- 1Guangdong Provincial Key Laboratory of Utilization and Conservation of Food and Medicinal Resources in Northern Region, Shaoguan University, Shaoguan, China
- 2College of Bioscience and Bioengineering, Jiangxi Agricultural University, Nanchang, Jiangxi, China
- 3Key Laboratory of Crop Physiology, Ecology and Genetic Breeding, Ministry of Education, Jiangxi Agricultural University, Nanchang, China
- 4Jiangxi Provincial Key Laboratory for Postharvest Storage and Preservation of Fruits & Vegetables, Jiangxi Agricultural University, Nanchang, China
1 Introduction
In Arabidopsis thaliana genome, a multitude of small coding genes have been identified, with 200 of them are likely to encode small signaling peptides (Takahashi et al., 2019). Small signaling peptides, typically comprising fewer than 100 amino acids, mediate cell-to-cell communications, and are also pivotal for plant growth and responses to biotic and abiotic stressors (Oh et al., 2018; Olsson et al., 2019; Han et al., 2022; Xie et al., 2022; Bashyal et al., 2024; Datta et al., 2024; Wen et al., 2024). The CLAVATA3/EMBRYO-SURROUNDING REGION (CLE) genes encode proteins with a signal peptide at the N-terminus, a variable central domain, and highly conserved CLE motifs at the C-terminus (Fletcher, 2020; Khan et al., 2021). Following proteolytic processing and post-translational modifications (PMTs) (Stührwohldt and Schaller, 2019), the functional CLE motif, consisting of 12 to 14 amino acids, is secreted to the apoplast via the endoplasmic reticulum (ER) and Golgi apparatus, where it executes its biological functions. A plethora of evidence underscores the pivotal roles of CLE peptides as key modulators of cell proliferation and differentiation in the shoot apical meristem (SAM) (Schlegel et al., 2021; Song et al., 2021; Wang et al., 2022; Takahashi et al., 2023), root apical meristem (RAM) (Stahl et al., 2009; Gutiérrez-Alanís et al., 2017; Berckmans et al., 2020; Breiden et al., 2021), and vascular cambium (Yuan and Wang, 2021; Carbonnel et al., 2023; Diaz-Ardila et al., 2023). The CLE peptides also mediate cellular responses to various environmental clues (Xie et al., 2022; Bashyal et al., 2024; Datta et al., 2024).
CLE peptides function as local or long-distance signaling molecules, and can interact with the extracellular domains of plasma membrane (PM)-localized leucine-rich-repeat receptor-like kinases (LRR-RLKs), and the CLE-receptor pair thus regulates a wide range of plant developmental and adaptive processes (Narasimhan and Simon, 2022; Xie et al., 2022; Furumizu and Aalen, 2023; Bashyal et al., 2024; Datta et al., 2024). Extensive in vitro investigations employing isolated extracellular domains of LRR-RLKs have demonstrated the binding dynamics between CLE peptides and their corresponding (co)receptors, revealing differential binding affinities across distinct LRR-RLK subfamilies (Zhang et al., 2016a, 2016b; Guo et al., 2010; Crook et al., 2020). Alteration of the C-terminal anchorage site of CLE peptides can significantly diminish their receptor binding efficacy (Li et al., 2017). Moreover, exchanging one or more N-terminal residues between INFLORESCENCE DEFICIENT IN ABSCISSION (IDA) and CLE9 peptides has been shown to reduce their cognate receptor affinity (Roman et al., 2022). Therefore, both the C-terminal and N-terminal residues of CLE motif are required for receptor interactions, with the N-terminal residues conferring binding specificity (Zhang et al., 2016a, 2016b; Guo et al., 2010; Li et al., 2017; Crook et al., 2020; Roman et al., 2022). However, these in vitro assays do not fully reflect in vivo conditions or may contradict the in vitro binding results (Shinohara and Matsubayashi, 2015). Hence, elucidating the intracellular dynamics and binding specificity of CLE peptides in vivo remains a challenge. Recently, a new toolbox has been developed to facilitate in vivo analysis of CLV3-receptor interactions specificity and to capture the spatiotemporal dynamics of CLV3 peptides (Narasimhan et al., 2024). This innovative tool offers new perspectives for future functionality research on small signaling peptides.
2 Design of fluorescently conjugated CLV3 peptide
Over the decades, a diverse array of small-molecule fluorescent probes has been created (Zhu et al., 2016; Pramanik and Das, 2021; Yin et al., 2021), and are extensively utilized in plants (Iwatate et al., 2020; Yagi et al., 2021). These fluorescent probes are highly sensitive and specific, rendering them indispensable for visualizing subcellular structures such as the plasma membrane (PM), endoplasmic reticulum (ER), and vacuole (Zhu et al., 2016; Yagi et al., 2021). The fluorescent probes have also been engineered to elucidate the impacts of phytohormones on plant growth and development as well as their subcellular dynamics (Lace and Prandi, 2016; Balcerowicz et al., 2021). Various fluorescent labeled phytohormones, including auxin (Hayashi et al., 2014; Sokołowska et al., 2014; Pařízková et al., 2021), cytokinin (Nishikawa et al., 2000; Kubiasová et al., 2020), abscisic acid (Asami et al., 1997; Yamazaki et al., 2003), strigolactone analogs (Prandi et al., 2013; Yao et al., 2018; Van Overtveldt et al., 2019), jasmonic acid (Liu et al., 2012; Liu and Sang, 2013), brassinosteroid (Irani et al., 2012), and gibberellin (Shani et al., 2013), have been developed for this purpose. It is worth noting that, the 5(6)-carboxyfluorescein (FAM) fluorescence probe has been harnessed to tag CLE25/CLE26 and CLE45, facilitating the examination of their respective roles in drought response and vascular tissue development (Endo et al., 2019; Endo and Fukuda, 2024).
