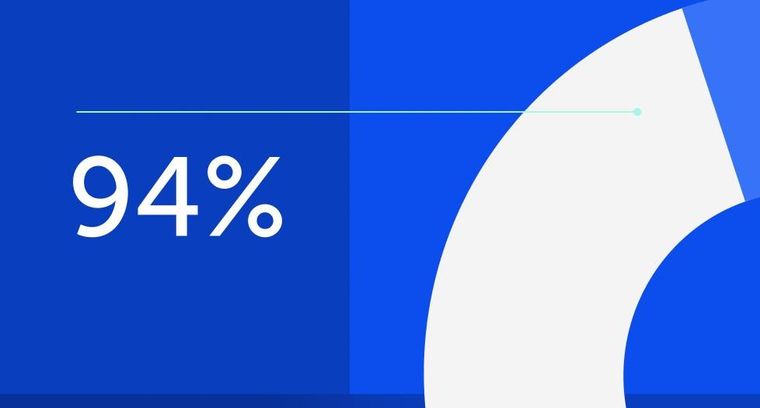
94% of researchers rate our articles as excellent or good
Learn more about the work of our research integrity team to safeguard the quality of each article we publish.
Find out more
ORIGINAL RESEARCH article
Front. Plant Sci., 02 May 2024
Sec. Plant Pathogen Interactions
Volume 15 - 2024 | https://doi.org/10.3389/fpls.2024.1339559
This article is part of the Research TopicInsights In Plant-Pathogen Interactions: 2023View all 19 articles
Iron- and reactive oxygen species (ROS)-dependent ferroptosis occurs in plant cells. Ca2+ acts as a conserved key mediator to control plant immune responses. Here, we report a novel role of cytoplasmic Ca2+ influx regulating ferroptotic cell death in rice immunity using pharmacological approaches. High Ca2+ influx triggered iron-dependent ROS accumulation, lipid peroxidation, and subsequent hypersensitive response (HR) cell death in rice (Oryza sativa). During Magnaporthe oryzae infection, 14 different Ca2+ influx regulators altered Ca2+, ROS and Fe2+ accumulation, glutathione reductase (GR) expression, glutathione (GSH) depletion and lipid peroxidation, leading to ferroptotic cell death in rice. High Ca2+ levels inhibited the reduction of glutathione isulphide (GSSG) to GSH in vitro. Ca2+ chelation by ethylene glycol-bis (2-aminoethylether)-N, N, N’, N’-tetra-acetic acid (EGTA) suppressed apoplastic Ca2+ influx in rice leaf sheaths during infection. Blocking apoplastic Ca2+ influx into the cytoplasm by Ca2+ chelation effectively suppressed Ca2+-mediated iron-dependent ROS accumulation and ferroptotic cell death. By contrast, acibenzolar-S-methyl (ASM), a plant defense activator, significantly enhanced Ca2+ influx, as well as ROS and iron accumulation to trigger ferroptotic cell death in rice. The cytoplasmic Ca2+ influx through calcium-permeable cation channels, including the putative resistosomes, could mediate iron- and ROS-dependent ferroptotic cell death under reduced GR expression levels in rice immune responses.
Plant cell death is an effective immune response to defend against microbial pathogens (Heath, 2000; Greenberg and Yao, 2004). Plant-pathogen interactions induce both pathogen-associated molecular patterns (PAMP)-triggered immunity (PTI) and effector-triggered immunity (ETI) in plant cells, depending on the mode of pathogen recognition (Jones and Dangl, 2006; Dangl et al., 2013; Ngou et al., 2021). Reactive oxygen species (ROS), such as superoxide, H2O2, and hydroxyl radical (•OH) are required to signal and execute plant cell death (Levine et al., 1994; Van Breusegem and Dat, 2006). ROS act as cellular signaling molecules that trigger PTI and ETI in plant cells after plants recognize pathogen infection (Jwa and Hwang, 2017). Robust ROS bursts are common signaling events that occur in hypersensitive response (HR) cell death (Van Breusegem and Dat, 2006; Jwa and Hwang, 2017). Virulent plant pathogens induce transient PTI with low levels of ROS. However, avirulent pathogens induce ETI with strong ROS bursts, leading to HR cell death (Grant and Loake, 2000). ETI is more potent in plant immunity than PTI and greatly limits the entry of microbial pathogens into plant cells via HR induced by intracellular nucleotide-binding leucine-rich repeat (NLR) receptors which can recognize pathogen effectors (Jones and Dangl, 2006). Plant pathogens have evolved to acquire a variety of effectors to suppress ROS bursts, which are key components of the plant immune response (Jwa and Hwang, 2017).
Ferroptosis is a nonapoptotic form of iron-dependent cell death first discovered in animal cells (Dixon et al., 2012; Stockwell et al., 2017) and then in plants, fungi, and bacteria (Distéfano et al., 2017; Dangol et al., 2019; Shen et al., 2020; Aguilera et al., 2022). Iron, ROS, and lipid hydroperoxides are directly involved in ferroptotic cell death (Stockwell et al., 2017; Dangol et al., 2019). The Fenton reaction (Fenton, 1894; Pierre and Fontecave, 1999) by iron ions (Fe2+) and ROS (H2O2) induces glutathione (GSH) depletion and iron- and ROS-dependent ferroptosis in the rice immune response (Dangol et al., 2019). Ca2+ is a conserved second messenger and a major mediator in plant immune responses (Köster et al., 2022). However, whether an abnormally high concentration of Ca2+ influx is directly associated with HR cell death is not fully understood, except for its role as a signal transducer in plant immunity (Moeder et al., 2019). Moreover, the role of Ca2+ in ferroptosis remains unclear.
Recently, it has been proposed that plant nucleotide-binding leucine-rich repeat receptors (NLRs) (Jones and Dangl, 2006; Dangl et al., 2013; Ngou et al., 2021) recognize pathogen effectors to form resistosome complexes as calcium-permeable cation channels in the plasma membrane (Bi et al., 2021). The ZAR1 (HOPZ-ACTIVATED RESISTANCE 1) resistosome (Lewis et al., 2020) is a membrane-localized Ca2+-permeable channel which can trigger immune signaling and cell death in Arabidopsis (Bi et al., 2021). The monocot wheat protein Sr35, which belongs to the CC-NLR class, has been demonstrated to assemble into a resistosome with a structure similar to ZAR1 (Förderer et al., 2022). Arabidopsis ‘helper’ immune NLRs form Ca2+-permeable cation channels, leading to cytoplasmic Ca2+ influx and subsequent cell death (Jacob et al., 2021). Ca2+-permeable cation channels of NLR-mediated resistosomes may induce a sustained high cytoplasmic Ca2+ influx during plant ETI (Jacob et al., 2021). Thus, the discovery of resistosomes that exhibit Ca2+ channel activity in plants provided a crucial clue to elucidate the common mechanism of plant cell death and immunity (Bi et al., 2021; Jacob et al., 2021).
The Ca2+ concentration in the apoplast (~1 mM) is approximately 10,000-fold higher than that in the cytoplasm (~100 nM) (Stael et al., 2012). The significant Ca2+ concentration gradient in the cell may be maintained by both passive impermeability of the plasma membrane to calcium ions and their active transport from the cytoplasm to the apoplast. Schanne et al. (1979) first demonstrated that perturbation of calcium homeostasis triggers toxic cell death in rat hepatocytes through cytoplasmic Ca2+ influx due to impaired membrane integrity, highlighting the pivotal role of Ca2+ in toxic cell death. During toxic cell death, disruption of Ca2+ homeostasis coincides with a decrease in glutathione peroxidase (GPX) and glutathione reductase (GR) activity, resulting in cellular damage due to the acute oxidative stress by glutathione depletion (Bellomo et al., 1982; Jewell et al., 1982). The other alternative hypothesis was that the cell death is not only due to ROS themselves, but also due to the generation of the hydroxyl radical, a more potent oxidizing species, through its reaction with iron (Starke and Farber, 1985; Farber, 1994). Reduced glutathione (GSH) plays a crucial role in controlling ROS in cells. ROS function as intracellular and extracellular signaling molecules. Complex crosstalks between ROS, oxidized glutathione (GSSG) and reduced glutathione (GSH), and the antioxidant enzyme glutathione reductase (GR) control the redox state inside the cell to be suitable for activation of programmed cell death (Couto et al., 2016). However, the roles of GPX and GR in plant HR cell death are largely unknown.
Rice (Oryza sativa)-Magnaporthe oryzae interaction is a good experimental system to analyze if Ca2+ mediates iron- and ROS-dependent ferroptotic cell death in plant immunity (Valent, 2021). In this study, Ca2+ sensor (35S::GCaMP6mC) transgenic rice lines, the Ca2+ indicator Fluo-5F AM, and the o-cresolphthalein complexone (o-CPC) method were used to visualize and quantify intracellular and apoplastic Ca2+ levels in rice leaf sheaths treated with multiple Ca2+ influx enhancers and/or inhibitors during M. oryzae infection. Taken together, our results suggest that Ca2+ influx from apoplast via calcium-permeable cation channels, triggers iron-dependent lipid-based reactive oxygen species (lipid ROS) accumulation by reduced Glutathione Reductase (GR) expression and glutathione depletion in rice cells, which acts as a critical redox switch for iron- and lipid ROS-dependent ferroptotic cell death.
Rice (Oryza sativa L.) cultivar Kitaake was used as the wild type (WT) in this study. Seeds of Kitaake were obtained from the National Institute of Crop Science, Jeonju, Korea (http://www.nics.go.kr). Plants were raised in a growth chamber at 30°C under 60% humidity and 16 h light/8 h dark photoperiod.
M. oryzae strains RO1-1 and 007 were obtained from the Center for Fungal Genetic Resources, Seoul National University, Korea (http://genebank.snu.ac.kr). M. oryzae RO1-1 is virulent to rice cultivar Kitaake, whereas M. oryzae 007 is avirulent. Both M. oryzae strains were stored at −20°C and cultured on rice bran agar medium (20 g of rice bran, 20 g of sucrose, and 20 g of agar in 1 L of water) at 25°C in the dark for 10–14 days (Singh et al., 2016). Sporulation of M. oryzae cultures was induced by incubating the culture plates under continuous light for 3–4 days. M. oryzae conidia were harvested from the sporulated culture plates using 0.025% Tween20 (Sigma-Aldrich) in sterile water (Kankanala et al., 2007). A conidial suspension of M. oryzae in 0.025% (v/v) Tween 20 was adjusted to appropriate conidial concentrations using a hemacytometer.
GCaMP6f was cloned into the plant expression vector pGWB554 using standard molecular techniques, as described previously (Choi et al., 2014; Weigand et al., 2021). The resultant construct was transformed into rice cultivar Kitaake using Agrobacterium tumefaciens strain LBA4404. Briefly, 35S::GCaMP6f-mCherry (GCaMP6fmC) was delivered into rice calli using Agrobacterium-mediated transformation (Hiei et al., 1994). Transformed calli were selected on the half-strength Murashige and Skoog (1/2 MS; Sigma-Aldrich) medium (2.15 g of MS salts, 15 g of sucrose, and 3.5 g of Gelrite [Duchefa Biochemie] in 1 L of water) supplemented with 20 µg·mL-1 hygromycin B (Duchefa Biochemie). After root and shoot formation, rice seedlings were transferred to water and acclimated for 2 days. Rice seedlings were then transferred into pots containing Baroker soil (Seoul Bio) and raised in a growth chamber.
The crystal structures of Ca2+-free GCaMP6 (ID: 3wlc), Ca2+-bound GCaMP6 (ID: 3wld), and mCherry (ID: 2h5q) were obtained from the RCSB Protein Data Bank (https://www.rcsb.org) (Shu et al., 2006; Ding et al., 2014). These three crystal structures were then used to construct crystal images of GCaMP6-mCherry using the PyMOL software (https://pymol.org) (Ding et al., 2014). The Ca2+ sensor construct GCaMP6f-mCherry (GCaMP6fmC) was cloned into the vector pGWB554 under the control of the Cauliflower mosaic virus (CaMV) 35S promoter (Supplementary Figure 1A).
The seeds of rice cultivar Kitaake and Ca2+ sensor (35S::GCaMP6fmC) transgenic rice lines were hulled, sterilized first with 100% ethanol for 1 min and then with 50% Clorox for 30 min, and washed three times with 3DW. The surface-sterilized seeds were then cultured on 1/2 MS medium (Sigma-Aldrich, St. Louis, MO) at 25°C under continuous light for 2 weeks. Leaves were collected from rice plants and subjected to genomic DNA extraction using the cetyltrimethylammonium bromide (CTAB) method. Plants were genotyped by PCR as described previously (Kim et al., 2011), and Ca2+ sensor (GCaMP6fmC) transgenic lines were identified using the hygromycin gene-specific and GCaMP6f-specific primers (Supplementary Table 1). Ca2+ sensor (GCaMP6fmC) expression was verified in the transgenic lines by PCR using hygromycin resistance (HygR) and GCaMP6fmC primers (Supplementary Figure 1B). Without Ca2+ binding, the GCaMP6fmC has low intrinsic fluorescence. However, the green fluorescence of GCaMP6fmC increases after binding of Ca2+ to the calmodulin domain (Figures 1A, B).
Figure 1 Ca2+ influx mediates ROS- and iron-dependent HR cell death in rice immune responses. (A) Schematic diagram of the Ca2+ sensor construct. When Ca2+ is free, the calmodulin (CaM) domain is dissociated from M13; however, the binding of Ca2+ to CaM promotes CaM-M13 binding, leading to increased eGFP fluorescence. mCherry (red fluorescence) serves as an internal fluorescence reference. (B) Time-course images of Ca2+ influx, ROS and Fe3+ accumulation, and HR cell death in the leaf sheaths of rice (Kitaake) Ca2+ sensor (GCaMP6fmC) transgenic line B during avirulent M. oryzae infection. Bars = 10 µm. (C) Time-course quantification of Ca2+ influx during virulent and avirulent M. oryzae infection. At least three regions of interest (ROIs) were selected to quantify Ca2+ changes by calculating the GCaMP6f/mCherry ratio at different time points (hpi) using the software ImageJ. The ratio values of GCaMP6f/mCherry were compared with those of the controls (0 hpi). (D) Quantification of disease phenotypes (HR/IH) (48 hpi) and ROS (36 hpi), GSH (48 hpi), and lipid (MDA) peroxidation (48 hpi) levels during virulent and avirulent M. oryzae infection. Data are represented as the mean ± SD (n = 4 leaf sheaths from different plants). Asterisks indicate statistically significant differences (*P< 0.05, **P< 0.01; Student’s t-test). hpi, hours post-inoculation; ns, non-significant; IH, invasive hyphae; HR, hypersensitive response; RLU, relative luminescent units; MDA, malondialdehyde.
