- 1Tropical Crops Genetic Resources Institute, Chinese Academy of Tropical Agricultural Sciences, Haikou, China
- 2School of Food Science and Engineering, Hainan University, Haikou, China
- 3Department of Plant Pathology, Agricultural College, Guizhou University, Guiyang, China
- 4Environment and Plant Protection Institute, Chinese Academy of Tropical Agricultural Sciences, Haikou, China
Postharvest litchi is susceptible to browning that limits the development of litchi industry. Hydrogen sulfide (H2S) is an important bioactive molecule that can regulate many physiological processes. This study examined the effects of exogenous H2S on pericarp browning and related physiological mechanisms in postharvest litchi. The results exhibited that exogenous H2S treatment delayed the browning of litchi pericarp and reduced the damage to cell membrane integrity during storage. This treatment inhibited the energy losses of litchi fruit by increasing the activities of H+-ATPase, Ca2+- ATPase, cytochrome C oxidase (CCO) and succinate dehydrogenase (SDH) and regulating the expression of energy metabolism-related genes, including LcAtpB, LcSnRK2, LcAAC1, LcAOX1 and LcUCP1. In addition, H2S treatment increased the levels of fructose, glucose, sucrose, inositol, galactose and sorbose in litchi fruit, and promoted sucrose synthesis by regulating the activities of sucrose phosphate synthase (SPS), sucrose synthase (SS), acid invertase (AI) and neutral invertase (NI). Based on the current findings, we suggest that exogenous H2S enhances the energy supply and antioxidant activity of litchi by modulating energy and sugar metabolism, thereby inhibiting fruit browning and senescence. These results indicated that H2S treatment is an effective approach to maintaining the quality of litchi fruit and extending its shelf life.
1 Introduction
Litchi (Litchi chinensis Sonn.) is a typical tropical fruit crop with high market acceptance because of its attractive red skin, sweet taste and rich nutrition (Pareek, 2016). However, the rapid pericarp browning after harvest seriously restricts the expansion of the litchi industry (Jiang et al., 2006). Fruit senescence is a programmed process involving multiple complex factors (Figueroa et al., 2021). Previous studies have reported that the oxidative damage to cell membrane is a key factor in causing litchi senescence and browning (Jiang et al., 2004; Deshi et al., 2020). Adenosine triphosphate (ATP) is a cellular “energy currency”, whose sufficient supply can enhance the antioxidant activity in cells and maintain normal physiological functions of cell membrane and cell wall in plants (Aghdam et al., 2018). A variety of researches have been confirmed that delay of fruit senescence and quality deterioration by various postharvest treatments could be involved in the improvement of energy state via regulation of energy metabolic enzymes and related genes, as demonstrated in litchi, longan, mango and pear fruits (Zhang et al., 2017; Wang et al., 2020a; Zhang et al., 2023a; Huang et al., 2024).
Sucrose, glucose and fructose are the foundational sources of energy in plants. They are not only responsible for the energy supply of fruit but also determine the flavor and nutritional value (Fan et al., 2021). Moreover, soluble sugars, as an important signaling molecules in plants, are also considered to be associated with abiotic stress responses, which are involved in osmotic adjustment and activation of antioxidant system (Saddhe et al., 2021). Some reports suggest that higher contents of sucrose, fructose, glucose due to 24-epibrassinolide and fibroin treatments could contribute to the improvement of energy state and stress resistance of fruit, hence aiding in the prevention of postharvest senescence in peach and banana fruit (Liu et al., 2019; Hu et al., 2023). Song et al. (2016) noted that chitosan/nano-silica treatment resulted in more accumulation of glucose and fructose in loquat in the low-temperature environment, which promoted the increase of antioxidant activity, reduced membrane damage, alleviating chilling injury of loquat fruit. For litchi, our recent research has confirmed that the response of litchi fruit to energy deficiency was involved in the modulation of sugar metabolic pathways (Zhao et al., 2024). However, the role of sugars in maintaining the quality of litchi fruit still requires further investigation.
Hydrogen sulfide (H2S), the third identified gaseous signaling molecule after hydrogen peroxide (H2O2) and nitric oxide (NO) in plants, plays undeniable roles in growth and development and response to environmental stresses (Arif et al., 2021). Emerging evidence indicates that H2S treatment at low concentration (μM) elicits beneficial effects on storability in postharvest crops. For example, exogenous H2S treatment (500 μM and 0.735 μM) was deemed to be capable of delaying senescence and improving stress resistance in postharvest fruits of banana and navel orange through enhancing cellular energy state, augmenting antioxidant capacity or regulating phenylpropanoid metabolism pathway (Li et al., 2016; Huang et al., 2023b). Moreover, H2S treatment, as a potential postharvest preservation technique, is attracting attention due to its straightforward operation, low cost, and the capability to be readily applied to large-scale fruit processing (Alhassan et al., 2022). Recent studies indicated that H2S treatment enhanced the activities of antioxidant enzymes in litchi, which led to reduced oxidative damage to the cell membrane and improved the fruit quality during storage (Deshi et al., 2020; Siddiqui et al., 2021). However, the influence of H2S treatment on postharvest litchi browning via energy and sugar metabolisms and its possible mechanism remain unclear. Therefore, this study aimed to elucidate the effects of exogenous H2S on postharvest browning of litchi in relation to membrane integrity, energy state, energy metabolism-related enzyme activities and gene expression, sugars content, and sucrose metabolism-related enzyme activities. The results might offer fresh insights into regulatory mechanisms of H2S effecting postharvest browning in litchi fruit.
2 Materials and methods
2.1 Fruit material and treatment
Litchi fruit (L. chinensis Sonn. cv. ‘A4 Wuhe’) with a commercial maturity were harvested in the litchi garden situated in Yongxing Town within Haikou, China. The picked fruit was transported to the laboratory within 2 hours. The selection criteria of litchi fruit for experiment were uniform size, color, and shape, without pericarp damage or disease symptoms. All fruit were treated with 0.1% (v/v) prochloraz for 3 min, washed with deionized water, then were randomly divided into two groups (576 fruit/group) after drying. The two groups of fruit were soaked in distilled water (control group) and 1.0 mM sodium hydrosulfide (H2S donor, experimental group) for 15 min, respectively. After drying, all fruit were placed in plastic boxes (without plastic covers), each group contained 48 boxes (12 fruit per box), then stored at 25 ± 1°C and 85 ± 5% relative humidity. During storage, samples were collected at 0, 12, 24, 48, 72, and 96 h. During each sampling opportunity, eight boxes were randomly selected from both the experimental and control groups, respectively, for the physiological investigation of fresh samples. This included the assessments of browning index (BI; 30 fruit defined as one replicate), respiration rate (20 fruit defined as one replicate) and membrane permeability (6 fruit defined as one replicate), with three replications for each parameter. Furthermore, pericarp samples from six litchi fruit were taken at each sampling point, frozen in liquid N2 and stored at -80°C for subsequent analysis. Each parameter underwent three measurements.
2.2 BI, respiration rate, membrane permeability, and malonaldehyde content
Pericarp browning was categorized into five scales depending on the proportion of browned area on the litchi surface (Wang et al., 2020a). 0, no browning; 1, ≤ 1/4 browning; 2, 1/4 - 1/2 browning; 3, 1/2 - 3/4 browning; 4, ≥ 3/4 browning. The BI was calculated as follows: BI = ∑(browning scale × number of fruit per class)/(total number of fruit per replicate of each treatment × maximum browning scale).
Respiration rate was assessed following the protocol outlined by Li et al. (2019). The litchi fruit were placed in a 4-L plastic containers for 20 min at 20°C, then obtaining the CO2 concentration using an infrared CO2 analyzer (CXH-3010E, Beijing, China). Respiration rate is the quantity of carbon dioxide generated per kilogram fresh weight (FW) every second (µmol kg−1 s−1).
The membrane permeability was reflected by relative electrolyte leakage (Zhang et al., 2017). Thirty discs of the pericarp were meticulously procured from each set of six fruit in every experimental replication, with a cork borer (8 mm) as the extraction tool. The discs were cleaned twice and incubated in 50 mL deionized water at 25°C for 30 min. The solution’s initial electrolyte conductivity (Ei) was detected using a conductivity meter. The total electrolyte value (Et) was determined after boiling the solution with discs for 20 min and then cooling it to 25°C. Relative electrolyte leakage was calculated as follows:
MDA content was analyzed using 3 g pericarp tissues following the procedure outlined by Li et al. (2019). MDA content was defined as µmol kg−1 FW.
2.3 Measurements of adenosine triphosphate, adenosine diphosphate (ADP), adenosine monophosphate (AMP) content and calculation of energy charge (EC)
The contents of ATP, ADP, and AMP in litchi pericarp were determined using the methodology outlined by Zhang et al. (2017).
