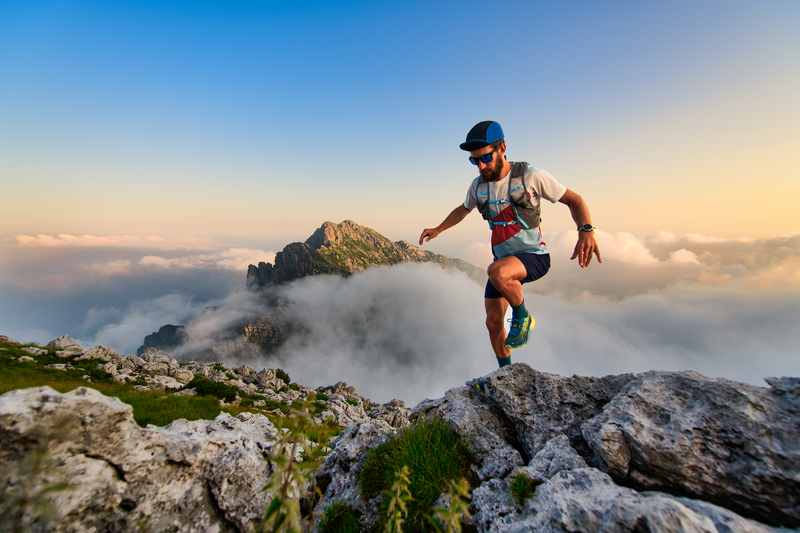
95% of researchers rate our articles as excellent or good
Learn more about the work of our research integrity team to safeguard the quality of each article we publish.
Find out more
BRIEF RESEARCH REPORT article
Front. Plant Sci. , 29 January 2024
Sec. Aquatic Photosynthetic Organisms
Volume 15 - 2024 | https://doi.org/10.3389/fpls.2024.1335085
Photosynthetic organisms often encounter phosphorus (P) limitation in natural habitats. When faced with P limitation, seed plants degrade nucleic acids and extra-plastid phospholipids to remobilize P, thereby enhancing their internal-P utilization efficiency. Although prokaryotic and eukaryotic photosynthetic organisms decrease the content of phosphatidylglycerol (PG) under P-limited conditions, it remains unclear whether PG is degraded for P remobilization. Moreover, information is limited on internal-P remobilization in photosynthetic microbes. This study investigates internal-P remobilization under P-starvation (-P) conditions in a cyanobacterium, Synechocystis sp. PCC 6803, focusing on PG and nucleic acids. Our results reveal that the PG content increases by more than double in the -P culture, indicating preferential PG synthesis among cellular P compounds. Simultaneously, the faster increases of glycolipids counteract this PG increase, which decreases the PG proportion in total lipids. Two genes, glpD and plsX, contribute to the synthesis of diacylglycerol moieties in glycerolipids, with glpD also responsible for the polar head group synthesis in PG. The mRNA levels of both glpD and plsX are upregulated during -P, which would cause the preferential metabolic flow of their P-containing substrates toward glycerolipid synthesis, particularly PG synthesis. Meanwhile, we find that RNA accounts for 62% of cellular P, and that rRNA species, which makes up the majority of RNA, are degraded under -P conditions to less than 30% of their initial levels. These findings emphasize the importance of PG in -P-acclimating cell growth and the role of rRNA as a significant internal-P source for P remobilization, including preferential PG synthesis.
Phosphorus (P), along with nitrogen (N) and sulfur (S), forms organic carbon compounds through covalent bonds. P-compounds include nucleic acids, phospholipids, and a variety of intermediate metabolites. Together with N and S, P is defined as a macronutrient in photosynthetic organisms (Kumar et al., 2021). Photosynthetic organisms often face nutrient deficiency stresses, with phosphate limitation, or P-limitation, being a common stressor. A series of P-limitation acclimation strategies, including improvements in external phosphate (Pi) acquisition ability and internal P use efficiency (PUE), have been developed in photosynthetic organisms (Plaxton and Tran, 2011; Heuer et al., 2017). Intriguingly, photosynthetic organisms exhibit milder symptoms in physiological processes with P-starvation (-P) compared to those with N or S-starvation, at least during the short-term stresses (Mao et al., 2018; Hirai et al., 2019; Teixeira et al., 2021). Notably, in a cyanobacterium, Synechocystis sp. PCC 6803, -P had a much smaller impact on cell growth compared to N or S-starvation (an 8-fold increase from the initial level with -P and a 2.5-fold increase with -N or -S, c.f., an 11-fold increase under nutrient-replete conditions; Hirai et al., 2019). The features of these photosynthetic organisms demonstrated that the utilization efficiency of a nutrient under starvation stress, relative to that under repletion, was much higher for P than for N or S.
The mechanism by which photosynthetic organisms establish high PUE under -P conditions have been investigated at the biochemical and molecular levels. In seed plants, PUE is improved through P remobilization between organs or tissues, such as from old leaves to young ones and growing roots (Rausch and Bucher, 2002). Nucleic acids generally make up 40-60% of organic P, with rRNA representing approximately 80% of nucleic-acid P (Veneklaas et al., 2012; Smith et al., 2015). Due to the abundance of rRNA and the induced expression of cytosolic RNase genes under P-limiting conditions, it is regarded that rRNA serves as a major source for P remobilization in seed plants acclimating to P-limitation stress (Młodzińska and Zboińska, 2016). Seed plants also remobilize P from chloroplast and mitochondrial DNA, which accounts for 6-9% of total nucleic acids (Takami et al., 2018). Concerning photosynthetic microbes, however, information is only fragmentary on nucleic-acid related PUE improvement: a green alga, Chlamydomonas reinhardti showed a decrease in the number of chloroplast nucleoids with P-limitation, with mRNA in chloroplasts enhanced in stability through the repression of the gene for PNPase, a phosphorylytic RNase (Yehudai-Resheff et al., 2007). It was therefore proposed that -P cells of C. reinhardtii degrade chloroplast DNA but not its RNA for P-remobilization.
Membrane lipid metabolism also plays a crucial role in increasing PUE in photosynthetic organisms. In green algae and seed plants, phosphatidylcholine (PC) and phosphatidylethanolamine (PE), major components of phospholipids that occupy 15-30% of total P, are markedly degraded in extra-plastid membranes under -P conditions through the actions of phospholipases C and/or D (Andersson et al., 2003; Cruz-Ramírez et al., 2006; Gaude et al., 2008; Nakamura et al., 2009; Oishi et al., 2022). This phospholipid losing process sequesters P from these phospholipids as an internal-P source and is accompanied by compensating increases in non-phospholipid contents. This replacement of phospholipids by non-phospholipids in extra-plastid membranes, therefore, contributes to the improvement in PUE.
