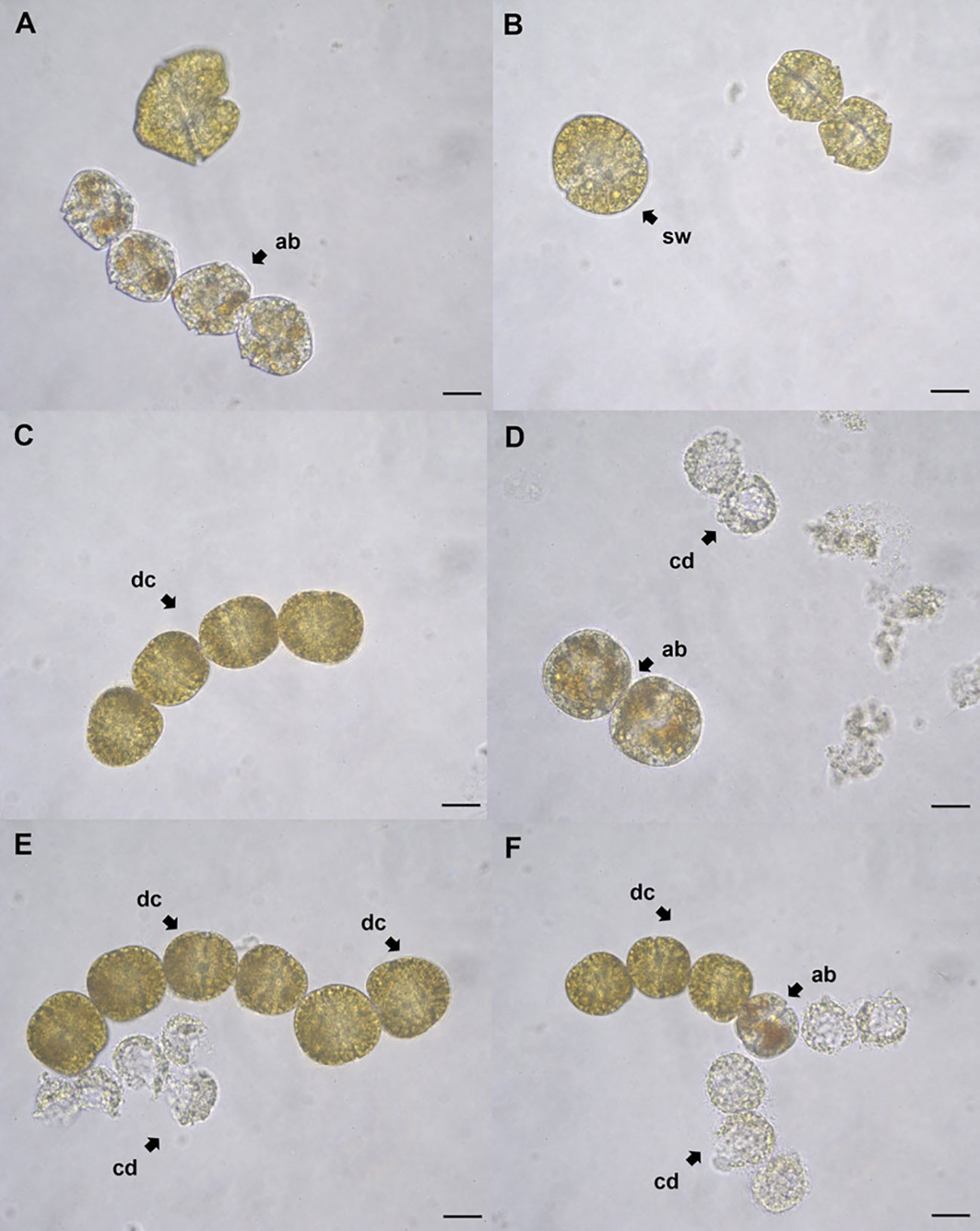
Figure 8 Gymnodinium catenatum cells exposed to stearidonic acid (SDA) at 48 h. Affected cells exposed to 0.5 mg L-1 (A), 1 mg L-1 (B), 2 mg L-1 (C) 3 mg L-1 (D), 4 mg L-1 (E), and 5 mg L-1 (F). Cell with orange-brown accumulation bodies (ab); cell swelling (sw); deformed chain-forming cells (dc); dead cells (cd). Scale bar = 20 μm.
