- 1Division of Plant Biology, Bose Institute, Kolkata, West Bengal, India
- 2Post Graduate Department of Botany, Ramakrishna Mission Vivekananda Centenary College (Autonomous), Rahara, Kolkata, India
- 3Faculty of Forestry and Wood Sciences, Czech University of Life Sciences, Prague, Czechia
Anthropogenic activities and subsequent global climate change instigate drastic crop productivity and yield changes. These changes comprise a rise in the number and severity of plant stress factors, which can arise simultaneously or sequentially. When abiotic stress factors are combined, their impact on plants is more substantial than that of a singleton stress factor. One such impact is the alteration of redox cellular homeostasis, which, in turn, can regulate downstream stress-responsive gene expression and resistance response. The epigenetic regulation of gene expression in response to varied stress factors is an interesting phenomenon, which, conversely, can be stable and heritable. The epigenetic control in plants in response to abiotic stress combinations and their interactions with cellular redox alteration is an emerging field to commemorate crop yield management under climate change. The article highlights the integration of the redox signaling pathways and epigenetic regulations as pivotal components in the complex network of plant responses against multi-combinatorial stresses across time and space. This review aims to lay the foundation for developing novel approaches to mitigate the impact of environmental stresses on crop productivity, bridging the gap between theoretical understanding and practical solutions in the face of a changing climate and anthropogenic disturbances.
1 Introduction
The collective influence of human activities on Earth over the last few decades has resulted in numerous severe environmental stress conditions within our ecosystems and agricultural areas (Rillig et al., 2019). These conditions encompass extreme and variable weather events attributable to climate change, such as heatwaves, frost, prolonged submergence, or drought. Additionally, they affect soil conditions, including salinity and varying pH, and they introduce various anthropogenic contaminants like heavy metals, microplastics, pesticides, antibiotics, and persistent organic pollutants into the environment. Other contributors to the complex environmental milieu include radiation (e.g., UV), restricted nutrient availability, and elevated concentrations of airborne molecules and gases such as ozone, particulate matter from combustion, and carbon dioxide (CO2).
In agriculture, the impact of these simultaneous stress conditions creates crop damage of different magnitudes. Plants often encounter a combination of abiotic stresses in their natural habitats, creating a dynamic and challenging environment that leads to a loss in crop productivity and yield. Optimizing crop yield is a paramount objective directly proportional to the formidable challenges posed by these stressors (Zhang et al., 2022). Abiotic stress, arising from non-living environmental factors, includes a spectrum of adversities such as extreme temperatures, drought, salinity, and pollutants. Each of these stressors, individually and collectively, profoundly influences plant physiological processes, thereby precipitating a discernible reduction in crop productivity. Drought conditions may coincide with elevated temperatures, salinity stress may accompany heavy metal toxicity, and fluctuating environmental factors may converge, presenting a complex matrix of challenges. Numerous reports and studies indicate the ill effects of individual stress factors. However, our knowledge of simultaneous stress remains rudimentary. Therefore, understanding how plants perceive, prioritize, and respond to these multi-combinatorial stresses at the molecular level requires a holistic approach. Multi-combinatorial stress denotes the simultaneous exposure of cells to various environmental challenges, necessitating sophisticated and adaptive cellular responses for endurance (Zandalinas and Mittler, 2022).
Redox signaling, characterized by the production and reception of reactive oxygen species (ROS), stands at the nexus of plant stress responses. The alteration of redox homoeostasis is considered the primary and essential cellular response against any stress. Once considered mere byproducts of cellular metabolism, ROS is now recognized as versatile signaling molecules that modulate various cellular processes. The integration of redox signaling in stress perception allows plants to sense and transduce signals in a dynamic and context-dependent manner, initiating a cascade of events that culminate in adaptive responses. In the context of cellular homeostasis, the interaction between epigenetic regulatory mechanisms and redox signaling has emerged as a focal point of investigation, particularly when confronted with multicombinatorial stressors simultaneously. Epigenetic control, characterized by heritable modifications to DNA, histones, and non-coding RNAs, orchestrates gene expression patterns without altering the underlying genomic sequence. Concurrently, redox signaling involves maintaining the delicate equilibrium between ROS and antioxidants, indispensable mediators in cellular signaling pathways. The amalgamation of these regulatory frameworks assumes heightened significance when cells are subjected to a confluence of environmental stressors, precipitating situation-dependent cellular response (Ueda and Seki, 2020).
The experimental work of Zandalinas et al. (2021) has introduced a crucial concept in plant biology: as the number and complexity of stressors on a plant increase, plant growth and survival decline significantly, even when each individual stressor is relatively mild. This synergistic effect of multifactorial stress was previously observed in soil microbiomes by Rillig et al. (2019), highlighting its potential impact on plant–microbiome interactions. In ecosystems with high biodiversity (3D forest ecosystems), outcomes of multifactorial stress can vary, but the effect is likely negative in low-biodiversity agroecosystems like crop fields. The plant’s resistance response to multifactorial stress is intricately linked to redox signaling and epigenetic regulation. Reactive oxygen species (ROS) play a critical role in signaling pathways that modulate stress responses, while epigenetic mechanisms regulate gene expression patterns without altering the DNA sequence. Understanding how these processes interact under combined stress conditions is essential for developing resilient crops. The review underscore the urgent need to limit the number and intensity of environmental stressors to prevent detrimental impacts on plant health and ecosystem stability. The initial observations by Rillig and Zandalinas call for further studies on multifactorial stress combinations across various plant species, microbiomes, and crops, with a focus on the roles of ROS and epigenetics. Addressing this issue requires a comprehensive approach that includes breeding and engineering plants for resilience, increasing crop diversity, and manipulating plant–microbiome interactions. Integrating laboratory and field experiments with genome-wide association studies and leveraging wild plant varieties and microbiomes can enhance crop resilience. Additionally, incorporating insights from material sciences, nanotechnology, physics, chemistry, and precision agriculture can help mitigate the effects of multifactorial stress. Despite the challenges posed by the increasing rate of anthropogenic activity, with concerted efforts, it is possible to develop strategies to counteract the negative impacts of multifactorial stress on crops and ecosystems, particularly through advancements in understanding ROS signaling and epigenetic modifications. Understanding the integration of redox signaling pathways and epigenetic control in plant responses to multiple abiotic stresses holds profound implications for crop improvement strategies (Suzuki et al., 2014). Unravelling the molecular underpinnings aids in developing targeted interventions to enhance stress tolerance and resilience in agriculturally important crops, ultimately contributing to global food security. In summary, this review explores the integration of the redox signaling pathways and epigenetic regulations as a central node in the complex web of plant responses to multiple abiotic stresses. By deciphering the molecular dialogues within this complex network, we aim to extend our knowledge of plant stress biology and pave the way for innovative strategies to bolster crop resilience in a changing world.
2 ROS and its cellular toxicity
ROS and reactive nitrogen species (RNS) are the most abundant active signaling intermediate in the cellular milieu. The molecules containing one or more lone pair of electrons are called free radicals. Oxygen and nitrogen are two vital molecules necessary for sustaining life and the generation of essential biomolecules. O2 can carry two lone pair of electrons, whereas N2 can bear three. The generation of active oxygen species (AOS) is common in aerobic life forms. ROS includes both radical and non-radical forms of active oxygen molecules generated through partial reduction of O2, e.g., oxygen radical or superoxide anion or superoxide radical (O2•–), hydroxyl radical (HO•) and hydrogen peroxide (H2O2), hydroxide ion (HO−), peroxide ion (O2−2) (Ray et al., 2012). H2O2 is not as reactive as the other reactive forms; it is generated in the chain reaction process. This category has also included the spontaneous generation of H2O2 from superoxide radicals. The radical form is more reactive due to reactive lone pairs and high reduction potential (Baek and Skinner, 2012). The triplet oxygen (O22•) and sometimes singlet oxygen (1O2) are also considered ROS (Pospíšil et al., 2019). The mystery behind the generation of active oxygen lies in its spin chemistry of lone pairs of electrons. The spin quantum numbers of this pair of electrons are the same; hence, it restricts the acceptance of electrons from another atom with similar spin. The oxidation of molecular O2 is only possible through one electron transfer to another paramagnetic center, usually transition metals (Fe and Cu) with unpaired electrons (Tripathy and Oelmüller, 2012). The source-to-sink transition of ROS relies on chain reactions depending on the redox potential of the elements (Pospíšil et al., 2019; Mansoor et al., 2022). The light-activated NADP+/NADPH system during photosynthesis in chloroplast and NAD+/NADH system during electron transport system in chloroplast can donate electrons to the molecular oxygen due to high reduction potential (-320 mV). The light-driven photoactivation of chlorophyll molecules into singlet and triplet forms is also parallel induction during photosynthesis. In this process, both peroxide ion (O2−2) and superoxide radicals (O2•−) are formed within plants. Gradually the antioxidant scavenger system, including superoxide dismutase (SOD) with an iron core and free iron (FeIII) plays a crucial role in a further reduction to form hydrogen peroxide and/or peroxide radicals (H2O2/HO•) before it is further reduced to O2 or water (Tripathy and Oelmüller, 2012; Pospíšil et al., 2019; Mansoor et al., 2022) (Figure 1).
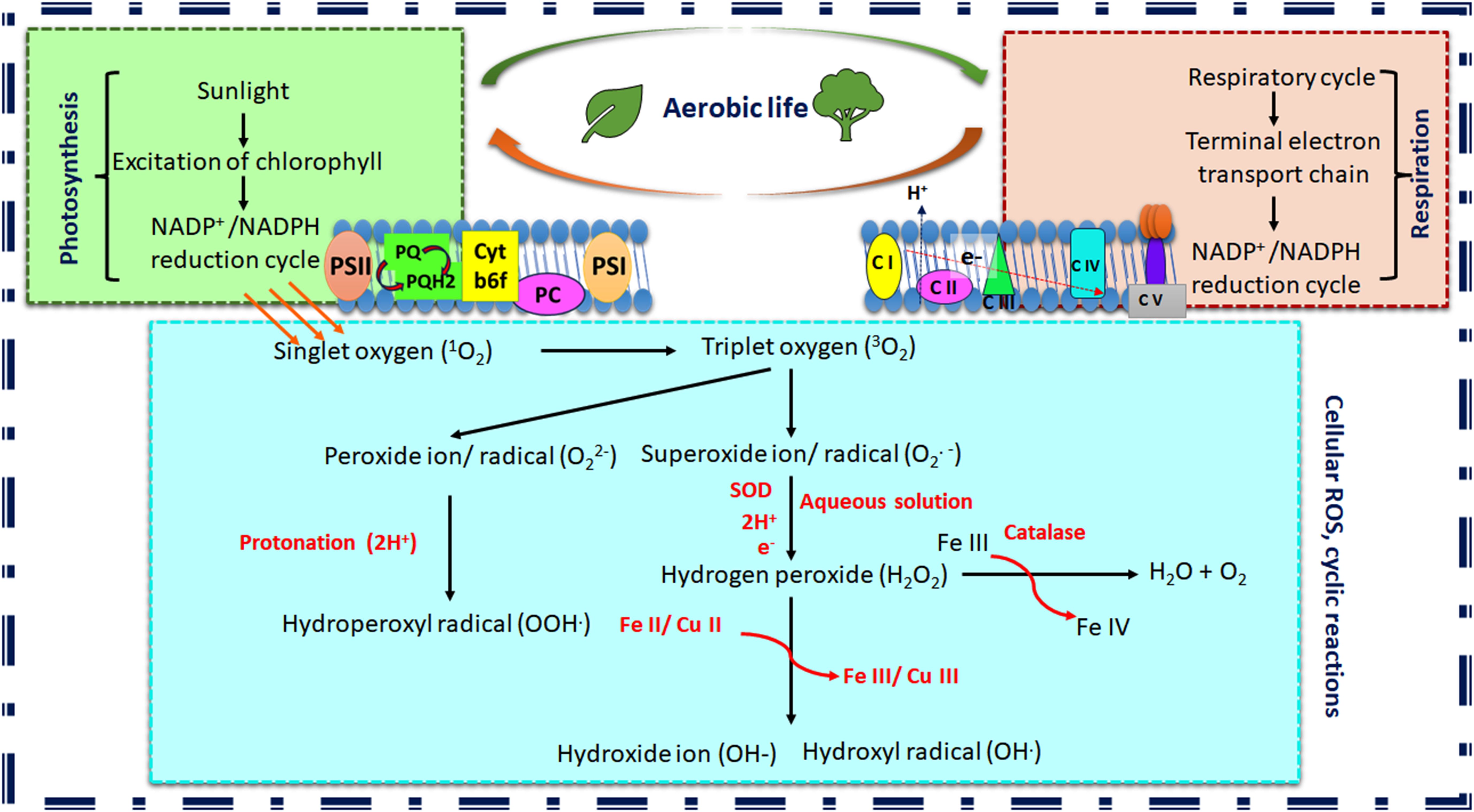
Figure 1. Schematic representation of cyclic reactions leading to reactive oxygen species (ROS) generation in plants. The figure depicts the apparent ROS production due to aerobic life. Photosynthesis and respiration are the primary biochemical cycles in plants that generate ROS as byproducts (PSI, PhotosystemI; PSII, PhotosystemII; PQ, Plastoquinone; Cyt-b6f, Cytochrome b6f complex; PC, Plastocyanine; CI-CV, Cytochrome I-V; SOD, Superoxide dismutase; NADPH, Nicotinamide Adenine Dinucleotide Phosphate Hydrogen.
Although highly reactive and toxic when accumulated, ROS are vital for normal plant growth, development, and stress signaling (Huang et al., 2019). The toxicity of ROS depends upon their longer half-life and extended diffusion capability within the cell (Mittler, 2017; Waszczak et al., 2018). The superoxide radicals (O2. −) are usually produced in mitochondrial ETS and can affect the mitochondrial genome. For instance, the light-grown maize seedlings showed high mitochondrial DNA damage due to excessive ROS production in developing mitochondria from non-pigmented meristematic cells (Tripathi et al., 2020). Singlet oxygen has almost similar transmission capability through the plasma membrane and has a greater affinity towards Trp, His, Tyr, and Cys residues of proteins (Dumanović et al., 2021). They can be transmitted from the chloroplast membrane to the cytosol and accumulate within the cell. The site-specific accumulation leads to programmed cell death (PCD) or hypersensitive response (HR) mediated cell death or stress response against varied abiotic factors (Dmitrieva et al., 2020). As the scavenging enzyme system of singlet oxygen is lacking, overproduction of this may be deleterious for the survivability of the plants. The delimited production of singlet oxygen may be involved in regulated cell death, controlling the normal development of plants (Bhatt et al., 2021). Recently, it has been observed that singlet oxygen governs pivotal signaling in the degradation of damaged chloroplasts (Lemke and Woodson, 2022). The mutant analysis with Arabidopsis fluorescent (flu) showed that SAFEGUARD1 (SAFE1), a chloroplast granum localized protein, is involved in the protection of chloroplast grana membrane in EXECUTER1 (EX1)-dependent retrograde signaling pathway (Wang et al., 2020). Recently, it has been demonstrated that EXECUTER 2 helps the EX1 signalosome to sense singlet oxygen in plastids (Dogra et al., 2022). The H2O2, instead, has a greater half-life and transmission time, hence targeting Cys and Met residues of proteins away from their origin. H2O2, after being produced by the activity of SOD in the chloroplast, mitochondria, plasma membrane NADPH oxidases, peroxisomal oxidases, type III peroxidases, etc., are transported within the cell by aquaporins. The accumulation of H2O2 leads to many toxic effects in plants but are readily detoxified by catalases, peroxiredoxin, glutathione peroxidases (GPX), and ascorbate peroxidases (APX) (Bhar et al., 2017). The accumulation of H2O2 may also lead to autophagy and PCD (Smirnoff and Arnaud, 2019). Fusarium-induced redox signaling was also observed in chickpeas during wilt disease. The susceptible plants showed extensive oxidative damage and membrane degradation due to poor antioxidative scavenging efficiency compared to the resistant chickpea plants (Gupta et al., 2013). Highly reactive HO• can modify almost all biomolecules (DNA, RNA, lipids, and proteins) in the cellular vicinity (Dumanović et al., 2021). The biotic and abiotic factors are responsible for the induction of ROS and target the biomolecules through varied transcription factors, e.g., NAC, Zinc finger, WRKY, ERF, MYB, DREB, and bZIP (Khedia et al., 2019). In most cases, ROS are accumulated and cause oxidative bursts in response to stress factors. Phenolic acids play critical roles in scavenging these heavy metal-induced ROS in Kandelia obovata and increase the bioavailability of metals (Chen et al., 2020). Soil aluminum (Al) induces oxidative bursts, interfering with plant water and nutrient uptake by affecting several nutrient uptake and aquaporin gene families (Chauhan et al., 2021). It has been concluded that uncontrolled production of ROS due to inefficient or damaged scavenging machinery leads to oxidative damage that includes degradation of biomolecules, membrane damage, disruption of cellular permeability, modification of metabolic enzymes, reduced carbon fixation, yield loss and cell death. However, balanced production of ROS is necessary for the normal functioning of plant cells. Hence, the toxicity of ROS is intricately dependent upon its cellular detoxification efficiency.
