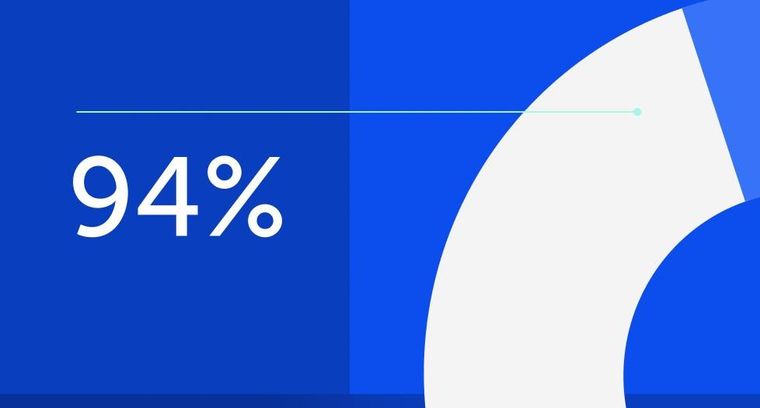
94% of researchers rate our articles as excellent or good
Learn more about the work of our research integrity team to safeguard the quality of each article we publish.
Find out more
REVIEW article
Front. Plant Sci., 06 February 2025
Sec. Plant Breeding
Volume 15 - 2024 | https://doi.org/10.3389/fpls.2024.1518123
This article is part of the Research TopicUtilizing Advanced Genomics and Biochemical Tools to Strengthen Crop Adaptation for Biotic and Abiotic StressesView all 4 articles
The challenges faced by today’s agronomists, plant breeders, and their managers encompass adapting sustainably to climate variability while working with limited budgets. Besides, managers are dealing with a multitude of issues with different organizations working on similar initiatives and projects, leading to a lack of a sustainable impact on smallholder farmers. To transform the current food systems as a more sustainable and resilient model efficient solutions are needed to deliver and convey results. Challenges such as logistics, labour, infrastructure, and equity, must be addressed alongside adapting to increasingly unstable climate conditions which affect the life cycle of transboundary pathogens and pests. In this context, transforming food systems go far beyond just farmers and plant breeders and it requires substantial contributions from industry, global finances, transportation, energy, education, and country developmental sectors including legislators. As a result, a holistic approach is essential for achieving sustainable and resilient food systems to sustain a global population anticipated to reach 9.7 billion by 2050 and 11.2 billion by 2100. As of 2021, nearly 193 million individuals were affected by food insecurity, 40 million more than in 2020. Meanwhile, the digital world is rapidly advancing with the digital economy estimated at about 20% of the global gross domestic product, suggesting that digital technologies are increasingly accessible even in areas affected by food insecurity. Leveraging these technologies can facilitate the development of climate-smart cultivars that adapt effectively to climate variation, meet consumer preferences, and address human and livestock nutritional needs. Most economically important traits in crops are controlled by multiple loci often with recessive alleles. Considering particularly Africa, this continent has several agro-climatic zones, hence crops need to be adapted to these. Therefore, targeting specific loci using modern tools offers a precise and efficient approach. This review article aims to address how these new technologies can provide a better support to smallholder farmers.
According to the FAO et al. (2019), over 820 million people globally live with hunger, which is a phenomenon rising in almost all subregions of Africa, and to a lesser extent in Latin America and Asia. Since 2014, food insecurity across the sub-Saharan Africa region, particularly South Sudan and Nigeria, has been exacerbated by human conflict as well as severe and long periods characterized by drought and sudden pests’ attacks, particularly affecting pastoralists (Anderson et al., 2021). Drought is known to be one of the main factors behind the undernourishment increase in this region (FAO et al., 2019) and has been consistently identified as a primary driver of famines (Maxwell and Hailey, 2020), especially in countries such as Ethiopia, Nigeria, and South Sudan.
Yet, alongside insufficient food intake, there is a contrasting issue where both children and adults suffer from obesity, diabetes, and other food-related diseases due to the consumption of low-quality and nutritionally poor food. In fact, obesity, along with overweight, contributes to approximately four million deaths annually worldwide. The connection between obesity and food insecurity is partly driven by high food costs and the widespread reliance on inexpensive sources of fats and sugars (GBD 2015 Obesity Collaborators et al., 2017). Despite the apparent stabilization in the global hunger trend observed throughout the 2021–2022 period, the concern remains regarding the significant number of women in rural areas who continue to struggle with accessing nutritious, safe, and sufficient food (FAO, 2023). Hunger remains a persistent challenge in least-developed regions, especially across all sub-regions of Africa (FAO, 2023) because substantial gaps between potential and actual crop yields continue to hinder food security (Figure 1). Extreme weather, particularly variation on temperature and rainfall, along with limited resource availability, further contribute to disparities in crop production across the different regions. For example, rainfed upland rice systems are more sensitive to soil moisture variability when compared to irrigated paddy rice systems (Stuecker et al., 2018). Thus, selecting cultivars that meet needs and expectations while providing stable incomes is quite challenging. The impact of heat and drought on crops varies by season and geography, with evidence showing that inter-annual climate variability can significantly affect harvested yields and overall production (Roberts et al., 2009; Lizumi and Ramankutty, 2016). Only climate-smart plant breeding can provide new cultivars that are tolerant to heat and drought.
Figure 1. Major field crop area vs. productivity in Africa, Asia, South America and world. The figure illustrates that in Africa, yam, cassava, plantain, millets and sorghum are crucial crops for maintaining food security sustaining the need for more breeding investments in these crops. Pigeonpeas, chickpeas, common beans, potato, but also maize, rice and wheat are examples of crops in which productivity exceeds the cultivated area. (Source: FAODATA, 2022).
Recent findings from the Agricultural Model Intercomparison and Improvement Project (AgMIP), and Inter-Sectoral Impact Model Intercomparison Project (ISIMIP) showed that higher temperatures generally result in lower grain yields for maize, soybean, and rice. Conversely wheat yields have been found to increase due to higher CO2 concentrations together with expanded high latitude regions (Jägermeyr et al., 2021). In the Iberian Peninsula, spring maximum temperatures contribute to significant grain yield losses in barley and rainfed wheat, but also for reducing the marketable tuber production in potatoes. However, northern regions are projected to experience increased grain yields due to early winter warming which promotes earlier growth of seedlings (Bento et al., 2021). These changes suggest that adjustments in agricultural practices, management and selection of crop species and cultivars are necessary.
The Paris Declaration endorsed the base development efforts on first-hand experience rather than simply conveying aid. It was supported by five main pillars: ownership, alignment, harmonization, result management, and accountability. However, despite these well-intentioned principles, the desire for quick results may have contributed to a lack of clarity and coordination in the development process, leading to incomplete fulfilment of providing nutritious and healthy food for all in a sustainable manner though current food systems. The impediments are many and may lie beyond ‘agricultural’ restrictions. Imbalances related to internal governance, either from the economic or territorial sides, do not convey the fairness of expected returns. Under a set of partnerships between the European Union (EU), the Food and Agriculture Organization of the United Nations (FAO/UN), the French Agricultural Research Centre for International Development (CIRAD), and national stakeholders, key points, including food security, nutrition and health were gathered for inclusive and sustainable solutions to transform food systems while preserving ecosystems and landscapes (FAO, 2021).
Africa, with its 55 countries, is the continent with the highest number of countries, and the highest population growth rate. By 2100, five of the 10 top-most populous countries in the world will be in Africa, accounting for over half of the projected global population growth through 2050. Additionally, the problem of inadequate access to food in countries affected by conflict is worsened by natural disasters, economic challenges, and public health issues (UN-DESA, 2022). This means that in Africa there is a high demand for increased productivity (Figure 1), and to counteract rising food prices, annual production needs to grow substantially. In these endowers should be acknowledged multiple efforts made in water management and some plant varieties adapted to low input conditions (Nyika and Dinka, 2023; CIMMYT, 2021).
Criticism was raised when it was pointed out that major efforts to improve water and nutrient efficiency in plants have not produced major results in the tropics, ignoring the fact that the advantages of crop improvement research and social protection programs are relative and dependent on the situation in a particular region (McIntire and Dobermann, 2023; Ginkel and Cherfas, 2023) (Figure 2).
Figure 2. Root cause analysis of the challenges faced by the African continent in agricultural development. Additional issues stated in the backbone diagram include emphasis in quick results, confusion among the stakeholders particularly when not involving the local communities in decision making and setting priorities, as well conflicts leading to lack of trust among partners.
In climate-smart plant breeding, the tackling of these challenges involves separate genetic selection for rainfed and irrigated conditions and for resistance to pests and pathogens. Additional actions include research efforts aimed at rejuvenating growth in crops that have reached a plateau yield improvement.
Besides the use of hybrid cultivars, the increase in grain yield in crops is known to be positively correlated with increased levels of nitrogen (N) fertilizers (Maheswari et al., 2017). Yet, despite N-fertilizers being a key determinant of yield, it also represents a significant cost for the farmers and in addition may have a negative environmental impact. An example is nitrate leaching from the field leading to soil and underground water contaminations as well as to greenhouse gas emissions. In sub-Saharan Africa, transformation in productivity can be achieved quickly through increased use of fertilizers and irrigation management. Yet, it is essential to consider that applying fertilizers without irrigation management has limited benefits, and the cost of irrigation can be a limiting factor (McIntire and Dobermann, 2023). Actions in this direction include breeding for nitrogen use efficiency (NUE: grain dry matter yield per unit of N availability) as well as tentative reduction of excess N-fertilizer input by management while maintaining an acceptable yield and quality. NUE is particularly important for smallholder farmers since they are known to have few resources at their disposal at any given time with which they work under very stringent financial constraints. Hence, this means that farmers cannot afford the luxury of buying large quantity of fertilizers as is always the case where one system is used for both feeding people and generating income. The trait NUE is controlled by many genes and fortunately many of these have in the recent years been genotyped and amenable for employing integrative tools for genetic enhancement of crop plants.
Optimization within breeding programs (BPs) is being led by management and optimization of the BP itself, using standardized tools and services. Yet, this may not necessarily lead to the desired improvements, as plant breeding is currently limited by the reliance on the selection of rare naturally occurring mutations in gene-regulatory regions and for traits that are controlled by multiple genes (Dwivedi et al., 2023). Rare alleles are mostly under-looked in the majority of quantitative genetic analyses since these are found in very few individuals of a population (Garcia-Oliveira et al., 2014), e.g. within less than a 2% frequency in a given population of a crop such as rice (Purugganan and Jackson, 2021).
Having a diverse set of alleles related to nitrogen uptake, assimilation, and utilization can contribute to improved NUE in plants. These alleles may also carry specific traits that make plants more adaptive to nitrogen-limited conditions. For example, in bread wheat, certain cultivars are more prone to aluminium stress because a panoply of physiological traits making plants more resistant to the damages incurred by aluminium in the soil (Garcia-Oliveira et al., 2016). In the case of N, the rare allele might encode a transporter protein with a higher affinity for nitrogen uptake from the soil, allowing the plant to acquire and utilize nitrogen more efficiently. If such rare alleles are identified and successfully incorporated in the target crop through breeding, it could result in a cultivar with improved NUE. Recently, Yoon et al. (2022) discovered that the nonsense-mutated GS3 gene, represented by the gs3 allele in the rice cultivar ‘Akita 63’, enhances yield production and grain size in rice, thereby improving harvest index and NUE in rice. With the newest mutational breeding tools the discovery can be transferred to other crop species swiftly.
When it comes to cultivar substitution and recommendation, it is important to consider inter-variability periodicity. Adjustment to identify main parameters including genotype nature (G), environment (E), and their interaction (G×E) would improve the predictions. The main aim is to make correct forecast and decisions that would result in maximum outcomes. Besides, the ploidy level, genotypic value, and allele frequency must also be taken into consideration. When considering G×E interaction parameters related to biotic and abiotic stress tolerance, a mean performance across different trials conducted under various field management (M), effects of the trial (E), repetition (e), genotype (G), interaction of genotype k and trial i (GkEi), residual error, number of trials and number of repetitions per trial should be taken into consideration in the model applied. Plant breeders focus on specific traits, also known as TPTs (Target Performance Traits), when developing new cultivars with improved tolerance, for example to abiotic stresses. Defining these traits, which form the basis for the ideotype concept (Donald, 1968), is crucial for effectively selecting and breeding plants that can withstand challenging environmental conditions. With reference to African climate and conditions, the G×E effects may be hugely underestimated for operation efficacy, along with a lack of resources. More precisely, four major agro-climatic zones, can be considered, and each characterized by distinct climate, soil, and vegetation types, namely the a) Arid and Semi-Arid Zone with low rainfall and high temperature (e.g. Sudano-Sahelian zone), the b) Sub-Humid Zone where the rainfall frequency is more reliable (e.g. coastal areas), the c) Humid zone characterized by high rainfall and supporting lush vegetation and diverse crops (e.g. the Central and southern parts of West Africa), and finally the d) Tropical high-altitude zone, typically comprised of highland areas characterized by cooler temperatures and distinct growing seasons (e.g. East African highlands of Ethiopia and Kenya as well the Rift valley of Tanzania). Studying these interactions may be very demanding, but principal component analysis (PCA) may help in this multifactorial-dependent process. Taken this continent as an example breeder’s face challenges specific to each climate zone where water scarcity, pests and disease pressure, and soil problems vary within these zones. Developing climate-smart cultivars, such as drought-resistant varieties for arid and semi-arid zones, involves more than just adaptation to a changing environment. It must first prioritize customization to the consumer’s needs and preferences. These market choices drive producers to use and harvest cultivars fitted to the unfavourable environment due to exposure to erratic edaphoclimatic conditions in terms of frequency and intensity (Table 1). For cultivar selection a significant genetic variation is needed, and where germplasm diversity is met by the high phenotypic variability provided by wild relatives, old cultivars, and landraces into elite lines with a known and stable yield production background (Garcia-Oliveira et al., 2009). Because climatic conditions evolve, cultivar replacement is thought to be a more frequent need when the primary focus is on supply-driven concerns. More focus should be put on adding locations (multi-site trials) as opposed to years (multi-year trials) (van Etten et al., 2019), through farmer-participatory on-farm trials. The advantages to this approach include that location variables tend to be more predictable than year variables, and one could target locations with different variable profiles to get well-adapted cultivars. Additionally, it attracts a greater number of participants and affords grower cooperators a meaningful presence in the discussions or decision-making processes. Yet, decisions from the breeding side must take into consideration their resource allocation of what is feasible in terms of number of years, number of sites, and how to choose sites to maximize adaption/climate-smartness.
Table 1. Predicted impact on global agricultural potential based on the projected trends in climatic change scenarios and its management through mitigation/adaptation practices.
Despite considerable advancement during the last decades, in terms of genomic tools and services, and marker utilization per se, still there is a low level of integration of molecular markers, in plant breeding programs. Several reasons could explain this, particularly in Africa, namely,
i. Establishment of genotyping facilities, accessible to all breeding programs is costly
ii. Lack of physical infrastructure such as uninterrupted power supply, centre for data storage, among others.
iii. Issues related to availability of qualified, cost-effective human resources
iv. High cost of genotyping due to underutilization of genotyping facilities
v. Inability to deliver high-quality genotyping data within a short period to meet breeding decision timelines
vi. Lack of comprehensive understanding of the potential of molecular markers in breeding programs
vii. Low or no funding for crop improvement from governments
To foster the integration of genomic data tools in breeding programs there must be a change in mindsets. This will require us to provide genotypic information consistently at a high-standard pro prompt and comprehensive interpretation, to assist in the implementation of advanced techniques such as marker-aided selection and genomic prediction of breeding values for further use in selection. It is very important to implement rigorous quality assurance processes that use Quality Control (QC) molecular markers for data integrity be maintained. Identifying more efficient methods of genotyping which will help in reducing the costs related with data generation and analysis. This will ensure that genotypic data is available within the breeding program workflow in time for decision-making. Additionally, it is important not to forget about embracing technological advancements in such areas as the utilization of drone-based imaging sensors and genetic algorithms based on artificial intelligence, for high-performance phenotyping since these breakthroughs can remarkably improve efficiency and precision of BPs. Concerning the implementation of genomic technologies, several questions should be posed before starting using genomic technology, including how the efficient and effective application of genomic technology can be leveraged to support BPs. Hence, there are three questions (3Qs) to address in planning a breeding program (Figure 3).
Figure 3. Example of workflow assessment for genotyping implementation in a breeding program (BP) as a part of a routine process in the breeding operations. Starting by defining the ideotype including trait target values, followed by accessing if genotyping is feasible, followed to understand where to perform the activity and the steps involved at the general level when outsourcing of genotyping is involved. Logistics and phytosanitary regulations are two major bottlenecks, with each country having different regulations for the same process.
Advanced technologies such as Kompetitive Allele-Specific PCR (KASP) markers, high-throughput genotyping, double haploid (DH) technology, mutational signature markers, and clustered regularly interspaced palindromic repeat (CRISPR)/CRISPR-associated endonuclease 9 (Cas9) technology must serve the purpose and be applicable in a breeding program. However, certain conditions must be met for this to occur. These prerequisites involve the availability of reliable markers linked to specific traits, the access to mutational signature markers, as well the presence of advanced laboratory infrastructure and equipment conducive to the efficient utilization of CRISPR technology.
