- 1Institute of Experimental Botany of the Czech Academy of Sciences, Centre of Plant Structural and Functional Genomics, Olomouc, Czechia
- 2Field Crops Research Institute, Agricultural Research Centre, Giza, Egypt
- 3Agricultural Institute, Hungarian Research Network (HUN-REN) Centre for Agricultural Research, Martonvásár, Hungary
- 4Genetics Department, Escuela Técnica Superior de Ingeniería Agronómica y de Montes (ETSIAM), Agrifood Campus of International Excellence (ceiA3), University of Córdoba, Córdoba, Spain
- 5Professor Emeritus, Kyoto University, Kyoto, Japan
Some species of the genus Aegilops, a wild relative of wheat, carry chromosomes that after introducing to wheat exhibit preferential transmission to progeny. Their selective retention is a result of the abortion of gametes lacking them due to induced chromosomal aberrations. These chromosomes are termed Gametocidal (Gc) and, based on their effects, they are categorized into three types: mild, intense or severe, and very strong. Gc elements within the same homoeologous chromosome groups of Aegilops (II, III, or IV) demonstrate similar Gc action. This review explores the intriguing dynamics of Gc chromosomes and encompasses comprehensive insights into their source species, behavioral aspects, mode of action, interactions, suppressions, and practical applications of the Gc system in wheat breeding. By delving into these areas, this work aims to contribute to the development of novel plant genetic resources for wheat breeding. The insights provided herein shed light on the utilization of Gc chromosomes to produce chromosomal rearrangements in wheat and its wild relatives, thereby facilitating the generation of chromosome deletions, translocations, and telosomic lines. The Gc approach has significantly advanced various aspects of wheat genetics, including the introgression of novel genes and alleles, molecular markers and gene mapping, and the exploration of homoeologous relationships within Triticeae species. The mystery lies in why gametes possessing Gc genes maintain their normality while those lacking Gc genes suffer abnormalities, highlighting an unresolved research gap necessitating deeper investigation.
1 Introduction
When introduced to common wheat (Triticum aestivum L., 2n = 6x = 42, AABBDD) some chromosomes of Aegilops species exhibit a surprisingly elevated rate of transmission to succeeding generations, leading to segregation distortion. These chromosomes are widely recognized as “Gametocidal (Gc)” and have been used in breeding programs aiming at widening genetic diversity of common wheat (Endo, 2015). The evolution of common wheat genome over thousands of years of domestication and breeding resulted in a narrow gene pool, including the loss of genes related to resistance and tolerance to biotic and abiotic stress, and quality traits. To address this challenge, breeders seek to introduce new genes and alleles by broadening the genetic diversity through interspecific or intergeneric hybridization with wild relatives. Crop wild relatives, particularly in the tribe Triticeae, offer a promising source of novel genes and alleles (Hajjar and Hodgkin, 2007). Despite their potential, the utilization of wild genetic diversity in wheat breeding faces hurdles like hybridization barriers, abnormalities, and sterility of F1 hybrids (Kishii, 2019). Reduced pairing during meiosis poses an additional challenge, especially when transferring genes from tertiary gene pool species (Qi et al., 2007).
Further challenges include linkage drag and insufficient compensation for substituted wheat chromatin. Hence, the integration of alien chromosome segments into the wheat genome necessitates induced chromosome rearrangements, achievable through methods such as meiotic manipulation (Copete-Parada et al., 2021; Türkösi et al., 2022; Liu et al., 2023), ionizing irradiation (Schubert et al., 2004; Guo et al., 2021; Kim et al., 2022), tissue culture (Lapitan et al., 1984; Li et al., 2000; Zhang et al., 2016), CRISPR (Clustered Regularly Interspaced Short Palindromic Repeats) (Kosicki et al., 2018; Schmidt et al., 2020) or the Gc system (Endo, 1985; Farkas et al., 2023; Türkösi et al., 2024). An approach that utilizes the Gc system enables the identification of alien chromosomal regions containing target genes and facilitates the analysis of their homoeologous relationships to overcome non-collinearity between donor and wheat chromosomes. This process is crucial for well-compensating translocations beneficial for wheat improvement (Qi et al., 2007; Han et al., 2014; Farkas et al., 2023; Türkösi et al., 2024). Numerous studies have successfully implemented the Gc action for this purpose to produce wheat aneuploid lines including deletions, translocations, and telosomic lines. This strategy made it possible to construct cytological chromosome maps, study homoeologous relationships, and localize chromosome breakpoints, genes, and DNA markers, where the lack of markers or genes in wheat aneuploids correlates with the absence of the chromatin segment (Endo and Gill, 1996; Nasuda et al., 2005; Sakata et al., 2010; Ishihara et al., 2014; Kwiatek et al., 2016, 2017; Farkas et al., 2023; Türkösi et al., 2024).
In the context of wheat breeding, Gc genes have been extensively studied, in terms of the transmission mode of the alien chromosomes, particularly concerning chromosome aberrations and segregation distortion in interspecific hybridizations with Aegilops species (Endo, 1982; Tsujimoto and Tsunewaki, 1984, 1985a; Endo, 2015). Segregation distortion occurs commonly in wide hybridization, wherein the allele(s) of a heterozygous locus segregate at frequencies divergent from the anticipated Mendelian ratios of 0.5 (Sandler and Novitski, 1957; Sandler et al., 1959; Endo, 2015; King et al., 2018). This deviation results from the preferential retention of chromosomal blocks carrying genes beneficial for reproductive viability (Niranjana, 2017). Whenever the transmission rate of a chromosome or a locus deviates from the expected Mendelian ratio, the resulting phenomenon is collectively referred to as “drive”. This term encompasses both transmission advantages and segregation distortion, reflecting deviations from the Mendelian principle of equal segregation (Houben, 2017). Segregation distortion observed in inter- and intra-specific hybrids mostly arises from, either pre-fertilization barriers like abortion of female or male gametes, such as pollen tubes competition in the style, or post-fertilization obstacles like abortion of zygote/embryo (Lyttle, 1993; Manabe et al., 1999). In this aspect, Gc factors distinguish themselves from other segregation distorters (Sds) in that their impact is evident in both male and female gametophytes, and they do not confer any reproductive advantage.
Nevertheless, the presence of Sds at specific loci can pose challenges in introgression breeding if they are closely linked to agronomically important genes. Likewise, gene transfer from Aegilops species carrying Gc factor(s) can lead to partial plant sterility. Therefore, during introgression breeding, the Gc genes need to be removed from the progenies to avoid segregation distortion of agronomically desirable genes linked to them and to avoid a decrease in fertility (Marais and Pretorius, 1996). Surprisingly, a pollen-killer (Ki) locus exhibiting dominant action was discovered on the long arm of wheat chromosome 6B with similar Gc action, but its effect is limited to only male gametophytes (Loegering and Sears, 1963; Kota and Dvorak, 1988). Another instance of genes with Gc-like action was reported in Thinopyrum ponticum (Podp.) Barkworth & D.R. Dewey [Syn. Agropyron elongatum (Host) P. Beauv., Lophopyrum ponticum (Popd.) A. Löve, Elytrigia pontica (Popd.) Holub] in which the chromosome carrying Sd: Sd1 and/or Sd2 gene(s) (Kota and Dvorak, 1988; Niranjana, 2017) exhibited preferential transmission through the female gametes but not through the male gametes (Kibirige-Sebunya and Knott, 1983). In homozygotes for the Sd genes, the seed set remains normal. However, a notable decrease in seed set occurs in heterozygotes, indicating the impact of the Sd genes on reproductive outcomes in these plants. The Sd1 locus was mapped proximal to a leaf rust resistance gene Lr19 (Zhang and Dvořák, 1990). While Gc, Ki, and Sd genes contribute to segregation distortion, the behavior of Gc elements is unique. Plants carrying Gc genes in the hemi- (Gc/-) or heterozygous (Gc/gc) form are semi-sterile, whereas homozygous (Gc/Gc) plants are fully fertile (Tsujimoto, 2005).
In this review, we present the narrative of the Gc elements, specifying species carrying them; provide an overview of their discovery, interactions, mechanisms, and suppressors; and discuss their principal applications in wheat breeding.
2 Discovery
During wheat breeding programs, scientists observed that certain chromosomes introduced from Aegilops species displayed an unexpectedly high transmission frequency to the next generation (Endo, 1990, 2015; Endo and Gill, 1996; Friebe et al., 1999). These chromosomes carry unique genes ensuring their persistence through selective abortion of gametes lacking them (Figure 1). The term “Gc” is derived from “Gamete” (egg or sperm) and “Cidal” (capable of killing), signifying gamete-killer (Endo, 1990, 2015). Gc genes, responsible for this action, distort Mendelian segregation in their favor without apparent phenotypic benefits, making them evolutionarily selfish genetic elements (Kwiatek et al., 2017). Originally coined by Maan (1975), the Gc term describes the preferential transmission of an alien chromosome into common wheat, leading to its rapid increase in frequency and eventual fixation in the population (Tsujimoto, 2005).
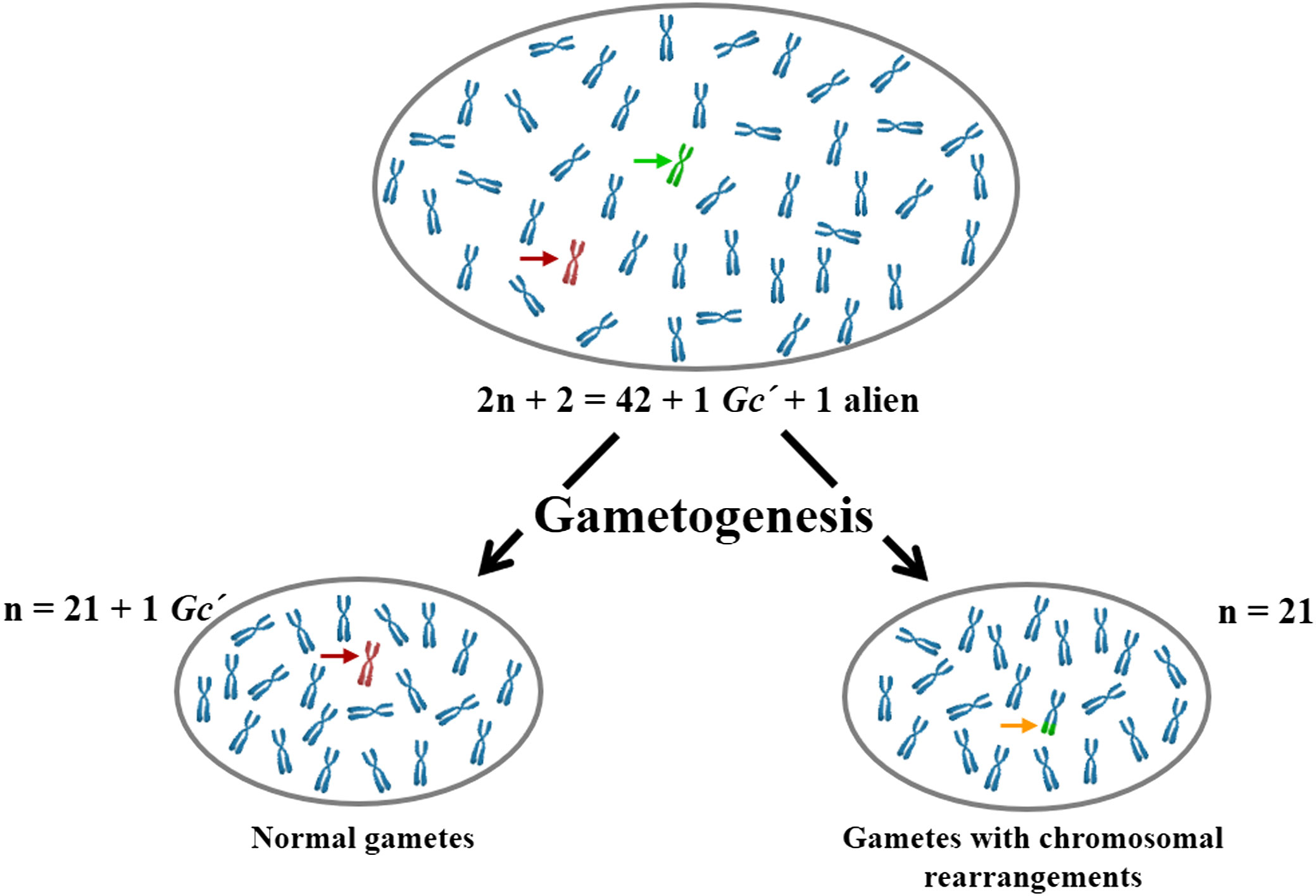
Figure 1 A schematic illustration depicting the Gc action on gametogenesis in wheat (2n + 2 = 42 + 1 Gc´ + 1 alien = 44 chromosomes). Both male and female gametes lacking the Gc genes experience failure or exhibit chromosome abnormalities. Green, brown, and orange arrows indicate alien, Gc, and wheat-alien translocated chromosomes, respectively. Gc´, stands for the Gc chromosome in a monosomic state.
