- Institute for Biosafety in Plant Biotechnology, Julius Kühn-Institut (JKI) - Federal Research Centre for Cultivated Plants, Quedlinburg, Germany
Currently transient expression is one of the preferred plant-based technologies for recombinant protein manufacturing, particularly in respect to pharmaceutically relevant products. Modern hybrid transient expression systems combine the features of Agrobacterium tumefaciens and viral vectors. However, host plant reaction to Agrobacterium-mediated delivery of gene of interest can negatively affect foreign protein accumulation. In this study, we investigated whether the modulation of plant immune response through knockdown of the Nb-SABP2 and Nb-COI1 N. benthamiana genes could improve recombinant protein yield. In plants, the SABP2 and COI1 proteins are involved in the salicylic acid and jasmonic acid metabolism, respectively. We exemplified the utility of this approach with the green fluorescence (GFP) and β nerve growth factor (βNGF) proteins: compared to the tobacco mosaic virus (TMV)-based vector the Nb-SABP2 and Nb-COI1-suppressed plants provided an increased recombinant protein accumulation. We also show that this strategy is extendable to the expression systems utilizing potato virus X (PVX) as the vector backbone: the enhanced amounts of βNGF were detected in the Nb-SABP2 and Nb-COI1-depleted leaves co-infiltrated with the PVX-βNGF. These findings suggest that modulating host plant reaction to agrodelivery of expression vectors could be useful for improving transient foreign protein production in N. benthamiana.
Introduction
Transient expression in N. benthamiana is an advanced technology, which has turned plants into commercial platform for recombinant protein production (Lomonossoff and D’Aoust, 2016; Schillberg et al., 2019; Schillberg and Finnern, 2021). This method uses the ability of Agrobacterium tumefaciens to transfer T-DNA carrying the expression vector with the gene of interest into plant cells (Kapila et al., 1997; Fischer et al., 1999). The major advances in this technique have centered on the development of hybrid expression systems combining features of A. tumefaciens and viral vectors (Gleba et al., 2005; Peyret and Lomonossoff, 2015). With a few exceptions the modern viral expression vectors rely on sequences of RNA (tobacco mosaic virus (TMV), potato virus X (PVX), cowpea mosaic virus (CPMV)) and DNA (bean yellow dwarf virus (BeYDV)) viruses (Gleba et al., 2007; Peyret and Lomonossoff, 2015). Among different species that have been tested for transient expression Nicotiana benthamiana is the preferred host due to a short life cycle, fast growth rate, suitable for massive infiltration leaf anatomy and a partly defective RNA silencing system (Goodin et al., 2008; Bally et al., 2018). Current research in the field of N. benthamiana-based transient expression is focused on the improvement of vector delivery systems and fine-tuning of plant expression host. A number of strategies have been developed to design plant cell conditions supporting foreign protein production. The first one is based on co-expression of cell cycle regulatory genes. In particular, several research groups reported about positive impact of virus- and plant-derived cell-cycle regulators on recombinant protein accumulation in N. benthamiana (Norkunas et al., 2018; Kopertekh and Reichardt, 2021, 2022). Another experimental approach of plant cell engineering has been described in publication of Wang et al. (2023). The authors showed that the application of a semi-dominant negative gain-of-function mutant form of plasmodesmata located protein 5 (PDLP) from Arabidopsis thaliana facilitated TMV-based vector movement and enhanced foreign protein production. One of the recent reports demonstrated that the depletion of the NbCORE receptor perceiving the cold shock protein of A. tumefaciens improves agroinfiltration productivity in N. benthamiana (Dodds et al., 2023). Finally, the attenuation of plant defense response against microbial components of transient vector delivery system has been also addressed to design a supportive cell environment for increased foreign protein accumulation. There are several examples in the literature showing that downregulation of endogenous plant genes involved in post-transcriptional gene silencing (PTGS) process can elevate recombinant protein accumulation in N. benthamiana host. Particularly, this approach has been shown to be feasible for the DCL2, DCL4 (Matsuo and Matsumura, 2017; Matsuo, 2022), RDR6 (Matsuo and Atsumi, 2019) and Arg2 (Ludman et al., 2017) genes encoding Dicer-like 2, Dicer-like 4, RNA-dependent RNA polymerase 6 and Argonaut 2 proteins, respectively.
One of the essential components of the plant pathogen response is the regulatory network of phytohormones. Salicylic acid (SA), jasmonic acid (JA) and ethylene (ET) are major plant hormones that orchestrate defense reaction (Li et al., 2019; Ngou et al., 2022). The phytohormones involved in plant response to biotic stress have been shown to play an essential role in both Agrobacterium- and virus-plant interactions. In respect to plant-Agrobacterium pathosystem it was demonstrated that the defective in SA accumulation Arabidopsis thaliana and N. bethamiana plants were more susceptible to infection, whereas mutants overproducing SA were relatively resistant (Yuan et al., 2007; Anand et al., 2008). It was suggested that SA shuts down the expression of the Vir regulon by the attenuation of the VirA protein kinase activity (Yuan et al., 2007). The role of JA and SA in plant-virus interaction has also been reported. For example, the exogenous application of SA and JA improved plant resistance to RNA viruses (Lee et al., 2011; Shang et al., 2011). Inversely, suppression of the SA and JA biosynthetic and signaling genes accelerated development of symptoms and accumulation of virus titters (Liu et al., 2004; Pacheco et al., 2012; García-Marcos et al., 2013; Zhu et al., 2014). Considering the negative impact of plant defense response on microbial component of transient vector delivery system, we supposed that the attenuation of defense reaction might have positive effect on agroinfiltration efficiency and subsequent recombinant protein accumulation. To proof our hypothesis we modulated pathogen defense in N. benthamiana by silencing two endogenous genes, Nb-SABP2 and Nb-COI1, which are involved in SA and JA metabolism, respectively. The Nb-SABP2 gene encodes a SA-binding protein 2 termed SABP2, which is essential for establishing systemic acquired resistance (SAR) (Kumar and Klessig, 2003; Forouhar et al., 2005). The SABP2 is involved in the conversion of biologically inactive methyl salicylate (MeSA) into active SA leading to activation of the SA-dependent defense response (Park et al., 2007). The coronatine insensitive 1 (COI1) gene encodes an F-box COI1 protein that determines the substrate specificity of the E3 ubiquitin ligase SCFCOI1 complex. This complex targets repressors of the JA-induced genes for degradation inducing their expression (Xie et al., 1998; Xu et al., 2002; Chini et al., 2009).
We report here that transient downregulation of the Nb-SABP2 and Nb-COI1 genes facilitated accumulation of viral vector and target protein transcripts, and resulted in enhanced recombinant protein production in N. benthamiana. These findings indicate that the attenuation of plant host response against biotic stress could have value in improving transient expression technology.
Materials and methods
Plasmid constructs
The pLH-35S-COI1-INT-COI1 and pLH-35S-SABP2-INT-SABP2 constructs were designed in several steps. The 186 bp region of the Nb-COI1 gene was amplified from N. benthamiana cDNA using two primer combinations, NcoI-COI1-forw/COI1-BamHI-rev and SpeI-COI1forw/COI1-XhoI-rev. The 230 bp fragment of the Nb-SABP2 gene was amplified from the same cDNA sample as for the Nb-COI1 gene by two primer pairs: the NcoI-SABP2-forw/SABP2-HindIII-rev and the XbaI-SABP2-forw/SABP2-SalI-rev. The second intron (IV2) of the potato ST-LS1 gene was amplified from the p35S-creINT plasmid (Mlynárová and Nap, 2003) by PCR using the BglII-ST-LS1-INT-forw/ST-LS1-INT-SalI-rev and HindIII-ST-LS1-INT-forw/STLS1-INT-SalI-rev primer combinations. The amplified fragments were cloned in pGEM-T Easy (Promega, Walldorf, Germany) vector and sequenced. The primers can be found in Supplementary Table S1. Next, the sense and antisense gene fragments together with the separating hairpin loop sequence were introduced between the 35S promoter and terminator in the pCK-GFP plasmid (Reichel et al., 1996). The pCK-35S-COI1-INT-COI1 construct was generated by the ligation of the NcoI-XbaI digested pCK-GFP with three restriction fragments, NcoI-COI1-BamHI, BglII-ST-LS1-INT-SalI and SpeI-COI1-XhoI, derived from the pGEM-NcoI-COI1-BamHI, pGEM-BglII-ST-LS1-INT-SalI and pGEM-SpeI-COI1-XhoI plasmids, respectively. The restriction enzymes used for cloning are defined at 5’ and 3’ ends of the restriction fragments. Finally, the Nb-COI1 RNAi cassette from the pCK-35S-COI1-INT-COI1 plasmid was cloned into PstI restriction site of the pLH7000 binary vector (Töpfer et al., 1993) resulting in the pLH-35S-COI1-INT-COI1 (Nb-COI1hpc).
Ligation of the NcoI/XbaI digested pCK-GFP plasmid with three restriction fragments, NcoI-SABP2-HindIII, HindIlI-ST-LS1-INT-SalI and XbaI-SABP2-SalI, yielded the pCK-35S-SABP2-INT-SABP2 plasmid. For the Nb-SABP2 gene, the NcoI-SABP2-HindIII, HindIlI-ST-LS1-INT-SalI and XbaI-SABP2-SalI restriction fragments were obtained from the pGEM-NcoI-SABP2-HindIII, pGEM-HindIlI-ST-LS1-INT-SalI and pGEM-XbaI-SABP2-SalI plasmids. Next, the silencing expression cassette from the pCK-35S-SABP2-INT-SABP2 intermediate construct was released by PstI and ligated to NsiI digested pLH7000 resulting in the pLH-35S-SABP2-INT-SABP2 (Nb-SABP2-hpc) final plasmid.
