- 1School of Biology and Environmental Science, Queensland University of Technology (QUT), Brisbane, QLD, Australia
- 2Centre for Agriculture and the Bioeconomy, Queensland University of Technology (QUT), Brisbane, QLD, Australia
- 3School of Chemistry and Molecular Biosciences, The University of Queensland, Brisbane, QLD, Australia
- 4Hawkesbury Institute for the Environment, Western Sydney University, Penrith, NSW, Australia
- 5Queensland Alliance for Agriculture and Food Innovation, The University of Queensland, Brisbane, QLD, Australia
- 6School of Agriculture and Food Sustainability, The University of Queensland, Brisbane, QLD, Australia
- 7The New Zealand Institute for Plant and Food Research Limited, Auckland, New Zealand
The challenges facing tree orchard production in the coming years will be largely driven by changes in the climate affecting the sustainability of farming practices in specific geographical regions. Identifying key traits that enable tree crops to modify their growth to varying environmental conditions and taking advantage of new crop improvement opportunities and technologies will ensure the tree crop industry remains viable and profitable into the future. In this review article we 1) outline climate and sustainability challenges relevant to horticultural tree crop industries, 2) describe key tree crop traits targeted for improvement in agroecosystem productivity and resilience to environmental change, and 3) discuss existing and emerging genomic technologies that provide opportunities for industries to future proof the next generation of orchards.
Introduction
Climate change is one of the major challenges of the 21st century. The Intergovernmental Panel on Climate Change (IPCC, 2023) predicts an increase in average global temperatures between 1.4 and 4.4°C by the end of this century. Increased global temperatures are predicted to have vast flow-on effects on global climate and local weather systems leading to more extreme and unpredictable temperatures, as well as increased extreme rainfall, drought, storm and fire events (IPCC, 2023). Many of these impacts are already being experienced around the world, with NASA declaring July 2023 the hottest month on record fuelling heat waves, wildfires, and floods across the Northern Hemisphere. These changes will affect every tree crop industry and region to varying degrees. Temperate tree crops are at particular risk due to their high reliance on seasonal variations in temperature for processes such as bud dormancy and flowering (Campoy et al., 2011), and pollen development and fruit set (Irenaeus and Mitra, 2014), which all in turn impact yield. Subtropical tree crops are also not immune to the effects of climate change with many reliant on cold winter temperatures to induce flowering (Wilkie et al., 2008), and temperature also significantly impacting pollen and fruit development (Dinesh and Reddy, 2012).
Another challenge facing humanity this century is increasing population size and demand for food. Taagepera and Nemčok (2023) predict the global population to increase to a peak of 11.2 ± 1.5 billion by the end of this century. Population increases during the 20th century were offset by the Green Revolution, a doubling in production of high-yielding varieties of several cereal crops (Khush, 1999). However, population growth since the 1990s has outstripped the rate of growth in food production requiring new strategies to increase cereal crop yield. Tree crops will require a more substantial increase in yield and reliability of yield as the Green Revolution gains in cereal crop productivity have not been implemented in tree crops. Adding to this challenge, the effects of climate change on yield are also uncertain, with changes in temperature, rainfall patterns and CO2 levels likely to significantly influence crop productivity (Aydinalp and Cresser, 2008).
One innovative change that is being implemented in some tree crop industries, e.g. olives (Olea europaea) in Europe (Lo Bianco et al., 2021), is the transition to higher density orchard plantings. This transition requires the use of small trees with low shoot vigour and, ideally, precocious flowering (i.e., flowering at a younger age). This is often achieved through orchard management but can also be achieved using varieties and/or rootstocks that confer these traits. However, some tree crops like avocado (Persea americana) and macadamia (Macadamia integrifolia, Macadamia tetraphylla, and hybrids) lack available varieties or rootstocks with these traits, and many horticultural industries have not made substantive use of available varieties and/or rootstocks. Planting at higher densities gives the potential for higher yield per land as seen in olive orchards (Sobreiro et al., 2023), however, this may not be applicable to all tree crops (Haque and Sakimin, 2022). The benefits of higher density plantings are increased if trees flower and produce high yield at a younger age as this improves the return on investment and allows for faster turn-around of plantings and quicker introduction of newer, improved varieties. Another advantage of higher density plantings is that they are more amenable to implementation of automation/robotics for crop management and harvest. This is especially pertinent in countries with high wages and labour scarcity (Rutledge and Taylor, 2023) that often cannot compete on a cost level with countries with much lower wages. The use of automation can dramatically reduce the ongoing costs associated with crop management and harvest to make these industries remain competitive on an international standing.
Although responding to these challenges will require a multi-pronged approach, it is imperative that genomic technologies are harnessed to complement traditional breeding efforts to enhance and accelerate the process. The advent of low-cost genome sequencing and associated increase in high quality genome assemblies and ability to re-sequence large-scale germplasm and hybrid populations (Okemo et al., 2022) has dramatically advanced the implementation of genomic approaches into breeding programs. In particular, high-throughput sequencing enables the use of genome-wide association studies (GWAS) and marker-assisted selection (MAS) for high-throughput assessment of breeding populations to identify preferred parental crosses and filter progeny for traits of interest. However, as many tree crops are highly heterozygous outcrossers (Miller and Gross, 2011), traditional crossing and selection remains challenging as many desirable traits can be lost during crossing. The ability to genetically manipulate crops for trait improvement using newer gene editing technologies such as CRISPR holds the promise of genetically engineering traits without the need for genetically modified (GM) cultivars (Hu and Gao, 2023). These technologies allow for the genetic manipulation of individual genes while maintaining other desirable traits, although, as we will discuss later, many challenges remain. And while these approaches have been extensively used in many cereal crops, their use in most tree crops has been relatively unexplored and underutilised to date.
In this review we describe several key traits and genetic targets (Figure 1) that are a focus for genetic improvement in tree crops in response to the challenges raised above. First, we explore the intensification of crop density through the modification of different aspects of tree architecture, including branching number, branching angle, and tree height. Next, we discuss bud dormancy in deciduous trees and how changes in temperature due to climate change may affect this important biological process. Following this we consider early, or precocious, flowering to accelerate tree crop breeding. And finally, we explore alternate bearing, a phenomenon experienced in many tree crops that influences yield predictability. While these traits are key targets for genetic improvement, there are many other important traits that are being targeted for improvement by breeding programs (see examples in Figure 2). In this review we also discuss existing and emerging genomic technologies that can be utilised for accelerating genetic improvement in tree crops. These rapid and precise genome-enabled technologies first require an understanding of the genetic basis of important traits in tree crops to inform marker assisted and genomic selection models or genetic manipulation of the tree crops through transgenics or gene editing.