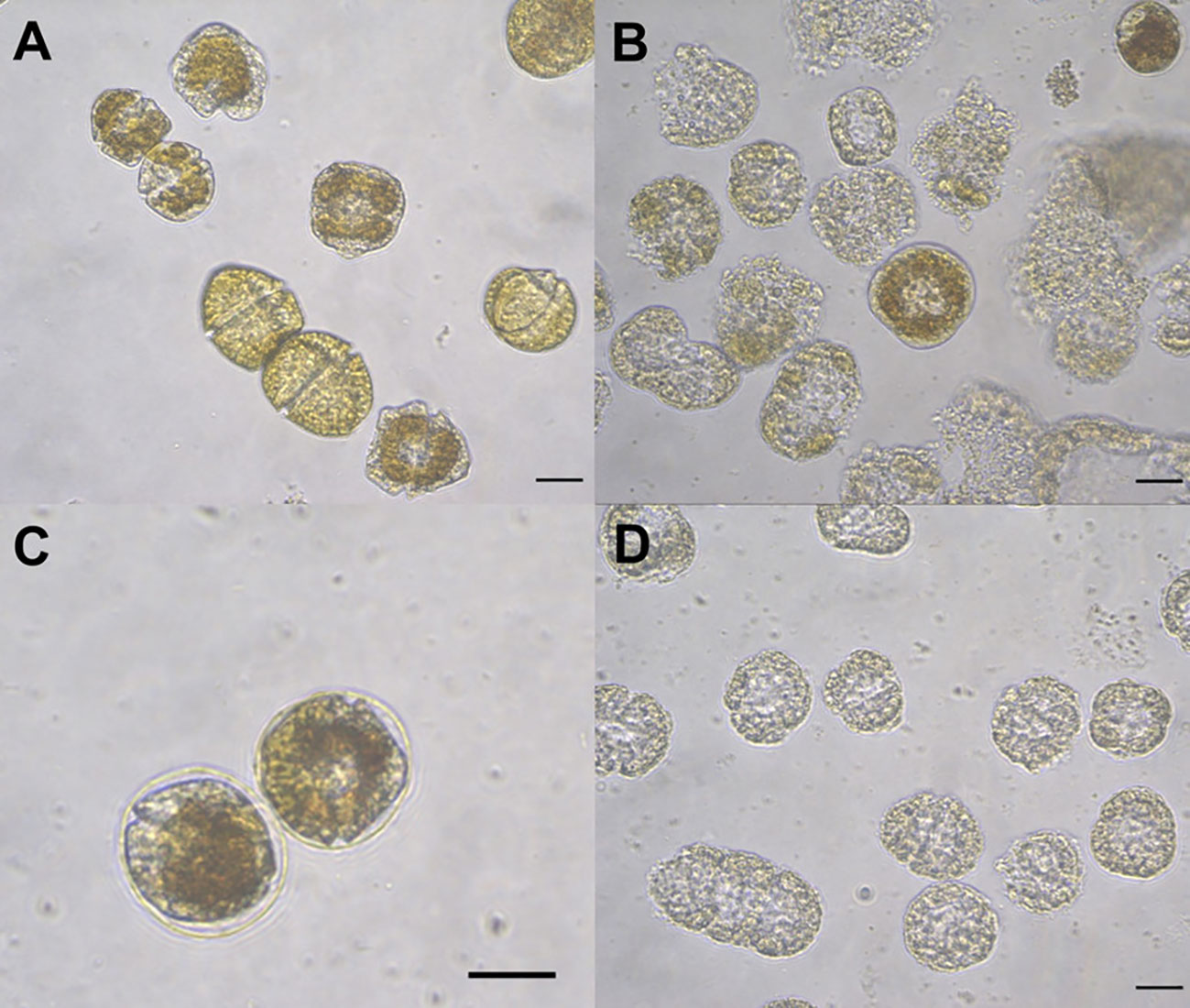
Figure 9 Gymnodinium catenatum cells exposed to hydrogen peroxide H2O2 at 24 h. Affected cells exposed to 100 μM showing deformation and membrane detachment (A), affected cells exposed to 200 μM showing deformation, chlorosis and cell death (B–D). Scale bar = 20 μm.
Exposure to concentrations above 2 mg L-1 EPA also caused evident changes in cell morphology at 24 h including swelling and vacuolization, the loss of motility and increased signs of cell lysis (Figure 7). Similar changes were observed in G. catenatum after exposure to SDA at 24 h, where the general loss of motility, the appearance of orange-brown accumulation bodies and swelling were also observed. Signs of lysis were more apparent at concentrations above 2 mg L-1 SDA; the presence of cells forming chains was also observed (Figure 8).
Cells of G. catenatum exposed to H2O2 showed similar effects on cell morphology as FFA, including the loss of motility, swelling, membrane detachment, lysis and chlorosis when exposed to the highest concentrations (180 – 200 μM H2O2, Figure 9). Few G. catenatum cells showing a similar deformation pattern were also found in mixed cultures with C. marina. These changes in cell morphology included swelling and vacuolization, loss of motility, presence of internal accumulation bodies and detachment of the external membrane.
4 Discussion
The results of this study show that PA, EPA, and SDA were the most abundant fatty acids in the strain of C. marina in exponential growth phase. Previous studies suggested these fatty acids are among the most abundant in raphidopyte strains of the species H. akashiwo, Fibrocapsa japonica, and C. marina from different geographic regions (Nichols et al., 1987; Marshall et al., 2002; Giner et al., 2008; Band-Schmidt et al., 2012; Dorantes-Aranda et al., 2013). The C. marina strain employed in the present study showed EPA as the most abundant fatty acid. This is in agreement with Band-Schmidt et al. (2012) who also suggested that EPA was the most abundant fatty acid (19.8 – 34.9%) in four strains of Chattonella and F. japonica from the Gulf of California, Mexico in exponential growth phase. Furthermore, Dorantes-Aranda et al. (2013) suggested the relative abundance of FFA in C. marina can vary; while some strains showed a higher abundance of FFA in the stationary phase, the strain CMCV-1 of C. marina from Bahía de La Paz showed a higher abundance in the exponential growth phase. The dinoflagellate G. catenatum contained a high proportion of PA (27.4 ± 0.93%), EPA (19.2 ± 0.51%), 22:6 ω-3 (DHA, 23.5 ± 1.72%) and 18:5 ω-3(9.0 ± 0.18%). A similar profile was reported by Hallegraeff et al. (1991) for laboratory cultures of G. catenatum and field samples, although the fatty acid 18:5 ω-3 was reported in low proportions (from 1.2 to 2.3%). This fatty acid has been reported to be quite variable among dinoflagellates (Mansour et al., 1999).
Negative effects of FFA and H2O2 on G. catenatum were observed in this study. Changes in cell density and signs of lysis in the experiments suggest the toxicity of these substances varies with the concentration and exposure time. Also, exposure to the highest concentrations of PA and H2O2 caused an apparent chlorosis in G. catenatum cells. It has been suggested that FFA are capable of interfering with the electron transport chain in chloroplasts (Venediktov and Krivoshejeva, 1983). It has also been suggested that FFA can cause membrane disruption and the detachment of pigments in freshwater microalgae (Wu et al., 1998; Wu et al., 2006). However, further studies are needed to elucidate the mechanisms involved.