Total RNA was extracted from rice plants using the TRIzol Reagent (Invitrogen) and used for cDNA synthesis. Transcript levels of rice Glutathione Reductase 1 (OsGR1), OsGR2, OsGR3, and Ubiquitin (OsUbi) genes were analyzed by reverse transcription polymerase chain reaction (RT-PCR) and real-time quantitative RT-PCR (real-time qRT-PCR) using gene-specific primers (Supplementary Table 1). Transcript levels of OsGR1–3 were normalized relative to that of OsUbi and presented as mean ± standard deviation (SD) of three biological replicates. The experiments were repeated three times.
To investigate Ca2+-mediated iron- and ROS-dependent ferroptotic cell death in rice during M. oryzae infection, Ca2+ influx inhibitors, including ethylene glycol-bis(2-aminoethylether)-N, N, N′, N′-tetra-acetic acid (EGTA; Sigma-Aldrich) (Atkinson et al., 1990; Cessna and Low, 2001), verapamil hydrochloride (verapamil; Sigma-Aldrich) (Beneloujaephajri et al., 2013), N-acetyl-cysteine (NAC; Sigma-Aldrich) (Sun et al., 2012), neomycin sulfate (neomycin; ChemCruz) (Franklin-Tong et al., 1996), LiCl (Tokyo Chemical Industry) (Moyen et al., 1998), AlCl3 (Sigma-Aldrich) (Kadota et al., 2005), and ruthenium red (RR; ChemCruz) (Bae et al., 2003), and Ca2+ influx enhancers, including acibenzolar-S-methyl (ASM; Sigma-Aldrich) (Brisset et al., 2000; Buonaurio et al., 2002), diamide (Sigma-Aldrich) (Kosower et al., 1969; Gilge et al., 2008), trifluoperazine hydrochloride (TFP; Sigma-Aldrich) (Kang et al., 2017), CaCl2/calcimycin (C/C; Sigma-Aldrich) (Verma et al., 2011), CaCl2/H2O2 (C/H; Sigma-Aldrich) (Rentel and Knight, 2004), rotenone (Sigma-Aldrich) (Li et al., 2003), and butylmalonic acid (BMA; Sigma-Aldrich) (Kamga et al., 2010), were applied onto rice leaf sheaths. All Ca2+ influx inhibitors and enhancers used in this study were treated on rice leaf sheaths with appropriate concentrations that never inhibited M. oryzae spore germination, appressorium formation and growth that are important for M. oryzae infection in rice. Among the Ca2+ influx enhancers, diamide and BMA were used to induce the depletion of reduced glutathione (GSH) (Gilge et al., 2008; Kamga et al., 2010), and rotenone was used to inhibit ROS burst by mitochondrial complex I (Li et al., 2003). Each Ca2+ influx inhibitor was applied onto a 5–7 cm long section of rice leaf sheath, together with avirulent M. oryzae 007 (5 × 105 conidia·mL–1) inoculation. Similarly, each Ca2+ influx enhancer (except TFP and rotenone) was applied onto a 5–7 cm long section of rice leaf sheath, together with virulent M. oryzae RO1-1 (5 × 105 conidia·mL–1) inoculation; TFP or rotenone was applied to rice sheath (5–7 cm long section) at 23 h post-inoculation (hpi) with virulent M. oryzae RO1-1. The Ca2+ influx inhibitor/enhancer treated and pathogen-inoculated rice leaf sheaths were incubated at 25°C under moist conditions. Rice leaf sheath samples were collected at different time points after inoculation with M. oryzae.
The epidermal cell layers excised from the inoculated rice leaf sheaths were observed under a microscope (Zeiss equipped with Axioplan 2; Campbell, CA), as described previously (Kankanala et al., 2007). The infected epidermal cells were counted and categorized into two types: cells with invasive hyphae (IH) and cells with hypersensitive response (HR) cell death. Infected cells of each infection type were quantified. The experiment was repeated three times.
Images of Ca2+ influx in rice sheath cells were taken using a microscope (Zeiss equipped with Axioplan2) with bright field filter, green fluorescence filter (excitation [Ex]/emission [Em] wavelengths: 450–490/515–565 nm), and red fluorescence filter (Ex/Em: 546/590 nm). At least three regions of interest (ROIs) were selected to quantify changes in Ca2+ levels by calculating the GCaMP6f/mCherry ratio at different time points or at 36 hpi using the ImageJ software. Green and red fluorescence signal intensities were measured using ImageJ installed with the Macro (Weigand et al., 2021). Data were exported into an Excel file, and the ratio of green to red fluorescence signal intensities was calculated. The values were compared with those of the mock control and expressed as relative fluorescence intensities (RFI). Images were taken using Leica TCS SP5 confocal microscope (Leica, Mannheim, Germany), with bright field, EGFP (Ex/Em: 488/500–540 nm), and mCherry (Ex/Em: 587/600–680 nm) filters, and merged.
Fluo-5F AM (Invitrogen) is a low affinity intracellular Ca2+ indicator suitable for detecting high intracellular Ca2+ levels ranging from 1 µM to 1 mM. Binding of Ca2+ to Fluo-5F is catalyzed by cellular esterases that break the ester bonds of Fluo-5F AM (Figure 2B). Intracellular Ca2+ dynamics in M. oryzae-infected rice sheaths were determined by the Ca2+ indicator Fluo-5F AM (Invitrogen) (Eaddy and Schnellmann, 2011). Fluo-5F AM was used to visualize intracellular Ca2+ ions during M. oryzae infection. Briefly, the epidermal layer recovered from the rice leaf sheaths was immersed in 0.5 M sucrose for 10 min for rapid plasmolysis. The leaf sheath epidermis was then incubated in a solution of Fluo-5F AM at a final concentration of 50 µM at 37°C for 1 h, followed by a rapid washing using 3DW. The epidermis was kept at room temperature for another 30 min to allow the reaction to occur. To visualize both Ca2+ and ROS simultaneously inside the same rice cell, a mixture of 50 µM Fluo-5F AM and 5 µM PO1 was used as described above. The above steps were performed under light-blocking conditions. Samples were observed under a fluorescence microscope (Zeiss equipped with an Axioplan 2; Campbell, CA) with a bright field (BF) filter and/or a green fluorescence (GF) filter. Fluorescence intensities of at least three regions of interest (ROIs) were selected to quantify changes in Ca2+ levels by measuring green fluorescence intensities at different time points using ImageJ software (Grossi et al., 2016). Corrected total cell fluorescence (CTCF) values were calculated as previously described (Jakic et al., 2017; Bora et al., 2021): CTCF = integrated fluorescence density – (ROI area × mean fluorescence of background readings).
Figure 2 Dynamics of Ca2+ influx in the leaf sheaths of rice Kitaake during Magnaprthe oryzae 007 (avirulent) and RO1-1 (virulent) infection. (A) Time-course images of Ca2+ influx in the rice leaf sheaths during M. oryzae infection. The rice leaf sheaths were inoculated with M. oryzae RO1-1 (virulent) and 007 (avirulent), and the Ca2+ dynamics were detected at 12, 24, 36, and 48 hpi. Rice leaf sheath cells were stained with Fluo-5F AM and were observed under a microscope (Zeiss equipped with Axioplan 2) using a bright field filter and/or the green fluorescence filter. Bars = 10 µm. WT, wild type; BF, bright field; GF, green fluorescence. hpi, hours post-inoculation. (B) Schematic diagram of Ca2+ detection by Fluo-5F AM. When Fluo-5F AM enters the cytoplasm from the apoplast, the AM is cut off by cellular esterases. When free Ca2+ binds to Fluo-5F, the Fluo-5F-Ca complex becomes fluorescent. (C) Time-course quantification of Ca2+ influx during M. oryzae infection. At least three regions of interest (ROIs) were selected to quantify Ca2+ changes by calculating the corrected total cell fluorescence (CTCF) at different time points (hpi) using the software ImageJ. Data are represented as the mean ± SD (n = 4 leaf sheaths from different plants). Asterisks above bars indicate significantly different means (**P< 0.01; Student’s t-test). hpi, hours post-inoculation; ns, non-significant.
Rice leaf sheaths were inoculated with avirulent M. oryzae 007 (4 × 105 conidia·mL-1) in 10 mM EGTA, and apoplastic washing fluid was prepared at 12, 24, 36, and 48 hpi as described previously (Rohringer et al., 1983), with some modifications. The M. oryzae-inoculated and/or EGTA-treated rice leaf sheaths were vacuum-infiltrated with 3DW (distilled water) and then centrifuged at 4,000× g. The supernatants were completely evaporated to dryness using an evaporator, and the residue was resuspended in a certain amount of 3DW. Apoplastic Ca2+ concentration in rice leaf sheaths was measured using the o-CPC method (Corns and Ludman, 1987), which is based on the reaction of Ca2+ with o-cresolphthalein complexone (o-CPC), resulting in the formation of an intense violet-colored complex. Briefly, 100 μL of intercellular fluid prepared from rice leaf sheath was added to a reaction solution containing 375 μL of 1 M ethanolamine (pH 10.6; Sigma-Aldrich), 71.6 μL of 100 mM 8-hydroxyquinoline (Sigma-Aldrich), 8.2 μL of 10 mM o-CPC (Sigma-Aldrich), 2.32 μL of 37% HCl (Samchun), and 3DW (up to a total volume of 1 mL). The absorbance of the Ca2+-o-CPC complex was measured at 575 nm using SP-2000 UV spectrophotometer (SmartPlus) (Corns and Ludman, 1987). Apoplastic Ca2+ concentration in rice leaf sheaths was calculated based on a standard curve obtained using 0–25 μM standard CaCl2 (Sigma-Aldrich).
ROS (H2O2) localization in M. oryzae-infected rice sheath cells was determined by Peroxy Orange 1 (PO1; Sigma-Aldrich) staining. The red fluorescent ROS indicator PO1 simultaneously visualizes Ca2+ (green fluorescence) and ROS accumulation inside the same infected rice cell through its distinct red fluorescence emission. Briefly, the epidermal cell layer peeled off from rice leaf sheaths was soaked in 5 µM PO1 for 40 min in the dark at room temperature (Muhlemann et al., 2018; Samuel et al., 2022). The samples were washed three times with 1× phosphate-buffered saline (PBS) and observed under a fluorescence microscope (Zeiss equipped with Axioplan 2; Campbell, CA) (Ex/Em: 546/590 nm).
A chemiluminescence assay (Singh et al., 2016; Chen et al., 2022) was performed to measure ROS levels in M. oryzae-infected rice leaf sheath cells. Briefly, small sections of epidermal cell layer (0.5 cm × 0.2 cm) were transferred into individual wells of a 96-well plate, with each well containing 100 µL of chemiluminescent solution (30 µL of luminol [Bio-Rad, Hercules, CA], 1 µL of 1 mg·mL–1 horseradish peroxidase [HRP; Jackson Immunoresearch, West Grove, PA], and 69 µL of Milli-Q water), and incubated in the dark for 5 min at room temperature. ROS chemiluminescence was detected using GloMax 96 Microplate Luminometer (Promega, Madison, WI) and expressed as relative luminescence units (RLU). Experiments were independently repeated three times.
Fe3+ accumulation in rice leaf sheath cells was detected and visualized by Prussian blue staining (Liu et al., 2007). Briefly, the epidermal layer of rice leaf sheath cells was isolated and then stained with a Prussian blue solution (7% potassium ferrocyanide [Sigma-Aldrich] and 2% HCl [Samchun], v:v = 1:1) for 15 h at room temperature and washed three times with 3DW. The stained epidermal cells were observed under a microscope (Zeiss equipped with Axioplan 2, Campbell, CA). Fe3+ were detected as a bright blue signal in sheath epidermal cells, because of their binding to ferric ferrocyanide in cells.
Lipid peroxidation in rice leaf sheath samples was determined by measuring the level of malondialdehyde (MDA), a product of unsaturated fatty acid peroxidation, using thiobarbituric acid (TBA). Rice leaf sheath tissue was ground in liquid nitrogen, and the powdered tissue was mixed with an equal amount of reaction solution (0.5% [w/v] TBA [Sigma-Aldrich], 20% [v/v] trichloroacetic acid [TCA; Sigma-Aldrich], and 0.25 mL of 175 mM NaCl in a total of 2 mL of 50 mM Tris-Cl [pH 8.0]). Samples were incubated in boiling water for 5 min and then centrifuged at 14,000 × g for 5 min at 4°C. The absorbance (optical density [OD]) of each supernatant was measured at 450, 532, and 600 nm with the SP-2000UV spectrophotometer (Woongki Science, Seoul), as previously described (Zhang et al., 2009). MDA concentration was calculated using the following equation (Dangol et al., 2019).
where CMDA is the concentration of MDA, and OD450, OD532, and OD600 represent the OD of the supernatant at 450, 532, and 600 nm, respectively.
The content of reduced glutathione (GSH) in rice leaf sheaths was measured spectrophotometrically. Freshly harvested conidial suspensions (4 × 105 conidia·mL-1) of M. oryzae strains, with or without Ca2+ influx inhibitor or enhancer, were used to inoculate rice leaf sheaths. The inoculated leaf sheaths were incubated for 48 h in the dark in a moistened box at 25°C and then ground in liquid nitrogen. Equal amounts of the powdered sample and 5% (w/v) metaphosphoric acid (Sigma-Aldrich) were mixed, and the homogenates were centrifuged at 21,000 × g for 20 min at 4°C. The supernatants were collected, and each supernatant was passed through a 0.45 µm nylon filter (Sigma-Aldrich). GSH quantification was performed as described previously (Griffith, 1980; Airaki et al., 2011). Briefly, 100 µL of the filtered supernatant was added to 600 µL of reaction buffer (100 mM sodium phosphate buffer [pH 7.5] and 1 mM EDTA [Sigma-Aldrich]). Then, 40 µL of 0.4% (w/v) 5,5’-dithiobis (2-nitrobenzoic acid) (DTNB, Sigma-Aldrich) and 350 µL of Milli-Q water were added to each sample, and the mixtures were incubated at room temperature for 5 min. To detect GSH, the absorbance of each mixture was measured at a wavelength of 412 nm using a spectrophotometer. The GSH content of each rice leaf sheath sample was quantified by constructing a calibration curve using a wide range of concentrations (0–25 µM) of standard GSH (Sigma-Aldrich).