To design the pLH-TMV-GFP expression construct the HindIII-KpnI digested pLH-Δbar-PacI (Kopertekh et al., 2004) plasmid was ligated with three restriction fragments, namely, KpnI-NcoI fragment of pICH17344, NcoI-SpeI fragment of pICH17344, and SpeI-HindIII fragment of pICH17344. The pLH-TMV-GFP was subsequently used to prepare the pLH-TMV-βNGF. At first, the βNGF gene containing XhoI and SwaI restriction sites at 5’ and 3’ ends, respectively, was synthesized by BioCat (Heidelberg, Germany). Then, XhoI-β-NGF-SwaI sequence digested with XhoI and SwaI enzymes was introduced into identical cloning sites of the pLH-TMV-GFP construct.
The pLH-PVX-βNGF expression vector was constructed as follows. At first, the βNGF gene containing NheI restriction site at the 5’ of the start codon and SalI restriction site at the 3’ end of the stop codon was synthesized by BioCat (Heidelberg, Germany). Following NheI-SalI restriction the βNGF sequence was ligated into equally digested pPVX201 (Chapman et al., 1992) producing the pPVX-βNGF. To transfer the PVX-βNGF sequence into the pLH-Δbar-PacI plasmid the pPVX-βNGF was linearized with SphI and, following removal of the single stranded termini with T4 polymerase digested with EheI. The SphI-EheI DNA fragment containing the PVX-βNGF sequence was ligated to pLH-Δbar-PacI plasmid restricted with StuI. The final plasmid was designated as pLH-PVX-βNGF.
The pLH7000, pLH-35S-COI1-INT-COI1, pLH-35S-SABP2-INT-SABP2, pLH-TMV-GFP, pLH-TMV-βNGF and pLH-PVX-βNGF expression vectors were introduced into A. tumefaciens (recently renamed to Rhizobium radiobacter) strain C58C1 by the freeze-thaw method (Holsters et al., 1978).
Agroinfiltration of N. benthamiana
A. tumefaciens cultures (strain C58C1) containing the expression vectors were grown overnight in LB medium supplemented with appropriate antibiotics (100 mg/L spectinomycin, 300 mg/L streptomycin, 50 mg/L rifampicin, 100 mg/L carbenicillin), pelleted by centrifugation and resuspended in agroinfiltration buffer (10 mM MES, 10 mM MgCl2, 150 µM acetosyringone, pH 5.6). The final optical density of A. tumefaciens cultures carrying the pLH-TMV-GFP, pLH-TMV-βNGF and pLH-PVX-βNGF vectors was adjusted to OD600 of 0.1, whereas the A. tumefaciens cultures carrying the pLH7000, pLH-35S-COI1-INT-COI1 and pLH-35S-SABP2-INT-SABP2 plasmids were brought to a final OD600 of 0.3. Agrobacterium suspensions were infiltrated into expanded leaves of 5-6 week-old N. benthamiana plants using a syringe without a needle. N. benthamiana plants were grown in the greenhouse at 24/22 0C day/night temperature with a 16 h light and 8 h dark photoperiod.
Gene expression analysis
Expression of the Nb-SABP2, Nb-COI1, TMV, GFP and βNGF was estimated by qPCR analysis. To this end, RNA from the agroinfiltrated and non-agroinfiltrated N. benthamiana plants was isolated using the BioSELL RNA Mini Kit (Bio&SELL, Feucht, Germany). One μg of RNA was used to synthesize cDNA by the random hexamer primer and Maxima Reverse Transcriptase according to the manufacturer’s protocol (Thermo Scientific, Waltham, USA). The qPCR has been carried out with the Nb-SABP2-forw/Nb-SABP2-rev, Nb-COI-forw/NbCOI-rev, gfp-forw/gfp-rev, βNGF-forw/βNGF-rev and TMV-CP-forw/TMV-CP-rev primers specific to the Nb-SABP2 (JX317629.1), Nb-COI1 (AY547493.1), GFP (KX458.181.2), βNGF (KF057035.1) and TMV coat protein (M34077.1) genes, respectively. The qPCR reactions were accomplished in Mastercycler® EP realplex (Eppendorf, Hamburg, Germany) utilizing Maxima SYBR Green qPCR Master Mix (Thermo Scientific, Waltham, USA). Relative quantifications were performed based on the ΔCT method using cyclophilin (cyp) (AY368274.1) gene as an internal standard. Primer sequences for target and reference genes are listed in Supplementary Table S1.
In total samples from 6-8 plants were subjected to RNA analysis. Each sample was pooled from three middle leaves of one plant. The number of samples is designated in figure legends as n. Three technical replicates were performed for each probe. The Mann-Whitney U test was applied to test if there were differences between wild type and silenced plants.
GFP imaging
For visual detection of GFP fluorescence N. benthamiana leaves were illuminated with a handheld UV lamp and photographed with the Canon digital camera EOS 300D.
Protein quantification
The GFP and βNGF proteins were quantified by specific ELISA. Plant samples were collected from the middle agroinfiltrated leaves at 3, 5 and 7 dpi. The description of the GFP quantification can be found in Kopertekh and Reichardt (2021). The amounts of the βNGF protein in N. benthamiana leaves were determined as follows. Leaf material (300 mg) was harvested, homogenized in two volumes (w/v) of TBS buffer (50 mM Tris, 150 mM NaCI, 0.05% Tween-20, pH 7.4) and clarified by centrifugation for 10 min at 4 °C. ELISA plates were coated overnight with capture antibody diluted in carbonate buffer (0.1 M NaHCO3, 0.1 M Na2CO3, pH 9.5). After incubation, the plates were washed three times with TBS buffer and blocked with blocking solution (TBS buffer supplemented with 1% BSA) for 1 h at room temperature. Following washing step as described above the plates were coated with the fresh prepared plant extracts and incubated overnight at 4 °C. Subsequently, the washing with TBS was repeated and a biotin anti-human NGF antibody in TBS buffer containing 1% BSA was added and incubated for 1 h at room temperature. After washing step performed as described, an avidine alkaline phosphatase was added and incubated for 30 minutes at room temperature. Finally, the plates were developed for 30 min with p-nitrophenyl phosphate as substrate and optical density was measured at 405 nm in a SUNRISE™ microplate reader (Tecan, Männedorf, Switzerland). All plates contained control βNGF protein diluted in TBS buffer for a standard curve.
Recombinant protein accumulation was analyzed in 6-8 biological and three technical replicates. The biological replicate represents a pooled sample from three middle leaves of one plant. The Mann-Whitney U test was performed to test if there were differences in recombinant protein accumulation between agroinfiltrated wild type and silenced plants.
Results
Expression of the Nb-SABP2 and Nb-COI1 genes in agroinfiltrated N. benthamiana leaves
To examine the involvement of the Nb-SABP2 and Nb-COI1 genes in N. benthamiana defense response to Agrobacterium-mediated delivery of virus-based expression vector the expression profile of these genes was investigated in agroinoculated leaf tissue. Following agroinfiltration of N. benthamiana plants with A. tumefaciens cultures carrying the pLH7000 and pLH-TMV-GFP (Figure 1) constructs the Nb-SABP2 and Nb-COI1 transcripts were quantified by qPCR at 2, 5 and 7 days after inoculation (dpi) and compared to that of non-treated plants. This analysis showed that the Nb-SABP2 gene was upregulated in response to A. tumefaciens (pLH7000) and TMV (pLH-TMV-GFP) at early infection stage, 2 dpi (Figure 2A). In the N. benthamiana leaves treated only with A. tumefaciens the accumulation of the Nb-SABP2 RNA was increased with a significant fold change value of 5.9 at 2 dpi, 6.3 at 5 dpi and 4.8, at 7 dpi. In presence of the pLH-TMV-GFP virus vector the Nb-SABP2 expression increased at levels 8.9, 8.7 and 6-fold of the means for the non-treated plant at 2, 5 and 7 dpi, respectively. These results suggest that the Nb-SABP2 gene is involved in N. benthamiana defense reaction against both components of transient gene delivery system, Agrobacterium and virus vector, and its upregulation starts at the early infection stage.
