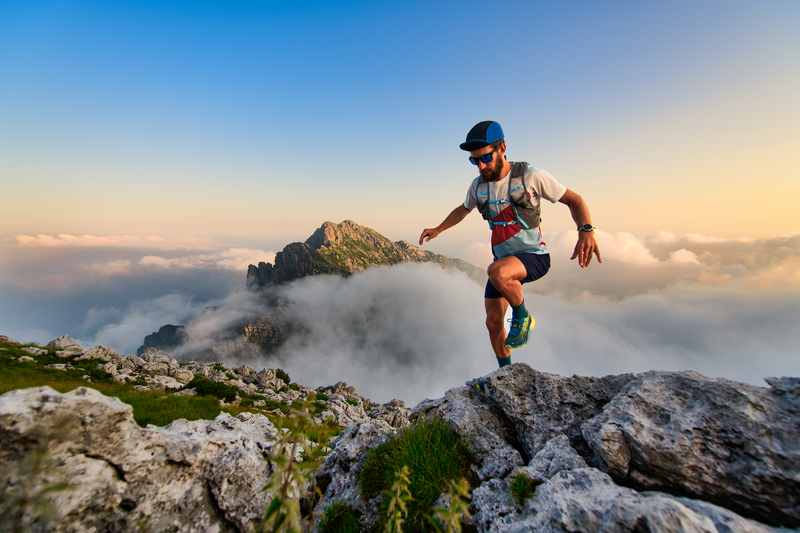
95% of researchers rate our articles as excellent or good
Learn more about the work of our research integrity team to safeguard the quality of each article we publish.
Find out more
ORIGINAL RESEARCH article
Front. Plant Sci. , 21 March 2025
Sec. Plant Nutrition
Volume 16 - 2025 | https://doi.org/10.3389/fpls.2025.1484734
Introduction: In high-latitude area, climate change has brought about recurrent chilling stress that adversely impacts the sustainable production of rice and alters the distribution of carbon (C) and nitrogen (N) in paddy ecosystems. A comprehensive understanding of how the paddy ecosystem’s C and N allocation responds to low-temperature stress during critical growth stages remains elusive.
Methods: A rice pot experiment of two varieties combined with 13C and 15N isotope labelling method was conducted to evaluate how low temperature stress at heading stage affects rice yield, and above- and belowground C and N partitioning.
Results and Discussion: Low-temperature stress significantly reduced rice grain yield of JN809 (sensitive to low-temperature stress) and J88 (tolerant to low-temperature stress) varieties by 27.6% and 21.4%, respectively, This stress tendency increased C and N accumulation in rice stems and leaves, while concurrently decreasing C and N accumulation in panicles. Specifically, under low-temperature stress, the 13C isotope content in stems and leaves was found to be 14.0% and 19.0% higher than in the control treatment, while the 13C and 15N isotope contents in their panicles were 29.3% and 22.5% lower, respectively. The low-temperature tolerant variety (J88) demonstrated a reduced effect of low-temperature stress on rice yield and C, N allocation due to efficient resource reallocation and stress tolerance mechanisms. The findings of this study provide a foundation for developing rice breeding and cultivation techniques that can enhance rice resilience and adaptability to climate change. Additionally, it informs strategies to optimize C and N sequestration practices in rice fields, ensuring high yields and efficient resource utilization.
Since the beginning of twenty-first century, the frequency and intensity of agricultural meteorological disasters have been on the rise due to climate change (Palmer, 2014; Ju et al., 2013; Chen et al., 2020). Meanwhile, the Fifth Assessment Report of the Intergovernmental Panel on Climate Change (IPCC_AR5) pointed out that as global climate warming and extreme weather events become more frequent, the risk of low-temperature stress may also increase, which to a certain extent increases the instability of agricultural production (Pachauri et al., 2014). Addressing agricultural meteorological disasters is urgent to maintain sustainable crop production (Gomez-Zavaglia et al., 2022).
Rice (Oryza sativa L.) is the most important staple food for more than half of the population across the world. It was estimated that global rice demand would increase from 644 million tons in 2007 to 827 million tons in 2050 (Alexandratos and Bruinsma, 2012). As the world’s largest producer and consumer, China plays a central role in meeting this increasing demand. Along with global warming, the rice cropping area in northeast China has increased rapidly, now accounting for more than 14% of China’s total production (National Bureau of Statistics of China, NBSC, 2023). Even more, nearly 75% of rice production in northeast China was for commodities. In recent years, extreme temperature events have occurred frequently in rice planting areas of China (Wang et al., 2019; Yuan et al., 2021), and cold damage has become the primary meteorological disaster affecting rice production, particularly in northeast China (Tao et al., 2013). Low-temperature stress is one of the major limiting factors affecting stable rice production in high-latitude areas (e.g. northeast China). Delayed-type or obstructive-type cold damage seriously affects rice growth, development, and grain filling (Tao et al., 2013). However, current research mainly focuses on the characteristics and mechanisms of the expected impact of rising atmospheric temperatures on C and N distribution in rice field systems (Tang et al., 2021, Tang et al., 2022; Haas et al., 2022; Xie et al., 2023), while extreme low-temperatures also affect rice growth and lead to differences in the distribution of C and N between rice crops, soil, and atmosphere (Deng et al., 2011).
Rice has a specific minimum suitable temperature at each growth and development stage, low-temperature stress is unfavorable for dry matter accumulation. Especially during the heading stage, rice exhibits its weakest tolerance to low-temperatures. Cold stress during this period can lead to floret degradation or pollen sterility, resulting in empty grains (Zhang et al., 2017; Siddik et al., 2019), reducing grain setting rate and thousand-grain weight, ultimately causing a significant decline in yield (Zhu et al., 2012; Sperotto et al., 2013). Furthermore, different varieties had varying tolerances to low-temperature stress (Wainaina et al., 2015; Geraldine et al., 2020). Low-temperature stress caused a certain degree of damage to the antioxidant system of flag leaves in rice. When the temperature was too low, the activity of protective enzymes in rice leaves was affected, leading to the impairment of rice photosynthesis and other functions, which in turn affected the stable formation of the final yield (Gazquez et al., 2018). At present, research on rice low-temperature damage mainly focuses on the degree of low-temperature damage, changes in rice physiological functions during low-temperatures, and the impact on yield components and quality (Bhattacharjees, 2013; Ren et al., 2017). However, there are limited reports on the effects of low-temperature damage and rice varieties on rice, particularly concerning the distribution of C and N and changes in soil C and N. Given the strong C sink function and outstanding C sequestration potential of the paddy ecosystems (Liu et al., 2023), understanding how low-temperature stress during critical growth stages and rice varieties affects C and N dynamics is crucial for addressing climate change.
Hereby, we hypothesized that (i) the biomass and yield of rice were affected by low-temperature during the rice heading stage; (ii) low-temperature significantly hindered the transportation of C assimilate and absorbed N from soil resulting in the re-distribution of C and N in paddy ecosystem. To test these hypotheses, an artificial climate chamber combined with a pot experiment and 13C and 15N isotope labeling methods were used to simulate extreme low-temperature conditions in the paddy ecosystem. The objectives of the present study are to evaluate the effects of low-temperature stress during the rice heading stage on C and N distribution in aboveground and underground parts of rice crops and changes in soil C and N in soils. It is anticipated to provide a basis for rice breeding and cultivation techniques that can enhance rice resilience and adaptability to climate change, as well as optimize C and N sequestration practices in the paddy ecosystem to ensure high yields and resilience to climate disaster risks.
