- 1Metabolome- and Proteome Research, Faculty of Biology & CeBiTec, Bielefeld University, Bielefeld, Germany
- 2Genetics and Genomics of Plants, Faculty of Biology, CeBiTec, Bielefeld University, Bielefeld, Germany
- 3Computational Metagenomics, Faculty of Technology & CeBiTec, Bielefeld University, Bielefeld, Germany
- 4AG 3 Anatomy and Cell Biology, Medical School OWL, Bielefeld, Germany
Hydrangea serrata, also knowen as the Japanese tea hortensia, is known for its sweet taste and health properties of bevarages produced from this plant. The H. serrata 3,4-dihydroisocoumarins, hydrangenol and phyllodulcin harbour a variety of biological activities and pharmacological properties. Therefore, a detailed understanding of dihydroisocoumarin biosynthesis in H. serrata is of major interest. Their biosynthesis is assumed to be enhanced by elicitors and mediated by polyketide synthases like in cases of phenylpropanoid derived phytoalexins. A de-novo transcriptome assembly of leaves and roots from the aluminium chloride treatment group versus the control group alongside with annotation was generated. Secondary plant metabolites were analysed by LC-MS. It revealed that a terpene synthase and a triterpenoid synthase gene as well as lignin biosynthesis encoding genes were upregulated in roots. Many genes for transporters, glycosyl, and other transferases as well as glycosylases were found to be differentially expressed in both organs. As no differentially expressed polyketide synthase gene homolog was found, the relative leaf and root 3,4-dihydroisocoumarin content was analysed by LC-MS measurement. Although Hydrangea species are known for their aluminium detoxification using phenylpropanoid-derived compounds, the levels of 3,4- dihydroisocoumarins were not enhanced. In this metabolite analysis, an organ- specific accumulation profile of hydrangenol, phyllodulcin, hydrangeic acid and their mono- and di-glycosides was figured out.
1 Introduction
The Japanese mountain Hydrangea (Hydrangea serrata) is an important cultural and medical plant due to the content of specialised metabolites. A traditional tea is prepared from the leaves. Health properties of H. serrata “Oamacha” and closely related cultivars are mainly due to 3,4-dihydroisocoumarins (DHICs) including hydrangenol (HYD), phyllodulcin (PHY) and stilbene carboxylic acids like hydrangeic acid (Hashimoto et al., 1979; Liu et al., 2013). They share similar positive health effects and anti-microbial properties with the group of isocoumarins (Saddiqa et al., 2017). In contrast to the mostly bitter-tasting isocoumarins, PHY tastes sweet and is traditionally consumed in tea and beverages (Yamato and Hashigaki, 1979). Within the genus Hydrangea, H. serrata the varieties “Oamacha” and “Amagi Amacha” (classified as a subspecies of H. macrophylla Thunbergii) is the only natural sweetener-producing member (Çiçek et al., 2018). The bioconversion of PHY-glycoside to the aglycon as high-intensity sweetener from Hydrangea macrophylla Thunbergii leaves is established (Jung et al., 2016).
PHY and HYD occur as glycosides or aglycons and have a structure and synthesis similar to stilbenoids or flavonoids (Asahina and Asano, 1929; Kuć and Rush, 1985; Liu et al., 2013). HYD and PHY are proposed to be derived from hydrangeic acid, a stilbene carboxylic acid (Akiyama et al., 1999; Eckermann et al., 2003; Schröder and Schröder, 1990). Like for other phenylpropanoids, the precursors phenylalanine and tyrosine are derived from the shikimate pathway (D’Auria and Gershenzon, 2005; Herrmann and Weaver, 1999). These aromatic amino acids are converted via phenylpropanoid pathway into p-coumarate-CoA (Hahlbrock and Scheel, 1989). An elongation of this intermediate with malonyl-CoA occurs 3× in addition to ring closure and is catalysed by a polyketide synthase (PKS) (Ibrahim and Towers, 1962). A type III PKS subgroup, the CHS family, includes chalcone synthases and stilbene synthases. Together they produce a large group of plant phenylpropanoids including many phytoalexins from specialised metabolism. Phytoalexins play important roles in plant defence, stress responses, or detoxification (Francini et al., 2019; Gundlach et al., 1992; Herrmann and Weaver, 1999). Their biosynthesis can be induced externally by abiotic stressors such as UV radiation, high light, drought, salinity, and toxic compounds such as metals and biotic stressors including certain signal molecules (elicitors) released upon attack by pathogenic organisms or herbivores (Adrian et al., 1996; Baier et al., 1999; Matern, 1991). The phytoalexin spectrum is huge. Terpenoids that are composed of isoprene subunits are non-phenylpropanoid derived phytoalexins (Chen et al., 2019; Ebel, 1986; Kuć and Rush, 1985; Li et al., 2015).
Hydrangea species are commonly known for their robustness against aluminium and also for their decorative flowers. H. serrata and H. macrophylla display a variation of sepal colour in response to soil pH change. The flowers are pink in alkaline and turn blue in acidic soil. Aluminium ions are complexed with two phenylpropanoids. This hydrangea blue complex is deposited in the sepals as means of detoxification (Ito et al., 2019; Schreiber et al., 2011). AlCl3 is toxic to plants according to other studies (Rahman and Upadhyaya, 2021). It changes gene expression upon stress response and especially effects the roots (Zhou et al., 2016). The response to treatment with AlCl3 on global changes in transcript levels was analysed in H. macrophylla which produces no DHICs (Chen et al., 2015). Other phenylpropanoids are inducible by AlCl3 or CuCl2 treatment (Adrian et al., 1996; Hanawa et al., 1992; Hossain et al., 2023; Schmidlin et al., 2008; Wang et al., 2022). The focus of our study was to observe whether phytoalexins or phenylpropanoids like DHICs in H. serrata can be induced by AlCl3 treatment. Phytoalexins in hydrangeas were not described. Some monoterpenoids, the iridoids-glycosides from hydrangeas were reviewed by Gousiadou et al. (2016). Secoiridoid glycosides and hydrangeosides A and C were isolated from H. serrata leaves (Inouye et al., 1980; Kikuchi et al., 2008).
In this study, we aim to describe changes in the H. serrata transcriptome under abiotic stress and compare the effects on roots and leaves. Aluminium chloride-induced alterations in leaf and root transcripts were analyzed to provide insights into specialized metabolism-related gene activity. Utilizing the Illumina NextSeq2000 technique, we employed a deep sequencing approach, which offers a solid foundation for further data analysis and comparisons using the established dataset. The H. serrata transcriptome data from this study includes differentially expressed sequences of terpenoid synthases and genes involved in monolignol biosynthesis. This dataset can be used to search for novel genes and gene functions. Additionally, our findings suggest that H. serrata is unlikely to produce phenylpropanoids in response to aluminium stress.
2 Materials and methods
2.1 Plant material
H. serrata (H. macrophylla sups. serrata “Oamacha”) plants of about 40 cm height (GartenBaumschule TIMM, Reppenstedt, Germany) were cultivated in the greenhouse at the Bielefeld University. The identity of the plants was confirmed by the production of characteristic secondary metabolites (see results) and by the morphology. According to the leaf and flower morphology the analysed species is H. serrata. We follow the taxonomical description of the Royal Botanical Garden Kew (https://powo.science.kew.org). Ten individual plants were purchased as one cultivar and divided into two groups. Five plants in the control group were treated daily with 100 mL of tap water for 7 days. The treatment group consisted of the other five plants, which were daily watered with 100 mL of 100 mM AlCl3 (Carl Roth, Karlsruhe, Germany) solution for 7 days. The plants were kept in 12 l containers with common potting soil. From every group, a pool sample of vital leaves was collected with N = 8 per plant. In parallel, a pool sample of about 2 g vital and rinsed roots were collected per individual plant of every group. All pool samples were immediately frozen in liquid nitrogen and stored at −80°C.
2.2 Total RNA extraction
The total RNA was extracted from 95 to 99 mg of frozen sample using the Spectrum Plant Total RNA Kit (Sigma-Aldrich, Taufkirchen, Germany) according to the supplier’s protocol. Sixteen samples were prepared, consisting of four biological replicates for each condition from leaves and roots. On-Column DNase I Digestion Set from Sigma-Aldrich (Taufkirchen, Germany) was used according to the supplier’s instructions. For RNA elution nuclease-free water was used (Merck, Darmstadt, Germany). The RNA quality was validated using a 2,100 Bioanalyzer system (Agilent, Santa Clara, CA, USA). Samples with RNA integrity number above 8 went into library construction.
2.3 Library construction and cDNA sequencing
Sequencing libraries were constructed from 3 µg of total RNA, following the TrueSeq v2 protocol (Illumina, Cambridge, UK). Paired-end sequencing was performed on an Illumina NextSeq2000 instrument at the Sequencing Core Facility of the Center for Biotechnology (CeBiTec) at Bielefeld University. A total of 24 samples libraries were applied on two lanes of a P3 flow cell. The paired-end read length was 150 bp and the total amount was 1.3 Mrd reads per sample.
2.4 Data processing and de-novo transcriptome assembly using Trinity
Illumina paired-end reads were processed analogous to the best practises guideline for a de-novo assembly using Trinity (Freedman and Weeks, 2020; Grabherr et al., 2011). All computational operations were calculated using the de.NBI cloud (https://www.denbi.de/cloud). First, read quality was examined by FastQC v0.11.3 (Andrews, 2010). Removal of erroneous k-mers was made by applying rCorrector v1.0.4 (Song and Florea, 2015). Adapter and low-quality regions were trimmed with TrimGalore! v0.6.6 (Krueger, 2015). Trimmed reads were obtained and a total number of 424,302,023 bases was assembled using Trinity v2.12.0 in genome independent- and paired-end mode, including normalisation to targeted maximum achievable coverage.