3 Plant immunity, ROS metabolism, and regulation
The plant immune response and signaling rely on biotic interaction. The pathogen-associated molecular pattern (PAMP) and host pattern recognition receptors (PRR) interact to instigate the first line of the immune response, pattern-triggered immunity (PTI). In the second line, the most specific and robust immune signaling takes place through the interaction of pathogen-secreted effector molecules (toxins) with the host resistance (R) gene, called effector-triggered immunity (ETI) (Jones and Dangle, 2006). The induction of ROS is an inevitable reaction in both PTI and ETI with varied magnitude (Yuan et al., 2021). This immune response may sometimes be impregnated as the genetic imprint and transgenerational, termed immunogenic memory (Bhar et al., 2021). The abiotic stress response does not always follow a generalized path, but oxidative burst is a common physiological effect that affects almost every abiotic stress factor. The host cells have specific receptors for abiotic stress signals. Membrane-bound receptor-like kinases (RLKs) play crucial roles in perceiving external stress signals in plants (Osakabe et al., 2013). Many biotic stress receptors may also act as abiotic stress receptors. Usually, the membrane damage associated with fluidity, integrity and the cell wall degradation product in response to both biotic and abiotic stress produces intermediates that act as damage/danger-associated molecular patterns (DAMPs). These DAMPs regulate many cellular responses, gene expressions, and hormonal cross-talk (Saijo and Loo, 2020). The receptor-mediated stress signals activated membrane-bound respiratory burst oxidase homolog (RBOH) or plant nicotinamide adenine dinucleotide phosphate oxidases (NADPH oxidases) (Liu and He, 2016). The receptor-mediated signal sometimes instigates calcium influx channels and generates a calcium signature, activating RBOH homologs (Bhar et al., 2023). The light-induced generation of singlet and triplet oxygen-mediated ROS production has also contributed to cellular redox homeostasis (Li and Kim, 2023). Despite their photodamaging behavior, they are also involved in cytosolic ROS signaling. Mitochondria, conversely, produces superoxide radicals during improper electron transport within the mitochondrial membrane. The abiotic stress and climate change induce elevated metabolism and produce proliferous ROS subsequently within mitochondria (Nadarajah, 2020). Alternatively, peroxisomal glycolate oxidases (GLO) play crucial roles in peroxisomal ROS production (Corpas, 2019). Climate change-induced heat stress and other abiotic stress combinations were reported to induce GLO-dependent H2O2 production in rice with elevated photosynthetic activity (Balfagón et al., 2020). The antioxidative scavenging machinery in plants actively detoxifies these ROS. The imbalanced production and scavenging system or inefficient detoxification led to oxidative stress in plants. The enzymatic scavengers include superoxide dismutase (SOD), catalase (CAT), ascorbate peroxidase (APX), glutathione peroxidase (GPX), monodehydroascorbate reductases (MDHAR), dehydroascorbate reductases (DHAR), glutathione reductase (GR) etc. The non-enzymatic scavengers encompass carotenoids, α-tocopherol, glutathione (GSH), ascorbic acid (AsA) and proline, which act on ROS to maintain cellular redox state (Mansoor et al., 2022). Thus, when the metabolic and stress-induced ROS surpasses the scavenging system, an oxidative burst takes place. Multiple abiotic stress stringently regulates acclimation using these redox alterations (Choudhury et al., 2017). The ROS leads to DNA damage, membrane degradation, lipid peroxidation etc. as oxidative stress markers (Bhar et al., 2023). Balanced ROS may act as a signaling intermediate and coordinates resistance gene expression by multiple induction of transcription factors (MYB, bZIP, WRKY, RAV, NAC, AP2/ERF, ZAT etc.) and cis acting elements (ARE, CORE, W-box, GCC box, as-1 like etc (Singh et al., 2019) (Figure 2).
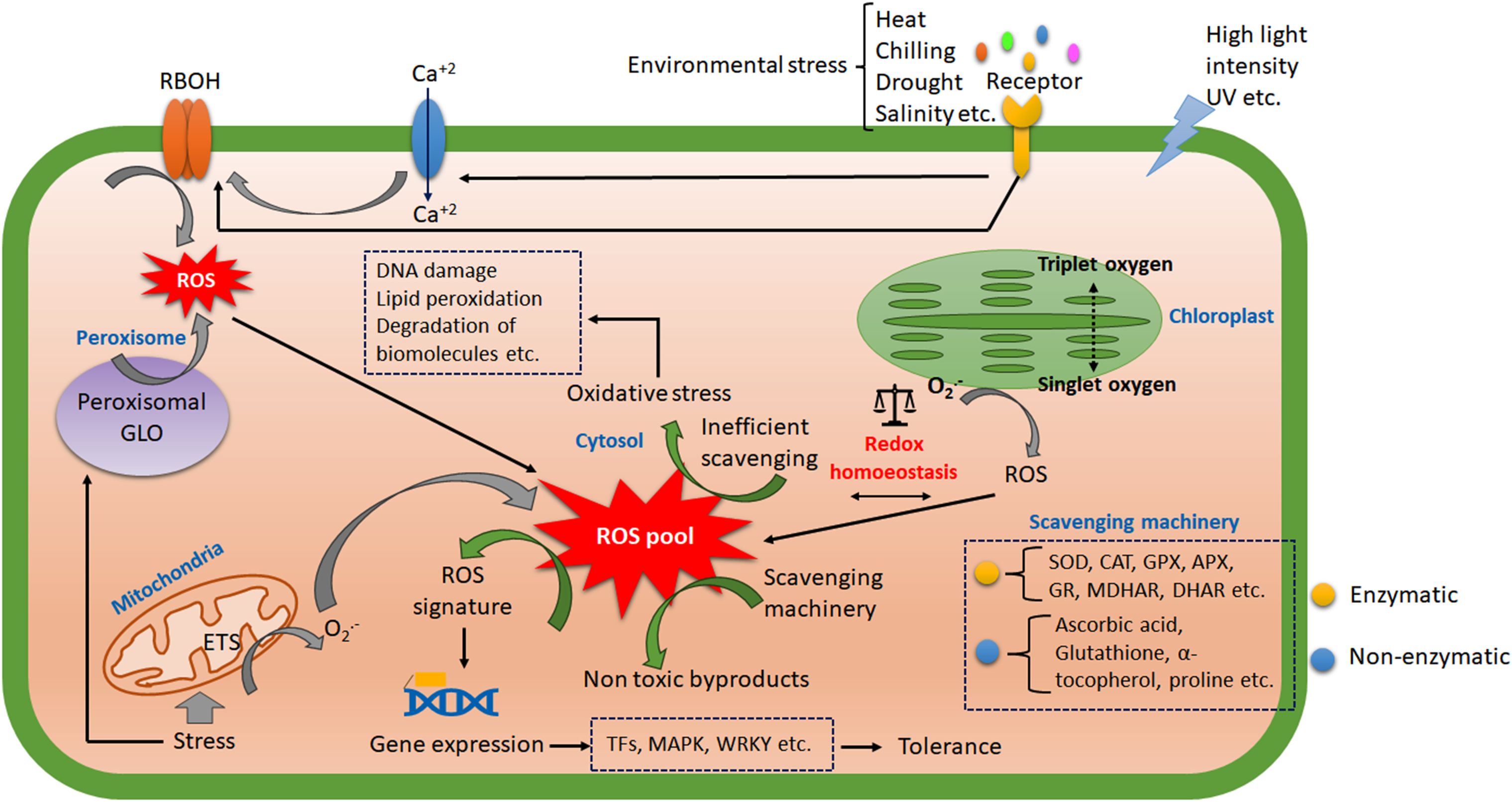
Figure 2. The overview of ROS signal transduction during abiotic stress. The receptors in the cell membrane perceive the major abiotic stress and transduce signals to several membrane-bound transporters. The calcium influx plays a crucial inducer of respiratory burst oxidase (RBOH) to generate ROS. The organelles also contribute to the intra-cellular ROS pool. The activity of enzymatic and non-enzymatic scavengers has balanced the cellular redox homoeostasis. The efficient scavenging produces a ROS signal that activates varying transcription factors and is responsible for stress-induced gene expression. On the other hand, inefficient scavenging leads to oxidative stress and macromolecular damage.
The network analysis of Arabidopsis ROS-regulated genes using STRING 12.0 (https://string-db.org/) (Szklarczyk et al., 2023) has revealed a considerable interactome consisting of 138 nodes and 395 edges with the PPI enrichment p-value, < 1.0e-16. The physical interaction-dependent subnetworks revealed clusters of genes involved in ROS metabolism. SOD homologs, mainly Mn-SOD, Fe SOD, and Cu-Zn SOD (MSD, CSD, FSD) produce an interacting cluster. Peroxisomal GLO produces another metabolic cluster with CATs. Thioredoxin reductase (NTRs) and peroxidases (PERs) produce independent metabolic networks. The regulation of ROS metabolism under abiotic stress demonstrated that gibberellic acid (GA) plays a critical role in Arabidopsis. Many GA-related genes and DELLA repressor-like proteins (GA, GASA, GAI, RGL, RGA) produce a common cluster with cytochrome-dependent proteins (CRY1, CRY 2). The transcription factor JUB1 (JUNGBRUNNEN), a negative leaf senescence regulator, interacts directly with the GA cluster. In addition, JUB1 modulates cellular H2O2 levels and enhances tolerance to various abiotic stresses by regulating DREB2A (Xu et al., 2020). JUB 1 interacts with SPINDLY (SPY). The SPY is an O-linked N-acetylglucosamine transferase (OGT) involved in various processes, such as the gibberellin (GA) signaling pathway and circadian clock. OGTs catalyze the addition of nucleotide-activated sugars directly onto the polypeptide through O-glycosidic linkage with the hydroxyl of serine or threonine. It probably acts by adding O-linked sugars to yet-unknown proteins. OGTs act as a repressor of the GA signaling pathway to inhibit hypocotyl elongation (Qin et al., 2011). Light-harvesting complexes (LHCB) regulate light-driven ROS generation, forming separate network clusters. Lipase-like phytoalexin deficient 4 (PAD4); required downstream of MPK4 pathway for accumulation of the plant defense-potentiating molecule, salicylic acid. The PAD4 is also considered the significant ROS regulator in Arabidopsis and enhanced disease susceptibility 1 (EDS1). Sodium/hydrogen exchanger (NHX), thylakoid formation 1 (THF), Sec-independent protein translocase protein (TATB), and Serine/threonine-protein kinase (SRK2E) are found to be other regulators of ROS metabolism in Arabidopsis (Figure 3; Supplementary Tables 1, 2).
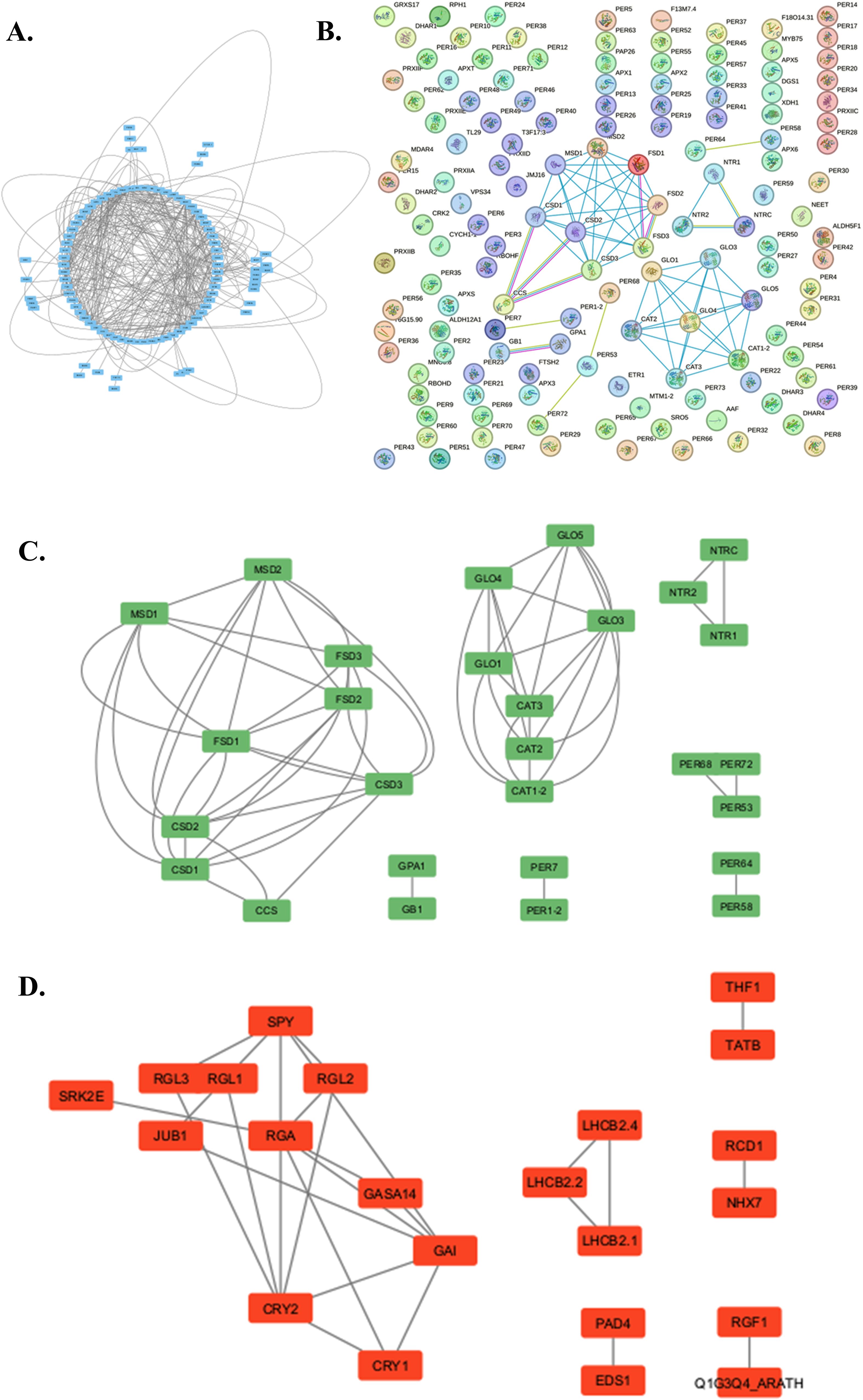
Figure 3. The metabolic network showing ROS-related regulation and metabolism in Arabidopsis thaliana analyzed by STRING (12.0) and further simplified and visualized through Cytoscape (3.10.1). (A) The entire ROS interactome illustrates intricate metabolic interactions. (B) The physical subnetwork analysis as visualized in STRING (12.0). (C) The simplified subnetwork shows the physical interaction of metabolic processes involving ROS (Cytoscape 3.10.1). (D) The simplified subnetwork demonstrates ROS metabolism regulation during stress (Cytoscape 3.10.1).
4 Sources of ROS production and its signaling during abiotic stress
As discussed earlier, ROS play a dual role in biological systems, serving as essential signaling molecules and potential mediators of oxidative damage. This largely depends on its production vs. scavenging efficiency. In the context of abiotic stress, a plethora of environmental factors, such as extreme temperatures, drought, salinity, and pollutants, can perturb cellular homeostasis, leading to an upsurge in ROS production. Hence, understanding the balance between ROS generation and signaling during abiotic stress is pivotal for deciphering the molecular mechanisms underlying plant stress responses. In plants, cellular ROS production is multifaceted as diverse sources contribute to their generation under abiotic stress conditions. From mitochondria and chloroplasts to peroxisomes and apoplast, each cellular compartment has distinct machinery for ROS production (Dietzel et al., 2008; Gilroy et al., 2016; Huang et al., 2016; Kerchev et al., 2016; Rodríguez-Serrano et al., 2016; Takagi et al., 2016). Elucidating the complex signaling pathways that transduce ROS-generated signals is critical for deciphering the adaptive strategies employed by organisms to cope with environmental challenges is essential. Furthermore, the redox status of individual ROS-producing organelles and cumulative cellular redox status will vary according to the stress condition faced by the plant. Different environmental factors, stress combinations, and permutations will result in distinct intracellular redox state subsets and a unique signature stress response.
