- 1Yuriev Plant Production Institute, National Academy of Agrarian Sciences of Ukraine, Kharkiv, Ukraine
- 2Institute of Food Biotechnology and Genomics, National Academy of Sciences of Ukraine, Kyiv, Ukraine
Melatonin is considered a multifunctional stress metabolite and a novel plant hormone affecting seed germination, root architecture, circadian rhythms, leaf senescence, and fruit ripening. Melatonin functions related to plant adaptation to stress stimuli of various natures are considered especially important. One of the key components of melatonin’s stress-protective action is its ability to neutralise reactive oxygen species (ROS) and reactive nitrogen species directly. However, many of its effects are related to its involvement in the signalling network of plant cells and its influence on the expression of a large number of genes important for adaptation to adverse factors. Insights into the functional relationships of melatonin with gasotransmitters (GT) – gaseous molecules performing signalling functions – are still emerging. This review has analysed and summarised the experimental data that testify to the participation of the main GTs – nitric oxide, hydrogen sulfide, and carbon monoxide – in the implementation of the protective effect of melatonin when plants are exposed to abiotic stimuli of various nature. In addition, modulation by melatonin of one of the most important components in the action of GTs and ROS – post-translational modifications of proteins and the influence of ROS and GTs on melatonin synthesis in plants under stress conditions and the specific physiological effects of exogenous melatonin and GTs have been reviewed. Finally, the prospects of the GTs’ practical application to achieve synergistic stress-protective effects on plants have been considered.
Introduction
World agricultural statistics show that abiotic stresses have become the main factors limiting crop production in recent decades. They are responsible for more than a 50% reduction in the productivity of most crops (Kul et al., 2019). Climate change trends increase the importance of research aimed at developing new biotechnological tools to improve plant resistance. Enhancing the resistance of plants is actively pursued combining selection-genetic (traditional methods of selection and new means of genetic engineering) and physiological approaches. The latter includes a wide range of plant hormones, their synthetic analogues, as well as signalling mediators and stress metabolites, which are often collectively referred to as ‘bioregulators’ (Srivastava et al., 2016; Zulfiqar and Ashraf, 2021). Expanding the knowledge of compounds involved in the regulation of plant adaptive responses opens new opportunities both for the use of effective exogenous treatments of plants with new physiologically active substances and the control of their synthesis in plants by genome editing methods (Raza et al., 2022).
The regulation of adaptive responses and plant growth under stress conditions is mediated by a highly complex network of molecules. These include plant hormones (auxins, gibberellic acid, cytokinins, abscisic acid (ABA), salicylic acid, jasmonates, ethylene) (Ali et al., 2024), key signalling mediators (calcium ions, reactive oxygen species (ROS), nitric oxide, cyclic nucleotides) (Srivastava et al., 2016; Roy Chowdhury et al., 2019), and several compounds that combine the properties of stress metabolites and plant hormones and/or signalling network participants. The last group of compounds is currently the subject of the most in-depth research, resulting in the accumulation of a huge amount of experimental data.
The main representatives of this group of compounds turned out to be the so-called plant neurotransmitters, substances that act as mediators of nerve impulse transmission in animals (melatonin, serotonin, dopamine, acetylcholine, and gamma-aminobutyric acid) (Akula and Mukherjee, 2020). Within this group of compounds, the greatest research interest has been focused on melatonin (N-acetyl-5-methoxytryptamine) (Colombage et al., 2023; Taboada et al., 2023). For instance, an analysis of publications in the Web of Science™ database revealed that melatonin was the fourth most studied exogenous substance for mitigating plant stress caused by the most common factor, drought, over the past 24 years, preceded only by the major plant stress hormone ABA, signalling mediator hydrogen peroxide, and antioxidant glutathione (Feng et al., 2024). Owing to its low toxicity and environmental safety, melatonin (Pardo-Hernández et al., 2021) is regarded as a promising natural biostimulant for sustainable crop production under adverse environmental conditions (Manzoor et al., 2023).
The impact of melatonin on various stages of plant ontogenesis, including circadian rhythms, seed germination, root development, leaf senescence, flowering, seed formation, and fruit maturation, has been demonstrated (Pan et al., 2023; Zhao and Hu, 2023; Wang et al., 2024). Numerous data have also been accumulated on increasing the resistance of plants to abiotic stresses of various types under the influence of exogenous melatonin. These data are the subject not only of analytical reviews but also of meta-analyses (Agathokleous et al., 2021; Wang et al., 2022b; Plokhovska et al., 2023).
Melatonin is involved in the complex signalling network of plant cells through several mechanisms. One of the key components of this network is gasotransmitters (GTs) (Yao et al., 2019; Kolupaev et al., 2022). This term unites small gaseous molecules that are synthesised by living organisms and perform signalling functions. In contrast to hormones, their effects are not receptor-dependent; rather, they act on multiple intracellular targets of protein nature (Dey et al., 2024). Nitric oxide (NO), carbon monoxide (CO) and hydrogen sulfide (H2S) are considered to be the main GTs of plant cells (Yao et al., 2019; Kolupaev et al., 2022; Dey et al., 2024).
Post-translational modification (PTM) of protein thiol groups is an important regulatory mechanism of GTs such as NO and H2S (Zhang and Liao, 2019; Aroca et al., 2021; Martí-Guillén et al., 2022). The same groups are easily oxidised by ROS, especially by H2O2 (Valderrama et al., 2019; Xuan et al., 2020). There are reasons to believe that melatonin, as a redox-active molecule, can affect protein PTM processes induced by ROS and GTs. However, despite the intensive accumulation of data on the involvement of both melatonin and GTs in the regulation of plant adaptive responses, the data on their functional interaction remain poorly systematised (Wang et al., 2022a). On the other hand, data on the relationship between melatonin and two other important GTs, H2S and CO, in the formation of adaptive responses in plants are still limited and have not been analysed from the point of view of the functioning of the signalling network as a whole. Taking into account the above mentioned, this paper presents a review and analysis of data on the mechanisms of participation of the main GTs in the realisation of the protective effects of melatonin under the influence of abiotic stresses on plants.
A brief summary of melatonin synthesis and metabolism in plant cells
In plants, melatonin is thought to be synthesised predominantly in chloroplasts (Zeng et al., 2022) (Figure 1). First, tryptophan decarboxylase (TDC) converts tryptophan to tryptamine by removing the carboxyl group. Then, under the influence of tryptamine 5-hydroxylase (T5H), tryptamine is hydroxylated to serotonin (5-hydroxytryptamine, 5-HT). The latter is converted to melatonin in two steps by serotonin N-acetyltransferase (SNAT) and N-acetylserotonin O-methyltransferase (ASMT, also known as caffeic acid O-methyltransferase, COMT) (Rehaman et al., 2021). However, the order of the transformations can vary depending on environmental conditions (Zeng et al., 2022). Under standard conditions, SNAT-catalysed acetylation of serotonin to N-acetyl 5-hydroxytryptamine (aHT) occurs first, followed by its O-methylation by ASMT/COMT, leading to melatonin (Ye et al., 2019). In contrast, abiotic stress induces the expression of different isoforms of ASMT/COMT so that serotonin is first O-methylated to 5-methoxytryptamine (5-MT). Subsequently, 5-MT is acetylated by SNAT to form melatonin (Tan and Reiter, 2020).
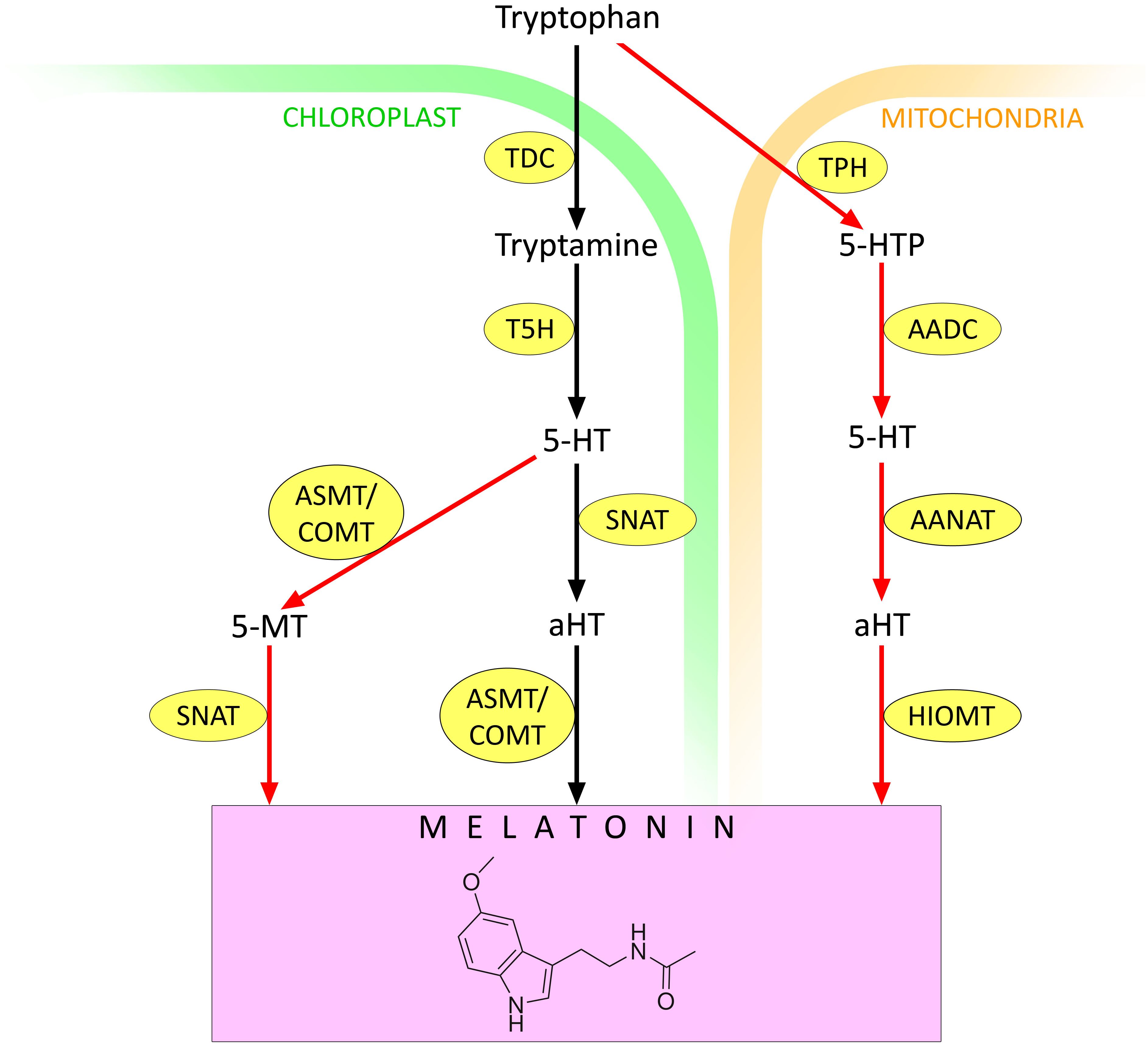
Figure 1. Melatonin synthesis in plants. 5-HT – 5-hydroxytryptamine (serotonin); 5-MT – 5-methoxytryptamine; AADC – aromatic amino acid decarboxylase; AANAT – arylalkylamine N-acetyltransferase (arylamine N-acetyltransferase); aHT – N-acetyl 5-hydroxytryptamine; ASMT – N-acetylserotonin O-methyltransferase; COMT – caffeic acid O-methyltransferase; HIOMT – hydroxyindole O-methyltransferase (N-acetylserotonin O-methyltransferase); SNAT – serotonin N-acetyltransferase; T5H – tryptamine 5-hydroxylase; TDC – tryptophan decarboxylase; TPH – tryptophan hydroxylase. Red arrows indicate melatonin synthesis pathways that are activated under stressful conditions. Other explanations in the text.
In the mitochondria, tryptophan can be converted to 5-hydroxytryptophan by the action of tryptophan hydroxylase (TPH). Then 5-HT is formed under the action of aromatic amino acid decarboxylase (AADC). 5-HT is converted to aHT by arylalkylamine N-acetyltransferase (AANAT), also known as arylamine N-acetyltransferase. Finally, aHT is converted to melatonin by the action of hydroxyindole O-methyltransferase (HIOMT), also known as N-acetylserotonin O-methyltransferase (Zeng et al., 2022) (Figure 1). There is evidence that the mitochondrial contribution to melatonin synthesis increases under stress conditions (Zeng et al., 2022). Therefore, depending on the environment, both the contribution of different chloroplast pathways to melatonin synthesis and the ratio of melatonin synthesis in chloroplasts and mitochondria may change.
To date, a rather extensive phenomenology of melatonin synthesis enhancement in plants in response to stress stimuli of different natures has been accumulated. For example, an almost twofold increase in melatonin content was observed in wheat and rice plant organs in response to heating (Byeon and Back, 2014; Buttar et al., 2020). Similar effects at high temperatures were found in Arabidopsis (Shi et al., 2015) and tomato plants (Xu et al., 2016). Under salt, osmotic, and heat stress, an increase in endogenous melatonin levels was detected in grapes, barley, and lupin (Hassan et al., 2022).
The mechanisms of melatonin perception by plant cells have not been fully studied. Plant cells, similar to animal cells, contain three subunits of the G-protein complex (Gα, Gβ, and Gγ) and several putative G-protein-coupled receptors (GPCR) (Cannon and Chapman, 2021). In plants, CAND2 and CAND7 proteins have been found to interact with GPCR (Jin et al., 2012). It is the CAND2 protein that has been proposed as the phytomelatonin receptor1 (PMTR1) in 2018 (Wei et al., 2018). Experimental data have been obtained that implicate the CAND2/PMTR1 protein in the activation of Ca2+ influx and K+ efflux during melatonin-induced stomatal closure. The Arabidopsis AtCand2 mutant, in contrast to wild-type plants, did not respond to melatonin treatment with stomatal closure (Wei et al., 2018). However, confocal microscopy data indicate that the CAND2 protein is localised in cytosol (Lee and Back, 2020). It is therefore, suggested that CAND2 might not be the G-protein that mediates melatonin-induced effects. Recently, receptor-like kinases (RLKs) have been considered as alternative candidates for melatonin receptors in plants (Back, 2021). Arabidopsis is known to have more than 600 RLK homologs. Since the MAP kinase cascade was activated in response to melatonin action, it is likely that one of the 600 RLKs in Arabidopsis functions as a melatonin receptor (Back, 2021). Nevertheless, recent experimental evidence points to a role for PMTR1 in melatonin perception and action in drought, salinity, and pathogen response in alfalfa, tobacco, and maize (Khan et al., 2023). For example, osmotic stress was shown to significantly increase PMTR1 transcript levels in plants in a mannitol dose-dependent manner (Wang et al., 2021). In addition, it was recently shown that overexpression of the MePMTR1 gene isolated from the tropical cassava plant (Manihot esculenta Crantz) in Arabidopsis plants resulted in an increased resistance to dark-induced senescence compared to the wild type (Cheng et al., 2024). However, the presence and cellular localisation of melatonin receptors in higher plants still need further investigation (Corpas et al., 2022a).
Functional interaction of melatonin with ROS
ROS are essential signalling molecules that play diverse roles in the rapid response of plants to environmental stimuli (Ahammed et al., 2024). However, ROS generally act as signalling mediators when their levels are increased in individual cell compartments in a short-term and cell-controlled manner (Kolupaev et al., 2019; Dvořák et al., 2021; Li and Kim, 2022). Excessive production of ROS can cause programmed cell death, and large-scale stochastic ROS production can lead to uncontrolled destructive cell changes (Kacperska, 2004; Hasanuzzaman et al., 2020).
ROS are generated in one-, two-, and three-electron oxygen reduction reactions as a result of spontaneous and enzymatic oxidation of various substrates, as well as in photoinduced reactions (Sachdev et al., 2021). In higher plant cells, the primary sources of ROS are the electron transfer chains present in chloroplasts and mitochondria, but various ROS-generating enzymes are present in subcellular compartments (Taboada et al., 2023).
ROS generation in chloroplasts
Photosynthesis is one of the major sources of ROS in green plant cells. Superoxide anion radicals O2•− are generated by photosystem I and II electron transport chain (ETC) function (Li and Kim, 2022). The photoinduced generation of ROS mainly depends on environmental conditions and the physiological state of photosynthetic apparatus (Foyer and Shigeoka, 2011). When CO2 fixation is limited under various stressors (e.g., drought, salinity, and high temperature), the NADPH pool is only slightly consumed, resulting in electron “leakage” from ferredoxin to molecular oxygen to form O2•−. Superoxide anion radicals formed in chloroplasts are readily converted to the more stable ROS, hydrogen peroxide H2O2, under the influence of superoxide dismutase (SOD).
Singlet oxygen is also formed in chloroplasts, primarily as a consequence of the transition of chlorophyll P680 to the triplet state within the reaction centre of photosystem II and/or the light-harvesting complex. The likelihood of singlet oxygen formation, as well as other ROS, increases when the ETC is over-reduced, which is characteristic of stressful conditions (Li and Kim, 2022).
ROS generation in mitochondria
Mitochondria, similar to chloroplasts, contain a large number of electron carriers. Their inadvertent interaction with molecular oxygen can lead to a one-electron reduction of O2 to O2•−. The main electron leakage sites in plants, as in animals, are considered to be complexes I and III of the ETC (Cvetkovska and Vanlerberghe, 2013). However, the ETC of plant mitochondria also involves an alternative electron transport pathway. It includes two molecules of NAD(P)H dehydrogenase on the outer and inner sides of the inner mitochondrial membrane, alternative oxidase, and an uncoupling protein, UCP, which limit ROS generation (Sachdev et al., 2021). The functions of these proteins are enhanced under stressful conditions.
ROS synthesis in peroxisomes
Peroxisomes are also among the compartments that generate significant amounts of ROS in processes such as photorespiration and β-oxidation of fatty acids mediated by acyl-CoA oxidase (Sachdev et al., 2021). ROS generation in peroxisomes may also be related to the activities of flavin oxidase, urate oxidase, xanthine oxidase and other enzymes (Hasanuzzaman et al., 2020). Under abiotic stress, photorespiration is initiated in the chloroplast because of the limited availability of CO2 and increased solubility of O2, which competitively accelerates the oxygenation of ribulose-1,5-biphosphate to form glycolate. The latter is exported to peroxisomes, where it is oxidized by glycolate oxidase to form H2O2 (del Río et al., 2003; Das and Roychoudhury, 2014).
