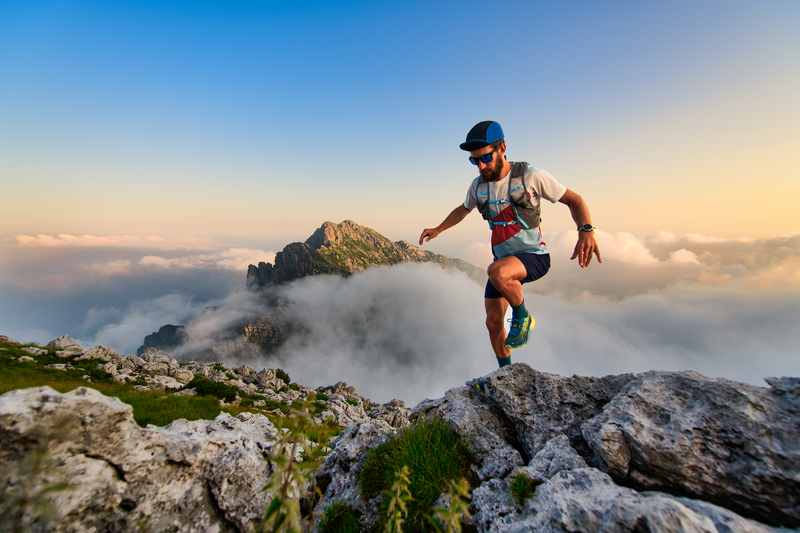
95% of researchers rate our articles as excellent or good
Learn more about the work of our research integrity team to safeguard the quality of each article we publish.
Find out more
ORIGINAL RESEARCH article
Front. Plant Sci. , 24 March 2025
Sec. Plant Abiotic Stress
Volume 16 - 2025 | https://doi.org/10.3389/fpls.2025.1549854
This article is part of the Research Topic Managing Metal Toxicity in Plants and Soil: Strategies for Stress Mitigation and Remediation View all 9 articles
Boron (B) is an essential micronutrient critical for crop growth and productivity. However, excessive boron concentrations can impair plant development, and detoxification remains a significant challenge. Understanding genetic variability and identifying tolerance mechanisms are crucial for developing boron-resistant cultivars. This study explores the physiological and molecular responses of two Actinidia species, namely kiwifruit (A.chinensis) and kiwiberry (A.arguta), to varying levels of excess B. Under excessive B conditions, B accumulation followed the order roots< stems< leaves, with maximum concentrations of 68.6 mg/kg, 105 mg/kg, and 160.7 mg/kg in AC, and 68.2 mg/kg, 107 mg/kg, and 196.9 mg/kg in AA, respectively. B toxicity symptoms appeared in AA when B levels exceeded 50 mg/kg, leading to a 15–20% reduction in dry weight across roots, stems, and leaves. AC exhibited greater sensitivity, with a 20–30% reduction in dry biomass. Both species showed significant declines in chlorophyll a and b content under B stress, with alterations in the chlorophyll a/b ratio and increased oxidative stress. Additionally, stress-responsive genes, including 1-aminocyclopropane-1-carboxylate synthase (Actinidia10066) and xyloglucan endotransglucosylase/hydrolase (Actinidia11948), were downregulated in response to B stress, suggesting potential disruptions in growth and development. These findings provide valuable insights into the differential physiological and molecular responses to excess boron in Actinidia species, laying a foundation for functional genomics research and the development of boron-tolerant kiwifruit cultivars.
Boron is a crucial element for the development and growth of vascular plants (Pereira et al., 2021; Bolaños et al., 2004; Shireen et al., 2018; Vera-Maldonado et al., 2024). It facilitates vital physiological processes, including carbohydrate distribution, plasma membrane transport, and cell wall stability (Chen et al., 2014; Liu et al., 2013; Shireen et al., 2018). Previous studies have established a strong correlation between optimal plant growth and adequate boron levels (Riaz et al., 2021). However, boron concentrations exceeding optimal levels can become toxic, negatively impacting plant health and growth (Miwa and Fujiwara, 2010). Different plant species exhibit varied tolerance to boron toxicity, with citrus being susceptible and some other species demonstrating higher resilience (Miwa and Fujiwara, 2010; Huang et al., 2014). Research into the morphological, physiological, and genetic responses in diverse species under excess boron stress provides critical insights for breeding programs that trigger the development of boron-tolerant varieties suitable for industrial purposes.
Excessive boron accumulation in soils presents a significant challenge, impeding growth and causing metabolic disruptions that can result in fruit deformation and progressive necrosis in leaves and stems (Reid et al., 2004; Landi et al., 2019). The primary phytotoxic effects of excess boron result from three key metabolic disturbances: (1) interference with cell division and development through binding with free ribose and RNA; (2) disruption of primary metabolism due to binding with ribose in ATP and NAD(P)H; and (3) reduction of cytosolic pH, affecting protein conformation and biosynthesis (Reid et al., 2004). The challenges in remediating boron toxicity, compounded by the limitations of current chemical and physical removal techniques, underscore the need for further research into alternative solutions (Laçin et al., 2015).
The toxic effects of excessive boron adversely affect various plant tissues, including leaves, stems, buds, fruits, and roots (Choi et al., 2007; Aquea et al., 2012; Esim et al., 2013). In leaves, boron toxicity can alter leaf thickness and disrupt the balance of photosynthetic pigments, including chlorophyll a and b, impairing photosynthetic efficiency (Zhang et al., 2023; Zou et al., 2024; Huang et al., 2014; Kayıhan et al., 2017). Reduced photosynthesis under high boron stress leads to increased reactive oxygen species (ROS) and elevated oxidative stress (Molassiotis et al., 2006; Cervilla et al., 2007; Ardıc et al., 2009; Çatav et al., 2018; Simón-Grao et al., 2019). Excess boron in stems induces the formation of cork and collenchyma cells, resulting in cell wall thickening in loquat (Papadakis et al., 2018; Wu X et al., 2019; Abdulnour et al., 2000; Wang et al., 2003), whereas it causes phloem cell wall thickening in citrus (Huang et al., 2014; Simón-Grao et al., 2018). Roots also exhibit adaptive responses, with decreased meristematic activity alongside increases in hypodermal thickening, cortical cell suberin deposition, and root lignification (Choi et al., 2007; Ghanati et al., 2002; Landi et al., 2019). These alterations indicate a detoxifying mechanism that prevents further uptake and reduces toxicity by sequestering excess boron in less sensitive tissues.
Kiwifruit (Actinidia spp.) is a vitamin C-rich fruit native to China, encompassing 54 species. The primary commercial varieties are A. chinensis, A. deliciosa, and A. arguta, which are widely cultivated in regions such as China, New Zealand, Italy, Greece, and Iran (Zhong et al., 2021). Previous studies have shown that appropriate levels of boron fertilization, such as foliar application of 0.3% sodium borate, can increase by 17.12% and dry matter content by 2.94% compared to controls (Long et al., 2015). Furthermore, boron application has been found to enhance chlorophyll content and dry matter accumulation in kiwifruit leaves, promoting photosynthesis (Ling et al., 2023). However, excessive boron fertilization can lead to B toxicity, which reduces both the yield and quality of kiwifruit. Understanding the biological role of boron, its effects, and its absorption mechanisms is thus crucial for managing boron toxicity in kiwifruit orchards (Chatzissavvidis and Antonopoulou, 2020). After boron toxicity occurs in kiwifruit, strategies such as improving irrigation practices and controlling industrial emissions has been proposed as potential methods to enhance boron tolerance in kiwifruit cultivation (Sotiropoulos et al., 2006; Zhao et al., 2024; Xia et al., 2021). Additionally, calcium application has been shown to reduce boron uptake in kiwifruit (Zhao et al., 2024; Liu et al., 2022). Numerous factors influence plant tolerance to boron toxicity, particularly those related to boron adsorption and translocation, which are crucial for improving tolerance in environments with excessive boron (Xia et al., 2021; Yıldırım and Uylaş, 2016). Research on resistance gene related to boron adsorption and translation in kiwifruit remains limited and needs systematic investigation.