Chattonella marina and M. polykrikoides have been suggested to produce ROS and FFA as metabolites involved in their ichthyotoxicity (Marshall et al., 2003; Dorantes-Aranda et al., 2009; Dorantes-Aranda et al., 2013). The role of ROS and FFA as potential allelochemicals produced by raphidophytes has been previously suggested by Marshall et al. (2003). Their results showed that ROS production is density dependent in raphidophytes. Additionally, the behavior of different strains of raphidophytes towards ROS production varied, including observations of autolysis in a strain of C. marina due to its ROS production, while other strains were resistant to higher concentrations of ROS. Their results also showed that EPA in its free form can be an allelochemical towards bacteria, an effect that is enhanced by the presence of superoxide. It has been reported that H2O2 production in C. marina takes place in the intracellular medium, while the production of O2•− takes place at the cell surface (Kim et al., 2007). The intracellular accumulation of H2O2 in raphidophyte cells has been suggested, as the measured H2O2 is higher in ruptured C. marina cell suspensions compared to intact cell suspensions (Oda et al., 1994; Oda et al., 1995). A mechanism for the exogenous release of FFA to the marine environment has not been suggested for C. marina. Cell rupture has been identified as a key factor in C. marina ichthyotoxicity, and an increase in FFA proportion has been observed in the profile of ruptured cells, suggesting that dying cells are more toxic due to the increased release of FFA and ROS (Dorantes-Aranda et al., 2013; Dorantes-Aranda et al., 2015). It is, therefore, possible that C. marina populations can benefit from their dying conspecifics upon releasing putative allelochemicals from ruptured cells. This hypothesis can be explored in future studies.
Supplementary Table S2 shows differences in measurements of H2O2 in Chattonella reported in the literature. Chattonella marina strains show substantial variability in H2O2 production. Laboratory culture conditions are suggested to play a significant role in ROS production in raphidophytes; particularly, different light intensities have been reported to cause differences in H2O2 production among C. marina strains (Dorantes-Aranda et al., 2013; Li et al., 2015). According to these measurements, an exceedingly high cell density of C. marina cells would be needed to reach the LD50 H2O2 concentrations estimated for G. catenatum in this study. Indicating that the release of H2O2 alone cannot be responsible for the allelopathic effects towards G. catenatum. Previous studies have suggested that the role of ROS on the ichthyotoxicity of harmful algae is not a direct one, and experiments that involve the chemical addition of ROS substances do not mimic the toxicity of live isolates (Marshall et al., 2003; Tang et al., 2005).
The effects of the FFA on G. catenatum in this study, suggest that the PUFA, EPA and SDA cause mortality at lower concentrations and in a shorter time than the saturated fatty acid PA. Particularly, SDA showed the most effective inhibitory capacity at 24 h of exposure. It has been previously suggested that fatty acid carbon chain length is related to the algicidal potential of these substances (Zhu et al., 2021). These observations are also in accordance with previous studies on the effect of FFA on freshwater microalgae. Chiang et al. (2004) reported that B. braunii can produce and release a mixture of FFA as allelochemicals, including PA which showed lower toxicity than the PUFA 18:3ω-3, 18:2ω-7 and 18:1ω-9. Moreover, Wu et al. (2006) reported that the toxicity of PA and EPA can vary among different species of microalgae, suggesting cells that lack an external covering structure beyond a thin mucilage layer are more susceptible to the effects of FFA. While it is suggested that G. catenatum has amphiesmal vesicles, it is generally recognized as a naked planktonic dinoflagellate that lacks cellulose plates (Morey-Gaines, 1982). This characteristic could explain its susceptibility to the effects of FFA.
The observed toxicity of FFA could suggest a relevant participation of the oxidized products from these substances (Aliotta et al., 1990). Previous experiments on the ichthyotoxicity of C. marina and other microalgae reported that the negative effects of fatty acids, particularly PUFA, can be enhanced by the presence of ROS (Marshall et al., 2003; Marshall et al., 2005; Mardones et al., 2015). Also, it has been suggested that the ichthyotoxicity among strains of C. marina is influenced mainly by differences in ROS production rather than by differences in fatty acid composition (Shikata et al., 2021). A synergistic role of different allelochemicals has also been reported in experiments with Cochlodinium geminatum (Wu et al., 2021). In this study, the strain of C. marina (CMBAPAZ-2) employed in mixed cultures with G. catenatum did not cause a significant decrease in cell density at the end of the experiments, as opposed to the C. marina var. marina strain previously reported to induce high mortalities of G. catenatum (Fernández-Herrera et al., 2016; Fernández-Herrera et al., 2021; Fernández-Herrera et al., 2022) which suggests that the allelopathic capabilities of C. marina can vary between strains or taxonomical varieties.
Our results show that low concentrations of PA (1 mg L-1) and H2O2 (60 μM) caused an increase in cell density of G. catenatum at punctual sampling times (48 h for PA; 6 h for H2O2). The presence of an initial stimulating phase has been reported in experiments with chlorellin exposure on freshwater species (DellaGreca et al., 2010). Vale (2018) also reported the presence of an initial stimulating phase in the cell density of G. catenatum exposed to low concentrations of H2O2. It has been suggested that ROS can have cell signaling capabilities related to growth processes at low concentrations (Holmström and Finkel, 2014). Additionally, Wang et al. (2023) reported that at low concentrations of the PUFA linoleic acid (0.1 mg L-1) promotes the growth of K. mikimotoi while higher concentrations (0.5 mg L-1) cause inhibitory effects on growth, carotenoid and polysaccharide biosynthesis, porphyrin, and sphingolipid metabolism, as well as an increase in ROS production.
Exposure to FFA and H2O2 in our experiments also produced changes in chain formation of G. catenatum, in which the decrease in cell density often coincided with an increase in single cell proportions. A similar response was also reported in the experiments by Vale (2018), in which concentrations above 245 μM of H2O2 under halogen light caused single cells to dominate in cultures of G. catenatum. It has been suggested that high irradiance conditions can enhance the toxicity of H2O2 in consequence to an increase in its transformation into hydroxyl radical (OH) (Drábková et al., 2007).