In this context, it is noteworthy that -P-stress additionally induces another type of lipid remodeling for PUE improvement, which involves decreases in anionic phospholipid, phosphatidylglycerol (PG), along with increases in anionic non-phospholipid, sulfoquinovosyl diacylglycerol (SQDG), in photosynthetic bacteria, cyanobacteria, and algal and seed-plant plastids (Benning et al., 1993; Güler et al., 1996; Essigmann et al., 1998; Riekhof et al., 2003; Awai et al., 2007; Van Mooy et al., 2009; Endo et al., 2016; Murakami et al., 2018; Peng et al., 2019; Oishi et al., 2022). This lipid remodeling, maintaining not only lipid contents in photosynthetic membranes but also their charge balance for photosynthesis functionality, appears to be conserved throughout the evolution of photosynthetic organisms (Sato, 2004). The molecular mechanism underlying -P-induced increases in SQDG includes upregulation of expression levels of the genes for SQDG synthesis (sqdB in cyanobacteria or UGP3/SQD1/SQD2 in algae and plants), with some of these genes found to be indispensable for maintenance of -P-acclimating growth of the organisms (Benning et al., 1993; Güler et al., 1996; Yu et al., 2002; Riekhof et al., 2003; Okazaki et al., 2009; Sato and Wada, 2009; Hung et al., 2015; Endo et al., 2016). However, the metabolic mechanism governing the decrease in PG and its physiological significance are yet to be elucidated, including whether or not PG is degraded for the acquisition of internal P.
Differing from seed plants, photosynthetic microbes, especially unicellular ones, need to carry out P remobilization in separate cells. We here investigated how cells of a unicellular cyanobactrium, Synechocystis sp. PCC 6803, remobilized intracellular P under -P conditions, focusing on the quantitative behavior of PG and nucleic acids. Our results demonstrated that under -P conditions, PG synthesis was promoted through the preferential incorporation of internal P, and that rRNA was degraded to provide a primary internal-P source for P remobilization.
Synechocystis sp. PCC 6803 cells were cultured at 30°C in tubes containing either a standard BG11 medium with phosphate or a modified BG11 medium in which phosphate was replaced with Cl- (Hiyoshi et al., 2021). The cultures were aerated with air and illuminated with fluorescence light (10 W·m-2). The optical density at 730 nm (OD730) and chlorophyll (Chl) content were determined using a spectrophotometer (DU 640, Beckman). For semi-continuous culturing under -P conditions, Synechocystis cells grown to the logarithmic phase under +P conditions were transferred to -P conditions and cultured for 36 h, maintaining the OD730 values of the culture between 0.1 and 0.5 through dilution with fresh BG11-P medium at specified intervals. Cells were harvested at specified times for DNA, RNA, or lipid isolation by centrifugation (3,000 rpm, 10 min). The OD730 value, and the chlorophyll (Chl) and phycobilisome (PBS) contents in the cultures were measured with a spectrophotometer (DU 640, Beckman, Hiyoshi et al., 2021).
DNA was isolated from Synechocystis cells with the use of NaI (Loparev et al., 1991). Harvested cells (less than 50 mg fresh weight) were resuspended in H2O and transferred to a microtube. The cells were pelleted by centrifugation (15,000 rpm, 10 min) and then resuspended in saturated NaI (200 μL) and left at 37°C for 30 min. The cell suspension was further resuspended with the addition of H2O (1 mL) and centrifuged (15,000 rpm, 2 min) to pellet the cells. This step was repeated once. The pellet was resuspended in a mixture (500 μL) containing 5 mg·mL-1 lysozyme, 50 mM Tris-HCl (pH 8.0), and 20 mM EDTA and incubated at 37°C for 30 min. The cells were then lysed through incubation for 2 h at 55°C after the addition of 10% SDS (50 μL) and 20 mg·mL-1 proteinase K (10.5 μL). DNA was extracted from the solution using phenol and a mixture of phenol and chloroform (1:1, vol.). The resultant DNA solution was treated with RNase, extracted with chloroform, and subjected to 2-propanol precipitation. DNA was dissolved in TE buffer and stored at -80°C until use.
RNA was isolated using a hot phenol extraction method (Aiba et al., 1981). Harvested cells of Synechocystis were resuspended at 65°C in a resuspension buffer containing 0.3 M sucrose, 10 mM sodium acetate, and 20 mM EDTA (125 μL) and lysed with a lysis buffer comprising 2% SDS and 10 mM sodium acetate (50 μL). The cell-lysis solution was transferred to a microtube and incubated at 65°C for 5 min after the addition of hot neutral phenol equilibrated with 100 mM Tris, pH 8.0 (50 μL), with vigorous shaking for 5 sec every other minute. The solution was incubated at 65°C for 5 min after the addition of acidic phenol equilibrated with H2O (250 μL), with vigorous shaking for 5 sec every other minute, left on ice for 5 min, and then centrifuged at 15,000 rpm for 5 min. The upper phase containing RNA with some DNA contamination was extracted with acidic phenol for RNA purification, which was repeated once. The recovered upper phase was extracted with a mixture of acidic phenol and chloroform (1:1, vol.), and finally with chloroform. The resultant solution was subjected to 2-propanol precipitation of RNA, followed by DNase I treatment. The DNase I-treated sample was subjected to RNA purification through phenol-chloroform extraction. RNA was precipitated with 2-propanol and dissolved in DEPC-treated H2O for storage at -80°C until use.
Total lipids were extracted from harvested cells of Synechocystis according to the method of Bligh and Dyer (1959). Total lipids were then separated into individual lipid classes by two-dimensional TLC for the measurement of their contents, as previously described (Sato et al., 2017a). The TLC plate was then sprayed with primulin and illuminated with UV light to detect separated lipid classes. Silica gels of individual lipid spots were scraped off and used for the preparation of methyl esters of their constituent fatty acids through treatment with 5% anhydrous methanolic HCl. The contents of fatty acid methyl esters in respective lipid classes were estimated by capillary GLC with arachidonic acid as an internal standard.
Intact Synechocystis cells suspended in H2O, or DNA or RNA solution, were mixed with potassium peroxodisulfate solution (40 g·L-1) in a ratio of 5 to 1. The resultant solution was autoclaved at 120°C for 30 min to release Pi from P-compounds. Pi contents in respective samples were determined colorimetrically by quantifying the malachite green/phosphomolybdate complex, as previously described (Hiyoshi et al., 2021). Meanwhile, rRNA contents were estimated through agarose gel electrophoresis of RNA. rRNA in the gel was stained with ethidium bromide to obtain a fluorescent image, which was analyzed with ImageJ (http://rsbweb.nih.gov/ij/).