Recently, a novel fluorescent labeled CLV3 peptide is successfully synthesized using a well-established solid phase peptide synthesis method (Wellings and Atherton, 1997; Narasimhan et al., 2024). In brief, the carboxyl terminus of the amino acid is in situ activated to form an active ester, facilitating its conjugation to a resin-bound amine group. Following the coupling, the fluorenylmethyloxycarbonyl (Fmoc) protecting group is selectively removed by piperidine, thus exposing the N-terminus for subsequent amino acid attachment. Through such iterative coupling cycles, the desired polypeptide sequence is assembled. Finally, the peptide is released from the resin, typically under acidic conditions, which also removes any protecting groups on the side chains (Wellings and Atherton, 1997; Narasimhan et al., 2024). Notably, the threonine at position 2 in CLV3 motif is replaced by lysine (Figure 1A) (Endo et al., 2019; Endo and Fukuda, 2024), as this amino acid is less conserved and can be modified without strongly influencing peptide activity (Ogawa et al., 2008; Song et al., 2012; Zhang et al., 2016a; Li et al., 2017). The lysine amino acid allows for the attachment of allyloxycarbonyl (Alloc) protecting group, which can be cleaved under specific conditions (Wojcik et al., 2012). Release of Alloc group leads to the introduction of the fluorophore 5-carboxytetramethylrhodamine (TAMRA) or fluorescein isothiocyanate (FITC) to the N-terminal of CLV3 peptide. Upon final cleavage, the fully deprotected CLV3-TAMRA and CLV3-FITC peptides are successfully synthesized (Figure 1A) (Narasimhan et al., 2024).
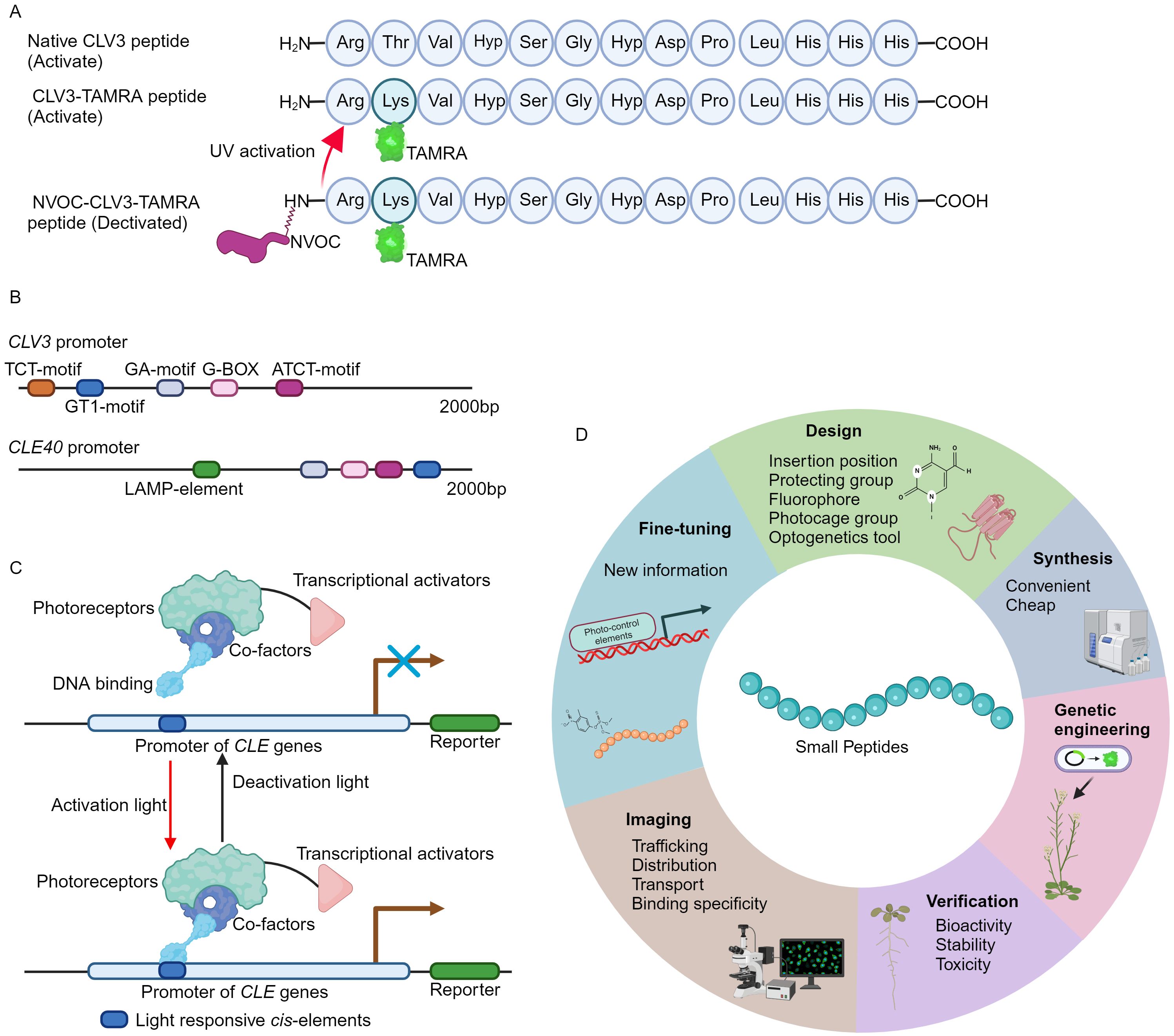
Figure 1. The development of fluorescence tagging and photo-activation of CLV3 peptide. (A) An overview of the structure of fluorescence labeling and photo-stimulation of the CLV3 peptide. (B) Numerous light-responsive cis-regulatory elements have been identified within the promoter regions of the CLV3 and CLE40 genes. The 2000 bp promoter sequences of CLV3 and CLE40 are obtained from TAIR 10 (https://www.arabidopsis.org/), and the PlantCARE database (https://bioinformatics.psb.ugent.be/webtools/plantcare/html/) is utilized to predict cis-regulatory elements. (C) An example of CLE pathway modulation through optogenetic techniques. Optogenetic engineering of CLE signaling involves the integration of photoreceptors, transcriptional activators, and DNA-binding domains into a functional complex. Upon exposure to specific activating wavelengths of light, this complex can interact with light-responsive elements within the promoter regions of CLE genes via its DNA-binding domains, thereby activating CLE signaling. Conversely, when exposed to deactivating wavelengths of light, the complex remains inactive, effectively terminating CLE signaling. (D) A summary of the basic principles for design of the fluorescence tagging and photo-activation of small peptides from other families.
