- 1Biodesign Institute, Arizona State University, Tempe, AZ, United States
- 2School of Life Sciences, Arizona State University, Tempe, AZ, United States
In 2022, the global outbreak of monkeypox virus (MPXV) with increased human-to-human transmission triggered urgent public health interventions. Plant-derived monoclonal antibodies (mAbs) are being explored as potential therapeutic strategies due to their diverse mechanisms of antiviral activity. MPXV produces two key infectious particles: the mature virion (MV) and the extracellular enveloped virion (EV), both essential for infection and spread. Effective therapies must target both to halt replication and transmission. Our prior research demonstrated the development of a potent neutralizing mAb against MPXV MV. This study focuses on developing a plant-derived mAb targeting MPXV EV, which is critical for viral dissemination within the host and generally resistant to antibody neutralization. Our findings reveal that the mAb (H2) can be robustly produced in Nicotiana benthamiana plants via transient expression. The plant-made H2 mAb effectively targets MPXV EV by binding specifically to the A35 MPXV antigen. Importantly, H2 mAb shows notable neutralizing activity against the infectious MPXV EV particle. This investigation is the first to report the development of a plant-derived anti-EV mAb for MPXV prevention and treatment, as well as the first demonstration of anti-MPXV EV activity by an mAb across any production platform. It highlights the potential of plant-produced mAbs as therapeutics for emerging infectious diseases, including the MPXV outbreak.
Introduction
Monkeypox virus (MPXV) is a zoonotic double-stranded DNA virus belonging to the Orthopoxvirus genus and shares close genetic and structural similarities with other genus members including Vaccinia virus (VACV), Variola virus (VARV, the causative agent of smallpox), and ectromelia virus (ECTV) (Lum et al., 2022). Endemic to central and western Africa for decades, MPXV experienced a global resurgence in 2022 through human-to-human transmission, leading to 109,699 confirmed cases worldwide and prompting an international health emergency declaration by the World Health Organization (WHO, 2024). MPXV exists in two infectious forms that facilitate infection: the mature virion (MV) and the extracellular enveloped virion (EV), each of which is responsible for the inter-host and intra-host dissemination, respectively (Schmidt et al., 2012).
Transmission of MPXV occurs through bodily fluids, respiratory droplets, direct contact with infected skin lesions, or contaminated fomites (Lum et al., 2022). The incubation period ranges from 5 to 21 days, and symptoms can include headache, fever, lymph node swelling, and muscle aches. Within 3 days of infection, a rash develops at the infection site, spreading to other bodily areas and resulting in papules or blisters (Lum et al., 2022). Currently, there are no specific approved treatments for MPXV. Antiviral therapies for other orthopoxviruses can be administered to treat MPXV infection under compassionate use policies (Siegrist and Sassine, 2022), although the clinical outcomes and risk-benefit ratio remain uncertain. Smallpox vaccines can also provide cross-protection against MPXV. However, due to the global eradication of smallpox in 1980, the vaccine is no longer routinely used (Gruber, 2022). Ongoing research and development efforts are focused on MPXV-specific treatments, underscoring the critical need for prophylactics and post-exposure therapeutics to protect immunocompromised individuals and those with severe allergic reactions to orthopoxvirus vaccines.
In the past few decades, monoclonal antibodies (mAbs) have emerged as promising protein-based biologics for combating a wide range of diseases due to their high specificity, versatility, and efficacy (Pantaleo et al., 2022). mAbs can be engineered to precisely target specific pathogens or cells, leading to better efficacy and fewer side effects compared to traditional therapies. Given the demonstrated effectiveness of neutralizing antibodies in protecting animals and humans from orthopoxvirus infections (Belyakov et al., 2003; Edghill-Smith et al., 2005; Sang et al., 2023), mAbs present a promising class of therapeutic candidates for combating the re-emerging MPXV epidemic. Our previous research demonstrated the development of a mAb that has potent neutralizing activity against the MV form of MPXV to curb the inter-host transmission (Esqueda et al., 2023). In contrast, there is still a need to develop more efficacious mAbs against the EV form of MPXV as it is generally more resistant to antibody neutralization due to the extra membrane that EVs possess (Vanderplasschen et al., 1998; Law and Smith, 2001). As a result, developing mAbs that can effectively target and neutralize the EV form of MPXV is crucial for achieving comprehensive protection and treatment of MPXV infection. The major viral antigens displayed by the EV of MPXV are A35 and B6, which are homologous to the VACV EV antigens of A33 and B5, respectively (Manes et al., 2008; Wang et al., 2023; Zhao et al., 2024). A35/A33 is particularly noteworthy for its role in viral dissemination within the host and anti-A33 antibodies have demonstrated protective efficacy in susceptible mice against lethal ECTV and VACV infections. Additionally, anti-A33 antibodies have been shown to reduce ECTV viral load in infected organs (Payne, 1980; Cohen et al., 2011; Tamir et al., 2024). Recently, a mAb (H2) has been isolated from A33-specific memory B cells of a volunteer vaccinated against smallpox over 40 years ago (Gu et al., 2022). The H2 mAb has been shown to bind to the A33 antigen of both VACV and ECTV, inhibit ECTV replication, and protect mice from VACV infection (Gu et al., 2022). However, the neutralizing activity of H2 mAb and other anti-A33/A35 mAbs against the EV form of MPXV has not been extensively characterized.
Currently, more than 100 mAbs are approved for therapeutic use (Mullard, 2021). The predominant platform for producing these biologics is based on mammalian cell culture systems, particularly Chinese hamster ovary (CHO) cells. CHO cells are favored for their ability to produce mAbs with high efficiency and human-like glycosylation patterns, which are crucial for antibody efficacy (Dahodwala and Lee, 2019). However, this production platform has several drawbacks, including high production costs and potential risks of contamination by mammalian pathogens. Furthermore, the glycosylation patterns in proteins produced by CHO cells can be heterogeneous, potentially hindering the development of mAbs with optimal effector functions (Sumit et al., 2019). Plants have emerged as a promising alternative platform for the production of mAbs (Chen, 2022). Utilizing plants as expression systems leverages their inherent capacity for rapid growth and biomass accumulation, which can significantly shorten production time and lower production costs compared to traditional mammalian cell culture methods (Chen and Davis, 2016; Nandi et al., 2016). Plant-based production of biologics also reduces the chance of introducing human pathogens during the manufacturing process (Chen, 2011). Furthermore, plant glycoengineering enables the generation of mAbs with a homogenous glycosylation profile, offering the capacity to modulate antibody Fc effector functions such as antibody-dependent cellular cytotoxicity (ADCC) activity and complement-dependent cytotoxicity (CDC) (Chen, 2016; Eidenberger et al., 2023; Esqueda and Chen, 2023).
In this study, we characterized a mAb H2, which was expressed in a glycoengineered Nicotiana benthamiana plant line (Strasser et al., 2008), targeting specifically the EV form of MPXV. The H2 mAb was successfully expressed and purified from N. benthamiana plants, achieving robust protein expression levels within 5-6 days post infiltration (DPI). Our findings reveal that plant-produced H2 mAb (p-H2 mAb) exhibits strong and specific binding to both MPXV-infected cells and its target antigen. Importantly, H2 mAb also demonstrated neutralizing activity against the EV form of MPXV. These results collectively suggest that plant-produced H2 mAb may be an effective candidate for eliminating MPVX EV virions, highlighting its potential therapeutic application in combating MPXV infections.
Materials and methods
Expression vector design and Agrobacterium tumefaciens infiltration
The heavy chain (HC) and light chain (LC) of the H2 mAb (Gu et al., 2022) were synthesized by Azenta Life Sciences (Burlington. MA, USA). For targeting the H2 mAb to the apoplast of leaf cells, the calreticulin signal peptide from Nicotiana plumbaginifolia (Giritch et al., 2006) was added to the N-terminus of both the LC and HC, without including any ER retention signals in either construct. Full sequences for the LC and HC can be found in the Supplementary Data Sheet 1. The synthesized HC and LC fragments were initially cloned into pBluescript KS+ and were then cloned into the one-module plant expression vector pBYR11eK2Md as described previously (Jugler et al., 2022a). Positive clones were verified by colony PCR using primers detailed in the Supplementary Material and were electroporated into Agrobacterium tumefaciens strain EHA105 (Esqueda and Chen, 2021). The EHA105 strain containing the H2 mAb construct was agroinfiltrated into the leaves of glycoengineered N. benthamiana plants using a needleless syringe as previously described (Leuzinger et al., 2013; Chen and Lai, 2014). Plants were grown in 65% humidity, at 25°C, with a 16-hour light and 8-hour dark cycle as described previously (Lai and Chen, 2012; Goulet et al., 2019).
H2 mAb extraction and purification
Leaves of glycoengineered N. benthamiana (~ 200 g per batch) were harvested at 5 DPI and homogenized in a buffer containing 1x phosphate-buffered saline (PBS) at pH 5.2, 10 mg/mL Sodium L-ascorbate, 1 mM ethylenediaminetetraacetic acid (EDTA), and 2 mM phenylmethylsulfonyl fluoride (PMSF) as previously described (Fulton et al., 2015; Jugler et al., 2020). The homogenate was filtered through a cheesecloth. The plant protein extract was centrifuged twice 15,000 x g at 4°C for 30 minutes and the pH of the supernatant was adjusted to 5.2 using 0.5N HCl. The protein extract was then incubated at 4°C overnight to precipitate host proteins. Following incubation, the plant extract was clarified by centrifuging three times at 15,000 x g at 4°C for 30 minutes. The pH of the clarified extract was adjusted to 7.0 using 0.5M NaOH and filtered using a 0.2-micron vacuum filter. The p-H2 mAb from plant extract was then purified using Protein A (MabSelect, Cytiva, Marlborough, MA, USA) affinity chromatography using a protocol provided by the manufacturer as we reported previously (Lai et al., 2010; Jugler et al., 2020). At least five batches of H2 mAb purification were conducted.
