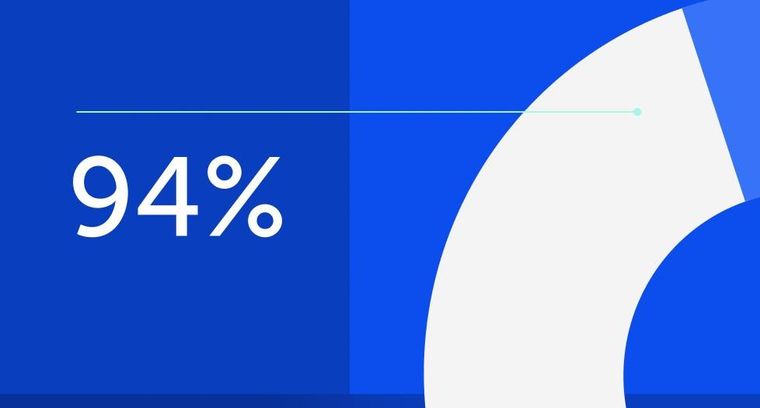
94% of researchers rate our articles as excellent or good
Learn more about the work of our research integrity team to safeguard the quality of each article we publish.
Find out more
ORIGINAL RESEARCH article
Front. Plant Sci., 15 April 2025
Sec. Plant Symbiotic Interactions
Volume 16 - 2025 | https://doi.org/10.3389/fpls.2025.1514416
Introduction: For low-fertile and degraded soils of sub-Saharan Africa, nitrogen (N) is often the most growth-limiting factor restricting crop yields. The often-suggested exploitation of advantageous rhizosphere traits such as enzyme secretion and/or the symbiosis with arbuscular mycorrhizal fungi (AMF) remains to be validated as a potential strategy to overcome N limitation, especially when N deficiency co-occurs with further abiotic stresses such as water scarcity.
Methods: Three sorghum genotypes were cultivated in soil mesocosms with a root-exclusion compartment, where only AMF could scavenge for nutrients under drought and optimal conditions. Plant carbon (C) investment into the rhizosphere and N uptake were tracked by 15N application coupled with 13CO2 labeling
Results: Under drought, uptake of mineral 15N by AMF from the root-exclusion compartment increased 4–12 times compared to well-watered conditions. In addition, water stress enhanced below-ground allocation of recently assimilated C into microbial biomass. Drought reduced the enzymatic potential (Vmax) of chitinase while increasing leucine aminopeptidase (LAP) activity. This suggests that N acquisition via protein mineralization in soil was relatively enhanced compared to that of chitin following moisture limitation. LAP substrate affinity (Km) was reduced by drought compared to that of chitinase with genotype-specific shifts in the rhizosphere enzyme systems observed.
Conclusion: Our findings suggest that below-ground C allocation activated AMF symbiosis and its associated microbiome. This not only led to a shift in enzyme-driven exploitation of distinct organic N sources but also induced a strong increase in AMF-based mineral N acquisition from the mycosphere. This trait plasticity in response to drought may be harnessed to stabilize food production from low-fertile soil under the increasingly negative impacts of droughts due to climate change.
The latest climate change models project continued warming and a decline in annual precipitation accompanied by frequent and severe drought events in many regions of the world, including sub-Saharan Africa (SSA) (Ayanlade et al., 2022; Conway et al., 2015; Field et al., 2011; Trisos et al., 2022). Consequently, a significant negative impact of climate change on food production is expected globally (Jägermeyr et al., 2021; IPCC, 2022), but particularly in SSA countries, whose economies and livelihoods depend on rainfed agriculture (FAO, 2020; Pickson and Boateng, 2022). Combined with infertile soils and often degraded soils (Baumgartner and Cherlet, 2015; Pasley et al., 2020), crops will suffer concurrent water and nutrient limitations. Among the nutrients, nitrogen (N) is of particular significance and its unavailability constrains plant growth and development in most terrestrial ecosystems (Fageria and Baligar, 2005; Harpole et al., 2011). Nitrogen deficiency is a major contributor to the large African crop yield gap (Falconnier et al., 2020; Gerber et al., 2024; Van Ittersum et al., 2016; Pasley et al., 2020). As water and nutrients are taken up from below ground, water and nutrient limitations primarily need to be overcome in soils. However, below-ground adaptive strategies have received less attention than above-ground strategies. Evident knowledge gaps, specifically relating to processes at the plant-soil interface—the rhizosphere—need to be explored. Among the below-ground traits, root properties, such as root length and rooting depth, play a critical role in the uptake of mobile resources such as water and N (Mommer et al., 2016). However, under drought, the interactions at the root and soil interface are critical in overcoming resource limitations, including N, since water and nutrients traverse the rhizosphere before being taken up by the plant (Abdalla et al., 2022; Cai et al., 2023). Thus, the rhizosphere is considered a critical interface governing resource acquisition by the plant (Vetterlein et al., 2020; Wang et al., 2022). Consequently, identifying effective rhizosphere traits is key in overcoming N scarcity while promoting sustainable agriculture (Hallett et al., 2022).
The interaction with and support of the microorganisms in the rhizosphere (the rhizo-microbiome), is one of the mechanisms by which plants enhance resource acquisition following drought (Jamil et al., 2022; Phour et al., 2020). Particularly, arbuscular mycorrhiza fungi (AMF), obligate symbionts with plant roots, are specialists among the rhizo-microbiome relying fully on their hosts for their C requirements (Chowdhury et al., 2022; Thirkell et al., 2020) in exchange for mineral nutrients (Thirkell et al., 2021; Wipf et al., 2019). Allocation of freshly assimilated C by the plant to symbiotic and freely associated members of the rhizo-microbiome is crucial for fueling the microbial activity and thus for enhancing plant nutrient uptake (Fellbaum et al., 2012; Kelly et al., 2022).
Previous studies have shown that the AMF hyphae-soil interface “the mycosphere” enhances the soil volume exploited for nutrient acquisition beyond the rhizosphere region (Faghihinia et al., 2023; Marschner, 2012; Miyata and Umehara, 2024; Wang et al., 2022). This subsequently improves the availability of nutrients with limited mobility in soils, such as phosphorus (P) (Liang et al., 2022; Qi et al., 2022; Tibbett et al., 2022). While AMF have been shown to enhance N uptake in their symbiotic plants (Thirkell et al., 2016; Shen et al., 2023; Wu et al., 2024; Xue et al., 2024), the mechanisms governing N acquisition, particularly within the rhizosphere, remain unclear and require further investigation. Disrupted connectivity of water films in dry soils reduces the mobility of all nutrient ions irrespective of their speciation (Xue et al., 2017; Bauke et al., 2022; Huntley, 2023). Thus, the role of AMF in bridging air gaps in dry soil presents an opportunity that might be exploited to increase their relevance for N transportation. One way, for example, lies in optimizing their N uptake by extending their hyphal networks into regions inaccessible by plant roots. The fungal hyphae, being much thinner than roots, penetrate small soil pores and maintain functionality in drier environments (Dodd et al., 2000; Zou et al., 2015; Diagne et al., 2020; Abdalla et al., 2023; Hammer et al., 2024). This allows them to access residual water and nutrients, such as N, that remain in the soil micropores despite overall soil drying (Clark and Zeto, 2000; Smith et al., 2010).
Besides their role in nutrient transport, AMF also modify the microbial community in the (rhizo-) hyphosphere (Faghihinia et al., 2023), which may further affect the mobilization of organic N by exoenzymes (Fall et al., 2022; Fellbaum et al., 2012; Jamil et al., 2022). Among the various organic N pools in soil, two key biomolecules, i.e., proteins and chitin, contain the majority of the hydrolyzable N pool, which is quantitatively important for crop N nutrition (Andersson and Berggren, 2005). They are derived from the two main organic matter sources, plant and microbial biomass. Proteins originate from both plants and microorganisms, whereas chitin and peptidoglycan are derived from the cell walls of the soil microbiome. Consequently, protease and chitinase activities play an important role in organic compound degradation and N cycling, especially under reduced N availability (Ndabankulu et al., 2022; Uwituze et al., 2022). Extracellular enzyme activities in soil depend strongly on the soil moisture status since i) water films are the medium in which substrates are transported to the active center of the enzymes or ii) enzymes increasingly adsorb to soil particles under shrinking water films (Geisseler et al., 2011; Tecon and Or, 2017; Schimel, 2018; Guber et al., 2022; Qu et al., 2023). However, it remains to be elucidated if drought-tolerant crops such as sorghum have developed specific strategies to efficiently exploit the soil organic N pool even under water scarcity. If so, it is still an open question to what degree this pool is of use for sorghum N nutrition.
Sorghum [Sorghum bicolor (L.) Moench] is one of the major staple food crops, ranked the fifth-most cultivated cereal after wheat, maize, rice, and barley (Ananda et al., 2020; Stefoska-Needham and Tapsell, 2020; Takanashi, 2023). It is the second most important cereal after maize in SSA (Haussmann et al., 2002; FAO, 2023; Yarnell, 2008) where unpredictable drought stress constitutes a major constraint for crop production (Dicko et al., 2006; Hadebe et al., 2017). Although sorghum is considered a drought-tolerant crop, water deficiency still affects its nutrient mobilization and uptake, with negative impacts on yield (Abreha et al., 2022; Ajeigbe et al., 2018; Yu et al., 2015). Previous studies have shown that AMF colonization of sorghum roots leads to enhanced N and P uptake (Deepadevi et al., 2010; Symanczik et al., 2018; Wang et al., 2019). However, an extensive understanding of the influence of water scarcity on AMF and associated enzyme activities is still limited. Thus, this study aims to fill the crucial gaps in understanding how sorghum responds to N limitation under drought.
To address these open questions, we designed a double-ring pot (DRP) experiment with two compartments separated by a 0.5 cm “gap” (Figure 1). Only AMF hyphae had access to the outer hyphal compartment, the mycosphere, whereas plant-roots and hyphae interacted jointly in the inner compartment of the pot, the mycorrhizosphere. We studied three sorghum genotypes, known to be adapted for cultivation under strong water deficit conditions and thus assumed to be well-adapted to nutrient uptake under drought conditions. We selected a local landrace Makueni local (Mkl), widely cultivated in Kenya; an open-pollinated variety Gadam (Gd), known to be highly drought tolerant; and a commercial hybrid IESH 22012 (IESH) that has been released by the International Crop Research Institute for Semi-Arid Tropics (ICRISAT), as a new drought-tolerant hybrid. The three sorghum genotypes were established at optimum [80% of water holding capacity (WHC)] and drought (30% of WHC) water levels.
Figure 1. Schematic diagram of (A) a double ring pot (DRP) showing the inner and outer compartments. (B) Aerial view of the DRP. (C) DRP with sorghum plants and the labeling chamber (transparent Plexiglas). (D) Isotopic labeling application.
This study aimed to 1) determine the effects of drought on N uptake in plant biomass of three sorghum genotypes; 2) assess the relative contribution of AMF to mineral N uptake of sorghum from mycorrhizosphere and mycosphere under optimal and drought conditions; 3) determine whether sorghum C allocation contributes to rhizosphere adaptation strategies that mitigate combined N and water stress; and, 4) investigate the possible shifts in activities of rhizo-(hyphosphere) enzymes degrading soil N polymers in response to drought. We hypothesized that reduced availability of mineral N under drought worsens plant N nutrition (H1) and that drought enhances AMF colonization of sorghum roots as a strategy to cope with water limitation (H2). This may be attributed to AMF hyphae’s ability to bridge air gaps between the disrupted water films inaccessible to roots, improving mineral N acquisition and transportation to the plant roots. We expect a relative increase in C allocation below ground under drought compared to well-watered conditions, which may support the microbiome in its functional contribution to plant nutrition (H3). We further hypothesized a decrease in enzyme activities and a concurrent impact on plant N nutrition under drought compared to optimal conditions (H4).
