- School of Life Sciences, Jiangsu University, Zhenjiang, China
Low temperature is a critical environmental stress factor that restricts crop growth and geographical distribution, significantly impacting crop quality and yield. When plants are exposed to low temperatures, a series of changes occur in their external morphology and internal physiological and biochemical metabolism. This article comprehensively reviews the alterations and regulatory mechanisms of physiological and biochemical indices, such as membrane system stability, redox system, fatty acid content, photosynthesis, and osmoregulatory substances, in response to low-temperature stress in plants. Furthermore, we summarize recent research on signal transduction and regulatory pathways, phytohormones, epigenetic modifications, and other molecular mechanisms mediating the response to low temperatures in higher plants. In addition, we outline cultivation practices to improve plant cold resistance and highlight the cold-related genes used in molecular breeding. Last, we discuss future research directions, potential application prospects of plant cold resistance breeding, and recent significant breakthroughs in the research and application of cold resistance mechanisms.
1 Introduction
The plant production process faces various adverse conditions, including salt-alkali, high temperature, low temperature, drought, pests, and other stresses. One of the common challenges is low temperature stress, which can be classified as cold damage (>0°C) and freezing damage (<0°C) (Ding et al., 2019). Both cold damage and freezing damage have detrimental effects on crop yield and quality (Aslam et al., 2022). They inhibit plant respiration and metabolism, reduce root water absorption rates, and lead to the yellowing of leaves and browning of fruits. Prolonged exposure to temperatures below 0°C damages the cell membrane, resulting in cell death. Even when the temperature is above 0°C and no ice is present, water balance and physiological activities can still be disrupted, causing injury and death of plants.
Plants in the growth stage are particularly sensitive to temperature, and low temperatures significantly reduce flowering and fruit yield in tomatoes (Yang et al., 2021; Iovane and Aronne, 2022). Studies have shown that Capsicum annuum is susceptible to cold damage during postharvest storage and transportation, leading to symptoms such as concave skin surface, concave spots, brown calyx, water, and nutrient loss (Ding and Wang, 2018; Kong et al., 2018). In the case of Solanum tuberosum, different low-temperature treatments have varying effects. Leaves of S. tuberosum above 0°C can maintain normal growth, but temperatures below −2°C cause serious damage, such as wilting, leaf drop, waterlogging, and even death (Yan et al., 2021). Low-temperature stress inhibits seed germination and increases seedling mortality in Brassica napus (Zhu et al., 2021). Cucumis sativus, a cold-sensitive plant, exhibits reduced germination rates, shriveled and yellowish leaves, decreased seed set rates, and increased susceptibility to rot during storage and transport after cold stress (Zhang et al., 2021). Solanum melongena leaves undergo elongation, become soft and atrophied, and curl completely under low-temperature stress, with light green leaf spots appearing. Zea mays growth is adversely affected by cold stress, resulting in inhibited germination, stunted grain development, and a significant decrease in yield (Peleg and Blumwald, 2011).
However, most plants have evolved a strategy known as cold acclimation to adapt to low-temperature environments. This strategy enhances their cold tolerance by triggering physiological changes and cold response gene expression through a cascade of molecular events (Browse and Xin, 2001). Currently, the molecular mechanism underlying plant resistance to low temperatures remains insufficiently understood. Therefore, there is an urgent need to analyze the regulatory mechanism of plants under low-temperature stress and develop technology to improve the plants’ resistance. This article comprehensively reviews the molecular mechanisms involved in plant responses to low-temperature stress, and the advances made in understanding the physiological, biochemical, and molecular signaling mechanisms underlying plant cold resistance. The roles of transcription factors (TFs), including C repeat binding factor (CBF), CBF expression inducer 1 (ICE1), APETALA2/ethylene-responsive factor (AP2/ERF), and dehydration-responsive element binding (DREB1), are extensively discussed in response to cold stress. It provides a theoretical basis for high-quality, high-yield, and stress-resistant plant cultivation and practical guidance for plant cold tolerance research.
Signaling pathway of plants’ response to low-temperature stress
2.1 Low-temperature perception and transduction of calcium signals
Calcium (Ca2+) serves as a universal second messenger in plant cells and plays a crucial role in plant growth, development, and responses to various biotic and abiotic stresses, such as cold, heat, and salt stress (Jiang et al., 2019). When exposed to low temperatures, changes in membrane fluidity and cytoskeletal rearrangement occur, leading to an influx of Ca2+ and an increase in intracellular Ca2+ levels (Dodd et al., 2010). The increased intracellular flow of Ca2+ is sensed by downstream calcium signal receptors and transmits Ca2+ signals further downstream, thus participating in signal transduction to induce the expression of cold response genes (Sanyal et al., 2016). Several calcium-responsive proteins, including calmodulin (CaM), CAM-like proteins (CML), calcium-dependent protein kinases (CDPK), and calcineurin B-like proteins (CBL), have been identified and play significant roles in responding to low-temperature stress (Reddy et al., 2011; Ma et al., 2015; Zhu, 2016). Studies conducted in A. thaliana have shown that the level of gene transcription induced by low temperature is positively correlated with the intensity of Ca2+ signals (Kleist and Luan, 2016). An increased concentration of intracellular Ca2+ promotes the production of inositol triphosphate (IP3), which amplifies the Ca2+ signal by regulating Ca2+ channels and further enhances the expression of cold response genes such as CBF and COR genes (Sulaiman et al., 2012). The specific components involved in the regulation of calcium signaling have been elucidated. For instance, the Ca2+ transporter AtANN1 contributes to cold-induced calcium signaling by positively regulating cold tolerance through its effects on Ca2+ influx and the downstream CBF-COR-dependent cold signal transduction pathway in A. thaliana (Liu et al., 2021c). Similarly, the low-temperature receptor protein COLD1 interacts with G proteins to activate Ca2+ channels and enhance cold tolerance in O. sativa (Nawaz et al., 2014). Furthermore, calcineurin B-like protein-interacting protein kinase (CIPK) is another crucial component of Ca2+ signaling in the stress response. CIPK specifically interacts with CBLs, which control the localization and activation of CIPK. The CBL-CIPK signaling pathway plays a critical role in Ca2+ signaling in plants and is involved in the response to cold stress (Ormancey et al., 2017). Furthermore, a study (Yang et al., 2010) demonstrated that CRLK1 is a positive regulator of the plant response to low-temperature stress through gene knockout and complementation experiments. CRLK1 interacts with MEKK1 to activate the MAPK cascade reaction. The mitogen-activated protein kinase (MAPK) cascade has been extensively studied for its role in the response to low-temperature stress. This cascade typically consists of three protein kinases: MAP kinases (MAPKKK, MAP3K, or MEKK), MAP kinases (MAP2K, MKK, or MEK), and MAP kinases (MAPK). MKK2 and MAPKKK kinase MEKK1 are simultaneously activated by cold stress, and MKK2 induces the expression of COR genes, thereby improving plant cold tolerance (Teige et al., 2004). The MAP2K/MKK2 signaling pathway is activated by low temperatures and regulates the expression of downstream COR genes (Teige et al., 2004). While significant progress has been made in understanding the Ca2+ regulation mechanism in the plant response to cold stress, further research is needed to explore the interaction between Ca2+-responsive transcription factors and the Ca2+ signaling pathway (Figure 1).
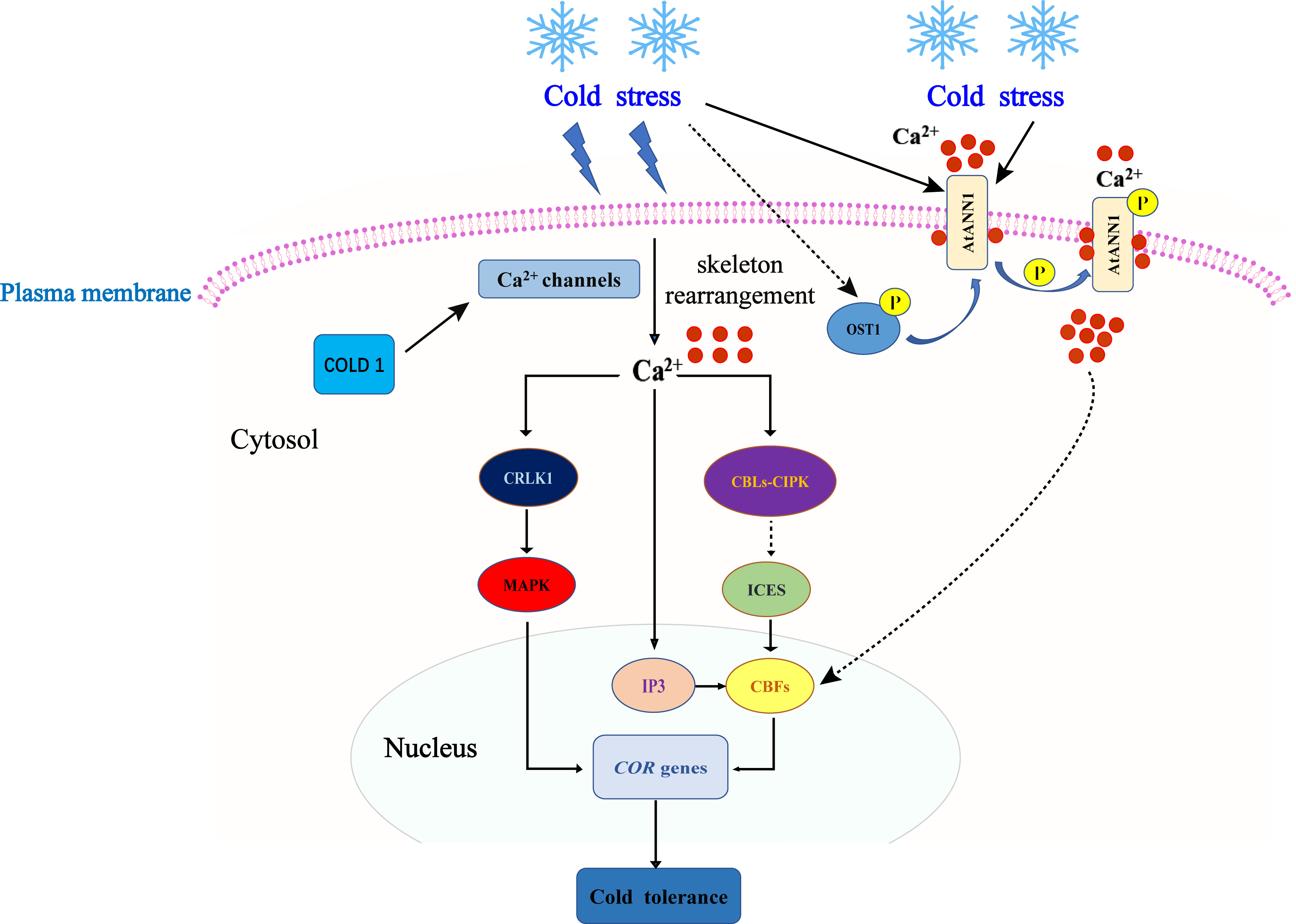
Figure 1 Ca2+ signal perception and transduction models. Cold stress induces extracellular Ca2+ influx, triggering the Ca2+ signal. Ca2+ promotes the production of IP3, further enhances the expression of the COR gene, and enhances plant cold tolerance. In Arabidopsis thaliana, OST1 kinase can regulate the expression of CBF and COR genes through phosphorylation of AtANN1 to enhance cold tolerance. Low temperature can activate the MAPK signaling pathway to regulate the expression of the downstream COR gene and enhance cold tolerance.