To investigate the effect of Ca2+ influx on the conversion of glutathione disulfide (GSSG; oxidized glutathione) into GSH (reduced glutathione) by GR, 30 µL of 1 mM GSSG (Sigma-Aldrich) and 20 µL of 4.8 mM NADPH (nicotinamide adenine dinucleotide phosphate, reduced form; Sigma-Aldrich) were added to 50 mM Tris-Cl (pH 7.5). Then, 10 µL (0.06 U) of rice GR (Koma Biotech) and yeast GR (Sigma-Aldrich) each was added to the mixture to initiate the reduction of GSSG, and the total volume of the reaction mixture was increased to 1 mL. According to the Beer-Lambert Law, 0.1 mM NADPH has an optical density which is equal to 0.622 through a 1 cm light path. Therefore, because of the consumption of NADPH during the reduction of GSSG, the OD340 of the sample was expected to decrease with a molar extinction coefficient of 6.22 mmol-1·cm-1 at 340 nm. The decrease in the absorbance of each sample was measured at 340 nm using SP-2000 UV spectrophotometer (SmartPlus). The reactions were monitored for 5 min at room temperature.
To perform the inhibition assay, different volumes of 100 mM CaCl2 were added to the reaction system so that the final concentrations of CaCl2 were 0, 10, 20, 30, and 50 mM. To investigate the inhibitory effect of different cations on GSSG reduction in vitro, 20 mM CaCl2 (Sigma-Aldrich), MgCl2 (Sigma-Aldrich), NaCl (Samchun), and KCl (Duksan) were added to the reaction system. The reaction was then monitored for 5 min by detecting the decrease in OD at 340 nm.
All the results are expressed as the mean ± standard deviation (SD). Statistical comparisons were performed by the least significant difference (LSD) test and Student’s t-test using GraphPad Prism 8 software (GraphPad Software, Inc., San Diego, CA, USA).
We first examined time-course images and levels of Ca2+ influx in the leaf sheaths of rice Kitaake during M. oryzae RO1-1 (virulent) and 007 (avirulent) infection (Figure 2). During virulent (compatible) M. oryzae RO1-1 infection, primary hyphae grew from the appressorium, differentiated into thicker, bulbous invasive hyphae (IH) in the invaded rice cell to spread into neighboring cells (Figure 2A). However, avirulent (incompatible) M. oryzae 007 infection induced severe HR death response with dark brown cellular aggregates at 48 h post-inoculation (hpi) in rice leaf sheath cells. We have used the Ca2+ indicator Fluo-5F AM to monitor time-course changes in cytoplasmic Ca2+ influx by visualizing and quantifying Ca2+ fluorophores in living rice cells (Eaddy and Schnellmann, 2011). Fluo-5F is initially non-fluorescent. However, it becomes fluorescent when it binds to free Ca2+ in cells. During avirulent M. oryzae 007 infection, cytoplasmic Ca2+ influx began to appear at 12 hpi, increased markedly at 24 hpi, and peaked at 36 hpi (Figures 2A, C). At 48 hpi, extensive HR cell death occurred with dark brown cellular aggregates; however, Ca2+ influx levels decreased rapidly. By contrast, cytoplasmic Ca2+ levels were rarely or not detected in rice cells during virulent M. oryzae RO1-1 infection. We also stained rice leaf sheaths with a mixture of Fluo-5F AM and Peroxy Orange 1 (PO1) to visualize localization of Ca2+ and ROS (H2O2) accumulation (36 hpi) during infection, respectively (Figure 3A). The interplay of Ca2+ and ROS during plant immunity is a well-known phenomenon, but its mechanisms are not fully understood. Interestingly, we observed a marked co-localization of Ca2+ and ROS accumulation around invasive hyphae (IH) as well as inside the invaded and neighboring cells of rice leaf sheaths at 36 hpi with avirulent M. oryzae 007 (Figure 3A). To determine the role of ferric ions (Fe3+) in HR cell death during M. oryzae infection, we further stained rice leaf sheath tissues with Prussian blue solution to detect Fe3+, as described previously (Dangol et al., 2019). Unlike Ca2+ or ROS accumulation, Fe3+ was observed (blue color) at hyphal invasion sites in the HR cell death response of rice leaf sheath cells at 48 hpi with avirulent M. oryzae 007 (Figure 3A). By contrast, Ca2+, ROS and iron (Fe3+) accumulation was not detected in the rice sheath cells infected by virulent M. oryzae RO1-1 (Figure 3A). Avirulent M. oryzae 007 infection, but not virulent M. oryzae RO1-1 infection, significantly induced Ca2+ and ROS accumulation (36 hpi), iron accumulation (48 hpi), HR cell death (48 hpi), reduced glutathione (GSH, γ-L-glutamyl-L-cysteinylglycine) depletion (48 hpi), and lipid peroxidation (48 hpi) in rice leaf sheath cells (Figures 3A, D).
Figure 3 Images and quantification of Ca2+ influx, ROS and iron accumulation, hypersensitive response (HR) cell death, reduced glutathione (GSH) depletion, and lipid peroxidation in rice leaf sheaths during Magnaporthe oryzae infection. (A) Images of Ca2+ influx and ROS and iron accumulation in rice leaf sheaths during M. oryzae infection. The leaf sheaths of Kitaake (WT) were inoculated with M. oryzae RO1-1 (virulent) and 007 (avirulent). Rice leaf sheath cells were stained with a mixture of Fluo-5F AM and Peroxy Orange 1 (PO1) to visualize Ca2+ influx and H2O2 accumulation in the rice cell during infection. Fe3+ accumulation was detected by Prussian blue staining. Images of Ca2+ influx, H2O2 accumulation, and Fe3+ accumulation in rice leaf sheaths were observed under a microscope (Zeiss equipped with Axioplan 2) using a bright field filter and/or fluorescence filters. Bars = 10 µm. Red arrows indicate invasive hyphae (IH). WT, wild type; BF, bright field. (B) Quantification of infection phenotypes, classified as cells showing invasive hyphal (IH) growth and HR cell death, in the leaf sheaths (48 hpi). (C) ROS quantification (36 hpi) in rice cells via a chemiluminescence assay using GloMax 96 Microplate Luminometer (Promega, Madison, WI). Values are mean ± standard deviation (SD; n = 10) of the total relative luminescence units (RLU) of different rice sheath discs. (D) Quantification of GSH levels in rice leaf sheaths (48 hpi). Values represent mean ± SD (n = 4) of GSH concentrations in the leaf sheaths of different plants. GSH reacts with 5,5’-dithiobis (2-nitrobenzoic acid) to generate 2-nitro-5-thiobenzoic acid, which was measured using the SP-2000UV spectrophotometer (Woongki Science, Seoul) at a wavelength of 412 nm. (E) Quantification of lipid peroxidation in rice leaf sheaths (48 hpi) by measuring malondialdehyde (MDA) levels. Values are mean ± SD (n = 4) of MDA concentrations in the leaf sheaths of different plants. Asterisks above bars indicate significantly different means (*P< 0.05, **P< 0.01; Student’s t-test). Experiments were repeated three times with similar results. hpi, hours post-inoculation.
The Ca2+ sensor was designed as the mCherry fused to the N-terminus of GCaMP6f and consisted of two tandem fluorescent proteins (Weigand et al., 2021). The protein-based Ca2+ sensor (GCaMP6fmC) was used as an alternative tool to compare two separate cytoplasmic Ca2+ influxes in the wild type and Ca2+ sensor transgenic rice lines. To investigate if Ca2+ mediates ferroptotic cell death in rice immunity, we next generated Ca2+ sensor rice (cultivar Kitaake) transgenic lines as an alternative Ca2+ visualization tool, in which GCaMP6f-mCherry fusion Ca2+ reporter (GCaMP6fmC) can be expressed to emit strong green fluorescence by binding of Ca2+ to the calmodulin domain (Figure 1A; Supplementary Figure 1). The Ca2+ sensor (GCaMP6fmC) made it possible to specifically detect and visualize intracellular Ca2+ changes inside living rice cells without any additional calcium indicator staining. Avirulent (incompatible) M. oryzae 007 grew poorly, with only a few invasive hyphae (IH) leading to HR cell death in rice leaf sheaths (Figures 2A, 1B, D). Ca2+ influx levels in the rice leaf sheaths were measured by confocal and fluorescence microscopy (Figure 2A, green fluorescence in the GF images; Figure 1B; Supplementary Figures 1C, 2). Peroxy Orange 1 and Prussian blue staining showed the accumulation of ROS (H2O2) and Fe3+, respectively, in leaf sheath cells (Figure 1B, red fluorescence in the RF images for ROS and blue-colored deposits for Fe3+). Ca2+ and ROS accumulation was significantly induced around the IH and within the invaded rice cells at 36-48 hpi with avirulent M. oryzae 007 (Figures 1B, D). By contrast, ROS accumulation was not detected in rice cells infected with virulent M. oryzae RO1-1. Avirulent M. oryzae 007, but not virulent M. oryzae RO1-1, significantly induced Fe3+ accumulation inside and around the IH in rice sheath cells at 36-48 hpi (Figure 1B). Fe3+ was observed as a blue color inside the IH and at the hyphal invasion sites in the HR cell death response where ROS accumulated. HR cell death was detected as vesicle-containing dark brown cellular aggregates inside the infected rice cells at 48 hpi (Figure 1B). During avirulent M. oryzae infection, abundant ROS bursts and HR cell death at 48 hpi were accompanied by significant depletion of reduced glutathione (GSH, γ-L-glutamyl-L-cysteinylglycine) and significant increase in lipid peroxidation [malondialdehyde (MDA) levels] (Figures 1B, D). GSH is one of the major water-soluble small molecule antioxidants that protect plant cells from oxidative damage (Airaki et al., 2011).
Ca2+ influx into the leaf sheath cells of Ca2+ sensor (GCaMP6fmC) transgenic line B was quantified over time during M. oryzae RO1-1 (virulent) and 007 (avirulent) infection (Figure 1C). During avirulent M. oryzae 007 infection, we observed M. oryzae appressorium formation at 12 hpi, hyphal invasion initiation at 24 hpi, strong Ca2+ influx at 18-36 hpi, abundant HR cell death at 48 hpi, along with ROS and iron accumulation, GSH depletion, and lipid peroxidation (Figures 1B, D). Overall, our data suggest that avirulent M. oryzae 007 infection induces higher levels of Ca2+ influx to mediate ROS- and iron-dependent ferroptotic cell death compared with virulent M. oryzae RO1-1 infection (Figures 2-1; Supplementary Figure 2).
During avirulent M. oryzae 007 infection, Ca2+-mediated ROS and iron accumulation in cells induced ferroptotic HR cell death (Figure 4). Avirulent M. oryzae infection induced high levels of Ca2+ influx and ROS and iron accumulation in rice leaf sheath cells, as visualized by confocal and fluorescence microscopy (Figure 4A). We applied the membrane-nonpermeable calcium chelator ethylene glycol-bis(2-aminoethylether)-N, N, N′, N′-tetra-acetic acid (EGTA) (Ellis-Davies and Kaplan, 1994) onto rice leaf sheaths to investigate whether EGTA blocks Ca2+ influx from the apoplast to the cytoplasm during rice HR cell death. EGTA (10 mM) treatment inhibited the accumulation of Ca2+, ROS and iron, and the induction of HR cell death by avirulent M. oryzae infection, which ultimately allowed the fungal hyphae to grow normally inside the leaf sheath cells (Figure 4A). We also quantified the infection phenotypes (IH and HR), Ca2+ influx, ROS production, and GSH and MDA levels in mock (water)- and EGTA-treated rice Kitaake and Ca2+ sensor (GCaMP6fmC) transgenic line B at different times after inoculation with avirulent M. oryzae 007 (Figures 4B, C). EGTA-treated leaf sheaths contained more pathogen-infected cells but fewer HR cells, compared to the mock (water)-treated leaf sheaths during avirulent M. oryzae 007 infection (Figure 4B). EGTA treatment significantly inhibited Ca2+ influx and ROS production, correlated with strong GSH production and reduced lipid peroxidation in avirulent M. oryzae 007-infected rice cells (Figure 4C).
Figure 4 EGTA suppresses Ca2+-mediated ROS- and iron-dependent HR cell death in rice immune responses. (A) Images of HR cell death (48 hpi), Ca2+ influx (36 hpi), ROS accumulation (36 hpi), and Fe3+ accumulation (48 hpi) in the leaf sheaths of rice Kitaake (WT) and Ca2+ sensor (GCaMP6fmC) transgenic line B during avirulent M. oryzae 007 infection after 10 mM EGTA treatment. Bars = 10 µm. (B) Quantification of IH/HR infection phenotypes (48 hpi). (C) Quantification of Ca2+ influx (36 hpi), ROS accumulation (36 hpi), reduced glutathione (GSH) levels (48 hpi), and lipid peroxidation (MDA) levels (48 hpi). For Ca2+ quantification, at least three ROIs were selected to calculate the GCaMP6f/mCherry ratio at 36 hpi using the software ImageJ. The ratio values of GCaMP6f/mCherry were compared with that of the control (Mock, Kitaake-M. oryzae 007). Data are represented as the mean ± SD (n = 4 leaf sheaths from different plants). Different letters above the bars indicate significantly different means, as determined by the least significant difference (LSD) test (P< 0.05). WT, wild type (Kitaake); EGTA, ethylene glycol-bis(2-aminoethylether)-N, N, N′, N′-tetra-acetic acid; hpi, hours post-inoculation; IH, invasive hyphae; HR, hypersensitive response; RLU, relative luminescent units; MDA, malondialdehyde.