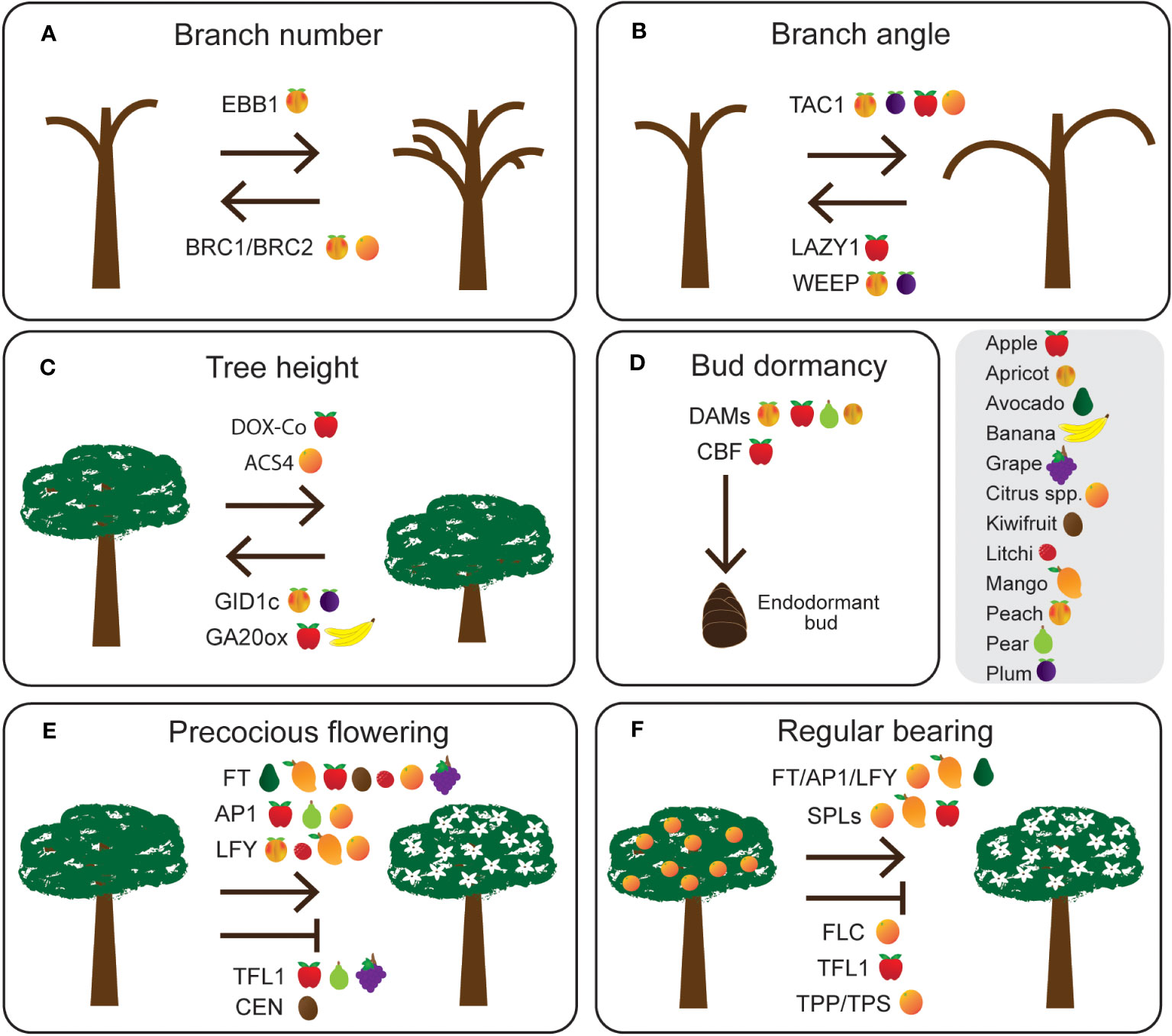
Figure 1 Potential gene targets controlling agronomically important traits such as (A) branch number, (B) branch angle, (C) tree height, (D) bud dormancy, (E) precocious flowering, and (F) regular bearing in tree crops. Species (grey box) with evidence of gene function or gene expression are indicated after each gene name.
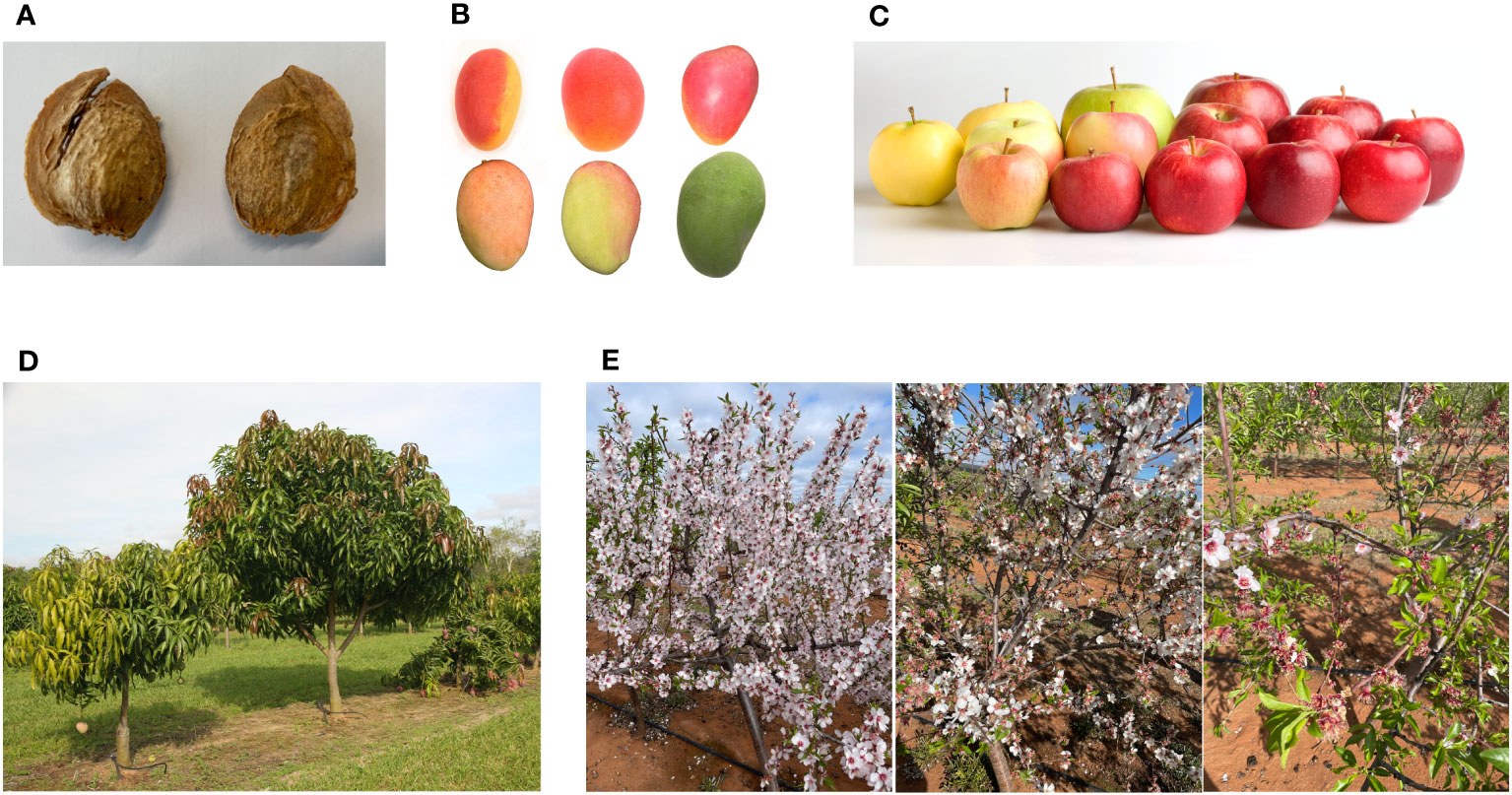
Figure 2 Pictures of several traits targeted for improvement in various tree crop breeding programs. (A) Nutshell seal in almond; The nut on the left demonstrates a variety with poor nutshell seal which increases risk of insect damage and diseases, while the nut on the right demonstrates a variety with good nutshell seal. (B) Fruit blush in mango; Consumer preference for fruit blush differs between countries and is mainly dependent on the main varieties available. In Australia a consistent red fruit blush (top) is preferred over inconsistent or no fruit blush (bottom). (C) Fruit characteristics in apple; fruit characteristics such as fruit peel colour and fruit size are also dependent on consumer preference. (D) Shoot architecture in mango; The tree on the right is a conventional mango tree with high vigour, while the tree on the left has low vigour with a small shoot architecture preferable for high density plantings. (E) Flowering time in almond; Almond varieties can be early (left), mid (middle) or late (right) flowering depending on their chill requirements during endodormancy, with ideal timing of flowering dependent on location and local climate.