Semi-quantitative reverse-transcriptase and quantitative real-time PCR (semi-qPCR and qPCR, respectively) analyses were performed as previously described by Sato et al. (2017b) using RNA prepared as described above. Supplementary Table S1 shows primer sets for the amplification of phoA, cdsA, pgsA, glpD, and plsX sequences, respectively, for semi-qPCR analysis. RNA was also subjected to qPCR using a Rotor-Gene Q PCR System (Qiagen) with primer for the genes glpD and plsX, respectively (Supplementary Table S2). The expression levels of the respective genes were normalized to that of rnpB, a subunit of ribonuclease P, using primer sets in Supplementary Tables S1 and S2 in semi-qPCR and qPCR analyses, respectively.
This study initially investigated lipid remodeling in Synechocystis cells responding to -P stress. Previous investigations of -P stress responses in photosynthetic microbes, including cyanobacteria, were primarily conducted in batch cultures. However, the batch culturing system inevitably involves nutrient shortages and shading as cell growth progresses. First, this study cultured Synechocystis cells semi-continuously, maintaining the OD730 values of the culture between 0.1 and 0.5 through dilution with fresh BG11-P medium (Supplementary Figure S1). This semi-continuous culturing system allowed for a focused examination of the impact of the sole stress of -P on cell physiology. Consistent with this approach, the semi-continuous -P culture of Synechocystis cells, in comparison to the batch culture, achieved higher levels of OD730, chlorophyll (Chl), and phycobilisomes (PBS) (Figures 1A, B). Moreover, when compared to batch culture, semi-continuous culture exhibited a delay in the decline of cellular Chl and PBS contents, i.e., a delay in the well-known symptoms of -P stress in cyanobacteria (Figures 1C, D; compare values at 12 h between the two culturing systems).
Figure 1 Cell growth and the accumulation of chlorophyll (Chl) and phycobilisomes (PBS) under -P conditions in semi-continuous or batch cultures of Synechocystis cells. (A) Cell growth, estimated based on OD730 values in the culture, relative to that at 0 h. The OD730 values at 0 h were 0.228 and 0.106 for semi-continuous and batch cultures, respectively. Open and closed circles represent semi-continuous and batch cultures, respectively. (B) Accumulation of Chl (circles) and PBS (triangles) in the culture, relative to that at 0 h. The Chl contents at 0 h were 1.41 and 0.677 μg·mL-1 for semi-continuous and batch cultures, respectively. The PBS contents at 0 h were 0.0587 and 0.0273 for semi-continuous and batch cultures, respectively. Open and closed symbols represent semi-continuous and batch cultures, respectively. Values in (A, B) in semi-continuous culture were estimated by multiplying them by the ratios of culture dilution with fresh BG11-P (Supplementary Figure S1). (C) Cellular Chl contents in semi-continuous cultures (open circles) and batch cultures (closed circles) were estimated by dividing the Chl content in (B) by OD730 values in (A). (D) Cellular PBS contents in semi-continuous cultures (open circles) and batch cultures (closed circles) were estimated by dividing the PBS contents in (B) by OD730 values in (A). The values in (C) or (D) are presented relative to that in +P cells at 0 h. The values shown are averages ± SE for three experiments. The significance of differences was evaluated by means of Student’s t-test. **P<0.01. Some error bars are hidden within symbols.
Next, four major membrane lipids were quantitatively analyzed in Synechocystis cells during 36 h of -P semi-continuous culturing (Figure 2A). PG decreased steadily from 10.9 to 4.6 mol% of total lipids, while SQDG increased from 29.8 to 33.7 mol%, with a minor dip at 12 h. Ultimately, Synechocystis exhibited the partial substitution of SQDG for PG, a phenomenon observed in various prokaryotic and eukaryotic photosynthetic organisms. Regarding galactolipids, monogalactosyl diacylglycerol (MGDG) experienced a slight decrease from 43.1 to 38.8 mol% over 36 h, with a minor peak at 12 h, while digalactosyl diacylglycerol (DGDG) increased steadily from 16.1 to 22.8 mol%. The analysis of lipid classes in the culture revealed constant increases under -P conditions, with DGDG exhibiting the most rapid increase (9.3-fold in 36 h), followed by SQDG (7.4-fold), MGDG (5.9-fold), and PG (2.7-fold) (Figures 2B, C). The steady increase in PG in the -P culture indicated the preferential synthesis of PG among P compounds. The decrease in PG relative to total lipids was thus attributed to its dilution by the more rapid increases in glycolipids. Meanwhile, similar to cellular Chl and PBS contents, the respective contents of cellular lipids decreased over time (Figure 2D). It was noteworthy that the cellular lipid decrease occurred even in the first 12 h, despite a simultaneous little decrease in cellular Chl or PBS (see semi-continuous culture in Figures 1C, D). This suggested that the reduction in lipid content in thylakoid membranes preceded that in the size of the photosynthetic machinery during the early phase of -P stress.
Figure 2 Quantitative changes in lipids in a semi-continuous culture of Synechocystis cells during -P. (A) Changes in lipid composition. (B) Accumulation of respective lipids in the culture. Respective lipid contents were estimated relative to the PG content at 0 h. (C) Lipid contents were respectively estimated after -P for 36 h, relative to their corresponding values at 0 h. White bars represent lipid contents in a semi-continuous culture, while a black bar represents the PG content in a batch culture. (D) Changes in cellular lipid contents were estimated by dividing the lipid contents in (B) by OD730 values, as represented in Figure 1A. The values are presented relative to that of MGDG at 0 h. The values shown are averages ± SE for three experiments. The significance of differences was evaluated by means of Student’s t-test. **P<0.01. Some error bars are hidden within symbols.
The facilitation of PG synthesis in -P cells, our key finding concerning the well-conserved SQDG substitution for PG, was also observed in the batch culture (Figure 2C). The batch culturing system, due to its simplicity, was then utilized to investigate the molecular mechanism behind this facilitation. Two genes, cdsA and pgsA, which encode CDP-diacylglycerol and phosphatidylglycerolphosphate synthases, respectively, participate in the formation of the polar head group of PG (Figure 3A). Semi-qPCR analysis revealed little change in the mRNA levels of cdsA or pgsA for at least 10 h under -P conditions, in contrast to the marked upregulation of phoA mRNA, as previously reported by Suzuki et al. (2004) (Figure 3B). The glpD gene, which encodes glycerol 3-phosphate dehydrogenase responsible for converting dihydroxyacetone phosphate (DHAP) to glycerol 3-phosphate. Glycerol 3-phosphate is essential for glycerolipid synthesis, and notably crucial for the formation of the polar head group of PG, as depicted in Figure 3A. Semi-qPCR analysis demonstrated that glpD was upregulated at the mRNA level under -P conditions (Supplementary Figure S2). Meanwhile, plsX, encoding phosphate acyltransferase and utilizing Pi for acyl-phosphate synthesis in glycerolipid synthesis, displayed a similar upregulation pattern to that of glpD (Supplementary Figure S2). Furthermore, it was confirmed that glpD and plsX were maximally upregulated by 4.3- and 3.0-fold, respectively, through qPCR (Figure 3C). In a situation where P-containing DHAP and Pi are limited in -P cells, the enhanced expression of glpD and plsX would contribute to their metabolic flow into glycerolipid synthesis, particularly PG synthesis.