2.5 Functional annotation and differential expression analysis
The transcriptome assembly quality was rated via (BUSCO v3.0.2) (Simão et al., 2015) using the reference dataset of embryophyte_odb9 containing 1,440 Benchmarking Universal Single-Copy Orthologs (BUSCOs) of 30 species. TransDecoder (Haas et al., 2013) was used to identify open reading frames (ORFs) and translate ORFs to peptide sequences with a minimum length of 100 amino acids. Genes were annotated by transferring the A. thaliana Araport11 (Cheng et al., 2016) functional annotation to the H. serrata gene models as described before (Schilbert et al., 2021). The quantitative transcriptome data were obtained from read counts per contiq. Therefore, Kallisto v.0.44 (Bray et al., 2016) was applied using default parameters to quantify transcript abundances and to generate count tables. The generated count tables were subjected to differential gene expression analysis via DESeq2 to obtain the log2 fold change for each gene (Love et al., 2014). Differentially expressed genes (DEGs) with a log2 fold change >2 and adjusted p-value <0.1 between leaves versus leaves_ AlCl3 and roots versus roots_ AlCl3 were identified. Identified DEGs were visualized by MapMan as follows: The functional annotations were sorted by biological categories of the proteins using Mercator4 v2.0 (Schwacke et al., 2019) and mapped together with the quantitative transcriptome data onto specialised metabolite pathways in MapMan 3.5.1R2 (Usadel et al., 2009). Pathway map files were adjusted to the biological categories using GIMP 2.10.18. The DEGs were displayed colour scaled on pathway maps and summarized in tables (Supplementary Material). The biological context was obtained from protein category bins using Mercator4 and MapMan. DEGs from the selected biological categories of interest were visualised in Venn diagrams using http://bioinformatics.psb.ugent.be/software.
2.6 UHPLC-ESI-QToF-MS analysis for DHIC profiling
Metabolite profiles were measured by UHPLC UltiMate 3000 and DAD (Thermo Scientific, Waltham, Massachusetts, USA) in combination with ESI Bruker MicroToFQ (Bruker Daltonics, Bremen, Germany) in negative ion mode. An Eurosphere II 100 mm × 2 mm C18 column (Knauer, Berlin Germany) was used for the separation in chromatography at a flow rate of 0.45 mL/min. The mobile phase was set to a gradient starting with 10% acetonitrile including 0.1% formic acid (B) and 90% water including 0.1% formic acid (A) for 0–5 min. Followed by 10%–25% B 5–10 min, 25%–45% B 10–20 min, 45%–65% B 20–30 min, 65%–100% B 30–40 min, hold 40–50 min, 100%–10% B 50–55 min. The wavelengths were set to 340 and 280 nm. HPLC grade water, solvents, and formic acid were purchased from TH Geyer (Höxter-Stahle, Germany). The technical settings were taken and modified from flavonoid-glycoside detection by Lin & Harnly (2007). Negative ions of sodium formate (VWR Chemicals, Haasrode, Belgium) was used for initial mass calibration in every first 60 s of sample acquisition. Mass spectra were taken for a range of 120–1000 m/z. Naringenin chalcone (Carl Roth, Karlsruhe, Germany) was used as internal standard. For mass identification of PHY and HYD a “Sweet Hydrangea Leaf Dihydroisocoumarin” reference was measured (FUJIFILM Wako Chemicals Europe, Neuss, Germany).
3 Results
H. serrata plants of about 40 cm height were grown in potting soil under optimal greenhouse conditions. The plants were treated with AlCl3 solution or with tap water as control. Leaves and roots were harvested, RNA was extracted and cDNA libraries were sequenced using the Illumina technology. The number of resulting 580,770 contigs includes isoforms, allelic variants and other sequences. The application of BUSCO revealed 96.2% complete BUSCO genes in the de-novo H. serrata transcriptome assembly. Among the complete BUSCOs, 10.4% were single-copy genes whereas 85.8% were duplicated. Moreover, 1.6% of all BUSCO genes were fragmented and only 2.2% were missing.
A differential expression analysis between AlCl3-treated organs and corresponding controls was made using DESeq. As displayed in volcano plots, a higher number of genes were differentially expressed in AlCl3-treated leaves compared to AlCl3-treated roots (Figure 1).
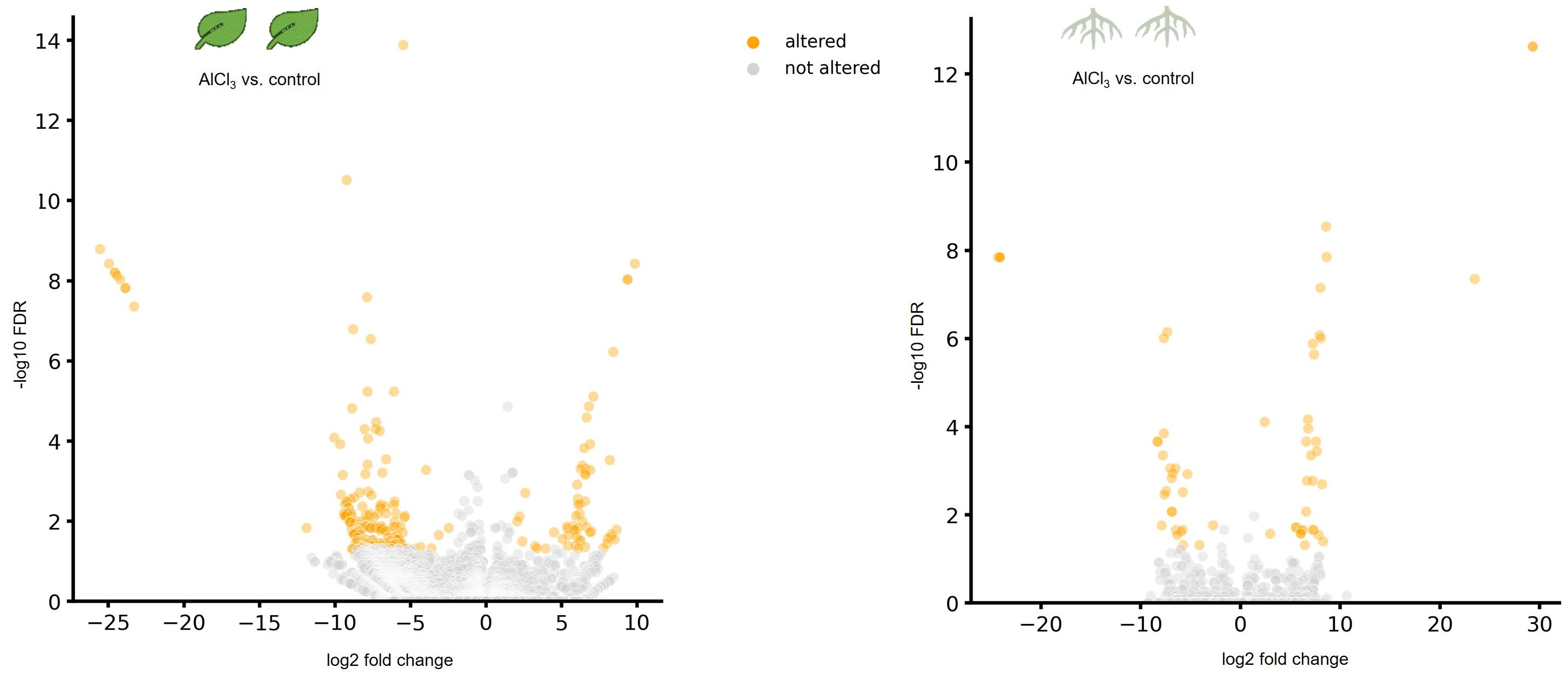
Figure 1. Volcano plots showing differentially expressed genes (DEGs) in leaves treated with aluminium chloride (AlCl3) compared to control leaves as well as in AlCl3-treated roots compared to control roots. DEGs with a log2 fold chance >2 and <−2 and an adjusted p-value of <0.05 are marked in orange. FDR is the false discovery rate of the adjusted p-value. The individual H. serrata plants were treated with tap water in the control group and with 100 mM AlCl3-solution in the treatment group for 5 days. Each condition group consists of four biological replicates.
A total of 119,756 representative peptide sequences were annotated via reciprocal best BLAST hits (RBHs) and best BLAST hits (BBHs) using Arabidopsis thaliana data. A total of 75,100 annotated sequences were sorted in biological categories plus 42,432 enzyme classification categorized in more than 50 bins using Mercator 4.0. Each bin contained second and third order subclasses or more for detailed specification.
3.1 Biological categorisation of differential expressed genes in response to aluminium chloride treatment
Categories of functional annotations were summarized using Mercator4. Tables 1, 2 show the strongest regulated genes, filtered for a log2 change <4>, and their annotations. Both H. serrata tissues showed transcriptional changes in the biological categories of solute transport, phytohormone action, external stimuli response, and specialised metabolism (Figures 2, 3). The most annotations were categorized into enzyme classification and solute transport followed by protein modification and vesicle trafficking (Figure 2). Many genes encoding for glycosyltransferases and oxidoreductases were regulated in both tissues. A higher number of glycosylases encoding genes were regulated in the roots (Figure 2; Tables 1, 2).