2.4 Enzymes activities related to energy metabolism
The activities of H+-ATPase, Ca2+-ATPase, cytochrome C oxidase (CCO) and succinate dehydrogenase (SDH) were assessed using the procedures outlined in Jin et al. (2013). Enzyme activities were quantified as U kg-1.
2.5 Determination of gene expression levels
Genes’ expression levels were assessed using real-time quantitative PCR (RT-qPCR). 1.5 g pericarp tissues was taken and used for the extract-purifying total RNA using Quick RNA isolation Kit (0416-50 GK; Huayueyang Biotech, Beijing, China). The cDNA fragment was obtained by reverse transcription through FastQuant RT Kit (KR106; Tiangen Biotech, Beijing, China) using the purified RNA template. The RT-qPCR primer sequences of LcAtpB (ATP synthase β-subunit), LcAOX1 (alternative oxidase 1), LcUCP1 (mitochondrial uncoupling protein 1), LcAAC1 (ADP/ATP carrier 1), and LcSnRK2 (sucrose non-fermenting-1-related kinase 2) were referred to the report of Wang et al. (2013). Fluorescence quantitative Kit uses SYBR® PremixEx Taq™ (Tli RNaseH Plus; Takara Biotech, Dalian, China). RT-PCR uses Life QuantStudio6 Flex with a 10 µL PCR reaction system (0.3 μL forward primer; 0.3 μL reverse primer; 0.2 μL ROX Reference Dye II; 1.0 μL cDNA; 8.2 μL ddH2O). The LcActin gene (GenBank ID: DQ990337.1) was employed as a reference for quantitative standardization during amplification. Gene expression levels were quantified applying the 2 −△△CT method.
2.6 Components and contents of sugars
Sugars were obtained and determined utilizing the protocol of Wu et al. (2016) with some changes. The litchi pericarp of 0.2 g was cooked in a microwave at 100°C for 30 s to disable the enzyme. The sample was homogenized in a mortar, extracted thrice with ethanol, and then diluted to 9 mL. The mixture was centrifuged at 13000 g for 15 min. The extraction solution was diluted 5-fold with deionized water. The 0.5 ml extraction solution was transferred into a 1.5 ml centrifuge tube and blow dry with nitrogen. A solution of 30 µL methylammonium chloride in pyridine (20 g L−1) was poured into the centrifuge tube and shaken at 650 rpm for 1.5 h at 37°C. The N, O-bis (trimethylsilyl) trifluoroacetamide with 1% trimethylchlorosilane (70 µL) was added and shaken at 650 rpm for one hour at 70°C. The supernatant (1 µL) was passed through the Agilent HP-5MS (30 m × 0.25 mm × 0.25 µm) after standing for 30 min at ambient temperature. The sugars in litchi were detected by gas chromatography using Agilent 7894A-5975C GC-MS (Agilent Technology, Palo Alto, CA, USA). An external standard solution was utilized to calculate the sugar concentration in litchi.
2.7 Activities of sucrose metabolic enzymes
The enzymatic activities of sucrose phosphate synthase (SPS), sucrose synthase synthesis (SS-s), sucrose synthase cleavage (SS-c), acid invertase (AI) and neutral invertase (NI) were evaluated using the methodologies specified in Sun et al. (2020). The enzymatic activity for SS-c, AI, and NI was ascertained based on the volume of enzyme that could yield 1 mg reducing sugar within a minute, with the absorbance for SS-c measured at 540 nm, and for AI and NI, at 510 nm. Conversely, the activity of SS-s and SPS was gauged by the amount required to synthesize 1 mg sucrose per minute, utilizing a wavelength of 480 nm for these assays. Enzyme activity were quantified as U kg-1.
2.8 Statistical analysis
The data is displayed as the mean ± standard error (SE). T-test analysis was conducted to compare the difference between control and experimental groups at the same day (*P < 0.05, **P < 0.01), using SPSS 27.0.1.
3 Results
3.1 Pericarp browning, respiration rate, membrane permeability, and MDA content
The control fruit did not show browning symptoms in first 24 h of storage, the BI sharply increased after 24 h and reached 0.83 ± 0.05 at 96 h of storage (Figures 1A, B). H2S treatment effectively delayed pericarp browning, in which the BI was still at a low level (0.34 ± 0.04) after storage for 96 h.
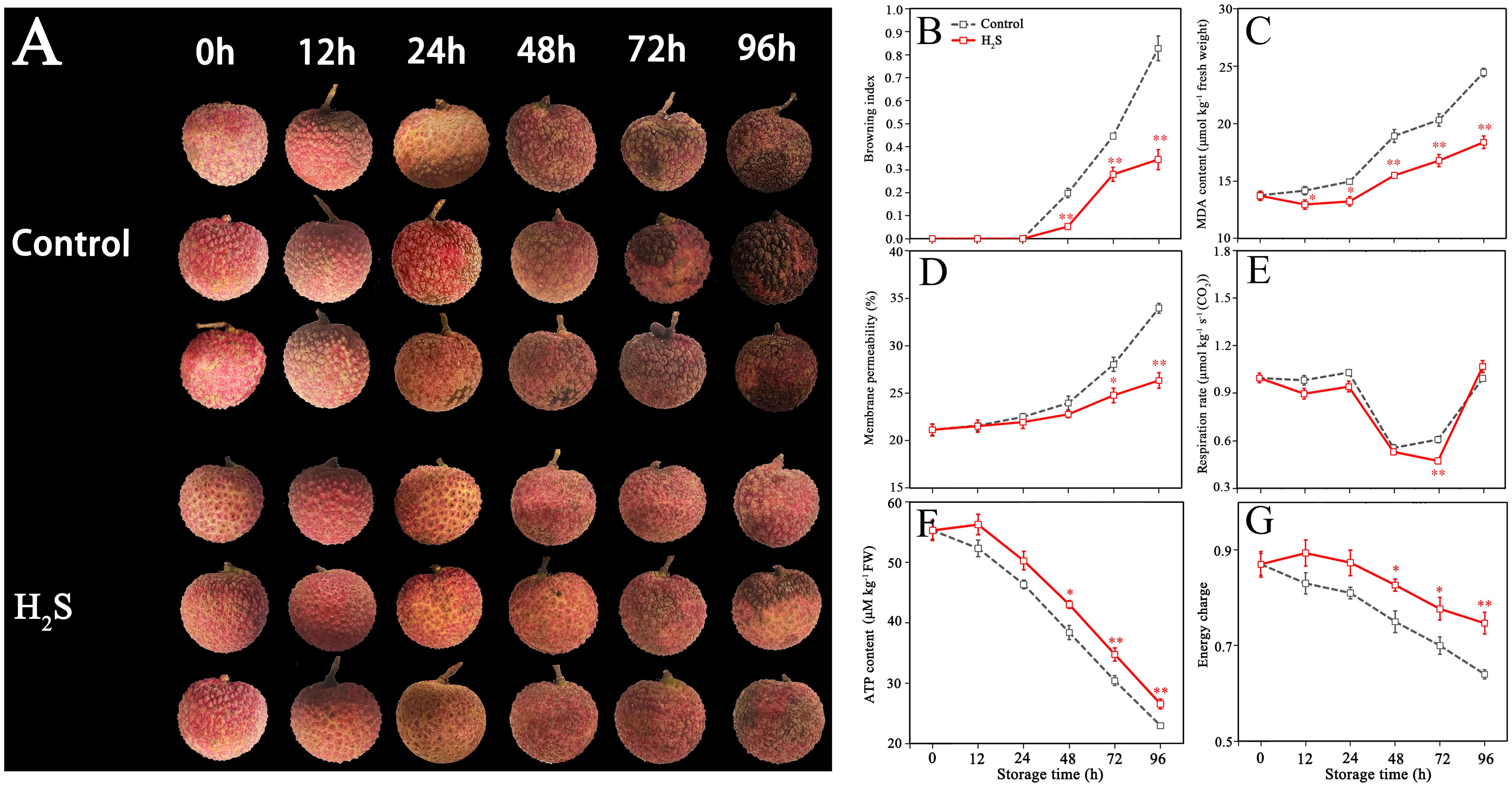
Figure 1. Appearance (A) of control and H2S treated fruit during storage, as well as corresponding changes in browning index (BI) (B), malonaldehyde (MDA) content (C), membrane permeability (D), respiration rate (E), adenosine triphosphate (ATP) (F), and energy charge (EC) (G). Data are the mean ± standard error (n=3). The asterisk represents the significant difference (* P < 0.05, ** P < 0.01) between the experimental and control groups.
The MDA content in control fruit increased continuously during storage with initial 13.8 ± 0.6 to final 24.5 ± 0.7 µmol kg−1 (Figure 1C). The MDA content of litchi pericarp was consistently 15% lower on average for the entire storage period due to H2S treatment, indicating that H2S treatment inhibited the oxidative damage to cell membrane.