Figure 3 Regulation of gene expression for PG synthesis promotion in Synechocystis cells under -P conditions. (A) The metabolic map of PG synthesis. Genes upregulated at their transcript levels and those little altered at the levels are shown in red and blue, respectively. (B) Semiquantitative PCR analysis of phoA encoding alkaline phosphatase, cdsA, and pgsA. Transcript levels were estimated relative to those of rnpB, as described in Materials and Methods. Note that the expression level of phoA was markedly increased under -P conditions, as previously reported (Suzuki et al., 2004). (C) Quantitative PCR analysis of glpD and plsX. The values shown are averages ± SE for three experiments. The significance of differences at 3, 6, or 10 h relative to at 0 h was evaluated by means of Student’s t-test. *P<0.05. Some error bars are hidden within symbols.
The quantitative behavior of P in nucleic acids, which typically constitute the majority of cellular P compounds, was investigated in Synechocystis cells following the transfer from +P to -P conditions (see the left panel of Figure 4A). Cells in culture retained 99.2% of the initial total P after 24 h of exposure to -P stress (142.9 μmol Pi·L-1 compared to 144.2 μmol Pi·L-1 at 0 h), indicating minimal leakage of Pi from the cells. In +P culture, RNA represented 89.4 μmol Pi·L-1 (62.0% of total Pi), which drastically decreased to 28.1 μmol Pi·L-1 (19.7% of total Pi) within 24 h under -P conditions. DNA accounted for 21.0 μmol Pi·L-1 (14.6% of total Pi) in +P culture, only slightly decreasing to 19.6 μmol Pi·L-1 (13.7% of total Pi) in -P culture. The increased Pi content in PG from 3.4 to 5.9 μmol Pi·L-1 (2.4 to 4.1% of total Pi) aligned with the results in Figures 2B, C. Meanwhile, the Pi content in a fraction of the other P-compounds ("Others" in Figure 4A) increased from 30.3 to 89.3 μmol Pi·L-1 (21.0 to 62.5% of total Pi). Collectively, RNA underwent significant degradation, decreasing to as low as 31.4% of the initial level (see the right panel of Figure 4B), in contrast to relatively stable DNA. Notably, the decreased Pi content in RNA (61.3 μmol Pi·L-1) was nearly equivalent to the increased Pi content in PG + others (61.5 μmol Pi·L-1), highlighting the significant role of RNA as an internal Pi supplier for the synthesis of these P compounds.
Figure 4 Remobilization of phosphorus (P) in a batch culture of Synechocystis cells under -P conditions. (A) Pi distribution to RNA, DNA, PG, and the rest (referred to as the other P-compounds) in +P or -P cells. Pi contents of cells, RNA, and DNA were estimated through calorimetric analysis of Pi, whereas Pi content of PG was estimated through fatty acid analysis by GC. Left panel: Pi contents in a culture of +P cells at OD730 = 0.2 (0 h) and those in a batch culture where these cells were grown for 24 h after the shift to -P conditions (24 h). Right panel: cellular Pi contents in +P (0 h) and -P cultures (24 h) estimated by dividing the data in the left panel by OD730 values of the cultures (0.20 and 0.94 ± 0.05 at 0 and 24 h, respectively). (B) Total RNA isolated from +P and -P cultures was utilized for rRNA analysis through agarose gel electrophoresis. Left panel: photograph of the gel, where 23S and 16S rRNAs were stained with ethidium bromide. Three lanes labeled with 10, 20, and 40% indicate the proportions of RNA samples from the +P culture used to create standard curves. Right panel: contents of respective rRNA species and total cellular RNA in -P culture, relative to their corresponding ones in +P culture. U, L, and 16S indicate the upper and lower bands of 23S rRNA and 16S rRNA while ‘total’ indicates total cellular RNA. The contents of 23S and 16S rRNA species were determined by measuring their fluorescence intensities, as described in Materials and Methods, followed by quantification using standard curves. The total cellular RNA content was estimated based on the data in (A). The values shown are averages ± SE for three experiments. The significance of differences was evaluated by means of Student’s t-test. **P<0.01. *P<0.05.
Cellular-P decreases were estimated in nmol P·OD730-1·mL-1 as follows (see the right panel of Figure 4A): from 716.6 to 138.7 in total P (i.e., to 19.4% of the initial level), from 431.0 to 27.2 (6.3%) in RNA, from 105.2 to 21.0 in DNA (20.0%), from 17.0 to 6.1 (35.9%) in PG, and from 163.5 to 84.4 (51.6%) in the other P-compounds. Concerning the retention ratio, DNA was almost equal to total P, which suggested that the cellular decrease in DNA was mainly due to cell division, i.e., DNA transfer to daughter cells. Meanwhile, PG was 1.8-fold higher than total P in the retention ratio, highlighting the physiological significance of PG in Synechocystis cells acclimating to -P stress.
rRNA, which generally predominates bacterial cellular RNA (more than 80%, Planson et al., 2020), was then measured in the culture by agarose gel electrophoresis of total RNA (Figure 4B). 23S rRNA species appeared as two bands in the gel (Mironov & Los, 2015), with degradation to 16.3% and 29.4% in the upper and lower bands, respectively, after -P for 24 h, relative to the initial levels. Additionally, 16S rRNA exhibited degradation to 24.4%. rRNA degradation was more pronounced than that in total RNA (31.4%). These results demonstrate that rRNA plays a major role as an internal-P source for P remobilization in Synechocystis cells.
