- 1Shanghai Key Laboratory of Plant Functional Genomics and Resources, Shanghai Chenshan Botanical Garden, Shanghai Chenshan Plant Science Research Center, Chinese Academy of Sciences, Shanghai, China
- 2State Key Laboratory of Plant Molecular Genetics, Chinese Academy of Sciences (CAS) Center for Excellence in Molecular Plant Sciences, Chinese Academy of Sciences, Shanghai, China
- 3University of Chinese Academy of Sciences, Beijing, China
Stachydrine, also known as proline betaine, is a prominent constituent of traditional Chinese herb Leonurus japonicus, renowned for its significant pharmacological effects. Widely distributed in plants like Leonurus and Citrus aurantium, as well as various bacteria, stachydrine serves pivotal physiological functions across animal, plant, and bacterial kingdoms. This review aims to summarizes diverse roles and mechanisms of stachydrine in addressing cardiovascular and cerebrovascular diseases, neuroprotection, anticancer activity, uterine regulation, anti-inflammatory response, obesity management, and respiratory ailments. Notably, stachydrine exhibits cardioprotective effects via multiple pathways encompassing anti-inflammatory, antioxidant, anti-apoptotic, and modulation of calcium handling functions. Furthermore, its anti-cancer properties inhibit proliferation and migration of numerous cancer cell types. With a bi-directional regulatory effect on uterine function, stachydrine holds promise for obstetrics and gynecology-related disorders. In plants, stachydrine serves as a secondary metabolite, contributing to osmotic pressure regulation, nitrogen fixation, pest resistance, and stress response. Similarly, in bacteria, it plays a crucial osmoprotective role, facilitating adaptation to high osmotic pressure environments. This review also addresses ongoing research on the anabolic metabolism of stachydrine. While the biosynthetic pathway remains incompletely understood, the metabolic pathway is well-established. A deeper understanding of stachydrine biosynthesis holds significance for elucidating its mechanism of action, advancing the study of plant secondary metabolism, enhancing drug quality control, and fostering new drug development endeavors.
1 Introduction
Stachydrine, also recognized as proline betaine and N, N-dimethyl-L-proline, represents an alkaloid characterized by the molecular structure of (2S)-1,1-dimethylpyrrolidine-2-carboxylic acid (shown in Figure 1). First isolated by Steenbock in 1918, stachydrine is noted for its biological activities and pharmaceutical potential (Connor et al., 1973). It is a key component of the traditional Chinese medicinal herb Leonurus, commonly known as motherwort, which is renowned for its pharmacological effectiveness (Kuchta et al., 2013).
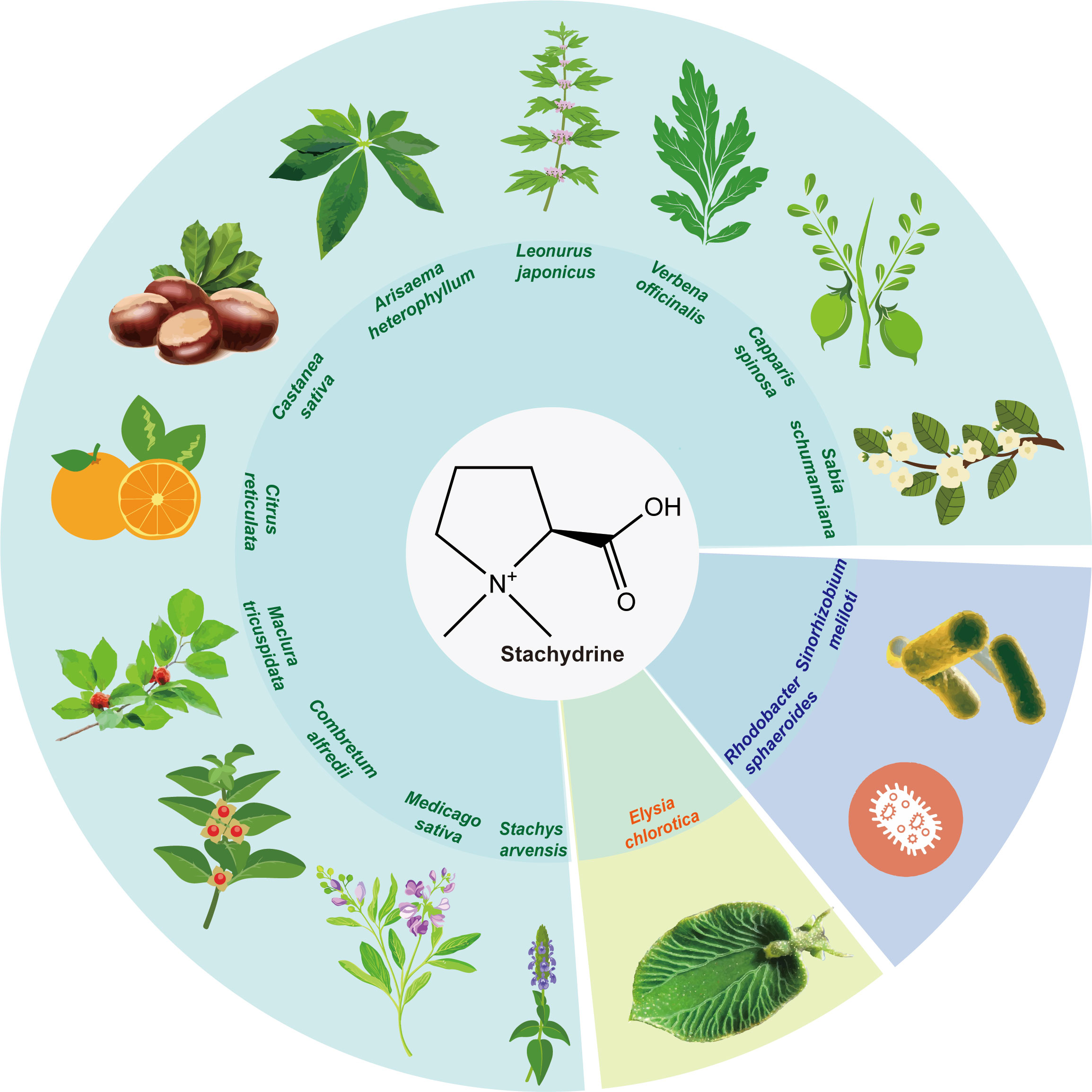
Figure 1 The natural occurrence of stachydrine. Stachydrine is found in the following plants: Leonurus, Capparis spinosa, Castanea sativa, Citrus reticulata, Medicago sativa, Sabia schumanniana, Maclura tricuspidata, Verbena officinalis, Arisaema heterophyllum, Combretum alfredii, and Stachys arvensis. In addition to these plants, stachydrine may also be found in other plants in the same genus as these plants. It is also found in the Elysia chlorotica and Sinorhizobium meliloti and Rhodobacter sphaeroides.
Leonurus, belonging to the Labiatae family, is rich in alkaloids, among which stachydrine constitutes a significant proportion, ranging from 0.59% to 1.72% (Dai et al., 2016a). Revered in ancient medical texts such as “Shennong’s Classic of a Hundred Herbs,” Leonurus, classified as ‘superior’ and non-toxic, is extensively used across China, Korea, and Japan, primarily for managing gynecological disorders. Furthermore, it has been employed for centuries in European countries to address neurological and functional heart conditions (Li et al., 2024). According to the Chinese Pharmacopoeia, Leonurus manifests various therapeutic effects, including blood circulation activation, menstruation regulation, diuretic and anti-inflammatory properties, and heat-clearing detoxification (The Pharmacopoeia Committee, 2020). Its widespread use is evidenced by the annual sales of Leonurus-related products in China, which amount to approximately one billion RMB. Chemical analysis has identified over 140 components from Leonurus, predominantly alkaloids, flavonoids, and terpenoids, supplemented by substantial potassium and vitamin content (Shang et al., 2014). Alkaloids, such as leonurine, stachydrine, betaine, and trigonelline, have been recognized as the principal bioactive compounds within Leonurus (Zhang et al., 2018; Li et al., 2022a).
Leonurus is not the only plant that produces stachydrine; it is also found in Capparis spinosa (Maresca et al., 2016), Castanea sativa (Servillo et al., 2016), Citrus reticulata (Heinzmann et al., 2010), Medicago sativa (Connor et al., 1973), Sabia schumanniana (Gong et al., 2012), Maclura tricuspidata (State Administration of Medicine Chinese herbal medicine information center station, 1986), Verbena officinalis (Cheng et al., 2010), Arisaema heterophyllum (Yu and Yu, 2007), Combretum alfredii (Wu et al., 2007), and Stachys arvensis (Cheng et al., 2020). Additionally, bacteria such as Sinorhizobium meliloti (Phillips et al., 1998) in alfalfa and Rhodobacter sphaeroides (Kumar et al., 2014) also produce stachydrine (Figure 1). Among these, Medicago sativa and Leonurus are the most extensively studied, containing approximately 0.1% and 1% dry weight (DW) of stachydrine, respectively (Connor et al., 1973; Kuchta et al., 2014). Citrus reticulata contains about 0.3% (DW), with lower levels reported in other plants (Sun et al., 2023).
In addition to being synthesized in plants, stachydrine may also be produced in animals. One study noted that a mollusk, the Elysia chlorotica, produces its own stachydrine, which regulates the size of its cells through stachydrine (Pierce et al., 1984) (Figure 1). It is also found in the human gut and kidneys and plays an important role in regulating osmotic pressure, which is likely derived from human food intake (Chambers and Kunin, 1987a).
