- 1School of Life Sciences, Yunnan Normal University, Kunming, China
- 2Engineering Research Center of Sustainable Development and Utilization of Biomass Energy, Ministry of Education, Kunming, China
- 3Key Laboratory of Biomass Energy and Environmental Biotechnology, Yunnan Province, Yunnan Normal University, Kunming, China
For the past 300 years, hydrogen sulfide (H2S) has been considered a toxic gas. Nowadays, it has been found to be a novel signaling molecule in plants involved in the regulation of cellular metabolism, seed germination, plant growth, development, and response to environmental stresses, including high temperature (HT) and low temperature (LT). As a signaling molecule, H2S can be actively synthesized and degraded in the cytosol, chloroplasts, and mitochondria of plant cells by enzymatic and non-enzymatic pathways to maintain homeostasis. To date, plant receptors for H2S have not been found. It usually exerts physiological functions through the persulfidation of target proteins. In the past 10 years, H2S signaling in plants has gained much attention. Therefore, in this review, based on that same attention, H2S homeostasis, protein persulfidation, and the signaling role of H2S in plant response to HT and LT stress were summarized. Also, the common mechanisms of H2S-induced HT and LT tolerance in plants were updated. These mechanisms involve restoration of biomembrane integrity, synthesis of stress proteins, enhancement of the antioxidant system and methylglyoxal (MG) detoxification system, improvement of the water homeostasis system, and reestablishment of Ca2+ homeostasis and acid-base balance. These updates lay the foundation for further understanding the physiological functions of H2S and acquiring temperature-stress-resistant crops to develop sustainable food and agriculture.
Introduction
Hydrogen sulfide (H2S) has long been deemed a toxic gas since its discovery as a sewer gas in the 1700s (Wang, 2012; Modolo and da-Silva, 2023). H2S has a high affinity for heme-containing proteins, such as hemoglobin, myoglobin, cytochrome oxidase, catalase (CAT), and peroxidase (POD), and acts through protein persulfidation. Persulfidation can change the conformation and activity of proteins, leading to disruption of cellular metabolism, and even cell death (Li et al., 2016a; Liu et al., 2021; Li et al., 2022; Yang et al., 2022; Huang and Xie, 2023). At the end of the Permian period, a large amount of H2S was accumulated on earth due to volcano eruptions, which caused a rapid decrease of oxygen (O2) in seawater. This decrease promoted the mass proliferation of anaerobic green sulfur bacteria, which used sulfate (SO42-) instead of O2 for respiration and released a mass of H2S. The released H2S entered the atmosphere and land, therefore leading to the extinction of 95% of marine species and 70% of terrestrial vertebrates (Wang, 2012; Li et al., 2016a, Li et al., 2022). In plants, H2S can result in necrosis, shedding, and growth inhibition of leaves and can inhibit the uptake of mineral elements, such as phosphate, by roots (Wang, 2012; Liu et al., 2021; Yang et al., 2022; Huang and Xie, 2023). Recently, Zhang et al. (2017) reported that H2S could inhibit taproot growth and regulate root system architecture in Arabidopsis plants by inducing reactive oxygen species (ROS) and nitric oxide (NO) to generate oxidative and nitrosative stress.
Promisingly, H2S has now been found to be a novel signaling molecule involved in many physiological processes in plants. These processes include cell metabolism and division, seed germination and dormancy, plant growth and development, organ maturation and senescence, and plant response to environmental stresses, including high temperature (HT) and low temperature (LT) (Li et al., 2016a; Liu et al., 2021; Wang et al., 2021; Yang et al., 2022; Huang et al., 2023). H2S, similar to other signaling molecules, such as calcium ion (Ca2+), hydrogen peroxide (H2O2), NO, methylglyoxal (MG), and glutamic acid, has a dual role, namely as a signaling molecule at low concentrations and as a toxic agent at high concentrations (Qiu et al., 2020; Iqbal et al., 2022; Pande et al., 2022; Huang and Xie, 2023; Mhamdi, 2023). Therefore, it must maintain homeostasis in plant cells under normal physiological and unfavorable environmental conditions. H2S homeostasis can be tightly regulated by enzymatic and non-enzymatic pathways, which are described as follows (Li et al., 2016a; Liu et al., 2021; Moseler et al., 2021; Li et al., 2022; Citi et al., 2023; Pedre et al., 2023).
In the past decade, H2S, as a pleiotropic signaling molecule in plants has gained much attention, especially in its metabolism and physiological function under HT and LT stress conditions (Li et al., 2016a; Liu et al., 2021; Moseler et al., 2021; Wang et al., 2021; Yang et al., 2022; Citi et al., 2023; Huang and Xie, 2023; Pedre et al., 2023). Therefore, in this review, based on that same attention, the enzymatic and non-enzymatic pathways of H2S homeostasis, protein persulfidation, and the signaling role of H2S in plant response to HT and LT stress were updated. This laid the groundwork for further understanding the physiological function of H2S and the acquisition of temperature-stress-resistant crops to develop sustainable food and agriculture.
H2S homeostasis
Because H2S is a toxic agent at high concentrations, it must maintain homeostasis in plant cells. Its homeostasis is tightly controlled by biosynthesis and degradation via enzymatic and non-enzymatic pathways (Figure 1). The mechanisms controlling H2S homeostasis in plant cells are detailed below.
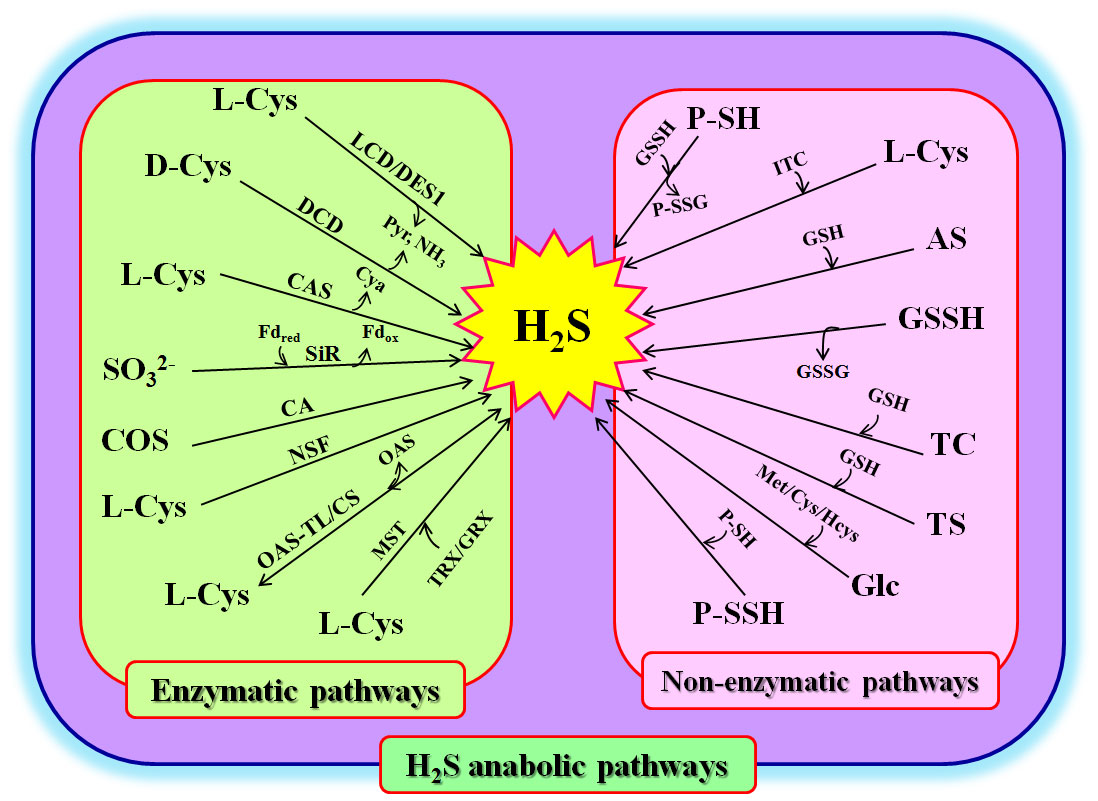
Figure 1 Hydrogen sulfide (H2S) anabolism in plants. AS, allyl sulfur; CA, carbonic anhydrase; L-Cys, L-cysteine; D-Cys, D-cysteine; CAS, β-cyanoalanine synthase; Cya, β-cyanoalanine; COS, carbonyl sulfur; CS, cysteine synthetase; LCD, L-cysteine desulfhydrase; DCD, D-cysteine desulfhydrase; DES1, cysteine desulfhydrase1; Fdred, reduced ferredoxin; Fdox, oxidized ferredoxin; Glc, glucose; GRX, glutaredoxin; GSH, glutathione; GSSH, glutathione persulfide; Hcys, homocysteine; ITC, thiocysteine; Met, methionine; MST, 3-mercaptopyruvate sulfur transferase; NH3, ammonia; NSF, nitrogenase Fe-S cluster; OAS-TL, O-acetylserine (thiol) lyase; P-SH, protein thiols; P-SSH, protein persulfidation P-SSG, glutathionylated proteins; Pyr, pyruvate; SiR, sulfite reductase; TC, isothiocyanate; TRX, thioredoxin; TS, thiosulfate.