2.2 Signaling pathway and regulatory mechanism in response to low-temperature stress
2.2.1 CBF-dependent low-temperature response signaling pathway
The CBF-dependent low-temperature response signaling pathway is an essential mechanism for plants to cope with cold stress. CBF, which stands for C-repeat binding factor, is a group of plant-specific transcription factors that play a crucial role in regulating the expression of COR (cold-regulated) genes (Stockinger et al., 1997). The signaling pathway of CBF in response to low-temperature stress in higher plants has been extensively investigated. In the model plant A. thaliana, CBF genes belong to the dehydration-responsive element-binding protein (DREB) subfamily. They are regulated by the upstream transcription factor ICE (inducer of CBF expression) (Li et al., 2020). Consequently, CBFs are also known as DREB1 genes, and their activation induces the downstream expression of COR genes to improve plant cold tolerance. This ICE-CBF-COR cascade is crucial in maintaining normal growth and development during cold stress. Several transcription factors, including the positive regulator CAMTA (calmodulin binding activator of transcription) and negative regulators such as MYB15 and HOS1, directly regulate CBF gene expression (Ding et al., 2019). When plants are exposed to cold stress, the signal transduction process is triggered, leading to the activation of ICE. ICE, in turn, promotes the transcription and expression of CBF1/2/3 genes, which enhances the expression of COR genes (Hwarari et al., 2022). The ICE-CBF protein is regulated by ubiquitin, which improves plant cold tolerance (Sharma et al., 2021). Research has shown that the ICE1 transcription factor is crucial for the expression of DREB1/CBF (Thomashow and Torii, 2020). ICE1, a structural protein of the basic helix–loop–helix (bHLH) domain of the MYC transcription factor, specifically recognizes cis-acting elements of MYC (CANNTG) in the promoter region of CBF3/DREB1A and binds to them, inducing the expression of CBF/DREB1 and activating the cold tolerance regulatory pathway (Lee et al., 2005a; Wang et al., 2008). A study of A. thaliana discovered that the ice1 mutation hampers CBF1 expression, leading to decreased downstream expression of the CBF gene, while overexpression of ICE1 effectively enhances CBF expression and improves cold tolerance in A. thaliana (Chinnusamy et al., 2003). Furthermore, PUB25 and PUB26 interact with ICE1 to ubiquitinate ICE1 and stabilize its protein levels. The stabilized ICE1 protein then interacts with MYB15 to inhibit its DNA-binding activity, ultimately activating CBF gene expression (Wang et al., 2023c). Furthermore, MYB15, ICE1, and HOS1 have been identified as direct regulators of CBF/DREB1 expression. ICE1 can interact with MYB15 and directly bind to the MYB15 promoter region, leading to the downregulation of MYB15 expression under cold stress (Agarwal et al., 2006b). The interaction between B1L (BYPASS1-LIKE) and TTL (TRANSTHYRETIN-LIKE) participates in the regulation of plant growth, development, and cold tolerance (Chen et al., 2020). The 14-3-3 proteins are a highly conserved family of acidic proteins widely expressed in eukaryotes and involved in various biological processes. B1L improves the stability of CBF by interacting with 14-3-3λ, thus inducing the expression of COR genes and improving cold tolerance in A. thaliana (Chen et al., 2019). ABI4 is a transcription factor of AP2/ERF (APETALA2/ethylene responsive factor). ABI4 can regulate downstream gene expression by recognizing promoter motifs (Chandrasekaran et al., 2020). MdABI4 interacts with MdICE1 to enhance the transcriptional regulation of the downstream target gene MdCBF1 by MdICE1. MdABI4 integrates jasmonic acid and abscisic acid signals to positively regulate cold tolerance in M. domestica through the JAZ-ABI4-ICE1-CBF cascade (An et al., 2022). PIF3 (phytochrome-interacting factors 3) is a bHLH transcription factor and a member of the PIF protein family (Ni et al., 1998). PIF3 acts as a negative regulator of plant cold tolerance by inhibiting the expression of the CBF gene. Two F-box proteins, EBF1 (EIN3-BINDING F-BOX 1) and EBF2 (EIN3-BINDING F-BOX 2), directly target PIF3 for degradation through the 26S proteasome pathway. This degradation leads to the activation of CBF gene expression (Jiang et al., 2017). Trx-h2 (Thioredoxin h2) interacts with CBFs at low temperatures to regulate CBF activity and protein structure. This interaction activates downstream COR gene expression and improves plant cold tolerance (Lee et al., 2021). Dehydrin, also known as LEA D-11 or LEA II (late embryogenesis abundant) protein induces cold acclimation in freezing-tolerant plants, enabling plants to survive under adverse conditions (Kosová et al., 2007). Dehydrin accumulates in plants when they are exposed to cold. Vernalization, the prolonged exposure of plants to low temperatures, is associated with a significant reduction in dehydrin accumulation, which delays the acquired frost tolerance of the plant (Kosová et al., 2011). There is a positive correlation between the relative accumulation of dehydrin protein and winter survival (WS) in winter wheat and barley (Vítámvás et al., 2019). Cold-induced dehydrin Lti30 binds to the membrane through its conserved K segment and reduces the membrane phase transition temperature (Eriksson et al., 2016). Lti30 binds to the lipid bilayer electrostatically, limiting the mobility of lipid and bound protein molecules and protecting the cell membrane by forming aggregates (Gupta et al., 2019). As a cold-induced CBF transcription factor in the A. thaliana genome, CBF1/2/3 can bind to the CRT/DRE/LTRE elements, thus increasing the tolerance to plant cold (Gilmour et al., 2004). Several dehydrin genes were identified in cold regulatory proteins whose promoters contained elements of CRT/DRE/LTRE (Zolotarov and Strömvik, 2015). Under cold stress, the ICE1-CBF cold response signaling pathway gene of the DIICE1 overexpression strain was significantly upregulated compared with the wild type (Yang et al., 2019). In conclusion, the CBF-dependent low-temperature response signaling pathway involves multiple transcription factors and protein interactions that collectively regulate the expression of COR genes and enhance plant cold tolerance (Figure 2).
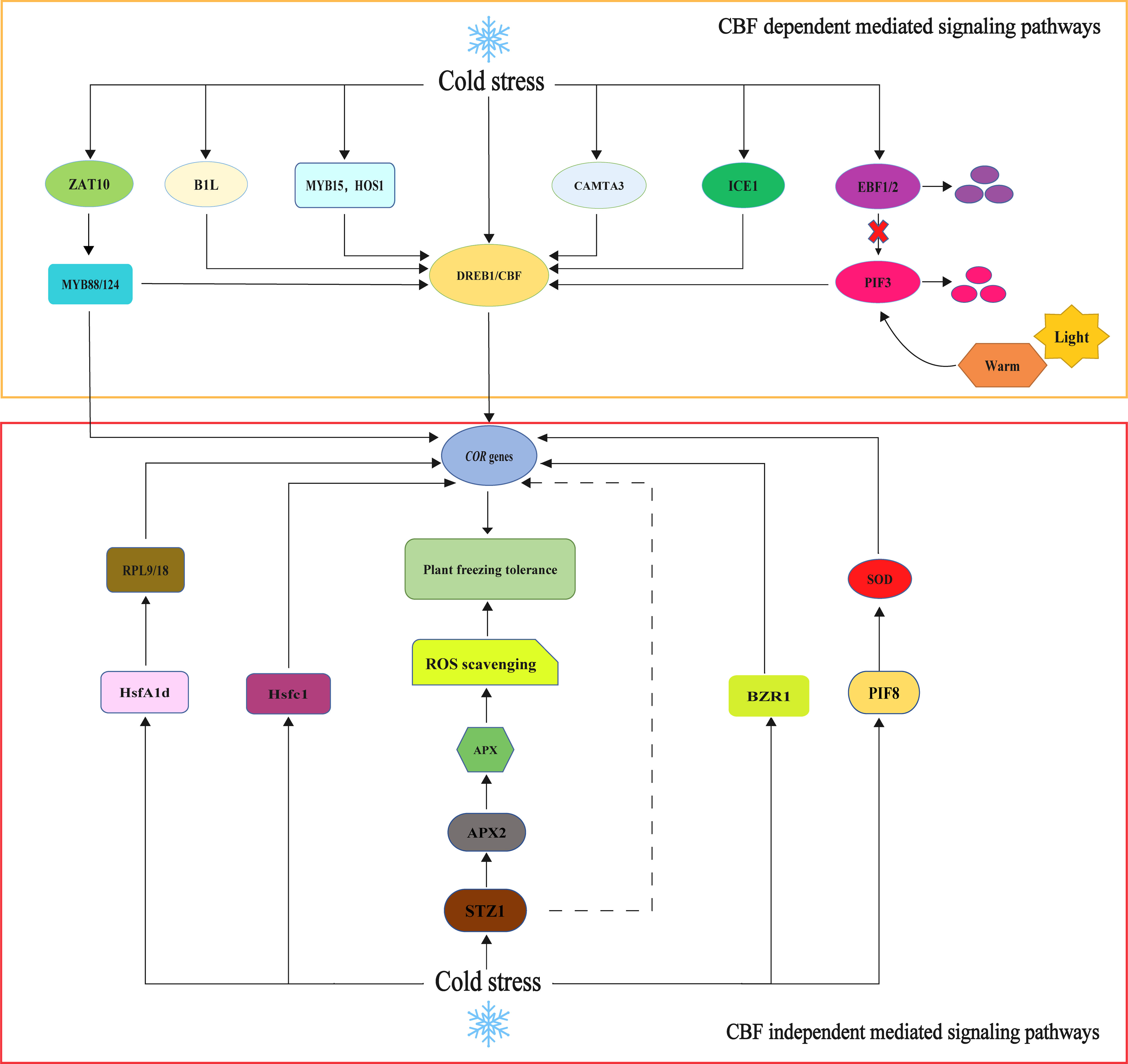
Figure 2 CBF-dependent and CBF-independent mediated signaling pathways models. Among the CBF-dependent pathways. The CBF-COR pathway is the primary cold stress signal regulation pathway. Cold stress can activate BIL, MYB15, HOS1, CAMTA3, ICE1, and ZAT10; regulate the expression of the CBF gene, thereby enhancing the expression of the COR gene; and regulate plant cold resistance. PIF3 can bind the promoter region of CBFs, inhibit the expression of CBFs and its downstream cold response genes, and negatively regulate the cold resistance of Arabidopsis thaliana. Under normal temperature and light conditions, EBF1/2 targets degrade PIF3 and induce CBF gene expression. Low-temperature and dark conditions promote the degradation of EBF protein, which makes PIF3 protein more stable. In the CBF-independent pathway, HsfA1d, Hsfc1, STZ1, BZR1, PIF8, and other transcription factors can directly regulate the expression of the COR gene independently of CBF and regulate plant cold tolerance.
2.2.2 CBF-independent signaling pathways for low-temperature response
The regulation of COR genes is crucial for enhancing plant tolerance to cold during cold acclimatization. However, recent studies have revealed that only 10%–20% of COR genes in plant cells are regulated by CBF, while the majority of them participate in cold tolerance signaling pathways independently of CBF (Ding et al., 2019). For instance, the overexpression of HsfC1 (heat shock transcription factor C1) in A. thaliana induces the expression of COR genes and improves cold tolerance (Jiao et al., 2022). A genome-wide association study on hypocotyl growth in A. thaliana demonstrated that HsfA1d (heat shock transcription factor A1d) promotes hypocotyl elongation under cold stress by binding to the promoters of two ribosomal protein genes, RPL9 and RPL18, thus enhancing plant tolerance to low temperatures (Liu et al., 2021a). Moreover, the transcriptional regulatory factor BZR1 in the brassinosteroid (BR) signaling pathway regulates the expression of CBF1 and CBF2 and also independently controls COR genes. BZR1 regulates plant cold tolerance through both CBF-dependent and CBF-independent pathways (Li et al., 2017b). Initially identified as a CBF-independent gene in the cold tolerance signaling pathway, ZAT10 is downstream of MAPK, APX, and CAT (Gong et al., 2020). ZAT10 induces the expression of COR genes by regulating the expression of MYB88/124, thus enhancing the cold tolerance of M. domestica (Li et al., 2023). The overexpression of CsPIF8 in S. lycopersicum and V. vinifera callus enhances citrus cold tolerance by increasing SOD activity and reducing O2 levels, indicating that CsPIF8 is involved in regulating cold tolerance independently of the CBF pathway (He et al., 2020). PeSTZ1 is induced under low temperature stress and directly binds to the PeAPX2 promoter, promoting its activation and reducing ROS accumulation under cold stress. This regulation of gene expression improves the cold resistance of Populus euphratica by regulating the expression of COR genes (He et al., 2019). Research has shown that BnHY5 regulates hypocotyl length in B. napus by regulating the downstream gene PIF4 in the IAA pathway. It is speculated that BnHY5 is a key gene involved in cold resistance and hypocotyl development in B. napus (Jin et al., 2022). Numerous CBF-independent signaling pathways for low-temperature response exist in plants, and further exploration is required to elucidate their regulatory mechanisms (Figure 2).
3 Low-temperature effect on the physiological and biochemical metabolism of plants
3.1 Effect of low temperature on membrane stability
The cell membrane, primarily composed of phospholipids, is highly sensitive to temperature and plays a crucial role in responding to low-temperature stimuli. It acts as the main sensor for abiotic stress signals, allowing plant cells to detect changes in cell membrane fluidity and protein conformation caused by low-temperature stress. Cell membrane fluidity, regulated by lipid composition and distribution, is a key factor in plant adaptation to temperature changes (Li et al., 2016). Prolonged exposure to cold temperatures can alter the lipid composition of plant cell membranes, causing a transition from a flexible, liquid crystal phase to a more rigid, solid gel phase. This decrease in membrane fluidity can impair its functionality and disrupt intracellular metabolism. Additionally, membrane and membrane-binding enzyme activities may be affected (Sanchez et al., 2019). During freezing stress, when the temperature drops below 0°C, ice crystals can form in plant tissues, potentially leading to plant death. The obstruction of the cell membrane prevents intracellular fluid from freezing while extracellular water molecules form ice crystals. This rapid increase in osmotic pressure of the extracellular fluid can damage the cell membrane and denature its proteins, ultimately resulting in the loss of cellular integrity (Wang et al., 2023d). Low-temperature stress induced by freezing or cold temperatures also directly affects membrane integrity, molecular transport, cell signaling, and respiratory functions during seed germination (Hassan et al., 2021). Exposure to cold stress can increase membrane permeability, causing the leakage of essential electrolytes and cellular substances, such as amino acids, carbohydrates, unsaturated fatty acids, and metabolic compounds. This disturbance in ion balance inside and outside the cell can cause cell damage or death. Studies have shown that a higher unsaturated fatty acid content in the membrane of A. hypogaea corresponds to a lower membrane lipid phase transition temperature and stronger cold resistance (Sui et al., 2018). Arabidopsis thaliana exhibited a significant reduction in membrane lipid composition and severe membrane structure damage under cold stress (Kenchanmane Raju et al., 2018). Membrane stability is crucial for the survival of plant seedlings under low-temperature stress, and cold acclimation is closely associated with dynamic changes in lipid composition (Zheng et al., 2011; Degenkolbe et al., 2012). Exposing plants to low-temperature conditions can lead to bursts of ROS, which can induce membrane lipid peroxidation and increase levels of malondialdehyde (MDA). MDA can react with proteins and nucleic acids, disabling their function (Morales and Munné-Bosch, 2019). This process significantly increases membrane permeability, causing electrolyte leakage and irreparable cell damage. Therefore, MDA levels are used as an evaluation marker for lipid peroxidation, reflecting the extent of cell membrane damage and plant stress resistance (Morales and Munné-Bosch, 2019).