2 Therapeutic roles of stachydrine in humans
2.1 Cardiovascular and neurological effects
Cardiovascular and cerebrovascular diseases, collectively termed cardiovascular diseases, are the leading causes of death globally according to the World Health Organization. Cardiovascular diseases encompass various pathophysiological states such as atherosclerosis, acute myocardial infarction, chronic heart failure, and vasospasm, while cerebrovascular diseases include conditions like ischemic stroke, craniocerebral trauma, and neurodegenerative disorders such as Alzheimer’s disease, Parkinson’s disease, and depression. The crucial roles of the heart and brain in overall health make their impairments significantly detrimental.
Current pharmacological treatments predominantly rely on chemically synthesized small molecule drugs, including nitrates, statins, beta-blockers, clopidogrel, aspirin, and ACE inhibitors/angiotensin receptor blockers (ARBs). Although these treatments are effective, they often come with considerable side effects and costs. It is crucial to explore safer and more efficient therapeutic alternatives and comprehensive treatment protocols to improve therapeutic outcomes and patient prognosis.
Recent research shows that stachydrine, a plant-derived natural product, is highly effective in treating cardiovascular and cerebrovascular diseases.
2.1.1 Cardiac cell regulation
It mitigates the enlargement of cardiac cells induced by various stimuli, such as norepinephrine and angiotensin II. Specifically, stachydrine impedes the calcium-modulated phosphatase/NFAT signaling cascade, thereby attenuating the aberrant cardiac hypertrophy elicited by adrenergic receptor activation. By disrupting the influence of these factors on cardiac cell signaling pathways, stachydrine effectively curtails pathological myocardial growth (Guo et al., 2012; Cao et al., 2017; Zheng et al., 2020). Furthermore, stachydrine confers cytoprotective effects by thwarting cardiomyocyte apoptosis triggered by hypoxia and regulating iron metabolism to mitigate iron-induced cell death (Liu et al., 2009; Liang et al., 2023). This protective mechanism is instrumental in combating conditions like myocardial ischemia and heart failure.
Stachydrine exerts regulatory control over calcium homeostasis within cardiac cells, thereby augmenting the efficiency of calcium transients while mitigating calcium leakage from cardiomyocytes. This regulatory action is essential for ensuring proper cardiac function during both the contraction and relaxation phases of the cardiac cycle (Li et al., 2022b).
Stachydrine augments the activity of key antioxidant enzymes, notably superoxide dismutase (SOD) and glutathione peroxidase (GSH-Px), while concurrently attenuating oxidative stress, lipid peroxidation, and the accumulation of reactive oxygen species (ROS). Through these mechanisms, stachydrine effectively counters the cellular damage induced by ischemia, reperfusion injury, or other oxidative insults. Consequently, stachydrine serves as a protective agent safeguarding the integrity of both the cardiovascular and cerebrovascular systems (Xie et al., 2018; Li et al., 2021; Lu et al., 2023).
2.1.2 Inhibition and amelioration of myocardial fibrosis
Stachydrine effectively inhibits the production and activity of key mediators involved in myocardial fibrosis, notably Angiotensin II (AngII) and Transforming Growth Factor β1 (TGFβ1). It achieves this by downregulating the expression of angiotensinogen (AGT) and angiotensin-converting enzyme (ACE) within cardiac tissues. These actions are crucial for halting the progression of cardiac fibrosis. TGFβ1, in particular, plays a central role in the pathogenesis of cardiac fibrosis. Stachydrine prevents the transformation of cardiac fibroblasts into myofibroblasts, a critical step in fibrosis, by interfering with this pathway. Importantly, stachydrine also modulates the TGF-β/Smad signaling axis, which is instrumental in activating fibroblasts and synthesizing collagen in the heart. By blocking the phosphorylation of Smad proteins, stachydrine reduces the expression of pro-fibrotic genes, thus diminishing the fibrotic response. Additionally, stachydrine directly inhibits cardiac fibroblast proliferation and activation triggered by AngII. This leads to a decrease in collagen production and other extracellular matrix components, thereby significantly alleviating myocardial fibrosis (Chen et al., 2017; Liu et al., 2019).
2.1.3 Inhibit inflammatory response
Stachydrine displays potent anti-inflammatory properties by decreasing the release of key inflammatory mediators, including interleukin-1β (IL-1β) and tumor necrosis factor-α (TNF-α). It also counteracts the NF-κB (nuclear factor κB) signaling pathway, a critical transcription factor that orchestrates intracellular inflammation. Stachydrine effectively blocks the phosphorylation and nuclear translocation of the NF-κB p65 subunit, thus reducing the production of inflammatory molecules. Additionally, it modulates the JAK/STAT (Janus kinase/signal transducer and activator of transcription) signaling pathway, which regulates cellular responses to cytokines and growth factors. Importantly, it inhibits the production of phosphorylated STAT3 (p-STAT3) and JAK2 (p-JAK2), reducing the transcriptional activity of inflammatory genes. This regulation of inflammation is crucial in managing cardiovascular diseases, as inflammation plays a central role in the development of atherosclerosis and other related complications. The comprehensive anti-inflammatory effects of stachydrine make it a promising candidate for treating inflammation-associated cardiovascular disorders (Zhao et al., 2017; Wu et al., 2020; Yu et al., 2023).
2.1.4 Improvement of homocysteine-induced endothelial dysfunction
Hyperhomocysteinemia (HHcy) is recognized as an independent risk factor for cardiovascular disease. Stachydrine has shown effectiveness in alleviating the detrimental effects of homocysteine (Hcy) on endothelial vasodilation across various arterial segments, such as the thoracic aorta, mesenteric arteries, and renal arteries in rat models. It enhances the production of tetrahydrobiopterin (BH4), a crucial cofactor for endothelial nitric oxide synthase (eNOS), by upregulating the expression of GTP cyclohydrolase 1 (GTPCH1) and dihydrofolate reductase (DHFR) within endothelial cells. This increase in eNOS activity highlights the essential role of BH4 in maintaining endothelial function. These findings emphasize stachydrine’s potential as a therapeutic agent for mitigating endothelial dysfunction caused by Hcy, providing vasoprotective benefits. Furthermore, the study reveals a novel molecular mechanism by which stachydrine exerts its vasoprotective effects, offering insights into its promising applications for managing cardiovascular diseases (Xie et al., 2018).
2.1.5 Promotes nitric oxide production and modulates n-glycosylation
Stachydrine boosts the production of nitric oxide (NO) by activating the AMPK and Akt signaling pathways, which in turn enhances the phosphorylation and activity of endothelial nitric oxide synthase (eNOS). Nitric oxide is a critical vasodilator that aids in the relaxation of blood vessels, thereby supporting healthy vascular function and preventing endothelial dysfunction (Xie et al., 2018; Xie et al., 2019).
Stachydrine modulates the N-glycosylation of the β1-adrenergic receptor (β1AR) by inhibiting α-1,6-fucosylation. This action preserves the normal functionality of β1AR and supports the excitation-contraction coupling process in the heart, crucial for maintaining cardiac rhythm and function (Hu et al., 2021).
2.1.6 Inhibits of platelet activation and thrombosis
Stachydrine effectively reduces the risk of thrombosis by mitigating platelet activation, aggregation, and secretion. Additionally, it attenuates the interactions between platelets and neutrophils, decreasing the likelihood of inflammatory and thrombotic complications. These mechanisms enable stachydrine to improve cardiac function, reduce cardiac load, counteract cardiac remodeling, and, to a certain extent, reverse cardiac dysfunction. Consequently, stachydrine emerges as a promising cardioprotective agent with significant potential in the treatment of cardiovascular diseases (Mansurov, 1972; Sun et al., 2022).
2.1.7 Neuroprotective actions
Stachydrine has demonstrated significant efficacy in reducing cell death, neurological impairment, and brain tissue damage in mouse models of traumatic brain injury (TBI). It notably decreases apoptosis, infarct volume, and brain water content in various experimental TBI models (Yu et al., 2018). Additionally, stachydrine promotes cellular growth, inhibits cell death, and alleviates inflammation, thus protecting neurons and ameliorating cognitive deficits in rat models of TBI (Yu et al., 2018). It also modulates key biomarkers of oxidative and inflammatory responses, including superoxide dismutase (SOD), malondialdehyde (MDA), interleukin-1β (IL-1β), and tumor necrosis factor-α (TNF-α). Furthermore, stachydrine reduces cerebral infarction size in rats subjected to middle cerebral artery occlusion and shields neurons from TBI-induced damage. These protective actions are mediated through the suppression of the PI3K/m-TOR/Akt and TLR4/NF-κB pathways, crucial regulators of cell survival, proliferation, and apoptosis. Through these mechanisms, stachydrine significantly enhances neurological function (Li et al., 2020).
2.2 Anticancer properties
Extensive research has consistently highlighted stachydrine’s potent antagonistic effects on various types of cancer, including astrocytoma (Liu et al., 2018), prostate cancer (Rathee et al., 2012), breast cancer (Bao et al., 2022), colon cancer (Zhao, 2018), gastric cancer (Ma et al., 2017), esophageal squamous cell carcinoma (Isozaki et al., 2014), chronic myeloid leukemia (CML) (Gu et al., 2022), hepatocellular carcinoma (HCC) (Chen and Yan, 2021), and others. Stachydrine’s anticancer activities primarily involve the inhibition of cell proliferation, induction of apoptosis, and blocking of cell migration and invasion. These effects are achieved through the modulation of multiple molecular pathways, providing a robust mechanistic foundation for its therapeutic potential against a wide array of cancers.