Membrane lipid remodeling is a crucial strategy for photosynthetic organisms to acclimate to environmental stress conditions (Yu et al., 2021). Specifically, the replacement of PG by SQDG in response to -P stress appears to be a conserved mechanism across prokaryotic and eukaryotic photosynthetic organisms (Güler et al., 1996; Yu et al., 2002; Riekhof et al., 2003; Sato and Wada, 2009; Van Mooy et al., 2009; Endo et al., 2016; Murakami et al., 2018; Oishi et al., 2022). However, it has remained unclear whether -P stress alone is sufficient to induce PG-SQDG remodeling. This study demonstrated that the sole -P stress indeed triggers this lipid remodeling in Synechocystis with the use of a semi-continuous culturing system, and uncovered the metabolic mechanisms underlying this phenomenon (Figure 2). Firstly, PG was preferentially synthesized among cellular P-compounds under -P conditions (Figures 2B, C). The increased metabolic flow of P into PG in -P cells, relative to +P cells, was previously suggested in a batch culture of another cyanobacterium, Thermosynechococcus elongatus BP-1 (Endo et al., 2016). Our detailed time-course experiments provided clear evidence that the sole -P stress induces the continuous remobilization of internal P into PG synthesis in Synechocystis cells, leading to its increase of up to more than twofold in the culture. Secondly, despite the stimulated PG synthesis, its content in total lipids decreased due to dilution by glycolipids, which were synthesized at much faster rates (Figures 2B, C). This phenomenon resulted in the partial replacement of PG by SQDG, improving PUE. Thirdly, the active synthesis of PG seemed to be achieved through the elevated expression of glpD and plsX genes involved in construction of the diacylglycerol moiety of membrane lipids, including PG, with plsX contributing also to the formation of its polar head group (Figure 3C). Protein amounts of GlpD and PlsX, together with their cellular activities, will be studied in the future. In contrast, the cdsA and pgsA genes, which are responsible specifically for PG synthesis, showed almost no elevation in mRNA levels (Figure 3B), suggesting that their mRNA levels in +P cells were already high enough to promote PG synthesis after shifting to -P conditions. Intriguingly, it appeared that both cdsA and pgsA mRNAs were present at excessively high levels in +P cells of Thermosynechococcus elongatus BP-1 since these mRNA levels were little altered in its SQDG-deficient mutant that elevated the PG content (Endo et al., 2016).
Cyanobacteria, including Synechocystis, accumulate glycogen and/or poly-β-hydroxybutyrate (PHB) under -P conditions (Kromkamp, 1987; Monshupanee and Incharoensakdi, 2014; Kaewbai-Ngam et al., 2016; Hirai et al., 2019). The synthesis of these C-storage compounds consumes surplus chemical energy, such as ATP, under -P conditions, thereby preventing the overreduction of the photosynthetic electron transport chain, and accordingly, the production of toxic reactive oxygen species (Ghirardi et al., 2018). Dihydroxyacetone phosphate (DHAP) and Pi are essential for glycogen and PHB synthesis, serving as a fixed carbon supplier and a substrate for ATP synthesis, respectively. The upregulation of glpD and plsX induced by -P supports the diversion of DHAP and Pi into PG synthesis, in competition with active glycogen and PHB synthesis. In the future, the metabolic and molecular mechanisms of -P-induced PG decrease in total lipids, including the possibility of stimulation of PG synthesis as observed in Synechocystis, will be examined in other cyanobacteria, algae, and seed plants.
When algae and seed plants are exposed to -P conditions, they undergo a different type of lipid remodeling in extraplastid membranes (Nakamura, 2013). This involves the degradation of PC and PE, along with compensatory increases in non-phospholipid contents in species-dependent manners, i.e., with an increase in DGDG in A. thaiana and an increase in a betaine lipid, diacylglyceryl-N,N,N-trimethylhomoserine, in a green alga, Chlorella kessleri (Härtel et al., 2000; Oishi et al., 2022). Notably, in C. kessleri, both PC and PE become undetectable in -P cells, being completely replaced by DGTS (Oishi et al., 2022). It is essential to emphasize that unlike PC and PE, PG does not undergo significant degradation in Synechocystis under -P conditions. Intriguingly, in green algae, SQDG, which constitutes 5-11% of total cellular sulfur (S), is almost entirely degraded in chloroplast membranes under sulfate-starvation conditions, with a simultaneous increase in PG (Sugimoto et al., 2007; Sugimoto et al., 2010; Sato et al., 2017a). This almost complete replacement of SQDG by PG seems to be established owing to the non-essential roles of SQDG in green algal cell growth (Sato et al., 1995a; Sato et al., 1995b; Sato, 2004). In contrast to SQDG in green algae, PG is essential for cell growth in cyanobacteria due to its involvement in various critical processes in cell membranes, including chlorophyll synthesis, efficient electron transfer from QA to QB, PSI and PSII construction, and cell division (Sato et al., 2000; Sato et al., 2004; Mizusawa and Wada, 2012; Kopečná et al., 2015; Kóbori et al., 2018). The -P-induced promotion of PG synthesis in Synechocystis may be necessary to provide PG molecules for -P acclimation processes. Future research should aim to further investigate this concept, including the identification of PG-demanding physiological processes in -P Synechocystis cells.
This study, through the quantification of inorganic phosphate (Pi) in major cellular P-compounds, specifically nucleic acids, demonstrated that rRNA degradation serves as a major intracellular-P source for P remobilization in Synechocystis under -P conditions (Figure 4). The degradation of rRNA likely reflected the reduced need for ribosomes in -P cells, where metabolic activities slowed down. Another potential intracellular-P source is polyphosphate, which is degraded under -P conditions in various bacteria, including Synechocystis (Li and Dittrich, 2019; Hiyoshi et al., 2021). However, Pi in polyphosphate accounted for only around 10% of total cellular P under normal conditions in Synechocystis, which was much smaller than the fraction of degraded rRNA (42.9%). Thus, we propose that under -P conditions, Synechocystis cells utilize rRNA as the sole major intracellular-P source for the synthesis of PG and other P-compounds that are yet to be identified.
The limited DNA degradation observed in -P culture of Synechocystis cells suggested that the reduction in cellular DNA content, i.e., genomic DNA copy number, primarily occurs through cell division (Figure 4). Consistent with this idea, a subset of Synechocystis strains was reported to decrease genome copy numbers under -P stress (Zerulla et al., 2016). In contrast, DNA in the chloroplast, which is the presumed descendant of endosymbiotic cyanobacteria, was positively degraded in -P cells of Chlamydomonas reinhartdtii to expand the internal-P pool (Yehudai-Resheff et al., 2007). Chloroplast DNA has only a restricted set of genes in accordance with its small size in C. reinhardtii (203 kbp; Maul et al., 2002) compared to chromosomal DNA in Synechocystis (3573 kbp; Kaneko and Tabata, 1997). Furthermore, chloroplast DNA typically had 80 copies in a C. reinhardtii cell, whereas chromosomal DNA in Synechocystis was present at most with 14 copies (Zerulla et al., 2016; Gallaher et al., 2018). These properties of chloroplast DNA may enable C. reinhardtii cells to utilize it as an internal-P source. On the contrary, chromosomal DNA appears to be spared from degradation in -P Synechocystis cells, given its extensive genome coverage and low copy number. Future studies would involve investigating whether other photosynthetic microbes utilize rRNA as the primary internal-P source for P-remobilization under -P conditions.
The original contributions presented in the study are included in the article/Supplementary Material, further inquiries can be directed to the corresponding author.
TH: Investigation, Methodology, Validation, Writing – review & editing. MH: Investigation, Methodology, Validation, Writing – review & editing. NS: Investigation, Methodology, Validation, Writing – review & editing, Conceptualization, Funding acquisition, Supervision, Writing – original draft.