Modification of crop density and tree architecture
Due to their extended lifespan, trees are in constant need of modifying their growth in response to the surrounding environment (Cooke et al., 2012). Tree architecture is an excellent example of this developmental plasticity, which is maintained via the regulation of axillary bud dormancy (Wang M. et al., 2019). Following establishment, axillary buds are maintained in a dormant state through a combination of various environmental and endogenous signals acting to ensure dormancy breaks at an appropriate time resulting in bud outgrowth and, if appropriate signals persist, in shoot branch formation (Wang M. et al., 2019). This high reliance of plant plasticity on environmental cues is increasingly becoming an issue in the face of rapidly changing, unreliable climatic conditions. Research focused on deciphering the genetic and molecular components/mechanisms regulating traits like shoot architecture and flowering is therefore critical to our ability to mitigate the impact of climate change on tree growth and productivity. Here, we will outline current knowledge of several key genetic components in tree crops regulating three specific shoot architecture traits: branching number, branching angle, and plant height. For horticultural crops, trees with shorter or less branches, and semi-dwarf phenotypes may be the ideal architecture allowing for higher planting density and reduced mechanical intervention like pruning (Scorza, 2005; Hollender and Dardick, 2015).
Shoot architecture has been a key target of domestication in many crops, with reduced branch number being an important component of the ideal shoot architecture (Figure 1A). In crops such as maize (Zea mays spp. mays), a decrease in tiller number correlates with yield increases (Doebley et al., 1997). The wild ancestor of maize (Zea mays spp. parviglumis) has an increased axillary branching phenotype (Doebley et al., 1995) and the gene controlling this trait was identified by Doebley et al. (1997) as TEOSINTE BRANCHED1 (TB1) which belongs to the TEOSINTE BRANCHED1, CYCLOIDEA, PROLIFERATING CELL FACTOR (TCP) family of transcription factors. Since then, orthologs have been identified and studied in fruit trees such as peach (Prunus persica) where overexpression of PpTCP18, a TB1/BRANCHED1 (BRC1) ortholog, in Arabidopsis thaliana caused a decrease in rosette branch numbers (Wang X. et al., 2023). Interestingly, in Carrizo citrange (Citrus sinensis x Poncirus trifoliata) the BRC1 ortholog THORN IDENTITY1 (TI1) acts partially redundantly with the BRC2 ortholog TI2 to control shoot branching alongside thorn identity (Zhang et al., 2020).
Shoot branch angle is another important aspect affecting overall shoot architecture which can lead to trees having an upright and erect architecture, termed pillar or broomy, or a spread apart architecture, termed weeping (Figure 1B). LAZY1 acts as a negative regulator of the polar auxin transport (PAT) to decrease the response to gravity, and loss-of-function mutations in rice promote a branching phenotype that is more spread apart (Li et al., 2007; Yoshihara and Iino, 2007). In apple (Malus domestica), the MdLAZY1A-W allele has a single nucleotide substitution that changes the amino acid from leucine to proline resulting in a dominant weeping phenotype (Dougherty et al., 2023). In contrast, an in-frame stop codon mutation in LAZY1 in silver birch (Betula pendula) leads to a recessive weeping phenotype (Salojärvi et al., 2017). LAZY1 belongs to the IGT gene family alongside another regulator of shoot branch angle, TILLER ANGLE CONTROL1 (TAC1). Mutations in rice (Oryza sativa) OsTAC1 result in a more compact phenotype (Yu et al., 2007; Jiang et al., 2012). The gene has since been identified in other species including the fruit trees peach (Dardick et al., 2013), plum (Prunus domestica; Hollender et al., 2018b), apple (Li et al., 2022), and sweet orange (Citrus sinensis; Dutt et al., 2022). In peach, the likely cause of the pillar phenotype in the ‘Italian pillar’ cultivar is an insertion causing a premature stop codon in PpeTAC1 (Dardick et al., 2013). Overexpression of PpeTAC1 in plum increased the branch angle, while RNA interference (RNAi) of PdoTAC1 in plum reduced the branch angle leading to a more upright architecture (Hollender et al., 2018b). Similarly, sweet orange CsTAC1 knockout transgenic lines displayed a more compact branch angle, and this was associated with low auxin levels and upregulation of CsBRC1 expression, following a similar expression pattern to Arabidopsis (Dutt et al., 2022). Another regulator of branching orientation identified in peach is the WEEP gene, encoding a sterile alpha motif (SAM) domain protein. The shoots on WEEP trees initially grow upwards but, after reaching a certain height (20 cm), start exhibiting a weeping phenotype (Hollender et al., 2018a). Grafting of buds from WEEP trees to wild-type rootstocks did not rescue the weeping phenotype of the scion suggesting that there is no requirement for a mobile or systemic signal for the weeping phenotype. Transcriptomic analysis showed differential expression of auxin-related genes like AUXIN/INDOLE-3-ACETIC ACID (Aux/IAAs) and AUXIN RESPONSE FACTORs (ARFs) indicating a role for auxin in the gravitropic response (Hollender et al., 2018a). RNAi of the WEEP gene in plum resulted in a weeping phenotype (Hollender et al., 2018a), showing conserved function of WEEP in a closely related tree species.
