- 1Department of Environmental Biology, State University of New York College of Environmental Science and Forestry, Syracuse, NY, United States
- 2InnovPlantProtect Collaborative Laboratory, Elvas, Portugal
- 3Centro de Investigação das Ferrugens do Cafeeiro, Instituto Superior de Agronomia, Universidade de Lisboa, Lisboa, Portugal
- 4Linking Landscape, Environment, Agriculture and Food, Associate Laboratory TERRA, Instituto Superior de Agronomia, Universidade de Lisboa, Lisboa, Portugal
- 5Instituto de Tecnologia Química e Biológica António Xavier (ITQB, Green-It Unit), Universidade NOVA de Lisboa, Oeiras, Portugal
- 6Instituto Nacional de Investigação Agrária e Veterinária I.P., Oeiras, Portugal
- 7Centro de Estudos Florestais, Associate Laboratory TERRA, Instituto Superior de Agronomia, Universidade de Lisboa, Lisboa, Portugal
Phytophthora cinnamomi Rands devastates forest species worldwide, causing significant ecological and economic impacts. The European chestnut (Castanea sativa) is susceptible to this hemibiotrophic oomycete, whereas the Asian chestnuts (Castanea crenata and Castanea mollissima) are resistant and have been successfully used as resistance donors in breeding programs. The molecular mechanisms underlying the different disease outcomes among chestnut species are a key foundation for developing science-based control strategies. However, these are still poorly understood. Dual RNA sequencing was performed in C. sativa and C. crenata roots inoculated with P. cinnamomi. The studied time points represent the pathogen’s hemibiotrophic lifestyle previously described at the cellular level. Phytophthora cinnamomi expressed several genes related to pathogenicity in both chestnut species, such as cell wall–degrading enzymes, host nutrient uptake transporters, and effectors. However, the expression of effectors related to the modulation of host programmed cell death (elicitins and NLPs) and sporulation-related genes was higher in the susceptible chestnut. After pathogen inoculation, 1,556 and 488 genes were differentially expressed by C. crenata and C. sativa, respectively. The most significant transcriptional changes occur at 2 h after inoculation (hai) in C. sativa and 48 hai in C. crenata. Nevertheless, C. crenata induced more defense-related genes, indicating that the resistant response to P. cinnamomi is controlled by multiple loci, including several pattern recognition receptors, genes involved in the phenylpropanoid, salicylic acid and ethylene/jasmonic acid pathways, and antifungal genes. Importantly, these results validate previously observed cellular responses for C. crenata. Collectively, this study provides a comprehensive time-resolved description of the chestnut–P. cinnamomi dynamic, revealing new insights into susceptible and resistant host responses and important pathogen strategies involved in disease development.
1 Introduction
Plants rely on two layers of their innate immune system to recognize and respond to pathogen infections (Jones and Dangl, 2006). Initially, the host recognizes pathogen-associated molecular patterns (PAMPs) by transmembrane pattern recognition receptors (PRRs) such as receptor-like kinases (RLKs) and receptor-like proteins (RLPs), leading to PTI (PAMP-triggered immunity) (Nicaise et al., 2009). PTI aims to block further colonization by inducing changes in ion fluxes across plasma membranes, releasing apoplastic reactive oxygen species (ROS) and cytosolic calcium influx, activation of mitogen-activated protein kinases (MAPKs) followed by the production of phytohormones [e.g., ethylene and salicylic acids (SAs)], stomatal closure, callose deposition, cell wall reinforcement, accumulation of phytoalexins, and transcriptomic/metabolic reprogramming (Jones and Dangl, 2006; van Loon et al., 2006; Saijo et al., 2018; Wang et al., 2020). As an evolutionary result, pathogens adapted to suppress PTI by secreting effectors (virulence factors) into the apoplast (the host–pathogen interface) or within the cytoplasm, resulting in effector-triggered susceptibility. This leads to the second plant-defensive layer: Effector-triggered immunity (ETI). The host recognizes the effectors by nucleotide-binding leucine-rich repeat protein products encoded by resistance (R) genes (Jones and Dangl, 2006; Wang et al., 2020). ETI is a faster and amplified reboot of PTI, leading to disease resistance and hypersensitive reaction (HR) at the infection site. Pathogen–host co-evolution is a result of consecutive infection cycles that allowed pathogens and plants to diversify genes encoding for effectors and R proteins, respectively (Jones and Dangl, 2006). In addition to networks of inducible host defenses, plants also signal distant organs to become primed against further infection, known as systemic acquired resistance (SAR). The establishment of SAR involves systemic SA accumulation and the induction of pathogenesis-related (PR) proteins along with other defense genes [reviewed by Gao et al. (2015)].
Ink disease, or root rot, is caused by Phytophthora cinnamomi Rands, one of the most virulent and devastating plant pathogens worldwide. It infects more than 5,000 plant species and causes severe economic and ecological damage, making it one of the world’s top 10 most destructive oomycetes (Kamoun et al., 2015). It is a hemibiotrophic pathogen [reviewed by Hardham and Blackman (2018)] that begins the infection by the chemotactic attraction of the zoospores (asexual spores) to the roots of suitable hosts (Carlile, 1983). After penetrating the root, it rapidly reaches the vascular system, obstructing the xylem vessels and consequently impairing water and nutrient uptake (Oßwald et al., 2014).
The mechanisms of Phytophthora spp. infection have been mainly studied in the model species P. infestans and P. sojae (Jiang and Tyler, 2012) and represent a valuable source of data for P. cinnamomi research [reviewed by Hardham and Blackman (2018)]. The apoplast effectors secreted by P. cinnamomi can be cell wall–degrading enzymes (CWDEs), elicitins, toxins, and inhibitors of plant enzymes. Some cytoplasmic effectors have been identified as suppressants of host defense responses, such as callose deposition and HR cell death; however, most of their functions remain unknown. Some effectors are involved in disease establishment during zoospore adhesion and root penetration (e.g., CWDE). Others facilitate attack and colonization, guaranteeing Phytophthora’s access to nutrients for growth and reproduction [e.g., elicitins, transglutaminases, Crinkler (CRN), Nep1-like proteins (NLPs), RxLR proteins, and Avirulence (Avr) Nudix hydrolases; reviewed by Hardham and Blackman (2018)]. Some of these effectors and cell wall components (e.g., β-1,3- and β-1,6-glucans) are known as oomycete PAMPs; however, this knowledge is still limited (Raaymakers and Van Den Ackerveken, 2016). The dichotomy of PTI/ETI in Phytophthora–plant interactions is blurred, and recent studies indicate that these phases are more of a continuum and not distinct (Naveed et al., 2020).
Phytophthora cinnamomi has severely affected European chestnut (Castanea sativa) production and threatens its sustainability in forests. In Southwest Europe, control strategies have yet to be effective. So far, the best way to prevent further chestnut decline is mainly by planting first-generation Euro-Asian hybrids that inherited P. cinnamomi resistance from the co-evolved Asian host species, Castanea mollissima (Chinese chestnut) and Castanea crenata (Japanese chestnut) (Crandall et al., 1945; Serdar et al., 2019). This comes as a sustainable solution for production; however, it may impact the genetic diversity of European chestnuts in forests (Alcaide et al., 2022). Additional methods, such as biotechnological and/or phytopharmaceutical tools, should be developed to mitigate P. cinnamomi’s consequences and potentially reduce its prevalence. The key question remains unanswered: What causes some plant species to survive P. cinnamomi infection? During the last decade, significant advances were made in genomic research to understand plant–P. cinnamomi interactions by using approaches such as transcriptomics (Serrazina et al., 2015; Meyer et al., 2016; Reeksting et al., 2016; Baldé et al., 2017; Evangelisti et al., 2017; Islam et al., 2018; van den Berg et al., 2018b), genotyping by sequencing, association mapping, and identification of quantitative trait loci (QTL; Santos et al., 2015b, 2017b; Zhebentyayeva et al., 2019). Plant defense strategies against P. cinnamomi seem to vary in different hosts. For example, callose deposition is proposed as an important response for avocado (van den Berg et al., 2018a) and maize (Cahill and Weste, 1983), but it does not seem to play a significant role in chestnuts (Fernandes et al., 2021). However, chestnut species have similar mechanisms of defense against P. cinnamomi challenge. The European and Japanese chestnuts both induce genes related to the Jasmonic acid (JA) pathway, anti-fungal metabolite synthesis, anti-fungal enzymes, and cell wall strengthening (Serrazina et al., 2015). Also, association mapping studies of Euro-Japanese and American-Chinese Castanea hybrids inoculated with the pathogen reported two overlapping QTL regions, suggesting shared allelic variants (Santos et al., 2017b; Zhebentyayeva et al., 2019). The suggested difference between resistant and susceptible responses seems to be related to a high constitutive expression of putative resistance genes in the Japanese chestnut and a delay of pathogen-induced gene expression in the European chestnut (Santos et al., 2017a). The fast response by the resistant species favors the blockage of some infections and consequently reduces the area of infection (Fernandes et al., 2021). However, understanding the molecular architecture of plant–P. cinnamomi interactions remain unclear.
By comparing the root transcript profiles of resistant (C. crenata) and susceptible chestnut species (C. sativa) after infection with P. cinnamomi, this study aims to (i) identify pathogenicity-related genes expressed by P. cinnamomi, (ii) identify candidate genes linked with host resistance, and (iii) provide new insights into the molecular mechanisms involved in resistant and susceptible host defense responses. This study’s selected time points (2 h, 48 h, and 72 h after inoculation) represent specific stages of the pathogen’s infection and plant responses previously described by Fernandes et al. (2021). We used dual RNA-seq to reveal the complex interplay between Castanea spp. and P. cinnamomi, as it allows the temporal determination of responses and changes in both organisms’ cellular networks (Westermann et al., 2012). Also, the recent availability of the P. cinnamomi reference genome (Engelbrecht et al., 2021) has revealed effectors that may have a role in chestnut invasion and, consequently, facilitate the identification of corresponding putative R genes for future validation. We also took advantage of the availability of optimized in vitro propagation protocols (Fernandes et al., 2020) and produced biological replicates for each condition, which were individually sequenced. The results suggest new Castanea genes involved in pathogen perception and activation of immune responses and set forward P. cinnamomi genes that could have pathogenicity roles in the interaction with chestnuts.
2 Materials and methods
2.1 Plant material, pathogen inoculation, and sampling
Plants of Castanea sativa (CS12) and Castanea crenata (CC14) (genotypes from TRAGSA-SEPI, Maceda, Spain) were produced as described by Fernandes et al. (2020) and grown in a peat:perlite:vermiculite (1:1:1) mixture for 3 months. The roots were carefully washed with water before inoculation to remove the substrate. Roots were inoculated with Phytophthora cinnamomi mycelium (GenBank, accession number OL901253; isolate IMI 340340 from the University of Trás-os-Montes and Alto Douro). Pathogen growth conditions and inoculation methods followed the described by Fernandes et al. (2021). Briefly, P. cinnamomi cultures were grown in vegetable juice (V8®) agar [20% (v/v) with CaCO3 (3 g/L) and agar (6 g/L)] in darkness at 24°C for 5 days. Each plant was placed into a container with a paper base and a cotton ball moistened with sterile distilled water to avoid desiccation. Several pieces of actively growing mycelium were placed, covering the roots. Approximately 80% of the root system was inoculated as the roots next to the collar were forced upward, preventing the inoculation in that area.
Based on previous microscopic analysis (Fernandes et al., 2021), three time points were selected, corresponding to key stages of P. cinnamomi infection and plant responses. (i) 2 h after inoculation (hai): Pathogen’s penetration and establishment of biotrophic growth in susceptible and resistant species; beginning of defense responses in resistant species (callose around intracellular hyphae, accumulation of phenolic-like compounds in the cell walls and cytoplasmic contents, and hypersensitive response). (ii) 48 hai: Biotrophic pathogen growth in both species, with a delay detected in the progression of infection in the resistant species, associated with lower areas of hyphae colonization and higher development of the defense responses compared to the susceptible species (where occasionally callose around some intracellular hyphae was observed). (iii) 72 hai: Switch to necrotrophic phase and formation of survival structures in the susceptible species, in contrast with more restricted pathogen growth areas in the resistant species, associated with defense responses. Three plants were inoculated, and three were non-inoculated (control) per time point. In total, nine inoculated and nine non-inoculated plants for each chestnut species (36 plants total) were used. Plants were maintained at 24°C at a natural photoperiod.
2.2 Light microscopic observation of fresh tissues
Light microscopic observations were performed according to Fernandes et al. (2021) to guarantee that sequenced samples were infected and time points corresponded to the previously described stages of infection. Segments of approximately 2 mm to 5 mm in length were randomly collected from inoculated and non-inoculated root tissue at the referred time points. Samples were fixed in a 1:1:18 FAA solution—formaldehyde (37% to 38%)/glacial acetic acid/ethanol (70%)—and stored at 4°C until used. Root segments were rehydrated with distilled water for 15 min before sectioning (20-µm to 25-µm thick) in a freezing microtome (Leica CM1850). Longitudinal root sections were stained and mounted in cotton blue lactophenol (Silva et al., 2002). Observations were performed using a Leica DM-2500 light microscope equipped with a LEICA DFC340FX camera. Images were acquired with the software Leica Application Suite Version 4.12 (Leica Microsystems, Switzerland).
2.3 RNA extraction, quantification, and quality assessment
Total RNA was extracted individually for all 36 root samples as described by Le Provost et al. (2007). All samples were treated with the Turbo DNA-free™ Kit (Ambion, Life Technologies, Ltd.) according to the manufacturer’s instructions. Total RNA concentration and purity were verified with a NanoDrop spectrophotometer ND-2000C (Thermo Scientific) by measuring absorbance at 260/280 and 260/230. Concentration was verified with Qubit 2.0. The integrity was checked by electrophoresis on 1% agarose gel (1× TBE), and the absence of genomic DNA was confirmed by PCR, using primers for a known gene in both species [Cast_Gnk2-like gene; primers and PCR conditions are described by McGuigan et al. (2020)].
2.4 mRNA Library preparation and sequencing
RNA was sequenced by NOVOGENE Co. (Cambridge, UK). The NEBNext® Ultra™ Directional RNA Library Prep Kit for Illumina® was used to prepare 36 strand-specific cDNA libraries. mRNA was purified from total RNA using oligo(dT) magnetic beads, and rRNA was removed using the Ribo-Zero kit. First, the mRNA is fragmented randomly by adding fragmentation buffer, and, then, the cDNA is synthesized by using mRNA template and random hexamers, after which a custom second-strand synthesis buffer (Illumina), deoxynucleotide triphosphates (dNTPs), ribonuclease (RNase) H, and DNA polymerase I are added to initiate the second-strand synthesis. Second, after a series of terminal repair, A ligation, and sequencing adaptor ligation, the double-stranded cDNA library is completed through size selection and PCR enrichment. Quality control of libraries was performed. The concentration was checked on a Qubit 2.0 fluorometer (Life Technologies), the insert size was checked on an Agilent 2100, and the quantification was performed through qPCR. Stranded libraries were sequenced on Illumina NovaSeq 6000 platform following the manufacturer’s recommendations, and approximately 67 million paired-end strand-specific reads of 150 bp were produced per sample.
2.5 Phytophthora cinnamomi differential gene expression analysis
RNA-seq read quality before and after trimming was assessed using FastQC v0.11.9 (https://www.bioinformatics.babraham.ac.uk/projects/fastqc/), and results were aggregated with MultiQC v1.11 (Ewels et al., 2016). Low-quality reads were filtered, and adapters were removed using Trimmomatic v0.39 (Bolger et al., 2014). Reads with less than 50 bp were removed, and reads with an average Q score below 20 were trimmed. Trimmed reads were aligned to the Phytophthora cinnamomi reference genome (Engelbrecht et al., 2021) using STAR aligner v2.7.9a (Dobin et al., 2013). Unmapped reads were collected for de novo assembly transcriptomes of both Castanea species. Counts per gene were summarized using featureCounts in the SubReads package v2.0.2 (Liao et al., 2014). Lowly expressed genes were filtered, and the resulting dataset was balanced following the trimmed mean of M-value method (Robinson and Oshlack, 2010) implemented in edgeR v3.34.0 (Robinson et al., 2010). Depth and gene length were normalized transforming pair-reads to fragments per kb per million counts with rpkm function. After dispersion between samples was evaluated, pairwise comparisons were performed with the exactTest function. Obtained p-values were re-adjusted (by the Benjamini–Hochberg procedure), and the differently expressed genes (DEGs) were filtered out by false discovery rate (FDR) of ≤0.05 and log2 fold change of ≥1.0 or ≤–1.0.
2.6 De novo assembly of Castanea sativa and Castanea crenata transcriptomes
Due to the absence of a reference genome of Castanea sativa, de novo transcriptome assembly of both Castanea species was performed using Trinity v2.12.0 (Grabherr et al., 2011) with the unmapped reads obtained previously. The representative isoform was selected as the longest isoform using the “get_longest_isoform_seq_per_trinity_gene.pl” utility in Trinity. Contigs with less than 250 bp were excluded. Weakly expressed contigs were also removed on the basis of their expression values (Haas et al., 2013) using the Trinity script “aling_and_estimate_abundance.pl” to estimate transcripts per million (TPM) values using Salmon (Patro et al., 2017) and then using “filter_low_expr_transcripts.pl” Trinity utility to exclude lowly expressed contigs (–min_expr_any 0.5). The transcriptome assembly was annotated using dammit! pipeline v1.2 (Scott, 2016), which was based on Pfam-A, Rfam, OrthoDB, and Castanea mollissima protein (GCA_000763605.2) databases. Contigs were taxonomy annotated using MG-RAST v4.0.3 (Meyer et al., 2008) with default parameters, and contigs assigned with Streptophyta phylum were retained. Benchmarking Universal Single-Copy Orthologs (BUSCO) v5.2.2 (Manni et al., 2021) was used to evaluate the assembly completeness using the embryophyta_odb10 database. To assess the read content of the assembly, reads were mapped back to the transcriptome assembly using Bowtie2 v2.4.5 (Langmead et al., 2019). Assembly statistics were obtained with Quast v5.0.2 (Mikheenko et al., 2018). Castanea spp. differential gene expression analysis was performed as previously for Phytophthora cinnamomi. DEGs were blasted against NCBI non-redundant (nr) protein database with Blast2GO v6.0.3 (Gotz et al., 2008), using a cutoff e-value of 1E−5 and an HSP-hit coverage of 80%. Blast2GO was also used to assign Kyoto Encyclopedia of Genes and Genomes (KEGG) metabolic pathways (Kanehisa and Goto, 2000). Genes putatively related to disease resistance were annotated with Disease Resistance Analysis and Gene Orthology (DRAGO 3) tool based on the Plant Resistance Genes database (García et al., 2022). OrthoFinder v2.5.4 (Emms and Kelly, 2019) was used to predict orthologs within both Castanea species. Differential gene expression analysis was performed as in the previous section.
2.7 Functional enrichment analysis
The lists of DEGs from Phytophthora cinnamomi and Castanea species were analyzed with enricher function from clusterProfiler v4.2.2 package (Wu et al., 2021) to identify functional categories significantly enriched according to Gene Ontology (GO) terms identified using Pannzer2 (Törönen et al., 2018). For Castanea species assemblies, protein sequence was translated from the transcriptome assembly with TransDecoder v5.5.0 (https://github.com/TransDecoder/TransDecoder). Enricher function uses a hypergeometric test, and the obtained Gene Ratio is defined as the ratio of the number of DEGs and total gene number in specific GO terms. Significantly enriched categories were selected considering the adjusted p-value ≤ 0.05 (Benjamini–Hochberg correction for multiple testing).
3 Results
All plants were alive before the root samples were collected for RNA extractions and histopathology. Slight root browning and oxidation were generally observed, but there were no signs of necrotic lesions. The efficacy of inoculation was verified microscopically by the presence of hyphae in the root tissue and by observing the progression of the pathogen’s infection stages (Supplementary Figure 1).
3.1 Phytophthora cinnamomi cellular progression of infection
The observed progress of infection followed the previously described by Fernandes et al. (2021). Phytophthora cinnamomi penetrated the root surface and continued to develop post-penetration in both chestnut species (Supplementary Figure 1). The switch to necrotrophy was observed in the susceptible Castanea sativa (Supplementary Figure 2). Figure 1 describes the observed infection process at each time point in both species and the previously described early-induced host responses (Fernandes et al., 2021).
