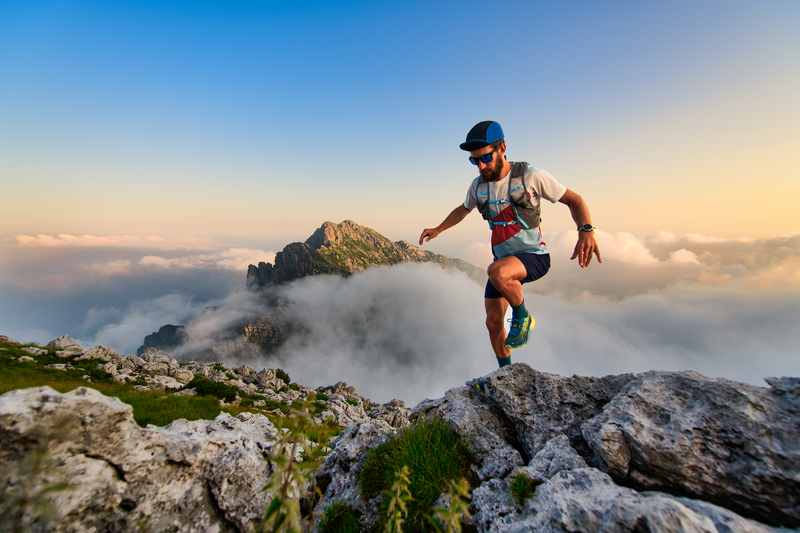
95% of researchers rate our articles as excellent or good
Learn more about the work of our research integrity team to safeguard the quality of each article we publish.
Find out more
REVIEW article
Front. Plant Sci. , 28 March 2024
Sec. Plant Nutrition
Volume 15 - 2024 | https://doi.org/10.3389/fpls.2024.1393458
This article is part of the Research Topic New Avenues of Silicon's Role in Plant Biology: Trends and Controversies View all 3 articles
Silicon (Si) is a widely recognized beneficial element in plants. With the emergence of nanotechnology in agriculture, silicon nanoparticles (SiNPs) demonstrate promising applicability in sustainable agriculture. Particularly, the application of SiNPs has proven to be a high-efficiency and cost-effective strategy for protecting plant against various biotic and abiotic stresses such as insect pests, pathogen diseases, metal stress, drought stress, and salt stress. To date, rapid progress has been made in unveiling the multiple functions and related mechanisms of SiNPs in promoting the sustainability of agricultural production in the recent decade, while a comprehensive summary is still lacking. Here, the review provides an up-to-date overview of the synthesis, uptake and translocation, and application of SiNPs in alleviating stresses aiming for the reasonable usage of SiNPs in nano-enabled agriculture. The major points are listed as following: (1) SiNPs can be synthesized by using physical, chemical, and biological (green synthesis) approaches, while green synthesis using agricultural wastes as raw materials is more suitable for large-scale production and recycling agriculture. (2) The uptake and translocation of SiNPs in plants differs significantly from that of Si, which is determined by plant factors and the properties of SiNPs. (3) Under stressful conditions, SiNPs can regulate plant stress acclimation at morphological, physiological, and molecular levels as growth stimulator; as well as deliver pesticides and plant growth regulating chemicals as nanocarrier, thereby enhancing plant growth and yield. (4) Several key issues deserve further investigation including effective approaches of SiNPs synthesis and modification, molecular basis of SiNPs-induced plant stress resistance, and systematic effects of SiNPs on agricultural ecosystem.
Silicon (Si) is a typical metalloid and the second most abundant element in the earth’s crust (Epstein, 1994). Due to its growth promotion effects in plants, particularly for those grown under stressful conditions, Si is widely recognized as a plant beneficial element (Epstein, 1999; Liang et al., 2015). In recent years, with the progressive integration of agriculture and nanotechnology, the application of nanoparticles (NPs) is shown to be an effective agronomic approach in crop production to address the escalating global food demand (Agathokleous et al., 2020; Wang et al., 2020; Sharma et al., 2023). Among the various NPs, silicon nanoparticles (SiNPs) demonstrate impressive advantages and applicability in nano-enabled agriculture for promoting plant stress resistance and ensuring stable crop yield (Rastogi et al., 2019; Dhakate et al., 2022).
Technically, SiNPs refer to fabricated Si particles at nanoscale, with the dimensions ranging from 1 to 100 nm. Based on the structures of SiNPs, they can be categorized into various types such as spheric, hollow, shaped (e.g., rod, cube), and porous (Mathur and Roy, 2020). SiNPs exhibit significant advantages over bulk Si sources including high surface/volume ratio, distinct charge properties and improved plant bioavailability (Jeelani et al., 2020). The characteristics and structures of SiNPs are commonly determined by the synthesizing process, which can be classified into physical, chemical, and biological synthesis (green synthesis), depending on the driving force (Naidu et al., 2023). The green synthesis of SiNPs with agricultural wastes as raw materials is gaining growing attention owing to its significant applicability in recycling and sustainable agricultural production (Mahawar et al., 2023). In plants subject to foliar or root application of SiNPs, due to the size effect and charge property, SiNPs can directly penetrate plant barriers such as epidermis, cell wall and plasma membrane, subsequently being accumulated and translocated in plants (Jeelani et al., 2020; Wang et al., 2022a). In addition to the direct penetration, plants also utilize SiNPs in the form of silicic acid after dissolution under the facilitation of Si channels and transporters (e.g., Lsi1, Lsi2, Lsi3 and Lsi6) (Ma and Yamaji, 2015; Mandlik et al., 2020).
The unique features and advantages of SiNPs including nanoscale sizes, nutritional effects, surface properties and porous nature, endowing them versatile functions in nano-enabled agriculture such as plant growth stimulator, nanocarrier, and soil conditioner (Figure 1; Ji et al., 2018; Rastogi et al., 2019; Mahawar et al., 2023). To date, numerous laboratory and field studies affirm that SiNPs, as plant growth stimulator, can enhance plant resistance to various biotic (e.g., insect pest, pathogen disease) and abiotic (e.g., metal stress, drought stress, salt stress) stress, thereby promoting plant growth, yield and quality (Bansal et al., 2022; Verma et al., 2022; Wang et al., 2022a). In addition, the porous nature of SiNPs makes them ideal carriers for delivering chemicals (e.g., fertilizer, pesticide, plant growth regulator) and bioactive molecules (e.g., DNA, protein) in agricultural production and plant biotechnology (Mathur and Roy, 2020; Zhang et al., 2023). Furthermore, SiNPs can also be applied for the improvement of soil properties, detection and monitoring of certain biochemical parameters relevant for agronomic production, and remediation of agricultural contamination (Giraldo et al., 2014; Kannan and Sujatha, 2022).
Overall, SiNPs show significant applicability to sustainable agricultural production, while rapid progress has been made to unveil the interaction between SiNPs and crops, especially for those under stressful conditions in the recent decade. This review provides an updated summary of the synthesis and application of SiNPs in agriculture, their uptake and accumulation in plants under foliar and root application, and the multiple roles and underlying mechanisms of SiNPs in protecting plants against biotic and abiotic stresses. In addition, several key issues related to the current limitations and future perspectives of SiNPs research and application in nano-enabled agriculture are highlighted.
The unique characteristics and attributes of SiNPs are predominantly dependent on precursors and methods employed in their synthesis processes (Jeelani et al., 2020). Presently, two strategies called top-to-down strategy and bottom-to-top strategy are mostly applied in the synthesis of NPs (Figure 2; Vetchinkina et al., 2019; Salami et al., 2022). The top-to-down strategy indicates the breakdown of larger materials into smaller particles using methods such as physical milling and chemical decomposition. Conversely, the bottom-to-up strategy involves the assemble of atomic or molecular precursors into complex nanostructures based on natural physical principles or external forces (Rahman and Padavettan, 2012; Tang et al., 2012).
According to the driven forces and precursors involved, the synthesis processes of NPs can be classified into physical, chemical, and biological (green synthesis) approaches (Figure 2; Usman et al., 2020; Zhao et al., 2020). Typically, physical and chemical synthesis are conducted using silicate precursors such as tetraethyl orthosilicate (TEOS) and tetramethyl orthosilicate (TMOS), while green synthesis usually utilizes plants and microorganisms (Tang et al., 2012). Physical methods include ball milling, ultrasonic peening, and laser ablation, while chemical methods encompass vapor condensation, microemulsion, co-precipitation, and sol-gel method (Debnath et al., 2012; Croissant et al., 2020). In most biogenic synthesis of SiNPs using plant materials, the process involves two steps including the extraction of silicate and the formation of SiNPs through the sol-gel method using inorganic salt neutralization with hydrochloric acid (Seroka et al., 2022).
Although the physical methods are relatively simple and direct with quick synthesis processes, they may be limited by prosomal material and usually result in nanoparticles with uncontrollable sizes. On the other hand, the chemical methods offer better control of particle size and functional manipulation, however, the usage of chemicals with potential toxicity could raise environmental risks (Figure 2). In contrast, the green synthesis using agricultural wastes such as rice straw and husk (Wang et al., 2012a; Gu et al., 2015; Bose et al., 2018), maize stalk (Adebisi et al., 2020; Piela et al., 2020), sugarcane bagasse (Alves et al., 2017), and coconut shell (Marousek et al., 2022) shows significant advantages over physical and chemical synthesis approaches. Especially, green synthesis demonstrates a significant potential for the large-scale production of SiNPs in recycling and sustainable agricultural production (Mahawar et al., 2023).
In the nature, Si mainly exists in the forms of SiO2 and silicates and silicic acid. As the soluble and sole form of Si that can be absorbed by plants root, mono-silicic acid would form silicates when the environmental pH > 9 (Epstein, 1994). In arable soils, the concentration of mono-silicic acid ranges from 0.1 to 0.6 mM (lower than its saturation solubility, about 2 mM), which is mainly determined by parent materials, development levels, and physical and chemical properties of soil (Liang et al., 2015; Yan et al., 2018). After being absorbed by root, most of Si (more than 90%) is loaded in xylem and transported from root to shoot in the form of silicic acid and then unloaded to parenchyma cells. In plants’ shoot, along with water loss driven by plant transpiration, silicic acid is polymerized to silica gel (SiO2·nH2O) and forms cuticle-silica double layers (Mandlik et al., 2020). In plants, the accumulation of Si in the above-ground part differs greatly, ranging from 0.1 to 10.0% on dry weight basis. Accordingly, plants have been artificially clarified into three categories including high accumulator (1.5-10%), moderate accumulator (0.2-1.5%), and low accumulator (lower than 0.2%, also called extruder). On the molecular level, the difference of existence, function and activity of Si transport proteins are shown to be responsible for the distinct abilities of Si accumulation among different plant species (Mitani-Ueno and Ma, 2021).
