- 1IRTA, Postharvest Programme, Edifici Fruitcentre, Parc Agrobiotech Lleida, Lleida, Catalonia, Spain
- 2Laboratory of Phytopathology, Wageningen University, Wageningen, Netherlands
- 3IRTA, Plant In Vitro Culture Laboratory, Fruticulture Program, Parc Agrobiotech Lleida, Lleida, Catalonia, Spain
Introduction: Brown rot is the most important fungal disease affecting stone fruit and it is mainly caused by Monilinia fructicola, M. laxa and M. fructigena. Monilinia spp. are necrotrophic plant pathogens with the ability to induce plant cell death by the secretion of different phytotoxic molecules, including proteins or metabolites that are collectively referred to as necrotrophic effectors (NEs).
Methods: We exploited the genomes of M. fructicola, M. laxa and M. fructigena to identify their common group of secreted effector proteins and tested the ability of a selected set of effectors to induce cell death in Nicotiana benthamiana, Solanum lycopersicum and Prunus spp. leaves.
Results: Fourteen candidate effector genes of M. fructicola, which displayed high expression during infection, were transiently expressed in plants by agroinfiltration using a modified Tobacco Rattle Virus (TRV)-based expression system. Some, but not all, effectors triggered leaf discoloration or cell death in N. benthamiana and S. lycopersicum, which are non-hosts for Monilinia and in Prunus spp., which are the natural hosts. The effector MFRU_030g00190 induced cell death in almost all Prunus genotypes tested, but not in the Solanaceous plants, while MFRU_014g02060, which is an ortholog to BcNep1, caused necrosis in all plant species tested.
Conclusion: This method provides opportunities for screening Prunus germplasm with Monilinia effector proteins, to serve as a tool for identifying genetic loci that confer susceptibility to brown rot disease.
1 Introduction
One of the most important fungal diseases affecting stone fruit is brown rot. It causes large economic losses, principally in peaches and nectarines, but also affects other stone fruit like cherries, apricots, and almonds (Ollero-Lara et al., 2019; Baró-Montel et al., 2019a). The infection occurs in the field, starting from overwintered mummies that generate the primary inoculum for emerging flowers and fruit during spring and summer (Villarino et al., 2010). Nevertheless, the main economic losses of brown rot appear during the postharvest handling, storage, and transportation (Casals et al., 2022).
The genus Monilinia are Ascomycetes included in the Sclerotiniaceae family and it contains 37 species, most of which are plant pathogens (Index Fungorum, 2023). The three species mainly responsible for stone fruit brown rot are Monilinia fructicola, M. laxa and M. fructigena. Out of these species, M. fructicola is considered to cause the most severe losses in stone fruit (Villarino et al., 2013). Occurrence and distribution of the three species has traditionally differed worldwide. While M. laxa and M. fructigena have long been the main causal agent of brown rot in stone fruit in Europe, M. fructicola has traditionally affected stone fruit in India, Japan, Oceania and the Americas. M. fructicola was first detected in Europe in fruit orchards in France in 2001 (Lichou et al., 2002) and it has since migrated into several European countries (Hrustić et al., 2015). In Spain it was first detected in Ebro Valley orchards in 2009 (De Cal et al., 2009).
Depending on the strategies developed to interact with the host, plant pathogenic fungi are classified as having either a necrotrophic, hemibiotrophic or biotrophic lifestyle (Tan et al., 2010). In a classical biotrophic lifestyle, the interaction between an effector (acting as an avirulence gene) and its specific plant receptor (encoded by an R-gene) leads to a defense response causing host cell death and stopping the infection process (Tan et al., 2015). By contrast, necrotrophic pathogens secrete cell death-inducing effectors with the capacity to trigger host programmed cell death (PCD), which is a key step in successful plant colonization and disease development (Tan et al., 2010; Kabbage et al., 2017; Faris and Friesen, 2020; Malvestiti and van Kan, in press).
Cell death-inducing effectors can either be secondary metabolites or relatively small proteins (Tan et al., 2010). Depending on their target in host plants, the effectors can be grouped as apoplastic or cytoplasmic effectors. Both are delivered into the plant, however, apoplastic effectors are secreted into the plant apoplast, where they interact with extracellular receptors, while cytoplasmic effectors are translocated inside the plant cell (Selin et al., 2016). In recent years, many studies have been devoted to host cell death-inducing effectors produced by necrotrophic pathogens affecting wheat, barley, and maize with the Parastagonospora nodorum-wheat pathosystem serving as a model for the interaction between necrotrophic fungi and their host (Friesen and Faris, 2021; Shao et al., 2021). Three fungal effector genes have been cloned along with their target genes in wheat: Tox1-Snn1, Tox3-Snn3 and ToxA-Tsn1 (Friesen and Faris, 2021). Three more effector genes (Tox4, Tox5 and Tox 267) have been cloned from P. nodorum and five wheat loci involved in recognition of these effectors were genetically mapped (reviewed in Malvestiti and van Kan, in press).
The best characterized necrotrophic pathogen from the Sclerotiniaceae family is Botrytis cinerea. A large number of cell death-inducing effectors have been studied and functionally characterized (Leisen et al., 2022; Bi et al., 2023). The availability of high-quality annotations of different Monilinia spp. genomes enables studies on effector proteins of these species (Landi et al., 2018; Naranjo-Ortíz et al., 2018; Rivera et al., 2018; De Miccolis Angelini et al., 2019; Marcet-Houben et al., 2021). Genome comparison among five Monilinia spp. highlighted the low variability in the CAZome, some common secondary metabolism clusters, and several potential candidate effector genes (Marcet-Houben et al., 2021). Despite the available genome information, molecular-genetic studies on the interaction between Monilinia spp. and Prunus spp. are in their infancy as compared to other Sclerotiniaceae. To investigate the role of Monilinia effectors in the infection process, it is desirable to have a tool to test their cell death-inducing capacity in Prunus spp. tissues. Previous studies in B. cinerea used heterologous expression in Pichia pastoris to produce a set of endopolygalacturonases (Kars et al., 2005), however, that is a time-consuming technique with relatively low efficiency. Functional screens with effector proteins of biotrophic pathogens often make use of transient expression systems using plant virus vectors as expression platform (Nasir et al., 2005; Vleeshouwers and Oliver, 2014). Potato Virus X (PVX) is often used for these purposes when screening germplasm of Solanaceae (Domazakis et al., 2017), however this virus was not reported to replicate in Prunus spp. Tobacco Rattle Virus (TRV), however, can replicate in a wide range of plant species, including Prunus spp. leaves (Bai et al., 2016) and this virus was considered to be potentially useful for expressing M. fructicola effector proteins in Prunus spp. leaves.
The aim of this study was to identify the common effectorome from the three most important species of Monilinia in stone fruit, M. fructicola, M. laxa and M. fructigena, and to study the cell death-inducing capacity of a subset of M. fructicola effectors using modified TRV constructs for expressing them in leaves of non-hosts and hosts of Monilinia spp.
2 Materials and methods
2.1 Identification of the common effectorome from three Monilinia species
The genomes of M. fructigena strain gena6 (BioProject Code PRJNA707424), M. laxa strain 8L (BioProject PRJNA433296; Naranjo-Ortíz et al., 2018) and M. fructicola strain CPMC6 (BioProject PRJNA503180; Vilanova et al., 2021) as well as the comparative genomic analysis (Marcet-Houben et al., 2021) were used for the identification of the effector genes for each species. Specifically, we used the effectorome data from Vilanova et al. (2021) for M. fructicola and the effectorome data from Marcet-Houben et al. (2021) for M. laxa and M. fructigena.
Each predicted effector gene from all three species was blasted to the other species one to one, using Genome Workbench and PhylomeDB (E-value cut-off 0.001 and query coverage ≥ 80%). The bidirectional best blast hits were listed and used for further analyses (Supplementary Table S1).
Transcriptionally inactive genes were eliminated based on RNA-Seq data from M. laxa- infected fruits (Balsells-Llauradó et al., 2020) and M. fructicola-infected leaves (Vilanova et al., unpublished).
2.2 Plant material and fungal culture
In this study, leaves from different plant species were used: Nicotiana benthamiana, Solanum lycopersicum, and Prunus spp. N. benthamiana and S. lycopersicum served as model plants to ensure the TRV system is well established. Additionally, N. benthamiana was used to detect non-host specific effectors while S. lycopersicum and Prunus spp. were used to identify host-specific effectors, with a particular focus on Prunus spp. as it is the actual host of Monilinia spp.