This study aimed to investigate the effects of elevated boron on kiwifruit production, focusing on physiological changes, boron concentration, chlorophyll levels, and antioxidant activity. Additionally, RNA sequencing and transcriptomic approaches were utilized to assess the molecular and physiological effects of varying boron concentrations in kiwifruit. The findings provide direct evidence to explore interspecies genetic variability and facilitate breeding varieties with improved resistance to boron-excessive soils.
This study was conducted at the National Actinidia Germplasm Repository, Wuhan Botanical Garden, Chinese Academy of Sciences, Wuhan, China (24°02’19” N, 109°23’48” E, 28 m elevation). Sixty kiwifruit seedlings, comprising 30 Actinidia chinensis cv. ‘Donghong’ and 30 Actinidia arguta cv. ‘Mizhan no.1’, were cultivated under varying boron (B) concentrations while maintaining consistent fertilizer and irrigation practices. Seedlings with uniform stem diameters and heights were selected. Each seedling was pruned to a small remnant to promote new stem and leaf formation in response to B treatment. Plants were grown in 6-inch plastic pots containing a 1:1 mixture of vermiculite and perlite and irrigated with Hoagland nutrient solution containing essential nutrients, including ZnSO₄·7H2O (8.6 mg/L), MgSO₄·7H2O (493 mg/L), Fe-EDTA (2.5 mL), KH2PO₄ (136 mg/L), K2HPO₄ (0.83 mg/L), KNO₃ (506 mg/L), Ca(NO₃)2·4H2O (945 mg/L), MnCl2·4H2O (22.3 mg/L), (NH₄)₆Mo₇O2₄ (80 mg/L), and CuSO₄·.5H2O (0.025 mg/L) (Hoagland and Arnon, 1950). All chemicals used were high purity, and deionized water was used for irrigation. The pH of the solution was maintained at 6.0 by adding 2 mL ultrapure water or NaOH every two weeks. Watering was carried out every three days throughout the experiment. Five different boron concentrations were applied: WT (0 mg/L), 0.6 mg/L, 3 mg/L, 6 mg/L, and 9 mg/L B, with boron derived from H₃BO₃. The 0 mg/L B concentration was considered the wild type (WT), while 0.6 and 3 mg/L B were deemed adequate, and 6 and 9 mg/L B represented excess levels. Each treatment was replicated six times per genotype, resulting in 60 seedlings.
Five boron treatments were applied to each of the two kiwifruit species in a completely randomized design with six replications. Boron treatments started on July 1, with the five B concentrations (WT, 0.6, 3, 6, and 9 mg/L B) applied over a week (Abdulnour et al., 2000). Leaf samples were collected from each genotype exhibiting toxicity symptoms to determine the B concentrations. Samples were oven-dried at 68°C for three days, then digested with 5 mL concentrated nitric acid and 1 mL hydrogen peroxide in a microwave system (ETHOS ONE, Milestone, Italy). The digested solution was neutralized, diluted to a final volume of 50 mL, and analyzed using inductively coupled plasma optical emission spectroscopy (ICP-OES, Optima 2000DV, Perkin-Elmer, USA) with a recovery rate of over 94% verified using control standards (GBW07604).
At the end of the experiment stem length, stem diameter, leaf length, and leaf width of each seedling were measured using a digital Vernier caliper (DL 91150) and a 30 cm steel ruler. Stem and root dry weights were recorded after the samples were dried. Leaf samples from each boron (B) treatment were prepared for scanning electron microscopy (SEM) analysis. This process involved drying the samples with a carbon dioxide critical point dryer (CPD300, Leica), coating them with gold using a sputter coater (MC1000, Hitachi), and imaging with a scanning electron microscope (TM3030, Hitachi) (Sotiropoulos et al., 2002).
Photosynthesis was measured using the SPAD-502 chlorophyll meter (Konica Minolta, Japan) after differences between wild-type (WT) and B-toxicity treatments were observed. Chlorophyll content was determined from a 0.20 g fresh leaf sample prepared according to standard protocols (Hiscox and Israelstam, 1979). Samples were incubated with dimethyl sulfoxide in an 8 mL centrifuge tube at 28°C in a dark environment with 200 rpm agitation for 72 hours. Absorbances at 470 nm, 645 nm, and 663 nm were recorded with an ultraviolet spectrometer, and chlorophyll a, chlorophyll b, total chlorophyll, and the chlorophyll a/b ratio were calculated as follows:
Where VT is the total volume (mL) of the extract, VS is the volume of the mixed-in cuvette (µL), and W is the sample fresh weight (g).
To analyze superoxide dismutase (SOD) and catalase (CAT) activity, freeze 0.1 g of leaf segments in liquid nitrogen. Mix the frozen pieces with 2 mL of ice-cold phosphate buffer (1 M KH2PO4, pH 6.7), 0.1 g of polyvinylpyrrolidone (PVP), and 20 µL of phenylmethylsulfonyl fluoride (PMSF, 1 mM). Blend the mixture and centrifuge it at 12000 g at 4°C for 10 minutes. Use the supernatant to measure SOD activity at 560 nm and CAT activity at 240 nm (Yan et al., 2021). A 0.1 g sample of fresh tissue was homogenized with 10% trichloroacetic acid (TCA) and centrifuged at 12000 g for 10 minutes. Then, 200 µL of the extract was combined with an equal volume of 20.0% (w/v) TCA and a solution containing 20.0% TCA plus 0.65% thiobarbituric acid (TBA). The mixture was heated at 95°C for 30 minutes, cooled in an ice bath, and centrifuged again at 12000 g for 10 minutes. Malondialdehyde (MDA) content was determined at 532 nm and 600 nm (Imran et al., 2020). Additionally, activities of ascorbate peroxidase (APX) at 290 nm and peroxidase (POD) at 470 nm were quantified using assay kits (Beijing Solarbio Science & Technology Co., Ltd.).
Total RNA was extracted from A. arguta leaves using the RePure Plant RNA Kit (Magen, Guangzhou, China). Following quality verification, cDNA synthesis was performed with the One-Step gDNA Removal and cDNA Synthesis SuperMix kit (TransGen, Beijing, China). RNA-seq libraries were prepared, and Illumina sequencing was conducted for wild-type (WT, 0 mg/L) and boron treatments (3 mg/L and 9 mg/L) in A. arguta. The RNA-seq reads were processed using Trimmomatic to remove low-quality sequences, and clean reads were aligned to the kiwifruit v3 genome reference (Wu H, et al., 2019). Differentially expressed genes (DEGs) were identified using DESeq2 (version 1.26.0) with thresholds of P-value< 0.05 and |log2FoldChange| ≥ 2 (Love et al., 2014). Gene Ontology (GO) (Ashburner et al., 2000) and KEGG Pathway (Kanehisa et al., 2004) enrichment analyses were performed using hypergeometric tests. Enrichment results were analyzed and visualized with the cluster Profiler software (version 3.14.3) in R (v4.0.2) (Yu et al., 2012). Heatmaps were generated using TBtools (Chen et al., 2020). Protein-protein interaction networks for boron-responsive genes were constructed and visualized in Cytoscape (v3.10.2). Quantitative PCR (qPCR) was conducted using the TransStart® Green qPCR SuperMix kit (TransGen) as described by Wang et al. (2018), with three biological replicates per treatment. Gene expression validation was performed using the ΔΔCt method, and primer sequences are provided in Supplementary Table 2. The RNA-seq data have been deposited in GenBank (NCBI) under accession number PRJNA1144175.
Statistical analyses were conducted using a completely randomized design, comprising five treatments with 60 plants, each was replicated six times. Gene Ontology (GO), Kyoto Encyclopedia of Genes and Genomes (KEGG), Volcano plots, Venn diagrams, and metabolic pathway data were executed using R software (version 4.4.0). The cluster heatmap was generated with TBtools (version 10.4.0), while protein-protein interaction networks were constructed using Cytoscape (version 3.10.2). Graphical representations were generated with GraphPad Prism (version 10.3.0), and tabular data were organized in Excel 2019. The statistical significance of observed effects was assessed using analysis of variance (ANOVA), with post-hoc comparisons performed utilizing t-tests. A significance threshold of P< 0.05 was applied (IBM SPSS Statistics 23) to evaluate differences between treatments and genotypes.
