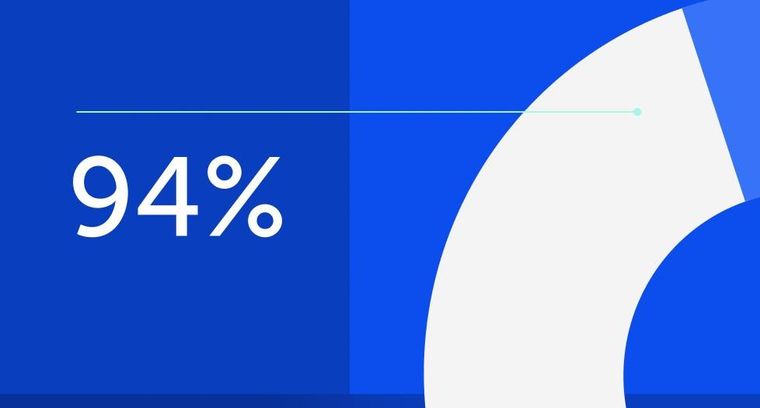
94% of researchers rate our articles as excellent or good
Learn more about the work of our research integrity team to safeguard the quality of each article we publish.
Find out more
REVIEW article
Front. Plant Sci., 06 February 2025
Sec. Sustainable and Intelligent Phytoprotection
Volume 16 - 2025 | https://doi.org/10.3389/fpls.2025.1492760
Crop production using greenhouse technology has become increasingly essential for intensifying agricultural output, particularly in regions with challenging climatic conditions. More so, greenhouses do not only support continuous crop supply but also provide a controlled environment crucial for studying plant-pathogen interaction. Likewise, pests and diseases are a constant threat to crop production, which requires innovative control methods. Providing a suitable and sustainable control method requires a detailed probe into the relationship between plants and biotic disturbance under controlled settings. Therefore this review explores the relationships between plants and pathogens, highlighting the impact of extreme greenhouse microclimates on plant pathology assays. Given the extreme weather conditions in the Arabian peninsula, the efficiency of greenhouses, especially during summer, is compromised without adequate cooling systems. This review discusses the current strategies employed to optimize greenhouse conditions in hot arid regions, aiming to enhance plant health by mitigating pathogen activity while minimizing energy, and water consumption. The review also provides an overview of how microclimatic parameters within greenhouses influence plant-pathogen dynamics, ensuring conditions that are conducive to managing both biotic and abiotic diseases. Additionally, the review aims to evaluate various cooling techniques available and most widely accepted in hot arid regions. Moreover, the performance indicators, principles, and effectiveness of each technique are discussed. Promising advances in the manipulations and combination of these techniques have proven to maintain an appropriate greenhouse microclimate with minimal resource use.
To meet the growing global demand for food while minimizing biodiversity loss, greenhouse technology has emerged as a sustainable and efficient crop production method (Aznar-Sánchez et al., 2020). Generally, greenhouses are employed to provide and regulate environmental conditions that support optimal plant growth in a controllable non-natural environment. Beyond enhancing productivity, greenhouses play a pivotal role in plant science research by enabling precise control of environmental conditions. This is particularly beneficial for investigating host-pathogen interactions, which are often influenced by climatic variables (Lahlali et al., 2024). In controlled settings, greenhouses facilitate the study of disease dynamics, allowing researchers to simulate conditions conducive to specific plant-pathogen interactions that would otherwise be difficult to reproduce in open fields.
Despite these advantages, plant diseases remain a persistent threat to agricultural productivity. More so, the spatial and temporal variability of pathogens (Dai et al., 2022), along with their ability to evolve into strains capable of overcoming host resistance (McLaren and Callahan, 2020), underscores the importance of understanding pathogen dynamics. Greenhouses offer an invaluable platform for such studies, providing the controlled environments necessary to probe the complexities of host-pathogen relationships. Nonetheless, conducting such research in greenhouses located in hot arid regions presents unique challenges, particularly in maintaining optimal conditions for both plants and pathogens.
Primarily, controlled environments are created by managing climatic variables and selecting appropriate covering materials. Despite the simplicity of the setup, the greenhouse presents dynamic processes that require delicate regulations. The controlled greenhouse has been considerably achieved in temperate climates whereas greenhouses in hot arid regions face technological challenges due to extreme weather conditions, especially in summer (Ghoulem et al., 2019). While greenhouses offer controlled environments for plant pathology research, they face significant challenges in hot arid regions, particularly in managing energy and water consumption.
Regions like the Arabian Peninsula, characterized by extreme weather conditions, present significant obstacles to greenhouse operations. High ambient temperatures, coupled with limited freshwater availability, strain conventional cooling systems, which are critical for creating favorable microclimates inside greenhouses. Consequently, without adequate cooling, the efficiency of greenhouses for both crop production and plant pathology research is severely compromised. All the same, the high energy and water demands of cooling systems (Abedrabboh et al., 2024) exacerbate the sustainability challenges in these resource-scarce regions.
Given these constraints, innovative approaches to greenhouse cooling are required to balance resource efficiency with the need for optimal conditions. Even though strategies such as evaporative cooling and the use of advanced materials for greenhouse structures have shown promise (Soussi et al., 2022), there are still obvious limitations, particularly in regions with saline water sources that clog cooling systems. Consequently, the efficiency of these strategies under the intense solar radiation of arid climates remains a critical research area. Taken together, these are necessities that need to be observed in controlling the high temperature inside greenhouses in hot arid regions with the minimal use of resources.
Therefore, the current review intends to bridge this gap, especially cooling the greenhouse for plant science studies. This review examines the interplay between greenhouse microclimates and plant-pathogen dynamics, focusing on the challenges faced in hot arid regions. It explores the current state of greenhouse cooling technologies, evaluates their effectiveness, and highlights opportunities for optimizing resource use. Special attention is given to identifying sustainable solutions tailored to the unique environmental conditions of the Arabian Peninsula. By addressing these gaps, this review aims to contribute to the development of resource-efficient greenhouses capable of supporting both agricultural productivity and advanced plant pathology research.
Plants are constantly influenced by biotic and abiotic factors (Chamard et al., 2024), which can either support or hinder their growth and health. The quality and yield of crops are determined by the complex interactions between plants, pathogens, and environmental conditions. Among these, the environment plays a pivotal role in shaping the outcomes of plant-pathogen interactions (Scholthof, 2007). Favorable environmental conditions often exacerbate pathogen activity (Lahlali et al., 2024), leading to devastating impacts on agriculture, significant economic losses, and threats to food security.
Diseases are manifested as symptoms in plants (Özkaya et al., 2024), signifying disturbance of their normal physiological functions. There is a need to curb the excesses of plant diseases on plants which can be achieved through a thorough knowledge of the causal pathogens, and how their activities bring about the disease situation. To control pathogens effectively, a comprehensive understanding of their disease-inducing activities is essential. Similarly, it’s important to understand their epidemiologic potential ensuing losses, which are relevant to mitigatory options. This knowledge forms the foundation of plant pathology, a field that has evolved from classical diagnostic methods to the incorporation of advanced tools like gene cloning, genetic engineering, and software-assisted modeling. These advancements enable precise disease management in both open-field and controlled environments, including greenhouses (Vidhyasekaran, 2004; Mojerlou, 2024). In the open field, disease incidence and density are determined by the host, the pathogen, and the environment (Mojerlou, 2024). Typically, a virulent pathogen interacting with a susceptible host in a conducive environment leads to disease. Understanding the dynamics of this interaction is essential for predicting outbreaks and implementing timely interventions. Furthermore, environmental factors such as temperature, humidity, and light are not only critical for disease progression but also for plant growth and productivity (Lahlali et al., 2024). Environmental conditions significantly impact disease progression and plant growth (Singh et al., 2020), necessitating innovative strategies like shielded cultivation to protect plants from biotic and abiotic stresses, ensuring optimal growth conditions. Generally, innovative strategies, such as protected cultivation systems, are increasingly used to mitigate the adverse impacts of biotic and abiotic stresses. Primarily, greenhouses, for instance, offer a controlled environment to optimize growth conditions while reducing exposure to unfavorable external factors. Altogether, the advancement provides a unique opportunity to study and manage plant-pathogen interactions, ensuring sustainable crop production even in regions with extreme climates. Thus, integrating environmental insights with advanced tools and cultivation techniques is vital for safeguarding plant health and productivity in the face of evolving agricultural challenges.
Protected cultivation has long been a cornerstone of agricultural innovation, offering a controlled environment to mitigate the effects of adverse weather conditions. Traditionally, farmers employed simple methods such as growing annual crops under the shade of perennial trees to regulate temperature and humidity during their tender stage (Beer et al., 1997). For example, a cultural practice among the cocoa farmers of southwestern Nigeria is spreading cocoa seeds under the shade of banana plants till seedling emergence after which the cocoa seedlings will be transplanted onto the open field (Famuwagun et al., 2018). This is done to prevent the seedling from environmental stress associated with direct sowing on the field. Similarly, elaborate bamboo stakes and palm fronds were used as shade structures for high-value crops, with shade levels tailored to the crop’s growth stage. In the nursery, about 70% shade level is required for grooming young cocoa plants while about 40% is required for mature cocoa plants (Asare and David, 2010). This is because the photosynthetic mechanisms of the leaves and flowers of cocoa plants are damaged when exposed to a high temperature above 30 °C (Famuwagun et al., 2018). This highlights the ingenuity of early agricultural systems in adapting to environmental challenges. Modern protected cultivation systems, such as greenhouses and screenhouses, have revolutionized agriculture by providing an optimal environment for plant growth and research. These systems not only enhance productivity and resource efficiency but also facilitate advanced studies in plant pathology by enabling precise control over environmental conditions.
Greenhouses represent one of the most advanced forms of protected cultivation (Hanan, 2017), offering a controlled setting for crop production and scientific research. They are indispensable in plant pathology research, providing an environment where plant-pathogen interactions can be meticulously studied. By isolating variables such as temperature, humidity, and light, researchers can investigate the effects of environmental factors on disease development and test mitigation strategies under controlled conditions.
Greenhouse technology is also critical for addressing global food security challenges (Khan et al., 2021). With the global population projected to reach 9.7 billion by 2050, agricultural production must increase by 70% to meet food demand (FAO, 2009). To meet this required estimated increment in world food production, the current amount of arable land needs to be extended or face an overbearing intensification (Alexander et al., 2015). However, the intensification of available arable land for agricultural purposes will require more input, such as agrochemicals, which pose additional environmental, human, and health-related hazards (Tilman, 1999) with a sizable amount of disturbances to many terrestrial and aquatic ecosystems. Taken together, we will agree that expanding arable land or intensifying agricultural practices often comes at the expense of biodiversity and ecosystem health (Aznar-Sánchez et al., 2019). Nonetheless, in as much as meeting the projected increase in food demand is important, a vital challenge for sustainable natural resources management is finding a balance between production and preservation of the natural ecosystem. Consequently, there is a dire need for greenhouse technology to mitigate this challenge. In achieving this goal, agricultural practices should shift from intensive to non-intensive through the adoption of improvement in agricultural technologies, minimal use of agrochemicals, and promotion of protected cultivation.