4.1 Abiotic stress-induced ROS production and signaling in chloroplasts
The green plants bear chloroplast as a unique organelle for photosynthesis. These chloroplasts play a significant role in generating ROS, serving as crucial signals in the cross-talk between chloroplasts and the nucleus. The photosynthetic electron transport chain’s (PETC) evolution is designed to control reactive oxygen species (ROS) generation, limit accidental injury, and support critical signaling pathways under abiotic stress conditions. In chloroplast, the abiotic stress, which constricts the availability of carbon dioxide by the closure of stomata, increases the production of ROS such as H2O2, O2·- and 1O2. This, in turn, disturbs the redox status, leading to the initiation of retrograde and anterograde signaling (Asada, 2006; Gill and Tuteja, 2010; Baniulis et al., 2013; Kleine and Leister, 2016; Mignolet-Spruyt et al., 2016). The stoichiometry of photosystems is also affected by stress-induced ROS production as the distribution of energy balance between PSI (Photosystem I) and PSII (Photosystem II) is altered (Dietzel et al., 2008; Vainonen et al., 2008; Pesaresi et al., 2009). The antioxidant systems in the thylakoid and stromal regions control the collection of ROS originating from photosynthesis, thereby governing environmental perception by adjusting oxidative signals exported from chloroplasts to the nucleus (Fichman et al., 2019; Gollan and Aro, 2020).
ROS form an integral part of a network of retrograde signals that modulate chloroplast functions based on prevailing metabolic and environmental conditions during organelle biogenesis and photosynthesis. Several chloroplast-to-nucleus signals, including those triggered by the accumulation of 3’-phosphoadenosine 5’-phosphate (Estavillo et al., 2011), methylerythritol cyclodiphosphate (Bjornson et al., 2017), dihydroxyacetone phosphate (Vogel et al., 2014), or heme (Espinas et al., 2012), have been identified. 3’-Phosphoadenosine 5’-phosphate, a product of sulfur metabolism, is generated through the transfer of the sulfate group from phosphoadenosine phosphosulphate (PAPS) by sulphotransferases, impacting molecules like desulphoglucosinolates and salicylic acid (Kopriva and Gigolashvili, 2016). The SAL1/FRY1 phosphatase prevents the accumulation of 3’-phosphoadenosine 5’-phosphate by degrading it to inorganic phosphate (Pi) and adenosine monophosphate (AMP) within chloroplasts and mitochondria (Chan et al., 2013, 2016). Under stress conditions, oxidative inactivation of SAL1 leads to increased 3’-phosphoadenosine 5’-phosphate accumulation. This pathway connects mitochondrion-to-nucleus and chloroplast-to-nucleus signaling, regulating transcription factors ANAC013 and ANAC017, which mediate a ROS-related retrograde signal from mitochondrial complex III (Shapiguzov et al., 2019). ANAC013 and ANAC017 functions are suppressed by the nuclear-localized RADICAL-INDUCED CELL DEATH1 (RCD1) protein, acting as a hub linking chloroplast and mitochondrial signaling to control metabolism in both organelles.
Chloroplast antioxidants, such as stromal ascorbate peroxidase (sAPX) and thylakoid ascorbate peroxidase (tAPX), may regulate retrograde signaling in plant responses to environmental stresses. tAPX, localized mainly in unstacked regions of the thylakoid membrane, efficiently removes H2O2 produced at PSI at the expense of ascorbate (Maruta et al., 2012). Ascorbate regeneration occurs at the thylakoid membrane and chloroplast stroma. tAPX, with higher turnover rates for H2O2 than peroxiredoxin (PRX), protects PSII more effectively from photooxidative damage (Dietz, 2016). The role of the glutathione (GSH) pool and dehydroascorbate reductases (DHARs) in regenerating ascorbate and detoxifying ROS is under scrutiny (Rahantaniaina et al., 2017). Nevertheless, recent research suggests GSH involvement in ascorbate recycling under high-light conditions (Terai et al., 2020). Production of these also curbs the ill effects of excessive ROS in chloroplast (Mittler et al., 2004). The production of ROS radicals also leads to nuclear reprogramming of gene expression, which leads to programmed cell death and chlorosis as a phenotype. It also activates a considerable subset of stress-related responsive genes. These gene expressions are executed by two chloroplast proteins associated with thylakoid membranes known as EXECUTER1 and EXECUTER2, both being transcribed in the nucleus (Wagner et al., 2004; Lee et al., 2007; Kleine and Leister, 2016). The accumulation of ROS during abiotic stress is managed by enzymes that can scavenge ROS and specific pathways like Fe- and Cu Zn-SODs and the Asada–Foyer–Halliwell pathway.
4.2 Abiotic stress-induced ROS production and signaling in mitochondria
Metabolism is the primary target of any type of stress, biotic or abiotic. The flow of electrons across the Electron Transport Chain (ETC) and during oxidative respiration generates ROS, including the superoxide ion and hydrogen peroxide (Moller, 2001; Murphy, 2009). The primary locations for generating ROS are ETC complexes I, II, and III (Andreyev et al., 2015). The ETC consistently produces superoxide and hydrogen peroxide in normal conditions called metabolic ROS. The various alterations in metabolism and stress-related conditions can induce a more reduced state in the ETC, resulting in heightened production of ROS, called stress-induced ROS. This phenomenon occurs, for example, when the ETC is inhibited or slowed down, particularly when stress reduces the availability of ADP. Consequently, respiratory inhibitors can impede ETC complexes, causing over-reduction in different segments of the ETC and resulting in excess superoxide production (Schwarzländer et al., 2009). Changes in mitochondrial ultrastructure triggered by stress, such as those observed in salt stress, have the potential to directly impact or impair the functioning of ETC and Oxidative Phosphorylation (OXPHOS) (Garcia de la Garma et al., 2015).
Particularly in response to abiotic stresses, the production of mitochondrial ROS increases significantly. Stress conditions disrupt the normal functioning of the respiratory electron transport chain in mitochondria, leading to the leakage of electrons from complex I and III and the formation of superoxide radicals (O2•-), which can be further converted to H2O2 by Mn-SOD (Quan et al., 2008; Huang et al., 2016). While superoxide has a brief half-life, the longer half-life of hydrogen peroxide allows it to traverse membranes through aquaporins, potentially functioning as a signaling molecule that connects mitochondria to other cellular compartments. The dynamic involvement of ROS from mitochondria has been connected to ROS signaling mechanisms (Ng et al., 2014). These mechanisms encompass oxidized intermediates, Ca2+ ions, and phosphorylation processes. Additionally, they are associated with transcriptional and post-transcriptional modifications in cell functioning, which can regulate growth and development, including programmed cell death (PCD) (Singh et al., 2016; Cui et al., 2019). Alternative oxidase (AOX), type II NAD(P)H dehydrogenase, and uncoupling proteins in the inner mitochondrial membrane can all help to slow down this process (Noctor et al., 2007; Rasmusson and Wallström, 2010). WRK15 binds to the promoter region of AOX1 to regulate the production of reactive oxygen species (Vanderauwera et al., 2012). Also, retrograde signaling between mitochondria and the nucleus is activated by altering ROS production in mitochondria during abiotic stress (Woodson and Chory, 2008).
Interestingly, mitochondrial redox alterations are also sometimes connected to hormonal imbalances. ROS signaling in mitochondria and the signaling of mitochondrial disturbances are connected to developmental processes controlled by auxins. Arabidopsis FtsH4 mutants, characterized by a deficiency in a mitochondrial protein-processing protease, exhibit an accumulation of mitochondrial hydrogen peroxide linked to the disruption of auxin homeostasis, dysregulation of the cell cycle, and impairment of meristem activity (Dolzblasz et al., 2018). The mutation of the splicing factor ABA-overly-sensitive-8 (ABO8), crucial for the accurate expression of the NAD4 component of complex I, leads to heightened production of mitochondrial ROS, increased sensitivity to abscisic acid (ABA), diminished auxin accumulation/signaling, and reduced meristem activity (Yang et al., 2014). The interaction among mitochondrial ROS, hormones, and developmental processes suggests that mitochondrial ROS signaling might be influenced, to some extent, by pathways associated with hormone signaling.
4.3 Abiotic stress-induced ROS production and signaling in peroxisomes
Peroxisomes are cellular organelles involved in various metabolic processes, including the breakdown of fatty acids and purines. During abiotic stress, peroxisomes can contribute to ROS production due to the activity of enzymes involved in these metabolic pathways, such as fatty acid beta-oxidation. Increased photo respiration causes increased hydrogen peroxide production by the enzyme glycolate oxidase in peroxisomes (Foyer and Noctor, 2009; Tripathy and Oelmüller, 2012; Kerchev et al., 2016). The antioxidant enzyme catalase (CAT) alleviates this over-accumulation of photo-respiratory reactive oxygen species. Hydrogen peroxide signaling during stress conditions is majorly understood by studying mutants deficient in peroxisomal CAT (Kerchev et al., 2016). Like other organelles, peroxisomal ROS causes cellular redox status changes and gene transcription in the nucleus (Vanderauwera et al., 2005).
There are at least four different processes through which ROS production in abiotic stress is mediated. Among these, NADPH Oxidases (Respiratory Burst Oxidase Homologs - RBOHs) are the most widely studied mechanism (Gilroy et al., 2014, 2016). This mechanism links ROS signaling and calcium signaling during stress and the production of superoxide molecules in the apoplast. RBOHs deficient mutants, for instance, RBOHD and RBOHF, for studying the interplay between redox status and abiotic stress tolerance. Hence, RBOHs have been shown to play an essential role in signaling mechanisms that assist in plant tolerance against abiotic stresses. Peroxidases are another enzyme mediating ROS generation in peroxisomes (O’Brien et al., 2012). Peroxidase-dependent ROS generation is shown to be involved in potassium deficiency response and regulation of root growth (Kim et al., 2010). Oxalate oxidase is another enzyme produced in peroxisome that regulates hydrogen peroxide production in the cell of roots and is shown as an essential protein in drought strengths (Voothuluru and Sharp, 2013). Ma et al. (2016) proposed another enzyme known as xanthine dehydrogenase that plays a vital role in stress signaling. Mitigation of ROS levels in apoplast is conducted by Cu/Zn-SODs, APXs, cell wall-bound peroxidases, and low levels of ascorbate and GSH. Compared to intracellular ROS production, apoplastic ROS production is ineffective, leading to imbalance. This disparity is a critical event in the signaling system and stress response.
5 ROS signaling in simultaneous abiotic stresses
Numerous research studies have been conducted to evaluate plant response against singular abiotic stress conditions. However, abiotic stress generally occurs in combination rather than independently in reality. Stress response at molecular, physiological, and metabolic levels tends to vary significantly in combinatorial abiotic stress compared to individual stress. Combinatorial abiotic stress in plants refers to the simultaneous occurrence of multiple stress factors that can negatively impact plant growth and development. These stress factors can include two or more abiotic stressors, creating a complex and challenging environment for plants (Table 1). Combinatorial stress response causes a major loss in crop productivity and yield (Mittler, 2006; Mittler and Blumwald, 2010; Suzuki et al., 2014). As a result, combination stressors are now the subject of more laboratory research since they are more damaging and have a greater detrimental effect on crop output. Additionally, distinct transcriptome signatures corresponding to combinations of abiotic stressors—for example, heat stress coupled with salinity or drought—are observed. These signatures comprise many transcripts that differ significantly from those of individual stress responses (Rizhsky et al., 2004). Besides, contrary to popular belief, some stress combinations even provide some amount of tolerance to another stress (abiotic or biotic) when it occurs after them.
From the redox point of view, much evidence indicates differences in ROS levels, gene expression of various ROS enzymes, and antioxidant systems when compared between combinatorial abiotic stress and individual stress application. Quantities of O2.-, H2O2, lipid peroxidation byproducts, expression of enzymes like SOD, APX, CAT, AOX, peroxidases, glutathione-S-transferase, glutathione reductase, and GPX, and accumulation of antioxidants like ascorbate, GSH, flavonols, phenolic compounds, alkaloids, tocopherol, and carotenoids, as well as osmo-protectants like proline, glycine betaine, trehalose, and sucrose, indicated these changes. Their expression patterns were observed to be distinct in response to multiple stressors. A specific redox status is likely a unique signature to abiotic stress combinations within the confinement of exclusive physiological responses to combinatorial stresses. The paramount example is to open the stomata to cool off and close it to avoid loss of excess water by the plants. However, seeing how these pressures interact with a plant will be intriguing (Rizhsky et al., 2002, 2004). Another interesting study by Koussevitzky et al., 2008 showed that Arabidopsis cytosolic APX1 (apx1) deficient mutants were more sensitive to drought heat stress than thylakoid APX, suggesting that hydrogen peroxide levels in the chloroplast are more critical for tolerance against this specific combinatorial stress. Similarly, Arabidopsis mutant deficit in ABA and ROS-regulator protein called PP2Cs were highly sensitive to salinity and heat stress as well as drought and heat stress, indicating the significance of interactions between abscisic acid and ROS signaling, which in turn is very important for plant stress tolerance (Suzuki et al., 2016). Based on research studies conducted on stress combination in plants, it was proposed that it is an important node in stress acclimation in differentially regulated ROS-responsive transcripts, as reviewed by Suzuki et al., 2014. The redox alteration is directly involved in the hypersensitive response mediated cell death in many cases (Liu and Zhang, 2021). Alternatively, it is also involved in downstream signaling. It has been found that in the case of the heat stress response (HSR), ROS are involved in the activation of different heat shock factors (HSFs) and heat shock proteins (HSPs) (Fortunato et al., 2023). In these signaling events, plant thiol peroxidases play critical roles in sensing and transducing the abiotic stress signals (Vogelsang and Dietz, 2022). Along with the induction of redox-mediated transcription factors (TFs), it is also involved in the epigenetic control of gene expression. It has been studied extensively in animals, and it was found that nuclear factor erythroid 2-related factor 2 (NRF2) is involved in this signal transition and epigenetic control. It is an emerging field in plant science research, and many interesting findings have been documented in recent years, comprehensively discussed in the following section.
6 Abiotic stress-mediated redox alteration: a trigger for epigenetic changes
Plants employ a sophisticated repertoire of molecular responses to navigate the complexities of abiotic stress. Central to this ensemble is the complex interaction between redox signaling and epigenetic modifications. This section provides an overview of the fundamental concepts, emphasizing the importance of studying the cross-talk between redox and epigenetic pathways. Epigenetic modifications are stable inheritable changes in gene expression that are not encoded in the DNA nucleotide sequence. It primarily includes DNA methylation, histone modifications, chromatin remodeling, and histone variants in plants. These changes can affect gene availability and activity, thus regulating many molecular functions, including gene transcription, DNA repair, and recombination. They are essential in controlling plant response to external stimuli, including abiotic stress. Since these modifications are reversible, they are controlled by various signals caused by developmental, environmental, and phytohormonal cues. The change in the redox status of cells caused by abiotic stress is highly significant among these signals. Abiotic stress triggers the production of ROS and disrupts cellular redox balance. Post-translational modification of gene product determines the fate of the final gene expression, which mediates the epigenetic pathway when introduced by oxidative stress. Considering the importance of such events, the following section explores the nuanced role of redox signaling in orchestrating stress responses, including the activation of defense mechanisms and the modulation of cellular processes. The nexus between redox changes and epigenetic modifications and how oxidative stress acts as a molecular trigger, influencing DNA methylation dynamics, histone modifications, and small RNA pathways, will also be discussed briefly.
6.1 DNA methylation-redox dynamics
Methylation and demethylation are interchangeable processes fundamental to epigenetic changes leading to altered gene expressions. Accumulation of evidence suggests that the presence of redox intermediates directs DNA methylome, which, in turn, regulates gene transcription. Generally, ROS accumulation leads to DNA hypomethylation, as confirmed by different studies. A comparative gene analysis study of Arabidopsis and its redox-compromised state transition 7 (stn7) mutant indicates that many nuclear genes were not expressed in their mutant form when treated with high light (Dietzel et al., 2008). However, its wild type had activated nuclear histone acetyltransferase (HAT) and histone deacetylase (HDAC), enzymes known to promote histone methylation and demethylation from the redox signals from their chloroplast.