Exogenous boron (B) application in kiwifruit species resulted in differential B accumulation across various tissues. Before treatment, soil B levels were deficient (0.070 mg/kg), and initial B concentrations in roots, stems, and older leaves were 0.26, 0.27, and 0.30 mg/kg, respectively. As B treatment levels increased, the most pronounced accumulation occurred between 3 mg/L and 6 mg/L, with B content in A. arguta leaves increasing 92-fold within this range. Under a 9 mg/L B treatment, B concentrations in AA tissues peaked at 78.2, 101, and 196.9 mg/kg in roots, stems, and older leaves, respectively (Figure 1B), representing increases of 78, 80, and 95 times compared to the wild type. Significant differences were also observed between the two kiwifruit species, A. chinensis and A. arguta. Under the same 9 mg/L treatment, B concentrations in AC tissues reached 70–98% of those in AA, with values of 73.4, 98.4, and 160.7 mg/kg in roots, stems, and older leaves, respectively (Figure 1C).
Figure 1. The proportion of B content in the leaf, stem, and root of (A) kiwifruit tree, (B) AA, and (C) AC kiwifruits were measured under five levels of B content. The line width indicates the proportion of stocks, and the box height indicates the percentage of total B content in each tissue.
Figure 1 illustrates the distribution of boron (B) in the leaves, stems, and roots of A. arguta and A. chinensis kiwifruit plants across five B treatment concentrations. The Sankey diagram highlights the leaves with the highest B levels under B stress. AA and AC leaves retained 55.86% and 48.48% of the total B, respectively. In contrast, B concentrations in AC stems and roots were 29.71% and 21.81%, while AA stems and roots contained lower proportions, at 25.21% and 18.94%, respectively. B translocation efficiency was assessed using transport factors (TFs) for root-to-stem (TF stem/root) and root-to-leaf (TF leaf/root) transport. AA exhibited a stronger TF leaf/root than AC, indicating a higher capacity for boron translocation to the leaves. However, AC demonstrated a greater TF stem/root (Supplementary Table 3). These findings suggest that both kiwifruit species, A. arguta and A. chinensis, are highly vulnerable to boron toxicity in their young organs. Conversely, the efficient leaf-to-root translocation in kiwifruit facilitates effective boron uptake from the soil, potentially aiding in the mitigation of soil contamination.
Boron toxicity significantly inhibited kiwifruit growth and development, with notable differences in growth parameters and dry matter accumulation across various B concentrations (Figures 2A, B). Symptoms of B toxicity, such as leaf chlorosis and growth inhibition, appeared within two weeks of treatment. Physiological parameters were significantly altered by B exposure over one month. At 3 mg/L B, growth was notably suppressed, with delayed leaf emergence and a color change in leaves from green to dark brown (Figures 2A, B). At 6 mg/L B, AC exhibited reductions in several growth parameters, including stem height (1.47 m), stem width (2.9 cm), stem dry weight (20.7 g), leaf length (13.5 cm), leaf width (8.9 cm), and root dry weight (9.5 g), showing declines of 20-50% compared to WT (Figure 3). Similarly, AA exhibited reductions in growth parameters (e.g., stem height, leaf length), but these were less pronounced (15-48%) than in AC. AA showed more excellent resistance to B toxicity than AC, as reflected by less overall growth inhibition.
Figure 2. The boron toxicity symptoms exhibited by Actinidia. arguta (A) and A. chinensis (B) plants under different boron concentrations after 15 and 30 days of treatment.
Figure 3. Kiwifruit seedling physiological parameters at different B concentrations. (A) Leaf length (cm) (B) Leave width (cm) (C) Stem weight (g-1 DW) (D) Stem length (cm) (E) Stem width (mm) (F) Root weight (g-1 DW). The Kiwifruit seedlings were treated with WT, 0.6, 3, 6, and 9 mg/L of B concentration of AC and AA. The significant difference among treatments by t-tests at P < 0.05.
Microscopic analysis of kiwifruit leaves under B stress revealed structural changes. Both wild-type AC and AA leaves showed typical epidermal cell sizes and well-organized palisade and spongy mesophyll cells. However, AC leaves treated with 0.6 mg/L and 3 mg/L B for 15 days exhibited a reduction in palisade and spongy mesophyll cell density and size (Figure 4A). In contrast, AA leaves maintained normal epidermal cell sizes, with only a slight reduction in mesophyll density, indicating milder toxicity symptoms (Figure 4B). At B concentrations exceeding 6 mg/L, both AC and AA leaves exhibited severe toxicity, including marked shrinkage and necrosis of cellular tissues, with AC leaves being more sensitive to boron toxicity at lower concentrations.
Figure 4. Micrographs of Cell Morphology in A. chinensis (A) and A. arguta (B) Leaves Exposed to Five Boron Concentrations (WT, 0.6, 3, 6, and 9 mg/L): Visualizing Upper Epidermis (UE), Palisade Cells (PC), and Lower Epidermis (LE), Scale Bar = 100 µm.
Excessive B treatments negatively impacted the chlorophyll content in kiwifruit leaves. Under prolonged irrigation, the chlorophyll concentrations in the AA and AC leaves remained low, with SPAD values of 16 and 13, respectively. The highest levels of chlorophyll a and b were observed with treatments of 0.6 and 3 mg/L B, which corresponded to SPAD values of 34 and 24 in the AA leaves. As B concentrations increased, chlorophyll a, b, and total chlorophyll levels declined progressively. The chlorophyll a/b ratio remained stable, indicating that both chlorophyll a and b were equally affected by boron toxicity (Figure 5). AC leaves exhibited higher overall chlorophyll concentrations compared to AA, likely due to species-specific traits. Both kiwifruit genotypes demonstrated tolerance to boron stress when subjected to treatment with 0.6 mg/L and 3 mg/L of boron.
Figure 5. Effects of Boron on SPAD Readings and Chlorophyll a and b Concentrations in AA and AC under Different Treatments. (A) SPAD-502 reading (B) Chlorophyll a (mg/kg FW) (C) Chlorophyll b (mg/kg FW) (D) Chlorophyll a/b (mg/kg FW) (E) Chlorophyll a+b (mg/kg FW). Error bars represent ± SD from six biological replicates (t-test, P < 0.05). WT (0), 0.6, and 3 mg/L represent optimal B levels; 6 and 9 mg/L represent excess B levels.
The antioxidant defense systems of the two kiwifruit species (AC and AA) exhibited similar physiological responses under boron stress. Notably, APX and CAT activities in both species sharply declined as boron concentration increased from 0.6 to 9 mg/L, with APX decreasing by 10.4% in AC and 2.9% in AA, and CAT decreasing by 7.2% in AC and 7.1% in AA at 9 mg/L B (Figure 6). Conversely, boron stress led to increased levels of MDA, POD, and SOD in both species (Tombuloglu et al., 2015). SOD activity rose by 28.66% in AC and 28.6% in AA, MDA levels increased by 28.7% in AC and 29.1% in AA, and POD levels increased by 31.7% in AC and 28.9% in AA under boron stress (Figure 6). These results suggest that AA is better adapted to boron stress than AC. The application of boron at concentrations of 0.6 mg/L and 3 mg/L significantly enhances antioxidant activity and increases tolerance to boron stress in both AC and AA.
Figure 6. Effects of B concentration on the antioxidant system of kiwifruits. (A) APX (U min-1 g-1 FW) (B) CAT (U g-1 FW) (C) POD (U min-1 g-1 FW) (D) SOD (U g-1 FW) (E) MDA (µmole g-1 FW). Kiwifruits were treated with WT, 0.6, 3, 6, and 9 mg/L B concentrations in AA and AC species. The error bar represents ± SD of six biological replicates at t-test P < 0.05. WT (0), 0.6 and 3 mg/L B as efficient B content, 6 and 9 mg/L B as excess B content.