It has been reported that a low number of G. catenatum cells can survive the allelopathic effect of C. marina, among these, chain forming cells show a higher growth rate, suggesting the survival strategy of G. catenatum involves chain formation (Fernández-Herrera et al., 2022). Our results also show that a low number of G. catenatum cells can survive exposure to the highest concentrations used of fatty acids and H2O2. In addition, treatments with the highest concentrations of SDA in our experiments showed an apparent growth stimulation at 48 h and 72 h. This was observed after a significant decrease in cell density at 24 h. Additionally, a decrease in single cells concomitant with an increase in chain formation was observed in treatments with concentrations of SDA above 2 mg L-1 at 48 h and 72 h, which suggests growing cells favor chain formation. An apparent stimulatory phase in a dose response curve may suggest that the surviving cells, after exposure to toxic agents, increase their growth rate in response to the inhibitory potential of the substances (Calabrese and Baldwin, 2001). The surviving cells exposed to the highest concentrations of SDA may be showing a compensatory response related to growth and chain formation which is observable at 48 h and 72 h after exposure.
The allelopathic effect of C. marina var. marina towards G. catenatum was reported by Fernández-Herrera et al. (2016). Their results showed that inhibition of G. catenatum was higher in mixed co-cultures of both species than in co-cultures with no direct contact and exposure to cell filtrates of C. marina. This suggested that C. marina can inhibit the growth of G. catenatum through the release of allelochemicals, although cell contact or proximity between the cells of both species can cause a higher inhibition towards G. catenatum. The changes observed in G. catenatum cells after exposure to FFA and H2O2 are similar, coinciding mainly in the loss of motility, swelling, detachment of the external membrane, and alterations in the length of chain formation. These effects are in accordance with the previous allelopathy experiments with C. marina var. marina, and the dinoflagellates M. polykrikoides and G. impudicum (Fernández-Herrera et al., 2016; Band-Schmidt et al., 2020; Fernández-Herrera et al., 2021; Fernández-Herrera et al., 2022).
Previous studies suggested that Chattonella marina var. antiqua can affect Akashiwo sanguinea via allelopathy (Qiu et al., 2011). Similar negative effects of allelopathy have been reported between other species; cells of Heterocapsa triquetra and Scrippsiella trochoidea exposed to cell-free filtrates of Alexandrium ostenfeldii, showed loss of motility (Tillmann et al., 2007; Hakanen et al., 2014). Studies in different species exposed to allelochemicals from Alexandrium leei reported that M. polykrikoides can be negatively affected showing alterations in chain formation, cell deformations and lysis, while the naked dinoflagellates G. catenatum, Karlodinium veneficum along with the haptophyte Isochrysis galbana were not dominated by A. leii (Shang et al., 2021). The precise identity of the allelochemicals produced by Alexandrium species remains unknown. However, Alexandrium tamarense has been suggested to produce lytic compounds targeting membrane related sterols (Ma et al., 2011).
Chain formation in dinoflagellates such as G. catenatum may constitute a survival strategy by enhancing motility and allowing displacement of cells (Doblin et al., 2006) out of the reach of allelochemicals in the marine environment; however, this strategy may be limited by the spatial restrictions of laboratory cultures. While being stressed, naked dinoflagellates could employ alternative defense mechanisms facing allelopathy, including chain formation or separation (Vale, 2018; Shang et al., 2021; Fernández-Herrera et al., 2022). Due to the spatial constraints of the experimental setup, the strategy of chain cell formation of G. catenatum as a means of escape to allelochemicals was not clearly observed in the presence of FFA and H2O2. Therefore, the effects on chain cell formation were not conclusive.
In conclusion, results from this study suggest that the fatty acids PA, EPA, and SDA along with H2O2 can individually cause deleterious effects on G. catenatum. Alterations in chain formation and cell morphology are similar to the expected effects of allelopathy towards G. catenatum by competing species, and suggest that these substances can be, at least partially, involved with allelopathic interactions in marine environments. Toxicity among the fatty acids varies, suggesting that PUFA with shorter carbon chains can potentially act as more effective allelochemical substances, causing a decrease in cell density at lower concentrations and within a shorter time than saturated fatty acids. However, the overall allelopathic effect of C. marina towards G. catenatum may be caused by a mixture of various compounds including ROS and FFA. It remains to be seen if other uncharacterized metabolites participate in this effect.
Data availability statement
The original contributions presented in the study are publicly available. This data can be found here: https://www.ncbi.nlm.nih.gov/; OR642744.
Author contributions
ME-Y: Conceptualization, Data curation, Formal analysis, Investigation, Methodology, Visualization, Writing – original draft. CB-S: Conceptualization, Funding acquisition, Investigation, Methodology, Project administration, Resources, Supervision, Validation, Visualization, Writing – review & editing. TZ-S: Conceptualization, Data curation, Methodology, Resources, Supervision, Validation, Writing – review & editing. IL-V: Data curation, Formal analysis, Investigation, Methodology, Supervision, Validation, Writing – review & editing. LFH: Methodology, Visualization, Writing – review & editing. EP-M: Data curation, Methodology, Resources, Validation, Writing – review & editing.
Funding
The author(s) declare financial support was received for the research, authorship, and/or publication of this article. ME-Y was a recipient of a student fellowship CONACyT-1106788 and BEIFI. CB-S is a COFFA-IPN and EDI-IPN fellow. This project was funded by institutional projects (SIP 20231019) and by the Consejo Nacional de Humanidades, Ciencias y Tecnologías (CONAHCyT, Ciencia básica A1-S-14968).
Acknowledgments
We are grateful for the technical support of O. Arjona-López for the analysis of fatty acids, O. Lugo-Lugo for assistance in analyses for oxidative stress indicators, and D.I. Rojas-Posadas for the molecular taxonomy methods.
Conflict of interest
The authors declare that the research was conducted in the absence of any commercial or financial relationships that could be construed as a potential conflict of interest.
The author(s) declared that they were an editorial board member of Frontiers, at the time of submission. This had no impact on the peer review process and the final decision.
