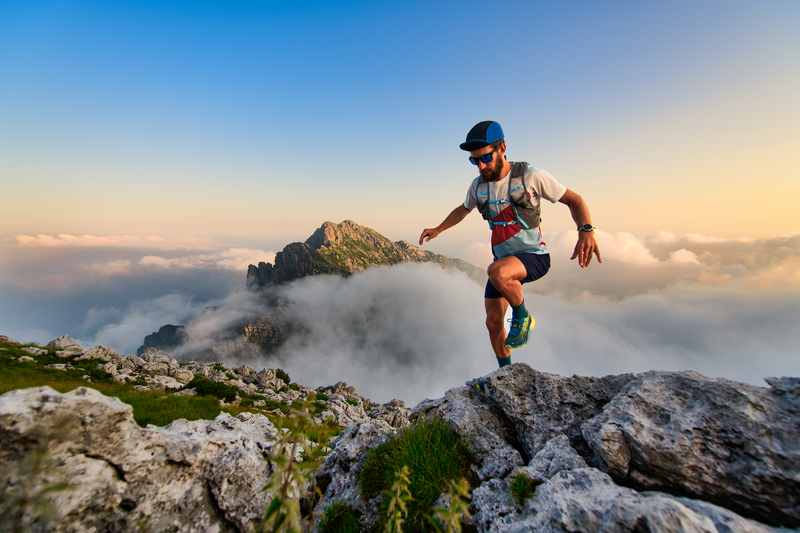
95% of researchers rate our articles as excellent or good
Learn more about the work of our research integrity team to safeguard the quality of each article we publish.
Find out more
ORIGINAL RESEARCH article
Front. Plant Sci. , 23 December 2024
Sec. Plant Breeding
Volume 15 - 2024 | https://doi.org/10.3389/fpls.2024.1507968
This article is part of the Research Topic From Classical Breeding to Modern Biotechnological Advancement in Horticultural Crops - Trait Improvement and Stress Resilience, Volume II View all 16 articles
Introduction: Chinese kale (Brassica oleracea var. alboglabra), is an annual herb belonging to the Brassica genus of Cruciferae, and is one of the famous specialty vegetables of southern China. Some varieties show bright green leaf (BGL) traits and have better commerciality. However, the genes responsible for this trait remain unidentified.
Methods: In this study, gene mapping was measured by BSR-Seq and molecular marker analysis. Gene expression analysis was performed qRT-PCR. Cloning and sequence analysis of candidate genes were also performed.
Results: Genetic analysis revealed that the bright green leaf trait is a dominant trait governed by a single pair of genes. BSR-seq and molecular marker validation mapped the candidate interval to about 1.5 Mb on chromosome C8. After expanding the BC1 population and analyzing recombinant individuals, the interval was refined to approximately 102 kb on chromosome C8 (50,787,908- 50,890,279 bp). There are 24 genes in this region, and after annotation and expression analysis, BolC8t52930H (BoCER1.C8), associated with wax synthesis, emerged as a key candidate for BoBGL. We cloned this gene from both parents, revealing significant differences in their promoter regions. A co-segregation primer was subsequently developed and validated in a segregated population, with results consistent with expectations.
Discussion: The gene BoCER1.C8 is a potential candidate for controlling the bright green leaf trait in Chinese kale, and its function needs to be validated next. Mapping and cloning this gene is crucial to understanding wax synthesis regulation and developing new bright green leafy varieties of Chinese kale.
Chinese kale (Brassica oleracea var. alboglabra, 2n=18), is an annual herb in the crucifer family. It is one of the famous specialty vegetables of South China. The main edible organs of Chinese kale are the tender, fleshy stalk and the tender leaves. The flesh is crisp, tender and sweet, rich in nutrients, and popular with consumers (Zeng et al., 2021; Zhao et al., 2021). Bright green Chinese kale, which features bright green and shiny leaves and stems, is a better-selling commodity than regular Chinese kale and is favored by consumers. However, the genes associated with the bright green leaf (BGL) properties of Chinese kale have not been mapped and cloned, since this an important trait, we would like to identify the genes controlling the trait, which will be useful for breeding.
Studies have shown that the appearance of bright green leaves is mostly caused by the reduction or loss of wax in the plant epidermis (Wang et al., 2023; Liu et al., 2017b). There have been significant recent advances in the study of wax-related genes and transport regulatory mechanisms in plants. Many genes involved in wax biosynthesis and metabolism have been cloned. These genes are involved in the three wax metabolism related processes of plant wax synthesis, transport processes, and regulatory pathways. The genes involved in wax biosynthesis include CER1, CER2, CER3, CER4, CER6, CER8, CER10, KCS1, KCS2, KCR1/KCR2, LACS2, FATB, MAH1, WAX2, etc (Lee and Suh, 2015, 2022). Genes such as CER5/ABCG12, PEC1/ABCG32 and WBC11 are involved in wax transport (McFarlane et al., 2010). Additionally, key regulatory genes like WIN1/SHN, MYB16, MYB30, MYB94, MYB96, MYB106, WRINKLED4, DEWAX, WAR3 and WAR4 have been highlighted in studies (Kannangara et al., 2007; Seo et al., 2011; Go et al., 2014; Bernard and Joubès, 2013; Lee and Suh, 2015, 2022).
