- 1Henan Collaborative Innovation Center of Modern Biological Breeding, Henan Institute of Science and Technology, Xinxiang, China
- 2State Key Laboratory of Crop Stress Adaptation and Improvement, College of Agriculture, Henan University, Kaifeng, China
- 3Institute of Molecular Biology and Biotechnology, The University of Lahore, Lahore, Pakistan
Climatic change and extreme weather events have become a major threat to global agricultural productivity. Plants coexist with microorganisms, which play a significant role in influencing their growth and functional traits. The rhizosphere serves as an ecological niche encompassing plant roots and is a chemically complex environment that supports the growth and development of diverse plant-interactive microbes. Although plant-microbe interactions have been extensively investigated however, limited exploration have been made how abiotic stresses affect the structure and assembly of microbial communities in the rhizosphere. This review highlights climate change influence on plant growth, functional traits, and microbial communities. It explores plant mechanisms for mitigating abiotic stress, such as removing reactive oxygen species (ROS), regulating antioxidant activity and indole-3-acetic acid (IAA) production, and controlling growth-inhibitory ethylene levels through colonization by bacteria producing ACC deaminase. Additionally, we elaborated the systematic communicatory network steered by hormonal crosstalk and root exudation, which can modulate and initiate the dialogues between plants and surrounding microbes. This network ultimately promotes the chemotactic movement of microbes towards the rhizosphere, facilitating their early colonization. Finally, we reviewed the recent advancements for understanding how plant-microbe interactions foster resilience under climate stress.
1 Introduction
Climatic trends show a significant increase in global mean temperature since 1970s, coupled with shift in precipitation patterns, and the frequency of extreme weather events (Capua and Rahmstorf, 2023). Agriculture sector is directly affected by climatic factors, experiencing a significant reduction in farm productivity. In addition, climate change could expand the range of pathogens and pests, leading to more frequent and severe disease outbreaks (De Wolf and Isard, 2007; Garrett et al., 2021). Recent findings has shown that global agricultural production is highly vulnerable to abiotic stresses (Bowerman et al., 2023). Furthermore, to meet the demand of growing human population, about 50% increase in agricultural production is needed by 2029 (Nawaz et al., 2024b). Consequently, a significant increase in deforestation and loss of natural habitats is occurring to acquire more land for cultivation (Foucher et al., 2024). To ensure food security with a limited expansion of agricultural land, a sustainable strategy involves improving the resilience of plants to climate change. Therefore, plant growth-promoting microorganisms represents one of the valuable resources to explore for increasing farm productivity (Bender et al., 2016).
Plants are associated with a diverse group of microorganisms, responsible for many essential functions e.g., plant growth, root development, nutrient use efficiency, and promoting resistance to abiotic and biotic stresses (Ojuederie et al., 2019). The rhizosphere serves as the central hub for the interactions among plant roots, soil, microorganisms, and environment (Trivedi et al., 2020). It is a specialized layer of soil around the root system, and the microbes in the soil are referred to as rhizosphere microorganisms (Rout and Southworth, 2013). Macro-nutrients such as nitrogen, phosphorous, and potassium, are assimilated by plants through the rhizosphere, enabling their integration into the nutrient cycle (Thepbandit and Athinuwat, 2024). Plants establish beneficial associations with various microorganisms in the rhizosphere to shape their community composition through rhizosphere deposition (Toju et al., 2018). Several important symbiotic microorganisms have been identified to promote plant growth by reducing the occurrence of different plant diseases, the regulation of phytohormones, and enhancing nutrient acquisition (Kuypers et al., 2018; Hu et al., 2020; Lopes et al., 2021). Plant growth-promoting rhizobacteria (PGPR) act as biofertilizers by enhancing the availability of both macro and micronutrients, thereby improving crop yield and soil fertility (Lopes et al., 2021). Therefore, a critical understanding of plant-microbe interactions in the rhizosphere, steered by hormonal crosstalk and root exudation, and their combined application for improving crop productivity is required.
Abiotic stresses impact not only plant physiology and metabolism but also soil microorganism activities. However, the impact of stress varies depending on the time, host plants, intensity, and other environmental factors (Georgieva and Vassileva, 2023). For instance, a significant reduction in growth and grain yield was observed in wheat grown under drought conditions, primarily due to the negative impact on photosynthesis, leaf area, seed set and weight (Rahimi-Moghaddam et al., 2023). In contrast, Thymus serphyllum exhibits increased production of osmolytes, such as proline, sorbitol, mannitol, and other amino acids, which confer tolerance to drought stress (Moradi et al., 2017). Furthermore, under salt stress, the rhizosphere of groundnuts exhibited a notable presence of Acidobacteria and Cyanobacteria, enhancing their salt tolerance (Xu et al., 2020). On the other hand, rice plants revealed significant yield penalties, including reduced growth, germination, and tillering which in turn affected plant biomass and height (Fang et al., 2023). Thus, interactions between plants and microbes during abiotic stress conditions are dynamic and complex. In order to harness the potential of plant microbiota in agriculture, it is essential to understand the impact of abiotic stressors on plants, microorganisms, and plant-microbe interactions (Fadiji et al., 2023). In the present review, we have summarized the effects of changing climatic conditions on plant-microbe interactions and highlighted the positive roles of plant associated microbes in enhancing agricultural production. Additionally, we discuss recent advancements in mitigating different stressors and their significance for achieving key objectives in future research.
2 Deciphering the coexisting relationship between plants and soil microbiota
Plants are associated with a varied and taxonomically organized community of microbiomes i.e., viruses, fungi, bacteria, and archaea that co-exist with plants in the rhizosphere (root-surrounding soil), endosphere (internal tissue), and phyllosphere (above-ground parts) to perform important activities regulating host health and fitness (Afridi et al., 2022). Among these micro-environments, rhizosphere is one of the most complex and diverse habitats for microbial communities. Plant-associated microorganisms may come from different sources, including soil, seeds, water, and other neighboring organisms i.e., insects and animals. Some of these organisms can develop a complex symbiotic relationship with plants (Fitzpatrick et al., 2018). Specifically, plant-associated microbiome form a symbiotic unit known as “holobiont” which can be influenced by environmental factors (Trivedi et al., 2020). A holobiont is a complex and interconnected system of organisms, living together in a close association in all types of ecosystems (Matthews, 2024). These microorganisms within the holobiont can significantly improve plant health by enhancing mineral solubility (Lemanceau et al., 2017), altering the signaling of phytohormones such as auxin (IAA), gibberellin (GA), and cytokinin (CK) (Spaepen and Vanderleyden, 2011), and directly providing nutrients (Saleem et al., 2024), alongside strengthening resistance against phytopathogens (Jin et al., 2024).
Plant-microbe crosstalk initiates with the release of chemical signals i.e., flavonoids and amino acids, establishing a favorable environment where microbes ultimately reside and assist plants in coping with stress and regulating growth (Stefan et al., 2018) (Figure 1). Therefore, plants recognize signals created by beneficial microbes during the early phases of symbiosis (Ravelo-Ortega et al., 2023). According to Fadiji et al. (2023), when plants receive adequate water and nutrient supply, rhizosphere microorganisms consistently aid in helping plants adapt to abiotic stresses, thereby potentially enhancing crop yield. At present, there are several studies on the isolation, identification, and application of useful symbiotic microorganisms as an alternative to chemical fertilizers, that are hazardous to humans, animals, aquatic lives, and environment (Kumar et al., 2022; Shahwar et al., 2023). Abdelaziz et al. (2019) demonstrated significant improvements in tomato growth and yield by introducing the root endophytic fungus (Piriformospora indica) into the soil. The interactions between plants and rhizobia are very specific and exist at a level of species and genotype, leading to the formation of effective symbiotic relationships (Wang et al., 2018). Overall, the rhizobia are the key members in the soil to produce and fix nitrogen compounds that help plants to grow and survive under unfavorable circumstances for better ecosystem productivity.
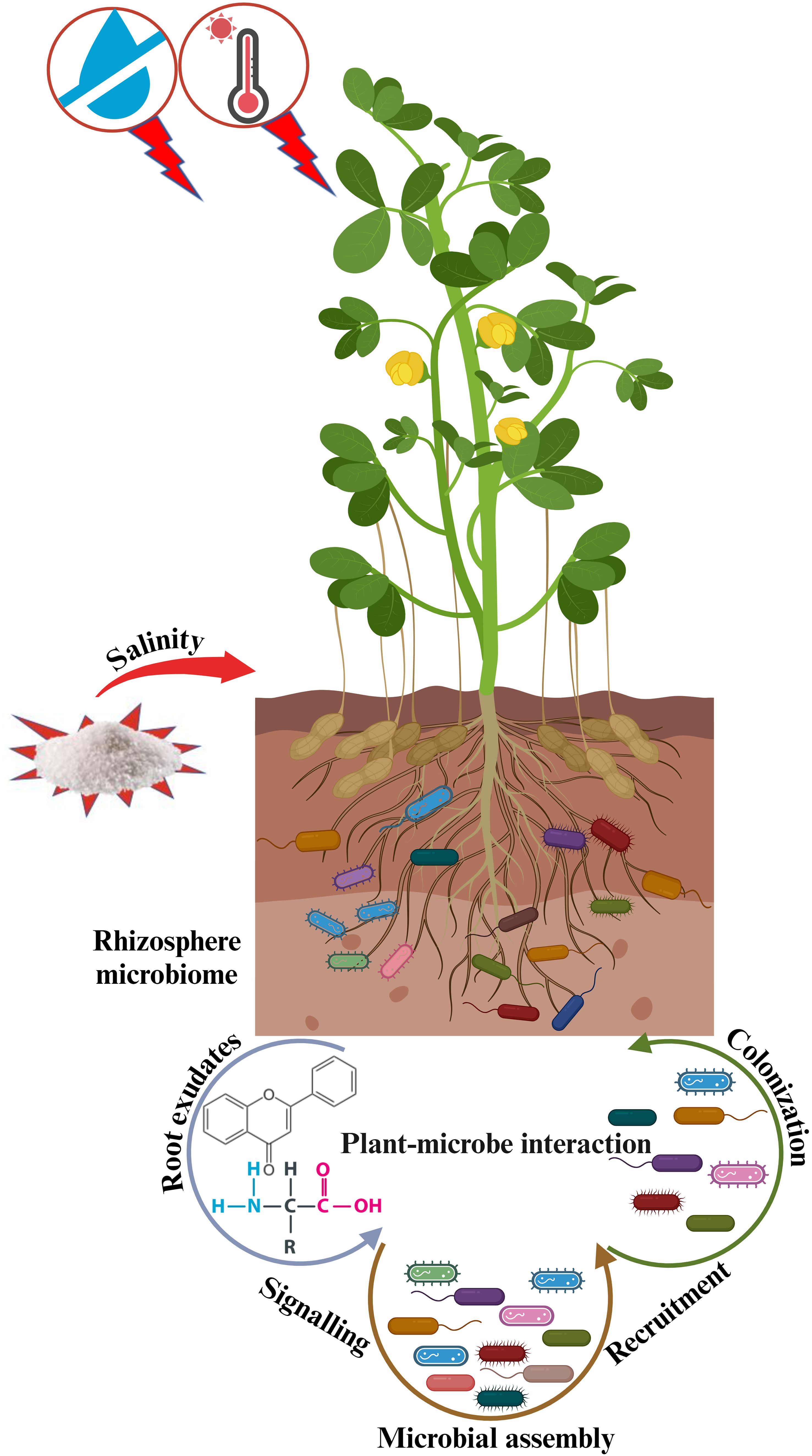
Figure 1. Systematic overview of plant-microbe interaction to mitigate abiotic stresses in changing climates. Plants release root exudates, such as flavonoids and amino acids that attract nearby microbes to their roots, helping the plants optimize water and nutrient allocation for better growth and survival in unfavorable circumstances.
3 Climate-associated abiotic stresses alter plants and their associated microbes in the rhizosphere
Plants are consistently exposed to the external environment that constantly changes in many ways (Nawaz et al., 2024a). Certain regions of the world are already facing extreme climatic shifts, such as drought, salinity, and extreme temperatures. These climatic stresses have detrimental effects on plant-associated microbial communities, which in turn influence plant growth and development (Afridi et al., 2022). In response to unfavorable conditions, plants induce a wide range of physiological and morphological modifications to adapt to these abrupt changes thus, experiencing significant growth and yield penalties (Xu et al., 2023). Abiotic stresses can affect rhizosphere microbial communities in various ways including the alteration in composition and function of microbial population in the rhizosphere. For instance, prolonged exposure to drought stress can lead to an increase in the abundance of Actinobacteria and Firmicutes (Vescio et al., 2021). Additionally, a proportionate rise in the abundance of Acidobacteria and Cyanobacteria was observed in the peanut rhizosphere, when exposed to salt stress (Xu et al., 2020). Recently, Dollete et al. (2024) revealed a reduction in symbiotic nitrogen fixation in forage legumes, thereby effecting plant growth and development. Furthermore, altered phosphate solubilizing efficiency of the biocontrol fungus Trichoderma sp. was observed under abiotic stress conditions such as pH, temperature, and heavy metals (Rawat and Tewari, 2011).