To the date, a molecular model of Si transport in higher plants responsible for Si uptake, translocation, distribution and accumulation has been sketchily established in rice, a typical Si accumulator and model plant in Si researches following the identification of Si channels and transporters including Lsi1, Lsi2, Lsi3 and Lsi6 based on mutant selection and forward genetics (Ma and Yamaji, 2015; Mitani-Ueno and Ma, 2021). OsLsi1 was the very first protein responsible for Si uptake in higher plants using a rice mutant (low silicon 1, lsi1), which belongs to the noduline-26 major intrinsic protein (NIP) family and act as an SI influx channel (Ma et al., 2002, Ma et al., 2006). Then, a Si efflux transporter (OsLsi2) was identified, which belongs to putative anion-channel transporter family (Ma et al., 2007). OsLsi1 and OsLsi2 are localized to the plasma membrane of exodermis and endodermis cells, while both proteins show polar localization (OsLsi1 at distal side and OsLsi2 at proximal side), and the cooperation of OsLsi1 and OsLsi2 facilitate Si uptake in rice root. OsLsi6, a homolog of OsLsi1, is localized at the adaxial side of xylem parenchyma cells and responsible for the unloading process of Si from xylem to arial parts (Yamaji et al., 2008; Yamaji and Ma, 2009). Beside the three proteins mentioned above, OsLsi3, a homolog of OsLsi2, takes the charge of controlling the distribution between panicles and flag leaves in cooperation with OsLsi2 and OsLsi6 in rice node (Yamaji et al., 2015).
The absorption and translocation of SiNPs significantly affects their efficacy as plant growth stimulator and nanocarrier in plants and agricultural production (Lombi et al., 2019; Usman et al., 2020). However, in contrast with the relatively well-understood mechanisms of Si transport, the uptake and translocation of SiNPs in plants, especially at the subcellular and molecular level, remains unclear. Moreover, the research on the SiNPs uptake and translocation in plants also lags those on metal NPs such as silver nanoparticles (AgNPs), gold nanoparticles (AuNPs), cerium nanoparticles (CeNPs), and iron nanoparticles (FeNPs). The issue of phytotoxicity of metal NPs has attracted much research attention; on the contrary, no convincing evidence has confirmed the toxicity of SiNPs (Miralles et al., 2012; Sharma et al., 2015; Ruttkay-Nedecky et al., 2017).
In general, NPs can directly enter plant shoot and root tissues due to their nanoscale sizes when supplied with foliar spray and root application, respectively (Figure 3; Lv et al., 2019; Rastogi et al., 2019). When supplied via foliar spray, NPs are usually absorbed through cuticle and/or stomata (Yeats and Rose, 2013). It has been estimated that the maximum size of NPs that can pass through cuticle is approximately 5 nm (Eichert and Goldbach, 2008), while the equivalent pore size of stomata is approximately 20 - 500 nm (Eichert et al., 2008). Given the fact that most of NPs used in agricultural practices are larger than 5 nm, the stomatal pathway could play a dominant role in NPs uptake under foliar application (Figure 3). In the case of root application, the uptake of NPs usually occurs in the immature parts of root such as root tips, root hairs, and lateral root junctions, where the physical barriers (e.g., Casparian strip, suberin lamella) are underdeveloped (Wang et al., 2022a). Additionally, SiNPs may be transformed into silicic acid in growth substance driven by geochemical, microbial, and plant biological factors, which can successively be taken up by plant roots through Si transport proteins (Ma and Yamaji, 2015; Mandlik et al., 2020). Once taken up by plant shoot or root, the shoot-to-root and root-to-shoot translocation of NPs subsequently occurs in phloem and xylem, respectively (Figure 3; Wang et al., 2012b; Ma et al., 2017). Moreover, it should be noted that most of the plant species are low Si accumulators with relatively poor ability in Si uptake and accumulation, and could benefit from Si application at relatively lower levels (Liang et al., 2015; Coskun et al., 2019). In contrast, due to different absorption and transport mechanisms with Si, the limitations would not exist under the application of SiNPs, since most of SiNPs are absorbed independently to Si transport proteins. Therefore, as a novel Si source, SiNPs show remarkable advantages and applicability, and may play more significant role in future, while the further research comparing SiNPs and bulk Si materials are still needed.
It can be concluded from previous literatures that, in essence, the uptake and translocation of NPs in plants is the process of NPs passing through plant biological barriers such as cuticle, stomata, cell wall and vascular vessels. Therefore, it is not surprising that both NPs properties (e.g., size and charge property) and plant factors (plant species, growth and development stage) would influence the uptake and distribution of NPs in plants (Figure 3; Ma et al., 2010; Tripathi et al., 2017). The size preference of plants in NPs uptake and translocation has been documented in different plant species (e.g., wheat, cucumber, and tobacco), that NPs with smaller size are more easily absorbed by plants (Judy et al., 2012; Hong et al., 2014). However, unlike that of size properties, the effects of charge properties of NPs on their absorption and translocation are more complicated, which also differs between root and foliar applications. Under root application, the positively charged NPs adhere to root surface more tightly, while those with negative charge are more efficiently translocated from root to shoot (Zhu et al., 2012; Avellan et al., 2017; Spielman-Sun et al., 2017). However, under foliar application, Hu et al. (2020) reported that NPs with positive charge showed the highest delivery efficiency into stomata and apoplastic space. Moreover, the uptake and translocation of NPs is also influenced by plant factors since several key parameters differ with plant species and development stages including (1) the contact area between plant and NPs based on plant morphological traits; (2) the amount of immature roots and leaves which are the major entrance of NPs into plants; (3) the equivalent pore sizes of physiological barriers such as cell wall, plasma membrane, cuticle, stomata, and vascular vessels) (Tripathi et al., 2017; Lv et al., 2019; Dhakate et al., 2022). Overall, current literature is largely based on the determination and/or observation of NPs in plants, while the mechanisms of NPs uptake and translocation, especially at cellular and molecular levels, remain unclear and deserve further investigation.
As an eco-friendly biocide, SiNPs restrain the growth and aggressiveness of pathogens and insect pests, thereby protecting plant against the attack of bacteria, fungi, and pests in agricultural production (Figure 4; Selvarajan et al., 2020; Goswami et al., 2022). For instance, it was shown that SiNPs induced significant antifungal effects against Rhizoctonia solani and Alternaria solani in the incubation experiments (Abdelrhim et al., 2021; Albalawi et al., 2022). In addition, Si/AgNPs (complex NPs of Si and Ag) also showed remarkable fungicidal and bactericidal effects against various plant pathogens such as Botrytis cinerea, Rhizoctonia solani, Pseudomonas syringae and Xanthomonas campestris (Park et al., 2006; Baka and El-Zahed, 2022). Furthermore, Khan et al. (2022) reported that both SiNPs and titanium nanoparticles (TiNPs) inhibited the growth of Phomopsis vexans and Ralstonia solanacearum, while SiNPs induced a more prominent recuction of pathogen growth than TiNPs. As for insect pests, in a surface contact and feeding experiment, Ayoub et al. (2017) found that SiNPs induced remarkable pesticidal effects in leafworm (Spodoptera littoralis), which was also influenced by their size and surface characteristics. Notably, SiNPs demonstrate unique advantage over traditional pesticides in that their pesticidal effects are based on the physical effects, implying pathogens and insect pests are unlikely to become resistant to SiNPs at physiological level through evolution.
In addition to the direct pesticidal effects, SiNPs treatment with seed priming and foliar application can enhance seed germination, and plant growth and yield under different biotic stresses (Figure 4; Naidu et al., 2023; Saw et al., 2023). Seed priming with SiNPs is an effective agronomic approach in enhancing seed germination and plant growth under the infection of pathogens. For example, SiNPs application via seed priming significantly promoted seed germination and seedling growth in wheat infected by Rhizoctonia solani (Abdelrhim et al., 2021) and watermelon infected by Fusarium oxysporum (Buchman et al., 2019). Besides, foliar application of SiNPs is more commonly used in agricultural practices in protecting plants against pathogens and pests. It has been reported that SiNPs foliar spray alleviated pathogen-induced growth inhibition and disease symptoms in plants under the infection of various pathogens and insect pests such as Ralstonia solanacearum (Khan et al., 2022; Wang et al., 2022b), Alternaria solani (Albalawi et al., 2022), Plasmopara viticola (Rashad et al., 2021), Fusarium oxysporum (Kang et al., 2021), Mythimna separata (Wang et al., 2021), Aphis craccivora and Spodoptera littoralis (Thabet et al., 2021).
The SiNPs-induced broad-spectrum biotic stress resistance in plants is largely based on the regulation of SiNPs on plant defense system at physiological and molecular levels such as enhancement of defense compounds metabolism, modulation of antioxidant system, and regulation of plant hormone signals (Figure 4). For instance, Suriyaprabha et al. (2014) reported that SiNPs was more effective than bulk Si in enhancing the resistance of maize to fungal pathogens including Fusarium oxysporum and Aspergillus niger through regulating the metabolism of phenolic compounds. As for insect pests, Wang et al. (2021) found that SiNPs enhanced the metabolism of chemical defense compounds such as chlorogenic acid and total phenolics, and protected maize against oriental armyworm. In a field test, SiNPs treatment reduced the population of three typical pests in faba bean and soybean via enhancing the attraction of the predators of pests, which could be due to the regulation of the metabolism of volatile compounds (Thabet et al., 2021). Furthermore, it has been documented that SiNPs regulated the activity of antioxidant enzyme and non-enzymatic antioxidant, thereby ensuring the homeostasis of reactive oxygen species (ROS) in eggplant under the infection by Alternaria solani (Albalawi et al., 2022) and wheat under the infection oby Rhizoctonia solani (Abdelrhim et al., 2021). Considering the dual roles of ROS in plants under stress including signal molecule and toxic radicals, the regulation of SiNPs on ROS balance could participate in both pathogen recognition and oxidative damage alleviation. Besides ROS, SiNPs can regulate the plant hormones signal pathways such as salicic acid (SA), jasmonic acid (JA) and ethylene, which play pivotal roles in biotic stress response and accilimation. It has been documented that SiNPs foliar spary enhanced SA metabolism via regulating the expression of related genes in tomato grown under the infection of Ralstonia solanacearum (Wang et al., 2022b). Moreover, Rashad et al. (2021) reported that SiNPs promoted the resistance of grapevine to Plasmopara viticola by regulating jasmonate and ethylene signal pathway, and enhacing the expression of pathogen defense-related genes. Particularly, SiNPs can trigger SA signal via relasing silicic acid or clogging stomata, thereby inducing systemic acquired resistance (SAR, a typical plant immune response under pathogen infection) and enhacing the resistance of Arabidopsis against Pseudomonas syringae (El-Shetehy et al., 2021).