With the advances in technology, particularly deep sequencing, the lowering of cost allowed the validation of other molecular panels to the crop community (Table 2). Several publicly available molecular marker platforms for plants include Gramene, Plant Markers Database (PMDB), and LegumeSSRdb. Gramene offers the access to SNP and SSR markers (https://www.gramene.org/; accessed November 3, 2024), whereas PMDB provides pathway-based markers for 82 plant genomes, including maize, rice, and sorghum (http://ppmdb.easyomics.org/; Mokhtar et al., 2021). In its turn, LegumeSSRdb is a comprehensive resource for SSRs in thirteen legume species (http://bioinfo.usu.edu/legumeSSRdb/; Duhan and Kaundal, 2021). Another straightforward example is the One CGIAR-CIMMYT marker platform. Initiated by the CGIAR Breeding Platform (https://excellenceinbreeding.org/toolbox/tools/kasp-low-density-genotyping-platform). This platform toolbox gathers several sets of useful markers developed for different traits and quality control (QC) in numerous crops to assess and ensure the quality and purity of breeding materials through sharing arrangements with dozens of scientists. Further, to validate the trait-specific KASP-based markers, the platform also includes the names of validated plant mid-density panels using Diversity Array Technology tag (DArTtag). Besides the above mentioned molecular marker types, there was a subsequent surge in the availability of similar platforms including the Bean Improvement Cooperative (http://www.bic.uprm.edu/?page_id=91) for common beans, the OZ Sorghum (https://aussorgm.org.au/sorghum-projects/) for description of quantitative trait loci (QTL) and providing resources for further marker sequence design, as well Primer3 (https://junli.netlify.app/apps/design-primers-with-primer3/) for free KASP markers design. A more recent, but equally interesting platform, is the Flex-Seq panels from Biosearch™ Technologies (https://www.biosearchtech.com/flex-seq-industry-standard-pre-designed-panels-8262) which allows different marker densities, and after made up of a standard panel for a crop any number of markers (sub-panels) can be used according to the needs of the breeding programs. Examples of these panels include maize, potato and soybean.
The basic steps of crop improvement include parental selection and crossing where there is a need for continuous genetic variation assessment and progeny selection. Without this yearly assessment, breeding programs cannot assure the suitability of their materials for trait improvement. Steps to integrate molecular markers include marker discovery, validation, and development (Figure 4) and many are the successful examples on how to apply them in BPs through diverse partnerships (Garcia-Oliveira et al., 2021, 2022, 2024).
Figure 4. Process to incorporate molecular markers into BP workflow. The discovery, validation and deployment of molecular markers is applicable across crop plant species.
In marker discovery, the genomic regions affecting the trait of interest for improvement are discovered through QTL mapping, including QTL identification and genome-associated mapping (GWAS), or by using reverse genetics. Once the relevant loci are revealed, they need to be validated in a wider range of targeted populations and optimized for technical efficiency. The marker validation process may involve the fine mapping of relevant loci. Finally, the validated markers are deployed in a cost-effective manner and integrated directly into the targeted breeding programs to enhance efficiency of genetic gain for specific trait.
The University of Minnesota’s (UoM, St. Paul, Minnesota, USA) Wheat Improvement Program uses markers in three main ways: 1) Parental genotyping to facilitate crossing decisions, 2) Enrichment of 3-way crosses for favourable marker alleles prior to generation advancement, and 3) Marker-assisted line purification during advanced breeding stages. Crossing parents are screened with KASP markers for approximately 50 genes influencing agronomic, end-use quality and disease-resistance related traits. Since parental genotypes are known, 3-way cross progeny can be screened and enriched for favourable alleles of segregating markers. In marker-assisted line purification, following generation advancement, lines are planted in plots of four head-rows, observed phenotypically, and screened with around a dozen KASP markers to select the most uniform and typical row to carry forward as the line. Markers are also used to screen bulks of large-scale seed increases, and if segregating markers are found, individual rows within the plot are screened to facilitate the grouping of impurities. In the case of marker-assisted line purification, special care is taken to precisely follow best experimental practices due to the importance of accurately identifying segregating rows. DNA is carefully normalized, and control genotypes for each allele, as well as negative controls, are included in each KASP experiment to facilitate accurate clustering (personal communication Dr. Emily Conley, UoM, Minnesota, USA).
In the breeding program of the International Potato Center (CIP, Lima, Perú), for the vegetatively propagated potato, DArTag markers (Endelman et al., 2024) are being used for genomic selection (GS), diversity analysis, and selection based on trait markers. KASP markers are used for traits with no available SNP markers in the panel array, as well to monitor the genetic identity of plants used in crossing plants (Kante et al., 2021). In Yam at the International Institute of Tropical Agriculture (IITA, Ibadan, Nigeria) KASP SNP markers are being used to distinguish different yam species (Diascorea alata, D. rotundata, D. praehensilis, D. cayenensis and D. abyssinica, and also tagged markers with the economically important traits such as plant vigour, sex distinguishing, flowering intensity, yam mosaic virus, among others (Agre et al., 2023). For marker discovery, other platforms with higher marker density can be of use.
In breeding, trait-associated haplotype identification is valuable in the context of genetic selection through the manipulation of population allele diversity. The inclusion of haplotype information in genomic selection models can enhance the accuracy of predicting an individual’s breeding value (Sallam et al., 2020). Haplotypes not only capture more extensive genetic variation but also provide a finer resolution of genomic variation compared to individual SNPs alone. KASP-haplotype genotyping allows the detection of multiple SNPs within a haplotype, thereby improving linkage disequilibrium between causal loci and marker haplotypes and leading to more precise estimates of marker effects for genomic selection.
However, to date, from QTL mapping, thousands of loci have been described for numerous traits, many of which control only a small proportion of the trait variance. Not only is the true proportion of phenotypic variance these KASP loci explain unknown but also these haplotypes of interest change from breeding cycle to breeding cycle due to recombination and introduction of new germplasm. The use of KASP-haplotypes can capture rare genetic variants that might not be detected or accurately imputed using individual SNP markers. Additionally, in the majority simply inherited traits, haplotypes are easy to find and quantify their contribution to the trait phenotype. Yet, the genetic complexity of the target trait, the genetic diversity of the population, the trait architecture, and the availability of genomic data, all factor into the ease of identifying and quantifying haplotypes of interest. A similar ‘in-house made’ method to KASP methodology is the “Amplifluor-like” method, and competitive against KASP methodology. A primary advantage of the Amplifluor-like method lies in its unparalleled flexibility, allowing for the modification or rearrangement of all its elements. This includes the ability to alter the structure and design of allele-specific primers and universal probes, as well as the amplification program, including parameters such as temperature and duration for each step (Khassanova et al., 2023). With the availability of knock-off mastermixes, that can be cheaper, and the primers ordered from whoever provides the cheapest oligo synthesis service in a particular region, one that has already an equipped and expert laboratory, such one at the UoM can design allele-specific assays (Allele 1, Allele 2 and reverse primer), add the hex tail on one primer and a fam tail on the other, and use PACE mastermix, which is commercially available but for about half the price of KASP (https://3crbio.com/). However, in breeding programs where laboratory facilities and consumables purchase are not competitive, using of shared services available is ideal.
Another motive to consider is the use of epialleles, as these can play a great role in the regulation and contribution to observed variation of quantitative traits (Gahlaut et al., 2020). Epigenetic modifications can influence gene expression levels, developmental processes, and responses to environmental cues, thereby impacting complex traits. Understanding the epigenetic regulation not only provides valuable insights into potential avenues for breeding, but also, hypothetically, and in the context of genomic selection, genomic selection models can potentially improve the accuracy of predicting breeding values, and therefore, enhancing selection efficiency, particularly at the level of environmental responsiveness and phenotypic plasticity (Figure 5). This approach may require a different type of marker platform entirely to get genome-wide coverage of SNPs in methylated as well as unmethylated regions. Current technologies could prove challenging, as most current high-throughput platforms tend to target unmethylated (presumably gene-rich) regions. Nevertheless, the incorporation of epialleles into genomic selection is still evolving, with the need to research effective methods for integrating these elements while understanding the complex interactions between epigenetics, quantitative traits, and breeding outcomes. Therefore, it is an exciting avenue for further improvement in breeding strategies.
Figure 5. Relevance of epialleles to genomic selection. 1. Including epigenetic information, such as DNA methylation patterns associated with quantitative traits, can provide additional information beyond the DNA sequence itself, 2. Epigenetic modifications can contribute to phenotypic stability across generations by providing an additional layer of heritable variation that is less affected by short-term environmental fluctuations. When considering epialleles associated with stable phenotypes, genomic selection can facilitate the breeding of more resilient and adaptable individuals. 3. Epigenetic modifications are sensitive to environmental cues and can mediate phenotypic plasticity. Incorporating information about epigenetic marks that respond to specific environmental conditions, genomic selection models can account for genotype-environment interactions and improve prediction accuracy in varying environments. Despite continuous technological advance, careful experimental design and computational analyses are still required to extract meaningful information for selection purposes, as in the case of DNA methylation sequencing. The signals (+) and (-) refers to advantages and drawbacks, respectively.
An effective KASP SNP result is achieved when the marker demonstrates clear differentiation between homozygous and heterozygous alleles in the genotyping output. However, not all the results are straightforward, often leading to complex outcomes where alleles cannot be assigned to specific allele groups. Also, in polyploid crops, information on allele dosage might not always be available. In such instances, a process of SNP recalling becomes necessary, which in some cases is a challenging task that demands technical expertise and is often carried out manually, consuming significant amounts of time and the need for knowledge expertise. Beyond assessing homozygosity/heterozygosity, KASP genotyping data can be used to predict target observable traits (phenotypes) associated with specific genetic variations and based on known associations between certain alleles or SNP markers and phenotypic traits of interest, established through genetic studies and BPs.
Another matter of importance is marker data imputation, either in diploid or polyploid crops, to fill in the missing or incomplete genetic information in a dataset. It involves predicting or estimating the allelic variants (genotypes) of individuals at specific physical positions in their DNA sequence where data is missing. Genotypic data can be incomplete for various reasons, such as genotyping errors, low sequencing coverage, or missing samples. This practice is particularly valuable to enhance statistical power and to improve the ability to identify genetic associations with traits. Common imputation tools and software packages used in genomics research include IMPUTE (Marchini et al., 2007), BEAGLE (Browning and Browning, 2009), LD-kNNi (Money et al., 2015) and Minimac (Howie et al., 2012). It is not uncommon to use the imputation replacing missing data with the average dosage for a specific site and population, noting that the accuracy of imputed genotypes depends on the quality and size of the reference panel and the specific imputation algorithm used.
It is important to acknowledge the challenges associated with obtaining accurate dosage information when employing KASP markers for QC purposes. These challenges encompass the optimization of assay conditions, including primer concentrations, annealing temperatures, and PCR conditions. Additionally, primer design and specificity must be carefully considered to prevent cross-reactivity with other genomic regions, which could potentially yield false results. The quality and quantity of DNA are crucial factors, as contaminants, degradation, or insufficient DNA concentrations may compromise the reliability of dosage information. Sample variability poses another challenge, emphasizing the necessity to confirm that the chosen marker is suitable for the specific genetic background under investigation. The absence of reliable reference materials or standards for use as control samples can further complicate dosage determination. The presence of PCR inhibitors in the DNA sample, if not addressed, can impact the efficiency of the reaction. Moreover, the calibration of equipment is paramount, as inaccuracies in temperature control and fluorescence detection can lead to unreliable results. The analysis of KASP data requires robust software capable of accurately calling genotypes and dosage information. Finally, incorporating replicates and positive/negative controls in experimental setups is essential for assessing assay reproducibility and detecting potential issues.
On the quality side, and to enhance reliability, the use of control genotypes is recommended. Yet, achieving marker stability is vital, given that the same marker may yield different calls across various plates, even when considering the same sample repetition. This could be due to poor DNA quality, or in the case of polyploids, failure to normalize DNA samples to a consistent concentration. It could also be due to a low level of true residual variation at some loci following generation advancement and line purification. Consistent marker performance over time is also a crucial characteristic of high-quality KASP markers. This necessitates the identification and selection of markers that amplify well and cluster cleanly, especially in the context of defining a limited set of QC markers, as demonstrated in the case of soybeans (Chander et al., 2021).
DNA mutations are the basis of all heritable variation in organisms, providing the raw material for natural selection and evolution. However, such naturally stable mutations are relatively rare in eukaryotes compared with prokaryotes, partly due to the presence of complex DNA repair mechanisms in the former. In plants, mutation rates vary across different regions of the genome, with lower frequencies observed in areas under strong functional constraints (Quiroz et al., 2023). Specifically, the mutation rates are reduced by 50% within gene bodies and by two-thirds within essential genes, as compared to non-essential genes (Monroe et al., 2022). This reduction is likely due to the elevated activity of targeted DNA repair mechanisms in these regions. A deeper understanding of mechanisms that promote specific DNA repair could help achieve critical goals in crop improvement programs through targeted mutagenesis. The application of mutation techniques by plant breeders has a long history, dating back to 1920’s when X-ray induced mutagenesis was first realized on fruit fly (Drosophila melanogaster) and cereal species (maize and barley) by Muller (1928) and Stadler (1928a, b), respectively. Muller’s groundbreaking research revealed that genes typically exhibit a mutation rate ranging from 10-5 to 10-6 per locus per generation. The advent of radiation-induced mutagenesis allowed scientists to achieve higher mutation rates than those occurring spontaneously leading its application in inducing novel genetic variability through radiation in plants. Over the past century, various physical agents like X- and gamma-rays, UV light radiations, as well as particle radiations including alpha- and beta-particles, neutrons, and protons, along with chemical substances such as alkylating agents (e.g., EMS - ethyl methanesulphonate, NEU - nitrosoethyl urea, MNU - N-methyl N-nitrosourea), colchicine, EI (ethylene imine), and sodium azide (NaZ), among others, have been extensively employed. These tools have not only been instrumental for studying gene function and DNA repair mechanisms but have also been pivotal in generating novel cultivars in various crops (Penna and Jain, 2023). Globally over 3400 cultivars derived from approximately 228 plant species have been developed in 75 countries to date, utilizing a range of mutagens (http://mvd.iaea.org accessed in January 2024). Among these, radiation-induced mutagenesis, particularly with gamma rays, remains one of the most prevalent methods.
More recently, the application of accelerated particles including heavy ions (C-, H-, and Ar- ions) or protons, has gained importance for developing novel cultivars with desired traits. These particles induce mutagenesis with high biological effectiveness at low radiation doses minimizing impacts on other phenotypes (Tanaka et al., 2010; Abe et al., 2015). The precision and specificity of accelerated particles along with their energy, reduce off-target mutagenesis making them a powerful tool in plant breeding. Similarly, space breeding conducted in environments beyond Earth’s atmosphere offers another promising avenue for creating novel mutants. This approach takes advantage of two unique factors: exposure to cosmic rays and microgravity (Liang et al., 2009; Zhao et al., 2010; Liu et al., 2021a).
In any of mutagenesis methods, it is crucial to consider the direct inheritance of the DNA damage caused by the mutagen as well as the persistence of the genetically induced alterations. This phenomenon, known as mutagen-induced genomic instability can occur in the progenies that have undergone irradiation (Ma et al., 2021). Compared to accelerated particles, X-rays induce a greater extent of DNA damage and offer fewer opportunities for repair by DNA polymerases. When DNA polymerases are less efficient at correcting errors, the mutation rate increases, particularly in the case of double-strand break (DSB) mutations. This can lead to chromosomal re-arrangements and larger deletions (Pastwa et al., 2003; Tsuruoka et al., 2008; Hirano et al., 2015). High-energy particles have been used to induce qualitative traits (sterility in fruit trees and flower appearance such as colour and shape in flowers), and quantitative agronomic traits in cereals and vegetable crops (Abe et al., 2012; Kato et al., 2016; Yamatani et al., 2018; Nishio et al., 2024). The new traits obtained through these methods are useful for gene function mining, gene mapping and the development of elite alleles. Besides seed-propagated crops, in vitro mutation breeding in vegetatively propagated crops has also resulted in lines exhibiting several desirable traits (Suprasanna et al., 2015; Maurya et al., 2022; Zhang et al., 2024). However, there is still much to be improve in this field of mutagenesis, including optimising physical radiation parameters such as dose and high linear energy transfer (LET), understanding the impact of different irradiated particles to decrease mutation randomness as well the integrating genomic and phenomics tools to enhance mutation breeding efficiency (Nielen et al., 2018).
The establishment of next-generation sequencing (NGS) platforms in the recent advances of technology has enhanced our capacity to sequence and map crop genomes, identify causal mutations and study gene regulation. However, it should be noted that the majority of these genomes are complex and characterized by a relatively high proportion of repetitive sequences and transposons. For larger genomes, the short sequence reads (<700bp) generated by second-generation sequencing platforms like Illumina, Roche, Solid, and others may not be ideal. The second-generation sequencing can be extremely useful but requires methods to reduce complexity and target informative regions. For example, Illumina NovaSeq platform can be used for routine genomic prediction in a breeding workflow, as it is low cost, despite requiring specialized knowledge to prepare libraries, access to core facilities with the sequencing instruments, and bioinformatics expertise to process the data. In cases where this is not possible long-read sequencing such as Oxford Nanopore and PacBio, also understood as the third-generation sequencing generation platforms, need to be used. These long-read sequencing platforms offer the capability to sequence longer fragments, enabling more comprehensive coverage of large genomes, especially in highly variable genomic regions which may facilitate the identification of epigenetic marks such as DNA methylation and gene expression analysis. However, these third-generation platforms are more expensive and generally less available than those of the second-generation. These are useful for discovery-type research, whereas second-generation would be more useful for routine breeding applications. For the detection of rare point mutations in plant genomes the use of NGS still presents challenges. As an alternative, the Simple, Multiplexed, PCR-based bar-coding of DNA (SiMsen-seq) system is an opportunity for selective mutation detection using sequencing, as it detects variants at or below 0.1% frequency with low DNA input (Ståhlberg et al., 2017).
Generally, mutation breeding operates outside of the typical regulatory controls. To make breeding more efficient, molecular markers can be used in selecting materials for specific traits of interest, which are then further identified in the field. Afterwards, characterization of these mutants can be carried out using advanced NGS pipelines. More importantly, it should be noted that genome editing technologies can play a significant role in trait pyramiding by enabling the introduction of multiple site mutations for different desired plant characteristics (Figure 6).
Figure 6. Use of high throughput genotyping and phenotyping for the development of desirable cultivars in plant mutation breeding. In forward genetics, after applying mutagenesis (such as radiation) and selecting M1 seeds, performance of precise phenotyping is done at the M2 generation. By the time the M3 generation is formed, a more refined phenotypic evaluations can be done, as the M3 plants are typically more stable and can provide clearer insights into the genetic basis of the traits of interest. This approach helps in identifying and confirming the relationship between specific mutations and the observed phenotypes. In reverse genetics, the focus is more on specific genes and their functions rather than broadly phenotyping entire populations like in forward genetics, therefore, CRISPR and gene editing can be used initially to create mutations in specific genes or may induce mutations through radiation. This is followed by phenotypic evaluation for change, starting at M2, and where the effects of mutations can be observed. By M3 generation, stable mutants can be characterised to confirm the function of targeted genes based on the phenotypes observed.
