- 1College of Life Sciences, Zaozhuang University, Zaozhuang, China
- 2National Key Laboratory of Wheat Improvement, College of Agronomy, Shandong Agricultural University, Tai’an, China
- 3Jinan Key Laboratory of Biological Breeding, Spring Valley Agriscience Co., Ltd., Jinan, China
All terrestrial plants possess a hydrophobic cuticle in the outermost layer of their aerial organs that is composed of cutin and wax. The cuticle serves as the first barrier between the plant and the surrounding environment and plays a key role in the resistance of plants to abiotic and biotic stressors. Additionally, they are closely associated with plant growth and development. Cuticular wax has attracted considerable attention as the main mediator of cuticular functions. In this review, we summarize the advances in the research investigating wheat cuticular wax, focusing on three aspects that include biosynthesis, genetics, and stress responses. Additionally, we discuss the applications of cuticular wax in wheat breeding.
1 Introduction
Wheat (Triticum aestivum), the primary grain crop worldwide, accounts for one-fifth of the total calories consumed by humans (Apples et al., 2018). The United Nations predicts that the global population will reach 9.3 billion by 2050 and surpass 10 billion by 2059. Therefore, food security is a significant global challenge (Lee, 2011; United Nations, 2022). Wheat cultivation is limited by multiple abiotic and biotic stressors that directly affect yield and quality (Song et al., 2024). For example, salt stress affects 20% of the world’s cultivated soils (Arora, 2019) and can lead to wheat yield losses of up to 45% (Ali et al., 2009), reduce the number of tillers (Abbas et al., 2013), and decrease the spikelet and grain weights (Frank et al., 1987). A 40% water reduction may result in a 20.6% loss in wheat yield. The threat of drought stress to wheat has been exacerbated by global warming (Lesk et al., 2016; Hickey et al., 2019). High- and low-temperature environments that occur due to climate change instability also affect wheat yield and quality (Jacott and Boden, 2020). Wheat stripe rust (WS) is a common disease caused by Puccinia striiformis f. sp. Tritici (Pst) that affects up to 4 million hectares of wheat annually in China (Zhang et al., 2019; Chen et al., 2022). Wheat powdery mildew is a widespread disease caused by Blumeria graminis f. sp. Tritici (Bgt) and accounts for approximately 5% of annual wheat yield loss (Savary et al., 2019; Xie et al., 2020). Therefore, considering the influence of abiotic and biotic stress factors, strategies must be developed to cope with high yields, resistance, and quality through genetic improvement.
Approximately 480 to 360 million years ago, ancient algae began to grow on land and became the first land plants (Kenrick and Crane, 1997; Bowman, 2022). These plants faced significant environmental challenges during their growth such as water deficiency, ultraviolet radiation, physical damage, and pathogenic infections (Kong et al., 2020a). In response to abiotic and biotic stresses, plants have evolved hydrophobic cuticles comprising an inner cutin polyester matrix and an outer layer of wax (Samuels et al., 2008; Yeats and Rose, 2013; Renault et al., 2017; Lee et al., 2020). As the supporting structure of the cuticle, cutin is a three-dimensional net polymeric structure composed of ω-hydroxyl groups, an intermediate chain, and C16 and C18 fatty acids and derivatives including hydroxy acids, dicarboxylic acids, and others (Nawrath, 2006; Jetter and Kunst, 2008). Wax is bluish-white (glaucous) in color and primarily composed of very-long-chain fatty acids (VLCFAs), their derivatives, triterpenoids, and certain secondary metabolites (Jetter and Kunst, 2008). The wax is divided into inner and outer epidermal wax in the cuticle. The inner epidermal wax was filled with a net structure formed by a cutin polyester matrix. In contrast, the outer epidermal wax covers the outermost layer of the cuticle and forms a layer of waxy crystals (Jetter and Kunst, 2008). As the dominant contributor to cuticular function, cuticular wax has attracted increasing attention (Kunst et al., 2006). In this review, we focus on the study of cuticular wax in wheat and discuss its future use in genetics and breeding.
2 Cuticular wax composition and its biosynthesis pathway in wheat
The cuticular wax of most plants is composed of VLCFAs and their derivatives (Figure 1) such as alkanes, alcohols, ketones, and aldehydes (Buschhaus and Jetter, 2011; Xue et al., 2017). However, wax composition varies from plant to plant and even from organ to organ. For example, β-diketone, the main component of wheat wax, is absent in Arabidopsis wax, and alkanes are lower in the waxes of corn and barley, while the waxes of soybean and alfalfa are richer in alkanes (Bergman et al., 1991; Post-Beittenmiller, 1996; Hen-Avivi et al., 2016). In addition to interspecies differences, cuticular wax differs across growth and developmental periods and even among different growing environments such as those characterized by distinct temperature and light conditions (Geyer and Schönherr, 1990; Domínguez et al., 2011; Xue et al., 2017). This indirectly indicates that different plants evolved wax biosynthesis genes under specific conditions.
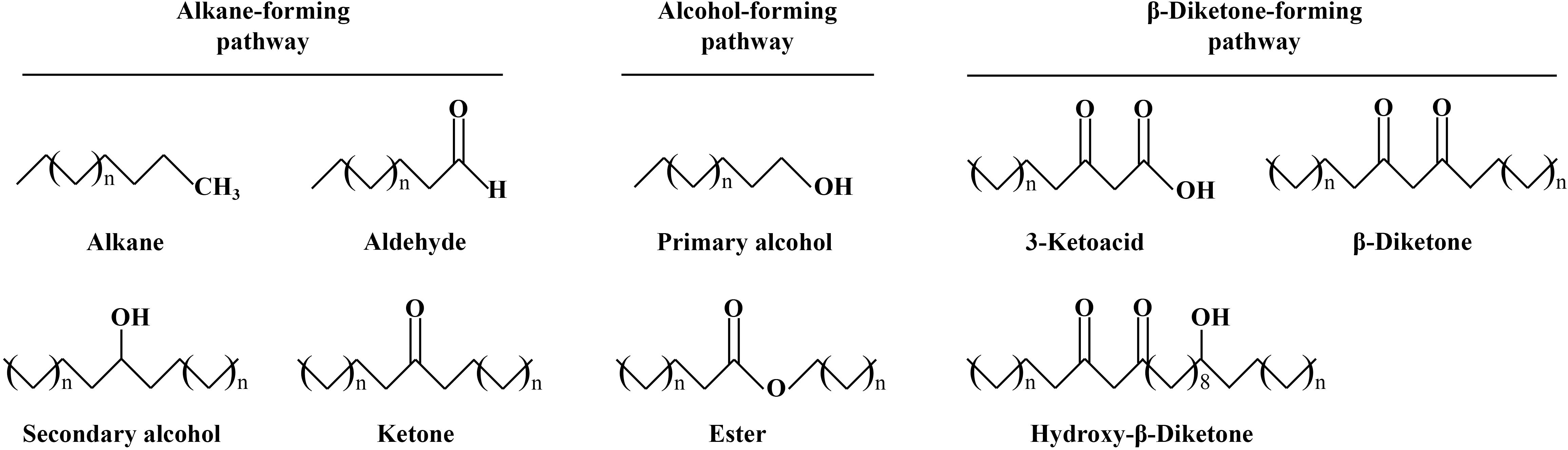
Figure 1. The cuticular wax is composed of many components in wheat. The role of different components depends on their chemical structure and properties.
In wheat, cuticular wax content and composition exhibited significant differences in different organs, stages, and environmental conditions. The wax of wheat leaves is predominantly composed of primary alcohol, the wax of wheat flag leaves is primarily composed of alcohol and β-diketone, and the wax of the leaf sheath, stem, and spike is primarily composed of β-diketone (Adamski et al., 2013; Zhang et al., 2013; Wang et al., 2015b). Wax at the wheat seedling stage is composed of fatty alcohols, whereas that at the adult plant stage is composed of alkanes (Yang et al., 2017). The cuticular wax content and composition also exhibit dynamic changes under different environmental conditions. When water is deficient, β-diketone accumulation will increase wax content to prevent water evaporation (Kuruparan et al., 2024). After pest infection, several wax biosynthesis-related genes are induced to enhance single-component accumulation and avoid further damage (Kosma et al., 2010).
Many physiological functions of cuticular wax biosynthesis have been conserved throughout the evolution of different plants (Kramer and Havens, 2009; Kong et al., 2020a; McWhite et al., 2020; Wang and Chang, 2022). Most conceptions of the wax biosynthesis pathway are based on research investigating model plants such as Arabidopsis. In recent years, the cuticular wax biosynthesis pathway in wheat has been gradually clarified based on studies involving Arabidopsis. For example, AtSHN1 was the first identified transcription factor involved in cuticular wax biosynthesis in Arabidopsis (Aharoni et al., 2004). As the homolog of AtSHN1, TaSHN1 has been identified in wheat (Bi et al., 2018). Similarly, the overexpression of TaSHN1 also altered wax accumulation in the cuticle, and the alkane content was higher in bread wheat (Bi et al., 2018).
Wax biosynthesis involves several metabolic pathways and protein complexes. It can be roughly divided into the following three steps that include synthesis of wax precursors (C16 and C18 fatty acids), synthesis of VLCFA acyl-CoAs, and synthesis, processing, and transport of wax derivatives (Figure 2; Table 1).