Although both bulk Si and SiNPs can alleviate biotic stress in plants acting as plant growth stimulator, SiNPs demonstrate distinct usage in plant protection. They can be used as vehicle in delivering pesticides in virtue of their porous nature, while the pesticide loaded SiNPs exhibit kinds of advantages over direct pesticide application (Figure 4). Generally, SiNPs can be applied to deliver pesticides directly or after certain modification in agricultural practices. The uptake efficiency and durability of pesticides in plants would be enhanced when loaded into SiNPs, therefore improving their pesticidal effects. For example, Bilal et al. (2020) reported that indoxacarb-loaded SiNPs exhibited better insecticidal activity than commercial indoxacarb in inhibiting Plutella xylostella when applied at the same dose. The usage of pectin coated SiNPs as carrier promoted the uptake, translocation, duration, and antifungal activity of prochloraz in rice (Abdelrahman et al., 2021). It has also been suggested that α-cyclodextrin anchored SiNPs enhanced the light- and thermal- shielding ability of avermectin after being loaded, thereby prolonging the duration of avermectin in controlling Plutella xylostellla (Kaziem et al., 2018). Furthermore, the surface modification of SiNPs using copper (Cu) or carboxymethyl chitosan enhanced their translocation in plants and extended the release period of azoxystrobin (Xu et al., 2018, Xu et al., 2020).
On the other hand, the application of SiNPs as a vehicle can improve the targeting precision and foster a controlled release of pesticide in plants or insect pests after specific modifications (Figure 4). For instance, Chen et al. (2016) fabricated a SiNPs-based chlorpyrifos release system with salicylaldehyde or Cu modification, which showed significant pH sensitivity and sustained pesticide release. Similarly, Gao et al. (2019) developed a pH-sensitive abamectin release system based on SiNPs after 3-(trimethoxysilyl)propyl methacrylate functionalization, which exhibited higher affinity for rice leaves, longer duration period of, and higher toxicity to the larvae of Cnaphalocrocis medinalis in contrast with commercial abamectin. In their further research, Gao et al. (2020) developed a temperature-responsive pesticide release formulation based on SiNPs using thermo-responsive copolymer, which showed stronger adhesion to rice leaves and long-term bioactivity of thiamethoxam. Moreover, Liang et al. (2020) modified SiNPs using functionalized starch with biodegradable disulfide-bridged structure, and then loaded avermectin into SiNPs. The results showed the modified SiNPs controlled the release of avermectin in response to glutathione and α-amylase, thereby enhancing the targeting pesticidal effects against Plutella xylostella. Analogously, the encapsulation of acetamiprid and decanethiol in SiNPs would also control the release of acetamiprid in response to glutathione and induce higher pesticidal effects in contrast with commercial acetamiprid (Ding et al., 2023). In the study of Bapat et al. (2020), the triethoxysilane-functionalized SiNPs was used as vehicle for delivering trypsin inhibitor, which would release pesticide once being transported to the gut of Helicoverpa armigera, thereby effectively inhibiting the activity of gut proteinase and the growth of this pests.
Notably, the excessive application of synthetic pesticide in agricultural practice threatens food safety and the sustainability of agricultural production. Under pesticide contamination in agriculture, SiNPs can decrease pesticide residues in edible parts, and be used for pesticide extraction and degradation in environmental remediation (Figure 4; Bapat et al., 2016). For instance, it has been reported that the usage of SiNPs as carrier in delivering prochloraz and spirotetramat decreased the final pesticide residue and related metabolites in the edible parts of cucumber (Zhao et al., 2018a, Zhao et al., 2018b). In the case of pesticide extraction and degradation, Korrani et al. (2016) reported that SiNPs effectively extracted three organic phosphorus pesticides including dicrotophos, chlorpyrifos and diazinon from water samples, which could be due to its mesoporous nature and high surface area. Amani et al. (2018) reported that SiNPs can be used for the removal of diazinon in solution, while the modification of SiNPs with propyl methacrylate enhanced the removal efficiency. Moreover, Yang et al. (2016) used SiNPs to immobilize laccase for degradation of 2,4-dichlorophenol, and the results indicated that the application of SiNPs enhanced the efficiency of degradation and reusability of laccase. However, it should be noted that most of the previous research using SiNPs for pesticide extraction and degradation were conducted in aqueous solution, while the potential application of SiNPs in agricultural soil for environmental remediation deserves further investigation.
Under the growing influence of human activities (e.g., mining, chemical fertilizer application and sewage irrigation) on agriculture, metal contamination has become one of the major threats in sustainable agricultural production and food safety (Clemens and Ma, 2016; Rai et al., 2019). Metal stresses induce oxidative damages, nutritional imbalance, photosynthesis system destruction, and plant growth inhibition (Clemens et al., 2002). As a promising tool in protecting plant against metal stress, SiNPs are proven to effectively ameliorate various metal toxicity such as cadmium (Cd) (Riaz et al., 2022a; Zhao et al., 2023), arsenic (As) (Gonzalez-Moscoso et al., 2022; Yang et al., 2022), mercury (Hg) (Li et al., 2020), lead (Pb) (Hussain et al., 2020), Cu (Riaz et al., 2022b), aluminum (Al) (de Sousa et al., 2019), and chromium (Cr) (Tripathi et al., 2015). Multiple mechanisms are involved behind the SiNPs-induced broad-spectrum metal stress tolerance (Figure 5).
SiNPs can decrease the accumulation of metals in plants, especially in arial or edible parts, under metal contamination (Okeke et al., 2023; Yadav et al., 2023). For example, in wheat grown under Cd exposure, SiNPs application through seed priming (Hussain et al., 2019), foliar spray, and soil application (Ali et al., 2019) significantly promoted plant growth and decreased Cd accumulation in wheat grains. In addition, Yang et al. (2022) reported that SiNPs application increased As accumulation in rice shoot and husk but decreased As content in grain under As contamination, while Tripathi et al. (2015) found that SiNPs reduced the accumulation of Cr in both shoot and root in pea seedling growth under Cr stress. As for the underlying mechanisms, it has been documented that SiNPs treatment reduced Cd accumulation in rice shoot via enhancing polysaccharides metabolism and cell wall retention (Riaz et al., 2022a). Moreover, Yan et al. (2023) indicated that SiNPs were more effective than Si in reducing apoplastic flow of Cd uptake, thereby decreased Cd accumulation tomato shoot exposed to Cd. By using suspension rice cells, Cui et al. (2017; 2020) investigated the effects of SiNPs on Cd and As accumulation and related mechanisms, and found that SiNPs regulated the expression of genes responsible for Cd transport (OsLCT1, OsNRAMP5 and OsHMA3) and chemical components of the cell wall, thereby reducing the accumulation of Cd and As in rice cells.
In addition to the reduction of toxic metal uptake and translocation, SiNPs can modulate the activity of antioxidant system and maintain mineral nutrients homeostasis, successively promoting plant growth and yield. Under Cd exposure, SiNPs application enhanced ROS scavenge and ameliorated oxidative injury in wheat and rapeseed via increasing the activity of antioxidant enzymes such as superoxide dismutase (SOD), catalase (CAT), ascorbate peroxidase(APX), and peroxidase (POD) (Adrees et al., 2022; Ahmed et al., 2023). Additionally, it has been shown that SiNPs alleviated Cd-induced oxidative damage in rapeseed by modulating the metabolism of antioxidants including ascorbate, glutathione, and proline (Zhao et al., 2023). The maintenance of mineral nutrient balance plays a key role in plant growth and development when grown under metal stresses, while it has been demonstrated that SiNPs was able to enhance mineral nutrition status including zinc (Zn), manganese (Mn), iron (Fe), magnesium (Mg), calcium (Ca), and potassium (K), resulting in promoted Cd stress resistance in barley (He et al., 2023). Similarly, in Phaseolus vulgaris under Cd stress, Koleva et al. (2022) reported that the application of SiNPs enhanced K uptake, polyamines biosynthesis and photosynthetic capacity, thereby promoting plant growth.
Salt stress is a major abiotic stress in agricultural production, affecting approximately 20% total arable land worldwide (Byrt and Munns, 2008). The alleviative effects of SiNPs on salt stress have been observed in various crops such as rice (Shalaby et al., 2021), wheat (Hajihashemi and Kazemi, 2022), maize (Rizwan et al., 2023), tomato (Sayed et al., 2022), cucumber (Alsaeedi et al., 2018), and potato (Gowayed et al., 2017). Under salt stress, the germination of plant seed is inhibited due to the water uptake limitation and ionic toxicity, while SiNPs priming can improve seed germination in cucumber (Alsaeedi et al., 2018), lentil (Alsaeedi et al., 2018), and maize (Naguib and Abdalla, 2019) which could be based on their regulation on K/Na ratio, ROS homeostasis and hormone metabolism in seeds. Besides seed germination, SiNPs can promote plant growth under salt stress through modulating Na/K homeostasis, antioxidant system, photosynthesis performance, and the expression of stress-response genes under salt stress (Etesami et al., 2021; Muhammad et al., 2022) (Figure 5).
In plant grown under saline condition, salt stress induces ion toxicity and osmotic constraint, thereby affecting plant growth, yield, and quality (Munns, 2005; Munns and Tester, 2008). The homeostasis of Na/K plays the dominant role in plant salt stress resistance, while it has been documented that SiNPs application regulated Na/K balance in rice and sweet orange via regulating the expression of Na/K transporter genes including HKT, SOS, and NHX (Mahmoud et al., 2022; Ijaz et al., 2023). Moreover, it was found that SiNPs eliminated the accumulation of MDA and H2O2 caused by salt stress, via enhancing the activity of antioxidant enzymes such as glutathione reductase (GR), APX, CAT, POD, SOD in squash (Cucurbita pepo L.) (Siddiqui et al., 2014) and pea (Ismail et al., 2022). In tomato grown under hydroponic condition, Haghighi and Pessarakli (2013) found that SiNPs can improve photosynthetic rate, mesophyll conductance, and photosynthetic water use efficiency, thereby promoting plant growth and salt stress resistance. In addition, Alam et al. (2022) reported that SiNPs promoted tomato growth, enhanced mineral nutrients accumulation (e.g., Mg, K, Fe, Mn, Zn) and photosynthesis performance, while foliar application was more effective in ameliorating salt stress in tomato than root dipping.
