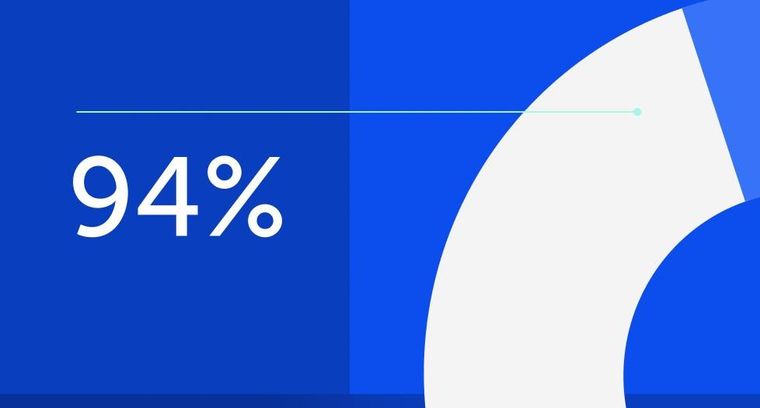
94% of researchers rate our articles as excellent or good
Learn more about the work of our research integrity team to safeguard the quality of each article we publish.
Find out more
ORIGINAL RESEARCH article
Front. Plant Sci., 08 October 2024
Sec. Functional Plant Ecology
Volume 15 - 2024 | https://doi.org/10.3389/fpls.2024.1463548
Exotic plant litter presents different chemical and physical properties relative to native plant litter and alters ecosystem processes and functions that may facilitate exotic plant dispersal. However, these effects are largely unknown, especially within wetland ecosystems. This study examines whether introducing litter from the exotic mangrove Laguncularia racemosa could result in (1) accelerated community litter decomposition rates and increased nutrient cycling rates and (2) microbial community structure changes in the invaded areas. A single decomposition experiment using litterbags was conducted to examine the short-term effects of L. racemosa litter in the native mangrove forest ecosystem. The soil nutrients and microbial communities of Rhizophora stylosa, L. racemosa, and mixed forests were also compared to explore the long-term cumulative effects of L. racemosa litter in native ecosystems. The results indicated that L. racemosa has lower-quality leaf litter than R. stylosa and a significantly faster decomposition rate. This may result from changes in the soil microbial community structure caused by L. racemosa leaf litter input, which favors the decomposition of its own litter. Both the short-term and cumulative effect experiments demonstrated that L. racemosa leaf litter significantly increased the relative abundance of microbes related to litter decomposition, such as Proteobacteria and Bdellovibrionota, and enhanced the alpha diversity of soil fungi, thus creating a microbial environment conducive to L. racemosa leaf litter decomposition. Moreover, the accumulation of soil nutrients was lower under L. racemosa than under R. stylosa over several years. This may be related to the more rapid growth of L. racemosa, which causes soil nutrient absorption and storage within the plant tissues, thereby reducing the soil nutrient content. Inputting exotic mangrove L. racemosa leaf litter reduced the soil blue carbon content, potentially adversely affecting global climate change. L. racemosa may employ a unique strategy to lower soil nutrient levels in native mangroves based on its low-quality leaf litter, thereby weakening the competitive ability of native plants that are intolerant to low-nutrient conditions and enhancing its own competitive advantage to further spread into these areas. In summary, the input of exotic L. racemosa leaf litter accelerates nutrient cycling in local mangroves.
Exotic plant invasion is a global phenomenon (Incerti et al., 2018) that threatens the biodiversity and stability of local ecosystems and profoundly impacts their functions and processes (Liao et al., 2008; Meisner et al., 2012; Woodworth et al., 2020). The mangrove Laguncularia racemosa, native to La Paz, Mexico (Zhong et al., 2011), is characterized by rapid growth, high reproductive capacity, and adaptability (Zhu et al., 2023). Since its introduction to Hainan, China, in 1999, it has rapidly spread and integrated into local mangrove communities (Liu et al., 2019). Numerous physiological and ecological studies have indicated that L. racemosa exhibits higher growth and reproductive abilities compared to native mangrove species in China, suggesting its potential invasiveness (Li et al., 2020; Feng et al., 2022). Research by Lang et al. (2022) also suggested that L. racemosa might invade higher latitudes and colder coastal regions of China. Additionally, Zhu et al. (2023) confirmed its invasion potential through field surveys and molecular studies, indicating that it may replace native mangrove species and reduce species diversity within local mangrove forests. Although studies have examined the potential invasiveness of L. racemosa from different perspectives, research focusing on associated changes in ecosystem functions remains lacking.
Exotic plants can alter the soil characteristics of invaded areas through their litter inputs, which impact the nutrient cycling within the ecosystem and create positive feedback for the spread of exotic plants (Meisner et al., 2012). Generally, exotic plant litter has higher quality and faster decomposition rates than native plant litter (Prescott and Zukswert, 2016; Woodworth et al., 2020; Hu et al., 2022), and its input often enhances the leaf litter quality in the invaded area, thereby accelerating leaf litter decomposition rates (Ehrenfeld, 2010), increasing soil nutrient contents (Liao et al., 2008), and accelerating nutrient cycling processes (Rothstein et al., 2004). Several studies have shown that these changes may promote fast-growing, nutrient-demanding exotic plants (Meisner et al., 2012) by creating favorable conditions for their spread (Allison and Vitousek, 2004). Additionally, changes in leaf litter quality due to exotic plant litter inputs have been shown to alter microbial communities in the soil, thereby affecting the detrital food web of the local ecosystem (Woodworth et al., 2020) and driving soil nutrient environments toward conditions more conducive to exotic plant growth.
Leaf litter decomposition plays a critical role in the global carbon cycle by regulating organic carbon exchange between the soil and atmosphere (Zhang et al., 2021; Bonanomi et al., 2023). The process of exotic plant invasion is often accompanied by the input of leaf litter with low carbon contents and high nitrogen and phosphorus contents (Kennedy and El-Sabaawi, 2017; Carrasco-Barea et al., 2022), which can impact the soil carbon content and nutrient cycling in the invaded area. Research has shown that the soil under exotic plants has a higher carbon content than soil under native plants (Sanon et al., 2012; Bray et al., 2017); however, studies on the effects of exotic plant litter on soil carbon content are limited. Moreover, although previous studies have primarily focused on how exotic plant invasion affects soil nutrient availability in terrestrial ecosystems, the effects of such invasions on the soil carbon content and nutrient cycling in wetland ecosystems have not been reported. The carbon content of mangrove ecosystems, known as blue carbon ecosystems, is among the highest of ecosystems on Earth (Lu et al., 2024). Since its introduction to China, L. racemosa has spread widely across the southeastern coastal provinces of China (Zhong et al., 2011) and invaded local mangrove communities (Liu et al., 2019). However, the impact of its litter input on soil nutrient cycling and blue carbon content in wetland ecosystems remains to be explored.
The impact of leaf litter decomposition on soil nutrients has been studied across various time scales, including the short-term effects of a single litter decomposition event (Meisner et al., 2012; Chen et al., 2020; Zhang et al., 2021) and the long-term effects of continued decomposition over several decades (Lin et al., 2003). However, few studies have integrated both short-term and long-term effects. Since the impacts of leaf litter decomposition on soil nutrients accumulate gradually, short-term experiments cannot provide insights into the effects over longer time scales (Liu and Peng, 2010). Thus, short-term impacts cannot be used to predict long-term effects. Understanding both short-term and long-term cumulative effects is crucial for investigating the impact and mechanisms of leaf litter decomposition on soil nutrient cycling. Therefore, both short-term and long-term effects should be examined to explore how the input of exotic mangrove L. racemosa litter affects nutrient cycling in mangrove ecosystems.
