- 1College of Forestry, Fujian Agriculture and Forestry University, Fuzhou, Fujian, China
- 2Service Center, Fujian Meteorological Bureau, Fuzhou, Fujian, China
- 3Production Technology Department, Fujian Minhou Baisha State-Owned Forest Farm, Fuzhou, Fujian, China
- 4Institute of Oceanography, Minjiang University, Fuzhou, Fujian, China
Introduction: Plant physiology response and adaptation to drought stress has become a hotspot in plant ecology and evolution. Cotoneaster multiflorus possesses high ecological, ornamental and economic benefits. It has large root system and tolerance to cold, drought and poor soil. Therefore, C. multiflorus is considered as one of the most important tree species for ecological restoration in arid and semi-arid areas. However, little is known about the physiological mechanisms, molecular mechanisms and drought strategies of how C. multiflorus responds to drought stress. Therefore, exploring the physiological response mechanisms, molecular mechanisms and adaptive strategies of C. multiflorus in response to drought is important for its growth in arid and semi-arid regions.
Methods: We investigated the response and coupling mechanisms of water status, photosynthetic properties and chloroplast fluorescence parameters in C. multiflorus in response to drought and rehydrated after drought, especially the importance of nocturnal sap flow and nocturnal water refilling to maintain its own water balance in response to drought stress. In addition, we studied the stress response of C. multiflorus transcriptome factors, and we also discussed drought adaptation strategies of C. multiflorus.
Results: C. multiflorus adapted to drought stress by a series of structural and physiological mechanisms, such as promoting closing stomata, increasing nocturnal sap flow. When rehydrated after undergoing severe drought stress, its physiological activities such as photosynthesis, water status, chlorophyll fluorescence parameters and other physiological activities have rapidly resumed. This showed C. multiflorus had strong tolerance to drought. In addition, water status, photosynthetic characteristics, and chloroplast fluorescence parameters of C. multiflorus were highly coupled. Nocturnal sap flow and nocturnal water refilling were very important for C. multiflorus to maintain its own water balance in response to drought stress. Finally, C. multiflorus will strengthen the drought defense mechanism by gene regulation of various metabolisms, such as promoting stomatal closure, reducing transpiration water loss, and vigorously regulating water balance. C. multiflorus responded to drought stress by avoiding or reducing water deficit in plant organs and tissues. Therefore, the shrub C. multiflorus is a drought-tolerant plant.
Discussion: We explored the response mechanisms of water status, photosynthetic characteristics, and chloroplast fluorescence parameters of C. multiflorus in drought and rehydrated after drought stress, especially the response mechanisms of nocturnal sap flow and nocturnal water refilling in response to drought stress, and identified the physiological coupling mechanisms, molecular mechanisms and drought types of C. multiflorus in response to drought.
1 Introduction
As global temperatures rise and precipitation patterns change, droughts have become increasingly frequent in recent years (Réjou-Méchain et al., 2021; Naumann et al., 2018). The trend of extreme drought frequency is even more pronounced (Gu et al., 2023; Christian et al., 2023; Batibeniz et al., 2023). These frequent droughts have had significant impacts on the local carbon balance and forest ecosystem services (Hartmann et al., 2022; Anderegg et al., 2020), including causing large-scale tree mortality at a regional level (Choat et al., 2018; Goulden and Bales, 2019; Zhang et al., 2023). Therefore, the response and adaptation of plants to drought stress have become a focal point in ecological and plant physiological research (Werner et al., 2021; Anderegg et al., 2018; Yao et al., 2023; Vinod et al., 2023; Wang et al., 2023a).
The causes, climatic thresholds and modelling of drought-induced tree mortality have been well studied (Pereira et al., 2024; Wion et al., 2022; Valerian et al., 2021), however, the underlying physiological mechanisms are still a subject of debate (Martinez-Vilalta et al., 2019; Grossiord et al., 2020; Trugman et al., 2018). During short-term and severe droughts, water availability is often the primary factor influencing plant mortality, while during extended periods of drought, the significance of carbon availability for critical survival functions tends to become more prominent (Arend et al., 2021; Wang et al., 2020). Nevertheless, it is essential to minimize the blockage of xylem conduits in order to enhance the transport of water to the canopy and regenerative tissues (apical and formative meristematic tissues) of plants. Among these mechanisms, stomatal regulation is the most crucial in preventing xylem embolism by regulating water transport and leaf transpiration (Chen et al., 2022). It is worth noting that the nighttime fluid flow has the effect of nighttime transpiration and stem hydration, and also helps to reduce the formation of xylem embolism and gas holes in plants, and avoid hydraulic failure and even death of plants due to drought (Klein et al., 2018; Wu et al., 2023). This is because night transpiration transporting the water replenishment of stems improves the low water potential of stems caused by daytime transpiration, prevents xylem embolism, repairs air holes to a certain extent, and avoids the death of plants caused by frequent hydraulic failure (Klein et al., 2018; Zeppel et al., 2019). In arid zones, these mechanisms are especially crucial for plant survival.
With global changes leading to shifts in climate patterns, the vegetation in arid zones is typically dominated by small trees, shrubs, or semi-shrubs, which act as pioneer species adapted to harsh conditions. These plants rely on efficient water transport mechanisms to withstand the challenges of arid environments and maintain their survival in the face of changing environmental conditions (Shen et al., 2022; Zhou et al., 2022; Davis et al., 2020). Cotoneaster multiflorus is a shrub belonging to the Cotoneaster genus in the apple subfamily (Maloideae) of the rose family (Rosaceae). This species is known for its robust characteristics, including a large root system, and its ability to withstand cold temperatures, drought conditions, and poor soil fertility. These traits make it well-suited for challenging environments and highlight its adaptability and resilience in adverse growing conditions (Chen et al., 2021). C. multiflorus is a pioneering species for vegetation restoration in the ecological transition zones of arid and semi-arid loess hills and gullies and bedrock hills (tufaceous mountainous areas), where it is extremely widespread. The distribution of C. multiflorus populations continue to expand under various scenarios in different future climates (Huang et al., 2024). Meanwhile, C. multiflorus has high ecological benefits, such as wind prevention and sand fixation, water, soil conservation (Zhao et al., 2022) and ornamental role (Yang et al., 2022). It also has great economic benefits, such as medicinal development (Chang and Jeon, 2003; Liu et al., 2018; Jia, 2022) and as a grafting rootstock for Malus pumila Mill (Wang et al., 1983). Therefore, to study leaf water status, photosynthetic characteristics and stem fluid flow of C. multiflorus can help to reveal the response mechanism of the shrub to the environment in arid and semi-arid zones.
However, plant adaptation to drought stress is not attributed to a single trait but is instead a combination of various traits that work together and are correlated with each other (McDowell et al., 2022; López et al., 2021). For example, plants may adjust photosynthetic physiological traits (reducing stomatal conductance, increasing water use efficiency, and reducing transpiration rate) and hydraulic traits (reducing xylem hydraulic conductivity and conduit diameter, etc.) to reduce water loss in response to drought conditions (Sevanto et al., 2014; Vastag et al., 2020). Therefore, further research is needed to explore the relationships among different traits in order to better understand the adaptive mechanisms and coping strategies that plants employ to tolerate drought stress.
At present, the coping strategies and molecular mechanism of C. multiflorus under drought stress are not well known. The research involved analysis of 25 different indicators and high-throughput sequencing to assess patterns of water status, photosynthetic characteristics, trunk runoff, and transcription factor in response to different drought intensities and rehydrated processes. Through the use of integrated analyses such as correlation analysis, principal component analysis, DEGs analysis and GO analysis, the study sought to provide insights into the adaptive strategies employed by C. multiflorus to withstand drought stress. The findings were expected to enhance our understanding of the growth patterns of C. multiflorus in arid and semi-arid regions and elucidate the strategies utilized by the species to cope with drought conditions, and further exploration of its drought resistance mechanism. The results of this study will improve our understanding of coping strategies under drought stress conditions and enhance the management of this important woody species in arid regions.
2 Materials and methods
2.1 Test materials and moisture control
The State Key Laboratory of Seedling Bioengineering (Ningxia Forestry Research Institute Co., Ltd.) provided 2-year-old C. multiflorus with robust growth, free of pests and diseases, and basically the same growth. On May 1, 2023, 350 pots (1 plant per pot) of C. multiflorus seedlings were cultivated in the greenhouse of Fujian Agriculture and Forestry University. The plastic pots used for the experiment were 20.5 cm (calibre) × 14.5 cm (ground diameter) × 18.5 cm (height). The potting soil was yellow soil, soil weight was 4.0 Kg/pot, in which the soil organic carbon (SOC), organic matter (SOM), available nitrogen (AN), available phosphorus (AP) and available potassium (AK) were 7.22 (g/Kg), 12.44 (g/Kg), 70.00 (mg/Kg), 7.90 (mg/Kg) and 7.72 (mg/Kg), respectively. During the experiment, the average daytime temperature and nighttime temperature were 38.8°C and 30.6°C, respectively, and the average daytime humidity and nighttime humidity were 52.5% and 69.1%, respectively.
From July 25 to 31, 2023, 350 test seedlings were subjected to different degrees of water deficit cultivation and partial normal water management. On August 1, 2023, 150 healthy C. multiflorus seedlings without diseases and insect pests, with similar growth potential and different water deficits were selected as experimental materials. The experiment was set up with 4 drought level treatments and 1 rehydrated treatment, a total of 5 treatments, each treatment was repeated 3 times, 10 plants per replicate, that was, 30 plants per treatment. Specific operations are as follows:
According to the soil relative water content (WCH) of 60% ~ 80%, 40% ~ 60%, and 20% ~ 40%, 30 seedlings were selected from 150 test seedlings as comparison (CK)、mild drought stress (LD)、moderate drought stress (MD), respectively. Finally, 60 plants with a WCH of 5% ~ 10% were selected. Among them, 30 plants were used as severe drought stress (SD), and the other 30 plants were replenished with water on August 6, 2023 and used as rehydrated treatment (RD).The assessment of each index began on August 8, 2023 and ended on August 10. Therefore, the drought stress time of CK, LD, MD, and SD was 7∼10 days, and the rehydrated lasts for 2∼5 days.
During the treatments, lost water was replaced daily by potting water control method (Luo et al., 2021; Zou et al., 2022), the pots were weighed and watered every 24h to maintain the corresponding soil moisture. The calculation formula of WCH is as follows (Wu, 2020):
WCH % is the relative water content of soil, is the original soil quality, and is the soil quality after drying.
2.2.1 Determination of leaf water potential and relative water content
The WP4C Dew Point PotentiaMeter was employed to measure the leaf water potential of C. multiflorus between 5:00 and 6:30 local time. Within each treatment group, 9 C. multiflorus plants were randomly chosen for analysis. From each selected plant, 10 mature leaves located in the middle of the plant were picked. In these 10 leaves, 5 were utilized to determine the leaf water potential using the WP4C Dew Point PotentiaMeter (Miao et al., 2017), the other 5 mature leaves were used to determine the relative water content of the leaves by the saturated weighing method (RWC) (Wang et al., 2023b).
2.2.2 Leaf stomatal determination
In the experiment, 3 plants of C. multiflorus from each treatment were randomly chosen. From each selected plant, 3 mature leaves were then randomly selected for stomatal determination. Stomatal characteristics were observed in 10 randomly selected fields of view of each leaf using a bench electron microscope (TM3030 Plus). The measurements of stomatal features including stomatal opening length, stomatal opening width, stomatal aperture, and stomatal density were obtained using ImageJ software. This analysis aimed to provide insights into the stomatal behavior of C. multiflorus under varying drought stress levels.
2.2.3 Trunk sap flow measurement
From August 9 to August 31, 2023, based on preliminary survey of sapwood thickness of C. multiflorus (Leng, 2020), each of the 12 C. multiflorus plants with similar growth was fitted with a probe (probe length 10 mm, probe diameter 1.2 mm). The probe was connected to the i-MIX data collector and the average value was calculated every 5 min and automatically stored in the data collector. Throughout the monitoring period, the soil water content was consistently monitored daily. The C. multiflorus experienced 3 times from the initial watering, to severe drought stress.
Additionally, the temperature variance between thermocouples was tracked using a probe to calculate the heat dissipation associated with sap flow. By establishing a relationship between the temperature difference and sap flow rate, the size of the sap flow rate was determined. This methodology aimed to provide insights into the plant’s response to varying soil moisture levels and their ability to adjust to changing environmental conditions.