In Brassica plants, most of the studies on wax synthesis and regulation focus on genetic analysis, and only a few wax-related genes are mapped or cloned. An example is BnaA.GL, a gene homologous to CER1 in Arabidopsis that is responsible for wax deficiency in B.napus, located at the end of chromosome A9 (Pu et al., 2013). The wax genes were located on chromosome A1 or A9, and the candidate genes included CER1 (Yang et al., 2022a), CER2 (Zhang et al., 2013; Li et al., 2023) and CER60 (Yang et al., 2022b). Fine mapping of some bright leaf genes in cabbage has also been reported. Candidate genes include CER1 homologous genes (Liu et al., 2017b; Ji et al., 2018); CER2 homologous genes (Han et al., 2021; Ji et al., 2021) and CER4 homologous gene (Liu et al., 2017a). Therefore, in general, there are few wax-related genes currently mapped and cloned in Brassica crops, which need to be further explored.
In this study, the bright green leaf mutant material ‘BGL’ of Chinese kale was used as the research object, which was crossed with the wild-type material to obtain the F1 and BC1 segregating population. Through bulk segregant analysis RNA sequencing (BSR-seq) and fine mapping, we identified the genes responsible for the bright green leaf trait. The establishment of co-segregated InDel markers will be helpful for molecular marker-assisted breeding of leaf type at seedling stage. This basic work will help to further analyze the wax synthesis regulation pathway of Chinese kale and the cultivation of new varieties of bright green leaf Chinese kale is of great significance.
The ‘BGL’ mutant (P1), characterized by its sterility and bright green leaves, was utilized as one parent, whereas the ‘WT’ parent (P2) exhibited fertility and a standard leaf phenotype (Figure 1). These two parental lines were crossed to produce the F1 hybrid generation (BGL×WT). Subsequently, Fifty F1 plants of bright green leaf type were used to obtain BC1F1 (F1×WT) generation. Leaf phenotypes of the F1 and BC1F1 generations were observed and recorded at the 7-leaf seedling stage. The bright green leaf bulk (Bulk-A) and the ordinary leaf bulk (Bulk-B) were selected from the BC1F1 generation based on leaf phenotype. An equal quantity of young leaves was promptly harvested, immediately placed in liquid nitrogen, and stored at -80°C for subsequent RNA extraction. The experimental materials were cultivated in a basic greenhouse at Gannan Normal University (N25°47′, E114°52′).
Figure 1. Phenotype and wax content of the studied materials. (A) Parent P1 (BGL); (B) Parent P2 (WT); (C) Comparison of leaf phenotypes between the two parents, scale = 5cm; (D) Appearance of leaf surface of BGL by SEM; (E) Appearance of leaf surface of WT by SEM; (F) Total wax content of BGL and WT, **Represents p <0.01.
Total RNA was extracted utilizing the Trizol Reagent (Invitrogen Life Technologies) and subsequently quantified using both the NanoDrop spectrophotometer (Thermo Scientific) and the Qubit 4.0 fluorometer. Quality control was conducted employing the Agilent 2100/4200 system (Agilent Technologies). Equivalent amounts of purified RNA from each of the 30 samples in Bulk-A and Bulk-B were pooled for RNA library construction. The libraries were constructed following the manufacturer’s protocols, and sequencing was carried out on the HiSeq 4000 platform. Raw sequencing reads were subjected to quality control using Fastp software (version 0.19.7). Clean reads were subsequently aligned to the B. oleracea reference genome (Braol_HDEM_V1.0) using BWA software. The BoBGL locus was mapped using MMAPPR software (Hill et al., 2013), briefly, Bam files were processed with the mpileup tool from the SAMtools package to generate a pileup file, which organizes the data into a position-based format displaying sequenced bases at each location. Reads were filtered based on specified minimum base and mapping quality thresholds, and allele frequencies were calculated. The resulting data were then analyzed in R for signal processing and peak identification. R plots the Loess fits and lists SNPs enriched in the mutant pool (allele frequency >0.75, Euclidean distance >0.5). These SNPs are filtered for nonsynonymous variants using Alleler and gene annotations, with results exported to a file. MMAPPR uses these optimized default values. The association analysis was conducted using the Δ(SNP-index) method with the identified SNPs and InDels.
Mature leaves were utilized for the quantification of wax content. Leaves of appropriate size were immersed in 15 mL of chloroform for 30 seconds to extract the total epidermal wax mixture. Subsequently, 20 μg of n-tetracosane (C24) was added as an internal standard. The extract was then dried under a nitrogen (N2) stream to a volume of 1 mL and transferred to a pre-weighed and recorded gas chromatography (GC) vial. Following the evaporation of the remaining liquid under nitrogen, 100 μL of trimethylsilyl reagent was added, and the sample was derivatized in a 70°C incubator for 60 minutes. All wax samples were converted into trimethylsilyl derivatives, subsequently dried using nitrogen gas, and then weighed. The total quantity of cuticular wax was normalized to unit leaf surface area. The experiment was conducted with three biological replicates.