Thus, this study aimed to explore the effects of L. racemosa leaf litter on nutrient cycling in native mangrove forests from an ecosystem function perspective. We investigated the mangrove forest at Dongzhai Port using the litterbag decomposition method and in situ direct sampling to examine the short-term and long-term effects of L. racemosa litter input on nutrient cycling in native mangrove ecosystems. We hypothesized that the introduction of litter from the exotic L. racemosa could (1) accelerate the decomposition rates of community litter and increase the nutrient cycling rates within the local ecosystem and (2) change the microbial community structure in the invaded areas.
The study was conducted at the Dongzhaigang National Nature Reserve, Haikou City, Hainan Province, China (19°51′ to 20°01′N, 110°32′ to 110°37′E). This region features a tropical monsoon maritime climate, with an annual average temperature of 23.3–23.8°C and an annual average rainfall of 1676.4 mm (He et al., 2019). The soil types in the area consist of saline sandy loam or saline marsh soil, with a thickness of approximately 1.0–1.5 m (Cai et al., 2022).
The spread of L. racemosa is accompanied by the input of its leaf litter. To better understand the role of L. racemosa leaf litter during its dispersal, we selected three types of mangrove forests within the Dongzhai Harbor Nature Reserve: a native mangrove area dominated by Rhizophora stylosa (R. stylosa forest), a mixed forest of naturally invaded L. racemosa and R. stylosa (mixed forest), and an experimentally planted mangrove forest dominated by L. racemosa (L. racemosa forest). The stages represented three processes of L. racemosa spread: a native mangrove area where L. racemosa has not invaded, a mixed forest during the spread, and a predominantly L. racemosa forest after replacement of the native mangrove.
By examining the long-term cumulative effects of L. racemosa leaf litter decomposition in the three forests and its short-term effects after a single decomposition event in R. stylosa forests, we explored the effects of L. racemosa leaf litter input on nutrient cycling, which is a key ecosystem function in native mangrove forests. The L. racemosa forests were established in 2013 (20 years of growth). Therefore, this study focused on the cumulative effects of L. racemosa litter decomposition over the 20-year period. Rhizophora stylosa is an evergreen tree in the Rhizophoraceae family, and it reaches heights of up to 8 m and is highly resistant to wind and wave impacts. It is one of the most representative mangrove species in China (Pan et al., 2018). Field surveys have reported its cooccurrence with the exotic species L. racemosa and Sonneratia apetala (Liang et al., 2021). We selected R. stylosa due to its widespread presence in various native mangrove communities, including those invaded by L. racemosa (Liu et al., 2019). Rhizophora stylosa covers a significant mangrove area on Hainan Island and accounts for 40% of the total mangrove area (Fang et al., 2022). Moreover, the probability of mixed growth between L. racemosa and R. stylosa is high, as was observed frequently during field surveys. Thus, the combination was highly representative in our study.
Both the long-term cumulative effects and short-term impacts of L. racemosa leaf litter decomposition on native mangrove ecosystems were studied to explore the influence of this species on nutrient cycling and soil microorganisms.
For the long-term cumulative effects study, we examined the impact of inputting L. racemosa litter on the long-term accumulation of soil nutrients and microorganisms in native mangrove soils by comparing soil nutrient elements and microorganisms across the three representative mangrove forest types in the study area: R. stylosa forest, mixed forest, and L. racemosa forest. In June 2023, three plots measuring 10 m × 10 m each were established in the understory of each forest type, with at least 10 m between plots. Within each plot, a five-point sampling method was employed to collect soil samples for nutrient and microorganism analysis. Soil samples from a depth of 0–15 cm were collected using a soil auger for nutrient analysis, which included measurements of organic C, total N (TN), total P (TP), and ammonium N (NH4+-N). Additionally, 5 g soil samples were collected in cryotubes, stored in liquid N, and subsequently analyzed to determine their bacterial and fungal communities.
For the short-term effects study after a single decomposition experiment, we conducted in situ decomposition experiments under the R. stylosa forests using litter decomposition bags. Fresh healthy leaves of L. racemosa and R. stylosa were collected, air-dried, and then placed into bags (1 mm mesh nylon bags at 20 cm × 25 cm) with varying types of litter: R. stylosa litter bags, R. stylosa and L. racemosa mixed litter bags, and L. racemosa litter bags. The bags represented scenarios with no L. racemosa litter input (R1), mix of L. racemosa and R. stylosa litter input (R2), and pure L. racemosa litter (R3), which replaced the native mangrove litter.
In October 2023, in situ decomposition experiments were conducted in three plots in the R. stylosa forest to explore the impact of L. racemosa leaf inputs on soil nutrients and microorganisms in native mangrove soils. In each plot, 27 bags of each type of litter were placed adjacent to each other, with 9 retrievals per plot and 3 replicates per retrieval, for a total of 243 litter decomposition bags across all plots. Each decomposition bag contained 15 g of air-dried leaves. After removing surface debris, the bags were secured on the soil surface with homemade stakes to prevent tidal displacement, thus ensuring that the bags were closely arranged without overlapping. Decomposition bags were retrieved at 0, 7, 14, 21, 28, 42, 70, 103, and 149 days. The bags were washed and dried to a constant weight, and the remaining mass of the litter was measured to investigate the decomposition rates and remaining percentages for the three types of litter. The chemical properties of the litter collected at 0 and 103 days were analyzed to assess the impact of L. racemosa litter input on the quality and nutrient release rate of the native mangrove litter substrate. At 103 days of decomposition, samples from a depth of 0–15 cm beneath the three types of litter bags were collected for nutrient analysis, whereas samples at the surface beneath the bags were collected for microbial analysis. These analyses aimed to investigate the short-term effects of L. racemosa litter input on soil nutrients and microorganisms in native mangrove soils. Since the remaining mass at the end of decomposition (149 days) was insufficient for analysis, samples from the 103-day mark were selected for detailed analysis.
Leaf and soil organic C contents were determined using the potassium dichromate-volumetric method. TN was quantified using the Kjeldahl method, and TP was assessed using the molybdenum antimony anti-colorimetric method. Soil NH4+-N was quantified using the indophenol blue colorimetric method (Bao, 2000). Leaf lignin contents and cellulose were quantified using a Multiskan™ GO multifunctional microplate reader (Thermo Fisher Scientific, Waltham, MA, USA) (Foster et al., 2010).
The soil bacterial and fungal community compositions were analyzed using the Illumina MiSeq sequencing platform. DNA was extracted from the soil samples using a MagPure Soil DNA LQ Kit (Magan). DNA concentration and purity were assessed using a NanoDrop 2000 spectrophotometer (Thermo Fisher Scientific) and agarose gel electrophoresis, respectively. For the bacterial diversity analysis, genomic DNA served as a template for amplifying the V3–V4 variable region of the 16S rRNA gene (using universal primers 343F and 798R). For the fungal diversity analysis, the ITS1 variable region of the ITS gene was amplified using the primers ITS1F and ITS2. The primer sequences were trimmed using Cutadapt software, and the data were processed using the default parameters of QIIME 2 software (version 2020.11). Representative sequences and amplicon-sequence variant abundance tables were obtained, and the classify-sklearn classifier was employed to annotate representative sequences against the SILVA (version 138; for 16S rRNA) and UNITE (for ITS rRNA) databases. To facilitate downstream diversity and composition analyses, each sample was rarefied to the minimum sequence depth. Species alignment annotations were further analyzed using QIIME 2 software (version 2022.2).