3.2 Influence of low temperature on fatty acid content
Lipids are essential components of biomembranes and play crucial roles in signal transduction and stress response (Suh et al., 2022). Moreover, they serve as fundamental building blocks for cell membranes, cuticle proteins, and wax constituents, forming a structural barrier against environmental stress (Upchurch, 2008). The activity of alpha-linolenic acid lipase contributes to the restoration of membrane fluidity (Grechkin, 1998), potentially regulating plant defense gene expression against cold stress and enhancing plant resilience (Kachroo et al., 2001). Fatty acids and their derivatives play crucial roles in various biological processes, including growth, development, and cellular metabolism in plants and related tissues (Lee et al., 2003). During the reproductive development of male flowers, fatty acids play a vital role in forming the anther cuticle and pollen wall, ensuring fertility (Wan et al., 2020). The plant cell membrane, consisting of a phospholipid bilayer in a liquid crystal phase, undergoes a temperature-dependent transformation of glycerol ester, closely related to the degree of fatty acid unsaturation. Cold-resistant plants have higher levels of unsaturated membrane lipids, resulting in a lower transition temperature compared to cold-sensitive plants(Lyons, 1973). Low temperatures significantly reduce membrane lipid fluidity and contraction, immobilizing membrane proteins and altering the controlled entry/exit of substances, which can induce plant metabolic disorders (Sevillano et al., 2009). The fluidity and stability of the cell membrane are closely associated with plant cold resistance. Generally, plants with greater membrane fluidity and stability exhibit higher cold tolerance (Wu et al., 2022). The presence of unsaturated fatty acids helps maintain the fluidity of the cell membrane in plants (Miquel et al., 1993; Upchurch, 2008). Plants primarily regulate fatty acid desaturase gene activity to influence the composition of membrane fatty acids. Lipid desaturation is a common stress response aimed at maintaining membrane fluidity under low temperature stress (Kenchanmane Raju et al., 2018). Correlation studies have shown that cold-tolerant varieties of Medicago sativa have higher total and unsaturated fatty acid contents than sensitive varieties under low temperature stress (Tian et al., 2022). Furthermore, S. tuberosum acclimated to cold storage stores linoleic acid in leaf tissue membrane glycerides (18:2) during cold stress (Vega et al., 2004). Hexadecanetrienoic acid (16:3) and linolenic acid (18:3) are important components of membrane lipids. Increasing the TA content in chloroplast membranes enhances plant tolerance to cold during early growth stages (Iba, 2002). Moreover, low-temperature cold stress treatment significantly increases galactosyl diglyceride (DGDG) content in cotyledon lipids of Phaseolus vulgaris varieties, enhancing cold resistance (Wang et al., 2020b). Lipidomic studies of Sorghum bicolor after cold stress revealed increased conservative and cooling-induced lipid unsaturation, increased phosphatidylcholine unsaturation during cooling, and higher galactoid unsaturation (Marla et al., 2017). Investigations into the effect of low temperature on fatty acid unsaturation in Lagenaria siceraria and cucumber roots measured membrane fluidity using the double bond index (DBI) and showed that excessive accumulation of linolenic acid may trigger a hyperbolic reaction. Additionally, cold stress upregulates lipoxygenase (LOX) activity in Ficus carica leaves and gourd roots. The level of unsaturated lipids in the plasma membranes of the root is closely associated with a plant’s cold tolerance capacity (Lee et al., 2005b). Linolenic acid serves as a precursor for synthesizing JA, which is formed after oxidation. Methyl jasmonate (MeJA) reduces oxidation and damage to plants during storage at low temperatures, thus promoting economic benefits (González-Aguilar et al., 2000; Ding et al., 2002; Gong et al., 2022). MeJA affects the expression of genes related to cold tolerance, protecting plants from low-temperature stress (Laura et al., 2018).
3.3 Influence of low temperature on organic osmolytes
Plants use two main mechanisms to accumulate organic osmolytes: uptake of inorganic ions from the external environment and synthesis of organic solutes within the cell. Osmotic regulation is crucial for plant protection against stress and relies on intracellular biosynthesis and absorption of specific substances. During abiotic stress responses, active solute accumulation and increased concentration of cell-soluble apoplastic fluid help maintain osmotic pressure, prevent excessive water loss, and protect against cell protoplast death, thereby enhancing plant stress resistance. The accumulation of soluble sugars (such as glucose, sucrose, fructose, and galactose) and soluble proteins is positively associated with plant cold resistance. These compounds help maintain osmotic potential, enhance water retention capacity, reduce the freezing point of cell fluids, alleviate damage to membrane structures under low-temperature stress, and prevent plant death. Free proline plays a role in maintaining the osmotic pressure balance between protoplasts and the surrounding environment. It promotes protein hydration after low-temperature stress, protects the spatial structure of enzymes, and improves plant resistance to low temperatures, thereby reducing damage (Fedotova and Dmitrieva, 2016). Proline promotes protein structural stability and membrane integrity through hydrogen bond interactions (Trovato et al., 2019). Studies have demonstrated that the contents of soluble sugars, proteins, and proline correlate with cold tolerance in plants (Li et al., 2012). A. hypogaea exhibits increased accumulation of organic osmolytes under cold stress, and cultivars with strong resistance to cold can accumulate higher levels of organic osmolytes to resist stress (Kavi Kishor and Sreenivasulu, 2014). Measuring proline and soluble sugar concentrations in O. sativa plants overexpressing OsLTPL159IL112 after low temperature treatment showed that this overexpression improved the cold tolerance of early O. sativa seedlings by promoting cellulose deposition and enhanced the accumulation of organic osmolytes (Zhao et al., 2020). On the other hand, disruption of the proline-rich protein encoded by OsPRP1 in O. sativa increased the plant’s sensitivity to low temperatures at the seedling stage (Nawaz et al., 2019). Proline levels are positively associated with plant cold tolerance and can indicate cold tolerance and low-temperature stress (Ghosh et al., 2022). Long-term low-temperature stress increased the osmotic pressure of maize leaves and reduced cell damage (Guo et al., 2022). The content of betaine can also serve as an index to evaluate the plant’s tolerance to cold. Betaine improves resistance to low temperature in plants under osmotic stress, maintains the stability of the structure and function of biological macromolecules (Huang et al., 2000), promotes seed germination (Park et al., 2004), enhances plant antioxidant capacity (Wang et al., 2021a), and plays a vital role in maintaining normal respiration and photosynthesis.
3.4 Influence of low temperature on photosynthesis
Photosynthesis, the fundamental process for crop and energy plant production, is highly sensitive to changes in environmental temperature (Chen et al., 2015). Research has shown that 90%–95% of a plant’s dry weight is derived from photosynthesis products, making them highly vulnerable to low-temperature stress (Chassot et al., 2001; Bilska and Sowinski, 2010). Many aspects of photosynthesis are affected by low temperature, including chloroplast structure, transpiration rate (Tr), photosynthetic pigment content, net photosynthetic efficiency (A), stomatal conductance (Gs), photochemical reactions, and the transport and distribution of photosynthetic products (Zhang et al., 2022a). For example, low-temperature treatment reduces the dry matter content of A. hypogaea seedlings, leads to a decrease in Gs and the transpiration rate of leaves, and causes a decrease in the photosynthetic rate (Pn) due to the interplay between stomatal and nonstomatal factors (Liu et al., 2013). Chloroplasts, which are one of the earliest organelles to sense low temperatures, play a vital role in plant growth and development (Liu et al., 2018). Low temperatures result in the destruction of organelle ultrastructure and affect the concentration of metabolites in chloroplasts (Ambavaram et al., 2014). Exposure to cold stress alters chloroplast membrane states and enzyme activities, leading to a decrease in photosynthetic effectiveness and the accumulation of excessive reactive oxygen species (ROS) and aberrant thylakoid morphology. Enzymatic reactions may slow down, and the high levels of ROS can damage membrane structures (Gan et al., 2019). These factors can lead to chloroplast dysfunction and degradation, ultimately affecting the activity of the light system (Liu et al., 2018). Under low-temperature stress, C3 plants, such as chrysanthemum, exhibit reduced maximum PSII photochemical efficiency (Fv/Fm), PSII quantum yield, light energy capture efficiency, and apparent quantum efficiency, which inhibits photosynthesis (Ozturk et al., 2013). Wheat leaves subjected to low temperatures also experience a decrease in the photosynthetic rate, stomatal conductance, and transpiration rate, which impacts wheat’s photosynthetic activity and leads to decreased yield (Zhang et al., 2022a). Low temperature diminishes the transfer of light energy and the conversion efficiency of PSII on the thylakoid membrane, reducing the capacity to absorb CO2 and the electron transport ability, ultimately affecting photosynthesis and the formation and transport of photosynthetic products (Paredes and Quiles, 2015). Additionally, following low-temperature treatment, genes related to photosynthetic pathways are downregulated in B. napus, inhibiting the photosynthetic system, reducing the maximum quantum efficiency of Fv/Fm, and affecting light response and the Calvin cycle (Hussain et al., 2022). In conclusion, low temperatures can impact the efficiency of light energy use in plants, leading to a decrease in enzyme activity and ultimately affecting plant photosynthesis.
3.5 Influence of low temperature on oxidative stress
Reactive oxygen species (ROS) are chemically reactive substances that contain oxygen, such as superoxide anion radical (O2•−), hydrogen peroxide (H2O2), hydroxyl radical (OH−), ozone (O3), and singlet oxygen (1O2) (Apel and Hirt, 2004). O2•− can be produced by NADPH oxidase (NOXs) or by the reduction in O2 by complexes I and III of the mitochondrial electron transport chain (ETC) (Murphy, 2009). Nicotinamide adenine dinucleotide phosphate (NADPH) produced through the pentose phosphate pathway is an important redox cofactor of the NOXs, which produce ROS (Sies and Jones, 2020). ROS produced by NADPH oxidase plays an important role in the plant’s participation in environmental response (Kawarazaki et al., 2013). Mitochondrial complexes III (CIII) and CI are necessary for ROS production (Fernández-Agüera et al., 2015). Studies have shown that Na can act as a second messenger to regulate the production of ROS by regulating the fluidity of the mitochondrial inner membrane (Hernansanz-Agustín et al., 2020). Excessive accumulation of ROS can be toxic, causing damage to carbohydrates, proteins, membranes, and genetic materials within plant cells. It inhibits normal plant growth, and development, and can even lead to plant death (Hossain et al., 2015; Jain and Gould, 2015). ROS production primarily occurs in chloroplasts and mitochondria through electron transport. Higher plants also produce ROS during metabolic processes such as fatty acid β-oxidation. Cold stress reduces the photosynthetic rate and affects photosynthetic electron transport, which affects ROS production (Bai et al., 2022). Living organisms position two antioxidant systems: enzymatic antioxidant systems and non-enzymatic antioxidant systems. Enzymatic antioxidant systems include peroxidase (POD), superoxide dismutase (SOD), catalase (CAT), ascorbate peroxidase (APX), and glutathione reductase (GR). Non-enzymatic antioxidant systems include flavonoids, phenols, carotenoids, vitamin C, vitamin E, glutathione (GSH), and ascorbic acid (ASA). Under normal temperature conditions, ROS production is kept at a low level in cell membranes, mitochondria, chloroplasts, and peroxisomes, with chloroplasts and mitochondria being the main sites of ROS production in plants (Neill et al., 2002). Studies on multiple plant species such as Oryza sativa (Guo et al., 2022), Z. mays (Zhao et al., 2022), Manihot esculenta Crantz (Liao et al., 2022), and Capsicum annuum (Wang et al., 2022) have shown that the activity of antioxidant enzymes is significantly enhanced under low temperature stress. In peanut seedlings, the activity of SOD and CAT shows a marked increase in response to cold stress, followed by an increase in the activity of POD before eventually decreasing. These antioxidant enzymes work together to maintain an appropriate level of active oxygen, which protects the plants against potential oxidative damage (Xue Yunyun et al., 2018). At low temperatures, full-length OsCYP20-2 in chloroplasts can improve the activity of superoxide dismutase OsFSD2, enhance the ability of cells to remove reactive oxygen species, and improve the cold tolerance of O. sativa (Ge et al., 2020a). In recent years, proteomic analysis of B. napus has shown that the molecular mechanism of the plant to enhance its tolerance to cold depends on the removal of ROS (Mi et al., 2021). It was reported that the activity of leaf antioxidant enzymes in plants under cold stress initially increased and then decreased over a certain period (Hongtao et al., 2017). Abscisic acid (ABA), an important plant hormone and growth regulator, has been found to improve cold tolerance by increasing the activity of antioxidant enzymes (Khorshidi and Nojavan, 2006). In the case of Triticum aestivum, treatment with ABA increases the activities of TaSOD, TaAPX, TaCAT, TaGR, TaDHAR, and TaMDHAR to enhance cold resistance (Yu et al., 2020). These findings highlight the importance of the redox system in plant response to low temperatures and the delicate balance between ROS production and scavenging for plant survival and adaptation to cold stress.