The experiment was conducted in Gongzhuling City, Jilin Province of China (124°48´E, 43°32´N) in 2019. Two-factor experiment with randomized block design was used to evaluate how air temperature stress and different rice varieties affect C and N allocation in above-ground and under-ground parts of rice, the changes in C and N in rhizosphere soil and bulk soil. The temperature treatments included the control treatment (CK, no low-temperature stress) and low-temperature stress treatment at the heading stage (LT). The rice varieties included Jijing 88 (J88, super japonica rice tolerant to low-temperature stress) and Jinongda 809 (JN809, sensitive to low-temperature stress) (https://www.ricedata.cn/; Zhang et al., 2021). The experiment adopted the pot planting method. There were sixteen pots for each treatment and a total of 64 pots for the experiment. Four pots, for each treatment and sampling date, were used for parameter measurement at the rice heading stage (5th August), grain filling stage (27th August), and harvesting stage (23rd September). The pot was 25 cm in height with a diameter of 20 cm. A nylon bag with a height of 10 cm and a diameter of 8 cm was buried in the basin as the root bag. The mesh size of the nylon bag is 37 μm, which ensures that the rice roots do not extend out of the root bag, but can ensure the exchange of nutrients between the root bag and soil in the pot. The total amount of soil in the basin and the root bag was 5.5 kg. The potting soil was a paddy soil developed from long-term conventional paddy fields and formed by red clay in quaternary soil. The soil is characterized as soil organic carbon of 21.3 g kg-1, total N of 2.0 g kg-1, alkali-hydrolyzed N of 176.1 mg kg-1, available phosphorus of 19.4 mg kg-1, available potassium of 64.1 mg kg-1, and pH of 5.6. Three uniformly growing rice seedlings (Rice with four leaves and one heart) are transplanted in each root bag at the seedling stage, maintaining a 3-4 cm water layer, and fertilized and managed according to conventional local practices, with N at 0.276 g kg-1, P2O5 at 0.272 g kg-1, and K2O at 0.185 g kg-1. The N fertilizer is urea (CO(NH2)2, containing 46% N), which is applied three times in the ratio of 3:4:3 as base fertilizer, tillering fertilizer, and grain filling fertilizer respectively. The P fertilizer was (NH4)2HPO4 (containing 53% P2O5), and the K fertilizer was K2SO4 (containing 54% K2O). Both P2O5 and K2O fertilizers are applied as base fertilizers at one time. The rice was grown under natural conditions except for the heading stage when low-temperature stress was conducted.
In order to differentiate the allocation of C and N assimilation in rice system, 13C and 15N isotope labelling methods were used in the present experiment. 13C marker is started at the booting stage. For labelling, rice was confined in a transparent labelling chamber (1 m length × 1 m width × 1 m height for each chamber, Supplementary Figure S1A) and after half an hour of confinement treatment, H2SO4 was dripped into 13C-labelled Na2CO3 (13C abundance >98%, Shanghai Research Institute of Chemical Technology, Shanghai, China) to produce 13CO2, which was aired after 4 hours of confinement and labelled continuously for 7 days (Supplementary Figure S1A). The 15N labelling was carried out simultaneously with 13C labelling by adding 15NH4Cl (15N abundance 99.9%, Shanghai Research Institute of Chemical Technology, Shanghai, China) in pots. The labelling amounts of 13C and 15N are 56.3 mg 13C pot-1 and 40.8 mg 15N pot-1, respectively. The control treatment applied the same amount of unlabeled NH4Cl. Fans and ice packs were placed inside for physical cooling during the marking period to prevent high temperatures in the labelling chamber.
An artificial climate chamber was used to simulate low-temperature stress at the rice heading stage. After the 13C and 15N labelling, half of the pots for each rice variety were moved into the artificial climate chamber (Supplementary Figure S1B, C), and the low-temperature treatment was conducted from 20: 00 pm to 8: 00 am the next day. The extreme low-temperature treatment was divided into four periods of 3 hours each, and the temperature of each period from the beginning to the end was 14°C, 11°C, 10°C and 13°C in order. The temperature of the control treatment from the beginning to the end was 25°C, 23°C, 22°C and 24°C in order (Supplementary Figure S1D). The artificial climate chamber was controlled to give potted plants light at 5:00 a.m. The extreme low-temperature treatment lasted for 7 days (from the night of July 30 to the morning of August 5). Figure 1 presents the effect of low-temperature treatment on air and soil temperatures. The mean air and soil temperatures from 20:00 to 8:00 under low-temperature treatment were 12.4~13.2°C, and 15.2~16.8 °C, respectively, which was suitable for simulating chilling stress in the experimental site. After the low-temperature treating completed, all the pots were moved from the artificial climate chamber to the natural conditions. After the low-temperature treatment, there were no differences in soil and air temperatures among all the treatments (Figure 1C).
Figure 1. Air (A) and soil (B) temperature during low-temperature treatment between CK and LT treatments, air and soil temperature (C) during the experiment period between CK and LT treatments. CK and LT denote control treatment and low-temperature treatment, respectively.
Plant and soil samples were collected three times at the rice heading stage (5th August, 2019), the grain filling stage (27th August, 2019), and the harvesting stage (23rd September, 2019). Four pots were collected at each sampling. The rice was pulled out with the root and the rhizosphere soil was collected by shaking method when sampling. The bulk soil was also sampled from the pot. The rice plants were separated according to the root, stem, leaf, and panicle, then put into the oven at 105°C for 30 min to kill, and then baked at 65°C until constant weight. After taking out, the dry matter weight of each part was weighed on a one-hundredth scale. The rhizosphere and bulk soil were placed in a cool and dry environment to dry naturally. The soil and plant samples were ground with a ball mill until they met the test criteria. The processed samples were determined by isotope mass spectrometry (Elementar Isoprime 100, Germany) to determine the values of total C and N as well as δ13C and δ15N for each fraction.
The contents of 13C and 15N in plants (root, stem, leaf and panicle) and soil (rhizosphere and bulk soils) can be calculated by the following formula (Equations 1, 2).
13Ci and 15Ni represent 13C and 15N contents (mg· pot-1), δ13Ci and 13Cn represent abundance of 13C in labeled and unlabeled samples (‰), δ15Ni and 15Nn represent abundance of 15N in labeled and unlabeled samples (‰), respectively. Ci and Ni represent the total C and N content (mg· pot-1) in plants (panicle, stem, leaf and root) and soil (rhizosphere and bulk soil).
The total assimilation of 13C and 15N was the sum of 13C and 15N of plants (root, stem, leaf, panicle) and soils (rhizosphere soil, bulk soil) measured at the end of labeling (Equations 3, 4).
The incorporation of 13C (% of assimilated 13C) and 15N (% of assimilated 15N) into panicle, stem, leaf, root, rhizosphere, and bulk soil at each sampling date were calculated as follows (Equations 5, 6):
The PROC GLM procedure in SAS (SAS Institute Inc., Version 9.2, Cary, NC) was used to analyze the effects of treatments on rice biomass, rice yield and its component factors, 15N and 13C contents, percentage distribution of the assimilated 15N and 13C. The parameters mentioned above were analyzed using the MIXED procedure of SAS, with sampling date, temperature treatment, and variety used as fixed effects Littell et al, 2006, and Compound symmetry covariance structure was used for repeated measures analysis. Means were compared using Fisher’s protected least significant difference (LSD) test at the 0.05 probability level. To assess the linear relationship between rice yield and the C13/N15 ratio, Pearson correlation analysis was employed.
Low-temperature treatment during the rice heading stage and different rice variety treatments both influenced dry matter accumulation and distribution across various growth stages (Figure 2). Low-temperature treatment generally reduced total dry matter accumulation in rice plants, whereas rice varieties affected leaf and panicle biomass. At the grain-filling stage, low-temperature treatment decreased root biomass of J88 by 7.2% compared to the control (CK). At the heading stage, stem biomass decreased by 12.5% in JN809 and by 6.5% in J88. However, at the grain-filling stage, stem biomass in JN809 increased by 12.3%. Low-temperature treatment reduced leaf biomass in both rice varieties. Leaf biomass decreased by 13.7% in JN809 and by 15.4% in J88 at the heading stage, and by 12.8% in JN809 and 22.1% in J88 at the grain-filling stage. Panicle biomass of J88 under low-temperature treatment at the grain-filling stage was 15.3% lower than CK. At the harvesting stage, panicle biomass under low-temperature treatment was 29.0% lower in JN809 and 25.0% lower in J88 compared to CK. At the heading stage, low-temperature treatment decreased total biomass by 11.6% in JN809 and by 6.5% in J88. At the grain-filling stage, total biomass in J88 decreased by 18.2%. At the harvesting stage, total biomass in J88 decreased by 8.4%.