Publisher’s note
All claims expressed in this article are solely those of the authors and do not necessarily represent those of their affiliated organizations, or those of the publisher, the editors and the reviewers. Any product that may be evaluated in this article, or claim that may be made by its manufacturer, is not guaranteed or endorsed by the publisher.
Supplementary material
The Supplementary Material for this article can be found online at: https://www.frontiersin.org/articles/10.3389/frpro.2024.1302560/full#supplementary-material
References
Adolf J. E., Bachvaroff T. R., Krupatkina D. N., Nonogaki H., Brown P. J. P., Lewitus A. J., et al. (2006). Species specificity and potential roles of Karlodinium micrum toxin. Afr. J. Mar. Sci. 28, 415–419. doi: 10.2989/18142320609504189
Aliotta G., Greca M. D., Monaco P., Pinto G., Pollio A., Previtera L. (1990). In vitro algal growth inhibition by phytotoxins of Typha latifolia L. J. Chem. Ecol. 16, 2637–2646. doi: 10.1007/BF00988075
Band-Schmidt C. J., Martínez-López A., Bustillos-Guzmán J. J., Carreón-Palau L., Morquecho L., Olguín-Monroy N. O., et al. (2012). Morphology, biochemistry, and growth of raphidophyte strains from the Gulf of California. Hydrobiologia 693, 81–97. doi: 10.1007/s10750-012-1088-y
Band-Schmidt C. J., Zumaya-Higuera M. G., López-Cortés D. J., Leyva-Valencia I., Quijano-Scheggia S. I., Hernández-Guerrero C. J. (2020). Allelopathic effects of Margalefidinium polykrikoides and Gymnodinium impudicum in the growth of Gymnodinium catenatum. Harmful Algae 96, 101846. doi: 10.1016/j.hal.2020.101846
Bustillos-Guzmán J. J., Band-Schmidt C. J., Durán-Riveroll L. M., Hernández-Sandoval F. E., López-Cortés D. J., Núñez-Vázquez E. J., et al. (2015). Paralytic toxin profile of the marine dinoflagellate Gymnodinium catenatum Graham from the Mexican Pacific as revealed by LC-MS/MS. Food Addit. Contam. Part. A 32, 381–394. doi: 10.1080/19440049.2014.1000978
Calabrese E. J., Baldwin L. A. (2001). U-shaped dose-responses in biology, toxicology, and public health. Annu. Rev. Public Health 22, 15–33. doi: 10.1146/annurev.publhealth.22.1.15
Campos A., Araújo P., Pinheiro C., Azevedo J., Osório H., Vasconcelos V. (2013). Effects on growth, antioxidant enzyme activity and levels of extracellular proteins in the green alga Chlorella vulgaris exposed to crude cyanobacterial extracts and pure microcystin and cylindrospermopsin. Ecotoxicol. Environ. Saf. 94, 45–53. doi: 10.1016/j.ecoenv.2013.04.019
Chaïb S., Pistevos J. C. A., Bertrand C., Bonnard I. (2021). Allelopathy and allelochemicals from microalgae: An innovative source for bio-herbicidal compounds and biocontrol research. Algal Res. 54, 102213. doi: 10.1016/j.algal.2021.102213
Chiang I. Z., Huang W. Y., Wu J. T. (2004). Allelochemicals of Botryococcus braunii (Chlorophyceae). J. Phycol. 40, 474–480. doi: 10.1111/j.1529-8817.2004.03096.x
DellaGreca M., Zarrelli A., Fergola P., Cerasuolo M., Pollio A., Pinto G. (2010). Fatty acids released by Chlorella vulgaris and their role in interference with Pseudokirchneriella subcapitata: Experiments and Modelling. J. Chem. Ecol. 36, 339–349. doi: 10.1007/s10886-010-9753-y
Doblin M. A., Thompson P. A., Revill A. T., Butler E. C. V., Blackburn S. I., Hallegraeff G. M. (2006). Vertical migration of the toxic dinoflagellate Gymnodinium catenatum under different concentrations of nutrients and humic substances in culture. Harmful Algae 5, 665–677. doi: 10.1016/j.hal.2006.02.002
Dorantes-Aranda J. J., García-de la Parra L. M. G., Alonso-Rodríguez R., Morquecho L. (2009). Hemolytic activity and fatty acids composition in the ichthyotoxic dinoflagellate Cochlodinium polykrikoides isolated from Bahía de la Paz, Gulf of California. Mar. pollut. Bull. 58, 1401–1405. doi: 10.1016/j.marpolbul.2009.06.007
Dorantes-Aranda J. J., Nichols P. D., David Waite T., Hallegraeff G. M. (2013). Strain variability in fatty acid composition of Chattonella marina (Raphidophyceae) and its relation to differing ichthyotoxicity toward rainbow trout gill cells. J. Phycol. 49, 427–438. doi: 10.1111/jpy.12053
Dorantes-Aranda J. J., Seger A., Mardones J. I., Nichols P. D., Hallegraeff G. M. (2015). Progress in understanding algal bloom-mediated fish kills: The role of superoxide radicals, phycotoxins and fatty acids. PloS One 10, e0133549. doi: 10.1371/journal.pone.0133549
Drábková M., Admiraal W., Maršálek B. (2007). Combined exposure to hydrogen peroxide and light-selective effects on cyanobacteria, green algae, and diatoms. Environ. Sci. Technol. 41, 309–314. doi: 10.1021/es060746i
Entzeroth M., Mead D. J., Patterson G. M. L., Moore R. E. (1985). A herbicidal fatty acid produced by Lyngbya aestuarii. Phytochemistry 24, 2875–2876. doi: 10.1016/0031-9422(85)80017-0
