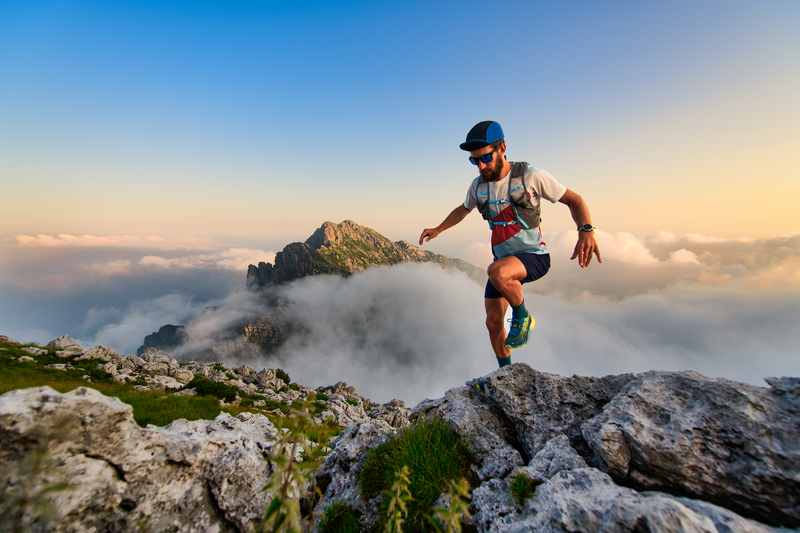
94% of researchers rate our articles as excellent or good
Learn more about the work of our research integrity team to safeguard the quality of each article we publish.
Find out more
ORIGINAL RESEARCH article
Front. Plant Sci. , 20 June 2024
Sec. Plant Physiology
Volume 15 - 2024 | https://doi.org/10.3389/fpls.2024.1417632
Introduction: Abscisic acid (ABA) can negatively regulate seed germination, but the mechanisms of ABA-mediated metabolism modulation are not well understood. Moreover, it remains unclear whether metabolic pathways vary with the different tissue parts of the embryo, such as the radicle, hypocotyl and cotyledon.
Methods: In this report, we performed the first comprehensive metabolome analysis of the radicle and hypocotyl + cotyledon in Pinus koraiensis seeds in response to ABA treatment during germination.
Results and discussion: Metabolome profiling showed that following ABA treatment, 67 significantly differentially accumulated metabolites in the embryo were closely associated with pyrimidine metabolism, phenylalanine metabolism, cysteine and methionine metabolism, galactose metabolism, terpenoid backbone biosynthesis, and glutathione metabolism. Meanwhile, 62 metabolites in the hypocotyl + cotyledon were primarily involved in glycerophospholipid metabolism and glycolysis/gluconeogenesis. We can conclude that ABA may inhibit Korean pine seed germination primarily by disrupting the biosynthesis of certain plant hormones mediated by cysteine and methionine metabolism and terpenoid backbone biosynthesis, as well as reducing the reactive oxygen species scavenging ability regulated by glutathione metabolism and shikimate pathway in radicle. ABA may strongly disrupt the structure and function of cellular membranes due to alterations in glycerophospholipid metabolism, and weaken glycolysis/gluconeogenesis in the hypocotyl + cotyledon, both of which are major contributors to ABA-mediated inhibition of seed germination. These results highlight that the spatial modulation of metabolic pathways in Pinus koraiensis seeds underlies the germination response to ABA.
Seed germination introduces seed plant into natural ecosystems, marking the beginning of their life cycle (Hao et al., 2022). This process is highly susceptible to diseases and environmental stresses (Liu et al., 2015). Thus, most plants have evolved seed dormancy mechanisms to prevent seedling emergence under unsuitable environmental conditions and initiate the new life cycle after sensing optimal environmental cues (Bentsink and Koornneef, 2008; Nonogaki et al., 2018). Based on the different times when seed dormancy occurs, seed dormancy can be classified into primary dormancy (developed during seed maturation) and secondary dormancy (induced after seed shedding) (Bewley et al., 2013). It is commonly known that ABA (abscisic acid) induces primary dormancy during seed maturation and inhibits germination during imbibition (Finkelstein et al., 2008). Therefore, it is of great theoretical and practical importance to reveal the mechanism by which ABA inhibits germination.
Although the time required for seed germination is relatively short compared with other stages in the plant life cycle, the underlying mechanism is complicated. Seed germination is a process during which the heterotrophic state is gradually transformed to an autotrophic state (Nonogaki et al., 2010; Shu et al., 2016; Carrera-Castaño et al., 2020), requiring a large amount of energy and carbon skeletons (Nonogaki et al., 2010; Weitbrecht et al., 2011). Metabolites can provide raw materials for diverse metabolic and cellular events, including DNA damage and repair, phytohormone metabolism and signal transduction, nutrient and energy metabolism, as well as cell wall remodeling and modification (Guo and Gan, 2005; Guo et al., 2021). Germinating seeds are in a highly active metabolic state. Undoubtedly, metabolites play key roles in seed germination. ABA can strongly affect the process of seed germination by modulating ABA-related metabolites. Thus, knowing the key metabolites as well as their pathways involved in modulation of ABA is important for understanding the mechanism of seed germination inhibited by this important exogenous phytohormone.
Transcriptomic, proteomic and metabolomic analyses are effective methods for examining simultaneous metabolic changes occurring during complex germination processes with high throughput and efficiency (Liu et al., 2015; Gorzolka et al., 2016; Kazmi et al., 2017; Silva et al., 2017; Chen et al., 2019). Along with the rapid development of systems biology and considerable progress in high-throughput sequencing, integrated omics have been increasingly used in studies on the mechanisms of seed development, dormancy and germination. In previous studies, transcriptomics, proteomics and/or metabolomics analysis of seed germination in the presence of ABA have been extensively conducted. Most studies have focused on herbaceous species, such as Arabidopsis (Garciarrubio et al., 1997; Pritchard et al., 2002; Wu et al., 2020a, b; Xue et al., 2021; Chen et al., 2022; Gong et al., 2022; Li et al., 2023), rice (Oryza sativa) (Kim et al., 2008; Liu et al., 2015; Wang et al., 2020), wheat (Triticum aestivum) (Yu et al., 2016), barley (Hordeum vulgare) (Huang et al., 2016), Medicago truncatula (Gimeno-Gilles et al., 2009), Astragalus membranaceus (Yang et al., 2018) and Panax notoginseng (Wang et al., 2023). By contrast, information on trees, such as pear (Pyrus pyrifolia (Burm.f.) Nakai) (Qi et al., 2022), Magnolia sieboldii (Lu et al., 2023), European beech (Fagus sylvatica) (Pawłowski, 2007), and Norway maple (Acer platanoides) (Pawłowski, 2009) remains limited. Based on these studies, it is concluded that ABA plays at least three roles in inhibiting seed germination. First, ABA prevents the mobilization of storage reserves, such as lipid, starch, and protein (Garciarrubio et al., 1997; Yu et al., 2016; Yang et al., 2018), and inhibits the activities of enzymes involved in carbohydrate and energy metabolic pathways, including glycolysis/gluconeogenesis, citric acid cycle, glyoxylate cycle, and oxidative phosphorylation) (Garciarrubio et al., 1997; Qi et al., 2022; Lu et al., 2023), thus resulting in a reduction in energy and nutrient supplies to the embryo (Pawłowski, 2009; Liu et al., 2015; Wang et al., 2023). Second, ABA promotes the biosynthesis of JA (jasmonic acid) and IAA (indoleacetic acid), while it downregulates GA (gibberellin) synthesis (Shi et al., 2019) and GA signal transduction (Wang et al., 2023). Furthermore, it blocks the biosynthesis pathway of ethylene precursors (Pawłowski, 2009; Liu et al., 2015). Third, ABA not only induces the accumulation of ROS (reactive oxygen species) but also decreases the ROS scavenging ability (Wu et al., 2020b; Gong et al., 2022; Li et al., 2023). In addition to three aspects described above explaining ABA-imposed germination inhibition, ABA is known to affect cell division (Liu et al., 2015), interfere with the purine metabolism pathway (Cornelius et al., 2011; Qi et al., 2022), inhibit amino acid synthesis (Wang et al., 2023), and interfere with cell wall loosening and expansion (Gimeno-Gilles et al., 2009). These conclusions have laid a solid foundation for deeper insights into the mechanisms by which ABA inhibits woody-plant seed germination. Furthermore, few studies reveal that there is a tissue-specific response of metabolic pathways to ABA sensitivity between the embryo and endosperm (Manz et al., 2005; Penfield et al., 2006; Yu et al., 2016). For example, the ABA-responsive metabolic pathways in the embryo included DNA synthesis, carbohydrate metabolism, hormone metabolism, and protein degradation, while in the endosperm the metabolism related to storage reserve mobilization, transport, biotic and abiotic stresses, hormone metabolism, cell wall metabolism, signaling, and development were more predominant (Yu et al., 2016). Compared with embryonic ABA, endospermic ABA seems to play minimal role in inhibiting seed germination (Izydorczyk et al., 2018). A recent and detailed study showed that ABA-mediated germination inhibition is due to the limit of local glucose supply in the hypocotyl region of embryo (Xue et al., 2021). Therefore, to gain an insight into the inhibition mechanism of ABA on germination, it is necessary to study the metabolic response to ABA in different embryo tissues, such as the radicle, hypocotyl and cotyledons.