Choi and Sano (2007) proposed that plants partially tolerate environmental stresses through epigenetic modifications. In their study, they found the activation of enzyme glycerophos- phodiesterase -like (NtGPDL) proteins in tobacco cells facing increased oxidative stress caused by the exposure of different inducers like paraquat, aluminum, cold stress, or salinity stress. Genomic loci of NtGPDL gene study, when further analyzed by methylation pattern study by bisulfite mapping, indicated selective demethylation of CG site of ORF of NtGPDL. The promoter region of the gene was also found to be demethylated. In another study, tobacco suspension culture, when treated with Juglone (5-hydroxy-1,4 naphthoquinone), showed increased over-accumulation of ROS species and subsequent hypo methylation of DNA (Poborilova et al., 2015). Similarly, Berglund (2017) also reported increased hypo methylation of DNA in Pisum sativum suspension culture when treated with nicotinamide (a precursor of NAD), known as redox intermediate. There was also an increase in the production of GSH. Although there are lots of studies in the animal kingdom regarding GSH-induced epigenetic changes, research remains scarce on plants. Ou et al. (2015) reported increased demethylation in rice seedlings treated with a NO donor such as sodium nitroprusside. It was seen that increased accumulation of ROS species by irradiation also causes epigenetic changes. A differential pattern of DNA methylation was observed in the aerial parts of Arabidopsis when exposed to irradiation concomitant with ROS accumulation (Ramakrishnan et al., 2022).
6.2 Histone acetylation-redox dynamics
Acetylation of histone is another type of post-translational modification that occurs in the nuclear region. However, unlike methylation, histone acetylation usually assists in the expression of genes. Conversely, there are few reports of DNA methylation acting as promoters of gene expressions. The fate of histone acetylation depends on the presence of a substrate known as acetyl CoA, which is managed by the opposing activity of two enzymes known as HAT and HDAC. These enzymes are also redox-regulated. There is abundant research indicating the mediation of histone acetylation by the redox status of cells in plants and animals. Mounting evidence indicates that redox components regulate histone acetylation by influencing the accumulation of acetyl CoA. It has been elucidated that the conversion of pyruvate to acetyl CoA is facilitated by the pyruvate dehydrogenase (PDH) complex, wherein NAD+ serves as a cofactor for its catalytic activity (Figure 4). In maize seedlings, caused by heat induction led to increased accumulation of ROS which further increases the hyperacetylation by increased expression of HAT. Causevic et al., 2006 reported different levels of expression of reactive oxygen species directly related to different levels of histone acetylation.
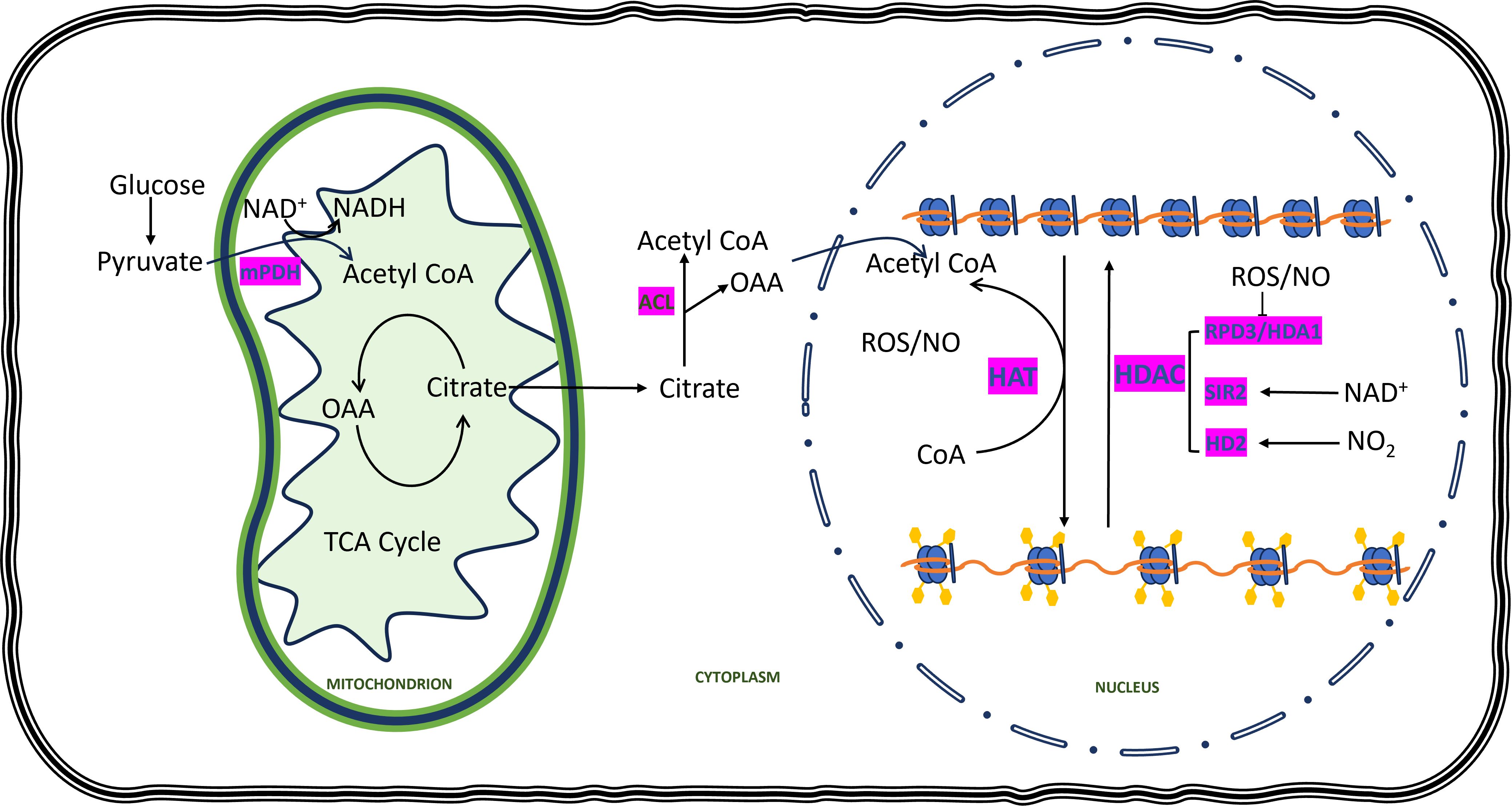
Figure 4. Redox components exert an influence on histone acetylation. In the cytoplasm, glucose undergoes breakdown to pyruvate, which enters the mitochondria and undergoes conversion to acetyl CoA by mitochondrial pyruvate dehydrogenase (mPDH), utilizing NAD+ for reduction. Acetyl CoA combines with oxaloacetate (OAA) generated in the TCA cycle to form citrate, which then enters the cytoplasm. Within the cytoplasm, citrate is converted back to OAA and acetyl CoA through ATP-citrate lyase (ACL). Acetyl CoA synthesized in the cytoplasm subsequently enters the nucleus, serving as the supplier of acetyl groups for the histone acetylation process. Histone acetyltransferases (HATs) utilize the acetyl group from acetyl CoA to introduce acetylation marks (Ac), yellow pentagons on the lysine residues of the histone tail, thereby weakening the interaction between DNA and histone and promoting gene expression. On the other hand, histone deacetylases (HDACs) remove histone acetyl groups, leading to chromatin compaction. Various HAT and HDAC enzymes are influenced by reactive oxygen species (ROS), nitric oxide (NO), and nicotinamide adenine dinucleotide (NAD+).
As mentioned earlier, HAT enzymes play an important role in epigenetic regulation, and activity is affected by oxidative stress. In combination with other factors, HAT enzyme forms are complex, and its activity is affected by oxidative stress. A component of the HAT complex known as elongator protein is reported to mediate anthocyanin biosynthesis and oxidative stress in Arabidopsis (Zhou et al., 2009). The induction of salinity stress on maize seedlings led to increased electrolyte leakage, which activated the antioxidant pathway genes (AbdElgawad et al., 2016). Additionally, there was increased production of gene expression of HAT enzymes Such as ZmHATB and ZmGCN5 and upregulation of cell wall synthesis genes, ZmEXPB2 (expansin-B2) and ZmXET (xyloglucan endotransglycosylase). Upon further examination, it was found that this accumulation was mediated by increased acetylation of their promoter region. This proves that the HAT enzyme mediates different stress responsive genes by altering their acetylation under stress.
6.3 Histone deacetylation-redox dynamics
As discussed earlier, gene expression increases with the higher acetylation level. Hence, stress-induced gene modulation primarily functions with the decreased action of histone deacetylase enzymes. Non-sirtuins [non- (SIRTs)] are a group of HDACs that regulate histone acetylation in cells. This becomes unrecruited from the repression of genes under oxidative stress. This action further drives increased acetylation and increased gene expression. In rice seedlings, it was reported that upon successful host-pathogen interactions, the transcript level of HDT701 was increased (Ding et al., 2012). When overexpressed in rice, this increases susceptibility to various abiotic stresses, including salinity and osmotic stress and decreased H4 acetylation. When the same gene was silenced, the whole pattern was reversed with increased expression of defense-related genes and increased H4 acetylation. Increased and enhanced acetylation marks on the promoter region of defense-related genes are generally linked to their increased expression. Non-sirtuins HDACs are sensitive to redox potential, and their production is connected to the presence or absence of oxidative stress. Under oxidative stress, these HDACs get inactivated, leading to the acetylation of histones in the promoter regions of stress-responsive genes, leading to their increased production and acclimation to abiotic stress tolerance (Luo et al., 2017). Many HDACs have been reported to possess redox switches for better oxidative stress tolerance (Jänsch et al., 2020).
Similarly, in Arabidopsis, cold stress induces degradation of HD2C by increasing acetylation levels of histone H3, which is promoted by CULLIN4-based ubiquitin E3 ligase. This increased acetylation activates COR or cold-responsive genes (Park et al., 2018). It further interacts with HDAC to modulate acetylation level and respective stress tolerance regulation. In double mutant Arabidopsis, HD2C directly interacted with HDA6, causing decreased demethylation of histone H3K9 and increased histone H3K9K14 acetylation. This activates ABA-responsive genes. Also, HD2C further interacts with the BRM -SWI/SNF chromatin remodeling complex, thereby regulating Arabidopsis response to heat stress (Luo et al., 2012).
7 Mechanism of redox and epigenetic alterations due to multiple abiotic stress responses in plants
While adaptive responses to single stress factors have been extensively studied under controlled laboratory conditions across various species (e.g (Ritonga and Chen, 2020; Takahashi et al., 2020; Zhao et al., 2020; Jethva et al., 2022; Zhang et al., 2022), research on the mechanisms underlying adaptation to multiple stresses in natural environments, such as combinatorial stress, remains limited. This gap in understanding is particularly evident in the context of flooding, where the molecular responses to simultaneous or sequential stresses, such as salinity or drought-induced soil conditions, are still not well-defined despite the frequent occurrence of these combined stressors in nature (Yin et al., 2023).
Flooding often coincides with other environmental stresses such as salinity, temperature extremes, and heavy metals, leading to complex interactions that can be antagonistic, additive, or synergistic. These interactions trigger a cellular energy crisis and activate transcriptional reprogramming through transcription factor families like ANAC and ERFVII. These factors, modulated by proteins like kinases, help integrate multiple stress responses, particularly in managing reactive oxygen species (ROS). The unique physiological responses resulting from these stress combinations often involve distinct gene and epigenetic regulation, with key signaling components like ERFVII and RBOHD playing central roles in coordinating the plant’s response to multiple stresses (Renziehausen et al., 2024).
Flooding challenges plants by causing oxygen deficiency, leading to hypoxia or anoxia, which is worsened by ethylene entrapment and reduced light during submergence (Voesenek and Bailey-Serres, 2015; Sasidharan et al., 2017). In response to hypoxia, plants undergo significant transcriptomic and metabolic changes, shifting from aerobic respiration to anaerobic glycolysis due to limited O2 availability, which substantially reduces energy production. Reactive oxygen species (ROS) signals, generated by both the mitochondrial electron transport chain (mtETC) and RESPIRATORY BURST OXIDASE HOMOLOG (RBOH) activity, play a critical role in hypoxic adaptation, triggering systemic responses to waterlogging (Fukao and Bailey-Serres, 2004; Hong et al., 2020). Transcription factors like ANAC013 and ANAC017 are central to mitochondrial retrograde signaling (MRS) and submergence tolerance, regulating key genes that mitigate ROS damage and enhance low-oxygen resilience (Bui et al., 2020; Eysholdt-Derzsó et al., 2023). Disruption of these pathways leads to excessive ROS accumulation, reduced anoxia tolerance, and impaired photosynthetic efficiency during reoxygenation (Van Aken et al., 2016; Jethva et al., 2023).
By decoupling mitochondrial electron transport chain (mtETC) activity from ATP production, UNCOUPLING PROTEIN 1 (UCP1) limits excessive reactive oxygen species (ROS) formation, thereby preventing over-reduction of mtETC proteins (Barreto et al., 2014, 2017, 2022). Although mitochondrial retrograde signaling (MRS) primarily relies on ROS signals, UCP1 enhances hypoxia-induced transcriptional changes, including the induction of ALTERNATIVE OXIDASE 1a (AOX1a). This effect is likely linked to UCP1’s role in stabilizing ETHYLENE RESPONSE FACTOR VII (ERFVII) transcription factors (TFs) by inhibiting the N-degron pathway, which otherwise marks proteins for degradation (]. In Arabidopsis, the ERFVII subfamily, comprising five members—RELATED TO APETALA 2.2 (RAP2.2), RAP2.3, RAP2.12, HYPOXIA RESPONSIVE ERF 1 (HRE1), and HRE2—plays a crucial role in low-oxygen sensing and subsequent gene regulation (Nakano et al., 2006). These TFs bind to the HYPOXIA-RESPONSIVE PROMOTER ELEMENT (HRPE) found in approximately 70% of hypoxia core gene (HCG) promoters, initiating transcriptional responses under hypoxic conditions (Zubrycka et al., 2023). RAP2.12, for instance, regulates the expression of RESPIRATORY BURST OXIDASE HOMOLOG D (RBOHD) and HYPOXIA RESPONSIVE UNIVERSAL STRESS PROTEIN 1 (HRU1), which together mediate ROS production under hypoxia (Gonzali et al., 2015; Yao et al., 2017).
ERFVII proteins possess a conserved cysteine residue in their N-termini, making them susceptible to degradation through the Arg/Cys branch of the N-degron pathway in an oxygen (O2)-and nitric oxide (NO)-dependent manner This degradation process involves sequential modifications: methionine is first removed by METHIONINE AMINOPEPTIDASES (MetAPs), followed by O2-dependent cysteine oxidation by PLANT CYSTEINE OXIDASES (PCOs), and then arginylation by ARGINYL TRANSFERASE ENZYME 1 (ATE1) and ATE2, which tags the TF for proteasomal degradation via PROTEOLYSIS 6 (PRT6) (Weits et al., 2014; White et al., 2017; Masson et al., 2019). However, in lowland rice, the ERFVII factor SUBMERGENCE 1A (SUB1A) is crucial for survival under flash flooding, as it escapes this degradation pathway by interacting its N-terminus with its C-terminus. Moreover, ethylene stabilizes ERFVII proteins during submergence by inducing PHYTOGLOBIN 1 (PGB1), a NO scavenger that prevents ERFVII degradation (Hartman et al., 2019). RAP2.12, specifically, avoids degradation under normal oxygen conditions by associating with ACYL-COA BINDING PROTEIN 1 (ACBP1) and ACBP2 at the plasma membrane and, upon hypoxia, dissociates to accumulate in the nucleus, thus contributing to the hypoxia response (Licausi et al., 2011; Kosmacz et al., 2015; Schmidt et al., 2018).
The combination of differential regulation of different redox sensors mentioned above and its role in epigenetic regulation to combat multicombinatorial stress is still naive. However, rapid acceleration in research to address the knowledge gaps in this field of study is also critical for our understanding.