Based on the observed data, Granier’s empirical formula (Granier, 1987) was used to derive the instantaneous trunk sap density (g/(m²·s)) :
Among them, (°C) is the instantaneous temperature difference between day and night; (°C) is the maximum instantaneous temperature difference between day and night. In general, at the time of maximum temperature difference, the density of nighttime trunk sap flow was zero.
2.2.4 Measurement of photosynthesis parameters
In each treatment group of 30 plants of C. multiflorus, three mature leaves were selected from each plant. The Li-6800 portable photosynthesis meter (LI-COR, USA) was used to measure the photosynthetic rate of plant leaves (), stomatal conductance (), transpiration rate()at the time of the day (9:00-11:00). And 3 replicates were performed for each leaf. Among them, 6800-01A chamber was used to determine the photosynthetic index; the carbon dioxide concentration in the reference chamber was set to 400 μmol·mol-1, RHcham was 67.94%, and flow rate was 500 μmol·s-1. Water use efficiency (WUE) calculation formula is as follow (Yan et al., 2022) :
2.2.5 Rapid determination of chlorophyll fluorescence parameters
In each treatment group of 30 C. multiflorus plants, 3 mature leaves were selected from each plant, and then chlorophyll fluorescence parameters were determined using a FluorPen (FP100) hand-held fluorometer, and the leaves were dark-adapted for 15 min before the determination (Ghaffar et al., 2023).
2.3 Transcriptome determination and analysis
2.3.1 Total RNA extraction and cDNA library construction
The young leaves of C. multiflorus of CK, SD and RD test groups were removed, frozen and ground with liquid nitrogen quickly. The total RNA of the samples was extracted by Trizol method. The integrity and purity of RNA was analyzed by agarose gel electrophoresis and ultra-micro spectrophotometer. Finally, the concentration and quality of RNA were measured by fluorescence quantifier.
2 μg RNA samples were taken to construct cDNA Library, using NEBNext® UltraTM RNA Library Prep Kit for Illumina kit (New England Biolabs). AMPure XP beads (Be control group man Coulter) were used to purify the cDNA samples (size between 100 ~ 200 bp), and the quality of the library was evaluated by Agilent 2100 biological analyzer.
2.3.2 Transcriptome sequencing and data quality control
After the quality inspection of the cDNA library was qualified, the different libraries were mixed according to the requirements and RNA-Seq sequencing was performed using the DNBSEQ-T7 sequencing platform. The obtained RNA sequencing data were subjected to quality control filtering through the fastp v0.19.3 platform (Vinod et al., 2023). The specific filtering criteria are as follows: 1) reads with adapters are not retained; 2) a read with N content more than 10% of its own base number is not retained; 3) a read with the number of low-quality bases more than 50% of its own base number is not retained. After quality control, clean reads were obtained for subsequent transcriptome analysis.
2.3.3 Clean reads comparison of reference genomes
Based on the HISAT2 software (v2.2.1) (Valeriano et al., 2021), the obtained clean reads were aligned to the assembled C. multiflorus genome. The software parameters were set by default.
2.3.4 Analysis of gene expression levels and differences
The FPKM (Fragments Per Kilobase of exon model per Million mapped fragments) for each gene was calculated using feature Counts v1.6.2 software (Wion et al., 2022). A single gene with FPKM ≥ 1 was retained as the screening basis for subsequent gene differential expression. The DESeq2 v1.22.1 package of R platform was called to perform gene differential expression analysis (Jung et al., 2023).
2.3.5 Functional annotation and GO enrichment analysis of differentially expressed genes
GO enrichment analysis was mainly composed of molecular function, biological process and cellular component. The obtained non-redundant transcript sequence was subjected to GO enrichment analysis using cluster Profiler (v4.0.5) (Martinez et al., 2019). Benjamini-Hochberg method was used as a multiple test method, and GO term with FDR < 0.05 was defined as significant enrichment.
2.4 Data processing
Data were organized using Microsoft excel 2019 software. Correlation analysis and principal component analysis were performed using DPS 7.5. ANOVA with multiple comparisons (Ducan’s method) were performed using SPSS. Graphs were plotted using Origin. Data used in the graphs were mean ± standard error.
3 Results
3.1 Effect of drought stress and rehydrated on water potential and relative water content (RWC) of C. multiflorus leaves
Both of them showed a downward and then an upward trend, leaf water potential decreased by -0.21 MPa, -1.77 MPa and -4.44 MPa under mild drought (LD), moderate drought (MD) and severe drought stresses (SD), respectively, compared to the control group (CK). After RD treatments, the leaf water potential of C. multiflorus increased by 0.85 MPa and 3.52 MPa compared to MD and SD, respectively (Figure 1A). Relative water content (RWC) decreased by 5.79%, 27.23% and 40.68%, respectively, compared to the CK (Figure 1B). After RD treatments, leaf RWC of C. multiflorus was elevated by 39.91% and 14.05%, respectively, compared to MD and SD.
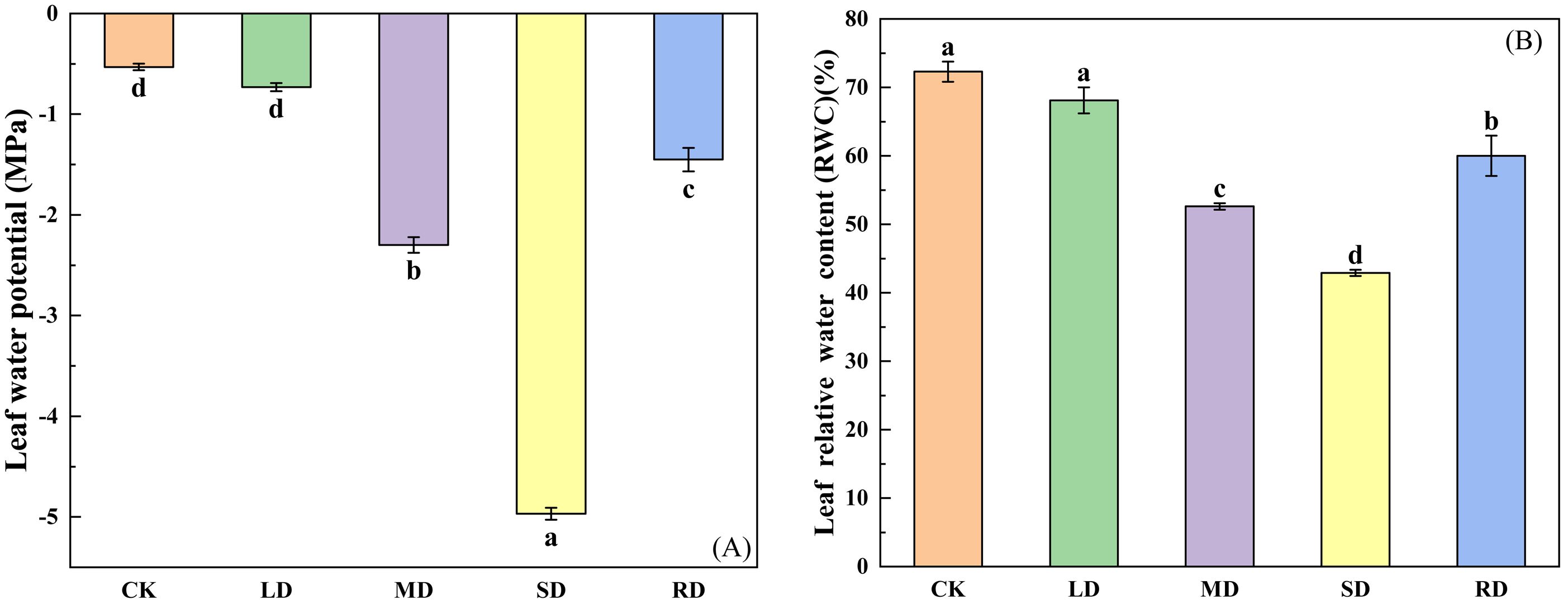
Figure 1. Effects of drought stress and rehydrated on leaf water potential and RWC of C. multiflorus. There was a significant difference between the different English letters marked in the figure (P < 0.05).
3.2 Effect of drought stress and rehydrated on stomatal characteristic of C. multiflorus leaves
As can be seen in Figure 2, as the degree of drought increased, the stomatal opening length, stomatal opening width, stomatal aperture and stomatal density (except LD) of C. multiflorus leaves were significantly reduced. Under SD, the above-mentioned variables were reduced by 23.27%, 21.22%, 37.86%, 16.83% and 48.71%, respectively, compared to the control group (CK). However, After RD treatments (48 h), those variables were able to return to the state between LD and MD.
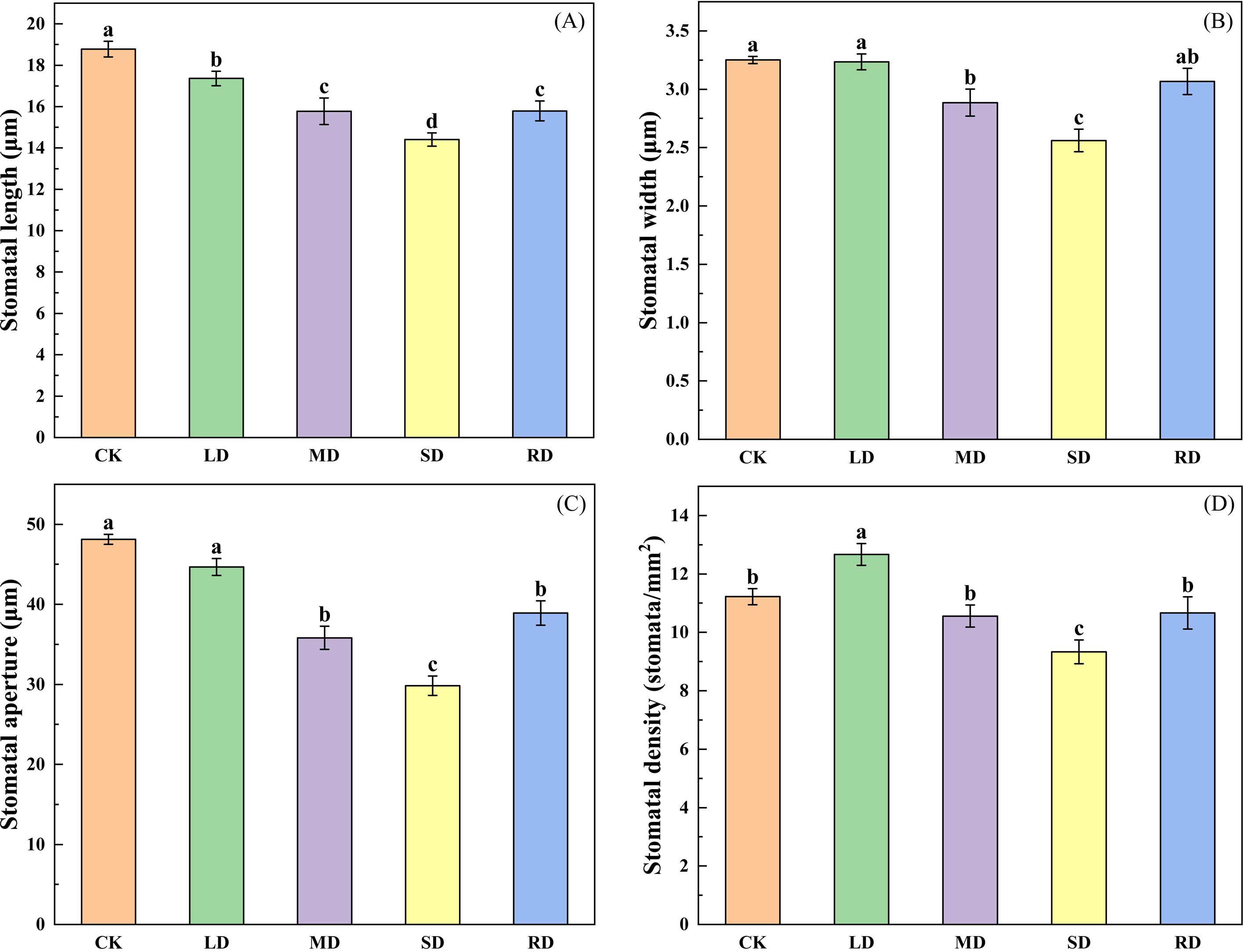
Figure 2. Effects of drought stress and rehydrated on stomatal characteristic of C. multiflorus. There was a significant difference between the different English letters marked in the figure (P < 0.05).