MicroRNAs (miRNAs) are a type of short (19-24 nucleotides in length) non-coding RNA molecules which are present within the inter and intragenic regions of genomic DNA. Since the discovery of first miRNA lin-4 in the nematode (Caenorhabditis elegans) about 30 years ago, much evidence has shown that miRNAs are ubiquitously present in eukaryotic genomes that regulate gene expression, mainly through sequence-specific cleavage and/or translation inhibition of the target mRNAs during or after transcription (Lee et al., 1993; Wightman et al., 1993; Voinnet, 2009; Sanei and Chen, 2015). As a result, miRNAs are emerging as master modulators of gene expression that could be utilized as next generation genomic tools for improvement of traits of interest in agronomically/economically important plants (Djami-Tchatchou et al., 2017; Tang and Chu, 2017; Raza et al., 2023). Most of functionally validated miRNA families are not only evolutionarily conserved among plant species, but also tend to have conserved targets which make more convenient of miRNAs for application in trait improvement in crop plants (Tang and Chu, 2017). Various types of miRNAs, including conserved miRNAs that facilitate comparative studies across species, tissue-specific miRNAs that inform on developmental processes or stress responses, and stress-responsive miRNAs that are upregulated or downregulated in response to stresses, have the ability to enhance their utility in breeding stress-resistant varieties. To date, the utilization of natural genetic variation in molecular marker-aided breeding mainly relied on either random DNA markers or so-called functional markers that derived from protein coding genes.
The continuous progress in DNA marker technology replaced previous PCR-based genotyping methods. High-throughput sequence-based single-nucleotide polymorphism (SNP) markers emerged as an attractive option because of low genotyping error rate, and amenability to automation, thereby resulting in a drastic reduction in cost per data point (Chander et al., 2021). SNPs in miRNA (MIR) genes and their target genes are widespread and can influence miRNA biogenesis and function by altering transcriptional patterns or miRNA-target interactions (Todesco et al., 2012; Liu et al., 2014). In rice, nucleotide polymorphism (GG/AA) in the terminal loop region of the osa-miR2923a precursor could be differentiated from japonica/indica cultivars which are also found to be significantly associated with grain length characteristic (Wang et al., 2013). Similarly, the naturally occurring nucleotide variation in OsmiR156h, exhibited reduced plant height, enhanced lodging resistance, increased tiller numbers per plant, and resulting in an increased grain yield (Zhao et al., 2015). Few recent examples, and exemplifying their outcome importance, include the miRNAs OsmiR168 and OSmiR395, targeting the Ago1 and ATP sulfurylase (OsAPS1) genes which enhanced the resistance and broad-spectrum resistance to rice blast fungus (Magnaporthe oryzae L.) and both pathovars Xanthomonas oryzae pv.oryzae (Xoo) and X. oryzae pv. oryzicola (Xoc), causing bacterial blight and leaf streak diseases in rice, respectively (Wang et al., 2021; Yang et al., 2022). Similarly, in alfalfa miR156 target combined stress modulators. The miR156 not only was found to module flooding tolerance by regulating physiological processes and SnRK1 gene expression (Feyissa et al., 2021) but also to influence heat, cold and drought tolerances by downregulating the SPL gene (Arshad et al., 2017a, b; Arshad and Hannoufa, 2022; Yadav et al., 2024). Therefore, the integration of NGS information together with various bioinformatics tools and transcriptomic studies may aid in the identification of the functional role of natural or induced variations at miRNAs and their target loci that could be utilized to develop molecular markers. For instance, in the case where the target gene has a desirable effect on the trait of interest but MIR serving as a negative regulator, selection of natural or induced miRNA-resistant target gene mutant strategy can be used. Contrarily, if a candidate MIR gene serve as a positive regulator and its native target gene has an undesirable effect on the trait of interest, the natural or induced MIR variant, such as the suggested isoMIR, can be utilized (Morin et al., 2008). It should be noted that compared to canonical miRNAs (https://www.mirbase.org/), their isoMIRs have often shown differential expressions, higher binding capacity, and efficiency in target cleavage in Arabidopsis thaliana (Jeong et al., 2013; Ahmed et al., 2014).
Using high-throughput sequencing (HTS), hundreds of miRNAs have been identified and annotated in different plants species (https://www.mirbase.org/), but their biological function still unknown. Like the study of protein-coding genes, following the identification of miRNAs-targets, their functional characterization is necessary by the creation of gain-of-function or loss-of-function analyses. To perform gain-of-function studies, miRNA genes or miRNA-resistant target genes could be overexpressed using various techniques such as traditional constitutive promoter, full-length cDNA of miRNA genes, or the two-hit artificial miRNA vector system, which is highly adaptable to plant transformation techniques (Teotia et al., 2020). As a result, most of miRNA studies have been conducted on Arabidopsis due to the ease of the floral-dip transformation method (Zhang and Unver, 2018).
While RNA interference (RNAi) and site-directed genome engineering techniques such as zinc finger nucleases (ZFNs) and transcription activator-like effector nucleases (TALENs) approaches were successfully employed for loss-of-function studies (Curtin et al., 2011; Li et al., 2012; Ainley et al., 2013; Liang et al., 2014). Compared to traditional genetic mutants of protein-coding genes, miRNA mutants are less effective due to the small size of miRNAs and have several members with overlapping functions. Thus, the simultaneous knockdown of all miRNAs in a family can be the best way to confirm the biological function of a specific miRNA family. To fill this lacune, different molecular techniques that knockdown of miRNAs by target decoys/mimics such as target mimics (TMs), short tandem target mimics (STTMs), molecular sponges (SPs) and artificial miRNAs (amiRNAs), have been demonstrated to be useful to underpin the role of these miRNAs. For instance, Franco-Zorrilla et al. (2007) identified an endogenous long non-protein coding gene INDUCED BY PHOSPHATE STARVATION 1 (IPS1) which altered the protein levels of PHOSPHATE2 (PHO2) by modulating the effects of miR399 in Arabidopsis.
It was observed that both IPS1 and PHO2 had highly conserved sequence motifs that contain complementary binding site for the phosphate (Pi) starvation–induced miRNA miR399. However, IPS1 showed three additional nucleotides bases, which provoke central mismatches in the miR399 binding site by forming of central “bulge” opposite the expected miRNA cleavage site, thus avoiding miR399-guided cleavage but instead sequesters of miR399. This endogenous regulatory mechanism of miRNA activity was termed as endogenous “target mimicry” or eTM which is commonly referred to as miRNA decoys/mimics technology. Based on the strategy of mimicking target transcripts, new methods to inhibit miRNAs, particularly Short Tandem Target MIMICs (STTMs)-based knockouts, have been developed in miRNA functional studies (Tang et al., 2012; Teotia et al., 2016). STTMs contain two miRNA-binding sites, which not only efficiently silence some highly abundant miRNAs but also can be used to study the interactions between two miRNAs by inserting two different miRNAs in the same STTM construct (Zhang et al., 2017; Peng et al., 2019).
In the area of in silico miRNA target prediction in plants, numerous bioinformatics tools can be used as web servers and freely accessed including TAPIR (https://ptarpmir.cu-bic.ca/), TarHunter (http://www.biosequencing.cn/TarHunter/), psRNATarget (https://www.zhaolab.org/psRNATarget/), psRobot (http://omicslab.genetics.ac.cn/psRobot/) and WPMIAS (https://cbi.njau.edu.cn/WPMIAS/), these three last lacking miRNA-initiated phasis information (Liu et al., 2021b). Furthermore, PHASIS (https://github.com/atulkakrana/PHASIS) and PhaseTank (https://phasetank.sourceforge.net/) were developed to support phasiRNAs prediction in plants. The database sRNAanno is presented as a comprehensive collection of phasiRNA loci in plants (http://plantsrnas.org/), but still, does not indicate which phasiRNA sites are triggered by miRNAs. More recently, TarDB (http://www.biosequencing.cn/TarDB/) surged as a web resource for exploring relatively high-confidence miRNA targets and miRNA-triggered phasiRNAs in plants RNA information (Liu et al., 2021b). An example of such specific miRNA targeting use can be exemplified by the recent 216 drought-responsive identified miRNAs (DRMs) based on 28 drought stress-specific sRNA datasets using the RiceMetaSys: Drought-miR database (Kumar et al., 2024). At a genome-wide scale, the high throughput degradome/PARE (Parallel analysis of RNA ends) sequencing techniques have enabled the characterization of miRNA cleavage sites. Computational pipelines such as CleaveLand, PARESnip and sPARTA can help further analyse the data sets originated through degradome/PARE-seq (Addo-Quaye et al., 2009; Kakrana et al., 2014; Folkes et al., 2012). Databases for plant miRNA such as miRBase (https://mirbase.org/) and PmiREN (https://www.pmiren.com/) can be utilised not only to archive and annotate miRNAs but also as a miRNA encyclopaedia with function and resource tools.
Over the past three decades, the unconditional success of genetically modified (GM) crops allowed the way for harnessing novel plant breeding technologies in crop improvement. Despite the widespread adoption of GM crops, the presence of foreign genes in them since their development has been central concern of public acceptance and potential biosafety issues in many parts of the world (Kumar et al., 2020). These concerns have driven the development of new plant breeding techniques, such as gene editing, which enable precise modification of plant genomes without introducing foreign DNA (Wang and Doudna, 2023).
The foundation of gene editing in plant was established with the discovery of I-SceI (meganuclease) induced double stranded breaks in a site-specific manner that enhanced homologous recombination in plants (Puchta et al., 1993). Meganucleases, also referred to as homing endonucleases, are sequence-specific endonucleases which found in prokaryotes, archaea, and unicellular eukaryotes. These endonucleases are often small proteins that are encoded by mobile genetic elements, either introns or inteins, and cleave relatively long sequences of DNA (ranging from 12-40 bp) resulting in higher specificity and lower off target cutting (Stoddard, 2014). The primary challenge facing meganuclease technology is the significant engineering efforts required to create enzymes with novel DNA specificity, particularly due to the overlapping nature of DNA-cleavage and DNA-binding domains in these homing endonucleases. Despite this hurdle, engineered meganucleases have demonstrated some success in various gene editing functions in plant species (Daboussi et al., 2015); however, the production of modified homing endonucleases is both time-consuming and lacks the necessary flexibility.
To address the problem associated with meganucleases, another class of site-specific nucleases (SSN) such as zinc finger nucleases (ZFNs) were developed by fusing an artificial DNA-binding domain (DBD) of a versatile class of eukaryotic transcription factors – zinc finger proteins (ZFPs) to the non-specific DNA cleavage domain of type II restriction endonuclease FokI (Kim et al., 1996). This achievement spawned from the discovery that the DNA-binding domain and the cleavage domain of the FokI function independently of each other (Li et al., 1992). The FokI nuclease operates as a dimer, thus necessitating the design of two ZFN molecules to target a single site. Each ZFN molecule binds itself to opposite strands of DNA molecule, causing a double-strand break. One notable development is that they have modified a vast variety of genomes in plants (Urnov et al., 2010). Yet, the only thing standing in the way is its challenges such as off-target cutting, and cytotoxicity which led to the development of a new class of nucleases: transcription activator-like effector nucleases (TALENs). TALENs, akin to ZFNs, arise from the fusion of catalytic domain of the FokI endonuclease with a DNA-binding domain called transcription activator-like effectors (TALEs), derived from the protein produced by plant pathogenic Xanthomonas spp. Bacterium (Christian et al., 2010; Bogdavone and Voytas, 2011).
Until a few years ago, both ZFNs and TALEN were the most powerful programmable site-specific technologies for genome engineering. These techniques are modular in form and function, comprised of DNA-binding domain fused to FokI cleavage domain that recognize target sequences by protein motifs and which requires the assemblance of specific proteins to each target, therefore having low specificity in recognizing and cleave the DNA targets (Gaj et al., 2016). Recently, clustered regularly interspaced short palindromic repeats/CRISPR-associated nuclease 9 (CRISPR/Cas9) techniques have been proved to be useful to genome editing because CRIPSR/Cas system has the advantage of recognizing targeted genomic sites by base complementarity between the single-stranded guide RNA (ssgRNA) and the target DNA. So far, CRISPR/Cas9 has been applied to introduce specific genetic modifications aimed at improving desirable traits in cereals, vegetables (including root and tuber crops) and fruit trees (Osakabe and Osakabe, 2015; Rodriguez-Leal et al., 2017; Jaganathan et al., 2018; Tripathi et al., 2020; Biswas et al., 2021; Ma et al., 2023).
Nonetheless, it is important to note that CRISPR/Cas is not a universal tool, as it has limitations related to the short sequence length and the specificity of the microRNA (MIR) genes. These limitations affect the design of guide RNAs (gRNAs) targeting MIR genes and their applicability, respectively. Precise genome editing requires accurate gene sequences and knowledge of their functions, which is facilitated by the whole-genome sequencing data currently being generated. Transgenic plants overexpressing cleavage-resistant targets such as miR164-resistant SlNAM2 (Hendelman et al., 2013) in tomato, and miR139-resistant OsTCP21 (Zhang et al., 2016a) and miR166-resistant OsHB4 (Zhang et al., 2018a) in rice, have been created and used to investigate miRNAs functions. Among the various loss-of-function systems available, miRNA decoy techniques [Target Mimic (TM) and Short Tandem Target Mimic (STTM)] and the CRISPR/Cas9 genome-editing system are the most utilized. As an example, the resistance to powdery mildew in barley accessions and mutant types was shown to be controlled by the mlo gene (the wild allele is Mlo) (Jørgensen, 1992). The loss of function of mlo mutants confers a durable and broad-spectrum resistance to powdery mildew. Yet, the mlo-associated resistance brings along growth and yield penalties, where the expression of Mlo in mlo genotypes is sufficient to confer single-cell susceptibility. Pleiotropic effects may explain this phenomenon, and many breeding programs may need to address it. In polyploid crops, this technique may require additional backcrossing cycles to eliminate any unwanted mutations (Figure 6).
In hexaploid wheat, the TALEN-generated Tamlo-R32 mutant targets the three wheat MLO1 genes (TaMLO-A1, TaMLO-B1 and TaMLO-D1) and is characterised by a 300kb pair targeted deletion in the MLO-B1 locus that retains crop growth and yields while conferring resistance to powdery mildew. Through epigenetic changes, this mutant exhibits a significant upregulation of TaTMT3B (Tonoplast monosaccharide transporter 3) locus transcript expression. The use of the CRISPR/Cas9 tool demonstrated that this genetic arrangement counteracts the negative effects associated with mlo mutations while maintaining strong disease resistance in wheat (Li et al., 2022).
In strawberry, fvesep3 mutated using CRISPR/Cas9 has produced the desirable trait of parthenocarpic fruits, which is highly sought in strawberry breeding programs (Liu et al., 2020b; Pi et al., 2021). Similarly, in polyploid and parthenocarpic bananas, where asexual breeding methods are less efficient, the utilization of CRISPR/Cas9 systems can enhance mutation efficiency and introduce traits of economic importance such as reduced plant height (Shao et al., 2020), improved quality (Kaur et al., 2020; Awasthi et al., 2022), and host plant resistance to pathogens (Tripathi et al., 2021).
Recently, and in response to some constraints presented by Cas9, Zheng et al. (2024) demonstrated in rice that CRISPR Cas12a is a more efficient tool compared to its Cas9 counterpart for generating knockout mutants of a miRNA gene. With this improvement, it seems possible to achieve editing efficiencies of up to 100%. Due to its ability to create larger deletions, which facilitates the generation of loss-of-function mutants of targeted genes, this new system appears better suited for developing genome-wide miRNA mutant libraries, at least in rice.
Improving crops by pyramiding of a few desired genes in a single elite line have been facilitated by marker-assisted selection (MAS). As shown for diverse crop species in the following sections, CRISPR take this a step further by the so-called multiplexing of a number of genes that have been mutated by CRISPR/cas in a single event. In fact, a mutant collection can be generated by use of CRISPR as demonstrated for maize in the BREEDIT pipeline by Lorenzo et al. (2023) where a first attempt of multiplexing 48 growth-related genes resulted in more than thousand mutant lines which can be screened for improvement in complex traits such as drought and heat tolerance.
In rice, noteworthy examples of precision editing include the knockout of three seed weight-related genes, namely GW2, GW5, and TGW6, which lead to an increase in grain weight (Xu et al., 2016). In this crop, the targeted disruption of the promoter region of the SWEET gene has been shown to enhance rice’s resistance to bacterial blight (Oliva et al., 2019). In quest to boost African rice’s agronomic potential, a method known as multiplex CRISPR/Cas9 was used to target HTD1, GS3, GW2, and GN1A loci, which bear domestication genes for the cultivation of rice. It has been established that mutations in these genes can lead to significant increase in seed output when tested in landraces of rice (Lacchini et al., 2020). In a different experiment, the GS3 locus was targeted alongside with the qSH1, An-1, and SD1 loci, that all together led to rapid domestication of wild allotetraploid rice (Yu et al., 2021). These examples highlight the effectiveness of multiplex CRISPR/Cas9 technique in rice that can lead to improve and advance crop domestication efforts.