N. benthamiana plants were grown in a climate chamber located at IRTA Fruitcentre in Lleida at 25°C under a 16 h light/8 h dark cycle, Solanum lycopersicum var. Moneymaker plants were grown in the greenhouses located at University of Lleida. Furthermore, Prunus spp. were included due to their role as the pathogen host. Initially, Prunus persica plants derived from directed crosses were employed to assess the viability of agroinfiltration in this plant species. Subsequently, five well-characterized genotypes were selected for further investigation. These individuals included: Cadaman (Prunus davidiana × Prunus persica), Prunus davidiana and three different genotypes denotated 37p15-13, 37p15-16, and 37p15-17. These three genotypes are a BC1 population derived from a cross between the peach cultivar Nectatop and Cadaman. This population has been previously generated and genotyped (Zaracho-Echagüe et al., 2022). All Prunus spp. plants were in vitro clonally propagated as described (Cantabella et al., 2021). Briefly, in vitro shoot tip cultures were established from field grown plants, propagated by axillary branching in MS (Murashige & Skoog) medium supplemented with 5 μM benzylaminopurine (BAP). Shoot elongation was achieved adding liquid MS media, without hormones, to the semisolid multiplication medium. Root induction on in vitro elongated shoots was conducted in half-strength MS media supplemented with 10 μM indole-3-acetic acid (IAA), followed by transfer to auxin-free medium amended with vermiculite, and afterwards acclimated to greenhouse conditions.
The M. fructicola strain CPMC6 belongs to the collection of the Postharvest Pathology group of IRTA (Lleida, Spain) and is deposited in the Spanish Culture Type Collection (CECT 21105). For long-term storage, the isolate was kept as a conidial suspension in 20% glycerol at -80°C. Cultures were grown in malt extract plates (MEA) and incubated at 25°C in darkness for 10-12 days before use.
2.3 RNA isolation and cDNA synthesis
M. fructicola was grown on cellophane on MEA incubated at 24°C for 10-12 days, and RNA was extracted from freeze-dried mycelium using RNeasy® Plant Mini Kit (Qiagen, Hilden, Germany) according to manufacturer´s recommendations. RNA from M. fructicola decayed stone fruit tissue was extracted following the methodology described by Baró-Montel et al. (2019c). For elimination of genomic DNA, RNA was treated with TURBO™ DNase (Invitrogen, ThermoFisher Scientific, Carlsbad, CA, USA). First strand cDNA was synthesized using the SuperScript™ IV First-Strand Synthesis Kit (Invitrogen, ThermoFisher Scientific, Carlsbad, CA, USA) and used for gene amplification.
2.4 Amplification of candidate effector genes
All oligonucleotides used are listed in Supplementary Table S2. Primers were designed using the NEBuilder Assembly Tool (New England Biolabs, MA, USA) to amplify two fragments for each selected gene. The coding sequence of the signal peptide of the pathogenesis-related protein from N. benthamiana (PR1) and the coding sequence (CDS) of the mature candidate effector excluding the N-terminal signal peptide were amplified. Fragments for MFRU002g05260 and MFRU030g00580 genes were amplified directly from the plasmids obtained by Vilanova et al. (2021). Fragment amplification for cloning was performed in a volume of 50 µL containing 1µM of both forward and reverse primers, 0.2 mM of each dNTP, 1X PFU buffer (Promega, Leiden, The Netherlands), 1.5 U PFU polymerase (Promega), and 2 µL of template (100 ng of cDNA from M. fructicola or 100 ng of plasmid). PCR program for both fragments of MFRU004g02710, MFRU004g02720, MFRU014g02060, MFRU048g00370, MFRU004g00620 and MFRU005g00910 was 95°C for 2 min; 10 cycles of 95°C for 30 s, 55°C for 30 s and 72°C for 1 min and 25 cycles with an annealing temperature of 60°C instead of 55°C, ending with a final step at 72°C for 5 min. PCR program for the PR1 fragment of MFRU004g02090, MFRU008g03430, MFRU010g02580, MFRU018g01470, MFRU027g00340 and MFRU030g00190 was 95°C for 2 min; 35 cycles of 95°C for 15 s, 55°C for 30 s, and 72°C for 30 s, ending with a final step at 72°C for 5 min. PCR program for the other fragments was 95°C for 2 min; 35 cycles of 95°C for 15 s, 52°C for 30 s, and 72°C for 80 s, ending with a final step at 72°C for 5 min. PCR products were visualized in a range of 1-3% agarose gel.
2.5 Vector construction and cloning
pTRV2 VIGS vector pYL156 (Liu et al., 2002) (kindly provided by Laurens Deurhof, Wageningen University) was modified by removing remainders of the TRV2b protein coding sequence and inserting a sequence encoding a plant secretion signal peptide (derived from the tobacco pathogenesis-related protein PR1a), joint in frame to the coding sequence of a mature Monilinia effector gene, lacking the fungal secretion signal peptide sequence. Constructs were made using the NEBuilder® HiFi DNA Assembly Cloning kit (New England BioLabs, MA, USA). The pTRV2 vector was linearized using restriction enzymes (New England BioLabs, MA, USA), either PvuII, EcoRI, AatII or BamHI (New England BioLabs, MA, USA) at 37°C, according to manufacturer’s recommendations. Each digestion was done one by one followed by a purification step. Fragments and the linearized vector were incubated with Clonexpress II one step cloning kit (Vazyme, Nanjing, China) or NEBuilder HiFi DNA Assembly Master Mix (New England BioLabs, MA, USA) following manufacturer´s recommendations (Supplementary Figure S1). From 10 ng to 1000 ng of ligated plasmid was transformed into ultra-competent DH5α E. coli cells. Cells were selected on LB medium with kanamycin and incubated overnight at 37°C. Plasmids were isolated using the QIAprep Spin Miniprep kit (Qiagen, Hilden, Germany) according to manufacturer’s recommendations. To check if the selected genes were integrated in the pTRV2 plasmids, PCR was performed using IBIAN®-Taq DNA Polymerase (Ibian Technologies, Zaragoza, Spain) in a total volume of 25 µL containing 0.5 µM of both forward and reverse primer, 0.2 mM of each dNTP, 1X IBIAN Buffer, 1.25 U IBIAN-Taq® DNA polymerase and 0.5 uL of the pTRV2 vector. PCR program was 94°C for 2 min; 30 cycles of 94°C for 10 s, 55°C for 20 s and 72°C for 1,5 min, ending with a final step of 72°C for 5 min. Those clones with similar size to the expected were sequenced for further confirmation.
2.6 Agrobacterium tumefaciens mediated-transient expression in Nicotiana benthamiana, Solanum lycopersicum and Prunus spp.
Fifty ng of plasmid were added to 50 μL of electro-competent Agrobacterium tumefaciens cells (strain GV3101). After electroporation, cells were plated in LB petri dishes supplemented with kanamycin and rifampicin and incubated at 28°C for two days. Agrobacterium cultures were transferred into 15 mL YEB medium containing 20 µM acetosyringone, 50 mg/L kanamycin and 20 mg/L rifampicin and they were grown during 22 h at 28°C and 200 rpm. Pellet was resuspended in MMAi (1 mM MES, 10 mM MgCl2, 200 µM acetosyringone) buffer at OD600 = 1.6. Each solution of the different pTRV2 strains were mixed 1:1 with pTRV1 (Liu et al., 2002) and incubated during 1 h at room temperature. The different mixes were infiltrated using a needleless 1 mL syringe in leaves of 4-6 weeks old N. benthamiana plants, 5-6 weeks old S. lycopersicum and in Prunus spp. plants with at least 10 leaves. Pictures of responses in all N. benthamiana and S. lycopersicum plants were taken 5 and 7 days postinfiltration, and for Prunus spp. leaves, the symptoms were observed 10 days postinfiltration. This experiment was replicated three times, and each Agrobacterium suspension was infiltrated in three different leaves from three different plants.
3 Results
3.1 Identification of the common effectorome from three Monilinia spp.
The identification of the common effector genes shared between the three main Monilinia spp. was based on the analysis of Marcet-Houben et al. (2021). Bidirectional blast analysis was performed between effector genes in M. fructicola, M. laxa and M. fructigena in all possible combinations resulting in 76 effector genes shared among the three Monilinia spp. (Figure 1; Supplementary Tables S3, S4).