Plant growth promoting microorganisms (PGPMs) have been frequently investigated to mitigate certain abiotic stresses while enhancing plants adaptation under unfavorable conditions (Chieb and Gachomo, 2023; Kibret et al., 2024). To sustain their growth under stressed conditions, plants employ various strategies i.e., induction of chemical signaling and regulation of phytohormones (biochemical adaptation), stomatal closure (physiological adaptation), changes in growth pattern (morphological adaptation) (Naamala and Smith, 2020) (Table 1). Climate-associated abiotic stresses may influence plants in different ways and at different growth stages, limiting plant performance (Lata et al., 2018). These abiotic stresses are discussed in detail to further elaborate their detrimental effects on plant adaptation, which may hinder the development of a sustainable ecosystem and reduce agricultural production.
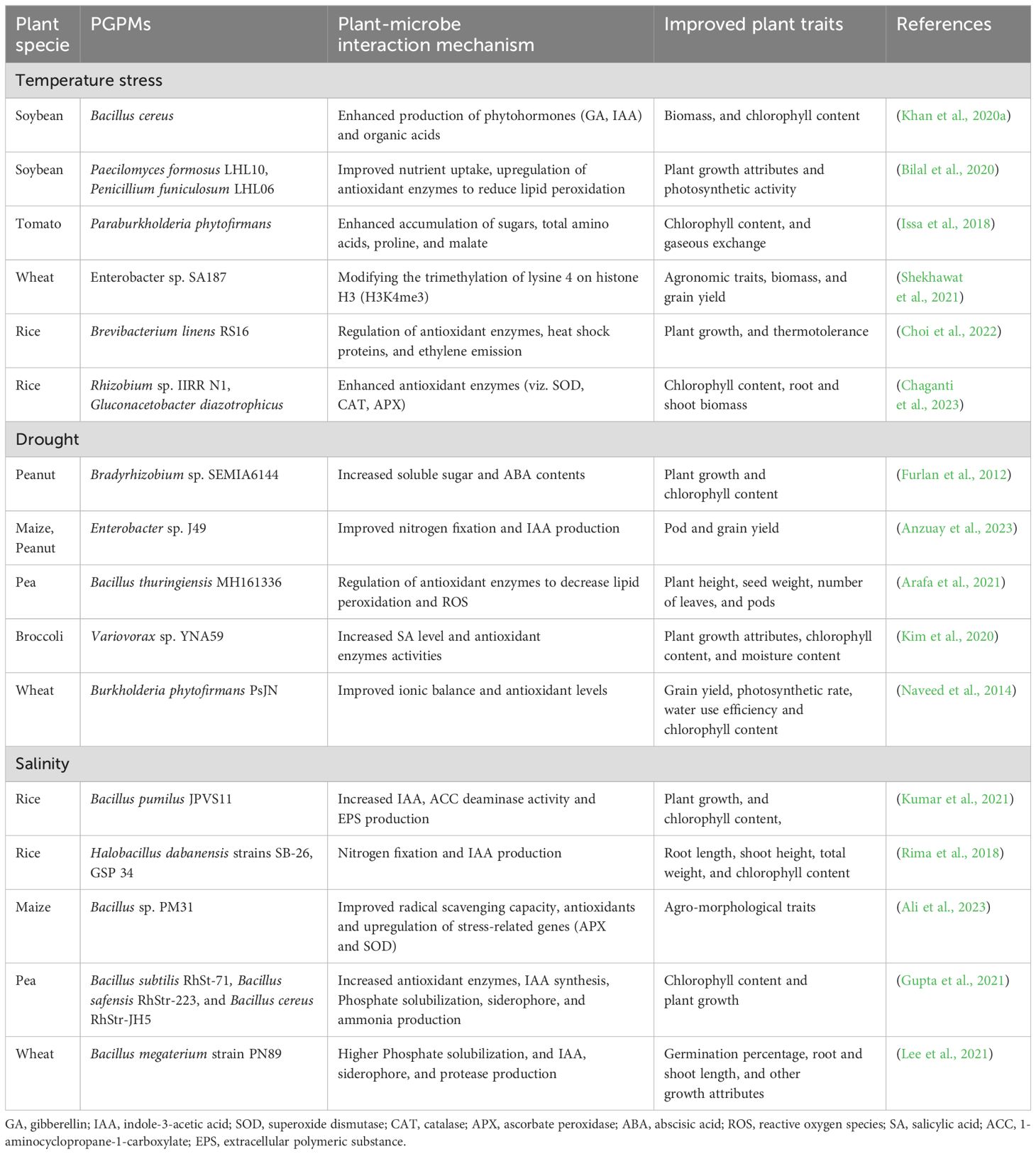
Table 1. Plant growth promoting microorganisms enhancing abiotic stress tolerance through various mechanisms.
3.1 Harnessing plant-microbes interplay to alleviate temperature stress
Temperature serves as a fundamental factor influencing plant development and phenological features together with shaping the microbial community associated with the plants (Kashyap et al., 2017). Compant et al. (2010) suggested a projected increase of about 1.8-3.6°C in global mean temperature by the year 2100 resulting water scarcity across several regions of the world which may significantly influence the composition, activities, and distribution of rhizosphere microbiome (Farooq et al., 2022). A substantial shift in microbial respiration rate in response to higher temperatures may accelerate their growth and abundance (Classen et al., 2015). Similarly, Vargas (2024) revealed that increased temperature may lead to an exponential increase in soil respiration. Furthermore, higher temperatures may influence the utilization of organic matter by microorganisms (Frey et al., 2013). Moreover, Velásquez et al. (2018) reported the connection between the pathogenicity of microorganisms with temperature changes. Higher temperatures may also mediate bacterial virulence, such as Pectobacterium atrosepticum causing the occurrences of soft rot disease and further affects the degradation of cell walls, resulting in an increased disease incidence in plants (Hasegawa et al., 2005).
Temperature stress greatly affects microbial activities as they require an optimal range of temperature for sustaining their growth, reproduction, and ability to cause diseases, unless they quickly acclimatize to cope with temperature changes (Wang et al., 2021a). For example, several microbes have developed specialized adaptations to counter extreme environmental conditions while supporting their host plants by efficiently utilizing carbon allocation (Vera-Gargallo et al., 2023). They also contain specific enzymes and complex regulatory networks of secondary metabolites to overcome the adverse effects of temperature stress (Raddadi et al., 2015). Several thermotolerant microbes i.e., endophytic bacteria and arbuscular mycorrhizal fungi (AMF), modify their structures to survive under high temperature and protect their host plants from extreme environmental conditions. Rasmussen et al. (2020), studied the impact of temperature on plant-associated AMF colonization and plant performance and found that under continuous temperature increase, both fungal colonization and plant growth increase. Several experimental studies reported similar findings about the increased colonization of AMF in response to rising temperatures (Compant et al., 2010; Xie et al., 2024). Khan et al. (2020a) revealed the positive effect of plant growth-promoting endophytic bacteria on crop production under higher temperatures and suggested their use as biofertilizers to mitigate heat stress damage in soybean plants. Additionally, other studies reported the use of thermotolerant bacteria enhancing the content of chlorophyll in rice and canola (Glick et al., 1997; Chaganti et al., 2023).
Moreover, certain microbes may help plants to cope with multiple stresses i.e., Burkholderia phytofirmans PsJN strain has been reported to enhance heat tolerance in tomatoes, salinity and freezing resistance in Arabidopsis, cold resilience in grapevines, and drought tolerance in wheat (Miotto-Vilanova et al., 2016; Issa et al., 2018). Similar findings have also been reported for other crops, indicating that heat-resistant bacteria could enhance crop growth and development in wheat, rice, tomato, potato, chickpea, sorghum, and canola plants under heat stress (Table 1). Altogether, it is crucial to understand the effects of elevated temperature on plant-microbe interactions, and to identify target microbes and their association with crops under a specific environment for sustainable ecosystem with improved agricultural production.
3.2 Drought and the role of plant-microbe symbiosis
Drought is one of the major causes of agricultural losses worldwide, threatening food security. Climate change and global warming will further intensify the situation with severe drought episodes, posing a greater threat to agricultural sustainability (Fadiji et al., 2023). One of the noticeable impacts of climate change is higher variation in precipitation patterns, which directly influences the moisture content in the atmosphere and soil, resulting in flood or drought conditions (Tabari, 2020). Water scarcity leads to several physiological and morphological responses in plants as a consequence of drought (Kumar and Verma, 2018). Drought limits the normal growth and development, disturbs water requirements, and reduces water use efficiency in plants. During the seedling stage, drought stress in plants maintains root development while limiting shoot growth, leading to a higher root/shoot ratio (Kou et al., 2022). Additionally, extreme water-limiting conditions cause retarded leaf development due to the shrinkage of the plant cells which ultimately reduces turgor pressure, resulting a decline in plant fresh weight (Fahad et al., 2017). The root morphology also adapts certain modifications such as shrinking, to optimize the distribution of water and nutrients to several parts of the plant under drought conditions, thus preventing the loss of water that might affect leaves potential to utilize photosynthesis II (Ma et al., 2020). Prolonged water scarcity affects cell wall integrity, resulting in the generation of ROS, causing premature leaf senescence, promoting ethylene accumulation, affecting chlorophyll content, and suppressing photosynthetic mechanisms (Kumar and Verma, 2018). In addition, drought impairs the dissolution and translocation of salts, leading to their accumulation in the rhizosphere soil, which ultimately causes salinity stress (Gupta et al., 2022).
Several plant species faced drought conditions for a long time have modified several traits to tolerate drought conditions, e.g. better utilization of phytohormones, increased synthesis of osmolytes, and heat-shock proteins to sustain growth and yield (Figure 2). These plants also activate cellular mechanisms to maintain water and salt balance by transporting excessive salt from specific cells to other parts of plant, producing suitable solutes that promote drought tolerance and increase several compatible antioxidant enzymes for scavenging excessive ROS (Gupta et al., 2022). During a metabolomic analysis, it was revealed that Thymus serphyllum exhibits increased production of osmolytes, such as proline, sorbitol, mannitol, and other amino acids conferring drought stress tolerance (Moradi et al., 2017). Drought significantly influences soil characteristics and the microbial community in the rhizosphere (Manzanera, 2021). The root system and rhizosphere microorganisms influence each other through mutual interactions (Figure 1).
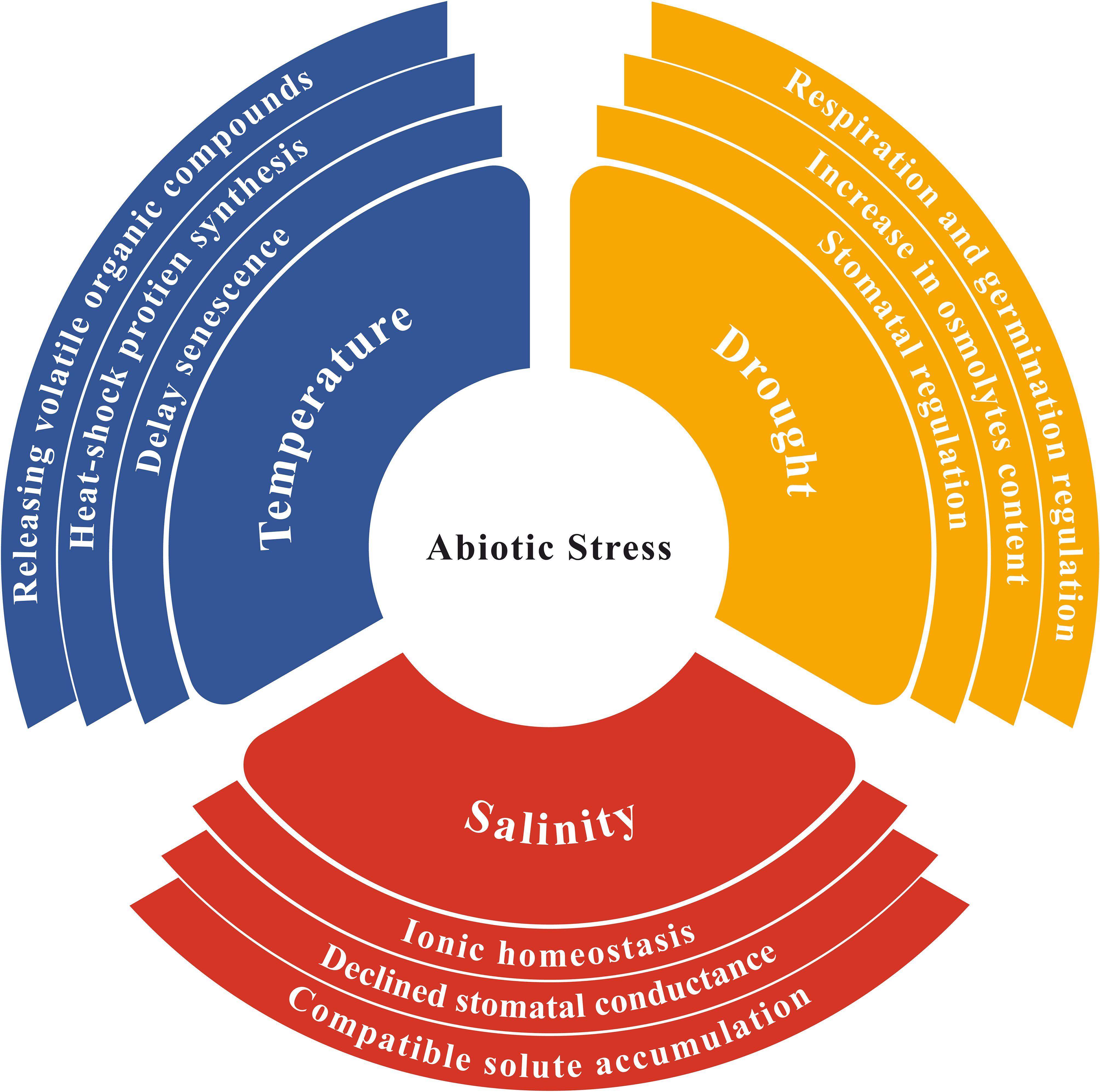
Figure 2. Plant adaptation and utilization of certain protective mechanisms against abiotic stresses in changing climates.