SDS-PAGE and western blot analysis
For SDS-PAGE analysis, purified p-H2 mAb was separated under both reducing and non-reducing conditions using 4-20% acrylamide gels and protein bands were visualized with Coomassie Blue R-250 staining as previously described (Jugler et al., 2022b). The purity of the H2 mAb was determined by imaging and quantifying Coomassie blue-stained protein bands on SDS-PAGE using a densitometer as described previously (Jugler et al., 2020). Western blot analysis was performed as previously described (Jugler et al., 2023). Briefly, total leaf soluble proteins were subjected to SDS-PAGE under reducing condition with 10% (v/v) β-mercaptoethanol and non-reducing condition on 4-12% or 12% acrylamide gels. The proteins were then transferred to PVDF membranes at 90 V for 90 minutes) in 1x transfer buffer (25mM Tris, 192 mM glycine, 10% Methanol, pH8.3). The membranes were blocked with 5% milk in 1X PBS containing 0.1% Tween-20 (PBS-T) and incubated for 1 hour at room temperature with goat anti-human kappa conjugated to horseradish peroxidase (HRP) for detecting the LC (Southern Biotech, Birmingham, AL, USA, 1:3,000 dilution) or goat anti-human gamma-HRP for the HC (Southern Biotech, Birmingham, AL, USA. 1:5,000 dilution). Detection was carried out using Pierce Western Blotting Substrate (Thermo Scientific, Waltham, MA, USA) for 5 minutes. Membranes were washed five times with 1X PBS-T at 5-minute intervals, and images were captured using the ImageQuant imaging system (Cytiva, Marlborough, MA, USA).
ELISA
1. P-H2 Binding to MPXV A35 Antigen
An ELISA was conducted to measure the specific binding of p-H2 mAb to the A35 antigen. The coding sequence of the antigen protein was cloned into the pET28a vector and expressed in E. coli strain BL21 as described previously (Fang et al., 2006; Yang et al., 2017). The soluble fraction of A35 was purified using immobilized metal affinity chromatography (IMAC) with Ni²⁺ resin, following the manufacturer’s instructions (Thermo Fisher Scientific, Waltham, MA, USA). Purified A35 (200 µl at 1µg/ml) was then coated onto a 96-well plate and incubated at 4°C overnight. The plate was blocked with 5% milk and followed by generic human IgG (SeraCare Life Sciences Inc., Milford, CT, USA, 50µg/ml). The plate was then incubated with p-H2 mAb (5µg/ml) conjugated to HRP using EZ-Link Plus Activated Peroxidase Kit (Thermo Fisher Scientific, Waltham, MA, USA). Detection of p-H2 mAb was performed using an HRP substrate (TMB, SeraCare Life Sciences Inc., Milford, CT, USA), after which the reaction was stopped with 1M H2SO4. The plate was then read on a spectrophotometer at 450 nm (Biotek Powerwave, Agilent Technologies., Santa Clara, CA, USA) and results were calculated and graphed using GraphPad Prism 10.2.2 (GraphPad, San Diego, CA, USA). The binding ELISA was conducted six times with technical triplicates.
2. Time Course of H2 mAb expression in plants
An ELISA that detects the fully assembled mAb was performed to investigate the temporal expression patten of H2 mAb in plants as described (Lai et al., 2014). Glycoengineered N. benthamiana plant leaves (at least 20 leaves per time point) were agroinfiltrated (OD600 = 0.2) with the expression vector of H2 mAb. Leaves were harvested at 4-9 DPI and stored at -80°C. Once all samples were collected, the leaves were ground for 5 minutes using a chilled mortar and pestle. Protein extracts from each DPI were isolated, clarified using the same extraction buffer and procedure as described in the “H2 mAb Extraction and Purification” section above. Throughout the isolation process, buffers, containers, and procedures were pre-chilled at 4°C and conducted at 4°C to ensure the stability of the H2 mAb. The extracts were then assayed on a 96-well plate coated with the capture antibody (goat anti-human HC, Southern Biotech, Birmingham, AL, USA, 200 µl at 2µg/ml). After 1 hour of incubation at 37°C, the plate was incubated with the detection antibody (a goat anti-human kappa IgG labeled with HRP, Southern Biotech, Birmingham, AL, USA, 0.25µg/ml) for 1 hour at 37°C. The plate was then developed using an HRP substrate (TMB, SeraCare Life Sciences Inc., Milford, CT, USA) and read on a spectrophotometer at 450 nm (Biotek Powerwave, Agilent Technologies., Santa Clara, CA, USA). For each ELISA, serial dilutions of an isotype IgG with known concentrations were included as standards to generate a standard curve, which we used to calculate the concentration of the H2 mAb in the leaves (μg of mAb per gram of fresh leaf weight, FLW). The H2 concentration was then graphed using GraphPad Prism 10.2.2 (GraphPad, San Diego, CA, USA). The time-course experiments were repeated three times. Proteins isolated from each experiment were assayed using ELISA, which was conducted at least twice with technical replicates.
Virus propagation and cell culture
MPXV strain WRAIR 7-61 (BEI Resources, NR-58622, Rockville, MD, USA) was propagated by infecting T175 cm² flasks of BSC40 cells (American Type Culture Collection (ATCC) CRL-2761, Manassas, VA, USA) at a multiplicity of infection (MOI) of 0.01 for one hour at 37°C in Eagle’s Minimal Essential Medium (MEM) containing 2% fetal bovine serum (FBS) (ThermoFisher, Waltham, MA, USA). After infection, the cells were overlaid with Dulbecco’s Modified-Minimal Essential Medium (DMEM) (ThermoFisher, Waltham, MA, USA) supplemented with 5% FBS. Upon observing viral cytopathic effect (CPE) in 75-100% of cells (approximately 3-4 days post-infection), the virus was harvested by scraping cells into media, centrifuging at 1,000 x g for 10 minutes, and resuspending the pellet in 10 mM Tris, pH 8.0. Crude viral stocks were prepared by freeze-thaw cycles (-80°C, thawed on ice for 30 minutes, then at 37°C for 10 minutes), removing cellular debris by centrifugation at 1,000 x g for 10 minutes, and collecting and aliquoting the supernatant. These crude viral stocks were titrated by infecting confluent monolayers of BSC40 cells with serial dilutions of the viral stock, staining the cells with 0.1% crystal violet in 20% ethanol (MilliporeSigma, Burlington, MA, USA) three days after infection, and then counting plaques. VACV strain Copenhagen (COP) VC2 (kindly provided by Virogenetics) (Jentarra et al., 2008) was prepared similarly as described for MPXV but cultivated in BHK21 cells (ATCC, CCL-10) in MEM with 5% FBS. Additionally, the virus was purified by centrifugation on a 36% sucrose pad at 18,000 x g for one hour at 4°C. Like MPXV, VACV stocks were titrated using BSC40 cells.
Immunofluorescence staining
BSC40 cells were seeded into a 96-well plate at 50% confluency, infected in triplicate with different dilutions of MPXV or VACV stocks, then fixed with 4% Paraformaldehyde (ThermoFisher, Waltham, MA, USA) in PBS for 15 minutes at room temperature by 24 or 48 hours post infection, respectively. After fixation, the cells were permeabilized with 0.1% Saponin (MilliporeSigma, Burlington, MA, USA) in PBS and stained with 5 µg/ml p-H2 mAb at 4°C overnight. The next day, cells were washed three times with PBS and stained with Alexa488 goat anti-human IgG Kappa (Southern Biotech, Birmingham, AL, USA) at a 1:500 dilution for 1.5 hours at room temperature. Images were captured using the EVOS Cell Imaging System (Thermo Fisher Scientific, Waltham, MA, USA). Experiments were independently repeated three times for both MPXV and VACV.
Preparation of extracellular enveloped virions
EVs were isolated by infecting a confluent monolayer of BSC40 cells in a 60 mm dish with the indicated virus (MPXV or VACV) at an MOI of 5. The monolayer was washed twice to remove input IMVs, and the cell media supernatant was collected 18-24 hours post-infection. The supernatant was centrifuged twice at 1,000 x g for 10 minutes to remove infected cells. This approach is comparable to, if not more stringent than, previously published protocols for EV isolation (Benhnia Mohammed et al., 2009b). EV stocks were used immediately or stored overnight at 4°C prior to subsequent neutralization assays.
MPXV and VACV neutralization assay
MPXV EVs were neutralized by diluting fresh MPXV and VACV EV stocks by 10-3 and 10-5, respectively, and then adding the appropriate volume of antibody or PBS to each dilution. The mixtures of diluted EVs and antibody/PBS were incubated at 37°C for one hour and then applied to confluent monolayers of BSC40 cells in a volume of 0.1mL for an additional hour at 37°C, with rocking every ten minutes. After the one-hour infection, monolayers were washed twice with fresh cell media and then incubated in cell media. MPXV and VACV-infected cells were stained with crystal violet 48 hours (VACV) and 72 hours (MPXV) post-infection. Percent (%) plaque reduction was calculated as: [(number of plaque per well without mAb) − (number of plaque per well with diluted mAb)/(number of plaque per well without mAb) × 100].
Statistical analyses
Statistical analyses were performed using GraphPad Prism software version 10.2.2. First, the data distribution was assessed using the D’Agostino & Pearson normality test, confirming a Gaussian distribution for all neutralization data. Subsequently, T-tests were employed to compare the neutralization activity of the p-H2 mAb against MPXV and VACV with data from at least two independent experiments with technical triplicates. A p-value of less than 0.05 was considered statistically significant.
Results
Expression of H2 mAb in Nicotiana benthamiana
The coding sequences of H2 mAb HC and LC were cloned into the plant expression vector and agroinfiltrated into leaves of a glycoengineered line of N. benthamiana plants that forgo the attachment of plant-specific xylose and fucose on their complex N-glycans (Strasser et al., 2008). Western blot analysis validated the expression of H2 mAb in plants, showing detection of the HC and LC at the expected molecular weights of 50kDa and 25 kDa respectively (Figure 1). Results obtained under non-reducing conditions indicated that p-H2 assembled into its tetrameric IgG format (Supplementary Figure S1), although there were minor, not-fully-assembled fragments that shared a similar banding pattern with the pharmaceutical-grade IgG isotype control (Supplementary Figure S1). Furthermore, the temporal expression pattern of p-H2 mAb was investigated by an ELISA that detects only the assembled form of IgG. Our results indicate that the fully assembled mAb accumulated quickly in plants with its peak expression (~ 150 μg of mAb per gram FLW) occurred at 5-6 DPI (Figure 2). These results indicate the robust production and assembly of p-H2 mAb in plants.