Stachydrine effectively inhibits several receptor tyrosine kinases, including BCR-ABL, which is crucial in the treatment of chronic myeloid leukemia (CML). By blocking these kinases, stachydrine disrupts proliferation and survival signaling in cancer cells, thereby curbing their growth and viability (Gu et al., 2022).
Stachydrine impacts multiple signaling pathways that are pivotal in cancer progression, such as PI3K/Akt, ERK/MAPK, and NF-κB. These pathways are integral to cell proliferation, survival, migration, and inflammatory responses. Stachydrine’s anticancer effects are mediated through the inhibition of key molecules within these pathways, including Akt, ERK, and IκBα, leading to reduced cancer cell activity (Liu et al., 2018).
Stachydrine promotes cancer cell apoptosis by activating pathways involved in programmed cell death, particularly the mitochondrial pathway. It lowers the mitochondrial membrane potential and activates key apoptotic proteins such as Bax and caspase-3, while simultaneously reducing the expression of the anti-apoptotic protein Bcl-2. This dual action both triggers apoptosis and inhibits survival signals within cancer cells (Bao et al., 2022; Zeng et al., 2023).
Stachydrine impedes the migration and invasive capabilities of tumor cells by inhibiting signaling pathways such as CXCR4/ERK and CXCR4/Akt. This inhibition is crucial for preventing the metastasis and spread of cancer, effectively blocking critical pathways involved in tumor cell dissemination (Liu et al., 2018).
Stachydrine modulates cell cycle progression, causing cancer cells to arrest in the G0/G1 phase, which hampers their proliferation. It has shown particular efficacy against corticoblastic astrocytoma (PA), inducing apoptosis and arresting cell cycle progression in human PA cells. These effects are mediated through the inhibition of CXCR4/Akt and CXCR4/ERK pathways and their downstream effectors, including CXCR4/Akt/MMP-9/2 and CXCR4/ERK/MMP-9/2, leading to decreased cell viability and reduced colony formation in PA cells (Liu et al., 2018; Zhai et al., 2024).
Stachydrine protects against oxidative stress by enhancing the activity of antioxidant enzymes such as superoxide dismutase (SOD). It also lowers serum lactate dehydrogenase levels, indicative of reduced oxidative damage. In studies on mice with gastric cancer induced by 1-methyl-3-nitro-1-nitrosoguanidine, stachydrine inhibited histone deacetylase (HDAC) activity in gastrointestinal tissues, significantly decreasing oxidative stress markers and cytokine levels. These findings suggest that stachydrine can mitigate oxidative damage in gastric cancer by inhibiting HDAC activity (Ma et al., 2017).
Despite its potent anticancer properties, stachydrine faces challenges in clinical implementation, particularly concerning bioavailability and pharmacokinetic properties. Researchers are actively working to enhance its therapeutic efficacy and safety profile through the synthesis of derivatives and the optimization of delivery techniques. These efforts aim to maximize stachydrine’s effectiveness in cancer treatment by improving its stability, absorption, and targeted delivery.
2.3 Effects of stachydrine on uterus
Leonurus has been traditionally used in the treatment of various obstetric and gynecological conditions. To evaluate its safety and efficacy, a study was conducted using a Leonurus injection, which contains 1 mL of solution with 18–22 mg of stachydrine hydrochloride, aimed at preventing post-abortion bleeding (Xia et al., 2020). The results confirmed its effectiveness in reducing post-abortion hemorrhage and promoting uterine retraction, thereby decreasing bleeding post-abortion. The injection also demonstrated a favorable safety profile, with no adverse reactions such as nausea, vomiting, diarrhea, or local reactions at the injection site, and no detrimental effects on liver and kidney functions were observed.
Stachydrine, a key compound found in Leonurus, exhibits a dual regulatory effect on uterine activity. In healthy guinea pigs, it enhances uterine contractions by increasing contraction intensity and decreasing contraction frequency (Dai et al., 2016b). Furthermore, it counteracts uterine contractions induced by oxytocin (Zheng and Wang, 2017). Stachydrine also inhibits abnormal proliferation of uterine smooth muscle cells (MSMC) induced by LPS stimulation, through the modulation of calcium-regulating proteins (Zeng, 2007). Its significant role in managing postpartum hemorrhage and aiding uterine recovery is highlighted by its ability to enhance uterine contraction and promote angiogenesis (He et al., 2018).
Despite the biological properties similar to those of Leonurus, research and application of stachydrine in obstetrics and gynecology remain relatively limited. Therefore, comprehensive future studies are essential to fully explore its potential applications and elucidate the underlying mechanisms in obstetric and gynecological contexts.
2.4 Anti-inflammatory effects of stachydrine
Research has identified stachydrine as an effective anti-arthritic and analgesic component in Capparis spinosa, demonstrating suitability for treating arthritis induced by Complete Freund’s Adjuvant (CFA) in rats (Feng et al., 2011). These findings support the anti-inflammatory properties of stachydrine. To further explore these properties, researchers have developed several inflammation models, especially those induced by Lipopolysaccharide (LPS). LPS, a major component of the cell wall of Gram-negative bacteria, triggers endothelial cells to overexpress inflammatory cytokines, initiating inflammation cascades that can lead to sepsis and multi-organ dysfunction (Dauphinee and Karsan, 2006).
Studies have shown that high doses of stachydrine can counteract endotoxin effects and inflammation, as evidenced by reduced hepatic and intestinal damage indices in mice with LPS-induced inflammation, without altering serum levels of LPS, TNF-α, and IL-1β. Additionally, stachydrine has been effective in inhibiting LPS-induced inflammatory bone loss by suppressing osteoclastogenesis both in vitro and in vivo. This effect is mediated by the inhibition of the NF-κB and AKT signaling pathways, activated by the receptor activator of NF-κB ligand (RANKL), highlighting its potential in treating conditions like osteoporosis (Meng et al., 2019).
Further, the anti-inflammatory activity of stachydrine was observed in various experimental models, including ear swelling in mice induced by xylene, granuloma formation from cotton ball implantation, and pleurisy in rats induced by carrageenan. These effects were linked to improvements in cell membrane permeability, suppression of inflammatory cytokine levels, and reduction in lipid peroxidation (Fang and Can, 2012).
Figure 2 illustrates the above pharmacological mechanisms of stachydrine.
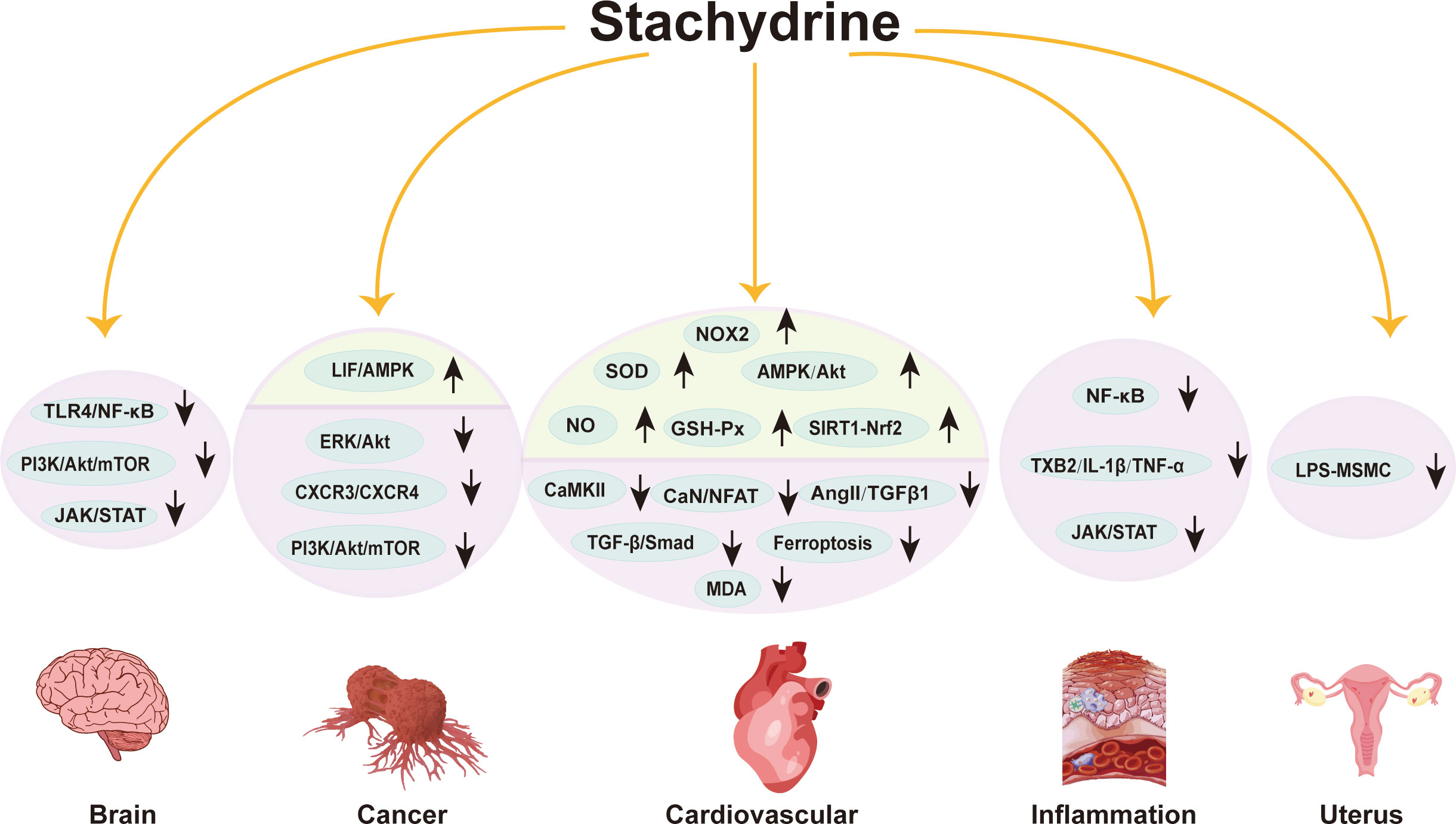
Figure 2 Pharmacological mechanisms of stachydrine. This figure outlines the pharmacological mechanisms of stachydrine, primarily affecting the cardiovascular system, cancer, inflammation, uterus, and nervous system. The direction of the arrows indicates the upregulation or downregulation of substances or signaling pathways.