ROS generation in the apoplast
The apoplast is the site of generation of a large amount of ROS, which may be a component of cell signalling. For example, NADPH oxidase, known as the Respiratory Burst Oxidase Homologs (RBOH), is localised on the plasma membrane (Marino et al., 2012). This enzyme complex reduces molecular oxygen to form superoxide anion radical. Arabidopsis genome contains 10 members of the RBOH membrane-bound (catalytic) subunit gene family, and the involvement of some of them in signalling processes has been confirmed by molecular genetic methods (Torres et al., 2002; Hasanuzzaman et al., 2020). A significant pool of signalling ROS appears to be also generated in the apoplast by the oxidase activity of class III heme peroxidases (Gautam et al., 2017).
Involvement of melatonin in ROS regulation
As shown previously, melatonin affects cellular content of ROS as a direct antioxidant and as a probable participant in the signalling network and inducer of other components of the antioxidant system (Ahammed et al., 2019).
Melatonin can directly interact with ROS such as •OH, H2O2, and 1O2 (Galano and Reiter, 2018). Melatonin has been reported to be more active than glutathione and mannitol in binding hydroxyl radicals (Arnao and Hernández-Ruiz, 2019). The chemical mechanisms of melatonin interaction with different ROS and their corresponding constants have been described in several reviews (Galano and Reiter, 2018; Arnao and Hernández-Ruiz, 2019).
The catabolism of melatonin by ROS is one of the mechanisms by which melatonin exerts its direct antioxidant action. In addition, melatonin metabolites also possess direct and indirect antioxidant properties (Taboada et al., 2023).
Melatonin catabolism can occur with or without enzyme involvement (Back, 2021; Zeng et al., 2022). One pathway involves the conversion of melatonin to cyclic N1-acetyl-N 2-formyl-5-methoxykynuramine (AFMK) by indoleamine 2,3-dioxygenase (IDO) (Wang et al., 2024) (Figure 2). AFMK is later converted to N1-acetyl-5-methoxykynuramine (AMK) by the action of melatonin 3-hydroxylase (M3H). Melatonin can also be converted to 2-hydroxymelatonin (2-OHM) and 3-hydroxymelatonin (3-OHM) by interaction with ROS; these processes involve M2H and M3H, respectively (Back, 2021). 2-OHM and 3-OHM are the predominant melatonin metabolites in plants (Back, 2021; Khan et al., 2023). In addition, the formation of 4-hydroxymelatonin and 6-hydroxymelatonin is possible under the influence of radical ROS, the latter being more characteristic of melatonin degradation in animals (Mannino et al., 2021).
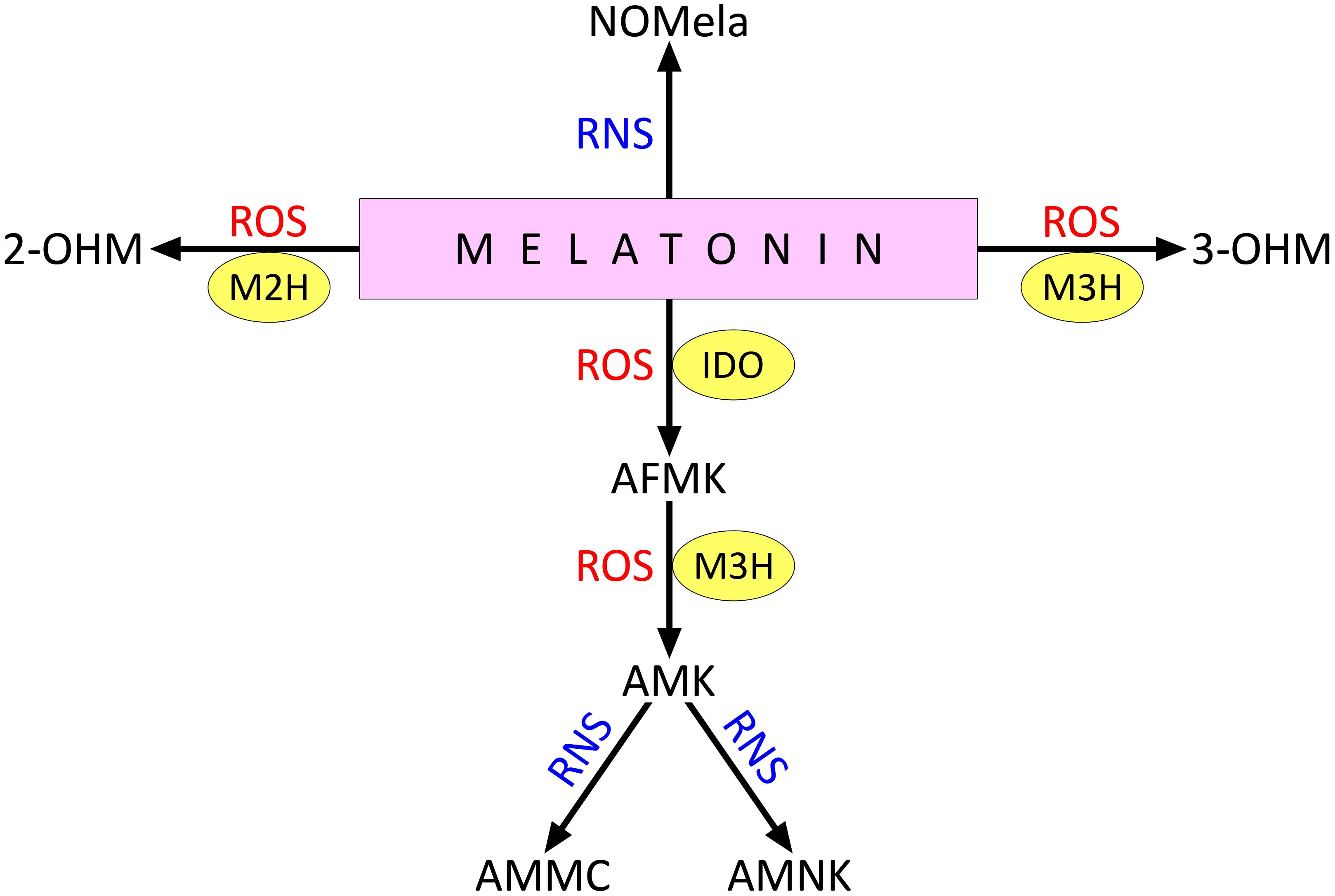
Figure 2. Direct interaction of melatonin with ROS and RNS. 2-OHM, 2-hydroxymelatonin; 3-OHM, 3-hydroxymelatonin; AFMK , cyclic N1-acetyl-N 2-formyl-5-methoxykynuramine; AMK , N1-acetyl-5-methoxykynuramine; AMMC , acetamidomethyl-6-methoxycinnolinone; AMNK , N1-acetyl-5-methoxy-3-nitrokynuramine; IDO , indoleamine 2,3-dioxygenase; M2H , melatonin 2-hydroxylase; M3H , melatonin 3-hydroxylase; NOMela , N-nitrosomelatonin; ROS , reactive oxygen species; RNS , reactive nitrogen species. Other explanations in the text.
Melatonin also interacts with RNS, particularly when it reacts with peroxynitrite or nitrogen dioxide to form N-nitrosomelatonin (NOMela) (Figure 2). The possible physiological functions of this compound are discussed below in the section on the functional interactions between melatonin and NO. In addition, AMK formed by the interaction of AMFK with ROS can react with RNS to form 3-acetamidomethyl-6-methoxycinnolinone (AMMC) or N1-acetyl-5-methoxy-3-nitrokynuramine (AMNK) (Mannino et al., 2021).
The melatonin hydroxymetabolites 2-OHM and 3-OHM are present in plant cells at much higher levels than melatonin itself. For example, it has been reported that in an intact rice leaf, the content of 3-OHM and 2-OHM exceeds the content of melatonin by 300 and 100 times, respectively (Byeon et al., 2015; Taboada et al., 2023). Even higher concentrations of melatonin hydroxymetabolites were recorded in rice under stress conditions, particularly under cadmium influence and salinity stress (Lee et al., 2017; Choi and Back, 2019).
The hydroxymetabolite 3-OHM, formed from melatonin as a result of M3H activity, has intrinsic antioxidant activity targeting hydroxyl and hydroperoxyl (•OOH) radicals (Tan et al., 2014). In reaction with 1,1-diphenyl-2-picrylhydrazyl, 3-OHM was shown to have 15 times more antioxidant activity than melatonin (Lee and Back, 2022).
The indirect effect of melatonin and its derivatives on the redox homeostasis of plant cells is even more complex. Several studies have reported increased gene expression and activity of antioxidant enzymes in plants of different taxonomic groups under normal and stress conditions (Magri and Petriccione, 2022; Zhang et al., 2022; Kolupaev et al., 2023a; Taboada et al., 2023). Such effects suggest the formation of ROS signalling under the influence of melatonin, which induces the antioxidant system (Figure 3). Indeed, some evidence has been obtained for the involvement of ROS generated by NADPH oxidase in the realisation of the stress-protective effect of melatonin (Gong et al., 2017; Wei et al., 2018; Sun et al., 2021). One of the mechanisms for the increase in NADPH oxidase activity under the action of melatonin may be related to the decrease in S-nitrosation of cysteine residues in its catalytic subunit (Gong et al., 2017). NO is known to participate in the S-nitrosation reaction of NADPH oxidase at Cys890, leading to its inhibition of ROS generation (Yun et al., 2011). Melatonin, which has the ability to bind NO, reduces S-nitrosation in NADPH oxidase molecules. Using Solanum lycopersicum as an example, it was found that treatment with exogenous melatonin first led to the accumulation of endogenous melatonin, which then removed the generated NO molecules, causing denitrosation of Cys890 residues in RBOH and increasing its activity (Gong et al., 2017) (Figure 3). Thus, activation of RBOH and increased ROS generation have been shown to be critical for melatonin to enhance plant resistance to drought, heat, and osmotic stress. Inhibition of RBOH or chemical removal of H2O2 by its scavenger significantly downregulated melatonin-induced plant defence responses, including decreased expression of several stress-related genes (CDPK1, MAPK1, TSPMS, ERF4, HSP80, and ERD15), and caused decreased activity of antioxidant enzymes (SOD, catalase, and ascorbate peroxidase) (Gong et al., 2017). The role of ROS generated by different molecular forms of NADPH oxidase in the stomatal closing effect induced by exogenous melatonin in Arabidopsis and dependent on the synthesis of NO was demonstrated (Wang et al., 2023a, see below).
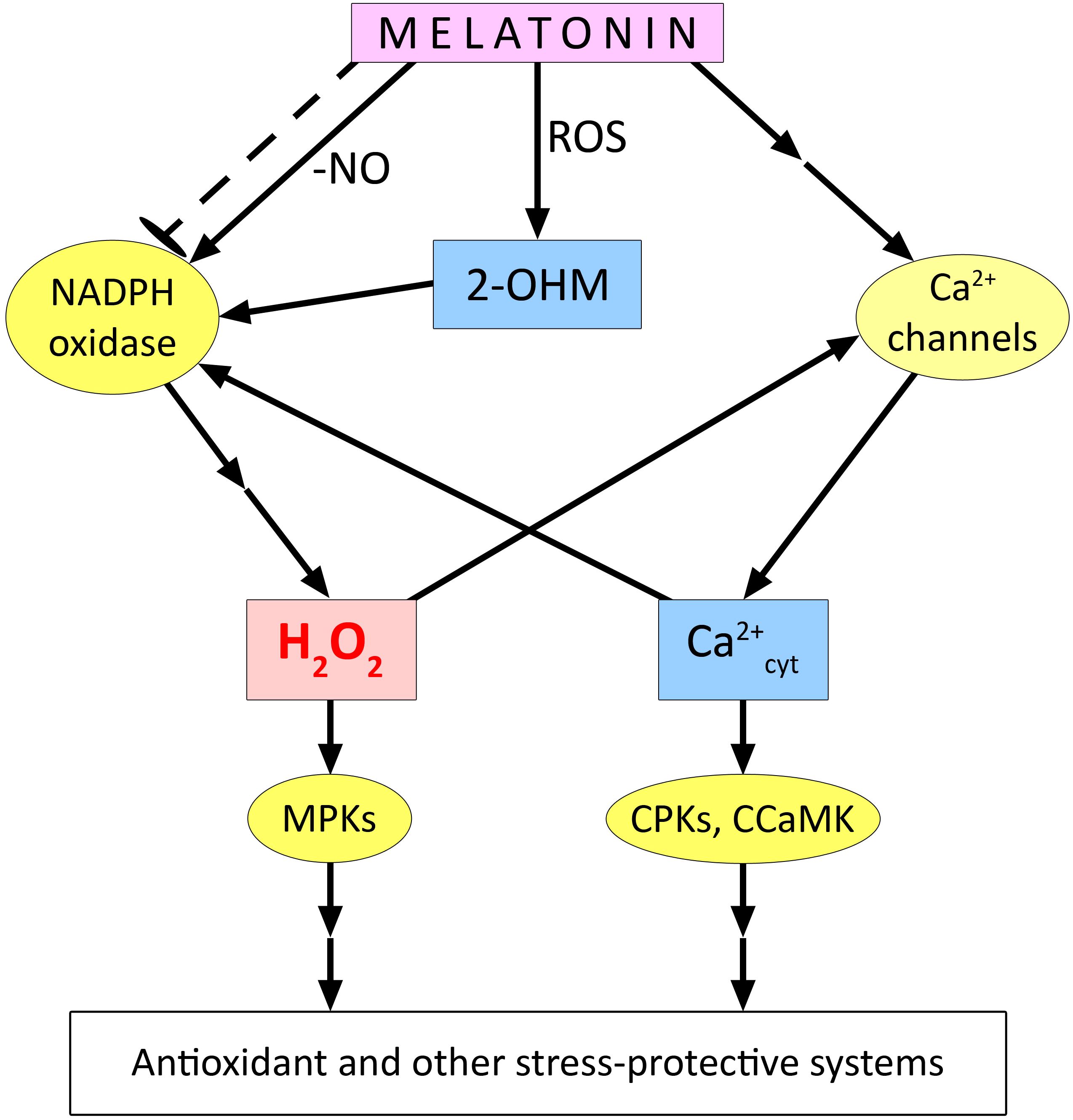
Figure 3. Functional interaction of melatonin with ROS in plant cells. 2-OHM – 2-hydroxymelatonin; CPKs, Ca2+-dependent protein kinases; CCaMK, Ca2+/calmodulin-dependent protein kinase; ROS, reactive oxygen species. The dashed arrow with blunt end indicates the possible inhibitory effect of melatonin on the expression of genes encoding certain molecular forms of NADPH oxidase. Other explanations in the text.
Nevertheless, the mechanisms underlying the increase in NADPH oxidase activity upon melatonin treatment of plants do not seem to be limited to its effect on S-nitrosation of Cys890 residues. In particular, there is reason to believe that melatonin may activate NADPH oxidase by affecting Ca2+ homeostasis. For example, in watermelon plants, it was shown that the recruitment of ROS generated by NADPH oxidase and calcium ions entering the cytosol through cyclic nucleotide-gated ion channels realise the protective effect of melatonin during cold stress (Chang et al., 2021). Our experiments showed that the melatonin-induced increase in heat tolerance of wheat seedlings was abolished by the H2O2 scavenger dimethylthiourea, the NADPH oxidase inhibitor imidazole, and various calcium antagonists (Kolupaev et al., 2024b). It is noteworthy that the activation of H2O2 accumulation under the action of melatonin was not manifested in the case of seedlings treated with EGTA, a chelator of extracellular calcium, or with neomycin, an inhibitor of phospholipase C, which is involved in formation of inositol 1,4,5-phosphate and thus in opening of intracellular Ca2+ channels. There is reason to believe that under the above experimental conditions, melatonin-induced changes in Ca2+ homeostasis are primordial in relation to activation of NADPH oxidase and increased generation of ROS, which act as signalling mediators (Figure 3). According to one model, calcium activates a Ca2+-dependent protein kinase that phosphorylates the N-terminal region of the membrane-associated subunit (RBOH) of NADPH oxidase and causes its conformational change to facilitate the binding of its cytosolic component, the Rop protein (GTPase). This results in the formation of an active dimer, leading to increased ROS generation (Wong et al., 2007). In a form of the membrane-bound subunit of rice NADPH oxidase (OsRBOHB), the presence of EF arms in the N-terminal region was found, indicating the formation of a dimer involving Ca2+ (Oda et al., 2010). Thus, the increase in NADPH oxidase activity with the involvement of calcium may be related not only to its activation of the protein kinase, but also to the direct interaction of Ca2+ with the catalytic subunit. In this context, it is possible that ROS and cytosolic Ca2+, as mediators of melatonin effects, may functionally interact according to the principle of a mutually reinforcing “signalling loop”.
In general, several experimental data were obtained on the participation of NADPH oxidase in signalling processes necessary for the realisation of the stress-protective effect of melatonin. For example, the role of NADPH oxidase in the melatonin-induced development of tolerance to salinity has been demonstrated in Arabidopsis. It was found that melatonin treatment could enhance antioxidant defence in stressed wild-type plants but not in the atrbohF mutant (Chen et al., 2017). Exogenous melatonin treatment significantly reduced the phytotoxic effects of the pesticide chlorothalonil on tomato plants. Moreover, the melatonin-induced increase in the activity of glutathione cycle enzymes involved in chlorothalonil detoxification was abolished by treating plants with NADPH oxidase inhibitor and H2O2 scavenger. This suggests a role for ROS generated by NADPH oxidase in the realisation of the stress-protective effect of melatonin (Peng et al., 2023). The activating effect of ROS and Ca2+ on the enzymatic antioxidant system is thought to be mediated mainly by MAP kinase signalling cascade, with the involvement of calmodulin-dependent protein kinases (Dvořák et al., 2021) (Figure 3). However, there is evidence for the involvement of the MAPK signalling cascade in shaping melatonin-mediated responses to abiotic and biotic stressors (Lee and Back, 2016; 2017; Mansoor et al., 2024). For example, the expression of MPK3 and MPK6 in Arabidopsis has been shown to be induced by melatonin and 2-OHM (Lee and Back, 2016). Thus, it is conceivable that different kinases are involved in the transduction of ROS and Ca2+ signals activated by melatonin and are required for its induction of the antioxidant system and possibly other plant defence responses (Figure 3).