3 CLV3-TAMRA peptide targets canonical CLE signaling pathway
A root length assay is performed to evaluate the bioactivity of CLV3-TAMRA and CLV3-FITC peptides. The synthesized native CLV3 peptide significantly inhibits root growth (Fiers et al., 2005; Blümke et al., 2021), whereas the CLV3-TAMRA peptide necessitates a higher concentration to exert a similar suppressive effect on root growth (Narasimhan et al., 2024). Notably, the CLV3-FITC peptide exhibits no obvious impact on root growth. Furthermore, the crn mutant shows no response to both synthesized native CLV3 and CLV3-TAMRA peptides, indicating that the CLV3-TAMRA peptide operates via the canonical CLE signaling pathway, albeit with low efficacy (Fletcher, 2020; Khan et al., 2021; Narasimhan et al., 2024). The differential bioactivity between the CLV3-TAMRA and CLV3-FITC peptides is likely attributable to structural variations in their fluorophores. The hydroxyl groups present in FITC might interact with the peptide backbone, thereby affecting its conformation and impeding receptor binding (Narasimhan et al., 2024).
Upon ligand binding, the receptors usually undergo endocytosis followed by lysosomal degradation, a process crucial for modulating signal transduction and specificity (Claus et al., 2018). After perception of CLE peptides, the CLAVATA1 (CLV1) receptor is internalized in the SAM (Nimchuk et al., 2011; Somssich et al., 2015; Wang et al., 2023). CLV3-TAMRA signal is detected at the cell plasma membrane in Arabidopsis roots and SAM, the signal then accumulates increasingly in the vacuole through the clathrin-mediated endocytosis (CME) trafficking pathway. Additionally, CLV3-TAMRA peptide treatment induces the endocytosis of CLV1 and BAM1 receptors but not PEPR1 receptor, suggesting the binding specificity of CLV3-TAMRA (Guo et al., 2010; Roman et al., 2022; Wang et al., 2023; Narasimhan et al., 2024).
In conclusion, the fluorescence labeled CLV3-TAMRA retain bioactivity, can be used to inspect the subcellular dynamics of CLV3 peptides in the cells and its binding specificity in vivo.
4 Photoactivation of CLV3 peptide
Caged compounds are usually chemically or biologically active molecules that are deactivated by coupling to a photocleavable protecting group (caging group), and can be rapidly released upon UV illumination (Ellis-Davies, 2007; Li et al., 2023). The caged molecules have their unique properties. For instance, the capability for rapid intracellular uncaging, the spatial and temporal resolution can be precisely controlled by optical instrumentations, and intracellular concentration is tightly controlled through modulation of light intensity and duration (Ellis-Davies, 2007; Li et al., 2023). The nitroveratryloxycarbonyl (NVOC) group, belonging to the 4,5- dimethoxy-2-nitrobenzyl (DMNB) type, is one of the most frequently used cages (Kohl-Landgraf et al., 2014; Kneuttinger, 2022). This approach has successfully yielded DMPNB-caged auxin (Kusaka et al., 2009). In order to attain spatiotemporal regulation of CLV3 peptide activity, the NVOC group is conjugated to the N-terminus of the CLV3-TAMRA peptide, resulting in a fluorescently tagged and photoactivatable NVOC-CLV3-TAMRA peptide (Figure 1A) (Narasimhan et al., 2024).
In the absence of UV exposure, the NVOC-CLV3-TAMRA peptide exhibits no impact on root growth. Upon UV irradiation, the NVOC-CLV3-TAMRA peptide suppresses root growth in wild-type Arabidopsis seedlings, but not in the clv2 mutant, indicating that the NVOC group is effectively cleaved, thereby releasing CLV3 peptide to repress root growth through the well-established CLE signaling pathway (Fletcher, 2020; Khan et al., 2021; Narasimhan et al., 2024). Additionally, fluorescence signals are predominantly localized in the apoplastic space before photoactivation in NVOC-CLV3-TAMRA peptide-treated Arabidopsis roots. After UV photoactivation, the fluorescence signals are observed at the plasma membrane, endosomal compartments, and vacuoles, suggesting a photo-activated trafficking of CLV3 peptide in the target cells (Narasimhan et al., 2024).