Figure 1D illustrates that the relative electrolyte leakage in the control group gradually increased from 21.13% to 33.96% during storage. Relative to the untreated specimens, the average values of relative electrolyte leakage for fruit treated with H2S exhibited a reduction of 12% during 24 to 96 h, indicating that H2S treatment effectively maintained the integrity and functionality of the cell membrane.
Figure 1E illustrates that the respiration rate of control fruit remained relatively stable within the first 24 h, decreased rapidly in 24-48 h, then increased sharply in 72-96 h. The respiration rate of fruit treated with H2S was inhibited during storage for 0-72 h.
3.2 Energy state
As shown in Figures 1F, G, ATP content and EC in litchi pericarp decreased sharply as storage time increased in the control fruit. H2S treatment prevented the reduction in ATP content and EC, with significant variations observed between 48 to 96 h.
3.3 Enzymatic activity involved in energy metabolism
Figures 2A, B showed that the activities of both H+- ATPase and Ca2+-ATPase initially increased and subsequently gradually declined over the storage period in the control group. CCO activity reduced somewhat from 0 to 12 hours, then gradually climbed, reaching its peak at 48 hours before decreasing (Figure 2C). SDH activity exhibited a change with fluctuation during storage (Figure 2D). Generally, H2S treatment increased the activities of H+- ATPase, Ca2+-ATPase, CCO and SDH during storage (Figures 2A–D), but its effects on Ca2+-ATPase and SDH were more profound (Figures 2B, D).
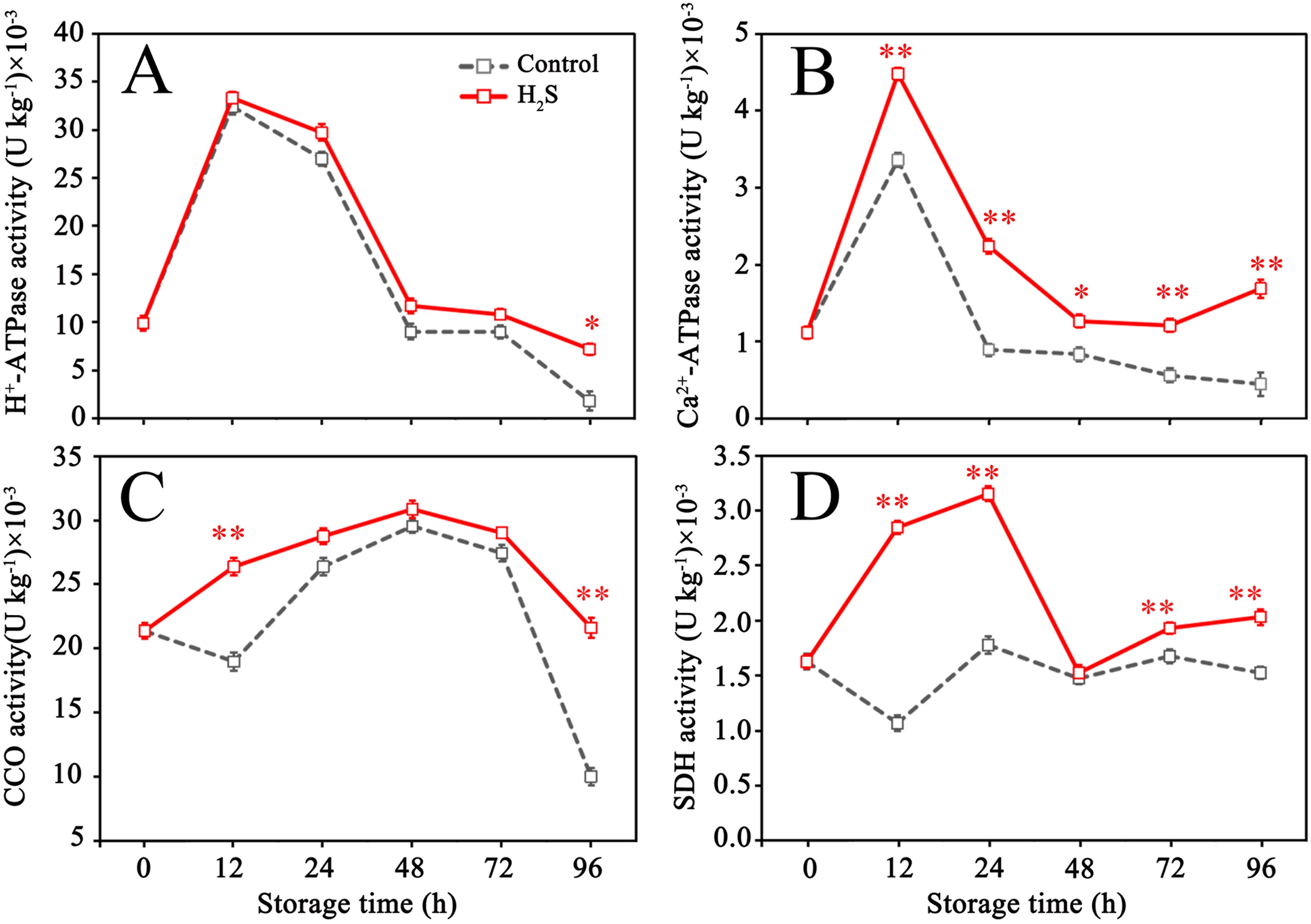
Figure 2. H+-ATPase (A), Ca2+-ATPase (B), cytochrome C oxidase (CCO) (C) and succinate dehydrogenase (SDH) (D) activities in control and H2S treated fruit during storage. Data are the mean ± standard error (n=3). The asterisk represents the significant difference (* P < 0.05, ** P < 0.01) between the experimental and control groups.
3.4 Expression of the energy metabolism-related gene
Figure 3 displayed the effects of H2S treatment on the expression of genes associated with energy metabolism pathways. Transcript abundances of LcAtpB, LcAOX1 and LcUCP1 in control fruit peaked at 12 hours and then experienced an overall decline throughout the remainder of the storage period (Figures 3A, C, E). H2S treatment remarkably enhanced LcAtpB expression at 24 h, promoted LcUCP1 expression within 24 to 96 h, and inhibited LcAOX1 expression during storage (Figures 3A, C, E). Expression of LcAAC1 in control fruit increased by 5.6-fold within 48 hours, then declined until the end of storage (Figure 3B). H2S treatment promoted LcAAC1 expression during storage, except for the value at 48 h (Figure 3B). LcSnRK2 expression in control fruit displayed a fluctuating changes, increased at 24 h and 72 h, but decreased at 48 h and 96 h (Figure 3D). Comparatively, higher levels of LcSnRK2 expression were detected in the litchi fruit treated with H2S during storage, especially at 12 h and 72 h (Figure 3D).