Greenhouses provide a sustainable alternative by optimizing resource use, reducing reliance on agrochemicals, minimizing environmental impact (Aznar-Sánchez et al., 2020),. In addition, greenhouses allow for off-season crop production, enabling tropical crops to be cultivated in temperate regions or arid climates where traditional farming methods are less viable (Lefers et al., 2020). However, despite their advantages, greenhouses in hot arid regions face significant challenges. Extreme temperatures, particularly during summer, demand substantial energy inputs for cooling (Bicer et al., 2022), complicating their sustainability. Addressing these challenges requires innovative cooling systems and efficient resource management to balance productivity with environmental conservation.
During summer, the main factor limiting protected cultivation in arid regions is the overheating of air in greenhouses. The daily outdoor average ambient air temperature often exceeds 45°C in the period from late April to late September. The greenhouse effect increases the air temperature in the uncooled greenhouse reaching over 60°C (Abdel-Ghany et al., 2015). Consequently, an efficient greenhouse cooling system is required to maintain plant growth-friendly temperatures. With natural ventilation, around 100 kJ of accumulated heat per m2 of floor area needs to be removed from the greenhouse every day (Abdel-Ghany et al., 2016). Thus, in regions with high ambient temperatures, such as the Arabian Peninsula, screen houses and plastic net houses offer an alternative to traditional greenhouses. Net houses offer several advantages, including reduced transpiration, lower pesticide use, and protection against wind, pests, and excessive solar radiation (Sotelo-Cardona et al., 2021).
These structures provide partial protection against environmental stressors while reducing the energy and water demands associated with greenhouse cooling. For instance, net houses have been shown to reduce water consumption by up to 13 kg m² day-¹ in summer and energy use by 0.26 kWh m² day-¹ compared to traditional greenhouses (Abdel-Ghany et al., 2016). However, their effectiveness is limited in extremely hot climates. While they mitigate some heat stress, they lack the full environmental control of greenhouses, resulting in higher daytime temperatures and lower photosynthetically active radiation (PAR) transmission compared to cooled greenhouses (Soussi et al., 2022; Alhelal et al., 2024). Consequently, while net houses are suitable for certain crops and conditions, greenhouses remain essential for cultivating high-value crops and conducting pathology research in hot arid regions.
Therefore, the net house serves as a form of complementary system to further enhance resource efficiency, earmarking them as a valuable unit in productive agricultural systems.
Crop performance requires optimal microclimatic parameters which should be catered for by the greenhouse control system, especially in arid regions where the outside weather parameters are at the extreme. Greenhouse cultivation is a popular adventure in the arid region with about 1300 ha of protected crops in Gulf countries alone (FAO, 2021). Greenhouses in this region not only enable water-efficient agriculture but also enhance pest and disease control, with productivity levels up to five times greater than open-field systems (Ghoulem et al., 2019). These attributes show the significance of greenhouse cultivation to the weather-disadvantaged Arabian Peninsula. However, these advantages come with high energy and water demands (Gorjian et al., 2021), especially during the summer months where energy expenditure presents the largest impact on the greenhouse systems and the environment (Ahemd et al., 2016). Addressing these challenges, thus, requires integrating climate-smart agriculture with advanced greenhouse management strategies. This will mitigate crop exposure to harsh internal conditions like heat scorching and the risk of a high disease incidence due to high relative humidity (RH).
Greenhouse temperature especially during the daytime builds up from the amount of solar radiation that is allowed into the greenhouse (Lamnatou and Chemisana, 2013; Colantoni et al., 2018). The temperature accrued is subsequently stored in mediums within the greenhouse and later serves as a source of heat during the nighttime (Papasolomontos et al., 2013). Daytime temperatures in a greenhouse are largely influenced by solar radiation, with the spectrum between 400 and 700 nm which is referred to as photosynthetic active radiation (PAR), being used for photosynthesis (Wells, 1997). The plant absorbs most of the PAR for use in its photosynthetic process, and heat is generated from the radiation balance. The PAR can be influenced by a variety of factors, including the characteristics of the specific greenhouse (Colantoni et al., 2018). For instance, the amount of radiation transmitted and available at the crop level in the greenhouse is influenced by the greenhouse covering material and incident irradiation. Also, a sufficient amount of light is required in the greenhouse for optimal plant performance (Paradiso and Proietti, 2022). However, in as much as light enhances photosynthesis and overall plant growth, its extreme can also negatively affect plant performance because its excess can cause heat stress. Particularly, in young plants, whose high rate of evaporation makes them vulnerable to scorching (Soussi et al., 2022). In essence, the greenhouse, particularly the design, plays a crucial role in temperature management. Studies have shown that actors such as covering materials, window placement, and orientation must optimize light transmission while minimizing heat buildup (Paradiso and Proietti, 2022; Zhang et al., 2022). For most crops, the optimal temperature range is 10–24°C (Subahi and Bouazza, 2020). Beyond this range, artificial cooling systems become necessary to prevent heat stress, which can weaken plants and increase susceptibility to pests and disease (Huot et al., 2017). For example, the root disease of clover has been shown to escalate under adverse soil temperatures (You et al., 2017). Despite the importance of temperature, disease outcome is however affected by combinations of different environmental factors including available moisture.
A very important parameter to be considered in maintaining healthy plant conditions in the greenhouse is the amount of water vapor therein otherwise referred to as relative humidity. Water stress is induced in crops at lower RH, and this low level can lead to a reduction in shoot development (Soussi et al., 2022). However, the reactions of crops to RH vary. It is observed through different Various studies have shown that low humidity can enhance plant quality in certain crops, while triggering opposite effects in some other crops (Mortensen, 2000). For example, the leaf area of cucumber has been observed to increase at high humidity while that of tomato decreases Nevertheless, maintaining an optimal RH level (45-85%) is critical for water regulation, leaf development, and tissue elongation (Fanourakis et al., 2020; Chowdhury et al., 2021).
High humidity can also be a factor in crop disease incidence in the greenhouse (Kruidhof and Elmer, 2020), thus affecting the quality of yield. High humidity can promote fungal diseases, such as Botrytis cinerea, by facilitating spore germination on leaf surfaces, which can be managed by lowering the high humidity in the greenhouse (Yunis et al., 1990). Controlled ventilation systems are key to managing RH, especially during the nighttime when restricted air exchange often leads to condensation and high humidity levels (Amani et al., 2020). However, certain situations, such as calcium pressurization or biocontrol application, may require elevated RH levels. Therefore, greenhouse systems must be adaptable to specific crop needs and environmental conditions.
In addition to water vapor, carbon dioxide (CO2) levels are a critical greenhouse parameter. Elevated concentration, driven by climate change, can initially enhance photosynthetic rates and plant biomass (Cooper et al., 2002). However, prolonged exposure, in the long run, can result in the downregulation of the net photosynthetic (Kirschbaum, 2011). Notably, elevated CO2 can also influence plant-pathogen interactions.
Biotrophic pathogens benefit from increased carbohydrate levels in the host tissue, leading to larger canopy size, and more biomass (Smith and Luna, 2023). However, elevated CO2 levels have been shown to accelerate disease progression in some crops while leaving others unaffected, such as pathogens adapted to dry soils (Hibberd et al., 1996; Mieslerová et al., 2022).
The direct effect of the microclimatic parameters may bring about changes in plants and/or the plant community, and the changes in the plant formation may affect infection (Burdon, 1987).
Temperature is an important environmental factor influencing the activities of pathogens on plants with a myriad of effects on plant-pathogen interaction (Li et al., 2013). Temperature is the most influencing microclimatic variable in greenhouse experiments in Saudi Arabia (Al-Askar et al., 2022). Temperature influences infection in diverse ways including the rate of pathogenesis and timing of infection (Figure 1). Consequently, the medium temperature influences and regulates the rate at which a pathogen will reproduce (Legler et al., 2012). In cases where the timing of infection is important for interactions between two pathogens, the form of competition may be shifted (Al-Naimi et al., 2005), and these changes may affect host susceptibility leading to difficulty in predictions of interactions. A temperature of -13 °C will kill all rust infections (Pfender and Vollmer, 1999), thereby causing a decline in rust survival and overwintering in such weather conditions. Despite this, some spores are suggested to survive better in uredinia (Barua et al., 2018). However, in the broader context of plant pathology, the impact of excessive temperature variations may present a significant obstacle to accurately estimating the frequency and establishment of disease.
Figure 1. Schematic representation of the plant infection cycle . This shows the plant infection cycle, depicting major stages from the production of inoculum to host colonization and expression of symptoms. The figure highlights interactions between the plant and the pathogen, emphasizing critical checkpoints where the infection process is initiated and possible mitigation of infective individual reproduction until multiplication and dissemination.
High temperature can also confer abiotic stress such as drought stress on the plant by increasing the soil environment temperature which can then affect plant predisposition to pathogen attack (Hunjan and Lore, 2020). Abiotic stress has been observed to contribute to plant susceptibility to pathogens or it may induce general defense pathways that increase resistance (Mittler, 2006). Generally, extreme temperature regimes have been observed to weaken plants’ resistance to disease (Sharath Chandran et al., 2021), and this situation may have influenced the pathogen attack and thwarted the actual measurement of virulence. For example, the virulence of Root-Knot Nematode (RKN) is a measurement of its reproductive factor, and in a situation whereby a plant immunity is weakened as a result of high temperature (Kim et al., 2022), then the RKN reproduction will be unnecessarily high. For this reason, it is noted that at 31°C, more juvenile RKN will penetrate the plants’ root than at 28°C (Yuan et al., 2018). Also, during high temperatures, plants may exhibit stress signs similar to water stress such as wilting, leaf burn, leaf folding, and abscission (Seleiman et al., 2021). Somehow, water stress can also hinder the resistance mechanism of plants (Atkinson and Urwin, 2012). For instance, the resistance mechanism in barley is disrupted when water is restored after drought stress (Guo et al., 2009; Frimpong et al., 2021).
Various studies have indicated that temperature regimes are pathosystem-specific. Moreover, if the greenhouse parameters are not well regulated, a high temperature within the greenhouse can influence the aggressiveness of a pathogen (Chen et al., 2017). Thus, a controllable environment is necessary for studies requiring a particular temperature. Studies have shown that Globodera pallida infection on potato plants is optimally favored at a temperature of 15°C (Jones et al., 2017), and rice is better infected by Xanthomonas oryzae at a maximum temperature of 35°C and a minimum of 27°C (Horino et al., 1982). High temperature can lead to strain dominance as certain strains that better adapt at higher temperature levels may be more supported (Mariette et al., 2016). New isolates of stripe rust caused by Puccinia striiformes f. sp. Tritici adapt better than older isolates at a higher temperature regime (Milus et al., 2009). Altogether, this indicates that experiments done in the hot greenhouse in a climate like that of Saudi Arabia can favor the establishment of a high-temperature inclined strain over others. Spores numbers and lesion size are amplified more under conditions of higher temperatures. In some cases of extreme or high temperatures, the establishment of fungal pathogens can be impeded and this can influence the outcome of research requiring the quantification of spores and lesion size. In a study involving Phakopsora pachyrhizi infection on soybean, urediniospores failed to develop at a temperature above 37 °C, likewise, no traces of lesion were observed (Bonde et al., 2012). This implies that disease incidence is delayed in the months when the temperature peaks. Greenhouses in Saudi Arabia feature this condition between June and August. All these are indices pointing to the importance of controllable greenhouse parameters in the arid region.