3.3 Effect of drought stress and rehydrated on stem sap flow of C. multiflorus
3.3.1 Diurnal variation of stem sap flow of C. multiflorus under drought stress and rehydrated
Figure 3A showed that C. multiflorus had a ‘lunch break’ from LD. The diurnal variation of stem sap flow rate appeared double or multiple peaks. As the degree of drought stress increased, the first peak appeared earlier and earlier. Under LD, MD and SD, the first peak of C. multiflorus appeared at about 11:00, 10:00 and 8:00, respectively. Even after RD treatments, C. multiflorus was not able to return to its initial state in a short time, and the phenomenon of ‘double peak’ still existed.
C. multiflorus showed a gradual decrease in all-day sap flow from LD. The opposite was true for nocturnal sap flow, which gradually increased with increasing drought stress levels, in the case of SD, nocturnal sap flow, on the other hand, was significantly lower than in the case of MD. However, after RD treatments, both all-day and nocturnal sap flow were elevated (Figure 3B). There was little difference in the ratio of nocturnal sap flow to daily between the LD and CK, which were 6.59% and 7.87%, respectively. However, this index at MD and SD was 2.60-fold and 1.56-fold higher than that of the CK. After RD treatments, the nocturnal sap flow of C. multiflorus accounted for 5.69% higher than that under SD.
3.3.2 Distributional characteristics of nocturnal sap flow of C. multiflorus under drought stress and rehydrated
Based on the integral area of each region (Figure 4), it was clear that C. multiflorus has both nocturnal water refilling and nocturnal transpiration at night. C. multiflorus responded rapidly to nocturnal water refilling under different drought stresses, increasing by 14.25%, 25.53% and 29.34%, respectively, compared to CK. The nocturnal water refilling under SD increased by only 3.04% compared to that under MD. But after RD treatments, the nocturnal water refilling of C. multiflorus was 7.50% lower than that under SD. Meanwhile, nocturnal transpiration of C. multiflorus gradually decreased with increasing drought stresses, accounting for 30.22%, 20.27%, 10.37%, 9.74% and 16.50% of nocturnal sap flow, respectively.
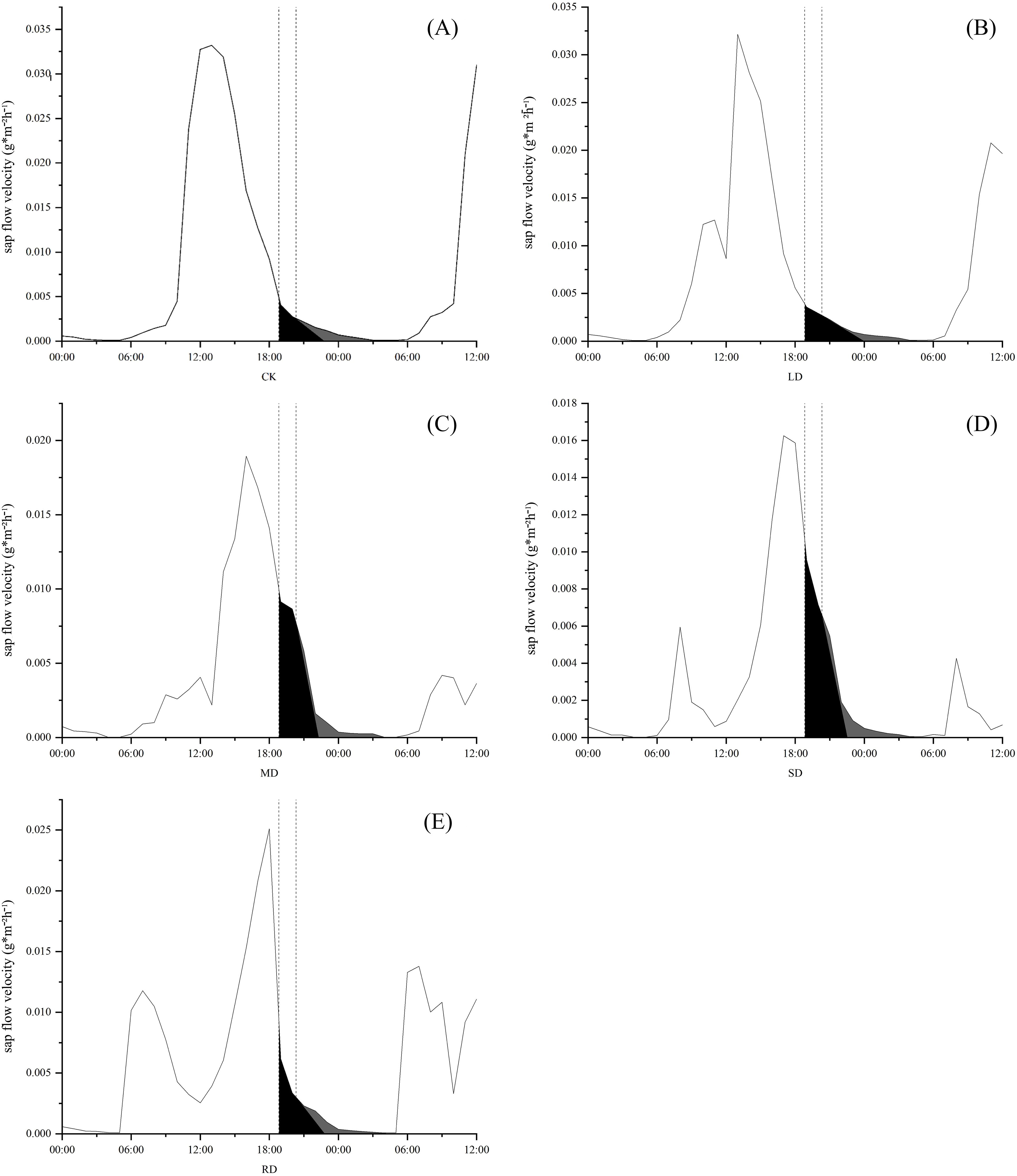
Figure 4. Distributional characteristics of sap flow of C. multiflorus under drought stress and rehydrated. The spacing between the two vertical gray dashed lines represents the lag time (80 minutes,18:50∼20:10) of sap flow activity and solar radiation changes. The black shaded area is nighttime stem rehydrated, and the gray shaded area is nighttime transpiration.
3.4 Effect of drought stress and rehydrated on photosynthetic characteristics of C.multiflorus
As can be seen from Figure 5, the , , and tended to decrease with increasing stress levels in all drought stress treatments, with decreasing by 12.73%, 34.32%, and 55.08% under LD, MD, and SD, respectively, compared with the CK. The decreased by 12.67%, 34.81% and 58.71%, while decreased by 12.52%, 29.84% and 47.60%, respectively, compared to the CK. The water use efficiency (WUE) under LD increased by 0.26% against the CK, while WUE under MD and SD decreased by 5.39% and 13.99% against the CK, respectively. After RD treatments, the , , , and WUE showed an increasing trend, which increased by 52.87%, 118.84%, 45.12%, and 6.43% compared to the CK.
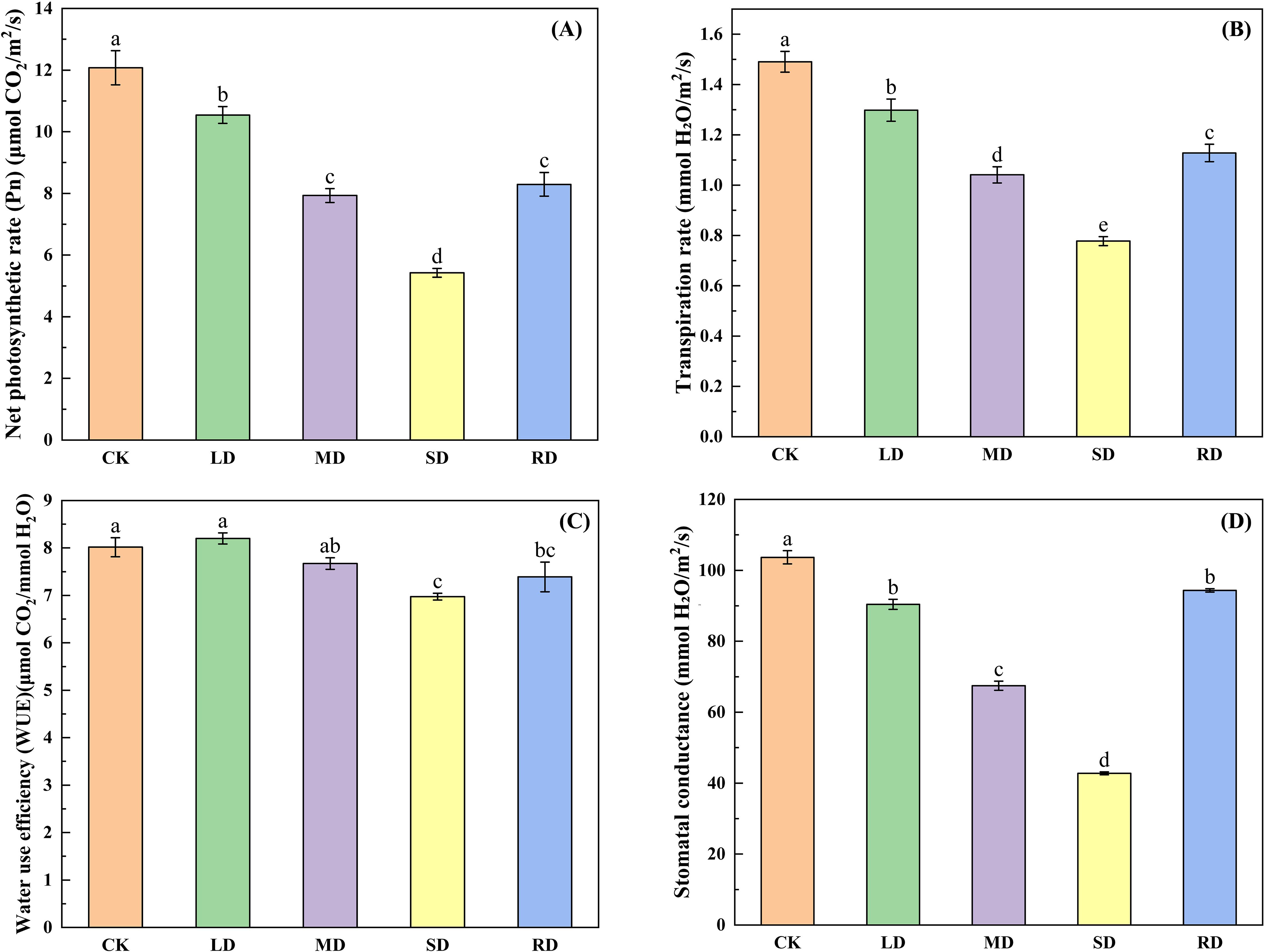
Figure 5. Effects of drought stress and rehydrated on photosynthetic characteristic of C. multiflorus. There was a significant difference between the different English letters marked in the figure (P < 0.05).
3.5 Effect of drought stress and rehydrated on chlorophyll fluorescence parameters of C. multiflorus
3.5.1 Changes in PSII reaction centers
From Figure 6A, the ABS/RC decreased by 11.55%, 26.29% and 41.16% under LD, MD and SD, respectively, compared to the CK. The decreased by 10.62%, 23.65% and 38.12%, respectively, compared to the CK. The decreased by 10.23%, 24.48% and 44.45%, respectively, compared to the CK. After RD treatments, the , and were elevated by 38.58%, 44.43% and 52.93%, respectively, compared to SD. The energy dissipated by heat () showed a tendency to increase and then decrease, with elevated by 9.92%, 30.51% and 70.58%, respectively, compared to the CK. It reduced by 30.51% after rehydrated, compared to SD.