The following formula was used to determine the remaining mass percentage (Hu et al., 2022):
where M0 is the initial litter mass and Mt is the remaining litter mass after time (t) of decomposition.
The litter decomposition rate was estimated using the exponential decay model proposed by Olson (1963) and defined as follows:
where X is the intercept, k is the litter-decomposition constant, y represents Mt/M0, a is the negative natural logarithm of X, and e is the base natural logarithm.
The formula for determining the mean relative decomposition rate (MRD) was derived from Patil et al. (2020):
where t1–t0 is the sampling interval in days.
The formulas used to calculate the time required for 50% and 95% litter decomposition (Bockheim et al., 1991; Zhang et al., 2021; Tao et al., 2023) were as follows:
The collected data were analyzed using IBM SPSS Statistics 22 (IBM Corp., Armonk, NY, USA) and MS Excel (Microsoft Corp., Redmond, WA, USA). Before conducting the statistical analyses, all data were assessed for normality and homogeneity of variance. One-way analysis of variance (ANOVA) or Kruskal–Wallis tests were employed to examine the effects of L. racemosa litter on the litter mass, decomposition rate, nutrient residues, and soil nutrients. A p-value < 0.05 was considered statistically significant. Pearson correlation analysis was used to examine the relationship between the average relative decomposition rate of litter and the quality of the litter substrate and determine the correlation between microbial phyla and soil nutrient content. SigmaPlot v15.0 (Grafiti LLC., Palo Alto, CA, USA) was used to fit the litter decomposition rates and their exponential decay models. The litter mass loss at each sampling time point was calculated as the difference between the initial and remaining weights. Bacterial and fungal α-diversity were computed using the stats package in R v3.3.1 (R Foundation for Statistical Computing, Vienna, Austria), alpha diversity was measured using the Shannon index, which considers both the richness and evenness of a sample, and calculated based on a rarefied feature table (QIIME diversity core-metrics-phylogenetic; > 2000 reads). Graphs were generated using Origin 2024 software (OriginLab Inc., Northampton, MA, USA).
The remaining mass percentages of leaf litter recovered at each time point in the single decomposition experiment are shown in Figure 1. All three litter types exhibited an initial rapid weight loss, followed by a relatively slower decomposition phase. From days 0 to 21 of decomposition, the remaining mass percentages of the three litter types were similar. During the later stages of decomposition (days 28–149, except for day 103), the remaining mass percentages of the L. racemosa litter were significantly lower than those of the R. stylosa litter. The mixed litter showed consistent patterns with L. racemosa except on day 70, when it exhibited significantly lower remaining percentages than R. stylosa litter. Based on the remaining mass-percentage data, during the later stages of decomposition, the decomposition rates of the L. racemosa and mixed litters were significantly higher than those of the R. stylosa litter.
Figure 1. Remaining leaf litter mass percentages from three different types of litter after decomposition in a R. stylosa forest. The decomposition constant (k) is depicted on the graph as the mean ± standard deviation. Lowercase letters indicate significant differences (p < 0.05) in the remaining mass percentage among the three types of leaf litter.
The various decomposition parameters observed via the litter decomposition-bag technique are presented in Table 1. An analysis of the annual average leaf litter decomposition rates showed that L. racemosa litter decomposed the fastest, R. stylosa litter decomposed the slowest, and mixed litter showed an intermediate rate. Curve fitting of the decomposition data for the three leaf litter types revealed a similar pattern of mass loss, with all single litter experiments exhibiting an exponential relationship and presenting R² values from 0.81 to 0.84 and slopes from 3.66 to 5.29. The leaf litter decomposition constant (k) and time required for the litter to lose 50% and 95% of its initial weight suggested that L. racemosa litter tended to decompose faster than R. stylosa litter, although this trend was not statistically significant.
Our analysis of the substrate quality of all three types of leaf litter is presented in Table 2. Laguncularia racemosa leaf litter exhibited significantly lower TN and TP contents and N:P ratios than R. stylosa leaf litter but significantly higher C:N, C:P, lignin:N, and lignin:P ratios. Correlation analysis (Supplementary Table S1) also indicated that the annual MRD was not significantly correlated with the initial litter quality, suggesting that the substrate quality of L. racemosa leaf litter could not explain its faster decomposition.
Basal chemical quality analyses of the litter indicated that after L. racemosa had begun to spread and replace R. stylosa (with all forest floor leaf litter being produced by L. racemosa), the quality of the forest floor leaf litter was significantly lower compared to that prior to the spread of L. racemosa into the area (i.e., no input of L. racemosa leaf litter). The findings suggest that adding L. racemosa litter led to a decline in the quality of native mangrove litter.
The nutrient content of the soil under all three forest types was determined to investigate the long-term impact of L. racemosa leaf litter input on soil nutrients in native mangrove forests. Figure 2A illustrates the nutrient content of the soil in all three forest types. The soil organic C, TN, and NH4+-N contents were significantly higher in the R. stylosa forests than the L. racemosa forests. Specifically, the organic C and TN contents in the R. stylosa forests were more than double those of the L. racemosa forests. R. stylosa forests, mixed-species forests, and L. racemosa forests were used to represent the three stages of L. racemosa invasion.
Figure 2. Elemental soil nutrient contents of the three vegetation types. (A) Soil element content beneath litter bags after decomposition, total contents (B), and content at the R1 sites (C) in grams per kilogram (g/kg) except for ammonium nitrogen, which was measured in milligrams per kilogram (mg/kg). In (B) ‘CK’ denotes the control soil site beneath R. stylosa, which was characterized by the absence of litter cover and decomposition. (D) Nutrient residual rates after litter decomposition. Different lowercase letters indicate significant differences at p < 0.05.
Comprehensive analysis of our data from all three sample sites beneath the R. stylosa forests (Figure 2B) revealed unexpected results. The nutrient element contents in the soil did not significantly differ (p > 0.05) for the three types of decomposed leaf litter. Furthermore, the nutrient contents in the soil after leaf litter decomposition did not differ significantly from those in the soil without leaf litter decomposition. Although not statistically significant, we did observe that the soil organic C, TN, and TP contents tended to be lower following the single decomposition of L. racemosa leaf litter than after the single decomposition of R. stylosa litter, with mixed litter showing an intermediate value.
However, we observed significant effects on decomposition at site R1, which was beneath the R. stylosa forest (Figure 2C). The soil organic C and TN contents at this site were significantly lower after L. racemosa and mixed leaf litter decomposition than after R. stylosa leaf litter decomposition.
Measuring the residual nutrient rates after short-term decomposition of the three types of leaf litter (Figure 2D) revealed that after 103 days of field decomposition, R. stylosa leaf litter exhibited the highest residual rates of organic C, P, and lignin (approximately 35, 35, and 45%, respectively), L. racemosa showed the lowest residual rates, and mixed leaf litter showed intermediate values. Notably, lignin, with its complex structure, is recalcitrant to decomposition. The significantly lower levels of residual lignin in L. racemosa leaf litter compared to R. stylosa leaf litter indicated that L. racemosa leaves decomposed more readily than R. stylosa leaves. Additionally, L. racemosa exhibited the lowest residual rates of organic C and P.
