- 1Cellular Differentiation and Molecular Genetics Section, Department of Botany, Jamia Hamdard, New Delhi, India
- 2Plant Production Department, College of Food and Agriculture Sciences, King Saud University, Riyadh, Saudi Arabia
- 3Research Institute of Nyíregyháza, Institutes for Agricultural Research and Educational Farm (IAREF), University of Debrecen, Nyíregyháza, Hungary
Digitalis purpurea L. is one of the important plant species of Nilgiris, Kashmir and Darjeeling regions of India, belonging to the family Plantaginaceae, with well-known pharmacological applications. In the present investigation, an in vitro culture technique of indirect shoot organogenesis of D. purpurea is being explored; the biochemical attributes, the antioxidant activities and the metabolomic analyses were made by utilizing untargeted Gas Chromatography-Mass Spectrometry (GC-MS) and Ultra Performance Liquid Chromatography coupled with electronspray ionization/quadrupole-time-of-flight-mass spectrometry (UPLC-ESI-QTOF-MS) approaches. Initially, the leaf explants were used for callus induction and proliferation and maximum callusing frequency (94.44%) and fresh biomass (4.9 g) were obtained on MS, fortified with 8.8 µM BAP (6-benzyl amino purine) + 0.9 µM 2,4-D (2,4-dichlorophenoxyacetic acid), subsequently shoot formation (indirect organogenesis) was noted on the same MS medium with a shoot induction frequency of 83.33%. Later on, the biochemical and antioxidant potential of in vivo-, in vitro grown leaf and leaf derived callus were assessed. Significantly higher total phenol, flavonoid, DPPH (2,2-diphenyl-1-picrylhydrazyl), POD (peroxidase) and SOD (superoxide dismutase) activities were noticed in in vitro grown callus and leaf tissues compared with field grown leaf. The GC-MS analysis of each methanolic extract (in vivo-, in vitro derived leaf and leaf derived callus) displayed the presence of more than 75 bioactive compounds viz loliolide, stigmasterin, alpha-tocopherol, squalene, palmitic acid, linoleic acid, beta-amyrin, campesterol etc. possessing immense therapeutic importance. The UPLC-MS based metabolite fingerprinting of each methanolic extracts were conducted in both positive and negative ionization mode. The obtained results revealed variation in phytochemical composition in field - and laboratory grown tissues, indicating the impact of in vitro culture conditions on plant tissues. The detected phytocompounds belongs to various classes such as flavonoids, steroids, terpenoids, carbohydrates, tannins, lignans etc. The medicinally important metabolites identified were 20, 22-dihydrodigoxigenin, digoxigenin monodigitoxoside, apigenin, luteolin, kaempferide, rosmarinic acid, nepitrin and others. The results of the present study suggest that in vitro culture of D. purpurea could successfully be utilized for the novel drug discovery by producing such important phytocompounds of commercial interest in shorter duration without harming the plants’ natural population.
1 Introduction
For decades, medicinal plants have been a crucial source for pharmaceutical sector as more than half of the world’s populations are still reliant on traditional medicine system (Gomathi et al., 2013). Synthetically designed chemical drugs are often associated with multiple side effects, whereas plant-based medicines are derived naturally and thus, are more sustainable with negligible side effects (Konappa et al., 2020). Medicinal plants are frequently used in traditional medicine, food, cosmetics and healthcare as the plants are enriched with diverse groups of bioactive compounds which can be extracted for industrial and commercial purposes (Shakya, 2016; Rad et al., 2021). Plants produce a vast array of therapeutically important secondary metabolites like alkaloids, flavonoids, lignans, terpenoids, steroids and anthocyanins, along with significant primary metabolites like lipids, sugars and amino acids (Mickymaray, 2019; Thakur et al., 2019) play not only a decisive role in plant growth, development and reproductive cycle but also in defensive mechanisms (Singh et al., 2020). Several bioactive compounds exhibit crucial biological activities such as antineoplastic, anti-proliferative, anti-aging, anti-inflammatory, anti-angiogenic, anti-microbial, antiviral properties etc (Altemimi et al., 2017; Anand et al., 2019).
Digitalis purpurea L. (Plantaginaceae family) is an important biannual herbaceous plant with both ornamental and medicinal values and is commonly known as foxglove (Al-Oqab et al., 2022). The plant is indigenous to Europe and is grown in the Nilgiris, Kashmir and Darjeeling regions (Rehman Nengroo and Rauf, 2020). The well-known feature of D. purpurea is its tall spike-borne, campanulate flowers with color ranging from purple, pink, yellow, or white (Wu et al., 2012). It is currently a popular source of characteristic bioactive compounds cardenolides (digoxin, digitoxin, gitaloxin, gitoxin, strospeside); flavonoids (digicitrin and cyaniding); anthraquinone (digiferruginol), phenylethanoids (cornoside and maxoside) etc (Kreis, 2017; Amiri et al., 2023). These plant-based compounds exhibit multiple impact on health such as neuro-, hepato- and cardioprotective, anti-diabetic, anti-viral, anti-cancerous and cytotoxic activities (Verma et al., 2016a; Nartop et al., 2021). Digoxin and digitoxin are significant cardiotonic glycosides that are used to treat atrial arrhythmia and congestive heart failure (CHF) (Bhusare et al., 2018), by modifying the heart muscles’ contractile force and aid in their inotropic actions (Patel, 2016).
The evaluation of such phytocompounds as potent novel drugs requires simple efficient extraction, compound identification, economical production, employment of host organisms, conduction of preclinical, clinical trials and quantitative structure-activity relationship (QSAR) studies and complete metabolomic profiling of source plants (Dey et al., 2020). However, several factors restricting commercial production of these phytocompounds from plants growing in the wild include poor accessibility, over-utilization, cultivation challenges, low production, seasonal fluctuations, complications in extraction, extent of impurities, and the financial cost associated with suitable screening biological assays (Halder et al., 2019). In such scenario, micropropagation techniques prove to be beneficial in producing elite clones that synthesize adequate amount of phytocompounds in a shorter period of time without harming natural habitat (Ajithan et al., 2019; Erişen et al., 2020). In vitro regenerated plantlets can be produced by two fascinating biotechnological methods: somatic embryogenesis and organogenesis (Bansal et al., 2022). The in vitro production of bioactive compounds can be obtained from callus, cell suspension, shootlets, roots etc. and the whole process consists of two steps: (i) biomass aggregation and (ii) phytocompounds biosynthesis and extraction (Chandran et al., 2020).
After transcriptomics and proteomics, the study of metabolites-related events in living organisms, or metabolomics has emerged as the third significant area of functional genomics (Deepalakshmi et al., 2016). Thus, the integration of in vitro plant cultures with metabolomics investigations opens significant avenues to analyze the bioactive profile of different plant samples qualitatively and quantitatively (Carla Guimarães Sobrinho et al., 2022). Furthermore, metabolomic information will offer exceptional perspectives on underlying characteristics of plant phenotypes concerning development, physiology, tissue identity, resistance, biodiversity, and so forth (Hall et al., 2002). A wide range of analytical techniques including Gas Chromatography-Mass Spectrometry (GC–MS), Nuclear Magnetic Resonance (NMR) (Abdelsalam et al., 2017), Ultra Performance Liquid Chromatography-Mass Spectrometry (UPLC–MS) (Oliveira et al., 2018), Capillary Electrophoresis (CE) and High-Performance Liquid Chromatography-Mass Spectrometry (HPLC–MS) (Marchetti et al., 2019) have been employed for high-throughput analysis of metabolites over the past ten years.
Because of the extremely diverse biochemistry of plants, and presence of several important bioactive groups, both the GC-MS and LC-MS techniques are most frequently used in detecting critical phytocompounds (El Sayed et al., 2020). In metabolomics analysis, the MS is used in conjunction with GC or LC to leverage the advantages of each technique-the robustness of MS detectors and the higher resolution and reproducibility of chromatographic system. The GC-MS is often used for the study of volatile organic compounds, lipids and derivatizable compounds, whereas LC-MS is typically used for the investigation of mostly semi-polar metabolites (Zeki et al., 2020). These techniques have recently been applied to different in vitro raised plant tissues like Leucojum aestivum, Saraca asoca and Pluchea lanceolata (Spina et al., 2021; Mamgain et al., 2022; Vignesh et al., 2022).
Considering all these aspects, the information regarding the metabolic profiling of in vitro raised tissues of D. purpurea is still unknown, so it would provide insights about the plants’ phytochemistry and pharmacological importance. Thus, in the present work, we report the comparative and untargeted metabolite profiling of leaf-callus and in vivo- and in vitro-raised leaf tissues of D. purpurea by GC-MS and LC-MS techniques. The biochemical attributes such as total phenolic content, total flavonoid content and anti-oxidant activities were also assessed in cultured tissues during metabolite accumulation in D. purpurea. This is the first report of its kind which may auger novel new drug development in future.
2 Materials and methods
2.1 Chemicals and reagents
All the chemicals and solvents used in this study were of analytical grade. MS medium (Murashige and Skoog, 1962), methanol, Folin-ciocalteu reagent, Gallic acid, Quercetin, 2,2-Diphenyl-1-picrylhydrazy (DPPH), hydrogen peroxide, Triton X-100, ethylenediaminetetraacetic acid (EDTA), polyvinylpyrollidone (PVP), methionine, Nitro blue tetrazolium (NBT), riboflavin and all other chemicals and reagents were purchased from Himedia (Mumbai, India), SRL (Mumbai, India) and Sigma Aldrich (USA).
2.2 Explant preparation and in vitro culture conditions
Healthy and young leaves of D. purpurea were collected and underwent surface sterilization following the protocol of Bansal et al. (2022). Under sterilized conditions of laminar air flow, the leaf explants (3-4 cm) were inoculated on MS medium containing 3% (w/v) sucrose and 0.8% (w/v) agar. After adjusting the pH to 5.8, the medium was sterilized for 15 min at 121°C at 1.06 kg/cm2 of pressure. The culture was maintained at 24 ± 2°C in the dark for initial two days, and then switched to a 16:8h photoperiod cycle (cool white fluorescent light with an intensity of 50 μmol/m2/s-1).
2.3 Callus induction and indirect organogenesis
For callus induction, the leaf explants of D. purpurea were cultured onto MS medium supplemented with a combination of 6-benzylaminopurine (BAP) and 2,4-dichlorophenoxy-acetic acid (2,4-D) at varying concentrations. After 4 weeks, the produced callus was sub-cultured on the same medium for another 4 weeks to obtain shoots (indirect organogenesis). Each treatment included five replicates (one explant/test tube), and every experiment was conducted thrice. The callus induction percentage (%), fresh biomass (g) as well as indirect shoot induction percentage (%) and the mean shoot numbers/callus mass were recorded after 4 weeks period.
2.4 Biochemical analyses
2.4.1 Sample preparation
The leaf derived calli and in vivo and in vitro (organogenic derived) grown leaf tissue of D. purpurea were collected and air dried at room temperature for 5 days. Using a mortar and pestle, around 1.0 g of each air-dried sample was crushed into a fine powder. Each sample was then separately extracted using 10 mL of methanol (MeOH) solvent on a rotary shaker for 2 days, followed by filtration of extracts with Whatman filter paper No. 1. The filtered samples were then centrifuged for five min at 12,000 rpm, and the recovered supernatant was stored at 4°C for further use.
2.4.2 Total phenolic content estimation
The TPC determination was carried out with Folin-Ciocalteu protocol (Baliyan et al., 2022). Firstly, 2.5 mL of 10% (v/v) Folin-Ciocalteu (FC) reagent ((Sigma-Aldrich, New York, NY, USA) was thoroughly mixed with approximately 0.5 mL of extract and kept at room temperature (RT) for 5 min. After that, 2 mL of 7% Na2C03 was added, followed by incubation for 90 min at RT. Then, the absorbance of each sample was measured at a wavelength of 765 nm by using a UV-Vis spectrophotometer (Biolinkk, BL-295, Delhi, India) against a blank. The experiment was conducted in triplicates and a calibration curve of gallic acid was prepared to determine the total phenolic content in each sample. Results were expressed as milligrams of Gallic acid equivalents per gram of Dry Weight (mg GAE/g DW).
2.4.3 Total flavonoid content estimation
The TFC determination was conducted following the protocol reported by Aryal et al. (2019). The first step involved mixing 1.0 mL of extract (sample) solutions with 0.2 mL of 10% AlCl3 and 0.2 mL of 1 M potassium acetate solution. Later, 3.6 mL of distilled water was added to the total reaction volume, which was then allowed to incubate at room temperature for 30 min. After fully mixing the aforementioned solution, the absorbance at 510 nm was measured using a UV-visible spectrophotometer against a blank. Every TFC determination was carried out in triplicate and a calibration curve of quercetin was plotted to determine the total flavonoid content in each sample. Results were expressed as milligrams of Quercetin Equivalents per gram of Dry Weight (mg QE/g DW).
2.4.4 DPPH radical scavenging activity assay
The free radical scavenging activity (FRSA) of extract samples of D. purpurea was analyzed using 2,2-diphenyl-1-picrylhydrazyl (DPPH) according to Baliyan et al. (2022) protocol. Approximately 100 µL of methanolic extracts (samples) were taken in separate test tubes and mixed with 3.0 mL DPPH (0.024% w/v). As a reference standard, 100 µL of methanol was mixed with 3.0 mL of DPPH. The samples were then incubated at room temperature for 90 min in complete darkness and the absorbance was checked at 517 nm wavelength. The following equation was used to determine the anti-oxidant potential of each sample (Khan H. et al., 2021):
where AC = recorded absorbance of control and AS = recorded absorbance of sample.
2.4.5 Determination of peroxidase (POD; EC: 1.11.1.7) activity
The sample preparation and POD assay were carried out in accordance with Bansal et al.’s (2024) procedure. 10 mL of 0.1M phosphate buffer (pH=6.0) was taken to homogenize 1.0g of fresh samples (in vivo-, in vitro-raised leaf tissues and leaf derived callus). The extracts were then filtered, centrifuged at 12000 rpm for about 30 min at 4°C and the supernatants were collected. The samples were preheated at 65°C for 50 secs and then refrigerated until needed. A reaction mixture containing 1.0 mL of 10 mM K-phosphate buffer (pH = 7.0), 500µL of 1% guaiacol solution, 500µL hydrogen peroxide solution (0.4%), 500µL of enzyme extract, and 2.5 mL of distilled water was used for the peroxidase enzyme assay. The control was prepared with all the above stated reagents, except the enzyme extract. The development of tetraguaiacol was then confirmed by measuring the increase in absorbance at 470 nm within 30 min. The following formula was used to determine the enzymatic activity:
Wherein A = absorbance, E = extinction coefficient (6.39 mM−1cm−1), L = path length (1.0 cm) and C = enzyme concentration (mM/g FW), and FW = fresh weight of samples.
2.4.6 Determination of superoxide dismutase (SOD; EC: 1.15.1.1) activity
Following the protocol provided by Mujib et al. (2022), the enzyme extract preparation and SOD assay were performed. Initially, 1.0 g of all the three fresh tissue samples were homogenized in 10 mL of 0.5 M sodium phosphate buffer (pH 7.3), containing 1.0% (v/v) Triton X-100, 3.0 mM ethylenediaminetetraacetic acid (EDTA), and 1.0% (w/v) polyvinylpyrollidone (PVP), to prepare the enzyme extracts. Finally, the supernatant was collected after the homogenate was filtered and centrifuged for 15 min at 4°C at 11,800 x g.
The SOD assay was conducted using a final reaction mixture of 3.0 mL, consisting of 50 mM K-phosphate buffer (pH 7.8), 45 µM methionine, 1.0 M Na2CO3, 2.25 mM Nitro blue tetrazolium (NBT) solution, 3.0 mM EDTA, 10 µM riboflavin, 10 µL of enzyme extract, and distilled water. A control group was established in which no enzyme extract was included. Subsequently, the mixture was subject to incubation at a temperature of 25°C for a duration of 10 min, while being exposed to fluorescent lamps with a power output of 15 W. The spectrophotometer was used to measure the absorbance of each sample at a wavelength of 560 nm. One unit of superoxide dismutase (SOD) activity is defined as the amount of enzyme required for 50% inhibition of NBT reduction. The activity is measured in units (U) per milligram of fresh weight (mg FW).
2.5 Metabolomics study using untargeted GC-MS approach
2.5.1 Preparation of extracts
For GC-MS analyses, approximately 1 g of each air-dried leaf tissue (in vivo- and in vitro grown) and air-dried leaf derived callus was finely grounded with a mortar and pestle. Each powdered sample was then individually macerated with MeOH at room temperature for 48h on an orbital shaker. The resulting extracts were filtered using Whatman No. 1 filter paper and centrifuged at 10,000 rpm for 5 min. Later, the supernatants were filtered using a syringe filter (0.22 μm, Genetix, New Delhi, India) and stored at 4°C for metabolite analyses.
2.5.2 GC-MS instrumentation and data analyses
The methanolic extracts of the samples were analyzed by GC-MS-QP-2010 equipment (Shimadzu, Tokyo, Japan), with the following parameters: The GC-MS separation was performed using the Rxi-5Sil MS GC Capillary Column (30 m, 0.25 mm ID, 0.25 μm film thickness). Helium was employed as the carrier gas at a consistent flow rate of 1.21 mL min−1. A GC-MS detection method utilized an electron impact ionization mode with ionization energy of 70 eV. The inlet temperature was set to 260°C, initial oven temperature was adjusted to 80°C and was programmed to increase to 280°C (hold time of 18 min) with a sample injection volume of 1 μl and scanning range of 40-600 m/z. For GC-MS analysis, the identification of phytocompounds present in each sample was done by comparing their retention times, peak area and peak area % to those of authenticated compounds listed in the database of NIST (National Institute of Standards and Technology) using GCMS solution software (Version 4.45 SP 1).
2.6 UPLC-ESI-QTOF-MS based metabolites profiling
2.6.1 Sample preparation and metabolite extraction
The samples were prepared by shade drying each plant material (in vivo-, in vitro grown and leaf derived calli) of D. purpurea for 10-12 days. The shade dried materials were then finely grounded into powder and 50 mg per sample was utilized for metabolites extraction for LC-MS analysis. The powdered samples were extracted with 1.0 mL methanol (MeOH), sonicated at 40 kHz for 15 min at room temperature, filtered using a 0.22µm syringe filter (Sigma-Aldrich, USA) and centrifuged at 14000 rpm for 10 min at 4°C. The supernatants were collected in glass vials and subjected to UPLC-ESI-QTOF-MS analyses.
2.6.2 LC-MS instrumentation
The determination of bioactive compounds in each sample (in vivo-, in vitro- derived callus and in vivo leaf tissues) was done by using UPLC-ESI-QTOF-MS technique. The analyses were performed by using a 2D nano ACQUITY Ultra-Performance Liquid Chromatography (UPLC) system (Waters Corporation, Milford, USA) online coupled with a SYNAPT G2-Si mass spectrometer (Waters Corporation, Milford, USA) via a NanoLockSpray dual electrospray ionization (ESI) source (Waters Corporation, Milford, USA). The separation of the bioactive compounds was carried out on acquity UPLC BEH C18 column (50x2.1mm, 1.7μm) operated at 35°C.The mobile phase comprised of two solvents: (A) 0.1% formic acid in water, and (B) acetonitrile. Gradient elution was done at a flow rate of 0.3 ml/min at room temperature and elution profile was represented in Table 1. The diode array detector was set at a wavelength range of 214-254 nm and the sample injection volume was 2 μl. The collision gas used was ultrahigh pure nitrogen gas. The mass data were acquired in both positive (+) and negative (-) electrospray ionization (ESI) modes with a scan range from m/z 100 to 1200 Da. The optimized parameters for positive mode were as follows: duration of the run was 25 min including 1766 cycles (0.4 secs each). For MS1 acquisition, ion spray voltage was set to 2500 V; turbo spray temperature of 500°C; collision gas, medium; nebulizer gas (GS1), heater gas (GS2) and curtain gas (CUR) rates were 50, 50 and 25 psi, respectively. For MS2 acquisition, a declustering potential of 80V; collision energy ranges from 20 to 45eV; and collision energy spread of 20V was applied as well. Negative ion mode had the same parameters but with an ion spray voltage of -2500 V.
