- Department of Plant Sciences, University of California Davis, Davis, CA, United States
Postharvest chilling injury (PCI) is a physiological disorder that often impairs tomato fruit ripening; this reduces fruit quality and shelf-life, and even accelerates spoilage at low temperatures. The CBF gene family confers cold tolerance in Arabidopsis thaliana, and constitutive overexpression of CBF in tomato increases vegetative chilling tolerance, in part by retarding growth, but, whether CBF increases PCI tolerance in fruit is unknown. We hypothesized that CBF1 overexpression (OE) would be induced in the cold and increase resistance to PCI. We induced high levels of CBF1 in fruit undergoing postharvest chilling by cloning it from S. lycopersicum and S. habrochaites, using the stress-inducible RD29A promoter. Harvested fruit were cold-stored (2.5°C) for up to three weeks, then rewarmed at 20°C for three days. Transgene upregulation was triggered during cold storage from 8.6- to 28.6-fold in SlCBF1-OE, and between 3.1- to 8.3-fold in ShCBF1-OE fruit, but developmental abnormalities in the absence of cold induction were visible. Remarkably, transgenic fruit displayed worsening of PCI symptoms, i.e., failure to ripen after rewarming, comparatively higher susceptibility to decay relative to wild-type (WT) fruit, lower total soluble solids, and the accumulation of volatile compounds responsible for off-odors. These symptoms correlated with CBF1 overexpression levels. Transcriptomic analysis revealed that the ripening and biotic and abiotic stress responses were altered in the cold-stored transgenic fruit. Seedlings grown from ‘chilled’ and ‘non-chilled’ WT fruit, in addition to ‘non-chilled’ transgenic fruit were also exposed to 0°C to test their photosynthetic response to chilling injury. Chilled WT seedlings adjusted their photosynthetic rates to reduce oxidative damage; ‘non-chilled’ WT seedlings did not. Photosynthetic parameters between transgenic seedlings were similar at 0°C, but SlCBF1-OE showed more severe photoinhibition than ShCBF1-OE, mirroring phenotypic observations. These results suggest that 1) CBF1 overexpression accelerated fruit deterioration in response to cold storage, and 2) Chilling acclimation in fructus can increase chilling tolerance in seedling progeny of WT tomato.
1 Introduction
Refrigeration is indisputably the most effective strategy to prolong shelf-life, preserve quality and delay the deterioration of many fruits and vegetables (Kader, 2002). However, in cold-sensitive commodities such as tomato (Solanum lycopersicum L.), storage at temperatures between 0-12°C induces the onset of molecular, biochemical, and physiological alterations known as postharvest chilling injury (PCI), which are manifested when fruit are rewarmed to room temperature (Lyons, 1973).
PCI is a complex and multilayered phenomenon. Its early stages are temperature-dependent and are mediated by physical changes in cellular membranes (Luengwilai et al., 2018). Loss of membrane stability triggers the activation of a signal transduction cascade that transmits the cold stimulus downstream through a series of molecular players, e.g., second messengers, eliciting symptoms characteristic of this disorder (Lyons, 1973). These symptoms include modifications in respiration and ethylene production, disruption in the synthesis of aroma volatiles, accumulation of reactive oxygen species (ROS), lipid peroxidation, and DNA and protein damage (Albornoz et al., 2022). These molecular and cellular processes ultimately lead to failures in fruit ripening, the development of surface pitting, seed browning and higher susceptibility to postharvest decay (Moline, 1976; Jackman et al., 1989; Biswas et al., 2016).
Most of what is known about the cold signal transduction pathway in plants, comes from studies of Arabidopsis thaliana, which is able to cold-acclimate and endure freezing temperatures (Gilmour et al., 1988). The C-Binding Factor (CBF) gene family (AtCBF1-3) of transcription factors are positive regulators of the cold response that interact with the cis-elements of downstream cold-responsive genes (Thomashow et al., 2001; Thomashow, 2010). These target genes, also known as the CBF-regulon, encode protective proteins and enzymes, and are involved in the synthesis of metabolites that enhance the plant’s fitness during cold stress (Thomashow et al., 2001; Thomashow, 2010).
In tomato fruit, SlCBF1-3 genes are also induced by cold (Zhao et al., 2009a; Zhang et al., 2016; Albornoz et al., 2019), but the size and types of genes comprising the CBF regulon are not the same as in Arabidopsis (Zhang et al., 2016). This might partly explain tomato’s inability to cold acclimate (Zhang et al., 2004). Different members of the Arabidopsis CBF gene family have been ectopically expressed in tomato plants under the control of the constitutive CaMV35S promoter, resulting in increased stress tolerance, but with concomitant growth reduction and flowering delay (Hsieh et al., 2002a, 2002; Zhang et al., 2004). This, due to CBF’s involvement in gibberellin (GA) repression, and DELLA protein accumulation (Achard et al., 2008; Zhou et al., 2017). A transgenic phenotype overexpressing AtCBF1 in tomato fruit was characterized, and revealed this gene influenced ripening as well as fruit’s response to postharvest cold stress (Albornoz et al., 2023).
In this work, we hypothesized that CBF1 overexpression in tomato cv. Micro-Tom fruit during postharvest chilling would enhance fruit tolerance to cold stress and reduce the incidence of PCI. We cloned this gene from two sources: cultivated tomato (SlCBF1) and the wild-tomato relative Solanum habrochaites (ShCBF1). S. habrochaites has been extensively studied due to its tolerance to cold stress (Patterson et al., 1984; Goodstal et al., 2005; Cao et al., 2014). ShCBF1 has been cloned and expressed into Arabidopsis plants conferring tolerance to freezing and salinity but displaying phenotypic abnormalities (Li et al., 2014). In this study, both ShCBF1 and SlCBF1 genes were driven by the stress-inducible promoter RD29A (Yamaguchi-Shinozaki and Shinozaki, 1994). Our goal was to specifically induce CBF1 expression in harvested fruit stored in the cold, as well as minimize pleiotropic effects caused by constitutive overexpression.
To test our hypothesis, transgenic fruit were cold-stored, which elicited ectopic CBF expression, and their postharvest performance was examined and compared to wild-type fruit under the same conditions. To broaden the scope of traditional studies of chilling injury beyond fruit postharvest, we also tested if the photosynthetic responses of CBF1-overexpressing seedlings would be affected by cold stress. The goal was to understand the physiological effects of additional CBF1 transcripts at different phases of the plant life cycle. Finally, we evaluated the influence of postharvest fruit chilling on seeds and seedling traits that were measured under control (room temperature) or cold conditions, to explore the concept of transgenerational adaptive mechanisms transmitted from fruit to progeny.
2 Materials and methods
2.1 Construct development
The stress-inducible promoter RD29A (GenBank Accession no. CS191722.1), the ShCBF1 gene (GenBank Accession no. KX890304.1) and the SlCBF1 gene (GenBank Accession no. NM_001247194.2) were separately cloned from Arabidopsis thaliana Col-0 genomic DNA, S. lycopersicum cv. Micro-Tom genomic DNA and S. habrochaites LA1777 (The C.M. Rick Tomato Genetics Resource Center at University of California, Davis) genomic DNA, respectively (Supplementary Table S1). The RD29A promoter and CBF1 genes were amplified separately, and the RD29A reverse primer was designed to include a 20 bp overlapping sequence with the forward primer of ShCBF1 or SlCBF1. A second PCR reaction was set up using the products of the first reactions as templates. The resulting assembly PCR product consisted of RD29A located at the 5’ end, having a SbfI restriction site and ShCBF1 or SlCBF1 at the 3’ end, having a HindIII restriction site. These products were cloned into pCAMBIA1300 SbfI and HindIII sites, and the absence of mutations was confirmed by sequencing. The resulting constructs were named pCAMBIA-RD29A::ShCBF1 and pCAMBIA1300-RD29A::SlCBF1 (Figure 1). The amino acid sequences of ShCBF1 and the native SlCBF1 gene share a 92% identity according to the Multiple Sequence Alignment by CLUSTALW tool (Kyoto University Bioinformatics Center). Constitutive overexpression of AtCBF1 (Hsieh et al., 2002a, 2002; Zhang et al., 2011) or AtCBF3 (Zhang et al., 2004) in tomato plants has been studied by others, therefore the amino acid alignment and phylogenetic relationships between these, and ShCBF1 and SlCBF1 proteins, are shown in Supplementary Figure S6.

Figure 1 The base constructs used for plant transformation. In this experiment, the CBF1 was cloned from S.lycopersicum, i.e., pCAMBIA-RD29A::SlCBF1 or from S.habrochaites, i.e., pCAMBIA-RD29A::ShCBF1. Elements from left to right: LB, Left T-DNA Border; RD29A pro, stress-inducible promoter from A. thaliana; SlCBF1 or ShCBF1, C-binding factor from either S. lycopersicum or S. habrochaites; NOS ter, terminator sequence; 35S, CaMV 35S promoter; HygR, hygromycin resistance gene; CaMV 35S poly A signal, termination signal; RB, right T-DNA Border. Vertical lines represent restriction sites.
2.2 Plant transformation and growing conditions
Tomato (Solanum lycopersicum L. cv. Micro-Tom) was chosen based on its short life cycle, ease of transformation and small size at full maturity (Meissner et al., 1997). The latter allowed growing a high density of plants, critical in postharvest studies, which require harvesting numerous fruit at the same maturity stage. Micro-Tom cotyledons and hypocotyls were transformed with Agrobacterium tumefaciens EHA105 cultures carrying either pCAMBIA-RD29A::ShCBF1 or pCAMBIA-RD29A::SlCBF1 constructs. Hygromycin B (15 mg/L) was used as selection agent, and transformants were confirmed by PCR. Rooted plants (T0 generation) were transferred to a greenhouse at UC Davis and grown at temperatures between 25-30°C. Two independent transformation events per construct were used in this study: ‘Sh-13’ (T2 generation) and ‘Sh-36’ (T2 generation) for pCAMBIA-RD29A::ShCBF1 (ShCBF1-OE lines), and ‘Sl-2’ (T3 generation) and ‘Sl-12’ (T2 generation) for pCAMBIA-RD29A::SlCBF1 (SlCBF1-OE lines). A null-segregant (‘NS’), a transformant that is negative for the transgene after segregation, was used as a genetic control, and was denoted here as the wild-type (‘WT’) event.
2.3 Transgene copy number determination
Genomic DNA isolation (Fulton et al., 1995) was carried out on two seedlings from each of the transgenic genotypes Sh-13 and Sl-2 using the Prosystemin (Prosys) gene as an endogenous control as previously reported (Albornoz et al., 2023). Primers were designed based on a conserved region between ShCBF1 and SlCBF1 (Supplementary Table S1). Transgene copy number was calculated by Real-Time Quantitative PCR (RT-qPCR) as the ratio of the copy number of SlCBF1 or ShCBF1 to Prosys, respectively (Shepherd et al., 2009).
2.4 Fruit harvest and phenotypic characterization
2.4.1 Fruit shape index
The width and height of 100 fruit per genotype were recorded. Fruit shape index (FSP) was determined as the ratio of maximum height to maximum width. When FSP = 1, this indicated a round fruit. In contrast, when FSP > 1 or FSP< 1, this indicates an elongated or squat fruit, respectively (Brewer et al., 2006).
2.4.2 Postharvest storage preparation
Fruit were randomly harvested from a group of 50 WT plants, 80 Sh-13 and Sl-2 plants, 26 Sh-36 plants and 76 Sl-12 individuals. Unblemished fruit were harvested at breaker stage (Figure 2), soaked in 0.25% (v/v) sodium hypochlorite for three minutes and gently blotted until dry with paper towels. For cold storage phenotyping, Sh-13, Sh-36, Sl-2, Sl-12, and WT fruit were stored at 2.5°C for one, two or three weeks, or transferred to 20°C for three days (‘rewarming’ or ‘RW’) to induce PCI. Characterization of fruit at 20°C was carried out for genotypes Sh-13, Sl-2, and WT for up to 14 days.
2.4.3 Chilling injury index (CII) and decay incidence
Fruit were removed from the 2.5°C room after one, two or three weeks of storage, transferred to 20°C for three days, and evaluated for CII based on the development of surface pitting and the presence of decay. Pitting severity was determined visually on each fruit based on the percentage of surface affected using a five-point scale as follows: 0 = no pitting, 1 =< 25%, 2 = 25-50%, 3 = 50-75%, 4 = > 75% (Aghdam et al., 2014). CII was calculated using the formula:
Table 1 shows the total fruit evaluated for each treatment.
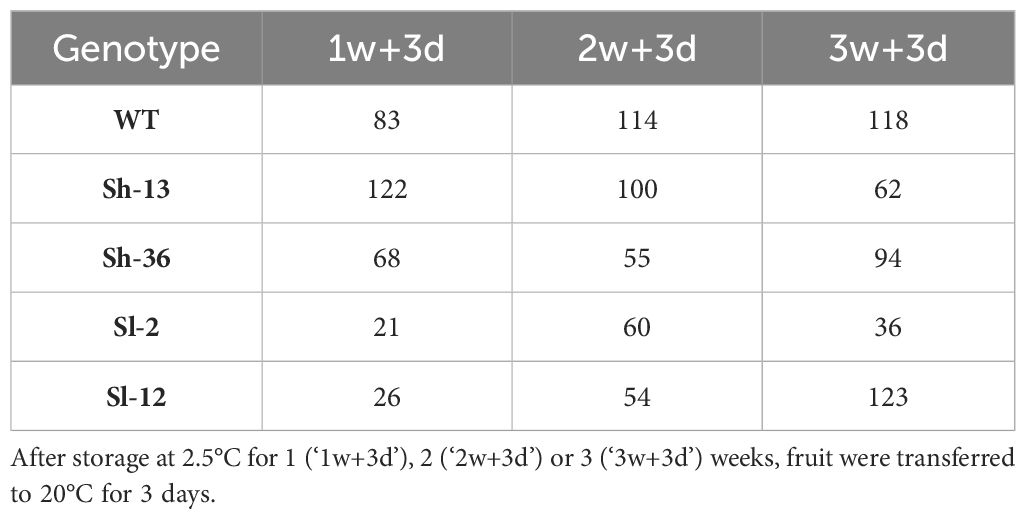
Table 1 Sample size for Chilling injury index and decay of CBF1-OE lines, and wild-type (‘WT’) tomato fruit.
2.4.4 Color determination
The Hue angle was used as a descriptor and recorded with a Konica Minolta colorimeter (Chroma Meter CR-400, Konica Minolta Sensing Americas, Ramsey, NJ, USA). A 2° observer and standard illuminant C setting in the L* a* b* scale was utilized. A total of 40 fruit per time point for each genotype were used. Lower Hue values are indicators of redder (riper) fruit, whereas higher values represent greener fruit. Table 2 shows the total fruit evaluated for each treatment.
2.4.5 Total soluble solids
The percentage of soluble solids was determined in Sh-13, Sl-2 and WT fruit juice using a digital refractometer HI-96801 (Hanna Instruments, Rhode Island, United States) in a total of 18 fruit per treatment for each genotype.
2.4.6 Respiration and ethylene evolution rates
Fruit from genotypes Sh-13, Sl-2 and WT were placed in 450 mL-jars connected to flow boards using capillary tubes to control flow rates. A total of six biological replicates were used for each of the genotypes. Each biological replicate corresponded to a jar containing 22 fruit. A total of 10 mL of gas samples for carbon dioxide (CO2) and ethylene were taken with a plastic syringe. Respiration rates were measured by injecting the gas sample into a high-range CO2 analyzer (Horiba VIA-510, Kyoto, Japan). A standard of 0.25% (v/v) CO2 was used for calibration. Ethylene concentration was measured with a gas chromatograph (Model 211, AGC Series 400; Hach-Carle Co., Colo., U.S.A.). Standards of 100 ppb or 1 ppm ethylene were used for calibration. All measurements took place at the corresponding storage temperatures (2.5 or 20°C) daily.