Drought stress is another major abiotic stress adversely affecting agricultural production. The alleviation effects of SiNPs on drought stress have been repeatedly documented, with diverse mechanisms involved (Figure 5). For instance, SiNPs promoted leaf area, chlorophyll content, and nitrogen assimilation, thereby enhancing plant growth and fruit yield in cucumber (Alsaeedi et al., 2019). Similarly, Aqaei et al. (2020) reported that foliar application of SiNPs on maize ameliorated drought stress-induced mineral nutrients imbalance and enhanced corn weight. In a field study, Namjoyan et al. (2020) indicated that SiNPs treatment at 1 mM improved shoot water status, enhanced photosynthesis rate and glycine betaine metabolism, and regulated the activities of antioxidant enzymes including SOD, CAT and GPX in sugar beet.
Moreover, the comparative effects of Si and SiNPs on drought stress tolerance in plants have been investigated in previous studies. Rai-Kalal et al. (2021) demonstrated that SiNPs priming was more efficient than that of bulk Si in promoting seed germination, seedling growth, chlorophyll fluorescence index in wheat under drought stress. In strawberry, Zahedi et al. (2023) reported that both Si and SiNPs improved drought stress tolerance through regulating photosynthesis performance and modulating the metabolism of carbon and plant hormone, while the regulatory effects differed between Si and SiNPs. Additionally, Ghorbanpour et al. (2020) compared the roles of Si and SiNPs in drought stress recovery in barley and found that SiNPs application more efficiently promoted barley growth, modulated antioxidant enzyme activity, and regulated the metabolism of osmolytes than bulk Si application.
In agricultural practice, SiNPs can also be applied along with other plant growth regulators such as plant growth promoting rhizobacteria (PGPR), plant hormones, other NPs, and plant growth stimulating chemicals, which is more effective in enhancing plant abiotic stress resistance (Figure 5). As for PGPR, Eltahawy et al. (2022) indicated that the integrated application of heavy metal-resistant bacteria and SiNPs more efficiently regulated antioxidant system and promoted spinach growth under metal contamination than individual application of SiNPs. In wheat grown under semi-arid condition, the combined treatment of SiNPs with CaCO3-precipitating bacteria induced more significant yield promotion than individual treatment with SiNPs or CaCO3-precipitating bacteria (Desoky et al., 2022). In addition, SiNPs can also be applied in conjunction with other NPs in alleviating abiotic stresses. It has been suggested that the combined application of SiNPs and selenium nanoparticles (SeNPs) promoted plant growth and alleviated stress symptoms in strawberry grown under drought stress (Zahedi et al., 2020) and rice grown under Pb exposure (Hussain et al., 2020). In wheat subject to Cd stress, the conjunct application of SiNPs, zinc nanoparticles (ZnNPs), and FeNPs was more effective than other treatments (individual NPs and combination of two NPs) in enhancing grain yield and reducing Cd accumulation (Hussain et al., 2021). In other cases, the synergistic effects of SiNPs and biochar (Alsamadany et al., 2022), indoleacetic acid (IAA) (Sharma et al., 2022), and methyl jasmonate (MeJA) (Moradi et al., 2022) have also been confirmed to be effective in alleviating abiotic stresses such as As stress, Cr stress and salt stress.
Overall, this review summarized recent progress of SiNPs application in nano-enabled agriculture, focusing on synthesis, uptake and translocation, and application of SiNPs against various biotic and abiotic stresses. Based on these literatures, it can be concluded that SiNPs application is a cost-effective and multifunctional agronomic approach that is applicable to sustainable agriculture. However, several key issues need further investigation for the more widespread and reasonable usage of SiNPs in agricultural production including: (1) more effective synthesis approach of SiNPs using agricultural wastes; (2) the detailed effects of plant factors (e.g. plant species, plant structures and developmental stages) and SiNPs properties (e.g. size, charge property and specific modification) on the uptake and translocation of SiNPs; (3) the physiological and molecular basis of SiNPs-induced broad-spectrum resistance; (4) the effects and mechanisms of SiNPs modification on their delivery efficiency; (5) the main concerns over potential phytotoxicity induced from the application of SiNPs in agricultural ecosystem.
GY: Conceptualization, Funding acquisition, Writing – original draft. QH: Writing – original draft. SZ: Writing – original draft. YX: Writing – original draft. YH: Writing – review & editing. MN: Conceptualization, Writing – review & editing. NN: Conceptualization, Writing – review & editing. YL: Conceptualization, Writing – review & editing. ZZ: Conceptualization, Funding acquisition, Writing – review & editing.
The author(s) declare that financial support was received for the research, authorship, and/or publication of this article. This work was supported by the Natural Science Foundation of Zhejiang Province (LQ22C150006 and LZ20C150001), the National Natural Science Foundation of China (32202583), and the Fundamental Research Funds for the Central Universities (226-2023-00077).
The authors declare that the research was conducted in the absence of any commercial or financial relationships that could be construed as a potential conflict of interest.
All claims expressed in this article are solely those of the authors and do not necessarily represent those of their affiliated organizations, or those of the publisher, the editors and the reviewers. Any product that may be evaluated in this article, or claim that may be made by its manufacturer, is not guaranteed or endorsed by the publisher.
Abdelrahman, T. M., Qin, X. Y., Li, D. L., Senosy, I. A., Mmby, M., Wan, H., et al. (2021). Pectinase-responsive carriers based on mesoporous silica nanoparticles for improving the translocation and fungicidal activity of prochloraz in rice plants. Chem. Eng. J. 404, 10. doi: 10.1016/j.cej.2020.126440
Abdelrhim, A. S., Mazrou, Y. S. A., Nehela, Y., Atallah, O. O., El-Ashmony, R. M., Dawood, M. F. A. (2021). Silicon dioxide nanoparticles induce innate immune responses and activate antioxidant machinery in wheat against Rhizoctonia solani. Plants 10, 2758. doi: 10.3390/plants10122758
Adebisi, J. A., Agunsoye, J. O., Bello, S. A., Haris, M., Ramakokovhu, M. M., Daramola, M. O., et al. (2020). Green production of silica nanoparticles from maize stalk. Particul. Sci. Technol. 38, 667–675. doi: 10.1080/02726351.2019.1578845
Adrees, M., Khan, Z. S., Rehman, M. Z. U., Rizwan, M., Ali, S. (2022). Foliar spray of silicon nanoparticles improved the growth and minimized cadmium (Cd) in wheat under combined Cd and water-limited stress. Environ. Sci. pollut. R. 29, 77321–77332. doi: 10.1007/s11356-022-21238-2
Agathokleous, E., Feng, Z. Z., Iavicoli, I., Calabrese, E. J. (2020). Nano-pesticides: a great challenge for biodiversity? the need for a broader perspective. Nano Today 30, 100808. doi: 10.1016/j.nantod.2019.100808
Ahmed, T., Masood, H. A., Noman, M., Al-Huqail, A. A., Alghanem, S. M. S., Khan, M. M., et al. (2023). Biogenic silicon nanoparticles mitigate cadmium (Cd) toxicity in rapeseed (Brassica napus L.) by modulating the cellular oxidative stress metabolism and reducing Cd translocation. J. Hazard. Mater. 459, 132070. doi: 10.1016/j.jhazmat.2023.132070
Alam, P., Arshad, M., Al-Kheraif, A. A., Azzam, M. A., Al Balawi, T. (2022). Silicon nanoparticle-induced regulation of carbohydrate metabolism, photosynthesis, and ROS homeostasis in Solanum lycopersicum subjected to salinity stress. ACS Omega 7, 31834–31844. doi: 10.1021/acsomega.2c02586
Albalawi, M. A., Abdelaziz, A. M., Attia, M. S., Saied, E., Elganzory, H. H., Hashem, A. H. (2022). Mycosynthesis of silica nanoparticles using Aspergillus Niger: control of Alternaria solani causing early blight disease, induction of innate immunity and reducing of oxidative stress in eggplant. Antioxidants 11, 20. doi: 10.3390/antiox11122323
Ali, S., Rizwan, M., Hussain, A., Rehman, M. Z. U., Ali, B., Yousaf, B., et al. (2019). Silicon nanoparticles enhanced the growth and reduced the cadmium accumulation in grains of wheat (Triticum aestivum L.). Plant Physiol. Biochem. 140, 1–8. doi: 10.1016/j.plaphy.2019.04.041
Alsaeedi, A., El-Ramady, H., Alshaal, T., El-Garawani, M., Elhawat, N., Al-Otaibi, A. (2018). Exogenous nanosilica improves germination and growth of cucumber by maintaining K+/Na+ ratio under elevated Na+ stress. Plant Physiol. Biochem. 125, 164–171. doi: 10.1016/j.plaphy.2018.02.006