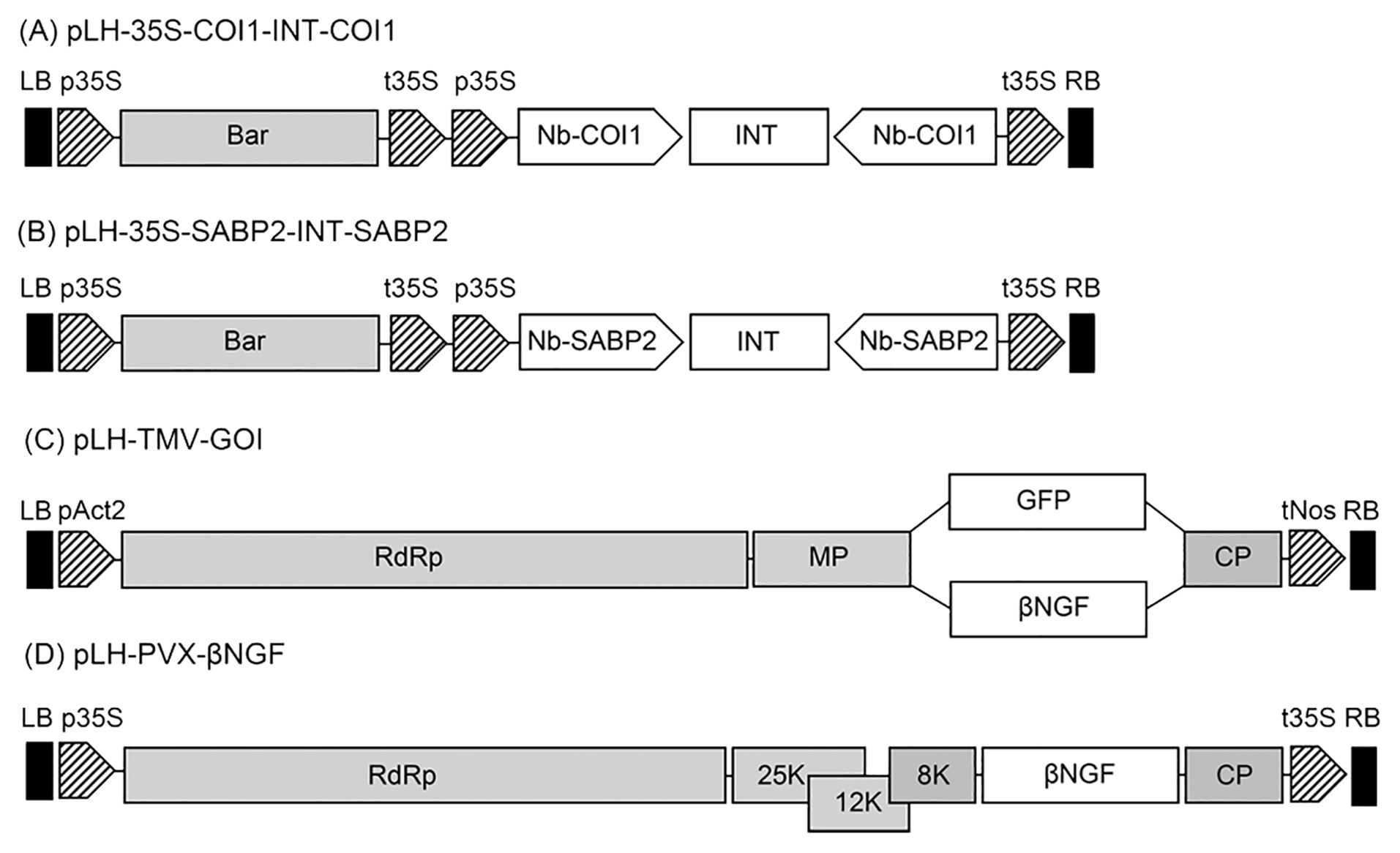
Figure 1. Schematic representation of expression constructs used in this study. (A) The pLH-35S-COI1-INT-COI1 RNAi construct. The pLH-35S-COI1-INT-COI1 plasmid contains the bar and Nb-COI1 RNAi expression cassettes. The components of the pLH-35S-COI1-INT-COI1 are designated as follows: bar, bar gene; Nb-COI1, the 186 bp fragment of the Nb-COI1 gene in sense and antisense orientation; INT, the second intron (IV2) of the potato ST-LS1 gene. (B) The pLH-35S-SABP2-INT-SABP2 RNAi construct. The pLH-35S-SABP2-INT-SABP2 plasmid contains the bar and Nb-SABP2 RNAi expression cassettes. The components of the pLH-35S-SABP2-INT-SABP2 are designated as follows: bar, bar gene; Nb-SABP2, the 230 bp fragment of the Nb-SABP2 gene in sense and antisense orientation; INT, the second intron (IV2) of the potato ST-LS1 gene. (C) The pLH-TMV-GOI expression vector. Features are as follows: RdRp, RNA-dependent RNA polymerase; MP, movement protein; genes of interest (GOI): βNGF (βNGF) and GFP (GFP); CP, coat protein encoding sequence; (D) The pLH-PVX-βNGF expression vector. Features are as follows: RdRp, RNA-dependent RNA polymerase; 25K, 12K, 8K, triple gene block; βNGF, βNGF gene; CP, coat protein encoding sequence. All expression constructs are based on the pLH7000 vector backbone. The regulatory elements used in expression vectors are pAct2, Act2 promoter from A thaliana; tNos, nopaline synthase terminator, p35S, CaMV 35S promoter; t35S, CaMV 35S terminator. LB, RB, left and right border of T-DNA, respectively.
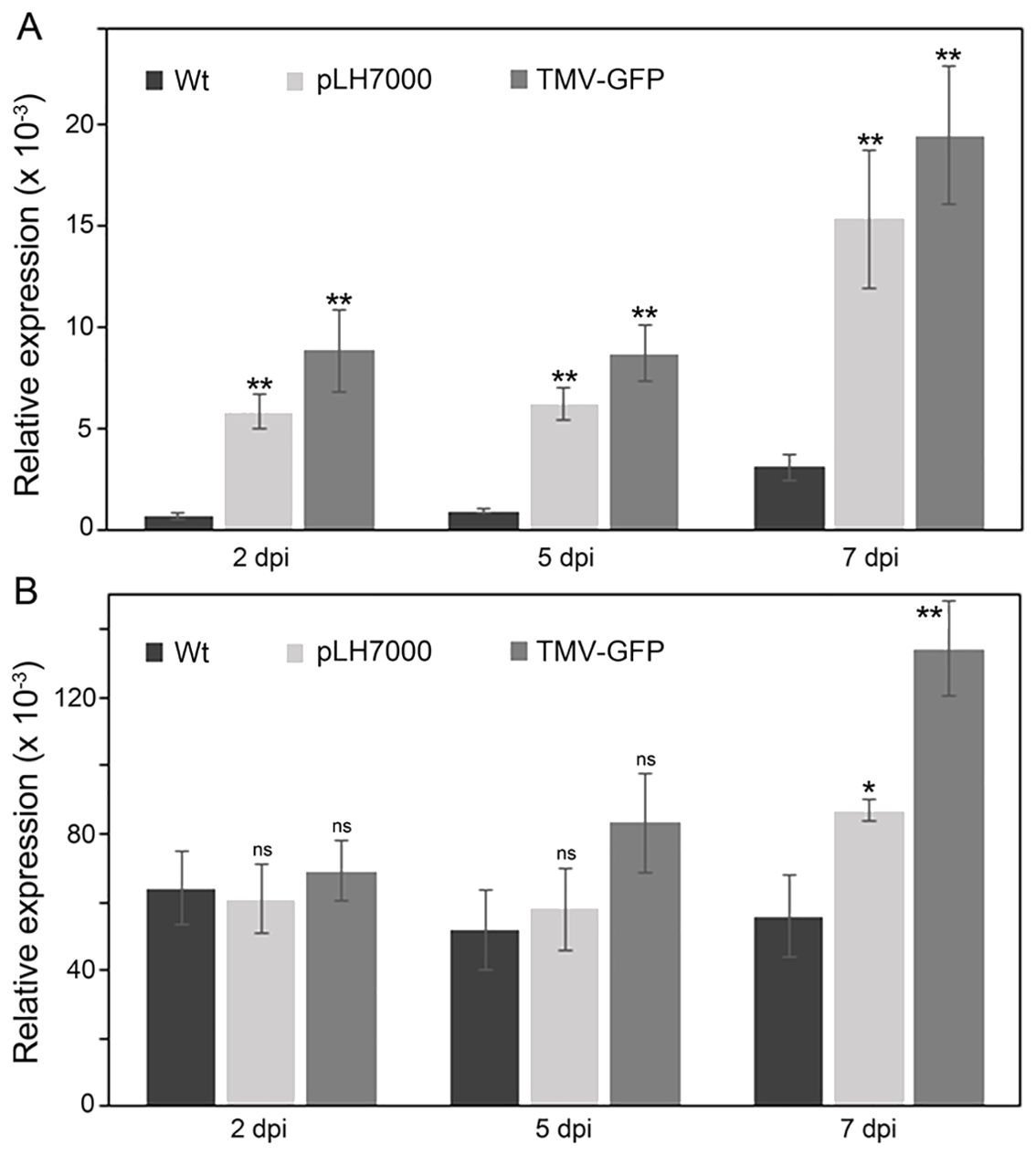
Figure 2. Expression of the Nb-SABP2 and Nb-COI1 genes in agroinfiltrated N. benthamiana leaves. N. benthamiana plants were agroinfiltrated with A. tumefaciens cultures carrying the pLH7000 and pLH-TMV-GFP expression vectors. The total RNA was isolated from leaf samples collected on 2, 5 and 7 dpi and subjected to qPCR analysis. The levels of the Nb-SABP2 (A) and Nb-COI1 (B) mRNA were quantified by gene-specific primers and normalized to cyp gene. Values represent the means with SE (n=8). Asterisks indicate significance as determined by the Mann-Whitney test, with *and ** denoting p < 0.05 and p < 0.01, respectively. Not significant values are determined as ns.
The expression profile of the Nb-COI1 gene differed from that of the Nb-SABP2 gene (Figure 2B). In comparison to non-inoculated control, no significant differences in the Nb-COI1 transcript accumulation were observed in agroinfiltrated N. benthamiana leaves at 2 and 5 dpi. The upregulation of the Nb-COI1 gene expression in treated leaves started late in the infection, at 7 dpi, achieving a 1.6-fold increase for Agrobacterium (pLH7000) and 2.4-fold increase for TMV (pLH-TMV-GFP). Therefore, the Nb-COI1 gene is also involved in plant defense response against Agrobacterium-mediated delivery of the TMV-based vector.
Downregulation of the Nb-SABP2 and Nb-COI1 genes facilitated TMV and foreign protein transcript accumulation
To determine whether downregulation of the Nb-SABP2 and Nb-COI1 endogenous genes can influence the TMV and foreign protein RNA accumulation the pLH-35S-COI1-INT-COI1 and pLH-35S-SABP2-INT-SABP2 constructs have been designed. Both construct are based on the pLH7000 vector backbone and utilize the CaMV 35S promoter and terminator to control the dsRNA expression unit. The Nb-SABP2 dsRNA expression cassette in the pLH-35S-SABP2-INT-SABP2 vector includes the Nb-SABP2 DNA fragment of 230 bp in sense and antisense orientation separated by the second intron of the potato ST-LS1 gene (Figure 1A). The pLH-35S-COI1-INT-COI1 silencing construct contained a 186 bp fragment of the Nb-COI1 gene in direct and indirect orientation and the same splitting intron sequence as in the case of the pLH-35S-SABP2-INT-SABP2 plasmid (Figure 1B).