The soil was obtained from the top 25 cm of an agricultural Acrisol (IUSS Working Group WRB, 2015) in Kitui (1° 22’ S and 37° 59’ E) located in lower eastern Kenya with a mean temperature of 24°CC and an annual rainfall ranging between 500–700 mm. The soil has a sandy and loamy texture with a total C content of 0.6%, a total N content of 0.07%, a pH (H2O) of 5.44, and a WHC of 32%. The soil was air-dried and passed through a 4-mm sieve prior to seed sowing. It is important to clarify that soil was not inoculated with AMF species but, before sampling, we made sure to select soil from a field site where frequent sorghum cultivation took place and where we expected a sufficient spore load in the soil for effective mycorrhization.
Double-ring pots (Figure 1) with a height of 30 cm were designed to separate the inner compartment (IC)—the mycorrhizosphere (12 cm diameter)—from the outer compartment (OC)—the mycosphere (1 cm width). The IC was separated from the OC by a 0.5 cm gap filled with hydrophobic plastic (2–3 mm) beads acting as a hydraulic barrier. Two layers of 20 µm polyamide gauze at the sides of the hydraulic barrier ensured that only hyphae and no fine roots could reach the OC. Sorghum (Sorghum bicolor MOENCH) seeds, obtained from ICRISAT, Kenya, were sterilized in a 10% hydrogen peroxide solution for 10 minutes (Gaume et al., 2001; Davoudpour et al., 2020). Three sorghum genotypes, i.e., Mkl, Gd, and IESH, representing different levels of breeding effort, were used. The genotypes (Mkl, Gd, and IESH) have varying levels of extensive selection to enhance their drought tolerance, with the landrace.
(Mkl) having the least, followed by the open-pollinated variety (Gd), while the hybrid (IESH) had the highest selection intensity. Five seeds of each genotype were sown in the IC of the double-ring pot and were thinned to one single plant when the third leaf (after 15 days) appeared.
At the beginning of the experiment, a half dose of the recommended seed starter fertilization in solid form of 135 mg NPK [0.1285 g KCl, 0.0251 g ammonium phosphate (NH4)(H2PO4), and 0.08 g urea CH4N2O] was mixed with the soil when filling each pot. This enabled germination and initial growth but then led to a transition towards nutrient deficiency, allowing the observation of the plant’s response to combined nutrient and drought stress. The fertilization was applied relative to the proportion of soil mass in the outer and inner compartments (3:1), so that the content of the applied fertilizer in mg/g soil was identical in both compartments. A total of 48 double-ring pots were prepared for a full factorial design with four replicates and two water levels: optimum (80% of WHC) and drought (30% of WHC). With 30% WHC, the drought-sensitive sorghum cultivars will fail to produce a yield, whereas the drought-tolerant ones may still produce a yield. This level was chosen as adaptive traits may have the highest impact in this range of soil moisture (Sauer et al., 2024; Loftus et al., 2025).
For the first week of growth, the WHC of all pots was maintained at 80%. We weighed each pot every second day and manually watered with deionized water the outer or inner compartments to ensure consistent moisture levels for optimal plant establishment. Plant-free control pots were also weighed to quantify the evaporation of water per gram of soil. This was assumed to equal the evaporation per gram of soil of the outer ring, which was then used to calculate the amount of water to be added to the outer ring to compensate for evaporation. The difference between the total weight loss and the amount of water added to the outer ring was, in consequence, assumed to be caused by evapotranspiration from the inner ring. This amount was then added, as described above, to the outer ring. After three weeks, 24 pots were no longer watered. The dry-down process was controlled by weighing and recording the soil moisture content (Supplementary Table S1) until it reached 30% WHC (drought treatment). The other half of the pots were maintained at optimal conditions (well-watered treatment). The plants were grown in a walk-in growth chamber (Department of Crop Science, University of Göttingen, Germany) with a photoperiod of 12-hr day and night at temperatures of 27°C and 17°C, respectively, and a light intensity of 500 µmol m−² s−¹ from LED fluorescent lamps.
To trace N acquisition from the IC relative to the OC, 15N isotopes (15NH4)2SO4 and 15N2-urea (99 at%, Sigma Aldrich, Munich, Germany) were applied. To minimize the nitrification that may occur due to high initial ammonium concentration, both ammonium and urea were applied together. This allows for a continued supply of ammonium ions through the slow mineralization of urea. Thus, 5 weeks after sowing, approximate concentrations of 1.67 mg ml-1 (15NH4)2SO4 and 0.85 mg ml-1 15N2-urea, along with the second half of the starter NPK fertilization in a single pulse, were applied. The tracer solution was applied in the form of eight single injections (eVol displacement pipette, SGE, Australia) of 0.5 ml each either into the IC or the OC, in a quadrilateral pattern to promote a homogeneous distribution of the tracer in the respective compartments.
At week 7, single-pot 13CO2 pulse labeling was carried out to quantify the below-ground C allocation of the individual plants (Kuzyakov and Domanski, 2000; Sommer et al., 2017; Stock et al., 2021). Silicone rubber (Henkel Teroson GmbH, Heidelberg, Germany) of about 5 mm was evenly and seamlessly applied on the soil surface a day prior to the labeling to allow sufficient time to dry and form a tight seal. This prevented the diffusion of the labeled CO2 into the soil, thereby avoiding hetero- or autotrophic microbial C fixation leading to an overestimation of the below-ground C respiration. Bespoke transparent polymethyl methacrylate domes with a height of approx. 50 cm and a volume of 10.7 l were placed on each pot and sealed to ensure an airtight system (Figure 1C). Before labeling, CO2 concentrations in the chambers were lowered by cycling the air through a sodium hydroxide trap (1 M) for 0.5 h. During labeling, the plants were exposed to 13C-enriched CO2 for 2 h produced by the reaction of 0.0045 g of Na213CO3 (99 at% 13C, Sigma Aldrich, Munich, Germany) with 1 M H3PO4 added dropwise per pulse, with two pulses applied over a period of 4 h. To ensure uniform circulation of 13CO2 during labeling, a battery-powered fan was operated inside the chamber. The chamber was opened before and after labeling, and unassimilated 13CO2 was captured by cycling the air for 0.5 h through 20 mL of a 1 M NaOH solution.
Two days after the 13C pulse labeling, the plants and soils were destructively sampled. Shoot and root biomass were separated. The roots were manually removed from the soil and washed with deionized water by sonification to remove the soil adhering to the roots. After this, the samples were freeze-dried and weighed to obtain dry weights. Soil samples were taken from each compartment, homogenized, and stored at 4°C prior to subsequent enzyme assay and microbial biomass 13C analysis.
To determine C and N content and their isotopic composition (δ13C and δ15N), the dry biomass of plant and soil samples were milled with MM2000 (Retsch, Haan, Germany), and a subsample of approximately 2 mg, 1.5 mg, and 30 mg of root, shoot and soil, was weighed in tin capsules, respectively. The C and N contents were determined by an elemental analyzer (Flash 2000, ThermoFisher Scientific, Bremen, Germany) coupled via a Conflow to an isotope ratio mass spectrometer (IRMS) (Delta V Plus, ThermoFisher Scientific, Bremen, Germany). Measurements were conducted at the Centre for Stable Isotope Research and Analysis (KOSI); University of Göttingen. To calculate the 15N recovery in plant material, the atom% 15N of sorghum plants not supplied with 15N served as a natural abundance control. The 15N recovery was calculated by Equation 1, as follows:
where [15N] acqui is the 15N amount taken up [15N in plant dry biomass (mg plant-1)], [N]lab is N amount of the labeled plant material [N in total plant dry biomass (mg plant-1)], at% 15Nlab is the at% 15N of the labeled plant material, and at%15Nnat and at% 15Ntracer are the unlabeled plant material and the injected tracer (99 at% 15N), respectively. The N amount [N]lab was derived by multiplying the weight of shoot or root biomass with the biomass N concentration [mg g-1] of the respective plant compartment.
The 15N recovery in the percentage of applied tracer per plant (derived from the inner or outer ring of the pot, respectively) was calculated by dividing [15N] acqui of the shoots and roots (in mg) by the 15N amount (mg) applied either to the inner or outer compartment and multiplied by 100.
The soil microbial biomass C (MBC) was extracted using the chloroform-fumigation extraction method (Potthoff et al., 2003; Wu et al., 1990). Briefly, two samples of 10 g fresh soil, stored at 4°C (for maximally 7 days), were taken; one sample was first fumigated in a chloroform atmosphere for 24 h in a desiccator to lyse the microbial cells membranes, while the other sample was left unfumigated. Water-dissolvable C was extracted from both samples by shaking them in 40 ml 0.05 M K2SO4 for 1.5 h. The samples were centrifuged at 2,000 rpm for 10 minutes, the supernatant filtered, and the solutions stored at -20°C until analysis on a N/C 2100 element analyzer (Jena Analytik, Jena, Germany). Afterward, the extracts were lyophilized and approximately 25 mg (fumigated) and 35 mg (unfumigated) freeze-dried powder were weighed into tin capsules and measured as described above (Section 2.6).
The 13Cacqui_fum/unfum was calculated according to the mixing model, which is shown in Equation 1 for N and was modified for C as shown in Equation 2. We first calculated the 13Caqui_fum and 13Cacqui_unfum as separate independent pools, using the 13C values of labeled and unlabeled microbial biomass extracts, respectively, and using the enrichment of 99 at% for the 13CO2 tracer, as added to the chamber.
where [13C]acqui is the 13C amount allocated below ground into the extractable pool with and without fumigation (µg pot-1), [C]lab(fum/unfum) is the amount of C extracted from the labeled fumigated and unfumigated samples and scaled per pot (µg pot-1) at% 13Clab, and at%13Cnat is the at% 13C value of the extract from the labeled and unlabeled pots, respectively, while at% 13Ctracer is the atom% value of the injected tracer (here 99 at% 13C), respectively.
Microbial biomass C content per µg of extracted soil was multiplied by the amount of soil in the respective ring to calculate the C pool [C]lab of the fumigated and unfumigated extracts from the soil of the respective rings. The 13C-MBC was calculated as the difference between 13C in fumigated and non-fumigated soil extracts and divided by a factor of 0.45 to correctly account for uncomplete cell lysis extraction efficiency (Wu et al., 1990), as shown in Equation 3.
where 13C acqui_fum and unfum indicate acquired 13C from fumigated and non-fumigated soil extracts, respectively.
After harvest, fresh roots were collected, washed, and weighed. A subsample of approximately 10% of root mass was taken to estimate AMF root colonization. Roots were stained according to the slightly modified method of Vierheilig et al. (1998) where the roots were subjected to a bleaching step by heating them in 5% KOH in a water bath at 95°C for 4 minutes (if needed, 4 minutes of bleaching were added). Thereafter, the roots were washed with tap water for 3 to 5 minutes and the water was changed once. All the roots were stained using 5% ink (Pelikan ink 4001®, Pelikan, Hannover) in an acetic acid solution for 5 minutes at a temperature of 95°C. Following this, the roots were de-stained in acidified water for 30 min. Roots were kept in 80% glycerol at 4°C until microscopic analysis (Stock et al., 2021). AMF colonization was quantified by counting vesicles, arbuscules, and intraradical hyphae under a light microscope (Olympus BX40 with Olympus CMOS-camera SC50M, 4.9 Mpx resolution) at a magnification of x150 (Olympus Ach x10/0.25 ph ∞ 0.17, ocular x15, Olympus Europe SE & Co. KG, Hamburg, Germany). Ten root segments, each of one cm length, were analyzed for each plant genotype. Grid squares were then classified and counted as either containing only root tissue (–) or containing root tissue and fungal structures (+). The percentage of roots colonized by AMF was calculated by the relation of squares containing root and fungi structures jointly to the total number of squares containing root segments with or without fungi. The results were expressed as a percentage of the root area colonized.