Enzymatic pathways for H2S biosynthesis
Involvement in multiple pathways is one of the characteristics of signaling molecules in plants (Liu et al., 2021; Wang et al., 2021). In general, H2S can be biosynthesized via enzymatic pathways in the cytosol, chloroplasts, and mitochondria of plants. These enzymes include L-cysteine (L-Cys) desulfhydrase (LCD), D-cysteine (D-Cys) desulfhydrase (DCD), O-acetyl-serine (thiol) lyase (OAS-TL), sulfite reductase (SiR), β-cyanoalanine synthase (CAS), and nitrogenase Fe-S cluster-like (NFS) (Liu et al., 2021; Wang et al., 2021; Huang and Xie, 2023) (Figure 1). In the cytosol, H2S can be produced from L-Cys or D-Cys under the catalysis of LCD or DCD, meanwhile releasing ammonia (NH3) and pyruvate (Pyr). In addition, L-Cys desulfhydrase 1 (DES1), an LCD homolog, can catalyze L-Cys to H2S, resulting in the production of NH3 and Pyr. H2S can also be used to synthesize L-Cys (by OAS-TL, also known as L-Cys synthetase, CS), whose reverse reaction can produce H2S and O-acetyl-serine (OAS) (Li et al., 2016a; Yang et al., 2022; Huang and Xie, 2023). In chloroplasts, sulfite (SO32-), which is reduced from SO42-, can be converted to H2S by SiR using ferredoxin (Fdred) as a cofactor. Similarly, NFS can use L-Cys to synthesize H2S (Aroca et al., 2020; Saud et al., 2022; Huang and Xie, 2023). In mitochondria, H2S can be formed under the catalysis of CAS using hydrogen cyanide (HCN) and L-Cys as substrates, along with the release of cyanoalanine (Cya) and the scavenging of HCN. Analogously, L-Cys can synthesize H2S via the catalysis of NFS, similar to the action of NFS in chloroplasts. OAS-TL can also catalyze L-Cys to H2S in mitochondria (Liu et al., 2021; Yang et al., 2022; Huang and Xie, 2023) (Figure 1).
In addition, carbonyl sulfide (COS), as an atmospheric trace gas, can enter the plant cells through stomata in association with carbon dioxide (CO2). Under the catalysis of carbonic anhydrase (CA), COS can be converted to H2S (Stimler et al., 2010; Li et al., 2022; Citi et al., 2023) (Figure 1). In addition, in Arabidopsis plants, 3-mercaptopyruvate sulfur transferase (MST) can be persulfidated by 3-mercaptopyruvate to form persulfidated MST (MST-SSH). The MST-SSH can release H2S by interacting with either thioredoxin (TRX) or glutaredoxin (GRX). Therefore, the redox systems, TRX and GRX, can not only regulate the activity of MST, but are also one of the biosynthetic pathways of H2S in plants (Moseler et al., 2021). Recently, MST was found to directly lead to the persulfidation of target proteins, it is considered a protein persulfidase (Pedre et al., 2023) (Figure 1).
Non-enzymatic pathways for H2S production
As mentioned above, MST is a protein persulfidase, and the sulfur in MST-SSH can be transferred to other proteins containing dithiols (two sulfydryl groups) to form protein persulfides (P-SSH). The P-SSH can be oxidized by oxidants, releasing H2S (Pedre et al., 2023) (Figure 1). MST-SSH can also be reduced to MST-SH and glutathione persulfide (GSSH) by glutathione (GSH). The GSSH is then converted to H2S and GSSG by the GSH. Similarly, P-SSH can interact with other proteins containing sulfydryl groups (P-SH) to form disulfide bonds between proteins (P-S-S-P) and release H2S (Pedre et al., 2023). Similarly, P-SH can be converted to glutathionylated proteins (P-SSG) and produce H2S under the action of GSSH. In addition, isothiocyanate (ITC), thiocysteine (TC), thiosulfate (TS), and allyl sulfur (AS), as major components of fertilizers and/or industrial pollutants, can release H2S under the action of L-Cys or GSH when they are taken up by plant roots (Nakajima et al., 2019; Yu et al., 2019; Moseler et al., 2021; Li et al., 2022; Citi et al., 2023). In addition to these, H2S can be released from glucose (Glc) under the influence of L-Cys, homocysteine, or methionine (Met) (Figure 1).
H2S degradation
To maintain homeostasis, the excess H2S in plant cells must be rapidly scavenged, degraded, or converted. As mentioned above, in the cytosol and mitochondria, CS can utilize H2S and OAS to synthesize Cys, which in turn forms peptides and/or proteins (Liu et al., 2021; Yang et al., 2022; Huang and Xie, 2023) (Figure 2). On the other hand, in mitochondria, sulfite quinone reductase (SQR) can catalyze H2S and GSH to GSSH in the presence of O2. The GSSH can be oxidized to SO32- by persulfide dioxygenase (ETHE1) (Gerush and Ferenchuk, 2019; Yang et al., 2022). Subsequently, SO32- is further oxidized to SO42- and S2O32- by sulfite oxidase (SO) and Rhodanese (namely thiosulfate sulfur transferase), respectively (Gerush and Ferenchuk, 2019; Yang et al., 2022) (Figure 2). Similarly, H2S can be methylated under the catalysis of thiol-S-methyltransferase (TMT) to form methanethiol (CH4S) and dimethyl sulfide (CH3SCH3), and then oxidized to SO42- by Rhodanese (Yang et al., 2022). H2S can also react with ROS, reactive nitrogen species (RNS), reactive halogen species (RHS), sulfenylated proteins (P-SOH), nitrosylated proteins (P-SNO), P-SSG, and Fe3+ to form persulfides and/or polysulfides (Yang et al., 2022) (Figure 2). These persulfides and polysulfides may act as signaling molecules to further execute the physiological functions of H2S in plants.
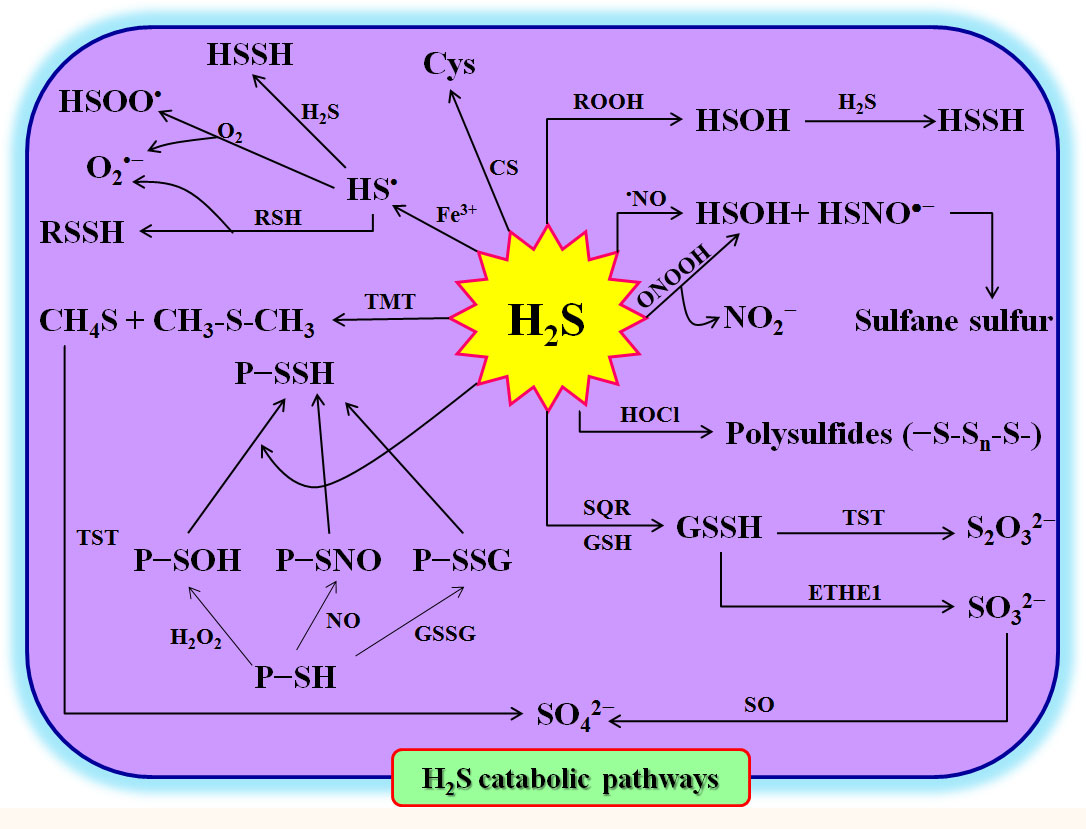
Figure 2 Hydrogen sulfide (H2S) catabolism in plants. Cys, L-cysteine; CS, cysteine synthetase; ETHE1, ethylmalonic encephalopathy 1 protein (persulfide dioxygenase); GSH, glutathione; GSSG, oxidized glutathione; GSSH, glutathione persulfide; H2O2, hydrogen peroxide; HOCl, hypochlorite; HS•, sulfhydryl radical; HSOH, hydrogen thioperoxide; HSOO•, hydroxysulfinyl radical; HSSH, hydrogen persulfide; NO, nitric oxide; ONOOH, peroxynitrous; P-SH, protein thiols; P-SNO, nitrosylated proteins; P-SOH, sulfenylated proteins; P-SSG, glutathionylated proteins; P-SSH, persulfidated proteins; RNS, reactive nitrogen species; ROOH, hydroperoxides; SO, sulfite oxidase; SQR, sulfite quinone reductase; TMT, thiol-S-methyl-transferase; TST, thiosulfate sulfur transferase; RSSH, hydropersulfides.
H2S response to HT stress
HT leads to multiple injuries at the molecular, physiological, biochemical, subcellular, cellular, and whole plant body levels (Wani and Kumar, 2020; Zhang et al., 2022; Huang et al., 2023; Kan et al., 2023). HT injury is implicated in biomembrane damage, protein denaturation, Ca2+ overload toxicity, ion and acid imbalance, and oxidative, osmotic, and MG stress (Kosová et al., 2021; Zhao et al., 2021; Zhang et al., 2022; Zhou et al., 2022a,; Huang et al., 2023; Kan et al., 2023; Wang et al., 2023c) (Figure 3). Therefore, plant HT tolerance is closely related to the repair of biomembrane (RBM), stress protein biosynthesis (SPB), Ca2+ ion equilibrium (CIE), ROS homeostasis (ROH), osmoregulation (OSR), MG balance (MGB), ion equilibrium (IEB), and pH value stability (PVS) in plants (Zhang et al., 2022; Zhou et al., 2022a, Zhou et al., 2022b; Huang et al., 2023; Kan et al., 2023; Wang et al., 2023c) (Figure 4; Table 1).