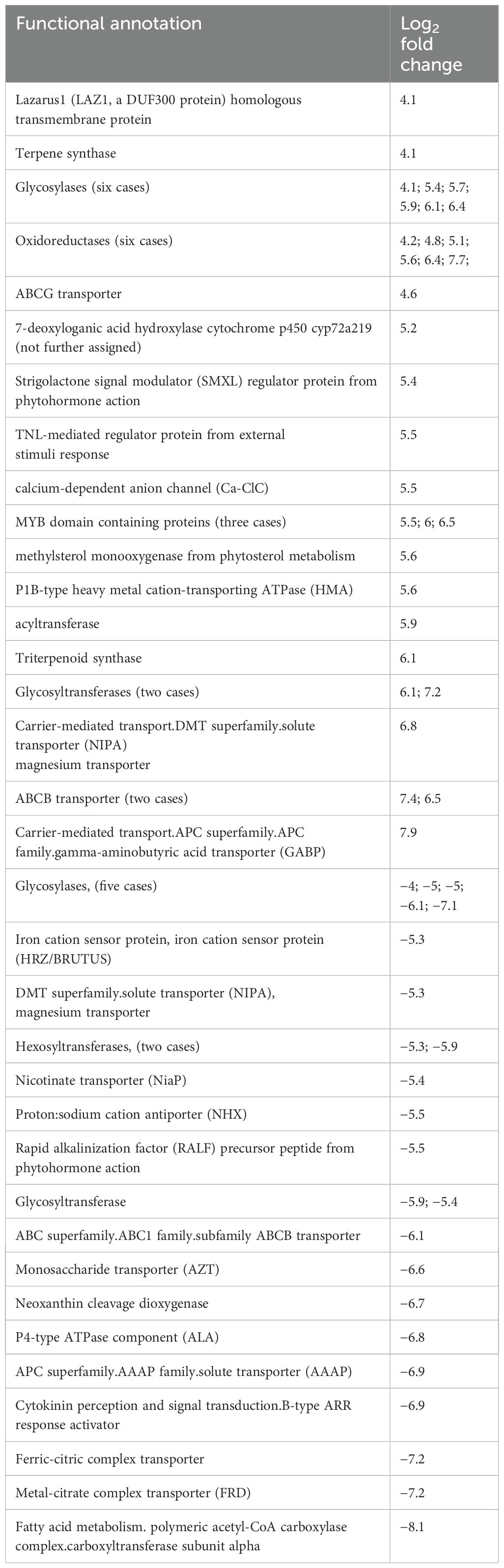
Table 1. Functional annotations of differential expressed genes from AlCl3-treated H. serrata roots versus control with a log2 fold change.
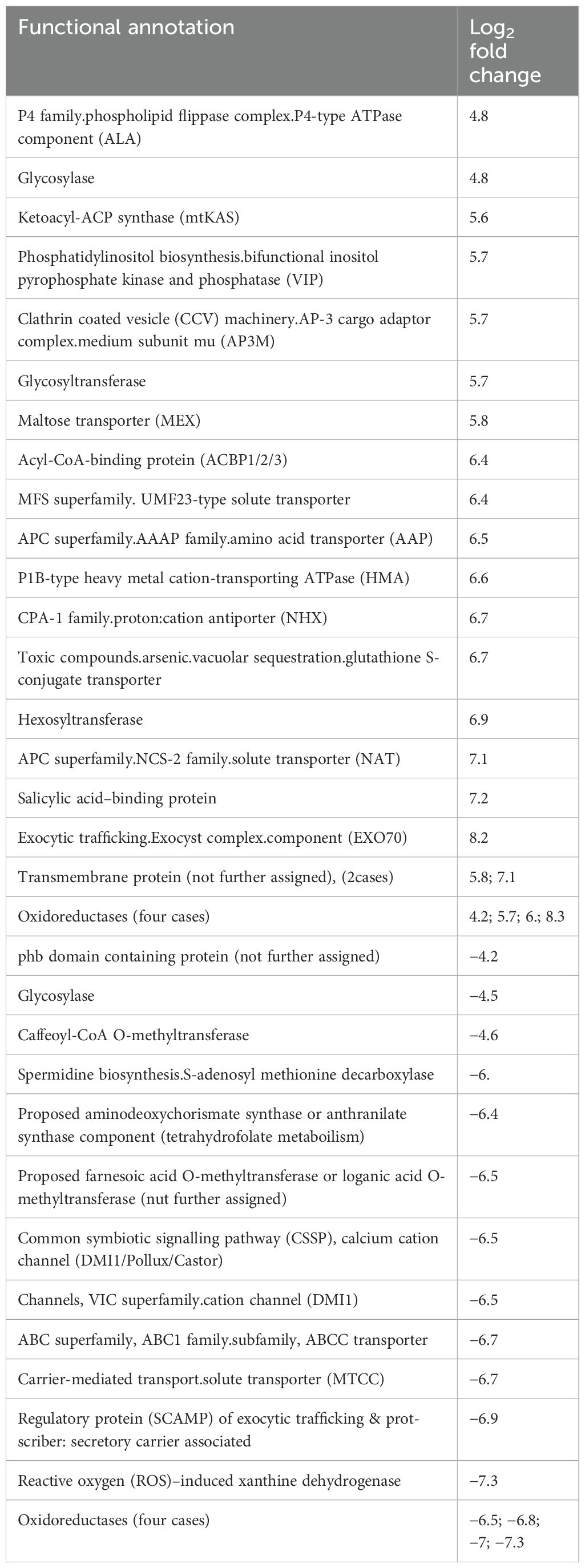
Table 2. Functional annotations of differential expressed genes from AlCl3-treated H. serrata leaves versus control with a log2 fold change.
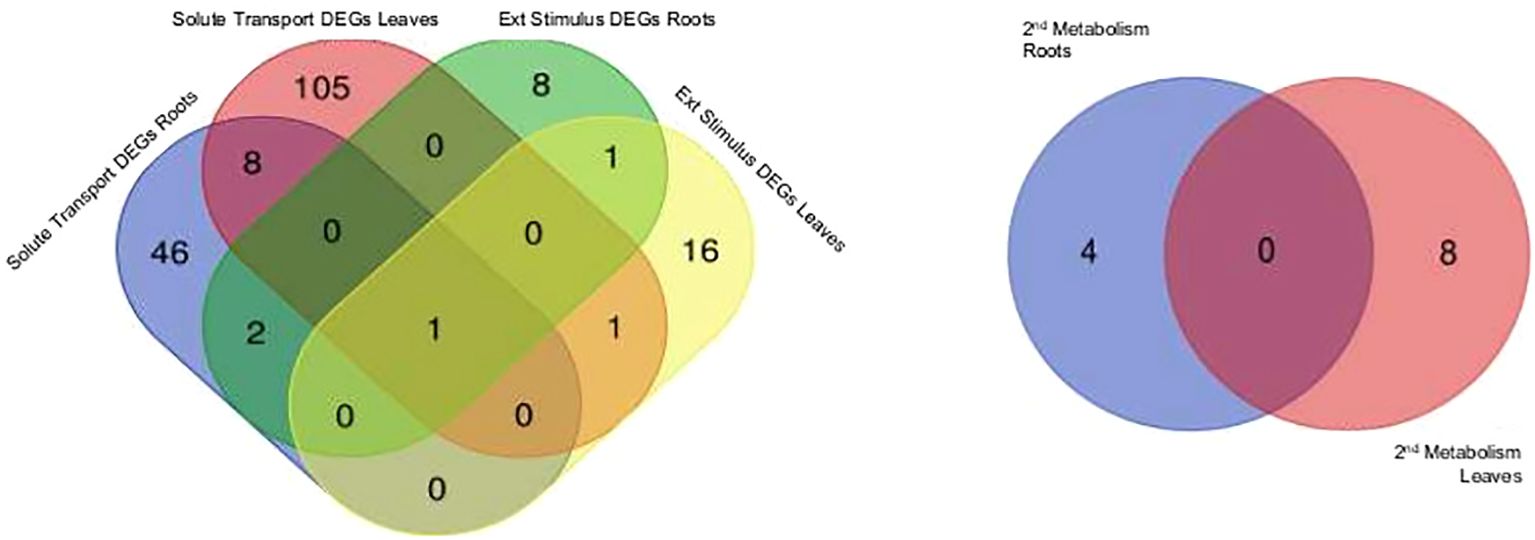
Figure 2. Differentially expressed genes (DEGs) are displayed for selected biologicals categories based on Mercator4 protein assignment to functional context. External stimuli response and solute transport in roots treated with aluminium chloride (AlCl3) compared to control roots as well as in leaves treated with AlCl3 compared to control leaves and specialised metabolism in roots treated with AlCl3 compared to control roots and in leaves treated with AlCl3 compared to control leaves. Both Venn diagrams show tissue specific DEG amounts, log2 fold change >2; <−2 and an adjusted p-value <0.1 from aluminium chloride (AlCl3) treated roots and leaves compared to the respective control. DEGs with same expression level are in the overlap.