2.5 Other effects of stachydrine in humans
Stachydrine has shown promising therapeutic effects against obesity and insulin resistance. Korean researchers have identified properties in a commonly consumed rice wine that contribute to weight reduction and attributed these effects to stachydrine. It promotes lipid breakdown and prevents lipid accumulation in 3T3-L1 adipocytes, reduces weight gain, and improves glucose tolerance and insulin sensitivity in mouse models. Notably, stachydrine significantly decreases adipsin mRNA levels in both liver and adipose tissue, while increasing adipsin levels in the bloodstream of mice compared to those on a high-fat diet alone. It also restores balance in endoplasmic reticulum function and modulates adipsin expression, highlighting its potential as a therapeutic agent against obesity and insulin resistance (Lee et al., 2022).
Stachydrine also exhibits osmoprotective effects on human kidneys and the surrounding microbiota. Although the precise mechanism of stachydrine formation in the human body remains unclear, its extraction from human urine and subsequent studies indicates its role in providing osmoprotection. This protective effect is crucial for renal health and maintaining microbial balance (Chambers and Kunin, 1987a).
Additionally, stachydrine offers notable respiratory benefits, particularly in reducing cough frequency. This has been demonstrated in a guinea pig cough model induced by citric acid, where stachydrine targets sensory nerve endings in the respiratory tract, reducing the sensitivity of the cough reflex and exerting an antitussive effect. Furthermore, when combined with synephrine, which activates the β2-adrenergic receptor (β2-AR) causing relaxation of bronchial smooth muscle, stachydrine enhances the bronchodilatory effect of synephrine, thus improving its efficacy in reducing airway spasms and supporting its use in asthma treatment (Shi et al., 2009).
2.6 Potential activity of stachydrine analogues
Stachydrine not only has numerous pharmacological effects but also holds promise through its derivatives, which may offer superior biological activities. Modifying stachydrine by adding different functional groups can amplify its medicinal properties, increase its lipophilicity, and significantly improve its bioavailability (Zeng et al., 2023). These advancements heighten the anticipation for future applications of stachydrine and its analogues.
3 Functions of stachydrine in plants and bacteria
3.1 Functions of stachydrine in plants
Stachydrine, a prevalent secondary metabolite in plants, plays a significant role in various physiological processes. It regulates osmotic pressure, aiding plants like alfalfa in swiftly accumulating stachydrine to counteract external salt stress. This accumulation enhances the resilience of plant against salt stress (Trinchant et al., 2004). Experiments with Arabidopsis thaliana have shown that adding appropriate amounts of stachydrine to growth media under salt stress significantly improves plant growth.
Stachydrine activates the NodD2 protein, a key regulatory element in rhizobium-plant symbiosis. This activation facilitates the expression of nodulation genes essential for rhizome formation and nitrogen fixation, bolstering the plant’s ability to absorb and utilize nitrogen efficiently (Phillips et al., 1992; Phillips et al., 1998).
Stachydrine also enhances plant resistance to specific pests. It has been shown to impede the growth and survival of larvae from pests such as the dance moth, providing a protective benefit against these invaders (Jiang et al., 2021).
Furthermore, stachydrine plays a role in the plant’s response to abiotic stresses, such as drought and salt stress. Its involvement helps plants adapt to adverse environmental conditions, thereby enhancing their overall resilience (Phillips et al., 1992; Randall et al., 1996; Hamilton and Heckathorn, 2001; Zhang et al., 2018; Jiang et al., 2021).
Stachydrine may influence the synthesis of important secondary metabolites, such as flavonoids, which are crucial for plant defense. It also acts as a regulator of plant growth, promoting development by modulating both primary and secondary metabolic pathways (Hamilton and Heckathorn, 2001; Wu et al., 2024).
Overall, stachydrine is instrumental in regulating various physiological and ecological processes within plants, contributing significantly to their growth, development, defense mechanisms, and stress responses.
3.2 Role of stachydrine in bacteria
Stachydrine exerts an osmoprotective effect not only in plants and animals but also in bacteria. Research has shown that stachydrine can significantly improve the growth of Escherichia coli under salt stress conditions when added to its culture medium, illustrating its protective role against osmotic stress (Hanson et al., 1994). Bacillus subtilis utilizes a dedicated transporter protein for stachydrine uptake, employing it as an osmoprotectant (Horn et al., 2006). Growth studies of Bacillus subtilis further underscore the osmoprotective effects of stachydrine, which also extend to strains of Staphylococcus aureus, Staphylococcus epidermidis, and Staphylococcus saprophyticus (Amin et al., 1995). Notably, in the human gut—which is characterized by high osmotic pressure—stachydrine helps maintain a thriving gut flora due to its osmoprotective action on these bacteria (Chambers and Kunin, 1987a).
Stachydrine functions as an effective osmoprotective compound in bacteria, supported by specific transport systems such as BetS, Prb, and others, which actively mediate the uptake or release of stachydrine. These systems often involve ATP-binding cassette (ABC) transporter proteins or Na+/stachydrine cotransporter proteins that respond to changes in osmolality to regulate intracellular osmoregulator levels. Acting as a compatible solute, stachydrine accumulates in the cytoplasm at high concentrations without disrupting cellular processes, aiding in maintaining cellular water balance and alleviating osmotic pressure induced by stress (Boscari et al., 2006; Horn et al., 2006).
Furthermore, the unique molecular structure of stachydrine allows it to interact with proteins and cellular membranes, potentially stabilizing protein structures through hydrophobic or electrostatic interactions. It may also act as a molecular chaperone, assisting in proper protein folding and preventing denaturation and aggregation under conditions of high salt or drought, thus preserving intracellular protein functionality during stress. Changes in osmotic pressure can influence the expression of genes involved in osmoregulator production and transportation in bacteria. The presence of stachydrine can modulate the activity of specific genes, either stimulating or suppressing their expression, thereby regulating osmoregulator synthesis and intracellular accumulation (Chambers and Kunin, 1987b; Hanson et al., 1994; Amin et al., 1995; Alloing et al., 2006; Bashir et al., 2014).
Through these mechanisms, stachydrine acts as a molecular shield for bacteria against osmotic stress, safeguarding the structural and functional integrity of cellular components.
Figure 3 illustrates the functions of stachydrine in humans, plants, and bacteria as described above.