Plant responses to external stimuli are spontaneous under a higher temperature regime (Obrepalska-Steplowska et al., 2015). A study by Zhang and co (Zhang et al., 2012) shows that the turnip crinkle virus (TCV) will replicate relentlessly under high temperatures. Similarly, the incidence of leaf blight of onion caused by Xanthomonas campestris will be relatively high during July and August when the temperature will be at its peak (Schwartz et al., 2003). This indicates that the bacterium infection is highly favored under high-temperature conditions. However, when the temperature continues to increase, the intensity of the blight begins to reduce because temperatures above 35°C are generally not favorable for the disease (Schwartz et al., 2003). We now have to imagine how the bacteria will behave on onions under temperatures conditions higher than 35°C which is prevalent during the summer months of July and August in Saudi Arabia. It may be difficult to get a proper understanding of the bacteria-onion interaction during these months. The implication is that the execution of experiments will be limited to a certain period of the year except if greenhouses are specially constructed and equipped such that the weather parameters are adequately controlled, and achieving this requires spending more on greenhouse technology.
Additionally, temperature can directly affect host plants genetically (Sbeiti et al., 2023). For example, the resistance conferred on tomato plants carrying the Mi-1 gene to Meloidogyne incognita is lost at a soil temperature above 32°C (Abdul-Baki et al., 1996; Devran and Özalp, 2018). M. incognita reproduced abundantly on the resistant variety exposed to high soil temperature, while the resistance persisted at a lower soil temperature. Moreover, the nematode sex allotment is influenced by temperature, therefore, high temperature will favor the determination of more nematode males which are not of much concern in the infection process (Papadopoulou and Traintaphyllou, 1982). This will hamper the reproduction cycle and overall infection process.
Experimental probes to help determine the temperature ranges at which resistance persists are necessary to determine resistance plant varieties under the plant pathogen management option. However, the Mi-1 gene effectiveness can be lost at temperatures above 28 °C (Abdul-Baki et al., 1996; Dropkin, 1969). Fortunately, the resistance capacity can be recovered when plants previously exposed to unfavorable high temperatures are subsequently maintained at a low temperature (Marques de Carvalho et al., 2015). Aside from this phenomenon in the pathosystem as a result of extreme temperature, symptom masking is another interesting observation in plant pathosystems.
Notably, there are instances when infections are not observed on plants due to high temperatures in the greenhouse, especially in plant-virus interactions. Studies based on of tobacco mosaic virus of Nicotiana benthamiana have demonstrated this. At 35°C, chlorosis appears instead of the typical necrotic local lesion that is typically associated with the disease (Szittya et al., 2003). Similarly, at higher temperatures, the wilting of tomatoes infected with Fusarium wilt was accompanied by leaf yellowing as opposed to lower temperatures when the wilting was absent (Larkin and Fravel, 2002; Thomidis et al., 2023). Conversely, higher temperatures trigger a defense response in plants infected with viruses leading to fewer symptoms due to RNA silencing (Chellappan et al., 2005). It is common to encounter the heat masking phenomenon whereby symptoms of infection especially in virus-plant interaction are lessened under high temperatures (Kassanis, 1957; Ghoshal and Sanfaçon, 2015; Tsai et al., 2022). It is this observation that informs the use of thermotherapy as a physical method of reducing the viral load of planting material (Manganaris et al., 2003). The symptoms of potato leaf roll on Physalis floridana fluctuate greatly at varying temperatures. The virus transit within the plant is highly affected by temperature which can lead to differential sampling results especially when only the inoculated leaves are sampled (Kassanis, 1957). Temperature also has a mark on the treatment aspect of an experiment such as fungicide. The effectiveness and duration of efficacy of fungicides are affected by increased temperature (Juroszek et al., 2022). Taken together, the temperature is considered an important factor in plant-pathogen interaction, and conditions leading to its fluctuations should be considerably managed. Despite all the important roles temperature has displayed in various pathosystems inside the greenhouse, the greenhouse moisture has carried an almost similar weight.
Disease prevalence is considerably influenced by moisture content. In the greenhouse, moisture supplies are mainly through irrigation to the surface of the plant and root environment, and evaporative cooling, if any. Moisture can affect plant-pathogen interaction in several ways. For instance, fungal spore growth is highly favored by moisture, promoting germ tube elongation, and overall host penetration (Roger et al., 1999). Similarly, bacteria and nematodes require moisture for various survival activities, such as motility and spread, on their host (Fujimoto et al., 2010). Likewise, certain bacteria, either through artificial inoculation or natural infection, require a substantial amount of humidity to facilitate a successful establishment. Implicatively, a moist environment is required for establishing a bacterial pathosystem. It is recommended that bacteria should get a film of water that is at least bigger than the bacteria’s size during proliferation on the plant surface. Primarily, the film provides a necessary aqueous environment that supports bacterial life and facilitates biofilm formation, which is essential for bacterial colonization and host interaction (Grinberg et al., 2019). Notably, Bacteria often face desiccation on plant leaves under fluctuating moisture levels. Moisture availability can influence the resistance mechanism of plants by altering the plant’s immune response and the pathogen’s ability to infect. High humidity inhibits plant immunity by altering the innate signaling pathways (Yao et al., 2023). Likewise, soil moisture levels can modulate microbial communities that directly influence the promotion or suppression of plant disease (Chen et al., 2023). For example, severe leaf symptoms are observed in Parthenocissus quinquefolia when infected with Xylella fastidiosa (McElrone et al., 2001), and regular watering has been proposed as a control for this disease. These findings highlight the importance of maintaining balanced moisture levels for effective disease management.
The amount of moisture can also directly affect host susceptibility. High moisture levels increase plant succulence, thus, making the plant more vulnerable to infection. However, excessively high moisture levels are often unfavorable for disease development. Gupta et al. (2019) suggested that a medium RH level of about 50% is usually favorable for disease development. For instance, downy mildew development has been shown to increase at maximum humidity (Sun et al., 2017), while onion foliar diseases are more severe at RH above 80% (Tho et al., 2019). Thus, it is apparent from different studies that plant pathogens require a persistent moisture period for development, highlighting the need for precise RH regulation in greenhouses. In arid regions, greenhouses may face challenges in providing consistent humidity levels due to the limited availability of water and fluctuating environmental conditions. For instance, Ascochyta blight caused by the fungus Mycosphaerella pinodes develops hastily (Kerling, 1949), especially under wet conditions (Roger et al., 1999), which also facilitates the ease of spread of the disease from one plant part to another part, and from plant to plants. Therefore, to optimize greenhouse conditions in arid regions, proper humidity control systems are essential. Essentially, achieving stable RH levels through evaporative cooling and moisture sensors can significantly enhance pathogen management. This approach is particularly recommended for arid climates, where experimental results and crop productivity can be compromised by extreme environmental conditions. In conclusion, by integrating precision technologies into greenhouse systems, consistent humidity and other factors in line with the requirement of specific pathosystems will be achieved, ensuring the generation of reliable data at optimal plant-pathogen relationship.
Greenhouses provide controlled environmental conditions essential for optimizing crop performance, especially in hot arid regions where external conditions are extreme. The efficiency of energy transfer and water vapor flux within a greenhouse depends on its design, cladding materials, shape and orientation, and microclimate control equipment (El Afou et al., 2015). Ensuring a proper greenhouse design and management is crucial to achieving favorable growing conditions and mitigating the unique challenges of arid climates. In arid regions with characteristics of high temperature and low humidity, effective cooling and ventilation systems are crucial. Natural ventilation has been a traditional method utilized in greenhouse cooling but it is most preferred in mild-climate countries and less effective under extreme heat (del Sagrado et al., 2016). In addressing this, advanced cooling techniques such as evaporative cooling and shading systems have been suggested as a suitable integration into the greenhouse structure. Additionally, constructing greenhouses with materials that can reflect solar radiation and that can retain thermal insulation can significantly reduce internal temperatures (Berroug et al., 2011). Primarily, energy and water efficiency are central to greenhouse operations in arid environments. Remarkably, automated and intelligent control systems that monitor and regulate temperature, humidity, and lighting in real-time are available to optimize resource use and operational cost (Çaylı et al., 2017; Zhang et al., 2020). These systems provide relevant precision in managing microclimates and ensuring that crops receive the optimal conditions for growth. By adopting these strategies, greenhouses in hot arid regions can achieve the balance required for effective conditions for an optimal crop production environment. There are nevertheless several ways to achieve this. For example, the appropriate greenhouse construction pattern for cooling is a necessity in hot arid regions.
Effective greenhouse design and orientation are critical for optimizing temperature and humidity control and minimizing energy use in hot arid regions. Remarkably, research has shown that the orientation of the greenhouse can be manipulated to control the environment inside the greenhouse (Dragicevic, 2011). Greenhouse orientation determines the exposure to solar radiation, which directly impacts internal temperatures. Research highlights that an East-West (E-W) orientation is ideal for reducing solar gain in high-temperature areas, such as Saudi Arabia, particularly at latitudes like 24 °N. This orientation minimizes summer radiation while maximizing winter sunlight, as confirmed by studies across various latitudes (Dragicevic, 2011; El-Maghlany et al., 2015). However, regions around the equator may require alternative alignment that adapts to their unique solar pattern, since it receives more solar radiation in the winter and less in the summer.
In addition to orientation, the shape of the greenhouse also influences its thermal efficiency. This greenhouse feature has offered a great effect in controlling greenhouse temperature (Choab et al., 2019). Many studies on the preferred shapes and forms of greenhouse have demonstrated that the uneven-span-shaped greenhouse can receive maximum radiation (Soussi et al., 2022). Minimum solar radiation has been demonstrated to be received by the Quonset and vinery-shaped greenhouse (Mobtaker et al., 2019). On considering the heating requirement of some common greenhouse shapes, Gupta and Chandra (2002) found that the Quonset shapes require the most heating due to their capacity for minimized radiation collection. The Quonset shape generally presents the least temperature and solar collection, unlike the uneven-span shape (Sethi, 2009; Choab et al., 2019). The single-span Quonset shape is predominantly used in the Arabian Peninsula (Al-Mulla, 2006), and the combination with the most appropriate covering material is most suitable for cooling greenhouses in arid regions.
The choice of covering material significantly influences the greenhouse microclimate, particularly in hot arid environments. Attempts have been made to identify the most suitable greenhouse cover by studying the properties of some covering materials as well as enhancing existing material with additional substances. Abdel-Ghany et al. (2012) highlighted the important requirements greenhouse cover under tough environments should complement. Ideal coverings should support a homogenous distribution of light and allow optimal light penetration while blocking excess heat and near-infrared radiation (NIR). Notably, glass covering is suggested to excel at transmitting photosynthetically active radiation (PAR) which is essential for plant growth (Kittas et al., 1999; Maraveas, 2019). However, despite the effectiveness of the glass cover, some plastic films and sheets possess favorable cover features, such as better NIR reflection, providing a conducive environment for plant activities (García-Alonso et al., 2007). As a result, it can be incorporated into the cooling system of greenhouses in hot, arid regions.
Advanced materials, such as fiberglass and polycarbonate sheets, are heat-resistant and durable (Briassoulis et al., 1997), making them suitable for regions like Saudi Arabia, where extreme temperatures and frequent sandstorms accelerate material degradation. Moreover, fiberglass and polycarbonate plastic sheets are strong, heat-resistant, and excellent at transmitting UV and PAR radiation (Al-Mahdouri et al., 2014). Fiberglass greenhouse supports more evaporative cooling and provides the desired environmental temperature for crops.