2.6.3 Data processing and identification of secondary metabolites
In LC-MS approach, each obtained peak was examined in relation to the Human Metabolome Database (HMDB, http://www.hmdb.ca/) and METLIN (http://metlin.scripps.edu/) databases. In order to identify secondary metabolites, masses were matched within a 500ppm mass accuracy range in the respective libraries. In addition, certain metabolites were identified by comparing the reported MS/MS spectra with their m/z values in the total ion count (TIC) profile. In the positive ionization mode, three parent ion adducts of [M+H]+,[M+Na]+ and [M+H-H2O]+; and in negative ionization mode, only one parent ion adduct of [M-H]- were considered in the databases search. The sturdiness of the compounds’ identification was validated using a comparison of the fragment masses obtained from the MS-MS spectra of every metabolite.
2.7 Statistical analysis
The in vitro culture experiment was conducted in a completely randomized manner in triplicates with six explants per treatment. The biochemical data were also statistically analyzed in triplicates. These data sets were presented as mean ± standard error. The statistical differences were tested using One-way ANOVA, followed by the post hoc analysis via Duncan’s Multiple Range Test (DMRT) (SPSS software, ver. 26.0) at p < 0.05 level.
3 Results
3.1 Callus induction and indirect organogenesis
Firstly, the healthy green leaf tissue of D. purpurea was employed as explants for callus induction. The induction was conducted on MS fortified with different concentrations of BAP and 2,4-D. Within about two weeks of culture, earliest signs of callus formation was noticed in all the tested media (Table 2). The calli were observed to be friable, with color varying from milky white to pale yellow (Figures 1A, B). All the tested media produced callus, with an overall rate of callus induction ranging from 94.44% to 22.21%. The best PGR combination was found to be 8.8 µM BAP and 0.9µM 2,4-D in which 94.44% callus induction and 4.9 g fresh biomass were noticed. This was followed by 8.8 µM BAP + 2.3µM 2,4-D and 4.4 µM BAP + 9.0 µM 2,4-D, which induced callus at the rates of 77.77 and 61.10 respectively. The lowest callusing frequency (22.21%) as well as fresh biomass (1.8 grams) was noted at 0.88µM BAP + 2.3µM 2,4-D. The sub-culturing of obtained callus on the same PGR amended MS medium resulted in shoots formation, i.e. indirect shoot organogenesis (Figures 2A, B). All the media produced plantlets on callus surface within 3-4 weeks of sub-culturing (Table 3). The highest shoot induction response was found to be 83.33% with maximum 4.7 ± 0.3 shoot numbers/callus mass was noted on BAP (8.8µM) and 2,4-D (0.9µM) added MS medium. On increasing 2,4-D concentrations, the rate of organogenesis gradually reduced. The lowest shoot development ability was observed at 0.88 µM BAP + 2.3µM 2,4-D, showing 11.10% shoot induction rate with 1.5 ± 0.3 mean shoot number/callus mass.
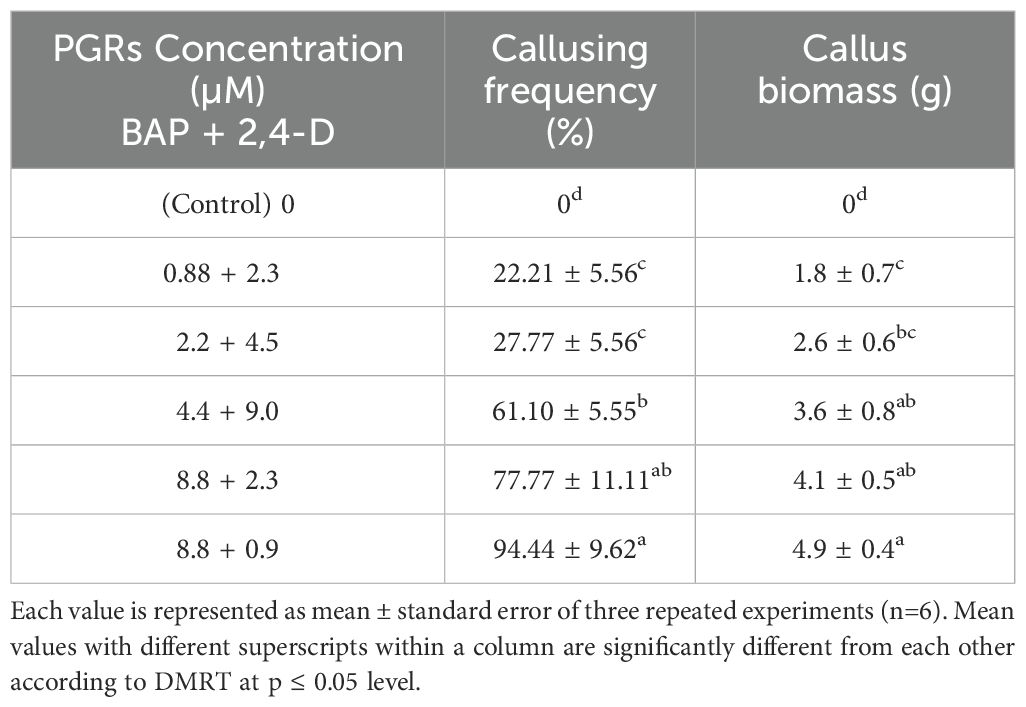
Table 2. Effect of different concentrations of BAP and 2,4-D on callus induction from leaf explants of D. purpurea L. after 4 weeks of inoculation.
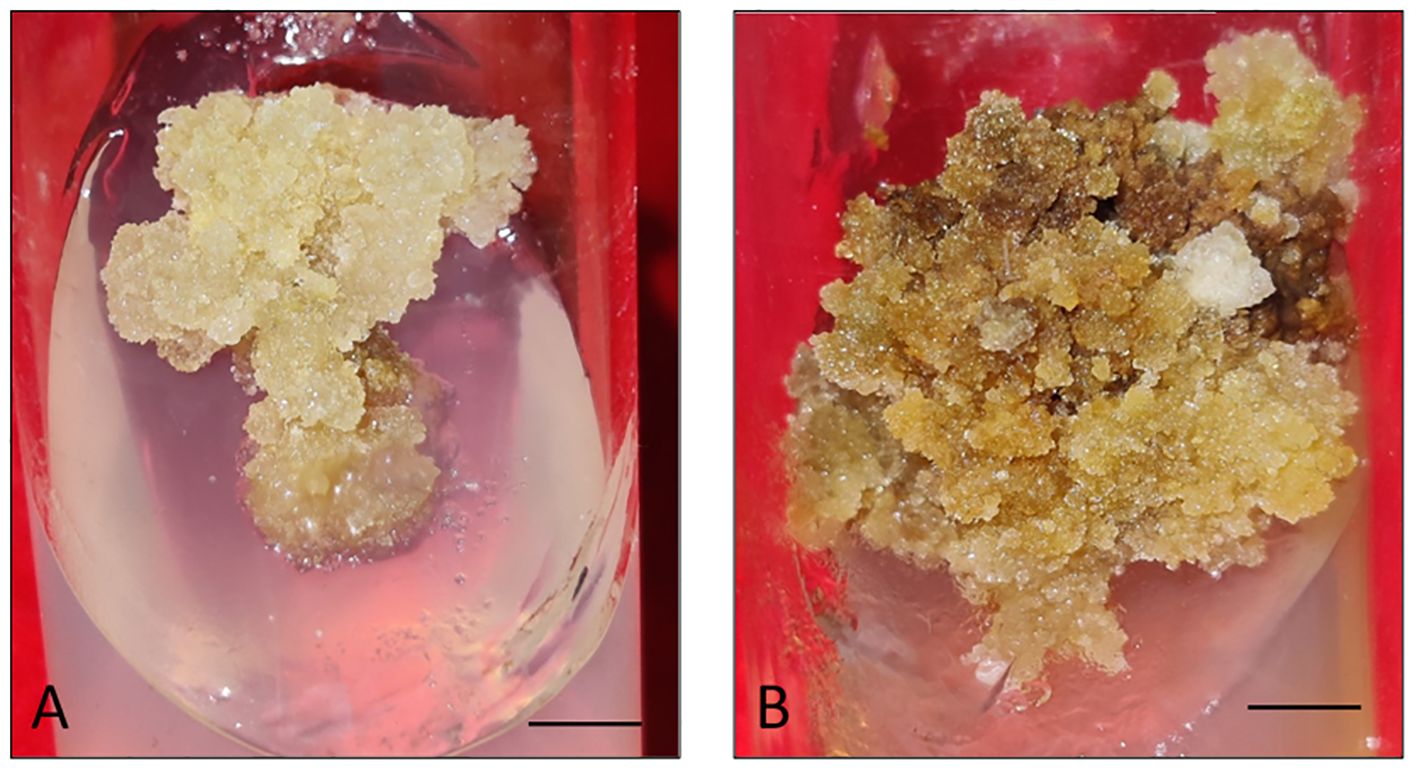
Figure 1. (A, B) Callus induction and proliferation from leaf explant of D. purpurea L. after 4 weeks of inoculation. (bars= 0.5 cm).
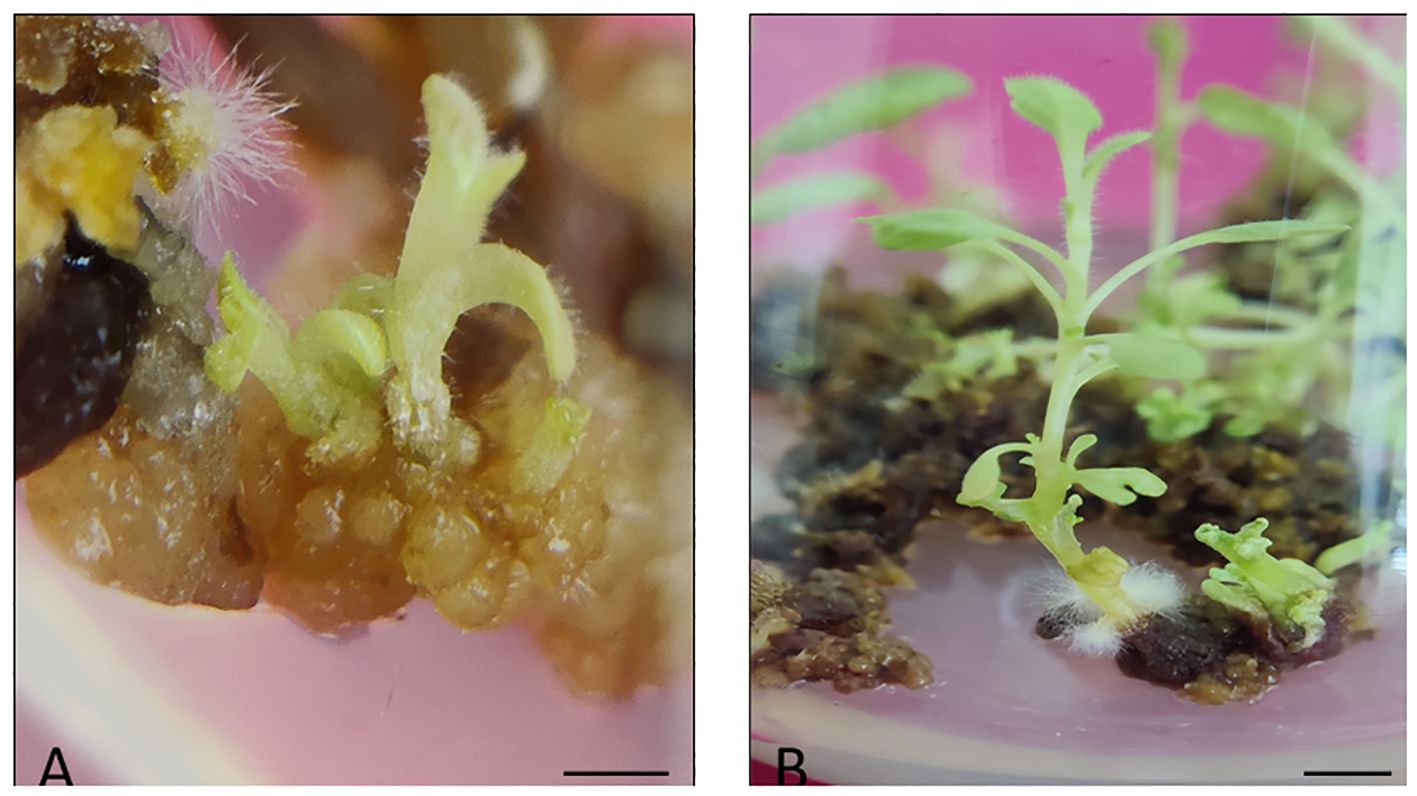
Figure 2. (A) Indirect shoot induction from leaf derived callus of D. purpurea L. after 4 weeks of sub-culturing (bar= 0.5 cm), (B) Elongation of indirect shootlet of D. purpurea L. on the same MS medium (bar= 1.0 cm).
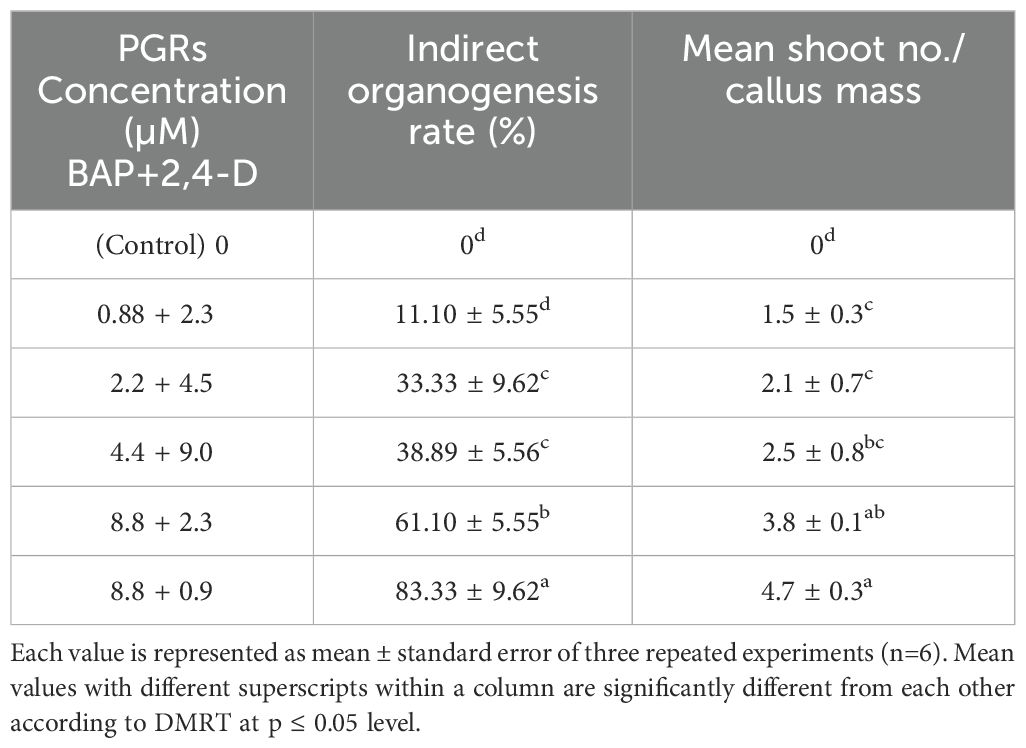
Table 3. Effect of BAP and 2,4-D on indirect organogenesis from leaf and hypocotyl explants of D. purpurea L. after 4 weeks of inoculation.
3.2 Biochemical analyses
3.2.1 Total phenolic content
The total phenolic content of MeOH extract of each sample was determined spectrophotometrically and the results are presented in Table 4. The TPC was found to be higher in in vitro developed callus and leaf samples than that of in vivo leaf sample. In vitro derived callus of D. purpurea had the highest phenolic content (5.43 ± 0.11 mg GAE/g DW), followed by in vitro regenerated leaf extract (4.47 ± 0.04 mg GAE/g DW) and in vivo derived leaf (3.64 ± 0.10 mg GAE/g DW). The results indicated that the callus sample accumulated more phenols than the other tested plant tissues.
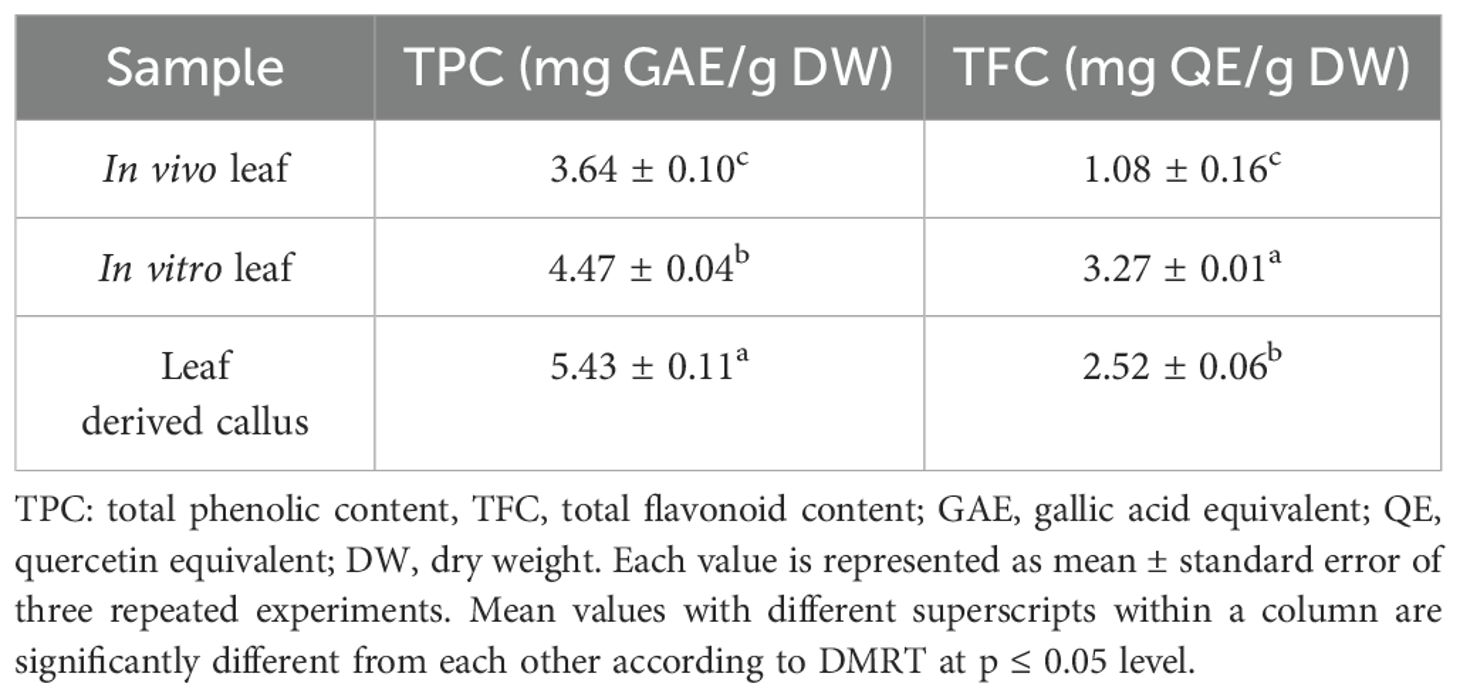
Table 4. Total phenolic content (TPC) and total flavonoid content (TFC) of callus and leaf tissues of D. purpurea L.