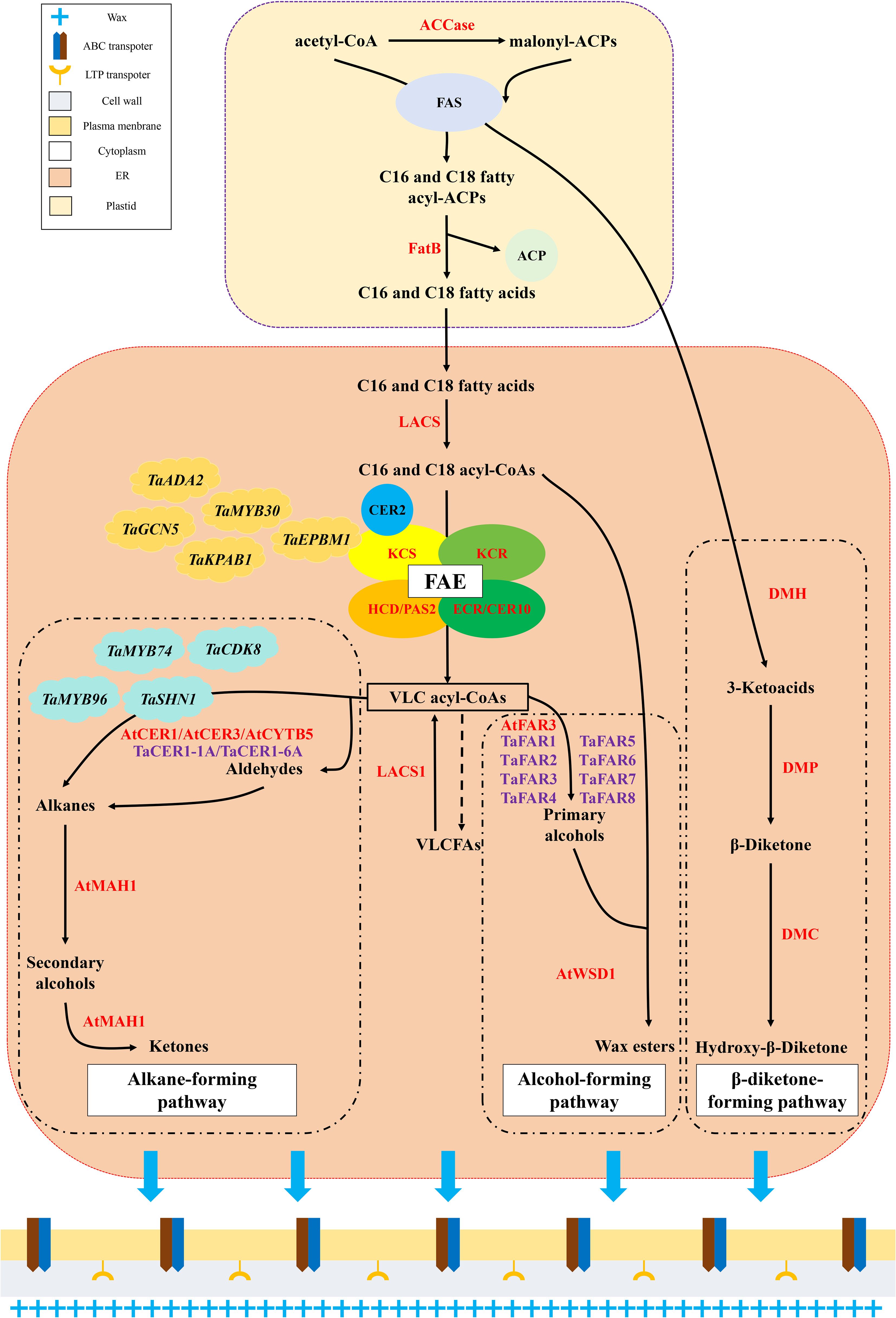
Figure 2. The pathway of cuticular wax biosynthesis in plants. The alcohol-forming pathway and alkane-forming pathway were ubiquitous in C3 and C4 plants. The β-diketone-forming pathway is only confirmed in C3 plants, and it was unique in wheat and other Triticeae Dumort plants.
Acetyl-CoA produces acyl–acyl carrier proteins (malonyl-ACPs) in the plastids of epidermal cells via the carboxylation of acetyl-CoA carboxylase (ACCase) and transacylation of acyl carrier protein (ACP) (Byers and Gong, 2007; Li-Beisson et al., 2013). Malonyl-ACP is a two-carbon donor involved in the synthesis of C16 and C18 fatty acyl ACPs. Subsequently, acetyl-CoA generates C16 and C18 fatty acyl-ACPs under the catalytic action of fatty acid synthetase (FAS) multi-enzyme complexes (Ohlrogge and Browse, 1995; Günenc et al., 2022). C16 and C18 fatty acyl-ACPs are hydrolyzed by fatty acyl–acyl ACP thioesterase B (FatB) to release ACPs and free C16 and C18 fatty acids (Li-Beisson et al., 2013). Finally, free C16 and C18 fatty acids are exported to the endoplasmic reticulum (ER) for the second stage of wax biosynthesis (Samuels et al., 2008).
The fatty acid elongase (FAE) complex plays a decisive role in the second stage of wax biosynthesis. It is composed of four different enzymes that include β-ketoacyl-CoA synthase (KCS), β-ketoacyl-CoA reductase (KCR), β-hydroxyacyl-CoA dehydratase (HCD/PAS2), and β-enoyl-CoA reductase (ECR/CER10) (Kim et al., 2022). After entering the ER, free C16 and C18 fatty acids are esterified to C16 and C18 acyl-CoA by long-chain acyl-CoA synthetase (LACS) proteins (Li-Beisson et al., 2013). Subsequently, C16 and C18 fatty acyl-CoAs undergo condensation, reduction, dehydration, and re-reduction cycles catalyzed by multimeric FAE complexes (Lee et al., 2009; Kim et al., 2022). In this cycle, an acyl-CoA precursor (C16 and C18 fatty acyl-CoA) and malonyl-CoA undergo a condensation reaction catalyzed by KCS to form β-ketoacyl-CoA. β-ketoacyl-CoA is then reduced to generate β-hydroxy acyl-CoA, and this is catalyzed by KCR. Subsequently, β-hydroxy acyl-CoA loses an H2O molecule under the catalysis of HCD/PAS2 to produce enoyl-CoA. ECR/CER10 catalyzes the reduction of enoyl-CoA to acyl-CoA. In each cycle, the final acyl-CoA is two carbons longer than the primary acyl-CoA precursor until it extends to very-long-chain acyl-CoAs (VLC acyl-CoAs) of > 20 carbons (Lee et al., 2009; Kunst and Samuels, 2009; Wang et al., 2017; Bai et al., 2022; Kim et al., 2022). Once the carbon chain length exceeds 28, the involvement of CER2-LIKE proteins in the BAHD superfamily of acyltransferases is indispensable (Haslam et al., 2015; Haslam and Kunst, 2021). To modify the chain length, CER2-LIKE proteins interact with KCS to adjust the chain length specificity of the elongase complex (Haslam et al., 2015; Haslam and Kunst, 2021). When VLC acyl-CoAs are produced, they are hydrolyzed by a hypothetical VLC acyl-CoA thioesterase to release VLCFAs (Lewandowska et al., 2020). A small proportion of VLCFAs may be released directly into the cuticular wax or reactivated back to VLC acyl-CoAs by LACS1. However, most VLC acyl-CoAs are further modified in the ER to synthesize wax derivatives (Kunst and Samuels, 2003; Samuels et al., 2008).
Wax derivatives can be produced via either alcohol-forming or alkane-forming pathways (Figure 2; Table 1). In the alcohol-forming pathway, most VLC acyl-CoAs are catalyzed by fatty acyl-CoA reductase 3 (FAR3) to produce primary alcohols (Wen and Jetter, 2009). In wheat, several FAR-like proteins have been demonstrated to participate in the synthesis of primary alcohols that are specifically involved in the production of C22–C30 very-long-chain primary alcohols (Wang et al., 2015a, 2015b, 2016; Chai et al., 2018). Wax synthetase/diacylglycerol acyltransferase 1 (WSD1) catalyzes the binding of primary alcohols to acyl-CoAs to form wax esters (Tomiyama et al., 2017; Li et al., 2008). Other derivatives, including aldehydes, alkanes, secondary alcohols, and ketones, were synthesized via the alkane-forming pathway. As vital products of this pathway, alkanes can be synthesized via direct generation by VLC acyl-CoAs (Jenks et al., 1995) or indirect generation, whereby VLC acyl-CoAs are first oxidized to aldehydes and then reduced to alkanes (Jenks et al., 1995). Both pathways are affected by an alkane synthesis protein complex comprising ECERIFERUM1 (CER1), CER1-LIKE1, CER3, and cytochrome B5 (Pascal et al., 2019). Two CER1 proteins have been identified in wheat. Both proteins are closely involved in the alkane-forming pathway and significantly affect alkane accumulation in wheat cuticular wax (Li et al., 2019; He et al., 2022). Moreover, alkanes can be hydroxylated to secondary alcohols by the CYP96A family cytochrome P450 enzyme that acts as a mid-chain alkane hydroxylase (MAH1), producing ketones via the same reaction (Greer et al., 2007). These wax constituents are transported from the ER to the plasma membrane through the combined action of ATP-binding cassette transporters and lipid-transfer proteins through the cell wall to the cell cuticle, where they undergo self-assembly to form wax crystals (Pighin et al., 2004; Rees et al., 2009; Wong et al., 2019).