In wheat, CRISPR/Cas-mediated genome editing had been targeted traits such as host plant resistance to powdery mildew (TaMLO & TaEDR1) (Shan et al., 2013; Wang et al., 2014; Zhang et al., 2017), seed yield (TaGW2 & TaGASR7) (Zhang et al., 2016, 2018b; Wang et al., 2018a), fusarium head blight resistance (TaHRC) (Su et al., 2019), herbicide resistance (TaALS & TaACC) (Zhang et al., 2019a, 2019), quality traits such as low gliadin (VIT2), starch and amylose (TaSBElla) contents (Jouanin et al., 2019; Liu et al., 2020; Li et al., 2020b), high haploid induction rate (TaCENH3α) (Lv et al., 2020), male sterility (TaMs1 and TaNP1) (Okada et al., 2019; Li et al., 2020c), increase of P uptake (TaPHO2-A1) (Ouyang et al., 2016) and storability (TaLOX2) (Shan et al., 2014; Zhang et al., 2016). These modifications were performed by using edits such as knockout, multiplexing, base editing, primer editing and HDR replicon (Li et al., 2021). In addition, the knockout of numerous conserved domains in approximately 100 α-gliadin family members facilitated the development of a low-gluten cultivar suitable for individuals with celiac disease (Sánchez-León et al., 2018). The existence of three sub-genomes (A, B, and D) in wheat makes the application of multiplex genome editing beneficial, although achieving precise editing remains challenging as current efforts predominantly focus on random mutations and knockouts through non-homologous end joining (NHEJ) repairing. In crops like wheat NHEJ is commonly exploited to induce these random changes in the DNA sequence, allowing researchers to disrupt or deactivate targeted genes for various purposes.
In tomato (Solanum lycopersicum L.), the multiplexing of the coding regions of SELF-PRUNING and SELF-PRUNING 5G, together with cis-regulatory regions of CLV3 and WUS or open reading frames (ORFs) of GGP1, allowed the generation of tomato fruits with compact plant architecture, synchronized fruit ripening, day length insensitivity, enlarged fruit size and increased vitamin C levels (Rodriguez-Leal et al., 2017; Li et al., 2018). Additionally, leveraging the multiplex capacity of CRISPR/Cas9, researchers successfully targeted five different genes associated with lycopene content accumulation in tomatoes (Li et al., 2018). More recently, in potatoes (Solanum tuberosum L.), the functional knockouts of several S-genes, namely StDND1, StCHL1, and DMG400000582 (StDDMR6-1), lead to the generation of tetraploid potatoes with increased resistance against late blight (Kieu et al., 2021). The mutation of DMR6 homologues indicates that StDMR6-1 and StDMR6-2 have two different biological functions, with the first involved in pathogen resistance whereas the second involved in plant growth. It was observed that Stdnd1 and Stchl1 mutants reduced infection lesion sites, whereas StDMR6-1 not only reduced the infection lesion sites but also the percentage of infected leaves. These results are very promising for the potato breeding since pathogen races in potatoes rapidly overcome the existing plant resistance.
CRISPR/Cas9 offers a potential solution to avoid the need for farmers to yearly seed purchase due to the phenomenon of heterosis. Heterosis, also known as hybrid vigour, is only maintained within the F1 generation, which means that the production of these superior offspring is laborious, costly, and often unaffordable to small scale farmers. Nevertheless, if CRISPR/Cas9 is used then it can help to overcome such limitations and allowing for the development of stable and improved seed cultivars that retain desirable traits across generations. This would mean that farmers would have a reducing dependence on purchasing new seeds every year, and, therefore, providing a more sustainable and cost-effective solution for farmers.
Through simultaneous editing of REC8, PAIR1, OSD1, and MTL genes, researchers have been able to fix the favourable F1 traits (Wang et al., 2019). When REC8, PAIR1, and OSD1 genes were knocked down simultaneously, and meiosis was replaced by mitosis, it was enabled the production of asexual hybrid rice seeds and the preservation of the hybrid vigour (Khanday et al., 2019). In strawberries, and other fruits crops, the knockdown of all the six anthocyanin transport genes RAP (REDUCED ANTHOCYANINS IN PETIOLES) using the CRISPR/CAS9 system has produced white octoploid fruits instead of the red-coloured typical berries (Gao et al., 2020). This demonstrates the simultaneous knockout of homoeologous alleles as a tool to breed polyploid plants.
Similarly, the generation of DH (double-haploid) homozygous lines is a labour-intensive and expensive endeavour that needs laboratory infrastructure and has variable efficiency. The timeline for the whole DH line production process takes from several months to a few years depending on factors such as the species involved, the haploid induction success rate and the chromosome doubling technique efficiency. However, targeted genome editing-mediated haploid induction has provided a more rapid option with lines being generated within one year (Hooghvorst and Nogués, 2020). Several key genes have been targeted for this purpose including MATL (MATRILINEAL), CENH3 (CENTROMERE-SPECIFIC HISTONE 3) and DMP (Domain Membrane Proteins) genes (Zhu et al., 2020; Kuppu et al., 2020; Zhong et al., 2019a). In wheat CRISPR/Cas9- mediated targeting of the MATL gene led to an inheritance rate of 18.9% haploid progeny (Liu et al., 2019a, b) while targeting maize DMP gene resulted in maternal haploids with an efficacy range of about 0.1–0.3% (Zhong et al., 2019a, b). An improved version of this system is the HI-editing technology (HI-Edit) which makes available transgene-free edited inbred lines lacking inducer parental DNA. By eliminating the necessity for introgression, this approach minimizes the time and expense involved (Kelliher et al., 2019). As technological advancement carries on, techniques like the CRISPR-Combo system which performs simultaneous gene activation and editing using CRISPR hold a lot of promise in terms of speeding up the identification process of markers-free genome-edited lines that are highly efficient. These techniques also offer an opportunity to screen mutants at both genome and transcriptional levels (Pan et al., 2022a). As these technologies continue to evolve, it’s expected that the process of obtaining and studying gene-edited lines will become more efficient and streamlined. Hence, we can look forward to easier and faster ways of getting and understanding these edited genetic lines in the future.
Ever since the first mention of CRISPR/Cas9 technology, back in 2013 (Li et al., 2013; Nekrasov et al., 2013; Shan et al., 2013) there have been some major improvements. In the past, this technology relied on using a DNA donor template to introduce the desired genetic changes. However, the most recent developments have allowed for the utilisation of CRISPR/Cas9 without the need for a DNA donor template, opening new possibilities for gene editing. This modified version of CRISPR/Cas9, instead of using a DNA donor, uses the Cas9 enzyme to produce targeted breaks in specific parts of the genome. These breaks then activate the cell’s natural DNA repair mechanisms, which can result in gene disruptions, insertions, or deletions. The CRISPR/Cas9 technology involves the cut of a specific sequence in the organism’s genome. This cutting action is triggered by an RNA called single-guide RNA (sgRNA), which is designed to match the target DNA sequence. Double-stranded breaks are generated when the homologous sequences of the guide RNA are in proximity to the specific protospacer adjacent motif (PAM) sequence. This allows for genetic modifications to occur during the subsequent repair mechanisms (Asmamaw and Zawdie, 2021; Singh et al., 2022). More recent advancements in CRISPR technology made it possible to combine an engineered reverse transcriptase and prime editing (PE) RNA with CRISPR/Cas9. This new approach eliminates the need for double-stranded breaks or donor templates and enable changing specific bases, deleting targeted sections, inserting, or even a combination of those. This methodology has been demonstrated on crops such as maize, lettuce, tobacco, rice, and bread wheat, and is a potential solution to tackle concerns raised by the public and regulatory bodies about CRISPR/Cas9-derived plants (Svitashev et al., 2015; Woo et al., 2015; Liang et al., 2017).
CRISPR/Cas9 prime-editors have become a powerful tool for precise editing of plant genomes. Prime-editing combines the Cas9 nuclease with an engineered reverse transcriptase and a prime editing RNA (pegRNA), which enables targeted base editing, insertions, deletions, and combinations without the need for double-stranded breaks or donor templates. Currently, the most usual editors used in the CRISPR system are the base editors, and characterised for making specific base changes, limited to A, C, G, and T. Instead, prime editors (PEs) offer a broader range of mutations, including all 12 possible transition and transversion mutations, as well as small insertions and deletions (INDELs), without the need for DNA double-strand breaks (Anzalone et al., 2019). One example of prime editing in plants is the modification of the acetyl-CoA carboxylase (ACCase) gene. This gene is crucial for fatty acid biosynthesis, and the loss-of-function mutations on it can lead to severe developmental abnormalities or even be lethal for plants (Baud et al., 2004). ACCase is also the target of many commercial herbicides. In rice, the OsACC gene confers herbicide resistance. Through base editing libraries, targeted mutations were introduced in both OsACC and acetolactate synthase (ALS) genes, which are targets of herbicides used for plant weed control in field crops (Garcia et al., 2017). Using this approach key amino acid residues that are related to resistance to herbicides were revealed (Liu et al., 2020; Kuang et al., 2020). In addition, various mutations in the acetyl-coenzyme A carboxylase 1(OsACC1) gene, have been discovered, resulting in herbicide resistance (Xu et al., 2021). These examples illustrate the significance of prime editing technology for crop improvement purposes specifically when it comes to development of herbicide-resistant cultivars and understanding the functional importance of specific substitutions of amino acids. Although its optimization is still underway, prime editing in plants promises precise genetic manipulation and therefore serves as a promising tool towards better agricultural achievements.
The access to complete DNA sequencing information and a thorough understanding of gene functions has facilitated the use of CRISPR-Cas9 technology for precise modulation of key genes. This also allows for the quick creation of new genetic resources to improve specific traits. Nevertheless, it’s crucial to note that direct sequencing methods might miss out on certain heterozygous alleles. To overcome this limitation, methods like TILLING (Targeting Induced Local Lesions IN Genomes) can be used to identify mutations at specific loci in the modified genetic material. Furthermore, DNA markers can be used to detect mutations at the early stages of plant growth. TILLING brings several benefits in this scenario. Libraries developed through TILLING contain preserved genetic resources, usually in the form of seeds, and the genomic DNA extracted from mutated materials can remain stable for many years. This allows for the identification of specific allele groups linked to different traits, which can be safeguarded and shared with the public. However, caution is advised when targeting traits controlled by multiple genes. The current landscape provides numerous prospects for breeders and scientists, depending on their gene preferences. When dealing with stable genes, hybridization is often the preferred strategy. Conversely, if the genes of interest are dominant, transformation approaches may be the most effective option. For recessive genes, mutation methodologies are frequently utilized. These strategies, based on our existing knowledge, are implemented in plant research to tackle urgent global challenges (Figure 7).
By employing refined NGS pipelines (Sahu et al., 2020) to characterize mutants generated through diverse chemical and irradiation techniques, new allelic variants in the genomes of various crops can be generated. This approach potentially may establish mutation breeding as a fundamental pillar in the efforts towards nutritional and food security. It is also an opportunity for the generation, validation, and removal of deleterious alleles, since these are mainly accumulated in the pericentromeric regions of chromosomes due to less selection efficiency (Dwivedi et al., 2023). Yet, one must keep in mind that these regions house a great amount of “housekeeping genes” that could produce deleterious effects if disturbed. Additionally, these regions might be protective of these types of genes. Whereas the CRISPR process transforms and introduces non-native genes, it can also generate transgene-free derivatives through genetic segregation via self-pollination. This is achieved by providing a fluorescent cassette marker genetic segregation, which signals the presence of the CRISPR/Cas9 construct. Another approach is to introduce suicidal genes (CMS2 and BARNASE genes) into the T0 plants, which selectively eliminate transgene-containing pollen and embryos through the crossing. These methods are, however, still not applicable for asexually propagated crops like potato, cassava, and banana. It’s important to note that while CRISPR is often associated with simple mutations, it can also facilitate the introduction of larger foreign DNA fragments, which is categorized as NGT 2 in the EU’s soft regulation framework (EC, 2023). This distinction is important, as it highlights that CRISPR’s capabilities extend beyond short, simple mutations typically seen with chemical-induced mutations, and therefore, enabling more complex genetic modifications.
Among the known challenges, it is worth mentioning the difficulty in predicting sgRNAs efficiencies, requiring the optimization of Cas protein derivates. The current delivery systems for Cas and sgRNAs have limitations, highlighting the need for improvement in the CRISPR specificity system, and the urgent need to expedite improvements in these delivery mechanisms (Table 3).
The abundance of genomic sequence data and the need to uncover target genes associated with essential agronomic traits highlights the importance of establishing precise links between genotypes and phenotypes. Therefore, better delivery systems in CRISPR technology are needed, including reducing the dependency on tissue culture methods and to facilitate the widespread adoption of this technology in agriculture.
While the technology seems useful for specific traits such as plant diseases and pests, or quality traits; when it comes to more complex traits, such as yield, the potential of CRISPR technology may be seen with some reservations. CRISPR technology seems to be a valuable tool to facilitate trait introgression and support the mitigation of linkage drag in the case of simply inherited traits. However, in both CRISPR and induced mutation, there is the need to address challenges of specific genetic variance generation for highly quantitative traits, which is crucial to achieve the necessary increases in production. We are likely to experience great innovations in crop genetics in a multitude of ways, yet, if accurate phenotyping will not accompany this evolution, it will be challenging to observe a rapid evolution in crop improvement. Integrating precise genotyping with accurate phenotyping will significantly contribute to the effective implementation of CRISPR technology and accelerate the progress in crop improvement efforts.
Regarding regulatory frameworks, currently, there are two regulatory frameworks that several countries have adopted to classify crops developed using CRISPR and other genome editing technologies: product-based and process-based regulations (Ishii and Araki, 2017). In the product-based framework, only the final product is controlled in terms of regulation. Consequently, if there are no exogenous transgenes in the commodity, then it is considered as a non-GMO. Conversely, under a process-based approach, any crop produced through CRISPR/Cas9 technology shall be considered a genetically modified organism (GMO). The choice between these two frameworks will determine how genome-edited crops should be managed and labelled. Different countries have adopted different approaches, and specific examples of countries following each framework can be found in related literature (Chen and Gao, 2020; Singh et al., 2022). Regulatory setups significantly influence commercialization as well as public reception of CRISPR/Cas9-derived crops across various regions globally. Despite technologies such as ZFN, TALEN, Meganucleases (MN), and CRISPR can be used for specific genome editing without the incorporation of foreign DNA into the target site, it is crucial to recognize that these tools are not perfect and sometimes irregularities occur during the process. The off-target effects are one major worry because they may result in cell death or cause genomic instabilities (Lazzarotto et al., 2020) while sequencing cannot detect or differentiate between natural and genetic engineered variability. Research demonstrates that prime editors (PEs) do not induce detectable pegRNA-independent off-target edits in plants (Jin et al., 2021). Using CRISPR to treat genetic diseases in humans, side effects such as chromosomal rearrangements can occur after genome editing (Raimondi et al., 2024), besides editing a gene may turn on or off other genes associated with that gene. In plants, off-targets and side effects may be segregated out during meiosis or discarded during the multigenerational field evaluation selection because of performance and therefor side effects may be of lesser practical problem in seed propagated plants. For asexually propagated plants, field assessments may be the only way of recognizing such effects. Nevertheless, continued research in the field of genome editing is needed to address these concerns and ensure the safe and precise application of these technologies in economically important crops. For small breeders that obtain royalties for their varieties, or payment for certified seeds and farmer saved seeds, outsourcing CRISPR technology is a good option for them to get access to specific traits without investing in costly laboratory facilities and staff with specialised skills.
The successful adoption and implementation of inclusive genomic innovations (IGIs) in the agriculture sector of the Global South, especially in low- and mid-income countries, continue to pose challenges. The primary obstacle stems from a lack of understanding and awareness regarding the specific needs and requirements of the target users (UNDP, 2022).
For instance, during the late 1990s, cotton crop was significantly affected by insecticide-resistant Lepidopteran pests in India resulting in excessive use of insecticides that caused not only a serious environmental and human health problem but also led to economic problems for cotton growers that contributed to farmer indebtedness. The introduction of genetically modified cotton [Bacillus thuringiensis (Bt) cotton] showed not only a direct positive impact on yield gains through reduced crop losses by good control of native bollworm Helicoverpa armigera, but indirectly also led to reductions in insecticide use (Gutierrez et al., 2020) resulting in 134% increase the income of smallholder farmers (Subramanian and Qaim, 2010). In China, the widespread planting of Bt cotton cultivars not only successfully controlled the effects of the polyphagous pest Helicoverpa armigera among multiple crops but also provided great advantages by providing a 10-fold increase in the products of Chinese farmers between 1996–2018 (Pray et al., 2011; Zhaozhi et al., 2022; ISAAA, 2020). Similarly, the adoption of Bt technology in other crops such as Bt brinjal contributed 22% higher revenues compared to non-Bt cultivars in Bangladesh (Shelton et al., 2020).
Despite these encouraging outcomes, public perception, concerns about potential risks and unintended consequences, and ethical considerations contribute to the ongoing debate. An alternative approach includes the use of ‘Fast Identification of Nucleotides variants by DigITal PCR’ known as FIND-IT, which offers a promising solution for rapid identification of pre-targeted genetic variants or rare alleles in large and very large populations. With this method libraries of 500,000 knockout barley mutant individuals, can be screened within only two weeks (Knudsen et al., 2021; Madsen et al., 2024). Contrary to CRISPR methodology, FIND-IT is not subjected to governmental regulations as a non-GM technique. Additionally, compared to TILLING methods, FINF-IT offers simpler technological requirements and greater sensitivity in detection. This high-throughput ddPCR method does not require transformation or tissue culture protocols making it a scalable and efficient approach for screening and targeting desired traits in crops with low mutation-density variant populations.
The innovative upfront genomic innovation (GI) technologies may not directly serve as tools for smallholder farmers in many countries but rather offer products that can benefit them. It is undeniable that smallholder farmers are a significant portion of the global agricultural workforce, operating, majority of the times, under resource constrains. Therefore, investments in high technologies such as genotyping are opportunities to improve yields, reduce production costs and even mitigate risks associated with the increased climate variability. The solution is to bridge the gap that exists between traditional farming methods and modern agricultural practices. By offering them tailored solutions to address their unique challenges, genomic innovations may make a difference. The information got from the genetic codes of crops, not only offers better cultivars that are better locally adapted but also facilitates the selection of the most desired traits and allows the farmers to produce higher-quality crops with fewer resources. Yet, to fully understand the potential of genomic innovations requires addressing issues such as access, affordability and capacity building to ensure equitable distribution of benefits across the farming communities. Besides the previous examples, in Sub-Saharan Africa, the development of drought-tolerant maize cultivars using MAS breeding techniques using genomic data (molecular markers) has helped farmers mitigate the adverse effects of climate change and improve food security (https://dtma.cimmyt.org/). The same was shown by the introduction of disease-resistant cassava cultivars in Nigeria and Uganda (https://www.nextgencassava.org/). With an exponential amount of information available, the future holds the opportunity to achieve tailored genomic technologies for smallholder farming. Such examples of this are MAS, GS and genome editing tools. One way to foster the integration of inclusive genomic innovations into agritech investments for smallholder farmers is to prioritize capacity building and knowledge transfer, including through training programs, extension services and farmer field schools.