5 Future perspectives
The pioneering approach of fluorescence tagging and photo-activation of the CLV3 peptide, alongside previously developed FAM and CdTe quantum dots (QDs) labeled CLE peptides (Yu et al., 2014; Endo et al., 2019; Endo and Fukuda, 2024; Narasimhan et al., 2024), provides crucial insights into the functional elucidation of CLE peptides. The CLV3-TAMRA and NVOC-CLV3-TAMRA peptides are easy to synthesis, stable, non-toxic to plants, and can be utilized to investigate the subcellular dynamic of CLV3 peptide and its receptor binding specificity in vivo (Yu et al., 2014; Narasimhan et al., 2024). Many different fluorescence groups have been created (Zhu et al., 2016; Pramanik and Das, 2021; Yin et al., 2021), however, not all of these fluorophores preserve their bioactivity when conjugated to the CLV3 motif (Narasimhan et al., 2024). Therefore, it is advisable to conduct computational modeling to mitigate their effects on peptide conformational alterations and receptor binding interactions. On the other hand, genetically engineered photo-activated CLE signaling systems remain undeveloped. In the promoter region of CLE genes, exampled by CLV3 and CLE40, multiple light responsive cis-regulatory elements can be identified (Figure 1B), suggesting the potential for genetic (de)activation via optogenetics. Over recent decades, a multitude of light receptors or sensors from bacteria, fungi, and plants have been discovered and integrated into optogenetic tools (Kolar et al., 2018; Zhang et al., 2024). These optogenetic systems have been successfully introduced into living cells to control spatio-temporal gene expression, protein stability, subcellular localization, and receptor activity through optical means (Banerjee and Mitra, 2020; Christie and Zurbriggen, 2021; Shikata and Denninger, 2022; Konrad et al., 2023). Additionally, these diverse optogenetic systems have been applied to visualize the subcellular distribution and dynamics of plant phytohormones such as auxin (Jásik et al., 2013; Salanenka et al., 2018) and gibberellin (Rizza et al., 2017). Based on the well-established design principles and optogenetic frameworks (Kolar et al., 2018; Banerjee and Mitra, 2020; Zhang et al., 2024), the engineering of genetic activation incorporating the promoters of CLE genes by a specific light wavelength will be achievable (Figure 1C), thereby spatio-temporally control CLE peptide signaling. Particularly, the plant usable light-switch elements (PULSE) system, which enables plant growth under normal light conditions, and is exclusively activated by red light stimuli (Ochoa-Fernandez et al., 2020).
Plants can produce various small signaling peptide families (Olsson et al., 2019; Takahashi et al., 2019). Among these small peptides, for example, C-TERMINALLY ENCODED PEPTIDE (CEP) is capable of being translocated from roots to aerial parts through long-distance transport mechanisms (Tabata et al., 2014). Remarkably, this long-distance translocation is achieved through the engineering of the Alexa Fluor 488 conjugated CEP1 peptide (Ohkubo et al., 2017). Subsequently, the leaf localized CEP RECEPTORs (CEPRs) can recognize CEP peptides, thus modulating plant development and stress responses accordingly (Taleski et al., 2024). Notably, the FITC conjugated Medicago CEP1 peptide is utilized to examine the ligand-receptor specificity between CEP receptors (Lee et al., 2021). Nevertheless, elucidating the intracellular localization and cellular dynamics of the majority of small peptides triggered by specific developmental cues or environmental stimuli, as well as their receptor binding affinities in vivo, still remains a significant challenge. In accordance with the methodologies for the synthesis of fluorescently labeled and photoreactive CLE peptides as well as CEP peptides (Figure 1D) (Yu et al., 2014; Ohkubo et al., 2017; Endo et al., 2019; Lee et al., 2021; Endo and Fukuda, 2024; Narasimhan et al., 2024), it is plausible to design and synthesize a variety of fluorescence-labeled and photo-activatable bioactive peptides derived from other small peptide family. These innovative tools will enable a more detailed investigation into the elusive biological functions of small signaling peptides in agronomic and horticultural plants, thus advancing the application of small peptides in modern agriculture.
Author contributions
YZ: Conceptualization, Funding acquisition, Writing – review & editing. JZ: Writing – original draft. HW: Writing – original draft. YY: Conceptualization, Writing – review & editing. HH: Conceptualization, Writing – review & editing, Funding acquisition.
Funding
The author(s) declare financial support was received for the research, authorship, and/or publication of this article. This work is supported by the Open Fund of the Guangdong Provincial Key Laboratory of Utilization and Conservation of Food and Medicinal Resources in Northern Region (FMR2023002M) and Natural Science Foundation of Jiangxi Province (20232BAB205039) to YZ; Natural Science Foundation of Jiangxi Province (20223BBF61017) to YY; Jiangxi Agricultural University (9232308314), Science and Technology Department of Jiangxi Province (20223BCJ25037) to HH.
Acknowledgments
We would like to thank the lab members, the reviewers and editor for their constructive comments. We apologize to those whose great work we are unable to include due to limited space.
Conflict of interest
The authors declare that the research was conducted in the absence of any commercial or financial relationships that could be construed as a potential conflict of interest.
Publisher’s note
All claims expressed in this article are solely those of the authors and do not necessarily represent those of their affiliated organizations, or those of the publisher, the editors and the reviewers. Any product that may be evaluated in this article, or claim that may be made by its manufacturer, is not guaranteed or endorsed by the publisher.