Wheat, as a member of the Triticeae Dumort family, exhibits another parallel crucial wax biosynthesis pathway responsible for β-diketone biosynthesis in addition to the two main wax biosynthesis pathways discussed above (Figure 2; Table 1). This pathway was first proposed in genetic studies of barley and later confirmed in wheat (Hen-Avivi et al., 2016; Wettstein-Knowles, 1995; Schneider et al., 2016). In this pathway, the β-diketone biosynthesis gene cluster that comprises three genes, diketone metabolism polyketide synthase (DMP), diketone hydrolase/carboxylesterase (DMH), and diketone cytochrome P450 (DMC), plays a predominant role (Hen-Avivi et al., 2016). The β-diketone biosynthesis pathway synthesizes β-diketone and related derivatives (Post-Beittenmiller, 1996). Based on previous studies of the β-diketone biosynthesis pathway, 3-ketoacyl-ACP produced in the FAS multienzyme complexes are first captured by DMH (Wettstein-Knowles, 1995; Zhang et al., 2013; Hen-Avivi et al., 2016). In the ER, 3-ketoacyl-ACP hydrolysis catalyzed by DMH results in the release of 3-ketoacid that is then converted to β-diketone and a hydroxylated derivative by the successive actions of DMP and DMC (Wettstein-Knowles, 1995; Zhang et al., 2013; Hen-Avivi et al., 2016). Finally, β-diketone and hydroxy-β-diketone are transported to the cuticle to assemble cuticular wax (Wettstein-Knowles, 1995; Zhang et al., 2013; Hen-Avivi et al., 2016).
A recent study has revealed the β-diketone-forming pathway in barley (Sun et al., 2023). As a core intermediate, 3-ketoacid was initially formed in the β-diketone-forming pathway through DMH. Sun et al. (2023) analyzed the origin and formation of the functional group of β-diketone and finally presented the head-to-head condensation hypothesis. An interesting catalytic reaction occurs during the DMP reaction. There was recarboxylative reaction to catalyze 3-ketoacids and fatty acyl-CoAs for head to head condensation into β-diketones (Sun et al., 2023). When β-diketones were formed, most of them would be transported to cuticular layer, while some would participate in the next DMC catalyzed reaction to produce hydroxy-β-diketones (Sun et al., 2023).
The β-diketone-forming pathway is different from the other two ubiquitous biosynthesis pathway. There are multiple enzymatic reactions in the other two pathways, while the β-diketone-forming pathway with only three enzymes can synthesize multiple wax components. Although a recent study was performed in barley (Sun et al., 2023), the details of β-diketone-forming pathway remain obscure in wheat. When the 3-ketoacids biosynthesis process begins, DMH must capture the intermediate 3-ketoacid-ACP. This action appears to compete with the production of C16 and C18 fatty acyl-ACPs and indirectly affects the other two ubiquitous biosynthesis pathways. Therefore, we speculate that β-diketone-forming pathway needs to be closely regulated. Moreover, as mentioned above, β-diketone is a major component of cuticular wax in wheat, but it is absent from the cuticular wax of the model plant Arabidopsis (Hen-Avivi et al., 2016). This may indicate that the wax biosynthesis pathway in Arabidopsis lacks genes encoding enzymes that catalyze the reaction to synthesize β-diketone or influence by other factors.
3 Genetic and regulatory mechanism of cuticular wax biosynthesis in wheat
Most commercial bread wheat contains wax on the surfaces of organs such as leaves, stems, and spikes (Figure 3), and glaucousness was observed after flowering. With the continuous in-depth study of cuticular wax over the last few decades, its genetic basis and regulatory mechanisms have gradually been revealed.
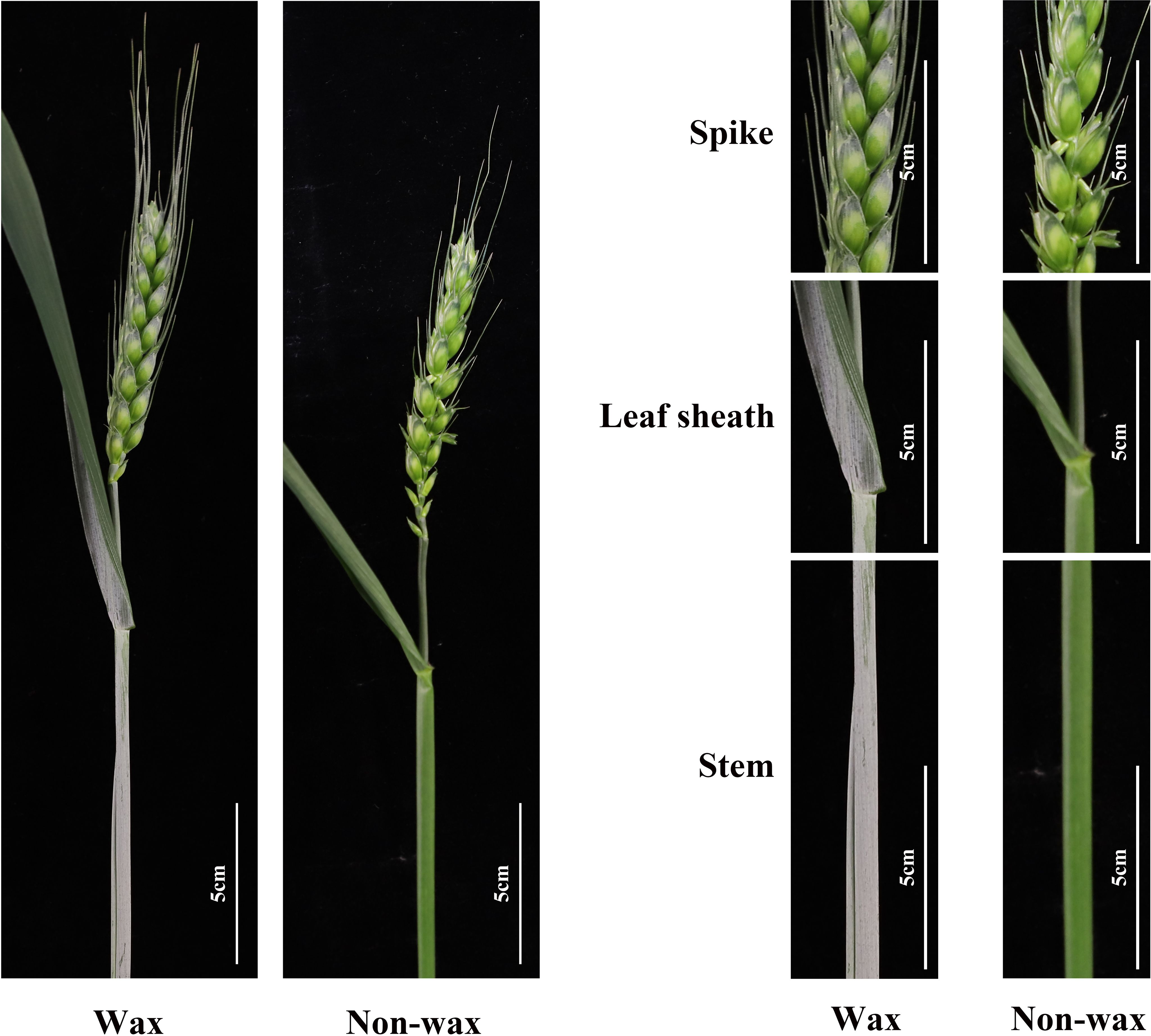
Figure 3. We utilized the wheat cultivar ‘Jimai38’ to create a mutant population in the previous study and investigated the wax-deficient phenotype of wheat at heading stages. The representative phenotypes of wax and wax-deficient plants were presented at single tiller, spike, leaf sheath, and stem.
3.1 Genetic basis of cuticular wax biosynthesis in wheat
The glaucousness trait of cuticular wax in wheat is controlled by two sets of dominant genes that include wax production loci (W1 and W2) and wax inhibition loci (Iw1 and Iw2) (Tsunewaki and Ebana, 1999). Genetic linkage analysis of W1 and Iw1 demonstrated that they are located on the short arm of chromosome 2BS and are closely linked at a genetic distance of 2 cM (Wu et al., 2013). W2 and Iw2 reside on the short arm of chromosome 2DS at a genetic distance of at least 130 cM (Tsunewaki and Ebana, 1999; Wu et al., 2013). W1, W2, Iw1, and Iw2 are the key genes that control cuticular wax biosynthesis (Allen and Vogel, 1960; Tsunewaki, 1962; Discoll and Jensen, 1964; Tsunewaki, 1964; Tsunewaki and Ebana, 1999; Wu et al., 2013). The dominant glaucousness production genes, W1 and W2, appear simultaneously or separately to produce a glaucousness phenotype. In contrast, the inhibition loci for glaucousness (Iw1 and Iw2) exhibit a dominant effect (Tsunewaki, 1962; Wu et al., 2013), as the presence of Iw1, Iw2 (or both) can inhibit the glaucousness phenotype (Tsunewaki, 1962; Wu et al., 2013). Schneider et al. identified three diverse cer genes on the short arm of barley chromosome 2H. Cer-c, -q and -u are tightly linked, forming a gene cluster known as Cer-cqu (Schneider et al., 2016). In wheat, the W1 locus is a gene cluster that affects β-diketone synthesis and is homologous to Cer-cqu in barley (Hen-Avivi et al., 2016; Schneider et al., 2016). The orthologs of Cer-cqu are W1-COE (DMH), W1-PKS (DMP), and W1-CYP (DMC) (Huang et al., 2017). As a miRNA precursor gene, Iw1 produces the miRNA miRW1 that targets the cleavage sites of W1-COE (DMH) to suppress the glaucousness phenotype (Huang et al., 2017).