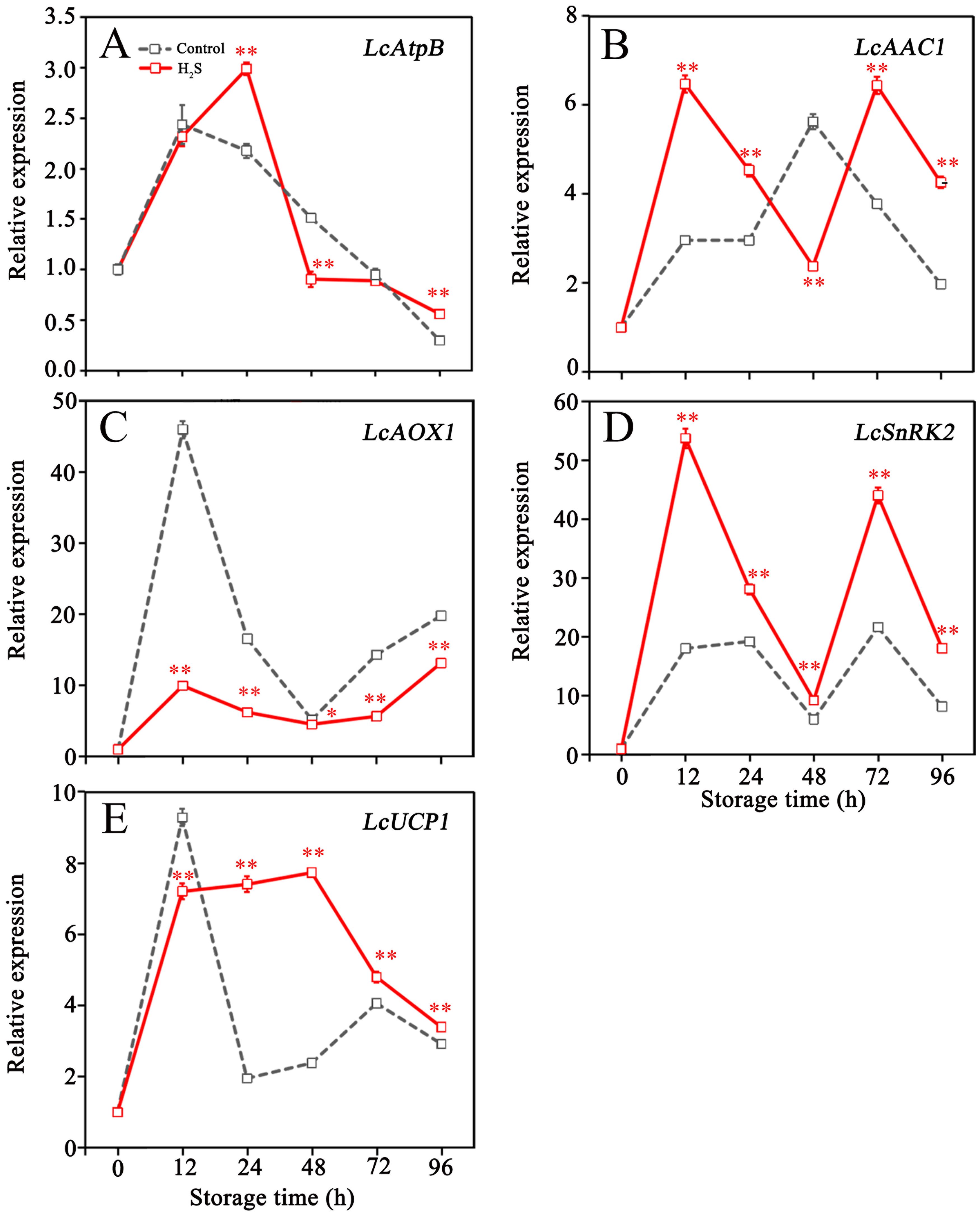
Figure 3. Relative expression of LcAtpB (A), LcAAC1 (B), LcAOX1 (C), LcSnRK2 (D) and LcUCP1 (E) in control and H2S treated litchi fruit during storage. Data are the mean ± standard error (n=3). The asterisk represents the significant difference (* P < 0.05, ** P < 0.01) between the experimental and control groups.
3.5 Components and content of sugars
In the litchi pericarp, six types of sugars were detected, with initial concentrations of 1263 mg kg−1 (fructose), 894 mg kg−1 (glucose), 611 mg kg−1 (sucrose), 58 mg kg−1 (inositol), 37 mg kg−1 (galactose), and 34 mg kg−1 (sorbose), respectively (Figure 4). The content of fructose, glucose and sucrose in control fruit rapidly decreased in the early stage of storage and reached the minimum at 48 h, followed by slight increases until the end of storage (Figures 4A–C). The H2S treatment delayed decrease in fructose, glucose and sucrose content, which reached the minimum after 72 h. The contents of inositol, galactose, and sorbose in litchi fruit of control were low and remained relatively stable during storage. However, a notable increase in the concentrations of these sugars was observed in fruit subjected to H2S treatment when contrasted with those that received no treatment (Figures 4D–F).
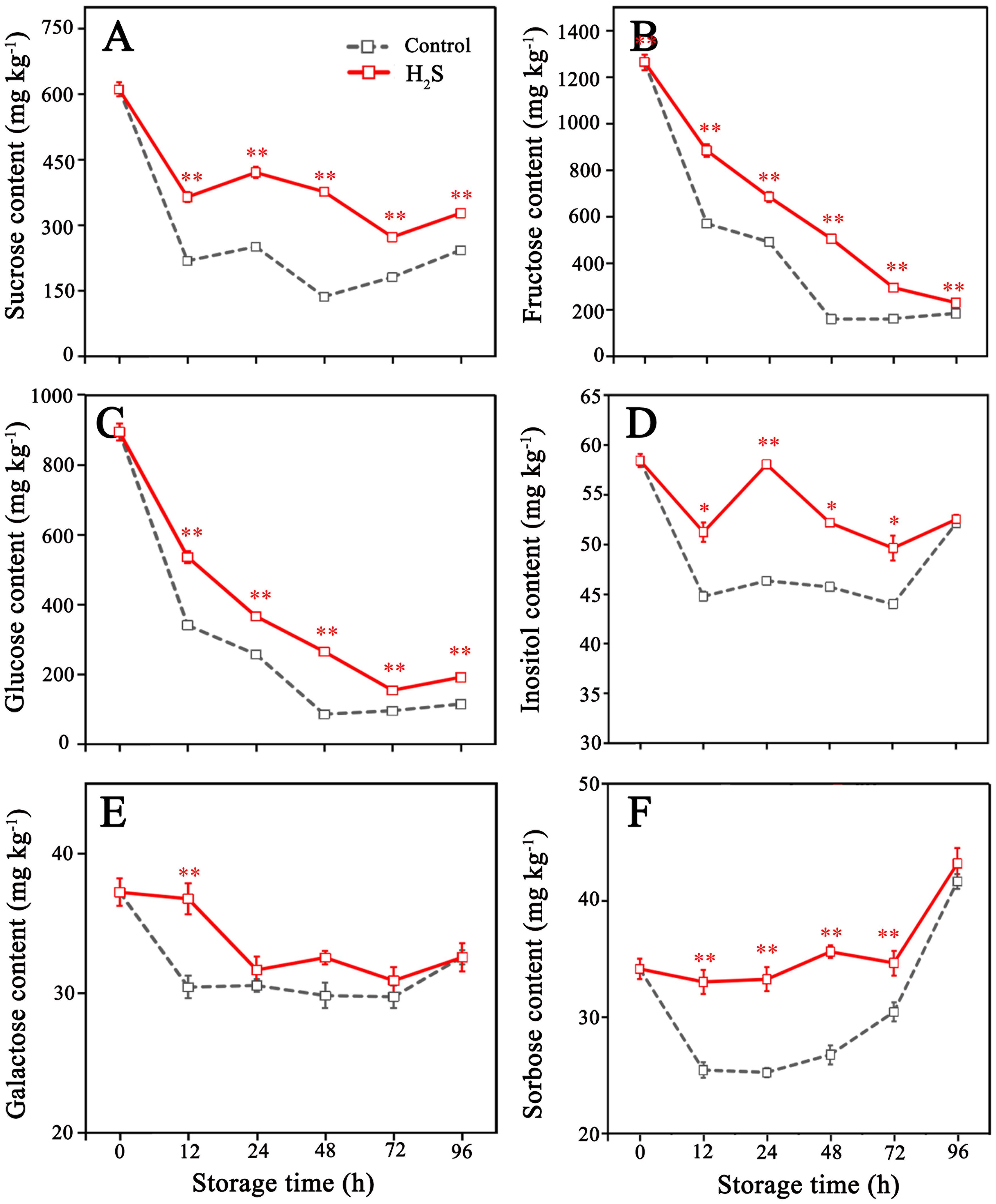
Figure 4. Contents of sucrose (A), fructose (B), glucose (C), inositol (D), galactose (E) and sorbose (F) in control and H2S treated litchi fruit during storage. Data are the mean ± standard error (n=3). The asterisk represents the significant difference (* P < 0.05, ** P < 0.01) between the experimental and control groups.
3.6 Enzymatic activity involved in sucrose metabolism
Figure 5 illustrates that the SPS activity in control fruit rose during the initial phase of storage, peaked at 48 h, and thereafter declined. AI activity in control fruit decreased within 0-24 h, then increased within 24-48 h and dropped after 48 h. SS-s activity declined within 0-24 h, then increased within 24-72 h and decreased again after 72 h. Contrary to the change of SS-s activity, SS-c activity climbed from 0 to 48 h, reduced within 48-72 h, then increased again. The activity of SS-s was higher than that of SS-c at the same period, as seen in Figures 5B, C. NI activity decreased after reaching the peak at 12 h, then continued to decrease after slightly increasing from 24 to 48 h. H2S treatment enhanced the activities of SPS and SS-s (0-48 h), while reduced the activities of SS-c (24-96 h), AI (24-96 h) and NI during storage.
