- 1Institute of Food Crops, Provincial Key Laboratory of Agrobiology, Jiangsu Academy of Agricultural Sciences, Nanjing, China
- 2Center for Tropical Crop Research, Research Institute of Tropical Roots and Tuber Crops (INIVIT), Santo Domingo, Cuba
- 3Xuzhou Institute of Agricultural Sciences, Chinese Academy of Agricultural Sciences, Xuzhou, China
Griffithsin (GRFT) is a highly effective, broad-spectrum, safe, and stable viral inhibitor used to suppress a variety of viruses. However, little information is available on whether GRFT can prevent plant viral diseases. In this study, we constructed a GRFT overexpression vector containing the sweetpotato storage cell signal peptide and generated exogenous GRFT overexpression lines through genetic transformation. The transgenic plants showed notable resistance to sweetpotato virus disease in the virus nursery. To verify the antiplant virus function of GRFT, transient expression in tobacco leaves showed that GRFT inhibited the sweetpotato leaf curl virus (SPLCV). The replication of SPLCV was entirely inhibited when the concentration of GRFT reached a certain level. The results of pulldown and BIFC assays showed that GRFT did not interact with the six components of SPLCV. In addition, the mutated GRFTD/A without the binding ability of carbohydrate and anticoronavirus function, in which three aspartate residues at carbohydrate binding sites were all mutated to alanine, also inhibited SPLCV. Quantitative reverse-transcription PCR analyses showed that the tobacco antiviral-related genes HIN1, ICS1, WRKY40, and PR10 were overexpressed after GRFT/GRFTD/A injection. Furthermore, HIN1, ICS1, and PR10 were more highly expressed in the leaves injected with GRFTD/A. The results suggest that sweetpotato is able to express GRFT exogenously as a bioreactor. Moreover, exogenous GRFT expression inhibits plant viruses by promoting the expression of plant antiviral genes.
1 Introduction
Lectins comprise a class of proteins with a distinct molecular structure. The structure is specific and reversible to enable recognition of, and binding to, carbohydrates without altering the carbohydrate portion (Van Damme et al., 1998). Based on the recognition and binding properties of lectins for specific carbohydrates, lectins play important roles in signal transduction, immune response, and plant defense, including resistance to pests and antiviral diseases, and induction of apoptosis. At present, lectins have broad application prospects in insect control, disease resistance, the biopharmaceutical industry, and medical detection and therapy. Therefore, strategies to increase the yield of lectins are of practical importance.
Griffithsin (GRFT), a lectin with a high affinity for mannose residues derived from the marine algae Griffithsia spp., is a widely used antiviral inhibitor. The protein comprises 121 amino acids folded into stable domain-exchanged homomeric dimers and targets mannose-terminal residues of high manno-oligosaccharides. GRFT has a total of six carbohydrate binding sites per homologous dimer (Ziólkowska et al., 2006; Ziólkowska et al., 2007), which may be one reason why it is highly effective against various viruses, such as human immunodeficiency virus (HIV), hepatitis C virus (HCV), Japanese encephalitis virus, herpes simplex virus (HSV), and porcine reproductive and respiratory syndrome virus (Meuleman et al., 2012; Ishag et al., 2013; Takebe et al., 2013; Levendosky et al., 2015; Lusvarghi and Bewley, 2016; Millet et al., 2016; Derby et al., 2018; Li et al., 2018; Lee, 2019; Nabeta et al., 2021).
The mechanisms of action of GRFT against animal viruses are diverse. Among the aforementioned viruses, GRFT inactivates HIV almost immediately after exposure to the virus (Emau et al., 2007). First, GRFT inhibits the binding of HIV to CD4 on the surface of lymphocytes by selectively binding to the mannose-rich glycan gp120 on the viral envelope glycoprotein (Balzarini, 2005; Alexandre et al., 2010; Lee, 2019). In addition, GRFT can inhibit the binding of dendritic cells (DC-SIGN) to HIV-1, thereby preventing the virus from infecting target cells (Van Damme et al., 1998; Mori et al., 2005). GRFT acts at an early stage of the HCV life cycle, acting in a genotype-independent manner by intervening in the interaction between the viral envelope protein E2 and the viral receptor CD81 (Meuleman et al., 2012). In addition, GRFT inhibits the severe acute respiratory syndrome coronavirus (SARS-CoV), but, unlike the preceding two viruses, GRFT binds to the SARS-CoV surface glycoprotein S but does not affect it, and virus recognition of Vero cells is indicative of the receptor (Ziólkowska et al., 2006). GRFT can inhibit HSV-2 infection after entry by blocking cell-to-cell diffusion, but virus invasion of cells is not affected (Nixon et al., 2013). GRFT inhibits the internalization stage of the human papillomavirus (HPV) by binding to the HPV receptor integrin α6 (Levendosky et al., 2015; Derby et al., 2018). The most important antiviral mechanism of GRFT is to bind to glycoproteins on the surface of the capsular virus to inhibit virus transmission (O’Keefe et al., 2009).
GRFT has been expressed in several exogenous expression systems because of its low biotoxicity and extensive antiviral capacity, for example, an Escherichia coli expression system based on the pET expression vector (Giomarelli et al., 2006), a transient expression system in tobacco based on the tobacco mosaic virus (TMV) vector (O’Keefe et al., 2009; Fuqua et al., 2015a; Fuqua et al., 2015b; Vafaee and Alizadeh, 2018), a chloroplast expression system in tobacco (Hoelscher et al., 2018), and a rice endosperm expression system (Vamvaka et al., 2016). Lectins expressed in plants are observed to have stronger antiviral activity and no biological toxicity (O’Keefe et al., 2009). Thus, we speculated that GRFT can be directly expressed in feed crops as an additive to prevent the occurrence of animal capsular viroid diseases.
Compared with other crops, sweetpotato has the following advantages as a bioreactor. First, sweetpotato is a natural animal feed that does not require prior purification. Second, sweetpotato is a crop that reproduces asexually. Under suitable growing conditions, the aboveground biomass of sweetpotato provides a vegetable for humans as well as feed for livestock. Lastly, extraction of GRFT from sweetpotatoes in combination with the starch isolation process would reduce costs and pollution.
Sweetpotatoes could be used as a bioreactor to produce high-value GRFT protein or as an additive to animal feed to prevent the incidence of animal capsular viroid infection and thereby increase the utilization efficiency and economic benefits of sweetpotatoes. In this study, the sequence of GRFT was optimized according to the sequence preference for sweetpotatoes without altering GRFT dimer formation. For storage of GRFT in the vacuole of sweetpotato cells for easy extraction, the endoplasmic reticulum signal peptide and vacuole sorting signal sequences were inserted at either end of the GRFT sequence, and the His-tag protein motif for purification was added to the 3’ end of the GRFT sequence. The designed sequences were synthesized directly by the company and transformed under the control of different promoters in the vectors. Transgenic lines harboring GRFT were obtained successfully by genetic transformation. The transformants showed distinct resistance to sweetpotato virus disease (SPVD) in the field. SPVD is a viral disease that can cause a severe reduction in sweetpotato production. At present, there are few reports of lectins conferring resistance to plant viruses. Transient expression of GRFT in tobacco leaves inhibited the sweetpotato leaf curl virus (SPLCV). Bimolecular fluorescence complementation (BIFC) and pulldown analysis showed that GRFT did not interact with the virus. By constructing GRFT transgenic plants, we showed that GRFT-conferred resistance to plant viruses was not dependent on the carbohydrate-binding ability of the lectin. Quantitative reverse-transcription PCR (RT-qPCR) analysis revealed that the mechanism of GRFT resistance to plant viruses was to induce the expression of antiviral genes. The mechanism of GRFT against SPVD represents a novel strategy for plant virus control.
2 Materials and methods
2.1 Plant materials and growth conditions
The sweetpotato cultivar ‘Xushu 29’ was obtained from the Xuzhou Institute of Agricultural Sciences in Jiangsu, Xuhuai district, Xuzhou, Jiangsu, China. Plants were propagated from stem cuttings, and the potted plants were transferred to a clean greenhouse maintained at 28°C with a 16 h/8 h (light/dark) photoperiod. The sweetpotato plants were transplanted to the field for growth at a suitable temperature, and tubers were harvested in early November.
2.2 GRFT expression vector construction
The pCAMBIA1305–2×35S/Super/Sp/Swap4-GRFT vector was constructed. First, the pCAMBIA1305 vector was used as the basic expression vector, into which different promoter sequences (2×CaMV35S, Super, Sp, and Swap4) were inserted. Super promoter stems from the pCAMBIA-Super1300-GFP vector. The super promoter consists of a trimer of the octopine synthase transcriptional activating element affixed to the mannopine synthase2′ (mas2′) transcriptional activating element plus a minimal promoter. Sp (GC534664.1) is the sporamin gene promoter in sweetpotatoes. Swap4 promoter is a multistress-induced peroxidase promoter from sweetpotato. The fragment of the designed GRFT gene was synthesized by Bioengineer Biotechnology. The following primers to amplify the fragment were designed using the homologous recombination method: GRFT-SmaI(F): 5′-GtctcgaggaccggtcccgggATGAAGGCTTTCACTCTTGCCT-3′ and GRFT-PmlI(R): 5′-ctggtcaccaattcacacgtgATACTGCTCGTAATAGATATCAAGGGA-3′.
