- 1Department of Plant Pathology and Microbiology, National Taiwan University, Taipei, Taiwan
- 2Department of Integrative Food, Bioscience and Biotechnology, Chonnam National University, Gwangju, Republic of Korea
- 3Department of Plant, Soil and Microbial Sciences, Michigan State University, East Lansing, MI, United States
- 4Institute of Plant and Microbial Biology, Academia Sinica, Taipei, Taiwan
- 5Master Program of Plant Medicine, National Taiwan University, Taipei, Taiwan
- 6Center of Biotechnology, National Taiwan University, Taipei, Taiwan
Rhizobacteria are capable of inducing defense responses via the expression of pathogenesis-related proteins (PR-proteins) such as chitinases, and many studies have validated the functions of plant chitinases in defense responses. Soybean (Glycine max) is an economically important crop worldwide, but the functional validation of soybean chitinase in defense responses remains limited. In this study, genome-wide characterization of soybean chitinases was conducted, and the defense contribution of three chitinases (GmChi01, GmChi02, or GmChi16) was validated in Arabidopsis transgenic lines against the soil-borne pathogen Fusarium oxysporum. Compared to the Arabidopsis Col-0 and empty vector controls, the transgenic lines with GmChi02 or GmChi16 exhibited fewer chlorosis symptoms and wilting. While GmChi02 and GmChi16 enhanced defense to F. oxysporum, GmChi02 was the only one significantly induced by Burkholderia ambifaria. The observation indicated that plant chitinases may be induced by different rhizobacteria for defense responses. The survey of 37 soybean chitinase gene expressions in response to six rhizobacteria observed diverse inducibility, where only 10 genes were significantly upregulated by at least one rhizobacterium and 9 genes did not respond to any of the rhizobacteria. Motif analysis on soybean promoters further identified not only consensus but also rhizobacterium-specific transcription factor-binding sites for the inducible chitinase genes. Collectively, these results confirmed the involvement of GmChi02 and GmChi16 in defense enhancement and highlighted the diverse inducibility of 37 soybean chitinases encountering F. oxysporum and six rhizobacteria.
Introduction
The composition of soil microbial communities varies depending on numerous factors, including the plant species or variety present. Plants release root exudates, which can attract beneficial microbes moving from bulk soil into the rhizosphere (Lugtenberg and Kamilova, 2009; Martinez-Medina et al., 2016; Lundberg and Teixeira, 2018). Many rhizobacteria, such as plant growth-promoting rhizobacteria (PGPR), can stimulate plant growth, and these rhizobacteria may enhance plant health by interacting directly or indirectly with soil-borne pathogens. The interactions can be generalized into three types: antagonism (Elnahal et al., 2022), parasitism (Tian et al., 2007), and induced systemic resistance (ISR) (Zhu et al., 2022). In terms of antagonism, rhizobacteria may secrete antibiotics or siderophores to antagonize or compete for nutrients with pathogens. Rhizobacteria such as Bacillus amyloliquefaciens and Streptomyces sp. exhibit these capabilities, and some strains have been developed into commercial products (Boubekri et al., 2022; Luo et al., 2022). Regarding parasitism, rhizobacteria may secrete enzymes such as chitinases that degrade fungal cell walls. Rhizobacteria such as Burkholderia ambifaria and Enterobacter sp. also possess predatory behaviors on fungi, thereby reducing fungal pathogens in the rhizosphere (Mousa et al., 2016; Stopnisek et al., 2016; Chang et al., 2021). Furthermore, due to the presence of microbe-associated molecular patterns (MAMPs) in most rhizobacteria, such as Pseudomonas fluorescens (Orozco-Mosqueda et al., 2023), pattern-triggered immunity (PTI) and ISR can be activated in the absence of soil-borne pathogens, leading to a phenomenon known as defense priming (Mauch-Mani et al., 2017; Salwan et al., 2023). Accordingly, the diverse mechanisms and interactions between plants and the rhizosphere microbes together contribute to the overall plant health and support agricultural sustainability.
The expression of pathogenesis-related protein (PR-protein) genes is one of the important responses in defense responses to combat pathogens, and many PR-protein genes have been confirmed to enhance defense responses in various mechanisms (Huang et al., 2016; Su et al., 2016; Luo et al., 2023; Wang Y et al., 2021). Among 17 families of PR-proteins (van Loon et al., 2006), the PR-3, PR-4, PR-8, and PR-11 proteins all encode plant chitinases, which contain the glycosyl hydrolases (GH) domain capable of breaking the β-1,4-glycosidic linkages of chitin, leading to disruption of fungal cell walls (Vaghela et al., 2022). These chitinases can be classified into GH18 and GH19 based on the similarity of their catalytic domains (CatD) (Kawase et al., 2004; Funkhouser and Aronson, 2007). The GH18 chitinases exhibit a barrel-like structure consisting of eight α-helices and eight β-sheets (Yang et al., 2010), and the GH19 chitinases possess a lysozyme-like domain composed of several α-helices (Kezuka et al., 2006). Plant chitinases have been further grouped into five classes (classes I–V) according to characteristics such as N-terminal sequences. While classes III and V belong to GH18, classes I, II, and IV belong to GH19 (Grover, 2012). Class I chitinases possess a chitin-binding domain (CBD) in their N-terminal region (Tang et al., 2004), and the C-terminal region of class I chitinases contains seven extended amino acids that facilitate their targeting to vacuolar and intracellular transport (Vaghela et al., 2022). Class II chitinases lack CBD in the N-terminal, and they are typically acidic proteins induced by pathogen infection and secreted to the extracellular space (Patil et al., 2000). Class III chitinases exhibit lysozyme activity without sequence similarity to classes I and II chitinases (Xu et al., 2016; Ma et al., 2017). Class IV chitinases possess both CBD and CatD similar to class I; however, due to deletions in the CBD and CatD domains, class IV chitinases are usually smaller than class I (Xu et al., 2016). Class V chitinases have a C-terminal extension for vacuolar targeting and may contain CBD (Taira et al., 2009; Grover, 2012). Accordingly, plant chitinases have evolved with diverse domains and variations.
The importance of plant chitinases in defense responses has been studied in several cases. For example, it was shown that 11 chitinase genes of rice were upregulated by R. solani infection. These rice chitinases were secreted into extracellular spaces, resulting in the degradation of fungal cell walls (Richa et al., 2016). Overexpression of the rice chitinase gene LOC_Os03g30470 enhanced defense against Botrytis cinerea and Diplocarpon rosae (Marchant et al., 1998; Núñez de Cáceres González et al., 2015). Overexpression of another rice chitinase gene, LOC_Os05g33130, increased defense responses to many diseases, and many studies have also demonstrated the defense contribution of various plant chitinases in different plant systems (Table 1). Other than the overexpression approach, gene silencing of the chili pepper chitinase gene CaChiIII7 resulted in larger foliar symptoms, less ROS accumulation in leaves, and reduced expression of defense-related genes (Ali et al., 2020). Collectively, the importance of plant chitinases in defense responses has been confirmed through overexpression and silencing approaches in different plant systems.
The advancement of high-throughput sequencing technology in the past decade has completed about 800 plant genomes (Marks et al., 2021; Sun et al., 2022), which speeded up the genome-wide characterization of plant chitinases in apple, Arabidopsis thaliana, Brassica rapa, cotton, cucumber, mulberry, rice, and tea (Grover, 2012; Xu et al., 2016; Chen et al., 2018; Bartholomew et al., 2019; Mir et al., 2020; Bordoloi et al., 2021; Haxim et al., 2022; Xin et al., 2022). For example, seven of the 24 chitinase genes discovered in the A. thaliana genome, such as AT1G19810, AT2G43570, AT2G43580, AT2G4359, and AT3G47540, were found to be upregulated upon infection by B. cinerea and Pseudomonas syringae. In the case of rice, 49 chitinase genes were characterized, and transcriptome analysis identified Os01g18400, Os01g19750, Os10g28050, and Os11g47510 being upregulated in response to Magnaporthe grisea infection (Grover, 2012). In the genomes of B. rapa and tea, 33 and 49 chitinase genes were discovered, respectively. Upregulation of several chitinase genes was also found during infections of the clubroot pathogen and three tea pathogens (Chen et al., 2018; Bordoloi et al., 2021). These findings demonstrate the power of high-throughput sequencing in genome-wide identification of plant chitinases, which also enable investigations for their expressions to different microbes.
Soybean (Glycine max) is one of the most important crops worldwide, and soybean diseases have been one of the major yield-limiting stresses for decades (Bandara et al., 2020; Bradley et al., 2021; Allen et al., 2022). However, there were limited studies on the functional validation of soybean chitinases (Lv et al., 2022) and their expressions induced by rhizobacteria. Therefore, this study performed a genome- and transcriptome-wide identification of soybean chitinases induced by B. ambifaria and validated the potential of soybean chitinases in defense against Fusarium oxysporum. In addition, transcriptomic analyses were conducted to profile soybean chitinases induced by six rhizobacteria, including B. amyloliquefaciens, Bradyrhizobium japonicum, B. ambifaria, Lysobacter enzymogenes, P. fluorescens, and Rhizobium rhizogenes (previously known as Agrobacterium rhizogenes). The study not only completed a comprehensive identification and validation of soybean chitinases induced by rhizobacteria but also highlighted the regulatory consensus and diversity among soybean chitinases to different rhizobacteria.
Results
Genome-wide identification of soybean chitinase genes
A search of the GH18 (PF00704) and GH19 (PF00182) domains identified 37 chitinase genes in the soybean genome, and all of them were predicted with an N-terminal signal peptide. Following the classification system of Arabidopsis chitinase genes, the soybean chitinase genes can be further divided into five genes in class I, four genes in class II, nineteen genes in class III, three genes in class IV, and six genes in class V (Figure 1). Using MEME analyses for characterizing motifs, the conserved GH18 motifs were identified in classes III and V, and the GH19 motifs were identified in classes I, II, and IV. As reported in the previous literature (Ma et al., 2017), class III chitinases harbor both GH18 motifs and GH19 lysozyme domain (motifs 3 and 8) as a classification signature. These results together confirmed that the HMMER method in genome-wide identification of soybean chitinase genes is robust and precise.
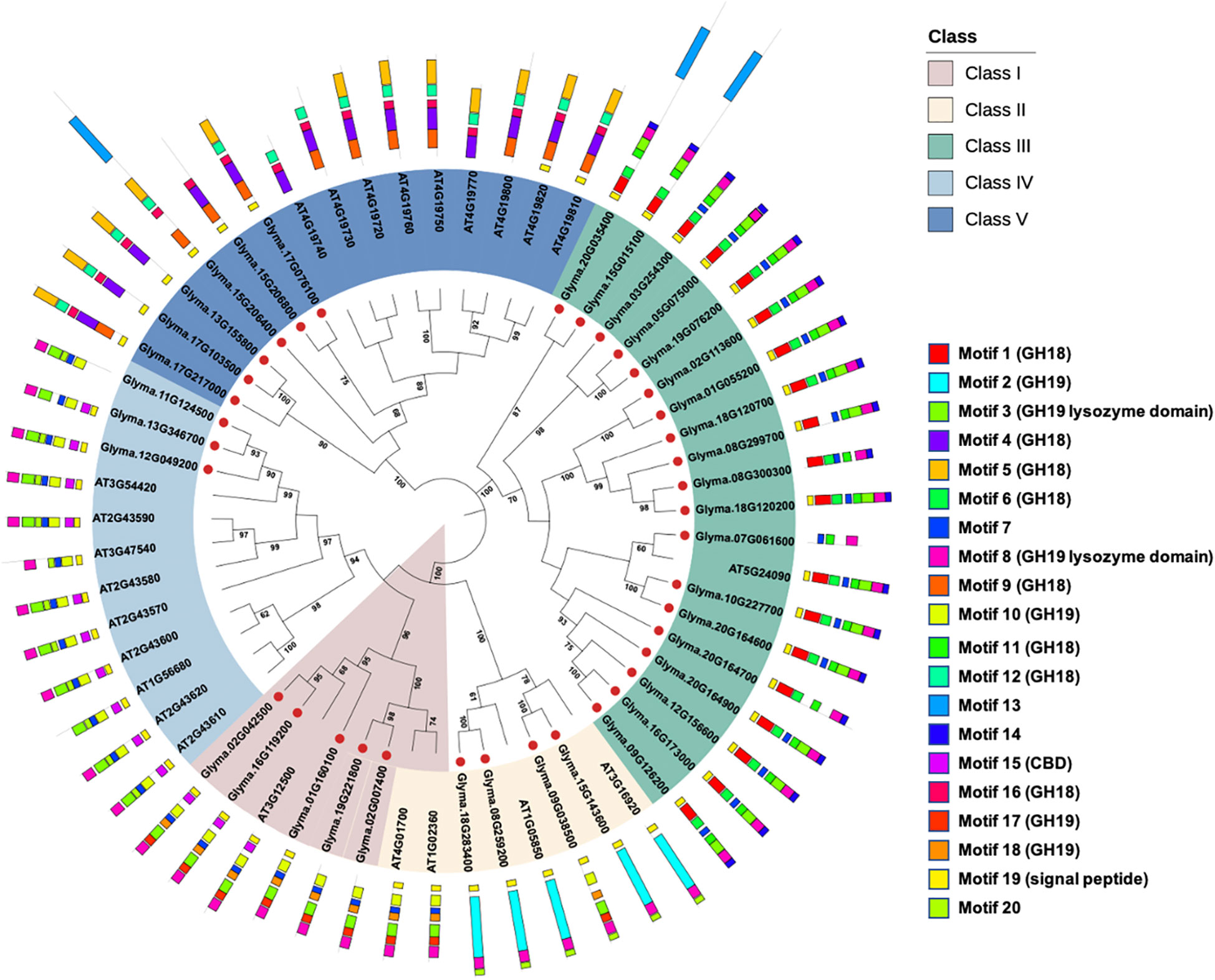
Figure 1 Genome-wide identification of soybean chitinase genes. The conserved HMM domains of the glycosyl hydrolase (GH) GH18 (PF00704) and GH19 (PF00182) chitinases were used as templates to identify soybean chitinases in the soybean reference genome. The 37 putative chitinases were further grouped using the neighbor-joining method into five classes according to the classification system built on Arabidopsis thaliana chitinases. There were 5, 4, 19, 3, and 6 chitinase genes in classes I, II, III, IV, and V, respectively, and there were 20 conserved motifs detected in the soybean chitinases. Bootstrap values above 60 were shown in the nodes.