Korean pine (Pinus koraiensis) is an ecologically and economically significant coniferous species that is widely distributed in China, Russia, Korea, and Japan (Wang, 1995). Korean pine could be used for the extraction of rosin, turpentine and pine needle oil (Wu et al., 1996). Moreover, Korean pine seeds possess high nutritional and certain medicinal value due to their high levels of vitamins, such as vitamin A and E (Shen, 2002; Xu et al., 2014), and unsaturated fatty acids, including linoleic acid, linolenic acid and pinolenic acid (Shen, 2002; Bao et al., 2020). Previous research has indicated that excessive logging of woody plants and the low germination percentage induced by seed dormancy have resulted in low regeneration of Korean pine individuals in the natural ecosystem (Zhu et al., 2007). Morphophysiological dormancy (physiological inhibiting factors in the undifferentiated or underdeveloped embryo) is observed in fresh Korean pine seeds when they disperse in autumn (Song et al., 2023). Moist chilling, where imbibed seeds were mixed with moist sand and then stored under low temperature conditions for approximately six months, is widely applied to reduce seed dormancy and promote seed germination of Korean pine (Wang, 1995). Although the inhibition of Korean pine seed germination and the regulation of seed dormancy status by ABA has been widely studied (Lai et al., 1989; Song et al., 2018), the underlying mechanisms remain incompletely understood. The objective of this study was to employ non-targeted metabolomics to identify the metabolic regulation mechanism underlying the inhibition of ABA on the germination of Pinus koraiensis seeds. We thus compared the metabolome of Korean pine seeds germinating at 25/15°C with that of Korean pine seeds whose germination was inhibited by treatment with exogenous ABA. We then identified the differentially accumulated compounds between untreated control and ABA-treated radicle and hypocotyl + cotyledons samples. The metabolic pathways associated with the ABA response were screened in radicle and hypocotyl + cotyledons, respectively. This information is useful for elucidating the spatial specificity of metabolomic regulation mechanisms of inhibiting seed germination by ABA.
In late-September (early-autumn) 2020, Korean pine seeds were collected from at least 50 Korean pine trees at the ripening stage in a mixed broad-leaved Korean pine forest (MBKPF) in Liangshui National Nature Reserve (47°6′49”N—47°16′10″N, 128°47′8″E—128°57′19”E) in northeastern China. Regional climate is characterized by temperate continental monsoon. The water content of the seed was less than approximately 10%, whereas its viability exceeded 90%. Seeds were then stored at -20°C for about one month to maintain their primary physiological dormancy status.
We previously showed that under natural conditions, the primary physiological dormancy of fresh Korean pine seeds falling off in early-autumn was progressively released during subsequent autumn and winter (Song et al., 2023). Based on this observation, in early October, Korean pine seeds were taken out of -20°C storage conditions and then buried between litterfall and soil in MBKPF for about eight months, aiming to release their primary physiological dormancy in the presence of moist cold conditions in autumn and winter.
The specific procedures were as follows. First, three sites, approximately 50 m apart from each other, were selected as seed burial points in MBPKF. Second, 5000 seeds were mixed with soil from MBKPF. The mixture of seeds and soil was then placed in a nylon bags (50 cm in length × 50 cm in width, with 3 mm aperture). Third, this nylon bag containing seeds and soil was then placed in a metal container to prevent predation from animals. One nylon bag was buried in each seed burial site, serving as a replicate. A total of three replicates was set up in the present study, with 5000 seeds in each replicate.
After eight months, in late May in the second year of seed burial, seeds were retrieved from each burial site and then used for germination tests to evaluate if these seeds had already been released from primary physiological dormancy. Seed dormancy release can be characterized by a gradual widening of the temperature range that permits germination to occur (Baskin and Baskin, 2014). Thus, four fluctuating temperature regimes mimicking the approximate mean maximum and minimum temperatures for each season in the Liangshui region were used for germination tests. 10/5°C, 20/10°C, 25/15°C and 30/20°C represent mid-spring/late-autumn, late-spring/early-autumn, early-summer and mid-summer temperature, respectively. Three replicates of 20 seeds each were incubated on five layers of filter paper moistened with 8 mL of distilled water in closed 100-mm diameter Petri dishes at four fluctuating temperature regimes for about eight weeks. For each fluctuating temperature regime, the duration at the maximum and minimum temperature was 14 and 10 h, respectively. Correspondingly, during the 14 h light period (200 μmol m-2 s-1), the temperature was set to the maximum, while during the 10 h dark period, the temperature was set to the minimum. Seeds were checked every two days, and a radicle length of 2 mm was used as the criterion for completion of germination. These germinated seeds were counted and removed from the petri dish. At the same time, distilled water was added as required to keep seeds moisture. Germination was considered complete when seeds did not germinate for three consecutive days. After the germination test was terminated, the ungerminated seeds were dissected to determine the viability of the embryo. Seeds with viable embryos were used to compute the seed germination percentage. Seeds containing white and firm embryos are considered alive. If embryos are soft and brown, these seeds are considered dead.
Seeds that had released their primary physiological dormancy were collected from seed burial sites in late-May in the second year of seed burial. These seeds were then treated with either distilled water (control) or 1 mM ABA (ABA-treatment). Five replicates, with 200 seeds per replicate, were set up for both the control and the ABA-treatment groups, respectively. Every 200 seeds were placed on a sterile medical cotton pad wetted with distilled water, in sealable, clear, plastic container (23 cm long × 20 cm wide × 5 cm high). The containers were then incubated at the optimal temperature of 25/15°C (day/night) under a 14/10-hour light/dark cycle, with a 14-hour photoperiod and approximately 200 μmol of photons m-2 s-1 for 2 weeks. After 2 weeks of incubation, the embryo of the control and ABA-treatment seeds were dissected into radicle and hypocotyl + cotyledon. The radicle and hypocotyl + cotyledon samples were frozen in liquid nitrogen and stored at -80°C until use.
After the samples were slowly thawed at 4°C, pre-cooled acetonitrile/methanol/aqueous solution (2:2:1, v/v/v) were added to the samples (Pi et al., 2018). The mixture of samples and extraction solution was first vortexed, and then subjected to cryogenic sonication treatment for 30 min (twice) (Pi et al., 2018). Then they were left to stand at -20°C for 60 min and subsequently centrifuged at 14,000 g at 4°C for 20 min to obtain the supernatant (Pi et al., 2018). The supernatant was lyophilized and stored at -80°C until further analysis. When liquid chromatography-mass spectrometry analysis was conducted, the freeze-dried powder was first redissolved in 100 μL of a 1:1 (v/v) acetonitrile:water solution, then vortexed, and subsequently centrifuged at 14000 g for 15 min at 4°C (Gu et al., 2018). After that the supernatant was taken for analysis.
LC-MS analysis was performed using an Agilent 1290 Infinity ultra-high performance liquid chromatography (Agilent Technologies, Santa Clara, CA, United States) coupled to a time-of-flight mass spectrometer (Triple TOF 6600, AB SCIEX, Framingham, MA, United States) (Wu et al., 2020a). The sample analytes were separated on a hydrophilic interaction liquid chromatography (HILIC) column (ACQUITY UPLC BEH Amide 2.1 mm × 100 mm column, internal diameter 1.7 μm, Waters, Ireland) at a constant flow rate of 0.5 mL·min-1 (Wu et al., 2020a). The column temperature was maintained at 25°C, and the sample injection volume was 2 μL (Wu et al., 2020a). The mobile phase comprised aqueous ammonium acetate (25 mM)/ammonia (25 mM) (A) and acetonitrile (B) (Wu et al., 2020a). The gradient elution program optimized for separation was as follows: 0–0.5 min, 95% B; 0.5–7 min, 95–65% B; 7–8 min, 65–40% B; 8–9 min, 40% B; 9–9.1 min, 40–95% B; 9.1–12 min, 95% B (Wu et al., 2020a). The samples were kept in a 4°C autosampler tray during the entire analysis process (Wu et al., 2020a).