Plant height is another key trait that influences overall shoot architecture (Figure 1C). In the 1960s, a specific type of architecture with short internodes and fewer axillary branches was observed in the mutant apple McIntosh Wijcik (Fisher, 1969). Further research identified a dominant 2-oxoglutarate-dependent dioxygenase (DOX) gene, Columnar (Co) or MdDOX-Co, as the likely candidate responsible for the mutant phenotype (Lapins, 1976; Okada et al., 2016). Overexpression of MdDOX-Co in tobacco (Nicotiana tabacum) resulted in a dwarfed phenotype with a shorter main stem and internodes (Okada et al., 2020). This was associated with decreased endogenous bioactive gibberellin (GA) levels and exogenous application of GA was able to rescue the dwarfed phenotype (Okada et al., 2020). Overexpressing MdDOX-Co in Arabidopsis led to the discovery that it regulates 12-hydroxylation of GA12, a precursor in the GA biosynthesis pathway, to reduce active GA levels resulting in the dwarf phenotype (Watanabe et al., 2021). Similar to the McIntosh Wijcik mutant, the peach dw mutants have a dwarfing phenotype associated with short internodes (Scorza, 1984). Mapping analysis identified a GA receptor gene GIBBERELLIN INSENSITIVE DWARF1c (GID1c) as the likely cause of the dw trait, and RNAi-induced silencing of PpeGID1c in plum led to varying degrees of dwarfing (Hollender et al., 2016). Rice breeding has for many years employed the use of the semidwarf (sd-1) allele, caused by a defective GA biosynthetic gene GIBBERELLIN 20-OXIDASE (GA20ox) to decrease overall size while increasing yield (Spielmeyer et al., 2002). Multiple GA biosynthesis genes were identified as differentially expressed between the ‘Williams’ banana (Musa acuminata) 8818-1 wild-type cultivar and its dwarf counterpart (Chen et al., 2016), and there are already cases of successfully using GA20ox gene(s) as a target to produce dwarf fruit varieties, such as via suppression of MpGA20ox1 in apple (Bulley et al., 2005) and via CRISPR-based silencing of five MaGA20ox2 genes in banana (Shao et al., 2020). Ethylene is another plant hormone that has been associated with plant height regulation. Overexpression of the lemon (Citrus limon) gene 1-AMINOCYCLOPROPANE-1-CARBOXYLIC ACID SYNTHASE 4 (CiACS4), involved in the ethylene biosynthesis pathway, in tobacco and lemon resulted in dwarf plants (Chu et al., 2023). CiACS4 was found to be directly upregulated by an ethylene response factor, CiERF023, and the CiACS4 protein also interacted with another ethylene response factor, CiERF3, to directly regulate the GA biosynthesis genes CiGa20ox1/2.
Here, we have discussed three main routes to shoot architecture manipulation: shoot branching numbers, branching angle, and/or overall plant height. The genetic regulation of these traits shows high levels of conservation between divergent plant species, thus providing several potential targets for improving architecture in other tree crops. Efforts to implement breeding and genomics-enabled approaches to alter these traits will provide significant inroads to achieving ideal tree architecture with maximised yield.
Regulation of bud dormancy in deciduous trees
Deciduous trees that grow in temperate regions experience fluctuating temperature and daylength due to annual seasonal changes and have evolved to adapt to these environmental conditions by modulating their annual cycle of active growth and growth cessation. In deciduous trees, both terminal and axillary buds (whether vegetative or reproductive) temporarily stop visible growth during winter and only resume growth and flowering when the season is favourable (Singh et al., 2017). Growth cessation, bud set, cold acclimatisation and establishment of bud dormancy occur in sequential order with some overlap (Singh et al., 2017) creating difficulty not only in delineating where growth cessation ends and dormancy starts, but also in differentiating phenotypes and genetic mechanisms that are associated with these processes. In the state of deepest dormancy commonly referred to as endodormancy (Figure 1D; Lang et al., 1985), growth is repressed and cannot be initiated by growth-promoting conditions until the buds accumulate a certain number of hours of cold temperature known as the chilling requirement. Although this is a gradual process (Cooke et al., 2012), the transition to the ability of the plant to resume growth in permissive conditions is often referred to as ecodormancy (Lang et al., 1985). Release from dormancy is followed by budbreak, primarily driven by accumulation of heat (Alburquerque et al., 2008). Thus, dormancy is an important physiological and adaptive process that helps plant buds survive harsh winter temperatures and is an important determinant of yield in deciduous tree crops.
Chilling and heat requirements are specific to the plant species and genotype, and reflect the long-term adaptation to specific growing conditions in their native or cultivated regions (Luedeling, 2012). For example, the specific phenology of the almond (Prunus dulcis) cultivar ‘Nonpareil’ grown in South Australia is depicted in Figure 3. Delayed budbreak and low yields arising as a consequence of reduced winter chilling in the warming climate are posing a global threat to agriculture and food security (Luedeling, 2012; Atkinson et al., 2013). For this reason, tree phenology has been a subject of extensive research, with significant progress made in understanding the environmental, physiological, genetic, epigenetic and hormonal regulation of dormancy (Lloret et al., 2017; Beauvieux et al., 2018; Liu and Sherif, 2019; Fadón et al., 2020; Pan et al., 2021; Yamane et al., 2021; Yang Q. et al., 2021; Nilsson, 2022). Not only do we need in-depth understanding of the molecular mechanisms regulating dormancy, but also taking advantage of next generation molecular techniques and translating these advancements to the orchard is crucial for plant biologists to develop new and improved cultivars better suited to the new climate dynamics.
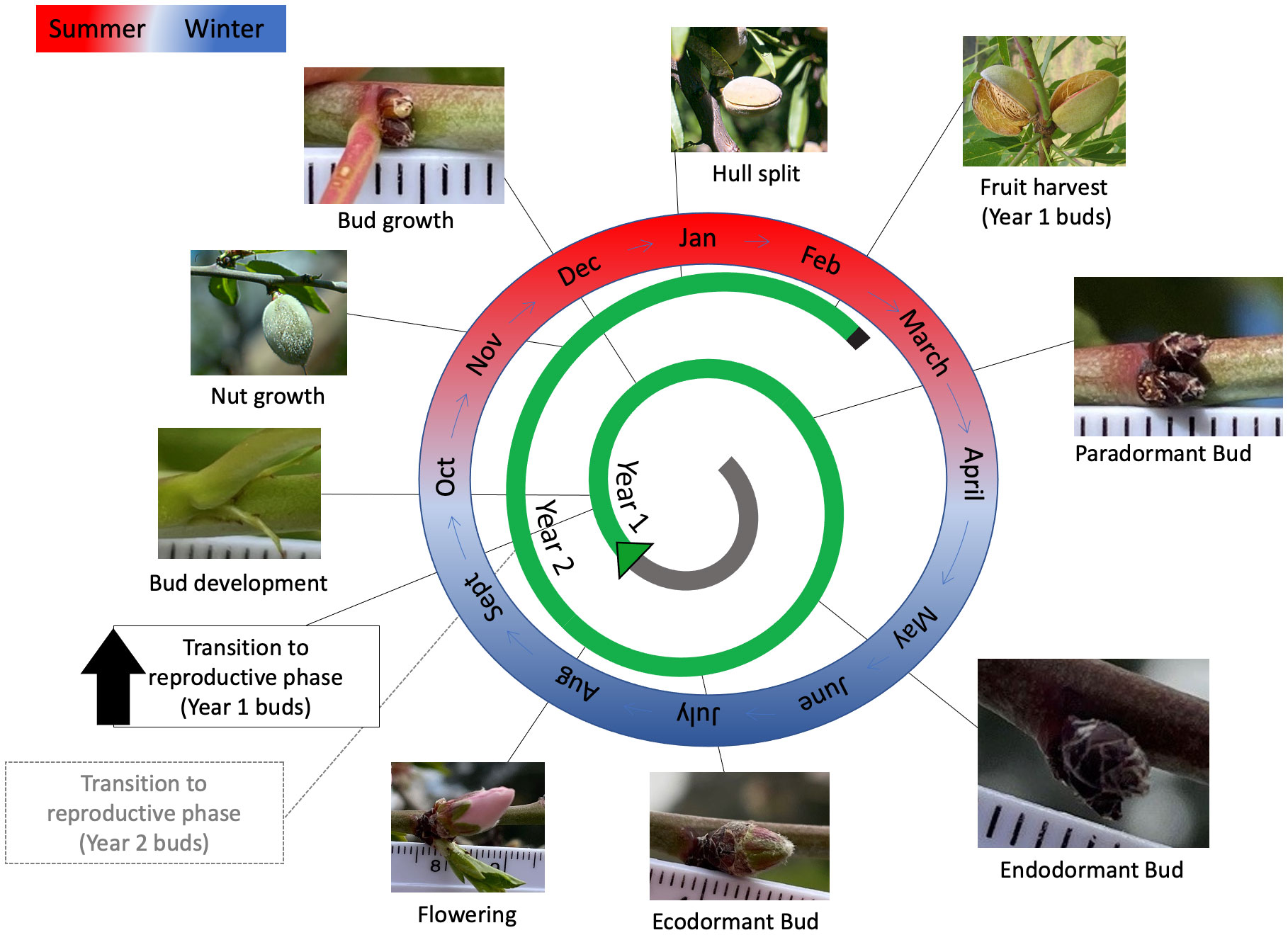
Figure 3 Phenology for the almond (Prunus dulcis) cultivar ‘Nonpareil’ grown in South Australia. The transition to flowering of new buds formed takes place in September 30-40 days after flowering. Buds are very small at this stage; however, the green, scale-like structures are visible at the nodes. The bud grows throughout summer. In the latter half of summer, buds are completely covered by layers of dark-brown scales. During the end of April and beginning of May, all almond leaves senesce, which signals the start of endodormancy. Buds accumulate chilling during May, June, and July. Once the chilling is complete, the bud enters ecodormancy. Ecodormant buds swell after some heat accumulation, then grow to develop flowers at the beginning of August. The fertilised ovaries grow throughout spring and summer to develop into fruit nuts. Once nut growth is complete, the hull starts to split in January. In February, the almond shell starts to desiccate, and the hull split widens indicating that the nuts are ready for harvest. The entire process from bud formation to harvest takes about 1.5 years; therefore, between September and February, almond trees have young buds from the current season and developing nuts from previous seasons’ buds.