Binding of Ca2+ to EGTA forms the EGTA-Ca complex (Figure 5A). To investigate if EGTA causes chelation of apoplastic Ca2+ in rice cells, we prepared apoplastic washing fluid from M. oryzae-inoculated and/or EGTA-treated rice leaf sheaths (Figure 5; Supplementary Figure 3). Ca2+ in the intercellular (apoplastic) fluid of rice leaf sheaths was detected using the calcium-o-cresolphthalein complexone (o-CPC) method (Supplementary Figure 3A). EGTA treatment markedly lowered apoplastic Ca2+ levels to block Ca2+ influx into the cytoplasm of rice leaf sheaths throughout avirulent M. oryzae 007 infection, ultimately suppressing ferroptotic HR cell death, but leading to normal fungal growth and susceptible disease (Figures 5B, C; Supplementary Figure 3B). Avirulent M. oryzae 007 infection resulted in 83.93% of HR cell death in rice sheaths. However, EGTA treatment significantly reduced HR cell death to 16.60%, which is comparable to virulent M. oryzae RO1-1 infection (Figure 5C). Together, our data suggest that the membrane-nonpermeable calcium chelator EGTA effectively blocks apoplastic Ca2+ influx into the cytoplasm to inhibit Ca2+-mediated iron-dependent ROS accumulation leading to the formation of normal M. oryzae hyphal structure and susceptible blast disease.
Figure 5 Ca2+ chelation by EGTA suppresses ferroptotic cell death in rice leaf sheaths during avirulent Magnaporthe oryzae 007 infection. (A) Schematic diagram of apoplastic Ca2+ chelation by EGTA. The binding of Ca2+ to EGTA forms the EGTA-Ca complex. (B) Quantification of apoplastic Ca2+ concentrations and HR cell death in rice (Kitaake) leaf sheaths treated with 10 mM EGTA during M. oryzae RO1-1 (virulent) and 007 (avirulent) infection. Data are represented as the mean ± SD (n = 4 leaf sheaths from different plants). Asterisks indicate significantly different means (**P< 0.01; Student’s t-test). Different letters above the bars indicate significantly different means, as determined by the least significant difference (LSD) test (P< 0.05). Experiments were repeated three times with similar results. (C) Schematic diagram of inhibition of ferroptotic cell death by apoplastic Ca2+ chelation using EGTA. During avirulent M. oryzae 007 infection, EGTA treatment effectively inhibits apoplastic Ca2+ influx into the cytoplasm to completely attenuate ferroptotic HR cell death in rice sheaths, which ultimately leads to the formation of normal hyphal structures and susceptible disease.
Acibenzolar-S-methyl (ASM) is one of the most effective plant activators that can induce systemic acquired resistance (SAR) against a broad range of plant pathogens (Matsuo et al., 2019). During virulent M. oryzae RO1-1 infection, fungal hyphae grew well inside the rice leaf sheath epidermal cells (Figure 6A). However, compared with the mock (water) treatment, ASM treatment effectively induced HR cell death and ROS and iron accumulation in virulent M. oryzae-infected rice cells (Figures 6A, B). ASM treatment reduced the number of cells with IH, but increased the number of cells with HR, in susceptible rice leaf sheaths infected (Figure 6B). ROS and iron accumulation in rice cells was detected by Peroxy Orange 1 and Prussian blue staining, respectively (Figure 6A). We also visualized Ca2+ influx under confocal and fluorescence microscopy (Figure 6A). The quantification of Ca2+ influx revealed that ASM treatment induced Ca2+ influx compared with the mock treatment (Figure 6C). A chemiluminescent assay showed that ASM treatment significantly induced the accumulation of ROS in rice leaf sheath cells at 36 hpi (Figure 6C). However, ASM treatment inhibited GSH accumulation, but enhanced lipid peroxidation (MDA levels), in rice leaf sheath cells at 48 h after inoculation with virulent M. oryzae RO1-1 (Figure 6C). Collectively, these results suggest that ROS and iron accumulation, GSH depletion and lipid peroxidation are required for ASM-induced Ca2+-mediated ferroptotic cell death during rice-M. oryzae interactions.
Figure 6 ASM induces Ca2+-mediated ROS- and iron-dependent HR cell death in rice immune responses. (A) Images of HR cell death (48 hpi), Ca2+ influx (36 hpi), ROS accumulation (36 hpi), and ferric ion accumulation (48 hpi) in the leaf sheaths of rice Kitaake (WT) and Ca2+ sensor (GCaMP6fmC) transgenic line B during virulent M. oryzae RO1-1 infection after 125 µM acibenzolar-S-methyl (ASM) treatment. Bars = 10 µm. (B) Quantification of IH/HR infection phenotypes (48 hpi). (C) Quantification of Ca2+ influx (36 hpi), ROS accumulation (36 hpi), reduced glutathione (GSH) levels (48 hpi), and lipid peroxidation (MDA) levels (48 hpi). For Ca2+ quantification, at least three ROIs were selected to calculate the GCaMP6f/mCherry ratio at 36 hpi using the software ImageJ. The ratio values of GCaMP6f/mCherry were compared with that of the control (Mock, Kitaake-M. oryzae RO1-1). Data are represented as the mean ± SD (n = 4 leaf sheaths from different plants). Different letters above the bars indicate significantly different means, as determined by the least significant difference (LSD) test (P< 0.05). WT, wild type; hpi, hours post-inoculation; IH, invasive hyphae; HR, hypersensitive response; RLU, relative luminescent units; MDA, malondialdehyde.
Based on the knowledge of pharmacological Ca2+ channel modulation identified in animal systems, we selected some cytoplasmic Ca2+ influx regulators to investigate whether exogenous chemicals control Ca2+-mediated iron- and ROS-dependent ferroptotic cell death in the rice immune response. Different Ca2+ influx inhibitors, including EGTA (Atkinson et al., 1990; Cessna and Low, 2001), verapamil hydrochloride (verapamil) (Beneloujaephajri et al., 2013), N-acetyl-cysteine (NAC) (Sun et al., 2012), neomycin sulfate (neomycin) (Franklin-Tong et al., 1996), lithium chloride (LiCl) (Moyen et al., 1998), aluminum chloride (AlCl3) (Kadota et al., 2005), and ruthenium red (RR) (Bae et al., 2003) were applied onto the rice leaf sheaths infected with avirulent M. oryzae 007 to compare their effects on Ca2+ influx, ROS accumulation, lipid peroxidation, and HR cell death (Figures 4, 7A; Supplementary Figure 4).
Figure 7 Ca2+ influx regulators differentially control Ca2+-mediated iron-and ROS-dependent ferroptotic cell death in rice immune responses. (A, B) Quantifications of Ca2+ influx (36 hpi), ROS accumulation (36 hpi), lipid peroxidation (MDA levels) (48 hpi), and HR cell death (48 hpi) in the leaf sheath cells of rice (Kitaake) Ca2+ sensor (GCaMP6fmC) transgenic line B infected with M. oryzae 007 (avirulent) and M. oryzae RO1-1 (virulent) and treated with Ca2+ influx inhibitors. (A) Ca2+ influx inhibitors included ethylene glycol-bis(2-aminoethylether)-N, N, N′, N′-tetra-acetic acid (EGTA), verapamil hydrochloride (verapamil), N-acetyl-cysteine (NAC), neomycin sulfate (neomycin), lithium chloride (LiCl), aluminum chloride (AlCl3) and ruthenium red (RR). (B) Ca2+ influx enhancers included acibenzolar-S-methyl (ASM), diamide, trifluoperazine dihydrochloride (TFP), CaCl2/calcimycin (C/C), CaCl2/H2O2 (C/H), rotenone and butylmalonic acid (BMA). For Ca2+ quantification, at least three ROIs were selected to calculate the GCaMP6f/mCherry ratio at 36 hpi using the software ImageJ. The ratio values of Ca2+ influx regulators were compared with those of the controls (M. oryzae 007 or M. oryzae RO1-1). Data are represented as the mean ± SD (n=4 leaf sheaths from different plants). Different letters above the bars indicate significantly different means, as determined by the LSD test (P< 0.05). hpi, hours post- inoculation; MDA, malondialdehyde.
Treatment with the calcium chelator EGTA dramatically inhibited Ca2+ influx, ROS and iron accumulation and HR cell death in rice cells compared with the other tested Ca2+ influx inhibitors during avirulent M. oryzae infection (Figures 4, 7A). However, other Ca2+ influx inhibitors showed variation in the suppression of Ca2+ influx, ROS accumulation, lipid peroxidation (MDA level) and HR cell death (Figure 7A; Supplementary Figure 4). Verapamil treatment is known to reduce cytoplasmic Ca2+ levels with attenuated ROS bursts in plant cells (Beneloujaephajri et al., 2013). Inhibition of Ca2+ influx by verapamil reduced cytoplasmic Ca2+ and ROS accumulation, lipid peroxidation and HR ferroptotic cell death, as detected by Prussian blue staining (Figure 7A; Supplementary Figure 4). NAC treatment increased GSH concentration and consequently reduced ROS accumulation, lipid peroxidation, and HR cell death, accompanied by decreased Ca2+ levels and increased M. oryzae infection (Figures 7A, 8D; Supplementary Figure 4). LiCl inhibits the release of intracellular Ca2+ from vacuoles (Moyen et al., 1998). The two-pore channel 1 (TPC1) channel family is a ROS-responsive Ca2+ channel, and aluminum is a specific blocker for TPC1, a voltage-dependent Ca2+ permeable channel (Kawano et al., 2004). TPC1 plays an important role in inducing ROS-dependent cytoplasmic Ca2+ influx during oxidative stress (Kadota et al., 2005). Neomycin, LiCl, AlCl3, and RR significantly inhibited Ca2+ influx during avirulent M. oryzae 007 infection (Figure 7A; Supplementary Figure 4). They also significantly reduced the ROS accumulation during avirulent M. oryzae 007 infection (Figure 7A; Supplementary Figure 4). They were relatively effective in limiting lipid peroxidation (MDA level) and HR cell death (Figure 7A; Supplementary Figure 4). The Ca2+ influx inhibitors also suppressed Fe3+ accumulation and HR cell death induced by avirulent M. oryzae 007 infection, which led to the successful colonization of IH as detected by Prussian blue staining (Supplementary Figure 4). These data indicate that different Ca2+ influx inhibitors significantly limit Ca2+-mediated ROS and iron accumulation, and lipid peroxidation, allowing the formation of normal hyphal structures of avirulent M. oryzae 007 in rice leaf sheaths and leading to blast disease (Figures 4, 7A; Supplementary Figure 4).
Figure 8 Ca2+ influx triggers reduced OsGR expression and GSH depletion during Magnaporthe oryzae infection. (A) Ca2+ inhibits GSSG reduction to GSH by glutathione reductase (GR). (B) Effects of CaCl2, MgCl2, KCl, and NaCl on the conversion of GSSG to GSH by rice GR. (C) Relative expression of rice Glutathione Reductase 2 (OsGR2) in the leaf sheaths of rice (Kitaake) during M. oryzae RO1-1 (virulent) and M. oryzae 007 (avirulent) infection. (D) Comparison of GSH levels in rice leaf sheaths treated with different Ca2+ influx regulators during M. oryzae infection. The ratio values of Ca2+ influx regulators were compared with those of the controls (M. oryzae 007 or M. oryzae RO1-1). Data are represented as the mean ± SD (n = 4 leaf sheaths from different plants). Asterisks indicate significantly different means (**P< 0.01; Student’s t-test). Different letters above the bars indicate significantly different means, as determined by the LSD test (P< 0.05). hpi, hours post-inoculation; GSSG, glutathione disulfide; GSH, reduced glutathione.
Different Ca2+ influx enhancers, including ASM (Brisset et al., 2000; Buonaurio et al., 2002), diamide (Kosower et al., 1969; Gilge et al., 2008), trifluoperazine dihydrochloride (TFP) (Kang et al., 2017), CaCl2/calcimycin (C/C) (Verma et al., 2011), CaCl2/H2O2 (C/H) (Rentel and Knight, 2004), rotenone (Li et al., 2003), and butylmalonic acid (BMA) (Kamga et al., 2010) were applied onto rice leaf sheaths infected with virulent M. oryzae RO1-1 to compare their effects on Ca2+ influx, ROS accumulation, lipid peroxidation, and HR cell death (Figures 6, 7B; Supplementary Figure 5). ASM treatment significantly enhanced Ca2+ influx, ROS and iron accumulation and HR cell death in rice leaf sheath cells during virulent M. oryzae infection compared with the other tested Ca2+ influx enhancers (Figures 6, 7B).
Other Ca2+ influx enhancers differentially induced Ca2+ influx, ROS accumulation, lipid peroxidation (MDA level) and HR cell death during virulent M. oryzae infection (Figure 7B; Supplementary Figure 5). Diamide, TFP, C/C, C/H, and BMA differentially induced Ca2+ influx; however, the induction of Ca2+ influx by rotenone was not significant. Diamide, TFP, C/C, C/H, and rotenone increased ROS levels by at least 15.98%. However, BMA did not affect ROS levels during infection. These Ca2+ influx enhancers also increased only slightly MDA levels in rice leaf sheath cells. However, diamide, TFP, C/C, C/H, rotenone, and BMA increased HR cell death up to 22.06-26.23% (Figure 7B). Prussian blue staining showed that Ca2+ influx enhancers effectively stimulated Fe3+ accumulation inside and around IH in rice cells during virulent M. oryzae infection (Supplementary Figure 5). These data indicate that Ca2+ influx enhancers trigger robust Ca2+ influx to varying degrees in compatible (susceptible) rice cells due to their different mode of actions, leading to iron- and ROS-dependent ferroptotic HR cell death in response to virulent M. oryzae infection (Figures 6, 7B; Supplementary Figure 5).
Glutathione reductase (GR) reduces glutathione disulfide (GSSG; oxidized glutathione) to produce reduced glutathione (GSH) in the presence of NADPH which can be converted to NADP+ in plant cells (Figure 8A). In vitro, increasing Ca2+ concentration from 10 mM to 50 mM gradually inhibited the activity of rice GR or yeast GR (Figure 8B; Supplementary Figures 6A, D), indicating that higher Ca2+ concentration more effectively inhibits the reduction of GSSG to GSH by GR. Lineweaver-Burt plot showed non-competitive inhibition of GSSG reduction to GSH through rice GR or yeast GR by increasing Ca2+ concentration from 10 mM to 50 mM (Supplementary Figures 6B, E). Several metal ions, including Ca2+, Mg2+, K+, and Na+, which are abundant intracellular cations in plant cells, exhibited different inhibitory effects on the reduction of GSSG to GSH by rice GR or yeast GR in vitro (Tandoğan and Ulusu, 2007); however, Ca2+ was the most effective in inhibiting the reduction of GSSG to GSH (Figure 8B; Supplementary Figures 6C, F). This suggests that Ca2+ may more specifically inhibit OsGR activity and deplete GSH production than the other ions Mg2+, K+, and Na+ in rice cells.