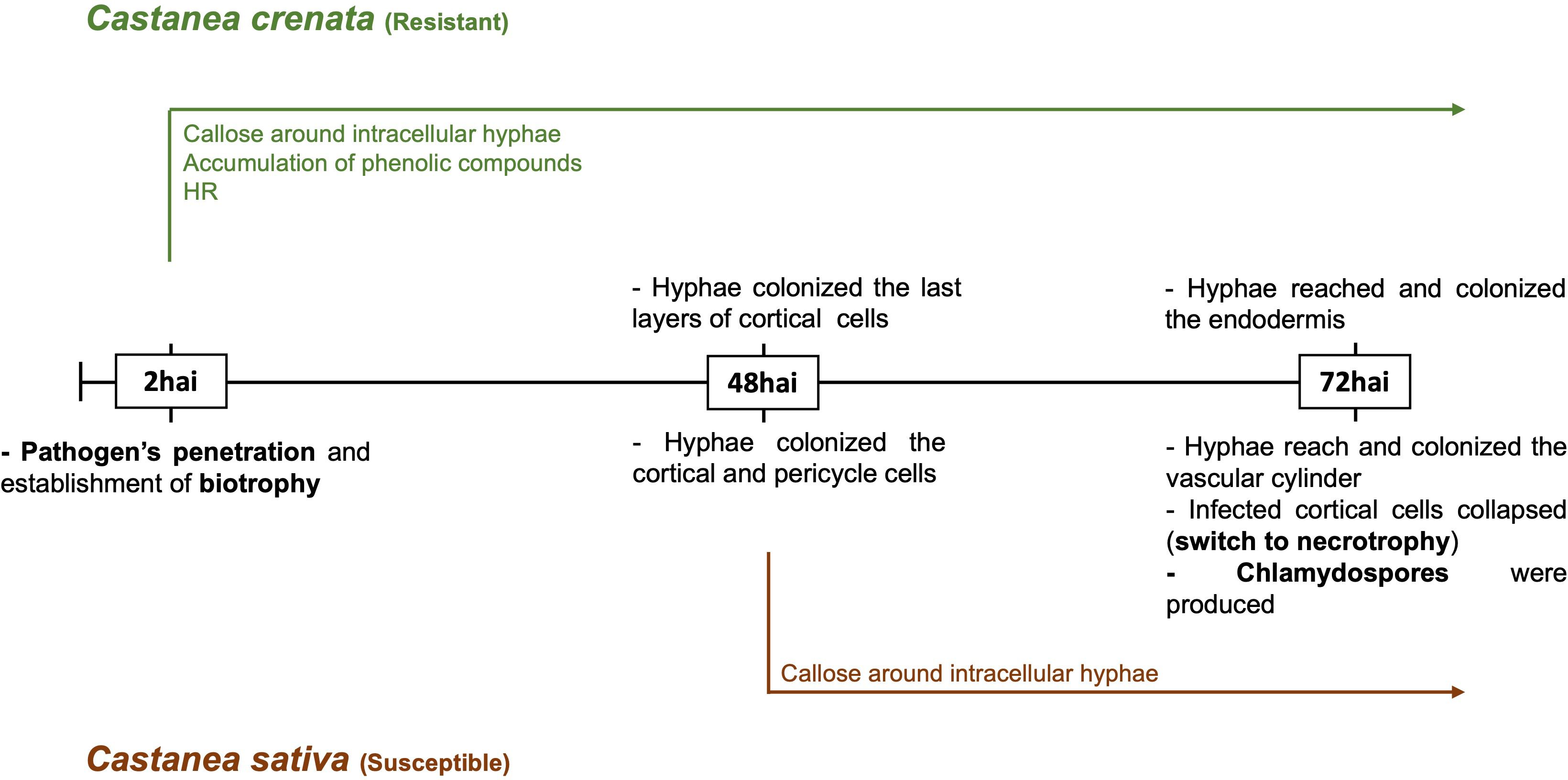
Figure 1. Time course of Phytophthora cinnamomi infection in roots of resistant and susceptible chestnut species. hai, hours after inoculation. The resistance was characterized by more restricted pathogen growth associated with the early activation of defense responses, such as callose around intracellular hyphae, accumulation of phenolic compounds in the cell walls and cytoplasmic contents, and the hypersensitive reaction (HR). In susceptible roots, callose deposition around some intracellular hyphae, observed from 48 hai, did not prevent the pathogen growth Fernandes et al. (2021).
3.2 RNA sequencing and transcriptome assembly
Approximately 33 million read pairs were obtained per sample, and 14.7% and 22.55% were mapped to Phytophthora cinnamomi’s genome in the Castanea crenata and Castanea sativa inoculated samples, respectively (Supplementary Table 1). A few reads from the control samples were mapped to P. cinnamomi genome (0.001%; Supplementary Table 1), which were considered conserved eukaryotic gene sequences. After filtering low-expression transcripts, 19,981 genes were annotated in P. cinnamomi transcriptome (Supplementary Table 2). The number of P. cinnamomi transcripts expressed during each condition (chestnut species and time point) is provided in Supplementary Table 2.
Castanea spp. de novo transcriptome assembly produced 98,389 and 95,820 transcripts for C. sativa and C. crenata, respectively. For further analysis, 14,733 and 15,314 transcripts for C. sativa and C. crenata, respectively, were retained after being taxonomically annotated to the Streptophyta phylum (Supplementary Table 3).
3.3 Phytophthora cinnamomi differential expression analysis in Castanea crenata vs. Castanea sativa highlighted enriched functions related to pathogen attack
Phytophthora cinnamomi transcript expression in Castanea crenata roots was compared to the expression in Castanea sativa roots at each time point after inoculation (Supplementary Table 4). The Multi-Dimensional Scaling (MDS) plots showed a good separation between samples at each time point (Supplementary Figure 3). In this analysis, upregulated genes correspond to P. cinnamomi genes that were more expressed in C. crenata roots than in C. sativa. Downregulated genes were genes less expressed in C. crenata, therefore more expressed in C. sativa roots. A total of 236 DEGs were identified, with the highest number of down- and upregulated genes occurring at 48 hai and 72 hai, respectively (Figure 2). No DEGs were common to all the time points (Supplementary Figure 4). Nine GO terms were enriched, most of them at 48 hai and 72 hai (Figure 3; Supplementary Table 5). Several GO terms related to pathogenicity, such as cysteine-type peptidase activity (2 hai) and modulation by symbiont of host defense-related programmed cell death (48 hai and 72 hai) were enriched in C. sativa samples (downregulated) and polygalacturonase activity (2 hai) in C. crenata (upregulated; Figure 3).
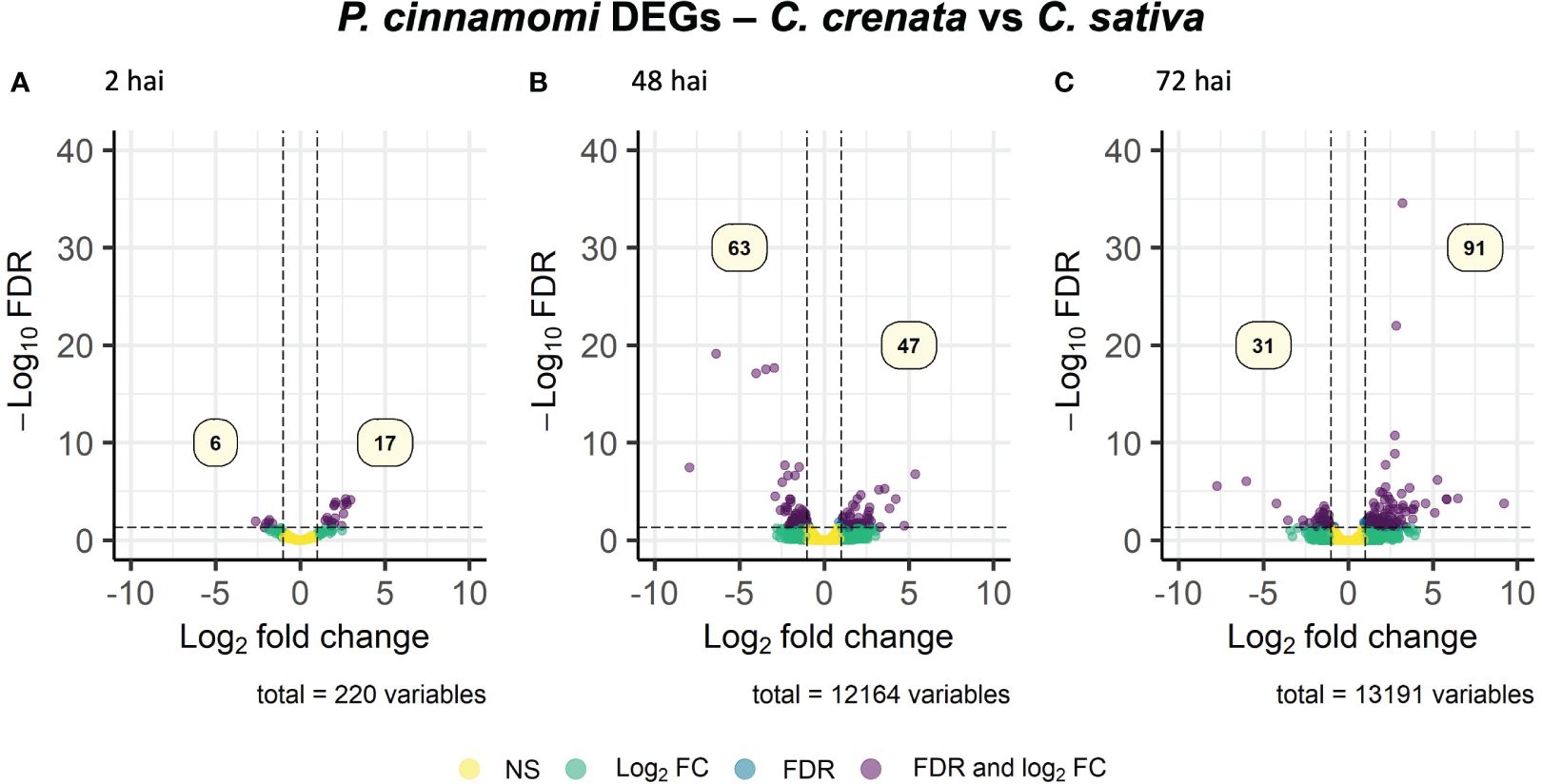
Figure 2. Volcano plots showing the dispersion of Phytophthora cinnamomi differentially expressed genes (DEGs) in C. crenata roots compared to C. sativa at 2 (A), 48 (B), and 72 (C) hours after inoculation (hai). DEGs were filtered out by false discovery rate (FDR) of ≤0.05 and log2 fold change (Log2 FC) of ≥1.0 or ≤–1.0. NS, not significant.
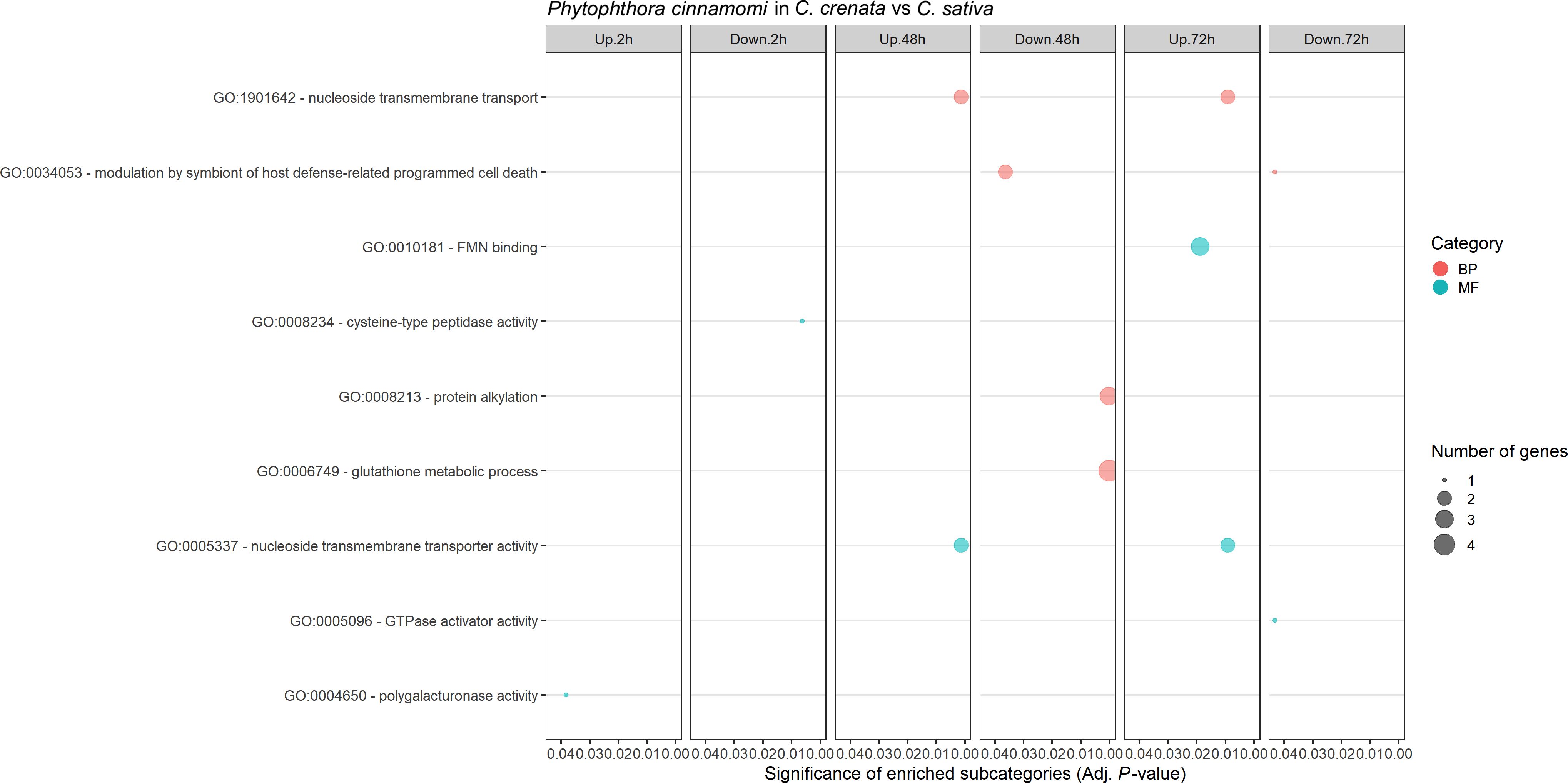
Figure 3. Gene Ontology (GO) enriched subcategories of Phytophthora cinnamomi differentially expressed genes in Castanea crenata roots compared with Castanea sativa, at 2 h, 48 h, and 72 h after inoculation. Analysis was performed using the hypergeometric statistical test, and significantly enriched categories were selected based on the adjusted P-value ≤ 0.05; BP, biological process, red circles; MF, molecular function, green circles. The complete dataset is provided in Supplementary Table 5.
Several transcripts encoding for P. cinnamomi effectors, such as proteins associated with pathogenicity, plant colonization, and transporters for organic compounds (e.g., sugars, amino acids, and nucleosides), ammonium, and phosphate were differentially expressed (DE) and selected for further analysis. Transporter selection was performed according to Abrahamian et al. (2016). A total of 15 effectors of pathogen attack strategies, 14 CWDE, 30 transporters, and two genes involved in hyphal growth were selected (Figure 4; Supplementary Table 6). Also included were two genes annotated for putative effectors of pathogen defense strategies, Kazal-like serine protease inhibitor and Catalase upregulated at 48 hai and 72 hai, respectively (Supplementary Table 6). Overall, there is an exponential increase throughout the time points of the number of P. cinnamomi genes more expressed in C. crenata tissues, unlike the downregulated genes (genes more expressed in C. sativa roots), which have a peek at 48 hai and a drastic decrease at 72 hai (Figure 4).
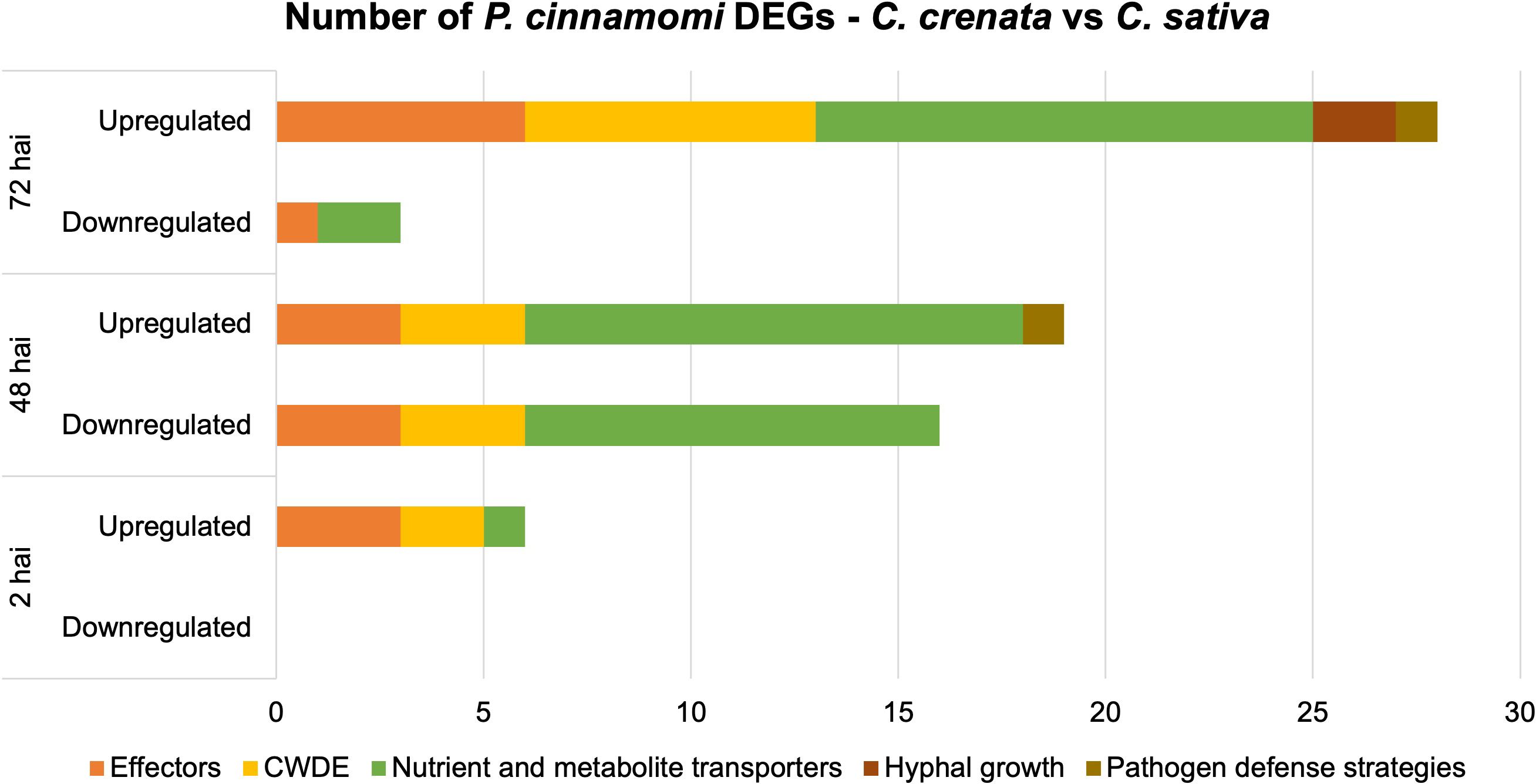
Figure 4. Number of differentially expressed genes (DEGs) by Phytophthora cinnamomi at 2 h, 48 h, and 72 h after inoculation (hai) in Castanea crenata roots compared to Castanea sativa. The selected DEGs represent several functional groups related to pathogen infection: effectors of pathogen attack, cell wall–degrading enzymes (CWDEs), nutrient and metabolite transporters, hyphal growth, and putative effectors of pathogen defense strategies. Upregulated: P. cinnamomi genes more expressed in C. crenata than in C. sativa roots. Downregulated: P. cinnamomi genes less expressed in C. crenata than in C. sativa roots and, therefore, more expressed in C. sativa.
At 2 hai, genes encoding for three effectors of pathogen attack, two CWDEs, and one transporter were upregulated (Figure 4). The upregulated effectors were Transglutaminase elicitor M81C, putative suppressor of necrosis 1, and Secretory protein OPEL (Supplementary Table 6). At 48 hai, all the DE CWDEs were mainly from the hydrolase family, but the type of effectors of pathogen attack and transporters differed between up- and downregulated genes. At this time point, downregulated effectors were primarily elicitins, and upregulated were Aldose 1-epimerase, Avr1b-1, RxLRs, and Crinkler (CRN)–like. Ammonium and sugar transporters were found to be upregulated, and phosphate, sodium, and amino acid/auxin permeases (AAAP) were mostly downregulated (Supplementary Table 6). At 72 hai, two transcripts encoding for Mucin-like proteins were upregulated in C. crenata tissues by 4.5 and 9.2 fold change compared to those in C. sativa.
For further insight into the differences in P. cinnamomi gene expression in both chestnut species, we assessed the top 10 most up- and downregulated genes at each time point with known or putative functions in pathogenicity, growth, and sporulation (Supplementary Table 7). Twenty-five of the predicted proteins show homology to transcripts with known functions in virulence and pathogenicity based on functional genetic assays performed with other pathogens. Twelve of these were not included in the assessment represented in Figure 4. Additionally, two downregulated transcripts related to Phytophthora zoospore development were identified: DEAD/DEAH box RNA helicase and Zinc finger, C2H2 (Supplementary Table 7).
3.4 Phytophthora cinnamomi differential gene expression at 72 h vs. 48 h after inoculation reveals different enriched functions in Castanea sativa and Castanea crenata
Expression of Phytophthora cinnamomi transcripts infecting Castanea crenata and Castanea sativa at 72 hai was compared to that at 48 hai. The MDS plots showed a good separation between the samples of the different time points for each species (Supplementary Figure 5). A total of 237 DEGs were identified, 209 in C. sativa, and 48 in C. crenata (Supplementary Table 8). Most of the DEGs were found to be downregulated at 72 h vs. 48 h in C. sativa roots (Figure 5). Nineteen downregulated genes were common to both species (Supplementary Figure 6). Four GO terms were enriched in C. sativa and 10 in C. crenata (Figure 6; Supplementary Table 9). GO terms related to pathogen attack were mainly identified in downregulated genes (genes more expressed at 48 hai) in both species. In C. sativa, the enriched terms were modulation by symbiont of host defense-related programmed cell death and, pectate lyase activity. In C. crenata, GO terms were mostly related to host cell wall degradation (pectin catabolic process, aspartyl esterase activity, and pectinesterase activity) and pathogen cell wall alteration (cell wall modification).