In addition to these key wax-related genes, numerous other genes affecting wax traits have been identified. Zhang et al. (2015) discovered a wax-deficient mutant in the wheat cultivar ‘Bobwhite’ and identified a new gene involved in β-diketone synthesis on chromosome 2BS, designated W3. Nishijima et al. cloned W4 from Aegilops tauschii Coss (D genome progenitor) located on chromosome 3DL (Nishijima et al., 2018). Li et al. disclosed a wax-deficient mutant W5 from the wheat cultivar ‘Jimai22’ and finely mapped it to a 194-kbp region on chromosome 7DL (Li et al., 2020). Similarly, another wax-affected gene, GLOSSY1, was identified and finely mapped to a 308.1-kbp region on chromosome 2DS (Li et al., 2021). Iw3, a new tissue-specific wax-inhibition locus, has been mapped to chromosome 1BS in Emmer wheat (Wang et al., 2014).
3.2 Regulatory mechanism of cuticular wax biosynthesis in wheat
Transcription factors (TFs) play important roles in the regulation of cuticular wax biosynthesis. SHINE1/WAX INDUCER1 (SHN1/WIN1) was the first TFs associated with wax regulation in Arabidopsis. AtSHN1 contains a highly conserved APETALA2 (AP2) domain (Aharoni et al., 2004). AtSHN1 overexpression drives the expression of a series of genes in Arabidopsis, such as CER1, KCS1, and CER2, that produce more wax (Broun et al., 2004). Moreover, SHN1 directly targets the promoter of LACS2 and modifies cuticle permeability (Kannangara et al., 2007). TaSHN1/WIN1 is a typical TF of the SHN1 family and is a homolog of AtSHN1 (Bi et al., 2018). The overexpression of TaSHN1 influences the components of cuticular wax and significantly increases alkane levels in leaves (Bi et al., 2018). Additionally, the knockdown of TaWIN1 expression weakened the accumulation of very-long-chain aldehydes and alkanes on wheat blade surfaces (Kong and Chang, 2018).
Previous studies have reported that Myeloblastosis (MYB) TFs are precisely regulated during wax biosynthesis in many plant species (Lee and Suh, 2015, 2022). Several MYB TFs such as TaMYB74, TaEPBM1, TaMYB96, and TaMYB30 have been demonstrated to regulate wax biosynthesis in wheat (Bi et al., 2016; Kong et al., 2020b; He et al., 2022; Liu et al., 2023). TaMYB74 specifically binds to the MYBR1 and MYBR2 cis-elements of the wax biosynthesis-related gene TaSHN1 under drought stress (Bi et al., 2016). TaEPBM1, an R2R3-type MYB TF, was isolated as a binding protein for TaECR that enhances TaECR expression levels (Kong et al., 2020b). TaMYB96, was observed to target the conserved motif “CAACCA” of three key wax biosynthesis genes, TaCER1-6A, TaCER1-1A, and TaFAR4 (He et al., 2022). Recently, TaMYB30, a novel MYB TF, was isolated from wheat (Liu et al., 2023). Similar to its homologous gene, AtMYB30 modulates VLCFA synthesis (Raffaele et al., 2008). TaMYB30 can directly bind to TaKCS1 and TaECR and accelerate wax biosynthesis (Liu et al., 2023). The basic helix-loop-helix (bHLH) transcription factor family regulates plant cuticle development (Wu et al., 2011; Li et al., 2016). TaKPAB1 binds directly to TaKCS6 and recruits TaCHR729 (Wang et al., 2019). Moreover, knockdown of TaKPAB1 reduces cuticular wax deposition in wheat (Wang et al., 2019).
Cuticular wax biosynthesis is also influenced by other pathways. Cyclin-dependent kinase 8 (CDK8) is a critical component of the eukaryotic mediator complex. In Arabidopsis, CDK8 interacts with AtSHN1 to regulate cuticle development, and a cdk8 mutant exhibits a notably different cuticle structure (Zhu et al., 2014). As a homolog of AtCDK8, TaCDK8 interacts with TaSHN1 to facilitate TaSHN1 transcription and regulate cuticular wax biosynthesis in wheat (Kong and Chang, 2018). Interestingly, a strong phosphorylation signal was detected in the immunocomplex kinase assay, demonstrating that TaCDK8-mediated phosphorylation increased the transcription-activating role of TaSHN1 (Kong and Chang, 2018). TaADA2-TaGCN5 histone acetyltransferase (HAT) complex regulates cuticular wax biosynthesis in wheat. The wheat TF TaEPBM1 can directly interact with the TaADA2-TaGCN5 HAT complex. This complex stimulates TaECR expression through histone modification, thus promoting the biosynthesis of cuticular wax components (Kong et al., 2020b).
4 Cuticular wax responses to abiotic and biotic stresses in wheat
As the first primary physical barrier, the cuticle plays an indispensable role in plant responses to abiotic and biotic stressors. Specifically, cuticular wax can limit non-stomatal water loss and protect plants from other stresses such as UV radiation, high temperatures, pathogens, and pests (Li et al., 2019; Long et al., 2003; Djemal and Khoudi, 2021; Skamnioti and Gurr, 2007; Zhou and Zhang, 2020). Previous studies have demonstrated that cuticular wax plays an important role in abiotic and biotic stresses tolerance in wheat (Table 2).
4.1 Cuticular wax responses to abiotic stresses in wheat
Drought, cold, heat, and salinity stresses are major environmental factors that affect the growth and development of wheat and threaten food security (Table 2). These environmental factors have become increasingly frequent due to climate change (Fedoroff et al., 2010; Zhu, 2016).
Heterologous overexpression of TaCER1-1A causes changes in the cuticular wax composition and confers significant drought resistance in Arabidopsis and rice (Li et al., 2019). Overexpression of TaSHN1 and TaCER1-6A induces cuticular wax accumulation, reduces cuticle permeability, and reinforces drought tolerance (Bi et al., 2018; He et al., 2022). Recently, eight FAR-like genes (TaFAR1, TaFAR2, TaFAR3, TaFAR4, TaFAR5, TaFAR6, TaFAR7, and TaFAR8) were demonstrated to be involved in cuticular wax biosynthesis (Wang et al., 2015a, 2015b, 2016; Chai et al., 2018). Transcriptional expression analysis revealed that TaFAR1, TaFAR2, TaFAR3, TaFAR4, TaFAR5, TaFAR6, TaFAR7, and TaFAR8 were induced under drought and cold stress. TaFAR2, TaFAR3, and TaFAR4 were positively regulated by salinity stress, and the expression levels of TaFAR6, TaFAR7, and TaFAR8 were significantly increased by heat stress (Wang et al., 2015a, 2015b, 2016; Chai et al., 2018). These results suggest that FAR-like genes are associated with cuticular wax biosynthesis and actively respond to multiple abiotic stressors.
4.2 Cuticular wax responses to biotic stresses in wheat
The cuticle is the first interface between the plant and the external environment and protects plants from pathogens and pests (Wang et al., 2020; Chen, 2021). Many studies have confirmed that pathogen or pest invasion can affect the expression of cuticular wax biosynthesis genes that regulate immune responses in wheat (Table 2).
Wheat powdery mildew caused by Bgt is a devastating disease that reduces global wheat yield (Sánchez-Martín et al., 2021; Zhu et al., 2022). Several studies have demonstrated that cuticular wax is closely associated with Bgt infections (Kong and Chang, 2018; Wang et al., 2019; Kong et al., 2020b). BSMV-VIGS induces TaCDK8 and TaWIN1 expression, resulting in reduced cuticular wax accumulation and the repression of Bgt germination (Kong and Chang, 2018). Similarly, the knockdown of TaKCS6, TaCHR729, TaKPAB1, TaECR, TaEPBM1, TaADA2, and TaGCN5 leads to a reduction in cuticular wax and germination of Bgt (Wang et al., 2019; Kong et al., 2020b). The hessian fly is a wheat pest that causes annual global crop losses (Smiley et al., 2004). After infestation of wheat with Hessian flies, the total amount of cuticular wax did not change significantly, more individual wax components were detected, and the transcript levels of CRE3, CER4, and KCS6 genes involved in wax synthesis were significantly upregulated in resistant plants (Kosma et al., 2010). These results suggest that cuticular wax plays an important role in the compatible and incompatible interactions between plants and pests, particularly in the permeability of the cuticle.
5 Perspectives on the role of cuticular wax in breeding applications
Germplasm resources are critical for breeding and genetic improvements (Liu et al., 2012). Creating mutant materials using chemical, physical, and biological methods is a reliable strategy for generating germplasm resources (Wang et al., 2024). Over the years, wax-deficient mutants have provided valuable genetic resources for mapping wax biosynthesis loci such as W3, W5, and GLOOSY1 in wheat (Zhang et al., 2015; Li et al., 2020, 2021). This remains the mainstream technique for exploring new wax sources using mutants. The application strategies include identification of wax mutant phenotypes, sequencing of trait-associated mutations (STAM) to acquire candidate genes (Ni et al., 2023), and molecular marker-assisted breeding. Thus, the “phenotype- STAM -molecular marker” model is considered as an effective engine for genetic improvement of wax traits.
