- 1Centro Nacional Instituto Español de Oceanografía (IEO-CSIC), Centro Oceanográfico de Vigo, Vigo, Spain
- 2Centro i~mar & CeBiB, Universidad de Los Lagos, Puerto Montt, Chile
Several Dinophysis species produce lipophilic toxins (diarrhetic shellfish poisoning, DSP and pectenotoxins PTX) which are transferred through the food web. Even at low cell densities (< 103 cell L-1), they can cause human illness and shellfish harvesting bans; toxins released into the water may kill early life stages of marine organisms. Dinophysis species are mixotrophs: they combine phototrophy (by means of kleptoplastids stolen from their prey) with highly selective phagotrophy on the ciliate Mesodinium, also a mixotroph which requires cryptophyte prey of the Teleaulax/Geminigera clade. Life cycle strategies, biological interactions and plastid acquisition and functioning in Dinophysis species make them exemplars of resilient holoplanktonic mixoplankters and of ongoing speciation and plastidial evolution. Nevertheless, 17 years after the first successful culture was established, the difficulties in isolating and establishing cultures with local ciliate prey, the lack of robust molecular markers for species discrimination, and the patchy distribution of low-density populations in thin layers, hinder physiological experiments to obtain biological measurements of their populations and slow down potential advances with next-generation technologies. The Omic’s age in Dinophysis research has only just started, but increased efforts need to be invested in systematic studies of plastidic diversity and culture establishment of ciliate and cryptophyte co-occurring with Dinophysis in the same planktonic assemblages.
1 Introduction
Dinoflagellate species of Dinophysis Ehrenberg are widely distributed in tropical, temperate and boreal waters, and in coastal, neritic and oceanic environments (Steidinger and Tangen, 1996). Until the late 1970s, interest in these species was focused on their taxonomy and their marked morphological variability (Jorgensen, 1923; Abé, 1967). Most taxonomists merged Dinophysis and Phalacroma based on plate tabulation criteria (Hallegraeff and Lucas, 1988) until genetic studies by Jensen and Daugbjerg (2009) supported the recognition of two separate genera. Dinophysis attracted special attention in the late 1970’s when severe gastroenteritis outbreaks in Tohoku, Japan, unrelated to bacteria, led to the description of a new seafood-borne syndrome, Diarrhetic Shellfish Poisoning (DSP), related to phytoplankton. Dinophysis fortii was identified as the source organism of the human illness after consumption of shellfish contaminated with its toxins (Yasumoto et al., 1978; Yasumoto et al., 1980). Ever since, Dinophysis species (main cause of shellfish harvesting closures in Europe) have been a target in monitoring programs aimed to safeguard public health and shellfish exploitations (Reguera et al., 2014). Dinophysis attracted further scrutiny when Schnepf and Elbrächter (1988) pointed to the orange autofluorescence and ultrastructure of its cryptophyte-like plastids. By the 1990s it was widely documented that initiation of Dinophysis blooms was closely related to the onset of thermohaline stratification (Maestrini, 1998) and that observations of ciliate remains in Dinophysis digestive vacuoles indicated their mixotrophic nature (Jacobson and Andersen, 1994).