2.3 Sweetpotato transformation and positive transformant identification
Xushu 29 was used as the transgene recipient using the following transformation process. Sweetpotato stem tips were used for callus induction on MS1D medium (4.4 g/L Murashige and Skoog salts (MS), 0.4 mg/L thiamin, 30 g/L sucrose, 0.1 g/L inositol, and 1 mg/L 2,4-dichlorophenoxyacetic acid). In total, 10–15 ml of Agrobacterium tumefaciens strain EHA105 harboring the GRFT expression vector was gathered. The Agrobacterium cells were resuspended in MS1D supplemented with 100 μM acetosyringone (AS) and mixed with the sweetpotato calli. The mixture was shaken slightly for 30 min and then ultrasonicated for 10 s. Next, the calli were transferred to a solid medium (MS1D supplemented with 100 μM AS) with filter paper. After incubation in the dark for 2–3 days, the calli were washed with sterile water to remove the Agrobacterium cells and transferred to a screening medium (MS1D supplemented with 10 mg/L tauramycin and 400 mg/L cefotaxime). The calli were incubated under controlled conditions for 4–6 weeks. The calli were then transferred to an MSCH regeneration medium (4.4 g/L MS, 10 mg/L chaotropic acid, and 200 mg/L cefotaxime). After the shoots had differentiated from the calli, the shoots were transferred to SBMC rooting medium (4.4 g/L MS, 200 mg/L cefotaxime, and 0.3 mg/L thiamin). Genomic DNA was extracted from the plantlets for the identification of transformants.
2.4 Tobacco transient expression system
Tobacco seedlings were transferred to pots and cultivated in a greenhouse maintained at 28°C with a 16-h/8-h (light/dark) photoperiod for 1 month. The cell suspension of Agrobacterium tumefaciens strain GV3101 was shaken overnight (> 14 h) at 200–230 rpm at 28°C until the optical density (OD) value was 0.6–1.2. The cell suspensions were centrifuged at 1,000×g for 10 min. The collected cells were resuspended in MMA buffer (10 mM MgCl2, 10 mM MES, and 150 μM MAS, pH 5.6) and adjusted to OD = 1.00. Three leaves were injected with 1 ml of the Agrobacterium suspension using a 1-ml syringe, and the infection site was circled with a marker pen.
2.5 Sweetpotato protein extraction
Fresh sweetpotato leaf or root tissue (50 mg) was ground in liquid nitrogen into powder. The powdered samples were transferred to 1.5 ml centrifuge tubes, to which extraction buffer was added (50 mM Tris-HCl [pH 7.5], 5 mM EDTA, 10% glycerol, and 1% Triton-X-100). The mixture was placed on ice for 30 min, shaken every 10 min, then centrifuged at 12,000×g for 10 min at 4°C, and finally the supernatant was collected and stored at −20°C. Phenylmethylsulfonyl fluoride or other protease inhibitors were added before the extract was stored to prevent degradation.
2.6 Purification of tagged proteins and pulldown analysis
For His-tagged protein purification, the plasmid His::GRFT was transfected into E. coli strain BL21 (DE3) cells, and expression was induced by isopropyl β-d-1-thiogalactopyranoside (IPTG) by incubation for 12 h at 16°C. The complex proteins generated were purified using Ni-charged MagBeads (GenScript, L00295). For GST-tagged protein purification, the plasmid GST::GRFT was transfected into E. coli strain BL21 (DE3) cells, and protein expression was induced by IPTG by incubation for 12 h at 16°C. The GST-tagged proteins were purified using the GST-Sefinose Kit (Sangon Biotech, C600913, Shanghai, China). The eluted proteins were analyzed directly by SDS-PAGE or stored at −20°C.
2.7 Western blot analysis
The protein extracts with 5 × SDS-PAGE sample loading buffer were denatured in a boiling water bath for 10 min. Polyacrylamide gel electrophoresis was performed using 5%–10% SDS-PAGE gels for 1 h. The gels and alcohol-activated polyvinylidene fluoride (PVDF) membranes (Millipore) were immersed in the transfer solution for 10 min. Subsequently, the proteins were transferred from the gels to the PVDF membranes using a protein wet-transfer apparatus for 1.5 h at room temperature on a shaker using 5% skimmed milk. The primary antibodies anti-His (1:5,000, mouse, Abmart, M20020S, Shanghai, China), antiactin (1:5,000, mouse, TransGen Biotech, HC201–01, Beijing, China), and anti-GFP (1:5000, Rabbit, Abcam, EPR14104, Shanghai, China) were incubated overnight at 4°C on a shaker, where antiactin was used as an internal reference control. The secondary antibodies, comprising antimouse (1:10,000, Invitrogen, A11003, California, USA) and antirabbit (1:10,000, Invitrogen, A11008), were applied separately for binding to the primary antibody. The membranes were treated with a chemiluminescent solution (ZOMANBIO, ZD310, Beijing, China) and inspected with a chemiluminescence imager (Tanon, Shanghai, China).
2.8 Bimolecular fluorescence complementation analysis
The six components of SPLCV (AV1, AV2, AC1, AC2, AC3, and AC4) were linked to the vector PCV-NYFP. GRFT was linked to the vector PCV-CYFP. The NYFP-SPLCVs and CYFP-GRFT plasmids were transformed into Agrobacterium strain EHA105. Bimolecular fluorescence complementation analysis of the interaction between SPLCV and GRFT was assessed in the leaves of 3-week-old tobacco plants. The Agrobacterium strains harboring SPLCV-NYFP, GRFT-CYFP, and P19, respectively, were shaken overnight (> 14 h) at 200–230 rpm at 28°C. The suspensions of SPLCV-NYFP, GRFT-CYFP, and P19 were mixed in equal volumes. The mixture was injected into tobacco leaves, which were incubated for 3–4 days in the dark. The leaves were observed with a fluorescence microscope.
2.9 RNA extraction and RT-qPCR analysis
RNA extraction and quantitative real-time PCR analyses were performed as described previously (Bian et al., 2022). In brief, total RNA was extracted with the RNAprep Pure Plant Kit (polysaccharides and polyphenolics-rich) (Tiangen, Beijing, China). A sample (1 mg) of the purified total RNA was reverse-transcribed using the FastKing gDNA Dispelling RT SuperMix Kit (Tiangen). Primer pairs specific to the tested genes were designed using the NCBI Primer-BLAST online tool. The qRT-PCR analysis was performed using a SYBR® Premix Ex Taq™ Kit (TaKaRa, Beijing, China) on an ABI Prism® 7900 Real-Time PCR System. The Nicotiana benthamiana Ubiquitin C (UBC) gene (GenBank: AB026056.1) was used as an internal reference. Relative changes in gene expression were calculated using the 2−ΔΔCt method. Primers used for qPCR analyses are listed in Supplementary Table S1.
3 Results
3.1 Construction of the GRFT gene expression vector
The monomer GRFT is composed of 121 amino acids, based on the complete sequence lodged in the GenBank database (accession no. FJ594069). According to the preferred plant base sequence, the sequences of GRFT were optimized without changing the amino acid sequence. Each GRFT monomer has three carbohydrate-binding sites, namely, D30, D70, and D112 (Xue et al., 2013). To improve protein stability, the GRFT protein was targeted for storage in vacuoles by inserting the endoplasmic reticulum signal peptide sequence at the N terminus and the vacuole sorting signal sequence at the C-terminus. For easy detection, the His-tag protein motif was added to the 3’ end of the GRFT sequence. The final sequence (516 bp, 172 aa) was driven by the promoters CaMV35S, a Super promoter, or a sweetpotato promoter (Sp or Swap4) (Figure 1). Figure 1A shows the linear vector map of different promoters activated. The final nucleic acid sequence and amino acid sequence are shown in Figures 1B, C, respectively.