We propose a rapid technological system for wheat breeding to improve the application efficiency of wax traits. This system was developed based on molecular marker selection, genome-wide liquid SNP chip development, and haploinduction technology. Taking the wax-dominant gene W1 as an example, the first step was to select a material containing the target gene W1 and an excellent wheat variety without wax traits. Second, W1-specific Kompetitive Allele-Specific PCR (KASP) markers were designed, and a genome-wide liquid SNP chip was developed for excellent wheat varieties. The two materials were then grown in a greenhouse. Considering the effect of breeding applications, the hybrid generation was backcrossed with the excellent wheat variety for to 3-4 generations. During backcrossing, the wax trait was investigated to identify the W1 gene of each hybrid generation. When the background of the hybrid generation recovered to approximately 95% of that of the excellent wheat variety, haploid induction was performed on the backcrossed generation to obtain homozygous lines. Finally, the wax phenotypes and agronomic traits were verified in the field. This system combines traditional breeding and genomic technologies.
From the perspective of natural evolution, cuticular wax plays an important role in combating biotic and abiotic stress. Therefore, it is necessary to cultivate the protective traits of waxes in crops. Future research should focus on three primary directions to support the breeding of effective cuticular waxes in wheat, including advanced genetic technologies, various wax germplasm resources, and effective utilization methods (Figure 4).
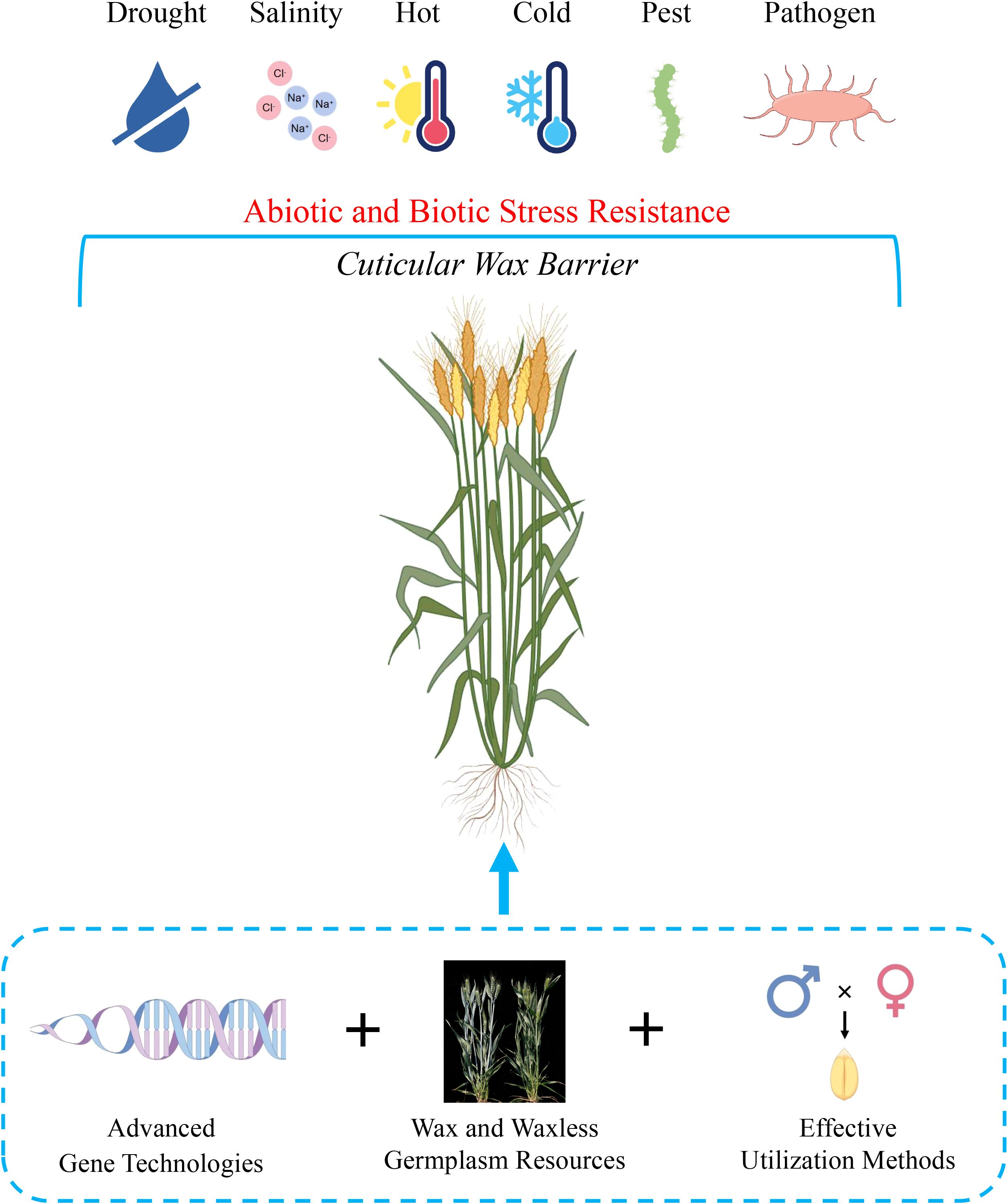
Figure 4. We purposed a model for future cuticular wax genetic improvement in wheat. As a protective barrier, the cuticular wax will confer a positive effect for wheat to withstand abiotic and biotic stress. Three key points were discussed in this review, including advanced gene technologies, wax and waxless germplasm resources, and effective utilization methods. The combination of three key points will accelerate application and development of wax traits in the context of genetic improvement. In addition to wax traits, the model can also be applied on other useful traits. The figure was created by figdraw.com.
6 Concluding remarks
In this review, we summarize recent research advances in wheat cuticular wax. Cuticular wax is a natural protective film that functions as a critical barrier in various stressful environments. Over the past few decades, remarkable progress has been made in the study of cuticular waxes. However, due to limited knowledge, the specific roles of cuticular wax components remain unclear, and the differences in wax composition between different species or organs require further investigation. Moreover, the regulatory mechanisms of cuticular wax biosynthesis and stress responses warrant further studies to deepen our understanding and improve the utilization efficiency of cuticular wax to enhance stress resistance in wheat.
Author contributions
RT: Writing – original draft. WL: Writing – original draft. YW: Writing – original draft, Writing – review & editing. WW: Funding acquisition, Resources, Writing – original draft, Writing – review & editing.
Funding
The author(s) declare that financial support was received for the research, authorship, and/or publication of this article. This work was supported by the Program for Youth Innovation team in Universities of Shandong (2022KJ276), the National Key Research and Development Program of China (2022YFF1002300), the Key Research and Development Program of Shandong (2024LZGCQY005), and the Quancheng ‘5150’ Talent Program (07962021047).
Conflict of interest
Authors RT, WL and WW was employed by the company Spring Valley Agriscience Co., Ltd.
The remaining author declare that the research was conducted in the absence of any commercial or financial relationships that could be construed as a potential conflict of interest.
Publisher’s note
All claims expressed in this article are solely those of the authors and do not necessarily represent those of their affiliated organizations, or those of the publisher, the editors and the reviewers. Any product that may be evaluated in this article, or claim that may be made by its manufacturer, is not guaranteed or endorsed by the publisher.
References
Abbas, G., Saqib, M., Rafique, Q., Rahman, A., Akhtar, J., Haq, M., et al. (2013). Effect of salinity on grain yield and grain quality of wheat (Triticum aestivum L.). Pakistan J. Agric. Res. 50, 185–189.
Adamski, N. M., Bush, M. S., Simmonds, J., Turner, A. S., Mugford, S. G., Jones, A., et al. (2013). The inhibitor of wax 1 locus (Iw1) prevents formation of β- and OH-β-diketones in wheat cuticular waxes and maps to a sub-cM interval on chromosome arm 2BS. Plant J. 74, 989–1002. doi: 10.1111/tpj.2013.74.issue-6
Aharoni, A., Dixit, S., Jetter, R., Thoenes, E., Arkel, G. V., Pereira, A. (2004). The SHINE clade of AP2 domain transcription factors activates wax biosynthesis, alters cuticle properties, and confers drought tolerance when overexpressed in Arabidopsis. Plant Cell 16, 2463–2480. doi: 10.1105/tpc.104.022897
Ali, A., Basra, S. M. A., Ahmad, R., Wahid, A. (2009). Optimizing silicon application to improve salinity tolerance in wheat. Soil Environ. 28, 136–144.
Allen, R. E., Vogel, O. A. (1960). F1 monosomic analysis involving a smooth-awn durum wheat. Wheat Inf. Serv 11, 3–4.