The bacterial community α-diversity (Shannon index) did not significantly differ among the three forest types in the cumulative effect experiments. However, the Shannon index for fungal communities in the soil under L. racemosa and mixed forests was significantly higher than that under R. stylosa (Figure 3A). Moreover, the bacterial α-diversity in the soil beneath the three types of leaf litter did not significantly differ after decomposition in the single decomposition experiment (Figure 3B). The Shannon index for soil fungi after R. stylosa leaf decomposition was significantly higher than that after mixed leaf decomposition, which was possibly due to the mixed effect of leaf litter reducing the α-diversity of soil fungi in the short term. However, the long-term cumulative effects of L. racemosa spreading into the area will likely reverse this trend and increase the soil fungal α-diversity.
Figure 3. Alpha (α)-diversity indices. (A) Understory soil samples from the three sites. (B) Soil beneath the litter after decomposition. Bacterial and fungal community α-diversity indices across the three sites, with different lowercase letters indicating significant differences among treatments (p < 0.05).
An analysis of the long-term cumulative effects on the bacterial communities in the soil under the three mangrove forest types revealed that 19 bacterial phyla had a relative abundance > 1% (defined as at least one treatment showing an average relative abundance > 1%). These phyla collectively represented over 96% of the total bacteria. Proteobacteria, Desulfobacterota, and Bacteroidetes were the dominant phyla in all three mangrove types (Supplementary Figure S2A). Among them, Proteobacteria was significantly more abundant in L. racemosa forests than in R. stylosa forests, with mixed forests exhibiting intermediate levels. Bdellovibrionota was significantly more abundant in L. racemosa forests than in R. stylosa forests. At the class level, differential analysis of the top 30 most abundant bacterial classes (Figure 4A) revealed that Alphaproteobacteria was significantly more abundant in L. racemosa forests than in the mixed and R. stylosa forests.
Figure 4. Differential bacterial and fungal class abundance. (A) Differences in bacterial (B) and fungal classes in the soil beneath the litter across all three sites. (C) Differential bacterial classes in the soil beneath the litter after decomposition. Different lowercase letters indicate significant differences among treatments (p < 0.05).
Regarding the fungal soil communities in the three mangrove forest types, four fungal phyla had a relative abundance > 1%. Ascomycota represented the dominant phylum common in all three mangrove types (Supplementary Figure S2B). Differential analysis of the 30 most abundant fungal phyla in the soil of all three mangrove forest types (Figure 5B) revealed that Ascomycota, known for its activity in the early decomposition stages, exhibited significantly higher relative abundance in R. stylosa and mixed forests than in L. racemosa forests. At the class level, Dothideomycetes was significantly more abundant in mixed and R. stylosa forests than in L. racemosa forests (Figure 4B).
Figure 5. Phylum abundance. (A) Differential bacterial (B) and fungal phyla in the soil beneath litter of all three forest types. (C) Differential bacterial (D) and fungal phyla in the soil beneath litter after decomposition. Different lowercase letters indicate significant differences among treatments (p < 0.05).
Correlation analysis of the microbial phyla (top 30 bacterial and fungal taxa based on relative abundance) and soil nutrient content in the three forest types (Supplementary Table S2, showing only microorganisms with significant correlations) revealed significant correlations between the microbial communities and soil nutrient contents. Furthermore, the results of the correlation and relative abundance analyses of differential microorganisms among the three forest types (Figure 5) were consistent with the results on the soil nutrient contents in the forests (Figure 2A).
An analysis of the short-term effects of the single decomposition litter experiment on the bacterial communities in the soil revealed 14 bacterial phyla with a relative abundance > 1%. These phyla collectively accounted for over 95% of the total bacterial population. Proteobacteria, Desulfobacterota, and Bacteroidota were the predominant phyla in the soil after litter decomposition (Supplementary Figure S2C). Differential analysis of the 30 most abundant bacterial phyla in the soil beneath the decomposed litter (Figure 5C) revealed significant differences in NB1-j and Bdellovibrionota across all three treatments. Bdellovibrionota, which aids in cellulose decomposition, was significantly more abundant in soils following L. racemosa litter decomposition than after R. stylosa litter decomposition. At the class level, differential analysis indicated that NB1-j was significantly less abundant in soils after L. racemosa litter decomposition than after R. stylosa and mixed litter decomposition.
The fungal community composition in the soil beneath the single decomposition experiment is illustrated in Supplementary Figure S2D. Differential analysis of the 30 most abundant fungal phyla across all three treatments (Figure 5D) revealed that Ascomycota, known for its abundant cellulase genes, was significantly more abundant in soils following L. racemosa and mixed litter decomposition than after R. stylosa litter decomposition. Moreover, fungal classes did not significantly differ across the three treatments. However, the number of bacterial and fungal species showing significant differences in the soils after the single decomposition experiments was lower than that observed after the cumulative effects multi-year decomposition experiments, indicating that the short-term impacts from small amounts of litter were relatively minor. The cumulative effects of multi-year L. racemosa litter decomposition shifted the bacterial and fungal communities in native mangrove soils toward faster decomposition.
Correlation analysis of the microbial phyla (the top 30 bacterial and fungal taxa by relative abundance) in the three soils after a single decomposition experiment revealed that certain soil microbial taxa associated with the decomposition of each leaf litter were significantly correlated with the MRD and soil nutrient content (Supplementary Table S3, listing only microorganisms with significant correlations). However, the microbial taxa exhibiting significant correlations in the soil after the single decomposition experiment were not entirely consistent with those exhibiting significant correlations in the understory of the three sites.
Many studies have shown that the input of exotic plant litter can accelerate the decomposition rate of litter in invaded areas (Liao et al., 2008; Poulette and Arthur, 2012; Chen et al., 2013). In our study, although the litter quality of L. racemosa was lower than that of R. stylosa, its litter input reduced the litter quality in the invaded sites, which led to accelerated decomposition rates (Table 1) and increased nutrient release rates (Figure 2D). This finding is consistent with the results of previous reports and supports our first hypothesis that the input of L. racemosa litter will accelerate the decomposition rate and nutrient cycling of invaded areas.
In terrestrial ecosystems, the litter quality of exotic plants is generally higher than that of native plants and thus enhances the local litter quality (Woodworth et al., 2020; Patil et al., 2020; Meisner et al., 2012; Zhang et al., 2021). Litter quality is a crucial factor affecting decomposition (Gao et al., 2016; Cheng et al., 2024), and higher-quality litter has been reported to decompose more easily than lower-quality litter (Kennedy and El-Sabaawi, 2017; Carrasco-Barea et al., 2022). However, whether this dynamic occurs in wetland ecosystems, particularly mangrove ecosystems, has not been previously clarified. Our findings on the exotic mangrove L. racemosa were inconsistent with those of previous studies. The litter of L. racemosa has higher C:N and C:P ratios than the litter of the native mangrove species R. stylosa, indicating that it has lower-quality litter. However, the low litter quality of L. racemosa accelerated its decomposition in the invaded area (Table 1), which is contrary to previous findings. Li et al. (2020) also highlighted the low-quality characteristics of L. racemosa litter. Under similar climatic conditions, litter decomposition is regulated by litter quality and decomposer communities (Patil et al., 2020; Zhang et al., 2021; Cheng et al., 2024). Thus, we sought to determine the factors underlying the faster decomposition rate of the lower-quality L. racemosa litter. An analysis of the soil microbial community structure revealed that the input of L. racemosa litter significantly increased the relative abundance of microbes related to litter decomposition, such as Proteobacteria and Bdellovibrionota, and enhanced the alpha diversity of soil fungi. Thus, a microbial environment conducive to the decomposition of L. racemosa leaf litter was established. This issue will be further discussed in the following section.