4 Molecular regulatory mechanism of plants’ response to low-temperature stress
4.1 Relations between plant hormones and cold resistance
Plant hormones are trace organic compounds produced by plants that play a crucial role in the regulation of their growth, development, and response to stress. These hormones include indole acetic acid (IAA), gibberellic acid (GA), cytokinin (CK), ABA, ethylene (ET), brassinosteroids (BR), JA, salicylic acid (SA), and strigolactones (SL). SA has been found to play a significant regulatory role in combating cold stress. A medium concentration of SA was applied to watermelon plants to induce cold tolerance by influencing the expression of the CBF and COR genes, improving the activity of antioxidant enzymes, and improving cold tolerance (Cheng et al., 2016). Combining SA and trisodium phosphate (TSP) improved fatty acid desaturation efficiency, improved water retention in C. annuum, and reduced membrane damage at low temperatures (Ge et al., 2020b). In Z. mays, O. sativa, and C. sativus leaves, treatment with 0.5 mM SA reduced cold-stress-induced electrolyte leakage and increased the activities of glutathione reductase and guaiacol peroxidase (G-POD) (Kang and Saltveit, 2002). ABA, which typically accumulates under cold stress, has been found to correlate with cold tolerance positively. The ABA content in cultivars with strong cold tolerance was significantly higher than in cold-sensitive cultivars (Huang et al., 2017; Agurla et al., 2018). The combination of low temperature and ABA induced the expression of CBF/DREB1 in Vitis vinifera seedlings and increased cold tolerance in dormant buds when ABA was applied to the leaves (Rubio et al., 2019). ABA enhances cold tolerance by increasing the activity of antioxidant enzymes and regulating stomatal opening and closing, thereby reducing CO2 fixation and inhibiting the accumulation of reactive oxygen species (Lim et al., 2015). ABA and GA are the main endogenous regulatory factors, and ABA and GA have antagonistic effects on seed germination (Miransari and Smith, 2014). ABA induces dormancy, and GA regulates dormancy and germination. Cold stress during seed development enhances ABA accumulation and decreases GA content (Kendall et al., 2011; He et al., 2014). Low temperature regulates seed germination by mediating ABA and GA metabolism and signaling, and the two hormones antagonistically promote seed coat and endosperm rupture (MacGregor et al., 2015). As a plant growth regulator (PGR) that releases ethylene, ethephon can delay the flowering of many plants, greatly reducing the chance of freezing damage (Pahwa and Ghai, 2015). Ethephon can delay flowering and prolong the dormant period of flower buds by increasing cooling and improving the cold tolerance of flower buds (Durner and Gianfagna, 1991). The study showed that the appropriate application of ethylene prolongs the cooling accumulation period of peach buds, improves cold resistance, and delays flowering (Liu et al., 2021b). Low-temperature treatment induced the release of ethylene and the expression of MdERF1B (encoding an ethylene signal activator) in M. domestica seedlings. Overexpression of MdERF1B significantly enhances the cold tolerance of M. domestica and A. thaliana seedlings (Wang et al., 2021b). Ethylene can positively regulate the cold tolerance of Poncirus trifoliata (Zhang et al., 2022b). A-type response regulator ZmRR1 in the CK signal transduction pathway is involved in the regulation of Z. mays cold tolerance, with natural variation in ZmRR1 leading to differences in cold tolerance among Z. mays inbred lines (Zeng et al., 2021). JA and its derivatives, collectively known as jasmonate (Jas), are important molecules in regulating plant responses to biotic and abiotic stresses (Ruan et al., 2019). JA positively regulates leaves senescence, CBF expression, and downstream cold response genes, increasing cold tolerance in O. sativa (Du et al., 2013; Hu et al., 2017). A B-box protein (BBX) MdBBX37 was identified as a positive regulator of M. domestica cold stress resistance mediated by JA, shedding light on its function in jasmonate-mediated cold resistance (An et al., 2021). MeJA treatment increases cold tolerance, activates genes related to JA synthesis, increases endogenous JA content, and significantly increases antioxidant-related substance content in C. annuum, alleviating cold stress damage (Seo et al., 2020). BR regulates plant tolerance to low-temperature stress. It enhances cold tolerance by accumulating dephosphorylated forms of BZR1 and BES1, induces the expression of CBF1 and CBF2, and positively regulates the cold tolerance of A. thaliana. BZR1 acts upstream of CBF1 and CBF2, directly regulating the expression of cold-tolerance-related genes (Li et al., 2017b). The phenylpropane group, including lignin, flavonoids, anthocyanins, and phenylpropane esters, is implicated in the response to cold stress. Upregulation of phenylpropanoid genes in A. thaliana seeds under cold stress is associated with a higher concentration of anthocyanins in the seed coat. Low temperatures can lead to cortical differences in tetrazolium, and low seed coat permeability or proanthocyanidin concentration can hinder seed dormancy after exposure to low temperatures (MacGregor et al., 2015). The early response genes of SL were identified in A. thaliana, and the molecular mechanism of SL anthocyanin accumulation and its regulation of plant growth and development was elucidated (Wang et al., 2020a). SL promotes the expression of the CBF gene and improves the cold tolerance of A. thaliana by promoting anthocyanin synthesis (Wang et al., 2023b). In summary, plant hormones such as SA, ABA, ethylene, CK, JA, BR, and SL play significant roles in the regulation of plant cold tolerance. Understanding the interactions between these hormones and their signaling pathways is crucial for enhancing plant cold resistance and improving crop productivity (Table 1).
4.2 Epigenetic mechanisms
Low-temperature stress has been shown to induce epigenetic changes in plants, such as DNA methylation, chromatin modification, histone modification, and microRNA (miRNA) regulation. These epigenetic modifications play a crucial role in plant response to cold stress. For example, miRNA-mediated gene expression has been shown to play a crucial role in the response of plants to cold stress (Megha et al., 2018). MiRNA, a small noncoding RNA, regulates physiological processes and can help alleviate the harmful effects of abiotic stress on plants. The overexpression of miR397a induced the expression of cold-regulated CBF genes and downstream COR genes, thus improving the cold tolerance of A. thaliana (Dong and Pei, 2014). Upregulation of miR393 under abiotic stress inhibits plant growth during stress, while cold stress leads to downregulation of miR2004 in A. thaliana (Sunkar and Zhu, 2004). Cold-responsive miRNAs in Z. mays can regulate the size of Z. mays leaves under cold stress by modulating developmental transcription factors and maintaining redox homeostasis in plant cells (Aydinoglu, 2020). In A. thaliana, the overexpression of miR402 can promote seed germination and enhance plant cold tolerance (Tiwari et al., 2020). OsmiR156 can enhance cold tolerance by targeting OsSPL3 to regulate the expression level of related transcription factor genes (Zhou and Tang, 2019). In O. sativa, OsmiR319 increases transcription levels of OsDREB1A by targeting OsPCF6 and OsTCP21, thereby clearing reactive oxygen species to improve plant cold tolerance (Wang et al., 2014). In general, only a few miRNAs may participate in plant cold tolerance through the CBF-COR pathway, laying a theoretical foundation for our study of the CBF-COR-independent pathway.
In A. thaliana, the highly expressed osmotic response protein HOS15 interacts with HD2C and binds to the promoter of COR genes, thus epigenetically regulating cold tolerance (Lim et al., 2020). Studies on O. sativa have shown that exposure to low temperatures can induce changes in the chromosome structure of the OsDREB1b promoter and upstream region. This can lead to histone H3 acetylation in the promoter regulatory region, altering the chromatin structure and impacting the cold tolerance of O. sativa (Roy et al., 2014). DNA methylation plays a crucial role in silencing endogenous gene transposons and regulating gene expression (Zhang et al., 2006). Cold stress can enhance the activity of transposons by altering the level of methylation of stress-responsive genes, leading to the induction of related cold resistance genes and enhanced cold tolerance. Significant changes in DNA methylation status have been observed in Solanum lycopersicum after exposure to low temperatures. Cold stress downregulates the expression of the plant DNA demethylase DML2, resulting in increased methylation levels in the promoter region. This leads to gene silencing, decreased flavor quality, and reduced volatile production (Zhang et al., 2016). Histone H3 trimethylation at lysine 27 (H3K27me3), a common chromatin modification, is pivotal in plant growth and stress resistance. V. vinifera leaves treated at 4°C exhibited changes in H3K27me3 modification. Genes targeted by H3K27me3 showed significantly reduced expression compared to non-targeted genes, indicating the suppressive effect of this modification on gene transcription across the entire genome. The methylation of relevant genomic proteins was detected through chip-seq binding transcriptome analysis, providing insights into the regulatory mechanism of H3K27me3 in response to cold stress (Zhu et al., 2023). The vernalization pathway plays an important role in the regulation of FLC. The histone demethylases JMJ30 and JMJ32 regulate the vernalization rate of A. thaliana by activating FLC (Maruoka et al., 2022). Therefore, understanding the molecular mechanisms of cold tolerance in plants, including epigenetic modifications and gene function, is a significant challenge that requires further research.
5 Summary and outlook of low-temperature resistance in plants
Temperature is a crucial environmental factor that significantly affects the growth of plants. Low temperatures can harm plants during winter, cause delays in sowing, and limit crop distribution. Therefore, it is imperative to enhance the cold resistance of plants and develop cold-resistant varieties for sustainable agricultural production in winter. Grafting is an alternative method to traditional breeding that can improve plants’ tolerance to environmental stress (Venema et al., 2008). Studying genes related to plant frost resistance is particularly significant because it allows us to use genetic engineering to enhance the frost resistance of non-hardy plants, with evident potential applications. Furthermore, using tolerant rootstocks during plant cultivation has been found to enhance resistance to low temperatures (Lee et al., 2010). However, there is still a lack of a comprehensive and efficient evaluation system for assessing cold tolerance in plants. Additionally, the molecular mechanism underlying the regulation of crop low-temperature and cold resistance regulatory proteins remains unclear.
Enhancing plant cold tolerance depends on various factors, including genes related to cold resistance, external environmental factors, and physiological mechanisms of cold resistance. Enriching germplasm resources for cold tolerance can be achieved by selecting varieties with excellent agronomic traits and quality and crossing them with varieties with strong cold resistance (Labroo et al., 2021). The determination of WCS120 protein content can also serve as a standard for selecting cold-resistant varieties (Vítámvás et al., 2010). The DHN5 protein, which is structurally similar to the WCS120 protein in wheat, can also be used as a marker for assessing frost tolerance (FT) in barley (Kosová et al., 2008; Kosová et al., 2013). The positive regulatory effect of CTB2 on cold tolerance in O. sativa has been confirmed through transgenic technology, contributing to the cultivation of cold-tolerant O. sativa germplasm resources (Li et al., 2021b). By closely examining QTLs associated with cold tolerance in O. sativa using genome-wide association studies (GWAS), researchers have conducted genetic analyses of cold tolerance-related genes, developed polymorphic molecular markers, and bred new cold-resistant varieties under cold stress conditions (Li et al., 2021a). Moreover, modern biotechnological advancements, including CRISPR/Cas9 technology, metabolomics, genomics, and proteomics, have facilitated genome sequencing in different cold-resistant plant varieties, enabling deeper investigations into the mechanisms of plant cold resistance. TALENs and CRISPR/Cas systems have revolutionized biological research, and their application to crop plants allows for targeting specific traits to improve crop productivity and ensure food security (Sedeek et al., 2019). Additionally, studies have shown that stimulating seeds with ROS nanoparticles (NPs) can enhance seed germination speed under stress conditions and improve seedling resistance to stress. Strategies based on this nanobiostimulant could promote sustainable agriculture by reducing the use of pesticides (Yan et al., 2023). This knowledge will facilitate the development of robust and resilient plant varieties that can withstand cold temperatures, ultimately addressing the issue of plant cold damage (Table 2).
Author contributions
YW: Writing – original draft, Writing – review & editing. JW: Writing – original draft, Writing – review & editing. RS: Writing – review & editing. WZ: Writing – review & editing. RG: Writing – review & editing. K-MZ: Writing – review & editing. X-LT: Writing – review & editing.
Funding
The author(s) declare financial support was received for the research, authorship, and/or publication of this article. This work was supported by the Jiangsu Agriculture Science and Technology Innovation Fund (CX (21) 2009) and Key R&D in the Jiangsu Province (BE2023342, BE2022340).
Conflict of interest
The authors declare that the research was conducted in the absence of any commercial or financial relationships that could be construed as a potential conflict of interest.
Publisher’s note
All claims expressed in this article are solely those of the authors and do not necessarily represent those of their affiliated organizations, or those of the publisher, the editors and the reviewers. Any product that may be evaluated in this article, or claim that may be made by its manufacturer, is not guaranteed or endorsed by the publisher.
