- College of Horticulture, South China Agricultural University, Guangzhou, China
Sustaining crop production and food security are threatened by a burgeoning world population and adverse environmental conditions. Traditional breeding methods for vegetable crops are time-consuming, laborious, and untargeted, often taking several years to develop new and improved varieties. The challenges faced by a long breeding cycle need to be overcome. The speed breeding (SB) approach is broadly employed in crop breeding, which greatly shortens breeding cycles and facilities plant growth to obtain new, better-adapted crop varieties as quickly as possible. Potential opportunities are offered by SB in plant factories, where optimal photoperiod, light quality, light intensity, temperature, CO2 concentration, and nutrients are precisely manipulated to enhance the growth of horticultural vegetable crops, holding promise to surmount the long-standing problem of lengthy crop breeding cycles. Additionally, integrated with other breeding technologies, such as genome editing, genomic selection, and high-throughput genotyping, SB in plant factories has emerged as a smart and promising platform to hasten generation turnover and enhance the efficiency of breeding in vegetable crops. This review considers the pivotal opportunities and challenges of SB in plant factories, aiming to accelerate plant generation turnover and improve vegetable crops with precision and efficiency.
1 Introduction
The current trajectory for crop productivity needs to produce 60% more food to sustainably nourish a burgeoning world population of 10 billion by 2050; therefore, there is an urgent need for higher-yielding varieties and accelerating crop production (Pardey et al., 2014). The ever-increasing human population, urbanization, declining agricultural lands, the rising frequency of destructive weather events, and the high incidence of pests and diseases pose grim threats to global food security (Shrivastava and Kumar, 2015; Alotaibi, 2023). In particular, during the coronavirus disease 2019 (COVID-19) pandemic era, improving crop productivity to alleviate food scarcity problems and ensuring food and nutritional security through modern breeding technologies are crucial for plant breeders and scientists.
2 The demand for faster vegetable crop breeding
The length of the breeding cycle is a major issue for crop genetic improvement (Saxena et al., 2017; Wanga et al., 2021). The development of genetically stable homozygous or novel cultivars with market-preferred traits of crops is time-consuming, which generally takes up to a decade or more via conventional breeding methodologies. Hence, shortening crop breeding cycles through promoting rapid plant growth and early flowering has greatly attracted the attention of plant breeders and researchers worldwide for crop production and productivity improvement (Watson et al., 2018; Bhatta et al., 2021; Guo et al., 2022; Liu et al., 2022). Efficient strategies are being employed to greatly reduce generation time including shuttle breeding, doubled haploid, targeting-induced local lesions in genomes (TILLING), and genome editing technologies (Table 1). However, these molecular and conventional breeding approaches cannot satisfy the ever-increasing demands for agricultural production and more available breeding approaches need to be explored.
In recent years, a system called speed breeding (SB) has been in the spotlight (Watson et al., 2018). It allows plant breeders to drastically shorten crop life cycles to produce more generations per year by optimized photoperiod, light quality, light intensity, day/night temperature and humidity, and CO2 concentration under controlled environments. This approach allows multiple generations of wheat, barley, rice, and legumes to be produced within a single year, significantly shortening the development cycles (Watson et al., 2018). Vegetable crops are integral to dietary diversity, nutritional sustenance, and economic development. While significant progress has been achieved in vegetable breeding, there remains a pressing need to expedite the development of green, environmentally resilient cultivars characterized by high yield and quality to meet the demands of the global population in a timely manner. Previous extensive reviews have summarized the methods, applications, potential advantages, and key challenges of SB techniques that can enhance crop quality and yield to meet the growing demand for food and ensure global food security. Certain reviews also focused on exploring the integration of SB with new breeding techniques (AI in crop breeding, genomics-assisted breeding, haplotype-based breeding, etc.) to efficiently and rapidly produce stable lines for both basic and applied research purposes. The current review mainly discusses the key technologies used in plant factories for efficient vegetable breeding and highlights the potential advantages of SB in plant factories as a smart and promising platform to hasten generation turnover.
3 Speed breeding technology utilizing plant factories
Plant factories are considered as multilayer closed plant production systems in which light, temperature, humidity, CO2, and nutrients are precisely manipulated through various sensors and artificial intelligence systems, allowing the precise control of physiological and developmental processes in plants (Kozai and Niu, 2015a) (Figure 1A). Crop production in plant factories can overcome many limitations of pathogens, pests, soil conditions, or climate change, allowing year-round cultivation at any location around the globe (Figure 1B). SB in plant factories paves a way to access four or more generations per year by providing optimal light and temperature, accelerating biological processes for rapid generation advancement (Ghosh et al., 2018; Watson et al., 2018). The shift from the vegetative to the flowering stage is one of the most crucial developmental milestones in the plant life cycle. Accelerating plant growth and development and promoting early flowering in plant factories, where various environmental factors, namely, photoperiod, temperature, and light quality and intensity, were regulated precisely and efficiently, are conducive to shortening the breeding cycles of vegetable crops Ghosh et al., 2018; Watson et al., 2018. Vegetables exhibiting short growth cycles and sensitivity to environmental cues are ideal candidates for SB, such as tomato, cucumbers, hot pepper, cabbage, spinach, and mustard greens. Their suitability for this method lies in their swift life cycles, enabling rapid generational turnover and efficient assessment of breeding characteristics. Furthermore, their adaptability to controlled environments permits precise adjustment of factors such as light, temperature, and humidity, facilitating accelerated vegetable growth rates.