References
Asami, T., Tao, L., Yamamoto, S., Robertson, M., Min, Y. K., Murofushi, N., et al. (1997). Fluorescence-labeled abscisic acid possessing abscisic acid-like activity in barley aleurone protoplasts. Biosci. Biotechnol. Biochem. 61, 1198–1199. doi: 10.1271/bbb.61.1198
Balcerowicz, M., Shetty, K. N., Jones, A. M. (2021). Fluorescent biosensors illuminating plant hormone research. Plant Physiol. 187, 590–602. doi: 10.1093/plphys/kiab278
Banerjee, S., Mitra, D. (2020). Structural basis of design and engineering for advanced plant optogenetics. Trends Plant Sci. 25, 35–65. doi: 10.1016/j.tplants.2019.10.002
Bashyal, S., Gautam, C. K., Müller, L. M. (2024). CLAVATA signaling in plant-environment interactions. Plant Physiol. 194, 1336–1357. doi: 10.1093/plphys/kiad591
Berckmans, B., Kirschner, G., Gerlitz, N., Stadler, R., Simon, R. (2020). CLE40 signaling regulates root stem cell fate. Plant Physiol. 182, 1776–1792. doi: 10.1104/pp.19.00914
Blümke, P., Schlegel, J., Gonzalez-Ferrer, C., Becher, S., Pinto, K. G., Monaghan, J., et al. (2021). Receptor-like cytoplasmic kinase MAZZA mediates developmental processes with CLAVATA1 family receptors in Arabidopsis. J. Exp. Bot. 72, 4853–4870. doi: 10.1093/jxb/erab183
Breiden, M., Olsson, V., Blümke, P., Schlegel, J., Gustavo-Pinto, K., Dietrich, P., et al. (2021). The cell fate controlling CLE40 peptide requires CNGCs to trigger highly localized Ca2+ transients in Arabidopsis thaliana root meristems. Plant Cell Physiol. 62, 1290–1301. doi: 10.1093/pcp/pcab079
Carbonnel, S., Cornelis, S., Hazak, O. (2023). The CLE33 peptide represses phloem differentiation via autocrine and paracrine signaling in Arabidopsis. Commun. Biol. 6, 588. doi: 10.1038/s42003-023-04972-2
Christie, J. M., Zurbriggen, M. D. (2021). Optogenetics in plants. New Phytol. 229, 3108–3115. doi: 10.1111/nph.17008
Claus, L. A. N., Savatin, D. V., Russinova, E. (2018). The crossroads of receptor-mediated signaling and endocytosis in plants. J. Integr. Plant Biol. 60, 827–840. doi: 10.1111/jipb.12672
Crook, A. D., Willoughby, A. C., Hazak, O., Okuda, S., VanDerMolen, K. R., Soyars, C. L., et al. (2020). BAM1/2 receptor kinase signaling drives CLE peptide-mediated formative cell divisions in Arabidopsis roots. Proc. Natl. Acad. Sci. U.S.A. 117, 32750–32756. doi: 10.1073/pnas.2018565117
Datta, T., Kumar, R. S., Sinha, H., Trivedi, P. K. (2024). Small but mighty: Peptides regulating abiotic stress responses in plants. Plant Cell Environ. 47, 1207–1223. doi: 10.1111/pce.14792
Diaz-Ardila, H. N., Gujas, B., Wang, Q., Moret, B., Hardtke, C. S. (2023). pH-dependent CLE peptide perception permits phloem differentiation in Arabidopsis roots. Curr. Biol. 33, 597–605.e3. doi: 10.1016/j.cub.2022.12.056
Ellis-Davies, G. C. (2007). Caged compounds: photorelease technology for control of cellular chemistry and physiology. Nat. Methods 4, 619–628. doi: 10.1038/nmeth1072
Endo, S., Fukuda, H. (2024). A cell-wall-modifying gene-dependent CLE26 peptide signaling confers drought resistance in Arabidopsis. PNAS Nexus 3, pgae049. doi: 10.1093/pnasnexus/pgae049
Endo, S., Iwai, Y., Fukuda, H. (2019). Cargo-dependent and cell wall-associated xylem transport in Arabidopsis. New Phytol. 222, 159–170. doi: 10.1111/nph.15540
Fiers, M., Golemiec, E., Xu, J., van der Geest, L., Heidstra, R., Stiekema, W., et al. (2005). The 14-amino acid CLV3, CLE19, and CLE40 peptides trigger consumption of the root meristem in Arabidopsis through a CLAVATA2-dependent pathway. Plant Cell 17, 2542–2553. doi: 10.1105/tpc.105.034009
Fletcher, J. C. (2020). Recent advances in arabidopsis CLE peptide signaling. Trends Plant Sci. 25, 1005–1016. doi: 10.1016/j.tplants.2020.04.014
Furumizu, C., Aalen, R. B. (2023). Peptide signaling through leucine-rich repeat receptor kinases: insight into land plant evolution. New Phytol. 238, 977–982. doi: 10.1111/nph.18827
Guo, Y., Han, L., Hymes, M., Denver, R., Clark, S. E. (2010). CLAVATA2 forms a distinct CLE-binding receptor complex regulating Arabidopsis stem cell specification. Plant J. 63, 889–900. doi: 10.1111/tpj.2010.63.issue-6
Gutiérrez-Alanís, D., Yong-Villalobos, L., Jiménez-Sandoval, P., Alatorre-Cobos, F., Oropeza-Aburto, A., Mora-Macías, J., et al. (2017). Phosphate starvation-dependent iron mobilization induces CLE14 expression to trigger root meristem differentiation through CLV2/PEPR2 signaling. Dev. Cell. 41, 555–570.e3. doi: 10.1016/j.devcel.2017.05.009
Han, H., Zhuang, K., Qiu, Z. (2022). CLE peptides join the plant longevity club. Trends Plant Sci. 27, 961–963. doi: 10.1016/j.tplants.2022.07.001
Hayashi, K., Nakamura, S., Fukunaga, S., Nishimura, T., Jenness, M. K., Murphy, A. S., et al. (2014). Auxin transport sites are visualized in planta using fluorescent auxin analogs. Proc. Natl. Acad. Sci. U.S.A. 111, 11557–11562. doi: 10.1073/pnas.1408960111