In Rosaceous plants like almond, peach, plum, pear (Pyrus spp.) and apple, DORMANCY ASSOCIATED MADS-BOX (DAMs) transcription factors are central regulators of bud dormancy during winter chilling (Figure 1D). DAMs were first identified in a natural mutant peach cultivar evergrowing, which fails to enter dormancy during winter (Bielenberg et al., 2008). Sequencing revealed six genes that were either partially or fully deleted in the mutant compared to wild-type peach and were termed as DAMs (Bielenberg et al., 2008). Various studies in apple (Wu et al., 2017), Japanese pear (Pyrus pyrifolia; Saito et al., 2013; Niu et al., 2016; Tuan et al., 2017) and Japanese apricot (Prunus mume; Yamane et al., 2019) have shown that overexpressing DAMs prolongs dormancy in these trees, and silencing or mutagenesis prevents dormancy in hybrid aspen (Populus tremula x P. tremuloides; Singh et al., 2019) and apple (Moser et al., 2020; Wu et al., 2021). A recent study by Falavigna et al. (2021) explored the gene regulatory networks controlled by DAMs during the dormancy cycle in apple in response to environmental cues and hormonal signalling pathways. Transcriptomic studies in various other species including WT-717 poplar hybrid, Japanese pear and raspberry (Rubus idaeus) show that DAMs, as well as important genes involved in abscisic acid (ABA) and GA synthesis and catabolism differentially accumulate in buds transitioning from growth cessation through the different stages of dormancy to growth resumption in spring (Mazzitelli et al., 2007; Ruttink et al., 2007; Niu et al., 2016). ABA has been shown to control dormancy by inhibiting genes involved in cell proliferation and growth, and activating catabolism genes of other hormones such as GAs (Yang Q. et al., 2023). However, the exact signalling cascades by which DAMs trigger ABA and GA pathways in response to environment are still obscure.
While DAMs play an important, conserved role in regulating bud dormancy in many temperate crops, other genes are also likely to play an important role in this process. For example, in apple the major quantitative trait locus (QTL) controlling bud dormancy does not contain any DAM genes suggesting other gene(s) are regulating bud dormancy in apple (Allard et al., 2016). The C-REPEAT BINDING FACTOR1 (PpCBF1) gene has been shown to play a role in bud dormancy when overexpressed in apple (Wisniewski et al., 2015). While CBF and other genes likely play a role in bud dormancy, the DAMs are clearly critical regulators of the onset and release of bud dormancy in Rosaceae species. Delving deeper into the downstream targets regulated by DAMs can reveal new targets towards modifying bud dormancy according to the geographical climates of orchard production.
Promoting earlier flowering (Precocity)
A critical developmental time in the life cycle of a tree is the vegetative to reproductive phase change, during which the tree attains the ability to flower and produce seeds. When compared to an annual plant, which can flower, produce seeds, and complete its life cycle within one year, a woody perennial tree will often not reach reproductive maturity for several years or even decades (Hackett, 1985). Following the first onset of flowering, trees flower annually in response to environmental signals but commit only some of the meristems to flowering, thereby maintaining the polycarpic growth habit (Amasino, 2009). The delayed maturity, long life span, and polycarpic growth of trees require a complex regulatory network to regulate the timing of developmental transitions and synchronise environmental signals with phenological events to ensure survival and successful reproduction (Brunner et al., 2017). While flowering gene homologs from woody perennials often show a conserved role in regulation of flowering in model annuals such as Arabidopsis and tobacco, functional studies in trees reveal shared and distinct functional aspects, highlighting the diverse roles of paralogs (Hsu et al., 2011; Sheng et al., 2022) and the pleiotropic effects of flowering genes on growth (André et al., 2022; Sheng et al., 2023), organ identity (Zhang et al., 2021), and phenology in trees (Ding and Nilsson, 2016). Therefore, targeting specific genes to reduce the years to reproduction in trees is a complex task that depends on the species in question. However, the types of genes that could be targeted to accelerate reproductive maturation are those regulating flowering time and floral meristem identity (Figure 1E).
Members of the phosphatidylethanolamine–binding protein (PEBP) family are crucial for floral transition. The divergence of PEBP genes into FLOWERING LOCUS T (FT) (predominantly activators of flowering) and TERMINAL FLOWER1 (TFL1)/CENTRORADIALIS (CEN) (predominantly repressors of flowering) (Wickland and Hanzawa, 2015) subgroups in angiosperms was critical for the reproductive success of flowering plants (Shalit et al., 2009; Karlgren et al., 2011). Further divergence through gene duplication events, followed by neo- and sub-functionalisation, gave rise to FT-like regulators implicated to have broader functions in plant development and adaptation (Pin and Nilsson, 2012). It is important to note that the specific functions and regulatory mechanisms of FT in perennial fruit trees can vary between different species and cultivars within the same species, but FT has generally maintained its role as an inducer of flowering. Early flowering can be induced in Arabidopsis by overexpressing FT orthologs from many diverse tree crops such as avocado (Ziv et al., 2014), mango (Mangifera indica; Fan et al., 2020), apple (Kotoda et al., 2010), kiwifruit (Actinidia chinensis; Voogd et al., 2017), and litchi (Litchi chinensis; Ding et al., 2015); while constitutive ectopic expression of FT promotes early flowering in tree crops such as apple (Kotoda et al., 2010), trifoliate orange (Poncirus trifoliata; Endo et al., 2005), and kiwifruit (Voogd et al., 2017). However, in some instances, overexpression of FT causes in vitro and abnormal flowering such as that observed in apple (Kotoda et al., 2010) and kiwifruit (Voogd et al., 2017; Moss et al., 2018). More recently, it was shown that FT chimeric proteins induce floral precocity without detrimental effects in citrus (Sinn et al., 2021) and kiwifruit (Herath et al., 2023) species, suggesting that this approach may be universally applicable for woody perennial crops.