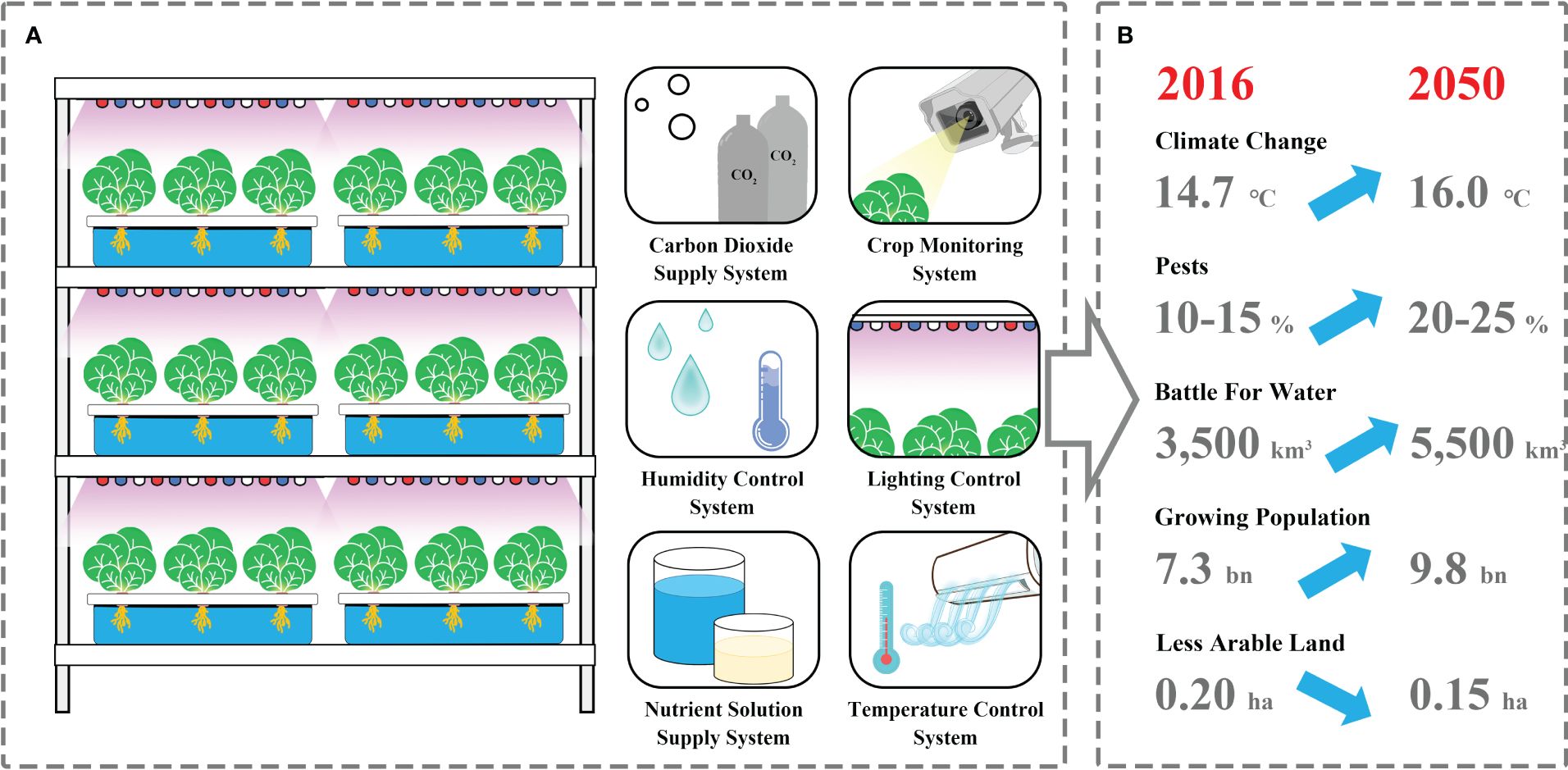
Figure 1 The primary structural components of a plant factory (A) are advantageous for coping with adverse factors (B) while simultaneously fulfilling the requirements for rapid crop breeding.
4 Opportunities for vegetable crops speed breeding in plant factories
4.1 Photoperiod
Photoperiod is a pivotal component of light environmental factors that has a prominent influence on vegetative and phenological development and physiological reactions. The effect of photoperiod on flowering time and seeding of plants varies on crop genotype or cultivar. Plants are classified into long-day, short-day, or day-neutral plants based on their sensitivity response to photoperiod (Jackson, 2009). For long-day plants, the time of flowering was frequently accelerated with a prolonged photoperiod, while in short-day plants, flowering is triggered when photoperiod becomes shorter than their critical photoperiod, or night time becomes longer than the critical night period (Jackson, 2009).
SB is an approach relying on prolonging the photoperiod, that is, providing a longer duration of lighted period to hasten plant growth and accelerate the plant life cycle (Watson et al., 2018). Efforts toward SB of vegetable crops mainly focus on accelerating the transition from vegetative to reproductive development. Optimum photoperiod is critical and responsible for vegetative and reproductive growth. Thus, manipulation of the photoperiod regime has been well applied in many plant species to stimulate early flowering and seeding and shorten breeding cycles, such as in wheat, canola, and hot pepper (Table 2). For long-day plants, although the days of flowering seem to decrease linearly with prolonging the photoperiod, some stress and damage such as foliar chlorosis, leaf burn, flower abortion, wilting, and mortality, caused by an artificially longer photoperiod, should not be neglected (Takahashi and Badger, 2011; Omar, 2020; Mitache et al., 2023). Short-day plants initiate flowering when the photoperiod is shorter than a critical day length, but to date, the sensitive period of short-day crops to flower is less unknown. Therefore, the optimum light/dark ratio that accelerates the plant growth of various stress-free crops still needs more exploration.
4.2 Light quality
Light quality, as another critical signal besides photoperiod, has broad effects on plant growth and developmental processes, including seed germination, photomorphogenesis, and flowering (Cerdán and Chory, 2003; Kami et al., 2010; Jones, 2018; Paradiso and Proietti, 2022). Rapid advances in LEDs make it possible to obtain vegetal material with desired traits through the application of specific light wavelengths and accelerate flowering and seeding to advance to the next breeding generation as quickly as possible (Brazaityte et al., 2016; Al Murad et al., 2021). Using optimal light quality could accelerate photosynthesis and flowering and shorten the length of the breeding cycle (Table 3).
Red and blue LEDs have been widely applied in horticulture, since these two spectral regions are regarded as particularly significant to photosynthetic CO2 assimilation (Chory, 2010). Both blue and red light are involved in the regulation of different processes through the plant life cycle (including seed germination and vegetative and reproductive growth). Blue light is a predominant signal for flower initiation; it can promote floral induction and flowering by regulating the level of hormones and gene expression associated with flowering (Yoshida et al., 2016; He et al., 2022; Wang et al., 2022; Nie et al., 2023). Inversely, red light has been recorded to promote both vegetative and reproductive growth but hinder flowering (Fukuda et al., 2016; Wang et al., 2022). However, it should be noted that flowering responses to blue and red light need to be further explored in various crop species since the research results often contain contradictory information to date (Giliberto et al., 2005; Monostori et al., 2018; Zhang et al., 2020; He et al., 2022).
Acceleration of flowering was well identified in many crops such as Arabidopsis thaliana, Hordeum vulgare, Petunia hybrida, and Zinnia elegans, in response to low R:FR ratios (Demotes-Mainard et al., 2016). In general, an approximate R:FR of 1.2, which occurred under natural sunlight, is suitable for plant growth and flowering (Holmes and Smith, 1975). However, the thresholds of R:FR ratio regarding flowering time vary among plant species and varieties, as well as growing conditions. For example, thresholds of R:FR ratio that promote or delay flowering were 3.5 and 5.3 in Eustoma grandiflora and lupin, respectively (Yamada et al., 2009; Croser et al., 2016). FR was beneficial for plants to facilitate light interception and tremendously accelerate plant growth and development responsible for the wide application in a controlled environment to induce early flowering and shorten breeding cycles (Liu et al., 2022; Song et al., 2022; Li et al., 2023). Besides R and FR light, blue light, in specific conditions, and some other light (i.e., green and ultraviolet-A) are also constructive for regulating the flowering of a few species such as petunia, tomato, and Chinese kale (Smith et al., 2017; Verdaguer et al., 2017; Kim and Hwang, 2019; Gao et al., 2022). To facilitate the optimal utilization of LEDs in the realm of horticultural crop production within plant factories, a comprehensive comprehension of the effects of light wavelengths on plant performance, encompassing the underlying mechanisms and temporal aspects, becomes imperative.