One controversial classification appeared for Glyma.01G160100, Glyma.02G007400, and Glyma.19G221800, which should be classified as class II for the absence of CBD (motif 15) if they followed the conventional classification for Arabidopsis chitinase genes (Patil et al., 2000). Although the presence or absence of CBD has been used to identify class I or IV chitinases (Patil et al., 2000; Grover, 2012; Xu et al., 2016), the MEME analyses found exceptions not only in soybean but also in Arabidopsis. For example, AT3G47540 was recognized as class IV chitinase because CBD was absent. In addition, although AT1G02360 and AT4G01700 were grouped as class II chitinases based on the absence of CBD, both phylogenetic and MEME analyses suggested that their sequences and motif structures were closer to the class I chitinases. Therefore, this study suggests a phylogeny-based classification for soybean chitinase genes (Figure 1), where classes II, III, and V chitinase genes do not contain CBD motif, while classes I and IV chitinase genes may contain CBD motif. According to such criteria, Glyma.01G160100, Glyma.02G007400, and Glyma.19G221800 were classified as class I chitinases.
Gene expression of soybean chitinases in a tritrophic RNA-Seq experiment
Among the soybean chitinase genes, 12 genes exhibited upregulation in response to inoculation with F. oxysporum (Figure 2A). The top 5 upregulated genes included Glyma.13G346700, Glyma.12G156600, Glyma.11G124500, Glyma.02G007400, and Glyma.02G024500, which displayed a log2 fold change of 7.27, 7.23, 6.44, 5.89, and 4.97, respectively. Meanwhile, three among these five genes (Glyma.13G346700, Glyma.11G124500, and Glyma.02G024500) were also upregulated by the inoculation of rhizobacterium B. ambifaria in the absence of F. oxysporum (Table 2). In addition, Glyma.16G173000 and Glyma.09G126200 were also induced by B. ambifaria, but the upregulation of these two genes by F. oxysporum was not as high as the others. These results highlighted that these five soybean chitinase genes (Glyma.02G024500, Glyma.09G126200, Glyma.11G124500, Glyma.13G346700, and Glyma.16G173000) participated in the defense responses induced by B. ambifaria, and three of the five genes were listed in the top 5 important chitinase genes in the defense responses to F. oxysporum infection (Figure 2B). Indeed, upon the co-inoculation of F. oxysporum and B. ambifaria, for which the biomass of F. oxysporum was reduced by the antagonism of B. ambifaria (Chang et al., 2021), the upregulation of Glyma.13G346700, Glyma.11G124500, and Glyma.02G024500 was about 20% to 33% reduced compared to inoculation with F. oxysporum alone (Table 2). Collectively, Glyma.13G346700, Glyma.11G124500, and Glyma.02G024500 became the research focus not only for their inducibility but also for their expression trends reflecting the biotic stress created by the inoculation of F. oxysporum.
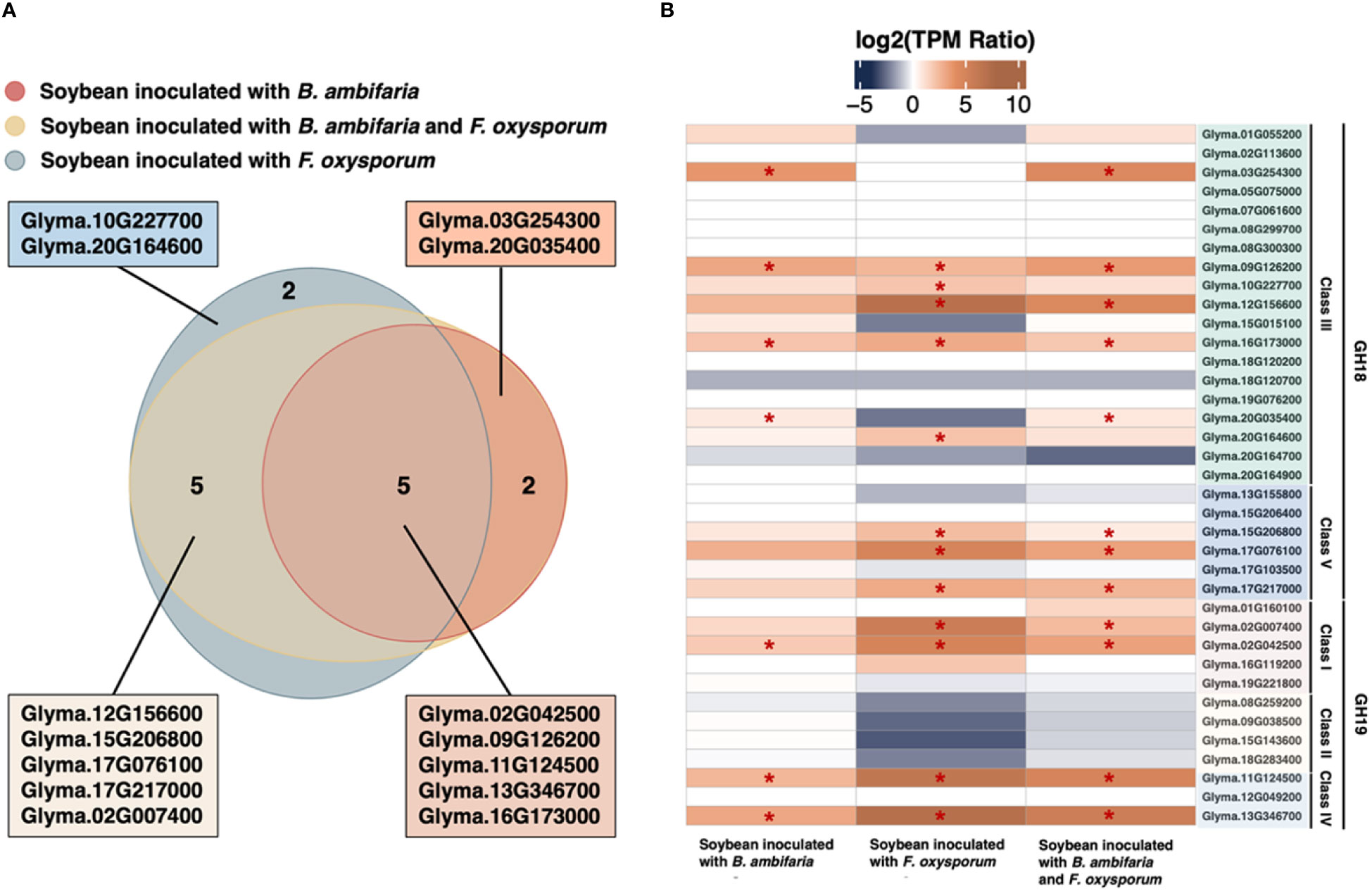
Figure 2 Soybean chitinase genes in response to the inoculation of Burkholderia ambifaria, Fusarium oxysporum, and co-inoculation. (A) Venn diagram suggests 12 chitinases being upregulated in response to F. oxysporum inoculation, while 7 chitinase genes were significantly induced by B. ambifaria in the absence of F. oxysporum. There were 5 consensus responding to the inoculation of B. ambifaria, F. oxysporum, and co-inoculation. (B) Gene expression of the 37 soybean chitinases, and the asterisk indicated the statistical significance of differential expression at a q-value of 0.05.
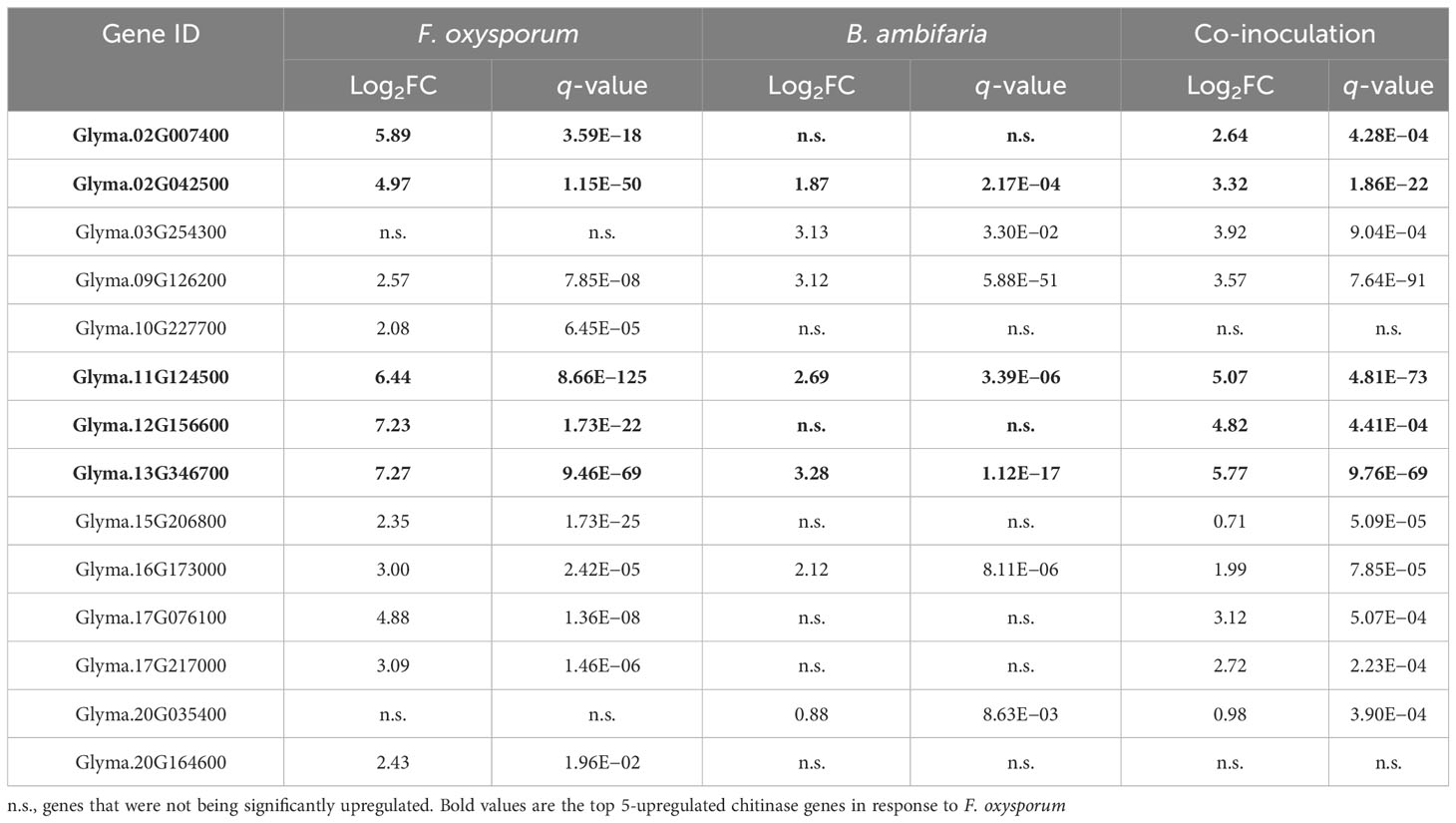
Table 2 Soybean chitinase genes induced by the inoculation of Fusarium oxysporum, Burkholderia ambifaria, and co-inoculation.
Phylogenetic analysis for soybean chitinases in defense responses
In order to assess the potentials of Glyma.13G346700, Glyma.11G124500, and Glyma.02G024500 in defense responses, a phylogenetic analysis for the 37 soybean chitinase genes was performed together with functionally validated plant chitinases from the literature (Table 1). For GH 18 chitinases, there were only three functionally validated plant chitinases, including cucumber, Chinese wild strawberry, and rice that can enhance defense responses to B. cinerea, Colletotrichum higginsianum, and R. solani, respectively (Kishimoto et al., 2004; Richa et al., 2017; Wen et al., 2020) (Figure 3A). There were more studies that confirmed the function of GH19 plant chitinases, and 27 functionally validated plant chitinases from apple, barley, cocoa, common bean, cucumber, maize, pepper, rice, and wheat were included in the phylogenetic analysis together with 12 GH19 soybean chitinases. These 12 soybean genes can be categorized into three groups (Figure 3B). The first group contains three soybean chitinase genes (Glyma.11G124500, Glyma.12G049200, and Glyma.13G346700), and the closest ortholog gene is the pepper CaChitIV, which enhances defense responses to Arabidopsis downy mildew (Kim et al., 2015). The second group includes four soybean chitinase genes, but only a strawberry gene, FvChi-14, which enhances Arabidopsis defense responses to C. higginsianum, is phylogenetically neighboring to these four genes (He et al., 2023). The last group with Glyma.01G160100, Glyma.02G024500, and Glyma.16G119200 clustered with apple, barley, bitter melon, cucumber, wild tomato, and zoysiagrass that were previously shown to contribute to the defense responses against multiple fungal pathogens, including many soil-borne fungi such as F. oxysporum, R. solani, and Verticillium dahliae (Table 1). In addition, two chitinase genes (Glyma.02G007400 and Glyma19G221800) were in the same clade but located distantly from the three soybean chitinase genes abovementioned. Among these genes, Glyma.02G024500 is the one that responded to the inoculation of F. oxysporum and B. ambifaria. On the other hand, the phylogenetically closed Glyma.01G160100 and Glyma.16G119200 did not seem to participate in the defense responses at least to F. oxysporum, nor be induced by B. ambifaria. The observation raised a question whether Glyma.01G160100, Glyma.02G024500, and Glyma.16G119200 (hereafter referred to a GmChi01, GmChi02, and GmChi16) all contain antifungal capability or if only Glyma.02G024500 remains antifungal. Accordingly, GmChi01, GmChi02, and GmChi16 were selected for functional validation.