Progress in understanding the dynamics of subsurface populations of holoplanktonic dinoflagellates (Dinophysis, Karenia) in the early 2000’s was achieved along with increased abilities to observe distributions and processes at different scales (Gentien et al., 2005; Berdalet et al., 2017). The first successful culture of Dinophysis (Park et al., 2006) opened possibilities for physiological studies, but maintaining the Dinophysis-Mesodinium-Teleaulax food chain in the laboratory has proven to be a cumbersome task undertaken by only a few groups. Measurements required to develop mechanistic models coupled to operational oceanography forecasts are far from being achieved. Targeted sampling of low biomass harmful algal blooms (HABs) of Dinophysis and laboratory experiments have revealed species-specific responses to fine scale differences in water column structure and resources (Figure 1J) (Díaz et al., 2013; Díaz et al., 2016; Baldrich et al., 2021; Baldich et al., 2023) (Table 1). But we have barely glimpsed ephemeral life history and feeding processes which occur in very narrow spatio-temporal windows. Here some morphological, life history and feeding behavior peculiarities of Dinophysis are analyzed, in particular those which have been controversial or are still unresolved.
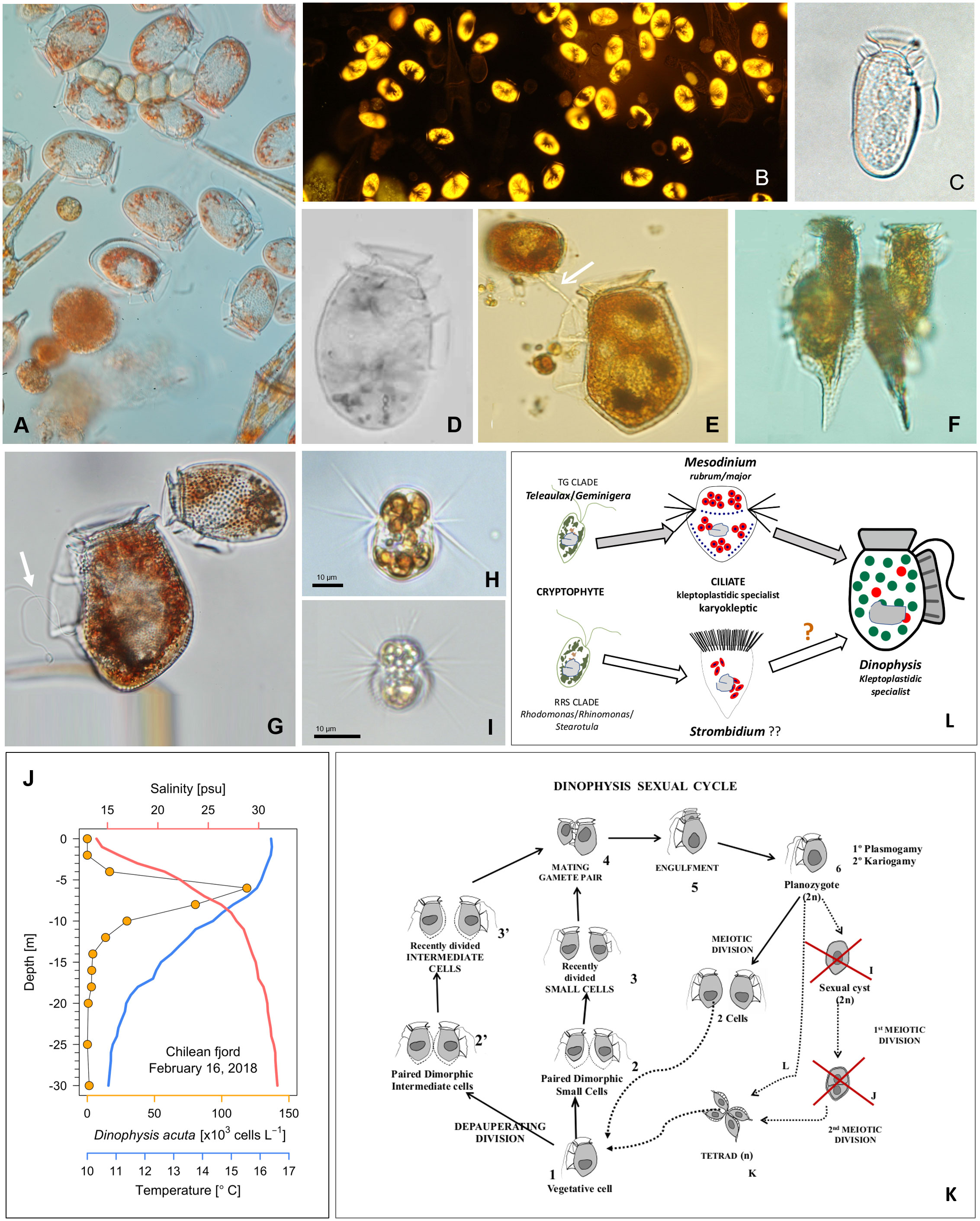
Figure 1 (A–G) Light micrographs of field specimens of Dinophysis spp. from the Galician Rías (NW Spain) (A) Net-haul sample of an autumn bloom of Dinophysis acuminata bloom (DIC, 400x) and (B). Natural autofluorescence of the same population (excitation 546 nm, emission 585 nm single-pass filter set, specific for phycoerithrin); (C) Dinophysis sacculus and (D) D. ovum, two morphospecies of the D. acuminata complex; (E) D. acuta with a towing filament (white arrow) to guide the small (D. dens-like) cell before mating; (F) A tetrad of small (D. diegensis-like) cells of D. caudata; (G) Planozygote of D. acuta with two trailing flagella (white arrow) and D. acuminata; (H) Live specimen of a Mesodinium rubrum strain for Denmark and (I) from Southwestern Spain; (J) Vertical distribution of D. acuta, in a Chilean fjord, aggregated in a thin layer above the depth of maximal density gradient (Díaz et al., 2021); (K) Sexual cycle of Dinophysis (modified from Escalera and Reguera, 2008) with red crosses marking wrong and dashed lines hypothetical life-cycle transitions; (L) Diagram of the cryptophyte-ciliate-dinoflagellate food chain used for mixotrophic cultures of Dinophysis (top) and a putative alternative (bottom).
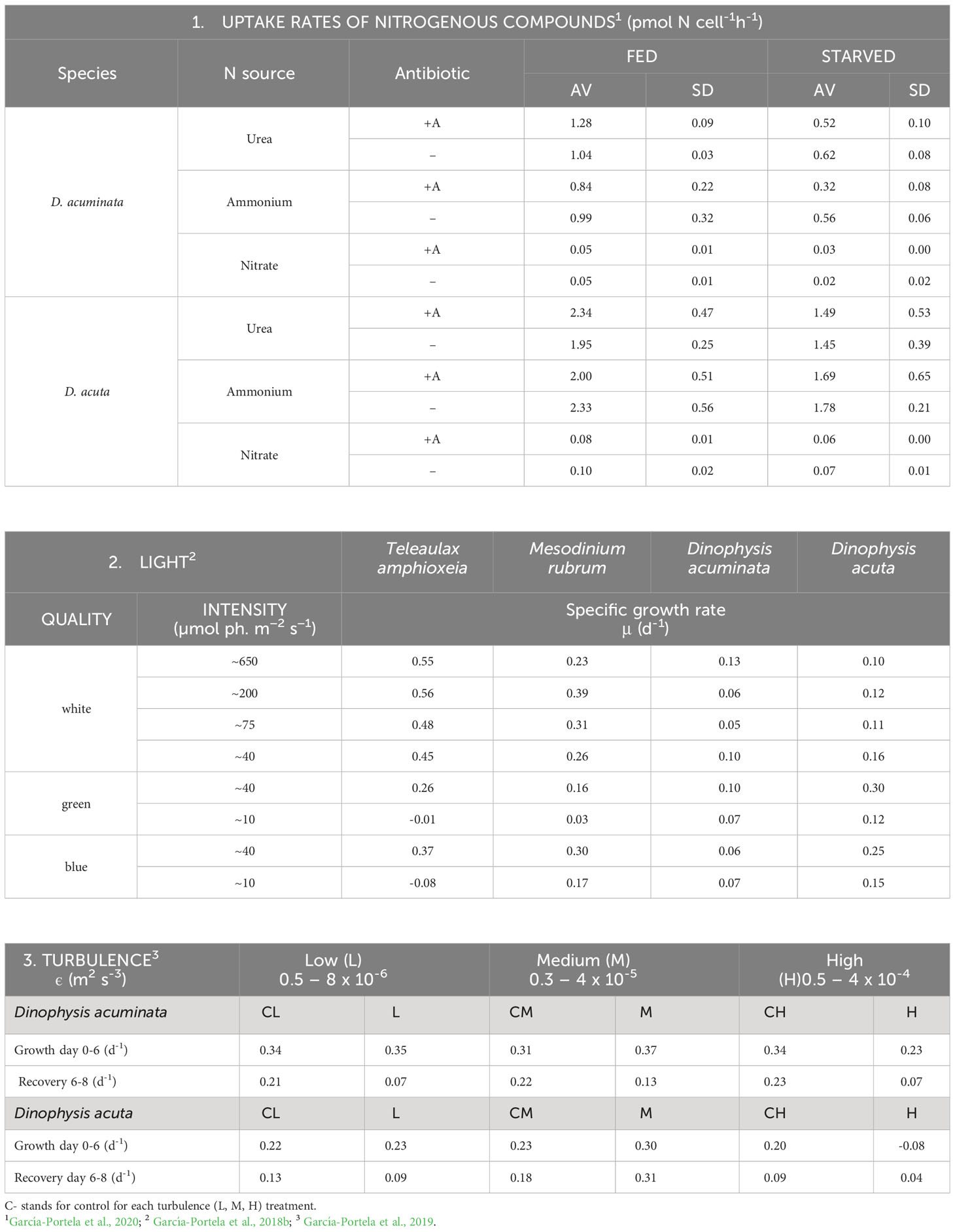
Table 1 Physiological traits in Dinophysis acuminata (VGO1349) and D. acuta (VGO1065) fed the ciliate Mesodinium rubrum (AND0711) fed Teleaulax amphioxeia (AND-0710) or Plagioselmis prolonga (CR10EHU) (3): 1. Uptake rates of inorganic and organic N sources; 2. Growth response (µ, d-1) to light intensity and quality and 3. Tolerance to low, medium and high levels of turbulence (ε, kinetic energy dissipation rate) generated with oscillating grids (Turbogen) in 4L cylinders.
2 Intraspecific morphological variability in Dinophysis
Classification of Dinophysis is largely based on the outline of their large hypothecal plates. Size and shape of these plates and their ornaments are affected by vegetative growth, sexual (life cycle) and feeding processes (Figure 1).
