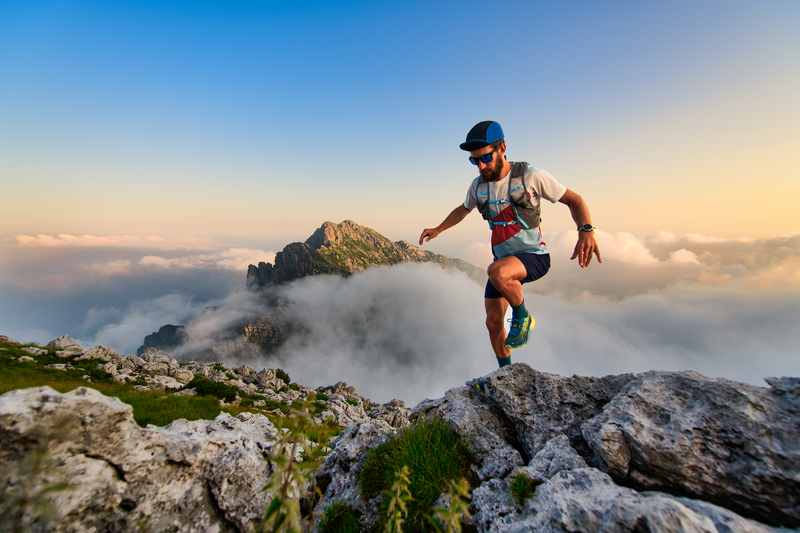
95% of researchers rate our articles as excellent or good
Learn more about the work of our research integrity team to safeguard the quality of each article we publish.
Find out more
ORIGINAL RESEARCH article
Front. Plant Sci. , 10 October 2024
Sec. Plant Genetics, Epigenetics and Chromosome Biology
Volume 15 - 2024 | https://doi.org/10.3389/fpls.2024.1475064
Introduction: Zicaitai (Brassica rapa var. purpuraria) is a Brassica variety renowned for its distinctive taste and rich nutritional profile. In recent years, the mitochondrial genomes of several Brassica species have been documented, but the mitogenome of Zicaitai remains unreported.
Methods: In this study, we characterized the Zicaitai mitogenome achieved through the assembly of sequencing reads derived from both the Oxford Nanopore and Illumina platforms. A detailed comparative analysis was carried out with other Brassica species to draw comparisons and contrasts. In-depth analyses of codon usage patterns, instances of RNA editing, and the prevalence of sequence repeats within the mitogenome were also conducted to gain a more nuanced understanding of its genetic architecture. A phylogenetic analysis was performed, utilizing the coding sequences (CDS) from the mitochondrial genome of Zicaitai and that of 20 closely related species/varieties to trace evolutionary connections.
Results: The Zicaitai mitogenome is characterized by a circular structure spanning 219,779 base pairs, and it encompasses a total of 59 genes. This gene set includes 33 protein-coding genes, 23 tRNA genes, and 3 rRNA genes, providing a rich foundation for further genomic study. An analysis of the Ka/Ks ratios for 30 protein-coding genes shared by the mitogenomes of Zicaitai and seven other Brassica species revealed that most of these genes had undergone purifying selection. Additionally, the study explored the migration of genes between the chloroplast and nuclear genomes and the mitogenome, offering insights into the dynamics of genetic exchange within the Brassica genus.
Discussion: The collective results in this study will serve as a foundational resource, aiding future evolutionary studies focused on B. rapa, and contributing to a broader understanding of the complexities of plant evolution.
Brassica is a critically important genus within the Brassicaceae family (Essoh et al., 2020). It encompasses six principal species: Brassica rapa (AA, 2n = 2x = 20), B. oleracea (CC, 2n = 2x = 18), B. nigra (BB, 2n = 2x = 16), B. napus (AACC, 2n = 4x = 38), B. juncea (AABB, 2n = 4x = 36) and B. carinata (BBCC, 2n = 4x = 34) (Koh et al., 2017; Zhang et al., 2022). The genetic interrelations among these species are often depicted as the ‘Triangle of U’ (Wu et al., 2022). Zicaitai (B. rapa var. purpuraria), a variety of B. rapa that originated in Central China as a result of extensive cultivation and domestication (Li et al., 2019), is widely cultivated in southern China. This variety is a popular vegetable nationwide, celebrated for its distinctive flavor profile and substantial nutritional benefits, particularly because of its rich anthocyanin content in the leaves and stalks (Podsędek, 2017; Guo et al., 2015). Anthocyanins, known for their diverse biological roles in plants and their health benefits to humans (Hayashi et al., 2010; Lee et al., 2017; Zhang and Jing, 2022; Nistor et al., 2022), have propelled zicaitai into the spotlight in recent years (Guo et al., 2015; Li et al., 2019; Zhang X. et al., 2020).
Mitochondria are semi-autonomous organelles found in the majority of eukaryotic cells (Ye et al., 2017) and perform a range of essential functions, including energy conversion, tricarboxylic acid cycling, oxidative phosphorylation, calcium ion storage, cell proliferation and metabolism, etc (Hamani and Giege, 2014; Møller et al., 2021). Plant mitochondrial genomes are considerably variable in length, gene arrangement, and gene content (Birky, 1995; Bi et al., 2020), and they contain large repeats that can result in isomerization within a species. Recent studies have also highlighted the frequent gene transfers between nuclear, mitochondrial, and chloroplast genomes as a common phenomenon during plant evolution (Chang et al., 2013; Zhao et al., 2019). Given the substantial impact of plant organellar genomes on metabolism and adaptation, a detailed analysis of their sequences and structures can shed light on the evolutionary history and the intricate interplay between these genomes (Pinard et al., 2019).