Figure 2. Root (A), stem (B), leaf (C), panicle (D) and total biomass (E) during different growing stages. CK and LT denote control treatment and low-temperature treatment, respectively. Bar means the standard error. “*” indicate significant differences between the CK and LT treatments, and between different varieties at the probability level of 0.05.
The low-temperature treatment impacted spikelets per panicle, percentage of grain filling and yield (Figure 3). While the rice panicles number per plot, spikelets per panicle, thousand-grain weight, percentage of grain filling and yield varied between varieties. The number of spikelets per panicle under low-temperature treatment was 20.2% and 15.1% lower in JN809 and J88 than in CK, respectively. The rice yield of JN809 and J88 under low-temperature treatment was 27.6% and 21.4% lower than those of CK, respectively. The lower-temperature treatment decreased the percentage of grain filling of JN809 and J88 by 38.5% and 24.8%, when compared with the CK treatment. The low-temperature treatment had no effect on the number of panicles and thousand-grain weight of rice. The spikelets per panicle and yield of JN809 were 57.4% and 32.3% lower than those of the J88 variety, respectively.
Figure 3. Grain yield and its component factors under different temperature treatments and varieties. (A) Panicle number per plot, (B) Spikelets per panicle, (C) Thousand-grain weight, (D) Percentage of grain filling, (E) Grain yield). CK and LT denote control treatment and low-temperature treatment, respectively. Bar means the standard error. Different lowercase letters for the same variety indicate significant differences between the CK and LT treatments at the probability level of 0.05, while different capital letters for the same temperature treatment indicate significant differences between different varieties at the probability level of 0.05.
The sampling period, low-temperature treatment, and rice variety all impacted nitrogen (N) uptake by rice (Figure 4). Low-temperature treatment reduced rhizosphere soil 15N content. At the heading stage, rhizosphere soil 15N content of JN809 and J88 under low-temperature was 50.1% and 20.0% lower than CK treatment, respectively. At the grain-filling stage, JN809’s rhizosphere soil 15N content under low-temperature was 49.7% lower than CK. However, at the harvesting stage, JN809’s rhizosphere soil 15N content under low-temperature was 36.2% higher than CK. Under low-temperature, J88’s rhizosphere soil 15N content was 66.7% higher than JN809 at the heading stage (Figure 4A). Low-temperature treatment increased bulk soil 15N content. At the heading stage, bulk soil 15N content under low-temperature was 41.4% and 19.9% higher in JN809 and J88, respectively, compared to CK. At the grain-filling stage, J88’s bulk soil 15N content under low-temperature was 173.7% higher than CK. At the harvesting stage, bulk soil 15N content under low-temperature was 30.5% and 24.4% higher in JN809 and J88, respectively, compared to CK. Under low-temperature, J88’s bulk soil 15N content was 115.5% higher at the grain-filling stage and 56.0% higher at the harvesting stage, compared to JN809 (Figure 4B). Low-temperature treatment also increased stem 15N content. At the heading stage, J88’s stem 15N content under low-temperature was 26.5% higher than CK. At the harvesting stage, J88’s stem 15N content under low-temperature was 15.5% higher than CK. Compared to JN809, J88’s stem 15N content under low-temperature was 42.2% higher at the heading stage, and 28.2% higher at the harvesting stage (Figure 4C).
Figure 4. The content of 15N retained in rice-soil system during different growing stages (Unit: mg pot-1). (A) Rhizosphere soil, (B) Bulk soil, (C) Stem, (D) Leaf, (E) Root, (F) Panicle). CK and LT denote control treatment and low-temperature treatment, respectively. Bar means the standard error. “*” indicate significant differences between the CK and LT treatments, and between different varieties at the probability level of 0.05.
Low-temperature treatment reduced leaf 15N content. At the heading stage, leaf 15N content of JN809 under low-temperature treatment was 26.6% lower than CK, while for J88, it was 32.3% lower. Under low-temperature, leaf 15N content of J88 was 20.5% higher than JN809 at the heading stage (Figure 4D). Root 15N content under low-temperature treatment was 27.5% lower in J88 at the heading stage, compared to CK, but 22.4% higher in JN809. At the harvesting stage, root 15N content of J88 under low-temperature was 32.2% higher than JN809 (Figure 4E). Panicle 15N content under low-temperature treatment was 103.4% higher in J88 at the heading stage, compared to CK. However, it was 18.0% lower in JN809 and 18.9% lower in J88 at the grain-filling stage. At the harvesting stage, panicle 15N content was 18.8% lower in JN809 and 26.1% lower in J88, compared to CK. Under low-temperature, panicle 15N content of J88 was 27.2% higher than JN809at the heading stage (Figure 4F).
The sampling period, low-temperature treatment, and rice variety all influenced the partitioning of photosynthetic products in rice (Figure 5). At the heading stage, rhizosphere soil 13C content under low-temperature treatment was 60.1% lower in JN809, compared to CK. At the grain-filling stage, it was 27.5% lower in JN809 and 38.9% lower in J88. At the harvesting stage, it was 37.8% lower in JN809 and 29.5% lower in J88 compared to CK. Under low-temperature, rhizosphere soil 13C content of J88 was 50.0% higher than JN809 at the heading stage (Figure 5A). Low-temperature treatment increased bulk soil 13C content. At the heading stage, bulk soil 13C content under low-temperature treatment was 25.8% higher in JN809 and 118.5% higher in J88, compared to CK. At the grain-filling stage, it was 62.1% higher in JN809, and at the harvesting stage, it was 72.6% higher in J88, compared to CK. Under low-temperature, bulk soil 13C content of J88 was 68.4% higher at the heading stage, but 30.4% lower at the grain-filling stage, compared to JN809 (Figure 5B). Stem 13C content under low-temperature treatment was 13.4% lower in JN809 at the heading stage. At the grain-filling stage, it was 27.4% higher in JN809 and 45.5% higher in J88. At the harvesting stage, it was 10.6% higher in JN809 and 17.3% higher in J88, compared to CK. Under low-temperature, leaf 13C content of J88 was 13.1% higher at the heading stage, but 15.4% lower at the harvesting stage, compared to JN809 (Figure 5C).
Figure 5. The content of 13C retained in the rice-soil system (Unit: mg pot-1). (A) Rhizosphere soil, (B) Bulk soil, (C) Stem, (D) Leaf, (E) Root, (F) Panicle). CK and LT denote control treatment and low-temperature treatment, respectively. Bar means the standard error. “*” indicate significant differences between the CK and LT treatments, and between different varieties at the probability level of 0.05.
Leaf 13C content under low-temperature treatment was 43.6% lower in J88 at the heading stage, 23.2% higher in JN809 at the grain-filling stage, and 16.3% higher in JN809 and 21.6% higher in J88 at the harvesting stage, compared to CK. Under low-temperature, leaf 13C content of J88 was 25.2% lower at the heading stage, and 41.9% lower at the grain-filling stage, compared to JN809 (Figure 5D). Root 13C content under low-temperature treatment was 23.0% higher in JN809 at the heading stage, 41.6% lower in J88 at the grain-filling stage, and 70.1% lower in JN809 and 56.7% lower in J88 at the harvesting stage, compared to CK. Under low-temperature, root 13C content of J88 was 26.7% lower at the heading stage, but 47.5% higher at the grain-filling stage, compared to JN809 (Figure 5E). Low-temperature treatment decreased panicle 13C content. At the heading stage, panicle 13C content under low-temperature treatment was 37.9% lower in JN809, and 69.2% lower in J88, compared to CK. At the grain-filling stage, it was 40.8% lower in JN809, and at the harvesting stage, it was 39.9% lower in JN809, compared to CK. Under low-temperature, panicle 13C content of J88 was 26.7% higher at the harvesting stage, compared to JN809 (Figure 5F).