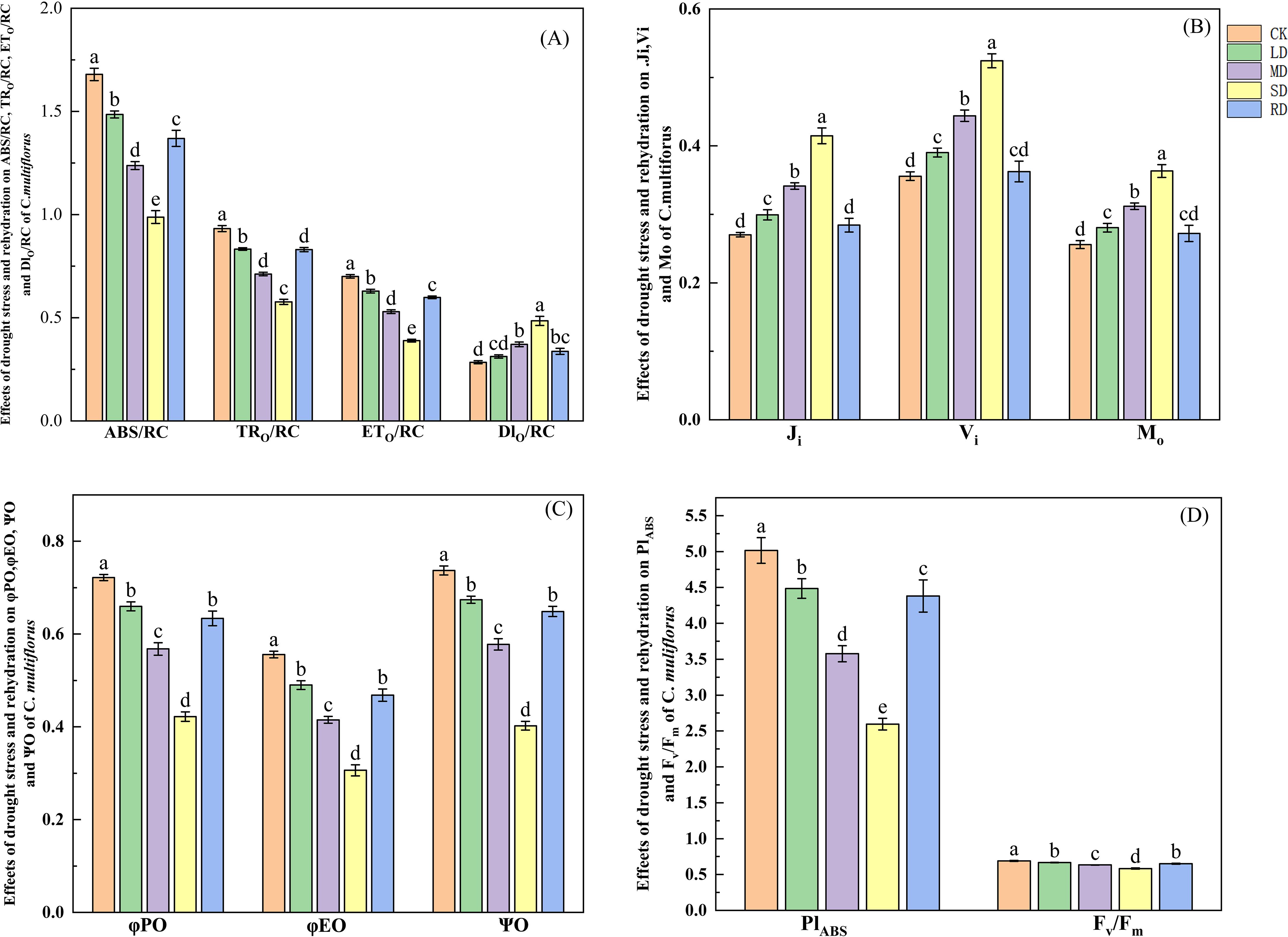
Figure 6. Effects of drought stress and rehydrated on chlorophyll fluorescence of C. multiflorus. There was a significant difference between the different English letters marked in the figure (P < 0.05).
3.5.2 Changes on the Acceptor Side of PSII
From Figure 6B, the increased by 9.57%, 21.80% and 41.95% under LD, MD and SD, compared to CK. The increased by 9.67%, 24.79% and 47.36%, respectively, compared to CK. The increased by 10.69%, 26.27% and 53.42%, respectively, compared to CK. After RD treatments, , and decreased by 25.10%, 30.84% and 31.44%, respectively, compared to that of SD. From Figure 6C, the decreased by 8.62%, 21.33% and 41.54% under LD, MD and SD, respectively, compared to CK. The decreased by 11.85%, 25.33% and 44.83% respectively, compared to CK. The decreased by 8.55%, 21.62% and 45.43% respectively, compared to CK. After RD treatments, , , and increased by 50.19%, 52.76% and 61.25% respectively, compared to that of SD.
3.5.3 Changes in blade performance index and
From Figure 6D, the decreased by 10.58%, 28.70% and 48.25% under LD, MD and SD, respectively, compared to CK. The decreased by 3.39%, 8.31% and 15.60%, respectively, compared to CK. After RD treatments, and increased by 68.80% and 11.65% respectively, compared to that of SD. It can be seen that the photosynthetic performance index of C. multiflorus leaves was more sensitive to drought stress than .
3.6 Correlation analysis of water status and photosynthetic characteristics of C. multiflorus under drought stress and rehydrated
As can be seen from Figure 7, there was a significant or extremely significant positive correlation between the water status indicators of C. multiflorus such as leaf water potential, RWC, average stomatal length, average stomatal width, average stomatal aperture, all-day sap flow, daytime sap flow and the photosynthetic characteristics indicators including net , stomatal conductance, , WUE, , , , , , and . There was a significant or highly significant negative correlation with both and . Leaf water potential, RWC, mean stomatal width, mean stomatal aperture and all-day sap flow were significantly or highly significantly negatively correlated with and . Mean stomatal length was negatively and significantly negatively correlated with and , respectively. Mean stomatal density and daytime sap flow were negatively correlated with and .
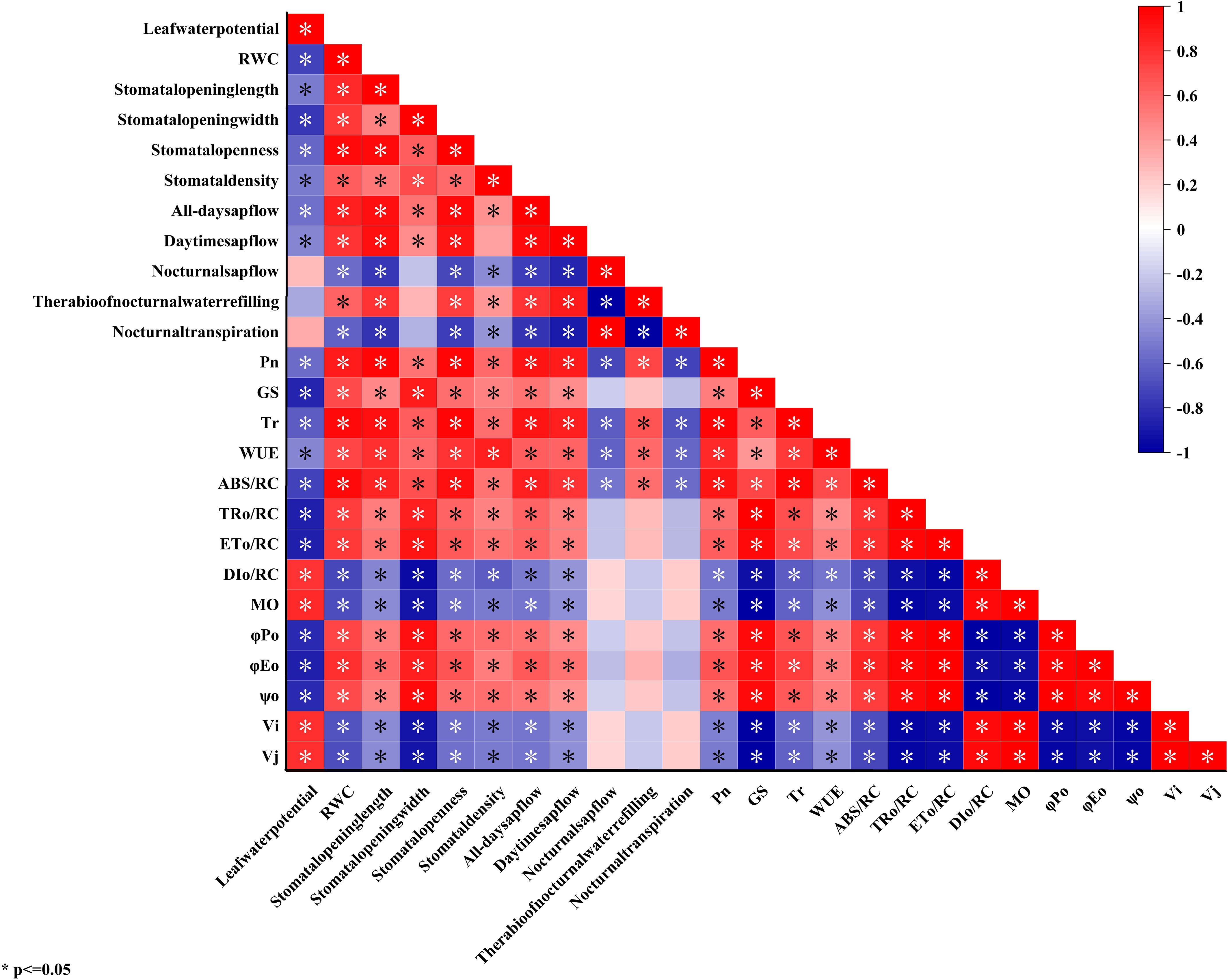
Figure 7. Correlation analysis of water status and photosynthetic characteristics of C. multiflorus under drought stress and rehydrated.
Significantly, a positive correlation was observed between the ratio of nocturnal water refilling and various plant parameters including leaf water potential, leaf relative water content, mean stomatal length, mean stomatal width, mean stomatal aperture, and mean stomatal density. Additionally, there was a notable positive correlation between the ratio of nocturnal water refilling and all-day as well as daytime sap flow. Interestingly, a significant negative correlation was found between the ratio of nocturnal water refilling and nocturnal sap flow. The ratio of nocturnal water refilling was positively correlated with the photosynthetic characteristics of , stomatal conductance, , WUE, , , , , , and negatively correlated with , , and .
3.7 Principal component analysis of indicators of water status and photosynthetic characteristics of C. multiflorus under drought stress and rehydrated
The 25 drought tolerance indicators of C. multiflorus under different drought levels and rehydrated states were analyzed by principal component analysis. The first 2 principal components had a cumulative contribution of 96.351% and an eigenvalue > 1, which was mainly representative (Table 1). The contribution rate of principal component I was 86.366%, and the loadings of the other 19 indicators in principal component I were 0.2022∼0.2148 (including negatively correlated indicators). The contribution of principal component II was 9.9856%, the loadings of nocturnal sap flow, the ratio of nocturnal water refilling and nocturnal transpiration on principal component II were higher, indicating that principal component II mainly reflected nocturnal water refilling and nocturnal transpiration of C. multiflorus under drought stress (Supplementary Table 1).
3.8 DEGs analysis and GO analysis under drought stress and rehydrated of C. multiflorus
The Spearman correlation of the leaves of C. multiflorus was calculated by selecting the genes with mean FPKM expression greater than 1. As shown in Figure 8B, the correlation between different treatments was high, indicating that the biological repetition was reliable. All DEGs (FC > 1, P < 0.01) were further processed by Z-Scrae method, and then cluster analysis was performed by machine learning K-Means method (parameter n = 5). A total of 5 categories were clustered (Figure 8A). Among them, in the second category (Figure 8C), the expression of DEGs was significantly up-regulated in SD, and was significantly down-regulated in RD. In the third category and the five categories (Figure 8D, E), the opposite was true, indicating that these three types of DEGs were related to drought in C. multiflorus.