The functionality of the pLH-35S-SABP2-INT-SABP2 and pLH-35S-COI1-INT-COI1 constructs has been tested for two vectors, pLH-TMV-GFP and pLH-TMV-βNGF (Figure 1C). For each expression vector N. bethamiana plants were agroinfiltrated with the A. tumefaciens cultures in following combinations: (i) pLH-TMV-GOI, (ii) pLH-TMV-GOI/pLH-35S-COI1-INT-COI1, (iii) pLH-TMV-GOI/pLH-35S-SABP2-INT-SABP2, (iv) pLH-TMV-GOI/pLH-35S-SABP2-INT-SABP2/pLH-35S-COI1-INT-COI1. The GOI designates the GFP and βNGF encoding sequences. The levels of the Nb-SABP2, Nb-COI1, TMV, GFP and βNGF transcripts were determined by qPCR analysis in the Nb-SABP2 and Nb-COI1 silenced leaves and compared with those of plants treated only with the pLH-TMV-GOI vector. The qPCR results for the pLH-TMV-GFP virus vector demonstrated that the Nb-SABP2 mRNA levels were reduced to 91% and 90% in the pLH-TMV-GFP/pLH-35S-SABP2-INT-SABP2, and pLH-TMV-GFP/pLH-35S-SABP2-INT-SABP2/pLH-35S-COI1-INT-COI1 agroinfiltrated leaves, respectively (Figure 3). The presence of the pLH-35S-COI1-INT-COI1 dsRNA construct resulted in the downregulation of the Nb-COI1 gene by 57% for the pLH-TMV-GFP/pLH-35S-COI1-INT-COI1 combination and by 68% for the TMV-GFP/pLH-35S-SABP2-INT-SABP2/pLH35S-COI1-INT-COI1 mixture of A. tumefaciens cultures. The content of TMV RNA elevated by 7, 9 and 6-fold in the Nb-COI1, Nb-SABP2 and Nb-COI1/Nb-SABP2-silenced plants, respectively. The accumulation of the GFP transcripts correlated with that of the TMV: it was increased by 5, 6 and 4-fold for the pLH-TMV-GFP/pLH-35S-COI1-INT-COI1, pLH-TMV-GFP/pLH-35S-SABP2-INT-SABP2, and pLH-TMV-GFP/pLH-35S-SABP2-INT-SABP2/pLH35S-COI1-INT-COI1 samples, correspondingly.
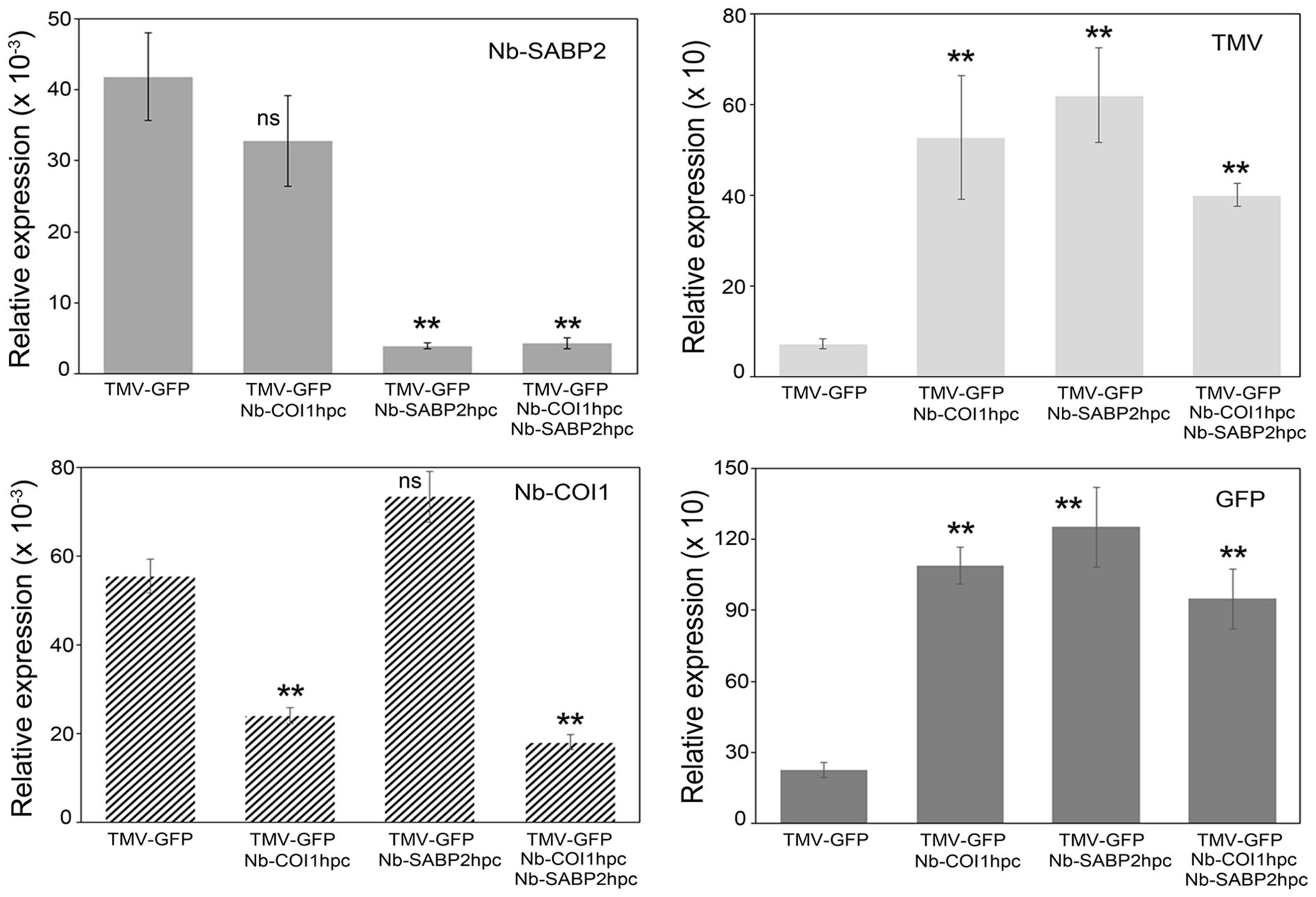
Figure 3. TMV and GFP RNA accumulation in Nb-COI1 and Nb-SABP2-silenced N. benthamiana leaves. N. benthamiana leaves were agroinfiltrated with four combinations of A. tumefaciens cultures, namely, pLH-TMV-GFP, pLH-TMV-GFP/pLH-35S-COI1-INT-COI1, pLH-TMV-GFP/pLH-35S-SABP2-INT-SABP2, pLH-TMV-GFP/pLH-35S-SABP2-INT-SABP2/pLH-35S-COI1-INT-COI1. Leaf samples for RNA isolation were collected at 6 dpi. RNA levels were quantified by qPCR using primers specific to the Nb-SABP2, Nb-COI1, TMV and GFP. Values represent the means with SE (n=6). Asterisks indicate significance as determined by the Mann-Whitney test, with ** denoting p < 0.01. Not significant values are determined as ns.
The qPCR data for the TMV vector carrying the βNGF coding sequence are shown in Figure 4. Similar to the pLH-TMV-GFP virus vector the Nb-SABP2 expression was reduced by 90% in leaves agroinfiltrated with the pLH-TMV-βNGF/pLH-35S-SABP2-INT-SABP2 culture mixture and by 86% in leaves agroinfiltrated with the pLH-TMV-βNGF/pLH-35S-SABP2-INT-SABP2/pLH-35S-COI1-INT-COI1 culture mixture. In leaves agroinfiltrated with the pLH-TMV-βNGF/pLH-35S-COI1-INT-COI1 and pLH-TMV-βNGF/pLH-35S-SABP2-INT-SABP2/pLH-35S-COI1-INT-COI1 combinations the Nb-COI1 mRNA levels were reduced by 60% and 59%, correspondingly. Downregulation of the Nb-SABP2 and Nb-COI1 genes resulted in increased accumulation of the TMV RNA at 3 fold in all tested combinations of constructs.
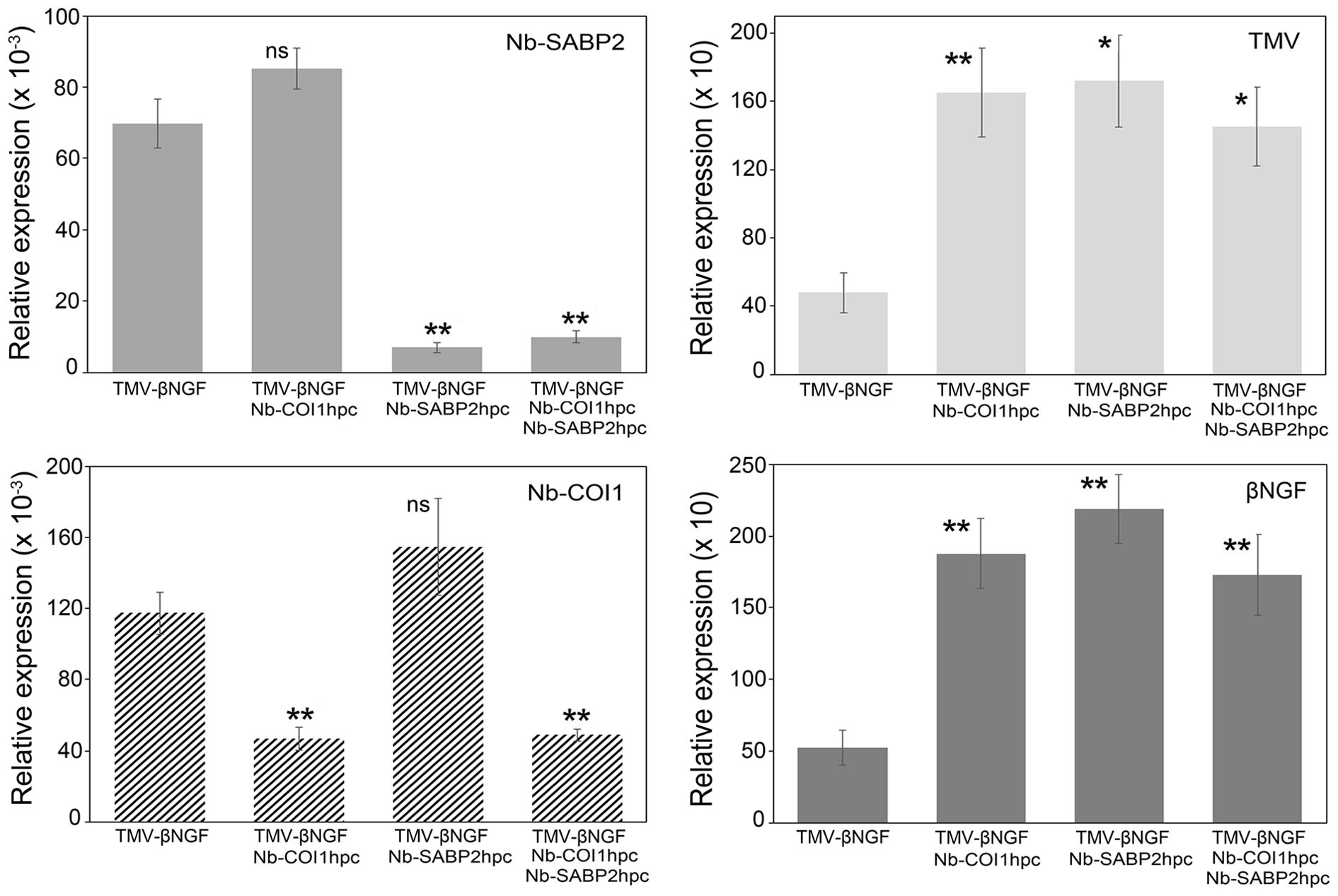
Figure 4. TMV and βNGF RNA accumulation in Nb-COI1 and Nb-SABP2-silenced N. benthamiana leaves. N. benthamiana leaves were agroinfiltrated with four combinations of A. tumefaciens cultures, namely, pLH-TMV-βNGF, pLH-TMV-βNGF/pLH-35S-COI1-INT-COI1, pLH-TMV-βNGF/pLH-35S-SABP2-INT-SABP2, pLH-TMV-βNGF/pLH-35S-SABP2-INT-SABP2/pLH-35S-COI1-INT-COI1. Leaf samples for RNA isolation were collected at 6 dpi. RNA levels were quantified by qPCR using primers specific to the Nb-SABP2, Nb-COI1, TMV and βNGF. Values represent the means with SE (n=6). Asterisks indicate significance as determined by the Mann-Whitney test, with * and ** denoting p < 0.05 and p < 0.01, respectively. Not significant values are determined as ns.