Leveraging the advancements in sequencing technology, numerous Brassica mitochondrial genomes have been characterized, including the mitogenomes of several subspecies in B. rapa (Handa, 2003; Chang et al., 2011; Chen et al., 2011; Wu et al., 2019; Shao et al., 2021). However, the situation among the subspecies/varieties of B. rapa remains confusing, especially for the Zicaitai, whose selection and domestication are still unknown. Studying the mitochondrial genome of Zicaitai is of significant value for understanding its evolutionary position within the species, genetic variation, phylogeny, and domestication history of the Chinese cabbage varieties. However, the mitochondrial genome of zicaitai remains largely uncharted territory. In this study, we sequenced and annotated the its mitogenome, conducting a detailed analysis of its genomic features, codon usage, RNA editing, sequence repeats, and comparative genomics. The findings provide an in-depth understanding of the zicaitai mitochondrial genome, which is expected to bolster ongoing research efforts into the evolution of B. rapa.
Zicaitai seeds, designated ‘Youxuan hongshan’ were sourced from the Guangzhou Academy of Agricultural Sciences. Germination of the seeds was initiated at 25°C within a growth chamber, followed by transplantation into a greenhouse under a 16/8-hour light-dark photoperiod at a temperature of 25°C, for a 6-week growth. Twenty grams of zicaitai leaf tissue from one single well-grown seedling was then harvested, promptly transported using dry ice, and dispatched to Genepioneer Biotechnologies Company in Nanjing, China. The integrity of the DNA samples was assessed via agarose gel electrophoresis, and their concentrations were quantified using a Nanodrop instrument (model 2000c UV-Vis). Samples meeting the quality criteria were subsequently submitted for sequencing using both Oxford Nanopore and Illumina platforms.
Given the high conservation of plant mitochondrial genes, including coding sequences (CDS) and ribosomal RNA (rRNA), the minimap2 software (version 2.1) (Li, 2018), a tool designed for tri-generational comparison, was utilized. This software compared the original tri-generational data against a reference gene sequence, with preference given to sequences exceeding 50 base pairs in length as candidate sequences. The seed sequence was chosen based on its enrichment in core genes and superior relative quality, indicating a more complete representation of the core genes. Subsequently, minimap2 was employed to align the original sequencing data with the seed sequence, and sequences with an overlap exceeding 1 kilobase and a similarity higher than 70% were integrated into the seed sequence. This iterative comparison of the original data with the seed sequence facilitated the acquisition of comprehensive mitochondrial genome data. The tri-generational assembly software Canu (Koren et al., 2017) was then applied to refine the obtained data. Bowtie2 (version 2.3.5.1) (Langmead and Salzberg, 2012) was used to align the second-generation data with the corrected sequence, followed by the application of Unicycler (version 0.4.8) (Wick et al., 2017) to align the second-generation data with the corrected tri-generational data. The final assembly was manually curated to determine the orientation of the branches. The mitochondrial genome map was generated using OGDRAW (https://chlorobox.mpimp-golm.mpg.de/OGDraw.html) (Lohse et al., 2007). Mitochondrial annotation proceeded through the following steps: (1) BLAST was employed to align published plant mitochondrial sequences with their respective encoded proteins and rRNA, with subsequent manual refinements based on related species; (2) Transfer RNA (tRNA) was annotated using tRNAscanSE (http://lowelab.ucsc.edu/tRNAscan-SE/) (Chan and Lowe, 2019); (3) Open Reading Frames (ORFs) were annotated with the Open Reading Frame Finder (http://www.ncbi.nlm.nih.gov/gorf/gorf.html), considering the shortest length of 102 base pairs. For the purpose of collinearity analysis, the nucmer (4.0.0beta2) software was executed utilizing the “–maxmatch” parameter to facilitate genomic alignments between the assembled mitochondrial sequence of zicaitai and those of related species or varieties, resulting in the creation of Dot-plot graphs.
Dispersed repeats were identified using the REPuter tool (Kurtz et al., 2001); tandem repeats were detected with the Tandem Repeats Finder software (trf409.linux64: 2 7 7 80 10 50 2000 -f -d -m) (Benson, 1999); and simple sequence repeats (SSRs) were analyzed using the MISA program (Beier et al., 2017), with a minimum distance between SSRs set at 100 base pairs and the motif size for one- to six-nucleotide SSRs set to 10, 5, 4, 3, 3, and 3, respectively.
The codon composition within the mitochondrial genome of zicaitai was examined using a custom Perl script, which was designed to identify unique coding sequences (CDS), determine the number of codons per gene, and calculate the Relative Synonymous Codon Usage (RSCU) for synonymous codons. RNA editing sites in the protein-coding genes (PCGs) of zicaitai and seven other Brassica mitogenomes (Brassica carinata, B. juncea, B. napus, B. oleracea, B. rapa subsp. Oleifera, B. nigra, and B. rapa subsp. Nipposinica) were predicted using the online resource http://prep.unl.edu/ (Mower, 2005).
The nonsynonymous (Ka) and synonymous (Ks) substitution rates for each PCG beteen zicaitai and seven other reported Brassica species (B. rapa subsp. nipposinica, B. rapa subsp. oleifera, B. juncea, B. napus, B. oleracea, B. carinata and B. nigra) were calculated using DnaSP (Librado, 2009). A total of 21 complete mitogenomes (Supplementary Table S5) were utilized to determine the phylogenetic position of zicaitai. The maximum likelihood evolutionary tree was constructed using 30 conserved mitochondrial PCG genes (atp1, atp4, atp6, atp8, atp9, ccmB, ccmC, ccmFc, ccmFn, cob, cox1, cox2, cox3, matR, nad1, nad2, nad3, nad4, nad4L, nad5, nad6, nad7, nad9, rpl2, rpl5, rps12, rps14(-), rps3, rps4, and rps7) present across the 21 analyzed species. The mafft software (version 7.427, –auto mode) was employed for multiple sequence alignment of inter-species sequences. The aligned sequences were concatenated, and trimAl (version 1.4, -0.7 gt) was used for refinement. The jmodeltest-2.1.10 software was then utilized to select the best-fit model, which was identified as the GTR model. Finally, the RAxML v8.2.10 software (https://cme.h-its.org/exelixis/software.html) was used with the GTRGAMMA model and a bootstrap value of 1000 to construct the maximum likelihood evolutionary tree.