Following boron concentration assessments in wild-type (WT) and boron-treated A. arguta leaves (Figure 1), RNA-seq analysis was conducted to identify gene expression changes under boron stress. After removing adaptor sequences and low-quality reads, WT, 3 mg/L B, and 9 mg/L B treatments generated 43,318,730, 42,063,369, and 43,335,754 clean reads, respectively. The kiwifruit reference transcriptome showed over 98% Q20 scores, over 95% Q30 scores, and a GC content exceeding 44%, indicating a high-quality sequencing library suitable for accurate gene expression analysis (Supplementary Table 1). Transcriptomic analysis revealed a clear separation between boron treatments and WT and boron-treated samples. Two critical comparisons were made: WT vs 3 mg/L B and WT vs 9 mg/L B. In the WT vs 3 mg/L B group, 3,573 differentially expressed genes (DEGs) were identified, with 2,010 upregulated and 1,563 downregulated genes. In WT vs 9 mg/L B, 12,146 DEGs were observed, with 5,763 upregulated and 6,383 downregulated genes (Figure 7C). Notably, the majority of DEGs were downregulated in the WT vs 9 mg/L B comparison, indicating a solid response to high boron stress. Venn diagram analysis showed that the highest number of DEGs (36,256) was found in the WT vs 3 mg/L B comparison, while the lowest number (35,682) was recorded in WT vs 9 mg/L B (Supplementary Figure 1F).
Figure 7. Analysis of differentially expressed genes (DEGs) related to boron (B) stress in A. arguta includes (A) KEGG enrichment of DEGs of WT vs 3 mg/L B, and (B) WT vs 9 mg/L B, (C) intraspecific pairwise comparison of kiwifruit treated with different B concentrations (D) Protein-protein interactions in kiwifruit genes affected by boron, with red for upregulated and yellow for downregulated genes. .
The KEGG pathway analysis identified 1,834 and 4,955 differentially expressed genes (DEGs) across 20 pathways for WT compared to 3 mg/L B and 9 mg/L B, respectively, metabolic pathways were the most enriched in both comparisons (Figures 7A, B). The heatmap showed gene expression changes in WT vs 3 mg/L B and WT vs 9 mg/L B. In the first comparison, Rhamnogalacturonate lyase (Actinidia06502) and AOP3 (Actinidia28035) were upregulated, while Cytochrome P450 (Actinidia12073) and Expansin (Actinidia38761) were downregulated. In the second comparison, Actinidia06502 and Actinidia28004 increased, whereas Xyloglucan endotransglucosylase (Actinidia11948) and Hexosyltransferase (Actinidia01673) decreased (Supplementary Figure 3A).
The Gene Ontology (GO) enrichment analysis of differentially expressed genes (DEGs) identified 62,032 annotated DEGs. Of these, 30,971 genes (49.93%) were associated with the comparison between wild type (WT) and 3 mg/L boron (B), while 31,061 genes (50.07%) were linked to the comparison between WT and 9 mg/L B (Supplementary Figures 1A, B). Key findings included prevalent Biological Process terms such as the regulation of transcription and translation. Significant Cellular Component terms highlighted integral membrane components and the nucleus, while important Molecular Functions included ATP and metal ion binding. Principal Component Analysis (PCA) was performed on the reliability among WT vs 3 mg/L B and WT vs 9 mg/L B DEGs (Supplementary Figure 3B). The volcano plot showed that in the WT vs 3 mg/L B comparison, there were 515 upregulated and 314 downregulated genes, while in the WT vs 9 mg/L B comparison, there were 1,522 upregulated and 486 downregulated genes (Supplementary Figures 1C, D). Lastly, the analysis of transcription factors (TFs) identified 21 families, with WRKY and NAC being upregulated and MYB and bHLH being downregulated (Supplementary Figure 1E).
The metabolic function of boron was investigated by examining boron-related pathways at WT vs 3 mg/L B and 9 mg/L B (Supplementary Figure 2). Several DEGs associated with cysteine/methionine metabolism, phenylpropanoid biosynthesis, inositol phosphate metabolism, and the MAPK signaling pathway were increased, as well as some genes related to protein processing in the endoplasmic reticulum under boron stress. On the other hand, DEGs involved in inositol phosphate metabolism, starch, and sucrose metabolism, plant hormone signal transduction, genetic information processing, and the MAPK signaling pathway were decreased by boron stress. Specifically, several DEGs involved in the MAPK signaling pathway were upregulated, such as the Pathogenesis-related protein 1 gene (Actinidia28004) and the Ethylene-responsive transcription factor 1 B-like gene (Actinidia40060). At the same time, most of the transcription factors MYC2 (i.e., Actinidia15025 and Actinidia28094) and FLS2 LRR receptor (i.e., Actinidia36397 and Actinidia37808) were downregulated by boron stress; this pathway contributes to boron tolerance. Additionally, plant hormone signal transduction genes (i.e., Actinidia11948, Actinidia37884), inositol phosphate metabolism genes (i.e., Actinidia11585 and Actinidia23530), and starch and sucrose metabolism genes (i.e., Actinidia19470, Actinidia28869) were also downregulated under boron stress (Supplementary Figure 2).
The protein-protein interaction (PPI) network analysis revealed 99 nodes and 113 edges. Significant differences in gene expression levels were observed. Notably, pectate lyase (Actinidia35948), Actinidia35179 (9-cis-epoxy carotenoid dioxygenase), Actinidia33909 (ferritin), and Actinidia40093 (Auxin-responsive protein) were identified as highly downregulated genes (Figure 7D). The auxin-responsive protein (Actinidia40093) demonstrated significant interactions with downregulated genes, supporting earlier research on auxin’s role in plant stress responses. Our findings indicate that genes related to boron stress and auxin interactions were significantly downregulated (Figure 7D).
Boron (B) is indispensable for plant growth and development, serving critical roles in cytoplasmic synthesis and cell wall formation by cross-linking rhamnogalacturonan II, a key cell wall component (Hu and Brown, 1994; O’Neill et al., 2001). However, excessive B uptake, particularly under conditions of high transpiration, disrupts osmotic balance, nutrient absorption, and the redistribution of essential metabolites such as starch and sugars (Macho-Rivero et al., 2018; Reid et al., 2004). This dual role of B as both an essential micronutrient and a stressor underscores its importance in agricultural systems. High B levels have been shown to significantly inhibit plant growth, as evidenced in rice and citrus rootstocks (de Abreu Neto et al., 2017; Wu et al., 2019). Our findings highlight that kiwifruit leaves accumulated the highest B concentrations among all treatments, consistent with the role of transpiration streams in B transport (Rees et al., 2011). Boron’s movement through roots and vascular tissues is critical for growth, but its excessive accumulation can impair growth, particularly in stems. Resistance mechanisms, such as efflux systems, help mitigate B toxicity. Similar trends have been reported in barley and wheat, where B toxicity resistance is associated with lower tissue B ratios (Sutton et al., 2007; Paull et al., 1991). Notably, low B treatments (0.6 and 3 mg/L B) improved growth in kiwifruit compared to the wild type (WT) and plants subjected to toxic conditions, aligning with findings in other crops (Sotiropoulos et al., 2002).
Excessive B induces physiological and biochemical disruptions, often accompanied by oxidative damage (Camacho-Cristóbal et al., 2015; Han et al., 2008; Kayıhan et al., 2016; Oiwa et al., 2013). Morphological changes, such as chlorosis and necrosis, were observed in kiwifruit leaves under B stress, particularly in the epidermal layers, leading to chlorosis at leaf tips that extended along the margins and culminated in significant leaf drop (Melnyk et al., 2005; Arredondo and Bonomelli, 2023). Root and stem development were also inhibited under high B conditions, manifesting as chlorosis and necrosis (Wimmer et al., 2015; Arredondo and Bonomelli, 2023). The observed toxicity symptoms in kiwifruit aligned with these phenotypes, confirming its sensitivity to excessive B. In addition, antioxidant enzyme activity and lipid peroxidation serve as indicators of plant sensitivity to B toxicity. Enhanced antioxidant activity under B stress has been documented in multiple crops (Zhou et al., 2017; Moustafa-Farag et al., 2016). In our study, Actinidia arguta (AA) demonstrated a more robust antioxidant response compared to Actinidia chinensis (AC). Wild-type (WT) plants exhibited reductions in superoxide dismutase (SOD), malondialdehyde (MDA), and catalase (CAT), whereas B toxicity led to increased MDA, peroxidase (POD), and SOD in both species, indicating the greater resilience of A. arguta (Riaz et al., 2021; Shah et al., 2017; Tombuloglu et al., 2015).