2.4.7 Volatile compounds analysis by solid phase microextraction (SPME) coupled with gas chromatography -mass spectrometry (GC-MS)
Breaker fruit from lines Sh-13, Sl-2, and WT stored at 2.5°C for 2 weeks, and RW for 3 days were removed from storage and kept at room temperature for 16 h before processing for volatile extraction. Tomato fruit were mixed with saturated calcium chloride solution (1:1, w/v) containing 2 μM 3-octanone as an internal standard, and then blended in a commercial blender for 1 min. Four milliliters of slurry of each sample were transferred into a 20 mL amber headspace vial, exposed to a SPME fiber (Supelco, USA), and stored at -80°C until analyzed on a GC-MS unit (Wang et al., 2019), except that a DB-WAX column (30 m x 0.25 mm, film thickness 0.25 μm) and a different oven program was used. The initial oven temperature was set at 40°C for 5 min, followed by an increase to 80°C at a rate of 5°C min-1, then an increase to 200°C at a rate of 10°C min-1, and another increase to 250°C at a rate of 20°C min-1. The AMDIS software (version 2.64) was used for spectral deconvolution (Stein, 1999) coupled with SpectConnect to consolidate the data (Styczynski et al., 2007). Compound identity was queried with NIST library (v. 2.0) and confirmed by matching the retention time in ChemStation (E.02.02.1431, Agilent, Santa Clara, CA, USA). A total of three biological replicates were used for each of the genotypes. Each biological replicate consisted of ~ 15 g of tomato fruit.
2.5 RNA isolation
Total RNA was isolated (Wang et al., 2009) from 100 mg of frozen fruit or leaf powder, depending on the experiment, and treated with the DNase TURBO DNA free Kit (Life Technologies, Carlsbad, CA, USA). RNA integrity and purity were assessed by agarose gel electrophoresis, and the A260/A280 and A260/A230 ratios.
2.6 Real-time quantitative PCR
Copy DNA (cDNA) was synthesized from 1 µg RNA. Cycling conditions for cDNA synthesis and RT-qPCR were the same as described in previous reports (Albornoz et al., 2019). Primers were designed based on the cDNA sequences available on GenBank (Supplementary Table S1).
The reaction efficiency was calculated through the standard curve method, using a cDNA dilution series (Taylor et al., 2010). The specificity of the primers was validated by melt-curve analysis (Taylor et al., 2010). The Pfaffl method was used for data normalization and relative quantification of transcript production (Pfaffl, 2012). A reference gene, actin (SlACT7) was used as the control for data normalization. Three biological and three technical replicates were used. The pericarp region of six fruit, or one true leaf per seedling corresponded to a biological replicate.
2.7 RNA sequencing
2.7.1 Messenger RNA isolation
Assessment was done on breaker fruit from lines Sh-13, Sl-2, and WT stored at 2.5°C for six hours or 1 week. Two biological replicates were used per genotype. A biological replicate consisted of the pericarp region of six fruit. The mRNA was isolated from 5 µg total RNA using the NEBNext® Poly(A) mRNA Magnetic Isolation Module.
2.7.2 3’ DGE RNA-Seq library construction and sequencing
Each treatment included two biological replicates. In total, 12 libraries were constructed using Strand-specific mRNA-library prep kits (Amaryllis Nucleics, Oakland, CA). Library concentration was assessed by a Qubit™ device (Invitrogen, Waltham, MA, USA), and the quality was checked by a bioanalyzer, following HiSeq PE150 sequencing in Novogene Co. (Beijing, China). The raw sequencing reads were trimmed according to quality score by FastQC (Andrews, 2010). The reads alignment was processed by the STAR software (Dobin et al., 2013) based on the tomato reference genome SL4.0 (Sol Genomics Network). The gene read counts were generated using the HTSeq package (Putri et al., 2022). Differentially expressed genes (DEGs) were extracted using the DEseq2 tool (Love et al., 2014). The Gene ontology (GO) functional enrichment analyses of DEGs were performed using the g:Profiler online tool, genome assembly SL3.0, accession GCA_000188115.
2.8 Vegetative stage tests
The effect of fruit storage conditions on the performance of seed and seedling progeny was investigated. Tests of percent germination, early growth and photosynthetic response in the WT and transgenic seeds, or seedlings obtained from PCI-affected or control fruit, were performed.
2.8.1 CBF1 expression in cold-exposed transgenic and wild-type seedlings
Three seedlings from the wild-type, and from transgenic Sh-13 and Sl-2 lines obtained from non-chilled fruit were grown until cotyledons were fully expanded, transplanted into a 36-cell polyethylene growth tray containing UC Mix substrate and transferred to the greenhouse until plants were at the six-(wild-type) or five-(transgenic) leaf stage. Plants were transferred to a room at 0°C for up to 24 hours. Leaf tissue was excised from each plant, and CBF1 expression was measured by RT-qPCR.
2.8.2 Seed color, germination, and seedling features
Seeds were collected from WT fruit that were harvested at breaker but stored at two conditions: a) room temperature until fully ripe (‘non-chilled’) or, b) 2.5°C for three weeks, followed by transfer to room temperature until fully ripe (‘chilled’).
Color (L* value or ‘lightness’) of dry seeds was recorded using a Konica Minolta colorimeter according to 2.4.4. When L* value = 0, it indicates darkness or absence of light (black), whereas when L* = 100, it represents the brightest white. Three replicates were used, each consisting of approximately 150 seeds. Seed weight was recorded, and three replicates were used, each consisting of a group of 50 dry seeds.
Seeds were soaked in 2.7% (v/v) sodium hypochlorite for one hour, rinsed thoroughly in running water, placed into Petri dishes with damp paper towels and located in a 20°C (± 2°C) room under 16/8 hour-photoperiod for one week. The number of germinated seeds from each type was recorded after one week to determine the germination percentage. Hypocotyls (‘shoots’) and roots were excised, and their fresh weight was recorded. Three replicates, each representing a Petri dish with 20 seeds for each type, were used.
2.8.3 Photosynthetic performance of seedlings under stress
Sixteen seedlings from the wild-type (‘chilled’ and ‘non-chilled’), and from transgenic Sh-13 and Sl-2 lines were grown as indicated in 2.8.1. Seedlings were placed at 2.5 or 0°C under a 16/8 photoperiod. Photosynthetic parameters: LEF (Linear Electron Flow), Phi2 (efficiency of Photosystem II, PSII), NPQt and PhiNPQ (estimates of Non-Photochemical Quenching), PhiNO (estimate of incoming light lost in non-regulated processes that can induce photodamage), relative chlorophyll, and PAR (Photosynthetically Active Radiation) were measured on the 4th leaf of each plant after 24, 48 and 72 hours with a MultispeQ device (PhotosynQ LLC, East Lansing, MI, USA) (Kuhlgert et al., 2016).
2.9 Statistical analysis
Experiments were carried out with a complete randomized design. Statistical analysis was performed using SAS software version 9.4 or Microsoft Excel. To detect significant differences between treatments, analysis of variance (ANOVA) or unpaired t-test (with either equal or unequal variance) were conducted. For mean comparison, Tukey’s test was used (α = 0.05). The z-score test for two population proportions was used to determine if CII values and decay, and germination % between treatments, differed significantly (p < 0.05). p-values were symbolized as follow: p < 0.05 (*), p ≤ 0.01 (**) and p ≤ 0.001 (***). Standard error (SE) was used as a measure of data variability.
3 Results
3.1 Transgenic plant and fruit characterization
The growth and development of the transgenic lines were altered by the expression of CBF1. Germination percentages were significantly reduced, and seedlings displayed high mortality (data not shown), suggesting that transgene homozygosis may be associated with a lethal phenotype. The inconclusive results from the transgene copy number estimation in lines Sh-13 and Sl-2 support this observation. ShCBF1-OE and SlCBF1-OE were stunted, with shorter internodes, and the leaves underwent accelerated senescence, produced less inflorescences, and consequently, less fruit, than wild-type individuals (Supplementary Figure S4).
Fruit morphology was altered in some of the transgenic lines (Supplementary Table S2). External color in freshly harvested fruit was similar in Sh-13, Sl-2, and WT fruit (Figure 3). Color differences between transgenic and non-transgenic lines at 20°C became significant after one week of storage and were maintained until the end of the experiment. As evidenced by higher Hue values, transgenic genotypes were unable to ripen to the same extent as WT fruit (Figure 3).
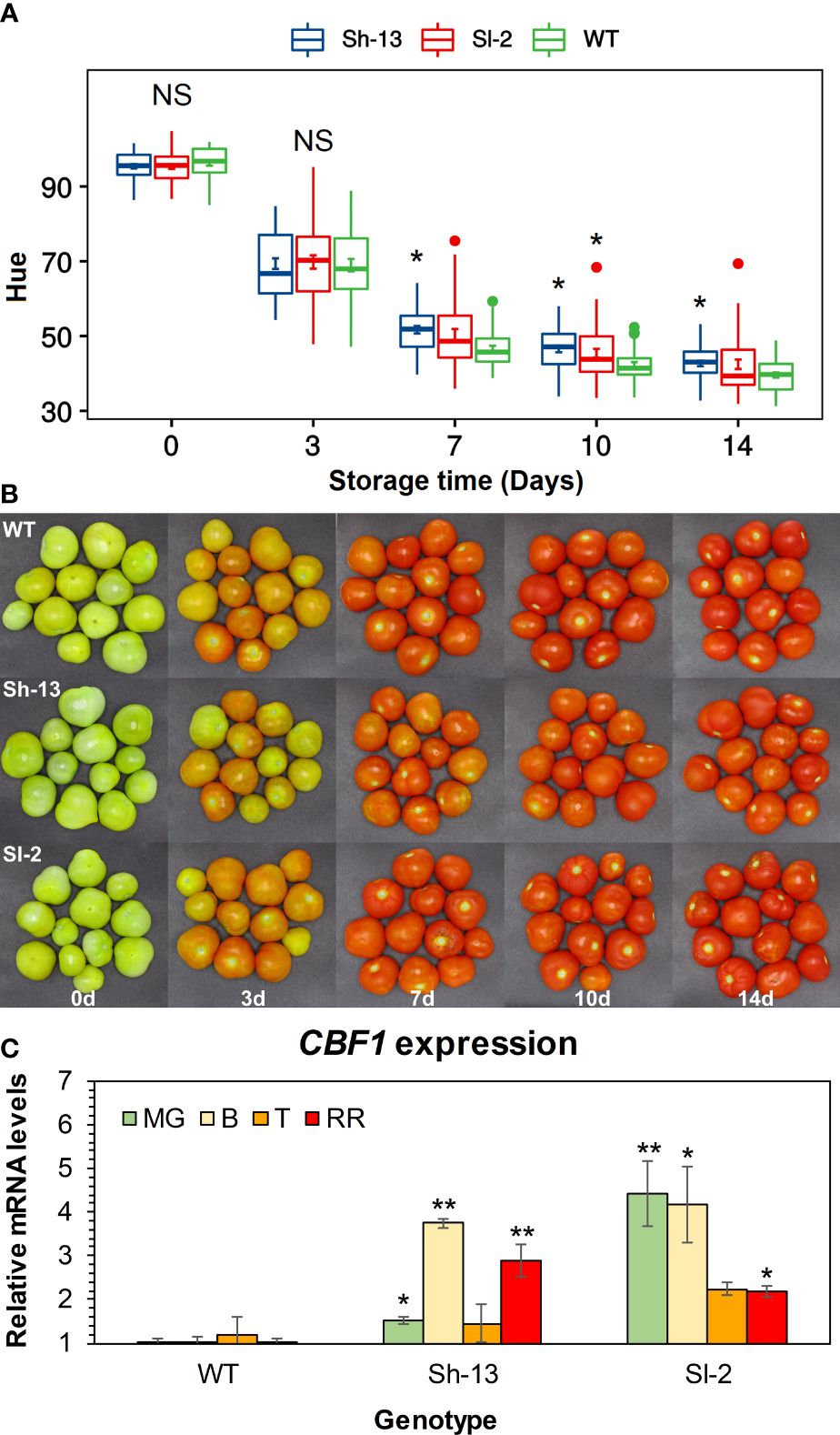
Figure 3 Characterization of CBF1-overexpression lines (Sh-13 and Sl-2) and WT fruit. (A) Hue angle of breaker fruit stored at 20°C for up to 14 days. Boxes with asterisks are significantly different (p< 0.05) than WT fruit by Dunnett’s test. Error bars are included. (B) External appearance of breaker fruit stored at 20°C for up to 14 days. (C) CBF1 expression of fruit harvested at different maturity stages. Transcript accumulation was determined by quantifying both native and transgenic expression of this gene at the mature green (MG), breaker (B), turning (T) and red ripe (RR) stages. WT fruit at each maturity stage were used as the calibrator. Columns with asterisks are significantly different (p< 0.05) to WT fruit at each stage by unpaired t-test. NS, Not significant.
The expression of CBF1 was characterized in fruit harvested at different stages of maturity, and in cold-exposed seedlings by RT-qPCR. In fruit, relative to the wild-type, all maturity stages had higher CBF1 expression, except for Turning fruit (Figure 3C). Seedlings from the WT and transgenic genotypes showed CBF1 upregulation after 12 or 24 hours of storage at 0°C, compared to samples kept at room temperature. In WT seedlings, CBF1 expression peaked after 24 h, showing a 936.4-fold increase, while Sh-13 and Sl-2 reached 41.4- and 15.2-fold increase, respectively (Supplementary Figure S1).
3.2 Cold storage induced transgene upregulation in fruit
Expression of CBF1 was assessed in two ways. First, CBF1 transcripts were monitored during fruit storage to determine potential changes over time (Supplementary Figure S2). Secondly, CBF1 transcripts were monitored at each timepoint in the engineered fruit and compared to that in WT, to determine upregulation due to the transgene. (Figure 4). Due to ShCBF1 and SlCBF1 sequence similarity, ShCBF1 transcript accumulation was determined by measuring the total CBF expression in lines Sh-13 and Sh-36. In Sh-13 and Sh-36, total CBF1 expression was stable over storage (Supplementary Figures S2C, S2E). Relative to WT fruit, transcripts were upregulated at all time points in Sh-36 (Figure 4A).