Alsaeedi, A., El-Ramady, H., Alshaal, T., El-Garawany, M., Elhawat, N., Al-Otaibi, A. (2019). Silica nanoparticles boost growth and productivity of cucumber under water deficit and salinity stresses by balancing nutrients uptake. Plant Physiol. Biochem. 139, 1–10. doi: 10.1016/j.plaphy.2019.03.008
Alsamadany, H., Alharby, H. F., Al-Zahrani, H. S., Alzahrani, Y. M., Almaghamsi, A. A., Abbas, G., et al. (2022). Silicon-nanoparticles doped biochar is more effective than biochar for mitigation of arsenic and salinity stress in Quinoa: insight to human health risk assessment. Front. Plant Sci. 13. doi: 10.3389/fpls.2022.989504
Alves, R. H., Reis, T. V. D., Rovani, S., Fungaro, D. A. (2017). Green synthesis and characterization of biosilica produced from sugarcane waste ash. J. Chem. 2017, 9. doi: 10.1155/2017/6129035
Amani, M. A., Latifi, A. M., Tahvildari, K., Karimian, R. (2018). Removal of diazinon pesticide from aqueous solutions using MCM-41 type materials: isotherms, kinetics and thermodynamics. Int. J. Environ. Sci. Te. 15, 1301–1312. doi: 10.1007/s13762-017-1469-x
Aqaei, P., Weisany, W., Diyanat, M., Razmi, J., Struik, P. C. (2020). Response of maize (Zea mays L.) to potassium nano-silica application under drought stress. J. Plant Nutr. 43, 1205–1216. doi: 10.1080/01904167.2020.1727508
Avellan, A., Schwab, F., Masion, A., Chaurand, P., Borschneck, D., Vidal, V., et al. (2017). Nanoparticle uptake in plants: gold nanomaterial localized in roots of Arabidopsis thaliana by X-ray computed nanotomography and hyperspectral imaging. Environ. Sci. Technol. 51, 8682–8691. doi: 10.1021/acs.est.7b01133
Ayoub, H. A., Khairy, M., Rashwan, F. A., Abdel-Hafez, H. F. (2017). Synthesis and characterization of silica nanostructures for cotton leaf worm control. J. Nanostructure Chem. 7, 91–100. doi: 10.1007/s40097-017-0229-2
Baka, Z. A., El-Zahed, M. M. (2022). Antifungal activity of silver/silicon dioxide nanocomposite on the response of faba bean plants (Vicia faba L.) infected by Botrytis cinerea. Bioresour. Bioprocess 9, 19. doi: 10.1186/s40643-022-00591-7
Bansal, K., Hooda, V., Verma, N., Kharewal, T., Tehri, N., Dhull, V., et al. (2022). Stress alleviation and crop improvement using silicon nanoparticles in agriculture: a review. Silicon 14, 10173–10186. doi: 10.1007/s12633-022-01755-y
Bapat, G., Labade, C., Chaudhari, A., Zinjarde, S. (2016). Silica nanoparticle based techniques for extraction, detection, and degradation of pesticides. Adv. Colloid Interface Sci. 237, 1–14. doi: 10.1016/j.cis.2016.06.001
Bapat, G., Zinjarde, S., Tamhane, V. (2020). Evaluation of silica nanoparticle mediated delivery of protease inhibitor in tomato plants and its effect on insect pest Helicoverpa armigera. Colloids Surf. B 193, 111079. doi: 10.1016/j.colsurfb.2020.111079
Bilal, M., Xu, C., Cao, L., Zhao, P., Cao, C., Li, F., et al. (2020). Indoxacarb-loaded fluorescent mesoporous silica nanoparticles for effective control of Plutella xylostella L. with decreased detoxification enzymes activities. Pest Manage. Sci. 76, 3749–3758. doi: 10.1002/ps.5924
Bose, S., Ganayee, M. A., Mondal, B., Baidya, A., Chennu, S., Mohanty, J. S., et al. (2018). Synthesis of silicon nanoparticles from rice husk and their use as sustainable fluorophores for white light emission. ACS Sustain. Chem. Eng. 6, 6203–6210. doi: 10.1021/acssuschemeng.7b04911
Buchman, J. T., Elmer, W. H., Ma, C. X., Landy, K. M., White, J. C., Haynes, C. L. (2019). Chitosan-coated mesoporous silica nanoparticle treatment of Citrullus lanatus (watermelon): enhanced fungal disease suppression and modulated expression of stress-related genes. ACS Sustain. Chem. Eng. 7, 19649–19659. doi: 10.1021/acssuschemeng.9b04800
Byrt, C. S., Munns, R. (2008). Living with salinity. New Phytol. 179, 903–905. doi: 10.1111/j.1469-8137.2008.02596.x
Chen, H. Y., Lin, Y. S., Zhou, H. J., Zhou, X. H., Gong, S., Xu, H. (2016). Synthesis and characterization of chlorpyrifos/copper(II) schiff base mesoporous silica with pH sensitivity for pesticide sustained release. J. Agric. Food Chem. 64, 8095–8102. doi: 10.1021/acs.jafc.6b03262
Clemens, S., Ma, J. F. (2016). Toxic heavy metal and metalloid accumulation in crop plants and foods. Annu. Rev. Plant Biol. 67, 489–512. doi: 10.1146/annurev-arplant-043015-112301
Clemens, S., Palmgren, M. G., Krämer, U. (2002). A long way ahead: understanding and engineering plant metal accumulation. Trends Plant Sci. 7, 309–315. doi: 10.1016/S1360-1385(02)02295-1
Coskun, D., Deshmukh, R., Sonah, H., Menzies, J. G., Reynolds, O., Ma, J. F., et al. (2019). The controversies of silicon’s role in plant biology. New Phytol. 221, 67–85. doi: 10.1111/nph.15343
Croissant, J. G., Butler, K. S., Zink, J. I., Brinker, C. J. (2020). Synthetic amorphous silica nanoparticles: toxicity, biomedical and environmental implications. Nat. Rev. Mater. 5, 886–909. doi: 10.1038/s41578-020-0230-0
Cui, J., Li, Y., Jin, Q., Li, F. (2020). Silica nanoparticles inhibit arsenic uptake into rice suspension cells via improving pectin synthesis and the mechanical force of the cell wall. Environ. Sci. Nano 7, 162–171. doi: 10.1039/C9EN01035A
Cui, J., Liu, T., Li, F., Yi, J., Liu, C., Yu, H. (2017). Silica nanoparticles alleviate cadmium toxicity in rice cells: mechanisms and size effects. Environ. pollut. 228, 363–369. doi: 10.1016/j.envpol.2017.05.014
Debnath, N., Mitra, S., Das, S., Goswami, A. (2012). Synthesis of surface functionalized silica nanoparticles and their use as entomotoxic nanocides. Powder Technol. 221, 252–256. doi: 10.1016/j.powtec.2012.01.009
Desoky, E. M., Rady, M. M., Nader, M. M., Mostafa, N. G., Elrys, A. S., Mathai, A., et al. (2022). Integrated application of bacterial carbonate precipitation and silicon nanoparticles enhances productivity, physiological attributes, and antioxidant defenses of wheat (Triticum aestivum L.) under semi-arid conditions. Front. Plant Sci. 13. doi: 10.3389/fpls.2022.947949
de Sousa, A., Saleh, A. M., Habeeb, T. H., Hassan, Y. M., Zrieq, R., Wadaan, M. A. M., et al. (2019). Silicon dioxide nanoparticles ameliorate the phytotoxic hazards of aluminum in maize grown on acidic soil. Sci. Total Environ. 693, 133636. doi: 10.1016/j.scitotenv.2019.133636
Dhakate, P., Kandhol, N., Raturi, G., Ray, P., Bhardwaj, A., Srivastava, A., et al. (2022). Silicon nanoforms in crop improvement and stress management. Chemosphere 305, 135165. doi: 10.1016/j.chemosphere.2022.135165
Ding, Y., Xiao, Z., Chen, F., Yue, L., Wang, C., Fan, N., et al. (2023). A mesoporous silica nanocarrier pesticide delivery system for loading acetamiprid: effectively manage aphids and reduce plant pesticide residue. Sci. Total Environ. 863, 160900. doi: 10.1016/j.scitotenv.2022.160900
Eichert, T., Goldbach, H. E. (2008). Equivalent pore radii of hydrophilic foliar uptake routes in stomatous and astomatous leaf surfaces - further evidence for a stomatal pathway. Physiol. Plant 132, 491–502. doi: 10.1111/j.1399-3054.2007.01023.x
Eichert, T., Kurtz, A., Steiner, U., Goldbach, H. E. (2008). Size exclusion limits and lateral heterogeneity of the stomatal foliar uptake pathway for aqueous solutes and water-suspended nanoparticles. Physiol. Plant 134, 151–160. doi: 10.1111/j.1399-3054.2008.01135.x
El-Shetehy, M., Moradi, A., Maceroni, M., Reinhardt, D., Petri-Fink, A., Rothen-Rutishauser, B., et al. (2021). Silica nanoparticles enhance disease resistance in Arabidopsis plants. Nat. Nanotechnol. 16, 344–353. doi: 10.1038/s41565-020-00812-0
Eltahawy, A. M. A. E., Awad, E.-S.A.M., Ibrahim, A. H. H., Merwad, A.-R.M.A., Desoky, E.-S. M. (2022). Integrative application of heavy metal-resistant bacteria, moringa extracts, and nano-silicon improves spinach yield and declines its contaminant contents on a heavy metal-contaminated soil. Front. Plant Sci. 13. doi: 10.3389/fpls.2022.1019014
Epstein, E. (1994). The anomaly of silicon in plant biology. Proc. Natl. Acad. Sci. U.S.A. 91, 11–17. doi: 10.1073/pnas.91.1.11
Epstein, E. (1999). Silicon. Annu. Rev. Plant Physiol. Plant Mol. Biol. 50, 641–664. doi: 10.1146/annurev.arplant.50.1.641
Etesami, H., Fatemi, H., Rizwan, M. (2021). Interactions of nanoparticles and salinity stress at physiological, biochemical and molecular levels in plants: a review. Ecotoxicol. Environ. Saf. 225, 112769. doi: 10.1016/j.ecoenv.2021.112769
Gao, Y. H., Xiao, Y. N., Mao, K. K., Qin, X. Y., Zhang, Y., Li, D. L., et al. (2020). Thermoresponsive polymer-encapsulated hollow mesoporous silica nanoparticles and their application in insecticide delivery. Chem. Eng. J. 383, 123169. doi: 10.1016/j.cej.2019.123169
Gao, Y. H., Zhang, Y. H., He, S., Xiao, Y. N., Qin, X. Y., Zhang, Y., et al. (2019). Fabrication of a hollow mesoporous silica hybrid to improve the targeting of a pesticide. Chem. Eng. J. 364, 361–369. doi: 10.1016/j.cej.2019.01.105
Ghorbanpour, M., Mohammadi, H., Kariman, K. (2020). Nanosilicon-based recovery of barley (Hordeum vulgare) plants subjected to drought stress. Environ. Sci. Nano 7, 443–461. doi: 10.1039/C9EN00973F