3.2.2 Total flavonoid content
The total flavonoid contents in different tissue samples of D. purpurea were quantified (Table 4). The results were as follows: in vitro leaves (3.27 ± 0.01 mg QE/g DW) > leaf derived callus (2.52 ± 0.06 mg QE/g DW) > in vivo leaves (1.08 ± 0.16mg QE/g DW). The content of TFC in D. purpurea leaves regenerated in vitro was the highest, showing a two-fold increment than the in vivo grown leaf tissues. The flavonoid content of callus extract was moderate in level.
3.2.3 DPPH radical scavenging activity assay
The methanolic extracts of in vivo-, in vitro raised leaf and leaf-callus were examined to determine the anti-oxidant potential using the DPPH assay. Results are summarized in Table 5. Both the in vitro raised tissues (callus and leaf) exhibited good anti-oxidant activity than the field grown leaf sample. The in vitro derived callus revealed the best free radical scavenging activity of 70.68 ± 0.57%, followed by in vitro regenerated leaf sample (60.59 ± 0.19%) and field grown leaf tissue (26.67 ± 1.11%). Thus, the lowest scavenging activity was displayed by in vivo grown leaf tissue.
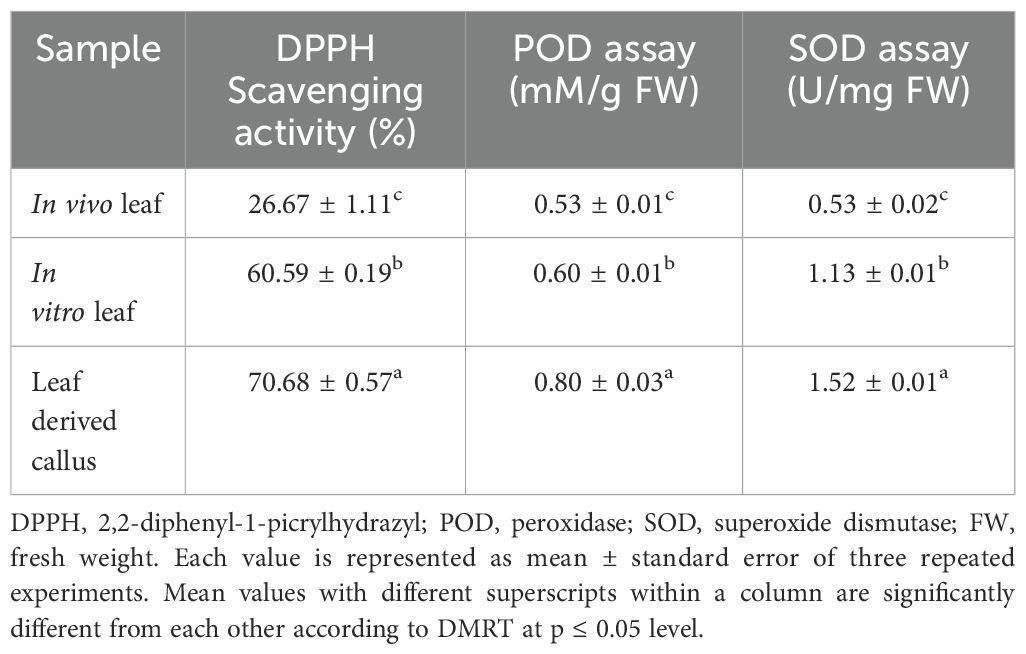
Table 5. DPPH, POD and SOD activities of in vivo-, in vitro- leaf and callus samples of D. purpurea L.
3.2.4 Activities of peroxidase and superoxide dismutase
The POD activity of in vivo-, in vitro grown leaf and leaf -callus sample of D. purpurea was assayed. It was observed that the POD activity was the highest in callus sample (0.80 ± 0.03 mM/g FW) than the other tested samples (Table 5). There is a minor difference in peroxidase activity in in vitro and in vivo derived leaf samples (0.60 ± 0.01mM/g FW and 0.53 ± 0.01 mM/g FW, respectively). The peroxidase activity thus showed this order: leaf derived callus > in vitro raised leaf > in vivo grown leaf. SOD assay was conducted to measure the anti-oxidant potential of each sample in D. purpurea. In this assay, a significant amount of anti-oxidant activity was noticed in all the extracts. The results revealed that the callus extract showed the maximum NBT degradation activity i.e. 1.52 ± 0.01 Units/mg FW (Table 5), while in vitro leaf displayed intermediate response of 1.13 ± 0.01 Units/mg FW, and the lowest activity (0.53 ± 0.02 Units/mg FW) was noted in in vivo grown leaf. Thus, the observation suggests that the highest SOD activity was observed in callus extract, followed by in vitro - and in vivo grown leaf tissue.
3.3 Metabolomics study using untargeted GC-MS approach
Metabolite profiling of methanolic extracts of field grown leaf, in vitro raised leaf and leaf-callus of D. purpurea were conducted by untargeted GC-MS technique. The phytocompounds in the extracts were identified by several parameters like retention time, fragment ions produced and comparison with the mass spectra of compounds indexed in the NIST library and the WILEY database. The retention time expressed in min, relative peak area percentage (%), molecular formula and molecular weight of compounds detected in each extract were listed in Tables 6–8 and the respective chromatograms are presented in Figure 3. The several compounds found in each extract are known to possess multiple biological activities and belongs to diverse groups of secondary metabolites such as alkaloids, tannins, saponins, fatty acids, terpenoids, flavonoids, sterols etc. The in vivo- and in vitro grown leaf extract showed the presence of 86 and 77 phytocompounds respectively. Among the compounds detected in methanolic leaf extract grown in vivo (Table 6; Figure 3A) are: Palmitic acid (15.09%), Neophytadiene (11.49%), (E)-Phytol (5.62%), linolenic acid (4.84%), stigmasterin (3.85%), 1,11-Undecanediol (3.52%), Phytyl octadecenoate (3.06%), Linoleic acid (2.44%), alpha-tocopherol (2.14%), Gamma-undecanolactone (1.71%), loliolide (0.68%) and others. The methanolic extract of in vitro raised leaf tissue of D. purpurea displayed a number of phytoconstituents (Table 7; Figure 3B) such as stigmasterin (12.68%), palmitic acid (11.91%), neophytadiene (8.29%), methyl ester of linoleic acid (2.20%), 4-Vinylguaiacol (2.09%), Pyranone (2.04%), Ledol (1.43%), (-)-Cedrenol (0.50%), Glycidyl palmitate (0.28%) etc.
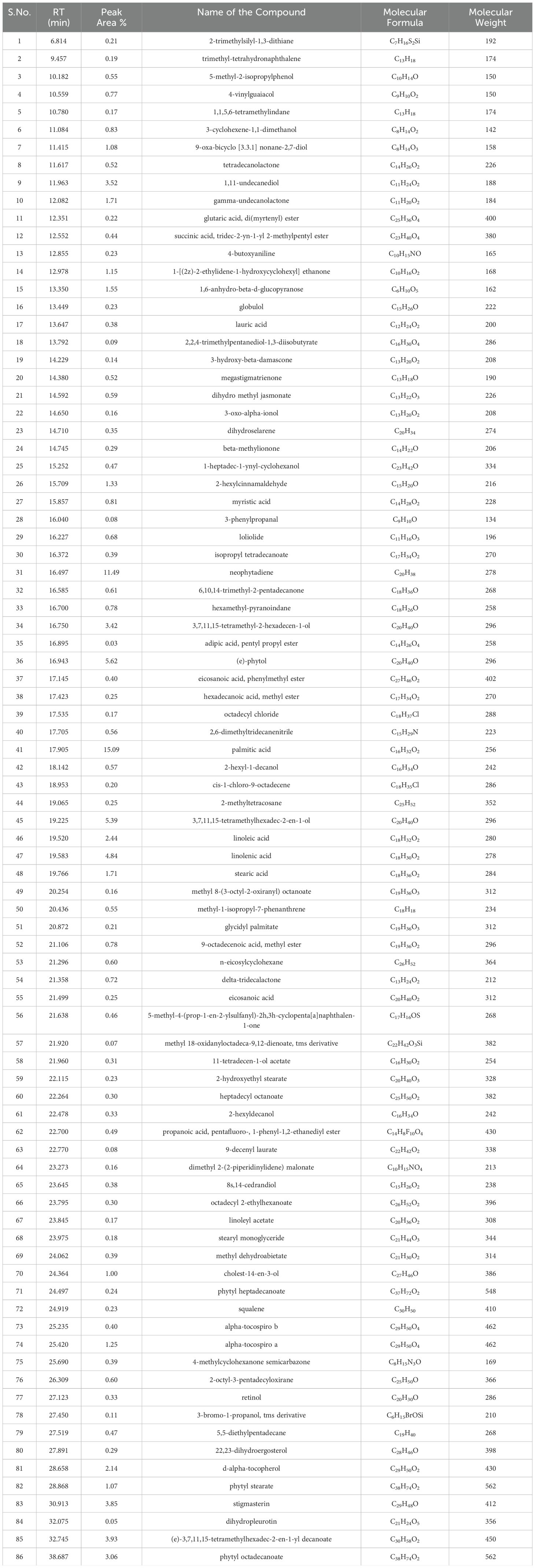
Table 6. List of phytocompounds identified in the methanolic extract of in vivo grown leaf tissue of D. purpurea L. by GC-MS.
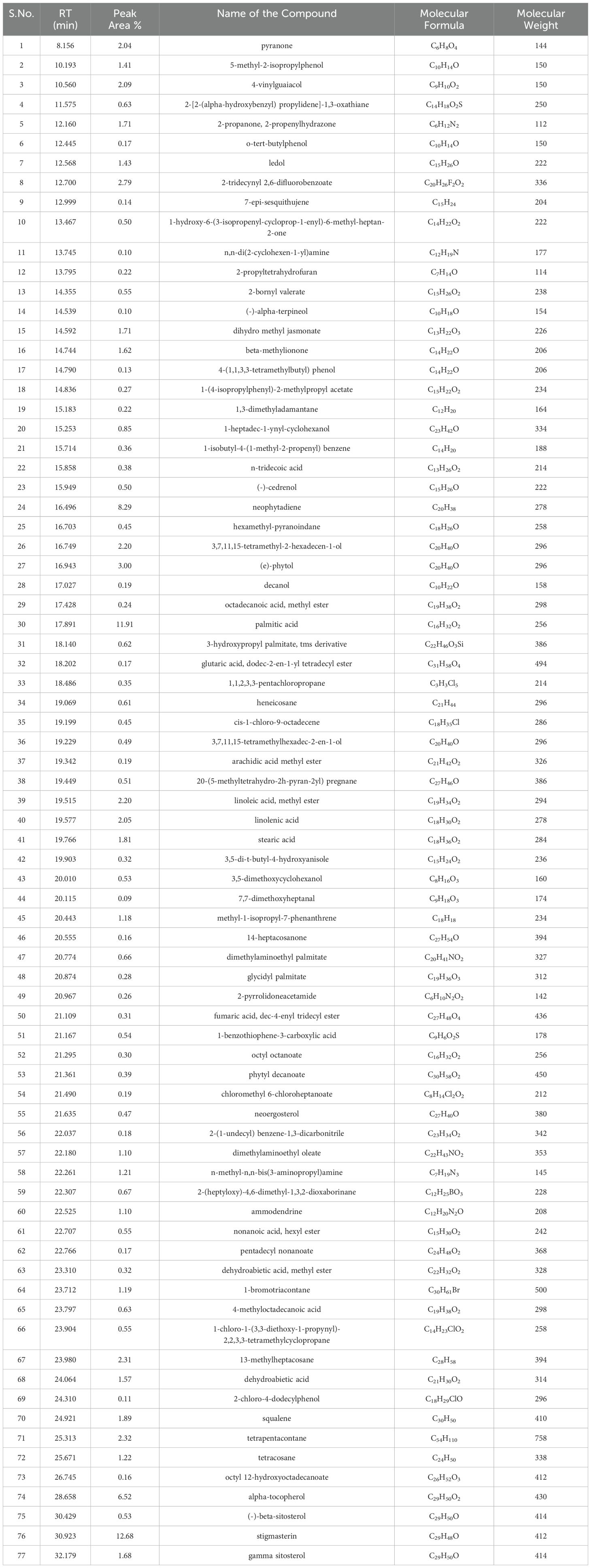
Table 7. List of phytocompounds identified in the methanolic extract of in vitro grown leaf tissue of D. purpurea L. by GC-MS.
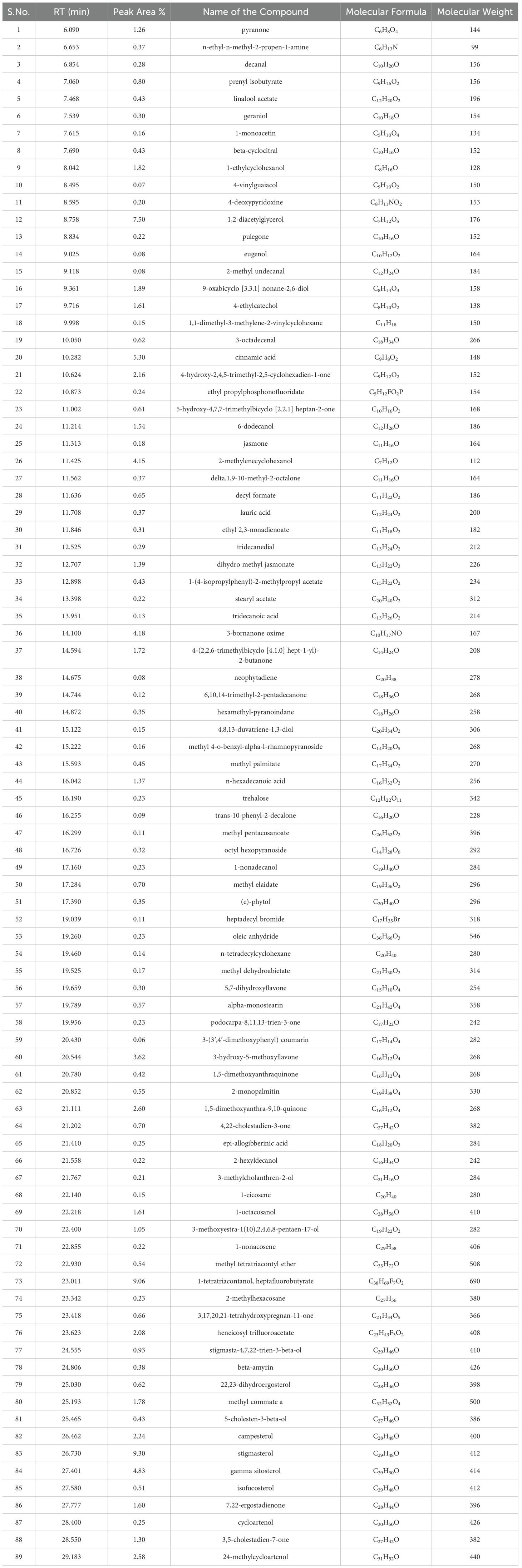
Table 8. List of phytocompounds identified in the methanolic extract of leaf derived callus of D. purpurea L. by GC-MS.
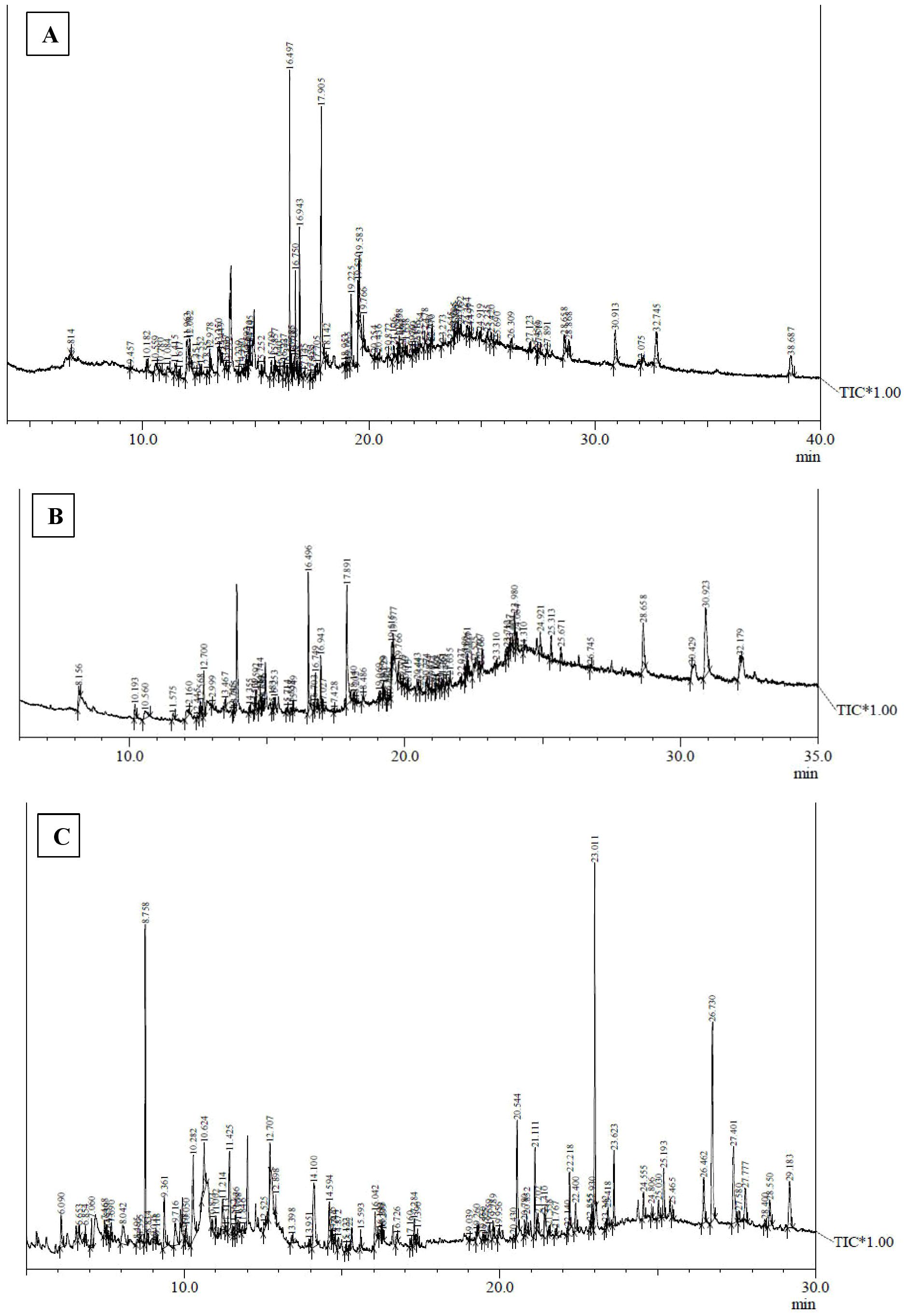
Figure 3. GC-MS chromatograms of methanolic extracts of (A) in vivo grown leaf tissue, (B) in vitro grown leaf tissue, and (C) leaf derived callus of D. purpurea L.