Glossary
References
Agarwal, M., Hao, Y., Kapoor, A., Dong, C.-H., Fujii, H., Zheng, X., et al. (2006a). A R2R3 type MYB transcription factor is involved in the cold regulation of CBF genes and in acquired freezing tolerance*. J. Biol. Chem. 281, 37636–37645. doi: 10.1074/jbc.M605895200
Agarwal, M., Hao, Y., Kapoor, A., Dong, C. H., Fujii, H., Zheng, X., et al. (2006b). A R2R3 type MYB transcription factor is involved in the cold regulation of CBF genes and in acquired freezing tolerance. J. Biol. Chem. 281, 37636–37645. doi: 10.1074/jbc.M605895200
Agurla, S., Gahir, S., Munemasa, S., Murata, Y., Raghavendra, A. S. (2018). Mechanism of stomatal closure in plants exposed to drought and cold stress. Adv. Exp. Med. Biol. 1081, 215–232. doi: 10.1007/978-981-13-1244-1_12
Ambavaram, M. M., Basu, S., Krishnan, A., Ramegowda, V., Batlang, U., Rahman, L., et al. (2014). Coordinated regulation of photosynthesis in rice increases yield and tolerance to environmental stress. Nat. Commun. 5, 5302. doi: 10.1038/ncomms6302
An, J. P., Wang, X. F., Zhang, X. W., You, C. X., Hao, Y. J. (2021). Apple B-box protein BBX37 regulates jasmonic acid mediated cold tolerance through the JAZ-BBX37-ICE1-CBF pathway and undergoes MIEL1-mediated ubiquitination and degradation. New Phytol. 229, 2707–2729. doi: 10.1111/nph.17050
An, J. P., Xu, R. R., Liu, X., Su, L., Yang, K., Wang, X. F., et al. (2022). Abscisic acid insensitive 4 interacts with ICE1 and JAZ proteins to regulate ABA signaling-mediated cold tolerance in apple. J. Exp. Bot. 73, 980–997. doi: 10.1093/jxb/erab433
Apel, K., Hirt, H. (2004). Reactive oxygen species: Metabolism, oxidative stress, and signal transduction. Annu. Rev. Plant Biol. 55, 373–399. doi: 10.1146/annurev.arplant.55.031903.141701
Aslam, M., Fakher, B., Ashraf, M. A., Cheng, Y., Wang, B., Qin, Y. (2022). Plant low-temperature stress: signaling and response. Agronomy 12, 702. doi: 10.3390/agronomy12030702
Aydinoglu, F. (2020). Elucidating the regulatory roles of microRNAs in maize (Zea mays L.) leaf growth response to chilling stress. Planta 251(2), 38. doi: 10.1007/s00425-019-03331-y
Bai, M. X., Zeng, W. J., Chen, F. Q., Ji, X. Z., Zhuang, Z. L., Jin, B. B., et al. (2022). Transcriptome expression profiles reveal response mechanisms to drought and drought-stress mitigation mechanisms by exogenous glycine betaine in maize. Biotechnol. Lett. 44, 367–386. doi: 10.1007/s10529-022-03221-6
Bilska, A., Sowinski, P. (2010). Closure of plasmodesmata in maize (Zea mays) at low temperature: a new mechanism for inhibition of photosynthesis. Ann. Bot. 106, 675–686. doi: 10.1093/aob/mcq169
Browse, J., Xin, Z. (2001). Temperature sensing and cold acclimation. Curr. Opin. Plant Biol. 4, 241–246. doi: 10.1016/S1369-5266(00)00167-9
Chandrasekaran, U., Luo, X., Zhou, W., Shu, K. (2020). Multifaceted signaling networks mediated by abscisic acid insensitive 4. Plant Commun. 1, 100040. doi: 10.1016/j.xplc.2020.100040
Chassot, A., Stamp, P., Richner, W. (2001). Root distribution and morphology of maize seedlings as affected by tillage and fertilizer placement. Plant Soil 231, 123–135. doi: 10.1023/A:1010335229111
Chen, J., Tan, R. K., Guo, X. J., Fu, Z. L., Wang, Z., Zhang, Z. Y., et al. (2015). Transcriptome analysis comparison of lipid biosynthesis in the leaves and developing seeds of brassica napus. PloS One 10, e0126250. doi: 10.1371/journal.pone.0126250
Chen, T., Chen, J. H., Zhang, W., Yang, G., Yu, L. J., Li, D. M., et al. (2019). BYPASS1-LIKE, A DUF793 family protein, participates in freezing tolerance via the CBF pathway in arabidopsis. Front. Plant Sci. 10, 807. doi: 10.3389/fpls.2019.00807
Chen, T., Zhang, W., Yang, G., Chen, J. H., Chen, B. X., Sun, R., et al. (2020). TRANSTHYRETIN-LIKE and BYPASS1-LIKE co-regulate growth and cold tolerance in Arabidopsis. BMC Plant Biol. 20, 332. doi: 10.1186/s12870-020-02534-w
Cheng, F., Lu, J., Gao, M., Shi, K., Kong, Q., Huang, Y., et al. (2016). Redox signaling and CBF-responsive pathway are involved in salicylic acid-improved photosynthesis and growth under chilling stress in watermelon. Front. Plant Sci. 7, 1519. doi: 10.3389/fpls.2016.01519
Chinnusamy, V., Ohta, M., Kanrar, S., Lee, B. H., Hong, X., Agarwal, M., et al. (2003). ICE1: a regulator of cold-induced transcriptome and freezing tolerance in Arabidopsis. Genes Dev. 17, 1043–1054. doi: 10.1101/gad.1077503
Degenkolbe, T., Giavalisco, P., Zuther, E., Seiwert, B., Hincha, D. K., Willmitzer, L. (2012). Differential remodeling of the lipidome during cold acclimation in natural accessions of Arabidopsis thaliana. Plant J. 72, 972–982. doi: 10.1111/tpj.12007
Ding, Y., Jia, Y., Shi, Y., Zhang, X., Song, C., Gong, Z., et al. (2018). OST1-mediated BTF3L phosphorylation positively regulates CBFs during plant cold responses. EMBO J. 37 (8), e98228. doi: 10.15252/embj.201798228
Ding, Y., Li, H., Zhang, X., Xie, Q., Gong, Z., Yang, S. (2015). OST1 kinase modulates freezing tolerance by enhancing ICE1 stability in Arabidopsis. Dev. Cell 32, 278–289. doi: 10.1016/j.devcel.2014.12.023
Ding, Y., Shi, Y., Yang, S. (2019). Advances and challenges in uncovering cold tolerance regulatory mechanisms in plants. New Phytol. 222, 1690–1704. doi: 10.1111/nph.15696
Ding, F., Wang, R. (2018). Amelioration of postharvest chilling stress by trehalose in pepper. Scientia Hortic. 232, 52–56. doi: 10.1016/j.scienta.2017.12.053
Ding, C. K., Wang, C. Y., Gross, K. C., Smith, D. L. (2002). Jasmonate and salicylate induce the expression of pathogenesis-related-protein genes and increase resistance to chilling injury in tomato fruit. Planta 214, 895–901. doi: 10.1007/s00425-001-0698-9
Dodd, A. N., Kudla, J., Sanders, D. (2010). The language of calcium signaling. Annu. Rev. Plant Biol. 61, 593–620. doi: 10.1146/annurev-arplant-070109-104628
Dong, C.-H., Pei, H. (2014). Over-expression of miR397 improves plant tolerance to cold stress in Arabidopsis thaliana. J. Plant Biol. 57, 209–217. doi: 10.1007/s12374-013-0490-y
Dong, Y., Tang, M., Huang, Z., Song, J., Xu, J., Ahammed, G. J., et al. (2022). The miR164a-NAM3 module confers cold tolerance by inducing ethylene production in tomato. Plant J. 111, 440–456. doi: 10.1111/tpj.15807
Du, H., Liu, H., Xiong, L. (2013). Endogenous auxin and jasmonic acid levels are differentially modulated by abiotic stresses in rice. Front. Plant Sci. 4, 397. doi: 10.3389/fpls.2013.00397
Durner, E., Gianfagna, T. (1991). Ethephon prolongs dormancy and enhances supercooling in peach flower buds. J. Am. Soc. Hortic. Sci. 116 (3), 500–506. doi: 10.21273/JASHS.116.3.500
Eriksson, S., Eremina, N., Barth, A., Danielsson, J., Harryson, P. (2016). Membrane-induced folding of the plant stress dehydrin lti30. Plant Physiol. 171(2), 932–943. doi: 10.1104/pp.15.01531
Fedotova, M. V., Dmitrieva, O. A. (2016). Proline hydration at low temperatures: its role in the protection of cell from freeze-induced stress. Amino Acids 48(7), 1685–1694. doi: 10.1007/s00726-016-2232-1
Fei, J., Wang, Y.-S., Cheng, H., Su, Y.-B., Zhong, Y.-J., Zheng, L. (2022). The Kandelia obovata transcription factor KoWRKY40 enhances cold tolerance in transgenic Arabidopsis. BMC Plant Biol. 22, 274. doi: 10.1186/s12870-022-03661-2
Fernández-Agüera, M. C., Gao, L., González-Rodríguez, P., Pintado, C. O., Arias-Mayenco, I., García-Flores, P., et al. (2015). Oxygen sensing by arterial chemoreceptors depends on mitochondrial complex I signaling. Cell Metab. 22, 825–837. doi: 10.1016/j.cmet.2015.09.004
Gan, P., Liu, F., Li, R., Wang, S., Luo, J. (2019). Chloroplasts- beyond energy capture and carbon fixation: tuning of photosynthesis in response to chilling stress. Int. J. Mol. Sci. 20(20), 5046. doi: 10.3390/ijms20205046
Ge, Q., Zhang, Y., Xu, Y., Bai, M., Luo, W., Wang, B., et al. (2020a). Cyclophilin OsCYP20-2 with a novel variant integrates defense and cell elongation for chilling response in rice. New Phytol. 225, 2453–2467. doi: 10.1111/nph.16324
Ge, W., Zhao, Y., Kong, X., Sun, H., Luo, M., Yao, M., et al. (2020b). Combining salicylic acid and trisodium phosphate alleviates chilling injury in bell pepper (Capsicum annuum L.) through enhancing fatty-acid desaturation efficiency and water retention. Food Chem. 327, 127057. doi: 10.1016/j.foodchem.2020.127057
Ghosh, U. K., Islam, M. N., Siddiqui, M. N., Cao, X., Khan, M. A. R. (2022). Proline, a multifaceted signalling molecule in plant responses to abiotic stress: understanding the physiological mechanisms. Plant Biol. (Stuttgart, Germany) 24(2), 227–239. doi: 10.1111/plb.13363
Gilmour, S. J., Fowler, S. G., Thomashow, M. F. (2004). Arabidopsis transcriptional activators CBF1, CBF2, and CBF3 have matching functional activities. Plant Mol. Biol. 54, 767–781. doi: 10.1023/B:PLAN.0000040902.06881.d4
Gong, D., Bi, Y., Li, Y., Wang, Y., Prusky, D., Alkan, N. (2022). Preharvest elicitors spray improves antioxidant activity, alleviates chilling injury, and maintains quality in harvested fruit. Horticulturae 8, 1208. doi: 10.3390/horticulturae8121208
Gong, Z., Xiong, L., Shi, H., Yang, S., Herrera-Estrella, L. R., Xu, G., et al. (2020). Plant abiotic stress response and nutrient use efficiency. Sci. China Life Sci. 63, 635–674. doi: 10.1007/s11427-020-1683-x
González-Aguilar, G. A., Fortiz, J., Cruz, R., Baez, R., Wang, C. Y. (2000). Methyl jasmonate reduces chilling injury and maintains postharvest quality of mango fruit. J. Agric. Food Chem. 48, 515–519. doi: 10.1021/jf9902806
Grechkin, A. (1998). Recent developments in biochemistry of the plant lipoxygenase pathway. Prog. Lipid Res. 37, 317–352. doi: 10.1016/S0163-7827(98)00014-9
Guo, Z., Cai, L., Liu, C., Chen, Z., Guan, S., Ma, W., et al. (2022). Low-temperature stress affects reactive oxygen species, osmotic adjustment substances, and antioxidants in rice (Oryza sativa L.) at the reproductive stage. Sci. Rep. 12, 6224. doi: 10.1038/s41598-022-10420-8
Gupta, A., Marzinek, J. K., Jefferies, D., Bond, P. J., Harryson, P., Wohland, T. (2019). The disordered plant dehydrin Lti30 protects the membrane during water-related stress by cross-linking lipids. J. Biol. Chem. 294, 6468–6482. doi: 10.1074/jbc.RA118.007163
Hassan, M. A., Xiang, C., Farooq, M., Muhammad, N., Yan, Z., Hui, X., et al. (2021). Cold stress in wheat: plant acclimation responses and management strategies. Front. Plant Sci. 12, 676884. doi: 10.3389/fpls.2021.676884
He, H., De Souza Vidigal, D., Snoek, L. B., Schnabel, S., Nijveen, H., Hilhorst, H., et al. (2014). Interaction between parental environment and genotype affects plant and seed performance in Arabidopsis. J. Exp. Bot. 65, 6603–6615. doi: 10.1093/jxb/eru378
He, F., Li, H. G., Wang, J. J., Su, Y., Wang, H. L., Feng, C. H., et al. (2019). PeSTZ1, a C2H2-type zinc finger transcription factor from Populus euphratica, enhances freezing tolerance through modulation of ROS scavenging by directly regulating PeAPX2. Plant Biotechnol. J. 17, 2169–2183. doi: 10.1111/pbi.13130
He, Z., Zhao, T., Yin, Z., Liu, J., Cheng, Y., Xu, J. (2020). The phytochrome-interacting transcription factor CsPIF8 contributes to cold tolerance in citrus by regulating superoxide dismutase expression. Plant Sci. 298, 110584. doi: 10.1016/j.plantsci.2020.110584