In the process of interspecific hybridization, the stable maintenance of alien chromosomes transferred into wheat depends on their ability to substitute homoeologous chromosomes of the host plant. Notably, they are eliminated from the offspring if they do not compensate for the loss of wheat chromosomes (Taketa et al., 1995; Molnár‐Láng et al., 2005; Szakács and Molnár-Láng, 2010). Conversely, chromosomes carrying Gc genes selfishly persist in host plants. Hence, upon the emergence of a Gc element through mutation or introgression, it undergoes swift proliferation within the population due to preferential transmission. These Gc factors quickly reach fixation and embed themselves in the genome, as they eliminate gametes devoid of them. In this context, Gc genes exhibit a pronounced selfish and parasitic nature toward the host species. Nevertheless, it remains uncertain whether Gc genes retain functionality within their original species. The concept of selfish genes inducing gamete abortion due to allelic interactions is not unique to wheat. Similar phenomena have been reported in various plant species, including tomato (Rick, 1966), tobacco (Cameron and Moav, 1957; Moav et al., 1968), and rice (Sano, 1990). Analogous DNA elements have been identified in the animal kingdom, such as the P element of the Sd system in Drosophila melanogaster (Lyttle, 1993) and t haplotypes in mice (Silver, 1985, 1993). Supernumerary B chromosomes are another group of selfish DNA elements, lacking apparent selective advantages, and have been reported across eukaryotic phyla (Jones, 1995; Jones and Houben, 2003; Jones et al., 2008; Aldrich et al., 2017; Houben, 2017; Ma et al., 2017; Blavet et al., 2021; Karafiátová et al., 2021).
3 Sources and behavioral aspects
Several species of the genus Aegilops carry Gc genes (Table 1), which were discovered in the offspring of wheat × Aegilops crosses. However, dissimilar behavior of Gc elements from various Aegilops species and different homoeologous chromosome groups of the species were observed (Endo, 1988a; Endo, 1988b; Endo, 2015). Thus, Gc effects may vary from (a) Mild, permitting slight anomalies in retained wheat chromosomes, through (b) Intense or Severe, where gametophytes lacking the alien chromosome may undergo severe chromosome abnormalities and become abortive, to (c) Very Strong, resulting in extensive chromosomal breakages when only gametes with the Gc factors are functional, leading to full transmission of the Gc carrier chromosome to the next generation. For instance, chromosome 2Ccy from Ae. cylindrica Host (jointed goatgrass) in wheat cv. Chinese Spring (CS) has a mild Gc effect ranging from lethal to semi-lethal. On the other hand, chromosome 3Ct of Ae. triuncialis L. (barb goatgrass) has an intense or severe Gc action in wheat CS, but mild or semi-lethal in other cultivars (Endo, 1988b; Tsujimoto, 2005; Niranjana et al., 2017). Moreover, it is considered that the Gc action of Ae. longissima Schw. et Musch., Ae. sharonensis Eig (Sharon goatgrass), and Ae. speltoides Tausch [syn. T. speltoides (Tausch) Gren.] is very strong, inducing extreme chromosomal mutations in gametophytes lacking the Gc chromosome, ensuring completely transmission of the Gc carrier chromosome (Miller et al., 1982; King et al., 1991a, 1991b; Nasuda et al., 1998; Friebe et al., 2003).
Thus, the mode of action of Gc elements from Ae. longissima, Ae. sharonensis, and Ae. speltoides differs from that of Gc genes of Ae. triuncialis and Ae. cylindrica and results in dissimilarities in terms of intensity and frequency of chromosome anomalies. Nevertheless, the detailed nuances of the Gc chromosome’s influence from Ae. caudata L. [syn. Ae. markgrafii (Greuter) Hammer], Ae. ovata L. [syn. Ae. geniculata Roth., T. ovatum (L.) Raspail], Ae. geniculata Roth (ovate goatgrass; syn. Ae. ovata L. pro parte), and Ae. biuncialis Vis. [syn. Ae. lorentii Hochst., Ae. macrochaeta Schuttl. et Huet, T. lorentii (Hochst), T. macrochaetum (Schuttl. et Huet) K. Richt, T. biunciale K. Richt] remain largely unexplored, particularly in terms of the intensity of the action.
4 Interactions
Some reports on the interaction between different Gc elements have been published, but the results are controversial. By observing double monosomic addition lines derived from three different Gc chromosome sources, Endo (1982) found that the Gc genes of Ae. triuncialis do not interfere with the Gc effects of Ae. longissima or Ae. sharonensis. In addition, he found that the Gc elements of Ae. longissima dominated the action of those from Ae. sharonensis, since only the Ae. longissima chromosome was necessary for gametes to function in double monosomic addition lines. Endo (1985) further reported that Gc factors located on chromosome 4Sl of Ae. longissima or 4Ssh of Ae. sharonensis are epistatic to those on chromosome 2Sl and 2Ssh, irrespective of the species.
Tsujimoto (1995) investigated the functional relationship between six Gc elements using plants carrying two different Gc factors and identified three functional groups. The First Group includes Gc elements located on chromosomes belonging to the Aegilops homoeologous group 2. For instance, Gc transferred from Ae. speltoides to chromosome 2B of common wheat showed similar function to those on chromosome 2Ssh of Ae. sharonensis. The Second Group includes Gc genes on chromosomes 4Ssh of Ae. sharonensis and 4Sl of Ae. longissima. These genes were epistatic to the Gc genes in the first group in terms of gamete abortion and preferential transmission (Endo, 1985). Although by themselves, the Gc elements in the first group cause chromosome breakage at low frequency (Tsujimoto and Tsunewaki, 1985b; Tsujimoto and Noda, 1989), these genes enhance breakage by the Gc genes of the second group. Conversely, the Gc genes in the second group may enhance breakage by those in the first group. The Third Group includes the Gc genes on chromosome 3Ct of Ae. triuncialis and is independent in terms of the action of the Gc factors in the first or second group. It is important to note that the activity of the second and third Gc element groups are partially suppressed by the Gc inhibitor genes located on chromosomes 4B and 3B, respectively, in certain common wheat strains (Tsujimoto and Tsunewaki, 1984, 1985a; Endo, 1988b, 1988a; King and Laurie, 1993).
Based on the interactions between the different Gc genes, Tsujimoto (1995) proposed the re-designation of the gene symbols following the rules for gene symbolization in wheat. Namely, Gc1, Gc2, and Gc3 for the Gc genes in the first, second, and third groups, respectively, followed by the genome symbol carrying the gene. The relationships between these Gc factors and those on chromosome 2Ccy of Ae. cylindrica, chromosome 4Mg of Ae. geniculata and chromosome 6Ss of Ae. speltoides have not yet been examined.
5 Mechanisms
The molecular mechanism by which Gc genes cause chromosome breakage and induce gamete abortion is not fully understood. The most frequent deletions are produced by a break of one arm of the chromosome followed by a loss of the acentric fragment distal to the breakpoint. This results in defective chromosomes (Werner et al., 1992) that may be stabilized by the action of telomerase. The effect of Gc in the male germline manifests itself as a mixture of normal and nonreproductive pollen, while that in the female germline appears as sporadic seed sets on spikes. Homozygotes for the Gc genes, that is, wheat disomic alien chromosome addition lines, do not show such gametic abortion because all gametes carry an alien chromosome with Gc elements (Endo and Tsunewaki, 1975; Endo, 1982, 2015; Endo and Gill, 1996).
The mode of Gc action differs from other Sd systems in two key aspects: (a) its selfish nature, which destroys gametes lacking Gc genes, and (b) its impact on both male and female gametogenesis. For instance, in plants monosomic for chromosome 4Ssh from Ae. sharonensis, approximately 50% of meiocytes at the first post-meiotic mitosis contained chromosome fragments and these fragments comprised a pair of equal-length segments of two sister chromatids (Finch et al., 1984). In monosomic conditions, the transmission frequency of chromosome 4Ssh through both the male and female gametes was shown to be at least 97.8% in various genetic backgrounds (King et al., 1991b). The ability of 4Ssh to cause chromosome fragmentation is reported not only in meiospores but also in developing embryos and endosperms. The types of aberration were similar to those seen at first pollen grain mitosis in plants monosomic for chromosome 4Ssh. Therefore, it was assumed that a single mechanism might be responsible for aberrations in meiocytes, embryos, and endosperm (King et al., 1991b).
The mode of Gc action is “sporophytic” in nature, as the genetic composition of the sporophyte dictates the sterility of the gametophyte that lacks the Gc genes (Maan, 1975; Niranjana et al., 2017). In the case of Gc factors with very strong gametocidal action in wheat CS and other wheat cultivars, such as chromosomes 4Ssh from Ae. sharonensis, 4Sl from Ae. longissima and 2Ss, 6Ss from Ae. speltoides, chromosome fragments in the form of single chromatid segments were observed during early embryo and endosperm development of plants carrying the Gc chromosome (King and Laurie, 1993; de Las Heras et al., 2001). Broken chromosome ends tend to fuse and form dicentric chromosomes and the break-fusion-bridge cycle is evident (McClintock, 1941; Werner et al., 1992). However, weaker Gc genes like the one located on Ae. cylindrica chromosome 2Ccy (Endo, 1988b), only induces moderate breakages, and the Gc chromosome is not always selectively retained. In the offspring of such plants, the recovery of chromosomal rearrangements is possible and allows for the production of deletion stocks in wheat. Endo (1988b) proposed that in cases of intense Gc action, gametophytes lacking the alien chromosome may experience significant chromosome breakages, leading to sterility and ensuring the exclusive transmission of the alien chromosome. On the contrary, when the Gc action is mild, gametophytes without the alien chromosome are fertilized, suffering slight chromosome damage, and develop into plants with chromosomal aberrations (Endo, 1988b; Tsujimoto, 2005; Niranjana et al., 2017). In case of chromosome 2Ccy, it has been suggested that the breaks occur mainly in the period between the end of meiosis and the interphase prior to the first mitosis of the pollen grain nucleus (Nasuda et al., 1998). The observation of deletions of similar size in sister chromatids at anaphase and telophase of the first pollen mitosis suggests that the breaks occur before DNA replication (Nasuda et al., 1998).
5.1 Induction-prevention phenomena
Two phenomena seem to be involved in the mechanism responsible for preferential transmission of the Gc chromosomes (Endo, 1990; Tsujimoto, 2005; Niranjana, 2017). The first of them is the induction of chromosome breakage, and the second one is the prevention of chromosome breakage. A breaking element is responsible for double-strand breaks in DNA resulting in deletions and translocations. It is conceivable that, when the breaking element alone is present, it induces too many double-strand breaks to be repaired by DNA repair mechanisms. When both breaking and preventive elements are present, the chromosome aberrations do not occur in gametes, because the Gc action is neutralized. The inhibitor may suppress the formation of double-strand breaks by efficient repair mechanisms (Niranjana, 2017). For instance, Friebe et al. (2003) documented the creation of a knockout wheat strain containing the Gc locus within chromosome 4Ssh of Ae. sharonensis. This strain lost its chromosome-breaking function while preserved the inhibitor element. Molecular marker mapping localized the Gc elements on a region proximal to a block of sub-telomeric heterochromatin on chromosome arm 4SshL (Knight et al., 2015; Niranjana, 2017).