Innovative financing mechanisms, including impact investing, venture capital and blended finance models may support resource mobilization and scale up the adoption of inclusive genomic innovations and therefore, accelerate their adoption by the farmer community. An example of this is the effort on biofortification through breeding by the HarvestPlus program (www.harcestplus.org) that over 20 years facilitated the release of more than 420 biofortified cultivars in different staple crops across Asia, Africa and Latin America (Dwivedi et al., 2023). So far, most of the biofortified cultivars developed through crossbreeding approaches, however, molecular breeding efforts using IGIs for the same purpose are gaining momentum, thus resulting in speed up the development of biofortified cultivars (Badu-Apraku et al., 2018; Sheoran et al., 2022). The molecular markers developed for biofortified traits such as pro-vitamin A, Fe and Zn content in edible parts of different staple crops could be utilized to improve locally adapted farmer-preferred cultivars in sub-Saharan Africa and Latin America.
Despite all the above-mentioned strengths in empowering smallholder farmers through genomic innovations, careful consideration must be given to potential risks including genetic erosion, biodiversity loss, and unintended environmental consequences. One must be aware that, beyond technology adoption, it is important to take into consideration that the use of CRISPR ultimately raises important concerns regarding Intellectual Property Rights (IPRs), particularly as these technologies can impact breeders’ rights, the ownership of genetically edited crops but also issues on the accessibility of the developed innovations in agricultural biotechnology. Potential solutions could involve not only the development of clear legal frameworks, encourage the open access to CRISPR technology findings to the breeders, develop fair and flexible licensing agreements so breeders can use CRISPR technology while providing compensations to innovators, improve communication and collaborations between the different stakeholders, as well promotion for fairer regulations that protect both innovators and breeders.
The future holds space not only to explore genome editing tools for unprecedented precision and efficiency but also the space to integrate the omics data technologies that promise deeper insights into gathering real-time data for real-time decision-making. A major contribution of these advanced technologies, from the breeder perspective, includes the implementation of multiple field trials, which should be emphasised for mutational and CRISPR-Cas generated parental lines after they have demonstrated success in greenhouse conditions.
To conclude, the integration of various approaches, whether it be through mutation breeding, gene editing, crossbreeding or other techniques, holds the potential for a more comprehensive and effective strategy in enhancing plant traits for increased production.
AG: Writing – original draft, Writing – review & editing. RO: Writing – review & editing. FS: Writing – review & editing. SR: Writing – review & editing. PA: Writing – review & editing. AA: Writing – review & editing. MK: Writing – review & editing. SC: Writing – review & editing.
The author(s) declare financial support was received for the research, authorship, and/or publication of this article. Bill and Melinda Gates Foundation for their support through the RTB Breeding Investment Project (INV-041105).
A.L.G.-O. would like to express gratitude to Dr. Emily Conley and Professor Jim Anderson from the Department of Agronomy and Plant Genetics at the University of Minnesota (UoM), Minnesota, U.S.A. for their valuable insights and support in this review. We also extend our appreciation to the Bill and Melinda Gates Foundation for their support through the RTB Breeding Investment Project (INV-041105).
The authors declare that the research was conducted in the absence of any commercial or financial relationships that could be construed as a potential conflict of interest.
The author(s) declare that no Generative AI was used in the creation of this manuscript.
All claims expressed in this article are solely those of the authors and do not necessarily represent those of their affiliated organizations, or those of the publisher, the editors and the reviewers. Any product that may be evaluated in this article, or claim that may be made by its manufacturer, is not guaranteed or endorsed by the publisher.
AgMIP: Agricultural Model Intercomparison and Improvement Project is a collaborative global research effort aiming to enhance agricultural models’s accuracy in predicting crop yields, food security assessment, and climate change impacts on agriculture and was established in 2010
CRISPR: Clustered Regularly Interspaced Short Palindromic Repeat system used to modify/edit a cultivar of plant species. It facilitates site-directed mutagenesis
CRISPR off-target: Accidental or unintentional alterations in different DNA regions, different from the intended spots, with similar but not exact sequences to the target site
Crop productivity: Efficiency of crop production, measured as yield per unit area. It can exceed 100% (as shown in Figure 1 of this paper), when yields surpass previous standards (global average) due to improved varieties, better management practices, or favourable conditions
DSB: Double-strand break (DSB) mutations and refers to a type of genetic alteration in which both strands of the DNA molecule are severed or broken at the same location. This disruption in the DNA structure can lead to various genetic changes, including mutations, deletions, insertions, and rearrangements
KASP™: Kompetitive Allele-Specific PCR, is a genotyping technology used to detect single nucleotide polymorphisms (SNPs) and small insertions or deletions in DNA samples
KASP haplotyping: Technology that allows the detection of SNPs in DNA sequences. It is a form of competitive PCR where allele-specific primers are designed to selectively amplify target DNA sequences. KASP haplotyping specifically focuses on identifying and characterizing haplotypes. Haplotypes are sets of closely linked genetic markers that tend to be inherited together. Both KASP haplotyping and MAS contribute to genomic selection by providing genetic information, KASP haplotyping specifically focuses on haplotype structures, whereas MAS encompasses a broader range of marker-assisted techniques for selecting individuals based on genetic markers associated with traits
IGIs: Inclusive genomic Innovations
IPRs: Intellectual Property Rights, are legal protections granted to individuals or entities for their creations of the mind or intellect
ISIMIP: The Inter-Sectoral Impact Model Intercomparison Project is an international research initiative aimed at assessing climate change’s potential impacts on diverse sectors, extending beyond agriculture to encompass water resources, ecosystems, health, economics, and more
Mutation breeding: The use of mutagens, such as radiation or chemicals, to induce genetic mutations in plants, leading to increased genetic variability generation of new allele variation for trait improvement
Mutational signature marker: These are specific patterns of mutations found in DNA indicating the underlying processes or exposures that caused those mutations. These signatures can be associated with various factors, including environmental influences and intrinsic factors, and adapted from Human genetics
NGT: New Genomic Technologies are a variety of techniques that alter the genetic material of an organism. Those considered equivalent to conventional plants (NGT 1 plants) would be exempted from most requirements of the GMO legislation. https://www.europarl.europa.eu/news/en/agenda/briefing/2024-02-05/2/new-genomic-techniques-debate-and-vote-on-new-eu-rules
NUE: Nitrogen Use Efficiency is the measure of how effectively plants utilize nitrogen from fertilizers, encompassing uptake for growth and minimizing losses through processes like leaching and volatilization
QC: Quality control ensures that the genetic data used in research is robust, reliable, and suitable for interpretation and analysis. By implementing QC measures, breeders can verify the genetic purity of the F1 offspring, thereby confirming these are genuine hybrids derived from the intended parental lines. Moreover, QC in breeding programs supports the monitorization of the genetic diversity within the populations. It ensures that breeding stocks retain a broad spectrum of alleles, and therefore, reducing the risk of inbreeding depression and preserving the potential for future genetic improvement
STTMs: Short Tandem Target Mimic are a class of synthetic small RNAs that are designed to regulate the expression of specific genes in plants by modulating microRNA (miRNA) activity
TALENs: Transcription Activator-Like Effector Nucleases, are a type of engineered proteins used for targeted genome editing in various organisms, including plants, animals, and even human cells
TILLING: Targeting Induced Local Lesions IN Genomes, is a high-throughput method used for identifying and characterizing mutations in specific genes of interest
Abe, T., Kazama, Y., Hirano, T. (2015). Ion beam breeding and gene discovery for function analysis using mutants. Nucl. Phys. News 25, 30–34. doi: 10.1080/10619127.2015.1104130
Abe, T., Ryuto, H., Fukunishi, N. (2012). “Ion beam radiation mutagenesis,” in Plant mutation breeding and biotechnology, eds. Shu, Q. Y., Forster, B. P., Nakagawa, H. (Vienna: Joint FAO/IAEA), 99–106. doi: 10.1079/9781780640853.0099
Addo-Quaye, C., Miller, W., Axtell, M. J. (2009). CleaveLand: a pipeline for using degradome data to find cleaved small RNA targets. Bioinformatics 25, 130–131. doi: 10.1093/bioinformatics/btn604
Agre, P. A., Clark, L. V., Garcia-Oliveira, A. L., Bohar, R., Adebola, P., Asiedu, R., et al. (2023). Identification of diagnostic KASP-SNP markers for routine breeding activities in yam (Dioscorea spp.). Plant Gen. 17, e20419. doi: 10.1002/tpg2.20419
Ahmed, F., Senthil-Kumar, M., Lee, S., Dai, X., Mysore, K. S., Zhao, P. X. (2014). Comprehensive analysis of small RNA-seq data reveals that combination of miRNA with its isomiRs increase the accuracy of target prediction in Arabidopsis thaliana, RNA. Biology 11, 1414–1429. doi: 10.1080/15476286.2014.996474
Ainley, W. M., Dent, S. L., Welter, M. E., Murray, M. G., Zeitler, B., Amora, R., et al. (2013). Trait stacking via targeted genome editing. Plant Biotechnol. J. 11, 1126–1134. doi: 10.1111/pbi.12107
Ali, A., Zafan, M. M., Farooq, Z., Ahmed, S. R., Ijaz, A., Anwar, Z., et al. (2023). Breakthrough in CRISPR/Cas system: Current and future directions and challenges. Biotechnol. J. 18, 2200642. doi: 10.1002/biot.202200642
Anderson, W., Taylor, C., McDermid, S., Ilboudo-Nebie, E., Seager, R., Schlenker, W., et al. (2021). Violent conflict exacerbated drought-related food insecurity between 2009 and 2019 in sub-Saharan Africa. Nat. Food 2, 603–615. doi: 10.1038/s43016-021-00327-4
Anzalone, A. V., Randolph, P. B., Davis, J. R., Sousa, A. A., Koblan, L. W., Levy, J. M., et al. (2019). Search-and-replace genome editing without double-strand breaks or donor DNA. Nature 576, 149–157. doi: 10.1038/s41586-019-1711-4
Arshad, M., Feyissa, B. A., Amyot, L., Aung, B., Hannoufa, A. (2017a). MicroRNA156 improves drought stress tolerance in alfalfa (Medicago sativa) by silencing SPL13. Plant Sci. 258, 122–136. doi: 10.1016/j.plantsci.2017.01.018
Arshad, M., Gruber, M. Y., Wall, K., Hannoufa, A. (2017b). An insight into microRNA156 role in salinity stress responses of alfalfa. Front. Plant Sci. 8. doi: 10.3389/fpls.2017.00356
Arshad, M., Hannoufa, A. (2022). Alfalfa transcriptome profiling provides insight into miR156-mediated molecular mechanisms of heat stress tolerance. Genome 65, 315–330. doi: 10.1139/gen-2021-0099
Asmamaw, M., Zawdie, B. (2021). Mechanism and applications of CRISPR/cas-9-mediated genome editing. Biologics 15, 353–361. doi: 10.2147/BTT.S326422
Awasthi, P., Khan, S., Lakhani, H., Chaturvedi, S., Shivani, N., Kaur, N., et al. (2022). Transgene-free genome editing supports CCD4 role as a negative regulator of beta-carotene in banana. J. Exp. Bot. 73, 3401–3416. doi: 10.1093/jxb/erac042
Badu-Apraku, B., Talabi, O. A., Garcia-Oliveira, A. L., Gedil, M. (2018). IITA scientists develop multiple stress tolerant maize hybrids with high levels of Pro-Vitamin A. IITA News 2463. 7th january 2019. Available online at: https://bulletin.iita.org (Accessed October 19, 2024).
Baud, S., Bellec, Y., Miquel, M., Bellini, C., Caboche, M., Lepiniec, L., et al. (2004). gurke and pasticcino3 mutants affected in embryo development are impaired in acetyl-CoA carboxylase. EMBO Rep. 5, 515–520. doi: 10.1038/sj.embor.7400124
Bento, V. A., Ribeiro, A. F. S., Russo, A., Gouveia, C. M., Carodo, R. M., Soares, P. M. M. (2021). The impact of climate change in wheat and barley yields in the Iberian Peninsula. Sci. Rep. 11, 15484. doi: 10.1038/s41598-021-95014-6
Biswas, S., Zhang, D., Shi, J. (2021). CRISPR/Cas systems: opportunities and challenges for crop breeding. Plant Cell Rep. 40, 979–998. doi: 10.1007/s00299-021-02708-2
Bogdavone, A. J., Voytas, D. F. (2011). TAL effectors: customizable protein for DNA targeting. Science 333, 1843–1846. doi: 10.1126/science.120409
Browning, B. L., Browning, S. R. (2009). A unified approach to genotype imputation and haplotype-phase inference for large data sets of trios and unrelated individuals. Am. J. Hum. Genet. 84, 210–223. doi: 10.1016/j.ajhg.2009.01.005
Cao, X., Xie, H., Song, M., Lu, J., Ma, P., Huang, B., et al. (2023). Cut-dip-budding delivery system enables genetic modifications in plants without tissue culture. Innovation (Camb) 4, 100345. doi: 10.1016/j.xinn.2022.100345
Chander, S., Garcia-Oliveira, A. L., Gedil, M., Shah, T., Otusanya, G. O., Asiedu, R., et al. (2021). Genetic diversity and population structure of soybean lines adapted to sub-saharan africa using single nucleotide polymorphism (SNP) markers. Agronomy 11, 604. doi: 10.3390/agronomy11030604
Chen, J. S., Dagdas, Y. S., Kleinstiver, B. P., Welch, M. M., Sousa, A. A., Harrington, L. B., et al. (2017). Enhanced proofreading governs CRISPR-Cas9 targeting accuracy. Nature 550, 407–410. doi: 10.1038/nature24268
Chen, K., Gao, C. (2020). Genome-edited crops: how to move them from laboratory to Market. Front. Agr. Sci. Eng. 7, 181–187. doi: 10.15302/J-FASE-2020332
Chen, P. J., Hussmann, J. A., Yan, J., Knipping, F., Ravisankar, P., Chen, P. F., et al. (2021). Enhanced prime editing systems by manipulating cellular determinants of editing outcomes. Cell 184, 5635–5652. doi: 10.1016/j.cell.2021.09.018
Christian, M., Cermak, T., Doyle, E. L., Schmidt, C., Zhang, F., Hummel, A., et al. (2010). Targeting DNA double-strand breaks with TAL effector nucleases. Genetics 186, 757–761. doi: 10.1534/genetics.110.120717
CIMMYT (2021). Drought-tolerant maize project pioneers a winning strategy for a world facing climate change. Available online at: https://www.cimmyt.org/news/drought-tolerant-maize-project-pioneers-a-winning-strategy-for-a-world-facing-climate-change/ (Accessed 19 October, 2024).
Curtin, S. J., Zhang, F., Sander, J. D., Haun, W. J., Starker, C., Baltes, N. J., et al. (2011). Targeted mutagenesis of duplicated genes in soybean with zinc-finger nucleases. Plant Physiol. 156, 466–473. doi: 10.1104/pp.111.172981
Daboussi, F., Stoddard, T. J., Zhang, F. (2015). “Engineering meganuclease for precise plant genome modification,” in Advances in new technology for targeted modification of plant genomes. Eds. Zhang, F., Puchta, H., Thomson, J. (Springer, New York, NY). doi: 10.1007/978-1-4939-2556-8_2
Djami-Tchatchou, A. T., Sanan-Mishra, N., Ntushelo, K., Dubery, I. A. (2017). Functional roles of microRNAs in agronomically important plants - potential as targets for crop improvement and protection. Front. Plant Sci. 8. doi: 10.3389/fpls.2017.00378
Donald, C. M. (1968). The breeding of crop ideotypes. Euphytica 17, 385–403. doi: 10.1007/BF00056241
Duhan, N., Kaundal, R. (2021). LegumeSSRdb: A comprehensive microsatellite marker database of legumes for germplasm characterization and crop improvement. Int. J. Mol. Sci. 22, 11350. doi: 10.3390/ijms222111350
Dwivedi, S. L., Heslop-Harrison, P., Spillane, C., McKeown, P. C., Edwards, D., Goldman, I., et al. (2023). Evolutionary dynamics and adaptive benefits of deleterious mutations in crop gene pools. Trends Plant Sci. 28, 685–697. doi: 10.1016/j.tplants.2023.01.006
EC. (2023). New techniques in biotechnology. Available online at: https://food.ec.europa.eu/plants/genetically-modified-organisms/new-techniques-biotechnology_en (Accessed 19 October 2024).
Endelman, J. B., Kante, M., Lindqvist-Kreuze, H., Kilian, A., Shannon, L. M., Caraza-Harter, M. V., et al. (2024). Targeted genotyping-by-sequencing of potato and data analysis with R/polyBreedR. Plant Gen. 17, e20484. doi: 10.1002/tpg2.20484
FAO. (2021). Food systems assessment – working towards the SDGs, FAO investment centre. Available online at: www.Fao.org/support-to-investment/our-ourk/projects/fsa2021/en (Accessed 17 November, 2024).
FAO. (2023). OVERVIEW - THE STATUS OF WOMEN IN AGRIFOOD SYSTEMS. Available online at: https://openknowledge.fao.org/server/api/core/bitstreams/317db554-c763-4654-a0d3-24a8488bbc3a/content/status-women-agrifood-systems-2023/overview.htmltop (Accessed 17 November, 2024).
FAO, IFAD, UNICEF, WFP, WHO. (2019). The State of Food Security and Nutrition in the World 2019. Safeguarding against economic slowdowns and downturns (Rome: FAO). Licence: CC BY-NC-SA 3.0 IGO.