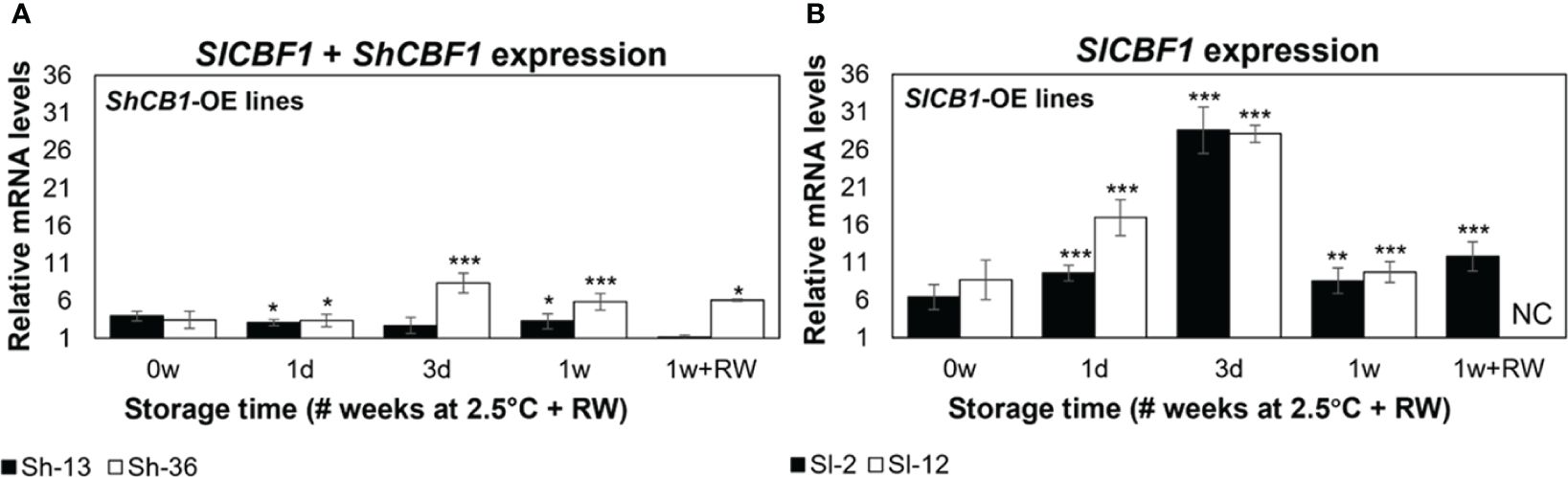
Figure 4 Relative CBF1 expression of transgenic fruit. Columns are the mean ± SE of breaker fruit stored at 2.5°C for up to 1 week, or followed by transfer to 20°C for 3 days (‘1w+RW’). (A) Expression of SlCBF1 and ShCBF1 in ShCBF1-OE lines. Due toSlCBF1 and ShCBF1 sequency similarity, total CBF1 expression was measured by designing primers to a conserved region between both genes. (B) SlCBF1 expression in SlCBF1-OE lines. Transcript accumulation was determined by quantifying both native and transgenic expression of this gene. ‘NC’ not calculated. WT fruit at each time point were used as the calibrator. Columns with asterisks are significantly different (p< 0.05) to the WT fruit at each time point by unpaired t-test.
SlCBF1-OE lines (Sl-2 and Sl-12) were developed to increase the number of transcripts of the native CBF1 gene. In these lines, transcript abundance decreased after one week of storage (Supplementary Figures S2D, S2F). Relative to freshly harvested fruit, CBF1 expression was upregulated at all time points in both lines, increasing from day 1, peaking at day 3, and declining after one week of cold exposure (Figure 4B).
In the ShCBF1-OE (Figure 4A) and SlCBF1-OE lines (Figure 4B), transcript accumulation did not change at 0w compared to the WT. Expression of SlCBF1 in the WT did not vary after one day of storage (Supplementary Figures S2A, S2B).
3.3 Deviation in color development between CBF1-OE lines and WT fruit
Fruit from wild-type and transgenic lines presented different objective color values during cold storage and after rewarming (Figure 5).
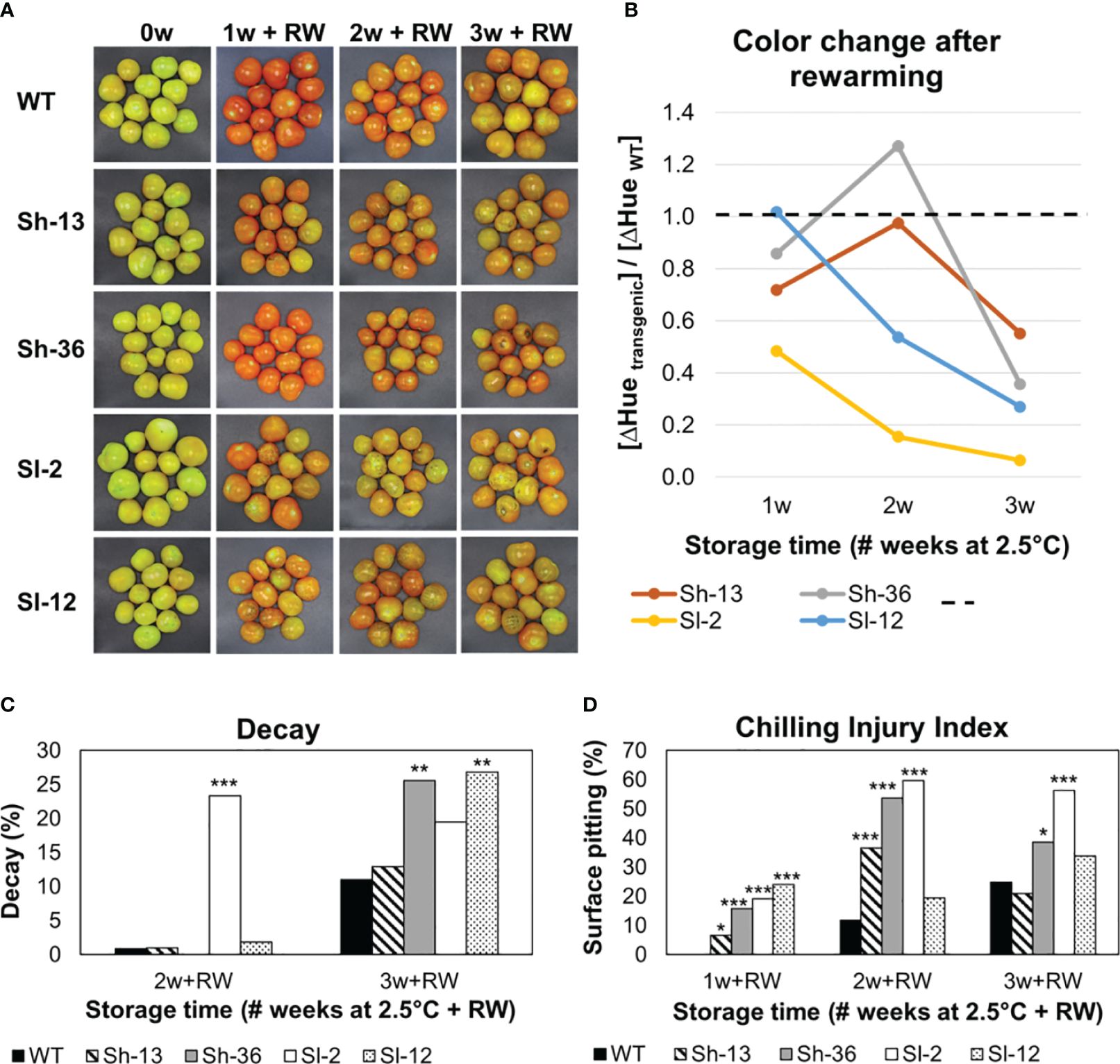
Figure 5 External appearance of cold-stored WT and transgenic tomato fruit. Breaker fruit were stored at 2.5°C for up to 3 weeks. After 1, 2 or 3 weeks of storage, fruit were rewarmed (RW). (A) Visual color changes. (B) Effect of RW on objective color change: the ratio of ΔHue in the transgenic vs. WT lines. ΔHue was calculated as the difference between the average of 1, 2 or 3 weeks of cold storage, and the same time followed by RW. WT fruit is represented by the dashed line. Values below 1 indicate that fruit did not redden to the same extent as the WT after rewarming. In contrast, fruit that turned as red or redder than the WT will present values equal to or greater than 1, respectively. (C) Decay %. (D) Chilling injury index. Scores were determined after RW. Columns with asterisks are significantly different (p< 0.05) to WT fruit by unpaired t-test.
Cold storage suppressed the green-to-red transition in all fruit (Figure 5A). Rewarming normally elicits fruit-reddening, but remarkably, reddening during rewarming was attenuated in the transgenic lines relative to the wild-type fruit as shown in Figure 5B.
3.4 Development of PCI symptoms was aggravated in transgenic fruit
Fruit were cold-stored and transferred to 20°C for three additional days to induce PCI. The presence of surface lesions or pitting (Chilling Injury Index, CII) and deterioration in the form of decay, were recorded. Wild-type fruit consistently had the lowest CII scores among all genotypes, maintaining levels below 30% after 2 or 3 weeks of cold storage, and rewarming (Figure 5A). In contrast, transgenic fruit developed pits after just one week at 2.5°C, especially in Sh-36 and Sl-2. There was a slight decrease in CII between weeks 2 and 3, which was linked to the pitted lesions becoming ‘swollen’ in appearance (Supplementary Figure S3G).
The highest incidence of decay was observed in Sh-36, Sl-2, and Sl-12 (Figure 5B). A myriad of symptoms was recorded, i.e., severe discoloration, wrinkles around the stem scar, and surface ‘translucency’ (Supplementary Figure S3), but they were absent or minimized in the WT.
Degradation of RNA samples obtained from the transgenic fruit was observed and verified during agarose gel electrophoresis (data not shown) and may be connected to the phenotypic deterioration induced by CBF1 overexpression.
Based on their contrasting PCI phenotype, further analyses were conducted on Sl-2 and Sh-13 fruit in addition to the WT. The total soluble solids (TSS) of the transgenic fruit was consistently lower than non-transgenic samples throughout cold storage, as well as after rewarming (Figure 6).
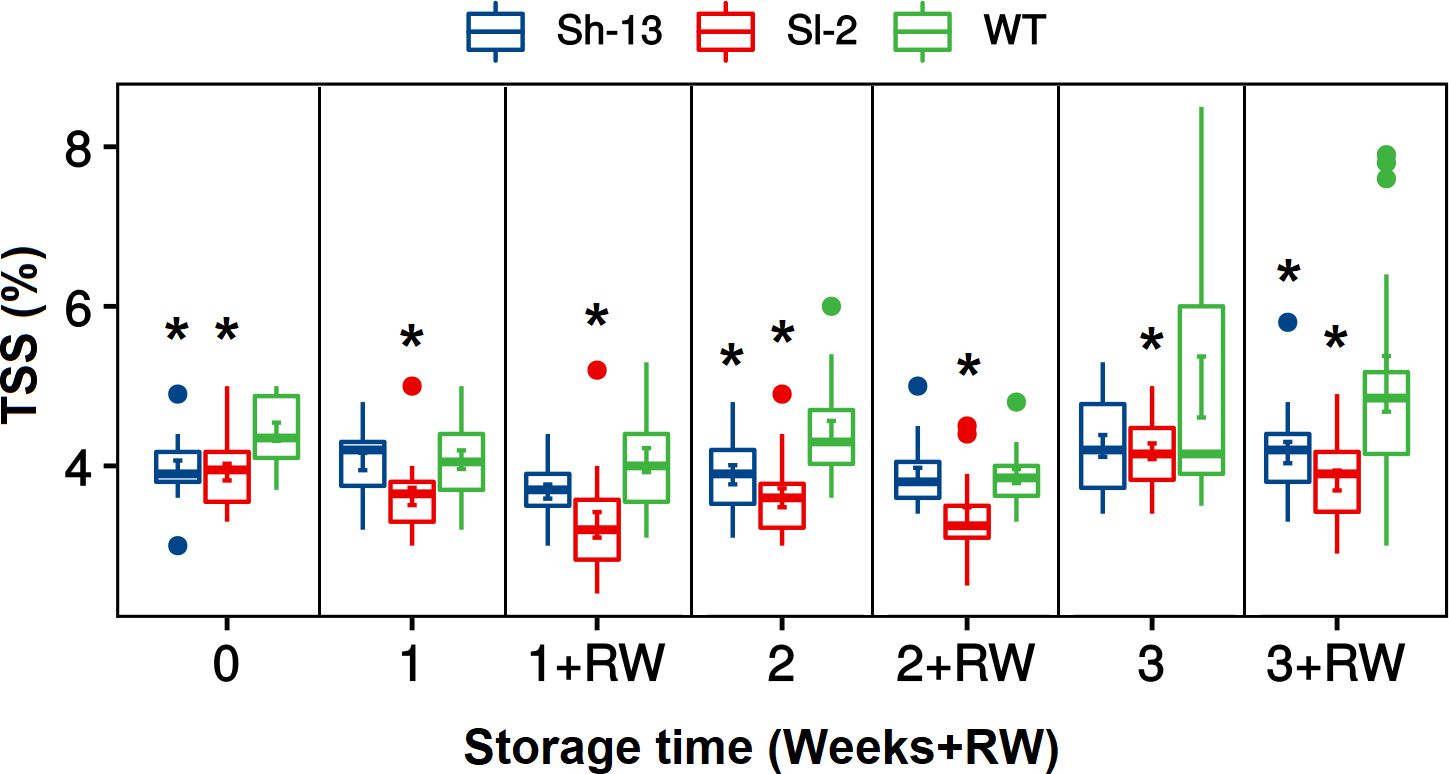
Figure 6 TSS changes of CBF1-overexpression lines (Sh-13 and Sl-2) and WT fruit. Breaker fruit were stored at 2.5°C for 7, 14 or 21 days, or rewarmed for three days. Boxes with asterisks are significantly different (p< 0.05) than WT fruit at the same time point by Dunnett’s test.
Respiration rates were significantly higher in Sh-13 compared to non-transgenic fruit throughout cold storage, except for day 4 (Figure 7A). However, after rewarming all three genotypes were the same. Interestingly, CO2 emission in Sh-13 only increased 512.8% which was lower than in Sl-2 (623.5%) and WT (744%) fruit.
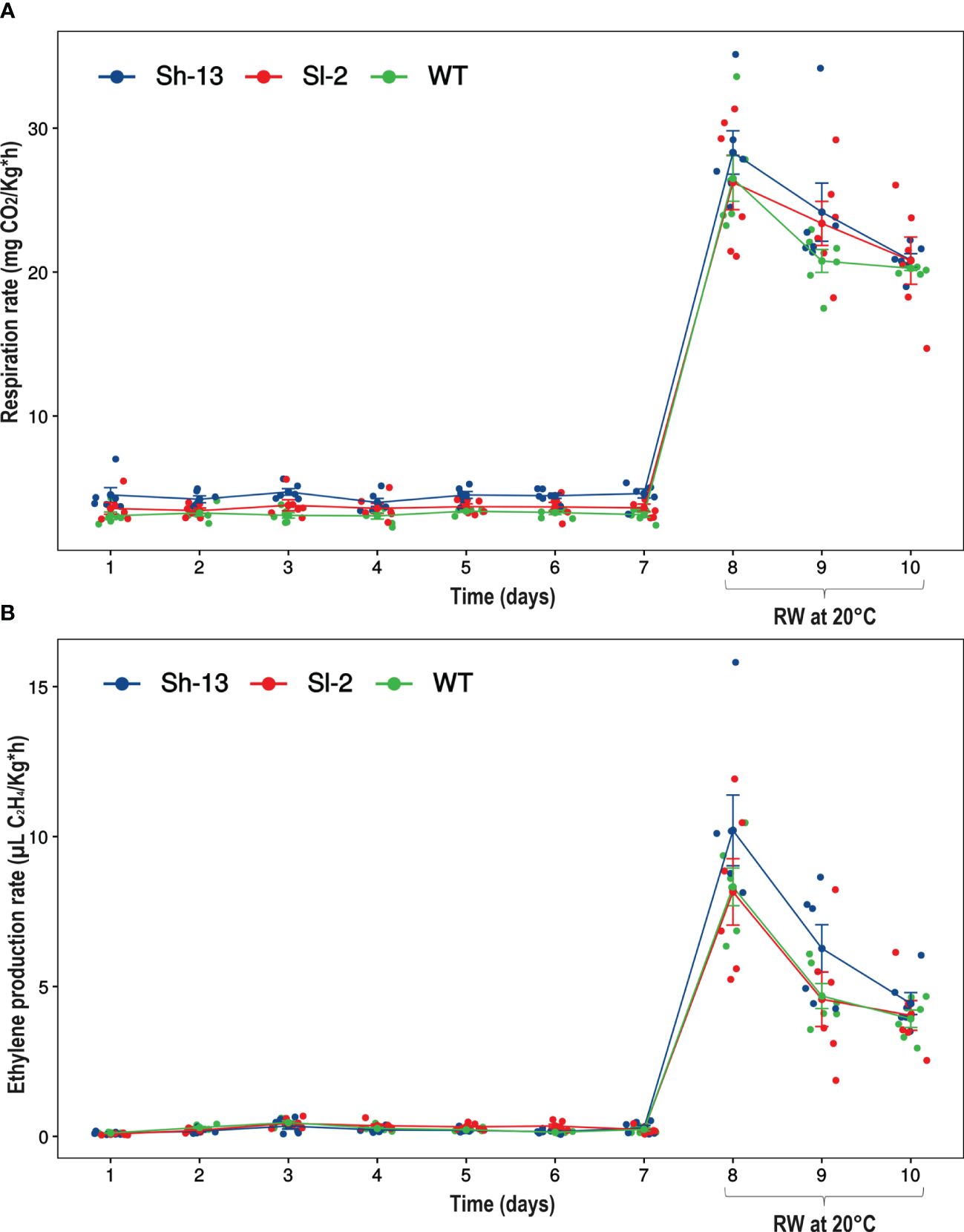
Figure 7 Gas production rates of CBF1-overexpression lines (Sh-13 and Sl-2) and WT fruit. (A) Respiration rate. (B) Ethylene production rate. Breaker fruit were stored at 2.5°C for up to 7 days, and then rewarmed for three days. Each point represents the mean ± SE.