Rhizosphere microorganisms through symbiotic interaction greatly reduce the effects of drought by improving root architecture and facilitating water and nutrient absorption. This association also promotes the release of certain beneficial metabolites that may help plants in enhancing the root/shoot ratio, promoting yield with greater biomass production, improving water absorption capacity, and enhancing nutrient availability (Figure 1) (He et al., 2021; Rawal et al., 2022). The host plant in turn, initiates root exudation, which generates other factors that affect the performance of these microorganisms (Gupta et al., 2022). Consequently, manipulating rhizosphere microbiomes can boost the capacity of crops in stress reduction (Xu and Coleman-Derr, 2019; de Vries et al., 2020). For instance, certain plant species can tolerate higher drought by allocating more endosphere Streptomyces (Fitzpatrick et al., 2018). Similarly, sorghum seedlings with Streptomyces colonization resulted in increased root growth under drought, while no noticeable effects were observed in well-watered conditions (Xu et al., 2018). Furthermore, AMF significantly contributes to the water availability of crops by utilizing external hyphae and glomalin, thereby enhancing crop drought tolerance (Wang et al., 2023). Likewise, AMF has a prominent role in root growth and development in citrus, and maize under arid soil conditions (Wu et al., 2013; Zhao et al., 2015). Several similar studies reported the role of PGPMs in plant defense against several abiotic stresses (Table 1). These findings revealed that plant health is greatly dependent on the composition and activity of plant-associated microbiome, thereby harnessing rhizosphere microorganisms under drought stress may have significant effects on crop yield by optimizing water and nutrient allocation.
3.3 Interactive mode of plant–microbiota in mediating salt tolerance
Soil salinity is one of the major abiotic stress, exerting adverse effects on crop growth and yield (Julkowska and Testerink, 2015). It has been estimated that approximately 7% of the total global fields (1 billion hectares of soil) are affected by salinization (Bayabil et al., 2021). More seriously, the area occupied by salinity-affected soils is increasing by around 10% per year due to climate change, owing to less precipitation and increased surface evaporation, improper agricultural practices (increased use of fertilizers and saline water irrigation, and industrial pollution) (Ouhibi et al., 2014; McFarlane et al., 2016). Naturally, plants are adapted to absorb a reasonable quantity of soluble salts resulting from mineral weathering. However, inadequate precipitation hinders salt leaching, causing the deposition of soluble salts in the root zone (Shrivastava and Kumar, 2015). Additionally, the intrusion of saltwater through surface or groundwater connections may have escalated the problem of salinity (Bayabil et al., 2021). Furthermore, the higher salt concentration in the rhizosphere influences water and nutrient absorption, leading to osmotic stress. This condition causes oxidative damage by inducing an increased number of ROS (Isayenkov and Maathuis, 2019; Rahman et al., 2024). Additionally, soil salinity has adverse effects on the nodulation process, reducing crop production and hindering nitrogen fixation by decreasing nitrogenase activity (Kumar and Verma, 2018).
When facing saline conditions, plants may develop several defensive strategies to safeguard themselves e.g., developing salt-releasing glands or trichomes (Yuan et al., 2016), restoring ionic homeostasis, osmotic and ROS levels (Yang and Guo, 2018), adjusting stomatal conductance (Li et al., 2020) and regulating certain growth patterns like flowering time (Kazan and Lyons, 2016) (Figure 2). Soil microorganisms greatly contribute to plant tolerance against salt and drought stresses through certain plant-mediated mechanisms (Sangiorgio et al., 2020) (Table 1). Microorganisms also accelerate antioxidant responses to protect plants from oxidative injury. These adaptive responses are prevalent in bacteria as they enable them to survive numerous harsh conditions (Liu et al., 2019). Among these features, extracellular polymeric substance (EPS) production regulates several services including mass transfer restriction, preventing water loss, and regulating essential biomolecules such as enzymes, nucleic acids and exopolysaccharides (López-Ortega et al., 2021). EPS-producing microbes can enhance salt tolerance in plants by absorbing sodium ions in the soil, making them less available to plants (Bhagat et al., 2021). Additionally, bacterial exopolysaccharides play a prominent role in improving soil structure under salt stress by producing micro and macro-aggregates (Grover et al., 2011).
Halophiles or halo-tolerant represent a diverse group of microorganisms with a remarkable ability to grow in a wide range of NaCl concentrations (Abbas et al., 2019). These microbes can be categorized ranging from highly halophilic (2.5-5.2 M) to halotolerant (0.3-0.5 M) through various adaptive mechanisms (López-Ortega et al., 2021). Some of the major contributions of these microbes include ACC deaminase activity, EPS production, nitrogen fixation, IAA production, biofilm development, building-up osmolytes in the cytoplasm of plant cells, maintaining turgor pressure in salt-stressed cells, and limiting osmotic and oxidative stress by producing plant hormones and antioxidants (Table 1) (Rajput et al., 2018; Rima et al., 2018; Nawaz et al., 2020; Kumar et al., 2021). According to Li et al. (2021), a diverse microbial community was recruited by plant roots in the rhizosphere of the salt-treated plants, enhancing plant salt tolerance. Similarly, the rhizosphere of groundnuts exhibited a notable presence of Acidobacteria and Cyanobacteria, when exposed to salt stress (Xu et al., 2020). Even though these findings reveal the importance of microbial communities in the defense mechanisms of plants under salt stress, further in-depth mechanistic investigation using different plant species under saline condition are required to fully understand the effects of salt stress on the plant-associated microorganisms.
4 Signaling and cross-talk involved in plant-microbe interactions
Plants in their natural habitat engage in dynamic crosstalk with various environmental signals, facilitating the integration of microbial communities. In such communicatory networks, plants possess the ability to efficiently sense and respond to interactive stimuli. However, upon recognizing of microbial substances, they have the potential to establish symbiosis or develop immune responses. Overlooking this complicated web of communication networks, the significance of chemical signaling in perception and modulation is highly pivotal for stationary organisms like plants (Afridi et al., 2022). Plants utilize chemical signals as stimuli to establish beneficial relationships with surrounding microorganisms, either aboveground (trunk, shoots, leaves) or belowground (roots). This sophisticated communicatory network is steered by hormonal crosstalk and root exudation, regulating the intricate interactions between plants and their diverse biotic and abiotic environments.
4.1 Role of root exudates in shaping rhizosphere microbiota
During various growth stages, plants exudate a wide array of metabolites including primary and secondary metabolites, through their roots. Primary metabolites regulate processes including growth, development, and nutrient acquisition. On the other hand, secondary metabolites are generally not involved in plant survival; however, they serve essential functions in plant defense and protection against insects and pests. Additionally, secondary metabolites are important for plant adaption to changing environments and resilience to different biotic and abiotic stresses. A diverse range of defensive functions are performed by these secondary metabolites under both biotic and abiotic stresses including photoprotection, signaling, antimicrobial ability and structural stabilization (Ingle et al., 2016). Moreover, plant secondary metabolites contribute to pest and disease tolerance, act as signaling molecules for plant-microbe interactions, and influence microbial communities associated with their hosts (Fakhri et al., 2022).
The chemical composition of root exudates is greatly dependent on the genetics and age of the plant species (Zhang et al., 2021) as well as the soil physico-chemical properties (Sasse et al., 2020). Variation in the root exudates among different plant species is mainly dependent on the intricate regulation of root system architecture (RSA) (Galindo-Castañeda et al., 2024). The area below the root cap is recognized as the major exudation site, although various root zones have been reported active in different plant species (Haldar and Sengupta, 2015). Furthermore, different root parts have different exudations. For instance, the root meristem and elongation site are responsible for asparagine and threonine, the root hair zone exudes glutamic acid, valine, leucine, and phenylalanine, whereas the whole root secretes aspartic acid (Haldar and Sengupta, 2015). Plant root exudation is the central source of nutrients in the rhizosphere, attracting indigenous microbes that thrive in that specific environment (Pascale et al., 2020). Root exudates not only function as signaling molecules, such as chemoattractants and stimulants, but in certain situations, they act as repellents and inhibitors. These chemicals continuously evolve with the changes in their immediate environment, modulating the initial dialogue between plant roots and soil microorganisms (Wiesenbauer et al., 2024). Chemotaxis and colonization are necessary for the establishment of a microbiome in the rhizosphere. Root exudates are considered the central source of signaling molecules for microorganisms, promoting their chemotactic movement toward the rhizosphere and facilitating early colonization. Thus, root exudates play key role in plant-soil feedback by modifying microbial communities.
4.2 Hormonal crosstalk in plant-microbe symbiosis for enhanced stress tolerance
Plants growing under natural conditions constantly interact with both biotic and abiotic environments. To ensure their survival and to sustain the effects of these diverse and often hostile conditions, plants developed a sophisticated and adaptable environmental signaling network steered by phytohormones (Jain et al., 2021). This elaborate hormonal crosstalk fine-tunes plant responses to extremely dynamic and varied circumstances. Plant hormones are organic substances produced in small amounts that serve as important regulators of plant developmental processes in response to external stimuli by triggering different physiological mechanisms (Hirayama and Mochida, 2022). Plants naturally synthesize a multitude of hormones including auxins, GA, CK, and ABA to oversee their growth and metabolism (Iqbal et al., 2022). Studies indicate that phytohormone application during stress greatly enhances plant functioning and metabolic processes. Among phytohormones, auxin and ABA have pivotal roles in alleviating abiotic stresses (Hu et al., 2013). However, researchers have also deciphered the potential roles of other phytohormones in numerous studies (Singh and Singh, 2017; Sharma et al., 2019). The crosstalk of ABA, SA, jasmonates, and ethylene with the major growth-2promoting hormones such as auxins, GA, and CK, greatly contributes to plant defense against several abiotic stresses (Figure 3).
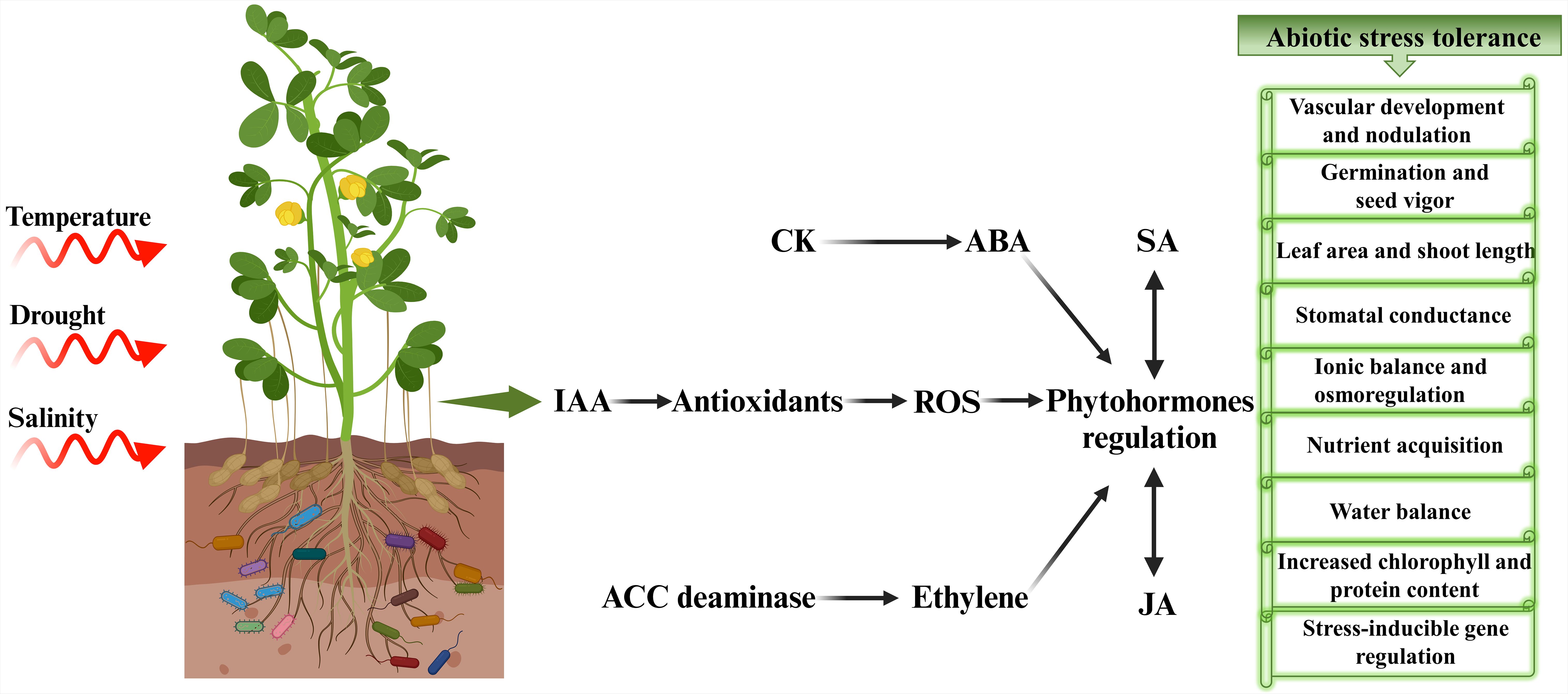
Figure 3. Hormonal crosstalk and signaling mediating abiotic stress tolerance in plants. IAA promotes antioxidant activity by neutralizing ROS. CK works with ABA to modulate stress responses. ABA is crucial for regulating stomatal conductance and promoting drought tolerance. SA interacts with other hormones like ABA and JA, influencing plant defense mechanisms. ACC deaminase modulates ethylene levels to mitigates stress effects. IAA, Indole acetic acid; ROS, reactive oxygen species; CK, cytokinins; ABA, abscisic acid; ACC, 1-aminocyclopropane-1-carboxylate; SA, salicylic acid; JA, jasmonic acid.