The sampling period, low-temperature treatment, and rice variety all affected the proportion of 15N partitioning (Figure 6). Under low-temperature treatment, the percentage distribution of soil 15N in the rice rhizosphere soil was 4.99 and 2.79 percentage points lower in JN809 and J88 at the heading stage, respectively, and 4.60 percentage points lower in JN809 at the grain-filling stage, compared to CK. At the heading stage, the percentage distribution of soil 15N in the rhizosphere soil of J88 was 6.17 percentage points higher than that of JN809 under low-temperature conditions (Figure 6A). Low-temperature treatment increased the percentage distribution of soil 15N in the rice bulk soil. At the heading stage, this distribution was 1.55 and 1.24 percentage points higher in JN809 and J88, respectively, compared to CK. At the grain-filling stage, it was 3.91 percentage points higher in J88, and at the harvesting stage, it was 0.43 and 0.76 percentage points higher in JN809 and J88, respectively, compared to CK. Under low-temperature conditions, the percentage distribution of soil 15N in the bulk soil of J88 was 2.20 percentage points higher at the heading stage, 4.04 percentage points higher at the grain-filling stage, and 2.01 percentage points higher at the harvesting stage compared to JN809 (Figure 6B). The percentage distribution of soil 15N in the rice stem also increased under low-temperature treatment. At the heading stage, it was 6.55 percentage points higher in J88 compared to CK, and at the harvesting stage, it was 5.82 percentage points higher in J88, compared to CK. Under low-temperature conditions, the percentage distribution of soil 15N in the stem of J88 was 14.86 percentage points higher at the heading stage, 5.13 percentage points higher at the grain-filling stage, and 18.11 percentage points higher at the harvesting stage, compared to JN809 (Figure 6C).
Figure 6. The percentage distribution of 15N (content at the end of labeling) in different parts of rice during different growth stages in rice-soil system (Unit: %). (A) Rhizosphere soil, (B) Bulk soil, (C) Stem, (D) Leaf, (E) Root, (F) Panicle). CK and LT denote control treatment and low-temperature treatment, respectively. Bar means the standard error. “*” indicate significant differences between the CK and LT treatments, and between different varieties at the probability level of 0.05.
Under low-temperature treatment, the percentage distribution of soil 15N in rice leaves was 7.35 percentage points lower in JN809 and 6.72 percentage points lower in J88 at the heading stage, compared to CK. However, at the harvesting stage, this distribution was 2.83 percentage points higher in J88 than CK. Additionally, the percentage distribution of soil 15N in the leaves of J88 was 12.46 percentage points higher at the heading stage, and 5.42 percentage points higher at the harvesting stage, compared to JN809 (Figure 6D).
In terms of rice roots, the percentage distribution of soil 15N under low-temperature treatment was 1.22 percentage points higher in JN809 at the grain-filling stage, and 3.79 percentage points higher in J88 at the harvesting stage, compared to CK. For the J88 variety, this distribution was 2.91 percentage points higher at the heading stage, 2.31 percentage points higher at the grain-filling stage, and 6.70 percentage points higher at the harvesting stage, compared to JN809 (Figure 6E). Regarding rice panicles, the percentage distribution of soil 15N under low-temperature treatment was 2.38 and 3.67 percentage points lower in JN809 and J88, respectively, at the grain-filling stage, compared to CK. However, at the harvesting stage, it was 2.56 percentage points higher in JN809, and 5.56 percentage points higher in J88, compared to CK. Under low-temperature conditions, the percentage distribution of soil 15N in the panicles of J88 was 4.20 percentage points higher at the heading stage, 4.84 percentage points higher at the grain-filling stage, and 4.64 percentage points higher at the harvesting stage compared to JN809 (Figure 6F).
The sampling period, low-temperature treatment, and rice variety all influenced the allocation of photosynthetic products in rice (Figure 7). Low-temperature treatment reduced the percentage distribution of 13C in rice rhizosphere soil. At the heading stage, this distribution was 2.09 percentage points lower in JN809 compared to CK. At the grain-filling stage, it was 0.77 percentage points lower in JN809 and 1.17 percentage points lower in J88. At the harvesting stage, it was 0.83 percentage points lower in JN809 and 0.63 percentage points lower in J88, compared to CK (Figure 7A). In rice bulk soil, the percentage distribution of 13C was 0.08 and 0.36 percentage points higher in JN809 and J88, respectively, at the heading stage compared to CK. At the grain-filling stage, it was 0.19 percentage points lower in JN809, and at the harvesting stage, it was 0.21 percentage points lower in J88 compared to CK. Under low-temperature conditions, the percentage distribution of 13C in the bulk soil of J88 was 0.17 percentage points lower at the grain-filling stage, compared to JN809 (Figure 7B). For rice stems, the percentage distribution of 13C was 7.35 and 8.74 percentage points higher in JN809 and J88, respectively, at the grain-filling stage compared to CK. At the harvesting stage, it was 5.93 percentage points higher in JN809 and 8.80 percentage points higher in J88 compared to CK. Under low-temperature conditions, the percentage distribution of 13C in the stems of J88 was 6.19 percentage points lower at the grain-filling stage, compared to JN809 (Figure 7C).
Figure 7. The percentage distribution of 13C (content at the end of labeling) in different parts of rice during different growth stages in rice-soil system (Unit: %). (A) Rhizosphere soil, (B) Bulk soil, (C) Stem, (D) Leaf, (E) Root, (F) Panicle). CK and LT denote control treatment and low-temperature treatment, respectively. Bar means the standard error. “*” indicate significant differences between the CK and LT treatments, and between different varieties at the probability level of 0.05.
In rice leaves, the percentage distribution of 13C was 14.73 percentage points lower in J88 at the heading stage, and 3.93 percentage points lower at the grain-filling stage, compared to CK. At the harvesting stage, this distribution was 1.90 percentage points higher in JN809, and 2.27 percentage points higher in J88, compared to CK. Under low-temperature conditions, the percentage distribution of 13C in rice leaves was 7.29 percentage points lower in J88 at the grain-filling stage, and 13.22 percentage points lower at the harvesting stage, compared to JN809 (Figure 7D). In rice roots, the percentage distribution of 13C was 7.80 percentage points lower in J88 at the heading stage, and 4.92 percentage points higher at the grain-filling stage, compared to CK. At the harvesting stage, it was 5.80 percentage points higher in JN809 and 5.07 percentage points higher in J88, compared to CK. Under low-temperature conditions, the percentage distribution of 13C in rice roots was 4.05 percentage points lower in J88 at the heading stage, and 5.01 percentage points higher at the grain-filling stage, compared to JN809 (Figure 7E). In rice panicles, the percentage distribution of 13C was 1.98 and 4.84 percentage points lower in JN809 and J88, respectively, at the heading stage compared to CK. At the grain-filling stage, it was 2.57 percentage points lower in JN809, and at the harvesting stage, it was 2.50 percentage points lower in JN809 compared to CK. Under low-temperature conditions, the percentage distribution of 13C in rice panicles was 1.10 percentage points lower in J88 at the heading stage, compared to JN809 (Figure 7F).
The research revealed a significant positive correlation between the soil’s C13/N15 ratio and rice yield. As the C13/N15 ratio in rice plants increased, the rice yield correspondingly rose. This trend was consistent: with higher C13/N15 ratios in the rice plants, the rice yield also increased (Figure 8).
Rice spikelet fertility, filling and setting rate are most susceptible to temperature extremes mainly in the last week before and during the rice heading stage, and low-temperatures during the rice heading stage resulted in reduced rice biomass and yield (Lü et al., 2013; Ren et al., 2017). In the present study, it was found that rice yield of JN809 and J88 treated with low-temperature during heading stage was 27.6% and 21.4% lower than CK, which is consistent with previous studies (Ghadirnezhad and Fallah, 2014; Zeng et al., 2017). The reasons might be that low temperature stress directly affected microspore differentiation and development, resulting in the reduction of the number of microspore differentiation and the dysplasia of differentiated microspores, resulting in poor or unable to crack anther, lower fertilization rate, and ultimately led to spikelet abortion, decreased the percentage of grain filling and reduced kernels per panicle and yield (Farrell et al., 2006; Li et al., 2014; Jia et al., 2019). Furthermore, low-temperature stress also reduced photosynthetic rate, chlorophyll fluorescence and dry matter accumulation, resulting in lower yield (Siddik et al., 2019; Ai et al., 2023). Moreover, the reduction in N uptake under low-temperature treatment could likewise lead to a decrease in leaf area index and net photosynthetic rate, which in turn inhibited normal panicle development (Jia et al., 2019).