Additionally, innovative solutions, such as liquid-filled roofs and reflective solid covers, are observed to further enhance cooling by absorbing or reflecting solar radiation. However, these systems can be resource-intensive, requiring significant water or material inputs (Abdel-Ghany et al., 2001). Similarly, biodegradable paper (Briassoulis and Giannoulis, 2018), shade clothes, and even brick walls (Zhang et al., 2017) have been adopted as covering materials for greenhouses in some regions. Altogether, an approach combining ventilation, evaporative cooling, and NIR-reflective films offers a practical solution for managing heat load while maintaining crop health (Figure 2).
Figure 2. Enhancing greenhouse microclimate with wet-pad and fans cooling system: A schematic guide to plant-pathogen interactions. This demonstrates the impact of a cooling system on mitigating extreme environmental conditions. the figure links improved microclimatic regulation to stress regulation on crops and suggests the modulation of plant-pathogen interaction, providing a conceptual framework for optimizing crop health in a protected and controlled environment. The greenhouse cover selectively filters solar radiation, to retain near-infrared radiation (NIR) and photosynthetically active radiation (PAR), which are essential for plant growth. This integrated approach to greenhouse technology in extreme arid conditions depicts the ultimate promotion of optimal environments required by crops and interactions with pathogens.
In addition to the greenhouse design and choice of cover material, important factors to consider during installation are the size of the greenhouse and the capacity of the cooling system (Dougka and Briassoulis, 2020). This consideration is a huge determinant of the efficiency and durability of the whole greenhouse system. To attain a suitable microclimate, the cooling system size to greenhouse size ratio should be appropriated. This is because excessive heat can build up when the cooling system provides less than what the greenhouse capacity requires.
Consequently, the necessity to provide a comfortable environment for crops inside greenhouses in hot climates has prompted the development of various cooling technologies that involve the replacement of the hot air present inside the greenhouse with the cooler air outside, through methods such as heat exchangers and evaporative cooling.
In mild climates, cooling the greenhouse can be a simple technique that regulates extremes of humidity and temperature through the management of natural ventilation (Abreu and Meneses, 1994). However, using just ventilation as a cooling strategy can be appropriate when there is a low outside temperature and a moderate inside temperature. In arid climates, where ambient temperatures often exceed 45 °C, cooling systems should be very efficient and sustainable. Besides, ventilative cooling in hot arid regions is hard to achieve due to the low relative humidity during summer in arid regions. Therefore, optional methods such as evaporative cooling can substitute ventilation in hot regions. Greenhouse temperature under evaporative cooling can be up to 12°C below the ambient level (Landsberg et al., 1979). Evaporative cooling is more efficient in hot climates because of the presence of dryer air which produces better cooling results than wet air. The principle of heat and mass exchange between air and water in the wet pad is utilized in evaporative cooling systems (Arbel et al., 1999; Patel and Sheth, 2015). When water that is exposed to evaporation moves into air space, the air temperature is reduced through the conversion of sensible heat to latent heat. Evaporative cooling systems can be the fan and pad system, fogging system, and roof evaporation cooling technique (von Zabeltitz, 2011; Soussi et al., 2022), the use of any of which will reduce the ambient temperature of greenhouses to a level suitable for great crop performance.
The fogging system entails pumping water through a fogging nozzle from above the greenhouse. This process humidifies the greenhouse’s air, bringing about the cooling effect (Montero et al., 1990). The system is cost-effective, ensuring uniform humidity and air temperature distribution (Abdel-Ghany and Kozai, 2006). Fogging efficiency depends on system design, such as high-pressure nozzles, 40 bars, producing 10-30 µm droplets, or low-pressure ones, (5 bars, producing 200 µm) (Kittas et al., 2012). Despite its benefits, the fogging system is water intensive and relies on fresh water, making it less suitable for arid regions like the Arabian Peninsula. It is thus not highly recommended for greenhouses in arid regions where the availability of fresh water is a huge concern. Additionally, it can lead to excessive water accumulation on plants’ surfaces, which can encourage disease development (Kamel et al., 2008). Also, in situations where less quality water is used, there is the possibility of salt precipitation deposition on the plants (Arbel et al., 1999). These limitations necessitate alternative cooling techniques, such as the fan and pad, which is also a popular greenhouse cooling technique in Saudi Arabia, especially during the summer. The fan and pad technique is considered an efficient greenhouse microclimate control method wherever the outside temperature is above 40°C (Soussi et al., 2022). In the simplest setup, it consists of a wet pad on one side of the greenhouse and electrically propelled fans on the opposite side, driving airflow across the pad. Cooling efficiency depends on pad material, thickness, and airflow rate (Liao and Chiu, 2002). Common pad materials include the aspen pad, plastic fiber, palm dates, and corrugated cellulose (Al-Helal, 2009). The appropriate size of the cooling pad depends on the type of pad material. It is recommended that the length of the pad should correspond to the length of the wall (Park et al., 2022). For cellulose material, an airflow of around 70 cubic meters per minute is required for each square meter of pad (Watson et al., 2019). Optimizing pad size and airflow is crucial for achieving desired cooling levels. For instance, Kittas et al. (2012) recommended 1 m2 of pad area per 20 – 30 m2 of the greenhouse, with an air flow of 120 to 150 m3/hour. For a standard 10 cm thick corrugated cellulose pad, a face velocity of 1.27 m/s is recommended by the American Society of Agricultural Engineering (ASAE, 2000). The outcome of the work of Al-Helal and Al-Toweejre. (2001) showed that the corrugated cellulose pad of 10 cm thickness provided a higher cooling efficiency than the same padding material but of 5 cm thickness. Remarkably, the system can lower greenhouse temperature by 4°C below the ambient temperature.
The pad becomes clogged due to salt accumulation during evaporation and the impact of accumulated dust from the surroundings. Therefore, maintenance and possible replacement costs may occur when the strength, water absorption capacity, and other physical properties have depreciated.
However, in arid regions, high salinity of impure water can lead to pad clogging due to salt accumulation during evaporation, reducing cooling efficiency and increasing energy consumption (Al-Helal, 2007). Over time, pad clogging and dust accumulation will necessitate frequent maintenance, and possible replacement may occur when the strength, water absorption capacity, and other physical properties have depreciated.
Evaporative cooling systems remain effective in arid conditions but require supplementation with shading techniques to mitigate excessive heating. Shading reduces solar radiation and heat load, addressing the high temperature of peak summer months (Abad et al., 2007; Ali and Albayati, 2017). Such strategies are crucial for optimizing greenhouse operations in hot arid regions of the Arabian Peninsula.
Shading is a system embrassed in greenhouses to maintain a suitable temperature by reducing transmitted solar radiation (Ahmed et al., 2019). The ideal greenhouse cover should be able to filter out unnecessary spectra components, allowing only the required light for plant growth. Plants require PAR for growth, while NIR contributes primarily to heat, making it less beneficial to the plant (Kempkes et al., 2012). Effective shading techniques aim to reduce NIR transmission to prevent overheating. Fortunately, UV-absorbing plastic films are widely available to filter UV radiation, leaving only the NIR as the primary source of unnecessary heat (Kempkes et al., 2009).
In arid regions, shading is crucial in reducing solar radiation load during peak temperatures (Abdel-Ghany et al., 2015; Hirich and Choukr-Allah, 2017; Fatnassi et al., 2023). This approach not only protects plants but also mitigates the rapid degradation of greenhouse covers as a result of the harsh climate (Abdel-Ghany et al., 2012). Traditional shading techniques include spraying an aqueous solution of hydrated calcium oxide on greenhouse exteriors (Mashonjowa et al., 2010), which can lower temperatures by up to 10°C (Omar et al., 2021). However, this method can have a mitigating effect on plant growth because it reduces both PAR and non-PAR radiation (Baille et al., 2001). Additionally, the method requires frequent application of the solution because its durability is limited especially during rainfall (Abdel-Ghany et al., 2012).
Other conventional shading methods include the use of fixed or movable curtains and plastic nets of various types and colors (Abdel-Ghany et al., 2015). Studies have shown a preference for white plastic nets for optimal crop performance (Medany et al., 2009). Typically, the mesh size is often considered during selection as it influences shading efficiency and microclimate regulation. Harmanto et al. (2006) recommended A mesh size of 52 due to its natable temperature control and pest prevention.
Depending on the desired effect, shading can be applied internally or externally. External shading is popular for heat reduction and improved ventilation. Abdel-Ghany et al. (2015) have confirmed that thermal reduction of up to 16°C in the greenhouse is possible with external shading.
Additionally, the available ventilation is another consideration when determining shading technique. Internal shading, although effective in maintaining temperature during the cooler season, can disturb natural ventilation and affect microclimate balance.
Similarly, different shading material color gives different crop growth levels (Mascarini et al., 2001). Thus, choosing the color that supports optimal efficiency is very crucial. For instance, white shading clothes at 40% shade effectively reduces the air temperature for optimal crop growth (Willits, 2001). Likewise, Tang et al. (2020) found that shading material influences light regulation and can enhance yield by up to 1.2 times compared to unshaded ones. Overall, Greenhouse technology continues to evolve, with ongoing advancement promising more efficient and sustainable solutions to optimize greenhouse microclimatic conditions for all greenhouse activities.
Greenhouse technology has witnessed tremendous improvement over the years and research to enhance the technology is ongoing. Greenhouse technology that initially focused on the distribution and design of lighting and ventilation structure types has now developed into the installation of automation systems and sensors (Aznar-Sánchez et al., 2020). The differences in external climatic conditions of greenhouses in different climatic areas are a reason why greenhouses face different challenges, and the automation systems put in place within them need to be modified to take into account these particular environmental aspects. For example, to enable optimal plant growth in colder places, greenhouse automation systems need to concentrate on effective heating mechanisms, insulation, and temperature management. In warmer climes, on the other hand, the focus can be on efficient cooling systems, shade management, and ventilation to avoid overheating and preserve a suitable habitat for plants, and other systems intended.
The challenging season for greenhouse cultivation in the desert of arid regions is the summer where high temperature and low humidity are the limiting factors. Currently, a popular corrective measure is the use of evaporative cooling which consequently has some issues with water use. However, recent advancements in greenhouse technologies propose suggestions to address issues associated with regulating extreme temperatures in desert region greenhouses. New greenhouse covering materials are being developed such that the optic properties of the material can be actively switchable. The work of Baeza et al. (2020) demonstrated, with the aid of simulation tools, the advantages switchable properties have over permanent properties where they were able to show considerable water saving for the pad system when a switchable cover was used. In the hot regions where available heat in the daytime is much, this can be exploited to provide a balanced heat requirement for the greenhouse through the day and night. It is suggested that the heat generated in the daytime can be stored in mediums that will release the stored heat at nighttime to warm up the greenhouse in winter (Soussi et al., 2022).
Knowledge-based methods for plant-pathogen-environment interaction studies have been deduced from integrating implemented sensors and machine learning into greenhouse technology (Kuska et al., 2022). This approach has presented the potential for quick and active identification of necessary parameters during plant-pathogen interaction inside the greenhouse. Intelligent technologies can be used to predict the greenhouse microclimate which can be translated into information necessary to maintain the required greenhouse conditions (Taki et al., 2018; Sedig et al., 2019). A convex bidirectional extreme learning machine (CB-ELM) has been developed to predict greenhouse temperature and humidity (Zou et al., 2017).