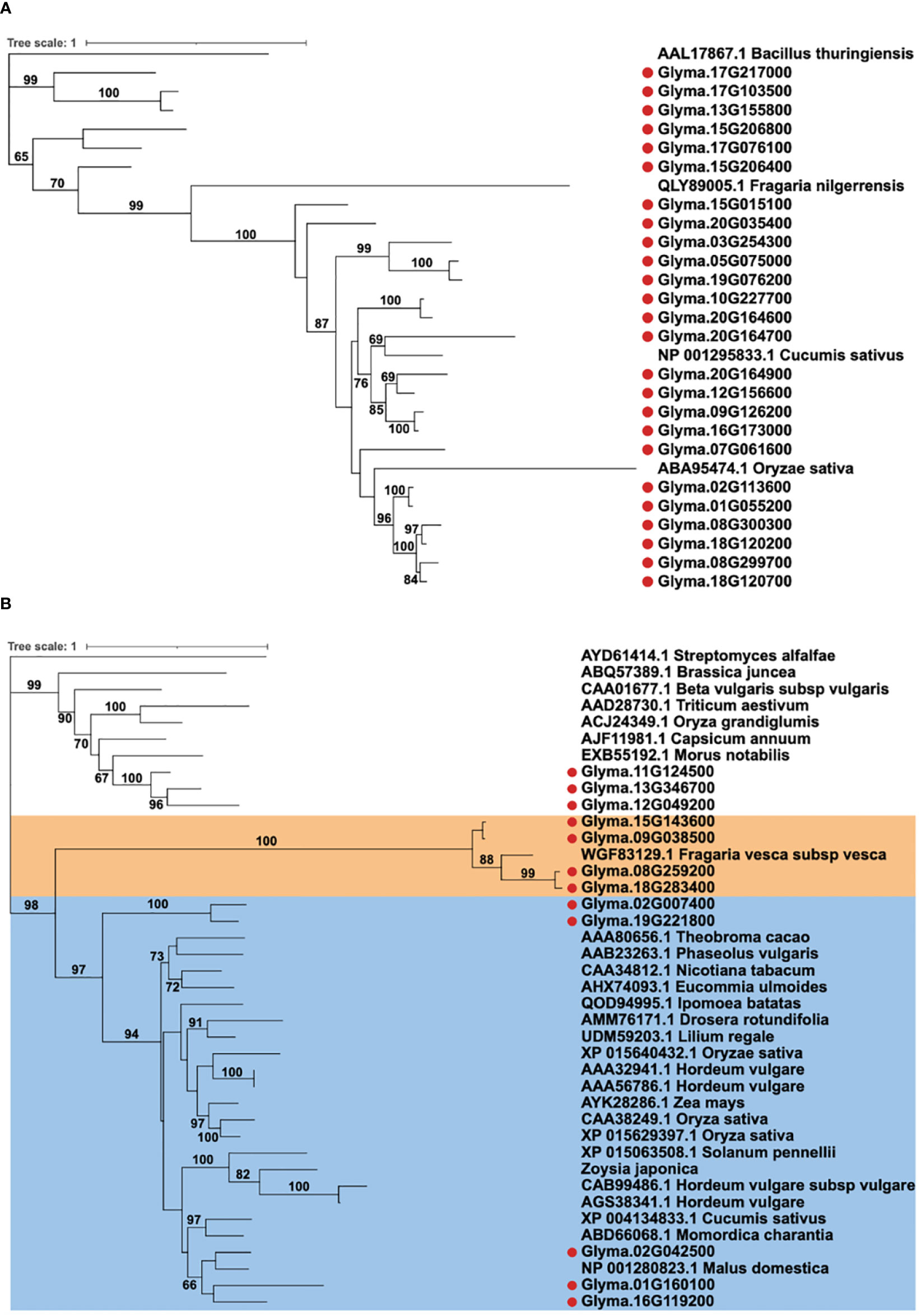
Figure 3 Phylogenetic analysis of soybean chitinase genes with functionally validated plant chitinases. (A) Soybean GH18 chitinases were analyzed with 3 functionally validated chitinases from Chinese wild strawberries, cucumber, and rice. (B) Soybean GH19 chitinases were analyzed with 27 functionally validated chitinases from multiple plant species. Soybean chitinase GmChi02, GmChi01, and GmChi16 (Glyma.02G042500, Glyma.01G160100, and Glyma.16G119200) are phylogenetically close to each other and grouped with most functionally validated plant chitinases. Unlike GmChi02, GmChi01 and GmChi16 were not upregulated by F. oxysporum nor induced by B. ambifaria. Bootstrap values above 60 in the Maximum likelihood tree are shown.
Functional validation for soybean chitinases in defense responses
The homozygous transgenic Arabidopsis lines overexpressing empty vector (EV), GmChi01, GmChi02, or GmChi16, respectively, were validated for the expression of soybean chitinases (Supplementary Figure S1) before using their T4 generation for experiments. For seedling root length, rosette leaves, and plant height, the transgenic lines (EV_6-8, GmChi01_6-8, GmChi01_7-1, GmChi02_3-7, GmChi02_6-3, GmChi16_4-3, and GmChi16_7-1) exhibited no difference in phenotypes (Figures 4A–C). However, the area under the disease progress curve (AUDPC) of these lines inoculated with F. oxysporum exhibited significant differences. The AUDPC of transgenic Arabidopsis overexpressing GmChi02 and GmChi16 were significantly lower than the controls (Figures 4D, E). Although transgenic Arabidopsis overexpressing GmChi01 exhibited reduced AUDPC in appearance, statistical analysis did not detect a significant difference. Meanwhile, identical results can be observed in soil inoculation with the conidial suspension of F. oxysporum, where transgenic Arabidopsis overexpressing GmChi02 and GmChi16 exhibited less seedling wilt. Transgenic Arabidopsis overexpressing GmChi01 again showed better survival in appearance, but the statistical analysis did not detect any significance (Figures 4F, G). These results indicate that GmChi02 and GmChi16 were indeed phylogenetically and functionally close, and these two soybean chitinases enhanced defense responses to F. oxysporum infection. However, the gene regulation of GmChi01, GmChi02, and GmChi16 appeared to be diversified, and only GmChi02 exhibited inducibility in response to B. ambifaria.
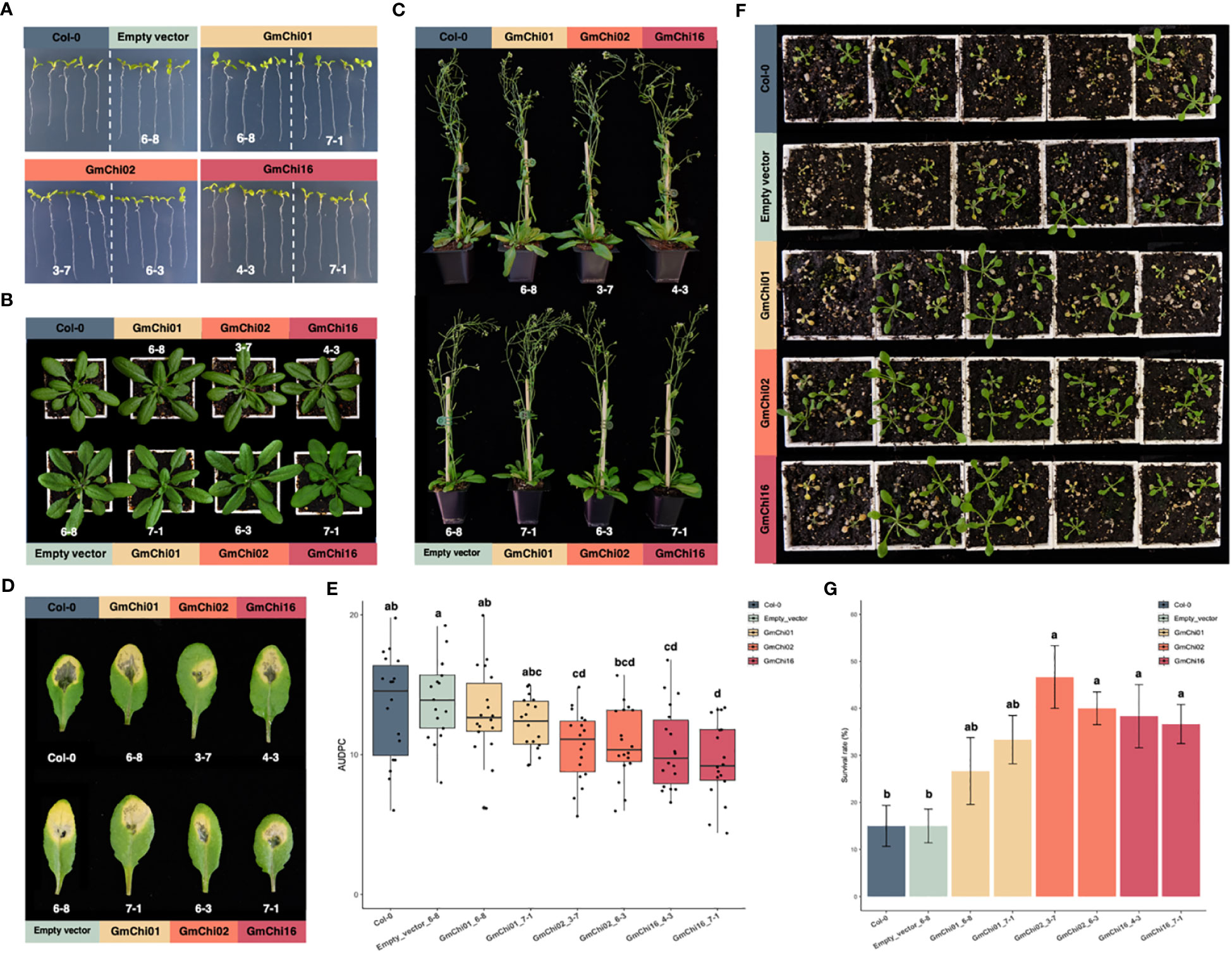
Figure 4 Phenotypes of Arabidopsis transgenic lines with GmChi01, GmChi02, or GmChi16. (A) Seedling root length, (B) Rosette leaves, and (C) Plant height of Arabidopsis transgenic lines exhibited no significant difference to the wild type Col-0 or Arabidopsis transgenic line with empty vector. (D) Arabidopsis transgenic lines with GmChi02 or GmChi16 exhibited mild symptoms after the inoculation of F. oxysporum. (E) Quantification of foliar symptoms after the inoculation of F. oxysporum. (F) Arabidopsis transgenic lines with GmChi02 or GmChi16 exhibited better seedling survival rates after the inoculation of F. oxysporum. (G) Quantification of seedling survival rates after the inoculation of F. oxysporum. Different letters indicate significant difference among the Arabidopsis transgenic lines (P< 0.05).
Gene preference induced by different rhizobacteria on soybean chitinases
In order to survey the inducibility of soybean chitinase genes, six rhizobacteria from different genera were applied to soybean taproot to characterize gene expressions. As a result, Bacillus amyloliquefaciens, Bradyrhizobium japonicum, B. ambifaria, Lysobacter enzymogenes, Pseudomonas fluorescens, and Rhizobium rhizogenes upregulated zero, one, eight, one, six, and zero chitinase genes, respectively. Although there were some chitinase genes showing upregulation based on the average log2 fold change, variation within biological replicates may reduce the confidence in detecting statistical significance for cases such as GmChi02 (Glyma.02G024500) in response to P. fluorescens. Nonetheless, the survey confirmed that soybean chitinase genes responded differently to various rhizobacteria, where the expression of 10 chitinase genes (Glyma.02G007400, Glyma.02G042500, Glyma.10G227700, Glyma.11G124500, Glyma.12G156600, Glyma.13G155800, Glyma.13G346700, Glyma.16G173000, Glyma.17G217000, and Glyma.20G035400) were significantly induced by at least one rhizobacterium, and the expression of nine chitinase genes (Glyma.07G061600, Glyma.08G299700, Glyma.08G300300, Glyma.12G049200, Glyma.15G206400, Glyma.15G206800, Glyma.17G076100, Glyma.18G120200, and Glyma.20G164900) remain unchanged to all rhizobacteria (Figure 5).
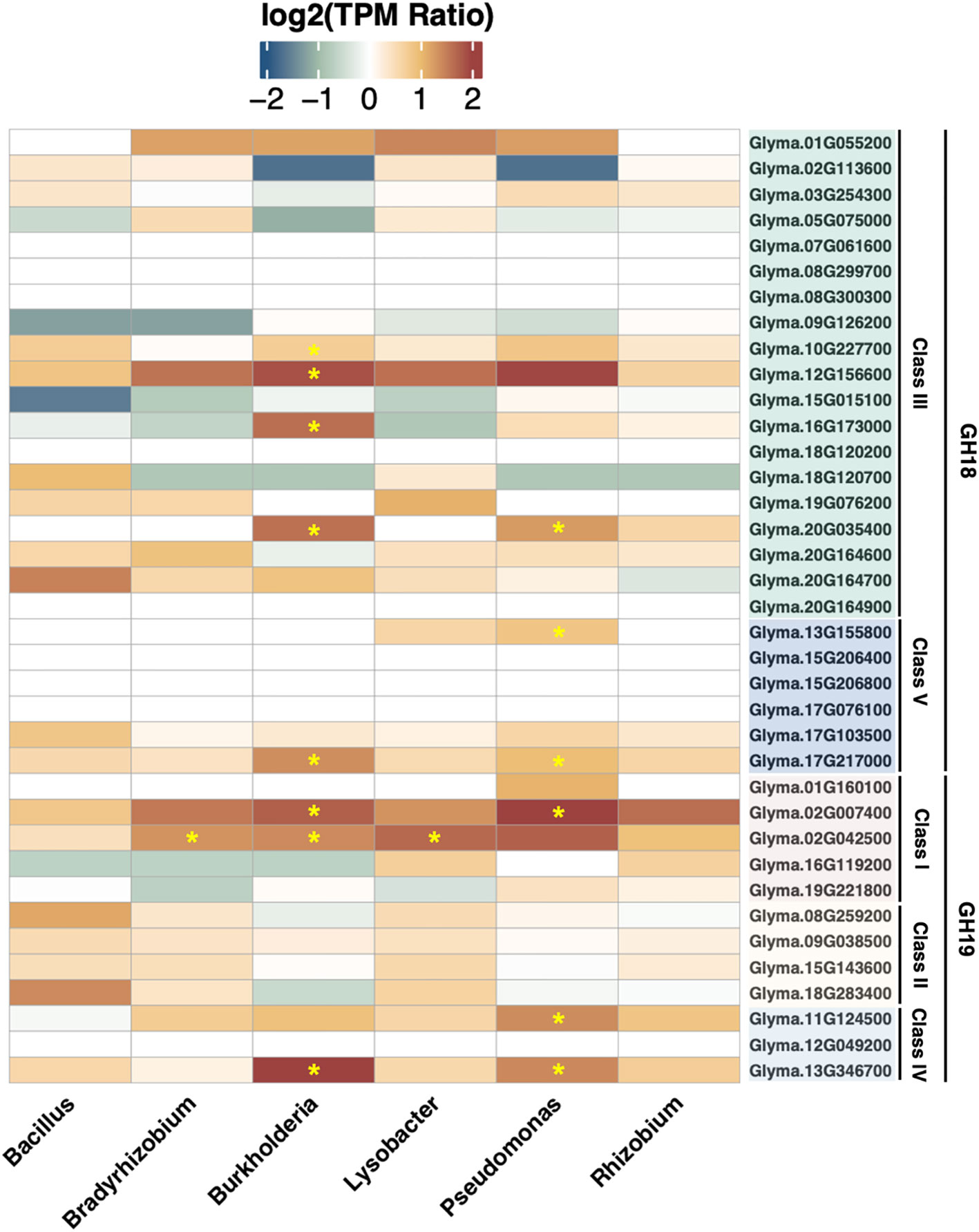
Figure 5 Gene expression of soybean chitinases in response to six rhizobacteria. Asterisks highlight the significant upregulation induced by the inoculation of each rhizobacterium. GmChi02 responded to all rhizobacteria, and the inoculation of B. japonicum, B. ambifaria, and L. enzymogenes reached statistical significance. GmChi01 only responded to P. fluorescens without statistical significance, and GmChi16 was upregulated by L. enzymogenes and R. rhizogenes without statistical significance.