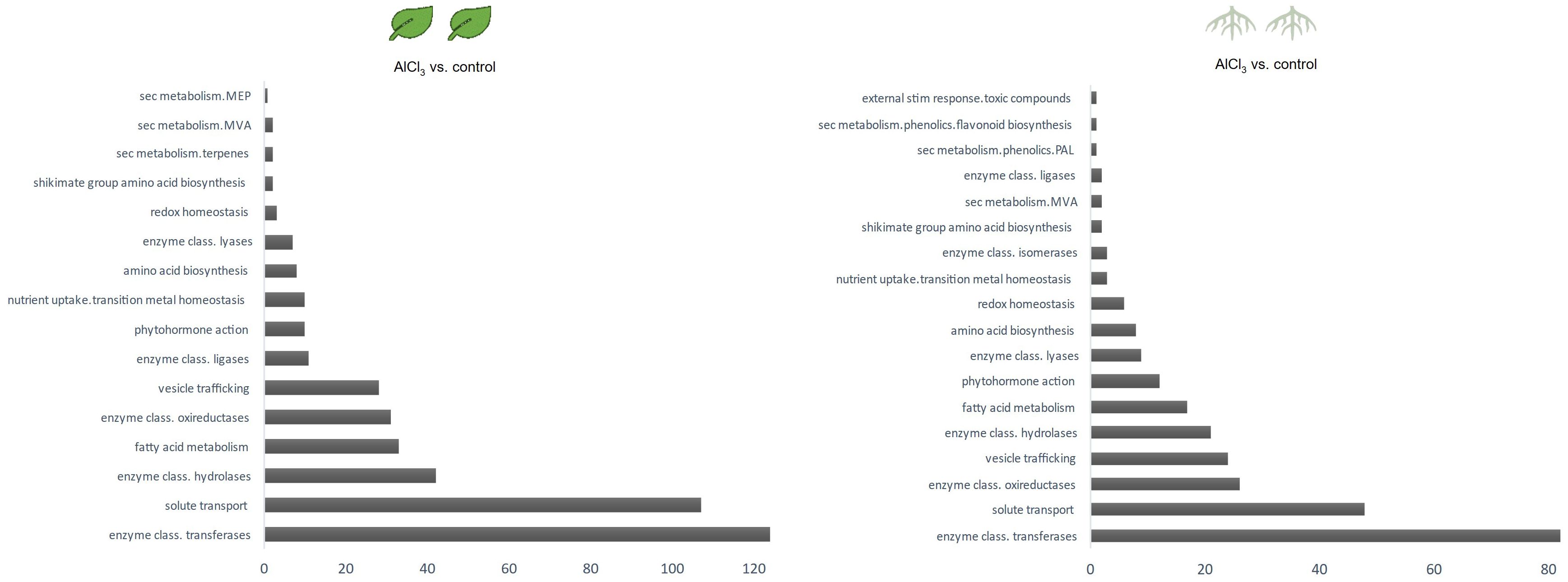
Figure 3. Counts of differentially expressed genes per biological category in leaves treated with aluminium chloride (AlCl3) compared to control leaves and in roots treated with AlCl3 compared to control roots. Functional annotated proteins were sorted in biologicals categories using Mercator4. The assigned functional protein categories were selected for specialised metabolism and the corresponding amount of DEGs having a log2 fold change >2; <−2 and adjusted p-value <0.1 was counted.
Among DEGs from AlCl3-treated roots (roots_AlCl3) versus control with a log2 fold change >4, several genes encode for enzymes such as oxidoreductases (six cases), glycosyltransferases (two cases), glycosylases (six cases), and one methylsterol monooxygenase from phytosterol metabolism. Additionally, differentially expressed sequences specialised metabolism include genes encoding terpene synthase and triterpenoid synthase. Other genes were annotated as transporter as well as ion channels.
Downregulated genes with log2 fold change <−4 were annotated as glycosylases (five cases), one glycosyltransferase, hexosyltransferases (two cases), and acyltransferases (two cases). Furthermore, downregulated genes encoded for iron cation sensor protein as well as many transporters. A polymeric acetyl-CoA carboxyltransferase subunit from the fatty acid metabolism encoding transcript was significantly downregulated (Table 1).
Genes for aluminium or general heavy metal ion response mechanisms like transporters were similarly differentially expressed, like Chen et al., 2015 reported for H. macrophylla. One interesting exception is the aluminium-activated malate transporter (QUAC/ALMT) which was slightly downregulated in roots (Supplementary Material). Also, in AlCl-treated roots of H. serrata the iron cation sensor protein HRZ/BRUTUS, ferric-citric complex transporter, and metal-citrate complex transporter FRD were downregulated.
DEGs in AlCl3-treated leaves (leaves_AlCl3) versus control with log2 fold change >4 was functionally annotated as transporters, transmembrane proteins (two cases), one acetyl-CoA binding protein, one salicylic acid binding protein, one glycosylase, oxidoreductases (three cases), one hexosyltransferase and one glycosyltransferase (Table 2).
Downregulated genes with log2 fold change <−4 encoded for other transporters, calcium cation channel, oxidoreductases (four cases), one glycosylase, and one ROS-induced xanthine dehydrogenase (Table 2).
3.2 Specialised metabolism-specific differential gene expression in response to aluminium chloride treatment
The biological categorisation of functional annotated DEGs were mapped onto pathways of the specialised metabolism. These MapMan images show which gene sets were regulated under the presence of AlCl3. The most significantly regulated gene sets covered the shikimate and MVA pathway, terpenoid, lignan, and lignin biosyntheses (Figures 4, 5). More of these genes were upregulated in roots_AlCl3 than in leaves_AlCl3 (Figure 4). One PAL isoform was downregulated in the roots (−2). Additionally, in the leaves an isoprenyl diphosphate synthase (−3.2) was downregulated, a triterpenoid synthase (6.1), a terpene synthase (4.1), and an MEP pathway reductase (2.3) were upregulated. Obviously, AlCl3 induced stress activated genes of the terpenoid pathway and genes encoding for enzymes of the lignin biosynthesis.
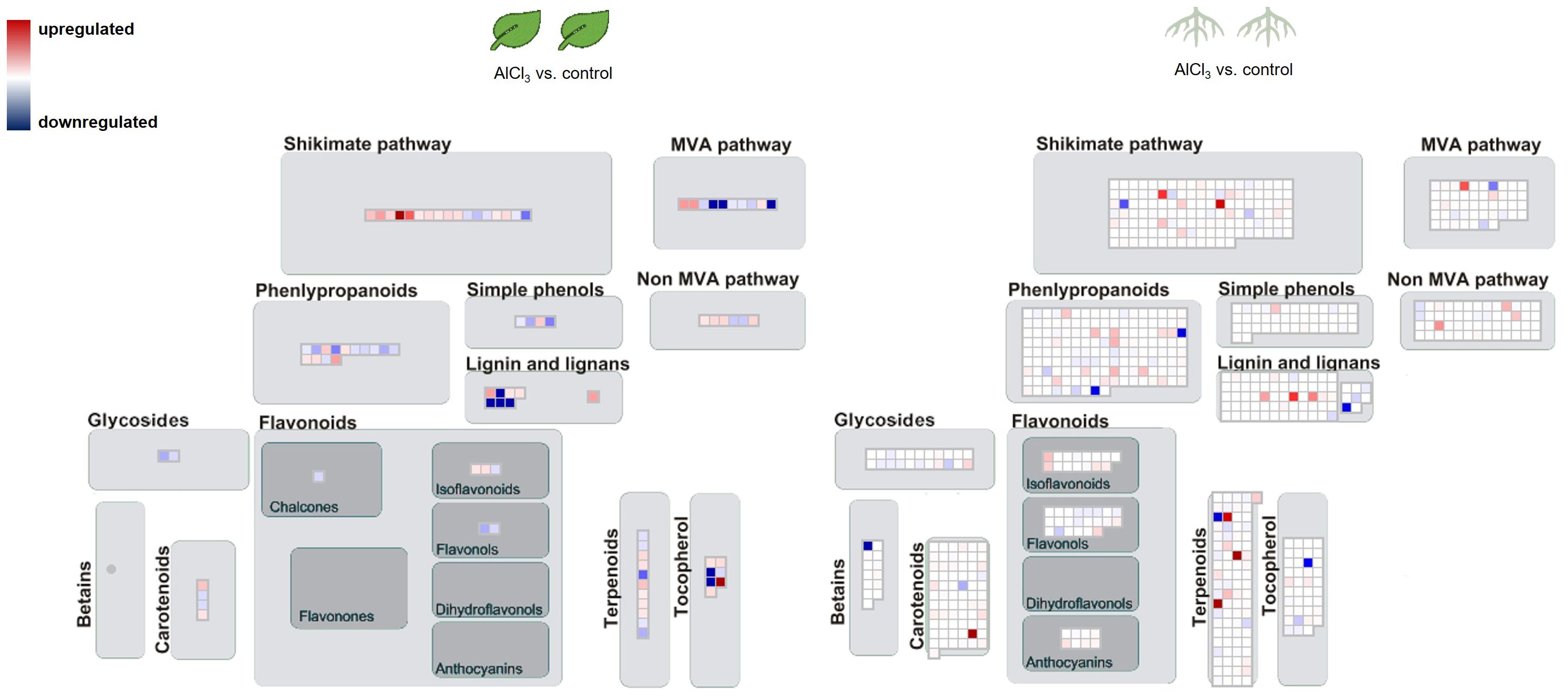
Figure 4. Pathway map for H. serrata specialised metabolism and differential expressed genes (DEGs) from roots treated with aluminium chloride (AlCl3) compared to control roots and from leaves treated with AlCl3 compared to control leaves. DEGs are shown as coloured squares in a MapMan-based image. Each square stands for one DEG or isoform. The genes were mapped on the pathway image based on biological categories of the encoded proteins which were summarized using Mercator4. The false colour scale represents log2 fold change >0 in red and <−0 in blue for adjusted p-value <1. Each condition group consists of four biological replicates from H. serrata. The plants were treated with tap water in the control group and with 100 mM AlCl3-solution in the treatment group for 5 days.