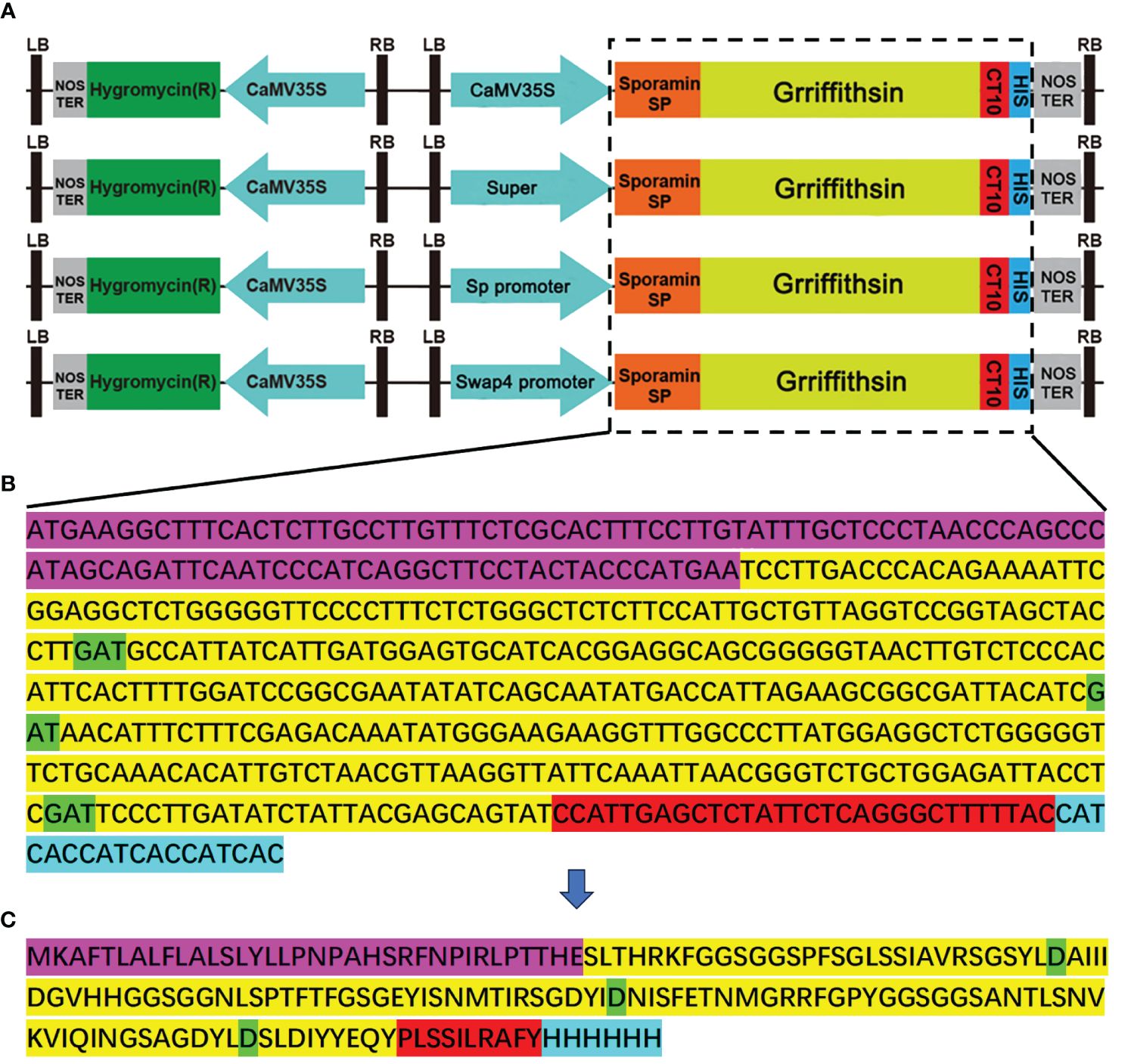
Figure 1 Construction of GRFT overexpression vectors. The pCambia1305-GFP was used as the skeleton to construct the vectors. (A) Linear vector map of different promoters activated. (B) Nucleic acid sequence of modified GRFT. (C) The amino acid sequence of GRFT. The purple-highlighted sequence is the sweetpotato endoplasmic reticulum signaling peptide. The yellow-highlighted sequence is GRFT. The green-highlighted aspartic acid residues are crucial sites for carbohydrate binding. The red-highlighted sequence is the sweetpotato vacuole sorting signal. The blue-highlighted sequence is the His-tag.
3.2 Confirmation of sweetpotato transformants expressing GRFT
The constructed vectors and empty vector (WT) were genetically transformed into the sweetpotato cultivar ‘Xushu 29’. Through hygromycin screening, the verified transgenic plants expressed GRFT driven by the CaMV35S and Super promoters rather than Sp or Swap4. Subsequently, the transgenic lines stably expressing GRFT were screened by western blot analysis with the anti-His antibody (Figure 2A). Owing to amino acids 15–19, GRFT usually exists as a homologous dimer, which is the optimal state for its antiviral function (Xue et al., 2013). In this study, four transformants were identified with bands larger than 25 kD, which is approximately twice the molecular weight of the GRFT monomer (12.7 kD). Therefore, the optimization of the GRFT sequence did not affect the structure of the GRFT protein. The transgenic lines expressing GRFT developed harvestable tubers similar to those of the wild type (Figure 2B).
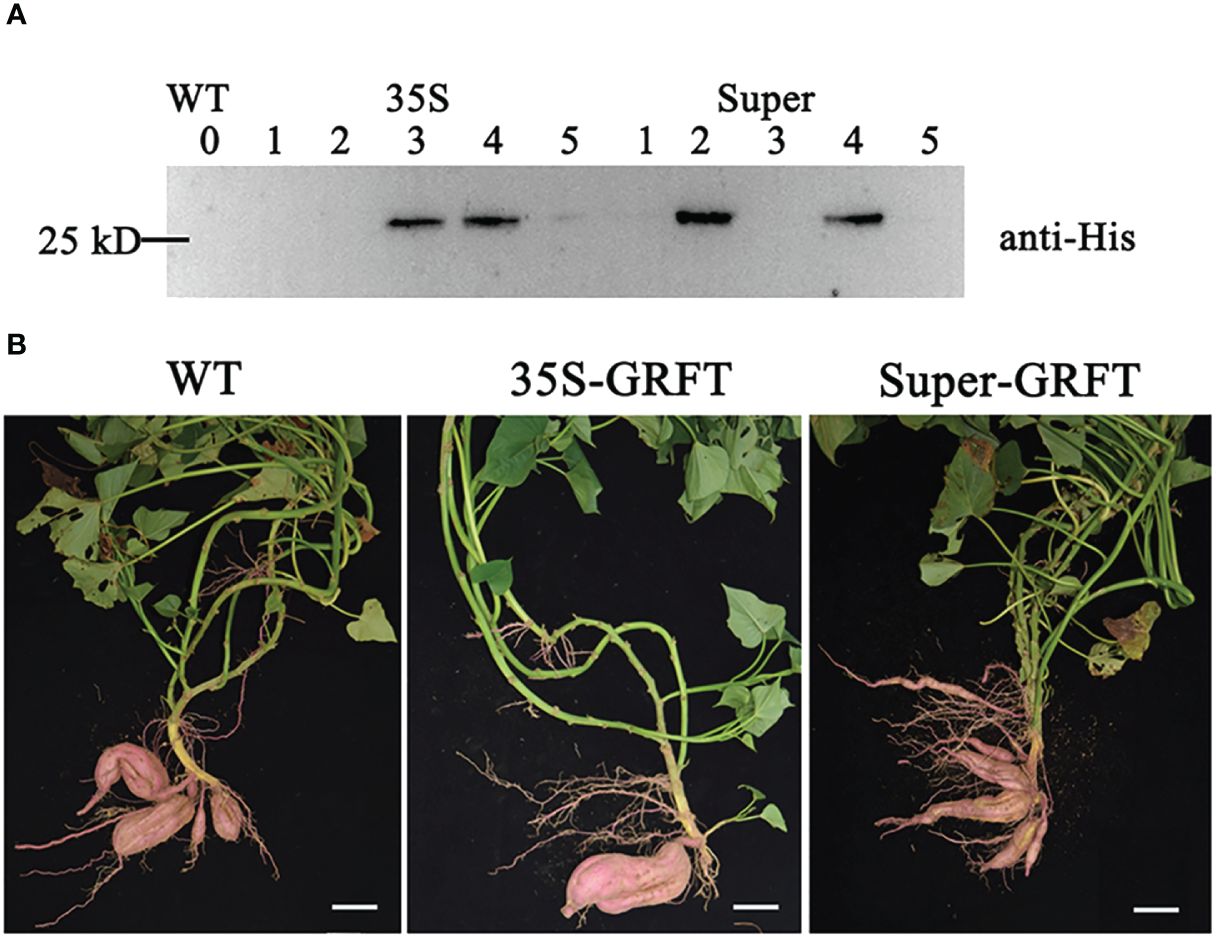
Figure 2 Sweetpotato transgenic lines expressing GRFT. The wild type (WT) was ‘Xushu 29’; 35S-GRFT and Super-GRFT are transgenic lines with GRFT driven by different promoters. (A) WT and transgenic lines were screened by Western blotting. (B) Tubers of the WT and transgenic lines after harvest. Scale bar: 5 cm.