The GC-MS profiling of D. purpurea callus extract revealed the presence of 89 bioactive compounds (Table 8; Figure 3C). the major bioactives identified by GC-MS analysis were Stigmasterol (9.30%), 1-Tetratriacontanol heptafluorobutyrate (9.06%), 1,2-Diacetylglycerol (7.50%), Cinnamic acid (5.30%), gamma sitosterol (4.83%), 3-bornanone oxime (4.18%), 3-Hydroxy-5-methoxyflavone (3.62%), Campesterol (2.24%), beta-Amyrin (0.38%), Lauric acid (0.37%) etc. The heatmap and the Venn diagram illustrated in the Figure 4 indicated the common phytocompounds present in all the three or two samples examined. In total, 5 metabolites had been found to be present in all the three extracts, these were 4-Vinylguaiacol, dihydro methyl jasmonate, Neophytadiene, hexamethyl-pyranoindane, (E)-phytol; whereas 13 bioactives were found to be common in both in vivo- and in vitro derived leaf tissue. There are 5 secondary metabolites present in field grown leaf and leaf derived callus, while only 3 phytocompounds were common in leaf-callus and in vitro raised leaf tissue.
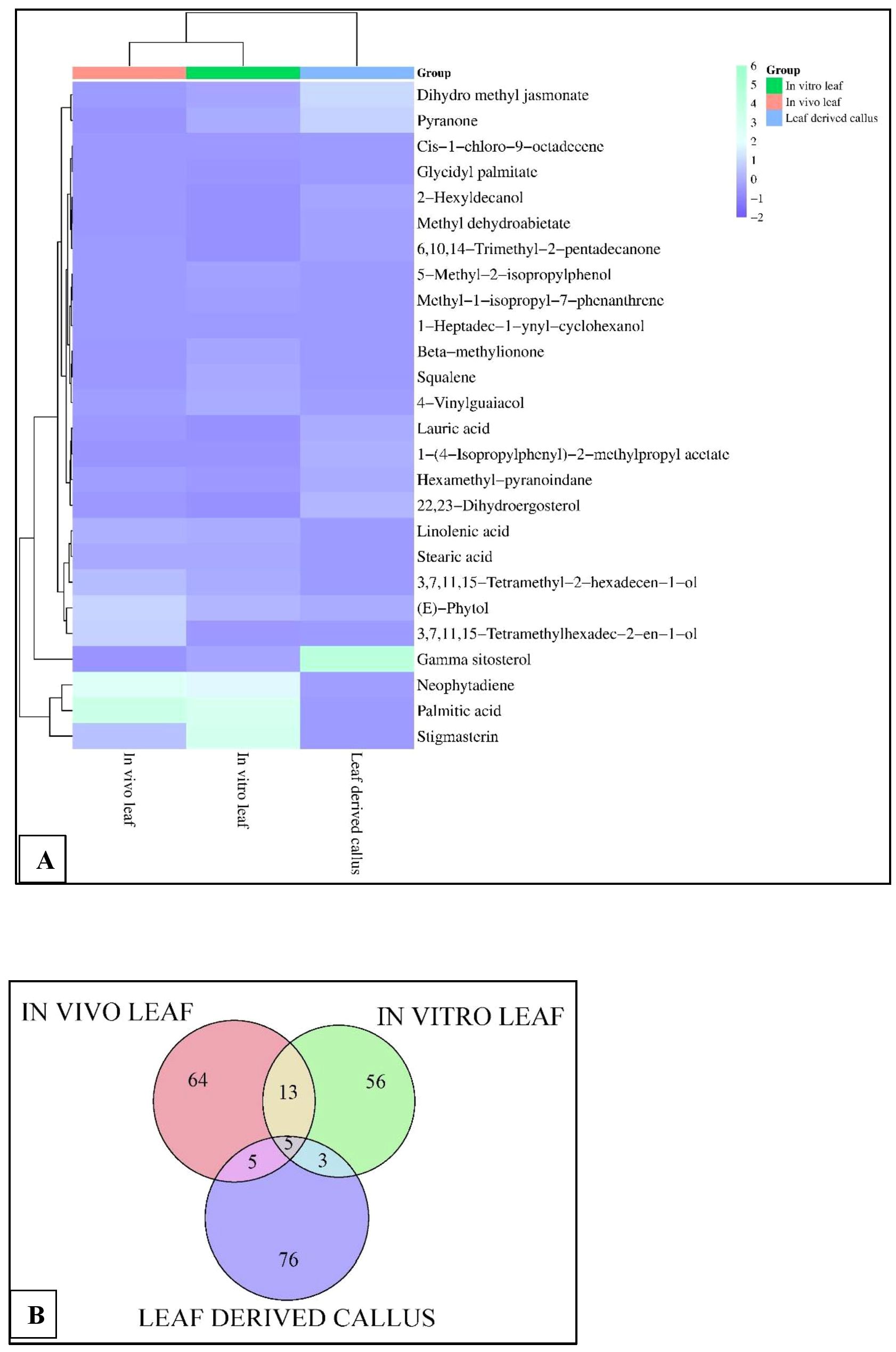
Figure 4. (A) Heatmap analysis, and (B) Venn diagram displaying the relative abundance of important phytocompounds in the in vivo-, in vitro- grown leaf and callus samples of D. purpurea L. detected by GC-MS.
3.4 LC-MS based metabolites profiling
Using UPLC-ESI-QTOF-MS method, the phytochemicals of field- and laboratory grown leaf and leaf-callus of D. purpurea were investigated in both the positive and negative ionization modes. The base peak chromatograms (positive and negative ion modes) of each sample are presented in Figures 5–10 with the retention time and measured m/z of the compounds. The phytocompounds were identified by comparing the [M+H]+, [M+Na]+ and [M+H-H2O]+ protonated molecule (for positive mode) and [M-H]- de-protonated molecule (for negative mode) and their fragmentation in MS/MS spectra with the data recorded in the HMDB and METLIN databases. The identified phytocompounds were tabulated in Tables 9–14 with their retention time, measured m/z, exact m/z, molecular formula and product ions (ms/ms).
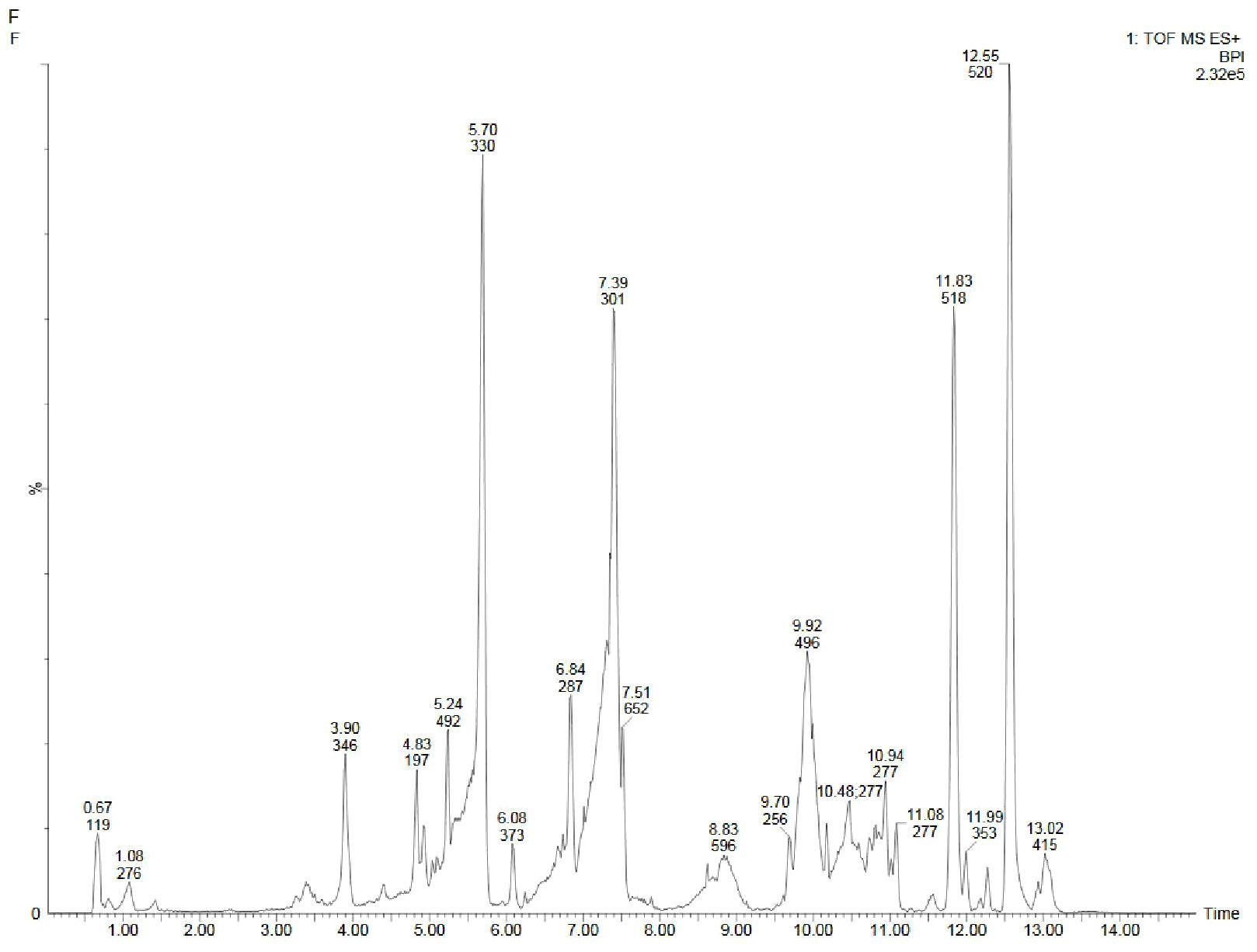
Figure 5. UPLC-ESI-QTOF-MS chromatogram of methanolic leaf extract of the in vivo grown D. purpurea L. in positive ionization mode.
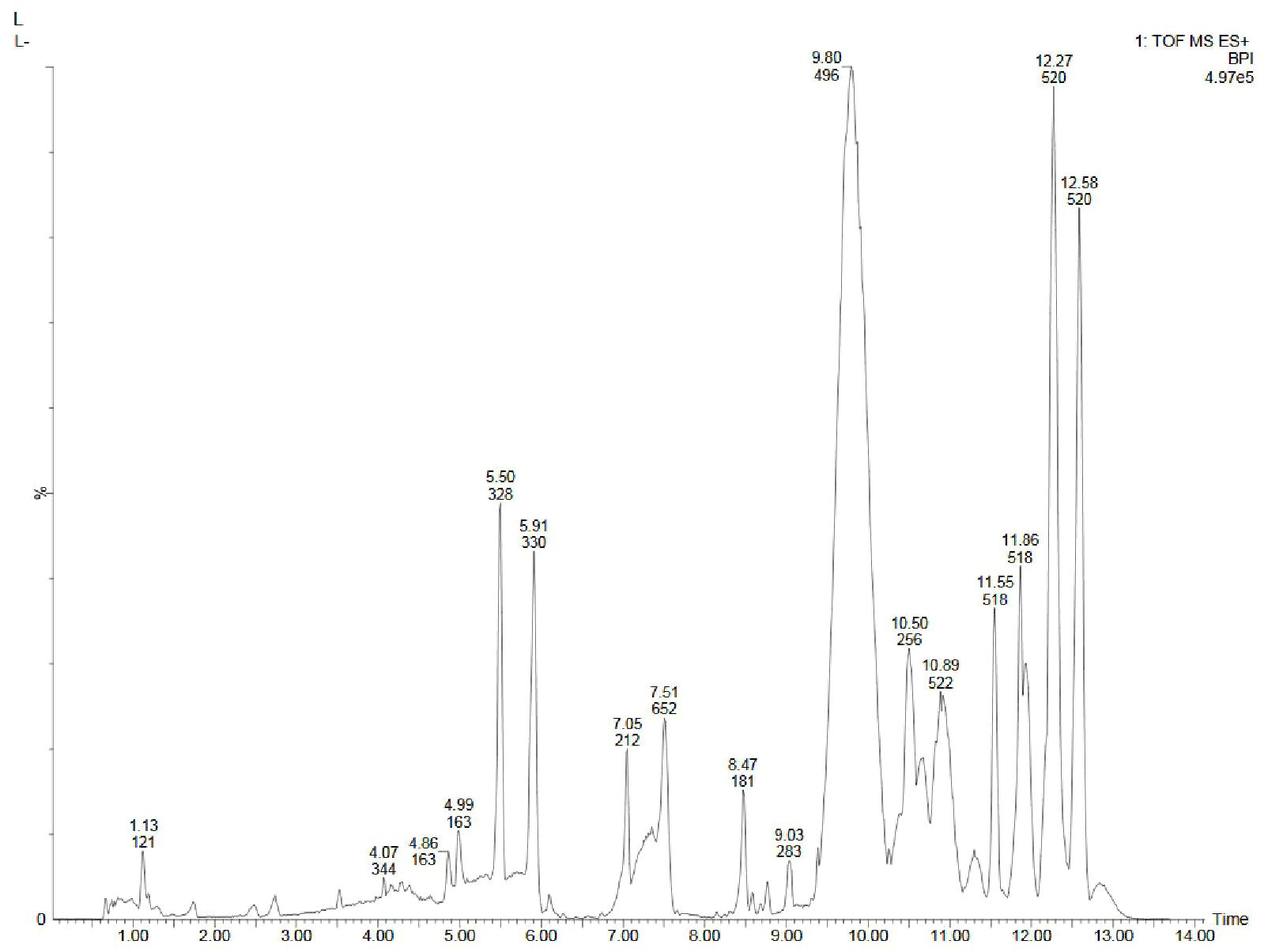
Figure 6. UPLC-ESI-QTOF-MS chromatogram of methanolic leaf extract of the in vitro grown D. purpurea L. in positive ionization mode.
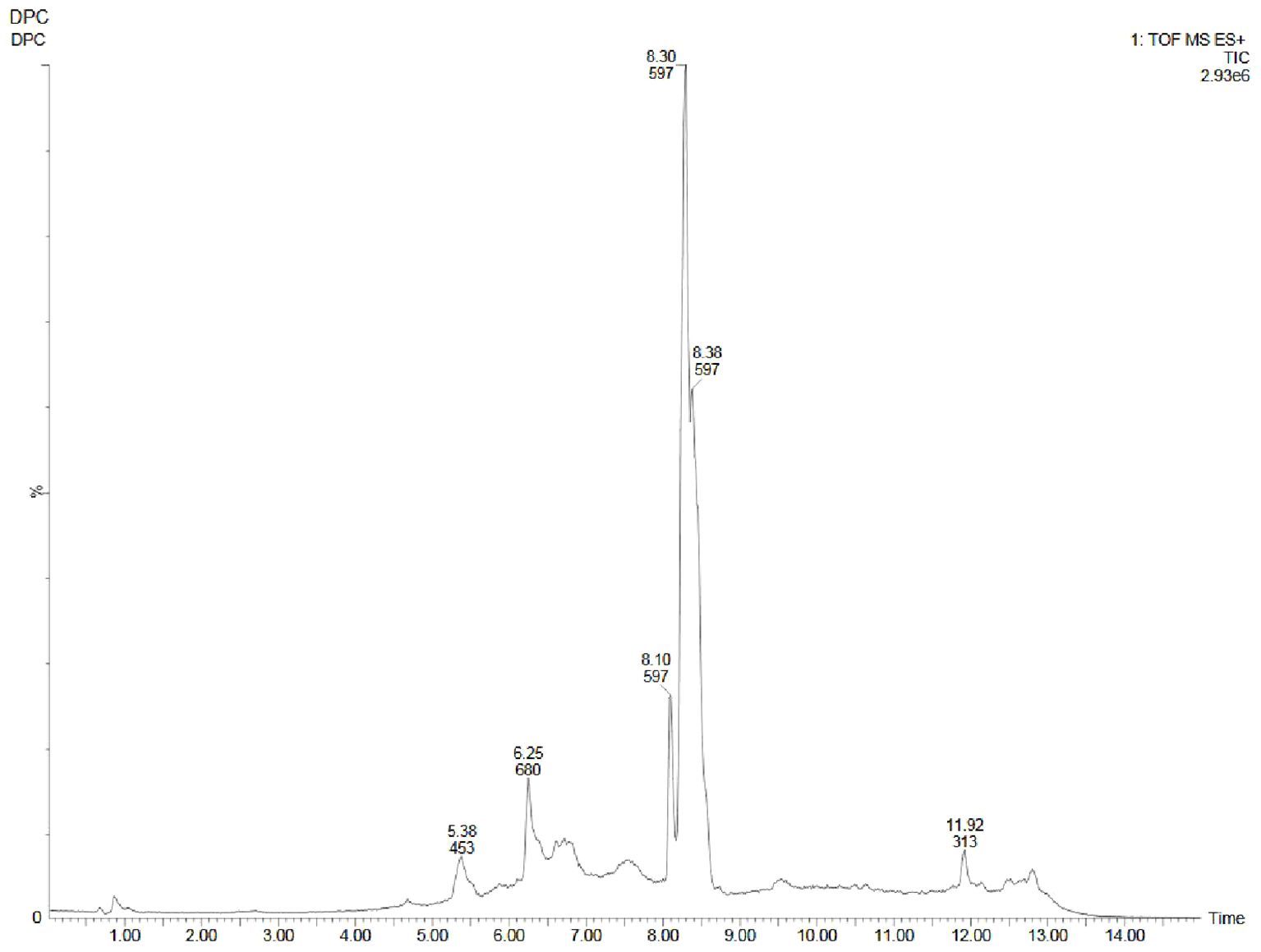
Figure 7. UPLC-ESI-QTOF-MS chromatogram of methanolic callus extract of D. purpurea L. in positive ionization mode.
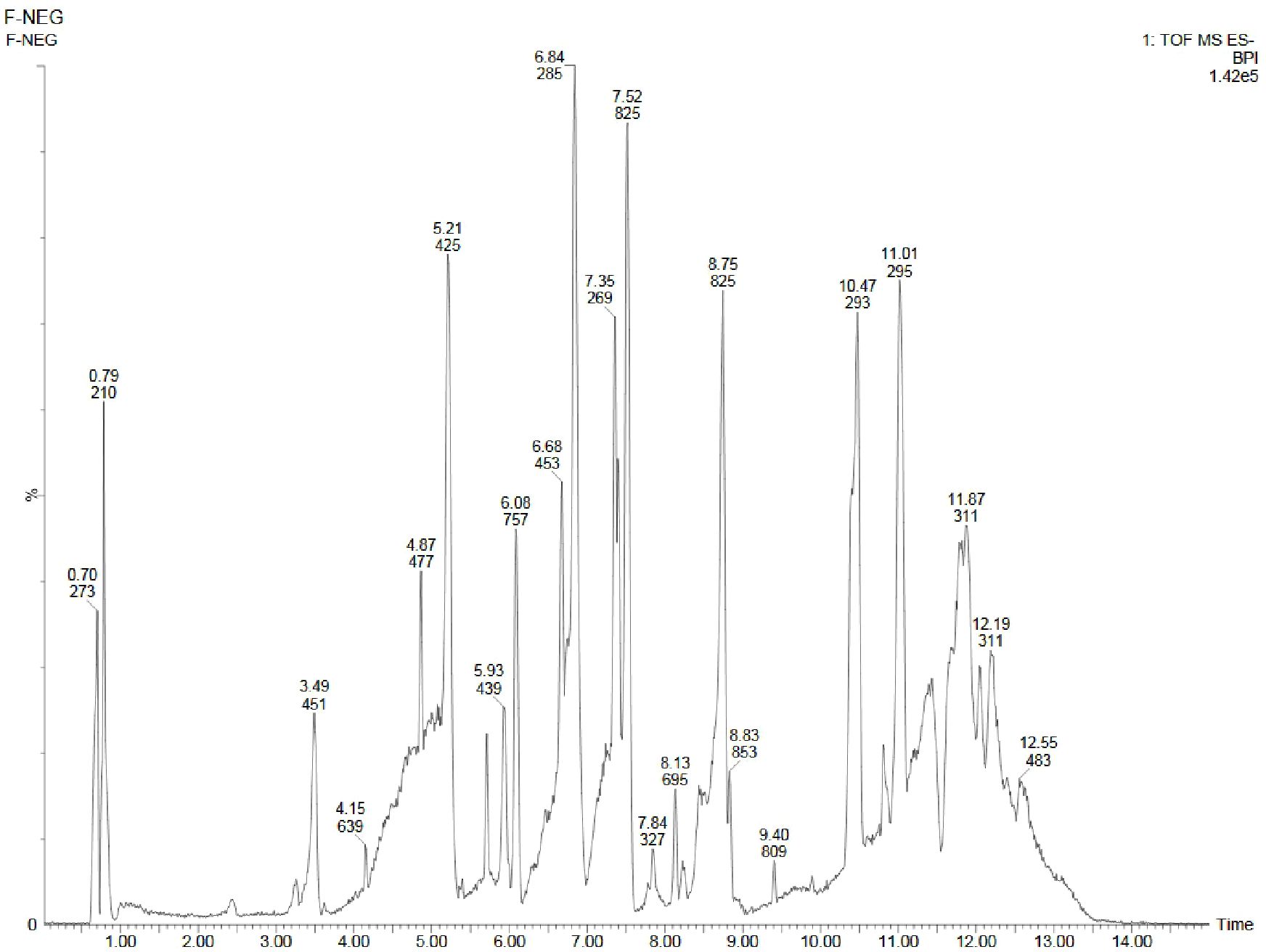
Figure 8. UPLC-ESI-QTOF-MS chromatogram of methanolic leaf extract of the in vivo grown D. purpurea L. in negative ionization mode.