Positive correlation was also found between the accumulation of viral and βNGF RNA. The βNGF gene showed significant fold change values of 3.6, 4.2 and 3.3 in the pLH-TMV-βNGF/pLH-35S-COI1-INT-COI1, pLH-TMV-βNGF/pLH-35S-SABP2-INT-SABP2 and pLH-TMV-βNGF/pLH-35S-SABP2-INT-SABP2/pLH-35S-COI1-INT-COI1 samples, respectively. These results suggest that the RNAi constructs for the Nb-SABP2 and Nb-COI1 are functional in a transient assay and the suppression of these genes has positive effect on foreign protein transcript accumulation.
Downregulation of the Nb-SABP2 and Nb-COI1 genes enhanced transient recombinant protein accumulation
Having identified the beneficial effect of the Nb-SABP2 and Nb-COI1 gene suppression on transient gene delivery system at RNA level (Figures 3, 4), we assessed the recombinant protein accumulation. Investigation of N. benthamiana leaves agroinfiltrated with the pLH-TMV-GFP under UV light revealed stronger GFP fluorescence in the presence of the Nb-SABP2 and Nb-COI1 RNAi silencing constructs (Figure 5A). Following a visual observation, the expression of GFP recombinant protein was analyzed over time in N. benthamiana leaves by specific ELISA. This analysis showed that the GFP accumulation increased steadily from day 3 to 7 and reached a peak on day 7 (Figure 5B). Compared to samples agroinfiltrated with the pLH-TMV-GFP alone, higher recombinant protein amounts were detected in leaf tissue co-infiltrated with the A. tumefaciens cultures containing the pLH-TMV-GFP and RNAi expression vectors. Production of GFP increased by 2.4-fold for the pLH-TMV-GFP/pLH-35S-COI1-INT-COI1 combination of expression constructs, by 2.6-fold for the pLH-TMV-GFP/pLH-35S-SABP2-INT-SABP2 combination of expression constructs and by 2.2-fold for the pLH-TMV-GFP/pLH-35S-SABP2-INT-SABP2/pLH-35S-COI1-INT-COI1 combination of expression constructs. The highest GFP accumulation level 560 ± 30 µg/g fresh weight was detected at 7 dpi in the N. benthamiana leaves co-infiltrated with the pLH-35S-SABP2-INT-SABP2 RNAi construct.
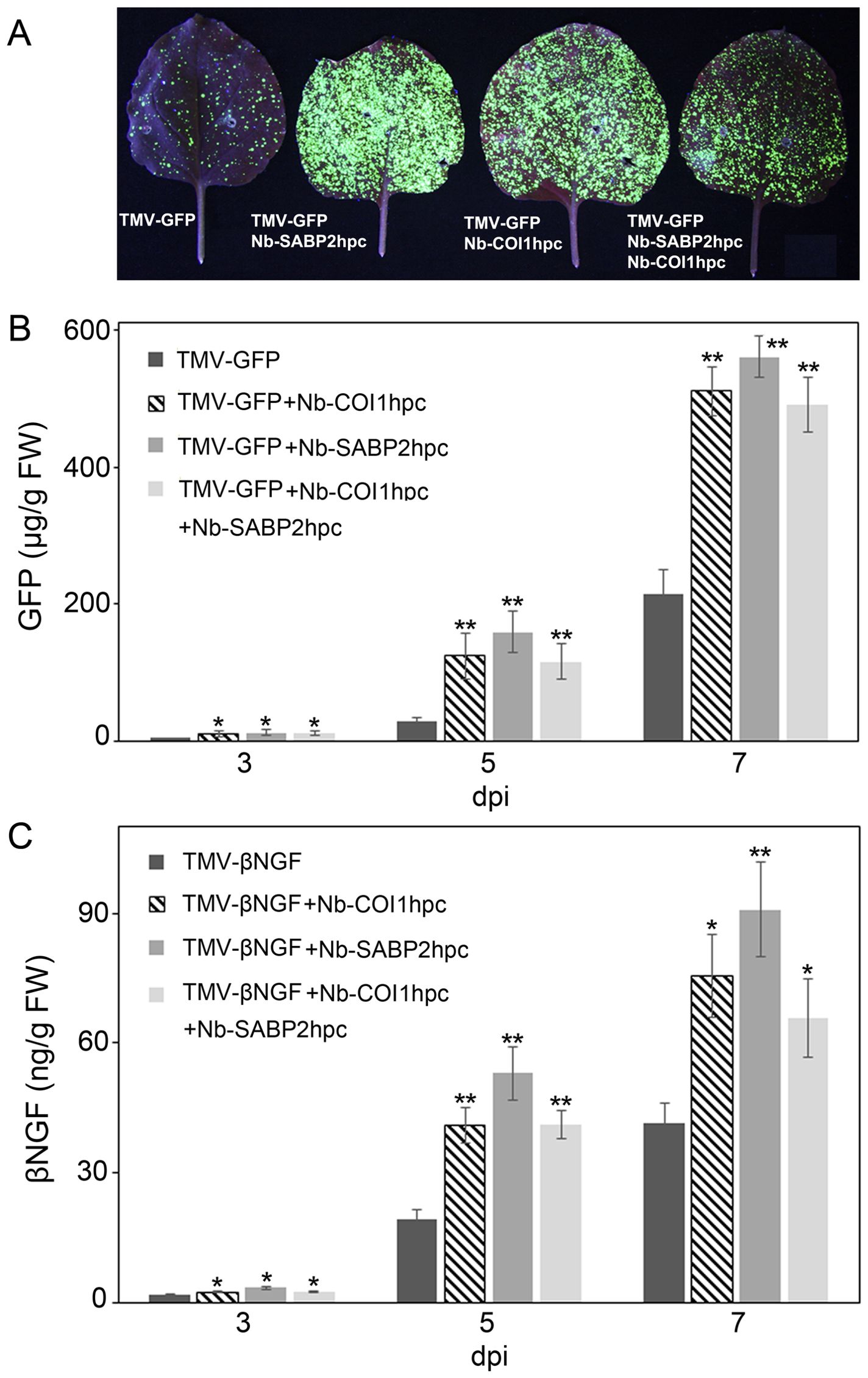
Figure 5. Transient production of GFP and βNGF recombinant proteins in Nb-COI1 and Nb-SABP2-silenced N. benthamiana leaves. (A) GFP visualization in agroinfiltrated leaves. N. benthamiana plants were co-agroinfiltrated with Nb-COI1 and Nb-SABP2 RNAi silencing constructs and pLH-TMV-GFP. Images were taken at 5 dpi. The representative leaves coinfiltrated with the pLH-TMV-GFP (1), pLH-TMV-GFP/pLH-35S-SABP2-INT-SABP2 (2), and pLH-TMV-GFP/pLH-35S-COI1-INT-COI1 (3), pLH-TMV-GFP/pLH-35S-SABP2-INT-SABP2/pLH-35S-COI1-INT-COI1 (4) are shown. (B) Time course analysis of GFP production. The leaves of N. benthamiana plants co-agroinfiltrated with the pLH-TMV-GFP, pLH-TMV-GFP/pLH-35S-SABP2-INT-SABP2 and pLH-TMV-GFP/pLH-35S-COI1-INT-COI1, pLH-TMV-GFP/pLH-35S-SABP2-INT-SABP2/pLH-35S-COI1-INT-COI1 A. tumefaciens cultures were sampled at 3, 5 and 7 dpi and analysed by specific ELISA for GFP accumulation. (C) Time course analysis of βNGF production. The leaves of N. benthamiana plants co-infiltrated with the pLH-TMV-βNGF, pLH-TMV-βNGF/pLH-35S-COI1-INT-COI1, pLH-TMV-βNGF/pLH-35S-SABP2-INT-SABP2, pLH-TMV-βNGF/pLH-35S-SABP2-INT-SABP2/pLH-35S-COI1-INT-COI1 A. tumefacines cultures were sampled at 3, 5 and 7 dpi and analysed by specific ELISA for βNGF accumulation. Values represent the means with SE (n=8). Asterisks indicate significance as determined by the Mann-Whitney test, with * and ** denoting p < 0.05 and p < 0.01, respectively.