4.3 Light intensity
To date, a considerable number of studies focus on clarifying the impact of light photoperiod and light quality on plants, while those that target plant responses to light intensity are relatively few, even though plants have developed a sophisticated mechanism to alter their morphological features in response to varying intensity. Generally, under the light saturation point, increasing light intensity and the photosynthesis rate, measured as photosynthetic oxygen evolution, increases linearly with the light, because in this phase, light is the limiting factor (Haliapas et al., 2008; Formighieri, 2015; Oh, 2015). Higher light intensity provides more photosynthetic energy required for the synthesis of flowering-promoting substances, which is conducive to the formation and growth of flower primordium (Wimalasekera, 2019). Thus, reasonable light intensity can promote photosynthesis, allowing more light assimilation substances to be distributed to seeds or fruits, leading to rapid growth, higher biomass, and greater seed production (Table 4). Advancements in LED technologies have significantly contributed to achieving optimal light intensity for facilitating plant growth, thereby expediting plant life cycles and enhancing the completion of multiple generations within a shorter time frame (Jähne et al., 2020; Liu et al., 2022). Notably, the augmentation of light intensity, concomitant with escalated energy expenditures, manifests only marginal alterations, necessitating a comprehensive evaluation of the balance between advantages and associated costs.
4.4 Temperature
Apart from the light environment, temperature is particularly important to manipulate plant reproductive development and accelerate plant flowering. Each species has a specific temperature regime (maximum and minimum temperatures) for growth and development. The temperature range conducive to the germination of most crops typically falls within 12°C to 30°C, while the optimal temperature range for growth, flowering, and seed setting is generally observed between 25°C and 30°C (Hatfield and Prueger, 2015). Within the temperature range that does not cause heat stress to plants, higher temperature might facilitate vegetative growth while lower temperature is more suitable for grain production in the reproductive stages (Hickey et al., 2019). Optimum temperature is beneficial to accelerate photosynthesis and flowering, coupled with early seed harvest to shorten the breeding cycle, such as the SB approach applied in peanut, chickpea, lupin, lentil, pea, soybean, and faba bean (O’Connor et al., 2013; Croser et al., 2016) (Table 5). Notably, some crops must undergo a period of low temperature to transition from vegetative growth to reproductive growth, that is, vernalization. For instance, to achieve typical flower initiation and formation and accelerate plant growth, winter wheat requires long exposure to low temperatures (Cha et al., 2022). Hence, overcoming the rapid vernalization of winter crops stands as a pivotal technological advancement for expediting breeding processes in plant factories.
4.5 CO2 concentrations
Carbon dioxide (CO2) serves as the principal substrate for both photosynthesis and photorespiration in higher plants, thereby playing a pivotal role as a predominant greenhouse gas that regulates crop growth and development. Plant growth and flowering exhibit sensitivity to varying CO2 levels, whose response depends on the species and environmental conditions (McGrath and Lobell, 2013). Overall, elevated concentrations of CO2 have generally facilitated expedited plant growth and hastened the transition from vegetative to reproductive stages across most species (Jagadish et al., 2016). This effect is particularly pronounced among C3 plants, which exhibit greater sensitivity to heightened CO2 levels compared to C4 plants. Supplemental CO2 or the remaining higher CO2 concentration in growth chambers contributes to the reduction in the days to flowering of rice and Arabidopsis (Ohnishi et al., 2011; Li et al., 2014; Tanaka et al., 2016) (Table 6). Regulation of CO2 level often combines with other optimal light, temperature, and innovative breeding technologies (i.e., embryo rescue, biotron breeding system, and using immature seeds) to shorten the breeding cycle length in the controlled environment (Tanaka et al., 2016; Nagatoshi and Fujita, 2019).
4.6 Density of plant populations
Planting density is an important agronomic management, and optimization of density will determine the productivity and economic returns of plant factory with artificial lighting (PFAL). Higher planting density promotes shoot positioned upward and leads to the elongation of stems and leaves to outcompete its neighbors for enough sunlight, ultimately accelerating the transition from a vegetative stage to a reproductive stage and triggering early flowering and maturation (Warnasooriya and Brutnell, 2014). Additionally, higher cultivation density provides more sufficient leaf area to absorb sunlight efficiently or improve symbiotic nitrogen fixation by root nodules, thus benefiting early flowering or improving grain yield (Abubaker, 2008; Makoi et al., 2009; Biosci et al., 2013; Rahmanet, 2019; Asemanrafat and Honar, 2023) (Table 7). In contrast, the greater availability of fertilizers per plant due to low plant density results in more vigorous vegetative growth and late flowering (Abubaker, 2008). However, biomass increase was not linear with the planting density. Excessive planting density results in an intra-specific competition for space, nutrients, and light, causing the source–sink ratio to be not reasonable and ultimately hinder plant growth and development (Khan et al., 2017).
4.7 Nutrition and hormones
Flowering is an important phase in the life cycle of plants, which is profoundly impacted by numerous exogenous and endogenous factors. Five different pathways, namely, photoperiod, vernalization, gibberellin acids (GAs), autonomous, and endogenous pathways, have been reported to regulate flowering time (Mouradov et al., 2002; Blümel et al., 2015). Five major types of plant hormones, namely, auxin, gibberellin, cytokinin, ethylene, and abscisic acid, are important to induce flowering and seeding as well as germination of immature seed in vitro working together or independently (Bermejo et al., 2016). Exogenous application of GAs accelerated flowering in wild-type Arabidopsis (Blázquez et al., 2015). Additionally, cytokinins (5.7 μM) in conjunction with auxin (2.3 μM) led to the highest rate of flowering (100%) and seeding (91%) with the shortest flowering time (32 d) in lentil and faba bean; this combination promoted plant flowering and seeding rates by 4.9 d in advance (Mobini et al., 2015). Moreover, applying 10–5 M of 6-benzylaminopurine (BAP) accelerated flowering in faba bean, with the generation time reduced by 20–24 days (Mobini et al., 2020). Despite the widespread demonstration of phytohormone-mediated flowering regulation potential, plant hormone application in breeding is constrained by several limitations. On the one hand, plant hormones exhibit intricate and extensive regulatory effects on growth and development, potentially leading to unanticipated physiological and morphological changes during the regulation of flowering. This complexity poses challenges for the precise control of hormone-mediated regulation in breeding and may result in unforeseen side effects. On the other hand, phytohormone-mediated flowering regulation is heavily influenced by environmental factors such as light, temperature, and water availability, leading to inconsistent effects in varying growth environments and limiting its stability and reliability in breeding practice. Additionally, the impact of hormones on genetic stability and safety further discourages their widespread use in breeding practices.