Transcriptomic analysis revealed that boron stress significantly altered gene expression in kiwifruit, highlighting pathways and genes associated with boron metabolism and stress responses. Under excessive B conditions, metabolic pathways related to cysteine, methionine, and phenylpropanoid biosynthesis were downregulated, while inositol phosphate metabolism was significantly activated (Supplementary Figure 2). Elevated expression of inositol oxidase 1 (Actinidia15291), involved in ascorbic acid synthesis, supports its role in enhancing antioxidant production under B stress (Michailidis et al., 2023; Lukaszewski and Blevins, 1996). Conversely, reduced expression of cinnamyl alcohol dehydrogenase (Actinidia35356), a key enzyme in lignin biosynthesis, indicated that B stress affects cell wall rigidity (Michailidis et al., 2023). Auxin-responsive genes, such as Actinidia40093, were implicated in growth adaptation under B stress. Auxins are critical for cellular elongation, division, and differentiation. Studies have shown that specific auxin response factors (ARFs) regulate distinct developmental processes. Our findings suggest that Actinidia40093 plays a pivotal role in mediating growth inhibition under B stress, underscoring its importance in plant adaptation to varying boron levels.
Identifying B-resistant genes or those encoding boric acid transporters, such as BOR1 and NIP5, is crucial for managing B toxicity (Takano et al., 2002, 2006). Previous research has demonstrated that Arabidopsis MYB transcription factors AtMYB13 and AtMYB69 enhance B tolerance without altering B levels (Nozawa et al., 2006). Similarly, suppressing the NAC-like factor BET-1 improved B tolerance in rice (Ochiai et al., 2011). Transcription factors such as WRKY42 and MYB44 regulate genes associated with B transport, ROS scavenging, and cellular homeostasis, playing vital roles in B-stress responses (Feng et al., 2021; Singhal et al., 2023; Wang et al., 2018).
Our transcriptomic analysis identified WRKY41 and NAC33 homologs in kiwifruit as potential regulators of B toxicity tolerance (Supplementary Figure 4). These findings, combined with the differential expression of metabolic genes such as Actinidia15025, Actinidia36397, and Actinidia11948, highlight the intricate regulatory networks governing B stress responses. Protein-protein interaction analyses further revealed Actinidia33909 and Actinidia40093 as central nodes, emphasizing their functional relevance in B toxicity tolerance.
Several strategies have been employed to mitigate boron toxicity in crops, including agronomic, chemical, and biological approaches. Effective irrigation and soil management practices, such as improving drainage and using low-boron irrigation water, help leach excess borron from the rhizosphere (Sotiropoulos et al., 2006). Adjusting soil pH can optimize boron availability (Zhao et al., 2024), while calcium amendments, such as calcium carbonate or sulfate, reduce boron uptake by altering soil ionic balance (Zhao et al., 2024; Liu et al., 2022). However, while these short-term interventions provide immediate relief, long-term solutions must focus on breeding boron-resistant crop varieties. Breeding such varieties is critical for enhancing agricultural productivity and ensuring the sustainability of industries reliant on boron-sensitive crops, such as kiwifruit, citrus, and grains. Advances in molecular genetics have identified genotypes with inherent tolerance to boron toxicity, particularly those exhibiting reduced boron uptake and efficient vacuolar sequestration (Princi et al., 2016; Hua et al., 2021). Our research has also identified variations in boron tolerance among different kiwifruit genotypes and uncovered boron toxicity response genes, laying the groundwork for biotechnological applications in breeding resistant varieties. In the future, molecular tools like Marker-Assisted Selection (MAS) will facilitate the identification of boron tolerance-related genotype and genes (Emebiri et al., 2009), supporting the development of resistant varieties.
This study demonstrates that excess boron (B) significantly impacts key physiological parameters in kiwifruit, including chlorophyll concentration, Boron accumulation, and antioxidant activity. The Actinidia chinensis genotype shows a higher sensitivity to boron (B) stress compared to Actinidia arguta, emphasizing the genotype-specific responses identified in this study. This research clarifies the physiological and molecular effects of boron excess in kiwifruit, highlighting the importance of dose-dependent reactions. Key transcription factors, such as WRKY41 and NAC33, along with stress-responsive genes like Actinidia17814 and Actinidia24652, play crucial roles in responding to boron (B) toxicity. Additionally, metabolic genes (e.g., Actinidia15025, Actinidia36397, Actinidia11948) and protein-protein interaction hubs (Actinidia33909 and Actinidia40093) highlight the complex regulatory networks that govern adaptation to B stress. These findings provide critical insights into the transcriptional and metabolic reprogramming triggered by B toxicity. They establish a foundation for future research to unravel specific regulatory mechanisms and interactions, ultimately enabling the development of B-tolerant kiwifruit cultivars. Such advancements will support sustainable crop production in high-B environments.
The datasets presented in this study can be found in online repositories. The names of the repository/repositories and accession number(s) can be found in the article/Supplementary Material.
DL: Conceptualization, Data curation, Formal Analysis, Funding acquisition, Investigation, Methodology, Project administration, Resources, Software, Supervision, Validation, Visualization, Writing – original draft, Writing – review & editing. GC: Conceptualization, Data curation, Formal Analysis, Investigation, Methodology, Resources, Software, Supervision, Validation, Visualization, Writing – original draft, Writing – review & editing. AM: Data curation, Formal Analysis, Methodology, Validation, Visualization, Writing – original draft, Writing – review & editing. MM: Writing – original draft, Writing – review & editing. EG: Writing – review & editing, Methodology, Formal analysis, Validation, Visualization, Software. XX: Conceptualization, Data curation, Formal Analysis, Funding acquisition, Investigation, Methodology, Project administration, Resources, Software, Supervision, Validation, Visualization, Writing – original draft, Writing – review & editing. XL: Writing – review & editing, Conceptualization, Data curation, Formal Analysis, Investigation, Methodology, Supervision, Validation, Writing – original draft. HL: Data curation, Investigation, Methodology, Supervision, Writing – review & editing. HT: Data curation, Investigation, Methodology, Supervision, Writing – review & editing. CZ: Conceptualization, Funding acquisition, Investigation, Project administration, Supervision, Writing – review & editing.
The author(s) declare that financial support was received for the research and/or publication of this article. This project was supported by National Key Research and Development Program of China (2024YFE0214500), Hubei Province Natural Science Fund Project of Outstanding Youth Project Awarded to Dawei Li (2023AFA075), the National Natural Science Foundation of China (32302474), CARS(CARS-26) and Hubei Province Natural Science and Technology resource database.
The authors declare that the research was conducted in the absence of any commercial or financial relationships that could be construed as a potential conflict of interest.
The author(s) declare that no Generative AI was used in the creation of this manuscript.
All claims expressed in this article are solely those of the authors and do not necessarily represent those of their affiliated organizations, or those of the publisher, the editors and the reviewers. Any product that may be evaluated in this article, or claim that may be made by its manufacturer, is not guaranteed or endorsed by the publisher.