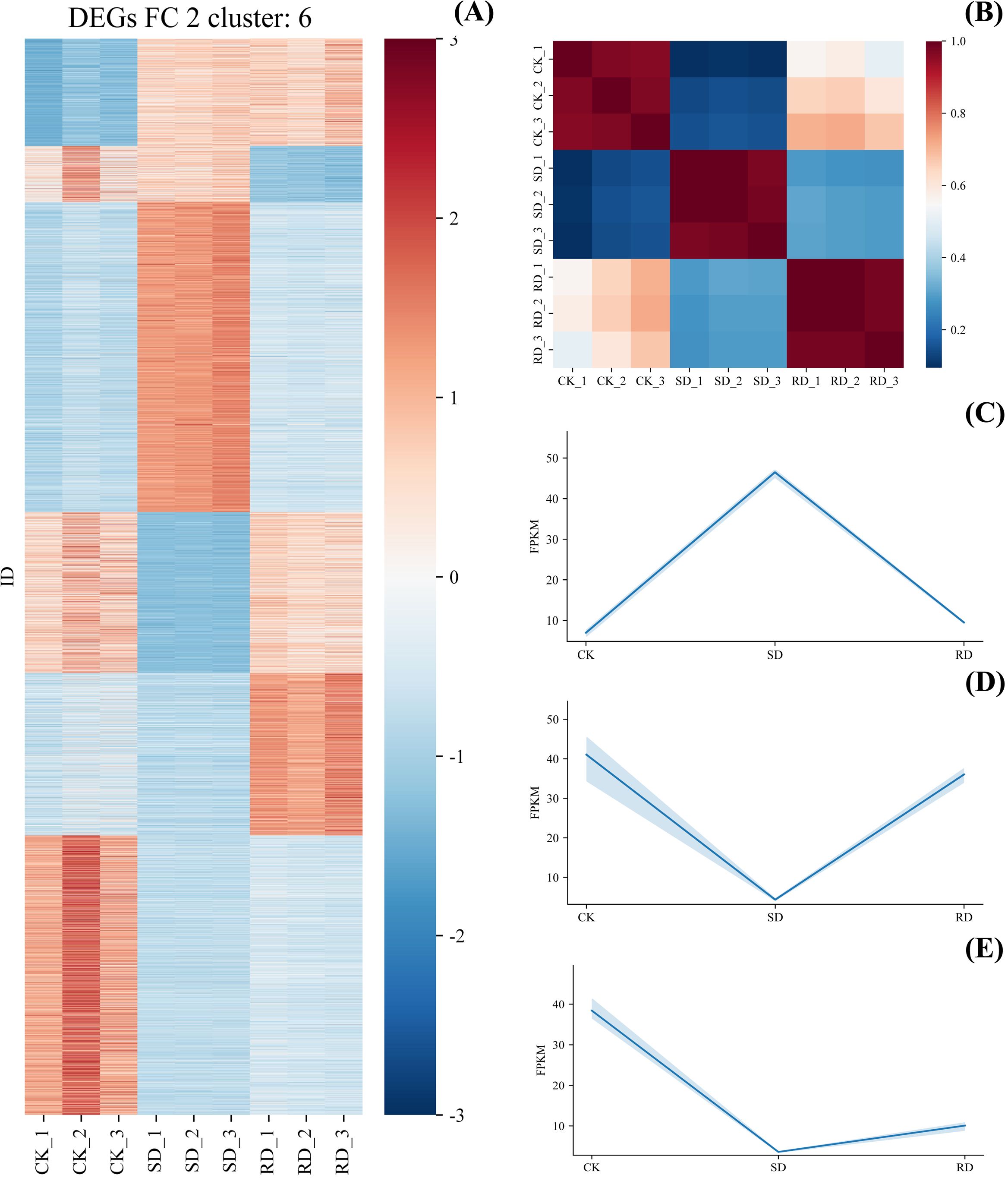
Figure 8. Spearman correlation analysis and DEGs cluster analysis of RNA expression in (C) multiflorus leaves under drought stress and rehydrated. (A) DEGs cluster analysis; (B) Spearman correlation analysis; (C) linear fitting of the second category; (D) linear fitting of the third category; (E) linear fitting of the fifth category.
GO analysis showed that the GO terms with significantly up-regulated DEGs expression in SD were mainly enriched in positive regulation of stress-activated MAPK cascade, regulation of JUN kinase activity, positive regulation of JUN kinase activity, positive regulation of JNK cascade, positive regulation of stress-activated protein kinase signaling cascade and innate immune response activating cell surface receptor signaling pathway (Figure 9A).
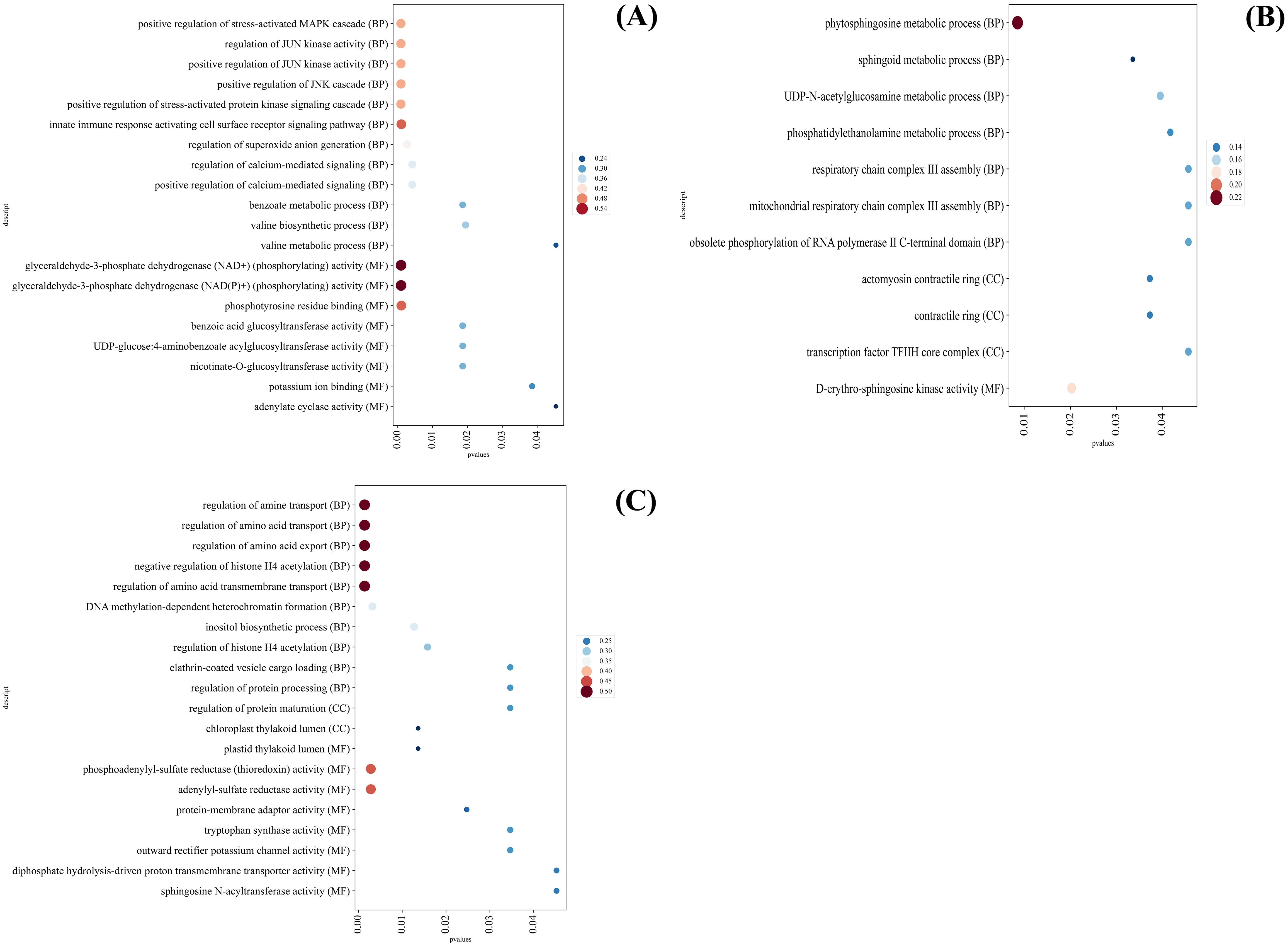
Figure 9. GO analysis of different categories of C. multiflorus leaves under drought stress and rehydrated. (A) GO analysis of the second category; (B) GO analysis of the third category; (C) GO analysis of the fifth category.
GO terms with significantly down-regulated DEGs expression in SD were mainly enriched in phytosphingosine metabolic process and D-erythro-sphingosine kinase activity (Figure 9B), regulation of amine transport, regulation of amino acid transport, regulation of amino acid export, negative regulation of histone H4 acetylation, and regulation of amino acid transmembrane transport (Figure 9C).
4 Discussion
4.1 Effect of drought-rehydrated on water status of C. multiflorus
With the intensification of drought stress, leaf water potential (Figure 1A), RWC (Figure 1B) and Tr (Figure 4) of C. multiflorus decreased significantly under different drought stresses. This is the same as the findings of other scholars (Song et al., 2022a; Ichie et al., 2023; Wang et al., 2021). The reason may be the significant reduction in leaf stomatal morphology (length, width and opening) in C. multiflorus during MD (Figure 3). This pathway reduces transpiration of C. multiflorus. (Figure 4B). However, under SD, the leaves had been severely dehydrated, stomata were forced to close, and the number of stomata was significantly reduced (Figure 3), leaf Tr was also reduced to a minimum, which is consistent with the findings of (Tardieu and Tuberosa, 2010; Xu et al., 2023). It is shown that C. multiflorus controls transpirational water consumption by changing stomatal morphology and density to exchange water with environment.
With the intensification of drought stress, the all-day and daytime stem sap flow of C. multiflorus decreased progressively (Figure 3). This may due to the fact that leaf water deficit occurs earlier when soil moisture is deficient, in the before noon, the stomatal aperture decreases significantly or partially closes, and the transpiration intensity decreases drastically as a result (Kang et al., 2007). C. multiflorus did not show a clear ‘double peak’ under LD and SD, while there was a clear ‘double peak’ under SD (Figure 3). It showed that C. multiflorus had an obvious ‘lunch break’ phenomenon under SD, and its physiology was severely stressed. It is shown that C. multiflorus can survive in soil relative water content above 5 to 10%(SD), but optimal growing conditions are when the soil contains more than 40% (LD) relative water.
During drought stress, the soil water content decreases and the conduits in the xylem of plants are under extreme negative pressure, which results in the failure of xylem function due to embolism of air pockets in the conduits, and water conduction will be limited (Guo et al., 2007). It is noteworthy that with increasing drought stress, C. multiflorus had both nocturnal stem rehydrated and nocturnal transpiration during the night, with a gradual increase in nocturnal sap flow in the stem and a gradual decrease in nocturnal transpiration. It was shown that C. multiflorus was able to alleviate its own water deficit by increasing nocturnal sap flow and reducing plant capacitance and air cavity formation as a way to enhance drought tolerance and avoid plant hydraulic failure or even death due to drought, which was the same as the study of Klein (Klein et al., 2018; Cochard and Delzon, 2013) and Zeppel (Zeppel et al., 2019).
When C. multiflorus was treated with rehydrated after SD, the leaf stomatal morphology and stomatal density indices were able to recover to the level between LD and MD; leaf water potential and RWC were able to recover to levels between MD and SD. In contrast, the variables of stem all-day sap flow, daily sap flow, nightly sap flow and nightly transpiration were not significantly different when compared with the SD. Possibly because when the tree is re-watered, the water potential increases, opening and restoring stomata (Liu et al., 2024), and leaf transpiration resumes again, thereby providing suction for water movement within the tree. However, under SD, xylem tracheal tissue of C. multiflorus may experience severe air pocket embolism, which renders the xylem functionally ineffective and limits water conduction (Dai et al., 2017). It was further shown that the stomata of C. multiflorus have a strong ability to ‘sense’ and regulate re-watering, and to control transpirational water consumption of C. multiflorus by changing the stomatal morphology and density for water exchange with the surrounding environment.
4.2 Effect of drought-rehydrated on photosynthetic characteristics of C. multiflorus
The results of the present study showed that the more severe the drought stress in C. multiflorus, the greater the degree of stomatal closing. This led to a significant decrease in and caused a significant limitation of , resulting in a significant inhibition of photosynthesis, which was the same as that of the studies conducted by Han (Han et al., 2021), Liu (Liu et al., 2024). Therefore, the self-protection mechanism adopted by C. multiflorus under drought stress is one of the main reasons for the limitation of photosynthesis (Wang et al., 2019; Zhang et al., 2019).
Plants can adapt to drought stress by rationally coordinating the relationship between photosynthetic carbon assimilation and water consumption, thereby regulating changes in leaf water use efficiency (WUE) (Mohammad et al., 2023). When under LD (RWC of 25 to 30%), stomata partially closed and both and began to decline, but the decline in was greater than the rate of decline in , resulting in an increase in WUE. It indicates that MD can increase the WUE of C. multiflorus leaves, which is the same as the findings of Song (Song et al., 2022a).
Drought stress injured both the PSII reaction center and the receptor side of C. multiflorus leaves and impeded photosynthetic electron transport (both and were decreased). This was mainly due to the fact that drought treatment resulted in the blockage of both electron transfer from the PS II receptor side to the secondary quinone receptor and acceptance of electrons by the plastoquinone pool in C. multiflorus (, increased). The degree of opening of active PSII reaction centers and the capacity of pools decreased, and the ability of the PSII receptor side of the to transfer electrons decreased (, and decreased, and increased). As well as degradation or inactivation of reaction centers and reduced capture of light energy by antenna pigments ( and decrease). This is the same as the findings of Mohammad (Mohammad et al., 2023) and Wu (Wu et al., 2019). However, when the reaction center of C. multiflorus was stressed, it allowed a decrease in trapped light energy () and induced an increase in heat dissipation (). This suggests that C. multiflorus initiated corresponding defense mechanisms after being subjected to drought stress, defending against excessive accumulation of light energy through reversible inactivation of PSII and reduction of light energy uptake, quantum yield and electron transfer on the one hand, and reducing the accumulation of excess excitation energy by which is the same as the findings of Song (Song et al., 2022b). This is consistent with the research results on the drought resistance mechanism of Vitis vinifera L (Shtai et al., 2024). and Jatropha curcas (Sapeta et al., 2023).