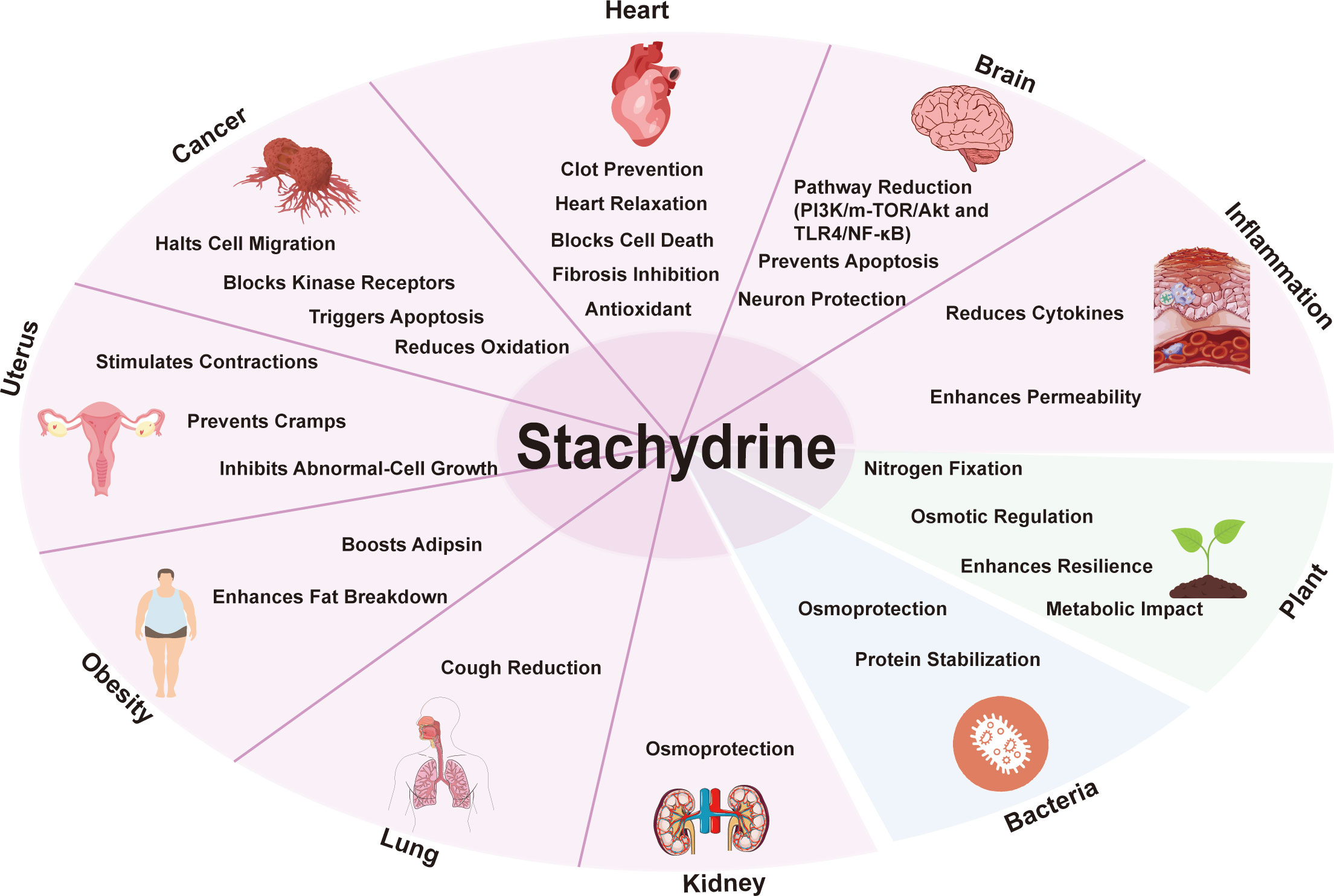
Figure 3 General overview of the biological activities of stachydrine. This figure illustrates the functions of stachydrine in the heart, brain, uterus, lungs, and kidneys, as well as its roles in cancer resistance and obesity; it also details the functions of stachydrine in plants and bacteria.
4 Progress in the anabolism of stachydrine
4.1 Possible synthetic pathways for stachydrine
Research into the production process of stachydrine has revealed insights, yet the precise biological mechanism of its synthesis remains elusive. Initially, studies suggested that ornithine in alfalfa could serve as a precursor to stachydrine. Experiments with isotope-labeled ornithine demonstrated its conversion into glutamic acid and then into proline, leading to stachydrine synthesis (Morgan and Marion, 1956). Additionally, methylation, particularly from the methyl group sourced from methionine, plays a crucial role in incorporating into stachydrine’s molecular structure. It has been observed that mature alfalfa plants at 12 weeks old produce stachydrine, unlike those aged 2–3 week (Leete et al., 1955; Wiehler and Marion, 1958; Robertson and Marion, 1960; Essery et al., 1962).
Chemically known as (2S)-1,1-dimethylpyrrolidine-2-carboxylic acid or N, N-dimethyl-L-proline, stachydrine is believed to be synthesized through a process involving the addition of two methyl groups to the N of proline (Figure 4). Various substrates, including iodomethane and dimethyl sulphate, have been used to react with L-proline to synthesize stachydrine, aiming to introduce two methyl groups to the N of proline (He and Wang, 2005a; He and Wang, 2005b). Experiments with proline containing a radioactive isotope in alfalfa plants led to the detection of radioactive N-methyl proline and stachydrine, suggesting the catalytic activity of N-methyltransferase (NMT) in this synthesis.
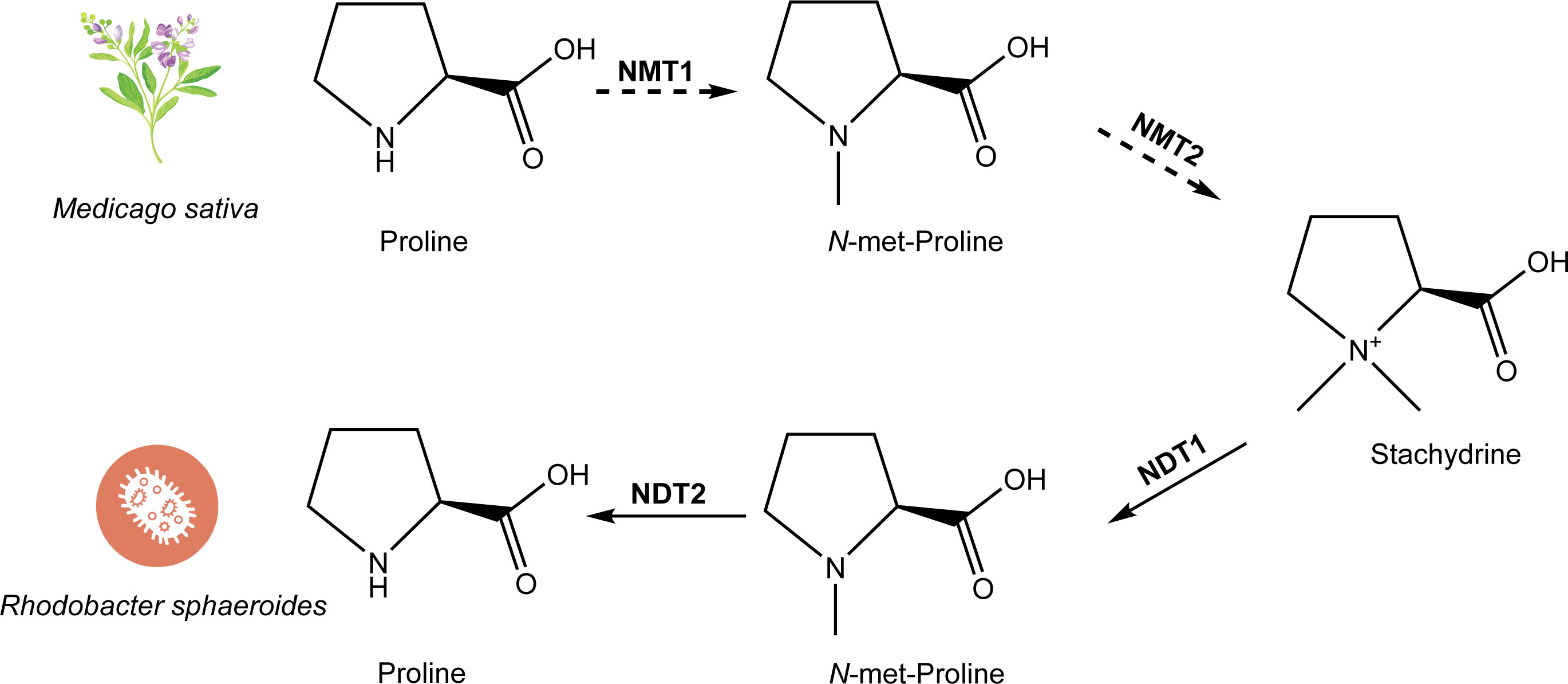
Figure 4 The biosynthetic and metabolic pathway of stachydrine. The biosynthetic pathway of stachydrine has been studied in Medicago sativa, where proline is catalyzed by NMT1 and NMT2 (which may be the same enzyme or different enzymes) through 1–2 step reactions to produce stachydrine. The degradation process of stachydrine has been studied in Rhodobacter sphaeroides, where stachydrine is hydrolyzed back to proline through a two-step reaction catalyzed by NDT1 and NDT2, respectively.
Despite advancements, studies into the biosynthesis of stachydrine are limited. Currently, stachydrine is produced either by extraction from plants or by chemical synthesis. Unraveling its biosynthetic pathway could significantly advance phytopharmacology, aiding in understanding stachydrine’s mechanism of action and interactions, potentially enhancing drug quality control, and facilitating the development of new pharmaceuticals through synthetic biology and genetic engineering.
4.2 Metabolic pathway of stachydrine
The metabolic pathways of stachydrine are largely understood, although its biosynthesis pathway remains unelucidated (Figure 4). Stachydrine is excreted by germinating seeds and plant roots into bacterial environments. Bacteria closely associated with plants have evolved mechanisms to utilize betaine for two primary purposes: as a protective shield against high osmotic pressure and as a source of carbon and nitrogen in the absence of osmotic stress. Recent research has identified the metabolic pathway of betaine in two strains of bacteria: Paracoccus denitrificans and Rhodobacter sphaeroides. Initially, stachydrine is enzymatically converted into N-methyl stachydrine by the action of the first N-desmethyltransferase enzyme (NDT1). Subsequently, proline is synthesized through the activity of the second N-desmethyltransferase enzyme (NDT2). Finally, proline undergoes further metabolism into glutamic acid (Kumar et al., 2014) (Figure 4). Notably, the metabolic pathway of stachydrine is essentially the reverse of the proposed synthetic pathway of stachydrine.
5 Outlook
Stachydrine, a versatile bioactive molecule, has garnered significant interest due to its promising medicinal properties. Ongoing research is dedicated to elucidating its precise mechanisms of action, refining its applications, and exploring its potential in pharmaceutical development. A key focus of these efforts is understanding the biosynthesis pathway of stachydrine. Despite the apparent simplicity of converting proline to stachydrine, research on this synthesis pathway in alfalfa has been challenging since the 1950s, primarily due to the lack of relevant enzyme identification. This challenge may be attributed to the limited availability of genomic data and the nascent stage of molecular biology techniques at that time. Alternatively, the complexity of the reaction in organisms may require more advanced genomic and multifaceted biochemical experiments for confirmation.
