- 1Graduate School of Integrative Science and Engineering, Tokyo City University, Setagata, Tokyo, Japan
- 2Department of Science and Engineering, Tokyo City University, Setagata, Tokyo, Japan
Adaptation of Farfugium japonicum (L.) Kitam. var. japonicum (Asteraceae) to the strong wind environment of coastal areas has been shown to reduce lamina size and shorten petioles; however, their effects on other traits of this species remain unknown. Our morphological analyses showed that shortening of the scape of this species is correlated with shortening of the petiole in coastal areas. The results suggested that when the height of the scapes became higher than that of the petioles, the wind stress on the scapes became stronger and their growth was suppressed. Therefore, the populations in coastal areas with strong winds had significantly shorter scapes than inland populations, and the height of petioles and scapes in the coastal populations were correlated. Further mechanical analysis by three-point bending tests revealed that the scapes had higher strength than the petioles. This species is evergreen and can produce new leaves regardless of the season, even if it loses its leaves by strong winds; however, because scapes only develop above ground for a limited period of the year, the loss of the scapes by strong winds has a significant impact on reproduction in that year. Therefore, even though the scapes were stronger than the petiole, shortening the scapes plays an important role in reducing strong wind stress in coastal areas.
1 Introduction
The adaptations of each plant organ to the environment have been central to the study of plant diversification. Fitness is an important means of evaluating plant adaptation to various environmental factors (Buonaiuto and Wolkovich, 2021; Creux et al., 2021), and serves as an index for evaluating the adaptability of plant morphological and anatomical characteristics in specific environments and the contribution of individual plants to the production of the next generation (Strauss, 1997). For example, plant groups that inhabit riverside areas have narrower leaves to resist and/or avoid flashing floods after heavy rains (Imaichi and Kato, 1992; Yamada et al., 2011; Ueda et al., 2012; Yokoyama et al., 2012; Ohga et al., 2012a; Matsui et al., 2013; Shiba et al., 2021; Shiba and Fukuda, 2024), and plants in serpentine areas exhibit severe morphological and anatomical limitations related to plant growth (Brady et al., 2005; Hayakawa et al., 2012; Ohga et al., 2012b; Kumekawa et al., 2013; Shiba et al., 2022a; 2022b). Wind is one of the most ubiquitous environmental stresses on many levels in natural ecosystems (Mylo and Speck, 2023), and the avoidance of wind stress using flexible and easily reconfigurable structures can be an alternative strategy that contributes to maintaining fitness (Biddington, 1986; Anten et al., 2009). How plants adapt or acclimate to such variable external forces depends on the intensity and frequency of stress as well as on plant structures. Some previous studies have indicated that plant responses to wind typically lowered photosynthesis (Ennos, 1997; Burgess et al., 2016), decreased leaf area (Anten et al., 2010; Shiba et al., 2023) and entailed inhibition of stem elongation and increased in stem diameter to stabilize the plant mechanically (Jaffe and Forbes, 1993; Telewski and Pruyn, 1998; Coutand and Moulia, 2000; Anten et al., 2010; Bang et al., 2010; Niez et al., 2019), and wind stress affects various aspects of plant life history such as development, growth, pollination, seed dispersal, insect herbivory and reproductive yield (Ennos, 1997; Wilcock and Neiland, 2002; Hodges et al., 2004; Fernandez and Hilker, 2007; Turbill, 2008; Takizawa et al., 2022; Shiba et al., 2022c, 2023).
Coastal areas are characterized by open, sandy, or rocky habitats with weakly developed soils. These characteristics can lead to the modification of the morphologies and plant species growing in the coastal regions of the world as they need to be adapted to an environment in which drought and wind strongly affect plant growth (Greenway and Munns, 1980). Some previous studies have been conducted on adaptation to drought (Tunala et al., 2012; Kumekawa et al., 2013; Ohga et al., 2013; Sunami et al., 2013; Takizawa et al., 2022; Shiba et al., 2022c, 2023) and wind stress (Meguro and Miyawaki, 1994; Shiba et al., 2023) in the coastal areas. Shiba et al. (2023) indicated that the leopard plant Farfugium japonicum (L.) Kitam. var. japonicum (Asteraceae) (Figure 1), an evergreen perennial herb that occurs from the margin of inland forests to near the coast in Japan (Koyama, 1995), has avoided an increase in wind speed outdoors by reducing the lamina area and petiole length per petiole cross-sectional area without mechanical changes of their petioles to the wind by using populations near meteorological recording stations of the Automated Meteorological Data Acquisition System (AMeDAS) of the Japan Meteorological Agency. However, this study was only part of the strategy against wind stress by linking the leaf morphology of F. japonicum and meteorological information in the field (Shiba et al., 2023), and it is questionable whether the reductions in these traits alone are sufficient to adapt F. japonicum var. japonicum to wind stress in coastal areas. The evaluation of other morphological traits except for these traits is also important for understanding the environmental adaptations of this plant. In this study, the traits of F. japonicum var. japonicum should be the focus of future studies. For example, Khan et al. (2016) reported that root architecture plays an important role in the anchorage capability to withstand toppling and overturning caused by wind loading. Wind not only induces thigmomorphogenesis in plants by altering root architecture (Chehab et al., 2009), but also improves the anchorage of plants by strengthening the development of roots (Stokes et al., 1995; Danjon et al., 2005; Tamasi et al., 2005; Štofko and Kodrík, 2008). In addition, Onoda and Anten (2011) claimed that strong winds could increase root biomass allocation, and other previous studies have indicated that wind affects root growth and biomass allocation to roots (Nicoll and Ray, 1996; Cleugh et al., 1998; Poorter et al., 2012; Gardiner et al., 2016; Feng et al., 2019). By considering these studies, does strong winds also affect the root architecture as in lamina and petiole of F. japonicum var. japonicum? However, it remains unclear whether F. japonicum var. japonicum in coastal areas increases root biomass allocation.
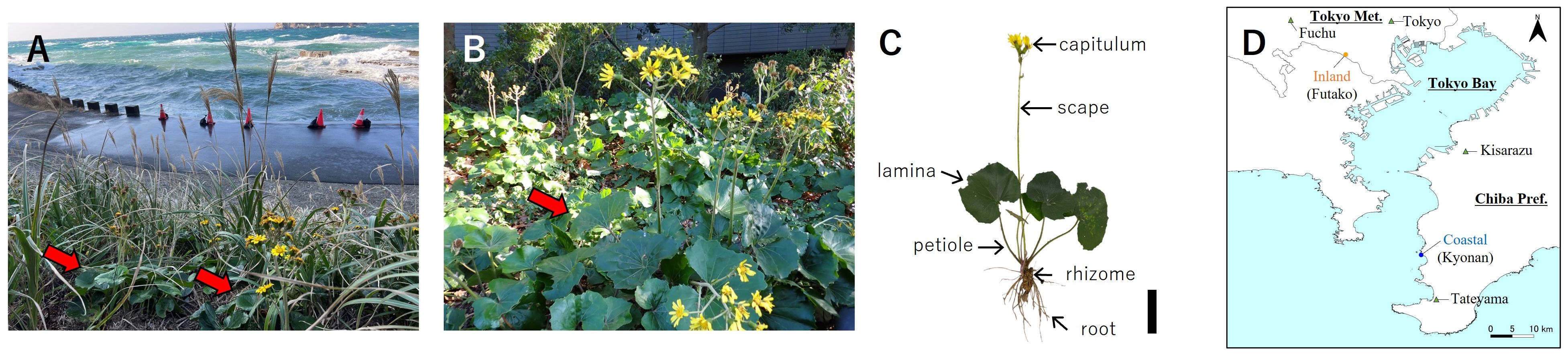
Figure 1. Farfugium japonicum var. japonicum (Asteraceae) at the Kyonan coast (A), and Futako inland (B). Arrows indicate F japonicum. Silhouette of F japonicum (C), scale bar = 10 cm. Sampling sites of F japonicum (D). Regarding sampling sites, orange squares indicate Futako inland and blue circles indicate Kyonan coast. The location of the AMeDAS is indicated by a green triangle.
Floral display is fundamental to plant fitness, which can affect the pollinator visitation rate and also total seed production (Bell, 1985), and is, therefore, under strong stabilizing selection, ensuring phenotypic homeostasis even under conditions of environmental and genetic perturbation (Givnish, 2002). F. japonicum var. japonicum have long scapes from 30 to 75 cm that support the capitulum, blooming yellow flowers from October to December, and an approximately 30 cm long petiole with a lamina approximately 10 cm long and 20 cm wide at the tip of the petiole all year round (Koyama, 1995). Although their radical leaves remain exposed to wind stress for a long period after appearing on the ground, the scape appears on the ground for a shorter period than the petioles. Does the difference in the emergence period reflect the difference in the adaptive traits between radical leaves and scapes? The scape extending from the rhizomes grows independently of the petioles, so even if the petioles become shorter with the same stiffness owing to the influence of strong winds over a long period, does this have a little effect on the morphological and mechanical traits of the scape? To answer this question, an investigation into the morphological and mechanical comparisons of the scapes and petioles, as well as scapes of coastal and inland populations of F. japonicum var. japonicum is needed in order to clarify the differences in petioles and scapes in response to strong winds in coastal areas. Several studies have been conducted on the strength of inflorescences using the model plant Arabidopsis thaliana (L.) Heynh (Yoshida et al., 2019; Nakata et al., 2020), the effect of strong winds on inflorescences or scapes remains unclear.
Many traits should be analyzed related to the adaption to the strong wind environment of coastal areas, in addition to lamina size and petiole length of F. japonicum var. japonicum, which have already been reported by Shiba et al. (2023). However, it is difficult to comprehensively analyze the various traits of F. japonicum var. japonicum. In general, plants are limited by the resources available in their natural environment; it is impossible for plants to achieve the best in all aspects, and they often have to adapt to their environment by making compromises (Funk and Vitousek, 2007). Therefore, as the resource allocation of one organ increases, that of another decreases, meaning that the cost of one organ can be measured by the resource consumption of the other (Reznick, 1985; Jösson and Tuomi, 1994; Wise and Abrahamson, 2017). Only by effectively adjusting resource allocation can plants achieve their optimal performance in complex environments and provide competitive advantages for survival and reproduction (Funk and Vitousek, 2007). Elucidating whether resource allocation is involved in shape differences would provide the basis for avoidance or tolerance to strong wind stress. Therefore, the evaluation of the above-mentioned traits of F. japonicum var. japonicum is one way to demonstrate the adaptation process in the strong wind environments of coastal areas by comparing their resource allocation patterns. The aim of this study is to clarify the effects of strong winds on the above-ground morphology of F. japonicum var. japonicum and its avoidance strategies from both morphological and mechanical aspects.
2 Materials and methods
The collection sites and nearby weather stations and their annual average wind speed are presented in in Figure 1D, Table 1. In the present study, Farfugium japonicum was collected from field populations in coastal and inland areas. The wind speed values are higher on the oceanside (Tateyama 3.40 m s-1, Kisarazu 2.88 m s-1, Tokyo 2.75 m s-1, Fuchu 1.71 m s-1), and the coastal sampling site Kyonan, located between Tateyama and Kisarazu, is assumed to have higher wind speed values than the inland sampling site Futako, which is located between Tokyo and Fuchu. Nomura et al. (2010) showed that there had no genetic differentiation among populations of F. japonicum based on some sequences of Internal Transcribed Spacer (ITS) of nuclear DNA and intergenic regions between trnL and trnF and between trnH and psbA of chloroplast DNA. The study was conducted from November to December during the flowering period of F. japonicum. Almost all roots were dug during collection. Another condition for collection was that the leaf blade and petiole were largely intact, and individuals of various sizes were collected. The field survey and sampling were conducted in areas where there were no collection restrictions under Japanese law.
2.1 Morphological analyses
For morphological analyses, the lamina area was analyzed using the graphic software Fiji (ImageJ Version 1.53e) for radical leaves. Petiole length lpetiole and scape length lscape were measured using digital calipers CD-15APX (Mitutoyo Corp., Kawasaki-shi, Kanagawa, Japan). Cross-sectional area A was calculated using the following formula to approximate it as an ellipse:
where a and b are the long and short diameters of the petiole and scape cross sections, respectively, measured using the digital calipers. The basal cross-sectional area Abase was calculated in the same manner as described in the aforementioned equation. In addition, the cross-sections of petioles and scapes were visually confirmed to be elliptical in shape and were compared for similar elliptical shapes based on the ratio of long/short diameter.
The number of radical leaves per plant was determined. The number of capitulae per scape were counted.
2.2 Dry mass analysis of organs
In general, the net increase in dry matter is the sum of the products of photosynthesis and mineral uptake minus the amount lost in respiration, leaching, guttation, leaf and twig abscission, and death of roots, and much of the discussion on the fitness costs associated with increased competitive ability has concentrated on the effects of dry matter partitioning within the plant (e.g., Poorter et al., 2012). Therefore, many studies have compared the dry weight of each organ to compare the allocation patterns, and we also used this method.
After morphological analyses, the cells were dried in an incubator FS-405 (ADVANTEC Co., Ltd., Tokyo, Japan) set at 75°C for at least 72 hours. The dried flowers (scapes and capitula), radical leaves (lamina and petiole), rhizomes, and roots were immediately weighed [g] using an analytical balance AG204 (Mettler-Toledo, Tokyo, Japan).