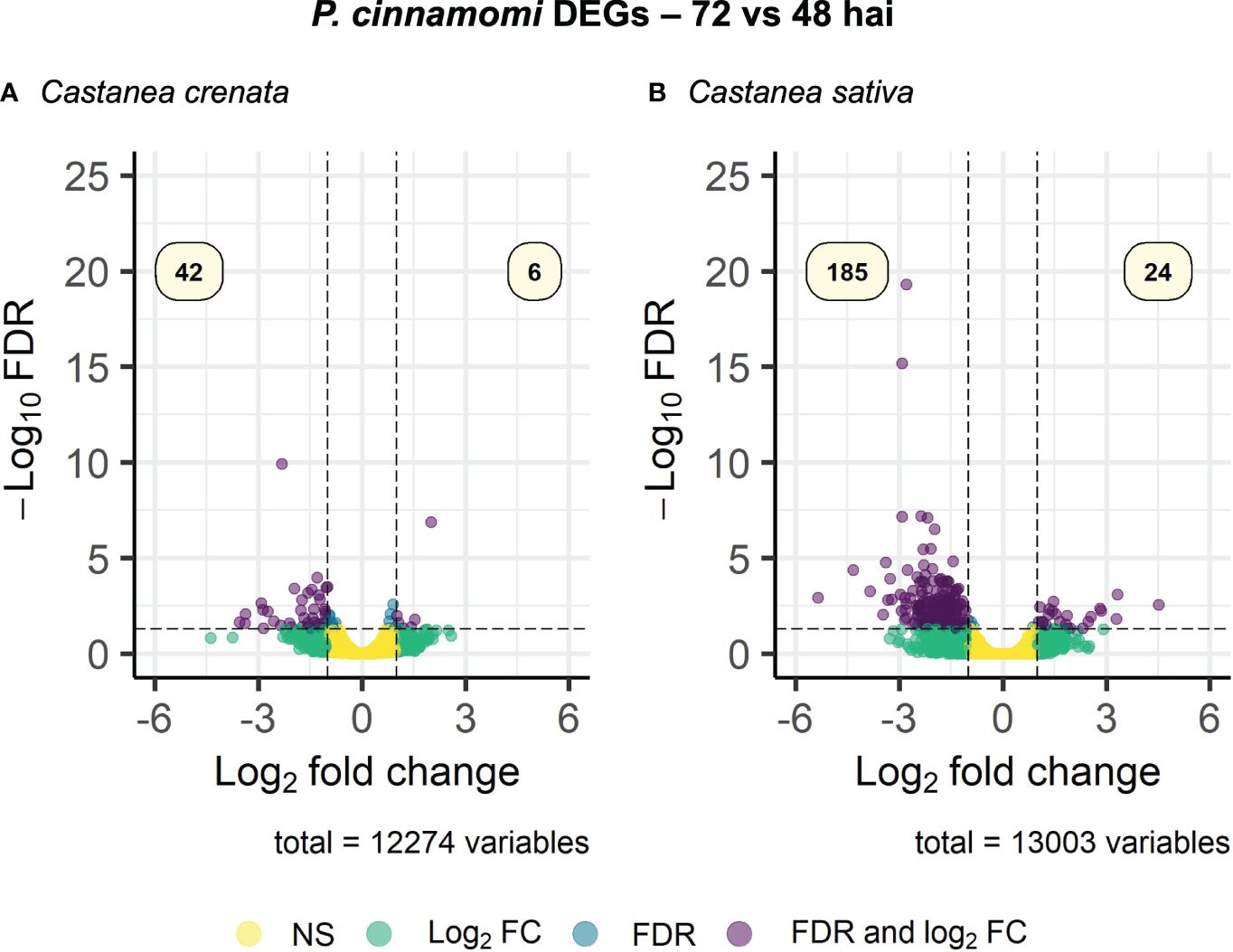
Figure 5. Volcano plot showing the dispersion of Phytophthora cinnamomi differentially expressed genes (DEGs) in Castanea crenata (A) and Castanea sativa (B) roots at 72 h after inoculation (hai) compared with 48 hai. DEGs were filtered out by false discovery rate (FDR) of ≤0.05 and log2 fold change (Log2 FC) of ≥1.0 or ≤–1.0. NS, not significant.
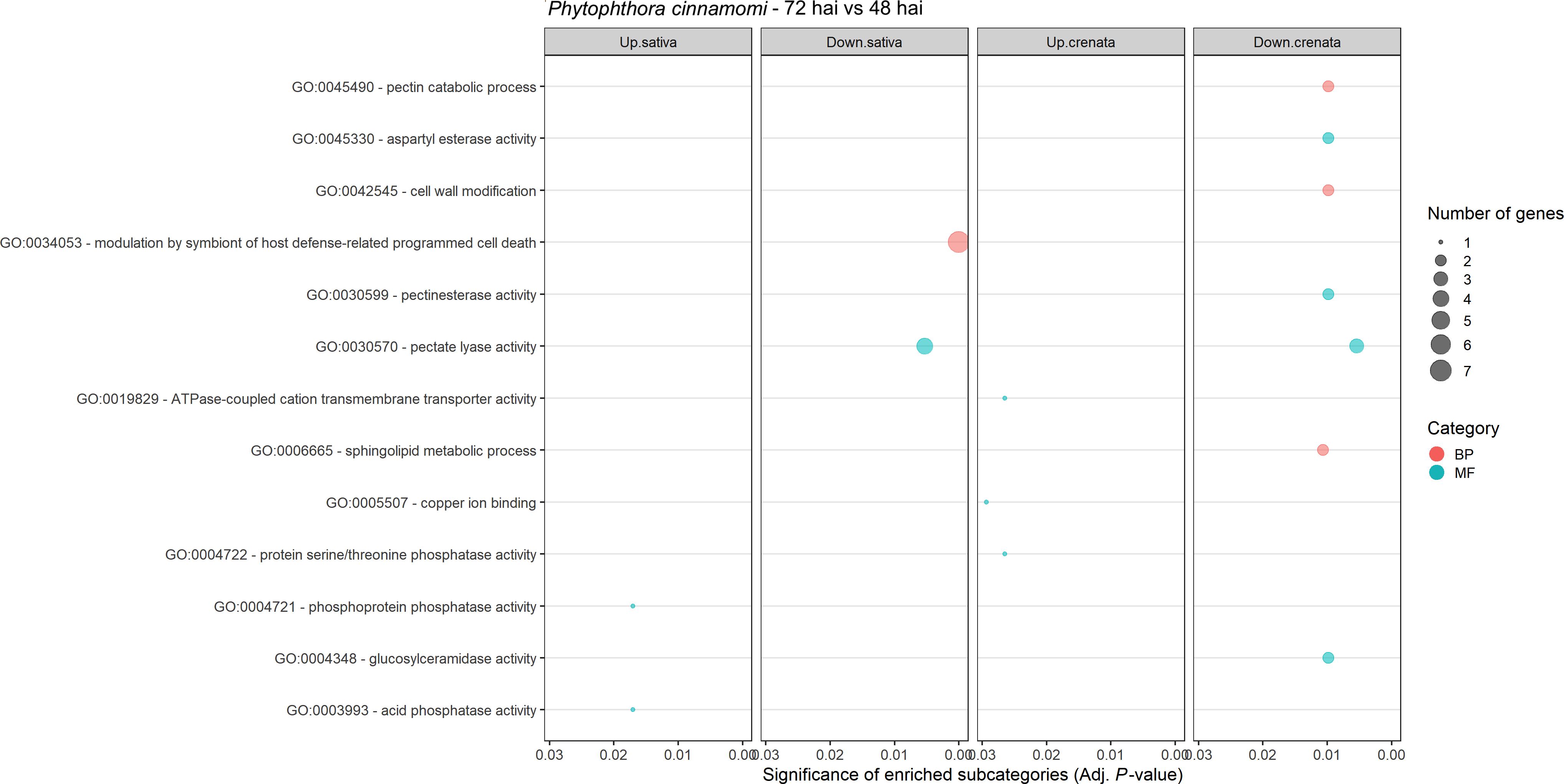
Figure 6. Gene Ontology (GO)–enriched terms of Phytophthora cinnamomi differentially expressed genes at 72 h after inoculation (hai) when compared to 48 hai in Castanea crenata and Castanea sativa roots. Analysis was performed using the hypergeometric statistical test, and significantly enriched categories were selected based on the adjusted p-value ≤ 0.05; BP, biological process, red circles; MF, molecular function, green circles. The complete dataset is provided in Supplementary Table 9.
Genes for effectors of pathogen attack, CWDE, nutrient and metabolite transporters, hyphal growth, and putative effectors of pathogen defense strategies were selected for further analysis, as described in the previous section (Supplementary Table 10; Figure 7). Only one gene was upregulated, corresponding to an effector of pathogen attack in C. crenata (Supplementary Table 10). Overall, the pathogen downregulated more genes at 72 hai in C. sativa compared to C. crenata, most being effectors and nutrient and metabolite transporters (Figure 7). This includes several Necrosis-inducing like proteins, Elicitins, RxLRs, Transglutaminase elicitor M81C, and putative suppressor of necrosis 1. The higher fold-change DEGs were Aldose 1-epimerase and Avr4-associated TDF-like protein with −3.84 and −3.21, respectively (Supplementary Table 10). From the 20 DE transporters identified in C. sativa, the most common were Amino Acid/Auxin Permease (AAAP) Family and Major Facilitator Superfamily (MFS) (Supplementary Table 10). Also downregulated in C. sativa roots were Catalase/peroxidase HPI and protease inhibitor Epi11, related to pathogen defense strategies (Supplementary Table 10).
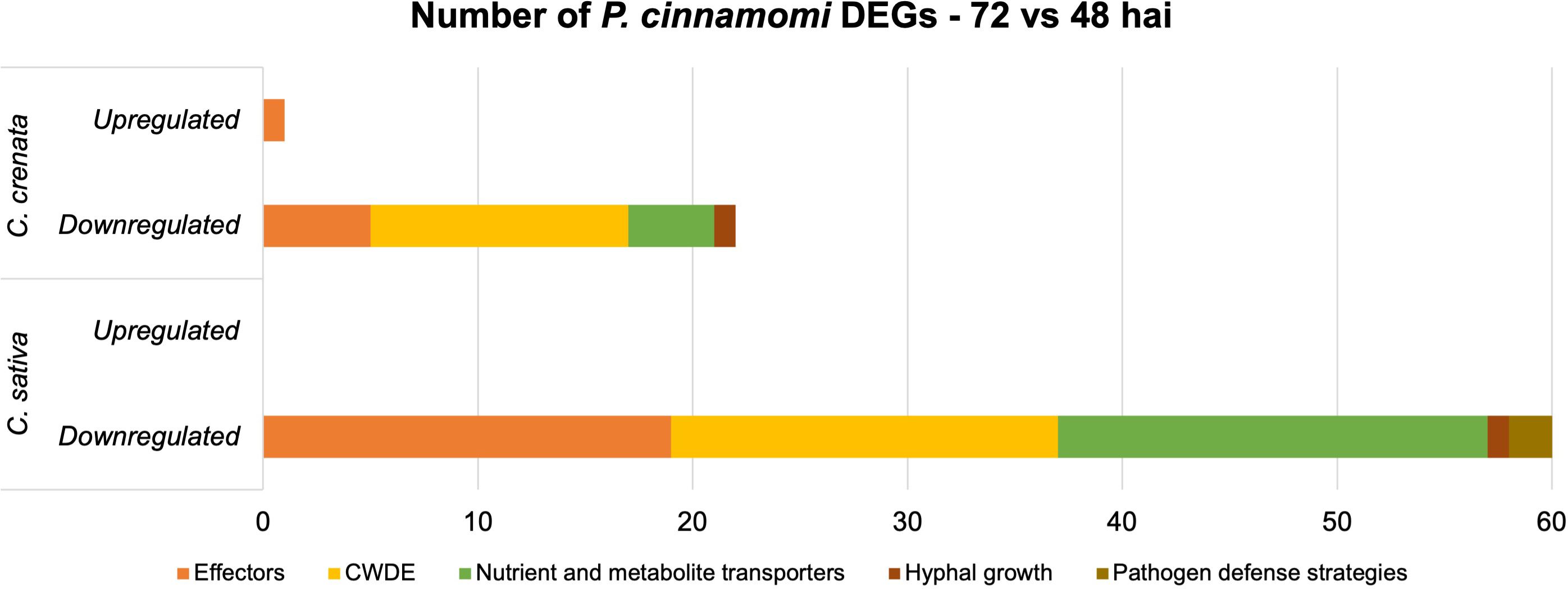
Figure 7. Number of differentially expressed genes (DEGs) expressed by Phytophthora cinnamomi 72 h after inoculation (hai) when compared to 48 hai in Castanea crenata and Castanea sativa roots. The genes represented in this graph are related to pathogen development and infection: effectors of pathogen attack, cell wall–degrading enzymes (CWDEs), nutrient and metabolite transporters, hyphal growth, and putative effectors of pathogen defense strategies.
We also looked into the top 10 most up- and downregulated in each species with known functions and/or with hits (coverage > 80%; E-value < 1E−3) in the PHI-BASE with the same selection criteria described in the previous section. However, all genes that fitted the criteria were already included in Supplementary Table 10, except for Transcript IUM83_01168, downregulated in C. crenata. This encodes for a pleiotropic drug resistance protein ABC superfamily (KAG6618853.1), which had a hit in the PHI-BASE with GPABC1 (Entry: 258; E-value: 1.37E−158), also an ABC transporter, that, when knocked down, reduces pathogen virulence.
3.5 Differentially expressed genes highlighted specific enriched functions in resistant and susceptible chestnuts after pathogen attack
Differential expression analysis in chestnut transcriptomes was performed by comparing inoculated with non-inoculated plants at three time points after inoculation. MDS plots showed separation between control and inoculated samples at all time points. This suggests that a Phytophthora cinnamomi infection influences Castanea sativa and Castanea crenata transcriptomic profiles, enabling discrimination between the control and inoculated samples (Supplementary Figure 7).
From 14,733 (C. sativa) and 15,314 (C. crenata) transcripts mapped for Streptophyta phylum, 488 (C. sativa) and 1,556 (C. crenata) were DE after P. cinnamomi inoculation, from which 406 and 1,293 had homology to Castanea mollissima protein database (Supplementary Tables 11, 12). From all the DEGs, 188 and 667 of C. sativa and C. crenata, respectively, were annotated with Blastx. The remaining genes did not show a minimum of 80% similarity to any reported proteins used by this algorithm (Supplementary Tables 11, 12); for that reason, the best dammit homology (lowest e-value < 1E−05) was considered. Castanea sativa upregulates the highest number of genes at 2 hai, and this number decreases in the subsequent time points (Figure 8A), whereas C. crenata with only one DE gene at 2 hai upregulates the highest number of genes at 48 hai, followed by a decrease at 72 hai (Figure 8B). Few DEG overlapped time points in both species (Supplementary Figure 8), and only Transcript_48468 was upregulated at all time points in C. sativa (Supplementary Figure 8A), which putatively encodes for a (+)-neomenthol dehydrogenase (Supplementary Table 11; dammit best homology).
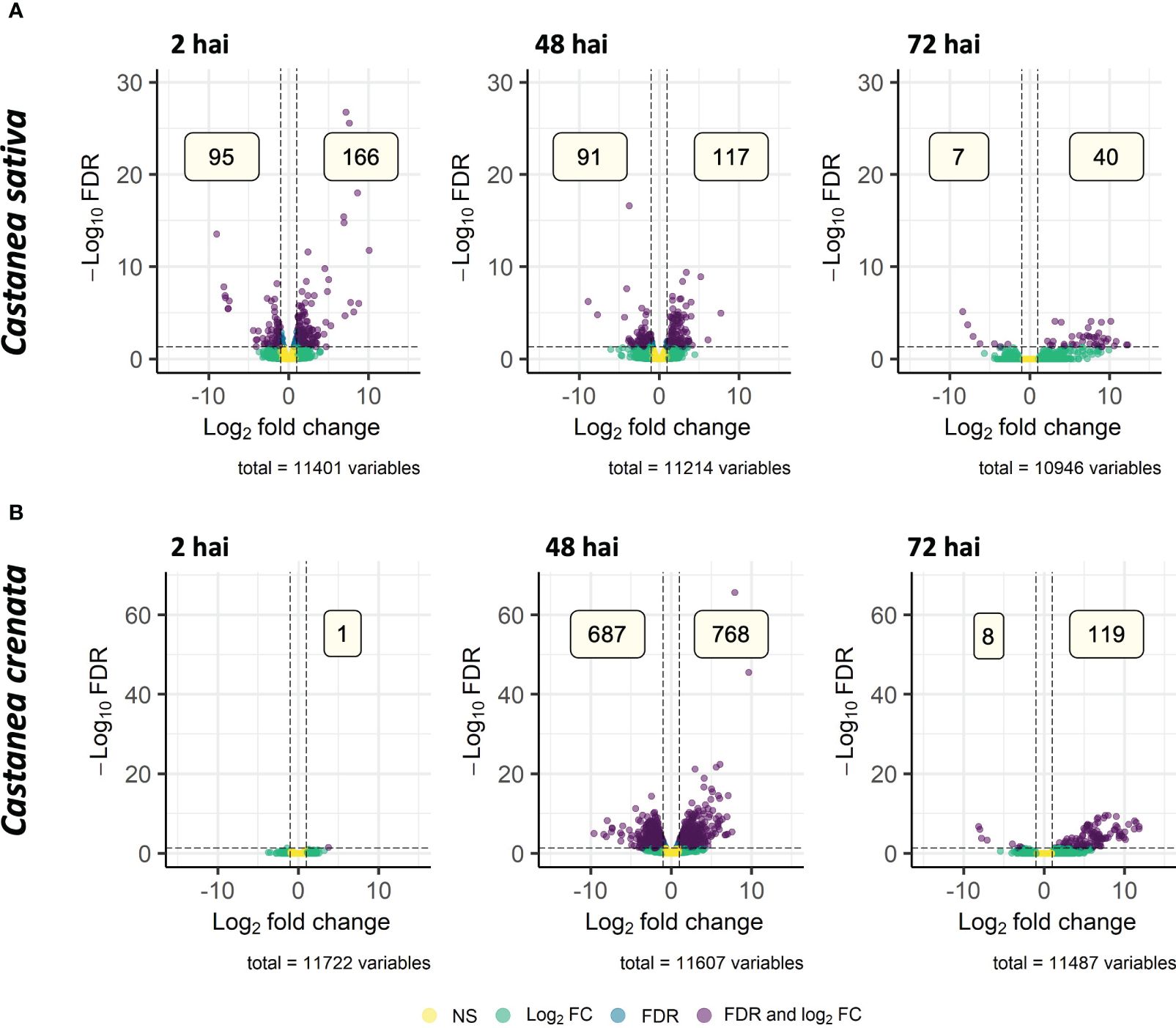
Figure 8. Volcano plots showing the dispersion of differentially expressed genes (DEGs) of Castanea sativa (A) and Castanea crenata (B) inoculated with Phytophthora cinnamomi when compared to non-inoculated controls at 2 h, 48 h, and 72 h after inoculation (hai). DEGs were filtered out by false discovery rate (FDR) of ≤0.05 and log2 fold change (Log2 FC) of ≥1.0 or ≤–1.0. NS, not significant.
A total of 25 and 42 GO terms were enriched in DEGs of susceptible (Figure 9) and resistant (Figure 10) chestnuts, respectively. Enriched GO terms related to biotic stress response, such as apoplast, plant-type cell wall organization, and ethylene-activated signaling pathway, were only enriched for C. crenata upregulated genes. Also, six GO terms related to cytoskeleton and microtubule organization were enriched in upregulated genes at 48 (actin filament organization) and 72 hai (structural constituent of cytoskeleton, microtubule organizing center, microtubule, cytoskeleton organization, and microtubule-based process) (Figure 10). Response to auxin was common to both species but at different time points (Figures 9, 10), and several GO terms enriched in C. sativa were related to the balance of the redox homeostasis, such as response to oxidative stress, peroxidase activity, superoxide dismutase activity, and hydrogen peroxide catabolic process. However, most of these terms are associated with downregulated genes, and the first two mentioned GO terms are enriched in upregulated genes at 2 hai and then in downregulated genes at 48 hai and 72 hai (Figure 9).
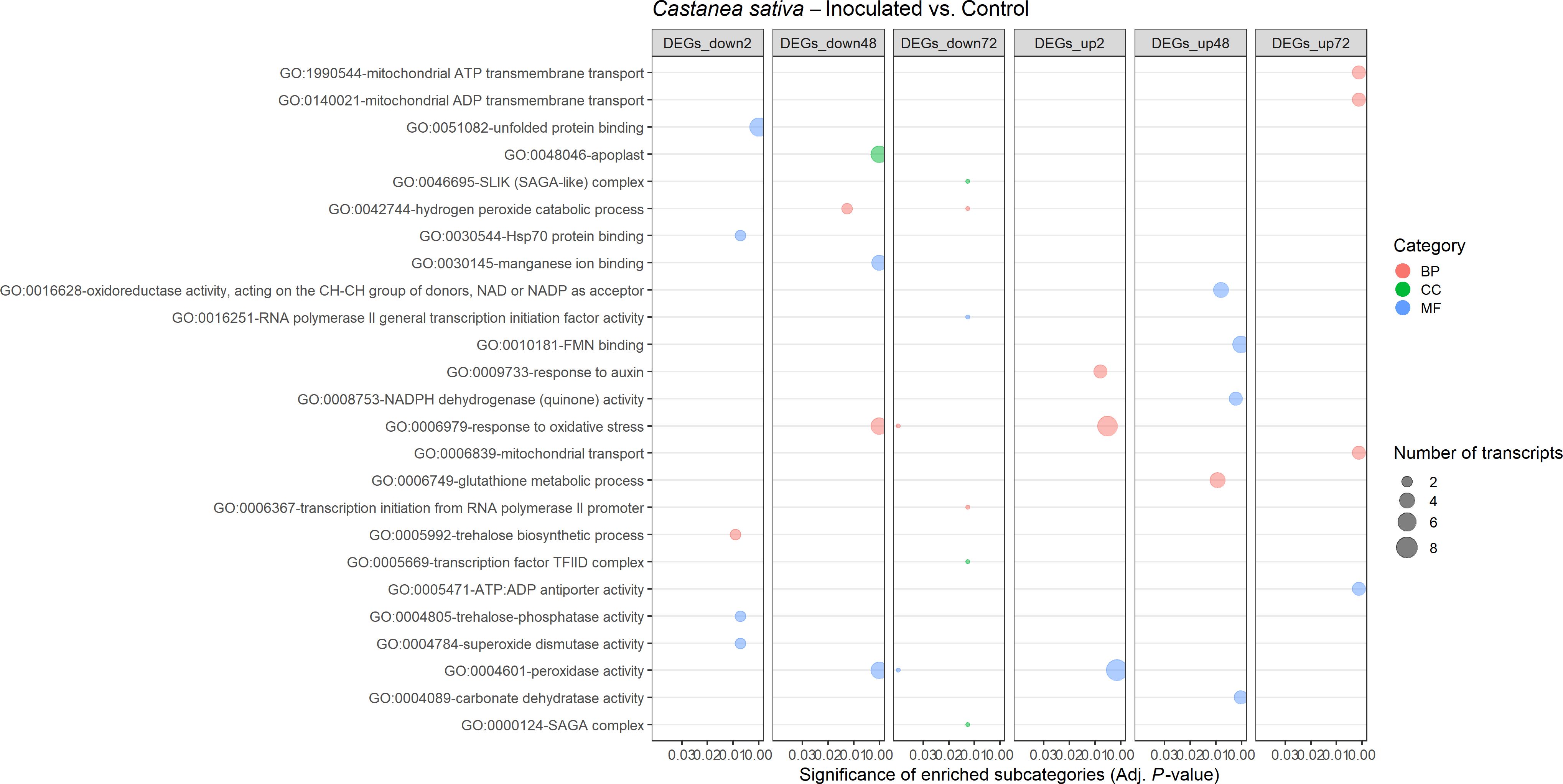
Figure 9. Gene Ontology (GO)–enriched terms in the differentially expressed genes of Castanea sativa at 2 h, 48 h, and 72 h after inoculation with Phytophthora cinnamomi. Analysis was performed using the hypergeometric statistical test, and significantly enriched categories were selected based on the adjusted p-value ≤ 0.05. Different categories are represented with different colors: red, biological process (BP); green, cellular component (CC); blue, molecular function (MF). The complete dataset is provided in Supplementary Table 13.