Microorganisms establish symbiotic relation with plants to protect them against abiotic and biotic stresses by triggering phytohormone signaling and activating defense mechanisms (Khan et al., 2020b). However, it is noteworthy that the influence of microbes on the regulation of phytohormones has significance not only in directly promoting plant growth but also in several other aspects of microbial effects on plants, such as enhancing nutrient acquisition and mediating stress resistance. Phytohormones play a significant role in mitigating abiotic stress by regulating vital functions in plants, including nutrient acquisition, water balance homeostasis, tolerance against pathogens, antioxidant activities, increased chlorophyll and protein content and stress-inducible gene regulation (Figure 3).
It is worth mentioning that microbes can modulate mineral acquisition through nitrogen fixation or phosphate solubilization, thereby indirectly influencing the concentration of phytohormones in plants, as the status of phytohormones partially contingent on the efficiency of nutrient acquisition (Kudoyarova et al., 2015). Moreover, the role of rhizosphere microorganisms in modulating plant hormonal status is likely involved in the majority of well-established mechanisms through which microbes promote plant growth. This could include activities like engineering the interplay of phytohormones or integrating microbial biocontrol solutions based on plant hormones (Shigenaga et al., 2017). Therefore, a detailed overview is required to better understand the multifaceted roles of phytohormones in regulating plant defenses and fitness (Vos et al., 2013; Guo et al., 2018). This will facilitate the optimized use of the phytohormone network for practical applications in plant cultivation and protection.
5 ACC deaminase mediated suppression of abiotic stress in plants
The enzyme 1-aminocyclopropane-1-carboxylate (ACC) deaminase is present in many PGPR, regulating plant growth by reducing the ethylene level produced in response to stress signals (Ali and Kim, 2018). Ethylene is a gaseous phytohormone that regulates plant growth at optimal concentrations; however, at higher concentrations, it affects various plant developmental processes, including root growth, nodulation, fruit ripening, flowering, and leaf senescence (Vanderstraeten et al., 2019). Prolonged exposure to various stresses can lead to increased accumulation of ethylene, which can significantly impact plant developmental processes (Dubois et al., 2018). IAA may elicit ACC synthesis, which severs as an intermediate precursor to ethylene biosynthesis and act as a key milestone in the regulation of ethylene production in plants.
When ACC is secreted by plant roots in the presence of high concentrations of ethylene, ACC deaminase-producing bacteria catalyze the breakdown of α-ketobutyrate and ammonia rather than ethylene, thereby reducing plant growth-inhibitory ethylene levels in developing or stressed plants (Ratnaningsih et al., 2023). Furthermore, IAA synthesis by these bacteria enhances root development and nutrient uptake, further supporting plant resilience against several abiotic stresses (Figure 4) (Etesami and Glick, 2024). Specifically, the role of IAA in promoting root and shoot growth significantly enhances plant adaptation to heavy metal stress (Shah et al., 2024). For instance, the availability of IAA has been shown to improve plant growth under heavy metal stress by increasing the phytoextraction of Pb, Zn, and Cd (Sytar et al., 2019). Similarly, the application of IAA can enhance plant growth in metal contaminated soil by mitigating the hazardous effects of heavy metals on plants (Sharif et al., 2022). Another study reported that the application of IAA-producing fungal endophyte Penicillium roqueforti greatly reduced the uptake of heavy metals by wheat plants (Ikram et al., 2018). Altogether, the synthesis of IAA by microbes plays significant role in enhancing plant growth and resilience, particularly in metal-contaminated soils.
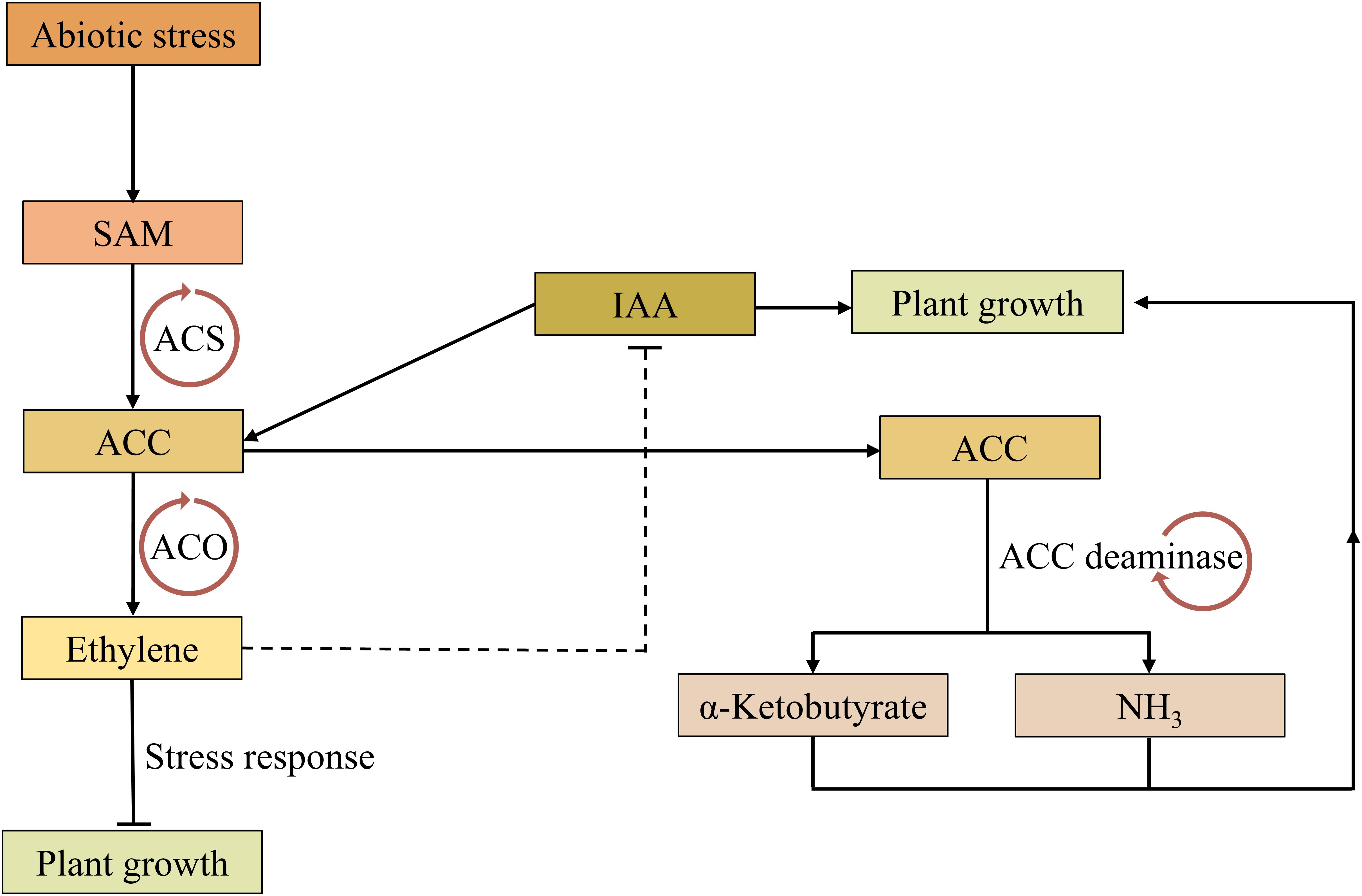
Figure 4. A schematic model proposing how ACC deaminase-producing bacteria promote plant growth by reducing ethylene concentration. The stress response in plants initiates with SAM conversion to ACC by the enzyme ACC synthase. ACC is subsequently converted to ethylene through ACC oxidase, which can hinder plant growth and restrict IAA biosynthesis. Meanwhile, ACC conversion in bacteria proceeding through ACC deaminase, resulting in the synthesis of α-ketubutyrate and ammonia, which in turn diminishes the synthesis of ethylene and promote plant growth. IAA biosynthesis is a complex and multi-enzymes/proteins process. Lines with an arrowhead represent a positive effect while those with flattened head represent inhibition. SAM, S-adenosyl-L-methionine; ACC, 1-aminocyclopropane-1-carboxylate; ACS, ACC synthase; ACO, ACC oxidase; IAA, indole-3-acetic acid.
Inoculating plants with ACC deaminase-producing bacteria provides protection against various stresses (Herpell et al., 2023; Roy Choudhury et al., 2023). Notably, Trichoderma strains containing ACC deaminase have been found to show phytopathogenic biocontrol and plant growth regulation activity in mangrove seedlings (Saravanakumar et al., 2018). Additionally, Increased ACC deaminase activity and IAA production was observed in the presence of Serratia K120 bacterium under heavy metal stress (Carlos et al., 2016). Similarly, it has been reported that Paraburkholderia dioscoreae Msb3, a novel bacterium designated strain, interacts with other symbionts, enhancing plant growth in tomato through ACC deaminase activity (Herpell et al., 2023). While the importance of ACC deaminase in plant growth promotion and abiotic stress resilience have been demonstrated, there is a lack of comprehensive studies on the plant root ACC exudation and microbial ACC deaminase activity under various abiotic stresses. In-depth investigation of the plant responses following the utilization of IAA- and ACC deaminase-producing bacteria would be rewarding, especially to reveal the best strategy for PGPR application in crop production, leading to more sustainable practices that reduce reliance on chemical fertilizers for better ecological safety.
6 Current challenges for optimizing plant-microbe interaction
Plants have been significantly affected due to the abrupt changes in climatic conditions, resulting in severe impacts on cellular homeostasis. These impacts eventually lead to stunted plant growth and development, highlighting the emerging need to shift from conventional breeding to more advanced and sustainable approaches. To address these challenges genetic engineering has emerged as a promising solution for developing microbial strains that are effective and possess extended lifespans to achieve crop yield under drought conditions. Recent developments in microbiology, molecular biology, and biotechnology have led to the discovery of novel genes linked to drought tolerance (Salvi et al., 2022). The integration of microbiotechnology concepts in agriculture should be leveraged to isolate microbial strains from the stress-affected soils, and further evaluation of these strains based on their stress tolerance may be useful in the process of bio-inoculation of crops cultivated in drylands (Kaushal and Wani, 2016).
Exploring diverse microbial communities poses a significant challenge in plant-microbe interaction research, as it is difficult to address important questions like the basic characteristics of a specific microbial community, the complex interplay among different community members, and how these members contribute to the survival of plants under such circumstances. It is now well-recognized that plants can regulate the recruitment of various root-associated microorganisms for required purposes (Bag et al., 2022). Moreover, the mechanisms of various signaling pathways in the rhizosphere that lead to the assembly and stability of intrinsic microbiome require investigation. Recent research breakthroughs in the area of plant-microbe interactions may have a significant impact on agricultural productivity. Research on these beneficial plant-microbe interactions in the rhizosphere will further elucidate how these microbes impact the nutritional composition of plant materials. However, it remains a challenging and demanding area of research to systematically exploit and utilize these beneficial microbial species living in the plant rhizosphere. Therefore, there is an urgent need to develop new and advanced scientific approaches to comprehend the complex interplay between plants and the microbiome under changing environmental conditions.
7 Recent innovations in plant-microbe interaction
The rhizosphere is an ever-changing environment, offering diverse and intriguing aspects to researchers. The advent of highly sophisticated molecular biology techniques has brought a new era for thoroughly investigating the complex plant-microbe crosstalk for green efficient production. The emergence and continuous updating of omics, gene-editing techniques, and high-throughput sequencing technology have opened up new ideas for studying the complex networks of plant-microbe interactions and plant resilience to different biotic and abiotic stresses (Kimotho and Maina, 2024). Genomics has proven to be an efficient tool in investigating and predicting plant-microbe interactions, as well as enhancing plant resilience to different stresses (Frantzeskakis et al., 2020). Multiple sequencing technologies, including prokaryotic 16S amplicon sequencing (Qin et al., 2024), fungal internal transcribed spacer (ITS) regions sequencing (Labouyrie et al., 2023), and metagenomics (Masuda et al., 2024) have been employed to analyze the extensive genetic variability present within the soil microbiome.
Next generation sequencing (NGS)-based transcriptomics is the most comprehensive and efficient approach to uncover the molecular background of plant-microbe interactions (Katara et al., 2024). It is mostly employed to evaluate plant performance under various stress conditions, revealing the physiological responses of plants to pathogens and elucidating the signaling mechanisms occurring in the rhizosphere (Roy et al., 2024). NGS has paved the way to investigate beneficial plant-microbe interactions and plant performance under abiotic stresses (Rehman et al., 2024). For instance, enhanced drought tolerance in wild soybean (Kim et al., 2024), the cold tolerance response in upland cotton (Wang et al., 2024), the impact of short-term flooding on the expression profile of orchard grass genes (Qiao et al., 2020), and altered gene expression level in Arabidopsis under cold stress (Zhang et al., 2024), have been revealed through NGS. Metagenome sequencing using oxford nanopore technologies (ONT) is currently the most effective strategy for pathogen detection (Yu et al., 2023). It is a robust and direct method of long-read sequencing without the need for an amplification step (Wang et al., 2021b). Due to its ability to directly detect pathogens except RNA viruses, it can be used without prior knowledge of pathogens (Javaran et al., 2023). The MinONTM technology has already been used for metagenome sequencing of bacteria, fungi, and viruses, affecting various crops (Jongman et al., 2020; Mechan Llontop et al., 2020). Overall, these modern molecular techniques have greatly improved agricultural productivity and sustainability with enhanced resilience to various ongoing climatic challenges.