Fresh mature leaf tissue was collected and fixed using a 2.5% glutaraldehyde solution for 2-4 hours. Subsequently, the samples were rinsed three times with 0.1 M phosphate buffer (pH 7.0) and then treated with a 1% glutaric acid solution in 0.1 M phosphate buffer (pH 7.0) for 1-3 hours. This was followed by three additional rinses with 0.1 M phosphate buffer (pH 7.0). The samples were then subjected to a dehydration series using ethanol concentrations of 50%, 70%, 80%, 90%, 95%, and 100% (twice) for 15 minutes each. After dehydration, the samples were soaked in a 1:1 mixture of 100% ethanol and isoamyl acetate for 30 minutes, followed by an overnight soak in pure isoamyl acetate. The samples were subsequently dried and transferred to a preparative chamber for vacuum coating. The samples were photographed using a scanning electron microscope (SEM) system (FEI Quanta 450).
Genomic DNA was extracted from young leaves using the CTAB method and diluted to 50 ng/µL with 1× TE solution. The InDels in the candidate interval from BSR-seq were used to design markers to narrow down the BoBGL locus. Primers were designed utilizing Primer3 software, the sequences corresponding to the candidate intervals were extracted, with the product size specified to range from 180 to 350 bp, allowing for one mismatched base. Primers were synthesized by Wuhan Tianyi Huayu Gene Technology Co., Ltd (Wuhan, China). The 10 µL PCR mixture included 2 µL of template DNA (50 ng/µL), 5 µL of 2× FineTaq® PCR SuperMix, 0.5 µL of each primer (10 µM), and 2 µL of sterilized ddH2O. The PCR program was: 94°C for 5min; 9 cycles of 94°C for 30s, 60°C for 30s (decreasing by 0.5°C per cycle), 72°C for 30s; 30 cycles of 94°C for 30s, 55°C for 30s, 72°C for 30s; and a final elongation at 72°C for 10min. PCR products were then separated on 6% polyacrylamide gel electrophoresis (PAGE).
The DNA of 940 BC1F1 recessive plants was subjected to amplification using polymorphic primers, followed by the screening of recombinant plants. Subsequently, the genetic distance between the markers was determined, and a genetic map was constructed. Two markers proximal to the target site were identified. A physical map was then developed by utilizing the physical locations of these two markers on the B. oleracea reference genome (Braol_HDEM_V1.0) to delineate the candidate interval. Genes in the target intervals were annotated, and key ones were analyzed for expression via qRT-PCR. Specific primers for the BoBGL gene were used to amplify sequences from two parental lines, BGL and WT, using Primer5. The PCR products were cloned into the pMDTM19-T vector and transformed into DH5α cells. The candidate gene was then sequenced using the Sanger method. Subsequently, DNA sequencing reactions were conducted by Wuhan Tianyi Huayu Gene Technology Co., Ltd (Wuhan, China). The primer sequences utilized for the molecular markers in this study are detailed in Supplementary Table S1.
P1 is a plant with bright green leaves (Figure 1A), and P2 is a plant with ordinary leaves (Figure 1B), and the wax content is significantly reduced in P2 (Figure 1F). The leaf surface of the hybrid F1 plants is characterized by bright green leaves. For the BC1F1 (F1×WT) offspring, the number of ordinary leaves and bright green leaves is 1:1. Furthermore, the number of common leaves and bright green leaves in BC2F1 was also 1:1 (Table 1). It can be judged that the bright green leaf trait of cabbage is completely apparent inheritance controlled by a pair of alleles.
Table 1. Genetic studies were conducted on the bright green leaf plants within various segregating populations.
The BoBGL locus was mapped using a mutation mapping pipeline for pooled RNA-seq (Hill et al., 2013). A total of 122,472,880 and 223,777,954 clean reads produced from the Bulk-A and Bulk-B RNA samples were mapped to the B. oleracea reference genome (Braol_HDEM_V1.0/, http://brassicadb.cn/#/Download/), respectively (Supplementary Table S1). Over 94% of the reads were mapped to unigenes, resulting in the discovery of 247,090 SNPs and 68,667 InDels between the two bulks. SNP-index was calculated according to the SNP of two progeny pools obtained by sequencing, which was used to map the gene of bright green leaf trait in Chinese kale. The Δ(SNP-index) of the two mixed pools was analyzed. The results showed that when the confidence level was 99%, the three regions C5, C7 and C8 distributed on the chromosome showed extremely significant peaks above the critical value level (Figure 2). The significant correlation intervals are: C5 (24.51-26.47Mb, ~1.96Mb), C7 (1.45-2.45Mb, ~1.00Mb), and C8 (49.36-51.42Mb, ~2.06Mb) (Table 2).
Figure 2. The chromosome-wise distribution of ΔSNP index values. Chromosome names (C1-C8) on the x-axis and colored points representing each SNP locus’s ΔSNP-index value. The black line shows the fitted ΔSNP-index value, and the red line marks the significance threshold.
Subsequently, we used the molecular marker method to further verify the candidate interval. Firstly, we examined the phenotypes (bright green leaves and ordinary leaves) of a BC1 segregating population containing 186 individuals. Secondly, we developed some InDel markers covering all candidate intervals. Genotyping of the 186 BC1 segregating plants was conducted with 9 pairs of polymorphic InDel markers (Supplementary Table S2), resulting in the creation of a genetic linkage map. The findings indicated that the gene responsible for the bright green leaf gene, BoGBL, was situated on chromosome C8 between markers INDEL109 and INDEL104 (Supplementary Figure S1), with positions on the reference genome at 49.43 Mb and 50.92 Mb, as shown in Figure 3A. Therefore, the bright green leaf gene BoGBL can be preliminarily located in about 1.5 Mb interval between 49.43Mb and 50.92Mb on chromosome C8 of B.oleracea.