Among essential nutrients, nitrogen (N), phosphorus (P), and potassium (K) are the most prominent nutrients required in greater amounts for the proper growth and development of crops (Ye et al., 2019). Nitrogen (N) as a most abundant essential nutrient plays critical roles in the process of flowering. The influence of N on flowering time greatly varies with different N forms and concentrations. In general, flowering is often accelerated by low N concentration, because limited N concentration enhances the export rate of assimilates from vegetative tissues to grains during the post-anthesis period. In contrast, excessive ammonium N supply delayed flowering by hindering N transport in the phloem at the flowering stage and decreased the efficiency of N remobilization (Marín et al., 2011; Zhang et al., 2021). A hypothetical U-shaped flowering curve was proposed, in that an appropriate concentration of nitrate promotes flowering, while both limited and excessive nitrate supply result in late flowering (Lin and Tsay, 2017). Phosphorus (P) fertilizer is a crucial nutrient for normal plant growth, development and yield, which is taken up by plant roots as inorganic phosphate (Pi) transporters (Muchhal et al., 1996). The flowering of rice and Arabidopsis grown under P deficiency conditions was prolonged (Kant et al., 2011; Jiaying et al., 2022). A longer time to flower was shown in low P levels, which might have contributed to the low gibberellin levels, where P level was positively correlated with gibberellin levels (Jiang et al., 2007). Notably, an antagonistic crosstalk was observed on the flowering between NO3− and Pi, in which low NO3− caused earlier flowering while low Pi postponed flowering time in Arabidopsis (Linkohr et al., 2002; Kant et al., 2011). Otherwise, the application of K fertilization led to flowering 1–3 days earlier in rice, and flowering time was faster as the application rate of K fertilizer increased (Ye et al., 2019). Thus, a robust and intelligent water and fertilizer control system needs to be created based on the demand for water and fertilizer for horticultural crops’ SB in a plant factory.
5 Challenges and perspectives
Remarkable breeding approaches in the last few decades have allowed researchers to shorten the breeding cycles, but they were limited in scale and cost and insufficient to cope with the ever-increasing population and radical changes under adverse environmental conditions. Integrating SB in plant factories with biology, information science, and breeding science to accelerate rapid growth, development, flowering, and seeding and establishing a rapid crop breeding technology system are a promising option to shorten the breeding cycle. However, every coin has two sides; thus, SB in plant factories has its advantages and limitations.
5.1 Challenges of speed breeding in plant factories
Tremendous advancements have been achieved in smart breeding recently, which is driven by big data, artificial intelligence, and integrated genomic–environmental prediction, but considerable challenges remain to be addressed for SB in plant factories. Firstly, the high cost of energy and considerable investment in setup and operation are major drawbacks of an artificially lighted plant factory. The electricity cost typically accounts for 25% of the total production cost (Kozai and Niu, 2015b), with the lighting, air conditioning, and other information control system depending on electricity. Advances in LED technology have provided more efficient power usage and reduced heat than other lighting types. Investing in solar panels is a good strategy to offset the erratic electricity supply in SB in plant factories.
Secondly, there is a lack of rapid vernalization techniques for long-day crops, and determining the short photoperiod for short-day crops remains challenging. Therefore, longer time needs to be taken to obtain the optimal environmental conditions (light intensity, light quality, temperature, etc.) for the rapid generation advancement of target crops.
The expression of the same phenotypes in both controlled environments and field conditions because of genotype × environment interactions is considered a challenge in SB. Thus, smart breeding driven by big data, artificial intelligence, and integrated genomic–enviromic prediction is an area that requires further enhancement in the future. Although phenotyping technology and platform have been widely applied in controlled environments for crop genetic improvement and breeding, compared with genomics, transcriptomics, and proteomics, phenomics is lagging behind. At present, a lack of in vivo dynamic detection technology for crop phenotypes, a poor ability to obtain high-throughput crop phenotypes, and the unclear relationship between genotype, phenotype, and environmental metrics limit the acquisition and analysis of crop phenotypes in plant factories. Therefore, with the targeted goal of horticultural crop breeding, a collaborative breeding framework of various branches of science is paramount to speed up breeding to accelerate crop improvement for a rapidly expanding global population.
5.2 Smart breeding: speed breeding in plant factories integrates with innovative breeding technologies
In the short-term or long-term future, SB in plant factories where the light environment (light quality, light intensity, and photoperiod), temperature, and nutrient are modulated accurately and efficiently holds promise to relieve the long-standing problem of lengthy crop breeding cycles. Notably, processes as diverse as seed germination, flower bud differentiation, flowering and fruiting, and seed development are closely regulated by light, temperature, phytohormone, and nutrition, and accelerating the above process is conducive to shortening the growth cycle of crops using the conditions provided by the smart system in plant factories.
Interdisciplinary strategies consisting of plant breeding, genome sequencing, genomic selection (GS), and CRISPR-based genome editing help plant breeders improve existing crops and develop new crops with good agronomic traits by minimizing time, cost, and space and maximizing resource efficiency. SB in plant factories is a promising approach that allows breeders to rapidly develop lines with the desired trait by advancing generations quickly within a controlled environment. Numerous steps are required for SB to effectively work in plant factories. Optimization of light formula and nutrient solution formula for species specificity and the establishment of rapid generation advancement technology within plant factories were the cornerstone for SB in plant factories. Moreover, overcoming physiological seed dormancy, combined with early seed harvest, is critical to reduce the length of the breeding cycle.
Phenotypic performance or target trait observation was the first step in plant breeding, and identifying desirable traits for selective breeding has become the crucial problem restricting crop breeding owing to the presence of masking. Recent advances in in vivo imaging, hyperspectral imaging, and graphic and spectral analysis technology, which dramatically help to measure crop phenotypic traits rapidly and nondestructively in controlled environments, make high-throughput phenotyping a powerful approach to solving future crop breeding problems.
Overall, the integration of innovative breeding technologies such as embryo rescue and immature seed harvesting, TILLING, gene editing, high-throughput phenotyping and genotyping, genomic selection, marker-assisted selection (MAS), machine learning, deep learning, and omics technologies to form a smart breeding platform offers an attractive opportunity to promote the transformation of breeding technology from the traditional “experience breeding” to a directional and more efficient “precision breeding” (Figure 2).