The concept of grafting on rootstocks engineered to overproduce FT to accelerate flowering of the scion has been explored as means of flowering control in horticultural and agricultural practices. However, the movement of FT and its ability to promote flowering in trees is still under debate (Putterill and Varkonyi-Gasic, 2016), with reports of movement across the graft union without precocity observed in juvenile apple, trifoliate orange, hybrid aspen or WT-717 poplar hybrid scions (Zhang et al., 2010; Wenzel et al., 2013; Freiman et al., 2015; Miskolczi et al., 2019; Wu et al., 2022), while precocious flowering was observed in Carrizo citrange (Soares et al., 2020) and Jatropha curcas scions (Ye et al., 2014). In various Jatropha spp., the distance between the graft union and bud is important for floral induction and increasing this distance can lead to a lower frequency of flowering (Tang et al., 2022). Therefore, grafting approaches may be dependent on many variables including the plant species, plant/scion size, as well as the FT gene and its regulatory promoter.
Plant viral vectors have also been used to either express FT for virus-induced flowering or silence TFL1 via virus-induced gene silencing (VIGS), and these approaches have induced early flowering in apple, Japanese pear, European pear (Pyrus communis), and Vitis spp. (Sasaki et al., 2011; Yamagishi et al., 2014; Velázquez et al., 2016; Yamagishi et al., 2016; Maeda et al., 2020). RNA silencing of TFL1/CEN has also induced early flowering in apple (Kotoda et al., 2006), WT-717 poplar hybrid (Mohamed et al., 2010) and European pear (Freiman et al., 2012). Recently, CRISPR/Cas9-mediated mutagenesis of one or two CEN genes induced fast flowering in several kiwifruit species (Varkonyi‐Gasic et al., 2019; Varkonyi-Gasic et al., 2021; Herath et al., 2023) and editing of TFL1 induced early flowering in apple and European pear (Charrier et al., 2019). However, editing of a blueberry (Vaccinium corymbosum) CEN gene did not result in precocious flowering (Omori et al., 2021), suggesting that some TFL1/CEN genes perform different roles. Indeed, mutagenesis of a kiwifruit BROTHER OF FT AND TFL1 (BFT) prevented the establishment of dormancy without affecting flowering (Herath et al., 2022), and in Carrizo citrange loss of CsCEN resulted in the conversion of axillary meristems to thorns, while ectopic CsCEN expression converted thorns to axillary meristems (Zhang et al., 2021).
Floral meristem identity genes perform as key outputs of FT-mediated floral transition or FT-independent flowering pathways. They include MADS-box proteins such as APETALA1 (AP1) and SUPPRESSOR OF OVEREXPRESSION OF CONSTANS1 (SOC1), necessary to initiate and maintain reproductive development, and LEAFY (LFY) acting as a pioneer transcription factor reprogramming vegetative meristematic cells into an inflorescence (Jin et al., 2021; Lai et al., 2021). LFY homologs from trees, including peach, litchi and mango, can induce flowering when overexpressed in annual models (An et al., 2012; Ding et al., 2018; Wang Y. et al., 2022). Ectopic overexpression of LFY also promoted flowering in Carrizo citrange (Peña et al., 2001), yet there were some inconsistencies in floral induction between various Populus hybrids that displayed altered flower development as well as sexual differentiation (Weigel and Nilsson, 1995; Rottmann et al., 2000). MADS-box genes have been important targets for selection during crop domestication and improvement as they play pivotal roles in every aspect of plant reproductive development, controlling flowering time, inflorescence architecture, determination of floral meristem and floral organ identity, and seed development (Schilling et al., 2018). The ability of AP1 homologs to induce flowering in a range of woody perennials, e.g. Carrizo citrange, silver birch and apple (Peña et al., 2001; Elo et al., 2007; Flachowsky et al., 2007), has been adopted for rapid cycle breeding approaches in apple and European pear (Flachowsky et al., 2011; Tomes et al., 2023). However, functional studies in hybrid aspen revealed that, like FT, homologs of MADS-box genes controlling the regulation of flowering time in annual plants have a role in control of vegetative phenology in trees (Azeez et al., 2014; Ding and Nilsson, 2016).
This section has highlighted several key genes and gene families that offer valuable tools for shaping and improving developmental and reproductive traits in crops. Their manipulation through targeted breeding approaches can contribute to the accelerated development of new crop varieties with enhanced characteristics (as demonstrated in Figure 4), ultimately benefiting horticultural food production.
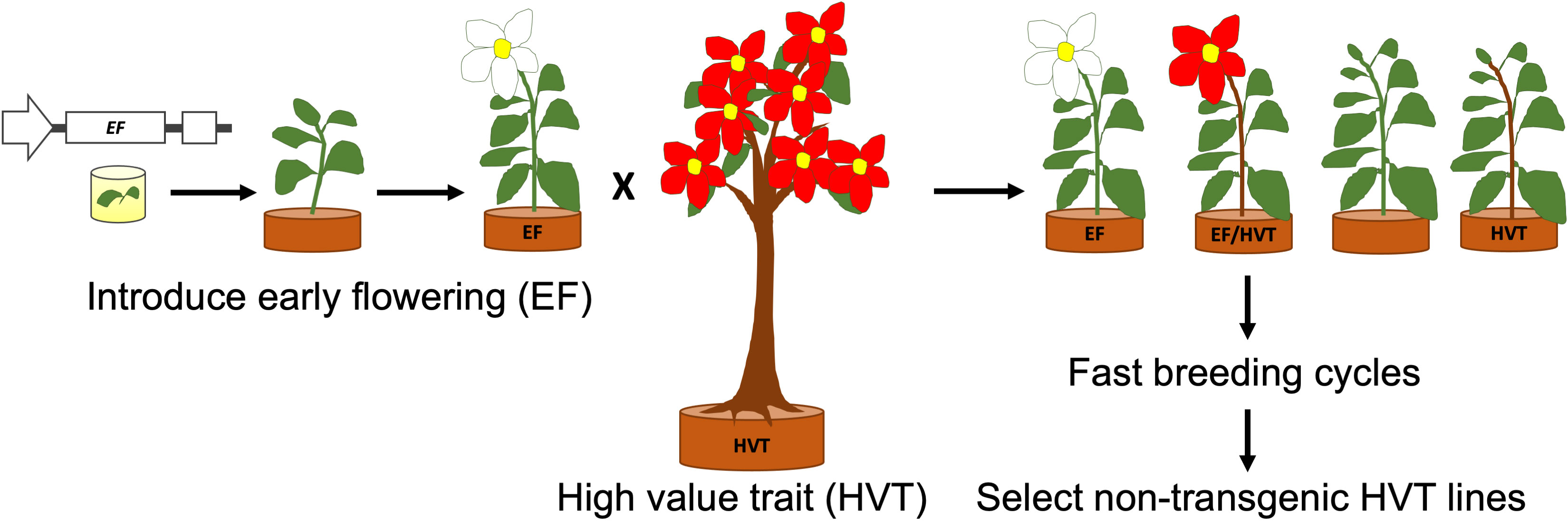
Figure 4 A schematic diagram representing fast breeding of tree crops using early flowering (EF) transgenic lines to introduce a high value trait (HVT). Half of the progeny is EF, with half of those also carrying the HVT gene(s). Non-transgenic lines are selected after a desired number of breeding cycles.