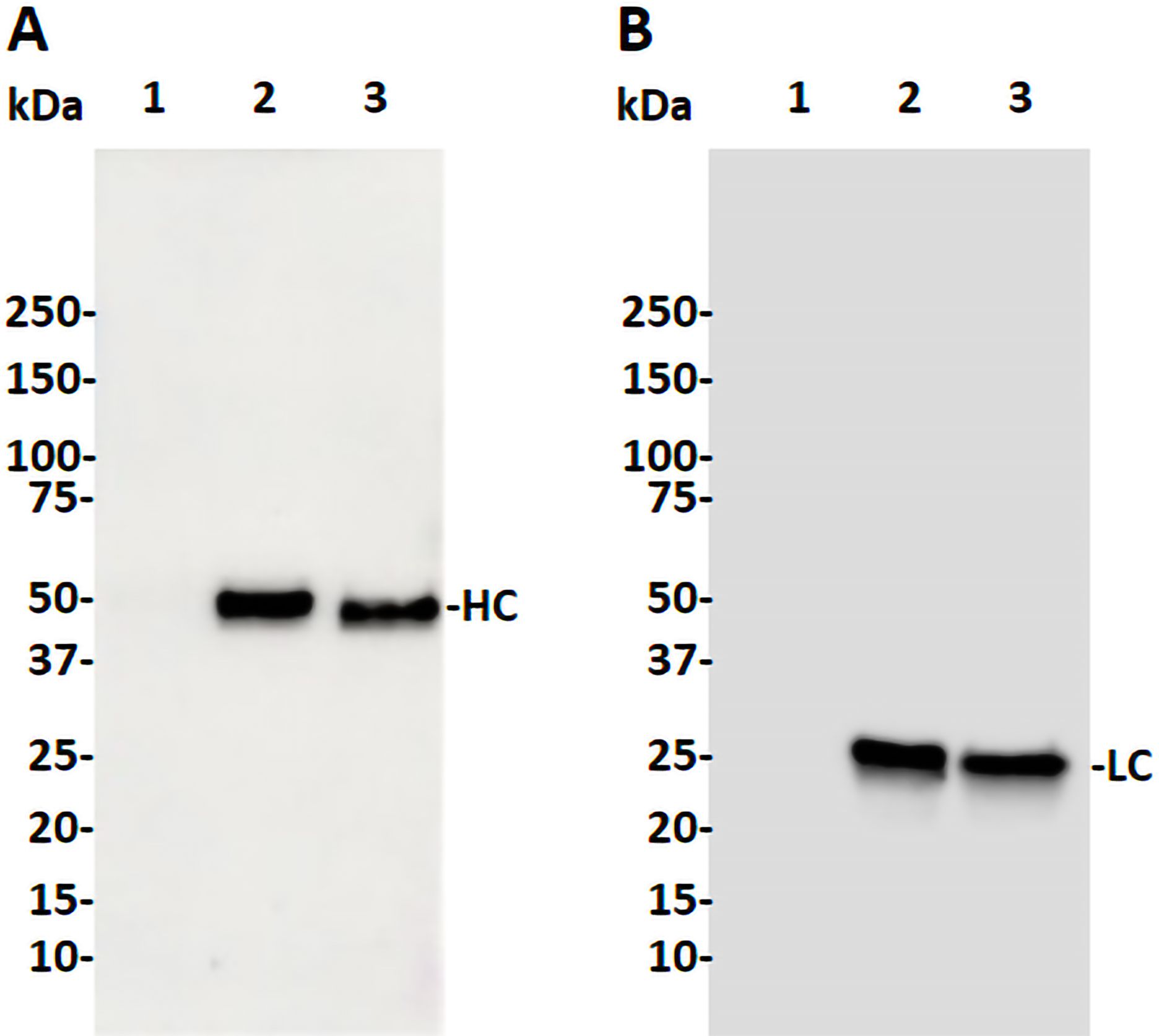
Figure 1. Western blot analysis of the H2 mAb produced in glycoengineered N. benthamiana plant. Total proteins were extracted from N. benthamiana leaves infiltrated with either the H2 mAb construct or buffer. Proteins were then separated by SDS-PAGE under reducing condition and transferred to PVDF membranes. Immunodetection was performed under reducing condition using antibodies against human gamma HC (A) or human kappa LC (B). Lane 1: 20 µg of total proteins from buffer-infiltrated leaves, serving as a negative control. Lane 2: 20 ng of isotype IgG, serving as both a positive control and a loading control. Lane 3: 20 µg of proteins from leaves infiltrated with the H2 mAb construct. One representative blot from multiple experiments is shown.
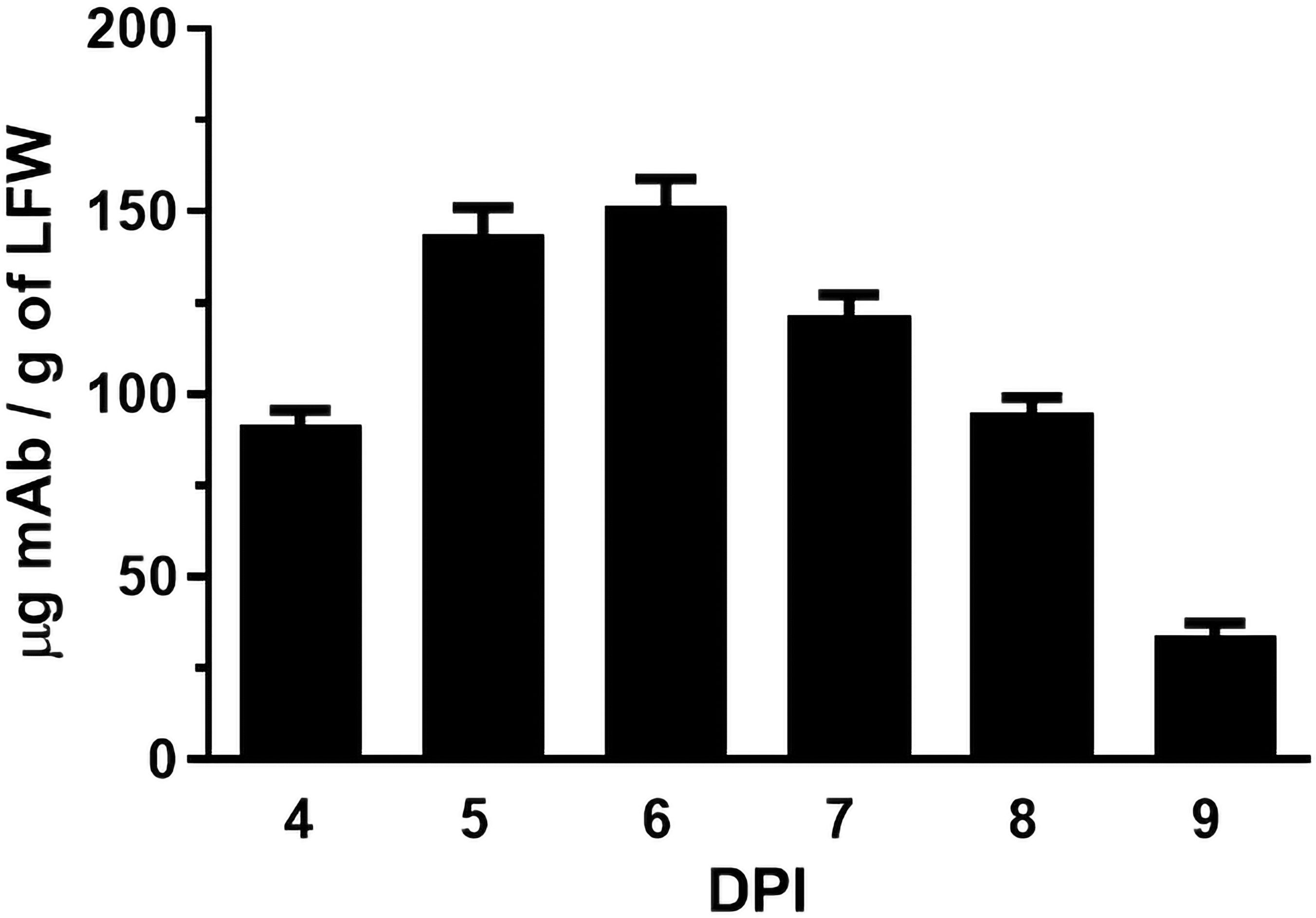
Figure 2. Time course of H2 mAb expression in N. benthamiana. Total protein was extracted from glycoengineered N. benthamiana leaves agroinfiltrated with the H2 mAb construct at 4, 5, 6, 7, 8, and 9 days post-infiltration. The Levels of H2 mAb were quantified by an ELISA that specifically detects assembled IgG. The data presented are derived from three independent infiltration experiments, with each ELISA repeated twice with technical replicates.
Assembly and purification of plant-produced H2 mAb
p-H2 mAb was purified by a two-step purification protocol previously developed in our laboratory. SDS-PAGE analysis of purified p-H2 mAb demonstrated that the mAb can be purified to greater than 90% homogeneity by this purification method, which is comparable to that of the control mAb produced in CHO cells (Figure 3). No significant degradation products were detected for either LC or HC (Figure 3, Lane 1), indicating the integrity of p-H2 mAb. Furthermore, p-H2 mAb was detected as a major band of approximately 170 kDa (Figure 3, Lane 3), confirming the assembly of the mAb as indicated by the western blot and ELISA results.