Irani, N. G., Di Rubbo, S., Mylle, E., Vanden Begin, J., Schneider-Pizon, J., Hniliková, J., et al. (2012). Fluorescent castasterone reveals BRI1 signaling from the plasma membrane. Nat. Chem. Biol. 8, 583–589. doi: 10.1038/nchembio.958
Iwatate, R. J., Yoshinari, A., Yagi, N., Grzybowski, M., Ogasawara, H., Kamiya, M., et al. (2020). Covalent self-labeling of tagged proteins with chemical fluorescent dyes in BY-2 cells and Arabidopsis seedlings. Plant Cell 32, 3081–3094. doi: 10.1105/tpc.20.00439
Jásik, J., Boggetti, B., Baluška, F., Volkmann, D., Gensch, T., Rutten, T., et al. (2013). PIN2 turnover in Arabidopsis root epidermal cells explored by the photoconvertible protein Dendra2. PloS One 8, e61403. doi: 10.1371/journal.pone.0061403
Khan, S. U., Khan, M. H. U., Ahmar, S., Fan, C. (2021). Comprehensive study and multipurpose role of the CLV3/ESR-related (CLE) genes family in plant growth and development. J. Cell Physiol. 236, 2298–2317. doi: 10.1002/jcp.30021
Kneuttinger, A. C. (2022). A guide to designing photocontrol in proteins: methods, strategies and applications. Biol. Chem. 403, 573–613. doi: 10.1515/hsz-2021-0417
Kohl-Landgraf, J., Buhr, F., Lefrancois, D., Mewes, J. M., Schwalbe, H., Dreuw, A., et al. (2014). Mechanism of the photoinduced uncaging reaction of puromycin protected by a 6-nitroveratryloxycarbonyl group. J. Am. Chem. Soc 136, 3430–3438. doi: 10.1021/ja410594y
Kolar, K., Knobloch, C., Stork, H., Žnidarič, M., Weber, W. (2018). OptoBase: A web platform for molecular optogenetics. ACS Synth. Biol. 7, 1825–1828. doi: 10.1021/acssynbio.8b00120
Konrad, K. R., Gao, S., Zurbriggen, M. D., Nagel, G. (2023). Optogenetic methods in plant biology. Annu. Rev. Plant Biol. 74, 313–339. doi: 10.1146/annurev-arplant-071122-094840
Kubiasová, K., Montesinos, J. C., Šamajová, O., Nisler, J., Mik, V., Semerádová, H., et al. (2020). Cytokinin fluoroprobe reveals multiple sites of cytokinin perception at plasma membrane and endoplasmic reticulum. Nat. Commun. 11, 4285. doi: 10.1038/s41467-020-17949-0
Kusaka, N., Maisch, J., Nick, P., Hayashi, K., Nozaki, H. (2009). Manipulation of intracellular auxin in a single cell by light with esterase-resistant caged auxins. Chembiochem 10, 2195–2120. doi: 10.1002/cbic.200900289
Lace, B., Prandi, C. (2016). Shaping small bioactive molecules to untangle their biological function: A focus on fluorescent plant hormones. Mol. Plant 9, 1099–1118. doi: 10.1016/j.molp.2016.06.011
Lee, H. C., Binos, S., Chapman, K., Pulsford, S. B., Ivanovici, A., Rathjen, J. P., et al. (2021). A new method to visualize CEP hormone-CEP receptor interactions in vascular tissue in vivo. J. Exp. Bot. 72, 6164–6174. doi: 10.1093/jxb/erab244
Li, Y., Wang, M., Wang, F., Lu, S., Chen, X. (2023). Recent progress in studies of photocages. Smart Molecules. 1, e20220003. doi: 10.1002/smo.20220003
Li, Z., Chakraborty, S., Xu, G. (2017). Differential CLE peptide perception by plant receptors implicated from structural and functional analyses of TDIF-TDR interactions. PloS One 12, e0175317. doi: 10.1371/journal.pone.0175317
Liu, S., Sang, R. (2013). Bioactive fluorescent jasmonate designed by molecular modeling and its migration in tomato visualized by fluorescent molecular imaging. Tetrahedron 69, 844–848. doi: 10.1016/j.tet.2012.10.105
Liu, S., Wang, W. H., Dang, Y. L., Fu, Y., Sang, R. (2012). Rational design and efficient synthesis of a fluorescent-labeled jasmonate. Tetrahedron Lett. 53, 4235e4239. doi: 10.1016/j.tetlet.2012.06.006
Narasimhan, M., Jahnke, N., Kallert, F., Bahafid, E., Böhmer, F., Hartmann, L., et al. (2024). Macromolecular tool box to elucidate CLAVATA3/Embryo Surrounding Region-Related-RLK binding, signaling and downstream effects. J. Exp. Bot., erae206. doi: 10.1093/jxb/erae206
Narasimhan, M., Simon, R. (2022). Spatial range, temporal span, and promiscuity of CLE-RLK signaling. Front. Plant Sci. 13, 906087. doi: 10.3389/fpls.2022.906087
Nimchuk, Z. L., Tarr, P. T., Ohno, C., Qu, X., Meyerowitz, E. M. (2011). Plant stem cell signaling involves ligand-dependent trafficking of the CLAVATA1 receptor kinase. Curr. Biol. 21, 345–352. doi: 10.1016/j.cub.2011.01.039
Nishikawa, S., Kurono, M., Shibayama, K., Okuno, S., Inagaki, M., Kashimura, N. (2000). Synthesis and cytokinin activity of fluorescent 7-phenylethynylimidazo[4,5-b]pyridine and its riboside. J. Agr. Food Chem. 48, 2559–2564. doi: 10.1021/jf0000225
Ochoa-Fernandez, R., Abel, N. B., Wieland, F. G., Schlegel, J., Koch, L. A., Miller, J. B., et al. (2020). Optogenetic control of gene expression in plants in the presence of ambient white light. Nat. Methods 17, 717–725. doi: 10.1038/s41592-020-0868-y
Ogawa, M., Shinohara, H., Sakagami, Y., Matsubayashi, Y. (2008). Arabidopsis CLV3 peptide directly binds CLV1 ectodomain. Science 319, 294. doi: 10.1126/science.1150083