Different varieties have different abilities to tolerate low-temperature stress (Wainaina et al., 2015; Geraldine et al., 2020). Low temperature stress could cause a certain degree of harm to the antioxidant system in rice flag leaves. Under low-temperature stress, with the continuous increase of reactive oxygen species, the activity of protective enzymes in rice flag leaves such as superoxide dismutase (SOD), catalase (CAT), and peroxidase (POD) increased, in order to maintain the free radicals at an appropriate level and reduce the damage to cells by free radicals (Li et al., 2014; Han et al., 2023). However, when the temperature was too low, the activity of protective enzymes in rice leaves was affected, leading to excessive accumulation of peroxides and a rapid increase in membrane unsaturation. The lipid membranes were extremely prone to oxidation. This led to damage to rice photosynthesis and other functions, affecting the stable formation of final yield. Studies found that cold- tolerant varieties were more likely to induce antioxidant enzyme activity under low-temperature treatment than non-cold- tolerant varieties (Guo et al., 2006; Gazquez et al., 2018). At the same time, previous studies have shown that overexpressing antioxidant enzymes in transgenic plants can better resist low-temperature stress (Kornyeyev et al., 2003; Wang et al., 2005). In this study, the yield decline of low-temperature tolerant variety J88 after low-temperature stress treatment was significantly lower than that of JN809 variety. This may be because the low-temperature tolerant variety J88, with higher physiological adaptive capacity, secreted more antioxidant enzymes from the leaves after being subjected to low-temperature stress to resist the adversity stress, thus slowing down the effect of low-temperature stress on the development of rice pollen mother cells and glumes (Shimono et al., 2007), which resulted in a lower rate of rice empty shells and a smaller decrease in yield (Susanti et al., 2019). This phenomenon was confirmed by the significantly higher kernel number of J88 variety than that of JN809 variety in the present study. Ali et al. (2021) similarly found that low-temperature tolerant varieties showed less yield reduction after exposure to low-temperature stress.
Nitrogen is one of the most important and active nutrient factors affecting the growth and yield of rice (Mi et al., 2017). Low-temperature cold damage interferes with plant physiological metabolic processes such as water metabolism, mineral nutrition, photosynthesis, respiration, and metabolism, and has a significant effect on their uptake of soil N (Lu et al., 2005; Zhao et al., 2015). Our results showed that the rhizosphere soil 15N contents of JN809 and J88 under low-temperature treatment were significantly lower than CK, while the bulk soil 15N contents were significantly higher than CK, respectively. These results indicate that low-temperature treatment reduced soil N uptake by rice root, which was mainly due to the weakened photosynthesis and reduced physiological metabolism of rice due to low-temperature, thus leading to lower nutrient demand of rice plants (Jia et al., 2019). Moreover, the total biomass of JN809 and J88 was lower under the low-temperature treatment than CK, respectively, which indicated that low-temperature could reduce the nutrient requirement of the rice plant. Rice yield is closely related to nitrogen absorption and utilization capacity of rice varieties (Fageria, 2003). In order to ensure normal ear development, it is necessary to maintain appropriate nitrogen content and reasonable balance of carbon and nitrogen metabolism during ear differentiation (Lawlor, 2002). Low-temperature stress leads to a reduction in the rate of N transport and a decrease in N accumulation in the rice panicle (Shimono et al., 2012). In this study, it was found that low-temperature stress during the rice heading stage led to a tendency for N accumulation in the bulk soil and stem and leaf parts, while the accumulation in the panicle was reduced (Figure 4). At rice harvest, the bulk soil 15N isotope contents of JN809 and J88 varieties treated at low-temperature stress were higher than CK, respectively, while the panicle 15N isotope contents were lower than CK, respectively. This suggests that low-temperature stress reduces N uptake by rice and affects N translocation to the rice panicle (Shimono et al., 2012; Liu et al., 2018). Jia et al. (2019) also found that low-temperature chilling reduced the ability of rice to absorb N and reduced rice N production by 4%-47%. The nitrogen absorption and utilization capacity of different rice varieties were different (Fageria, 2003). Low-temperature tolerant rice varieties can mitigate the impact of low-temperature stress on N absorption by rice. This study found that the 15N isotope content in both the aboveground and underground parts of the low-temperature tolerant variety J88 was higher than that of the conventional rice variety JN809. The reason may be that the low-temperature tolerant rice variety J88 had stronger physiological adaptability and genetic basis, which could maintain high growth activity and nutrient absorption capacity in low-temperature environments. At the same time, low-temperature tolerant rice varieties may also have better stress resistance mechanisms, which could alleviate the adverse effects of low-temperature on nutrient absorption and distribution by adjusting their internal physiological processes and metabolic pathways (Liu et al., 2015; Wu et al., 2017).
Plant photosynthetic C is an important component of the atmosphere-plant-soil C cycle in terrestrial ecosystems (Schlesinger and Andrews, 2000). Plants convert CO2 into organic matter through the photosynthetic C assimilation pathway, and the resulting photosynthetic C is transported through the plant phloem and distributed to the plant-soil system. A portion of this photosynthetic C is imported into the below-ground part for root growth, while C is imported to the soil in the form of root deposits (Uchida et al., 2010). The other part is stored in the above-ground part of the plant. Low-temperature treatment affected photosynthetic C allocation in rice (Jia et al., 2019, Jia et al., 2022). In this study, we found that at the end of the low-temperature treatment, the soil 13C isotope contents of the low-temperature treated JN809 and J88 varieties were 26.7% and 113.3% higher than CK, while the rice panicle 13C isotope contents of JN809 and J88 were significantly lower than CK, respectively. This may be due to the low-temperature treatment slowing down the fertility process in rice. The proportion of photosynthetic C allocated to the root system and soil was high in the vegetative growth stage of the rice-soil system (Werth and Kuzyakov, 2010). The proportion of photosynthetic C allocated to the root system and soil tended to decrease as the reproductive period progressed (Deng et al., 2017; Zang et al., 2019), and photosynthetic C tended to be allocated more toward the panicle portion. In addition, the reduction in soil N uptake by rice plants under the low-temperature treatment led to a decrease in leaf area index and net photosynthetic rate, which inhibited normal panicle development (Jia et al., 2019), then likewise resulted in reduced photosynthetic C transport to the panicle in rice. The 13C isotope contents of rice stem, leaf and root of different varieties under low-temperature treatment during rice harvest were higher than CK, and the 13C isotope content of rice panicle of J809 variety was lower than CK. This could also indicate that the low-temperature treatment affected the photosynthetic C transport in rice, leading to a reduction in photosynthetic C accumulation in the panicle, which in turn affected the formation of rice yield.
Low-temperature tolerant rice varieties effectively mitigate the impact of low-temperature stress on the distribution of photosynthetic carbon within the rice-soil system. According to our research, during the grain filling stage, the 13C isotope partitioning ratios in the stems and leaves of the low-temperature tolerant variety J88 were lower than JN809. However, during the harvesting stage, the 13C isotope partitioning ratio in the rice panicles of J88 was higher than that of JN809 (Figure 7). This difference was likely attributed to the unique genetic traits of the low-temperature tolerant varieties, which allowed them to maintain robust growth activity and nutrient absorption even under low-temperature conditions (Liu et al., 2015). As this study was only carried out once for 13C isotope labelling prior to the low-temperature treatment, it could not reveal the transport pathways of the newly transformed photosynthetic C after the low-temperature treatment. Follow-up studies could be carried out several times after the end of the low-temperature treatment for 13C isotope labelling, which could provide more clarity on the allocation of photosynthetic C to rice by the low-temperature treatment.