Dry mass per unit volume of petioles and scapes was calculated using the following equation:
where Vfresh is the volume of the petioles or scapes measured by the dry mass.
Vfresh was calculated using the following equation:
where h is the height of petioles or scapes. The dry mass per unit volume is an indicator of the cell-wall content of the analyzed organ. The relationship between the dry mass per unit volume and cross-sectional area was analyzed.
In addition, the flowers and leaves were divided into the aboveground part organs and rhizomes and roots were divided into below ground part organs, and the above/below ground part dry mass ratio was analyzed.
2.3 Mechanical analyses of petioles and scapes
A three-point bending test was used for the mechanical testing (Figure 2). Samples used for mechanical testing were processed fresh within 12 hours of collection. The test setup consisted of a tabletop tensile and compression testing machine MCT-1150 (A&D Company, Ltd., Tokyo, Japan) and either an R5 bending test fixture JM-B1-500N(A&D Company, Ltd., Tokyo, Japan) or an R2 bending test fixture JM-B-500N (A&D Company, Ltd., Tokyo, Japan) if the average sample diameter was less than 3 mm. The test speed was set to 10 mm min-1. The termination criterion for the test was set when the displacement of the upper fixture reached 3 mm. The specimens had to be cut for mechanical testing, and the average diameter was measured before the test on the non-tapered portion of the petiole or scape, and the length of the specimen and the distance between spans were set so that the ratio of span/average diameter was “16”. This span-to-diameter ratio condition was expected to yield mechanical results when the general bending theory was applied (Shah et al., 2017).
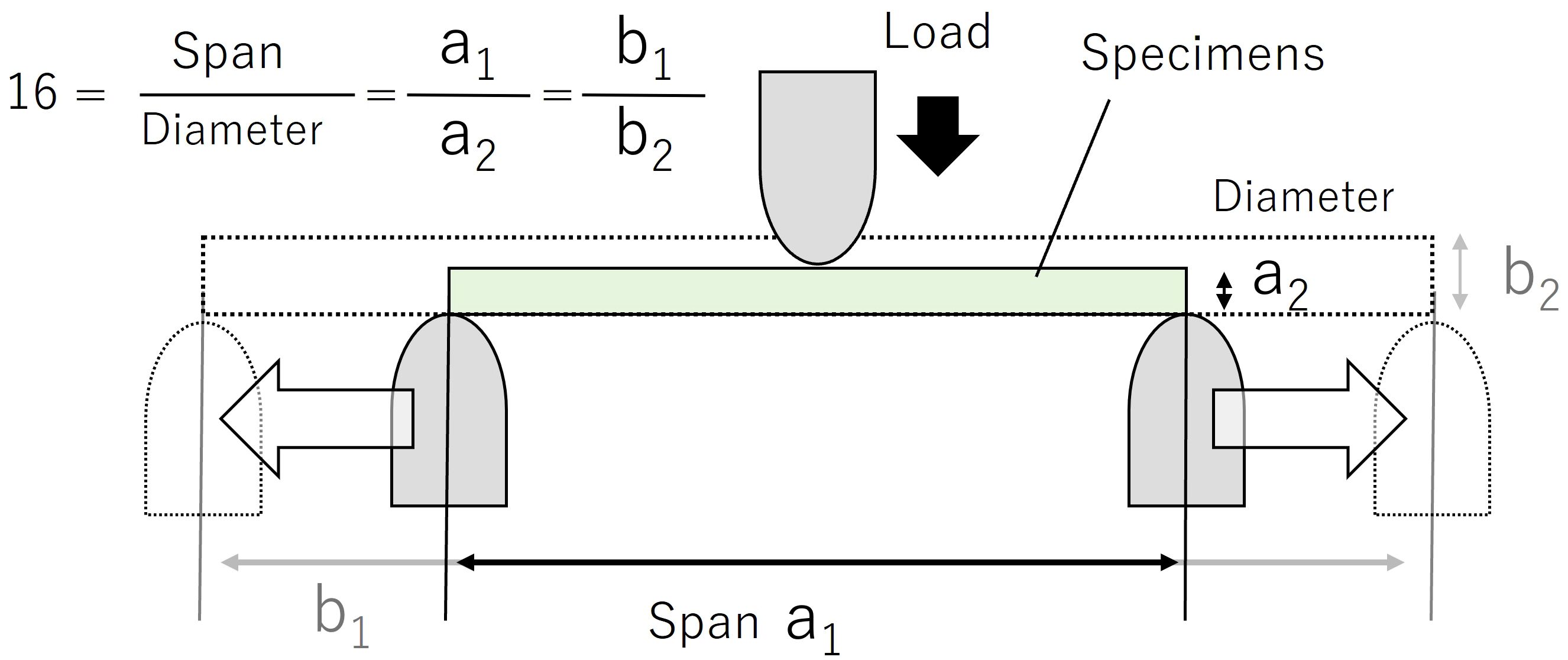
Figure 2. Set-up diagram for a three-point bending test. Move the support pin so that the span/diameter ratio is 16. The load is applied to the center of the specimen at constant velocity.
The mechanical tests in this study were conducted on simply supported beams, so the values of bending stress σ and strain ϵ were calculated using the following equations:
and
where P is the load measured in the three-point bending test, M is bending moment at concentrated load at mid-span, L is the span length, Z is the section modules, δ is the deflection measured in the three-point bending test, and I is the second moment of area. In this study, the dimensionless strain ϵ is converted to %.
The following equations were used to calculate the elliptical section coefficient Z and the axial second moment of area I:
and
The bending modulus of elasticity E was calculated by multiplying the slope of the approximately straight linear part of the stress–strain curve by 100. In addition, the flexural rigidity EI was calculated using the following equation:
Data on specimen sizes are presented in Supplementary Table 1.
2.4 Anatomical analysis
Cross-sectional tissues of petioles and scapes were observed. A manual microtome CMK (Kenis, Ltd., Osaka city, Osaka, Japan) was used to prepare cross-sectional sections of approximately 20 µm thickness. One section of the petiole and scape bases was stained with a 0.05% toluidine blue O solution (pH 7.0) for 1 min and then washed thoroughly with distilled water. Toluidine blue O stains lignified tissue, corky tissue, tannins blue-green, and non-lignified cell walls red-purple (Sakai, 1973). Stained sections were photographed using an optical microscope CX43 (Olympus Corp., Tokyo, Japan) equipped with a digital microscope camera Moticam X3-12V (Shimadzu Rika Corp., Tokyo, Japan).
2.5 Statistical analysis
Statistical analyses were performed using the GNU R 4.3.3 software (R Core Team, 2024). These analyses involved various statistical tests to compare the different groups and examine the relationships between variables. Specifically, we utilized the Shapiro-Wilk test (p<0.05) to assess the normality of the data distribution. When the assumption of normality was violated, we employed the Mann-Whitney U test (p<0.05) to compare two independent groups or the Kruskal-Wallis test (p<0.05) to compare four independent groups. Welch’s t-test (p<0.05) was used when data followed a normal distribution. Additionally, we conducted an analysis of covariance to examine the relationship between the variables while controlling for covariates, we conducted an analysis of covariance (ANCOVA). The correlation coefficient and the coefficient of determination when fitting a linear regression were examined. These statistical methods were selected based on the characteristics of the data and the specific hypotheses tested.
3 Results
3.1 Morphological analysis
Comparison of the longest petiole length (PL) and basal petiole cross-sectional areas (PCA) of individuals indicated no significant difference between the coastal and inland areas (PL; p = 0.22, mean; coastal = 320.85, inland = 362.28: PCA; p = 0.19, mean; coastal = 39.60, inland = 45.76), indicating that petiole length and cross-sectional area were comparable between the two populations (Figures 3A, B, Table 2). We investigated whether the influence of petiole cross-sectional area on petiole length varied depending on the habitat. Analysis of covariance indicated no correlation between petiole cross-sectional area and habitat (p = 0.46). However, significant differences were observed in the intercepts (p< 0.001: Figure 3C).
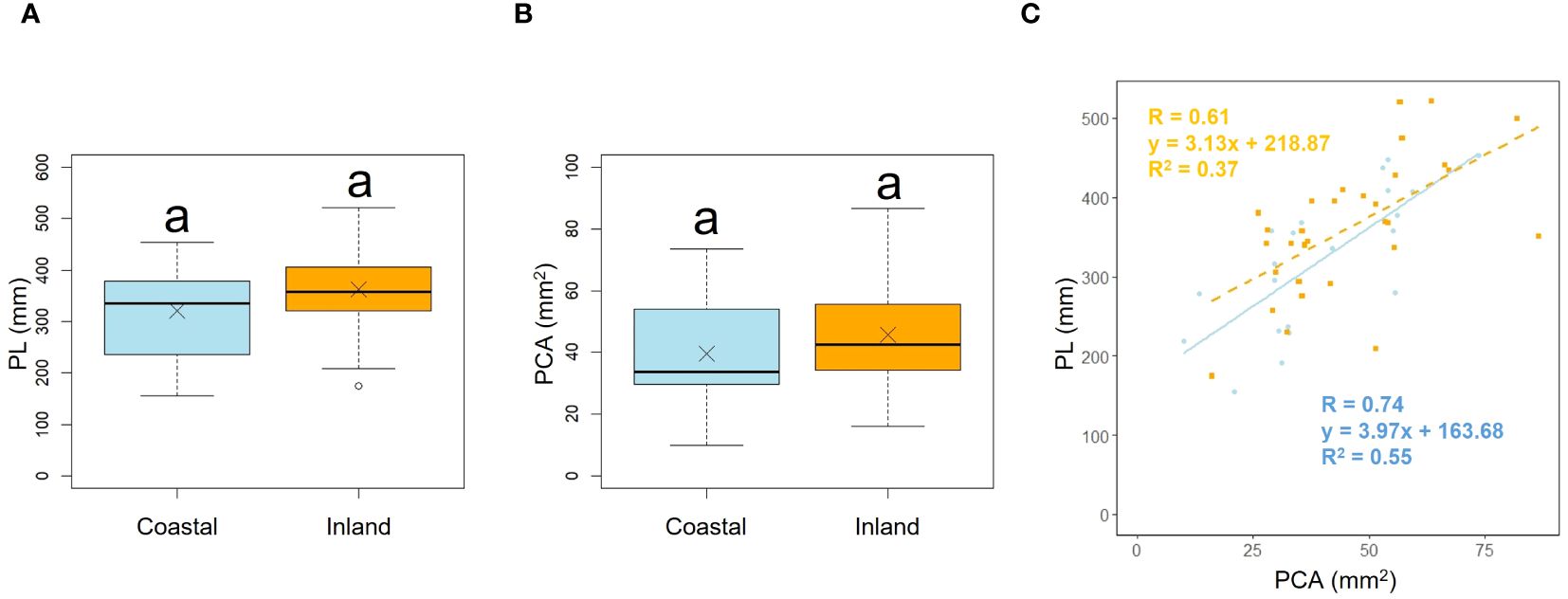
Figure 3. Comparison of the petiole length (PL) (A) and petiole cross-sectional area (PCA) (B) between coastal and inland populations. Following the results of the Shapiro-Wilk test (p< 0.05), the petiole length was compared using the Mann-Whitney U test (p = 0.22), while the petiole cross-sectional area was compared using Welch’s t-test (p = 0.19). Sample numbers of coastal and inland, ncoastal = 21, ninland =31. Box-whisker plots, median, quartiles, outliers; crosses indicate the position of the mean. Same letters indicate no statistically significant difference. PCA vs PL for coastal (blue circle and solid line) and inland (orange square and dashed line) plant samples (PCA: Habitatp = 0.46) (C).
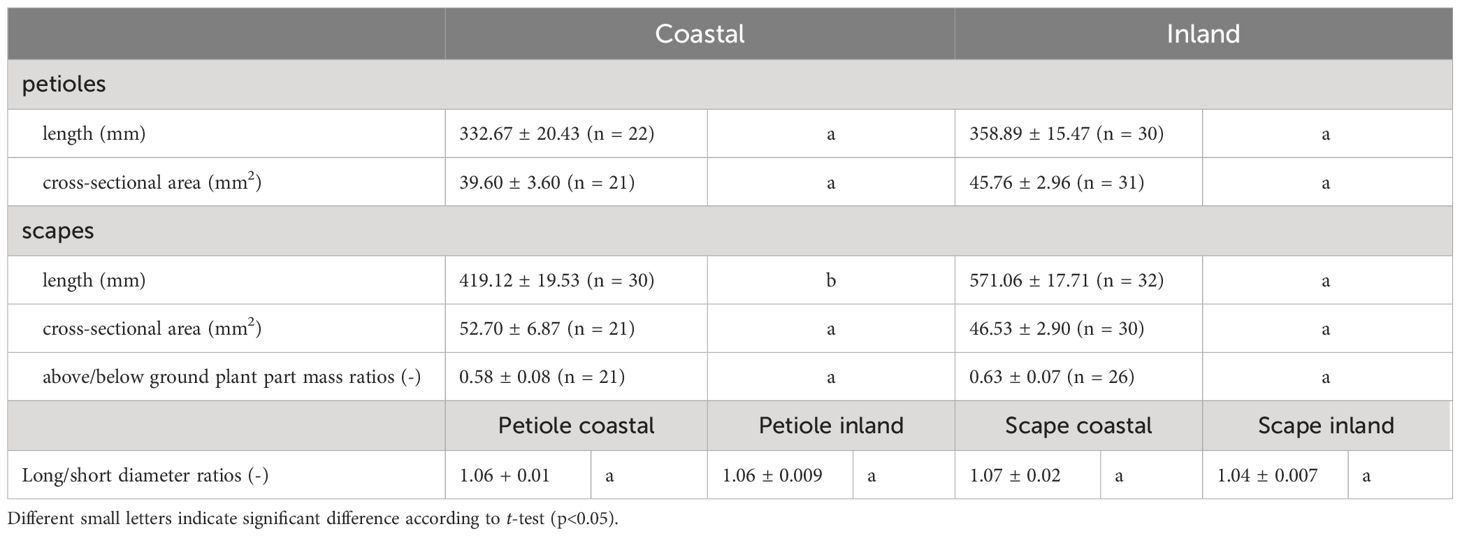
Table 2. Morphological measurements and above/below ground plant part mass ratios (mean ± standard error) of petioles and scapes in Farfugium japonicum.