5.2 Restriction-modification system
Tsujimoto and Tsunewaki (1985b) noted that the phenomena associated with Gc action in wheat are similar to hybrid dysgenesis observed in fruit fly Drosophila. Hybrid dysgenesis includes sterility, lethality, mutation, chromosome breakage, male recombination, or segregation distortion, and appears only in the F1 progeny of a cross between P or I strain of males and the M or R strain of females (Crow, 1983; Kidwell, 1983; Tsujimoto, 2005). Later, Tsujimoto and Noda (1989, 1990) and Tsujimoto (2005) mentioned the similarity between the nature of Gc action and the restriction-modification systems found in many bacteria. In bacteria, a restriction endonuclease in the host cuts alien DNA at/or around a particular base sequence. On the contrary, host DNA is protected from digestion through methylation. This restriction-modification system might explain chromosome breakage caused by Gc genes in gametogenesis and zygotic cells in wheat. Tsujimoto (2005) proposed a model of Gc action in which the Gc genes produce both a restriction enzyme (RE) and a modification enzyme (ME) like DNA methylase. RE cleaves the specific restriction sites that it recognizes. However, if the sites are protected by DNA methylation, RE cannot cleave. This would be the case in homozygotes for the Gc genes, where no chromosome breakage appears. If the ME function is incomplete and cannot protect all restriction sites, which is likely soon after DNA replication, chromosome breakage may appear with some frequency. After the meiosis of heterozygotes and hemizygotes for a Gc element, haploid cells without the Gc genes are generated. Prior to the first mitotic division in the gametogenesis, DNA is replicated. In cells lacking ME, restriction sites on one of the strands of the replicated DNA are not modified. If RE remains in the cell longer than ME, or if RE is supplied from other cells, for example, the pollen mother cells (PMCs), the unmodified restriction sites are broken by RE. In the following mitoses, unmodified DNA is broken in the same manner. Thus, the gametes without the Gc factor become nonreproductive. In this model, the hemi-modified or hemi-methylated DNA must be deduced to cut by RE because chromosome breakage is observed in the first pollen mitosis. This model can explain chromosome breakage in zygotic cells, as outlined in Tsujimoto (2005). This means that, when pollen carrying the Gc genes fertilizes an egg cell without the Gc genes, unmodified DNA in the egg is exposed to RE from pollen; thus, chromosomes are broken as a result. However, ME soon modifies the DNA derived from the egg and protects against RE. Thus, chromosome breakage ceases soon after fertilization. A diagram illustrating the proposed mechanism of how Gc likely works is presented in Figure 2.
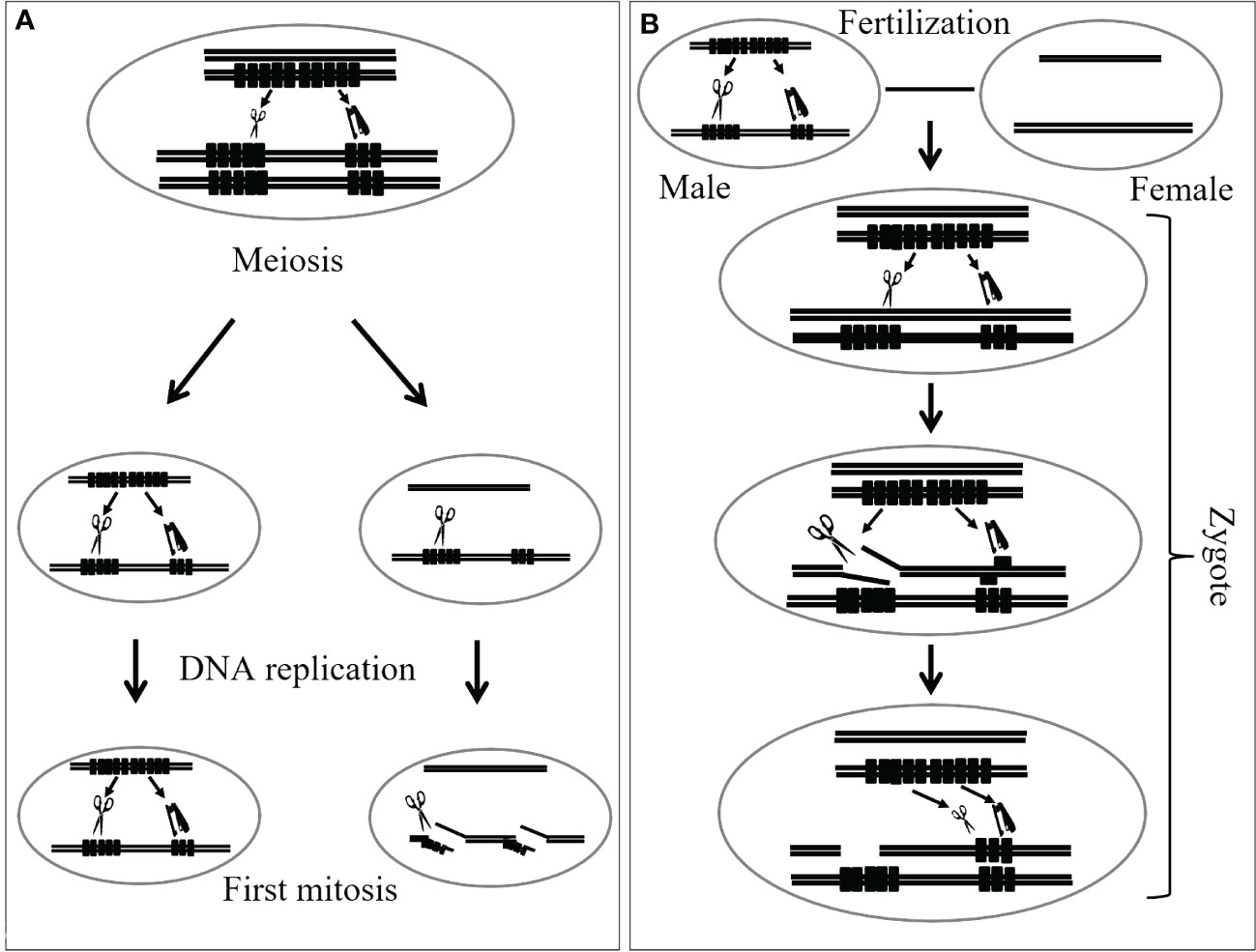
Figure 2 A diagram demonstrates how the restriction-modification system explains chromosome breakage occurring during both gametogenesis (A) and in zygotic cells (B) of wheat, adapted from Tsujimoto (2005) with modifications. RE represents the gene for the restriction enzyme (acting like a scissor), while ME represents the gene for the modification enzyme (acting like a stapler). The RE acts by cleaving specific recognition sites on DNA. However, when these sites are shielded by DNA methylation facilitated by the ME, the RE is unable to cleave. This scenario typically occurs in individuals homozygous for the Gc gene, where chromosome breakage does not occur. The incomplete function of ME can result in the inability to protect all restriction sites, leading to chromosome breakage. Following the meiosis of hemizygotes for the Gc gene, haploid cells lacking the Gc gene are produced. Before the initial mitotic division during gametogenesis, DNA undergoes replication. As these cells lack ME, one strand of the replicated DNA remains unmodified at restriction sites. If the RE persists in the cell longer than ME, or if RE is introduced by other cells, it can cleave the unmodified restriction sites. In the following mitosis, unmodified DNA is cleaved similarly. Consequently, gametes lacking the Gc gene become non-viable. In this model, hemi-modified or hemi-methylated DNA is hypothesized to be susceptible to cleavage by RE, as evidenced by chromosome breakage observed during the first pollen mitosis.
In brief, these chromosomes harbor Gc genes, and the current understanding suggests that Gc genes likely engage DNA methylation and may mimic restriction-modification systems to selectively induce chromosome breaks in gametes without Gc genes. This highlights a role for DNA methylation, with the Gc chromosome carrying the modification machinery that protects its own DNA and triggers breaks in non-methylated gametes, which lack Gc genes. The mechanism likely involves the recognition of the non-carrier chromosomes, followed by the activation of DNA repair pathways that result in double-strand breaks (Tsujimoto et al., 1997; Tsujimoto, 2005). The recognition of non-carrier chromosomes probably involves specific-sequences or structural feature interactions that distinguish the Gc chromosomes from others. Upon recognition, the process occurs through the action of specific genetic elements present on the Gc chromosome. When non-carrier gametes encounter Gc chromosomes during meiosis, the Gc genes on the chromosome can trigger DNA breaks in the non-carrier chromosomes during gametogenesis (Endo, 2007).
6 Suppressors
Based on the current knowledge, Gc action is triggered by chromosomes from particular Aegilops species. However, Kihara (1959) produced wheat alloplasmic lines with Ae. caudata cytoplasm without any Gc chromosomes as discussed in Tsunewaki (2015), while Friebe et al. (1992) reported the whole set of wheat-Ae. caudata disomic addition lines. Also, Feldman (1979) generated a series of seven wheat addition lines for all Ae. longissima chromosomes. These facts may indicate that these strains of the Aegilops species did not carry Gc genes. However, it is known that certain cultivars of common wheat possess genes that partially suppress the function of the Gc factors. If such cultivars with the suppressor were used as the nucleus donors of the alloplasmic lines and the recipients of the alien chromosomes, the Gc genes would not have been noticed. Moreover, if the alien species has the suppressor, Gc effect will be removed in the early backcross generations (Tsujimoto, 2005). It was reported that chromosome 3B partially inhibits the Gc action of 3Ct from Ae. triuncialis (Tsujimoto and Tsunewaki, 1984, 1985a), while 4B incompletely suppresses Gc effects of 4Ssh from Ae. sharonensis or 4Sl from Ae. longissima (Endo, 1988b, 1988a; King and Laurie, 1993).
For instance, plants with monosomic addition of chromosome 3Ct from Ae. triuncialis in the genetic background of common wheat cultivars Jones Fife (JF) and CS showed both male and female semi-sterility. However, semi-sterility did not appear in the common wheat cultivar Norin 26 (N26). Chromosome 3Ct is preferentially transmitted to the next generation from both male and female sides in JF, but only from the female side in CS (Endo and Katayama, 1978; Endo, 2007; Chen et al., 2008; Yamano et al., 2010). In the JF genetic background, both male and female gametes without chromosome 3Ct were unsuccessful whereas, in the CS background, pollen without the Gc chromosome was functional. This result suggested the existence of an incomplete suppressor in the wheat CS background.
The recovery of fertility in the 3Ct monosomic addition in N26 suggested that chromosome 3Ct is transmitted as other alien monosomes without Gc (Endo and Katayama, 1978; Yamano et al., 2010). Tsujimoto and Tsunewaki (1984, 1985a) analyzed the genetic factor in N26 that suppresses the Gc function of 3Ct. The data indicated that a single dominant suppressor gene (Igc1) controls the suppression of Gc action of the 3Ct. Through monosomic analysis, Igc1 was mapped to chromosome 3B of the N26 variety. Moreover, pollen grains carrying Igc1 had a slight advantage during fertilization over pollen grains carrying igc1. The fact that both the Gc genes and the suppressor were located on the chromosomes of the same homoeologous group and that Igc1 is located in the B genome, which originated from an outcrossing species, suggest that Igc1 also has part of the Gc properties. On the other hand, Tsujimoto (2005) reported that Igc1 cannot suppress the Gc genes that exhibit very strong actions, such as those from Ae. sharonensis, Ae. longissima or Ae. speltoides and that no Gc suppressors for these Gc genes were discovered among hundreds of common wheat cultivars tested.
Endo (1988a) reported chromosome breakage in the F1 progeny of a cross between CS monosomic 4B and disomic alien addition lines for chromosome 4Ssh from Ae. sharonensis or 4Sl from Ae. longissima. Since mutations occurred more frequently when the monosomic plant was female than when euploid CS was female, chromosome 4B in the egg cell may partially suppress chromosome breakage (Endo, 1988a). However, King and Laurie (1993) observed chromosome anomaly in early zygotic and endosperm cells of the F1 progeny of monosomic 4B (female) crossed with the substitution line of the chromosome 4Ssh for 4B. Similarly, Nasuda et al. (1998) observed chromosome breakage in a line possessing the Gc chromosome from Ae. speltoides. These findings suggest that 4B might not be an effective suppressor in such instances. The chromosomal abnormalities appeared to be specifically associated with gametes lacking the Gc factor.
Friebe et al. (2003) further substantiated these findings through direct demonstration via fluorescence in-situ hybridization (FISH). They utilized a probe targeting a repetitive DNA sequence specific to the Gc chromosome, revealing that chromosome breakage during pollen mitosis occurred exclusively in gametes without the Gc factors. Moreover, Friebe et al. (2003) produced a mutation of the Ae. sharonensis Gc element (Gcmut), which does not induce gametophytic chromosomal breakage in hemizygous (Gcmut/-) or heterozygous (Gcmut/Gc) conditions, where the plants had fully fertile spikes. The result clearly indicated that Gc encoded two agents behaving like the abovementioned RE and ME systems for chromosome breakage and DNA protection, respectively. Because Gcmut lost the function of the RE, Gcmut/- plants did not show semi-sterility or chromosome breakage; in addition, because of the ME-like function of Gcmut, Gcmut/Gc plants were fertile and showed no induction of chromosome breakage. The function of Gcmut is similar to that of the Igc1 suppressor.