Hernansanz-Agustín, P., Choya-Foces, C., Carregal-Romero, S., Ramos, E., Oliva, T., Villa-Piña, T., et al. (2020). Na(+) controls hypoxic signalling by the mitochondrial respiratory chain. Nature 586, 287–291. doi: 10.1038/s41586-020-2551-y
Hongtao, X., Tongtong, W., Dianfeng, Z., Lizhi, W., Yanjiang, F., Yu, L., et al. (2017). ABA pretreatment enhances the chilling tolerance of a chilling-sensitive rice cultivar. Braz. J. Bot. 40, 853–860. doi: 10.1007/s40415-017-0409-9
Hossain, M. A., Bhattacharjee, S., Armin, S. M., Qian, P., Xin, W., Li, H. Y., et al. (2015). Hydrogen peroxide priming modulates abiotic oxidative stress tolerance: insights from ROS detoxification and scavenging. Front. Plant Sci. 6, 420. doi: 10.3389/fpls.2015.00420
Hu, Y., Jiang, Y., Han, X., Wang, H., Pan, J., Yu, D. (2017). Jasmonate regulates leaf senescence and tolerance to cold stress: crosstalk with other phytohormones. J. Exp. Bot. 68, 1361–1369. doi: 10.1093/jxb/erx004
Huang, X., Cao, L., Fan, J., Ma, G., Chen, L. (2022). CdWRKY2-mediated sucrose biosynthesis and CBF-signalling pathways coordinately contribute to cold tolerance in Bermudagrass. Plant Biotechnol. J. 20, 660–675. doi: 10.1111/pbi.13745
Huang, J., Hirji, R., Adam, L., Rozwadowski, K. L., Hammerlindl, J. K., Keller, W. A., et al. (2000). Genetic engineering of glycinebetaine production toward enhancing stress tolerance in plants: metabolic limitations. Plant Physiol. 122, 747–756. doi: 10.1104/pp.122.3.747
Huang, X., Shi, H., Hu, Z., Liu, A., Amombo, E., Chen, L., et al. (2017). ABA is involved in regulation of cold stress response in Bermudagrass. Front. Plant Sci. 8, 1613. doi: 10.3389/fpls.2017.01613
Hussain, M. A., Luo, D., Zeng, L., Ding, X., Cheng, Y., Zou, X., et al. (2022). Genome-wide transcriptome profiling revealed biological macromolecules respond to low temperature stress in Brassica napus L. Front. Plant Sci. 13, 1050995. doi: 10.3389/fpls.2022.1050995
Hwarari, D., Guan, Y., Ahmad, B., Movahedi, A., Min, T., Hao, Z., et al. (2022). ICE-CBF-COR signaling cascade and its regulation in plants responding to cold stress. Int. J. Mol. Sci. 23 (3), 1549. doi: 10.3390/ijms23031549
Iba, K. (2002). Acclimative response to temperature stress in higher plants: Approaches of gene engineering for temperature tolerance. Annu. Rev. Plant Biol. 53, 225–245. doi: 10.1146/annurev.arplant.53.100201.160729
Iovane, M., Aronne, G. (2022). High temperatures during microsporogenesis fatally shorten pollen lifespan. Plant Reprod. 35, 9–17. doi: 10.1007/s00497-021-00425-0
Jain, G., Gould, K. S. (2015). Are betalain pigments the functional homologues of anthocyanins in plants? Environ. Exp. Bot. 119, 48–53. doi: 10.1016/j.envexpbot.2015.06.002
Jiang, B., Shi, Y., Zhang, X., Xin, X., Qi, L., Guo, H., et al. (2017). PIF3 is a negative regulator of the CBF pathway and freezing tolerance in Arabidopsis. Proc. Natl. Acad. Sci. U.S.A. 114, E6695–e6702. doi: 10.1073/pnas.1706226114
Jiang, Z., Zhou, X., Tao, M., Yuan, F., Liu, L., Wu, F., et al. (2019). Plant cell-surface GIPC sphingolipids sense salt to trigger Ca(2+) influx. Nature 572, 341–346. doi: 10.1038/s41586-019-1449-z
Jiao, S.-Z., Guo, C., Yao, W.-K., Zhang, N.-B., Zhang, J.-Y., Xu, W.-R. (2022). An Amur grape VaHsfC1 is involved in multiple abiotic stresses. Scientia Hortic. 295, 110785. doi: 10.1016/j.scienta.2021.110785
Jin, J., Sun, W., Wu, J., Fang, Y., Li, X., Ma, L., et al. (2022). Hypocotyl elongation based on HY5 transcription factor in cold resistant winter rapeseed (Brassica napus L.). Oil Crop Sci. 7, 40–52. doi: 10.1016/j.ocsci.2022.02.005
Kachroo, P., Shanklin, J., Shah, J., Whittle, E. J., Klessig, D. F. (2001). A fatty acid desaturase modulates the activation of defense signaling pathways in plants. Proc. Natl. Acad. Sci. U. S. A. 98 (16), 9448–9453. doi: 10.1073/pnas.151258398
Kang, H. M., Saltveit, M. E. (2002). Chilling tolerance of maize, cucumber and rice seedling leaves and roots are differentially affected by salicylic acid. Physiol. Plant 115, 571–576. doi: 10.1034/j.1399-3054.2002.1150411.x
Kavi Kishor, P. B., Sreenivasulu, N. (2014). Is proline accumulation per se correlated with stress tolerance or is proline homeostasis a more critical issue? Plant Cell Environ. 37, 300–311. doi: 10.1111/pce.12157
Kawarazaki, T., Kimura, S., Iizuka, A., Hanamata, S., Nibori, H., Michikawa, M., et al. (2013). A low temperature-inducible protein AtSRC2 enhances the ROS-producing activity of NADPH oxidase AtRbohF. Biochim. Biophys. Acta (BBA) - Mol. Cell Res. 1833 (12), 2775–2780. doi: 10.1016/j.bbamcr.2013.06.024
Kenchanmane Raju, S. K., Barnes, A. C., Schnable, J. C., Roston, R. L. (2018). Low-temperature tolerance in land plants: Are transcript and membrane responses conserved? Plant Sci. 276, 73–86. doi: 10.1016/j.plantsci.2018.08.002
Kendall, S. L., Hellwege, A., Marriot, P., Whalley, C., Graham, I. A., Penfield, S. (2011). Induction of dormancy in arabidopsis summer annuals requires parallel regulation of DOG1 and hormone metabolism by low temperature and CBF transcription factors. Plant Cell 23, 2568–2580. doi: 10.1105/tpc.111.087643
Khorshidi, M., Nojavan, A. M. (2006). The effects of abscisic acid and CaCl2 on the activities of antioxidant enzymes under cold stress in maize seedlings in the dark. Pakistan J. Biol. Sci. 9, 54–59. doi: 10.3923/pjbs.2006.54.59
Kleist, T. J., Luan, S. (2016). Constant change: dynamic regulation of membrane transport by calcium signalling networks keeps plants in tune with their environment. Plant Cell Environ. 39, 467–481. doi: 10.1111/pce.12599
Kong, X. M., Wei, B. D., Gao, Z., Zhou, Y., Shi, F., Zhou, X., et al. (2018). Changes in membrane lipid composition and function accompanying chilling injury in bell peppers. Plant Cell Physiol. 59, 167–178. doi: 10.1093/pcp/pcx171
Kosová, K., Holková, L., Prášil, I. T., Prášilová, P., Bradáčová, M., Vítámvás, P., et al. (2008). Expression of dehydrin 5 during the development of frost tolerance in barley (Hordeum vulgare). J. Plant Physiol. 165, 1142–1151. doi: 10.1016/j.jplph.2007.10.009
Kosová, K., Vítámvás, P., Prášil, I. T. (2007). The role of dehydrins in plant response to cold. Biol. Plantarum 51, 601–617. doi: 10.1007/s10535-007-0133-6
Kosová, K., Vítámvás, P., Prášil, I. T. (2011). Expression of dehydrins in wheat and barley under different temperatures. Plant Sci. 180, 46–52. doi: 10.1016/j.plantsci.2010.07.003
Kosová, K., Vítámvás, P., Prášilová, P., Prášil, I. T. (2013). Accumulation of WCS120 and DHN5 proteins in differently frost-tolerant wheat and barley cultivars grown under a broad temperature scale. Biol. Plantarum 57, 105–112. doi: 10.1007/s10535-012-0237-5
Labroo, M. R., Studer, A. J., Rutkoski, J. E. (2021). Heterosis and hybrid crop breeding: A multidisciplinary review. Front. Genet. 12, 643761. doi: 10.3389/fgene.2021.643761
Laura, B., Silvia, P., Francesca, F., Benedetta, S., Carla, C. (2018). Epigenetic control of defense genes following MeJA-induced priming in rice (O. sativa). J. Plant Physiol. 228, 166–177. doi: 10.1016/j.jplph.2018.06.007
Lee, S. H., Ahn, S. J., Im, Y. J., Cho, K., Chung, G.-C., Cho, B.-H., et al. (2005b). Differential impact of low temperature on fatty acid unsaturation and lipoxygenase activity in figleaf gourd and cucumber roots. Biochem. Biophys. Res. Commun. 330, 1194–1198. doi: 10.1016/j.bbrc.2005.03.098
Lee, B.-H., Henderson, D. A., Zhu, J.-K. (2005a). The arabidopsis cold-responsive transcriptome and its regulation by ICE1. Plant Cell 17, 3155–3175. doi: 10.1105/tpc.105.035568
Lee, J. M., Kubota, C., Tsao, S.-J. J., Bie, Z., Echevarría, P. H., Morra, L., et al. (2010). Current status of vegetable grafting: Diffusion, grafting techniques, automation. Scientia Hortic. 127, 93–105. doi: 10.1016/j.scienta.2010.08.003
Lee, C. H., Olson, P., Evans, R. M. (2003). Minireview: Lipid metabolism, metabolic diseases, and peroxisome proliferator-activated receptors. Endocrinology 144, 2201–2207. doi: 10.1210/en.2003-0288
Lee, E. S., Park, J. H., Wi, S. D., Kang, C. H., Chi, Y. H., Chae, H. B., et al. (2021). Redox-dependent structural switch and CBF activation confer freezing tolerance in plants. Nat. Plants 7, 914–922. doi: 10.1038/s41477-021-00944-8
Li, C., Chen, S., Xu, W., Li, D.-S., Gu, X.-K., Zhu, X., et al. (2012). Effect of Low Temperature at Seedling Stage on Antioxidation Enzymes and Cytoplasmic Osmoticum of Leaves in Wheat Cultivar Yangmai 16: Effect of Low Temperature at Seedling Stage on Antioxidation Enzymes and Cytoplasmic Osmoticum of Leaves in Wheat Cultivar Yangmai 16. Acta Agronomica Sin. 37, 2293–2298. doi: 10.3724/SP.J.1006.2011.02293
Li, H., Ding, Y., Shi, Y., Zhang, X., Zhang, S., Gong, Z., et al. (2017a). MPK3- and MPK6-mediated ICE1 phosphorylation negatively regulates ICE1 stability and freezing tolerance in arabidopsis. Dev. Cell 43, 630–642.e634. doi: 10.1016/j.devcel.2017.09.025
Li, X., Liang, X., Li, W., Yao, A., Liu, W., Wang, Y., et al. (2022a). Isolation and Functional Analysis of MbCBF2, a Malus baccata (L.) Borkh CBF Transcription Factor Gene, with Functions in Tolerance to Cold and Salt Stress in Transgenic Arabidopsis thaliana. Int. J. Mol. Sci. 23, 9827. doi: 10.3390/ijms23179827
Li, C., Liu, J., Bian, J., Jin, T., Zou, B., Liu, S., et al. (2021a). Identification of cold tolerance QTLs at the bud burst stage in 211 rice landraces by GWAS. BMC Plant Biol. 21, 542. doi: 10.1186/s12870-021-03317-7
Li, X., Liu, C., Zhao, Z., Ma, D., Zhang, J., Yang, Y., et al. (2020). COR27 and COR28 are novel regulators of the COP1-HY5 regulatory hub and photomorphogenesis in arabidopsis. Plant Cell 32, 3139–3154. doi: 10.1105/tpc.20.00195
Li, X. L., Meng, D., Li, M. J., Zhou, J., Yang, Y. Z., Zhou, B. B., et al. (2023). Transcription factors MhDREB2A/MhZAT10 play a role in drought and cold stress response crosstalk in apple. Plant Physiol. 192 (3), 2203–2220. doi: 10.1093/plphys/kiad147
Li, Q., Shen, W., Zheng, Q., Fowler, D. B., Zou, J. (2016). Adjustments of lipid pathways in plant adaptation to temperature stress. Plant Signal Behav. 11, e1058461. doi: 10.1080/15592324.2015.1058461
Li, X., Yang, Q., Liao, X., Tian, Y., Zhang, F., Zhang, L., et al. (2022b). A natural antisense RNA improves chrysanthemum cold tolerance by regulating the transcription factor DgTCP1. Plant Physiol. 190, 605–620. doi: 10.1093/plphys/kiac267
Li, H., Ye, K., Shi, Y., Cheng, J., Zhang, X., Yang, S. (2017b). BZR1 positively regulates freezing tolerance via CBF-dependent and CBF-independent pathways in arabidopsis. Mol. Plant 10, 545–559. doi: 10.1016/j.molp.2017.01.004
Li, J., Zeng, Y., Pan, Y., Zhou, L., Zhang, Z., Guo, H., et al. (2021b). Stepwise selection of natural variations at CTB2 and CTB4a improves cold adaptation during domestication of japonica rice. New Phytol. 231, 1056–1072. doi: 10.1111/nph.17407
Liao, W., Cai, J., Xu, H., Wang, Y., Cao, Y., Ruan, M., et al. (2022). The transcription factor MebHLH18 in cassava functions in decreasing low temperature-induced leaf abscission to promote low-temperature tolerance. Front. Plant Sci. 13, 1101821. doi: 10.3389/fpls.2022.1101821
Lim, C. W., Baek, W., Jung, J., Kim, J. H., Lee, S. C. (2015). Function of ABA in stomatal defense against biotic and drought stresses. Int. J. Mol. Sci. 16, 15251–15270. doi: 10.3390/ijms160715251
Lim, C. J., Park, J., Shen, M., Park, H. J., Cheong, M. S., Park, K. S., et al. (2020). The histone-modifying complex PWR/HOS15/HD2C epigenetically regulates cold tolerance. Plant Physiol. 184, 1097–1111. doi: 10.1104/pp.20.00439