Following HILIC chromatographic separation, the electrospray ionization (ESI) source operation parameters were set as follows: ion source gas1 (Gas1) and ion source gas2 (Gas2) were both set to 60, curtain gas (CUR) to 30, and the source temperature to 600°C. The IonSapary Voltage Floating (ISVF) was ± 5500 V for both positive and negative ion mode. The TOF MS scan m/z range was set from 60 to 1000 Da, while the product ion scan m/z range was from 25 to 1000 Da. The TOF MS scan accumulation time was 0.20 s per spectrum, and for product ion scan accumulation time, it was 0.05 s per spectrum (Fan et al., 2018). MS/MS data was acquired using information-dependent acquisition (IDA) with high sensitivity mode selected (Fan et al., 2018). The parameters were set as follows: the collision energy (CE) was fixed at 35 ± 15 eV, the declustering potential (DP) was ± 60 V (positive and negative ion mode), isotopes within 4 Da were excluded and 10 candidate ions were monitored per cycle (Fan et al., 2018).
The raw data (wiff.scan files) were converted to MzXML files by ProteoWizard MSConvert. Peak alignment, retention time correction and peak area extraction were conducted with the XCMS program. The structure of metabolite was identified by matching the mass-to-charge ratio, retention time, molecular mass (molecular mass error within < 25 ppm), secondary fragmentation spectra, and collision energy of the sample metabolite with the metabolite in in-house database (Shanghai Applied Protein Technology) (Gu et al., 2018).
Partial least squares-discriminant analysis (PLS-DA) was carried out on the control and ABA-treatment samples to reveal the difference in metabolite profiles among the radicle of control embryos (abbreviated R) and ABA-treated embryos (abbreviated RA), the hypocotyl + cotyledon of control embryos (abbreviated HC) and ABA-treated embryos (abbreviated HCA). The metabolite data were log transformed (generalized logarithm transformation) and auto scaled (mean-centered and divided by the standard deviation of each variable) for normalization before performing PLS-DA analysis. PLS-DA was also conducted to determine the differentially expressed metabolites between R and RA, as well as between HC and HCA. The metabolite data were pareto scaled (mean-centered and divided by the square root of the standard deviation of each variable) for normalization before performing PLS-DA. The key differentially expressed metabolites were selected based on two criteria: variable importance in the projection (VIP) values greater than 1 and P-values from Student’s t-test less than 0.05. Fold change in the relative contents of these differentially expressed metabolites was calculated between R and RA, and also between HC and HCA. Subsequently, those metabolites with VIP greater than 1 and P-value less than 0.05 were also subjected to metabolic pathway analysis to determine the metabolic pathways in which they are enriched. PLS-DA, the calculation of fold change and the metabolic pathways analysis were all conducted using the MetaboAnalyst 5.0. For the metabolic pathways analysis, the corresponding parameters were set according to the methods described by Song and Zhu (2019). The most relevant metabolic pathways were identified by applying the criteria of a P-value less than 0.05 and an impact-value threshold greater than 0.1 (Wang et al., 2012).
A total of 66.67 ± 10.41%, 83.33 ± 7.64% and 56.67 ± 15.28% of seeds germinated at 20/10°C, 25/15°C and 30/20°C, respectively. However, only about 15.00% of seeds germinated at 10/5°C. Notably, none of the seeds treated with 1 mM ABA germinated under any of these four temperature regimes.
PLS-DA of the total metabolite data revealed differences in metabolites among samples. The PLS-DA of all the measured metabolites demonstrated that the five replicates of control and ABA-treated radicle (or hypocotyl + cotyledon) were clustered together separately (Figure 1), indicating similarity among replicates within each group. The first principal component (pca1) separated the radicle and hypocotyl + cotyledon groups, with a contribution value reaching 27.4%. The second principal component (pca2) had a contribution value of 14.9% (Figure 1). The clear distinction was observed on the second principal component between the control and ABA-treated radicle groups, as well as between the control and ABA-treated hypocotyl + cotyledon groups (Figure 1).
Figure 1 Principal least squares-discriminant analysis of measured metabolites in the radicle of control seeds (R), the radicle of ABA-treated seeds (RA), the hypocotyl + cotyledon of control seeds (HC), and the hypocotyl + cotyledon of ABA-treated seeds (HCA).
When seeds were germinated for 2 weeks at 25/15°C, which is the optimal temperature for Korean pine seed germination, a total of 67 metabolites were identified as having significantly altered relative contents in the ABA-treated radicle compared to the control radicle (Table 1 and Supplementary Table S1). It was found that the relative amounts of 20 metabolites were significantly higher in the ABA-treated radicle compared to the control (Table 1). These metabolites that showed significant increases in relative levels were mainly classified into nucleosides, nucleotides, and analogues (nine in total), lipids and lipid-like molecules (three in total), and organic acids and derivatives (three in total). After ABA treatment, the ABA relative level in the radicle was approximately 10-fold higher. Additionally, 47 metabolites exhibited relatively lower contents in the ABA-treated radicle compared to the control radicle (Table 1). Specifically, the most numerous metabolites were organic acids and derivatives (14 in total), followed by lipids and lipid-like molecules (eight in total), and carbohydrates and carbohydrate conjugates (five in total), organoheterocyclic compounds (five in total) and benzenoids (three in total). Six organic acids and derivatives, including L-leucine, 5-L-glutamyl-L-alanine, gamma-glutamyl-L-methionine, L-valine, (R)-mevalonic acid 5-phosphate and 3-hydroxycapric acid, were observed to show at least a 1.5-fold decrease. S-methyl-5’-thioadenosine, a member of the nucleosides, nucleotides, and their analogues, exhibited the most significant reduction, with a 3.20-fold drop.
Table 1 Information on 67 metabolites with VIP > 1 and P < 0.05 is presented for the comparison between the radicle of control seeds and the radicle of ABA-treated seeds.
A total of 62 ABA-responsive metabolites in the hypocotyl + cotyledon were identified when comparing the germination of Korean pine seeds treated with ABA to germination in distilled water, both at 25/15°C, after 2 weeks (Table 2 and Supplementary Table S2). Our results revealed that seven metabolites were accumulated and 55 metabolites decreased in the hypocotyl + cotyledon of ABA-treated seeds compared to the control group. The relative content of ABA in the hypocotyl + cotyledon was increased by at least 10-fold in ABA-treated seeds, in comparison to the control group. Furthermore, there was also a 1- to 3-fold increase in the relative levels of methylmalonic acid, dodecanoic acid, isobutyric acid, meso-tartaric acid, myristic acid, and (R)-mevalonic acid 5-phosphate following ABA treatment. Fifty-five metabolites with a significant decrease in relative levels were classified into lipids and lipid-like molecules (25), organic acids and derivatives (seven), carbohydrates and carbohydrate conjugates (six), nucleosides, nucleotides, and analogues (five), benzenoids and phenylpropanoids (two), and polyketides (two). Furthermore, the relative levels of approximately 12 lipids and lipid-like molecules, including cholesteryl sulfate, 15-Keto-prostaglandin E1, eicosapentaenoic acid, eicosadienoic acid, oleanolic acid, tetracosanoic acid, tricosanoic acid, behenic acid, eicosatrienoic acid, arachidic acid, 2-hydroxy-butanoic acid and heneicosanoic acid were reduced by more than 2-fold. Seven organic acids and derivatives mainly comprised three amino acids, namely gamma-glutamyl-L-methionine, L-isoleucine, and argininosuccinic acid, along with (S)-Lactate and phosphoenolpyruvate. Other carbohydrates and carbohydrate conjugates that were found to decrease significantly mainly comprised threonic acid, L-iditol, alpha-D-glucose, 2’-deoxy-D-ribose, 3,3’,4,5-tetrahydroxy-trans-stilbene, and L-ribulose, with decreases ranging from 1.5- to over 3.4-fold.
Table 2 Information on 62 metabolites with VIP > 1 and P < 0.05 is presented for the comparison between the hypocotyl + cotyledon of control seeds and the hypocotyl + cotyledon of ABA-treated seeds.
In the radicle, differentially expressed metabolites were enriched in eight metabolic pathways, including pyrimidine metabolism, phenylalanine metabolism, nicotinate and nicotinamide metabolism, cysteine and methionine metabolism, phenylalanine, tyrosine and tryptophan biosynthesis, terpenoid backbone biosynthesis, galactose metabolism, and glutathione metabolism (Figures 2A, 3). Two metabolic pathways, namely glycerophospholipid metabolism and glycolysis/gluconeogenesis, were identified in the hypocotyl + cotyledon of ABA-treated seeds (Figures 2B, 4).
Figure 2 A Metabolome view depicts the altered metabolic pathways in (A) the radicle and (B) the hypocotyl + cotyledon of control seeds compared to ABA-treated seeds. Each node stands for a metabolic pathway. The color of the node signifies the P-value associated with each metabolic pathway, and the size (or radius) of the node represents the impact value of each metabolic pathway. Dark red, large circles located in the top right corner of the “metabolome view” indicate the primary altered pathways, in contrast to the yellow, small circles positioned on the left side of the graph represent the metabolic pathways that are less affected by ABA.