Figure 3. Mapping of the candidate gene controlling the bright green leaf trait. (A) BoBGL was initially located in the range of 49.88-50.92 Mb. (B) The positioning interval for the candidate region was narrowed using three InDel markers, and the flanking markers were Indel150 and Indel78. (C) The candidate interval contained 24 genes.
In order to further narrow the candidate interval, combined with the results of preliminary mapping, 940 BC1 populations (all ordinary leaf type) were screened for recombinant plants based on flanking markers (INDEL109, INDEL104), and a total of 81 recombinant plants were obtained. At the same time, four polymorphic InDel markers were used to genotype 81 recombinant plants and finely map the bright green leaf gene BoBGL in B.oleracea. The BoBGL locus was more precisely identified to be within a 102-kb region on chromosome C8 of the B. oleracea reference genome, located between the markers INDEL150 (50 787 908bp) and INDEL78 (50 890 279bp) (Figure 3B; Supplementary Figure S1).
According to the location of the BoBGL locus, there were 24 genes within these 102-kb region in the reference B. oleracea genome (Figure 3C). To identify the key candidates, we conducted gene annotation on 24 genes contained in this region. The results showed that among the 24 genes, only the candidate gene BolC8t52930H, named as BoCER1.C8, was related to the biosynthesis of plant cuticle wax (Table 3). The gene is highly homologous to the Arabidopsis wax synthesis-related gene AtCER1 (Table 3). In addition, qRT-PCR showed the gene BolC8t52930H was significantly differentially expressed in the two parents (Figure 4). In summary, the gene BolC8t52930H was identified as a key candidate gene for BoBGL.
Table 3. The annotated genes in the candidate genomic regions associated with the bright green leaf trait.
Figure 4. qRT-PCR validation of candidate gene. BolC8t52930H expression levels determined by qRT-PCR between BGL and WT. BoActin is used as an equal loading control. Error bars indicate standard errors from three biological replicates. ** denotes significant differences (p<0.01).
To confirm our speculation, we cloned the genomic sequence and coding sequence of BolC8t52930H from two parental plants. The findings indicated that the coding sequences in the parental organisms were completely consistent, so as the protein sequence, despite minor variations in their genomic sequences (Figure 5A; Supplementary Figure S2). We also cloned the promoter sequence of BolC8t52930H from about 3K before the onset codon from both parents. There are some differences between the two promoters in the range of -280bp to -1500bp, with the largest being -282bp to -325bp (Figure 5A). The interval of about 40bp is significantly different from the insertion and deletion, and the differences in other intervals are mainly SNPs (about 30 ones), and 4 gaps (Figure 5A; Supplementary Figure S2). In summary, there are significant sequence differences in promoter regions between the two parents, and therefore, we speculate that variations in the promoter region of BolC8t52930H may be responsible for the changes in gene expression in bright green leaf material.
Figure 5. The structure of BolC8t52930H in the two parents and verification of co-segregation in BC1 generation. (A) Gene structure of BolC8t52930H and its mutation in BGL. Yellow boxes represent promoters, green boxes represent exons, black boxes between green boxes represent introns, green triangles indicate insert mutations, brown triangles indicate delete mutations, and dashed lines indicate SNP mutations. (B) The developed polymorphic InDel marker was used to analyze the co-segregation of BC1 genotypes.
Next, we developed an polymorphic InDel marker using the sequence differences in the interval (-282bp to -325bp) with the largest difference in gene regions between parents to analyze whether BoBGL is co-segregated with bright green leaf traits. As we expected, the co-segregation analysis of 184 BC1 plants showed that their genotypes were completely co-segregated with their phenotypes, that is, bright green leaf individuals can amplify a 184bp fragment, while ordinary leaf individuals cannot (Figure 5B). Therefore, we developed a useful functional marker that can be used for marker-assisted selection of bright green leaf traits in B. oleracea.
Bright green leaf Chinese kale has shiny leaves and stems that are more commercially viable than ordinary Chinese kale. This study revealed a monogenic inheritance pattern for the bright green leaf trait in Chinese kale. Through BSR-seq and fine mapping, we mapped the gene for the bright green leaf trait of Chinese kale to a 102-kb region of 50 787 908- 50 890 279 bp on the C8 chromosome of B.oleracea. There are 24 genes annotated in this region, of which only BolC8t52930H is related to wax synthesis. It is a homologous gene of AtCER1 in A.thaliana, so it is named BoCER1.C8. Gene expression analysis also confirmed that it showed strong differential expression between the two parents. Cloning BoCER1.C8 revealed notable variations in sequence between the parental strains in the promoter regions. Finally, we developed an InDel marker and used it to verify the co-segregation of BoCER1.C8 with bright green leaf traits. Finally, we concluded that BoCER1.C8 is the gene corresponding to the bright green leaf trait in Chinese kale.
