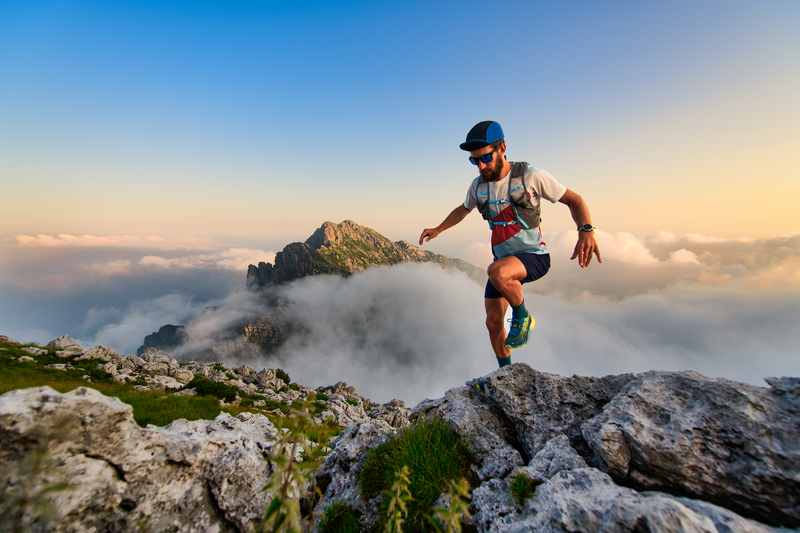
95% of researchers rate our articles as excellent or good
Learn more about the work of our research integrity team to safeguard the quality of each article we publish.
Find out more
ORIGINAL RESEARCH article
Front. Plant Sci. , 16 May 2024
Sec. Plant Abiotic Stress
Volume 15 - 2024 | https://doi.org/10.3389/fpls.2024.1392425
This article is part of the Research Topic Surviving and Thriving: How Crops Perceive and Respond to Temperature Stress View all 13 articles
Backgrounds: As a conserved signaling pathway, mitogen-activated protein kinase (MAPK) cascade regulates cellular signaling in response to abiotic stress. High temperature may contribute to a significant decrease in economic yield. However, research into the expression patterns of StMAPKK family genes under high temperature is limited and lacks experimental validation regarding their role in supporting potato plant growth.
Methods: To trigger heat stress responses, potato plants were grown at 35°C. qRT-PCR was conducted to analyze the expression pattern of StMAPKK family genes in potato plants. Plant with StMAPKK5 loss-of-function and gain-of-function were developed. Potato growth and morphological features were assessed through measures of plant height, dry weight, and fresh weight. The antioxidant ability of StMAPKK5 was indicated by antioxidant enzyme activity and H2O2 content. Cell membrane integrity and permeability were suggested by relative electrical conductivity (REC), and contents of MDA and proline. Photosynthetic capacity was next determined. Further, mRNA expression of heat stress-responsive genes and antioxidant enzyme genes was examined.
Results: In reaction to heat stress, the expression profiles of StMAPKK family genes were changed. The StMAPKK5 protein is located to the nucleus, cytoplasm and cytomembrane, playing a role in controlling the height and weight of potato plants under heat stress conditions. StMAPKK5 over-expression promoted photosynthesis and maintained cell membrane integrity, while inhibited transpiration and stomatal conductance under heat stress. Overexpression of StMAPKK5 triggered biochemical defenses in potato plant against heat stress, modulating the levels of H2O2, MDA and proline, as well as the antioxidant activities of CAT, SOD and POD. Overexpression of StMAPKK5 elicited genetic responses in potato plants to heat stress, affecting heat stress-responsive genes and genes encoding antioxidant enzymes.
Conclusion: StMAPKK5 can improve the resilience of potato plants to heat stress-induced damage, offering a promising approach for engineering potatoes with enhanced adaptability to challenging heat stress conditions.
● In response to heat stresses, expression patterns of StMAPKKs are altered in different potato tissues;
● StMAPKK5 influences potato morphological characteristics, including plant weight and height under heat stress conditions;
● StMAPKK5 regulates the physiological index of potato plants responding to heat stress.
● StMAPKK5 regulates photosynthesis and transpiration in response to heat stress;
● StMAPKK5 leads to altered expression of heat stress-responsive genes and antioxidant enzyme genes in potato plants under heat stress conditions.
Over the past century, the Earth’s average surface temperature has been rising (Hansen et al., 2022; Katlane et al., 2023). Evidence indicates that extreme weather events, including heat waves, are increasing in both frequency and intensity (Walsh et al., 2020). Extreme temperatures confer negative roles in photosynthesis, flower pollination, and fruit setting, which leads to lower crop yields and affects food availability (Beillouin et al., 2020; Dalhaus et al., 2020; Nicholson and Egan, 2020; Wi et al., 2020). As the fourth most important crop in agricultural production, potatoes (Solanum tuberosum L.) are susceptible to high temperature or adverse water supply (Singh et al., 2020). Due to climate change, tuber growth is inhibited above 33°C and high temperature above 25°C induces leaf senescence (Rykaczewska, 2015). We need to better understand how heat stress affects plant growth and development as global temperatures rise in the future. This could offer valuable insights into breeding programs to improve plant heat-stress tolerance.
Protein kinases (PK) constitute a vast and crucial superfamily of enzymes involved in cellular signaling, regulating a wide array of biological processes (Chen et al., 2021a). The mitogen-activated protein kinases (MAPKs) are important and evolutionarily conserved subfamily of protein kinases that play a central role in transducing signals from the cell surface to the nucleus responding to a variety of stimuli, for instance, heat stress (He et al., 2020; Kumar et al., 2020). The hierarchical organization of MAPK signaling cascades, encompassing the MAPKs, MAPK kinases (MAPKKs or MEKs), and MAPKKs kinases (MAPKKKs or MEKKs), forms a crucial framework for transmitting external signals, amplifying stress signaling, and ultimately facilitating communication between the cellular environment and transcriptional responses (Zwerger and Hirt, 2001; Taj et al., 2010; Zhang and Zhang, 2022). Heat stress can indeed trigger various signaling mechanisms within biological systems in three pathways, including Ca2+-dependent salt overlay sensitive signaling and osmotic/oxidative stress signaling (Rodriguez et al., 2010). The role of oxidative stress signaling in promoting osmolyte accumulation through the MAPK cascade, involving G-protein receptors, histidine kinases, and protein tyrosine kinases, aligns with known cellular responses to stress (Ara and Sinha, 2014; Röhm et al., 2021).
Genome-wide analyses have progressively identified multiple MAPKKs genes in various plant species. Yin et al. identified 23 MAPKKs in the Gossypiumhirsutum genome and divided into 4 groups. Most of these genes feature the active site motif-D(I/L)K-, which includes two conserved residues, K (lysine) and D (aspartic acid) (Yin et al., 2021). The cucumber genome contains 6 MAPKK genes classified into 4 groups (A-D) (Wang et al., 2015). Ten MAPKK genes have been reported in Arabidopsis genome, which are also classified into 4 groups (A-D) (Danquah et al., 2014; Choi et al., 2017). Bioinformatic analysis of maize genome revealed 9 MAPKK genes and identified 11 subdomains of protein kinases showing serine/threonine specificity (Kong et al., 2013). From the available genome, 5 MAPKK genes were identified in tomato (Wu et al., 2014) and 7 in rice (Kumar et al., 2008), respectively. However, systematic studies of the MAPKK gene family are still required for potato plant.
The MAPKK factors, as the components of the MAPK signaling pathway, exhibit evolutionary conservation across various organisms and regulate a wide array of functions, such as stomatal development (Bergmann et al., 2004), plant architecture (Yu et al., 2014), chlorophyll synthesis (Li et al., 2022), glucose metabolism (Chardin et al., 2017a), apical meristem (Lee et al., 2019), and flower development (Lafleur et al., 2015). However, studies on how StMAPKKs respond to heat stress in potato plants are scarce. This study aimed to describe the expression features of StMAPKKs under heat stress situations. Then, our study analyzed the functional aspects of StMAPKK5, including its roles in potato plant growth, antioxidation, cell membrane integrity, photosynthesis capacity, and mRNA expression of heat stress-responsive genes and antioxidant enzyme genes. The potato cultivar “Atlantic” is a cultivar introduced and popularised in Guangdong Province. As a high-quality and high-yield potato variety, “Atlantic” has been studied rarely under heat stress. Meanwhile, this study aimed to screen heat tolerance genes, which may provide an effective basis for its molecular breeding for heat stress tolerance.