The MapMan image (Figure 6) depicts a larger overview of the genes which were regulated in roots_AlCl3 compared to leaves_AlCl3. Differential expression with log2 fold change <−2 and >2 and adjusted p-value <0.05 occurred in gene sets related to metabolism of terpenes, flavonoids, and other phenylpropanoids. Many shikimate, MVA pathway, carotenoid, tocopherol, and phenylpropanoid synthesis genes were downregulated, same as in the flavanone, flavanol, dihydroflavonol, glycoside, and for some genes in chalcone, tocopherol, and carotenoid syntheses. Betain, a few carotenoid, terpenoid, lignin, one lignan, isoflavonoid, and anthocyanin synthesis-related genes were upregulated (Figure 5). Two interesting genes were upregulated. They encode for one lignin, monolignol biosynthesis involved caffeoyl-CoA 3-O-methyltransferase (2.3) and one (CCoA-OMT) terpenoid biosynthesis, mevalonate pathway involved acetyl-CoA C-acyltransferase (2.2).
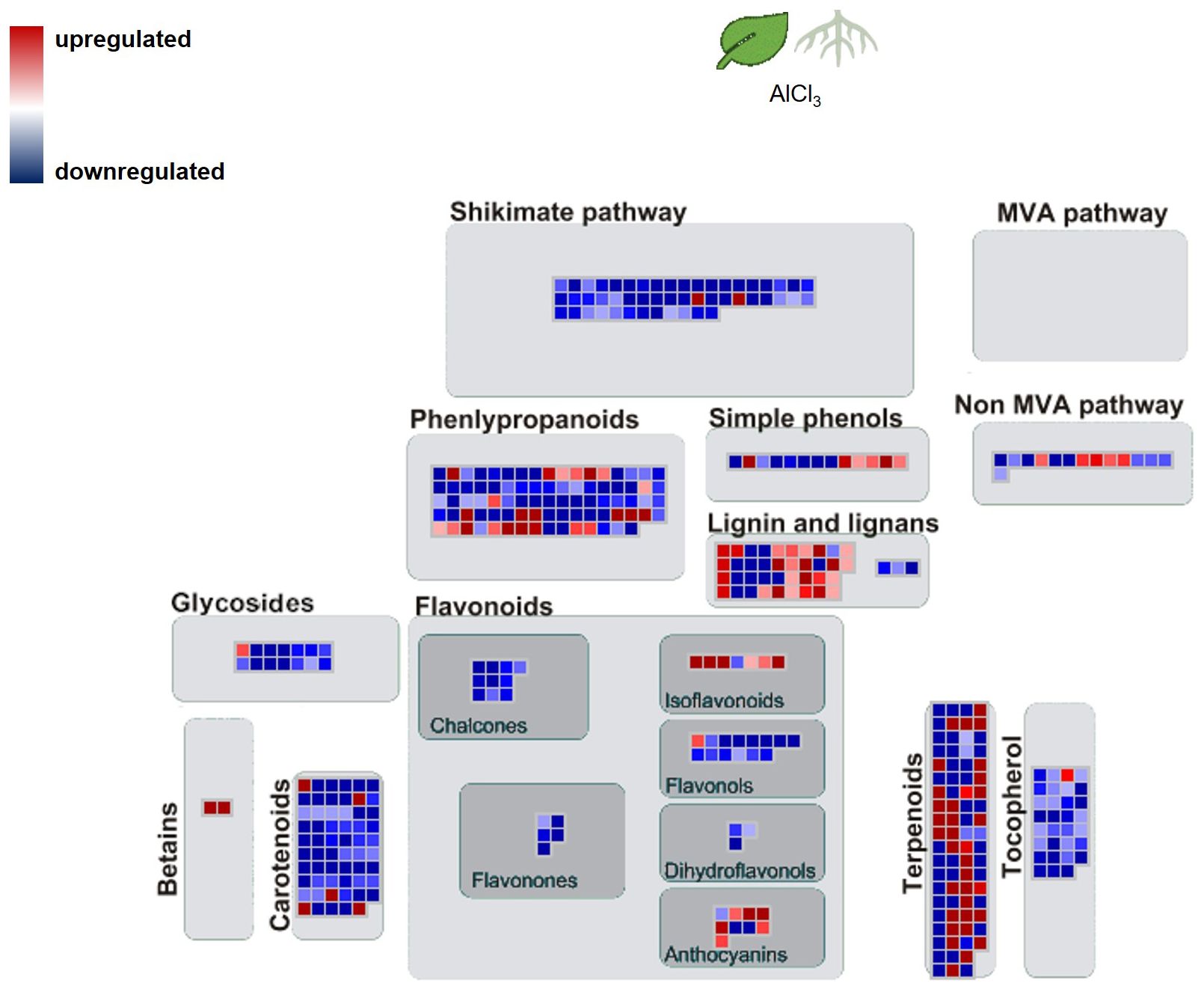
Figure 5. Pathway map of H. serrata specialised metabolism and differential expressed genes (DEGs) from and roots compared to leaves aluminium chloride (AlCl3) treatment group. Coloured squares are shown in MapMan-based image. Each square stands for one differentially regulated gene or isoform. The genes were mapped on the pathway image based on biological categories of the encoded proteins which were summarized using Mercator4. The false colour scale represents log2 fold change >2 in red and <−2 in blue for p-value adjusted <0.05.
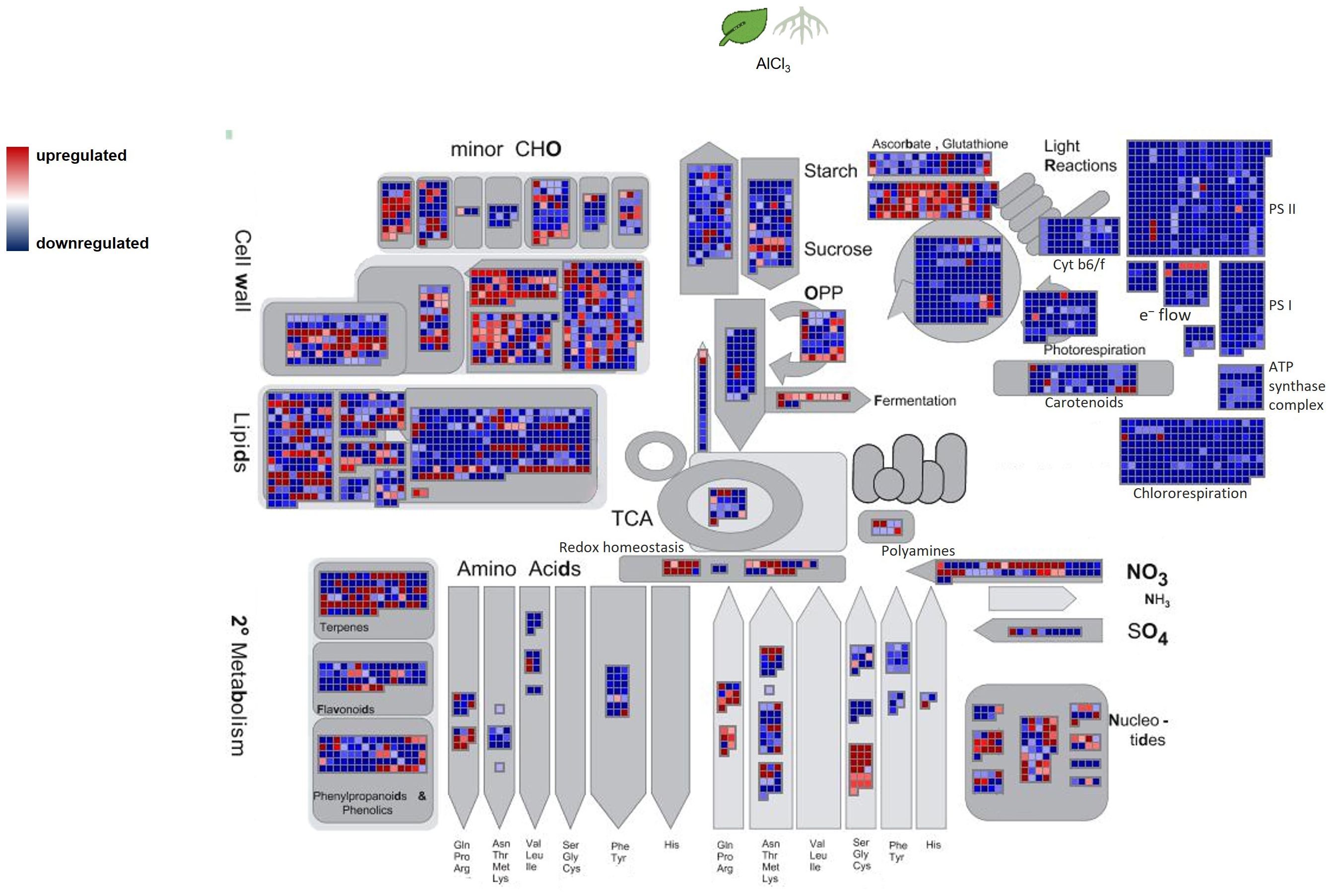
Figure 6. Overview of H. serrata metabolism and its involved differentially expressed genes (DEGs) from roots compared to leaves. DEGs are shown as coloured squares in a MapMan-based image. Each square stands for one DEG or isoform. The genes were mapped on the pathway image based on biological categories of the encoded proteins which were summarized using Mercator4. The. The false colour scale represents log2 fold change >2 in red and <−2 in blue for p-value adjusted <0.05.
3.3 LC-MS analysis of organ specific dihydroisocoumarin levels
The Illumina NextSeq2000 cDNA sequencing was followed by a general transcriptome analysis. In DHIC biosynthesis or among PKS homologues, no differential gene expression was observed. It was the aim of this study to decipher differences on DHIC level.