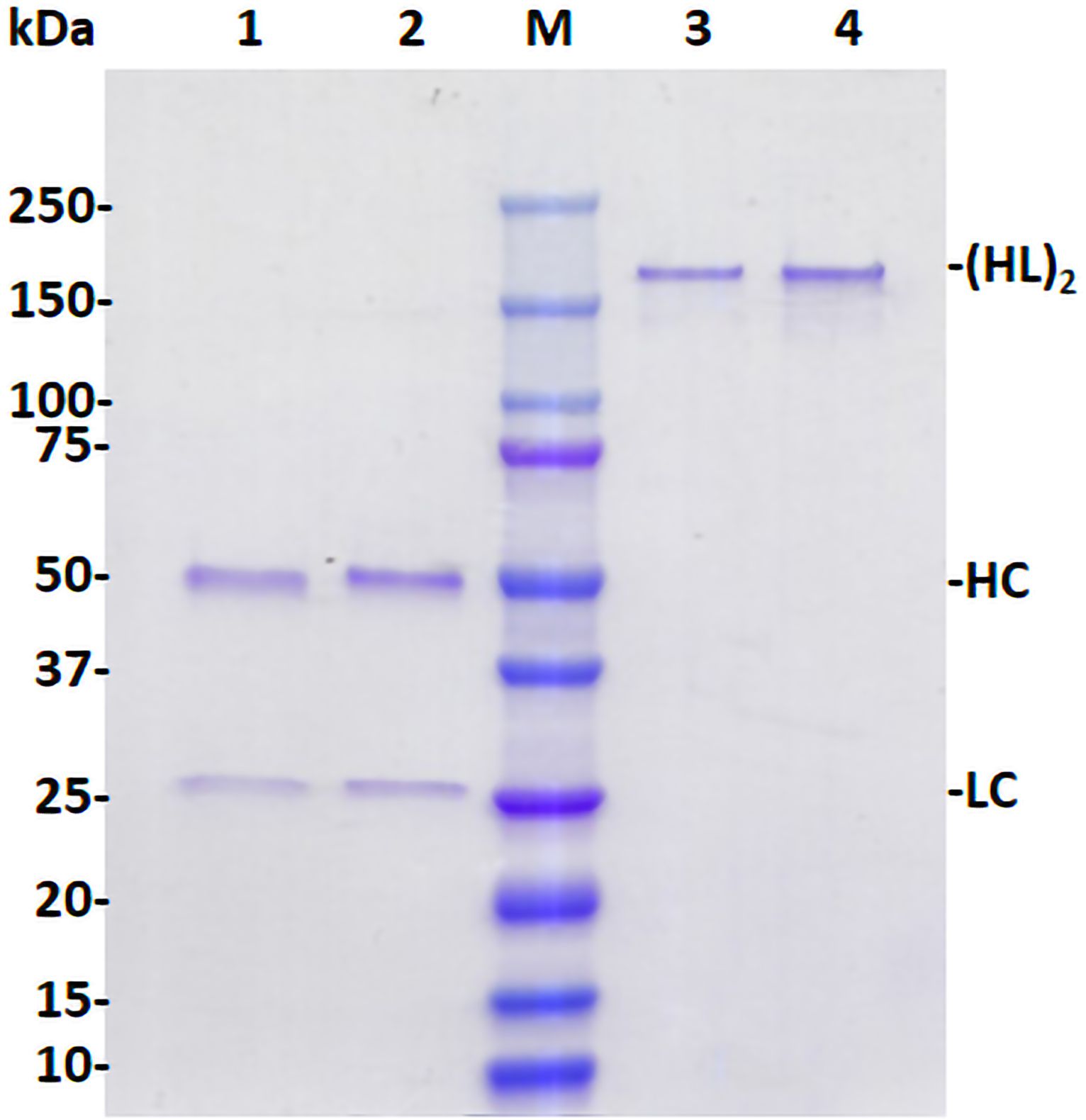
Figure 3. Assembly and purification of plant-produced H2 mAb. The p-H2 mAb was extracted from leaves of glycoengineered N. benthamiana plants and purified using Protein A affinity chromatography. Purified p-H2 mAb was analyzed by SDS-PAGE under both reducing (Lanes 1 and 2) and non-reducing conditions (Lanes 3 and 4), and subsequently stained with Coomassie blue. Lanes 1 (3 µg protein) and 3 (1 µg protein) show the plant-produced H2 mAb, while Lanes 2 (3 µg protein) and 4 (1 µg protein) show the Isotype IgG reference produced in CHO cells, serving as both a positive control and a loading control. A single representative result from multiple experiments is presented.
p-H2 mAb specifically binds to the target Monkeypox virus antigen
The specific recognition of p-H2 mAb to its cognate antigen was investigated by two independent methods. We first measured its binding to the specific MPXV antigen displayed on the authentic virus. Our results demonstrated that p-H2 mAb exhibits targeted binding to MPXV-infected BSC40 cells (Figure 4A), but with no binding observed to uninfected cells (Figure 4B) or cells detected with only the secondary antibody (Figure 4C). As shown in Figures 4D–F, similar cell numbers and distributions were observed under bright-field settings, regardless of whether the cells were infected, uninfected, stained sequentially with primary and secondary antibodies, or stained with only the secondary antibody. Similarly, the p-H2 mAb also demonstrated specific binding to cells infected with the closely related VACV (Figure 5). To confirm that p-H2 mAb specifically recognized its target antigen (A35) displayed on MPXV EV, an ELISA was performed with purified A35. As shown in Figure 6, p-H2 mAb indeed showed specific binding to A35 antigen. These results demonstrated that p-H2 mAb displayed Fab domains with authentic conformation that can recognize its target antigen in purified form as well as when displayed on the surface of the virion.
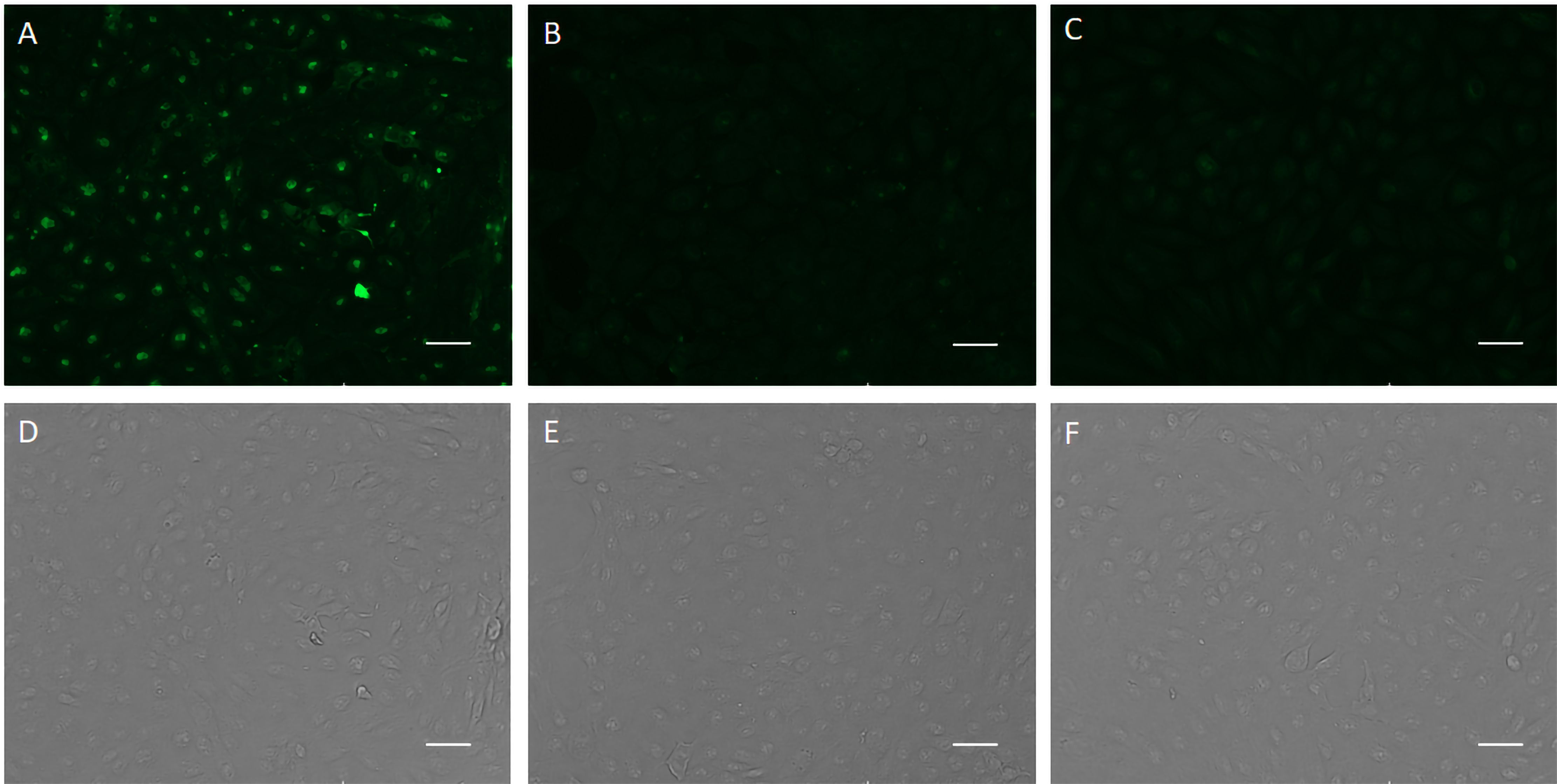
Figure 4. Recognition of viral antigen in MPXV-infected BSC40 cells by p-H2 mAb using immunofluorescence microscopy. MPXV-infected (A, D) or uninfected [(B, E), negative control] BSC40 cells in a 96-well plate were fixed and permeabilized, then incubated with p-H2 mAb and subsequently stained with Alexa488-conjugated goat anti-human IgG Kappa. Panels (C, F) show MPXV-infected BSC40 cells stained directly with Alexa488-conjugated goat anti-human IgG Kappa, serving as a secondary-antibody-only negative control. Images were captured using an EVOS Cell Imaging System with the EVOS AlexaFluor 488 light cube filter (A–C) or under white light settings (D–F). Scale bar represents 50 µm.
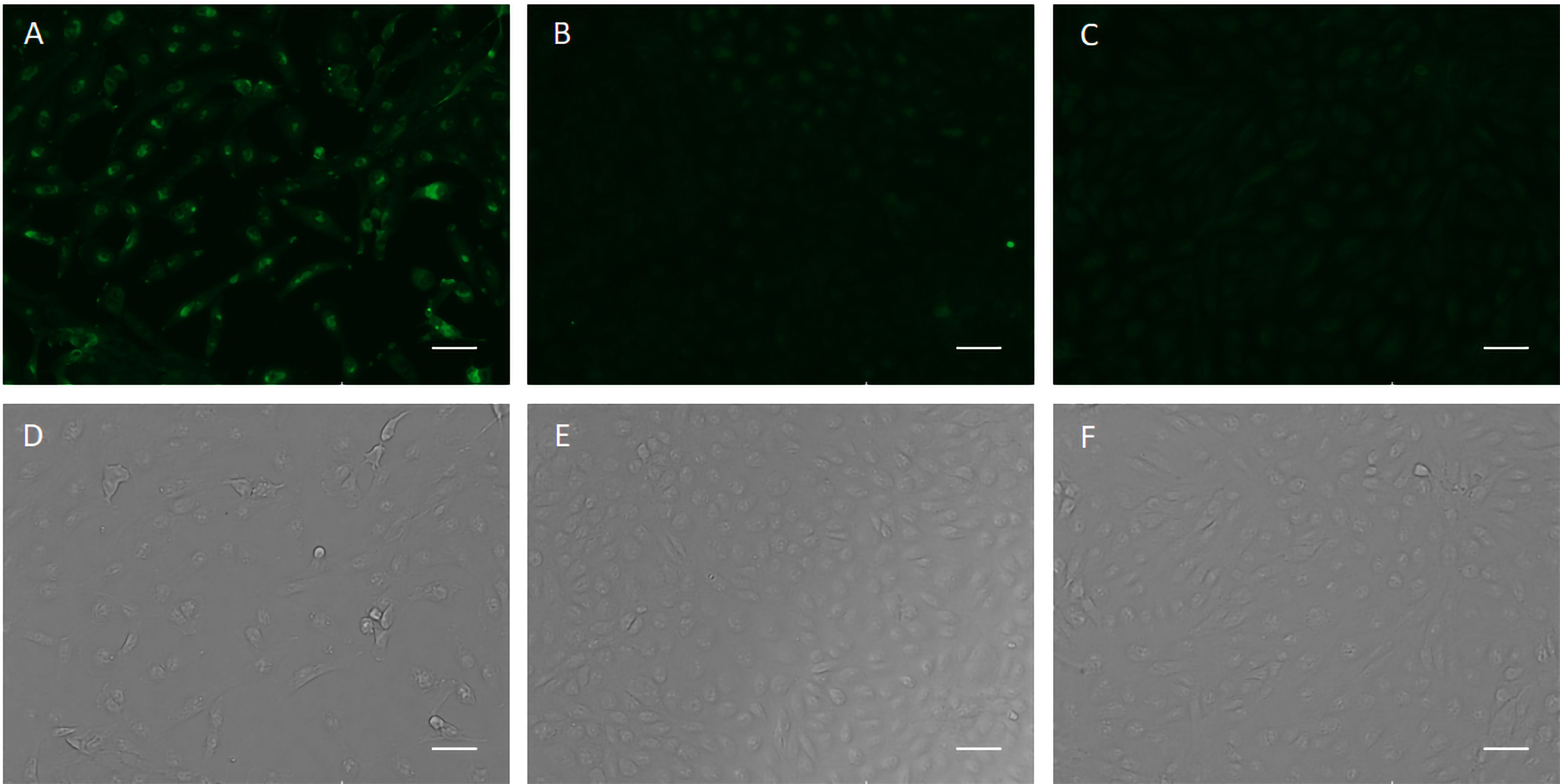
Figure 5. p-H2 mAb recognizes viral antigen in VACV-infected BSC40 cells. BSC40 cells infected with VACV were stained sequentially with p-H2 mAb and the secondary antibody Alexa488-conjugated goat anti-human IgG Kappa (A, D) or with the secondary antibody only [(C, F), negative control]. Uninfected BSC40 cells stained with p-H2 mAb and Alexa488-conjugated goat anti-human IgG Kappa (B, E) served as an additional negative control. Immunostained images are shown in panels (A–C), with corresponding bright field images in panels (D–F).