The zicaitai mitogenome was compared against the chloroplast genome of zicaitai (OP729397.1) using BLASTN 2.9.0+ with the following criteria: a matching rate of ≥70%, an E-value of ≤1e-5, and a sequence length of ≥40 base pairs (Wick et al., 2017). To identify potential regions of nuclear origin within the zicaitai mitogenome, a BLASTN search (with a maximum E-value of 1e-50) was also conducted against all contigs from the previously sequenced zicaitai nuclear genome. Sequences longer than 250 base pairs with a pairwise similarity of >80% were subjected to annotation in the GenBank database to identify their potential nuclear origins.
The mitogenome of zicaitai, as determined through sequencing with both the Oxford Nanopore and Illumina platforms, has been deposited in the GenBank database under the accession number OP729396.1. This complete mitogenome spans a length of 219,779 base pairs (bp) and exhibits the characteristic circular conformation, as depicted in Figure 1. The mitochondrial DNA (mtDNA) nucleotide composition is comprised of adenine (A) at 27.45%, guanine (G) at 22.32%, cytosine (C) at 22.92%, and thymine (T) at 27.31%, with a GC content of 45.24% (Table 1). Protein-coding genes (PCGs), introns, tRNA genes, rRNA genes and non-coding regions account for 13.22%, 12.86%, 0.79%, 2.34% and 70.79% of the whole mitogenome, respectively.
Figure 1. Circular map of the zicaitai mitogenome. Genes shown on the outside and inside of the circle are transcribed clockwise and counterclockwise, respectively. The dark gray region in the inner circle depicts GC content.
The zicaitai mitogenome contains 977 open reading frames (ORFs) and 59 genes, including 33 protein-coding genes, 23 tRNA genes, and 3 rRNA genes (Table 2). The protein-coding genes are categorized into 9 functional groups: 5 for ATP synthases, 4 for cytochrome c biogenesis, 1 for ubiquinol cytochrome c reductase, 3 for cytochrome c oxidase, 1 for maturase, 1 for transport membrane protein, 9 for NADH dehydrogenase, 4 for LSU ribosomal proteins, and 5 for SSU ribosomal proteins. Additionally, three tRNA genes (trnH-GTG, trnM-CAT, trnY-GTA) occur in two or three copies and are located within repeat sequences (Figure 1).
Mitogenomic sequence-based collinearity analysis has uncovered a high degree of collinearity between the mitogenome of zicaitai and those of B. rapa subsp. nipposinica, B. rapa subsp. oleifera, and notably, B. juncea, which is a distinct species from zicaitai, exhibiting minimal genomic rearrangement characteristics. In contrast, substantial rearrangements were observed when comparing zicaitai with other Brassica species (Supplementary Figure S1).
The zicaitai mitogenome’s protein-coding genes (PCGs) span a total of 29,055 bp. The majority of these PCGs initiate with the standard ATG start codon and terminate with the common stop codons TAA, TGA, or TAG. Exceptions include the nad1 gene with an ACG start codon and the ccmFc gene with a CGA stop codon; both exhibit the phenomenon of C to U RNA editing (Table 2).
Additionally, we examined the relative synonymous codon usage (RSCU) for the 33 PCGs in the zicaitai mitogenome (Figure 2). Excluding termination codons, these 33 PCGs encode for 9,652 codons. The analysis indicated that leucine (Leu) (10.91%), serine (Ser) (8.87%), and arginase (Arg) (6.48%) are the most frequently used amino acids, as shown in Supplementary Table S1. The RSCU values for nearly all NNT and NNA codons exceeded 1.0, with the exceptions of Ala (GCA, 0.96), Thr (ACA, 0.91), Leu (CUA, 0.89), and Ile (AUA, 0.84). A strong bias toward T (U) or A at the third position of the codons in the zicaitai can be observed, a pattern commonly found in land plant mitogenomes (Ma et al., 2022).
Figure 2. Relative synonymous codon usage (RSCU) in the zicaitai mitogenome. Codon families are shown on the x-axis. RSCU values represent the observed frequency of a specific codon in relation to the expected frequency if synonymous codons were used uniformly.
In the field of genetics, the ratio of nonsynonymous to synonymous substitutions (Ka/Ks) serves as a valuable tool for elucidating the evolutionary dynamics of genes. In this study, we calculated the Ka/Ks ratio for 30 protein-coding genes (PCGs) shared by zicaitai and seven other Brassica species (B. rapa subsp. nipposinica, B. rapa subsp. oleifera, B. juncea, B. napus, B. oleracea, B. carinata and B. nigra) (Figure 3; Supplementary Table S2). The PCGs were found to be highly homologous among zicaitai, B. rapa subsp. nipposinica, B. rapa subsp. oleifera, and B. oleracea, as evidenced by a Ka/Ks ratio of zero for these 30 PCGs. Moreover, the majority of Ka/Ks ratios were below 1.0, implying that these genes have been predominantly under the influence of purifying selection throughout their evolutionary history. In contrast, a single gene, rpl2, exhibited a Ka/Ks ratio exceeding 1.0, indicating that it has been subject to positive selection. Lastly, two genes, atp6 and nad2, displayed Ka/Ks ratios approaching 1, suggesting that they have undergone neutral evolution since the time of their common ancestor’s divergence.
Figure 3. Ka/Ks ratios of 30 protein-coding genes in zicaitai in comparison with B. rapa subsp. nipposinica, B. rapa subsp. Oleifera, B. juncea, B. napus, B. oleracea, B. carinata and B. nigra, respectively.
An analysis of long repeats in the zicaitai mitogenome identified 252 dispersed repeats, comprising 108 forward (42.86%) and 144 palindromic (57.14%) repeats; no reverse or complementary repeats were detected. The total length of these long repeats was 16,251 bp, accounting for 7.39% of the length of the complete mitogenome. The average length of the repeats was 64.49 bp, with the longest repeat spanning 2,427 bp. The majority of the repeats (153 repeats, 60.71%) were within the range of 30 to 50 bp in length (Supplementary Table S3).