To determine the potential activities of C- and N-cycling exoenzymes, we measured leucine aminopeptidase (LAP) and poly-N-acetyl-glucosaminidase (chitinase) activities. The LAP cleaves the N-terminal from proteins and peptides (Greenfield et al., 2021; Matsui et al., 2006); while chitinases hydrolyze chitin to low molecular weight chitooligomers (Hoang et al., 2016; Zhang et al., 2020). Both are significant N- and C-acquiring enzymes and broadly representative of C/N cycling activities (Cenini et al., 2016). Fluorogenically labeled substrates (all from Sigma Aldrich, Germany), 4-MUF-N-acetyl-b-D-glucosaminide (MUF-NAG) and L-Leucine-7-amino-4-methylcomarin hydrochloride (AMC-LAP), were used to assess the enzyme activities for chitinase and LAP respectively (German et al., 2011; Marx et al., 2001). The enzymes’ activities were determined over a range of substrate concentrations from low to high (0, 5, 10, 15, 20, 25, and 75 μmol g-1 soil) at room temperature. Approximately 1 g of fresh soil was mixed with 50 ml of sterile water and placed on a horizontal shaker for 30 minutes. After low-energy sonification for 2 minutes, the 50 μL soil solution and 50 μL MES and Trizma buffer for chitinase and LAP, respectively, were pipetted into 96-well black microtiter plates. Finally, 100 μL of each substrate in a series of 0, 5, 10, 15, 20, 25, and 75 μmol g-1 soil were added to the wells. The fluorescence was repeatedly measured at 0, 1 hour, and 2 hours after adding the soil suspension, substrate, and buffer at an excitation wavelength of 360 nm and an emission wavelength of 450 nm, on a fluorescent microplate reader (Victor3 1420-050 multi label counter PerkinElmer, USA) (Razavi et al., 2015). Enzyme activities were expressed as MUF/AMC release in nmol g-1 soil h-1. The assays of each enzyme at each substrate concentration were performed in three analytical replicates. The Michaelis–Menten equation (Equation 4) was used to calculate the parameters of enzyme activities Vmax and Km.
where Vmax is the maximal rate of enzymatic activity, Km is the half-substrate concentration at the half-maximal rate and S is the substrate concentration.
Statistical analyses were performed using statistical software R, version 4.2.0. (R Core Team, 2022). Prior to running analyses, data were checked for normality and homogeneity of variance using Shapiro–Wilk and Levene’s test, respectively. The data violating normal distribution were first logarithmically transformed before further analysis. Data with eight replicates were tested by two-way ANOVA, where the water content and genotypes were used as predictor variables for the plant biomass, 13C allocation, and enzyme data analyses, while three-way with only four replicates ANOVA was performed on 15N recovery with root presence as the third predictor. Subsequently, Tukey’s HSD post hoc test was performed at a significance level of α = 0.05 to separate the means. Simple linear regression analysis was conducted to assess the relationship between N acquisition and enzyme activity, assuming linearity, normal distribution of residuals, and homoscedasticity.
Drought substantially decreased (p<0.0001) plant biomass compared to growth under optimal water conditions (Figures 2A, B). Shoot dry weights of the three sorghum genotypes were considerably reduced under drought stress (Figure 2A), with IESH showing the highest reduction of 50.7%, followed by Gd and Mkl with 44% and 30%, respectively. Similarly, the dry weight of the roots decreased under drought but was significant only for IESH with a 46% decrease (Figure 2B). Under drought, the root:shoot ratio significantly increased for Mkl while that of Gd and IESH remained relatively unaffected (Table 1).
Figure 2. (A) Shoot and (B) root biomass, (C) shoot and (D) root total nitrogen (TN) content, and (E) N concentration per g dry weight in shoot and (F) root of sorghum genotypes under well-watered (green) and drought (brown) conditions. Data are presented as means ± SE (n=8). Different letters indicate significant differences (p<0.05). Sorghum genotypes: Mkl, Makueni local; Gd, Gadam; IESH, IESH 22012. P-values of ANOVA of water availability, genotypes, and their interactions are indicated as ***p<0.001, **p<0.01, and *p<0.05, and ns indicates not significant.
Table 1. Root/shoot ratio and percentage of AMF root colonization in sorghum genotypes under well-watered and drought conditions.
Drought decreased (p<0.0001) total nitrogen (TN) content by 13%–17.5% (Figure 2C) and N concentration per dry weight in shoot biomass (Figure 2E). However, only IESH showed a significant reduction of its N content with a water deficit in root tissue, while that of Gd and Mkl remained unchanged (Figure 2D). Biomass and N content among the studied genotypes did not vary under drought conditions (Figures 2A–D). All individuals were colonized by AMF. Water availability significantly influenced (p<0.0001) the degree of colonization (% of colonized root area) (Table 1, ANOVA). Drought conditions significantly decreased root colonization for IESH, while Gd and Mkl were not significantly affected (Table 1). The genotype significantly influenced (p<0.001) AMF colonization. Mkl roots showed the lowest colonization of all genotypes irrespective of soil moisture levels (Table 1).
Water availability, genotype, and root presence/absence in the compartment of the 15N application, and their interactions significantly affected 15N recovery in both above- and below-ground biomass (Table 2). All genotypes displayed significantly higher recoveries of the mineral 15N applied in the mycorrhizosphere compared to that from the mycosphere. For example, the uptake of 15N from the mycorrhizosphere into the shoots was 7–17 times higher than the uptake from the mycosphere. In roots, 15N uptake from the mycorrhizosphere was 11–33 times higher than uptake from the mycosphere.
Table 2. Effects of water availability, genotype, soil compartment (where the tracer was applied), and their interactions on 15N uptake into shoot and root biomass of the sorghum genotypes as analyzed by three-way ANOVA.
In contrast, a significantly higher contribution of the mycosphere to plant 15N recovery was observed under drought conditions for all genotypes. While under well-watered conditions only approximately 1% of the 15N uptake resulted from the outer mycosphere relative to the inner mycorrhizosphere compartment, the contribution of the mycosphere compartment increased to 9% –44% of the 15N uptake under drought. The highest recovery was obtained by the genotype Gd.
In shoots, Mkl and IESH showed a 4-fold and Gd a12-fold higher 15N recovery from the outer mycosphere compartment under drought than under well-watered conditions. In the roots, Mkl had a 5-fold, IESH had a 9-fold, and Gd had a 10-fold higher 15N recovery from the outer mycosphere compartment under drought compared to well-watered conditions, respectively (Figures 3A, B, for at% data, see Supplementary Table S2). The sorghum genotypes showed a significant variation in their 15N recovery from the mycosphere in both shoot and root biomass (Figures 3A, B). Gd showed higher recovery than Mkl, while IESH was not significantly different from either of the other two genotypes.
Figure 3. 15N recovery of applied tracer N in (A) shoot and (B) root of sorghum genotypes under well-watered (green) and drought (brown) conditions when labelled in the mycorrhizosphere (left) and mycosphere (right). Data are presented as means ± SE (n=4). Different letters indicate significant difference (p<0.05). Sorghum genotypes; Mkl-Makueni local, Gd-Gadam and IESH 22012.
Drought stress resulted in a significant increase (p<0.0001) in the allocation of recently assimilated 13C into the microbial biomass pool, both in the mycorrhizosphere and mycosphere compared to the well-watered pots with no difference among the three genotypes (Figures 4A, B). The amount of 13C incorporated in microbial biomass under drought was 5- to 7-fold higher than in the optimal moisture treatment in the mycorrhizosphere (Figure 4A). Although there was a lower recovery of recently assimilated C in the microbial biomass of the mycosphere compared to mycorrhizosphere by a factor of 50, drought stress also boosted the incorporation of freshly assimilated C in this mycosphere compartment, reaching increases of 2.5 to 6-fold (Figure 4B).
Figure 4. 13C allocation into the microbial biomass pool (A) in the mycorrhizosphere and (B) in the mycosphere of the three sorghum genotypes under well-watered (green) and drought (brown) conditions. Data are presented as means ± SE (n=8). Different letters indicate significant differences (p<0.05). Sorghum genotypes: Mkl, Makueni local; Gd, Gadam; IESH, IESH 22012. P-values of ANOVA of water availability, genotypes, and their interactions are indicated as ***p<0.001, **p<0.01, and *p<0.05, and ns indicates not significant.
Water content, genotypes, and their interactions had no effect on maximal potential enzyme activities (Vmax) of chitinase in the mycorrhizosphere (Figure 5A). Chitinase Vmax was significantly higher (p<0.001) under optimal compared to drought conditions in the mycosphere of Gd and IESH (Figure 5B). However, in the mycorrhizosphere, the maximal chitinase activity of the genotypes did not differ depending on water availability (Figure 5A). Root presence resulted in higher (p<0.0001) soil LAP Vmax values of all the genotypes under drought (Figure 5C). However, there was no water effect on the maximal enzyme activity for LAP in the mycosphere apart from for Gd (Figure 5D). Water availability, genotype, and their interactions indicated no effect on substrate affinity (Km values) of chitinase in the mycorrhizosphere (Figure 5E). However, in the hyphal compartment, drought reduced and raised the chitinase Km values for Gd and Mkl, respectively, while that of IESH remained unaffected (Figure 5F). For IESH, drought significantly lowered the affinity of LAP in both compartments (Figures 5G, H). Mycosphere Km values of LAP were significantly lower compared to that of the mycorrhizosphere (Figures 5G, H).
Figure 5. (A, B) Chitinase and (C, D) leucine aminopeptidase maximal activity (Vmax, nmol g-1 soil h-1). (E, F) Chitinase and (G, H) leucine aminopeptidase Michaelis–Menten constants (Km, μmol g-1 soil) of the mycorrhizosphere and mycosphere soil for the three sorghum genotypes under optimal water (green) supply and drought (brown) conditions. Data are presented as means ± SE (n=8). Asterisks indicate significant differences between the compartments, i.e., the mycorrhizosphere and mycosphere. Sorghum genotypes: Mkl, Makueni local; Gd, Gadam; IESH, IESH 22012. P-values of ANOVA of water availability, genotypes, and their interactions are indicated as, ***p<0.001, **p<0.01, and *p<0.05, and ns indicates not significant.
Shoot N content showed a positive correlation with chitinase enzyme activity (Vmax) (r (24) =0.67, p<0.001) (Figure 6B) under optimal water conditions in the mycosphere soil but not in the mycorrhizosphere soil (Figure 6A). In contrast, under drought, shoot N content was positively correlated with LAP activity in the mycorrhizosphere (r = 0.60, p<0.01) and the mycosphere (r = 0.46, p<0.05) (Figures 6C, D). 15N recovery in shoot biomass from mycosphere soil under drought was positively correlated with the degree of root AMF colonization (r =0.66), p<0.05) (Figure 6F), whereas there was no correlation observed under optimal conditions (Figure 6E).