Author contributions
RH: Conceptualization, Methodology, Writing – original draft, Writing – review & editing. JJ: Conceptualization, Data curation, Validation, Writing – original draft. KL: Conceptualization, Methodology, Writing – original draft. JS: Conceptualization, Supervision, Validation, Writing – review & editing. XL: Conceptualization, Methodology, Writing – original draft. YL: Conceptualization, Methodology, Writing – original draft. SZ: Data curation, Investigation, Methodology, Writing – original draft. MZ: Conceptualization, Methodology, Writing – review & editing. YH: Conceptualization, Methodology, Writing – original draft. HL: Conceptualization, Funding acquisition, Investigation, Methodology, Project administration, Resources, Supervision, Writing – review & editing.
Funding
The author(s) declare financial support was received for the research, authorship, and/or publication of this article. This research is supported by a grant from the Key Research and Development Program of Ningxia (2021BBF02024) and the National Key Research and Development Program of China (2017YFE0131000).
Conflict of interest
The authors declare that the research was conducted in the absence of any commercial or financial relationships that could be construed as a potential conflict of interest.
Publisher’s note
All claims expressed in this article are solely those of the authors and do not necessarily represent those of their affiliated organizations, or those of the publisher, the editors and the reviewers. Any product that may be evaluated in this article, or claim that may be made by its manufacturer, is not guaranteed or endorsed by the publisher.
References
Abubaker, S. (2008). Effect of plant density on flowering date, yield and quality attribute of bush beans (Phaseolus Vulgaris L.) under center pivot iirrigation system. Am. J. Agric. Biol. Sci. 3, 666–668. doi: 10.3844/ajabssp.2008.666.668
Al Murad, M., Razi, K., Jeong, B. R., Samy, P. M. A., Muneer, S. (2021). Light emitting diodes (LEDs) as agricultural lighting: Impact and its potential on improving physiology, flowering, and secondary metabolites of crops. Sustain 13, 1–25. doi: 10.3390/su13041985
Alotaibi, M. (2023). Climate change, its impact on crop production, challenges, and possible solutions. Not. Bot. Horti Agrobot. Cluj-Napoca. 51, 1–39. doi: 10.15835/nbha51113020
Asemanrafat, M., Honar, T. (2023). Effect of plant density and different irrigation strategieson crop yield and canopy cover of red beans, Phaseolusvulgaris L. cv. Akhtar. Iran Agric. Res. 36, 13–22. doi: 10.22099/iar.2017.4145
Bermejo, C., Gatti, I., Cointry, E. (2016). In vitro embryo culture to shorten the breeding cycle in lentil (Lens culinaris Medik). Plant Cell. Tissue Organ Cult. 127, 585–590. doi: 10.1007/s11240-016-1065-7
Bhatta, M., Sandro, P., Smith, M. R., Delaney, O., Voss-Fels, K. P., Gutierrez, L., et al. (2021). Need for speed: manipulating plant growth to accelerate breeding cycles. Curr. Opin. Plant Biol. 60, 101986. doi: 10.1016/j.pbi.2020.101986
Biosci, I. J., Ardakani, L. G., Farajee, H. (2013). The effect of water stress and plant density on yield and some physiologic traits of spotted bean (Phaseolus vulgaris L.), cultivar Talash in Yasouj region. Int. J. Biosci. 3, 175–184. doi: 10.12692/ijb/3.9.175-184
Blázquez, M. A., Green, R., Nilsson, O., Sussman, M. R., Weigel, D., The, S., et al. (2015). Gibberellins promote flowering of Arabidopsis by activating the LEAFY promoter. Plant Cell 10, 791–800. doi: 10.1105/tpc.10.5.791
Blümel, M., Dally, N., Jung, C. (2015). Flowering time regulation in crops-what did we learn from Arabidopsis? Curr. Opin. Biotechnol. 32, 121–129. doi: 10.1016/j.copbio.2014.11.023
Brazaityte, A., Viršile, A., Samuoliene, G., Jankauskiene, J., Sakalauskiene, S., Sirtautas, R., et al. (2016). Light quality: Growth and nutritional value of microgreens under indoor and greenhouse conditions. Acta Hortic. 1134, 277–284. doi: 10.17660/ActaHortic.2016.1134.37
Cerdán, P. D., Chory, J. (2003). Regulation of flowering time by light quality. Nature 423, 881–885. doi: 10.1038/nature01636
Cha, J. K., O’Connor, K., Alahmad, S., Lee, J. H., Dinglasan, E., Park, H., et al. (2022). Speed vernalization to accelerate generation advance in winter cereal crops. Mol. Plant 15, 1300–1309. doi: 10.1016/j.molp.2022.06.012
Chen, L., Hao, L., Parry, M. A. J., Phillips, A. L., Hu, Y. G. (2014). Progress in TILLING as a tool for functional genomics and improvement of crops. J. Integr. Plant Biol. 56, 425–443. doi: 10.1111/jipb.12192
Chory, J. (2010). Light signal transduction: An infinite spectrum of possibilities. Plant J. 61, 982–991. doi: 10.1111/j.1365-313X.2009.04105.x
Croser, J. S., Pazos-Navarro, M., Bennett, R. G., Tschirren, S., Edwards, K., Erskine, W., et al. (2016). Time to flowering of temperate pulses in vivo and generation turnover in vivo–in vitro of narrow-leaf lupin accelerated by low red to far-red ratio and high intensity in the far-red region. Plant Cell. Tissue Organ Cult. 127, 591–599. doi: 10.1007/s11240-016-1092-4
Demotes-Mainard, S., Péron, T., Corot, A., Bertheloot, J., Le Gourrierec, J., Pelleschi-Travier, S., et al. (2016). Plant responses to red and far-red lights, applications in horticulture. Environ. Exp. Bot. 121, 4–21. doi: 10.1016/j.envexpbot.2015.05.010
Formighieri, C. (2015). “Light saturation of photosynthesis,” in Solar-To-Fuel Conversion in Algae and Cyanobacteria (Springer, Cham, Switzerland), 55–58. doi: 10.1007/978-3-319-16730-5
Fukuda, N., Ajima, C., Yukawa, T., Olsen, J. E. (2016). Antagonistic action of blue and red light on shoot elongation in petunia depends on gibberellin, but the effects on flowering are not generally linked to gibberellin. Environ. Exp. Bot. 121, 102–111. doi: 10.1016/j.envexpbot.2015.06.014