The expression profile of βNGF protein was similar to that of the GFP protein with the accumulation peak at 7 dpi (Figure 5C). The ELISA data revealed that co-expression of the pLH-TMV-βNGF/pLH-35S-COI1-INT-COI1, pLH-TMV-βNGF/pLH-35S-SABP2-INT-SABP2 and pLH-TMV-βNGF/pLH-35S-SABP2-INT-SABP2/pLH-35S-COI1-INT-COI1 constructs increased βNGF accumulation over the pLH-TMV-βNGF control in 1.8, 2.2 and 1.6-folds, respectively. The highest βNGF production 91 ± 10 ng/g fresh weight was observed at 7 dpi when the pLH-TMV-βNGF and pLH-35S-SABP2-INT-SABP2 were combined.
To investigate whether RNAi-mediated suppression of the Nb-SABP2 and Nb-COI1 plant defense related genes can facilitate the accumulation of βNGF delivered by the PVX-based expression vector N. benthamiana plants were agroinfiltrated with the A. tumefaciens cultures carrying following constructs: the pLH-PVX-βNGF/pLH-35S-COI1-INT-COI1, pLH-PVX-βNGF/pLH-35S-SABP2-INT-SABP2 and pLH-PVX-βNGF/pLH-35S-SABP2-INT-SABP2/pLH-35S-COI1-INT-COI1. Five days after inoculation the agroinfiltrated leaves were examined using ELISA assay to determine the βNGF production. The results of this analysis are shown in Figure 6. In line with the results obtained for the pLH-TMV-βNGF expression vector knockdown of the Nb-SABP2 and Nb-COI1 genes had positive impact on the βNGF accumulation. Co-infiltration of the pLH-35S-COI1-INT-COI1 caused 2.6-fold enhancement of the βNGF amount. Combination of the pLH-PVX-βNGF and pLH-35S-SABP2-INT-SABP2 led to 3.3-fold increase in βNGF content. Finally, simultaneous suppression of Nb-SABP2 and Nb-COI1 gene expression by the corresponding RNAi constructs resulted in 2.4 fold increase in βNGF accumulation. The best performing combination (pLH-PVX-βNGF/pLH-35S-SABP2-INT-SABP2) yielded 129 ± 25 ng/g fresh weight of βNGF.
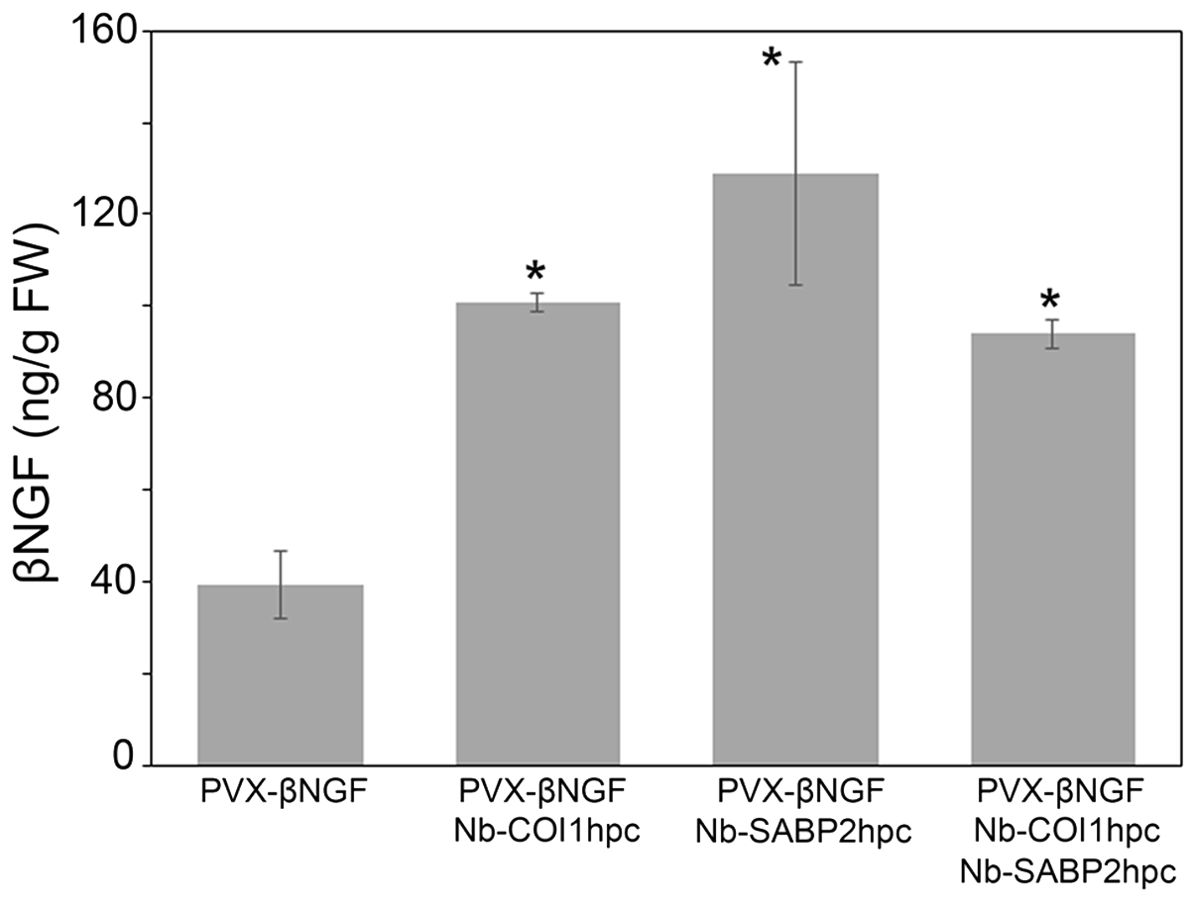
Figure 6. Transient production of the βNGF recombinant protein delivered by the PVX-based vector in the Nb-COI1 and Nb-SABP2-silenced N. benthamiana leaves. The leaves of N. benthamiana plants co-infiltrated with the pLH-PVX-βNGF, pLH-PVX-βNGF/pLH-35S-COI1-INT-COI1, pLH-PVX-βNGF/pLH-35S-SABP2-INT-SABP2, pLH-PVX-βNGF/pLH-35S-SABP2-INT-SABP2/pLH-35S-COI1-INT-COI1 A. tumefacines cultures were sampled at 5 dpi and analyzed by specific ELISA for βNGF accumulation. Values represent the means with SE (n=6). Asterisks indicate significance as determined by the Mann-Whitney test, with * denoting p < 0.05.
The size and relative abundance of GFP and βNGF were estimated using Western blot analysis in total protein extracts from agroinfiltrated plant tissue. In the Nb-SABP2 and Nb-COI1 downregulated plants as well as in the control plants agoinfiltrated only with the pLH-PVX-βNGF and pLH-TMV-GFP the produced proteins had the expected size of 27 kDa for GFP and 35-37 kDa for βNGF (Supplementary Figure S1).
Discussion
The success of transient expression depends on both host plant and expression vector delivery component. Recent overview of the molecular changes in agroinfiltrated N. benthamiana leaves demonstrated that hybrid expression systems consisting of A. tumefaciens and binary vector trigger the plant defense response and this can limit the recombinant protein yield (Hamel et al., 2023). In this study we suppressed N. benthamiana immunity through knockdown of endogenous genes involved in plant hormone metabolism. The expression pattern of the Nb-SABP2 and Nb-COI1 genes assessed by qPCR analysis revealed that both genes were upregulated upon agroinfiltration conferring their involvement in plant immune reaction. The association of the SABP2 with SA-dependent plant defense response to biotic stress has been shown by several research groups. In Nicotiana tabacum the Nt-SABP2 gene expression was induced after TMV inoculation (Kumar and Klessig, 2003). Similarly, the functional homologs of the Nt-SABP2 gene from A. thaliana, At-MES1, At-MES7 and At-MES9 were transcriptionally upregulated during infection with Pseudomonas syringae (Vlot et al., 2008). Our data are also consistent with the experiments demonstrating the positive role of a key regulator of JA signaling COI1 in plant defense. For example, in Sacharum sp the expression levels of the Ss-COI1–4b and Ss-COI1–3b genes were increased in smut-resistant cultivar YC05–179 and downregulated in smut-susceptible cultivar ROC22 (Sun et al., 2022). Similarly, the higher Ta-COI1 transcript amounts were observed during early response to Blumeria graminis in resistant Triticum aestivum variety in comparison to susceptible one (Liu et al., 2018).
To examine whether suppression of plant immune system can facilitate the TMV multiplication we targeted the Nb-SABP2 and Nb-COI1 genes with corresponding dsRNAs. Downregulation of these genes increased susceptibility to TMV virus carrying GFP and βNGF sequences, which was manifested by the enhanced viral RNA accumulation in agroinfiltrated leaves. Our data are in agreement with the previous reports demonstrating that SA functions as a positive regulator of TMV resistance. For instance, Kumar and Klessig (2003) showed that the Nt-SABP2-silenced N. tabacum plants accumulated higher levels of TMV coat protein in comparison to control plants. In N. benthamiana knockdown of SA-metabolism-related genes including Nb-SABP2, Nb-ICS1 (isochorismate synthase), Nb-NPR1 (non-expresser of PR gene 1), Nb-SAMT (SA methyl transferase) strongly increased susceptibility to TMV (Zhu et al., 2014). The role of JA in TMV resistance has not been fully clarified. Our data are in accordance with the report showing the positive role of the JA biosynthetic and signaling genes in antiviral response: downregulation of the Nb-COI1, Nb-OPR3 (12-oxo-phytodienoic acid reductase), NbJMT (jasmonic acid carboxyl methyltransferase) genes boosted accumulation of TMV (Zhu et al., 2014). In contrast, Oka and co-workers demonstrated that silencing of the JA receptor COI1 resulted in resistance against TMV in tobacco containing N gene (Oka et al., 2013). These authors suggested that the JA signaling is indirectly responsible for TMV susceptibility through modification of SA-dependent and SA-independent resistance pathways.