Fernández-Herrera L. J., Band-Schmidt C. J., López-Cortés D. J., Hernández-Guerrero C. J., Bustillos-Guzmán J. J., Núñez-Vázquez E. (2016). Allelopathic effect of Chattonella marina var. marina (Raphidophyceae) on Gymnodinium catenatum (Dinophycea). Harmful Algae 51, 1–9. doi: 10.1016/j.hal.2015.10.009
Fernández-Herrera L. J., Band-Schmidt C. J., Zenteno-Savín T., Leyva-Valencia I., Hernández-Guerrero C. J., Hernández-Sandoval F. E., et al. (2022). Changes in Toxin Production, Morphology and Viability of Gymnodinium catenatum Associated with Allelopathy of Chattonella marina var. marina and Gymnodinium impudicum. Toxins (Basel) 14, 616. doi: 10.3390/toxins14090616
Fernández-Herrera L. J., Band-Schmidt C. J., Zenteno-Savín T., Leyva-Valencia I., Hernández-Guerrero C. J., Muñoz-Ochoa M. (2021). Cell death and metabolic stress in Gymnodinium catenatum induced by allelopathy. Toxins (Basel) 13, 506. doi: 10.3390/toxins13070506
Gantar M., Berry J. P., Thomas S., Wang M., Perez R., Rein K. S. (2008). Allelopathic activity among Cyanobacteria and microalgae isolated from Florida freshwater habitats. FEMS Microbiol. Ecol. 64, 55–64. doi: 10.1111/j.1574-6941.2008.00439.x
Giner J. L., Zhao H., Tomas C. (2008). Sterols and fatty acids of three harmful algae previously assigned as Chattonella. Phytochemistry 69, 2167–2171. doi: 10.1016/j.phytochem.2008.05.013
Gleason F. H., Jephcott T. G., Küpper F. C., Gerphagnon M., Sime-Ngando T., Karpov S. A., et al. (2015). Potential roles for recently discovered chytrid parasites in the dynamics of harmful algal blooms. Fungal. Biol. Rev. 29, 20–33. doi: 10.1016/j.fbr.2015.03.002
Granéli E., Weberg M., Salomon P. S. (2008). Harmful algal blooms of allelopathic microalgal species: The role of eutrophication. Harmful Algae 8, 94–102. doi: 10.1016/j.hal.2008.08.011
Guillard R. R. L., Ryther J. H. (1962). Studies of marine planktonic diatoms. I. Cyclotella nana Hustedt, and Detonula confervacea (Cleve) Gran. Can. J. Microbiol. 1962 (8), 229–239. doi: 10.1139/m62-029
Hagmann L., Jüttner F. (1996). Fischerellin A, a novel photosystem-II-inhibiting allelochemical of the cyanobacterium Fischerella muscicola with antifungal and herbicidal activity. Tetrahedron Lett. 37, 6539–6542. doi: 10.1016/0040-4039(96)01445-1
Hakanen P., Suikkanen S., Kremp A. (2014). Allelopathic activity of the toxic dinoflagellate Alexandrium ostenfeldii: Intra-population variability and response of co-occurring dinoflagellates. Harmful Algae 39, 287–294. doi: 10.1016/j.hal.2014.08.005
Hallegraeff G. M., Nichols P. D., Volkman J. K., Blackburn S. I., Everitt D. A. (1991). Pigments, fatty acids, and sterols of the toxic dinoflagellate Gymnodinium catenatum. J. Phycol. 27 (5), 591–599. doi: 10.1111/j.0022-3646.1991.00591.x
Holmström K. M., Finkel T. (2014). Cellular mechanisms and physiological consequences of redox-dependent signalling. Nat. Rev. Mol. Cell. Biol. 15, 411–421. doi: 10.1038/nrm3801
Hosoi-Tanabe S., Otake I., Sako Y. (2006). Phylogenetic analysis of noxious red tide flagellates Chattonella antiqua, C. marina, C. ovata, and C. verruculosa (Raphidophyceae) based on the rRNA gene family. Fish. Sci. 72, 1200–1208. doi: 10.1111/j.1444-2906.2006.01277.x
Katano T., Yoshida M., Lee J., Han M. S., Hayami Y. (2009). Fixation of Chattonella antiqua and C. marina (Raphidophyceae) using Hepes-buffered paraformaldehyde and glutaraldehyde for flow cytometry and light microscopy. Phycologia 48, 473–479. doi: 10.2216/08-102.1
Kearns K. D., Hunter M. D. (2001). Toxin-producing Anabaena flos-aquae induces settling of Chlamydomonas reinhardtii, a competing motile alga. Microb. Ecol. 42, 80–86. doi: 10.1007/s002480000086
Keating K. I. (1977). Allelopathic influence on blue-green bloom sequence in a eutrophic lake. Science 196 (4292), 885–887. doi: 10.1126/science.196.4292.885
Kim D., Nakashima T., Matsuyama Y., Niwano Y., Yamaguchi K., Oda T. (2007). Presence of the distinct systems responsible for superoxide anion and hydrogen peroxide generation in red tide phytoplankton Chattonella marina and Chattonella ovata. J. Plankton Res. 29, 241–247. doi: 10.1093/plankt/fbm011
Leão P. N., Pereira A. R., Liu W. T., Ng J., Pevzner P. A., Dorrestein P. C., et al. (2010). Synergistic allelochemicals from a freshwater cyanobacterium. Proc. Natl. Acad. Sci. U.S.A. 107, 11183–11188. doi: 10.1073/pnas.0914343107
Legrand C., Rengefors K., Fistarol G. O., Granéli E. (2003). Allelopathy in phytoplankton - Biochemical, ecological and evolutionary aspects. Phycologia 42, 406–419. doi: 10.2216/i0031-8884-42-4-406.1
Li X., Liu T., Wang K., Waite T. D. (2015). Light-induced extracellular electron transport by the marine raphidophyte Chattonella marina. Environ. Sci. Technol. 49, 1392–1399. doi: 10.1021/es503511m
López-Cortés D. J., Band-Schmidt C. J., Gárate-Lizárraga I., Bustillos-Guzmán J. J., Hernández-Sandoval F. E., Núñez-Vázquez E. J. (2011). Co-ocurrencia de Chattonella marina y Gymnodinium catenatum en la Bahía de la Paz, Golfo de California (primavera 2009). Hidrobiologica 21, 185–196.