3.3 Identification of the antiplant virus function of GRFT
It is well known that the anti-animal virus function of lectins is mainly affected by their binding to viral surface glycoproteins. Plant viruses lack glycoproteins, and currently, there are few reports of lectins effective against plant viruses. Interestingly, the transgenic plants expressing GRFT showed obvious resistance to SPVD in the virus disease nursery (Figure 3A). SPVD is a contagious viral disease caused by coinfection with the sweetpotato feather mottle virus (SPFMV) and the sweetpotato chlorotic stunt virus (SPCSV). Almost all sweetpotato plants in the virus nursery were infected with SPVD, except for GRFT-transgenic plants. Therefore, we speculated that GRFT may provide broad-spectrum resistance to plant viruses. SPLCV is a single-component bipartite virus that can infect a variety of dicotyledonous plants, including sweetpotato, tobacco, and petunia. To test this hypothesis, CaMV35S:GRFT and CaMV35S:SPLCV-GFP vectors were transfected via Agrobacterium tumefaciens strain GV3101 and used to simultaneously infect tobacco leaves according to different ratios (Figure 3B). At a ratio of 4:1, the green fluorescence signal was slightly weaker than that observed after injection of the same concentration of the viral expression vector. When the relative concentration of GRFT was 19 times that of SPLCV-GFP, the green fluorescence signal was weak. No green fluorescence was visible when the concentration of GRFT was 99 times that of SPLCV-GFP (Figure 3B). The protein at the infection site was extracted for western blot analysis with the anti-GFP antibody. The results showed that the GFP signal gradually weakened with the increase in GRFT proportion (Figure 3C). Thus, the results of transient expression of GRFT in tobacco were consistent with the western blotting results. This phenomenon suggests that GRFT can inhibit infection by SPLCV and that GRFT may provide broad-spectrum resistance to plant viruses.
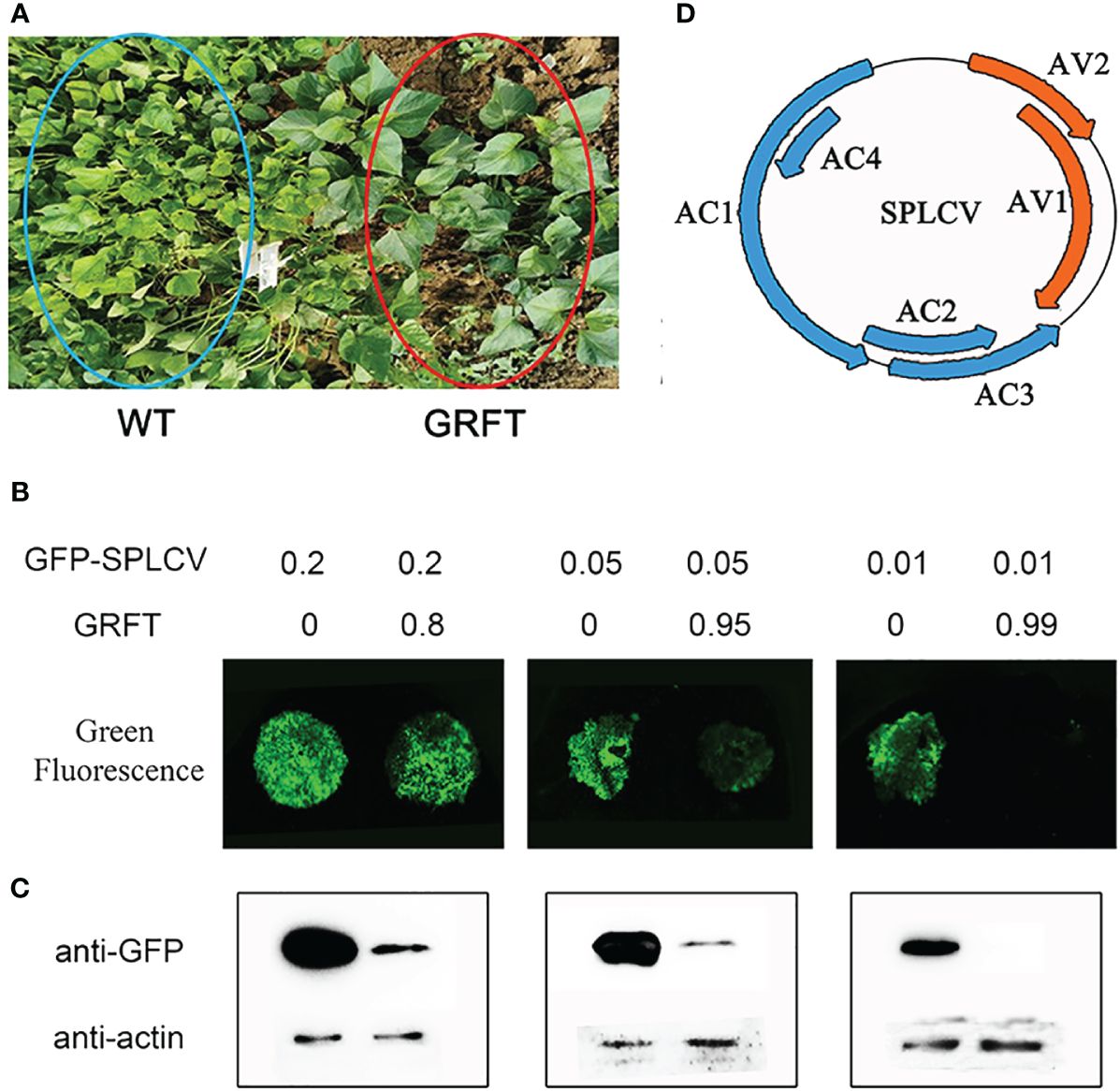
Figure 3 Resistance to plant viruses conferred by GRFT. (A) Transgenic lines were grown in the virus nursery for SPVD outbreaks. (B) Transient expression of GFP-SPLCV and 35S-GRFT in tobacco leaves. The infection solution (concentration OD = 1) was mixed in different proportions. (C) After transient expression, the protein was extracted from the tobacco leaves for Western blotting analysis. (D) The six components of SPLCV.
3.4 Mechanism of GRFT resistance to plant viruses
GRFT is known to provide resistance to coronaviruses by binding to the surface coat protein of the virus, thus inhibiting the transmission of the virus. The shells of plant viruses lack glycoproteins, so GRFT may inhibit plant viruses by binding to other components of plant viruses. The SPLCV genome is approximately 2.8 kb in length and consists of six open reading frames (Figure 3D). The six components are AV1 (29.36 kD), AV2 (13.34 kD), AC1 (40.88 kD), AC2 (16.60 kD), AC3 (16.70 kD), and AC4 (9.48 kD), of which AV1 is the coat protein (CP). The pCMV-C-YFP vectors associated with these six components were coinjected into Nicotiana benthamiana leaves with pCMV-N-YFP : GRFT. No fluorescence signal was observed (Figure 4A). These results suggested that GRFT does not bind to SPLCV. To verify this conclusion, GST-GRFT and His-tagged SPLCV components were purified for pulldown in vitro (Figures 4B, C). Similarly, no component of SPLCV was combined with GRFT (Figure 4D). In conclusion, GRFT was indicated to combat plant viruses by a different mechanism than how it inhibits animal viruses. Three aspartic acid residues associated with sugar binding in GRFT were simultaneously point-mutated to alanine (Figure 5A). GRFTD30,70,112A with the His-tag and signal peptide was attached to the CaMV35S vector. GRFTD/A inhibited SPLCV expression as effectively as GRFT in the tobacco leaf transient expression experiments (Figure 5B). SPLCV was not suppressed by the negative control (the empty CaMV35S vector). Similarly, GRFTD/A did not interact with SPLCV (Figure 4D). These results further indicated that the antiviral function of GRFT in plants was independent of its sugar-binding activity.