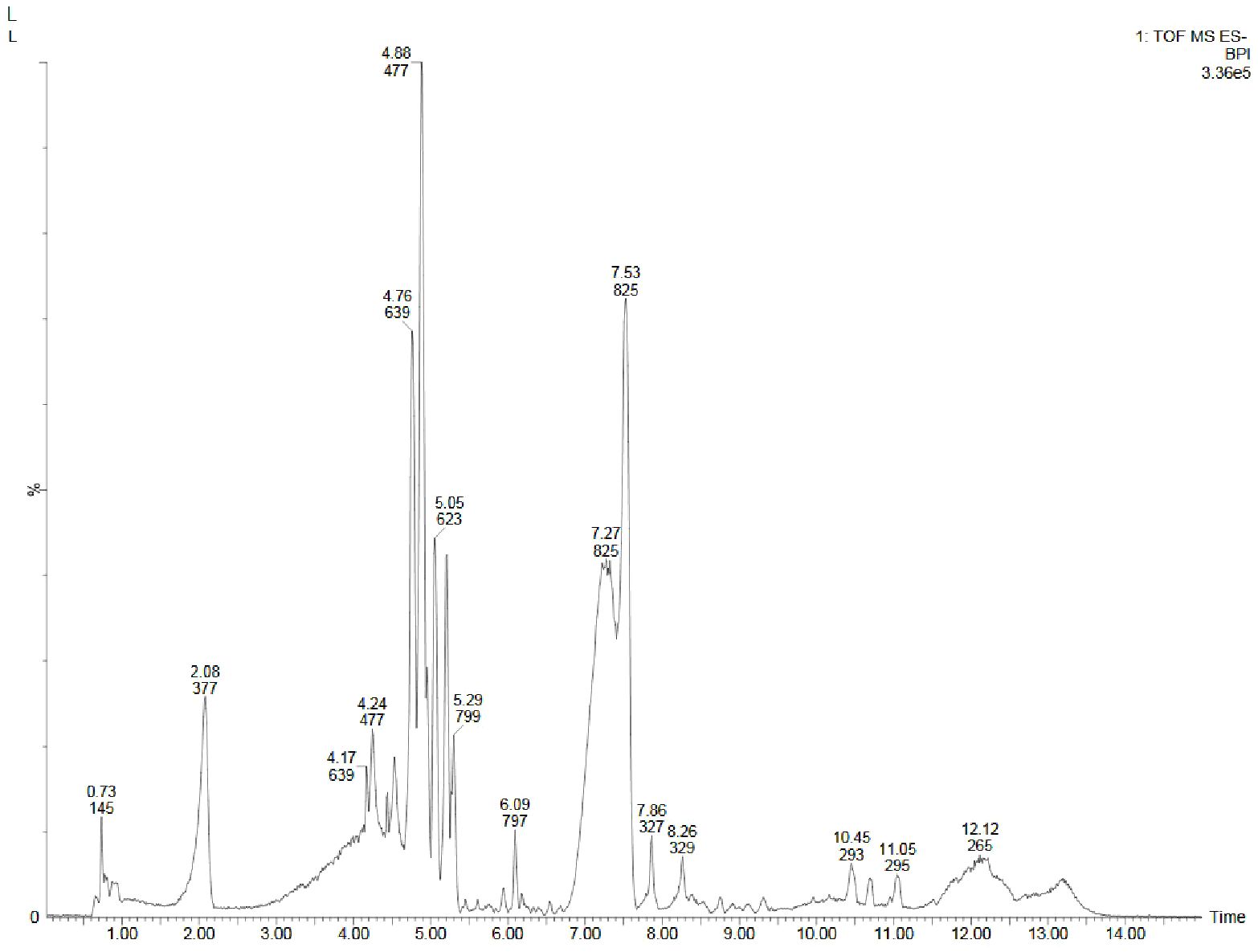
Figure 9. UPLC-ESI-QTOF-MS chromatogram of methanolic leaf extract of the in vitro grown D. purpurea L. in negative ionization mode.
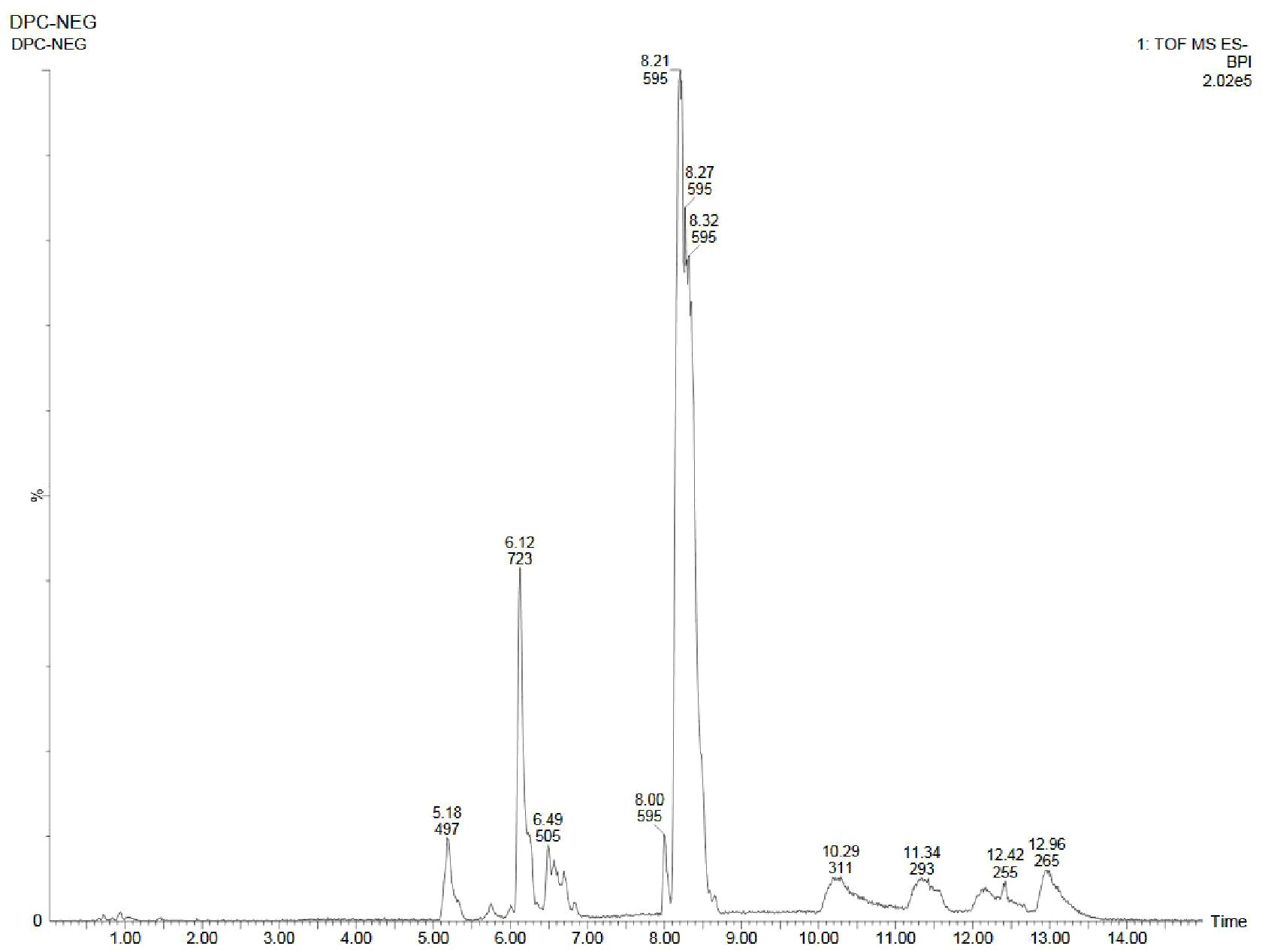
Figure 10. UPLC-ESI-QTOF-MS chromatogram of methanolic callus extract of D. purpurea L. in negative ionization mode.
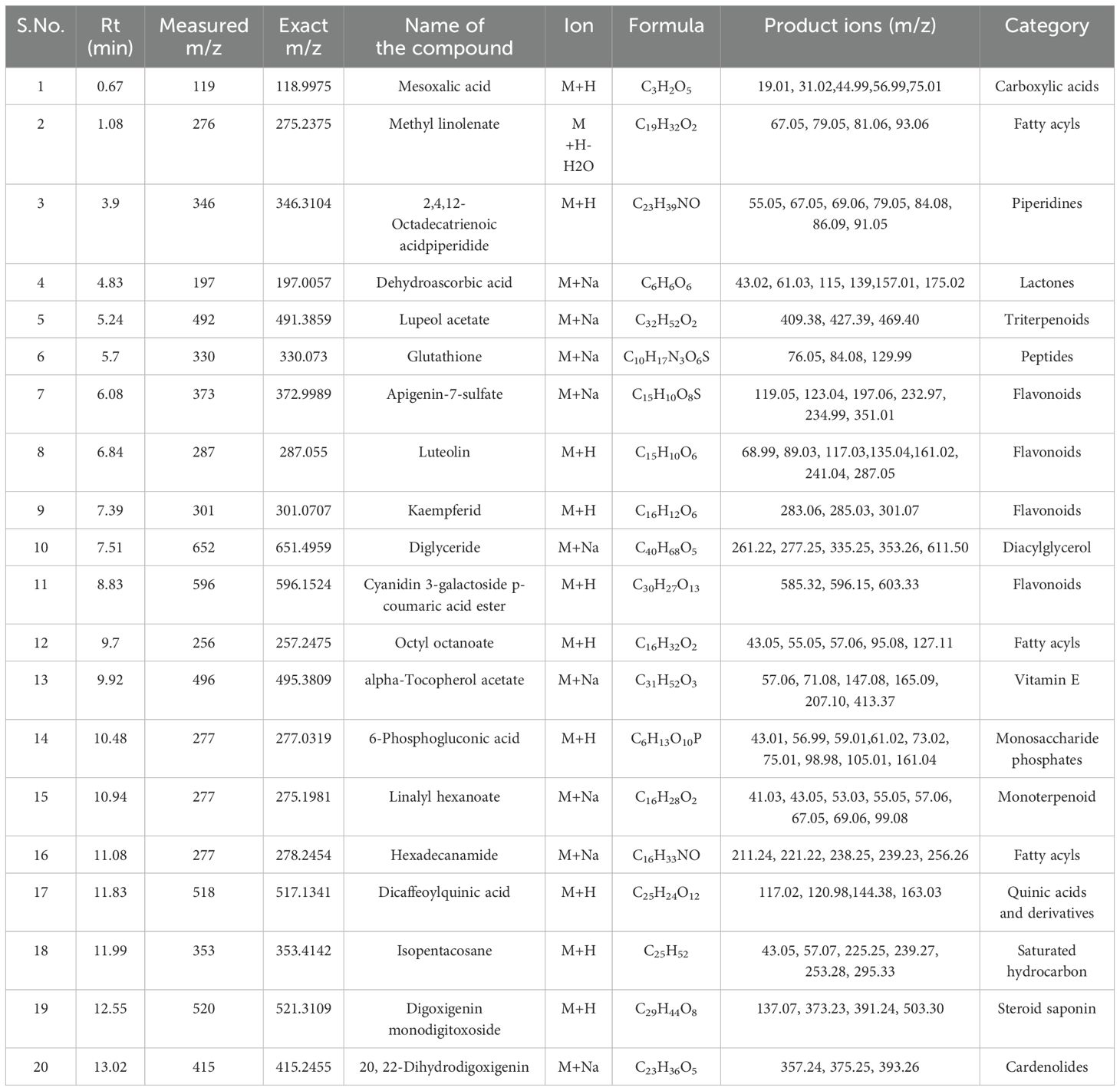
Table 9. Identification of phytocompounds in the methanolic leaf extract of in vivo grown D. purpurea L. by UPLC-ESI-QTOF-MS (positive ion mode) analysis.
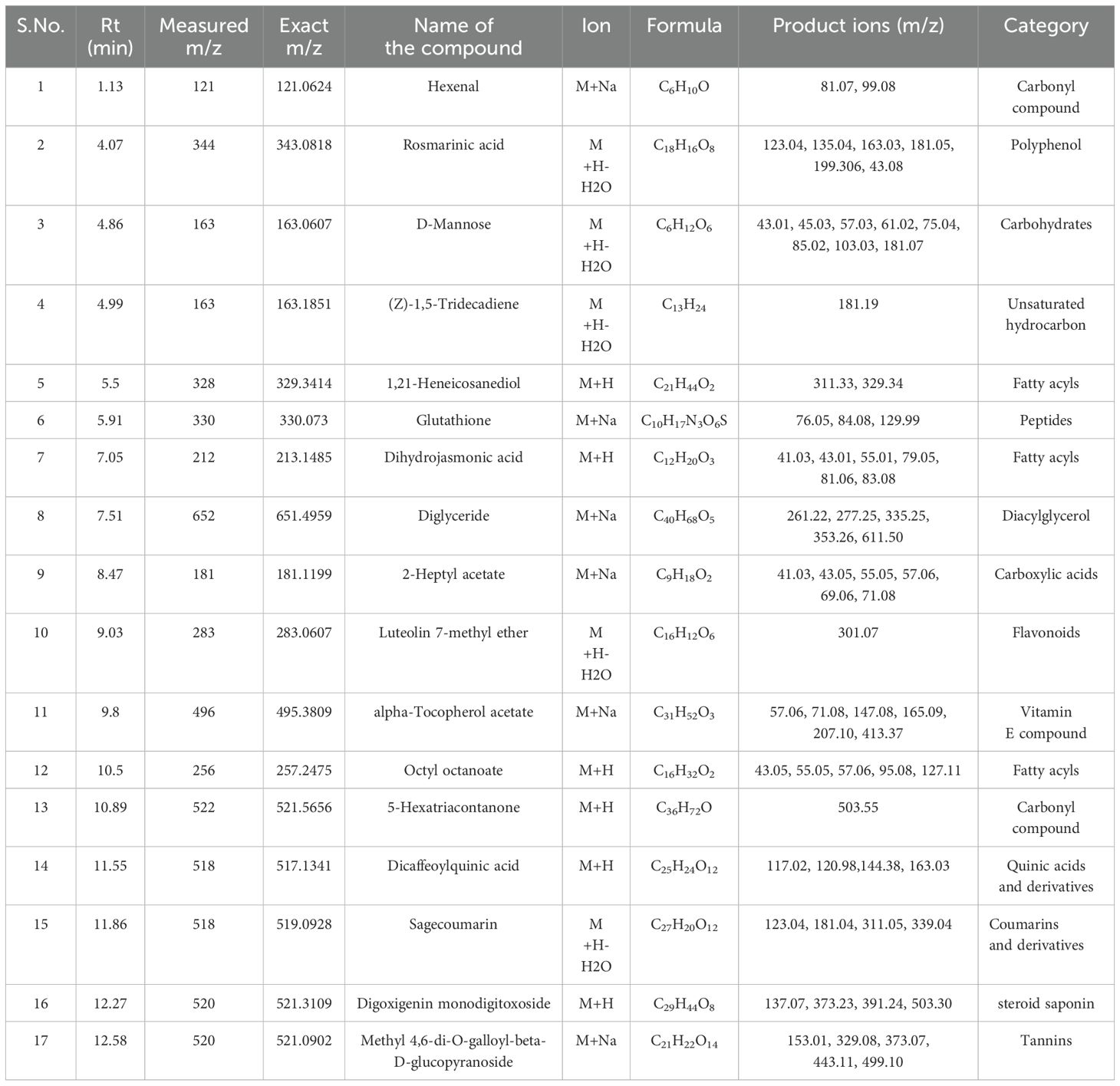
Table 10. Identification of phytocompounds in the methanolic leaf extract of in vitro grown D. purpurea L. by UPLC-ESI-QTOF-MS (positive ion mode) analysis.
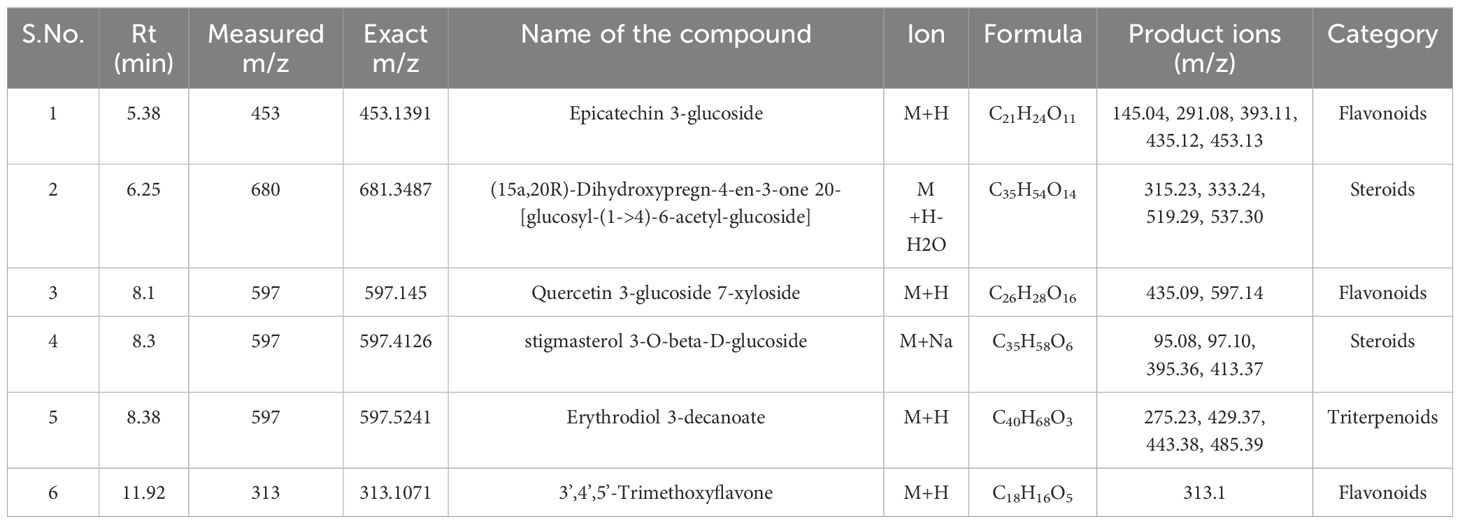
Table 11. Identification of phytocompounds in the methanolic callus extract of D. purpurea L. by UPLC-ESI-QTOF-MS (positive ion mode) analysis.