The Supplementary Material for this article can be found online at: https://www.frontiersin.org/articles/10.3389/fpls.2025.1549854/full#supplementary-material
Abdulnour, J. E., Donnelly, D. J., Barthakur, N. N. (2000). The effect of boron on calcium uptake and growth in micropropagated potato plantlets. Potato Res. 43, 287–295. doi: 10.1007/bf02358088
Aquea, F., Federici, F., Moscoso, C., Vega, A., Jullian, P., Haseloff, J. I. M., et al. (2012). A molecular framework for the inhibition of Arabidopsis root growth in response to boron toxicity. Plant Cell Environ. 35, 719–734. doi: 10.1111/j.1365-3040.2011.02446
Ardıc, M., Sekmen, A. H., Tokur, S., Ozdemir, F., Turkan, I. (2009). Antioxidant responses of chickpea plants subjected to boron toxicity. Plant Biol. 11, 328–338. doi: 10.1111/j.1438-8677.2008.00132
Arredondo, G., Bonomelli, C. (2023). Effect of three boron concentrations in soil on growth and physiology in sweet cherry trees. Plants 12, 1240. doi: 10.3390/plants12061240
Ashburner, M., Ball, C. A., Blake, J. A., Botstein, D., Butler, H., Cherry, J. M., et al. (2000). Gene ontology: tool for the unification of biology. Nat. Genet. 25, 25–29. doi: 10.1038/75556
Bolaños, L., Lukaszewski, K., Bonilla, I., Blevins, D. (2004). Why boron? Plant Physiol. Biochem. 42, 907–912. doi: 10.1016/j.plaphy.2004.11.002
Camacho-Cristóbal, J. J., Martín-Rejano, E. M., Herrera-Rodríguez, M. B., Navarro-Gochicoa, M. T., Rexach, J., González-Fontes, A. (2015). Boron deficiency inhibits root cell elongation via an ethylene/auxin/ROS-dependent pathway in Arabidopsis seedlings. J. Exp. Bot. 66, 3831–3840. doi: 10.1093/jxb/erv186
Çatav, Ş.S., Genç, T. O., Kesik Oktay, M., Küçükakyüz, K. (2018). Effect of boron toxicity on oxidative stress and genotoxicity in wheat (Triticum aestivum L.). Bulletin of Environmental Contamination and Toxicology. 100, 502–508. doi: 10.1007/s00128-018-2292-x
Cervilla, L. M., Blasco, B., Ríos, J. J., Romero, L., Ruiz, J. M. (2007). Oxidative stress and antioxidants in tomato (Solanum lycopersicum) plants subjected to boron toxicity. Ann. Bot. 100, 747–756. doi: 10.1093/aob/mcm156
Chatzissavvidis, C., Antonopoulou, C. (2020). “Chapter 16,” in Boron toxicity in fruit crops: Agronomic and physiological implications. Eds. Srivastava, A. K., Hu, C. B. T.-F. C. (Elsevier), 211–221. doi: 10.1016/B978-0-12-818732-6.00016-2
Chen, C., Chen, H., Zhang, Y., Thomas, H. R., Frank, M. H., He, Y., et al. (2020). TBtools: an integrative toolkit developed for interactive analyses of big biological data. Mol. Plant 13, 1194–1202. doi: 10.1016/j.molp.2020.06.009
Chen, M., Mishra, S., Heckathorn, S. A., Frantz, J. M., Krause, C. (2014). Proteomic analysis of Arabidopsis thaliana leaves in response to acute boron deficiency and toxicity reveals effects on photosynthesis, carbohydrate metabolism, and protein synthesis. J. Plant Physiol. 171, 235–242. doi: 10.1016/j.jplph.2013.07.008
Choi, E., Kolesik, P., McNeill, A. N. N., Collins, H., Zhang, Q., Huynh, B., et al. (2007). The mechanism of boron tolerance for maintenance of root growth in barley (Hordeum vulgare L.). Plant Cell Environ. 30, 984–993. doi: 10.1111/j.1365-3040.2007.01693.x
de Abreu Neto, J. B., Hurtado-Perez, M. C., Wimmer, M. A., Frei, M. (2017). Genetic factors underlying boron toxicity tolerance in rice: genome-wide association study and transcriptomic analysis. J. Exp. Bot. 68, 687–700. doi: 10.1093/jxb/erw423
Emebiri, L. C., Michael, P., Moody, D. B. (2009). Enhanced tolerance to boron toxicity in two-rowed barley by marker-assisted introgression of favourable alleles derived from Sahara 3771. Plant Soil 314, 77–85. doi: 10.1007/s11104-008-9707-0
Esim, N., Tiryaki, D., Karadagoglu, O., Atici, O. (2013). Toxic effects of boron on growth and antioxidant system parameters of maize (Zea mays L.) roots. Toxicol. Ind. Health 29, 800–805. doi: 10.1177/0748233712442729
Feng, Y., Cui, R., Huang, Y., Shi, L., Wang, S., Xu, F. (2021). Repression of transcription factor AtWRKY47 confers tolerance to boron toxicity in Arabidopsis thaliana. Ecotoxicology Environ. Saf. 220, 112406. doi: 10.1016/j.ecoenv.2021.112406
Ghanati, F., Morita, A., Yokota, H. (2002). Induction of suberin and increase of lignin content by excess boron in tobacco cells. Soil Sci. Plant Nutr. 48, 357–364. doi: 10.1080/00380768.2002.10409212
Han, S., Chen, L.-S., Jiang, H.-X., Smith, B. R., Yang, L.-T., Xie, C.-Y. (2008). Boron deficiency decreases growth and photosynthesis, and increases starch and hexoses in leaves of citrus seedlings. J. Plant Physiol. 165, 1331–1341. doi: 10.1016/j.jplph.2007.11.002
Hiscox, J. D., Israelstam, G. F. (1979). A method for the extraction of chlorophyll from leaf tissue without maceration. Can. J. Bot. 57, 1332–1334. doi: 10.1139/b79-163
Hoagland, D. R., Arnon, D. I. (1950). The water-culture method for growing plants without soil. Circular. California Agric. Experiment Station 347. doi: 10.1016/j.jplph.2007.11.002
Hu, H., Brown, P. H. (1994). Localization of boron in cell walls of squash and tobacco and its association with pectin (evidence for a structural role of boron in the cell wall). Plant Physiol. 105, 681–689. doi: 10.1104/pp.105.2.681
Hua, T., Zhang, R., Sun, H., Liu, C. (2021). Alleviation of boron toxicity in plants: Mechanisms and approaches. Crit. Rev. Environ. Sci. Technol. 51, 2975–3015. doi: 10.1080/10643389.2020.1807451
Huang, J.-H., Cai, Z.-J., Wen, S.-X., Guo, P., Ye, X., Lin, G.-Z., et al. (2014). Effects of boron toxicity on root and leaf anatomy in two citrus species differing in boron tolerance. Trees 28, 1653–1666. doi: 10.1007/s00468-014-1075-1
Imran, M., Sun, X., Hussain, S., Ali, U., Rana, M. S., Rasul, F., et al. (2020). Molybdenum application regulates oxidative stress tolerance in winter wheat under different nitrogen sources. J. Soil Sci. Plant Nutr. 20, 1827–1837. doi: 10.1007/s42729-020-00254-6
Kanehisa, M., Goto, S., Kawashima, S., Okuno, Y., Hattori, M. (2004). The KEGG resource for deciphering the genome. Nucleic Acids Res. 32, 277–280. doi: 10.1093/nar/gkh063
Kayıhan, D. S., Kayıhan, C., Çiftçi, Y. Ö. (2016). Excess boron responsive regulations of antioxidative mechanism at physio-biochemical and molecular levels in Arabidopsis thaliana. Plant Physiology and Biochemistry, 109, 337–345. doi: 10.1016/j.plaphy.2016.10.016
Kayıhan, C., Öz, M. T., Eyidoğan, F., Yücel, M., Öktem, H. A. (2017). Physiological, biochemical, and transcriptomic responses to boron toxicity in leaf and root tissues of contrasting wheat cultivars. Plant Mol. Biol. Rep. 35, 97–109. doi: 10.1007/s11105-016-1008-9
Laçin, B., Ertit Taştan, B., Dönmez, G. (2015). Detection of boron removal capacities of different microorganisms in wastewater and effective removal process. Water Sci. Technol. 72, 1832–1839. doi: 10.2166/wst.2015.409
Landi, M., Margaritopoulou, T., Papadakis, I. E., Araniti, F. (2019). Boron toxicity in higher plants: an update. Planta 250, 1011–1032. doi: 10.1007/s00425-019-03220-4