Additionally, recommendations have been made on manipulating the resources unique to the Arabian Peninsula such as intense sunlight and saline sea water into emerging technologies for advanced greenhouse technology concepts. An interesting outlook is the probable use of transparent infrared harvesting solar cells that will allow low-energy cooling, temperature reduction, and even energy generation (Lefers et al., 2020). This effort will lead to reduced freshwater requirement; which is of primary consideration in the Arabian Peninsula. With this concept, there is hope for greenhouses that are energy efficient in the provision of a controlled environment in the Arabian Peninsula.
Greenhouse technology plays a great role in modern agriculture, particularly in regions with extreme climatic conditions, by offering a controlled environment for continuous crop production and detailed study of plant-pathogen interactions. The success of greenhouse cultivation especially for plant pathology research in hot arid regions lies in the ability to maintain optimal microclimatic conditions that support plant health while effectively managing pests and diseases.
This review has highlighted the critical need for innovative cooling strategies in greenhouses to ameliorate the harsh summer conditions of the Arabian peninsula. Through an extensive examination of various cooling techniques, principles, and effectiveness, we have identified promising approaches that can create a controlled environment conducive to plant, pathogen control, and plant pathology studies. The integration and manipulation of these techniques offer the potential for maintaining a balanced microclimate with minimal resource consumption, thereby ensuring sustainable and efficient greenhouse operations.
In conclusion, the interplay between greenhouse microclimates and plant pathology is crucial for advancing agricultural productivity. Future research and technological advancement should focus on refining these cooling systems to enhance greenhouse functions that optimize plant pathology research output. By doing so, we can ensure that greenhouses offer a viable and effective solution to the challenges facing plant pathology research in particular and crop production in generation in the hot arid region.
AY: Conceptualization, Writing – original draft, Writing – review & editing. FA-Y: Writing – review & editing. AS: Writing – review & editing. AA-G: Writing – review & editing.
The author(s) declare that no financial support was received for the research, authorship, and/or publication of this article.
The authors declare that the research was conducted in the absence of any commercial or financial relationships that could be construed as a potential conflict of interest.
All claims expressed in this article are solely those of the authors and do not necessarily represent those of their affiliated organizations, or those of the publisher, the editors and the reviewers. Any product that may be evaluated in this article, or claim that may be made by its manufacturer, is not guaranteed or endorsed by the publisher.
Abad, D., López, J. C., Garrido, J., Baeza, E., Pérez-Parra, J., Zaragoza, G. (2007). A comparison of three different cooling systems in parral type greenhouses in Almería. Span. J. Agric. Res. 5, 285–292. doi: 10.5424/sjar/2007053-5341
Abdel-Ghany, A. M., Al-Helal, I. M., Alzahrani, S. M., Alsadon, A. A., Ali, I. M., Elleithy, R. M. (2012). Covering materials incorporating radiation-preventing techniques to meet greenhouse cooling challenges in arid regions: A review. Sci. World J. 2012, e906360. doi: 10.1100/2012/906360
Abdel-Ghany, A. M., Al-Helal, I. M., Picuno, P., Shady, M. R. (2016). Modified plastic net-houses as alternative agricultural structures for saving energy and water in hot and sunny regions. Renew. Energy 93, 332–339. doi: 10.1016/j.renene.2016.02.084
Abdel-Ghany, A. M., Kozai, T. (2006). Cooling efficiency of fogging systems for greenhouses. Biosyst. Eng. 94, 97–109. doi: 10.1016/j.biosystemseng.2006.02.008
Abdel-Ghany, A. M., Kozai, T., Abdel-Shafi, N. Y., Taha, I. S., Huzayyin, A. S. (2001). Dynamic simulation modeling of heat and water vapor transfer in a fluid-roof greenhouse. J. Agric. Meteorol. 57, 169–182. doi: 10.2480/agrmet.57.169
Abdel-Ghany, A. M., Picuno, P., Al-Helal, I., Alsadon, A., Ibrahim, A., Shady, M. (2015). Radiometric characterization, solar and thermal radiation in a greenhouse as affected by shading configuration in an arid climate. Energies 8, 13928–13937. doi: 10.3390/en81212404
Abdul-Baki, A. A., Haroon, S. A., Chitwood, D. J. (1996). Temperature effects on resistance to meloidogyne spp. in excised tomato roots. HortScience 31, 147–149. doi: 10.21273/HORTSCI.31.1.147
Abedrabboh, O., Koç, M., Biçer, Y. (2024). Comparative thermoeconomic assessment of renewable-driven hybrid-cooled sustainable greenhouses for subtropical regions. Energy Convers. Manage. 300, 117990. doi: 10.1016/j.enconman.2023.117990
Abreu, P. E., Meneses, J. F. (1994). Climatic characterization of two plastic covered greenhouses under different natural ventilation methods, with a cool season tomato crop. Acta Hortic. 366, 183–194. doi: 10.17660/ActaHortic.1994.366.22
Ahemd, H. A., Al-Faraj, A. A., Abdel-Ghany, A. M. (2016). Shading greenhouses to improve the microclimate, energy and water saving in hot regions: A review. Sci. Hortic. 201, 36–45. doi: 10.1016/j.scienta.2016.01.030
Ahmed, H., Al-Faraj, A., Abdel-Ghany, A. (2019). Spatial distribution of air temperature and relative humidity in the greenhouse as affected by external shading in arid climates. J. Integr. Agric. 18, 2869–2882. doi: 10.1016/S2095-3119(19)62598-0
Al-Askar, A. A., Ghoneem, K. M., Hafez, E. E., Saber, W. I. A. (2022). A case study in Saudi Arabia: biodiversity of maize seed-borne pathogenic fungi in relation to biochemical, physiological, and molecular characteristics. Plants 11, 829. doi: 10.3390/plants11060829
Alexander, P., Rounsevell, M. D. A., Dislich, C., Dodson, J. R., Engström, K., Moran, D. (2015). Drivers for global agricultural land use change: The nexus of diet, population, yield and bioenergy. Glob. Environ. Change 35, 138–147. doi: 10.1016/j.gloenvcha.2015.08.011
Al-Helal, I. (2007). Effects of ventilation rate on the environment of a fan-pad evaporatively cooled, shaded greenhouse in extreme arid climates. Applied Engineering in Agriculture. 23, 221–230. doi: 10.13031/2013.22605
Al-Helal, I. M. (2009). A pilot-scale study for evaluating the performance of a fan-pad cooling system under different climatic conditions of Saudi Arabia. Misr J. Agric. Eng. 26, 514–533. doi: 10.21608/mjae.2020.110169
Alhelal, I. M., Albadawi, A. A., Alsadon, A. A., Alenazi, M. M., Ibrahim, A. A., Shady, M., et al. (2024). Effects of shading nets color on the internal environmental conditions, light spectral distribution, and strawberry growth and yield in greenhouses. Plants 13, 2318. doi: 10.3390/plants13162318
Al-Helal, I. M., Al-Toweejre., H. (2001). Evaporative cooling for palm dates fiber pads 159 and cross-flutted cellulose pads under arid conditions. Misr J. Agric. Eng. 18, 469–483.
Ali, I. M., Albayati, H. D. R. (2017). Influence of Covering Materials and Shading on the Greenhouse Cooling in Iraq. Available online at: https://www.semanticscholar.org/paper/Influence-of-Covering-Materials-and-Shading-on-the-Ali-Albayati/0484a039ab02ac8b8dc76ad1a431aa17ff7b5b17 (Accessed October 9, 2022).
Al-Mahdouri, A., Gonome, H., Okajima, J., Maruyama, S. (2014). Theoretical and experimental study of solar thermal performance of different greenhouse cladding materials. Sol. Energy 107, 314–327. doi: 10.1016/j.solener.2014.05.006
Al-Mulla, Y. A. (2006). Cooling greenhouses in the arabian peninsula. Acta Hortic. 719, 499–506. doi: 10.17660/ActaHortic.2006.719.57
Al-Naimi, F. A., Garrett, K. A., Bockus, W. W. (2005). Competition, facilitation, and niche differentiation in two foliar pathogens. Oecologia 143, 449–457. doi: 10.1007/s00442-004-1814-x
Amani, M., Foroushani, S., Sultan, M., Bahrami, M. (2020). Comprehensive review on dehumidification strategies for agricultural greenhouse applications. Appl. Therm. Eng. 181, 115979. doi: 10.1016/j.applthermaleng.2020.115979
Arbel, A., Yekutieli, O., Barak, M. (1999). Performance of a fog system for cooling greenhouses. J. Agric. Eng. Res. 72, 129–136. doi: 10.1006/jaer.1998.0351
Asare, R., David, S. (2010). Planting, replanting and tree diversification in cocoa systems-learning about sustainable cocoa production: a guide for participatory farmer training. Forest and Landscape Denmark. Available at: https://www.cabdirect.org/cabdirect/abstract/20103200773.
Atkinson, N. J., Urwin, P. E. (2012). The interaction of plant biotic and abiotic stresses: from genes to the field. J. Exp. Bot. 63, 3523–3543. doi: 10.1093/jxb/ers100
Aznar-Sánchez, J. A., Piquer-Rodríguez, M., Velasco-Muñoz, J. F., Manzano-Agugliaro, F. (2019). Worldwide research trends on sustainable land use in agriculture. Land Use Policy 87, 104069. doi: 10.1016/j.landusepol.2019.104069
Aznar-Sánchez, J. A., Velasco-Muñoz, J. F., López-Felices, B., Román-Sánchez, I. M. (2020). An analysis of global research trends on greenhouse technology: towards a sustainable agriculture. Int. J. Environ. Res. Public. Health 17, 664. doi: 10.3390/ijerph17020664
Baeza, E., Hemming, S., Stanghellini, C. (2020). Materials with switchable radiometric properties: Could they become the perfect greenhouse cover? Biosyst. Eng. 193, 157–173. doi: 10.1016/j.biosystemseng.2020.02.012
Baille, A., Kittas, C., Katsoulas, N. (2001). Influence of whitening on greenhouse microclimate and crop energy partitioning. Agric. For. Meteorol. 107, 293–306. doi: 10.1016/S0168-1923(01)00216-7
Barua, P., You, M. P., Bayliss, K. L., Lanoiselet, V., Barbetti, M. J. (2018). Extended survival of Puccinia graminis f. sp. tritici urediniospores: implications for biosecurity and on-farm management. Plant Pathol. 67, 799–809. doi: 10.1111/ppa.12794
Beer, J., Muschler, R., Kass, D., Somarriba, E. (1997). Shade management in coffee and cacao plantations. Agrofor. Syst. 38, 139–164. doi: 10.1023/A:1005956528316
Berroug, F., Lakhal, E. K., El Omari, M., Faraji, M., El Qarnia, H. (2011). Thermal performance of a greenhouse with a phase change material north wall. Energy Build. 43, 3027–3035. doi: 10.1016/j.enbuild.2011.07.020
Bicer, Y., Sajid, M. U., Al-Breiki, M. (2022). Optimal spectra management for self-power producing greenhouses for hot arid climates. Renew. Sustain. Energy Rev. 159, 112194. doi: 10.1016/j.rser.2022.112194
Bonde, M. R., Nester, S. E., Berner, D. K. (2012). Effects of daily temperature highs on development of phakopsora pachyrhizi on soybean. Phytopathology® 102, 761–768. doi: 10.1094/PHYTO-01-12-0011-R
Briassoulis, D., Giannoulis, A. (2018). Evaluation of the functionality of bio-based plastic mulching films. Polym. Test. 67, 99–109. doi: 10.1016/j.polymertesting.2018.02.019
Briassoulis, D., Waaijenberg, D., Gratraud, J., von Elsner, B. (1997). Mechanical properties of covering materials for greenhouses part 2: quality assessment. J. Agric. Eng. Res. 67, 171–217. doi: 10.1006/jaer.1997.0155
Burdon, J. J. (1987). Diseases and plant population biology (Cambridge [Cambridgeshire]: Cambridge University Press).