Scape length (SL) and basal cape cross-sectional area (SCA) were compared. The results indicated that scape length was significantly smaller in the coastal region (SL; p< 0.001, mean; coastal = 434.54, inland = 572.64: Figure 4A, Table 2). However, there was no significant difference in the cross-sectional area of the scape, indicating a similar thickness (SCA; p = 0.90, mean; coastal = 51.69, inland = 46.52: Figure 4B, Table 2). We investigated whether the influence of scape cross-sectional area on scape length varied depending on the habitat. Analysis of covariance indicated no correlation between cross-sectional area and habitat (p = 0.36). However, significant differences were observed in the intercept (p< 0.001: Figure 4C). We examined whether the effect of scape length on capitulum number (CN) varied depending on habitat. The results of comparing the fitting of a linear regression model with a non-linear regression model indicated that the non-linear regression model was a better fit. Analysis of covariance results indicated no correlation between scape length and habitat (p = 0.80: Figure 5).
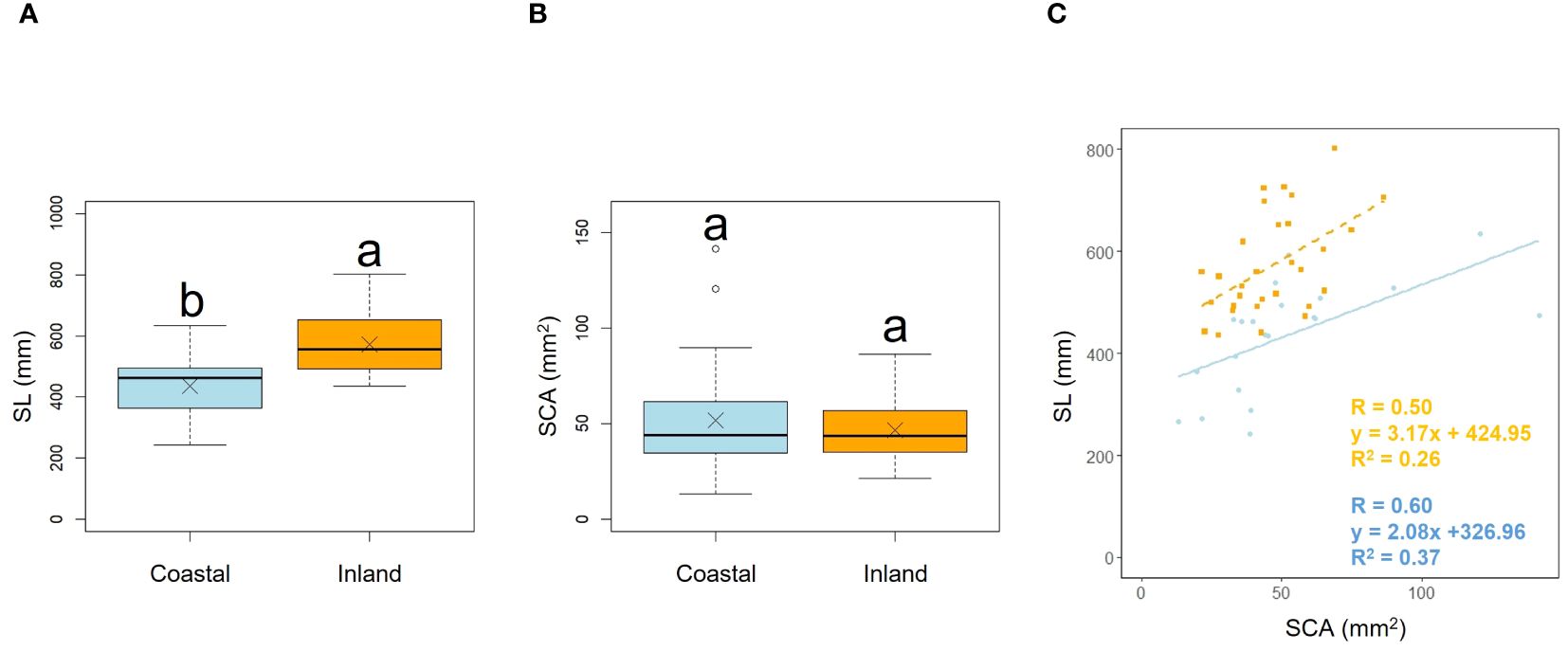
Figure 4. Comparison of scape length (SL) (A) and scape cross-sectional area (SCA) (B) between coastal and inland populations. Following the results of the Shapiro-Wilk test (p< 0.05), the scape length was compared using Welch’s t-test (p< 0.001), while the scape cross-sectional area was compared using the Mann-Whitney U test (p = 0.90). Sample numbers of coastal and inland, ncoastal = 21, ninland =30. Box-whisker plots, median, quartiles, outliers; crosses indicate the position of the mean. Same letters indicate no statistically significant difference. SCA vs. SL for coastal (blue circle and solid line) and inland (orange square and dashed line) plant samples (SCA: Habitat, p = 0.36) (C).
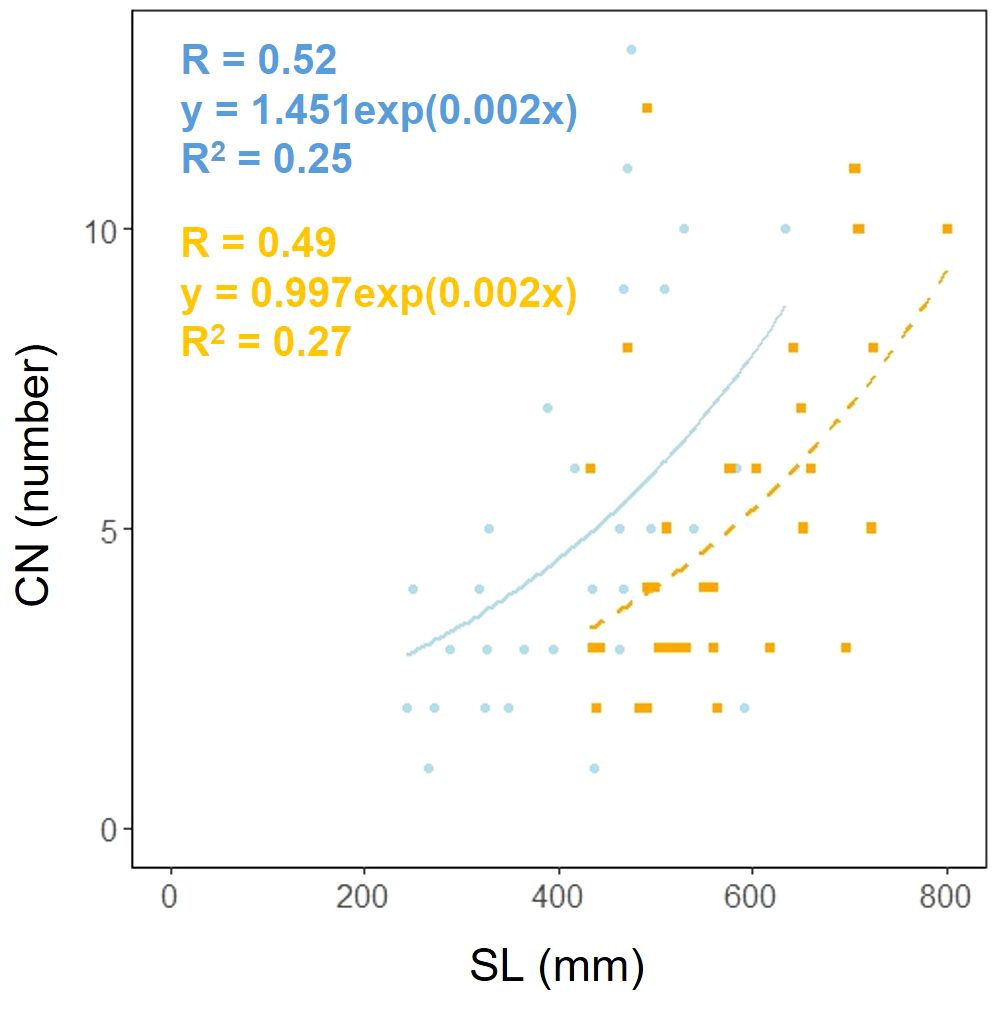
Figure 5. Capitulum number (CN) vs. scape length (SL) for coastal (blue circle and solid line) and inland (orange square and dashed line) plant samples (SL: Habitat, p = 0.80). Sample numbers of coastal and inland, ncoastal = 30, ninland =32.
A comparison of long/short diameter ratios in the cross-sections of petioles and scapes indicated no significant differences between the two populations (p = 0.49, mean; petiole coastal = 1.06, inland = 1.06, scape coastal = 1.07, inland = 1.04), indicating a similar elliptical shape (Figure 6, Table 2).
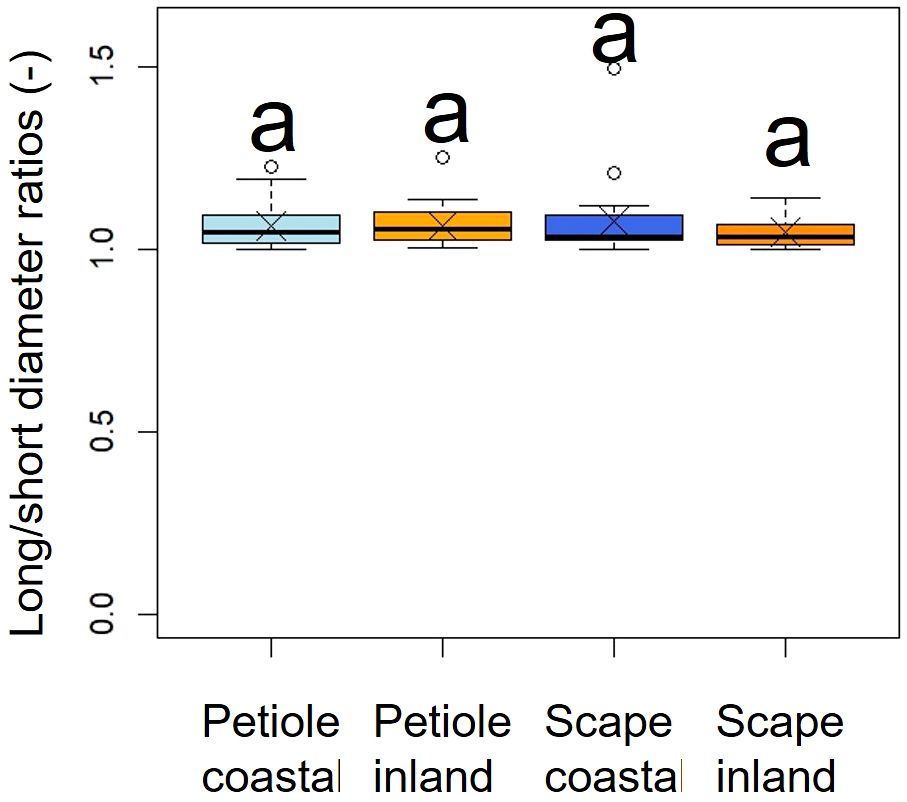
Figure 6. Comparison of long/short diameter ratios in the cross sections of petioles and scapes. The Kruskal-Wallis test indicated no significant difference (p = 0.49); indicated by same letters. Crosses indicate the position of the mean. Petiole; ncoastal = 21, ninland = 31, Scape; ncoastal = 21, ninland = 30. Box-whisker plots, mean, quartiles, outliers; crosses indicate the position of the mean. Same letters indicate no statistically significant difference.
We investigated whether the effect of scape length (SL) on petiole length (PL) varied depending on the habitat. Analysis of covariance showed that there was a correlation between petiole length and habitat (p< 0.05), indicating that different habitats influence the relationship between petiole length and petiole length (Figure 7).