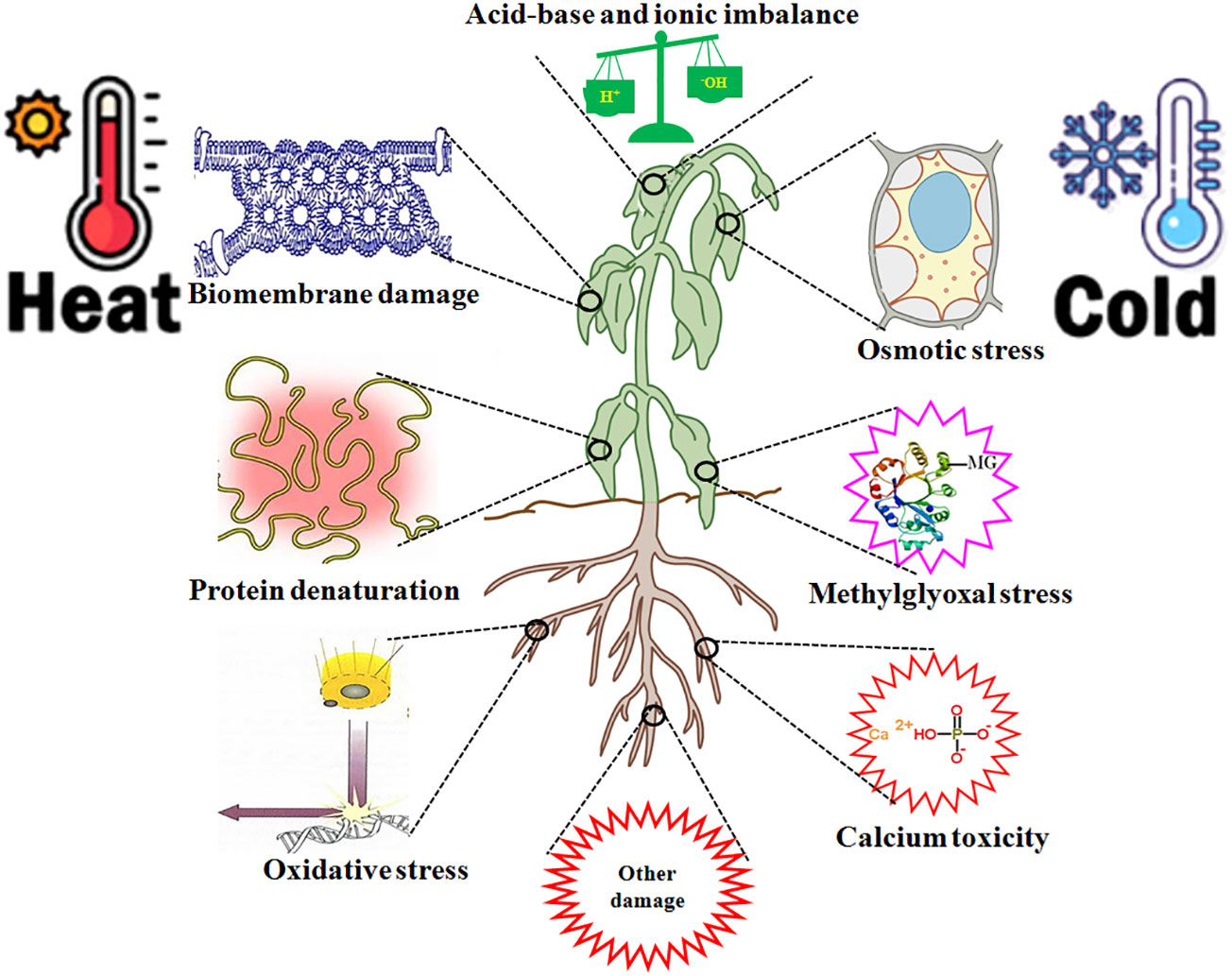
Figure 3 high temperature (HT) and low temperature (LT) stress injury to plants. HT and LT stress commonly cause biomembrane damage, protein denaturation, oxidative stress, osmotic stress, methylglyoxal (MG) stress, calcium overload toxicity (mainly calcium phosphate precipitation), acid-base and ion imbalance, and other damage in plants.
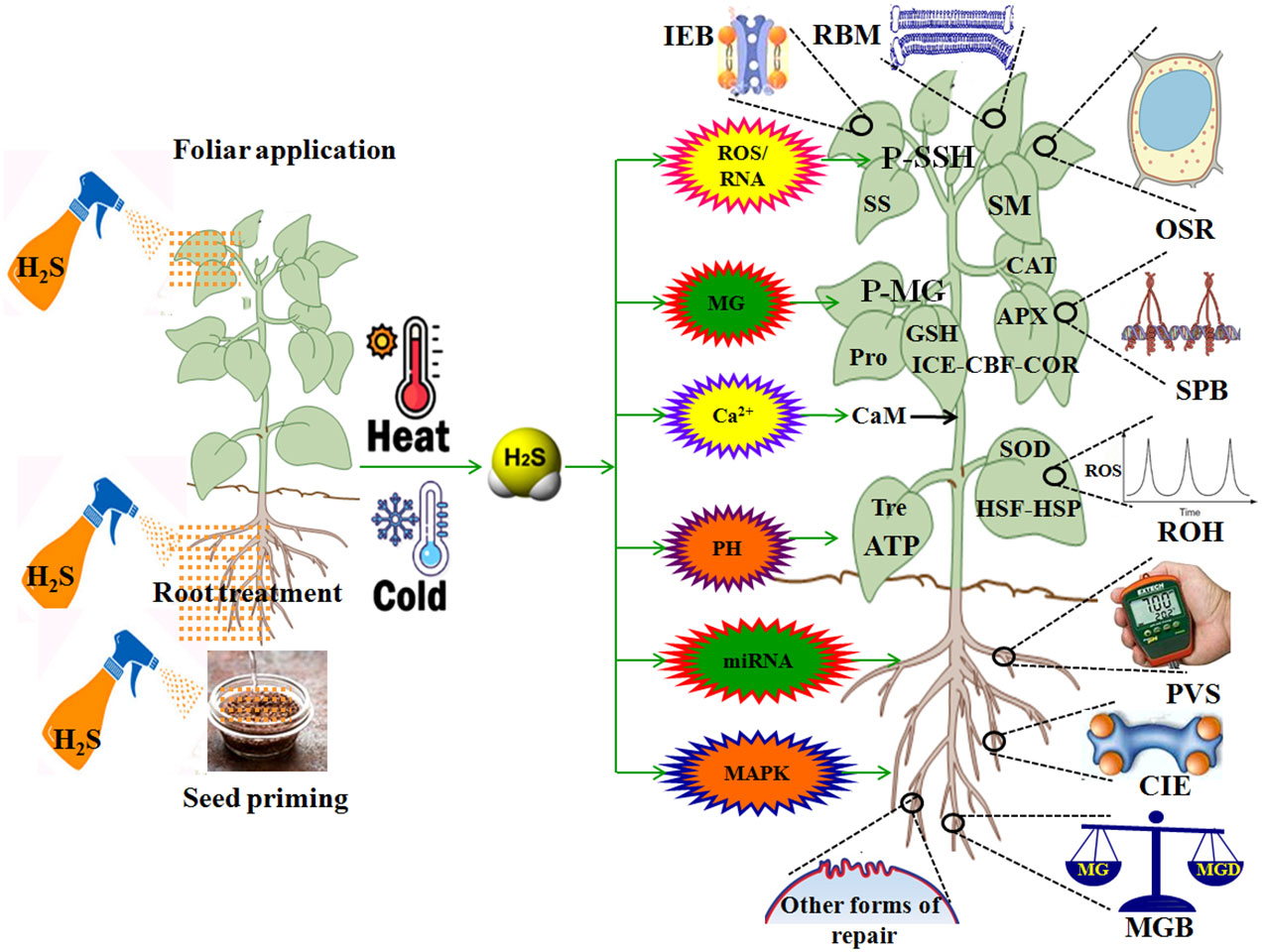
Figure 4 Role of hydrogen sulfide (H2S) in plant response to high temperature (HT) and low temperature (LT) stress. APX, ascorbate peroxidase; CBF, C-repeat binding factor; Ca2+, calcium ion; CaM, calmodulin; CAT, catalase; CIE, calcium ion equilibrium; COR, cold regulated proteins; DREB, dehydration response element binding proteins; GSH, glutathione; HSF, heat shock factors; HSP, heat shock proteins; ICE, inducer of CBF expression; IEB, ion equilibrium; MG, methylglyoxal; MGB, MG balance; MAPK, mitogen-activated protein kinase; miRNA, microRNA; OSR, osmoregulation; P-SSG, protein persulfidation; P-MG, protein methylglyoxalation; PVS, pH value stability; RBM, repair of biomembrane; Pro, proline; ROS, reactive oxygen species; ROH, ROS homeostasis; RNS, reactive nitrogen species; SM, secondary metabolites; SOD, superoxide dismutase; SPB, stress protein biosynthesis; SS, soluble sugars; Tre, trehalose.