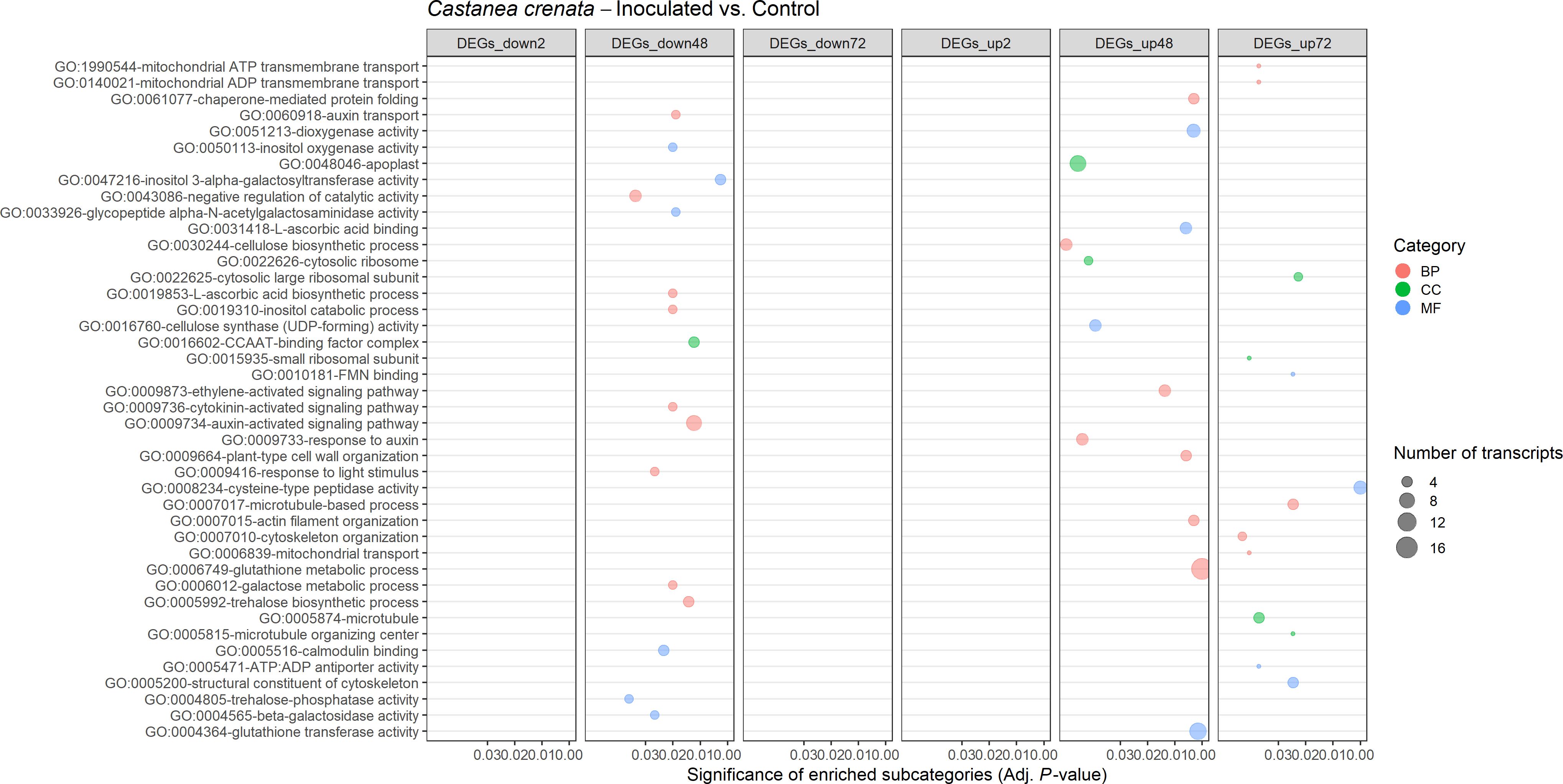
Figure 10. Gene Ontology (GO)–enriched terms in the differentially expressed genes of Castanea crenata at 2 h, 48 h, and 72 h after inoculation with Phytophthora cinnamomi. Analysis was performed by using the hypergeometric statistical test and significantly enriched categories were selected based on the adjusted p-value ≤ 0.05. Different categories are represented with different colors: red, biological process (BP); green, cellular component (CC); blue, molecular function (MF). The complete dataset is provided in Supplementary Table 14.
OrthoFinder was used to identify orthologs within Castanea species (Supplementary Tables 15, 16) and 515 DE single-copy orthologs (SCOs) were filtered and classified according to their expression at each time point (upregulated and downregulated in both species, only in C. crenata, and only in C. sativa; Figure 11; Supplementary Table 17). Two-hundred and fourteen SCOs were upregulated only in C. crenata (Supplementary Table 18).
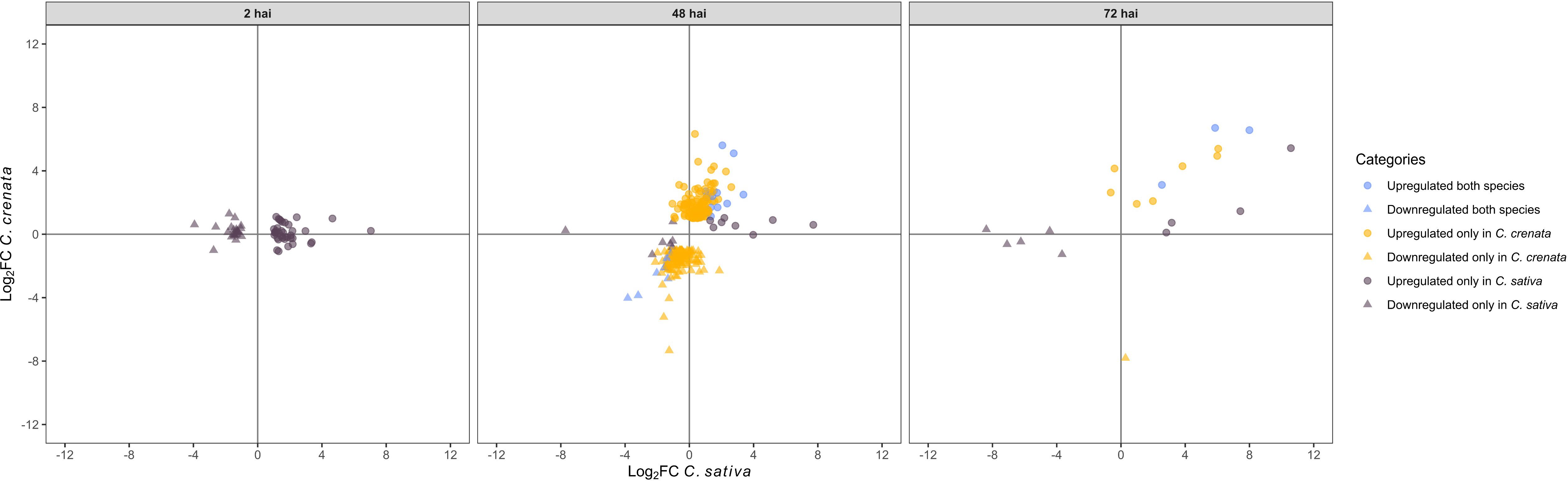
Figure 11. Scatterplot showing single-copy orthologs upregulated and downregulated in both species, only in Castanea crenata and only in Castanea sativa after inoculation with Phytophthora cinnamomi. DEGs were filtered out by false discovery rate (FDR) of ≤0.05 and log2 fold change (Log2 FC) of ≥1.0 or ≤–1.0.
3.6 Castanea crenata differentially expresses more putative resistance genes after inoculation compared to Castanea sativa
The analysis with Drago3 identified 35 and 7 DEGs from Castanea crenata and Castanea sativa, respectively, putatively encoding for resistance proteins with domains such as LRRs or kinase (Santana Silva and Micheli, 2020; Calle García et al., 2022; Supplementary Tables 19, 20). Several ectodomains have been described as ligand-binding, such as LRR, LysMs, lectin-like motifs, and epidermal growth factor (EGF)–like domains (Saijo et al., 2018). An additional 43 and 3 DEGs for C. crenata and C. sativa, respectively, encoding for LRR, Kinase, LysM, and PAN-like domains were identified with the dammit algorithm. Of all the putative resistance genes identified for both species, several had corresponding orthologs, but only one was DE after inoculation (Supplementary Tables 19, 20). Upregulated genes were considered candidate resistance genes. Castanea crenata upregulated 49 transcripts, among which 12 were SCOs exclusively upregulated in the resistant chestnut (highlighted in Supplementary Table 19); several putatively encode for PRRs such as G-type lectin S-receptor–like serine/threonine-protein kinase RLK1 (chitin receptor; Brotman et al., 2012), cysteine-rich RLP kinases, and leucine-rich repeat RLP kinase. Other genes encoding proteins involved in phosphorylation (serine/threonine-protein kinase STY13), PRR signal transmission (calcium-dependent protein kinase 10; Saijo et al., 2018), and protein degradation (26S proteasome regulatory subunit 4 homolog A; Adams and Spoel, 2018) were also exclusively upregulated by C. crenata. Multiple genes that did not have an ortholog in the susceptible species were also upregulated. Some examples putatively encode for the PRR chitin receptor Protein LYK5 (Cao et al., 2014), LRR receptor kinase BAK1, and the PRR signal transmission MAPK kinase kinase 17 (Saijo et al., 2018) (Supplementary Table 19). Castanea sativa upregulated only seven protein-coding genes, which also included PRRs (e.g., Cysteine-rich RLP kinase 10), MAPKs (Mitogen-activated protein kinase 9), and transporters (e.g., Pleiotropic drug resistance protein 1) (Supplementary Table 20). In both species, most genes are upregulated at 48 hai with the exception of Cysteine-rich receptor-like protein kinase 10 at 2 hai in C. sativa, and Rust resistance kinase Lr10, 26S proteasome regulatory subunit 4 homolog A, and a non-annotated gene in C. crenata at 72 hai (Supplementary Tables 19, 20).
3.7 The induction of genes involved in the plant defense network was higher in Castanea crenata
Upregulated genes from both species with GO terms related to innate immune response, SAR, defense response, transcription factors, different phytohormone response and signaling pathways, cell wall, callose, calcium-binding, antifungal, chitin, and proteolysis were selected for analysis. Upregulated genes previously proposed as putatively involved in European and Japanese chestnuts’ susceptibility/resistance to Phytophthora cinnamomi [e.g., Santos et al. (2017a, 2017b), Serrazina et al. (2021), and Pavese et al. (2021)] that did not show in the previous selection were hand-searched. Selected genes were organized into categories related to their putative function suggested by the attributed GO terms and published literature and were further analyzed. A total of 41 and 90 genes were selected for Castanea sativa and Castanea crenata, respectively (Tables 1, 2). Both species upregulated genes related to the induction of similar defense responses and signaling pathways such as elicitors of immune response (e.g., PAMP-induced secreted peptides), genes involved in recognition of Ca2+ signals stimulated by biotic stress and translation into cellular responses (calcium-binding proteins; Cheval et al., 2013), involved in SA, JA, and ET signaling regulation (e.g., ethylene-responsive transcription factors and SA-binding proteins), anti-fungal activity (e.g., thaumatin-like proteins), cell wall reinforcement (e.g., pectinesterases), lignin biosynthesis (e.g., dirigent protein-like), proteolysis (e.g., Cysteine proteinases), and oxidative stress response (e.g., peroxidases). However, C. crenata presents higher fold changes and upregulates more genes from all categories. Indeed, 25 selected genes were SCOs only upregulated in C. crenata such as VQ motif-containing protein 1 and transcription factors (e.g., bHLH162-like and NAC domain-containing protein) (Table 2). Examples of genes induced by C. crenata that did not have orthologs in C. sativa include the PR proteins from families 2 (e.g., glucan endo-1,3-beta-D-glucosidase-like) and 4 (pathogenesis-related protein PR-4-like), the auxin transporter auxin efflux carrier component 5 (PIN5) (Adamowski and Friml, 2015), and the HR promoter Alpha-dioxygenase 1 (De León et al., 2002; Table 2). Some genes possibly related to susceptibility were identified in both species. In C. sativa, two expansins, which loosen the plant cell wall (Bellincampi et al., 2014) and the auxin efflux carrier component 4 (PIN4) responsible for auxin concentration at the root tip (Adamowski and Friml, 2015), were upregulated at 2 hai; and BON1-associated protein 2-like (BAP2-like), an inhibitor of programmed cell death (Yang et al., 2007), was upregulated at 48 hai (Table 1). Castanea crenata also upregulated two expansins at 48 hai and 72 hai and Downy mildew resistance 6 (DMR6) at 48 hai, a negative regulator of SA pathway (Pavese et al., 2021). However, C. crenata upregulated 11 chestnut genes currently proposed as candidates for P. cinnamomi resistance, including four SCOs uniquely upregulated in this species (Table 2). These genes putatively regulate defense responses (transcription factor MYB4-like; Santos et al., 2017a), induce pathogen osmotic rupture (thaumatin-like protein 1; Corredoira et al., 2012), secrete anti-fungal compounds (Cysteine-rich repeat secretory protein 38; Santos et al., 2017a), deposit pectates in the cell wall (Pectinesterase 2; Santos et al., 2017a), and synthesize cellulose in the cell wall (Cellulose synthase-like proteins; Santos et al., 2017b). Only one candidate resistance gene for P. cinnamomi resistance was identified in C. sativa (thaumatin-like protein 1; Corredoira et al., 2012; Table 1).
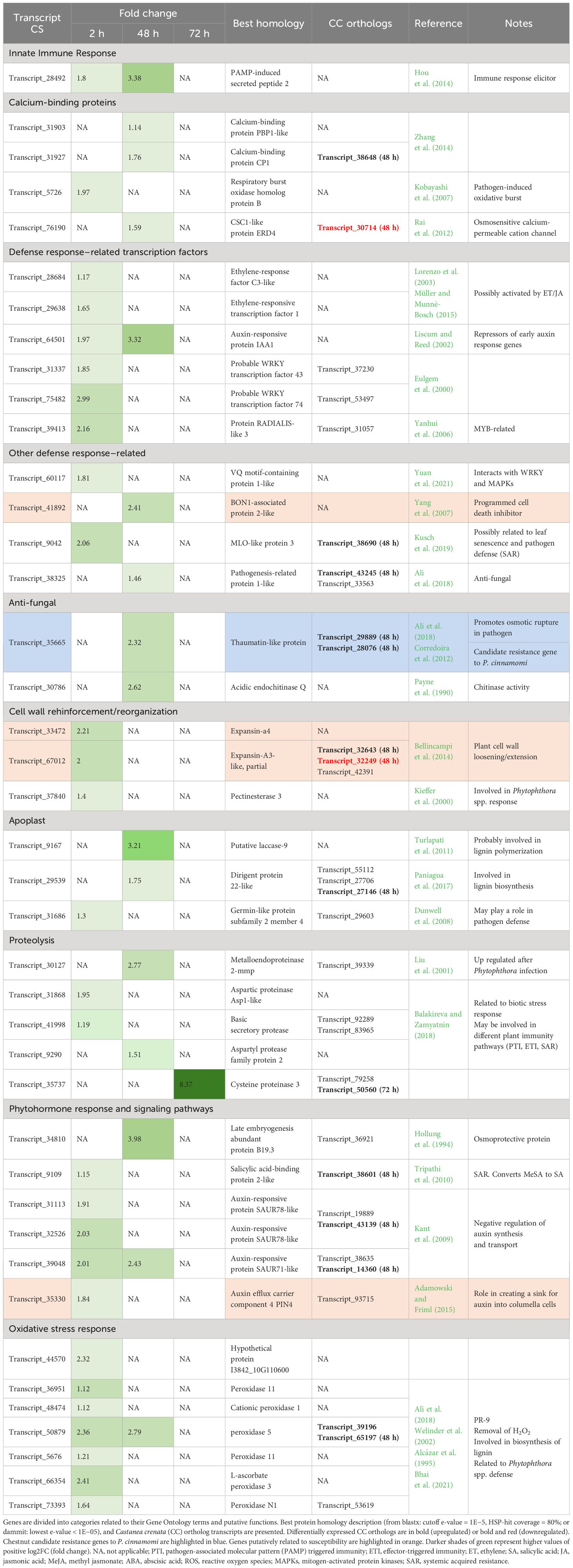
Table 1. Castanea sativa genes related to pathogen defense overexpressed at 2 h, 48 h, and 72 h after inoculation with Phytophthora cinnamomi.

Table 2. Castanea crenata (CC) genes related to pathogen defense overexpressed at 2 h, 48 h, and 72 h after inoculation with Phytophthora cinnamomi.
Castanea sativa upregulated 28, 15, and 1 genes at 2 hai, 48 hai, and 72 hai, respectively. Only four genes were upregulated at more than one time point (2 hai and 48 hai) and putatively encode for an elicitor of immune response (PAMP-induced secreted peptide 2; Hou et al., 2014), an auxin repressor (Auxin-responsive protein IAA1; Liscum and Reed, 2002), a negative regulator of auxin synthesis and transport (auxin-responsive protein SAUR71-like; Kant et al., 2009), and a protease with several roles in biotic stress response such as removal of hydrogen peroxide (H2O2) and lignin polymerization (peroxidase 5; Welinder et al., 2002). At 2 hai, C. sativa is mainly upregulating transcription factors known to mediate gene expression related to biotic stress responses, such as ethylene-response factors (ERFs; Lorenzo et al., 2003; Müller and Munné-Bosch, 2015), WRKYs (Eulgem et al., 2000), and protein radialis-like 3 (MYB-related; Yanhui et al., 2006). It also upregulates genes involved in phytohormone response and signaling pathways (mostly negative regulators of auxin synthesis and transport), oxidative stress response, and cell wall reinforcement or reorganization. A calcium-dependent NADPH oxidase that generates superoxide—respiratory burst oxidase homolog protein B (RBOHB; Kobayashi et al., 2007)—was also upregulated at 2 hai. The genes upregulated at 48 hai are anti-fungal (pathogenesis-related protein 1-like; Ali et al., 2018; Acidic endochitinase Q; Payne et al., 1990; and thaumatin-like protein, Corredoira et al., 2012), are probably involved in lignin synthesis (putative laccase-9; Turlapati et al., 2011; and dirigent protein 22-like; Paniagua et al., 2017), and encode Ca2+-binding proteins. The gene with the highest fold change (8.37) was the protease Cysteine proteinase 3, the only gene upregulated at 72 hai. From the 41 genes, only 11 had orthologs in C. crenata also being upregulated (Table 1).
Castanea crenata only upregulated VQ motif-containing protein 1 (interacts with WRKY and MAPKs; Yuan et al., 2021) at 2 hai, followed by a transcriptional burst at 48 hai of 86 genes from all categories (Table 2). At 72 hai, the number of upregulated genes decreased to 10 which included two elicitors of immune response (PAMP-induced secreted peptide 2-like isoform X2; Hou et al., 2014), one component of microtubule-organization center (Caltractin; Veeraraghavan et al., 2002), three anti-fungal (basic form of pathogenesis-related protein 1-like; thaumatin-like protein 1; Ali et al., 2018), one cell wall loosener (expansin-A4; Bellincampi et al., 2014), two proteases (Cysteine proteinase 5 and serine carboxypeptidase-like 50 isoform X1; Balakireva and Zamyatnin, 2018), and one oxidative stress reducer (Cationic peroxidase 1; Welinder et al., 2002). Several genes with distinct functions in the defense process (e.g., cell wall reinforcement, proteolysis, and oxidative stress response) had a fold change higher than 5 (highlighted in darker green in Table 2). From the total 90 genes, 15 had orthologs in C. sativa being upregulated (Table 2).