The rehydrated of C. multiflorus after severe drought can photosynthesis and chlorophyll fluorescence to the level of MD. This indicated that the photosynthetic reaction center PSII of C. multiflorus had been repaired to a certain extent after the damage, but it had not been restored to the level before drought stress. The photosynthetic reaction center of C. multiflorus was still photoinhibited. This is the same as the findings of Wei (Wei et al., 2018).
4.3 Coupling of water status and photosynthetic carbon assimilation in C. multiflorus and adaptation strategies
The leaf water potential of C. multiflorus at SD was -4.97 Mpa, which was 4.44 MPa lower than that of CK, while the , , and all-day sap flow of C. multiflorus at SD were 44.92%, 41.29%, 52.39% and 57.65% of CK, respectively. It may be because the leaf water potential was delayed by 2 days compared with the photosynthetic index measurement, which led to a further reduction of soil moisture and a more serious water stress on C. multiflorus. When the stem sap flow and leaf water potential were measured on a different day, the environmental factors (temperature, air humidity, etc.) would affect the sap flow. However, the water status parameters of C. multiflorus (except for nocturnal sap flow, the ratio of nocturnal water refilling, and nocturnal transpiration) showed significant or highly significant correlations with photosynthetic characteristics and chlorophyll fluorescence parameters. In particular, stomatal aperture and stomatal conductance of C. multiflorus leaves showed significant or highly significant correlations with water status parameters (except for nocturnal sap flow, the ratio of nocturnal water refilling, and the ratio of nocturnal transpiration), photosynthetic characteristics, and chlorophyll fluorescence parameters. This was because stomatal function was capable of controlling plant water status and photosynthesis at the same time (Peláez-Vico et al., 2024). When C. multiflorus was subjected to drought stress, the decrease in its hydraulic conductivity, which caused a decrease in the stomatal parameters, further reducing the activity of the photosynthetic apparatus and reducing CO2 influx, ultimately leading to a decline in photosynthesis (Han et al., 2022; Bonoso et al., 2020). This suggests a highly coupled relationship between water status parameters and photosynthesis parameters in C. multiflorus (Sebastià et al., 2014; Reddy et al., 2019; Limousin et al., 2013).
From the principal component analysis of 25 drought tolerance variables of C. multiflorus under different drought degrees and rehydrated status (Table 1), it can be seen that the cumulative contribution of principal component I and principal component II reached 96.3513%, and the eigenvalue was >1, which was mainly representative. Although nocturnal sap flow, the ratio of nocturnal water refilling, and nocturnal transpiration of C. multiflorus had lower loadings on principal component I, they had higher loadings on principal component II. And with the intensification of drought stress, the proportion of nocturnal sap flow in all-day sap flow and the ratio of nocturnal water refilling tended to increase, reflecting the strategy and ability of C. multiflorus to cope with drought changes by compensating for tree sap, which was the same as that in the studies of Li et al (Li et al., 2023), Liu et al (Liu et al., 2023a). This is because the residual effects of water stress are mainly caused by hydraulic dysfunction (Gori et al., 2023), whereas nocturnal sap flow compensates for the internal water deficit of the tree and transports nutrients essential for plant growth and development (Zhang et al., 2022). At the same time, it increased the stem and leaf water potential of the plant before dawn (Fang et al., 2018). In addition, nocturnal sap flow helps to reduce plant xylem embolism and aerial cavity formation, avoiding the phenomenon of plant water failure or even death due to drought (Zeppel et al., 2019). Therefore, nocturnal sap flow cannot be ignored in the study of drought stress in plants.
When C. multiflorus is subjected to drought stress, on the one hand, C. multiflorus leaves reduce transpiration rate and water loss by changing stomatal morphology and stomatal density, and also maintain their own water balance by increasing the nocturnal sap flow rate and nocturnal water refilling to maintain their physiological and metabolic activities. On the other hand, with the intensification of drought, the of C. multiflorus leaves showed an overall decreasing trend, and stomatal restriction led to the reduction of photosynthetic performance. But stomata have a strong ability to ‘sense’ and regulate the rehydrated after SD. They exchange water vapour and gas with the surroundings by changing stomatal morphology and stomatal density, thereby control the water consumption of C. multiflorus. In addition, when subjected to drought stress, C. multiflorus will have activated the appropriate defence mechanisms to defend against excessive accumulation of light energy and promote heat dissipation, protecting the photosynthetic performance of C. multiflorus leaves as much as possible. This is the same as drought-avoidant plants such as Nitraria tangutorum (Liu and Zheng, 2024), Genista versicolor (Albrecht et al., 2024), and deciduous oak (Kaproth et al., 2023). During drought stress, plant morphology and cell metabolism are rearranged (e.g., stomatal closure and leaf rolling, etc.) to avoid or reduce water deficit in plant organs and tissues (Hassan et al., 2023; Perlikowski and Kosmala, 2020). Therefore, to some extent, it can be tentatively assumed that C. multiflorus also belongs to drought-avoiding plants.
4.4 Stress response of transcriptome factors of C. multiflorus under different drought stress and rehydrated conditions
The abundance of transcripts changed significantly in SD, and the number of up-regulated or down-regulated DEGs was relatively large. It was indicated that under SD, the normal physiological function of the leaves of C. multiflorus were seriously disturbed, and the gene expression pattern changed dramatically. But C. multiflorus had strong tolerance and recovery ability, which was the same as that of Carya illinoinensis (Wu et al., 2022), and Cerasus humilis (Vastag et al., 2020).
The gene expression of phytosphingosine metabolic process, D-erythro-sphingosine kinase activity, respiratory chain complex III assembly and mitochondrial respiratory chain complex III assembly were significantly down-regulated in SD. It was indicated that SD might lead to the decrease of antioxidant capacity and the inhibition of respiration in C. multiflorus (Figure 9B), which was the same as the inhibition of antioxidant enzyme system and respiration in Atractylodes lancea (Thunb.) DC. under SD (Zhang et al., 2021). The gene expression of Chloroplast thylakoid lumen, tryptophan synthase activity, plastid thylakoid lumen, and tryptophan synthase activity were significantly down-regulated, indicating that SD might cause photosynthesis to be blocked (Figure 9C), which was the same as Xanthoceras sorbifolium bunge (Liu et al., 2023b).
Although severe drought severely inhibited the respiration, photosynthesis and antioxidant capacity of C. multiflorus, it could activate the regulation of stress-activated MAPK cascade and JUN kinase activity. The expression of the gene regulating osmotic pressure and closing stomatal (glyceraldehyde-3-phosphate dehydrogenase, NAD+) was up-regulated, which indicated that C. multiflorus would strengthen the drought defense mechanism, promote stomatal closure, reduce transpiration water loss, and vigorously regulate water balance in the body, exhibiting a series of “self-saving” behaviors.
5 Conclusion
In conclusion, we systematically investigated the response,coupling mechanisms and molecular mechanisms of water status, photosynthetic characteristics, chloroplast fluorescence parameters and other indicators of C. multiflorus in response to drought and rehydrated after drought stress. Our results showed that water status of C. multiflorus was highly coupled with photosynthetic characteristics and chloroplast fluorescence parameters during drought stress. C. multiflorus could regulate transpiration and photosynthesis by regulating stomatal status and initiating a series of physiological activities through gene regulation. Such as defending against overaccumulation of light energy and promoting heat dissipation, to achieve a balance between hydraulic conductance in response to drought stress. In addition, C. multiflorus maintains its own water balance by increasing nocturnal sap flow and nocturnal water refilling to avoid or reduce water deficit in plant organs and tissues. Therefore, the shrub C. multiflorus is a drought-tolerant plant.
Data availability statement
The original contributions presented in the study are included in the article/Supplementary Material. Further inquiries can be directed to the corresponding author.
Author contributions
QH: Conceptualization, Data curation, Formal Analysis, Investigation, Methodology, Writing – original draft, Writing – review & editing. MZ: Formal Analysis, Investigation, Writing – original draft. CL: Formal Analysis, Investigation, Writing – original draft. BL: Formal Analysis, Investigation, Writing – original draft. SZ: Investigation, Writing – original draft. YY: Investigation, Writing – original draft. YC: Investigation, Writing – original draft. aZ: Formal Analysis, Writing – original draft. HL: Investigation, Writing – original draft. WL: Formal Analysis, Writing – original draft. ZH: Investigation, Writing – original draft. MC: Investigation, Writing – original draft. ZY: Formal Analysis, Writing – original draft. GZ: Conceptualization, Project administration, Writing – original draft, Writing – review & editing.
Funding
The author(s) declare financial support was received for the research, authorship, and/or publication of this article. This study was supported by the Fujian Science and Technology Plan Project (2022I0008), East West Collaborative Project (KH180062A, 11891008004, KH190315A), Science and Technology Innovation Fund of Fujian Agriculture and Forestry University (CXZX2019046), Ningxia Hui Autonomous Region Science and Technology Department Project (KJA19H01A).
Conflict of interest
The authors declare that the research was conducted in the absence of any commercial or financial relationships that could be construed as a potential conflict of interest.
Publisher’s note
All claims expressed in this article are solely those of the authors and do not necessarily represent those of their affiliated organizations, or those of the publisher, the editors and the reviewers. Any product that may be evaluated in this article, or claim that may be made by its manufacturer, is not guaranteed or endorsed by the publisher.