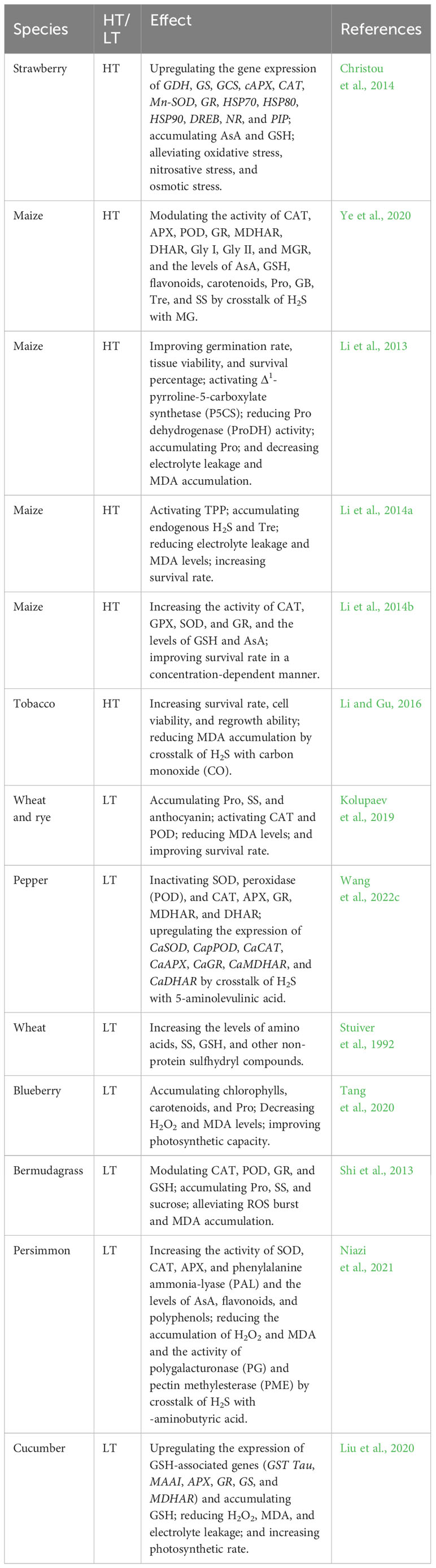
Table 1 Effect of hydrogen sulfide (H2S) on high temperature (HT) and low temperature (LT) stress tolerance in plants.
H2S improves plant HT tolerance by enhancing the antioxidant system and water homeostasis systems, biosynthesizing heat shock proteins (HSPs), and reducing biomembrane damage. In wheat (Triticum aestivum L.) seedlings, foliar application of sodium hydrosulfide (NaHS), an H2S donor, promoted HT tolerance by reducing malonaldehyde (MDA) accumulation in a concentration-dependent manner (Yang et al., 2016). H2S pretreatment also increased the activity of superoxide dismutase (SOD), CAT, ascorbate peroxidase (APX), and the levels of endogenous H2S and soluble sugars (SS). In addition, H2S upregulated the expression of Fe-SOD, Mn-SOD, Cu/Zn-SOD, mAPX, tAPX, and CAT, and decreased the levels of H2O2 and MDA in wheat seedlings under HT stress (Yang et al., 2016). Similarly, in strawberry (Fragaria x ananassa cv. ‘Camarosa’) plants, root treatment with H2S increased the levels of ascorbic acid (AsA) and GSH. The gene expression of AsA and GSH biosynthetic enzymes (GDH, GS, and GCS), antioxidant enzymes (cAPX, CAT, Mn-SOD, and GR), HSPs (HSP70, HSP80, and HSP90), transcription factor (DREB), NO biosynthesis (NR), and aquaporins (PIP) was also upregulated by H2S (Christou et al., 2011, Christou et al., 2014) (Table 1). Therefore, these molecular and physiological effects protected strawberry plants from HT damage by mitigating ROS-induced oxidative stress, NO-induced nitrosative stress, and water deficit-induced osmotic stress under HT stress conditions. Interestingly, aquaporins (AQPs) could be nitrosylated (-SNO) by NO, sulfenylated (-SOH) by H2O2, and persulfidated (-SSH) by H2S. These posttranslational modifications (PTMs) could change the conformation and activity of proteins, thus regulating water homeostasis in plant cells (Liu et al., 2021; Mukherjee et al., 2024). The above PTMs also indicate the crosstalk of H2O2, NO, and H2S in plants under HT stress conditions. In maize (Zea mays L.), seed priming with H2S increased the seed germination rate, in addition to shoot length, root length, and fresh weight of seedlings under HT stress conditions (Zhou et al., 2018). Moreover, H2S activated APX, SOD, CAT, glutathione reductase (GR), POD, 1-pyrroline-5-carboxylate synthetase (P5CS), proline (Pro) dehydrogenase (ProDH), ornithine aminotransferase, glycine betaine (GB) aldehyde dehydrogenase (GDH), and trehalose (Tre)-6-phosphate phosphatase (TPP) in maize seedlings (Zhou et al., 2018). The activation of these enzymes further accumulated the antioxidants AsA and GSH, and the osmolytes Pro, GB, and Tre (Zhou et al., 2018). In contrast, H2S-primed HT tolerance was separately enhanced by exogenous Pro, GB, and Tre, and attenuated by gabaculine, disulfiram, and sodium citrate, which are the inhibitors of osmolyte biosynthetic enzymes (Zhou et al., 2018).
H2S modulates HT tolerance through crosstalk with other signaling molecules. As discussed above, a large number of signaling molecules and their interactions are involved in the development of HT tolerance (Li et al., 2021; Wang et al., 2021; Zhang et al., 2022). For H2S-Ca2+ crosstalk in tobacco (Nicotiana tabacum) suspension-cultured cells, H2S pre-treatment improved HT tolerance by increasing cell viability and survival rate and decreasing electrolyte leakage and MDA accumulation (Li et al., 2012). H2S-induced HT tolerance was enhanced by Ca2+ and its ionophore A23187, whereas it was repaired by the Ca2+ chelator ethylene glycol-bis(β-aminoethyl-ether)-N,N,N’,N’-tetraacetic acid, the plasma membrane channel blocker La3+, and the calmodulin (CaM) antagonists chlorpromazine and trifluoperazine (Li et al., 2012). This study is the first to report that H2S interacts with Ca2+ in the acquisition of HT tolerance in plants. Similarly, in tobacco cells, the activity of the H2S-generating enzyme LCD could be activated by Ca2+ and CaM (Li et al., 2015a). Interestingly, H2S could persulfidate DES1 at Cys44 and Cys205, which in turn enhanced its activity to achieve self-amplification of H2S signaling (Shen et al., 2020). H2S was also able to regulate Ca2+ homeostasis in animal cells through the persulfidation of Ca2+ channels (Zhang et al., 2015), but this regulatory mechanism needs to be further investigated in the plant system in the future.
For H2S-NO crosstalk, in wheat seedlings, H2S and NO alone or in combination could reduce the photosynthetic suppression induced by glucose accumulation. The reduced photosynthetic suppression was closely associated with the activation of the AsA-GSH cycle and antioxidant system (CAT; SOD; APX; GR; AsA; GSH; monodehydroascorbate reductase, MDHAR; and dehydroascorbate reductase, DHAR) under HT stress conditions (Iqbal et al., 2021b). These effects were exacerbated by the NO scavenger 2-4-carboxyphenyl-4,4,5,5-tetramethylimidazoline-1-oxyl-3-oxide (cPTIO) and the H2S scavenger hypotaurine via the accumulation of H2O2 and MDA (Iqbal et al., 2021b). Furthermore, NO-induced HT tolerance was enhanced by H2S, while being reduced by hypotaurine, suggesting that H2S is downstream of NO to trigger the development of HT tolerance (Iqbal et al., 2021b). Similar crosstalk between H2S and NO was observed in the development of HT tolerance in poplar (Populus trichocarpa) (Cheng et al., 2018) and maize seedlings (Li et al., 2013). Interestingly, the activities of CAT1, APX1, POD5, and ROS-generating enzyme oxidase homolog protein D (RBOHD) could be separately regulated by H2S and NO via persulfidation (-SSH) and nitrosylation (-SNO) (Yun et al., 2011; Begara-Morales et al., 2014; Li et al., 2020; Shen et al., 2020; Terron-Camero et al., 2020). Protein persulfidation (-SSH) and nitrosylation (-SNO) could activate APX1, while inactivating CAT1 (Begara-Morales et al., 2014; Li et al., 2020; Terron-Camero et al., 2020). RBOHD could also be activated by persulfidation (-SSH), but inactivated by nitrosylation (-SNO) (Yun et al., 2011; Shen et al., 2020; Kosová et al., 2021). The persulfidation (-SSH) and nitrosylation (-SNO) of antioxidant and ROS-generating enzymes also support the crosstalk of H2S with NO and H2O2 in plants.
For the H2S-phytohormone crosstalk, in rice (Oryza sativa L.) cultivars, ethylene (ETH), NO, and H2S increased the dry weight of shoots and roots, the levels of Pro, GB, Tre, and SS, and the activity of SOD, CAT, and GR in leaves under normal and HT stress conditions. Also, the treatment improved photosynthetic parameters, such as actual, maximal, and intrinsic efficiency of PSII, in addition to photochemical quenching, non-photochemical quenching, and electron transport rate (Gautam et al., 2022). Similarly, the levels of these signaling molecules and the relative expression of psbA, psbB, Mn-SOD, Fe-SOD, Cu/Zn-SOD, and APX were upregulated by exogenous application (Gautam et al., 2022). These effects of ETH or NO were enhanced by H2S, while reversed by the H2S scavenger hypotaurine, further supporting that the ameliorative effects of ETH or NO involve H2S (Gautam et al., 2022). Similar signaling crosstalk of H2S with ETH, salicylic acid (SA), abscisic acid (ABA), and melatonin (MT) has been reported in maize seedlings (Li et al., 2015b; Wang et al., 2023b), tobacco cells (Li and Jin, 2016), and wheat seedlings (Iqbal et al., 2021a). Fortunately, the activity of 1-aminocyclopropane-1-carboxylic acid oxidase1,2 (ACO1, ACO2), N-acetylserotonin methyltransferase (ASMT), serotonin N-acetyltransferase (SNAT), abscisic acid insensitive4 (ABI4), mitogen-activated protein kinase4 (MAPK4), and SNF1-related protein kinase2.6 (SNRK2.6) could be regulated by H2S via protein persulfidation (-SSH) (Jia et al., 2018; Shen et al., 2020; Du et al., 2021; Zhou et al., 2021a; Wang et al., 2022b). Protein persulfidation inhibited the activity of ACO1 and ACO2, while activating ASMT, SNAT, ABI4, and MAPK4, further implying the signaling crosstalk of H2S with ETH, MT, and ABA in plants.