8 Future perspectives
Plants, and specifically the rhizosphere are bustling with microorganisms. When plants encounter unfavorable conditions, they can recruit beneficial microbes to help mitigate these stresses by releasing a range of chemical signals, which is known as the ‘cry for help’ strategy (Bai et al., 2022). To understand the mechanistic background of ‘cry for help’ strategy of plants, it is important to investigate these molecular aspects of plant-microbe interactions in the rhizosphere (Zancarini et al., 2021). Identifying genes with specific functions is mandatory for understanding the signaling cascades that regulate plant growth and stress responses (Depuydt and Vandepoele, 2021). Understanding the interplay between plant functional genes and rhizosphere microbes will be essential for regulating plant growth and development processes, including root architecture, microbial abundance, phytohormones, secondary metabolites, nutrient acquisition, and plant immune responses (Liu et al., 2023). For instance, the MYB72 transcription factor in Arabidopsis, recognized for its prominent role in the induced systematic response (ISR) mediated by beneficial microbes, represents a promising area for further investigation (Vlot et al., 2021). Additionally, the roles of members of the plant multidrug and toxic compound extrusion (MATE) family in transporting phytohormones and secondary metabolites require further exploration (Wang et al., 2022). Moreover, the co-expression of the Arabidopsis gene AVP1, the rice gene OsSIZ1, and the cyanobacterium flavodoxin gene FId revealed their important contributions to plant growth and resilience to multiple environmental adversities through delicately fine-tuned their genetic and epigenetic regulation (Zhao et al., 2024).
Innovations in plant-microbe interactions at the molecular level offer a new direction for genetic breeding (Nerva et al., 2022). Exploring this relationship will help us develop crop varieties with enhanced adaptability to various stresses. Future research using cutting-edge technologies such as multi-omics, NGS, and imaging techniques as discussed in section 7, will enhance our understanding of the microbes that mutually interact with host genes is expected to provide new germplasm resources. Furthermore, the molecular and mechanistic background of microbial ACC deaminase activity in response to root-exuded ACC under various abiotic stresses is still at a preliminary stage. In-depth investigation of plant responses to IAA- and ACC deaminase-producing bacteria would be rewarding, especially in revealing the best strategies for PGPR application in crop production, leading to more sustainable practices that reduce reliance on chemical fertilizers for better ecological safety.
9 Conclusions
Climate change, with increased adversity, is a concurrent global concern that leads to implications for worldwide food security, negatively impacting both plant and microbial growth. Identifying innovative approaches to meet the growing demand for food in a changing climate is challenging, particularly as plant stresses and diseases greatly affect crop production and sustainability. Improving plant adaptations to these stressors while increasing agricultural production is one of the potential demands. In this context, the current review explores comprehensive insights into how plants and their microbiomes enhance resilience to stresses like drought, salinity, and temperature variations. We highlight key microbial strategies that mediate plant tolerance, including ROS scavenging, antioxidant regulation, and IAA synthesis. Additionally, we emphasize the role of ACC deaminase-producing bacteria in regulating ethylene levels, suggesting that future research should focus on the interaction between plant root ACC exudation and microbial ACC deaminase activity for effective ethylene mitigation during stress. Recent advancements in molecular techniques, such as next-generation sequencing and multi-omics approaches, are discussed to optimize these interactions, along with the chemotactic movement of microbes driven by hormonal crosstalk and root exudation.
Author contributions
AM: Writing – original draft, Project administration, Funding acquisition, Data curation, Conceptualization. XK: Writing – review & editing, Data curation, Conceptualization. SZ: Writing – review & editing, Project administration, Formal analysis. NB: Writing – review & editing, Visualization, Methodology. LL: Writing – review & editing, Software, Methodology. MHUK: Writing – review & editing, Validation, Software. SF: Writing – review & editing, Visualization, Methodology. ZZ: Writing – review & editing, Validation, Supervision, Resources, Funding acquisition, Conceptualization.
Funding
The author(s) declare that financial support was received for the research, authorship, and/or publication of this article. This work was partially supported by the Henan Center of Outstanding Overseas Scientists (Award # GZS 2024018), the International Science and Technology Cooperation Project of Henan Province (242102521050), the Henan Province High-Talent Foreign Experts Introduction Plan (HNGD2024030), and the Key R&D and Promotion Projects of Henan Province (222102110303 and 232102110221). We gratefully acknowledge Professor Xiaoping Pan from East Carolina University for her great input, comments, and proofreading of this paper.
Conflict of interest
The authors declare that the research was conducted in the absence of any commercial or financial relationships that could be construed as a potential conflict of interest.
Publisher’s note
All claims expressed in this article are solely those of the authors and do not necessarily represent those of their affiliated organizations, or those of the publisher, the editors and the reviewers. Any product that may be evaluated in this article, or claim that may be made by its manufacturer, is not guaranteed or endorsed by the publisher.
Abbreviations
IAA, Indole-3-acetic acid; GA, Gibberellin; CK, Cytokinin; ROS, Reactive oxygen species; ET, Ethylene; SA, Salicylic acid; SOD, Superoxide dismutase; CAT, Catalase; APX, Ascorbate peroxidase; ACC, 1-aminocyclopropane-1-carboxylate; EPS, Extracellular polymeric substance; AMF, Arbuscular mycorrhizal fungi; ABA, Abscisic acid; WUE, Water use efficiency; RSA, Root system architecture; ITS, Internal transcribed spacer regions; ONT, Oxford nanopore technologies; NGS, Next generation sequencing.
References
Abbas, R., Rasul, S., Aslam, K., Baber, M., Shahid, M., Mubeen, F., et al. (2019). Halotolerant PGPR: A hope for cultivation of saline soils. J. King Saud University-Science 31, 1195–1201. doi: 10.1016/j.jksus.2019.02.019
Abdelaziz, M. E., Abdelsattar, M., Abdeldaym, E. A., Atia, M. A., Mahmoud, A. W. M., Saad, M. M., et al. (2019). Piriformospora indica alters Na+/K+ homeostasis, antioxidant enzymes and LeNHX1 expression of greenhouse tomato grown under salt stress. Scientia Hortic 256, 108532. doi: 10.1016/j.scienta.2019.05.059
Afridi, M. S., Javed, M. A., Ali, S., De Medeiros, F. H. V., Ali, B., Salam, A., et al. (2022). New opportunities in plant microbiome engineering for increasing agricultural sustainability under stressful conditions. Front. Plant Sci 13, 899464. doi: 10.3389/fpls.2022.899464
Ali, B., Hafeez, A., Afridi, M. S., Javed, M. A., Suleman, F., Sumaira Suleman, F., et al. (2023). Bacterial-Mediated Salinity Stress Tolerance in Maize (Zea mays L.): A Fortunate Way toward Sustainable Agriculture. ACS omega. 8 (23), 20471–20487. doi: 10.1021/acsomega.3c00723
Ali, S., Kim, W.-C. (2018). Plant growth promotion under water: decrease of waterlogging-induced ACC and ethylene levels by ACC deaminase-producing bacteria. Front. Microbiol 9, 1096. doi: 10.3389/fmicb.2018.01096
Anzuay, M. S., Prenollio, A., Ludueña, L. M., Morla, F. D., Cerliani, C., Lucero, C., et al. (2023). Enterobacter sp. J49: A native plant growth-promoting bacteria as alternative to the application of chemical fertilizers on peanut and maize crops. Curr. Microbiol 80, 85. doi: 10.1007/s00284-023-03181-8
Arafa, S. A., Attia, K. A., Niedbała, G., Piekutowska, M., Alamery, S., Abdelaal, K., et al. (2021). Seed priming boost adaptation in pea plants under drought stress. Plants 10, 2201. doi: 10.3390/plants10102201
Bag, S., Mondal, A., Majumder, A., Mondal, S. K., Banik, A. (2022). Flavonoid mediated selective cross-talk between plants and beneficial soil microbiome. Phytochem. Rev 21, 1739–1760. doi: 10.1007/s11101-022-09806-3
Bai, B., Liu, W., Qiu, X., Zhang, J., Zhang, J., Bai, Y. (2022). The root microbiome: Community assembly and its contributions to plant fitness. J. Integr. Plant Biol 64, 230–243. doi: 10.1111/jipb.13226
Bayabil, H. K., Li, Y., Tong, Z., Gao, B. (2021). Potential management practices of saltwater intrusion impacts on soil health and water quality: a review. J. Water Climate Change 12, 1327–1343. doi: 10.2166/wcc.2020.013
Bender, S. F., Wagg, C., van der Heijden, M. G. (2016). An underground revolution: biodiversity and soil ecological engineering for agricultural sustainability. Trends Ecol. Evol 31, 440–452. doi: 10.1016/j.tree.2016.02.016
Bhagat, N., Raghav, M., Dubey, S., Bedi, N. (2021). Bacterial exopolysaccharides: Insight into their role in plant abiotic stress tolerance. J. Microbiol. Biotechnol 31, 1045. doi: 10.4014/jmb.2105.05009
Bilal, S., Shahzad, R., Imran, M., Jan, R., Kim, K. M., Lee, I.-J. (2020). Synergistic association of endophytic fungi enhances Glycine max L. resilience to combined abiotic stresses: Heavy metals, high temperature and drought stress. Ind. Crops Products 143, 111931. doi: 10.1016/j.indcrop.2019.111931
Bowerman, A. F., Byrt, C. S., Roy, S. J., Whitney, S. M., Mortimer, J. C., Ankeny, R. A., et al. (2023). Potential abiotic stress targets for modern genetic manipulation. Plant Cell 35, 139–161. doi: 10.1093/plcell/koac327
Capua, G. D., Rahmstorf, S. (2023). Extreme weather in a changing climate. Environ. Res. Lett 18, 102001. doi: 10.1088/1748-9326/acfb23
Carlos, M.-H. J., Stefani, P.-V. Y., Janette, A.-M., Melani, M.-S. S., Gabriela, P.-O. (2016). Assessing the effects of heavy metals in ACC deaminase and IAA production on plant growth-promoting bacteria. Microbiological Res 188, 53–61. doi: 10.1016/j.micres.2016.05.001
Chaganti, C., Phule, A. S., Chandran, L. P., Sonth, B., Kavuru, V. P. B., Govindannagari, R., et al. (2023). Silicate solubilizing and plant growth promoting bacteria interact with biogenic silica to impart heat stress tolerance in rice by modulating physiology and gene expression. Front. Microbiol 14. doi: 10.3389/fmicb.2023.1168415
Chieb, M., Gachomo, E. W. (2023). The role of plant growth promoting rhizobacteria in plant drought stress responses. BMC Plant Biol 23, 407. doi: 10.1186/s12870-023-04403-8
Choi, J., Roy Choudhury, A., Walitang, D. I., Lee, Y., Sa, T. (2022). ACC deaminase-producing Brevibacterium linens RS16 enhances heat-stress tolerance of rice (Oryza sativa L.). Physiologia Plantarum 174, e13584. doi: 10.1111/ppl.13584
Classen, A. T., Sundqvist, M. K., Henning, J. A., Newman, G. S., Moore, J. A., Cregger, M. A., et al. (2015). Direct and indirect effects of climate change on soil microbial and soil microbial-plant interactions: What lies ahead? Ecosphere 6, 1–21. doi: 10.1890/ES15-00217.1