Liu, Q., Ding, Y., Shi, Y., Ma, L., Wang, Y., Song, C., et al. (2021c). The calcium transporter ANNEXIN1 mediates cold-induced calcium signaling and freezing tolerance in plants. EMBO J. 40, e104559. doi: 10.15252/embj.2020104559
Liu, Y.-F., Han, X.-R., Zhan, X.-M., Yang, J.-F., Wang, Y.-Z., Song, Q.-B., et al. (2013). Regulation of calcium on peanut photosynthesis under low night temperature stress. J. Integr. Agric. 12, 2172–2178. doi: 10.1016/S2095-3119(13)60411-6
Liu, J., Islam, M. T., Sapkota, S., Ravindran, P., Kumar, P. P., Artlip, T. S., et al. (2021b). Ethylene-mediated modulation of bud phenology, cold hardiness, and hormone biosynthesis in peach (Prunus persica). Plants (Basel) 10(7), 1266. doi: 10.3390/plants10071266
Liu, M., Yang, G., Zhou, W., Wang, X., Han, Q., Wang, J., et al. (2023). Transcriptomic Analysis Reveals CBF-Dependent and CBF-Independent Pathways under Low-Temperature Stress in Teak (Tectona grandis). Genes 14, 2098. doi: 10.3390/genes14112098
Liu, H., Zhang, Y., Lu, S., Chen, H., Wu, J., Zhu, X., et al. (2021a). HsfA1d promotes hypocotyl elongation under chilling via enhancing expression of ribosomal protein genes in Arabidopsis. New Phytol. 231, 646–660. doi: 10.1111/nph.17413
Liu, X., Zhou, Y., Xiao, J., Bao, F. (2018). Effects of chilling on the structure, function and development of chloroplasts. Front. Plant Sci. 9, 1715. doi: 10.3389/fpls.2018.01715
Lyons, J. M. (1973). Chilling injury in plants. Annu. Rev. Plant Physiol. 24, 445–466. doi: 10.1146/annurev.pp.24.060173.002305
Ma, Y., Dai, X., Xu, Y., Luo, W., Zheng, X., Zeng, D., et al. (2015). COLD1 confers chilling tolerance in rice. Cell 160, 1209–1221. doi: 10.1016/j.cell.2015.01.046
Ma, X., Gai, W.-X., Li, Y., Yu, Y.-N., Ali, M., Gong, Z.-H. (2021). The CBL-interacting protein kinase CaCIPK13 positively regulates defence mechanisms against cold stress in pepper. J. Exp. Bot. 73, 1655–1667. doi: 10.1093/jxb/erab505
MacGregor, D. R., Kendall, S. L., Florance, H., Fedi, F., Moore, K., Paszkiewicz, K., et al. (2015). Seed production temperature regulation of primary dormancy occurs through control of seed coat phenylpropanoid metabolism. New Phytol. 205, 642–652. doi: 10.1111/nph.13090
Marla, S. R., Shiva, S., Welti, R., Liu, S., Burke, J. J., Morris, G. P. (2017). Comparative transcriptome and lipidome analyses reveal molecular chilling responses in chilling-tolerant sorghums. Plant Genome 10 (3), 10.3835/plantgenome2017.03.0025. doi: 10.3835/plantgenome2017.03.0025
Maruoka, T., Gan, E. S., Otsuka, N., Shirakawa, M., Ito, T. (2022). Histone demethylases JMJ30 and JMJ32 modulate the speed of vernalization through the activation of FLOWERING LOCUS C in arabidopsis thaliana. Front. Plant Sci. 13, 837831. doi: 10.3389/fpls.2022.837831
Megha, S., Basu, U., Kav, N. N. V. (2018). Regulation of low temperature stress in plants by microRNAs. Plant Cell Environ. 41, 1–15. doi: 10.1111/pce.12956
Mei, C., Yang, J., Mei, Q., Jia, D., Yan, P., Feng, B., et al. (2023). MdNAC104 positively regulates apple cold tolerance via CBF-dependent and CBF-independent pathways. Plant Biotechnol. J. 21, 2057–2073. doi: 10.1111/pbi.14112
Mi, W., Liu, Z., Jin, J., Dong, X., Xu, C., Zou, Y., et al. (2021). Comparative proteomics analysis reveals the molecular mechanism of enhanced cold tolerance through ROS scavenging in winter rapeseed (Brassica napus L.). PloS One 16, e0243292. doi: 10.1371/journal.pone.0243292
Miquel, M., James, D., Jr., Dooner, H., Browse, J. (1993). Arabidopsis requires polyunsaturated lipids for low-temperature survival. Proc. Natl. Acad. Sci. U.S.A. 90, 6208–6212. doi: 10.1073/pnas.90.13.6208
Miransari, M., Smith, D. L. (2014). Plant hormones and seed germination. Environ. Exp. Bot. 99, 110–121. doi: 10.1016/j.envexpbot.2013.11.005
Morales, M., Munné-Bosch, S. (2019). Malondialdehyde: facts and artifacts. Plant Physiol. 180(3), 1246–1250. doi: 10.1104/pp.19.00405
Murphy, M. P. (2009). How mitochondria produce reactive oxygen species. Biochem. J. 417, 1–13. doi: 10.1042/BJ20081386
Nawaz, G., Han, Y., Usman, B., Liu, F., Qin, B., Li, R. (2019). Knockout of OsPRP1, a gene encoding proline-rich protein, confers enhanced cold sensitivity in rice (Oryza sativa L.) at the seedling stage. 3 Biotech. 9, 254. doi: 10.1007/s13205-019-1787-4
Nawaz, Z., Kakar, K. U., Saand, M. A., Shu, Q. Y. (2014). Cyclic nucleotide-gated ion channel gene family in rice, identification, characterization and experimental analysis of expression response to plant hormones, biotic and abiotic stresses. BMC Genomics 15, 853. doi: 10.1186/1471-2164-15-853
Neill, S., Desikan, R., Hancock, J. (2002). Hydrogen peroxide signalling. Curr. Opin. Plant Biol. 5, 388–395. doi: 10.1016/S1369-5266(02)00282-0
Ni, M., Tepperman, J. M., Quail, P. H. (1998). PIF3, a phytochrome-interacting factor necessary for normal photoinduced signal transduction, is a novel basic helix-loop-helix protein. Cell 95, 657–667. doi: 10.1016/S0092-8674(00)81636-0
Ormancey, M., Thuleau, P., Mazars, C., Cotelle, V. (2017). CDPKs and 14-3-3 proteins: emerging duo in signaling. Trends Plant Sci. 22, 263–272. doi: 10.1016/j.tplants.2016.11.007
Ozturk, I., Ottosen, C.-O., Ritz, C. (2013). The effect of temperature on photosynthetic induction under fluctuating light in Chrysanthemum morifolium. Acta Physiologiae Plantarum 35, 1179–1188. doi: 10.1007/s11738-012-1157-x
Pahwa, K., Ghai, N. (2015). Effect of ethylene on physiological and biochemical parameters in different crop plants-A review. J. Appl. Natural Sci. 7, 1064–1069. doi: 10.31018/jans.v7i2.732
Paredes, M., Quiles, M. J. (2015). The effects of cold stress on photosynthesis in hibiscus plants. PloS One 10, e0137472. doi: 10.1371/journal.pone.0137472
Park, E. J., Jeknić, Z., Sakamoto, A., Denoma, J., Yuwansiri, R., Murata, N., et al. (2004). Genetic engineering of glycinebetaine synthesis in tomato protects seeds, plants, and flowers from chilling damage. Plant J. 40, 474–487. doi: 10.1111/j.1365-313X.2004.02237.x
Peleg, Z., Blumwald, E. (2011). Hormone balance and abiotic stress tolerance in crop plants. Curr. Opin. Plant Biol. 14, 290–295. doi: 10.1016/j.pbi.2011.02.001
Qiu, Y.-M., Guo, J., Jiang, W.-Z., Ding, J.-H., Song, R.-F., Zhang, J.-L., et al. (2023). HbBIN2 Functions in Plant Cold Stress Resistance through Modulation of HbICE1 Transcriptional Activity and ROS Homeostasis in Hevea brasiliensis. Int. J. Mol. Sci. 24, 15778. doi: 10.3390/ijms242115778
Reddy, A. S., Ali, G. S., Celesnik, H., Day, I. S. (2011). Coping with stresses: roles of calcium- and calcium/calmodulin-regulated gene expression. Plant Cell 23, 2010–2032. doi: 10.1105/tpc.111.084988
Roy, D., Paul, A., Roy, A., Ghosh, R., Ganguly, P., Chaudhuri, S. (2014). Differential acetylation of histone H3 at the regulatory region of OsDREB1b promoter facilitates chromatin remodelling and transcription activation during cold stress. PloS One 9, e100343. doi: 10.1371/journal.pone.0100343
Ruan, J., Zhou, Y., Zhou, M., Yan, J., Khurshid, M., Weng, W., et al. (2019). Jasmonic acid signaling pathway in plants. Int. J. Mol. Sci. 20(10), 2479. doi: 10.3390/ijms20102479
Rubio, S., Noriega, X., Pérez, F. J. (2019). Abscisic acid (ABA) and low temperatures synergistically increase the expression of CBF/DREB1 transcription factors and cold-hardiness in grapevine dormant buds. Ann. Bot. 123, 681–689. doi: 10.1093/aob/mcy201
Sanchez, J., Mangat, P. K., Angeles-Shim, R. B. (2019). Weathering the cold: modifying membrane and storage fatty acid composition of seeds to improve cold germination ability in upland cotton (Gossypium hirsutum L.). Agronomy-Basel 9, 684. doi: 10.3390/agronomy9110684
Sanyal, S. K., Rao, S., Mishra, L. K., Sharma, M., Pandey, G. K. (2016). Plant stress responses mediated by CBL-CIPK phosphorylation network. Enzymes 40, 31–64. doi: 10.1016/bs.enz.2016.08.002
Sedeek, K. E. M., Mahas, A., Mahfouz, M. (2019). Plant genome engineering for targeted improvement of crop traits. Front. Plant Sci. 10, 114. doi: 10.3389/fpls.2019.00114
Seo, J., Yi, G., Lee, J. G., Choi, J. H., Lee, E. J. (2020). Seed browning in pepper (Capsicum annuum L.) fruit during cold storage is inhibited by methyl jasmonate or induced by methyl salicylate. Postharvest Biol. Technol. 166, 111210. doi: 10.1016/j.postharvbio.2020.111210
Sevillano, L., Sanchez-Ballesta, M. T., Romojaro, F., Flores, F. B. (2009). Physiological, hormonal and molecular mechanisms regulating chilling injury in horticultural species. Postharvest technologies applied to reduce its impact. J. Sci. Food Agric. 89, 555–573. doi: 10.1002/jsfa.3468
Sharma, S., Prasad, A., Sharma, N., Prasad, M. (2021). Role of ubiquitination enzymes in abiotic environmental interactions with plants. Int. J. Biol. Macromol 181, 494–507. doi: 10.1016/j.ijbiomac.2021.03.185
Sies, H., Jones, D. P. (2020). Reactive oxygen species (ROS) as pleiotropic physiological signalling agents. Nat. Rev. Mol. Cell Biol. 21, 363–383. doi: 10.1038/s41580-020-0230-3
Song, J., Lin, R., Tang, M., Wang, L., Fan, P., Xia, X., et al. (2023). SlMPK1- and SlMPK2-mediated SlBBX17 phosphorylation positively regulates CBF-dependent cold tolerance in tomato. New Phytol. 239, 1887–1902. doi: 10.1111/nph.19072
Song, J., Wu, H., He, F., Qu, J., Wang, Y., Li, C., et al. (2021). Citrus sinensis CBF1 functions in cold tolerance by modulating putrescine biosynthesis through regulation of arginine decarboxylase. Plant Cell Physiol. 63, 19–29. doi: 10.1093/pcp/pcab135
Stockinger, E. J., Gilmour, S. J., Thomashow, M. F. (1997). Arabidopsis thaliana CBF1 encodes an AP2 domain-containing transcriptional activator that binds to the C-repeat/DRE, a cis-acting DNA regulatory element that stimulates transcription in response to low temperature and water deficit. Proc. Natl. Acad. Sci. U. S. A. 94, 1035–1040. doi: 10.1073/pnas.94.3.1035
Suh, M. C., Uk Kim, H., Nakamura, Y. (2022). Plant lipids: trends and beyond. J. Exp. Bot. 73, 2715–2720. doi: 10.1093/jxb/erac125
Sui, N., Wang, Y., Liu, S. S., Yang, Z., Wang, F., Wan, S. B. (2018). Transcriptomic and physiological evidence for the relationship between unsaturated fatty acid and salt stress in peanut. Front. Plant Sci. 9, 7. doi: 10.3389/fpls.2018.00007
Sulaiman, Y., Knight, M., Kataky, R. (2012). Non-invasive monitoring of temperature stress in Arabidopsis thaliana roots, using ion amperometry. Anal. Methods 4, 1656–1661. doi: 10.1039/c2ay05747f
Sunkar, R., Zhu, J. K. (2004). Novel and stress-regulated microRNAs and other small RNAs from Arabidopsis. Plant Cell 16, 2001–2019. doi: 10.1105/tpc.104.022830
Teige, M., Scheikl, E., Eulgem, T., Dóczi, R., Ichimura, K., Shinozaki, K., et al. (2004). The MKK2 pathway mediates cold and salt stress signaling in Arabidopsis. Mol. Cell 15, 141–152. doi: 10.1016/j.molcel.2004.06.023
Thomashow, M. F., Torii, K. U. (2020). SCREAMing twist on the role of ICE1 in freezing tolerance[OPEN]. Plant Cell 32, 816–819. doi: 10.1105/tpc.20.00124
Tian, J., Tian, L., Chen, M., Chen, Y., Wei, A. (2022). Low temperature affects fatty acids profiling and key synthesis genes expression patterns in zanthoxylum bungeanum maxim. Int. J. Mol. Sci. 23, 2319. doi: 10.3390/ijms23042319