Figure 3 Metabolites were enriched in the eight altered pathway in the radicle of control seeds (R) compared to the radicle of ABA-treated seeds (RA). The relative contents of differentially expressed metabolites were first log transformed (using a generalized logarithm transformation) and then auto scaled (mean-centered and divided by the standard deviation of each variable) for normalization purposes. The normalized values are presented on the Y-axis. Since only nicotinamide adenine dinucleotide is enriched in nicotinate and nicotinamide metabolism, and phenylpyruvate is enriched in phenylalanine metabolism, these two pathways are not included.
Figure 4 Metabolites were enriched in the two altered metabolic pathways in the hypocotyl + cotyledon of control seeds (HC) compared to the hypocotyl + cotyledon of ABA-treated seeds (HCA). The relative contents of differentially expressed metabolites were pareto scaled (mean-centered and divided by the square root of the standard deviation of each variable) for normalization purposes. The normalized values are presented on the Y-axis.
Approximately 70% of the metabolites with VIP > 1 and P < 0.05 were decreased under ABA treatment, indicating that ABA inhibited the metabolism of a large number of compounds in the radicle of Korean pine seeds. These metabolites were enriched in eight metabolic pathways, suggesting that these metabolic pathways may be affected by the ABA treatment. Furthermore, most of the metabolites involved in these pathways decreased under ABA treatment. These results further confirm that ABA may play an important role in the inhibition of Korean pine seed germination by disrupting certain pathways in the radicle, which mainly include phenylalanine, tyrosine and tryptophan biosynthesis, glutathione metabolism, terpenoid backbone biosynthesis, and cysteine and methionine metabolism (Figure 5).
Figure 5 A Schematic diagram of metabolic regulation of ABA inhibition of Korean pine seed germination. The red upward arrows indicate upregulated metabolites, whereas the downward arrows represent the downregulated metabolites.
It has been well documented that SAM (S-adenosylmethionine) functions as the universal methyl-group donor affecting the expression of genes related to seed dormancy breaking (Liu et al., 2015; Lu et al., 2018). In addition, SAM is also a precursor for the synthesis of various compounds, including the plant hormone ethylene, the vitamin biotin, the polyamines spermidine and spermine, as well as the iron chelator nicotianamine (Ravanel et al., 2008; Takahashi et al., 2011). Ethylene can antagonize ABA effects, thus promoting the breaking of seed dormancy and germination (Beaudoin et al., 2000; Lee et al., 2010b). The germination-promoting role of polyamines has been widely reported (Huang et al., 2017; Chen et al., 2019). S-adenosylmethionine synthetase catalyzes the biosynthesis of SAM from methionine and adenosine triphosphate (Gimeno-Gilles et al., 2009). During the biosynthesis of ethylene and polyamines, SAM produces S-methyl-5’-thioadenosine (MTA) as a byproduct. This MTA is then recycled to methionine via the Yang cycle (Miyazaki and Yang, 1987). Previous investigations have indirectly indicated that the metabolic process catalyzed by S-adenosylmethionine synthetase has a significant regulatory function when Arabidopsis seeds shift from a quiescent to a highly active state (Gallardo et al., 2003; Bouton et al., 2005).
In the present research, three metabolites related to the synthesis and breakdown of SAM have been identified in the metabolism of cysteine and methionine. The apparent accumulation of precursor of SAM biosynthesis, namely L-methionine, coupled with a significant reduction in the relative content of the product of SAM breakdown, MTA, in response to ABA, suggests that the SAM-related biosynthetic and catabolic pathways may be influenced by ABA. Moreover, the observed decline in 3-phosphoserine, the precursor of L-methionine, further supports the notion that the accumulation of L-methionine in ABA-treated radicles can be primarily attributed to the inhibition of SAM biosynthesis pathway. This observation led to the hypothesis that ABA inhibits Korean pine seed germination by preventing the biosynthesis of SAM, blocking methylation reactions in which SAM participates, or disrupting sulfur, ethylene, and polyamine biosynthesis. Our results resembled those of Pawłowski, who found that exogenously applied ABA down-regulates SAM synthetase and proposed that the negative regulation of ethylene synthesis caused by ABA-mediated processes is related to the inhibition of seed dormancy breaking in Norway maple (Pawłowski, 2009). A similar mechanism imposing an ABA requirement to inhibit germination was also reported in other plant species. For instance, a previous study on rice revealed that ABA treatment inhibited germination and reduced the abundance of proteins related to methionine metabolism (Liu et al., 2015). Mathur and Sachar (1991) reported that ABA significantly inhibited the increase in S-adenosylmethionine synthetase activity in dwarf pea (Pisum sativum). The position of the action of ABA is the epicotyls in the above research. It was also found that the proteins associated with s-adenosylmethionine synthetase accumulated in the hypocotyl during seed germination (Gimeno-Gilles et al., 2009). However, our metabolomics data suggest that ABA may inhibit the SAM metabolic pathway specifically in the radicle, rather than in the hypocotyl or cotyledon. The observed inconsistencies in these experiments could potentially be attributed to species differences, though this requires further confirmation through additional studies.
Several plant hormones, including ABA, ethylene, GA, auxin, cytokinins, and brassinosteroids play an important role in regulating seed germination (Miransari and Smith, 2014). ABA negatively regulates seed germination (Nambara et al., 2010), while the others induce it (Hermann et al., 2007). It is well known that ABA, GA, and all other isoprenoids compounds such as brassinolide and zeatin are produced from IPP (isopentenyl diphosphate), which is synthesized from mevalonic acid (Nagata et al., 2003; Pawłowski, 2009; Bajguz and Piotrowska-Niczyporuk, 2023). Mevalonate kinase catalyzes the phosphorylation of mevalonic acid to (R)-5-phosphomevalonate, which can then can converted to IPP by decarboxylation and isomerization (Senbagalakshmi et al., 2019). The data from our study revealed a 2.0-fold and 2.6-fold reduction, respectively, in the relative contents of mevalonic acid and (R)-5-phosphomevalonate under ABA-treatment. This was also accompanied by a 1.3-fold decrease in the endogenous relative contents of trans-zeatin. These observations imply that ABA may restrict the biosynthesis of certain plant hormones during germination of Korean pine seed. This notion was supported by the fact that the GA and ethylene signaling pathways in Arabidopsis seeds were suppressed by ABA (Ghassemian et al., 2000; Seo et al., 2006). Similarly, it has been observed that ABA treatment decreased GA levels in Panax notoginseng seeds (Wang et al., 2023). It has been reported that exogenous ABA treatment increased the content of endogenous ABA (Hu et al., 2010), and promoted the expression of key genes in ABA synthesis pathway and signal transduction pathway (Hu et al., 2010; Wang et al., 2023). However, it is not clear in this study whether exogenous ABA promotes or inhibits the ABA metabolic pathway, due to the lack of measurement of the expression levels of key genes in ABA synthesis, catabolism, and signal transduction pathways. But we supposed that exogenous ABA treatment may potentially affect ABA metabolism by decreasing the levels of precursors for the synthesis of ABA.
ROS, such as H2O2, O2−, hydroxyl radicals, and superoxide radicals, are unavoidable byproducts of aerobic metabolism (Yu et al., 2016). The regulation of seed germination by ROS is concentration-dependent, with relatively low levels of ROS enhancing seed germination by promoting ABA catabolism (Choudhury et al., 2017), whereas high levels of ROS inhibit seed germination by leading to oxidative damage of cell membranes (Liu et al., 2010; Suzuki et al., 2013). The establishment of the balance between ROS production and scavenging is very important to seed germination (Wu et al., 2020b). Seeds can regulate ROS contents through metabolism of ascorbate and glutathione (Tommasi et al., 2001). In glutathione metabolism, ROS could be scavenged by the oxidation of GSH (glutathione) to GSSG (oxidized glutathione) (Hao et al., 2021). In addition, GSH could also be converted to 5-L-glutamyl-L-alanine and L-pyroglutamic acid. L-pyroglutamic acid can be further metabolized to L-glutamate, which can then be used for the regeneration of glutathione. The significant increase in the levels of these metabolites in glutathione metabolism may accelerate the GSH-GSSG cycle, which is conducive to ROS scavenging. However, in this study, the GSH amount did not significantly change, but the relative contents of GSSG increased 1.3-fold in the presence of ABA. Moreover, 5-L-glutamyl-L-alanine and L-pyroglutamic acid were found to dramatically decline. These results indicate that GSH is mainly consumed in converting to its oxidation product GSSG, rather than entering the following metabolic pathway that leads to 5-L-glutamyl-L-alanine, L-pyroglutamic acid, and ultimately L-glutamate. Thus, we supposed that the disruption of GSH-GSSG function could result in a decline in ROS scavenging ability in the radicle of ABA-treated Korean pine seeds. It is reasonable to hypothesize that the radicle of the ABA-treated seeds may be subjected to oxidative stress. These results are in line with the previous study that found that ABA inhibited Arabidopsis thaliana seed germination by stimulating the accumulation of ROS (Chen et al., 2022; Gong et al., 2022). Similarly, a reduction of the glutathione S-transferase associated with glutathione metabolism was observed during ABA-inhibition of germination in Arabidopsis thaliana (Pawłowski, 2007). In addition to glutathione metabolism, shikimic acid has been reported to have antioxidant functions, thus improving seed germination capacity (El-Soud et al., 2013; Guo et al., 2021). The relative contents of shikimate and 3-dehydroshikimic acid were decreased more than 2-fold in the radicle of ABA-treated embryos, indicating that exogenous ABA treatment disrupts antioxidative activity. In addition, chorismate, the end product of the shikimate pathway, is an important precursor for the synthesis of salicylic acid (Mishra and Baek, 2021). As a hormone, salicylic acid can promote seed germination (Lee et al., 2010a). A significant change (1.69-fold decrease in radicle and 1.92-fold decrease in hypocotyl + cotyledon) in the relative levels of salicylic acid in ABA treatment further indicate that shikimate pathway plays an important role in Korean pine seed germination.