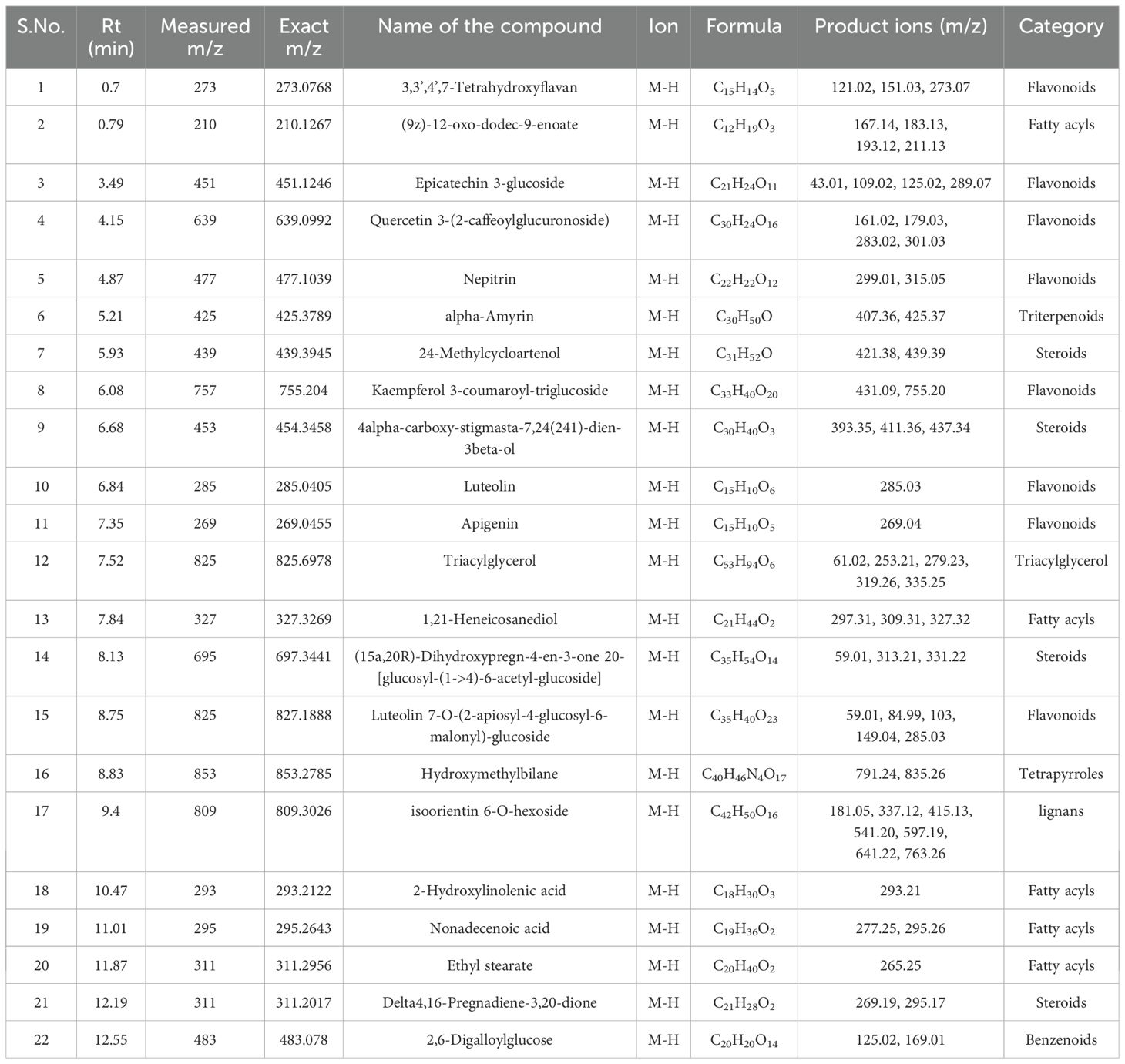
Table 12. Identification of phytocompounds in the methanolic leaf extract of in vivo grown D. purpurea L. by UPLC-ESI-QTOF-MS (negative ion mode) analysis.
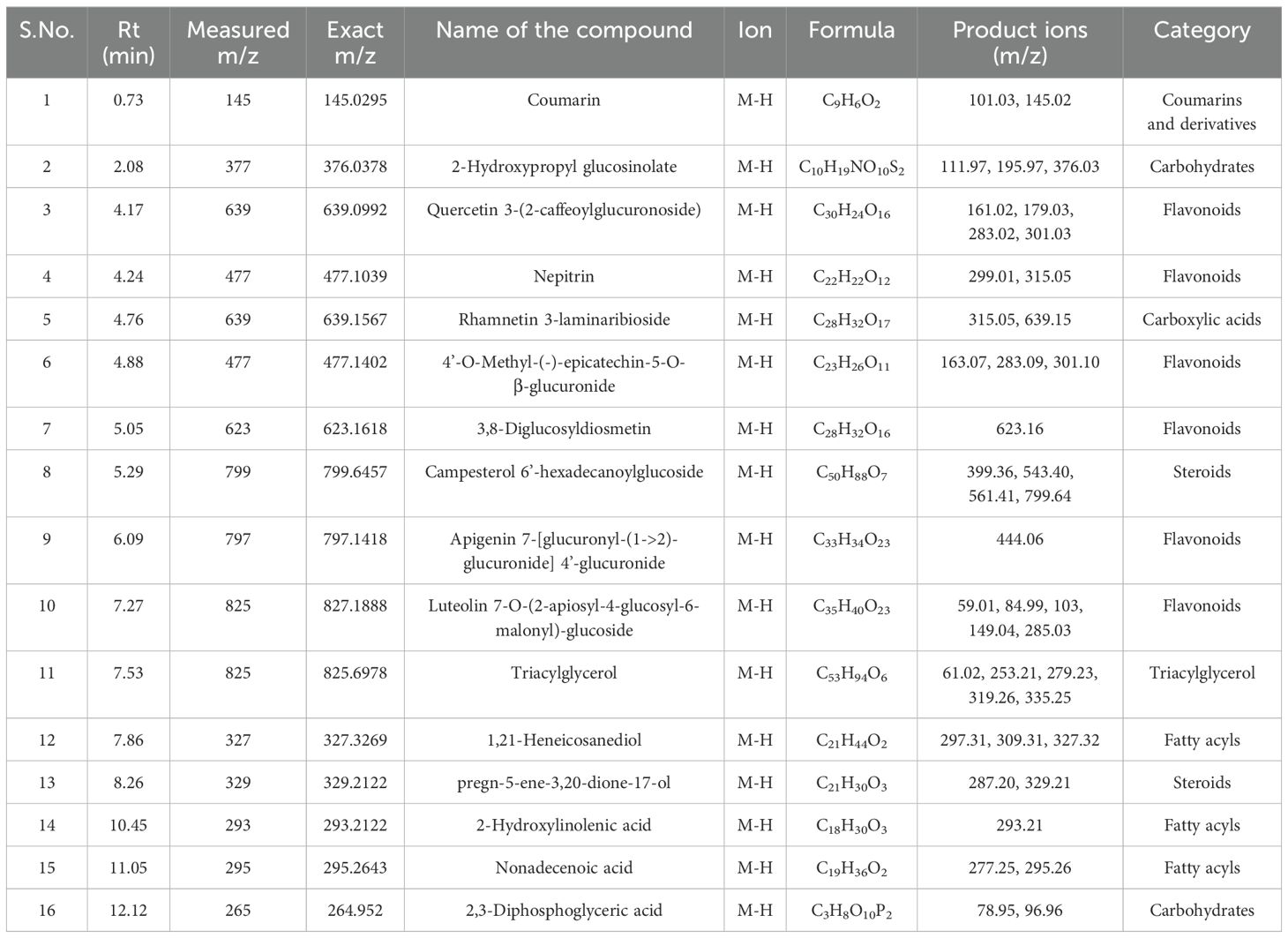
Table 13. Identification of phytocompounds in the methanolic leaf extract of in vitro grown D. purpurea L. by UPLC-ESI-QTOF-MS (negative ion mode) analysis.
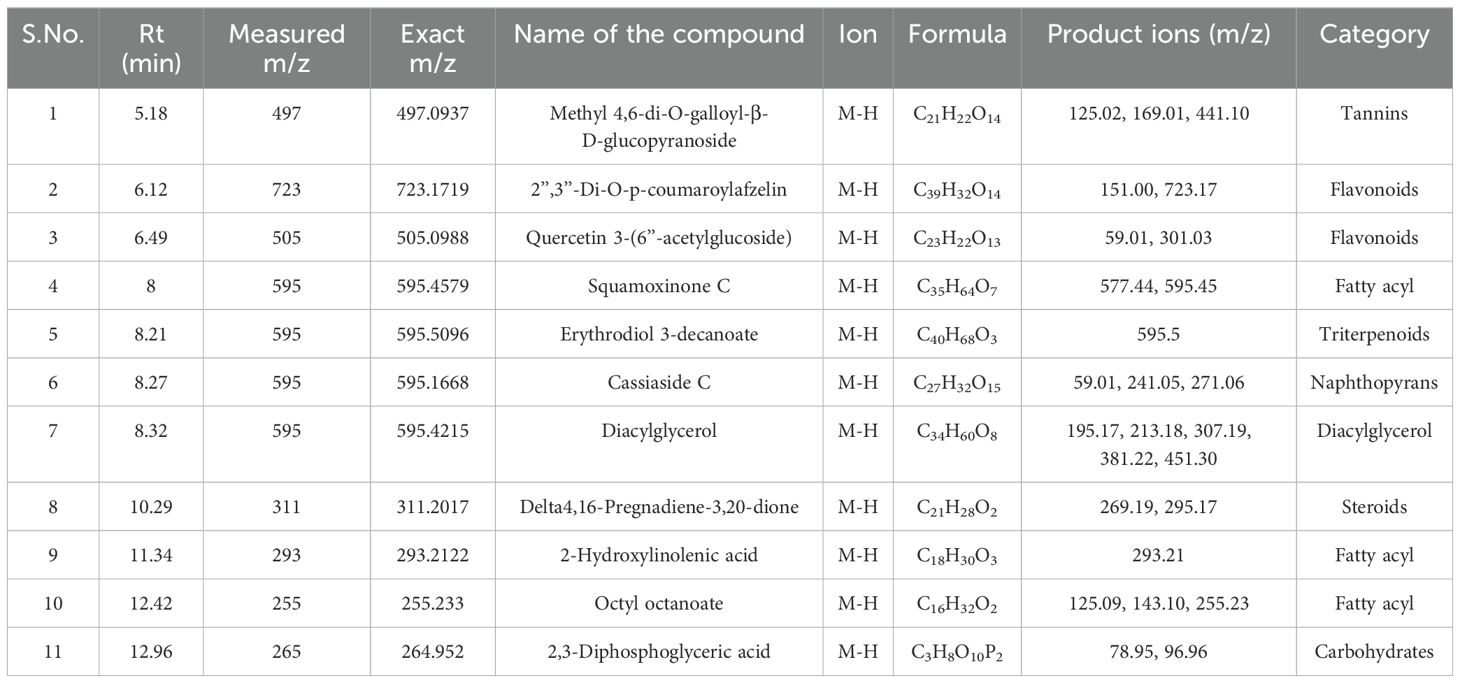
Table 14. Identification of phytocompounds in the methanolic callus extract of D. purpurea L. by UPLC-ESI-QTOF-MS (negative ion mode) analysis.
3.4.1 Analyses in the positive-ion mode
The in vivo grown leaf sample contained a total of 20 metabolites in 40 min elution time, mostly of which were flavonoids and fatty acyl groups. The first eluted compound was mesoxalic acid with a mass of 119 m/z at 0.67 min (Table 9; Figure 5). Various flavonoids such as apigenin-7-sulfate (6.08 min), luteolin (6.84 min) and kaempferide (7.39 min) were identified with the mass of 372.99 m/z, 287.05 m/z and 301.07 m/z, respectively. Also, certain fatty acyls like methyl linolenate (1.08 min), octyl octanoate (9.70 min) and hexadecanamide (11.08 min) were detected having masses of 275.23 m/z, 257.24 m/z and 278.24 m/z, respectively. An important cardenolide (20,22-Dihydrodigoxigenin) was also found in in vivo leaf extract at 13.02 min with the mass of 415.24 m/z. The LC-MS analysis of in vitro leaf extract of D. purpurea revealed the presence of 17 phytocompounds of diverse classes like carbohydrates, fatty acyls, flavonoids, tannins etc. (Table 10; Figure 6). The first compound showed the characteristic protonated [M+Na]+ molecule at m/z 121.06, corresponding to hexenal (C6H10O), other major compounds detected were rosmarinic acid (polyphenol), digoxigenin monodigitoxoside (a steroidal saponin), methyl 4,6-di-O-galloyl-beta-D-glucopyranoside (a tannin) and others. One coumarin derivative named sagecoumarin (C27H20O12) was also detected at 11.86 min with a mass of 519.09 m/z. On the contrary, the methanolic extract of leaf-callus indicated the presence of only 6 phytoconstituents (Table 11; Figure 7). Among the compounds identified, 3 compounds belong to flavonoid group, 2 from steroidal group and one from terpenoid class. The compounds successfully identified were: Epicatechin 3-glucoside (453.13 m/z, [M+H]+); (15a,20R)-Dihydroxypregn-4-en-3-one 20-[glucosyl-(1->4)-6-acetyl-glucoside] (681.34 m/z, [M+H-H2O]+); Quercetin 3-glucoside 7-xyloside (597.14 m/z, [M+H]+); stigmasterol 3-O-beta-D-glucoside (597.41 m/z, [M+Na]+); Erythrodiol 3-decanoate (597.52 m/z, [M+H]+) and 3’,4’,5’-Trimethoxyflavone (313.10 m/z, [M+H]+).
3.4.2 Analyses in the negative-ion mode
Similar to positive ion, all the three samples were analyzed in negative ionization mode. Firstly, the field grown leaf sample was investigated and it showed some 22 bioactive compounds, more than observed in positive ion mode. The first compound eluted was 3,3’,4’,7-Tetrahydroxyflavan (flavonoid) at 0.70 min with the mass 273.07 m/z (Table 12; Figure 8). Likewise, multiple flavonoids such as epicatechin 3-glucoside (3.49 min), nepitrin (4.87 min), apigenin (7.35 min) were detected with the masses of 451.12 m/z, 477.10 m/z and 269.04 m/z, respectively. A base peak at m/z 425 was noticed, corresponding to the alpha-amyrin (a triterpenoid) at 5.21 min. Further analyses revealed that methanolic leaf (in vivo) extract contained several phytocompounds involving fatty acyls, steroids, triacyl glycerols, lignans and benzenoids. In a similar manner, the in vitro regenerated leaf extract was known to possess 16 phytoconstituents belonging to varied metabolite classes. 2-Hydroxypropyl glucosinolate (376.03 m/z), nepitrin (477.10 m/z), rhamnetin 3-laminaribioside (639.15 m/z), pregn-5-ene-3,20-dione-17-ol (329.21 m/z) and 2,3-Diphosphoglyceric acid (264.95 m/z) were some of the major compounds (Table 13; Figure 9). Finally, the callus extract of D. purpurea was also analyzed in negative ion mode and 11 bioactives were noted (Table 14; Figure 10). The characteristic compounds identified were squamoxinone C (fatty acyl) at 8.00 min, Delta4,16-Pregnadiene-3,20-dione at (steroid) at 10.29 min, 2,3-Diphosphoglyceric acid (carbohydrate) at 12.96 min with the masses corresponding to 595.45 m/z, 311.20 m/z and 264.95 m/z, respectively. Overall, the LC-MS analysis (both positive and negative ion mode) of the field grown leaf sample of D. purpurea had shown the presence of flavonoids in the major proportion (28.57%), followed by fatty acyl groups (19.04%), steroids (9.52%), triterpenoids (4.76%) and other compounds (26.21%) viz. the monosaccharide, saturated hydrocarbon, benzenoid etc. (Figure 11A). The in vitro raised leaf extract had the flavonoids as the highest occurring bioactive group (21.21%), followed by fatty acyls (18.18%), carbohydrates (9.09%), carbonyl compounds, carboxylic acids, coumarins and steroids (all at 6.06%), tannins (3.03%) etc. (Figure 11B). The major metabolite groups noted in callus extract followed the same trend as was noticed in vivo- and in vitro grown leaf tissue, with flavonoid being the top group (29.42%), then fatty acyl (17.65%), steroids (17.65%), triterpenoids (11.76%) and tannins (5.88%) (Figure 11C).
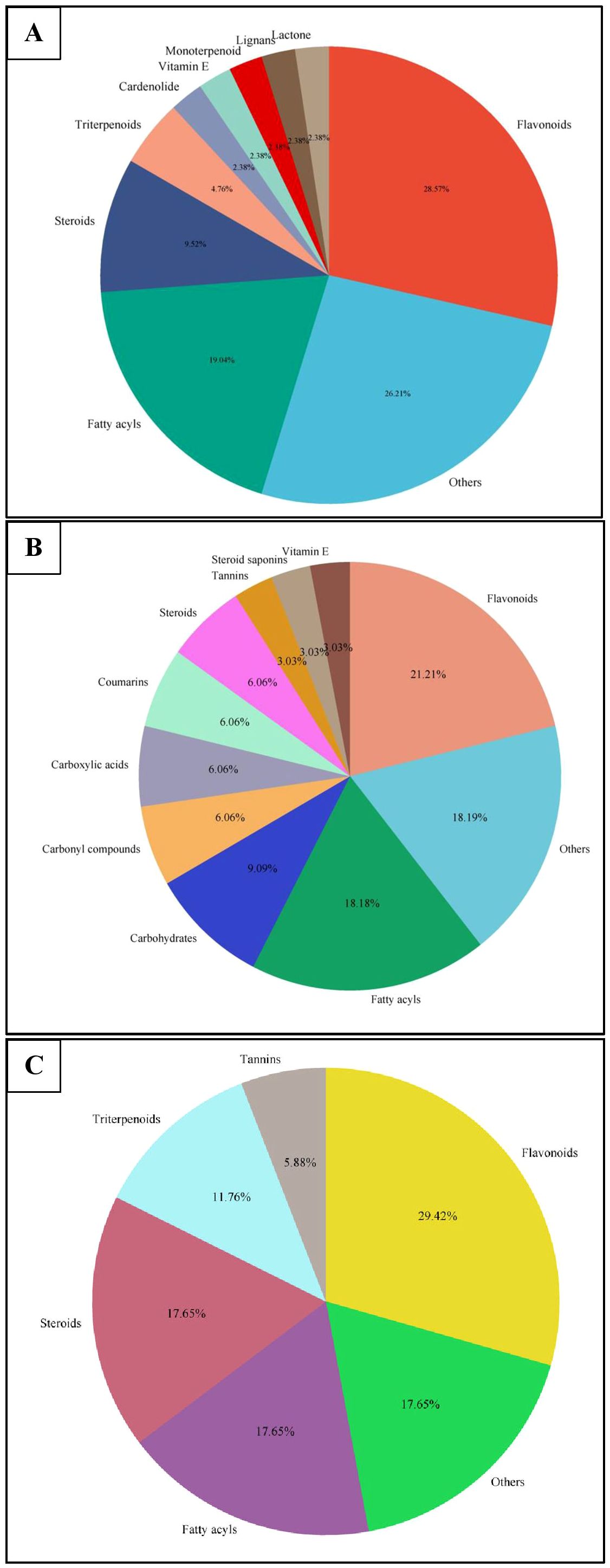
Figure 11. Pie-charts showing the proportions of the most abundant categories of phytocompounds detected in UPLC-ESI-QTOF-MS analysis of (A) in vivo grown leaf tissue, (B) in vitro grown leaf tissue, and (C) leaf derived callus of D. purpurea L.