Due to the quick advancement of high-throughput sequencing technology, BSR-seq has the potential to serve as a valuable resource for initial gene mapping. BSR-seq is a commonly utilized technique in various crops for quickly and cost-effectively mapping genes of interest in plants. For example, wheat (Shi et al., 2021; Wang et al., 2022), soybean (Huang et al., 2024), Sugarcane (Cheng et al., 2022; Wu et al., 2022), the Black Lemma (Liu et al., 2023), foxtail millet (Tian et al., 2022), etc. In this study, we used BSR-seq to quickly lock the gene BoBGL, which controls the bright leaf trait, in the interval of about 1.5-Mb on the C8 chromosome, and obtained a large number of inter-parental SNP and InDel variations for marker development. This strategy greatly improves the efficiency of locating genes of interest.
The generation of bright leaf traits is usually caused by the loss or reduction of wax. In Brassica plants, some bright color trait genes have been mapped or cloned, which are basically related to wax synthesis and regulation (Pu et al., 2013; Zhang et al., 2013; Liu et al., 2017c, 2017; Ji et al., 2021; Han et al., 2021; Yang et al., 2022b; Li et al., 2023; Wang et al., 2023). Several genes associated with bright leaf characteristics in B.oleracea have been identified through mapping or cloning, such as Bol018504 (CER1) (Liu et al., 2017b, 2017c; Ji et al., 2018), Bo1g039030 (BoCER2) (Ji et al., 2021; Han et al., 2021), Bol013612 (CER4) (Liu et al., 2017a), and Bol026949 (Wang et al., 2023). In the current study, the primary candidate gene was identified on the CER1 gene located on the C8 chromosome, a finding that aligns with several previous studies (Liu et al., 2017b, 2017c; Ji et al., 2018). However, our research proposes that the bright leaf trait in Chinese kale is regulated by a dominant gene, a contrast to other reports suggesting control by a recessive gene (Liu et al., 2017b, 2017c; Ji et al., 2018). Notably, a 2,722-bp insertion in the first intron of Bol018504 (CER1) in the glossy mutant was found to result in an alteration in the RNA splice site (Liu et al., 2017c). Furthermore, the fourth intron of BoCER1 in the glossy mutant was observed to incorporate a 252-bp insertion (Ji et al., 2018). In this study, we found some base variations in the promoter region, which may lead to dominant inheritance, but the specific mechanism is not clear. Dominance is a genetic interaction between alleles at a locus, often included in gene expression models based on molecular networks. It can occur at various integration levels, from allele-specific expression biases to organismal traits, involving diverse molecular interactions and physiological and developmental characteristics (Billiard et al., 2021). For this study, a possible mechanism is the trans-action of sRNA encoded by the flanking region of the dominant allele to induce transcriptional silencing of the recessive allele (Tarutani et al., 2010).
The gene CER1 is responsible for producing an enzyme that creates alkanes, potentially interacting with CER3 and various cytochrome b5 (CYTB5s) isoforms to facilitate the production of extremely long alkanes (Bernard et al., 2012; Pascal et al., 2019; Bourdenx et al., 2011). In Arabidopsis, the overexpression of the CER1 gene has been shown to enhance the biosynthesis of very long-chain alkanes (Bourdenx et al., 2011). The cer1-1 mutant, on the other hand, is distinguished by a pronounced reduction in the products of the alkane formation pathway, including alkanes, secondary alcohols, and ketones, with a concurrent increase in the corresponding aldehydes (Aarts et al., 1995). Furthermore, the role of CER1 in the synthesis of long-chain alkanes has been substantiated in other plant species as well, such as cucumber (Wang et al., 2015), rice (Ni et al., 2018), Brachypodium distachyon (Wu et al., 2019), and tomato (Wu et al., 2022). In Brassica species, CER1 was also proposed as the candidate gene for the glassy trait (Pu et al., 2013; Liu et al., 2017b, 2017c; Ji et al., 2018; Yang et al., 2022a). Specifically, in B.napus, the gene BnaA.GL has been precisely mapped to chromosome A09, with BnCER1 identified as the candidate gene (Pu et al., 2013). Furthermore, in B.rapa, the cuticular wax biosynthesis gene, BrWax2, was identified through map-based cloning, proposing Bra032670 (CER1) as the candidate gene (Yang et al., 2022a). In this study, we narrowed the interval to approximately 102-kb by fine mapping, including 24 candidate genes, including the CER1 gene. The level of expression of the CER1 gene was significantly reduced in the bright green leaf mutant. Sequence analysis also revealed a distinct sequence variation in the promoter region of the bright green leaf mutant, which may be responsible for the down-regulation of CER1 gene expression. Therefore, we believe that the CER1 gene is the target gene for the current status of the bright leaves of Chinese kale.
In conclusion, we identified a candidate gene, BolC8t52930H (BoCER1.C8), associated with the bright green leaf trait in Brassica oleracea on chromosome C8. This identification was achieved using a genetic linkage map constructed through the re-sequencing of a BC1 segregating population derived from hybridization and backcrossing with the wild type (WT). We subsequently cloned this gene from both parental lines, uncovering significant differences in their promoter regions. A co-segregation marker was then developed and validated within a segregating population, successfully yielding the anticipated results. Consequently, the gene BoCER1.C8 emerges as a potential candidate for regulating the bright green leaf trait in Chinese kale.