Figure 6. Correlations between shoot N content and chitinase (A, B) and leucine aminopeptidase (C, D) enzyme activity (Vmax) in the mycorrhizosphere and mycosphere, and between the 15N recovery in shoots (%) and the AMF hyphae colonization (E, F) under optimal (green) and drought (brown) conditions. P-values, R2 coefficients of determination, and correlation coefficients (r) are indicated.
Drought significantly affects various processes of plant growth (Goche et al., 2020; Seleiman et al., 2021), e.g., it reduced both shoot and root biomass and decreased TN content and N concentration per dry weight in shoot biomass (Figures 2A–E). To reduce the loss of water, plants respond to drought through stomatal closure, a primary factor for reduced photosynthesis (Abdalla et al., 2022; Agurla et al., 2018; Buckley, 2019), resulting in a decrease of plant biomass (Hosseini et al., 2022; Khalil et al., 2020; Sigua et al., 2018). Although drought generally reduced sorghum biomass formation in all the genotypes (Figures 2A, B), the increased root/shoot ratio (Table 1) revealed resource investment in root growth. Root biomass accumulation was particularly enhanced in Mkl and partly in Gd in response to the water deficit (Figure 2B). Moreover, the relative increase or absence of change in N allocation in root tissue (Figure 2D, F), apart from that in IESH under drought (Figure 2D), is an often-observed adaptation strategy of plants to maintain root activity and enhance water and nutrient acquisition from soils under resource-limited conditions (Eziz et al., 2017; Wilschut and van Kleunen, 2021).
Soil moisture availability significantly supports the nutrient uptake of plants (Bista et al., 2018; Liu et al., 2023). Drought not only reduced sorghum biomass but also reduced overall N content in the plant tissue (Figures 2C, D), confirming our first hypothesis (H1). One underlying reason may be that the mobile nutrients, including mineral N, are co-transported with the water mass flow to the roots. It is likely that restricted mobility of N ions occurs if the water mass flow is reduced by drought (Araus et al., 2020; He and Dijkstra, 2014). Our findings are consistent with other studies showing that soil moisture deficit conditions suppress root growth, as in IESH genotype (Asif et al., 2020; Kou et al., 2022). Additionally, reduced N content in plant tissues could be due to the loss of contact of the root with surrounding soil particles. Root and root hairs shrink under drought (Duddek et al., 2022), impeding water and nutrient accessibility (Cai et al., 2023; Saengwilai et al., 2021).
Moreover, we observed a reduced Vmax of chitinase under drought (Figure 5A). Soil drying alters the functioning of the microbes and their enzymes (Ahmed et al., 2018) by, e.g., confining substrates in thin water films (Goebel et al., 2011), leading to their sorption to the mineral phase (Singh et al., 2021). Drying also induces enzyme denaturation due to high ion strength or strong interactions in thin water films and reduced enzyme diffusion (Davidson and Janssens, 2006; Guber et al., 2018) This is of special relevance in the iron mineral-rich Acrisol used in this experiment, which has a very high sorption capacity (Kaiser and Guggenberger, 2007; Zhou et al., 2023).
In agreement with our fourth hypothesis (H4), chitinase activity decreased under drought. In contrast, moisture limitation induced an increase of the Vmax of LAP in comparison to optimal conditions (Figure 5B)—a phenomenon already observed in previous studies on LAP (Sanaullah et al., 2011; Zhang et al., 2021). We observed its potential activities to be more pronounced in the mycorrhizosphere than in the mycosphere (Figure 5B). Such high maximal LAP activities under drought in root proximity may have been either aided by root priming of the rhizosphere microbiome (Shahzad et al., 2015) or may have been derived from the direct release of LAP from roots (Greenfield et al., 2020, 2021). Reduced tissue N content may have enhanced LAP production to counteract a potential N deficiency, as confirmed by a significant correlation of shoot N content with LAP enzyme activity under drought (Figures 6C, D).
The additional release of enzymes upon root and microbial cell death and lysis under drought may perhaps further result in higher enzyme activities of LAP (Turner et al., 2003). Drought consistently lowered the affinity of LAP for its substrates while the response of substrate affinity of chitinase to water scarcity was genotype-specific (Figures 5G, H). The change in Km under drought indicates the presence of enzyme isoforms different to those under optimal conditions (Kuzyakov and Blagodatskaya, 2015). This indication suggests a shift in microbial community structure and/or modification or shift of the enzyme system (released by roots, hyphae, or microorganisms) itself (Bradford, 2013) as an essential strategy to enhance drought tolerance. The difference in these enzyme-to-substrate affinities suggests conformational shifts at the root-soil and hyphae interface in the extracellular enzyme-substrate interactions. This could be a survival strategy employed by sorghum genotypes to enhance drought tolerance.
As expected, a much higher proportion of the mineral 15N tracer was acquired from the mycorrhizosphere compared to the root-free mycosphere compartment (Figures 3A, B) This pattern was visible in shoot and root 15N recovery, confirming that mineral 15N, taken up by roots, is highly mobile in the plant biomass (White, 2011). Besides the key role of roots in taking up mineral N (Mäder et al., 2000; Miransari, 2011), there was a detectable contribution to the 15N uptake from the mycosphere irrespective of the moisture. This confirms that mycorrhizal hyphae also contributed to the 15N acquisition. This might have been attributed to the hyphae’s extremely narrow diameter, which enhances their ability to infiltrate very tiny pores and gain access to nutrient resources that are generally concealed from the roots’ direct access (Bahadur et al., 2019; Smith et al., 2010).
Mycorrhizal hyphae contributed a much lower degree of plant mineral N nutrition under well-watered conditions. Conversely, under drought, an enhanced contribution of 15N uptake by AMF hyphae from the mycosphere compartment was observed (Figures 3A, B). This contribution was between 4 to 11.5 times higher than in the well-watered plants. AMF can establish extensive hyphal networks, which tremendously increase the absorptive capacity of the roots and thus enhance water and nutrient uptake (Xiao et al., 2023). Our findings support observations by Kakouridis et al. (2022) that AMF hyphae may have the ability to bridge air gaps in soils to reach out for disconnected soil resources. We lack any imaging confirmation of such bridging of air gaps. However, for AMF to bridge the compartment between the inner and outer ring, filled with hydrophobic pearls with no water film on their surface, a bridging of air gaps to reach out for the other compartment seems unavoidable. The relatively higher contribution of the mycosphere to sorghum’s N nutrition under drought suggests that this ability of AMF may be highly relevant in supporting N acquisition during periods of water scarcity.
The higher affinity of AMF than of plant roots for the less mobile NH4+ (Pérez-Tienda et al., 2012) could also be a possible explanation for the increased N recovery under drought (Xiao et al., 2023). This holds not only true for mineral N, but AMF can also stimulate their mycosphere microbiome to secrete hydrolytic enzymes and transport via the hyphae the released monomers or mineralized N forms to the plant (Kaur and Suseela, 2020). The increased LAP activity of Gd’s mycosphere under drought (Figure 5D) suggests that this induction of protein mining by AMF seems to be a key drought adaptation strategy, at least for this genotype. In this study, we observed a far higher transport of the 15N applied to the mycosphere soil to the soil of the mycorrhizosphere compartment than we observed transport in the opposite direction, i.e. from the mycorrhizosphere to the mycosphere compartment (Supplementary Figure S1). This suggests that we did not observe a randomized N transport through filamentous microbiome members, just from one soil volume to another, but that our largely unidirectional transport can specifically be attributed to AMF, which allocate 15N from the outer ring to the plant compartment, and then also use it in the soil, e.g., for their own biomass or for the supportive hyphosphere microbiome.
The ability of filamentous soil microorganisms to bridge air gaps is particularly crucial with discontinuous water films in dry soils (Smith et al., 2010; Li et al., 2019) where AMF hyphae may still reach water-filled isolated soil micro-structures. In contrast, roots only have access to macro- and mesopores, which could result in shrinkage and occasional root death in the absence of moisture (Kakouridis et al., 2022). This strongly buttresses the significant role of AMF in N acquisition under moisture-limited conditions when mass flow and diffusion of ions are restricted (Tobar et al., 1994), and thus supports our hypothesis that AMF access N pools unavailable to roots under drought and convey it to the plants. In this context, it was rather astonishing that drought-stressed plants showed much lower AMF root colonization compared to the well-watered plants (Table 1)—a phenomenon already observed by Begum et al. (2019) and Neumann and George (2004). Nevertheless, water limitation strongly enhanced 15N acquisition, via the AMF pathway, as also observed in the studies of Gholamhoseini et al. (2013) and Symanczik et al. (2018), suggesting that mycorrhizal fungi colonization boosted N uptake under drought irrespective of the lower colonization rate.
The significant positive correlation between 15N uptake by sorghum genotypes from the mycosphere and the rate of AMF colonization under drought (Figure 6F) suggests that AMF hyphae might have played a role in facilitating the acquisition of N from the outer soil compartment. In contrast, under optimal conditions, the lack of correlation between 15N recovery and root colonization by AMF possibly indicates that high colonization under adequate moisture levels does not necessarily translate to high mycorrhizal N uptake or play a supportive role in plant nutrition. Whereas, under optimal conditions, the 15N uptake by AMF was very low for all sorghum genotypes, the genotypes displayed significant plasticity for 15N uptake under drought from the mycosphere. Under water-limited conditions, Gd showed the highest mineral 15N recovery in shoots and roots from the mycosphere (Figures 3A, B), suggesting that Gd’s N uptake might have resulted from an optimized activation and exploitation of its associated microbial partners, specifically AMF. The slightly higher mycorrhizal root colonization of Gd compared to the other genotypes under drought (Table 1) suggests that genotype-specific differences in colonization rates may serve as an indicator of AMF contribution to mineral nitrogen uptake (Figure 3).
As already shown in Zea mays (Somasundaram et al., 2009), Festuca arundinacea and Medicago sativa (Sanaullah et al., 2012), and Trifolium repens and Lolium perene (Qiao et al., 2022), a water deficit increases plant-derived C allocation into microbial biomass (Figures 4A, B). In response to restrictions in below-ground resource access, plants tend to direct a greater portion of their photosynthetic products directly to their roots or to their AMF partners, which are jointly in charge of obtaining the scarce resources—water and nutrients—under drought (Johnson, 2010).
Besides the rhizosphere microbiome and AMF, the AMF microbiome partners in the mycosphere profited strongly from the higher photoassimilate allocation below ground under drought (Figure 4B). Such an enhanced C transfer by the host plant to the AMF hyphae and its mycosphere microbiome very likely stimulates N mineralization in the soils and its transfer via AMF to the plant (Fellbaum et al., 2012; Kiers et al., 2011; Wang et al., 2021). It is feasible that a significant increase in assimilated 13C in the extractable microbial biomass of the mycosphere compartment could be a result of a direct transfer of plant-derived C to the AMF and their supporting hyphal microbiome (Kaiser et al., 2015; Kakouridis et al., 2024) to support resource acquisition under drought.
To cope with the effects of water scarcity, plants adapt their enzymatic N mining activity in the rhizosphere. Maintaining constant chitinase activity in the mycorrhizosphere and additionally increasing LAP activity under drought may be an important strategy to overcome increasing N limitation under water scarcity. Thereby, genotype‐specific variations were observed in their responsiveness to AMF symbiosis and N acquisition following water limitation. Gd showed more pronounced N acquisition from the mycosphere under drought, potentially resulting from an enhanced interaction with AMF. However, there were no indications that modern sorghum breeding had negative effects on mycorrhizal growth receptiveness and/or the role of the tested N hydrolyzing enzymes for the N nutrition of sorghum. Irrespective of the genotype, we identified generic responses of sorghum’s N acquisition strategy to drought, which are strongly related to its interaction with the mycorrhizal partner. Overall, both AMF symbiosis and shifts in enzymatic N mining play a crucial role in sorghum’s N acquisition strategy, particularly under drought stress, by enhancing nutrient mobilization and availability in the rhizosphere (Figure 7).