4 Discussion
To the best of our knowledge, this is the first study in characterizing metabolomes of cultured tissues in Digitalis purpurea by employing untargeted GC-MS and LC-MS approaches. Thus, in the current study, the leaf explants of D. purpurea were successfully used for callus induction and subsequent shoot regeneration (organogenesis) was achieved by amending MS medium with two most frequently used PGRs i.e. BAP and 2,4-D at varying concentrations. It was observed that a high BAP (8.8 µM) and low 2,4-D (0.9 µM) concentration exerted the best stimulatory effect on callus biomass production. Similar responses have earlier been reported in D. purpurea and several other related species (Verma et al., 2016b; Rad et al., 2021). On further sub-culturing, the callus became organogenic and produced green shoots on the same medium. Similarly, a relative higher concentration of BAP (compared to 2,4-D) was found to trigger organogenesis in various plant species such as Pancratium maritimum (Yasemin et al., 2023), P. angulata and P. chenopodifolia (Romo-Paz et al., 2023). On the contrary, several reports are available where promotive effects of BAP and NAA were noted for shoot organogenesis (de Oliveira et al., 2022; Raju et al., 2022; Liu et al., 2023).
Various internal and external factors like genetic makeup, used agronomic techniques, environmental conditions and in vitro culture practices often alter biochemical attributes in the wilds as well as in vitro raised plants (Phuyal et al., 2020).Thus, The biochemical and antioxidant markers levels were checked between mother (donor) plant and different in vitro raised tissues in D. purpurea. From biochemical assays, it was clearly evident that the in vitro grown tissues (both callus and organogenic derived shoot leaf) accumulated more phenolics and flavonoids than the donor D. purpurea plant. Higher content of phenols and flavonoids in the micropropagated tissues (compared to field grown plant) have also been reported in Scrophularia takesimensis (Jeong and Sivanesan, 2015) and Dendrobium nobile (Bhattacharyya et al., 2016). As PGRs utilized in in vitro culture system, regulate biosynthesis pathway genes, the plant tissue culture system plays a significant role in the production of phenols and flavonoids (Ghosh et al., 2018). The role of clonal propagation is receiving a lot of attention since the primary significance of medicinal plants as a natural source of antioxidants is realized (Bose et al., 2015). Plant tissue, rich in phenols and flavonoids is considered to be a good source of antioxidants as there is a positive correlation of phenolic, flavonoids with antioxidant activities (Khorasani Esmaeili et al., 2015). The antioxidant potential of mother tissues/plants and laboratory grown plants was evaluated by conducting DPPH, POD and SOD assays. DPPH is an easy, reliable and popular method for assessing the ability of plant extracts to scavenge radicals by quenching the stable purple colored DPPH radical into yellow colored DPPH (Aryal et al., 2019). SOD scavenges superoxide radical and manages the levels of H2O2, while POD helps in oxidation and decomposition of H2O2 (Bansal et al., 2024). In our study, it was observed that the laboratory grown tissues (callus and in vitro leaf) possessed higher antioxidative activity than the field grown plant parts in all the three assays performed. These findings indicate a much greater antioxidant activity in micropropagated plant tissues compared to the mother plant, consistent with previous experimental observations from other researchers (Zayova et al., 2016; Ali et al., 2018; Mamgain et al., 2023).
Large-scale metabolite identification and/or quantification from one or more samples is referred to as untargeted metabolomic study. The metabolite profile strategy, otherwise called as top-down approach, examines the whole metabolomic profile of a given complicated sample rather than the requirement of a previous comprehensive hypothesis on a particular set of metabolites (de Souza et al., 2022). These can be achieved by performing untargeted GC-MS and LC-MS based metabolite profiling. The GC-MS technique has been applied in several plant species like Tanacetum sinaicum (Adel et al., 2021) and Catharanthus roseus (Bansal et al., 2023). Till date, there has been no previous untargeted metabolomics investigations in D. purpurea that examined in vitro regenerated callus and leaf tissue using GC-MS and LC-MS combined approach. In the present study, a comparative metabolite profile of in vivo-, in vitro grown leaf and leaf-callus has been made by using GC-MS and LC-MS techniques. The methanolic extracts of studied samples revealed the presence of more than 75 phytoconstituents belonging to various classes, like alkaloids, phytosterols, terpenoids, steroids, phenols, sugars etc. These detected bioactive compounds confer this plant diverse therapeutic importance. The major metabolites found in methanolic leaf extract of in vivo plant were 4-vinylguaiacol, lauric acid, myristic acid, loliolide, palmitic acid, squalene, stigmasterin, d-alpha-tocopherol etc. Loliolide is a type of monoterpenoid hydroxylactones, exhibiting anti-proliferative, anti-bacterial, anti-fungal and allelochemic activities and is reported in various plant species such as Rauvolfia yunnanensis,
Veronica persica, Salvia divinorum etc (Grabarczyk et al., 2015). Stigmasterin (or Stigmasterol) shows diverse range of pharmacological effects like anti-diabetic, immunomodulatory, antiparasitic, anticancer, anti-osteoarthritis, anti-inflammatory, antifungal properties (Bakrim et al., 2022). Squalene, a triterpenoid, possess antioxidant, anti-inflammatory, anti-neoplastic and anti-atherosclerotic properties (Lou-Bonafonte et al., 2018).
Similarly, the methanolic leaf extract of in vitro raised plant had 77 phytocompounds such as neophytadiene, phytol, beta-methylionone, linolenic acid, stearic acid, campesterol, gamma sitosterol, cycloartenol etc. Neophytadiene and phytol are diterpenoid compounds with anti-microbial, anti-inflammatory, anti-cancerous and antipyretic activities, which have been detected by GC-MS in various plant species (Willie et al., 2020; Banni and Jayaraj, 2022). Beta-sitosterol is widely known for its anti-diabetic, anti-inflammatory, cytotoxic and immunosuppressive properties (Salazar et al., 2020), campesterol (another sterol) has been associated with cancer prevention, anti-fungal and cholesterol lowering activities (Uttu et al., 2023). Callus extract on the other hand, showed a more varied range of metabolites such as linalool acetate, eugenol, cinnamic acid, jasmone, alpha-monostearin, 3-hydroxy-5-methoxyflavone, beta-amyrin, cycloartenol etc. in varied quantities. Eugenol (volatile phenolic compound) has been detected in several plant species, namely Eugenia caryophyllata, Myristica fragrans, Ocimum basilicum and act as antifungal, analgesic, anticancer, antiparasitic, antioxidant and antimicrobial agent (Abdou et al., 2021). Cinnamic acid is a key compound of aromatic carboxylic acid group, possessing anti-diabetic, anti-inflammatory, anti-cancerous and anti-microbial activity and is widely found in a number of plant varieties like Cinnamomum cassia, Panax ginseng etc (Ruwizhi and Aderibigbe, 2020). Beta-amyrin (a triterpenoid) demonstrates analgesic, anti-inflammatory, gastroprotective, hepatoprotective, anticonvulsant, antidepressive, antipancreatitic effects (Nogueira et al., 2018). This differential presence of metabolites in in vitro regenerated tissues (as compared to the mother plant parts) may be attributed to specific ecotype, genotype, explant, relative humidity, photoperiod, temperature, PGRs exposure and other variables in cultured conditions (Khan A. et al., 2021).
Finally, the methanolic extracts of each sample were subject to LC-MS analyses and the observation revealed that the Digitalis purpurea is enriched with a wide variety of phytocompounds such as flavonoids, fatty acyls, terpenoids, saponins, cardenolides, sugars, steroids, tannins and lignans, thereby increases plant’s medicinal potential. Several detected compounds in this present work, were reported previously in other Plantaginaceae members. These are like apigenin (269.04 m/z), Dicaffeoylquinic acid (517.13 m/z), luteolin (285.04 m/z), Quercetin 3-(2-caffeoylglucuronoside) (639.09 m/z) etc (Nedime et al., 2023; Bouali et al., 2024). The most significant phytocompound groups identified in both positive and negative ionization modes perhaps were flavonoids, fatty acyl and steroids. Major flavonoids detected in the current study include 3,3’,4’,7-tetrahydroxyflavan, nepitrin, luteolin, apigenin and kaempferid. Nepitrin has earlier been isolated from Rosmarinus officinalis and Salvia plebeia (Slimestad et al., 2022), both belonging to the same order Lamiales just like Digitalis purpurea. Kaempferid (299 m/z), luteolin (285 m/z) and apigenin (269 m/z) were also identified in the leaf extracts of Digitalis trojana. Flavonoids represent the most extensive category of naturally occurring compounds, with over 9000 phenolic chemicals identified in plants (Shomali et al., 2022). The application of flavonoids is quite widespread which includes antitumor, neuroprotective, anticancer, antibacterial, antiviral, antiangiogenic, antioxidant, and anti-proliferative activities (Ullah et al., 2020).
Fatty acyl followed by steroids group was the next most abundant metabolites detected in the present work. The fatty acyl group includes 2-hydroxylinolenic acid, ethyl stearate, octyl octanoate, dihydrojasmonic acid. Dihydrojasmonic acid is well known for its anti-cancerous, anti-depressant, anti-inflammatory, anti-nociceptive, anti-parasitic activities (Ghasemi Pirbalouti et al., 2014). 24-methylcycloartenol, delta4, 16-pregnadiene-3,20-dione, pregn-5-ene-3,20-dione-17-ol, campesterol 6’-hexadecanoylglucoside, stigmasterol 3-o-beta-d-glucoside were some of the major steroidal compounds identified in the tested samples. Campesterol and stigmasterol are the important phytosterols, found to be accumulated in D. purpurea tissues and act as precursor sterol for cardenolide biosynthesis (Carroll et al., 2023; Kunert et al., 2023). Two interesting derivatives of digoxigenin (and digoxin metabolite) i.e., digoxigenin monodigitoxoside and 20,22-dihydrodigoxigenin were detected in positive ion modes in in vivo- and in vitro grown leaf samples of D. purpurea. Their presence indicated that the cardenolides biosynthetic pathway was operational in studied experimental leaf tissue of both field grown and laboratory grown D. purpurea. Some other important metabolites identified were alpha-amyrin, rosmarinic acid, 2,3-diphosphoglyceric acid. Rosamarinic acid is a polyphenol, exhibiting antioxidant, anti-bacterial, anti-viral and anti-inflammatory activities (Andrade et al., 2018). In general, the callus extract showed the least number of phytocompounds in both positive and negative ionization modes as compared to the in vivo- and in vitro-grown leaves. This may be due to the fact that the callus is a simple tissue, some degree of cellular differentiation and tissue organization are necessary in controlling synthesis and accumulation of secondary metabolites (Karakas and Turker, 2016). These studies may pave the way for broad-spectrum drug development after ascertaining the bioactivity, toxicity and clinical trials of identified bioactive.
5 Conclusions
A comparative analysis of biochemical parameters, antioxidant activities and metabolite profiles of mother/donor plant and in vitro regenerated leaf tissues and leaf-callus were studied. To our knowledge, the current work represents the first metabolic profiling study of in vitro cultured tissues in Digitalis purpurea by GC-MS and UPLC-ESI-QTOF-MS techniques. The biochemical and antioxidant attributes showed that the in vitro derived tissues (callus and leaf samples) had higher level of phenols, flavonoids as well as antioxidant activities than the field grown (mother) plant. A variety of phytocompounds were identified and quantified in each sample by using GC-MS approach, revealing diverse pharmacological effects of this plant. The phytochemical composition of methanolic extracts of tissues were assayed by using UPLC-ESI-QTOF-MS in positive and negative modes. Major phytoconstituents detected were flavonoids, fatty acyls, steroids, triterpenoids, carbohydrates etc. The variation in metabolites of studied sample may be attributed to a number of factors like explant/tissue specific, genotype, used PGRs concentration, in vitro culture conditions etc. These analyses confirmed diverse therapeutic value of D. purpurea; the in vitro culture may therefore, be exploited for production of important bioactive compounds for pharmaceutical industry. Furthermore, studies like molecular docking and bio-prospecting, could be performed to deduce the ligand-protein interaction and biological properties of these therapeutically important phytocompounds, which will lead to pre-clinical and clinical trials in the later stages.
Data availability statement
The original contributions presented in the study are included in the article/supplementary material. Further inquiries can be directed to the corresponding author.
Author contributions
YB: Conceptualization, Data curation, Investigation, Methodology, Writing – original draft, Writing – review & editing. AM: Conceptualization, Investigation, Supervision, Writing – review & editing. JM: Data curation, Investigation, Methodology, Writing – review & editing. RS: Data curation, Investigation, Project administration, Writing – review & editing. MM: Data curation, Writing – review & editing. AN: Formal analysis, Writing – review & editing. YD: Formal analysis, Methodology, Writing – review & editing. NM-D: Investigation, Methodology, Writing – review & editing.
Funding
The author(s) declare financial support was received for the research, authorship, and/or publication of this article. This research work is funded by the Department of Biotechnology (DBT/2020/JH/1336), New Delhi, India and researchers supporting project (RSP-2024R375), King Saud University, Riyadh, Saudi Arabia.
Acknowledgments
The first author is thankful to the Department of Biotechnology (DBT) for financial support given as a Senior Research Fellowship (SRF). The authors are also grateful to the laboratory facilities provided by the Department of Botany, Jamia Hamdard, New Delhi. The authors acknowledge the researchers supporting project number (RSP-2024R375), King Saud University, Riyadh, Saudi Arabia.
Conflict of interest
The authors declare that the research was conducted in the absence of any commercial or financial relationships that could be construed as a potential conflict of interest.
Publisher’s note
All claims expressed in this article are solely those of the authors and do not necessarily represent those of their affiliated organizations, or those of the publisher, the editors and the reviewers. Any product that may be evaluated in this article, or claim that may be made by its manufacturer, is not guaranteed or endorsed by the publisher.
References
Abdelsalam, A., Mahran, E., Chowdhury, K., Boroujerdi, A., El-Bakry, A. (2017). NMR-based metabolomic analysis of wild, greenhouse, and in vitro regenerated shoots of Cymbopogon schoenanthus subsp. proximus with GC–MS assessment of proximadiol. Physiol. Mol. Biol. Plants 23, 369–383. doi: 10.1007/s12298-017-0432-0
Abdou, A., Elmakssoudi, A., El Amrani, A., JamalEddine, J., Dakir, M. (2021). Recent advances in chemical reactivity and biological activities of eugenol derivatives. Med. Chem. Res. 30, 1011–1030. doi: 10.1007/s00044-021-02712-x
Adel, R., Abdel-Ghani, A. E., Abouelenein, D. D., El-Dahmy, S. I. (2021). Variation in the Volatile Constituents of Wild and In Vitro Propagated Tanacetum sinaicum Del. Ex DC through GC-MS Chemical Fingerprint. Ind. J. Nat. Prod. Res. 12, 238–246. Available online at: http://nopr.niscpr.res.in/handle/123456789/57755.