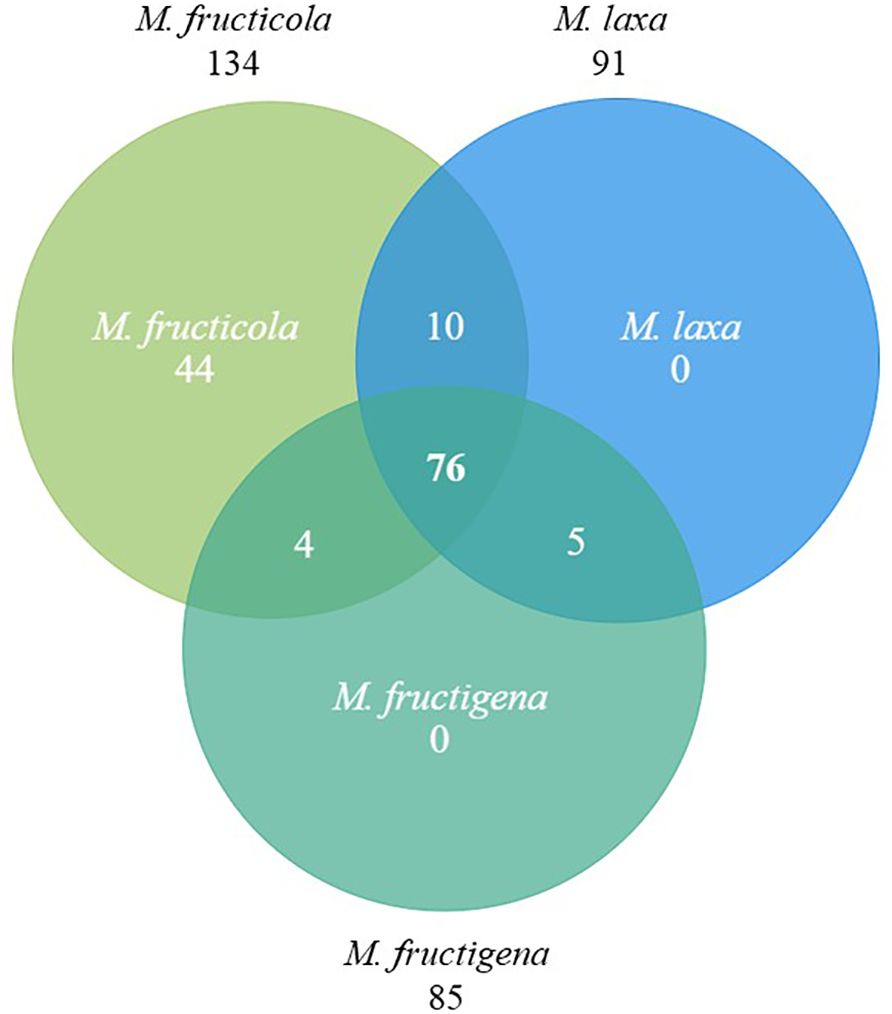
Figure 1. Venn diagram showing the effector genes shared among Monilinia fructicola, M. laxa and M. fructigena. Each circle represents the set of effectors for each species, with overlapping areas indicating shared effector genes between species.
For these 76 common effectors, information about their expression during the infection of M. fructicola in leaves (Vilanova et al., 2021) and of M. laxa in nectarines (Balsells-Llauradó et al., 2020) was used to eventually select 14 effector genes for transient expression analysis of cell death-induction in different plant species (Table 1).
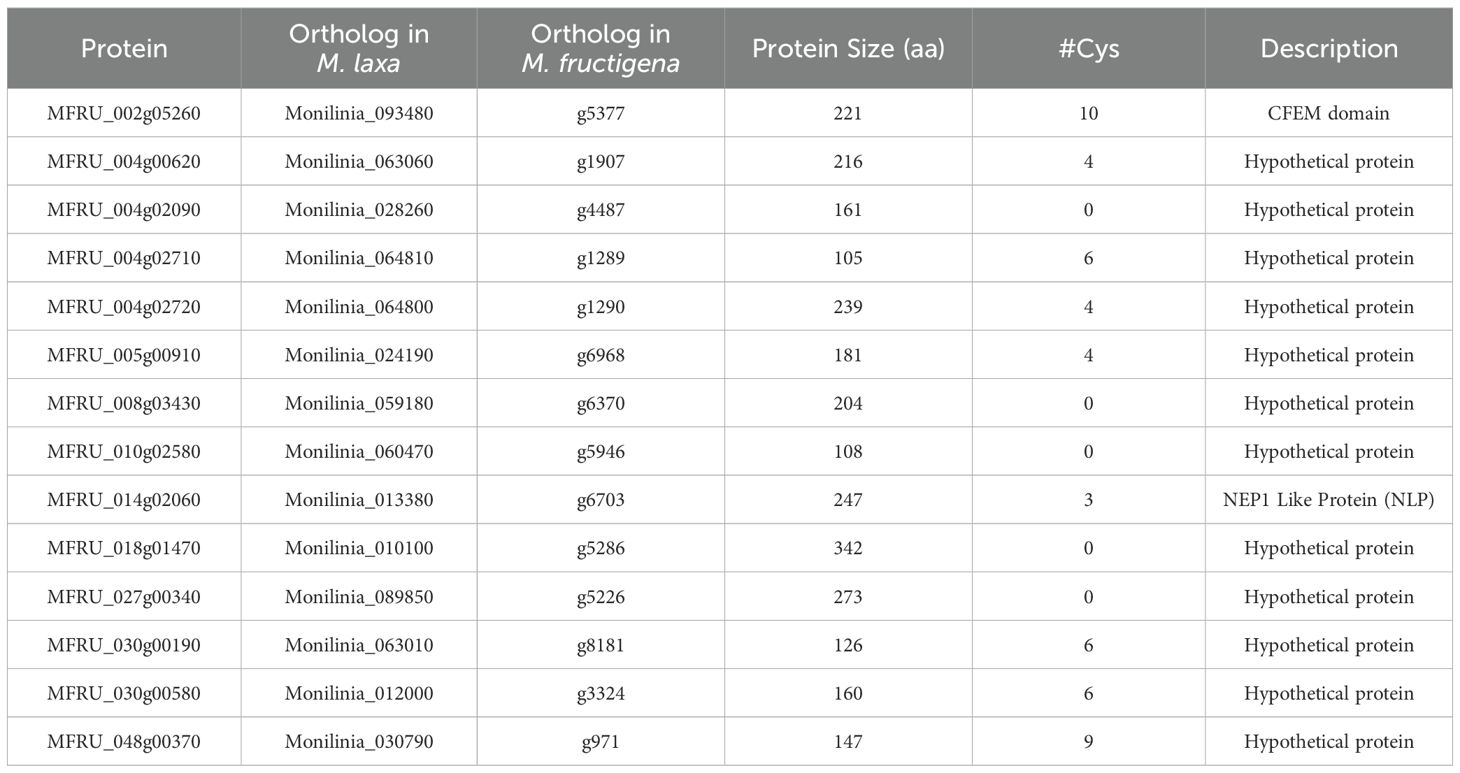
Table 1. Monilinia fructicola effector genes tested for cell death-induction by Agrobacterium tumefaciens transient expression (ATTA) in plants.
3.2 Response to transient expression in Nicotiana benthamiana and Solanum lycopersicum
The cell death-inducing capacity of each of the 14 candidate effector genes from M. fructicola was tested by ATTA using a modified binary vector containing the Tobacco Rattle Virus (pTRV2 mixed 1:1 with pTRV1) in three different plant species (Supplementary Figure S2). MFRU_014g02060 is an ortholog to BcNep1, which was previously shown to have a strong necrotizing activity in N. benthamiana leaves (Schouten et al., 2008), and it was used as a positive control. As negative control, we used an empty TRV2 vector combined with TRV1 (Figure 2).