FAODATA. (2022). Available online at: https://www.fao.org/faostat/en/homev (Accessed 17 November, 2024).
Feyissa, B. A., Amyot, L., Nasrollahi, V., Papadopoulos, Y., Kohalmi, S. E., Hannoufa, A. (2021). Involvement of the miR156/SPL module in flooding response in medicago sativa. Sci. Rep. 11, 3243. doi: 10.1038/s41598-021-82450-7
Folkes, L., Moxon, S., Woolfenden, H. C., Stocks, M. B., Szittya, G., Dalmay, T., et al. (2012). PAREsnip: a tool for rapid genome-wide discovery of small RNA/target interactions evidenced through degradome sequencing. Nucleic Acids Res. 40, e103. doi: 10.1093/nar/gks277
Franco-Zorrilla, J. M., Valli, A., Todesco, M., Mateos, I., Puga, M. I., Rubio-Somoza, I., et al. (2007). Target mimicry provides a new mechanism for regulation of microRNA activity. Nat. Genet. 39, 1033–1037. doi: 10.1038/ng2079
Gahlaut, V., Zinta, G., Jaiswal, V., Kumar, S. (2020). Quantitative epigenetics: a new avenue for crop improvement. Epigenomes 4, 25. doi: 10.3390/epigenomes4040025
Gaj, T., Sirk, S. J., Shui, S. L., Liu, J. (2016). Genome-editing technologies: Principles and applications. Cold Spring Harb Perspect. Biol. 8 (12), a023754. doi: 10.1101/cshperspect.a023754
Gao, C. (2021). Genome engineering for crop improvement and future agriculture. Cell 184, 1621–1635. doi: 10.1016/j.cell.2021.01.005
Gao, Q., Luo, H., Li, Y., Liu, Z., Kang, C. (2020). Genetic modulation of RAP alters fruit coloration in both wild and cultivated strawberry. Plant Biotechnol. J. 18, 1550–1561. doi: 10.1111/pbi.13317
Garcia, M. D., Nouwens, A., Lonhienne, T. G., Guddat, L. W. (2017). Comprehensive understanding of acetohydroxyacid synthase inhibition by different herbicide families. Proc. Natl. Acad. Sci. U.S.A. 114, E1091–E1100. doi: 10.1073/pnas.1616142114
Garcia-Oliveira, A. L., Ayele, L., Uwimana, B., Storr, S., Taye, T., Ng, E. H., et al. (2021). Access to genetic analysis accelerates banana breeding in East Africa. (CGIAR: CIMMYT). Available online at: https://excellenceinbreeding.org/news/access-genetic-analysis-accelerates-banana-breeding-east-africa.
Garcia-Oliveira, A. L., Miguel, M., Acacio, J., Mutaliano, J., Abade, H., Chauque, P., et al. (2022). Advanced genotyping in Mozambican breeding programs to accelerate genetic improvement, conservation, and agronomy. (CGIAR: CIMMYT). Available online at: https://www.bing.com/search?q=Advanced+genotyping+in+Mozambican+breeding+programs+to+accelerate+genetic+improvement%2C+conservation%2C+and+agronomy&form=ANNH01&refig=5b80812031b9454ab2cd8828b4efdefd&pc=LCTS.
Garcia-Oliveira, A. L., Federico, M. L., Guimarães, C., Adu, G. B., Simões, F., Soares, C., et al. (2024). The reshaping of crop breeding programs: How CGIAR genotyping and global partnerships are bridging gaps. (CGIAR). Available online at: https://www.cgiar.org/news-events/news/the-reshaping-of-crop-breeding-programs-how-cgiar-genotyping-and-global-partnerships-are-bridging-gaps/.
Garcia-Oliveira, A. L., Martins-Lopes, P., Tolrà, R., Poschenrieder, C., Guedes-Pinto, H., Benito, C. (2016). Differential Physiological Responses of Portuguese Bread Wheat (Triticum aestivum L.) Genotypes under Aluminium Stress. Diversity 8, 26. doi: 10.3390/d8040026
Garcia-Oliveira, A. L., Martins-Lopes, P., Tolrá, R., Poschenrieder, C., Tarquis, M., Guedes-Pinto, H., et al. (2014). Molecular characterization of the citrate transporter gene TaMATE1 and expression analysis of upstream genes involved in organic acid transport under Al stress in bread wheat (Triticum aestivum). Physiol. Plant 152, 441–452. doi: 10.1111/ppl.12179
Garcia-Oliveira, A. L., Miguel, M., Acacio, J., Mutaliano, J., et al. (2022b). Advanced genotyping in Mozambican breeding programs to accelerate genetic improvement, conservation, and agronomy (CGIAR). Available at: https://www.bing.com/search?q=Advanced+genotyping+in+Mozambican+breeding+programs+to+accelerate+genetic+improvement%2C+conservation%2C+and+agronomy&form=ANNH01&refig=5b80812031b9454ab2cd8828b4efdefd&pc=LCTS.
Garcia-Oliveira, A. L., Tan, L., Fu, Y., Sun, Q. (2009). Genetic identification of quantitative trait loci for contents of mineral nutrients in rice grain. J. Integr. Plant Biol. 51, 84–92. doi: 10.1111/j.1744-7909.2008.00730.x
GBD 2015 Obesity Collaborators, Afshin, A., Forouzanfar, M.H., Reitsma, M.B., Sur, P., Estep, K., et al. (2017). Health effects of overweight and obesity in 195 countries over 25 years. NEJM 377, 13–27. doi: 10.1056/NEJMoa1614362
Ginkel, M.V., Cherfas, J. (2023). What is wrong with biofortification. Glob. Food Secur. 37, 100689. doi: 10.1016/j.gfs.2023.100689
Gutierrez, A. P., Ponti, L., Kranthi, K. R., Baumgartner, J., Kenmore, P., Gilioli, G., et al. (2020). Bio-economics of Indian hybrid Bt cotton and farmer suicides. Environ. Sci. Eur. 32, 1–15. doi: 10.1186/s12302-020-00445-z
Hendelman, A., Stav, R., Zemach, H., Arazi, T. (2013). The tomato NAC transcription factor SlNAM2 is involved in flower-boundary morphogenesis. J. Exp. Bot. 64, 5497–5507. doi: 10.1093/jxb/ert324
Hirano, T., Kazama, Y., Ishii, K., Ohbu, S., Shirakawa, Y., Abe, T. (2015). Comprehensive identification of mutations induced by heavy-ion beam irradiation in Arabidopsis thaliana. Plant J. 82, 93–104. doi: 10.1111/tpj.12793
Hooghvorst, I., Nogués, S. (2020). Chromosome doubling methods in doubled haploid and haploid inducer-mediated genome-editing systems in major crops. Plant Cell Rep. 40, 255–270. doi: 10.1007/s00299-020-02605-0
Howie, B., Fuchsberger, C., Stephens, M., Marchini, J., Abecasis, G. R. (2012). Fast and accurate genotype imputation in genome-wide association studies through pre-phasing. Nat. Genet. 44, 8, 955–8, 959. doi: 10.1038/ng.2354
ISAAA (2020). International service for the acquisition of agri-biotech applications. Bt-Cotton Increased Farmers’ produce by 10-Fold in China. Available online at: https://www.isaaa.org/kc/cropbiotechupdate/article/default.asp?ID=1844 (Accessed April 23, 2024).
Ishii, T., Araki, M. (2017). A future scenario of the global regulatory landscape regarding genome-edited crops. GM Crops Food 8, 44–56. doi: 10.1080/21645698.2016.1261787
Jaganathan, D., Ramasamy, K., Sellamuthu, G., Jayabalan, S., Venkataraman, G. (2018). CRISPR for crop improvement: an update review. Front. Plant Sci. 9. doi: 10.3389/fpls.2018.00985
Jägermeyr, J., Müller, C., Ruane, A. C., Elliot, E., Balkovic, J., Castillo, O., et al. (2021). Climate impacts on global agriculture emerge earlier in new generation of climate and crop models. Nat. Food 2 (11), 873–885. doi: 10.1038/s43016-021-00400-y
Jeong, I. S., Aksoy, E., Fukudome, A., Akhter, S., Hiraguri, A., Fukuhara, T., et al. (2013). Arabidopsis C-terminal domain phosphatase-like 1 functions in miRNA accumulation and DNA methylation. PloS One 8, e74739. doi: 10.1371/journal.pone.0074739
Jin, S., Fei, H., Zhu, Z., Luo, Y., Liu, J., Gao, S., et al. (2020a). Rationally designed APOBEC3B Cytosine Base editors with improved specificity. Mol. Cell. 79, 728–740.e726. doi: 10.1016/j.molcel.2020.07.005
Jin, S., Lin, Q., Luo, Y., Zhu, Z., Liu, G., Chen, K., et al. (2021). Genome-wide specificity of prime editors in plants. Nat. Biotechnol. 39, 1292–1299. doi: 10.1038/s41587-021-00891-x
Jørgensen, J. H. (1992). Discovery, characterization and exploitation of Mlo powdery mildew resistance in barley. Euphytica 63, 141–152. doi: 10.1007/BF00023919
Jouanin, A., Schaart, J. G., Boyd, L. A., Cockram, J., Leigh, F. J., Bates, R., et al. (2019). Outlook for coeliac disease patients: towards bread wheat with hypoimmunogenic gluten by gene editing of alpha- and gammagliadin gene families. BMC Plant Biol. 19, 333. doi: 10.1186/s12870-019-1889-5
Kakrana, A., Hammond, R., Patel, P., Nakano, M., Meyers, B. C. (2014). sPARTA: a parallelized pipeline for integrated analysis of plant miRNA and cleaved mRNA data sets, including new miRNA target-identification software. Nucleic Acids Res. 42, e139. doi: 10.1093/nar/gku693
Kante, M., Lindqvist-Kreuze, H., Portal, L., David, M., Gastelo, M. (2021). Kompetitive allele specific PCR (KASP) markers for potato: an effective tool for increased genetic gains. Agron. 11, 2315. doi: 10.3390/agronomy11112315
Kato, M., Masamura, N., Shono, J., Okamoto, D., Abe, T., Imai, S. (2016). Production and characterization of tearless and non-pungent onion. Sci. Rep. 6, 23779. doi: 10.1038/srep23779
Kaur, N., Alok, A., Shivani, K. P., Kaur, N., Awasthi, P., Chaturvedi, S., et al. (2020). CRISPR/Cas9 directed editing of lycopene epsilon-cyclase modulates metabolic fux for betacarotene biosynthesis in banana fruit. Metab. Eng. 59, 76–86. doi: 10.1016/j.ymben.2020.01.008
Kelliher, T., Starr, D., Su, X., Tang, G., Chen, Z., Carter, J., et al. (2019). One-step genome editing of elite crop germplasm during haploid induction. Nat. Biotechnol. 37, 287–292. doi: 10.1038/s41587-019-0038-x
Khanday, I., Skinner, D., Yang, B., Mercier, R., Sundaresan, V. (2019). A male-expressed rice embryogenic trigger redirected for asexual propagation through seeds. Nature 565, 91–95. doi: 10.1038/s41586-018-0785-8
Khassanova, G., Khalbayeva, S., Serikbay, D., Mazkirat, S., Bulatova, K., Utebayev, M., et al. (2023). “SNP genotyping with amplifluor-like method,” in Plant genotyping: methods and protocols, methods in molecular biology. ed. Shavrukov, Y. (New York, NY: Humana), 2638. doi: 10.1007/978-1-0716-3024-2_14
Kieu, N. P., Lenman, M., Wang, E. S., Petersen, B. L., Andreasson, E. (2021). Mutations introduced in susceptibility genes through CRISPR/Cas9 genome editing confer increased late blight resistance in potatoes. Sci. Rep. 11, 4487. doi: 10.1038/s41598-021-83972-w
Kim, Y. G., Cha, J., Chandrasegaran, S. (1996). Hybrid restriction enzymes: zinc finger fusions to Fok I cleavage domain. Proc. Natl. Acad. Sci. U.S.A. 93, 1156–1160. doi: 10.1073/pnas.93.3.1156
Knudsen, S., Wendt, T., Dockter, C., Thomsen, H. C., Rasmussen, M., Jørgensen, M. E, et al. (2021). FIND-IT: Ultrafast mining of genome diversity. bioRxiv. 2021.05.20.444969, 1–22. doi: 10.1101/2021.05.20.444969
Kuang, Y., Li, S., Ren, B., Yan, F., Spetz, C., Li, X., et al. (2020). Base-editing-mediated artificial evolution of osALS1 in planta to develop novel herbicide-tolerant rice germplasms. Mol. Plant 13, 565–572. doi: 10.1016/j.molp.2020.01.010
Kumar, K., Gambhir, G., Dass, A., Tripathi, A. K., Singh, A., Jha, A. K., et al. (2020). Genetically modified crops: current status and future prospects. Planta 251, 91. doi: 10.1007/s00425-020-03372-8
Kumar, D., Venkadesan, S., Prabha, R., Begam, S., Dutta, B., Mishra, D. C., et al. (2024). RiceMetaSys: Drought-miR, a one-stop solution for drought responsive miRNAs-mRNA module in rice. Database baae076, 1–12. doi: 10.1093/database/baae076
Kuppu, S., Ron, M., Marimuthu, M. P. A., Li, G., Huddleson, A., Siddeek, M. H., et al. (2020). A variety of changes, including CRISPR/Cas9-mediated deletions, in CENH3 lead to haploid induction on outcrossing. Plant Biotechnol. J. 18, 2068–2080. doi: 10.1111/pbi.13365
Lacchini, E., Kiegle, E., Castellani, M., Adam, H., Jouannic, S., Gregis, V., et al. (2020). CRISPR-mediated accelerated domestication of African rice landraces. PloS One 15, e0229782. doi: 10.1371/journal.pone.0229782
Laforest, L. C., Nadakuduti, S. S. (2022). Advances in delivery mechanisms of CRISPR gene editing reagents in plants. Front. Genome Ed. 4. doi: 10.3389/fgeed.2022.830178
Lazzarotto, C. R., Malinin, N. L., Li, Y., Zhang, R., Yang, Y., Lee, G., et al. (2020). CHANGE-seq reveals genetic and epigenetic effects on CRISPR-Cas9 genome-wide activity. Nat. Biotechnol. 38, 1317–1327. doi: 10.1038/s41587-020-0555-7
Lee, R. C., Feinbaum, R. L., Ambros, V. (1993). The C. elegans heterochronic gene lin-4 encodes small RNAs with antisense complementarity to lin-14. Cell 75, 843–854. doi: 10.1016/0092-8674(93)90529-Y
Li, J., Jiao, G., Sun, Y., Chen, J., Zhong, Y., Yan, L., et al. (2020b). Modification of starch composition, structure and properties through editing of TaSBEIIa in both winter and spring wheat varieties by CRISPR/Cas9. Plant Biotechnol. J. 19, 937–951. doi: 10.1111/pbi.13519
Li, S., Lin, D., Zhang, Y., Deng, M., Chen, Y., Lv, B., et al. (2022). Genome-edited powdery mildew resistance in wheat without growth penalties. Nature 602, 455–460. doi: 10.1038/s41586-022-04395-9
Li, T., Liu, B., Spalding, M. H., Weeks, D. P., Yang, B. (2012). High-efficiency TALEN-based gene editing produces disease-resistant rice. Nat. Biotechnol. 30, 390–392. doi: 10.1038/nbt.2199
Li, J. F., Norville, J. E., Aach, J., McCormack, M., Zhang, D., Bush, J., et al. (2013). Multiplex and homologous recombination-mediated genome editing in Arabidopsis and Nicotiana benthamiana using guide RNA and Cas9. Nat. Biotech. 31, 688–691. doi: 10.1038/nbt.2654
Li, J., Wang, Z., He, G., Ma, L., Deng, X. W. (2020c). CRISPR/Cas9-mediated disruption of TaNP1 genes results in complete male sterility in bread wheat. J. Genet. Genomics 47, 263–272. doi: 10.1016/j.jgg.2020.05.004
Li, X., Wang, Y., Sha, C., Tian, H., Daqi, F., Benzhong, Z., et al. (2018). Lycopene is enriched in tomato fruit by CRISPR/cas9-mediated multiplex genome editing. Front. Plant Sci. 9. doi: 10.3389/fpls.2018.00559
Li, L., Wu, L. P., Chandrasegaran, S. (1992). Functional domains in FokI restriction endonuclease. Proc. Natl. Acad. Sci. U.S.A. 89, 4275–4279. doi: 10.1073/pnas.89.10.4275
Li, S., Zhang, C., Li, J., Yan, L., Wang, N., Xia, L. (2021). Present and future prospects for wheat improvement through genome editing and advanced technologies. Plant Commun. 2, 100211. doi: 10.1016/j.xplc.2021.100211
Liang, Z., Chen, K., Li, T., Zhang, Y., Wang, Y., Zhao, Q., et al. (2017). Efficient DNA-free genome editing of bread wheat using CRISPR/Cas9 ribonucleoprotein complexes. Nat. Commun. 8, 14261. doi: 10.1038/ncomms14261
Liang, X., Huang, X., Mo, M. (2009). Feasibility analysis of mulberry breeding by aerospace mutation. Guanxy Sericult. 46, 32–36.