The potato (Solanum tuberosum L.) cultivar “Atlantic” was cultivated in vitro using Murashige and Skoog (MS) medium with a pH of 5.8–6.0. The medium was supplemented with 8% sucrose and the plants were cultivated for 4 weeks. Next, four-week seedlings were cultured in the dark for 30 days to induce tuber generation. Approximately 1 g of potato tubers with 1 mm germinated buds were transferred into containers (19 cm ×25 cm ×26 cm) filled with soil-vermiculite mixture (1:1, v/v) and cultivated for 5 weeks in Zhanjiang located at latitude 21°11’43’’N and longitude 110°34’56’’E. Soil water holding capacity ranged from 75% to 80%, supplemented with 100 mL of nutrient solution (pH: 5.8; 0.20 mmol/L FeSO4, 2.57 mmol/L KH2PO4, 2.08 mmol/L MgSO4, 1.29 mmol/L (NH4)2SO4, and 9.89 mmol/L KNO3) every 7 days. Potato plants in each group exhibiting uniform growth appearance were next cultivated under 35°C. Physiological and photosynthetic indexes were assayed at 0 h, 8 h, 12 h, 24 h, and 48 h after cultivation under heat stress conditions. The control plants were cultivated at 22°C. Each group was prepared with three biological replicates and three technical replicates.
To examine the mRNA expression of MAPKK family genes in various plant tissues, the potted plants were continually cultured. Flower, root, stem, leaf, petiole, stolon, and shoot were collected at the developmental stage, and tuber was collected at the maturity stage. Next, the plants were cultivated under 35°C, and StMAPKKs expression in leaf, stem, and root were examined at 0 h, 1 h, 2 h, 4 h, 8 h, 12 h, 24 h, and 48 h after cultivation under heat stress conditions.
The gene encoding the StMAPKK5 protein was cloned into the pBI121-EGFP plasmid using a previously described method (Li et al., 2020), to develop StMAPKK5-overexpressing plants (OE for short). Agrobacterium tumefaciens strains LBA4404 was used for transformation experiments. Potato plants low expressing StMAPKK5 (RNAi or Ri for short) were established with a previously described method (Lu et al., 2019). The primers utilized for plasmid construction are listed in Table 1.
Agrobacterium containing plasmids were cultured for about 48 h in LB medium in addition with 50 mg/L gentamicin and 50 mg/L spectinomycin at 28°C, next harvested by centrifugation (5,000 rpm, 10 min), and re-suspended in MS medium (OD600 = 0.3). The sterile seedling stems (2 cm) were incubated in Agrobacterium suspension for 10 min, and then grown in MS medium (pH: 5.8) containing 7.4 g/L agar, 30 g/L sucrose, 0.5 mg/L 6-BA, 2.0 mg/L ZT, 0.2 mg/L GA3, and 1.0 mg/L IAA and maintained in the dark for 48–72 h. Next, the plants were transferred into differentiation media (MS, 7.4 g/L agar, 30 g/L sucrose, 300 mg/L Timentin, 100 mg/L kan, 0.5 mg/L 6-BA, 2.0 mg/L ZT, 0.2 mg/L GA3, and 1.0 mg/L IAA; PH: 5.8), with media changed every 2 weeks. After induction of adventitious bud, the resistant adventitious buds were transferred to formulated root medium (MS + 7.4 g/L agar+ 30 g/L sucrose + 300 mg/L Timentin + 100 mg/L kan, pH=5.8) until adventitious roots were induced.
TRIzol RNA Extraction kit (Invitrogen, Carlsbad, CA, USA) was used to extract total RNA from the collected samples. The first-strand cDNA of the target genes were synthesized using the First-Strand cDNA Synthesis Kit (TransGen Biotech, Beijing, China). qPCR was conducted using a LightCycler 480 II real-time PCR system (Roche, Rotkreuz, Switzerland) with the reaction mixture comprising 0.8 μL of specific primers (0.5 μM), 100 ng of cDNA (Table 1), and 10 μL of SYBR Premix Ex Taq (2 ×) (Takara, Tokyo, Japan). The reactions underwent an initial incubation at 94°C for 3 min, followed by the procedures: 36 cycles of 94°C for 45 s, 59°C for 34 s, and 72°C for 1 min. The relative transcription levels were determined using the 2-△△Ct method.
The expression vector pPBI121-EGFP carrying protein-coding sequence of StMAPKK5 gene was transformed into Agrobacterium tumefaciens GV3101. The primers utilized for plasmid construction are detailed in Table 1. Following the method described by Sparkes et al. (2006), tobacco epidermal cells were infiltrated with the transformed strain. A confocal laser scanning microscope (Olympus, Tokyo, Japan) was used to observe the sample. For the autofluorescence of chlorophyll in chloroplasts, the excitation wavelength and transmission range for emission were set at 640 nm and 675 nm, and 488 nm and 510 nm for the GFP-tagged StMAPKK5 protein. The software Olympus Fluovie was used for imaging processing.
MEGA 5.05 software was utilized to construct a phylogenetic tree with a neighbor-joining algorithm. DNAman tool (Lynnon Biosoft, San Ramon, CA, USA) was applied to multiple-sequence alignment of the conserved subdomains.
Non-transgenic (NT) and transgenic plantlets, uniformly measuring 2 cm in height, were planted in MS medium under controlled conditions in an incubator with an 8 h-dark cycle and 16 h-photoperiod at 22°C and 3,000 Lx. To induce heat stress, plants were cultivated under 35°C. Four weeks after heat stress treatment, we measured root fresh/dry weight, plant fresh/dry weight, and plant height.
Four-week-old potted plants were cultivated under 35°C. After 0 h, 8 h, 16 h, 24 h, and 48 h, we examined stomatal conductance, transpiration rate, and net photosynthetic rate. Stomatal conductance, transpiration rate, and net photosynthetic rate were assessed using a portable LI-6400XT system (Li-COR, Lincoln, NE, USA) between 9:30–11:30. The fourth fully expanded functional leaf was collected from the plant top. The parameters were configured as follows, 50%-70% of relative humidity in leaf chamber, 1,500 μmol·m-2·s-1 of the photon flux density, and 400 μmol/mol of CO2.
Four-week-old potted plants were cultivated under 35°C. Following exposure to heat stress for durations of 0 h, 8 h, 16 h, 24 h, and 48 h, the potted plants were assayed for the levels of proline, malondialdehyde (MDA), and hydrogen peroxide (H2O2), and activities of peroxidase (POD), superoxide dismutase (SOD), and catalase (CAT), superoxide dismutase (SOD), and peroxidase (POD) activities with methodologies previously established in our research (Zhu et al., 2021). Description of detailed experimental procedures are provided in Supplementary Methods.
Relative electrical conductivity (REC) and chlorophyll content were assayed according to our previous methods (Zhu et al., 2023). Description of detailed experimental procedures are provided in Supplementary Methods.
Statistical analysis was performed using GraphPad Prism Software (GraphPad, San Diego, CA, USA) and IBM SPSS 19.0 Statistical Software (IBM, Chicago, IL, USA). Results are presented as mean ± standard deviation. Histograms and line charts were generated using GraphPad Prism software. For multiple comparisons, one-way ANOVA with Tukey test or Dunnett’s T3 for post-hoc analysis or two-way ANOVA corrected by Sidak’s multiple comparisons test were employed.
After phylogenetic analysis of the amino acid sequences of the reported plant MAPKK homologs, we found StMAPKK5 was homologous with SlMAPKK5, AtMAPKK3 and OsMAPKK3 (Figure 1A). There was a high degree of homology between StMAPKK4 and SlMAPKK4, StMAPKK2 and SlMAPKK2, StMAPKK3 and SlMAPKK3, as well as StMAPKK1 and SlMAPKK1. A comparison of the MAPKK proteins from Solanum tuberosum (St) (StMAPKK1–5), Arabidopsis thaliana (At) (AtMAPKK1–10), Solanum lycopersicum (Sl) (SlMAPKK1–5), and Oryza sativa (Os) (1, 3, 4, 5, 6, 10–1 and 10–2) showed the presence of the highly conserved domains (Figure 1B). StMAPKK5 contains 11 conserved catalytic subdomains typical of MAPKKs and the conserved consensus motif GXGXXG.