The original contributions presented in the study are included in the article/Supplementary Material. Further inquiries can be directed to the corresponding author.
QZ: Data curation, Investigation, Methodology, Validation, Visualization, Writing – original draft. CW: Data curation, Investigation, Methodology, Validation, Visualization, Writing – original draft. JS: Data curation, Investigation, Validation, Writing – review & editing. LG: Data curation, Funding acquisition, Validation, Writing – review & editing. WS: Investigation, Methodology, Writing – review & editing. YL: Data curation, Investigation, Writing – review & editing. DC: Conceptualization, Data curation, Funding acquisition, Validation, Writing – original draft, Writing – review & editing. CT: Conceptualization, Data curation, Funding acquisition, Project administration, Validation, Writing – original draft, Writing – review & editing.
The author(s) declare financial support was received for the research, authorship, and/or publication of this article. The study was supported by the National Natural Science Foundation of China (32260469), the Natural Science Foundation of Jiangxi Province (20224BAB215016 and 20212BAB215003), Ganpo Talent Support Program of Jiangxi Province (20232BCJ23089), and the Science and Technology Foundation of Education Department of Jiangxi Province for Young Scholar (GJJ201450).
The authors declare that the research was conducted in the absence of any commercial or financial relationships that could be construed as a potential conflict of interest.
The author(s) declare that no Generative AI was used in the creation of this manuscript.
All claims expressed in this article are solely those of the authors and do not necessarily represent those of their affiliated organizations, or those of the publisher, the editors and the reviewers. Any product that may be evaluated in this article, or claim that may be made by its manufacturer, is not guaranteed or endorsed by the publisher.
The Supplementary Material for this article can be found online at: https://www.frontiersin.org/articles/10.3389/fpls.2024.1507968/full#supplementary-material
Supplementary Figure 1 | Genetic linkage maps. (A) Preliminary mapping of genetic linkage map. (B) Fine mapping genetic linkage map.
Supplementary Figure 2 | Sequence alignment analysis of candidate gene BoCER1.C8. (A) Amino acid sequence alignment diagram of coding region between the two parents. (B) Promoter sequence alignment between the two parents.
Aarts, M. G., Keijzer, C. J., Stiekema, W. J., Pereira, A. (1995). Molecular characterization of the CER1 gene of arabidopsis involved in epicuticular wax biosynthesis and pollen fertility. Plant Cell 7, 2115–2127. doi: 10.1105/tpc.7.12.2115
Bernard, A., Domergue, F., Pascal, S., Jetter, R., Renne, C., Faure, J. D., et al. (2012). Reconstitution of plant alkane biosynthesis in yeast demonstrates that arabidopsis ECERIFERUM1 and ECERIFERUM3 are core components of a very-long-chain alkane synthesis complex. Plant Cell 24, 3106–3118. doi: 10.1105/tpc.112.099796
Bernard, A., Joubès, J. (2013). Arabidopsis cuticular waxes: advances in synthesis, export and regulation. Prog. Lipid Res. 52, 110–129. doi: 10.1016/j.plipres.2012.10.002
Billiard, S., Castric, V., Llaurens, V. (2021). The integrative biology of genetic dominance. Biol. Rev. Cambridge Philos. Soc. 96, 2925–2942. doi: 10.1111/brv.12786
Bourdenx, B., Bernard, A., Domergue, F., Pascal, S., Leger, A., Roby, D., et al. (2011). Overexpression of arabidopsis ECERIFERUM1 promotes wax very-long-chain alkane biosynthesis and influences plant response to biotic and abiotic stresses. Plant Physiol. 156, 29–45. doi: 10.1104/pp.111.172320
Cheng, W., Wang, Z. T., Xu, F., Lu, G. L., Su, Y. C., Wu, Q. B., et al. (2022). Screening of candidate genes associated with brown stripe resistance in sugarcane via BSR-seq analysis. Int. J. Mol. Sci. 23, (24). doi: 10.3390/ijms232415500
Go, Y. S., Kim, H., Kim, H. J., Suh, M. C. (2014). Arabidopsis cuticular wax biosynthesis is negatively regulated by the DEWAX gene encoding an AP2/ERF-type transcription factor. Plant Cell 26, 1666–1680. doi: 10.1105/tpc.114.123307
Han, F., Huang, J., Xie, Q., Liu, Y., Fang, Z., Yang, L., et al. (2021). Genetic mapping and candidate gene identification of BoGL5, a gene essential for cuticular wax biosynthesis in broccoli. BMC Genomics 22, 811. doi: 10.1186/s12864-021-08143-7