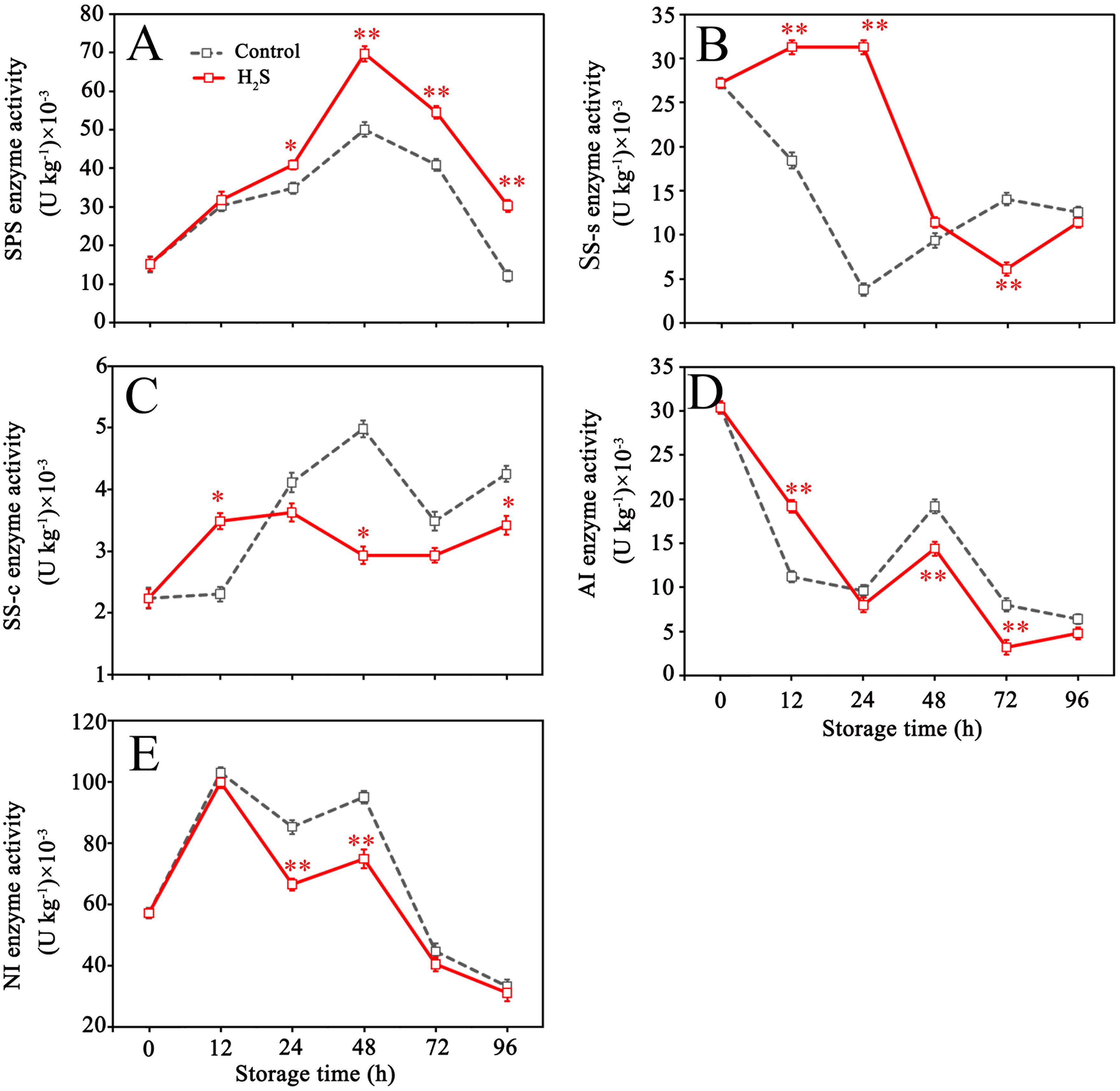
Figure 5. Sucrose phosphate synthase (SPS) (A), sucrose synthase synthesis (SS-s) (B), sucrose synthase cleavage (SS-c) (C), acid invertase (AI) (D) and neutral invertase (NI) (E) activities in control and H2S treated fruit during storage. Data are the mean ± standard error (n=3). The asterisk represents the significant difference (* P < 0.05, ** P < 0.01) between the experimental and control groups.
4 Discussion
4.1 Effect of H2S on pericarp browning in litchi in relation to energy state
Rapid pericarp browning in harvested litchi fruit significantly reduces shelf life and compromises fruit quality, which limits the development of the litchi industry (Huang et al., 2023a). Recently, many alternative methods have been emerged to alleviate litchi browning, including radiation processing, modified atmosphere packaging, edible coating, organic acid treatment, and plant extracts treatment (Zhang et al., 2023a). In the present study, we observed that the browning symptoms of litchi were alleviated following treatment with H2S (Figure 1A). The results were consistent with the reports on H2S delaying quality deterioration in apples (Chen et al., 2021), bananas (Ge et al., 2017) and grapes (Ni et al., 2016).
During storage of fruit, the reactive oxygen species (ROS) in cells constantly accumulate, which leads to oxidative damage of biomacromolecules (e.g., DNA, proteins and lipids), destroy the function of membrane systems and organelles, thereby triggering browning and senescence in fruit pericarp (Chen and Yang, 2020; Xu et al., 2023). When sufficient energy is available in fruit, the activities of enzymatic/non-enzymatic antioxidant systems can be maintained, contributing to inhibition of ROS accumulation, maintenance of cell membrane integrity and delayed browning in litchi fruit (Yi et al., 2010). Therefore, ensuring the energy requirements of cells is of paramount importance for safeguarding the normal physiological functions of the fruit and improving its quality (Hu et al., 2023). The research on H2S functions has confirmed that endogenous H2S as a regulatory factor in the energy metabolism of eukaryotes, can facilitate the cell’s energy production under adverse conditions and fulfill the demands for normal growth and development (Fu et al., 2012). Additionally, research has shown that H2S treatment actively contributes to reducing oxidative damage to cell membranes and enhancing the overall antioxidant capacity of litchi fruit (Deshi et al., 2020). According to the outcomes of experiments, H2S treatment maintained a higher energy state of litchi fruit and alleviated the membrane integrity damage, as reflected by the enhancement of ATP and EC levels, and the reduction of MDA content and membrane permeability. The data above suggest that H2S-delayed litchi pericarp browning may be associated with the improvement of energy state, which is consistent with the report of He et al. (2022).
4.2 Effect of H2S on activities of energy metabolic enzymes
Previous research studies demonstrated that energy state is associated with the activities of energy metabolism-related enzyme, mainly involving H+-ATPase, Ca2+-ATPase, CCO and SDH (Ali et al., 2020). H+-ATPase and Ca2+-ATPase can catalyze ATP decomposition to release energy and form ADP and free phosphate ion (Wang et al., 2020a). SDH and CCO are both key enzymes in the tricarboxylic acid (TCA) cycle and electron transport chain (ETC). SDH facilitates the conversion of succinate to fumarate in TCA cycle, while promoting the reduction of ubiquinone to ubiquinol in the ETC. CCO as a terminal oxidase in the ETC, provides energy for cells through coupling oxidative phosphorylation (Wang et al., 2020a). Aghdam et al. (2018) found that the reduction in the activities of energy metabolism-related enzymes had a negative effect on mitochondrial function, which could worsen fruit quality. For example, the reduction in H+-ATPase activity disrupts cellular ionic and pH homeostasis, exacerbating the senescence process of fruits (Zhang et al., 2017). Similarly, the inhibition of Ca2+-ATPase activity increases the Ca2+ accumulation, which is also closely associated with the senescence process of fruits (Zhang et al., 2017). Furthermore, CCO and SDH play active roles in energy production (Li et al., 2016). Their activities directly influence the energy state of fruits and are crucial for maintaining their metabolic vitality. The results showed that H2S treatment increased the activities of H+-ATPase, Ca2+-ATPase, CCO and SDH during litchi storage, indicating that H2S might improve energy state by upregulating the catalytic activity of energy-related enzymes, thereby inhibiting pericarp browning. Consistent with this finding, H2S application to bananas significantly enhanced the activities of H+-ATPase, Ca2+-ATPase, CCO and SDH, therefore maintaining the energy charge, which helped to improve fruit quality and extend the shelf life of fruit (Li et al., 2016).
4.3 Effect of H2S on the expression of energy metabolism-related genes
AtpB is one of the core components in F1Fo-ATP synthase and the function significantly influences the process of oxidative phosphorylation in respiration pathway (Brandt et al., 2013). In addition, the role of AtpB extends beyond energy production, it is also identified as a protein that induces apoptosis in response to various stress signals, can trigger the cell programmed death (Chivasa et al., 2011). In the context of pericarp browning, litchi fruit senescence has been associated with a fast increase in the expression of the AtpB gene, as documented by Liu et al. (2015). The results in this study revealed that the application of H2S can delay the surge in LcAtpB expression while reducing the browning index, suggesting that ability of H2S prolonging the shelf life of litchi may be attributed to its regulation on LcAtpB expression.
As a cellular energy sensor, SnRK can perceive energy state of the cell, and promote energy production and reduce energy consumption when energy shortage occurs (Nietzsche et al., 2014). Meanwhile, the activation of SnRK2 is thought to be in concert with the expression of genes involved in energy metabolism, which may cause an increase in ATP synthesis and a delay in fruit senescence (Aghdam et al., 2018). The results showed that the LcSnRK2 expression was upregulated in litchi during early storage, which might be a response to the ATP deficiency; this is consistent with findings by Zhang et al. (2017). The LcSnRK2 expression was enhanced following H2S treatment, suggesting that this treatment could modulate the energy supply of litchi by influencing the expression of LcSnRK2.