In this study, we primarily investigated the effects of low-temperature stress on the allocation of carbon (C) and nitrogen (N) in different rice varieties. However, we recognize that the regulatory mechanisms of C and N allocation under low-temperature stress are multifaceted. Among these, key metabolic enzymes play an indispensable role (Nunes-Nesi et al., 2010). For instance, sucrose phosphate synthase (SPS) and sucrose synthase (SUSY) are key enzymes in regulating carbohydrate metabolism, playing important roles in the synthesis and breakdown of sucrose within plants (Li J. et al., 2024). Sucrose, as the main form of C transport in plants, experiences changes in its metabolic levels under low-temperature stress, which directly affects the plant’s C allocation and biomass accumulation (Koch, 2004). In leaves, the activities of SPS and SUSY may be closely related to the transport and storage of photosynthetic products, thereby influencing the allocation of C to other organs, such as grains (Li J. et al., 2024). Additionally, enzymes related to N assimilation (such as glutamine synthetase and glutamate synthase) play a central role in the absorption, transformation, and transport of N (Dubey et al., 2021). The rational allocation of N is crucial for plant growth, development, and yield formation, especially during grain development, where the effective supply and allocation of N directly affect protein synthesis and grain quality (Fageria, 2014). Although we were unable to measure the activities of these metabolic enzymes and the levels of related metabolites in this study, future research could consider a more in-depth analysis of the activities of these enzymes under low-temperature stress and their correlation with biomass accumulation. This would help to provide a more comprehensive understanding of the regulatory mechanisms of C and N allocation in different rice varieties under low-temperature stress.
Low-temperature stress is one of the major limiting factors affecting stable rice production in high-latitude areas (e.g. northeast China). Delayed-type or obstructive-type cold damage seriously affects rice growth, development, and grain filling (Tao et al., 2013). Due to the low temperature stress reduced the absorption of N by rice root system and the transfer of nitrogen to rice panicles, which disrupted the transportation of photosynthetic C in rice. However, the low-temperature tolerant varieties could effectively alleviate the influence of low temperature stress on the distribution of photosynthetic C and N absorption in rice-soil system, thus reducing the adverse effects on the growth and development of rice panicles. By understanding the distribution and transformation of C and N in plants and soil under low-temperature stress, appropriate measures can be taken to cope with the adverse effects of climate change on rice. For example, rational fertilization and irrigation, application of plant growth regulators, adjusting the sowing date, and improving the stress resistance of rice (Huang et al., 2019; Wang et al., 2023; Yang et al., 2024). In addition, using modern biotechnology methods such as gene editing to cultivate low-temperature tolerant rice varieties and improve their stress resistance is also the most important measure to cope with climate change (Li K. et al., 2024).
Low-temperature stress during the rice heading stage reduced biomass and yield, mainly by decreasing the number of rice panicles and lowering the setting rate. This effect was primarily due to reduced N uptake by rice roots, decreased N translocation to the rice panicles, and disrupted photosynthetic C transport in rice under low-temperature treatment. In contrast, the low-temperature tolerance varieties effectively mitigated the impact of low-temperature stress on photosynthetic C distribution and N uptake within the rice-soil system, thereby reducing the adverse effects on panicle growth and development. Considering the limitations of the pot experiment, further field trials are needed to validate these findings and to better understand the mechanisms involved. This will help in developing more effective countermeasures for rice encountering low-temperature damage.
The original contributions presented in the study are included in the article/Supplementary Material. Further inquiries can be directed to the corresponding author.
TS: Data curation, Funding acquisition, Investigation, Visualization, Writing – original draft, Writing – review & editing. JR: Data curation, Investigation, Visualization, Writing – original draft. TC: Conceptualization, Methodology, Supervision, Writing – review & editing. LY: Funding acquisition, Writing – review & editing, Formal analysis. ZZ: Formal Analysis, Methodology, Supervision, Writing – review & editing. JZ: Formal analysis, Methodology, Writing – review & editing, Conceptualization. JL: Data curation, Investigation, Writing – original draft. AD: Project administration, Supervision, Writing – review & editing. HC: Methodology, Visualization, Writing – review & editing. XG: Funding acquisition, Project administration, Supervision, Writing – review & editing. ZS: Conceptualization, Funding acquisition, Methodology, Project administration, Supervision, Validation, Writing – review & editing.
The author(s) declare that financial support was received for the research and/or publication of this article. This work was funded by the National Natural Science Founds of China (grant no. 32172129), the Natural Science Foundation of the Shandong Province (grant no. ZR2023QC064), the Modern Agro-industry Technology Research System of Shandong Province-Ecological Agriculture (Grant No. SDAIT-30-15), the Agricultural Scientific and Technological Innovation Project of Shandong Academy of Agricultural Sciences (grant no. CXGC2024A06, CXGC2024F04), and the Modern Agro-industry Technology Research System-Green manure (grant no. CARS-22-G-16).
The authors declare that the research was conducted in the absence of any commercial or financial relationships that could be construed as a potential conflict of interest.
All claims expressed in this article are solely those of the authors and do not necessarily represent those of their affiliated organizations, or those of the publisher, the editors and the reviewers. Any product that may be evaluated in this article, or claim that may be made by its manufacturer, is not guaranteed or endorsed by the publisher.
The Supplementary Material for this article can be found online at: https://www.frontiersin.org/articles/10.3389/fpls.2025.1484734/full#supplementary-material
Ai, X., Xiong, R., Tan, X., Wang, H., Zeng, Y., Huang, S., et al. (2023). Low-temperature and light combined stress after heading on starch fine structure and physicochemical properties of late-season indica rice with different grain quality in southern China. Food Res. Int. 164, 112320. doi: 10.1016/j.foodres.2022.112320
Alexandratos, N., Bruinsma, J. (2012). “World agriculture towards 2030/2050: the 2012 revision,” in ESA working paper no. 12-03 (FAO, Rome).
Ali, I., Tang, L., Dai, J., Kang, M., Mahmood, A., Wang, W., et al. (2021). Responses of grain yield and yield related parameters to post-heading low-temperature stress in japonica rice. Plants 10, 1425. doi: 10.3390/plants10071425
Bhattacharjees, S. (2013). Heat and chilling induced disruption of redox homeostasis and its regulation by hydrogen peroxide in germinating rice seeds (Oryza sativa L, cultivar Ratna). Physiol. Mol. Biol. Pla. 19 , 199–207. doi: 10.1007/s12298-012-0159-x
Chen, X., Wang, L., Niu, Z., Zhang, M., Li, C., Li, J. (2020). The effects of projected climate change and extreme climate on maize and rice in the Yangtze River Basin, China. Agr. For. Meteorol. 282–283, 107867. doi: 10.1016/j.agrformet.2019.107867
Deng, H., Che, F., Xiao, Y., Tang, W., Pan, Y., Liu, Z., et al. (2011). Effects of low-temperature stress during flowering period on pollen characters and flag leaf physiological and biochemical characteristics of rice. Chin. J. @ Appl. Ecol. 22, 66–72. doi: 10.13287/j.1001-9332.2011.0022
Deng, Y. W., Tang, C., Yuan, H. C., Ning, Z., Zou, G. F., Wang, J. R., et al. (2017). The 13C-CO2 pulsing labeling method: distribution of rice photosynthetic carbon in plant-soil system during different rice growth stages. Acta Ecol. Sin. 37, 6466–6471. doi: 10.5846/stxb201607241506
Dubey, R. S., Srivastava, R. K., Pessarakli, M. (2021). “Physiological mechanisms of nitrogen absorption and assimilation in plants under stressful conditions,” in Handbook of plant and crop physiology (Boca Raton: CRC Press), 579–616.