The antioxidant enzyme glutathione reductase (GR) is responsible for maintaining the supply of reduced glutathione (GSH) for the cellular control of ROS inside cells (Couto et al., 2016). GR plays an important role in scavenging ROS to regulate the redox state of glutathione in plants (Wang et al., 2018). During avirulent M. oryzae infection, the expression of rice cytoplasmic OsGR2 was effectively reduced relative to chloroplast OsGR1 and OsGR3 in leaf sheath cells compared with virulent M. oryzae infection (Figure 8C; Supplementary Figure 7). This indicates that avirulent M. oryzae infection significantly downregulates cytoplasmic OsGR expression to inhibit the reduction of GSSG to GSH. GSH depletion and lipid peroxidation are essential signaling events leading to iron- and lipid ROS-dependent ferroptotic cell death during avirulent M. oryzae infection (Dangol et al., 2019). During avirulent M. oryzae infection, treatment with the Ca2+ influx inhibitors EGTA and verapamil effectively rescued GSH depletion, increasing GSH content by 32.2%, and 28.3%, respectively (Figure 8D). The other Ca2+ influx inhibitors also more significantly increased GSH content compared with that of the control M. oryzae infection alone. However, during virulent M. oryzae infection, the Ca2+ influx enhancer ASM significantly decreased GSH production by 30.2% (Figure 8D). The other Ca2+ influx enhancers also more significantly reduced GSH production compared with that of the control M. oryzae infection alone.
Iron- and ROS-dependent ferroptotosis occurs not only in animals (Dixon et al., 2012), but also in plants (Dangol et al., 2019; Nguyen et al., 2022). Fe2+ present in the cell is highly reactive with ROS (H2O2), which subsequently produces Fe3+ and hydroxyl radicals (·OH) (Fenton, 1894; Pierre and Fontecave, 1999). Ferric ions (Fe3+) and ROS accumulated in rice tissues undergoing HR cell death during avirulent M. oryzae infection (Dangol et al., 2019). Iron- and ROS-dependent signaling is required for the ferroptotic cell death pathway in rice to disrupt M. oryzae infection. Rice iron storage protein ferritin 2 (OsFER2) could positively regulate rice ferroptosis and immune responses against M. oryzae infection (Nguyen et al., 2022). Mitogen-activated protein kinase (MAPK) signaling cascades are involved in plant immunity and HR cell death responses to pathogen infection (Meng and Zhang, 2013; Thulasi Devendrakumar et al., 2018). Rice MAP kinase (OsMEK2 and OsMPK1) expression triggered iron- and ROS-dependent ferroptotic cell death (Dangol et al., 2021).
Calcium (Ca2+) is a secondary messenger that mediates diverse signaling pathways in eukaryotic cells (Zhao et al., 2021). In plants, Ca2+ influx is required for HR cell death in immune responses (Atkinson et al., 1990; Grant and Loake, 2000; Moeder et al., 2019). The ZAR1 resistosome is a calcium-permeable channel triggering plant immune signaling (Bi et al., 2021). Given the key role of calcium in plant immune responses and the emerging role of resistosomes as novel Ca2+ channels in plants, we hypothesized that Ca2+ acts as a key trigger of iron- and ROS-dependent ferroptotic cell death in rice immunity. In this study, we suggest that cytoplasmic Ca2+ influx through calcium-permeable cation channels, including the putative resistosomes, mediates iron- and lipid ROS-dependent ferroptotic cell death under reduced OsGR expression levels in rice immune responses.
To study the role of cytoplasmic Ca2+ influx in plant cells, it is absolutely necessary to accurately monitor fine spatial and temporal changes in intracellular Ca2+ concentration (Choi et al., 2012). The discovery and application of the Ca2+-sensitive bioluminescent photoprotein aequorin, made it possible to detect changes in cytoplasmic Ca2+ in the submicromolar range (Shimomura et al., 1963). However, despite advances in the Ca2+ detection techniques, the difficult detection and imaging of the weak luminescence signal of aequorin have been a limiting factor for its use in Ca2+ research (Hepler, 2005). Ca2+ indicators used for intracellular Ca2+ monitoring are mainly small molecules or proteins with highly specific sensitivity and responsiveness to intracellular Ca2+ (Kanchiswamy et al., 2014). The emerging role of resistosomes as Ca2+-permeable channels has stimulated the study of intracellular Ca2+ in plant immunity (Bi et al., 2021; Jacob et al., 2021). Accurate spatial and temporal Ca2+ imaging approaches are required to monitor resistsome-mediated Ca2+ influx into living rice cells during M. oryzae infection. Genetically encoded Ca2+ sensors that can be expressed inside the transgenic plant cells are preferably used because they do not require a staining step for Ca2+ detection.
In this study, we used fluorescence-based chemical Ca2+ indicator (Fluo-5F AM) and protein Ca2+ sensor (GCaMP6fmC), which are relatively more reliable compared to luminescence, to detect Ca2+ changes in living cells during cell death in rice immunity. The non-ratiometric Fluo Ca2+ indicator was first introduced to monitor Ca2+ influx in Lima bean leaf cells in response to herbivore attack (Maffei et al., 2004). Here, we selected Fluo-5F AM as a low-affinity intracellular Ca2+ indicator suitable for detecting high intracellular Ca2+ levels in the range of 1 μM to 1 mM in rice cells. We next used a genetically encoded ratiometric Ca2+ sensor (GCaMP6fmC) (Weigand et al., 2021) to investigate cytoplasmic Ca2+ influx in rice sheath cell during M. oryzae infection. The two different fluorescence-based Ca2+ detection tools were first shown to be highly reliable for detecting and quantifying changes in cytoplasmic Ca2+ levels in living rice cells during M. oryzae infection. In parallel with Ca2+ imaging, staining with Peroxy Orange 1 (PO1), a red fluorescent ROS indicator, revealed that the ROS burst occurs in the same region of the rice cell where Ca2+ influx occurs during avirulent M. oryzae infection. The red fluorescent ROS indicator PO1 could simultaneously visualize Ca2+ (green fluorescence) and ROS accumulation inside the same rice cell through its distinct red fluorescence emission.
In the current study, we found that avirulent M. oryzae infection triggers a robust Ca2+ influx, which mediates iron- and ROS-dependent ferroptotic cell death in rice. The strong ROS burst is a cellular signaling event that occurs in HR cell death in plant immunity (Van Breusegem and Dat, 2006; Jwa and Hwang, 2017). Pathogen effectors can interact with NLR receptors of host plants, leading to ROS burst and HR cell death in plant immune responses (Jones and Dangl, 2006; Dangl et al., 2013; Han and Hwang, 2017). The ZAR1 resistosome has recently been proposed to be a calcium-permeable channel triggering plant immunity and cell death (Bi et al., 2021). Ca2+ influx from the apoplast to the cytoplasm may mediate iron- and ROS-dependent ferroptotic HR cell death in rice immunity. Plant cells maintain a steady state Ca2+ concentration of 100-200 nM in contrast to the Ca2+ concentration in the extracellular space at 1-10 mM (Martins et al., 2013). The steep Ca2+ concentration gradient between the cytoplasm (~100 nM) and apoplast (~1 mM) (Stael et al., 2012) may rapidly increase cytoplasmic Ca2+ influx through the resistosome, a membrane-localized Ca2+-permeable channel, which triggers calcium signaling of ROS- and iron-dependent ferroptotic cell death in rice immunity. Avirulent M. oryzae infection sustained high iron and ROS accumulation in ferroptotic HR cell death, with a layer of high Ca2+ concentration near the cell membrane, GSH depletion, and lipid peroxidation. The maintenance of a persistently high Ca2+ concentration gradient near the cell membrane is an ionic stress, which can have a significant impact on ambient ROS burst, iron accumulation, and antioxidant defense machineries. Ferroptotic HR cell death may only be caused by prolonged Ca2+/ROS bursts during ETI, but not transient Ca2+/ROS bursts during PTI (Grant and Loake, 2000). It is thus likely that continuous Ca2+ influx into the cytoplasm through the calcium-permeable cation channels, including the putative NLR resistosomes, mediates iron- and ROS-dependent ferroptotic HR cell death in the rice immune response.
Different Ca2+ influx inhibitors such as EGTA, verapamil, NAC, neomycin, LiCl, AlCl3, and RR significantly suppressed cytoplasmic Ca2+ influx, ROS accumulation, glutathione (GSH) depletion, and lipid peroxidation, leading to reduced iron- and ROS-dependent ferroptotic HR cell death during avirulent M. oryzae infection. Notably, the membrane-impermeable Ca2+ chelator EGTA (Ellis-Davies and Kaplan, 1994; Cessna and Low, 2001) and the Ca2+ channel blocker verapamil (Beneloujaephajri et al., 2013) more effectively suppressed GSH depletion in rice sheaths than other Ca2+ influx inhibitors during avirulent M. oryzae infection. Ca2+ chelation by EGTA blocked Ca2+ influx from the apoplastic environment into the cytosol of rice cells during infection. EGTA can deplete extracellular free Ca2+ sources through high-affinity Ca2+ chelation, which essentially blocks Ca2+ influx from the apoplast. Resistosomes are thought to act as Ca2+-permeable channels that connect the cytoplasm to a high-level extracellular Ca2+ pool (Bi et al., 2021). The binding of Ca2+ to EGTA may prevent Ca2+ from freely diffusing across the cell membrane and consequently lower cytoplasmic Ca2+ levels.
Verapamil limits the transport of extracellular Ca2+ across the plasma membrane into the cytosol (Bergson et al., 2011). However, the inhibitory effect of verapamil on ferroptotic cell death was significantly lower than that of Ca2+ chelation with EGTA. NAC is a precursor of the antioxidant glutathione, which inhibits ROS levels and the oxidation of cellular glutathione (Sun et al., 2012). Neomycin acts as an inhibitor of polyphosphoinositide hydrolysis in animals and also inhibits Ca2+-dependent polyphosphoinositide-specific phospholipase C (PLC) activity in plants (Franklin-Tong et al., 1996). RR, a non-competitive inhibitor of the mitochondrial uniporter, is responsible for Ca2+ uptake into mitochondria in animal cells (Bae et al., 2003), and it also inhibits plant cell cation channels in a voltage-independent manner (White, 1996). Taken together, the seven different Ca2+ influx inhibitors tested in this study significantly inhibit Ca2+-mediated ROS, iron accumulation, GSH depletion, and lipid peroxidation, which ultimately increase the growth of invasive hyphae (IH) inside rice cells during avirulent M. oryzae infection leading to blast disease.
Plant cells have high Ca2+ levels in both the apoplast and internal stores, and the cytoplasmic Ca2+ influx can increase intracellular Ca2+ concentration (Stael et al., 2012). Different Ca2+ influx enhancers ASM, diamide, TFP, CaCl2/calcimycin (C/C), CaCl2/H2O2 (C/H), rotenone, and BMA differentially induced Ca2+ influx, ROS accumulation, GSH depletion, lipid peroxidation (MDA level) leading to iron- and ROS-dependent ferroptotic HR cell death during virulent M. oryzae infection. The plant defense activator ASM, a salicylic acid (SA) analogue, induces systemic acquired resistance (SAR) against a broad spectrum of plant pathogens and is widely used for crop protection (Buonaurio et al., 2002; Matsuo et al., 2019). ASM significantly enhanced Ca2+ influx, as well as iron and ROS accumulation leading to ferroptotic cell death in rice even during virulent M. oryzae infection. ASM induced the highest increase in cytoplasmic Ca2+ level among the seven Ca2+ enhancers tested and effectively inhibited virulent M. oryzae infection. These results suggest that the enhancement of Ca2+ influx by ASM may activate SAR via resistosomes in plants, thereby contributing to disease control.
Diamide is a cell-permeable chemical oxidant that can specifically target thiols of GSH and free SH groups in proteins (Kosower et al., 1969; Gilge et al., 2008). Diamide treatment reduced intracellular GSH concentration as expected. The increase in Ca2+ levels induced a decrease in GSH, which increased ROS accumulation, lipid peroxidation, and HR cell death, resulting in a decrease in M. oryzae growth. These results suggest that reduced cytoplasmic GSH levels play a crucial role in iron- and lipid ROS-dependent HR cell death in rice immunity. TFP directly dissociates calmodulin (CaM) from IP3R [IP3 (inositol 1,4,5-trisphosphate) receptor] by interacting at the TFP-binding site of CaM and opens IP3R to release large amounts of Ca2+ from intracellular stores such as the endoplasmic reticulum (ER) in animals (Kang et al., 2017). Calcium ionophore A23187 (calcimycin), a hydrophobic molecule, can selectively bind to Ca2+ and permeate the hydrophobic interior of lipid bilayers, increasing cell permeability to Ca2+ (Verma et al., 2011). H2O2 is known to induce a biphasic cytoplasmic Ca2+ response in Arabidopsis seedling plants (Rentel and Knight, 2004). The recent discovery of a novel plant receptor that covalently regulates Ca2+ channel activity by H2O2 is a good example of direct activation of Ca2+ influx by H2O2 (Wu et al., 2020). Rotenone specifically targets mitochondrial complex 1 of the electron transport chain and causes impaired ATP production and oxidative stress, leading to cellular dysfunction and ultimately cell death (Li et al., 2003). BMA inhibits the dicarboxylate transporter (DIC), which transports glutathione into mitochondria in animal cells (Kamga et al., 2010). Taken together, Ca2+ influx enhancers could effectively promote cytoplasmic Ca2+ influx to trigger iron- and ROS-dependent ferroptotic cell death in rice plants during virulent M. oryzae infection.
Ca2+ may operate more like an essential switch to signal plant cell death and immunity (Scrase-Field and Knight, 2003). Glutathione reductase (GR) catalyzes the NADPH-dependent reduction of oxidized glutathione (GSSG) to reduced glutathione (GSH) (Tandoğan and Ulusu, 2007). Robust Ca2+ influx into the cytoplasm triggered reduced OsGR expression and GSH depletion during avirulent M. oryzae infection. Inhibition of cytoplasmic OsGR2 expression by avirulent M. oryzae infection may cause GSH depletion, leading to rice ferroptotic cell death. The intracellular concentration of glutathione can indicate the condition of oxidative stress in cells (Pastore et al., 2001). Within the cell, glutathione exists in a reduced state (GSH) and an oxidized state (GSSG). Glutathione, an important cellular antioxidant, plays a crucial role in disease resistance in plants by helping to regulate intracellular ROS homeostasis (Hwang et al., 1992; Parisy et al., 2007). GSH depletion disrupts intracellular ROS homeostasis and leads to iron- and ROS-dependent ferroptotic cell death (Dangol et al., 2019). GSH depletion is a common phenomenon observed during both plant and animal ferroptosis (Stockwell et al., 2017; Dangol et al., 2019).