However, melatonin may not only have an activating effect on NADPH oxidase and ROS generation. It has been shown that melatonin can also suppress the expression of genes encoding plasma membrane-bound NADPH oxidase (TaRbohD, TaRbohF) in wheat. Such data were obtained while studying the effect of priming wheat seeds and seedlings of Brassica juncea with melatonin, which mitigated the subsequent toxic effects of chromium (Lei et al., 2021; Kour et al., 2024). The authors consider this effect as an additional mechanism to mitigate oxidative stress. Similar effects were found in a study on the protective effects of melatonin on Vicia faba plants exposed to arsenic toxicity (Siddiqui et al., 2020). Melatonin treatment reduced the effects of increased NADPH oxidase activity and O2•− and H2O2 generation induced by the treatment. At the same time, no inhibitory effect of melatonin on NADPH oxidase activity and ROS generation was observed in the absence of stress stimuli. It is still unclear how the effects of NADPH oxidase activation and suppression by melatonin recorded in different objects relate to each other. A different set of mediators may be involved in the regulation of NADPH oxidase under stress conditions than under optimal conditions. Moreover, the regulation of redox homeostasis during the action of exogenous melatonin on plants may be even more complex because of its transformation into hydroxy derivatives. For example, Lee and Back (2021) obtained experimental data suggesting a more important role of 2-OHM in enhancing ROS generation than melatonin. It was shown that treatment of tobacco and Arabidopsis leaves with 2-OHM resulted in a significantly greater enhancement of superoxide anion radical generation compared to melatonin treatment.
Recently, more significant effects of 2-OHM on Arabidopsis seed germination than melatonin have been reported (Lee and Back, 2022). These effects are attributed to ROS-mediated induction of gibberellin synthesis. Molecular genetic studies using the knockout mutant m2h and plants with overexpression of the M2H gene confirmed the importance of increasing ROS generation in the implementation of the effect of 2-OHM on the expression of the gibberellin synthesis enzyme gene and seed germination (Lee and Back, 2022). Undoubtedly, data on the participation of 2-OHM and other metabolites of melatonin in cellular signalling processes are still insufficient. It is still very difficult to distinguish the physiological effects of these compounds from those of melatonin itself.
Functional relationships between melatonin and NO
GT NO, together with ROS, is considered a very important signalling mediator of plant cells, somehow related to almost all known signalling molecules (Santolini et al., 2017). One of the main ways of realising the signalling potential of NO molecules is through the PTM of proteins. The most common NO-mediated PTM is S-nitrosation, a reversible redox modification based on the addition of a nitroso group to the thiol group (SH) of a specific cysteine residue (Cys) to form S-nitrosothiol (SNO), which can induce conformational changes and, consequently, alter the activity or function of the corresponding protein (Lamotte et al., 2015). To date, hundreds of proteins that are regulated by S-nitrosation have been identified (Sánchez-Vicente et al., 2019). The second most abundant NO-mediated PTM is tyrosine nitration (Blume et al., 2013; Sánchez-Vicente et al., 2019; Zhu et al., 2019).
The biological significance of this process is still difficult to interpret, as it is considered one of the markers of nitrosative stress (Ischiropoulos, 2003). It is likely that tyrosine nitration is also important in plants for regulating the activity of individual proteins. For example, nitration of tyrosine residues in molecules of the major microtubule protein α-tubulin has been found, which may be related to the regulation of their dynamic properties and participation in the growth and division of plant cells (Blume et al., 2013).
NO is considered to be one of the main regulators of Ca2+ homeostasis. It affects several types of Ca2+ channels and promotes Ca2+ entry into the cytosol from the extracellular space and intracellular compartments (Jeandroz et al., 2013; Santolini et al., 2017). NO, through S-nitrosation, affects the functional activity of many proteins involved in Ca2+ signalling, including protein kinases (Courtois et al., 2008) and calmodulin (Astier et al., 2012; Jeandroz et al., 2013). It is also evident that the targets of NO-mediated PTM are also proteins involved in Ca2+ channel opening. Studies using agonists or antagonists of cGMP and cADP-ribose (cADPR) have shown that these secondary cytosolic messengers play a central role in the realisation of the effects of NO on Ca2+ channels (Santolini et al., 2017). There is also considerable experimental evidence for NO activation of the mitogen-activated protein kinase (MAPK) cascade, which in turn is tightly coupled to many other components of the signalling network (Zhu et al., 2019).
The signalling potential of NO is also realised by its functional and chemical interaction with ROS, in particular with O2•− and H2O2. This interaction of key signalling mediators can be either synergistic or antagonistic (Farnese et al., 2016). NO-induced PTMs of individual protein molecules can induce rapid and long-lasting effects that may have different directions. For example, inhibition of individual antioxidant enzymes by the direct action of NO may lead to the formation of a signal that induces gene expression of these enzymes (Corpas et al., 2022b; Kolupaev et al., 2023b). On the other hand, NO-induced PTMs can reduce the activity of enzymes involved in ROS generation, such as glycolate oxidase and NADPH oxidase, allowing the cell to maintain redox homeostasis (Farnese et al., 2016). To date, there is evidence that NO is also involved in the realisation of the physiological effects of most known plant hormones (Zhou et al., 2019; Shang et al., 2022; Kolupaev et al., 2024a). Melatonin is a new plant hormone, but there is already considerable evidence of its functional and chemical interactions with NO (He and He, 2020). This interaction is manifested by melatonin binding NO to form nitrosomelatonin, influencing S-nitrosation processes, and inducing NO synthesis (Corpas et al., 2022a; Ahmad et al., 2023).
Pathways of nitric oxide synthesis in plants
It is now generally accepted that there are two main pathways for the synthesis of NO in plants: a reductive pathway from nitrates and nitrites, which is mainly driven by nitrate reductase (NR) activity, and an oxidative pathway associated with the conversion of L-arginine by an enzyme with activity similar to NO synthase (NOS), so called because its activity requires the same biochemical conditions as animal NOS (Astier et al., 2018; Corpas et al., 2022a). In higher plants, the main cellular compartments of NO generation are the cytosol, peroxisomes, chloroplasts, and mitochondria. Experimental data indicate that NO can be synthesized in the cytosol with the participation of NR, a multifunctional enzyme involved in nitrogen assimilation and metabolism. In vivo and in vitro experiments have shown that NR can catalyse the reduction of nitrate to NO and its derivative peroxynitrite (ONOO−) (Khator et al., 2024). The activity of different molecular forms of NR can be regulated by phosphorylation involving MAP kinases, whose activity can be modulated by ROS (Wang et al., 2010; Zhu et al., 2019).
NO can be synthesized in mitochondrial membranes via the reductive pathway, but with the participation of other catalytic systems. NR as well as the enzymatic complexes of the electron transport chain cytochrome oxidase (CIII) and cytochrome reductase (CIV) can be involved in this process (Gupta and Kaiser, 2010; Farnese et al., 2016; Khator et al., 2024). The oxidative pathway of NO synthesis is considered to be as important as the reductive pathway, although the nature of the enzymatic systems that provide this pathway in higher plants has been debated for nearly three decades (Farnese et al., 2016). Nevertheless, enzymatic oxidation of L-arginine to citrulline and NO has been shown to be possible in leaf peroxisomes and chloroplasts of green algae and vascular plants (Hancock and Neill, 2019). This enzymatic activity has been named NOS-like because, as in the case of the animal enzyme, it has been reported to be strictly dependent on the presence of arginine and NADPH as well as several NOS cofactors (NADPH, FAD, FMN, Ca2+, and calmodulin) (Corpas and Barroso, 2014; Farnese et al., 2016). However, molecular genetic evidence of the presence of the corresponding protein in higher plants is still lacking (Astier et al., 2018). Currently, there is a hypothesis that there are polypeptides with redox-active domains that can be assembled into a single enzymatic complex that catalyses the reactions of arginine-dependent NO formation in higher plants (Kolbert et al., 2019). Partial overlap of cellular compartments where NO is synthesised with those for melatonin synthesis (chloroplasts and mitochondria) is considered by some authors as a fact that indirectly indicates a possible relationship between melatonin and NO (Wang et al., 2022a).
Nitric oxide conjugates and their functional relationship to melatonin
A peculiarity of NO is its short half-life (approximately 30 s) (Martínez-Lorente et al., 2022). In this respect, its physiological effects depend largely on its transformation into more stable conjugated compounds. Among these, S-nitrosothiols are well known. The most abundant S-nitrosothiol is S-nitrosoglutathione (GSNO), which is formed by the interaction of NO with reduced glutathione (GSH) (Corpas et al., 2013). GSNO levels are regulated by S-nitrosoglutathione reductase, which reduces GSNO to glutathione sulfinamide (GS(O)NH2) using NADH (Martínez-Lorente et al., 2022). Melatonin may affect the GSNO pool by inhibiting S-nitrosoglutathione reductase (Wen et al., 2016).
An even more important mechanism of interaction between NO and melatonin may be the formation of NOMela, which occurs in an aerobic environment and at physiological pH values (Martínez-Lorente et al., 2022). This interaction is considered one of the mechanisms of NO binding to prevent the development of nitrosative stress (Singh et al., 2016). At the same time, NOMela is considered one of the natural NO donors, like GSNO or S-nitrosocysteine (Mukherjee, 2019). Both nitrosothiols and NOMela can participate in protein PTM as NO sources. The ability of NOMela to efficiently transnitrosate cysteine residues in proteins has been demonstrated using an in vitro system (Singh et al., 2016). In particular, NOMela was found to be 10 times more effective than S-nitrocysteine in the reaction of S-nitrosation of catalytically active cysteine residues by glyceraldehyde-3-phosphate dehydrogenase (Kirsch and de Groot, 2008).
NOMela may be a conjugate that enables long-distance transport of both melatonin and NO at the whole-plant level (Mukherjee, 2019). It has been suggested that GSNO and NOMela may compete for binding sites for long-distance transport in plants, although direct experimental evidence for this hypothesis is still lacking (Singh et al., 2016). However, NOMela has been shown to be more efficient than GSNO in transporting NO from roots to leaves of Arabidopsis in a model experiment (Singh et al., 2021).
Effect of melatonin on NO synthesis in the regulation of plant resistance to stress
Several studies have reported an increase in NO synthesis during melatonin treatment of plants and the role of this effect in the induction of plant resistance to various stress stimuli (Table 1; Figure 4). For example, treatment of tomato plants with melatonin, which induces the development of heat tolerance, resulted in an NR-dependent increase in leaf NO content (Jahan et al., 2019). Data on the participation of NO as a mediator of the action of melatonin were also obtained when studying the induction of cold resistance in plants, in particular rape seedlings (Ma et al., 2022). The increase in NO and cytosolic Ca2+ was more significant in melatonin-treated leaves in response to cold than in untreated leaves (Table 1). Notably, co-treatment of melatonin-treated plants with NO or Ca2+ antagonists also inhibited melatonin-induced MAPK3/6 expression under cold stress conditions (Ma et al., 2022). Thus, there is reason to believe that Ca2+ and NO are at the beginning of the melatonin-induced signalling cascade leading to the development of cold tolerance in rapeseed.
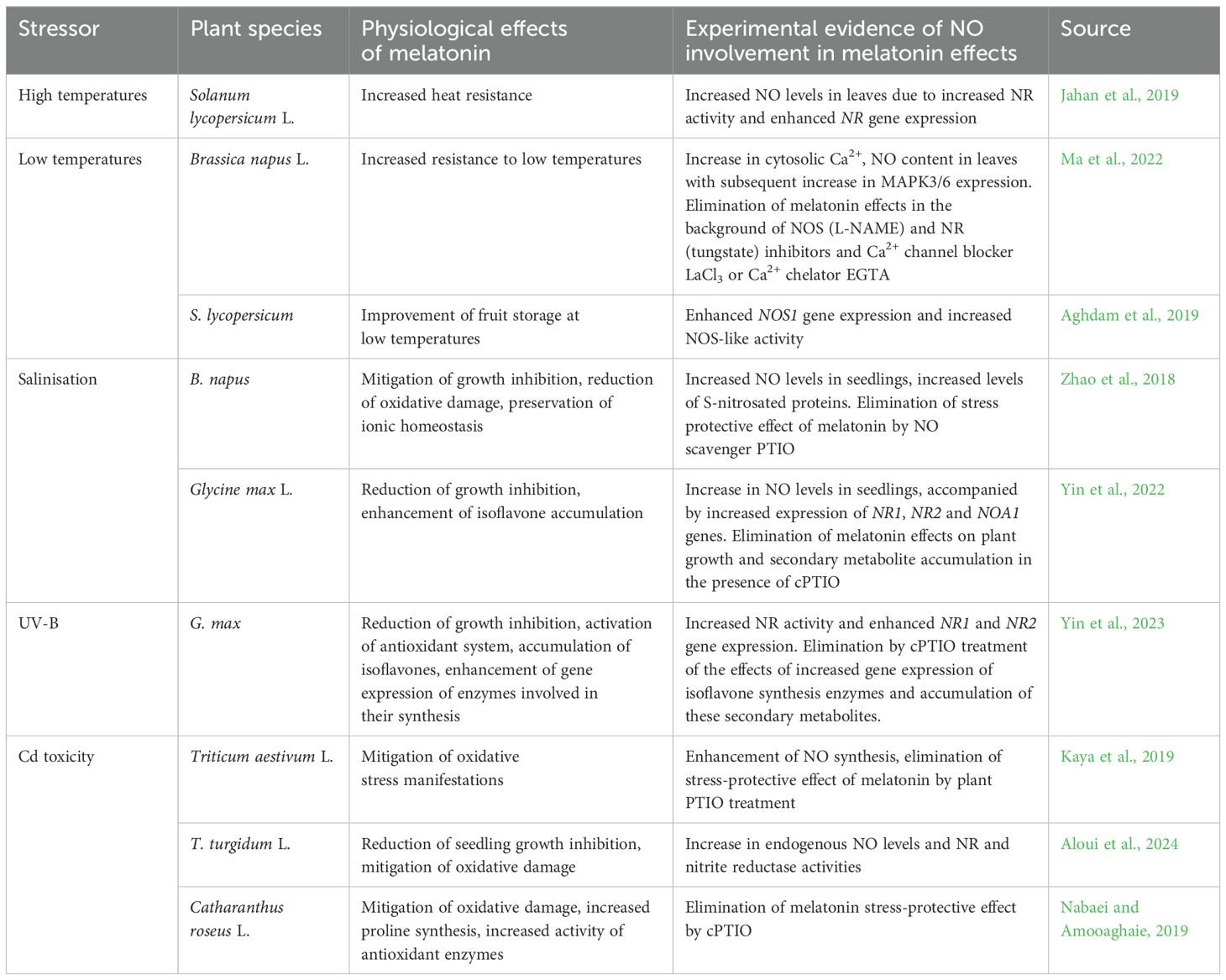
Table 1. Examples of the involvement of nitric oxide in the realisation of the effect of melatonin on plant resistance to abiotic stresses.
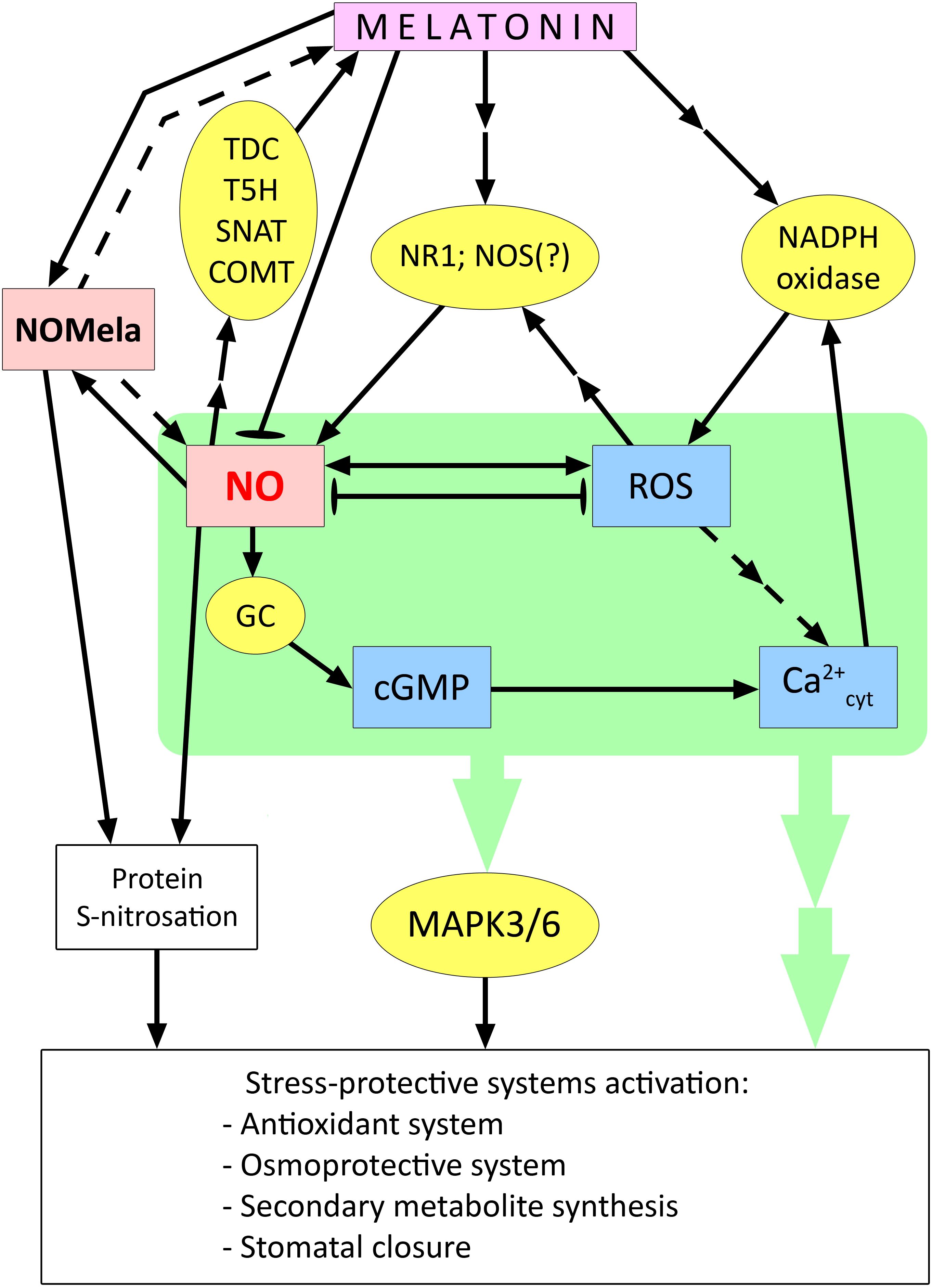
Figure 4. Involvement of NO in the activation of stress protection systems in plant cells under the action of melatonin. cGMP – cyclic guanosine monophosphate; COMT – caffeic acid O-methyltransferase; GC – guanylate cyclase; MAPK3/6 – mitogen activated protein kinases 3/6; NOMela – N-nitrosomelatonin; NOS – NO synthase; NR1 – nitrate reductase 1; ROS – reactive oxygen species; SNAT – serotonin N-acetyltransferase; T5H – tryptamine 5-hydroxylase; TDC – tryptophan decarboxylase. Dashed arrows indicate connections between signalling mediators without clear experimental evidence; blunt-ended arrows indicate antagonistic interactions between signalling mediators. Other explanations in the text.