Fageria, N. K. (2003). Plant tissue test for determination of optimum concentration and uptake of nitrogen at different growth stages in lowland rice. Commun. Soil Sci. Plan. 34, 259–270. doi: 10.1081/css-120017430
Farrell, T. C., Fox, K. M., Williams, R. L., Fukai, S. (2006). Genotypic variation for cold tolerance during reproductive development in rice: Screening with cold air and cold water. Field Crop Res. 98, 178–194. doi: 10.1016/j.fcr.2006.01.003
Gazquez, A., Vilas, J. M., Colman Lerner, J. E., Santiago, J., Calzadilla, P. I., Menéndez, A. B., et al. (2018). Rice tolerance to suboptimal low temperatures relies on the maintenance of the photosynthetic capacity. Plant Physiol. Bioch. 127, 537–552. doi: 10.1016/j.plaphy.2018.04.035
Geraldine, A., Junior, A., Gastmann, R., Adamski, J. M., Sperotto, R. A. (2020). Root responses of contrasting rice genotypes to low-temperature stress. J. Plant Physiol. 255, 153307. doi: 10.1016/j.jplph.2020.153307
Ghadirnezhad, R., Fallah, A. (2014). Temperature effect on yield and yield components of different rice cultivars in flowering stage. Int. J. Agron. 2014, 846707. doi: 10.1155/2014/846707
Gomez-Zavaglia, A., Mejuto, J. C., Simal-Gandara, J. (2022). Mitigation of emerging implications of climate change on food production system. Food Res. Int. 134, 109256. doi: 10.1016/j.foodres.2020.109256
Guo, Z., Ou, W., Lu, S., Zhong, Q. (2006). Differential responses of antioxidative system to chilling and drought in four rice cultivars differing in sensitivity. Plant Physiol. Biochem. 44, 828–836. doi: 10.1016/j.plaphy.2006.10.024
Haas, E., Carozzi, M., Massad, R. S., Butterbach-Bahl, K., Scheer, C. (2022). Long term impact of residue management on soil organic carbon stocks and nitrous oxide emissions from European croplands. Sci. Total Environ. 836, 154932. doi: 10.1016/j.scitotenv.2022.154932
Han, R., Wang, Y., Li, C., Wu, Z. (2023). Dephosphorylation of nitrate reductase protein regulates growth of rice and adaptability to low temperature. J. Plant Growth Regul. 42, 6920–6932. doi: 10.1007/s00344-023-10985-2
Huang, M., Fang, S., Shan, S., Zou, Y. (2019). Delayed transplanting reduced grain yield due to low temperature stress at anthesis in machine-transplanted late-season rice. Exp. Agr. 55, 843–848. doi: 10.1017/S001447971800042X
Jia, Y., Liu, H., Wang, H., Zou, D., Qu, Z., Wang, J., et al. (2022). Effects of root characteristics on panicle formation in japonica rice under low-temperature water stress at the reproductive stage. Field Crop Res. 277, 108395. doi: 10.1016/j.fcr.2021.108395
Jia, Y., Wang, J., Qu, Z., Zou, D., Sha, H., Liu, H., et al. (2019). Effects of low water temperature during reproductive growth on photosynthetic production and nitrogen accumulation in rice. Field Crop Res. 242, 107587. doi: 10.1016/j.fcr.2019.107587
Ju, H., Marijn, V., Lin, E., Xiong, W., Li, Y. (2013). The impacts of climate change on agricultural production systems in China. Climatic Change 120, 313–324. doi: 10.1007/s10584-013-0803-7
Koch, K. (2004). Sucrose metabolism: regulatory mechanisms and pivotal roles in sugar sensing and plant development. Curr. Opin. Plant Biol. 7, 235–246. doi: 10.1016/j.pbi.2004.03.014
Kornyeyev, D., Logan, B. A., Allen, R. D., Holaday, A. S. (2003). Effect of chloroplastic overproduction of ascorbate peroxidase on photosynthesis and photoprotection in cotton leaves subjected to low temperature photoinhibition. Plant Sci. 165, 1033–1041. doi: 10.1016/S0168-9452(03)00294-2
Lawlor, D. W. (2002). Carbon and nitrogen assimilation in relation to yield: mechanisms are the key to understanding production systems. J. Exp. Bot. 53, 773–787. doi: 10.1093/jexbot/53.370.773
Li, K., Hassan, M. A., Guo, J., Zhao, X., Gan, Q., Lin, C., et al. (2024). Analysis of genome-wide association studies of low-temperature germination in Xian and Geng rice. Front. Plant Sci. 15. doi: 10.3389/fpls.2024.1404879
Li, J., Hu, Y., Hu, J., Xie, Q., Chen, X., Qi, X. (2024). Sucrose synthase: an enzyme with multiple roles in plant physiology. J. Plant Physiol. 303, 154352. doi: 10.1016/j.jplph.2024.154352
Li, L. J., Huo, Z. G., Wu, L. J., Zhu, Q. H., Hu, F. (2014). Effects of low temperature on grain yield of rice and its physiological mechanism at the booting stage. Chin. J. Rice Sci. 28, 277–288. doi: 10.3969/j.issn.1001-7216.2014.03.007
Littell, R. C., Milliken, G. A., Stroup, W. W., Wolfnger, R. D., Schabenberger, O. (2006). SAS system for mixed models. 2nd ed (Cary, NC: SAS Inst).
Liu, Y., Ge, T., Wang, P., Groenigen, K. J., Xu, X., Cheng, K., et al. (2023). Residence time of carbon in paddy soils. J. Clean Prod. 400, 136707. doi: 10.1016/j.jclepro.2023.136707
Liu, Z., Tao, L., Liu, T., Zhang, X., Wang, W., Song, J., et al. (2018). Nitrogen application after low-temperature exposure alleviates tiller decrease in rice. Environ. Exp. Bot. 158, 205–214. doi: 10.1016/j.envexpbot.2018.11.001
Liu, T., Zhao, J., Sun, T., He, X., Li, C., Zhu, Y. (2015). The different response mechanisms of traditional and modern rice varieties under low temperature. Mol. Plant Breed. 13, 269–275. doi: 10.13271/j.mpb.013.000269
Lü, G. H., Wu, Y. F., Bai, W. B., Ma, B., Wang, C. Y., Song, J. Q. (2013). Influence of high temperature stress on net photosynthesis, dry matter partitioning and rice grain yield at flowering and grain filling Stages. J. Integr. Agr. 12, 603–609. doi: 10.1016/S2095-3119(13)60278-6
Lu, B., Yuan, Y., Zhang, C., Ou, J., Zhou, W., Lin, Q. (2005). Modulation of key enzymes involved in ammonium assimilation and carbon metabolism by low-temperature in rice (Oryza sativa L.) root. Plant Sci. 169, 295–302. doi: 10.1016/j.plantsci.2004.09.031
Mi, W. H., Zheng, S. Y., Yang, X., Wu, L. H., Liu, Y. L., Chen, J. Q. (2017). Comparison of yield and nitrogen use efficiency of different types of nitrogen fertilizers for different rice cropping systems at subtropical monsoon climate in China. Eur. J. Agron. 90, 78–86. doi: 10.1016/j.eja.2017.07.013
National Bureau of Statistics of China (NBSC) (2023). China statistics yearbook (Beijing: China Statistics Press).
Nunes-Nesi, A., Fernie, A. R., Stitt, M. (2010). Metabolic and signaling aspects underpinning the regulation of plant carbon nitrogen interactions. Mol. Plant 3, 973–996. doi: 10.1093/mp/ssq049
Pachauri, R. K., Meyer, L., Plattner, G. K., Stocker, T. (2014). IPC: climate change 2014: synthesis report,in Contribution of working groups I, II and III to the fifth assessment report of the intergovernmental panel on climate change geneva (IPCC, Switzerland).