Supplementary material
The Supplementary Material for this article can be found online at: https://www.frontiersin.org/articles/10.3389/fpls.2024.1457955/full#supplementary-material
References
Albrecht, E. C., Dobbert, S., Pape, R., Loffler, J. (2024). Patterns, timing, and environmental drivers of growth in twocoexisting green-stemmed Mediterranean alpine shrubs species. New Phytologist. 241, 114–130. doi: 10.1111/nph.19285
Anderegg, W. R. L., Konings, A. G., Trugman, A. T., Yu, K. L., Bowling, D. R., Gabbitas, R., et al. (2018). Hydraulic diversity of forests regulates ecosystem resilience during drought. Nature. 561, 538–541. doi: 10.1038/s41586-018-0539-7
Anderegg, W. R. L., Trugman, A. T., Badgley, G., Konings, A. G., Shaw, J. D. (2020). Divergent forest sensitivity to repeated extreme droughts. Nat. Clim. Change 10, 1091–1095. doi: 10.1038/s41558-020-00919
Arend, M., Link, R. M., Patthey, R., Hocha, G., Schuldt, B., Kahmen, A. (2021). Rapid hydraulic collapse as cause of drought-induced mortality in conifers. PNAS. 118, 1–6. doi: 10.1073/pnas.2025251118
Batibeniz, F., Hauser, M., Seneviratne, S. I. (2023). Countries most exposed to individual and concurrent extremes and near-permanent extreme conditions at different global warming levels. Earth. Syst. Dynam. 14, 485–505. doi: 10.5194/esd-14-485-2023
Bonoso, S. E., Rosa, S. L., Cristina, L. H., Victor M, G. S., María, A. C., Ana María, M. A., et al. (2020). Responses and Differences in Tolerance to Water Shortage under Climatic Dryness Conditions in Seedlings from Quercus spp. and Andalusian Q. ilex Populations. Forests. 11, 707–725. doi: 10.3390/f11060707
Chang, C. S., Jeon, J. I. (2003). Leaf flavonoids in Cotoneaster wilsonii (Rosaceae) from the island Ulleung-do, Korea. Biochem. Systematics Ecology. 31, 171–179. doi: 10.1016/S0305-1978(02)00064-9
Chen, Z. C., Li, S., Wan, X. C., Liu, S. R. (2022). Strategies of tree species to adapt to drought from leaf stomatal regulation and stem embolism resistance to root properties. Front. Plant Sci. 13. doi: 10.3389/fpls.2022.926535
Chen, G., Wang, Y. M., Lv, D., Zhao, X. P., Wang, B., Yan, K. L., et al. (2021). Introduction Adaptability of Cotoneaster in Zhangye City in arid and semi-arid region of Hexi. For. Sci. Technology. 8, 32–35. doi: 10.13456/j.cnki.lykt.2020.08021.0001
Choat, B., Brodribb, T. J., Brodersen, C. R., Duursma, R. A., López, R., Medlyn, B. E. (2018). Triggers of tree mortality under drought. Nature. 558, 531–539. doi: 10.1038/s41586-018-0240-x
Christian, J. I., Martin, E. R., Basara, J. B., Furtado, J. C., Otkin, J. A., Lowman, L. E. L., et al. (2023). Global projections of flash drought show increased risk in a warming climate. Commun. Earth. Environ. 4, 165–174. doi: 10.1038/s43247-023-00826-1
Cochard, H. H., Delzon, S. S. (2013). Hydraulic failure and repair are not routine in trees. Ann. For. Science. 70, 659–661. doi: 10.1007/s13595-013-0317-5
Dai, Y. X., Wang, L., Wan, X. C. (2017). Effects of Shading and Girdling on Carbon Allocation and Hydraulic Architecture of Robinia pseudoacacia and Platycladus orientalis Seedlings. Scientia Silvae Sinicae. 53, 37–44. doi: 10.11707/j.1001-7488.20170704
Davis, E., Trant, A., Hermanutz, L., Way, R. G., Lewkowicz, A. G., Collier, L. S., et al. (2020). Plant-environment interactions in the low Arctic Torngat Mountains of Labrador. Ecosystems. 24, 1038–1058. doi: 10.1007/s10021-020-00577-6
Fang, W. W., Lü, N., Fu, B. J. (2018). Research advances in nighttime sap flow density, its physiological implications, and influencing factors in plants. Acta Ecologica Sinica. 38, 7521–7529. doi: 10.5846/stxb201802080337
Ghaffar, A., Hussain, N., Ajaj, R., Shahin, S. M., Bano, H., Javed, M., et al. (2023). Photosynthetic activity and metabolic profiling of bread wheat cultivars contrasting in drought tolerance. Front. Plant Sci. 14. doi: 10.3389/fpls.2023.1123080
Gori, A., Moura, B. B., Sillo, F., Alderotti, F., Pasquini, D., Balestrini, R., et al. (2023). Unveiling resilience mechanisms of Quercus ilex seedlings to severe water stress: Changes in non-structural carbohydrates, xylem hydraulic functionality and wood anatomy. Sci. Total Environment. 878, 163124–163137. doi: 10.1016/j.scitotenv.2023.163124
Goulden, M. L., Bales, R. C. (2019). California forest die-off linked to multi-year deep soil drying in 2012-2015 drought. Nat. Geosci. 12, 632–637. doi: 10.1038/s41561-019-0388-5
Granier, A. (1987). Evaluation of transpiration in a Douglas-fir stand by means of sap flow measurements. Tree Physiol. 3, 309–320. doi: 10.1093/treephys/3.4.309
Grossiord, C., Buckley, T. N., Cernusak, L. A., Novick, K. A., Poulter, B., Siegwolf, R. T. W., et al. (2020). Plant responses to rising vapor pressure deficit. New Phytologist. 226, 1550–1566. doi: 10.1111/nph.16485
Gu, L., Yin, J. B., Slater, L. J., Chen, J., Do, H. X., Wang, H. M., et al. (2023). Intensification of global hydrological droughts under anthropogenic climate warming. Water. Resour. Res. 59, 32997–33021. doi: 10.1029/2022WR032997
Guo, W. H., Li, B., Zhang, X. S., Wang, R. Q. (2007). The impact of water stress on transpiration indices in Hippophae rhamnoides and Caragana intermedia. Acta Ecologica Sinica. 27, 4132–4140. doi: 10.1007/s10457-010-9337-4
Han, Y. R., Shan, W., Xu, D. W., Zhang, W. Y. (2021). Response of photosynthetic characteristics and water use efficiency to drought stress of caragana korshinskii in desert. J. Southwest Forestry Univesity. 41, 37–44. doi: 10.11929/j.swfu.202001031
Han, X. L., Zhao, M. S., Wang, Z. Y., Ye, L. F., Lu, S. T., Chen, S., et al. (2022). Adaptation of xylem structure and function of three gymnosperms to different habitats. Chin. J. Plant Ecology. 46, 440–450. doi: 10.17521/cjpe.2021.0186
Hartmann, H., Bastos, A., Das, A. J., Esquivel-Muelbert, A., Hammond, W. M., Martínez-Vilalta, J., et al. (2022). Climate change risks to global forest health: emergence of unexpected events of elevated tree mortality worldwide. Annu. Rev. Of Plant Biol. 73, 673–702. doi: 10.1146/annurev-arplant-102820-012804
Hassan, M. A., Dahu, N., Hongning, T., Qian, Z., Yueming, Y., Li, Y. R., et al. (2023). Drought stress in rice: morpho-physiological and molecular responses and marker-assisted breeding. Front. Plant Sci. 14. doi: 10.3389/fpls.2023.1215371
Huang, Q. L., Liu, H. Y., Li, C. S., Zhu, X. R., Yuan, Z. S., Lai, J. L., et al. (2024). Predicting the geographical distribution and niche characteristics of Cotoneaster multiflorus based on future climate change. Front. Plant Sci. 15. doi: 10.3389/fpls.2024.1360190
Ichie, T., Igarashi, S., Tanimoto, T., Inoue, Y., Mohizah, M., Kenz, T. (2023). Ecophysiological responses of seedlings of six dipterocar species to short-term drought in Borneo. Front. For. Glob. Change 6. doi: 10.3389/ffgc.2023.1112852
Jia, J. (2022). Ionic liquid-based microwave-assisted extraction of oligomeric proanthocyanidins from Cotoneaster Multiflorus pulp. Heilongjiang Med. J. 35, 37–40. doi: 10.14035/J.cnki.hljyy.2022.01.013
Jung, H. M. Y., Park, H. J., Jo, S. H., Lee, A. R., Lee, H. J., Kim, H. S., et al. (2023). Nuclear OsFKBP20-1b maintains SR34 stability and promotes the splicing of retained introns upon ABA exposure in rice[J. New Phytologist. 238, 2476–2494. doi: 10.1111/nph.18892
Kang, H. Z., Zhu, J. J., Xu, M. L. (2007). Study on water physiological properties of the artificially-planned saplings of Pinus sylvestris var. mongolica in the Horqin Sandland. Arid Zone Res. 24, 15–22. doi: 10.1016/S1872-5791(07)60026-8
Kaproth, M. A., Fredericksen, B. W., González-Rodríguez, A., Hipp, A. L., Cavender-Bares, J. (2023). Drought response strategies are coupled with leaf habit in 35 evergreen and deciduous oak (Quercus) species across a climatic gradient in the Americas. New Phytologist. 239, 888–904. doi: 10.1111/nph.19019
Klein, T., Zeppel, M. J. B., Anderegg, W. R. L., Bloemen, J., De Kauwe, M. G., Hudson, P., et al. (2018). Xylem embolism refilling and resilience against droughtinduced mortality in woody plants: Processes and tradeoffs. Ecol. Res. 33, 839–855. doi: 10.1007/s11284-018-1588-y
Leng, B., Cao, K (2020). The sap flow ofsix tree species and stand water use of a mangrove forest in hainan, China. Glob Ecol. Conserv. 24, 1233–1245. doi: 10.1016/j.gecco.2020.e01233
Li, M. Y., Dang, H. Z., Chen, S., Liu, C. Y., Yang, W., Qiao, Y. N. (2023). Sap flow variation in Pinus sylvestris var. mongolica plantation at the southern margin of Mu Us sandy land and its response to environmental factors. J. Arid Land Resour. Environment. 37, 153–162. doi: 10.13448/j.cnki.Jalre.2023.098
Limousin, J. M., Bickford, C. P., Dickman, L. T., Pangle, R. E., Hudson, P. J., Boutz, A. L., et al. (2013). Regulation and acclimation of leaf gas exchange in a piñon-juniper woodland exposed to three different precipitation regimes. Plant Cell Environment. 36, 1812–1825. doi: 10.1111/pce.12089
Liu, S. Y., Zheng, J (2024). Adaptive strategies based on shrub leafstem anatomy and their environmental interpretations in the eastern Qaidam Basin. BMC Plant Biol. 24, 323–338. doi: 10.1186/s12870-024-05026-3
Liu, D., Guo, H. L., Yan, L. P., Gao, L., Zhai, S. S., Xu, Y. (2024). Physiological, photosynthetic and stomatal ultrastructural responses of quercus acutissima seedlings to drought stress and rewatering. Forests. 15, 71–87. doi: 10.3390/f15010071
Liu, X. P., Jia, J., Jing, X. M., Li, G. L. (2018). Antioxidant activities of extracts from sarcocarp of cotoneaster multiflorus. J. Chem. 1, 1–7. doi: 10.1155/2018/4619768
Liu, Y., Ma, X., Di, N., Zeng, Z. H., Fu, H. M., Li, X., et al. (2023a). Root sap flow and hydraulic redistribution of. Populus tomentosa. Chin. J. Plant Ecology. 47, 123–133. doi: 10.17521/cjpe.2021.0492
Liu, Y., Zhang, L. N., Liu, X. H., Zeng, X. M., Jia, R. X. (2023b). Research progress from individual plant physiological response to ecological model prediction under drought stress. Acta Ecologica Sinica. 43, 10042–10053. doi: 10.20103/j.stxb.202304070703
López, R., Cano, F. J., Martin-StPaul, N. K., Cochard, H., Choat, B. (2021). Coordination of stem and leaf traits define different strategies to regulate water loss and tolerance ranges to aridity. New Phytologist. 230, 497–509. doi: 10.1111/nph.17185
Luo, H., Duan, M., Kong, L., He, L., Chen, Y., Wang, Z., et al. (2021). The regulatory mechanism of 2-acetyl-1-pyrroline biosynthesis in fragrant rice (Oryza sativa L.) under different soil moisture contents. Front. Plant Sci. 12. doi: 10.3389/fpls.2021.772728
Martinez-Vilalta, J., Anderegg, W. R. L., Sapes, G., Sala, A. (2019). Greater focus on water pools may improve our ability to understand and anticipate drought-induced mortality in plants. New Phytologist. 223, 22–32. doi: 10.1111/nph.15644
McDowell, N. G., Sapes, G., Pivovaroff, A., Adams, H. D., Allen, C. D., Anderegg, W. R. L., et al. (2022). Mechanisms of woody-plant mortality under rising drought, CO2 and vapour pressure deficit. Nat. Rev. Earth Environ. 3, 294–308. doi: 10.1038/s43017-022-00272-1
Miao, L. F., Zhang, L. J., Pu, Y. J., Yang, F. (2017). Factors that affect measurement using the WP4C dewpoint potential meter to determine water potential-Illustrated by the case of. Dalbergia odorifera. Plant Sci. J. 35, 93–98. doi: 10.11913/PSJ.2095-0837.2017.10093
Mohammad, M. A., Hossein, A., Sasan, A., Ali, M. B., Ahmad, E., Mohammad, S. H., et al. (2023). Natural variation in photosynthesis and water use efficiency of locally adapted Persian walnut populations under drought stress and recovery. Plant Physiol. Biochem. 201, 107859–107877. doi: 10.1016/j.plaphy.2023.107859