Ethylene emission rates were similar between transgenic lines and the WT, with changes on days 2 and 6. After transfer to 20°C, values increased ~3,500% (Figure 7B).
3.5 Volatile compounds
The level of volatile organic compounds (VOCs) in Sh-13, Sl-2, and WT fruit chilled at 2.5°C for 2 weeks followed by RW was determined by solid phase microextraction (SPME) coupled with gas chromatography–mass spectrometry (GC-MS). A total of 34 VOCs were identified from the fruit headspace (Supplementary Table S6; Supplementary Figure S7). Principal component analysis (PCA) revealed 52.8% and 15.3% of the variance was explained by the first and second principal components, respectively. Samples from the transgenic fruit did not separate from those of the WT (Figure 8A). However, transgenic fruit had a lower relative abundance of the monoterpenes α- terpineol, 3,7-dimethyl-1,6-octadien-3-ol, and β- damascenone, higher levels of the fatty acid 3-methylbutanoic acid (Figure 8B).
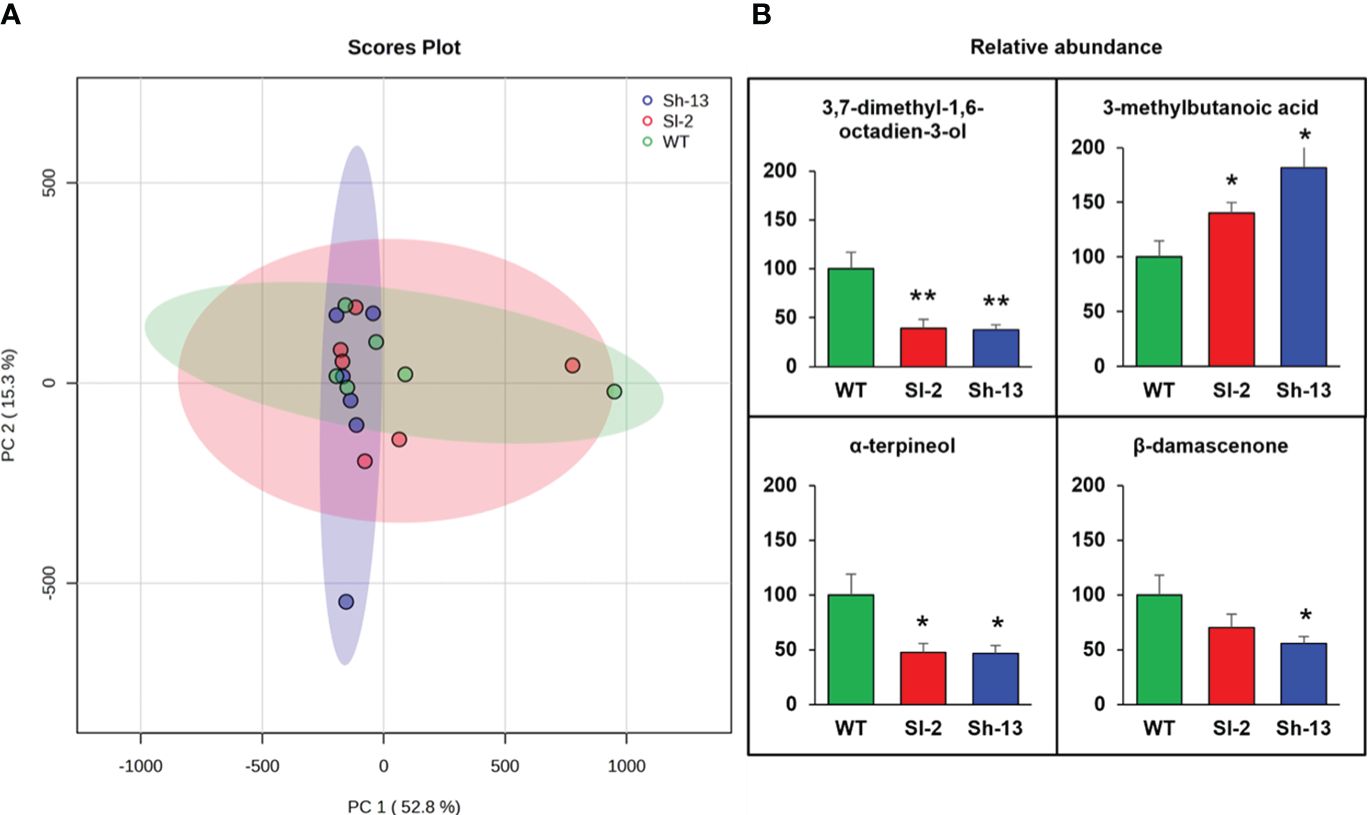
Figure 8 Volatile compounds assayed in the CBF1-overexpression lines (Sh-13 and Sl-2) and WT fruit. Breaker fruit were stored at 2.5°C for 14 days, and then rewarmed for three days. (A) PCA generated from SPME/GC-MS analysis of volatile compounds. (B) Relative abundance of volatile compounds with statistical significance across genotypes. For each compound, average levels of WT were arbitrarily set to 100 for comparison. Bars are the mean ± SE of fruit. Columns with asterisks are significantly different (p< 0.05) than WT fruit by t-test.
3.6 Transcriptomic analysis by RNA-Seq
Differentially expressed genes (DEGs) in the Sh-13, Sl-2 transgenic fruit vs. the WT after storage at 2.5°C for either 6 hours or 1 week were identified. The data from the WT fruit were compared against the pooled data of the transgenics.
After 6 hours and 1 week of cold storage, 5,169 genes were induced collectively in the transgenics, whereas 5,007 genes were repressed (Figure 9A). In Sh-13 and Sl-2 fruit, a range of 5,832-7,141 DEGs were shared by both genotypes in the cold (Supplementary Figure S8). The similarity of these results strengthened the justification for treating the combined results from the CBF1-OE lines as biological replicates of the same genotype. Among the greatest upregulated annotated genes were ARGINASE2 (ARG2), aquaporins PIP1-7 and PIP2-1, protein LURP-one-related 14-like, and Cytokinin riboside 5’-monophosphate phosphoribohydrolase LONELY GUY 1 (TLOG1). As for the downregulated genes, AT-hook motif nuclear-localized protein 17 (AHL17), RIPENING INHIBITOR (RIN), Ripening regulated protein DDTFR18, Acyl-CoA-binding domain-containing protein 3 (ACBD3), Vestitone reductase-like, Expansin-like B1 (EXLB1) and a chloroplastic Protein CutA were identified (Figure 9B; Supplementary Table S7).
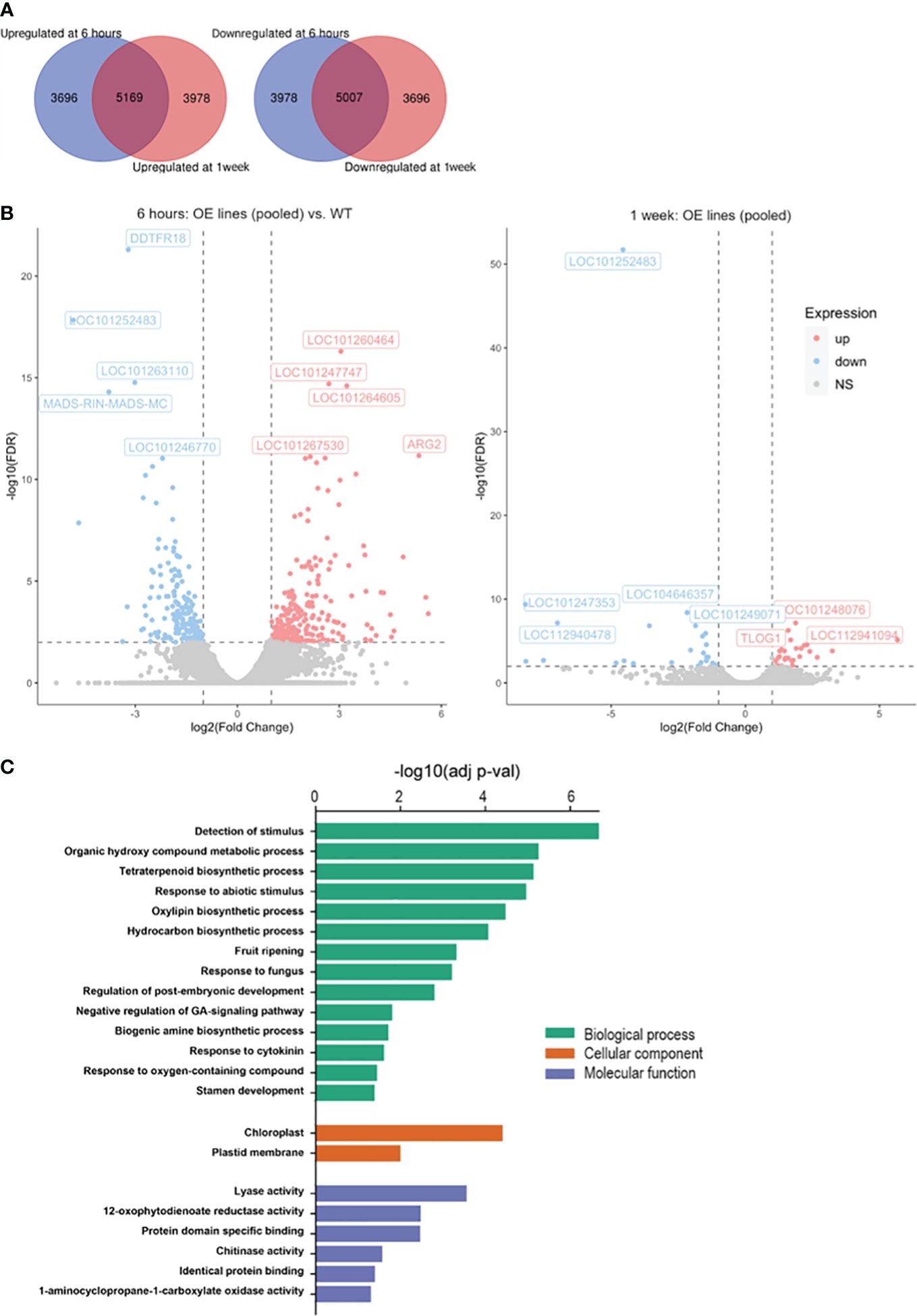
Figure 9 RNA-Seq data of CBF1-overexpression lines (Sh-13 and Sl-2) and WT fruit. Breaker fruit were stored at 2.5°C for 6 hours or 1 week. (A) Venn diagrams representing the up- (left) or downregulated (right) genes of pooled Sh-13 and Sl-2 samples in relation to WT fruit. (B) Volcano plot representing the highest up- or downregulated genes in pooled transgenic samples (OE lines) compared to WT fruit after 6 h (left) or 1 w (right) of cold storage. FDR: False discovery rate; NS: non-significant. FDR< 0.01, fold change > 2. (C) Gene ontology enrichment analysis indicating biological processes (BP), cellular component (CC), and molecular function of differentially expressed genes (DEGs) in pooled transgenic samples compared to WT fruit. Adjusted p-value<0.05. Analysis was carried out using the g:Profiler online tool, and S. lycopersicum as a reference organism.
To identify the most relevant gene groups affected by CBF overexpression in cold stored fruit, gene ontology (GO) enrichment analysis was conducted. Some of the biological processes altered include the detection and response to abiotic stimulus and fungus, and fruit ripening. Among the cellular compartments involved were the chloroplast and plastid membrane, whereas lyase and chitinase activities, and the ethylene biosynthesis pathway were shown as modulated molecular functions in CBF1-OE fruit compared to the WT (Figure 9C).
3.7 Fruit chilling affected wild-type seed and seedling features
To develop a more holistic and integrative view of chilling injury in the context of a continuum between fruit and progeny responses to cold stress, we explored if fruit cold response would influence the germination of tomato seeds derived from those fruit. This information would allow us to establish a baseline to assess the vegetative cold tolerance of the CBF1-OE lines.
WT seeds were collected from wild-type fruit at breaker stage, induced to PCI (‘chilled’), or kept at room temperature (‘non-chilled’). The L* value, assesses sample brightness and was lower (darker) in chilled compared to non-chilled seeds (Figure 10A). Seed weight was higher (p > 0.05) in chilled (3.9 ± 0.2 mg/seed) compared to non-chilled (2.8 ± 0.4 mg/seed) seeds.