As a vital mitochondrial carrier protein, the primary function of AAC is to facilitate the exchange of ADP and ATP and meet the energy needs of the cell (Klingenberg, 2008). Similar to the function of AtpB, AAC also plays a significant role in the process of programmed cell death (Huang et al., 2014). The research reveals that the function dysfunction of AAC can lead to various diseases, which are related to the disruption of mitochondrial energy production (Clémençon et al., 2013). In the results of this study, H2S treatment upregulated the LcAAC1 expression, which might help maintain the energy state of litchi fruit and contribute to delayed pericarp browning.
AOX and UCP are energy dissipation systems that are universally present in plant mitochondria (Pu et al., 2015). In the electron transfer chain, AOX can receive electrons and transfer them to oxygen, bypassing complexes III and IV, and reducing EC levels, while UCP can suppress ATP production through dissipating the proton electrochemical gradient produced by the ETC (Pu et al., 2015). Both AOX and UCP serve crucial roles in defending cells from oxidative damage and maintaining energy balance (Borecký et al., 2006). For example, AOX and UCP have been shown to regulate the energy state and ethylene release during the senescence of papaya fruit (Oliveira et al., 2015). Li et al. (2020a) found that reducing the expression of certain AOX and UCP genes can extend the shelf life of longan fruit, which was attributed to decrease in the energy dissipation. In this research, the expression of LcAOX1 and LcUCP1 in the control litchi fruit decreased overall after 12 h, indicating that the function of energy dissipation system could be inhibited when energy state declined. In contrast to the effects on LcAOX1 expression, H2S treatment enhanced the LcUCP1 expression during litchi storage for 24-96 h. In fruit, the functions of UCP and AOX have been demonstrated to be complementary, with the expression levels being jointly regulated by external stress and cellular signaling (Li et al., 2020a). The upregulation of LcUCP1 expression suggested that it might participate in regulating the fruit’s energy balance and mitigate oxidative damage as the major energy dissipation system when energy state in litchi improved in response to H2S.
4.4 Effect of H2S on the sugar metabolism
The content of soluble sugars is a key parameter for assessing fruit quality, not only determining the sweetness of fruit but also having a significant impact on the energy provision, cellular matrix stability, and stress tolerance (Wang et al., 2020b). In litchi fruit, the exceptional sweetness is a direct result of high sugar content, which accounts for 15–20% of the fresh weight, primarily consisting of sucrose, fructose, and glucose (Wang et al., 2006). In the present study, the application of H2S effectively preserved the levels of six sugars in the litchi fruit during postharvest storage, while reducing the respiration rate. Previous research indicated that H2S treatment could improve the ratio of pentose phosphate pathway in fruit, while reducing the Embden-Meyerhof-Parnas-tricarboxylic acid cycle in respiratory pathways. This regulation reduced the respiration rate and the consumption of respiratory substrates, ensuring energy supply, mitigating oxidative damage, and maintaining the integrity of the cell membrane, thereby enhancing the fruit’s resistance to stress (Wang et al., 2023).
Sucrose is an important form of sugar accumulation, closely related to fruit quality, energy metabolism, osmotic regulation, and stress resistance (Zhang et al., 2023b). Sucrose and hexose in cells can maintain a dynamic equilibrium through mutual conversion under the catalysis of multiple enzymes, which integrate sucrose into key sugar metabolism pathways like the pentose phosphate pathway and the TCA (Li et al., 2020b). It has been confirmed that the enzymes such as SS-s and SPS are instrumental in catalyzing the biosynthesis and storage of sucrose, whereas sucrose-consuming enzymes, like SS-c, AI, and NI, are implicated in the hydrolytic breakdown of sucrose into glucose and fructose (Cao et al., 2013). The results demonstrated that H2S treatment enhanced the enzymatic activities of SS-s and SPS in litchi fruit over the initial 48 hours, along with decrease in the activities of SS-c, AI, and NI. This may be caused by the improvement of the energy state in the fruit (Sun et al., 2020). The enzymatic modulation led to a higher sucrose synthesis, which is maintained throughout the intermediate and later phases of storage. Concurrent studies have also corroborated the notion that sustaining elevated sucrose levels can be advantageous in decelerating fruit senescence by securing a robust energy supply and strengthening antioxidant defenses in diverse fruit species, including peaches (Wang et al., 2023), apples (Chen et al., 2019), and raspberries (Shi et al., 2019). In light of these findings, the improvement of litchi browning by exogenous H2S treatment may be partly attributed to sucrose synthesis through regulating SPS, SS, AI and NI activities during storage.
Interestingly, as the essential substrates of respiratory metabolism, the content of fructose, glucose, and sucrose in the control fruit marginally increased after 48 h of storage, accompanied by the energy deficit of fruit. Past researches have demonstrated that under carbohydrate deficiency, alternative respiratory substrates such as proteins and lipids will participate in cellular respiration to regulate energy state (Araújo et al., 2011), which may lead to the maintenance or marginal increase of sugar pools in the plant tissue (King and Morris, 1994). The application of H2S has been observed to forestall the emergence of this metabolic shift, potentially attributable to the role of H2S in mitigating the depletion of sugar reserves in litchi. This observation suggests that H2S may exert a protective effect on the energy metabolism of litchi by preserving the endogenous sugar levels and reducing oxidative damage to the cell membrane, thereby preventing the premature decomposition of non-carbohydrate substrates in the respiratory process.
5 Conclusion
In conclusion, H2S treatment successfully reduced the postharvest pericarp browning in litchi fruit. H2S treatment enhanced energy state by modulating the expression of LcAtpB, LcAOX1, LcUCP1, LcAAC1 and LcSnRK2, and the activities of H+-ATPase, Ca2+-ATPase, CCO and SDH. Moreover, H2S treatment reduced soluble sugar consumption in litchi fruit, promoted sucrose synthesis by controlling the activities of SPS, SS, AI and NI that are associated with sucrose metabolism, ensuring the energy supply and alleviating oxidative stress. These findings suggest that H2S treatment can delay the pericarp browning by enhancing the energy supply and antioxidant activity of litchi fruit. Further research is needed to determine the effects of H2S on other regulatory factors (e.g., signaling molecules and transcription factors) associated with sugar and energy metabolisms. Our results provide crucial insights into the mechanism of H2S inhibiting postharvest pericarp browning of litchi, providing a theoretical foundation for application of H2S in litchi preservation.
Data availability statement
The raw data supporting the conclusions of this article will be made available by the authors, without undue reservation.
Author contributions
ZG: Funding acquisition, Project administration, Writing – original draft. KZ: Data curation, Formal analysis, Methodology, Visualization, Writing – original draft. ZZ: Validation, Writing – review & editing. MN: Formal analysis, Writing – review & editing. SL: Methodology, Software, Writing – review & editing. ML: Resources, Writing – review & editing. DG: Investigation, Writing – review & editing. JW: Conceptualization, Supervision, Validation, Writing – review & editing. MH: Investigation, Resources, Supervision, Visualization, Writing – review & editing.
Funding
The author(s) declare financial support was received for the research, authorship, and/or publication of this article. This work was supported by the National Key R & D Program of China (NO. 2023YFD2300805), the Major Science & Technology project of Hainan Province (ZDKJ2021006) and the China Agriculture Research System (NO. CARS-32-20).
Conflict of interest
The authors declare that the research was conducted in the absence of any commercial or financial relationships that could be construed as a potential conflict of interest.
Publisher’s note
All claims expressed in this article are solely those of the authors and do not necessarily represent those of their affiliated organizations, or those of the publisher, the editors and the reviewers. Any product that may be evaluated in this article, or claim that may be made by its manufacturer, is not guaranteed or endorsed by the publisher.