Figure 7. Schematic illustration of rhizosphere dynamics and growth performance of the three sorghum genotypes: Makueni local (Mkl), Gadam (Gd), and IESH 22012 (IESH) under optimal (left) and drought (right) conditions. The diagram depicts shoot and root biomass, mineral 15N uptake from the mycorrhizosphere (IC) and mycosphere (OC), relative 13C allocation in microbial biomass carbon (MBC), and the activity of enzymes [chitinase and leucine aminopeptidase (LAP)]. Variation in N uptake, 13C in MBC, and enzymatic activities are illustrated by triangular shapes. The triangles show the overall drought effects without consideration of individual genotypes, while the arrows indicate relative differences between the genotypes within each water treatment. Green arrows indicate carbon fluxes, while blue arrows represent mineral 15N fluxes, with arrow sizes corresponding to the relative quantities of 13C and mineral 15 N. Further details are explained in the main text.
Drought substantially reduced N uptake and AMF root colonization. However, the support of the mycorrhizal symbiosis in mineral N acquisition was enhanced for all sorghum genotypes under drought stress (Figure 7). Under optimal moisture content, sorghum genotypes use more C to form the above-ground biomass and N uptake largely occurred via the roots. However, in response to water limitation, there was a considerable shift toward investment into root C and a higher C supply for the below-ground microbial partners, the latter most likely to activate the AMF and the associated microbiome, and both jointly contribute to enhanced N mobilization and uptake. Therefore, we can conclude that enhanced C allocation below ground, and specifically to microbial biomass (including AMF), appears to be a key drought mitigation strategy of sorghum to cope with combined water and N limitation. AMF notably enhanced the mineral N uptake by the plant from the mycosphere, indicating their critical role in N acquisition from soil compartments not accessible by roots (Abdalla et al., 2023).
The raw data supporting the conclusions of this article will be made available by the authors, without undue reservation.
RM: Data curation, Formal Analysis, Methodology, Writing – original draft, Writing – review & editing. OM: Formal Analysis, Methodology, Writing – original draft, Writing – review & editing. SL: Data curation, Formal Analysis, Methodology, Writing – original draft, Writing – review & editing. CB: Investigation, Methodology, Supervision, Writing – review & editing. RR: Supervision, Validation, Writing – review & editing. EB: Conceptualization, Methodology, Resources, Validation, Writing – review & editing. BM: Resources, Writing – review & editing. KM: Resources, Validation, Writing – review & editing. DO: Supervision, Validation, Writing – review & editing. MA: Conceptualization, Investigation, Methodology, Supervision, Validation, Visualization, Writing – review & editing. MD: Conceptualization, Funding acquisition, Investigation, Methodology, Project administration, Resources, Supervision, Visualization, Writing – review & editing.
The author(s) declare that financial support was received for the research and/or publication of this article. We are grateful to Robert-Bosch Junior Professorship 2017 for funding this study. We are also grateful to the German Academic Exchange Service (DAAD) for financial support for RM and some of the laboratory consumables. We acknowledge support from the Open Access Publication Fund of the University of Tübingen.
We gratefully specifically acknowledge Henry Olojong and Eric Manyasa from ICRISAT, Kenya, for the provision of sorghum seeds used in the experiment. We are also grateful to the technical support of the Department of Biogeochemistry of Agroecosystems, University of Goettingen, and particularly Karin Schmidt and Susan Enzmann for microbial biomass C and enzyme activity determination. We also thank the team at KOSI (Centre for Stable Isotope Analysis) for the 13C and 15N analyses.
The authors declare that the research was conducted in the absence of any commercial or financial relationships that could be construed as a potential conflict of interest.
The author(s) declare that no Generative AI was used in the creation of this manuscript.
All claims expressed in this article are solely those of the authors and do not necessarily represent those of their affiliated organizations, or those of the publisher, the editors and the reviewers. Any product that may be evaluated in this article, or claim that may be made by its manufacturer, is not guaranteed or endorsed by the publisher.
The Supplementary Material for this article can be found online at: https://www.frontiersin.org/articles/10.3389/fpls.2025.1514416/full#supplementary-material
Abdalla, M., Ahmed, M. A., Cai, G., Wankmüller, F., Schwartz, N., Litig, O., et al. (2022). Stomatal closure during water deficit is controlled by below-ground hydraulics. Ann. Bot. 129, 161–170. doi: 10.1093/aob/mcab141
Abdalla, M., Bitterlich, M., Jansa, J., Püschel, D., Ahmed, M. A. (2023). The role of arbuscular mycorrhizal symbiosis in improving plant water status under drought. J. Exp. Bot. 74, 4808–4824. doi: 10.1093/jxb/erad249
Abreha, K. B., Enyew, M., Carlsson, A. S., Vetukuri, R. R., Feyissa, T., Motlhaodi, T., et al. (2022). Sorghum in dryland: morphological, physiological, and molecular responses of sorghum under drought stress. Planta 255, 1–23. doi: 10.1007/s00425-021-03799-7
Agurla, S., Gahir, S., Munemasa, S., Murata, Y., Raghavendra, A. S. (2018). Mechanism of stomatal closure in plants exposed to drought and cold stress. Adv. Exp. Med. Biol. 1081, 215–232. doi: 10.1007/978-981-13-1244-1_12
Ahmed, M. A., Sanaullah, M., Blagodatskaya, E., Mason-Jones, K., Jawad, H., Kuzyakov, Y., et al. (2018). Soil microorganisms exhibit enzymatic and priming response to root mucilage under drought. Soil Biol. Biochem. 116, 410–418. doi: 10.1016/j.soilbio.2017.10.041
Ajeigbe, H. A., Akinseye, F. M., Ayuba, K., Jonah, J. (2018). Productivity and Water Use Efficiency of Sorghum [Sorghum bicolor (L.) Moench] Grown under Different Nitrogen Applications in Sudan Savanna Zone, Nigeria. Int. J. Agron. 2018, 1–9. doi: 10.1155/2018/7676058
Ananda, G. K. S., Myrans, H., Norton, S. L., Gleadow, R., Furtado, A., Henry, R. J. (2020). Wild sorghum as a promising resource for crop improvement. Front. Plant Sci. 11. doi: 10.3389/fpls.2020.01108
Andersson, P., Berggren, D. (2005). Amino acids, total organic and inorganic nitrogen in forest floor soil solution at low and high nitrogen input. Water. Air. Soil pollut. 162, 369–384. doi: 10.1007/s11270-005-7372-y
Araus, V., Swift, J., Alvarez, J. M., Henry, A., Coruzzi, G. M. (2020). A balancing act: How plants integrate nitrogen and water signals. J. Exp. Bot. 71, 4442–4451. doi: 10.1093/jxb/eraa054
Asif, I., Qiang, D., Xiangru, W., Huiping, G., Hengheng, Z., Xiling, Z., et al. (2020). High nitrogen enhance drought tolerance in cotton. Plants 9, 2–22. doi: 10.3390/plants9020178
Ayanlade, A., Oluwaranti, A., Ayanlade, O. S., Borderon, M., Sterly, H., Sakdapolrak, P., et al. (2022). Extreme climate events in sub-Saharan Africa: A call for improving agricultural technology transfer to enhance adaptive capacity. Clim. Serv. 27, 100311. doi: 10.1016/j.cliser.2022.100311
Bahadur, A., Batool, A., Nasir, F., Jiang, S., Mingsen, Q., Zhang, Q., et al. (2019). Mechanistic insights into arbuscular mycorrhizal fungi-mediated drought stress tolerance in plants. Int. J. Mol. Sci. 20, 1–18. doi: 10.3390/ijms20174199
Bauke, S. L., Amelung, W., Bol, R., Brandt, L., Brüggemann, N., Kandeler, E., et al. (2022). Soil water status shapes nutrient cycling in agroecosystems from micrometer to landscape scales. J. Plant Nutr. Soil Sci. 185, 773–792. doi: 10.1002/jpln.202200357
Baumgartner, P., Cherlet, J. (2015). Institutional framework of (in) action against land degradation. (Cham, Switzerland: Springer International). doi: 10.1007/978-3-319-19168-3_3
Begum, H., Ahanger, M. A., Su, Y., Lei, Y., Mustafa, N. S. A., Ahmad, P., et al. (2019). Improved drought tolerance by AMF inoculation in maize (Zea mays) involves physiological and biochemical implications. Plants 8, 579. doi: 10.3390/plants8120579
Bista, D. R., Heckathorn, S. A., Jayawardena, D. M., Mishra, S., Boldt, J. K. (2018). Effects of drought on nutrient uptake and the levels of nutrient-uptake proteins in roots of drought-sensitive and -tolerant grasses. Plants 7, 1–16. doi: 10.3390/plants7020028
Bradford, M. A. (2013). Thermal adaptation of decomposer communities in warming soils. Front. Microbiol. 4. doi: 10.3389/fmicb.2013.00333
Buckley, T. N. (2019). How do stomata respond to water status? New Phytol. 224, 21–36. doi: 10.1111/nph.15899
Cai, A., Tang, S., Waqas, M. A., Wang, B., Tian, D., Zhang, Y., et al. (2023). Magnitude, direction, and drivers of rhizosphere effect on soil nitrogen and phosphorus in global agroecosystem. Int. Soil Water Conserv. Res. 11, 482–493. doi: 10.1016/j.iswcr.2022.07.004
Cenini, V. L., Fornara, D. A., McMullan, G., Ternan, N., Carolan, R., Crawley, M. J., et al. (2016). Linkages between extracellular enzyme activities and the carbon and nitrogen content of grassland soils. Soil Biol. Biochem. 96, 198–206. doi: 10.1016/j.soilbio.2016.02.015
Chowdhury, S., Lange, M., Malik, A. A., Goodall, T., Huang, J., Griffiths, R. I., et al. (2022). Plants with arbuscular mycorrhizal fungi efficiently acquire Nitrogen from substrate additions by shaping the decomposer community composition and their net plant carbon demand. Plant Soil 475, 473–490. doi: 10.1007/s11104-022-05380-x
Clark, R. B., Zeto, S. K. (2000). Mineral acquisition by arbuscular mycorrhizal plants. J. Plant Nutr. 23, 867–902. doi: 10.1080/01904160009382068
Conway, D., Archer van Garderen, E., Deryng, D., Dorling, S., Krueger, T., Landman, W., et al (2015). Climate and southern Africa’s water–energy–food nexus. Nat. Clim. Change 5, 837–846. doi: 10.1038/nclimate2735
Davidson, E. A., Janssens, I. A. (2006). Temperature sensitivity of soil carbon decomposition and feedbacks to climate change. Nature 440, 165–173. doi: 10.1038/nature04514
Davoudpour, Y., Schmidt, M., Calabrese, F., Richnow, H. H., Musat, N. (2020). High resolution microscopy to evaluate the efficiency of surface sterilization of Zea Mays seeds. PloS One 15, 1–21. doi: 10.1371/journal.pone.0242247
Deepadevi, M., Basu, M. J., Santhaguru, K. (2010). Response of Sorghum bicolor (L.) Moench to dual inoculation with Glomus fasciculatum and Herbaspirillum seropedicae. Gen. Appl. Plant Physiol. 36, 176–182.