Hill, J. T., Demarest, B. L., Bisgrove, B. W., Gorsi, B., Su, Y. C., Yost, H. J. (2013). MMAPPR: mutation mapping analysis pipeline for pooled RNA-seq. Genome Res. 23, 687–697. doi: 10.1101/gr.146936.112
Huang, C. C., Lin, C. H., Lin, Y. C., Chang, H. X. (2024). Application of bulk segregant RNA-Seq (BSR-Seq) and allele-specific primers to study soybean powdery mildew resistance. BMC Plant Biol. 24, (1). doi: 10.1186/s12870-024-04822-1
Ji, J. L., Cao, W. X., Dong, X., Liu, Z. Z., Fang, Z. Y., Zhuang, M., et al. (2018). A 252-bp insertion in BoCER1 is responsible for the glossy phenotype in cabbage (Brassica oleracea L. var. capitata). Mol. Breed. 38, (11). doi: 10.1007/s11032-018-0888-9
Ji, J., Cao, W., Tong, L., Fang, Z., Zhang, Y., Zhuang, M., et al. (2021). Identification and validation of an ECERIFERUM2- LIKE gene controlling cuticular wax biosynthesis in cabbage (Brassica oleracea L. var. capitata L.). Theor. Appl. Genet. 134, 4055–4066. doi: 10.1007/s00122-021-03947-3
Kannangara, R., Branigan, C., Liu, Y., Penfield, T., Rao, V., Mouille, G., et al. (2007). The transcription factor WIN1/SHN1 regulates Cutin biosynthesis in Arabidopsis thaliana. Plant Cell 19, 1278–1294. doi: 10.1105/tpc.106.047076
Lee, S. B., Suh, M. C. (2015). Advances in the understanding of cuticular waxes in and crop species. Plant Cell Rep. 34, 557–572. doi: 10.1007/s00299-015-1772-2
Lee, S. B., Suh, M. C. (2022). Regulatory mechanisms underlying cuticular wax biosynthesis. J. Exp. Bot. 73, 2799–2816. doi: 10.1093/jxb/erab509
Li, B., Yue, Z., Ding, X., Zhao, Y., Lei, J., Zang, Y., et al. (2023). A BrLINE1-RUP insertion in BrCER2 alters cuticular wax biosynthesis in Chinese cabbage (Brassica rapa L. ssp. pekinensis). Front. Plant Sci. 14. doi: 10.3389/fpls.2023.1212528
Liu, Y. J., Chen, P. Z., Li, W. S., Liu, X. C., Yu, G. W., Zhao, H., et al. (2023). Conjunctive analyses of BSA-seq and BSR-seq to identify candidate genes controlling the black lemma and pericarp trait in barley. Int. J. Mol. Sci. 24, (11). doi: 10.3390/ijms24119473
Liu, Z., Fang, Z., Zhuang, M., Zhang, Y., Lv, H., Liu, Y., et al. (2017c). Fine-Mapping and Analysis of Cgl1, a Gene Conferring Glossy Trait in Cabbage (Brassica oleracea L. var. capitata). Front. Plant Sci. 8. doi: 10.3389/fpls.2017.00239
Liu, D., Tang, J., Liu, Z., Dong, X., Zhuang, M., Zhang, Y., et al. (2017a). Cgl2 plays an essential role in cuticular wax biosynthesis in cabbage (Brassica oleracea L. var. capitata). BMC Plant Biol. 17, 223. doi: 10.1186/s12870-017-1162-8
Liu, D., Tang, J., Liu, Z., Dong, X., Zhuang, M., Zhang, Y., et al. (2017b). Fine mapping of BoGL1, a gene controlling the glossy green trait in cabbage (Brassica oleracea L. Var. capitata). Mol. Breed. 37, 69. doi: 10.1007/s11032-017-0674-0
McFarlane, H. E., Shin, J. J., Bird, D. A., Samuels, A. L. (2010). Arabidopsis ABCG transporters, which are required for export of diverse cuticular lipids, dimerize in different combinations. Plant Cell 22, 3066–3075. doi: 10.1105/tpc.110.077974
Ni, E. D., Zhou, L. Y., Li, J., Jiang, D. G., Wang, Z. H., Zheng, S. Y., et al. (2018). OsCER1 plays a pivotal role in very-long-chain alkane biosynthesis and affects plastid development and programmed cell death of tapetum in rice (Oryza sativa L.). Front. Plant Sci. 9. doi: 10.3389/fpls.2018.01217
Pascal, S., Bernard, A., Deslous, P., Gronnier, J., Fournier-Goss, A., Domergue, F., et al. (2019). Arabidopsis CER1-LIKE1 functions in a cuticular very-long-chain alkane-forming complex. Plant Physiol. 179, 415–432. doi: 10.1104/pp.18.01075
Pu, Y., Gao, J., Guo, Y., Liu, T., Zhu, L., Xu, P., et al. (2013). A novel dominant glossy mutation causes suppression of wax biosynthesis pathway and deficiency of cuticular wax in Brassica napus. BMC Plant Biol. 13, 215. doi: 10.1186/1471-2229-13-215
Seo, P. J., Lee, S. B., Suh, M. C., Park, M. J., Go, Y. S., Park, C. M. (2011). The MYB96 transcription factor regulates cuticular wax biosynthesis under drought conditions in arabidopsis. Plant Cell 23, 1138–1152. doi: 10.1105/tpc.111.083485
Shi, X. H., Wu, P. P., Hu, J. H., Qiu, D., Qu, Y. F., Li, Y. H., et al. (2021). Molecular characterization of all-stage and adult-plant resistance loci against powdery mildew in winter wheat cultivar liangxing 99 using BSR-seq technology. Plant Dis. 105, 3443–3450. doi: 10.1094/Pdis-03-21-0664-Re