The usefulness of strategy based on plant defense response modification for biotechnological purposes was demonstrated for GFP and βNGF recombinant proteins: the enhanced accumulation of TMV correlated with the increased amounts of GFP and βNGF transcripts. Furthermore, ELISA investigation confirmed this observation at protein level. For TMV-based expression vector, the downregulation of the Nb-SABP2, Nb-COI1 and Nb-SABP2/Nb-COI1 genes enhanced GFP accumulation by 2.4, 2.6 and 2.2 fold, respectively. Co-expression of the pLH-TMV-βNGF and RNAi constructs increased βNGF yield by 1.8-fold for the pLH-TMV-βNGF/pLH-35S-COI1-INT-COI1 combination, 2.2-fold for the pLH-TMV-βNGF/pLH-35S-SABP2-INT-SABP2 combination and 1.6-fold for the pLH-TMV-βNGF/pLH-35S-SABP2-INT-SABP2/pLH-35S-COI1-INT-COI1 combination. These results are in content with data that have been reported for a number of strategies for plant host optimization. In particular, transient co-delivery of virus-derived cell cycle regulator genes elevated GUS accumulation about 2-3 fold (Norkunas et al., 2018). Similar increase in production of GFP and scFv-TM43-E10 antibody fragment was observed in N. benthamiana leaves, which were co-infiltrated with the At-CycD2 and At-CDC27a plant cell regulators and TMV-based vector containing the GFP and scFvTM43-E10 sequences (Kopertekh and Schiemann, 2019). Modification of gene silencing machinery through simultaneous knockout of Nb-DCL2 and Nb-DCL4 genes using CRISPR/Cas9 technology enhanced the production of human fibroblast growth factor by 9 fold (Matsuo, 2022). In the same manner, the CRISPR/Cas9-mediated editing of the Nb-RDR6 gene elevated GFP accumulation in N. benthamiana by 2.5-fold (Matsuo and Atsumi, 2019).
A combined downregulation of multiple genes may have an additive effect to maximize the recombinant protein yield in transient expression. The simultaneous silencing of the Nb-SABP2 and Nb-COI1 genes did not result in synergistic effect compared to the suppression of the Nb-SABP2 and Nb-COI1 alone: we observed lower RNA and protein accumulation levels for TMV, GFP and βNGF in double-silenced plants. Data reported in several papers indicated the importance of the crosstalk between JA and SA to determine the degree of TMV resistance (Oka et al., 2013; Zhu et al., 2014). It can be suggested that in the Nb-SABP2/Nb-COI1-suppressed leaves the balance between endogenous JA and SA did not provide the superior cellular conditions for TMV infection in comparison to the individual silencing of the Nb-SABP2 and Nb-COI1 genes. Therefore, separated knockdown of the Nb-SABP2 and Nb-COI1 genes is more effective for enhanced recombinant protein production.
In summary, we demonstrated in this study that the components of Agrobacterium-mediated vector delivery system upregulate the Nb-SABP2 and Nb-COI1 genes, which take part in SA and JA-mediated plant defense response. The suppression of plant immunity through silencing of these genes improved recombinant protein yield as was exemplified by the full TMV-based expression vector. We suppose that this strategy can be useful for several commercial transient expression systems including GENEWARE® (Shivprasad et al., 1999), TRBO (Lindbo, 2007), magnICON® (Gleba et al., 2007), TMV launch vector (Musiychuk et al., 2007) utilizing TMV as the vector backbone. This approach might be extended to other virus-based expression vectors given that other plant-virus-based expression systems are limited by the SA- and JA-mediated virus resistance. Indeed, we demonstrated the increased βNGF production in the presence of the Nb-SABP2 and Nb-COI1 RNAi silencing constructs when the βNGF encoding sequence was delivered by the PVX-based virus vector.
It is important to note that this strategy is might be incompatible with the application of expression vectors containing strong suppressors of gene silencing such as P19 protein of Tomato bushy stunt virus (TBSV) (Scholthof, 2006). However, the commercial plant virus-based expression vectors listed above do not utilize strong silencing suppressors.
Data availability statement
The raw data supporting the conclusions of this article will be made available by the author, without undue reservation.
Author contributions
LK: Writing – review & editing.
Funding
The author(s) declare financial support was received for the research, authorship, and/or publication of this article. This research was financed in frame of the self-funded JKI project by the German Federal Ministry of Food and Agriculture (BMEL).
Acknowledgments
The technical support of Cornelia Freyer and Dominik Meindl is greatly acknowledged.
Conflict of interest
The author declares that the research was conducted in the absence of any commercial or financial relationships that could be construed as a potential conflict of interest.
Publisher’s note
All claims expressed in this article are solely those of the authors and do not necessarily represent those of their affiliated organizations, or those of the publisher, the editors and the reviewers. Any product that may be evaluated in this article, or claim that may be made by its manufacturer, is not guaranteed or endorsed by the publisher.
Supplementary material
The Supplementary Material for this article can be found online at: https://www.frontiersin.org/articles/10.3389/fpls.2024.1453930/full#supplementary-material
References
Anand, A., Uppalapati, S. R., Ryu, C. M., Allen, S. N., Kang, L., Tang, Y., et al. (2008). Salicylic acid and systemic acquired resistance play a role in attenuating crown gall disease caused by Agrobacterium tumefaciens. Plant Physiol. 146, 703–715. doi: 10.1104/pp.107.111302
Bally, J., Jung, H., Mortimer, C., Naim, F., Philips, J. G., Hellens, R., et al. (2018). The rise and rise of Nicotiana benthamiana: a plant for all reasons. Annu. Rev. Phytopathol. 56, 405–426. doi: 10.1146/annurev-phyto-080417050141
Chapman, S., Kavanagh, T., Baulcombe, D. (1992). Potato virus X as a vector for gene expression in plants. Plant J. 2, 549–557. doi: 10.1046/j.1365-313x.1992.t01-24-00999.x
Chini, A., Boter, M., Solano, R. (2009). Plant oxylipins: COI1/JAZs/MYC2 as the core jasmonic acid-signalling module. FEBS J. 276, 4682–4692. doi: 10.1111/j.1742-4658.2009.07194.x
Dodds, I., Chen, C., Buscaill, P., van der Hoorn, R. A. L. (2023). Depletion of the NbCORE receptor drastically improves agroinfiltration productivity in older Nicotiana benthamiana plants. Plant Biotechnol. J. 21, 1103–1105. doi: 10.1111/pbi.14037
Fischer, R., Vaquero-Martin, C., Sack, M., Drossard, J., Emans, N., Commandeur, U. (1999). Towards molecular farming in the future: transient protein expression in plants. Biotechnol. Appl. Biochem. 30, 113–116. doi: 10.1111/j.1470-8744.1999.tb00900.x
Forouhar, F., Yang, Y., Kumar, D., Chen, Y., Fridman, E., Park, S. W., et al. (2005). Structural and biochemical studies identify tobacco SABP2 as a methyl salicylate esterase and implicate it in plant innate immunity. Proc. Natl. Acad. Sci. U. S. A. 102, 1773–1778. doi: 10.1073/pnas.0409227102
García-Marcos, A., Pacheco, R., Manzano, A., Aguilar, E., Tenllado, F. (2013). Oxylipin biosynthesis genes positively regulate programmed cell death during compatible infections with the synergistic pair potato virus X-potato virus Y and Tomato spotted wilt virus. J. Virol. 87, 5769–5783. doi: 10.1128/JVI.03573-12
Gleba, Y., Klimyuk, V., Marillonnet, S. (2005). Magnifection - a new platform for expressing recombinant vaccines in plants. Vaccine 23, 2042–2048. doi: 10.1016/j.vaccine.2005.01.006
Gleba, Y., Klimyuk, V., Marillonnet, S. (2007). Viral vectors for the expression of proteins in plants. Curr. Opin. Biotechnol. 18, 134–141. doi: 10.1016/j.copbio.2007.03.002
Goodin, M. M., Zaitlin, D., Naidu, R. A., Lommel, S. A. (2008). Nicotiana benthamiana: its history and future as a model for plant-pathogen interactions. Mol. Plant Microbe Interact. 2, 1015–1026. doi: 10.1094/MPMI-21-8-1015
Hamel, L. P., Tardif, R., Poirier-Gravel, F., Rasoolizadeh, A., Brosseau, C., Giroux, G., et al. (2023). Molecular responses of agroinfiltrated Nicotiana benthamiana leaves expressing suppressor of silencing P19 and influenza virus-like particles. Plant Biotechnol. J. 22, 1078–1100. doi: 10.1111/pbi.14247
Holsters, M., de Waele, D., Depicker, A., Messens, E., van Montagu, M., Schell, J. (1978). Transfection and transformation of A. tumefaciens. Mol. Gen. Genet. 163, 181–187. doi: 10.1007/BF00267408
Kapila, J., De Rycke, R., Van Montagu, M., Angenon, G. (1997). An Agrobacterium-mediated transient gene expression system for intact leaves. Plant Sci. 122, 101–108. doi: 10.1016/S0168-9452(96)04541-4
Kopertekh, L., Jüttner, G., Schiemann, J. (2004). PVX-Cre-mediated marker gene elimination from transgenic plants. Plant Mol. Biol. 55, 491–500. doi: 10.1007/s11103-004-0237-8
Kopertekh, L., Reichardt, S. (2021). At-CycD2 enhances accumulation of above-ground biomass and recombinant proteins in transgenic Nicotiana benthamiana plants. Front. Plant Sci. 12, 712438. doi: 10.3389/fpls.2021.712438