The author(s) declare financial support was received for the research, authorship, and/or publication of this article. This work was financially supported by Grants-in-Aid for Scientific Research (C) from the Japan Society for the Promotion of Science (19K12384 and 23K11481, NS).
The authors declare that the research was conducted in the absence of any commercial or financial relationships that could be construed as a potential conflict of interest.
All claims expressed in this article are solely those of the authors and do not necessarily represent those of their affiliated organizations, or those of the publisher, the editors and the reviewers. Any product that may be evaluated in this article, or claim that may be made by its manufacturer, is not guaranteed or endorsed by the publisher.
The Supplementary Material for this article can be found online at: https://www.frontiersin.org/articles/10.3389/fpls.2024.1335085/full#supplementary-material
Aiba, H., Adhya, S., de Crombrugghe, B. (1981). Evidence for two functional gal promoters in intact Escherichia coli cells. J. Biol. Chem. 256, 11905–11910. doi: 10.1016/S0021-9258(19)68491-7
Andersson, M. X., Stridh, M. H., Larsson, K. E., Liljenberg, C., Sandelius, A. S. (2003). Phosphate-deficient oat replaces a major portion of the plasma membrane phospholipids with the galactolipid digalactosyldiacylglycerol. FEBS Lett. 537, 128–132. doi: 10.1016/S0014-5793(03)00109-1
Awai, K., Watanabe, H., Benning, C., Nishida, I. (2007). Digalactosyldiacylglycerol is required for better photosynthetic growth of Synechocystis sp. PCC6803 under phosphate limitation. Plant Cell Physiol. 48, 1517–1523. doi: 10.1093/pcp/pcm134
Benning, C., Beatty, J. T., Prince, R. C., Somerville, C. R. (1993). The sulfolipid sulfoquinovosyldiacylglycerol is not required for photosynthetic electron transport in Rhodobacter sphaeroides but enhances growth under phosphate limitation. Proc. Natl. Acad. Sci. U.S.A. 90, 1561–1565. doi: 10.1073/pnas.90.4.1561
Bligh, E. G., Dyer, W. J. (1959). A rapid method of total lipid extraction and purification. Can. J. Biochem. Physiol. 37, 911–917. doi: 10.1139/y59-099
Cruz-Ramírez, A., Oropeza-Aburto, A., Razo-Hernández, F., Ramírez-Chávez, E., Herrera-Estrella, L. (2006). Phospholipase DZ2 plays an important role in extraplastidic galactolipid biosynthesis and phosphate recycling in Arabidopsis roots. Proc. Natl. Acad. Sci. U.S.A. 103, 6765–6770. doi: 10.1073/pnas.0600863103
Endo, K., Kobayashi, K., Wada, H. (2016). Sulfoquinovosyldiacylglycerol has an essentialrole in Thermosynechococcus elongatus BP-1 under phosphate-deficient conditions. Plant Cell Physiol. 57, 2461–2471. doi: 10.1093/pcp/pcw159
Essigmann, B., Güler, S., Narang, R. A., Linke, D., Benning, C. (1998). Phosphate availability affects the thylakoid lipid composition and the expression of SQD1, a gene required for sulfolipid biosynthesis in Arabidopsis thaliana. Proc. Natl. Acad. Sci. U.S.A. 95, 1950–1955. doi: 10.1073/pnas.95.4.1950
Gallaher, S. D., Fitz-Gibbon, S. T., Strenkert, D., Purvine, S. O., Pellegrini, M., Merchant, S. S. (2018). High-throughput sequencing of the chloroplast and mitochondrion of Chlamydomonas reinhardtii to generate improved de novo assemblies, analyze expression patterns and transcript speciation, and evaluate diversity among laboratory strains and wild isolates. Plant J. 93, 545–565. doi: 10.1111/tpj.13788
Gaude, N., Nakamura, Y., Scheible, W. R., Ohta, H., Dörmann, P. (2008). Phospholipase C5 (NPC5) is involved in galactolipid accumulation during phosphate limitation in leaves of Arabidopsis. Plant J. 56, 28–39. doi: 10.1111/j.1365-313X.2008.03582.x
Ghirardi, M., Morgan, J. A., Yu, J. (2018). Glycogen synthesis and metabolite overflow contribute to energy balancing in cyanobacteria. Cell Rep. 23, 667–672. doi: 10.1016/j.celrep.2018.03.083
Güler, S., Seeliger, A., Härtel, H., Renger, G., Benning, C. (1996). A null mutant of Synechococcus sp. PCC7942 deficient in the sulfolipid sulfoquinovosyl diacylglycerol. J. Biol. Chem. 271, 7501–7507. doi: 10.1074/jbc.271.13.7501
Härtel, H., Dormann, P., Benning, C. (2000). DGD1-independent biosynthesis of extraplastidic galactolipids after phosphate deprivation in Arabidopsis. Proc. Natl. Acad. Sci. U.S.A. 97, 10649–10654. doi: 10.1073/pnas.180320497
Heuer, S., Gaxiola, R., Schilling, R., Herrera-Estrella, L., López-Arredondo, D., Wissuwa, M., et al. (2017). Improving phosphorus use efficiency: a complex trait with emerging opportunities. Plant J. 90, 868–885. doi: 10.1111/tpj.13423
Hirai, K., Nojo, M., Sato, Y., Tsuzuki, M., Sato, N. (2019). Contribution of protein synthesis depression to poly-β-hydroxybutyrate accumulation in Synechocystis sp. PCC 6803 under nutrient-starved conditions. Sci. Rep. 27, 19944. doi: 10.1038/s41598-019-56520-w
Hiyoshi, T., Oyanagi, K., Niki, T., Fujiwara, S., Sato, N. (2021). Requirement of the exopolyphosphatase gene for cellular acclimation to phosphorus starvation in a cyanobacterium, Synechocystis sp. PCC 6803. Biochem. Biophys. Res. Commun. 540, 16–21. doi: 10.1016/j.bbrc.2020.12.095
Hung, C. H., Endo, K., Kobayashi, K., Nakamura, Y., Wada, H. (2015). Characterization of Chlamydomonas reinhardtii phosphatidylglycerophosphate synthase in Synechocystis sp. PCC 6803. Front. Microbiol. 6, 842. doi: 10.3389/fmicb.2015.00842
Kaewbai-Ngam, A., Incharoensakdi, A., Monshupanee, T. (2016). Increased accumulation of polyhydroxybutyrate in divergent cyanobacteria under nutrient-deprived photoautotrophy: An efficient conversion of solar energy and carbon dioxide to polyhydroxybutyrate by Calothrix scytonemicola TISTR 8095. Bioresour. Technol. 212, 342–347. doi: 10.1016/j.biortech.2016.04.035
Kaneko, T., Tabata, S. (1997). Complete genome structure of the unicellular cyanobacterium Synechocystis sp. PCC6803. Plant Cell Physiol. 38, 1171–1176. doi: 10.1093/oxfordjournals.pcp.a029103
Kóbori, T. O., Uzumaki, T., Kis, M., Kovács, L., Domonkos, I., Itoh, S., et al. (2018). Phosphatidylglycerol is implicated in divisome formation and metabolic processes of cyanobacteria. J. Plant Physiol. 223, 96–104. doi: 10.1016/j.jplph.2018.02.008