Ling, Q., Ha., S., Luo, H., Miao, C., Wang, Y., Wang, R. (2023). Effects of different types of boron on growth, photosynthetic characteristics and fruit quality of kiwifruit. Northern Horticulture 2023, 10–16. doi: 10.11937/bfyy.20221896
Liu, Y., Riaz, M., Lei, Y., Yu, Z., Jiang, C. (2022). Exogenous calcium reduces boron toxicity by regulating efficient antioxidant system and boron forms in trifoliate rootstock. J. Plant Nutr. 45, 1601–1613. doi: 10.1080/01904167.2021.2003396
Liu, G.-D., Wang, R.-D., Liu, L.-C., Wu, L.-S., Jiang, C.-C. (2013). Cellular boron allocation and pectin composition in two citrus rootstock seedlings differing in boron-deficiency response. Plant Soil 370, 555–565. doi: 10.1007/s11104-013-1659-3
Long, Y. H., Zhang, C., Wu, X. M., Yin, X. H., Zhao, W. (2015). Effect of foliar application of boron nutrition on kiwifruit quality and yield. Northern Horticulture 5, 9–12. doi: 10.11937/bfyy.201505004
Love, M. I., Huber, W., Anders, S. (2014). Moderated estimation of fold change and dispersion for RNA-seq data with DESeq2. Genome Biol. 15, 1–21. doi: 10.1186/s13059-014-0550-8
Lukaszewski, K. M., Blevins, D. G. (1996). Root growth inhibition in boron-deficient or aluminum-stressed squash may be a result of impaired ascorbate metabolism. Plant Physiol. 112, 1135–1140. doi: 10.1104/pp.112.3.1135
Macho-Rivero, M. A., Herrera-Rodríguez, M. B., Brejcha, R., Schäffner, A. R., Tanaka, N., Fujiwara, T., et al. (2018). Boron toxicity reduces water transport from root to shoot in Arabidopsis plants. Evidence for a reduced transpiration rate and expression of major PIP aquaporin genes. Plant Cell Physiol. 59, 841–849. doi: 10.1093/pcp/pcy026
Melnyk, L., Goncharuk, V., Butnyk, I., Tsapiuk, E. (2005). Boron removal from natural and waste waters using combined sorption/membrane process. Desalination 185, 147–157. doi: 10.1016/j.desal.2005.02.076
Michailidis, M., Bazakos, C., Kollaros, M., Adamakis, I. S., Ganopoulos, I., Molassiotis, A., et al. (2023). Boron stimulates fruit formation and reprograms developmental metabolism in sweet cherry. Physiologia Plantarum 175, e13946. doi: 10.1111/ppl.13946
Miwa, K., Fujiwara, T. (2010). Boron transport in plants: co-ordinated regulation of transporters. Ann. Bot. 105, 1103–1108. doi: 10.1093/aob/mcq044
Molassiotis, A., Sotiropoulos, T., Tanou, G., Diamantidis, G., Therios, I. (2006). Boron-induced oxidative damage and antioxidant and nucleolytic responses in shoot tips culture of the apple rootstock EM 9 (Malus domestica Borkh). Environ. Exp. Bot. 56, 54–62. doi: 10.1016/j.envexpbot.2005.01.002
Moustafa-Farag, M., Bingsheng, F., Malangisha Guy, K., Hu, Z., Yang, J., Zhang, M. (2016). Activated antioxidant enzymes-reduced malondialdehyde concentration, and improved mineral uptake-promoted watermelon seedlings growth under boron deficiency. J. Plant Nutr. 39, 1989–2001. doi: 10.1080/01904167.2015.1105263
Nozawa, A., Miwa, K., Kobayashi, M., Fujiwara, T. (2006). Isolation of Arabidopsis thaliana cDNAs that confer yeast boric acid tolerance. Bioscience Biotechnology Biochem. 70, 1724–1730. doi: 10.1271/bbb.60065
O’Neill, M. A., Eberhard, S., Albersheim, P., Darvill, A. G. (2001). Requirement of borate cross-linking of cell wall rhamnogalacturonan II for Arabidopsis growth. Science 294, 846–849. doi: 10.3390/plants12040777
Ochiai, K., Shimizu, A., Okumoto, Y., Fujiwara, T., Matoh, T. (2011). Suppression of a NAC-like transcription factor gene improves boron-toxicity tolerance in rice. Plant Physiol. 156, 1457–1463. doi: 10.1104/pp.110.171470
Oiwa, Y., Kitayama, K., Kobayashi, M., Matoh, T. (2013). Boron deprivation immediately causes cell death in growing roots of Arabidopsis thaliana (L.) Heynh. Soil Sci. Plant Nutr. 59, 621–627. doi: 10.1080/00380768.2013.813382
Papadakis, I. E., Tsiantas, P. I., Tsaniklidis, G., Landi, M., Psychoyou, M., Fasseas, C. (2018). Changes in sugar metabolism associated to stem bark thickening partially assist young tissues of Eriobotrya japonica seedlings under boron stress. J. Plant Physiol. 231, 337–345. doi: 10.1016/j.jplph.2018.10.012
Paull, J. G., Rathjen, A. J., Cartwright, B. (1991). Major gene control of tolerance of bread wheat (Triticum aestivum L.) to high concentrations of soil boron. Euphytica 55, 217–228. doi: 10.1007/bf00021242
Pereira, G. L., Siqueira, J. A., Batista-Silva, W., Cardoso, F. B., Nunes-Nesi, A., Araújo, W. L. (2021). Boron: More than an essential element for land plants? Front. Plant Sci. 11. doi: 10.3389/fpls.2020.610307
Princi, M. P., Lupini, A., Araniti, F., Longo, C., Mauceri, A., Sunseri, F., et al. (2016). “Chapter 5,” in Boron toxicity and tolerance in plants: Recent advances and future perspectives. Ed. Ahmad, P. B. T.-P. M. I. (Elsevier), 115–147. doi: 10.1016/B978-0-12-803158-2.00005-9
Rees, R., Robinson, B. H., Menon, M., Lehmann, E., Günthardt-Goerg, M. S., Schulin, R. (2011). Boron accumulation and toxicity in hybrid poplar (Populus nigra× euramericana). Environ. Sci. Technol. 45, 10538–10543. doi: 10.1021/es201100b
Reid, R. J., Hayes, J. E., Post, A., Stangoulis, J. C. R., Graham, R. D. (2004). A critical analysis of the causes of boron toxicity in plants. Plant Cell Environ. 27, 1405–1414. doi: 10.1111/j.1365-3040.2004.01243.x
Riaz, M., Kamran, M., El-Esawi, M. A., Hussain, S., Wang, X. (2021). Boron-toxicity induced changes in cell wall components, boron forms, and antioxidant defense system in rice seedlings. Ecotoxicology Environ. Saf. 216, 112192. doi: 10.1016/j.ecoenv.2021.112192
Shah, A., Wu, X., Ullah, A., Fahad, S., Muhammad, R., Yan, L., et al. (2017). Deficiency and toxicity of boron: alterations in growth, oxidative damage and uptake by citrange orange plants. Ecotoxicology Environ. Saf. 145, 575–582. doi: 10.1016/j.ecoenv.2017.08.003
Shireen, F., Nawaz, M. A., Chen, C., Zhang, Q., Zheng, Z., Sohail, H., et al. (2018). Boron: functions and approaches to enhance its availability in plants for sustainable agriculture. In Int. J. Mol. Sci. 19, 1856. doi: 10.3390/ijms19071856
Simón-Grao, S., Nieves, M., Martínez-Nicolás, J. J., Alfosea-Simón, M., Cámara-Zapata, J. M., Fernández-Zapata, J. C., et al. (2019). Arbuscular mycorrhizal symbiosis improves tolerance of Carrizo citrange to excess boron supply by reducing leaf B concentration and toxicity in the leaves and roots. Ecotoxicology Environ. Saf. 173, 322–330. doi: 10.1016/j.ecoenv.2019.02.030
Simón-Grao, S., Nieves, M., Martínez-Nicolás, J. J., Cámara-Zapata, J. M., Alfosea-Simón, M., García-Sánchez, F. (2018). Response of three citrus genotypes used as rootstocks grown under boron excess conditions. Ecotoxicology Environ. Saf. 159, 10–19. doi: 10.1016/j.ecoenv.2018.04.042
Singhal, R. K., Fahad, S., Kumar, P., Choyal, P., Javed, T., Jinger, D., et al. (2023). Beneficial elements: New Players in improving nutrient use efficiency and abiotic stress tolerance. Plant Growth Regul. 100, 237–265. doi: 10.1007/s10725-022-00843-8