An increase in free Ca2+ levels in apoplasts during avirulent M. oryzae infection may cause Ca2+ influx into the cytoplasm via calcium-permeable cation channels, including the putative NLR-activated resistosomes. High cytoplasmic Ca2+ influx can inhibit the reduction of oxidized glutathione (GSSG) to GSH by GR, leading to GSH depletion in vitro. Cytoplasmic Ca2+ influx from the apoplast through the putative resistosomes induces GSH depletion, which leads to the production of lipid ROS, thus promoting iron-dependent ferroptotic cell death in rice. Robust Ca2+, ROS and iron accumulation occurred together in the vicinity of the plasma membrane, where resistosomes are likely localized, during avirulent M. oryzae infection. This highlights the importance of GSH depletion for the initiation of Ca2+-mediated ferroptotic cell death in rice during M. oryzae infection. Strong Ca2+ influx into the cytoplasm leading to GSH depletion may be due primarily to the irreversible influx of apoplastic Ca2+ through the putative resistosomes, Ca2+-permeable selective channels (Bi et al., 2021), in the plasma membrane. It is thus likely that the irreversible Ca2+ influx acts as a driving force for lipid ROS production that induces ferroptotic cell death.
Our data collectively support a model of Ca2+-mediated iron- and lipid ROS-dependent ferroptotic cell death by calcium-permeable cation channels, including the putative NLR-activated resistosomes and consequent GSH depletion during M. oryzae infection in rice (Figure 9). Recently, multiple major NLR genes, including PigmR, which confer broad-spectrum resistance to M. oryzae have been identified in the rice genome (Deng et al., 2017; Wang L. et al., 2019; Zhai et al., 2019). Plant NLRs form resistosomes upon the recognition of pathogen effectors (Bi et al., 2021); however, there is still no experimental evidence that rice NLRs such as PigmR form resistosomes that recognize M. oryzae effectors. The NLR resistosomes (Wang J. et al., 2019) act as irreversible Ca2+-permeable channels in the plasma membrane to trigger HR cell death in plant immunity (Bi et al., 2021). Cytoplasmic Ca2+ influx by calcium-permeable cation channels, including the putative resistosome induces GSH depletion and eventually triggers a ROS burst at the same site where Ca2+ accumulation occurs. During virulent M. oryzae infection, NLR-activated resistosome formation and GSH depletion do not occur due to increased expression of rice glutathione reductase (OsGR), and M. oryzae hyphae grow invasively inside rice cells, causing disease without ferroptotic cell death. Blast disease (susceptibility)-related cell death is ROS-dependent but iron-independent in the compatible rice–M. oryzae interaction (Dangol et al., 2021). However, during avirulent M. oryzae infection, strong cytoplasmic Ca2+ influx through calcium-permeable cation channels, including the putative NLR-activated resistosomes triggers iron- and ROS-dependent ferroptotic cell death owing to the increase in Fe2+, ROS and lipid ROS levels as well as GSH depletion by repressed OsGR2 expression. Overall, our results suggest that cytoplasmic Ca2+ influx from the apoplast through calcium-permeable cation channels, including the putative NLR resistosomes (Wang J. et al., 2019) inhibits the reduction of GSSG to GSH under reduced OsGR expression levels, leading to iron- and lipid ROS-dependent ferroptotic cell death in the rice immune response.
Figure 9 Model of Ca2+-mediated iron- and lipid ROS-dependent ferroptotic cell death by the putative NLR-activated resistosomes in rice immune responses. Glutathione is an important and potent antioxidant that regulates intracellular ROS homeostasis through the continuous redox. In compatible rice-M. oryzae (virulent) interactions, massive cytoplasmic Ca2+-influx does not occur due to inactivation of NLR, ROS homeostasis is well maintained by redox of glutathione due to increased expression of glutathione reductase (GR), and M. oryzae hyphae grow normally and successfully infect rice. By contrast, during incompatible rice-M. oryzae (avirulent) interactions, plant NLR-pathogen effector recognition leads to resistosome formation, and resistosome-mediated massive influx of cytoplasmic Ca2+ from the apoplast sharply represses GR expression in rice cells during the early infection stage. Consequently, reduced GR activity exacerbates glutathione depletion, leading to iron- and lipid ROS-dependent ferroptotic cell death in the rice immune response. GR, glutathione reductase; GSSG, glutathione disulfide; GSH, reduced glutathione; NLR, nucleotide-binding leucine-rich repeat receptor.
The original contributions presented in the study are included in the article/Supplementary Materials, further inquiries can be directed to the corresponding author/s.
JW: Conceptualization, Data curation, Formal analysis, Investigation, Methodology, Visualization, Writing – original draft, Writing – review & editing. W-GC: Formal analysis, Funding acquisition, Investigation, Methodology, Software, Validation, Visualization, Writing – review & editing. NKN: Data curation, Formal analysis, Investigation, Methodology, Validation, Visualization, Writing – review & editing. DPL: Data curation, Formal analysis, Investigation, Methodology, Validation, Visualization, Writing – review & editing. S-HK: Formal analysis, Investigation, Methodology, Validation, Writing – review & editing. DYL: Formal analysis, Methodology, Validation, Writing – review & editing. BKH: Conceptualization, Formal analysis, Methodology, Supervision, Validation, Visualization, Writing – original draft, Writing – review & editing. N-SJ: Conceptualization, Data curation, Formal analysis, Funding acquisition, Investigation, Methodology, Project administration, Resources, Software, Supervision, Validation, Visualization, Writing – original draft, Writing – review & editing.
The author(s) declare financial support was received for the research, authorship, and/or publication of this article. This work was supported by the National Research Foundation (NRF) of Korea (grant no. 2023R1A2C1003099 to N-SJ) and the National Science Foundation of United States (MCB grant no. 2016143 to W-GC).
We thank B. R. Stockwell (Columbia University), S. Gilroy (University of Wisconsin, Madison), and J. F. Harper (University of Nevada, Reno) for critical reading and commenting on the manuscript. We also thank the Sejong University Research Facility Center Confocal Microscopy Team for technical support and J-S Ha for fluorescence microscopy imaging.
The authors declare that the research was conducted in the absence of any commercial or financial relationships that could be construed as a potential conflict of interest.
The author(s) declared that they were an editorial board member of Frontiers, at the time of submission. This had no impact on the peer review process and the final decision.
All claims expressed in this article are solely those of the authors and do not necessarily represent those of their affiliated organizations, or those of the publisher, the editors and the reviewers. Any product that may be evaluated in this article, or claim that may be made by its manufacturer, is not guaranteed or endorsed by the publisher.
The Supplementary Material for this article can be found online at: https://www.frontiersin.org/articles/10.3389/fpls.2024.1339559/full#supplementary-material
Supplementary Figure 1 | Analysis of the effect of Ca2+ influx on the immunity of avirulent Magnaprthe oryzae 007-inoculated rice using the transgenic approach. (A) Schematic diagram of the Ca2+ sensor construct. The Ca2+ sensor GCaMP6fmC is composed of the calmodulin (CaM)-binding site M13, a circularly permutated enhanced green fluorescent protein (cp-eGFP), and mCherry-fused CaM. The Ca2+ sensor construct GCaMP6f-mCherry (GCaMP6fmC) was cloned into the vector pGWB554 under the control of the Cauliflower mosaic virus (CaMV) 35S promoter. LB, left border; RB, right border. (B) PCR confirmation of Ca2+ sensor (GCaMP6fmC) transgenic lines of the rice cultivar Kitaake using hygromycin resistance (HygR) and GCaMP6fmC primers. (C) Detection of Ca2+ influx in rice leaf sheaths during avirulent M. oryzae 007 infection. The leaf sheaths of wild-type (WT) plants and Ca2+ sensor (GCaMP6fmC) transgenic lines A, B, and C were inoculated with avirulent M. oryzae 007, and Ca2+ influx in leaf sheath cells was visualized by fluorescence microscopy at 36 h post-inoculation (hpi). Images were taken using a microscope (Zeiss equipped with Axioplan 2) with bright field filter, green fluorescence filter (Ex/Em: 450–490/515–565 nm), and red fluorescence filter (Ex/Em: 546/590 nm). Bars = 10 µm. BF, bright field; GF, green fluorescence; RF, red fluorescence.
Supplementary Figure 2 | Images of Ca2+ influx and ROS and iron accumulation in the leaf sheaths of rice Kitaake (WT) and 35S::GCamP6fmC line B plants during Magnaporthe oryzae infection. The leaf sheaths of WT and Ca2+ sensor (GCaMP6fmC) transgenic line B plants were inoculated with M. oryzae RO1-1 (virulent) and 007 (avirulent), and the influx of Ca2+, accumulation of ROS (H2O2), and accumulation of ferric ions (Fe3+) were measured at 36, 40, and 48 hpi, respectively. Rice leaf sheath cells were stained with Peroxy Orange 1 (PO1) to visualize H2O2 accumulation around the site of infection. Fe3+ accumulation was detected by Prussian blue staining. Ca2+ influx, H2O2 accumulation, and Fe3+ accumulation in rice leaf sheaths were observed under a microscope (Zeiss equipped with Axioplan 2) using a bright field filter and/or fluorescence filters. Bars = 10 µm. Red arrows indicate invasive hyphae. WT, wild type; BF, bright field; GF, green fluorescence; RF, red fluorescence. hpi, hours post-inoculation.
Supplementary Figure 3 | Ca2+ chelation by EGTA suppresses apoplastic Ca2+ influx in rice leaf sheaths during avirulent Magnaporthe oryzae 007 infection. (A) Schematic diagram of apoplastic Ca2+ detection using the calcium-o-cresolphthalein complexone (o-CPC) method. The binding of Ca2+ to o-CPC results in the formation of an intense violet-colored complex, which can be quantified by measuring the absorbance of the intercellular fluid of the rice leaf sheath at 575 nm using the SP-2000UV spectrophotometer. (B) Quantitative determination of apoplastic Ca2+ concentrations in rice leaf sheaths treated with 10 mM EGTA during avirulent M. oryzae 007 infection. The leaf sheaths of rice (Kitaake) plants were inoculated with avirulent M. oryzae 007 (4 × 105 conidia·mL-1) supplemented with 10 mM EGTA. Ca2+ in the intercellular fluid of rice leaf sheath was detected using the o-CPC method, and Ca2+ concentration was measured at 575 nm using the SP-2000UV spectrophotometer. Data are represented as the mean ± SD (n = 4 leaf sheaths from different plants). Asterisks above bars indicate significantly different means (*P< 0.05, **P< 0.01; Student’s t-test). Experiments were repeated three times with similar results.
Supplementary Figure 4 | Different Ca2+ influx inhibitors significantly limit Ca2+-mediated iron- and ROS-dependent ferroptotic cell death in rice immune responses. Images show HR cell death (48 hpi), Ca2+ influx (36 hpi), ROS accumulation (36 hpi), and Fe3+ accumulation (48 hpi) in the leaf sheath cells of rice (Kitaake) Ca2+ sensor (GCaMP6fmC) transgenic line B infected with M. oryzae 007 (avirulent), supplemented with Ca2+ influx inhibitors, including verapamil hydrochloride (verapamil), N-acetyl-cysteine (NAC), neomycin sulfate (neomycin), lithium chloride (LiCl), aluminum chloride (AlCl3), and ruthenium red (RR). HR, hypersensitive response; BF, bright field; GF, green fluorescence; RF, red fluorescence; hpi, hours post-inoculation. Bars = 10 µm. Different Ca2+ influx inhibitors significantly limit Ca2+-mediated iron- and ROS-dependent ferroptotic cell death in rice immune responses. Images show HR cell death (48 hpi), Ca2+ influx (36 hpi), ROS accumulation (36 hpi), and Fe3+ accumulation (48 hpi) in the leaf sheath cells of rice (Kitaake) Ca2+ sensor (GCaMP6fmC) transgenic line B infected with M. oryzae 007 (avirulent), supplemented with Ca2+ influx inhibitors, including verapamil hydrochloride (verapamil), N-acetyl-cysteine (NAC), neomycin sulfate (neomycin), lithium chloride (LiCl), aluminum chloride (AlCl3), and ruthenium red (RR). HR, hypersensitive response; BF, bright field; GF, green fluorescence; RF, red fluorescence; hpi, hours post-inoculation. Bars = 10 µm.
Supplementary Figure 5 | Different Ca2+ influx enhancers significantly trigger Ca2+-mediated iron- and ROS-dependent ferroptotic cell death in rice immune responses. Images show HR cell death (48 hpi), Ca2+ influx (36 hpi), ROS accumulation (36 hpi), and Fe3+ accumulation (48 hpi) in the leaf sheath cells of rice (Kitaake) Ca2+ sensor (GCaMP6fmC) transgenic line B infected with M. oryzae RO1-1 (virulent), supplemented with Ca2+ influx enhancers, including diamide, trifluoperazine dihydrochloride (TFP), CaCl2/calcimycin (C/C), CaCl2/H2O2 (C/H), rotenone and butylmalonic acid (BMA). HR, hypersensitive response; BF, bright field; GF, green fluorescence; RF, red fluorescence; hpi, hours post inoculation. Bars = 10 µm.
Supplementary Figure 6 | High concentrations of CaCl2 inhibit the reduction of GSSG to GSH. GSSG can be reduced to GSH by glutathione reductase (GR) using NADPH as a cofactor, which is converted to NADP+ during the reaction. The decrease in the absorbance of NADPH at 340 nm was measured spectrophotometrically for 5 min at room temperature. (A, D) Effect of the increase in CaCl2 concentration on the inhibition of GSSG reduction to GSH by rice GR (A) or yeast GR (D). Values represent mean ± SD of OD340 at different time points. (B, E) Linerweaver-Burt plot showing non-competitive inhibition of the GSSG reduction reaction by rice GR (B) or yeast GR (E). The x-axis represents the inverse of the substrate concentration (1/S), and the y-axis represents the inverse of the initial reaction velocity (1/V0). (C, F) Inhibitory effects of CaCl2, MgCl2, KCl, and NaCl on GSSG reduction to GSH by rice GR (C) or yeast GR (F). Values represent mean ± SD of OD340 values at different time points.