Specifically, GmChi02 can be significantly induced by B. diazoefficiens, B. ambifaria, and L. enzymogenes. On the other hand, GmChi01 or GmChi16 did not reach statistical significance for any rhizobacteria. As for other chitinase genes such as Glyma.13G346700, Glyma.12G156600, Glyma.11G124500, and Glyma.02G007400 that were induced by F. oxysporum infection (Figure 2B), Glyma.13G346700 and Glyma.02G007400 can be significantly induced by B. ambifaria and P. fluorescens. On the other hand, Glyma.12G156600 was upregulated by B. ambifaria, while Glyma.11G124500 was upregulated by P. fluorescens. These results suggest that soybean chitinase genes upregulated in the defense responses to F. oxysporum infection all interacted with at least one of the six rhizobacteria. Therefore, transcription factor-binding sites may have emerged during the co-evolution between soybeans and these rhizobacteria.
Identification of transcription factor and transcription factor-binding sites for the rhizobacteria-inducible soybean chitinase genes
In order to identify the potential regulatory motifs, the 5′ UTR and 3′ UTR of soybean chitinase genes that responded to the six rhizobacteria were analyzed. There were 94, 62, 76, 59, 125, and 90 soybean transcription factor-binding sites (TFBSs) associated with transcription factors (TFs) for soybean chitinase genes induced by B. amyloliquefaciens, B. japonicum, B. ambifaria, L. enzymogenes, P. fluorescens, and R. rhizogenes, respectively (Figure 6A). Among these genes, there were 55 TFBSs associated with TFs consensually identified for all rhizobacteria, while there were only 11, zero, three, zero, 66, and six TFs exhibiting a specificity to B. amyloliquefaciens, B. japonicum, B. ambifaria, L. enzymogenes, P. fluorescens, and R. rhizogenes, respectively. Focusing on the TF enriched for interacting with B. ambifaria, there were three unique TFs, including two homeobox domain TFs (Glyma.01G240100 and Glyma.07G076800) and one SQUAMOSA promoter-binding protein (SBP)-box TF (Glyma03g29900) (Figures 6B–E).
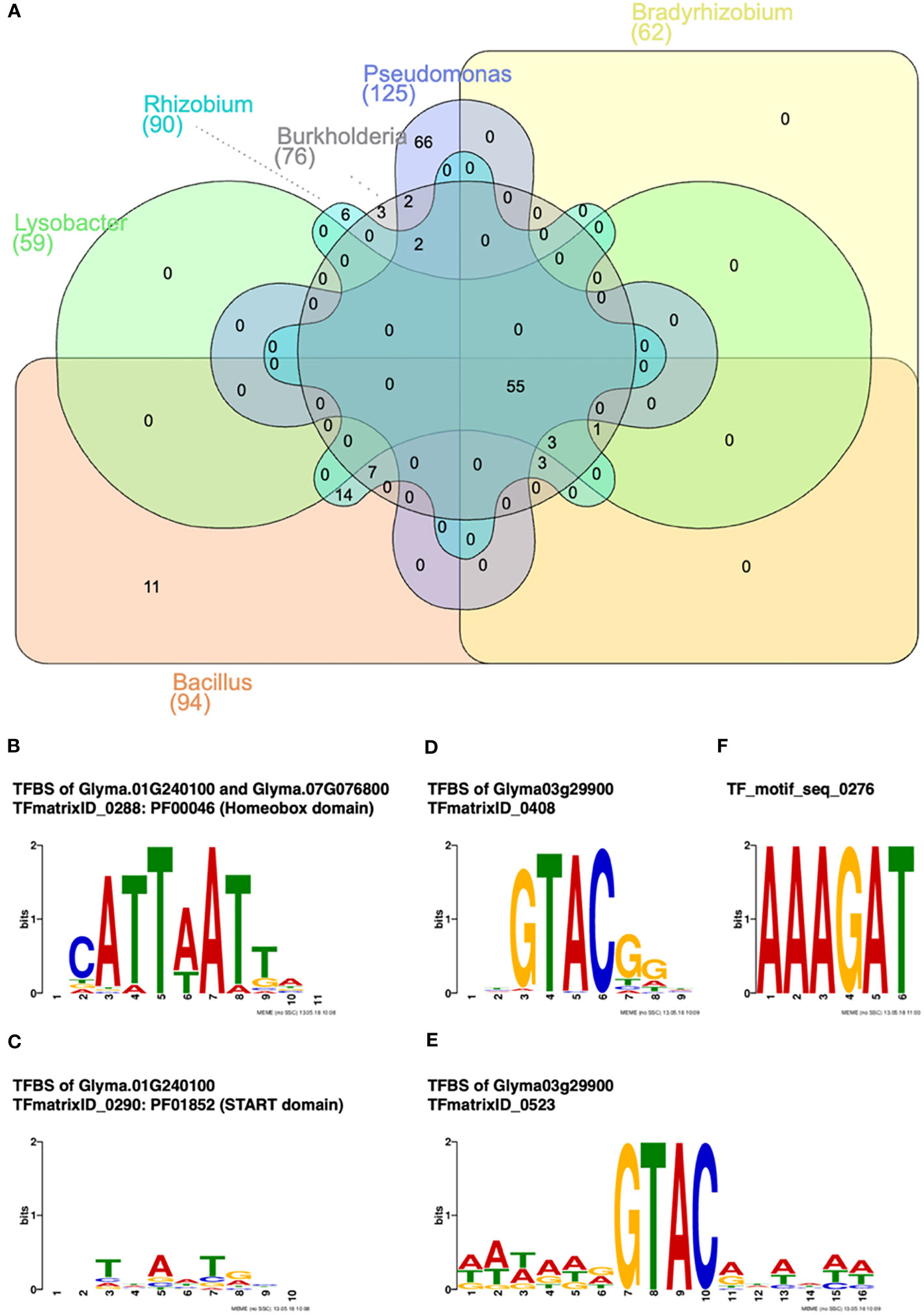
Figure 6 Transcription factor (TF) and TF-binding site (TFBS) analysis for rhizobacteria-inducible soybean chitinase genes. (A) Venn diagram of inducible soybean chitinase genes by Bacillus amyloliquefaciens, Bradyrhizobium japonicum, Burkholderia ambifaria, Lysobacter enzymogenes, Pseudomonas fluorescens, and Rhizobium rhizogenes. Only three soybean TFs responded to B. ambifaria, specifically. (B) Two TFs containing the homeobox domain in response to the inoculation of B. ambifaria, specifically. (C) One TF containing the START domain in response to the inoculation of B. ambifaria, specifically. (D, E) One TF containing the SQUAMOSA promoter-binding (SPB) domain in response to the inoculation of B. ambifaria, specifically. (F) The nodule-specific cis-regulatory motif was found in response to B. amyloliquefaciens, B. japonicum, B. ambifaria, L. enzymogenes, and R. rhizogenes.
As for TFBS without associated TFs, only one cis-regulatory element, NODCON1GM1, was found for B. amyloliquefaciens, B. japonicum, B. ambifaria, L. enzymogenes, and R. rhizogenes in contrast to the inducible and noninducible chitinase genes (Figure 6F). Based on the consensus and diversified inducibility of soybean chitinase genes, the results indicated that the regulatory mechanism of chitinase genes may have co-evolved with soybean–rhizobacteria interaction.
Discussion
The benefits of rhizobacteria for plant health have been greatly recognized in different aspects. Other than direct antagonism against soil-borne pathogens, rhizobacteria may stimulate defense responses to provide sustainable plant protection. However, some scholars have pointed out that crops grown in fields may already be in a constant defense priming and/or induced systemic resistance (ISR) state because they are persistently interacting with rhizobacteria, and simply applying or exposure to rhizobacteria may not be sufficient to combat pathogens (Pieterse et al., 2014). Other literature indicates the density of rhizobacteria is a crucial key for defense priming, or ISR. For example, the minimum bacterial density required for P. fluorescens alone to induce ISR in laboratory conditions required 105 (Raaijmakers, 1995), and it may need to be higher in field conditions. Therefore, it could be challenging to achieve a sufficient population density of rhizobacteria for defense priming or ISR throughout the entire growing season (Walters et al., 2013). An alternative strategy is molecular breeding for important defense genes to bypass the reliance on bacterial density. For example, Rushanaedy et al. (2012) found the koa tree chitinase genes AKchit1a and AKchit1b were significantly upregulated in the resistant cultivars against F. oxysporum compared to susceptible cultivars, providing a screening criterion for disease resistance. Another application of plant chitinase genes is the early detection of biotic stresses. For example, the chitinase activity of papaya fruits was significantly increased upon Colletotrichum gloeosporioides infection during both the preharvest and storage stages. Since C. gloeosporioides is a pathogen exhibiting a latent infection stage, the expression of chitinase genes may serve as diagnostic biomarkers for asymptomatic fruits (Lucas-Bautista et al., 2020). Regardless of being selected as breeding targets or diagnosis biomarkers, the characterization of plant chitinase genes can provide novel insights and a comprehensive understanding of defense responses for a plant species.
This study performed genome- and transcriptome-wide identifications of soybean chitinases and functionally validated three phylogenetically close-related genes (GmChi01, GmChi02, and GmChi16) for their involvement in defense responses. The results showed that GmChi02 and GmChi16 enhanced defense responses to F. oxysporum, but only GmChi02 can be induced by B. ambifaria. In the transcriptomic characterization of GmChi02 in different rhizobacteria, the results confirmed a significant upregulation by B. ambifaria and P. fluorescens, and the expression of GmChi02 also responded to the inoculation of B. amyloliquefaciens, B. japonicum, L. enzymogenes, as well as R. rhizogenes. The observation indicates that GmChi02 may have co-evolved with multiple rhizobacteria to induce defense responses against soil-borne pathogens. On the other hand, although GmChi16 exhibited an equivalent defense effect as GmChi02, the expression of GmChi16 only responded to the inoculation of L. enzymogenes and R. rhizogenes. Collectively, these observations indicate that the regulatory mechanism of soybean chitinase genes may have diversity not only in the coding sequence level for functionality but also in the expression level in terms of inducibility.
Several TFs and TFBSs have been shown to regulate plant chitinase expression. For example, the homeodomain leucine zipper III TF CsHB15 of cucumber was found to bind the promoter of CsChi23 and induce gene expression in response to F. oxysporum (Bartholomew et al., 2022). The R2R3-MYB TF of brown mustard was shown to recognize the W-box-like-4 (Wbl-4) element to activate BjCHI1 in response to B. cinerea (Gao et al., 2016). Another example is the LrWRKY2 of lily, which induced LrCHI2 expression in response to F. oxysporum (Li et al., 2021). However, whether plant chitinase genes harbor conserved TF and TFBS in response to rhizobacteria has not been assessed. In this study, several TF genes and motifs were highlighted by contrasting the TFs and TFBS motifs between the inducible and non-inducible soybean chitinase genes in each rhizobacterium (Supplementary Table S2). One with particular interest would be the NODCON1GM, which has been known to be a nodule-specific regulatory element (Wang et al., 2022). Mutation and deletion of NODCON1GM (5′-AAAGAT) or another regulatory element, NODCON2GM (5′-CTCTT), were shown to decrease the number of nodule formations (Jørgensen et al., 1991). The presence of NODCON1GM in the promoters of rhizobacteria-inducible chitinase genes suggests a possibility that the regulatory mechanism to drive chitinase genes may rely on a similar manner as the regulatory element NODCON1GM. Additional studies on the emergence of NODCON1GM in some but not all soybean chitinase genes, along with studies on the presence of NODCON1GM in the promoters of other soybean PR-protein genes, may further illuminate the evolution of rhizobacteria-induced defense responses.
Research has shown that soybean has diverse rhizobacteria, including Bradyrhizobium, Bacillus, Burkholderia, and Rhizobium species (Biate et al., 2014; Zhong et al., 2019; Han et al., 2020; Yamazaki et al., 2021), that could affect soybean yield and disease incidence (Chang et al., 2017; Hussain et al., 2018). Future studies may focus on the selection pressure derived from soybean rhizobacteria on the expressions of PR-protein genes and the regulatory mechanisms of defense responses induced by different rhizobacteria. These research advances may provide a broad knowledge of the application of beneficial rhizobacteria to enhance plant health.
Materials and methods
Plant and microbial materials
For routine cultivation of Arabidopsis thaliana, the seeds were surface-sterilized using 70% ethanol and 50% Clorox bleach (Oakland, CA, USA). After rinsing five times with sterile water, the seeds were placed in the dark at 4°C for 48 h for vernalization. Subsequently, the seeds were planted in a soil mixture (peat moss:vermiculite:perlite = 6:1:1) and cultured in a long-day condition (16 h light/8 h dark) at 22°C.