Gao, M., Li, Y., Jiang, H., He, R., Shi, R., Song, S., et al. (2022). UVA-radiation exposure of different Durations promoted the growth, phytochemicals and glucosinolate biosynthesis of Chinese Kale. Int. J. Mol. Sci. 23, 7619. doi: 10.3390/ijms23147619
Germanà, M. A. (2011). Anther culture for haploid and doubled haploid production. Plant Cell. Tissue Organ Cult. 104, 283–300. doi: 10.1007/s11240-010-9852-z
Ghosh, S., Watson, A., Gonzalez-Navarro, O. E., Ramirez-Gonzalez, R. H., Yanes, L., Mendoza-Suárez, M., et al. (2018). Speed breeding in growth chambers and glasshouses for crop breeding and model plant research. Nat. Protoc. 13, 2944–2963. doi: 10.1038/s41596-018-0072-z
Giliberto, L., Perrotta, G., Pallara, P., Weller, J. L., Fraser, P. D., Bramley, P. M., et al. (2005). Manipulation of the blue light photoreceptor cryptochrome 2 in tomato affects vegetative development, flowering time, and fruit antioxidant content. Plant Physiol. 37, 199–208. doi: 10.1104/pp.104.051987
Guo, X., Xue, X., Chen, L., Li, J., Wang, Z., Zhang, Y. (2022). Effects of LEDs light spectra on the growth, yield, and quality of winter wheat (Triticum aestivum L.) cultured in plant factory. J. Plant Growth Regul. 42, 2530–2544. doi: 10.1007/s00344-022-10724-z
Haliapas, S., Yupsanis, T. A., Syros, T. D., Kofidis, G., Economou, A. S. (2008). Petunia x hybrida during transition to flowering as affected by light intensity and quality treatments. Acta Physiol. Plant 30, 807–815. doi: 10.1007/s11738-008-0185-z
Harrison, D., Da Silva, M., Wu, C., De Oliveira, M., Ravelombola, F., Florez-Palacios, L., et al. (2021). Effect of light wavelength on soybean growth and development in a context of speed breeding. Crop Sci. 61, 917–928. doi: 10.1002/csc2.20327
Hatfield, J. L., Prueger, J. H. (2015). Temperature extremes: Effect on plant growth and development. Weather Clim. Extrem. 10, 4–10. doi: 10.1016/j.wace.2015.08.001
He, R., Wei, J., Zhang, J., Tan, X., Li, Y., Gao, M., et al. (2022). Supplemental blue light frequencies improve ripening and nutritional qualities of tomato fruits. Front. Plant Sci. 13. doi: 10.3389/fpls.2022.888976
Hickey, L. T., Hafeez, N., Robinson, H., Jackson, S. A., Leal-Bertioli, S. C. M., Tester, M., et al. (2019). Breeding crops to feed 10 billion. Nat. Biotechnol. 37, 744–754. doi: 10.1038/s41587-019-0152-9
Holmes, M. G., Smith, H. (1975). Erratum: The function of phytochrome in plants growing in the natural environment. Nature 256, 72. doi: 10.1038/256072a0
Jackson, S. D. (2009). Plant responses to photoperiod. New Phytol. 181, 517–531. doi: 10.1111/j.1469-8137.2008.02681.x
Jagadish, S. V. K., Bahuguna, R. N., Djanaguiraman, M., Gamuyao, R., Prasad, P. V. V., Craufurd, P. Q. (2016). Implications of high temperature and elevated CO2 on flowering time in plants. Front. Plant Sci. 7. doi: 10.3389/fpls.2016.00913
Jähne, F., Hahn, V., Würschum, T., Leiser, W. L. (2020). Speed breeding short-day crops by LED-controlled light schemes. Theor. Appl. Genet. 133, 2335–2342. doi: 10.1007/s00122-020-03601-4
Jiang, C., Gao, X., Liao, L., Harberd, N. P., Fu, X. (2007). Phosphate starvation root architecture and anthocyanin accumulation responses are modulated by the gibberellin-DELLA signaling pathway in Arabidopsis. Plant Physiol. 145, 1460–1470. doi: 10.1104/pp.107.103788
Jiaying, M., Tingting, C., Jie, L., Weimeng, F., Baohua, F., Guangyan, L., et al. (2022). Functions of nitrogen, phosphorus and potassium in energy status and their influences on rice growth and development. Rice Sci. 29, 166–178. doi: 10.1016/j.rsci.2022.01.005
Jones, M. A. (2018). Using light to improve commercial value. Hortic. Res. 5, 47. doi: 10.1038/s41438-018-0049-7
Kami, C., Lorrain, S., Hornitschek, P., Fankhauser, C. (2010). Light-regulated plant growth and development. Curr. Topics Dev. Biol. 91, 29–66. doi: 10.1016/S0070-2153(10)91002-8
Kant, S., Peng, M., Rothstein, S. J. (2011). Genetic regulation by NLA and microRNA827 for maintaining nitrate-dependent phosphate homeostasis in Arabidopsis. PloS Genet. 7, e1002021. doi: 10.1371/journal.pgen.1002021
Khan, A., Najeeb, U., Wang, L., Tan, D. K. Y., Yang, G., Munsif, F., et al. (2017). Planting density and sowing date strongly influence growth and lint yield of cotton crops. F. Crop Res. 209, 129–135. doi: 10.1016/j.fcr.2017.04.019
Kigoni, M., Choi, M., Arbelaez, J. D. (2023). ‘Single-Seed-SpeedBulks:’ a protocol that combines ‘speed breeding’ with a cost-efficient modified single-seed descent method for rapid-generation-advancement in oat (Avena sativa L.). Plant Methods 19, 1–9. doi: 10.1186/s13007-023-01067-1
Kim, H. M., Hwang, S. J. (2019). The growth and development of ‘mini chal’ tomato plug seedlings grown under various wavelengths using light emitting diodes. Agronomy 9, 190–205. doi: 10.3390/agronomy9030157
Kozai, T., Niu, G. (2015a). Role of the plant factory with artificial lighting (PFAL) in urban areas. Plant Factory 2016, 7–33. doi: 10.1016/B978-0-12-801775-3.00002-0
Kozai, T., Niu, G. (2015b). Plant Factory as a resource-efficient closed pplant pproduction ssystem. Plant factory: an indoor vertical farming system for efficient quality food production. Plant Factory 16, 69–90. doi: 10.1016/B978-0-12-801775-3.00004-4
Li, Y., Jiang, H., Gao, M., He, R., Liu, X., Su, W., et al. (2023). Far-red-light-induced morphology changes, phytohormone, and transcriptome reprogramming of Chinese kale (Brassica alboglabra Bailey). Int. J. Mol. Sci. 24, 5563. doi: 10.3390/ijms24065563