Diagne, N., Ngom, M., Djighaly, P. I., Fall, D., Hocher, V., Svistoonoff, S. (2020). Roles of arbuscular mycorrhizal fungi on plant growth and performance: importance in biotic and abiotic stressed regulation. Diversity 12, 1–25. doi: 10.3390/d12100370
Dicko, M. H., Gruppen, H., Traoré, A. S., Voragen, A. G. J., Van Berkel, W. J. H. (2006). Sorghum grain as human food in Africa: Relevance of content of starch and amylase activities. Afr. J. Biotechnol. 5, 384–395.
Dodd, J. C., Boddington, C. L., Rodriguez, A., Gonzalez-Chavez, C., Mansur, I. (2000). Mycelium of arbuscular mycorrhizal fungi (AMF) from different genera: Form, function and detection. Plant Soil 226, 131–151. doi: 10.1023/A:1026574828169
Duddek, P., Carminati, A., Koebernick, N., Ohmann, L., Lovric, G., Delzon, S., et al. (2022). The impact of drought-induced root and root hair shrinkage on root–soil contact. Plant Physiol. 189, 1232–1236. doi: 10.1093/plphys/kiac144
Eziz, A., Yan, Z., Tian, D., Han, W., Tang, Z., Fang, J. (2017). Drought effect on plant biomass allocation: A meta-analysis. Ecol. Evol. 7, 11002–11010. doi: 10.1002/ece3.3630
Fageria, N. K., Baligar, V. C. (2005). Enhancing nitrogen use efficiency in crop plants. Adv. Agron. 88, 97–185. doi: 10.1016/S0065-2113(05)88004-6
Faghihinia, M., Jansa, J., Halverson, L. J., Staddon, P. L. (2023). Hyphosphere microbiome of arbuscular mycorrhizal fungi: a realm of unknowns. Biol. Fertil. Soils 59, 17–34. doi: 10.1007/s00374-022-01683-4
Falconnier, G. N., Corbeels, M., Boote, K. J., Affholder, F., Adam, M., MacCarthy, D. S., et al. (2020). Modelling climate change impacts on maize yields under low nitrogen input conditions in sub-Saharan Africa. Glob. Change Biol. 26, 5942–5964. doi: 10.1111/gcb.15261
Fall, A. F., Nakabonge, G., Ssekandi, J., Founoune-Mboup, H., Apori, S. O., Ndiaye, A., et al. (2022). Roles of arbuscular mycorrhizal fungi on soil fertility: contribution in the improvement of physical, chemical, and biological properties of the soil. Front. Fungal Biol. 3. doi: 10.3389/ffunb.2022.723892
FAO (2020). The State of Food and Agriculture: Overcoming Water Challenges in Agriculture. (Cham, Switzerland: Springer International).
FAO (2023). Agricultural production statistics Agricultural production statistics 2000-2022. Faostat Anal. Br. 79.
Fellbaum, C. R., Gachomo, E. W., Beesetty, Y., Choudhari, S., Strahan, G. D., Pfeffer, P. E., et al. (2012). Carbon availability triggers fungal nitrogen uptake and transport in arbuscular mycorrhizal symbiosis. Proc. Natl. Acad. Sci. U. S. A. 109, 2666–2671. doi: 10.1073/pnas.1118650109
Field, C. B., Barros, V., Stocker, T. F., Dahe, Q., Dokken, D. J., Ebi, K. L., et al. (2011). Special Report of the Intergovernmental Panel on Climate Change Edited. (Cham, Switzerland: Springer International).
Gaume, A., Mächler, F., De León, C., Narro, L., Frossard, E. (2001). Low-P tolerance by maize (Zea mays L.) genotypes: Significance of root growth, and organic acids and acid phosphatase root exudation. Plant Soil 228, 253–264. doi: 10.1023/A:1004824019289
Geisseler, D., Horwath, W. R., Scow, K. M. (2011). Soil moisture and plant residue addition interact in their effect on extracellular enzyme activity. Pedobiologia (Jena). 54, 71–78. doi: 10.1016/j.pedobi.2010.10.001
Gerber, J. S., Ray, D. K., Makowski, D., Butler, E. E., Mueller, N. D., West, P. C., et al (2024). Global spatially explicit yield gap time trends reveal regions at risk of future crop yield stagnation. Nat. Food 5, 125–135. doi: 10.1038/s43016-024-00870-5
German, D. P., Weintraub, M. N., Grandy, A. S., Lauber, C. L., Rinkes, Z. L., Allison, S. D. (2011). Optimization of hydrolytic and oxidative enzyme methods for ecosystem studies. Soil Biol. Biochem. 43, 1387–1397. doi: 10.1016/j.soilbio.2011.03.017
Gholamhoseini, M., Ghalavand, A., Dolatabadian, A., Jamshidi, E., Khodaei-Joghan, A. (2013). Effects of arbuscular mycorrhizal inoculation on growth, yield, nutrient uptake and irrigation water productivity of sunflowers grown under drought stress. Agric. Water Manage. 117, 106–114. doi: 10.1016/j.agwat.2012.11.007
Goche, T., Shargie, N. G., Cummins, I., Brown, A. P., Chivasa, S., Ngara, R. (2020). Comparative physiological and root proteome analyses of two sorghum varieties responding to water limitation. Sci. Rep. 10, 1–18. doi: 10.1038/s41598-020-68735-3
Goebel, M. O., Bachmann, J., Reichstein, M., Janssens, I. A., Guggenberger, G. (2011). Soil water repellency and its implications for organic matter decomposition - is there a link to extreme climatic events? Glob. Change Biol. 17, 2640–2656. doi: 10.1111/j.1365-2486.2011.02414.x
Greenfield, L. M., Hill, P. W., Paterson, E., Baggs, E. M., Jones, D. L. (2020). Do plants use root-derived proteases to promote the uptake of soil organic nitrogen? Plant Soil 456, 355–367. doi: 10.1007/s11104-020-04719-6
Greenfield, L. M., Razavi, B. S., Bilyera, N., Zhang, X., Jones, D. L. (2021). Root hairs and protein addition to soil promote leucine aminopeptidase activity of Hordeum vulgare L. Rhizosphere 18, 1–7. doi: 10.1016/j.rhisph.2021.100329
Guber, A., Blagodatskaya, E., Kravchenko, A. (2022). Are enzymes transported in soils by water fluxes? Soil Biol. Biochem. 168, 108633. doi: 10.1016/j.soilbio.2022.108633
Guber, A., Kravchenko, A., Razavi, B. S., Uteau, D., Peth, S., Blagodatskaya, E., et al. (2018). Quantitative soil zymography: Mechanisms, processes of substrate and enzyme diffusion in porous media. Soil Biol. Biochem. 127, 156–167. doi: 10.1016/j.soilbio.2018.09.030
Hadebe, S. T., Modi, A. T., Mabhaudhi, T. (2017). Drought tolerance and water use of cereal crops: A focus on sorghum as a food security crop in sub-saharan africa. J. Agron. Crop Sci. 203, 177–191. doi: 10.1111/jac.12191
Hallett, P. D., Marin, M., Bending, G. D., George, T. S., Collins, C. D., Otten, W. (2022). Building soil sustainability from root–soil interface traits. Trends Plant Sci. 27, 688–698. doi: 10.1016/j.tplants.2022.01.010
Hammer, E. C., Arellano-Caicedo, C., Mafla-Endara, P. M., Kiers, E. T., Shimizu, T., Ohlsson, P., et al. (2024). Hyphal exploration strategies and habitat modification of an arbuscular mycorrhizal fungus in microengineered soil chips. Fungal Ecol. 67, 101302. doi: 10.1016/j.funeco.2023.101302
Harpole, W. S., Ngai, J. T., Cleland, E. E., Seabloom, E. W., Borer, E. T., Bracken, M. E. S., et al. (2011). Nutrient co-limitation of primary producer communities. Ecol. Lett. 14, 852–862. doi: 10.1111/j.1461-0248.2011.01651.x
Haussmann, B. I. G., Mahalakshmi, V., Reddy, B. V. S., Seetharama, N., Hash, C. T., Geiger, H. H. (2002). QTL mapping of stay-green in two sorghum recombinant inbred populations. Theor. Appl. Genet. 106, 133–142. doi: 10.1007/s00122-002-1012-3
He, M., Dijkstra, F. A. (2014). Drought effect on plant nitrogen and phosphorus: A meta-analysis. New Phytol. 204, 924–931. doi: 10.1111/nph.12952
Hoang, D. T. T., Razavi, B. S., Kuzyakov, Y., Blagodatskaya, E. (2016). Earthworm burrows: Kinetics and spatial distribution of enzymes of C-, N- and P- cycles. Soil Biol. Biochem. 99, 94–103. doi: 10.1016/j.soilbio.2016.04.021
Hosseini, S. S., Lakzian, A., Razavi, B. S. (2022). Reduction in root active zones under drought stress controls spatial distribution and catalytic efficiency of enzyme activities in rhizosphere of wheat. Rhizosphere 23, 1–9. doi: 10.1016/j.rhisph.2022.100561
Huntley, B. J. (2023). Ecology of Angola. (Cham, Switzerland: Springer International). doi: 10.1007/978-3-031-18923-4
IPCC (2022). “Climate Change 2022: Impacts, Adaptation and Vulnerability,” in Contribution of Working Group II to the Sixth Assessment Report of the Intergovernmental Panel on Climate Change. eds. Pörtner, H.-O., Roberts, D. C., Tignor, M., Poloczanska, E. S., Mintenbeck, K., Alegría, A., et al (Cambridge, UK and New York, NY, USA: Cambridge University Press), 3056 pp. doi: 10.1017/9781009325844
IUSS Working Group WRB (2015). “World Reference Base for Soil Resources 2014,” in update 2015: International soil classification system for naming soils and creating legends for soil maps. World Soil Resources Reports No. 106. Rome: FAO.