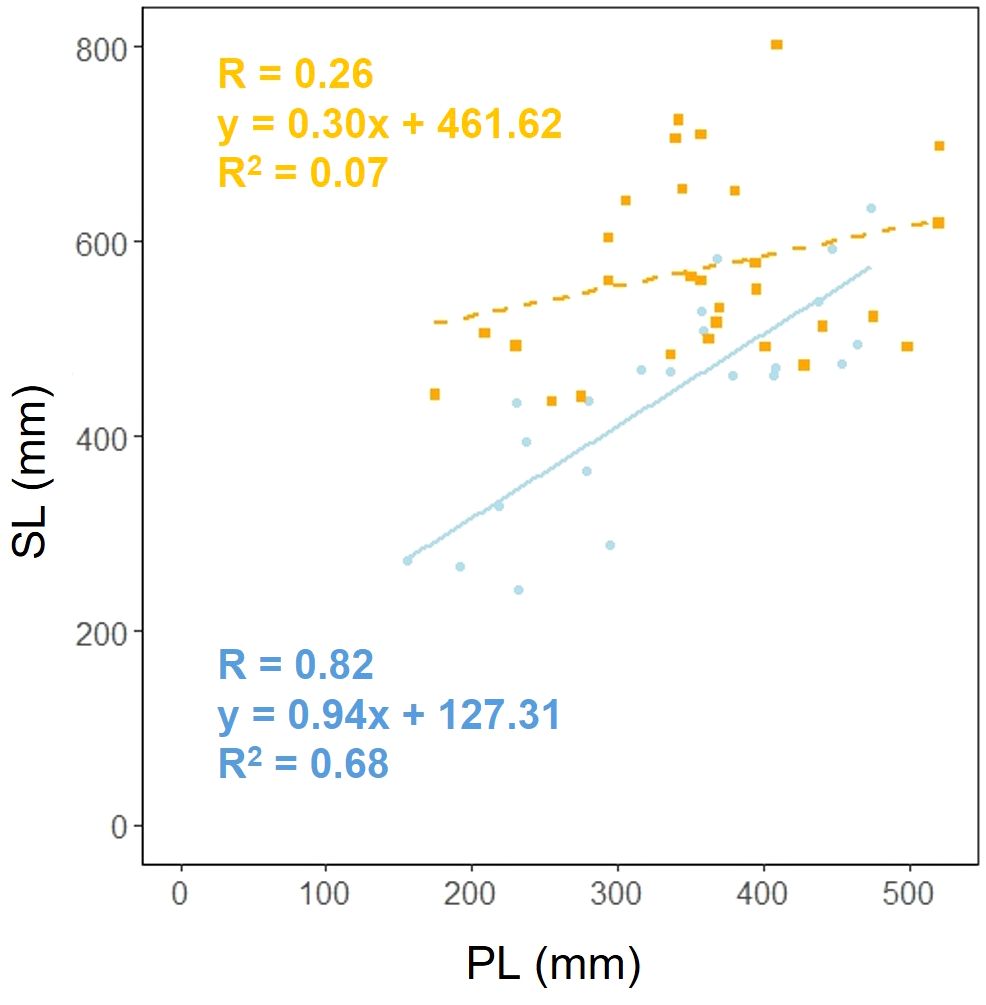
Figure 7. Petiole length (PL) vs. scape length (SL) for coastal (blue circle and solid line) and inland (orange square and dashed line) plant samples (PL: Habitat, p< 0.05). Sample numbers of coastal and inland, ncoastal = 22, ninland = 30.
3.2 Relationship between total dry mass and each morphological trait
A comparison of above/below ground part mass ratios indicated no significant difference between the two populations (p = 0.51, mean; coastal = 0.58, inland = 0.63), indicating similar dry mass ratios (Figure 8, Table 2).
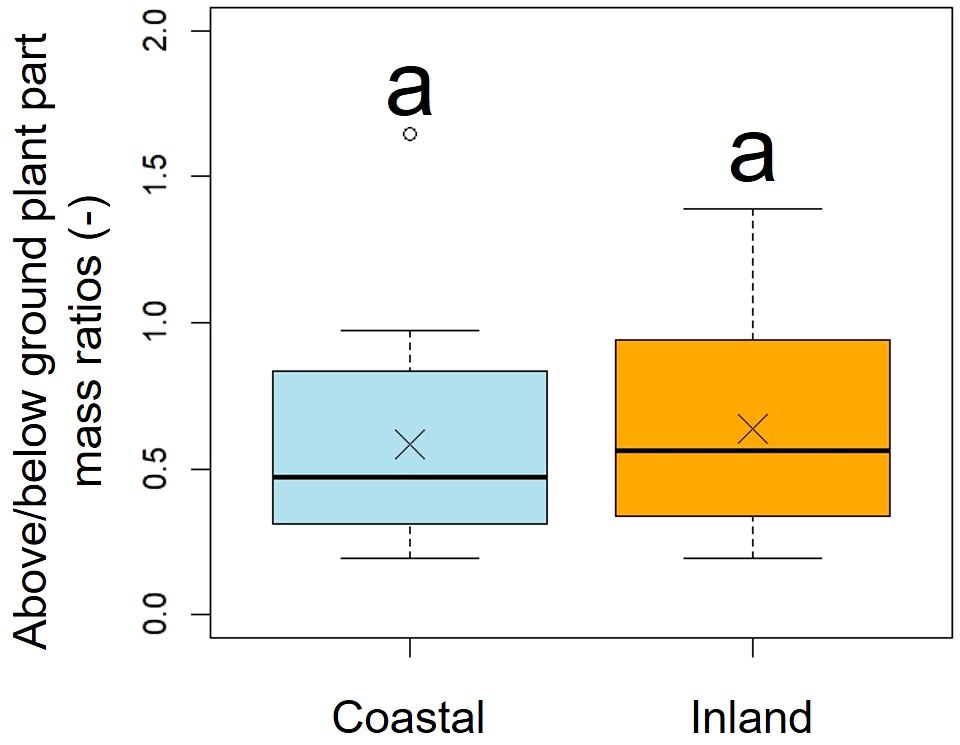
Figure 8. Comparison of the above/below ground plant part mass ratios. Followed the results of the Shapiro-Wilk test (p< 0.05), the above/below ground plant part mass ratios were compared using the Mann-Whitney U test (p = 0.51). Sample numbers of coastal and inland, ncoastal = 21, ninland =26. Box-whisker plots, median, quartiles, outliers; crosses indicate the position of the mean. Same letters indicate no statistically significant difference.
We examined whether the effect of leaf number (LN) on total dry mass varied depending on habitat. Analysis of covariance showed no correlation between total dry mass and habitat (p = 0.90), suggesting that the effect of habitat on the relationship between total dry mass and leaf number is additive (Figure 9A). We investigated whether the effect of total petiole length (TPL) on total dry mass varied depending on habitat. Analysis of covariance showed no correlation between total dry mass and habitat (p = 0.24), indicating that the effect of habitat on the relationship between total dry mass and petiole length is additive (Figure 9B).
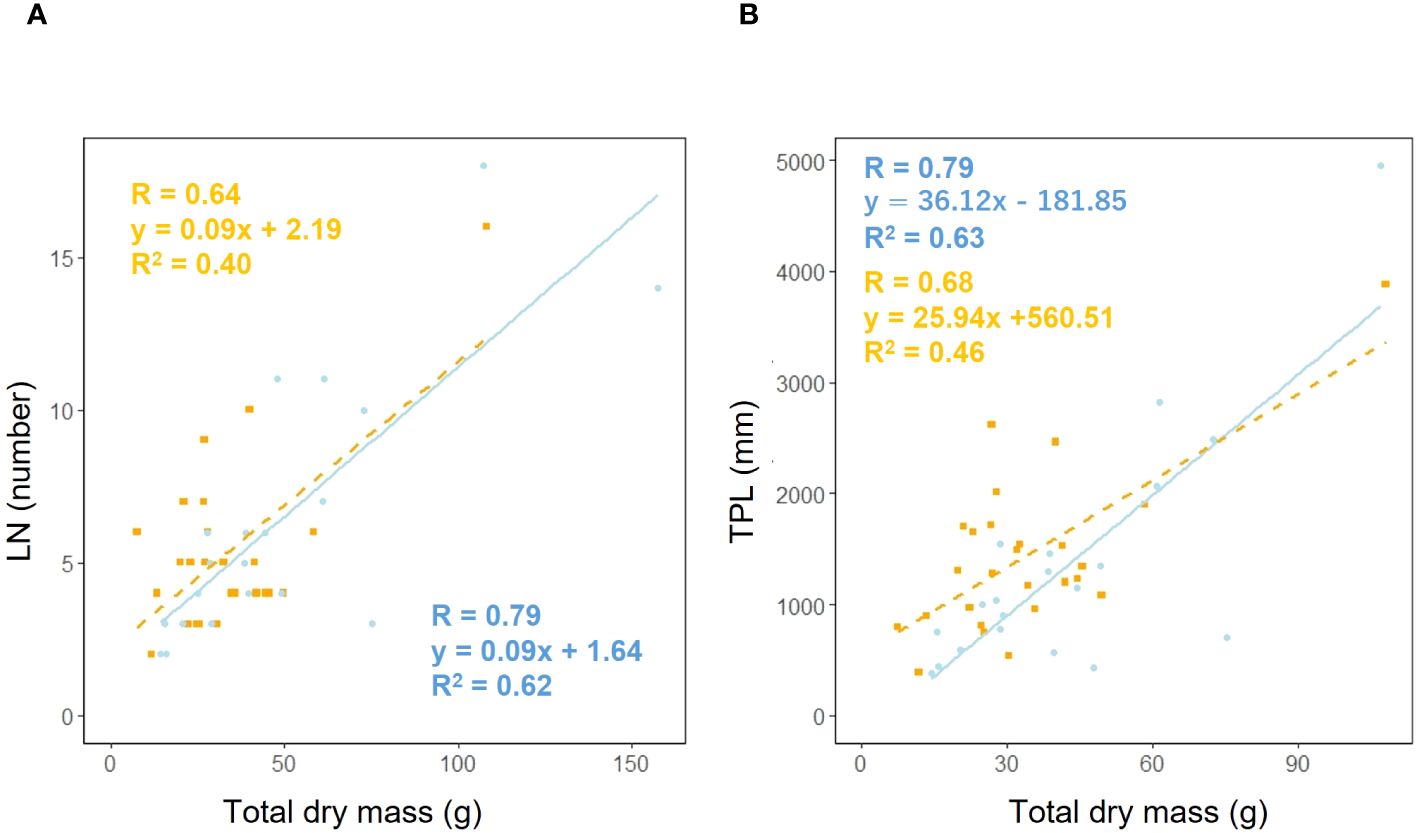
Figure 9. Leaf number (LN) vs. total dry mass (A) for coastal (blue circle and solid line) and inland (orange square) plant samples (total dry mass: Habitat, p = 0.90). Sample numbers of coastal and inland, ncoastal = 21, ninland =26. Total petiole length (TPL) vs. total dry mass (B) for coastal (blue circle and solid line) and inland (orange square and dashed line) plant samples (total dry mass: Habitat, p = 0.24). Sample numbers of coastal and inland, ncoastal = 20, ninland =26.
We examined whether the effect of the cross-sectional area on the dry mass per unit volume ρ in the petioles and scapes varied depending on the organ. The results of comparing the fitting of a linear regression model with a non-linear regression model indicated that the non-linear regression model was a better fit. The exponential approximation formula was also the best fit for the non-linear regression model. Analysis of covariance results indicated no correlation between cross-sectional area and organ (p = 0.90: Figure 10).
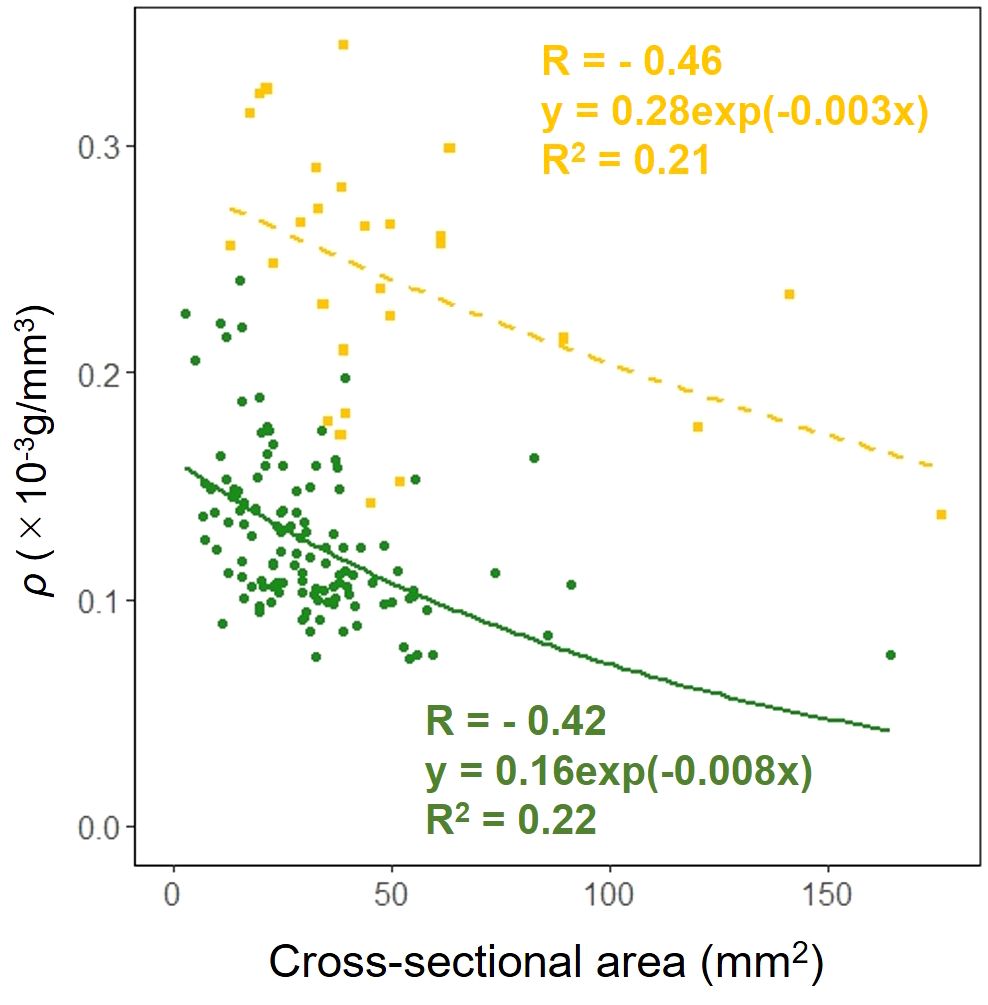
Figure 10. Cell wall mass per unit petiole and scape volume ρ vs base cross-sectional area of petiole (green circle and solid line) and scape (yellow square and dashed line) (cross-sectional area: Habitat, p = 0.90). Sample numbers of petiole and scape, npetiole=123, nscape=28.