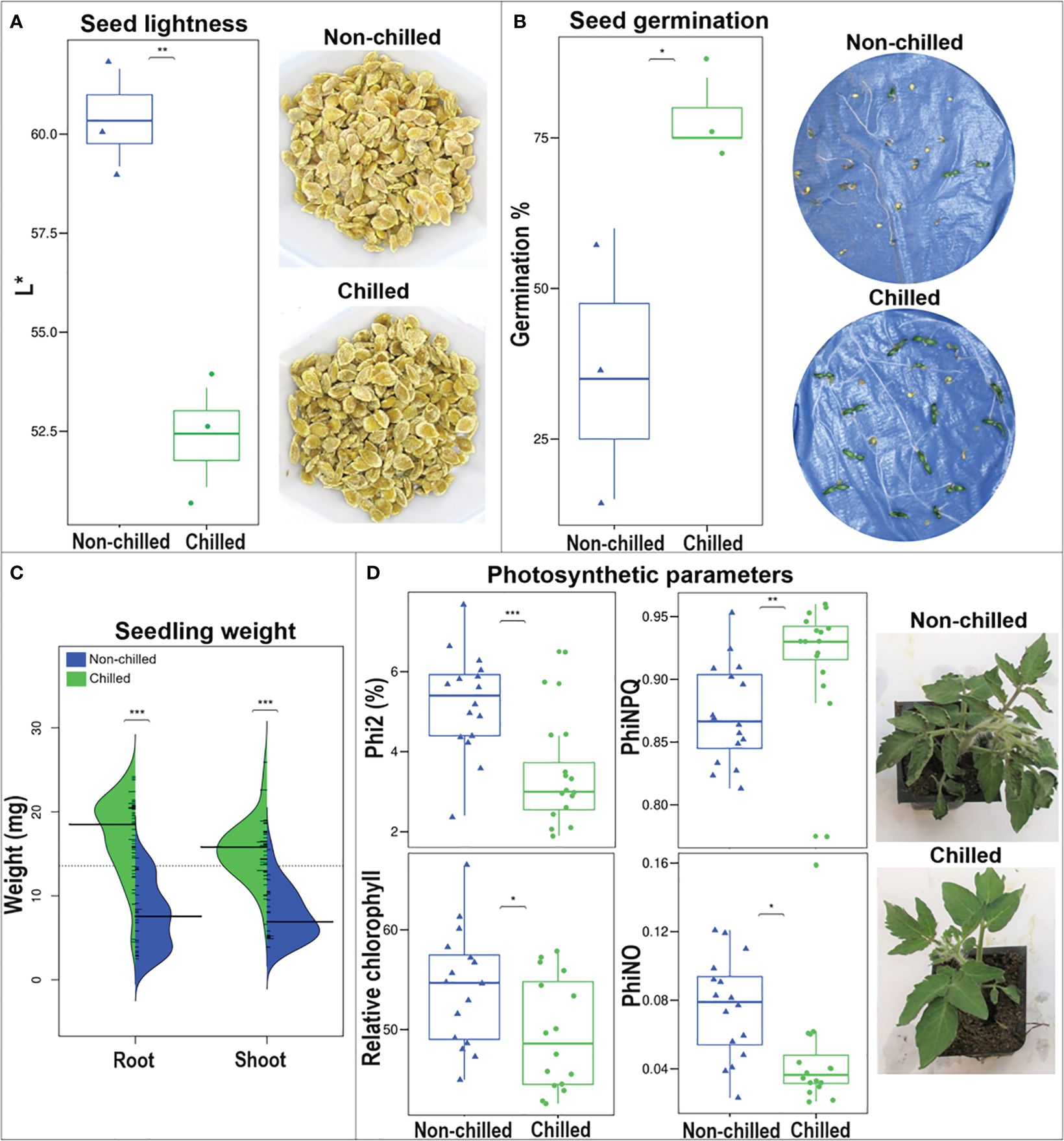
Figure 10 Seed and seedling physiological parameters of WT tomato. Seeds were collected from ‘non-chilled’ and ‘chilled’ fruit. (A) L* value box plot and seed appearance. (B) Germination percentage box plot after 7 d at 20°C. (C) Shoot and root fresh weight violin plot after 1 week at 20°C and seedling appearance after 5 days at 20°C. (D) Photosynthetic performance and appearance of plants after 72 hours at 0°C. Asterisks indicate significant difference (p< 0.05) by unpaired t-test.
A higher percentage of chilled seeds germinated (78.3%) compared to the non-chilled samples (36.7%) (Figure 10B), and the fresh mass of ‘chilled’ hypocotyls (shoot) and roots was also superior after 7 days of germination at room temperature (Figure 10C).
3.8 Photosynthetic performance of wild-type seedlings was affected by cold stress
Seedlings at the six-leaf stage, obtained from WT chilled and non-chilled fruit, were stored at 2.5 or 0°C for up to 72 hours, and their photosynthetic performance was monitored every 24 hours on the fourth leaf. No differences were detected between the 2.5°C and control seedlings (Supplementary Table S3), however, photosynthetic responses differed after storage at 0°C, and particularly after 72 hours (Figure 10D; Supplementary Table S4). Responses to cold were heterogeneous among parameters. Some were lower in chilled, compared to non-chilled seedlings: 1) quantum yield of PSII, or Phi2, which is the percentage of incoming light directed into PSII; 2) Linear Electron Flow (LEF) or the flow of electrons from antennae complexes into PSII; 3) PhiNO, a ratio of processes that inhibit photosynthesis, when absorbed light is not dissipated by non-photochemical quenching (Kuhlgert et al., 2016).
Two parameters related to Non-Photochemical Quenching (NPQ) were also recorded i.e., NPQt and PhiNPQ (Supplementary Table S4; Figure 10D). They denote the ratio of incoming light in terms of excess energy that is dissipated as heat (Müller et al., 2001). These two variables progressively increased in both ‘chilled’ and ‘non-chilled’ samples over the course of the experiment, however, after 72 hours, they were higher in ‘chilled’ compared to ‘non-chilled’ seedlings.
The relative chlorophyll content of seedlings was consistently higher in ‘non-chilled’ compared to ‘chilled’ seedlings after 24, 48, and 72 hours at 0°C, matching qualitative observations (Figure 10D). However, at 2.5°C, values did not differ (Supplementary Table S3). Overall, visual attributes of non-chilled seedlings were more affected by cold storage after three days than chilled samples. Wilting and leaf curling was accentuated in non-chilled seedlings.
3.9 Transgenic CBF1-OE lines had similar photosynthetic performance during cold storage
Of the engineered genotypes studied, Sl-2 and Sh-13 fruit had the highest and lowest incidence of PCI symptoms, respectively (Figure 5), and Sl-12 showed more aberrant vegetative growth compared to Sh-13 (Supplementary Figure S4). Therefore, Sl-2 and Sh-13 were selected to characterize their photosynthetic performance during cold storage at the seedling stage. The aim of this test was to determine if fruit and seedling response to cold stress were correlated, and it was instructive to choose lines with distinct PCI responses.
Seedlings from Sh-13 and Sl-2 lines at the 6th-leaf stage, obtained from non-chilled fruit, were exposed to 0°C for up to 72 hours. Photosynthetic parameters were monitored every 24 hours on the fourth leaf. LEF progressively decreased over time in both lines and reached the lowest level on day three, with Sl-2 being higher than Sh-13 (Supplementary Table S5). The rest of the parameters were similar between genotypes at all time points, but indicators of non-photochemical quenching were higher in Sh-13 (p > 0.05). Phenotypically, Sl-2, which showed the highest severity PCI symptoms in the fruit, had a greater incidence of leaf curling and wilting after cold-storage than Sh-13 (Supplementary Figure S5).
Absolute parallels between the responses of wild-type and transgenic seedlings cannot be established, due to differences in light intensity between experiments.
4 Discussion
4.1 The use of the RD29A promoter did not ameliorate pleiotropic effects due to CBF1 overexpression
Constitutive overexpression of AtCBF1 (Hsieh et al., 2002a, 2002), AtCBF3 (Zhang et al., 2004) or SlCBF1 (Zhang et al., 2004) in tomato increased abiotic stress tolerance, but at the cost of growth and development. The use of stress-inducible promoters yielded variable results. In ABRC1::AtCBF1 and RD29A::AtCBF1 plants, developmental abnormalities were absent, but it is unclear if growth chambers or greenhouse were used in these studies (Lee et al., 2003; Singh et al., 2011). Growth chamber-grown RD29A::AtCBF3 plants had a phenotype consistent with CBF overexpression (Hu et al., 2015), whereas dexamethasone-inducible lines overexpressing AtCBF1 did not manifest abnormalities during vegetative growth (Albornoz et al., 2023). In this study, we used RD29A as a cold-inducible promoter to preferentially overexpress ShCBF1 or SlCBF1 in harvested fruit under chilling, to minimize plant pleiotropic effects associated with constitutive overexpression. The RD29A promoter has been extensively studied in Arabidopsis and widely used in ectopic expression systems, including tomato (Singh et al., 2011), potato (Pino et al., 2007) and tobacco (Qiu et al., 2012). In this study, RD29A was selected as a promoter based on its responsiveness to cold stress, and the minimized leakiness reported by other authors.
Our plants were phenotypically abnormal, similar to transgenic lines constitutively expressing CBF1 (Hu et al., 2015). Therefore, our greenhouse led to variable, ‘stressful’ conditions, that activated RD29A and the transcription of CBF1 in the absence of an imposed, designed stress, i.e., cold. The RD29A promoter contains cis-elements that are responsive to various abiotic stresses involving changes in osmotic potential, low temperature, dehydration, salinity, and ABA application (Yamaguchi-Shinozaki and Shinozaki, 1994). In soybean, the activity of the transgenic RD29A promoter showed a strong induction with low soil moisture; however, under optimally-watered conditions, its activity decreased but it was not completely suppressed (Bihmidine et al., 2013). In petunia (Estrada-Melo et al., 2015), tobacco (Kasuga et al., 2004), Arabidopsis (Chen et al., 2010), and potato (Huynh et al., 2014), leaky induction of this promoter was also documented.
Leaky induction of CBF1 could be associated with changes at the whole plant level, unrelated to the phenomenon under study, i.e., cold stress response in fruit. Nevertheless, the postharvest responses of cold-stored fruit from the transgenic lines and independent transformation events confirmed that the phenotype was consistent across genotypes.
4.2 CBF1 overexpression aggravated PCI symptoms and altered VOCs in transgenic fruit
The CBF regulon in tomato plants constitutively overexpressing AtCBF3 or SlCBF1 (formerly LeCBF1), consisted of two dehydrins (with key roles in cryoprotection), and one putative proteinase inhibitor (Zhang et al., 2004). A higher synthesis of protective molecules may explain increases in cold tolerance due to CBF constitutive overexpression (Jaglo-Ottosen et al., 1998; Hsieh et al., 2002a).
We hypothesized that the induction of additional CBF1 transcripts in tomato fruit during postharvest chilling, would increase cold tolerance and ameliorate PCI symptoms due to increased synthesis of transcripts associated with protective proteins. Furthermore, the regulated overexpression of CBF1 would circumvent pleiotropic effects during plant growth and development.
We were able to induce CBF1 overexpression in fruit postharvest chilling, consistent with previous reports (Albornoz et al., 2023), thus validating the use of this regulated-expression approach. Expression of CBF1 peaked after three days of cold exposure relative to wild-type fruit: 8-fold in ShCBF1-OE, and 28-fold higher in SlCBF1-OE lines.
Remarkably, CBF1 overexpression aggravated PCI symptoms. Fruit were unable to complete the ripening process, and deterioration was accelerated when compared to wild-type fruit under the same conditions. Lines Sl-2, Sl-12 and Sh-36 had the highest incidence of pitting and decay, which correlated with CBF1 overexpression data. These observations corroborate the connection between CBF1 upregulation and worsening of PCI symptoms. Expression and phenotypic differences between transformation events of the same transgenic construct may be due to position effects (van Leeuwen et al., 2001), somaclonal variation due to chromosomal rearrangements during tissue culture (Manchanda et al., 2024), or transgene copy number variations. Insertion of multiple copies of a transgene could lead to silencing and hinder the interpretation of progeny segregation ratios (Collier et al., 2017). Previous reports altering the expression of CBFs in tomato fruit have revealed mixed results. The absence of a severe phenotype during fruit postharvest chilling of AtCBF1-overexpression lines may be due to the limited storage length used (Albornoz et al., 2023). On the other hand, fruit from slcbf1 mutants showed aggravated PCI symptoms after rewarming, compared to the wild-type (Yu et al., 2024).
Fruit decay susceptibility is commonly associated with pathogen accessibility to cell wall components, which is facilitated by ripening (Cantu et al., 2008). However, CBF1 transgenic fruit were ripening-impaired, yet had a higher incidence of decay. It is tempting to hypothesize that CBF1 regulates cell wall degradation via a ripening-independent pathway.
Higher decay and deterioration of transgenic fruit mirrored our volatile data. CBF1-overexpression fruit had a higher relative abundance of 3-methylbutanoic acid, which is associated with an unpleasant ‘cheesy’, ‘sweaty’ aroma in tomato pomace samples fermented by yeast, compared to unfermented samples (Bartoshuk and Klee, 2013; Güneser et al., 2015). Other compounds were less abundant in the transgenics than in WT fruit. α-terpineol is a defense VOC over-emitted in tomato plants that resisted a bacterial disease (López-Gresa et al., 2017), and it is perceived as a pine-like, woody aroma, valued in the flavors and fragrances industry (Sales et al., 2020). This correlates with the lower decay incidence observed in WT fruit. Synthesis of β-damascenone and 3,7-dimethyl-1,6-octadien-3-ol (linalool) was greater in ripe fruit (Mir et al., 2004; Rambla et al., 2014) and it is associated with the lower color development in our chilled transgenic samples compared to the WT.
The CBF family of transcription factors integrate environmental and hormonal signals, to promote plant survival under stresses (Kurepin et al., 2013). There is evidence of an interaction between ethylene biosynthesis and SlCBF1 expression in response to postharvest chilling stress, and, during ripening (Albornoz et al., 2019). Exogenous application of ethylene in addition to cold storage, led to SlCBF1 upregulation (Zhao et al., 2009b). Further, the suppression of ethylene biosynthesis was linked to lower SlCBF1 transcript accumulation and an increase in chilling sensitivity in tomato fruit (Yu et al., 2019). Our results are consistent with an increase in fruit susceptibility to chilling due to CBF1 upregulation, but they did not correlate with ethylene biosynthetic rates.
CBFs repress the cellular content of the hormone gibberellin (GA), and this may lead to pleiotropic effects seen in CBF1 transgenic lines. Lower GA levels have been shown to underscore the dwarfism observed in transgenic lines overexpressing CBF cloned from Arabidopsis (Achard et al., 2008; Zhou et al., 2017). This phenotype has also been observed in potato (Pino et al., 2008), rice (Lee et al., 2004; Moon et al., 2019), apple (Wisniewski et al., 2011), and in our transgenic plants. When we treated CBF1-OE seedlings with 5 µL L-1 GA they grew taller than water-treated samples but not to WT levels (results not shown). GAs may also influence fruit ripening, although the mechanism is not yet clear (Park and Malka, 2022). Mature green Micro-Tom fruit treated with exogenous GA ripened slower than the non-treated fruit (Li et al., 2019). In our lines, fruit GA levels were not assessed, however, fruit sensitivity and responsivity to altered GA may differ, causing differential responses in fruit ripening.
Based on our results, we propose that CBFs are involved in critical processes in plant growth and development. The higher rate of fruit deterioration and susceptibility to postharvest decay suggest CBFs are important regulators in fruit ripening and senescence under stress conditions. Further research should address its interplay with plant hormones and other key players in the cold stress response pathway.
4.3 Transcriptomic changes in CBF1-OE lines were consistent with phenotypic data
Lines Sh-13 (ShCBF1-OE) and Sl-2 (SlCBF1-OE) were selected for RNASeq analysis after short- (6 h) or long-(1 w) term cold storage as they developed similar PCI symptoms.
Upregulation of genes involved in abiotic and biotic stress response and hormone biosynthesis was detected after cold storage. ARGINASE2 (ARG2) is involved in arginine metabolism and has been linked to methyl jasmonate-induced defense responses to Botrytis cinerea (Min et al., 2020), and PCI tolerance in heat-shock exposed tomato fruit (Zhang et al., 2013). Modulation of aquaporin expression has been seen in tomato plants exposed to abiotic stresses, including salt, drought, and elevated carbon dioxide (Fang et al., 2019; Jia et al., 2020). The LURP1 gene is involved in biotic stress responses in Arabidopsis (Knoth and Eulgem, 2008; Bektas et al., 2016). Interestingly, TLOG1 codes for a cytokinin-activating enzyme associated with tuber formation in tomato plants overexpressing this gene (Eviatar-Ribak et al., 2013) and suggests that hormone homeostasis was compromised in our transgenic lines.