3.8 Castanea crenata upregulated several transcripts involved in phenolic compounds’ metabolic pathways
The KEGG metabolic pathways analysis identified 20 and 71 pathways that were assigned to 19 and 81 DEGs of Castanea sativa and Castanea crenata, respectively (Supplementary Tables 21, 22). Phenolic compounds are important for early resistant defense responses against Phytophthora cinnamomi (Fernandes et al., 2021). Metabolic pathways related to the biosynthesis of phenolic compounds were assigned to several C. crenata upregulated DEGs at 48 hai: 1) flavonoid biosynthesis [five upregulated transcripts; e.g., chalcone isomerase and anthocyanidin reductase ((2S)-flavan-3-ol-forming)]; 2) flavone and flavonol biosynthesis (one upregulated transcript, cytochrome P450 CYP736A12-like); 3) phenylpropanoid biosynthesis (one upregulated transcript, caffeoyl-CoA O-methyltransferase); 4) phenylalanine, tyrosine, and tryptophan biosynthesis (six upregulated transcripts; e.g., L-aspartate oxidase, chloroplastic and Bifunctional 3-dehydroquinate dehydratase/shikimate dehydrogenase, chloroplastic); and 5) rerpenoid backbone biosynthesis (2 upregulated transcripts; putative dihydroflavonol 4-reductase and geranylgeranyl pyrophosphate synthase, chloroplastic-like; Supplementary Tables 12, 22). No phenolic compound pathway was assigned to C. sativa DEGs (Supplementary Table 21).
4 Discussion
Dual-transcriptomics can capture both host and pathogen transcriptomes (Westermann et al., 2012) when it is unfeasible to separate the interacting organisms. Studying the associated gene expression changes simultaneously has allowed a more comprehensive understanding of the dynamics of several plant-pathogen interactions (Hayden et al., 2014; Meyer et al., 2016; Evangelisti et al., 2017; Liao et al., 2019).
4.1 Phytophthora cinnamomi during chestnut infection
4.1.1 PAMPs and effectors putatively linked to chestnut defense response
The recently available reference genome of Phytophthora cinnamomi (Engelbrecht et al., 2021) represents a valuable resource that provides insight into pathogenicity determinants in this species, as previously demonstrated for other Phytophthora species, such as P. infestans, P. sojae, and P. ramorum [reviewed by Jiang and Tyler (2012)]. Several oomycete PAMPs were expressed by P. cinnamomi in the roots of susceptible and resistant chestnuts. Secretory OPEL protein is present in the cell wall of Phytophthora spp. and can induce the expression of several SA-responsive genes (e.g., PR1 and PR5) and callose deposition (Chang et al., 2015). These host responses were detected in Castanea crenata and Castanea sativa, which may indicate that both species could recognize Secretory OPEL protein. This protein can also induce cell death and ROS accumulation (Chang et al., 2015). OPEL was more expressed in C. crenata than in C. sativa at 2 hai and was not DE between 72 hai and 48 hai in either chestnut species. This may indicate that this PAMP is more expressed during penetration and establishment of biotrophy in this interaction. Indeed, OPEL is highly expressed by P. parasitica after plant inoculation, reaching its peak at 24 h and declining at 48 hai and 72 hai (Chang et al., 2015). Transglutaminase elicitor M81C was also more expressed in the resistant chestnut at 2 hai. M81C displays elicitor-like activity in P. infestans due to the presence of a 100% conserved region of 13 amino acids (Pep-13) initially identified as a PAMP in P. sojae (Nürnberger et al., 1994; Fabritius and Judelson, 2003). Pep-13 could be responsible for a quick increase in cytosolic Ca2+ concentration, as previously reported to occur in parsley (Blume et al., 2000). The putative early perception of OPEL and M81C by C. crenata may be inducing HR and callose deposition observed after P. cinnamomi penetration and biotrophy establishment (Fernandes et al., 2021).
Phytophthora spp. are an example of a pathogenic and evolutionary success because they quickly evolved diverse effector gene complements. RxLR proteins [including Avirulence (Avr) proteins] and CRN are cytoplasmic effectors that are targeted to the plant nucleus and suppress host defenses, promoting disease in susceptible plants. However, only RxLRs are currently known to activate avirulence activity in resistant plants [reviewed by Naveed et al. (2020)]. In C. sativa, RxLR-like genes were upregulated at 48 compared to 72 hai, as the rest of the effectors of pathogen attack, possibly contributing to the susceptibility of the host as indicated by the enriched GO modulation by symbiont of host defense-related programmed cell death. CRNs can cause crinkling and necrosis (Hardham and Blackman, 2018), two symptoms associated with P. cinnamomi–induced root rot in chestnuts (Santos et al., 2015a). Suppressor of necrosis 1 can also be translocated to the plant nucleus and suppress the induction of programmed cell death, as previously reported during the biotrophy of tomato–P. infestans interaction (Kelley et al., 2010). Interestingly, putative suppressor of necrosis 1 is more expressed in C. crenata roots at 2 hai and 72 hai than that in C. sativa. Still, HR-related cell death was observed in the resistant chestnut (Fernandes et al., 2021).
4.1.2 Nutrient uptake during chestnut infection
The apoplast plays an important role in nutrient uptake and signal exchange, and it is where the initial interactions between plants and pathogens occur (Aung et al., 2018). The apoplastic effector aldose 1-epimerase (AEP1) is an oomycete PAMP and also a virulence factor that mediates P. sojae extracellular cellular sugar uptake (Xu et al., 2021). Hemibiotrophic oomycetes need a biotrophy phase to acquire nutrients from the host living cells before switching to a necrotrophic lifestyle in which nutrients are obtained from killing host cells. Indeed, the allocation of energy-rich compounds is very important during the biotrophic growth of P. cinnamomi (Oßwald et al., 2014) and other Phytophthora spp (Xu et al., 2021). Results suggest that transporters potentially involved in Phytophthora nutrition are more expressed by P. cinnamomi in both chestnut species at 48 hai. It is likely that the pathogen was uptaking nutrients to increase growth and colonize roots. There are limited studies on oomycete nutrition; however, it has been previously reported that Phytophthora spp. can assimilate nutrients from their plant host and have metabolic switches during host infection (Rodenburg et al., 2019).
4.1.3 Biotrophy to necrotrophy switch
After 3 to 6 days, Phytophthora spp. biotrophy is followed by a necrotrophic phase when necrotic lesions appear, and sporangia develop (Van West et al., 1998; Maia et al., 2012; Crone et al., 2013; Redondo et al., 2015; Ah-Fong et al., 2017). By 72 hai, P. cinnamomi developed resistance structures (chlamydospores) and switched to necrotrophic growth in C. sativa’s roots. Elicitins are oomycete PAMPs that, when not recognized by the host, induce structural changes in plant cells and participate in sterol scavenging, helping with pathogen growth and disease development (Hardham and Blackman, 2018). Knocking out the expression of elicitins resulted in reduced pathogen penetration and colonization in cork oak and European chestnut (Horta et al., 2010; Maia et al., 2012). Data suggest that genes encoding for elicitins/elicitins-like are mostly expressed in C. sativa at 48 hai and 72 hai. Necrosis-inducing like protein (NLP) genes were also found mainly upregulated in C. sativa at 48 hai and can act in the host cytoplasm as toxins, causing plant necrosis by plasma membrane destruction and cytolysis (Oßwald et al., 2014; Hardham and Blackman, 2018). In previous reports, the expression of NLP npp1 (necrosis-inducing Phytophthora protein 1) in C. sativa radicles increased at 36 hai (Martins et al., 2019). NLPs can be recognized during PTI and ETI [reviewed by Naveed et al. (2020)] and have been related to the switch to necrotrophy and acceleration of host cell death during this stage of the pathogen’s growth in Phytophthora spp (Qutob et al., 2002; Jupe et al., 2013).
4.1.4 Sporulation-related genes
The successful colonization of an oomycete during a compatible host interaction usually ends in sporulation, which has been previously detected a few days after infection (e.g., 72 hai and 96 hai) in plant–Phytophthora spp. interactions (Hardham, 2007; Judelson et al., 2009; Jupe et al., 2013). DEAD/DEAH box RNA helicase and Zinc finger, C2H2, two genes related to sporulation in Phytophthora spp. (Walker et al., 2008; Wang et al., 2009) were found more expressed by P. cinnamomi in the roots of the susceptible chestnut. During host infection, P. infestans starts upregulating sporulation-related genes pre-sporulation (48 hai and 72 hai) and reaches a peak post-sporulation (96 hai; Judelson et al., 2009). Sporangium development was not observed in infected chestnuts in this work or in the work of Fernandes et al. (2021); however, future studies should be developed to verify if and when sporulation is achieved in chestnut species.
4.2 Chestnut transcriptomes during Phytophthora cinnamomi infection
By using the Phytophthora cinnamomi genome, reads of the pathogen were separated from the host, and the development of single de novo assemblies for the two hosts was possible. After filtering de novo transcriptomes for Streptophyta, the number of remaining transcripts in both species was higher than the previously reported transcriptomes of chestnut roots inoculated with this pathogen (approximately 14,000 vs. 8,000 transcripts; Serrazina et al., 2015). The number of DEGs was also higher (488 vs. 305 in Castanea sativa; 1,556 vs. 283 in Castanea crenata; Serrazina et al., 2015). This was expected because the 2015 transcriptomes were developed with 454 sequencing, which typically results in lower-depth datasets when compared to Illumina (Ward et al., 2012). In a general analysis, the results reported in this work suggest similar defense responses as previously reported (Serrazina et al., 2015), such as recognition of the pathogen by both species and upregulation of genes related to HR, SA, and ET/JA-dependent signaling pathways, antifungal compounds and enzymes, and cell wall reinforcement.
4.2.1 Chestnut’s recognition of Phytophthora cinnamomi
Plants recognize Phytophthora spp. by sensing several elicitors [reviewed by Naveed et al. (2020)]. Although this knowledge is still scarce, a few Phytophthora PAMPs that can induce PTI have been identified, and plant perception models have been proposed [reviewed by Naveed et al. (2020)]. The recognition of some Phytophthora PAMPs is done by a PRR that associates with the LRR RLK BAK1 (BRI1-associated kinase-1), a known hub in defense responses (Heese et al., 2007). The recognition of elicitins, NLPs [reviewed by Naveed et al. (2020)], and AEP1 (Xu et al., 2021) is BAK1-dependent. So far, there are only two BAK1 cognate PRRs known to recognize Phytophthora PAMPs, the Elicitin response receptor (ELR) and RLP23, which recognize the INF1 elicitin and nlp20, respectively [reviewed by Naveed et al. (2020)]. However, these were not identified in this work. BAK1 was upregulated by C. crenata at 48 hai, suggesting PRR-BAK1 association as a part of the Japanese chestnut machinery to perceive P. cinnamomi’s PAMPs. Castanea crenata also upregulates several genes encoding putative PRRs that might interact with BAK1 during pathogen perception and PTI induction. Castanea sativa had no BAK1 ortholog detected in the transcriptome and few putative PRR genes DE during infection. A higher number of PRRs was expected in C. crenata since it co-evolved with P. cinnamomi (Crandall et al., 1945). The selection pressure ultimately led to the evolution of PRRs to recognize PAMPs/effectors as danger signals. A gene putatively encoding the lysin motif RLK (LysM-RLK) LYK5 is also overexpressed by C. crenata at 48 hai. In Arabidopsis, LYK5 receptor forms a chitin-inducible complex with CERK1 to induce host immunity (Cao et al., 2014). Cheng et al. (2019) proposed that chitin may act as a PAMP in Phytophthora spp. and is recognized by LYK5-CERK1. Although the presence of chitin is yet to be reported in Phytophthora spp., chitin synthases (enzymes responsible for chitin biosynthesis) are present in their genomes and expressed during some developmental stages and after infection (Cheng et al., 2019). It has also been recently shown that CERK1 can bind to synthetic β-1,3-glucan oligosaccharides (components of oomycete cell walls; Wang et al., 2018). Since CERK1 and LYK5 are very similar receptors (Cao et al., 2014), they might share the capability of attaching to β-1,3-glucan. Nevertheless, if C. crenata LYK5 were able to recognize chitin or β-1,3-glucan, then its signaling would have to be transduced downstream by associating with another receptor since its kinase domain is inactive (Cao et al., 2014).
4.2.2 Castanea sativa defense response to the pathogen
The earliest host events reported after receptor-mediated perception of Phytophthora PAMPs involve Ca2+ influx (within 30 s after PAMP perception) and ROS bursts (within minutes). Also, calcium, MAPK, and hormonal signaling (JA, SA, and ET) pathways are activated, leading to transcriptional reprogramming and activation of PTI defenses that include callose deposition, HR, expression of defense genes, accumulation of antimicrobials, and SAR [reviewed by Naveed et al. (2020)]. Castanea sativa seems to have a smaller but quicker transcriptional change in response to P. cinnamomi than C. crenata. At 2 hai, the susceptible chestnut upregulates several genes related to resistance responses such as pathogen-induced ROS burst (RBOHB; Kobayashi et al., 2007), ET/JA (ERFs; Lorenzo et al., 2003; Müller and Munné-Bosch, 2015) and SA signaling pathways [salicylic acid-binding protein 2-like (SABP2-like); Tripathi et al., 2010]. However, the decrease of upregulated defense-related genes throughout the time points suggests a decline in the response, unlike the delayed response to the pathogen previously proposed by Santos et al. (2017a). At 48 hai, C. sativa continued to upregulate several transcripts encoding for proteins putatively involved in Phytophthora spp. defense, such as metalloendoproteinase 2-mmp, peroxidase 5, and thaumatin-like. Metalloendoproteinase 2-mmp leads to the production of antimicrobial peptides and is highly induced in soybean after Phytophthora infection (Liu et al., 2001); peroxidase activity was suggested as a marker for Phytophthora resistance in Black pepper (Bhai et al., 2021), and thaumatin-like may induce osmotic rupture in the pathogen and it is proposed as a chestnut candidate resistance gene to P. cinnamomi (Corredoira et al., 2012). PAMP-induced secreted peptide 2 (PIP2, elicitors of immune response in Arabidopsis; Hou et al., 2014), MAPKs, and several calcium-binding proteins are also upregulated at 48 hai, which could be players on PTI-induced defenses. These results raise the hypothesis that P. cinnamomi may not be able to suppress the classic host defense pathways induced by the susceptible chestnut during the early stages of infection. However, C. sativa transcriptome suggests a lack of defenses when the oomycete begins necrotrophic growth. Similar results have been previously reported by Vargas et al. (2012) for the interaction of maize with the hemibiotroph Colletotricum graminicola. In this interaction, C. graminicola is exposed to detrimental conditions during the biotrophic phase due to the host immune system responses (e.g., ROS burst). The switch to necrotrophy is interpreted as a mechanism to avoid contact with the defense molecules produced by the host’s living cells and, therefore, achieve full pathogenicity (Vargas et al., 2012). Nevertheless, other factors may be contributing to the European chestnut’s defense decrease such as the overexpression by 2.4 fold change of BAP2 at 48 hai. This gene’s product might be suppressing attempts of programmed cell death induced by C. sativa after pathogen attack, a function demonstrated with Arabidopsis BAP2 loss of function mutants (Yang et al., 2007).
4.2.3 Castanea crenata initial defense
Castanea crenata only upregulated one gene at 2 hai by 3.75 fold change, VQ motif-containing protein 1. This gene’s product may be interacting with WRKY transcription factors and MAPK signaling cascades (Yuan et al., 2021), two well-known participants of the plant immune system (Meng and Zhang, 2013; Wani et al., 2021). Indeed, VQ motif-containing proteins were suggested to control plant response to P. parasitica by having their transcripts overexpressed immediately after pathogen penetration, enabling the host to cope with effector-induced cellular reprogramming (Le Berre et al., 2017). The low number of DEGs at 2 hai may indicate that C. crenata’s constitutive levels of gene expression could be sufficient as a first defense after pathogen penetration. Indeed, at this time point, the Japanese chestnut presented callose deposition around intracellular hyphae, accumulation of phenolic compounds on the cell wall, and HR-related cell death (Fernandes et al., 2021). This was hypothesized by Santos et al. (2017a) after verifying in non-inoculated Japanese chestnuts, high expression levels of several genes related to resistance responses such as pathogen recognition (Cast_LRR-RLK), transcription factors (Cast_Myb4), antifungal activity (Cast_Gnk2-like), and cell wall strengthening (Cast_PE-2). These genes were also found to be upregulated at 48 hai (Santos et al., 2017a), as reported in this work.
4.2.4 Antifungal activity
Cysteine-rich repeat secretory protein 38 (Cast_Gnk2-like) encodes for an antifungal secretory protein first identified in Ginkgo biloba seeds, also known as Ginkgobilobin-2 (Wang and Ng, 2000; Sawano et al., 2007; Santos et al., 2017a). This protein may also activate actin-dependent cell death (Gao et al., 2016). Cast_Gnk2-like was linked to the constitutive defense of C. crenata due to putatively preventing the pathogen’s growth through chemical properties or by inducing HR-like cell death (Santos et al., 2017a). This gene was upregulated in C. crenata in this work, and no ortholog was identified in the susceptible species transcriptome. This is probably due to the extremely low expression of this gene described by Santos et al. (2017a). The functional validation of Cast_Gnk2-like as a resistance gene is ongoing through genetic transformation of susceptible species (McGuigan et al., 2020; Serrazina et al., 2022) and the study of the putative anti-oomycete activity of the encoded protein (Colavolpe et al., 2023).
Genes encoding for proteins with antifungal activity (basic form of PR protein 1-like; thaumatin-like protein 1; Corredoira et al., 2012; Ali et al., 2018) were still highly upregulated (3 to 4 fold change) at 72 hai in C. crenata. Also, at this time point, P. cinnamomi is expressing a Catalase in C. crenata 2.22 fold more than in C. sativa, which may indicate the presence of ROS. Catalases are suggested to act as Phytophthora effectors against plant defenses by breaking down H2O2 into water and oxygen (Hardham and Blackman, 2018).
4.2.5 Phenylpropanoid pathway and cell wall reinforcement
Lignin and phenylpropanoid biosynthesis involvement is well-known during plant defense against biotic stresses (Miedes et al., 2014). Castanea crenata had a considerably higher upregulation of genes related to the biosynthesis of these compounds compared to C. sativa. The resistant chestnut overexpressed several genes encoding for enzymes and MYB transcription factors involved in the phenylpropanoid metabolism and several dirigent proteins involved in lignin biosynthesis (Paniagua et al., 2017). The accumulation of phenolic compounds in the cell walls is detected 30 min after inoculation in C. crenata and 72 hai in C. sativa (Fernandes et al., 2021). The buildup of phenolic compounds leads to lignin polymerization (Pomar et al., 2002), which has been shown to be an active defense against P. cinnamomi in later stages of the infection in Eucalyptus spp (Cahill and McComb, 1992), avocado (van den Berg et al., 2018a), and chestnut Euro-Asian hybrids [P. Fernandes and M. C. Silva, Unpublished data, as cited in the work of Fernandes et al. (2021)]. Two pectinesterase 2 and five cellulose synthase-like were upregulated at 48 hai by C. crenata and are putative resistance genes to P. cinnamomi acting on cell wall reinforcement (Santos et al., 2017a, b). Interestingly, mutating a cellulose synthase gene in Arabidopsis reduced the cellulose, stimulating the production of lignin and consequently increasing the resistance to powdery mildew pathogens [reviewed by Bellincampi et al. (2014)].
The accumulation of phenolic compounds reinforces C. crenata cell walls and blocks of P. cinnamomi hyphae, which were occasionally found dead at 72 hai (Fernandes et al., 2021). The Japanese chestnut cells possibly induced defenses that created a hostile environment for the oomycete, such as phenolic compound accumulation, antimicrobial compounds, and ROS. Many phenolic compounds from chestnuts may exhibit antimicrobial activity (Silva et al., 2020).
4.2.6 Microtubule and cytoskeleton organization
Reorganization of the cytoskeleton and architecture changes can be players in the plant immune system (Li and Staiger, 2018). It has been previously shown that plants are able to focus actin microfilaments, aggregate ER membrane, and accumulate Golgi bodies near oomycete penetration sites (Takemoto et al., 2003), suggesting a rapid relocation of defenses. Actin filament organization is an enriched GO term at 48 hai in C. crenata, which may suggest relocation of defenses after P. cinnamomi infection. Several other GO terms related to cytoskeleton and microtubule processes are enriched at 72 hai. However, Takemoto et al. (2003) did not find microtubules focused on the penetration site suggesting a different function for the genes related to these GO terms during the late stages of P. cinnamomi infection. Indeed, microtubule and cytoskeleton organization have been previously related to HR cell death in plants infected with the pathogen cowpea rust fungus (Škalamera and Heath, 1998). The same authors also suggested that microtubules are involved in the deposition of phenolics by adjacent living cells. Both HR and phenolic compound deposition were observed in C. crenata cells infected with P. cinnamomi (Fernandes et al., 2021). However, cytoskeleton dynamics, namely, actin and microtubule changes, should be addressed microscopically to study these hypotheses.
4.2.7 SA, JA, and ET signaling
Intersignaling between the stress-induced hormones SA, JA, and ET is considered the core of immunity, and its importance has been established for a long time (Pieterse et al., 2012). In previous reports, specific roles have been given to each hormone pathway during pathogen attacks. The SA pathway is associated with the response against several biotrophic and hemibiotrophic pathogens. ET/JA pathway seems to be recognized as the main defense against necrotrophic pathogens, wounding, and herbivores. However, studies with P. cinnamomi show the complex interplay between these phytohormones. So far, no predominant pathway is responsible for restraining this pathogen’s growth [reviewed by van den Berg et al. (2021)]. Actually, signature genes related to SA (e.g., pathogenesis-related 1-like and pathogenesis-related 5; Ali et al., 2018) and to JA (e.g., pathogenesis-related 4; Ali et al., 2018) signaling pathways were upregulated at the same time in C. crenata, along with transcription factors that might have been participating in SA/JA regulation (e.g., WRKY51; Gao et al., 2011; and WRKY41; Higashi et al., 2008). ERFs are activated by ethylene and jasmonate (Lorenzo et al., 2003) and have been shown to confer resistance to Phytophthora spp. by participating in the reprogramming of gene expression (Yu et al., 2020) and upregulation of PR genes in overexpressing mutants (Zhao et al., 2017). ERFs were considered involved in the regulation of defense responses in chestnuts by Serrazina et al. (2015).