No significant alteration as a response to aluminium chloride treatment, in terms of DHICs was detected, in the LC-MS analysis of the methanolic extracts. The metabolite analysis shows an organ specific accumulation profile of DHICs and mono- as well as di-glycosidic versions (Table 3). Also, a small elicitor study using yeast extract solution on H. serrata leaves, as well as a drought experiment and jasmonic acid treatment neither enhanced HYD nor PHY in the LC-MS measurement of leaf or root extracts (data not shown). Interestingly, the plants showed signs of stress response in specialised metabolites. One sign was the accumulation of anthocyanins, which was visible to the eye in the experiment that combined high light and drought. Another observation was the formation of necrosis in leaves treated with yeast-elicitor solution (data not shown). The DHIC content was not affected in the described experiments (data not shown).
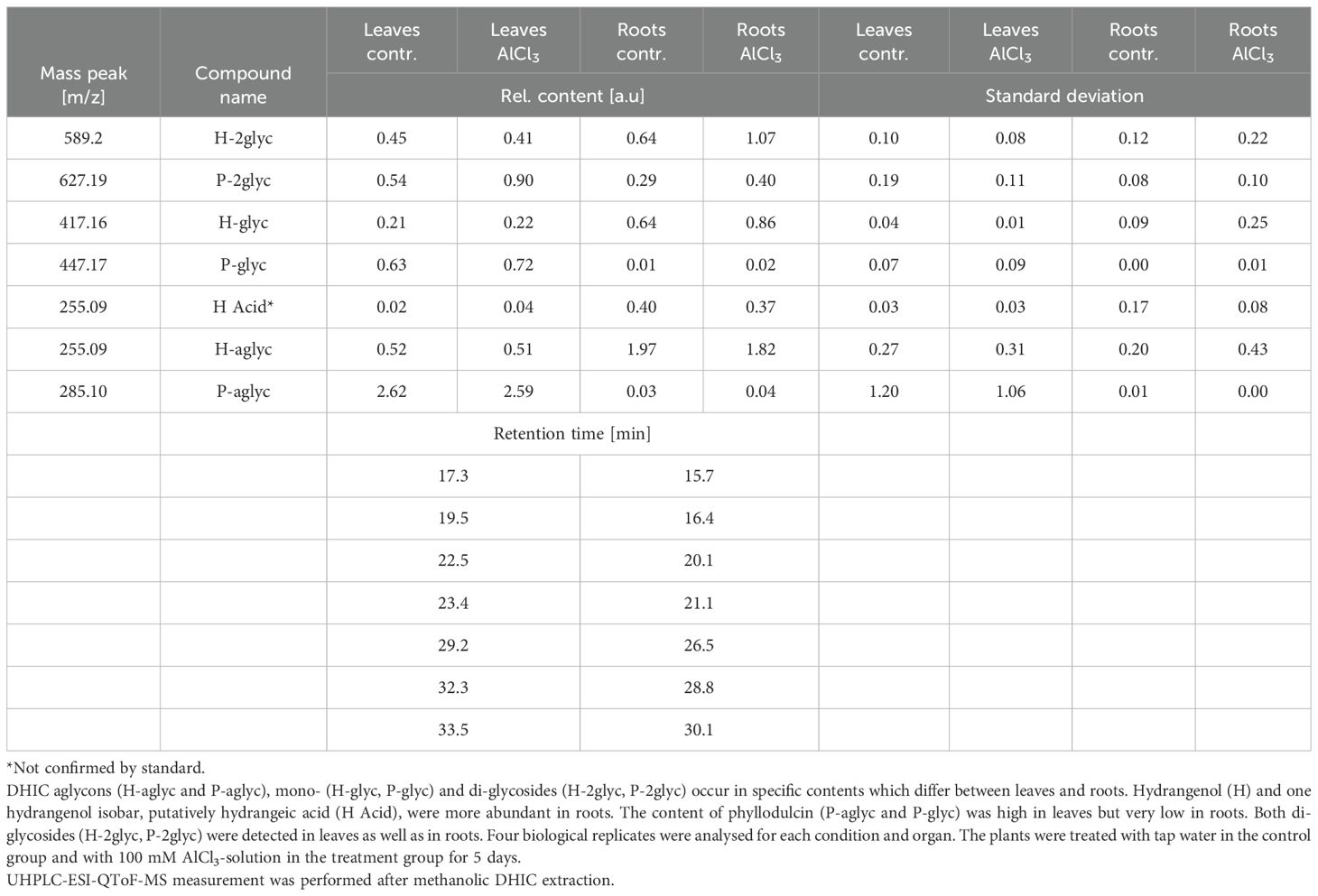
Table 3. Relative content of dihydroisocoumarins (DHICs) in extracts from aluminium chloride (AlCl3) treated and control Hydrangea serrata leaves and roots.
4 Discussion
Illumina NextSeq 2000 sequencing of cDNAs is a powerful technique to gain insight into differences in gene expression related to plant specialised metabolism under abiotic stress. This study is based on paired-end reads which were sequenced in two technical replicates from sixteen cDNA libraries, consisting of four biological replicates for each condition applied on two H. serrata organs. A total of 380,000 different transcripts was proposed as genes by Trinity out of 580,770 assembled contigs from H. serrata leaf and root sequencing data. For roots from H. macrophylla 256,127 unigenes of 401,215 contigs were reported (Chen et al., 2015). This transcriptome study examined two tissues under aluminium influence and we saw that a higher number of genes were expressed in leaves than in roots. AlCl3, in some studies, has been reported to be toxic to plants (Rahman and Upadhyaya, 2021). It changed gene expression as a stress response and especially effected the roots (Zhou et al., 2016). A response of H. serrata under AlCl3 treatment detected as DEGs was found in roots and additionally in leaves. Similar genes encoding transporter proteins which were found in H. serrata were described for H. macrophylla roots and studies on other plants (Chen et al., 2015; Wu et al., 2023; Zhang et al., 2019; Zhou et al., 2016).
The transcriptional changes under aluminium chloride treatment were visualized using the MapMan software. Combined with the high-quality functional annotation via data from A. thaliana and a Mercator4 summarization, the DEGs were categorized according to the biological context of their functional annotation. Signs of DHIC biosynthesis were not visible in differential gene expression against our expectation. In contrast, genes encoding for enzymes of the terpenoid biosynthesis and cell wall lignification were upregulated.
From the context of specialised metabolism many genes were downregulated in roots and only a few were upregulated related to isoflavonol, anthocyanin, carotene, and betaine synthesis. Additionally, lignin- and lignan biosynthesis related genes were upregulated in both tissues. Enhanced lignin synthesis is known as a part of stress defence in plants (Moura et al., 2010; Sasaki et al., 1996; Vanholme et al., 2012). Metal ions cause oxidative stress as ROS in plants. The data show evidence that ROS detoxifying mechanisms were activated or enhanced. Genes from the sectors of glutathione synthesis, sulfur containing amino acids, and redox homeostasis were upregulated in roots, but not in leaves.
Tissue-specific alterations in roots_ AlCl3 versus control roots showed that genes encoding for genes related to betain, tocopherol, and phenylpropanoid metabolisms were downregulated. Genes related to MVA, MEP, non-MVA, carotenoid, and shikimate pathway were upregulated. Phenylpropanoid synthesis-involved genes were less expressed than expected. It is remarkable that no homologue of Type III PKS was among DEGs under AlCl3 treatment. Moreover, genes from terpenoid and lignin metabolism were upregulated. Upregulated DEGs from leaves_ AlCl3 versus control leaves belong to MVA, shikimate, tocopherol, and minor to phenylpropanoid pathways. In addition to various phenylpropanoids, the terpenoids are known to have phytoalexin functions (Chen et al., 2019; Li et al., 2015).
The external stimulation for H. serrata DHICs seems difficult because little is known about the biological purpose in the plant. Studies on highlight or shading experiments did not effectuate a change in the DHIC content in leaves (Moll et al., 2021). Also, herbivore attacks did not enhance the DHIC content (Ujihara et al., 1995). Aluminium chloride is another stressor that did not affect the DHIC biosynthesis. A recent study shows that neither ethylene nor salicylic acid had an influence on the HYD and PHY accumulation in H. serrata leaves. The HYD and PHY contents in leaves were enhanced due to a methyl jasmonic acid treatment (Preusche et al., 2022). So, methyl jasmonic acid seems to be the right elicitor for the induction of the DHIC biosynthesis and it needs further investigation in the discovery of genes involved in the DHIC biosynthesis and the biological assignment of DHICs.
Important bioactive terpenoids were isolated from hydrangeas, but they were not investigated as phytoalexins. The isolated terpenoids from H. macrophylla were identified as secoiridoid glycosides, loganin, secologanin, secologanic acid, and sweroside (Gousiadou et al., 2016; Yoshikawa et al., 1994). Secoiridoid glycosides and hydrangeosides A and C were isolated from H. serrata leaves (Inouye et al., 1980; Kikuchi et al., 2008). Other terpenoids then described in literature may exist as inducible phytoalexins. The use of terpenoids as phytoalexins in response to aluminium chloride in H. serrata would explain that less transcriptional changes related to phenylpropanoid metabolism were detected in this study. Even this extensive transcriptome dataset for this study on H. serrata was generated, its analysis in terms of DHIC was limited. The most limiting factor is the rareness of 3,4-DHICs in Hydrangea species and in plant kingdom at all and therefore a lack of suitable reference enzymes in forms of annotated PKS.
5 Conclusion
Many aspects about the main mechanisms of aluminium chloride detoxification in hydrangea which were described in other studies were observed in this study as well. It is important to mention that transporter and cell wall stability related genes were differentially expressed as a similar study on H. macrophylla showed. This work was more focussed on the specialised metabolites and especially in DHICs. Interestingly, H. serrata in contrast to H. macrophylla showed changes in the terpenoid biosynthesis as response to aluminium chloride. In terms of DHIC biosynthesis little references on genetic level are available to fully explore the differential gene expression.