In addition, the involvement of NO in the preservation of melatonin-treated tomato fruits during storage at low temperatures was demonstrated (Aghdam et al., 2019).
One of the effects of exogenous melatonin, which is important for plant resistance to drought and other stresses associated with plant desiccation, is stomatal closure. These processes depend on NO and its functional interaction with ROS. In Arabidopsis plants, it was shown that exogenous melatonin-induced stomatal closure was reversed by the NO scavenger cPTIO (carboxy-phenyl-4,4,5,5-tetramethylimidazoline-1-oxyl-3-oxide) (Wang et al., 2023a). In addition, lines mutant for the genes for NO synthesis enzymes, nitrate reductase 1 and 2 (nia1/nia2) and NO-associated 1 (noa1), were found to be unable to close stomata when exposed to melatonin. Melatonin-induced NO production was also impaired in plants mutant for NADPH oxidase genes (rhohC and rbohD/F). This indicates that NO is downstream of ROS in the melatonin-inducible signalling chain (Wang et al., 2023a) (Figure 4).
NO may also mediate the induction of salt tolerance by exogenous melatonin in rapeseed (Table 1). In addition, it was shown that mutants defective in NR genes had increased sensitivity to salt stress and that melatonin did not affect their salt tolerance (Zhao et al., 2018).
Using soybeans as an example, it has been shown that when melatonin induces salt tolerance and UV-B resistance NO is involved in an important defence response such as the accumulation of isoflavones (Yin et al., 2022, 2023) (Table 1).
The involvement of NO was also shown in the protective effect of melatonin on plants of two wheat species and Catharanthus roseus exposed to cadmium toxicity (Table 1). Notably, treatment of C. roseus plants with an NO donor, as with melatonin, increased proline content and antioxidant enzyme activities in roots under Cd stress (Nabaei and Amooaghaie, 2019). These melatonin-induced responses were inhibited by the NO scavenger, cPTIO. However, different results were obtained when the effect of melatonin on the Cd resistance in Chinese cabbage (Brassica rapa subsp. pekinensis) was studied (Wang et al., 2021). It was shown that treatment of the plants with melatonin significantly reduced the Cd content in them. At the same time, treatment with the NO scavenger cPTIO had the same effect on the Cd content in the plants. The authors showed that NO enhances the expression of the Cd transporter gene IRT1, thereby increasing Cd uptake and aggravating stress in plants, while exogenously added melatonin can inhibit NO synthesis, thereby reducing Cd levels and alleviating stress caused by its toxic effect (Wang et al., 2021).
The effect of reducing NO levels in plants treated with melatonin has also been reported in some other studies. There are reasons to believe that melatonin, in addition to inducing the synthesis of NO as a signalling mediator necessary for the activation of stress-protective systems, can prevent the accumulation of excessive amounts of NO and the development of nitrosative stress in plants.
Apparently, there are complex direct and inverse relationships between melatonin and NO. In addition to the facts of melatonin modulation of NO synthesis discussed above, data have also been obtained on the ability of NO to act as a signal inducing melatonin synthesis. Thus, it has been shown that NO mediated by cGMP can activate the expression of genes for the melatonin synthesis enzymes TDC, T5H, SNAT, and COMT, which leads to an increase in the endogenous level of melatonin (Wang et al., 2022a; Aghdam and Arnao, 2024). The NO scavenger cPTIO was found to disrupt Cd-induced melatonin synthesis by decreasing the expression of TDC and COMT genes in rice (He and He, 2020). Thus, plants may possess mechanisms for the mutual enhancement of melatonin and NO synthesis. At the same time, melatonin, which is synthesized in chloroplasts and mitochondria, may directly reduce the toxic effect of RNS in these organelles (Wang et al., 2022a).
Summarising the available data, it can be assumed that cytosolic Ca2+- and ROS-dependent enhancement of NO formation is a tool for melatonin activation of other components of the signalling network (in particular, the MAP-kinase cascade) and subsequent changes in the expression of genes that control rather universal stress-protective systems (antioxidant complex, synthesis of osmoprotectants, secondary metabolites, stomatal closure, etc (He and He, 2020; Khan et al., 2023; Plokhovska et al., 2023; Aghdam and Arnao, 2024) (Figure 4). On the other hand, the functional interaction between melatonin and NO also includes mechanisms that limit the effects of NO, in particular the formation of NOMela conjugate, as well as the negative effect of melatonin on the gene expression of NO synthesis enzymes, the mechanisms of which remain unclear. Thus, melatonin may prevent the development of nitrosative stress in plants. At the same time, it remains unclear how the transition from a synergistic interaction between melatonin and NO to an antagonistic one can occur. The complexity of the functional interaction between melatonin and NO is further complicated by the ambiguous and not yet well-understood role of NOMela, which may be both a product of excess NO binding and a source of NO for S-nitrosation processes of some proteins (Figure 4).
Functional relationships of melatonin and hydrogen sulfide in plants
Hydrogen sulfide (H2S) in animal cells is considered the third GT in terms of time of discovery and the importance after NO and CO (Liu et al., 2024). However, in the context of plant cell function, evidence for the role of H2S and related signalling processes is accumulating more dynamically than for the action of CO. Numerous studies have shown that H2S acts as a gaseous signalling molecule that enhances plant adaptation/tolerance to various abiotic stresses, including drought, salinity, temperature extremes, heavy metal effects, and other adverse factors (Zhang et al., 2021; Liu et al., 2024). H2S has also been found to be directly or indirectly involved in a wide range of physiological processes that occur under normal conditions, including seed germination, root development, stomatal movement, and fruit ripening (Corpas and Palma, 2020). At the same time, H2S is closely related to other components of the signalling network, primarily ROS, calcium ions, and NO (Corpas et al., 2022b; Khan et al., 2022; Liu et al., 2024). One of the most studied ways in which H2S is involved in signalling processes is through a PTM of proteins known as persulfidation (Liu et al., 2024). This process involves the conversion of protein thiol groups (RSH) to persulfide groups (RSSH). It is suggested that such a reversible PTM is not only important for signalling processes but is also one of the mechanisms of the direct protective effect of H2S on proteins under conditions of oxidative stress (Wang et al., 2021). The mechanism of protein persulfidation is not fully understood. It is believed that H2S or its ionic forms, HS– and S2–, cannot react directly with protein thiols. Such interaction requires the presence of oxidizing agents (Aroca et al., 2021).
Persulfidation is likely a part of the toolkit for gene expression regulation. A transcriptome study performed on Arabidopsis plants showed that treatment with exogenous H2S induced significant changes in the expression of genes encoding different transcriptional regulators (Aroca et al., 2017). Persulfidation is also one of the mechanisms regulating the activity of several antioxidant enzymes, and it can cause both increased and decreased catalytic activity and protect protein molecules from oxidative degradation (Li et al., 2020; Shivaraj et al., 2020). In addition, the same enzymatic proteins can be targets for persulfidation and S-nitrosation, which is a prerequisite for functional interaction between H2S and NO (Kolupaev et al., 2023b). For example, the possibility of cross-regulation of activity by persulfidation and nitrosation has been demonstrated for several molecular forms of ascorbate peroxidase (Kolupaev et al., 2023b; Li et al., 2024). It was also shown that persulfidation of two cysteine residues increased the activity of one of the molecular forms of NADPH oxidase (Shen et al., 2020), suggesting a functional interaction between H2S and ROS in signalling processes. On the other hand, as mentioned above, NO can inhibit NADPH oxidase through S-nitrosylation. Thus, ROS, NO, and H2S form a complex signalling and regulatory network that controls redox modifications of proteins (Kolupaev et al., 2023b).
Another integral component of such a network is calcium as a universal intracellular messenger. In particular, the possibility of persulfidation of Ca2+ channels with an increase in the concentration of H2S in the submembrane space is considered (Li et al., 2024). In many works, the dependence of induction of physiological reactions by exogenous H2S (its donors) on Ca2+ homeostasis has been shown by inhibitor methods (Li et al., 2012; Kolupaev et al., 2017).
Brief overview of H2S synthesis in plants
In plant cells, H2S synthesis occurs predominantly in chloroplasts and to a lesser extent in other subcellular spaces such as the cytoplasm and mitochondria (Pandey et al., 2024). L-cysteine desulfhydrase (LCD) is considered to be the major enzyme of H2S synthesis. It is localised in cytoplasm, plastids and mitochondria as reported by (Riemenschneider et al., 2005). The synthesis of H2S from D-cysteine is also possible through the action of D-cysteine desulfhydrase localised in the cytoplasm (Guo et al., 2016). In recent years, the desulfhydrase AtDES1 has been considered another novel H2S synthesising enzyme in the Arabidopsis cytoplasm, which is believed to be a protein similar to cysteine synthase, but mainly exhibits activity related to the degradation of L-cysteine, accompanied by the formation of H2S (Zhang et al., 2021). Experimental evidence has been provided for the role of this enzyme in the synthesis of H2S in response to abiotic stresses, particularly drought (Zhang et al., 2021).
In chloroplasts, H2S can also be synthesized by sulfite reduction involving sulfite reductase when the photosynthetic sulfate assimilation pathway is active (Pandey et al., 2024). In mitochondria, H2S formation is possible during cyanide detoxification. This process is mediated by β-cyanoalanine synthase, an enzyme that catalyses the conversion of cyanide to β-cyanoalanine with the consumption of cysteine (Feng et al., 2022). The putative compartmentalisation of the synthesis of a significant pool of H2S in mitochondria and chloroplasts, compartments in which melatonin is mainly synthesised, is considered the basis for studying the functional interaction between melatonin and H2S (Wang et al., 2022b).
Involvement of H2S in realisation of stress-protective effect of melatonin on plants
At present, there is a lot of data indicating the participation of H2S as a mediator in the realisation of the protective effects of melatonin when plants are exposed to stress factors of various nature (Table 2). In particular, it was shown that the increase in heat resistance of wheat plants under the influence of melatonin was eliminated by the H2S scavenger hypotaurine (Iqbal et al., 2021). The dependence of melatonin enhancement for drought tolerance in tomato plants on H2S synthesis has also been reported (Khan et al., 2024a) (Table 2).
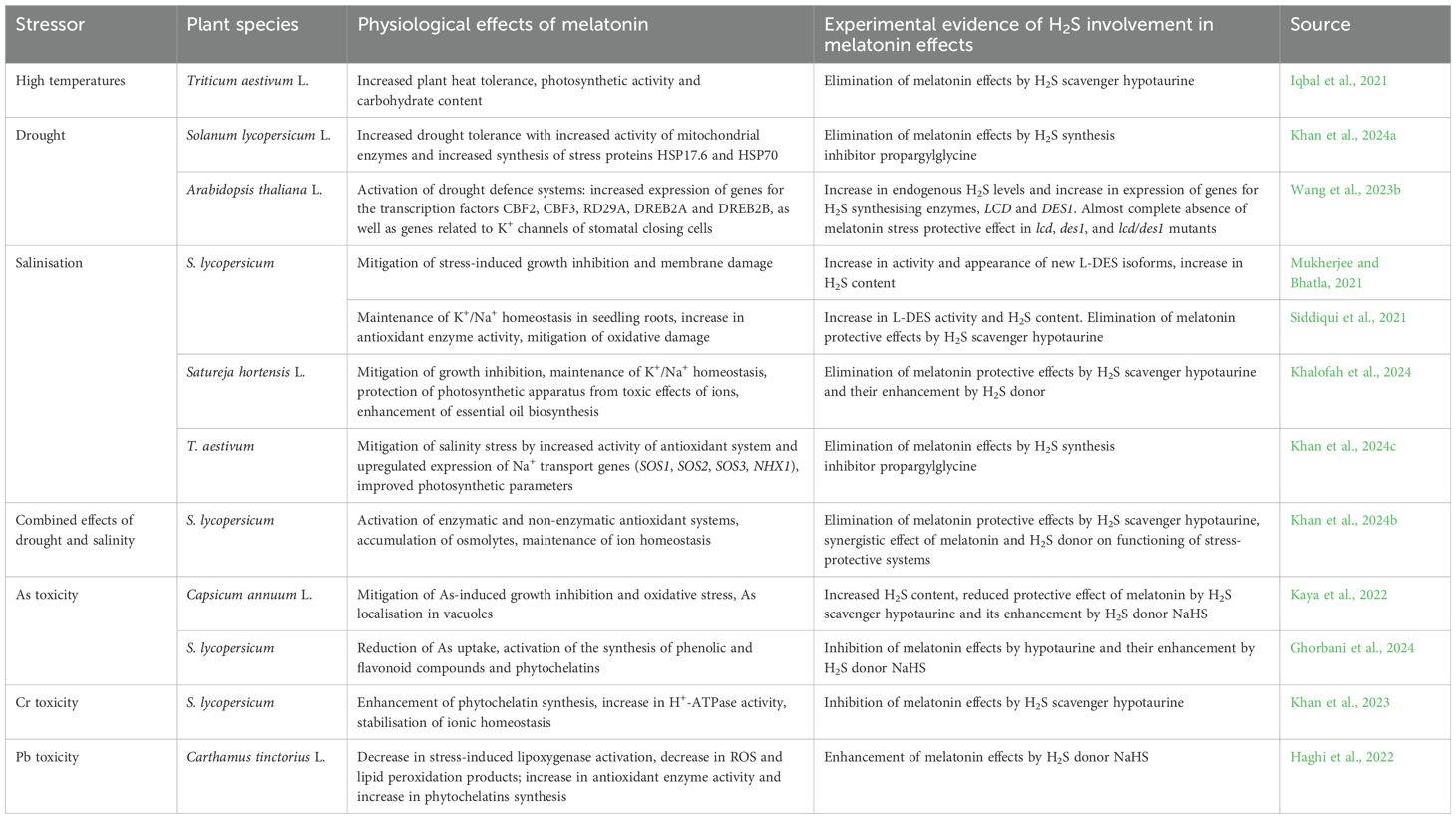
Table 2. Examples of the involvement of hydrogen sulfide in the realisation of the effect of melatonin on plant resistance to abiotic stresses.
In Arabidopsis plants, molecular genetic methods have shown that H2S is involved in many of the stress-protective effects of melatonin under drought (Wang et al., 2023b) (Table 2). It was also found that both melatonin and H2S affected the level of transcription of genes associated with K+ channels involved in maintaining stomatal closure (Wang et al., 2023b).
There is reason to believe that not only the effect of melatonin on stomatal status depends on H2S synthesis, but conversely, H2S-induced stomatal closure depends on melatonin synthesis (Wang et al., 2022a). For example, Arabidopsis plants showed that exogenous H2S increased transcript levels of the melatonin synthesis enzymes SNAT, ASMT, and COMT1 in leaves and caused persulfidation of SNAT and ASMT. At the same time, H2S was found to have little effect on the condition of stomata in mutants of the melatonin synthesis enzymes snat, comt1, and asmt (Wang et al., 2022a). These findings suggest that there may be some kind of signal amplifying loop between melatonin and H2S (Figure 5).
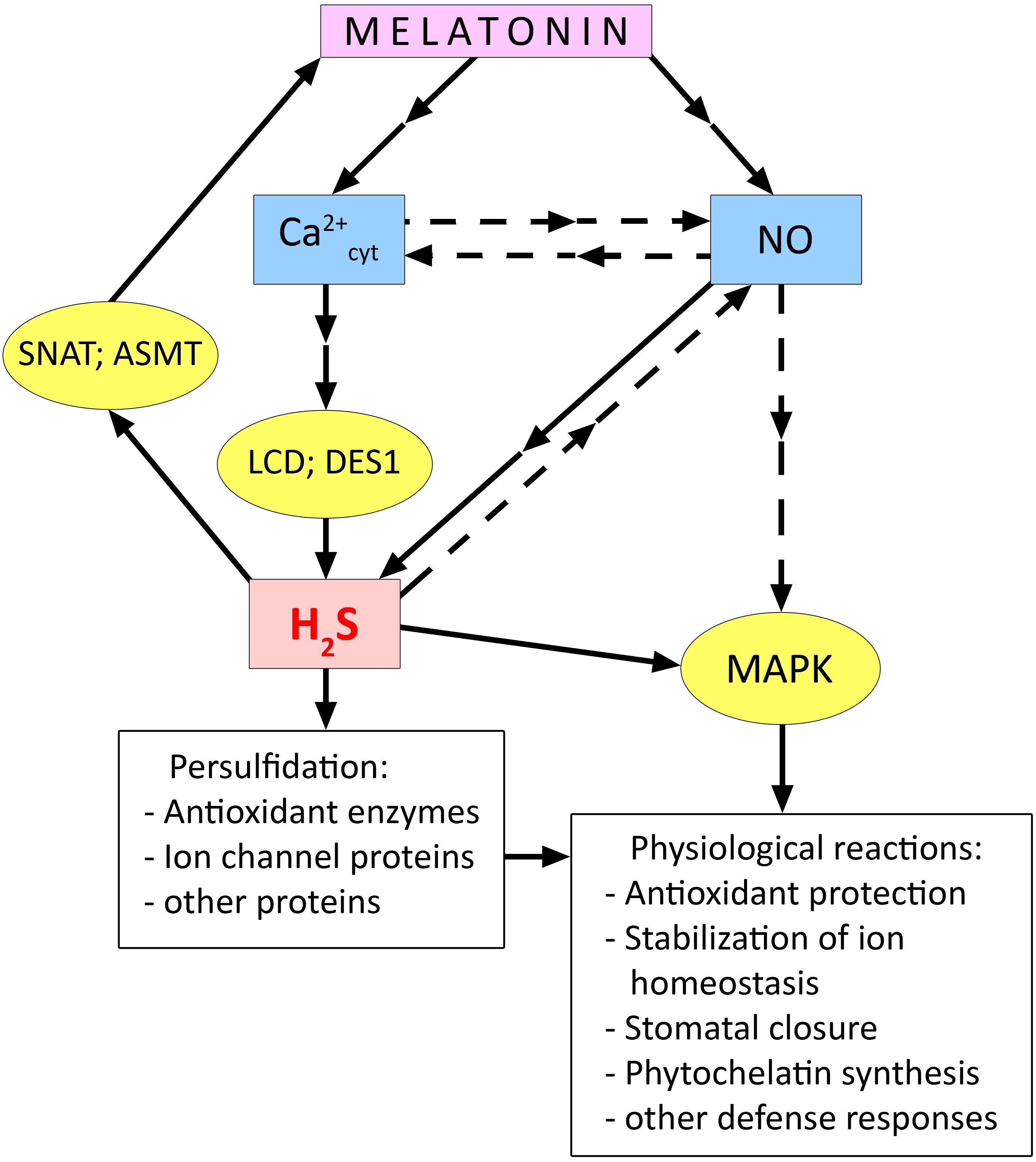
Figure 5. Relationship between melatonin and hydrogen sulfide during activation of plant cell stress defence systems. ASMT, N-acetylserotonin O-methyltransferase; DES1, cysteine synthase-like desulfhydrase 1; LCD, L-cysteine desulfhydrase; MAPK, mitogen activated protein kinases; SNAT, serotonin N-acetyltransferase. Dashed arrows indicate connections between signalling mediators without clear experimental evidence. Other explanations in the text.