For routine growth of rhizobacteria, Bradyrhizobium japonicum USDA6 (BCRC 80814T) was cultured in yeast mannitol broth (0.2 g/L K2HPO4, 0.2 g/L MgSO4·7H2O, 10.0 g/L mannitol, 0.05 g/L NaCl, 0.3 g/L yeast extract; pH 6.2). The other bacterial species, including Bacillus amyloliquefaciens ATCC23350 (BCRC 11601T), Burkholderia ambifaria AMMD ATCCBAA-244 (NRRL B-23395T), Lysobacter enzymogenes ATCC29487 (BCRC 11654T), Pseudomonas fluorescens ATCC 13525 (BCRC 11028T), and Rhizobium rhizogenes K599 (Lifeasible, Shirley, NY 11967, USA) were cultured in Nutrient Broth (HiMedia, Mumbai, India). All rhizobacteria were cultured at 28°C with 125 rpm shaking. To establish the correlation between optical density (OD) 600 and colony-forming units (CFU), bacterial suspensions at OD600 value of 0.5 were diluted and quantified on plates, and linear regression was applied in the later experiment for estimating CFU of bacterial suspensions.
For routine growth of Fusarium oxysporum f.sp. rapae (BCRC FU31513), the fungus was subcultured on potato dextrose agar (PDA) at 28°C without light every 7 days. For producing conidia, the fungus was cultured in synthetic nutrient-poor broth (SNB) (0.5 g/L MgSO4·7H2O, 1 g/L KH2PO4, 1 g/L KNO3, 0.5 g/L KCl, 0.2 g/L glucose, and 0.2 g/L sucrose) (Moura et al., 2020) in the dark at 28°C and 125 rpm for 7 days. The conidia suspension was adjusted to a concentration of 1 × 106 conidia/ml.
Genome-wide identification and phylogenetic analysis of soybean chitinases
To identify chitinase genes in the soybean genome, the HMMs of the GH18 (PF00704) and GH19 (PF00182) protein domains were downloaded from the Pfam database (Mistry et al., 2021). Subsequently, HMMER v3.3.2 was applied to search PF00704 and PF00182 in the ‘Williams 82’ (W82) (Gmax_508_Wm82.a4.v1.protein) at a threshold of 1−10 E-value (Finn et al., 2011). The presence of GH18 or GH19 domain was double-checked using the NCBI Conserved Domain Database at a threshold of 1−20 E-value. In addition, protein tertiary structure was assessed by predicting the folded structure of each soybean chitinase gene protein sequence using ColabFold (Mirdita et al., 2022). Furthermore, MEME v5.4.1 was utilized at a setting of a maximum motif length of 300 and a number of motifs of 20 to identify conserved motifs within the protein sequences (Bailey and Elkan, 1994). The Protparam (Gasteiger et al., 2005), SignalP5.0 (Almagro Armenteros et al., 2019), and DeepLoc-1.0 (Almagro Armenteros et al., 2017) webtools were employed to investigate the amino acid composition, molecular weight, and isoelectric point of soybean chitinase proteins.
The protein sequences of soybean chitinases were aligned with 24 Arabidopsis thaliana chitinases sourced from the TAIR database (Lamesch et al., 2012). Alignment was performed using MAFFT v7 (Katoh et al., 2019), and the phylogenetic tree was constructed using the neighbor-joining (NJ) method in MEGA-X (Kumar et al., 2018). Additionally, the protein sequences of soybean chitinases were aligned with functionally validated chitinase sequences from 21 plant species (Table 1). The phylogenetic tree was constructed using the maximum likelihood (ML) method in IQ-TREE v2.2.0 (Nguyen et al., 2015). The visualization of the phylogenetic trees was generated using iTOL (Letunic and Bork, 2021).
Transcriptomic analysis of soybean chitinases
The tritrophic RNA-Seq data were obtained from a previous study on the gene expression of F. oxysporum in the roots of the soybean variety ‘Jack’ under the influence of the antagonistic bacterium B. ambifaria (Chang et al., 2021). The data can be categorized into four treatments: (1) soybean roots without B. ambifaria or F. oxysporum, (2) soybean roots inoculated with B. ambifaria, (3) soybean roots inoculated with F. oxysporum, and (4) soybean roots simultaneously inoculated with both B. ambifaria and F. oxysporum. Each treatment consisted of three biological replicates, totaling 12 samples. The RNA-Seq was performed using the Illumina HiSeq 4000 platform (Illumina, San Diego, CA, USA). The raw data underwent quality control to keep reads with a Phred score ≥ 30 using the FASTQC and FASTX-ToolKit v0.0.14. The soybean W82 transcriptome (Gmax_508_Wm82.a4.v1.cds.fa) was used as a template for Kallisto v0.46.1 (Bray et al., 2016). Subsequently, differential gene expression analysis was conducted using the R package Sleuth v0.30 (Pimentel et al., 2017) at a threshold of 0.05 q-value. Transcript per million (TPM) measurements of the 37 soybean chitinase genes were presented in a heatmap using the R package ComplexHeatmap v2.13.1 (Gu et al., 2016).
In the RNA-Seq experiment of soybean root inoculated by six rhizobacteria, the W82 soybean seeds were sterilized in 1% bleach for 15 min, followed by five rinses with sterile water. The sterilized seeds were vernalized in sterile water at 28°C without light to better synchronize the germination rate. The next day, the seed coats were removed, and the seeds were placed on 1.5% water agar plates in a growth chamber at 28°C without light for 3 days. After the seeds germinated and the hypocotyls elongated to approximately 3 cm to 5 cm, the seedlings were transferred to new water agar (WA) plates, where 100 µl (approximately 1 × 107 CFU/ml) of bacterial suspension was inoculated onto the soybean hypocotyls. The control group was inoculated with ddH2O.
The inoculated soybean seedlings were further incubated in a growth chamber at 28°C without light. After incubating for 2 days, the frozen taproot samples were homogenized in liquid nitrogen with Invitrogen™ TRIzol™ Reagent (Thermo Fisher Scientific, Waltham, MA, USA), followed by the extraction workflow using chloroform and isopropanol. With two biological replicates per rhizobacteria and control, a total of 14 samples were sent to RNA-Seq using the Illumina NovaSeq 6000 platform in a 150-bp pair-ended platform (Biotools, New Taipei City, Taiwan).
Molecular cloning of GmChi01, GmChi02, and GmChi16
Three chitinase genes, namely Glyma.01G160100 (GmChi01), Glyma.02G042500 (GmChi02), and Glyma.16G119200 (GmChi16), were PCR amplified from the soybean W82 genomic DNA using primers with a SpeI site at the 3′ end (GmChi01_F_SpeI/GmChi01_R_SpeI; GmChi02_F_SpeI/GmChi02_R_SpeI; GmChi16_F_SpeI/GmChi16_R_SpeI) (Supplementary Table S1) via the Phusion® High-Fidelity DNA Polymerase (New England Biolabs, Ipswich, MA, USA). The PCR sizes of three chitinase genes were 2227 bp (GmChi01), 2243 bp (GmChi02), and 1417 bp (GmChi16), and the amplicons were treated with SpeI before being cleaned up using the GenepHlowTW Gel/PCR Kit (Geneaid, New Taipei City, Taiwan). The T4 DNA Ligase (NEB) was used to ligate chitinase amplicons into the pCAMBIA1302 vector pretreated with shrimp alkaline phosphatase (rSAP) (NEB). The ligation mixture was heat-shock transformed into Escherichia coli DH5α competent cells (Yeastern Biotech, New Taipei City, Taiwan) and selected on kanamycin. Colony PCR was performed using specific primers for each chitinase gene (Table 2) using the SMB All-1 DNA Polymerase Premix (StarMoonBio, New Taipei City, Taiwan). The constructs (pCAMBIA1302::GmChi01, pCAMBIA1302::GmChi02, and pCAMBIA1302::GmChi16) were purified using the EasyPure Plasmid DNA Mini Kit (Bioman, New Taipei City, Taiwan) before being sent for Sanger sequencing (Genomics Co., New Taipei City, Taiwan).
Generation of Arabidopsis transgenic lines using Agrobacterium floral dipping
The Agrobacterium tumefaciens GV3101 was cultured in the 523 liquid medium (8 g/L casein hydrolysate, 2 g/L K2HPO4, 0.3 g/L MgSO4·7H2O, 10 g/L sucrose, 4 g/L yeast extract; pH 6.9) supplemented with rifampicin (50 mg/L) and streptomycin (100 mg/L) at 125 rpm shaking for 24 h at 28°C. Upon the optical density (OD600) reaching 1.0 to 1.5, the Agrobacterium suspension was centrifuged at 4,500 rpm at 4°C. The bacterial pellet was resuspended in 20 mM CaCl2 as competent cells. Three soybean chitinase constructs and an empty vector were individually transformed into A. tumefaciens GV3101 using the freeze–thaw method, including a 30-s liquid nitrogen immersion and a 37°C water bath for 5 min. The transformed bacterial cells were selected by kanamycin (50 mg/L). Colony PCR, using gene-specific primer pairs, was used for validation. The Agrobacterium strains were stored in 523/Kan+/Rif+/Strep+ medium with 50% glycerol at −80°C.
For Agrobacterium floral dipping, the desired Agrobacterium strains were freshly prepared in the 523/Kan+/Rif+/Strep+ medium, and the bacterial pellets were resuspended in a 5% sucrose solution containing 0.02% Silwet L-77 (PhytoTech Lab, Lenexa, KS, USA) to OD600 = 0.6 as inoculum. The floral dipping procedure followed the protocol by Zhang et al. (2006) with slight modifications; in brief, the siliques and pollinated flowers were removed from 6-week-old A. thaliana ecotype Col-0, and the unopened Arabidopsis inflorescences were immersed in the Agrobacterium inoculum for 20 s. After immersion, the plants were kept in humid chambers before being routinely cultured at 22°C.
The Arabidopsis seeds harvested after floral dipping represented the T1 generation. The T1 seeds were selected on the MS medium containing 40 ppm hygromycin. The T1 plants with hygromycin resistance were further PCR-confirmed before generating the T2 seeds. The T2 seeds were selected on hygromycin to estimate the Mendelian segregation (3:1) for each T1 lineage. T1 lineages with a single T-DNA insertion were propagated into the T3 generation. Approximately 100 T3 seeds of each lineage were screened on hygromycin. If the T3 germination rate was approximately 100%, the lineage was considered to be homozygous. On the other hand, if the germination rate was around 75%, the lineage was considered to be heterozygous at the T2 generation. Phenotyping and pathogenicity assay were only performed using the progenies of homozygous T2 lineages (Supplementary Figure S1).
For the Arabidopsis transgenic lines, the expressions of soybean chitinase (GmChi01, GmChi02, or GmChi16) were confirmed via RT-qPCR. In brief, foliar RNA of transgenic lines was extracted by the TRIzol procedure described above. The raw RNA was treated with the TURBO DNase (Thermo Fisher Scientific) before cDNA synthesis using the SuperScript IV Reverse Transcriptase (Thermo Fisher Scientific) and oligo d(T)18 primer (Bioman). RT-qPCR was performed using the iQ™ SYBR® Green Supermix (Bio-RAD, Hercules, CA, USA) with the primers (GmChi01_qPCR; GmChi02_qPCR; GmChi16_qPCR; AtACT7) (Supplementary Table S1) on the CFX ConnectTM Real-Time PCR Detection System (Bio-RAD). Three-step thermocycling conditions were set: initial denaturation at 95°C for 5 min, 40 cycles of denaturation at 95°C for 15 s, annealing at 62°C for 10 s, and extension at 72°C for 10 s. The gene expression was presented using the formula ΔCt = Cttarget gene − CtAtACT7. The melty curve of each RT-qPCR amplicon was assessed to confirm specificity, and the amplification efficiencies of primers were optimized to ensure the use of 2−ΔCt (Livak and Schmittgen, 2001).
Phenotyping and pathogenicity assay on Arabidopsis transgenic lines
The hypocotyl length, radical length, rosette area, and stem length were measured for the wild-type A. thaliana Col-0 and the Arabidopsis transgenic lines. Hypocotyl and radical lengths were measured after 1 week of growth on MS medium, while rosette area was calculated using the software Easy Leaf Area (Easlon and Bloom, 2014) after another 3 weeks in pots. Stem length measurements were conducted at the 6-week growth stage. The experiments were repeated twice, and there were 15 biological replicates each time. These data were collected for statistical analyses.
The detached leaf assay was applied to evaluate the defense responses of Arabidopsis lines. A 5-mm-diameter PDA plug with the mycelial edge of F. oxysporum f.sp. rapae was inoculated onto Arabidopsis leaves with a needle wound on the leaf surface. The inoculated leaves were grown for 4 weeks. An ordinal disease index (DI) was measured daily for 1 week, for which the index at 0, 1, 2, 3, 4, and 5 indicates 0%, 1%–10%, 11%–25%, 25%–50%, 51%–75%, and 76%–100% of leaf yellowing, and index at 6 indicates a complete wilt and dead leaf (Supplementary Figure S2). The area under the disease progress curve (AUDPC) was calculated (Sparks et al., 2008). The experiments were repeated three times, and there were nine biological replicates each time.
In addition, soil inoculation was performed by spreading the conidial suspension of F. oxysporum f. sp. rapae onto the 1-week-old Arabidopsis lines. After inoculation, the pots were covered with plastic lids to maintain humidity and placed in the greenhouse at room temperature (25°C ± 2°C). The plastic lids were removed after 10 days postinoculation. The experiments were repeated three times, and there were four biological replicates each time. These data were collected for statistical analyses.
Statistical analysis for phenotypic data
The R v4.0.5 environment and RStudio v1.4.17 were used for statistical analyses. All data were analyzed using the nonparametric Kruskal–Wallis rank sum test, and Dunn’s test was applied for mean separation at a threshold of α = 0.05.
Identification of TF and TFBS for the rhizobacteria-inducible soybean chitinase genes
Soybean chitinase genes were grouped into two categories, including rhizobacteria-inducible chitinase genes (regardless of up- or downregulation) and nonrhizobacteria-inducible chitinase genes (Supplementary Table S2). The upstream 2,000 bp 5′UTR and downstream 500 bp 3′UTR of these genes were subjected to PlantPAN3.0 analysis (Chow et al., 2019) using soybean as the model plant for searching TF and TFBS at 90% frequency of support.
Data availability statement
The RNA-Seq data were deposited in the NCBI BioProject PRJNA987518 and the analyses also included the previously published data in the NCBI BioProject PRJNA512928.
Author contributions
J-YC: Conceptualization, Data curation, Formal analysis, Investigation, Methodology, Resources, Software, Validation, Visualization, Writing – original draft, Writing – review & editing. HS: Conceptualization, Investigation, Methodology, Writing – review & editing. MC: Conceptualization, Investigation, Methodology, Writing – review & editing. C-HW: Conceptualization, Investigation, Methodology, Writing – review & editing. H-XC: Conceptualization, Data curation, Funding acquisition, Investigation, Methodology, Project administration, Supervision, Visualization, Writing – original draft, Writing – review & editing.