In radicle, in addition to above-mentioned metabolism, pyrimidine metabolism, phenylalanine metabolism and galactose metabolism were also found to be influenced by ABA treatment in Korean pine seeds. However, we were unable to identify whether these three metabolic pathways are downregulated due to the detection of fewer metabolites and the varying trends observed in the presence of ABA. But these metabolic pathways are still worthy of attention for future study on ABA inhibition of tree seed germination. The involvement of pyrimidine metabolism, phenylalanine metabolism and galactose metabolism in germination inhibition induced by ABA has been reported in numerous studies (Cornelius et al., 2011; Qi et al., 2022; Wang et al., 2023).
It was found that approximately 89% of the metabolites with VIP > 1 and P < 0.05 in hypocotyl + cotyledon were decreased under ABA treatment. These metabolites were majorly enriched in two metabolic pathways, including glycerophospholipid and glycolysis/gluconeogenesis. Furthermore, the metabolites that are involved in these two pathways were decreased under ABA treatment, suggesting that ABA might inhibit Korean pine seed germination by repressing glycerophospholipid metabolism and glycolysis/gluconeogenesis in hypocotyl + cotyledon (Figure 5).
The maintenance of cell membrane stability and integrity is critical for controlling regular cell metabolism and physiological processes (Ling et al., 2022). Glycerophospholipid is an important component of the cell membrane (Calzada et al., 2016). The metabolites of the glycerophospholipid pathway, such as phosphatidic acids (i.e., a basic class of phospholipids) and phosphatidylcholine (i.e., a structural lipid with a very high content in the membrane), probably maintain the stability of cell membranes (Furse and de Kroon, 2015; Colin and Jaillais, 2020; Felczak et al., 2022). It has been reported that the down-regulation of glycerophospholipid metabolism can significantly damage the stability and permeability of the cell membrane, leading to the leakage of intracellular inclusions (Wang et al., 2019). By contrast, the level of glycerophospholipid (1-palmitoyl-sn-glycero-3-phosphocholine) was significantly increased in the root system of Brassica napus in response to salt stress (Wang et al., 2022). Sun et al. (2023) found that phosphatidylcholine enhanced the resistance of peach seedlings to salt stress. After Korean pine seeds were incubated with ABA for 2 weeks, we observed a remarkably strong decrease in three glycerophospholipids, including phosphatidate, phosphatidylcholine, and 1-acyl-sn-glycero-3-phosphocholine. From these data, it would appear that ABA may damage the cell membrane system in the hypocotyl + cotyledon of Korean pine seeds, presumably through the downregulation of glycerophospholipid metabolism. Relatively few investigations have attempted to characterize the role of ABA-mediated glycerophospholipid metabolism in seed germination so far. However, most studies have focused on the involvement of phosphatidic acids in ABA signal pathways, influencing the germination process (Zhang et al., 2004; Ling et al., 2022).
Seed germination is an energy-consuming process that primarily relies on glycolysis, the pentose phosphate pathway, and the tricarboxylic acid cycle for energy production, given the absence of mineral absorption systems and photosynthetic apparatus during this stage (Bonora et al., 2012; Huang et al., 2021). In the hypocotyl + cotyledon of ABA-treated seeds, the relative contents of three metabolites involved in glycolysis/gluconeogenesis, namely alpha-D-glucose, phosphoenolpyruvate, and (S)-Lactate, were significantly reduced, suggesting a suppression of the glycolysis/gluconeogenesis pathway. Until now, most researchers have demonstrated that ABA inhibits seed germination by weaking four main respiratory metabolism pathways, yet they have paid insufficient attention to the specific site of ABA’s action within the embryo. Recently, an investigation showed that ABA limited the availability of glucose in the hypocotyl region of Arabidopsis thaliana seed, and thus led to the inhibition of seed germination (Xue et al., 2021). Our study also uncovered an attenuated glycolysis/gluconeogenesis in the hypocotyl + cotyledon, but not in the radicle, following ABA treatment. The disparity in respiratory metabolism pathways between the hypocotyl and cotyledon merits further exploration.
Korean pine seeds exhibit differential metabolic processes in response to ABA treatment between the radicle and the hypocotyl + cotyledon during seed germination. Taken together, the available literature and the present results suggest that ABA treatment may inhibit Korean pine seed germination by influencing ten metabolic pathways. These metabolic pathways included pyrimidine metabolism, phenylalanine metabolism, nicotinate and nicotinamide metabolism, cysteine and methionine metabolism, phenylalanine, tyrosine and tryptophan biosynthesis, terpenoid backbone biosynthesis galactose metabolism and glutathione metabolism in the radicle, as well as glycerophospholipid metabolism and glycolysis/gluconeogenesis in the hypocotyl + cotyledon. ABA may inhibit Korean pine seed germination primarily by disrupting the biosynthesis of certain plant hormones that is mediated by cysteine and methionine metabolism and terpenoid backbone biosynthesis, as well as reducing the ROS scavenging ability, which is regulated by glutathione metabolism and the shikimate pathway in the radicle. ABA may strongly destroy the structure and function of cellular membranes by affecting glycerophospholipid metabolism, and weaken glycolysis/gluconeogenesis in the hypocotyl + cotyledon, which are also major contributors to ABA-mediated inhibition of seed germination. This study has the potential to provide fundamental insights into the metabolic mechanisms that underlie the seed germination process of Korean pine, serving as a valuable reference for understanding seed dormancy in other trees exhibiting profound physiological dormancy.
The raw data supporting the conclusions of this article will be made available by the authors, without undue reservation.
YS: Funding acquisition, Methodology, Writing – original draft, Writing – review & editing. XL: Investigation, Writing – original draft. MZ: Data curation, Writing – original draft. CX: Writing – review & editing.
The author(s) declare financial support was received for the research, authorship, and/or publication of this article. This study was supported by the National Natural Science Foundation of China (No. 31901300) and Guizhou Provincial Science and Technology Projects (No. ZK(2024)ZD060).
We thank Chuanzhao Liu for his field support.
The authors declare that the research was conducted in the absence of any commercial or financial relationships that could be construed as a potential conflict of interest.
All claims expressed in this article are solely those of the authors and do not necessarily represent those of their affiliated organizations, or those of the publisher, the editors and the reviewers. Any product that may be evaluated in this article, or claim that may be made by its manufacturer, is not guaranteed or endorsed by the publisher.
The Supplementary Material for this article can be found online at: https://www.frontiersin.org/articles/10.3389/fpls.2024.1417632/full#supplementary-material
Bajguz, A., Piotrowska-Niczyporuk, A. (2023). Biosynthetic pathways of hormones in plants. Metabolites 13, 884. doi: 10.3390/metabo13080884
Bao, S., Wang, X., Yang, Y., Wu, X., Zhao, C., Fu, Y. (2020). Enrichment and purification technology of pinolenic acid from Pinus koraiensis seed oil. Chem. Ind. For. Prod. 40, 23–29. doi: 10.3969/j.issn.0253–2417.2020.06.004
Baskin, C. C., Baskin, J. M. (2014). Seeds: ecology, biogeography, and evolution of dormancy and germination. 2nd edn (San Diego: Elsevier/Academic Press).