Tandem repeat sequences, also known as satellite DNAs, are formed by the sequential arrangement of short sequences that serve as repeating units, typically ranging from 1 to 200 bases in length. As detailed in Table 3, a total of 12 tandem repeats were identified in the zicaitai mitogenome, with lengths varying from 12 to 39 bp, and all of them exhibited a match degree exceeding 95%.
Microsatellites, also known as simple sequence repeats (SSRs), are DNA fragments ranging from 1 to 6 bp in length and are useful molecular markers for studying genetic diversity and identifying species (Ma et al., 2022). In our research, we identified 55 SSRs in the zicaitai mitogenome, comprising 20 mono-, 11 di-, 5 tri-, 18 tetra-, and 1 pentanucleotide repeat (Table 4). Over 89% of these SSRs were mono-, di-, or pentanucleotide types. Detailed analysis showed that the A/T repeat accounted for 32.73% (18/55) of the SSRs, followed by the AG/CT repeat at 12.73% (7/55) and the AATG/ATTC repeat at 9.09% (5/55). This is different from the most abundant SSR types in B. rapa’s nuclear genome, where A/T and AT/TA repeats are predominant (Gao et al., 2022).
A total of 378 RNA editing sites were identified in the zicaitai mitogenome, all featuring C-to-U changes (Table 5). Of these sites, 30.42% (115/378) were located at the first position and 65.34% (247/378) at the second position of codons, while a small fraction, 4.23% (16/378), were found at both positions. There was significant variation in the number of RNA editing sites across different genes, with the highest counts observed in the NADH dehydrogenase genes (nad4, nad5, nad1, nad2, and nad7) and those involved in cytochrome c biogenesis (ccmB and ccmC). In contrast, no RNA editing sites were detected in the atp8 and cox1 genes of zicaitai (Supplementary Table S4).
We examined RNA editing sites across 33 protein-coding genes (PCGs) present in the mitogenomes of zicaitai and seven other Brassica species (Figure 4), and predicted a total of 2,823 RNA editing sites, with counts varying from 308 in B. nigra to 397 in both B. rapa subsp. Oleifera and B. oleracea. Zicaitai and B. juncea had the most similar counts, with 378 and 375 sites respectively.
Figure 4. Statistic of RNA editing sites in 33 protein-coding genes present in the mitogenomes of zicaitai and other Brassica species.
To ascertain the phylogenetic position of zicaitai, we retrieved 20 plant mitogenomes from GenBank (https://www.ncbi.nlm.nih.gov/genome/browse/) and constructed a phylogenetic tree using a set of 30 conserved single-copy orthologous genes that are present across all 21 analyzed mitogenomes (Supplementary Table S5). As depicted in Figure 5, 13 out of 18 nodes within the constructed tree had bootstrap support values exceeding 70%, with 9 nodes achieving full support at 100%. The phylogenetic analysis robustly endorses the close phylogenetic ties between species in genus Brassica. Furthermore, it also indicated a close relationship between B. rapa and B. napus, aligning with the findings of Chang et al. (2011), who suggested that B. napus possesses a primary mitotype highly similar to that of B. rapa. Collectively, our mitogenomic analysis lays a valuable groundwork for future studies on the phylogenetic relationships within the Brassica genus.
Figure 5. Maximum-likelihood phylogenetic tree based on the sequences of 30 single-copy orthologous genes shared by 21 species in genus Brassica. Numbers at nodes are bootstrap support values. The position of zicaitai (B. rapa var. Purpuraria) is indicated in bold.
There are six potential directions for gene transfer between nuclear, mitochondrial and chloroplast genomes during plant evolution. To gain a deeper understanding of the sequence transfer events in zicaitai, we conducted a search using the zicaitai mitogenome sequences as queries against the zicaitai nuclear and chloroplast genomes. Our search yielded 1040 hits, encompassing 267,131 base pairs (bp) of sequences shared by both the nuclear genome and the mitogenome (Supplementary Table S6). Based on the alignment between the nuclear and mitochondrial genomes, we observed hits across every chromosome of zicaitai (Figure 6). However, the total lengths of these hits and their respective coverage percentages varied among the chromosomes. Notably, Chromosome 6 exhibited the longest cumulative length of hits (137,674 bp), significantly surpassing the lengths found on the other chromosomes.
Figure 6. Homologous fragments identified between zicaitai mitogenome and chromosomes (left) and between the mitogenome and chloroplast genome (right). Zicaitai’s ten chromosomes are labeled numerically from 1 to 10 on the left circle. Both circles depict the linkage of homologous fragments across various genome types, connected by curves.
The zicaitai mitogenome sequence, spanning 219,779 bp, is approximately 1.43 times the length of its chloroplast genome (153,621 bp). We identified 22 fragments totaling 13,323 bp, representing 6.06% of the mitogenome, that appear to be the result of gene exchange between the chloroplast genome and the mitogenome of zicaitai (Table 6). These fragments encompass seven complete chloroplast genes: ycf15, trnL-CAA, trnN-GUU, trnW-CCA, trnD-GUC, trnM-CAU, and trnI-CAU. The remaining fragments consist of partial gene sequences or intergenic spacer regions in the chloroplast genome. Interestingly, our analysis revealed that DNA migration frequently occurred within the inverted repeat region of the zicaitai chloroplast genome.