4.2.8 Auxin signaling
Understanding the role of growth-promoting hormones (auxins, cytokinins, gibberellic acid, and abscisic acid) in homeostasis regulation during plant-pathogen interactions is far behind when compared to SA, ET, and JA. The importance of auxin signaling pathways during plant–Phytophthora interactions has been previously proposed (Evangelisti et al., 2013; Eshraghi et al., 2014). The present results suggest a response to auxin and downregulation of auxin-signaling pathways at 48 hai in the resistant chestnut. In addition to the GO-enriched terms in downregulated genes (auxin-activated signaling pathway; auxin transport), C. crenata is overexpressing several transcripts that are rapidly and transiently induced in response to auxins such as the Aux/IAA (auxin-responsive protein IAA20-like) and Small auxin-up RNAs (SAURs; Hagen and Guilfoyle, 2002). Aux/IAA proteins are short-lived transcription factors that repress auxin early responses (Liscum and Reed, 2002). The function of the SAUR family is not very well known; however, some members have been proposed as negative regulators for auxin synthesis and transport (Kant et al., 2009) and may play a role in the recovery or control of stress response levels caused by auxin (Markakis et al., 2013). A similar overexpression seems to be happening in the susceptible species but with fewer genes and earlier in the infection process (2 hai/48 hai). Castanea crenata was also overexpressing PIN5 (auxin efflux carrier component 5), frequently localized in the endoplasmic reticulum and likely mediating auxin transport into this cellular compartment (Mravec et al., 2009). PIN5 appears to be necessary for fine-tuning auxin function and is most likely regulating the hormone’s homeostasis by subcellular auxin compartmentalization in the ER lumen (Mravec et al., 2009). Contrary, C. sativa is overexpressing PIN4 (auxin efflux carrier component 4), which plays a role in creating a sink for auxin in the columella cells (Adamowski and Friml, 2015). The interpretation of these results is not clear; however, it may suggest a temporary decrease of root growth as a trade-off between defense and a resistance response in C. crenata to avoid P. cinnamomi manipulation of this phytohormone pathway as reported for Arabidopsis–P. parasitica (Evangelisti et al., 2013). During penetration P. parasitica transiently upregulates the RXLR effector PSE1, which is suggested to modulate auxin content in the root by inducing the host to overexpress PIN4 and PIN7 transporters, leading to an accumulation of auxin in the columella. This may facilitate pathogen penetration and suppress plant cell death (Evangelisti et al., 2013).
4.2.9 Protein degradation
The 26S regulatory subunit plays a central role in the degradation of plant proteins through the ubiquitin/26S proteasome system and can be responsible for the degradation of several substrates involved in hormone signaling and plant defense (Dreher and Callis, 2007; Dielen et al., 2010). Some subunits may also inhibit pathogen effectors and trigger PAMP and ETI responses (Park et al., 2012; Üstün and Börnke, 2014). Given its important regulatory role in plant defense, it is a target for utilization by pathogens such as P. infestans, which inhibits plant immunity by modulating proteasome activity (Bos et al., 2010). 26S proteasome subunits have been previously related to host resistance to P. cinnamomi after observation of increased susceptibility in Arabidopsis defective mutants (Eshraghi et al., 2014). It was also related to soybean-enhanced resistance to P. sojae, where overexpressing a 26S proteasome subunit gene improved the activity of antioxidant enzymes and decreased ROS. In contrast, knockdowns increased ROS accumulation and inhibition of antioxidant enzymes (Liu et al., 2021). The high fold change of a 26S proteasome subunit gene in C. crenata and several proteases may indicate an important role in protein degradation during P. cinnamomi infection. Indeed, this pathogen overexpresses in C. crenata tissues (compared to C. sativa) a Kazal-like serine protease inhibitor, a protease inhibitor usually expressed as a defense against host responses (Hardham and Blackman, 2018).
4.2.10 Similarity to chestnut blight defense
Another serious threat to chestnuts is chestnut blight, which is caused by the fungi Cryphonectria parasitica (Murr.) Barr. Candidate resistance genes for C. parasitica obtained from studies with C. mollissima (Cm) and C. seguinii (Cse) are reviewed by Nelson et al. (2014). Several of these genes are similar to the ones in this work including Laccase-like protein/diphenol oxidase (Cm), Ethylene Transcription Factor (Cm), Thaumatin-like protein (Cm), Caffeoyl-CoA-O-methyltransferase (Cm), Peroxidase (Cm), Subtilisin-like protease (Cse), and Glucan endo-1,3-glucosidase (Cm) (Nelson et al., 2014). Indeed, Serrazina et al. (2015) suggested that responses of European and Japanese chestnuts to P. cinnamomi, and American and Chinese chestnuts to C. parasitica were similar. The DEGs after pathogen attack were involved in common defenses such as pathogen recognition, JA signaling, gene regulation by MYB, and ethylene-responsive transcription factors, HR and cell lignification, and anti-fungal proteins (Barakat et al., 2009, 2012; Serrazina et al., 2015).
4.3 Conclusions
The generalized lack of symptomology and high survival in Asian chestnut species and hybrids resistant to Phytophthora cinnamomi is far from being unraveled. However, this work reveals new and valuable contributions to help enlighten these interactions.
Phytophthora cinnamomi had a higher expression of different effectors in resistant and susceptible chestnuts. RxLRs and CRLs were more expressed in Castanea crenata and elicitins and NLPs in Castanea sativa. Considering the low number of potential PRR genes overexpressed in C. sativa after infection and the undetected BAK1, it is likely that elicitins and NLPs have a significant role in the cellular decline of this chestnut species. Additionally, P. cinnamomi’s switch to necrotrophy in the susceptible chestnut might be associated with the evasion of host responses from living cells followed by a pathogenicity increase. Genes related to sporulation were only overexpressed in C. sativa, whereas genes related to pathogen defense against host responses are more expressed in C. crenata.
The hypothesis of P. cinnamomi PAMPs perception and PTI activation via PRR-BAK1 should be explored. The main difference between non-resistant and resistant host response in chestnut–P. cinnamomi interactions might be more quantitative than qualitative, as previously suggested by Serrazina et al. (2015) and Zhebentyayeva et al. (2019). This has been recorded in other plant–Phytophthora interactions (Vleeshouwers et al., 2000) and chestnut-Cryphonectria interactions (Huang, 1996; Kubisiak et al., 1997, 2013). Castanea sativa is able to recognize P. cinnamomi and upregulate genes involved in defense responses, which include at 2 hai: ROS burst, regulation of defense genes by WRKY and possibly MAPKs (through VQ1), ET/JA signaling regulation by ERFs, SA signaling regulation by SABP2-like, negative regulation of auxin by SAUR-like, proteases, and oxidative stress response; at 48 hai: possibly Ca2+ signaling and MAPK signaling cascades, antifungal proteins, lignin metabolism, and proteases; and at 72 hai: most defenses are possibly disabled. However, the number of genes upregulated to induce a specific response was much lower than the induced by the resistant chestnut. Therefore, the defenses induced by C. sativa after P. cinnamomi attack are not enough to restrain the pathogen’s colonization, which is able to start the necrotrophic growth at 72 hai. The pathogen possibly manipulates C. sativa by translocation of effectors to the plant nucleus, which, together with the host expression of BAP2, might halt the induction of programmed cell death and several other defense mechanisms. The role of BAP2 as a putative suppressor of PCD in chestnuts should be considered for further studies.
The resistant chestnut possibly has constitutive levels of gene expression that do not require upregulation of several genes for a first defense response. Castanea crenata probably recognizes most of the pathogen PAMPs/effectors, considering the high number of PRR genes upregulated, and induces PTI-, ETI-, and SAR-related defense responses due to a transcriptional burst at 48 hai. This includes Ca2+ and MAPK signaling cascades, HR-cell death, regulation of ET/JA and SA signaling pathways, repression of auxin, phenolic compound accumulation, cell wall reinforcement, antifungal proteins, proteases, and oxidative stress response. At 72 hai, it maintains some active defenses that continue to restrain the pathogen and, consequently, reduce the area of infection.
The outcomes of this work reveal new information about chestnut–P. cinnamomi interaction and indicate several new genes as potential targets for functional validation, such as several PRRs from C. crenata, susceptibility genes from C. sativa, and pathogen effectors. These genes could inform ongoing programs to improve the resistance of susceptible chestnuts by providing new baselines for the development of molecular markers (fast genomic selection), and host and pathogen genes for genetic transformation (overexpression/knockout; induced gene silencing by RNA interference). These results will also be useful in understanding the interaction of P. cinnamomi with other plant species, mainly woody species belonging to the Fagaceae family.
Data availability statement
The datasets generated in this study have been deposited in the NCBI BioProject database (https://www.ncbi.nlm.nih.gov/bioproject/), BioProject accession number PRJNA1108966.
Author contributions
PaF: Conceptualization, Data curation, Formal analysis, Investigation, Methodology, Writing – original draft, Writing – review & editing. DP: Data curation, Formal analysis, Software, Writing – original draft, Writing – review & editing. RR: Data curation, Formal analysis, Software, Writing – review & editing. MS: Conceptualization, Formal analysis, Funding acquisition, Investigation, Methodology, Supervision, Writing – review & editing. PeF: Conceptualization, Funding acquisition, Supervision, Writing – review & editing. RC: Conceptualization, Funding acquisition, Project administration, Supervision, Writing – review & editing.
Funding
The author(s) declare financial support was received for the research, authorship, and/or publication of this article. This work was supported by FCT -Fundação para a Ciência e Tecnologia, I.P. under the project 414103 FCT-Lx-FEDER-28760, Ph.D. grant SFRH/BD/115424/2016 (awarded to PaF), project reference UIDB/00239/2020 of the Forest Research Centre and DOI identifier 10.54499/UIDB/00239/2020, and project reference UIDB/04129/2020 of LEAF (Linking Landscape, Environment, Agriculture and Food) Research Center and DOI identifier 10.54499/UIDB/04129/2020.
Acknowledgments
The authors would like to thank Helena Machado (INIAV) for assisting during inoculations, Ehsan Valiollahi (InovPlantProtect) for releasing the data to NCBI and Tetyana Zhebentyayeva (PSU) for reviewing the manuscript. This work was part of a PhD thesis (Fernandes, 2022).
Conflict of interest
The authors declare that the research was conducted in the absence of any commercial or financial relationships that could be construed as a potential conflict of interest.
Publisher’s note
All claims expressed in this article are solely those of the authors and do not necessarily represent those of their affiliated organizations, or those of the publisher, the editors and the reviewers. Any product that may be evaluated in this article, or claim that may be made by its manufacturer, is not guaranteed or endorsed by the publisher.
Supplementary material
The Supplementary Material for this article can be found online at: https://www.frontiersin.org/articles/10.3389/fpls.2024.1439380/full#supplementary-material
References
Abrahamian, M., Ah-Fong, A. M. V., Davis, C., Andreeva, K., Judelson, H. S. (2016). Gene expression and silencing studies in phytophthora infestans reveal infection-specific nutrient transporters and a role for the nitrate reductase pathway in plant pathogenesis. PloS Pathog. 12, e1006097. doi: 10.1371/JOURNAL.PPAT.1006097
Adamowski, M., Friml, J. (2015). PIN-dependent auxin transport: action, regulation, and evolution. Plant Cell 27, 20. doi: 10.1105/TPC.114.134874
Adams, E. H. G., Spoel, S. H. (2018). The ubiquitin-proteasome system as a transcriptional regulator of plant immunity. J. Exp. Bot. 69, 4529–4537. doi: 10.1093/jxb/ery216
Ah-Fong, A. M. V., Shrivastava, J., Judelson, H. S. (2017). Lifestyle, gene gain and loss, and transcriptional remodeling cause divergence in the transcriptomes of Phytophthora infestans and Pythium ultimum during potato tuber colonization. BMC Genomics 18, 1–28. doi: 10.1186/S12864-017-4151-2/FIGURES/6
Alcaide, F., Solla, A., Cuenca, B., Martín, M.Á. (2022). Molecular evidence of introgression of Asian germplasm into a natural Castanea sativa forest in Spain. Forestry.: Int. J. For. Res. 95, 95–104. doi: 10.1093/FORESTRY/CPAB030
Alcázar, M. D., Egea, C., Espín, A., Candela, M. E. (1995). Peroxidase isoenzymes in the defense response of Capsicum annuum to Phytophthora capsici. Physiol. Plant 94, 736–742. doi: 10.1111/J.1399-3054.1995.TB00992.X
Ali, S., Ganai, B. A., Kamili, A. N., Bhat, A. A., Mir, Z. A., Bhat, J. A., et al. (2018). Pathogenesis-related proteins and peptides as promising tools for engineering plants with multiple stress tolerance. Microbiol. Res. 212–213, 29–37. doi: 10.1016/J.MICRES.2018.04.008
Ambawat, S., Sharma, P., Yadav, N. R., Yadav, R. C. (2013). MYB transcription factor genes as regulators for plant responses: an overview. Physiol. Mol. Biol. Plants 19, 307. doi: 10.1007/S12298-013-0179-1
Aung, K., Jiang, Y., He, S. Y. (2018). The role of water in plant-microbe interactions. Plant J. 93, 771. doi: 10.1111/TPJ.13795
Balakireva, A. V., Zamyatnin, A. A. (2018). Indispensable role of proteases in plant innate immunity. Int. J. Mol. Sci. 19 (2), 629. doi: 10.3390/IJMS19020629
Baldé, A., Neves, D., García-Breijo, F. J., Pais, M. S., Cravador, A. (2017). De novo assembly of Phlomis purpurea after challenging with Phytophthora cinnamomi. BMC Genomics 18, 700. doi: 10.1186/s12864-017-4042-6
Barakat, A., Diloreto, D. S., Zhang, Y., Smith, C., Baier, K., Powell, W. A., et al. (2009). Comparison of the transcriptomes of American chestnut (Castanea dentata) and Chinese chestnut (Castanea mollissima) in response to the chestnut blight infection. BMC Plant Biol. 9, 51. doi: 10.1186/1471-2229-9-51
Barakat, A., Staton, M., Cheng, C.-H., Park, J., Yassin, N. B. M., Ficklin, S., et al. (2012). Chestnut resistance to the blight disease: Insights from transcriptome analysis. BMC Plant Biol. 12, 38. doi: 10.1186/1471-2229-12-38
Bellincampi, D., Cervone, F., Lionetti, V. (2014). Plant cell wall dynamics and wall-related susceptibility in plant-pathogen interactions. Front. Plant Sci. 5. doi: 10.3389/FPLS.2014.00228/BIBTEX
Bhai, R. S., Alex, R., Vadivukkarasi, P., Shivakumar, M. S. (2021). Peroxidase activity as a marker to evaluate resistance in Black pepper against Phytophthora infection. Indian Phytopathol. 74, 1099–1104. doi: 10.1007/S42360-021-00335-1/TABLES/3
Blume, B., Nurnberger, T., Nass, N., Scheel, D. (2000). Receptor-mediated increase in cytoplasmic free calcium required for activation of pathogen defense in parsley. Plant Cell 12, 1425. doi: 10.1105/TPC.12.8.1425
Bolger, A. M., Lohse, M., Usadel, B. (2014). Trimmomatic: A flexible trimmer for Illumina sequence data. Bioinformatics 30, 2114–2120. doi: 10.1093/bioinformatics/btu170
Bos, J. I. B., Armstrong, M. R., Gilroy, E. M., Boevink, P. C., Hein, I., Taylor, R. M., et al. (2010). Phytophthora infestans effector AVR3a is essential for virulence and manipulates plant immunity by stabilizing host E3 ligase CMPG1. Proc. Natl. Acad. Sci. U.S.A. 107, 9909–9914. doi: 10.1073/PNAS.0914408107/-/DCSUPPLEMENTAL
Bowler, C., Alliotte, T., De Loose, M., Van Montagu, M., Inzé, D. (1989). The induction of manganese superoxide dismutase in response to stress in Nicotiana plumbaginifolia. EMBO J. 8, 31–38. doi: 10.1002/J.1460-2075.1989.TB03345.X
Brotman, Y., Landau, U., Pnini, S., Lisec, J., Balazadeh, S., Mueller-Roeber, B., et al. (2012). The LysM receptor-like kinase LysM RLK1 is required to activate defense and abiotic-stress responses induced by overexpression of fungal chitinases in Arabidopsis plants. Mol. Plant 5, 1113–1124. doi: 10.1093/MP/SSS021
Cahill, D. M., McComb, J. A. (1992). A comparison of changes in phenylalanine ammonia-lyase activity, lignin and phenolic synthesis in the roots of Eucalyptus calophylla (field resistant) and E. marginata (susceptible) when infected with Phytophthora cinnamomi. Physiol. Mol. Plant Pathol. 40, 315–332. doi: 10.1016/0885-5765(92)90014-M
Cahill, D., Weste, G. (1983). Formation of callose deposits as a response to infection with Phytophthora cinnamomi. Trans. Br. Mycological. Soc. 80, 23–29. doi: 10.1016/S0007-1536(83)80161-2
Calle García, J., Guadagno, A., Paytuvi-Gallart, A., Saera-Vila, A., Amoroso, C. G., D’Esposito, D., et al. (2022). PRGdb 4.0: an updated database dedicated to genes involved in plant disease resistance process. Nucleic Acids Res. 50, D1483–D1490. doi: 10.1093/nar/gkab1087
Cao, Y., Liang, Y., Tanaka, K., Nguyen, C. T., Jedrzejczak, R. P., Joachimiak, A., et al. (2014). The kinase LYK5 is a major chitin receptor in Arabidopsis and forms a chitin-induced complex with related kinase CERK1. Elife 3, 3766. doi: 10.7554/ELIFE.03766
Carlile, M. J. (1983). Motility, taxis, and tropism in Phytophthora. in Phytophthora: its Biology, Ecology, and Pathology. St. Paul, MN, USA: The American Phytopathological Society, eds Erwin, D. C., Barticki-Garcia, sS., Tsao, P. H. 95–107. doi: 10.3/JQUERY-UI.JS
Ceccardi, T. L., Barthe, G. A., Derrick, K. S. (1998). A novel protein associated with citrus blight has sequence similarities to expansin. Plant Mol. Biol. 38, 775–783. doi: 10.1023/A:1006039016393
Chang, Y. H., Yan, H. Z., Liou, R. F. (2015). A novel elicitor protein from Phytophthora parasitica induces plant basal immunity and systemic acquired resistance. Mol. Plant Pathol. 16, 123–136. doi: 10.1111/MPP.12166
Cheng, W., Lin, M., Qiu, M., Kong, L., Xu, Y., Li, Y., et al. (2019). Chitin synthase is involved in vegetative growth, asexual reproduction and pathogenesis of Phytophthora capsici and Phytophthora sojae. Environ. Microbiol. 21, 4537–4547. doi: 10.1111/1462-2920.14744
Cheval, C., Aldon, D., Galaud, J. P., Ranty, B. (2013). Calcium/calmodulin-mediated regulation of plant immunity. Biochim. Biophys. Acta (BBA). - Mol. Cell Res. 1833, 1766–1771. doi: 10.1016/J.BBAMCR.2013.01.031
Colavolpe, M. B., Vaz Dias, F., Serrazina, S., Malhó, R., Lourenço Costa, R. (2023). Castanea crenata Ginkbilobin-2-like Recombinant Protein Reveals Potential as an Antimicrobial against Phytophthora cinnamomi, the Causal Agent of Ink Disease in European Chestnut. Forests 14, 785. doi: 10.3390/f14040785
Corredoira, E., San José, M. C., Vieitez, A. M., Allona, I., Aragoncillo, C., Ballester, A. (2016). Agrobacterium-mediated transformation of European chestnut somatic embryos with a Castanea sativa (Mill.) endochitinase gene. New For. (Dordr). 47, 669–684. doi: 10.1007/s11056-016-9537-5
Corredoira, E., Valladares, S., Allona, I., Aragoncillo, C., Vieitez, A. M., Ballester, A. (2012). Genetic transformation of European chestnut somatic embryos with a native thaumatin-like protein (CsTL1) gene isolated from Castanea sativa seeds. Tree Physiol. 00, 1–14. doi: 10.1093/treephys/tps098
Crandall, B. S., Gravatt, G. F., Ryan, M. M. (1945). Root disease of Castanea species and some coniferous and broadleaf nursery stocks, caused by Phytophthora cinnamomi. Phytopathology 35, 162–180.