8 Cross-talks between redox pathway and epigenetic regulations during multiple abiotic stress
Alteration of the cellular redox state is inevitable in response to any abiotic stress factor. The multiple abiotic stress impacts cellular redox state more dramatically. As discussed earlier, cellular redox homeostasis is contributed by the complex interaction between chloroplast-mitochondra-nucleus (Locato et al., 2018). The cellular antioxidative system efficiently contributes to modulating this homeostasis. The efficiency of these antioxidative systems determines ROS-induced hypersensitive response (ROS HR) or ROS-induced signaling in response to stress (Gupta et al., 2013). The redox state also directly controls the gene expression by DNA methylation, histone acetylation, and histone methylation. The perception of stress depends upon the signal that originates from chloroplasts and mitochondria and is transmitted to the nucleus. The antagonistic and synergistic effect regulates the controlled expression of genes in response to multiple abiotic stress (Locato et al., 2018). The major redox-regulated proteins and non-proteins e.g., NADPH, glutathione, gluteredoxin, peroxiredoxins, ascorbate, thioredoxin, ferredoxin etc. participate in the generation of redox signatures, which in turn also controls the priming against multiple abiotic stress (Tripathi et al., 2024) This also triggers the site-directed epigenetic modification that also restrains the concurrent stress situations in plants significantly (Harris et al., 2023). The epigenetic imprints may also be involved in transgenerational memory development and resistance phenomenon in subsequent generations (Bhar et al., 2021). Substantial supportive studies are required to understand the exact mechanism of plant transgenerational memory development. The redox alteration also modulates the metabolism in plants in terms of the deregulation of several proteins and metabolite synthesis (Shen et al., 2016). This regulation may involve an anterograde trafficking system from the nucleus to the cytosol and then mitochondria and chloroplast. The primary signal of this retrograde signaling is ROS. The chloroplast and mitochondrial genome have a significant level of rearrangements during the course of different abiotic stresses (Dobrogojski et al., 2020; Meng et al., 2022). More research is necessary to unveil the crosstalk between the nuclear, chloroplast and mitochondrial genomes. Additionally, it has recently been demonstrated that the crosstalk between cellular redox homeostasis and epigenetic regulation occurs through the involvement of melatonin (Ahmad et al., 2023). The major roles of melatonin in scavenging cellular ROS, along with the signaling intermediates of different signal transduction warrants its significant contribution to varied abiotic stress tolerance mechanisms (Colombage et al., 2023). Further investigation may open up new redox and epigenetic control directions in response to multiple abiotic stress tolerance with the hormonal crosstalk in plants.
Studying plant responses to stress combinations is complex and costly, yet crucial for developing resilient crops in the face of climate change. The controlled variable approach, which isolates one stress factor while keeping others constant, has revealed how stress combinations can trigger unique molecular defenses in plants. Advances in genomic technologies, such as multi-omics approaches, have deepened our understanding of these responses, but translating laboratory findings to field environments remains challenging. Integrating lab and field research with multidisciplinary technologies like nanobiotechnology and genome editing offers promising avenues to enhance crop resilience by fine-tuning stress signaling pathways and physiological adaptations. To further strengthen research, it is essential to identify key survival thresholds and nodes in plants under stress combinations and systematically integrate existing data to uncover the mechanisms behind these responses. Developing efficient research methods and exploring how plants prioritize and adapt to multiple stresses will be critical as future climate conditions become more unpredictable. By leveraging advanced technologies like machine learning, gene editing, and remote sensing, and by breeding multi-resistant plant varieties, we can better equip crops to withstand the increasingly complex stress environments of the future, ensuring food security and sustainable agriculture.
9 Conclusion and future directions
The reactive oxygen species is inevitable in any stress events in plants. The ROS generation in response to multiple abiotic stress regulates the stress tolerance mechanism in plants like a two-edged sword. The excessive production of ROS leads to oxidative stress and damage to the plant’s cellular machinery. Alternatively, an effective scavenging system may detoxify the ROS and produce a balanced redox signature. This will lead to downstream signaling by activating stress-responsive transcription factors and gene activation. Epigenetic regulation, on the other hand, demonstrates significant controlling gateways for multiple abiotic stress tolerance mechanisms. The cross-talk between the ROS pathway and epigenetic control involves a complex network of molecular events, e.g., histone modification, DNA methylation, DNA acetylation, deacetylation, etc. These regulatory nexuses modulate the gene expression, physiological modulations, antioxidative defense, etc., to enable the plants to adapt towards multiple abiotic cues. This emerging field warrants many questions for future research to unravel specific interactions between ROS and epigenetic modification in the context of abiotic stress tolerance. Some of the relevant future directions are summarized below,
1. Further research is necessary to unveil the duality of ROS signaling in response to abiotic stress combinations. Any stress situations instigate cellular redox alterations. The efficient scavenging mechanism leads to the generation of “signature ROS”, which helps in many defense gene regulations. In response to abiotic stress, the cellular ROS is generated as a first line of defense signal, which in turn, after effective scavenging, leads to the “balanced ROS” helping in retrograde signaling. This duality of ROS signal in response to the multiple abiotic stress factors should be deciphered so that such signaling can be used effectively in sustainable stress management.
2. The synergy between epigenetic control and ROS should be decoded. Although the interplay between the ROS and epigenetic control has been established, more intriguing study is necessary to uncover the synergy ultimately.
3. Unveiling the cross-talk between ROS, epigenetic modification, and hormonal regulation at the molecular level can provide a better understanding of multi-stress tolerance strategies in crops.
4. The transgenerational impact of ROS-induced epigenetic modifications must be evaluated to develop a sustainable crop management system against multiple abiotic stresses. The epigenetic control and development of transgenerational memory are currently under scientific investigation. The epigenetic imprint developed under multiple abiotic stresses under a changing cellular redox milieu would provide significant gateways for future agricultural applications.
5. More emphasis is required to integrate advanced genome editing tools, e.g.), Clustered Regularly Interspaced Short Palindromic Repeats (CRISPR)/CRISPR-associated (Cas) technology to develop multiple abiotic stress-tolerant climate-smart crops.
6. The major challenge is judicially utilizing all the information obtained for crop genome tailoring to overcome the future environmental crisis. The compete gene expressional blue print under multiple abiotic stress responses is the prior requirement for the successful implication of site-specific gene editing technology.
Author contributions
SS: Data curation, Investigation, Visualization, Writing – original draft. AB: Conceptualization, Investigation, Software, Visualization, Writing – review & editing. AR: Conceptualization, Funding acquisition, Supervision, Writing – review & editing.
Funding
The author(s) declare that financial support was received for the research, authorship, and/or publication of this article. AR acknowledges the funding support from the Excellent Team Grant, FLD, CZU.
Conflict of interest
The authors declare that the research was conducted in the absence of any commercial or financial relationships that could be construed as a potential conflict of interest.
Publisher’s note
All claims expressed in this article are solely those of the authors and do not necessarily represent those of their affiliated organizations, or those of the publisher, the editors and the reviewers. Any product that may be evaluated in this article, or claim that may be made by its manufacturer, is not guaranteed or endorsed by the publisher.
Supplementary material
The Supplementary Material for this article can be found online at: https://www.frontiersin.org/articles/10.3389/fpls.2024.1456414/full#supplementary-material
References
AbdElgawad, H., Zinta, G., Hegab, M. M., Pandey, R., Asard, H., Abuelsoud, W. (2016). High salinity induces different oxidative stress and antioxidant responses in maize seedlings organs. Front. Plant Sci. 7. doi: 10.3389/fpls.2016.00276
Ahmad, N., Naeem, M., Ali, H., Alabbosh, K. F., Hussain, H., Khan, I., et al. (2023). From challenges to solutions: The impact of melatonin on abiotic stress synergies in horticultural plants via redox regulation and epigenetic signaling. Sci. Hortic. 321, 112369. doi: 10.1016/j.scienta.2023.112369
Ahmed, I. M., Cao, F., Zhang, M., Chen, X., Zhang, G., Wu, F. (2013). Difference in yield and physiological features in response to drought and salinity combined stress during anthesis in tibetan wild and cultivated barleys. PloS One 8, e77869. doi: 10.1371/journal.pone.0077869
Aliyeva, N., Nasibova, A., Mammadov, Z., Eftekhari, A., Khalilov, R. (2023). Individual and combinative effect of NaCl and γ-radiation on NADPH-generating enzymes activity in corn (Zea mays L.) sprouts. Heliyon 9, e22126. doi: 10.1016/j.heliyon.2023.e22126
Andreyev, A. Y., Kushnareva, Y. E., Murphy, A. N., Starkov, A. A. (2015). Mitochondrial ROS metabolism: 10 years later. Biochem. Biokhimiia 80, 517–531. doi: 10.1134/S0006297915050028
Asada, K. (2006). Production and scavenging of reactive oxygen species in chloroplasts and their functions. Plant Physiol. 141, 391–396. doi: 10.1104/pp.106.082040
Attia, H., Alamer, K. H., Ouhibi, C., Oueslati, S., Lachaal, M. (2020). Interaction between salt stress and drought stress on some physiological parameters in two pea cultivars. Int. J. Bot. 16, 1–8. doi: 10.3923/ijb.2020.1.8
Baek, K.-H., Skinner, D. Z. (2012). Production of reactive oxygen species by freezing stress and the protective roles of antioxidant enzymes in plants. J. Agric. Chem. Environ. 01, 34–40. doi: 10.4236/jacen.2012.11006
Balfagón, D., Zandalinas, S. I., Mittler, R., Gómez-Cadenas, A. (2020). High temperatures modify plant responses to abiotic stress conditions. Physiol. Plant 170, 335–344. doi: 10.1111/ppl.13151
Baniulis, D., Hasan, S. S., Stofleth, J. T., Cramer, W. A. (2013). Mechanism of enhanced superoxide production in the cytochrome b(6)f complex of oxygenic photosynthesis. Biochemistry 52, 8975–8983. doi: 10.1021/bi4013534
Bao, G., Tang, W., An, Q., Liu, Y., Tian, J., Zhao, N., et al. (2020). Physiological effects of the combined stresses of freezing-thawing, acid precipitation and deicing salt on alfalfa seedlings. BMC Plant Biol. 20, 204. doi: 10.1186/s12870-020-02413-4
Barreto, P., Dambire, C., Sharma, G., Vicente, J., Osborne, R., Yassitepe, J., et al. (2022). Mitochondrial retrograde signaling through UCP1-mediated inhibition of the plant oxygen-sensing pathway. Curr. Biol. 32, 1403–1411.e4. doi: 10.1016/j.cub.2022.01.037
Barreto, P., Okura, V. K., Neshich, I. A. P., Maia, I. D. G., Arruda, P. (2014). Overexpression of UCP1 in tobacco induces mitochondrial biogenesis and amplifies a broad stress response. BMC Plant Biol. 14, 144. doi: 10.1186/1471-2229-14-144
Barreto, P., Yassitepe, J. E. C. T., Wilson, Z. A., Arruda, P. (2017). Mitochondrial Uncoupling Protein 1 Overexpression Increases Yield in Nicotiana tabacum under Drought Stress by Improving Source and Sink Metabolism. Front. Plant Sci. 8. doi: 10.3389/fpls.2017.01836
Becker, V. I., Goessling, J. W., Duarte, B., Caçador, I., Liu, F., Rosenqvist, E., et al. (2017). Combined effects of soil salinity and high temperature on photosynthesis and growth of quinoa plants (Chenopodium quinoa). Funct. Plant Biol. 44, 665–678. doi: 10.1071/FP16370
Benali, A., El Haddad, N., Patil, S. B., Goyal, A., Hejjaoui, K., El Baouchi, A., et al. (2023). Impact of terminal heat and combined heat-drought stress on plant growth, yield, grain size, and nutritional quality in chickpea (Cicer arietinum L.). Plants Basel Switz. 12, 3726. doi: 10.3390/plants12213726
Berglund, T., Wallström, A., Nguyen, T.-V., Laurell, C., Ohlsson, A. B. (2017). Nicotinamide; antioxidative and DNA hypomethylation effects in plant cells. Plant Physiology and Biochemistry 118, 551–560. doi: 10.1016/j.plaphy.2017.07.023
Bhar, A., Chakraborty, A., Roy, A. (2021). Plant responses to biotic stress: old memories matter. Plants 11, 84. doi: 10.3390/plants11010084
Bhar, A., Chakraborty, A., Roy, A. (2023). The captivating role of calcium in plant-microbe interaction. Front. Plant Sci. 14. doi: 10.3389/fpls.2023.1138252
Bhar, A., Gupta, S., Chatterjee, M., Das, S. (2017). “Redox regulatory networks in response to biotic stress in plants: A new insight through chickpea- fusarium interplay,” in Mechanism of plant hormone signaling under stress. Ed. Pandey, G. K. (United States: Wiley), 23–43. doi: 10.1002/9781118889022.ch20
Bhatt, M., Pandey, S. S., Tiwari, A. K., Tiwari, B. S. (2021). Plastid-mediated singlet oxygen in regulated cell death. Plant Biol. 23, 686–694. doi: 10.1111/plb.13260
Bjornson, M., Balcke, G. U., Xiao, Y., de Souza, A., Wang, J.-Z., Zhabinskaya, D., et al. (2017). Integrated omics analyses of retrograde signaling mutant delineate interrelated stress-response strata. Plant J. Cell Mol. Biol. 91, 70–84. doi: 10.1111/tpj.13547
Brand, D., Wijewardana, C., Gao, W., Reddy, K. R. (2016). Interactive effects of carbon dioxide, low temperature, and ultraviolet-B radiation on cotton seedling root and shoot morphology and growth. Front. Earth Sci. 10, 607–620. doi: 10.1007/s11707-016-0605-0