Çaylı, A., Akyüz, A., Baytorun, A. N., Boyacı, S., Üstün, S., Kozak, F. B. (2017).Sera Çevre Koşullarının Nesnelerin İnterneti Tabanlı İzleme ve Analiz Sistemi ile Denetlenmesi. In: Control of Greenhouse Environmental Conditions with IOT Based Monitoring and Analysis System. Available online at: http://openaccess.ahievran.edu.tr/xmlui/handle/20.500.12513/1069 (Accessed August 8, 2022).
Chamard, J., Faticov, M., Blanchet, F. G., Chagnon, P.-L., Laforest-Lapointe, I. (2024). Interplay of biotic and abiotic factors shapes tree seedling growth and root-associated microbial communities. Commun. Biol. 7, 1–13. doi: 10.1038/s42003-024-06042-7
Chellappan, P., Vanitharani, R., Ogbe, F., Fauquet, C. M. (2005). Effect of temperature on geminivirus-induced RNA silencing in plants. Plant Physiol. 138, 1828–1841. doi: 10.1104/pp.105.066563
Chen, F., Duan, G.-H., Li, D.-L., Zhan, J. (2017). Host resistance and temperature-dependent evolution of aggressiveness in the plant pathogen zymoseptoria tritici. Front. Microbiol. 8. doi: 10.3389/fmicb.2017.01217
Chen, W., Modi, D., Picot, A. (2023). Soil and phytomicrobiome for plant disease suppression and management under climate change: A review. Plants 12, 2736. doi: 10.3390/plants12142736
Choab, N., Allouhi, A., El Maakoul, A., Kousksou, T., Saadeddine, S., Jamil, A. (2019). Review on greenhouse microclimate and application: Design parameters, thermal modeling and simulation, climate controlling technologies. Sol. Energy 191, 109–137. doi: 10.1016/j.solener.2019.08.042
Chowdhury, M., Kiraga, S., Islam, M. N., Ali, M., Reza, M. N., Lee, W.-H., et al. (2021). Effects of temperature, relative humidity, and carbon dioxide concentration on growth and glucosinolate content of kale grown in a plant factory. Foods 10, 1524. doi: 10.3390/foods10071524
Colantoni, A., Monarca, D., Marucci, A., Cecchini, M., Zambon, I., Di Battista, F., et al. (2018). Solar radiation distribution inside a greenhouse prototypal with photovoltaic mobile plant and effects on flower growth. Sustainability 10, 855. doi: 10.3390/su10030855
Cooper, R. N., Houghton, J. T., McCarthy, J. J., Metz, B. (2002). Climate change 2001: the scientific basis. Foreign Aff. 81, 208. doi: 10.2307/20033020
Dai, Y., Wu, X., Wang, H., Li, W., Cai, L., Li, J., et al. (2022). Spatio-temporal variation in the phyllospheric microbial biodiversity of alternaria alternata-infected tobacco foliage. Front. Microbiol. 13. doi: 10.3389/fmicb.2022.920109
del Sagrado, J., Sánchez, J. A., Rodríguez, F., Berenguel, M. (2016). Bayesian networks for greenhouse temperature control. J. Appl. Log. 17, 25–35. doi: 10.1016/j.jal.2015.09.006
Devran, Z., Özalp, T. (2018). Response of tomato plants carrying Mi-1 gene to Meloidogyne incognita (Kofoid & White 1919) Chitwood 1949 under high soil temperatures. Turk. J. Entomol. 42 (4), 313–322. doi: 10.16970/entoted.467189
Dougka, G., Briassoulis, D. (2020). Load carrying capacity of greenhouse covering films under wind action: Optimising the supporting systems of greenhouse films. Biosyst. Eng. 192, 199–214. doi: 10.1016/j.biosystemseng.2020.01.020
Dragicevic, S. (2011). Determining the optimum orientation of a greenhouse on the basis of the total solar radiation availability. Therm. Sci. 15, 215–221. doi: 10.2298/TSCI100220057D
Dropkin, V. (1969). necrotic reaction of tomatoes and other hosts resistant to Meloidogyne: reversal by temperature. Phytopathology 59, 1632–1637.
El Afou, Y., Msaad, A. A., Kousksou, T., Mahdaoui, M. (2015). “Predictive control of temperature under greenhouse using LQG strategy,” in 2015 3rd International Renewable and Sustainable Energy Conference (IRSEC). IEEE. 1–5. doi: 10.1109/IRSEC.2015.7454975
El-Maghlany, W. M., Teamah, M. A., Tanaka, H. (2015). Optimum design and orientation of the greenhouses for maximum capture of solar energy in North Tropical Region. Energy Convers. Manage. 105, 1096–1104. doi: 10.1016/j.enconman.2015.08.066
Famuwagun, I. B., Agele, S. O., Aiyelari, O. P. (2018). Shade effects on growth and development of cacao following two years of continuous dry season irrigation. Int. J. Fruit Sci. 18, 153–176. doi: 10.1080/15538362.2017.1416326
Fanourakis, D., Aliniaeifard, S., Sellin, A., Giday, H., Körner, O., Rezaei Nejad, A., et al. (2020). Stomatal behavior following mid- or long-term exposure to high relative air humidity: A review. Plant Physiol. Biochem. 153, 92–105. doi: 10.1016/j.plaphy.2020.05.024
FAO (2009). Food and Agriculture Organization of the United Nations. Available online at: https://scholar.google.com/scholar_lookup?title=High+Level+Expert+Forum%E2%80%94How+to+Feed+the+World+in+2050&publication_year=2009& (Accessed July 11, 2022).
FAO (2021). Unlocking the potential of protected agriculture in the countries of the Gulf Cooperation Council - Saving water and improving nutrition (Cairo, Egypt: FAO). doi: 10.4060/cb4070en
Fatnassi, H., Zaaboul, R., Elbattay, A., Molina-Aiz, F. D., Valera, D. L. (2023). Protected agriculture systems in the UAE: challenges and opportunities. Acta Hortic., 495–502. doi: 10.17660/ActaHortic.2023.1377.60
Frimpong, F., Windt, C. W., van Dusschoten, D., Naz, A. A., Frei, M., Fiorani, F. (2021). A wild allele of pyrroline-5-carboxylate synthase1 leads to proline accumulation in spikes and leaves of barley contributing to improved performance under reduced water availability. Front. Plant Sci. 12. doi: 10.3389/fpls.2021.633448
Fujimoto, T., Hasegawa, S., Otobe, K., Mizukubo, T. (2010). The effect of soil water flow and soil properties on the motility of second-stage juveniles of the root-knot nematode ( Meloidogyne incognita). Soil Biol. Biochem. - Soil Biol. Biochem. 42, 1065–1072. doi: 10.1016/j.soilbio.2010.03.003
García-Alonso, Y., Espi, E., Salmerón, A., Fontecha, A., Gonzalez Benavente-Garcia, A., López-Marin, J. (2007). New cool plastic films for greenhouse covering in tropical and subtropical areas. Acta Hortic. 719, 131–137. doi: 10.17660/ActaHortic.2006.719.12
Ghoshal, B., Sanfaçon, H. (2015). Symptom recovery in virus-infected plants: revisiting the role of RNA silencing mechanisms. Virology 479, 167–179. doi: 10.1016/j.virol.2015.01.008
Ghoulem, M., El Moueddeb, K., Nehdi, E., Boukhanouf, R., Kaiser Calautit, J. (2019). Greenhouse design and cooling technologies for sustainable food cultivation in hot climates: Review of current practice and future status. Biosyst. Eng. 183, 121–150. doi: 10.1016/j.biosystemseng.2019.04.016
Gorjian, S., Calise, F., Kant, K., Ahamed, M. S., Copertaro, B., Najafi, G., et al. (2021). A review on opportunities for implementation of solar energy technologies in agricultural greenhouses. J. Clean. Prod. 285, 124807. doi: 10.1016/j.jclepro.2020.124807
Grinberg, M., Orevi, T., Steinberg, S., Kashtan, N. (2019). Bacterial survival in microscopic surface wetness. eLife 8, e48508. doi: 10.7554/eLife.48508
Guo, P., Baum, M., Grando, S., Ceccarelli, S., Bai, G., Li, R., et al. (2009). Differentially expressed genes between drought-tolerant and drought-sensitive barley genotypes in response to drought stress during the reproductive stage. J. Exp. Bot. 60, 3531–3544. doi: 10.1093/jxb/erp194
Gupta, M. J., Chandra, P. (2002). Effect of greenhouse design parameters on conservation of energy for greenhouse environmental control. Energy 27, 777–794. doi: 10.1016/S0360-5442(02)00030-0
Gupta, M., Leharwan, M., Shukla, A. (2019). Effect of temperature and moisture levels on disease development of stem gall of coriander. Agric. Sci. Dig. - Res. J. 38 (4), 307–309. doi: 10.18805/ag.D-4745
Hanan, J. (2017). Greenhouses: Advanced Technology for Protected Horticulture. doi: 10.1201/9780203719824
Harmanto, Tantau, H. J., Salokhe, V. M. (2006). Microclimate and air exchange rates in greenhouses covered with different nets in the humid tropics. Biosyst. Eng. 94, 239–253. doi: 10.1016/j.biosystemseng.2006.02.016
Hibberd, J. M., Whitbread, R., Farrar, J. F. (1996). Effect of 700 μmol mol –1CO2 and infection with powdery mildew on the growth and carbon partitioning of barley. New Phytol. 134, 309–315. doi: 10.1111/j.1469-8137.1996.tb04635.x
Hirich, A., Choukr-Allah, R. (2017). “Water and Energy Use Efficiency of Greenhouse and Net house Under Desert Conditions of UAE: Agronomic and Economic Analysis,” in Water Resources in Arid Areas: The Way Forward. Eds. Abdalla, O., Kacimov, A., Chen, M., Al-Maktoumi, A., Al-Hosni, T., Clark, I. (Cham: Springer International Publishing), 481–499. doi: 10.1007/978-3-319-51856-5_28
Horino, O., Mew, T. W., Yamada, T. (1982). The effect of temperature on the development of bacterial leaf blight on rice. Jpn. J. Phytopathol. 48, 72–75. doi: 10.3186/jjphytopath.48.72
Hunjan, M. S., Lore, J. S. (2020). “Climate Change: Impact on Plant Pathogens, Diseases, and Their Management,” in Crop Protection Under Changing Climate. Eds. Jabran, K., Florentine, S., Chauhan, B. S. (Cham: Springer International Publishing), 85–100. doi: 10.1007/978-3-030-46111-9_4
Huot, B., Castroverde, C. D. M., Velásquez, A. C., Hubbard, E., Pulman, J. A., Yao, J., et al. (2017). Dual impact of elevated temperature on plant defence and bacterial virulence in Arabidopsis. Nat. Commun. 8, 1808. doi: 10.1038/s41467-017-01674-2
Jones, L. M., Koehler, A.-K., Trnka, M., Balek, J., Challinor, A. J., Atkinson, H. J., et al. (2017). Climate change is predicted to alter the current pest status of Globodera pallida and G. rostochiensis in the United Kingdom. Glob. Change Biol. 23, 4497–4507. doi: 10.1111/gcb.13676
Juroszek, P., Laborde, M., Kleinhenz, B., Mellenthin, M., Racca, P., Sierotzki, H. (2022). A review on the potential effects of temperature on fungicide effectiveness. Plant Pathol. 71, 775–784. doi: 10.1111/ppa.13531
Kamel, A., Nicot, P., Guechi, A., Bardin, M., Mohamed, C. (2008). Grey mould development in greenhouse tomatoes under drip and furrow irrigation. Agron. Sustain. Dev. 28, 403–409. doi: 10.1051/agro:2008016
Kassanis, B. (1957). “Effects of Changing Temperature on Plant Virus Diseases,” in Advances in Virus Research. Eds. Smith, K. M., Lauffer, M. A. (Academic Press), 221–241. doi: 10.1016/S0065-3527(08)60600-4
Kempkes, F., Hemming, S., Vitali, M., Baeza, E. (2012). Calculation of NIR effect on greenhouse climate in various conditions. Acta Hortic. 927, 543–550. doi: 10.17660/ActaHortic.2012.927.66
Kempkes, F. L. K., Stanghellini, C., Hemming, S. (2009). Cover materials excluding near infrared radiation: what is the best strategy in mild climates? Acta Hortic., 67–72. doi: 10.17660/ActaHortic.2009.807.5
Kerling, L. C. P. (1949). Aantasting van erwten door Mycosphaerella pinodes (Berk. et Blox.) Stone. Tijdschr. Plantenziekten 55, 41–68. doi: 10.1007/BF01991842
Khan, N., Ray, R. L., Sargani, G. R., Ihtisham, M., Khayyam, M., Ismail, S. (2021). Current progress and future prospects of agriculture technology: gateway to sustainable agriculture. Sustainability 13, 4883. doi: 10.3390/su13094883
Kim, J. H., Castroverde, C. D. M., Huang, S., Li, C., Hilleary, R., Seroka, A., et al. (2022). Increasing the resilience of plant immunity to a warming climate. Nature 607, 339–344. doi: 10.1038/s41586-022-04902-y
Kirschbaum, M. U. F. (2011). Does enhanced photosynthesis enhance growth? Lessons learned from CO2 enrichment studies. Plant Physiol. 155, 117–124. doi: 10.1104/pp.110.166819
Kittas, C., Baille, A., Giaglaras, P. (1999). Influence of covering material and shading on the spectral distribution of light in greenhouses. J. Agric. Eng. Res. 73, 341–351. doi: 10.1006/jaer.1999.0420
Kittas, C., Katsoulas, N., Bartzanas, T. (2012). Greenhouse climate control in mediterranean greenhouses. Cuadernos de estudios agroalimentarios 26(3), 89–114.