2.1 Cell cycle related morphological variability in Dinophysis
The volume of microalgal vegetative cells between two consecutive generations (a cell cycle) ranges from a minimum in recently divided specimens to a maximum in mitotic cells before division. Dinophysoid thecate dinoflagellates divide by desmoschisis, Von Stosch (1973) which involves sharing the mother theca between the two daughter cells and formation of a new complimentary moiety (Taylor, 1973). Upon cellular fission, wall elements (wings, spines) which were not symmetrically distributed allow recognition and enumeration of mitotic and recently divided cells. These morphological marks together with double nucleated cells, of cell-cycle terminal events can be recognized with light and epifluorescence microscopy, and quantified to estimate in situ division rates (Carpenter et al., 1995; Garcés et al., 1997; Gisselson et al., 1999; Reguera et al., 2003). There is only one study involving quantification of cell-cycle phases using synchronized cultures of D. acuminata (Jia et al., 2019), and no attempts reported of application of flow cytometry (with cell-sorting) to study cell-cycle response to environmental stressors.
2.2 Life cycle related morphological variability in Dinophysis
Modelling bloom dynamics of target species is constrained by poor abilities to recognize different life-cycle stages and identify the environmental and intrinsic factors triggering transitions between stages (Garcés et al., 2002). Dinoflagellates are protists with complex, heteromorphic life cycles with transitions between stages with differing ploidy and morphology (Figueroa et al., 2018). Differentiation of vegetative cells into gametes may proceed through a “depauperating division” (Von Stosch, 1973), each mother cell producing two daughter cells with lower biomass, poor pigmentation and distinct swimming behavior (Figures 1E–G). Dinophysis species are holoplanktonic with a (presumably haplontic) polymorphic life cycle which includes: small gamete-like cells formed by depauperating division; dimorphic mating gamete pairs connected by their ventral margin with a mating tube, engulfment and gamete fusion to produce a planozygote, which differs from the vegetative cell in having two (instead of one) trailing flagella. A single serendipitous field observation showed that well phased sexual division before sunset (Figure 1 in Mackenzie, 1992) and vegetative division (after sunrise) are triggered by different cues. Current developments in electron microscopy and molecular tools have unveiled frequent cases of small cells misclassified as different taxa. An extreme case is illustrated with field and culture specimens of Dinophysis caudata and D. tripos (Reguera et al., 2007; Rodríguez et al., 2012). But information is biased by our current focus on a few toxigenic species of Dinophysis (D. acuminata complex, D. acuta, D. caudata, D. fortii and D. norvegica) which reach bloom numbers (> 103 cells L-1) in coastal waters. A thorough revision of the genus with (still inexistent) robust molecular probes would probably lead to a large reduction of the about 100 species recognized in Gómez (2005) checklist. Flow cytometry, a potent tool to monitor DNA changes through the cell cycle (with cell sorter in field populations) has never been applied to study sexual processes (i.e. ploidy, gametogenesis, phased gamete pairing) with Dinophysis cultures.
A Dinophysis life cycle model was proposed by Reguera and González-Gil (2001) and some misinterpretations clarified later (Koike et al., 2006; Escalera and Reguera, 2008) (Figures 1E–G, K). For example, the mating anisogamous gamete pair, united by their ventral margin, are not undergoing conjugation, i.e. transfer of nuclear material from a donor to a receptor through a conjugation tube. Instead, a tube from the large cell guides the small cell to the cingulum to be engulfed. Nuclear fusion takes place following engulfment and cellular fusion; planozygotes with two longitudinal flagella can divide without going through a resting cyst stage. It is not known if cells grouped in tetrads result from division of planozygotes, from normal vegetative cells, or from both. All these forms, plus the first remark about the ciliate Mesodinium entangled in mucilage in the bottom of Dinophysis culture vessels (Nagai et al., 2008) are well illustrated by Nagai et al. (2020). Putative resting cysts turned out to be pellicle cysts of Fragilidium after eating Dinophysis. A thin and translucid harpoon-like tube, difficult to illustrate, has been described for Dinophysis to catch prey in addition to a feeding peduncle (Hansen and Tillmann, 2020). Early observations of the mating process were interpreted as an act of cannibalism. Indeed, the large cell guides the small one to be engulfed in the same fashion as the towing peduncle used by heterotrophic protists (e.g. Protoperidinium species) with their prey (Figure 1E). How many tubes do Dinophysis cell have? Is Dinophysis sexual division a living heirloom of the “cannibal origin of sex” proposed by Sagan and Margulis (1987)?
2.3 Identification problems: controversial “Dinophysis acuminata complex”
This complex refers to a group of morphologically similar species of Dinophysis difficult to separate when their blooms, with small and intermediate morphotypes, co-occur (Figures 1C, D) (Séchet et al., 2021). Sequencing the ITSrDNA- region of single cell isolates with a new technique showed a 99% similarity between D. acuminata and D. sacculus, two frequent species of this complex (Marín et al., 2001). Later, the apparent success of using the mitochondrial cox1 gene to discriminate between D. acuminata and D. ovum (Raho et al., 2008) turned out to be a mistake in the alignment of a D. acuminata strain (AM931587). Recently the impossibility to separate D. acuminata from D. ovum with the available sequences (SSU rDNA, ITS1, ITS2 and cox1) was confirmed (Park et al., 2019). These sequences are the best to group toxigenic species of Dinophysis in several clades, such as the D. acuminata complex and the D. caudata group.
Dinophysis acuminata and D. ovum are well distinguished in field samples by monitoring experts in Southern Europe on the basis of their size and contour. In eastern USA they also show very different toxic potential. Their distribution shows latitudinal and seasonal differences in Atlantic and Mediterranean coastal waters in Europe (Séchet et al., 2021) as well as those from Eastern USA and the Gulf of Mexico (Wolny et al., 2020; Ayache et al., 2023).
Park et al. (2019) found that shape in single-cell incubations of D. acuminata complex specimens from Korea (all images resembling the D. ovum morphotype) changed after weeks of incubation to forms corresponding to descriptions of the two (D. acuminata and D. ovum) morphospecies. It is important to note within this context that armored dinoflagellate specimens in culture may display a smoother wall texture and their tapered antapical ends lose sharpness (e.g. cells of D. acuta in culture may end with the appearance of D. fortii). Some authors group all morphologically close morphotypes and their small cells in their routine cell counts. This is unfortunate, because whether they consider them to be one or two species, different life forms are revealing adaptations to environmental conditions (Margalef, 1978). Valuable ecological information is being missed. In the case of recognizable small cells, e.g. D. skagii, the small cell of D. acuminata, their detection and quantification provide valuable parameterization for models including life cycle transitions. The continuum of shapes between species of the D. acuminata complex may provide a model of ongoing speciation.