Lu Z., Sha J., Tian Y., Zhang X., Liu B., Wu Z. (2017). Polyphenolic allelochemical pyrogallic acid induces caspase-3(like)-dependent programmed cell death in the cyanobacterium Microcystis aeruginosa. Algal. Res. 21, 148–155. doi: 10.1016/j.algal.2016.11.007
Ma H., Krock B., Tillmann U., Bickmeyer U., Graeve M., Cembella A. (2011). Mode of action of membrane-disruptive lytic compounds from the marine dinoflagellate Alexandrium tamarense. Toxicon 58, 247–258. doi: 10.1016/j.toxicon.2011.06.004
Mansour M. P., Volkman J. K., Jackson A. E., Blackburn S. I. (1999). The fatty acid and sterol composition of five marine dinoflagellates. J. Phycol. 35, 710–720. doi: 10.1046/j.1529-8817.1999.3540710.x
Mardones J. I., Dorantes-Aranda J. J., Nichols P. D., Hallegraeff G. M. (2015). Fish gill damage by the dinoflagellate Alexandrium catenella from Chilean fjords: Synergistic action of ROS and PUFA. Harmful Algae 49, 40–49. doi: 10.1016/j.hal.2015.09.001
Marshall J. A., Nichols P. D., Hallegraeff G. M. (2002). Chemotaxonomic survey of sterols and fatty acids in six marine raphidophyte algae. J. Appl. Phycol. 14, 255–265. doi: 10.1023/A:1021101203543
Marshall J. A., Nichols P. D., Hamilton B., Lewis R. J., Hallegraeff G. M. (2003). Ichthyotoxicity of Chattonella marina (Raphidophyceae) to damselfish (Acanthochromis polycanthus): The synergistic role of reactive oxygen species and free fatty acids. Harmful Algae 2, 273–281. doi: 10.1016/S1568-9883(03)00046-5
Marshall J. A., Ross T., Pyecroft S., Hallegraeff G. (2005). Superoxide production by marine microalgae: II. Towards understanding ecological consequences and possible functions. Mar. Biol. 147, 541–549. doi: 10.1007/s00227-005-1597-6
Mazzillo F. F. M., Ryan J. P., Silver M. W. (2011). Parasitism as a biological control agent of dinoflagellate blooms in the California Current System. Harmful Algae 10, 763–773. doi: 10.1016/j.hal.2011.06.009
Morey-Gaines G. (1982). Gymnodinium catenatum Graham (Dinophyceae): morphology and affinities with armoured forms. Phycologia 21, 154–163. doi: 10.2216/i0031-8884-21-2-154.1
Nichols P. D., Volkman J. K., Hallegraeff G. M., Blackburn S. I. (1987). Sterols and fatty acids of the red tide flagellates Heterosigma akashiwo and Chattonella antiqua (Raphidophyceae). Phytochemistry 26, 2537–2541. doi: 10.1016/S0031-9422(00)83871-6
Oda T., Moritomi J., Kawano I., Hamaguchi S., Muramatsu T., Ishimatsu A. (1995). Catalase- and superoxide dismutase-induced morphological changes and growth inhibition in the red tide phytoplankton Chattonella marina. Biosci. Biotechnol. Biochem. 59, 2044–2048. doi: 10.1271/bbb.59.2044
Oda T., Takeshita S., Muramatsu T., Ishimatsu A. (1994). Hydrogen peroxide production by the red-tide flagellate Chattonella marina. Biosci. Biotechnol. Biochem. 58, 957–958. doi: 10.1271/bbb.58.957
Qian H., Xu X., Chen W., Jiang H., Jin Y., Liu W., et al. (2009). Allelochemical stress causes oxidative damage and inhibition of photosynthesis in Chlorella vulgaris. Chemosphere 75, 368–375. doi: 10.1016/j.chemosphere.2008.12.040
Qiu X., Yamasaki Y., Shimasaki Y., Gunjikake H., Matsubara T., Nagasoe S., et al. (2011). Growth interactions between the raphidophyte Chattonella antiqua and the dinoflagellate Akashiwo sanguinea. Harmful Algae 11, 81–87. doi: 10.1016/j.hal.2011.08.001
R. Core Team (2021). R: A Language and Environment for Statistical Computing. R Foundation for Statistical Computing. (Vienna, Austria) v4.2.1. https://www.r-project.org/.
Ritz C., Baty F., Streibig J. C., Gerhard D. (2015). Dose-response analysis using R. PloS One 10, e0146021. doi: 10.1371/journal.pone.0146021
Rizvi S. J. H., Haque H., Singh V. K., Rizvi V. (1992). “A discipline called allelopathy,” in Allelopathy. Eds. Rizvi S. J. H., Rizvi V. (Dordrecht: Springer), 1–10.