7 Application in wheat breeding
Given the ability of the Gc system to trigger chromosome aberrations, numerous scientists took advantage of this approach to produce wheat pre-breeding materials. Endo and Gill (1996) identified 436 wheat chromosome deletions in the progeny of a monosomic Ae. cylindrica 2Ccy addition line of wheat CS. Thus, about 80% of the deletions were established as homozygous stocks. Svačina et al. (2020) developed a set of 113 deletion lines for chromosome 3D in wheat CS by using the 2Ccy Gc system. The deletion stocks have been extensively utilized for physical mapping of DNA markers (Gill et al., 1996; Qi et al., 2003) and genes (Nomura et al., 2003) to specific sub-arm chromosome regions of wheat chromosomes. For instance, wheat chromosomal mutants induced by the Gc effect enabled positional cloning of Pairing homoeologous 2 (Ph2) from a 121.16 Mb candidate region on 3DS (Serra et al., 2021). Based on the analysis of a set of specifically created 3DS deletion mutants using Gc genes action (Svačina et al., 2020), combined with exome sequencing and transcriptome analysis of ph2a and ph2b mutants versus wild-type, Serra et al. (2021) identified TaMSH7-3D, a gene encoding a plant specific DNA mismatch repair protein.
The Gc system was applied to induce chromosomal changes not only in euploid common wheat but also in wheat-alien chromosome addition lines (Figures 3, 4). Besides chromosome deletions, the Gc-induced chromosome breaks lead to translocations, including intergenomic translocations as well. Structural rearrangements of cultivated barley (Hordeum vulgare L.) chromosomes were obtained in common wheat by the Gc system (Shi and Endo, 1999). The rearranged alien chromosomes, including deletions and wheat-alien translocations, were used for the physical mapping of molecular markers on chromosome 7H (Serizawa et al., 2001; Masoudi-Nejad et al., 2005), 5H (Ashida et al., 2007), 3H (Sakai et al., 2009), and 4H (Sakata et al., 2010). Friebe et al. (2000) used the Gc system of chromosome 2Ccy to induce and study the nature of chromosomal rearrangements in rye chromosomes added to wheat. Following backcrossing and selfing, 33 deletions were identified, in either homozygous or heterozygous states and covering all rye chromosomes except 7R. The Gc system was also used to produce chromosome rearrangements between Ae. ovata and hexaploid triticale by expression of the Gc action located on chromosome 4Mg from Ae. ovata (Kwiatek et al., 2016). Similarly, using the Gc mechanism located on chromosome 4Mg from Ae. geniculata Kwiatek et al. (2017) produced 41 triticale lines and seventeen of them carried chromosome aberrations.
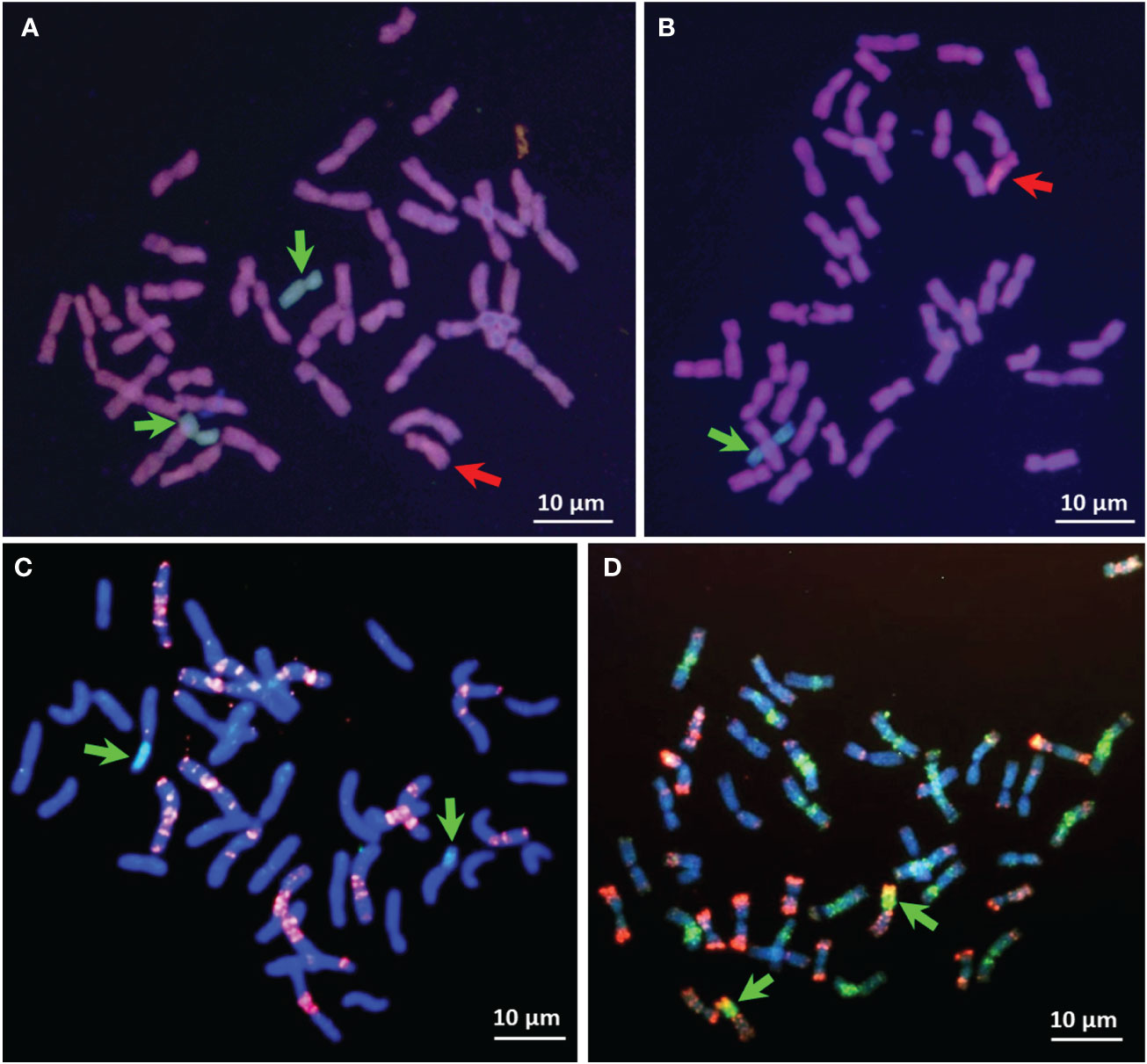
Figure 3 Application of Gc action via chromosome 2Ccy from Ae. cylindrica to induce chromosomal breakage in H. chilense in the background of common wheat. GISH on metaphase spreads showing Ae. cylindrica (red arrows) chromosome 2Ccy (A, B) and H. chilense (green arrows) chromosomes 2Hch and 7Hch (A) and 7Hch (B) in CS genetic background. No chromosomal aberrations showed in the cells carrying monosomic 2Ccy (A, B). However, after selfing or backcrossing, mutations are expected in the following generations in the zygotes lacking the 2Ccy chromosome. Homozygous centromeric translocation (green arrows) 7HchS·5AL (C), adapted from Mattera et al. (2015) with modifications, and Robertsonian translocation (green arrows) 2HchS·2DL (D), adapted from Palomino and Cabrera (2019) with modifications. FISH red signals are from probes GAA-satellite sequence (C) and repetitive sequence pAs1 (D). Chromosomes were counterstained with DAPI (blue color).
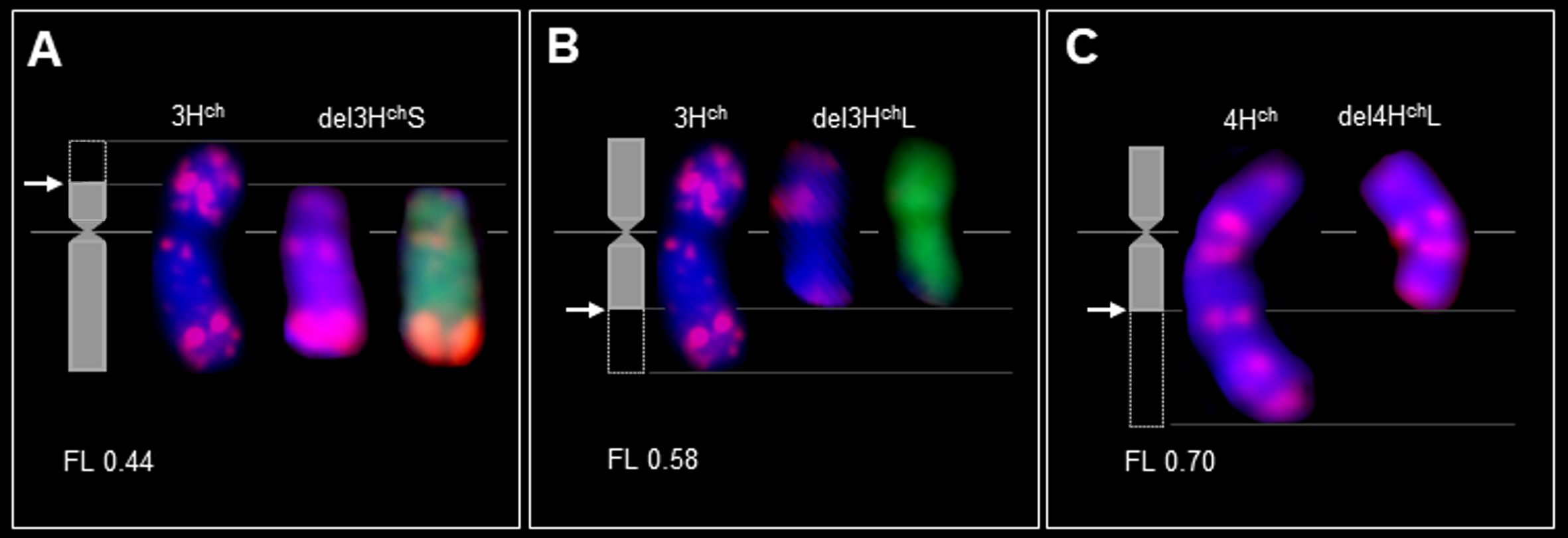
Figure 4 Breakage by Gc action in chromosomes from wild barley (H. chilense) as alien disomic additions in wheat; adapted from Said and Cabrera (2009) and Said et al. (2012) with modifications. The idiograms (left) in (A-C) show the breakpoints (arrows). Double FISH with the pAs1 (red) and GISH (green) probes on mitotic metaphase of chromosome 3Hch and its deletions in the genetic background of wheat (A, B). FISH with pAs1 (red) probe on mitotic metaphase of chromosome 4Hch and its deletion in the genetic background of wheat (C) (Said et al., unpublished). The chromosomes were counterstained with DAPI (blue). Chromosome deletion (del), fraction length (FL), short and long arms (S and L, respectively).
Similarly, Farkas et al. (2023) employed the Gc mechanism located on chromosome 4Mb of Ae. biuncialis to create various wheat cv. Mv9kr1 lines, including Mv9kr1-Ae. biuncialis disomic 4Ub addition, 4Mb(4D), and 5Mb(5D) substitutions, as well as several introgression lines, leading to the establishment of species-specific molecular markers and positively influencing the morphology of spikes and seeds. These newly developed cytogenetic stocks could prove valuable as a genetic resource for introducing wild alleles of crucial genes that govern significant agronomic traits into wheat through chromosome engineering. Using Gc action of chromosome 2Ccy from Ae. cylindrica, structural changes were obtained for wild barley (H. chilense Roem. et Schult, 2n = 2x=14, HchHch) chromosomes 1Hch (Cherif-Mouaki et al., 2011), 3Hch (Said et al., 2012), 4Hch (Said and Cabrera, 2009), 2Hch, and 7Hch in wheat (Mattera et al., 2015; Palomino and Cabrera, 2019). Initially, chromosomes 2Hch and 7Hch, along with 2Ccy, were simultaneously acquired within the wheat genetic background (Figure 3A). Subsequently, chromosomes 2Hch and 7Hch were separated into different plants, each still accompanied by 2Ccy (Figure 3B However, the emergence of chromosome breakages and translocations is anticipated in subsequent generations, as depicted in the schematic illustration of the Gc process (Figure 1). Indeed, the wheat chromosome translocations with 7Hch by Mattera et al. (2015) (Figure 3C) and with 2Hch were obtained by Palomino and Cabrera (2019) (Figure 3D). Moreover, illustrations by genomic in-situ hybridization (GISH) and FISH are provided for chromosome deletions, breakpoints, and Fraction length (FL) resulting from the Gc action of 2Ccy on chromosomes 3Hch (Figures 4A, B) and 4Hch (Figure 4C).