Giraldo, J. P., Landry, M. P., Faltermeier, S. M., McNicholas, T. P., Iverson, N. M., Boghossian, A. A., et al. (2014). Plant nanobionics approach to augment photosynthesis and biochemical sensing. Nat. Mater. 13, 400–408. doi: 10.1038/nmat3890
Gonzalez-Moscoso, M., Juarez-Maldonado, A., Cadenas-Pliego, G., Meza-Figueroa, D., SenGupta, B., Martinez-Villegas, N. (2022). Silicon nanoparticles decrease arsenic translocation and mitigate phytotoxicity in tomato plants. Environ. Sci. pollut. R. 29, 34147–34163. doi: 10.1007/s11356-021-17665-2
Goswami, P., Mathur, J., Srivastava, N. (2022). Silica nanoparticles as novel sustainable approach for plant growth and crop protection. Heliyon 8, e09908. doi: 10.1016/j.heliyon.2022.e09908
Gowayed, S. M. H., Al-Zahrani, H. S. M., Metwali, E. M. R. (2017). Improving the salinity tolerance in potato (Solanum tuberosum) by exogenous application of silicon dioxide nanoparticles. Int. J. Agric. Biol. 19, 183–192. doi: 10.17957/IJAB/15.0262
Gu, S., Zhou, J. S., Yu, C. J., Luo, Z. Y., Wang, Q. H., Shi, Z. L. (2015). A novel two-staged thermal synthesis method of generating nanosilica from rice husk via pre-pyrolysis combined with calcination. Ind. Crop Prod. 65, 1–6. doi: 10.1016/j.indcrop.2014.11.045
Haghighi, M., Pessarakli, M. (2013). Influence of silicon and nano-silicon on salinity tolerance of cherry tomatoes (Solanum lycopersicum L.) at early growth stage. Sci. Hortic. 161, 111–117. doi: 10.1016/j.scienta.2013.06.034
Hajihashemi, S., Kazemi, S. (2022). The potential of foliar application of nano-chitosan-encapsulated nano-silicon donor in amelioration the adverse effect of salinity in the wheat plant. BMC Plant Biol. 22, 148. doi: 10.1186/s12870-022-03531-x
He, S., Lian, X., Zhang, B., Liu, X., Yu, J., Gao, Y., et al. (2023). Nano silicon dioxide reduces cadmium uptake, regulates nutritional homeostasis and antioxidative enzyme system in barley seedlings (Hordeum vulgare L.) under cadmium stress. Environ. Sci. pollut. R. 30, 67552–67564. doi: 10.1007/s11356-023-27130-x
Hong, J., Peralta-Videa, J. R., Rico, C., Sahi, S., Viveros, M. N., Bartonjo, J., et al. (2014). Evidence of translocation and physiological impacts of foliar applied CeO2 nanoparticles on cucumber (Cucumis sativus) plants. Environ. Sci. Technol. 48, 4376–4385. doi: 10.1021/es404931g
Hu, P., An, J., Faulkner, M. M., Wu, H., Li, Z., Tian, X., et al. (2020). Nanoparticle charge and size control foliar delivery efficiency to plant cells and organelles. ACS Nano 14, 7970–7986. doi: 10.1021/acsnano.9b09178
Hussain, B., Lin, Q., Hamid, Y., Sanaullah, M., Di, L., Hashmi, M., et al. (2020). Foliage application of selenium and silicon nanoparticles alleviates Cd and Pb toxicity in rice (Oryza sativa L.). Sci. Total Environ. 712, 136497. doi: 10.1016/j.scitotenv.2020.136497
Hussain, A., Rizwan, M., Ali, Q., Ali, S. (2019). Seed priming with silicon nanoparticles improved the biomass and yield while reduced the oxidative stress and cadmium concentration in wheat grains. Environ. Sci. pollut. R. 26, 7579–7588. doi: 10.1007/s11356-019-04210-5
Hussain, A., Rizwan, M., Ali, S., Rehman, M. Z. U., Qayyum, M. F., Nawaz, R., et al. (2021). Combined use of different nanoparticles effectively decreased cadmium (Cd) concentration in grains of wheat grown in a field contaminated with Cd. Ecotoxicol. Environ. Saf. 215, 112139. doi: 10.1016/j.ecoenv.2021.112139
Ijaz, U., Ahmed, T., Rizwan, M., Noman, M., Shah, A. A., Azeem, F., et al. (2023). Rice straw based silicon nanoparticles improve morphological and nutrient profile of rice plants under salinity stress by triggering physiological and genetic repair mechanisms. Plant Physiol. Biochem. 201, 107788. doi: 10.1016/j.plaphy.2023.107788
Ismail, L. M., Soliman, M. I., Abd El-Aziz, M. H., Abdel-Aziz, H. M. M. (2022). Impact of silica ions and nano silica on growth and productivity of pea plants under salinity stress. Plants 11, 494. doi: 10.3390/plants11040494
Jeelani, P. G., Mulay, P., Venkat, R., Ramalingam, C. (2020). Multifaceted application of silica nanoparticles. a review. Silicon 12, 1337–1354. doi: 10.1007/s12633-019-00229-y
Ji, X. Y., Wang, H. Y., Song, B., Chu, B. B., He, Y. (2018). Silicon nanomaterials for biosensing and bioimaging analysis. Front. Chem. 6. doi: 10.3389/fchem.2018.00038
Judy, J. D., Unrine, J. M., Rao, W., Wirick, S., Bertsch, P. M. (2012). Bioavailability of gold nanomaterials to plants: importance of particle size and surface coating. Environ. Sci. Technol. 46, 8467–8474. doi: 10.1021/es3019397
Kang, H., Elmer, W., Shen, Y., Zuverza-Mena, N., Ma, C., Botella, P., et al. (2021). Silica nanoparticle dissolution rate controls the suppression of Fusarium wilt of watermelon (Citrullus lanatus). Environ. Sci. Technol. 55, 13513–13522. doi: 10.1021/acs.est.0c07126
Kannan, G., Sujatha, E. R. (2022). A review on the choice of nano-silica as soil stabilizer. Silicon 14, 6477–6492. doi: 10.1007/s12633-021-01455-z
Kaziem, A. E., Gao, Y. H., Zhang, Y., Qin, X. Y., Xiao, Y. N., Zhang, Y. H., et al. (2018). α-Amylase triggered-carriers based on cyclodextrin anchored hollow mesoporous silica for enhancing insecticidal activity of avermectin against Plutella xylostella. J. Hazard. Mater. 359, 213–221. doi: 10.1016/j.jhazmat.2018.07.059
Khan, M., Siddiqui, Z. A., Parveen, A., Khan, A. A., Moon, I. S., Alam, M. (2022). Elucidating the role of silicon dioxide and titanium dioxide nanoparticles in mitigating the disease of the eggplant caused by Phomopsis vexans, Ralstonia solanacearum, and root-knot nematode Meloidogyne incognita. Nanotechnol. Rev. 11, 1606–1619. doi: 10.1515/ntrev-2022-0097
Koleva, L., Umar, A., Yasin, N. A., Shah, A. A., Siddiqui, M. H., Alamri, S., et al. (2022). Iron oxide and silicon nanoparticles modulate mineral nutrient homeostasis and metabolism in cadmium-stressed Phaseolus vulgaris. Front. Plant Sci. 13. doi: 10.3389/fpls.2022.806781
Korrani, Z. S., Ibrahim, W. A. W., Nodeh, H. R., Aboul-Enein, H. Y., Sanagi, M. M. (2016). Simultaneous preconcentration of polar and non-polar organophosphorus pesticides from water samples by using a new sorbent based on mesoporous silica. J. Sep. Sci. 39, 1144–1151. doi: 10.1002/jssc.201500896
Li, Y. Y., Zhu, N. L., Liang, X. J., Bai, X., Zheng, L. R., Zhao, J. T., et al. (2020). Silica nanoparticles alleviate mercury toxicity via immobilization and inactivation of Hg(II) in soybean (Glycine max). Environ. Sci. Nano 7, 1807–1817. doi: 10.1039/D0EN00091D
Liang, Y., Gao, Y. H., Wang, W. C., Dong, H. Q., Tang, R., Yang, J. L., et al. (2020). Fabrication of smart stimuli-responsive mesoporous organosilica nano-vehicles for targeted pesticide delivery. J. Hazard. Mater. 389, 11. doi: 10.1016/j.jhazmat.2020.122075
Liang, Y. C., Nikolic, M., Belanger, R., Gong, H. J., Song, A. L. (2015). Silicon in agriculture: from theory to practice (Dordrecht: Springer). doi: 10.1007/978-94-017-9978-2
Lombi, E., Donner, E., Dusinska, M., Wickson, F. (2019). A One Health approach to managing the applications and implications of nanotechnologies in agriculture. Nat. Nanotechnol. 14, 523–531. doi: 10.1038/s41565-019-0460-8
Lv, J., Christie, P., Zhang, S. (2019). Uptake, translocation, and transformation of metal-based nanoparticles in plants: recent advances and methodological challenges. Environ. Sci. Nano 6, 41–59. doi: 10.1039/C8EN00645H
Ma, X., Geiser-Lee, J., Deng, Y., Kolmakov, A. (2010). Interactions between engineered nanoparticles (ENPs) and plants: phytotoxicity, uptake and accumulation. Sci. Total Environ. 408, 3053–3061. doi: 10.1016/j.scitotenv.2010.03.031
Ma, Y., He, X., Zhang, P., Zhang, Z., Ding, Y., Zhang, J., et al. (2017). Xylem and phloem based transport of CeO2 nanoparticles in hydroponic cucumber plants. Environ. Sci. Technol. 51, 5215–5221. doi: 10.1021/acs.est.6b05998
Ma, J. F., Tamai, K., Ichii, M., Wu, G. F. (2002). A rice mutant defective in Si uptake. Plant Physiol. 130, 2111–2117. doi: 10.1104/pp.010348
Ma, J. F., Tamai, K., Yamaji, N., Mitani, N., Konishi, S., Katsuhara, M., et al. (2006). A silicon transporter in rice. Nature 440, 688–691. doi: 10.1038/nature04590
Ma, J. F., Yamaji, N. (2015). A cooperative system of silicon transport in plants. Trends Plant Sci. 20, 435–442. doi: 10.1016/j.tplants.2015.04.007
Ma, J. F., Yamaji, N., Mitani, N., Tamai, K., Konishi, S., Fujiwara, T., et al. (2007). An efflux transporter of silicon in rice. Nature 448, 209–212. doi: 10.1038/nature05964
Mahawar, L., Ramasamy, K. P., Suhel, M., Prasad, S. M., Zivcak, M., Brestic, M., et al. (2023). Silicon nanoparticles: comprehensive review on biogenic synthesis and applications in agriculture. Environ. Res. 232, 116292. doi: 10.1016/j.envres.2023.116292
Mahmoud, L. M., Shalan, A. M., El-Boray, M. S., Vincent, C. I., El-Kady, M. E., Grosser, J. W., et al. (2022). Application of silicon nanoparticles enhances oxidative stress tolerance in salt stressed ‘Valencia’ sweet orange plants. Sci. Hortic. 295, 110856. doi: 10.1016/j.scienta.2021.110856