Funding
The author(s) declare financial support was received for the research, authorship, and/or publication of this article. This project was supported by the Yushan Young Scholar Program (Ministry of Education, Taiwan) to Dr. Hao-Xun Chang.
Conflict of interest
The authors declare that the research was conducted in the absence of any commercial or financial relationships that could be construed as a potential conflict of interest.
Publisher’s note
All claims expressed in this article are solely those of the authors and do not necessarily represent those of their affiliated organizations, or those of the publisher, the editors and the reviewers. Any product that may be evaluated in this article, or claim that may be made by its manufacturer, is not guaranteed or endorsed by the publisher.
Supplementary material
The Supplementary Material for this article can be found online at: https://www.frontiersin.org/articles/10.3389/fpls.2024.1341181/full#supplementary-material
Supplementary Figure 1 | RT-PCR validation for the expression of GmChi01, GmChi02, and GmChi16 in the Arabidopsis transgenic lines.
Supplementary Figure 2 | The disease index scale for detached leaf assay.
Supplementary Table 1 | Primer sequences.
Supplementary Table 2 | Soybean chitinase genes inducible or non-inducible by six rhizobacteria.
References
Ali, M., Li, Q.-H., Zou, T., Wei, A.-M., Gombojab, G., Lu, G., et al. (2020). Chitinase gene positively regulates hypersensitive and defense responses of pepper to Colletotrichum acutatum infection. Int. J. Mol. Sci. 21, 6624. doi: 10.3390/ijms21186624
Allen, T., Mueller, D., Sisson, A. (2022). Soybean disease loss estimates from the United States and Ontario (Canada: Crop Protection Network). doi: 10.31274/cpn-20230421-1
Almagro Armenteros, J. J., Sønderby, C. K., Sønderby, S. K., Nielsen, H., Winther, O. (2017). DeepLoc: prediction of protein subcellular localization using deep learning. Bioinformatics 33, 3387–3395. doi: 10.1093/bioinformatics/btx431
Almagro Armenteros, J. J., Tsirigos, K. D., Sønderby, C. K., Peterson, T. N., Winther, O., Brunak, S., et al. (2019). SignalP 5.0 improves signal peptide predictions using deep neural networks. Nat. Biotechnol. 37, 420–423. doi: 10.1038/s41587-019-0036-z
Asao, H., Nishizawa, Y., Arai, S., Sato, T., Hirai, M., Yoshida, K., et al. (1997). Enhanced resistance against a fungal pathogen Sphaerotheca humuli in transgenic strawberry expressing a rice chitinase gene. Plant Biotechnol. 14, 145–149. doi: 10.5511/plantbiotechnology.14.145
Bailey, T. L., Elkan, C. (1994). Fitting a mixture model by expectation maximization to discover motifs in biopolymers. Proc. Int. Conf Intell. Syst. Mol. Biol. 2, 28–36.
Bandara, A. Y., Weerasooriya, D. K., Bradley, C. A., Allen, T. W., Esker, P. D. (2020). Dissecting the economic impact of soybean diseases in the United States over two decades. PloS One 15, e0231141. doi: 10.1371/journal.pone.0231141
Bartholomew, E. S., Black, K., Feng, Z., Liu, W., Shan, N., Zhang, X., et al. (2019). Comprehensive analysis of the chitinase gene family in cucumber (Cucumis sativus L.): From gene identification and evolution to expression in response to Fusarium oxysporum. Int. J. Mol. Sci. 20, 5309. doi: 10.3390/ijms20215309
Bartholomew, E. S., Xu, S., Zhang, Y., Yin, S., Feng, Z., Chen, S., et al. (2022). A chitinase CsChi23 promoter polymorphism underlies cucumber resistance against Fusarium oxysporum f. sp. cucumerinum. New Phytol. 236, 1471–1486. doi: 10.1111/nph.18463
Benhamou, N., Broglie, K., Chet, I., Broglie, R. (1993). Cytology of infection of 35S-bean chitinase transgenic canola plants by Rhizoctonia solani: cytochemical aspects of chitin breakdown in vivo. Plant J. 4, 295–305. doi: 10.1046/j.1365-313X.1993.04020295.x
Biate, D. L., Kumar, L. V., Ramadoss, D., Kumari, A., Naik, S., Reddy, K. K., et al. (2014). “Genetic diversity of soybean root nodulating bacteria,” in Bacterial diversity in sustainable agriculture, sustainable development and biodiversity. Ed. Maheshwari, D. K. (Cham: Springer International Publishing), 131–145.
Bordoloi, K. S., Krishnatreya, D. B., Baruah, P. M., Borah, A. K., Mondal, T. K., Agarwala, N. (2021). Genome-wide identification and expression profiling of chitinase genes in tea (Camellia sinensis (L.) O. Kuntze) under biotic stress conditions. Physiol. Mol. Biol. Plants 27, 369–385. doi: 10.1007/s12298-021-00947-x
Boubekri, K., Soumare, A., Mardad, I., Lyamlouli, K., Ouhdouch, Y., Hafidi, M., et al. (2022). Multifunctional role of Actinobacteria in agricultural production sustainability: A review. Microbiol. Res. 261, 127059. doi: 10.1016/j.micres.2022.127059
Bradley, C. A., Allen, T. W., Sisson, A. J., Bergstrom, G. C., Bissonnette, K. M., Bond, J., et al. (2021). Soybean yield loss estimates due to diseases in the United States and Ontario, Canada, from 2015 to 2019. Plant Health Progress. 22, 483–495. doi: 10.1094/PHP-01-21-0013-RS
Bray, N. L., Pimentel, H., Melsted, P., Pachter, L. (2016). Near-optimal probabilistic RNA-seq quantification. Nat. Biotechnol. 34, 525–527. doi: 10.1038/nbt.3519
Brogue, K., Chet, I., Holliday, M., Cressman, R., Biddle, P., Knowlton, S., et al. (1991). Transgenic plants with enhanced resistance to the fungal pathogen. Rhizoctonia solani. Science. 254, 1194–1197. doi: 10.1126/science.254.5035.1194
Chalavi, V., Tabaeizadeh, Z., Thibodeau, P. (2003). Enhanced resistance to Verticillium dahliae in transgenic strawberry plants expressing a Lycopersicon Chilense chitinase gene. J. Am. Soc Hortic. Sci. 128, 747–753. doi: 10.21273/JASHS.128.5.0747
Chang, H.-X., Haudenshield, J. S., Bowen, C. R., Hartman, G. L. (2017). Metagenome-wide association study and machine learning prediction of bulk soil microbiome and crop productivity. Front. Microbiol. 8, 519. doi: 10.3389/fmicb.2017.00519
Chang, H.-X., Noel, Z. A., Chilvers, M. I. (2021). A β-lactamase gene of Fusarium oxysporum alters the rhizosphere microbiota of soybean. Plant J. 106, 1588–1604. doi: 10.1111/tpj.15257
Chen, J., Piao, Y., Liu, Y., Li, X., Piao, Z. (2018). Genome-wide identification and expression analysis of chitinase gene family in Brassica rapa reveals its role in clubroot resistance. Plant Sci. 270, 257–267. doi: 10.1016/j.plantsci.2018.02.017
Chopra, R., Saini, R. (2014). Transformation of blackgram (Vigna mungo (L.) Hepper) by barley chitinase and ribosome-inactivating protein genes towards improving resistance to Corynespora leaf spot fungal disease. Appl. Biochem. Biotechnol. 174, 2791–2800. doi: 10.1007/s12010-014-1226-2
Chow, C.-N., Lee, T.-Y., Hung, Y.-C., Li, G.-Z., Tseng, K.-C., Liu, Y.-H., et al. (2019). PlantPAN3.0: a new and updated resource for reconstructing transcriptional regulatory networks from ChIP-seq experiments in plants. Nucleic Acids Res. 47, D1155–D1163. doi: 10.1093/nar/gky1081
Das, D. K., Rahman, A. (2018). Expression of a rice chitinase gene enhances antifungal response in transgenic litchi (cv. Bedana). Am. J. Plant Sci. 9, 2256–2275. doi: 10.4236/ajps.2018.911163
Datta, K., Tu, J., Oliva, N., Ona, I., Velazhahan, R., Mew, T. W., et al. (2001). Enhanced resistance to sheath blight by constitutive expression of infection-related rice chitinase in transgenic elite indica rice cultivars. Plant Sci. 160, 405–414. doi: 10.1016/S0168-9452(00)00413-1
Dong, X., Zhao, Y., Ran, X., Guo, L., Zhao, D. G. (2017). Overexpression of a new chitinase gene EuCHIT2 enhances resistance to Erysiphe cichoracearum DC. @ in Tobacco Plants. Int. J. Mol. Sci. 18, 2361. doi: 10.3390/ijms18112361
Dowd, P. F., Naumann, T. A., Price, N. P. J., Johnson, E. T. (2018). Identification of a maize (Zea mays) chitinase allele sequence suitable for a role in ear rot fungal resistance. Agric. Gene. 7, 15–22. doi: 10.1016/j.aggene.2017.10.001
Durechova, D., Jopcik, M., Rajninec, M., Moravcikova, J., Libantova, J. (2019). Expression of Drosera rotundifolia chitinase in transgenic tobacco plants enhanced their antifungal potential. Mol. Biotechnol. 61, 916–928. doi: 10.1007/s12033-019-00214-1
Easlon, H. M., Bloom, A. J. (2014). Easy leaf area: Automated digital image analysis for rapid and accurate measurement of leaf area. App. Plant Sci. 2, 1400033. doi: 10.3732/apps.1400033
Eissa, H. F., Hassanien, S. E., Ramadan, A. M., El-Shamy, M. M., Saleh, O. M., Shokry, A. M., et al. (2017). Developing transgenic wheat to encounter rusts and powdery mildew by overexpressing barley chi26 gene for fungal resistance. Plant Methods 13, 41. doi: 10.1186/s13007-017-0191-5
Elnahal, A. S. M., El-Saadony, M. T., Saad, A. M., Desoky, E.-S. M., El-Tahan, A. M., Rady, M. M., et al. (2022). The use of microbial inoculants for biological control, plant growth promotion, and sustainable agriculture: A review. Eur. J. Plant Pathol. 162, 759–792. doi: 10.1007/s10658-021-02393-7
Finn, R. D., Clements, J., Eddy, S. R. (2011). HMMER web server: interactive sequence similarity searching. Nucleic Acids Res. 39, W29–W37. doi: 10.1093/nar/gkr367
Funkhouser, J. D., Aronson, N. N. (2007). Chitinase family GH18: evolutionary insights from the genomic history of a diverse protein family. BMC Evol. Biol. 7, 96. doi: 10.1186/1471-2148-7-96
Gao, Y., Jia, S., Wang, C., Wang, F., Wang, F., Zhao, K. (2016). BjMYB1, a transcription factor implicated in plant defence through activating BjCHI1 chitinase expression by binding to a W-box-like element. J. Exp. Bot. 67, 4647–4658. doi: 10.1093/jxb/erw240
Gasteiger, E., Hoogland, C., Gattiker, A., Duvaud, S., Wilkins, M. R., Appel, R. D., et al. (2005). “Protein identification and analysis tools on the ExPASy server,” in The proteomics protocols handbook. Eds. Walker, J. M., Totowa, N. J. (Totowa, NJ: Humana Press US), 571–607. doi: 10.1385/1-59259-890-0:571
Girhepuje, P. V., Shinde, G. B. (2011). Transgenic tomato plants expressing a wheat endochitinase gene demonstrate enhanced resistance to Fusarium oxysporum f. sp. lycopersici. Plant Cell Tiss. Organ. Cult. 105, 243–251. doi: 10.1007/s11240-010-9859-5
Grover, A. (2012). Plant chitinases: Genetic diversity and physiological roles. Crit. Rev. Plant Sci. 31, 57–73. doi: 10.1080/07352689.2011.616043
Gu, Z., Eils, R., Schlesner, M. (2016). Complex heatmaps reveal patterns and correlations in multidimensional genomic data. Bioinform. 32, 2847–2849. doi: 10.1093/bioinformatics/btw313
Han, Q., Ma, Q., Chen, Y., Tian, B., Xu, L., Bai, Y., et al. (2020). Variation in rhizosphere microbial communities and its association with the symbiotic efficiency of rhizobia in soybean. ISME J. 14, 1915–1928. doi: 10.1038/s41396-020-0648-9
Haxim, Y., Kahar, G., Zhang, X., Si, Y., Waheed, A., Liu, X., et al. (2022). Genome-wide characterization of the chitinase gene family in wild apple (Malus sieversii) and domesticated apple (Malus domestica) reveals its role in resistance to Valsa Mali. Front. Plant Sci. 13, 1007936. doi: 10.3389/fpls.2022.1007936
He, T., Fan, J., Jiao, G., Liu, Y., Zhang, Q., Luo, N., et al. (2023). Bioinformatics and expression analysis of the chitinase genes in strawberry (Fragaria vesca) and functional study of FvChi-14. Plants. 12, 1543. doi: 10.3390/plants12071543
Huang, L.-F., Lin, K.-H., He, S.-L., Chen, J.-L., Jiang, J.-Z., Chen, B.-H., et al. (2016). Multiple patterns of regulation and overexpression of a ribonuclease-like pathogenesis-related protein gene, OsPR10a, conferring disease resistance in rice and Arabidopsis. PloS One 11, e0156414. doi: 10.1371/journal.pone.0156414
Huang, X., Wang, J., Du, Z., Zhang, C., Li, L., Xu, Z. (2013). Enhanced resistance to stripe rust disease in transgenic wheat expressing the rice chitinase gene RC24. Transgenic Res. 22, 939–947. doi: 10.1007/s11248-013-9704-9