Crone, M., McComb, J. A., O’Brien, P. A., Hardy, G. E. S. J. (2013). Survival of Phytophthora cinnamomi as oospores, Stromata, And thick-walled chlamydospores in roots of symptomatic and asymptomatic annual and herbaceous perennial plant species. Fungal Biol. 117, 112–123. doi: 10.1016/j.funbio.2012.12.004
Cui, J., Luan, Y., Jiang, N., Bao, H., Meng, J. (2017). Comparative transcriptome analysis between resistant and susceptible tomato allows the identification of lncRNA16397 conferring resistance to Phytophthora infestans by co-expressing glutaredoxin. Plant J. 89, 577–589. doi: 10.1111/tpj.13408
De León, I. P., Sanz, A., Hamberg, M., Castresana, C. (2002). Involvement of the Arabidopsis α-DOX1 fatty acid dioxygenase in protection against oxidative stress and cell death. Plant J. 29, 61–72. doi: 10.1046/J.1365-313X.2002.01195.X
Devoto, A., Hartmann, H. A., Piffanelli, P., Elliott, C., Simmons, C., Taramino, G., et al. (2003). Molecular phylogeny and evolution of the plant-specific seven-transmembrane MLO family. J. Mol. Evol. 56, 77–88. doi: 10.1007/S00239-002-2382-5
Dielen, A. S., Badaoui, S., Candresse, T., German-Retana, S. (2010). The ubiquitin/26S proteasome system in plant–pathogen interactions: a never‐ending hide‐and‐seek game. Mol. Plant Pathol. 11, 293. doi: 10.1111/J.1364-3703.2009.00596.X
Dobin, A., Davis, C. A., Schlesinger, F., Drenkow, J., Zaleski, C., Jha, S., et al. (2013). STAR: Ultrafast universal RNA-seq aligner. Bioinformatics 29, 15–21. doi: 10.1093/bioinformatics/bts635
Dreher, K., Callis, J. (2007). Ubiquitin, hormones and biotic stress in plantsDreher and callis — Ubiquitin, hormones and biotic stress in plantsDreher and callis — Ubiquitin, hormones and biotic stress in plants. Ann. Bot. 99, 787–822. doi: 10.1093/AOB/MCL255
Dunwell, J. M., Gibbings, J. G., Mahmood, T., Saqlan Naqvi, S. M. (2008). Germin and germin-like proteins: evolution, structure, and function. CRC. Crit. Rev. Plant Sci. 27, 342–375. doi: 10.1080/07352680802333938
Emms, D. M., Kelly, S. (2019). OrthoFinder: phylogenetic orthology inference for comparative genomics. Genome Biol. 20, 238. doi: 10.1186/s13059-019-1832-y
Engelbrecht, J., Duong, T. A., Prabhu, S. A., Seedat, M., van den Berg, N. (2021). Genome of the destructive oomycete Phytophthora cinnamomi provides insights into its pathogenicity and adaptive potential. BMC Genomics 22, 302. doi: 10.1186/s12864-021-07552-y
Eshraghi, L., Anderson, J. P., Aryamanesh, N., McComb, J. A., Shearer, B., Hardy, G. S. J. E. (2014). Suppression of the auxin response pathway enhances susceptibility to Phytophthora cinnamomi while phosphite-mediated resistance stimulates the auxin signalling pathway. BMC Plant Biol. 14, 68. doi: 10.1186/1471-2229-14-68
Eulgem, T., Rushton, P. J., Robatzek, S., Somssich, I. E. (2000). The WRKY superfamily of plant transcription factors. Trends Plant Sci. 5, 199–206. doi: 10.1016/S1360-1385(00)01600-9
Evangelisti, E., Gogleva, A., Hainaux, T., Doumane, M., Tulin, F., Quan, C., et al. (2017). Time-resolved dual transcriptomics reveal early induced Nicotiana benthamiana root genes and conserved infection-promoting Phytophthora palmivora effectors. BMC Biol. 15, 1–24. doi: 10.1186/s12915-017-0379-1
Evangelisti, E., Govetto, B., Minet-Kebdani, N., Kuhn, M. L., Attard, A., Ponchet, M., et al. (2013). The Phytophthora parasitica RXLR effector Penetration-Specific Effector 1 favours Arabidopsis thaliana infection by interfering with auxin physiology. New Phytol. 199, 476–489. doi: 10.1111/NPH.12270
Ewels, P., Magnusson, M., Lundin, S., Käller, M. (2016). MultiQC: Summarize analysis results for multiple tools and samples in a single report. Bioinformatics 32, 3047–3048. doi: 10.1093/bioinformatics/btw354
Fabritius, A. L., Judelson, H. S. (2003). A mating-induced protein of Phytophthora infestans is a member of a family of elicitors with divergent structures and stage-specific patterns of expression. Mol. Plant Microbe Interact. 16, 926–935. doi: 10.1094/MPMI.2003.16.10.926
Fernandes, P. (2022). Molecular and histological approaches to unravel Castanea spp. responses to Phytophthora cinnamomi infection. (PhD dissertation). Oeiras, Portugal: ITQB-NOVA University of Lisbon.
Fernandes, P., Machado, H., do Céu Silva, M., Costa, R. L. (2021). A histopathological study reveals new insights into responses of chestnut (Castanea spp.) to root infection by Phytophthora cinnamomi. Phytopathology 111, 345–355. doi: 10.1094/PHYTO-04-20-0115-R
Fernandes, P., Tedesco, S., da Silva, I. V., Santos, C., MaChado, H., Costa, R. L. (2020). A new clonal propagation protocol develops quality root systems in chestnut. Forests 11, 826. doi: 10.3390/F11080826
Figueiredo, A., Monteiro, F., Sebastiana, M. (2014). Subtilisin-like proteases in plant–pathogen recognition and immune priming: A perspective. Front. Plant Sci. 5. doi: 10.3389/FPLS.2014.00739/BIBTEX
Fukamatsu, Y., Yabe, N., Hasunuma, K. (2003). Arabidopsis NDK1 is a component of ROS signaling by interacting with three catalases. Plant Cell Physiol. 44, 982–989. doi: 10.1093/PCP/PCG140
Gao, Q. M., Venugopal, S., Navarre, D., Kachroo, A. (2011). Low oleic acid-derived repression of jasmonic acid-inducible defense responses requires the WRKY50 and WRKY51 proteins. Plant Physiol. 155, 464–476. doi: 10.1104/PP.110.166876
Gao, N., Wadhwani, P., Mühlhäuser, P., Liu, Q., Riemann, M., Ulrich, A. S., et al. (2016). An antifungal protein from Ginkgo biloba binds actin and can trigger cell death. Protoplasma 253, 1159–1174. doi: 10.1007/s00709-015-0876-4
Gao, Q. M., Zhu, S., Kachroo, P., Kachroo, A. (2015). Signal regulators of systemic acquired resistance. Front. Plant Sci. 6. doi: 10.3389/FPLS.2015.00228/BIBTEX
Goossens, J., Mertens, J., Goossens, A. (2017). Role and functioning of bHLH transcription factors in jasmonate signalling. J. Exp. Bot. 68, 1333–1347. doi: 10.1093/JXB/ERW440
Gotz, S., Garcia-Gomez, J. M., Terol, J., Williams, T. D., Nagaraj, S. H., Nueda, M. J., et al. (2008). High-throughput functional annotation and data mining with the Blast2GO suite. Nucleic Acids Res. 36, 3420–3435. doi: 10.1093/nar/gkn176
Grabherr, M. G., Haas, B. J., Yassour, M., Levin, J. Z., Thompson, D. A., Amit, I., et al. (2011). Full-length transcriptome assembly from RNA-Seq data without a reference genome. Nat. Biotechnol. 29, 644–652. doi: 10.1038/NBT.1883
Haas, B. J., Papanicolaou, A., Yassour, M., Grabherr, M., Blood, P. D., Bowden, J., et al. (2013). De novo transcript sequence reconstruction from RNA-seq using the Trinity platform for reference generation and analysis. Nat. Protoc. 8, 1494–1512. doi: 10.1038/nprot.2013.084
Hagen, G., Guilfoyle, T. (2002). Auxin-responsive gene expression: genes, promoters and regulatory factors. Plant Mol. Biol. 49, 373–385. doi: 10.1023/A:1015207114117
Hardham, A. R. (2007). Cell biology of plant-oomycete interactions. Cell Microbiol. 9, 31–39. doi: 10.1111/j.1462-5822.2006.00833.x
Hardham, A. R., Blackman, L. M. (2018). Phytophthora cinnamomi. Mol. Plant Pathol. 19, 260–285. doi: 10.1111/mpp.12568
Hayden, K. J., Garbelotto, M., Knaus, B. J., Cronn, R. C., Rai, H., Wright, J. W. (2014). Dual RNA-seq of the plant pathogen Phytophthora ramorum and its tanoak host. Tree Genet. Genomes 10, 489–502. doi: 10.1007/S11295-014-0698-0
Heese, A., Hann, D. R., Gimenez-Ibanez, S., Jones, A. M. E., He, K., Li, J., et al. (2007). The receptor-like kinase SERK3/BAK1 is a central regulator of innate immunity in plants. Proc. Natl. Acad. Sci. U.S.A. 104, 12217–12222. doi: 10.1073/PNAS.0705306104
Higashi, K., Ishiga, Y., Inagaki, Y., Toyoda, K., Shiraishi, T., Ichinose, Y. (2008). Modulation of defense signal transduction by flagellin-induced WRKY41 transcription factor in Arabidopsis thaliana. Mol. Genet. Genomics 279, 303–312. doi: 10.1007/S00438-007-0315-0/FIGURES/6
Hollung, K., Espelund, M., Jakobsen, K. S. (1994). AnotherLea B19 gene (Group1Lea) from barley containing a single 20 amino acid hydrophilic motif. Plant Mol. Biol. 25, 559–564. doi: 10.1007/BF00043884
Horta, M., Caetano, P., Medeira, C., Maia, I., Cravador, A. (2010). Involvement of the β -cinnamomin elicitin in infection and colonisation of cork oak roots by Phytophthora cinnamomi. Eur. J. Plant Pathol. 127, 427–436. doi: 10.1007/s10658-010-9609-x
Hou, S., Wang, X., Chen, D., Yang, X., Wang, M., Turrà, D., et al. (2014). The secreted peptide PIP1 amplifies immunity through receptor-like kinase 7. PloS Pathog. 10, e1004331. doi: 10.1371/JOURNAL.PPAT.1004331
Huang, H. (1996). Evaluation of chinese chestnut cultivars for resistance to cryphonectria parasitica. Plant Dis. 80, 45. doi: 10.1094/PD-80-0045
Huang, Y., Chen, X., Liu, Y., Roth, C., Copeland, C., McFarlane, H. E., et al. (2013). Mitochondrial AtPAM16 is required for plant survival and the negative regulation of plant immunity. Nat. Commun. 4, 1–13. doi: 10.1038/ncomms3558
Islam, M. T., Hussain, H. I., Rookes, J. E., Cahill, D. M. (2018). Transcriptome analysis, using RNA-Seq of Lomandra longifolia roots infected with Phytophthora cinnamomi reveals the complexity of the resistance response. Plant Biol. 20, 130–142. doi: 10.1111/plb.12624
Jiang, R. H. Y., Tyler, B. M. (2012). Mechanisms and evolution of virulence in oomycetes. Annu. Rev. Phytopathol. 50, 295–318. doi: 10.1146/annurev-phyto-081211-172912
Jones, J. D. G., Dangl, J. L. (2006). The plant immune system. Nature 444, 323–329. doi: 10.1038/nature05286
Judelson, H. S., Narayan, R. D., Ah-Fong, A. M. V., Kim, K. S. (2009). Gene expression changes during asexual sporulation by the late blight agent Phytophthora infestans occur in discrete temporal stages. Mol. Genet. Genomics 281, 193–206. doi: 10.1007/S00438-008-0407-5
Jupe, J., Stam, R., Howden, A. J. M., Morris, J. A., Zhang, R., Hedley, P. E., et al. (2013). Phytophthora capsici-tomato interaction features dramatic shifts in gene expression associated with a hemi-biotrophic lifestyle. Genome Biol. 14, R63. doi: 10.1186/gb-2013-14-6-r63
Kamoun, S., Furzer, O., Jones, J. D. G., Judelson, H. S., Ali, G. S., Dalio, R. J. D., et al. (2015). The Top 10 oomycete pathogens in molecular plant pathology. Mol. Plant Pathol. 16, 413–434. doi: 10.1111/mpp.12190
Kanehisa, M., Goto, S. (2000). KEGG: kyoto encyclopedia of genes and genomes. Nucleic Acids Res. 28, 27–30. doi: 10.1093/NAR/28.1.27
Kant, S., Bi, Y. M., Zhu, T., Rothstein, S. J. (2009). SAUR39, a small auxin-up RNA gene, acts as a negative regulator of auxin synthesis and transport in rice. Plant Physiol. 151, 691–701. doi: 10.1104/PP.109.143875
Kelley, B. S., Lee, S. J., Damasceno, C. M. B., Chakravarthy, S., Kim, B. D., Martin, G. B., et al. (2010). A secreted effector protein (SNE1) from Phytophthora infestans is a broadly acting suppressor of programmed cell death. Plant J. 62, 357–366. doi: 10.1111/J.1365-313X.2010.04160.X
Kieffer, F., Lherminier, J., Simon-Plas, F., Nicole, M., Paynot, M., Elmayan, T., et al. (2000). The fungal elicitor cryptogein induces cell wall modifications on tobacco cell suspension. J. Exp. Bot. 51, 1799–1811. doi: 10.1093/JEXBOT/51.352.1799
Kobayashi, M., Ohura, I., Kawakita, K., Yokota, N., Fujiwara, M., Shimamoto, K., et al. (2007). Calcium-dependent protein kinases regulate the production of reactive oxygen species by potato NADPH oxidase. Plant Cell 19, 1065–1080. doi: 10.1105/TPC.106.048884
Kubisiak, T. L., Hebard, F. V., Nelson, C. D., Zhang, J., Bernatzky, R., Huang, H., et al. (1997). Molecular mapping of resistance to blight in an interspecific cross in the genus castanea. Phytopathology 87, 751–759. doi: 10.1094/PHYTO.1997.87.7.751
Kubisiak, T. L., Nelson, C. D., Staton, M. E., Zhebentyayeva, T., Smith, C., Olukolu, B. A., et al. (2013). A transcriptome-based genetic map of Chinese chestnut (Castanea mollissima) and identification of regions of segmental homology with peach (Prunus persica). Tree Genet. Genomes 9, 557–571. doi: 10.1007/s11295-012-0579-3
Kusch, S., Thiery, S., Reinstädler, A., Gruner, K., Zienkiewicz, K., Feussner, I., et al. (2019). Arabidopsis mlo3 mutant plants exhibit spontaneous callose deposition and signs of early leaf senescence. Plant Mol. Biol. 101, 21–40. doi: 10.1007/s11103-019-00877-z
Landrieu, I., Da Costa, M., De Veylder, L., Dewitte, F., Vandepoele, K., Hassan, S., et al. (2004). A small CDC25 dual-specificity tyrosine-phosphatase isoform in Arabidopsis thaliana. Proc. Natl. Acad. Sci. U.S.A. 101, 13380–13385. doi: 10.1073/PNAS.0405248101
Langmead, B., Wilks, C., Antonescu, V., Charles, R. (2019). Scaling read aligners to hundreds of threads on general-purpose processors. Bioinformatics 35, 421–432. doi: 10.1093/bioinformatics/bty648
Le Berre, J. Y., Gourgues, M., Samans, B., Keller, H., Panabières, F., Attard, A. (2017). Transcriptome dynamic of Arabidopsis roots infected with Phytophthora parasitica identifies VQ29, a gene induced during the penetration and involved in the restriction of infection. PloS One 12, e0190341. doi: 10.1371/JOURNAL.PONE.0190341
Le Gall, H., Philippe, F., Domon, J. M., Gillet, F., Pelloux, J., Rayon, C. (2015). Cell wall metabolism in response to abiotic stress. Plants 4, 112–166. doi: 10.3390/PLANTS4010112
Le Provost, G., Herrera, R., Paiva, J. A., Chaumeil, P., Salin, F., Plomion, C. (2007). A micromethod for high throughput RNA extraction in forest trees. Biol. Res. 40, 291–297. doi: 10.4067/S0716-97602007000400003
Li, L., He, Z., Pandey, G. K., Tsuchiya, T., Luan, S. (2002). Functional cloning and characterization of a plant efflux carrier for multidrug and heavy metal detoxification. J. Biol. Chem. 277, 5360–5368. doi: 10.1074/JBC.M108777200
Li, J., Staiger, C. J. (2018). Understanding cytoskeletal dynamics during the plant immune response. Annu. Rev. Phytopathol. 56, 513–533. doi: 10.1146/annurev-phyto-080516-035632
Liao, Z. X., Ni, Z., Wei, X. L., Chen, L., Li, J. Y., Yu, Y. H., et al. (2019). Dual RNA-seq of Xanthomonas oryzae pv. oryzicola infecting rice reveals novel insights into bacterial-plant interaction. PloS One 14, e0215039. doi: 10.1371/JOURNAL.PONE.0215039
Liao, Y., Smyth, G. K., Shi, W. (2014). FeatureCounts: An efficient general purpose program for assigning sequence reads to genomic features. Bioinformatics 30, 923–930. doi: 10.1093/bioinformatics/btt656
Liscum, E., Reed, J. W. (2002). Genetics of Aux/IAA and ARF action in plant growth and development. Plant Mol. Biol. 49, 387–400. doi: 10.1023/A:1015255030047
Liu, Y., Dammann, C., Bhattacharyya, M. K. (2001). The matrix metalloproteinase gene GmMMp2 is activated in response to pathogenic infections in soybean. Plant Physiol. 127, 1788–1797. doi: 10.1104/PP.010593
Liu, T., Wang, H., Liu, Z., Pang, Z., Zhang, C., Zhao, M., et al. (2021). The 26S proteasome regulatory subunit gmPSMD promotes resistance to phytophthora sojae in soybean. Front. Plant Sci. 12. doi: 10.3389/fpls.2021.513388
Liu, H., Wang, X., Zhang, H., Yang, Y., Ge, X., Song, F. (2008). A rice serine carboxypeptidase-like gene OsBISCPL1 is involved in regulation of defense responses against biotic and oxidative stress. Gene 420, 57–65. doi: 10.1016/J.GENE.2008.05.006
Lorenzo, O., Piqueras, R., Sánchez-Serrano, J. J., Solano, R. (2003). ETHYLENE RESPONSE FACTOR1 integrates signals from ethylene and jasmonate pathways in plant defense. Plant Cell 15, 165–178. doi: 10.1105/TPC.007468
Maia, I., Horta, M., Cravador, A., Medeira, C. (2012). “Loss of aggressiveness of Phytophthora cinnamomi (beta-cinnamomin silenced strain) in the infection of Castanea sativa,” in Microscopy and Microanalysis, 17–18. doi: 10.1017/S1431927612012743
Manni, M., Berkeley, M. R., Seppey, M., Simão, F. A., Zdobnov, E. M. (2021). BUSCO update: novel and streamlined workflows along with broader and deeper phylogenetic coverage for scoring of eukaryotic, prokaryotic, and viral genomes. Mol. Biol. Evol. 38, 4647–4654. doi: 10.1093/molbev/msab199
Markakis, M. N., Boron, A. K., Van Loock, B., Saini, K., Cirera, S., Verbelen, J. P., et al. (2013). Characterization of a small auxin-up RNA (SAUR)-like gene involved in arabidopsis thaliana development. PloS One 8, e82596. doi: 10.1371/JOURNAL.PONE.0082596
Marshall, S. D. G., Putterill, J. J., Plummer, K. M., Newcomb, R. D. (2003). The carboxylesterase gene family from arabidopsis thaliana. J. Mol. Evol. 57, 487–500. doi: 10.1007/S00239-003-2492-8
Martins, I. M., Meirinho, S., Costa, R., Cravador, A., Choupina, A. (2019). Cloning, characterization, in vitro and in planta expression of a necrosis-inducing Phytophthora protein 1 gene npp1 from Phytophthora cinnamomi. Mol. Biol. Rep. 46, 6453–6462. doi: 10.1007/s11033-019-05091-0
McGuigan, L., Fernandes, P., Oakes, A., Stewart, K., Powell, W. (2020). Transformation of American chestnut (Castanea dentata (marsh.) borkh) using RITA® temporary immersion bioreactors and we vitro containers. Forests 11, 1–15. doi: 10.3390/f11111196
Meng, X., Zhang, S. (2013). MAPK cascades in plant disease resistance signaling. Annu. Rev. Phytopathol. 51, 245–266. doi: 10.1146/annurev-phyto-082712-102314
Meyer, F., Paarmann, D., D’Souza, M., Olson, R., Glass, E. M., Kubal, M., et al. (2008). The metagenomics RAST server - a public resource for the automatic phylogenetic and functional analysis of metagenomes. BMC Bioinf. 9, 386. doi: 10.1186/1471-2105-9-386
Meyer, F. E., Shuey, L. S., Naidoo, S., Mamni, T., Berger, D. K., Myburg, A. A., et al. (2016). Dual RNA-sequencing of Eucalyptus nitens during Phytophthora cinnamomi challenge reveals pathogen and host factors influencing compatibility. Front. Plant Sci. 7. doi: 10.3389/fpls.2016.00191
Miedes, E., Vanholme, R., Boerjan, W., Molina, A. (2014). The role of the secondary cell wall in plant resistance to pathogens. Front. Plant Sci. 5. doi: 10.3389/FPLS.2014.00358/BIBTEX
Mikheenko, A., Prjibelski, A., Saveliev, V., Antipov, D., Gurevich, A. (2018). Versatile genome assembly evaluation with QUAST-LG. Bioinformatics 34, i142–i150. doi: 10.1093/bioinformatics/bty266
Minic, Z. (2007). Physiological roles of plant glycoside hydrolases. Planta 227, 723–740. doi: 10.1007/S00425-007-0668-Y
Mravec, J., Skůpa, P., Bailly, A., Hoyerová, K., Křeček, P., Bielach, A., et al. (2009). Subcellular homeostasis of phytohormone auxin is mediated by the ER-localized PIN5 transporter. Nature 459, 1136–1140. doi: 10.1038/nature08066
Müller, M., Munné-Bosch, S. (2015). Focus on ethylene: ethylene response factors: A key regulatory hub in hormone and stress signaling. Plant Physiol. 169, 32. doi: 10.1104/PP.15.00677
Naveed, Z. A., Wei, X., Chen, J., Mubeen, H., Ali, G. S. (2020). The PTI to ETI continuum in phytophthora-plant interactions. Front. Plant Sci. 11. doi: 10.3389/FPLS.2020.593905/BIBTEX
Nelson, C. D., Powell, W. A., Merkle, S. A., Carlson, J. E., Hebard, F. V., Islam-Faridi, N., et al. (2014). “Biotechnology of Trees: Chestnut,” in Tree Biotechnology. Eds. Ramawat, K. G., Mérillon, J. M., Ahuja, M. R. (CRC News, Boca Raton, FL, USA), 3–35.