For H2S-ROS-NO crosstalk, H2S increased H2O2 and NO accumulation, in addition to NR activity in wheat plants. The increased NO was suppressed by the NR inhibitor sodium tungstate, but not by the NG-nitro-L-arginine methyl ester (NO synthase inhibitor) (Karpets et al., 2020). Similarly, NR activity and NO levels were abolished by the H2O2 scavenger dimethylthiourea and the NADPH oxidase inhibitor imidazole, whereas H2S-induced H2O2 levels were only weakly affected by the NO scavenger cPTIO and sodium tungstate (Karpets et al., 2020). Furthermore, H2S-induced HT tolerance was abolished by dimethylthiourea, imidazole, cPTIO, and tungstate (Karpets et al., 2020). This study demonstrates the signaling crosstalk of H2S with H2O2 and NO in the development of HT tolerance in wheat plants. A similar H2S-ROS-NO crosstalk was found in rice seedlings under HT stress (Gautam et al., 2022). Interestingly, in rice seedlings, NIA2 (an NR isoform), a NO-generating enzyme, could be persulfidated by H2S, which in turn decreased the enzyme activity, thus regulating nitrate metabolism and NO levels (Zhou et al., 2021b), further supporting the crosstalk of H2S with NO in plants.
H2S response to LT stress
LT also results in multiple injuries in plants (Ruelland et al., 2009; Ding et al., 2019; Ritonga and Chen, 2020; Manasa et al., 2022; Gusain et al., 2023; Wen et al., 2023) (Figure 3), similar to that of HT (Zhang et al., 2022; Huang et al., 2023; Kan et al., 2023). Therefore, the acquisition of LT and HT tolerance in plants has some common mechanisms (Figure 4).
H2S improves LT tolerance in fruits by enhancing the ROS-scavenging system and maintaining the integrity of biomembranes and cell structures. In hawthorn (Crateagus pinnatifida) fruit, H2S fumigation increased the endogenous levels of H2S by activating LCD and DCD, which in turn increased the activity of SOD, CAT, APX, and phenylalanine ammonia lyase. The levels of phenols, flavonoids, anthocyanins, and AsA in fruits were also increased by H2S. The enhanced antioxidant system further reduced the accumulation of MDA and H2O2 under LT stress conditions, thus ameliorating LT injury in fruits (Aghdam et al., 2018). Similarly, H2S increased endogenous H2S levels by enhancing the activity of LCD, DCD, OAS-TL, and serine acetyltransferase (SAT) in peach (Prunus persica L.) fruits, which in turn accumulated AsA and GSH. In addition, the activity of APX, GR, CAT, and SOD was increased by H2S, thereby decreasing the levels of ROS (mainly H2O2 and superoxide radicals) under LT stress conditions (Wang et al., 2022a). In the same way, H2S decreased the activity of pectin methylesterase, polygalacturonase, β-glucosidase, carboxymethylcellulose, and β-galactosidase in peach fruits under LT stress conditions. The reduced enzyme activity inhibited the degradation of cell-wall polysaccharide fractions, thus maintaining the integrity of the cell structure (Wang et al., 2022a). The identical effects of H2S were found in banana (Musa paradisiaca L.) (Luo et al., 2015; Li et al., 2016b) and peach (Wang et al., 2023a) fruits under LT stress conditions through regulation of energy and Pro metabolism.
H2S enhances LT tolerance in plants through the inducer of C-repeat-binding factor (CBF) expression (ICE)−CBF−cold regulated proteins (COR) signaling pathways. In grape (Vitis vinifera L) seedlings, foliar application of H2S increased SOD activity and VvICE1 and VvCBF3 gene expression in leaves, and reduced superoxide anion radical and MDA levels and biomembrane damage under LT stress conditions (Fu et al., 2013). These effects were reversed by the H2S scavenger hypotaurine (Fu et al., 2013), suggesting that H2S could improve LT tolerance in grape seedlings. In the same way, H2S pre-treatment could improve LT tolerance of cucumber (Cucumis sativus L) seedlings through the CsARF5-CsDREB3 module (Zhang et al., 2021), similar to H2S-induced HT tolerance in strawberry (Christou et al., 2011, Christou et al., 2014). In cucumber seedlings, foliar application of H2S also increased the activity of APX, CAT, and POD, in addition to the levels of SS, Pro, and GSH in leaves. The enhanced ROS-scavenging system further reduced the accumulation of MDA and H2O2 and electrolyte leakage under LT stress conditions, thus improving LT tolerance of cucumber (Nasibi et al., 2020).
H2S modulates LT tolerance in plants through crosstalk with other signaling molecules. For H2S-NO-ROS crosstalk, in cucumber seedlings, H2S increased plasma membrane H+-ATPase (PMA) activity by upregulating the expression of PMA genes (CsHA2, CsH4, CsH8, CsH9, and CsHA10) under LT stress conditions (Janicka et al., 2018). Similarly, NO and H2O2 upregulated the expression of the gene CsHA2, which in turn slightly increased PMA activity in cucumber under LT stress conditions. More interestingly, NO could nitrosylate PMA, thus promoting its phosphorylation and increasing the H+/ATP coupling ratio under LT stress conditions (Janicka et al., 2018). This study suggests that under LT stress conditions PMA plays a key role in the development of LT tolerance in plants by maintaining PVS via the crosstalk of H2S with NO and H2O2. Similar effects have been observed in cucumber seedlings (Wu et al., 2022), peach fruits (Geng et al., 2019), and other plant species (Kolupaev et al., 2023) through H2S-H2O2 crosstalk. Interestingly, in Arabidopsis plants, H2S could persulfidate PMA, which in turn enhanced its activity, thus promoting H+ efflux and maintaining pH homeostasis in plant cells (Ma et al., 2023).
For the H2S-MAPK crosstalk, in the model plant Arabidopsis thaliana, H2S upregulated the gene expression of MPK4 under normal and LT stress conditions. The upregulation of MPK4 further activated the transcription of ICE1, CBF3, COR15A, and COR15B, which inhibited stomatal opening and alleviated LT injury (Du et al., 2017). Further studies found that H2S-alleviated LT tolerance was attenuated in mpk4 mutants, but not in the upstream mek2 and crlk1 mutants (Du et al., 2021). More interestingly, MPK4, which has a basal persulfidation, could be further persulfidated by H2S, which in turn enhanced its activity nearly tenfold, whereas MEK2 was not persulfidated by H2S (Du et al., 2021). These data further support the fact that H2S alleviates LT tolerance through the MPK4 and ICE-CBF-COR signaling pathways, as discussed above.
For the H2S-phytohormone crosstalk, in cucumber seedlings, SA upregulated the gene expression of LCD and DCD, which in turn increased the endogenous levels of H2S under normal and LT stress conditions (Pan et al., 2020). This effect was blocked by paclobutrazol and 2-aminoindan-2-phosphonic acid (inhibitors of SA biosynthesis). In contrast, H2S and its scavenger hypotaurine and inhibitor DL-propargylglycine had no significant effect on endogenous SA levels in cucumber seedlings under normal and LT stress conditions (Pan et al., 2020). This study suggests that H2S plays a signaling role downstream of SA. SA and NaHS also upregulated the expression of chilling response genes (ICE, CBF1, and COR) and antioxidant enzymes (SOD, POD, CAT, APX, and GR). The upregulation of gene expression further increased enzyme activity and AsA and GSH levels. The enhanced antioxidant system reduced ROS and MDA accumulation and electrolyte leakage in cucumber seedlings under LT stress conditions, thus alleviating LT injury in cucumber (Pan et al., 2020). These data further support the fact that H2S enhances LT tolerance through the ICE−CBF−COR signaling pathway in grape (Fu et al., 2013), cucumber (Janicka et al., 2018; Zhang et al., 2021), and Arabidopsis (Du et al., 2021), as discussed above. Similarly, Zhang et al. (2020) reported that H2S increased the transcription and activity of flavin monooxygenase-like proteins (YUCCA2), thereby increasing indoleacetic acid (IAA) levels in cucumber seedlings under LT stress conditions. However, IAA treatment had no significant effect on LCD and DCD activities or H2S levels in cucumber seedlings under LT stress conditions (Zhang et al., 2020), indicating that H2S plays a signaling role in the upstream of IAA. In addition, H2S and IAA improved LT tolerance of cucumber seedlings by reducing electrolyte leakage and ROS accumulation. The H2S-induced tolerance was reduced by the IAA polar transport inhibitor (1-naphthylphthalamic acid), while the H2S scavenger hypotaurine had little effect on the IAA-induced tolerance (Zhang et al., 2020). These data further support the fact that IAA plays a signaling role downstream of H2S in plant LT tolerance through auxin response factor (ARF)−dehydration-responsive element-binding (DREB) protein signaling pathways. However, whether the ICE−CBF−COR module and plant hormone signaling proteins can be persulfidated by H2S needs to be further elucidated in the future.
Conclusion and prospects
Overall, H2S, as a novel signaling molecule in plants, plays a pivotal role in plant growth and response to HT and LT stress through crosstalk with other signaling molecules (Shen et al., 2020; Liu et al., 2021; Wang et al., 2021; Yang et al., 2022; Mukherjee et al., 2024). As discussed above, HT and LT jointly result in biomembrane damage, protein denaturation, Ca2+ overload toxicity, acid-base imbalance, and oxidative, osmotic, and MG stress (Figure 3). To survive, plants have developed the mechanisms of tolerance to HT and LT stress by repairing the biomembrane, biosynthesizing stress proteins, enhancing the ROS-/MG-detoxification and water homeostasis systems, and maintaining Ca2+ and H+ balance (Figure 4). Hopefully, the tolerance mechanisms can be triggered by the application of H2S in the form of seed priming, foliar application, and root treatments (Figure 4). Briefly, under HT and LT stress conditions, the repairing of biomembrane is involved in the change of membrane lipid components, saturation, and chain length. The denatured proteins can be renatured or replaced by stress proteins, such as HSPs and cold shock proteins. Also, oxidative damage can be alleviated by detoxification systems, mainly the ROS detoxification system and the MG detoxification system. The ROS detoxification system consists of enzymes (SOD, APX, CAT, GR, DHAR, and MDHAR) and non-enzymatic antioxidants (AsA, GSH, phenols, flavonoids, and anthocyanins). The MG detoxification system includes the glyoxylase system (glyoxalase I, Gly I; Gly II; and Gly III) and the non-glyoxalase system (MG reductase, MGR; aldose/aldehyde reductase, ALR; aldo-keto reductase, AKR; and lactate dehydrogenase, LDH) (Table 1). Similarly, osmotic stress can be alleviated by the water homeostasis system consisting of osmolytes (Pro, GB, Tre, SS, and soluble proteins) and their metabolic enzymes (P5CS, ProDH, GDH, and TPP) and water transporters (AQPs). In addition, Ca2+ and H+ balance can be maintained by the synergistic effect of Ca2+–ATPase, Ca2+ channels, PMAs, vacuolar H+–ATPase, and vacuolar pyrophosphatase (Cosse and Seidel, 2021) (Figure 4).