Mitochondria are the energy-producing factories essential for life processes. Plant mitogenomes have more complex structures than those of animals due to size variation, the presence of repeated sequences and other factors (Kozik et al., 2019; Cheng et al., 2021). The availability of rapid and cost-effective genome sequencing technologies has significantly advanced our understanding of mitogenomes. In this study, we characterized for the first time the complete mitogenome of zicaitai, a distinctive B. rapa variety. We found that the zicaitai mitogenome has a similar size and GC content to that of other Brassica species (Handa, 2003; Chang et al., 2011) and exhibits high degree of collinearity with the mitogenomes of B. rapa subsp. nipposinica, B. rapa subsp. oleifera, and a distinct species, B. juncea, distinguishing zicaitai from other Brassica species (Supplementary Figure S1). Similar to most other mitogenomes, most sequences in the zicaitai mitogenome are non-coding. The zicaitai mitogenome, like many others, predominantly consists of non-coding sequences, with protein-coding genes (PCGs) making up 13.22%. This is similar to B. rapa subsp. nipposinica (12.36%), B. juncea (14.85%), and B. oleracea (13.44%), yet significantly lower than the 20-23% found in other closely related Brassica species/varieties. Intriguingly, zicaitai, although belonging to the same species as B. rapa subsp. oleifera, exhibits a lower proportion of protein-encoding sequences compared to the latter (22.99%), suggesting an ongoing process of genetic differentiation within the species, likely driven by domestication.
Our research showed that in the zicaitai mitogenome, all protein-coding genes (PCGs) initiate with the standard ATG start codon, with the sole exception of the nad1 gene, which starts with ACG - a common occurrence in angiosperms due to RNA editing (Ye et al., 2017; Bi et al., 2020; Ma et al., 2022). In plants, RNA editing is crucial for gene expression, particularly with the cytidine (C) to uridine (U) transition, which is prevalent in mitochondrial and chloroplast genomes (Ma et al., 2022). We also identified the cox1 gene, which is known to involve in horizontal gene transfer among angiosperms and vary in its copy number across species as well as populations within a species (Zhang C. et al., 2020). Further studies are needed to clarify the role played by the cox1 gene in Brassica evolution. A single gene (rpl2) was discovered in this study to present a Ka/Ks ratio exceeding 1.0, consistent with the previous research on other mitochondrial genes with similarly elevated ratios (Ye et al., 2017; Bi et al., 2016, 2020). The significance of the rpl2 gene in gene selection and the evolutionary process of Brassica species/varieties warrants further investigation.
Repeats, encompassing tandem, short, and large types, are crucial for identifying markers in population and evolutionary studies and are prevalent in mitogenomes (Bi et al., 2016; Ma et al., 2017; Liu et al., 2021). They play key roles in intermolecular recombination within mitochondrial DNA, leading to structural variations and diverse mitogenome sizes (Alverson et al., 2010; Guo et al., 2016). In this study, we found an abundance of repeats in the zicaitai mitogenome, notably one large repeat of 2,427 bp. This repeat is identical in size to the one found in the B. oleracea mitogenome, suggesting a close genetic link between B. rapa and B. oleracea, as depicted in Figure 5. Our discovery of SSRs in zicaitai’s organelle genome provides a valuable complement to Gao et al. (2022)’s research, which identified 173,892 SSRs within the nuclear genome of B. rapa.
Despite variations in RNA editing site counts across zicaitai and various Brassica species or varieties, genes for NADH dehydrogenase and cytochrome c biogenesis consistently exhibit the highest editing frequencies among these species/varieties (Figure 6). This pattern mirrors observations from previous studies on the mitogenomes of other plant species (Ma et al., 2022). Furthermore, all identified RNA editing sites in the zicaitai mitogenomes are situated at the first and/or second codon positions. It has been hypothesized by previous researchers that the absence of editing sites at the third codon position may be attributed to the limitations of the PREP-Mt predictive method, rather than their non-existence (Mower, 2005; Bi et al., 2020). Consequently, further investigation employing experimental techniques is warranted to clarify this issue.
Previous research has identified the predominant gene transfer in angiosperms as from organellar to nuclear genomes, with secondary importance given to transfers between nuclear, plastid, and mitochondrial genomes (Martin et al., 1998; Rice et al., 2013; Zhao et al., 2018, 2019; Grewe et al., 2014). In higher plants, the total length of transferred DNA varies from 50 kb in Arabidopsis thaliana to 1.1 Mb in rice (Smith et al., 2011). Our study has identified a cumulative 267 kb of DNA sequences transferred from 10 chromosomes into the zicaitai mitogenome, with variations in total length and coverage among the chromosomes. Chromosome 6 contributes the largest portion, amounting to 138 kb, which is 62.6% of the mitogenome’s total length. In contrast, sequences from the chloroplast genome make up only 6.1% of the zicaitai mitogenome. Future studies should explore the coevolution of this chromosome with the mitogenome and its potential implications.
In this study, we found 11 tRNA and 3 rRNA genes common to both the nuclear and mitochondrial genomes in zicaitai (Supplementary Table S6) and six tRNA genes shared by the chloroplast genome and the mitogenome. While nuclear-to-mitogenome transfers of tRNA genes have been documented in other plants (Chang et al., 2013; Ma et al., 2022), and chloroplast-to-mitogenome transfers are typical in angiosperms (Bergthorsson et al., 2003; Cheng et al., 2021; Bi et al., 2016), the more extensive migration of these genes in zicaitai suggests a more fluid genetic exchange between different cellular compartments than previously thought. Future research should delve into the mechanisms and evolutionary implications of this genetic fluidity.
We for the first time conducted a comprehensive characterization of the zicaitai mitogenome, which spans a length of 219,779 bp and encompasses a total of 59 genes, including 33 protein-coding genes, 23 tRNA genes, and 3rRNA genes. The protein-coding genes (PCGs), cis introns, tRNA genes, and rRNA genes constitute 13.22%, 12.86% 0.79% and 2.34%, of the zicaitai mitogenome, respectively. The Ka/Ks analysis showed that among 30 shared mitochondrial PCGs in zicaitai and other Brassica species, most have experienced purifying selection. Only the rpl2 gene showed signs of positive selection, and two genes, atp6 and nad2, exhibited neutral evolution. Phylogenetic analysis based on these 30 PCGs from zicaitai and 20 related species/varieties indicated that B. rapa is most closely related to B. napus, with B. oleracea being the next closest. Furthermore, we detected the migration of genes between the chloroplast and nuclear genomes and the zicaitai mitogenome. The collective findings in this study offer an in-depth understanding of the zicaitai mitogenome, which is instrumental for advancing evolutionary studies within the species B. rapa.