Sotiropoulos, T. E., Dimassi, K. N., Tsirakoglou, V. (2006). Effect of boron and methionine on growth and ion content in kiwifruit shoots cultured in vitro. Biol. Plantarum 50, 300–302. doi: 10.1007/s10535-006-0025-1
Sotiropoulos, T. E., Therios, I. N., Dimassi, K. N., Bosabalidis, A., Kofidis, G. (2002). Nutritional status, growth, CO2 assimilation, and leaf anatomical responses in two kiwifruit species under boron toxicity. J. Plant Nutr. 25, 1249–1261. doi: 10.1081/pln-120004386
Sutton, T., Baumann, U., Hayes, J., Collins, N. C., Schnurbusch, T., Hay, A., et al. (2007). Boron-toxicity tolerance in barley arising from efflux transporter amplification. Science 318, 1446–1449. doi: 10.1126/science.1146853
Takano, J., Noguchi, K., Yasumori, M., Kobayashi, M., Gajdos, Z., Miwa, K., et al. (2002). Arabidopsis boron transporter for xylem loading. Nature 420, 337–340. doi: 10.1038/nature01139
Takano, J., Wada, M., Ludewig, U., Schaaf, G., von Wirén, N., Fujiwara, T. (2006). The Arabidopsis major intrinsic protein NIP5; 1 is essential for efficient boron uptake and plant development under boron limitation. Plant Cell 18, 1498–1509. doi: 10.1105/tpc.106.041640
Tombuloglu, G., Tombuloglu, H., Sakcali, M. S., Unver, T. (2015). High-throughput transcriptome analysis of barley (Hordeum vulgare) exposed to excessive boron. Gene 557, 71–81. doi: 10.1016/j.gene.2014.12.012
Vera-Maldonado, P., Aquea, F., Reyes-Díaz, M., Cárcamo-Fincheira, P., Soto-Cerda, B., Nunes-Nesi, A., et al. (2024). Role of boron and its interaction with other elements in plants. Front. Plant Sci. 15. doi: 10.3389/fpls.2024.1332459
Wang, H., Wang, Y., Du, C., Xu, F., Yang, Y. (2003). Effects of boron and calcium supply on calcium fractionation in plants and suspension cells of rape cultivars with different boron efficiency. J. Plant Nutr. 26, 789–806. doi: 10.1081/pln-120018565
Wang, Z., Wang, S., Li, D., Zhang, Q., Li, L., Zhong, C., et al. (2018). Optimized paired-sgRNA/Cas9 cloning and expression cassette triggers high-efficiency multiplex genome editing in kiwifruit. Plant Biotechnol. J. 16, 1424–1433. doi: 10.1111/pbi.12884
Wimmer, M. A., Goldberg, S., Gupta, U. C. (2015). “8 boron,” in Handbook of Plant Nutrition, vol. 305. . Available at: https://www.ars.usda.gov/research/publications/publication/?seqNo115=300733.
Wu, X., Lu, X., Riaz, M., Yan, L., Jiang, C. (2019). Boron toxicity induced specific changes of cell ultrastructure and architecture of components in leaf center and tip of trifoliate orange [Poncirus trifoliata (L.) Raf. J. Environ. Manage. 246, 426–433. doi: 10.1016/j.jenvman.2019.05.148
Wu, H., Ma, T., Kang, M., Ai, F., Zhang, J., Dong, G., et al. (2019). A high-quality Actinidia chinensis (kiwifruit) genome. Horticulture Res. 6, 117. doi: 10.1038/s41438-019-0202-y
Xia, J., Hua, T., Xue, Y., Zhao, L., Sun, H., Liu, C. (2021). Myriophyllum elatinoides: A potential candidate for the phytoremediation of water with low level boron contamination. J. Hazardous Materials 401, 123333. doi: 10.1016/j.jhazmat.2020.123333
Yan, L., Riaz, M., Liu, J., Liu, Y., Zeng, Y., Jiang, C. (2021). Boron reduces aluminum deposition in alkali-soluble pectin and cytoplasm to release aluminum toxicity. J. Hazardous Materials 401, 123388. doi: 10.1016/j.jhazmat.2020.123388
Yıldırım, K., Uylaş, S. (2016). Genome-wide transcriptome profiling of black poplar (Populus nigra L.) under boron toxicity revealed candidate genes responsible in boron uptake, transport and detoxification. Plant Physiol. Biochem. 109, 146–155. doi: 10.1016/j.plaphy.2016.09.015
Yu, G., Wang, L.-G., Han, Y., He, Q.-Y. (2012). clusterProfiler: an R package for comparing biological themes among gene clusters. Omics: A J. Integr. Biol. 16, 284–287. doi: 10.1089/omi.2011.0118
Zhang, Q., Ackah, M., Wang, M., Amoako, F. K., Shi, Y., Wang, L., et al. (2023). The impact of boron nutrient supply in mulberry (Morus alba) response to metabolomics, enzyme activities, and physiological parameters. Plant Physiol. Biochem. 200, 107649. doi: 10.1016/j.plaphy.2023.107649
Zhao, S., Huq, M. E., Fahad, S., Kamran, M., Riaz, M. (2024). Boron toxicity in plants: understanding mechanisms and developing coping strategies; a review. Plant Cell Rep. 43, 238. doi: 10.1007/s00299-024-03317-5
Zhong, C., Huang, H., Li, D., Zhang, Q., Li, L. (2021). Analysis of the development of the world kiwifruit industry and fresh fruit trade dynamics. China Fruits, 101–108. Available at: https://scholar.google.com/scholar?cluster=257819080453347695&hl=de&as_sdt=2005&sciodt=0,5.
Zhou, T., Hua, Y., Xu, F. (2017). Involvement of reactive oxygen species and Ca2+ in the differential responses to low-boron in rape seed genotypes. Plant Soil 419, 219–236. doi: 10.1007/s11104-017-3337-3
Zou, J., Zhang, Q., Amoako, F. K., Ackah, M., Li, H., Shi, Y., et al. (2024). Genome-wide transcriptome profiling of mulberry (Morus alba) response to boron deficiency and toxicity reveal candidate genes associated with boron tolerance in leaves. Plant Physiol. Biochem. 207, 108316. doi: 10.1016/j.plaphy.2023.108316
Keywords: kiwifruit, B function, boron stress, physiological response, gene regulation
Citation: Chalchisa G, Muzahid ANM, Mollah MDA, Gasura E, Xie X, Liu X, Lv H, Tian H, Zhong C and Li D (2025) Kiwifruit sensitivity to boron: impact on physiological and molecular responses. Front. Plant Sci. 16:1549854. doi: 10.3389/fpls.2025.1549854
Received: 22 December 2024; Accepted: 24 February 2025;
Published: 24 March 2025.
Edited by:
Yousif Abdelrahman Yousif Abdellah, Chinese Academy of Sciences (CAS), ChinaReviewed by:
Shanshan Sun, Heilongjiang University, ChinaCopyright © 2025 Chalchisa, Muzahid, Mollah, Gasura, Xie, Liu, Lv, Tian, Zhong and Li. This is an open-access article distributed under the terms of the Creative Commons Attribution License (CC BY). The use, distribution or reproduction in other forums is permitted, provided the original author(s) and the copyright owner(s) are credited and that the original publication in this journal is cited, in accordance with accepted academic practice. No use, distribution or reproduction is permitted which does not comply with these terms.
*Correspondence: Dawei Li, bGlkYXdlaUB3YmdjYXMuY24=; Caihong Zhong, emhvbmdjaEB3YmdjYXMuY24=
†These authors share first authorship
Disclaimer: All claims expressed in this article are solely those of the authors and do not necessarily represent those of their affiliated organizations, or those of the publisher, the editors and the reviewers. Any product that may be evaluated in this article or claim that may be made by its manufacturer is not guaranteed or endorsed by the publisher.
Research integrity at Frontiers
Learn more about the work of our research integrity team to safeguard the quality of each article we publish.