Over the past decade, great progress has been made in understanding H2S signaling in plants from seed germination to plant HT and LT tolerance. However, several open questions need to be addressed in the future. For example, the metabolism of H2S, especially the non-enzymatic pathways, is not fully understood. Also, H2S is an emerging signaling molecule and its receptors have not been found in plants. Moreover, the exact mechanisms of H2S-induced HT and LT tolerance remain to be elucidated using molecular, physiological, biochemical, omics, and multi-omics approaches.
Author contributions
Z-GL: Writing – review & editing, Writing – original draft, Conceptualization. J-RF: Writing – original draft. S-JB: Writing – original draft.
Funding
The author(s) declare that financial support was received for the research, authorship, and/or publication of this article. This research was funded by the National Natural Science Foundation of China (32160065).
Conflict of interest
The authors declare that the research was conducted in the absence of any commercial or financial relationships that could be construed as a potential conflict of interest.
Publisher’s note
All claims expressed in this article are solely those of the authors and do not necessarily represent those of their affiliated organizations, or those of the publisher, the editors and the reviewers. Any product that may be evaluated in this article, or claim that may be made by its manufacturer, is not guaranteed or endorsed by the publisher.
References
Aghdam, M. S., Mahmoudi, R., Razavi, F., Rabiei, V., Soleimani, A. (2018). Hydrogen sulfide treatment confers chilling tolerance in hawthorn fruit during cold storage by triggering endogenous H2S accumulation, enhancing antioxidant enzymes activity and promoting phenols accumulation. Sci. Hortic. 238, 264–271. doi: 10.1016/j.scienta.2018.04.063
Aroca, A., Gotor, C., Bassham, D. C., Romero, L. C. (2020). Hydrogen sulfide: From a toxic molecule to a key molecule of cell life. Antioxidants 9, 621. doi: 10.3390/antiox9070621
Begara-Morales, J. C., Sanchez-Calvo, B., Chaki, M., Valderrama, R., Mata-Perez, C., Lopez-Jaramillo, J., et al. (2014). Dual regulation of cytosolic ascorbate peroxidase (APX) by tyrosine nitration and S-nitrosylation. J. Exp. Bot. 65, 527–538. doi: 10.1093/jxb/ert396
Cheng, T. L., Shi, J. S., Dong, Y. N., Ma, Y., Peng, Y., Hu, X. Y., et al. (2018). Hydrogen sulfide enhances poplar tolerance to high temperature stress by increasing S-nitrosoglutathione reductase (GSNOR) activity and reducing reactive oxygen/nitrogen damage. Plant Growth Regul. 84, 11–23. doi: 10.1007/s10725-017-0316-x
Christou, A., Filippou, P., Manganaris, G. A., Fotopoulos, V. (2014). Sodium hydrosulfide induces systemic thermotolerance to strawberry plants through transcriptional regulation of heat shock proteins and aquaporin. BMC Plant Biol. 14, 42. doi: 10.1186/1471-2229-14-42
Christou, A., Manganaris, G., Papadopoulos, I., Fotopoulos, V. (2011). “The importance of hydrogen sulfide as a systemic priming agent in strawberry plants grown under key abiotic stress factors,” in 4th International Workshop - Cost action FA0605 Plant Abiotic Stress: From Systems Biology to Sustainable Agriculture, Limassol Cyprus. Vol. 47.
Citi, V., Passerini, M., Calderone, V., Testai, L. (2023). Plants and pushrooms as possible new sources of H2S releasing sulfur compounds. Int. J. Mol. Sci. 24, 11886. doi: 10.3390/ijms241511886
Cosse, M., Seidel, T. (2021). Plant proton pumps and cytosolic pH-homeostasis. Front. Plant Sci. 12, 672873. doi: 10.3389/fpls.2021.672873
Ding, Y., Shi, Y., Yang, S. (2019). Advances and challenges in uncovering cold tolerance regulatory mechanisms in plants. New Phytol. 222, 1690–1704. doi: 10.1111/nph.15696
Du, X., Jin, Z., Liu, D., Yang, G., Pei, Y. (2017). Hydrogen sulfide alleviates the cold stress through MPK4 in Arabidopsis thaliana. Plant Physiol. Biochem. 120, 112–119. doi: 10.1016/j.plaphy.2017.09.028
Du, X., Jin, Z., Liu, Z., Liu, D., Zhang, L., Ma, X., et al. (2021). H2S persulfidated and increased kinase activity of MPK4 to response cold stress in Arabidopsis. Front. Mol. Biosci. 8, 635470. doi: 10.3389/fmolb.2021.635470
Fu, P. N., Wang, W. J., Hou, L. X., Liu, X. (2013). Hydrogen sulfide is involved in the chilling stress response in Vitis vinifera L. Acta Soc Bot. Pol. 82, 295–302. doi: 10.5586/asbp.2013.031
Gautam, H., Fatma, M., Sehar, Z., Mir, I. R., Khan, N. A. (2022). Hydrogen sulfide, ethylene, and nitric oxide regulate redox homeostasis and protect photosynthetic metabolism under high temperature stress in rice plants. Antioxidants 11, 1478. doi: 10.3390/antiox11081478
Geng, B., Huang, D. D., Zhu, S. H. (2019). Regulation of hydrogen sulfide metabolism by nitric oxide inhibitors and the quality of peaches during cold storage. Antioxidants 8, 401. doi: 10.3390/antiox8090401
Gerush, I. V., Ferenchuk, Y. O. (2019). Hydrogen sulfide and mitochondria. Biopolym. Cell 35, 3–15. doi: 10.7124/bc
Gusain, S., Joshi, S., Joshi, R. (2023). Sensing, signalling, and regulatory mechanism of cold-stress tolerance in plants. Plant Physiol. Biochem. 197, 107646. doi: 10.1016/j.plaphy.2023.107646
Huang, Y., An, J., Sircar, S., Bergis, B., Lopes, C. D., He, X., et al. (2023). HSFA1a modulates plant heat stress responses and alters the 3D chromatin organization of enhancer-promoter interactions. Nat. Commun. 14, 469. doi: 10.1038/s41467-023-36227-3
Huang, J., Xie, Y. (2023). Hydrogen sulfide signaling in plants. Antioxid. Redox Signal. 39, 40–58. doi: 10.1089/ars.2023.0267
Iqbal, N., Fatma, M., Gautam, H., Umar, S., Sofo, A., D’ippolito, I., et al. (2021a). The crosstalk of melatonin and hydrogen sulfide determines photosynthetic performance by regulation of carbohydrate metabolism in wheat under heat stress. Plants 10, 1778. doi: 10.3390/plants10091778
Iqbal, Z., Memon, A. G., Ahmad, A., Iqbal, M. S. (2022). Calcium mediated cold acclimation in plants: Underlying signaling and molecular mechanisms. Front. Plant Sci. 13, 855559. doi: 10.3389/fpls.2022.855559
Iqbal, N., Umar, S., Khan, N. A., Corpas, F. J. (2021b). Nitric oxide and hydrogen sulfide coordinately reduce glucose sensitivity and decrease oxidative stress via ascorbate-glutathione cycle in heat-stressed wheat (Triticum aestivum L.) plants. Antioxidants 10, 108. doi: 10.3390/antiox10010108
Janicka, M., Reda, M., Czyzewska, K., Kabala, K. (2018). Involvement of signalling molecules NO, H2O2 and H2S in modification of plasma membrane proton pump in cucumber roots subjected to salt or low temperature stress. Funct. Plant Biol. 45, 428–439. doi: 10.1071/FP17095
Jia, H., Chen, S., Liu, D., Liesche, J., Shi, C., Wang, J., et al. (2018). Ethylene-induced hydrogen sulfide negatively regulates ethylene biosynthesis by persulfidation of ACO in tomato under osmotic stress. Front. Plant Sci. 9, 1517. doi: 10.3389/fpls.2018.01517
Kan, Y., Mu, X. R., Gao, J., Lin, H. X., Lin, Y. (2023). The molecular basis of heat stress responses in plants. Mol. Plant 16, 1612–1634. doi: 10.1016/j.molp.2023.09.013
Karpets, Y. V., Kolupaev, Y. E., Lugovaya, A. A., Shvidenko, N. V., Shkliarevskyi, M. A., Yastreb, T. O. (2020). Functional interaction of ROS and nitric oxide during induction of heat resistance of wheat seedlings by hydrogen sulfide donor. Russ. J. Plant Physiol. 67, 653–660. doi: 10.1134/S1021443720030140
Kolupaev, Y. E., Horielova, E. I., Yastreb, T. O., Ryabchun, N. I., Kirichenko, V. V. (2019). Stress protective responses of wheat and rye seedlings whose chilling resistance was induced with a donor of hydrogen sulfide. Russ. J. Plant Physiol. 66, 540–547. doi: 10.1134/S1021443719040058
Kolupaev, Y. E., Yemets, A. I., Yastreb, T. O., Blume, Y. B. (2023). The role of nitric oxide and hydrogen sulfide in regulation of redox homeostasis at extreme temperatures in plants. Front. Plant Sci. 14, 1128439. doi: 10.3389/fpls.2023.1128439
Kosová, K., Vítámvás, P., Prášil, I. T., Klíma, M., Renaut, J. (2021). Plant proteoforms under environmental stress: Functional proteins arising from a single gene. Front. Plant Sci. 12, 793113. doi: 10.3389/fpls.2021.793113