The Gc system proved to be effective in inducing structural rearrangements in alien chromosomes added to common wheat from Haynaldia villosa (L.) (Chen et al., 2008), Leymus racemosus (Lam.) Tzvelev (Chen et al., 2005) and Agropyron cristatum (L.) Gaertn (2n = 4x = 28, PPPP) (Liu et al., 2010; Luan et al., 2010; Copete-Parada et al., 2021). The production of germplasms with chromosomal rearrangements allowed the location of genes and/or markers on specific sub-arm chromosome of wheat and wild relatives (Said and Cabrera, 2009; Cherif-Mouaki et al., 2011; Said et al., 2012; Ochoa et al., 2015; Said et al., 2019b; Farkas et al., 2023). In this case, the absence of markers can be directly associated with the chromosomal fragment that has been lost (Figures 3–5). These lines also provide information on the homoeology and structure of the chromosomes and can be useful for the identification of functionally important chromosomal regions, particularly for the location of genes that determine interesting traits in agriculture, as well as to transfer new alleles from alien species to wheat.
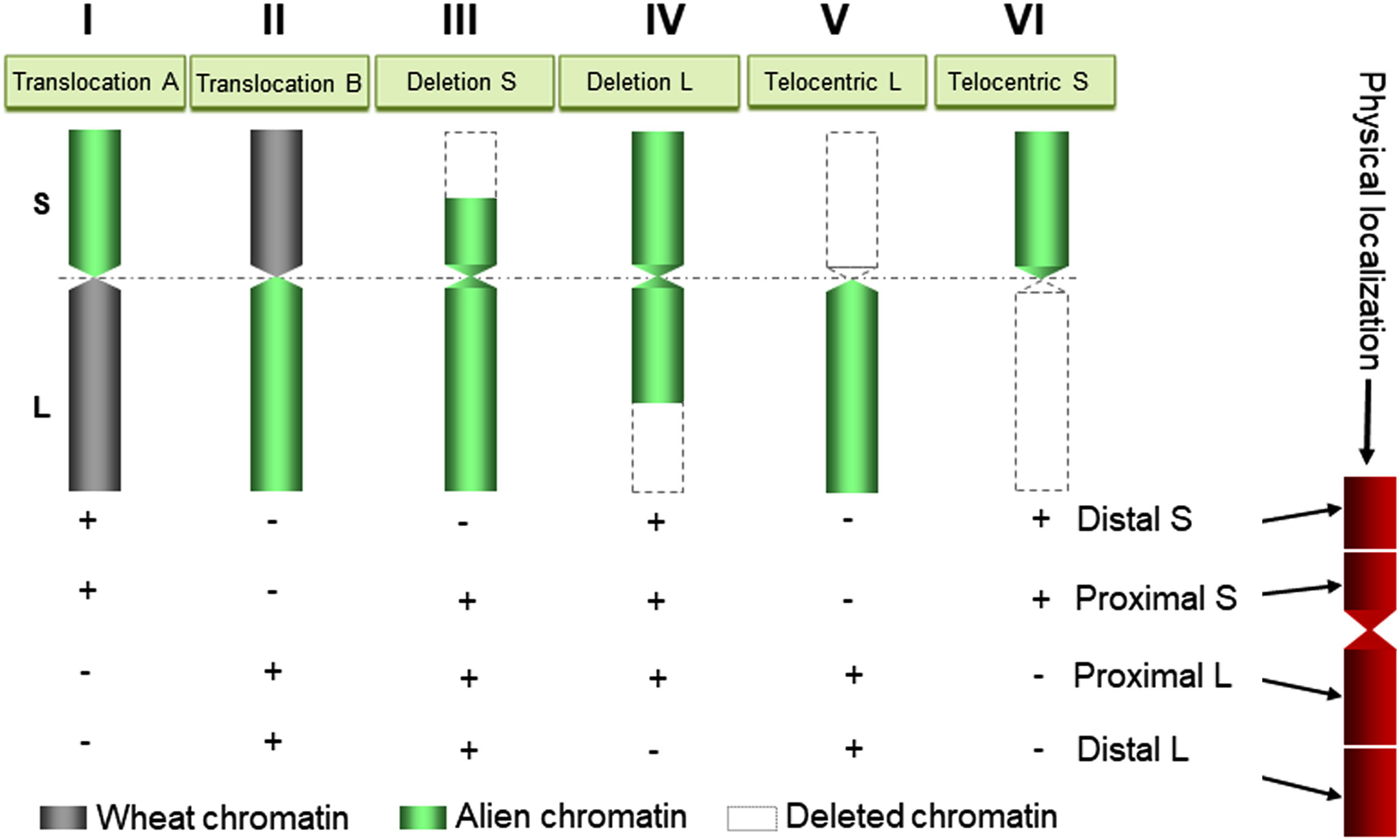
Figure 5 A diagram showing the use of wheat aneuploids carrying rearranged alien chromosomes generated by Gc action for physical mapping of DNA markers/genes on alien chromosomes in wheat. Idiograms I, II, III, IV, V, and VI illustrate translocation between alien short (S)/wheat long (L) arms, translocation between wheat short (S)/alien long (L) arms, deletion in the short arm, deletion in the long arm, telocentric long arm, and telocentric short arm, respectively. The + or – signs indicate the presence or absence, respectively, of markers/genes in the wheat aneuploids, which is directly related to the existent or missing chromatin region.
8 Discussion
This paper reviews the principles and use of the Gc system to induce chromosomal rearrangements in wheat with alien chromosomes originating from its wild relatives. This is crucial because maintaining and enriching genetic diversity of elite germplasm by crossbreeding is an indispensable prerequisite for adapting one of the world’s key food security crops to the demands of farmers and consumers and adapting it to the changing climate. Genetic diversity of the secondary and tertiary gene pool, including wheat ancestors and wild relatives, serves as a precious resource for this purpose.
This review is an update of the previous reviews treatises on the Gc system (Endo, 1990, 2007) and synthesizes the main characteristics of the topic that have changed over time to give rise to current understandings about the mode of action, interactions, suppressions, and practical applications of Gc genes in wheat breeding. Furthermore, it fills a gap in a recent review by Boehm and Cai (2024), which lacks a reference to the Gc system, by reviewing the current knowledge on alien introgressions in wheat breeding. The work also outlines essential strategies for leveraging alien introgression to diversify the wheat genome, providing indispensable guidance for researchers and breeders in their pursuit of crop improvement.
Several approaches can be employed to induce chromosomal aberrations. They differ in terms of mechanisms, affected cell populations, heritability, safety concerns, and applications. Moreover, each method has its own set of advantages and disadvantages.
Ionizing irradiation and chemical agents causing chromosomal breaks (clastogenes) affect a broad range of cells by directly damaging DNA in both somatic and germ cells, causing various forms of DNA damage, including single- and double-strand breaks, and nucleotide base modifications (Schubert et al., 2004; Durante and Formenti, 2018; Kim et al., 2020, 2022; Guo et al., 2021). Ionizing irradiation and clastogens induce chromosomal rearrangements, encompassing duplications, and inversions, among others, causing more infertility. Chromosomal aberrations can be heritable if arising in germ cells but not if occurring in somatic cells (Durante and Formenti, 2018; Kim et al., 2020, 2022; Guo et al., 2021). Furthermore, the irradiation method generally poses greater safety concerns due to its non-specificity and potential for widespread genetic damage. It is crucial to mention that the outcomes of irradiation and chemicals are unpredictable, constituting a completely random process (Schubert et al., 2004; Durante and Formenti, 2018; Kim et al., 2022).
In contrast, Gc chromosomes, being naturally occurring, act selectively during gametogenesis, precisely targeting germ cells to induce double-strand DNA breaks, but not affecting the somatic cells of the plant (Endo, 2015; King et al., 2018). The resulting chromosomal aberrations, such as deletions and translocations, are always heritable as they arise in reproductive cells and are passed to offspring (Nasuda et al., 2005; Sakata et al., 2010; Ishihara et al., 2014; Farkas et al., 2023; Türkösi et al., 2024). Thus, using Gc chromosomes is encouraged due to its perceived safety and efficacy in producing inherited chromosomal rearrangements (Tsujimoto, 2005; Sakata et al., 2010; Ishihara et al., 2014). The number of chromosome breaks induced by the Gc system per pollen can vary depending on various factors such as Gc chromosome category, the specific wheat genotype, environmental conditions, the presence of suppressors and other genetic modifiers (Endo and Katayama, 1978; Endo, 1988b, 2007; Tsujimoto, 2005; Chen et al., 2008; Yamano et al., 2010; Niranjana et al., 2017).
Contrasting with the approaches discussed above, recently developed CRISPR/Cas technology is renowned for its precision in genome editing and can induce chromosome aberrations at particular loci and induce double-strand breaks in somatic and germ cells (Kosicki et al., 2018). The breaks can lead to various chromosomal rearrangements such as deletions, inversions, duplications, or translocations depending on repair mechanisms and cellular context (Symington, 2016; Kosicki et al., 2018; Schmidt et al., 2020). Researchers have devised methods to use CRISPR to induce chromosomal rearrangements for studying chromosomal structure, genetic engineering, and disease modeling (Zuo et al., 2017; Kosicki et al., 2018; Schmidt et al., 2020). CRISPR’s ability to manipulate genetic material at the chromosomal level offers powerful tools beyond traditional genome editing applications (Symington, 2016; Zuo et al., 2017; Haapaniemi et al., 2018; Kosicki et al., 2018). However, despite CRISPR technology’s significant advancements in animal and medical research, its use for inducing chromosomal rearrangements in plants remains limited (Schmidt et al., 2020).
The Gc system is a highly valuable and versatile approach with a wide range of uses for wheat gene mapping and breeding. It is an efficient tool to produce cytogenetic stocks, such as deletions, translocations, and telocentrics of either wheat or alien chromosomes introgressed into wheat. This plant material could be used for gene tracking, DNA markers physical mapping, comparative genome analysis and to study homologous relationships. It has been indispensable in widening genetic diversity of cereals and improving the crop by insertion of alien chromatin segments with traits of interest into the wheat genome. The method provides remarkably novel breeding material carrying new genes or alleles delivered from wild relatives. Producing breeding material using the Gc strategy is a long-term investment, but it is essential for developing new varieties that can meet the needs of a growing population in a changing climate.
In plant breeding, accurately assessing the effects of Gc chromosomes is essential for understanding their impact on chromosomal rearrangements, genome stability, fertility, and overall breeding objectives. The most popular cytogenetic FISH-based techniques rely on hybridization of labeled probes to particular DNA sequences and allow identification of chromosome breaks (Molnár et al., 2016; King et al., 2017; Said et al., 2021). The probes are typically designed to target specific chromosome regions or whole alien chromosomes as in case of GISH probes. However, the resolution of FISH techniques may not be high enough to detect subtle chromosome rearrangements meticulously. This may lead to underestimation of the actual number of breaks induced by Gc chromosomes (Badaeva et al., 1996, 2004; Molnár et al., 2009, 2011, 2016). In case of GISH, which specifically targets alien chromosomes, it is essential to recognize its limitations in precisely quantifying induced chromosome breaks or rearrangements. If the rearrangements induced by a Gc chromosome involve wheat chromosomes rather than the alien chromosomes, they will not be captured (Said and Cabrera, 2009; Mattera et al., 2015; Ochoa et al., 2015; Palomino and Cabrera, 2019; Said et al., 2019a; Said et al., 2019b).
Given these limitations, it is crucial to consider the potential underestimation of chromosome breaks or rearrangements in the plant breeding process, especially if Gc chromosomes or similar elements are involved. Alternative techniques with higher resolution and sensitivity, such as molecular markers targeting specific chromosome regions, or whole-genome sequencing (Cherif-Mouaki et al., 2011; Said et al., 2019b, 2021), may be needed to complement FISH to provide a more comprehensive understanding of chromosomal alterations induced by Gc chromosome. Clearly, incorporating multiple analytical approaches to detect and characterize chromosomal rearrangements ensures a more robust evaluation of breeding materials and facilitates the development of improved cultivars with desired traits.