Apples, R., Eversole, K., Stein, N., Feuillet, C., Keller, B., Rogers, J., et al. (2018). Shifting the limits in wheat research and breeding using a fully annotated reference genome. Science 361, eaar7191. doi: 10.1126/science.aar7191
Arora, N. K. (2019). Impact of climate change on agriculture production and its sustainable solutions. Environ. Sustainability 2, 95–96. doi: 10.1007/s42398-019-00078-w
Bai, F., Yu, L., Shi, J., Li-Beisson, Y., Liu, J. (2022). Long-chain acyl-CoA synthetases activate fatty acids for lipid synthesis, remodeling and energy production in Chlamydomonas. New Phytol. 233, 823–837. doi: 10.1111/nph.v233.2
Bergman, D. K., Dillwith, J. W., Zarrabi, A. A., Berberet, R. C. (1991). Epicuticular lipids of alfalfa leaves relative to position on the stem and their correlation with aphid (Homoptera: Aphididae) distributions. Environ. Entomol 20, 781–785. doi: 10.1093/ee/20.3.781
Bi, H., Luang, S., Li, Y., Bazanova, N., Morran, S., Song, Z., et al. (2016). Identification and characterization of wheat drought-responsive MYB transcription factors involved in the regulation of cuticle biosynthesis. J. Exp. Bot. 67, 5363–5380. doi: 10.1093/jxb/erw298
Bi, H., Shi, J., Kovalchuk, N., Luang, S., Bazanova, N., Chirkova, L., et al. (2018). Overexpression of the TaSHN1 transcription factor in bread wheat leads to leaf surface modifications, improved drought tolerance, and no yield penalty under controlled growth conditions. Plant Cell Environ. 41, 2549–2566. doi: 10.1111/pce.v41.11
Bowman, J. L. (2022). The origin of a land flora. Nat. Plants 8, 1352–1369. doi: 10.1038/s41477-022-01283-y
Broun, P., Poindexter, P., Osborne, E., Jiang, C., Riechmann, J. L. (2004). WIN1, a transcriptional activator of epidermal wax accumulation in Arabidopsis. Proc. Natl. Acad. Sci. U.S.A. 101, 4706–4711. doi: 10.1073/pnas.0305574101
Buschhaus, C., Jetter, R. (2011). Composition differences between epicuticular and intracuticular wax substructures: how do plants seal their epidermal surfaces? J. Exp. Bot. 62, 841–853. doi: 10.1093/jxb/erq366
Byers, D. M., Gong, H. (2007). Acyl carrier protein: structure-function relationships in a conserved multifunctional protein family. Biochem. Cell Biol. 85, 649–662. doi: 10.1139/O07-109
Chai, G., Li, C., Xu, F., Li, Y., Shi, X., Wang, Y., et al. (2018). Three endoplasmic reticulum-associated fatty acyl-coenzyme a reductases were involved in the production of primary alcohols in hexaploid wheat (Triticum aestivum L.). BMC Plant Biol. 18, 41. doi: 10.1186/s12870-018-1256-y
Chen, C., Hao, W., Wu, J., Si, H., Xia, X., Ma, C. (2022). Fine mapping of stripe-rust-resistance gene YrJ22 in common wheat by BSR-Seq and MutMap-Based Sequencing. Plants (Basel) 11, 3244. doi: 10.3390/plants11233244
Chen, M. (2021). The tea plant leaf cuticle: From plant protection to tea quality. Front. Plant Sci. 12, 751547. doi: 10.3389/fpls.2021.751547
Discoll, C. J., Jensen, N. F. (1964). Chromosomes associated with waxlessness, awnedness and time of maturity of common wheat. Can. J. Gent Cytol 6, 324–333. doi: 10.1139/g64-041
Djemal, R., Khoudi, H. (2021). The barley SHN1-type transcription factor HvSHN1 imparts heat, drought and salt tolerances in transgenic tobacco. Plant Physiol. Biochem. 164, 44–53. doi: 10.1016/j.plaphy.2021.04.018
Domínguez, E., Heredia-Guerrero, J. A., Heredia, A. (2011). The biophysical design of plant cuticles: an overview. New Phytol. 189, 938–949. doi: 10.1111/j.1469-8137.2010.03553.x
Fedoroff, N. V., Battisti, D. S., Beachy, R. N., Cooper, P. J., Fischhoff, D. A., Hodges, C. N., et al. (2010). Radically rethinking agriculture for the 21st century. Science 327, 833–834. doi: 10.1126/science.1186834
Frank, A. B., Bauer, A., Black, A. L. (1987). Effects of air temperature and water stress on apex development in spring wheat. Crop Sci. 27, 113–116. doi: 10.2135/cropsci1987.0011183X002700010028x
Geyer, U., Schönherr, J. (1990). The effect of the environment on the permeability and composition of Citrus leaf cuticles: I. Water permeability of isolated cuticular membranes. Planta 180, 147–153. doi: 10.1007/BF00193989
Greer, S., Wen, M., Bird, D., Wu, X., Samuels, L., Kunst, L., et al. (2007). The cytochrome P450 enzyme CYP96A15 is the midchain alkane hydroxylase responsible for formation of secondary alcohols and ketones in stem cuticular wax of Arabidopsis. Plant Physiol. 145, 653–667. doi: 10.1104/pp.107.107300
Günenc, A. N., Graf, B., Stark, H., Chari, A. (2022). Fatty acid synthase: structure, function, and regulation. Subcell Biochem. 99, 1–33. doi: 10.1007/978-3-031-00793-4_1
Haslam, T. M., Haslam, R., Thoraval, D., Pascal, S., Delude, C., Domergue, F., et al. (2015). ECERIFERUM2-LIKE proteins have unique biochemical and physiological functions in very-long-chain fatty acid elongation. Plant Physiol. 167, 682–692. doi: 10.1104/pp.114.253195
Haslam, T. M., Kunst, L. (2021). Arabidopsis ECERIFERUM2-LIKEs are mediators of condensing enzyme function. Plant Cell Physiol. 61, 2126–2138. doi: 10.1093/pcp/pcaa133
He, J., Li, C., Hu, N., Zhu, Y., He, Z., Sun, Y., et al. (2022). ECERIFERUM1-6A is required for the synthesis of cuticular wax alkanes and promotes drought tolerance in wheat. Plant Physiol. 190, 1640–1657. doi: 10.1093/plphys/kiac394
Hen-Avivi, S., Savin, O., Racovita, R. C., Lee, W. S., Adamski, N. M., Malitsky, S., et al. (2016). A metabolic gene cluster in the wheat W1 and the barley Cer-cqu loci determines β-Diketone biosynthesis and glaucousness. Plant Cell 28, 1440–1460. doi: 10.1105/tpc.16.00197
Hickey, L. T., Hafeez, A. N., Robinson, H., Jackson, S. A., Leal-Bertioli, S. C. M., Tester, M., et al. (2019). Breeding crops to feed 10 billion. Nat. Biotechnol. 37, 744–754. doi: 10.1038/s41587-019-0152-9
Huang, D., Feurtado, J. A., Smith, M. A., Flatman, L. K., Koh, C., Cutler, A. J. (2017). Long noncoding miRNA gene represses wheat β-diketone waxes. Proc. Natl. Acad. Sci. U.S.A. 114, E3149–E3158. doi: 10.1073/pnas.1617483114
Jacott, C. N., Boden, S. A. (2020). Feeling the heat: developmental and molecular responses of wheat and barley to high ambient temperatures. J. Exp. Bot. 1, 5740–5751. doi: 10.1093/jxb/eraa326
Jenks, M. A., Tuttle, H. A., Eigenbrode, S. D., Feldmann, K. A. (1995). Leaf epicuticular waxes of the eceriferum mutants in Arabidopsis. Plant Physiol. 108, 369–377. doi: 10.1104/pp.108.1.369
Jetter, R., Kunst, L. (2008). Plant surface lipid biosynthesis pathways and their utility for metabolic engineering of waxes and hydrocarbon biofuels. Plant J. 54, 670–683. doi: 10.1111/j.1365-313X.2008.03467.x
Kannangara, R., Branigan, C., Liu, Y., Penfield, T., Rao, V., Mouille, G., et al. (2007). The transcription factor WIN1/SHN1 regulates Cutin biosynthesis in Arabidopsis thaliana. Plant Cell 19, 1278–1294. doi: 10.1105/tpc.106.047076
Kenrick, P., Crane, P. (1997). The origin and early evolution of plants on land. Nature 389, 33–39. doi: 10.1038/37918
Kim, J., Kim, R. J., Lee, S. B., Suh, M. C. (2022). Protein-protein interactions in fatty acid elongase complexes are important for very-long-chain fatty acid synthesis. J. Exp. Bot. 73, 3004–3017. doi: 10.1093/jxb/erab543
Kong, L., Chang, C. (2018). Suppression of wheat TaCDK8/TaWIN1 interaction negatively affects germination of Blumeria graminis f.sp. tritici by interfering with very-long-chain aldehyde biosynthesis. Plant Mol. Biol. 96, 165–178. doi: 10.1007/s11103-017-0687-4
Kong, L., Liu, Y., Zhi, P., Wang, X., Xu, B., Gong, Z., et al. (2020a). Origins and evolution of cuticle biosynthetic machinery in land plants. Plant Physiol. 184, 1998–2010. doi: 10.1104/pp.20.00913
Kong, L., Zhi, P., Liu, J., Li, H., Zhang, X., Xu, J., et al. (2020b). Epigenetic activation of enoyl-CoA reductase by an acetyltransferase complex triggers wheat wax biosynthesis. Plant Physiol. 183, 1250–1267. doi: 10.1104/pp.20.00603
Kosma, D. K., Nemacheck, J. A., Jenks, M. A., Williams, C. E. (2010). Changes in properties of wheat leaf cuticle during interactions with Hessian fly. Plant J. 63, 31–43. doi: 10.1111/j.1365-313X.2010.04229.x
Kramer, A. T., Havens, K. (2009). Plant conservation genetics in a changing world. Trends Plant Sci. 14, 599–607. doi: 10.1016/j.tplants.2009.08.005
Kunst, L., Jetter, R., Samuels, A. L. (2006). Biosynthesis and transport of plant cuticular waxes. Annu. Plant Rev. 23, 182–215. doi: 10.1002/9781119312994.apr0233
Kunst, L., Samuels, A. L. (2003). Biosynthesis and secretion of plant cuticular wax. Prog. Lipid Res. 42, 51–80. doi: 10.1016/S0163-7827(02)00045-0
Kunst, L., Samuels, L. (2009). Plant cuticles shine: advances in wax biosynthesis and export. Curr. Opin. Plant Biol. 12, 721–727. doi: 10.1016/j.pbi.2009.09.009