Beaudoin, N., Serizet, C., Gosti, F., Giraudat, J. (2000). Interactions between abscisic acid and ethylene signaling cascades. Plant Cell. 12, 1103–1115. doi: 10.1105/tpc.12.7.1103
Bentsink, L., Koornneef, M. (2008). Seed dormancy and germination. Arabidopsis Book 6, e0119. doi: 10.1199/tab.0119
Bewley, J. D., Bradford, K. J., Hilhorst, H. W. M., Nonogaki, H. (2013). Seeds: Physiology of Development, Germination and Dormancy. 3rd Edn (New York, NY: Springer). doi: 10.1007/978-1-4614-4693-4
Bonora, M., Patergnani, S., Rimessi, A., De Marchi, E., Suski, J. M., Bononi, A., et al. (2012). ATP synthesis and storage. Purinerg. Signal 8, 343–357. doi: 10.1007/s11302-012-9305-8
Bouton, S., Viau, L., Lelièvre, E., Limami, A. M. (2005). A gene encoding a protein with a proline-rich domain (MtPPRD1), revealed by suppressive subtractive hybridization (SSH), is specifically expressed in the Medicago truncatula embryo axis during germination. J. Exp. Bot. 56, 825–832. doi: 10.1093/jxb/eri077
Calzada, E., Onguka, O., Claypool, S. M. (2016). Phosphatidylethanolamine metabolism in health and disease. Int. Rev. Cell Mol. Biol. 321, 29–88. doi: 10.1016/bs.ircmb.2015.10.001
Carrera-Castaño, G., Calleja-Cabrera, J., Pernas, M., Gómez, L., Oñate-Sánchez, L. (2020). An updated overview on the regulation of seed germination. Plants 9, 703. doi: 10.3390/plants9060703
Chen, Q., Wang, W., Zhang, Y., Zhan, Q., Liu, K., Botella, J. R., et al. (2022). Abscisic acid-induced cytoplasmic translocation of constitutive photomorphogenic 1 enhances reactive oxygen species accumulation through the HY5-ABI5 pathway to modulate seed germination. Plant Cell Environ. 45, 1474–1489. doi: 10.1111/pce.14298
Chen, L., Wu, J., Li, Z., Liu, Q., Zhao, X., Yang, H. (2019). Metabolomic analysis of energy regulated germination and sprouting of organic mung bean (Vigna radiata) using NMR spectroscopy. Food Chem. 286, 87–97. doi: 10.1016/j.foodchem.2019.01.183
Choudhury, F. K., Rivero, R. M., Blumwald, E., Mittler, R. (2017). Reactive oxygen species, abiotic stress and stress combination. Plant J. 90, 856–867. doi: 10.1111/tpj.13299
Colin, L. A., Jaillais, Y. (2020). Phospholipids across scales: lipid patterns and plant development. Curr. Opin. Plant Biol. 53, 1–9. doi: 10.1016/j.pbi.2019.08.007
Cornelius, S., Witz, S., Rolletschek, H., Möhlmann, T. (2011). Pyrimidine degradation influences germination seedling growth and production of Arabidopsis seeds. J. Exp. Bot. 62, 5623–5632. doi: 10.1093/jxb/err251
El-Soud, W. A., Hegab, M. M., AbdElgawad, H., Zinta, G., Asard, H. (2013). Ability of ellagic acid to alleviate osmotic stress on chickpea seedlings. Plant Physiol. Bioch. 71, 173–183. doi: 10.1016/j.plaphy.2013.07.007
Fan, W., Ge, G., Liu, Y., Wang, W., Liu, L., Jia, Y. (2018). Proteomics integrated with metabolomics: analysis of the internal causes of nutrient changes in alfalfa at different growth stages. BMC Plant Biol. 18, 1–15. doi: 10.1186/s12870-018-1291-8
Felczak, A., Zawadzka, K., Bernat, P., Nowak-Lange, M., Lisowska, K. (2022). Effect of quinoline on the phospholipid profile of Curvularia lunata and its microbial detoxification. Molecules 27, 2081. doi: 10.3390/molecules27072081
Finkelstein, R., Reeves, W., Ariizumi, T., Steber, C. (2008). Molecular aspects of seed dormancy. Ann. Rev. Plant Biol. 59, 387–415. doi: 10.1146/annurev.arplant.59.032607.092740
Furse, S., de Kroon, A. I. (2015). Phosphatidylcholine’s functions beyond that of a membrane brick. Mol. Membr. Biol. 32, 117–119. doi: 10.3109/09687688.2015.1066894
Gallardo, K., Le Signor, C., Vandekerckhove, J., Thompson, R. D., Burstin, J. (2003). Proteomics of Medicago truncatula seed development establishes the time frame of diverse metabolic processes related to reserve accumulation. Plant Physiol. 133, 664–682. doi: 10.1104/pp.103.025254
Garciarrubio, A., Legaria, J. P., Covarrubias, A. A. (1997). Abscisic acid inhibits germination of mature Arabidopsis seeds by limiting the availability of energy and nutrients. Planta 203, 182–187. doi: 10.1007/s004250050180
Ghassemian, M., Nambara, E., Cutler, S., Kawaide, H., Kamiya, Y., McCourt, P. (2000). Regulation of abscisic acid signaling by the ethylene response pathway in Arabidopsis. Plant Cell. 12, 1117–1126. doi: 10.1105/tpc.12.7.1117
Gimeno-Gilles, C., Lelièvre, E., Viau, L., Malik-Ghulam, M., Ricoult, C., Niebel, A., et al. (2009). ABA-mediated inhibition of germination is related to the inhibition of genes encoding cell-wall biosynthetic and architecture: modifying enzymes and structural proteins in Medicago truncatula embryo axis. Mol. Plant 2, 108–119. doi: 10.1093/mp/ssn092
Gong, C., Yin, X., Ye, T., Liu, X., Yu, M., Dong, T., et al. (2022). The F-Box/DUF295 Brassiceae specific 2 is involved in ABA-inhibited seed germination and seedling growth in Arabidopsis. Plant Sci. 323, 111369. doi: 10.1016/j.plantsci.2022.111369
Gorzolka, K., Kölling, J., Nattkemper, T. W., Niehaus, K. (2016). Spatio-temporal metabolite profiling of the barley germination process by MALDI MS imaging. PloS One 11, e0150208. doi: 10.1371/journal.pone.0150208
Gu, Z., Li, L., Tang, S., Liu, C., Fu, X., Shi, Z., et al. (2018). Metabolomics reveals that crossbred dairy buffaloes are more thermotolerant than Holstein cows under chronic heat stress. J. Agric. Food Chem. 66, 12889–12897. doi: 10.1021/acs.jafc.8b02862
Guo, Y., Gan, S. (2005). Leaf senescence: signals, execution, and regulation. Curr. Top. Dev. Biol. 71, 83–112. doi: 10.1016/S0070-2153(05)71003-6
Guo, H., Lyv, Y., Zheng, W., Yang, C., Li, Y., Wang, X., et al. (2021). Comparative metabolomics reveals two metabolic modules affecting seed germination in rice (Oryza sativa). Metabolites 11, 880. doi: 10.3390/metabo11120880
Hao, Y., Hong, Y., Guo, H., Qin, P., Huang, A., Yang, X., et al. (2022). Transcriptomic and metabolomic landscape of quinoa during seed germination. BMC Plant Biol. 22, 237–250. doi: 10.1186/s12870-022-03621-w
Hao, Y., Xu, S., Lyu, Z., Wang, H., Kong, L., Sun, S. (2021). Comparative analysis of the glutathione S-transferase gene family of four Triticeae species and transcriptome analysis of GST genes in common wheat responding to salt stress. Int. J. Genom. 2021, 6289174. doi: 10.1155/2021/6289174
Hermann, K., Meinhard, J., Dobrev, P., Linkies, A., Pesek, B., Heß, B., et al. (2007). 1-Aminocyclopropane-1-carboxylic acid and abscisic acid during the germination of sugar beet (Beta vulgaris L.): a comparative study of fruits and seeds. J. Exp. Bot. 58, 3047–3060. doi: 10.1093/jxb/erm162
Hu, B., Wan, X., Liu, X., Guo, D., Li, L. (2010). Abscisic acid (ABA)-mediated inhibition of seed germination involves a positive feedback regulation of ABA biosynthesis in Arachis hypogaea L. Afri. J. Biotechnol. 9, 1578–1586. doi: 10.5897/AJB10.1819
Huang, Y., Cai, S., Ye, L., Hu, H., Li, C., Zhang, G. (2016). The effects of GA and ABA treatments on metabolite profile of germinating barley. Food Chem. 192, 928–933. doi: 10.1016/j.foodchem.2015.07.090
Huang, Y., Lin, C., He, F., Li, Z., Guan, Y., Hu, Q., et al. (2017). Exogenous spermidine improves seed germination of sweet corn via involvement in phytohormone interactions, H2O2 and relevant gene expression. BMC Plant Biol. 17, 1. doi: 10.1186/s12870-016-0951-9
Huang, X., Tian, T., Chen, J., Wang, D., Tong, B., Liu, J. (2021). Transcriptome analysis of Cinnamomum migao seed germination in medicinal plants of Southwest China. BMC Plant Biol. 21, 270. doi: 10.1186/s12870-021-03020-7
Izydorczyk, C., Nguyen, T. N., Jo, S., Son, S., Tuan, P. A., Ayele, B. T. (2018). Spatiotemporal modulation of abscisic acid and gibberellin metabolism and signaling mediates the effect of suboptimal and supraoptimal temperatures on seed germination in wheat (Triticum aestivum L.). Plant Cell Environ. 41, 1022–1037. doi: 10.1111/pce.12949
Kazmi, R. H., Willems, L. A. J., Joosen, R. V. L., Khan, N., Ligterink, W., Hilhorst, H. W. M. (2017). Metabolomic analysis of tomato seed germination. Metabolomics 13, 145. doi: 10.1007/s11306-017-1284-x
Kim, S. T., Kang, S. Y., Wang, Y., Kim, S. G., Hwang, D. H., Kang, K. (2008). Analysis of embryonic proteome modulation by GA and ABA from germinating rice seeds. Proteomics 8, 3577–3587. doi: 10.1002/pmic.200800183
Lai, L., Zheng, G., Xing, H. (1989). Relationship between seed coat of Korean pine and its dormancy. Acta Bot. Sin. 31, 928–933.