3.3 Mechanical properties of petioles and scapes
Comparative results of mechanical properties are shown in Figure 11, Table 3. A comparison of the E values of the scapes were significantly higher than those of the petioles (p < 0.001, mean; petiole = 45.90, scape = 102.00). A comparison of the I values of the scapes were significantly higher than those of the petioles (p < 0.01, mean; petiole = 62.26, scape = 238.59). A comparison of the EI values of the scapes were significantly higher than those of the petioles (p < 0.001, mean; petiole = 2678.84, scape = 24354.46).
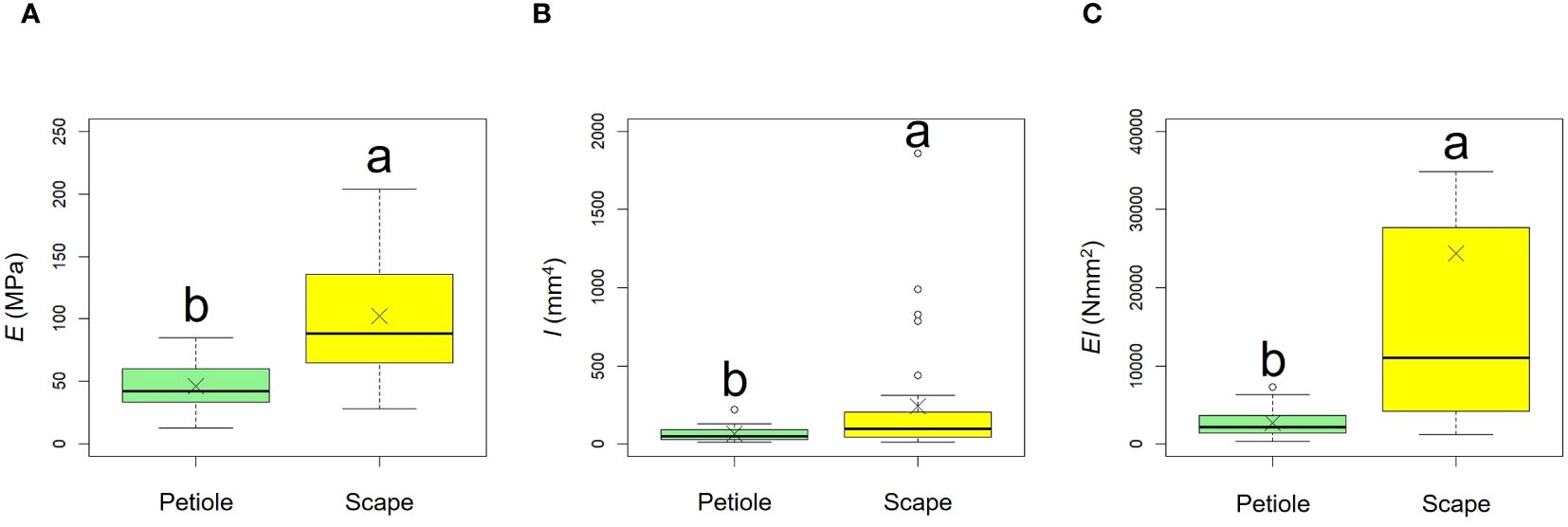
Figure 11. Comparison of the bending modulus of elasticity E (A), axial second moment of area I (B), and flexural rigidity EI (C) between petiole and scape. Following the results of the Shapiro-Wilk test (p< 0.05), the E, I, and EI were compared using the Mann-Whitney U test (E, I, EI: p<0.05). Sample numbers of petiole and scape, npetiole = 35, nscape = 33. Box-whisker plots, mean, quartiles, outliers; crosses indicate the position of the mean. Same letters indicate no statistically significant difference.

Table 3. Mechanical properties (mean ± standard error) measured for the petioles and scapes in Farfugium japonicum.
3.4 Histological structure on petiole and scape cross-section
Histological analysis revealed that the petiole stelar type was an atactostele (Figure 12A), and the scape stelar type was an eustele (Figure 12B). Mucilage channels were observed in petiole. Scape had several vascular arrangements outside of the annular vascular bundle. Toluidine blue staining revealed lignification near the epidermis and vascular bundles in both organs, while parenchyma was non-lignified. Within the vascular bundles, xylem stained blue more than phloem, indicating that it was more lignified.
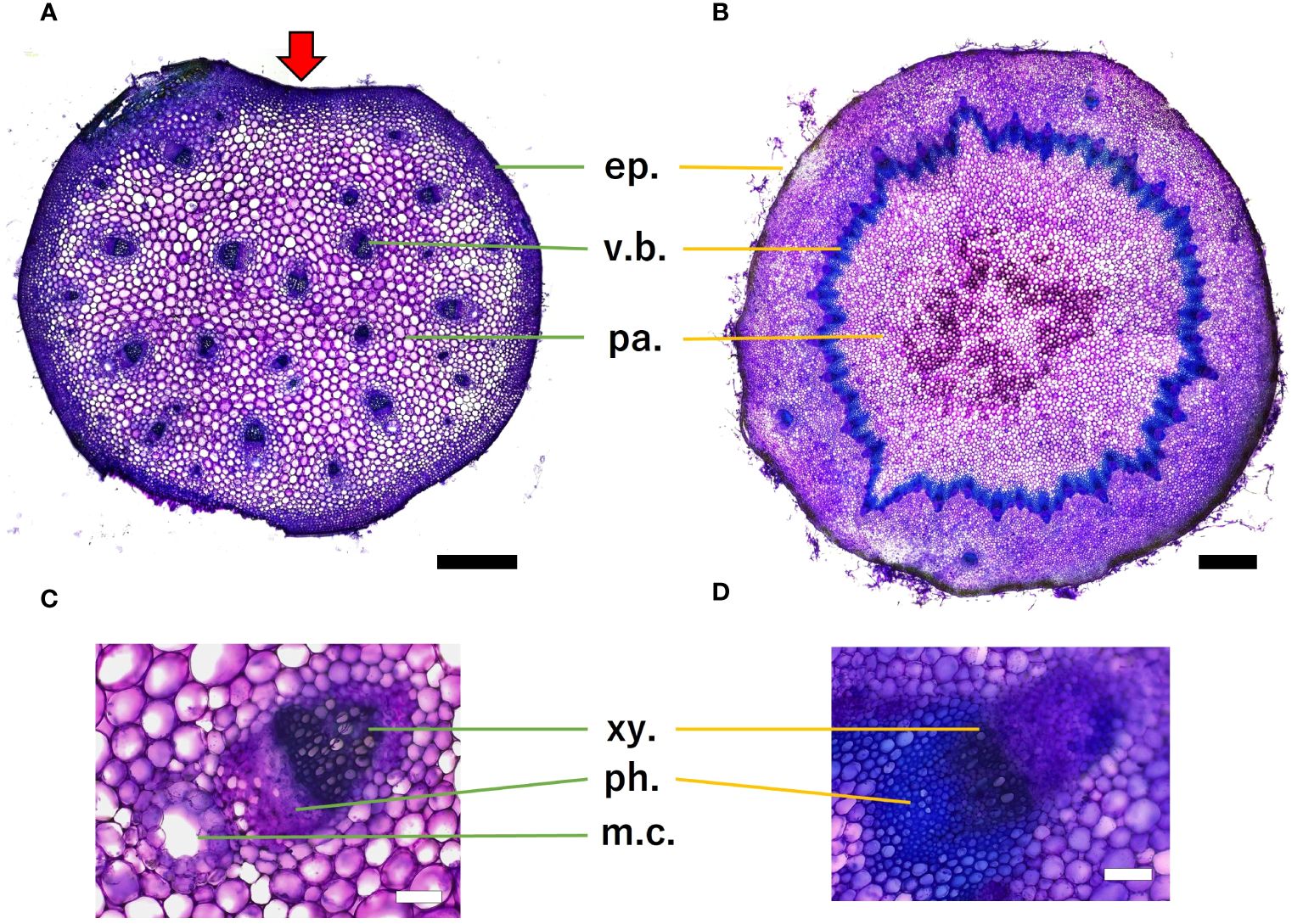
Figure 12. Microscopic images of petiole (A) and scape (B) cross-section, scale bar = 0.5 mm. Red arrows indicate micro canal-shaped grooves. Vascular bundles of petiole (C) and scape (D), scale bar = 100 µm. The epidermis (ep.), the vascular bundle (v.b.), parenchyma (pa.), xylem (xy.), phloem (ph.), and mucilage channels (m.c.) are shown. Toluidine blue staining showed that blue-green stained lignified tissue and purple stained non-lignified cell walls.
In terms of cross-sectional shape, the present study assumed an elliptical shape for both organs in the analysis of mechanical properties, but cross-sectional observation of the petiole revealed a “micro canal-shaped grooves” that developed on the vertical side of the petiole relative to the adaxial side of the lamina.
4 Discussion
Plant growth responses to thigmomorphogenesis include changes in branch and foliar development, stem shape and mass, leaf size, branch size, and stem height, all of which are restricted by the mechanical action of wind (Telewski and Pruyn, 1998). In our study, similar to the findings of Shiba et al. (2023), there were no significant differences found in the morphological characteristics of the lamina and petiole between inland and coastal habitats (Figures 3 4). However, significant differences were observed in scape length between habitats (Figure 4A), and differences in the distribution of data between habitats were evident in the relationship between scape cross-sectional area and scape length (Figure 4C). Perennial plants are comprised of individuals at various growth stages, and individual size and reproductive investment increase with growth (Samson and Werk, 1986). The number of flowers and inflorescences per individual can influence plant fitness by increasing pollinator attraction (Ohashi and Yahara, 1999; Makino et al., 2007). In addition, the taller the scape, the more capitula and flowers it can hold and the greater its influence on the frequency of flower visits by pollinators (Levin and Kerster, 1973; Pyke, 1981). In our study, there were no differences in the aboveground and below ground dry mass ratios of F. japonicum between the habitats (Figure 8). However, coastal F. japonicum exhibited shorter scape lengths, and also significant differences were observed in the distribution of data concerning the relationship between scape length and capitulum number, indicating that when attaching a similar number of capitulae, inland plants were able to position them higher than coastal plants (Figure 5). Furthermore, petiole length in the coastal populations of F. japonicum var. japonicum were correlated with those of the scape (Figure 7). The scapes of inland populations with low wind stress were significantly higher than those of coastal populations, regardless of petiole length. Why do the lengths of petioles and scapes correlate with strong wind stress in coastal areas? Nagashima and Hikosaka (2011) indicated that plants reduce their height growth to maintain similarity to neighboring plants, because artificially lifted individuals in the population have reduced plant height growth compared to others, contributing to the avoidance of strong wind stress, which has been reported in several plants since this study (Nagashima and Hikosaka, 2012; Masaki et al., 2013; Thomas et al., 2015; Gruntman et al., 2017). Based on these findings, if the escape lengths of F. japonicum var. japonicum far exceeded that of the petioles, this meant that the scape would be strongly affected by strong wind stress in coastal areas. This was alleviated by petioles and laminas, with the expectation that the risk of breakage of the scape would increase. Therefore, we considered that the length of the petioles and the scapes were correlated because the growth of the scapes repeatedly decreased owing to the influence of strong winds in coastal areas when the scapes exceeded the height of the petioles and laminas. Similar shortening of petioles and scapes under strong winds was expected to have similar mechanical strengths. Mechanical analysis of the scape and petioles is presented below.