Kuruparan, A., Gao, P., Soolanayakanahally, R., Kumar, S., Gonzales-Vigil, E. (2024). [amp]]beta;-diketone accumulation in response to drought stress is weakened in modern bread wheat varieties (Triticum aestivum L.). Front. Plant Sci. 15, 1401135. doi: 10.3389/fpls.2024.1401135
Lee, R. (2011). The outlook for population growth. Science 333, 569–573. doi: 10.1126/science.1208859
Lee, S. B., Jung, S. J., Go, Y. S., Kim, H. U., Kim, J. K., Cho, H. J., et al. (2009). Two Arabidopsis 3-ketoacyl CoA synthase genes, KCS20 and KCS2/DAISY, are functionally redundant in cuticular wax and root suberin biosynthesis, but differentially controlled by osmotic stress. Plant J. 60, 462–475. doi: 10.1111/j.1365-313X.2009.03973.x
Lee, S. B., Suh, M. C. (2015). Advances in the understanding of cuticular waxes in Arabidopsis thaliana and crop species. Plant Cell Rep. 34, 557–572. doi: 10.1007/s00299-015-1772-2
Lee, S. B., Suh, M. C. (2022). Regulatory mechanisms underlying cuticular wax biosynthesis. J. Exp. Bot. 73, 2799–2816. doi: 10.1093/jxb/erab509
Lee, S. B., Yang, S. U., Pandey, G., Kim, M. S., Hyoung, S., Choi, D., et al. (2020). Occurrence of land-plant-specific glycerol-3-phosphate acyltransferases is essential for cuticle formation and gametophore development in Physcomitrella patens. New Phytol. 225, 2468–2483. doi: 10.1111/nph.v225.6
Lesk, C., Rowhani, P., Ramankutty, N. (2016). Influence of extreme weather disasters on global crop production. Nature 529, 84–87. doi: 10.1038/nature16467
Lewandowska, M., Keyl, A., Feussner, I. (2020). Wax biosynthesis in response to danger: its regulation upon abiotic and biotic stress. New Phytol. 227, 698–713. doi: 10.1111/nph.v227.3
Li, L., Chai, L., Xu, H., Zhai, H., Wang, T., Zhang, M., et al. (2021). Phenotypic characterization of the glossy1 mutant and fine mapping of GLOSSY1 in common wheat (Triticum aestivum L.). Theor. Appl. Genet. 134, 835–847. doi: 10.1007/s00122-020-03734-6
Li, L., Qi, Z., Chai, L., Chen, Z., Wang, T., Zhang, M., et al. (2020). The semidominant mutation w5 impairs epicuticular wax deposition in common wheat (Triticum aestivum L.). Theor. Appl. Genet. 133, 1213–1225. doi: 10.1007/s00122-020-03543-x
Li, T., Sun, Y., Liu, T., Wu, H., An, P., Shui, Z., et al. (2019). TaCER1-1A is involved in cuticular wax alkane biosynthesis in hexaploid wheat and responds to plant abiotic stresses. Plant Cell Environ. 42, 3077–3091. doi: 10.1111/pce.v42.11
Li, S., Wang, X., He, S., Li, J., Huang, Q., Imaizumi, T., et al. (2016). CFLAP1 and CFLAP2 are two bHLH transcription factors participating in synergistic regulation of AtCFL1-mediated cuticle development in Arabidopsis. PloS Genet. 12, e1005744. doi: 10.1371/journal.pgen.1005744
Li, F., Wu, X., Lam, P., Bird, D., Zheng, H., Samuels, L., et al. (2008). Identification of the wax ester synthase/acyl-coenzyme A: diacylglycerol acyltransferase WSD1 required for stem wax ester biosynthesis in Arabidopsis. Plant Physiol. 148, 97–107. doi: 10.1104/pp.108.123471
Li-Beisson, Y., Shorrosh, B., Beisson, F., Andersson, M. X., Arondel, V., Bates, P. D., et al. (2013). Acyl-lipid metabolism. Arabidopsis Book 11, e0161. doi: 10.1199/tab.0161
Liu, L., Li, H., Wang, X., Chang, C. (2023). Transcription factor TaMYB30 activates wheat wax biosynthesis. Int. J. Mol. Sci. 24, 10235. doi: 10.3390/ijms241210235
Liu, S., Yeh, C. T., Tang, H., Nettleton, D., Schnable, P. S. (2012). Gene mapping via bulked segregant RNA-Seq (BSR-Seq). PloS One 7, e36406. doi: 10.1371/journal.pone.0036406
Long, L. M., Patel, H. P., Cory, W. C., Stapleton, A. E. (2003). The maize epicuticular wax layer provides UV protection. Funct. Plant Biol. 30, 75–81. doi: 10.1071/FP02159
McWhite, C. D., Papoulas, O., Drew, K., Cox, R. M., June, V., Dong, O. X., et al. (2020). A Pan-plant protein complex map reveals deep conservation and novel assemblies. Cell 181, 460–474. doi: 10.1016/j.cell.2020.02.049
Nawrath, C. (2006). Unraveling the complex network of cuticular structure and function. Curr. Opin. Plant Biol. 9, 281. doi: 10.1016/j.pbi.2006.03.001
Ni, F., Zheng, Y., Liu, X., Yu, Y., Zhang, G., Epstein, L., et al. (2023). Sequencing trait-associated mutations to clone wheat rust-resistance gene YrNAM. Nat. Commun. 14, 4353. doi: 10.1038/s41467-023-39993-2
Nishijima, R., Tanaka, C., Yoshida, K., Takumi, S. (2018). Genetic mapping of a novel recessive allele for non-glaucousness in wild diploid wheat Aegilops tauschii: implications for the evolution of common wheat. Genetica 146, 249–254. doi: 10.1007/s10709-018-0012-4
Ohlrogge, J., Browse, J. (1995). Lipid biosynthesis. Plant Cell 7, 957–970. doi: 10.1105/tpc.7.7.957
Pascal, S., Bernard, A., Deslous, P., Gronnier, J., Fournier-Goss, A., Domergue, F., et al. (2019). Arabidopsis CER1-LIKE1 functions in a cuticular very-long-chain alkane-forming complex. Plant Physiol. 179, 415–432. doi: 10.1104/pp.18.01075
Pighin, J. A., Zheng, H., Balakshin, L. J., Goodman, I. P., Western, T. L., Jetter, R., et al. (2004). Plant cuticular lipid export requires an ABC transporter. Science 306, 702–704. doi: 10.1126/science.1102331
Post-Beittenmiller, D. (1996). Biochemistry and molecular biology of wax production in plants. Annu. Rev. Plant Physiol. Plant Mol. Biol. 47, 405–430. doi: 10.1146/annurev.arplant.47.1.405
Raffaele, S., Vailleau, F., Léger, A., Joubès, J., Miersch, O., Huard, C., et al. (2008). A MYB transcription factor regulates very-long-chain fatty acid biosynthesis for activation of the hypersensitive cell death response in Arabidopsis. Plant Cell 20, 752–767. doi: 10.1105/tpc.107.054858
Rees, D. C., Johnson, E., Lewinson, O. (2009). ABC transporters: the power to change. Nat. Rev. Mol. Cell Biol. 10, 218–227. doi: 10.1038/nrm2646
Renault, H., Alber, A., Horst, N. A., Basilio Lopes, A., Fich, E. A., Kriegshauser, L., et al. (2017). A phenol-enriched cuticle is ancestral to lignin evolution in land plants. Nat. Commun. 8, 14713. doi: 10.1038/ncomms14713
Samuels, L., Kunst, L., Jetter, R. (2008). Sealing plant surfaces: cuticular wax formation by epidermal cells. Annu. Rev. Plant Biol. 59, 683–707. doi: 10.1146/annurev.arplant.59.103006.093219
Sánchez-Martín, J., Widrig, V., Herren, G., Wicker, T., Zbinden, H., Gronnier, J., et al. (2021). Wheat Pm4 resistance to powdery mildew is controlled by alternative splice variants encoding chimeric proteins. Nat. Plants 7, 327–341. doi: 10.1038/s41477-021-00869-2
Savary, S., Willocquet, L., Pethybridge, S. J., Esker, P., McRoberts, N., Nelson, A. (2019). The global burden of pathogens and pests on major food crops. Nat. Ecol. Evol. 3, 430–439. doi: 10.1038/s41559-018-0793-y
Schneider, L. M., Adamski, N. M., Christensen, C. E., Stuart, D. B., Vautrin, S., Hansson, M., et al. (2016). The Cer-cqu gene cluster determines three key players in a β-diketone synthase polyketide pathway synthesizing aliphatics in epicuticular waxes. J. Exp. Bot. 67, 2715–2730. doi: 10.1093/jxb/erw105
Skamnioti, P., Gurr, S. J. (2007). Magnaporthe grisea cutinase2 mediates appressorium differentiation and host penetration and is required for full virulence. Plant Cell 19, 2674–2689. doi: 10.1105/tpc.107.051219
Smiley, R. W., Gourlie, J. A., Whittaker, R. G., Easley, S. A., Kidwell, K. K. (2004). Economic impact of Hessian fly (Diptera: Cecidomyiidae) on spring wheat in Oregon and additive yield losses with Fusarium crown rot and lesion nematode. J. Econ. Entomol. 97, 397–408. doi: 10.1093/jee/97.2.397
Song, M. Y., Xu, X., Dong, Y., Bimpong, D., Liu, L. J., Li, Y. L., et al. (2024). The Roles of Glutaredoxins in Wheat (Triticum aestivum L.) under Biotic and Abiotic Stress Conditions, including Fungal and Hormone Treatments. Agronomy 14, 2057. doi: 10.3390/agronomy14092057
Sun, Y. L., Ruiz Orduna, A., Zhang, Z. H., Feakins, S. J., Jetter, R. (2023). Biosynthesis of barley wax β-diketones: a type-III polyketide synthase condensing two fatty acyl units. Nat. Commun. 14, 7284. doi: 10.1038/s41467-023-42917-9
Tomiyama, T., Kurihara, K., Ogawa, T., Maruta, T., Ogawa, T., Ohta, D., et al. (2017). Wax ester synthase/diacylglycerol acyltransferase isoenzymes play a pivotal role in wax ester biosynthesis in Euglena gracilis. Sci. Rep. 7, 13504. doi: 10.1038/s41598-017-14077-6
Tsunewaki, K. (1962). Monosomic analysis of synthesized hexaploid wheats. Jap J. Genet. 37, 155–168. doi: 10.1266/jjg.37.155
Tsunewaki, K. (1964). Genetic studies of a 6x-derivative from an 8x triticale. Can. J. Gent Cytol 6, 1–11. doi: 10.1139/g64-001
Tsunewaki, K., Ebana, K. (1999). Production of near-isogenic lines of common wheat for glaucousness and genetic basis of this trait clarifed by their use. Genes Genet. Syst. 74, 33–41. doi: 10.1266/ggs.74.33
United Nations (2022). “World population prospects 2022: summary of results,” in United Nations. Available at: https://www.un.org/development/desa/pd/content/World-Population-Prospects-2022.