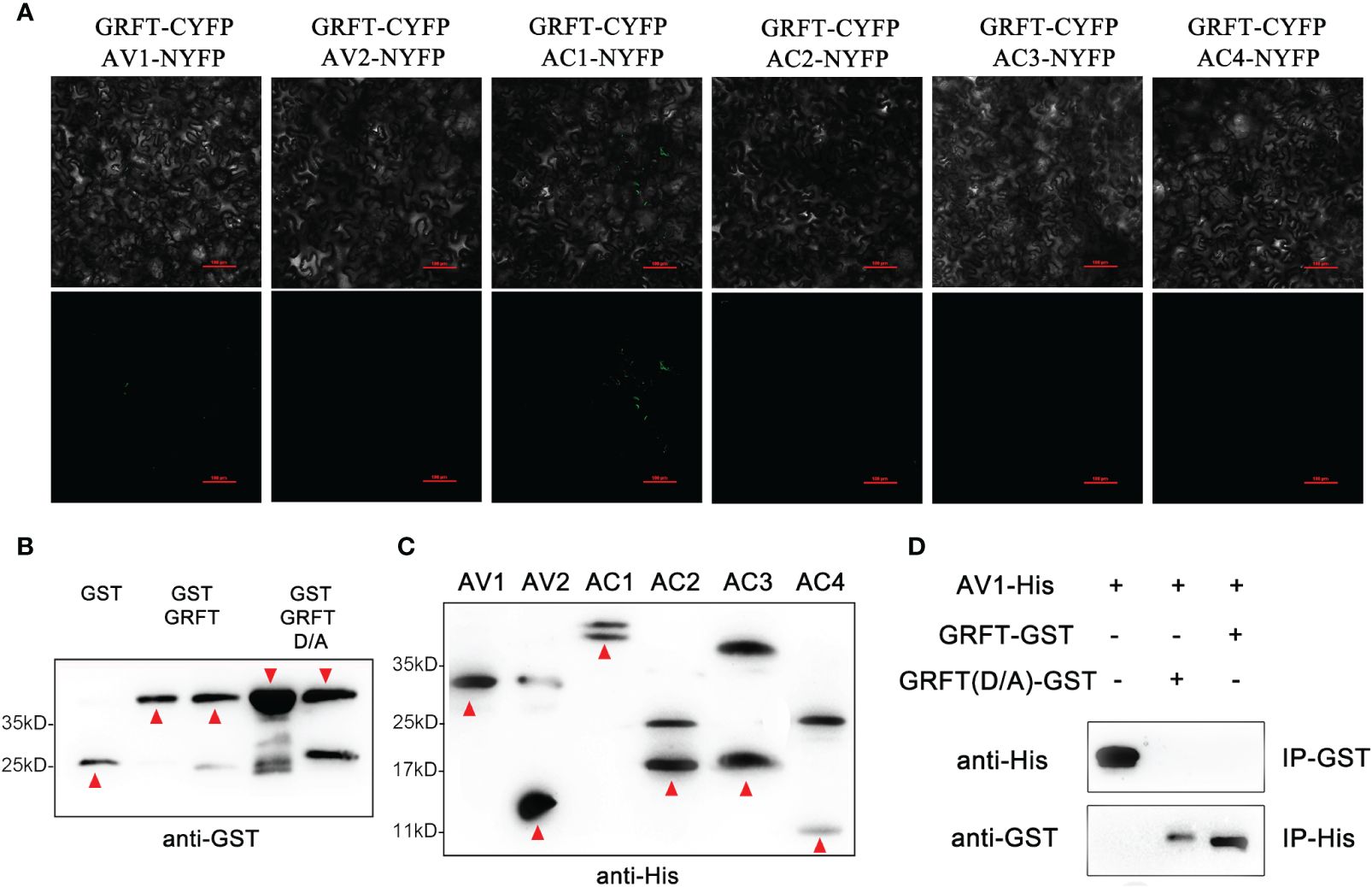
Figure 4 Interaction between GRFT and SPLCV verified by BIFC and pulldown assays. (A) Bimolecular fluorescence complementation assay revealing noninteraction between GRFT and SPLCVs. SPLCVs and GRFT were fused to the N- or C-terminal portion of YFP, and the fusion proteins were co-expressed in Nicotiana benthamiana leaves. Images were obtained using a confocal laser-scanning microscope at 3 dpi. The experiment was performed three times with similar results. Scale bars: 10 μm. (B) Purified His-tagged proteins GST-GRFT and GST-GRFTD/A were analyzed by Western blotting with the anti-GST antibody. Red arrowheads point to the correct protein band. (C) Purified His-tagged proteins His-SPLCVs (AV1–AC4) were analyzed by Western blotting with the anti-His antibody. Red arrowheads point to the correct protein band. (D) SPLCVs and GRFT do not interact in vitro. GST-GRFT or GST-GRFTD/A were immobilized on glutathione sepharose beads, incubated with the His-AV1(CP) protein, and subjected to immunoblot analysis with an anti-His antibody. The His-AV1(CP) protein was immobilized on Ni-charged MagBeads, incubated with GST-GRFT or GST-GRFTD/A, and subjected to immunoblot analysis with an anti-GST antibody. The results for the other components of SPLCV were consistent and were not shown.
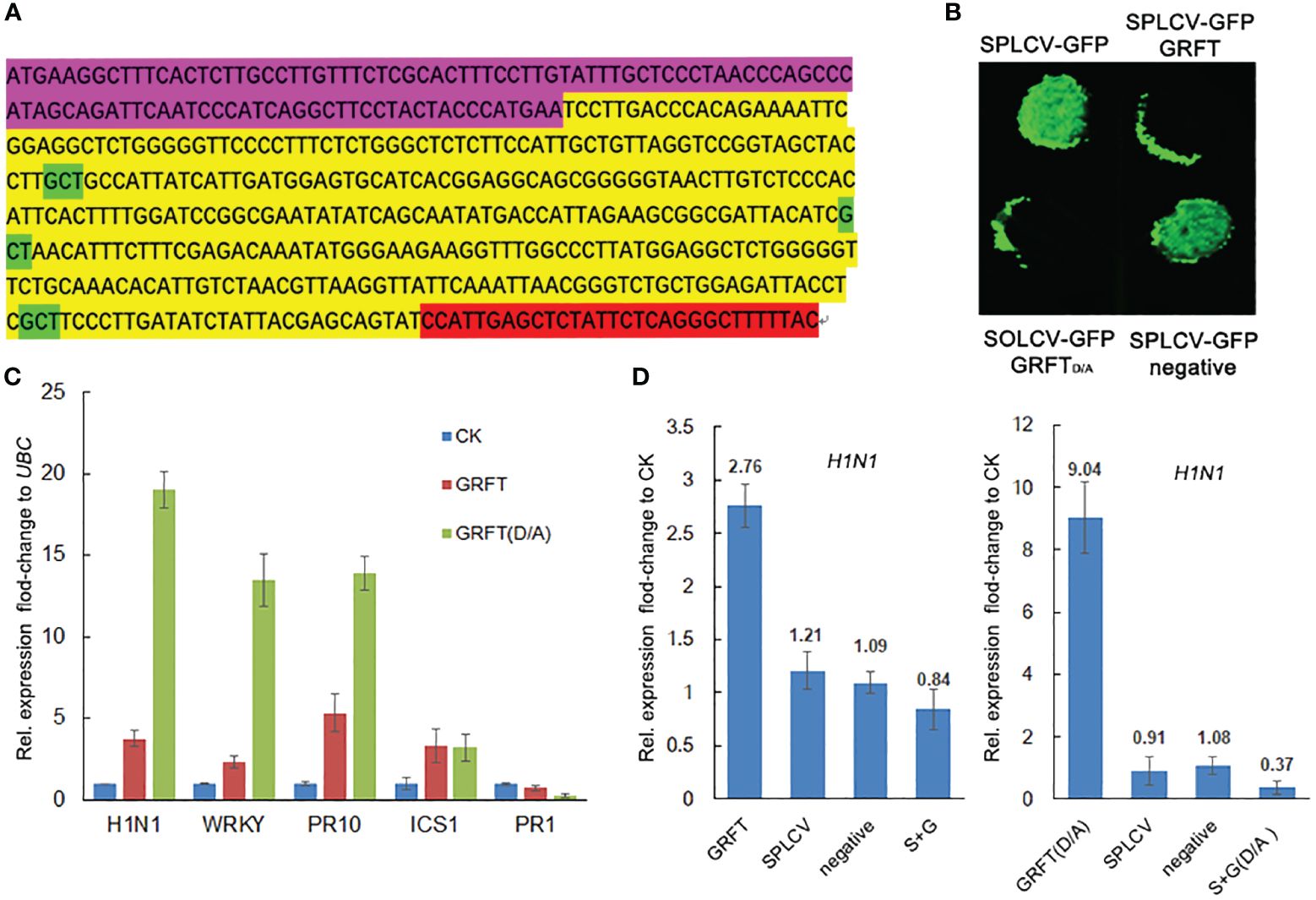
Figure 5 Mechanism of inhibition of SPLCV by GRFT. (A) Three aspartate residues of GRFT were replaced by alanine to generate GRFTD/A. (B) Four combinations of constructs were transiently expressed in the leaves of Nicotiana benthamiana, namely, SPLCV-GFP, SPLCV-GFP+GRFT, SPLCV-GFP+GRFTD/A, and SPLCV-GFP+negative. The infection solution (concentration OD = 0.5) was mixed. Negative is the empty 35S vector. (C) Expression of antiviral genes detected using qRT-PCR in N. benthamiana leaves infected with GRFT or GRFTD/A for 3 days. CK is untreated leaves. Bars and error bars represent the mean and standard error of the fold-change in expression level relative to UBC calculated from three independent experiments each with three biological replicates. (D) H1N1 expression in N. benthamiana leaves infected with GRFT, SPLCV, negative, or GRFT+SPLCV (or GRFTD/A+SPLCV). Bars and error bars represent the mean and standard error of the fold-change in expression level relative to the CK calculated from three independent experiments each with three biological replicates. CK is untreated leaves. Statistical significance in (C, D) was assessed using one-way ANOVA followed by Tukey’s test.
3.5 Expression of antiviral genes in tobacco was induced by GRFT
Given that GRFT did not bind to SPLCV, we hypothesized that GRFT induced the expression of antiviral genes in the plant. To date, many genes with antiviral functions have been identified in tobacco, such as hairpin-induced 1 (HIN1), isochorismate synthase I (ICS1), WRKY40, pathogenesis-related 1 (PR1), and PR10 (van Verk et al., 2011; Hao et al., 2018; Peng et al., 2019; Jiang et al., 2021). The expression of these genes was examined in tobacco leaves injected with 35S:GRFT or 35S:GRFTD/A or no construct (CK). Compared with the CK, the expression levels of HIN1, ICS1, WRKY40, and PR10 in leaves infected by GRFT or GRFTD/A were significantly increased. The changes in expression of HIN1, WRKY40, and PR10 caused by GRFTD/A were more strongly significant (Figure 5C). 35S:GRFT/GRFTD/A, SPLCV, the negative control (empty vector), and both constructs (35S:GRFT/GRFTD/A+SPLCV) were injected separately on the same leaf. 35S:GRFT increased HIN1 expression; 35S:GRFTD/A had a stronger effect; but SPLCV did not cause any change in expression. It was interesting that the expression of H1N1 decreased in leaves injected with both GRFT and SPLCV (Figure 5D). This phenomenon suggests that GRFT may inhibit SPLCV by inducing the expression of antiviral genes.