Mandlik, R., Thakral, V., Raturi, G., Shinde, S., Nikolic, M., Tripathi, D. K., et al. (2020). Significance of silicon uptake, transport, and deposition in plants. J. Exp. Bot. 71, 6703–6718. doi: 10.1093/jxb/eraa301
Marousek, J., Marouskova, A., Periakaruppan, R., Gokul, G. M., Anbukumaran, A., Bohata, A., et al. (2022). Silica nanoparticles from coir pith synthesized by acidic sol-gel method improve germination economics. Polymers 14, 266. doi: 10.3390/polym14020266
Mathur, P., Roy, S. (2020). Nanosilica facilitates silica uptake, growth and stress tolerance in plants. Plant Physiol. Biochem. 157, 114–127. doi: 10.1016/j.plaphy.2020.10.011
Miralles, P., Church, T. L., Harris, A. T. (2012). Toxicity, uptake, and translocation of engineered nanomaterials in vascular plants. Environ. Sci. Technol. 46, 9224–9239. doi: 10.1021/es202995d
Mitani-Ueno, N., Ma, J. F. (2021). Linking transport system of silicon with its accumulation in different plant species. Soil Sci. Plant Nutr. 67, 10–17. doi: 10.1080/00380768.2020.1845972
Moradi, P., Vafaee, Y., Mozafari, A. A., Tahir, N. A. R. (2022). Silicon nanoparticles and methyl jasmonate improve physiological response and increase expression of stress-related genes in strawberry cv. Paros under salinity stress. Silicon 14, 10559–10569. doi: 10.1007/s12633-022-01791-8
Muhammad, H. M. D., Abbas, A., Ahmad, R. (2022). Fascinating role of silicon nanoparticles to mitigate adverse effects of salinity in fruit trees: a mechanistic approach. Silicon 14, 8319–8326. doi: 10.1007/s12633-021-01604-4
Munns, R. (2005). Genes and salt tolerance: bringing them together. New Phytol. 167, 645–663. doi: 10.1111/j.1469-8137.2005.01487.x
Munns, R., Tester, M. (2008). Mechanisms of salinity tolerance. Annu. Rev. Plant Biol. 59, 651–681. doi: 10.1146/annurev.arplant.59.032607.092911
Naguib, D. M., Abdalla, H. (2019). Metabolic status during germination of nano silica primed Zea mays seeds under salinity stress. J. Crop Sci. Biotechnol. 22, 415–423. doi: 10.1007/s12892-019-0168-0
Naidu, S., Pandey, J., Mishra, L. C., Chakraborty, A., Roy, A., Singh, I. K., et al. (2023). Silicon nanoparticles: synthesis, uptake and their role in mitigation of biotic stress. Ecotoxicol. Environ. Saf. 255, 114783. doi: 10.1016/j.ecoenv.2023.114783
Namjoyan, S., Sorooshzadeh, A., Rajabi, A., Aghaalikhani, M. (2020). Nano-silicon protects sugar beet plants against water deficit stress by improving the antioxidant systems and compatible solutes. Acta Physiol. Plant 42, 157. doi: 10.1007/s11738-020-03137-6
Okeke, E. S., Nweze, E. J., Ezike, T. C., Nwuche, C. O., Ezeorba, T. P. C., Nwankwo, C. E. I. (2023). Silicon-based nanoparticles for mitigating the effect of potentially toxic elements and plant stress in agroecosystems: a sustainable pathway towards food security. Sci. Total Environ. 898, 165446. doi: 10.1016/j.scitotenv.2023.165446
Park, H. J., Kim, S. H., Kim, H. J., Choi, S. H. (2006). A new composition of nanosized silica-silver for control of various plant diseases. Plant Pathol. J. 22, 295–302. doi: 10.5423/PPJ.2006.22.3.295
Piela, A., Zymanczyk-Duda, E., Brzezinska-Rodak, M., Duda, M., Grzesiak, J., Saeid, A., et al. (2020). Biogenic synthesis of silica nanoparticles from corn cobs husks. Dependence of the productivity on the method of raw material processing. Bioorg. Chem. 99, 13. doi: 10.1016/j.bioorg.2020.103773
Rahman, I. A., Padavettan, V. (2012). Synthesis of silica nanoparticles by sol-gel: size-dependent properties, surface modification, and applications in silica-polymer nanocomposites - a review. J. Nanomater. 2012, 132424. doi: 10.1155/2012/132424
Rai, P. K., Lee, S. S., Zhang, M., Tsang, Y. F., Kim, K. H. (2019). Heavy metals in food crops: health risks, fate, mechanisms, and management. Environ. Int. 125, 365–385. doi: 10.1016/j.envint.2019.01.067
Rai-Kalal, P., Tomar, R. S., Jajoo, A. (2021). Seed nanopriming by silicon oxide improves drought stress alleviation potential in wheat plants. Funct. Plant Biol. 48, 905–915. doi: 10.1071/FP21079
Rashad, Y. M., El-Sharkawy, H. H. A., Belal, B. E. A., Abdel Razik, E. S., Galilah, D. A. (2021). Silica nanoparticles as a probable anti-oomycete compound against Downy mildew, and yield and quality enhancer in grapevines: field evaluation, molecular, physiological, ultrastructural, and toxicity investigations. Front. Plant Sci. 12. doi: 10.3389/fpls.2021.763365
Rastogi, A., Tripathi, D. K., Yadav, S., Chauhan, D. K., Zivcak, M., Ghorbanpour, M., et al. (2019). Application of silicon nanoparticles in agriculture. 3 Biotech. 9, 90. doi: 10.1007/s13205-019-1626-7
Riaz, M., Kamran, M., Fahad, S., Wang, X. (2022a). Nano-silicon mediated alleviation of Cd toxicity by cell wall adsorption and antioxidant defense system in rice seedlings. Plant Soil 486, 103–117. doi: 10.1007/s11104-022-05588-x
Riaz, M., Zhao, S., Kamran, M., Ur Rehman, N., Mora-Poblete, F., Maldonado, C., et al. (2022b). Effect of nano-silicon on the regulation of ascorbate-glutathione contents, antioxidant defense system and growth of copper stressed wheat (Triticum aestivum L.) seedlings. Front. Plant Sci. 13. doi: 10.3389/fpls.2022.986991
Rizwan, A., Zia-ur-Rehman, M., Rizwan, M., Usman, M., Anayatullah, S., Alharby, H. F., et al. (2023). Effects of silicon nanoparticles and conventional Si amendments on growth and nutrient accumulation by maize (Zea mays L.) grown in saline-sodic soil. Environ. Res. 227, 115740. doi: 10.1016/j.envres.2023.115740
Ruttkay-Nedecky, B., Krystofova, O., Nejdl, L., Adam, V. (2017). Nanoparticles based on essential metals and their phytotoxicity. J. Nanobiotechnology 15, 33. doi: 10.1186/s12951-017-0268-3
Salami, B. A., Oyehan, T. A., Gambo, Y., Badmus, S. O., Tanimu, G., Adamu, S., et al. (2022). Technological trends in nanosilica synthesis and utilization in advanced treatment of water and wastewater. Environ. Sci. pollut. R. 29, 42560–42600. doi: 10.1007/s11356-022-19793-9
Saw, G., Nagdev, P., Jeer, M., Murali-Baskaran, R. K. (2023). Silica nanoparticles mediated insect pest management. Pestic. Biochem. Physiol. 194, 105524. doi: 10.1016/j.pestbp.2023.105524
Sayed, E. G., Mahmoud, A. W. M., El-Mogy, M. M., Ali, M. A. A., Fahmy, M. A. M., Tawfic, G. A. (2022). The effective role of nano-silicon application in improving the productivity and quality of grafted tomato grown under salinity stress. Horticulturae 8, 293. doi: 10.3390/horticulturae8040293
Selvarajan, V., Obuobi, S., Ee, P. L. R. (2020). Silica nanoparticles-a versatile tool for the treatment of bacterial infections. Front. Chem. 8. doi: 10.3389/fchem.2020.00602
Seroka, N. S., Taziwa, R. T., Khotseng, L. (2022). Extraction and synthesis of silicon nanoparticles (SiNPs) from sugarcane bagasse ash: a mini-review. Appl. Sci. 12, 2310. doi: 10.3390/app12052310
Shalaby, T. A., Abd-Alkarim, E., El-Aidy, F., Hamed, E.-S., Sharaf-Eldin, M., Taha, N., et al. (2021). Nano-selenium, silicon and H2O2 boost growth and productivity of cucumber under combined salinity and heat stress. Ecotoxicol. Environ. Saf. 212, 111962. doi: 10.1016/j.ecoenv.2021.111962
Sharma, V. K., Filip, J., Zboril, R., Varma, R. S. (2015). Natural inorganic nanoparticles-formation, fate, and toxicity in the environment. Chem. Soc Rev. 44, 8410–8423. doi: 10.1039/C5CS00236B
Sharma, B., Tiwari, S., Kumawat, K. C., Cardinale, M. (2023). Nano-biofertilizers as bio-emerging strategies for sustainable agriculture development: potentiality and their limitations. Sci. Total Environ. 860, 160476. doi: 10.1016/j.scitotenv.2022.160476
Sharma, A., Vishwakarma, K., Singh, N. K., Prakash, V., Ramawat, N., Prasad, R., et al. (2022). Synergistic action of silicon nanoparticles and indole acetic acid in alleviation of chromium (CrVI) toxicity in Oryza sativa seedlings. J. Biotechnol. 343, 71–82. doi: 10.1016/j.jbiotec.2021.09.005
Siddiqui, M. H., Al-Whaibi, M. H., Faisal, M., Al Sahli, A. A. (2014). Nano-silicon dioxide mitigates the adverse effects of salt stress on Cucurbita pepo L. Environ. Toxicol. Chem. 33, 2429–2437. doi: 10.1002/etc.2697
Spielman-Sun, E., Lombi, E., Donner, E., Howard, D., Unrine, J. M., Lowry, G. V. (2017). Impact of surface charge on cerium oxide nanoparticle uptake and translocation by wheat (Triticum aestivum). Environ. Sci. Technol. 51, 7361–7368. doi: 10.1021/acs.est.7b00813
Suriyaprabha, R., Karunakaran, G., Kavitha, K., Yuvakkumar, R., Rajendran, V., Kannan, N. (2014). Application of silica nanoparticles in maize to enhance fungal resistance. Iet Nanobiotechnol 8, 133–137. doi: 10.1049/iet-nbt.2013.0004
Tang, F. Q., Li, L. L., Chen, D. (2012). Mesoporous silica nanoparticles: synthesis, biocompatibility and drug delivery. Adv. Mater. 24, 1504–1534. doi: 10.1002/adma.201104763
Thabet, A. F., Boraei, H. A., Galal, O. A., El-Samahy, M. F. M., Mousa, K. M., Zhang, Y. Z., et al. (2021). Silica nanoparticles as pesticide against insects of different feeding types and their non-target attraction of predators. Sci. Rep. 11, 14484. doi: 10.1038/s41598-021-93518-9