Wang, X., Chang, C. (2022). Exploring and exploiting cuticle biosynthesis for abiotic and biotic stress tolerance in wheat and barley. Front. Plant Sci. 13, 1064390. doi: 10.3389/fpls.2022.1064390
Wang, W., Guan, X., Gan, Y., Liu, G., Zou, C., Wang, W., et al. (2024). Creating large EMS populations for functional genomics and breeding in wheat. J. Integr. Agr 23, 484–493. doi: 10.1016/j.jia.2023.05.039
Wang, X., Guan, Y., Zhang, D., Dong, X., Tian, L., Qu, L. (2017). A β-Ketoacyl-CoA synthase is involved in rice leaf cuticular wax synthesis and requires a CER2-LIKE protein as a cofactor. Plant Physiol. 173, 944–955. doi: 10.1104/pp.16.01527
Wang, X., Kong, L., Zhi, P., Chang, C. (2020). Update on cuticular wax biosynthesis and its roles in plant disease resistance. Int. J. Mol. Sci. 21, 5514. doi: 10.3390/ijms21155514
Wang, J., Li, W., Wang, W. (2014). Fine mapping and metabolic and physiological characterization of the glume glaucousness inhibitor locus Iw3 derived from wild wheat. Theor. Appl. Genet. 127, 831–841. doi: 10.1007/s00122-014-2260-8
Wang, Y., Wang, M., Sun, Y., Hegebarth, D., Li, T., Jetter, R., et al. (2015a). Molecular characterization of taFAR1 involved in primary alcohol biosynthesis of cuticular wax in hexaploid wheat. Plant Cell Physiol. 56, 1944–1961. doi: 10.1093/pcp/pcv112
Wang, Y., Wang, M., Sun, Y., Wang, Y., Li, T., Chai, G., et al. (2015b). FAR5, a fatty acyl-coenzyme A reductase, is involved in primary alcohol biosynthesis of the leaf blade cuticular wax in wheat (Triticum aestivum L.). J. Exp. Bot. 66, 1165–1178. doi: 10.1093/jxb/eru457
Wang, M., Wang, Y., Wu, H., Xu, J., Li, T., Hegebarth, D., et al. (2016). Three TaFAR genes function in the biosynthesis of primary alcohols and the response to abiotic stresses in Triticum aestivum. Sci. Rep. 6, 25008. doi: 10.1038/srep25008
Wang, X., Zhi, P., Fan, Q., Zhang, M., Chang, C. (2019). Wheat CHD3 protein TaCHR729 regulates the cuticular wax biosynthesis required for stimulating germination of Blumeria graminis f.sp. tritici. J. Exp. Bot. 70, 701–713. doi: 10.1093/jxb/ery377
Wen, M., Jetter, R. (2009). Composition of secondary alcohols, ketones, alkanediols, and ketols in Arabidopsis thaliana cuticular waxes. J. Exp. Bot. 60, 1811–1821. doi: 10.1093/jxb/erp061
Wettstein-Knowles, P. V. (1995). “Biosynthesis and genetics of waxes,” in Waxes: Chemistry, Molecular Biology and Functions. Ed. Hamilton, R. J. (The Oily Press, Ayr, Scotland), 91–129.
Wong, L. H., Gatta, A. T., Levine, T. P. (2019). Lipid transfer proteins: the lipid commute via shuttles, bridges and tubes. Nat. Rev. Mol. Cell Biol. 20, 85–101. doi: 10.1038/s41580-018-0071-5
Wu, R., Li, S., He, S., Wassmann, F., Yu, C., Qin, G., et al. (2011). CFL1, a WW domain protein, regulates cuticle development by modulating the function of HDG1, a class IV homeodomain transcription factor, in rice and Arabidopsis. Plant Cell 23, 3392–3411. doi: 10.1105/tpc.111.088625
Wu, H., Qin, J., Han, J., Zhao, X., Ouyang, S., Liang, Y., et al. (2013). Comparative high-resolution mapping of the wax inhibitors Iw1 and Iw2 in hexaploid wheat. PloS One 8, e84691. doi: 10.1371/journal.pone.0084691
Xie, J., Guo, G., Wang, Y., Hu, T., Wang, L., Li, J., et al. (2020). A rare single nucleotide variant in Pm5e confers powdery mildew resistance in common wheat. New Phytol. 228, 1011–1026. doi: 10.1111/nph.v228.3
Xue, D., Zhang, X., Lu, X., Chen, G., Chen, Z. (2017). Molecular and evolutionary mechanisms of cuticular wax for plant drought tolerance. Front. Plant Sci. 8, 621. doi: 10.3389/fpls.2017.00621
Yang, H., Shi, X., Xia, L., Wang, Y., Wang, Z., Li, C. (2017). Analysis on composition and content of cuticular waxes on spikes of different wheat varieties (Lines). J. Triticeae Crops 37, 403–408.
Yeats, T. H., Rose, J. K. C. (2013). The formation and function of plant cuticles. Plant Physiol. 163, 5–20. doi: 10.1104/pp.113.222737
Zhang, C., Huang, L., Zhang, H., Hao, Q., Lyu, B., Wang, M., et al. (2019). An ancestral NB-LRR with duplicated 3’UTRs confers stripe rust resistance in wheat and barley. Nat. Commun. 10, 4023. doi: 10.1038/s41467-019-11872-9
Zhang, Z., Wang, W., Li, W. (2013). Genetic interactions underlying the biosynthesis and inhibition of β-diketones in wheat and their impact on glaucousness and cuticle permeability. PloS One 8, e54129. doi: 10.1371/journal.pone.0054129
Zhang, Z., Wei, W., Zhu, H., Challa, G. S., Bi, C., Trick, H. N., et al. (2015). W3 is a new wax locus that is essential for biosynthesis of β-Diketone, development of glaucousness, and reduction of cuticle permeability in common wheat. PloS One 10, e0140524. doi: 10.1371/journal.pone.0140524
Zhou, J., Zhang, Y. (2020). Plant Immunity: Danger perception and signaling. Cell 181, 978–989. doi: 10.1016/j.cell.2020.04.028
Zhu, J. (2016). Abiotic stress signaling and responses in plants. Cell 167, 313–324. doi: 10.1016/j.cell.2016.08.029
Zhu, K., Li, M., Wu, H., Zhang, D., Dong, L., Wu, Q., et al. (2022). Fine mapping of powdery mildew resistance gene MlWE74 derived from wild emmer wheat (Triticum turgidum ssp. dicoccoides) in an NBS-LRR gene cluster. Theor. Appl. Genet. 135, 1235–1245. doi: 10.1007/s00122-021-04027-2
Keywords: wheat, cuticular wax, biosynthesis, genetics, stress response
Citation: Tian R, Liu W, Wang Y and Wang W (2024) Cuticular wax in wheat: biosynthesis, genetics, and the stress response. Front. Plant Sci. 15:1498505. doi: 10.3389/fpls.2024.1498505
Received: 19 September 2024; Accepted: 11 November 2024;
Published: 03 December 2024.
Edited by:
Hang Zhao, Qufu Normal University, ChinaReviewed by:
Xianpeng Yang, Shandong Normal University, ChinaHuayan Yin, Qingdao Agricultural University, China
Copyright © 2024 Tian, Liu, Wang and Wang. This is an open-access article distributed under the terms of the Creative Commons Attribution License (CC BY). The use, distribution or reproduction in other forums is permitted, provided the original author(s) and the copyright owner(s) are credited and that the original publication in this journal is cited, in accordance with accepted academic practice. No use, distribution or reproduction is permitted which does not comply with these terms.
*Correspondence: Wenqiang Wang, d2FuZ3dlbnFpYW5nODgxMjAyQDE2My5jb20=; Yuhai Wang, eWh3YW5nOTJAMTYzLmNvbQ==