Compant, S., van der Heijden, M. G., Sessitsch, A. (2010). Climate change effects on beneficial plant–microorganism interactions. FEMS Microbiol. Ecol 73, 197–214. doi: 10.1111/j.1574-6941.2010.00900.x
Depuydt, T., Vandepoele, K. (2021). Multi-omics network-based functional annotation of unknown Arabidopsis genes. Plant J 108, 1193–1212. doi: 10.1111/tpj.v108.4
de Vries, F. T., Griffiths, R. I., Knight, C. G., Nicolitch, O., Williams, A. (2020). Harnessing rhizosphere microbiomes for drought-resilient crop production. Science 368, 270–274. doi: 10.1126/science.aaz5192
De Wolf, E. D., Isard, S. A. (2007). Disease cycle approach to plant disease prediction. Annu. Rev. Phytopathol 45, 203–220. doi: 10.1146/annurev.phyto.44.070505.143329
Dollete, D., Lumactud, R. A., Carlyle, C. N., Szczyglowski, K., Hill, B., Thilakarathna, M. S. (2024). Effect of drought stress on symbiotic nitrogen fixation, soil nitrogen availability and soil microbial diversity in forage legumes. Plant Soil 495, 445–467. doi: 10.1007/s11104-023-06348-1
Dubois, M., Van den Broeck, L., Inzé, D. (2018). The pivotal role of ethylene in plant growth. Trends Plant Sci 23, 311–323. doi: 10.1016/j.tplants.2018.01.003
Etesami, H., Glick, B. R. (2024). Bacterial indole-3-acetic acid: A key regulator for plant growth, plant-microbe interactions, and agricultural adaptive resilience. Microbiological Res 281, 127602. doi: 10.1016/j.micres.2024.127602
Fadiji, A. E., Yadav, A. N., Santoyo, G., Babalola, O. O. (2023). Understanding the plant-microbe interactions in environments exposed to abiotic stresses: An overview. Microbiological Res 271, 127368. doi: 10.1016/j.micres.2023.127368
Fahad, S., Bajwa, A. A., Nazir, U., Anjum, S. A., Farooq, A., Zohaib, A., et al. (2017). Crop production under drought and heat stress: plant responses and management options. Front. Plant Sci 8, 1147. doi: 10.3389/fpls.2017.01147
Fakhri, S., Moradi, S. Z., Farzaei, M. H., Bishayee, A. 2022 “Modulation of dysregulated cancer metabolism by plant secondary metabolites: A mechanistic review,” in Seminars in cancer biology (England: Elsevier Ltd). 80, 276–305. doi: 10.1016/j.semcancer.2020.02.007
Fang, X., Mo, J., Zhou, H., Shen, X., Xie, Y., Xu, J., et al. (2023). Comparative transcriptome analysis of gene responses of salt-tolerant and salt-sensitive rice cultivars to salt stress. Sci. Rep 13, 19065. doi: 10.1038/s41598-023-46389-1
Farooq, T. H., Rafay, M., Basit, H., Shakoor, A., Shabbir, R., Riaz, M. U., et al. (2022). Morpho-physiological growth performance and phytoremediation capabilities of selected xerophyte grass species toward Cr and Pb stress. Front. Plant Sci 13, 997120. doi: 10.3389/fpls.2022.997120
Fitzpatrick, C. R., Copeland, J., Wang, P. W., Guttman, D. S., Kotanen, P. M., Johnson, M. T. (2018). Assembly and ecological function of the root microbiome across angiosperm plant species. Proc. Natl. Acad. Sci 115, E1157–E1165. doi: 10.1073/pnas.1717617115
Foucher, A., Evrard, O., Rabiet, L., Cerdan, O., Landemaine, V., Bizeul, R., et al. (2024). Uncontrolled deforestation and population growth threaten a tropical island’s water and land resources in only 10 years. Sci. Adv 10, eadn5941. doi: 10.1126/sciadv.adn5941
Frantzeskakis, L., Di Pietro, A., Rep, M., Schirawski, J., Wu, C. H., Panstruga, R. (2020). Rapid evolution in plant–microbe interactions–a molecular genomics perspective. New Phytol 225, 1134–1142. doi: 10.1111/nph.v225.3
Frey, S. D., Lee, J., Melillo, J. M., Six, J. (2013). The temperature response of soil microbial efficiency and its feedback to climate. Nat. Climate Change 3, 395–398. doi: 10.1038/nclimate1796
Furlan, A., Llanes, A., Luna, V., Castro, S. (2012). Physiological and biochemical responses to drought stress and subsequent rehydration in the symbiotic association peanut-Bradyrhizobium sp. ISRN Agron 2012, 1–8. doi: 10.5402/2012/318083
Galindo-Castañeda, T., Hartmann, M., Lynch, J. P. (2024). Location: root architecture structures rhizosphere microbial associations. J. Exp. Bot 75, 594–604. doi: 10.1093/jxb/erad421
Garrett, K. A., Nita, M., De Wolf, E., Esker, P. D., Gomez-Montano, L., Sparks, A. H. (2021). “Plant pathogens as indicators of climate change,” in Climate change (Netherlands: Elsevier), 499–513. doi: 10.1016/B978-0-12-821575-3.00024-4
Georgieva, M., Vassileva, V. (2023). Stress management in plants: examining provisional and unique dose-dependent responses. Int. J. Mol. Sci 24, 5105. doi: 10.3390/ijms24065105
Glick, B. R., Liu, C., Ghosh, S., Dumbroff, E. B. (1997). Early development of canola seedlings in the presence of the plant growth-promoting rhizobacterium Pseudomonas putida GR12-2. Soil Biol. Biochem 29, 1233–1239. doi: 10.1016/S0038-0717(97)00026-6
Grover, M., Ali, S. Z., Sandhya, V., Rasul, A., Venkateswarlu, B. (2011). Role of microorganisms in adaptation of agriculture crops to abiotic stresses. World J. Microbiol. Biotechnol 27, 1231–1240. doi: 10.1007/s11274-010-0572-7
Guo, Q., Major, I. T., Howe, G. A. (2018). Resolution of growth–defense conflict: mechanistic insights from jasmonate signaling. Curr. Opin. Plant Biol 44, 72–81. doi: 10.1016/j.pbi.2018.02.009
Gupta, A., Bano, A., Rai, S., Mishra, R., Singh, M., Sharma, S., et al. (2022). Mechanistic insights of plant-microbe interaction towards drought and salinity stress in plants for enhancing the agriculture productivity. Plant Stress 4, 100073. doi: 10.1016/j.stress.2022.100073
Gupta, A., Rai, S., Bano, A., Khanam, A., Sharma, S., Pathak, N. (2021). Comparative evaluation of different salt-tolerant plant growth-promoting bacterial isolates in mitigating the induced adverse effect of salinity in pisum sativum. Biointerface Res. Appl. Chem 11, 13141–13154. doi: 10.33263/BRIAC
Haldar, S., Sengupta, S. (2015). Plant-microbe cross-talk in the rhizosphere: insight and biotechnological potential. Open Microbiol. J 9, 1. doi: 10.2174/1874285801509010001
Hasegawa, H., Chatterjee, A., Cui, Y., Chatterjee, A. (2005). Elevated temperature enhances virulence of Erwinia carotovora subsp. carotovora strain EC153 to plants and stimulates production of the quorum sensing signal, N-acyl homoserine lactone, and extracellular proteins. Appl. Environ. Microbiol 71, 4655–4663. doi: 10.1128/AEM.71.8.4655-4663.2005
He, A., Niu, S., Yang, D., Ren, W., Zhao, L., Sun, Y., et al. (2021). Two PGPR strains from the rhizosphere of Haloxylon ammodendron promoted growth and enhanced drought tolerance of ryegrass. Plant Physiol. Biochem 161, 74–85. doi: 10.1016/j.plaphy.2021.02.003
Herpell, J. B., Alickovic, A., Diallo, B., Schindler, F., Weckwerth, W. (2023). Phyllosphere symbiont promotes plant growth through ACC deaminase production. ISME J 17, 1267–1277. doi: 10.1038/s41396-023-01428-7
Hirayama, T., Mochida, K. (2022). Plant hormonomics: a key tool for deep physiological phenotyping to improve crop productivity. Plant Cell Physiol 63, 1826–1839. doi: 10.1093/pcp/pcac067
Hu, J., Wei, Z., Kowalchuk, G. A., Xu, Y., Shen, Q., Jousset, A. (2020). Rhizosphere microbiome functional diversity and pathogen invasion resistance build up during plant development. Environ. Microbiol 22, 5005–5018. doi: 10.1111/1462-2920.15097
Hu, Y. F., Zhou, G., Na, X. F., Yang, L., Nan, W. B., Liu, X., et al. (2013). Cadmium interferes with maintenance of auxin homeostasis in Arabidopsis seedlings. J. Plant Physiol 170, 965–975. doi: 10.1016/j.jplph.2013.02.008
Ikram, M., Ali, N., Jan, G., Jan, F. G., Rahman, I. U., Iqbal, A., et al. (2018). IAA producing fungal endophyte Penicillium roqueforti Thom., enhances stress tolerance and nutrients uptake in wheat plants grown on heavy metal contaminated soils. PloS One 13, e0208150. doi: 10.1371/journal.pone.0208150
Ingle, K., Padole, D., VC, K. (2016). Secondary metabolites for plant growth promotion and plant protection. Adv. Life Sci 5, 10888–10891.
Iqbal, S., Wang, X., Mubeen, I., Kamran, M., Kanwal, I., Díaz, G. A., et al. (2022). Phytohormones trigger drought tolerance in crop plants: outlook and future perspectives. Front. Plant Sci 12, 799318. doi: 10.3389/fpls.2021.799318
Isayenkov, S. V., Maathuis, F. J. (2019). Plant salinity stress: many unanswered questions remain. Front. Plant Sci 10, 80. doi: 10.3389/fpls.2019.00080
Issa, A., Esmaeel, Q., Sanchez, L., Courteaux, B., Guise, J.-F., Gibon, Y., et al. (20181397). Impacts of Paraburkholderia phytofirmans strain PsJN on tomato (Lycopersicon esculentum L.) under high temperature. Front. Plant Sci 9. doi: 10.3389/fpls.2018.01397
Jain, A., Singh, H. B., Das, S. (2021). Deciphering plant-microbe crosstalk through proteomics studies. Microbiological Res 242, 126590. doi: 10.1016/j.micres.2020.126590
Javaran, V. J., Poursalavati, A., Lemoyne, P., Ste-Croix, D. T., Moffett, P., Fall, M. L. (2023). NanoViromics: long-read sequencing of dsRNA for plant virus and viroid rapid detection. Front. Microbiol 14, 1192781. doi: 10.3389/fmicb.2023.1192781
Jin, X., Jia, H., Ran, L., Wu, F., Liu, J., Schlaeppi, K., et al. (2024). Fusaric acid mediates the assembly of disease-suppressive rhizosphere microbiota via induced shifts in plant root exudates. Nat. Commun 15, 5125. doi: 10.1038/s41467-024-49218-9
Jongman, M., Carmichael, P. C., Bill, M. (2020). Technological advances in phytopathogen detection and metagenome profiling techniques. Curr. Microbiol 77, 675–681. doi: 10.1007/s00284-020-01881-z
Julkowska, M. M., Testerink, C. (2015). Tuning plant signaling and growth to survive salt. Trends Plant Sci 20, 586–594. doi: 10.1016/j.tplants.2015.06.008
Kashyap, A. S., Pandey, V. K., Manzar, N., Kannojia, P., Singh, U. B., Sharma, P. (2017). Role of plant growth-promoting rhizobacteria for improving crop productivity in sustainable agriculture. Plant-Microbe Interact. Agro-Ecological Perspectives: Volume 2: Microbial Interact. Agro-Ecological Impacts 2, 673–693. doi: 10.1007/978-981-10-6593-4_28
Katara, A., Chand, S., Chaudhary, H., Chaudhry, V., Chandra, H., Dubey, R. C. (2024). Evolution and applications of Next Generation Sequencing and its intricate relations with chromatographic and spectrometric techniques in modern day sciences. J. Chromatogr. Open 5, 100121. doi: 10.1016/j.jcoa.2024.100121
Kaushal, M., Wani, S. P. (2016). Plant-growth-promoting rhizobacteria: drought stress alleviators to ameliorate crop production in drylands. Ann. Microbiol 66, 35–42. doi: 10.1007/s13213-015-1112-3
Kazan, K., Lyons, R. (2016). The link between flowering time and stress tolerance. J. Exp. Bot 67, 47–60. doi: 10.1093/jxb/erv441
Khan, M. A., Asaf, S., Khan, A. L., Jan, R., Kang, S.-M., Kim, K.-M., et al. (2020a). Thermotolerance effect of plant growth-promoting Bacillus cereus SA1 on soybean during heat stress. BMC Microbiol 20, 1–14. doi: 10.1186/s12866-020-01822-7
Khan, N., Bano, A., Ali, S., Babar, M. A. (2020b). Crosstalk amongst phytohormones from planta and PGPR under biotic and abiotic stresses. Plant Growth Regul 90, 189–203. doi: 10.1007/s10725-020-00571-x
Kibret, M., Devkota, K., Bakrim, W. B., Ezzariai, A., Terefe, H., Karouach, F., et al. (2024). Plant growth promoting rhizobacteria mitigate drought and salinity stresses, and improve the physiological and agronomic performances in crops: A systematic review. CABI Rev 19, 1. doi: 10.1079/cabireviews.2024.0025
Kim, T., Hwang, H., Bang, G., Ha, J., Park, Y.-J., Kim, J. Y. (2024). Understanding the molecular mechanisms of drought tolerance in wild soybean (Glycine soja) through multi-omics-based alternative splicing predictions. Environ. Exp. Bot 225, 105872. doi: 10.1016/j.envexpbot.2024.105872