Tripathi, D. K., Shweta, Singh, S., Singh, S., Pandey, R., Singh, V. P., et al. (2017). An overview on manufactured nanoparticles in plants: uptake, translocation, accumulation and phytotoxicity. Plant Physiol. Biochem. 110, 2–12. doi: 10.1016/j.plaphy.2016.07.030
Tripathi, D. K., Singh, V. P., Prasad, S. M., Chauhan, D. K., Dubey, N. K. (2015). Silicon nanoparticles (SiNp) alleviate chromium (VI) phytotoxicity in Pisum sativum (L.) seedlings. Plant Physiol. Biochem. 96, 189–198. doi: 10.1016/j.plaphy.2015.07.026
Usman, M., Farooq, M., Wakeel, A., Nawaz, A., Cheema, S. A., Rehman, H. U., et al. (2020). Nanotechnology in agriculture: current status, challenges and future opportunities. Sci. Total Environ. 721, 137778. doi: 10.1016/j.scitotenv.2020.137778
Verma, K. K., Zeng, Y., Song, X. P., Singh, M., Wu, K. C., Rajput, V. D., et al. (2022). Nanosilicon: an approach for abiotic stress mitigation and sustainable agriculture. Front. Plant Sci. 13. doi: 10.3389/fpls.2022.1025974
Vetchinkina, E., Loshchinina, E., Kupryashina, M., Burov, A., Nikitina, V. (2019). Shape and size diversity of gold, silver, selenium, and silica nanoparticles prepared by green synthesis using fungi and bacteria. Ind. Eng. Chem. Res. 58, 17207–17218. doi: 10.1021/acs.iecr.9b03345
Wang, W. X., Martin, J. C., Fan, X. T., Han, A. J., Luo, Z. P., Sun, L. Y. (2012a). Silica nanoparticles and frameworks from rice husk biomass. Acs. Appl. Mater. Inter. 4, 977–981. doi: 10.1021/am201619u
Wang, L., Ning, C., Pan, T., Cai, K. (2022a). Role of silica nanoparticles in abiotic and biotic stress tolerance in plants: a review. Int. J. Mol. Sci. 23, 1947. doi: 10.3390/ijms23041947
Wang, L., Pan, T., Gao, X., An, J., Ning, C., Li, S., et al. (2022b). Silica nanoparticles activate defense responses by reducing reactive oxygen species under Ralstonia solanacearum infection in tomato plants. NanoImpact 28, 100418. doi: 10.1016/j.impact.2022.100418
Wang, Z. Y., Xie, X. Y., Zhao, J., Liu, X. Y., Feng, W. Q., White, J. C., et al. (2012b). Xylem- and phloem-based transport of CuO nanoparticles in maize (Zea mays L.). Environ. Sci. Technol. 46, 4434–4441. doi: 10.1021/es204212z
Wang, Z. Y., Yue, L., Dhankher, O. P., Xing, B. S. (2020). Nano-enabled improvements of growth and nutritional quality in food plants driven by rhizosphere processes. Environ. Int. 142, 105831. doi: 10.1016/j.envint.2020.105831
Wang, Z., Zhu, W., Chen, F., Yue, L., Ding, Y., Xu, H., et al. (2021). Nanosilicon enhances maize resistance against oriental armyworm (Mythimna separata) by activating the biosynthesis of chemical defenses. Sci. Total Environ. 778, 146378. doi: 10.1016/j.scitotenv.2021.146378
Xu, C. L., Cao, L. D., Zhao, P. Y., Zhou, Z. L., Cao, C., Li, F. M., et al. (2018). Emulsion-based synchronous pesticide encapsulation and surface modification of mesoporous silica nanoparticles with carboxymethyl chitosan for controlled azoxystrobin release. Chem. Eng. J. 348, 244–254. doi: 10.1016/j.cej.2018.05.008
Xu, C. L., Shan, Y. P., Bilal, M., Xu, B., Cao, L. D., Huang, Q. L. (2020). Copper ions chelated mesoporous silica nanoparticles via dopamine chemistry for controlled pesticide release regulated by coordination bonding. Chem. Eng. J. 395, 13. doi: 10.1016/j.cej.2020.125093
Yadav, M., George, N., Dwibedi, V. (2023). Emergence of toxic trace elements in plant environment: insights into potential of silica nanoparticles for mitigation of metal toxicity in plants. Environ. pollut. 333, 122112. doi: 10.1016/j.envpol.2023.122112
Yamaji, N., Ma, J. F. (2009). A transporter at the node responsible for intervascular transfer of silicon in rice. Plant Cell 21, 2878–2883. doi: 10.1105/tpc.109.069831
Yamaji, N., Mitatni, N., Ma, J. F. (2008). A transporter regulating silicon distribution in rice shoots. Plant Cell 20, 1381–1389. doi: 10.1105/tpc.108.059311
Yamaji, N., Sakurai, G., Mitani-Ueno, N., Ma, J. F. (2015). Orchestration of three transporters and distinct vascular structures in node for intervascular transfer of silicon in rice. Proc. Natl. Acad. Sci. U.S.A. 112, 11401–11406. doi: 10.1073/pnas.1508987112
Yan, G. C., Jin, H., Yin, C., Hua, Y. C., Huang, Q. Y., Zhou, G. F., et al. (2023). Comparative effects of silicon and silicon nanoparticles on the antioxidant system and cadmium uptake in tomato under cadmium stress. Sci. Total Environ. 904, 166819. doi: 10.1016/j.scitotenv.2023.166819
Yan, G. C., Nikolic, M., Ye, M. J., Xiao, Z. X., Liang, Y. C. (2018). Silicon acquisition and accumulation in plant and its significance for agriculture. J. Interg. Agric. 17, 2138–2150. doi: 10.1016/S2095-3119(18)62037-4
Yang, J., Sun, M., Chen, Z., Xiao, Y., Wei, H., Zhang, J., et al. (2022). Effect of foliage applied chitosan-based silicon nanoparticles on arsenic uptake and translocation in rice (Oryza sativa L.). J. Hazard. Mater. 433, 128781. doi: 10.1016/j.jhazmat.2022.128781
Yang, Y. X., Xu, Y., Yang, Y. W., Yang, H., Yuan, H. M., Huang, Y., et al. (2016). Laccase immobilized on mesoporous SiO2 and its use for degradation of chlorophenol pesticides. Russ. J. Phys. Chem. A 90, 2044–2054. doi: 10.1134/S0036024416100307
Yeats, T. H., Rose, J. K. C. (2013). The formation and function of plant cuticles. Plant Physiol. 163, 5–20. doi: 10.1104/pp.113.222737
Zahedi, S. M., Hosseini, M. S., Fahadi Hoveizeh, N., Kadkhodaei, S., Vaculík, M. (2023). Comparative morphological, physiological and molecular analyses of drought-stressed strawberry plants affected by SiO2 and SiO2-NPs foliar spray. Sci. Hortic. 309, 111686. doi: 10.1016/j.scienta.2022.111686
Zahedi, S. M., Moharrami, F., Sarikhani, S., Padervand, M. (2020). Selenium and silica nanostructure-based recovery of strawberry plants subjected to drought stress. Sci. Rep. 10, 17672. doi: 10.1038/s41598-020-74273-9
Zhang, J., Kothalawala, S., Yu, C. Z. (2023). Engineered silica nanomaterials in pesticide delivery: challenges and perspectives. Environ. pollut. 320, 14. doi: 10.1016/j.envpol.2023.121045
Zhao, P. Y., Cao, L. D., Ma, D. K., Zhou, Z. L., Huang, Q. L., Pan, C. P. (2018a). Translocation, distribution and degradation of prochloraz-loaded mesoporous silica nanoparticles in cucumber plants. Nanoscale 10, 1798–1806. doi: 10.1039/C7NR08107C
Zhao, S., Kamran, M., Rizwan, M., Ali, S., Yan, L., Alwahibi, M. S., et al. (2023). Regulation of proline metabolism, AsA-GSH cycle, cadmium uptake and subcellular distribution in Brassica napus L. under the effect of nano-silicon. Environ. pollut. 335, 122321. doi: 10.1016/j.envpol.2023.122321
Zhao, L., Lu, L., Wang, A., Zhang, H., Huang, M., Wu, H., et al. (2020). Nano-biotechnology in agriculture: use of nanomaterials to promote plant growth and stress tolerance. J. Agric. Food Chem. 68, 1935–1947. doi: 10.1021/acs.jafc.9b06615
Zhao, P. Y., Yuan, W. L., Xu, C. L., Li, F. M., Cao, L. D., Huang, Q. L. (2018b). Enhancement of spirotetramat transfer in cucumber plant using mesoporous silica nanoparticles as carriers. J. Agric. Food Chem. 66, 11592–11600. doi: 10.1021/acs.jafc.8b04415
Keywords: silicon nanoparticles (SiNPs), synthesis, uptake and translocation, biotic and abiotic stress, plant growth stimulator, nanocarrier, sustainable agriculture
Citation: Yan G, Huang Q, Zhao S, Xu Y, He Y, Nikolic M, Nikolic N, Liang Y and Zhu Z (2024) Silicon nanoparticles in sustainable agriculture: synthesis, absorption, and plant stress alleviation. Front. Plant Sci. 15:1393458. doi: 10.3389/fpls.2024.1393458
Received: 29 February 2024; Accepted: 18 March 2024;
Published: 28 March 2024.
Edited by:
Haijun Gong, Northwest A&F University, ChinaReviewed by:
Meng Xu, Chinese Academy of Agricultural Sciences, ChinaCopyright © 2024 Yan, Huang, Zhao, Xu, He, Nikolic, Nikolic, Liang and Zhu. This is an open-access article distributed under the terms of the Creative Commons Attribution License (CC BY). The use, distribution or reproduction in other forums is permitted, provided the original author(s) and the copyright owner(s) are credited and that the original publication in this journal is cited, in accordance with accepted academic practice. No use, distribution or reproduction is permitted which does not comply with these terms.
*Correspondence: Zhujun Zhu, emh1empAemFmdS5lZHUuY24=
†These authors have contributed equally to this work and share first authorship
Disclaimer: All claims expressed in this article are solely those of the authors and do not necessarily represent those of their affiliated organizations, or those of the publisher, the editors and the reviewers. Any product that may be evaluated in this article or claim that may be made by its manufacturer is not guaranteed or endorsed by the publisher.
Research integrity at Frontiers
Learn more about the work of our research integrity team to safeguard the quality of each article we publish.