We found that the input of L. racemosa leaf litter altered the soil microbial community in native mangroves and stimulated the growth of certain dominant bacteria and fungi associated with litter decomposition, such as Proteobacteria, Bdellovibrionota, and Ascomycota. These microbial groups play a crucial role in accelerating leaf decomposition (Gladkov et al., 2022; Andronov et al., 2022; Kimeklis et al., 2023) and presented a higher relative abundance after the input of L. racemosa litter; thus, they accelerated the decomposition of the L. racemosa litter. The long-term cumulative effects indicated that L. racemosa litter decomposition also increased the soil fungal α-diversity. Higher microbial diversity supports elevated levels of ecosystem functions, which further facilitate the decomposition of L. racemosa litter. Correlation analyses further revealed that certain soil bacteria and fungi were significantly related to the MRD and nutrient content of the soil after litter decomposition (Supplementary Tables S2, S3), indicating a correlation between the faster decomposition rates of L. racemosa leaf litter and the structure of the soil decomposer community. This supports our hypothesis that the input of L. racemosa litter alters the microbial community structure in the invaded area. Additionally, these results suggest that a key reason for the faster decomposition rate of L. racemosa litter compared to R. stylosa litter may be changes in the soil microbial community structure caused by the input of L. racemosa litter. One of the ecological impacts of exotic plant dispersal is the alteration of soil microbial communities, which affects the diversity, abundance, and functionality of microbes, thereby leading to changes in litter decomposition rates and soil nutrient availability (Torres et al., 2021). Specific types of litter have distinct morphological and chemical characteristics that can act as ecological filters that select for or exclude certain microbial groups from the shared soil pool. Variations in the chemical exudates from litter can also directly influence soil microbial communities (Waldrop and Firestone, 2004). Therefore, the input of litter from exotic plants with different morphological and chemical traits leads to alterations in the local soil microbial community composition. Microbial communities with higher abundance and diversity often support higher levels of ecosystem functions, such as biomass decomposition, by facilitating interactions and resource allocation among microbial species (Zhang et al., 2023), thereby accelerating the decomposition process.
A meta-analysis of the effects of invasive plants on soil microbial communities revealed that exotic plants can enhance soil nutrient cycling by altering soil microbes, thus promoting invasion success (Hou et al., 2022). Our study revealed that after the input of L. racemosa leaf litter, the α-diversity of soil fungi increased. Changes in the relative abundance of dominant bacteria and fungi associated with leaf litter decomposition led to increased decomposition rates and nutrient release from native mangrove leaf litter. These findings are consistent with those of previous studies on invasive plants (Arthur et al., 2012; Yan et al., 2018; Zhang et al., 2023) and suggest that L. racemosa shares similar characteristics with invasive species in terms of modifying soil microbes to enhance soil nutrient cycling. This finding indicates the potential invasiveness of L. racemosa.
Research on the impact of exotic plants on soil nutrient cycling is important for evaluating the effects of these plants (Ehrenfeld, 2003). Exotic plants often have higher leaf litter quality and decomposition rates, leading to increased nutrient content in the soil through leaf decomposition, which alters ecosystem nutrient cycling and promotes exotic plant adaptation and growth (Meisner et al., 2012; Patil et al., 2020; Rothstein et al., 2004). However, our experiments showed that the short-term and long-term inputs of L. racemosa leaf litter resulted in decreased levels of carbon, nitrogen, and ammonium nitrogen in the soil of the invaded native mangrove forests, which is inconsistent with the increase in nutrients typically observed in studies of exotic plant litter (Sanon et al., 2012; Bray et al., 2017).
An area of research is why L. racemosa exhibits rapid litter decomposition but causes lower soil nutrient contents. Although the leaf litter of L. racemosa decomposes quickly, it has low quality and nutrient contents. However, L. racemosa is a tall fast-growing species, suggesting that its rapid growth requires substantial nutrients. Thus, the high amount of nutrients released from the decomposing leaf litter may be absorbed by L. racemosa plants to support their growth and development. This hypothesis was validated by Zhu et al. (2023), who performed genomic analyses of the leaves of L. racemosa from Dongzhaigang (Hainan Province) and discovered convergent evolution in genes responsible for nutrient absorption, which enhanced N and P uptake and transport capacity. The results of Liu et al. (2020) further supported the hypothesis that the introduced mangrove L. racemosa predominantly absorbs and enriches nutrients in plant tissues. They observed significantly higher richness in rhizospheric and endophytic diazotrophic communities as well as rhizospheric nutrient components (such as TN) in L. racemosa mangroves than in native mangroves, thus providing evidence based on the rhizospheric microbiota and nutrition. In our study, we also discovered that Alphaproteobacteria, which facilitates N fixation in plants, was significantly more abundant in L. racemosa forests than in mixed and R. stylosa forests, thus providing further support for our hypothesis. Therefore, the rapid decomposition of L. racemosa litter and lower soil nutrient contents might be attributed to the retention of nutrient elements in L. racemosa plants to facilitate their own rapid growth and development. In summary, inputting exotic mangrove L. racemosa litter accelerated nutrient cycling within mangrove ecosystems. The rapid decomposition of L. racemosa litter and the lower soil nutrient contents could reflect the absorption and retention of released nutrients to promote their rapid growth and development. Ecosystem nutrient cycling is a continuous process involving leaf litter decomposition, soil nutrient increase, plant growth, and subsequent leaf litter decomposition. The soil nutrient contents depend not only on the amount of nutrients released during leaf litter decomposition but also on the amount of nutrients absorbed and fixed within the plant biomass, thus reflecting a balance between nutrient inputs and outputs. Due to the rapid growth and high biomass of exotic plants, more nutrients are sequestered in their larger structures, leading to reduced nutrient levels in the soil. Compared to native plants, these larger exotic plants can gain a competitive advantage based on their enhanced absorption of sunlight and uptake of soil nutrients, which further facilitate their spread into new areas.
Many studies have assumed that the impact of exotic plants on soil nutrient cycling enhances the competitive advantage of these plants within ecosystems (Yelenik and Levine, 2011) because their high-quality litter increases soil nutrients, thereby promoting their own biomass accumulation and reproductive growth. Such increased nutrient availability may bolster their competitive ability and contribute to their successful dispersal (Patil et al., 2020; Hou et al., 2022). However, contrary to reports on other exotic plants (Meisner et al., 2012; Rothstein et al., 2004), our experiments revealed that litter inputs from L. racemosa actually led to a decrease in soil nutrient levels. Similar outcomes were observed for the invasive plant Agropyron cristatum (Christian and Wilson, 1999). This finding raises the questions of how the reduction in soil nutrients caused by L. racemosa litter aids the spread of this plant and what advantages are conferred by this strategy. In low-resource environments, the competitive ability of invasive plants is often influenced by their ability to enhance resource availability and tolerate low-resource conditions (Funk, 2013). Adaptations to low-resource ecosystems are frequently associated with traits related to resource acquisition or conservation (Chapin, 1980; Craine, 2009). Previous research indicates that the genetic and rhizosphere microbial characteristics of L. racemosa enhance its ability to acquire nutrients and tolerate low-nutrient conditions (Zhu et al., 2023; Liu et al., 2020), potentially strengthening its competitive ability in nutrient-poor environments. Therefore, we hypothesized that L. racemosa may employ a unique invasion strategy in which it reduces soil nutrient levels in native mangroves through low-quality litter inputs. This reduction in quality reduces the competitive advantage of native mangrove species intolerant to low-nutrient conditions but enhances the competitive advantage of L. racemosa, which is tolerant of low-nutrient environments (Liu et al., 2020). Similarly, Leary et al. (2006) found that litter of the invasive plant Ulex europaeus causes lower soil nutrient contents than that of native plants; thus, U. europaeus exhibits a competitive advantage over Panicum virgatum under nutrient-poor conditions.