Figure 1 The phylogenetic relationship and protein sequence alignment of MAPKK proteins with MAPKK proteins. (A) Dendrogram of MAPKK proteins from Solanum tuberosum (St) (StMAPKK1–5), Arabidopsis thaliana (At) (AtMAPKK1–10), Solanum lycopersicum (Sl) (SlMAPKK1–5), and Oryza sativa (Os) (1,3,4,5,6,10–1 and 10–2); Bootstrap values were calculated from 500 resampled datasets with a 50% cut-off; MEGA-X software was used to establish the neighbor-joining phylogenetic tree. (B) Protein sequence of MAPKK proteins were aligned using DNAman software; Identical and similar amnio acids were marked in the same colors. The conserved subdomains were indicated on the bottom, using the Roman numbers (I-XI). The dark line marks the active site motif. Red asterisks indicate substrate specificity, and the black dots above the sequences represent activating sites. The conserved consensus motif GXGXXG is marked as I.
A variety of plant tissues (flower, root, stem, leaf, petiole, stolon, tuber, and shoot) were collected to analyze the expression features of StMAPKK family genes. StMAPKK1 showed the highest level of expression in root (Figure 2A). StMAPKK2 displayed the maximum expression in leaf (Figure 2B), StMAPKK3 in stem and shoot (Figure 2C), StMAPKK4 in root (Figure 2D), stem and leaf, and StMAKK5 in leaf (Figure 2E). These results represented the tissue-specific expression profiles of StMAPKK family genes. We speculated genes within the StMAPKKs family may play a crucial role in regulating the growth and development of different tissues and organs in potato.
Figure 2 mRNA expression of StMAPKK family genes of the various tissues of potato. Relative expression of (A) StMAPKK1, (B) StMAPKK2, (C) StMAPKK3, (D) StMAPKK4, and (E) StMAPKK5 at mRNA levels, in flower, root, stem, leaf, petiole, stolon, tuber, and shoot. Data were the means ± standard deviation. Different letters indicated significant difference between two groups (p-value less than 0.05, calculated by one-way ANOVA, followed by LSD and Duncan or Dunnett’s T3).
Potato leaves, stems and roots were chosen for quantification because the relative expression of StMAPKK5 gene was found to be higher than in other tissues. At 0 h, 1 h, 2 h, 4 h, 8 h, 16 h, 24 h, or 48 h after exposure to heat stress (35°C), potato leaves, stem and roots were obtained. qRT-PCR was then performed to assay the expression features of StMAPKK family genes. The expression of StMAPKK1 increased steadily with the duration of heat stress (p < 0.05) (Figure 3A). However, we observed that the expression of StMAPKK2 (Figure 3B), StMAPKK3 (Figure 3C), and StMAPKK4 (Figure 3D) in leaves, stems and roots changed in a disordered manner in response to heat stress (p < 0.05). Likewise, we noted that heat stress conditions resulted in a consistent increase in the transcript levels of StMAPK5 in potato leaves, stems, and roots (p < 0.05) (Figure 3E). Considering that after treatment, StMAPKK5 transcript levels were up-regulated at a higher rate than StMAPKK1–4 (p < 0.05). Therefore, we speculated that in response to heat stress, StMAPKK5 gene may perform crucial molecular functions.
Figure 3 Expression profiles of StMAPKK family genes at mRNA level in potato leaves, stem and roots in response to heat stress. Relative mRNA levels of (A) StMAPK1, (B) StMAPKK2, (C) StMAPKK3, (D) StMAPKK4, and (E) StMAPKK5 in leaves, stem, and roots. Four-week-old normally grown plants were exposed to heat stress (35°C) for 0 h, 1 h, 2 h, 4 h, 8 h, 16 h, 24 h, or 48 h. Data were the means ± standard deviation. Different letters indicated significant difference between two groups (p-value less than 0.05, calculated by one-way ANOV, followed by LSD and Duncan or Dunnett’s T3).
We generated StMAPKK5 overexpression plants by introducing pBI121-EGFP-StMAPKK5 and create loss-of-function plants through the induction of pART-StMAPKK5-RNAi. In transgenic plants, StMAPKK5 mRNA expression was significantly elevated or decreased relative to the wild-type plant (p < 0.001) (Figures 4A, B). Within the under-expression lines, Ri-2, Ri-3, and Ri-5 were chosen as notable under-expressors, while OE-2, OE-3 and OE-6 were identified as significant over-expressors for subsequent functional analysis under heat stress conditions. In order to better assess the location of StMAPKK5 protein, we examined by tagging StMAPKK5 protein with GFP and dissecting the green fluorescence, with the red autofluorescence of chlorophyll as a location reference. The green fluorescence is evenly distributed in the nucleus, cytoplasm and cytomembrane (Figure 4C), which was consistent with our previous result (Luo et al., 2024). In comparison with the NT plants, StMAPKK5 overexpression significantly enhanced the thermotolerance of potato plants as evidenced by the increases in plant height, dry weight, dry root weight, fresh weight, and root fresh weight, under normal and heat stress conditions after 4 weeks of cultivation (p < 0.001) (Figures 4D, E). In contrast, under normal or heat stress conditions, RNAi-mediated silencing of StMAPKK5 apparently resulted in the inhibition of plant growth, compared to non-transgenic plants (p < 0.001).
Figure 4 StMAPKK5 overexpression and under-expression altered the growth of potato plant under heat stress condition. StMAPKK5 transcription levels in (A) StMAPKK5 overexpression plants and (B) StMAPKK5 under-expression plants. (C) Fluorescence images showed StMAPKK5-encoding protein located in the nucleus, cytoplasm and cytomembrane; bars = 50 μm; StMAPKK5 protein was tagged with EGFP (enhanced green fluorescent protein); red fluorescence derives from the chlorophyll. (D) Growth morphology of potato plant cultivated under normal and heat stress conditions. (E) Plant growth indexes were assayed, including plant height, dry root weight, dry weight, fresh root weight, and fresh weight. Transgenic or non-transgenic plants were measured 0 h, 8 h, 16 h, 24 h, and 48 h after cultivation under normal or heat stress conditions. NT, non-transgenic plants; Ri, pART-StMAPKK5-RNAi-transgenic plants (RNAi-2, RNAi-3, and RNAi-5); OE, pBI121-EGFP-StMAPKK5-transgenic lines (OE-2, OE-3, and OE-6); Data were the means ± standard deviation; p-values (*p < 0.05, **p < 0.01, ***p < 0.001) were calculated by ordinary two-way ANOVA, followed by Tukey’s multiple comparisons test (n = 9).
Heat stresses led to a significant increase in H2O2 in non-transgenic plants, and it continually increased with prolonged duration of heat stress (p < 0.001) (Figure 5A). StMAPKK5 transgenic lines showed significantly reduced H2O2 production at 8 h, 16 h, 24 h, and 48 h following cultivation under heat stress conditions, compared to non-transgenic plants, while RNAi-mediated silencing of StMAPKK5 reversely increased the accumulation of H2O2 (p < 0.001). MAD generated in non-transgenic plant was found significantly increased as the extension of heat stress (p < 0.001) (Figure 5B). Compared to non-transgenic plants, MAD content was decreased in StMAPKK5 transgenic lines (OE-2, OE-3, and OE-6 after heat stress. In contrast, as compared to non-transgenic plants, knockdown of StMAPKK5 resulted in the accumulation of MDA under heat stress. The level of proline, as a consequence of heat stress, was increased in non-transgenic plants in a time-dependent manner (Figure 5C). The transgenic lines (OE-2, OE-3, and OE-6) accumulated more proline compared to non-transgenic plants under heat stress condition, while StMAPKK5-silencing plants were detected with decreased proline content. Under heat stress conditions, the specific activities of CAT, SOD, and POD were consistently higher in non-transgenic plants (p < 0.001) (Figures 5D–F). After 48 h of heat stress, the activity of CAT, SOD, and POD was found higher in StMAPKK5-transgenic lines compared to non-transgenic plants, respectively. However, compared to non-transgenic plants, StMAPKK5-silencing plants displayed decreased activities of CAT, SOD, and POD, 48 h after heat stress.