F. japonicum var. japonicum increases the number of flowers and capitula to attract pollinators; however, this also increases the load on the scape as the tip of the scape becomes heavier by increasing number of them. In the present study, significant differences were observed in the distribution of data on the relationship between scape length and capitulum number. Specifically, when attaching a similar number of capitulae, inland plants were able to position themselves higher than coastal plants (Figure 5). However, our results do not provide insights into the specific arrangement of the capitula within the scape. Plant species have acquired unique strengths to support their aboveground organs (Shiba et al., 2024), and their resistance to breakage or overturning under strong wind conditions depends largely on structural modifications in terms of morphology, anatomy, and mechanical strength (Shiba et al., 2023). Mechanical studies have focused on agricultural crops and woody products (Gibson et al., 1988; Kokubo et al., 1989; Skubisz, 2001, 2002; Green et al., 2006; Lim et al., 2011; Christoforo et al., 2012; Slater and Ennos, 2013; Lemloh et al., 2014; Sekhon et al., 2020), and there are some studies on plant adaptation (Spatz et al., 1998; Anten et al., 2010; Hesse et al., 2020; Wolff-Vorbeck et al., 2021; Langer et al., 2022; Mylo et al., 2022; Shiba et al., 2023, 2024; Shiba and Fukuda, 2024). These previous studies have shown that analyses from a mechanical perspective are necessary not only for petioles, but also for scapes of F. japonicum var. japonicum. In particular, the maintenance of the scape during the flowering period of F. japonicum var. japonicum is important for the production of the next generation, and if scape is lost, the flowers and seeds of this species cannot be produced until the following year. Our results showed that the scapes of F. japonicum var. japonicum was stronger than in the petiole (Figure 11). The mechanism by which F. japonicum var. japonicum can increase the strength of scape through the petiole? The petioles and scapes of F. japonicum var. japonicum exhibited similar elliptical cross-sectional shapes (Figure 6). However, the axial second moment of the area, which depends on the diameter, was significantly higher in the scapes, indicating greater resistance to bending. Moreover, our results showed that the dry mass per unit volume of scape was significantly greater than that of petioles (Figure 10), indicating that the former contained more cell walls than the latter. Niklas and Paolillo (1997) and Shah et al. (2017) reported that an increase in the number of cell walls per unit volume reflected strengths such as the bending strength, elastic modulus, and plastic deformation of the organ. Moreover, plants have different types of cells and tissues, and there are tissues that support the plant structure: the epidermis of the petiole is smooth and has a slightly thick cuticle; however, the outer layer of the cortical layer inside the epidermis is mainly sclerotic and also it is extensive, while the inner zone is composed of thin-walled parenchyma, which has flexible cellulose cell walls (Karam and Gibson, 1994). Kohler and Spatz (2002) showed that the outer strengthening tissues have an elastic modulus and strength approximately four times higher than the core tissues. In addition, the relative amounts of these tissues may play an important role in petiole flexibility during bending or standing under strong pressure; however, it is very difficult to measure the exact properties of different tissues and cell types (Karam and Gibson, 1994). Our anatomical results using toluidine blue O staining showed that the vascular bundle arrangement was more circular in scapes than in petioles, with blue-stained xylem arranged more regularly than in petioles (Figure 12). These tissues contain lignin in their walls, which cannot be bent or broken, and the relative amount of these tissues determines whether the plant will bend or stand under strong pressure (Moysset and Simón, 1991; Paiva and Machado, 2003; Leroux, 2012). Therefore, the scape of F. japonicum var. japonicum achieved a greater strength than the petiole by increasing the number of cell walls and arranging a pattern of supportive tissues. Although the period when the scapes are exposed to strong winds on the ground is shorter than that of leaves, the former were shown to be stronger than the latter and maintained their structure throughout the flowering period, from October to December.
F. japonicum var. japonicum is a cold-resistant evergreen perennial species (Koyama, 1995), and its leaves are continuously exposed to wind stress for long periods. The capitulum of F. japonicum var. japonicum consists of female ray flowers on the outside and bisexual tubular flowers in the center. The capitulum has yellow blooms on the long scape from autumn to winter that makes fluff like dandelions after flowering (Koyama, 1995). The period of exposure of the scapes to wind stress was shorter than that of the leaves. Nevertheless, why did the scape of F. japonicum var. japonicum need greater strength than the petiole even though it was shorter under strong winds like the petiole? Considering the surface area exposed to the wind, the area of capitula at the tips of F. japonicum var. japonicum are clearly smaller than that of the leaves (Figure 1C) and they do not receive as much drag from the wind as the leaves; therefore, it seems that something other than wind is involved in the stiffness of the scape. Attracting animal pollinators is essential for the reproductive success of the majority of angiosperm species, and flowers have been described as sensory billboards, catching the attention of potential pollinators and advertising the presence of nectar and pollen rewards within (Raguso, 2004). Capitulum of F. japonicum var. japonicum has been pollinated by bees, house flies, syrphids, and butterflies, and Colletes perforator Smith are identified as the pollinator involved in the hybridization of F. japonicum var. japonicum and F. hiberniflorum (Makino) Kitam (Yahara et al., 1986). These pollinators collect pollen while holding it on to flowers and move freely between flowers in the capitulum. Loads are placed on the scape when these pollinators are stationary or move within and among the capitulum, and even more so when many pollinators arrive simultaneously. Therefore, the scape of F. japonicum var. japonicum may be stiffer than the petiole to withstand not only wind stress, but also the sudden and unexpected load on the scape by pollinators, suggesting that these were reflected excessively in the strength of the scape of F. japonicum var. japonicum. F. japonicum var. japonicum could produce new leaves regardless of the season, even if this species loses its leaves due to strong winds; however, since scapes only develop above ground for a limited period of time, their loss due to strong winds has a significant impact on reproduction in that year, suggesting that the scapes not only have higher strength than the petioles, but also shorten them to reduce the bending moment caused by strong winds. In contrast, leaves attract herbivorous insects that add weight to the leaves. Leaves have been protected not only by direct defense against various chemical substances, but also by indirect defense, which recruits natural enemies and exterminates them through the diffusion of substances induced into the leaves by herbivorous insects (Karban et al., 1997; Agrawal and Rutter, 1998; Baldwin and Preston, 1999; Dicke et al., 2003). However, the larvae of the moth Ostrinia sp. fed on the lamina of F. japonicum var. japonicum because some of the chemical compounds in this species are sex pheromones for Ostrinia sp., as determined by analyses using gas chromatography coupled to a mass spectrometer and an electroantennographic detector (Tabata et al., 2008). Although wind stress was alleviated by the lamina area of F. japonicum var. japonicum being reduced, as the lamina was likely to be eaten, the weight of the larvae makes the leaves heavier; therefore, it will be interesting to elucidate the reproductive strategy under such conditions in the future. In addition, the large lamina of this species have the effect of reducing the wind speed around the individual, and so that the reduced wind speed had experienced by the flowers and inflorescences in populations with shortened scapes in coastal areas. Eaton et al. (2020) revealed that as the wind speed increased, the number of bees visiting flowers decreased significantly because the number of times bees hesitated to fly out of flowers increased significantly, and therefore, the microclimatic variation created by the large lamina may affect pollination in coastal populations with shortened scapes. Future research should take such points into consideration. In addition, Onoda et al. (2008) indicated that the mechanical properties of the leaf blade of Plantago major varied in different light environments. The comparison of mechanical properties between petiole, scape and leaf loss individuals under different photosynthetic conditions using e.g. forest floor ecotypes of F. japonicum is an interesting subject.
Our study has clarified the adaptation process of each aboveground organ in F. japonicum var. japonicum under strong wind stress in coastal areas. Morphological changes in plant species occur in the shape of individual roots when they had been subjected to certain mechanical stresses in the aboveground organs (Cannell and Coutts, 1988; Stokes et al., 1997). F. japonicum var. japonicum is a perennial herb (Koyama, 1995) and the size of its roots increases as it grows, allowing it to reproduce in the following year. Roots of F. japonicum var. japonicum also responds to the strong wind stress experienced by its aboveground organs in coastal areas. Our comparison of dry mass between the aboveground and below ground organs indicated that there was no significant difference between the coastal and inland populations of F. japonicum var. japonicum (Figure 8). This suggests that the effects of aboveground mechanical stress on F. japonicum var. japonicum did not differ between coastal and inland populations. Shiba et al. (2023) reported that in regions with higher wind speeds than those in this study, the aboveground morphology of F. japonicum var. japonicum tended to be smaller, suggesting potential impacts on below ground organs in these coastal populations. In contrast, F. japonicum var. japonicum typically grows on rocky substrates in areas with high wind velocities, making it difficult to collect individual below ground organs; thus, they could not be included in the measurements in this study.
Among the varieties of F. japonicum, F. japonicum var. luchuense could provide insights into the morphological, anatomical, and genetic mechanisms of local adaptation because the narrow leaves of F. japonicum var. luchuense was subjected to flash floods after heavy rain as a strong selective pressure in the area along rivers and streams, and was gained by the decreased number of leaf cells across the width of the leaf (Usukura et al., 1994). Plant groups including F. japonicum var. luchuense with such morphological leaf characteristics inhabiting riverbank habitats, are called rheophytes (van Steenis, 1981) and some previous studies have reported the convergent evolution of many phylogenetically distinct taxa of ferns and angiosperms (Imaichi and Kato, 1992, 1993; Setoguchi and Kajimura, 2004; Yamada et al., 2011; Ueda et al., 2012; Yokoyama et al., 2012; Ohga et al., 2012a; Matsui et al., 2013; Kato, 2017; Shiba et al., 2021). It would be interesting to evaluate whether the adaptation of F. japonicum var. luchuense under water stress showed the same pattern as F. japonicum var. japonicum in response to strong winds. Furthermore, a recent study revealed that petioles of the rheophytic species Osmunda lancea Thunb. (Osmundaceae), exhibit flexibility by increasing the size of their supportive cells, which helps avoid strong water stress along rivers (Shiba and Fukuda, 2024). By considering the adaptation of plant species to physical stress, such as strong winds and strong flash floods, there is endless interest in the diversification process of F. japonicum group, including the giant F. japonicum var. giganteum in coastal areas, as mentioned previously.
Data availability statement
The original contributions presented in the study are included in the article/Supplementary Material. Further inquiries can be directed to the corresponding author.
Author contributions
MS: Writing – review & editing, Writing – original draft, Methodology, Investigation, Formal analysis, Funding acquisition. SA: Writing – review & editing, Methodology, Investigation. SH: Writing – review & editing, Methodology, Software, Investigation. TF: Investigation, Writing – review & editing, Writing – original draft, Funding acquisition.
Funding
The author(s) declare financial support was received for the research, authorship, and/or publication of this article. This work was supported by JSPS KAKENHI Grant Numbers JP21K06324, JP24KJ2045.
Acknowledgments
We thank Drs. Marui Y, Sato R, and Yasuda T for their advice regarding our study.
Conflict of interest
The authors declare that the research was conducted in the absence of any commercial or financial relationships that could be construed as a potential conflict of interest.
Correction note
A correction has been made to this article. Details can be found at: 10.3389/fpls.2025.1633304.
Publisher’s note
All claims expressed in this article are solely those of the authors and do not necessarily represent those of their affiliated organizations, or those of the publisher, the editors and the reviewers. Any product that may be evaluated in this article, or claim that may be made by its manufacturer, is not guaranteed or endorsed by the publisher.