The fundamental question of how gametes with Gc genes stay normal while those without Gc do not remains unanswered, representing a current research gap that requires further investigation. Nevertheless, the abovementioned behavior aligns with the Gc chromosomes’ self-preservation strategy, wherein they eliminate gametes lacking them to ensure their own survival. This performance mirrors their actions in their original species, where they function as normal chromosomes without causing gametic damage.
Although the accurate molecular mechanism is still under investigation and the exact course may vary, research in this area continues to uncover the complexities of Gc behavior in plants. However, further efforts are required to fully clarify the intricate details of this process.
Author contributions
MS: Conceptualization, Data curation, Formal analysis, Investigation, Methodology, Project administration, Resources, Software, Supervision, Validation, Visualization, Writing – original draft, Writing – review & editing. EG: Data curation, Formal analysis, Investigation, Methodology, Validation, Visualization, Writing – review & editing. AF: Formal analysis, Investigation, Methodology, Validation, Visualization, Writing – review & editing. IM: Conceptualization, Data curation, Formal analysis, Investigation, Methodology, Validation, Visualization, Writing – review & editing. JB: Conceptualization, Data curation, Formal analysis, Funding acquisition, Investigation, Project administration, Resources, Validation, Visualization, Writing – review & editing. JD: Conceptualization, Data curation, Formal analysis, Funding acquisition, Investigation, Project administration, Resources, Validation, Visualization, Writing – review & editing. AC: Conceptualization, Data curation, Formal analysis, Investigation, Methodology, Validation, Visualization, Writing – review & editing. TE: Data curation, Formal analysis, Investigation, Validation, Visualization, Writing – review & editing.
Funding
The author(s) declare financial support was received for the research, authorship, and/or publication of this article. This work was supported by the project TowArds Next GENeration Crops, reg. no. CZ.02.01.01/00/22_008/0004581 of the ERDF Programme Johannes Amos Comenius, the Hungarian National Research, Development and Innovation Office (PD145915, K135057, TKP2021-NKTA-06 and 2019-2.1.11-TÉT-2019-00074), and by the Spanish State Research Agency (Spanish Ministry of Science and Innovation), co-financed by the European Regional Development Fund (FEDER) from the European Union, grant numbers RTI2018-093367-B-I00 and PID2021-122530OB-I00.
Acknowledgments
Figure 1 was created with the help of BioRender.com.
Conflict of interest
The authors declare that the research was conducted in the absence of any commercial or financial relationships that could be construed as a potential conflict of interest.
The author(s) declared that they were an editorial board member of Frontiers, at the time of submission. This had no impact on the peer review process and the final decision.
Publisher’s note
All claims expressed in this article are solely those of the authors and do not necessarily represent those of their affiliated organizations, or those of the publisher, the editors and the reviewers. Any product that may be evaluated in this article, or claim that may be made by its manufacturer, is not guaranteed or endorsed by the publisher.
References
Aldrich, J. C., Leibholz, A., Cheema, M. S., Ausiό, J., Ferree, P. M. (2017). A “selfish” B chromosome induces genome elimination by disrupting the histone code in the jewel wasp Nasonia vitripennis. Sci. Rep. 7, 42551. doi: 10.1038/srep42551
Ashida, T., Nasuda, S., Sato, K., Endo, T. R. (2007). Dissection of barley chromosome 5H in common wheat. Genes Genet. Syst. 82, 123–133. doi: 10.1266/ggs.82.123
Badaeva, E. D., Amosova, A. V., Samatadze, T. E., Zoshchuk, S. A., Shostak, N. G., Chikida, N. N., et al. (2004). Genome differentiation in Aegilops. 4. Evolution of the U-genome cluster. Plant Syst. Evol. 246, 45–76. doi: 10.1007/s00606-003-0072-4
Badaeva, E. D., Friebe, B., Gill, B. S. (1996). Genome differentiation in Aegilops. 1. Distribution of highly repetitive DNA sequences on chromosomes of diploid species. Genome 39, 293–306. doi: 10.1139/g96-040
Blavet, N., Yang, H., Su, H., Solanský, P., Douglas, R. N., Karafiátová, M., et al. (2021). Sequence of the supernumerary B chromosome of maize provides insight into its drive mechanism and evolution. PNAS 118, 1–11. doi: 10.1073/pnas.2104254118
Boehm, J., Cai, X. (2024). Enrichment and diversification of the wheat genome via alien introgression. Plants 13, 339. doi: 10.3390/plants13030339
Cameron, D. R., Moav, R. M. (1957). Inheritance in Nicotiana tabacum XXVII. Pollen killer, an alien genetic locus inducing abortion of microspores not carrying it. Genetics 42, 326–335. doi: 10.1093/genetics/42.3.326
Chen, P., Liu, W., Yuan, J., Wang, X., Zhou, B., Wang, S., et al. (2005). Development and characterization of wheat- Leymus racemosus translocation lines with resistance to Fusarium Head Blight. Theor. Appl. Genet. 111, 941–948. doi: 10.1007/s00122-005-0026-z
Chen, Q., Cao, A., Qi, Z., Zhang, W., Chen, P. (2008). Structural Changes of 2V Chromosome of Haynaldia villosa Induced by Gametocidal Chromosome 3C of Aegilops triuncialis. Agric. Sci. China 7, 804–811. doi: 10.1016/S1671-2927(08)60117-0
Cherif-Mouaki, S., Said, M., Alvarez, J. B., Cabrera, A. (2011). Sub-arm location of prolamin and EST-SSR loci on chromosome 1H(ch) from Hordeum Chilense. Euphytica 178, 63–69. doi: 10.1007/s10681-010-0268-y
Copete-Parada, A., Palomino, C., Cabrera, A. (2021). Development and characterization of wheat-Agropyron cristatum introgression lines induced by gametocidal genes and wheat ph1b mutant. Agronomy 11, 277. doi: 10.3390/agronomy11020277
Crow, J. F. (1983). Hybrid dysgenesis and the P factor in Drosophila. Japanese J. Genet. 58, 621–625. doi: 10.1266/jjg.58.621
de Las Heras, J. I., King, I. P., Parker, J. S. (2001). 5-azacytidine induces chromosomal breakage in the root tips of wheat carrying the cuckoo chromosome 4SL from Aegilops sharonensis. Heredity 87, 474–479. doi: 10.1046/j.1365-2540.2001.00931.x
Durante, M., Formenti, S. C. (2018). Radiation-induced chromosomal aberrations and immunotherapy: micronuclei, cytosolic DNA, and interferon-production pathway. Front. Oncol. 8. doi: 10.3389/fonc.2018.00192
Endo, T. R. (1979). Selective gametocidal action of a chromosome of Aegilops cylindrica in a cultivar of common wheat. Wheat Inf. service 50, 24–28.
Endo, T. R. (1982). Gametocidal chromosomes of three Aegilops species in common wheat. Can. J. Genet. Cytol. 24, 201–206. doi: 10.1139/g82-020
Endo, T. R. (1985). Two types of gametocidal chromosome of Aegilops sharonensis and Ae. longissima. Japanese J. Genet. 60, 125–135. doi: 10.1266/jjg.60.125
Endo, T. R. (1988a). “Chromosome mutations induced by gametocidal chromosomes in common wheat,” in Proceedings of the seventh international wheat genetics symposium, Cambridge, UK, 13-19 July 1988, CABI Digital Library. 259–265.
Endo, T. R. (1988b). Induction of chromosomal structural changes by a chromosome of Aegilops cylindrica L. @ in common wheat. J. Hered 79, 366–370. doi: 10.1093/oxfordjournals.jhered.a110529
Endo, T. R. (1990). Gametocidal chromosomes and their induction of chromosome mutations in wheat. Japanese J. Genet. 65, 135–152. doi: 10.1266/jjg.65.135
Endo, T. R. (2007). The gametocidal chromosome as a tool for chromosome manipulation in wheat. Chromosome Res. 15, 67–75. doi: 10.1007/s10577-006-1100-3
Endo, T. R. (2015). “Gametocidal Genes,” in Alien Introgression in Wheat: Cytogenetics, Molecular Biology, and Genomics. Eds. Molnár-Láng, M., Ceoloni, C., Doležel, J. (Springer International Publishing, Cham), 121–131. doi: 10.1007/978-3-319-23494-6_5
Endo, T. R., Gill, B. S. (1996). The deletion stocks of common wheat. J. Heredity 87, 295–307. doi: 10.1093/oxfordjournals.jhered.a023003
Endo, T. R., Katayama, Y. (1978). Finding of a selectively retained chromosome of Aegilops caudata L. in common wheat. Wheat Inf. Serv. 47/48, 32–35.
Endo, T. R., Tsunewaki, K. (1975). Sterility of common wheat with Aegilops triuncialis cytoplasm. J. Heredity 66, 13–18. doi: 10.1093/oxfordjournals.jhered.a108562
Farkas, A., Gaál, E., Ivanizs, L., Blavet, N., Said, M., Holušová, K., et al. (2023). Chromosome genomics facilitates the marker development and selection of wheat-Aegilops biuncialis addition, substitution and translocation lines. Sci. Rep. 13, 20499. doi: 10.1038/s41598-023-47845-8
Feldman, M. (1979). “New evidence on the origin of the B genome of wheat,” in Proceedings of the Fifth International Wheat Genetics Symposium, New Delhi, India: Indian Society of Genetics & Plant Breeding, February 23-28, 1978. 120–132.
Finch, R. A., Miller, T. E., Bennett, M. D. (1984). “Cuckoo” Aegilops addition chromosome in wheat ensures its transmission by causing chromosome breaks in meiospores lacking it. Chromosoma 90, 84–88. doi: 10.1007/BF00352282
Friebe, B., Kynast, R. G., Gill, B. S. (2000). Gametocidal factor-induced structural rearrangements in rye chromosomes added to common wheat. Chromosome Res. 8, 501–511. doi: 10.1023/A:1009219722418
Friebe, B., Schubert, V., Blüthner, W. D., Hammer, K. (1992). C-banding pattern and polymorphism of Aegilops caudata and chromosomal constitutions of the amphiploid T. aestivum — Ae. caudata and six derived chromosome addition lines. Theoret. Appl. Genet. 83, 589–596. doi: 10.1007/BF00226902
Friebe, B. R., Tuleen, N. A., Gill, B. S. (1999). Development and identification of a complete set of Triticum aestivum - Aegilops geniculata chromosome addition lines. Genome 42, 374–380. doi: 10.1139/g99-011
Friebe, B., Zhang, P., Gill, B. S., Nasuda, S. (2003). Characterization of a knock-out mutation at the Gc2 locus in wheat. Chromosoma 111, 509–517. doi: 10.1007/s00412-003-0234-8
Gill, K. S., Gill, B. S., Endo, T. R., Boyko, E. V. (1996). Identification and high-density mapping of gene-rich regions in chromosome group 5 of wheat. Genetics 143, 1001–1012. doi: 10.1093/genetics/143.2.1001
Guo, W., Comai, L., Henry, I. M. (2021). Chromoanagenesis from radiation-induced genome damage in Populus. PloS Genet. 17, e1009735. doi: 10.1371/journal.pgen.1009735
Haapaniemi, E., Botla, S., Persson, J., Schmierer, B., Taipale, J. (2018). CRISPR–Cas9 genome editing induces a p53-mediated DNA damage response. Nat. Med. 24, 927–930. doi: 10.1038/s41591-018-0049-z
Hajjar, R., Hodgkin, T. (2007). The use of wild relatives in crop improvement: a survey of developments over the last 20 years. Euphytica 156, 1–13. doi: 10.1007/s10681-007-9363-0
Han, H., Bai, L., Su, J., Zhang, J., Song, L., Gao, A., et al. (2014). Genetic rearrangements of six wheat–Agropyron cristatum 6P addition lines revealed by molecular markers. PloS One 9, e91066. doi: 10.1371/journal.pone.0091066
Houben, A. (2017). B chromosomes – A matter of chromosome drive. Front. Plant Sci. 8. doi: 10.3389/fpls.2017.00210
Ishihara, A., Mizuno, N., Islam, R. A. K. M., Doležel, J., Endo, T. R., Nasuda, S. (2014). Dissection of barley chromosomes 1H and 6H by the gametocidal system. Genes Genet. Syst. 89, 203–214. doi: 10.1266/ggs.89.203
Jones, R. N. (1995). B chromosomes in plants. NewPhytol 131, 411–434. doi: 10.1111/j.1469-8137.1995.tb03079.x
Jones, N., Houben, A. (2003). B chromosomes in plants: escapees from the A chromosome genome? Trends Plant Sci. 8, 417–423. doi: 10.1016/S1360-1385(03)00187-0
Jones, R. N., Viegas, W., Houben, A. (2008). A century of B chromosomes in plants: so what? Ann. Bot. 101, 767–775. doi: 10.1093/aob/mcm167
Karafiátová, M., Bednářová, M., Said, M., Čížková, J., Holušová, K., Blavet, N., et al. (2021). The B chromosome of Sorghum purpureosericeum reveals the first pieces of its sequence. J. Exp. Bot. 72, 1606–1616. doi: 10.1093/jxb/eraa548
Kibirige-Sebunya, I., Knott, D. R. (1983). Transfer of stem rust resistance to wheat from an Agropyron chromosome having a gametocidal effect. Can. J. Genet. Cytol. 25, 215–221. doi: 10.1139/g83-035
Kidwell, M. G. (1983). Evolution of hybrid dysgenesis determinants in Drosophila melanogaster. Proc. Natl. Acad. Sci. 80, 1655–1659. doi: 10.1073/pnas.80.6.1655
Kihara, H. (1959). Fertility and morphological variation in the substitution backcrosses of the hybrid Triticum vulgare × Aegilops caudata. Proc. 10th Int. Congr. Genet. 1, 142–171.