New portions of the genome need to be sequenced to develop more robust molecular tools for species identification. In the meantime, we should keep different forms separated or name them adding the letter “f” (form), followed by the epithet (acuminata, ovum, sacculus) that best fits their shape, as done in the past to distinguish three morphotypes of D. caudata: abbreviata, allieri and pedunculata (reviewed in Reguera et al., 2007).
Recently, promising results have been obtained by comparing transcribed sequences of D. acuminata (Atlantic coast of US) and D. ovum (Gulf of Mexico) (Gaonkar and Campbell, 2023). Dinophysis ovum has some nuclear encoded genes (not present in D. acuminata) affecting the synthesis of plastidial pigments (phycoerythrin). These findings have potential to solve the design of a robust probe for the acuminata complex. It will also contribute to understand the Dinophysis species-specific response to light.
3 Dinophysis nutritional sources
A large majority of free living dinoflagellates are mixoplanktonic, i.e. they have the ability to combine phototrophy with phagotrophy (Jeong et al., 2010; Hansen and Tillmann, 2020). Photosynthesis is performed either using permanent (constitutive) or temporary (non-constitutive) plastids stolen (kleptoplastids) from a variety (generalist) or from a very selected group of prey (plastidic specialist) (Mitra et al., 2016; Mitra et al., 2023). Most phototrophic dinoflagellates have secondary plastids which contain chlorophyll c and peridinin, but some genera (e.g. Dinophysis, Karenia, Lepidodinium) contain plastids with pigments other than peridinin. Dinophysis and Amylax species use second hand cryptophyte-like plastids acquired from ciliate prey Mesodinium, which in turn utilize kleptoplastids derived from their cryptophyte prey Teleaulax (Figure 1L) (Koike and Takishita, 2008; Kim et al., 2012; Park et al., 2013), Additional haptophyte and cyanophyte-like (presumably from cyanobionts) plastids were found in field specimens of D. miles (Qiu et al., 2011). But cultures of this species, and of Phalacroma mitra, a pigmented exception among Phalacroma species with haptophyte-like plastids (Nishitani et al., 2012), have not yet been established.
3.1 Dissolved inorganic/organic nitrogen sources
In addition to live prey, Dinophysis needs light and dissolved nutrients to perform photosynthesis. Uptake rates of N15 labeled compounds during blooms of several HAB species in a coastal upwelling system showed Dinophysis had a clear preference for regenerated nitrogen (ammonium and urea). Unlike Pseudo-nitzschia australis and Alexandrium catenella, which are able to take up very fast the nitrates in the upwelled water (“high uptake velocity strategists”) D. acuminata is a “high affinity strategist”, i.e., is able to use very efficiently low concentrations of nutrients that would be limiting for the other two species (Seeyave et al., 2009). Laboratory incubations of D. acuminata yielded very low or even below detection uptake rates of nitrate, but rapid assimilation of ammonia and urea (Hattenrath-Lehmann and Gobler, 2015). Dinophysis acuta, a species 3 times larger, exhibited uptakes rates 2-3 times higher than D. acuminata. Unlike phototrophic species, starvation did not boost uptake rates which were higher in well fed cultures (García-Portela et al., 2020) (Table 1). Nitrate reductase membrane transporters from 30 dinoflagellate species (Keeling et al., 2014) showed a paucity of these transporters in D. acuminata comparable with the amount found in the red Noctiluca heterotroph. New experimental transcriptomic and isotopic data revealed the central role of NH4 (Hattenrath-Lehmann et al., 2021).
3.2 Difficulties to cultivate highly selective Dinophysis and Mesodinium
Since Schnepf and Elbrächter (1988) drew attention to the cryptophyte-like plastids in Dinophysis until the first culture of D. acuminata was established, advances in molecular biology were essential for the final success, preceded by the cultivation of the ciliate Mesodinium rubrum (Dinophysis prey) fed cryptophytes. These cryptophytes are the source of kleptoplastids for the phototrophic ciliate Mesodinium (Gustafson et al., 2000) (Figure 1L). Mesodinium rubrum, grown in the laboratory with Teleaulax amphioxeia, T. gracilis, T. minuta and Plagioselmis prolonga, is considered to be genus-specific about its selected prey (Peltomaa and Johnson, 2017). Plagioselmis prolonga, only 1 bp different from T. amphioxeia, was found to be a haploid stage in the diplohaplontic life cycle of the latter (Altenburger et al., 2020), so we should change to “TG” the old TPG clade. But Mesodinium growth rate and yield varied with different prey and optimal results were obtained only if strains of ciliate and its cryptophyte prey had been isolated from the same location (Hernández-Urcera et al., 2018). Likewise, Dinophysis growth was not the same with different strains of Mesodinium (Figures 1H, I) or with different quality and size of the same species/strain (García-Portela et al., 2018; Fiorendino et al., 2020).
Spatio-temporal matching of Dinophysis and Mesodinium field populations (both mixotrophs with different optimal environmental windows)(Fiorendino et al., 2020) is the key factor constraining Dinophysis growth. The apparent strain-level selectivity of the cryptophyte prey by Mesodinium, and strain-level preferences of Dinophysis for its ciliate prey may explain the fact that only a few laboratories have been able to grow Mesodinium from their own locality. Three strains of Mesodinium − MBL-DK2009 from Danish waters, AND-0711 from Southwest Spain, and JAMR-2007 from eastern Japan, are the only three shared strains which have been used in most Dinophysis experiments carried out to date; they have been fed with Teleaulax amphioxeia strains from the same locations, Danish K-0434, Spanish AND-0710 and Japanese JATA, with two exceptions using Geminigera cryophyla or a Teleaulax strain from the Gulf of Mexico (GoMTA) to feed a foreign Mesodinium. There were also Korean strains of ciliate and prey of restrained use under a patent (Yih et al., 2012). In short, a large proportion of research in the last 15 years, with Dinophysis fed foreign strains of Mesodinium and cryptophyte, has been carried out with cultures growing in suboptimal conditions. A coincidence of plastidic sequences in local Dinophysis species with those from M. rubrum and M. major have been found in the Galician Rias (Rial et al., 2015; Herfort et al., 2017). The latter was the dominant Mesodinium species in samples from Argentina and Chile (Johnson et al., 2017). Recently, cultures of Mesodinium major fed the same strain of T. amphioxeia as M. rubrum have been established. The former reached a biovolume 4 times higher and exhibited better adaptations to high light intensities than M. rubrum. This is the first case of a Mesodinium culture other than M rubrum. Most likely, M. major will constitute a more appropriate prey for the large sized summer – blooming D. acuta. Furthermore, predominance of identical crytophyte plastid sequences belonging to clade V (Rhodomonas/Rhinomonas/Storeatula) were found in several species of Dinophysis, in ciliates of the genus Strombidium and in co-occurring heliozoans in fjordic and oceanic waters off Los Lagos and Aysén, Chile (Díaz et al., 2020). But there are only a few studies where seasonal variability in Dinophysis, ciliate and cryptophytes plastids of the same location have been systematically explored.