Managing alternate bearing for more consistent yields
Alternate bearing, also known as biennial bearing, is a significant issue faced by the horticultural industry and has been reported to affect mango, cranberry (Vaccinium macrocarpon), avocado, olive, apple, European pear, plum, apricot (Prunus armeniaca), coffee (Coffea arabica), Citrus spp., litchi and nut producing trees (Monselise and Goldschmidt, 1982). Alternate bearing is initiated by an abnormally heavy fruit load one year, decreasing the ability of the tree to undergo floral initiation and resulting in a light fruit load the following season. This pattern of alternating high then low crop production years can differ in intensity between cultivars within a species, and can also be strongly influenced by environmental factors (Monselise and Goldschmidt, 1982). These inconsistent patterns of bearing and yield have major implications for growers and for responding to the need for reliable food sources into the future.
Studies have demonstrated that fruit load affects flowering the following season by repressing the expression of genes involved in floral initiation (Figure 1F). FT, AP1 and LFY are shown to be highly expressed in the absence of fruit and have minimal to no expression in the presence of a heavy crop load in avocado (Ziv et al., 2014), mango (Nakagawa et al., 2012; Das et al., 2019), and ‘Moncada’ mandarin (Citrus clementina x Kara mandarin (C. unshiu x C. nobilis); Muñoz-Fambuena et al., 2011). In avocado, expression of floral initiation genes was suppressed in shoots with high fruit load, and the transition from vegetative to reproductive structure was completely repressed with young leaves appearing instead (Ziv et al., 2014). In Citrus spp., CiFT2 expression is downregulated in trees with high fruit load by CcMADS19, an ortholog of the floral repressor FLOWERING LOCUS C (FLC), while in trees with a low fruit load, low CcMADS19 expression results in an increase in CiFT2 expression (Agustí et al., 2020). Expression of CcMADS19 itself was shown to be correlated with epigenetic changes (Agustí et al., 2020). Fluctuations in activity of SQUAMOSA PROMOTER BINDING PROTEIN-LIKE (SPL) genes have also been observed in response to fruit load in mandarin (Citrus reticulata; Shalom et al., 2012), mango (Sharma et al., 2020), and apple (Guitton et al., 2016).
Studies in ‘Murcott’ mandarin (Citrus reticulata x C. sinensis) and olive have shown the possible involvement of auxin (IAA) in alternate bearing, with auxin transmitting a heavy-fruit-load signal to the shoot apical meristem to repress floral initiation, and thus playing a role in determining whether vegetative or floral development proceeds (Haim et al., 2021). Peaks in transcript levels of IAA transporter genes were also associated with the induction of the flowering-inhibition signal (Haim et al., 2021). It has been shown that auxin induces the biosynthesis of GA during fruit set in tomato (Solanum lycopersicum; de Jong et al., 2009; Hu et al., 2018) and woodland strawberry (Fragaria vesca; Liao et al., 2018), and GA has been widely shown to promote vegetative growth and inhibit flower formation in tree crops. Heavy fruit load increases GA levels in ‘Satsuma’ mandarin (Citrus unshiu) leaves (Koshita et al., 1999), and fruit load affects the expression patterns of GA metabolism genes in mango (Nakagawa et al., 2012; Sharma et al., 2020). Exogenous application of GA also leads to a significant increase in TFL1 expression in apple (Haberman et al., 2016) and reduced FT expression in ‘Orri’ mandarin (Citrus reticulata x C. temple; Goldberg-Moeller et al., 2013), apple (Elsysy and Hirst, 2019), and mango (Krishna et al., 2017). Therefore, in fruit trees, auxin likely plays a role in mediating fruit-load inhibition of flowering by increasing GA levels to regulate expression of both floral promoter and repressor genes.
Another important factor regulating alternate bearing is resource availability, in particular, carbohydrate levels. Competition for carbohydrates between developing fruit and nearby apical buds leads to depletion of carbon levels and reduced cellular activity in vegetative meristems, blocking the onset of floral development and potentially inducing bud dormancy (Tarancón et al., 2017; Martín-Fontecha et al., 2018). Tree starch content is significantly depleted by a heavy crop load resulting in fewer flowers and a lower crop load the following year (Nakagawa et al., 2012). Sugars are increasingly recognised as important signalling molecules (Fichtner and Lunn, 2021) with several sugar signalling pathways associated with bud outgrowth. In mandarin, buds on branches with high fruit-load showed induction of the trehalose metabolism enzymes, TREHALOSE PHOSPHATE PHOSPHATASE (TPP) and TREHALOSE PHOSPHATE SYNTHASE (TPS), which play a role in flowering in model species (van Dijken et al., 2004), suggesting a possible role for trehalose and/or its biosynthetic pathway in the transition to flowering (Shalom et al., 2012). The promotion or repression of flowering may be regulated by changes in sugar signalling which reflects changes in resource availability i.e., when many fruit are present, buds are deprived of photoassimilates, preventing flower bud formation.
By improving our understanding of the molecular regulation of alternate bearing we can continue to develop and optimise targeted tree management strategies, such as plant growth regulator application, nutritional management and regulating carbohydrate depletion, to ensure the consistent promotion of flowering and fruit development. Additionally, the significant variation in susceptibility to alternate bearing observed between cultivars offers potential targets for genetic manipulation to reduce alternate bearing and the need for often time-consuming tree management strategies.
Fast-forwarding technologies towards precision tree crop production
Plant breeding has been transformed in recent years through the advent of rapid and precise genome-enabled breeding technologies. These cutting-edge methods have enabled breeding programs to accelerate genetic gain using techniques that accelerate the development of superior crop varieties (Table 1). In the following section we examine the spectrum of genome-enabled plant breeding methods. First, we delve into purely genomic techniques, such as MAS and genomic selection (GS). Both methods use the power of molecular markers and genomic data to identify and select plants with desirable traits more efficiently. By doing so, they can dramatically shorten breeding cycles and reduce the size of resource-intensive progeny trials. Moving beyond MAS and GS, we explore how advances in functional genomics and computational biology enable plant scientists to unravel the complex web of genetic interactions governing plant traits. Greater understanding of gene regulatory networks provides precise information into which genes are best to target for genetic modification, allowing molecular breeders to fine-tune desirable traits with precision. Finally, we review genetic manipulation in plants, evaluating the potential for transgenic and gene editing approaches to precisely modify traits in elite crop varieties. Most of these technologies are reliant on, or aided by, a sound understanding of the genetic basis of traits of interest, such as described in the previous sections.