Figure 5 Elevated performance of StMAPKK5 transgenic plants in scavenging ROS in response to heat stress. (A) H2O2 accumulation, (B) MDA content, (C) proline content, antioxidant enzyme activity of (D) CAT, (E) SOD, and (F) POD, (G) chlorophyll content, and (H) REC of potato plant cultivated under normal and heat stress conditions. Transgenic or non-transgenic plants were measured 0 h, 8 h, 16 h, 24 h, and 48 h after cultivated under heat stress conditions. NT, non-transgenic plants; Ri, pART-StMAPKK5-RNAi-transgenic plants (RNAi-2, RNAi-3, and RNAi-5); OE, pBI121-EGFP-StMAPKK5-transgenic lines (OE-2, OE-3, and OE-6); Data were the means ± standard deviation; p-values (***p < 0.001) were calculated by ordinary two-way ANOVA, followed by Tukey’s multiple comparisons test (n = 9).
In general, chlorophyll synthesis is sensitive to heat stress, serving as an indicator of the extent of heat stress injury. It was observed that heat stress had an adverse effect on chlorophyll in potato plants (Figure 5G). Overexpression of StMAPKK5 may increase the heat stress tolerance of potato plants by maintaining the total chlorophyll content, compared to non-transgenic plants (p < 0.001). StMAPKK5-silencing lines appeared to be more susceptible to heat stress, evidenced by a higher reduction in total chlorophyll content than non-transgenic plants (p < 0.001). REC have commonly been examined to reflect stress-induced injury of cell membrane. Heat stress significantly exacerbated REC with the extension of time (Figure 5H). StMAPKK5 overexpression profoundly inhibited REC while StMAPKK5 silence significantly increased REC, compared to the NT plants (p < 0.001).
Comparisons of measured rates of net photosynthesis suggested that direct suppression of photosynthesis happens at temperatures higher than about 35°C. Cultivation under heat stress contributed to the inhibition of net photosynthetic rate for the NT plants (p < 0.05) (Figure 6A). However, compared to the NT plants, StMAPKK5-overexpressing plants showed increased net photosynthetic rates in response to heat stress (p < 0.001). Potato plants lowly expressing StMAPKK5 failed to maintain net photosynthetic rate in comparison with the NT plants (p < 0.001). Transpiration is an essential physiological process, during which course water and mineral nutrients are transferred from soils to plants to dissipate heat. In this study, the transpiration rate of the plants decreased as the duration of thermal incubation increased (Figure 6B). StMAPKK5 overexpression further inhibited transpiration in potato plants, whereas StMAPKK5 low expression promoted transpiration in instead (p < 0.001). Leaf stomata manages plant CO2 absorption through transpiration and photosynthesis. It was shown that stomatal conductance was decreased responding to increased temperatures (Figure 6C). In transgenic plants, compared to the NT plants, stomatal conductance of StMAPKK5 overexpression plants decreased markedly (p < 0.001). Reversely, StMAPKK5 silence maintained the stomatal conductance relative to the NT plants (p < 0.001). These results indicated that in response to heat stresses, overexpression of StMAPKK5 increased photosynthetic rate and decreased transpiration and stomatal conductance.
Figure 6 Stomatal apertures and photosynthesis in plants with overexpression or under-expression of StMAPKK5 responding to heat stresses. (A) Net photosynthetic rate, (B) transpiration rate and (C) stomatal conductance of potato leaves. Transgenic or non-transgenic plants were measured 0 h, 8 h, 16 h, 24 h, and 48 h after cultivated under heat stress conditions. NT, non-transgenic plants; Ri, pART-StMAPKK5-RNAi-transgenic plants (RNAi-2, RNAi-3, and RNAi-5); OE, pBI121-EGFP-StMAPKK5-transgenic lines (OE-2, OE-3, and OE-6). Mean ± standard deviation. p-values (***p < 0.001) were calculated by ordinary two-way ANOVA, followed by Tukey’s multiple comparisons test (n = 9).
To protect the structure and operation of plant cells from the damaging effects of oxidative stress caused by heat stress, the antioxidant defense system is generated, consisting of antioxidant enzymes, like FeSOD, MnSOD, and CuZnSOD, in chloroplasts, mitochondria, and peroxisomes. Under heat stress conditions, NT plants showed a significant increase in mRNA expression of StFeSOD2, StFeSOD, StMnSOD, StCuZnSOD1, and StCuZnSOD2 (Figures 7A–E). StMAPKK5-transgenic plants (OE-2, OE-3, and OE-6 lines) exhibited increased sensitivity to heat stress, suggesting a higher mRNA expression of StFeSOD2, StFeSOD3, StMnSOD, StCuZnSOD1 and StCuZnSOD2, while Ri-2, Ri-3, and Ri-5 plants exhibited a decreased expressed compared to the NT plants, after 48 h of cultivation under heat stress condition (p < 0.001). However, StMAPKK5-low expressing plants showed decreased gene expression (p < 0.001). Peroxidases, encoded by StPOD66, StPOD47, and StPOD12 are enzymes involved in scavenging ROS, in response to heat stress. The CAT genes encode for catalase, such as StCAT1 and StCAT2, playing a vital role in the antioxidant defense system by scavenging ROS generated under heat stress situations. In the NT plants, StPOD66 (Figure 7F), StPOD47 (Figure 7G), StPOD1 (Figure 7H), StCAT1 (Figure 7I), and StCAT2 (Figure 7J) was highly expressed in response to heat stress (p < 0.001). Compared to the NT plants, the transgenic potato lines (OE-2, OE-3, and OE-6) increased the gene expression, while the RNAi plants profoundly decreased the mRNA expression of peroxidases, in response to heat stress (p < 0.001). The StHSFA3-encoded protein belongs to the plant heat shock transcription factor family, orchestrating the transcription of genes that encode heat shock proteins (HSP) assisting the plant in coping with heat-induced damage (Friedrich et al., 2021). After 48 h of cultivation under heat stress condition, the NT plants displayed increased mRNA expression of StHSFA3 (Figure 7K), StHsp20–20 (Figure 7L), StHsp20–33 (Figure 7M), StHsp20–44 (Figure 7N), StHsp70 (Figure 7O), StHsp90.2 (Figure 7P), and StHsp90.4 (Figure 7Q). In contrast to the NT plants, the OE lines showed enhanced transcriptional levels, while the Ri plants exhibited decreased expression of the genes, under heat stress conditions (p < 0.001). The StP5CS gene, which encodes △1-pyrroline-5-carboxylate synthetase, plays a critical role in proline biosynthesis, and proline is an important amino acid involved in maintaining cellular osmotic balance and scavenging ROS. Consistently, the NT plants increased the mRNA expression of StP5CS, 48 h after cultivation under heat stress conditions (p < 0.001) (Figure 7R). In comparison with the NT plants, the mRNA expression of StP5CS was evidently enhanced in the OE lines, while reduced in the Ri plants, in response to heat stress. Our results suggested that overexpression of StMAPKK5 triggered genetic responses of potato plant to combat heat stress.
Figure 7 Alterations in mRNA expression of antioxidant enzyme genes and heat-stress responsive genes in plants with overexpression or under-expression of StMAPKK5 under heat stress conditions. mRNA expression of (A) StFeSOD2, (B) StFeSOD2, (C) StPOD, (D) StCuZnSOD1, (E) StCuZnSOD2, (F) StPOD66, (G) StPOD47, (H) StPOD12, (I) StCAT1, (J) StCAT2, (K) StHSFA3, (L) StHsp20–20, (M) StHsp20–33, (N) StHsp20–44, (O) StHsp70, (P) StHsp90.2, (Q) StHsp90.4, and (R) StP5CS in potato leaves were estimated 0 h, 8 h, 16 h, 24 h, and 48 h after cultivation under 35°C. NT, non-transgenic plants; Ri, pART-StMAPKK5-RNAi-transgenic plants (RNAi-2, RNAi-3, and RNAi-5); OE, pBI121-EGFP-StMAPKK5-transgenic lines (OE-2, OE-3, and OE-6). Mean ± standard deviation. p-values (*p < 0.05, **p < 0.01, ***p < 0.001) were calculated by ordinary two-way ANOVA, followed by Tukey’s multiple comparisons test (n = 9).