Nicaise, V., Roux, M., Zipfel, C. (2009). Recent Advances in PAMP-Triggered Immunity against Bacteria: Pattern Recognition Receptors Watch over and Raise the Alarm. Plant Physiol. 150, 1638–1647. doi: 10.1104/PP.109.139709
Nürnberger, T., Nennstiel, D., Jabs, T., Sacks, W. R., Hahlbrock, K., Scheel, D. (1994). High affinity binding of a fungal oligopeptide elicitor to parsley plasma membranes triggers multiple defense responses. Cell 78, 449–460. doi: 10.1016/0092-8674(94)90423-5
Oßwald, W., Fleischmann, F., Rigling, D., Coelho, A. C., Cravador, A., Diez, J., et al. (2014). Strategies of attack and defence in woody plant-Phytophthora interactions. For. Pathol. 44, 169–190. doi: 10.1111/efp.12096
Paniagua, C., Bilkova, A., Jackson, P., Dabravolski, S., Riber, W., Didi, V., et al. (2017). Dirigent proteins in plants: modulating cell wall metabolism during abiotic and biotic stress exposure. J. Exp. Bot. 68, 3287–3301. doi: 10.1093/JXB/ERX141
Park, C. H., Chen, S., Shirsekar, G., Zhou, B., Khang, C. H., Songkumarn, P., et al. (2012). The magnaporthe oryzae effector avrPiz-t targets the RING E3 ubiquitin ligase APIP6 to suppress pathogen-associated molecular pattern–triggered immunity in rice. Plant Cell 24, 4748–4762. doi: 10.1105/TPC.112.105429
Park, S. C., Lee, J. R., Shin, S. O., Park, Y., Lee, S. Y., Hahm, K. S. (2007). Characterization of a heat-sxxx protein with antimicrobial activity from Arabidopsis thaliana. Biochem. Biophys. Res. Commun. 362, 562–567. doi: 10.1016/J.BBRC.2007.07.188
Park, M. R., Yun, K. Y., Mohanty, B., Herath, V., Xu, F., Wijaya, E., et al. (2010). Supra-optimal expression of the cold-regulated OsMyb4 transcription factor in transgenic rice changes the complexity of transcriptional network with major effects on stress tolerance and panicle development. Plant Cell Environ. 33, 2209–2230. doi: 10.1111/J.1365-3040.2010.02221.X
Patro, R., Duggal, G., Love, M. I., Irizarry, R. A., Kingsford, C. (2017). Salmon provides fast and bias-aware quantification of transcript expression. Nat. Methods 14, 417–419. doi: 10.1038/nmeth.4197
Pavese, V., Moglia, A., Gonthier, P., Marinoni, D. T., Cavalet-Giorsa, E., Botta, R. (2021). Identification of Susceptibility Genes in Castanea sativa and Their Transcription Dynamics following Pathogen Infection. Plants 10, 913. doi: 10.3390/PLANTS10050913
Payne, G., Ahl, P., Moyer, M., Harper, A., Beck, J., Meins, F., et al. (1990). Isolation of complementary DNA clones encoding pathogenesis-related proteins P and Q, two acidic chitinases from tobacco. Proc. Natl. Acad. Sci. U.S.A. 87, 98–102. doi: 10.1073/PNAS.87.1.98
Pieterse, C. M. J., van der Does, D., Zamioudis, C., Leon-Reyes, A., Van Wees, S. C. M. (2012). Hormonal modulation of plant immunity. Annu. Rev. Cell Dev. Biol. 28, 489–521. doi: 10.1146/annurev-cellbio-092910-154055
Pomar, F., Merino, F., Barceló, A. R. (2002). O-4-linked coniferyl and sinapyl aldehydes in lignifying cell walls are the main targets of the Wiesner (phloroglucinol-HCl) reaction. Protoplasma 220, 17–28. doi: 10.1007/s00709-002-0030-y
Qin, Y. M., Hu, C. Y., Pang, Y., Kastaniotis, A. J., Hiltunen, J. K., Zhu, Y. X. (2007). Saturated very-long-chain fatty acids promote cotton fiber and arabidopsis cell elongation by activating ethylene biosynthesis. Plant Cell 19, 3692–3704. doi: 10.1105/TPC.107.054437
Qutob, D., Kamoun, S., Gijzen, M. (2002). Expression of a Phytophthora sojae necrosis-inducing protein occurs during transition from biotrophy to necrotrophy. Plant J. 32, 361–373. doi: 10.1046/J.1365-313X.2002.01439.X
Raaymakers, T. M., Van Den Ackerveken, G. (2016). Extracellular recognition of oomycetes during biotrophic infection of plants. Front. Plant Sci. 7. doi: 10.3389/FPLS.2016.00906
Rai, A., Suprasanna, P., D’Souza, S. F., Kumar, V. (2012). Membrane topology and predicted RNA-binding function of the ‘Early responsive to dehydration (ERD4)’ Plant protein. PloS One 7, e32658. doi: 10.1371/JOURNAL.PONE.0032658
Redondo, M.Á., Pérez-Sierra, A., Abad-Campos, P., Torres, L., Solla, A., Reig-Armiñana, J., et al. (2015). Histology of Quercus ilex roots during infection by Phytophthora cinnamomi. Trees - Structure. Funct. 29, 1943–1957. doi: 10.1007/s00468-015-1275-3
Reeksting, B. J., Olivier, N. A., van den Berg, N. (2016). Transcriptome responses of an ungrafted Phytophthora root rot tolerant avocado (Persea americana) rootstock to flooding and Phytophthora cinnamomi. BMC Plant Biol. 16, 205. doi: 10.1186/s12870-016-0893-2
Robinson, M. D., McCarthy, D. J., Smyth, G. K. (2010). edgeR: A Bioconductor package for differential expression analysis of digital gene expression data. Bioinformatics 26, 139–140. doi: 10.1093/bioinformatics/btp616
Robinson, M. D., Oshlack, A. (2010). A scaling normalization method for differential expression analysis of RNA-seq data. Genome Biol. 11, R25. doi: 10.1186/gb-2010-11-3-r25
Rodenburg, S. Y. A., Seidl, M. F., Judelson, H. S., Vu, A. L., Govers, F., De Ridder, D. (2019). Metabolic model of the Phytophthora infestans-tomato interaction reveals metabolic switches during host colonization. mBio 10. doi: 10.1128/mBio.00454-19
Rodriguez, L., Gonzalez-Guzman, M., Diaz, M., Rodrigues, A., Izquierdo-Garcia, A. C., Peirats-Llobet, M., et al. (2014). C2-domain abscisic acid-related proteins mediate the interaction of PYR/PYL/RCAR abscisic acid receptors with the plasma membrane and regulate abscisic acid sensitivity in arabidopsis. Plant Cell 26, 4802. doi: 10.1105/TPC.114.129973
Saijo, Y., Loo, E. P., Yasuda, S. (2018). Pattern recognition receptors and signaling in plant-microbe interactions. Plant J. 93, 592–613. doi: 10.1111/tpj.13808
Santana Silva, R. J., Micheli, F. (2020). RRGPredictor, a set-theory-based tool for predicting pathogen-associated molecular pattern receptors (PRRs) and resistance (R) proteins from plants. Genomics 112, 2666–2676. doi: 10.1016/J.YGENO.2020.03.001
Santos, C., Duarte, S., Tedesco, S., Fevereiro, P., Costa, R. L. (2017a). Expression profiling of Castanea genes during resistant and susceptible interactions with the oomycete pathogen Phytophthora cinnamomi reveal possible mechanisms of immunity. Front. Plant Sci. 8. doi: 10.3389/fpls.2017.00515
Santos, C., MaChado, H., Correia, I., Gomes, F., Gomes-Laranjo, J., Costa, R. (2015a). Phenotyping Castanea hybrids for Phytophthora cinnamomi resistance. Plant Pathol. 64, 901–910. doi: 10.1111/ppa.12313
Santos, C., Nelson, C. D., Zhebentyayeva, T., MaChado, H., Gomes-Laranjo, J., Costa, R. L. (2017b). First interspecific genetic linkage map for Castanea sativa x Castanea crenata revealed QTLs for resistance to Phytophthora cinnamomi. PloS One 12, e0184381. doi: 10.1371/journal.pone.0184381
Santos, C., Zhebentyayeva, T., Serrazina, S., Nelson, C. D., Costa, R. (2015b). Development and characterization of EST-SSR markers for mapping reaction to Phytophthora cinnamomi in Castanea spp. Sci. Hortic. 194, 181–187. doi: 10.1016/j.scienta.2015.07.043
Sawano, Y., Miyakawa, T., Yamazaki, H., Tanokura, M., Hatano, K. I. (2007). Purification, characterization, and molecular gene cloning of an antifungal protein from Ginkgo biloba seeds. Biol. Chem. 388, 273–280. doi: 10.1515/BC.2007.030
Scott, C. (2016). dammit: an open and accessible de novo transcriptome annotator. Available online at: http://dib-lab.github.io/dammit/.
Serdar, Ü., Saito, T., Cuenca, B., Akyüz, B., Laranjo, J. G., Beccaro, G., et al. (2019). Advances in cultivation of chestnuts (Cambridge, UK: Burleigh Dodds Science Publishing Limited), 349–388. doi: 10.19103/as.2018.0042.22
Serrazina, S., MaChado, H., Costa, R. L., Duque, P., Malhó, R. (2021). Expression of Castanea crenata Allene Oxide Synthase in Arabidopsis Improves the Defense to Phytophthora cinnamomi. Front. Plant Sci. 12. doi: 10.3389/fpls.2021.628697
Serrazina, S., Martínez, M. T., Cano, V., Malhó, R., Lourenço Costa, R., Corredoira, E. (2022). Genetic transformation of quercus ilex somatic embryos with a gnk2-like protein that reveals a putative anti-oomycete action. Plants 11, 304. doi: 10.3390/plants11030304
Serrazina, S., Santos, C., MaChado, H., Pesquita, C., Vicentini, R., Pais, M. S., et al. (2015). Castanea root transcriptome in response to Phytophthora cinnamomi challenge. Tree Genet. Genomes 11, 6. doi: 10.1007/s11295-014-0829-7
Silva, V., Falco, V., Dias, M. I., Barros, L., Silva, A., Capita, R., et al. (2020). Evaluation of the Phenolic Profile of Castanea sativa Mill. By-Products and Their Antioxidant and Antimicrobial Activity against Multiresistant Bacteria. Antioxidants 9, 87. doi: 10.3390/ANTIOX9010087
Silva, M. C., Nicole, M., Guerra-Guimarães, L., Rodrigues, C. J. (2002). Hypersensitive cell death and post-haustorial defence responses arrest the orange rust (Hemileia vastatrix) growth in resistant coffee leaves. Physiol. Mol. Plant Pathol. 60, 169–183. doi: 10.1006/pmpp.2002.0389
Škalamera, D., Heath, M. C. (1998). Changes in the cytoskeleton accompanying infection-induced nuclear movements and the hypersensitive response in plant cells invaded by rust fungi. Plant J. 16, 191–200. doi: 10.1046/J.1365-313X.1998.00285.X
Tachi, H., Fukuda-Yamada, K., Kojima, T., Shiraiwa, M., Takahara, H. (2009). Molecular characterization of a novel soybean gene encoding a neutral PR-5 protein induced by high-salt stress. Plant Physiol. Biochem. 47, 73–79. doi: 10.1016/J.PLAPHY.2008.09.012
Takemoto, D., Jones, D. A., Hardham, A. R. (2003). GFP-tagging of cell components reveals the dynamics of subcellular re-organization in response to infection of Arabidopsis by oomycete pathogens. Plant J. 33, 775–792. doi: 10.1046/J.1365-313X.2003.01673.X
Törönen, P., Medlar, A., Holm, L. (2018). PANNZER2: a rapid functional annotation web server. Nucleic Acids Res. 46, W84–W88. doi: 10.1093/NAR/GKY350
Tripathi, D., Jiang, Y. L., Kumar, D. (2010). SABP2, a methyl salicylate esterase is required for the systemic acquired resistance induced by acibenzolar-S-methyl in plants. FEBS Lett. 584, 3458–3463. doi: 10.1016/J.FEBSLET.2010.06.046
Turlapati, P. V., Kim, K. W., Davin, L. B., Lewis, N. G. (2011). The laccase multigene family in Arabidopsis thaliana: Towards addressing the mystery of their gene function(s). Planta 233, 439–470. doi: 10.1007/S00425-010-1298-3/FIGURES/13
Tyurenkov, I. N., Ozerov, A. A., Kurkin, D. V., Gao, L. (2019). Structure analysis of a pathogenesis-related 10 protein from gardenia jasminoides. IOP. Conf. Ser. Earth Environ. Sci. 242, 42005. doi: 10.1088/1755-1315/242/4/042005
Üstün, S., Börnke, F. (2014). Interactions of Xanthomonas type-III effector proteins with the plant ubiquitin and ubiquitin-like pathways. Front. Plant Sci. 5. doi: 10.3389/FPLS.2014.00736/BIBTEX
van den Berg, N., Christie, J. B., Aveling, T. A. S., Engelbrecht, J. (2018a). Callose and β-1,3-glucanase inhibit Phytophthora cinnamomi in a resistant avocado rootstock. Plant Pathol. 67, 1150–1160. doi: 10.1111/PPA.12819
van den Berg, N., Mahomed, W., Olivier, N. A., Swart, V., Crampton, B. G. (2018b). Transcriptome analysis of an incompatible Persea americana-Phytophthora cinnamomi interaction reveals the involvement of SA- and JA-pathways in a successful defense response. PloS One 13, e0205705. doi: 10.1371/journal.pone.0205705
van den Berg, N., Swart, V., Backer, R., Fick, A., Wienk, R., Engelbrecht, J., et al. (2021). Advances in Understanding Defense Mechanisms in Persea americana Against Phytophthora cinnamomi. Front. Plant Sci. 12. doi: 10.3389/FPLS.2021.636339
van Loon, L. C., Rep, M., Pieterse, C. M. J. (2006). Significance of inducible defense-related proteins in infected plants. Annu. Rev. Phytopathol. 44, 135–162. doi: 10.1146/annurev.phyto.44.070505.143425
Van West, P., De Jong, A. J., Judelson, H. S., Emons, A. M. C., Govers, F. (1998). The ipiO Gene of Phytophthora infestans Is Highly Expressed in Invading Hyphae during Infection. Fungal Genet. Biol. 23, 126–138. doi: 10.1006/FGBI.1998.1036
Vargas, W. A., Sanz Martín, J. M., Rech, G. E., Rivera, L. P., Benito, E. P., Díaz-Mínguez, J. M., et al. (2012). Plant Defense Mechanisms Are Activated during Biotrophic and Necrotrophic Development of Colletotricum graminicola in Maize. Plant Physiol. 158, 1342–1358. doi: 10.1104/PP.111.190397
Veeraraghavan, S., Fagan, P. A., Hu, H., Lee, V., Harper, J. F., Huang, B., et al. (2002). Structural independence of the two EF-hand domains of caltractin. J. Biol. Chem. 277, 28564–28571. doi: 10.1074/JBC.M112232200
Vleeshouwers, V. G. A. A., Van Dooijeweert, W., Govers, F., Kamoun, S., Colon, L. T. (2000). The hypersensitive response is associated with host and nonhost resistance to Phytophthora infestans. Planta 210, 853–864. doi: 10.1007/s004250050690
Walker, C. A., Köppe, M., Grenville-Briggs, L. J., Avrova, A. O., Horner, N. R., McKinnon, A. D., et al. (2008). A putative DEAD-box RNA-helicase is required for normal zoospore development in the late blight pathogen Phytophthora infestans. Fungal Genet. Biol. 45, 954–962. doi: 10.1016/J.FGB.2008.03.004
Wang, Y., Dou, D., Wang, X., Li, A., Sheng, Y., Hua, C., et al. (2009). The PsCZF1 gene encoding a C2H2 zinc finger protein is required for growth, development and pathogenesis in Phytophthora sojae. Microb. Pathog. 47, 78–86. doi: 10.1016/J.MICPATH.2009.04.013
Wang, W., Feng, B., Zhou, J. M., Tang, D. (2020). Plant immune signaling: Advancing on two frontiers. J. Integr. Plant Biol. 62, 2–24. doi: 10.1111/JIPB.12898
Wang, H., He, H., Qi, Y., McLellan, H., Tian, Z., Birch, P. R. J., et al. (2018). The oomycete microbe-associated molecular pattern Pep-13 triggers SERK3/BAK1-independent plant immunity. Plant Cell Rep. 38, 173–182. doi: 10.1007/S00299-018-2359-5
Wang, H. H., Ng, T. B. (2000). Ginkbilobin, a novel antifungal protein from Ginkgo biloba seeds with sequence similarity to embryo-abundant protein. Biochem. Biophys. Res. Commun. 279, 407–411. doi: 10.1006/bbrc.2000.3929
Wani, S. H., Anand, S., Singh, B., Bohra, A., Joshi, R. (2021). WRKY transcription factors and plant defense responses: latest discoveries and future prospects. Plant Cell Rep. 40, 1071–1085. doi: 10.1007/S00299-021-02691-8/xxxS/1
Ward, J. A., Ponnala, L., Weber, C. A. (2012). Strategies for transcriptome analysis in nonmodel plants. Am. J. Bot. 99, 267–276. doi: 10.3732/ajb.1100334
Welinder, K. G., Justesen, A. F., Kjærsgård, I. V. H., Jensen, R. B., Rasmussen, S. K., Jespersen, H. M., et al. (2002). Structural diversity and transcription of class III peroxidases from Arabidopsis thaliana. Eur. J. Biochem. 269, 6063–6081. doi: 10.1046/J.1432-1033.2002.03311.X
Westermann, A. J., Gorski, S. A., Vogel, J. (2012). Dual RNA-seq of pathogen and host. Nat. Rev. Microbiol. 10, 618–630. doi: 10.1038/nrmicro2852
Wu, T., Hu, E., Xu, S., Chen, M., Guo, P., Dai, Z., et al. (2021). clusterProfiler 4.0: A universal enrichment tool for interpreting omics data. Innovation (N. Y). 2, 100141. doi: 10.1016/j.xinn.2021.100141
Xie, Y. D., Li, W., Guo, D., Dong, J., Zhang, Q., Fu, Y., et al. (2010). The Arabidopsis gene SIGMA FACTOR-BINDING PROTEIN 1 plays a role in the salicylate- and jasmonate-mediated defence responses. Plant Cell Environ. 33, 828. doi: 10.1111/J.1365-3040.2009.02109.X
Xu, Y., Zhang, Y., Zhu, J., Sun, Y., Guo, B., Liu, F., et al. (2021). Phytophthora sojae apoplastic effector AEP1 mediates sugar uptake by mutarotation of extracellular aldose and is recognized as a MAMP. Plant Physiol. 187, 321–335. doi: 10.1093/PLPHYS/KIAB239
Yang, H., Yang, S., Li, Y., Hua, J. (2007). The Arabidopsis BAP1 and BAP2 genes are general inhibitors of programmed cell death. Plant Physiol. 145, 135–146. doi: 10.1104/PP.107.100800
Yanhui, C., Xiaoyuan, Y., Kun, H., Meihua, L., Jigang, L., Zhaofeng, G., et al. (2006). The MYB transcription factor superfamily of arabidopsis: expression analysis and phylogenetic comparison with the rice MYB family. Plant Mol. Biol. 60, 107–124. doi: 10.1007/S11103-005-2910-Y
Yoo, S. Y., Kim, Y., Kim, S. Y., Lee, J. S., Ahn, J. H. (2007). Control of flowering time and cold response by a NAC-domain protein in arabidopsis. PloS One 2, e642. doi: 10.1371/JOURNAL.PONE.0000642
Yu, J., Chai, C., Ai, G., Jia, Y., Liu, W., Zhang, X., et al. (2020). A Nicotiana benthamiana AP2/ERF transcription factor confers resistance to Phytophthora parasitica. Phytopathol. Res. 2, 1–13. doi: 10.1186/S42483-020-0045-3
Yuan, G., Qian, Y., Ren, Y., Guan, Y., Wu, X., Ge, C., et al. (2021). The role of plant-specific VQ motif-containing proteins: An ever-thickening plot. Plant Physiol. Biochem. 159, 12–16. doi: 10.1016/J.PLAPHY.2020.12.005
Zhang, L., Du, L., Poovaiah, B. W. (2014). Calcium signaling and biotic defense responses in plants. Plant Signal Behav. 9 (11), e973818. doi: 10.4161/15592324.2014.973818
Zhao, Y., Chang, X., Qi, D., Dong, L., Wang, G., Fan, S., et al. (2017). A novel soybean ERF transcription factor, gmERF113, increases resistance to phytophthora sojae infection in soybean. Front. Plant Sci. 8. doi: 10.3389/FPLS.2017.00299
Zhebentyayeva, T. N., Sisco, P. H., Georgi, L. L., Jeffers, S. N., Perkins, M. T., James, J. B., et al. (2019). Dissecting resistance to phytophthora cinnamomi in interspecific hybrid chestnut crosses using sequence-based genotyping and QTL mapping. Phytopathology 109, 1594–1604. doi: 10.1094/PHYTO-11-18-0425-R
Keywords: chestnut, immune response, ink disease, pattern recognition receptors, PAMP, resistance, susceptibility, oomycete
Citation: Fernandes P, Pimentel D, Ramiro RS, Silva MdC, Fevereiro P and Costa RL (2024) Dual transcriptomic analysis reveals early induced Castanea defense-related genes and Phytophthora cinnamomi effectors. Front. Plant Sci. 15:1439380. doi: 10.3389/fpls.2024.1439380
Received: 27 May 2024; Accepted: 05 July 2024;
Published: 12 August 2024.
Edited by:
Nicolas Rispail, Spanish National Research Council (CSIC), SpainReviewed by:
Jared W. Westbrook, The American Chestnut Foundation, United StatesAlfredo Cravador, University of Algarve, Portugal
Copyright © 2024 Fernandes, Pimentel, Ramiro, Silva, Fevereiro and Costa. This is an open-access article distributed under the terms of the Creative Commons Attribution License (CC BY). The use, distribution or reproduction in other forums is permitted, provided the original author(s) and the copyright owner(s) are credited and that the original publication in this journal is cited, in accordance with accepted academic practice. No use, distribution or reproduction is permitted which does not comply with these terms.
*Correspondence: Patrícia Fernandes, cG1vcmFpc2ZAZXNmLmVkdQ==; Rita Lourenço Costa, cml0YS5sY29zdGFAaW5pYXYucHQ=