Supplementary material
The Supplementary Material for this article can be found online at: https://www.frontiersin.org/articles/10.3389/fpls.2024.1407127/full#supplementary-material
References
Agrawal, A. A. and Rutter, M. T. (1998). Dynamic anti-herbivore defense in ant-plants: the role of induced responses. Oikos. 83, 227–236. doi: 10.2307/3546834
Anten, N. P. R., Alcalá-Herrera, R., Schieving, F., and Onoda, Y. (2010). Wind and mechanical stimuli differentially affect leaf traits in Plantago major. New Phytol. 188, 554–564. doi: 10.1111/j.1469-8137.2010.03379.x
Anten, N. P. R., von Wettberg, E. J., Pawlowski, M., and Huber, H. (2009). Interactive effects of spectral shading and mechanical stress on the expression and costs of shade avoidance. Am. Nat. 173, 241–255. doi: 10.1086/595761
Baldwin, I. T. and Preston, C. A. (1999). The eco-physiological complexity of plant responses to insect herbivores. Planta. 208, 137–145. doi: 10.1007/s004250050543
Bang, C., Sabo, J. L., and Faeth, S. H. (2010). Reduced wind speed improves plant growth in a desert city. PloS One 5, e11061. doi: 10.1371/journal.pone.0011061
Bell, G. (1985). On the function of flowers. Proc. R. Soc Lond. B. 22, 223–265. doi: 10.1098/rspb.1985.0031
Biddington, N. L. (1986). The effects of mechanically induced stress in plants—a review. Plant Growth Regul. 4, 103–123. doi: 10.1007/BF00025193
Brady, K. U., Kruckeberg, A. R., and Bradshaw, H. D., Jr. (2005). Evolutionary ecology of plant adaptation to serpentine soils. Annu. Rev. Ecol. Evol. Syst. 36, 243–266. doi: 10.1146/annurev.ecolsys.35.021103.105730
Buonaiuto, D. M. and Wolkovich, E. M. (2021). Differences between flower and leaf phenological responses to environmental variation drive shifts in spring phenological sequences of temperate woody plants. J. Ecol. 109, 2922–2933. doi: 10.1111/1365-2745.13708
Burgess, A. J., Retkute, R., Preston, S. P., Jensen, O. E., Pound, M. P., Pridmore, T. P., et al. (2016). The 4-dimensional plant: effects of wind-induced canopy movement on light fluctuations and photosynthesis. Front. Plant Sci. 7. doi: 10.3389/fpls.2016.01392
Chehab, E., Eich, E., and Braam, J. (2009). Thigmomorphogenesis: a complex plant response to mechano-stimulation. J. Exp. Bot. 60, 43–56. doi: 10.1093/jxb/ern315
Christoforo, A., Lahr, F., Morales, E., Zangiacomo, A., and Panzera, T. (2012). Influence of displacements on calculus of the longitudinal module of elasticity of Pinus carbaea structural round timber beams. Int. J. Agric. For. 2, 157–160. doi: 10.5923/j.ijaf.20120204.04
Cleugh, H. A., Miller, J. M., and Böhm, M. (1998). Direct mechanical effects of wind on crops. Agrofor. Syst. 41, 85–112. doi: 10.1023/A:1006067721039
Coutand, C. and Moulia, B. (2000). Biomechanical study of the effect of a controlled bending on tomato stem elongation: local strain sensing and spatial integration of the signal. J. Exp. Bot. 51, 1824–1842. doi: 10.1093/jexbot/51.352.1825
Creux, N. M., Brown, E. A., Garner, A. G., Saeed, S., Scher, C. L., Holalu, S. V., et al. (2021). Flower orientation influences floral temperature, pollinator visits and plant fitness. New Phytol. 232, 868–879. doi: 10.1111/nph.17627
Danjon, F., Fourcaud, T., and Bert, D. (2005). Root architecture and wind-firmness of mature Pinus pinaster. New Phytol. 168, 387–400. doi: 10.1111/j.1469-8137.2005.01497.x
Dicke, M., van Poecke, R. M. P., and de Boer, J. G. (2003). Inducible indirect defence of plants: from mechanism to ecological functions. Basic Appl. Ecol. 4, 27–42. doi: 10.1078/1439-1791-00131
Eaton, C., Wright, P., Jackson, E., Goulson, D., and Ratnieks, F. F. L. W. (2020). Gone with the wind: effects of wind on honey bee visit rate and foraging behaviour. Anim. Behav. 161, 23–31. doi: 10.1016/j.anbehav.2019.12.018
Ennos, A. R. (1997). Wind as an ecological factor. Trends Ecol. Evol. 12, 108–111. doi: 10.1016/S0169-5347(96)10066-5
Feng, J., Huang, P., and Wan, X. (2019). Interactive effects of wind and light on growth and architecture of poplar saplings. Ecol. Res. 34, 94–105. doi: 10.1111/1440-1703.1013
Fernandez, P. and Hilker, M. (2007). Host plant location by Chrysomelidae. Basic Appl. Ecol. 8, 97–116. doi: 10.1016/j.baae.2006.05.001
Funk, J. L. and Vitousek, P. M. (2007). Resource-use efficiency and plant invasion in low-resource systems. Nature. 446, 1079–1081. doi: 10.1038/nature05719
Gardiner, B., Berry, P., and Moulia, B. (2016). Review: wind impacts on plant growth, mechanics and damage. Plant Sci. 245, 94–118. doi: 10.1016/j.plantsci.2016.01.006
Gibson, L. J., Ashby, M. F., and Easterling, K. E. (1988). Structure and mechanics of the iris leaf. J. Mater. Sci. 23, 3041–3048. doi: 10.1007/BF00551271
Givnish, T. J. (2002). Ecological constraints on the evolution of plasticity in plants. Evol. Ecol. 16, 213–242. doi: 10.1023/A:1019676410041
Green, D. W., Gorman, T. M., Evans, J. W., and Murphy, J. F. (2006). Mechanical grading of round timber beams. J. Mater. Civ. Eng. 18, 1–10. doi: 10.1061/(ASCE)0899-1561(2006)18:1(1
Greenway, H. and Munns, R. (1980). Mechanisms of salt tolerance in non-halophytes. Annu. Rev. Plant Physiol. 22, 131–160. doi: 10.1146/annurev.pp.31.060180.001053
Gruntman, M., Groß, D., Májeková, M., and Tielbörger, K. (2017). Decision-making in plants under competition. Nat. Commun. 8, 2235. doi: 10.1038/s41467-017-02147-2
Hayakawa, H., Tunala, Minamiya, Y., Ito, K., Gale, S. W., Yokoyama, J., et al. (2012). Comparative study of leaf morphology in Aster hispidus Thunb. var. leptocladus (Makino) Okuyama (Asteraceae). Am. J. Plant Sci. 3, 110–113. doi: 10.4236/ajps.2012.31011
Hesse, L., Kampowski, T., Leupold, J., Caliaro, S., Speck, T., and Speck, O. (2020). Comparative analyses of the self-sealing mechanisms in leaves of Delosperma cooperi and Delosperma ecklonis (Aizoaceae). Int. J. Mol. Sci. 21, 5768. doi: 10.3390/ijms21165768
Hodges, L., Suratman, M. N., Brandle, J. R., and Hubbard, K. G. (2004). Growth and yield of snap beans as affected by wind protection and microclimate changes due to shelterbelts and planting dates. HortSci. 39, 996–1004. doi: 10.21273/HORTSCI.39.5.996
Imaichi, R. and Kato, M. (1992). Comparative leaf development of Osmunda lancea and O. japonica (Osmundaceae): heterochronic origin of rheophytic stenophylly. Bot. Mag. Tokyo. 105, 199–213. doi: 10.1007/BF02489415
Imaichi, R. and Kato, M. (1993). Comparative leaf morphology of young sporophytes of rheophytic Osmunda lancea and dryland O. japonica. J. Plant Res. 106, 37–45. doi: 10.1007/BF02344371
Jaffe, M. J. and Forbes, S. (1993). Thigmomorphogenesis: the effect of mechanical perturbation on plants. Plant Growth Regul. 12, 313–324. doi: 10.1007/BF00027213
Jösson, K. I. and Tuomi, J. (1994). Costs of reproduction in a historical perspective. Trends Ecol. Evol. 9, 304–307. doi: 10.1016/0169-5347(94)90042-6
Karam, G. N. and Gibson, L. J. (1994). Biomimicking of animal quills and plant stems: natural cylindrical shells with foam cores. Mater. Sci. Eng. C. 2, 113–132. doi: 10.1016/0928-4931(94)90039-6
Karban, R., Agrawal, A. A., and Mangel, M. (1997). The benefits of induced defenses against herbivores. Ecology. 78, 1351–1355. doi: 10.1890/0012-9658(1997)078[1351:TBOIDA]2.0.CO;2
Khan, M. A., Gemenet, D. C., and Villordon, A. (2016). Root system architecture and abiotic stress tolerance: current knowledge in root and tuber crops. Front. Plant Sci. 7. doi: 10.3389/fpls.2016.01584
Kohler, L. and Spatz, H. C. (2002). Micromechanics of plant tissues beyond the linear-elastic range. Planta 215, 33–40. doi: 10.1007/s00425-001-0718-9
Kokubo, A., Kuraishi, S., and Sakurai, N. (1989). Culm strength of barley: correlation among maximum bending stress, cell wall dimensions, and cellulose content. Plant Physiol. 91, 876–882. doi: 10.1104/pp.91.3.876
Koyama, H. (1995). Farfugium, in flora of Japan IIIb. Eds. Iwatsuki, K., Yamazaki, T., Boufford, D. E., and Ohba, H. (Tokyo: Kodansha), 37–38.
Kumekawa, Y., Miyata, H., Ohga, K., Hayakawa, H., Yokoyama, J., Ito, K., et al. (2013). Comparative analyses of stomatal size and density among ecotypes of Aster hispidus (Asteraceae). Am. J. Plant Sci. 4, 524–527. doi: 10.4236/ajps.2013.43067
Langer, M., Hegge, E., Speck, T., and Speck, O. (2022). Acclimation to wind loads and/or contact stimuli? A biomechanical study of peltate leaves of Pilea peperomioides. J. Exp. Bot. 73, 1236–1252. doi: 10.1093/jxb/erab541
Lemloh, M., Pohl, A., Weber, E., Zeiger, M., Bauer, P., Weiss, I. M., et al. (2014). Structure-property relationships in mechanically stimulated Sorghum bicolor stalks. Bioinspired Mater. 1, 1–11. doi: 10.2478/bima-2014-0001
Leroux, O. (2012). Collenchyma: a versatile mechanical tissue with dynamic cell walls. Ann. Bot. 110, 1083–1098. doi: 10.1093/aob/mcs186
Levin, D. A. and Kerster, H. W. (1973). Assortative pollination for stature in Lythrum salicaria. Evolution. 27, 144–152. doi: 10.1111/j.1558-5646.1973.tb05926.x
Lim, J., Yeo, H., and Lee, J. (2011). Study on the evaluation of performance for pitch pine round timbers as safety barrier beam members. J. Korean Wood Sci. Technol. 39, 390–397. doi: 10.5658/WOOD.2011.39.5.390
Makino, T. T., Ohashi, K., and Sakai, S. (2007). How do floral display size and the density of surrounding flowers influence the likelihood of bumble-bee revisitation to a plant? Funct. Ecol. 21, 87–95. doi: 10.1111/j.1365-2435.2006.01211.x
Masaki, T., Hitsuma, G., Yagihashi, T., Noguchi, M., Shibata, M., and Takata, M. (2013). How do thinning intensities affects long-term growth of tree height in a Jappanese ceder plantation. J. Jpn. For. Soc 95, 227–233. doi: 10.4005/jjfs.95.227
Matsui, R., Takei, S., Ohga, K., Hayakawa, H., Yoshida, M., Yokoyama, J., et al. (2013). Morphological and anatomical variations in rheophytic ecotype of violet, Viola mandshurica var. ikedaeana (Violaceae). Am. J. Plant Sci. 4, 859–865. doi: 10.4236/ajps.2013.44106
Meguro, S. and Miyawaki, A. (1994). A study of the relationship between mechanical characteristics and the coastal vegetation among several broad-leaf trees in Miura Peninsula in Japan. Vegetatio. 112, 101–111. doi: 10.1007/BF00044685
Moysset, L. and Simón, E. (1991). Secondary pulvinus of Robinia pseudoacacia (Leguminosae)- structural and ultrastructural features. Am. J. Bot. 78, 1467–1486. doi: 10.1002/j.1537-2197.1991.tb11426.x
Mylo, M. D., Hoppe, A., Pastewka, L., Speck, T., and Speck, O. (2022). Elastic property and fracture mechanics of lateral branch-branch junctions in cacti: a case study of Opuntia ficus-indica and Cylindropuntia bigelovii. Front. Plant Sci. 13. doi: 10.3389/fpls.2022.950860
Mylo, M. D. and Speck, O. (2023). Longevity of system functions in biology and biomimetics: a matter of robustness and resilience. Biomimetics 8, 173. doi: 10.3390/biomimetics8020173
Nagashima, H. and Hikosaka, K. (2011). Plants in a crowded stand regulate their height growth so as to maintain similar heights to neighbours even when they have potential advantages in height growth. Ann. Bot. 108, 207–214. doi: 10.1093/aob/mcr109
Nagashima, H. and Hikosaka, K. (2012). Not only light quality but also mechanical stimuli are involved in height convergence in crowded Chenopodium album stands. New Phytol. 195, 803–811. doi: 10.1111/j.1469-8137.2012.04218.x
Nakata, M. T., Nakao, M., Denda, A., Onoda, Y., Ueda, H., and Demura, T. (2020). Estimating the flexural rigidity of Arabidopsis inflorescence stems: free-vibration test vs. three-point bending test. Plant Biotechnol. (Tokyo). 37, 471–474. doi: 10.5511/plantbiotechnology.20.1214a
Nicoll, B. C. and Ray, D. (1996). Adaptive growth of the tree root system in response to wind action and site conditions. Tree Physiol. 16, 891–898. doi: 10.1093/treephys/16.11-12.891
Niez, B., Dlouha, J., Moulia, B., and Badel, E. (2019). Water-stressed or not, the mechanical acclimation is a priority requirement for trees. Trees 33, 279–291. doi: 10.1007/s00468-018-1776-y
Niklas, K. and Paolillo, D. (1997). The role of the epidermis as a stiffening agent in Tulipa (Liliaceae) stems. Am. J. Bot. 84, 735. doi: 10.2307/2445809
Nomura, N., Takaso, T., Peng, C., Kono, Y., Oginuma, K., Mitsui, Y., et al. (2010). Molecular phylogeny and habitat diversification of the genus Farfugium (Asteraceae) based on nuclear rDNA and plastid DNA. Ann. Bot. 106, 467–482. doi: 10.1093/aob/mcq139
Ohashi, K. and Yahara, T. (1999). How long to stay on, and how of ten to visit a flowering plant? – a model for foraging strategy when floral displays vary in size. Oikos. 86, 386–392. doi: 10.2307/3546457
Ohga, K., Muroi, M., Hayakawa, H., Yokoyama, J., Ito, K., Tebayashi, S., et al. (2012a). Comparative morphology and anatomy of non-rheophytic and rheophytic types of Adenophora triphylla var. japonica (Campanulaceae). Am. J. Plant Sci. 3, 805–809. doi: 10.4236/ajps.2012.36097
Ohga, K., Muroi, M., Hayakawa, H., Yokoyama, J., Ito, K., Tebayashi, S., et al. (2012b). Morphological and anatomical analyses of the serpentine ecotype of Adenophora triphylla var. japonica (Campanulaceae). J. Plant Stud. 1, 180–187. doi: 10.5539/jps.v1n2p180
Ohga, K., Muroi, M., Hayakawa, H., Yokoyama, J., Ito, K., Tebayashi, S., et al. (2013). Coastal adaptation of Adenophora triphylla var. japonica (Campanulaceae). Am. J. Plant Sci. 4, 596–601. doi: 10.4236/ajps.2013.43078
Onoda, Y. and Anten, N. P. R. (2011). Challenges to understand plant responses to wind. Plant Signal. Behav. 6, 1057–1059. doi: 10.4161/psb.6.7.15635
Onoda, Y., Schieving, F., and Anten, N. P. R. (2008). Effects of light and nutrient availability on leaf mechanical properties of Plantago major: a conceptual approach. Ann. Bot. 101, 727–736. doi: 10.1093/aob/mcn013
Paiva, E. A. S. and MaChado, S. R. (2003). Collenchyma in Panicum maximum (Poaceae): localisation and possible role. Aust. J. Bot. 51, 69–73. doi: 10.1071/BT02046
Poorter, H., Niklas, K. J., Reich, P. B., Oleksyn, J., Poot, P., and Mommer, L. (2012). Biomass allocation to leaves, stems and roots: meta-analyses of interspecific variation and environmental control. New Phytol. 193, 30–50. doi: 10.1111/j.1469-8137.2011.03952.x
Pyke, G. H. (1981). Effects of inflorescence height and number of flowers per inflorescence on fruit set in waratahs (Telopea speciosissima). Aust. J. Bot. 29, 419–424. doi: 10.1071/BT9810419
Raguso, R. A. (2004). Flowers as sensory billboards: progress towards an integrated understanding of floral advertisement. Curr. Opin. Plant Biol. 7, 434–440. doi: 10.1016/j.pbi.2004.05.010
R Core Team. (2024). R: A language and environment for statistical computing (Vienna, Austria: R Foundation for Statistical Computing). Available at: https://www.R-project.org/.