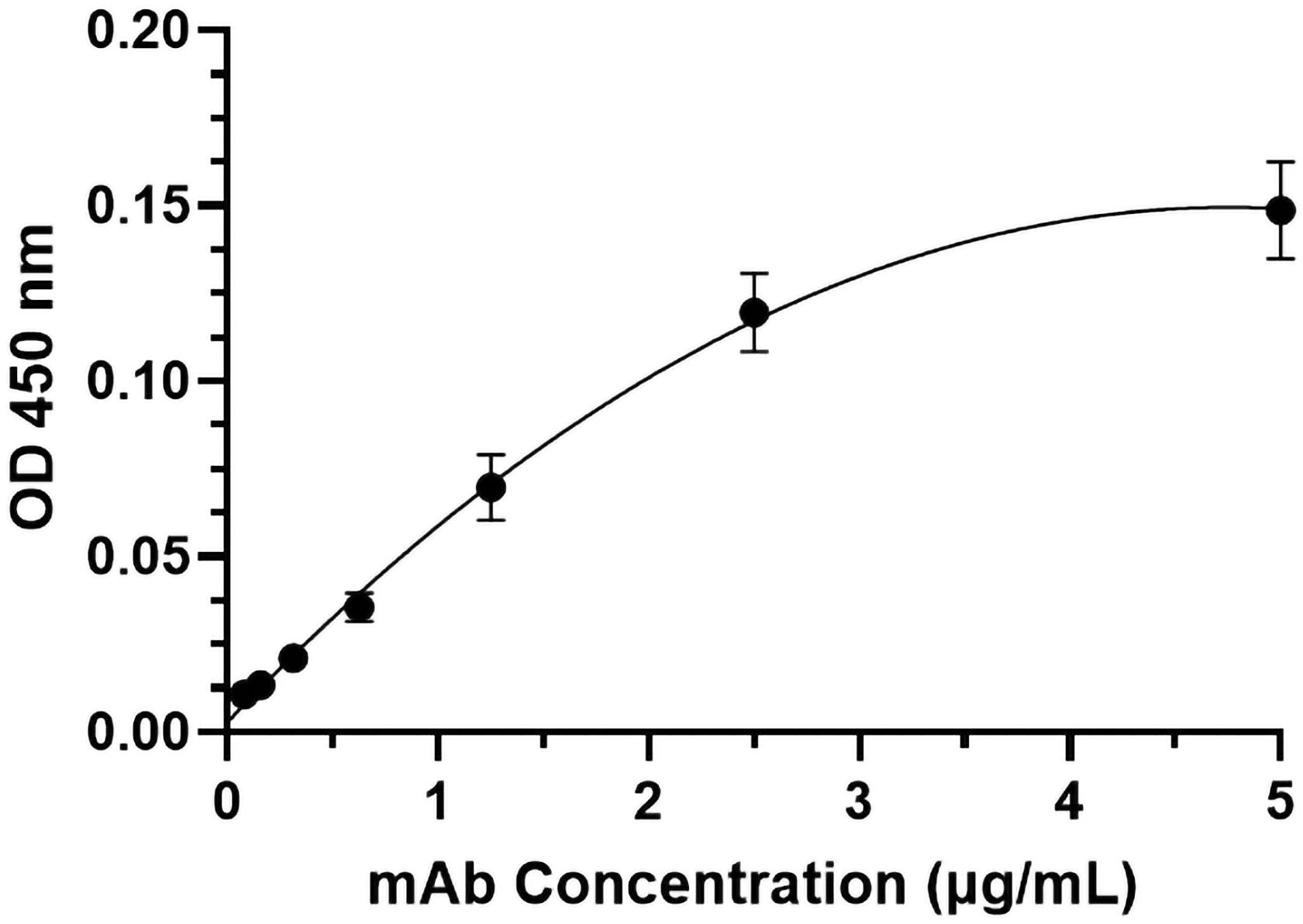
Figure 6. Specific binding of p-H2 mAb to the MPXV A35 antigen. MPXV A35 antigen was immobilized on ELISA plates. After blocking with 5% milk and generic human IgG, the plates were incubated with serial dilutions of HRP-conjugated p-H2 mAb. The mean and standard deviation of absorbance values at 450 nm were measured from six independent experiments, each with technical triplicates, and the data were plotted using GraphPad Prism 10.2.2.
p-H2 mAb neutralizes extracellular enveloped Monkeypox virion
The neutralization potential of p-H2 mAb against the EV virion of MPXV was investigated by a plaque assay as described previously (Denzler et al., 2011; Arndt et al., 2016). As shown in Figure 7A, treatment with p-H2 mAb resulted in significant neutralization of MPXV compared to the negative control achieving a 56% plaque reduction (p = 0.0001 compared to the negative control). Additionally, the p-H2 mAb also exhibited significant neutralizing activity against VACV, which is similar to that of MPXV (p = 0.14 compared to MPXV), resulting in a 45% plaque reduction when treated with p-H2 mAb (p < 0.0001 compared to the negative control) (Figure 7B). These results indicate that p-H2 mAb are functional in vitro against the EV form of MPXV as well as that of VACV.
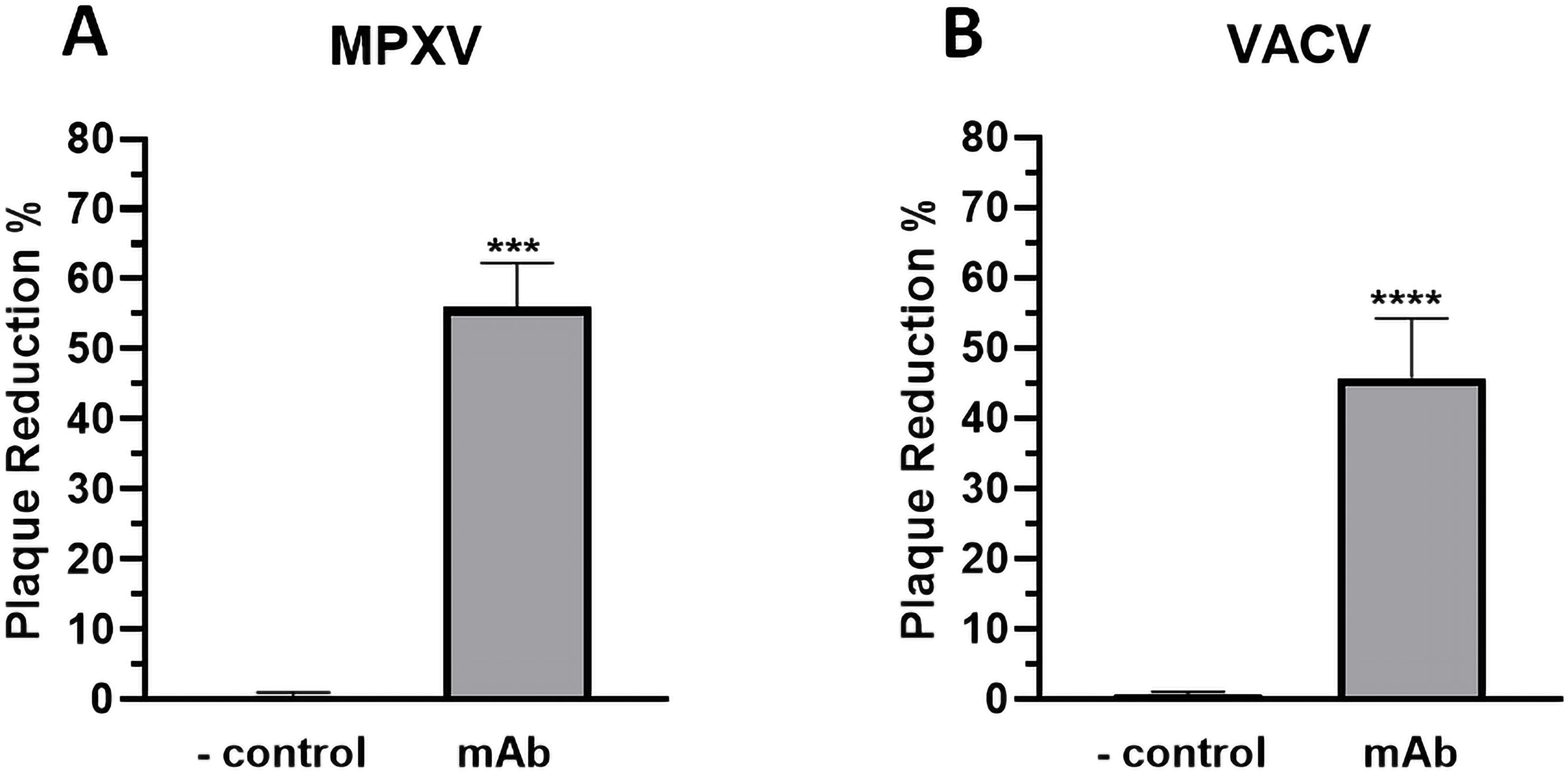
Figure 7. Neutralization of extracellular virion of MPXV and VACV by p-H2 mAb. EVs of MPXV (A) or VACV (B) were incubated with either PBS (negative control) or p-H2 mAb (25 µg/ml) before being added to BSC40 cells. MPXV- or VACV-infected cells were stained with crystal violet three days or two days later, respectively. Plaque numbers were counted, and plaque reduction percentage (%) was calculated. The results represent data from at least two independent experiments with technical triplicates. *** and **** indicate p values of 0.0001 and < 0.0001, respectively, from T test analysis.