Kopečná, J., Pilný, J., Krynická, V., Tomčala, A., Kis, M., Gombos, Z., et al. (2015). Lack of phosphatidylglycerol inhibits chlorophyll biosynthesis at multiple sites and limits chlorophyllide reutilization in Synechocystis sp. strain PCC 6803. Plant Physiol. 169, 1307–1317. doi: 10.1104/pp.15.01150
Kromkamp, J. (1987). Formation and functional significance of storage products in cyanobacteria. New Z. J. Mar. Freshw. Res. 21, 457–465. doi: 10.1080/00288330.1987.9516241
Kumar, S., Kumar, S., Mohapatra, T. (2021). Interaction between macro- and micro-nutrients in plants. Front. Plant Sci. 12, 665583. doi: 10.3389/fpls.2021.665583
Li, J., Dittrich, M. (2019). Dynamic polyphosphate metabolism in cyanobacteria responding to phosphorus availability. Environ. Microbiol. 21, 572–583. doi: 10.1111/1462-2920.14488
Loparev, V. N., Cartas, M. A., Monken, C. E., Velpandi, A., Srinivasan, A. (1991). An efficient and simple method of DNA extraction from whole blood and cell lines to identify infectious agents. J. Virol. Methods 34, 105–112. doi: 10.1016/0166-0934(91)90126-K
Mao, X., Wu, T., Sun, D., Zhang, Z., Chen, F. (2018). Differential responses of the green microalga Chlorella zofingiensis to the starvation of various nutrients for oil and astaxanthin production. Bioresour. Technol. 249, 791–798. doi: 10.1016/j.biortech.2017.10.090
Maul, J. E., Lilly, J. W., Cui, L., dePamphilis, C. W., Miller, W., Harris, E. H., et al. (2002). The Chlamydomonas reinhardtii plastid chromosome: islands of genes in a sea of repeats. Plant Cell 14, 2659–2679. doi: 10.1105/tpc.006155
Mironov, K. S., Los, D. A. (2015). RNA isolation from Synechocystis. Bio-protocol 5. doi: 10.21769/BioProtoc.1428
Mizusawa, N., Wada, H. (2012). The role of lipids in photosystem II. Biochim. Biophys. Acta 1817, 194–208. doi: 10.1016/j.bbabio.2011.04.008
Młodzińska, E., Zboińska, M. (2016). Phosphate uptake and allocation – a closer look at Arabidopsis thaliana L. and Oryza sativa L. Front. Plant Sci. 7, 1198. doi: 10.3389/fpls.2016.01198
Monshupanee, T., Incharoensakdi, A. (2014). Enhanced accumulation of glycogen, lipids and polyhydroxybutyrate under optimal nutrients and light intensities in the cyanobacterium Synechocystis sp. PCC 6803. J. Appl. Microbiol. 116, 830–838. doi: 10.1111/jam.12409
Murakami, H., Nobusawa, T., Hori, K., Shimojima, M., Ohta, H. (2018). Betaine lipid is crucial for adapting to low temperature and phosphate deficiency in Nannochloropsis. Plant Physiol. 177, 181–193. doi: 10.1104/pp.17.01573
Nakamura, Y. (2013). Phosphate starvation and membrane lipid remodeling in seed plants. Prog. Lipid. Res. 52, 43–50. doi: 10.1016/j.plipres.2012.07.002
Nakamura, Y., Koizumi, R., Shui, G., Shimojima, M., Wenk, M. R., Ito, T., et al. (2009). Arabidopsis lipins mediate eukaryotic pathway of lipid metabolism and cope critically with phosphate starvation. Proc. Natl. Acad. Sci. U.S.A. 106, 20978–20983. doi: 10.1073/pnas.0907173106
Oishi, Y., Otaki, R., Iijima, Y., Kumagai, E., Aoki, M., Tsuzuki, M., et al. (2022). Diacylglyceryl-N,N,N-trimethylhomoserine-dependent lipid remodeling in a green alga, Chlorella kessleri. Commun. Biol. 5, 19. doi: 10.1038/s42003-021-02927-z
Okazaki, Y., Shimojima, M., Sawada, Y., Toyooka, K., Narisawa, T., Mochida, K., et al. (2009). A chloroplastic UDP-glucose pyrophosphorylase from Arabidopsis is the committed enzyme for the first step of sulfolipid biosynthesis. Plant Cell 21, 892–909. doi: 10.1105/tpc.108.063925
Peng, Z., Feng, L., Wang, X., Miao, X. (2019). Adaptation of Synechococcus sp. PCC 7942 to phosphate starvation by glycolipid accumulation and membrane lipid remodeling. Biochim. Biophys. Acta 1864, 158522. doi: 10.1016/j.bbalip.2019.158522
Planson, A. G., Sauveplane, V., Dervyn, E., Jules, M. (2020). Bacterial growth physiology and RNA metabolism. Biochim. Biophys. Acta 1863, 194502. doi: 10.1016/j.bbagrm.2020.194502
Plaxton, W. C., Tran, H. T. (2011). Metabolic adaptations of phosphate-starved plants. Plant Physiol. 156, 1006–1015. doi: 10.1104/pp.111.175281
Rausch, C., Bucher, M. (2002). Molecular mechanisms of phosphate transport in plants. Planta 216, 23–37. doi: 10.1007/s00425-002-0921-3
Riekhof, W. R., Ruckle, M. E., Lydic, T. A., Sears, B. B., Benning, C. (2003). The sulfolipids 2'-O-acyl-sulfoquinovosyldiacylglycerol and sulfoquinovosyldiacylglycerol are absent from a Chlamydomonas reinhardtii mutant deleted in SQD1. Plant Physiol. 133, 864–874. doi: 10.1104/pp.103.029249
Sato, N. (2004). Roles of the acidic lipids sulfoquinovosyl diacylglycerol and phosphatidylglycerol in photosynthesis: their specificity and evolution. J. Plant Res. 117, 495–505. doi: 10.1007/s10265-004-0183-1
Sato, N., Ebiya, Y., Kobayashi, R., Nishiyama, Y., Tsuzuki, M. (2017a). Disturbance of cell-size determination by forced overproduction of sulfoquinovosyl diacylglycerol in the cyanobacterium Synechococcus elongatus PCC 7942. Biochem. Biophys. Res. Commun. 487, 734–739. doi: 10.1016/j.bbrc.2017.04.129
Sato, N., Hagio, M., Wada, H., Tsuzuki, M. (2000). Requirement of phosphatidylglycerol for photosynthetic function in thylakoid membranes. Proc. Natl. Acad. Sci. U.S.A. 97, 10655–10660. doi: 10.1073/pnas.97.19.10655
Sato, N., Kamimura, R., Kaneta, K., Yoshikawa, M., Tsuzuki, M. (2017b). Species-specific roles of sulfolipid metabolism in acclimation of photosynthetic microbes to sulfur-starvation stress. PloS One 12, e0186154. doi: 10.1371/journal.pone.0186154
Sato, N., Sonoike, K., Tsuzuki, M., Kawaguchi, A. (1995a). Impaired photosystem II in a mutant of Chlamydomonas reinhardtii defective in sulfoquinovosyl diacylglycerol. Eur. J. Biochem. 234, 16–23. doi: 10.1111/j.1432-1033.1995.016_c.x
Sato, N., Suda, K., Tsuzuki, M. (2004). Responsibility of phosphatidylglycerol for biogenesis of the PSI complex. Biochim. Biophys. Acta 1658, 235–243. doi: 10.1016/j.bbabio.2004.06.008
Sato, N., Tsuzuki, M., Matsuda, Y., Ehara, T., Osafune, T., Kawaguchi, A. (1995b). Isolation and characterization of mutants affected in lipid metabolism of Chlamydomonas reinhardtii. Eur. J. Biochem. 230, 987–993. doi: 10.1111/j.1432-1033.1995.tb20646.x
Sato, N., Wada, H. (2009). “Lipid biosynthesis and its regulation in cyanobacteria,” in Lipids in Photosynthesis. Advances in Photosynthesis and Respiration, vol. 30 . Eds. Wada, H., Murata, N. (Dordrecht: Springer).