The original mitochondrial genome presented in the study are publicly available. This data can be found in NCBI (https://www.ncbi.nlm.nih.gov/) under the GenBank: OP729396.1 (https://www.ncbi.nlm.nih.gov/nuccore/OP729396.1). The data are publicly available. The datasets presented in this study can be found in NCBI.
WX: Data curation, Formal analysis, Investigation, Writing – original draft. XW: Data curation, Investigation, Writing – original draft. XZ: Investigation, Writing – original draft. JZ: Formal analysis, Funding acquisition, Writing – original draft. JH: Data curation, Writing – review & editing. XD: Funding acquisition, Supervision, Writing – original draft. HR: Conceptualization, Data curation, Writing – original draft. DX: Conceptualization, Writing – review & editing.
The author(s) declare financial support was received for the research, authorship, and/or publication of this article. This work is supported by Guangdong Seed Industry Revitalization Project (2022-NJS-03-001), Guangdong Vegetable Germplasm Resource Bank (Nursery) Operation and Maintenance (2023-KYXM221), and Quality Supervision and Inspection of Crop Seeds in Guangzhou City in 2024 (2024-KYXM726).
The authors declare that the research was conducted in the absence of any commercial or financial relationships that could be construed as a potential conflict of interest.
All claims expressed in this article are solely those of the authors and do not necessarily represent those of their affiliated organizations, or those of the publisher, the editors and the reviewers. Any product that may be evaluated in this article, or claim that may be made by its manufacturer, is not guaranteed or endorsed by the publisher.
The Supplementary Material for this article can be found online at: https://www.frontiersin.org/articles/10.3389/fpls.2024.1475064/full#supplementary-material
Supplementary Figure 1 | Collinearity analysis of mitogenomic sequences between zicatai and some related species/varieties. In each box, the horizontal axis represents the assembled zicaitai’s mitogenomic sequence, and the vertical axis represents the that of related species/varieties. The red lines within the boxes indicate forward alignments, while the blue lines indicate reverse complement alignments.
Alverson, A. J., Wei, X., Rice, D. W., Stern, D. B., Barry, K., Palmer, J. D. (2010). Insights into the evolution of mitochondrial genome size from complete sequences of Citrullus lanatus and Cucurbita pepo (Cucurbitaceae). Mol. Biol. Evol. 27, 1436–1448. doi: 10.1093/molbev/msq029
Beier, S., Thiel, T., Münch, T., Scholz, U., Mascher, M. (2017). MISA-web: a web server for microsatellite prediction. Bioinformatics 33, 2583–2585. doi: 10.1093/bioinformatics/btx198
Benson, G. (1999). Tandem repeats finder: a program to analyze DNA sequences. Nucleic Acids Res. 27, 573–580. doi: 10.1093/nar/27.2.573
Bergthorsson, U., Adams, K., Thomason, B., Palmer, J. (2003). Widespread horizontal transfer of mitochondrial genes in flowering plants. Nature 424, 197–201. doi: 10.1038/nature01743
Bi, C., Lu, N., Xu, Y., He, C. (2020). Characterization and analysis of the mitochondrial genome of common bean (Phaseolus vulgaris) by comparative genomic approaches. Int. J. Mol. Sci. 21, 3778. doi: 10.3390/ijms21113778
Bi, C., Paterson, A., Wang, X., Xu, Y., Wu, D., Qu, Y., et al. (2016). Analysis of the complete mitochondrial genome sequence of the diploid cotton Gossypium raimondii by comparative genomics approaches. BioMed. Res. Int. 2016, 5040598. doi: 10.1155/2016/5040598
Birky, C. (1995). Uniparental inheritance of mitochondrial and chloroplast genes: mechanisms and evolution. PNAS 92, 11331–11338. doi: 10.1073/pnas.92.25.11331
Chan, P. P., Lowe, T. M. (2019). tRNAscan-SE: searching for tRNA genes in genomic sequences. Methods Mol. Biol. 1962, 1–14. doi: 10.1007/978-1-4939-9173-0_1
Chang, S., Wang, Y., Lu, J., Gai, J., Li, J., Chu, P., et al. (2013). The mitochondrial genome of soybean reveals complex genome structures and gene evolution at intercellular and phylogenetic levels. PloS One 8, e56502. doi: 10.1371/journal.pone.0056502
Chang, S., Yang, T., Du, T., Huang, Y., Chen, J., Yan, J., et al. (2011). Mitochondrial genome sequencing helps show the evolutionary mechanism of mitochondrial genome formation in Brassica. BMC Genomics 12, 497. doi: 10.1186/1471-2164-12-497
Chen, J., Guan, R., Chang, S., Du, T., Zhang, H., Xing, H. (2011). Substoichiometrically different mitotypes coexist in mitochondrial genomes of Brassica napus L. PloS One 6, e17662. doi: 10.1371/journal.pone.0017662
Cheng, Y., He, X., Priyadarshani, S., Wang, Y., Ye, L., Qin, Y. (2021). Assembly and comparative analysis of the complete mitochondrial genome of Suaeda glauca. BMC Genomics 22, 167. doi: 10.1186/s12864-021-07490-9
Essoh, A. P., Monteiro, F., Pena, A. R., Pais, M. S., Moura, M., Romeiras, M. M. (2020). Exploring glucosinolates diversity in Brassicaceae: a genomic and chemical assessment for deciphering abiotic stress tolerance. Plant Physiol. Biochem. 150, 151–161. doi: 10.1016/j.plaphy.2020.02.032