Discussion
The global MPXV outbreak in 2022 was the largest and most widespread in history, with a significant increase in human-to-human transmission highlighting the urgent need for prophylactics and therapeutics (Lum et al., 2022). These measures are critical to prevent further virus spread and to protect and treat individuals who are allergic to existing MPXV vaccines or unable to mount a protective response to vaccination, especially given the current lack of an FDA-approved therapy specifically for MPXV. mAbs are promising candidates for treating MPXV infection, as their protective efficacy against related orthopoxviruses has been demonstrated in animal models, although research on MPXV remains limited (Belyakov et al., 2003; Edghill-Smith et al., 2005). MPXV produces two distinct types of infectious particles: the MV and the EV. MV, characterized by a single lipid membrane, is predominantly released from infected cells upon cell lysis (Roberts and Smith, 2008; Lum et al., 2022). In contrast, EV buds off from infected cells, acquiring an additional outer lipid envelope that confers resistance to the host’s immune responses, facilitates more efficient viral spread within the host, and helps the virus evade immune detection (Payne, 1980; Schmidt et al., 2012). Consequently, MVs primarily establish initial infection and facilitate host-to-host transmission, while EVs are crucial for viral spread and immune evasion within the host (Schmidt et al., 2012). Accordingly, a successful mAb-based therapy for MPXV should target both MVs and EVs to ensure effective virus neutralization during early infection stages and control viral spread within the host. Failure to target both forms may result in incomplete viral containment, leading to ongoing viral replication and transmission.
Previously, we developed a mAb with potent neutralizing activity against the MV form of MPXV, aimed at preventing inter-host transmission (Esqueda et al., 2023). In this study, we sought to characterize an EV-specific mAb to inhibit viral spreading within the infected host, focusing on the EV-specific antigen A35 from MPXV. The A35 antigen, a homolog of VACV A33, is an envelope glycoprotein, contributing to the formation of action-containing microvilli and facilitating the effective viral dissemination within the host (Perdiguero and Blasco, 2006; Wang et al., 2023). We specifically selected the H2 mAb for further evaluation as a candidate against MPXV EV due to its demonstrated ability to inhibit VACV infection and its recognition of a conserved epitope on both VACV A33 and MPXV A35 (Gu et al., 2022; Yefet et al., 2023). Here, we demonstrated that H2 mAb was rapidly expressed in glycoengineered N. benthamiana plants, reaching an accumulation level of approximately 150 μg/gram FLW within 5-6 days post-gene delivery. This expression level is comparable to that of other plant-expressed mAbs driven by the same expression vector (Jugler et al., 2021; Sun et al., 2023). However, further yield enhancement of p-H2 mAb in plants can be achieved through several strategies, such as employing improved expression vectors (Klimyuk et al., 2014) or co-expressing H2 mAb with chaperones (Margolin et al., 2020). Our results also confirm that p-H2 mAb correctly assembled into the expected IgG structure and was purified to a high degree of homogeneity, with no detectable degradation or truncation occurring during its accumulation or purification from plants. We validated the specific binding of p-H2 mAb using two distinct assays. First, we assessed its binding to the MPXV antigen on the authentic virus and found that p-H2 mAb specifically bound to MPXV-infected cells. Similarly, it bound to cells infected with VACV. To further confirm that p-H2 mAb recognizes its target antigen on MPXV EV, we conducted an ELISA using purified A35 EV antigen. The results confirmed that p-H2 mAb specifically binds to the A35 antigen, indicating proper folding and recognition of its target antigen in both purified form and on the surface of the virion. Importantly, p-H2 mAb exhibits neutralization activity against both live MPXV and VACV, with comparable potency to each other and to that reported for ECTV (Gu et al., 2022). Given that the mammalian cell-produced H2 mAb has shown significant protective efficacy against lethal VACV infection in mice (Gu et al., 2022), the similar neutralizing potency against MPXV suggests that p-H2 mAb may also be effective in vivo as a prophylactic or therapeutic agent for preventing or treating MPXV infection.
Neutralizing MPXV EV with antibodies is challenging primarily due to the EV’s additional outer lipid envelope. Studies with VACV have shown that direct antibody neutralization of EVs is inefficient even at high antibody concentrations (Vanderplasschen et al., 1998; Galmiche et al., 1999; Law and Smith, 2001; Bell et al., 2004; Viner and Isaacs, 2005). However, further studies reveal that anti-EV antibodies can provide protection through mechanisms beyond neutralization, such as Fc effector functions. For example, polyclonal antibodies against A33 and B5 have been shown to engage the complement system, eliminating VACV EVs via CDC activity through opsonization and virolysis (Lustig et al., 2004; Cohen et al., 2011). Additionally, Fc receptor engagement and ADCC activity of anti-EV antibodies have been documented (Benhnia Mohammed et al., 2009a, Benhnia Mohammed et al., 2009b; Cohen et al., 2011). Given that Fc effector functions depend on the N-glycosylation of the antibody’s Fc domain (Kellner et al., 2017; Steffen et al., 2020), this evidence suggests that our plant-based system may provide an opportunity to enhance the efficacy of anti-MPXV EV mAbs. Plant glycoengineering has enabled the production of mAbs with a homogeneous N-glycosylation profile, a level of uniformity that current mammalian-cell-based systems cannot achieve (Chen, 2016). Since p-H2 mAb was produced in the glycoengineered GnGn plant line, it is expected to exhibit the same uniform GnGn N-glycosylation structure as other mAbs produced in this line of N. benthamiana (Jugler et al., 2022a, Jugler et al., 2023; Sun et al., 2023). Afucosylated GnGn glycoforms have been shown to enhance CDC and ADCC activity via increased binding to Fc-gamma receptors (Sun et al., 2019; Yu et al., 2021; Yang et al., 2023). Thus, p-H2 mAb may offer additional mechanisms of MPXV elimination in vivo in addition to neutralization, supporting the hypothesis that p-H2 mAb might have better in vivo efficacy compared to mammalian cell-produced H2 mAb due to enhanced Fc effector functions. While plant host engineering has produced plant lines with several advantages over mammalian systems for producing mAbs with defined human N-glycans, challenges remain. One such challenge is underglycosylation, which is occasionally observed in plant-produced IgGs (Chen, 2016). This issue can be addressed by co-expressing oligosaccharyltransferases—enzymes that transfer preassembled oligosaccharides to polypeptides in the endoplasmic reticulum (Chen, 2016). Additionally, as a relatively new production platform, there has been uncertainty regarding regulatory hurdles in approving glycoprotein biologics made in plants. Fortunately, results from human clinical trials of plant-made glycoproteins have shown that they are not particularly immunogenic, and even the presence of plant-specific glycans does not induce any unwanted side effects (Shaaltiel and Tekoah, 2016; Rup et al., 2017).
This study is the first to report the development of an anti-EV mAb in plants for preventing and treating MPXV infection, as well as the first demonstration of anti-MPXV EV activity by an mAb across any production platform. In the next phase of the study, we plan to perform comparative analyses of the CDC and ADCC activities of various H2 mAb glycovariants, including those produced in mammalian cells, and assess their efficacy in animal models. These studies will provide valuable insights into the full potential of plant-produced H2 mAb. It would also be worthwhile to investigate potential synergistic effects with a therapeutic cocktail comprising p-H2 anti-EV mAb and anti-MV mAb against MPXV. These analyses could provide valuable insights into how different viral proteins dictate the requirements for the host to eliminate the virus using various mechanisms and how Fc effector functions contribute to protection in vivo. As H2 mAb was isolated from a volunteer vaccinated with the VARV vaccine and has demonstrated inhibitory activity against both VACV and ECTV, our findings on its activity against MPXV further expand its effectiveness against another orthopoxvirus, supporting its potential as a broad-spectrum, pan-orthopoxvirus therapeutic. In August of 2024, the WHO re-declared that the MPXV epidemic a Public Health Emergency of International Concern. As MPXV outbreaks continue, up to 90% of cases occur in people with human immunodeficiency virus (HIV) (Saldana et al., 2023), who experience in more severe symptoms than those without HIV (Chastain et al., 2023). Therefore, it is crucial to monitor emerging mutants and quickly develop effective therapeutics against them. We propose that the plant-based system may be one of the best platforms to rapidly develop therapeutic mAbs that maintain efficacy against potential mutants via Fc effector functions and quickly screen for mAb cocktails with synergistic interactions. In summary, this study demonstrates the potential of using anti-EV mAbs as therapeutics against MPXV infection and highlights the utility of plant biotechnology in developing biologics against viral infections.
Data availability statement
The original contributions presented in the study are included in the article/Supplementary Material. Further inquiries can be directed to the corresponding author/s.
Author contributions
JM: Writing – original draft, Methodology, Investigation, Formal analysis, Data curation. HS: Writing – review & editing, Supervision, Methodology, Investigation, Formal analysis, Data curation. JB: Writing – review & editing, Methodology, Investigation, Formal analysis, Data curation. QC: Writing – original draft, Supervision, Funding acquisition, Conceptualization.
Funding
The author(s) declare financial support was received for the research, authorship, and/or publication of this article. This research was supported in part by a grant (FP00019102) from Greenbio to QC.
Acknowledgments
The authors would like to thank Dr. Herta Steinkellner at the University of Natural Resources and Life Sciences, Vienna (BOKU), for providing the seeds of the GnGn plant line. Additionally, we acknowledge Daniel Tran for his assistance with the maintenance of N. benthamiana.
Conflict of interest
The authors have no conflicts of interest to disclose concerning this manuscript. Q.C is a shareholder of Greenbio, a biotechnology company. However, he has no conflict of interest pertaining to the research conducted or the results discussed in this article.