How Mesodinium and Dinophysis recognize their local (optimal) prey is not known. One possibility may be a sympatric coevolution of predator and prey similar to that suggested between planktonic parasites and hosts, i.e. interactions between the predator and prey genotypes.
3.3 Dinophysis plastids
Following the first mixotrophic culturing of D. acuminata (Park et al., 2006), there was a controversy as to whether Dinophysis performed photosynthesis with its own (constitutive) plastids or with those kept from prey (García-Cuetos et al., 2010). Kim et al. (2012) showed that after ingestion of Mesodinium, the retained plastids in D. caudata suffered some transformation and lost two of the four surrounding membranes. Thus, Dinophysis reproduces in a few hours a process which may have taken years of evolution for ancestral heterotrophic dinoflagellates to acquire permanent plastids.
Further questions were raised about the potential control of Dinophysis over its kleptoplastids, which in D. acuta cultures were found to display photoregulation (Hansen et al., 2016). Since Dinophysis do not keep the nuclei of their prey, this observation suggested some role for the host in controlling the kleptoplastid physiology. Rusterholz et al. (2017) demonstrated that D. acuta and D. acuminata were able to divide their kleptoplastids during cell division; decreases in total kleptoplastid volume and their number were not associated with dilution by cellular divisions. To date, Dinophysis represents the only kleptoplastidic protist showing plastidial division in the absence of the prey nucleus. Is this an example of ongoing evolutionary transition towards permanent possession of chloroplasts of cryptophyte origin?
Transcriptomic analysis showed that products of some nuclear encoded genes transported by kleptoplastids are acquired in D. acuminata by Lateral Gene Transfer (LGT), a process which does not involve vertical genetic inheritance from fucoxanthin dinoflagellates, haptophytes and cryptophytes (Wisecaver and Hackett, 2010). More recently, Hongo et al. (2019) studied the origin of genes encoding D. fortii proteins involved in photosynthesis (including the biosynthesis of porphyrins, chlorophylls and isoprenoids). A total of 58 proteins involved in these processes were identified, 30 of which were traced to peridinin dinoflagellates, 21 to other groups/species (from fucoxanthin dinoflagellates, haptophytes, chlorarachniophytes, cyanobacteria and cryptophytes) as a result of LGT, and 7 from unknown sources.
An interpretation of these results is that since the ancestral Dinophysis engulfed haptophytes and/or fucoxanthin containing dinoflagellates, the original peridinin plastid has been reduced (as in D. acuminata) (Janouškovec et al., 2017). During evolution, photosynthetic species of Dinophysis began feeding on M. rubrum and using its derived plastid, with a more recent transition to retention of plastids obtained from cryptophytes.
The OMICs age in Dinophysis research has only just started. Molecular tools unblocked the bottleneck (identification of the prey) that slowed progress in knowledge of Dinophysis physiology. Present challenges include prediction of the response of individual HAB species to environmental change. New breakthroughs can be expected with the help of OMIC technologies and in situ imaging platforms. BUT before, special efforts need to be invested in modest artisanal activities aimed to enlarge the meagre list of ciliate prey strains available and to carry out systematic observations on plastidial diversity in local populations of the ciliate and cryptophyte communities supporting Dinophysis.
Author contributions
BR: Conceptualization, Writing – original draft, Writing – review & editing. MG: Investigation, Methodology, Writing – review & editing. EV: Methodology, Writing – review & editing. PR: Methodology, Writing – review & editing. LE: Methodology, Writing – review & editing. PD: Writing – review & editing, Project administration. FR: Conceptualization, Writing – review & editing, Project administration.
Funding
The author(s) declare financial support was received for the research, authorship, and/or publication of this article. Funding was provided by the EU INTERREG Atlantic Area project PRIMROSE (EAPA 182/2016), and National Spanish BIOTOX (PID2021-125643OB-C22; Proyectos de generación de conocimiento 2021, MICINN). PD was funded by ANID-FONDECYT 1231220 from the National Research and Development Agency (ANID), Chile.
Acknowledgments
This is a contribution to SCOR WG #165 MixONET which is supported by grant OCE-214035 from the National Science Foundation to the Scientific Committee on Oceanic Research (SCOR) and contributions from SCOR National Committees.
Conflict of interest
The authors declare that the research was conducted in the absence of any commercial or financial relationships that could be construed as a potential conflict of interest.
Publisher’s note
All claims expressed in this article are solely those of the authors and do not necessarily represent those of their affiliated organizations, or those of the publisher, the editors and the reviewers. Any product that may be evaluated in this article, or claim that may be made by its manufacturer, is not guaranteed or endorsed by the publisher.
References
Abé T. H. (1967). The armoured Dinoflagellata: II. Prorocentridae and Dinophysidae (B) - Dinophysis and its allied genera. Publications Seto Mar. Biol. Lab. 2, 37–78. doi: 10.5134/175455
Altenburger A., Blossom H. E., García-Cuetos L., Jakobsen H. H., Carstensen J., Lundholm N., et al. (2020). Dimorphism in cryptophytes — The case of Teleaulax amphioxeia/Plagioselmis prolonga and its ecological implications. Sci. Adv. 6, eabb1611. doi: 10.1126/sciadv.abb1611
Ayache N., Bill B. D., Brosnahan M. L., Campell L., Deeds J. R., Fiorendino J. D., et al. (2023). A survey of Dinophysis spp. and their potential to cause diarrhetic shellfish poisoning in coastal waters of the United States. J. Phycol. 59, 658–680. doi: 10.1111/jpy.13331