Data availability statement
The original contributions presented in the study are publicly available. This data can be found at the National Center for Biotechnology Information (NCBI) using accession number PRJNA1061562.
Author contributions
AS: Conceptualization, Methodology, Project administration, Writing – review & editing, Data curation, Formal analysis, Investigation, Visualization, Writing – original draft. HS: Data curation, Formal analysis, Software, Writing – review & editing. PV: Writing – review & editing, Formal analysis. BW: Writing – review & editing, Resources, Supervision, Validation. MB: Formal analysis, Writing – review & editing, Software. JN: Writing – review & editing, Visualization. HB: Writing – review & editing, Funding acquisition, Project administration, Supervision. KN: Conceptualization, Methodology, Project administration, Writing – review & editing, Funding acquisition, Resources, Supervision.
Funding
The author(s) declare financial support was received for the research, authorship, and/or publication of this article. This work was funded by the state of North Rhine Westphalia (NRW) and the “European Regional Development Fund (EFRE)”, Project “ Cluster Industrial Biotechnology (CLIB) Kompetenzzentrum Biotechnologie (CKB)” 34.EFRE- 0300095/1703FI04.
Acknowledgments
The authors would like to acknowledge and thank the gardeners of the Faculty of Biology at University Bielefeld (Bielefeld, Germany) for assistance in plant care. Viktoria Fuchs helped as student coworker to collect and prepare samples for LC-MS measurement and total RNA extraction in the workgroup Proteome and Metabolome Research, Faculty of Biology at University Bielefeld (Bielefeld, Germany). We thank the Center for Biotechnology (CeBiTec) at Bielefeld University for providing an environment to perform the computational analyses.
Conflict of interest
The authors declare that the research was conducted in the absence of any commercial or financial relationships that could be construed as a potential conflict of interest.
Publisher’s note
All claims expressed in this article are solely those of the authors and do not necessarily represent those of their affiliated organizations, or those of the publisher, the editors and the reviewers. Any product that may be evaluated in this article, or claim that may be made by its manufacturer, is not guaranteed or endorsed by the publisher.
Supplementary material
The Supplementary Material for this article can be found online at: https://www.frontiersin.org/articles/10.3389/fpls.2024.1412189/full#supplementary-material
References
Adrian, M., Jeandet, P., Bessis, R., Joubert, J. M. (1996). Induction of phytoalexin (Resveratrol) synthesis in grapevine leaves treated with aluminum chloride (AlCl 3 ). J. Agric. Food Chem. 44, 1979–1981. Available at: https://pubs.acs.org/sharingguidelines.
Akiyama, T., Shibuya, M., Liu, H. M., Ebizuka, Y. (1999). p-Coumaroyltriacetic acid synthase, a new homologue of chalcone synthase, from Hydrangea macrophylla var. thunbergii. Eur. J. Biochem. 263, 834–839. doi: 10.1046/j.1432-1327.1999.00562.x
Andrews, S. (2010). FastQC: A quality control tool for high throughput sequence data. Available at: http://www.bioinformatics.babraham.ac.uk/projects/fastqc/
Asahina, Y., Asano, J. (1929). Über die Konstitution von Hydrangenol und Phyllodulcin. Eur. J. Inorg. Chem. 62, 171–177.
Baier, R., Schiene, K., Kohring, B., Flaschel, E., Niehaus, K. (1999). Alfalfa and tobacco cells react differently to chitin oligosaccharides and Sinorhizobium meliloti nodulation factors. Planta 210, 157–164. doi: 10.1007/s004250050665
Bray, N. L., Pimentel, H., Melsted, P., Pachter, L. (2016). Near-optimal probabilistic RNA-seq quantification. Nat. Biotechnol. 34, 525–527. doi: 10.1038/nbt.3519
Chen, H., Lu, C., Jiang, H., Peng, J. (2015). Global transcriptome analysis reveals distinct aluminum-tolerance pathways in the al-accumulating species hydrangea macrophylla and marker identification. PLoS One, 101–20. doi: 10.1371/journal.pone.0144927
Chen, X., Liu, F., Liu, L., Qiu, J., Fang, D., Wang, W., et al. (2019). Characterization and evolution of gene clusters for terpenoid phytoalexin biosynthesis in tobacco. Planta 250, 1687–1702. doi: 10.1007/s00425-019-03255-7
Cheng, C.-Y., Krishnakumar, V., Chan, A. P., ßoise Thibaud-Nissen, F., Schobel, S., Town, C. D. (2016). Araport11: a complete reannotation of the Arabidopsis thaliana reference genome. Plant J. 89, 789–804. doi: 10.1111/tpj.13415
Çiçek, S. S., Vitalini, S., Zidorn, C. (2018). Natural phenyldihydroisocoumarins: sources, chemistry and bioactivity. Natural Product Commun. 13, 279–288. doi: 10.1177/1934578X1801300306
D’Auria, J. C., Gershenzon, J. (2005). The secondary metabolism of Arabidopsis thaliana: growing like a weed. Curr. Opin. Plant Biol. 8, 308–316. doi: 10.1016/J.PBI.2005.03.012
Ebel, J. (1986). Phytoalexin synthesis: the biochemical analysis of the induction process. Ann. Rev. Phytopathol. 24. Available at: www.annualreviews.org.
Eckermann, C., Schröder, G. S., Eckermann, S., Strack, D., Schmidt, J. J., Schneider, B., et al. (2003). Stilbenecarboxylate biosynthesis: a new function in the family of chalcone synthase-related proteins. Phytochemistry 62, 271–286. Available at: www.elsevier.com/locate/phytochem.
Francini, A., Giro, A., Ferrante, A. (2019). Biochemical and molecular regulation of phenylpropanoids pathway under abiotic stresses. Plant Signaling Molecules: Role Regul. under Stressful Environments, 183–192. doi: 10.1016/B978-0-12-816451-8.00011-3
Freedman, A., Weeks, N. (2020). Best Practices for De Novo Transcriptome Assembly with Trinity. Harvard 462 FAS InfomaricsHarvard 462 FAS Infomarics. Available at: https://informatics.fas.harvard.edu/best-practices-for-de-novo-transcriptome-463assembly-with-trinity.html
Gousiadou, C., Li, H. Q., Gotfredsen, C., Jensen, S. R. (2016). Iridoids in Hydrangeaceae. Biochemical 465 Systematics and Ecology 64, 122–130. doi: 10.1016/j.bse.2015.12.002
Grabherr, M. G., Haas, B. J., Yassour, M., Levin, J. Z., Thompson, D. A., Amit, I., et al. (2011). Full-length transcriptome assembly from RNA-Seq data without a reference genome. Nat. Biotechnol. 29, 644–652. doi: 10.1038/nbt.1883
Gundlach, H., Muller, M. J., Kutchan, T. M., Zenk, M. H. (1992). Jasmonic acid is a signal transducer in elicitor-induced plant cell cultures. Plant Biol. 89. doi: 10.1073/pnas.89.6.2389
Haas, B. J., Papanicolaou, A., Yassour, M., Grabherr, M., Blood, P. D., Bowden, J., et al. (2013). De novo transcript sequence reconstruction from RNA-seq using the Trinity platform for reference generation and analysis. Nat. Protoc. 8, 1494–1512. doi: 10.1038/nprot.2013.084
Hahlbrock, K., Scheel, D. (1989). Physiology and molecular biology of phenylpropanoid metabolism. Annu. Rev. Plant Physiol. Plant Mol. Biol. 40, 347–369. doi: 10.1146/annurev.pp.40.060189.002023
Hanawa, F., Tahara, S., Mizijtani, J. (1992). Antifungal stress compounds from veratrum grandiflorum leaves treated with cupric chloride. Phytochemistry 31. doi: 10.1016/0031-9422(92)83436-3
Hashimoto, Y., Moriyasu -, M., Kanai, Y., Mirase, M. (1979). High-performance liquid chromatographic determination of the sweet component in sweet hydrangea. Phytochemistry. 26, 3323–3330. doi: 10.1016/S0021-9673(01)95343-9
Herrmann, K. M., Weaver, L. M. (1999). THE SHIKIMATE PATHWAY. Annu. Rev. Plant Physiol. Plant Mol. Biol. 50. Available at: www.annualreviews.org.
Hossain, A., Maitra, S., Sarker, S., Al Mahmud, A., Ahmad, Z., Emon, R. M., et al. (2023). “Aluminium stress in plants: Consequences and mitigation mechanisms,” in Beneficial Chemical Elements of Plants: Recent Developments and Future Prospects (wiley), 123–168. doi: 10.1002/9781119691419.ch6
Ibrahim, R. K., Towers, G. H. N. (1962). Studies of hydrangenol in Hydrangea macrophylla Ser. II. Biosynthesis of hydrangenol from C14-labelled compounds. BCan. J. Biochem. Physiol. 40, 449–453.
Inouye, H., Takeda, Y., Uesato, S., Uobe, K., Hashimoto, T., Shingu, T. (1980). A novel type secoiridoid glucoside, hydrangenoside a from hydrangea macrophylla. Tetrahedron 21, 1051–1062.
Ito, T., Aoki, D., Fukushima, K., Yoshida, K. (2019). Direct mapping of hydrangea blue-complex in sepal tissues of Hydrangea macrophylla. Sci. Rep. 9. doi: 10.1038/s41598-019-41968-7
Jung, C. H., Kim, Y., Kim, M. S., Lee, S., Yoo, S. H. (2016). The establishment of efficient bioconversion, extraction, and isolation processes for the production of phyllodulcin, a potential high intensity sweetener, from sweet hydrangea leaves (Hydrangea macrophylla Thunbergii). Phytochemical Anal. 27, 140–147. doi: 10.1002/pca.2609
Kikuchi, M., Kakuda, R., Kikuchi, M., Yaoita, Y. (2008). Three New Glycosides from the Leaves of 506 Hydrangea macrophylla subsp. serrata (THUNB.) MAKINO 1). Chem. Pharm. Bull. 56, 610–611.