Bui, L. T., Shukla, V., Giorgi, F. M., Trivellini, A., Perata, P., Licausi, F., et al. (2020). Differential submergence tolerance between juvenile and adult Arabidopsis plants involves the ANAC017 transcription factor. Plant J. 104, 979–994. doi: 10.1111/tpj.14975
Causevic, A., Gentil, M.-V., Delaunay, A., El-Soud, W. A., Garcia, Z., Pannetier, C., et al. (2006). Relationship between DNA methylation and histone acetylation levels, cell redox and cell differentiation states in sugarbeet lines. Planta. 224, 812–827. doi: 10.1007/s00425-006-0267-3
Chan, K. X., Mabbitt, P. D., Phua, S. Y., Mueller, J. W., Nisar, N., Gigolashvili, T., et al. (2016). Sensing and signaling of oxidative stress in chloroplasts by inactivation of the SAL1 phosphoadenosine phosphatase. Proc. Natl. Acad. Sci. U. S. A. 113, E4567–E4576. doi: 10.1073/pnas.1604936113
Chan, K. X., Wirtz, M., Phua, S. Y., Estavillo, G. M., Pogson, B. J. (2013). Balancing metabolites in drought: the sulfur assimilation conundrum. Trends Plant Sci. 18, 18–29. doi: 10.1016/j.tplants.2012.07.005
Chauhan, D. K., Yadav, V., Vaculík, M., Gassmann, W., Pike, S., Arif, N., et al. (2021). Aluminum toxicity and aluminum stress-induced physiological tolerance responses in higher plants. Crit. Rev. Biotechnol. 41, 715–730. doi: 10.1080/07388551.2021.1874282
Chen, S., Lin, R., Lu, H., Wang, Q., Yang, J., Liu, J., et al. (2020). Effects of phenolic acids on free radical scavenging and heavy metal bioavailability in kandelia obovata under cadmium and zinc stress. Chemosphere 249, 126341. doi: 10.1016/j.chemosphere.2020.126341
Chen, Y.-E., Zhang, C.-M., Su, Y.-Q., Ma, J., Zhang, Z.-W., Yuan, M., et al. (2017). Responses of photosystem II and antioxidative systems to high light and high temperature co-stress in wheat. Environ. Exp. Bot. 135, 45–55. doi: 10.1016/j.envexpbot.2016.12.001
Choi, C.-S., Sano, H. (2007). Abiotic-stress induces demethylation and transcriptional activation of a gene encoding a glycerophosphodiesterase-like protein in tobacco plants. Mol. Genet. Genomics 277, 589–600. doi: 10.1007/s00438-007-0209-1
Choudhury, F. K., Rivero, R. M., Blumwald, E., Mittler, R. (2017). Reactive oxygen species, abiotic stress and stress combination. Plant J. 90, 856–867. doi: 10.1111/tpj.13299
Colombage, R., Singh, M. B., Bhalla, P. L. (2023). Melatonin and abiotic stress tolerance in crop plants. Int. J. Mol. Sci. 24, 7447. doi: 10.3390/ijms24087447
Corpas, F. J. (2019). Peroxisomes in higher plants: an example of metabolic adaptability. Bot. Lett. 166, 298–308. doi: 10.1080/23818107.2019.1619196
Costa, A. C., Rezende-Silva, S. L., Megguer, C. A., Moura, L. M. F., Rosa, M., Silva, A. A. (2015). The effect of irradiance and water restriction on photosynthesis in young jatobá-do-cerrado (Hymenaea stigonocarpa) plants. Photosynthetica 53, 118–127. doi: 10.1007/s11099-015-0085-6
Cui, F., Brosché, M., Shapiguzov, A., He, X.-Q., Vainonen, J. P., Leppälä, J., et al. (2019). Interaction of methyl viologen-induced chloroplast and mitochondrial signaling in Arabidopsis. Free Radic. Biol. Med. 134, 555–566. doi: 10.1016/j.freeradbiomed.2019.02.006
Dietz, K.-J. (2016). Thiol-based peroxidases and ascorbate peroxidases: why plants rely on multiple peroxidase systems in the photosynthesizing chloroplast? Mol. Cells 39, 20–25. doi: 10.14348/molcells.2016.2324
Dietzel, L., Bräutigam, K., Pfannschmidt, T. (2008). Photosynthetic acclimation: state transitions and adjustment of photosystem stoichiometry–functional relationships between short-term and long-term light quality acclimation in plants. FEBS J. 275, 1080–1088. doi: 10.1111/j.1742-4658.2008.06264.x
Ding, B., Bellizzi, M. D. R., Ning, Y., Meyers, B. C., Wang, G.-L. (2012). HDT701, a histone H4 deacetylase, negatively regulates plant innate immunity by modulating histone H4 acetylation of defense-related genes in rice. Plant Cell 24, 3783–3794. doi: 10.1105/tpc.112.101972
Dmitrieva, V. A., Tyutereva, E. V., Voitsekhovskaja, O. V. (2020). Singlet oxygen in plants: generation, detection, and signaling roles. Int. J. Mol. Sci. 21, 3237. doi: 10.3390/ijms21093237
Dobrogojski, J., Adamiec, M., Luciński, R. (2020). The chloroplast genome: a review. Acta Physiol. Plant 42, 98. doi: 10.1007/s11738-020-03089-x
Dogra, V., Singh, R. M., Li, M., Li, M., Singh, S., Kim, C. (2022). EXECUTER2 modulates the EXECUTER1 signalosome through its singlet oxygen-dependent oxidation. Mol. Plant 15, 438–453. doi: 10.1016/j.molp.2021.12.016
Dolzblasz, A., Gola, E. M., Sokołowska, K., Smakowska-Luzan, E., Twardawska, A., Janska, H. (2018). Impairment of meristem proliferation in plants lacking the mitochondrial protease atFTSH4. Int. J. Mol. Sci. 19, 853. doi: 10.3390/ijms19030853
Dugasa, M. T., Cao, F., Ibrahim, W., Wu, F. (2019). Differences in physiological and biochemical characteristics in response to single and combined drought and salinity stresses between wheat genotypes differing in salt tolerance. Physiol. Plant 165, 134–143. doi: 10.1111/ppl.12743
Dumanović, J., Nepovimova, E., Natić, M., Kuča, K., Jaćević, V. (2021). The significance of reactive oxygen species and antioxidant defense system in plants: A concise overview. Front. Plant Sci. 11. doi: 10.3389/fpls.2020.552969
Esmailpourmoghadam, E., Salehi, H., Moshtaghi, N. (2023). Differential gene expression responses to salt and drought stress in tall fescue (Festuca arundinacea schreb.). Mol. Biotechnol. doi: 10.1007/s12033-023-00888-8
Espinas, N. A., Kobayashi, K., Takahashi, S., Mochizuki, N., Masuda, T. (2012). Evaluation of unbound free heme in plant cells by differential acetone extraction. Plant Cell Physiol. 53, 1344–1354. doi: 10.1093/pcp/pcs067
Estavillo, G. M., Crisp, P. A., Pornsiriwong, W., Wirtz, M., Collinge, D., Carrie, C., et al. (2011). Evidence for a SAL1-PAP chloroplast retrograde pathway that functions in drought and high light signaling in Arabidopsis. Plant Cell 23, 3992–4012. doi: 10.1105/tpc.111.091033
Eysholdt-Derzsó, E., Renziehausen, T., Frings, S., Frohn, S., Von Bongartz, K., Igisch, C. P., et al. (2023). Endoplasmic reticulum–bound ANAC013 factor is cleaved by RHOMBOID-LIKE 2 during the initial response to hypoxia in Arabidopsis thaliana. Proc. Natl. Acad. Sci. 120, e2221308120. doi: 10.1073/pnas.2221308120
Fichman, Y., Miller, G., Mittler, R. (2019). Whole-plant live imaging of reactive oxygen species. Mol. Plant 12, 1203–1210. doi: 10.1016/j.molp.2019.06.003
Fortunato, S., Lasorella, C., Dipierro, N., Vita, F., De Pinto, M. C. (2023). Redox signaling in plant heat stress response. Antioxidants 12, 605. doi: 10.3390/antiox12030605
Foyer, C. H., Noctor, G. (2009). Redox regulation in photosynthetic organisms: signaling, acclimation, and practical implications. Antioxid. Redox Signal. 11, 861–905. doi: 10.1089/ars.2008.2177
Fukao, T., Bailey-Serres, J. (2004). Plant responses to hypoxia – is survival a balancing act? Trends Plant Sci. 9, 449–456. doi: 10.1016/j.tplants.2004.07.005
Gao, Y., Zheng, W., Zhang, C., Zhang, L., Xu, K. (2019). High temperature and high light intensity induced photoinhibition of bayberry (Myrica rubra Sieb. et Zucc.) by disruption of D1 turnover in photosystem II. Sci. Hortic. 248, 132–137. doi: 10.1016/j.scienta.2019.01.007
Garcia de la Garma, J., Fernandez-Garcia, N., Bardisi, E., Pallol, B., Asensio-Rubio, J. S., Bru, R., et al. (2015). New insights into plant salt acclimation: the roles of vesicle trafficking and reactive oxygen species signalling in mitochondria and the endomembrane system. New Phytol. 205, 216–239. doi: 10.1111/nph.12997
Garg, N., Chandel, S. (2012). Role of arbuscular mycorrhizal (AM) fungi on growth, cadmium uptake, osmolyte, and phytochelatin synthesis in cajanus cajan (L.) millsp. Under naCl and cd stresses. J. Plant Growth Regul. 31, 292–308. doi: 10.1007/s00344-011-9239-3
Garg, R., Verma, M., Agrawal, S., Shankar, R., Majee, M., Jain, M. (2014). Deep transcriptome sequencing of wild halophyte rice, Porteresia coarctata, provides novel insights into the salinity and submergence tolerance factors. DNA Res. Int. J. Rapid Publ. Rep. Genes Genomes 21, 69–84. doi: 10.1093/dnares/dst042
Gill, S. S., Tuteja, N. (2010). Reactive oxygen species and antioxidant machinery in abiotic stress tolerance in crop plants. Plant Physiol. Biochem. PPB 48, 909–930. doi: 10.1016/j.plaphy.2010.08.016
Gilroy, S., Białasek, M., Suzuki, N., Górecka, M., Devireddy, A. R., Karpiński, S., et al. (2016). ROS, calcium, and electric signals: key mediators of rapid systemic signaling in plants. Plant Physiol. 171, 1606–1615. doi: 10.1104/pp.16.00434
Gilroy, S., Suzuki, N., Miller, G., Choi, W.-G., Toyota, M., Devireddy, A. R., et al. (2014). A tidal wave of signals: calcium and ROS at the forefront of rapid systemic signaling. Trends Plant Sci. 19, 623–630. doi: 10.1016/j.tplants.2014.06.013
Gollan, P. J., Aro, E.-M. (2020). Photosynthetic signalling during high light stress and recovery: targets and dynamics. Philos. Trans. R. Soc Lond. B. Biol. Sci. 375, 20190406. doi: 10.1098/rstb.2019.0406
Gong, B., Qiu, H., Van Gestel, C. A. M., Peijnenburg, W. J. G. M., He, E. (2022). Increasing temperatures potentiate the damage of rare earth element yttrium to the crop plant triticum aestivum L. J. Agric. Food Chem. 70, 16390–16400. doi: 10.1021/acs.jafc.2c05883
Gonzali, S., Loreti, E., Cardarelli, F., Novi, G., Parlanti, S., Pucciariello, C., et al. (2015). Universal stress protein HRU1 mediates ROS homeostasis under anoxia. Nat. Plants 1, 15151. doi: 10.1038/nplants.2015.151
Gupta, S., Bhar, A., Chatterjee, M., Das, S. (2013). Fusarium oxysporum f.sp. ciceri Race 1 Induced Redox State Alterations Are Coupled to Downstream Defense Signaling in Root Tissues of Chickpea (Cicer arietinum L.). PloS One 8, e73163. doi: 10.1371/journal.pone.0073163
Haddad, R., Kamangar, A. (2015). The ameliorative effect of silicon and potassium on drought stressed grape (Vitis vinifera L.) leaves. Iran. J. Genet. Plant Breed. 4, 48–58.
Hamidi-Moghaddam, A., Arouiee, H., Moshtaghi, N., Azizi, M., Shoor, M., Sefidkon, F. (2019). Visual quality and morphological responses of rosemary plants to UV-B radiation and salinity stress. J. Ecol. Eng. 20, 34–43. doi: 10.12911/22998993/94920
Harris, C. J., Amtmann, A., Ton, J. (2023). Epigenetic processes in plant stress priming: Open questions and new approaches. Curr. Opin. Plant Biol. 75, 102432. doi: 10.1016/j.pbi.2023.102432
Hartman, S., Liu, Z., Van Veen, H., Vicente, J., Reinen, E., Martopawiro, S., et al. (2019). Ethylene-mediated nitric oxide depletion pre-adapts plants to hypoxia stress. Nat. Commun. 10, 4020. doi: 10.1038/s41467-019-12045-4
Hong, C.-P., Wang, M.-C., Yang, C.-Y. (2020). NADPH oxidase rbohD and ethylene signaling are involved in modulating seedling growth and survival under submergence stress. Plants 9, 471. doi: 10.3390/plants9040471
Huang, H., Ullah, F., Zhou, D.-X., Yi, M., Zhao, Y. (2019). Mechanisms of ROS regulation of plant development and stress responses. Front. Plant Sci. 10. doi: 10.3389/fpls.2019.00800
Huang, S., Van Aken, O., Schwarzländer, M., Belt, K., Millar, A. H. (2016). The roles of mitochondrial reactive oxygen species in cellular signaling and stress response in plants. Plant Physiol. 171, 1551–1559. doi: 10.1104/pp.16.00166
Jampoh, E. A., Sáfrán, E., Babinyec-Czifra, D., Kristóf, Z., Krárné Péntek, B., Fábián, A., et al. (2023). Morpho-anatomical, physiological and biochemical adjustments in response to heat and drought co-stress in winter barley. Plants 12, 3907. doi: 10.3390/plants12223907
Jänsch, N., Sugiarto, W. O., Muth, M., Kopranovic, A., Desczyk, C., Ballweg, M., et al. (2020). Switching the switch: ligand induced disulfide formation in HDAC8. Chem. – Eur. J. 26, 13249–13255. doi: 10.1002/chem.202001712
Jethva, J., Lichtenauer, S., Schmidt-Schippers, R., Steffen-Heins, A., Poschet, G., Wirtz, M., et al. (2023). Mitochondrial alternative NADH dehydrogenases NDA1 and NDA2 promote survival of reoxygenation stress in Arabidopsis by safeguarding photosynthesis and limiting ROS generation. New Phytol. 238, 96–112. doi: 10.1111/nph.18657
Jethva, J., Schmidt, R. R., Sauter, M., Selinski, J. (2022). Try or die: dynamics of plant respiration and how to survive low oxygen conditions. Plants 11, 205. doi: 10.3390/plants11020205
Johnson, S. M., Lim, F.-L., Finkler, A., Fromm, H., Slabas, A. R., Knight, M. R. (2014). Transcriptomic analysis of Sorghum bicolor responding to combined heat and drought stress. BMC Genomics 15, 456. doi: 10.1186/1471-2164-15-456
Jones, J. D. G., Dangl, J. L. (2006). The plant immune system. Nature 444, 323–329. doi: 10.1038/nature05286
Joshi, N. C., Yadav, D., Ratner, K., Kamara, I., Aviv-Sharon, E., Irihimovitch, V., et al. (2020). Sodium hydrosulfide priming improves the response of photosynthesis to overnight frost and day high light in avocado (Persea americana Mill, cv. ‘Hass’). Physiol. Plant 168, 394–405. doi: 10.1111/ppl.13023
Kerchev, P., Waszczak, C., Lewandowska, A., Willems, P., Shapiguzov, A., Li, Z., et al. (2016). Lack of GLYCOLATE OXIDASE1, but not GLYCOLATE OXIDASE2, attenuates the photorespiratory phenotype of CATALASE2-deficient arabidopsis. Plant Physiol. 171, 1704–1719. doi: 10.1104/pp.16.00359
Khedia, J., Agarwal, P., Agarwal, P. K. (2019). Deciphering hydrogen peroxide-induced signalling towards stress tolerance in plants. Biotech 9, 395. doi: 10.1007/s13205-019-1924-0
Kim, M. J., Ciani, S., Schachtman, D. P. (2010). A peroxidase contributes to ROS production during Arabidopsis root response to potassium deficiency. Mol. Plant 3, 420–427. doi: 10.1093/mp/ssp121
Kleine, T., Leister, D. (2016). Retrograde signaling: Organelles go networking. Biochim. Biophys. Acta 1857, 1313–1325. doi: 10.1016/j.bbabio.2016.03.017
Kopriva, S., Gigolashvili, T. (2016). “Glucosinolate synthesis in the context of plant metabolism,” in Advances in botanical research (United Kingdom: Elsevier), 99–124. doi: 10.1016/bs.abr.2016.07.002
Kosmacz, M., Parlanti, S., Schwarzländer, M., Kragler, F., Licausi, F., Van Dongen, J. T. (2015). The stability and nuclear localization of the transcription factor RAP 2.12 are dynamically regulated by oxygen concentration. Plant Cell Environ. 38, 1094–1103. doi: 10.1111/pce.12493
Koussevitzky, S., Suzuki, N., Huntington, S., Armijo, L., Sha, W., Cortes, D., et al. (2008). Ascorbate peroxidase 1 plays a key role in the response of Arabidopsis thaliana to stress combination. J. Biol. Chem. 283, 34197–34203. doi: 10.1074/jbc.M806337200
Kumar, D., Datta, R., Hazra, S., Sultana, A., Mukhopadhyay, R., Chattopadhyay, S. (2015). Transcriptomic Profiling of Arabidopsis thaliana Mutant pad2.1 in Response to Combined Cold and Osmotic Stress. PloS One 10, e0122690. doi: 10.1371/journal.pone.0122690