References
Aghdam, M. S., Jannatizadeh, A., Luo, Z., Paliyath, G. (2018). Ensuring sufficient intracellular ATP supplying and friendly extracellular ATP signaling attenuates stresses, delays senescence and maintains quality in horticultural crops during postharvest life. Trends Food Sci. Technol. 76, 67–81. doi: 10.1016/j.tifs.2018.04.003
Alhassan, N., Wills, R. B., Bowyer, M. C., Pristijono, P., Golding, J. B. (2022). Comparative study of the auxins 2, 4-D, fluroxypyr, dicamba, MCPA and hydrogen sulphide to inhibit postharvest calyx senescence and maintain internal quality of Valencia oranges. N. Z. J. Crop Hortic. Sci. 50, 131–142. doi: 10.1080/01140671.2021.2017984
Ali, M., Raza, M. A., Li, S., Huan, C., Zheng, X. (2020). 1-Methylcyclopropene treatment controls ethanol accumulation associated with regulation of mitochondrial energy metabolism in kiwifruit (Actinidia deliciosa) cv. “Bruno” during storage at room temperature. J. Food Biochem. 44, e13273. doi: 10.1111/jfbc.13273
Araújo, W. L., Tohge, T., Ishizaki, K., Leaver, C. J., Fernie, A. R. (2011). Protein degradation–an alternative respiratory substrate for stressed plants. Trends Plant Sci. 16, 489–498. doi: 10.1016/j.tplants.2011.05.008
Arif, Y., Hayat, S., Yusuf, M., Bajguz, A. (2021). Hydrogen sulfide: A versatile gaseous molecule in plants. Plant Physiol. Biochem. 158, 372–384. doi: 10.1016/j.plaphy.2020.11.045
Borecký, J., Nogueira, F. T., De Oliveira, K. A., Maia, I. G., Vercesi, A. E., Arruda, P. (2006). The plant energy-dissipating mitochondrial systems: depicting the genomic structure and the expression profiles of the gene families of uncoupling protein and alternative oxidase in monocots and dicots. J. Exp. Bot. 57, 849–864. doi: 10.1093/jxb/erj070
Brandt, K., Maiwald, S., Herkenhoff-Hesselmann, B., Gnirss, K., Greie, J. C., Dunn, S. D., et al. (2013). Individual interactions of the subunits within the stator of the Escherichia coli ATP synthase. J. Biol. Chem. 288, 24465–24479. doi: 10.1074/jbc.M113.465633
Cao, S., Yang, Z., Zheng, Y. (2013). Sugar metabolism in relation to chilling tolerance of loquat fruit. Food Chem. 136, 139–143. doi: 10.1016/j.foodchem.2012.07.113
Chen, C., Jiang, A., Liu, C., Wagstaff, C., Zhao, Q., Zhang, Y., et al. (2021). Hydrogen sulfide inhibits the browning of fresh-cut apple by regulating the antioxidant, energy and lipid metabolism. Postharvest Biol. Tec. 175, 111487. doi: 10.1016/j.postharvbio.2021.111487
Chen, Q., Yang, G. (2020). Signal function studies of ROS, especially RBOH-dependent ROS, in plant growth, development and environmental stress. J. Plant Growth Regul. 39, 157–171. doi: 10.1007/s00344-019-09971-4
Chen, Y., Ge, Y., Zhao, J., Wei, M., Li, C., Hou, J., et al. (2019). Postharvest sodium nitroprusside treatment maintains storage quality of apple fruit by regulating sucrose metabolism. Postharvest Biol. Tec. 154, 115–120. doi: 10.1016/j.postharvbio.2019.04.024
Chivasa, S., Tome, D. F., Hamilton, J. M., Slabas, A. R. (2011). Proteomic analysis of extracellular ATP-regulated proteins identifies ATP synthase β-subunit as a novel plant cell death regulator. Mol. Cell. Proteomics. 10, 3. doi: 10.1074/mcp.M110.003905
Clémençon, B., Babot, M., Trézéguet, V. (2013). The mitochondrial ADP/ATP carrier (SLC25 family): pathological implications of its dysfunction. Mol. Aspects Med. 34, 485–493. doi: 10.1016/j.mam.2012.05.006
Deshi, V., Siddiqui, M. W., Homa, F., Singh, J. P. (2020). Postharvest hydrogen sulfide infiltration modulates antioxidative metabolism and increases shelf life of litchi. Acta Physiol. Plant 42, 67. doi: 10.1007/s11738-020-03056-6
Fan, S., Wang, D., Xie, H., Wang, H., Qin, Y., Hu, G., et al. (2021). Sugar transport, metabolism and signaling in fruit development of Litchi chinensis sonn: A review. Int. J. Mol. Sci. 22, 11231. doi: 10.3390/ijms222011231
Figueroa, C. R., Jiang, C. Z., Torres, C. A., Fortes, A. M., Alkan, N. (2021). Editorial: Regulation of fruit ripening and senescence. Front. Plant Sci. 12. doi: 10.3389/fpls.2021.711458
Fu, M., Zhang, W., Wu, L., Yang, G., Li, H., Wang, R. (2012). Hydrogen sulfide (H2S) metabolism in mitochondria and its regulatory role in energy production. PNAS. 109, 2943–2948. doi: 10.1073/pnas.1115634109
Ge, Y., Hu, K. D., Wang, S. S., Hu, L. Y., Chen, X. Y., Li, Y. H., et al. (2017). Hydrogen sulfide alleviates postharvest ripening and senescence of banana by antagonizing the effect of ethylene. PloS One 12, e0180113. doi: 10.1371/journal.pone.0180113
He, M., Xie, L., Wu, Y., Hong, M., Jiang, Y., Li, T. (2022). Fluopyram inhibits tricarboxylic acid cycle but induces γ-aminobutyric acid-shunt pathway to prolong postharvest shelf life of litchi fruit. Postharvest Biol. Tec. 192, 112036. doi: 10.1016/j.postharvbio.2022.112036
Hu, S., Hou, Y., Zhao, L., Zheng, Y., Jin, P. (2023). Exogenous 24-epibrassinolide alleviates chilling injury in peach fruit through modulating PpGATA12-mediated sucrose and energy metabolisms. Food Chem. 400, 133996. doi: 10.1016/j.foodchem.2022.133996
Huang, K., Fu, D., Jiang, Y., Liu, H., Shi, F., Wen, Y., et al. (2023a). Storability and linear regression models of pericarp browning and decay in fifty litchi (Litchi chinensis Sonn.) cultivars at room temperature storage. Foods. 12, 1725. doi: 10.3390/foods12081725
Huang, T., Li, Y., Luo, J., Wang, J., Cai, Z., Shen, Y., et al. (2023b). Hydrogen sulfide enhances resistance to Penicillium italicum by activating phenylpropanoid metabolism in postharvest navel orange fruit. Postharvest Biol. Tec. 198, 112259. doi: 10.1016/j.postharvbio.2023.112259
Huang, T., Liu, G., Zhu, L., Liu, J., Xiang, Y., Xu, X., et al. (2024). Mitigation of chilling injury in mango fruit by methyl jasmonate is associated with regulation of antioxidant capacity and energy homeostasis. Postharvest Biol. Tec. 211, 112801. doi: 10.1016/j.postharvbio.2024.112801
Huang, Z., Guo, L., Wang, H., Qu, H., Ma, S., Liu, Y., et al. (2014). Energy status of kiwifruit stored under different temperatures or exposed to long-term anaerobic conditions or pure oxygen. Postharvest Biol. Tec. 98, 56–64. doi: 10.1016/j.postharvbio.2014.07.008
Jiang, Y., Duan, X., Joyce, D., Zhang, Z., Li, J. (2004). Advances in understanding of enzymatic browning in harvested litchi fruit. Food Chem. 88, 443–446. doi: 10.1016/j.foodchem.2004.02.004
Jiang, Y. M., Wang, Y., Song, L., Liu, H., Lichter, A., Kerdchoechuen, O., et al. (2006). Postharvest characteristics and handling of litchi fruit-an overview. Aust. J. Exp. Agric. 46, 1541–1556. doi: 10.1071/ea05108
Jin, P., Zhu, H., Wang, J., Chen, J., Wang, X., Zheng, Y. (2013). Effect of methyl jasmonate on energy metabolism in peach fruit during chilling stress. J. Sci. Food Agric. 93, 1827–1832. doi: 10.1002/jsfa.5973
King, G. A., Morris, S. C. (1994). Early compositional changes during postharvest senescence of broccoli. J. Am. Soc Hortic. Sci. 119, 1000–1005. doi: 10.21273/JASHS.119.5.1000
Klingenberg, M. (2008). The ADP and ATP transport in mitochondria and its carrier. Bba-biomembranes. 1778, 1978–2021. doi: 10.1016/j.bbamem.2008.04.011
Li, D., Limwachiranon, J., Li, L., Du, R., Luo, Z. (2016). Involvement of energy metabolism to chilling tolerance induced by hydrogen sulfide in cold-stored banana fruit. Food Chem. 208, 272–278. doi: 10.1016/j.foodchem.2016.03.113
Li, M., Zheng, Q., Lin, H., Lin, M., Chen, Y., Lin, Y., et al. (2020a). The influence of ATP treatment on energy dissipation system in postharvest longan fruit during senescence. Postharvest Biol. Tec. 164, 111154. doi: 10.1016/j.postharvbio.2020.111154