Oh, E., Seo, P. J., Kim, J. (2018). Signaling peptides and receptors coordinating plant root development. Trends Plant Sci. 23, 337–351. doi: 10.1016/j.tplants.2017.12.007
Ohkubo, Y., Tanaka, M., Tabata, R., Ogawa-Ohnishi, M., Matsubayashi, Y. (2017). Shoot-to-root mobile polypeptides involved in systemic regulation of nitrogen acquisition. Nat. Plants 3, 17029. doi: 10.1038/nplants.2017.29
Olsson, V., Joos, L., Zhu, S., Gevaert, K., Butenko, M. A., De Smet, I. (2019). Look closely, the beautiful may be small: precursor-derived peptides in plants. Annu. Rev. Plant Biol. 70, 153–186. doi: 10.1146/annurev-arplant-042817-040413
Pařízková, B., Žukauskaitė, A., Vain, T., Grones, P., Raggi, S., Kubeš, M. F., et al. (2021). New fluorescent auxin probes visualise tissue-specific and subcellular distributions of auxin in Arabidopsis. New Phytol. 230, 535–549. doi: 10.1111/nph.17183
Pramanik, S. K., Das, A. (2021). Fluorescent probes for imaging bioactive species in subcellular organelles. Chem. Commun. (Camb). 57, 12058–12073. doi: 10.1039/D1CC04273D
Prandi, C., Rosso, H., Lace, B., Occhiato, E. G., Oppedisano, A., Tabasso, S., et al. (2013). Strigolactone analogs as molecular probes in chasing the (SLs) receptor/s: design and synthesis of fluorescent labeled molecules. Mol. Plant 6, 113–127. doi: 10.1093/mp/sss133
Rizza, A., Walia, A., Lanquar, V., Frommer, W. B., Jones, A. M. (2017). In vivo gibberellin gradients visualized in rapidly elongating tissues. Nat. Plants 3, 803–813. doi: 10.1038/s41477-017-0021-9
Roman, A. O., Jimenez-Sandoval, P., Augustin, S., Broyart, C., Hothorn, L. A., Santiago, J. (2022). HSL1 and BAM1/2 impact epidermal cell development by sensing distinct signaling peptides. Nat. Commun. 13, 876. doi: 10.1038/s41467-022-28558-4
Salanenka, Y., Verstraeten, I., Löfke, C., Tabata, K., Naramoto, S., Glanc, M., et al. (2018). Gibberellin DELLA signaling targets the retromer complex to redirect protein trafficking to the plasma membrane. Proc. Natl. Acad. Sci. U.S.A. 115, 3716–3721. doi: 10.1073/pnas.1721760115
Schlegel, J., Denay, G., Wink, R., Pinto, K. G., Stahl, Y., Schmid, J., et al. (2021). Control of Arabidopsis shoot stem cell homeostasis by two antagonistic CLE peptide signalling pathways. eLife 10, e70934. doi: 10.7554/eLife.70934.sa2
Shani, E., Weinstain, R., Zhang, Y., Castillejo, C., Kaiserli, E., Chory, J., et al. (2013). Gibberellins accumulate in the elongating endodermal cells of Arabidopsis root. Proc. Natl. Acad. Sci. U.S.A. 110, 4834–4839. doi: 10.1073/pnas.1300436110
Shikata, H., Denninger, P. (2022). Plant optogenetics: Applications and perspectives. Curr. Opin. Plant Biol. 68, 102256. doi: 10.1016/j.pbi.2022.102256
Shinohara, H., Matsubayashi, Y. (2015). Reevaluation of the CLV3-receptor interaction in the shoot apical meristem: dissection of the CLV3 signaling pathway from a direct ligand-binding point of view. Plant J. 82, 328–336. doi: 10.1111/tpj.12817
Sokołowska, K., Kizińska, J., Szewczuk, Z., Banasiak, A. (2014). Auxin conjugated to fluorescent dyes–a tool for the analysis of auxin transport pathways. Plant Biol. (Stuttg) 16, 866–877. doi: 10.1111/plb.12144
Somssich, M., Ma, Q., Weidtkamp-Peters, S., Stahl, Y., Felekyan, S., Bleckmann, A., et al. (2015). Real-time dynamics of peptide ligand-dependent receptor complex formation in planta. Sci. Signal. 8, ra76. doi: 10.1126/scisignal.aab0598
Song, X. F., Hou, X. L., Liu, C. M. (2021). CLE peptides: critical regulators for stem cell maintenance in plants. Planta 255, 5. doi: 10.1007/s00425-021-03791-1
Song, X. F., Yu, D. L., Xu, T. T., Ren, S. C., Guo, P., Liu, C. M. (2012). Contributions of individual amino acid residues to the endogenous CLV3 function in shoot apical meristem maintenance in Arabidopsis. Mol. Plant 5, 515–523. doi: 10.1093/mp/ssr120
Stahl, Y., Wink, R. H., Ingram, G. C., Simon, R. (2009). A signaling module controlling the stem cell niche in Arabidopsis root meristems. Curr. Biol. 19, 909–914. doi: 10.1016/j.cub.2009.03.060
Stührwohldt, N., Schaller, A. (2019). Regulation of plant peptide hormones and growth factors by post-translational modification. Plant Biol. (Stuttg) 1, 49–63. doi: 10.1111/plb.12881
Tabata, R., Sumida, K., Yoshii, T., Ohyama, K., Shinohara, H., Matsubayashi, Y. (2014). Perception of root-derived peptides by shoot LRR-RKs mediates systemic N-demand signaling. Science 346, 343–346. doi: 10.1126/science.1257800
Takahashi, F., Hanada, K., Kondo, T., Shinozaki, K. (2019). Hormone-like peptides and small coding genes in plant stress signaling and development. Curr. Opin. Plant Biol. 51, 88–95. doi: 10.1016/j.pbi.2019.05.011
Takahashi, G., Kiyosue, T., Hirakawa, Y. (2023). Control of stem cell behavior by CLE-JINGASA signaling in the shoot apical meristem in Marchantia polymorpha. Curr. Biol. 33, 5121–5131.e6. doi: 10.1016/j.cub.2023.10.054