This hypothesis is also supported by data on the synergistic interaction between melatonin and H2S, obtained in the study of plant adaptation to various stresses. Thus, the involvement of endogenous H2S and the LCD enzyme was found in the melatonin-induced increase in salt tolerance of tomato (Table 2). Synergism of the joint protective effect of melatonin and H2S was also observed, which was expressed in the prevention of the development of oxidative cell damage by increasing the activity of antioxidant enzymes (Siddiqui et al., 2021). Data indicating the involvement of H2S as a mediator in the realisation of stress-protective effects of melatonin were also obtained when studying its effect on salt tolerance of Satureja hortensis L. (Table 2).
The protective effect of melatonin on the state of antioxidant and osmoprotective systems of tomato plants under the combined action of drought and salinity was not manifested in the presence of the H2S scavenger hypotaurine but was enhanced by simultaneous treatment of plants with a hydrogen sulfide donor (Khalofah et al., 2024; Table 2).
H2S also appears to be involved in realising the protective effects of melatonin on pepper and tomato plants exposed to arsenic toxicity (Table 2). These studies (Kaya et al., 2022; Ghorbani et al., 2024) also found not only attenuation of the stress-protective effect of melatonin by the H2S scavenger, but also enhancement of the protective effects when melatonin was used together with the H2S donor NaHS.
Data were obtained on the participation of H2S as a mediator in the development of specific protective reactions of plants induced by melatonin and in adaptation to the action of other heavy metals (Table 2). Thus, the melatonin-induced increase in the resistance of tomato seedlings was attenuated by an H2S scavenger (Khan et al., 2023). Treatment of safflower plants with melatonin and the H2S donor NaHS attenuated the toxic effect of lead (Haghi et al., 2022). The combined action of melatonin and NaHS was particularly effective (Table 2), with a significant increase in the activity of enzymes responsible for the ascorbate-glutathione cycle and increased synthesis of metal-binding ligands.
It is still unclear how H2S as a participant of signal transduction induced by melatonin is related to other key signal mediators. At the same time, some experimental data suggest the role of interaction of H2S with Ca2+ and NO in the manifestation of melatonin-induced stress-protective responses. Mukherjee et al. (2023) showed that enhancement of synthesis of H2S required for melatonin to strengthen sunflower resistance to salt was suppressed in the presence of the Ca2+ chelator EGTA and the Ca2+ channel blocker verapamil. The involvement of Ca2+ signalling in the activation of H2S synthesis was also confirmed in the study of melatonin activation of seed germination in plants of different species (Wang et al., 2024).
Exogenous melatonin caused an increase in salt tolerance of cucumber seedlings, improved the function of the photosynthetic apparatus and antioxidant system, and increased MAPK activity under stress conditions (Sun et al., 2021). All the above-mentioned stress-protective effects of melatonin were eliminated by treating plants with NO scavenger (cPTIO) and H2S scavenger (hypotaurine). In contrast, the MAPK inhibitor (U0126) had no effect on H2S and NO synthesis. The authors suggest that H2S acts as a mediator of the physiological effects of melatonin and is upstream of the MAPK cascade in the signalling chain (Figure 5). Kaya et al. (2020) provided evidence that the melatonin-induced increase in H2S synthesis in pepper (Capsicum annuum L.) plants was mediated by an increase in NO levels. The authors found that the melatonin-induced increase in H2S content was suppressed by the action of both the H2S scavenger hypotaurine and the NO scavenger cPTIO. In contrast, hypotaurine did not eliminate the effect of increased NO in plants. This work also showed the involvement of NO and H2S in the realisation of the protective effect of foliar treatment of pepper plants with melatonin under iron deficiency and salinity stress (Kaya et al., 2020).
Thus, few experimental data indicate the joint involvement of NO and H2S in transducing melatonin-induced signals leading to the development of plant resistance to certain stresses such as salinity (Kaya et al., 2020; Sun et al., 2021) (Figure 5). At the same time, it has been shown that treatment of etiolated seedlings of Triticum turgidum L. with melatonin, which increases their resistance to cadmium, was accompanied by an increase in NO levels but a decrease in H2S levels and the activity of its synthetic enzymes L- and D-cysteine desulfhydrases (Aloui et al., 2024). It is difficult to interpret such data on the antagonistic interaction between melatonin and H2S because they are sporadic and may be due to specific features of the object of study or the experimental design. In this regard, it should be noted that a recent work by Zulfiqar et al. (2024) reported a synergistic effect of melatonin and the H2S donor NaHS on the resistance of Matthiola incana L. plants to the toxic effect of cadmium. When used together, melatonin and H2S donor more effectively protected plants from the development of oxidative stress by increasing the activity of antioxidant enzymes and one of the key enzymes of the synthesis of secondary metabolites phenylalanine ammonia-lyase.
Possible relationship of melatonin and carbon monoxide in plant adaptation to abiotic stresses
The mechanisms underlying the biological activity of CO are significantly different from those of NO and H2S. It is assumed that they are largely due to the formation of coordination bonds between CO and metals in the active centres of proteins, primarily heme-containing proteins (Feelisch and Olson, 2013). In general, however, the question of the molecular targets of CO action involved in the manifestation of certain physiological effects in plants remains open. Nevertheless, it is now clear that CO has a positive effect on seed germination, root development, fruit ripening and maturation, and stomatal closure (Cao et al., 2007; Zhang et al., 2014; Gahir et al., 2020; Hong et al., 2024). In recent years, the involvement of CO in the adaptation of plants to the action of stress factors of different nature and the possibility of increasing plant resistance with the help of CO donors have been studied particularly intensively (Yao et al., 2019; Kolupaev et al., 2022).
Hemoxygenase, which catalyses the stereospecific conversion of heme to biliverdin-IXα (BV-IXα) with the release of Fe2+ and CO, is considered to be the major enzyme responsible for CO synthesis in both animals and plants (Shekhawat and Verma, 2010). This reaction requires NADPH as an electron source and molecular oxygen (Bilban et al., 2008). Plant hemoxygenases are represented by a family of four genes, of which HO-1 is the most highly expressed (Matsumoto et al., 2004). In plant cells, the enzymatic protein HO-1 is found in chloroplasts and mitochondria (Shekhawat and Verma, 2010; Dixit et al., 2014). Thus, the localisation of CO synthesis, as well as other GTs, coincides with the subcellular localisation of melatonin formation in plants.
The data on the mechanisms of CO signal transduction in the genetic apparatus of plant cells, as well as on its relationship with other signal mediators, are still insufficient. It is known that CO can bind to the Fe atom of the heme fragment of guanylate cyclase in animal cells, thereby activating the enzyme and the synthesis of the secondary intracellular messenger cGMP (He and He, 2014). The presence of guanylate cyclase activity and cGMP in cells has been demonstrated in a number of plant species in recent years. It has been suggested that CO, like NO (Neill et al., 2008), may affect Ca2+ homeostasis via cGMP.
There are also data in the literature suggesting a functional relationship between CO and NO. For example, enhancement of lateral root formation in rapeseed induced by CO donor treatment was dependent on NO synthesis (Cao et al., 2007). Root hair development under the action of exogenous CO was accompanied by an increase in endogenous NO (Guo et al., 2009). It was also shown that stomatal closure effect induced by exogenous CO was accompanied by an increase in NO in the closing cells and was eliminated by inhibitors of NO synthesis (Song et al., 2008). The increase in heat resistance of wheat seedlings induced by the CO donor hemin was accompanied by a transient increase in NO levels in root cells associated with NR activation (Shkliarevskyi et al., 2021). Subsequently, H2O2 generation was enhanced by activation of extracellular peroxidase. However, both the effect of increased NO and the effect of increased H2O2 were supressed by various Ca2+ antagonists. It has been suggested that Ca2+ and NO are upstream of ROS in the signalling chain when heat resistance of wheat seedlings is induced by CO (Shkliarevskyi et al., 2020; 2021).
The relationship between CO and melatonin in plants has not been experimentally confirmed until recently. A review by Wang et al. (2022a) suggested a possible effect of melatonin on HO-1 gene expression by analogy with the existence of such a mechanism in animal cells. More recently, the involvement of CO as a signalling mediator in melatonin-induced drought tolerance in tomato was investigated (Liu et al., 2024). It was shown that melatonin-induced alleviation of the growth inhibitory effects of drought was eliminated by the CO scavenger hemoglobin. Hemoglobin also eliminated the beneficial effect of melatonin on the expression of several genes related to chlorophyll and heme synthesis. Thus, based on the inhibitor analysis data, the authors suggest that CO is involved in the realisation of the stress-protective effect of melatonin on plants under drought. However, there are no direct experimental evidence confirming the effect of melatonin on HO-1 gene expression and activity of this enzyme in plants. The connection of CO as a possible mediator in the realisation of melatonin action with other signalling molecules (ROS, NO, Ca2+ ions), which, as mentioned above, are involved in the signal transduction of CO when it induces stress-protective reactions in plants, remains unexplored. The potential effect of CO on melatonin synthesis in plants also remains unexplored. However, it has been shown in animal models that CO can stimulate pineal gland cells to produce melatonin (Romerowicz-Misielak et al., 2018).
Conclusion and prospects
Melatonin is now considered a novel plant hormone and a promising regulator of plant growth and adaptation (Aghdam, and Arnao, 2024; Arnao et al., 2022). At the same time, as the authors of a recent review wittily pointed out (Aghdam and Arnao, 2024), only a decade and a half of intensive research has moved phytomelatonin from being studied as a key player in intracellular signalling to being a player in the global horticulture market. The number of articles on phytomelatonin in leading journals has increased from 33 in 2007 to over 500 in 2023 (Aghdam and Arnao, 2024).
The accumulated data show that melatonin exerts its stress-protective effect mainly through its involvement in the general signalling network of plant cells (Murch and Erland, 2021). Data have been obtained indicating the ability of melatonin to influence the state of Ca2+ channels, and the melatonin-induced increase in Ca2+ influx into the cytosol from the extracellular space and intracellular compartments may be one of the first stages of its involvement in the signalling network. Equally important appears to be the involvement of melatonin in ROS signalling. Despite the potent direct antioxidant effect of melatonin, its exogenous entry into plant cells results in a transient increase in ROS generation, which is likely primarily associated with the activation of NADPH oxidase.
The involvement of GTs in plant cell signalling and adaptation to various stresses has become one of the main directions in experimental plant biology, along with the topic of melatonin (Yao et al., 2019; Kolupaev et al., 2022). However, it is only in the last few years that knowledge has begun to accumulate, which allows us to build, at least in a general way, models of the functional interaction between melatonin and GTs. Thus, in a review published two years ago (Wang et al., 2022a), the discussion of possible functional relationships between melatonin and GTs in plant cells was mainly based on analogies with the mechanisms of their interaction in animal cells. At present, experimental data have been obtained indicating that for the manifestation of many stress-protective effects of melatonin it is necessary to enhance NO synthesis in plants. Using inhibitor analysis and partly molecular genetic methods, information on the involvement of H2S in the realisation of melatonin effects has also been obtained.
At the same time, the elucidation of the functional interaction of the two most important GTs, H2S and NO, in the realisation of melatonin’s regulatory effects is only beginning. The ability of both GTs to induce protein PTM and the ability of melatonin to bind NO and ROS, which react with thiol groups (Figure 6), suggest that melatonin may act as a regulator of the PTM of at least some proteins. However, the effects of melatonin on these processes are far from clear. For example, as mentioned above, NOMela formed by the interaction of NO with melatonin may be more efficient than NO in S-nitrosation reactions of thiol groups. On the other hand, the reaction product of melatonin with ROS 2-OHM can significantly activate one of the main ROS-generating enzymes, NADPH oxidase (Figure 3). Considering that groups previously subjected to S-nitrosation or oxidation are involved in persulfidation reactions by H2S (Aroca et al., 2021), it is conceivable that melatonin may also play a prominent role in modulating the process of protein persulfidation. The hypothetical pathways outlined above only consider the direct interaction of melatonin with PTM inducers. However, there is no doubt that these in vivo processes are superimposed by the involvement of melatonin in the general signalling network and, as a consequence, its influence on the gene expression of many proteins. Unfortunately, the regulatory functions of melatonin as an agent potentially affecting protein PTMs involving ROS, NO and H2S remain largely unexplored. Individual examples of proteins whose state is affected by melatonin and its derivatives (NADPH oxidase and glyceraldehyde-3-phosphate dehydrogenase) have been given above. On the other hand, the functional interaction of NO and H2S GTs in protein PTM is better understood and there are considerably more examples of proteins regulated by them, including in a competitive manner (for reviews, see Arora and Bhatla, 2015; Correa-Aragunde et al., 2015; Corpas et al., 2019a, 2019; Mishra et al., 2021; Kolupaev et al., 2023b). Thus, a detailed study of the effect of melatonin on the processes of protein PTM by GTs may become one of the important areas of research that can contribute to the elucidation of the mechanisms of its stress-protective action in plant cells.
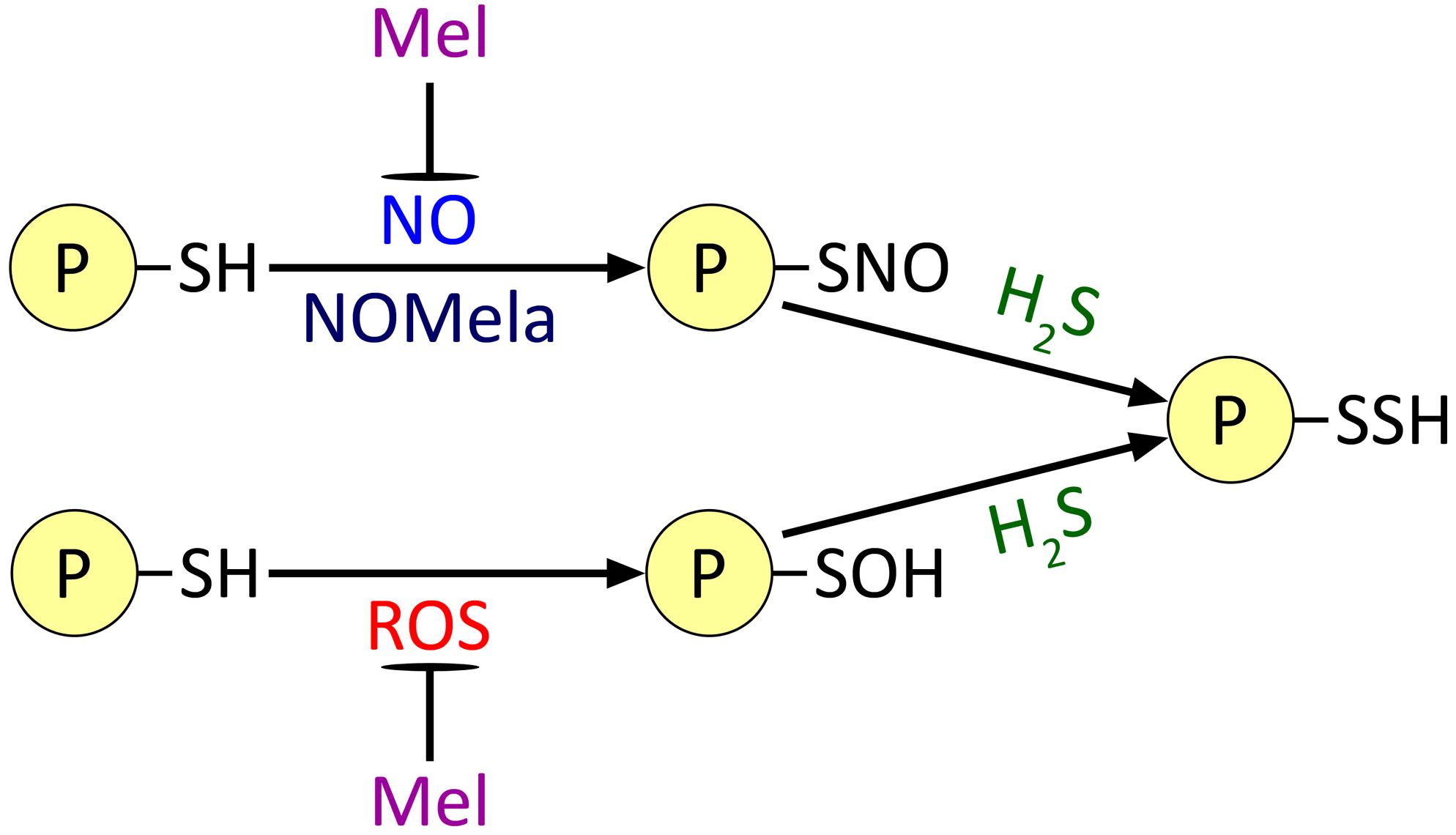
Figure 6. Possible effect of melatonin on the processes of post-translational modification of proteins by gasotransmitters and ROS. Mel, melatonin; NOMela, N-nitrosomelatonin; ROS, reactive oxygen species. Other explanations in the text.
Finally, there is a clear lack of experimental data on the functional interaction between melatonin and CO in plant cells. The latter is a recognised GT of plant cells, but due to the lack of cheap and available CO donors, it has not yet found practical application in crop production. Nevertheless, elucidation of the role of CO as a likely link in the physiological effects of melatonin in plants is also necessary to fully understand the mechanisms of action of this new plant hormone.