The initial exploration of the biosynthesis route of stachydrine is now being modeled using bioinformatics tools and systems biology approaches. This theoretical analysis helps predict unidentified intermediates and key enzymes, providing a foundation for experimental design. Radioisotope or stable isotope labeling techniques are also employed to trace the biosynthetic pathway of stachydrine, offering a detailed understanding of each step in the synthesis process.
Understanding the biochemical pathway of stachydrine paves the way for using genetic engineering to manipulate or enhance the genes involved in its biosynthesis in plants, potentially increasing stachydrine production. Synthetic biology opens possibilities for creating microbial cell factories that express plant-derived enzymes, enabling the production of stachydrine or its precursors. Metabolic engineering strategies aim to optimize metabolic pathways in plants or microorganisms to improve stachydrine biosynthesis, reduce by-product formation, and enhance the yield and purity of desired molecules.
Furthermore, the exploration of stachydrine and its derivatives through high-throughput screening and natural product mining may uncover novel analogues from other plants or microorganisms. These analogues might exhibit improved pharmacological activities or decreased toxicity compared to stachydrine itself. Additionally, integrating biosynthetic pathways from diverse organisms could lead to the generation of novel stachydrine derivatives using combinatorial biosynthesis techniques, thus expanding the spectrum of chemical variations and potential therapeutic applications.
In summary, a deeper understanding of the biosynthetic process of stachydrine promises to significantly advance its utilization in medicine, health, and agriculture, opening new avenues for research and development in these fields.
Author contributions
ZH: Conceptualization, Data curation, Formal analysis, Investigation, Methodology, Project administration, Resources, Software, Validation, Visualization, Writing – original draft, Writing – review & editing. PL: Writing – review & editing, Methodology, Project administration, Resources. PL: Writing – review & editing. PX: Data curation, Formal analysis, Funding acquisition, Project administration, Resources, Supervision, Visualization, Writing – review & editing, Methodology, Writing – original draft.
Funding
The author(s) declare financial support was received for the research, authorship, and/or publication of this article. This work was funded by the National Natural Science Foundation of China (32170349 to PX), the Ministry of Science and Technology of the People’s Republic of China (YDZX20223100001003 to YH), and the Chenshan Special Fund for the Shanghai Landscaping Administration Bureau Program (G232402 to PX).
Conflict of interest
The authors declare that the research was conducted in the absence of any commercial or financial relationships that could be construed as a potential conflict of interest.
Publisher’s note
All claims expressed in this article are solely those of the authors and do not necessarily represent those of their affiliated organizations, or those of the publisher, the editors and the reviewers. Any product that may be evaluated in this article, or claim that may be made by its manufacturer, is not guaranteed or endorsed by the publisher.
References
Alloing, G., Travers, I., Sagot, B., Le Rudulier, D., Dupont, L. (2006). Proline betaine uptake in Sinorhizobium meliloti: Characterization of Prb, an opp-like ABC transporter regulated by both proline betaine and salinity stress. J. Bacteriol 188, 6308–6317. doi: 10.1128/JB.00585-06
Amin, U. S., Lash, T. D., Wilkinson, B. J. (1995). Proline betaine is a highly effective osmoprotectant for Staphylococcus aureus. Arch. Microbiol. 163, 138–142. doi: 10.1007/BF00381788
Bao, X., Liu, Y., Huang, J., Yin, S., Sheng, H., Han, X., et al. (2022). Stachydrine hydrochloride inhibits hepatocellular carcinoma progression via LIF/AMPK axis. Phytomedicine 100, 154066. doi: 10.1016/j.phymed.2022.154066
Bashir, A., Hoffmann, T., Kempf, B., Xie, X., Smits, S. H. J., Bremer, E. (2014). Plant-derived compatible solutes proline betaine and betonicine confer enhanced osmotic and temperature stress tolerance to Bacillus subtilis. Microbiol. (Reading) 160, 2283–2294. doi: 10.1099/mic.0.079665-0
Boscari, A., Van de Sype, G., Le Rudulier, D., Mandon, K. (2006). Overexpression of BetS, a Sinorhizobium meliloti high-affinity betaine transporter, in bacteroids from Medicago sativa nodules sustains nitrogen fixation during early salt stress adaptation. Mol. Plant Microbe Interact. 19, 896–903. doi: 10.1094/MPMI-19-0896
Cao, T. T., Chen, H. H., Dong, Z., Xu, Y. W., Zhao, P., Guo, W., et al. (2017). Stachydrine protects against pressure overload-induced cardiac hypertrophy by suppressing autophagy. Cell Physiol. Biochem. 42, 103–114. doi: 10.1159/000477119
Chambers, S. T., Kunin, C. M. (1987a). Osmoprotective activity for Escherichia coli in mammalian renal inner medulla and urine. Correlation of glycine and proline betaines and sorbitol with response to osmotic loads. J. Clin. Invest. 80, 1255–1260. doi: 10.1172/JCI113200
Chambers, S. T., Kunin, C. M. (1987b). Isolation of glycine betaine and proline betaine from human urine. Assessment of their role as osmoprotective agents for bacteria and the kidney. J. Clin. Invest. 79, 731–737. doi: 10.1172/JCI112878
Chen, H.-H., Zhao, P., Zhao, W.-X., Tian, J., Guo, W., Xu, M., et al. (2017). Stachydrine ameliorates pressure overload-induced diastolic heart failure by suppressing myocardial fibrosis. Am. J. Transl. Res. 9, 4250–4260.
Chen, X., Yan, N. (2021). Stachydrine inhibits TGF-β1-induced epithelial-mesenchymal transition in hepatocellular carcinoma cells through the TGF-β/Smad and PI3K/Akt/mTOR signaling pathways. Anticancer Drugs 32, 786–792. doi: 10.1097/CAD.0000000000001066
Cheng, X., Meng, Y., Zhang, L. (2010). Progress in the study of chemical composition and pharmacological effects of Verbena officinalis. Hebei Med. J. (32), 2089–2091. doi: 10.3969/j.issn.1002-7386.2010.15.066
Cheng, F., Zhou, Y., Wang, M., Guo, C., Cao, Z., Zhang, R., et al. (2020). A review of pharmacological and pharmacokinetic properties of stachydrine. Pharmacol. Res. 155, 104755. doi: 10.1016/j.phrs.2020.104755
Connor, M. A., Stark, J. B., Fritz, J. C., Kohler, G. O. (1973). Stachydrine. Content in alfalfa and biological activity in chicks. J. Agric. Food Chem. 21, 195–198. doi: 10.1021/jf60186a043
Dai, L. P., Dong, Y. H., Hu, T. T., Xie, X. F., Peng, C. (2016a). Research advances in pharmacological effects of stachydrine. Chin. J. Exp. Traditional Med. Formulae 22 (19), 189–193. doi: 10.13422/j.cnki.syfjx.2016190189
Dai, L. P., Xie, X. F., Sun, C., Ao, H., Yan-Hong, D., Cheng, P. (2016b). Effect of alkaloid monomer of yimucao injection on isolated smooth muscle of uterus. Natural Product Res. Dev. 28 (10), 1633. doi: 10.16333/j.1001-6880.2016.10.025
Dauphinee, S. M., Karsan, A. (2006). Lipopolysaccharide signaling in endothelial cells. Lab. Invest. 86, 9–22. doi: 10.1038/labinvest.3700366
Essery, J. M., McCaldint, D. J., Marion, L. (1962). The biogenesis of stachydrine. Phytochemistry 1, 209–213. doi: 10.1016/S0031-9422(00)82824-1
Fang, W., Can, W. (2012). Study on the Anti-inflammatory Activity of Stachydrine (Chongqing: Medicine China Pharmacy).
Feng, X., Lu, J., Xin, H., Zhang, L., Wang, Y., Tang, K. (2011). Anti-arthritic active fraction of capparis spinosa L. Fruits and its chemical constituents. Yakugaku Zasshi 131, 423–429. doi: 10.1248/yakushi.131.423
Gong, Y. F., Zheng, Y. S., Chen, D. H., Zhang, Z. B. (2012). Organic Chemistry (Wuhan City, Hubei Province: Huazhong University of Science and Technology Press).
Gu, R., Zhang, W., Xu, D. (2022). Stachydrine is effective and selective against blast phase chronic myeloid leukaemia through inhibition of multiple receptor tyrosine kinases. Pharm. Biol. 60, 700–707. doi: 10.1080/13880209.2022.2044862
Guo, W., Zhang, C., Liao, Y.-L., Lv, R., Wei, H.-C. (2012). [Effect of Leonurus stachydrine on myocardial cell hypertrophy. J. Chin. Med. Mat. 35, 940–943.