Baldrich A. M., Díaz P. A., Álvarez G., Pérez-Santos I., Schwerter C., Díaz M., et al. (2023). Dinophysis acuminata or Dinophysis acuta: What Makes the Difference in Highly Stratified Fjords? Mar. Drugs 21, 64. doi: 10.3390/md21020064
Baldrich A., Pérez-Santos I., Álvarez G., Reguera B., Fernández-Pena C., Rodríguez-Villegas C., et al. (2021). Niche differentiation of Dinophysis acuta and D. acuminata in a stratified fjord. Harmful Algae 103, 102010. doi: 10.1016/j.hal.2021.102010
Berdalet E., Montresor M., Reguera B., Roy S., Yamazaki H., Cembella A., et al. (2017). Harmful algal blooms in fjords, coastal embayments, and stratified systems: Recent progress and future research. Oceanography 30, 46–57. doi: 10.5670/oceanog.2017.109
Carpenter E. J., Janson S., Boje R., Pollehne F., Chang J. (1995). Dinophysis norvegica: biological and ecological observations in the Baltic Sea. Eur. J. Phycol. 30, 1–10. doi: 10.1080/09670269500650751
Díaz P. A., Fernández-Pena C., Pérez-Santos I., Baldrich A., Díaz M., Rodríguez F. (2020). Dinophysis Ehrenberg (Dinophyceae) in Southern Chile harbours red cryptophyte plastids from Rhodomonas/Storeatula clade. Harmful Algae 99, 101907. doi: 10.1016/j.hal.2020.101907
Díaz P. A., Pérez-Santos I., Álvarez G., Garreaud R., Pinilla E., Díaz M., et al. (2021). Multiscale physical background to an exceptional harmful algal bloom of Dinophysis acuta in a fjord system. Sci. Total Environ. 773, 145621. doi: 10.1016/j.scitotenv.2021.145621
Díaz P. A., Reguera B., Ruiz-Villarreal M., Pazos Y., Velo-Suárez L., Berger H., et al. (2013). Climate variability and oceanographic settings associated with interannual variability in the initiation of Dinophysis acuminata blooms. Mar. Drugs 11, 2964–2981. doi: 10.3390/md11082964
Díaz P. A., Ruiz-Villarreal M., Pazos Y., Moita M. T., Reguera B. (2016). Climate variability and Dinophysis acuta blooms in an upwelling system. Harmful Algae 53, 145–159. doi: 10.1016/j.hal.2015.11.007
Escalera L., Reguera B. (2008). Planozygote division and other observations on the sexual cycle of several species of Dinophysis (Dinophyceae, Dinophysiales). J. Phycol. 44, 1425–1436. doi: 10.1111/j.1529-8817.2008.00610.x
Figueroa R. I., Estrada M., Garcés E. (2018). Life histories of microalgal species causing harmful blooms: Haploids, diploids and the relevance of benthic stages. Harmful Algae 73, 44–57. doi: 10.1016/j.hal.2018.01.006
Fiorendino J. M., Smith J. L., Campbell L. (2020). Growth response of Dinophysis, Mesodinium, and Teleaulax cultures to temperature, irradiance, and salinity. Harmful Algae 98, 101896. doi: 10.1016/j.hal.2020.101896
Gaonkar C. C., Campbell L. (2023). De novo transcriptome assembly and gene annotation for the toxic dinoflagellate Dinophysis. Sci. Data 10, 345. doi: 10.1038/s41597-023-02250-8
Garcés E., Delgado M., Camp J. (1997). Phased cell division in a natural population of Dinophysis sacculus and the in situ measurement of potential growth rate. J. Plankton Res. 19, 2067–2077. doi: 10.1093/plankt/19.12.2067
Garcés E., Zingone A., Montresor M., Reguera B., Dale B. (2002). LIFEHAB: Life Histories of Microalgal Species Causing Harmful Blooms (Luxembourg: Office for the Official Publications of the European Commission).
García-Cuetos L., Moestrup Ø., Hansen P. J., Daugbjerg N. (2010). The toxic dinoflagellate Dinophysis acuminata harbors permanent chloroplasts of cryptomonad origin, not kleptochloroplasts. Harmful Algae 9, 25–38. doi: 10.1016/j.hal.2009.07.002
García-Portela M., Reguera B., Gago J., Le Gac M., Rodríguez F. (2020). Uptake of inorganic and organic nitrogen sources by Dinophysis acuminata and D. acuta. Microorganisms 8, 187. doi: 10.3390/microorganisms8020187
García-Portela M., Reguera B., Ribera d’Alcalá M., Rodríguez F., Montresor M. (2019). Short term effect of small-scale turbulence in two species of Dinophysis. Harmful Algae 89, 101654. doi: 10.1016/j.hal.2019.101654
García-Portela M., Reguera B., Sibat M., Altenburger A., Rodríguez F., Hess P. (2018a). Metabolomic profiles of Dinophysis acuminata and Dinophysis acuta. Using non-targeted High-Resolution Mass Spectrometry: Effect of nutritional status and prey. Mar. Drugs 16, 143. doi: 10.3390/md16050143
García-Portela M., Riobó P., Reguera B., Garrido J.L., Blanco J., Rodríguez F., et al. (2018b). Comparative ecophysiology of Dinophysis acuminata and D. acuta (Dinophyceae, Dinophysiales): effect of light intensity and quality on growth, cellular toxin content and photosynthesis. J. Phycol. 54, 899–917. doi: 10.1111/jpy.12794
Gentien P., Donaghay P., Yamazaki H., Raine R., Reguera B., Osborn T. (2005). Harmful algal blooms in stratified environments. Oceanography 18, 152–163. doi: 10.5670/oceanog.2005.52
Gisselson L., Granéli E., Carlsson P. (1999). Using cell cycle analysis to estimate in situ growth rate of the dinoflagellate Dinophysis acuminata drawback of the DNA quantification method. Mar. Ecol. Prog. Ser. 184, 55–62. doi: 10.3354/meps184055
Gómez F. (2005). A list of free-living dinoflagellate species in the world’s oceans. Acta Botanica Croata 64, 129–212.
Gustafson D. E., Stoecker D. K., Johnson M. D., Van Heukelem W. F., Sneider K. (2000). Cryptophyte algae are robbed of their organelles by the marine ciliate Mesodinium rubrum. Nature 405, 1049–1052. doi: 10.1038/35016570
Hallegraeff G., Lucas I. (1988). The marine dinoflagellate genus Dinophysis (Dinophyceae):photosynthetic, neritic and non-photosynthetic, oceanic species. Phycologia 27, 25–42. doi: 10.2216/i0031-8884-27-1-25.1
Hansen P. J., Ojamäe K., Berge T., Trampe E. C. L., Nielsen L. T., Lips I., et al. (2016). Photoregulation in a kleptochloroplastidic dinoflagellate, Dinophysis acuta. Front. Microbiol. 7, 785. doi: 10.3389/fmicb.2016.00785
Hansen P. J., Tillmann U. (2020). “Mixotrophy in dinoflagellates: prey selection, physiology and ecological importance,” in Dinoflagellates: Classification, Evolution, Physiology and Ecological Significance. Ed. Subba Rao D. (New York, USA: Nova Science Publishers, Inc), 201–260.