Li, Z. G., Gong, M., Xie, H., Yang, L., Li, J. (2012). Hydrogen sulfide donor sodium hydrosulfide-induced heat tolerance in tobacco (Nicotiana tabacum L.) suspension cultured cells and involvement of Ca2+ and calmodulin. Plant Sci. 185–186, 185–189. doi: 10.1016/j.plantsci.2011.10.006
Li, Z. G., Gu, S. P. (2016). Hydrogen sulfide as a signal molecule in hematin-induced heat tolerance of tobacco cell suspension. Biol. Plant 60, 595–600. doi: 10.1007/s10535-016-0612-8
Li, Z. G., Jin, J. Z. (2016). Hydrogen sulfide partly mediates abscisic acid-induced heat tolerance in tobacco (Nicotiana tabacum L.) suspension cultured cells. Plant Cell Tissue Organ. Cult. 125, 207–214. doi: 10.1007/s11240-015-0939-4
Li, D., Limwachiranon, J., Li, L., Du, R., Luo, Z. (2016b). Involvement of energy metabolism to chilling tolerance induced by hydrogen sulfide in cold-stored banana fruit. Food Chem. 208, 272–278. doi: 10.1016/j.foodchem.2016.03.113
Li, Z. G., Long, W. B., Yang, S. Z., Chen, C. B., Sun, Y. Y., Wang, J. Q., et al. (2022). Hydrogen sulfide: From toxic gas to plant signaling molecule. J. Yunnan Normal Univ. (Nat. Sci. Ed.) 42, 1–10. doi: 10.7699/j.ynnu.ns-2022-001
Li, Z. G., Long, W. B., Yang, S. Z., Wang, Y. C., Tang, J. H., Wen, L., et al. (2015a). Endogenous hydrogen sulfide regulated by calcium is involved in thermotolerance in tobacco Nicotiana tabacum L. suspension cell cultures. Acta Physiol. Plant 37, 219. doi: 10.1007/s11738-015-1971-z
Li, Z. G., Luo, L. J., Zhu, L. P. (2014a). Involvement of trehalose in hydrogen sulfide donor sodium hydrosulfide-induced the acquisition of heat tolerance in maize (Zea mays L.) seedlings. Bot. Stud. 55, 20. doi: 10.1186/1999-3110-55-20
Li, Z. G., Min, X., Zhou, Z. H. (2016a). Hydrogen sulfide: A signal molecule in plant cross-adaptation. Front. Plant Sci. 7, 1621. doi: 10.3389/fpls.2016.01621
Li, J., Shi, C., Wang, X., Liu, C., Ding, X., Ma, P., et al. (2020). Hydrogen sulfide regulates the activity of antioxidant enzymes through persulfidation and improves the resistance of tomato seedling to Copper Oxide nanoparticles (CuO NPs)-induced oxidative stress. Plant Physiol. Biochem. 156, 257–266. doi: 10.1016/j.plaphy.2020.09.020
Li, Z. G., Xiang, R. H., Wang, J. Q. (2021). Hydrogen sulfide-phytohormone interaction in plants under physiological and stress conditions. J. Plant Growth Regul. 40, 2476–2484. doi: 10.1007/s00344-021-10350-1
Li, Z. G., Xie, L. R., Li, X. J. (2015b). Hydrogensulfide acts as a downstream signal molecule in salicylic acid-induced heat tolerance in maize (Zea mays L.) seedlings. J. Plant Physiol. 177, 121–127. doi: 10.1016/j.jplph.2014.12.018
Li, Z. G., Yang, S. Z., Long, W. B., Yang, G. X., Shen, Z. Z. (2013). Hydrogen sulfide may be a novel downstream signal molecule in nitric oxide-induced heat tolerance of maize (Zea mays L.) seedlings. Plant Cell Environ. 36, 1564–1572. doi: 10.1111/pce.12092
Li, Z. G., Yi, X. Y., Li, Y. T. (2014b). Effect of pretreatment with hydrogen sulfide donor sodium hydrosulfide on heat tolerance in relation to antioxidant system in maize (Zea mays) seedlings. Biologia 69, 1001–1009. doi: 10.2478/s11756-014-0396-2
Liu, H., Wang, J., Liu, J., Liu, T., Xue, S. (2021). Hydrogen sulfide (H2S) signaling in plant development and stress responses. aBIOTECH 2, 32–63. doi: 10.1007/s42994-021-00035-4
Liu, F., Zhang, X., Cai, B., Pan, D., Fu, X., Bi, H., et al. (2020). Physiological response and transcription profiling analysis reveal the role of glutathione in H2S-induced chilling stress tolerance of cucumber seedlings. Plant Sci. 291, 110363. doi: 10.1016/j.plantsci.2019.110363
Luo, Z., Li, D., Du, R., Mou, W. (2015). Hydrogen sulfide alleviates chilling injury of banana fruit by enhanced antioxidant system and proline content. Sci. Hortic. 183, 144–151. doi: 10.1016/j.scienta.2014.12.021
Ma, Y., Li, F., Yi, Y., Wang, X., Li, T., Wang, X., et al. (2023). Hydrogen sulfide improves salt tolerance through persulfidation of PMA1 in Arabidopsis. Plant Cell Rep. 42, 1265–1277. doi: 10.1007/s00299-023-03029-2
Manasa, L., Panigrahy, M., Panigrahi, K. C. S., Rout, G. R. (2022). Overview of cold stress regulation in plants. Bot. Rev. 88, 359–387. doi: 10.1007/s12229-021-09267-x
Mhamdi, A. (2023). Hydrogen peroxide in plants. Adv. Bot. Res. 105, 43–75. doi: 10.1016/bs.abr.2022.11.002
Modolo, L. V., da-Silva, C. J. (2023). H2S in plants: Past, present and beyond (London: Academic Press).
Moseler, A., Dhalleine, T., Rouhier, N., Couturier, J. (2021). Arabidopsis thaliana 3-mercaptopyruvate sulfurtransferases interact with and are protected by reducing systems. J. Biol. Chem. 296, 100429. doi: 10.1016/j.jbc.2021.100429
Mukherjee, S., Roy, S., Corpas, F. J. (2024). Aquaporins: A vital nexus in H2O2-gasotransmitter signaling. Trends Plant Sci. 29. in press. doi: 10.1016/j.tplants.2023.11.021
Nakajima, T., Kawano, Y., Ohtsu, I., Maruyuama-Nakashita, A., Allahham, A., Sato, M., et al. (2019). Effects of thiosulfate as a sulfur source on plant growth, metabolites accumulation and gene expression in Arabidopsis and rice. Plant Cell Physiol. 60, 1683–1701. doi: 10.1093/pcp/pcz082
Nasibi, F., Kalantari, K. M., Tavakoli, Z. M. (2020). Effects of hydrogen sulfide on cold-induced oxidative damage in Cucumis sativus L. Int. J. Hortic. Sci. Technol. 7, 199–211. doi: 10.22059/ijhst.2020.284285.301
Niazi, Z., Razavi, F., Khademi, O., Aghdam, M. S. (2021). Exogenous application of hydrogen sulfide and γ-aminobutyric acid alleviates chilling injury and preserves quality of persimmon fruit (Diospyros kaki, cv. Karaj) during cold storage. Sci. Hortic. 258, 110198. doi: 10.1016/j.scienta.2021.110198
Pan, D. Y., Fu, X., Zhang, X. W., Liu, F. J., Bi, H. G., Ai, X. Z. (2020). Hydrogen sulfide is required for salicylic acid–induced chilling tolerance of cucumber seedlings. Protoplasma 257, 1543–1557. doi: 10.1007/s00709-020-01531-y
Pande, A., Mun, B. G., Khan, M., Rahim, W., Lee, D. S., Lee, G. M., et al. (2022). Nitric oxide signaling and its association with ubiquitin-mediated proteasomal degradation in plants. Int. J. Mol. Sci. 23, 1657. doi: 10.3390/ijms23031657
Pedre, B., Talwar, D., Barayeu, U., Schilling, D., Luzarowski, M., Sokolowski, M., et al. (2023). 3-Mercaptopyruvate sulfur transferase is a protein persulfidase. Nat. Chem. Biol. 19, 507–517. doi: 10.1038/s41589-022-01244-8
Qiu, X. M., Sun, Y. Y., Ye, X. Y., Li, Z. G. (2020). Signaling role of glutamate in plants. Front. Plant Sci. 10, 1743. doi: 10.3389/fpls.2019.01743
Ritonga, F. N., Chen, S. (2020). Physiological and molecular mechanism involved in cold stress tolerance in plants. Plants (Basel). 9, 560. doi: 10.3390/plants9050560
Ruelland, E., Vaultier, M. N., Zachowski, A., Hurry, V. (2009). Cold signaling and cold acclimation in plants. Adv. Bot. Res. 49, 35–150. doi: 10.1016/S0065-2296(08)00602-2
Saud, S., Hassan, S., Xiong, L., Sun, X., Andleeb, S., Fahad, S. (2022). The physiological function and molecular mechanism of hydrogen sulfide resisting abiotic stress in plants. Braz. J. Bot. 45, 563–572. doi: 10.1007/s40415-022-00785-5
Shen, J., Zhang, J., Zhou, M., Zhou, H., Cui, B., Gotor, C., et al. (2020). Persulfidation-based modification of cysteine desulfhydrase and the NADPH oxidase RBOHD controls guard cell abscisic acid signaling. Plant Cell 32, 1000–1017. doi: 10.1105/tpc.19.00826
Shi, H., Ye, T., Chan, Z. (2013). Exogenous application of hydrogen sulfide donor sodium hydrosulfide enhanced multiple abiotic stress tolerance in Bermudagrass (Cynodon dactylon (L). Pers.). Plant Physiol. Biochem. 71, 226–234. doi: 10.1016/j.plaphy.2013.07.021
Stimler, K., Montzka, S. A., Berry, J. A., Rudich, Y., Yakir, D. (2010). Relationships between carbonyl sulfide (COS) and CO2 during leaf gas exchange. New Phytol. 186, 869–878. doi: 10.1111/j.1469-8137.2010.03218.x
Stuiver, C. E. E., De Kok, L. J., Kuiper, P. J. C. (1992). Freezing tolerance and biochemical changes in wheat shoots as affected by H2S fumigation. Plant Physiol. Biochem. 30, 47–55.