The carbon content of mangroves is among the highest relative to other ecosystems (Friess et al., 2021; Macreadie et al., 2021); thus, mangroves play a crucial role in global carbon capture and storage (Lu et al., 2024). Nutrients from leaf litter decomposition are a primary source of organic matter in mangrove soils (Kristensen et al., 2008). Our single decomposition experiment and cumulative effect experiments demonstrated that the input of L. racemosa leaf litter decreased the soil organic carbon content. This reduction in soil organic carbon may be due to changes in microbial community structure caused by L. racemosa leaf litter, which enhanced microbial respiration and accelerated the conversion of soil carbon to carbon dioxide (CO2), leading to a decrease in organic carbon sequestration in the soil. Exotic plants, especially invasive ones, typically have high biomass and rapid growth rates, and L. racemosa is no exception (Li et al., 2020; Feng et al., 2022). These high-biomass, fast-growing plants return more organic carbon to the soil each year, potentially leading to increased carbon sequestration within the soil ecosystem (Cheng et al., 2006). Traditionally, such plants have been considered high carbon-sequestration species. However, in addition to plant biomass, the decomposition rates and patterns of leaf litter are also crucial factors in determining soil carbon storage. If high-biomass plants experience elevated levels of mineralization during litter decomposition, then additional carbon will be released back into the atmosphere, resulting in less organic carbon retained in the soil (Yan et al., 2018). Studies on terrestrial and wetland ecosystems indicate that the input of exotic plant litter can alter the soil microbial community structure by increasing the proportion of microbial communities responsible for mineralization (e.g., Proteobacteria), which can affect organic carbon decomposition patterns. This change can lead to increased soil respiration and CO2 emissions, thereby reducing soil organic carbon storage (Meisner et al., 2012; Spirito et al., 2014; Zhang et al., 2014; Yan et al., 2018). Our results indirectly support this mechanism.
The results of our study confirm our hypotheses that L. racemosa leaf litter input accelerates nutrient cycling in native mangrove forests while reducing soil nutrient contents and altering soil microbial communities. Compared with the native mangrove species (R. stylosa), the exotic mangrove L. racemosa exhibited lower leaf litter quality and faster decomposition rates. Laguncularia racemosa leaf litter input decreased the quality of the native mangrove soil and shifted the microbial communities toward those conducive to accelerated leaf decomposition rates and increased nutrient release rates, which accelerated nutrient cycling. Changes in the soil microbial community structure induced by L. racemosa leaf litter are likely responsible for the higher leaf litter decomposition rates in the invaded native mangrove forests compared with that observed for the native species because L. racemosa leaf litter is of lower quality. The soil nutrient content accumulated over several years under L. racemosa was lower than that under R. stylosa. This may be because nutrients released from decomposing leaf litter are absorbed by L. racemosa plants to support their growth and development. Furthermore, adding exotic mangrove L. racemosa leaf litter reduced the soil blue carbon content, which may adversely affect global climate change. The results of our study suggest that the spread of L. racemosa may differ from that of other exotic plants because it lowers the soil nutrient levels through lower-quality leaf litter, thereby reducing the competitive ability of native mangrove species that are intolerant to low-nutrient conditions. The enhanced competitiveness of L. racemosa under low-nutrient conditions likely contributed to its successful spread in the study area.
The datasets presented in this study can be found in online repositories. The names of the repository/repositories and accession number(s) can be found below: https://www.ncbi.nlm.nih.gov/, PRJNA1132499.
HL: Writing – original draft, Writing – review & editing. CC: Investigation, Writing – review & editing. JZ: Investigation, Writing – review & editing. HB: Methodology, Writing – review & editing. SZ: Methodology, Writing – review & editing. QL: Conceptualization, Funding acquisition, Writing – review & editing.
The author(s) declare that financial support was received for the research, authorship, and/or publication of this article. This study was supported by the National Natural Science Foundation of China (grant number 31960281).
We acknowledge our research team partners for their collaboration and assistance provided throughout this study.
The authors declare that the research was conducted in the absence of any commercial or financial relationships that could be construed as a potential conflict of interest.
All claims expressed in this article are solely those of the authors and do not necessarily represent those of their affiliated organizations, or those of the publisher, the editors and the reviewers. Any product that may be evaluated in this article, or claim that may be made by its manufacturer, is not guaranteed or endorsed by the publisher.
The Supplementary Material for this article can be found online at: https://www.frontiersin.org/articles/10.3389/fpls.2024.1463548/full#supplementary-material
Allison, S. D., Vitousek, P. M. (2004). Rapid nutrient cycling in leaf litter from invasive plants in Hawai'i. Oecologia 141, 612–619. doi: 10.1007/s00442-004-1679-z
Andronov, E. E. (2022). The Structure of Stable Cellulolytic Consortia Isolated from Natural Lignocellulosic Substrates. Internat J. M. Sci. 23, 10779. doi: 10.3390/ijms231810779
Arthur, M. A., Bray, S. R., Kuchle, C. R., McEwan, R. W. (2012). The influence of the invasive shrub, Lonicera maackii, on leaf decomposition and microbial community dynamics. Plant Ecol. 213, 1571–1582. doi: 10.1007/s11258-012-0112-7
Bockheim, J. G., Jepsen, E. A., Heisey, D. M. (1991). Nutrient dynamics in decomposing leaf litter of four tree species on a sandy soil in northwestern Wisconsin. Can. J. For. Res. 21, 803–812. doi: 10.1139/x91-113
Bonanomi, G., Motti, R., De Marco, A., Idbella, M. (2023). Temperature sensitivity and decomposition rate of 101 leaf litter types from Mediterranean ecosystems. Sci. Total Environ. 894, 165026. doi: 10.1016/j.scitotenv.2023.165026
Bray, S. R., Hoyt, A. M., Yang, Z., Arthur, M. A. (2017). Non-native liana, Euonymus fortunei, associated with increased soil nutrients, unique bacterial communities, and faster decomposition rate. Plant Ecol. 218, 329–343. doi: 10.1007/s11258-016-0689-3
Cai, R., Ding, R., Yan, X., Li, C., Sun, J., Tan, H., et al. (2022). Adaptive response of Dongzhaigang mangrove in China to future sea level rise. Sci. Rep. 12, 11495. doi: 10.1038/s41598-022-15774-7
Carrasco-Barea, L., Llorens, L., Romaní, A. M., Gispert, M., Verdaguer, D. (2022). Litter decomposition of three halophytes in a Mediterranean salt marsh: Relevance of litter quality, microbial activity and microhabitat. Sci. Total Environ. 838, 155743. doi: 10.1016/j.scitotenv.2022.155743
Chapin, F. S. III. (1980). The mineral nutrition of wild plants. Annu. Rev. Ecol. Syst. 11, 233–260.