Gao, Y., Li, N., Li, X., Lu, Y., Feng, D., Zhang, X., et al. (2022). Genome-wide development and utilization of Simple Sequence Repeats in Chinese cabbage (Brassica rapa L.ssp.pekinensis). Vegetable Res. 2, 9. doi: 10.48130/VR-2022-0009
Grewe, F., Edger, P. P., Keren, I., Sultan, L., Pires, J. C., Ostersetzer-Biran, O., et al. (2014). Comparative analysis of 11 Brassicales mitochondrial genomes and the mitochondrial transcriptome of Brassica oleracea. Mitochondrion 19 Part B, 135–143. doi: 10.1016/j.mito.2014.05.008
Guo, N., Wu, J., Zheng, S. (2015). Anthocyanin profile characterization and quantitative trait locus mapping in zicaitai (Brassica rapa L. ssp. chinensis var. purpurea). Mol. Breed. 35, 113. doi: 10.1007/s11032-015-0237-1
Guo, W., Felix, G., Fan, W., Young, G. (2016). Ginkgo and Welwitschia Mitogenomes reveal extreme contrasts in gymnosperm mitochondrial evolution. Mol. Biol. Evol. 33, 1448–1460. doi: 10.1093/molbev/msw024
Hamani, K., Giege, P. (2014). RNA metabolism in plant mitochondria. Trends Plant Sci. 19, 380–389. doi: 10.1016/j.tplants.2013.12.008
Handa, H. (2003). The complete nucleotide sequence and RNA editing content of the mitochondrial genome of rapeseed (Brassica napus L.): comparative analysis of the mitochondrial genomes of rapeseed and Arabidopsis thaliana. Nucleic Acids Res. 31, 5907–5916. doi: 10.1093/nar/gkg795
Hayashi, K., Matsumoto, S., Tsukazaki, H., Kondo, T. (2010). Mapping of a novel locus regulating anthocyanin pigmentation in Brassica rapa. Breed. Sci. 60, 76–80. doi: 10.1270/jsbbs.60.76
Koh, J. C. O., Barbulescu, D. M., Norton, S., Redden, B., Salisbury, P. A., Kaur, S., et al. (2017). A multiplex PCR for rapid identification of Brassica species in the triangle of U. Plant Methods 13, 49. doi: 10.1186/s13007-017-0200-8
Koren, S., Walenz, B. P., Berlin, K., Miller, J. R., Bergman, N. H., Phillippy, A. M. (2017). Canu: scalable and accurate long-read assembly via adaptive k-mer weighting and repeat separation. Genome Res. 27, 722–736. doi: 10.1101/gr.215087.116
Kozik, A., Rowan, B., Lavelle, D., Berke, L., Schranz, M., Michelmore, R. (2019). The alternative reality of plant mitochondrial DNA: one ring does not rule them all. PloS Genet. 15, e1008373. doi: 10.1371/journal.pgen.1008373
Kurtz, S., Choudhuri, J., Enno, O., Chris, S., Jens, S., Robert, G. (2001). REPuter: the manifold applications of repeat analysis on a genomic scale. Nucleic Acids Res. 29, 4633–4642. doi: 10.1093/nar/29.22.4633
Langmead, B., Salzberg, S. (2012). Fast gapped-read alignment with Bowtie 2. Nat. Methods 9, 357–359. doi: 10.1038/nmeth.1923
Lee, Y. M., Yoon, Y., Yoon, H., Park, H. M., Song, S., Yeum, K. J. (2017). Dietary anthocyanins against obesity and inflammation. Nutrients 9, 1089. doi: 10.3390/nu9101089
Li, G. H., Chen, H. C., Liu, J. L., Luo, W. L., Xie, D. S., Luo, S. B., et al. (2019). A high-density genetic map developed by specific-locus amplified fragment (SLAF) sequencing and identification of a locus controlling anthocyanin pigmentation in stalk of Zicaitai (Brassica rapa L. ssp. chinensis var. purpurea). BMC Genomics 20, 343. doi: 10.1186/s12864-019-5693-2
Li, H. (2018). Minimap2: pairwise alignment for nucleotide sequences. Bioinformatics 34, 3094–3100. doi: 10.1093/bioinformatics/bty191
Librado, R. J. (2009). DnaSP v5: a software for comprehensive analysis of DNA polymorphism data. Bioinformatics 25, 1451–1452. doi: 10.1093/bioinformatics/btp187
Liu, L., Fan, X., Tan, P., Wu, J., Zhang, H., Han, C., et al. (2021). The development of SSR markers based on RNA-sequencing and its validation between and within Carex L. species. BMC Plant Biol. 21, 17. doi: 10.1186/s12870-020-02792-8
Lohse, M., Drechsel, O., Bock, R. (2007). OrganellarGenomeDRAW (OGDRAW): a tool for the easy generation of high-quality custom graphical maps of plastid and mitochondrial genomes. Curr. Genet. 52, 267–274. doi: 10.1007/s00294-007-0161-y
Ma, Q., Li, S., Bi, C., Hao, Z., Sun, C., Ye, N. (2017). Complete chloroplast genome sequence of a major economic species, Ziziphus jujuba (Rhamnaceae). Curr. Genet. 63, 117–129. doi: 10.1007/s00294-016-0612-4
Ma, Q., Wang, Y., Li, S., Wen, J., Zhu, L., Yan, K., et al. (2022). Assembly and comparative analysis of the first complete mitochondrial genome of Acer truncatum Bunge: a woody oil-tree species producing nervonic acid. BMC Plant Biol. 22, 29. doi: 10.1186/s12870-021-03416-5
Martin, W., Stoebe, B., Goremykin, V., Hansmann, S., Hasegawa, M., Kowallik, K. V. (1998). Gene transfer to the nucleus and the evolution of chloroplasts. Nature 393, 162–165. doi: 10.1038/30234
Møller, I. M., Rasmusson, A. G., Van Aken, O. (2021). Plant mitochondria - past, present and future. Plant J. 108, 912–959. doi: 10.1111/tpj.15495
Mower, J. (2005). PREP-Mt: predictive RNA editor for plant mitochondrial genes. BMC Bioinf. 6, 96. doi: 10.1186/1471-2105-6-96