Li, Y., Xu, J., Haq, N. U., Zhang, H., Zhu, X. G. (2014). Was low CO2 a driving force of C4 evolution: Arabidopsis responses to long-term low CO2 stress. J. Exp. Bot. 65, 3657–3667. doi: 10.1093/jxb/eru193
Lin, Y. L., Tsay, Y. F. (2017). Influence of differing nitrate and nitrogen availability on flowering control in Arabidopsis. J. Exp. Bot. 68, 2603–2609. doi: 10.1093/jxb/erx053
Linkohr, B. I., Williamson, L. C., Fitter, A. H., Leyser, H. M. O. (2002). Nitrate and phosphate availability and distribution have different effects on root system architecture of Arabidopsis. Plant J. 29, 751–760. doi: 10.1046/j.1365-313X.2002.01251.x
Liu, K., He, R., He, X., Tan, J., Chen, Y., Li, Y., et al. (2022). Speed breeding scheme of hot pepper through light environment modification. Sustain 14, 1–9. doi: 10.3390/su141912225
Makoi, J. H. J. R., Chimphango, S. B. M., Dakora, F. D. (2009). Effect of legume plant density and mixed culture on symbiotic N2 fixation in five cowpea (Vigna unguiculata L. Walp.) genotypes in South Africa. Symbiosis 48, 57–67. doi: 10.1007/BF03179985
Marín, I. C., Loef, I., Bartetzko, L., Searle, I., Coupland, G., Stitt, M., et al. (2011). Nitrate regulates floral induction in Arabidopsis, acting independently of light, gibberellin and autonomous pathways. Planta 233, 539–552. doi: 10.1007/s00425-010-1316-5
Mba, C. (2013). Induced mutations unleash the potentials of plant genetic resources for food and agriculture. Agronomy 3, 200–231. doi: 10.3390/agronomy3010200
McGrath, J. M., Lobell, D. B. (2013). Regional disparities in the CO2 fertilization effect and implications for crop yields. Environ. Res. Lett. 8, 14054. doi: 10.1088/1748-9326/8/1/014054
Meng, Q., Runkle, E. S. (2019). Far-red radiation interacts with relative and absolute blue and red photon flux densities to regulate growth, morphology, and pigmentation of lettuce and basil seedlings. Sci. Hortic. (Amsterdam) 255, 269–280. doi: 10.1016/j.scienta.2019.05.030
Mitache, M., Baidani, A., Houasli, C., Khouakhi, K., Bencharki, B., Idrissi, O. (2023). Optimization of light/dark cycle in an extended photoperiod-based speed breeding protocol for grain legumes. Plant Breed. 4, 1–14. doi: 10.1111/pbr.13112
Mobini, S., Khazaei, H., Warkentin, T. D., Vandenberg, A. (2020). Shortening the generation cycle in faba bean (Vicia faba) by application of cytokinin ands cold stress to assist speed breeding. Plant Breed. 139, 1181–1189. doi: 10.1111/pbr.12868
Mobini, S. H., Lulsdorf, M., Warkentin, T. D., Vandenberg, A. (2015). Plant growth regulators improve in vitro flowering and rapid generation advancement in lentil and faba bean. Vitr. Cell. Dev. Biol. - Plant 51, 71–79. doi: 10.1007/s11627-014-9647-8
Monostori, I., Heilmann, M., Kocsy, G., Rakszegi, M., Simon-sarkadi, L., Harnos, N., et al. (2018). LED lighting–modification of growth, metabolism, yield and flour composition in wheat by spectral quality and intensity. Front. Plant Sci. 9. doi: 10.3389/fpls.2018.00605
Mouradov, A., Cremer, F., Coupland, G. (2002). Control of flowering time: Interacting pathways as a basis for diversity. Plant Cell. 14, 111–130. doi: 10.1105/tpc.001362
Muchhal, U. S., Pardo, J. M., Raghothama, K. G. (1996). Phosphate transporters from the higher plant Arabidopsis thaliana. Proc. Natl. Acad. Sci. U. S. A. 93, 10519–10523. doi: 10.1073/pnas.93.19.10519
Nagatoshi, Y., Fujita, Y. (2019). Accelerating soybean breeding in a CO2-supplemented growth chamber. Plant Cell Physiol. 60, 77–84. doi: 10.1093/pcp/pcy189
Nie, W. F., Li, Y., Chen, Y., Zhou, Y., Yu, T., Zhou, Y., et al. (2023). Spectral light quality regulates the morphogenesis, architecture, and flowering in pepper (Capsicum annuum L.). J. Photochem. Photobiol. B Biol. 241, 112673. doi: 10.1016/j.jphotobiol.2023.112673
O’Connor, D. J., Wright, G. C., Dieters, M. J., George, D. L., Hunter, M. N., Tatnell, J. R., et al. (2013). Development and application of speed breeding technologies in a commercial peanut breeding program. Peanut Sci. 40, 107–114. doi: 10.3146/PS12-12.1
Oh, W. (2015). Effects of temperature, photoperiod and light intensity on growth and flowering in Eustoma grandiflorum. Korean J. Hortic. Sci. Technol. 33, 349–355. doi: 10.7235/hort.2015.15023
Ohnishi, T., Yoshino, M., Yamakawa, H., Kinoshita, T. (2011). The biotron breeding system: A rapid and reliable procedure for genetic studies and breeding in rice. Plant Cell Physiol. 52, 1249–1257. doi: 10.1093/pcp/pcr066
Omar, I. (2020). Application of extended photoperiod in lentil: Towards acceleratedgenetic gain in breeding for rapid improved variety development. Moroccan J. Agric. Sci. 1, 14–19.
Ortiz, R., Trethowan, R., Ferrara, G. O., Iwanaga, M., Dodds, J. H., Crouch, J. H., et al. (2007). High yield potential, shuttle breeding, genetic diversity, and a new international wheat improvement strategy. Euphytica 157, 365–384. doi: 10.1007/s10681-007-9375-9
Paradiso, R., Proietti, S. (2022). Light-quality manipulation to control plant growth and photomorphogenesis in greenhouse horticulture: The state of the art and the opportunities of modern LED systems. J. Plant Growth Regul. 41, 742–780. doi: 10.1007/s00344-021-10337-y
Pardey, P. G., Beddow, J. M., Hurley, T. M., Beatty, T. K. M., Eidman, V. R. (2014). A bounds analysis of world food futures: Global agriculture through to 2050. Aust. J. Agric. Resour. Econ. 58, 571–589. doi: 10.1111/1467-8489.12072
Park, Y. G., Muneer, S., Soundararajan, P., Manivnnan, A., Jeong, B. R. (2017). Light quality during night interruption affects morphogenesis and flowering in geranium. Hortic. Environ. Biotechnol. 58, 212–217. doi: 10.1007/s13580-017-0246-6
Rahmanet, M. A. (2019). Field rapid generation advance: An effective technique for industrial scale rice breeding program. Exp 47, 2659–2670.