Other genes related to biotic and abiotic stress response, and developmental processes, were downregulated in CBF1-OE fruit in response to cold. For instance, AHL genes are positive regulators of cold tolerance in trifoliate orange (Dahro et al., 2022). Acyl-CoA-binding domain-containing proteins (ACBPs) and EXLB1 are involved in stress responses and ripening in tomato (Chatterjee et al., 2007), Arabidopsis (Xue et al., 2014), and apple (Chen et al., 2022). Vestitone reductase-like proteins participate in the biosynthesis of the defense compounds phytoalexins (Jeandet et al., 2013). Repression of these genes is consistent with the higher chilling sensitivity and impaired stress response presented by our transgenic fruit.
Downregulation of the transcription factor and master ripening regulator, RIN, (Li et al., 2020) can partly explain the inability of CBF1-OE fruit to resume ripening to WT levels after cold storage, and its early repression (6 h) suggests it may be a candidate for predicting PCI onset. Consistent with this, DDTFR18, with putative roles in ripening and ethylene response (Giovannoni et al., 1999) was also repressed. In WT Micro-Tom fruit, DDTFR18 was cold-induced (Weiss and Egea-Cortines, 2009), but ectopic CBF1 overexpression may have altered this response.
4.4 Accelerated fruit deterioration due to CBF1 overexpression may be advantageous for seed dispersal
Ripening is an early stage in the continuous process leading to senescence (Oeller et al., 1991; Klee and Giovannoni, 2011), and it may include changes in fruit color, firmness, and flavor. PCI compromises the normal transition between chloroplast and chromoplast during ripening (Gomez et al., 2009), even after transfer to warmer conditions (Albornoz et al., 2019). However, in the current study, CBF1 overexpression not only accentuated the inability of fruit to resume ripening after rewarming that was already observed in WT fruit, but it also increased susceptibility to decay, thus triggering accelerated deterioration and tissue disassembly.
Fruits evolved as vehicles for seed dispersal (Tanksley, 2004; Knapp and Litt, 2013). During ripening, a complex and dynamic set of changes that modify fruit appearance (Khudairi, 1972), firmness (Shackel et al., 1991), aroma (Zou et al., 2018) and flavor (Klee and Giovannoni, 2011) take place (Giovannoni, 2004). This reconfiguration makes the fruit more attractive for seed-dispersing animals, including humans, and is known as the dispersal syndrome (Herrera, 2002). As ripening progresses, fruit becomes more susceptible to pathogen infestation (Gapper et al., 2013; Alkan and Fortes, 2015). Since color development is terminated early in CBF1-overexpression lines, we propose that the aggravation of surface pitting, decay, and other symptoms of global fruit disassembly, might constitute a strategy to facilitate seed dispersal in absence of other traits normally elicited by ripening. Understanding the basis of fruit deterioration caused by PCI might help to develop solutions for maintaining fruit quality during refrigeration (Figure 11).
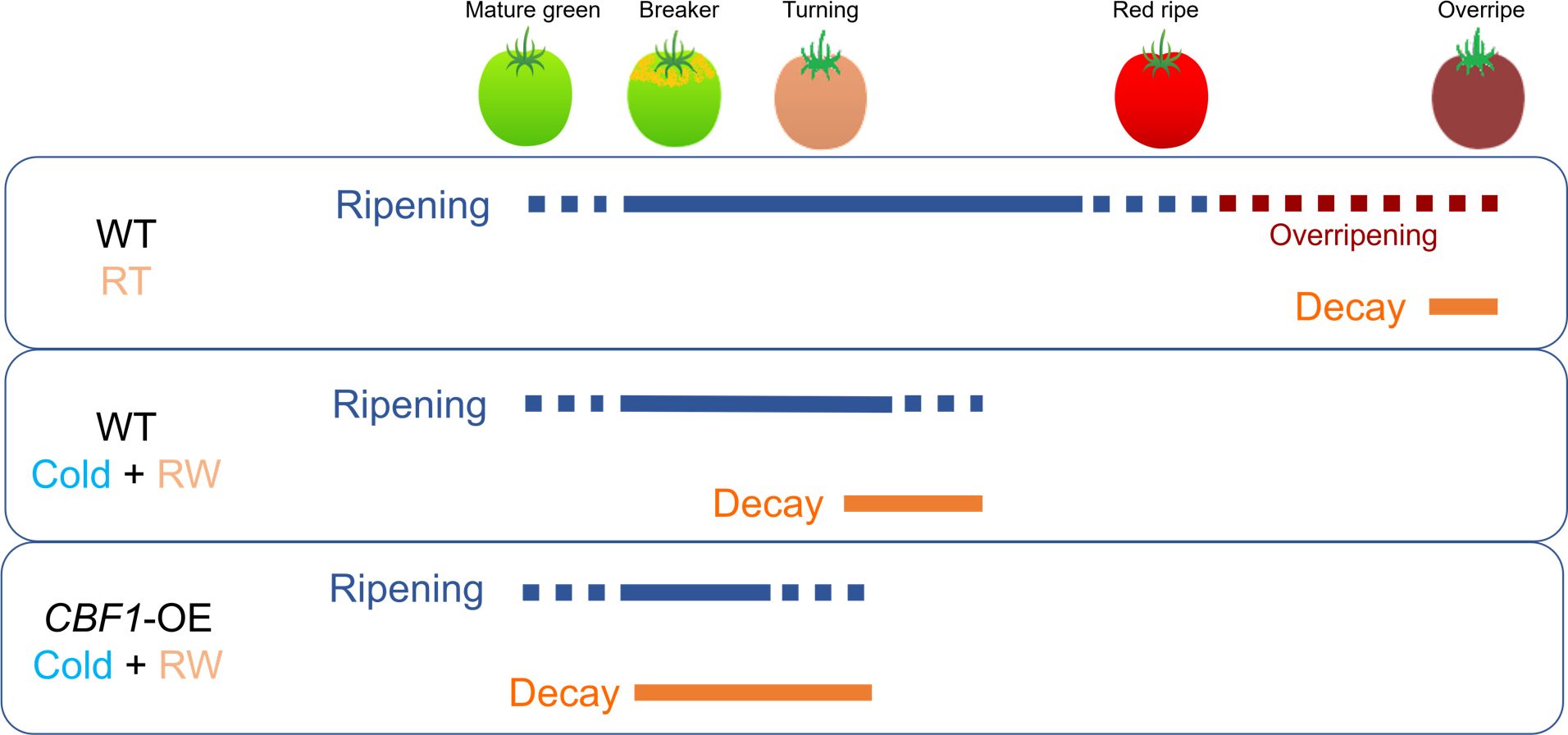
Figure 11 Schematic representation of ripening and decay processes in postharvest fruit at different stages. Shown are wild-type (‘WT’) and CBF1-overexpression (‘CBF1-OE’) lines. RT, room temperature; RW, rewarming of fruit at 20°C. The initiation and period over which decay occurs vary due to genotype, maturity stage at harvest, and environment.
4.5 Chilling in wild-type fruit improved progeny fitness under control and cold stress conditions
To explore a potentially advantageous role of PCI in the next plant generation, we collected seeds from WT fruit that were allowed to ripen at room temperature (‘non-chilled’), and from cold-stored fruit followed by RW (‘chilled’). Seed color and weight, seedling germination percentages and fresh weight were recorded at room temperature.
Cold storage caused seed browning and discoloration, triggered by cell decompartmentalization (Boonsiri et al., 2007; Massolo et al., 2011; Albornoz et al., 2019). The higher weight (p > 0.05) in chilled seeds could be associated with greater sugar translocation into the seeds during rewarming due to enhanced fruit respiration (Kitano et al., 1998). We observed similar enhanced respiration in fruit rewarmed after 2.5°C-storage compared to fruit at 12.5°C (Albornoz et al., 2019). Thus, chilled seeds could also have experienced higher sugar translocation from the fruit after rewarming.
Stratification is the process of disrupting seed dormancy by exposure to moist chilling conditions (Bentsink and Koornneef, 2008). Treatments like thermal hardening (Nawaz et al., 2009; Yari et al., 2012), can support our observations of enhanced germination percentage and seedling weight in samples from chilled fruit. These treatments alter hormonal signaling and also interact with mechanisms crucial to the perception of environmental cues (Bentsink and Koornneef, 2008).
In the presence of light, chilling stress in cold-sensitive species causes the desynchronization of antenna complexes and PSII (Garstka et al., 2007), causing photoinhibition and damage (Garstka et al., 2007; Knight and Knight, 2012; Onaga and Wydra, 2016). To better understand how cold acclimation of the seeds in fructa affects the cold tolerance of the resultant seedlings, we exposed WT ‘chilled’ and ‘non-chilled’ seedlings to 0°C for three days and compared their photosynthetic responses. We observed that seed chilling tolerance was enhanced when contained in a maternal organ (fruit), thus suggesting that an adaptive mechanism to cope with cold stress was transmitted across generations from the maternal tissue to the embryo. Evidence of chilling acclimation exists, but with the primary stress occurring on seedlings, not fruit-contained seeds (Zhou et al., 2012; Barrero-Gil et al., 2016). Photosynthetic results suggest 1) a reduction of the flow of energy and electrons directed towards PSII, as well as the proportion of photosynthetic inhibition due to excess energy, and relative chlorophyll content, and 2) an increase in mechanisms associated with non-photochemical quenching to minimize overexcitation. Chilling-acclimated seedlings may have reduced the amount of light used in photosynthesis to minimize damage, and reducing total chlorophyll is an adaptive mechanism to lower absorbed light (Barrero-Gil et al., 2016).
4.6 Transgenic fruit and seedling responses to chilling stress highlight the heterogeneity of CBF1 roles in different developmental stages
To gain insight into the response of CBF1-OE lines to cold stress during early development, seedlings from lines Sh-13 and Sl-2 were exposed to 0°C for three days. A trend towards higher light absorption (p < 0.05) and lower non-photochemical quenching (p > 0.05) was observed in Sl-2 compared to Sh-13. This revealed that Sl-2 experienced more photoinhibition and excitation pressure than Sh-13, suggesting that high levels of SlCBF1 transcript might have weakened the mechanisms against chilling injury in some vegetative tissues.
Compared to Sh-13, line Sl-2 exhibited higher ectopic CBF1 expression and PCI incidence in fruit, along with severe dwarfism and reduced photosynthetic performance in seedlings. Elevated CBF1 expression can be beneficial in vegetative tissues, but in excess, it may suppress the plant’s capacity to cope with cold stress. Further, CBF role in the cold response might be tissue-specific and controlled by different mechanisms. While it minimizes photoinhibition and oxidative damage in vegetative tissues, it enhances fruit senescence to facilitate seed dispersal.
5 Conclusions
Postharvest chilling injury (PCI) is a complex physiological disorder that leads to quantitative and qualitative losses. Knowledge of the CBF1 pathway in the cold-sensitive tomato and molecular mechanisms involved in the development of cold tolerance is needed to develop effective solutions; however, it is still limited compared with that in the cold-tolerant Arabidopsis.
In the current study, transgenic tomato lines overexpressing the CBF1 gene from Solanum habrochaites (ShCBF1) and cultivated tomato (SlCBF1) driven by the RD29A promoter, were generated. Ectopic CBF1 expression data by RT-qPCR confirmed its induction in fruit during cold storage and upregulation for up to one week. Transgenic fruit showed aggravation of PCI symptoms, failure to ripen normally, reduced soluble solids content, abnormal volatile profile and accelerated decay when compared with wild-type fruit, and this correlated with transgenic CBF1 expression. Transcriptomic data suggested that CBF1 overexpression in the transgenic lines led to alterations in the ripening process and biotic and abiotic stress responses compared to WT cold stored fruit. Wild-type seedlings originating from chilled fruit displayed signs of acclimation relative to non-chilled samples. Transgenic seedlings photosynthetic performance differed between genotypes, and additional studies are needed to determine their response to cold stress in relation to wild-type seedlings.
Data availability statement
The data presented in the study are deposited in the NCBI BioProject database, accession number PRJNA1088148.
Author contributions
KA: Writing – original draft, Visualization, Validation, Methodology, Investigation, Formal analysis, Writing – review & editing, Resources, Funding acquisition, Conceptualization. JZ: Writing – review & editing, Methodology, Investigation, Formal analysis. FZ: Methodology, Investigation, Formal analysis, Writing – review & editing. JG: Funding acquisition, Methodology, Investigation, Formal analysis, Writing – review & editing. MW: Writing – review & editing, Methodology, Investigation, Formal analysis. DB: Writing – original draft, Supervision, Resources, Project administration, Funding acquisition, Formal analysis, Conceptualization, Writing – review & editing.
Funding
The author(s) declare financial support was received for the research, authorship, and/or publication of this article. KA: Chilean National Agency for Research and Development (ANID), and the Horticulture and Agronomy Graduate Group at UC Davis for Ph.D. funding. The material described here are the basis for work supported by NIFA/USDA, under project number SC-1700674. JZ: China Scholarship Council and the Horticulture and Agronomy Graduate Group at UC Davis. JG: Provost Undergraduate Fellowship at UC Davis. DB: Hatch Project CA-D-PLS-2404-H and a UC Advance Fellowship.
Acknowledgments
We are grateful to Dr. David M. Tricoli and Bailey Van Bockern, who assisted with plant transformation and regeneration. We thank Esmeralda Segura, Gareth Gabb, and Megan Shih (UC Davis undergraduate interns), and Victor Johnson (UC Davis Young Scholar’s Program), who helped with postharvest characterization of fruit, RNA extraction, germination assays and measurement of photosynthetic parameters.
Conflict of interest
The authors declare that the research was conducted in the absence of any commercial or financial relationships that could be construed as a potential conflict of interest.
Publisher’s note
All claims expressed in this article are solely those of the authors and do not necessarily represent those of their affiliated organizations, or those of the publisher, the editors and the reviewers. Any product that may be evaluated in this article, or claim that may be made by its manufacturer, is not guaranteed or endorsed by the publisher.
Supplementary material
The Supplementary Material for this article can be found online at: https://www.frontiersin.org/articles/10.3389/fpls.2024.1429321/full#supplementary-material
References
Achard, P., Gong, F., Cheminant, S., Alioua, M., Hedden, P., Genschik, P. (2008). The cold-inducible CBF1 factor-dependent signaling pathway modulates the accumulation of the growth-repressing DELLA proteins via its effect on gibberellin metabolism. Plant Cell 20, 2117–2129. doi: 10.1105/tpc.108.058941
Aghdam, M. S., Asghari, M., Khorsandi, O., Mohayeji, M. (2014). Alleviation of postharvest chilling injury of tomato fruit by salicylic acid treatment. J. Food Sci. Tech Mys 51, 2815–2820. doi: 10.1007/s13197-012-0757-1
Albornoz, K., Cantwell, M. I., Zhang, L., Beckles, D. M. (2019). Integrative analysis of postharvest chilling injury in cherry tomato fruit reveals contrapuntal spatio-temporal responses to ripening and cold stress. Sci. Rep. 9, 1–14. doi: 10.1038/s41598-019-38877-0
Albornoz, K., Zhou, J., Beckles, D. M. (2023). Chemical induction of the Arabidopsis thaliana CBF1 gene in transgenic tomato fruit to study postharvest chilling injury. Curr. Plant Biol. 33, 100275. doi: 10.1016/j.cpb.2023.100275
Albornoz, K., Zhou, J. Q., Yu, J. W., Beckles, D. M. (2022). Dissecting postharvest chilling injury through biotechnology. Curr. Opin. Biotech. 78. doi: 10.1016/j.copbio.2022.102790
Alkan, N., Fortes, A. M. (2015). Insights into molecular and metabolic events associated with fruit response to post-harvest fungal pathogens. Front. Plant Sci. 6, 889. doi: 10.3389/fpls.2015.00889
Andrews, S. (2010). FastQC: A Quality Control Tool for High Throughput Sequence Data [Online]. Available at: http://www.bioinformatics.babraham.ac.uk/projects/fastqc/.