Chen, B. M., Peng, S. L., D'Antonio, C. M., Li, D. J., Ren, W. T. (2013). Non-Additive effects on decomposition from mixing litter of the invasive Mikania micrantha H.B.K. with native plants. PloS One 8, e66289. doi: 10.1371/journal.pone.0066289
Chen, Z., Wang, C., Chen, X., Yuan, Z., Song, H., Li, C. (2020). Heterogeneous leaves of predominant trees species enhance decomposition and nutrient release in the riparian zone of the Three Gorges Reservoir. Sci. Rep. 10, 17382. doi: 10.1038/s41598-020-74062-4
Cheng, C., Liu, Z., Zhang, Y., He, Q., Li, B., Wu, J. (2024). Leaf litter decomposition and its drivers differ between an invasive and a native plant: Management implications. Ecol. applications: Publ. Ecol. Soc. America 34, e2739. doi: 10.1002/eap.2739
Cheng, X., Luo, Y., Chen, J., Lin, G., Chen, J., Li, B. (2006). Short-term C4 plant Spartina alterniflora invasions change the soil carbon in C3 plant-dominated tidal wetlands on a growing estuarine Island. Soil Biol. Biochem. 38, 3380–3386. doi: 10.1016/j.soilbio.2006.05.016
Christian, J. M., Wilson, S. D. (1999). Long-term ecosystem impacts of an introduced grass in the northern Great Plains. Ecology 80, 2397–2407. doi: 10.1890/0012-9658(1999)080[2397:LTEIOA]2.0.CO;2
Craine, J. M. (2009). Chapter 10. the low-water and low-co2 strategies: resource strategies of wild plants. J. Developmental Behav. Pediatrics 35(12), 107–107.
Ehrenfeld, J. G. (2003). Effects of exotic plant invasions on soil nutrient cycling processes. Ecosystems 6, 503–523. doi: 10.1007/s10021-002-0151-3
Ehrenfeld, J. G. (2010). Ecosystem consequences of biological invasions. Annu. Rev. Ecol. Evol. Systematics 41, 59–80. doi: 10.1146/annurev-ecolsys-102209-144650
Fang, F. Z., Li, Z. J., Gui, H. Y. (2022). Investigation and research of mangrove forest in Hainan. Trop. Forestry, 42–49.
Feng, H., Gu, X., Tang, T., Lin, Q., Ratul, S. B., Wang, X., et al. (2022). Determining the effects of salinity and light on key ecophysiological traits of two nonnative mangrove species in China. J. Coast. Res. 38, 361–368.
Foster, C. E., Martin, T. M., Pauly, M. (2010). Comprehensive compositional analysis of plant cell walls (Lignocellulosic biomass) part I: lignin. J. Visualized Experiments: JoVE, 1745. doi: 10.3791/1745
Friess, D. A., Sasmito, S. D., Sanderman, J., Sievers, M., Buelow, C., Kauffman, J. B., et al. (2021). Future carbon emissions from global mangrove forest loss. Global Change Biol. 27(12), 2856–2866. doi: 10.1111/gcb.15571
Funk, J. L. (2013). The physiology of invasive plants in low-resource environments. Conserv. Physiol. 1, cot026. doi: 10.1093/conphys/cot026
Gao, J., Kang, F., Han, H. (2016). Effect of litter quality on leaf-litter decomposition in the context of home-field advantage and non-additive effects in temperate forests in China. Polish J. Environ. Stud. 25(5), 1911–1920. doi: 10.15244/pjoes/62822
Gladkov, G. V., Kimeklis, A. K., Afonin, A. M., Lisina, T. O., Orlova, O. V., Aksenova, T. S., et al. (2022). The structure of stable cellulolytic consortia isolated from natural lignocellulosic substrates. Int. J. Mol. Sci. 23, 10779. doi: 10.3390/ijms231810779
He, Y., Guan, W., Xue, D., Liu, L., Peng, C., Liao, B., et al. (2019). Comparison of methane emissions among invasive and native mangrove species in Dongzhaigang, Hainan Island. Sci. Total Environ. 697, 133945. doi: 10.1016/j.scitotenv.2019.133945
Hou, Q. C., Feng, Y. L., Zhou, Y. J., Ao, Y. M., Chen, C. X., Xing, Y. J., et al. (2022). Main hypotheses on mechanisms underlying plant invasion: A review. J. Appl. Ecol. 33, 3105–3115. doi: 10.13287/j.1001-9332.202211.005
Hu, X., Arif, M., Ding, D., Li, J., He, X., Li, C. (2022). Invasive plants and species richness impact litter decomposition in riparian zones. Front. Plant Sci. 13. doi: 10.3389/fpls.2022.955656
Incerti, G., Cartenì, F., Cesarano, G., Sarker, T. C., Abd El-Gawad, A. M., D'Ascoli, R., et al. (2018). Faster N release, but not C loss, from leaf litter of invasives compared to native species in mediterranean ecosystems. Front. Plant Sci. 9. doi: 10.3389/fpls.2018.00534
Kennedy, K. T., El-Sabaawi, R. W. (2017). A global meta-analysis of exotic versus native leaf decay in stream ecosystems. Freshw. Biol. 62, 977–989. doi: 10.1111/fwb.2017.62.issue-6
Kimeklis, A. K., Gladkov, G. V., Orlova, O. V., Afonin, A. M., Gribchenko, E. S., Aksenova, T. S., et al. (2023). The succession of the cellulolytic microbial community from the soil during oat straw decomposition. Int. J. Mol. Sci. 24, 6342. doi: 10.3390/ijms24076342
Kristensen, E., Bouillon, S., Dittmar, T., Marchand, C. (2008). Organic carbon dynamics in mangrove ecosystems: a review. Aquat. Bot. 89, 201–219. doi: 10.1016/j.aquabot.2007.12.005
Lang, T., Tang, Y., Tam, N. F., Gan, K., Wu, J., Wu, W., et al. (2022). Microcosm study on cold adaptation and recovery of an exotic mangrove plant, Laguncularia racemosa in China. Mar. Environ. Res. 176, 105611. doi: 10.1016/j.marenvres.2022.105611
Leary, J. K., Hue, N. V., Singleton, P. W., Borthakur, D. (2006). The major features of an infestation by the invasive weed legume gorse (Ulex europaeus) on volcanic soils in Hawaii. Biol. Fertility Soils 42, 215–223. doi: 10.1007/s00374-005-0018-9
Li, F. L., Zhong, L., Cheung, S. G., Wong, Y. S., Shin, P. K. S., Lei, A. P., et al. (2020). Is Laguncularia racemosa more invasive than Sonneratia apetala in northern Fujian, China in terms of leaf energetic cost? Mar. pollut. Bull. 152, 110897. doi: 10.1016/j.marpolbul.2020.110897
Liang, Z., Huang, Z., Peng, T., Su, M., Shu, F., Zhang, B. (2021). Current status and conservation strategies of mangrove resources in Huidong County. J. Zhejiang Forestry Sci. Technol. 41, 108–112. doi: 10.3969/j.issn.1001-3776.2021.01.017
Liao, C., Peng, R., Luo, Y., Zhou, X., Wu, X., Fang, C., et al. (2008). Altered ecosystem carbon and nitrogen cycles by plant invasion: a meta-analysis. New Phytol. 177, 706–714. doi: 10.1111/j.1469-8137.2007.02290.x
Lin, B., Liu, Q., Wu, Y., Pang, X., He, H. (2003). Effects of alpine coniferous forest litter on soil physical and chemical properties in western Sichuan, China. Chin. J. Appl. Environ. Biol. 04), 346–351.