The author(s) declared that they were an editorial board member of Frontiers, at the time of submission. This had no impact on the peer review process and the final decision.
Publisher’s note
All claims expressed in this article are solely those of the authors and do not necessarily represent those of their affiliated organizations, or those of the publisher, the editors and the reviewers. Any product that may be evaluated in this article, or claim that may be made by its manufacturer, is not guaranteed or endorsed by the publisher.
Supplementary material
The Supplementary Material for this article can be found online at: https://www.frontiersin.org/articles/10.3389/fpls.2024.1481452/full#supplementary-material
References
Arndt, W. D., White, S. D., Johnson, B. P., Huynh, T., Liao, J., Harrington, H., et al. (2016). Monkeypox virus induces the synthesis of less dsRNA than vaccinia virus, and is more resistant to the anti-poxvirus drug, IBT, than vaccinia virus. Virology 497, 125–135. doi: 10.1016/j.virol.2016.07.016
Bell, E., Shamim, M., Whitbeck, J. C., Sfyroera, G., Lambris, J. D., Isaacs, S. N. (2004). Antibodies against the extracellular enveloped virus B5R protein are mainly responsible for the EEV neutralizing capacity of vaccinia immune globulin. Virology 325, 425–431. doi: 10.1016/j.virol.2004.05.004
Belyakov, I. M., Earl, P., Dzutsev, A., Kuznetsov, V. A., Lemon, M., Wyatt, L. S., et al. (2003). Shared modes of protection against poxvirus infection by attenuated and conventional smallpox vaccine viruses. Proc. Natl. Acad. Sci. 100, 9458–9463. doi: 10.1073/pnas.1233578100
Benhnia Mohammed, R.-E.-I., Mccausland Megan, M., Laudenslager, J., Granger Steven, W., Rickert, S., Koriazova, L., et al. (2009a). Heavily isotype-dependent protective activities of human antibodies against vaccinia virus extracellular virion antigen B5. J. Virol. 83, 12355–12367. doi: 10.1128/JVI.01593-09
Benhnia Mohammed, R.-E.-I., Mccausland Megan, M., Moyron, J., Laudenslager, J., Granger, S., Rickert, S., et al. (2009b). Vaccinia virus extracellular enveloped virion neutralization in vitro and protection in vivo depend on complement. J. Virol. 83, 1201–1215. doi: 10.1128/JVI.01797-08
Chastain, D. B., Motoa, G., Ortiz-Martínez, Y., Gharamti, A., Henao-Martínez, A. F. (2023). Characteristics and clinical manifestations of monkeypox among people with and without HIV in the United States: a retrospective cohort. Aids 37, 611–616. doi: 10.1097/QAD.0000000000003449
Chen, Q. (2011). “Expression and manufacture of pharmaceutical proteins in genetically engineered horticultural plants,” in Transgenic Horticultural Crops: Challenges and Opportunities - Essays by Experts. Eds. Mou, B., Scorza, R. (Taylor & Francis, Boca Raton), 83–124.
Chen, Q. (2016). Glycoengineering of plants yields glycoproteins with polysialylation and other defined N-glycoforms. Proc. Natl. Acad. Sci. 113, 9404–9406. doi: 10.1073/pnas.1610803113
Chen, Q. (2022). Development of plant-made monoclonal antibodies against viral infections. Curr. Opin. Virol. 52, 148–160. doi: 10.1016/j.coviro.2021.12.005
Chen, Q., Davis, K. (2016). The potential of plants as a system for the development and production of human biologics. F1000Research 5. doi: 10.12688/f11000research.18010.12681
Chen, Q., Lai, H. (2014). Gene delivery into plant cells for recombinant protein production. BioMed. Res. Int. 2014, 10. doi: 10.1155/2015/932161
Cohen, M. E., Xiao, Y., Eisenberg, R. J., Cohen, G. H., Isaacs, S. N. (2011). Antibody against extracellular vaccinia virus (EV) protects mice through complement and Fc receptors. PLoS One 6, e20597. doi: 10.1371/journal.pone.0020597
Dahodwala, H., Lee, K. H. (2019). The fickle CHO: a review of the causes, implications, and potential alleviation of the CHO cell line instability problem. Curr. Opin. Biotechnol. 60, 128–137. doi: 10.1016/j.copbio.2019.01.011
Denzler, K. L., Schriewer, J., Parker, S., Werner, C., Hartzler, H., Hembrador, E., et al. (2011). The attenuated NYCBH vaccinia virus deleted for the immune evasion gene, E3L, completely protects mice against heterologous challenge with ectromelia virus. Vaccine 29, 9691–9696. doi: 10.1016/j.vaccine.2011.09.108
Edghill-Smith, Y., Golding, H., Manischewitz, J., King, L. R., Scott, D., Bray, M., et al. (2005). Smallpox vaccine-induced antibodies are necessary and sufficient for protection against monkeypox virus. Nat. Med. 11, 740–747. doi: 10.1038/nm1261
Eidenberger, L., Kogelmann, B., Steinkellner, H. (2023). Plant-based biopharmaceutical engineering. Nat. Rev. Bioengineering 1, 426–439. doi: 10.1038/s44222-023-00044-6
Esqueda, A., Chen, Q. (2021). Development and expression of subunit vaccines against viruses in plants. Methods Mol. Biol. 2225, 25–38. doi: 10.1007/978-1-0716-1012-1_2
Esqueda, A., Chen, Q. (2023). “Producing biologics with defined N-glycosylation in plants,” in Chemokine-Glycosaminoglycan Interactions: Methods and Protocols. Ed. Lucas, A. R. (Springer US, New York, NY), 235–250.
Esqueda, A., Sun, H., Bonner, J., Lai, H., Jugler, C., Kibler, K. V., et al. (2023). A monoclonal antibody produced in glycoengineered plants potently neutralizes monkeypox virus. Vaccines 11, 1179. doi: 10.3390/vaccines11071179
Fang, M., Cheng, H., Dai, Z., Bu, Z., Sigal, L. J. (2006). Immunization with a single extracellular enveloped virus protein produced in bacteria provides partial protection from a lethal orthopoxvirus infection in a natural host. Virology 345, 231–243. doi: 10.1016/j.virol.2005.09.056
Fulton, A., Lai, H., Chen, Q., Zhang, C. (2015). Purification of monoclonal antibody against Ebola GP1 protein expressed in Nicotiana benthamiana. J. Chromatogr. A 1389, 128–132. doi: 10.1016/j.chroma.2015.02.013
Galmiche, M. C., Goenaga, J., Wittek, R., Rindisbacher, L. (1999). Neutralizing and protective antibodies directed against vaccinia virus envelope antigens. Virology 254, 71–80. doi: 10.1006/viro.1998.9516
Giritch, A., Marillonnet, S., Engler, C., Van Eldik, G., Botterman, J., Klimyuk, V., et al. (2006). Rapid high-yield expression of full-size IgG antibodies in plants coinfected with noncompeting viral vectors. Proc. Natl. Acad. Sci. United States America 103, 14701–14706. doi: 10.1073/pnas.0606631103
Goulet, M.-C., Gaudreau, L., Gagné, M., Maltais, A.-M., Laliberté, A.-C., Éthier, G., et al. (2019). Production of biopharmaceuticals in nicotiana benthamiana—Axillary stem growth as a key determinant of total protein yield. Front. Plant Sci. 10. doi: 10.3389/fpls.2019.00735
Gruber, M. F. (2022). Current status of monkeypox vaccines. NPJ Vaccines 7, 94. doi: 10.1038/s41541-022-00527-4
Gu, X., Zhang, Y., Jiang, W., Wang, D., Lu, J., Gu, G., et al. (2022). Protective human anti-poxvirus monoclonal antibodies are generated from rare memory B cells isolated by multicolor antigen tetramers. Vaccines (Basel) 10. doi: 10.3390/vaccines10071084
Jentarra, G. M., Heck, M. C., Youn, J. W., Kibler, K., Langland, J. O., Baskin, C. R., et al. (2008). Vaccinia viruses with mutations in the E3L gene as potential replication-competent, attenuated vaccines: Scarification vaccination. Vaccine 26, 2860–2872. doi: 10.1016/j.vaccine.2008.03.044
Jugler, C., Grill, F. J., Eidenberger, L., Karr, T. L., Grys, T. E., Steinkellner, H., et al. (2022a). Humanization and expression of IgG and IgM antibodies in plants as potential diagnostic reagents for Valley Fever. Front. Plant Sci. 13. doi: 10.3389/fpls.2022.925008
Jugler, C., Joensuu, J., Chen, Q. (2020). Hydrophobin-protein A fusion protein produced in plants efficiently purified an anti-west nile virus monoclonal antibody from plant extracts via aqueous two-phase separation. Int. J. Mol. Sci. 21, 2140. doi: 10.3390/ijms21062140
Jugler, C., Sun, H., Chen, Q. (2021). SARS-CoV-2 spike protein-induced interleukin 6 signaling is blocked by a plant-produced anti-interleukin 6 receptor monoclonal antibody. Vaccines 9, 1365. doi: 10.3390/vaccines9111365
Jugler, C., Sun, H., Grill, F., Kibler, K., Esqueda, A., Lai, H., et al. (2022b). Potential for a plant-made SARS-CoV-2 neutralizing monoclonal antibody as a synergetic cocktail component. Vaccines 10. doi: 10.3390/vaccines10050772
Jugler, C., Sun, H., Nguyen, K., Palt, R., Felder, M., Steinkellner, H., et al. (2023). A novel plant-made monoclonal antibody enhances the synergetic potency of an antibody cocktail against the SARS-CoV-2 Omicron variant. Plant Biotechnol. J. 21, 549–559. doi: 10.1111/pbi.13970
Kellner, C., Otte, A., Cappuzzello, E., Klausz, K., Peipp, M. (2017). Modulating cytotoxic effector functions by fc engineering to improve cancer therapy. Transfus Med. Hemother 44, 327–336. doi: 10.1159/000479980
Klimyuk, V., Pogue, G., Herz, S., Butler, J., Haydon, H. (2014). Production of recombinant antigens and antibodies in Nicotiana benthamiana using 'magnifection' technology: GMP-compliant facilities for small- and large-scale manufacturing. Curr. Top. Microbiol. Immunol. 375, 127–154. doi: 10.1007/82_2012_212
Lai, H., Chen, Q. (2012). Bioprocessing of plant-derived virus-like particles of Norwalk virus capsid protein under current Good Manufacture Practice regulations. Plant Cell Rep. 31, 573–584. doi: 10.1007/s00299-011-1196-6