The effects of high temperatures are now evident in the reduced yield, nutrient depletion, and diminished quality of potatoes in an aberrant environment (Lee et al., 2020; Singh et al., 2020). The MAPK cascade is a crucial signaling pathway that plays a key module in transducing signals from environmental factors such as heat. The cascade has been extensively investigated and detailed in model plants like Arabidopsis, and crops such as rice owing to their relatively well-established research resources (Kumar et al., 2020; Chen et al., 2021). However, understanding the MAPK cascade in response to heat stress has been more challenging due to the complexity of its genome. Here, transgenic potato plants overexpressing StMAPKK5 displayed an improved heat stress tolerance in comparison to the non-transformed controls. Overexpression of StMAPKK5 triggered antioxidant enzyme genes, such as StFeSOD2, which was then implicated in regulating potato growth and physiology. As a result, StMAPKK5 can be used to create varieties that are more resistant to heat stress.
Numerous MAPKKs have been detected in a variety of plant species, including Arabidopsisthaliana (MAPK group, 2002), Populus trichocarpa (Nicole et al., 2006), Capsicum annuum (Liu et al., 2015), Zea mays (Kong et al., 2013), and Oryza sativa (Kumar et al., 2008). We analyzed the phylogenetic relationship of MAPKK proteins from Solanum tuberosum, Arabidopsis thaliana, Solanum lycopersicum, and Oryza sativa, and performed multiple alignment, to provide a systematic phylogenetic analysis of MAPKK proteins. Plant MAPKKs feature the phosphorylation site motif S/T-X5-S/T and a presumptive MAPK-docking domain characterized by the sequence K/R-K/R-K/R-X1–6-LX-L/V/I (Chae et al., 2010). In StMAPKK1, StMAPKK2, StMAPKK3, StMAPKK4, and StMAPKK5, we found S/G-X5-S/T/G/R motif, while K/R-K/R-K/R-X1–6-LX-L/V/I was not noted. Sequence alignment placed the potato MAPKKs into four groups, A (StMAPKK1 and StMAPKK3), B (StMAPKK5), C (StMAPKK2), and D (StMAPKK4).
The modification of MAPKK protein expression under abiotic and biotic stress conditions appear to be a plant strategy for adapting and defending against challenging survival environments. Yang et al. revealed that in response to heat stress, the transcription level of OsMKK1 in Xanthomonas oryzae was apparently up-regulated (Yang et al., 2024). As for the abiotic stress, Kumar et al. OsMAPKK1, OsMAPKK3, OsMAPKK4, OsMAPKK6, and OsMAPKK10–2 expression levels are differentially regulated by heat, cold, drought, and salinity stresses (Kumar et al., 2008). In Prunus mume under exposure to cold, the expression of 4 PmMKK genes (PmMKK5, PmMKK6, PmMKK20, and PmMKK3) is reduced with prolonged treatment (Wen et al., 2023). The expression patterns of MKK genes were determined in different tissues of poplar by Wang et al., and the expression of MKK2a was altered after cultivation under salt tress (Wang et al., 2022). However, limited studies have explored the expression patterns of StMAPKKs under heat stress conditions. Considering that plant MAPKKs have crucial functions responding to abiotic stresses, and their expressions are regulated by heat stress (Kumar et al., 2008; Kumar and Sinha, 2013), we firstly investigated StMAPKKs expression distribution in different organs of potato plant and expression profile under heat stress conditions. The expression of StMAPKK1, StMAPKK2, StMAPKK3, StMAPKK4, and StMAPKK5 was distinctly distributed in flower, root, stem, leaf, petiole, stolon, tuber, and shoot. Furthermore, mRNA expression of the five genes in leaf, stem and root were affected to different extent. It is noteworthy that the increase in StMAPKK5 expression was relatively high and stable. Subsequently, we further constructed StMAPKK5 overexpression and low-expression plants to analyze its biological functions.
It’s concerning to see the visible impact of aberrant environmental conditions on potato production, nutrient levels, and quality. Transgenic technology has been explored in creating varieties with traits like resistance to tolerance to environmental stressors like high temperature. Chen et al. concluded that the interaction between SaMKK2 and SaMAPK4/7 positively induces the expression of the downstream genes (SLD2, OPR2, and CBFs), thereby endowing potatoes with innate cold resistance (Chen et al., 2022). StMKK1 is involved in potato defense against the potato late-blight pathogen Phytophthora infestans, the gray-mold fungal pathogen Botrytis cinerea, and the bacterial wilt pathogen Ralstonia solanacearum (Chen et al., 2021b). However, it is still unknown whether StMAPKK5 mediates the growth of potato plant under heat stress. Our results showed that in response to heat stress, StMAPKK5 overexpression maintained or even fostered the plant growth, while StMAPKK5 down-regulation even decreased the growth compared to the non-transgenic plants. Duan et al. suggested a potential relationship between OsMKK4 and grain size or growth (Duan et al., 2014). GhMKK3 overexpression facilitates root growth in transgenic N. benthamiana (Wang et al., 2016). These findings are consistent with our results, providing a rationale for the involvement of MAPKK genes in plant growth.
Plants activate various defense mechanisms to alleviate the harmful impacts of oxidative stress or consequential injury to cells or tissues in response to abiotic stresses (drought, cold, heat, and salinity stresses) (Sachdev et al., 2021). These defense mechanisms include various non-enzymatic antioxidants and antioxidant enzymes (SOD, CAT, and POD) to neutralize ROS and maintain cellular homeostasis (Ahmad et al., 2009; Caverzan et al., 2016). High temperature affects multiple cellular processes, including photosynthesis, respiration and protein stability, and the disruption of these normal physiological activities leads to an overproduction of ROS and causes oxidative stress (Kumar, 2012; Posch et al., 2019; Zahra et al., 2023). Previous work has reported that in response to heat stress, MAPK cascade is involved in signal transduction, which affects plant physiological processes, such as photosynthesis, respiration, transpiration, nutrient uptake, tropisms, and senescence (Lee et al., 2016; Chardin et al., 2017b; Gouda et al., 2020; Liu et al., 2020). In this study, there was a reduction in contents of H2O2 and MDA, and an increase in antioxidant activities, induced by StMAPKK5 overexpression under heat stress conditions. Chlorophyll content and REC are regarded as the physiological parameters mostly affected by heat and drought stress (Rehman et al., 2016). From our findings, it can be concluded that StMAPKK5 overexpression can alleviate the adverse effects on photosynthetic efficiency and cell membrane integrity.
SOD functions in the primary defense against oxidative damage in cells, and participates in the detoxification, neutralization, scavenging, and converting of superoxide radicals (Alscher et al., 2002; Mishra and Sharma, 2019). There are three main types of SOD, each containing a different metal cofactor, including FeSOD, MnSOD, and CuZnSOD (Mishra and Sharma, 2019). Catalase detoxifies hydrogen peroxide and maintains redox homeostasis responding to heat stress (Dat et al., 1998). Peroxidase prevents the accumulation of hydrogen peroxide and reduce oxidative stress, working in coordination with other antioxidants like SOD and catalase (Veal and Day, 2011). StMAPKK5 overexpression significantly enhances the mRNA expression of SOD, catalase and peroxidase responding to heat stress. HSFA protein activate HSP expression, which are molecular chaperones that assist in protein refolding and prevent protein denaturation under heat stress conditions (Bourgine and Guihur, 2021; Khan et al., 2021). From our results, the increase in StHSFA3 mRNA expression and the induction of StHsp20–20, StHsp20–33, StHsp20–44, StHsp70, StHsp90.2, and StHsp90.4 seems to be related to StMAPKK5 overexpression. P5CS gene is involved in the biosynthesis of proline that serves as an osmoprotectant, maintaining osmotic balance during heat stress (Liu and Wang, 2020). StMAPKK5 overexpression-induced heat stress resistance may be through the regulation of StP5CS gene. Thus, StMAPKK5 overexpression modulated relative expression of heat-stress responsive genes in response to heat stress.