Kim, Y.-N., Khan, M. A., Kang, S.-M., Hamayun, M., Lee, I.-J. (2020). Enhancement of drought-stress tolerance of Brassica oleracea var. italica L. by newly isolated Variovorax sp. YNA59. J. Microbiol. Biotechnol 30, 1500. doi: 10.4014/jmb.2006.06010
Kimotho, R. N., Maina, S. (2024). Unraveling plant–microbe interactions: can integrated omics approaches offer concrete answers? J. Exp. Bot 75, 1289–1313. doi: 10.1093/jxb/erad448
Kou, X., Han, W., Kang, J. (2022). Responses of root system architecture to water stress at multiple levels: A meta-analysis of trials under controlled conditions. Front. Plant Sci 13, 1085409. doi: 10.3389/fpls.2022.1085409
Kudoyarova, G. R., Dodd, I. C., Veselov, D. S., Rothwell, S. A., Yu. Veselov, S. (2015). Common and specific responses to availability of mineral nutrients and water. J. Exp. Bot 66, 2133–2144. doi: 10.1093/jxb/erv017
Kumar, S., Sindhu, S. S., Kumar, R. (2022). Biofertilizers: An ecofriendly technology for nutrient recycling and environmental sustainability. Curr. Res. Microbial Sci 3, 100094. doi: 10.1016/j.crmicr.2021.100094
Kumar, A., Singh, S., Mukherjee, A., Rastogi, R. P., Verma, J. P. (2021). Salt-tolerant plant growth-promoting Bacillus pumilus strain JPVS11 to enhance plant growth attributes of rice and improve soil health under salinity stress. Microbiological Res 242, 126616. doi: 10.1016/j.micres.2020.126616
Kumar, A., Verma, J. P. (2018). Does plant—microbe interaction confer stress tolerance in plants: a review. Microbiological Res 207, 41–52. doi: 10.1016/j.micres.2017.11.004
Kuypers, M. M., Marchant, H. K., Kartal, B. (2018). The microbial nitrogen-cycling network. Nat. Rev. Microbiol 16, 263–276. doi: 10.1038/nrmicro.2018.9
Labouyrie, M., Ballabio, C., Romero, F., Panagos, P., Jones, A., Schmid, M. W., et al. (2023). Patterns in soil microbial diversity across Europe. Nat. Commun 14, 3311. doi: 10.1038/s41467-023-37937-4
Lata, R., Chowdhury, S., Gond, S. K., White, J. J. F. (2018). Induction of abiotic stress tolerance in plants by endophytic microbes. Lett. Appl. Microbiol 66, 268–276. doi: 10.1111/lam.2018.66.issue-4
Lee, D. G., Lee, J. M., Choi, C. G., Lee, H., Moon, J. C., Chung, N. (2021). Effect of plant growth-promoting rhizobacterial treatment on growth and physiological characteristics of Triticum aestivum L. under salt stress. Appl. Biol. Chem 64, 1–10. doi: 10.1186/s13765-021-00663-w
Lemanceau, P., Blouin, M., Muller, D., Moënne-Loccoz, Y. (2017). Let the core microbiota be functional. Trends Plant Sci 22, 583–595. doi: 10.1016/j.tplants.2017.04.008
Li, H., La, S., Zhang, X., Gao, L., Tian, Y. (2021). Salt-induced recruitment of specific root-associated bacterial consortium capable of enhancing plant adaptability to salt stress. ISME J 15, 2865–2882. doi: 10.1038/s41396-021-00974-2
Li, S., Li, X., Wei, Z., Liu, F. (2020). ABA-mediated modulation of elevated CO2 on stomatal response to drought. Curr. Opin. Plant Biol 56, 174–180. doi: 10.1016/j.pbi.2019.12.002
Liu, C., Baffoe, D. K., Zhan, Y., Zhang, M., Li, Y., Zhang, G. (2019). Halophile, an essential platform for bioproduction. J. microbiological Methods 166, 105704. doi: 10.1016/j.mimet.2019.105704
Liu, Q., Cheng, L., Nian, H., Jin, J., Lian, T. (2023). Linking plant functional genes to rhizosphere microbes: a review. Plant Biotechnol. J 21, 902–917. doi: 10.1111/pbi.13950
Lopes, M., Dias-Filho, M. B., Gurgel, E. S. C. (2021). Successful plant growth-promoting microbes: inoculation methods and abiotic factors. Front. Sustain. Food Syst 5, 606454. doi: 10.3389/fsufs.2021.606454
López-Ortega, M. A., Chavarría-Hernández, N., del Rocío López-Cuellar, M., Rodríguez-Hernández, A. I. (2021). A review of extracellular polysaccharides from extreme niches: an emerging natural source for the biotechnology. From the adverse to diverse! Int. J. Biol. macromolecules 177, 559–577. doi: 10.1016/j.ijbiomac.2021.02.101
Ma, Y., Dias, M. C., Freitas, H. (2020). Drought and salinity stress responses and microbe-induced tolerance in plants. Front. Plant Sci 11, 591911. doi: 10.3389/fpls.2020.591911
Manzanera, M. (2021). Dealing with water stress and microbial preservation. Environ. Microbiol 23, 3351–3359. doi: 10.1111/1462-2920.15096
Masuda, Y., Mise, K., Xu, Z., Zhang, Z., Shiratori, Y., Senoo, K., et al. (2024). Global soil metagenomics reveals distribution and predominance of Deltaproteobacteria in nitrogen-fixing microbiome. Microbiome 12, 95. doi: 10.1186/s40168-024-01812-1
Matthews, J. L. (2024). Holobiont interactions. Front. Media SA. 12, 1382169. doi: 10.3389/fevo.2024.1382169
McFarlane, D., George, R., Barrett-Lennard, E., Gilfedder, M. (2016). Salinity in dryland agricultural systems: challenges and opportunities. Innov. dryland Agric, 521–547. doi: 10.1007/978-3-319-47928-6_19
Mechan Llontop, M. E., Sharma, P., Aguilera Flores, M., Yang, S., Pollok, J., Tian, L., et al. (2020). Strain-level identification of bacterial tomato pathogens directly from metagenomic sequences. Phytopathology 110, 768–779. doi: 10.1094/PHYTO-09-19-0351-R
Miotto-Vilanova, L., Jacquard, C., Courteaux, B., Wortham, L., Michel, J., Clément, C., et al. (20161236). Burkholderia phytofirmans PsJN confers grapevine resistance against Botrytis cinerea via a direct antimicrobial effect combined with a better resource mobilization. Front. Plant Sci 7. doi: 10.3389/fpls.2016.01236
Moradi, P., Ford-Lloyd, B., Pritchard, J. (2017). Metabolomic approach reveals the biochemical mechanisms underlying drought stress tolerance in thyme. Analytical Biochem 527, 49–62. doi: 10.1016/j.ab.2017.02.006
Naamala, J., Smith, D. L. (2020). Relevance of plant growth promoting microorganisms and their derived compounds, in the face of climate change. Agronomy 10, 1179. doi: 10.3390/agronomy10081179
Naveed, M., Hussain, M. B., Zahir, Z. A., Mitter, B., Sessitsch, A. (2014). Drought stress amelioration in wheat through inoculation with Burkholderia phytofirmans strain PsJN. Plant Growth Regul 73, 121–131. doi: 10.1007/s10725-013-9874-8
Nawaz, T., Gu, L., Fahad, S., Saud, S., Bleakley, B., Zhou, R. (2024a). Exploring sustainable agriculture with nitrogen-fixing cyanobacteria and nanotechnology. Molecules 29, 2534. doi: 10.3390/molecules29112534
Nawaz, T., Saud, S., Gu, L., Khan, I., Fahad, S., Zhou, R. (2024b). Cyanobacteria: harnessing the power of microorganisms for plant growth promotion, stress alleviation, and phytoremediation in the Era of sustainable agriculture. Plant Stress 11, 100399. doi: 10.1016/j.stress.2024.100399
Nawaz, A., Shahbaz, M., Asadullah, Imran, A., Marghoob, M. U., Imtiaz, M., et al. (2020). Potential of salt tolerant PGPR in growth and yield augmentation of wheat (Triticum aestivum L.) under saline conditions. Front. Microbiol 11, 2019. doi: 10.3389/fmicb.2020.02019
Nerva, L., Sandrini, M., Moffa, L., Velasco, R., Balestrini, R., Chitarra, W. (2022). Breeding toward improved ecological plant–microbiome interactions. Trends Plant Sci 27, 1134–1143. doi: 10.1016/j.tplants.2022.06.004
Ojuederie, O. B., Olanrewaju, O. S., Babalola, O. O. (2019). Plant growth promoting rhizobacterial mitigation of drought stress in crop plants: Implications for sustainable agriculture. Agronomy 9, 712. doi: 10.3390/agronomy9110712
Ouhibi, C., Attia, H., Rebah, F., Msilini, N., Chebbi, M., Aarrouf, J., et al. (2014). Salt stress mitigation by seed priming with UV-C in lettuce plants: Growth, antioxidant activity and phenolic compounds. Plant Physiol. Biochem 83, 126–133. doi: 10.1016/j.plaphy.2014.07.019
Pascale, A., Proietti, S., Pantelides, I. S., Stringlis, I. A. (2020). Modulation of the root microbiome by plant molecules: the basis for targeted disease suppression and plant growth promotion. Front. Plant Sci 10, 1741. doi: 10.3389/fpls.2019.01741
Qiao, D., Zhang, Y., Xiong, X., Li, M., Cai, K., Luo, H., et al. (2020). Transcriptome analysis on responses of orchardgrass (Dactylis glomerata L.) leaves to a short term flooding. Hereditas 157, 1–16. doi: 10.1186/s41065-020-00134-0
Qin, S., Zhang, D., Wei, B., Yang, Y. (2024). Dual roles of microbes in mediating soil carbon dynamics in response to warming. Nat. Commun 15, 6439. doi: 10.1038/s41467-024-50800-4
Raddadi, N., Cherif, A., Daffonchio, D., Neifar, M., Fava, F. (2015). Biotechnological applications of extremophiles, extremozymes and extremolytes. Appl. Microbiol. Biotechnol 99, 7907–7913. doi: 10.1007/s00253-015-6874-9
Rahimi-Moghaddam, S., Deihimfard, R., Nazari, M. R., Mohammadi-Ahmadmahmoudi, E., Chenu, K. (2023). Understanding wheat growth and the seasonal climatic characteristics of major drought patterns occurring in cold dryland environments from Iran. Eur. J. Agron 145, 126772. doi: 10.1016/j.eja.2023.126772
Rahman, M. M., Ghosh, P. K., Akter, M., Al Noor, M. M., Rahman, M. A., Keya, S. S., et al. (2024). Green vanguards: Harnessing the power of plant antioxidants, signal catalysts, and genetic engineering to combat reactive oxygen species under multiple abiotic stresses. Plant Stress, 100547. doi: 10.1016/j.stress.2024.100547
Rajput, L., Imran, A., Mubeen, F., Hafeez, F. Y. (2018). Wheat (Triticum aestivum L.) growth promotion by halo-tolerant PGPR-consortium. Soil Environ 37, 178–189. doi: 10.25252/SE/18/61522
Rasmussen, P. U., Bennett, A. E., Tack, A. J. (2020). The impact of elevated temperature and drought on the ecology and evolution of plant–soil microbe interactions. J. Ecol 108, 337–352. doi: 10.1111/1365-2745.13292
Ratnaningsih, H. R., Noviana, Z., Dewi, T. K., Loekito, S., Wiyono, S., Gafur, A., et al. (2023). IAA and ACC deaminase producing-bacteria isolated from the rhizosphere of pineapple plants grown under different abiotic and biotic stresses. Heliyon 9 (6), e16306. doi: 10.1016/j.heliyon.2023.e16306
Ravelo-Ortega, G., Raya-González, J., López-Bucio, J. (2023). Compounds from rhizosphere microbes that promote plant growth. Curr. Opin. Plant Biol 73, 102336. doi: 10.1016/j.pbi.2023.102336
Rawal, R., Scheerens, J. C., Fenstemaker, S. M., Francis, D. M., Miller, S. A., Benitez, M.-S. (2022). Novel Trichoderma isolates alleviate water deficit stress in susceptible tomato genotypes. Front. Plant Sci 13, 869090. doi: 10.3389/fpls.2022.869090
Rawat, R., Tewari, L. (2011). Effect of abiotic stress on phosphate solubilization by biocontrol fungus Trichoderma sp. Curr. Microbiol 62, 1521–1526. doi: 10.1007/s00284-011-9888-2
Rehman, A., Tian, C., He, S., Li, H., Lu, S., Du, X., et al. (2024). Transcriptome dynamics of Gossypium purpurascens in response to abiotic stresses by Iso-seq and RNA-seq data. Sci. Data 11, 477. doi: 10.1038/s41597-024-03334-9
Rima, F. S., Biswas, S., Sarker, P. K., Islam, M. R., Seraj, Z. I. (2018). Bacteria endemic to saline coastal belt and their ability to mitigate the effects of salt stress on rice growth and yields. Ann. Microbiol 68, 525–535. doi: 10.1007/s13213-018-1358-7
Rout, M. E., Southworth, D. (2013). The root microbiome influences scales from molecules to ecosystems: the unseen majority1. Am. J. Bot 100, 1689–1691. doi: 10.3732/ajb.1300291
Roy, T., Yadav, P., Chaudhary, A., Yadav, K., Singh, K. (2024). “Rhizosphere microbiome-assisted approaches for biotic stress management,” in Plant-microbe interaction and stress management. Eds. Chauhan, P.S., Tewari, S. K., Misra, S. (Springer Nature Singapore, Singapore), 135–158.