Lai, H., Engle, M., Fuchs, A., Keller, T., Johnson, S., Gorlatov, S., et al. (2010). Monoclonal antibody produced in plants efficiently treats West Nile virus infection in mice. Proc. Natl. Acad. Sci. United States America 107, 2419–2424. doi: 10.1073/pnas.0914503107
Lai, H., He, J., Hurtado, J., Stahnke, J., Fuchs, A., Mehlhop, E., et al. (2014). Structural and functional characterization of an anti-West Nile virus monoclonal antibody and its single-chain variant produced in glycoengineered plants. Plant Biotechnol. J. 12, 1098–1107. doi: 10.1111/pbi.2014.12.issue-8
Law, M., Smith, G. L. (2001). Antibody neutralization of the extracellular enveloped form of vaccinia virus. Virology 280, 132–142. doi: 10.1006/viro.2000.0750
Leuzinger, K., Dent, M., Hurtado, J., Stahnke, J., Lai, H., Zhou, X., et al. (2013). Efficient agroinfiltration of plants for high-level transient expression of recombinant proteins. J. Visualized Experiments 77. doi: 10.3791/50521
Lum, F.-M., Torres-Ruesta, A., Tay, M. Z., Lin, R. T. P., Lye, D. C., Rénia, L., et al. (2022). Monkeypox: disease epidemiology, host immunity and clinical interventions. Nat. Rev. Immunol. 22, 597–613. doi: 10.1038/s41577-022-00775-4
Lustig, S., Fogg, C., Whitbeck, J. C., Moss, B. (2004). Synergistic neutralizing activities of antibodies to outer membrane proteins of the two infectious forms of vaccinia virus in the presence of complement. Virology 328, 30–35. doi: 10.1016/j.virol.2004.07.024
Manes, N. P., Estep, R. D., Mottaz, H. M., Moore, R. J., Clauss, T. R., Monroe, M. E., et al. (2008). Comparative proteomics of human monkeypox and vaccinia intracellular mature and extracellular enveloped virions. J. Proteome Res. 7, 960–968. doi: 10.1021/pr070432+
Margolin, E., Oh, Y. J., Verbeek, M., Naude, J., Ponndorf, D., Meshcheriakova, Y. A., et al. (2020). Co-expression of human calreticulin significantly improves the production of HIV gp140 and other viral glycoproteins in plants. Plant Biotechnol. J. 18, 2109–2117. doi: 10.1111/pbi.v18.10
Mullard, A. (2021). FDA approves 100th monoclonal antibody product. Nat. Rev. Drug Discovery 20, 491–495. doi: 10.1038/d41573-021-00079-7
Nandi, S., Kwong, A. T., Holtz, B. R., Erwin, R. L., Marcel, S., Mcdonald, K. A. (2016). Techno-economic analysis of a transient plant-based platform for monoclonal antibody production. mAbs 8, 1456–1466. doi: 10.1080/19420862.2016.1227901
Pantaleo, G., Correia, B., Fenwick, C., Joo, V. S., Perez, L. (2022). Antibodies to combat viral infections: development strategies and progress. Nat. Rev. Drug Discovery 21, 676–696. doi: 10.1038/s41573-022-00495-3
Payne, L. G. (1980). Significance of extracellular enveloped virus in the in vitro and in vivo dissemination of vaccinia. J. Gen. Virol. 50, 89–100. doi: 10.1099/0022-1317-50-1-89
Perdiguero, B., Blasco, R. (2006). Interaction between vaccinia virus extracellular virus envelope A33 and B5 glycoproteins. J. Virol. 80, 8763–8777. doi: 10.1128/JVI.00598-06
Roberts, K. L., Smith, G. L. (2008). Vaccinia virus morphogenesis and dissemination. Trends Microbiol. 16, 472–479. doi: 10.1016/j.tim.2008.07.009
Rup, B., Alon, S., Amit-Cohen, B. C., Brill Almon, E., Chertkoff, R., Tekoah, Y., et al. (2017). Immunogenicity of glycans on biotherapeutic drugs produced in plant expression systems-The taliglucerase alfa story. PLoS One 12, e0186211. doi: 10.1371/journal.pone.0186211
Saldana, C. S., Kelley, C. F., Aldred, B. M., Cantos, V. D. (2023). Mpox and HIV: a narrative review. Curr. HIV/AIDS Rep. 20, 261–269. doi: 10.1007/s11904-023-00661-1
Sang, Y., Zhang, Z., Liu, F., Lu, H., Yu, C., Sun, H., et al. (2023). Monkeypox virus quadrivalent mRNA vaccine induces immune response and protects against vaccinia virus. Signal Transduction Targeted Ther. 8, 172. doi: 10.1038/s41392-023-01432-5
Schmidt, F. I., Bleck, C. K. E., Mercer, J. (2012). Poxvirus host cell entry. Curr. Opin. Virol. 2, 20–27. doi: 10.1016/j.coviro.2011.11.007
Shaaltiel, Y., Tekoah, Y. (2016). Plant specific N-glycans do not have proven adverse effects in humans. Nat. Biotech. 34, 706–708. doi: 10.1038/nbt.3556
Siegrist, E. A., Sassine, J. (2022). Antivirals with activity against mpox: A clinically oriented review. Clin. Infect. Dis. 76, 155–164. doi: 10.1093/cid/ciac622
Steffen, U., Koeleman, C. A., Sokolova, M. V., Bang, H., Kleyer, A., Rech, J., et al. (2020). IgA subclasses have different effector functions associated with distinct glycosylation profiles. Nat. Commun. 11, 120. doi: 10.1038/s41467-019-13992-8
Strasser, R., Stadlmann, J., Schahs, M., Stiegler, G., Quendler, H., Mach, L., et al. (2008). Generation of glyco-engineered Nicotiana benthamiana for the production of monoclonal antibodies with a homogeneous human-like N-glycan structure. Plant Biotechnol. J. 6, 392–402. doi: 10.1111/j.1467-7652.2008.00330.x
Sumit, M., Dolatshahi, S., Chu, A. A., Cote, K., Scarcelli, J. J., Marshall, J. K., et al. (2019). Dissecting N-glycosylation dynamics in chinese hamster ovary cells fed-batch cultures using time course omics analyses. iScience 12, 102–120. doi: 10.1016/j.isci.2019.01.006
Sun, H., Yang, M., Lai, H., Neupane, B., Teh, A. Y.-H., Jugler, C., et al. (2023). A dual-approach strategy to optimize the safety and efficacy of anti-zika virus monoclonal antibody therapeutics. Viruses 15, 1156. doi: 10.3390/v15051156
Sun, P., Williams, M., Nagabhushana, N., Jani, V., Defang, G., Morrison, B. J. (2019). NK cells activated through antibody-dependent cell cytotoxicity and armed with degranulation/IFN-γ Production suppress antibody-dependent enhancement of dengue viral infection. Sci. Rep. 9, 1109. doi: 10.1038/s41598-018-36972-2
Tamir, H., Noy-Porat, T., Melamed, S., Cherry-Mimran, L., Barlev-Gross, M., Alcalay, R., et al. (2024). Synergistic effect of two human-like monoclonal antibodies confers protection against orthopoxvirus infection. Nat. Commun. 15, 3265. doi: 10.1038/s41467-024-47328-y
Vanderplasschen, A., Mathew, E., Hollinshead, M., Sim, R. B., Smith, G. L. (1998). Extracellular enveloped vaccinia virus is resistant to complement because of incorporation of host complement control proteins into its envelope. Proc. Natl. Acad. Sci. 95, 7544–7549. doi: 10.1073/pnas.95.13.7544
Viner, K. M., Isaacs, S. N. (2005). Activity of vaccinia virus-neutralizing antibody in the sera of smallpox vaccinees. Microbes infection 7, 579–583. doi: 10.1016/j.micinf.2005.02.004
Wang, Y., Yang, K., Zhou, H. (2023). Immunogenic proteins and potential delivery platforms for mpox virus vaccine development: A rapid review. Int. J. Biol. Macromolecules 245, 125515. doi: 10.1016/j.ijbiomac.2023.125515
WHO. (2024). 2022-2024 Monkeypox Outbreak: Global Trends. Available online at: https://worldhealthorg.shinyapps.io/mpx_global/ (Accessed October 10, 2024).
Yang, M., Dent, M., Lai, H., Sun, H., Chen, Q. (2017). Immunization of Zika virus envelope protein domain III induces specific and neutralizing immune responses against Zika virus. Vacccine 35, 4287–4294. doi: 10.1016/j.vaccine.2017.04.052
Yang, M., Sun, H., Lai, H., Neupane, B., Bai, F., Steinkellner, H., et al. (2023). Plant-produced anti-zika virus monoclonal antibody glycovariant exhibits abrogated antibody-dependent enhancement of infection. Vaccines 11, 755. doi: 10.3390/vaccines11040755
Yefet, R., Friedel, N., Tamir, H., Polonsky, K., Mor, M., Cherry-Mimran, L., et al. (2023). Monkeypox infection elicits strong antibody and B cell response against A35R and H3L antigens. iScience 26, 105957. doi: 10.1016/j.isci.2023.105957
Yu, L., Liu, X., Ye, X., Su, W., Zhang, X., Deng, W., et al. (2021). Monoclonal Antibodies against Zika Virus NS1 Protein Confer Protection via Fcγ Receptor-Dependent and -Independent Pathways. mBio 12. doi: 10.1128/mbio.03179-03120
Keywords: Monkeypox virus (MPXV), extracellular enveloped virion (EV), monoclonal antibody, plant-made biologics, plant-made antibody
Citation: Melendez JA, Sun H, Bonner J and Chen Q (2024) Characterization of a plant-derived monoclonal antibody targeting extracellular enveloped virions of Monkeypox virus. Front. Plant Sci. 15:1481452. doi: 10.3389/fpls.2024.1481452
Received: 15 August 2024; Accepted: 10 October 2024;
Published: 01 November 2024.
Edited by:
Kevin Yueju Wang, University of Pikeville, United StatesReviewed by:
Bhabesh Borphukan, Washington State University, United StatesMd Salik Noorani, Jamia Hamdard University, India
Balamurugan Shanmugaraj, Chulalongkorn University, Thailand
Copyright © 2024 Melendez, Sun, Bonner and Chen. This is an open-access article distributed under the terms of the Creative Commons Attribution License (CC BY). The use, distribution or reproduction in other forums is permitted, provided the original author(s) and the copyright owner(s) are credited and that the original publication in this journal is cited, in accordance with accepted academic practice. No use, distribution or reproduction is permitted which does not comply with these terms.
*Correspondence: Qiang Chen, cWlhbmcuY2hlbi40QGFzdS5lZHU=
†These authors have contributed equally to this work