Kruidhof, H. M., Elmer, W. H. (2020). ““Cultural Methods for Greenhouse Pest and Disease Management,”,” in Integrated Pest and Disease Management in Greenhouse Crops. Eds. Gullino, M. L., Albajes, R., Nicot, P. C. (Cham: Springer International Publishing), 285–330. doi: 10.1007/978-3-030-22304-5_10
Kuska, M. T., Heim, R. H. J., Geedicke, I., Gold, K. M., Brugger, A., Paulus, S. (2022). Digital plant pathology: a foundation and guide to modern agriculture. J. Plant Dis. Prot. Sci. J. Ger. Phytomedical Soc DPG 129, 457–468. doi: 10.1007/s41348-022-00600-z
Lahlali, R., Taoussi, M., Laasli, S.-E., Gachara, G., Ezzouggari, R., Belabess, Z., et al. (2024). Effects of climate change on plant pathogens and host-pathogen interactions. Crop Environ. 3, 159–170. doi: 10.1016/j.crope.2024.05.003
Lamnatou, C., Chemisana, D. (2013). Solar radiation manipulations and their role in greenhouse claddings: Fluorescent solar concentrators, photoselective and other materials. Renew. Sustain. Energy Rev. 27, 175–190. doi: 10.1016/j.rser.2013.06.052
Landsberg, J. J., White, B., Thorpe, M. R. (1979). Computer analysis of the efficacy of evaporative cooling for glasshouses in high energy environments. J. Agric. Eng. Res. 24, 29–39. doi: 10.1016/0021-8634(79)90058-1
Larkin, R. P., Fravel, D. R. (2002). Effects of varying environmental conditions on biological control of fusarium wilt of tomato by nonpathogenic fusarium spp. Phytopathology® 92, 1160–1166. doi: 10.1094/PHYTO.2002.92.11.1160
Lefers, R. M., Tester, M., Lauersen, K. J. (2020). Emerging technologies to enable sustainable controlled environment agriculture in the extreme environments of middle East-North Africa coastal regions. Front. Plant Sci. 11.
Legler, S. E., Caffi, T., Rossi, V. (2012). A nonlinear model for temperature-dependent development of Erysiphe necator chasmothecia on grapevine leaves. Plant Pathol. 61, 96–105. doi: 10.1111/j.1365-3059.2011.02498.x
Li, J., Lin, X., Chen, A., Peterson, T., Ma, K., Bertzky, M., et al. (2013). Global priority conservation areas in the face of 21st century climate change. PloS One 8, e54839. doi: 10.1371/journal.pone.0054839
Liao, C.-M., Chiu, K.-H. (2002). Wind tunnel modeling the system performance of alternative evaporative cooling pads in Taiwan region. Build. Environ. 37, 177–187. doi: 10.1016/S0360-1323(00)00098-6
Manganaris, G. A., Economou, A. S., Boubourakas, I. N., Katis, N. I. (2003). Elimination of PPV and PNRSV through thermotherapy and meristem-tip culture in nectarine. Plant Cell Rep. 22, 195–200. doi: 10.1007/s00299-003-0681-y
Maraveas, C. (2019). Environmental sustainability of greenhouse covering materials. Sustainability 11. doi: 10.3390/su11216129
Mariette, N., Androdias, A., Mabon, R., Corbière, R., Marquer, B., Montarry, J., et al. (2016). Local adaptation to temperature in populations and clonal lineages of the Irish potato famine pathogen Phytophthora infestans. Ecol. Evol. 6, 6320–6331. doi: 10.1002/ece3.2282
Marques de Carvalho, L., Benda, N. D., Vaughan, M. M., Cabrera, A. R., Hung, K., Cox, T., et al. (2015). Mi-1-mediated nematode resistance in tomatoes is broken by short-term heat stress but recovers over time. J. Nematol. 47, 133–140.
Mascarini, L., Mascarini, A., Goldberg, M., Landini, A., Orden, S., Vilella, F. (2001). Effect of greenhouse shading materials on the foliar area and flowering of two cyclamen persicum hybrids. Acta Hortic., 211–216. doi: 10.17660/ActaHortic.2001.559.30
Mashonjowa, E., Ronsse, F., Mhizha, T., Milford, J. R., Lemeur, R., Pieters, J. G. (2010). The effects of whitening and dust accumulation on the microclimate and canopy behaviour of rose plants (Rosa hybrida) in a greenhouse in Zimbabwe. Sol. Energy 84, 10–23. doi: 10.1016/j.solener.2009.09.004
McElrone, A. J., Sherald, J. L., Forseth, I. N. (2001). Effects of Water Stress on Symptomatology and Growth of Parthenocissus quinquefolia Infected by Xylella fastidiosa. Plant Dis. 85, 1160–1164. doi: 10.1094/PDIS.2001.85.11.1160
McLaren, M. R., Callahan, B. J. (2020). Pathogen resistance may be the principal evolutionary advantage provided by the microbiome. Philos. Trans. R. Soc B Biol. Sci. 375, 20190592. doi: 10.1098/rstb.2019.0592
Medany, M. A., Hassanein, M. K., Farag, A. A. (2009). Effect of black and white nets as alternative covers to sweet pepper production under greenhouses in Egypt. Acta Hortic., 121–126. doi: 10.17660/ActaHortic.2009.807.14
Mieslerová, B., Cook, R. T., Wheater, C. P., Lebeda, A. (2022). Ecology of powdery mildews–influence of abiotic factors on their development and epidemiology. Crit. Rev. Plant Sci. 41, 365–390.
Milus, E. A., Kristensen, K., Hovmøller, M. S. (2009). Evidence for Increased Aggressiveness in a Recent Widespread Strain of Puccinia striiformis f. sp. tritici Causing Stripe Rust of Wheat. Phytopathology® 99, 89–94. doi: 10.1094/PHYTO-99-1-0089
Mittler, R. (2006). Abiotic stress, the field environment and stress combination. Trends Plant Sci. 11, 15–19. doi: 10.1016/j.tplants.2005.11.002
Mobtaker, H. G., Ajabshirchi, Y., Ranjbar, S. F., Matloobi, M. (2019). Simulation of thermal performance of solar greenhouse in north-west of Iran: An experimental validation. Renew. Energy 135, 88–97. doi: 10.1016/j.renene.2018.10.003
Mojerlou, S. (2024). “Recent Advances in Management of Diseases and Pests of Common Occurrence Under Protected Cultivation,” in Protected Cultivation (Apple Academic Press).
Montero, J. I., Anton, A., Biel, C., Franquet, A. (1990). Cooling of greenhouses with compressed air fogging nozzles. Acta Hortic., 199–210. doi: 10.17660/ActaHortic.1990.281.22
Mortensen, L. M. (2000). Effects of air humidity on growth, flowering, keeping quality and water relations of four short-day greenhouse species. Sci. Hortic. 86, 299–310. doi: 10.1016/S0304-4238(00)00155-2
Obrepalska-Steplowska, A., Renaut, J., Planchon, S., Przybylska, A., Wieczorek, P., Barylski, J., et al. (2015). Effect of temperature on the pathogenesis, accumulation of viral and satellite RNAs and on plant proteome in peanut stunt virus and satellite RNA-infected plants. Front. Plant Sci. 6. doi: 10.3389/fpls.2015.00903
Omar, M. N., Taha, A. T., Samak, A. A., Keshek, M. H., Gomaa, E. M., Elsisi, S. F. (2021). Simulation and validation model of cooling greenhouse by solar energy (P V) integrated with painting its cover and its effect on the cucumber production. Renew. Energy 172, 1154–1173. doi: 10.1016/j.renene.2021.03.092
Özkaya, S., Soylu, S., Kara, M., Gümüş, Y., Soylu, E. M., Teke, İ., et al. (2024). Disease prevalence, incidence, morphological and molecular characterisation of Lasiodiplodia pseudotheobromae causing collar rot disease on peanut plants in Turkey. J. Plant Dis. Prot. 131, 1639–1651. doi: 10.1007/s41348-024-00933-x
Papadopoulou, J., Traintaphyllou, A. C. (1982). Sex differentiation in meloidogyne incognita and anatomical evidence of sex reversal. J. Nematol. 14, 549–566.
Papasolomontos, A., Baudoin, W., Lutaladio, N., Castilla, N., Baeza, E., Montero, J. I., et al. (2013). Good Agricultural Practices for greenhouse vegetable crops Principles for Mediterranean climate areas.