Roy Choudhury, A., Trivedi, P., Choi, J., Madhaiyan, M., Park, J. H., Choi, W., et al. (2023). Inoculation of ACC deaminase-producing endophytic bacteria down-regulates ethylene-induced pathogenesis-related signaling in red pepper (Capsicum annuum L.) under salt stress. Physiologia Plantarum 175, e13909. doi: 10.1111/ppl.v175.2
Saleem, A., Anwar, S., Nawaz, T., Fahad, S., Saud, S., Ur Rahman, T., et al. (2024). Securing a sustainable future: the climate change threat to agriculture, food security, and sustainable development goals. J. Umm Al-Qura Univ. Appl. Sci, 1–17. doi: 10.1007/s43994-024-00177-3
Salvi, P., Mahawar, H., Agarrwal, R., Kajal, Gautam, V., Deshmukh, R. (2022). Advancement in the molecular perspective of plant-endophytic interaction to mitigate drought stress in plants. Front. Microbiol 13, 981355. doi: 10.3389/fmicb.2022.981355
Sangiorgio, D., Cellini, A., Donati, I., Pastore, C., Onofrietti, C., Spinelli, F. (2020). Facing climate change: application of microbial biostimulants to mitigate stress in horticultural crops. Agronomy 10, 794. doi: 10.3390/agronomy10060794
Saravanakumar, K., MubarakAli, D., Kathiresan, K., Wang, M.-H.. (2018). An evidence of fungal derived 1-aminocyclopropane-1-carboxylate deaminase promoting the growth of mangroves. Beni-Suef University journal of basic and applied sciences. 7 (4), 446–451. doi: 10.1016/j.bjbas.2018.03.013
Sasse, J., Kosina, S. M., de Raad, M., Jordan, J. S., Whiting, K., Zhalnina, K., et al. (2020). Root morphology and exudate availability are shaped by particle size and chemistry in Brachypodium distachyon. Plant Direct 4, e00207. doi: 10.1002/pld3.v4.7
Shah, N., Irshad, M., Murad, W., Hamayun, M., Qadir, M., Hussain, A., et al. (2024). IAA is more effective than EDTA in enhancing phytoremediation potential for cadmium and copper contaminated soils. BMC Plant Biol 24, 815. doi: 10.1186/s12870-024-05329-5
Shahwar, D., Mushtaq, Z., Mushtaq, H., Alqarawi, A. A., Park, Y., Alshahrani, T. S., et al. (2023). Role of microbial inoculants as bio fertilizers for improving crop productivity: A review. Heliyon 9 (6), e16134. doi: 10.1016/j.heliyon.2023.e16134
Sharif, R., Su, L., Chen, X., Qi, X. (2022). Involvement of auxin in growth and stress response of cucumber. Vegetable Res 2, 1–9. doi: 10.48130/VR-2022-0013
Sharma, A., Shahzad, B., Kumar, V., Kohli, S. K., Sidhu, G. P. S., Bali, A. S., et al. (2019). Phytohormones regulate accumulation of osmolytes under abiotic stress. Biomolecules 9, 285. doi: 10.3390/biom9070285
Shekhawat, K., Saad, M. M., Sheikh, A., Mariappan, K., Al-Mahmoudi, H., Abdulhakim, F., et al. (2021). Root endophyte induced plant thermotolerance by constitutive chromatin modification at heat stress memory gene loci. EMBO Rep 22, e51049. doi: 10.15252/embr.202051049
Shigenaga, A. M., Berens, M. L., Tsuda, K., Argueso, C. T. (2017). Towards engineering of hormonal crosstalk in plant immunity. Curr. Opin. Plant Biol 38, 164–172. doi: 10.1016/j.pbi.2017.04.021
Shrivastava, P., Kumar, R. (2015). Soil salinity: A serious environmental issue and plant growth promoting bacteria as one of the tools for its alleviation. Saudi J. Biol. Sci 22, 123–131. doi: 10.1016/j.sjbs.2014.12.001
Singh, R., Singh, G. (2017). Traditional agriculture: a climate-smart approach for sustainable food production. Energy Ecol. Environ 2, 296–316. doi: 10.1007/s40974-017-0074-7
Spaepen, S., Vanderleyden, J. (2011). Auxin and plant-microbe interactions. Cold Spring Harbor Perspect. Biol 3, a001438. doi: 10.1101/cshperspect.a001438
Stefan, A., Van Cauwenberghe, J., Rosu, C. M., Stedel, C., Labrou, N. E., Flemetakis, E., et al. (2018). Genetic diversity and structure of Rhizobium leguminosarum populations associated with clover plants are influenced by local environmental variables. Systematic Appl. Microbiol 41, 251–259. doi: 10.1016/j.syapm.2018.01.007
Sytar, O., Kumari, P., Yadav, S., Brestic, M., Rastogi, A. (2019). Phytohormone priming: regulator for heavy metal stress in plants. J. Plant Growth Regul 38, 739–752. doi: 10.1007/s00344-018-9886-8
Tabari, H. (2020). Climate change impact on flood and extreme precipitation increases with water availability. Sci. Rep 10, 13768. doi: 10.1038/s41598-020-70816-2
Thepbandit, W., Athinuwat, D. (2024). Rhizosphere microorganisms supply availability of soil nutrients and induce plant defense. Microorganisms 12, 558. doi: 10.3390/microorganisms12030558
Toju, H., Peay, K. G., Yamamichi, M., Narisawa, K., Hiruma, K., Naito, K., et al. (2018). Core microbiomes for sustainable agroecosystems. Nat. Plants 4, 247–257. doi: 10.1038/s41477-018-0139-4
Trivedi, P., Leach, J. E., Tringe, S. G., Sa, T., Singh, B. K. (2020). Plant–microbiome interactions: from community assembly to plant health. Nat. Rev. Microbiol 18, 607–621. doi: 10.1038/s41579-020-0412-1
Vanderstraeten, L., Depaepe, T., Bertrand, S., van der Straeten, D. (2019). The ethylene precursor ACC affects early vegetative development independently of ethylene signaling. Front. Plant Sci 10, 1591. doi: 10.3389/fpls.2019.01591
Vargas, R. (2024). Beyond a deterministic representation of the temperature dependence of soil respiration. Sci. Total Environ 912, 169391. doi: 10.1016/j.scitotenv.2023.169391
Velásquez, A. C., Castroverde, C. D. M., He, S. Y. (2018). Plant–pathogen warfare under changing climate conditions. Curr. Biol 28, R619–R634. doi: 10.1016/j.cub.2018.03.054
Vera-Gargallo, B., Hernández, M., Dumont, M. G., Ventosa, A. (2023). Thrive or survive: prokaryotic life in hypersaline soils. Environ. microbiome 18, 17. doi: 10.1186/s40793-023-00475-z
Vescio, R., Malacrinò, A., Bennett, A. E., Sorgonà, A. (2021). Single and combined abiotic stressors affect maize rhizosphere bacterial microbiota. Rhizosphere 17, 100318. doi: 10.1016/j.rhisph.2021.100318
Vlot, A. C., Sales, J. H., Lenk, M., Bauer, K., Brambilla, A., Sommer, A., et al. (2021). Systemic propagation of immunity in plants. New Phytol 229, 1234–1250. doi: 10.1111/nph.v229.3
Vos, I. A., Pieterse, C. M., Van Wees, S. C. (2013). Costs and benefits of hormone-regulated plant defences. Plant Pathol 62, 43–55. doi: 10.1111/ppa.2013.62.issue-S1
Wang, S., Chen, K., Zhang, J., Wang, J., Li, H., Yang, X., et al. (2022). Genome-wide characterization of MATE family members in Cucumis melo L. and their expression profiles in response to abiotic and biotic stress. Hortic. Plant J 8, 474–488. doi: 10.1016/j.hpj.2022.05.004
Wang, J., Gong, Z., Zheng, J., Li, Z., Zhou, G., Xu, Y., et al. (2024). Genomic and epigenomic insights into the mechanism of cold response in upland cotton (Gossypium hirsutum). Plant Physiol. Biochem 206, 108206. doi: 10.1016/j.plaphy.2023.108206
Wang, Y.-J., He, X.-H., Meng, L.-L., Zou, Y.-N., Wu, Q.-S. (2023). Extraradical mycorrhizal hyphae promote soil carbon sequestration through difficultly extractable glomalin-related soil protein in response to soil water stress. Microbial Ecol 86, 1023–1034. doi: 10.1007/s00248-022-02153-y
Wang, Q., Liu, J., Zhu, H. (2018). Genetic and molecular mechanisms underlying symbiotic specificity in legume-rhizobium interactions. Front. Plant Sci 9, 313. doi: 10.3389/fpls.2018.00313
Wang, C., Morrissey, E. M., Mau, R. L., Hayer, M., Piñeiro, J., Mack, M. C., et al. (2021a). The temperature sensitivity of soil: microbial biodiversity, growth, and carbon mineralization. ISME J 15, 2738–2747. doi: 10.1038/s41396-021-00959-1
Wang, Y., Zhao, Y., Bollas, A., Wang, Y., Au, K. F. (2021b). Nanopore sequencing technology, bioinformatics and applications. Nat. Biotechnol 39, 1348–1365. doi: 10.1038/s41587-021-01108-x
Wiesenbauer, J., König, A., Gorka, S., Marchand, L., Nunan, N., Kitzler, B., et al. (2024). A pulse of simulated root exudation alters the composition and temporal dynamics of microbial metabolites in its immediate vicinity. Soil Biol. Biochem 189, 109259. doi: 10.1016/j.soilbio.2023.109259
Wu, Q.-S., Zou, Y.-N., Huang, Y.-M. (2013). The arbuscular mycorrhizal fungus Diversispora spurca ameliorates effects of waterlogging on growth, root system architecture and antioxidant enzyme activities of citrus seedlings. Fungal Ecol 6, 37–43. doi: 10.1016/j.funeco.2012.09.002
Xie, T., Lin, Y., Li, X. (2024). Responses of the arbuscular mycorrhizal fungi community to warming coupled with increased drought in an arid desert region. Geoderma 441, 116744. doi: 10.1016/j.geoderma.2023.116744
Xu, L., Coleman-Derr, D. (2019). Causes and consequences of a conserved bacterial root microbiome response to drought stress. Curr. Opin. Microbiol 49, 1–6. doi: 10.1016/j.mib.2019.07.003
Xu, W., Miao, Y., Kong, J., Lindsey, K., Zhang, X., Min, L. (2023). ROS signaling and its involvement in abiotic stress with emphasis on heat stress-driven anther sterility in plants. Crop Environment. 3 (2), 65–74. doi: 10.1016/j.crope.2023.12.002
Xu, L., Naylor, D., Dong, Z., Simmons, T., Pierroz, G., Hixson, K. K., et al. (2018). Drought delays development of the sorghum root microbiome and enriches for monoderm bacteria. Proc. Natl. Acad. Sci 115, E4284–E4293. doi: 10.1073/pnas.1717308115
Xu, Y., Zhang, G., Ding, H., Ci, D., Dai, L., Zhang, Z. (2020). Influence of salt stress on the rhizosphere soil bacterial community structure and growth performance of groundnut (Arachis hypogaea L.). Int. Microbiol 23, 453–465. doi: 10.1007/s10123-020-00118-0
Yang, Y., Guo, Y. (2018). Unraveling salt stress signaling in plants. J. Integr. Plant Biol 60, 796–804. doi: 10.1111/jipb.12689
Yu, P.-L., Fulton, J. C., Hudson, O. H., Huguet-Tapia, J. C., Brawner, J. T. (2023). Next-generation fungal identification using target enrichment and Nanopore sequencing. BMC Genomics 24, 581. doi: 10.1186/s12864-023-09691-w
Yuan, F., Leng, B., Wang, B. (2016). Progress in studying salt secretion from the salt glands in recretohalophytes: how do plants secrete salt. Front. Plant Sci 7, 977. doi: 10.3389/fpls.2016.00977
Zancarini, A., Westerhuis, J. A., Smilde, A. K., Bouwmeester, H. J. (2021). Integration of omics data to unravel root microbiome recruitment. Curr. Opin. Biotechnol 70, 255–261. doi: 10.1016/j.copbio.2021.06.016
Zhang, Y., Dong, Q., Wang, Z., Liu, Q., Yu, H., Sun, W., et al. (2024). A fine-scale Arabidopsis chromatin landscape reveals chromatin conformation-associated transcriptional dynamics. Nat. Commun 15, 3253. doi: 10.1038/s41467-024-47678-7
Zhang, W., Gao, W., Whalley, W. R., Ren, T. (2021). Physical properties of a sandy soil as affected by incubation with a synthetic root exudate: Strength, thermal and hydraulic conductivity, and evaporation. Eur. J. Soil Sci 72, 782–792. doi: 10.1111/ejss.13007
Zhao, R., Guo, W., Bi, N., Guo, J., Wang, L., Zhao, J., et al. (2015). Arbuscular mycorrhizal fungi affect the growth, nutrient uptake and water status of maize (Zea mays L.) grown in two types of coal mine spoils under drought stress. Appl. Soil Ecol 88, 41–49. doi: 10.1016/j.apsoil.2014.11.016
Keywords: climate factors, rhizosphere, nutrient acquisition, phytohormones, sustainable ecosystem
Citation: Muhammad A, Kong X, Zheng S, Bai N, Li L, Khan MHU, Fiaz S and Zhang Z (2024) Exploring plant-microbe interactions in adapting to abiotic stress under climate change: a review. Front. Plant Sci. 15:1482739. doi: 10.3389/fpls.2024.1482739
Received: 18 August 2024; Accepted: 28 October 2024;
Published: 15 November 2024.
Edited by:
Milan Kumar Lal, National Rice Research Institute (ICAR), IndiaReviewed by:
Afeez Adesina Adedayo, Western Illinois University, United StatesMallesham Bulle, Texas Tech University, United States
Shobhit Raj Vimal, University of Allahabad, India
Copyright © 2024 Muhammad, Kong, Zheng, Bai, Li, Khan, Fiaz and Zhang. This is an open-access article distributed under the terms of the Creative Commons Attribution License (CC BY). The use, distribution or reproduction in other forums is permitted, provided the original author(s) and the copyright owner(s) are credited and that the original publication in this journal is cited, in accordance with accepted academic practice. No use, distribution or reproduction is permitted which does not comply with these terms.
*Correspondence: Zhiyong Zhang, el96eTEyM0AxMjYuY29t