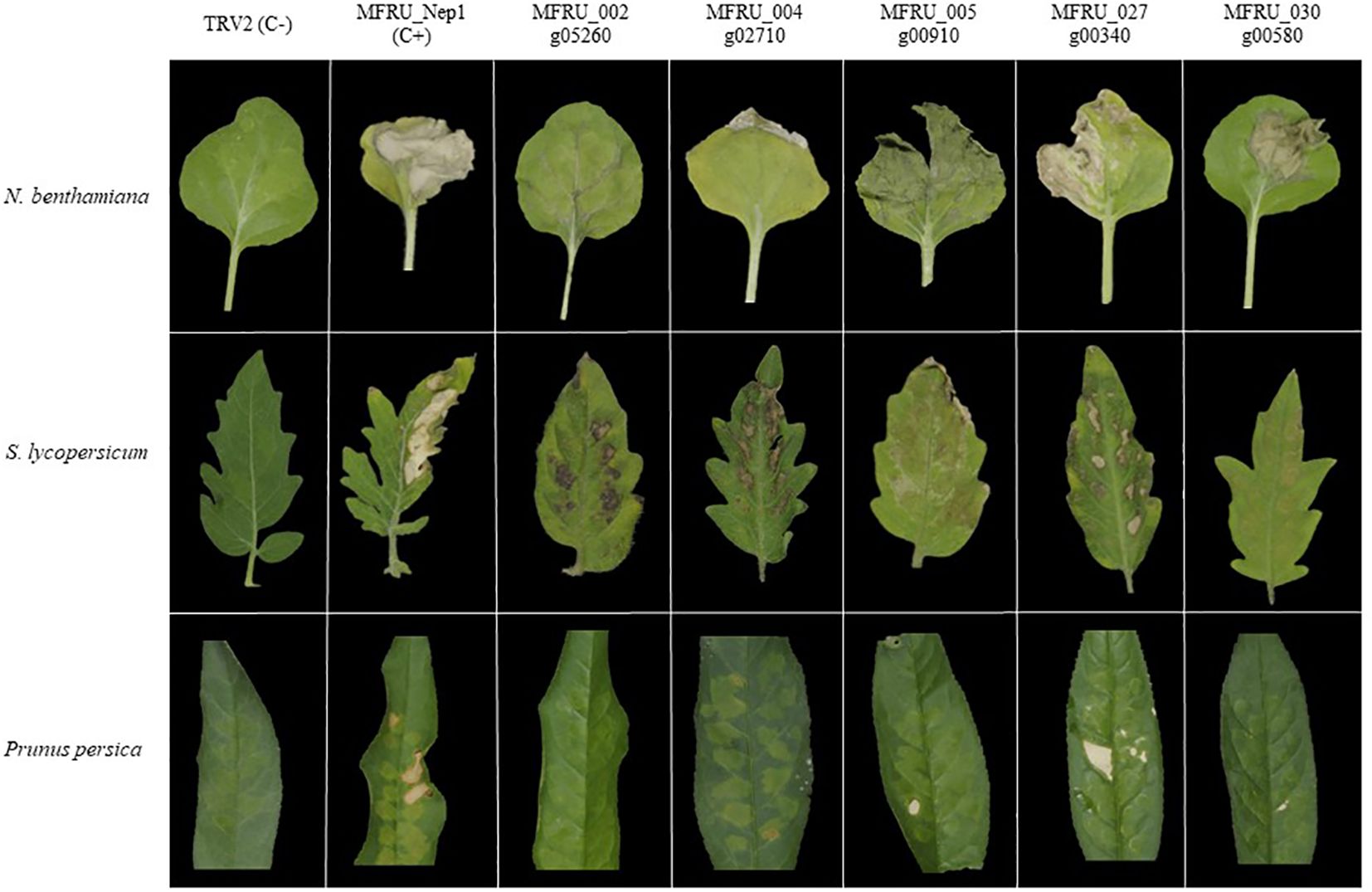
Figure 2. Agrobacterium tumefaciens mediated-transient expression (ATTA) using modified TRV binary vector for expressing several Monilinia fructicola candidate effector proteins in N. benthamiana (top row) and S. lycopersicum (middle row) and Prunus persica (bottom row). First column is the positive control, and the second column is the negative control. Pictures were taken 7 days postinfiltration.
In N. benthamiana leaves, six of the 14 candidate genes caused necrosis in the infiltrated area at five days postinfiltration (Table 2), with the genes MFRU_027g00340, MFRU_030g00580 and MFRU_005g00910 showing the strongest cell death-inducing activity (Figure 2).
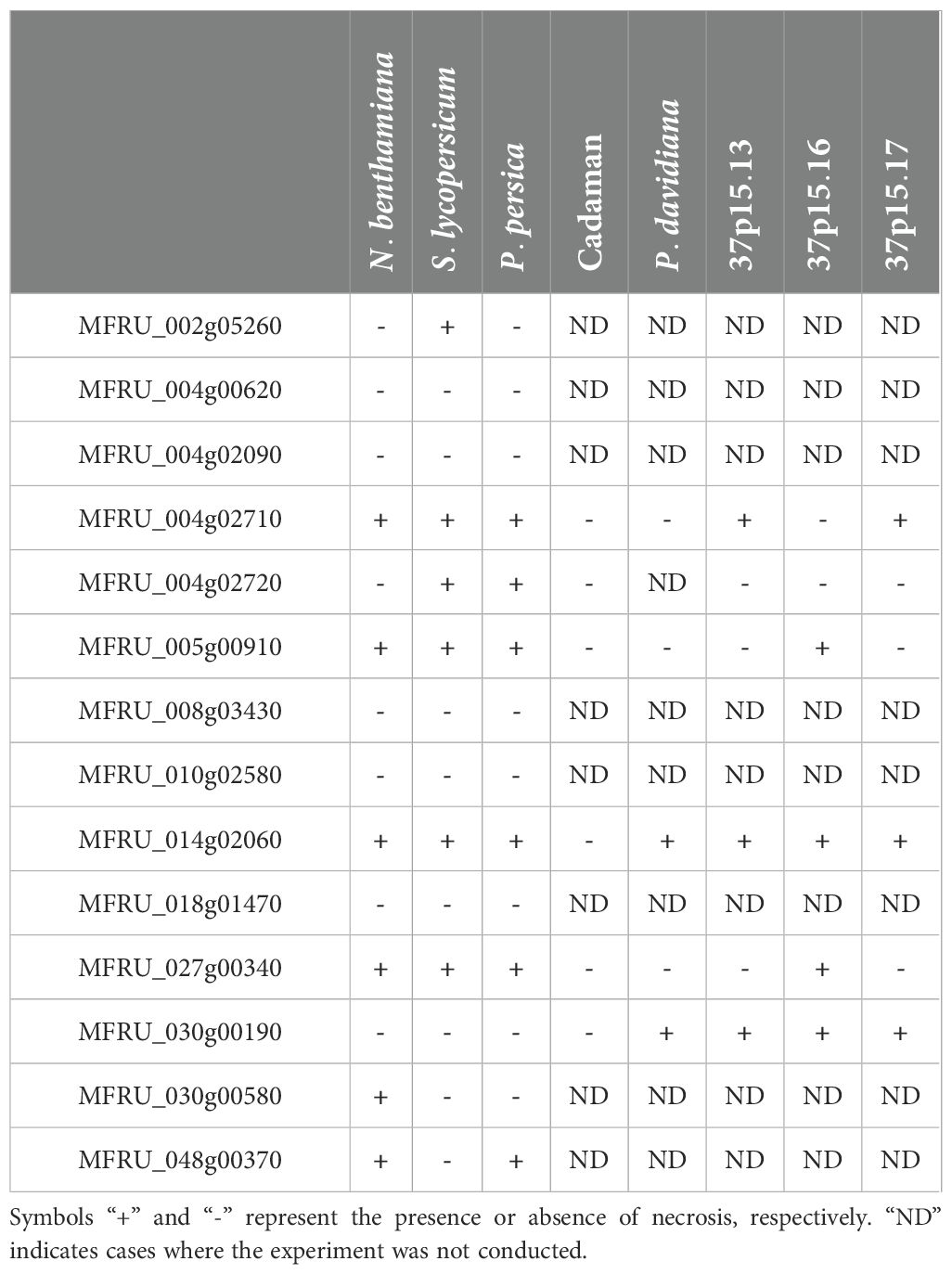
Table 2. Summary of Agrobacterium infiltration and necrosis observation in various plant species for Monilinia fructicola proteins.
Similar experiments were conducted in S. lycopersicum leaves and some differences were observed. Compared to N. benthamiana, the same number of candidate genes induced necrosis in the infiltrated area (Table 2), but these genes were different. MFRU_014g02060, MFRU_004g02710, MFRU_005g00910 and MFRU_027g00340 induced necrosis in the infiltrated area in both tomato and N. benthamiana leaves (Figure 2). No necrosis was observed in tomato leaves upon infiltration with the MFRU_030g00580 construct, while this produced significant necrosis in N. benthamiana. In contrast, clear necrosis was observed after infiltrating MFRU_002g05260 and MFRU_004g02710 in tomato, whereas no necrosis were noted in N. benthamiana. The cell death-inducing capacity of the MFRU_027g00340 construct exhibited the closest response to that of the positive control in both species.
3.3 Effect of transient gene expression in Prunus spp. leaves
As stone fruit is the real host of M. fructicola, the next step was to test the fourteen selected effector genes in host-specific tissues, specifically stone fruit leaves, using the transient TRV-mediated expression system developed in this work.