Reznick, D. (1985). Costs of reproduction: an evaluation of the empirical evidence. Oikos. 44, 257–267. doi: 10.2307/3544698
Sakai, W. S. (1973). Simple method for differential staining of paraffin embedded plant material using toluidine blue o. Stain Technol. 48, 247–249. doi: 10.3109/10520297309116632
Samson, D. A. and Werk, K. S. (1986). Size-dependent effects in the analysis of reproductive effort in plants. Am. Nat. 127, 667–680. doi: 10.1086/284512
Sekhon, R. S., Joyner, C. N., Ackerman, A. J., McMahan, C. S., Cook, D. D., and Robertson, D. J. (2020). Stalk bending strength is strongly associated with maize stalk lodging incidence across multiple environments. Field Crops Res. 249, 107737. doi: 10.1016/j.fcr.2020.107737
Setoguchi, H. and Kajimura, G. (2004). Leaf morphology of the rheophyte, Rhododendron indicum f. otakumi (Ericaceae). Acta Phytotaxon. Geobot. 55, 45–54. doi: 10.18942/apg.KJ00004622803
Shah, D. U., Reynolds, T. P. S., and Ramage, M. H. (2017). The strength of plants: theory and experimental methods to measure the mechanical properties of stems. J. Exp. Bot. 68, 4497–4516. doi: 10.1093/jxb/erx245
Shiba, M. and Fukuda, T. (2024). Rheophytic Osmunda lancea (Osmundaceae) exhibits large flexibility in the petiole. Sci. Rep. 14, 2866. doi: 10.1038/s41598-024-53406-4
Shiba, M., Mizuno, T., and Fukuda, T. (2023). Effect of strong wind on laminas and petioles of Farfugium japonicum (L.) Kitam. var. japonicum (Asteraceae). Front. Plant Sci. 14. doi: 10.3389/fpls.2023.1182266
Shiba, M., Sato, R., and Fukuda, T. (2024). A comparison of mechanical characteristics among Setaria viridis var. minor, Setaria italica, and Setaria × Pycnocoma species of the family Poaceae. Plant Species Biol. 39, 51–58. doi: 10.1111/1442-1984.12435
Shiba, M., Tate, T., and Fukuda, T. (2021). Rheophytic adaptation of Eurya japonica Thunb. (Ternstroemiaceae). Int. J. Biol. 13, 65–73. doi: 10.5539/ijb.v13n2p65
Shiba, M., Tate, T., and Fukuda, T. (2022a). Adaptative leaf morphology of Eurya japonica Thunb. (Ternstroemiaceae) in serpentine areas. J. Plant Stud. 11, 10–18. doi: 10.5539/jps.v11n1p10
Shiba, M., Tate, T., and Fukuda, T. (2022b). Serpentine adaptative of Ligustrum japonicum Thunb. (Oleaceae) based on morphological and anatomical approaches. Int. J. Biol. 14, 10–18. doi: 10.5539/ijb.v14n2p10
Shiba, M., Tate, T., and Fukuda, T. (2022c). Leaf adaptation of Eurya japonica Thunb. (Pentaphylacaceae) in coastal area. J. Plant Stud. 11, 31–41. doi: 10.5539/jps.v11n1p31
Skubisz, G. (2001). Development of studies on the mechanical properties of winter rape stems. Int. Agrophys. 15, 197–200.
Skubisz, G. (2002). Method for the determination of the mechanical properties of pea stems. Int. Agrophys. 16, 73–77.
Slater, D. and Ennos, A. R. (2013). Determining the mechanical properties of hazel forks by testing their component parts. Trees. 27, 1515–1524. doi: 10.1007/s00468-013-0898-5
Spatz, H.-C. H., Köhler, L., and Speck., T. (1998). Biomechanics and functional anatomy of hollow stemmed sphenopsids: I. Equisetum giganteum (Equisetaceae). Am. J. Bot. 85, 305–314. doi: 10.2307/2446321
Štofko, P. and Kodrík, M. (2008). Comparison of root system architecture between windthrown and undamaged spruces growing in poorly drained sites. J. For. Sci. 54, 150–160. doi: 10.17221/3101-JFS
Stokes, A., Fitter, A. H., and Courts, M. P. (1995). Responses of young trees to wind and shading: effects on root architecture. J. Exp. Bot. 46, 1139–1146. doi: 10.1093/jxb/46.9.1139
Stokes, A., Nicoll, B. C., Coutts, M. P., and Fitter, A. H. (1997). Responses of young Sitka spruce clones to mechanical perturbation and nutrition: effects on biomass allocation, root development, and resistance to bending. Can. J. For. Res. 27, 1049–1057. doi: 10.1139/x97-041
Strauss, S. Y. (1997). Floral characters link herbivores, pollinators, and plant fitness. Ecology. 78, 1640–1645. doi: 10.1890/0012-9658(1997)078[1640:FCLHPA]2.0.CO;2
Sunami, T., Ohga, K., Muroi, M., Hayakawa, H., Yokoyama, J., Ito, K., et al. (2013). Comparative analyses of hairless-leaf and hairy-leaf type individuals in Aster hispidus var. insularis (Asteraceae). J. Plant Stud. 2, 1–6. doi: 10.5539/jps.v2n1p1
Tabata, J., Huang, Y., Ohno, S., Yoshiyasu, Y., Sugie, H., Tatsuki, S., et al. (2008). Sex pheromone of Ostrinia sp. newly found on the leopard plant Farfugium japonicum. J. Appl. Entomol. 132, 566–574. doi: 10.1111/j.1439-0418.2008.01295.x
Takizawa, E., Shiba, M., Yoshizaki, S., and Fukuda, T. (2023). Stomatal Study of Introduced Species, Ligustrum lucidum Aiton (Oleaceae), in Coastal Areas of Japan. J. Plant Stud. 12, 24–36. doi: 10.5539/jps.v12n1p24
Takizawa, E., Tate, T., Shiba, M., Ishii, C., Yoshizaki, S., and Fukuda, T. (2022). Coastal adaptation of Ligustrum japonicum Thunb. (Oleaceae): a case study of stomatal adaptation pattern into coastal forests. J. Jpn. Soc Coast. Forest. 21, 1–87. doi: 10.60398/kaiganrin.21.1_1
Tamasi, E., Stokes, A., Lasserre, B., Danjon, F., Berthier, S., Fourcaud, T., et al. (2005). Influence of wind loading on root system development and architecture in oak (Quercus robur L.) seedlings. Trees. 19, 374–384. doi: 10.1007/s00468-004-0396-x
Telewski, F. W. and Pruyn, M. L. (1998). Thigmomorphogenesis: a dose response to flexing in Ulmus americana seedlings. Tree Physiol. 18, 65–68. doi: 10.1093/treephys/18.1.65
Thomas, S. C., Martin, A. R., and Mycroft, E. E. (2015). Tropical trees in a wind-exposed island ecosystem: height-diameter allometry and size at onset of maturity. J. Ecol. 103, 594–605. doi: 10.1111/1365-2745.12378
Tunala, H. H., Minamiya, Y., Gale, S. W., Yokoyama, J., Arakawa, R., and Fukuda, T. (2012). Foliar adaptations in Aster hispidus var. insularis (Asteraceae). J. Plant Stud. 1, 19–25. doi: 10.5539/jps.v1n2p19
Turbill, C. (2008). Winter activity of Australian tree-roosting bats: influence of temperature and climatic patterns. J. Zool. 276, 285–290. doi: 10.1111/j.1469-7998.2008.00487.x
Ueda, R., Minamiya, Y., Hirata, A., Hayakawa, H., Muramatsu, Y., Saito, M., et al. (2012). Morphological and anatomical analyses of rheophytic Rhododendron ripense Makino (Ericaceae). Plant Species Biol. 27, 233–240. doi: 10.1111/j.1442-1984.2011.00345.x
Usukura, M., Imaichi, R., and Kato, M. (1994). Leaf morphology of a facultative rheophyte,Farfugium japonicum var. luchuense (Compositae). J. Plant Res. 107, 263–267. doi: 10.1007/BF02344253
van Steenis, C. G. G. J. (1981). Rheophytes of the world (Alpen Aan Den Rijn: Sijhoff and Noordhoff). doi: 10.1007/978-94-009-8588-9
Wilcock, C. and Neiland, R. (2002). Pollination failure in plants: why it happens and when it matters. Trends Plant Sci. 7, 270–277. doi: 10.1016/S1360-1385(02)02258-6
Wise, M. J. and Abrahamson, W. G. (2017). Constraints on the evolution of resistance to gall flies in Solidago altissima: resistance sometimes costs more than it is worth. New Phytol. 215, 423–433. doi: 10.1111/nph.14583
Wolff-Vorbeck, S., Speck, O., Speck, T., and Dondl, P. W. (2021). Influence of structural reinforcements on the twist-to-bend ratio of plant axes: a case study on Carex pendula. Sci. Rep. 11, 21232. doi: 10.1038/s41598-021-00569-z
Yahara, T., Yamaguchi, H., and Yumoto, T. (1986). “Biology of hybridization between Farfugium japonica and F. hiberniflorum (Compositae),” in Modern aspects of species. Eds. In, I. K., Raven, P. H., and Bock, W. J. (University of Tokyo Press, Tokyo), 183–193.
Yamada, Y., Hayakawa, H., Minamiya, Y., Ito, K., Shibayama, Z., Arakawa, R., et al. (2011). Comparative morphology and anatomy of rheophytic Aster microcephalus (Miq.) Franch. et Sav. Var. Ripensis Makino (Asteraceae). J. Phytogeogr. Taxon. 59, 35–42. doi: 10.24517/00053453
Yokoyama, N., Hayakawa, H., Matsuyama, K., Muroi, M., Ohga, K., Ito, K., et al. (2012). Morphological and molecular analyses of rheophytic Rhododendron ripense and its allied dryland species R. macrosepalum (Ericaceae). Environ. Control Biol. 50, 305–312. doi: 10.2525/ecb.50.305
Keywords: Farfugium japonicum, mechanical properties, petiole, scape, wind conditions, thigmomorphogenesis
Citation: Shiba M, Arihara S, Harada S and Fukuda T (2024) Impact on the scape of Farfugium japonicum var. japonicum (Asteraceae) under strong wind conditions based on morphological and mechanical analyses. Front. Plant Sci. 15:1407127. doi: 10.3389/fpls.2024.1407127
Received: 26 March 2024; Accepted: 17 July 2024;
Published: 06 August 2024; Corrected: 25 June 2025.
Edited by:
Jun Ni, Nanjing Agricultural University, ChinaReviewed by:
Dagmar Voigt, Technische Universität Dresden, GermanyMax David Mylo, Cluster of Excellence livMatS @ FIT – Freiburg Center for Interactive Materials and Bioinspired Technologies, Germany
Eric Badel, INRAE Clermont-Auvergne-Rhône-Alpes, France
Copyright © 2024 Shiba, Arihara, Harada and Fukuda. This is an open-access article distributed under the terms of the Creative Commons Attribution License (CC BY). The use, distribution or reproduction in other forums is permitted, provided the original author(s) and the copyright owner(s) are credited and that the original publication in this journal is cited, in accordance with accepted academic practice. No use, distribution or reproduction is permitted which does not comply with these terms.
*Correspondence: Masayuki Shiba, bXN5a3NoaWJhNDhAZ21haWwuY29t