Kopertekh, L., Reichardt, S. (2022). Effect of the At-CDC27a gene on Nicotiana benthamiana phenotype and accumulation of recombinant proteins. Front. Plant Sci. 13, 1042446. doi: 10.3389/fpls.2022.1042446
Kopertekh, L., Schiemann, J. (2019). Enhanced foreign protein accumulation in Nicotiana benthamiana leaves co-infiltrated with a TMV vector and plant cell cycle regulator genes. Transgenic Res. 28, 411–417. doi: 10.1007/s11248-019-00128-3
Kumar, D., Klessig, D. F. (2003). High-affinity salicylic acid-binding protein 2 is required for plant innate immunity and has salicylic acid-stimulated lipase activity. Proc. Natl. Acad. Sci. U. S. A. 100, 16101–16106. doi: 10.1073/pnas.0307162100
Lee, W. S., Fu, S. F., Verchot-Lubicz, J., Carr, J. P. (2011). Genetic modification of alternative respiration in Nicotiana benthamiana affects basal and salicylic acid-induced resistance to potato virus X. BMC Plant Biol. 11, 41. doi: 10.1186/1471-2229-11-41
Li, N., Han, X., Feng, D., Yuan, D., Huang, L.-J. (2019). Signaling crosstalk between salicylic acid and ethylene/jasmonate in plant defense: Do we understand what they are whispering? Int. J. Mol. Sci. 20, 671. doi: 10.3390/ijms20030671
Lindbo, J. A. (2007). TRBO: a high-efficiency tobacco mosaic virus RNA-based overexpression vector. Plant Physiol. 145, 1232–1240. doi: 10.1104/pp.107.106377
Liu, X., Wang, J., Fan, B., Shang, Y., Sun, Y., Dang, C., et al. (2018). A COI1 gene in wheat contributes to the early defence response against wheat powdery mildew. J. Phytopathol. 166, 116–122. doi: 10.1111/jph.12667
Liu, Y., Schiff, M., Dinesh-Kumar, S. P. (2004). Involvement of MEK1 MAPKK, NTF6 MAPK, WRKY/MYB transcription factors, COI1 and CTR1 in N-mediated resistance to tobacco mosaic virus. Plant J. 38, 800–809. doi: 10.1111/j.1365-313X.2004.02085.x
Lomonossoff, G. P., D’Aoust, M. A. (2016). Plant-produced biopharmaceuticals: A case of technical developments driving clinical deployment. Science 353, 1237–1240. doi: 10.1126/science.aaf6638
Ludman, M., Burgyán, J., Fátyol, K. (2017). Crispr/Cas9 mediated inactivation of argonaute 2 reveals its differential involvement in antiviral responses. Sci. Rep. 7, 1010. doi: 10.1038/s41598-017-01050-6
Matsuo, K. (2022). CRISPR/Cas9-mediated knockout of the DCL2 and DCL4 genes in Nicotiana benthamiana and its productivity of recombinant proteins. Plant Cell Rep. 41, 307–317. doi: 10.1007/s00299-021-02809-y
Matsuo, K., Atsumi, G. (2019). CRISPR/Cas9-mediated knockout of the RDR6 gene in Nicotiana benthamiana for efficient transient expression of recombinant proteins. Planta 250, 463–473. doi: 10.1007/s00425-019-03180-9
Matsuo, K., Matsumura, T. (2017). Repression of the DCL2 and DCL4 genes in Nicotiana benthamiana plants for the transient expression of recombinant proteins. J. Biosci. Bioeng. 124, 215–220. doi: 10.1016/j.jbiosc.2017.02.019
Mlynárová, L., Nap, J. P. (2003). A self-excising Cre recombinase allows efficient recombination of multiple ectopic heterospecific lox sites in transgenic tobacco. Transgenic Res. 12, 45–57. doi: 10.1023/a:1022112221427
Musiychuk, K., Stephenson, N., Bi, H., Farrance, C. E., Orozovic, G., Brodelius, M., et al. (2007). A launch vector for the production of vaccine antigens in plants. Influenza other Respir. viruses 1, 19–25. doi: 10.1111/j.1750-2659.2006.00005.x
Ngou, B. P. M., Jones, J. D. G., Ding, P. (2022). Plant immune networks. Trends Plant Sci. 27, 255273. doi: 10.1016/j.tplants.2021.08.012
Norkunas, K., Harding, R., Dale, J., Dugdale, B. (2018). Improving agroinfiltration-based transient gene expression in Nicotiana benthamiana. Plant Methods 14, 71. doi: 10.1186/s13007-018-0343-2
Oka, K., Kobayashi, M., Mitsuhara, I., Seo, S. (2013). Jasmonic acid negatively regulates resistance to Tobacco mosaic virus in tobacco. Plant Cell Physiol. 54, 1999–2010. doi: 10.1093/pcp/pct137
Pacheco, R., García-Marcos, A., Manzano, A., de Lacoba, M. G., Camañes, G., García-Agustín, P., et al. (2012). Comparative analysis of transcriptomic and hormonal responses to compatible and incompatible plant-virus interactions that lead to cell death. Mol. Plant Microbe Interact. 25, 709–723. doi: 10.1094/MPMI-11-11-0305
Park, S. W., Kaimoyo, E., Kumar, D., Mosher, S., Klessig, D. F. (2007). Methyl salicylate is a critical mobile signal for plant systemic acquired resistance. Science 318, 113–116. doi: 10.1126/science.1147113
Peyret, H., Lomonossoff, G. P. (2015). When plant virology met Agrobacterium: the rise of the deconstructed clones. Plant Biotechnol. J. 13, 1121–1135. doi: 10.1111/pbi.12412
Reichel, C., Mathur, J., Eckes, P., Langenkemper, K., Koncz, C., Schell, J., et al. (1996). Enhanced green fluorescence by the expression of an Aequorea victoria green fluorescent protein mutant in mono- and dicotyledonous plant cells. Proc. Natl. Acad. Sci. U. S. A. 93, 5888–5893. doi: 10.1073/pnas.93.12.5888
Schillberg, S., Finnern, R. (2021). Plant molecular farming for the production of valuable proteins - Critical evaluation of achievements and future challenges. J. Plant Physiol. 258-259, 153359. doi: 10.1016/j.jplph.2020.153359
Schillberg, S., Raven, N., Spiegel, H., Rasche, S., Buntru, M. (2019). Critical analysis of the commercial potential of plants for the production of recombinant proteins. Front. Plant Sci. 10. doi: 10.3389/fpls.2019.00720
Scholthof, H. B. (2006). The Tombusvirus-encoded P19: from irrelevance to elegance. Nat. Rev. Microbiol. 4, 405–411. doi: 10.1038/nrmicro1395
Shang, J., Xi, D. H., Xu, F., Wang, S. D., Cao, S., Xu, M. Y., et al. (2011). A broad-spectrum, efficient and nontransgenic approach to control plant viruses by application of salicylic acid and jasmonic acid. Planta 233, 299–308. doi: 10.1007/s00425-010-1308-5
Shivprasad, S., Pogue, G. P., Lewandowski, D. J., Hidalgo, J., Donson, J., Grill, L. K., et al. (1999). Heterologous sequences greatly affect foreign gene expression in tobacco mosaic virus-based vectors. Virology 255, 312–323. doi: 10.1006/viro.1998.9579
Sun, T., Meng, Y., Cen, G., Feng, A., Su, W., Chen, Y., et al. (2022). Genome-wide identification and expression analysis of the coronatine-insensitive 1 (COI1) gene family in response to biotic and abiotic stresses in Saccharum. BMC Genomics 23, 38. doi: 10.1186/s12864-021-08255-0
Töpfer, R., Maas, C., Horicke-Grandpierre, C., Schell, J., Steinbiss, H. H. (1993). Expression vectors for high-level gene expression in dicotyledonous and monocotyledonous plants. Meth. Enzymol. 217, 67–87. doi: 10.1016/0076-6879(93)17056-b
Vlot, A. C., Liu, P. P., Cameron, R. K., Park, S. W., Yang, Y., Kumar, D., et al. (2008). Identification of likely orthologs of tobacco salicylic acid-binding protein 2 and their role in systemic acquired resistance in Arabidopsis thaliana. Plant J. 56, 445–456. doi: 10.1111/j.1365-313X.2008.03618.x
Wang, X., Prokhnevsky, A. I., Skarjinskaia, M., Razzak, M. A., Streatfield, S. J., Lee, J. Y. (2023). Facilitating viral vector movement enhances heterologous protein production in an established plant system. Plant Biotechnol. J. 21, 635–645. doi: 10.1111/pbi.13977
Xie, D. X., Feys, B. F., James, S., Nieto-Rostro, M., Turner, J. G. (1998). COI1: an Arabidopsis gene required for jasmonate-regulated defense and fertility. Science 280, 1091–1094. doi: 10.1126/science.280.5366.1091
Xu, L., Liu, F., Lechner, E., Genschik, P., Crosby, W. L., Ma, H., et al. (2002). The SCF(COI1) ubiquitin-ligase complexes are required for jasmonate response in Arabidopsis. Plant Cell. 14, 1919–1935. doi: 10.1105/tpc.003368
Yuan, Z. C., Edlind, M. P., Liu, P., Saenkham, P., Banta, L. M., Wise, A. A., et al. (2007). The plant signal salicylic acid shuts down expression of the vir regulon and activates quormone-quenching genes in Agrobacterium. Proc. Natl. Acad. Sci. U. S. A. 104, 11790–11795. doi: 10.1073/pnas.0704866104
Keywords: Nicotiana benthamiana, Nb-COI1, Nb-SABP2, plant defense response, recombinant proteins, transient expression
Citation: Kopertekh L (2024) Improving transient expression in N. benthamiana by suppression of the Nb-SABP2 and Nb-COI1 plant defence response related genes. Front. Plant Sci. 15:1453930. doi: 10.3389/fpls.2024.1453930
Received: 24 June 2024; Accepted: 12 August 2024;
Published: 09 September 2024.
Edited by:
Kevin Yueju Wang, University of Pikeville, United StatesReviewed by:
Jinping Zhao, Texas A and M University, United StatesBeatriz Xoconostle-Cázares, National Polytechnic Institute of Mexico (CINVESTAV), Mexico
Copyright © 2024 Kopertekh. This is an open-access article distributed under the terms of the Creative Commons Attribution License (CC BY). The use, distribution or reproduction in other forums is permitted, provided the original author(s) and the copyright owner(s) are credited and that the original publication in this journal is cited, in accordance with accepted academic practice. No use, distribution or reproduction is permitted which does not comply with these terms.
*Correspondence: Lilya Kopertekh, bGlseWEua29wZXJ0ZWtoQGp1bGl1cy1rdWVobi5kZQ==