Kim, S. H., Kim, S. W., Ryu, J., Kang, S.-Y., Kang, B.-C., Kim, J.-B. (2020). Dark/light treatments followed by γ-irradiation increase the frequency of leaf-color mutants in cymbidium. Plants 9, 532. doi: 10.3390/plants9040532
Kim, Y. J., Lee, J. W., Cho, Y. H., Choi, Y. J., Lee, Y., Chung, H. W. (2022). Chromosome damage in relation to recent radiation exposure and radiation quality in nuclear power plant workers. Toxics 10, 94. doi: 10.3390/toxics10020094
King, J., Grewal, S., Yang, C.-Y., Hubbart, S., Scholefield, D., Ashling, S., et al. (2017). A step change in the transfer of interspecific variation into wheat from Amblyopyrum muticum. Plant Biotechnol. J. 15, 217–226. doi: 10.1111/pbi.12606
King, J., Grewal, S., Yang, C., Hubbart Edwards, S., Scholefield, D., Ashling, S., et al. (2018). Introgression of Aegilops speltoides segments in Triticum aestivum and the effect of the gametocidal genes. Ann. Bot. 121, 229–240. doi: 10.1093/aob/mcx149
King, I. P., Koebner, R. M. D., Schlegel, R., Reader, S. M., Miller, T. E., Law, C. N. (1991a). Exploitation of a preferentially transmitted chromosome from Aegilops sharonensis for the elimination of segregation for height in semidwarf bread wheat varieties. Genome 34, 944–949. doi: 10.1139/g91-146
King, I. P., Laurie, D. A. (1993). Chromosome damage in early embryo and endosperm development in crosses involving the preferentially transmitted 4S1 chromosome of Aegilops sharonensis. Heredity 70, 52–59. doi: 10.1038/hdy.1993.7
King, I. P., Miller, T. E., Koebner, R. M. D. (1991b). Determination of the transmission frequency of chromosome 4S (l) of Aegilops sharonensis in a range of wheat genetic backgrounds. Theoret. Appl. Genet. 81, 519–523. doi: 10.1007/BF00219443
Kishii, M. (2019). An update of recent use of Aegilops species in wheat breeding. Front. Plant Sci. 10. doi: 10.3389/fpls.2019.00585
Knight, E., Binnie, A., Draeger, T., Moscou, M., Rey, M.-D., Sucher, J., et al. (2015). Mapping the ‘breaker’ element of the gametocidal locus proximal to a block of sub-telomeric heterochromatin on the long arm of chromosome 4Ssh of Aegilops sharonensis. Theor. Appl. Genet. 128, 1049–1059. doi: 10.1007/s00122-015-2489-x
Kosicki, M., Tomberg, K., Bradley, A. (2018). Repair of double-strand breaks induced by CRISPR–Cas9 leads to large deletions and complex rearrangements. Nat. Biotechnol. 36, 765–771. doi: 10.1038/nbt.4192
Kota, R. S., Dvorak, J. (1988). Genomic instability in wheat induced by chromosome 6b(s) of Triticum speltoides. Genetics 120, 1085–1094. doi: 10.1093/genetics/120.4.1085
Kwiatek, M., Majka, M., Ślusarkiewicz-Jarzina, A., Ponitka, A., Pudelska, H., Belter, J., et al. (2016). Transmission of the Aegilops ovata chromosomes carrying gametocidal factors in hexaploid triticale (×Triticosecale Wittm.) hybrids. J. Appl. Genet. 57, 305–315. doi: 10.1007/s13353-015-0332-3
Kwiatek, M. T., Wiśniewska, H., Ślusarkiewicz-Jarzina, A., Majka, J., Majka, M., Belter, J., et al. (2017). Gametocidal factor transferred from Aegilops geniculata Roth can be adapted for large-scale chromosome manipulations in cereals. Front. Plant Sci. 8. doi: 10.3389/fpls.2017.00409
Kynast, R. G., Friebe, B., Gill, B. S. (2000). Fate of multicentric and ring chromosomes induced by a new gametocidal factor located on chromosome 4Mg of Aegilops geniculata. Chromosome Res. 8, 133–139. doi: 10.1023/A:1009294519798
Lapitan, N. L. V., Sears, R. G., Gill, B. S. (1984). Translocations and other karyotypic structural changes in wheat x rye hybrids regenerated from tissue culture. Theoret. Appl. Genet. 68, 547–554. doi: 10.1007/BF00285012
Li, H.-J., Guo, B.-H., Li, Y.-W., Du, L.-Q., Jia, X., Chu, C.-C. (2000). Molecular cytogenetic analysis of intergeneric chromosomal translocations between wheat (Triticum aestivum L.) and Dasypyrum villosum arising from tissue culture. Genome 43, 756–762. doi: 10.1139/g00-020
Liu, C., Guo, W., Wang, Y., Fu, B., Doležel, J., Liu, Y., et al. (2023). Introgression of sharp eyespot resistance from Dasypyrum villosum chromosome 2VL into bread wheat. Crop J. 11, 1512–1520. doi: 10.1016/j.cj.2023.04.013
Liu, W.-H., Luan, Y., Wang, J.-C., Wang, X.-G., Su, J.-J., Zhang, J.-P., et al. (2010). Production and identification of wheat – Agropyron cristatum (1·4P) alien translocation lines. Genome 53, 472–481. doi: 10.1139/G10-023
Loegering, W. Q., Sears, E. R. (1963). Distorted inheritance of stem-rust resistance of timstein wheat caused by a pollen-killing gene. Can. J. Genet. Cytol. 5, 65–72. doi: 10.1139/g63-010
Luan, Y., Wang, X., Liu, W., Li, C., Zhang, J., Gao, A., et al. (2010). Production and identification of wheat-Agropyron cristatum 6P translocation lines. Planta 232, 501–510. doi: 10.1007/s00425-010-1187-9
Lyttle, T. W. (1993). Cheaters sometimes prosper: distortion of mendelian segregation by meiotic drive. Trends Genet. 9, 205–210. doi: 10.1016/0168-9525(93)90120-7
Ma, W., Gabriel, T. S., Martis, M. M., Gursinsky, T., Schubert, V., Vrána, J., et al. (2017). Rye B chromosomes encode a functional Argonaute-like protein with in vitro slicer activities similar to its A chromosome paralog. New Phytol. 213, 916–928. doi: 10.1111/nph.14110
Maan, S. S. (1975). Exclusive preferential transmission of an alien chromosome in common wheat. Crop Sci. 15, cropsci1975.0011183X001500030002x. doi: 10.2135/cropsci1975.0011183X001500030002x
Manabe, M., Ino, T., Kasaya, M., Takumi, S., Mori, N., Ohtsuka, I., et al. (1999). Segregation distortion through female gametophytes in interspecific hybrids of tetraploid wheat as revealed by RAPD analysis. Hereditas 131, 47–53. doi: 10.1111/j.1601-5223.1999.00047.x
Marais, G. F., Pretorius, Z. A. (1996). Gametocidal effects and resistance to wheat leaf rust and stem rust in derivatives of a Triticum turgidum ssp. durum/Aegilops speltoides hybrid. Euphytica 88, 117–124. doi: 10.1007/BF00032442
Masoudi-Nejad, A., Nasuda, S., Bihoreau, M.-T., Waugh, R., Endo, T. R. (2005). An alternative to radiation hybrid mapping for large-scale genome analysis in barley. Mol. Genet. Genomics 274, 589–594. doi: 10.1007/s00438-005-0052-1
Mattera, M. G., Ávila, C. M., Atienza, S. G., Cabrera, A. (2015). Cytological and molecular characterization of wheat-Hordeum chilense chromosome 7Hch introgression lines. Euphytica 203, 165–176. doi: 10.1007/s10681-014-1292-0
McClintock, B. (1941). The stability of broken ends of chromosomes in Zea mays. Genetics 26, 234–282. doi: 10.1093/genetics/26.2.234
Miller, T. E., Hutchinson, J., Chapman, V. (1982). Investigation of a preferentially transmitted Aegilops sharonensis chromosome in wheat. Theoret. Appl. Genet. 61, 27–33. doi: 10.1007/BF00261506
Moav, J., Moav, R., Zohary, D. (1968). Spontaneous morphological alterations of chromosomes in Nicotiana hybrids. Genetics 59, 57–63. doi: 10.1093/genetics/59.1.57
Molnár, I., Benavente, E., Molnár-Láng, M. (2009). Detection of intergenomic chromosome rearrangements in irradiated Triticum aestivum-Aegilops biuncialis amphiploids by multicolour genomic in situ hybridization. Genome 52, 156–165. doi: 10.1139/g08-114
Molnár, I., Cifuentes, M., Schneider, A., Benavente, E., Molnár-Láng, M. (2011). Association between simple sequence repeat-rich chromosome regions and intergenomic translocation breakpoints in natural populations of allopolyploid wild wheats. Ann. Bot. 107, 65–76. doi: 10.1093/aob/mcq215
Molnár, I., Vrána, J., Burešová, V., Cápal, P., Farkas, A., Darkó, É., et al. (2016). Dissecting the U, M, S and C genomes of wild relatives of bread wheat (Aegilops spp.) into chromosomes and exploring their synteny with wheat. Plant J. 88, 452–467. doi: 10.1111/tpj.13266
Molnár-Láng, M., Novotny, C., Linc, G., Naoy, E. D. (2005). Changes in the meiotic pairing behaviour of a winter wheat-winter barley hybrid maintained for a long term in tissue culture, and tracing the barley chromatin in the progeny using GISH and SSR markers. Plant Breed. 124, 247–252. doi: 10.1111/j.1439-0523.2005.01097.x
Nasuda, S., Friebe, B., Gill, B. S. (1998). Gametocidal genes induce chromosome breakage in the interphase prior to the first mitotic cell division of the male gametophyte in wheat. Genetics 149, 1115–1124. doi: 10.1093/genetics/149.2.1115
Nasuda, S., Kikkawa, Y., Ashida, T., Islam, A. K. M. R., Sato, K., Endo, T. R. (2005). Chromosomal assignment and deletion mapping of barley EST markers. Genes Genet. Syst. 80, 357–366. doi: 10.1266/ggs.80.357
Niranjana, M. (2017). Gametocidal genes of Aegilops: segregation distorters in wheat–Aegilops wide hybridization. Genome 60, 639–647. doi: 10.1139/gen-2017-0023
Niranjana, M., Vinod, Sharma, J. B., Mallick, N., Tomar, S. M. S., Jha, S. K. (2017). Cytogenetic analysis and mapping of leaf rust resistance in Aegilops speltoides Tausch derived bread wheat line Selection2427 carrying putative gametocidal gene(s). Genome 60, 1076–1085. doi: 10.1139/gen-2017-0107
Nomura, T., Ishihara, A., Imaishi, H., Ohkawa, H., Endo, T. R., Iwamura, H. (2003). Rearrangement of the genes for the biosynthesis of benzoxazinones in the evolution of Triticeae species. Planta 217, 776–782. doi: 10.1007/s00425-003-1040-5
Ochoa, V., Madrid, E., Said, M., Rubiales, D., Cabrera, A. (2015). Molecular and cytogenetic characterization of a common wheat-Agropyron cristatum chromosome translocation conferring resistance to leaf rust. Euphytica 201, 89–95. doi: 10.1007/s10681-014-1190-5
Palomino, C., Cabrera, A. (2019). Development of wheat—Hordeum chilense Chromosome 2Hch Introgression Lines Potentially Useful for Improving Grain Quality Traits. Agronomy 9, 493. doi: 10.3390/agronomy9090493
Qi, L., Echalier, B., Friebe, B., Gill, B. S. (2003). Molecular characterization of a set of wheat deletion stocks for use in chromosome bin mapping of ESTs. Funct. Integr. Genomics 3, 39–55. doi: 10.1007/s10142-002-0063-5