Jägermeyr, J., Müller, C., Ruane, A. C., Elliott, J., Balkovic, J., Castillo, O., et al. (2021). Climate impacts on global agriculture emerge earlier in new generation of climate and crop models. Nat. Food 2, 873–885. doi: 10.1038/s43016-021-00400-y
Jamil, F., Mukhtar, H., Fouillaud, M., Dufossé, L. (2022). Rhizosphere signaling: insights into plant–rhizomicrobiome interactions for sustainable agronomy. Microorganisms 10, 1–26. doi: 10.3390/microorganisms10050899
Johnson, N. C. (2010). Resource stoichiometry elucidates the structure and function of arbuscular mycorrhizas across scales. New Phytol. 185, 631–647. doi: 10.1111/j.1469-8137.2009.03110.x
Kaiser, K., Guggenberger, G. (2007). Sorptive stabilization of organic matter by microporous goethite: Sorption into small pores vs. surface complexation. Eur. J. Soil Sci. 58, 45–59. doi: 10.1111/j.1365-2389.2006.00799.x
Kaiser, C., Kilburn, M. R., Clode, P. L., Fuchslueger, L., Koranda, M., Cliff, J. B., et al. (2015). Exploring the transfer of recent plant photosynthates to soil microbes: Mycorrhizal pathway vs direct root exudation. New Phytol. 205, 1537–1551. doi: 10.1111/nph.13138
Kakouridis, A., Hagen, J. A., Kan, M. P., Mambelli, S., Feldman, L. J., Herman, D. J., et al. (2022). Routes to roots: direct evidence of water transport by arbuscular mycorrhizal fungi to host plants. New Phytol. 236, 210–225. doi: 10.1111/nph.18281
Kakouridis, A., Yuan, M., Nuccio, E. E., Hagen, J. A., Fossum, C. A., Moore, M. L., et al. (2024). Arbuscular mycorrhiza convey significant plant carbon to a diverse hyphosphere microbial food web and mineral-associated organic matter. New Phytol. 242, 1661–1675. doi: 10.1111/nph.19560
Kaur, S., Suseela, V. (2020). Unraveling arbuscular mycorrhiza-induced changes in plant primary and secondary metabolome. Metabolites 10, 1–30. doi: 10.3390/metabo10080335
Kelly, C., Haddix, M. L., Byrne, P. F., Cotrufo, M. F., Schipanski, M., Kallenbach, C. M., et al. (2022). Divergent belowground carbon allocation patterns of winter wheat shape rhizosphere microbial communities and nitrogen cycling activities. Soil Biol. Biochem. 165, 108518. doi: 10.1016/j.soilbio.2021.108518
Khalil, A. M., Murchie, E. H., Mooney, S. J. (2020). Quantifying the influence of water deficit on root and shoot growth in wheat using X-ray Computed Tomography. AoB Plants 12, 1–13. doi: 10.1093/aobpla/plaa036
Kiers, E. T., Duhamel, M., Beesetty, Y., Mensah, J. A., Franken, O., Verbruggen, E., et al. (2011). Reciprocal rewardsStabilize cooperation in theMycorrhizal symbiosis. Science 333, 880–882. doi: 10.1126/science.1208473
Kou, X., Han, W., Kang, J. (2022). Responses of root system architecture to water stress at multiple levels: A meta-analysis of trials under controlled conditions. Front. Plant Sci. 13. doi: 10.3389/fpls.2022.1085409
Kuzyakov, Y., Blagodatskaya, E. (2015). Microbial hotspots and hot moments in soil: Concept & review. Soil Biol. Biochem. 83, 184–199. doi: 10.1016/j.soilbio.2015.01.025
Kuzyakov, Y., Domanski, G. (2000). Carbon input by plants into the soil. Review. J. Plant Nutr. Soil Sci. 163, 421–431. doi: 10.1002/1522-2624(200008)163:4<421::AID-JPLN421>3.0.CO;2-R
Li, J., Meng, B., Chai, H., Yang, X., Song, W., Li, S., et al. (2019). Arbuscular Mycorrhizal Fungi Alleviate Drought Stress in C3 (Leymus chinensis) and C4 (Hemarthria altissima) Grasses via Altering Antioxidant Enzyme Activities and Photosynthesis. Front. Plant Sci. 10. doi: 10.3389/fpls.2019.00499
Liang, L., Liu, B., Huang, D., Kuang, Q., An, T., Liu, S., et al. (2022). Arbuscular mycorrhizal fungi alleviate low phosphorus stress in maize genotypes with contrasting root systems. Plants 11, 1–18. doi: 10.3390/plants11223105
Liu, C., Siri, M., Li, H., Ren, C., Huang, J., Feng, C., et al. (2023). Drought is threatening plant growth and soil nutrients of grassland ecosystems: A meta-analysis. Ecol. Evol. 13, 1–10. doi: 10.1002/ece3.10092
Loftus, S., Sauer, A. M., Schneider, E. M., Erugoti, L. K., Tharanya, M., Rötter, R. P., et al. (2025). Distinct water and phosphorus extraction patterns are key to maintaining the productivity of sorghum under drought and limited soil resources. Sci. Rep. 15, 1–15. doi: 10.1038/s41598-025-88705-x
Mäder, P., Vierheilig, H., Streitwolf-Engel, R., Boller, T., Frey, B., Christie, P., et al. (2000). Transport of 15N from a soil compartment separated by a polytetrafluoroethylene membrane to plant roots via the hyphae of arbuscular mycorrhizal fungi. New Phytol. 146, 155–161. doi: 10.1046/j.1469-8137.2000.00615.x
Marschner, P. (2012). Marschner’s mineral nutrition of higher plants. (Cham, Switzerland: Springer International).
Marx, M. C., Wood, M., Jarvis, S. C. (2001). A microplate fluorimetric assay for the study of enzyme diversity in soils. Soil Biol. Biochem. 33, 1633–1640. doi: 10.1016/S0038-0717(01)00079-7
Matsui, M., Fowler, J. H., Walling, L. L. (2006). Leucine aminopeptidases: Diversity in structure and function. Biol. Chem. 387, 1535–1544. doi: 10.1515/BC.2006.191
Miransari, M. (2011). Arbuscular mycorrhizal fungi and nitrogen uptake. Arch. Microbiol. 193, 77–81. doi: 10.1007/s00203-010-0657-6
Miyata, K., Umehara, M. (2024). Roles of arbuscular mycorrhizal fungi for essential nutrient acquisition under nutrient deficiency in plants. Arbuscular Mycorrhizal Fungi High. Plants, 123–148. doi: 10.1007/978-981-99-8220-2
Mommer, L., Hinsinger, P., Prigent-Combaret, C., Visser, E. J. W. (2016). Advances in the rhizosphere: stretching the interface of life. Plant Soil 407, 1–8. doi: 10.1007/s11104-016-3040-9
Ndabankulu, K., Egbewale, S. O., Tsvuura, Z., Magadlela, A. (2022). Soil microbes and associated extracellular enzymes largely impact nutrient bioavailability in acidic and nutrient poor grassland ecosystem soils. Sci. Rep. 12, 1–11. doi: 10.1038/s41598-022-16949-y
Neumann, E., George, E. (2004). Colonisation with the arbuscular mycorrhizal fungus Glomus mosseae (Nicol. & Gerd.) enhanced phosphorus uptake from dry soil in Sorghum bicolor (L.). Plant Soil 261, 245–255. doi: 10.1023/B:PLSO.0000035573.94425.60
Pasley, H. R., Camberato, J. J., Cairns, J. E., Zaman-Allah, M., Das, B., Vyn, T. J. (2020). Nitrogen rate impacts on tropical maize nitrogen use efficiency and soil nitrogen depletion in eastern and southern Africa. Nutr. Cycl. Agroecosystems 116, 397–408. doi: 10.1007/s10705-020-10049-x
Pérez-Tienda, J., Valderas, A., Camañes, G., García-Agustín, P., Ferrol, N. (2012). Kinetics of NH4+uptake by the arbuscular mycorrhizal fungus Rhizophagus irregularis. Mycorrhiza 22, 485–491. doi: 10.1007/s00572-012-0452-0
Phour, M., Sehrawat, A., Sindhu, S. S., Glick, B. R. (2020). Interkingdom signaling in plant-rhizomicrobiome interactions for sustainable agriculture. Microbiol. Res. 241, 126589. doi: 10.1016/j.micres.2020.126589
Pickson, R. B., Boateng, E. (2022). Climate change: a friend or foe to food security in Africa? Environ. Dev. Sustain. 24, 4387–4412. doi: 10.1007/s10668-021-01621-8
Potthoff, M., Loftfield, N., Buegger, F., Wick, B., John, B., Joergensen, R. G., et al. (2003). The determination of δ13C in soil microbial biomass using fumigation-extraction. Soil Biol. Biochem. 35, 947–954. doi: 10.1016/S0038-0717(03)00151-2
Qi, S., Wang, J., Wan, L., Dai, Z., da Silva Matos, D. M., Du, D., et al. (2022). Arbuscular mycorrhizal fungi contribute to phosphorous uptake and allocation strategies of solidago canadensis in a phosphorous-deficient environment. Front. Plant Sci. 13. doi: 10.3389/fpls.2022.831654
Qiao, C., Wang, X., Shirvan, M. B., Keitel, C., Cavagnaro, T. R., Dijkstra, F. A. (2022). Drought and interspecific competition increase belowground carbon allocation for nitrogen acquisition in monocultures and mixtures of Trifolium repens and Lolium perenne. Plant Soil 481, 269–283. doi: 10.1007/s11104-022-05636-6
Qu, Q., Wang, Z., Gan, Q., Liu, R., Xu, H. (2023). Impact of drought on soil microbial biomass and extracellular enzyme activity. Front. Plant Sci. 14. doi: 10.3389/fpls.2023.1221288
R Core Team (2022). R: A language and environment for statistical computing. (Vienna, Austria: R Foundation for Statistical Computing). Available online at: https://www.R-project.org/.