Smith, A. P., Fontenot, E. B., Zahraeifard, S., DiTusa, S. F. (2015). Molecular components that drive phosphorus-remobilisation during leaf senescence. Annu. Plant Rev. 48, 159–186. doi: 10.1002/9781118958841.ch6
Sugimoto, K., Sato, N., Tsuzuki, M. (2007). Utilization of a chloroplast membrane sulfolipid as a major internal sulfur source for protein synthesis in the early phase of sulfur starvation in Chlamydomonas reinhardtii. FEBS Lett. 581, 4519–4522. doi: 10.1016/j.febslet.2007.08.035
Sugimoto, K., Tsuzuki, M., Sato, N. (2010). Regulation of synthesis and degradation of a sulfolipid under sulfur-starved conditions and its physiological significance in Chlamydomonas reinhardtii. New Phytol. 185, 676–686. doi: 10.1111/j.1469-8137.2009.03115.x
Suzuki, S., Ferjani, A., Suzuki, I., Murata, N. (2004). The SphS-SphR two component system is the exclusive sensor for the induction of gene expression in response to phosphate limitation in Synechocystis. J. Biol. Chem. 279, 13234–13240. doi: 10.1074/jbc.M313358200
Takami, T., Ohnishi, N., Kurita, Y., Iwamura, S., Ohnishi, M., Kusaba, M., et al. (2018). Organelle DNA degradation contributes to the efficient use of phosphate in seed plants. Nat. Plants 4, 1044–1055. doi: 10.1038/s41477-018-0291-x
Teixeira, G. C. M., Prado, R. M., Oliveira, K. S., Buchelt, A. C., Rocha, A. M. S., Santos, M. S. (2021). Nutritional deficiency in scarlet eggplant limits its growth by modifying the absorption and use efficiency of macronutrients. PloS One 16, e0252866. doi: 10.1371/journal.pone.0252866
Van Mooy, B. A., Fredricks, H. F., Pedler, B. E., Dyhrman, S. T., Karl, D. M., Koblízek, M., et al. (2009). Phytoplankton in the ocean use non-phosphorus lipids in response to phosphorus scarcity. Nature 458, 69–72. doi: 10.1038/nature07659
Veneklaas, E. J., Lambers, H., Bragg, J., Finnegan, P. M., Lovelock, C. E., Plaxton, W. C., et al. (2012). Opportunities for improving phosphorus-use efficiency in crop plants. New Phytol. 195, 306–320. doi: 10.1111/j.1469-8137.2012.04190.x
Yehudai-Resheff, S., Zimmer, S. L., Komine, Y., David, B. S. (2007). Integration of chloroplast nucleic acid metabolism into the phosphate deprivation response in Chlamydomonas reinhardtii. Plant Cell 19, 1023–1038. doi: 10.1105/tpc.106.045427
Yu, B., Xu, C., Benning, C. (2002). Arabidopsis disrupted in SQD2 encoding sulfolipid synthase is impaired in phosphate-limited growth. Proc. Natl. Acad. Sci. U.S.A. 99, 5732–5737. doi: 10.1073/pnas.082696499
Yu, L., Zhou, C., Fan, J., Shanklin, J., Xu, C. (2021). Mechanisms and functions of membrane lipid remodeling in plants. Plant J. 107, 37–53. doi: 10.1111/tpj.15273
Keywords: cyanobacteria, phosphatidylglycerol, phosphorus starvation, rRNA, Synechocystis
Citation: Hiyoshi T, Haga M and Sato N (2024) Preferential phosphatidylglycerol synthesis via phosphorus supply through rRNA degradation in the cyanobacterium, Synechocystis sp. PCC 6803, under phosphate-starved conditions. Front. Plant Sci. 15:1335085. doi: 10.3389/fpls.2024.1335085
Received: 08 November 2023; Accepted: 08 January 2024;
Published: 29 January 2024.
Edited by:
Michael Hippler, University of Münster, GermanyReviewed by:
Martin Hagemann, University of Rostock, GermanyCopyright © 2024 Hiyoshi, Haga and Sato. This is an open-access article distributed under the terms of the Creative Commons Attribution License (CC BY). The use, distribution or reproduction in other forums is permitted, provided the original author(s) and the copyright owner(s) are credited and that the original publication in this journal is cited, in accordance with accepted academic practice. No use, distribution or reproduction is permitted which does not comply with these terms.
*Correspondence: Norihiro Sato, bnNhdG9AbHMudG95YWt1LmFjLmpw
Disclaimer: All claims expressed in this article are solely those of the authors and do not necessarily represent those of their affiliated organizations, or those of the publisher, the editors and the reviewers. Any product that may be evaluated in this article or claim that may be made by its manufacturer is not guaranteed or endorsed by the publisher.
Research integrity at Frontiers
Learn more about the work of our research integrity team to safeguard the quality of each article we publish.