Taleski, M., Jin, M., Chapman, K., Taylor, K., Winning, C., Frank, M., et al. (2024). CEP hormones at the nexus of nutrient acquisition and allocation, root development, and plant-microbe interactions. J. Exp. Bot. 75, 538–552. doi: 10.1093/jxb/erad444
Van Overtveldt, M., Braem, L., Struk, S., Kaczmarek, A. M., Boyer, F. D., Van Deun., R., et al. (2019). Design and visualization of second-generation cyanoisoindole-based fluorescent strigolactone analogs. Plant J. 98, 165–180. doi: 10.1111/tpj.14197
Wang, W., Hu, C., Li, X., Zhu, Y., Tao, L., Cui, Y., et al. (2022). Receptor-like cytoplasmic kinases PBL34/35/36 are required for CLE peptide-mediated signaling to maintain shoot apical meristem and root apical meristem homeostasis in Arabidopsis. Plant Cell 34, 1289–1307. doi: 10.1093/plcell/koab315
Wang, J., Jiang, Q., Pleskot, R., Grones, P., Bahafid, E., Denay, G., et al. (2023). TPLATE complex-dependent endocytosis attenuates CLAVATA1 signaling for shoot apical meristem maintenance. EMBO Rep. 4, e54709. doi: 10.15252/embr.202254709
Wellings, D. A., Atherton, E. (1997). Standard fmoc protocols. Methods Enzymol. 289, 44–67. doi: 10.1016/S0076-6879(97)89043-X
Wen, Y., Yang, Y., Liu, J., Han, H. (2024). CLV3-CLV1 signaling governs flower primordia outgrowth across environmental temperatures. Trends Plant Sci. 29, 400–402. doi: 10.1016/j.tplants.2023.12.004
Wojcik, F., Mosca, S., Hartmann, L. (2012). Solid-phase synthesis of asymmetrically branched sequence-defined poly/oligo(amidoamines). J. Org. Chem. 77, 4226–4234. doi: 10.1021/jo202561k
Xie, H., Zhao, W., Li, W., Li, W., Zhang, Y., Hajný, J., et al. (2022). Small signaling peptides mediate plant adaptions to abiotic environmental stress. Planta 255, 72. doi: 10.1007/s00425-022-03859-6
Yagi, N., Yoshinari, A., Iwatate, R. J., Isoda, R., Frommer, W. B., Nakamura, M. (2021). Advances in synthetic fluorescent probe labeling for live-cell imaging in plants. Plant Cell Physiol. 62, 1259–1268. doi: 10.1093/pcp/pcab104
Yamazaki, D., Yoshida, S., Asami, T., Kuchitsu, K. (2003). Visualization of abscisic acid-perception sites on the plasma membrane of stomatal guard cells. Plant J. 35, 129–139. doi: 10.1046/j.1365-313X.2003.01782.x
Yao, J., Mashiguchi, K., Scaffidi, A., Akatsu, T., Melville, K. T., Morita, R., et al. (2018). An allelic series at the KARRIKIN INSENSITIVE 2 locus of Arabidopsis thaliana decouples ligand hydrolysis and receptor degradation from downstream signalling. Plant J. 96, 75–89. doi: 10.1111/tpj.14017
Yin, J., Huang, L., Wu, L., Li, J., James, T. D., Lin, W. (2021). Small molecule based fluorescent chemosensors for imaging the microenvironment within specific cellular regions. Chem. Soc Rev. 50, 12098–12150. doi: 10.1039/D1CS00645B
Yu, G., Tan, Y., He, X., Qin, Y., Liang, J. (2014). CLAVATA3 dodecapeptide modified CdTe nanoparticles: a biocompatible quantum dot probe for in vivo labeling of plant stem cells. PloS One 9, e89241. doi: 10.1371/journal.pone.0089241
Yuan, B., Wang, H. (2021). Peptide signaling pathways regulate plant vascular development. Front. Plant Sci. 12, 719606. doi: 10.3389/fpls.2021.719606
Zhang, H., Han, Z., Song, W., Chai, J. (2016a). Structural insight into recognition of plant peptide hormones by receptors. Mol. Plant 9, 1454–1463. doi: 10.1016/j.molp.2016.10.002
Zhang, Z., Li, F., Duan, Z., Shi, C., Wang, X., Zhu, F., et al. (2024). OPTICS: An interactive online platform for photosensory and bio-functional proteins in optogenetic systems. Comput. Biol. Med. 178, 108687. doi: 10.1016/j.compbiomed.2024.108687
Zhang, H., Lin, X., Han, Z., Qu, L. J., Chai, J. (2016b). Crystal structure of PXY-TDIF complex reveals a conserved recognition mechanism among CLE peptide-receptor pairs. Cell Res. 26, 543–555. doi: 10.1038/cr.2016.45
Keywords: CLV3 peptide, fluorescent labeling, photoactivation, endocytosis, binding specificity
Citation: Zhou Y, Zheng J, Wu H, Yang Y and Han H (2024) A novel toolbox to record CLE peptide signaling. Front. Plant Sci. 15:1468763. doi: 10.3389/fpls.2024.1468763
Received: 22 July 2024; Accepted: 31 July 2024;
Published: 14 August 2024.
Edited by:
Thorsten Seidel, Bielefeld University, GermanyReviewed by:
Daisuke Kurihara, Nagoya University, JapanCopyright © 2024 Zhou, Zheng, Wu, Yang and Han. This is an open-access article distributed under the terms of the Creative Commons Attribution License (CC BY). The use, distribution or reproduction in other forums is permitted, provided the original author(s) and the copyright owner(s) are credited and that the original publication in this journal is cited, in accordance with accepted academic practice. No use, distribution or reproduction is permitted which does not comply with these terms.
*Correspondence: Huibin Han, aHVpYmluaGFuQGp4YXUuZWR1LmNu; Youxin Yang, eWFuZ3lvdXhpbkBqeGF1LmVkdS5jbg==