Tang, X. D., An, B. Y., Cao, D. M., Xu, R., Wang, S. Y., Zhang, Z. D., et al. (2020). Improving photosynthetic capacity, alleviating photosynthetic inhibition and oxidative stress under low temperature stress with exogenous hydrogen sulfide in blueberry seedlings. Front. Plant Sci. 11, 108. doi: 10.3389/fpls.2020.00108
Terron-Camero, L. C., Rodriguez-Serrano, M., Sandalio, L. M., Romero-Puertas, M. C. (2020). Nitric oxide is essential for cadmium-induced peroxule formation and peroxisome proliferation. Plant Cell Environ. 43, 2492–2507. doi: 10.1111/pce.13855
Wang, R. (2012). Physiological implications of hydrogen sulfide: a whiff exploration that blossomed. Physiol. Rev. 92, 791–896. doi: 10.1152/physrev.00017.2011
Wang, L., Chen, S., Shao, J., Zhang, C., Mei, L., Wang, K., et al. (2022a). Hydrogen sulfide alleviates chilling injury in peach fruit by maintaining cell structure integrity via regulating endogenous H2S, antioxidant and cell wall metabolisms. Food Chem. 391, 133283. doi: 10.1016/j.foodchem.2022.133283
Wang, C., Deng, Y., Liu, Z., Liao, W. (2021). Hydrogen sulfide in plants: Crosstalk with other signal molecules in response to abiotic stresses. Int. J. Mol. Sci. 22, 12068. doi: 10.3390/ijms222112068
Wang, L., Huang, X., Liu, C., Zhang, C., Shi, K., Wang, M., et al. (2023a). Hydrogen sulfide alleviates chilling injury by modulating respiration and energy metabolisms in cold-stored peach fruit. Postharv. Biol. Technol. 199, 112291. doi: 10.1016/j.postharvbio.2023.112291
Wang, H., Liu, Z., Li, J., Luo, S., Zhang, J., Xie, J. (2022c). Hydrogen sulfide interacts with 5-aminolevulinic acid to enhance the antioxidant capacity of pepper (Capsicum annuum L.) seedlings under chilling stress. Agronomy 12, 572. doi: 10.3390/agronomy12030572
Wang, Z., Mu, Y., Hao, X., Yang, J., Zhang, D., Jin, Z., et al. (2022b). H2S aids osmotic stress resistance by S-sulfhydration of melatonin production-related enzymes in Arabidopsis thaliana. Plant Cell Rep. 41, 365–376. doi: 10.1007/s00299-021-02813-2
Wang, X., Tan, N. W. K., Chung, F. Y., Yamaguchi, N., Gan, E. S., Ito, T. (2023c). Transcriptional regulators of plant adaptation to heat stress. Int. J. Mol. Sci. 24, 13297. doi: 10.3390/ijms241713297
Wang, J. Q., Xiang, R. H., Li, Z. G. (2023b). The essential role of H2S-ABA crosstalk in maize thermotolerance through the ROS-scavenging system. Int. J. Mol. Sci. 24, 12264. doi: 10.3390/ijms241512264
Wani, S. H., Kumar, V. (2020). Heat stress tolerance in plants: physiological, molecular and genetic prospectives (Hoboken: Wiley). 2020.
Wen, Y., Ye, Q., Román-Palacios, C., Liu, H., Wu, G. (2023). Physiological cold tolerance evolves faster than climatic niches in plants. Front. Plant Sci. 14,1257499. doi: 10.3389/fpls.2023.1257499
Wu, G., Li, S., Dong, Y., Bi, H., Ai, X. (2022). Exogenous hydrogen sulfde improves chilling tolerance by regulating hydrogen peroxide production in cucumber seedlings. Hortic. Environ. Biotechnol. 63, 651–663. doi: 10.1007/s13580-022-00433-7
Yang, M., Qin, B. P., Ma, X. L., Wang, P., Li, M. L., Chen, L. L., et al. (2016). Foliar application of sodium hydrosulfide (NaHS), a hydrogen sulfide (H2S) donor, can protect seedlings against heat stress in wheat (Triticum aestivum L.). J. Integr. Agr. 15, 2745–2758. doi: 10.1016/S2095-3119(16)61358-8
Yang, Z., Wang, X., Feng, J., Zhu, S. (2022). Biological functions of hydrogen sulfide in plants. Int. J. Mol. Sci. 23, 15107. doi: 10.3390/ijms232315107
Ye, X. Y., Qiu, X. M., Sun, Y. Y., Li, Z. G. (2020). Interplay between hydrogen sulfide and methylglyoxal initiates thermotolerance in maize seedlings by modulating reactive oxidative species and osmolyte metabolism. Protoplasma 257, 1415–1432. doi: 10.1007/s00709-020-01516-x
Yu, X. Z., Lin, Y. J., Shen, P. P., Zhang, Q., Gupta, D. K. (2019). Molecular evidence on transport of thiocyanate into rice seedlings and assimilation by 13C and 15N labeling and gene expression analyses. Int. Biodeter. Biodegr. 139, 11–17. doi: 10.1016/j.ibiod.2019.02.003
Yun, B. W., Feechan, A., Yin, M., Saidi, N. B., Le Bihan, T., Yu, M., et al. (2011). S-nitrosylation of NADPH oxidase regulates cell death in plant immunity. Nature 478, 264–268. doi: 10.1038/nature10427
Zhang, X., Fu, X., Liu, F., Wang, Y., Bi, H., Ai, X. (2021). Hydrogen sulfide improves the cold stress resistance through the CsARF5-CsDREB3 module in cucumber. Int. J. Mol. Sci. 22, 13229. doi: 10.3390/ijms222413229
Zhang, X. W., Liu, F. J., Zhai, J., Li, F. D., Bi, H. G., Ai, X. Z. (2020). Auxin acts as a downstream signaling molecule involved in hydrogen sulfide-induced chilling tolerance in cucumber. Planta 251, 69. doi: 10.1007/s00425-020-03362-w
Zhang, P., Luo, Q., Wang, R., Xu, J. (2017). Hydrogen sulfide toxicity inhibits primary root growth through the ROS-NO pathway. Sci. Rep. 7, 868. doi: 10.1038/s41598-017-01046-2
Zhang, W., Xu, C., Yang, G., Wu, L., Wang, R. (2015). Interaction of H2S with calcium permeable channels and transporters. Oxid. Med. Cell. Longev. 2015, 323269. doi: 10.1155/2015/323269
Zhang, H., Zhu, J., Gong, Z., Zhu, J. K. (2022). Abiotic stress responses in plants. Nat. Rev. Genet. 23, 104–119. doi: 10.1038/s41576-021-00413-0
Zhao, J., Lu, Z., Wang, L., Jin, B. (2021). Plant responses to heat stress: physiology, transcription, noncoding RNAs, and epigenetics. Int. J. Mol. Sci. 22, 117. doi: 10.3390/ijms22010117
Zhou, X., Joshi, S., Patil, S., Khare, T., Kumar, V. (2022a). Reactive oxygen, nitrogen, carbonyl and sulfur species and their roles in plant abiotic stress responses and tolerance. J. Plant Growth Reg. 41, 119–142. doi: 10.1007/s00344-020-10294-y
Zhou, Z. H., Wang, Y., Ye, X. Y., Li, Z. G. (2018). Signaling molecule hydrogen sulfide improves seed germination and seedling growth of maize (Zea mays L.) under high temperature by inducing antioxidant system and osmolyte biosynthesis. Front. Plant Sci. 9, 1288. doi: 10.3389/fpls.2018.01288
Zhou, Y., Xu, F., Shao, Y., He, J. (2022b). Regulatory mechanisms of heat stress response and thermomorphogenesis in plants. Plants 11, 3410. doi: 10.3390/plants11243410
Zhou, M., Zhang, J., Shen, J., Zhou, H., Zhao, D., Gotor, C., et al. (2021a). Hydrogen sulfide-linked persulfidation of ABI4 controls ABA responses through the transactivation of MAPKKK18 in Arabidopsis. Mol. Plant 14, 921–936. doi: 10.1016/j.molp.2021.03.007
Keywords: hydrogen sulfide, high and low temperature, protein persulfidation, temperature stress, stress tolerance
Citation: Li Z-G, Fang J-R and Bai S-J (2024) Hydrogen sulfide signaling in plant response to temperature stress. Front. Plant Sci. 15:1337250. doi: 10.3389/fpls.2024.1337250
Received: 12 November 2023; Accepted: 22 February 2024;
Published: 13 March 2024.
Edited by:
Anna N. Stepanova, North Carolina State University, United StatesReviewed by:
Viktória Kovács, Centre for Agricultural Research, HungaryKlára Kosová, Crop Research Institute (CRI), Czechia
Copyright © 2024 Li, Fang and Bai. This is an open-access article distributed under the terms of the Creative Commons Attribution License (CC BY). The use, distribution or reproduction in other forums is permitted, provided the original author(s) and the copyright owner(s) are credited and that the original publication in this journal is cited, in accordance with accepted academic practice. No use, distribution or reproduction is permitted which does not comply with these terms.
*Correspondence: Zhong-Guang Li, emhvbmdndWFuZ19saUAxNjMuY29t