Li, T., Shi, D., Wu, Q., Zhang, Z., Qu, H., Jiang, Y. (2019). Sodium para-aminosalicylate delays pericarp browning of litchi fruit by inhibiting ROS-mediated senescence during postharvest storage. Food Chem. 278, 552–559. doi: 10.1016/j.foodchem.2018.11.099
Li, X., Li, C., Sun, J., Jackson, A. (2020b). Dynamic changes of enzymes involved in sugar and organic acid level modification during blueberry fruit maturation. Food Chem. 309, 125617. doi: 10.1016/j.foodchem.2019.125617
Liu, J., Li, F., Li, T., Yun, Z., Duan, X., Jiang, Y. (2019). Fibroin treatment inhibits chilling injury of banana fruit via energy regulation. Sci. Hortic. 248, 8–13. doi: 10.1016/j.scienta.2018.12.052
Liu, T., Wang, H., Kuang, J., Sun, C., Shi, J., Duan, X., et al. (2015). Short-term anaerobic, pure oxygen and refrigerated storage conditions affect the energy status and selective gene expression in litchi fruit. LWT. 60, 1254–1261. doi: 10.1016/j.lwt.2014.09.003
Ni, Z. J., Hu, K. D., Song, C. B., Ma, R. H., Li, Z. R., Zheng, J. L., et al. (2016). Hydrogen sulfide alleviates postharvest senescence of grape by modulating the antioxidant defenses. Oxid. Med. Cell. Longevity. 2016, 0–4715651. doi: 10.1155/2016/4715651
Nietzsche, M., Schiessl, I., Bornke, F. (2014). The complex becomes more complex: protein-protein interactions of SnRK1 with DUF581 family proteins provide a framework for cell and stimulus type-specific SnRK1 signaling in plants. Front. Plant Sci. 5. doi: 10.3389/fpls.2014.00054
Oliveira, M. G., Mazorra, L. M., Souza, A. F., Silva, G. M. C., Correa, S. F., Santos, W. C., et al. (2015). Involvement of AOX and UCP pathways in the post-harvest ripening of papaya fruits. J. Plant Physiol. 189, 42–50. doi: 10.1016/j.jplph.2015.10.001
Pareek, S. (2016). Nutritional and biochemical composition of lychee (Litchi chinensis Sonn.) cultivars. Nutritional composition of fruit cultivars, (Elsevier, Amsterdam, Netherlands), 395–418. doi: 10.1016/B978-0-12-408117-8.00017-9
Pu, X., Lv, X., Tan, T., Fu, F., Qin, G., Lin, H. (2015). Roles of mitochondrial energy dissipation systems in plant development and acclimation to stress. Ann. Bot. 116, 583–600. doi: 10.1093/aob/mcv063
Saddhe, A. A., Manuka, R., Penna, S. (2021). Plant sugars: Homeostasis and transport under abiotic stress in plants. Physiol. Plant 171, 739–755. doi: 10.1111/ppl.13283
Shi, K., Liu, Z., Wang, J., Zhu, S., Huang, D. (2019). Nitric oxide modulates sugar metabolism and maintains the quality of red raspberry during storage. Sci. Hortic. 256, 108611. doi: 10.1016/j.scienta.2019.108611
Siddiqui, M. W., Deshi, V., Homa, F., Aftab, M. A., Aftab, T. (2021). Inhibitory effects of hydrogen sulfide on oxidative damage and pericarp browning in harvested litchi. J. Plant Growth Regul. 40, 2560–2569. doi: 10.1007/s00344-021-10300-x
Song, H., Yuan, W., Jin, P., Wang, W., Wang, X., Yang, L., et al. (2016). Effects of chitosan/nano-silica on postharvest quality and antioxidant capacity of loquat fruit during cold storage. Postharvest Biol. Tec. 119, 41–48. doi: 10.1016/j.postharvbio.2016.04.015
Sun, L., Li, C., Zhu, J., Jiang, C., Li, Y., Ge, Y. (2020). Influences of postharvest ATP treatment on storage quality and enzyme activity in sucrose metabolism of Malus domestica. Plant Physiol. Biochem. 156, 87–94. doi: 10.1016/j.plaphy.2020.09.004
Wang, H. C., Huang, H. B., Huang, X. M., Hu, Z. Q. (2006). Sugar and acid compositions in the arils of Litchi chinensis Sonn.: cultivar differences and evidence for the absence of succinic acid. J. Hortic. Sci. Biotechnol. 81, 57–62. doi: 10.1080/14620316.2006.11512029
Wang, H., Qian, Z., Ma, S., Zhou, Y., Patrick, J. W., Duan, X., et al. (2013). Energy status of ripening and postharvest senescent fruit of litchi (Litchi chinensis Sonn.). BMC Plant Biol. 13, 1–16. doi: 10.1186/1471-2229-13-55
Wang, J., Dong, S., Jiang, Y., He, H., Liu, T., Lv, M., et al. (2020b). Influence of long-term cold storage on phenylpropanoid and soluble sugar metabolisms accompanied with peel browning of ‘Nanguo’ pears during subsequent shelf life. Sci. Hortic. 260, 108888. doi: 10.1016/j.scienta.2019.108888
Wang, L., Huang, X., Liu, C., Zhang, C., Shi, K., Wang, M., et al. (2023). Hydrogen sulfide alleviates chilling injury by modulating respiration and energy metabolisms in cold-stored peach fruit. Postharvest Biol. Tec. 199, 112291. doi: 10.1016/j.postharvbio.2023.112291
Wang, T., Hu, M., Yuan, D., Yun, Z., Gao, Z., Su, Z., et al. (2020a). Melatonin alleviates pericarp browning in litchi fruit by regulating membrane lipid and energy metabolisms. Postharvest Biol. Tec. 160, 111066. doi: 10.1016/j.postharvbio.2019.111066
Wu, Z. C., Yang, Z. Y., Li, J. G., Chen, H. B., Huang, X. M., Wang, H. C. (2016). Methyl-inositol, γ-aminobutyric acid and other health benefit compounds in the aril of litchi. Int. J. Food Sci. Nutr. 67, 762–772. doi: 10.1080/09637486.2016.1198888
Xu, P., Huber, D. J., Gong, D., Yun, Z., Pan, Y., Jiang, Y., et al. (2023). Amelioration of chilling injury in ‘Guifei’ mango fruit by melatonin is associated with regulation of lipid metabolic enzymes and remodeling of lipidome. Postharvest Biol. Tec. 198, 112233. doi: 10.1016/j.postharvbio.2022.112233
Yi, C., Jiang, Y. M., Shi, J., Qu, H. X., Xue, S., Duan, X. W., et al. (2010). ATP-regulation of antioxidant properties and phenolics in litchi fruit during browning and pathogen infection process. Food Chem. 118, 42–47. doi: 10.1016/j.foodchem.2009.04.074
Zhang, W., Pan, Y., Jiang, Y., Zhang, Z. (2023a). Advances in control technologies and mechanisms to treat peel browning in postharvest fruit. Sci. Hortic. 311, 111798. doi: 10.1016/j.scienta.2022.111798
Zhang, Y., Tang, H., Lei, D., Zhao, B., Zhou, X., Yao, W., et al. (2023b). Exogenous melatonin maintains postharvest quality in kiwiberry fruit by regulating sugar metabolism during cold storage. LWT. 174, 114385. doi: 10.1016/j.lwt.2022.114385
Zhang, Z., Hu, M., Yun, Z., Wang, J., Feng, G., Gao, Z., et al. (2017). Effect of tea seed oil treatment on browning of litchi fruit in relation to energy status and metabolism. Postharvest Biol. Tec. 132, 97–104. doi: 10.1016/j.postharvbio.2017.05.010
Keywords: litchi, browning, hydrogen sulfide, energy, sugars
Citation: Gao Z, Zhao K, Zhang Z, Nizamani MM, Li S, Li M, Gong D, Wang J and Hu M (2024) Hydrogen sulfide alleviates pericarp browning in lichi fruit by modulating energy and sugar metabolisms. Front. Plant Sci. 15:1421203. doi: 10.3389/fpls.2024.1421203
Received: 22 April 2024; Accepted: 19 August 2024;
Published: 03 September 2024.
Edited by:
Vittorio Farina, University of Palermo, ItalyReviewed by:
Yihui Chen, Fujian Agriculture and Forestry University, ChinaJianzhao Li, Ludong University, China
María Serrano, Miguel Hernández University of Elche, Spain
Copyright © 2024 Gao, Zhao, Zhang, Nizamani, Li, Li, Gong, Wang and Hu. This is an open-access article distributed under the terms of the Creative Commons Attribution License (CC BY). The use, distribution or reproduction in other forums is permitted, provided the original author(s) and the copyright owner(s) are credited and that the original publication in this journal is cited, in accordance with accepted academic practice. No use, distribution or reproduction is permitted which does not comply with these terms.
*Correspondence: Jiabao Wang, d2FuZ2ppYWJhb0BjYXRhcy5jbg==; Meijiao Hu, aHVtZWlqaWFvMzIwQHNpbmEuY29t
†These authors have contributed equally to this work and share first authorship