Razavi, B. S., Blagodatskaya, E., Kuzyakov, Y. (2015). Nonlinear temperature sensitivity of enzyme kinetics explains canceling effect-A case study on loamy haplic Luvisol. Front. Microbiol. 6. doi: 10.3389/fmicb.2015.01126
Saengwilai, P., Strock, C., Rangarajan, H., Chimungu, J., Salungyu, J., Lynch, J. P. (2021). Root hair phenotypes influence nitrogen acquisition in maize. Ann. Bot. 128, 849–858. doi: 10.1093/aob/mcab104
Sanaullah, M., Blagodatskaya, E., Chabbi, A., Rumpel, C., Kuzyakov, Y. (2011). Drought effects on microbial biomass and enzyme activities in the rhizosphere of grasses depend on plant community composition. Appl. Soil Ecol. 48, 38–44. doi: 10.1016/j.apsoil.2011.02.004
Sanaullah, M., Chabbi, A., Rumpel, C., Kuzyakov, Y. (2012). Carbon allocation in grassland communities under drought stress followed by 14C pulse labeling. Soil Biol. Biochem. 55, 132–139. doi: 10.1016/j.soilbio.2012.06.004
Sauer, A. M., Loftus, S., Schneider, E. M., Sudhabindu, K., Hajjarpoor, A., Sivasakthi, K., et al. (2024). Sorghum landraces perform better than a commonly used cultivar under terminal drought, especially on sandy soil. Plant Stress 13, 1–15. doi: 10.1016/j.stress.2024.100549
Schimel, J. P. (2018). Life in dry soils: Effects of drought on soil microbial communities and processes. Annu. Rev. Ecol. Evol. Syst. 49, 409–432. doi: 10.1146/annurev-ecolsys-110617-062614
Seleiman, M. F., Al-Suhaibani, N., Ali, N., Akmal, M., Alotaibi, M., Refay, Y., et al. (2021). Drought stress impacts on plants and different approaches to alleviate its adverse effects. Plants 10, 1–25. doi: 10.3390/plants10020259
Shahzad, T., Chenu, C., Genet, P., Barot, S., Perveen, N., Mougin, C., et al. (2015). Contribution of exudates, arbuscular mycorrhizal fungi and litter depositions to the rhizosphere priming effect induced by grassland species. Soil Biol. Biochem. 80, 146–155. doi: 10.1016/j.soilbio.2014.09.023
Shen, Y., Zhang, F., Yang, Y., Lu, G., Yang, X. (2023). Arbuscular mycorrhizal fungi alter plant N and P resorption of dominant species in a degraded grassland of northern China. Ecol. Indic. 150, 110195. doi: 10.1016/j.ecolind.2023.110195
Sigua, G. C., Stone, K. C., Bauer, P. J., Szogi, A. A. (2018). Biomass and nitrogen use efficiency of grain sorghum with nitrogen and supplemental irrigation. Agron. J. 110, 1119–1127. doi: 10.2134/agronj2017.09.0533
Singh, S., Mayes, M. A., Shekoofa, A., Kivlin, S. N., Bansal, S., Jagadamma, S. (2021). Soil organic carbon cycling in response to simulated soil moisture variation under field conditions. Sci. Rep. 11, 1–13. doi: 10.1038/s41598-021-90359-4
Smith, S. E., Facelli, E., Pope, S., Smith, F. A. (2010). Plant performance in stressful environments: Interpreting new and established knowledge of the roles of arbuscular mycorrhizas. Plant Soil 326, 3–20. doi: 10.1007/s11104-009-9981-5
Somasundaram, S., Rao, T. P., Tatsumi, J., Iijima, M. (2009). Rhizodeposition of mucilage, root border cells, carbon and water under combined soil physical stresses in Zea mays L. Plant Prod. Sci. 12, 443–448. doi: 10.1626/pps.12.443
Sommer, J., Dippold, M. A., Zieger, S. L., Handke, A., Scheu, S., Kuzyakov, Y. (2017). The tree species matters: Belowground carbon input and utilization in the myco-rhizosphere. Eur. J. Soil Biol. 81, 100–107. doi: 10.1016/j.ejsobi.2017.07.001
Stefoska-Needham, A., Tapsell, L. (2020). Considerations for progressing a mainstream position for sorghum, a potentially sustainable cereal crop, for food product innovation pipelines. Trends Food Sci. Technol. 97, 249–253. doi: 10.1016/j.tifs.2020.01.012
Stock, S. C., Koester, M., Boy, J., Godoy, R., Nájera, F., Matus, F., et al. (2021). Plant carbon investment in fine roots and arbuscular mycorrhizal fungi: A cross-biome study on nutrient acquisition strategies. Sci. Total Environ. 781, 146748. doi: 10.1016/j.scitotenv.2021.146748
Symanczik, S., Lehmann, M. F., Wiemken, A., Boller, T., Courty, P. E. (2018). Effects of two contrasted arbuscular mycorrhizal fungal isolates on nutrient uptake by Sorghum bicolor under drought. Mycorrhiza 28, 779–785. doi: 10.1007/s00572-018-0853-9
Takanashi, H. (2023). Genetic control of morphological traits useful for improving sorghum. Breed. Sci. 73, 57–69. doi: 10.1270/jsbbs.22069
Tecon, R., Or, D. (2017). Biophysical processes supporting the diversity of microbial life in soil. FEMS Microbiol. Rev. 41, 599–623. doi: 10.1093/femsre/fux039
Thirkell, T. J., Cameron, D. D., Hodge, A. (2016). Resolving the ‘nitrogen paradox’ of arbuscular mycorrhizas: fertilization with organic matter brings considerable benefits for plant nutrition and growth. Plant Cell Environ. 39, 1683–1690. doi: 10.1111/pce.12667
Thirkell, T. J., Campbell, M., Driver, J., Pastok, D., Merry, B., Field, K. J. (2021). Cultivar-dependent increases in mycorrhizal nutrient acquisition by barley in response to elevated CO2. Plants People Planet 3, 553–566. doi: 10.1002/ppp3.10174
Thirkell, T. J., Pastok, D., Field, K. J. (2020). Carbon for nutrient exchange between arbuscular mycorrhizal fungi and wheat varies according to cultivar and changes in atmospheric carbon dioxide concentration. Glob. Change Biol. 26, 1725–1738. doi: 10.1111/gcb.14851
Tibbett, M., Daws, M. I., Ryan, M. H. (2022). Phosphorus uptake and toxicity are delimited by mycorrhizal symbiosis in P-sensitive Eucalyptus marginata but not in P-tolerant Acacia celastrifolia. AoB Plants 14, 1–9. doi: 10.1093/aobpla/plac037
Tobar, R., Azcon, R., Barea, J. M. (1994). Improved nitrogen uptake and transport from N-labelled nitrate by external of arbusculat mycorrhiza nder water-stressed condition. New Phytol. 126, 119–122. doi: 10.1111/j.1469-8137.1994.tb07536.x
Trisos, C. H., Adelekan, I. O., Totin, E., Ayanlade, A., Efitre, J., Gemeda, A., et al. (2022). “Africa,” in Climate Change 2022: Impacts, Adaptation, and Vulnerability. Contribution of Working Group II to the Sixth Assessment Report of the Intergovernmental Panel on Climate Change. Eds. Pörtner, H.-O., Roberts, D. C., Tignor, M., Poloczanska, E. S., Mintenb, K.. (Cham, Switzerland: Springer International). doi: 10.1017/9781009325844.011.1286
Turner, B. L., Driessen, J. P., Haygarth, P. M., Mckelvie, I. D. (2003). Potential contribution of lysed bacterial cells to phosphorus solubilisation in two rewetted Australian pasture soils. Soil Biol. Biochem. 35, 187–189. doi: 10.1016/S0038-0717(02)00244-4
Uwituze, Y., Nyiraneza, J., Fraser, T. D., Dessureaut-Rompré, J., Ziadi, N., Lafond, J. (2022). Carbon, nitrogen, phosphorus, and extracellular soil enzyme responses to different land use. Front. Soil Sci. 2. doi: 10.3389/fsoil.2022.814554
Van Ittersum, M. K., Van Bussel, L. G. J., Wolf, J., Grassini, P., Van Wart, J., Guilpart, N., et al. (2016). Can sub-Saharan Africa feed itself? Proc. Natl. Acad. Sci. U. S. A. 113, 14964–14969. doi: 10.1073/pnas.1610359113
Vetterlein, D., Carminati, A., Kögel-Knabner, I., Bienert, G. P., Smalla, K., Oburger, E., et al. (2020). Rhizosphere spatiotemporal organization–A key to rhizosphere functions. Front. Agron. 2. doi: 10.3389/fagro.2020.00008
Vierheilig, H., Coughlan, A. P., Wyss, U., Piché, Y. (1998). Ink and vinegar, a simple staining technique for arbuscular-mycorrhizal fungi. Appl. Environ. Microbiol. 64, 5004–5007. doi: 10.1128/aem.64.12.5004-5007.1998
Wang, R., Cavagnaro, T. R., Jiang, Y., Keitel, C., Dijkstra, F. A. (2021). Carbon allocation to the rhizosphere is affected by drought and nitrogen addition. J. Ecol. 109, 3699–3709. doi: 10.1111/1365-2745.13746
Wang, F., Sun, Y., Shi, Z. (2019). Arbuscular mycorrhiza enhances biomass production and salt tolerance of sweet sorghum. Microorganisms 7, 1–14. doi: 10.3390/microorganisms7090289
Wang, F., Zhang, L., Zhou, J., Rengel, Z., George, T. S., Feng, G. (2022). Exploring the secrets of hyphosphere of arbuscular mycorrhizal fungi: processes and ecological functions. Plant Soil 481, 1–22. doi: 10.1007/s11104-022-05621-z
White, P. J. (2011). Long-distance transport in the Xylem and Phloem (London, UK: Elsevier Ltd). doi: 10.1016/B978-0-12-384905-2.00003-0
Wilschut, R. A., van Kleunen, M. (2021). Drought alters plant-soil feedback effects on biomass allocation but not on plant performance. Plant Soil 462, 285–296. doi: 10.1007/s11104-021-04861-9
Wipf, D., Krajinski, F., van Tuinen, D., Recorbet, G., Courty, P. E. (2019). Trading on the arbuscular mycorrhiza market: from arbuscules to common mycorrhizal networks. New Phytol. 223, 1127–1142. doi: 10.1111/nph.15775
Wu, Y., Chen, C., Wang, G. (2024). Inoculation with arbuscular mycorrhizal fungi improves plant biomass and nitrogen and phosphorus nutrients: a meta-analysis. BMC Plant Biol. 24, 960. doi: 10.1186/s12870-024-05638-9
Wu, J., Joergensen, R. G., Pommerening, B., Chaussod, R., Brookes, P. C. (1990). Measurement of soil microbial biomass C by fumigation-extraction-an automated procedure. Soil Biol. Biochem. 22, 1167–1169. doi: 10.1016/0038-0717(90)90046-3
Xiao, X., Liao, X., Yan, Q., Xie, Y., Chen, J., Liang, G., et al. (2023). Arbuscular Mycorrhizal Fungi Improve the Growth, Water Status, and Nutrient Uptake of Cinnamomum migao and the Soil Nutrient Stoichiometry under Drought Stress and Recovery. J. Fungi 9, 1–23. doi: 10.3390/jof9030321
Xue, J., Guo, L., Li, L., Zhang, Z., Huang, M., Cai, J., et al. (2024). Effects of arbuscular mycorrhizal fungi on uptake, partitioning and use efficiency of nitrogen in wheat. F. Crop Res. 306, 109244. doi: 10.1016/j.fcr.2023.109244
Xue, R., Shen, Y., Marschner, P. (2017). Soil water content during and after plant growth influence nutrient availability and microbial biomass. J. Soil Sci. Plant Nutr. 17, 702–715. doi: 10.4067/S0718-95162017000300012
Yu, S. M., Lo, S. F., Ho, T. H. D. (2015). Source-sink communication: regulated by hormone, nutrient, and stress cross-signaling. Trends Plant Sci. 20, 844–857. doi: 10.1016/j.tplants.2015.10.009
Zhang, A., Mo, X., Zhou, N., Wang, Y., Wei, G., Chen, J., et al. (2020). A novel bacterial β-N-acetyl glucosaminidase from Chitinolyticbacter meiyuanensis possessing transglycosylation and reverse hydrolysis activities. Biotechnol. Biofuels 13, 1–14. doi: 10.1186/s13068-020-01754-4
Zhang, X., Myrold, D. D., Shi, L., Kuzyakov, Y., Dai, H., Thu Hoang, D. T., et al. (2021). Resistance of microbial community and its functional sensitivity in the rhizosphere hotspots to drought. Soil Biol. Biochem. 161, 108360. doi: 10.1016/j.soilbio.2021.108360
Zhou, R., Zhou, J., Jia, L., Wei, J., Yan, J., Ji, J., et al. (2023). Polyphosphate hydrolysis, sorption, and conversion in two different soils. Eur. J. Soil Sci. 74, 1–13. doi: 10.1111/ejss.13341
Keywords: enzyme activity, mycorrhiza, photoassimilate use, moisture limitation, nitrogen mobilization, sorghum
Citation: Munene R, Mustafa O, Loftus S, Banfield CC, Rötter RP, Bore EK, Mweu B, Mganga KZ, Otieno DO, Ahmed MA and Dippold MA (2025) Contribution of arbuscular mycorrhiza and exoenzymes to nitrogen acquisition of sorghum under drought. Front. Plant Sci. 16:1514416. doi: 10.3389/fpls.2025.1514416
Received: 20 October 2024; Accepted: 20 March 2025;
Published: 15 April 2025.
Edited by:
Sabine Dagmar Zimmermann, IPSiM Institute of Plant Science in Montpellier CNRS UMR5004, FranceReviewed by:
Nieves Goicoechea, University of Navarra, SpainCopyright © 2025 Munene, Mustafa, Loftus, Banfield, Rötter, Bore, Mweu, Mganga, Otieno, Ahmed and Dippold. This is an open-access article distributed under the terms of the Creative Commons Attribution License (CC BY). The use, distribution or reproduction in other forums is permitted, provided the original author(s) and the copyright owner(s) are credited and that the original publication in this journal is cited, in accordance with accepted academic practice. No use, distribution or reproduction is permitted which does not comply with these terms.
*Correspondence: Rosepiah Munene, cm96eW11bmVuZUBnbWFpbC5jb20=
†These authors share first authorship
Disclaimer: All claims expressed in this article are solely those of the authors and do not necessarily represent those of their affiliated organizations, or those of the publisher, the editors and the reviewers. Any product that may be evaluated in this article or claim that may be made by its manufacturer is not guaranteed or endorsed by the publisher.
Research integrity at Frontiers
Learn more about the work of our research integrity team to safeguard the quality of each article we publish.