Tiwari, B., Habermann, K., Arif, M. A., Weil, H. L., Garcia-Molina, A., Kleine, T., et al. (2020). Identification of small RNAs during cold acclimation in Arabidopsis thaliana. BMC Plant Biol. 20, 298. doi: 10.1186/s12870-020-02511-3
Trovato, M., Forlani, G., Signorelli, S., Funck, D. (2019). Proline Metabolism and Its Functions in Development and Stress Tolerance. In: Hossain, M., Kumar, V., Burritt, D., Fujita, M., Mäkelä, P. (eds) Osmoprotectant-Mediated Abiotic Stress Tolerance in Plants. Springer, Cham. doi: 10.1007/978-3-030-27423-8_2
Upchurch, R. G. (2008). Fatty acid unsaturation, mobilization, and regulation in the response of plants to stress. Biotechnol. Lett. 30, 967–977. doi: 10.1007/s10529-008-9639-z
Vega, S. E., Del Rio, A. H., Bamberg, J. B., Palta, J. P. (2004). Evidence for the up-regulation of stearoyl-ACP (Δ9) desaturase gene expression during cold acclimation. Am. J. Potato Res. 81, 125–135. doi: 10.1007/BF02853610
Venema, J. H., Dijk, B. E., Bax, J. M., Van Hasselt, P. R., Elzenga, J. T. M. (2008). Grafting tomato (Solanum lycopersicum) onto the rootstock of a high-altitude accession of Solanum habrochaites improves suboptimal-temperature tolerance. Environ. Exp. Bot. 63, 359–367. doi: 10.1016/j.envexpbot.2007.12.015
Vítámvás, P., Kosová, K., Musilová, J., Holková, L., Mařík, P., Smutná, P., et al. (2019). Relationship between dehydrin accumulation and winter survival in winter wheat and barley grown in the field. Front. Plant Sci. 10, 7. doi: 10.3389/fpls.2019.00007
Vítámvás, P., Kosová, K., Prášilová, P., Prášil, I. T. (2010). Accumulation of WCS120 protein in wheat cultivars grown at 9°C or 17°C in relation to their winter survival. Plant Breed. 129, 611–616. doi: 10.1111/j.1439-0523.2010.01783.x
Wan, X. Y., Wu, S. W., Li, Z. W., An, X. L., Tian, Y. H. (2020). Lipid metabolism: critical roles in male fertility and other aspects of reproductive development in plants. Mol. Plant 13, 955–983. doi: 10.1016/j.molp.2020.05.009
Wang, Y., Chen, K., Yao, Q., Wang, W., Zhu, Z. (2008). The basic helix-loop-helix transcription factor family in the honey bee, Apis mellifera. J. Insect Sci. 8, 1–12. doi: 10.1673/031.008.4001
Wang, X., Ding, Y., Li, Z., Shi, Y., Wang, J., Hua, J., et al. (2019). PUB25 and PUB26 promote plant freezing tolerance by degrading the cold signaling negative regulator MYB15. Dev. Cell 51, 222–235.e225. doi: 10.1016/j.devcel.2019.08.008
Wang, Y., Gao, C., Wang, S., He, X. (2020b). Changes in photoinhibition and fatty acid composition in the thylakoid membrane of kidney bean leaves under low temperature and weak light stress. Acta Prataculturae Sin. 29, 116–125. doi: 10.11686/cyxb2020072
Wang, Y., Jiang, H., Mao, Z., Liu, W., Jiang, S., Xu, H., et al. (2021b). Ethylene increases the cold tolerance of apple via the MdERF1B-MdCIbHLH1 regulatory module. Plant J. 106, 379–393. doi: 10.1111/tpj.15170
Wang, X., Li, Z., Shi, Y., Liu, Z., Zhang, X., Gong, Z., et al. (2023b). Strigolactones promote plant freezing tolerance by releasing the WRKY41-mediated inhibition of CBF/DREB1 expression. EMBO J. 42(19), e112999. doi: 10.15252/embj.2022112999
Wang, Y., Samarina, L., Mallano, A. I., Tong, W., Xia, E. (2023d). Recent progress and perspectives on physiological and molecular mechanisms underlying cold tolerance of tea plants. Front. Plant Sci. 14, 1145609. doi: 10.3389/fpls.2023.1145609
Wang, S., Shen, Y., Deng, D., Guo, L., Zhang, Y., Nie, Y., et al. (2023a). Orthogroup and phylotranscriptomic analyses identify transcription factors involved in the plant cold response: A case study of Arabidopsis BBX29. Plant Commun. 4, 100684. doi: 10.1016/j.xplc.2023.100684
Wang, S. T., Sun, X. L., Hoshino, Y., Yu, Y., Jia, B., Sun, Z. W., et al. (2014). MicroRNA319 positively regulates cold tolerance by targeting OsPCF6 and OsTCP21 in rice (Oryza sativa L.). PloS One 9, e91357. doi: 10.1371/journal.pone.0091357
Wang, F., Sun, H., Rong, L., Li, Z., An, T., Hu, W., et al. (2021a). Genotypic-dependent alternation in D1 protein turnover and PSII repair cycle in psf mutant rice (Oryza sativa L.), as well as its relation to light-induced leaf senescence. Plant Growth Regul. 95, 121–136. doi: 10.1007/s10725-021-00730-8
Wang, L., Wang, B., Yu, H., Guo, H., Lin, T., Kou, L., et al. (2020a). Transcriptional regulation of strigolactone signalling in Arabidopsis. Nature 583, 277–281. doi: 10.1038/s41586-020-2382-x
Wang, Z., Zhang, Y., Hu, H., Chen, L., Zhang, H., Chen, R. (2022). CabHLH79 acts upstream of caNAC035 to regulate cold stress in pepper. Int. J. Mol. Sci. 23 (5), 2537. doi: 10.3390/ijms23052537
Wang, X., Zhang, X., Song, C., Gong, Z., Yang, S., Ding, Y. (2023c). PUB25 and PUB26 dynamically modulate ICE1 stability via differential ubiquitination during cold stress in Arabidopsis. Plant Cell. 35 (9), 3585–3603. doi: 10.1093/plcell/koad159
Wu, J., Nadeem, M., Galagedara, L., Thomas, R., Cheema, M. (2022). Recent insights into cell responses to cold stress in plants: Signaling, defence, and potential functions of phosphatidic acid. Environ. Exp. Bot. 203, 105068. doi: 10.1016/j.envexpbot.2022.105068
Xue Yunyun, B. D., Yuexia, T., Baoquan, Q. (2018). Cold tolerance identification for 24 peanut resources from Shanxi Province at the stage of germination and seedling. J. Nucl. Agric. Sci. 32, 582–590. doi: 10.11869/j.issn.100-8551.2018.03.0582
Yan, X., Chen, S., Pan, Z., Zhao, W., Rui, Y., Zhao, L. (2023). AgNPs-triggered seed metabolic and transcriptional reprogramming enhanced rice salt tolerance and blast resistance. ACS Nano 17, 492–504. doi: 10.1021/acsnano.2c09181
Yan, C. C., Zhang, N., Wang, Q. Q., Fu, Y. Y., Wang, F., Su, Y. B., et al. (2021). The effect of low temperature stress on the leaves and microRNA expression of potato seedlings. Front. Ecol. Evol. 9. doi: 10.3389/fevo.2021.727081
Yang, E. Y., Rajametov, S. N., Cho, M. C., Jeong, H. B., Chae, W. B. (2021). Factors affecting tolerance to low night temperature differ by fruit types in tomato. Agriculture-Basel 11, 681. doi: 10.3390/agriculture11070681
Yang, T., Shad Ali, G., Yang, L., Du, L., Reddy, A. S., Poovaiah, B. W. (2010). Calcium/calmodulin-regulated receptor-like kinase CRLK1 interacts with MEKK1 in plants. Plant Signal Behav. 5, 991–994. doi: 10.4161/psb.5.8.12225
Yang, X., Wang, R., Hu, Q., Li, S., Mao, X., Jing, H., et al. (2019). DlICE1, a stress-responsive gene from Dimocarpus longan, enhances cold tolerance in transgenic Arabidopsis. Plant Physiol. Biochem. 142, 490–499. doi: 10.1016/j.plaphy.2019.08.007
Yu, J., Cang, J., Lu, Q., Fan, B., Xu, Q., Li, W., et al. (2020). ABA enhanced cold tolerance of wheat 'dn1' via increasing ROS scavenging system. Plant Signal Behav. 15, 1780403. doi: 10.1080/15592324.2020.1780403
Yu, Q., Zheng, Q., Shen, W., Li, J., Yao, W., Xu, W. (2022). Grape CIPK18 acts as a positive regulator of CBF cold signaling pathway by modulating ROS homeostasis. Environ. Exp. Bot. 203, 105063. doi: 10.1016/j.envexpbot.2022.105063
Zeng, R., Li, Z., Shi, Y., Fu, D., Yin, P., Cheng, J., et al. (2021). Natural variation in a type-A response regulator confers maize chilling tolerance. Nat. Commun. 12, 4713. doi: 10.1038/s41467-021-25001-y
Zhang, X., Fu, X., Liu, F., Wang, Y., Bi, H., Ai, X. (2021). Hydrogen sulfide improves the cold stress resistance through the CsARF5-CsDREB3 module in cucumber. Int. J. Mol. Sci. 22 (24), 13229. doi: 10.3390/ijms222413229
Zhang, Z., Li, J., Li, F., Liu, H., Yang, W., Chong, K., et al. (2017). OsMAPK3 phosphorylates OsbHLH002/OsICE1 and inhibits its ubiquitination to activate OsTPP1 and enhances rice chilling tolerance. Dev. Cell 43, 731–743.e735. doi: 10.1016/j.devcel.2017.11.016
Zhang, Y., Liu, L., Chen, X., Li, J. (2022). Effects of low-temperature stress during the anther differentiation period on winter wheat photosynthetic performance and spike-setting characteristics. Plants (Basel) 11 (3), 389. doi: 10.3390/plants11030389
Zhang, Y., Ming, R., Khan, M., Wang, Y., Dahro, B., Xiao, W., et al. (2022b). ERF9 of Poncirus trifoliata (L.) Raf. undergoes feedback regulation by ethylene and modulates cold tolerance via regulating a glutathione S-transferase U17 gene. Plant Biotechnol. J. 20, 183–200. doi: 10.1111/pbi.13705
Zhang, B., Tieman, D. M., Jiao, C., Xu, Y., Chen, K., Fei, Z., et al. (2016). Chilling-induced tomato flavor loss is associated with altered volatile synthesis and transient changes in DNA methylation. Proc. Natl. Acad. Sci. U.S.A. 113, 12580–12585. doi: 10.1073/pnas.1613910113
Zhang, X., Yazaki, J., Sundaresan, A., Cokus, S., Chan, S. W., Chen, H., et al. (2006). Genome-wide high-resolution mapping and functional analysis of DNA methylation in arabidopsis. Cell 126, 1189–1201. doi: 10.1016/j.cell.2006.08.003
Zhao, J., Wang, S., Qin, J., Sun, C., Liu, F. (2020). The lipid transfer protein OsLTPL159 is involved in cold tolerance at the early seedling stage in rice. Plant Biotechnol. J. 18, 756–769. doi: 10.1111/pbi.13243
Zhao, X., Zhao, C., Niu, Y., Chao, W., He, W., Wang, Y., et al. (2022). Understanding and comprehensive evaluation of cold resistance in the seedlings of multiple maize genotypes. Plants (Basel) 11 (14), 1881. doi: 10.3390/plants11141881
Zheng, G. W., Tian, B., Zhang, F. J., Tao, F. Q., Li, W. Q. (2011). Plant adaptation to frequent alterations between high and low temperatures: remodelling of membrane lipids and maintenance of unsaturation levels. Plant Cell Environ. 34, 1431–1442. doi: 10.1111/j.1365-3040.2011.02341.x
Zhou, M., Tang, W. (2019). MicroRNA156 amplifies transcription factor-associated cold stress tolerance in plant cells. Mol. Genet. Genomics 294, 379–393. doi: 10.1007/s00438-018-1516-4
Zhu, J. K. (2016). Abiotic stress signaling and responses in plants. Cell 167, 313–324. doi: 10.1016/j.cell.2016.08.029
Zhu, Z., Li, Q., Gichuki, D. K., Hou, Y., Liu, Y., Zhou, H., et al. (2023). Genome-wide profiling of histone H3 lysine 27 trimethylation and its modification in response to chilling stress in grapevine leaves. Hortic. Plant J. 9, 496–508. doi: 10.1016/j.hpj.2023.03.002
Zhu, J., Wang, W., Jiang, M., Yang, L., Zhou, X. (2021). QTL mapping for low temperature germination in rapeseed. Sci. Rep. 11, 23382. doi: 10.1038/s41598-021-02912-w
Keywords: low-temperature stress, physiological and biochemical metabolism, signal transduction, hormones, cold resistance mechanism
Citation: Wang Y, Wang J, Sarwar R, Zhang W, Geng R, Zhu K-M and Tan X-L (2024) Research progress on the physiological response and molecular mechanism of cold response in plants. Front. Plant Sci. 15:1334913. doi: 10.3389/fpls.2024.1334913
Received: 08 November 2023; Accepted: 10 January 2024;
Published: 30 January 2024.
Edited by:
Tangchun Zheng, Beijing Forestry University, ChinaCopyright © 2024 Wang, Wang, Sarwar, Zhang, Geng, Zhu and Tan. This is an open-access article distributed under the terms of the Creative Commons Attribution License (CC BY). The use, distribution or reproduction in other forums is permitted, provided the original author(s) and the copyright owner(s) are credited and that the original publication in this journal is cited, in accordance with accepted academic practice. No use, distribution or reproduction is permitted which does not comply with these terms.
*Correspondence: Xiao-Li Tan, eGx0YW5AdWpzLmVkdS5jbg==
†These authors have contributed equally to this work