Shang L., Xu Y., Leaw C. P., Lim P. T., Wang J., Chen J., et al. (2021). Potent allelopathy and non-PSTs, non-spirolides toxicity of the dinoflagellate Alexandrium leei to phytoplankton, finfish and zooplankton observed from laboratory bioassays. Sci. Total Environ. 780, 146484. doi: 10.1016/j.scitotenv.2021.146484
Shikata T., Yuasa K., Kitatsuji S., Sakamoto S., Akita K., Fujinami Y., et al. (2021). Superoxide production by the red tide-producing Chattonella marina complex (Raphidophyceae) correlates with toxicity to aquacultured fishes. Antioxidants 10, 1635. doi: 10.3390/antiox10101635
Śliwińska-Wilczewska S., Wiśniewska K., Konarzewska Z., Cieszyńska A., Barreiro Felpeto A., Lewandowska A. U., et al. (2021). The current state of knowledge on taxonomy, modulating factors, ecological roles, and mode of action of phytoplankton allelochemicals. Sci. Total Environ. 773, 145681. doi: 10.1016/j.scitotenv.2021.145681
Solé J., Garcia-Ladona E., Estrada M. (2006). The role of selective predation in harmful algal blooms. J. Mar. Syst. 62, 46–54. doi: 10.1016/j.jmarsys.2006.04.002
Song H., Lavoie M., Fan X., Tan H., Liu G., Xu P., et al. (2017). Allelopathic interactions of linoleic acid and nitric oxide increase the competitive ability of Microcystis aeruginosa. ISME J. 11, 1865–1876. doi: 10.1038/ismej.2017.45
Sugg L. M., VanDolah F. M. (1999). No evidence for an allelopathic role of okadaic acid among ciguatera-associated dinoflagellates. J. Phycol. 35, 93–103. doi: 10.1046/j.1529-8817.1999.3510093.x
Tang J. Y., Anderson D. M., Au D. W. (2005). Hydrogen peroxide is not the cause of fish kills associated with Chattonella marina: cytological and physiological evidence. Aquat Toxicol. 72, 351–360. doi: 10.1016/j.aquatox.2005.01.007
Tang Y. Z., Gobler C. J. (2010). Allelopathic effects of Cochlodinium polykrikoides isolates and blooms from the estuaries of Long Island, New York, on co-occurring phytoplankton. Mar. Ecol. Prog. Ser. 406, 19–31. doi: 10.3354/meps08537
Tillmann U., John U., Cembella A. (2007). On the allelochemical potency of the marine dinoflagellate Alexandrium ostenfeldii against heterotrophic and autotrophic protists. J. Plankton. Res. 29, 527–543. doi: 10.1093/plankt/fbm034
Uchida A., Shimada A., Ishida Y. (1988). Antibacterial and antialgal substances produced by the dinoflagellate Peridinium bipes. Nippon Suisan Gakkaishi (Japanese Edition) 54, 1941–1945. doi: 10.2331/suisan.54.1941
Vale P. (2018). Resistance to hydrogen peroxide highlights Gymnodinium catenatum (Dinophyceae) sensitivity to geomagnetic activity. Photochem. Photobiol. 94, 95–104. doi: 10.1111/php.12815
Venediktov P. S., Krivoshejeva A. A. (1983). The mechanisms of fatty-acid inhibition of electron transport in chloroplasts. Planta 159, 411–414. doi: 10.1007/BF00392076
Wang C., Wang R., Hu L., Xi M., Wang M., Ma Y., et al. (2023). Metabolites and metabolic pathways associated with allelochemical effects of linoleic acid on Karenia mikimotoi. J. Hazard. Mater. 447, 130815. doi: 10.1016/j.jhazmat.2023.130815
Wang R., Wu J., Zhou S., Cao R., Chan L. L. (2020). A preliminary study on the allelopathy and toxicity of the dinoflagellate Karlodinium veneficum. Mar. pollut. Bull. 158, 111400. doi: 10.1016/j.marpolbul.2020.111400
Weir T. L., Park S. W., Vivanco J. M. (2004). Biochemical and physiological mechanisms mediated by allelochemicals. Curr. Opin. Plant Biol. 7, 472–479. doi: 10.1016/j.pbi.2004.05.007
Windust A. J., Wright J. L. C., McLachlan J. L. (1996). The effects of the diarrhetic shellfish poisoning toxins, okadaic acid and dinophysistoxin-1, on the growth of microalgae. Mar. Biol. 126, 19–25. doi: 10.1007/BF00571373
Wu X., Cai Y., Shi F., Duan L., Zhang Q., Xu N. (2021). Characterization of allelopathic compounds from the harmful dinoflagellate, Cochlodinium geminatum. Harmful Algae 107, 102069. doi: 10.1016/j.hal.2021.102069
Wu J. T., Chiang Y. R., Huang W. Y., Jane W. N. (2006). Cytotoxic effects of free fatty acids on phytoplankton algae and cyanobacteria. Aquat. Toxicol. 80, 338–345. doi: 10.1016/j.aquatox.2006.09.011
Wu J. T., Kuo-Huang L. L., Lee J. (1998). Algicidal effect of Peridinium bipes on Microcystis aeruginosa. Curr. Microbiol. 37, 257–261. doi: 10.1007/s002849900375
Zhu X., Dao G., Tao Y., Zhan X., Hu H. (2021). A review on control of harmful algal blooms by plant-derived allelochemicals. J. Hazard Mater. 401, 123403. doi: 10.1016/j.jhazmat.2020.123403
Keywords: allelopathy, dinoflagellate, phytoplankton, reactive oxygen species, fatty acids
Citation: Encinas-Yánez MF, Band-Schmidt CJ, Zenteno-Savín T, Leyva-Valencia I, Fernández Herrera LJ and Palacios-Mechetnov E (2024) Deleterious effects of free fatty acids and hydrogen peroxide towards the dinoflagellate Gymnodinium catenatum. Front. Protistol. 2:1302560. doi: 10.3389/frpro.2024.1302560
Received: 26 September 2023; Accepted: 15 January 2024;
Published: 07 February 2024.
Edited by:
Shauna Ann Murray, University of Technology Sydney, AustraliaReviewed by:
Gustaaf Marinus Hallegraeff, University of Tasmania, AustraliaMarilou P. Sison-Mangus, University of California, United States
Copyright © 2024 Encinas-Yánez, Band-Schmidt, Zenteno-Savín, Leyva-Valencia, Fernández Herrera and Palacios-Mechetnov. This is an open-access article distributed under the terms of the Creative Commons Attribution License (CC BY). The use, distribution or reproduction in other forums is permitted, provided the original author(s) and the copyright owner(s) are credited and that the original publication in this journal is cited, in accordance with accepted academic practice. No use, distribution or reproduction is permitted which does not comply with these terms.
*Correspondence: Martín Fernando Encinas-Yánez, bWZlbmNpbmFzeUBnbWFpbC5jb20=; Christine Johanna Band-Schmidt, Y2JhbmRzQGlwbi5teA==