Barrero-Gil, J., Huertas, R., Rambla, J. L., Granell, A., Salinas, J. (2016). Tomato plants increase their tolerance to low temperature in a chilling acclimation process entailing comprehensive transcriptional and metabolic adjustments. Plant Cell Environ. 39, 2303–2318. doi: 10.1111/pce.12799
Bartoshuk, L. M., Klee, H. J. (2013). Better fruits and vegetables through sensory analysis. Curr. Biol. 23, R374–R378. doi: 10.1016/j.cub.2013.03.038
Bektas, Y., Rodriguez-Salus, M., Schroeder, M., Gomez, A., Kaloshian, I., Eulgem, T. (2016). The synthetic elicitor DPMP (2,4-dichloro-6-{(E)-[(3-methoxyphenyl)imino]methyl}phenol) triggers strong immunity in arabidopsis thaliana and tomato. Sci. Rep. 6, 29554. doi: 10.1038/srep29554
Bentsink, L., Koornneef, M. (2008). “Seed Dormancy and Germination,” in The Arabidopsis Book, vol. 6. doi: 10.1199/tab.0119
Bihmidine, S., Lin, J., Stone, J. M., Awada, T., Specht, J. E., Clemente, T. E. (2013). Activity of the Arabidopsis RD29A and RD29B promoter elements in soybean under water stress. Planta 237, 55–64. doi: 10.1007/s00425-012-1740-9
Biswas, P., East, A. R., Hewett, E. W., Heyes, J. A. (2016). Chilling injury in tomato fruit. Hortic. Rev. 44, 229–278. doi: 10.1002/9781119281269.ch5
Boonsiri, K., Ketsa, S., van Doorn, W. G. (2007). Seed browning of hot peppers during low temperature storage. Postharvest Biol. Technol. 45, 358–365. doi: 10.1016/j.postharvbio.2007.03.014
Brewer, M. T., Lang, L., Fujimura, K., Dujmovic, N., Gray, S., van der Knaap, E. (2006). Development of a controlled vocabulary and software application to analyze fruit shape variation in tomato and other plant species. Plant Physiol. 141, 15–25. doi: 10.1104/pp.106.077867
Cantu, D., Vicente, A., Greve, L., Dewey, F., Bennett, A., Labavitch, J., et al. (2008). The intersection between cell wall disassembly, ripening, and fruit susceptibility to Botrytis cinerea. Proc. Natl. Acad. Sci. 105, 859–864. doi: 10.1073/pnas.0709813105
Cao, X., Wu, Z., Jiang, F., Zhou, R., Yang, Z. (2014). Identification of chilling stress-responsive tomato microRNAs and their target genes by high-throughput sequencing and degradome analysis. BMC Genomics 15, 1130. doi: 10.1186/1471-2164-15-1130
Chatterjee, A., Cui, Y., Hasegawa, H., Chatterjee, A. K. (2007). PsrA, thePseudomonas Sigma Regulator, Controls Regulators of Epiphytic Fitness, Quorum-Sensing Signals, and Plant Interactions inPseudomonas syringae pv. tomato Strain DC3000. Appl. Environ. Microbiol. 73, 3684–3694. doi: 10.1128/AEM.02445-06
Chen, Y.-h., Xie, B., An, X.-h., Ma, R.-p., Zhao, D.-y., Cheng, C.-g., et al. (2022). Overexpression of the apple expansin-like gene MdEXLB1 accelerates the softening of fruit texture in tomato. J. Integr. Agric. 21, 3578–3588. doi: 10.1016/j.jia.2022.08.030
Chen, Q.-J., Xie, M., Ma, X.-X., Dong, L., Chen, J., Wang, X.-C. (2010). MISSA is a highly efficient in vivo DNA assembly method for plant multiple-gene transformation. Plant Physiol. 153, 41–51. doi: 10.1104/pp.109.152249
Collier, R., Dasgupta, K., Xing, Y. P., Hernandez, B. T., Shao, M., Rohozinski, D., et al. (2017). Accurate measurement of transgene copy number in crop plants using droplet digital PCR. Plant J. 90, 1014–1025. doi: 10.1111/tpj.13517
Dahro, B., Wang, Y., Khan, M., Zhang, Y., Fang, T., Ming, R., et al. (2022). Two AT-Hook proteins regulate A/NINV7 expression to modulate sucrose catabolism for cold tolerance in Poncirus trifoliata. New Phytol. 235, 2331–2349. doi: 10.1111/nph.18304
Dobin, A., Davis, C. A., Schlesinger, F., Drenkow, J., Zaleski, C., Jha, S., et al. (2013). STAR: ultrafast universal RNA-seq aligner. Bioinformatics 29 (1), 15–21. doi: 10.1093/bioinformatics/bts635
Estrada-Melo, A. C., Reid, M. S., Jiang, C.-Z. (2015). Overexpression of an ABA biosynthesis gene using a stress-inducible promoter enhances drought resistance in petunia. Horticulture Res. 2, 15013. doi: 10.1038/hortres.2015.13
Eviatar-Ribak, T., Shalit-Kaneh, A., Chappell-Maor, L., Amsellem, Z., Eshed, Y., Lifschitz, E. (2013). A cytokinin-activating enzyme promotes tuber formation in tomato. Curr. Biol. 23, 1057–1064. doi: 10.1016/j.cub.2013.04.061
Fang, L., Abdelhakim, L. O. A., Hegelund, J. N., Li, S., Liu, J., Peng, X., et al. (2019). ABA-mediated regulation of leaf and root hydraulic conductance in tomato grown at elevated CO2 is associated with altered gene expression of aquaporins. Horticulture Res. 6, 104. doi: 10.1038/s41438-019-0187-6
Fulton, T. M., Chunwongse, J., Tanksley, S. D. (1995). Microprep protocol for extraction of DNA from tomato and other herbaceous plants. Plant Mol. Biol. Rep. 13, 207–209. doi: 10.1007/BF02670897
Gapper, N. E., McQuinn, R. P., Giovannoni, J. J. (2013). Molecular and genetic regulation of fruit ripening. Plant Mol. Biol. 82, 575–591. doi: 10.1007/s11103-013-0050-3
Garstka, M., Venema, J. H., Rumak, I., Gieczewska, K., Rosiak, M., Koziol-Lipinska, J., et al. (2007). Contrasting effect of dark-chilling on chloroplast structure and arrangement of chlorophyll–protein complexes in pea and tomato: plants with a different susceptibility to non-freezing temperature. Planta 226, 1165. doi: 10.1007/s00425-007-0562-7
Gilmour, S. J., Hajela, R. K., Thomashow, M. F. (1988). Cold acclimation in Arabidopsis thaliana. Plant Physiol. 87, 745–750. doi: 10.1104/pp.87.3.745
Giovannoni, J. J. (2004). Genetic regulation of fruit development and ripening. Plant Cell 16, S170–S180. doi: 10.1105/tpc.019158
Giovannoni, J., Yen, H., Shelton, B., Miller, S., Vrebalov, J., Kannan, P., et al. (1999). Genetic mapping of ripening and ethylene-related loci in tomato. Theor. Appl. Genet. 98, 1005–1013. doi: 10.1007/s001220051161
Gomez, P., Ferrer, M. A., Fernandez-Trujillo, J. P., Calderon, A., Artes, F., Egea-Cortines, M., et al. (2009). Structural changes, chemical composition and antioxidant activity of cherry tomato fruits (cv. Micro-Tom) stored under optimal and chilling conditions. J. Sci. Food Agr 89, 1543–1551. doi: 10.1002/jsfa.3622
Goodstal, F. J., Kohler, G. R., Randall, L. B., Bloom, A. J., Clair, D. A. S. (2005). A major QTL introgressed from wild Lycopersicon hirsutum confers chilling tolerance to cultivated tomato (Lycopersicon esculentum). Theor. Appl. Genet. 111, 898–905. doi: 10.1007/s00122-005-0015-2
Güneser, O., Demirkol, A., Yüceer, Y. K., Togay, S.Ö., Hosoglu, M. I., Elibol, M. (2015). Bioflavour production from tomato and pepper pomaces by Kluyveromyces marxianus and Debaryomyces hansenii. Bioproc Biosyst. Eng. 38, 1143–1155. doi: 10.1007/s00449-015-1356-0
Herrera, C. M. (2002). Seed dispersal by vertebrates. In Plant–animal interactions: an evolutionary approach. (Wiley-Blackwell, Hoboken, New Jersey, United States: Blackwell Science).
Hsieh, T.-H., Lee, J.-T., Charng, Y.-Y., Chan, M.-T. (2002a). Tomato plants ectopically expressing Arabidopsis CBF1 show enhanced resistance to water deficit stress. Plant Physiol. 130, 618–626. doi: 10.1104/pp.006783
Hsieh, T.-H., Lee, J.-T., Yang, P.-T., Chiu, L.-H., Charng, Y.-y., Wang, Y.-C., et al. (2002b). Heterology expression of the arabidopsisC-repeat/dehydration response element binding factor 1 gene confers elevated tolerance to chilling and oxidative stresses in transgenic tomato. Plant Physiol. 129, 1086–1094. doi: 10.1104/pp.003442
Hu, Y., Wu, Q., Sprague, S. A., Park, J., Oh, M., Rajashekar, C., et al. (2015). Tomato expressing Arabidopsis glutaredoxin gene AtGRXS17 confers tolerance to chilling stress via modulating cold responsive components. Horticulture Res. 2, 15051. doi: 10.1038/hortres.2015.51
Huynh, H. D., Shimazaki, T., Kasuga, M., Yamaguchi-Shinozaki, K., Kikuchi, A., Watanabe, K. N. (2014). In vitro evaluation of dehydration tolerance in AtDREB1A transgenic potatoes. Plant Biotechnol. 31, 77–81. doi: 10.5511/plantbiotechnology.13.1208a
Jackman, R. L., Yada, R. Y., Marangoni, A., Parkin, K. L., Stanley, D. W. (1989). Chilling injury - A review of quality aspects. J. Food Qual. 11, 253–278. doi: 10.1111/j.1745-4557.1988.tb00887.x
Jaglo-Ottosen, K. R., Gilmour, S. J., Zarka, D. G., Schabenberger, O., Thomashow, M. F. (1998). Arabidopsis CBF1 overexpression induces COR genes and enhances freezing tolerance. Science 280, 104–106. doi: 10.1126/science.280.5360.104
Jeandet, P., Clément, C., Courot, E., Cordelier, S. (2013). Modulation of phytoalexin biosynthesis in engineered plants for disease resistance. Int. J. Mol. Sci. 14, 14136–14170. doi: 10.3390/ijms140714136
Jia, J., Liang, Y., Gou, T., Hu, Y., Zhu, Y., Huo, H., et al. (2020). The expression response of plasma membrane aquaporins to salt stress in tomato plants. Environ. Exp. Bot. 178, 104190. doi: 10.1016/j.envexpbot.2020.104190
Kader, A. (2002). “Postharvest Biology and Technology: An Overview,” in Postharvest Technology for Horticultural Crops. Ed. Kader, A. (UCANR, Oakland, CA), 39–47.
Kasuga, M., Miura, S., Shinozaki, K., Yamaguchi-Shinozaki, K. (2004). A combination of the Arabidopsis DREB1A gene and stress-inducible rd29A promoter improved drought-and low-temperature stress tolerance in tobacco by gene transfer. Plant Cell Physiol. 45, 346–350. doi: 10.1093/pcp/pch037
Khudairi, A. K. (1972). The ripening of tomatoes: A molecular ecological approach to the physiology of fruit ripening. Am. Scientist 60, 696–707. Available at: https://www.jstor.org/stable/27843462.
Kitano, M., Araki, T., Eguchi, H. (1998). Temperature dependence of postphloem transport regulated by respiration in tomato fruits. Biotronics 27, 33–39.
Klee, H. J., Giovannoni, J. J. (2011). Genetics and control of tomato fruit ripening and quality attributes. Annu. Rev. Genet. 45, 41–59. doi: 10.1146/annurev-genet-110410-132507
Knapp, S., Litt, A. (2013). “Fruit-an angiosperm innovation,” in The Molecular Biology and Biochemistry of Fruit Ripening (Hoboken, New Jersey, United States: John Wiley & Sons, Inc).