Hamilton, E. W., Heckathorn, S. A. (2001). Mitochondrial adaptations to NaCl. Complex I is protected by anti-oxidants and small heat shock proteins, whereas complex II is protected by proline and betaine. Plant Physiol. 126, 1266–1274. doi: 10.1104/pp.126.3.1266
Hanson, A. D., Rathinasabapathi, B., Rivoal, J., Burnet, M., Dillon, M. O., Gage, D. A. (1994). Osmoprotective compounds in the Plumbaginaceae: a natural experiment in metabolic engineering of stress tolerance. Proc. Natl. Acad. Sci. 91, 306–310. doi: 10.1073/pnas.91.1.306
He, Y. L., Shi, J. Y., Peng, C., Hu, L. J., Liu, J., Zhou, Q. M., et al. (2018). Angiogenic effect of motherwort (Leonurus japonicus) alkaloids and toxicity of motherwort essential oil on zebrafish embryos. Fitoterapia 128, 36–42. doi: 10.1016/j.fitote.2018.05.002
He, L. Q., Wang, X. S. (2005a). Study on synthesis technology of stachydrine. West China J. Pharm. Sci. (1), 50–51. doi: 10.13375/j.cnki.wcjps.2005.01.021
He, L. Q., Wang, X. S. (2005b). Synthesis of stachydrine. Huaxue Shijie (Chemical World) (5), 296–298. doi: 10.19500/j.cnki.0367-6358.2005.05.013
Heinzmann, S. S., Brown, I. J., Chan, Q., Bictash, M., Dumas, M.-E., Kochhar, S., et al. (2010). Metabolic profiling strategy for discovery of nutritional biomarkers: proline betaine as a marker of citrus consumption. Am. J. Clin. Nutr. 92, 436–443. doi: 10.3945/ajcn.2010.29672
Horn, C., Sohn-Bösser, L., Breed, J., Welte, W., Schmitt, L., Bremer, E. (2006). Molecular determinants for substrate specificity of the ligand-binding protein OpuAC from Bacillus subtilis for the compatible solutes glycine betaine and proline betaine. J. Mol. Biol. 357, 592–606. doi: 10.1016/j.jmb.2005.12.085
Hu, P., Guo, S., Yang, S., Wang, S., Wang, S., Shan, X., et al. (2021). Stachytine hydrochloride improves cardiac function in mice with ISO-induced heart failure by inhibiting the α-1,6-fucosylation on N-glycosylation of β1AR. Front. Pharmacol. 12, 834192. doi: 10.3389/fphar.2021.834192
Isozaki, Y., Hoshino, I., Akutsu, Y., Hanari, N., Mori, M., Nishimori, T., et al. (2014). Screening of alternative drugs to the tumor suppressor miR-375 in esophageal squamous cell carcinoma using the connectivity map. Oncology 87, 351–363. doi: 10.1159/000365592
Jiang, D., Tan, M., Wu, S., Zheng, L., Wang, Q., Wang, G., et al. (2021). Defense responses of arbuscular mycorrhizal fungus-colonized poplar seedlings against gypsy moth larvae: a multiomics study. Hortic. Res. 8, 245. doi: 10.1038/s41438-021-00671-3
Kuchta, K., Ortwein, J., Hennig, L., Rauwald, H. W. (2014). 1H-qNMR for direct quantification of stachydrine in Leonurus japonicus and L. cardiaca. Fitoterapia 96, 8–17. doi: 10.1016/j.fitote.2014.03.023
Kuchta, K., Volk, R. B., Rauwald, H. W. (2013). Stachydrine in Leonurus cardiaca, Leonurus japonicus, Leonotis leonurus: detection and quantification by instrumental HPTLC and 1H-qNMR analyses. Pharmazie 68 (7), 534–540.
Kumar, R., Zhao, S., Vetting, M., Wood, B., Sakai, A., Cho, K., et al. (2014). Prediction and biochemical demonstration of a catabolic pathway for the osmoprotectant proline betaine. mBio 5 (1), e00933–13. doi: 10.1128/mBio.00933-13
Lee, E., Kang, S., Lee, A.-R., Kim, J. H., Kim, T. W., Lee, J. E., et al. (2022). Stachydrine derived from fermented rice prevents diet-induced obesity by regulating adipsin and endoplasmic reticulum homeostasis. J. Nutr. Biochem. 107, 109036. doi: 10.1016/j.jnutbio.2022.109036
Leete, E., Marion, L., Spenser, I. D. (1955). The biogenesis of alkaloids. XIII. The role of ornithine in the biosynthesis of stachydrine. J. Biol. Chem. 214, 71–77. doi: 10.1016/S0021-9258(18)70944-7
Li, Z., Chen, K., Rose, P., Zhu, Y. Z. (2022a). Natural products in drug discovery and development: Synthesis and medicinal perspective of leonurine. Front. Chem. 10, 1036329. doi: 10.3389/fchem.2022.1036329
Li, X.-Q., Lu, S., Xia, L., Shan, X.-L., Zhao, W.-X., Chen, H.-H., et al. (2022b). Stachydrine hydrochloride ameliorates cardiac hypertrophy through CaMKII/HDAC4/MEF2C signal pathway. Am. J. Transl. Res. 14, 3840–3853.
Li, L., Sun, L., Qiu, Y., Zhu, W., Hu, K., Mao, J. (2020). Protective effect of stachydrine against cerebral ischemia-reperfusion injury by reducing inflammation and apoptosis through P65 and JAK2/STAT3 signaling pathway. Front. Pharmacol. 11, 64. doi: 10.3389/fphar.2020.00064
Li, P., Yan, M. X., Liu, P., Yang, D. J., He, Z. K., Gao, Y. (2024). Multiomics analyses of two Leonurus species illuminate leonurine biosynthesis and its evolution. Mol. Plant 17, 158–177. doi: 10.1016/j.molp.2023.11.003
Li, F., Zhu, S., Jiang, Q., Hou, C., Pang, T., Zhang, L., et al. (2021). Novel stachydrine-leonurine conjugate SL06 as a potent neuroprotective agent for cerebral ischemic stroke. ACS Chem. Neurosci. 12, 2478–2490. doi: 10.1021/acschemneuro.1c00200
Liang, Y., Xia, L., Lu, S., Yang, S., Guo, S., Shan, X., et al. (2023). A new mechanism of therapeutic effect of stachydrine on heart failure by inhibiting myocardial ferroptosis. Eur. J. Pharmacol. 954:175881. doi: 10.1016/j.ejphar.2023.175881
Liu, X., Shan, X., Chen, H., Li, Z., Zhao, P., Zhang, C., et al. (2019). Stachydrine ameliorates cardiac fibrosis through inhibition of angiotensin II/transformation growth factor β1 fibrogenic axis. Front. Pharmacol. 10, 538. doi: 10.3389/fphar.2019.00538
Liu, Y., Wei, S., Zou, Q., Luo, Y. (2018). Stachydrine suppresses viability & migration of astrocytoma cells via CXCR4/ERK & CXCR4/Akt pathway activity. Future Oncol. 14, 1443–1459. doi: 10.2217/fon-2017-0562
Liu, X.-H., Xin, H., Hou, A.-J., Zhu, Y.-Z. (2009). Protective effects of leonurine in neonatal rat hypoxic cardiomyocytes and rat infarcted heart. Clin. Exp. Pharmacol. Physiol. 36, 696–703. doi: 10.1111/j.1440-1681.2008.05135.x
Lu, S., Liang, Y., Yang, S., Fu, M., Shan, X., Zhang, C., et al. (2023). Stachydrine hydrochloride regulates the NOX2-ROS-signaling axis in pressure-overload-induced heart failure. Int. J. Mol. Sci. 24, 14369. doi: 10.3390/ijms241814369
Ma, N., Wei, F.-Q., Guo, J. (2017). Anti tumor activity of stachydrine by inhibiting histone diacetylase enzyme in gastric cancer. (Biomed Res-India. London: Allied Acad) 28 (2), 802–806. Available online at: https://www.researchgate.net/publication/316506450
Mansurov, M. M. (1972). The effect of stachydrine alkaloid on the blood coagulation system. Farmakol Toksikol 35 (6), 715–717.
Maresca, M., Micheli, L., Di Cesare Mannelli, L., Tenci, B., Innocenti, M., Khatib, M., et al. (2016). Acute effect of Capparis spinosa root extracts on rat articular pain. J. Ethnopharmacol 193, 456–465. doi: 10.1016/j.jep.2016.09.032
Meng, J., Zhou, C., Zhang, W., Wang, W., He, B., Hu, B., et al. (2019). Stachydrine prevents LPS-induced bone loss by inhibiting osteoclastogenesis via NF-κB and Akt signalling. J. Cell Mol. Med. 23, 6730–6743. doi: 10.1111/jcmm.14551
Morgan, A., Marion, L. (1956). The biogenesis of alkaloids: xvii. further study of the role of ornithine in the biogenesis of stachydrine. Can. J. Chem. 34, 1704–1708. doi: 10.1139/v56-221
Phillips, D. A., Joseph, C. M., Maxwell, C. A. (1992). Trigonelline and stachydrine released from alfalfa seeds activate NodD2 protein in rhizobium meliloti. Plant Physiol. 99, 1526–1531. doi: 10.1104/pp.99.4.1526
Phillips, D. A., Sande, E. S., Vriezen, J. A. C., de Bruijn, F. J., Le Rudulier, D., Joseph, C. M. (1998). A new genetic locus in sinorhizobium meliloti is involved in stachydrine utilization. Appl. Environ. Microbiol. 64, 3954–3960. doi: 10.1128/AEM.64.10.3954-3960.1998
Pierce, S. K., Edward, S. C., Mazzocchi, P. H., Klingle, L. J., Warre, M. K. (1984). Proline betaine: a unique osmolyte in an extremely euryhaline osmoconformer. Biol. Bull. 167, 495–500. doi: 10.2307/1541294
Randall, K., Lever, M., Peddie, B. A., Chambers, S. T. (1996). Accumulation of natural and synthetic betaines by a mammalian renal cell line. Biochem. Cell Biol. 74, 283–287. doi: 10.1139/o96-030
Rathee, P., Rathee, D., Rathee, D., Rathee, S. (2012). In vitro anticancer activity of stachydrine isolated from Capparis decidua on prostate cancer cell lines. Nat. Prod. Res. 26 (18), 1737–1740.