Extreme high temperature leads to lower crop yields and confers negative roles in food availability. Here, we found the constructed StMAPKK5-transgenic potato plants exhibited an intensified heat stress tolerance compared to the non-transgenic plants. However, StMAPKK5 deficiency showed relatively poor thermal stress resistance. The adverse effects of heat stress on antioxidant activities POD, CAT, and SOD, photosynthetic efficiency and cell membrane integrity were alleviated by StMAPKK5 overexpression. The enhanced heat stress resistance in plants due to StMAPKK5 overexpression may be attributed to alterations in the relative expression of antioxidant enzyme genes and heat-stress responsive genes.
The original contributions presented in the study are included in the article/Supplementary Material, further inquiries can be directed to the corresponding author/s. The MAPKK protein sequences discussed in this article can be accessed at the National Center for Biotechnology Information (NCBI) website (https://www.ncbi.nlm.nih.gov/) under the specified accession number: AtMKK1 (NP_194337.1), AtMKK2 (NP_001031751.1), AtMKK3 (NP_001318713.1), AtMKK4 (NP_175577.1), AtMKK5 (NP_001319606.1), AtMKK6 (NP_200469.1), AtMKK7 (NP_173271.1), AtMKK8 (NP_187274.1), AtMKK9 (NP_177492.1), AtMKK10 (NP_174510.1), SlMAPKK1 (NP_001304158.1), SlMAPKK2 (NP_001234588.1), SlMAPKK3 (NP_001234591.1), SlMAPKK4 (NP_001234595.1), SlMAPKK5 (XP_010317547.1), StMAPKK1 (XP_006353547.1), StMAPKK2 (NP_001275406.1), StMAPKK3 (NP_001305558.1), StMAPKK4 (XP_006363984.1), StMAPKK5 (XP_006351529.1), OsMAPKK1 (XP_015644525.1), OsMAPKK3 (XP_015643944.1), OsMAPKK4 (XP_015627603.1), OsMAPKK5 (XP_015643444.1), OsMAPKK6 (XP_015621394.1), OsMAPKK10–1 (XP_015624529.2), OsMAPKK10–2 (XP_015628376. 1), OsMAPKK10–3 (XP_015629634.1).
XZ: Writing – original draft, Writing – review & editing. WL: Writing – original draft, Writing – review & editing. NZ: Writing – original draft, Writing – review & editing. HJ: Writing – original draft, Writing – review & editing. HD: Writing – original draft, Writing – review & editing. ZC: Writing – original draft, Writing – review & editing. SC: Writing – original draft, Writing – review & editing. QW: Writing – original draft, Writing – review & editing. JT: Writing – original draft, Writing – review & editing. JZ: Writing – original draft, Writing – review & editing. YZ: Writing – original draft, Writing – review & editing. HS: Writing – original draft, Writing – review & editing.
The author(s) declare financial support was received for the research, authorship, and/or publication of this article. This Research Program was financially supported by the National Natural Science Foundation of China (No. 32360459), the Hainan Provincial Natural Science Foundation of China (No. 322MS116, 323MS095, 324MS098, 324QN327), and Central Public-interest Scientific Institution Basal Research Fund for Chinese Academy of Tropical Agricultural Sciences (No. 1630062024005).
We thank Rongkai Wang (Bioediates, Shaanxi, China) for providing the plasmids pBI121-EGFP, pHANNIBAL, and pART.
The authors declare that the research was conducted in the absence of any commercial or financial relationships that could be construed as a potential conflict of interest.
All claims expressed in this article are solely those of the authors and do not necessarily represent those of their affiliated organizations, or those of the publisher, the editors and the reviewers. Any product that may be evaluated in this article, or claim that may be made by its manufacturer, is not guaranteed or endorsed by the publisher.
The Supplementary Material for this article can be found online at: https://www.frontiersin.org/articles/10.3389/fpls.2024.1392425/full#supplementary-material
Ahmad, P., Jaleel, C. A., Azooz, M. M., Nabi, G. (2009). Generation of ROS and non-enzymatic antioxidants during abiotic stress in plants. Bot. Res. Int. 2, 11–20.
Alscher, R. G., Erturk, N., Heath, L. S. (2002). Role of superoxide dismutases (SODs) in controlling oxidative stress in plants. J. Exp. Bot. 53, 1331–1341. doi: 10.1093/jexbot/53.372.1331
Ara, H., Sinha, A. K. (2014). Conscientiousness of mitogen activated protein kinases in acquiring tolerance for abiotic stresses in plants. Proc. Ind. Natl. Sci. Acad. 80, 211–219. doi: 10.16943/ptinsa/2014/v80i2/1
Beillouin, D., Schauberger, B., Bastos, A., Ciais, P., Makowski, D. (2020). Impact of extreme weather conditions on European crop production in 2018. Philos. Trans. R Soc. Lond B Biol. Sci. 375, 20190510. doi: 10.1098/rstb.2019.0510
Bergmann, D. C., Lukowitz, W., Somerville, C. R. (2004). Stomatal development and pattern controlled by a MAPKK kinase. Science 304, 1494–1497. doi: 10.1126/science.1096014
Bourgine, B., Guihur, A. (2021). Heat shock signaling in land plants: From plasma membrane sensing to the transcription of small heat shock proteins. Front. Plant Sci. 12, 710801. doi: 10.3389/fpls.2021.710801
Caverzan, A., Casassola, A., Brammer, S. P. (2016). Reactive oxygen species and antioxidant enzymes involved in plant tolerance to stress. Abiotic biotic Stress plants-recent Adv. Future Perspect. 17, 463–480.
Chae, L., Pandey, G. P., Luan, S., Cheong, Y. H. (2010). Protein kinases and phosphatases for stress signal transduction in plants. Abiotic Stress adaptation plants: physiological Mol. genomic foundation p, 123–163.
Chardin, C., Schenk, S. T., Hirt, H., Colcombet, J., Krapp, A. (2017a). Review: Mitogen-Activated Protein Kinases in nutritional signaling in Arabidopsis. Plant Sci. 260, 101–108. doi: 10.1016/j.plantsci.2017.04.006
Chardin, C., Schenk, S. T., Hirt, H., Colcombet, J., Krapp, A. (2017b). Mitogen-activated protein kinases in nutritional signaling in Arabidopsis. Plant Sci. 260, 101–108. doi: 10.1016/j.plantsci.2017.04.006
Chen, X., Ding, Y., Yang, Y., Song, C., Wang, B., Yang, S., et al. (2021a). Protein kinases in plant responses to drought, salt, and cold stress. J. Integr. Plant Biol. 63, 53–78. doi: 10.1111/jipb.13061
Chen, X., Wang, W., Cai, P., Wang, Z., Li, T., Du, Y. (2021b). The role of the MAP kinase–kinase protein StMKK1 in potato immunity to different pathogens. Horticulture Res. 8, 117. doi: 10.1038/s41438-021-00556-5
Chen, Y., Chen, L., Sun, X., Kou, S., Liu, T., Dong, J., et al. (2022). The mitogen-activated protein kinase MKK2 positively regulates constitutive cold resistance in the potato. Environ. Exp. Bot. 194, 104702. doi: 10.1016/j.envexpbot.2021.104702
Chen, J., Wang, L., Yuan, M. (2021). Update on the roles of rice MAPK cascades. Int. J. Mol. Sci. 22, 1679. doi: 10.3390/ijms22041679
Choi, S. W., Lee, S. B., Na, Y. J., Jeung, S. G., Kim, S. Y. (2017). Arabidopsis MAP3K16 and other salt-inducible MAP3Ks regulate ABA response redundantly. Mol. Cells 40, 230–242. doi: 10.14348/molcells.2017.0002
Dalhaus, T., Schlenker, W., Blanke, M. M., Bravin, E., Finger, R. (2020). The effects of extreme weather on apple quality. Sci. Rep. 10, 7919. doi: 10.1038/s41598-020-64806-7
Danquah, A., Zelicourt, A. D., Colcombet, J., Hirt, H. (2014). The role of ABA and MAPK signaling pathways in plant abiotic stress responses. Biotechnol. Adv. 32, 40–52. doi: 10.1016/j.biotechadv.2013.09.006
Dat, J. F., Lopez-Delgado, H., Foyer, C. H., Scott, I. M. (1998). Parallel changes in H2O2 and catalase during thermotolerance induced by salicylic acid or heat acclimation in mustard seedlings. Plant Physiol. 116, 1351–1357. doi: 10.1104/pp.116.4.1351
Duan, P., Rao, Y., Zeng, D., Yang, Y., Xu, R., Zhang, B., et al. (2014). SMALL GRAIN 1, which encodes a mitogen-activated protein kinase 4, influences grain size in rice. Plant J. 77, 547–557. doi: 10.1111/tpj.12405
Friedrich, T., Oberkofler, V., Trindade, I., Altmann, S., Brzezinka, K., Lamke, J., et al. (2021). Heteromeric HSFA2/HSFA3 complexes drive transcriptional memory after heat stress in Arabidopsis. Nat. Commun. 12, 3426. doi: 10.1038/s41467-021-23786-6
Gouda, M. B., Zhang, C., Wang, J., Peng, S. (2020). ROS and MAPK cascades in the post-harvest senescence of horticultural products. J. Proteomics Bioinform. 13, 1–7.