Lee, K. P., Kim, C., Landgraf, F., Apel, K. (2007). EXECUTER1- and EXECUTER2-dependent transfer of stress-related signals from the plastid to the nucleus of Arabidopsis thaliana. Proc. Natl. Acad. Sci. U. S. A. 104, 10270–10275. doi: 10.1073/pnas.0702061104
Lee, J. K., Woo, S. Y., Kwak, M. J., Park, S. H., Kim, H. D., Lim, Y. J., et al. (2020). Effects of elevated temperature and ozone in brassica juncea L.: growth, physiology, and ROS accumulation. Forests 11, 68. doi: 10.3390/f11010068
Lemke, M. D., Woodson, J. D. (2022). Targeted for destruction: degradation of singlet oxygen-damaged chloroplasts. Plant Signal. Behav. 17, 2084955. doi: 10.1080/15592324.2022.2084955
León-Chan, R. G., López-Meyer, M., Osuna-Enciso, T., Sañudo-Barajas, J. A., Heredia, J. B., León-Félix, J. (2017). Low temperature and ultraviolet-B radiation affect chlorophyll content and induce the accumulation of UV-B-absorbing and antioxidant compounds in bell pepper (Capsicum annuum) plants. Environ. Exp. Bot. 139, 143–151. doi: 10.1016/j.envexpbot.2017.05.006
Li, X., Cai, J., Liu, F., Dai, T., Cao, W., Jiang, D. (2014). Physiological, proteomic and transcriptional responses of wheat to combination of drought or waterlogging with late spring low temperature. Funct. Plant Biol. FPB 41, 690–703. doi: 10.1071/FP13306
Li, M., Kim, C. (2023). “Singlet oxygen in plants: From genesis to signaling,” in Advances in botanical research (United Kingdom: Elsevier), 1–42. doi: 10.1016/bs.abr.2022.08.023
Licausi, F., Kosmacz, M., Weits, D. A., Giuntoli, B., Giorgi, F. M., Voesenek, L. A. C. J., et al. (2011). Oxygen sensing in plants is mediated by an N-end rule pathway for protein destabilization. Nature 479, 419–422. doi: 10.1038/nature10536
Lin, H.-H., Lin, K.-H., Chen, S.-C., Shen, Y.-H., Lo, H.-F. (2015). Proteomic analysis of broccoli (Brassica oleracea) under high temperature and waterlogging stresses. Bot. Stud. 56, 18. doi: 10.1186/s40529-015-0098-2
Liu, Y., He, C. (2016). Regulation of plant reactive oxygen species (ROS) in stress responses: learning from AtRBOHD. Plant Cell Rep. 35, 995–1007. doi: 10.1007/s00299-016-1950-x
Liu, B., Wang, R., Gong, J., Zhu, T., Long, S., Guo, H., et al. (2023). Combined cold and drought stress-induced response of photosynthesis and osmotic adjustment in elymus nutans griseb. Agronomy 13, 2368. doi: 10.3390/agronomy13092368
Liu, Y., Zhang, H. (2021). Reactive oxygen species and nitric oxide as mediators in plant hypersensitive response and stomatal closure. Plant Signal. Behav. 16, 1985860. doi: 10.1080/15592324.2021.1985860
Locato, V., Cimini, S., De Gara, L. (2018). ROS and redox balance as multifaceted players of cross-tolerance: epigenetic and retrograde control of gene expression. J. Exp. Bot. 69, 3373–3391. doi: 10.1093/jxb/ery168
Lu, T., Meng, Z., Zhang, G., Qi, M., Sun, Z., Liu, Y., et al. (2017). Sub-high temperature and high light intensity induced irreversible inhibition on photosynthesis system of tomato plant (Solanum lycopersicum L.). Front. Plant Sci. 8. doi: 10.3389/fpls.2017.00365
Luo, M., Cheng, K., Xu, Y., Yang, S., Wu, K. (2017). Plant responses to abiotic stress regulated by histone deacetylases. Front. Plant Sci. 8. doi: 10.3389/fpls.2017.02147
Luo, M., Wang, Y.-Y., Liu, X., Yang, S., Lu, Q., Cui, Y., et al. (2012). HD2C interacts with HDA6 and is involved in ABA and salt stress response in Arabidopsis. J. Exp. Bot. 63, 3297–3306. doi: 10.1093/jxb/ers059
Ma, X., Wang, W., Bittner, F., Schmidt, N., Berkey, R., Zhang, L., et al. (2016). Dual and opposing roles of xanthine dehydrogenase in defense-associated reactive oxygen species metabolism in arabidopsis. Plant Cell 28, 1108–1126. doi: 10.1105/tpc.15.00880
Mansoor, S., Ali Wani, O., Lone, J. K., Manhas, S., Kour, N., Alam, P., et al. (2022). Reactive oxygen species in plants: from source to sink. Antioxidants 11, 225. doi: 10.3390/antiox11020225
Maruta, T., Noshi, M., Tanouchi, A., Tamoi, M., Yabuta, Y., Yoshimura, K., et al. (2012). H2O2-triggered retrograde signaling from chloroplasts to nucleus plays specific role in response to stress. J. Biol. Chem. 287, 11717–11729. doi: 10.1074/jbc.M111.292847
Masson, N., Keeley, T. P., Giuntoli, B., White, M. D., Puerta, M. L., Perata, P., et al. (2019). Conserved N-terminal cysteine dioxygenases transduce responses to hypoxia in animals and plants. Science 365, 65–69. doi: 10.1126/science.aaw0112
Meng, X., Li, L., Pascual, J., Rahikainen, M., Yi, C., Jost, R., et al. (2022). GWAS on multiple traits identifies mitochondrial ACONITASE3 as important for acclimation to submergence stress. Plant Physiol. 188, 2039–2058. doi: 10.1093/plphys/kiac011
Mignolet-Spruyt, L., Xu, E., Idänheimo, N., Hoeberichts, F. A., Mühlenbock, P., Brosché, M., et al. (2016). Spreading the news: subcellular and organellar reactive oxygen species production and signalling. J. Exp. Bot. 67, 3831–3844. doi: 10.1093/jxb/erw080
Mittler, R. (2006). Abiotic stress, the field environment and stress combination. Trends Plant Sci. 11, 15–19. doi: 10.1016/j.tplants.2005.11.002
Mittler, R., Blumwald, E. (2010). Genetic engineering for modern agriculture: challenges and perspectives. Annu. Rev. Plant Biol. 61, 443–462. doi: 10.1146/annurev-arplant-042809-112116
Mittler, R., Vanderauwera, S., Gollery, M., Van Breusegem, F. (2004). Reactive oxygen gene network of plants. Trends Plant Sci. 9, 490–498. doi: 10.1016/j.tplants.2004.08.009
Moller, I. M. (2001). PLANT MITOCHONDRIA AND OXIDATIVE STRESS: electron transport, NADPH turnover, and metabolism of reactive oxygen species. Annu. Rev. Plant Physiol. Plant Mol. Biol. 52, 561–591. doi: 10.1146/annurev.arplant.52.1.561
Murphy, M. P. (2009). How mitochondria produce reactive oxygen species. Biochem. J. 417, 1–13. doi: 10.1042/BJ20081386
Nadarajah, K. K. (2020). ROS homeostasis in abiotic stress tolerance in plants. Int. J. Mol. Sci. 21, 5208. doi: 10.3390/ijms21155208
Nakano, T., Suzuki, K., Fujimura, T., Shinshi, H. (2006). Genome-wide analysis of the ERF gene family in arabidopsis and rice. Plant Physiol. 140, 411–432. doi: 10.1104/pp.105.073783
Nayyar, H., Awasthi, R., Kaushal, N., Vadez, V., Turner, N., Berger, J., et al. (2014). Individual and combined effects of transient drought and heat stress on carbon assimilation and seed filling in chickpea. Funct. Plant Biol. 41, 1148–1167. doi: 10.1071/FP13340
Ng, S., De Clercq, I., Van Aken, O., Law, S. R., Ivanova, A., Willems, P., et al. (2014). Anterograde and retrograde regulation of nuclear genes encoding mitochondrial proteins during growth, development, and stress. Mol. Plant 7, 1075–1093. doi: 10.1093/mp/ssu037
Noctor, G., De Paepe, R., Foyer, C. H. (2007). Mitochondrial redox biology and homeostasis in plants. Trends Plant Sci. 12, 125–134. doi: 10.1016/j.tplants.2007.01.005
O’Brien, J. A., Daudi, A., Finch, P., Butt, V. S., Whitelegge, J. P., Souda, P., et al. (2012). A peroxidase-dependent apoplastic oxidative burst in cultured Arabidopsis cells functions in MAMP-elicited defense. Plant Physiol. 158, 2013–2027. doi: 10.1104/pp.111.190140
Ors, S., Suarez, D. L. (2017). Spinach biomass yield and physiological response to interactive salinity and water stress. Agric. Water Manage. 190, 31–41. doi: 10.1016/j.agwat.2017.05.003
Osakabe, Y., Yamaguchi-Shinozaki, K., Shinozaki, K., Tran, L.-S. P. (2013). Sensing the environment: key roles of membrane-localized kinases in plant perception and response to abiotic stress. J. Exp. Bot. 64, 445–458. doi: 10.1093/jxb/ers354
Osthoff, A., Donà Dalle Rose, P., Baldauf, J. A., Piepho, H.-P., Hochholdinger, F. (2019). Transcriptomic reprogramming of barley seminal roots by combined water deficit and salt stress. BMC Genomics 20, 325. doi: 10.1186/s12864-019-5634-0
Ou, X., Zhuang, T., Yin, W., Miao, Y., Wang, B., Zhang, Y., et al. (2015). DNA methylation changes induced in rice by exposure to high concentrations of the nitric oxide modulator, sodium nitroprusside. Plant Mol. Biol. Rep. 33, 1428–1440. doi: 10.1007/s11105-014-0843-9
Park, J., Lim, C. J., Shen, M., Park, H. J., Cha, J.-Y., Iniesto, E., et al. (2018). Epigenetic switch from repressive to permissive chromatin in response to cold stress. Proc. Natl. Acad. Sci. 115, E5400–E5409. doi: 10.1073/pnas.1721241115
Pesaresi, P., Hertle, A., Pribil, M., Kleine, T., Wagner, R., Strissel, H., et al. (2009). Arabidopsis STN7 kinase provides a link between short- and long-term photosynthetic acclimation. Plant Cell 21, 2402–2423. doi: 10.1105/tpc.108.064964
Poborilova, Z., Ohlsson, A. B., Berglund, T., Vildova, A., Provaznik, I., Babula, P. (2015). DNA hypomethylation concomitant with the overproduction of ROS induced by naphthoquinone juglone on tobacco BY-2 suspension cells. Environ. Exp. Bot. 113, 28–39. doi: 10.1016/j.envexpbot.2015.01.005
Pospíšil, P., Prasad, A., Rác, M. (2019). Mechanism of the formation of electronically excited species by oxidative metabolic processes: role of reactive oxygen species. Biomolecules 9, 258. doi: 10.3390/biom9070258
Prasch, C. M., Sonnewald, U. (2013). Simultaneous application of heat, drought, and virus to arabidopsis plants reveals significant shifts in signaling networks. Plant Physiol. 162, 1849–1866. doi: 10.1104/pp.113.221044
Qin, F., Kodaira, K.-S., Maruyama, K., Mizoi, J., Tran, L.-S. P., Fujita, Y., et al. (2011). SPINDLY, a negative regulator of gibberellic acid signaling, is involved in the plant abiotic stress response. Plant Physiol. 157, 1900–1913. doi: 10.1104/pp.111.187302
Quan, L., Zhang., B., Shi., W. W., Li., H. Y. (2008). Hydrogen peroxide in plants: a versatile molecule of the reactive oxygen species network. J. Integr. Plant Biol. 50, 2–18. doi: 10.1111/j.1744-7909.2007.00599.x
Rahantaniaina, M.-S., Li, S., Chatel-Innocenti, G., Tuzet, A., Mhamdi, A., Vanacker, H., et al. (2017). Glutathione oxidation in response to intracellular H2O2: Key but overlapping roles for dehydroascorbate reductases. Plant Signal. Behav. 12, e1356531. doi: 10.1080/15592324.2017.1356531
Rahman, M. M., Mostofa, M. G., Keya, S. S., Ghosh, P. K., Abdelrahman, M., Anik, T. R., et al. (2023). Jasmonic acid priming augments antioxidant defense and photosynthesis in soybean to alleviate combined heat and drought stress effects. Plant Physiol. Biochem. PPB 206, 108193. doi: 10.1016/j.plaphy.2023.108193
Ramakrishnan, M., Papolu, P. K., Satish, L., Vinod, K. K., Wei, Q., Sharma, A., et al. (2022). Redox status of the plant cell determines epigenetic modifications under abiotic stress conditions and during developmental processes. J. Adv. Res. 42, 99–116. doi: 10.1016/j.jare.2022.04.007
Rasmusson, A. G., Wallström, S. V. (2010). Involvement of mitochondria in the control of plant cell NAD(P)H reduction levels. Biochem. Soc Trans. 38, 661–666. doi: 10.1042/BST0380661
Ray, P. D., Huang, B.-W., Tsuji, Y. (2012). Reactive oxygen species (ROS) homeostasis and redox regulation in cellular signaling. Cell. Signal. 24, 981–990. doi: 10.1016/j.cellsig.2012.01.008
Renziehausen, T., Frings, S., Schmidt-Schippers, R. (2024). Against all floods’: plant adaptation to flooding stress and combined abiotic stresses. Plant J. 117, 1836–1855. doi: 10.1111/tpj.16614
Rillig, M. C., Ryo, M., Lehmann, A., Aguilar-Trigueros, C. A., Buchert, S., Wulf, A., et al. (2019). The role of multiple global change factors in driving soil functions and microbial biodiversity. Science 366, 886–890. doi: 10.1126/science.aay2832
Ritonga, F. N., Chen, S. (2020). Physiological and molecular mechanism involved in cold stress tolerance in plants. Plants 9, 560. doi: 10.3390/plants9050560
Rivero, R. M., Mestre, T. C., Mittler, R., Rubio, F., Garcia-Sanchez, F., Martinez, V. (2014). The combined effect of salinity and heat reveals a specific physiological, biochemical and molecular response in tomato plants. Plant Cell Environ. 37, 1059–1073. doi: 10.1111/pce.12199
Rizhsky, L., Liang, H., Mittler, R. (2002). The combined effect of drought stress and heat shock on gene expression in tobacco. Plant Physiol. 130, 1143–1151. doi: 10.1104/pp.006858
Rizhsky, L., Liang, H., Shuman, J., Shulaev, V., Davletova, S., Mittler, R. (2004). When defense pathways collide. The response of Arabidopsis to a combination of drought and heat stress. Plant Physiol. 134, 1683–1696. doi: 10.1104/pp.103.033431
Rodríguez-Serrano, M., Romero-Puertas, M. C., Sanz-Fernández, M., Hu, J., Sandalio, L. M. (2016). Peroxisomes extend peroxules in a fast response to stress via a reactive oxygen species-mediated induction of the peroxin PEX11a. Plant Physiol. 171, 1665–1674. doi: 10.1104/pp.16.00648
Rollins, J. A., Habte, E., Templer, S. E., Colby, T., Schmidt, J., von Korff, M. (2013). Leaf proteome alterations in the context of physiological and morphological responses to drought and heat stress in barley (Hordeum vulgare L.). J. Exp. Bot. 64, 3201–3212. doi: 10.1093/jxb/ert158
Saijo, Y., Loo, E. P. (2020). Plant immunity in signal integration between biotic and abiotic stress responses. New Phytol. 225, 87–104. doi: 10.1111/nph.15989
Samsone, I., Andersone-Ozola, U., Karlsons, A., Ievinsh, G. (2020). Light conditions affect naCl-induced physiological responses in a clonal plant species. Proc. Latv. Acad. Sci. Sect. B Nat. Exact Appl. Sci. 74, 335–343. doi: 10.2478/prolas-2020-0050
Sasidharan, R., Bailey-Serres, J., Ashikari, M., Atwell, B. J., Colmer, T. D., Fagerstedt, K., et al. (2017). Community recommendations on terminology and procedures used in flooding and low oxygen stress research. New Phytol. 214, 1403–1407. doi: 10.1111/nph.14519
Schmidt, R. R., Fulda, M., Paul, M. V., Anders, M., Plum, F., Weits, D. A., et al. (2018). Low-oxygen response is triggered by an ATP-dependent shift in oleoyl-CoA in Arabidopsis. Proc. Natl. Acad. Sci. 115, 2098–2106. doi: 10.1073/pnas.1809429115
Schwarzländer, M., Fricker, M. D., Sweetlove, L. J. (2009). Monitoring the in vivo redox state of plant mitochondria: effect of respiratory inhibitors, abiotic stress and assessment of recovery from oxidative challenge. Biochim. Biophys. Acta 1787, 468–475. doi: 10.1016/j.bbabio.2009.01.020
Shaar-Moshe, L., Blumwald, E., Peleg, Z. (2017). Unique physiological and transcriptional shifts under combinations of salinity, drought, and heat. Plant Physiol. 174, 421–434. doi: 10.1104/pp.17.00030
Shapiguzov, A., Vainonen, J. P., Hunter, K., Tossavainen, H., Tiwari, A., Järvi, S., et al. (2019). Arabidopsis RCD1 coordinates chloroplast and mitochondrial functions through interaction with ANAC transcription factors. eLife 8, e43284. doi: 10.7554/eLife.43284
Sharif, P., Seyedsalehi, M., Paladino, O., Van Damme, P., Sillanpää, M., Sharifi, A. A. (2018). Effect of drought and salinity stresses on morphological and physiological characteristics of canola. Int. J. Environ. Sci. Technol. 15, 1859–1866. doi: 10.1007/s13762-017-1508-7