Ribalta, F. M., Pazos-Navarro, M., Nelson, K., Edwards, K., Ross, J. J., Bennett, R. G., et al. (2017). Precocious floral initiation and identification of exact timing of embryo physiological maturity facilitate germination of immature seeds to truncate the lifecycle of pea. Plant Growth Regul. 81, 345–353. doi: 10.1007/s10725-016-0211-x
Saxena, K., Saxena, R. K., Varshney, R. K. (2017). Use of immature seed germination and single seed descent for rapid genetic gains in pigeonpea. Plant Breed. 136, 954–957. doi: 10.1111/pbr.12538
Shrivastava, P., Kumar, R. (2015). Soil salinity: A serious environmental issue and plant growth promoting bacteria as one of the tools for its alleviation. Saudi J. Biol. Sci. 22, 123–131. doi: 10.1016/j.sjbs.2014.12.001
Sidhu, V., Bernier-English, V., Lamontagne-Drolet, M., Gravel, V. (2022). Effect of light quality and extended photoperiod on flower bud induction during transplant production of day-neutral strawberry cultivars. Can. J. Plant Sci. 102, 356–367. doi: 10.1139/cjps-2021-0081
Singh, V., Nguyen, C. T., van Oosterom, E. J., Chapman, S. C., Jordan, D. R., Hammer, G. L. (2015). Sorghum genotypes differ in high temperature responses for seed set. F. Crop Res. 171, 32–40. doi: 10.1016/j.fcr.2014.11.003
Smith, H. L., Mcausland, L., Murchie, E. H. (2017). Don’t ignore the green light: Exploring diverse roles in plant processes. J. Exp. Bot. 68, 2099–2110. doi: 10.1093/jxb/erx098
Song, Y., Duan, X., Wang, P., Li, X., Yuan, X., Wang, Z., et al. (2022). Comprehensive speed breeding: a high-throughput and rapid generation system for long-day crops. Plant Biotechnol. J. 20, 13–15. doi: 10.1111/pbi.13726
Stetter, M. G., Zeitler, L., Steinhaus, A., Kroener, K., Biljecki, M., Schmid, K. J. (2016). Crossing methods and cultivation conditions for rapid production of segregating populations in three grain amaranth species. Front. Plant Sci. 7. doi: 10.3389/fpls.2016.00816
Takahashi, S., Badger, M. R. (2011). Photoprotection in plants: A new light on photosystem II damage. Trends Plant Sci. 16, 53–60. doi: 10.1016/j.tplants.2010.10.001
Tanaka, J., Hayashi, T., Iwata, H. (2016). A practical, rapid generation-advancement system for rice breeding using simplified biotron breeding system. Breed. Sci. 66, 542–551. doi: 10.1270/jsbbs.15038
Verdaguer, D., Jansen, M. A. K., Llorens, L., Morales, L. O., Neugart, S. (2017). UV-A radiation effects on higher plants: Exploring the known unknown. Plant Sci. 255, 72–81. doi: 10.1016/j.plantsci.2016.11.014
Wang, S., Liu, X., Liu, X., Xue, J., Ren, X., Zhai, Y., et al. (2022). The red/blue light ratios from light-emitting diodes affect growth and flower quality of Hippeastrum hybridum ‘Red Lion.’. Front. Plant Sci. 13. doi: 10.3389/fpls.2022.1048770
Wanga, M. A., Shimelis, H., Mashilo, J., Laing, M. D. (2021). Opportunities and challenges of speed breeding: A review. Plant Breed. 140, 185–194. doi: 10.1111/pbr.12909
Warnasooriya, S. N., Brutnell, T. P. (2014). Enhancing the productivity of grasses under high-density planting by engineering light responses: From model systems to feedstocks. J. Exp. Bot. 65, 2825–2834. doi: 10.1093/jxb/eru221
Watson, A., Ghosh, S., Williams, M. J., Cuddy, W. S., Simmonds, J., Rey, M. D., et al. (2018). Speed breeding is a powerful tool to accelerate crop research and breeding. Nat. Plants 4, 23–29. doi: 10.1038/s41477-017-0083-8
Wimalasekera, R. (2019). Effect of light intensity on photosynthesis. Photosynthesis productivity Environ. stress 5, 65–73. doi: 10.1002/9781119501800
Yamada, A., Tanigawa, T., Suyama, T., Matsuno, T., Kunitake, T. (2009). Red:far-red light ratio and far-red light integral promote or retard growth and flowering in Eustoma grandiflorum (Raf.) Shinn. Sci. Hortic. (Amsterdam) 120, 101–106. doi: 10.1016/j.scienta.2008.09.009
Ye, T., Li, Y., Zhang, J., Hou, W., Zhou, W., Lu, J., et al. (2019). Nitrogen, phosphorus, and potassium fertilization affects the flowering time of rice (Oryza sativa L.). Glob. Ecol. Conserv. 20, e00753. doi: 10.1016/j.gecco.2019.e00753
Yoshida, H., Mizuta, D., Fukuda, N., Hikosaka, S., Goto, E., et al. (2016). Effects of varying light quality from single-peak blue and red light-emitting diodes during nursery period on flowering , photosynthesis , growth , and fruit yield of everbearing strawberry. Plant Biol. 276, 267–276. doi: 10.5511/plantbiotechnology.16.0216a
Zhan, X., Lu, Y., Zhu, J. K., Botella, J. R. (2021). Genome editing for plant research and crop improvement. J. Integr. Plant Biol. 63, 3–33. doi: 10.1111/jipb.13063
Zhang, J., Zhang, Y., Song, S., Su, W., Hao, Y., Liu, H. (2020). Supplementary red light results in the earlier ripening of tomato fruit depending on ethylene production. Environ. Exp. Bot. 175, 104044. doi: 10.1016/j.envexpbot.2020.104044
Keywords: vegetable, speed breeding, plant factory, smart breeding, breeding cycle
Citation: He R, Ju J, Liu K, Song J, Zhang S, Zhang M, Hu Y, Liu X, Li Y and Liu H (2024) Technology of plant factory for vegetable crop speed breeding. Front. Plant Sci. 15:1414860. doi: 10.3389/fpls.2024.1414860
Received: 09 April 2024; Accepted: 18 June 2024;
Published: 11 July 2024.
Edited by:
Sergio Ruffo Roberto, State University of Londrina, BrazilReviewed by:
Shinsuke Agehara, University of Florida, United StatesMarzena Małgorzata Kurowska, University of Silesia in Katowice, Poland
Copyright © 2024 He, Ju, Liu, Song, Zhang, Zhang, Hu, Liu, Li and Liu. This is an open-access article distributed under the terms of the Creative Commons Attribution License (CC BY). The use, distribution or reproduction in other forums is permitted, provided the original author(s) and the copyright owner(s) are credited and that the original publication in this journal is cited, in accordance with accepted academic practice. No use, distribution or reproduction is permitted which does not comply with these terms.
*Correspondence: Houcheng Liu, bGl1aGNoQHNjYXUuZWR1LmNu