Hansen, J., Sato, M., Ruedy, R. (2022). Global temperature in 2021 Vol. 1 (Diponível em, Washington D.C). Available at: http://www.columbia.edu/~jeh1/mailings/2022/Temperature2021.13January2022.pdf.
He, X., Wang, C., Wang, H., Li, L., Wang, C. (2020). The function of MAPK cascades in response to various stresses in horticultural plants. Front. Plant Sci. 11, 952. doi: 10.3389/fpls.2020.00952
Katlane, R., Kilani, B. E., Dhaoui, O., Kateb, F., Chehata, N. (2023). Monitoring of sea surface temperature, chlorophyll, and turbidity in Tunisian waters from 2005 to 2020 using MODIS imagery and the Google Earth Engine. Regional Stud. Mar. Sci. 66, 103143. doi: 10.1016/j.rsma.2023.103143
Khan, S., Jabeen, R., Deeba, F., Waheed, U. (2021). Heat shock proteins: classification, functions and expressions in plants during environmental stresses. J. Bioresource Manage. 8, 85–97. doi: 10.35691/JBM.1202.0183
Kong, X., Pan, J., Zhang, D., Jiang, S., Cai, G., Wang, L., et al. (2013). Identification of mitogen-activated protein kinase gene family and MKK-MAPK interaction network in maize. Biochem. Biophys. Res. Commun. 441, 964–969. doi: 10.1016/j.bbrc.2013.11.008
Kumar, K., Rao, K. P., Sharma, P., Sinha, A. K. (2008). Differential regulation of rice mitogen activated protein kinase (MKK) by abiotic stress. Plant Physiol. Biochem. 46, 891–897. doi: 10.1016/j.plaphy.2008.05.014
Kumar, R. R. (2012). Protection against heat stress in wheat involves change in cell membrane stability, antioxidant enzymes, osmolyte, H2O2 and transcript of heat shock protein. Int. J. Plant Physiol. Biochem. 4, 83–91.
Kumar, R. R., Arora, K., Goswami, S., Sakhare, A., Singh, B., Chinnusamy, V., et al. (2020). MAPK enzymes: a ROS activated signaling sensors involved in modulating heat stress response, tolerance and grain stability of wheat under heat stress. 3 Biotech. 10, 380. doi: 10.1007/s13205-020-02377-0
Kumar, K., Raina, S. K., Sultan, S. M. (2020). Arabidopsis MAPK signaling pathways and their cross talks in abiotic stress response. J. Plant Biochem. Biotechnol. 29, 700–714. doi: 10.1007/s13562-020-00596-3
Kumar, K., Sinha, A. K. (2013). Overexpression of constitutively active mitogen activated protein kinase 6 enhances tolerance to salt stress in rice. Rice (N Y) 6, 25. doi: 10.1186/1939-8433-6-25
Lafleur, E., Kapfer, C., Joly, V., Liu, Y., Tebbji, F., Daigle, C., et al. (2015). The FRK1 mitogen-activated protein kinase (MAPKKK) from Solanum chacoense is involved in embryo sac and pollen development. J. Exp. Bot. 66, 1833–1843. doi: 10.1093/jxb/eru524
Lee, Y., Kim, Y. J., Kim, M. H., Kwak, J. M. (2016). MAPK cascades in guard cell signal transduction. Front. Plant Sci. 7, 80. doi: 10.3389/fpls.2016.00080
Lee, H., Lee, H., Jun, Y. S., Cha, O. K., Sheen, J. (2019). Mitogen-activated protein kinases MPK3 and MPK6 are required for stem cell maintenance in the Arabidopsis shoot apical meristem. Plant Cell Rep. 38, 311–319. doi: 10.1007/s00299-018-2367-5
Lee, Y.-H., Sang, W. G., Baek, J. K., Kim, J. H., Shin, P., Seo, M. C., et al. (2020). The effect of concurrent elevation in CO2 and temperature on the growth, photosynthesis, and yield of potato crops. PloS One 15, e0241081. doi: 10.1371/journal.pone.0241081
Li, G., Cao, C., Yang, H., Wang, J., Wei, W., Zhu, D., et al. (2020). Molecular cloning and potential role of DiSOC1s in flowering regulation in Davidia involucrata Baill. Plant Physiol. Biochem. 157, 453–459. doi: 10.1016/j.plaphy.2020.11.003
Li, C., Zhu, J., Cheng, Y., Hou, J., Sun, L., Ge, Y. (2022). Acibenzolar-S-methyl activates mitogen-activated protein kinase cascade to mediate chlorophyll and carotenoid metabolisms in the exocarp of Docteur Jules Guyot pears. J. Sci. Food Agric. 102, 4435–4445. doi: 10.1002/jsfa.11797
Liu, Z., Shi, L., Liu, Y., Tang, Q., Shen, L., Yang, S., et al. (2015). Genome-wide identification and transcriptional expression analysis of mitogen-activated protein kinase and mitogen-activated protein kinase genes in Capsicum annuum. Front. Plant Sci. 6, 780. doi: 10.3389/fpls.2015.00780
Liu, J., Wang, X., Yang, L., Nan, W., Ruan, M., Bi, Y. (2020). Involvement of active MKK9-MAPK3/MAPK6 in increasing respiration in salt-treated Arabidopsis callus. Protoplasma 257, 965–977. doi: 10.1007/s00709-020-01483-3
Liu, J., Wang, Y.-S. (2020). Proline metabolism and molecular cloning of AmP5CS in the mangrove Avicennia marina under heat stress. Ecotoxicology 29, 698–706. doi: 10.1007/s10646-020-02198-0
Lu, H., Klocko, A. M., Brunner, A. M., Ma, C., Magnuson, A. C., Howe, G. T., et al. (2019). RNA interference suppression of AGAMOUS and SEEDSTICK alters floral organ identity and impairs floral organ determinacy, ovule differentiation, and seed-hair development in Populus. New Phytol. 222, 923–937. doi: 10.1111/nph.15648
Luo, Y., et al. (2024). StMAPKK5 positively regulates response to drought and salt stress in potato. Int. J. Mol. Sci. 25, 3662. doi: 10.3390/ijms25073662
MAPK group. (2002). Mitogen-activated protein kinase cascades in plants: a new nomenclature. Trends Plant Sci. 7, 301–308. doi: 10.1016/S1360-1385(02)02302-6
Mishra, P., Sharma, P. (2019). Superoxide Dismutases (SODs) and their role in regulating abiotic stress induced oxidative stress in plants. Reactive oxygen nitrogen sulfur species plants: production metabolism Signaling defense Mech. p, 53–88. doi: 10.1002/9781119468677.ch3
Nicholson, C. C., Egan, P. A. (2020). Natural hazard threats to pollinators and pollination. Glob Chang Biol. 26, 380–391. doi: 10.1111/gcb.14840
Nicole, M.-C., Hamel, L. P., Morency, M. J., Beaudoin, N., Ellis, B. E., Seguin, A. (2006). MAP-ping genomic organization and organ-specific expression profiles of poplar MAP kinases and MAP kinase kinases. BMC Genomics 7, 223. doi: 10.1186/1471-2164-7-223
Posch, B. C., Kariyawasam, B. C., Bramley, H., Coast, O., Richards, R. A., Reynolds, M. P., et al. (2019). Exploring high temperature responses of photosynthesis and respiration to improve heat tolerance in wheat. J. Exp. Bot. 70, 5051–5069. doi: 10.1093/jxb/erz257
Rehman, S. U., Bilal, M., Rana, R. M., Tahir, M. N., Shah, M. K., Ayalew, H., et al. (2016). Cell membrane stability and chlorophyll content variation in wheat (Triticum aestivum) genotypes under conditions of heat and drought. Crop Pasture Sci. 67, 712–718. doi: 10.1071/CP15385
Rodriguez, M. C., Petersen, M., Mundy, J. (2010). Mitogen-activated protein kinase signaling in plants. Annu. Rev. Plant Biol. 61, 621–649. doi: 10.1146/annurev-arplant-042809-112252
Röhm, S., Krämer, A., Knapp, S. (2021). Function, structure and topology of protein kinases (Springer, Cham).