Ajithan, C., Vasudevan, V., Sathish, D., Sathish, S., Krishnan, V., Manickavasagam, M. (2019). The influential role of polyamines on the in vitro regeneration of pea (Pisum sativum L.) and genetic fidelity assessment by SCoT and RAPD markers. Plant Cell Tissue Organ Culture (PCTOC) 139, 547–561. doi: 10.1007/s11240-019-01699-z
Al-Oqab, M. A., Zaid, S., Ammouri, Y. (2022). Effect of nutrient media enhanced with plant-growth regulators on indirect somatic embryogenesis induction for the tissue culture of Digitalis purpurea. J. Appl. Biol. Biotechnol. 10, 44–50. doi: 10.7324/jabb.2022.100605
Ali, A. M. A., El-Nour, M. E. M., Yagi, S. M. (2018). Total phenolic and flavonoid contents and antioxidant activity of ginger (Zingiber officinale Rosc.) rhizome, callus and callus treated with some elicitors. J. Genet. Eng. Biotechnol. 16, 677–682. doi: 10.1016/j.jgeb.2018.03.003
Altemimi, A., Lakhssassi, N., Baharlouei, A., Watson, D. G., Lightfoot, D. A. (2017). Phytochemicals: extraction, isolation, and identification of bioactive compounds from plant extracts. Plants 6, 42. doi: 10.3390/plants6040042
Amiri, F., Moghadam, A., Tahmasebi, A., Niazi, A. (2023). Identification of key genes involved in secondary metabolite biosynthesis in Digitalis purpurea. PloS One 18, e0277293. doi: 10.1371/journal.pone.0277293
Anand, U., Jacobo-Herrera, N., Altemimi, A., Lakhssassi, N. (2019). A comprehensive review on medicinal plants as antimicrobial therapeutics: potential avenues of biocompatible drug discovery. Metabolites 9, 258. doi: 10.3390/metabo9110258
Andrade, J. M., Faustino, C., Garcia, C., Ladeiras, D., Reis, C. P., Rijo, P. (2018). Rosmarinus officinalis L.: an update review of its phytochemistry and biological activity. Future Sci. OA 4, FSO283. doi: 10.4155/fsoa-2017-0124
Aryal, S., Baniya, M. K., Danekhu, K., Kunwar, P., Gurung, R., Koirala, N. (2019). Total phenolic content, flavonoid content and antioxidant potential of wild vegetables from western Nepal. Plants 8, 96. doi: 10.3390/plants8040096
Bakrim, S., Benkhaira, N., Bourais, I., Benali, T., Lee, L.-H., El Omari, N., et al. (2022). Health benefits and pharmacological properties of stigmasterol. Antioxidants 11, 1912. doi: 10.3390/antiox11101912
Baliyan, S., Mukherjee, R., Priyadarshini, A., Vibhuti, A., Gupta, A., Pandey, R. P., et al. (2022). Determination of antioxidants by DPPH radical scavenging activity and quantitative phytochemical analysis of ficus religiosa. Molecules 27, 1326. doi: 10.3390/molecules27041326
Banni, M., Jayaraj, M. (2022). Identification of bioactive compounds of leaf extracts of sida cordata (Burm.f.) borss.Waalk. by GC/MS analysis. Appl. Biochem. Biotechnol. 195, 556–572. doi: 10.1007/s12010-022-04115-z
Bansal, Y., Mujib, A., Mamgain, J., Dewir, Y. H., Rihan, H. Z. (2023). Phytochemical composition and detection of novel bioactives in anther callus of catharanthus roseus L. Plants 12, 2186. doi: 10.3390/plants12112186
Bansal, Y., Mujib, A., Mamgain, J., Kumar, S., Dewir, Y. H., Magyar-Tábori, K. (2024). Synthesis and accumulation of phytocompounds in field-, tissue-culture grown (Stress) root tissues and simultaneous defense response activity in glycyrrhiza glabra L. Sustainability 16, 1613. doi: 10.3390/su16041613
Bansal, Y., Mujib, A., Siddiqui, Z. H., Mamgain, J., Syeed, R., Ejaz, B. (2022). Ploidy status, nuclear DNA content and start codon targeted (SCoT) genetic homogeneity assessment in digitalis purpurea L., regenerated in vitro. Genes 13, 2335. doi: 10.3390/genes13122335
Bhattacharyya, P., Kumaria, S., Tandon, P. (2016). High frequency regeneration protocol for Dendrobium nobile : A model tissue culture approach for propagation of medicinally important orchid species. South Afr. J. Bot. 104, 232–243. doi: 10.1016/j.sajb.2015.11.013
Bhusare, B. P., John, C. K., Bhatt, V. P., Nikam, T. D. (2018). In vitro propagation of Digitalis lanata Ehrh. through direct shoot regeneration – A source of cardiotonic glycosides. Ind. Crops Prod. 121, 313–319. doi: 10.1016/j.indcrop.2018.05.019
Bose, B., Kumaria, S., Choudhury, H., Tandon, P. (2015). Assessment of genetic homogeneity and analysis of phytomedicinal potential in micropropagated plants of Nardostachys jatamansi, a critically endangered, medicinal plant of alpine Himalayas. Plant Cell Tissue Organ Culture (PCTOC) 124, 331–349. doi: 10.1007/s11240-015-0897-x
Bouali, A., Spissu, Y., Barberis, A., Fadda, A., Azara, E., Orrù, G., et al. (2024). Phytochemical evaluation and exploration of some biological activities of aqueous and ethanolic extracts of two species of the genus Plantago L. PloS One 19, e0298518. doi: 10.1371/journal.pone.0298518
Carla Guimarães Sobrinho, A., Silva Corpes, R., Maria Menezes Barra, I., Kiyoshi Miyagawa, H., Silva Santos, A. (2022). Untargeted GC-MS Metabolomics applied to wild leaves and callus produced by plant tissue culture of Hibiscus sabdariffa L. Arabian J. Chem. 15, 104103. doi: 10.1016/j.arabjc.2022.104103
Carroll, E., Ravi Gopal, B., Raghavan, I., Mukherjee, M., Wang, Z. Q. (2023). A cytochrome P450 CYP87A4 imparts sterol side-chain cleavage in digoxin biosynthesis. Nat. Commun. 14, 4042. doi: 10.1038/s41467-023-39719-4
Chandran, H., Meena, M., Barupal, T., Sharma, K. (2020). Plant tissue culture as a perpetual source for production of industrially important bioactive compounds. Biotechnol. Rep. 26, e00450. doi: 10.1016/j.btre.2020.e00450
Deepalakshmi, P. D., Odgerel, K., Thirugnanasambantham, P., Yungeree, O., Khorolragchaa, A., Senthil, K. (2016). Metabolite profiling of in vitro cultured and field grown rhizomes of acorus calamus from Mongolia using GC–MS. Chromatographia 79, 1359–1371. doi: 10.1007/s10337-016-3152-7
de Oliveira, L. S., Brondani, G. E., Molinari, L. V., Dias, R. Z., Teixeira, G. L., Gonçalves, A. N., et al. (2022). Optimal cytokinin/auxin balance for indirect shoot organogenesis of Eucalyptus cloeziana and production of ex vitro rooted micro-cuttings. J. Forestry Res. 33, 1573–1584. doi: 10.1007/s11676-022-01454-9
de Souza, D. P., de Carvalho Gonçalves, J. F., de Carvalho, J. C., da Silva, K. K. G., Fernandes, A. V., de Oliveira Nascimento, G., et al. (2022). Untargeted metabolomics used to describe the chemical composition and antimicrobial effects of the essential oil from the leaves of Guatteria citriodora Ducke. Ind. Crops Prod. 186, 115180. doi: 10.1016/j.indcrop.2022.115180
Dey, A., Nandy, S., Nongdam, P., Tikendra, L., Mukherjee, A., Mukherjee, S., et al. (2020). Methyl jasmonate and salicylic acid elicit indole alkaloid production and modulate antioxidant defence and biocidal properties in Rauvolfia serpentina Benth. ex Kurz. in vitro cultures. South Afr. J. Bot. 135, 1–17. doi: 10.1016/j.sajb.2020.07.020
El Sayed, A. M., Basam, S. M., El-Naggar, E.-M., Bellah, A., Marzouk, H. S., El-Hawary, S. (2020). LC–MS/MS and GC–MS profiling as well as the antimicrobial effect of leaves of selected Yucca species introduced to Egypt. Sci. Rep. 10. doi: 10.1038/s41598-020-74440-y
Erişen, S., Kurt-Gür, G., Servi, H. (2020). In vitro propagation of Salvia sclarea L. by meta-Topolin, and assessment of genetic stability and secondary metabolite profiling of micropropagated plants. Ind. Crops Prod. 157, 112892. doi: 10.1016/j.indcrop.2020.112892
Ghasemi Pirbalouti, A., Sajjadi, S. E., Parang, K. (2014). ChemInform abstract: A review (Research and patents) on jasmonic acid and its derivatives. ChemInform 45, 229–239. doi: 10.1002/chin.201423288
Ghosh, A., Igamberdiev, A. U., Debnath, S. C. (2018). Thidiazuron-induced somatic embryogenesis and changes of antioxidant properties in tissue cultures of half-high blueberry plants. Sci. Rep. 8, 16978. doi: 10.1038/s41598-018-35233-6
Gomathi, D., Kalaiselvi, M., Ravikumar, G., Devaki, K., Uma, C. (2013). GC-MS analysis of bioactive compounds from the whole plant ethanolic extract of Evolvulus alsinoides (L.) L. J. Food Sci. Technol. 52, 1212–1217. doi: 10.1007/s13197-013-1105-9
Grabarczyk, M., Wińska, K., Mączka, W., Potaniec, B., Anioł, M. (2015). Loliolide - the most ubiquitous lactone. Folia Biologica Oecologica 11, 1–8. doi: 10.1515/fobio-2015-0001
Halder, M., Sarkar, S., Jha, S. (2019). Elicitation: A biotechnological tool for enhanced production of secondary metabolites in hairy root cultures. Eng. Life Sci. 19, 880–895. doi: 10.1002/elsc.201900058
Hall, R., Beale, M., Fiehn, O., Hardy, N., Sumner, L., Bino, R. (2002). Plant metabolomics. Plant Cell 14, 1437–1440. doi: 10.1105/tpc.140720
Jeong, B. R., Sivanesan, I. (2015). Direct adventitious shoot regeneration, in vitro flowering, fruiting, secondary metabolite content and antioxidant activity of Scrophularia takesimensis Nakai. Plant Cell Tissue Organ Culture (PCTOC) 123, 607–618. doi: 10.1007/s11240-015-0864-6
Karakas, F. P., Turker, A. U. (2016). Improvement of shoot proliferation and comparison of secondary metabolites in shoot and callus cultures of Phlomis Armeniaca by LC-ESI-MS/MS analysis. In Vitro Cell. Dev. Biol. - Plant 52, 608–618. doi: 10.1007/s11627-016-9792-3
Khan, A., Shah, A. H., Ali, N. (2021). In-vitro propagation and phytochemical profiling of a highly medicinal and endemic plant species of the Himalayan region (Saussurea costus). Sci. Rep. 11, 23575. doi: 10.1038/s41598-021-03032-1
Khan, H., Khan, T., Ahmad, N., Zaman, G., Khan, T., Ahmad, W., et al. (2021). Chemical elicitors-induced variation in cellular biomass, biosynthesis of secondary cell products, and antioxidant system in callus cultures of fagonia indica. Molecules 26, 6340. doi: 10.3390/molecules26216340
Khorasani Esmaeili, A., Mat Taha, R., Mohajer, S., Banisalam, B. (2015). Antioxidant activity and total phenolic and flavonoid content of various solvent extracts from in vivo and in vitro grown trifolium pratense L. (Red clover). BioMed. Res. Int. 2015, 1–11. doi: 10.1155/2015/643285
Konappa, N., Udayashankar, A. C., Krishnamurthy, S., Pradeep, C. K., Chowdappa, S., Jogaiah, S. (2020). GC–MS analysis of phytoconstituents from Amomum nilgiricum and molecular docking interactions of bioactive serverogenin acetate with target proteins. Sci. Rep. 10, 16438. doi: 10.1038/s41598-020-73442-0
Kreis, W. (2017). The foxgloves (Digitalis) revisited. Planta Med. 83, 962–976. doi: 10.1055/s-0043-111240
Kunert, M., Langley, C., Lucier, R., Ploss, K., Rodríguez López, C. E., Serna Guerrero, D. A., et al. (2023). Promiscuous CYP87A enzyme activity initiates cardenolide biosynthesis in plants. Nat. Plants 9, 1607–1617. doi: 10.1038/s41477-023-01515-9
Liu, Y., Zhan, Y., Fu, Q., Li, S., Sun, X., Wang, Y., et al. (2023). Plant Regeneration via Somatic Embryogenesis and Indirect Organogenesis in Blue Honeysuckle (Lonicera caerulea L.). Horticulturae 9, 996. doi: 10.3390/horticulturae9090996
Lou-Bonafonte, J. M., Martínez-Beamonte, R., Sanclemente, T., Surra, J. C., Herrera-Marcos, L. V., Sanchez-Marco, J., et al. (2018). Current insights into the biological action of squalene. Mol. Nutr. Food Res. 62, 1800136. doi: 10.1002/mnfr.201800136
Mamgain, J., Mujib, A., Bansal, Y., Gulzar, B., Zafar, N., Syeed, R., et al. (2023). Elicitation induced α-amyrin synthesis in tylophora indica in vitro cultures and comparative phytochemical analyses of in vivo and micropropagated plants. Plants 13, 122. doi: 10.3390/plants13010122
Mamgain, J., Mujib, A., Syeed, R., Ejaz, B., Malik, M. Q., Bansal, Y. (2022). Genome size and gas chromatography-mass spectrometry (GC–MS) analysis of field-grown and in vitro regenerated Pluchea lanceolata plants. J. Appl. Genet. 64, 1–21. doi: 10.1007/s13353-022-00727-7
Marchetti, L., Pellati, F., Graziosi, R., Brighenti, V., Pinetti, D., Bertelli, D. (2019). Identification and determination of bioactive phenylpropanoid glycosides of Aloysia polystachya (Griseb. et Moldenke) by HPLC-MS. J. Pharm. Biomed. Anal. 166, 364–370. doi: 10.1016/j.jpba.2019.01.033
Mickymaray, N. (2019). Efficacy and mechanism of traditional medicinal plants and bioactive compounds against clinically important pathogens. Antibiotics 8, 257. doi: 10.3390/antibiotics8040257
Mujib, A., Fatima, S., Malik, M. Q. (2022). Gamma ray–induced tissue responses and improved secondary metabolites accumulation in Catharanthus roseus. Appl. Microbiol. Biotechnol. 106, 6109–6123. doi: 10.1007/s00253-022-12122-7
Murashige, T., Skoog, F. (1962). A revised medium for rapid growth and bio assays with tobacco tissue cultures. Physiologia Plantarum 15, 473–497. doi: 10.1111/j.1399-3054.1962.tb08052.x
Nartop, P., Altan, A. D., Titrek, A. (2021). Modeling of in vitro biomass production of digitalis purpurea under the effects of biosynthetic silver nanoparticles. Iranian J. Sci. Technol. Trans. A: Sci. 45, 775–783. doi: 10.1007/s40995-021-01105-4
Nedime, D., Yasar, D., Nursel, I., Seray, B., Emir, Z. H., Berrin, B. (2023). HPLC determination of polyphenols of the flowers of Digitalis lamarckii, Xeranthemum annuum, Epilobium hirsutum and Silene compacta from Bolu (Turkey). J. Med. Plants Res. 17, 164–179. doi: 10.5897/jmpr2022.7282
Nogueira, A. O., Oliveira, Y. I. S., Adjafre, B. L., de Moraes, M. E. A., Aragão, G. F. (2018). Pharmacological effects of the isomeric mixture of alpha and beta amyrin from Protium heptaphyllum: a literature review. Fundam. Clin. Pharmacol. 33, 4–12. doi: 10.1111/fcp.12402
Oliveira, J. P. S., Hakimi, O., Murgu, M., Koblitz, M. G. B., Ferreira, M. S. L., Cameron, L. C., et al. (2018). Tissue culture and metabolome investigation of a wild endangered medicinal plant using high definition mass spectrometry. Plant Cell Tissue Organ Culture (PCTOC) 134, 153–162. doi: 10.1007/s11240-018-1408-7
Patel, S. (2016). Plant-derived cardiac glycosides: Role in heart ailments and cancer management. Biomed. Pharmacother. 84, 1036–1041. doi: 10.1016/j.biopha.2016.10.030
Phuyal, N., Jha, P. K., Raturi, P. P., Rajbhandary, S. (2020). Total phenolic, flavonoid contents, and antioxidant activities of fruit, seed, and bark extracts of zanthoxylum armatum DC. Sci. World J. 2020, 1–7. doi: 10.1155/2020/8780704
Rad, M. M., Abdossi, V., Moradi, P., Rakhshandehroo, F., Mehrafarin, A. (2021). Phytochemical changes of Digitalis purpurea L. @ in response to polyamines and methyl jasmonate application in callus culture. J. Plant Biochem. Biotechnol. 31, 310–319. doi: 10.1007/s13562-021-00678-w
Raju, R. I., Hashi, A. K., Jazib, A., Hossain, M. T. (2022). Micropropagation of Alocasia amazonica through Indirect Shoot Organogenesis. Plant Tissue Culture Biotechnol. 32, 13–20. doi: 10.3329/ptcb.v32i1.60468
Rehman Nengroo, Z., Rauf, A. (2020). Inula racemosa and Digitalis purpurea from Kashmir: Fatty acid composition, antioxidant, antibacterial activities, and functional group evaluation. Flavour Fragrance J. 35, 653–665. doi: 10.1002/ffj.3604
Romo-Paz, F., de, J., Orozco-Flores, J. D., Delgado-Aceves, L., Zamora-Natera, J. F., Salcedo-Pérez, E., et al. (2023). Micropropagation of Physalis angulata L. and P. chenopodifolia Lam. (Solanaceae) via indirect organogenesis. In Vitro Cell. Dev. Biol. - Plant 59, 497–506. doi: 10.1007/s11627-023-10363-3
Ruwizhi, N., Aderibigbe, B. A. (2020). Cinnamic acid derivatives and their biological efficacy. Int. J. Mol. Sci. 21, 5712. doi: 10.3390/ijms21165712
Salazar, J. R., Loza-Mejía, M. A., Soto-Cabrera, D. (2020). Chemistry, biological activities and in silico bioprospection of sterols and triterpenes from mexican columnar cactaceae. Molecules 25, 1649. doi: 10.3390/molecules25071649
Shakya, A. K. (2016). Medicinal plants: Future source of new drugs. Int. J. Herb. Med. 4, 59–64. doi: 10.13140/RG.2.1.1395.6085
Shomali, A., Das, S., Arif, N., Sarraf, M., Zahra, N., Yadav, V., et al. (2022). Diverse physiological roles of flavonoids in plant environmental stress responses and tolerance. Plants 11, 3158. doi: 10.3390/plants11223158
Singh, T., Sharma, U., Agrawal, V. (2020). Isolation and optimization of plumbagin production in root callus of Plumbago zeylanica L. augmented with chitosan and yeast extract. Ind. Crops Prod. 151, 112446. doi: 10.1016/j.indcrop.2020.112446
Slimestad, R., Johny, A., Thomsen, M. G., Karlsen, C. R., Rosnes, J. T. (2022). Chemical profiling and biological activity of extracts from nine norwegian medicinal and aromatic plants. Molecules 27, 7335. doi: 10.3390/molecules27217335
Spina, R., Saliba, S., Dupire, F., Ptak, A., Hehn, A., Piutti, S., et al. (2021). Molecular identification of endophytic bacteria in leucojum aestivum in vitro culture, NMR-based metabolomics study and LC-MS analysis leading to potential amaryllidaceae alkaloid production. Int. J. Mol. Sci. 22, 1773. doi: 10.3390/ijms22041773
Thakur, M., Bhattacharya, S., Khosla, P. K., Puri, S. (2019). Improving production of plant secondary metabolites through biotic and abiotic elicitation. J. Appl. Res. Med. Aromat. Plants 12, 1–12. doi: 10.1016/j.jarmap.2018.11.004
Ullah, A., Munir, S., Badshah, S. L., Khan, N., Ghani, L., Poulson, B. G., et al. (2020). Important flavonoids and their role as a therapeutic agent. Molecules 25, 5243. doi: 10.3390/molecules25225243
Uttu, A. J., Sallau, M. S., Ibrahim, H., Iyun, O. R. A. (2023). Isolation, characterization, and docking studies of campesterol and β-sitosterol from Strychnos innocua (Delile) root bark. J. Taibah Univ. Med. Sci. 18, 566–578. doi: 10.1016/j.jtumed.2022.12.003
Verma, S. K., Das, A. K., Cingoz, G. S., Gurel, E. (2016a). In vitro culture of Digitalis L. (Foxglove) and the production of cardenolides: An up-to-date review. Ind. Crops Prod. 94, 20–51. doi: 10.1016/j.indcrop.2016.08.031
Verma, S. K., Sahin, G., Gurel, E. (2016b). Somatic embryogenesis, pigment accumulation, and synthetic seed production in Digitalis davisiana Heywood. PubMed 54, 245–253. Available at: https://pubmed.ncbi.nlm.nih.gov/27295921.
Vignesh, A., Selvakumar, S., Vasanth, K. (2022). Comparative LC-MS analysis of bioactive compounds, antioxidants and antibacterial activity from leaf and callus extracts of Saraca asoca. Phytomedicine Plus 2, 100167. doi: 10.1016/j.phyplu.2021.100167
Willie, P., Uyoh, E. A., Aikpokpodion, P. O. (2020). Gas chromatography-mass spectrometry (GC-MS) assay of bio- active compounds and phytochemical analyses in three species of apocynaceae. Pharmacognosy J. 13, 383–392. doi: 10.5530/pj.2021.13.49
Wu, B., Li, Y., Yan, H., Ma, Y., Luo, H., Yuan, L., et al. (2012). Comprehensive transcriptome analysis reveals novel genes involved in cardiac glycoside biosynthesis and mlncRNAs associated with secondary metabolism and stress response in Digitalis purpurea. BMC Genomics 13, 1–22. doi: 10.1186/1471-2164-13-15
Yasemin, S., Koksal, N., Buyukalaca, S. (2023). Indirect organogenesis and in vitro bulb formation of Pancratium maritimum. Plant Cell Tissue Organ Culture (PCTOC) 154, 713–727. doi: 10.1007/s11240-023-02545-z
Zayova, E., Nikolova, M., Dimitrova, L., Petrova, M. (2016). Comparative study of in vitro, ex vitro and in vivo propagated salvia hispanica (Chia) plants: morphometric analysis and antioxidant activity. AgroLife Sci. J. 5, 166–173. Available online at: https://agrolifejournal.usamv.ro/index.php/agrolife/article/view/681.
Keywords: metabolomics, indirect organogenesis, biochemical, SOD, POD, GC-MS, UPLC-ESI-QTOF-MS, cardenolides
Citation: Bansal Y, Mujib A, Mamgain J, Syeed R, Mohsin M, Nafees A, Dewir YH and Mendler-Drienyovszki N (2024) Integrated GC-MS and UPLC-ESI-QTOF-MS based untargeted metabolomics analysis of in vitro raised tissues of Digitalis purpurea L. Front. Plant Sci. 15:1433634. doi: 10.3389/fpls.2024.1433634
Received: 16 May 2024; Accepted: 01 August 2024;
Published: 22 August 2024.
Edited by:
Marcos Edel Martinez-Montero, University of Ciego de Ávila, CubaReviewed by:
Vishnu Mishra, University of Delaware, United StatesAnwar Shahzad, Aligarh Muslim University, India
Asifa Khan, University of Massachusetts Medical School, United States
Copyright © 2024 Bansal, Mujib, Mamgain, Syeed, Mohsin, Nafees, Dewir and Mendler-Drienyovszki. This is an open-access article distributed under the terms of the Creative Commons Attribution License (CC BY). The use, distribution or reproduction in other forums is permitted, provided the original author(s) and the copyright owner(s) are credited and that the original publication in this journal is cited, in accordance with accepted academic practice. No use, distribution or reproduction is permitted which does not comply with these terms.
*Correspondence: A. Mujib, YW11amliM0B5YWhvby5jby5pbg==