Paradiso, R., Proietti, S. (2022). Light-quality manipulation to control plant growth and photomorphogenesis in greenhouse horticulture: the state of the art and the opportunities of modern LED systems. J. Plant Growth Regul. 41, 742–780. doi: 10.1007/s00344-021-10337-y
Park, D. Y., Park, B., Choi, S. G. (2022). Comparative analysis of cooling effect by cooling technologies applied to smart greenhouses in the UAE. Case Stud. Therm. Eng. 36, 102207. doi: 10.1016/j.csite.2022.102207
Patel, R. R., Sheth, B. (2015). Evaporative cooling of water for indirect air cooling. In Afro-Asian International Conference on Science, Engineering & Technology (pp. 266–269).
Pfender, W. F., Vollmer, S. S. (1999). Freezing Temperature Effect on Survival of Puccinia graminis subsp. graminicola in Festuca arundinacea and Lolium perenne. Plant Dis. 83, 1058–1062. doi: 10.1094/PDIS.1999.83.11.1058
Roger, C., Tivoli, B., Huber, L. (1999). Effects of temperature and moisture on disease and fruit body development of Mycosphaerella pinodes on pea (Pisum sativum). Plant Pathol. 48, 1–9. doi: 10.1046/j.1365-3059.1999.00312.x
Sbeiti, A. A. L., Mazurier, M., Ben, C., Rickauer, M., Gentzbittel, L. (2023). Temperature increase modifies susceptibility to Verticillium wilt in Medicago spp and may contribute to the emergence of more aggressive pathogenic strains. Front. Plant Sci. 14. doi: 10.3389/fpls.2023.1109154
Scholthof, K.-B. G. (2007). The disease triangle: pathogens, the environment and society. Nat. Rev. Microbiol. 5, 152–156. doi: 10.1038/nrmicro1596
Schwartz, H. F., Otto, K. L., Gent, D. H. (2003). Relation of temperature and rainfall to development of xanthomonas and pantoea leaf blights of onion in colorado. Plant Dis. 87, 11–14. doi: 10.1094/PDIS.2003.87.1.11
Sedig, V., Samuelsson, E., Gumaelius, N., Lindgren, A. (2019). Greenhouse Climate Optimization using Weather Forecasts and Machine Learning. Available online at: http://urn.kb.se/resolve?urn=urn:nbn:se:uu:diva-391045 (Accessed November 15, 2022).
Seleiman, M. F., Al-Suhaibani, N., Ali, N., Akmal, M., Alotaibi, M., Refay, Y., et al. (2021). Drought stress impacts on plants and different approaches to alleviate its adverse effects. Plants 10, 259. doi: 10.3390/plants10020259
Sethi, V. P. (2009). On the selection of shape and orientation of a greenhouse: Thermal modeling and experimental validation. Sol. Energy 83, 21–38. doi: 10.1016/j.solener.2008.05.018
Sharath Chandran, U. S., Tarafdar, A., Mahesha, H. S., Sharma, M. (2021). Temperature and soil moisture stress modulate the host defense response in chickpea during dry root rot incidence. Front. Plant Sci. 12. doi: 10.3389/fpls.2021.653265
Singh, R. P., Tiwari, S., Singh, M., Singh, A., Singh, A. (2020). Important diseases of greenhouse crops and their integrated management: A review. J. Entomol. Zool. Stud. 8, 962–970.
Smith, F., Luna, E. (2023). Elevated atmospheric carbon dioxide and plant immunity to fungal pathogens: do the risks outweigh the benefits? Biochem. J. 480, 1791–1804. doi: 10.1042/BCJ20230152
Sotelo-Cardona, P., Lin, M.-Y., Srinivasan, R. (2021). Growing tomato under protected cultivation conditions: overall effects on productivity, nutritional yield, and pest incidences. Crops 1, 97–110. doi: 10.3390/crops1020010
Soussi, M., Chaibi, M. T., Buchholz, M., Saghrouni, Z. (2022). Comprehensive review on climate control and cooling systems in greenhouses under hot and arid conditions. Agronomy 12, 626. doi: 10.3390/agronomy12030626
Subahi, A. F., Bouazza, K. E. (2020). An intelligent ioT-based system design for controlling and monitoring greenhouse temperature. IEEE Access 8, 125488–125500. doi: 10.1109/ACCESS.2020.3007955
Sun, S., Lian, S., Feng, S., Dong, X., Wang, C., Li, B., et al. (2017). Effects of temperature and moisture on sporulation and infection by pseudoperonospora cubensis. Plant Dis. 101, 562–567. doi: 10.1094/PDIS-09-16-1232-RE
Szittya, G., Silhavy, D., Molnár, A., Havelda, Z., Lovas, A., Lakatos, L., et al. (2003). Low temperature inhibits RNA silencing-mediated defence by the control of siRNA generation. EMBO J. 22, 633–640. doi: 10.1093/emboj/cdg74
Taki, M., Abdanan Mehdizadeh, S., Rohani, A., Rahnama, M., Rahmati-Joneidabad, M. (2018). Applied machine learning in greenhouse simulation; new application and analysis. Inf. Process. Agric. 5, 253–268. doi: 10.1016/j.inpa.2018.01.003
Tang, Y., Ma, X., Li, M., Wang, Y. (2020). The effect of temperature and light on strawberry production in a solar greenhouse. Sol. Energy 195, 318–328. doi: 10.1016/j.solener.2019.11.070
Tho, K. E., Brisco-McCann, E., Wiriyajitsomboon, P., Hausbeck, M. K. (2019). Effects of temperature, relative humidity, and plant age on bacterial disease of onion plants. Plant Health Prog. 20, 200–206. doi: 10.1094/PHP-05-19-0038-RS
Thomidis, T., Prodromou, I., Paresidou, M., Damos, P. (2023). Effects of temperature and leaf wetness duration on pathogens causing preharvest fruit rots on tomato. J. Plant Pathol. 105, 1431–1448. doi: 10.1007/s42161-023-01443-9
Tilman, D. (1999). Global environmental impacts of agricultural expansion: The need for sustainable and efficient practices. Proc. Natl. Acad. Sci. U. S. A. 96, 5995–6000. doi: 10.1073/pnas.96.11.5995
Tsai, W.-A., Shafiei-Peters, J. R., Mitter, N., Dietzgen, R. G. (2022). Effects of elevated temperature on the susceptibility of capsicum plants to capsicum chlorosis virus infection. Pathogens 11, 200. doi: 10.3390/pathogens11020200
Vidhyasekaran, P. (2004). Concise Encyclopedia of Plant Pathology (Boca Raton: CRC Press). doi: 10.1201/9781482277951
von Zabeltitz, C. (2011). Integrated Greenhouse Systems for Mild Climates (Berlin, Heidelberg: Springer). doi: 10.1007/978-3-642-14582-7
Watson, J., Gómez, C., Bucklin, R., Leary, J., Mcconnell, D. (2019). Fan and pad greenhouse evaporative cooling systems. doi: 10.13140/RG.2.2.20202.88004
Wells, C. M. (1997). Greenhouse Climate Control: an integrated approach. Eds. Bakker, J. C., Bot, G. P. A., Challa, H., Braak, N. J. V. d (Wageningen: Wageningen Pers), 279. doi: 10.1016/S0168-1699(97)01315-X
Willits, D. H. (2001). SE—Structures and environment: the effect of cloth characteristics on the cooling performance of external shade cloths for greenhouses. J. Agric. Eng. Res. 79, 331–340. doi: 10.1006/jaer.2001.0702
Yao, L., Jiang, Z., Wang, Y., Hu, Y., Hao, G., Zhong, W., et al. (2023). High air humidity dampens salicylic acid pathway and NPR1 function to promote plant disease. EMBO J. 42, e113499. doi: 10.15252/embj.2023113499
You, M. P., Rensing, K., Renton, M., Barbetti, M. J. (2017). Modeling effects of temperature, soil, moisture, nutrition and variety as determinants of severity of pythium damping-off and root disease in subterranean clover. Front. Microbiol. 8. doi: 10.3389/fmicb.2017.02223
Yuan, W., Holbrook, C. C., Chu, Y., Ozias-Akins, P., Dickson, D. W. (2018). Influence of temperature on susceptibility of CVS. Tifguard and Georgia-06G peanut to meloidogyne arenaria. J. Nematol. 50, 33–40. doi: 10.21307/jofnem-2018-006
Yunis, H., Elad, Y., Mahrer, Y. (1990). Effects of air temperature, relative humidity and canopy wetness on gray mold of cucumbers in unheated greenhouses. Phytoparasitica 18, 203–215. doi: 10.1007/BF02980990
Zhang, S., Guo, Y., Zhao, H., Wang, Y., Chow, D., Fang, Y. (2020). Methodologies of control strategies for improving energy efficiency in agricultural greenhouses. J. Clean. Prod. 274, 122695. doi: 10.1016/j.jclepro.2020.122695
Zhang, J., Wang, J., Guo, S., Wei, B., He, X., Sun, J., et al. (2017). Study on heat transfer characteristics of straw block wall in solar greenhouse. Energy Build. 139, 91–100. doi: 10.1016/j.enbuild.2016.12.061
Zhang, M., Yan, T., Wang, W., Jia, X., Wang, J., Klemeš, J. J. (2022). Energy-saving design and control strategy towards modern sustainable greenhouse: A review. Renew. Sustain. Energy Rev. 164, 112602. doi: 10.1016/j.rser.2022.112602
Zhang, X., Zhang, X., Singh, J., Li, D., Qu, F. (2012). Temperature-dependent survival of turnip crinkle virus-infected arabidopsis plants relies on an RNA silencing-based defense that requires DCL2, AGO2, and HEN1. J. Virol. 86, 6847–6854. doi: 10.1128/JVI.00497-12
Keywords: greenhouse technology, pathosystems, plant-pathogen interaction, sustainable agriculture, climate change, disease control, arid regions
Citation: Yusuf AG, Al-Yahya FA, Saleh AA and Abdel-Ghany AM (2025) Optimizing greenhouse microclimate for plant pathology: challenges and cooling solutions for pathogen control in arid regions. Front. Plant Sci. 16:1492760. doi: 10.3389/fpls.2025.1492760
Received: 09 September 2024; Accepted: 13 January 2025;
Published: 06 February 2025.
Edited by:
Lorena Parra, Universitat Politècnica de València, SpainReviewed by:
Jesús Muñoz-Rojas, Meritorious Autonomous University of Puebla, MexicoCopyright © 2025 Yusuf, Al-Yahya, Saleh and Abdel-Ghany. This is an open-access article distributed under the terms of the Creative Commons Attribution License (CC BY). The use, distribution or reproduction in other forums is permitted, provided the original author(s) and the copyright owner(s) are credited and that the original publication in this journal is cited, in accordance with accepted academic practice. No use, distribution or reproduction is permitted which does not comply with these terms.
*Correspondence: Abdulmujib G. Yusuf, eXVzdWZhYmR1bG11amliQHlhaG9vLmNvbQ==
Disclaimer: All claims expressed in this article are solely those of the authors and do not necessarily represent those of their affiliated organizations, or those of the publisher, the editors and the reviewers. Any product that may be evaluated in this article or claim that may be made by its manufacturer is not guaranteed or endorsed by the publisher.
Research integrity at Frontiers
Learn more about the work of our research integrity team to safeguard the quality of each article we publish.