Liu, X., Yang, C., Yu, X., Yu, H., Zhuang, W., Gu, H., et al. (2020). Revealing structure and assembly for rhizophyte-endophyte diazotrophic community in mangrove ecosystem after introduced Sonneratia apetala and Laguncularia racemosa. Sci. Total Environ. 721, 137807. doi: 10.1016/j.scitotenv.2020.137807
Liu, Q., Zhang, Y., Zhong, C. R., Yang, Y., Li, D. L., Zhang, S. J., et al. (2019). Study on invasiveness of exotic mangrove species Laguncularia racemosa. Hubei Agric. Sci. 58, 60–64.
Lu, Z., Qin, G., Gan, S., Liu, H., Macreadie, P. I., Cheah, W., et al. (2024). Blue carbon sink capacity of mangroves determined by leaves and their associated microbiome. Global Change Biol. 30, e17007. doi: 10.1111/gcb.17007
Macreadie, P. I., Costa, M. D., Atwood, T. B., Friess, D. A., Kelleway, J. J., Kennedy, H., et al. (2021). Blue carbon as a natural climate solution. Nat. Rev. Earth Environ. 2, 826–839. doi: 10.1038/s43017-021-00224-1
Meisner, A., de Boer, W., Cornelissen, J. H., van der Putten, W. H. (2012). Reciprocal effects of litter from exotic and congeneric native plant species via soil nutrients. PloS One 7, e31596. doi: 10.1371/journal.pone.0031596
Olson, J. S. (1963). Energy storage and the balance of producers and decomposers in ecological systems. Ecology 44, 322–331. doi: 10.2307/1932179
Pan, L., Shi, X., Zeng, C., Chen, Y. (2018). Plant types of mangroves in Guangxi. Guangxi Sci. 04), 352–362. doi: 10.13656/j.cnki.gxkx.20180813.002
Patil, M., Kumar, A., Kumar, P., Cheema, N. K., Kaur, R., Bhatti, R., et al. (2020). Comparative litter decomposability traits of selected native and exotic woody species from an urban environment of north-western Siwalik region, India. Sci. Rep. 10, 7888. doi: 10.1038/s41598-020-64576-2
Poulette, M. M., Arthur, M. A. (2012). The impact of the invasive shrub Lonicera maackii on the decomposition dynamics of a native plant community. Ecol. applications: Publ. Ecol. Soc. America 22, 412–424. doi: 10.1890/11-1105.1
Prescott, C. E., Zukswert, J. M. (2016). Invasive plant species and litter decomposition: time to challenge assumptions. New Phytol. 209, 5–7. doi: 10.1111/nph.13741
Rothstein, D. E., Vitousek, P. M., Simmons, B. L. (2004). An exotic tree alters decomposition and nutrient cycling in a Hawaiian montane forest. Ecosystems 7, 805–814. doi: 10.1007/s10021-004-0009-y
Sanon, A., Beguiristain, T., Cébron, A., Berthelin, J., Sylla, S. N., Duponnois, R. (2012). Differences in nutrient availability and mycorrhizal infectivity in soils invaded by an exotic plant negatively influence the development of indigenous Acacia species. J. Environ. Manage. 95 Suppl, S275–S279. doi: 10.1016/j.jenvman.2011.01.025
Spirito, F., Yahdjian, L., Tognetti, P. M., Chaneton, E. J. (2014). Soil ecosystem function under native and exotic plant assemblages as alternative states of successional grasslands. Acta Oecologica 54, 4–12. doi: 10.1016/j.actao.2012.10.004
Tao, J., Lei, Z. Y., Wang, G. C., Zhou, F. Y., Bai, W. Q. (2023). Decomposition and nutrient release of leaf litter in Pinus sylvestris var. mongolica plantations. J. Arid Land Resour. Environ. 12), 131–139.
Torres, N., Herrera, I., Fajardo, L., Bustamante, R. O. (2021). Meta-analysis of the impact of plant invasions on soil microbial communities. BMC Ecol. Evol. 21, 172. doi: 10.1186/s12862-021-01899-2
Waldrop, M. P., Firestone, M. K. (2004). Microbial community utilization of recalcitrant and simple carbon compounds: impact of oak-woodland plant communities. Oecologia 138, 275–284. doi: 10.1007/s00442-003-1419-9
Woodworth, G. R., Ward, J. N., Carr, D. E. (2020). Exotic tree and shrub invasions alter leaf-litter microflora and arthropod communities. Oecologia 193, 177–187. doi: 10.1007/s00442-020-04657-1
Yan, J., Wang, L., Hu, Y., Tsang, Y. F., Zhang, Y., Wu, J., et al. (2018). Plant litter composition selects different soil microbial structures and in turn drives different litter decomposition pattern and soil carbon sequestration capability. Geoderma 319, 194–203. doi: 10.1016/j.geoderma.2018.01.009
Yelenik, S. G., Levine, J. M. (2011). The role of plant-soil feedbacks in driving native-species recovery. Ecology 92, 66–74. doi: 10.1890/10-0465.1
Zhang, L., Li, J., Wang, Z., Zhang, D., Liu, H., Wang, J., et al. (2023). Litter mixing promoted decomposition and altered microbial community in common bean root litter. BMC Microbiol. 23, 148. doi: 10.1186/s12866-023-02871-4
Zhang, J., Li, H., Zhang, H., Zhang, H., Tang, Z. (2021). Responses of litter decomposition and nutrient dynamics to nitrogen addition in temperate shrublands of north China. Front. Plant Sci. 11. doi: 10.3389/fpls.2020.618675
Zhang, L., Wang, H., Zou, J., Rogers, W. E., Siemann, E. (2014). Non-native plant litter enhances soil carbon dioxide emissions in an invaded annual grassland. PloS One 9, e92301. doi: 10.1371/journal.pone.0092301
Zhong, C. R., Li, S. C., Yang, Y., Zhang, Y., Lin, Z. W. (2011). Analysis of the introduction effect of a mangrove species laguncularia racemosa. J. Fujian Forestry Sci. Technol. 03), 96–99.
Keywords: exotic plant, Laguncularia racemosa, leaf litter, nutrient cycling, soil microorganisms, microbial decomposers
Citation: Li H, Chen C, Zhou J, Bai H, Zhang S and Liu Q (2024) Exotic mangrove Laguncularia racemosa litter input accelerates nutrient cycling in mangrove ecosystems. Front. Plant Sci. 15:1463548. doi: 10.3389/fpls.2024.1463548
Received: 12 July 2024; Accepted: 23 September 2024;
Published: 08 October 2024.
Edited by:
Runguo Zang, Chinese Academy of Forestry, ChinaReviewed by:
Yuelin Li, Chinese Academy of Sciences (CAS), ChinaCopyright © 2024 Li, Chen, Zhou, Bai, Zhang and Liu. This is an open-access article distributed under the terms of the Creative Commons Attribution License (CC BY). The use, distribution or reproduction in other forums is permitted, provided the original author(s) and the copyright owner(s) are credited and that the original publication in this journal is cited, in accordance with accepted academic practice. No use, distribution or reproduction is permitted which does not comply with these terms.
*Correspondence: Qiang Liu, aG5zeWxxQDE2My5jb20=
Disclaimer: All claims expressed in this article are solely those of the authors and do not necessarily represent those of their affiliated organizations, or those of the publisher, the editors and the reviewers. Any product that may be evaluated in this article or claim that may be made by its manufacturer is not guaranteed or endorsed by the publisher.
Research integrity at Frontiers
Learn more about the work of our research integrity team to safeguard the quality of each article we publish.