Palmer, T. (2014). Record-breaking winters and global climate change. Science 344, 803–804. doi: 10.1126/science.1255147
Ren, H., Jing, P., Chen, Y., Hu, Y., Chen, M., Huo, Z. (2017). Effects of low-temperature at booting stage on growth and yield formation of rice. Chin. Rice 23, 56–62. doi: 10.16819/j.1001-7216.2022.210602
Schlesinger, W. H., Andrews, J. A. (2000). Soil respiration and the global carbon cycle. Biogeochemistry 48 , 7–20. doi: 10.1023/A:1006247623877
Shimono, H., Fujimura, S., Nishimura, T., Hasegawa, T. (2012). Nitrogen uptake by rice (Oryza sativa L.) exposed to low water temperatures at different growth stages. J. Agron. Crop Sci. 198, 145–151. doi: 10.1111/j.1439-037X.2011.00503.x
Shimono, H., Okada, M., Kanda, E., Arakawa, I. (2007). Low-temperature-induced sterility in rice: Evidence for the effects of temperature before panicle initiation. Field Crop Res. 101, 221–231. doi: 10.1016/j.fcr.2006.11.010
Siddik, M. A., Zhang, J., Chen, J., Qian, H., Jiang, Y., kareem, R. A., et al. (2019). Responses of indica rice yield and quality to extreme high and low-temperatures during the reproductive period. Eur. J. Agron. 106, 30–38. doi: 10.1016/j.eja.2019.03.004
Sperotto, R. A., Cruz, R., Cargnelutti, D., Adamski, J. M., Fett, J. P. (2013). Avoiding damage and achieving cold tolerance in rice plants. Food Energy Secur. 2, 96–119. doi: 10.1002/fes3.25
Susanti, Z., Snell, P., Fukai, S., Mitchell, J. H. (2019). Importance of anther dehiscence for low-temperature tolerance in rice at the young microspore and flowering stages. Crop Pasture Sci. 70 , 113–120. doi: 10.1071/CP18212
Tang, S., Cheng, W., Hu, R., Guigue, J., Hattori, S., Tawaraya, K., et al. (2021). Five-year soil warming changes soil C and N dynamics in a single rice paddy field in Japan. Sci. Total Environ. 756, 143845. doi: 10.1016/j.scitotenv.2020.143845
Tang, S., Yuan, P., Tawaraya, K., Tokida, T., Fukuoka, M., Yoshimoto, M., et al. (2022). Winter nocturnal warming affects the freeze-thaw frequency, soil aggregate distribution, and the contents and decomposability of C and N in paddy fields. Sci. Total Environ. 802, 149870. doi: 10.1016/j.scitotenv.2021.149870
Tao, F., Zhang, S., Zhang, Z. (2013). Changes in rice disasters across China in recent decades and the meteorological and agronomic causes. Reg. Environ. Change. 13, 743–759. doi: 10.1007/s10113-012-0357-7
Uchida, Y., Hunt, J. E., Barbour, M. M., Clough, T. J., Kelliher, F. M., Sherlock, R. R. (2010). Soil properties and presence of plants affect the temperature sensitivity of carbon dioxide production by soils. Plant Soil 337 , 375–387. doi: 10.1007/s11104-010-0533-9
Wainaina, C. M., Inukai, Y., Masinde, P. W., Ateka, E. M., Murage, H., Kano-Nakata, M., et al. (2015). Evaluation of cold tolerance in NERICAs compared with Japanese standard rice varieties at the reproductive stage. J. Agron. Crop Sci. 201, 461–472. doi: 10.1111/jac.12125
Wang, P., Hu, T., Kong, F., Xu, J., Zhang, D. (2019). Rice exposure to cold stress in China: how has its spatial pattern changed under climate change? Eur. J. Agron. 103, 73–79. doi: 10.1016/j.eja.2018.11.004
Wang, Y. J., Wisniewski, M., Meilan, R., Cui, M. G., Webb, R., Fuchigami, L. (2005). Overexpression of cytosolic ascorbate peroxidase in tomato confers tolerance to chilling and salt stress. J. Am. Soc Hortic. Sci. 130, 167–173. doi: 10.1046/j.1365-2109.2001.00538.x
Wang, H., Zhong, L., Fu, X., Huang, S., Zhao, D., He, H., et al. (2023). Physiological analysis reveals the mechanism of accelerated growth recovery for rice seedlings by nitrogen application after low temperature stress. Front. Plant Sci. 14. doi: 10.3389/fpls.2023.1133592
Werth, M., Kuzyakov, Y. (2010). 13C fractionation at the root-microorganisms-soil interface: a review and outlook for partitioning studies. Soil Biol. Biochem. 42, 1372–1384. doi: 10.1016/j.soilbio.2010.04.009
Wu, C., Cui, K., Wang, W., Qian, L., Shah, F., Hu, Q., et al. (2017). Heat-induced cytokinin transportation and degradation are associated with reduced panicle cytokinin expression and fewer spikelets per panicle in rice. Front. Plant Sci. 8. doi: 10.3389/fpls.2017.00371
Xie, Y., Shen, Q., Li, F., Ni, S., Yu, J. (2023). Temperature response of plants and heat tolerance in Rice: A review. Adv. Agron. 179, 135–203. doi: 10.1016/bs.agron.2023.01.003
Yang, J., Zhang, X., Wang, D., Wu, J., Xu, H., Xiao, Y., et al. (2024). The deterioration of starch physiochemical and minerals in high-quality indica rice under low-temperature stress during grain filling. Front. Plant Sci. 14. doi: 10.3389/fpls.2023.1295003
Yuan, M., Cai, C., Wang, C. Z., Li, G., Wu, G., Wang, J., et al. (2021). Warm air temperatures increase photosynthetic acclimation to elevated CO2 concentrations in rice under field conditions. Field Crop Res. 262, 108036. doi: 10.1016/j.fcr.2020.108036
Zang, H., Xiao, M., Wang, Y., Ling, N., Wu, J., Ge, T., et al. (2019). Allocation of assimilated carbon in paddies depending on rice age, chase period and N fertilization: Experiment with 13CO2 labelling and literature synthesis. Plant Soil 445, 113–123. doi: 10.1007/s11104-019-03995-1
Zeng, Y., Zhang, Y., Xiang, J., Uphoff, N. T., Pan, X., Zhu, D. (2017). Effects of low-temperature stress on spikelet-related parameters during anthesis in indica–japonica hybrid rice. Front. Plant Sci. 8. doi: 10.3389/fpls.2017.01350
Zhang, Z., Chen, Y., Wang, C., Wang, P., Tao, F. (2017). Future extreme temperature and its impact on rice yield in China. Int. J. Climatol. 37 , 4814–4827. doi: 10.1002/joc.5125
Zhang, Z., Dang, S., Long, G., Luo, D., Gong, X., Gao, Q. (2021). Study on cold tolerance of rice varieties in Jilin. Chin. Rice 27, 95–99. doi: 10.3969/j.issn.1006-8082.2021.06.017
Zhao, L., Li, M., Zheng, D., Wang, S., Gu, C., Na, Y., et al. (2015). Research progress of physiological changes and regulation of exogenous substance of chilling injury on plant. Chin. Agr. Sci. Bull. 31 , 217–223. doi: 10.11924/j.issn.1000-6850.2014-2411
Keywords: rice, chilly stress, carbon and nitrogen partition, isotope labelling, high latitude area
Citation: Sun T, Ruan J, Cao T, Yao L, Zhao Z, Zhang J, Li J, Deng A, Chen H, Gao X and Song Z (2025) Effects of low-temperature stress during rice heading stage on carbon and nitrogen allocation in paddy eco-system of northeastern China. Front. Plant Sci. 16:1484734. doi: 10.3389/fpls.2025.1484734
Received: 22 August 2024; Accepted: 03 March 2025;
Published: 21 March 2025.
Edited by:
Xiaorong Fan, Nanjing Agricultural University, ChinaReviewed by:
Krishan K. Verma, Guangxi Academy of Agricultural Sciences, ChinaCopyright © 2025 Sun, Ruan, Cao, Yao, Zhao, Zhang, Li, Deng, Chen, Gao and Song. This is an open-access article distributed under the terms of the Creative Commons Attribution License (CC BY). The use, distribution or reproduction in other forums is permitted, provided the original author(s) and the copyright owner(s) are credited and that the original publication in this journal is cited, in accordance with accepted academic practice. No use, distribution or reproduction is permitted which does not comply with these terms.
*Correspondence: Zhenwei Song, c29uZ3poZW53ZWlAY2Fhcy5jbg==
†These authors have contributed equally to this work
Disclaimer: All claims expressed in this article are solely those of the authors and do not necessarily represent those of their affiliated organizations, or those of the publisher, the editors and the reviewers. Any product that may be evaluated in this article or claim that may be made by its manufacturer is not guaranteed or endorsed by the publisher.
Research integrity at Frontiers
Learn more about the work of our research integrity team to safeguard the quality of each article we publish.