Tarutani, Y., Shiba, H., Iwano, M., Kakizaki, T., Suzuki, G., Watanabe, M., et al. (2010). Trans-acting small RNA determines dominance relationships in Brassica self-incompatibility. Nature 466, 983–986. doi: 10.1038/nature09308
Tian, B. H., Zhang, L. X., Hu, J. H., Liu, Y. L., Zhou, L. L., Ping, W. C., et al. (2022). Genetic characterization of hull color using BSR-Seq and genome re-sequencing approaches in foxtail millet. Front. Plant Sci. 13. doi: 10.3389/fpls.2022.1019496
Wang, J. M., Li, Y. H., Xu, F., Xu, H. X., Han, Z. H., Liu, L. L., et al. (2022). Candidate powdery mildew resistance gene in wheat landrace cultivar Hongyoumai discovered using SLAF and BSR-seq. BMC Plant Biol. 22, (1). doi: 10.1186/s12870-022-03448-5
Wang, P., Li, Z., Zhu, L., Cheng, M., Chen, X., Wang, A., et al. (2023). Fine Mapping and Identification of a Candidate Gene for the Glossy Green Trait in Cabbage (Brassica oleracea var. capitata). Plants (Basel) 12, (18). doi: 10.3390/plants12183340
Wang, W. J., Zhang, Y., Xu, C., Ren, J. J., Liu, X. F., Black, K., et al. (2015). Cucumber ECERIFERUM1 (CsCER1), which influences the cuticle properties and drought tolerance of cucumber, plays a key role in VLC alkanes biosynthesis. Plant Mol. Biol. 87, 219–233. doi: 10.1007/s11103-014-0271-0
Wu, H., Shi, S., Lu, X., Li, T., Wang, J., Liu, T., et al. (2019). Expression analysis and functional characterization of CER1 family genes involved in very-long-chain alkanes biosynthesis in brachypodium distachyon. Front. Plant Sci. 10. doi: 10.3389/fpls.2019.01389
Wu, Q. B., Su, Y. C., Pan, Y. B., Xu, F., Zou, W. H., Que, B. B., et al. (2022). Genetic identification of SNP markers and candidate genes associated with sugarcane smut resistance using BSR-Seq. Front. In Plant Sci. 13. doi: 10.3389/fpls.2022.1035266
Yang, S., Liu, H., Wei, X., Zhao, Y., Wang, Z., Su, H., et al. (2022a). BrWAX2 plays an essential role in cuticular wax biosynthesis in Chinese cabbage (Brassica rapa L. ssp. pekinensis). Theor. Appl. Genet. 135, 693–707. doi: 10.1007/s00122-021-03993-x
Yang, S., Tang, H., Wei, X., Zhao, Y., Wang, Z., Su, H., et al. (2022b). BrWAX3, encoding a β-ketoacyl-coA synthase, plays an essential role in cuticular wax biosynthesis in Chinese cabbage. Int. J. Mol. Sci. 23, (18). doi: 10.3390/ijms231810938
Zeng, W., Tao, H., Li, Y., Wang, J., Xia, C., Li, S., et al. (2021). The flavor of Chinese kale sprouts is affected by genotypic variation of glucosinolates and their breakdown products. Food Chem. 359, 129824. doi: 10.1016/j.foodchem.2021.129824
Zhang, X., Liu, Z. Y., Wang, P., Wang, Q. S., Yang, S., Feng, H. (2013). Fine mapping of BrWax1, a gene controlling cuticular wax biosynthesis in Chinese cabbage (Brassica rapa L. ssp. pekinensis). Mol. Breed. 32, 867–874. doi: 10.1007/s11032-013-9914-0
Keywords: bright green leaf, Brassica oleracea, Chinese kale, fine mapping, wax biosynthesis
Citation: Zhang Q, Wang C, Song J, Gao L, Shen W, Liu Y, Chen D and Tan C (2024) Fine mapping and identification of the bright green leaf gene BoBGL in Chinese kale (Brassica oleracea var. alboglabra). Front. Plant Sci. 15:1507968. doi: 10.3389/fpls.2024.1507968
Received: 08 October 2024; Accepted: 10 December 2024;
Published: 23 December 2024.
Edited by:
Mohammad Irfan, Cornell University, United StatesReviewed by:
Dengfeng Hong, Huazhong Agricultural University, ChinaCopyright © 2024 Zhang, Wang, Song, Gao, Shen, Liu, Chen and Tan. This is an open-access article distributed under the terms of the Creative Commons Attribution License (CC BY). The use, distribution or reproduction in other forums is permitted, provided the original author(s) and the copyright owner(s) are credited and that the original publication in this journal is cited, in accordance with accepted academic practice. No use, distribution or reproduction is permitted which does not comply with these terms.
*Correspondence: Chen Tan, dGFuY2hlbjY2NkAxNjMuY29t
†These authors have contributed equally to this work
Disclaimer: All claims expressed in this article are solely those of the authors and do not necessarily represent those of their affiliated organizations, or those of the publisher, the editors and the reviewers. Any product that may be evaluated in this article or claim that may be made by its manufacturer is not guaranteed or endorsed by the publisher.
Research integrity at Frontiers
Learn more about the work of our research integrity team to safeguard the quality of each article we publish.