4 Discussion
4.1 Sweetpotato is suitable as a bioreactor for the exogenous expression of GRFT
GRFT is an efficient, broad-spectrum, safe, and stable anti-animal virus inhibitor and is currently the main lectin candidate drug used in the clinical prevention of a variety of viruses (Barton et al., 2014; Derby et al., 2018). Therefore, establishing a stable and high-yielding GRFT expression system is important. At present, researchers have experimented with a variety of exogenous expression systems, such as E. coli (Giomarelli et al., 2006), tobacco (Hoelscher et al., 2018), and rice (Vamvaka et al., 2016). However, exogenous expression of GRFT in sweetpotatoes is advantageous. First, the tubers and leaves of sweetpotatoes can be directly utilized as natural feed for livestock, which is not possible without the processing of rice. The use of sweetpotato-expressing GRFT as a feed additive will help to prevent the incidence of animal capsular viroid diseases. Second, sweetpotatoes can be continuously grown under suitable greenhouse conditions, providing fresh material for GRFT extraction at any time. In the present study, we successfully obtained GRFT transgenic lines expressing storage cell signaling peptides. In the following study, chloroplast expression systems and other promoters will be attempted to generate sweetpotato transgenic lines with a higher GRFT content. The use of sweetpotatoes as a bioreactor will be of considerable importance to increasing the yield of GRFT.
4.2 GRFT functions in resistance to plant viruses
As devastating plant pathogens, plant viruses pose a serious threat to global food security and economic development (Savary et al., 2019; He and Krainer, 2020). To date, more than 2,000 different types of plant viruses have been reported, and, with the rapid development of virus identification and diagnostic technologies, this number is expected to continue to increase. Research on resistance to plant viruses has mainly investigated three aspects: autophagy, plant hormones, and resistance genes. Autophagy is a process in which eucaryotes perform the turnover of intracellular substances. Through autophagy, plants can “consume” viruses (Yang and Liu, 2022). In recent years, the glycoprotein of the rice stripe mosaic virus was found to directly interact with OsSnRK1B, thus activating autophagy and hindering virus proliferation (Huang et al., 2024). Similarly, C1 proteins from the Tomato Frizzy-Yunnan virus and several other geminiviridaes have been targeted for autophagy degradation through interaction with the ATG8h protein (Li et al., 2022). Plant hormones and their signaling regulatory networks play an important role in regulating plant–virus interactions (Alazem and Lin, 2015; Zhao and Li, 2021). Li et al. revealed that the jasmonic acid signaling pathway in rice can promote the immune mechanism against the rice stripe virus (RSV) virus by regulating RNA silencing. Interestingly, rice plants infected with RSV show abscisic acid accumulation, which further promotes viral infection (Cui et al., 2021). Nucleotide oligomerization and binding domain-like receptors (NLRs) are typical antiviral genes in many plant species, effective against soybean mosaic virus, potato virus Y (PVY), and TMV (Yin et al., 2021). The first monocotyledon NLR gene to be cloned was from Brachypodium (Wu et al., 2022). Phytolectins are widely used for their antiviral properties, but this function has rarely been reported in plants. This is mainly because the antiviral function of lectins is achieved through their specific binding to viral surface carbohydrates, whereas plant viruses lack envelope structures containing glycoproteins. Nevertheless, some studies of lectins inhibiting plant viruses have been reported. For example, at certain concentrations, poplar mushroom lectin can inhibit TMV (Sun et al., 2003). Certain mushroom lectins can inhibit plant viruses, such as Paxillus involutus lectins, which reduce the toxicity of TMV (Peumans and Vandamme, 1995). However, the mechanism of lectin-conferred resistance to plant viruses has not been elucidated previously. In the current study, exogenous expression of GRFT in sweetpotatoes inhibited SPVD infection. In addition, GRFT inhibited SPLCV expression. SPVD is caused by two RNA viruses, namely, SPFMV and SPSCV. SPLCV is a DNA virus. Therefore, we speculate that GRFT also has a broad-spectrum antiviral function in plants. Confirmation of this conclusion will provide a novel strategy for future plant antiviral research.
4.3 Mechanism of GRFT resistance to plant viruses
Plant viruses lack envelope proteins that can interact with GRFT but do have coat proteins that control viral proliferation. In the present study, BIFC and pulldown assays determined that GRFT did not interact with the six components of SPLCV, including the coat protein (AV2). Further investigation showed that the simultaneous site-specific mutation of three important sugar-binding sites of GRFT did not influence its antiviral effect. These results suggested that GRFT resistance to plant viruses does not depend on its ability to bind to carbohydrates. In recent years, certain studies have reported that lectins can interact with glycoproteins or extracellular glycans on the cell wall to indirectly resist infection by plant viruses. Ayouba et al. (Ayouba et al., 1994) point out that the seed lectins of several legumes can strongly interact with cell wall peptide polymers. Lectins extracted from potatoes can distinguish between non-toxic and toxic strains of Raleella soliculosa and prevent the toxic strains from being adsorbed on the cell wall. Other lectins block the spread of viruses by killing the pests that transmit them. Galanthus nivalis agglutinin has a strong toxic effect on rice brown planthoppers and black-tailed leafhoppers and can also inhibit the growth of aphids (Du et al., 2000; Wang et al., 2005; Michiels et al., 2010). Neither the interaction with the cell wall nor the killing of pests is consistent with the mechanism of GRFT resistance against plant viruses. Therefore, we speculate that GRFT may induce the expression of antiviral genes in plants. To date, many genes with antiviral functions have been mined in plants. For example, NbHIN1 expression induced by exogenous Harpin protein induces allergic reactions in plants, and its overexpression in tobacco inhibits TMV (Peng et al., 2019). Overexpression of NbWRKY40 increased resistance to tomato mosaic virus (ToMV), whereas knockdown of NbWRKY40 increased the susceptibility of NbWRKY-silenced plants to ToMV infection (Jiang et al., 2021). ICS1 promotes salicylic acid production after a tobacco infection with the virus. Pathogenesis-related gene expression can be induced by SA (Baebler et al., 2014; Liang et al., 2024). Similarly, in Arabidopsis thaliana, vanisulfane can trigger salicylic acid accumulation, upregulate the expression of ICS1 and PR1, and induce resistance to PVY in transgenic Arabidopsis (Zhang et al., 2023). Treatment of Arabidopsis leaves with benzazol-S-methyl acid significantly increased the expression of the systemic acquired resistance-related genes PR1, SID2, and ALD1 and inhibited Plantago mosaic virus infection after 1 day (Ito et al., 2024). In the present study, the expression levels of the antiviral genes HIN1, ICS1, WRKY40, and PR10 were significantly increased in tobacco 3 days after GRFT injection. Therefore, we hypothesized that exogenous GRFT resistance to plant viruses is induced by the expression of plant antiviral genes. Interestingly, the expression levels of HIN1, WRKY40, and PR10 were higher in tobacco injected with GRFTD/A. This finding suggests that the ability of GRFT to bind to carbohydrates is an inhibitor, rather than a booster, of resistance to plant viruses. Confusingly, in tobacco leaves injected with both GRFT and SPLCV, the expression of H1N1 was reduced. We hypothesize that H1N1 is not a major anti-SPLCV gene. GRFT may induce a major anti-SPLCV protein product, which inhibits the expression of the virus and H1N1 by interacting with SPLCV. Because GRFT can induce additional antiviral substances, the simultaneous injection of GRFT mutations and SPLCV makes the inhibition of H1N1 more pronounced. In summary, the present results imply that exogenous expression of GRFT can lead to resistance to plant viral diseases by inducing the expression of endogenous antiviral genes in the plant.
Data availability statement
The original contributions presented in the study are included in the article/Supplementary Material. Further inquiries can be directed to the corresponding author.
Author contributions
SL: Conceptualization, Data curation, Formal Analysis, Funding acquisition, Investigation, Methodology, Software, Validation, Visualization, Writing – original draft, Writing – review & editing. YY: Data curation, Writing – review & editing, Funding acquisition, Methodology, Software. KG: Writing – review & editing, Data curation, Formal Analysis. QZ: Investigation, Writing – review & editing. ZJ: Writing – review & editing, Formal Analysis, Methodology, Software. MA: Writing – review & editing, Investigation, Funding acquisition. PM: Writing – review & editing, Methodology. HX: Data curation, Writing – review & editing. XB: Conceptualization, Data curation, Formal Analysis, Funding acquisition, Project administration, Resources, Supervision, Validation, Writing – original draft, Writing – review & editing.