Author contributions
YK: Formal analysis, Writing – original draft. AY: Methodology, Writing – review & editing, Writing – original draft. TY: Formal analysis, Writing – original draft, Writing – review & editing. YB: Conceptualization, Supervision, Writing – original draft, Writing – review & editing.
Funding
The author(s) declare that financial support was received for the research, authorship, and/or publication of this article. This work was carried out (AY) partially under the financial support of the National Academy of Sciences of Ukraine (NASU) (budget program 6541030, 2024-2028, state registration number 0124U002424) and the National Academy of Agrarian Sciences of Ukraine (budget program 14.00.02.06.P, state registration number of work 0124U000126).
Acknowledgments
The authors are grateful to Dr. V. Kyrylenko (Institute of Food Biotechnology and Genomics, NASU) for final improving the English version of the manuscript.
Conflict of interest
The authors declare that the research was conducted in the absence of any commercial or financial relationships that could be construed as a potential conflict of interest.
The author(s) declared that they were an editorial board member of Frontiers, at the time of submission. This had no impact on the peer review process and the final decision.
Generative AI statement
The author(s) declare that no Generative AI was used in the creation of this manuscript.
Publisher’s note
All claims expressed in this article are solely those of the authors and do not necessarily represent those of their affiliated organizations, or those of the publisher, the editors and the reviewers. Any product that may be evaluated in this article, or claim that may be made by its manufacturer, is not guaranteed or endorsed by the publisher.
References
Agathokleous, E., Zhou, B., Xu, J., Ioannou, A., Feng, Z., Saitanis, C. J., et al. (2021). Exogenous application of melatonin to plants, algae, and harvested products to sustain agricultural productivity and enhance nutritional and nutraceutical value: A meta-analysis. Environ. Res. 200, 111746. doi: 10.1016/j.envres.2021.111746
Aghdam, M. S., Arnao, M. B. (2024). Phytomelatonin: from intracellular signaling to global horticulture market. J. Pineal. Res. 76, e12990. doi: 10.1111/jpi.12990
Aghdam, M. S., Luo, Z., Jannatizadeh, A., Sheikh-Assadi, M., Sharafi, Y., Farmani, B., et al. (2019). Employing exogenous melatonin applying confers chilling tolerance in tomato fruits by upregulating ZAT2/6/12 giving rise to promoting endogenous polyamines, proline, and nitric oxide accumulation by triggering arginine pathway activity. Food Chem. 275, 549–556. doi: 10.1016/j.foodchem.2018.09.157
Ahammed, G. J., Li, Z., Chen, J., Dong, Y., Qu, K., Guo, T., et al. (2024). Reactive oxygen species signaling in melatonin-mediated plant stress response. Plant Physiol. Biochem. 207, 108398. doi: 10.1016/j.plaphy.2024.108398
Ahammed, G. J., Xu, W., Liu, A., Chen, S. (2019). Endogenous melatonin deficiency aggravates high temperature-induced oxidative stress in Solanum lycopersicum L. Environ. Exp. Bot. 161, 303–311. doi: 10.1016/j.envexpbot.2018.06.006
Ahmad, I., Song, X., Hussein Ibrahim, M. E., Jamal, Y., Younas, M. U., Zhu, G., et al. (2023). The role of melatonin in plant growth and metabolism, and its interplay with nitric oxide and auxin in plants under different types of abiotic stress. Front. Plant Sci. 14. doi: 10.3389/fpls.2023.1108507
Akula, R., Mukherjee, S. (2020). New insights on neurotransmitters signaling mechanisms in plants. Plant Signal. Behav. 15, 1737450. doi: 10.1080/15592324.2020.1737450
Ali, M. A. A., Nasser, M. A., Abdelhamid, A. N., Ali, I. A. A., Saudy, H. S., Hassan, K. M. (2024). Melatonin as a key factor for regulating and relieving abiotic stresses in harmony with phytohormones in horticultural plants — a review. J. Soil Sci. Plant Nutr. 24, 54–73. doi: 10.1007/s42729-023-01586-9
Aloui, N., Kharbech, O., Mahjoubi, Y., Chaoui, A., Karmous, I. (2024). Exogenous melatonin alleviates cadmium toxicity in wheat (Triticum turgidum L.) by modulating endogenous nitric oxide and hydrogen sulfide metabolism. J. Soil Sci. Plant Nutr. 24, 2535–2552. doi: 10.1007/s42729-024-01675-3
Arnao, M. B., Cano, A., Hernández-Ruiz, J. (2022). Phytomelatonin: an unexpected molecule with amazing performances in plants. J. Exp. Bot. 73, 5779–5800. doi: 10.1093/jxb/erac009
Arnao, M., Hernández-Ruiz, J. (2019). Melatonin and reactive oxygen and nitrogen species: a model for the plant redox network. Melatonin. Res. 2, 152. doi: 10.32794/11250036
Aroca, A., Benito, J. M., Gotor, C., Romero, L. C. (2017). Persulfidation proteome reveals the regulation of protein function by hydrogen sulfide in diverse biological processes in Arabidopsis. J. Exp. Bot. 68, 4915–4927. doi: 10.1093/jxb/erx294
Aroca, A., Zhang, J., Xie, Y., Romero, L. C., Gotor, C. (2021). Hydrogen sulfide signaling in plant adaptations to adverse conditions: molecular mechanisms. J. Exp. Bot. 72, 5893–5904. doi: 10.1093/jxb/erab239
Arora, D., Bhatla, S. C. (2015). Nitric oxide triggers a concentration-dependent differential modulation of superoxide dismutase (FeSOD and Cu/ZnSOD) activity in sunflower seedling roots and cotyledons as an early and long. Plant Signal. Behav. 10, e1071753. doi: 10.1080/15592324.2015.1071753
Astier, J., Gross, I., Durner, J. (2018). Nitric oxide production in plants: an update. J. Exp. Bot. 69, 3401–3411. doi: 10.1093/jxb/erx420
Astier, J., Kulik, A., Koen, E., Besson-Bard, A., Bourque, S., Jeandroz, S., et al. (2012). Protein S-nitrosylation: what’s going on in plants? Free Radical Biol. Med. 53, 1101–1110. doi: 10.1016/j.freeradbiomed.2012.06.032
Back, K. (2021). Melatonin metabolism, signaling and possible roles in plants. Plant J. 105, 376–391. doi: 10.1111/tpj.14915
Bilban, M., Haschemi, A., Wegiel, B., Chin, B. Y., Wagner, O., Otterbein, L. E. (2008). Heme oxygenase and carbon monoxide initiate homeostatic signaling. J. Mol. Med. 86, 267–279. doi: 10.1007/s00109-007-0276-0
Blume, Y. B., Krasylenko, Y. A., Demchuk, O. M., Yemets, A. I. (2013). Tubulin tyrosine nitration regulates microtubule organization in plant cells. Front. Plant Sci. 4. doi: 10.3389/fpls.2013.00530
Buttar, Z. A., Wu, S. N., Arnao, M. B., Wang, C., Ullah, I. (2020). Melatonin suppressed the heat stress-induced damage in wheat seedlings by modulating the antioxidant machinery. Plants (Basel). 9, 809. doi: 10.3390/plants9070809
Byeon, Y., Back, K. (2014). Melatonin synthesis in rice seedlings in vivo is enhanced at high temperatures and under dark conditions due to increased serotonin N-acetyltransferase and N-acetylserotonin methyltransferase activities. J. Pineal. Res. 56, 189–195. doi: 10.1111/jpi.12111
Byeon, Y., Tan, D. X., Reiter, R. J., Back, K. (2015). Predominance of 2-hydroxymelatonin over melatonin in plants. J. Pineal. Res. 59, 448–454. doi: 10.1111/jpi.12274
Cannon, A. E., Chapman, K. D. (2021). Lipid signaling through G proteins. Trends Plant Sci. 26, 720–728. doi: 10.1016/j.tplants.2020.12.012
Cao, Z., Xuan, W., Liu, Z., Li, X., Zhao, N., Xu, P., et al. (2007). Carbon monoxide promotes lateral root formation in rapeseed. J. Integr. Plant Biol. 49, 1070–1079. doi: 10.1111/j.1672-9072.2007.00482.x
Chang, J., Guo, Y., Li, J., Su, Z., Wang, C., Zhang, R., et al. (2021). Positive interaction between H2O2 and Ca2+ mediates melatonin-induced CBF pathway and cold tolerance in watermelon (Citrullus lanatus L.). Antioxidants 10, 1457. doi: 10.3390/antiox10091457
Chen, Z., Xie, Y., Gu, Q., Zhao, G., Zhang, Y., Cui, W., et al. (2017). The AtrbohF-dependent regulation of ROS signaling is required for melatonin-induced salinity tolerance in Arabidopsis. Free Radic. Biol. Med. 108, 465–477. doi: 10.1016/j.freeradbiomed.2017.04.009
Cheng, X., Zheng, Y., Liu, X., Xu, L., An, S., Liu, Y., et al. (2024). Overexpression of cassava melatonin receptor PMTR1 plays dual roles in development under light and dark conditions in Arabidopsis. Plant Cell Rep. 43, 153. doi: 10.1007/s00299-024-03246-3
Choi, G. H., Back, K. (2019). Suppression of melatonin 2-hydroxylase increases melatonin production leading to the enhanced abiotic stress tolerance against cadmium, senescence, salt, and tunicamycin in rice plants. Biomolecules 9, 589. doi: 10.3390/biom9100589
Colombage, R., Singh, M. B., Bhalla, P. L. (2023). Melatonin and abiotic stress tolerance in crop plants. Int. J. Mol. Sci. 24, 7447. doi: 10.3390/ijms24087447
Corpas, F. J., Alché, J. D., Barroso, J. B. (2013). Current overview of S-nitrosoglutathione (GSNO) in higher plants. Front. Plant Sci. 4. doi: 10.3389/fpls.2013.00126
Corpas, F. J., Barroso, J. B. (2014). Peroxisomal plant nitric oxide synthase (NOS) protein is imported by peroxisomal targeting signal type 2 (PTS2) in a process that depends on the cytosolic receptor PEX7 and calmodulin. FEBS Lett. 588, 2049–2054. doi: 10.1016/j.febslet.2014.04.034
Corpas, F. J., Barroso, J. B., González-Gordo, S., Muñoz-Vargas, M. A., Palma, J. M. (2019a). Hydrogen sulfide: A novel component in arabidopsis peroxisomes which triggers catalase inhibition. J. Integr. Plant Biol. 61, 871–883. doi: 10.1111/jipb.12779
Corpas, F. J., Del Río, L. A., Palma, J. M. (2019b). Plant peroxisomes at the crossroad of NO and H2O2 metabolism. J. Integr. Plant Biol. 61, 803–816. doi: 10.1111/jipb.12772
Corpas, F. J., González-Gordo, S., Rodríguez-Ruiz, M., Muñoz-Vargas, M. A., Palma, J. M. (2022b). Thiol-based oxidative posttranslational modifications (OxiPTMs) of plant proteins. Plant Cell Physiol. 63, 889–900. doi: 10.1093/pcp/pcac036
Corpas, F. J., Palma, J. M. (2020). H2S signaling in plants and applications in agriculture. J. Adv. Res. 24, 131–137. doi: 10.1016/j.jare.2020.03.011
Corpas, F. J., Rodríguez-Ruiz, M., Muñoz-Vargas, M. A., González-Gordo, S., Reiter, R. J., Palma, J. M. (2022a). Interactions of melatonin, reactive oxygen species, and nitric oxide during fruit ripening: an update and prospective view. J. Exp. Bot. 73, 5947–5960. doi: 10.1093/jxb/erac128
Correa-Aragunde, N., Foresi, N., Lamattina, L. (2015). Nitric oxide is a ubiquitous signal for maintaining redox balance in plant cells: Regulation of ascorbate peroxidase as a case study. J. Exp. Bot. 66, 2913–2921. doi: 10.1093/jxb/erv073
Courtois, C., Besson, A., Dahan, J., Bourque, S., Dobrowolska, G., Pugin, A., et al. (2008). Nitric oxide signalling in plants: interplays with Ca2+ and protein kinases. J. Exp. Bot. 59, 155–163. doi: 10.1093/jxb/erm197
Cvetkovska, M., Vanlerberghe, G. C. (2013). Alternative oxidase impacts the plant response to biotic stress by influencing the mitochondrial generation of reactive oxygen species. Plant Cell Environ. 36, 721–732. doi: 10.1111/pce.12009
Das, K., Roychoudhury, A. (2014). Reactive oxygen species (ROS) and response of antioxidants as ROS-scavengers during environmental stress in plants. Front. Environ. Sci. 2. doi: 10.3389/fenvs.2014.00053
del Río, L. A., Corpas, F. J., Sandalio, L. M., Palma, J. M., Barroso, J. B. (2003). Plant peroxisomes, reactive oxygen metabolism and nitric oxide. IUBMB Life. 55, 71–81. doi: 10.1002/tbmb.718540875
Dey, P., Pattanaik, D., Mohapatra, D., Saha, D., Dash, D., Mishra, A., et al. (2024). Gasotransmitters signaling and their crosstalk with other signaling molecules under diverse stress conditions in plants. South Afr. J. Bot. 169, 119–133. doi: 10.1016/j.sajb.2024.03.041
Dixit, S., Verma, K., Shekhawat, G. S. (2014). In vitro evaluation of mitochondrial-chloroplast subcellular localization of heme oxygenase1 (HO1) in Glycine max. Protoplasma 251, 671–675. doi: 10.1007/s00709-013-0569-9
Dvořák, P., Krasylenko, Y., Zeiner, A., Šamaj, J., Takáč, T. (2021). Signaling toward reactive oxygen species-scavenging enzymes in plants. Front. Plant Sci. 11. doi: 10.3389/fpls.2020.61883
Farnese, F. S., Menezes-Silva, P. E., Gusman, G. S., Oliveira, J. A. (2016). When bad guys become good ones: The key role of reactive oxygen species and nitric oxide in the plant responses to abiotic stress. Front. Plant Sci. 7. doi: 10.3389/fpls.2016.00471
Feelisch, M., Olson, K. R. (2013). Embracing sulfide and CO to understand nitric oxide biology. Nitric. Oxide 35, 2–4. doi: 10.1016/j.niox.2013.06.004
Feng, Y. X., Li, C. Z., Lin, Y. J., Yu, X. Z. (2022). Involvement of β-cyanoalanine synthase (β-CAS) and sulfurtransferase (ST) in cyanide (CN–) assimilation in rice seedlings. Chemosphere 294, 133789. doi: 10.1016/j.chemosphere.2022.133789
Feng, D., Liu, W., Chen, K., Ning, S., Gao, Q., Chen, J., et al. (2024). Exogenous substances used to relieve plants from drought stress and their associated underlying mechanisms. Int. J. Mol. Sci. 25, 9249. doi: 10.3390/ijms25179249
Foyer, C. H., Shigeoka, S. (2011). Understanding oxidative stress and antioxidant functions to enhance photosynthesis. Plant Physiol. 155, 93–100. doi: 10.1104/pp.110.166181
Gahir, S., Bharath, P., Raghavendra, A. S. (2020). The role of gasotransmitters in movement of stomata: mechanisms of action and importance for plant immunity. Biol. Plant 64, 623–632. doi: 10.32615/bp.2020.071
Galano, A., Reiter, R. J. (2018). Melatonin and its metabolites vs oxidative stress: from individual actions to collective protection. J. Pineal. Res. 65, e12514. doi: 10.1111/jpi.12514
Gautam, V., Kaur, R., Kohli, S. K., Verma, V., Kaur, P., Singh, R., et al. (2017). “ROS compartmentalization in plant cells under abiotic stress condition,” in Reactive oxygen species and antioxidant systems in plants: Role and regulation under abiotic stress (Springer, Singapore), 89–114. doi: 10.1007/978-981-10-5254-5_4
Ghorbani, A., Pehlivan, N., Zargar, M., Chen, M. (2024). Synergistic role of melatonin and hydrogen sulfide in modulating secondary metabolites and metal uptake/sequestration in arsenic-stressed tomato plants. Sci. Horticult. 331, 113159. doi: 10.1016/j.scienta.2024.113159
Gong, B., Yan, Y., Wen, D., Shi, Q. (2017). Hydrogen peroxide produced by NADPH oxidase: a novel downstream signaling pathway in melatonin-induced stress tolerance in Solanum lycopersicum. Physiol. Plant 160, 396–409. doi: 10.1111/ppl.12581
Guo, K., Kong, W. W., Yang, Z. M. (2009). Carbon monoxide promotes root hair development in tomato. Plant Cell Environ. 32, 1033–1045. doi: 10.1111/j.1365-3040.2009.01986.x
Guo, H., Xiao, T., Zhou, H., Xie, Y., Shen, W. (2016). Hydrogen sulfide: A versatile regulator of environmental stress in plants. Acta Physiol. Plant 38, 16. doi: 10.1007/s11738-015-2038-x
Gupta, K. J., Kaiser, W. M. (2010). Production and scavenging of nitric oxide by barley root mitochondria. Plant Cell Physiol. 51, 576–584. doi: 10.1093/pcp/pcq022
Haghi, V., Namdjoyan, S., Soorki, A. A. (2022). Interactive effects of exogenous melatonin and hydrogen sulfide in alleviating lead toxicity in safflower seedlings. Ind. Crops Prod. 187, 115523. doi: 10.1016/j.indcrop.2022.115523
Hancock, J. T., Neill, S. J. (2019). Nitric oxide: Its generation and interactions with other reactive signaling compounds. Plants (Basel). 8, 41. doi: 10.3390/plants8020041
Hasanuzzaman, M., Bhuyan, M. H. M., Zulfiqar, F., Raza, A., Mohsin, S. M., Mahmud, J. A., et al. (2020). Reactive oxygen species and antioxidant defense in plants under abiotic stress: revisiting the crucial role of a universal defense regulator. Antioxidants 9, 681. doi: 10.3390/antiox9080681
Hassan, M. U., Ghareeb, R. Y., Nawaz, M., Mahmood, A., Shah, A. N., Abdel-Megeed, A., et al. (2022). Melatonin: A vital protectant for crops against heat stress: mechanisms and prospects. Agronomy 12, 1116. doi: 10.3390/agronomy12051116
He, H., He, L. (2014). The role of carbon monoxide signaling in the responses of plants to abiotic stresses. Nitric. Oxide 42, 40–43. doi: 10.1016/j.niox.2014.08.011
He, H., He, L. F. (2020). Crosstalk between melatonin and nitric oxide in plant development and stress responses. Physiol. Plant 170, 218–226. doi: 10.1111/ppl.13143
Hong, K., Radani, Y., Ahmad, W., Li, P., Luo, Y. (2024). Carbon monoxide modulates auxin transport and nitric oxide signaling in plants under iron deficiency stress. Phyton-Int. J. Exp. Bot. 93, 45–61. doi: 10.32604/phyton.2023.046389
Iqbal, N., Fatma, M., Gautam, H., Umar, S., Sofo, A., D’ippolito, I., et al. (2021). The crosstalk of melatonin and hydrogen sulfide determines photosynthetic performance by regulation of carbohydrate metabolism in wheat under heat stress. Plants (Basel). 10, 1778. doi: 10.3390/plants10091778