Hussain, M., Hamid, M. I., Tian, J., Hu, J., Zhang, X., Chen, J., et al. (2018). Bacterial community assemblages in the rhizosphere soil, root endosphere and cyst of soybean cyst nematode-suppressive soil challenged with nematodes. FEMS Microbiol. Ecol. 94, fiy142. doi: 10.1093/femsec/fiy142
Ignacimuthu, S., Ceasar, S. A. (2012). Development of transgenic finger millet (Eleusine coracana (L.) Gaertn.) resistant to leaf blast disease. J. Biosci. 37, 135–147. doi: 10.1007/s12038-011-9178-y
Iqbal, M. M., Nazir, F., Ali, S., Asif, M. A., Zafar, Y., Iqbal, J., et al. (2012). Over expression of rice chitinase gene in transgenic peanut (Arachis hypogaea L.) improves resistance against leaf spot. Mol. Biotechnol. 50, 129–136. doi: 10.1007/s12033-011-9426-2
Jabeen, N., Chaudhary, Z., Gulfraz, M., Rashid, H., Mirza, B. (2015). Expression of rice chitinase gene in genetically engineered tomato confers enhanced resistance to Fusarium wilt and early blight. Plant Pathol. J. 31, 252–258. doi: 10.5423/PPJ.OA.03.2015.0026
Jach, G., Görnhardt, B., Mundy, J., Logemann, J., Pinsdorf, E., Leah, R., et al. (1995). Enhanced quantitative resistance against fungal disease by combinatorial expression of different barley antifungal proteins in transgenic tobacco. Plant J. 8, 97–109. doi: 10.1046/j.1365-313X.1995.08010097.x
Jørgensen, J. E., Stougaard, J., Marcker, K. A. (1991). A two-component nodule-specific enhancer in the soybean N23 gene promoter. Plant Cell 3, 819–827. doi: 10.1105/tpc.3.8.819
Kang, J.-N., Park, M.-Y., Kim, W.-N., Kang, H.-G., Sun, H.-J., Yang, D.-H., et al. (2017). Resistance of transgenic zoysiagrass overexpressing the zoysiagrass class II chitinase gene Zjchi2 against Rhizoctonia solani AG2-2 (IV). Plant Biotechnol. Rep. 11, 229–238. doi: 10.1007/s11816-017-0445-8
Katoh, K., Rozewicki, J., Yamada, K. D. (2019). MAFFT online service: multiple sequence alignment, interactive sequence choice and visualization. Brief. Bioinform. 20, 1160–1166. doi: 10.1093/bib/bbx108
Kawase, T., Saito, A., Sato, T., Kanai, R., Fujii, T., Nikaidou, N., et al. (2004). Distribution and phylogenetic analysis of family 19 chitinases in Actinobacteria. Appl. Environ. Microbiol. 70, 1135–1144. doi: 10.1128/AEM.70.2.1135-1144.2004
Kezuka, Y., Ohishi, M., Itoh, Y., Watanabe, J., Mitsutomi, M., Watanabe, T., et al. (2006). Structural studies of a two-domain chitinase from Streptomyces griseus HUT6037. J. Mol. Biol. 358, 472–484. doi: 10.1016/j.jmb.2006.02.013
Khan, A., Nasir, I. A., Tabassum, B., Aaliya, K., Tariq, M., Rao, A. Q. (2017). Expression studies of chitinase gene in transgenic potato against Alternaria solani. Plant Cell Tiss Organ Cult. 128, 563–576. doi: 10.1007/s11240-016-1134-y
Kim, D. S., Kim, N. H., Hwang, B. K. (2015). The Capsicum annuum class IV chitinase ChitIV interacts with receptor-like cytoplasmic protein kinase PIK1 to accelerate PIK1-triggered cell death and defence responses. J. Exp. Bot. 66, 1987–1999. doi: 10.1093/jxb/erv001
Kishimoto, K., Nishizawa, Y., Tabei, Y., Hibi, T., Nakajima, M., Akutsu, K. (2002). Detailed analysis of rice chitinase gene expression in transgenic cucumber plants showing different levels of disease resistance to gray mold (Botrytis cinerea). Plant Sci. 162, 655–662. doi: 10.1016/S0168-9452(01)00602-1
Kishimoto, K., Nishizawa, Y., Tabei, Y., Nakajima, M., Hibi, T., Akutsu, K. (2004). Transgenic cucumber expressing an endogenous class III chitinase gene has reduced symptoms from Botrytis cinerea. J. Gen. Plant Pathol. 70, 314–320. doi: 10.1007/s10327-004-0152-5
Kovács, G., Sági, L., Jacon, G., Arinaitwe, G., Busogoro, J.-P., Thiry, E., et al. (2013). Expression of a rice chitinase gene in transgenic banana (‘Gros Michel’, AAA genome group) confers resistance to black leaf streak disease. Transgenic Res. 22, 117–130. doi: 10.1007/s11248-012-9631-1
Kumar, S., Stecher, G., Li, M., Knyaz, C., Tamura, K. (2018). MEGA X: Molecular evolutionary genetics analysis across computing platforms. Mol. Biol. Evol. 35, 1547–1549. doi: 10.1093/molbev/msy096
Lamesch, P., Berardini, T. Z., Li, D., Swarbreck, D., Wilks, C., Sasidharan, R., et al. (2012). The Arabidopsis information resource (TAIR): Improved gene annotation and new tools. Nucleic Acids Res. 40, D1202–D1210. doi: 10.1093/nar/gkr1090
Letunic, I., Bork, P. (2021). Interactive tree of life (iTOL) v5: An online tool for phylogenetic tree display and annotation. Nucleic Acids Res. 49, W293–W296. doi: 10.1093/nar/gkab301
Li, P., Pei, Y., Sang, X., Ling, Y., Yang, Z., He, G. (2009). Transgenic indica rice expressing a bitter melon (Momordica charantia) class I chitinase gene (McCHIT1) confers enhanced resistance to Magnaporthe grisea and Rhizoctonia solani. Eur. J. Plant Pathol. 125, 533–543. doi: 10.1007/s10658-009-9501-8
Li, S., Hai, J., Wang, Z., Deng, J., Liang, T., Su, L., et al. (2021). Lilium regale Wilson WRKY2 regulates chitinase gene expression during the response to the root rot pathogen Fusarium oxysporum. Front. Plant Sci. 12, 741463. doi: 10.3389/fpls.2021.741463
Liu, M., Gong, Y., Sun, H., Zhang, J., Zhang, L., Sun, J., et al. (2020). Characterization of a novel chitinase from sweet potato and its fungicidal effect against Ceratocystis fimbriata. J. Agric. Food Chem. 68, 7591–7600. doi: 10.1021/acs.jafc.0c01813
Livak, K. J., Schmittgen, T. D. (2001). Analysis of relative gene expression data using real-time quantitative PCR and the 2–ΔΔCT method. Methods. 25, 402–408. doi: 10.1006/meth.2001.1262
Lucas-Bautista, J. A., Ventura-Aguilar, R. I., Bautista-Baños, S., Corona-Rangel, M. L., Guillén-Sánchez, D. (2020). Evaluation of the chitinase activity in papaya fruit at different phenological stages as a possible biomarker for the detection of Colletotrichum gloeosporioides infection. Curr. Plant Biol. 23, 100165. doi: 10.1016/j.cpb.2020.100165
Lugtenberg, B., Kamilova, F. (2009). Plant-growth-promoting rhizobacteria. Annu. Rev. Microbiol. 63, 541–556. doi: 10.1146/annurev.micro.62.081307.162918
Lundberg, D. S., Teixeira, P. J. P. L. (2018). Root-exuded coumarin shapes the root microbiome. PNAS. 115, 5629–5631. doi: 10.1073/pnas.1805944115
Luo, X., Tian, T., Feng, L., Yang, X., Li, L., Tan, X., et al. (2023). Pathogenesis-related protein 1 suppresses oomycete pathogen by targeting against AMPK kinase complex. J. Adv. Res. 43, 13–26. doi: 10.1016/j.jare.2022.02.002
Luo, L., Zhao, C., Wang, E., Raza, A., Yin, C. (2022). Bacillus amyloliquefaciens as an excellent agent for biofertilizer and biocontrol in agriculture: An overview for its mechanisms. Microbiol. Res. 259, 127016. doi: 10.1016/j.micres.2022.127016
Lv, P., Zhang, C., Xie, P., Yang, X., El-Sheikh, M. A., Hefft, D. I., et al. (2022). Genome-wide identification and expression analyses of the chitinase gene family in response to white mold and drought stress in soybean (Glycine max). Life 12, 1340. doi: 10.3390/life12091340
Ma, X. L., Milne, R. I., Zhou, H. X., Fang, J. Y., Zha, H. G. (2017). Floral nectar of the obligate outcrossing Canavalia gladiata (Jacq.) DC. (Fabaceae) contains only one predominant protein, a class III acidic chitinase. Plant Biol. 19, 749–759. doi: 10.1111/plb.12583
Marchant, R., Davey, M. R., Lucas, J. A., Lamb, C. J., Dixon, R. A., Power, J. B. (1998). Expression of a chitinase transgene in rose (Rosa hybrida L.) reduces development of blackspot disease (Diplocarpon rosae Wolf). Mol. Breeding. 4, 187–194. doi: 10.1023/A:1009642707505
Marks, R. A., Hotaling, S., Frandsen, P. B., VanBuren, R. (2021). Representation and participation across 20 years of plant genome sequencing. Nat. Plants 7, 1571–1578. doi: 10.1038/s41477-021-01031-8
Mauch-Mani, B., Baccelli, I., Luna, E., Flors, V. (2017). Defense priming: An adaptive part of induced resistance. Annu. Rev. Plant Biol. 68, 485–512. doi: 10.1146/annurev-arplant-042916-041132
Martinez-Medina, A., Flors, V., Heil, M., Mauch-Mani, B., Pieterse, C. M. J., Pozo, M. J., et al. (2016). Recognizing Plant Defense Priming. Trends Plant Sci. 21, 818–822. doi: 10.1016/j.tplants.2016.07.009
Maximova, S. N., Marelli, J.-P., Young, A., Pishak, S., Verica, J. A., Guiltinan, M. J. (2006). Over-expression of a cacao class I chitinase gene in Theobroma cacao L. enhances resistance against the pathogen, Colletotrichum gloeosporioides. Planta. 224, 740–749. doi: 10.1007/s00425-005-0188-6
Mir, Z. A., Ali, S., Shivaraj, S. M., Bhat, J. A., Singh, A., Yadav, P., et al. (2020). Genome-wide identification and characterization of chitinase gene family in Brassica juncea and Camelina sativa in response to Alternaria brassicae. Genomics. 112, 749–763. doi: 10.1016/j.ygeno.2019.05.011
Mir, Z. A., Ali, S., Singh, A., Yadav, P., Tyagi, A., Chaturani, G. D. G., et al. (2021). In silico analysis and overexpression of chitinase class IV gene in Brassica juncea improves resistance against Alternaria brassicae. Ind. Crops Prod. 169, 113555. doi: 10.1016/j.indcrop.2021.113555
Mirdita, M., Schütze, K., Moriwaki, Y., Heo, L., Ovchinnikov, S., Steinegger, M. (2022). ColabFold: making protein folding accessible to all. Nat. Methods 19, 679–682. doi: 10.1038/s41592-022-01488-1
Mistry, J., Chuguransky, S., Williams, L., Qureshi, M., Salazar, G. A., Sonnhammer, E. L. L., et al. (2021). Pfam: The protein families database in 2021. Nucleic Acids Res. 49, D412–D419. doi: 10.1093/nar/gkaa913
Moura, R. D., de Castro, L. A. M., Culik, M. P., Fernandes, A. A. R., Fernandes, P. M. B., Ventura, J. A. (2020). Culture medium for improved production of conidia for identification and systematic studies of Fusarium pathogens. J. Microbiol. Meth. 173, 105915. doi: 10.1016/j.mimet.2020.105915
Mousa, W. K., Shearer, C., Limay-Rios, V., Ettinger, C. L., Eisen, J. A., Raizada, M. N. (2016). Root-hair endophyte stacking in finger millet creates a physicochemical barrier to trap the fungal pathogen Fusarium graminearum. Nat. Microbiol. 1, 1–12. doi: 10.1038/nmicrobiol.2016.167
Nguyen, L.-T., Schmidt, H. A., von Haeseler, A., Minh, B. Q. (2015). IQ-TREE: A fast and effective stochastic algorithm for estimating maximum-likelihood phylogenies. Mol. Biol. Evol. 32, 268–274. doi: 10.1093/molbev/msu300
Nirala, N. K., Das, D. K., Srivastava, P. S., Sopory, S. K., Upadhyaya, K. C. (2010). Expression of a rice chitinase gene enhances antifungal potential in transgenic grapevine (Vitis vinifera L.). Vitis. 49 (4), 181–187. doi: 10.5073/vitis.2010.49.181-187
Nishizawa, Y., Nishio, Z., Nakazono, K., Soma, M., Nakajima, E., Ugaki, M., et al. (1999). Enhanced resistance to blast (Magnaporthe grisea) in transgenic Japonica rice by constitutive expression of rice chitinase. Theor. Appl. Genet. 99, 383–390. doi: 10.1007/s001220051248
Núñez de Cáceres González, F. F., Davey, M. R., Cancho Sanchez, E., Wilson, Z. A. (2015). Conferred resistance to Botrytis cinerea in Lilium by overexpression of the RCH10 chitinase gene. Plant Cell Rep. 34, 1201–1209. doi: 10.1007/s00299-015-1778-9
Orozco-Mosqueda, M d.C., Fadiji, A. E., Babalola, O. O., Santoyo, G. (2023). Bacterial elicitors of the plant immune system: An overview and the way forward. Plant Stress. 7, 100138. doi: 10.1016/j.stress.2023.100138
Pak, J.-H., Chung, E.-S., Shin, S.-H., Jeon, E.-H., Kim, M.-J., Lee, H.-Y., et al. (2009). Enhanced fungal resistance in Arabidopsis expressing wild rice PR-3 (OgChitIVa) encoding chitinase class IV. Plant Biotechnol. Rep. 3, 147–155. doi: 10.1007/s11816-009-0084-9
Pappinen, A., Degefu, Y., Syrjälä, L., Keinonen, K., von Weissenberg, K. (2002). Transgenic silver birch (Betula pendula) expressing sugarbeet chitinase 4 shows enhanced resistance to Pyrenopeziza betulicola. Plant Cell Rep. 20, 1046–1051. doi: 10.1007/s00299-002-0449-9
Pasonen, H.-L., Seppänen, S.-K., Degefu, Y., Rytkönen, A., von Weissenberg, K., Pappinen, A. (2004). Field performance of chitinase transgenic silver birches (Betula pendula): Resistance to fungal diseases. Theor. Appl. Genet. 109, 562–570. doi: 10.1007/s00122-004-1650-8