The constructs for the expression of the fourteen effector genes were tested in leaves of Prunus persica, and symptoms became apparent for some constructs 10 days postinfiltration (Supplementary Figure S2). Six of the fourteen genes induced necrosis in the infiltrated area and surroundings (Table 2). These genes were: MFRU_004g02710, MFRU_004g02720, MFRU_005g00910, MFRU_014g02060 (MFRU_Nep1), MFRU_027g00340, and MFRU_48g00370. In contrast with N. benthamiana and tomato, respectively, no necrosis symptoms were observed after the infiltration of MFRU_002g05260 construct and MFRU_030g00580 in Prunus leaves. The constructs that were able to induce necrosis symptoms in all three plant species after infiltration were MFRU_004g02710, MFRU_005g0910, MFRU_014g02060 and MFRU_027g00340 constructs (Table 2).
Subsequently, we tested the cell death-inducing capacity of six candidate effector genes in five different Prunus genotypes: Cadaman, Prunus davidiana and three genotypes called 37p15-13, 37p15-16, and 37p15-17 (Figure 3). Particularly, genotype 37p15-16 showed the most pronounced necrosis after the infiltration of all six candidate effector genes, except for MFRU_004g02710 and MFRU_004g02720, which only caused mild discoloration (Supplementary Figure S3, Table 2). In this genotype, the gene that produced more necrosis in the infiltrated area and surroundings was MFRU_005g00910, together with MFRU_014g02060 (Figure 3). On the other hand, no response was observed after the infiltration of any construct in the hybrid Cadaman. P. davidiana was the species in which symptoms of necrosis only appeared after the infiltration of MFRU_014g02060 and MFRU_030g00190. These 2 candidate effector genes, together with MFRU_004g02710, also induced necrosis and cell death in the leaves of the 37P15-17 and 37P15-13 genotypes (Figure 3).
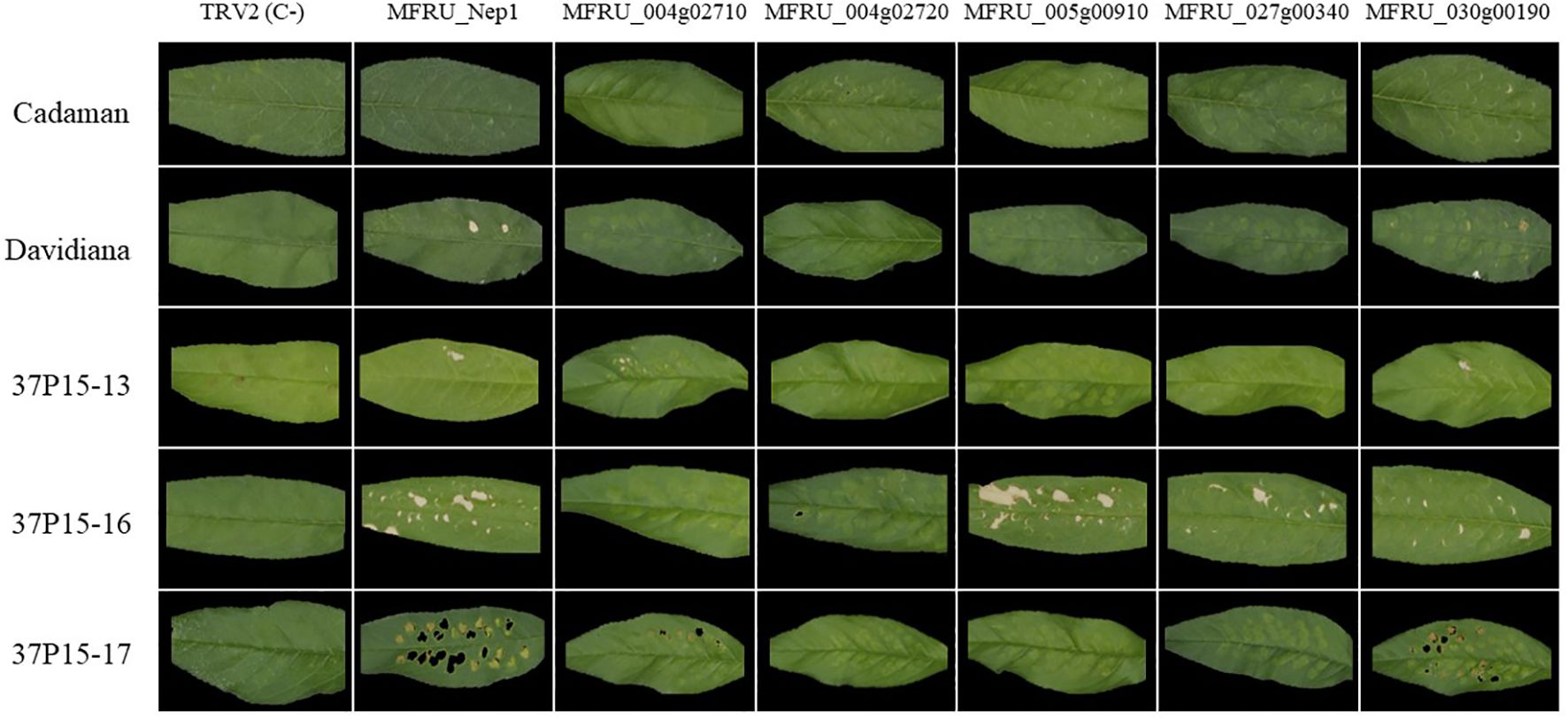
Figure 3. Agrobacterium mediated-transient expression (ATTA) using modified TRV construct for expressing several M. fructicola candidate effector proteins in different Prunus genotypes leaves. Columns contain the different gene constructs and rows contain are the different Prunus genotypes. Pictures were taken 10 days postinfiltration.
4 Discussion
M. fructicola is considered to be the most economically damaging Monilinia spp. in stone fruit in Europe, even more than endemic Monilinia spp. such as M. fructigena and M. laxa. Its ability to produce more conidia than other Monilinia spp. may help it succeed as an invasive species (Villarino et al., 2013). In an effort to gain insights into the pathogenic mechanisms of M. fructicola, several groups have produced genome data for Monilinia spp. in recent years (Naranjo-Ortíz et al., 2018; Rivera et al., 2018; De Miccolis Angelini et al., 2019; Vilanova et al., 2021).
Valero-Jiménez et al. (2019) tried to identify species-specific effectors that were determinant in host specificity, by comparing the effector set of nine different Botrytis spp. No host-specific relation was found between the different genes found in the different species and its hosts, and furthermore, all effectors that were previously functionally characterized in B. cinerea were also present in the other species analyzed. Taking this into account, the approach of the present study was the opposite, identifying the common effectors shared among three Monilinia species responsible for causing brown rot in stone fruit. In the case of M. fructicola, the number of effector genes identified by Marcet-Houben et al. (2021) was different when compared to the number of effector genes defined by Vilanova et al. (2021). The numbers of effector genes that we used differ from the study by De Miccolis Angelini et al. (2022) which had fewer effector genes in all three species, and the study by Akhoon et al. (2023), which had significantly more effector genes in all three species. This discrepancy is due to the use of distinct criteria to determine what constitutes an effector and to differences in prediction tools and choices in the stringency of prediction parameters. Moreover, manual curation efforts as conducted by Vilanova et al. (2021) may further contribute to variability in gene numbers and lists. The strategy used in this study for identifying effectors shared between all three Monilinia species was based on bidirectional blasts of the effector repertoire of each species to the other species, one by one. After all comparisons, the number of effector genes shared among the three Monilinia spp. genomes in this study was 76. The effectors described by De Miccolis Angelini et al. (2022) and Akhoon et al. (2023) that are not represented in our effector lists might be excellent candidates for testing using the TRV-based expression system.
Viral vectors are used in effectoromic screenings, but those studies are mostly done with Potato Virus X on Solanaceae (Nasir et al., 2005; Vleeshouwers and Oliver, 2014). As PVX was not reported to infect Prunus, we decided to switch to a virus that is reported to infect and replicate in Prunus, namely Tobacco Rattle Virus (TRV). TRV-based expression systems are commonly used in plants for the purpose of achieving virus-induced gene silencing (VIGS). It serves as a high-throughput tool to investigate gene function in several plant species (Hartl et al., 2008). In this study, a TRV vector designed for VIGS (Liu et al., 2002) was modified to enable the transient expression of fungal effector genes in plants. The virus possesses a bipartite genome, with RNA2 encoding two proteins redundant for viral replication but essential for viral transmission by nematodes (Macfarlane, 2010). Liu et al. (2002) crafted a binary vector construct that includes T-DNA borders flanking an expression cassette containing TRV RNA2 sequences and a multiple cloning site for the insertion of desired sequences. Agrobacterium tumefaciens carrying this binary vector construct can be mixed with another A. tumefaciens containing TRV RNA1 sequences and then infiltrated into plants. The mixture of these Agrobacterium strains allows for the transformation of plant cells, facilitating the expression and subsequent replication of both viral RNAs. These RNAs can disperse throughout the host plant, enabling the expression of heterologous genes, particularly fungal effectors in this case.