Nistor, M., Pop, R., Daescu, A., Pintea, A., Socaciu, C., Rugina, D. (2022). Anthocyanins as key phytochemicals acting for the prevention of metabolic diseases: an overview. Molecules 27, 4254. doi: 10.3390/molecules27134254
Pinard, D., Myburg, A. A., Mizrachi, E. (2019). The plastid and mitochondrial genomes of Eucalyptus grandis. BMC Genomics 20, 132. doi: 10.1186/s12864-019-5444-4
Podsędek, A. (2017). Natural antioxidants and antioxidant capacity of Brassica vegetables: a review. LWT - Food Sci. Tech. 40, 1–11. doi: 10.1016/j.lwt.2005.07.023
Rice, D., Alverson, A., Richardson, A., Young, G., Sanchez-Puerta, M., Munzinger, J., et al. (2013). Horizontal transfer of entire genomes via mitochondrial fusion in the angiosperm Amborella. Science 342, 1468–1473. doi: 10.1126/science.1246275
Shao, D., Ma, Y., Li, X., Ga, S., Ren, Y. (2021). The sequence structure and phylogenetic analysis by complete mitochondrial genome of kohlrabi (Brassica oleracea var. gongylodes L.). Mitochondrial DNA B Resour. 6, 2714–2716. doi: 10.1080/23802359.2021.1966341
Smith, D., Crosby, K., Lee, R. (2011). Correlation between nuclear plastid DNA abundance and plastid number supports the limited transfer window hypothesis. Genome Biol. Evol. 3, 365–371. doi: 10.1093/gbe/evr001
Wick, R. R., Judd, L. M., Gorrie, C. L., Holt, K. E. (2017). Unicycler: Resolving bacterial genome assemblies from short and long sequencing reads. PloS Comput. Biol. 13, e1005595. doi: 10.1371/journal.pcbi.1005595
Wu, J., Liang, J., Lin, R., Cai, X., Zhang, L., Guo, X., et al. (2022). Investigation of Brassica and its relative genomes in the post-genomics era. Hortic. Res. 9, uhac182. doi: 10.1093/hr/uhac182
Wu, Z., Hu, K., Yan, M., Song, L., Wen, J., Ma, C., et al. (2019). Mitochondrial genome and transcriptome analysis of five alloplasmic male-sterile lines in Brassica juncea. BMC Genomics 20, 348. doi: 10.1186/s12864-019-5721-2
Ye, N., Wang, X., Li, J., Bi, C. (2017). Assembly and comparative analysis of complete mitochondrial genome sequence of an economic plant Salix suchowensis. PeerJ 5, e3148. doi: 10.7717/peerj.3148
Zhang, C., Ma, H., Sanchez-Puerta, M. V., Li, L., Xiao, J., Liu, Z., et al. (2020). Horizontal gene transfer has impacted cox1 gene evolution in Cassytha fliformis. J. Mol. Evol. 88, 361–371. doi: 10.1007/s00239-020-09937-1
Zhang, L., Li, X., Chang, L., Wang, T., Liang, J., Lin, R., et al. (2022). Expanding the genetic variation of Brassica juncea by introgression of the Brassica rapa genome. Hortic. Res. 9, uhab054. doi: 10.1093/hr/uhab054
Zhang, N., Jing, P. (2022). Corinthians in Brassicaceae: composition, stability, bioavailability, and potential health benefits. Crit. Rev. Food Sci. Nutr. 62, 2205–2220. doi: 10.1080/10408398.2020.1852170
Zhang, X., Zhang, K., Wu, J., Guo, N., Liang, J., Wang, X., et al. (2020). QTL-seq and sequence assembly rapidly mapped the gene brMYBL2.1 for the purple trait in brassica rapa. Sci. Rep. 10, 2328. doi: 10.1038/s41598-020-58916-5
Zhao, N., Grover, C., Chen, Z., Wendel, J., Hua, J. (2019). Intergenomic gene transfer in diploid and allopolyploid Gossypium. BMC Plant Biol. 19, 492. doi: 10.1186/s12870-019-2041-2
Keywords: zicaitai, Brassica, mitochondrial genome, phylogenetic analysis, gene transfer
Citation: Xiao W, Wu X, Zhou X, Zhang J, Huang J, Dai X, Ren H and Xu D (2024) Assembly and comparative analysis of the first complete mitochondrial genome of zicaitai (Brassica rapa var. Purpuraria): insights into its genetic architecture and evolutionary relationships. Front. Plant Sci. 15:1475064. doi: 10.3389/fpls.2024.1475064
Received: 02 August 2024; Accepted: 25 September 2024;
Published: 10 October 2024.
Edited by:
Miloslava Fojtova, Masaryk University, CzechiaReviewed by:
Fengqing Han, Chinese Academy of Agricultural Sciences, ChinaCopyright © 2024 Xiao, Wu, Zhou, Zhang, Huang, Dai, Ren and Xu. This is an open-access article distributed under the terms of the Creative Commons Attribution License (CC BY). The use, distribution or reproduction in other forums is permitted, provided the original author(s) and the copyright owner(s) are credited and that the original publication in this journal is cited, in accordance with accepted academic practice. No use, distribution or reproduction is permitted which does not comply with these terms.
*Correspondence: Jianghua Huang, amhodWFuZ3prQHpoa3UuZWR1LmNu; Xiuchun Dai, MTg5MjIxMjkwMDlAMTYzLmNvbQ==; Hailong Ren, cmVuaGFpbG9uZ18yMDA2QDE2My5jb20=; Donglin Xu, aWNlam95MjAwOUBpY2xvdWQuY29t
†These authors have contributed equally to this work
Disclaimer: All claims expressed in this article are solely those of the authors and do not necessarily represent those of their affiliated organizations, or those of the publisher, the editors and the reviewers. Any product that may be evaluated in this article or claim that may be made by its manufacturer is not guaranteed or endorsed by the publisher.
Research integrity at Frontiers
Learn more about the work of our research integrity team to safeguard the quality of each article we publish.