Rykaczewska, K. (2015). The effect of high temperature occurring in subsequent stages of plant development on potato yield and tuber physiological defects. Am. J. Potato Res. 92, 339–349. doi: 10.1007/s12230-015-9436-x
Sachdev, S., Ansari, S. A., Ansari, M. I., Fujita, M., Hasanuzzaman, M. (2021). Abiotic stress and reactive oxygen species: Generation, signaling, and defense mechanisms. Antioxidants 10, 277. doi: 10.3390/antiox10020277
Singh, B., Kukreja, S., Goutam, U. (2020). Impact of heat stress on potato (Solanum tuberosum L.): Present scenario and future opportunities. J. Hortic. Sci. Biotechnol. 95, 407–424. doi: 10.1080/14620316.2019.1700173
Sparkes, I. A., Runions, J., Kearns, A., Hawes, C. (2006). Rapid, transient expression of fluorescent fusion proteins in tobacco plants and generation of stably transformed plants. Nat. Protoc. 1, 2019–2025. doi: 10.1038/nprot.2006.286
Taj, G., Agarwal, P., Grant, M., Kumar, A. (2010). MAPK machinery in plants: recognition and response to different stresses through multiple signal transduction pathways. Plant Signal Behav. 5, 1370–1378. doi: 10.4161/psb.5.11.13020
Veal, E., Day, A. (2011). Hydrogen peroxide as a signaling molecule. Antioxidants Redox Signaling 15, 147–151. doi: 10.1089/ars.2011.3968
Walsh, J. E., Ballinger, T. H., Euskirchen, E. S., Hanna, E. (2020). Extreme weather and climate events in northern areas: A review. Earth-Science Rev. 209, 103324. doi: 10.1016/j.earscirev.2020.103324
Wang, J., Pan, C., Wang, Y., Ye, L., Wu, J., Chen, L., et al. (2015). Genome-wide identification of MAPK, MAPKK, and MAPKKK gene families and transcriptional profiling analysis during development and stress response in cucumber. BMC Genomics 16, 386. doi: 10.1186/s12864-015-1621-2
Wang, C., Lu, W., He, X., Wang, F., Zhou, Y., Guo, X., et al. (2016). The cotton mitogen-activated protein kinase 3 functions in drought tolerance by regulating stomatal responses and root growth. Plant Cell Physiol. 57, 1629–1642. doi: 10.1093/pcp/pcw090
Wang, J., Sun, Z., Chen, C., Xu, M. (2022). The MKK2a gene involved in the MAPK signaling cascades enhances populus salt tolerance. Int. J. Mol. Sci. 23, 10185. doi: 10.3390/ijms231710185
Wen, Z., Li, M., Meng, J., Miao, R., Liu, X, Fan, D., et al. (2023). Genome-wide identification of the MAPK and MAPKK gene families in response to cold stress in prunus mume. Int. J. Mol. Sci. 24, 8829. doi: 10.3390/ijms24108829
Wi, S. H., Lee, H. J., An, S., Kim, S. K. (2020). Evaluating growth and photosynthesis of Kimchi cabbage according to extreme weather conditions. Agronomy 10, 1846. doi: 10.3390/agronomy10121846
Wu, J., Wang, J., Pan, C., Guan, X., Wang, Y., Liu, S., et al. (2014). Genome-wide identification of MAPKK and MAPKKK gene families in tomato and transcriptional profiling analysis during development and stress response. PloS One 9, e103032. doi: 10.1371/journal.pone.0103032
Yang, Z., Zhu, Z., Guo, Y., Lan, J., Zhang, J., Chen, S., et al. (2024). OsMKK1 is a novel element that positively regulates the Xa21-mediated resistance response to Xanthomonas oryzae pv. oryzae in rice. Plant Cell Rep. 43, 31. doi: 10.1007/s00299-023-03085-8
Yin, Z., Zhu, W., Zhang, X., Chen, X., Wang, W., Lin, H., et al. (2021). Molecular characterization, expression and interaction of MAPK, MAPKK and MAPKKK genes in upland cotton. Genomics 113, 1071–1086. doi: 10.1016/j.ygeno.2020.11.004
Yu, S., Zhang, L., Chen, C., Li, J., Ye, S., Liu, G., et al. (2014). Isolation and characterization of BnMKK1 responsive to multiple stresses and affecting plant architecture in tobacco. Acta physiologiae plantarum 36, 1313–1324. doi: 10.1007/s11738-014-1510-3
Zahra, N., Hafeez, M. B., Ghaffar, A., Kausar, A., Zeidi, M. A., Siddiaue, K. H., et al. (2023). Plant photosynthesis under heat stress: Effects and management. Environ. Exp. Bot. 206, 105178. doi: 10.1016/j.envexpbot.2022.105178
Zhang, M., Zhang, S. (2022). Mitogen-activated protein kinase cascades in plant signaling. J. Integr. Plant Biol. 64, 301–341. doi: 10.1111/jipb.13215
Zhu, X., Hong, X., Liu, X., Li, S., Yang, J., Wang, F., et al. (2021). Calcium-dependent protein kinase 32 gene maintains photosynthesis and tolerance of potato in response to salt stress. Scientia Hortic. 285, 110179. doi: 10.1016/j.scienta.2021.110179
Zhu, X., Duan, H., Zhang, G., Jin, H., Xu, C., Chen, S., et al. (2023). StMAPK1 functions as a thermos-tolerant gene in regulating heat stress tolerance in potato (Solanum tuberosum). Front. Plant Sci. 14, 1218962. doi: 10.3389/fpls.2023.1218962
Keywords: potato, heat stress, StMAPKK5, transpiration, photosynthesis
Citation: Zhu X, Li W, Zhang N, Jin H, Duan H, Chen Z, Chen S, Wang Q, Tang J, Zhou J, Zhang Y and Si H (2024) StMAPKK5 responds to heat stress by regulating potato growth, photosynthesis, and antioxidant defenses. Front. Plant Sci. 15:1392425. doi: 10.3389/fpls.2024.1392425
Received: 27 February 2024; Accepted: 29 April 2024;
Published: 16 May 2024.
Edited by:
Yi Wang, Chinese Academy of Sciences (CAS), ChinaReviewed by:
Jing Li, Hainan University, ChinaCopyright © 2024 Zhu, Li, Zhang, Jin, Duan, Chen, Chen, Wang, Tang, Zhou, Zhang and Si. This is an open-access article distributed under the terms of the Creative Commons Attribution License (CC BY). The use, distribution or reproduction in other forums is permitted, provided the original author(s) and the copyright owner(s) are credited and that the original publication in this journal is cited, in accordance with accepted academic practice. No use, distribution or reproduction is permitted which does not comply with these terms.
*Correspondence: Jiannan Zhou, emhvdWppYW5uYW5AYWxpeXVuLmNvbQ==; Yu Zhang, emhhbmd5dUBjYXRhcy5jbg==; Huaijun Si, aGpzaUBnc2F1LmVkdS5jbg==
†These authors have contributed equally to this work
Disclaimer: All claims expressed in this article are solely those of the authors and do not necessarily represent those of their affiliated organizations, or those of the publisher, the editors and the reviewers. Any product that may be evaluated in this article or claim that may be made by its manufacturer is not guaranteed or endorsed by the publisher.
Research integrity at Frontiers
Learn more about the work of our research integrity team to safeguard the quality of each article we publish.