With this modified virus construct, the cell death-inducing capacity of putative effector genes of M. fructicola could be tested in leaf tissues of different plants, including its host, Prunus. The development of a high-throughput screening method using a modified TRV expression vector offers a good perspective in disease resistance breeding against plant pathogens. The strategy can be applied to study the response to fungal effector proteins in plants that can be infected by this virus such as strawberry, wheat, maize, and others (Zeng et al., 2019). This methodology provides an alternative strategy to heterologous protein production in bacteria or yeasts, which is more labour- and time-intensive. Besides this, these kind of effector assays could be complemented with infection assays and field trials (Vleeshouwers and Oliver, 2014) to test whether stone fruit, which do not show necrosis when infiltrating by fungal effectors, are less susceptible to Monilinia spp. In recent years, several genetic and quantitative genetic studies have revealed QTLs or markers related to brown rot resistance in peach (Baró-Montel et al., 2019b). In the case of Monilinia spp., knowledge about the capacity of its effectors to induce cell death and promote virulence can be exploited to genetically map and eventually clone susceptibility genes with the use of genomic tools (Martínez-García et al., 2013; Pacheco et al., 2014).
This study has identified several M. fructicola effector genes that induce cell death in its host plants leaf tissue. Necrosis was observed in leaves of almost all Prunus spp. tested upon infiltration of MFRU_030g00190, while neither N. benthamiana nor tomato responded to this protein. We hypothesize that the recognition of necrotrophic effectors (NEs) secreted by Monilinia species by receptor proteins of Prunus leads to host cell death and thereby confers susceptibility. If a pathogen does not produce a particular effector, or if the host does not express the susceptibility gene, the pathogen cannot invade the host, resulting in resistance (Faris and Friesen, 2020). This susceptibility mechanism is well-studied in the interaction between wheat and the necrotrophic fungi Parastagonospora nodorum and Pyrenophora tritici-repentis (Tan et al., 2012; Virdi et al., 2016; Faris and Friesen, 2020). Another well-characterized pathosystem involves fungi from the Cochliobolus genus, which produce cell death-inducing metabolites that are crucial for pathogenicity. In particular, C. victoriae produces victorin, conferring pathogenicity to oat genotypes carrying the Vb locus as a susceptibility determinant (reviewed in Malvestiti and van Kan, in press). Within the Sclerotiniaceae family, Botrytis cinerea stands out as the most extensively studied necrotrophic pathogen. It secretes several cell wall degrading enzymes (CWDEs) with NE activity, as well as NEs that lack an annotated enzymatic domain (for example BcNep1 and BcNep2), and secondary metabolites that contribute to virulence (Malvestiti and van Kan, in press; Bi et al., 2023).
In contrast with what it was observed with MFRU_030g00190, two genes (MFRU_005g00910 and MFRU_014g02060) were shown to cause necrosis in all three plant species tested. The MFRU_014g02060 protein is the ortholog to NEP1 proteins from Botrytis spp. NEP1-like proteins (NLPs) have been identified in plant pathogenic fungi, bacteria, and oomycetes, despite there has been ambiguity regarding their host range. While functionally characterized cytolytic NLPs have traditionally shown broad activity exclusively on dicotyledonous plant species (Seidl and Van den Ackerveken, 2019), recent reports revealed sensitivity of several monocotyledonous plants to NLPs as well (Steentjes et al., 2022). This underscores the importance of further investigating the host range and functional roles of these effectors. To do so, the generation of knockout mutants and even multigenic knockout mutants for the most interesting effectors identified in this study would be a promising approach. As demonstrated in B. cinerea, this approach would provide valuable insights into the function of each effector and its contribution to disease development (Leisen et al., 2022). By employing marker-free transformation methods using CRISPR/Cas and telomere vectors recently developed in B. cinerea (Leisen et al., 2020), we could systematically dissect the role of these effectors in the virulence of M. fructicola. This would enhance our understanding of the molecular mechanisms underlying pathogenicity and aid in the development of targeted strategies for disease management. As part of future experiments, it is also planned to produce pure effectors related to virulence to elucidate plant receptors and further enhance our understanding of plant-pathogen interactions.
The use of purified effectors is very promising as a base for genetic analysis allowing us to explore the complexities of plant responses and uncover the underlying mechanisms involved. This approach facilitates the identification of genetic loci associated with susceptibility to the disease, offering valuable insights into how plants interact with pathogens. Such insights are crucial for developing effective strategies to enhance crop resilience. This perspective aligns with successful instances, such as the P. nodorum-wheat interaction, where strategically excluding effector-sensitive genotypes from breeding programs has contributed to bolstering the partial resistance of wheat to P. nodorum (Friesen et al., 2007; Liu et al., 2012; Shi et al., 2016; Downie et al., 2018; Cowger et al., 2020) Emphasizing the parallels between these strategies, our study encourages the adoption of refined breeding approaches that exclude susceptible genotypes, providing breeders and growers with a powerful tool to fortify crops against the targeted disease. The methodology developed in this study, enables large-scale trials to be conducted, testing various effectors in non-model plants. This advancement opens the door to assessing germplasm for differences in cell death induction, which may serve as an indicator of susceptibility to brown rot. By employing advanced techniques such as marker-assisted selection breeders can more precisely identify and eliminate susceptible genotypes, resulting in the development of crop varieties with enhanced resistance.
Data availability statement
The original contributions presented in the study are included in the article/Supplementary Material. Further inquiries can be directed to the corresponding authors.
Author contributions
AL: Methodology, Writing – original draft, Writing – review & editing. JK: Conceptualization, Methodology, Supervision, Writing – review & editing. HB: Methodology, Writing – review & editing. RD-S: Methodology, Writing – review & editing. NT: Funding acquisition, Writing – review & editing. RT: Funding acquisition, Supervision, Writing – original draft, Writing – review & editing. LV: Conceptualization, Funding acquisition, Methodology, Supervision, Writing – original draft, Writing – review & editing.
Funding
The author(s) declare financial support was received for the research, authorship, and/or publication of this article. This work was supported by national project PID2020-115702RB-C22/AEI/10.13039/501100011033 from the Spanish Government (MINECO) and by funding received from the CERCA Programme SGR-01477/Generalitat de Catalunya. LV received funding from the postdoctoral fellowships programme Beatriu de Pinós, funded by the Secretary of Universities and Research (Government of Catalonia) and by the Horizon 2020 programme of the European Union (MSCA grant 801370) and AL is recipient of a IRTA Sponsored Fellowship 2022.
Acknowledgments
The authors gratefully acknowledge Dr. Iban Eduardo for providing part of the plant material.
Conflict of interest
The authors declare that the research was conducted in the absence of any commercial or financial relationships that could be construed as a potential conflict of interest.
Publisher’s note
All claims expressed in this article are solely those of the authors and do not necessarily represent those of their affiliated organizations, or those of the publisher, the editors and the reviewers. Any product that may be evaluated in this article, or claim that may be made by its manufacturer, is not guaranteed or endorsed by the publisher.