Shen, Y., Issakidis-Bourguet, E., Zhou, D.-X. (2016). Perspectives on the interactions between metabolism, redox, and epigenetics in plants. J. Exp. Bot. 67, 5291–5300. doi: 10.1093/jxb/erw310
Shu, S., Tang, Y., Yuan, Y., Sun, J., Zhong, M., Guo, S. (2016). The role of 24-epibrassinolide in the regulation of photosynthetic characteristics and nitrogen metabolism of tomato seedlings under a combined low temperature and weak light stress. Plant Physiol. Biochem. 107, 344–353. doi: 10.1016/j.plaphy.2016.06.021
Silva, E. N., Vieira, S. A., Ribeiro, R. V., Ponte, L. F. A., Ferreira-Silva, S. L., Silveira, J. A. G. (2013). Contrasting physiological responses of jatropha curcas plants to single and combined stresses of salinity and heat. J. Plant Growth Regul. 32, 159–169. doi: 10.1007/s00344-012-9287-3
Singh, A., Kumar, A., Yadav, S., Singh, I. K. (2019). Reactive oxygen species-mediated signaling during abiotic stress. Plant Gene 18, 100173. doi: 10.1016/j.plgene.2019.100173
Singh, S. K., Reddy, K. R., Reddy, V. R., Gao, W. (2014). Maize growth and developmental responses to temperature and ultraviolet-B radiation interaction. Photosynthetica 52, 262–271. doi: 10.1007/s11099-014-0029-6
Singh, R., Singh, S., Parihar, P., Mishra, R. K., Tripathi, D. K., Singh, V. P., et al. (2016). Reactive oxygen species (ROS): beneficial companions of plants’ Developmental processes. Front. Plant Sci. 7. doi: 10.3389/fpls.2016.01299
Smirnoff, N., Arnaud, D. (2019). Hydrogen peroxide metabolism and functions in plants. New Phytol. 221, 1197–1214. doi: 10.1111/nph.15488
Su, L., Dai, Z., Li, S., Xin, H. (2015). A novel system for evaluating drought–cold tolerance of grapevines using chlorophyll fluorescence. BMC Plant Biol. 15, 82. doi: 10.1186/s12870-015-0459-8
Suszek-Łopatka, B., Maliszewska-Kordybach, B., Klimkowicz-Pawlas, A., Smreczak, B. (2021). The multifactorial assessment of the Zn impact on high and low temperature stress towards wheat seedling growth under diverse moisture conditions (optimal and wet) in three soils. J. Hazard. Mater. 416, 126087. doi: 10.1016/j.jhazmat.2021.126087
Suzuki, N., Bassil, E., Hamilton, J. S., Inupakutika, M. A., Zandalinas, S. I., Tripathy, D., et al. (2016). ABA is required for plant acclimation to a combination of salt and heat stress. PloS One 11, e0147625. doi: 10.1371/journal.pone.0147625
Suzuki, N., Rivero, R. M., Shulaev, V., Blumwald, E., Mittler, R. (2014). Abiotic and biotic stress combinations. New Phytol. 203, 32–43. doi: 10.1111/nph.12797
Szklarczyk, D., Kirsch, R., Koutrouli, M., Nastou, K., Mehryary, F., Hachilif, R., et al. (2023). The STRING database in 2023: protein-protein association networks and functional enrichment analyses for any sequenced genome of interest. Nucleic Acids Res. 51, D638–D646. doi: 10.1093/nar/gkac1000
Szucs, A., Jäger, K., Jurca, M. E., Fábián, A., Bottka, S., Zvara, A., et al. (2010). Histological and microarray analysis of the direct effect of water shortage alone or combined with heat on early grain development in wheat (Triticum aestivum). Physiol. Plant 140, 174–188. doi: 10.1111/j.1399-3054.2010.01394.x
Takagi, D., Takumi, S., Hashiguchi, M., Sejima, T., Miyake, C. (2016). Superoxide and singlet oxygen produced within the thylakoid membranes both cause photosystem I photoinhibition. Plant Physiol. 171, 1626–1634. doi: 10.1104/pp.16.00246
Takahashi, F., Kuromori, T., Urano, K., Yamaguchi-Shinozaki, K., Shinozaki, K. (2020). Drought stress responses and resistance in plants: from cellular responses to long-distance intercellular communication. Front. Plant Sci. 11. doi: 10.3389/fpls.2020.556972
Tan, S., Dong, F., Yang, Y., Zeng, Q., Chen, B., Jiang, L. (2017). Effects of waterlogging and cadmium on ecophysiological responses and metal bio-accumulation in Bermuda grass (Cynodon dactylon). Environ. Earth Sci. 76, 719. doi: 10.1007/s12665-017-7060-4
Terai, Y., Ueno, H., Ogawa, T., Sawa, Y., Miyagi, A., Kawai-Yamada, M., et al. (2020). Dehydroascorbate reductases and glutathione set a threshold for high-light-induced ascorbate accumulation. Plant Physiol. 183, 112–122. doi: 10.1104/pp.19.01556
Tripathi, R., Agrawal, S. B. (2013). Interactive effect of supplemental ultraviolet B and elevated ozone on seed yield and oil quality of two cultivars of linseed (Linum usitatissimum L.) carried out in open top chambers. J. Sci. Food Agric. 93, 1016–1025. doi: 10.1002/jsfa.5838
Tripathi, D. K., Bhat, J. A., Antoniou, C., Kandhol, N., Singh, V. P., Fernie, A. R., et al. (2024). Redox regulation by priming agents toward a sustainable agriculture. Plant Cell Physiol. 65, 1087–1102. doi: 10.1093/pcp/pcae031
Tripathi, D., Nam, A., Oldenburg, D. J., Bendich, A. J. (2020). Reactive oxygen species, antioxidant agents, and DNA damage in developing maize mitochondria and plastids. Front. Plant Sci. 11. doi: 10.3389/fpls.2020.00596
Tripathi, R., Rai, K., Singh, S., Agrawal, M., Agrawal, S. B. (2019). Role of supplemental UV-B in changing the level of ozone toxicity in two cultivars of sunflower: growth, seed yield and oil quality. Ecotoxicol. Lond. Engl. 28, 277–293. doi: 10.1007/s10646-019-02020-6
Tripathy, B. C., Oelmüller, R. (2012). Reactive oxygen species generation and signaling in plants. Plant Signal. Behav. 7, 1621–1633. doi: 10.4161/psb.22455
Ueda, M., Seki, M. (2020). Histone modifications form epigenetic regulatory networks to regulate abiotic stress response. Plant Physiol. 182, 15–26. doi: 10.1104/pp.19.00988
Vainonen, J. P., Sakuragi, Y., Stael, S., Tikkanen, M., Allahverdiyeva, Y., Paakkarinen, V., et al. (2008). Light regulation of CaS, a novel phosphoprotein in the thylakoid membrane of Arabidopsis thaliana. FEBS J. 275, 1767–1777. doi: 10.1111/j.1742-4658.2008.06335.x
Van Aken, O., De Clercq, I., Ivanova, A., Law, S. R., Van Breusegem, F., Millar, A. H., et al. (2016). Mitochondrial and chloroplast stress responses are modulated in distinct touch and chemical inhibition phases. Plant Physiol. 171, 2150–2165. doi: 10.1104/pp.16.00273
Vanderauwera, S., Vandenbroucke, K., Inzé, A., Van De Cotte, B., Mühlenbock, P., De Rycke, R., et al. (2012). AtWRKY15 perturbation abolishes the mitochondrial stress response that steers osmotic stress tolerance in Arabidopsis. Proc. Natl. Acad. Sci. 109, 20113–20118. doi: 10.1073/pnas.1217516109
Vanderauwera, S., Zimmermann, P., Rombauts, S., Vandenabeele, S., Langebartels, C., Gruissem, W., et al. (2005). Genome-wide analysis of hydrogen peroxide-regulated gene expression in Arabidopsis reveals a high light-induced transcriptional cluster involved in anthocyanin biosynthesis. Plant Physiol. 139, 806–821. doi: 10.1104/pp.105.065896
Vincent, C., Schaffer, B., Rowland, D. (2018). Water-deficit priming of papaya reduces high-light stress through oxidation avoidance rather than anti-oxidant activity. Environ. Exp. Bot. 156, 106–119. doi: 10.1016/j.envexpbot.2018.04.016
Voesenek, L. A. C. J., Bailey-Serres, J. (2015). Flood adaptive traits and processes: an overview. New Phytol. 206, 57–73. doi: 10.1111/nph.13209
Vogel, M. O., Moore, M., König, K., Pecher, P., Alsharafa, K., Lee, J., et al. (2014). Fast retrograde signaling in response to high light involves metabolite export, MITOGEN-ACTIVATED PROTEIN KINASE6, and AP2/ERF transcription factors in Arabidopsis. Plant Cell 26, 1151–1165. doi: 10.1105/tpc.113.121061
Vogelsang, L., Dietz, K.-J. (2022). Plant thiol peroxidases as redox sensors and signal transducers in abiotic stress acclimation. Free Radic. Biol. Med. 193, 764–778. doi: 10.1016/j.freeradbiomed.2022.11.019
Voothuluru, P., Sharp, R. E. (2013). Apoplastic hydrogen peroxide in the growth zone of the maize primary root under water stress. I. Increased levels are specific to the apical region of growth maintenance. J. Exp. Bot. 64, 1223–1233. doi: 10.1093/jxb/ers277
Wagner, D., Przybyla, D., Op den Camp, R., Kim, C., Landgraf, F., Lee, K. P., et al. (2004). The genetic basis of singlet oxygen-induced stress responses of Arabidopsis thaliana. Science 306, 1183–1185. doi: 10.1126/science.1103178
Wang, L., Leister, D., Guan, L., Zheng, Y., Schneider, K., Lehmann, M., et al. (2020). The Arabidopsis SAFEGUARD1 suppresses singlet oxygen-induced stress responses by protecting grana margins. Proc. Natl. Acad. Sci. 117, 6918–6927. doi: 10.1073/pnas.1918640117
Waszczak, C., Carmody, M., Kangasjärvi, J. (2018). Reactive oxygen species in plant signaling. Annu. Rev. Plant Biol. 69, 209–236. doi: 10.1146/annurev-arplant-042817-040322
Weits, D. A., Giuntoli, B., Kosmacz, M., Parlanti, S., Hubberten, H.-M., Riegler, H., et al. (2014). Plant cysteine oxidases control the oxygen-dependent branch of the N-end-rule pathway. Nat. Commun. 5, 3425. doi: 10.1038/ncomms4425
White, M. D., Klecker, M., Hopkinson, R. J., Weits, D. A., Mueller, C., Naumann, C., et al. (2017). Plant cysteine oxidases are dioxygenases that directly enable arginyl transferase-catalysed arginylation of N-end rule targets. Nat. Commun. 8, 14690. doi: 10.1038/ncomms14690
Wolfe, M. D., Tonsor, S. J. (2014). Adaptation to spring heat and drought in northeastern Spanish Arabidopsis thaliana. New Phytol. 201, 323–334. doi: 10.1111/nph.12485
Woodson, J. D., Chory, J. (2008). Coordination of gene expression between organellar and nuclear genomes. Nat. Rev. Genet. 9, 383–395. doi: 10.1038/nrg2348
Xu, P., Chen, H., Cai, W. (2020). Transcription factor CDF4 promotes leaf senescence and floral organ abscission by regulating abscisic acid and reactive oxygen species pathways in Arabidopsis. EMBO Rep. 21, e48967. doi: 10.15252/embr.201948967
Xu, L., Pan, R., Shabala, L., Shabala, S., Zhang, W.-Y. (2019). Temperature influences waterlogging stress-induced damage in Arabidopsis through the regulation of photosynthesis and hypoxia-related genes. Plant Growth Regul. 89, 143–152. doi: 10.1007/s10725-019-00518-x
Yang, L., Zhang, J., He, J., Qin, Y., Hua, D., Duan, Y., et al. (2014). ABA-mediated ROS in mitochondria regulate root meristem activity by controlling PLETHORA expression in Arabidopsis. PloS Genet. 10, e1004791. doi: 10.1371/journal.pgen.1004791
Yao, Y., He, R. J., Xie, Q. L., Zhao, X. H., Deng, X. M., He, J. B., et al. (2017). ETHYLENE RESPONSE FACTOR 74 (ERF74) plays an essential role in controlling a respiratory burst oxidase homolog D (RbohD)-dependent mechanism in response to different stresses in Arabidopsis. New Phytol. 213, 1667–1681. doi: 10.1111/nph.14278
Yildirim, E., Agar, G., Ors, S., Yuksel, E. A., Aydin, M., Ekinci, M., et al. (2023). Growth, physiological, biochemical and DNA methylation responses to cadmium stress of bean (phaseolus vulgaris L) grown under different irrigation levels. Plant Growth Regul. 101, 537–556. doi: 10.1007/s10725-023-01039-4
Yin, J., Gao, Y., Chen, R., Yu, D., Wilby, R., Wright, N., et al. (2023). Flash floods: why are more of them devastating the world’s driest regions? Nature 615, 212–215. doi: 10.1038/d41586-023-00626-9
Yuan, M., Ngou, B. P. M., Ding, P., Xin, X.-F. (2021). PTI-ETI crosstalk: an integrative view of plant immunity. Curr. Opin. Plant Biol. 62, 102030. doi: 10.1016/j.pbi.2021.102030
Zafar, M. M., Chattha, W. S., Khan, A. I., Zafar, S., Subhan, M., Saleem, H., et al. (2023). Drought and heat stress on cotton genotypes suggested agro-physiological and biochemical features for climate resilience. Front. Plant Sci. 14. doi: 10.3389/fpls.2023.1265700
Zandalinas, S. I., Mittler, R. (2022). Plant responses to multifactorial stress combination. New Phytol. 234, 1161–1167. doi: 10.1111/nph.18087
Zandalinas, S. I., Sengupta, S., Fritschi, F. B., Azad, R. K., Nechushtai, R., Mittler, R. (2021). The impact of multifactorial stress combination on plant growth and survival. New Phytol. 230, 1034–1048. doi: 10.1111/nph.17232
Zhang, L.-J., Zhong, T.-X., Xu, L.-X., Han, L., Zhang, X. (2015). Water deficit irrigation impacts on antioxidant metabolism associated with freezing tolerance in creeping bentgrass. J. Am. Soc Hortic. Sci. 140, 323–332. doi: 10.21273/JASHS.140.4.323
Zhang, H., Zhu, J., Gong, Z., Zhu, J.-K. (2022). Abiotic stress responses in plants. Nat. Rev. Genet. 23, 104–119. doi: 10.1038/s41576-021-00413-0
Zhao, C., Zhang, H., Song, C., Zhu, J.-K., Shabala, S. (2020). Mechanisms of plant responses and adaptation to soil salinity. Innovation 1, 100017. doi: 10.1016/j.xinn.2020.100017
Zheng, Y., Cheng, D., Simmons, M. (2014). Ozone pollution effects on gas exchange, growth and biomass yield of salinity-treated winter wheat cultivars. Sci. Total Environ. 499, 18–26. doi: 10.1016/j.scitotenv.2014.08.049
Zheng, C., Wang, Y., Ding, Z., Zhao, L. (2016). Global transcriptional analysis reveals the complex relationship between tea quality, leaf senescence and the responses to cold-drought combined stress in camellia sinensis. Front. Plant Sci. 7. doi: 10.3389/fpls.2016.01858
Zhou, X., Hua, D., Chen, Z., Zhou, Z., Gong, Z. (2009). Elongator mediates ABA responses, oxidative stress resistance and anthocyanin biosynthesis in Arabidopsis. Plant J. 60, 79–90. doi: 10.1111/j.1365-313X.2009.03931.x
Zhou, R., Yu, X., Li, X., Mendanha dos Santos, T., Rosenqvist, E., Ottosen, C.-O. (2020). Combined high light and heat stress induced complex response in tomato with better leaf cooling after heat priming. Plant Physiol. Biochem. 151, 1–9. doi: 10.1016/j.plaphy.2020.03.011
Keywords: abiotic stress, crop resilience, epigenetic regulation, histone modification, reactive oxygen species, anthropogenic disturbances
Citation: Shriti S, Bhar A and Roy A (2024) Unveiling the role of epigenetic mechanisms and redox signaling in alleviating multiple abiotic stress in plants. Front. Plant Sci. 15:1456414. doi: 10.3389/fpls.2024.1456414
Received: 28 June 2024; Accepted: 30 August 2024;
Published: 19 September 2024.
Edited by:
Sunil Kumar Sahu, Beijing Genomics Institute (BGI), ChinaReviewed by:
Qingming Li, Chinese Academy of Agricultural Sciences, ChinaMuhammad Shahid Iqbal, Ayub Agricultural Research Institute, Pakistan
Copyright © 2024 Shriti, Bhar and Roy. This is an open-access article distributed under the terms of the Creative Commons Attribution License (CC BY). The use, distribution or reproduction in other forums is permitted, provided the original author(s) and the copyright owner(s) are credited and that the original publication in this journal is cited, in accordance with accepted academic practice. No use, distribution or reproduction is permitted which does not comply with these terms.
*Correspondence: Anirban Bhar, YW5pcmJhbmJoYXJAcmttdmNjcmFoYXJhLmFjLmlu; Amit Roy, cm95QGZsZC5jenUuY3o=