Naumann, G., Alfieri, L., Wyser, K., Mentaschi, L., Betts, R. A., Carrao, H., et al. (2018). Global changes in drought conditions under different levels of warming. Geophysical. Res. Letters. 45, 3285–3296. doi: 10.1002/2017GL076521
Peláez-Vico, M. A., Zandalinas, S. I., Devireddy, A. R., Sinha, R., Mittler, R. (2024). Systemic stomatal responses in plants: Coordinating development, stress, and pathogen defense under a changing climate. Plant Cell Environment. 47, 1171–1184. doi: 10.1111/pce.14797
Pereira, T. S., Oliveira, L. A., Andrade, M. T., Haverroth, E. J., Cardoso, A. A., Martins, S. C. V., et al. (2024). Linking water-use strategies with drought resistance across herbaceous crops. Physiologia Plantarum. 176, 14114–14126. doi: 10.1111/ppl.14114
Perlikowski, D., Kosmala, A. (2020). Mechanisms of drought resistance in introgression forms of Lolium multiflorum/Festuca arundinacea. Biol. Plantarum. 64, 497–503. doi: 10.32615/bp.2020.076
Reddy, K. S., Sekhar, K. M., Sreeharsha, R. V., Reddy, A. R. (2019). Hydraulic dynamics and photosynthetic performance facilitate rapid screening of field grown mulberry (Morus spp.) genotypes for drought tolerance. Environ. Exp. Botany. 157, 320–330. doi: 10.1016/j.envexpbot.2018.10.038
Réjou-Méchain, M., Mortier, F., Bastin, J. F., Cornu, G., Barbier, N., Bénédet, F., et al. (2021). Unveiling african rainforest composition and vulnerability to global change. Nature. 593, 90–94. doi: 10.1038/s41586-021-03483-6
Sapeta, H., Yokono, M., Takabayashi, A., Ueno, Y., Cordeiro, A. M., Hara, T., et al. (2023). Reversible down-regulation of photosystems I and II leads to fast photosynthesis recovery after long-term drought inJatropha curcas[J. J. Exp. Botany. 74, 336–351. doi: 10.1093/jxb/erac423
Sebastià, M., Antonio, D. E., Hipólito, M., Marilyn, C. B., Brendan, C. (2014). Rapid hydraulic recovery in Eucalyptus pauciflora after drought: linkages between stem hydraulics and leaf gas exchange. Plant Cell Environment. 37), 617–626. doi: 10.1111/pce.12182
Sevanto, S., McDowell, N. G., Dickman, L. T., Pangle, R., Pockman, W. T. (2014). How do trees die? A test of the hydraulic failure and carbon starvation hypotheses. Plant Cell Environ. 37, 153–161. doi: 10.1111/pce.12141
Shen, X. J., Liu, Y. W., Liu, B. H., Zhang, J. Q., Wang, L., Lu, X. G., et al. (2022). Effect of shrub encroachment on land surface temperature in semi-arid areas of temperate regions of the Northern Hemisphere. Agric. For. Meteorology. 320, 108943–108950. doi: 10.1016/j.agrformet.2022.108943
Shtai, W., Asensio, D., Kadison, A. E., Schwarz, M., Raifer, B., Andreotti, C., et al. (2024). Soil water availability modulates the response of grapevine leaf gas exchange and PSII traits to a simulated heat wave[J. Plant Soil. 501, 537–554. doi: 10.1007/s11104-024-06536-7
Song, K. C., Hu, H. Y., Xie, Y. Z., Fu, L. (2022a). The effect of soil water deficiency on water use strategies and response mechanisms of glycyrrhiza uralensis fisch. Plants. 11, 1464–1480. doi: 10.3390/plants11111464
Song, Y. G., Hwang, J. E., An, J., Kim, P. B., Park, H. B., Park, H. J., et al. (2022b). The Growth and Physiological Characteristics of the Endangered CAM Plant,Nadopungnan (Sedirea japonica), under Drought and Climate Change Scenarios. Forests. 13, 1823–1843. doi: 10.3390/f13111823
Tardieu, F., Tuberosa, R. (2010). Dissection and modelling of abiotic stress tolerance in plants. Curr. Opin. Plant Biol. 13, 206–212. doi: 10.1016/j.pbi.2009.12.012
Trugman, A. T., Detto, M., Bartlett, M. K., Medvigy, D., Anderegg, W. R. L., Schwalm, C., et al. (2018). Tree carbon allocation explains forest drought-kill and recovery patterns. Ecol. Letters. 21, 1552–1560. doi: 10.1111/ele.13136
Valeriano, C., Gazol, A., Colangelo, M., De-Andres, E. G., Camarero, J. J. (2021). Modeling climate impacts on tree growth to assess tree vulnerability to drought during forest dieback. Front. Plant Sci. 12672855. doi: 10.3389/fpls.2021.672855
Vastag, E., Orlović, S., Konôpková, A., Kurjak, D., Cocozza, C., Pšidová, E., et al. (2020). Magnolia grandiflora L. shows better responses to drought than Magnolia × soulangeana in urban environment. Forest. 13, 575–583. doi: 10.3832/ifor3596-013
Vinod, N., Slot, M., McGregor, I. R., Ordway, E. M., Smith, M. N., Taylor, T. C., et al. (2023). Thermal sensitivity across forest vertical profiles: patterns, mechanisms, and ecological implications. New phytologist 237 (1), 22–47. doi: 10.1111/nph.18539
Wang, W. Z., English, N. B., Grossiord, C., Gessler, A., Das, A. J., Stephenson, N. L., et al. (2020). Mortality predispositions of conifers across western USA. New phytologist. 229, 831–844. doi: 10.1111/nph.16864
Wang, X., Fan, Y. L., Zhang, C. C., Zhao, Y. H., Du, G. Y., Li, M., et al. (2023a). From comfort zone to mortality: sequence of physiological stress thresholds in robinia pseudoacacia seedlings during progressive drought. Front. Plant Sci. 14. doi: 10.3389/fpls.2023.1149760
Wang, S., Hou, Y. X., Feng, L. J., Lu, Q. Q., Zhou, L (2023b). Effect of drought stress on anatomical structure of leaves in table grape varieties. J. Agric. Sci. Technol. 25, 40–49. doi: 10.13304/j.nykjdb.2021.0818
Wang, C. X., Liu, H. Y., Xia, J. B., Xing, X. S., Zhang, S. Y. (2021). Critical effects on the photosynthetic efficiency and stem sap flow of poplar in the Yellow River Delta in response to soil water. J. Forestry Res. 32, 2485–2498. doi: 10.1007/s11676-020-01281-w
Wang, Z. Y., Xie, S. M., Yang, P. F., Wang, G. (1983). Apple intergeneric dwarf rootstock hairy Cotoneaster submultiflorus Popov and Cotoneaster multiflorus Bunge. J. shanxi Agric. university. 3, 33–42. doi: 10.13842/j.cnki.issn16718151.1983.02.006
Wei, Q. J., Feng, F. F., Ma, Z. Z., Su, S. T., Ning, S. J., Gu, Q. Q. (2018). Effects of drought and rewatering on leaf photosynthesis, chlorophyll fluorescence, and root architecture of citrus seedlings. Chin. J. Appl. Ecology. 29, 2485–2492. doi: 10.13287/j.1001-9332.201808.028
Werner, C., Meredith, L. K., Ladd, S. N., Ingrisch, J., Kübert, A., van Haren, J., et al. (2021). Ecosystem fluxes during drought and recovery in an experimental forest. Science. 374, 1514–1518. doi: 10.1126/science.abj6789
Wion, A. P., Breshears, D. D., Carroll, C. J. W., Cobb, N. S., Hart, S. J., Law, D. J., et al. (2022). Dead again: predictions of repeat tree die-off under hotter droughts confirm mortality thresholds for a dryland conifer species. Environ. Res. Lett. 17, 74031–74151. doi: 10.1088/1748-9326/ac7968
Wu, G. X. (2020).Soil Relative Water Content Change in High Cold Forestation Area. Forest Science and Technology 5, 45–4810.
Wu, M., Deng, P., Zhao, Y., Zhao, S. H., Chen, J. N., Shu, Y., et al. (2019). Effects of drought on leaf growth and chlorophyll fluorescence kinetics parameters in Cyclobalanopsis glauca seedlings of Karst areas. J. Appl. Ecology. 30, 4071–4081. doi: 10.13287/j.1001-9332.201912.001
Wu, S., Gu, X., Zheng, Y., Chen, L. (2023). Nocturnal sap flow as compensation for water deficits: an implicit water-saving strategy used by mangroves in stressful environments. Front. Plant Sci. 14. doi: 10.3389/fpls.2023.1118970
Wu, T., Tan, N., Tissue, D. T., Huang, J., Duan, H. L., Su, W., et al. (2022). Physiological traits and response strategies of four subtropical tree species exposed to drought[J. Environ. Exp. Botany. 203, 105046–105058. doi: 10.1016/j.envexpbot.2022.105046
Xu, Z., Tian, Y., Liu, Z. W., Xia, X. R. (2023). Comprehensive effects of atmosphere and soil drying on stomatal behavior of different plant types. Water. 15, 1675–1691. doi: 10.3390/w15091675
Yan, S. P., Chong, P. F., Zhao, M. (2022). Effect of salt stress on thephotosynthetic characteristics and endogenous hormones, and: A comprehensive evaluation of salt tolerance in Reaumuria soongorica seedlings. Plant Signaling Behavior. 17, 2031782–2031797. doi: 10.1080/15592324.2022.2031782
Yang, J., Kim, S. H., Pak, J. H., Kim, S. C. (2022). Infrageneric plastid genomes of cotoneaster (Rosaceae): implications for the plastome evolution and origin of C.wilsonii on ulleung island. Genes. 13, 728–728. doi: 10.3390/genes13050728
Yao, Y., Liu, Y. X., Zhou, S., Song, J. X., Fu, B. J. (2023). Soil moisture determines the recovery time of ecosystems from drought. Glob. Change. Biol. 29, 3562–3574. doi: 10.1111/gcb.16620
Zeppel, M. J. B., Anderegg, W. R. L., Adams, H. D., Hudson, P., Cook, A., Rumman, R., et al. (2019). Embolism recovery strategies and nocturnal water loss across species influenced by biogeographic origin. Ecol. Evol. 9, 5348–5361. doi: 10.1002/ece3.5126
Zhang, R., Bi, H. X., Jiao, Z. H., Wang, N., Zhao, D. Y., Yun, H. Y., et al. (2022). Diurnal and nocturnal changes in stem sap flow of Robinia pseudoacacia during growing season and its response to meteorological factors. J. Zhejiang A&F University. 39, 1238–1246. doi: 10.11833/j.issn.2095-0756.20220159
Zhang, A. Q., Liu, M. G., Gu, W., Chen, Z. Y., Gu, Y. C., Pei, L. F., et al. (2021). Efect of drought on photosynthesis, total antioxidant capacity, bioactive component accumulation, and the transcriptome of. Atractylodes lancea. BMC Plant Biol. 21, 293–307. doi: 10.1186/s12870-021-03048-9
Zhang, X., Tong, C. Z., Fang, D. M., Mei, T. T., Li, Y. (2023). Different hydraulic and photosynthetic responses to summer drought between newly sprouted and established moso bamboo culms. Front. Plant Sci. 14. doi: 10.3389/fpls.2023.1252862
Zhang, Y. A., Zhang, W., Tian, K., Sun, M., Dai, Q., Guan, D. X., et al. (2019). Correlation analysis of stomatal and photosynthetic characteristics of brasenia schreberi leaves under different water levels. J. Southwest Forestry Univesity. 39, 35–42. doi: 10.11929/j.swfu.201812017
Zhao, H. L., Cheng, H., Xie, Q. M., Hou, H., Ni, X. L. (2022). Evaluation of drought resistance of six shrubs in arid area and selection of identification indexes. J. Northwest Forestry University. 37, 24–29. doi: 10.3969/J.issn.1001-7461.2022.03.04
Zhou, J., Yang, X. D., Wang, Y. Y., Long, Y. X., Wang, Y., Li, B. R., et al. (2022). Difference in adaptation strategy between Haloxylon ammodendron and Alhagi sparsifolia to drought. Chin. J. Plant Ecology. 46, 1064–1076. doi: 10.17521/cjpe.2021.0338
Keywords: Cotoneaster multiflorus, drought stress, photosynthetic characteristics, water status, nocturnal sap flow, high-throughput sequencing, high-strategy
Citation: Huang Q-l, Zhang M-m, Li C-s, Li B-y, Zhuo S-l, Yang Y-s, Chen Y-d, Zhong A-n, Liu H-y, Lai W-f, Huang Z-b, Cao M-h, Yuan Z-s and Zhang G-f (2025) Response mechanism of water status and photosynthetic characteristics of Cotoneaster multiflorus under drought stress and rehydrated conditions. Front. Plant Sci. 15:1457955. doi: 10.3389/fpls.2024.1457955
Received: 01 July 2024; Accepted: 13 December 2024;
Published: 14 January 2025.
Edited by:
PingWu Liu, Hainan University, ChinaReviewed by:
Alla Yemets, National Academy of Sciences of Ukraine (NAN Ukraine), UkraineFrancisco Javier Cano, Spanish National Research Council (CSIC), Spain
Copyright © 2025 Huang, Zhang, Li, Li, Zhuo, Yang, Chen, Zhong, Liu, Lai, Huang, Cao, Yuan and Zhang. This is an open-access article distributed under the terms of the Creative Commons Attribution License (CC BY). The use, distribution or reproduction in other forums is permitted, provided the original author(s) and the copyright owner(s) are credited and that the original publication in this journal is cited, in accordance with accepted academic practice. No use, distribution or reproduction is permitted which does not comply with these terms.
*Correspondence: Guo-fang Zhang, Zmp6Z2Z6Z2ZAMTYzLmNvbQ==