Robertson, A. V., Marion, L. (1960). The biogenesis of alkaloids: xxv. the role of hygric acid in the biogenesis of stachydrine. Can. J. Chem. 38, 396–398. doi: 10.1139/v60-055
Servillo, L., Giovane, A., Casale, R., Balestrieri, M. L., Cautela, D., Paolucci, M., et al. (2016). Betaines and related ammonium compounds in chestnut (Castanea sativa Mill.). Food Chem. 196, 1301–1309. doi: 10.1016/j.foodchem.2015.10.070
Shang, X., Pan, H., Wang, X., He, H., Li, M. (2014). Leonurus japonicus Houtt.: ethnopharmacology, phytochemistry and pharmacology of an important traditional Chinese medicine. J. Ethnopharmacol 152, 14–32. doi: 10.1016/j.jep.2013.12.052
Shi, Q., Liu, Z., Yang, Y., Geng, P., Zhu, Y., Zhang, Q., et al. (2009). Identification of anti-asthmatic compounds in Pericarpium citri reticulatae and evaluation of their synergistic effects. Acta Pharmacol. Sin. 30, 567–575. doi: 10.1038/aps.2009.36
State Administration of Medicine Chinese herbal medicine information center station. (1986). Handbook of Active Ingredients in Botanicals (Peking: People’s medical publishing house).
Sun, Y., Xia, X., Yuan, G., Zhang, T., Deng, B., Feng, X., et al. (2023). Stachydrine, a bioactive equilibrist for synephrine, identified from four citrus chinese herbs. Molecules 28, 3813. doi: 10.3390/molecules28093813
Sun, X., Zhou, M., Pu, J., Wang, T. (2022). Stachydrine exhibits a novel antiplatelet property and ameliorates platelet-mediated thrombo-inflammation. BioMed. Pharmacother. 152, 113184. doi: 10.1016/j.biopha.2022.113184
The Pharmacopoeia Committee (2020). Pharmacopoeia of the People’s Republic of China 2020 Edition (Bei Jing: China Medical Science and Technology Press).
Trinchant, J. C., Boscari, A., Spennato, G., Van de Sype, G., Le Rudulier, D. (2004). Proline betaine accumulation and metabolism in alfalfa plants under sodium chloride stress. Exploring Its Compartmentalization Nodules. Plant Physiol. 135, 1583–1594. doi: 10.1104/pp.103.037556
Wiehler, G., Marion, L. (1958). The biogenesis of alkaloids. XX. The induced biogenesis of stachydrine. J. Biol. Chem. 231, 799–805. doi: 10.1016/S0021-9258(18)70443-2
Wu, X. P., Cheng, G. Y., Jiang, C. Y. (2007). Research advances in the chemical constituents and pharmacological functions of Combretum. J. Hainan Normal Univ. (Natural Science) (1), 63–68. doi: 10.3969/j.issn.1674-4942.2007.01.017
Wu, C., Yang, Y., Wang, Y., Zhang, W., Sun, H. (2024). Colonization of root endophytic fungus Serendipita indica improves drought tolerance of Pinus taeda seedlings by regulating metabolome and proteome. Front. Microbiol. 15, 1294833. doi: 10.3389/fmicb.2024.1294833
Wu, H., Zhang, M., Li, W., Zhu, S., Zhang, D. (2020). Stachydrine attenuates IL-1β-induced inflammatory response in osteoarthritis chondrocytes through the NF-κB signaling pathway. Chem. Biol. Interact. 326, 109136. doi: 10.1016/j.cbi.2020.109136
Xia, W. T., Zhou, H., Wang, Y., Hu, H. Q., Xu, Z. H., Li, S. W., et al. (2020). Motherwort injection in preventing post-abortion hemorrhage after induced abortion: A multi-center, prospective, randomized controlled trial. Explore (NY). 16, 110–115. doi: 10.1016/j.explore.2019.08.004
Xie, X., Yang, C., Cui, Q., Ma, W., Liu, J., Yao, Q., et al. (2019). Stachydrine mediates rapid vascular relaxation: activation of endothelial nitric oxide synthase involving AMP-activated protein kinase and akt phosphorylation in vascular endothelial cells. J. Agric. Food Chem. 67, 9805–9811. doi: 10.1021/acs.jafc.9b03501
Xie, X., Zhang, Z., Wang, X., Luo, Z., Lai, B., Xiao, L., et al. (2018). Stachydrine protects eNOS uncoupling and ameliorates endothelial dysfunction induced by homocysteine. Mol. Med. 24, 10. doi: 10.1186/s10020-018-0010-0
Yu, N., Hu, S., Hao, Z. (2018). Benificial effect of stachydrine on the traumatic brain injury induced neurodegeneration by attenuating the expressions of akt/mTOR/PI3K and TLR4/NFκ-B pathway. Transl. Neurosci. 9, 175–182. doi: 10.1515/tnsci-2018-0026
Yu, J., Ke, L., Zhou, J., Ding, C., Yang, H., Yan, D., et al. (2023). Stachydrine relieved the inflammation and promoted the autophagy in diabetes retinopathy through activating the AMPK/SIRT1 signaling pathway. Diabetes Metab. Syndr. Obes. 16, 2593–2604. doi: 10.2147/DMSO.S420253
Yu, Q., Yu, Y. (2007). Overview of studies on the chemical composition and pharmacological effects of Amanita phalloides. Inf. Traditional Chin. Med. (5), 26–28. doi: CNKI:SUN:ZYXN.0.2007-05-013
Zeng, R. Q. (2007). Effects of Leonurus on MSMC’s Proliferation, Apoptosisa and Expression of Calponin Induced by LPS [Master’s thesis]. (Changsha, Hunan Province: Hunan University of Traditional Chinese Medicine).
Zeng, H., Xu, D., Song, Y., Tian, S., Qiao, J., Li, Z., et al. (2023). Synthesis, characterization and anti-breast cancer activities of stachydrine derivatives. Eur. J. Med. Chem. 259, 115679. doi: 10.1016/j.ejmech.2023.115679
Zhai, Z., Mu, T., Zhao, L., Zhu, D., Zhong, X., Li, Y., et al. (2024). Stachydrine represses the proliferation and enhances cell cycle arrest and apoptosis of breast cancer cells via PLA2G2A/DCN axis. Chem. Biol. Drug Des. 103, e14429. doi: 10.1111/cbdd.14429
Zhang, R.-H., Liu, Z.-K., Yang, D.-S., Zhang, X.-J., Sun, H.-D., Xiao, W.-L. (2018). Phytochemistry and pharmacology of the genus Leonurus: The herb to benefit the mothers and more. Phytochemistry 147, 167–183. doi: 10.1016/j.phytochem.2017.12.016
Zhao, L.-G. (2018). Stachydrine inhibits the growth of colon cancer by regulating the expression of ACTG2. Chin. Pharm. J., 1077–1082. doi: 10.11669/cpj.2018.13.008
Zhao, L., Wu, D., Sang, M., Xu, Y., Liu, Z., Wu, Q. (2017). Stachydrine ameliorates isoproterenol-induced cardiac hypertrophy and fibrosis by suppressing inflammation and oxidative stress through inhibiting NF-κB and JAK/STAT signaling pathways in rats. Int. Immunopharmacol 48, 102–109. doi: 10.1016/j.intimp.2017.05.002
Zheng, J., Tian, J., Wang, S., Hu, P., Wu, Q., Shan, X., et al. (2020). Stachydrine hydrochloride suppresses phenylephrine-induced pathological cardiac hypertrophy by inhibiting the calcineurin/nuclear factor of activated T-cell signalling pathway. Eur. J. Pharmacol. 883, 173386. doi: 10.1016/j.ejphar.2020.173386
Keywords: stachydrine, Leonurus japonicus, pharmacological effects, cardioprotective, osmoprotective, biosynthesis pathway
Citation: He Z, Li P, Liu P and Xu P (2024) Exploring stachydrine: from natural occurrence to biological activities and metabolic pathways. Front. Plant Sci. 15:1442879. doi: 10.3389/fpls.2024.1442879
Received: 03 June 2024; Accepted: 08 July 2024;
Published: 06 August 2024.
Edited by:
Sheng-Hong Li, Kunming Institute of Botany, Chinese Academy of Sciences (CAS), ChinaReviewed by:
Chunmao Yuan, Key Laboratory of Chemistry for Natural Products of Guizhou Province (CAS), ChinaRufeng Wang, Shanghai University of Traditional Chinese Medicine, China
Copyright © 2024 He, Li, Liu and Xu. This is an open-access article distributed under the terms of the Creative Commons Attribution License (CC BY). The use, distribution or reproduction in other forums is permitted, provided the original author(s) and the copyright owner(s) are credited and that the original publication in this journal is cited, in accordance with accepted academic practice. No use, distribution or reproduction is permitted which does not comply with these terms.
*Correspondence: Ping Xu, eHVwaW5nMDFAc2licy5hYy5jbg==; Peng Li, cGVuZ2xlZTk4QDE2My5jb20=