Knight, M. R., Knight, H. (2012). Low-temperature perception leading to gene expression and cold tolerance in higher plants. New Phytol. 195, 737–751. doi: 10.1111/j.1469-8137.2012.04239.x
Knoth, C., Eulgem, T. (2008). The oomycete response gene LURP1 is required for defense against Hyaloperonospora parasitica in Arabidopsis thaliana. Plant J. 55, 53–64. doi: 10.1111/j.1365-313X.2008.03486.x
Kuhlgert, S., Austic, G., Zegarac, R., Osei-Bonsu, I., Hoh, D., Chilvers, M. I., et al. (2016). MultispeQ Beta: a tool for large-scale plant phenotyping connected to the open PhotosynQ network. R. Soc. Open Sci. 3, 160592. doi: 10.1098/rsos.160592
Kurepin, L., Dahal, K., Savitch, L., Singh, J., Bode, R., Ivanov, A., et al. (2013). Role of CBFs as integrators of chloroplast redox, phytochrome and plant hormone signaling during cold acclimation. Int. J. Mol. Sci. 14, 12729–12763. doi: 10.3390/ijms140612729
Lee, S.-C., Huh, K.-W., An, K., An, G., Kim, S.-R. (2004). Ectopic expression of a cold-inducible transcription factor, CBF1/DREB1b, in transgenic rice (Oryza sativa L.). Molecules Cells 18. doi: 10.1016/S1016-8478(23)13088-3
Lee, J. T., Prasad, V., Yang, P. T., Wu, J. F., Ho, T. H. D., Charng, Y. Y., et al. (2003). Expression of Arabidopsis CBF1 regulated by an ABA/stress inducible promoter in transgenic tomato confers stress tolerance without affecting yield. Plant Cell Environ. 26, 1181–1190. doi: 10.1046/j.1365-3040.2003.01048.x
Li, H., Wu, H., Qi, Q., Li, H., Li, Z., Chen, S., et al. (2019). Gibberellins play a role in regulating tomato fruit ripening. Plant Cell Physiol. 60, 1619–1629. doi: 10.1093/pcp/pcz069
Li, Z., Zhang, L., Li, J., Xu, X., Yao, Q., Wang, A. (2014). Isolation and functional characterization of the ShCBF1 gene encoding a CRT/DRE-binding factor from the wild tomato species Solanum habrochaites. Plant Physiol. Biochem. 74, 294–303. doi: 10.1016/j.plaphy.2013.11.024
Li, S., Zhu, B., Pirrello, J., Xu, C., Zhang, B., Bouzayen, M., et al. (2020). Roles of RIN and ethylene in tomato fruit ripening and ripening-associated traits. New Phytol. 226, 460–475. doi: 10.1111/nph.16362
López-Gresa, M. P., Lisón, P., Campos, L., Rodrigo, I., Rambla, J. L., Granell, A., et al. (2017). A non-targeted metabolomics approach unravels the VOCs associated with the tomato immune response against. Front. Plant Sci. 8. doi: 10.3389/fpls.2017.01188
Love, M. I., Huber, W., Anders, S. (2014). Moderated estimation of fold change and dispersion for RNA-seq data with DESeq2. Genome Biol. 15, 1–21. doi: 10.1186/s13059-014-0550-8
Luengwilai, K., Beckles, D. M., Roessner, U., Dias, D. A., Lui, V., Siriphanich, J. (2018). Identification of physiological changes and key metabolites coincident with postharvest internal browning of pineapple (Ananas comosus L.) fruit. Postharvest Biol. Technol. 137, 56–65. doi: 10.1016/j.postharvbio.2017.11.013
Lyons, J. M. (1973). Chilling injury in plants. Annu. Rev. Plant Physiol. Plant Mol. Biol. 24, 445–466. doi: 10.1146/annurev.pp.24.060173.002305
Manchanda, P., Sharma, D., Kaur, G., Kaur, H., Vanshika (2024). Exploring the significance of somaclonal variations in horticultural crops. Mol. Biotechnol., 1–19. doi: 10.1007/s12033-024-01214-6
Massolo, J. F., Concellón, A., Chaves, A. R., Vicente, A. R. (2011). 1-Methylcyclopropene (1-MCP) delays senescence, maintains quality and reduces browning of non-climacteric eggplant (Solanum melongena L.) fruit. Postharvest Biol. Technol. 59, 10–15. doi: 10.1016/j.postharvbio.2010.08.007
Meissner, R., Jacobson, Y., Melamed, S., Levyatuv, S., Shalev, G., Ashri, A., et al. (1997). A new model system for tomato genetics. Plant J. 12, 1465–1472. doi: 10.1046/j.1365-313x.1997.12061465.x
Min, D., Ai, W., Zhou, J., Li, J., Zhang, X., Li, Z., et al. (2020). SlARG2 contributes to MeJA-induced defense responses to Botrytis cinerea in tomato fruit. Pest Manag Sci. 76, 3292–3301. doi: 10.1002/ps.5888
Mir, N., Canoles, M., Beaudry, R., Baldwin, E., Mehla, C. P. (2004). Inhibiting tomato ripening with 1-methylcyclopropene. J. Am. Soc. Hortic. Sci. 129, 112–120. doi: 10.21273/JASHS.129.1.0112
Moline, H. (1976). Ultrastructural changes associated with chilling of tomato fruit [Injuries]. Phytopathology. 66, 617–624. doi: 10.1094/Phyto-66-617
Moon, S.-J., Min, M. K., Kim, J.-A., Kim, D. Y., Yoon, I. S., Kwon, T. R., et al. (2019). Ectopic expression of OsDREB1G, a member of the OsDREB1 subfamily, confers cold stress tolerance in rice. Front. Plant Sci. 10. doi: 10.3389/fpls.2019.00297
Müller, P., Li, X.-P., Niyogi, K. K. (2001). Non-photochemical quenching. A response to excess light energy. Plant Physiol. 125, 1558–1566. doi: 10.1104/pp.125.4.1558
Nawaz, A., Amjad, M., Iqbal, J. (2009). Effect of thermal hardening on germination and seedling vigour of tomato. J. Res. (Science) Bahauddin Zakariya Univ. 20-21, 39–49.
Oeller, P. W., Lu, M., Taylor, L. P., Pike, D. A., Theologis, A. (1991). Reversible inhibition of tomato fruit senescence by antisense RNA. Science 254, 437–439. doi: 10.1126/science.1925603
Onaga, G., Wydra, K. (2016). Advances in plant tolerance to abiotic stresses, Plant Genomics (London, United Kingdom: IntechOpen). doi: 10.5772/60746
Park, M. H., Malka, S. K. (2022). Gibberellin delays metabolic shift during tomato ripening by inducing auxin signaling. Front. Plant Sci. 13, 1045761. doi: 10.3389/fpls.2022.1045761
Patterson, B. D., Macrae, E. A., Ferguson, I. B. (1984). Estimation of hydrogen-peroxide in plant-extracts using titanium (IV). Anal. Biochem. 139, 487–492. doi: 10.1016/0003-2697(84)90039-3
Pfaffl, M. W. (2012). Quantification strategies in real-time polymerase chain reaction. Ed. Bustin, S. A. (La Jolla, CA, USA: A-Z of Quantitative PCR International University Line).
Pino, M. T., Skinner, J. S., Park, E. J., Jeknić, Z., Hayes, P. M., Thomashow, M. F., et al. (2007). Use of a stress inducible promoter to drive ectopic AtCBF expression improves potato freezing tolerance while minimizing negative effects on tuber yield. Plant Biotechnol. J. 5 (5), 591–604. doi: 10.1111/j.1467-7652.2007.00269.x
Pino, M. T., Skinner, J. S., Jeknic, Z., Hayes, P. M., Soeldner, A. H., Thomashow, M. F., et al. (2008). Ectopic AtCBF1 over-expression enhances freezing tolerance and induces cold acclimation-associated physiological modifications in potato. Plant Cell Environ. 31, 393–406. doi: 10.1111/j.1365-3040.2008.01776.x
Putri, G. H., Anders, S., Pyl, P. T., Pimanda, J. E., Zanini, F. (2022). Analysing high-throughput sequencing data in Python with HTSeq 2.0. Bioinformatics 38 (10), 2943–2945. doi: 10.1093/bioinformatics/btac166
Qiu, W., Liu, M., Qiao, G., Jiang, J., Xie, L., Zhuo, R. (2012). An isopentyl transferase gene driven by the stress-inducible rd29A promoter improves salinity stress tolerance in transgenic tobacco. Plant Mol. Biol. Rep. 30, 519–528. doi: 10.1007/s11105-011-0337-y
Rambla, J. L., Tikunov, Y. M., Monforte, A. J., Bovy, A. G., Granell, A. (2014). The expanded tomato fruit volatile landscape. J. Exp. Bot. 65, 4613–4623. doi: 10.1093/jxb/eru128
Sales, A., Felipe, L. D. O., Bicas, J. L. (2020). Production, properties, and applications of α-terpineol. Food bioprocess Technol. 13 (8), 1261–1279. doi: 10.1007/s11947-020-02461-6
Shackel, K. A., Greve, C., Labavitch, J. M., Ahmadi, H. (1991). Cell turgor changes associated with ripening in tomato pericarp tissue. Plant Physiol. 97, 814–816. doi: 10.1104/pp.97.2.814
Shepherd, C. T., Moran Lauter, A. N., Scott, M. P. (2009). Determination of transgene copy number by real-time quantitative PCR. Methods Mol. Biol. 526, 129–134. doi: 10.1007/978-1-59745-494-0_11
Singh, S., Rathore, M., Goyary, D., Singh, R. K., Anandhan, S., Sharma, D. K., et al. (2011). Induced ectopic expression of AtCBF1 in marker-free transgenic tomatoes confers enhanced chilling tolerance. Plant Cell Rep. 30, 1019–1028. doi: 10.1007/s00299-011-1007-0
Stein, S. E. (1999). An integrated method for spectrum extraction and compound identification from gas chromatography/mass spectrometry data. J. Am. Soc. Mass Spectrometry 10, 770–781. doi: 10.1016/S1044-0305(99)00047-1
Styczynski, M. P., Moxley, J. F., Tong, L. V., Walther, J. L., Jensen, K. L., Stephanopoulos, G. N. (2007). Systematic identification of conserved metabolites in GC/MS data for metabolomics and biomarker discovery. Analytical Chem. 79, 966–973. doi: 10.1021/ac0614846
Tanksley, S. D. (2004). The genetic, developmental, and molecular bases of fruit size and shape variation in tomato. Plant Cell 16, S181–S189. doi: 10.1105/tpc.018119
Taylor, S., Wakem, M., Dijkman, G., Alsarraj, M., Nguyen, M. (2010). A practical approach to RT-qPCR—publishing data that conform to the MIQE guidelines. Methods 50, S1–S5. doi: 10.1016/j.ymeth.2010.01.005
Thomashow, M. F. (2010). Molecular basis of plant cold acclimation: insights gained from studying the CBF cold response pathway. Plant Physiol. 154, 571–577. doi: 10.1104/pp.110.161794
Thomashow, M. F., Gilmour, S. J., Stockinger, E. J., Jaglo-Ottosen, K. R., Zarka, D. G. (2001). Role of the Arabidopsis CBF transcriptional activators in cold acclimation. Physiologia Plantarum 112, 171–175. doi: 10.1034/j.1399-3054.2001.1120204.x
van Leeuwen, W., Ruttink, T., Borst-Vrenssen, A. W., van der Plas, L. H., van der Krol, A. R. (2001). Characterization of position-induced spatial and temporal regulation of transgene promoter activity in plants. J. Exp. Bot. 52, 949–959. doi: 10.1093/jexbot/52.358.949
Wang, H. M., Yin, W. C., Wang, C. K., To, K. Y. (2009). Isolation of functional RNA from different tissues of tomato suitable for developmental profiling by microarray analysis. Bot. Stud. 50, 115–125. doi: 10.5555/20093147241
Wang, M., Zhang, L., Boo, K. H., Park, E., Drakakaki, G., Zakharov, F. (2019). PDC1, a pyruvate/α-ketoacid decarboxylase, is involved in acetaldehyde, propanal and pentanal biosynthesis in melon (Cucumis melo L.) fruit. Plant J. 98, 112–125. doi: 10.1111/tpj.14204
Weiss, J., Egea-Cortines, M. (2009). Transcriptomic analysis of cold response in tomato fruits identifies dehydrin as a marker of cold stress. J. Appl. Genet. 50, 311–319. doi: 10.1007/BF03195689
Wisniewski, M., Norelli, J., Bassett, C., Artlip, T., Macarisin, D. (2011). Ectopic expression of a novel peach (Prunus persica) CBF transcription factor in apple (Malus× domestica) results in short-day induced dormancy and increased cold hardiness. Planta 233, 971–983. doi: 10.1007/s00425-011-1358-3
Xue, Y., Xiao, S., Kim, J., Lung, S. C., Chen, L., Tanner, J. A., et al. (2014). Arabidopsis membrane-associated acyl-CoA-binding protein ACBP1 is involved in stem cuticle formation. J. Exp. Bot. 65, 5473–5483. doi: 10.1093/jxb/eru304
Yamaguchi-Shinozaki, K., Shinozaki, K. (1994). A novel cis-acting element in an Arabidopsis gene is involved in responsiveness to drought, low-temperature, or high-salt stress. Plant Cell 6, 251–264. doi: 10.1105/tpc.6.2.251
Yari, L., Zareyan, A., Sheidaie, S., Khazaei, F. (2012). Influence of high and low temperature treatments on seed germination and seedling vigor of rice (Oryza sativa L.). World Appl. Sci. J. 16, 1015–1018.
Yu, W., Sheng, J., Zhao, R., Wang, Q., Ma, P., Shen, L. (2019). Ethylene biosynthesis is involved in regulating chilling tolerance and SlCBF1 gene expression in tomato fruit. Postharvest Biol. Technol. 149, 139–147. doi: 10.1016/j.postharvbio.2018.11.012
Zhang, X., Fowler, S. G., Cheng, H., Lou, Y., Rhee, S. Y., Stockinger, E. J., et al. (2004). Freezing-sensitive tomato has a functional CBF cold response pathway, but a CBF regulon that differs from that of freezing-tolerant Arabidopsis. Plant J. 39, 905–919. doi: 10.1111/j.1365-313X.2004.02176.x
Zhang, X., Shen, L., Li, F., Meng, D., Sheng, J. (2013). Arginase induction by heat treatment contributes to amelioration of chilling injury and activation of antioxidant enzymes in tomato fruit. Postharvest Biol. Technol. 79, 1–8. doi: 10.1016/j.postharvbio.2012.12.019
Zhang, B., Tieman, D. M., Jiao, C., Xu, Y., Chen, K., Fei, Z., et al. (2016). Chilling-induced tomato flavor loss is associated with altered volatile synthesis and transient changes in DNA methylation. Proc. Natl. Acad. Sci. 113, 12580–12585. doi: 10.1073/pnas.1613910113
Zhang, Y. J., Yang, J. S., Guo, S. J., Meng, J. J., Zhang, Y. L., Wan, S. B., et al. (2011). Over-expression of the Arabidopsis CBF1 gene improves resistance of tomato leaves to low temperature under low irradiance. Plant Biol. 13, 362–367. doi: 10.1111/j.1438-8677.2010.00365.x
Zhao, D., Shen, L., Fan, B., Liu, K., Yu, M., Zheng, Y., et al. (2009a). Physiological and genetic properties of tomato fruits from 2 cultivars differing in chilling tolerance at cold storage. J. Food Sci. 74, C348–C352. doi: 10.1111/j.1750-3841.2009.01156.x
Zhao, D., Shen, L., Fan, B., Yu, M., Zheng, Y., Lv, S., et al. (2009b). Ethylene and cold participate in the regulation of LeCBF1 gene expression in postharvest tomato fruits. FEBS Lett. 583, 3329–3334. doi: 10.1016/j.febslet.2009.09.029
Zhou, M., Chen, H., Wei, D., Ma, H., Lin, J. (2017). Arabidopsis CBF3 and DELLAs positively regulate each other in response to low temperature. Sci. Rep. 7, 39819. doi: 10.1038/srep39819
Zhou, J., Wang, J., Shi, K., Xia, X. J., Zhou, Y. H., Yu, J. Q. (2012). Hydrogen peroxide is involved in the cold acclimation-induced chilling tolerance of tomato plants. Plant Physiol. Biochem. 60, 141–149. doi: 10.1016/j.plaphy.2012.07.010
Zou, J., Chen, J., Tang, N., Gao, Y., Hong, M., Wei, W., et al. (2018). Transcriptome analysis of aroma volatile metabolism change in tomato (Solanum lycopersicum) fruit under different storage temperatures and 1-MCP treatment. Postharvest Biol. Technol. 135, 57–67. doi: 10.1016/j.postharvbio.2017.08.017
Keywords: postharvest chilling injury, Solanum lycopersicum, C-binding factor (CBF1), chilling acclimation, cold response, fruit senescence
Citation: Albornoz K, Zhou J, Zakharov F, Grove J, Wang M and Beckles DM (2024) Ectopic overexpression of ShCBF1 and SlCBF1 in tomato suggests an alternative view of fruit responses to chilling stress postharvest. Front. Plant Sci. 15:1429321. doi: 10.3389/fpls.2024.1429321
Received: 07 May 2024; Accepted: 09 July 2024;
Published: 05 August 2024.
Edited by:
Mohammad Irfan, Cornell University, United StatesReviewed by:
Selman Uluisik, Mehmet Akif Ersoy University, TürkiyePankaj Kumar, Dr. Yashwant Singh Parmar University of Horticulture and Forestry, India
Copyright © 2024 Albornoz, Zhou, Zakharov, Grove, Wang and Beckles. This is an open-access article distributed under the terms of the Creative Commons Attribution License (CC BY). The use, distribution or reproduction in other forums is permitted, provided the original author(s) and the copyright owner(s) are credited and that the original publication in this journal is cited, in accordance with accepted academic practice. No use, distribution or reproduction is permitted which does not comply with these terms.
*Correspondence: Diane M. Beckles, ZG1iZWNrbGVzQHVjZGF2aXMuZWR1
†Present address: Karin Albornoz, Department of Food, Nutrition, and Packaging Sciences, Coastal Research and Education Center, Clemson University, Charleston, SC, United States