Liang, Z., Zhang, K., Chen, K., Gao, C. (2014). Targeted mutagenesis in Zea mays using TALENs and the CRISPR/Cas system. J. Genet. Genom 41, 63–68. doi: 10.1016/j.jgg.2013.12.001
Liu, Y., Li, G., Zhang, Y., Chen, L. (2019b). Current advances on CRISPR/Cas genome editing technologies in plants. J. South China Agric. Univ. 40, 38–49. doi: 10.7671/j.issn.1001-411X.201905058
Liu, L., Xie, Y., Guo, H., Zhao, L., Xiong, H., Gu, J. (2021a). “New mutation techniques for crop improvement in China,” in Mutation breeding, genetic diversity and crop adaptation to climate change. ed Sivasankar, S. (International Atomic Energy Agency), 47–52. doi: 10.1079/9781789249095.000
Liu, Z., Ma, H., Jung, S., Main, D., Guo., L. (2020b). Developmental mechanisms of fleshy fruit diversity in Rosaceae. Annu. Rev. Plant Biol. 71, 547–573. doi: 10.1146/annurev-arplant-111119-021700
Liu, X., Qin, R., Li, J., Liao, S., Shan, T., Xu, R., et al. (2020). A CRISPR–Cas9-mediated domain-specific base-editing screen enables functional assessment of ACCase variants in rice. Plant Biotechnol. J. 18, 13348. doi: 10.1111/pbi.13348
Liu, N., Tu, L., Tang, W., Gao, W., Lindsey, K., Zhang, X. (2014). Small RNA and degradome profiling reveals a role for miRNAs and their targets in the developing fibbers of Gossypium barbadense. TPJ 80, 331–344. doi: 10.1111/tpj.12636
Liu, H., Wang, K., Jia, Z., Gong, Q., Lin, Z., Du, L., et al. (2019a). Efficient induction of haploid plants in wheat by editing of TaMTL using an optimized Agrobacterium-mediated CRISPR system. J. Exp. Bot. 71, 1337–1349. doi: 10.1093/jxb/erz529
Liu, J., Liu, X., Zhang, S., Liang, S., Luan, W., Ma, X. (2021b). TarDB: an online database for plant miRNA targets and miRNA-triggered phased siRNAs. BMC Gen. 22 (1), 348. doi: 10.1186/s12864-021-07680-5
Lizumi, T., Ramankutty, N. (2016). Changes in yield variability of major crops for 1981–2010 explained by climate change. Environ. Res. Lett. 11, 34003. doi: 10.1088/1748-9326/11/3/034003
Long, L., Guo, D. D., Gao, W., Yang, W. W., Hou, L. P., Ma, X. N., et al. (2018). Optimization of CRISPR/Cas9 genome editing in cotton by improved sgRNA expression. Plant Methods 14, 85. doi: 10.1186/s13007-018-0353-0
Lorenzo, C. D., Debray, K., Herwegh, D., Develtere, W., Impens, L., Schaumont, D., et al. (2023). BREEDIT: a multiplex genome editing strategy to improve complex quantitative traits in maize. Plant Cell 35, 218–238. doi: 10.1093/plcell/koac243
Lu, Y., Tian, Y., Shen, R., Yao, Q., Wang, M., Chen, M., et al. (2020). Targeted, efficient sequence insertion and replacement in rice. Nat. Biotechnol. 38, 1402–1407. doi: 10.1038/s41587-020-0581-5
Lv, J., Yu, K., Wei, J., Gui, H., Liu, C., Liang, D., et al. (2020). Generation of paternal haploids in wheat by genome editing of the centromeric histone CENH3. Nat. Biotechnol. 38, 1397–1401. doi: 10.1038/s41587-020-0728-4
Ma, L., Kong, F., Sun, K., Wang, T., Guo, T. (2021). From classical radiation to modern radiation: Past, Present and Future of Radiation mutation breeding. Front. Public Health 9. doi: 10.3389/fpubh.2021.768071
Ma, Z., Ma, L., Zhou, J. (2023). Applications of CRISPR/Cas genome editing in economically important fruit crops: recent advances and future directions. Mol. Hortic. 3, 1. doi: 10.1186/s43897-023-00049-0
Ma, X., Zhang, X., Liu, H., Li, Z. (2020). Highly efficient DNA-free plant genome editing using virally delivered CRISPR-Cas9. Nat. Plants 6, 773–779. doi: 10.1038/s41477-020-0704-5
Madsen, C. K., Brearley, C. A., Harholt, J., Brinch-Pedersen, H. (2024). Optimized barley phytase gene expression by focused FIND-IT screening for mutations in cis-acting regulatory elements. Front. Plant Sci. 15. doi: 10.3389/fpls.2024.1372049
Maheswari, M., Murthy, A. N. G., Shanker, A. K. (2017). “Nitrogen nutrition in crops and its importance in crop quality. Nitrogen nutrition in crops and its importance in crop quality,” in The Indian nitrogen assessment. eds. Abrol, Y. P., Adhya, T. K., Aneja, V. P., Raghuram, N., Pathak, H., Kulshrestha, U., Sharma, C., Singh, B. (Elsevier Inc.), 175–186. doi: 10.1016/B978-0-12-811836-8.00012-4
Mahmoud, L. M., Kaur, P., Stanton, D., Grosser, J. W., Dutt, M. (2022). A cationic lipid-mediated CRISPR/Cas9 technique for the production of stable genome edited citrus plants. Plant Methods 18, 33. doi: 10.1186/s13007-022-00870-6
Malabarba, J., Chevreau, E., Dousset, N., Veillet, F., Moizan, J., Vergne, E. (2020). New strategies to overcome present CRISPR/Cas9 limitations in apple and pear: Efficient Dechimerization and Base Editing. Int. J. Mol. Sci. 22, 319–337. doi: 10.3390/ijms22010319
Marchini, J., Howie, B., Myers, S., McVean, G., Donnelly, P. (2007). A new multipoint method for genome-wide association studies by imputation of genotypes. Nat. Genet. 39, 906–913. doi: 10.1038/ng2088
Maurya, P., Sagore, B., Jain, S., Saini, S., Ingole, A., Meena, R., et al. (2022). Mutational breeding in fruit crops: A review. Pharma. Innov. SP-11, 1631–1637. Available online at: https://www.thepharmajournal.com/archives/2022/vol11issue6S/PartU/S-11-6-177-726.pdf.
Maxwell, D., Hailey, P. (2020). The politics of information and analysis in famines and extreme emergencies: findings from six case studies (Feinstein International Center). Available online at: https://fic.tufts.edu/wp-content/uploads/Politics-of-Information-and-Analysis_6-5-2020.pdf (Accessed 19 October, 2024).
McIntire, J., Dobermann, A. (2023). The CGIAR needs a revolution. GFSI 38, 100712. doi: 10.1016/j.gfs.2023.100712
Ming, M., Ren, Q., Pan, C., He, Y., Zhang, Y., Liu, S., et al. (2020). CRISPR-Cas12b enables efficient plant genome engineering. Nat. Plants 6, 202. doi: 10.1038/s41477-020-0614-6
Mokhtar, M. M., El Allali, A., Hegazy, M. E. F., Atia, M.A.M. (2021). PlantPathMarks (PPMdb): an interactive hub for pathways-based markers in plant genomes. Sci. Rep. 11, 21300. doi: 10.1038/s41598-021-00504-2
Money, D., Gardner, K., Migicovsky, Z., Schwaninger, H., Zhing, G.-Y., Myles, S. (2015). LinkImpute: fast and accurate genotype imputation for nonmodel organisms. G3-GENES GENOM Genet. 5, 2383–2390. doi: 10.1534/g3.115.021667
Monroe, J. G., Srikant, T., Carbonell-Bejerano, P., Becker, B., Lensink, M., Exposito-Alonso, M., et al. (2022). Mutation bias reflects natural selection in Arabidopsis thaliana. Nature 602, 101–105. doi: 10.1038/s41586-021-04269-6
Morin, R. D., O’Connor, M. D., Griffith, M., Kuchenbauer, F., Delaney, F. A., Prabhu, A.-L., et al. (2008). Application of massively parallel sequencing to microRNA profiling and discovery in human embryonic stem cells. Genome Res. 18, 610–621. doi: 10.1101/gr.7179508
Muller, H. J. (1928). The measurement of gene mutation rate in Drosophila, its high variability, and its dependence upon temperature. Genetics 13, 279–357. doi: 10.1093/genetics/13.4.279
Nekrasov, V., Staskawicz, B., Weigel, D., Jones, J. D., Kamoun, S. (2013). Targeted mutagenesis in the model plant Nicotiana benthamiana using Cas9 RNA-guided endonuclease. Nat. Biotech. 31, 691–693. doi: 10.1038/nbt.2655
Nelson, J. W., Randolph, P. B., Shen, S. P., Everette, K. A., Chen, P. J., Anzalone, A. V., et al. (2022). Engineered pegRNAs improve prime editing efficiency. Nat. Biotechnol. 40, 402–410. doi: 10.1038/s41587-021-01039-7
Nielen, S., Forster, B. P., Heslop-Harrison, J. S. (2018). “Mutagen effects in the first generation after seed treatment: biological effects of mutation treatments,” in Manual on mutation breeding, 3rd ed. Eds. Spencer-Lopes, M. M., Forster, B. P., Jankuloski, L. (Food and Agriculture Organization of the United Nations, Rome, Italy), 301.
Nishio, S., Shirasawa, K., Nishimura, R., Takeuchi, Y., Imai, A., Mase, N., et al. (2024). A self-compatible pear mutant derived from g-irradiated pollen carries an 11-Mb duplication in chromosome 17. Front. Plant Sci. 15. doi: 10.3389/fpls.2024.1360185
Nyika, J., Dinka, M. O. (2023). Water challenges in rural and urban sub-saharan africa and their management (SpringerBriefs in Water Science and Technology). doi: 10.1007/978-3-031-26271-5_4
Okada, A., Arndell, T., Borisjuk, N., Sharma, N., Watson-Haigh, N. S., Tucker, E. J., et al. (2019). CRISPR/Cas9-mediated knockout of Ms1 enables the rapid generation of male-sterile hexaploid wheat lines for use in hybrid seed production. Plant Biotechnol. J. 17, 1905–1913. doi: 10.1111/pbi.13106
Oliva, R., Ji, C., Atienza-Grande, G., Huguet-Tapia, J. C., Perez-Quintero, A., Li, T., et al. (2019). Broad-spectrum resistance to bacterial blight in rice using genome editing. Nat. Biotech. 37, 1344–1350. doi: 10.1038/s41587-019-0267-z
Osakabe, Y., Osakabe, K. (2015). Genome editing with engineered nucleases in plants. Plant Cell Physiol. 56, 389–400. doi: 10.1093/pcp/pcu170
Ouyang, X., Hong, X., Zhao, X., Zhang, W., He, X., Ma, W., et al. (2016). Knock out of the PHOSPHATE 2 gene TaPHO2-A1 improves phosphorus uptake and grain yield under low phosphorus conditions in common wheat. Sci. Rep. 6, 29850. doi: 10.1038/srep29850
Pan, W., Cheng, Z., Han, Z., Yang, H., Zhang, W., Zhang, H. (2022b). Efficient genetic transformation and CRISPR/Cas9-mediated genome editing of watermelon assisted by genes encoding developmental regulators. J. Zhejiang Univ. Sci. B. 23, 339–344. doi: 10.1631/jzus.B2200119
Pan, C., Li, G., Malzahn, A. A., Cheng, Y., Leyson, B., Sretenovic, S., et al. (2022a). Boosting plant genome editing with a versatile CRISPR-Combo system. Nat. Plants 8, 513–525. doi: 10.1038/s41477-022-01151-9
Pan, C., Wu, X., Markel, K., Malzahn, A. A., Kundagrami, N., Sretenovic, S., et al. (2021). CRISPR-Act3.0 for highly efficient multiplexed gene activation in plants. Nat. Plants. 7, 942–953. doi: 10.1038/s41477-021-00953-7
Pastwa, E., Neumann, R. D., Mezhevaya, K., Winters, T. A. (2003). Repair of radiation-induced DNA double-strand breaks is dependent upon radiation quality and the structural complexity of double-strand breaks. Radiat. Res. 159, 251–261. doi: 10.1667/0033-7587(2003)159[0251:roridd]2.0.co;2
Peng, T., Teotia, S., Tang, G. A.-O., Zhao, Q. (2019). MicroRNAs meet with quantitative trait loci: small powerful players in regulating quantitative yield traits in rice. WIREs RNA 10, 1556. doi: 10.1002/wrna.1556
Penna, S., Jain, S. M. (Eds.) (2023). Mutation breeding for sustainable food production and climate resilience (Singapure: Springer Nature), 809. doi: 10.1007/978-981-16-9720-3
Pi, M., Hu, S., Cheng, L., Zhong, R., Cai, Z., Liu, Z., et al. (2021). The MADS-box gene FveSEP3 plays essential roles in fower organogenesis and fruit development in woodland strawberry. Hortic. Res. 8, 247. doi: 10.1038/s41438-021-00673-1
Pompili, V., Dalla Costa, L., Piazza, S., Pindo, M., Malnoy, M. (2020). Reduced fre blight susceptibility in apple cultivars using a high-efficiency CRISPR/Cas9- FLP/FRT-based gene editing system. Plant Biotechnol. J. 18, 845–858. doi: 10.1111/pbi.13253
Pray, C. E., Nagarajan, L., Huang, J., Hu, R., Ramaswami, B. (2011). “The impact of bt cotton and the potential impact of biotechnology on other crops in China and India,” in Genetically modified food and global welfare (Frontiers of economics and globalization, vol. 10). Eds. Carter, C. A., Moschini, G., Sheldon, I. (Emerald Group Publishing Limited, Bingley), 83–114. doi: 10.1108/S1574-8715(2011)0000010009
Puchta, H., Dujon, B., Hohn, B. (1993). Homologous recombination in plant cells is enhanced by in vivo induction of double-strand breaks into DNA by a site-specific endonuclease. Nucleic Acids Res. 21, 5034–5040. doi: 10.1093/nar/21.22.5034
Purugganan, M. D., Jackson, S. A. (2021). Advancing crop genomics from lab to field. Nat. Genet. 53, 595–601. doi: 10.1038/s41588-021-00866-3
Quiroz, D., Lensink, M., Kliebenstein, D. J., Monroe, J. G. (2023). Causes of mutation rate variability in plant genomes. Annu. Rev. Plant Biol. 74, 751–775. doi: 10.1146/annurev-arplant-070522-054109
Raimondi, F., Siow, K. M., Wrona, D., Fuster-Garcia, C., Pastukhov, O., Schmitz, M., et al. (2024). Gene editing of NCF1 loci is associated with homologous recombination and chromosomal rearrangements. Commun. Biol. 7, 1291. doi: 10.1038/s42003-024-06959-z
Raza, A., Charagh, S., Karikari, B., Sharif, R., Yadav, V., Mubarik, M. S., et al. (2023). miRNAs for crop improvement. Plant Physiol. Biochem. 201, 107857. doi: 10.1016/j.plaphy.2023.107857
Ren, C., Liu, Y., Guo, Y., Duan, W., Fan, P., Li, S., et al. (2021). Optimizing the CRISPR/Cas9 system for genome editing in grape by using grape promoters. Hortic. Res. 8. doi: 10.3389/fpls.2019.00612
Ren, F., Ren, C., Zhang, Z., Duan, W., Lecourieux, D., Li, S., et al. (2019). Efficiency optimization of CRISPR/Cas9-mediated targeted mutagenesis in grape. Front. Plant Sci. 10. doi: 10.3389/fpls.2019.00612
Roberts, M. G., Dawe, D., Falcon, W. P., Naylor, R. L. (2009). El Niño-Southern Oscillation impacts on rice production in Luzon, the Philippines. J. Appl. Meteorol. Climatol. 48, 1718–1724. doi: 10.1175/2008JAMC1628.1
Rodriguez-Leal, D., Lemmon, Z. H., Man, J., Bartlett, M. E., Lippman, Z. B. (2017). Engineering quantitative trait variation for crop improvement by genome editing. Cell 171, 470–480. doi: 10.1016/j.cell.2017.08.030
Sahu, P. K., Sao, R., Mondal, S., Vishwakarma, G., Gupta, S. K., Kumar, V., et al. (2020). Next generation sequencing based forward genetic approaches for identification and mapping of causal mutations in crop plants: A comprehensive review. Plants 9, 1355. doi: 10.3390/plants9101355
Sallam, A. H., Conley, E., Prakapenka, D., Da, Y., Anderson, J. A. (2020). Improving prediction accuracy using multi-allelic haplotype prediction and training population optimization in wheat. G3 (Bethesda) 10, 2265–2273. doi: 10.1534/g3.120.401165
Sánchez-León, S., Gil-Humanes, J., Ozuna, C. V., Giménez, M. J., Sousa, C., Voytas, D. F., et al. (2018). Low-gluten, nontransgenic wheat engineered with CRISPR/Cas9. Plant Biotechnol. J. 16, 902–910. doi: 10.1111/pbi.12837
Sanei, M., Chen, X. (2015). Mechanisms of microRNA turnover. Curr. Opin. Plant Biol. 27, 199–206. doi: 10.1016/j.pbi.2015.07.008
Shan, Q., Wang, Y., Li, J., Gao, C. (2014). Genome editing in rice and wheat using the CRISPR/Cas system. Nat. Protoc. 9, 2395–2410. doi: 10.1038/nprot.2014.157
Shan, Q., Wang, Y., Li, J., Zhang, Y., Chen, K., Liang, Z., et al. (2013). Targeted genome modification of crop plants using a CRISPR-Cas system. Nat. Biotechnol. 31, 686–688. doi: 10.1038/nbt.2650
Shao, X., Wu, S., Dou, T., Zhu, H., Hu, C., Huo, H., et al. (2020). Using CRISPR/Cas9 genome editing system to create MaGA20ox2 gene-modified semi-dwarf banana. Plant Biotechnol. J. 18, 17–19. doi: 10.1111/pbi.13216
Shelton, A. M., Sarwer, S. H., Hossain, M., Brookes, G., Paranjape, V. (2020). Impact of bt brinjal cultivation in the market value chain in five districts of Bangladesh. Front. Bioeng. Biotechnol. 8. doi: 10.3389/fbioe.2020.00498
Sheoran, S., Kumar, S., Ramtekey, V., Kar, P., Meena, R. S., Jangir, C. K. (2022). Current status and potential of biofortification to enhance crop nutritional quality: and overview. Sustainability 14, 3301. doi: 10.3390/su14063301
Singh, J., Sharma, D., Brar, G. S., Sandhu, K. S., Wani, S. H., Kashyap, R., et al. (2022). CRISPR/Cas tool designs for multiplex genome editing and its applications in developing biotic and abiotic stress-resistant crop plants. Mol. Biol. Rep. 49, 11443–11467. doi: 10.1007/s11033-022-07741-2
Stadler, L. J. (1928a). Mutations in barley induced by x-rays and radium. Science 69, 186–187. doi: 10.1126/science.68.1756.186
Stadler, L. J. (1928b). Genetics effects of x-rays in maize. PNAS 14, 69–75. doi: 10.1073/pnas.14.1.69
Ståhlberg, A., Krzyzanowski, P. M., Egyud, M., Filges, S., Stein, L., Godfrey, T. E. (2017). Simple multiplexed PCR-based barcoding of DNA for ultrasensitive mutation detection by next-generation sequencing. Nat. Protoc. 12, 664–682. doi: 10.1038/nprot.2017.006
Stoddard, B. L. (2014). Homing endonucleases from mobile group I introns: discovery to genome engineering. Mob. DNA 5, 1–16. doi: 10.1186/1759-8753-5-7
Stuecker, M. F., Tigchelaar, M., Kantar, M. B. (2018). Climate variability impacts on rice production in the Philippines. PloS One 13, e0201426. doi: 10.1371/journal.pone.0201426
Su, Z., Bernardo, A., Tian, B., Chen, H., Wang, S., Ma, H., et al. (2019). A deletion mutation in TaHRC confers Fhb1 resistance to Fusarium head blight in wheat. Nat. Genet. 51, 1099–1105. doi: 10.1038/s41588-019-0425-8
Subramanian, A., Qaim, M. (2010). The impact of bt cotton on poor households in rural India. J. Dev. Stud. 46, 2. doi: 10.1080/00220380903002954
Suprasanna, P., Mirajkar, S. J., Bhagwat, S. G. (2015). “Induced mutations and crop improvement,” in Plant biology and biotechnology. Eds. Bahadur, B., Venkat Rajam, M., Sahijram, L., Krishnamurthy, K. (Springer, New Delhi). doi: 10.1007/978-81-322-2286-6_23
Svitashev, S., Schwartz, C., Lenderts, B., Young, J. K., Cigan, A. M. (2015). Cas9-gRNA directed genome editing in maize. Plant Physiol. se169, 931–945. doi: 10.1038/ncomms13274
Tanaka, A., Shikazono, N., Hase, Y. (2010). Studies on biological effects of ions beams on lethality, molecular nature of mutation, mutation rate, and spectrum of mutation phenotype for mutation breeding in higher plants. J. Radiat. Res. 51, 223–233. doi: 10.1269/jrr.09143
Tang, J., Chu, C. (2017). MicroRNAs in crop improvement: fine-tuners for complex traits. Nat. Plants 3, 17077. doi: 10.1038/nplants.2017.77
Tang, G., Yan, J., Gu, Y., Qiao, M., Fan, R., Mao, Y., et al. (2012). Construction of short tandem target mimic (STTM) to block the functions of plant and animal microRNAs. Methods 58, 118–125. doi: 10.1016/j.ymeth.2012.10.006
Teotia, S., Singh, D., Tang, G. (2020). “Technologies to address plant microRNA functions,” in Plant microRNAs concepts and strategies in plant sciences. Eds. Miguel, C., Dalmay, T., Chaves, I. (Springer, Cham.), 25–43. doi: 10.1007/978-3-030-35772-6_2
Teotia, S., Singh, D., Tang, X., Tang, G. (2016). Essential RNA-based technologies and their applications in plant functional genomics. Trends Biotechnol. 34, 106–123. doi: 10.1016/j.tibtech.2015.12.001
Todesco, M., Balasubramanian, S., Cao, J., Ott, F., Sureshkumar, S., Schneeberger, K., et al. (2012). Natural variation in biogenesis efficiency of individual Arabidopsis thaliana microRNAs. Curr. Biol. 22, 166–170. doi: 10.1016/j.cub.2011.11.060
Tripathi, J. N., Ntui, V. O., Shah, T., Tripathi, L. (2021). CRISPR/Cas9-mediated editing of DMR6 orthologue in banana (Musa spp.) confers enhanced resistance to bacterial disease. Plant Biotechnol. J. 19, 1291–1293. doi: 10.1111/pbi.13614
Tripathi, L., Ntui, V. O., Tripathi, J. N. (2020). CRISPR/Cas9-based genome editing of banana for disease resistance. Curr. Opin. Plant Biol. 56, 118–126. doi: 10.1016/j.pbi.2020.05.003
Tsuruoka, C., Suzuki, M., Handle, M. P., Furusawa, Y., Anzai, K., Okayasu, R. (2008). The difference in LET and ion species dependence for induction of initially measured and non-rejoined chromatin breaks in normal human fibroblasts. Radiat. Res. 170, 163–171. doi: 10.1667/RR1279.1
UN-DESA (2022). World population prospects 2022: summary of results. UN DESA/POP/2022/TR/NO. 3. Available online at: https://population.un.org/wpp/Download/Standard/MostUsed/ (Accessed 21 October, 2024).