Hattenrath-Lehmann T., Gobler C. J. (2015). The contribution of inorganic and organic nutrients to the growth of a North American isolate of the mixotrophic dinoflagellate, Dinophysis acuminata. Limmol. Oceanogr. 60, 1588–1603. doi: 10.1002/lno.10119
Hattenrath-Lehmann T. K., Nanjappa D., Zhang H., Yu L., Goleski J. A., Lin S., et al. (2021). Transcriptomic and isotopic data reveal central role of ammonium in facilitating the growth of the mixotrophic dinoflagellate, Dinophysis acuminata. Harmful Algae 104, 102031. doi: 10.1016/j.hal.2021.102031
Herfort L., Maxey K., Voorhees I., Simon H. M., Grobler K., Peterson T. D., et al. (2017). Use of highly specific molecular markers reveals positive correlation between abundances of Mesodinium cf. major and Its preferred prey, Teleaulax amphioxeia, during red water blooms in the Columbia River Estuary. J. Eukaryot. Microbiol. 64, 740-745. doi: 10.1111/jeu.12407
Hernández-Urcera J., Rial P., García-Portela M., Lourés P., Kilcoyne J., Rodríguez F., et al. (2018). Notes on the cultivation of two mixotrophic Dinophysis species and their ciliate prey Mesodinium rubrum. Toxins 10, 505. doi: 10.3390/toxins10120505
Hongo Y., Yabuki A., Fujikura K., Nagai S. (2019). Genes functioned in kleptoplastids of Dinophysis are derived from haptophytes rather than from cryptophytes. Sci. Rep. 9, 9009. doi: 10.1038/s41598-019-45326-5
Jacobson D., Andersen R. (1994). The discovery of mixotrophy in photosynthetic species of Dinophysis (Dinophyceae): light and electro microscopical observations of food vacuales in Dinophysis acuminata, D. norvegica and two heterotrophic dinophysoid dinoflagellates. Phycologia 33, 97–110. doi: 10.2216/i0031-8884-33-2-97.1
Janouškovec J., Gavelis G. S., Burki F., Dinh D., Bachvaroff T. R., Gornik S. G., et al. (2017). Major transitions in dinoflagellate evolution unveiled by phylotranscriptomics. Proc. Natl. Acad. Sci. 114, E171–E180. doi: doi: 10.1073/pnas.1614842114
Jensen M., Daugbjerg N. (2009). Molecular phylogeny of selected species of the order dinophysiales (Dinophyceae) testing the hypothesis of a dinophysioid radiation. J. Phycol. 45, 1136–1152. doi: 10.1111/j.1529-8817.2009.00741.x
Jeong H. J., Yoo Y. D., Kim J. S., Seong K. A., Kang N. S., Kim T. H. (2010). Growth, feeding and ecological roles of the mixotrophic and heterotrophic dinoflagellates in marine planktonic food webs. Ocean Sci. J. 45, 65–91. doi: 10.1007/s12601-010-0007-2
Jia Y., Gao H., Tong M., Anderson D.M. (2019). Cell cycle regulation of the mixotrophic dinoflagellate Dinophysis acuminata: Growth, photosynthetic efficiency and toxin production. Harmful Algae 89, 101672. doi: 10.1016/j.hal.2019.101672
Johnson M. D., Beaudoin D. J., Laza-Martinez A., Dyhrman S. T., Fensin E., Lin S., et al. (2017). The genetic diversity of Mesodinium and associated cryptophytes. Front. Microbiol. 7. doi: 10.3389/fmicb.2016.02017
Keeling P. J., Burki F., Wilcox H. M., Allam B., Allen E. E., Amaral-Zettler L. A., et al. (2014). The Marine Microbial Eukaryote Transcriptome Sequencing Project (MMETSP): Illuminating the functional diversity of eukaryotic life in the oceans through transcriptome sequencing. PloS Biol. 12(6):e1001889. doi: 10.1371/journal.pbio.1001889
Kim M., Nam S. W., Shin W., Coats W., Park M. G. (2012). Dinophysis caudata (Dinophyceae) sequesters and retains plastids from the mixotrophic ciliate prey Mesodinium rubrum. J. Phycol. 48, 569–579. doi: 10.1111/j.1529-8817.2012.01150.x
Koike K., Nicshiyama N., Saitoh K., Imai K., Koike K., Kobiyama A., et al. (2006). Mechanism of gamete fusion in Dinophysis fortii (Dinophyceae, Dinophyta): light microscopic and ultrastrutural observations. J. Phycol. 42, 1247–1256. doi: 10.1111/j.1529-8817.2006.00288.x
Koike K., Takishita K. (2008). Anucleated cryptophyte vestiges in the gonyaulacalean dinoflagellates Amylax buxus and Amylax triacantha (Dinophyceae). Phycol. Res. 56, 301–311. doi: 10.1111/j.1440-1835.2008.00512.x
Mackenzie L. (1992). Does Dinophysis (Dinophyceae) have a sexual life cycle? J. Phycol. 28, 399–406. doi: 10.1111/j.0022-3646.1992.00399.x
Maestrini S. (1998). “Bloom dynamics and ecophysiology of Dinophysis spp,” in Physiological ecology of harmful algal blooms. Eds. Anderson D., Cembella A., Hallegraeff G. (Berlin, Heildelburg, New York: NATO ASI Ser Ser G Ecol Sci, Springer-Verlag), 243–265.
Margalef R. (1978). Life forms of phytoplankton as survival alternatives in an unstable environment. Oceanol. Acta 1, 493–509.
Marín I., Aguilera A., Reguera B., Abad J. P. (2001). Preparation of DNA suitable for PCR amplification from fresh or fixed single dinoflagellate cells. Biotechniques 30, 88–93. doi: 10.2144/01301st05
Mitra A., Caron D. A., Faure E., Flynn K. J., GonçAlves Leles S., Hansen P. J., et al. (2023). The Mixoplankton Database (MDB): Diversity of photo-phago-trophic plankton in form, function, and distribution across the global ocean. J. Eukarryot. Microbiol. 70, e12972. doi: 10.1111/jeu.12972
Mitra A., Flynn K., Tillmann U., Ja. R., Caron D., Stoecker D., et al. (2016). Defining planktonic protist functional groups on mechanisms for energy and nutrient acquisition: Incorporation of diverse mixotrophic strategies. Protist 167, 106–120. doi: 10.1016/j.protis.2016.01.003
Nagai S., Nitshitani G., Tomaru Y., Sakiyama S., Kamiyama T. (2008). Predation by the toxic dinoflagellate Dinophysis fortii on the ciliate Myrionecta rubra and observation of sequestration of ciliate chloroplasts. J. Phycol. 44, 909–922. doi: 10.1111/j.1529-8817.2008.00544.x
Nagai S., Sildever S., Suzuki T., Nishitani G., Basti L., Kamiyama T. (2020). “Growth and feeding behavior of mixotrophic species in laboratory cultures,” in Dinoflagellates: Classification, Evolution, Physiology and Ecological Significance. Ed. Subba Rao D. (New York, USA: Nova Science Publishers, Inc), 129–166.