Qi, L., Friebe, B., Zhang, P., Gill, B. S. (2007). Homoeologous recombination, chromosome engineering and crop improvement. Chromosome Res. 15, 3–19. doi: 10.1007/s10577-006-1108-8
Rick, C. M. (1966). Abortion of male and female gametes in the tomato determined by allelic interaction. Genetics 53, 85–96. doi: 10.1093/genetics/53.1.85
Said, M., Cabrera, A. (2009). A physical map of chromosome 4Hch from H. chilense containing SSR, STS and EST-SSR molecular markers. Euphytica 167, 253–259. doi: 10.1007/s10681-009-9895-6
Said, M., Holušová, K., Farkas, A., Ivanizs, L., Gaál, E., Cápal, P., et al. (2021). Development of DNA Markers From Physically Mapped Loci in Aegilops comosa and Aegilops umbellulata Using Single-Gene FISH and Chromosome Sequences. Front. Plant Sci. 12. doi: 10.3389/fpls.2021.689031
Said, M., Kubaláková, M., Karafiátová, M., Molnár, I., Doležel, J., Vrána, J. (2019a). Dissecting the complex genome of crested wheatgrass by chromosome flow sorting. Plant Genome 12, 180096. doi: 10.3835/plantgenome2018.12.0096
Said, M., Parada, A. C., Gaál, E., Molnár, I., Cabrera, A., Doležel, J., et al. (2019b). Uncovering homeologous relationships between tetraploid Agropyron cristatum and bread wheat genomes using COS markers. Theor. Appl. Genet. 132, 2881–2898. doi: 10.1007/s00122-019-03394-1
Said, M., Recio, R., Cabrera, A. (2012). Development and characterisation of structural changes in chromosome 3Hch from Hordeum chilense in common wheat and their use in physical mapping. Euphytica 188, 429–440. doi: 10.1007/s10681-012-0712-2
Sakai, K., Nasuda, S., Sato, K., Endo, T. R. (2009). Dissection of barley chromosome 3H in common wheat and a comparison of 3H physical and genetic maps. Genes Genet. Syst. 84, 25–34. doi: 10.1266/ggs.84.25
Sakata, M., Nasuda, S., Endo, T. R. (2010). Dissection of barley chromosome 4H in common wheat by the gametocidal system and cytological mapping of chromosome 4H with EST markers. Genes Genet. Syst. 85, 19–29. doi: 10.1266/ggs.85.19
Sandler, L., Hiraizumi, Y., Sandler, I. (1959). Meiotic drive in natural populations of Drosophila melanogaster. I. the cytogenetic basis of segregation-distortion. Genetics 44, 233–250. doi: 10.1093/genetics/44.2.233
Sandler, L., Novitski, E. (1957). Meiotic drive as an evolutionary force. Am. Nat. 91, 105–110. doi: 10.1086/281969
Sano, Y. (1990). The genic nature of gamete eliminator in rice. Genetics 125, 183–191. doi: 10.1093/genetics/125.1.183
Schmidt, C., Schindele, P., Puchta, H. (2020). From gene editing to genome engineering: restructuring plant chromosomes via CRISPR/Cas. aBIOTECH 1, 21–31. doi: 10.1007/s42994-019-00002-0
Schubert, I., Pecinka, A., Meister, A., Schubert, V., Klatte, M., Jovtchev, G. (2004). DNA damage processing and aberration formation in plants. Cytogenetic Genome Res. 104, 104–108. doi: 10.1159/000077473
Serizawa, N., Nasuda, S., Shi, F., Endo, T. R., Prodanovic, S., Schubert, I., et al. (2001). Deletion-based physical mapping of barley chromosome 7H. Theor. Appl. Genet. 103, 827–834. doi: 10.1007/s001220100703
Serra, H., Svačina, R., Baumann, U., Whitford, R., Sutton, T., Bartoš, J., et al. (2021). Ph2 encodes the mismatch repair protein MSH7-3D that inhibits wheat homoeologous recombination. Nat. Commun. 12, 803. doi: 10.1038/s41467-021-21127-1
Shi, F., Endo, T. R. (1999). Genetic induction of structural changes in barley chromosomes added to common wheat by a gametocidal chromosome derived from Aegilops cylindrica. Genes Genet. Syst. 74, 49–54. doi: 10.1266/ggs.74.49
Silver, L. M. (1985). MOUSE tau HAPLOTYPES. Annu. Rev. Genet. 19, 179–208. doi: 10.1146/annurev.ge.19.120185.001143
Silver, L. M. (1993). The peculiar journey of a selfish chromosome: mouse t haplotypes and meiotic drive. Trends Genet. 9, 250–254. doi: 10.1016/0168-9525(93)90090-5
Svačina, R., Karafiátová, M., Malurová, M., Serra, H., Vítek, D., Endo, T. R., et al. (2020). Development of deletion lines for chromosome 3D of bread wheat. Front. Plant Sci. 10. doi: 10.3389/fpls.2019.01756
Symington, L. S. (2016). Mechanism and regulation of DNA end resection in eukaryotes. Crit. Rev. Biochem. Mol. Biol. 51, 195–212. doi: 10.3109/10409238.2016.1172552
Szakács, E., Molnár-Láng, M. (2010). Molecular cytogenetic evaluation of chromosome instability in Triticum aestivum-Secale cereale disomic addition lines. J. Appl. Genet. 51, 149–152. doi: 10.1007/BF03195723
Taketa, S., Kato, J., Takeda, K. (1995). High crossability of wild barley (Hordeum spontaneum C. Koch) with bread wheat and the differential elimination of barley chromosomes in the hybrids. Theor. Appl. Genet. 91, 1203–1209. doi: 10.1007/BF00220930
Tsujimoto, H. (1995). Gametocidal genes in wheat and its relatives. IV. Functional relationships between six gametocidal genes. Genome 38, 283–289. doi: 10.1139/g95-035
Tsujimoto, H. (2005). Gametocidal genes in wheat as the inducer of chromosome breakage. Wheat Inf Serv. 100, 33–48.
Tsujimoto, H., Noda, K. (1989). Structure of chromosome 5A of wheat speltoid mutants induced by the gametocidal genes of Aegilops speltoides. Genome 32, 1085–1090. doi: 10.1139/g89-558
Tsujimoto, H., Noda, K. (1990). Deletion mapping by gametocidal genes in common wheat: position of speltoid suppression (Q) and β-amylase (β-Amy-A2) genes on chromosome 5A. Genome 33, 850–853. doi: 10.1139/g90-128
Tsujimoto, H., Tsunewaki, K. (1984). Gametocidal genes in wheat and its relatives. I. Genetic analyses in common wheat of a gametocidal gene derived from Aegilops speltoides. Can. J. Genet. Cytol. 26, 78–84. doi: 10.1139/g84-013
Tsujimoto, H., Tsunewaki, K. (1985a). Gametocidal genes in wheat and its relatives. II. Suppressor of the chromosome 3C gametocidal gene of Aegilops triuncialis. Can. J. Genet. Cytol. 27, 178–185. doi: 10.1139/g85-027
Tsujimoto, H., Tsunewaki, K. (1985b). Hybrid dysgenesis in common wheat caused by gametocidal genes. Japanese J. Genet. 60, 565–578. doi: 10.1266/jjg.60.565
Tsujimoto, H., Tsunewaki, K. (1988). Gametocidal genes in wheat and its relatives. III. Chromosome location and effects of two Aegilops speltoides-derived gametocidal genes in common wheat. Genome 30, 239–244. doi: 10.1139/g88-041
Tsujimoto, H., Yamada, T., Sasakuma, T. (1997). Molecular structure of a wheat chromosome end healed after gametocidal gene-induced breakage. Proc. Natl. Acad. Sci. 94, 3140–3144. doi: 10.1073/pnas.94.7.3140
Tsunewaki, K. (2015). “Prof. H. Kihara’s Genome Concept and Advancements in Wheat Cytogenetics in His School,” in Advances in Wheat Genetics: From Genome to Field. Eds. Ogihara, Y., Takumi, S., Handa, H. (Springer Japan, Tokyo), 3–11. doi: 10.1007/978-4-431-55675-6_1
Türkösi, E., Ivanizs, L., Farkas, A., Gaál, E., Kruppa, K., Kovács, P., et al. (2022). Transfer of the ph1b Deletion Chromosome 5B From Chinese Spring Wheat Into a Winter Wheat Line and Induction of Chromosome Rearrangements in Wheat-Aegilops biuncialis Hybrids. Front. Plant Sci. 13. doi: 10.3389/fpls.2022.875676
Türkösi, E., Szakács, É., Ivanizs, L., Farkas, A., Gaál, E., Said, M., et al. (2024). A chromosome arm from Thinopyrum intermedium × Thinopyrum ponticum hybrid confers increased tillering and yield potential in wheat. Mol. Breed. 44, 7. doi: 10.1007/s11032-024-01439-y
Werner, J. E., Kota, R. S., Gill, B. S., Endo, T. R. (1992). Distribution of telomeric repeats and their role in the healing of broken chromosome ends in wheat. Genome 35, 844–848. doi: 10.1139/g92-128
Yamano, S., Nitta, M., Tsujimoto, H., Ishikawa, G., Nakamura, T., Endo, T. R., et al. (2010). Molecular mapping of the suppressor gene Igc1 to the gametocidal gene Gc3-C1 in common wheat. Genes Genet. Syst. 85, 43–53. doi: 10.1266/ggs.85.43
Zhang, H.-B., Dvořák, J. (1990). Characterization and distribution of an interspersed repeated nucleotide sequence from Lophopyrum elongatum and mapping of a segregation-distortion factor with it. Genome 33, 927–936. doi: 10.1139/g90-139
Zhang, R., Sun, B., Chen, J., Cao, A., Xing, L., Feng, Y., et al. (2016). Pm55, a developmental-stage and tissue-specific powdery mildew resistance gene introgressed from Dasypyrum villosum into common wheat. Theor. Appl. Genet. 129, 1975–1984. doi: 10.1007/s00122-016-2753-8
Keywords: wheat, Triticum, Aegilops, gametocidal, Gc factors/elements/genes, pollen-killer, segregation distorter
Citation: Said M, Gaál E, Farkas A, Molnár I, Bartoš J, Doležel J, Cabrera A and Endo TR (2024) Gametocidal genes: from a discovery to the application in wheat breeding. Front. Plant Sci. 15:1396553. doi: 10.3389/fpls.2024.1396553
Received: 05 March 2024; Accepted: 02 April 2024;
Published: 22 April 2024.
Edited by:
Leonor Morais-Cecilio, University of Lisbon, PortugalReviewed by:
Andreas Houben, Leibniz Institute of Plant Genetics and Crop Plant Research (IPK), GermanyBernd Hackauf, Julius Kühn-Institut, Germany
Copyright © 2024 Said, Gaál, Farkas, Molnár, Bartoš, Doležel, Cabrera and Endo. This is an open-access article distributed under the terms of the Creative Commons Attribution License (CC BY). The use, distribution or reproduction in other forums is permitted, provided the original author(s) and the copyright owner(s) are credited and that the original publication in this journal is cited, in accordance with accepted academic practice. No use, distribution or reproduction is permitted which does not comply with these terms.
*Correspondence: Mahmoud Said, c2FpZEB1ZWIuY2FzLmN6
†ORCID: Mahmoud Said, orcid.org/0000-0002-6169-8655
Eszter Gaál, orcid.org/0000-0002-1081-9656
András Farkas, orcid.org/0000-0002-9545-0332
István Molnár, orcid.org/0000-0002-7167-9319
Jan Bartoš, orcid.org/0000-0002-4154-8895
Jaroslav Doležel, orcid.org/0000-0002-6263-0492
Adoración Cabrera, orcid.org/0000-0002-3612-0245