Ischiropoulos, H. (2003). Biological selectivity and functional aspects of protein tyrosine nitration. Biochem. Biophys. Res. Commun. 305, 776–783. doi: 10.1016/S0006-291X(03)00814-3
Jahan, M. S., Shu, S., Wang, Y., Chen, Z., He, M., Tao, M., et al. (2019). Melatonin alleviates heat-induced damage of tomato seedlings by balancing redox homeostasis and modulating polyamine and nitric oxide biosynthesis. BMC Plant Biol. 19, 414. doi: 10.1186/s12870-019-1992-7
Jeandroz, S., Lamotte, O., Astier, J., Rasul, S., Trapet, P., Besson-Bard, A., et al. (2013). There’s more to the picture than meets the eye: nitric oxide cross talk with Ca2+ signaling. Plant Physiol. 163, 459–470. doi: 10.1104/pp.113.220624
Jin, G., Liu, F., Ma, H., Hao, S., Zhao, Q., Bian, Z., et al. (2012). Two G-protein-coupled-receptor candidates, Cand2 and Cand7, are involved in Arabidopsis root growth mediated by the bacterial quorum-sensing signals N-acyl-homoserine lactones. Biochem. Biophys. Res. Commun. 417, 991–995. doi: 10.1016/j.bbrc.2011.12.066
Kacperska, A. (2004). Sensor types in signal transduction pathways in plant cells responding to abiotic stressors: do they depend on stress intensity. Physiol. Plant 122, 159–168. doi: 10.1111/j.0031-9317.2004.00388.x
Kaya, C., Higgs, D., Ashraf, M., AlYemeni, M. N., Ahmad, P. (2020). Integrative roles of nitric oxide and hydrogen sulfide in melatonin-induced tolerance of pepper (Capsicum annuum L.) plants to iron deficiency and salt stress alone or in combination. Physiol. Plant 168, 256–277. doi: 10.1111/ppl.12976
Kaya, C., Okant, M., Ugurlar, F., AlYemeni, M. N., Ashraf, M., Ahmad, P. (2019). Melatonin-mediated nitric oxide improves tolerance to cadmium toxicity by reducing oxidative stress in wheat plants. Chemosphere 225, 627–638. doi: 10.1016/j.chemosphere.2019.03.026
Kaya, C., Ugurlar, F., Ashraf, M., AlYemeni, M. N., Bajguz, A., Ahmad, P. (2022). The involvement of hydrogen sulphide in melatonin-induced tolerance to arsenic toxicity in pepper (Capsicum annuum L.) plants by regulating sequestration and subcellular distribution of arsenic, and antioxidant defense system. Chemosphere 309, 136678. doi: 10.1016/j.chemosphere.2022.136678
Khalofah, A., Bamatov, I., Zargar, M. (2024). Interaction of melatonin and H2S mitigates NaCl toxicity summer savory (Satureja hortensis L.) through Modulation of biosynthesis of secondary metabolites and physio-biochemical attributes. Environ. Sci. pollut. Res. 31, 47757–47770. doi: 10.1007/s11356-024-34356-w
Khan, M. S. S., Ahmed, S., Ikram, A. U., Hannan, F., Yasin, M. U., Wang, J., et al. (2023). Phytomelatonin: A key regulator of redox and phytohormones signaling against biotic/abiotic stresses. Redox Biol. 64, 102805. doi: 10.1016/j.redox.2023.102805
Khan, M. N., AlSolami, M. A., Siddiqui, Z. H., AlOmrani, M. A. M., Kalaji, H. M. (2024b). Regulation of Na+/H+ antiport system and nitrogen metabolism by melatonin and endogenous hydrogen sulfide confers resilience to drought and salt stress. South Afr. J. Bot. 164, 152–166. doi: 10.1016/j.sajb.2023.11.033
Khan, S., Alvi, A. F., Fatma, M., Al-Hashimi, A., Sofo, A., Khan, N. A. (2024c). Relative effects of melatonin and hydrogen sulfide treatments in mitigating salt damage in wheat. Front. Plant Sci. 15. doi: 10.3389/fpls.2024.1406092
Khan, M. N., Siddiqui, M. H., AlSolami, M. A., Siddiqui, Z. H. (2024a). Melatonin-regulated heat shock proteins and mitochondrial ATP synthase induce drought tolerance through sustaining ROS homeostasis in H2S-dependent manner. Plant Physiol. Biochem. 206, 108231. doi: 10.1016/j.plaphy.2023.108231
Khan, M. N., Siddiqui, Z. H., Naeem, M., Abbas, Z. K., Ansari, M. W. (2022). “Nitric oxide and hydrogen sulfide interactions in plants under adverse environmental conditions,” in Emerging plant growth regulators in agriculture (Academic Press, New York), 215–244. doi: 10.1016/B978-0-323-91005-7.00015-1
Khator, K., Parihar, S., Jasik, J., Shekhawat, G. S. (2024). Nitric oxide in plants: an insight on redox activity and responses toward abiotic stress signaling. Plant Signal. Behav. 19, 2298053. doi: 10.1080/15592324.2023.2298053
Kirsch, M., de Groot, H. (2008). N-nitrosomelatonin outcompetes S-nitrosocysteine in inhibiting glyceraldehyde 3-phosphate dehydrogenase: first evidence that N-nitrosomelatonin can modify protein function. J. Pineal. Res. 44, 244–249. doi: 10.1111/j.1600-079X.2007.00517.x
Kolbert, Z., Barroso, J. B., Brouquisse, R., Corpas, F. J., Gupta, K. J., Lindermayr, C., et al. (2019). A forty year journey: the generation and roles of NO in plants. Nitric. Oxide 93, 53–70. doi: 10.1016/j.niox.2019.09.006
Kolupaev, Y. E., Firsova, E. N., Yastreb, T. O., Lugovaya, A. A. (2017). The participation of calcium ions and reactive oxygen species in the induction of antioxidant enzymes and heat resistance in plant cells by hydrogen sulfide donor. Appl. Biochem. Microbiol. 53, 573–579. doi: 10.1134/S0003683817050088
Kolupaev, Y. E., Karpets, Y. V., Kabashnikova, L. F. (2019). Antioxidative system of plants: Cellular compartmentalization, protective and signaling functions, mechanisms of regulation (Review). Appl. Biochem. Microbiol. 55, 441–459. doi: 10.1134/S0003683819050089
Kolupaev, Y. E., Karpets, Y. V., Shkliarevskyi, M. A., Yastreb, T. O., Plohovska, S. H., Yemets, A. I., et al. (2022). Gasotransmitters in plants: Mechanisms of participation in adaptive responses. Open Agricult. J. 16, e187433152207050. doi: 10.2174/18743315-v16-e2207050
Kolupaev, Y. E., Shkliarevskyi, M. A., Pyshchalenko, M. A., Dmitriev, A. P. (2024a). Nitric oxide: functional interaction with phytohormones and applications in crop production. Agric. Forestry. 70, 379–411. doi: 10.17707/AgricultForest.70.1.24
Kolupaev, Y. E., Taraban, D. A., Karpets, Y. V., Kokorev, A. I., Yastreb, T. O., Blume, Y. B., et al. (2024b). Involvement of ROS and calcium ions in developing heat resistance and inducing antioxidant system of wheat seedlings under melatonin’s effects. Protoplasma 261, 975–989. doi: 10.1007/s00709-024-01952-z
Kolupaev, Y. E., Taraban, D. A., Karpets, Y. V., Makaova, B. E., Ryabchun, N. I., Dyachenko, A. I., et al. (2023a). Induction of cell protective reactions of Triticum aestivum and Secale cereale to the effect of high temperatures by melatonin. Cytol. Genet. 57, 117–127. doi: 10.3103/S0095452723020068
Kolupaev, Y. E., Yemets, A. I., Yastreb, T. O., Blume, Y. B. (2023b). The role of nitric oxide and hydrogen sulfide in regulation of redox homeostasis at extreme temperatures in plants. Front. Plant Sci. 14. doi: 10.3389/fpls.2023.1128439
Kour, J., Bhardwaj, T., Chouhan, R., Singh, A. D., Gandhi, S. G., Bhardwaj, R., et al. (2024). Phytomelatonin maintained chromium toxicity induced oxidative burst in Brassica juncea L. through improving antioxidant system and gene expression. Environ. pollut. 356, 124256. doi: 10.1016/j.envpol.2024.124256
Kul, R., Esringü, A., Dadasoglu, E., Sahin, Ü., Turan, M., Örs, S., et al. (2019). “Melatonin: role in increasing plant tolerance in abiotic stress conditions,” in Abiotic and Biotic Stress in Plants (IntechOpen, London). doi: 10.5772/intechopen.82590
Lamotte, O., Bertoldo, J. B., Besson-Bard, A., Rosnoblet, C., Aimé, S., Hichami, S., et al. (2015). Protein S-nitrosylation: Specificity and identification strategies in plants. Front. Chem. 2, 114. doi: 10.3389/fchem.2014.00114
Lee, H. Y., Back, K. (2016). Mitogen-activated protein kinase pathways are required for melatonin-mediated defense responses in plants. J. Pineal. Res. 60, 327–335. doi: 10.1111/jpi.12314
Lee, H. Y., Back, K. (2017). Melatonin is required for H2O2-and NO-mediated defense signaling through MAPKKK 3 and OXI 1 in Arabidopsis thaliana. J. Pineal. Res. 62, e12379. doi: 10.1111/jpi.12379
Lee, H. Y., Back, K. (2020). The phytomelatonin receptor (PMRT1) Arabidopsis Cand2 is not a bona fide G protein–coupled melatonin receptor. Melatonin. Res. 3, 177–186. doi: 10.32794/mr11250055
Lee, H. Y., Back, K. (2021). 2-Hydroxymelatonin, rather than melatonin, is responsible for RBOH-dependent reactive oxygen species production leading to premature senescence in plants. Antioxidants 10, 1728. doi: 10.3390/antiox10111728
Lee, H. Y., Back, K. (2022). The antioxidant cyclic 3-hydroxymelatonin promotes the growth and flowering of Arabidopsis thaliana. Antioxidants 11, 1157. doi: 10.3390/antiox11061157
Lee, K., Choi, G. H., Back, K. (2017). Cadmium-induced melatonin synthesis in rice requires light, hydrogen peroxide, and nitric oxide: key regulatory roles for tryptophan decarboxylase and caffeic acid O-methyltransferase. J. Pineal. Res. 63, e12441. doi: 10.1111/jpi.12441
Lei, K., Sun, S., Zhong, K., Li, S., Hu, H., Sun, C., et al. (2021). Seed soaking with melatonin promotes seed germination under chromium stress via enhancing reserve mobilization and antioxidant metabolism in wheat. Ecotoxicol. Environ. Saf. 220, 112241. doi: 10.1016/j.ecoenv.2021.112241
Li, Z. G., Fang, J. R., Bai, S. J. (2024). Hydrogen sulfide signaling in plant response to temperature stress. Front. Plant Sci. 15. doi: 10.3389/fpls.2024.1337250
Li, Z. G., Gong, M., Xie, H., Yang, L., Li, J. (2012). Hydrogen sulfide donor sodium hydrosulfide-induced heat tolerance in tobacco (Nicotiana tabacum L.) suspension cultured cells and involvement of Ca2+ and calmodulin. Plant Sci. 185/186, 185–189. doi: 10.1016/j.plantsci.2011.10.006
Li, M., Kim, C. (2022). Chloroplast ROS and stress signaling. Plant Commun. 3. doi: 10.1016/j.xplc.2021.100264
Li, J., Shi, C., Wang, X., Liu, C., Ding, X., Ma, P., et al. (2020). Hydrogen sulfide regulates the activity of antioxidant enzymes through persulfidation and improves the resistance of tomato seedling to copper oxide nanoparticles (CuO NPs)-induced oxidative stress. Plant Physiol. Biochem. 156, 257–266. doi: 10.1016/j.plaphy.2020.09.020
Liu, Y., Xu, J., Lu, X., Huang, M., Mao, Y., Li, C., et al. (2024). Carbon monoxide is involved in melatonin-enhanced drought resistance in tomato seedlings by enhancing chlorophyll synthesis pathway. BMC Plant Biol. 24, 97. doi: 10.1186/s12870-024-04793-3
Ma, C., Pei, Z. Q., Bai, X., Feng, J. Y., Zhang, L., Fan, J. R., et al. (2022). Involvement of NO and Ca2+ in the enhancement of cold tolerance induced by melatonin in winter turnip rape (Brassica rapa L.). Plant Physiol. Biochem. 190, 262–276. doi: 10.1016/j.plaphy.2022.09.011
Magri, A., Petriccione, M. (2022). Melatonin treatment reduces qualitative decay and improves antioxidant system in highbush blueberry fruit during cold storage. J. Sci. Food Agric. 102, 4229–4237. doi: 10.1002/jsfa.11774
Mannino, G., Pernici, C., Serio, G., Gentile, C., Bertea, C. M. (2021). Melatonin and phytomelatonin: Chemistry, biosynthesis, metabolism, distribution and bioactivity in plants and animals—An overview. Int. J. Mol. Sci. 22, 9996. doi: 10.3390/ijms22189996
Mansoor, S., Farooq, I., Wani, O. A., Ahmad, P., Reiter, R. J., Boo, K. H., et al. (2024). Melatonin as a modulator of MAPK cascade and ROS-RNS feedforward loop during plant pathogen interaction. Physiol. Mol. Plant Pathol. 133, 102367. doi: 10.1016/j.pmpp.2024.102367
Manzoor, M. A., Xu, Y., Xu, J., Wang, Y., Sun, W., Liu, X., et al. (2023). Melatonin: A multi-functional regulator of fruit crop development and abiotic stress response. Sci. Horticult. 321, 112282. doi: 10.1016/j.scienta.2023.112282
Marino, D., Dunand, C., Puppo, A., Pauly, N. (2012). A burst of plant NADPH oxidases. Trends Plant Sci. 17, 9–15. doi: 10.1016/j.tplants.2011.10.001
Martí-Guillén, J. M., Pardo-Hernández, M., Martínez-Lorente, S. E., Almagro, L., Rivero, R. M. (2022). Redox post-translational modifications and their interplay in plant abiotic stress tolerance. Front. Plant Sci. 13. doi: 10.3389/fpls.2022.1027730
Martínez-Lorente, S. E., Pardo-Hernández, M., Martí-Guillén, J. M., López-Delacalle, M., Rivero, R. M. (2022). Interaction between melatonin and NO: Action mechanisms, main targets, and putative roles of the emerging molecule NOmela. Int. J. Mol. Sci. 23, 6646. doi: 10.3390/ijms23126646
Matsumoto, F., Obayashi, T., Sasaki-Sekimoto, Y., Ohta, H., Takamiya, K.-I., Masuda, T. (2004). Gene expression profiling of the tetrapyrrole metabolic pathway in Arabidopsis with a mini-array system. Plant Physiol. 135, 2379–2391. doi: 10.1104/pp.104.042408
Mishra, V., Singh, P., Tripathi, D. K., Corpas, F. J., Singh, V. P. (2021). Nitric oxide and hydrogen sulfide: An indispensable combination for plant functioning. Trends Plant Sci. 26, 1270–1285. doi: 10.1016/j.tplants.2021.07.016
Mukherjee, S. (2019). Insights into nitric oxide-melatonin crosstalk and N-nitrosomelatonin functioning in plants. J. Exp. Bot. 70, 6035–6047. doi: 10.1093/jxb/erz375
Mukherjee, S., Bhatla, S. C. (2021). Exogenous melatonin modulates endogenous H2S homeostasis and L-cysteine desulfhydrase activity in salt-stressed tomato (Solanum lycopersicum L. var. cherry) seedling cotyledons. J. Plant Growth Regul. 40, 2502–2514. doi: 10.1007/s00344-020-10261-7
Mukherjee, S., Khan, M. N., Mathur, P. (2023). Exogenous calcium (Ca2+) and verapamil-sensitive Ca2+ channel activity differentially modulates melatonin-mediated regulation of endogenous hydrogen sulphide (H2S) homeostasis, and L-cysteine desulfhydrase (LDES) activity in NaCl-stressed etiolated sunflower seedlings. South Afr. J. Bot. 152, 124–135. doi: 10.1016/j.sajb.2022.11.029
Murch, S. J., Erland, L. A. E. (2021). A systematic review of melatonin in plants: An example of evolution of literature. Front. Plant Sci. 12. doi: 10.3389/fpls.2021.683047
Nabaei, M., Amooaghaie, R. (2019). Nitric oxide is involved in the regulation of melatonin-induced antioxidant responses in Catharanthus roseus roots under cadmium stress. Botany 97, 681–690. doi: 10.1139/cjb-2019-0107
Neill, S., Barros, R., Bright, J., Desikan, R., Hancock, J., Harrison, J., et al. (2008). Nitric oxide, stomatal closure and abiotic stress. J. Exp. Bot. 59, 165–176. doi: 10.1093/jxb/erm293
Oda, T., Hashimoto, H., Kuwabara, N., Akashi, S., Hayashi, K., Kojima, C., et al. (2010). Structure of the N-terminal regulatory domain of a plant NADPH oxidase and its functional implications. J. Biol. Chem. 285, 1435–1445. doi: 10.1074/jbc.M109.058909
Pan, Y., Xu, X., Li, L., Sun, Q., Wang, Q., Huang, H., et al. (2023). Melatonin-mediated development and abiotic stress tolerance in plants. Front. Plant Sci. 14. doi: 10.3389/fpls.2023.1100827
Pandey, A. K., Borokotoky, S., Tripathi, K., Gautam, A. (2024). “Interplay of hydrogen sulfide and plant metabolites under environmental stress,” in H2S in Plants. Past, Present and Beyond (New York: Elsevier Inc), 297–317. doi: 10.1016/B978-0-323-99035-6.00004-X
Pardo-Hernández, M., López-Delacalle, M., Martí-Guillen, J. M., Martínez-Lorente, S. E., Rivero, R. M. (2021). ROS and NO phytomelatonin induced signaling mechanisms under metal toxicity in plants: A review. Antioxidants 10, 775. doi: 10.3390/antiox10050775
Peng, X., Wang, N., Sun, S., Geng, L., Guo, N., Liu, A., et al. (2023). Reactive oxygen species signaling is involved in melatonin-induced reduction of chlorothalonil residue in tomato leaves. J. Hazard. Mater. 443, 130212. doi: 10.1016/j.jhazmat.2022.130212
Plokhovska, S. H., Kravets, E. A., Yemets, A. I., Blume, Y. B. (2023). “Crosstalk between melatonin and nitric oxide in plant development and UV-B stress response,” in UV-B Radiation and Crop Growth, Plant Life and Environment Dynamics (Springer, Singapore), 319–339. doi: 10.1007/978-981-193620-3_15
Raza, A., Salehi, H., Rahman, M. A., Zahid, Z., Madadkar Haghjou, M., Najafi-Kakavand, S., et al. (2022). Plant hormones and neurotransmitter interactions mediate antioxidant defenses under induced oxidative stress in plants. Front. Plant Sci. 13. doi: 10.3389/fpls.2022.961872
Rehaman, A., Mishra, A. K., Ferdose, A., Per, T. S., Hanief, M., Jan, A. T., et al. (2021). Melatonin in plant defense against abiotic stress. Forests 12, 1404. doi: 10.3390/f12101404
Riemenschneider, A., Wegele, R., Schmidt, A., Papenbrock, J. (2005). Isolation and characterization of a D-cysteine desulfhydrase protein from Arabidopsis thaliana. FEBS J. 272, 1291–1304. doi: 10.1111/j.1742-4658.2005.04567.x
Romerowicz-Misielak, M., Koziorowska, A., Kusak, O., Górka, A., Broda, D., Waszkiewicz, E. M., et al. (2018). Carbon monoxide modulates melatonin synthesis in porcine pineal cells in vitro: a preliminary study. J. Biol. Regul. Homeost. Agents 32, 1479–1483.