Supplementary Figure 7 | RT-PCR and q-RT-PCR analyses of the expression levels of rice glutathione reductase (OsGR) genes in the leaf sheaths of rice (Kitaake) plants during Magnaporthe oryzae infection. (A) Reverse transcription PCR (RT-PCR) analysis of the expression levels of OsGR1, OsGR2, OsGR3, and Ubiquitin (OsUbiquitin) in rice leaf sheaths at different time points after inoculation with M. oryzae RO1-1 (virulent) and 007 (avirulent). (B) Real-time quantitative RT-PCR (qRT-PCR) analysis of the expression levels of OsGR1, OsGR3, and OsUbiquitin in rice leaf sheaths at different time points after inoculation with M. oryzae RO1-1 (virulent) and 007 (avirulent). Transcription levels of OsGR genes were normalized relative to that of the internal reference gene OsUbiquitin. hpi, hours post-inoculation.
Aguilera, A., Berdun, F., Bartoli, C., Steelheart, C., Alegre, M., Bayir, H., et al. (2022). C-ferroptosis is an iron-dependent form of regulated cell death in cyanobacteria. J. Cell Biol. 221, e201911005. doi: 10.1083/jcb.201911005
Airaki, M., Sánchez-Moreno, L., Leterrier, M., Barroso, J. B., Palma, J. M., Corpas, F. J. (2011). Detection and quantification of S-nitrosoglutathione (GSNO) in pepper (Capsicum annuum L.) plant organs by LC-ES/MS. Plant Cell Physiol. 52, 2006–2015. doi: 10.1093/pcp/pcr133
Atkinson, M. M., Keppler, L. D., Orlandi, E. W., Baker, C. J., Mischke, C. F. (1990). Involvement of plasma membrane calcium influx in bacterial induction of the k/h and hypersensitive responses in tobacco. Plant Physiol. 92, 215–221. doi: 10.1104/pp.92.1.215
Bae, J. H., Park, J. W., Kwon, T. K. (2003). Ruthenium red, inhibitor of mitochondrial Ca2+ uniporter, inhibits curcumin-induced apoptosis via the prevention of intracellular Ca2+ depletion and cytochrome c release. Biochem. Biophys. Res. Commun. 303, 1073–1079. doi: 10.1016/s0006-291x(03)00479-0
Bellomo, G., Jewell, S. A., Thor, H., Orrenius, S. (1982). Regulation of intracellular calcium compartmentation: studies with isolated hepatocytes and t-butyl hydroperoxide. Proc. Natl. Acad. Sci. U. S. A. 79, 6842–6846. doi: 10.1073/pnas.79.22.6842
Beneloujaephajri, E., Costa, A., L’Haridon, F., Métraux, J. P., Binda, M. (2013). Production of reactive oxygen species and wound-induced resistance in Arabidopsis thaliana against Botrytis cinerea are preceded and depend on a burst of calcium. BMC Plant Biol. 13, 160. doi: 10.1186/1471-2229-13-160
Bergson, P., Lipkind, G., Lee, S. P., Duban, M. E., Hanck, D. A. (2011). Verapamil block of T-type calcium channels. Mol. Pharmacol. 79, 411–419. doi: 10.1124/mol.110.069492
Bi, G., Su, M., Li, N., Liang, Y., Dang, S., Xu, J., et al. (2021). The ZAR1 resistosome is a calcium-permeable channel triggering plant immune signaling. Cell 184, 3528–3541.e12. doi: 10.1016/j.cell.2021.05.003
Bora, P., Gahurova, L., Mašek, T., Hauserova, A., Potěšil, D., Jansova, D., et al. (2021). p38-MAPK-mediated translation regulation during early blastocyst development is required for primitive endoderm differentiation in mice. Commun. Biol. 4, 788. doi: 10.1038/s42003-021-02290-z
Brisset, M. N., Cesbron, S., Thomson, S. V., Paulin, J. P. (2000). Acibenzolar-S-methyl induces the accumulation of defense-related enzymes in apple and protects from fire blight. Eur. J. Plant Pathol. 106, 529–536. doi: 10.1023/A:1008728119087
Buonaurio, R., Scarponi, L., Ferrara, M., Sidoti, P., Bertona, A. (2002). Induction of systemic acquired resistance in pepper plants by acibenzolar-S-methyl against bacterial spot disease. Eur. J. Plant Pathol. 108, 41–49. doi: 10.1023/A:1013984511233
Cessna, S. G., Low, P. S. (2001). Activation of the oxidative burst in aequorin-transformed Nicotiana tabacum cells is mediated by protein kinase- and anion channel-dependent release of Ca2+ from internal stores. Planta 214, 126–134. doi: 10.1007/s004250100596
Chen, Y., Wang, J., Nguyen, N. K., Hwang, B. K., Jwa, N. S. (2022). The NIN-like protein OsNLP2 negatively regulates ferroptotic cell death and immune responses to Magnaporthe oryzae in rice. Antioxidants 11, 1795. doi: 10.3390/antiox11091795
Choi, W. G., Swanson, S. J., Gilroy, S. (2012). High-resolution imaging of Ca2+, redox status, ROS and pH using GFP biosensors. Plant J. 70, 118–128. doi: 10.1111/j.1365-313X.2012.04917.x
Choi, W. G., Toyota, M., Kim, S. H., Hilleary, R., Gilroy, S. (2014). Salt stress-induced Ca2+ waves are associated with rapid, long-distance root-to-shoot signaling in plants. Proc. Natl. Acad. Sci. U. S. A. 111, 6497–6502. doi: 10.1073/pnas.1319955111
Corns, C. M., Ludman, C. J. (1987). Some observations on the nature of the calcium-cresolphthalein complexone reaction and its relevance to the clinical laboratory. Ann. Clin. Biochem. 24, 345–351. doi: 10.1177/000456328702400403
Couto, N., Wood, J., Barber, J. (2016). The role of glutathione reductase and related enzymes on cellular redox homoeostasis network. Free Radic. Biol. Med. 95, 27–42. doi: 10.1016/j.freeradbiomed.2016.02.028
Dangl, J. L., Horvath, D. M., Staskawicz, B. J. (2013). Pivoting the plant immune system from dissection to deployment. Science 341, 746–751. doi: 10.1126/science.1236011
Dangol, S., Chen, Y., Hwang, B. K., Jwa, N. S. (2019). Iron- and reactive oxygen species-dependent ferroptotic cell death in rice-Magnaporthe oryzae interactions. Plant Cell. 31, 189–209. doi: 10.1105/tpc.18.00535
Dangol, S., Nguyen, N. K., Singh, R., Chen, Y., Wang, J., Lee, H. G., et al. (2021). Mitogen-activated protein kinase OsMEK2 and OsMPK1 signaling is required for ferroptotic cell death in rice-Magnaporthe oryzae interactions. Front. Plant Sci. 12. doi: 10.3389/fpls.2021.710794
Deng, Y., Zhai, K., Xie, Z., Yang, D., Zhu, X., Liu, J., et al. (2017). Epigenetic regulation of antagonistic receptors confers rice blast resistance with yield balance. Science 355, 962–965. doi: 10.1126/science.aai8898
Ding, J., Luo, A. F., Hu, L., Wang, D., Shao, F. (2014). Structural basis of the ultrasensitive calcium indicator GCaMP6. Sci. China Life Sci. 57, 269–274. doi: 10.1007/s11427-013-4599-5
Distéfano, A. M., Martin, M. V., Córdoba, J. P., Bellido, A. M., D’Ippólito, S., Colman, S. L., et al. (2017). Heat stress induces ferroptosis-like cell death in plants. J. Cell Biol. 216, 463–476. doi: 10.1083/jcb.201605110
Dixon, S. J., Lemberg, K. M., Lamprecht, M. R., Skouta, R., Zaitsev, E. M., Gleason, C. E., et al. (2012). Ferroptosis: an iron-dependent form of nonapoptotic cell death. Cell 149, 1060–1072. doi: 10.1093/jxb/eraa42510.1016/j.cell.2012.03.042
Eaddy, A. C., Schnellmann, R. G. (2011). Visualization and quantification of endoplasmic reticulum Ca2+ in renal cells using confocal microscopy and Fluo5F. Biochem. Biophys. Res. Commun. 404, 424–427. doi: 10.1093/jxb/eraa42510.1016/j.bbrc.2010.11.137
Ellis-Davies, G. C., Kaplan, J. H. (1994). Nitrophenyl-EGTA, a photolabile chelator that selectively binds Ca2+ with high affinity and releases it rapidly upon photolysis. Proc. Natl. Acad. Sci. U. S. A. 91, 187–191. doi: 10.1093/jxb/eraa42510.1073/pnas.91.1.187
Farber, J. L. (1994). Mechanisms of cell injury by activated oxygen species. Environ. Health Perspect. 102, 17–24. doi: 10.1289/ehp.94102s1017
Fenton, H. J. H. (1894). Oxidation of tartaric acid in presence of iron. J. Chem. Soc. Trans. 65, 899–910. doi: 10.1039/CT8946500899
Förderer, A., Li, E., Lawson, A. W., Deng, Y. N., Sun, Y., Logemann, E., et al. (2022). A wheat resistosome defines common principles of immune receptor channels. Nature 610, 532–539. doi: 10.1038/s41586-022-05231-w
Franklin-Tong, V. E., Drobak, B. K., Allan, A. C., Watkins, P., Trewavas, A. J. (1996). Growth of pollen tubes of Papaver rhoeas is regulated by a slow-moving calcium wave propagated by inositol 1,4,5-trisphosphate. Plant Cell. 8, 1305–1321. doi: 10.1105/tpc.8.8.1305
Gilge, J. L., Fisher, M., Chai, Y. C. (2008). The effect of oxidant and the non-oxidant alteration of cellular thiol concentration on the formation of protein mixed-disulfides in HEK 293 cells. PloS One 3, e4015. doi: 10.1371/journal.pone.0004015
Grant, J. J., Loake, G. J. (2000). Role of reactive oxygen intermediates and cognate redox signaling in disease resistance. Plant Physiol. 124, 21–29. doi: 10.1104/pp.124.1.21
Greenberg, J. T., Yao, N. (2004). The role and regulation of programmed cell death in plant-pathogen interactions. Cell Microbiol. 6, 201–211. doi: 10.1111/j.1462-5822.2004.00361.x
Griffith, O. W. (1980). Determination of glutathione and glutathione disulfide using glutathione reductase and 2-vinylpyridine. Anal. Biochem. 106, 207–212. doi: 10.1016/0003-2697(80)90139-6
Grossi, M., Morgunova, M., Cheung, S., Scholz, D., Conroy, E., Terrile, M., et al. (2016). Lysosome triggered near-infrared fluorescence imaging of cellular trafficking processes in real time. Nat. Commun. 7, 10855. doi: 10.1038/ncomms10855
Han, S. W., Hwang, B. K. (2017). Molecular functions of Xanthomonas type III effector AvrBsT and its plant interactors in cell death and defense signaling. Planta 245, 237–253. doi: 10.1007/s00425-016-2628-x
Heath, M. C. (2000). Hypersensitive response-related death. Plant Mol. Biol. 44, 321–334. doi: 10.1023/a:1026592509060
Hepler, P. K. (2005). Calcium: a central regulator of plant growth and development. Plant Cell. 17, 2142–2155. doi: 10.1105/tpc.105.032508
Hiei, Y., Ohta, S., Komari, T., Kumashiro, T. (1994). Efficient transformation of rice (Oryza sativa L.) mediated by Agrobacterium and sequence analysis of the boundaries of the T-DNA. Plant J. 6, 271–282. doi: 10.1046/j.1365-313x.1994.6020271.x
Hwang, C., Sinskey, A. J., Lodish, H. F. (1992). Oxidized redox state of glutathione in the endoplasmic reticulum. Science 257, 1496–1502. doi: 10.1126/science.1523409
Jacob, P., Kim, N. H., Wu, F., El-Kasmi, F., Chi, Y., Walton, W. G., et al. (2021). Plant “helper” immune receptors are Ca2+-permeable nonselective cation channels. Science 373, 420–425. doi: 10.1126/science.abg7917
Jakic, B., Buszko, M., Cappellano, G., Wick, G. (2017). Elevated sodium leads to the increased expression of HSP60 and induces apoptosis in HUVECs. PloS One 12, e0179383. doi: 10.1371/journal.pone.0179383
Jewell, S. A., Bellomo, G., Thor, H., Orrenius, S., Smith, M. (1982). Bleb formation in hepatocytes during drug metabolism is caused by disturbances in thiol and calcium ion homeostasis. Science 217, 1257–1259. doi: 10.1126/science.7112127
Jones, J. D., Dangl, J. L. (2006). The plant immune system. Nature 444, 323–329. doi: 10.1038/nature05286
Jwa, N. S., Hwang, B. K. (2017). Convergent evolution of pathogen effectors toward reactive oxygen species signaling networks in plants. Front. Plant Sci. 8. doi: 10.3389/fpls.2017.01687
Kadota, Y., Furuichi, T., Sano, T., Kaya, H., Gunji, W., Murakami, Y., et al. (2005). Cell-cycle-dependent regulation of oxidative stress responses and Ca2+ permeable channels NtTPC1A/B in tobacco BY-2 cells. Biochem. Biophys. Res. Commun. 336, 1259–1267. doi: 10.1016/j.bbrc.2005.09.004
Kamga, C. K., Zhang, S. X., Wang, Y. (2010). Dicarboxylate carrier-mediated glutathione transport is essential for reactive oxygen species homeostasis and normal respiration in rat brain mitochondria. Am. J. Physiol. Cell Physiol. 299, 497–505. doi: 10.1152/ajpcell.00058.2010
Kanchiswamy, C. N., Malnoy, M., Occhipinti, A., Maffei, M. E. (2014). Calcium imaging perspectives in plants. Int. J. Mol. Sci. 15, 3842–3859. doi: 10.3390/ijms15033842
Kang, S., Hong, J., Lee, J. M., Moon, H. E., Jeon, B., Choi, J., et al. (2017). Trifluoperazine, a well-known antipsychotic, inhibits glioblastoma invasion by binding to calmodulin and disinhibiting calcium release channel IP3R. Mol. Cancer Ther. 16, 217–227. doi: 10.1158/1535-7163.MCT-16-0169-T
Kankanala, P., Czymmek, K., Valent, B. (2007). Roles for rice membrane dynamics and plasmodesmata during biotrophic invasion by the blast fungus. Plant Cell. 19, 706–724. doi: 10.1105/tpc.106.046300