Krueger, F. (2015). Trim galore. A wrapper tool around Cutadapt and FastQC to consistently apply quality and adapter trimming to FastQ files. Available at: https://github.com/FelixKrueger/TrimGalore
Kuć, J., Rush, J. S. (1985). Phytoalexins. Arch. OF Biochem. AND BIOPHYSICS 236, 455–472. doi: 10.1016/0003-9861(85)90648-4
Li, R., Tee, C. S., Jiang, Y. L., Jiang, X. Y., Venkatesh, P. N., Sarojam, R., et al. (2015). A terpenoid phytoalexin plays a role in basal defense of Nicotiana benthamiana against Potato virus X. Sci. Rep. 5. doi: 10.1038/srep09682
Liu, J., Nakamura, S., Zhuang, Y., Yoshikawa, M., Mohamed, G., Hussein, E., et al. (2013). Medicinal Flowers. XXXX. 1) Structures of Dihydroisocoumarin Glycosides and Inhibitory Effects on Aldose Reducatase from the Flowers of Hydrangea macrophylla var. thunbergii. Chem. Pharm. Bull. 61. doi: 10.1248/cpb.c13-00160
Love, M. I., Huber, W., Anders, S. (2014). Moderated estimation of fold change and dispersion for RNA-seq data with DESeq2. Genome Biol. 15. doi: 10.1186/s13059-014-0550-8
Matern, U. (1991). Coumarins and other phenylpropanoid compounds in the defense response of plant cells. Planta Med. 57, 15–20. doi: 10.1055/s-2006-960224
Moll, M. D., Vieregge, A. S., Wiesbaum, C., Blings, M., Vana, F., Hillebrand, S., et al. (2021). Dihydroisocoumarin content and phenotyping of hydrangea macrophylla subsp. Serrata cultivars under different shading regimes. Agronomy 11. doi: 10.3390/agronomy11091743
Moura, J. C. M. S., Bonine, C. A. V., de Oliveira Fernandes Viana, J., Dornelas, M. C., Mazzafera, P. (2010). Abiotic and biotic stresses and changes in the lignin content and composition in plants. J. Integr. Plant Biol. 52, 360–376. doi: 10.1111/j.1744-7909.2010.00892.x
Preusche, M., Ley, J., Schulz, M., Hillebrand, S., Blings, M., Theisen, A., et al. (2022). Culture methods for high hydrangenol and phyllodulcin contents in Hydrangea macrophylla subsp. serrata (Thunb.) Makino. Eur. J. Hortic. Sci. 87, 1–12. doi: 10.17660/eJHS.2022/057
Rahman, R., Upadhyaya, H. (2021). Aluminium toxicity and its tolerance in plant: A review. J. Plant Biol. 64, 101–121. doi: 10.1007/s12374-020-09280-4. Springer Science and Business Media Deutschland GmbH.
Saddiqa, A., Usman, M., Çakmak, O. (2017). Isocoumarins and 3,4-dihydroisocoumarins, amazing natural products: A review. Turkish J. Chem. 41, 153–178. doi: 10.3906/kim-1604-66
Sasaki, M., Yamamoto, Y., Matsumoto, H. (1996). Lignin deposition induced by aluminum in wheat (Triticum aestivum) roots. Physiologia Plantarum 96, 193–198. doi: 10.1111/j.1399-3054.1996.tb00201.x
Schilbert, H. M., Schöne, M., Baier, T., Busche, M., Viehöver, P., Weisshaar, B., et al. (2021). Characterization of the Brassica napus flavonol synthase gene family reveals bifunctional flavonol synthases. Front. Plant Sci. 12. doi: 10.3389/fpls.2021.733762
Schmidlin, L., Poutaraud, A., Claudel, P., Mestre, P., Prado, E., Santos-Rosa, M., et al. (2008). A stress-inducible resveratrol O-methyltransferase involved in the biosynthesis of pterostilbene in grapevine. Plant Physiol. 148, 1630–1639. doi: 10.1104/pp.108.126003
Schreiber, H. D., Jones, A. H., Lariviere, C. M., Mayhew, K. M., Cain, J. B. (2011). Role of aluminum in red-to-blue color changes in Hydrangea macrophylla sepals. Biometals 24, 1005–1015. doi: 10.1007/s10534-011-9458-x
Schröder, J., Schröder, G. (1990). Stilbene and chalcone synthases: related enzymes with key functions in plant-specific pathways. Z. Naturforsch. 45, 1–8. doi: 10.1515/znc-1990-1-202
Schwacke, R., Ponce-Soto, G. Y., Krause, K., Bolger, A. M., Arsova, B., Hallab, A., et al. (2019). MapMan4: A refined protein classification and annotation framework applicable to multi-omics data analysis. Mol. Plant 12, 879–892. doi: 10.1016/J.MOLP.2019.01.003
Simão, F. A., Waterhouse, R. M., Ioannidis, P., Kriventseva, E. V., Zdobnov, E. M. (2015). BUSCO: Assessing genome assembly and annotation completeness with single-copy orthologs. Bioinformatics 31, 3210–3212. doi: 10.1093/bioinformatics/btv351
Song, L., Florea, L. (2015). Rcorrector: efficient and accurate error correction for Illumina RNA-seq reads. GigaScience 4, 48. doi: 10.1186/s13742-015-0089-y
Ujihara, M., Shinozaki, M., Kato, M. (1995). Accumulation of phyllodulcin in sweet-leaf plants of hydrangea serrata and its neutrality in the defence against a specialist leafmining herbivore. Popul. Ecol. 37. doi: 10.1007/BF02515827
Usadel, B., Poree, F., Nagel, A., Lohse, M., Czedik-Eysenberg, A., Stitt, M. (2009). A guide to using MapMan to visualize and compare Omics data in plants: A case study in the crop species, Maize. Plant Cell Environ. 32, 1211–1229. doi: 10.1111/j.1365-3040.2009.01978.x
Vanholme, R., Storme, V., Vanholme, B., Sundin, L., Christensen, J. H., Goeminne, G., et al. (2012). A systems biology view of responses to lignin biosynthesis perturbations in Arabidopsis W. Plant Cell 24, 3506–3529. doi: 10.1105/tpc.112.102574
Wang, R., Duan, D., Metzger, C., Zhu, X., Riemann, M., Pla, M., et al. (2022). Aluminum can activate grapevine defense through actin remodeling. Horticulture Res. 9. doi: 10.1093/hr/uhab016
Wu, B. S., Huang, W. T., Rao, R. Y., Chen, W. S., Hua, D., Lai, N. W., et al. (2023). Integrated analysis of transcriptome, metabolome and physiology revealed the molecular mechanisms for elevated pH-mediated-mitigation of aluminum-toxicity in Citrus sinensis leaves. Scientia Hortic. 322. doi: 10.1016/j.scienta.2023.112391
Yamato, M., Hashigaki, K. (1979). Chemical structure and sweet taste of isocoumarins and related compounds. Chem. Senses Flavour 4, 35–47. Available at: https://academic.oup.com/chemse/article-abstract/4/1/35/345737.
Yoshikawa, M., Matsuda, H., Shimoda, H. (1994). Development of bioactive functions in hydrangeae dulcis folium. III. On the antiallergic and antimicrobial principles of hydrangeae dulcis folium. Thunberginols A, B, an. Phd F. Chemarm. Bull. 42, 2225–2230.
Zhang, F., Yan, X., Han, X., Tang, R., Chu, M., Yang, Y., et al. (2019). A defective vacuolar proton pump enhances aluminum tolerance by reducing vacuole sequestration of organic acids 1. Plant Physiol. 181, 743–761. doi: 10.1104/pp.19.00626
Keywords: Hydrangea serrata, aluminium stress, differential transcriptome analysis, secondary metabilites, dihydrocoumarin
Citation: Scholpp A-C, Schilbert HM, Viehöver P, Weisshaar B, Beckstette M, Neumann JM, Bednarz H and Niehaus K (2024) Differential gene expression in leaves and roots of Hydrangea serrata treated with aluminium chloride. Front. Plant Sci. 15:1412189. doi: 10.3389/fpls.2024.1412189
Received: 09 April 2024; Accepted: 02 August 2024;
Published: 03 September 2024.
Edited by:
Dev Mani Pandey, Birla Institute of Technology, Mesra, IndiaReviewed by:
Gursharn Singh Randhawa, Sardar Bhagwan Singh Post Graduate Institute of Biomedical Science & Research, IndiaGholamreza Khaksar, Chulalongkorn University, Thailand
María Ayelén Pagani, National Scientific and Technical Research Council (CONICET), Argentina
Copyright © 2024 Scholpp, Schilbert, Viehöver, Weisshaar, Beckstette, Neumann, Bednarz and Niehaus. This is an open-access article distributed under the terms of the Creative Commons Attribution License (CC BY). The use, distribution or reproduction in other forums is permitted, provided the original author(s) and the copyright owner(s) are credited and that the original publication in this journal is cited, in accordance with accepted academic practice. No use, distribution or reproduction is permitted which does not comply with these terms.
*Correspondence: Karsten Niehaus, a25pZWhhdXNAY2ViaXRlYy51bmktYmllbGVmZWxkLmRl
†ORCID: Bernd Weisshaar, orcid.org/0000-0002-7635-3473
Karsten Niehaus, orcid.org/0000-0003-4078-9870