Lee, S., Kim, S., Park, C. (2010a). Salicylic acid promotes seed germination under high salinity by modulating antioxidant activity in Arabidopsis. New Phytol. 188, 626–637. doi: 10.1111/j.1469-8137.2010.03378.x
Lee, K. P., Piskurewicz, U., Tureckova, V., Strnad, M., Lopez-Molina, L. (2010b). A seed coat bedding assay shows that RGL2-dependent release of abscisic acid by the endosperm controls embryo growth in Arabidopsis dormant seeds. PNAS 107, 19108–19113. doi: 10.1073/pnas.1012896107
Li, L., Li, L., Cui, S., Qian, D., Lyu, S., Liu, W., et al. (2023). PDC1 is activated by ABF4 and inhibits seed germination by promoting ROS accumulation in Arabidopsis. Environ. Exp. Bot. 206, 105188. doi: 10.1016/j.envexpbot.2022.105188
Ling, J., Xia, Y., Hu, J., Zhu, T., Wang, J., Zhang, H., et al. (2022). Integrated lipidomic and transcriptomic analysis reveals phospholipid changes in somatic embryos of Picea asperata in response to partial desiccation. Int. J. Mol. Sci. 23, 6494. doi: 10.3390/ijms23126494
Liu, S., Xu, H., Wang, W., Li, N., Wang, W., Møller, I. M., et al. (2015). A proteomic analysis of rice seed germination as affected by high temperature and ABA treatment. Physiol. Plantarum 154, 142–161. doi: 10.1111/ppl.12292
Liu, Y., Ye, N., Liu, R., Chen, M., Zhang, J. (2010). H2O2 mediates the regulation of ABA catabolism and GA biosynthesis in Arabidopsis seed dormancy and germination. J. Exp. Bot. 61, 2979–2990. doi: 10.1093/jxb/erq125
Lu, X., Zeng, W., Wang, L., Zhang, X. (2023). Transcriptomic insights into the effects of abscisic acid on the germination of Magnolia sieboldii K. Koch seed. Gene 853, 147066. doi: 10.1016/j.gene.2022.147066
Lu, Q., Zhang, Z., Zhan, R., He, R. (2018). Proteomic analysis of Zanthoxylum nitidum seeds dormancy release: influence of stratification and gibberellin. Ind. Crop Prod. 122, 7–15. doi: 10.1016/j.indcrop.2018.05.044
Manz, B., Müller, K., Kucera, B., Volke, F., Leubner-Metzger, G. (2005). Water uptake and distribution in germinating tobacco seeds investigated in vivo by nuclear magnetic resonance imaging. Plant Physiol. 138, 1538–1551. doi: 10.1104/pp.105.061663
Mathur, M., Sachar, R. C. (1991). Phytohormonal regulation of S-adenosylmethionine synthetase and S-adenosylmethionine levels in dwarf pea epicotyls. FEBS Lett. 287, 113–117. doi: 10.1016/0014-5793(91)80028-2
Miransari, M., Smith, D. L. (2014). Plant hormones and seed germination. Environ. Exp. Bot. 99, 110–121. doi: 10.1016/j.envexpbot.2013.11.005
Mishra, A. K., Baek, K. H. (2021). Salicylic acid biosynthesis and metabolism: a divergent pathway for plants and bacteria. Biomolecules 11, 705. doi: 10.3390/biom11050705
Miyazaki, J. H., Yang, S. (1987). The methionine salvage pathway in relation to ethylene and polyamine biosynthesis. Physiol. Plant 69, 366–370. doi: 10.1111/j.1399-3054.1987.tb04302.x
Nagata, N., Suzuki, M., Yoshida, S., Muranaka, T. (2003). “Action of mevalonic acid-derived isoprenoids in Arabidopsis,” in Advanced research on plant lipids. Eds. Murata, N., Yamada, M., Nishida, I., Okuyama, H., Sekiya, J., Hajime, W. (Springer, Dordrecht).
Nambara, E., Okamoto, M., Tatematsu, K., Yano, R., Seo, M., Kamiya, Y. (2010). Abscisic acid and the control of seed dormancy and germination. Seed Sci. Res. 20, 55–67. doi: 10.1017/S0960258510000012
Nonogaki, H., Barrero, J. M., Li, C. (2018). Seed dormancy, germination, and pre-harvest sprouting. Front. Plant Sci. 9. doi: 10.3389/fpls.2018.01783
Nonogaki, H., Bassel, G. W., Bewley, J. D. (2010). Germination-still a mystery. Plant Sci. 179, 574–581. doi: 10.1016/j.plantsci.2010.02.010
Pawłowski, T. A. (2007). Proteomics of European beech (Fagus sylvatica L.) seed dormancy breaking: influence of abscisic and gibberellic acids. Proteomics 7, 2246–2257. doi: 10.1002/pmic.200600912
Pawłowski, T. A. (2009). Proteome analysis of Norway maple (Acer platanoides L.) seeds dormancy breaking and germination: influence of abscisic and gibberellic acids. BMC Plant Biol. 9, 48–61. doi: 10.1186/1471-2229-9-48
Penfield, S., Li, Y., Gilday, A. D., Graham, S., Graham, I. A. (2006). Arabidopsis ABAINSENSITIVE4 regulates lipid mobilization in the embryo and reveals repression of seed germination by the endosperm. Plant Cell. 18, 1887–1899. doi: 10.1105/tpc.106.041277
Pi, E., Zhu, C., Fan, W., Huang, Y., Qu, L., Li, Y., et al. (2018). Quantitative phosphoproteomic and metabonomic analyses reveal GmMYB173 optimizes flavonoid metabolism in soybean under salt stress. Mol. Cell. Proteomics 17, 1209–1224. doi: 10.1074/mcp.RA117.000417
Pritchard, S. L., Charlton, W. L., Baker, A., Graham., I. A. (2002). Germination and storage reserve mobilization are regulated independently in Arabidopsis. Plant J. 31, 639–647. doi: 10.1046/j.1365-313X.2002.01376.x
Qi, K., Wu, X., Gao, X., Li, L., Sun, X., Xie, Z., et al. (2022). Metabolome and transcriptome analyses unravel the inhibition of embryo germination by abscisic acid in pear. Sci. Hortic 292, 110652. doi: 10.1016/j.scienta.2021.110652
Ravanel, S., Gakière, G., Job, D., Douce, R. (2008). The specific features of methionine biosynthesis and metabolism in plants. PNAS 95, 7805–7812. doi: 10.1073/pnas.95.13.7805
Senbagalakshmi, P., Muthukrishnan, S., Jebasingh, T., Senthil Kumar, T., Rao, M. V. (2019). Squalene, biosynthesis and its role in production of bioactive compounds, a Proper Scientific Challenge – A Review. J. Emerg. Technol. Innov. Res. 6, 505–526.