Funding
This work was supported by the National Key R&D Program of China (Grant No. 2023YFE0111700), National Natural Science Foundation of China (Grant No. 32101787), the Jiangsu Provincial Agricultural Science and Technology Independent Innovation Fund (Grant No. CX(22)2012), the Project of Seed Industry Revitalization in Jiangsu Province (Grant No. JBGS (2021)010), the earmarked fund for China Agriculture Research System (Grant No. CARS-10-Sweetpotato), and the Natural Science Foundation of Jiangsu Province (Grant No. BK20231383).
Acknowledgments
We thank Robert McKenzie, PhD, from Liwen Bianji (Edanz) (www.liwenbianji.cn) for editing a draft of this manuscript.
Conflict of interest
The authors declare that the research was conducted in the absence of any commercial or financial relationships that could be construed as a potential conflict of interest.
Publisher’s note
All claims expressed in this article are solely those of the authors and do not necessarily represent those of their affiliated organizations, or those of the publisher, the editors and the reviewers. Any product that may be evaluated in this article, or claim that may be made by its manufacturer, is not guaranteed or endorsed by the publisher.
Supplementary material
The Supplementary Material for this article can be found online at: https://www.frontiersin.org/articles/10.3389/fpls.2024.1421244/full#supplementary-material
References
Alazem, M., Lin, N. S. (2015). Roles of plant hormones in the regulation of host-virus interactions. Mol. Plant Pathol. 16, 529–540. doi: 10.1111/mpp.12204
Alexandre, K. B., Gray, E. S., Lambson, B. E., Moore, P. L., Choge, I. A., Mlisana, K., et al. (2010). Mannose-rich glycosylation patterns on HIV-1 subtype C gp120 and sensitivity to the lectins, griffithsin, cyanovirin-N and scytovirin. Virology 402, 187–196. doi: 10.1016/j.virol.2010.03.021
Ayouba, A., Causse, H., Vandamme, E. J. M., Peumans, W. J., Bourne, Y., Cambillau, C., et al. (1994). Interactions of plant-lectins with the components of the bacterial-cell wall peptidoglycan. Biochem. Syst. Ecol. 22, 153–159. doi: 10.1016/0305-1978(94)90005-1
Baebler, S., Witek, K., Petek, M., Stare, K., Tusek-Znidaric, M., Pompe-Novak, M., et al. (2014). Salicylic acid is an indispensable component of the Ny-1 resistance-gene-mediated response against Potato virus Y infection in potato. J. Exp. Bot. 65, 1095–1109. doi: 10.1093/jxb/ert447
Balzarini, O. (2005). Targeting the glycans of gp120: a novel approach aimed at the Achilles heel of HIV. Lancet Infect. Dis. 5, 726–731. doi: 10.1016/S1473-3099(05)70271-1
Barton, C., Kouokam, J. C., Lasnik, A. B., Foreman, O., Cambon, A., Brock, G., et al. (2014). Activity of and effect of subcutaneous treatment with the broad-spectrum antiviral lectin griffithsin in two laboratory rodent models. Antimicrob. Agents Ch. 58, 120–127. doi: 10.1128/AAC.01407-13
Bian, X. F., Kim, H. S., Kwak, S. S., Zhang, Q., Liu, S., Ma, P. Y., et al. (2022). Different Functions of IbRAP2.4, a drought-responsive AP2/ERF transcription factor, in regulating root development between Arabidopsis and Sweetpotato. Front. Plant Sci. 13. doi: 10.3389/fpls.2022.820450
Cui, W. J., Wang, S., Han, K. L., Zheng, E. S., Ji, M. F., Chen, B. H., et al. (2021). Ferredoxin 1 is downregulated by the accumulation of abscisic acid in an ABI5-dependent manner to facilitate rice stripe virus infection in Nicotiana benthamiana and rice. Plant J. 107, 1183–1197. doi: 10.1111/tpj.15377
Derby, N., Lal, M., Aravantinou, M., Kizima, L., Barnable, P., Rodriguez, A., et al. (2018). Griffithsin carrageenan fast dissolving inserts prevent SHIV HSV-2 and HPV infections in vivo. Nat. Commun. 9, 3881. doi: 10.1038/s41467-018-06349-0
Du, J. P., Foissac, X., Carss, A., Gatehouse, A. M. R., Gatehouse, J. A. (2000). Ferritin acts as the most abundant binding protein for snowdrop lectin in the midgut of rice brown planthoppers (Nilaparvata lugens). Insect Biochem. Molec. 30, 297–305. doi: 10.1016/S0965-1748(99)00130-7
Emau, P., Tian, B., O’Keefa, B. R., Mori, T., McMahon, J. B., Palmer, K. E., et al. (2007). Griffithsin, a potent HIV entry inhibitor, is an excellent candidate for anti-HIV microbicide. J. Med. Primatol. 36, 244–253. doi: 10.1111/j.1600-0684.2007.00242.x
Fuqua, J. L., Hamorsky, K., Khalsa, G., Matoba, N., Palmer, K. E. (2015a). Bulk production of the antiviral lectin griffithsin. Plant Biotechnol. J. 13, 1160–1168. doi: 10.1111/pbi.12433
Fuqua, J. L., Wanga, V., Palmer, K. E. (2015b). Improving the large scale purification of the HIV microbicide, griffithsin. BMC Biotechnol. 15, 12. doi: 10.1186/s12896-015-0120-5
Giomarelli, B., Schumacher, K. M., Taylor, T. E., Sowder, R. C., Hartley, J. L., McMahon, J. B., et al. (2006). Recombinant production of anti-HIV protein, griffithsin, by auto-induction in a fermentor culture. Protein Expres Purif. 47, 194–202. doi: 10.1016/j.pep.2005.10.014
Hao, Q. Q., Wang, W. Q., Han, X. L., Wu, J. Z., Lyu, B., Chen, F. J., et al. (2018). Isochorismate-based salicylic acid biosynthesis confers basal resistance to Fusarium graminearum in barley. Mol. Plant Pathol. 19, 1995–2010. doi: 10.1111/mpp.12675
He, S., Krainer, K. M. C. (2020). Pandemics of people and plants: which is the greater threat to food security? Mol. Plant 13, 933–934. doi: 10.1016/j.molp.2020.06.007
Hoelscher, M., Tiller, N., Teh, A. Y. H., Wu, G. Z., Ma, J. K. C., Bock, R. (2018). High-level expression of the HIV entry inhibitor griffithsin from the plastid genome and retention of biological activity in dried tobacco leaves. Plant Mol. Biol. 97, 357–370. doi: 10.1007/s11103-018-0744-7
Huang, X. Q., Wang, J. K., Chen, S. P., Liu, S. Y., Li, Z. B., Wang, Z. Y., et al. (2024). Rhabdovirus encoded glycoprotein induces and harnesses host antiviral autophagy for maintaining its compatible infection. Autophagy 20, 275–294. doi: 10.1080/15548627.2023.2252273
Ishag, H. Z. A., Li, C., Huang, L., Sun, M. X., Wang, F. J., Ni, B., et al. (2013). Griffithsin inhibits Japanese encephalitis virus infection in vitro and in vivo. Arch. Virol. 158, 349–358. doi: 10.1007/s00705-012-1489-2
Ito, S., Sakugawa, K., Novianti, F., Arie, T., Komatsu, K. (2024). Local application of Acibenzolar-S-Methyl treatment induces antiviral responses in distal leaves of Arabidopsis thaliana. Int. J. Mol. Sci. 25, 1808. doi: 10.3390/ijms25031808
Jiang, Y. Y., Zheng, W. R., Li, J., Liu, P., Zhong, K. L., Jin, P., et al. (2021). NbWRKY40 positively regulates the response of Nicotiana benthamiana to tomato mosaic virus via salicylic acid signaling. Front. Plant Sci. 11. doi: 10.3389/fpls.2020.603518
Lee, C. (2019). Griffithsin, a highly potent broad-spectrum antiviral lectin from red algae: from discovery to clinical application. Mar. Drugs 17, 567. doi: 10.3390/md17100567
Levendosky, K., Mizenina, O., Martinelli, E., Jean-Pierre, N., Kizima, L., Rodriguez, A., et al. (2015). Griffithsin and carrageenan combination to target herpes simplex virus 2 and human papillomavirus. Antimicrob. Agents Chemother. 59, 7290–7298. doi: 10.1128/AAC.01816-15
Li, F. F., Qiao, R., Yang, X. L., Gong, P., Zhou, X. P. (2022). Occurrence, distribution, and management of tomato yellow leaf curl virus in China. Phytopathol. Res. 4, 1–12. doi: 10.1186/s42483-022-00133-1
Li, L., Tian, X. N., Chen, J., Li, P. C., Zheng, Q. S., Hou, J. B. (2018). Griffithsin inhibits porcine reproductive and respiratory syndrome virus infection in vitro. Arch. Virol. 163, 3317–3325. doi: 10.1007/s00705-018-4029-x