Nishitani G., Nagai S., Hayakawa S., Kosaka Y., Sakurada K., Kamiyama T., et al. (2012). Multiple plastids collected by the dinoflagellate Dinophysis mitra through kleptoplastidy. Appl. Environ. Microbiol. 78, 813–821. doi: 10.1128/AEM.06544-11
Park J. H., Kim M., Jeong H. J., Park M. G. (2019). Revisiting the taxonomy of the “Dinophysis acuminata complex’’ (Dinophyta). Harmful Algae 88, 101657. doi: 10.1016/j.hal.2019.101657
Park M. G., Kim M., Kang M. (2013). A dinoflagellate Amylax triacantha with plastids of the cryptophyte origin: phylogeny, feeding mechanism, and growth and grazing responses. J. Eukaryot. Microbiol. 60, 363–376. doi: 10.1111/jeu.12041
Park M., Kim S., Kim H., Myung G., Kang Y., Yih W. (2006). First successful culture of the marine dinoflagellate Dinophysis acuminata. Aquat. Microb. Ecol. 45, 101–106. doi: 10.3354/ame045101
Peltomaa E., Johnson M. D. (2017). Mesodinium rubrum exhibits genus-level but not species level cryptophyte prey selection. Aquat. Microb. Ecol. 78, 147–159. doi: 10.3354/ame01809
Qiu D., Huang L., Liu S., Lin S. (2011). Nuclear, mitochondrial and plastid gene phylogenies of Dinophysis miles (Dinophyceae): Evidence of variable types of chloroplasts. PloS One 6, e29398. doi: 10.1371/journal.pone.0029398
Raho N., Pizarro G., Escalera L., Reguera B., Marín I. (2008). Morphology, toxin composition and molecular analysis of Dinophysis ovum Schütt, a dinoflagellate of the ‘‘Dinophysis acuminata complex’’. Harmful Algae 7:839-848. doi: 10.1016/j.hal.2008.04.006
Reguera B., Garcés E., Pazos Y., Bravo I., Ramilo I., González-Gil S. (2003). Cell cycle patterns and estimates of in situ división rates of dinoflagellates of the genus Dinophysis by a postmitotic index. Mar. Ecol. Prog. Ser. 249, 117–131. doi: 10.3354/meps249117
Reguera B., Gonzáles-Gil S., Delgado M. (2007). Dinophysis diegensis is a life history stage of Dinophysis caudata (Dinophyceae, Dinophysiales). J. Phycol. 43, 1083–1093. doi: 10.1111/j.1529-8817.2007.00399.x
Reguera B., González-Gil S. (2001). Small cell and intermediate cell formation in species of Dinophysis (Dinophyceae, Dinophysiales). J. Phycol. 37:318-333. doi: 10.1046/j.1529-8817.2001.037002318.x
Reguera B., Riobó P., Rodríguez F., Díaz P. A., Pizarro G., Paz B., et al. (2014). Dinophysis toxins: causative organisms, distribution and fate in shellfish. Mar. Drugs 12, 394–461. doi: 10.3390/md12010394
Rial P., Laza-Martínez A., Reguera B., Raho N., Rodríguez F. (2015). Origin of cryptophyte plastids in Dinophysis from Galician waters: results from field and culture experiments. Aquat. Microb. Ecol. 76:433–437. doi: 10.3354/ame01774
Rodríguez F., Escalera L., Reguera B., Rial P., Riobó P., Silva T. J. (2012). Morphological variability, toxinology and genetics of the dinoflagellate Dinophysis tripos (Dinophysiaceae, Dinophysiales). Harmful Algae 13, 26–33. doi: 10.1016/j.hal.2011.09.012
Rusterholz P. M., Hansen P. J., Daugbjerg N. (2017). Evolutionary transition towards permanent chloroplasts? - Division of kleptochloroplasts in starved cells of two species of Dinophysis (Dinophyceae). PloS One 12, e0177512. doi: 10.1371/journal.pone.0177512
Schnepf E., Elbrächter M. (1988). Cryptophycean-like double membrane-bound chloroplast in the dinoflagellate, Dinophysis Ehrenb: evolutionary, phylogenetic and toxicological implitations. Botanica Acta 101, 196–203. doi: 10.1111/j.1438-8677.1988.tb00033.x
Séchet V., Sibat M., Billien G., Carpentier L., Rovillon G. A., Raimbault V., et al. (2021). Characterization of toxin-producing strains of Dinophysis spp. (Dinophyceae) isolated from French coastal waters, with a particular focus on the D. acumuinata-complex. Harmful Algae 107, 101974. doi: 10.1016/j.hal.2021.101974
Seeyave S., Probyn T. A., Pitcher G. C., Lucas M. I., Purdie D. A. (2009). Nitrogen nutrition in assemblages dominated by Pseudo-nitzschia spp., Alexandrium catenella and Dinophysis acuminata off the west coast of South Africa. Mar. Ecol. Prog. Ser. 379, 91–107. doi: 10.3354/meps07898
Steidinger K., Tangen K. (1996). “Dinoflagellates,” in Identifying Marine Phytoplankton. Ed. Tomas C. R. (New York, USA: Academic Press), 387–584.
Taylor F. (1973). Topography of cell division in the structurally complex dinoflagellate genus Ornithocercus. J. Phycol. 9, 1–10. doi: 10.1111/j.0022-3646.1973.00001.x
Von Stosch H. A. (1973). Observations on vegetative reproduction and sexual life cycles of two freshwater dinoflagellates, Gymnodinium pseudopalustre Schiller and Woloszynskia apiculata sp. nov. Br. Phycol. J. 8:105-134.
Wisecaver J. H., Hackett J. D. (2010). Transcriptome analysis reveals nuclear-encoded proteins for the maintenance of temporary plastids in the dinoflagellate Dinophysis acuminata. BMC Genomics 11, 366. doi: 10.1186/1471-2164-11-366
Wolny J. L., Egerton T. A., Handy S. M., Stutts W. L., Smith J. L., Whereat E. B., et al. (2020). Characterization of Dinophysis spp. (Dinophyceae, Dinophysiales) from the mid-Atlantic region of the United States. J. Phycol. 56, 404–424. doi: 10.1111/jpy.12966
Yasumoto T., Oshima Y., Sugawara W., Fukuyo Y., Oguri H., Igarashi T., et al. (1980). Identification of Dinophysis fortii as the causative organism of diarrhetic shellfish poisoning. Bull. Jpn. Soc Sci. Fish. 46, 1405–1411. doi: 10.2331/suisan.46.1405
Yasumoto T., Oshima Y., Yamagushi M. (1978). Occurrence of a new type of shellfish poisoning in the Tohoku district. Bull. Jpn. Soc Fish. 44, 1249–1255. doi: 10.2331/suisan.44.1249
Keywords: Dinophysis, toxic HABs, morphological variability, life history stages, plastidic specialist, mixoplankton
Citation: Reguera B, García-Portela M, Velasco-Senovilla E, Rial P, Escalera L, Díaz PA and Rodríguez F (2024) Dinophysis, a highly specialized mixoplanktonic protist. Front. Protistol. 1:1328026. doi: 10.3389/frpro.2023.1328026
Received: 26 October 2023; Accepted: 04 December 2023;
Published: 08 January 2024.
Edited by:
Panagiota Katikou, Veterinary Research Institute Greek Agricultural Organization Demeter, GreeceReviewed by:
Živana Ninčević Gladan, Institute of Oceanography and Fisheries (IZOR), CroatiaCopyright © 2024 Reguera, García-Portela, Velasco-Senovilla, Rial, Escalera, Díaz and Rodríguez. This is an open-access article distributed under the terms of the Creative Commons Attribution License (CC BY). The use, distribution or reproduction in other forums is permitted, provided the original author(s) and the copyright owner(s) are credited and that the original publication in this journal is cited, in accordance with accepted academic practice. No use, distribution or reproduction is permitted which does not comply with these terms.
*Correspondence: Beatriz Reguera, YmVhdHJpei5yZWd1ZXJhQGllby5jc2ljLmVz