Roy Chowdhury, A., Ghosh, M., Lal, M., Pal, A., Hazra, K. K., Acharya, S. S., et al. (2019). Efficacy of calcium chloride and arginine foliar spray in alleviating terminal heat stress in late-sown wheat (Triticum aestivum L.). J. Agricult. Sci. 157, 533–549. doi: 10.1017/S002185961900087X
Sachdev, S., Ansari, S. A., Ansari, M. I., Fujita, M., Hasanuzzaman, M. (2021). Abiotic stress and reactive oxygen species: generation, signaling, and defense mechanisms. Antioxidants 10, 277. doi: 10.3390/antiox10020277
Sánchez-Vicente, I., Fernández-Espinosa, M. G., Lorenzo, O. (2019). Nitric oxide molecular targets: reprogramming plant development upon stress. J. Exp. Bot. 70, 4441–4460. doi: 10.1093/jxb/erz339
Santolini, J., André, F., Jeandroz, S., Wendehenne, D. (2017). Nitric oxide synthase in plants: where do we stand? Nitric. Oxide 63, 30–38. doi: 10.1016/j.niox.2016.09.005
Shang, J.-X., Li, X., Li, C., Zhao, L. (2022). The role of nitric oxide in plant responses to salt stress. Int. J. Mol. Sci. 23, 6167. doi: 10.3390/ijms23116167
Shekhawat, G. S., Verma, K. (2010). Haem oxygenase (HO): an overlooked enzyme of plant metabolism and defence. J. Exp. Bot. 61, 2255–2270. doi: 10.1093/jxb/erq074
Shen, J., Zhang, J., Zhou, M., Zhou, H., Cui, B., Gotor, C., et al. (2020). Persulfidation-based modification of cysteine desulfhydrase and the NADPH oxidase RBOHD controls guard cell abscisic acid signaling. Plant Cell 32, 1000–1017. doi: 10.1105/tpc.19.00826
Shi, H., Tan, D. X., Reiter, R. J., Ye, T., Yang, F., Chan, Z. (2015). Melatonin induces class A1 heat-shock factors (HSFA1s) and their possible involvement of thermotolerance in Arabidopsis. J. Pineal. Res. 58, 335–342. doi: 10.1111/jpi.12219
Shivaraj, S. M., Vats, S., Bhat, J. A., Dhakte, P., Goyal, V., Khatri, P., et al. (2020). Nitric oxide and hydrogen sulfide crosstalk during heavy metal stress in plants. Physiol. Plant 168, 437–455. doi: 10.1111/ppl.13028
Shkliarevskyi, M. A., Karpets, Y. V., Kolupaev, Y. E., Lugovaya, A. A., Dmitriev, A. P. (2020). Calcium-dependent changes in cellular redox homeostasis and heat resistance of wheat plantlets under influence of hemin (carbon monoxide donor). Cytol. Genet. 54, 522–530. doi: 10.3103/S0095452720060109
Shkliarevskyi, M. A., Kolupaev, Y. E., Karpets, Y. V., Lugovaya, A. A., Bessonova, V. P. (2021). Involvement of nitrate reductase and nitric oxide (NO) in implementation of the stress-protective action of a carbon monoxide (CO) donor on wheat seedlings under hyperthermy. Russ. J. Plant Physiol. 68, 688–695. doi: 10.1134/S1021443721040166
Siddiqui, M. H., Alamri, S., Nasir Khan, M., Corpas, F. J., Al-Amri, A. A., Alsubaie, Q. D., et al. (2020). Melatonin and calcium function synergistically to promote the resilience through ROS metabolism under arsenic-induced stress. J. Hazard. Mater. 398, 122882. doi: 10.1016/j.jhazmat.2020.122882
Siddiqui, M. H., Khan, M. N., Mukherjee, S., Basahi, R. A., Alamri, S., Al Amri, A. A., et al. (2021). Exogenous melatonin-mediated regulation of K+/Na+ transport, H+-ATPase activity and enzymatic antioxidative defence operate through endogenous hydrogen sulphide signalling in NaCl-stressed tomato seedling roots. Plant Biol. 23, 797–805. doi: 10.1111/plb.13296
Singh, N., Jain, P., Gupta, S., Khurana, J. M., Bhatla, S. C. (2021). N-Nitrosomelatonin, an efficient nitric oxide donor and transporter in Arabidopsis seedlings. Nitric. Oxide 113–114, 50–56. doi: 10.1016/j.niox.2021.05.001
Singh, N., Kaur, H., Yadav, S., Bhatla, S. C. (2016). Does N-nitrosomelatonin compete with S-nitrosothiols as a long distance nitric oxide carrier in plants? Biochem. Anal. Biochem. 5, 262. doi: 10.4172/2161-1009.1000262
Song, X. G., She, X. P., Zhang, B. (2008). Carbon monoxide-induced stomatal closure in Vicia faba is dependent on nitric oxide synthesis. Physiol. Plant 132, 514–525. doi: 10.1111/j.1399-3054.2007.01026.x
Srivastava, A. K., Pasala, R., Minhas, P. S., Suprasanna, P. (2016). Plant bioregulators for sustainable agriculture: integrating redox signaling as a possible unifying mechanism. Adv. Agron. 137, 237–278. doi: 10.1016/bs.agron.2015.12.002
Sun, Y., Ma, C., Kang, X., Zhang, L., Wang, J., Zheng, S., et al. (2021). Hydrogen sulfide and nitric oxide are involved in melatonin-induced salt tolerance in cucumber. Plant Physiol. Biochem. 167, 101–112. doi: 10.1016/j.plaphy.2021.07.023
Taboada, J., Reiter, R. J., Palma, J. M., Corpas, F. J. (2023). “Melatonin and the metabolism of reactive oxygen species (ROS) in higher plants,” in Melatonin: Role in Plant Signaling, Growth and Stress Tolerance. Plant in Challenging Environments, vol. vol 4. (Springer, Cham), 3–25. doi: 10.1007/978-3-031-40173-2_1
Tan, D. X., Hardeland, R., Manchester, L. C., Galano, A., Reiter, R. J. (2014). Cyclic-3-hydroxymelatonin (C3HOM), a potent antioxidant, scavenges free radicals and suppresses oxidative reactions. Curr. Med. Chem. 21, 1557–1565. doi: 10.2174/0929867321666131129113146
Tan, D. X., Reiter, R. J. (2020). An evolutionary view of melatonin synthesis and metabolism related to its biological functions in plants. J. Exp. Bot. 71, 4677–4689. doi: 10.1093/jxb/eraa235
Torres, M. A., Dangl, J. L., Jones, J. D. (2002). Arabidopsis gp91phox homologues AtrbohD and AtrbohF are required for accumulation of reactive oxygen intermediates in the plant defense response. Proc. Natl. Acad. Sci. U.S.A. 99, 517–522. doi: 10.1073/pnas.012452499
Valderrama, R., Begara-Morales, J. C., Chaki, M., Mata-Pérez, C., Padilla, M. N., Barroso, J. B. (2019). “Hydrogen peroxide (H2O2)- and nitric oxide (NO)-derived posttranslational modifications,” in Nitric oxide and hydrogen peroxide signaling in higher plants (Springer, Switzerland), 37–67. doi: 10.1007/978-3-030-11129-8_3
Wang, Y., Cheng, P., Zhao, G., Li, L., Shen, W. (2022a). Phytomelatonin and gasotransmitters: a crucial combination for plant physiological functions. J. Exp. Bot. 73, 5851–5862. doi: 10.1093/jxb/erac159
Wang, P., Du, Y., Li, Y., Ren, D., Song, C. P. (2010). Hydrogen peroxide-mediated activation of MAP kinase 6 modulates nitric oxide biosynthesis and signal transduction in Arabidopsis. Plant Cell 22, 2981–2998. doi: 10.1105/tpc.109.072959
Wang, Y., Gun, S., Li, Y., Qu, L. (2022b). Meta-analysis of effects of melatonin treatment on plant drought stress alleviation. Agriculture 12, 1335. doi: 10.3390/agriculture12091335
Wang, Z., Li, L., Khan, D., Chen, Y., Pu, X., Wang, X., et al. (2023a). Nitric oxide acts downstream of reactive oxygen species in phytomelatonin receptor 1 (PMTR1)-mediated stomatal closure in Arabidopsis. J. Plant Physiol. 282, 153917. doi: 10.1016/j.jplph.2023.153917
Wang, L. F., Li, T.-T., Zhang, Y., Guo, J.-X., Lu, K.-K., Liu, W.-C. (2021). CAND2/PMTR1 is required for melatonin-conferred osmotic stress tolerance in Arabidopsis. Int. J. Mol. Sci. 22, 4014. doi: 10.3390/ijms22084014
Wang, Z., Mu, Y., Zhang, L., Liu, Z., Liu, D., Jin, Z., et al. (2023b). Hydrogen sulfide mediated the melatonin induced stoma closure by regulating the K+ channel in Arabidopsis thaliana. Environ. Exp. Bot. 205, 105125. doi: 10.1016/j.envexpbot.2022.105125
Wang, L., Tanveer, M., Wang, H., Arnao, M. B. (2024). Melatonin as a key regulator in seed germination under abiotic stress. J. Pineal. Res. 76, e12937. doi: 10.1111/jpi.12937
Wei, J., Li, D. X., Zhang, J. R., Shan, C., Rengel, Z., Song, Z. B., et al. (2018). Phytomelatonin receptor PMTR1-mediated signaling regulates stomatal closure in Arabidopsis thaliana. J. Pineal. Res. 65, e12500. doi: 10.1111/jpi.12500
Wen, D., Gong, B., Sun, S., Liu, S., Wang, X., Wei, M., et al. (2016). Promoting roles of melatonin in adventitious root development of Solanum lycopersicum L. by regulating auxin and nitric oxide signaling. Front. Plant Sci. 7. doi: 10.3389/fpls.2016.00718
Wong, H. L., Pinontoan, R., Hayashi, K., Tabata, R., Yaeno, T., Hasegawa, K., et al. (2007). Regulation of rice NADPH oxidase by binding of Rac GTPase to its N-terminal extension. Plant Cell 19, 4022–4034. doi: 10.1105/tpc.107.055624
Xu, W., Cai, S. Y., Zhang, Y., Wang, Y., Ahammed, G. J., Xia, X. J., et al. (2016). Melatonin enhances thermotolerance by promoting cellular protein protection in tomato plants. J. Pineal. Res. 61, 457–469. doi: 10.1111/jpi.12359
Xuan, L., Li, J., Wang, X., Wang, C. (2020). Crosstalk between hydrogen sulfide and other signal molecules regulates plant growth and development. Int. J. Mol. Sci. 21, 4593. doi: 10.3390/ijms21134593
Yao, Y., Yang, Y., Li, C., Huang, D., Zhang, J., Wang, C., et al. (2019). Research progress on the functions of gasotransmitters in plant responses to abiotic stresses. Plants (Basel). 8, 605. doi: 10.3390/plants8120605
Ye, T., Yin, X., Yu, L., Zheng, S. J., Cai, W. J., Wu, Y., et al. (2019). Metabolic analysis of the melatonin biosynthesis pathway using chemical labeling coupled with liquid chromatography-mass spectrometry. J. Pineal. Res. 66, e12531. doi: 10.1111/jpi.12531
Yin, Y., Hu, J., Tian, X., Yang, Z., Fang, W. (2022). Nitric oxide mediates melatonin-induced isoflavone accumulation and growth improvement in germinating soybeans under NaCl stress. J. Plant Physiol. 279, 153855. doi: 10.1016/j.jplph.2022.153855
Yin, Y., Zhang, R., Tian, X., Yang, Z., Fang, W. (2023). Nitric oxide affects melatonin mediates enrichment of isoflavones and physiological biochemistry in germinated soybeans under Ultraviolet-B stress. Plant Growth Regul. 100, 657–666. doi: 10.1007/s10725-022-00958-y
Yun, B. W., Feechan, A., Yin, M., Saidi, N. B., Le Bihan, T., Yu, M., et al. (2011). S-nitrosylation of NADPH oxidase regulates cell death in plant immunity. Nature 478, 264–268. doi: 10.1038/nature10427
Zeng, W., Mostafa, S., Lu, Z., Jin, B. (2022). Melatonin-mediated abiotic stress tolerance in plants. Front. Plant Sci. 13. doi: 10.3389/fpls.2022.847175
Zhang, S., Li, Q., Mao, Y. (2014). Effect of carbon monoxide on active oxygen metabolism of postharvest Jujube. J. Food Technol. Res. 1, 146–155. doi: 10.18488/journal.58/2014.1.2/58.2.146.155
Zhang, J., Liao, W. (2019). Protein S-nitrosylation in plant abiotic stresses. Funct. Plant Biol. 47, 1–10. doi: 10.1071/FP19071
Zhang, J., Zhou, M., Zhou, H., Zhao, D., Gotor, C., Romero, L. C., et al. (2021). Hydrogen sulfide, a signaling molecule in plant stress responses. J. Integr. Plant Biol. 63, 146–160. doi: 10.1111/jipb.13022
Zhang, Z., Liu, L., Li, H., Zhang, S., Fu, X., Zhai, X., et al. (2022). Exogenous melatonin promotes the salt tolerance by removing active oxygen and maintaining ion balance in wheat (Triticum aestivum L.). Front. Plant Sci. 12. doi: 10.3389/fpls.2021.787062
Zhao, J., Hu, J. (2023). Melatonin: Current status and future perspectives in horticultural plants. Front. Plant Sci. 14. doi: 10.3389/fpls.2023.1140803
Zhao, G., Zhao, Y., Yu, X., Kiprotich, F., Han, H., Guan., R., et al. (2018). Nitric oxide is required for melatonin-enhanced tolerance against salinity stress in rapeseed (Brassica napus L.) seedlings. Int. J. Mol. Sci. 19, 1912. doi: 10.3390/ijms19071912
Zhou, J., Ran, Z. F., Yang, X. T., Li, J. (2019). Postharvest UV-B Irradiation stimulated ginsenoside Rg1 biosynthesis through nitric oxide (NO) and jasmonic acid (JA) in Panax quinquefolius roots. Molecules 24, 1462. doi: 10.3390/molecules24081462
Zhu, Y., Gao, H., Lu, M., Hao, C., Pu, Z., Guo, M., et al. (2019). Melatonin-nitric oxide crosstalk and their roles in the redox network in plants. Int. J. Mol. Sci. 20 (24), 6200. doi: 10.3390/ijms20246200
Zulfiqar, F., Ashraf, M. (2021). Bioregulators: unlocking their potential role in regulation of the plant oxidative defense system. Plant Mol. Biol. 105, 11–41. doi: 10.1007/s11103-020-01077-w
Keywords: melatonin, ROS, nitric oxide, hydrogen sulfide, carbon monoxide, cell signalling, protein post-translational modifications, abiotic stress
Citation: Kolupaev YE, Yemets A, Yastreb TO and Blume Y (2024) Functional interaction of melatonin with gasotransmitters and ROS in plant adaptation to abiotic stresses. Front. Plant Sci. 15:1505874. doi: 10.3389/fpls.2024.1505874
Received: 03 October 2024; Accepted: 26 November 2024;
Published: 12 December 2024.
Edited by:
Karl H. Hasenstein, University of Louisiana at Lafayette, United StatesReviewed by:
Alfonso Ortega Garrido, Universidad de Extremadura, SpainIslam Frahat Hassan, National Research Centre, Egypt
Copyright © 2024 Kolupaev, Yemets, Yastreb and Blume. This is an open-access article distributed under the terms of the Creative Commons Attribution License (CC BY). The use, distribution or reproduction in other forums is permitted, provided the original author(s) and the copyright owner(s) are credited and that the original publication in this journal is cited, in accordance with accepted academic practice. No use, distribution or reproduction is permitted which does not comply with these terms.
*Correspondence: Yuriy E. Kolupaev, cGxhbnRfYmlvbG9neUB1a3IubmV0
†ORCID: Yuriy E. Kolupaev, orcid.org/0000-0001-7151-906X
Alla Yemets, orcid.org/0000-0001-6887-0705
Tetiana O. Yastreb, orcid.org/0000-0003-3604-9028
Yaroslav Blume, orcid.org/0000-0001-7078-7548