Liang, Y. P., Wan, J. F., Zhang, X., Li, K. M., Gui, M., Li, Y. Z., et al. (2024). Comprehensive phytohormone metabolomic and transcriptomic analysis of tobacco (Nicotiana tabacum) infected by tomato spotted wilt virus (TSWV). Virus Res. 342, 199334. doi: 10.1016/j.virusres.2024.199334
Lusvarghi, S., Bewley, C. A. (2016). Griffithsin: an antiviral lectin with outstanding therapeutic potential. Viruses-Basel 8, 296. doi: 10.3390/v8100296
Meuleman, P., Albecka, A., Belouzard, S., Vercauteren, K., Verhoye, L., Wychowski, C., et al. (2012). The lectin griffithsin has antiviral activity against hepatitis C virus in vitro and in vivo. J. Hepatol. 56, S335–S3S6. doi: 10.1016/S0168-8278(12)60873-3
Michiels, K., Van Damme, E. J. M., Smagghe, G. (2010). Plant-insect interactions: what can we learn from plant lectins? Arch. Insect Biochem. 73, 193–212. doi: 10.1002/arch.20351
Millet, J. K., Séron, K., Labitt, R. N., Danneels, A., Palmer, K. E., Whittaker, G. R., et al. (2016). Middle East respiratory syndrome coronavirus infection is inhibited by griffithsin. Antivir. Res. 133, 1–8. doi: 10.1016/j.antiviral.2016.07.011
Mori, T., O’Keefe, B. R., Sowder, R. C., Bringans, S., Gardella, R., Berg, S., et al. (2005). Isolation and characterization of griffithsin, a novel HIV-inactivating protein, from the red alga Griffithsia sp. J. Biol. Chem. 280, 9345–9353. doi: 10.1074/jbc.M411122200
Nabeta, H. W., Kouokam, J. C., Lasnik, A. B., Fuqua, J. L., Palmer, K. E. (2021). Novel antifungal activity of q-griffithsin, a broad-spectrum antiviral lectin. Microbiol. Spectr. 9, e0095721. doi: 10.1128/Spectrum.00957-21
Nixon, B., Stefanidou, M., Mesquita, P. M. M., Fakioglu, E., Segarra, T., Rohan, L., et al. (2013). Griffithsin protects mice from genital herpes by preventing cell-to-cell spread. J. Virol. 87, 6257–6269. doi: 10.1128/JVI.00012-13
O’Keefe, B. R., Vojdani, F., Buffa, V., Shattock, R. J., Montefiori, D. C., Bakke, J., et al. (2009). Scaleable manufacture of HIV-1 entry inhibitor griffithsin and validation of its safety and efficacy as a topical microbicide component. P Natl. Acad. Sci. U.S.A. 106, 6099–6104. doi: 10.1073/pnas.0901506106
Peng, H. R., Pu, Y. D., Yang, X., Wu, G. T., Qing, L., Ma, L. S., et al. (2019). Overexpression of a pathogenesis-related gene NbHIN1 confers resistance to tobacco mosaic virus in Nicotiana benthamiana by potentially activating the jasmonic acid signaling pathway. Plant Sci. 283, 147–156. doi: 10.1016/j.plantsci.2019.02.018
Peumans, W. J., Vandamme, E. J. M. (1995). Lectins as plant defense proteins. Plant Physiol. 109, 347–352. doi: 10.1104/pp.109.2.347
Savary, S., Willocquet, L., Pethybridge, S. J., Esker, P., McRoberts, N., Nelson, A. (2019). The global burden of pathogens and pests on major food crops. Nat. Ecol. Evol. 3, 430–439. doi: 10.1038/s41559-018-0793-y
Sun, H., Zhao, C. G., Tong, X., Qi, Y. P. (2003). A lectin with mycelia differentiation and antiphytovirus activities from the edible mushroom. J. Biochem. Mol. Biol. 36, 214–222. doi: 10.5483/BMBRep.2003.36.2.214
Takebe, Y., Saucedo, C. J., Lund, G., Uenishi, R., Hase, S., Tsuchiura, T., et al. (2013). Antiviral lectins from red and blue-green algae show potent and activity against hepatitis C virus. PloS One 8, e64449. doi: 10.1371/journal.pone.0064449
Vafaee, Y., Alizadeh, H. (2018). Heterologous production of recombinant anti-HIV microbicide griffithsin in transgenic lettuce and tobacco lines. Plant Cell Tiss Org. 135, 85–97. doi: 10.1007/s11240-018-1445-2
Vamvaka, E., Arcalis, E., Ramessar, K., Evans, A., O’Keefe, B. R., Shattock, R. J., et al. (2016). Rice endosperm is cost-effective for the production of recombinant griffithsin with potent activity against HIV. Plant Biotechnol. J. 14, 1427–1437. doi: 10.1111/pbi.12507
Van Damme, E. J. M., Peumans, W. J., Barre, A., Rougé, P. (1998). Plant lectins: A composite of several distinct families of structurally and evolutionary related proteins with diverse biological roles. Crit. Rev. Plant Sci. 17, 575–692. doi: 10.1080/07352689891304276
van Verk, M. C., Bol, J. F., Linthorst, H. J. M. (2011). WRKY transcription factors involved in activation of SA biosynthesis genes. BMC Plant Biol. 11, 89. doi: 10.1186/1471-2229-11-89
Wang, Z. Y., Zhang, K. W., Sun, X. F., Tang, K. X., Zhang, J. R. (2005). Enhancement of resistance to aphids by introducing the snowdrop lectin gene gna into maize plants. J. Biosci. 30, 627–638. doi: 10.1007/BF02703563
Wu, Q. H., Cui, Y., Jin, X. J., Wang, G. X., Yan, L. J., Zhong, C. C., et al. (2022). The CC-NB-LRR protein BSR1 from Brachypodium confers resistance to Barley stripe mosaic virus in gramineous plants by recognising TGB1 movement protein. New Phytol. 236, 2233–2248. doi: 10.1111/nph.18457
Xue, J., Hoorelbeke, B., Kagiampakis, I., Demeler, B., Balzarini, J., LiWang, P. J. (2013). The griffithsin dimer is required for high-potency inhibition of HIV-1: evidence for manipulation of the structure of gp120 as part of the griffithsin dimer mechanism. Antimicrob. Agents Ch. 57, 3976–3989. doi: 10.1128/AAC.00332-13
Yang, M., Liu, Y. L. (2022). Autophagy in plant viral infection. FEBS Lett. 596, 2152–2162. doi: 10.1002/1873-3468.14349
Yin, J. L., Wang, L. Q., Jin, T. T., Nie, Y., Liu, H., Qiu, Y. L., et al. (2021). A cell wall-localized NLR confers resistance to Soybean mosaic virus by recognizing viral- encoded cylindrical inclusion protein. Mol. Plant 14, 1881–1900. doi: 10.1016/j.molp.2021.07.013
Zhang, S. X., Wei, C. L., Yu, L., Song, B. A. (2023). Vanisulfane induced plant resistance toward Potato Virus Y via the salicylic-depended acid signaling pathway. J. Agr. Food Chem. 71, 14527–14538. doi: 10.1021/acs.jafc.3c05838
Zhao, S. S., Li, Y. (2021). Current understanding of the interplays between host hormones and plant viral infections. PloS Pathog. 17, e1009242. doi: 10.1371/journal.ppat.1009242
Ziólkowska, N. E., O’Keefe, B. R., Mori, T., Zhu, C., Giomarelli, B., Vojdani, F., et al. (2006). Domain-swapped structure of the potent antiviral protein griffithsin and its mode of carbohydrate binding. Structure 14, 1127–1135. doi: 10.1016/j.str.2006.05.017
Ziólkowska, N. E., Shenoy, S. R., O’Keefe, B. R., McMahon, J. B., Palmer, K. E., Dwek, R. A., et al. (2007). Crystallographic, thermodynamic, and molecular modeling studies of the mode of binding of oligosaccharides to the potent antiviral protein griffithsin. Proteins 67, 661–670. doi: 10.1002/prot.21336
Keywords: griffithsin, sweetpotato (Ipomoea batatas (L.) Lam), sweetpotato virus disease, sweetpotato leaf curl virus, antiviral genes
Citation: Liu S, Yu Y, Guo K, Zhang Q, Jia Z, Alfredo MR, Ma P, Xie H and Bian X (2024) Expression and antiviral application of exogenous lectin (griffithsin) in sweetpotatoes. Front. Plant Sci. 15:1421244. doi: 10.3389/fpls.2024.1421244
Received: 15 May 2024; Accepted: 20 June 2024;
Published: 16 July 2024.
Edited by:
Xiangjin Wei, China National Rice Research Institute (CAAS), ChinaReviewed by:
Feng Zhou, Chinese Academy of Sciences (CAS), ChinaLinglong Liu, Nanjing Agricultural University, China
Copyright © 2024 Liu, Yu, Guo, Zhang, Jia, Alfredo, Ma, Xie and Bian. This is an open-access article distributed under the terms of the Creative Commons Attribution License (CC BY). The use, distribution or reproduction in other forums is permitted, provided the original author(s) and the copyright owner(s) are credited and that the original publication in this journal is cited, in accordance with accepted academic practice. No use, distribution or reproduction is permitted which does not comply with these terms.
*Correspondence: Xiaofeng Bian, YmlhbnhpYW9mZW5nMkAxNjMuY29t