Supplementary material
The Supplementary Material for this article can be found online at: https://www.frontiersin.org/articles/10.3389/fpls.2024.1428613/full#supplementary-material
References
Akhoon, B. A., Gupta, S. K., Dhar, M. K. (2023). Dissecting the genome, secretome, and effectome repertoires of Monilinia spp.: The causal agent of brown rot disease: A comparative analysis. Postharvest Biol. Technol. 195, 112120. doi: 10.1016/j.postharvbio.2022.112120
Bai, S., Tuan, P. A., Tatsuki, M., Yaegaki, H., Ohmiya, A., Yamamizo, C., et al. (2016). Knockdown of carotenoid cleavage dioxygenase 4 (CCD4) via virus-induced gene silencing confers yellow coloration in peach fruit: evaluation of gene function related to fruit traits. Plant Mol. Biol. Rep. 34, 257–264. doi: 10.1007/s11105-015-0920-8
Balsells-Llauradó, M., Silva, C. J., Usall, J., Vall-llaura, N., Serrano-Prieto, S., Teixidó, N., et al. (2020). Depicting the battle between nectarine and Monilinia laxa: the fruit developmental stage dictates the effectiveness of the host defenses and the pathogen’s infection strategies. Hortic. Res. 7, 167. doi: 10.1038/s41438-020-00387-w
Baró-Montel, N., Eduardo, I., Usall, J., Casals, C., Arús, P., Teixidó, N., et al. (2019a). Exploring sources of resistance to brown rot in an interspecific almond × peach population. J. Sci. Food Agric. 99, 4105–4113. doi: 10.1002/jsfa.9640
Baró-Montel, N., Torres, R., Casals, C., Teixidó, N., Segarra, J., Usall, J. (2019b). Developing a methodology for identifying brown rot resistance in stone fruit. Eur. J. Plant Pathol. 154, 287–303. doi: 10.1007/s10658-018-01655-1
Baró-Montel, N., Vall-llaura, N., Giné-Bordonaba, J., Usall, J., Serrano-Prieto, S., Teixidó, N., et al. (2019c). Double-sided battle: The role of ethylene during Monilinia spp. infection in peach at different phenological stages. Plant Physiol. Biochem. 144, 324–333. doi: 10.1016/j.plaphy.2019.09.048
Bi, K., Liang, Y., Mengiste, T., Sharon, A. (2023). Killing softly: a roadmap of Botrytis cinerea pathogenicity. Trends Plant Sci. 28, 211–222. doi: 10.1016/j.tplants.2022.08.024
Cantabella, D., Teixidó, N., Segarra, G., Torres, R., Casanovas, M., Dolcet-Sanjuan, R. (2021). Rhizosphere microorganisms enhance in vitro root and plantlet development of Pyrus and Prunus rootstocks. Planta 253, 78. doi: 10.1007/s00425-021-03595-3
Casals, C., Torres, R., Teixidó, N., de Cal, A., Segarra, J., Usall, J. (2022). Brown rot on stone fruit: From epidemiology studies to the development of effective control strategies. Sci. Hortic. 301, 111096. doi: 10.1016/j.scienta.2022.111096
Cowger, C., Ward, B., Brown-Guedira, G., Brown, J. K. M. (2020). Role of effector-sensitivity gene interactions and durability of quantitative resistance to Septoria nodorum blotch in eastern U.S. wheat. Front. Plant Scien. 11. doi: 10.3389/fpls.2020.00155
De Cal, A., Usall, J., Melgarejo, P. (2009). First report of brown rot caused by Monilinia fructicola in peach orchards in Ebro valley, Spain. Plant Dise 93, 763–763. doi: 10.1094/PDIS-93-7-0763A
De Miccolis Angelini, R. M., Landi, L., Raguseo, C., Pollastro, S., Faretra, F., Romanazzi, G. (2022). Tracking of diversity and evolution in the brown rot fungi Monilinia fructicola, Monilinia fructigena, and Monilinia laxa. Front. Microbiol. 13, 854852. doi: 10.3389/fmicb.2022.854852
De Miccolis Angelini, R. M., Romanazzi, G., Pollastro, S., Rotolo, C., Faretra, F., Landi, L. (2019). New high-quality draft genome of the brown rot fungal pathogen Monilinia fructicola. Genome Biol. Evol. 11, 2850–2855. doi: 10.1093/gbe/evz207
Domazakis, E., Lin, X., Aguilera-Galvez, C., Wouters, D., Bijsterbosch, G., Wolters J., P., et al. (2017). Effectoromics-based identification of cell surface receptors in potato. Methods Mol. Biol. 1578, 337–353. doi: 10.1007/978-1-4939-6859-6_29
Downie, R. C., Bouvet, L., Furuki, E., Gosman, N., Gardner, K. A., Mackay, I. J., et al. (2018). Assessing European wheat sensitivities to Parastagonospora nodorum necrotrophic effectors and fine-mapping the Snn3-B1 locus conferring sensitivity to the effector SnTox3. Front. Plant Sci. 9. doi: 10.3389/fpls.2018.00881
Faris, J. D., Friesen, T. L. (2020). Plant genes hijacked by necrotrophic fungal pathogens. Curr. Opin. Plant Biol. 56, 74–80. doi: 10.1016/j.pbi.2020.04.003
Friesen, T. L., Faris, J. D. (2021). Characterization of effector-target interactions in necrotrophic pathosystems reveals trends and variation in host manipulation. Annu. Rev. Phytopathol. 59, 77–98. doi: 10.1146/annurev-phyto-120320
Friesen, T. L., Meinhardt, S. W., Faris, J. D. (2007). The Stagonospora nodorum-wheat pathosystem involves multiple proteinaceous host-selective toxins and corresponding host sensitivity genes that interact in an inverse gene-for-gene manner. Plant J. 51, 681–692. doi: 10.1111/j.1365-313X.2007.03166.x
Hartl, M., Merker, H., Schmidt, D. D., Baldwin, I. T. (2008). Optimized virus-induced gene silencing in Solanum nigrum reveals the defensive function of leucine aminopeptidase against herbivores and the shortcomings of empty vector controls. New Phytol. 179, 356–365. doi: 10.1111/j.1469-8137.2008.02479.x
Hrustić, J., Delibašić, G., Stanković, I., Grahovac, M., Krstić, B., Bulajić, A., et al. (2015). Monilinia spp. causing brown rot of stone fruit in Serbia. Plant Dis. 99, 709–717. doi: 10.1094/PDIS-07-14-0732-RE
Index Fungorum (2023). Monilinia species list. Available online at: http://www.indexfungorum.org.
Kabbage, M., Kessens, R., Bartholomay, L. C., Williams, B. (2017). The life and death of a plant cell. Annu. Rev. Plant Biol. 68, 375–404. doi: 10.1146/annurev-arplant-043015
Kars, I., Krooshof, G. H., Wagemakers, L., Joosten, R., Benen, J. A. E., van Kan, J. A. L. (2005). Necrotizing activity of five Botrytis cinerea endopolygalacturonases produced in Pichia pastoris. Plant J. 43, 213–225. doi: 10.1111/j.1365-313X.2005.02436.x
Landi, L., De Miccolis Angelini, R. M., Pollastro, S., Abate, D., Faretra, F., Romanazzi, G. (2018). Genome sequence of the brown rot fungal pathogen Monilinia fructigena. BMC Res. Notes 11, 758. doi: 10.1186/s13104-018-3854-z
Leisen, T., Bietz, F., Werner, J., Wegner, A., Schaffrath, U., Scheuring, D., et al. (2020). CRISPR/Cas with ribonucleoprotein complexes and transiently selected telomere vectors allows highly efficient marker-free and multiple genome editing in Botrytis cinerea. PloS Pathog. 16, e1008326. doi: 10.1371/journal.ppat.1008326
Leisen, T., Werner, J., Pattar, P., Safari, N., Ymeri, E., Sommer, F., et al. (2022). Multiple knockout mutants reveal a high redundancy of phytotoxic compounds contributing to necrotrophic pathogenesis of Botrytis cinerea. PloS Pathog. 18, e1010367. doi: 10.1371/journal.ppat.1010367
Lichou, J., Mandrin, J. F., Breniaux, D., Mercier, V., Giauque, P., Desbrus, D., et al. (2002). A new, powerful monilia: Monilia fructicola chooses stone-fruit trees for its attacks. Phytoma 547, 22–25.
Liu, Y., Schiff, M., Marathe, R., Dinesh-Kumar, S. P. (2002). Tobacco Rar1, EDS1 and NPR1/NIM1 like genes are required for N-mediated resistance to tobacco mosaic virus. Plant J. 30, 415–429. doi: 10.1046/j.1365-313X.2002.01297.x
Liu, Z., Zhang, Z., Faris, J. D., Oliver, R. P., Syme, R., McDonald, M. C., et al. (2012). The cysteine rich necrotrophic effector SnTox1 produced by Stagonospora nodorum triggers susceptibility of wheat lines harboring Snn1. PloS Pathog. 8, e1002467. doi: 10.1371/journal.ppat.1002467
Macfarlane, S. A. (2010). Tobraviruses—plant pathogens and tools for biotechnology. Mol. Plant Pathol. 11, 577–583. doi: 10.1111/j.1364-3703.2010.00617.x
Malvestiti, M. C., van Kan, J. A. L. “Your death, my life: understanding the success story of necrotrophic plant pathogenic Ascomycota,” in The MYCOTA, Agricultural and Industrial Applications. Eds. Kempken, F., Grüttner, S., Kollath-Leiss, K. (Springer Nature Publishers). in press.