UNDP (2022). Fostering agritech innovation: learnings from cultiv@te (Singapore: UNDP global centre for technology, innovation and sustainable development 2022). Available online at: file:///C:/Users/aoliveira/Downloads/UNDP-Cultiv@te-FinalReport-June2022-compressed.pdf (Accessed 21 October, 2024).
Urnov, F. D., Rebar, E. J., Holmes, M. C., Zhang, H. S., Gregory, P. D. (2010). Genome editing with engineered zinc finger nucleases. Nat. Rev. Gent. 11, 636–646. doi: 10.1038/ngr2842
van Etten, J., de Sousa, K., Aguilarc, A., Barriosc, M., Steinke, J. (2019). Crop variety management for climate adaptation supported by citizen science. PNAS 116, 4194–4199. doi: 10.1073/pnas.1813720116
Voinnet, O. (2009). Origin, biogenesis, and activity of plant microRNAs. Cell 136, 669–687. doi: 10.1016/j.cell.2009.01.046
Wang, Y., Cheng, X., Shan, Q., Zhang, Y., Liu, J., Gao, C., et al. (2014). Simultaneous editing of three homoeoalleles in hexaploid bread wheat confers heritable resistance to powdery mildew. Nat. Biotechnol. 32, 947–951. doi: 10.1038/nbt.2969
Wang, J. Y., Doudna, J. A. (2023). CRISPR technology: A decade of genome editing is only the beginning. Science 379, eadd8643. doi: 10.1126/science.add86
Wang, J., He, Z., Wang, G., Zhang, R., Duan, R., Gao, P., et al. (2022c). Efficient targeted insertion of large DNA fragments without DNA donors. Nat. Methods 19, 331–340. doi: 10.1038/s41592-022-01399-1
Wang, H., Li, Y., Chern, M., Zhu, Y., Zhang, L., Lu, J., et al. (2021). Suppression of rice miR168 improves yield, flowering time and immunity. Nat. Plants 7, 129–136. doi: 10.1016/j.molp.2021.12.013
Wang, C., Liu, Q., Shen, Y., Hua, Y., Wang, J., Lin, J., et al. (2019). Clonal seeds from hybrid rice by simultaneous genome engineering of meiosis and fertilization genes. Nat. Biotechnol. 37, 283–286. doi: 10.1038/s41587-018-0003-0
Wang, W., Simmonds, J., Pan, Q., Davidson, D., He, F., Battal, A., et al. (2018a). Gene editing and mutagenesis reveal inter-cultivar differences and additivity in the contribution of TaGW2 homoeologues to grain size and weight in wheat. Theor. Appl. Genet. 131, 2463–2475. doi: 10.1007/s00122-018-3166-7
Wang, Z., Wang, S., Li, D., Zhang, Q., Li, L., Zhong, C., et al. (2018b). Optimized paired-sgRNA/Cas9 cloning and expression cassette triggers high-efficiency multiplex genome editing in kiwifruit. Plant Biotechnol. J. 16, 1424–1433. doi: 10.1111/pbi.12884
Wang, C., Ye, J., Tang, W., Liu, Z., Zhu, C., Wang, M., et al. (2013). Loop nucleotide polymorphism in a putative miRNA precursor associated with seed length in rice (Oryza sativa L.). Int. J. Biol. Sci. 9, 578–586. doi: 10.7150/ijbs.6357
Wang, Z. P., Zhang, Z. B., Zheng, D. Y., Zhang, T. T., Li, X. L., Zhang, C., et al. (2022b). Efficient and genotype independent maize transformation using pollen transfected by DNA-coated magnetic nanoparticles. J. Integr. Plant Biol. 64, 1145–1156. doi: 10.1111/jipb.13263
Wightman, B., Ha, I., Ruvkun, G. (1993). Posttranscriptional regulation of the heterochronic gene lin-14 by lin-4 mediates temporal pattern formation in C. elegans. Cell 75, 855–862. doi: 10.1016/0092-8674(93)90530-4
Wilson, C., Chen, P. J., Miao, Z., Liu, D. R. (2020). Programmable m(6) A modifcation of cellular RNAs with a Cas13-directed methyltransferase. Nat. Biotechnol. 38, 1431–1440. doi: 10.1038/s41587-020-0572-6
Woo, J. W., Kim, J., Kwon, S. I., Corvalán, C., Cho, S. W., Kim, H., et al. (2015). DNA-free genome editing in plants with preassembled CRISPR-Cas9 ribonucleoproteins. Nat. Biotech. 33, 1162–1164. doi: 10.1038/nbt.3389
Xu, R., Liu, X., Li, J., Qin, R., Wei, P. (2021). Identification of herbicide resistance OsACC1 mutations via in planta prime-editing-library screening in rice. Nat. Plants 7, 888–892. doi: 10.1038/s41477-021-00942-w
Xu, R., Yang, Y., Qin, R., Li, H., Qiu, C., Li, L., et al. (2016). Rapid improvement of grain weight via highly efficient CRISPR/Cas9-mediated multiplex genome editing in rice. JGG 43, 529–532. doi: 10.1016/j.jgg.2016.07.003
Yadav, A., Mathan, J., Dubey, A. K., Singh, A. (2024). The emerging role of non-coding RNAs (ncRNAs) in plant growth, development, and stress response signaling. Non-Coding RNA 10, 13. doi: 10.3390/ncrna10010013
Yamatani, H., Kohzuma, K., Nakano, M., Takami, T., Kato, Y., Hayashi, Y., et al. (2018). Impairment of Lhca4, a subunit of LHCI, causes high accumulation of chlorophyll and the stay-green phenotype in rice. J. Exp. Bot. 69, 1027–1035. doi: 10.1093/jxb/erx468
Yang, Z., Hui, S., Lv, Y., Zhang, M., Chen, D., Tian, J., et al. (2022). miR395-regulated sulfate metabolism exploits pathogen sensitivity to sulfate to boost immunity in rice. Mol. Plant 15, 671–688. doi: 10.1016/j.molp.2021.12.013
Yoon, D.-K., Sugamani, M., Ishiyama, K., Kagawa, T., Tanaka, M., Nagao, R., et al. (2022). The gs3allele from a large-grain rice cultivar, Akita63, increases yield and improves nitrogen-use efficiency. Plant Direct 6, e417. doi: 10.1002/pld3.417
Yu, H., Lin, T., Meng, X., Du, H., Zhang, J., Liu, G., et al. (2021). A route to de novo domestication of wild allotetraploid rice. Cell 184, 1156–1170. doi: 10.1016/j.cell.2021.01.013
Zhang, Y., Bai, Y., Wu, G., Zou, S., Chen, Y., Gao, C., et al. (2017). Simultaneous modification of three homoeologs of TaEDR1 by genome editing enhances powdery mildew resistance in wheat. Plant J. 91, 714–724. doi: 10.1111/tpj.13599
Zhang, C., Ding, Z., Wu, K., Yang, L., Li, Y., Yang, Z., et al. (2016a). Suppression of jasmonic acid-mediated defense by viral-inducible microRNA319 facilitates virus infection in rice. Mol. Plant 9, 1302–1314. doi: 10.1016/j.molp.2016.06.014
Zhang, Y., Li, D., Zhang, D., Zhao, X., Cao, X., Dong, L., et al. (2018b). Analysis of the functions of TaGW2 homoeologs in wheat grain weight and protein content traits. Plant J. 94, 857–866. doi: 10.1111/tpj.13903
Zhang, Y., Liang, Z., Zong, Y., Wang, Y., Liu, J., Chen, K., et al. (2016). Efficient and transgene-free genome editing in wheat through transient expression of CRISPR/Cas9 DNA or RNA. Nat. Commun. 7, 12617. doi: 10.1038/ncomms12617
Zhang, R., Liu, J., Chai, Z., Chen, S., Bai, Y., Zong, Y., et al. (2019a). Generation of herbicide tolerance traits and a new selectable marker in wheat using base editing. Nat. Plants 5, 480–485. doi: 10.1038/s41477-019-0405-0
Zhang, Y., Malzahn, A. A., Sretenovic, S., Qi, Y. (2019). The emerging and uncultivated potential of CRISPR technology in plant science. Nat. Plants. 5, 778–794. doi: 10.1038/s41477-019-0461-5
Zhang, B., Unver, T. (2018). A critical and speculative review on microRNA technology in crop improvement: Current challenges and future directions. Plant Sci. 274, 193–200. doi: 10.1016/j.plantsci.2018.05.031
Zhang, Y., Wang, H., Du, Y., Zhang, L., Li, X., Guo, H., et al. (2024). Biological responses of an elite centipedegrass [Eremochloa ophiuroides (Munro) Hack.] cultivar (Ganbei) to carbon ion beam irradiation. Front. Plant Sci. 15. doi: 10.3389/fpls.2024.1433121
Zhang, S., Wu, S., Hu, C., Yang, Q., Dong, T., Sheng, O., et al. (2022). Increased mutation efficiency of CRISPR/Cas9 genome editing in banana by optimized construct. Peer J. 10, e12664. doi: 10.7717/peerj.12664
Zhang, J., Zhang, H., Srivastava, A. K., Pan, Y., Bai, J., Fang, J., et al. (2018a). Knockdown of rice microRNA166 confers drought resistance by causing leaf rolling and altering stem xylem development. Plant Physiol. 176, 2082–2094. doi: 10.1104/pp.17.01432
Zhao, H., Guo, H., Gu, J., Zhao, S., Li, J., Liu, L. (2010). A temperature-sensitive winter wheat chlorophyll mutant derived from space mutated. J. Nucl. Agric. 24, 1110–1116.
Zhao, M., Liu, B., Wu, K., Ye, Y., Huang, S., Wang, S., et al. (2015). Regulation of osmiR156h through alternative polyadenylation improves grain yield in rice. PloS One 10, e0126154. doi: 10.1371/journal.pone.0126154
Zhaozhi, L., Xiaojie, H., XiaoXian, L., Chunhong, Y., Downes, S., Parry, H., et al. (2022). Quo vadis Bt cotton: a dead-end trap crop in the post Bt era in China? Entomol. Gen. 42, 649–654. doi: 10.1127/entomologia/2021/1355
Zheng, X., Tang, X., Wu, Y., Zheng, X., Zhou, J., Han, Q., et al. (2024). An efficient CRISPR-Cas12a-mediated MicroRNA knockout strategy in plants. Plant Biotech. J., 1–13. doi: 10.1111/pbi.14484
Zhong, Y., Blennow, A., Kofoed-Enevoldsen, O., Jiang, D., Hebelstrup, K. H. (2019b). Protein Targeting to Starch 1 is essential for starchy endosperm development in barley. J. Exp. Bot. 70, 485–496. doi: 10.1093/jxb/ery398
Zhong, Y., Liu, C., Qi, X., Jiao, Y., Wang, D., Wang, Y., et al. (2019a). Mutation of ZmDMP enhances haploid induction in maize. Nat. Plant 5, 575–580. doi: 10.1038/s41477-019-0443-7
Zhou, J., Wang, G., Liu, Z. (2018). Efficient genome editing of wild strawberry genes, vector development and validation. Plant Biotechnol. J. 16, 1868–1877. doi: 10.1111/pbi.12922
Keywords: agriculture, marker genotyping, mutation breeding, NGT, CRISPR, legislation, climate-smart cultivars
Citation: Garcia-Oliveira AL, Ortiz R, Sarsu F, Rasmussen SK, Agre P, Asfaw A, Kante M and Chander S (2025) The importance of genotyping within the climate-smart plant breeding value chain – integrative tools for genetic enhancement programs. Front. Plant Sci. 15:1518123. doi: 10.3389/fpls.2024.1518123
Received: 29 October 2024; Accepted: 25 November 2024;
Published: 06 February 2025.
Edited by:
Simardeep Kaur, The ICAR Research Complex for North Eastern Hill Region (ICAR RC NEH), IndiaReviewed by:
Sonu Shekhawat, Indian Agricultural Research Institute (ICAR), IndiaCopyright © 2025 Garcia-Oliveira, Ortiz, Sarsu, Rasmussen, Agre, Asfaw, Kante and Chander. This is an open-access article distributed under the terms of the Creative Commons Attribution License (CC BY). The use, distribution or reproduction in other forums is permitted, provided the original author(s) and the copyright owner(s) are credited and that the original publication in this journal is cited, in accordance with accepted academic practice. No use, distribution or reproduction is permitted which does not comply with these terms.
*Correspondence: Ana Luísa Garcia-Oliveira, YS5vbGl2ZWlyYUBjZ2lhci5vcmc=
†ORCID: Ana Luísa Garcia-Oliveira, orcid.org/0000-0001-8561-4172
Rodomiro Ortiz, orcid.org/0000-0002-1739-7206
Fatma Sarsu, orcid.org/0000-0003-2771-7418
Søren K. Rasmussen, orcid.org/0000-0001-6169-2504
Paterne Agre, orcid.org/0000-0003-1231-2530
Asrat Asfaw, orcid.org/0000-0002-4859-0631
Moctar Kante, orcid.org/0000-0003-4669-3132
Subhash Chander, orcid.org/0000-0001-5353-6317
Disclaimer: All claims expressed in this article are solely those of the authors and do not necessarily represent those of their affiliated organizations, or those of the publisher, the editors and the reviewers. Any product that may be evaluated in this article or claim that may be made by its manufacturer is not guaranteed or endorsed by the publisher.
Research integrity at Frontiers
Learn more about the work of our research integrity team to safeguard the quality of each article we publish.