Patil, R. S., Ghormade, V., Deshpande, M. V. (2000). Chitinolytic enzymes: An exploration. Enzyme Microb. Tech. 26, 473–483. doi: 10.1016/S0141-0229(00)00134-4
Pieterse, C. M. J., Zamioudis, C., Berendsen, R. L., Weller, D. M., Van Wees, S. C. M., Bakker, P. A. H. M. (2014). Induced systemic resistance by beneficial microbes. Annu. Rev. Phytopathol. 52, 347–375. doi: 10.1146/annurev-phyto-082712-102340
Pimentel, H., Bray, N. L., Puente, S., Melsted, P., Pachter, L. (2017). Differential analysis of RNA-seq incorporating quantification uncertainty. Nat. Methods 14, 687–690. doi: 10.1038/nmeth.4324
Prasad, K., Bhatnagar-Mathur, P., Waliyar, F., Sharma, K. K. (2013). Overexpression of a chitinase gene in transgenic peanut confers enhanced resistance to major soil borne and foliar fungal pathogens. J. Plant Biochem. Biotechnol. 22, 222–233. doi: 10.1007/s13562-012-0155-9
Raaijmakers, J. M., Leeman, M., van Oorschot, M. M. P., van der Sluis, I., Schippers, B., Bakker, P. A. H. M. (1995). Dose-response relationships in biological control of Fusarium wilt of radish by Pseudomonas spp. Phytopathology 85, 1075–1081. doi: 10.1094/Phyto-85-1075
Rajesh, T., Maruthasalam, S., Kalpana, K., Poovannan, K., Kumar, K. K., Kokiladevi, E., et al. (2016). Stability of sheath blight resistance in transgenic ASD16 rice lines expressing a rice chi11 gene encoding chitinase. Biol. Plant 60, 749–756. doi: 10.1007/s10535-016-0594-6
Richa, K., Tiwari, I. M., Devanna, B. N., Botella, J. R., Sharma, V., Sharma, T. R. (2017). Novel chitinase gene LOC_Os11g47510 from indica rice Tetep provides enhanced resistance against sheath blight pathogen Rhizoctonia solani in rice. Front. Plant Sci. 8, 596. doi: 10.3389/fpls.2017.00596
Richa, K., Tiwari, I. M., Kumari, M., Devanna, B. N., Sonah, H., Kumari, A., et al. (2016). Functional characterization of novel chitinase genes present in the sheath blight resistance QTL: qSBR11-1 in rice line Tetep. Front. Plant Sci. 7, 244. doi: 10.3389/fpls.2016.00244
Rohini, V. K., Sankara Rao, K. (2001). Transformation of peanut (Arachis hypogaea L.) with tobacco chitinase gene: variable response of transformants to leaf spot disease. Plant Sci. 160, 889–898. doi: 10.1016/S0168-9452(00)00462-3
Rushanaedy, I., Jones, T. C., Dudley, N. S., Liao, R. J. F., Agbayani, R., Borthakur, D. (2012). Chitinase is a potential molecular biomarker for detecting resistance to Fusarium oxysporum in Acacia koa. Trop. Plant Biol. 5, 244–252. doi: 10.1007/s12042-012-9108-7
Salwan, R., Sharma, M., Sharma, A., Sharma, V. (2023). Insights into plant beneficial microorganism-triggered induced systemic resistance. Plant Stress 7, 100140. doi: 10.1016/j.stress.2023.100140
Shin, S., Mackintosh, C. A., Lewis, J., Heinen, S. J., Radmer, L., Dill-Macky, R., et al. (2008). Transgenic wheat expressing a barley class II chitinase gene has enhanced resistance against Fusarium graminearum. J. Exp. Bot. 59, 2371–2378. doi: 10.1093/jxb/ern103
Sparks, A. H., Esker, P. D., Bates, M., Dall' Acqua, W., Guo, Z., Segovia, V., et al. (2008). Ecology and epidemiology in R: Disease progress over time. Plant Health Instructor. doi: 10.1094/PHI-A-2008-0129-02
Stopnisek, N., Zühlke, D., Carlier, A., Barberán, A., Fierer, N., Becher, D., et al. (2016). Molecular mechanisms underlying the close association between soil Burkholderia and fungi. ISME J. 10, 253–264. doi: 10.1038/ismej.2015.73
Su, Y., Wang, Z., Liu, F., Li, Z., Peng, Q., Guo, J., et al. (2016). Isolation and characterization of ScGluD2, a new sugarcane beta-1,3-glucanase D family gene induced by Sporisorium scitamineum, ABA, H2O2, NaCl, and CdCl2 stresses. Front. Plant Sci. 7, 1348. doi: 10.3389/fpls.2016.01348
Sun, Y., Shang, L., Zhu, Q.-H., Fan, L., Guo, L. (2022). Twenty years of plant genome sequencing: achievements and challenges. Trends Plant Sci. 27, 391–401. doi: 10.1016/j.tplants.2021.10.006
Tabaeizadeh, Z., Agharbaoui, Z., Harrak, H., Poysa, V. (1999). Transgenic tomato plants expressing a Lycopersicon Chilense chitinase gene demonstrate improved resistance to Verticillium dahliae race 2. Plant Cell Rep. 19, 197–202. doi: 10.1007/s002990050733
Tabei, Y., Kitade, S., Nishizawa, Y., Kikuchi, N., Kayano, T., Hibi, T., et al. (1998). Transgenic cucumber plants harboring a rice chitinase gene exhibit enhanced resistance to gray mold (Botrytis cinerea). Plant Cell Rep. 17, 159–164. doi: 10.1007/s002990050371
Taira, T., Hayashi, H., Tajiri, Y., Onaga, S., Uechi, G., Iwasaki, H., et al. (2009). A plant class V chitinase from a cycad (Cycas revoluta): Biochemical characterization, cDNA isolation, and posttranslational modification. Glycobiol. 19, 1452–1461. doi: 10.1093/glycob/cwp119
Takahashi, W., Fujimori, M., Miura, Y., Komatsu, T., Nishizawa, Y., Hibi, T., et al. (2005). Increased resistance to crown rust disease in transgenic Italian ryegrass (Lolium multiflorum Lam.) expressing the rice chitinase gene. Plant Cell Rep. 23, 811–818. doi: 10.1007/s00299-004-0900-1
Takatsu, Y., Nishizawa, Y., Hibi, T., Akutsu, K. (1999). Transgenic chrysanthemum (Dendranthema grandiflorum (Ramat.) Kitamura) expressing a rice chitinase gene shows enhanced resistance to gray mold (Botrytis cinerea). Sci. Hortic. 82, 113–123. doi: 10.1016/S0304-4238(99)00034-5
Tang, C. M., Chye, M.-L., Ramalingam, S., Ouyang, S.-W., Zhao, K.-J., Ubhayasekera, W., et al. (2004). Functional analyses of the chitin-binding domains and the catalytic domain of Brassica juncea chitinase BjCHI1. Plant Mol. Biol. 56, 285–298. doi: 10.1007/s11103-004-3382-1
Tariq, M., Khan, A., Tabassum, B., Toufiq, N., Bhatti, M., Riaz, S., et al. (2018). Antifungal activity of chitinase II against Colletotrichum falcatum Went. causing red rot disease in transgenic sugarcane. Turk. J. Biol. 42, 45–53. doi: 10.3906/biy-1709-17
Tian, B., Yang, J., Zhang, K.-Q. (2007). Bacteria used in the biological control of plant-parasitic nematodes: populations, mechanisms of action, and future prospects. FEMS Microbiol. Ecol. 61, 197–213. doi: 10.1111/j.1574-6941.2007.00349.x
Tohidfar, M., Mohammadi, M., Ghareyazie, B. (2005). Agrobacterium-mediated transformation of cotton (Gossypium hirsutum) using a heterologous bean chitinase gene. Plant Cell Tiss. Organ. Cult. 83, 83–96. doi: 10.1007/s11240-004-6155-2
Vaghela, B., Vashi, R., Rajput, K., Joshi, R. (2022). Plant chitinases and their role in plant defense: A comprehensive review. Enzyme Microb. Tech. 159, 110055. doi: 10.1016/j.enzmictec.2022.110055
van Loon, L. C., Rep, M., Pieterse, C. M. J. (2006). Significance of inducible defense-related proteins in infected plants. Annu. Rev. Phytopathol. 44, 135–162. doi: 10.1146/annurev.phyto.44.070505.143425
Vellicce, G. R., Ricci, J. C. D., Hernández, L., Castagnaro, A. P. (2006). Enhanced resistance to Botrytis cinerea mediated by the transgenic expression of the chitinase gene ch5B in strawberry. Transgenic Res. 15, 57–68. doi: 10.1007/s11248-005-2543-6
Vierheilig, H., Alt, M., Neuhaus, J. M., Boller, T., Wiemken, A. (1993). Colonization of transgenic Nicotiana sylvestris plants, expressing different forms of Nicotiana tabacum chitinase, by the root pathogen Rhizoctonia solani and by the mycorrhizal symbiont Glomus mosseae. MPMI. 6, 261–264. doi: 10.1094/MPMI-6-261
Walters, D. R., Ratsep, J., Havis, N. D. (2013). Controlling crop diseases using induced resistance: challenges for the future. J. Exp. Bot. 64, 1263–1280. doi: 10.1093/jxb/ert026
Wang, X., Chen, K., Zhou, M., Gao, Y., Huang, H., Liu, C., et al. (2022). GmNAC181 promotes symbiotic nodulation and salt tolerance of nodulation by directly regulating GmNINa expression in soybean. New Phytol. 236, 656–670. doi: 10.1111/nph.18343
Wang, Y., Liu, M., Wang, X., Zhong, L., Shi, G., Xu, Y., et al. (2021). A novel β-1,3-glucanase Gns6 from rice possesses antifungal activity against Magnaporthe oryzae. J. Plant Physiol. 265, 153493. doi: 10.1016/j.jplph.2021.153493
Wang, F., Yang, S., Wang, Y., Zhang, B., Zhang, F., Xue, H., et al. (2021). Overexpression of chitinase gene enhances resistance to Colletotrichum gloeosporioides and Alternaria alternata in apple (Malus × domestica). Sci. Hortic. 277, 109779. doi: 10.1016/j.scienta.2020.109779
Wen, Z., Bai, J., Wang, L., Yao, L., Ahmad, B., Hanif, M., et al. (2020). Over expression of a chitinase 2 gene from Chinese wild strawberry improves resistance to anthracnose disease in transgenic Arabidopsis thaliana. Plant Biotechnol. Rep. 14, 725–736. doi: 10.1007/s11816-020-00648-z
Xiao, Y.-H., Li, X.-B., Yang, X.-Y., Luo, M., Hou, L., Guo, S.-H., et al. (2007). Cloning and characterization of a balsam pear class I chitinase gene (Mcchit1) and its ectopic expression enhances fungal resistance in transgenic plants. Biosci. Biotechnol. Biochem. 71, 1211–1219. doi: 10.1271/bbb.60658
Xin, Y., Wang, D., Han, S., Li, S., Gong, N., Fan, Y., et al. (2022). Characterization of the chitinase gene family in mulberry (Morus notabilis) and MnChi18 involved in resistance to Botrytis cinerea. Genes. 13, 98. doi: 10.3390/genes13010098
Xu, J., Xu, X., Tian, L., Wang, G., Zhang, X., Wang, X., et al. (2016). Discovery and identification of candidate genes from the chitinase gene family for Verticillium dahliae resistance in cotton. Sci. Rep. 6, 29022. doi: 10.1038/srep29022
Yamamoto, T., Iketani, H., Ieki, H., Nishizawa, Y., Notsuka, K., Hibi, T., et al. (2000). Transgenic grapevine plants expressing a rice chitinase with enhanced resistance to fungal pathogens. Plant Cell Rep. 19, 639–646. doi: 10.1007/s002999900174
Yamazaki, S., Mardani-korrani, H., Kaida, R., Ochiai, K., Kobayashi, M., Nagano, A. J., et al. (2021). Field multi-omics analysis reveals a close association between bacterial communities and mineral properties in the soybean rhizosphere. Sci. Rep. 11, 8878. doi: 10.1038/s41598-021-87384-8
Yang, J., Gan, Z., Lou, Z., Tao, N., Mi, Q., Liang, L., et al. (2010). Crystal structure and mutagenesis analysis of chitinase CrChi1 from the nematophagous fungus Clonostachys rosea in complex with the inhibitor caffeine. Microbiol. 156, 3566–3574. doi: 10.1099/mic.0.043653-0
Zhang, X., Henriques, R., Lin, S.-S., Niu, Q.-W., Chua, N.-H. (2006). Agrobacterium-mediated transformation of Arabidopsis thaliana using the floral dip method. Nat. Protoc. 1, 641–646. doi: 10.1038/nprot.2006.97
Zhong, Y., Yang, Y., Liu, P., Xu, R., Rensing, C., Fu, X., et al. (2019). Genotype and rhizobium inoculation modulate the assembly of soybean rhizobacterial communities. Plant Cell Environment. 42, 2028–2044. doi: 10.1111/pce.13519
Keywords: Bacillus amyloliquefaciens, Bradyrhizobium japonicum, Burkholderia ambifaria, Lysobacter enzymogenes, Pseudomonas fluorescens, Rhizobium rhizogenes, Glycine max
Citation: Chen J-Y, Sang H, Chilvers MI, Wu C-H and Chang H-X (2024) Characterization of soybean chitinase genes induced by rhizobacteria involved in the defense against Fusarium oxysporum. Front. Plant Sci. 15:1341181. doi: 10.3389/fpls.2024.1341181
Received: 20 November 2023; Accepted: 08 January 2024;
Published: 09 February 2024.
Edited by:
Choong-Min Ryu, Korea Research Institute of Bioscience and Biotechnology (KRIBB), Republic of KoreaReviewed by:
Ahsan Rizvi, Institute of Advanced Research (IAR), IndiaVeerendra Sharma, Kansas State University, United States
Copyright © 2024 Chen, Sang, Chilvers, Wu and Chang. This is an open-access article distributed under the terms of the Creative Commons Attribution License (CC BY). The use, distribution or reproduction in other forums is permitted, provided the original author(s) and the copyright owner(s) are credited and that the original publication in this journal is cited, in accordance with accepted academic practice. No use, distribution or reproduction is permitted which does not comply with these terms.
*Correspondence: Hao-Xun Chang, hxchang@ntu.edu.tw