Marcet-Houben, M., Villarino, M., Vilanova, L., de Cal, A., van Kan, J. A. L., Usall, J., et al. (2021). Comparative genomics used to predict virulence factors and metabolic genes among Monilinia species. J. Fungi 7, 464. doi: 10.3390/jof7060464
Martínez-García, P. J., Parfitt, D. E., Bostock, R. M., Fresnedo-Ramírez, J., Vazquez-Lobo, A., Ogundiwin, E. A., et al. (2013). Application of genomic and quantitative genetic tools to identify candidate resistance genes for brown rot resistance in peach. PloS One 8, e78634. doi: 10.1371/journal.pone.0078634
Naranjo-Ortíz, M. A., Rodríguez-Píres, S., Torres, R., De Cal, A., Usall, J., Gabaldón, T. (2018). Genome sequence of the brown rot fungal pathogen Monilinia laxa. Geno. Announc. 6, 10–1128. doi: 10.1128/genomeA.00214-18
Nasir, K. H., Takahashi, Y., Ito, A., Saitoh, H., Matsumura, H., Kanzaki, H., et al. (2005). High-throughput in planta expression screening identifies a class II ethylene-responsive element binding factor-like protein that regulates plant cell death and non-host resistance. Plant J. 43, 491–505. doi: 10.1111/j.1365-313X.2005.02472.x
Ollero-Lara, A., Agustí-Brisach, C., Lovera, M., Roca, L. F., Arquero, O., Trapero, A. (2019). Field susceptibility of almond cultivars to the four most common aerial fungal diseases in southern Spain. Crop Prot. 121, 18–27. doi: 10.1016/j.cropro.2019.03.005
Pacheco, I., Bassi, D., Eduardo, I., Ciacciulli, A., Pirona, R., Rossini, L., et al. (2014). QTL mapping for brown rot (Monilinia fructigena) resistance in an intraspecific peach (Prunus persica L. Batsch) F1 progeny. Tree Genet. Genmes. 10, 1223–1242. doi: 10.1007/s11295-014-0756-7
Rivera, Y., Zeller, K., Srivastava, S., Sutherland, J., Galvez, M., Nakhla, M., et al. (2018). Draft genome resources for the phytopathogenic fungi Monilinia fructicola, M. fructigena, M. polystroma, and M. laxa, the causal agents of brown rot. Phytopathology 108, 1141–1142. doi: 10.1094/PHYTO-12-17-0418-A
Schouten, A., van Baarlen, P., van Kan, J. A. L. (2008). Phytotoxic Nep1-like proteins from the necrotrophic fungus Botrytis cinerea associate with membranes and the nucleus of plant cells. New Phytol. 177, 493–505. doi: 10.1111/j.1469-8137.2007.02274.x
Seidl, M. F., Van den Ackerveken, G. (2019). Activity and phylogenetics of the broadly occurring family of microbial Nep1-like proteins. Annu.l Rev. Phytopathol. 57, 367–386. doi: 10.1146/annurev-phyto-082718
Selin, C., de Kievit, T. R., Belmonte, M. F., Fernando, W. G. D. (2016). Elucidating the role of effectors in plant-fungal interactions: Progress and challenges. Front. Microbiol. 7, 600. doi: 10.3389/fmicb.2016.00600
Shao, D., Smith, D. L., Kabbage, M., Roth, M. G. (2021). Effectors of plant necrotrophic fungi. Front. Plant Sci. 12. doi: 10.3389/fpls.2021.687713
Shi, G., Zhang, Z., Friesen, T. L., Raats, D., Fahima, T., Brueggeman, R. S., et al. (2016). The hijacking of a receptor kinase-driven pathway by a wheat fungal pathogen leads to disease. Sci. Adv. 2, e1600822. doi: 10.1126/sciadv.1600822
Steentjes, M. B. F., Valderrama, A. L. H., Fouillen, L., Bahammou, D., Leisen, T., Albert, I., et al. (2022). Cytotoxic activity of Nep1-like proteins on monocots. New Phytol. 235, 690–700. doi: 10.1111/nph.18146
Tan, K.-C., Ferguson-Hunt, M., Rybak, K., Waters, O. D. C., Stanley, W. A., Bond, C. S., et al. (2012). Quantitative variation in effector activity of ToxA isoforms from Stagonospora nodorum and Pyrenophora tritici-repentis. Mol. Plant Microbe Interact. 25, 515–522. doi: 10.1094/MPMI
Tan, K. C., Oliver, R. P., Solomon, P. S., Moffat, C. S. (2010). Proteinaceous necrotrophic effectors in fungal virulence. Funct. Plant Biol. 37, 907–912. doi: 10.1071/FP10067
Tan, K. C., Phan, H. T. T., Rybak, K., John, E., Chooi, Y. H., Solomon, P. S., et al. (2015). Functional redundancy of necrotrophic effectors – consequences for exploitation for breeding. Front. Plant Sci. 6, 501. doi: 10.3389/fpls.2015.00501
Valero-Jiménez, C. A., Veloso, J., Staats, M., van Kan, J. A. L. (2019). Comparative genomics of plant pathogenic Botrytis species with distinct host specificity. BMC Genomic. 20, 203. doi: 10.1186/s12864-019-5580-x
Vilanova, L., Valero-Jiménez, C. A., Van Kan, J. A. L. (2021). Deciphering the Monilinia fructicola genome to discover effector genes possibly involved in virulence. Genes 12, 568. doi: 10.3390/genes12040568
Villarino, M., Egüen, B., Lamarca, N., Segarra, J., Usall, J., Melgarejo, P., et al. (2013). Occurrence of Monilinia laxa and M. fructigena after introduction of M. fructicola in peach orchards in Spain. Eur. J. Plant Pathol. 137, 835–845. doi: 10.1007/s10658-013-0292-6
Villarino, M., Melgarejo, P., Usall, J., Segarra, J., De Cal, A. (2010). Primary inoculum sources of Monilinia spp. in Spanish peach orchards and their relative importance in brown rot. Plant Dis. 94, 1048–1054. doi: 10.1094/PDIS-94-8-1048
Virdi, S. K., Liu, Z., Overlander, M. E., Zhang, Z., Xu, S. S., Friesen, T. L., et al. (2016). New insights into the roles of host gene-necrotrophic effector interactions in governing susceptibility of durum wheat to tan spot and Septoria nodorum blotch. G3: Genes Genomes. Genet. 6, 4139–4150. doi: 10.1534/g3.116.036525
Vleeshouwers, V. G. A. A., Oliver, R. P. (2014). Effectors as tools in disease resistance breeding against biotrophic, hemibiotrophic, and necrotrophic plant pathogens. Mol. Plant Microbe Interact. 27, 196–206. doi: 10.1094/MPMI-10-13-0313-IA
Zaracho-Echagüe, N. H., Arús, P., Eduardo, I. (2022). Construction of a NIL collection of P. davidiana into the peach genetic background. Acta Hortic. 1352, 263–270. doi: 10.17660/ActaHortic.2022.1352.35
Keywords: Monilinia fructicola, Prunus spp., transient expression, NLPs, necrotrophic fungi
Citation: López A, van Kan JAL, Beenen HG, Dolcet-Sanjuan R, Teixidó N, Torres R and Vilanova L (2024) Evaluation of cell death-inducing activity of Monilinia spp. effectors in several plants using a modified TRV expression system. Front. Plant Sci. 15:1428613. doi: 10.3389/fpls.2024.1428613
Received: 06 May 2024; Accepted: 29 July 2024;
Published: 16 August 2024.
Edited by:
Rachid Lahlali, Ecole Nationale d’Agriculture de Meknès, MoroccoReviewed by:
David Vela-Corcia, University of Malaga, SpainLucia Landi, Marche Polytechnic University, Italy
Copyright © 2024 López, van Kan, Beenen, Dolcet-Sanjuan, Teixidó, Torres and Vilanova. This is an open-access article distributed under the terms of the Creative Commons Attribution License (CC BY). The use, distribution or reproduction in other forums is permitted, provided the original author(s) and the copyright owner(s) are credited and that the original publication in this journal is cited, in accordance with accepted academic practice. No use, distribution or reproduction is permitted which does not comply with these terms.
*Correspondence: Laura Vilanova, bGF2aXRvODJAZ21haWwuY29t; Rosario Torres, cm9zYXJpby50b3JyZXNAaXJ0YS5jYXQ=