Seo, M., Hanada, A., Kuwahara, A., Endo, A., Okamoto, M., Yamauchi, Y., et al. (2006). Regulation of hormone metabolism in Arabidopsis seeds: phytochrome regulation of abscisic acid metabolism and abscisic acid regulation of gibberellin metabolism. Plant J. 48, 354–366. doi: 10.1111/j.1365-313X.2006.02881.x
Shen, H. (2002). Korean Pine as a nut production species in China-present situation and future development. Acta Hortic. 620, 187–191. doi: 10.17660/ActaHortic.2003.620.20
Shi, J., Wang, J., Wang, N., Zhou, H., Xu, Q., Yan, G. (2019). Overexpression of StGA2ox1 gene increases the tolerance to abiotic stress in transgenic potato (Solanum tuberosum L.) Plants. Appl. Biochem. Biotech. 187, 1204–1219. doi: 10.1007/s12010-018-2848-6
Shu, K., Liu, X., Xie, Q., He, Z. (2016). Two faces of one seed: Hormonal regulation of dormancy and germination. Mol. Plant 9, 34–45. doi: 10.1016/j.molp.2015.08.010
Silva, A. T., Ligterink, W., Hilhorst, H. W. M. (2017). Metabolite profiling and associated gene expression reveal two metabolic shifts during the seed-to-seedling transition in Arabidopsis thaliana. Plant Mol. Biol. 95, 481–496. doi: 10.1007/s11103-017-0665-x
Song, Y., Zhang, M., Guo, Y., Gao, X. (2023). Change in seed dormancy status controls seasonal timing of seed germination in Korean pine (Pinus koraiensis). J. Plant Ecol. 16, rtac067. doi: 10.1093/jpe/rtac067
Song, Y., Zhu, J. (2019). The roles of metabolic pathways in maintaining primary dormancy of Pinus koraiensis seeds. BMC Plant Biol. 19, 1–15. doi: 10.1186/s12870-019-2167-2
Song, Y., Zhu, J., Yan, Q., Wang, G. (2018). Korean pine seed: linking changes in dormancy to germination in the two years following dispersal. Forestry 91, 98–109. doi: 10.1093/forestry/cpx037
Sun, M., Liu, X., Zhang, B., Yu, W., Xiao, Y., Peng, F. (2023). Lipid metabolomic and transcriptomic analyses reveal that phosphatidylcholine enhanced the resistance of peach seedlings to salt stress through phosphatidic acid. J. Agric. Food Chem. 71, 8846–8858. doi: 10.1021/acs.jafc.3c01383
Suzuki, N., Miller, G., Salazar, C., Mondal, H. A., Shulaev, E., Cortes, D. F., et al. (2013). Temporal-spatial interaction between reactive oxygen species and abscisic acid regulates rapid systemic acclimation in plant. Plant Cell. 25, 3553–3569. doi: 10.1105/tpc.113.114595
Takahashi, H., Kopriva, S., Giordano, M., Saito, K., Hell, R. (2011). Sulfur assimilation in photosynthetic organisms: molecular functions and regulations of transporters and assimilatory enzymes. Annu. Rev. Plant Biol. 62, 157–184. doi: 10.1146/annurev-arplant-042110-103921
Tommasi, F., Paciolla, C., de Pinto, M. C., De Gara, L. (2001). A comparative study of glutathione and ascorbate metabolism during germination of Pinus pinea L. seeds. J. Exp. Bot. 52, 1647–1654. doi: 10.1093/jexbot/52.361.1647
Wang, Y., Hou, Y., Qiu, J., Wang, H., Wang, S., Tang, L., et al. (2020). Abscisic acid promotes jasmonic acid biosynthesis via a ‘SAPK10-bZIP72-AOC’ pathway to synergistically inhibit seed germination in rice (Oryza sativa). New Phytol. 228, 1336–1353. doi: 10.1111/nph.16774
Wang, Y., Ji, D., Chen, T., Li, B., Zhang, Z., Qin, G., et al. (2019). Production, signaling, and scavenging mechanisms of reactive oxygen species in fruit–pathogen interactions. Int. J. Mol. Sci. 20, 2994. doi: 10.3390/ijms20122994
Wang, W., Pang, J., Zhang, F., Sun, L., Yang, L., Siddique, K. H. (2022). Transcriptomic and metabolomics-based analysis of key biological pathways reveals the role of lipid metabolism in response to salt stress in the root system of Brassica napus. Plant Growth Regul. 97, 127–141. doi: 10.1007/s10725-021-00788-4
Wang, Q., Yang, L., Ge, N., Jia, J., Huang, R., Chen, C., et al. (2023). Exogenous abscisic acid prolongs the dormancy of recalcitrant seed of Panax notoginseng. Front. Plant Sci. 14. doi: 10.3389/fpls.2023.1054736
Wang, X., Yang, B., Zhang, A., Sun, H., Yan, G. (2012). Potential drug targets on insomnia and intervention effects of Jujuboside a through metabolic pathway analysis as revealed by UPLC/ESI-SYNAPT-HDMS coupled with pattern recognition approach. J. Proteome. 75, 1411–1427. doi: 10.1016/j.jprot.2011.11.011
Weitbrecht, K., Müller, K., Leubner-Metzger, G. (2011). First off the mark: early seed germination. J. Exp. Bot. 62, 3289–3309. doi: 10.1093/jxb/err030
Wu, X., Shen, S., Han, S., Wu, H., Kong, L. (1996). The processing of ready-to-eat and fresh of Korean pine seeds. For. By-prod. Speciality China 2, 29–30. doi: 10.13268/j.cnki.fbsic.1996.02.016
Wu, X., Song, H., Guan, C., Zhang, Z. (2020a). Boron alleviates cadmium toxicity in Brassica napus by promoting the chelation of cadmium onto the root cell wall components. Sci. Total Environ. 728, 138833. doi: 10.1016/j.scitotenv.2020.138833
Wu, J., Zhang, N., Liu, Z., Liu, S., Liu, C., Lin, J., et al. (2020b). The AtGSTU7 gene influences glutathione-dependent seed germination under ABA and osmotic stress in Arabidopsis. Biochem. Bioph. Res. Co. 528, 538–544. doi: 10.1016/j.bbrc.2020.05.153
Xu, X., Mao, W., Liu, G., He, J., Zhang, Z., Zhang, T. (2014). Nutritional composition of pine nuts and physicochemical properties, physiological active components of pine nut oils. Acta Nutrimenta Sin. 36, 99–101. doi: 10.13325/j.cnki.acta.nutr.sin.2014.01.025
Xue, X., Yu, Y., Wu, Y., Xue, H., Chen, L. (2021). Locally restricted glucose availability in the embryonic hypocotyl determines seed germination under ABA treatment. New Phytol. 231, 1832–1844. doi: 10.1111/nph.17513
Yang, N., Guo, X., Wu, Y., Hu, X., Ma, Y., Zhang, Y., et al. (2018). The inhibited seed germination by ABA and MeJA is associated with the disturbance of reserve utilizations in Astragalus membranaceus. J. Plant Interact. 13, 388–397. doi: 10.1080/17429145.2018.1483034
Yu, Y., Zhen, S., Wang, S., Wang, Y., Cao, H., Zhang, Y., et al. (2016). Comparative transcriptome analysis of wheat embryo and endosperm responses to ABA and H2O2 stresses during seed germination. BMC Genomics 17, 97. doi: 10.1186/s12864-016-2416-9
Zhang, W., Qin, C., Zhao, J., Wang, X. (2004). Phospholipase Dα1-derived phosphatidic acid interacts with ABI1 phosphatase 2C and regulates abscisic acid signaling. PNAS 101, 9508–9513. doi: 10.1073/pnas.0402112101
Keywords: seed germination, abscisic acid, metabolism, radicle, hypocotyl, cotyledon
Citation: Song Y, Li X, Zhang M and Xiong C (2024) Spatial specificity of metabolism regulation of abscisic acid-imposed seed germination inhibition in Korean pine (Pinus koraiensis sieb et zucc). Front. Plant Sci. 15:1417632. doi: 10.3389/fpls.2024.1417632
Received: 15 April 2024; Accepted: 07 June 2024;
Published: 20 June 2024.
Edited by:
Magda Pál, HUN-REN Centre for Agricultural Research (HUN-REN CAR), HungaryReviewed by:
Sylva Prerostova, Academy of Sciences of the Czech Republic, CzechiaCopyright © 2024 Song, Li, Zhang and Xiong. This is an open-access article distributed under the terms of the Creative Commons Attribution License (CC BY). The use, distribution or reproduction in other forums is permitted, provided the original author(s) and the copyright owner(s) are credited and that the original publication in this journal is cited, in accordance with accepted academic practice. No use, distribution or reproduction is permitted which does not comply with these terms.
*Correspondence: Yuan Song, c29uZ3l1YW5faW4yMDAwQHllYWgubmV0
Disclaimer: All claims expressed in this article are solely those of the authors and do not necessarily represent those of their affiliated organizations, or those of the publisher, the editors and the reviewers. Any product that may be evaluated in this article or claim that may be made by its manufacturer is not guaranteed or endorsed by the publisher.
Research integrity at Frontiers
Learn more about the work of our research integrity team to safeguard the quality of each article we publish.