Kawano, T., Kadono, T., Fumoto, K., Lapeyrie, F., Kuse, M., Isobe, M., et al. (2004). Aluminum as a specific inhibitor of plant TPC1 Ca2+ channels. Biochem. Biophys. Res. Commun. 324, 40–45. doi: 10.1016/j.bbrc.2004.09.015
Kim, S. R., Jeon, J. S., An, G. (2011). Development of an efficient inverse PCR method for isolating gene tags from T-DNA insertional mutants in rice. Methods Mol. Biol. 678, 139–146. doi: 10.1007/978-1-60761-682-5_11
Kosower, N. S., Kosower, E. M., Wertheim, B., Correa, W. S. (1969). Diamide, a new reagent for the intracellular oxidation of glutathione to the disulfide. Biochem. Biophys. Res. Commun. 37, 593–596. doi: 10.1016/0006-291x(69)90850-x
Köster, P., DeFalco, T. A., Zipfel, C. (2022). Ca2+ signals in plant immunity. EMBO J. 41, e110741. doi: 10.15252/embj.2022110741
Levine, A., Tenhaken, R., Dixon, R., Lamb, C. (1994). H2O2 from the oxidative burst orchestrates the plant hypersensitive disease resistance response. Cell 79, 583–593. doi: 10.1016/0092-8674(94)90544-4
Lewis, J. D., Wu, R., Guttman, D. S., Desveaux, D. (2010). Allele-specific virulence attenuation of the Pseudomonas syringae HopZ1a type III effector via the Arabidopsis ZAR1 resistance protein. PloS Genet. 6, e1000894. doi: 10.1371/journal.pgen.1000894
Li, N., Ragheb, K., Lawler, G., Sturgis, J., Rajwa, B., Melendez, J. A., et al. (2003). Mitochondrial complex I inhibitor rotenone induces apoptosis through enhancing mitochondrial reactive oxygen species production. J. Biol. Chem. 278, 8516–8525. doi: 10.1074/jbc.M210432200
Liu, G., Greenshields, D. L., Sammynaiken, R., Hirji, R. N., Selvaraj, G., Wei, Y. (2007). Targeted alterations in iron homeostasis underlie plant defense responses. J. Cell Sci. 120, 596–605. doi: 10.1242/jcs.001362
Maffei, M., Bossi, S., Spiteller, D., Mithöfer, A., Boland, W. (2004). Effects of feeding Spodoptera littoralis on lima bean leaves. I. Membrane potentials, intracellular calcium variations, oral secretions, and regurgitate components. Plant Physiol. 134, 1752–1762. doi: 10.1104/pp.103.034165
Martins, T. V., Evans, M. J., Woolfenden, H. C., Morris, R. J. (2013). Towards the physics of calcium signalling in plants. Plants 2, 541–588. doi: 10.3390/plants2040541
Matsuo, Y., Novianti, F., Takehara, M., Fukuhara, T., Arie, T., Komatsu, K. (2019). Acibenzolar-S-methyl restricts infection of Nicotiana benthamiana by plantago asiatica mosaic virus at two distinct stages. Mol. Plant Microbe Interact. 32, 1475–1486. doi: 10.1094/MPMI-03-19-0087-R
Meng, X., Zhang, S. (2013). MAPK cascades in plant disease resistance signaling. Annu. Rev. Phytopathol. 51, 245–266. doi: 10.1146/annurev-phyto-082712-102314
Moeder, W., Phan, V., Yoshioka, K. (2019). Ca2+ to the rescue - Ca2+ channels and signaling in plant immunity. Plant Sci. 279, 19–26. doi: 10.1016/j.plantsci.2018.04.012
Moyen, C., Hammond-Kosack, K. E., Jones, J., Knight, M. R., Johannes, E. (1998). Systemin triggers an increase of cytoplasmic calcium in tomato mesophyll cells: Ca2+ mobilization from intra- and extracellular compartments. Plant Cell Environ. 21, 1101–1111. doi: 10.1046/j.1365-3040.1998.00378.x
Muhlemann, J. K., Younts, T. L. B., Muday, G. K. (2018). Flavonols control pollen tube growth and integrity by regulating ROS homeostasis during high-temperature stress. Proc. Natl. Acad. Sci. U. S. A. 115, E11188–E11197. doi: 10.1073/pnas.1811492115
Ngou, B. P. M., Ding, P., Jones, J. D. G. (2021). Channeling plant immunity. Cell 184, 3358–3360. doi: 10.1016/j.cell.2021.05.035
Nguyen, N. K., Wang, J., Liu, D., Hwang, B. K., Jwa, N. S. (2022). Rice iron storage protein ferritin 2 (OsFER2) positively regulates ferroptotic cell death and defense responses against Magnaporthe oryzae. Front. Plant Sci. 13. doi: 10.3389/fpls.2022.1019669
Parisy, V., Poinssot, B., Owsianowski, L., Buchala, A., Glazebrook, J., Mauch, F. (2007). Identification of PAD2 as a γ-glutamylcysteine synthetase highlights the importance of glutathione in disease resistance of Arabidopsis. Plant J. 49, 159–172. doi: 10.1111/j.1365-313X.2006.02938.x
Pastore, A., Piemonte, F., Locatelli, M., Russo, A. L., Gaeta, L. ,. M., Tozzi, G., et al. (2001). Determination of blood total, reduced, and oxidized glutathione in pediatric subjects. Clin. Chem. 47, 1467–1469. doi: 10.1093/clinchem/47.8.1467
Pierre, J. L., Fontecave, M. (1999). Iron and activated oxygen species in biology: the basic chemistry. Biometals 12, 195–199. doi: 10.1023/a:1009252919854
Rentel, M. C., Knight, M. R. (2004). Oxidative stress-induced calcium signaling in Arabidopsis. Plant Physiol. 135, 1471–1479. doi: 10.1104/pp.104.042663
Rohringer, R., Ebrahim-Nesbat, F., Wolf, G. (1983). Proteins in intercellular washing fluids from leaves of barley (Hordeum vulgare L.). J. Exp. Bot. 34, 1589–1605. doi: 10.1093/jxb/34.12.1589
Samuel, A. M., Mather, A. K., Arun, G., Norma, A. C., Ricarda, H., Jiamei, L., et al. (2022). Cadmium interference with iron sensing reveals transcriptional programs sensitive and insensitive to reactive oxygen species. J. Exp. Bot. 73, 324–338. doi: 10.1093/jxb/erab393
Schanne, F. A., Kane, A. B., Young, E. E., Farber, J. L. (1979). Calcium dependence of toxic cell death: a final common pathway. Science 206, 700–702. doi: 10.1126/science.386513
Scrase-Field, S. A., Knight, M. R. (2003). Calcium: just a chemical switch? Curr. Opin. Plant Biol. 6, 500–506. doi: 10.1016/s1369-5266(03)00091-8
Shen, Q., Liang, M., Yang, F., Deng, Y. Z., Naqvi, N. I. (2020). Ferroptosis contributes to developmental cell death in rice blast. New Phytol. 227, 1831–1846. doi: 10.1111/nph.16636
Shimomura, O., Johnson, F. H., Saiga, Y. (1963). Further data on the bioluminescent protein, aequorin. J. Cell Comp. Physiol. 62, 1–8. doi: 10.1002/jcp.1030620102
Shu, X., Shaner, N. C., Yarbrough, C. A., Tsien, R. Y., Remington, S. J. (2006). Novel chromophores and buried charges control color in mFruits. Biochemistry 45, 9639–9647. doi: 10.1021/bi060773l
Singh, R., Dangol, S., Chen, Y., Choi, J., Cho, Y. S., Lee, J. E., et al. (2016). Magnaporthe oryzae effector AVR-Pii helps to establish compatibility by inhibition of the rice NADP-malic enzyme resulting in disruption of oxidative burst and host innate immunity. Mol. Cells 39, 426–438. doi: 10.14348/molcells.2016.0094
Stael, S., Wurzinger, B., Mair, A., Mehlmer, N., Vothknecht, U. C., Teige, M. (2012). Plant organellar calcium signalling: an emerging field. J. Exp. Bot. 63, 1525–1542. doi: 10.1093/jxb/err394
Starke, P. E., Farber, J. L. (1985). Ferric iron and superoxide ions are required for the killing of cultured hepatocytes by hydrogen peroxide. Evidence for the participation of hydroxyl radicals formed by an iron-catalyzed Haber-Weiss reaction. J. Biol. Chem. 260, 10099–10104. doi: 10.1016/S0021-9258(17)39218-9
Stockwell, B. R., Friedmann Angeli, J. P., Bayir, H., Bush, A. I., Conrad, M., Dixon, S. J., et al. (2017). Ferroptosis: A regulated cell death nexus linking metabolism, redox biology, and disease. Cell 171, 273–285. doi: 10.1016/j.cell.2017.09.021
Sun, L., Gu, L., Wang, S., Yuan, J., Yang, H., Zhu, J., et al. (2012). N-acetylcysteine protects against apoptosis through modulation of group I metabotropic glutamate receptor activity. PloS One 7, e32503. doi: 10.1371/journal.pone.0032503
Tandoğan, B., Ulusu, N. N. (2007). The inhibition kinetics of yeast glutathione reductase by some metal ions. J. Enzyme Inhib. Med. Chem. 22, 489–495. doi: 10.1080/14756360601162147
Thulasi Devendrakumar, K., Li, X., Zhang, Y. (2018). MAP kinase signalling: interplays between plant PAMP- and effector-triggered immunity. Cell Mol. Life Sci. 75, 2981–2989. doi: 10.1007/s00018-018-2839-3
Valent, B. (2021). The impact of blast disease: past, present, and future. Methods Mol. Biol. 2356, 1–18. doi: 10.1007/978-1-0716-1613-0_1
Van Breusegem, F., Dat, J. F. (2006). Reactive oxygen species in plant cell death. Plant Physiol. 141, 384–390. doi: 10.1104/pp.106.078295
Verma, A., Bhatt, A. N., Farooque, A., Khanna, S., Singh, S., Dwarakanath, B. S. (2011). Calcium ionophore A23187 reveals calcium related cellular stress as “I-Bodies”: an old actor in a new role. Cell Calcium. 50, 510–522. doi: 10.1016/j.ceca.2011.08.007
Wang, J., Hu, M., Wang, J., Qi, J., Han, Z., Wang, G., et al. (2019). Reconstitution and structure of a plant NLR resistosome conferring immunity. Science 364, eaav5870. doi: 10.1126/science.aav5870
Wang, Q., Pu, Y., Yang, D., Yin, X., He, Z., Yang, Y., et al. (2018). Molecular cloning and characterization of the glutathione reductase gene from Stipa purpurea. Biochem. Biophys. Res. Commun. 495, 1851–1857. doi: 10.1016/j.bbrc.2017.12.054
Wang, L., Zhao, L., Zhang, X., Zhang, Q., Jia, Y., Wang, G., et al. (2019). Large-scale identification and functional analysis of NLR genes in blast resistance in the Tetep rice genome sequence. Proc. Natl. Acad. Sci. U. S. A. 116, 18479–18487. doi: 10.1073/pnas.1910229116
Weigand, C., Kim, S. H., Brown, E., Medina, E., Mares, M., Miller, G., et al. (2021). A ratiometric calcium reporter CGf reveals calcium dynamics both in the single cell and whole plant levels under heat stress. Front. Plant Sci. 12. doi: 10.3389/fpls.2021.777975
White, P. J. (1996). Specificity of ion channel inhibitors for the maxi cation channel in rye root plasma membranes. J. Exp. Bot. 47, 713–716. doi: 10.1093/jxb/47.5.713
Wu, F., Chi, Y., Jiang, Z., Xu, Y., Xie, L., Huang, F., et al. (2020). Hydrogen peroxide sensor HPCA1 is an LRR receptor kinase in Arabidopsis. Nature 578, 577–581. doi: 10.1038/s41586-020-2032-3
Zhai, K., Deng, Y., Liang, D., Tang, J., Liu, J., Yan, B., et al. (2019). RRM transcription factors interact with NLRs and regulate broad-spectrum blast resistance in rice. Mol. Cell. 74, 996–1009.e7. doi: 10.1016/j.molcel.2019.03.013
Zhang, W., Zhou, R. G., Gao, Y. J., Zheng, S. Z., Xu, P., Zhang, S. Q., et al. (2009). Molecular and genetic evidence for the key role of AtCaM3 in heat-shock signal transduction in Arabidopsis. Plant Physiol. 149, 1773–1784. doi: 10.1104/pp.108.133744
Keywords: iron, ROS, Ca2+ influx, GR expression, ferroptotic cell death, rice, Magnaporthe oryzae
Citation: Wang J, Choi W-G, Nguyen NK, Liu D, Kim S-H, Lim D, Hwang BK and Jwa N-S (2024) Cytoplasmic Ca2+ influx mediates iron- and reactive oxygen species-dependent ferroptotic cell death in rice immunity. Front. Plant Sci. 15:1339559. doi: 10.3389/fpls.2024.1339559
Received: 16 November 2023; Accepted: 17 April 2024;
Published: 02 May 2024.
Edited by:
Choong-Min Ryu, Korea Research Institute of Bioscience and Biotechnology (KRIBB), Republic of KoreaReviewed by:
Nicolás M. Cecchini, National University of Cordoba (CIQUIBIC), ArgentinaCopyright © 2024 Wang, Choi, Nguyen, Liu, Kim, Lim, Hwang and Jwa. This is an open-access article distributed under the terms of the Creative Commons Attribution License (CC BY). The use, distribution or reproduction in other forums is permitted, provided the original author(s) and the copyright owner(s) are credited and that the original publication in this journal is cited, in accordance with accepted academic practice. No use, distribution or reproduction is permitted which does not comply with these terms.
*Correspondence: Nam-Soo Jwa, bnNqd2FAc2Vqb25nLmFjLmty
†These authors have contributed equally to this work
Disclaimer: All claims expressed in this article are solely those of the authors and do not necessarily represent those of their affiliated organizations, or those of the publisher, the editors and the reviewers. Any product that may be evaluated in this article or claim that may be made by its manufacturer is not guaranteed or endorsed by the publisher.
Research integrity at Frontiers
Learn more about the work of our research integrity team to safeguard the quality of each article we publish.