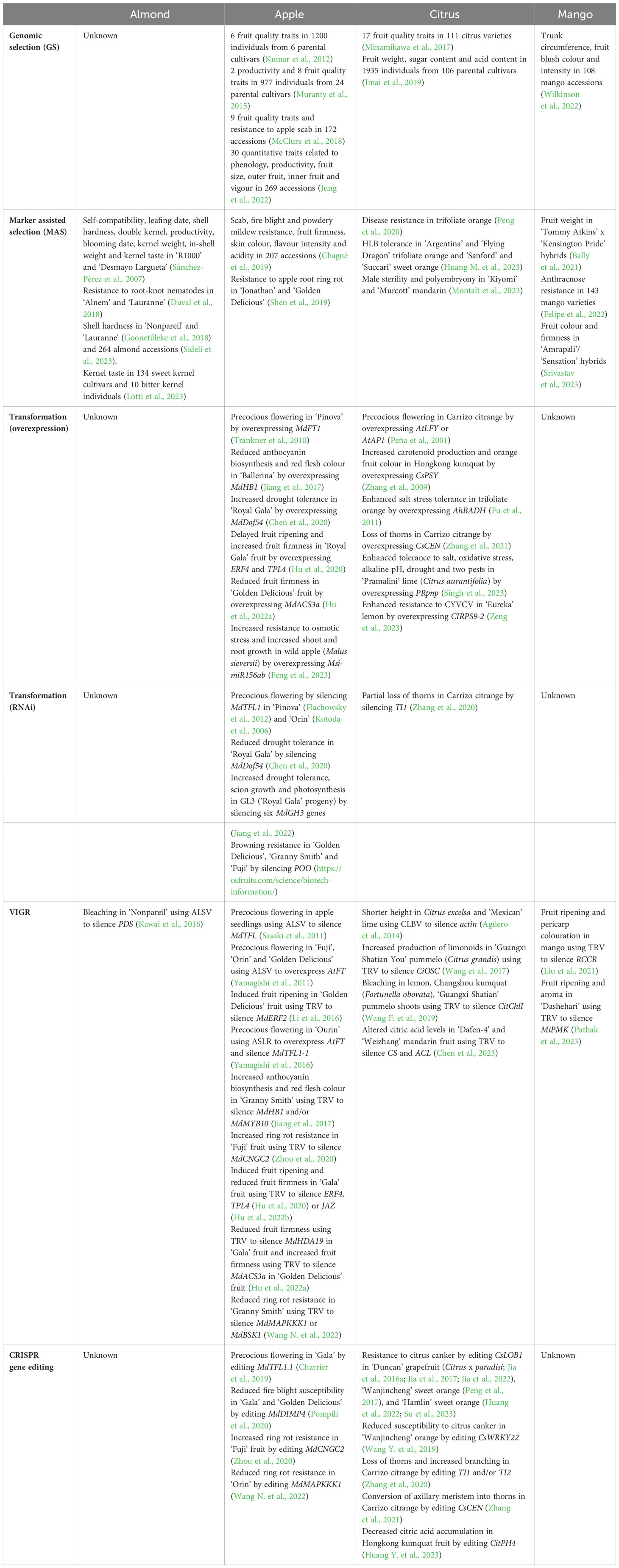
Table 1 Selected examples of the use of different genomics approaches for trait improvement in several tree crops.
Genomics approaches
The improvement of horticultural tree crops has been modernised with the advent of high-throughput genomic technologies, i.e., high-throughput microarray genotyping and next generation sequencing, which have played pivotal roles in enhancing the precision, speed, and efficiency of tree breeding programs. These technologies and new selection methods have enabled tree breeders to accelerate genetic gain and the development of superior tree varieties with desirable trait combinations. High-throughput genotyping methods assay thousands of genetic markers simultaneously at a relatively modest cost and when analysed in segregating or reference populations have enabled the identification of key genetic variants associated with traits of interest, such as disease resistance, fruit quality, and plant architecture, among others (Peng et al., 2020; Wang L. et al., 2022; Huang M. et al., 2023; Srivastav et al., 2023). Comprehensive knowledge of the genetic basis of multiple agronomically important traits has allowed breeders to make informed decisions on the selection of parent trees for crossing in breeding programs, generating new hybrid varieties with improved trait combinations.
In tree species characterised by a long juvenile phase, traditional breeding methods can be slow and labour intensive. The use of MAS, which employs genetic markers closely associated with traits of interest, has emerged as a game-changer in this context. MAS allows breeders to screen large populations during the seedling stage and select those with the potential to exhibit the desired traits at maturity. For example, in citrus a hybrid population of ‘Kiyomi’ and ‘Murcott’ tangors segregating for male sterility and polyembryony was used to develop and validate markers tightly linked to both traits to be used for MAS in breeding programs (Montalt et al., 2023). In macadamia, the identification of markers tightly linked to nut quality characteristics could be used to screen for elite progeny potentially reducing the selection process by seven years in the breeding program (O’Connor et al., 2019). And examples in Rosaceae fruit trees include screening apricot seedlings for markers associated with plum pox resistance (Zuriaga et al., 2018; Polo-Oltra et al., 2020), apple seedlings for markers associated with fruit quality and disease resistance (Chagné et al., 2019; Petiteau et al., 2023), and peach seedlings for markers linked with fruit colour (Adami et al., 2013) increasing the efficiency of these breeding programs. This approach significantly reduces the time required for traditional phenotypic evaluation which for many traits can be cumbersome, thus accelerating the breeding cycle in tree crops. Moreover, MAS has the added benefit of eliminating individuals without specific alleles, streamlining the selection process and conserving resources by focusing only on candidates with the highest potential for success.
GS is another analysis method that relies on an alternative approach based on analysis of all QTL effects, regardless of their significance, which may improve tree breeding by increasing the accuracy and efficiency of variety selection. It enables breeders to focus their efforts on candidates with the highest genetic potential, resulting in more targeted and successful breeding outcomes. Unlike traditional breeding methods that rely on the phenotypic evaluation of individual trees, GS leverages comprehensive genomic data to predict the breeding value of trees based on their genetic makeup. GS has been applied with great success in cereal and livestock breeding (Xu et al., 2021) but is still in its infancy for horticultural trees. For example, a recent study in apple determined the genetic architecture of 30 important traits and set out a strategy to implement GS in apple breeding programs to improve selection strategies (Jung et al., 2022). Another example from macadamia demonstrated that using GS models could increase genetic gain for yield, which is more than double that achieved from traditional breeding (O’Connor et al., 2021). Consequently, GS has the potential to enable breeders to accurately estimate the performance of trees before they reach maturity, allowing for early identification of top-performing individuals.
Regulation of crop trait genetic interactions
The recent and fast-paced advancements in high-throughput next generation sequencing technologies have also aided in the rapid increase of omics-based experiments to collect expression data. These transcriptomic data can be used to infer gene co-expression networks using a mathematical approach for transcriptomic data analysis resulting in gene co-expression network inference (Lee et al., 2015; Haque et al., 2019; Jiang et al., 2023). The network can be broken down into two components; nodes (genes and their products) represented by shapes, and edges representing interactions between the nodes. The network follows a guilt by association principle whereby genes that observe similar expression patterns are clustered together implying a potential (co)-regulatory relationship within the endogenous system (Lee et al., 2015; Haque et al., 2019). This approach is a relatively simple way to identify many of the genetic components underlying important agricultural traits and has already been implemented to study different traits for apple (Ding et al., 2021), peach (Khan et al., 2022), apricot (Yu et al., 2020), sweet cherry (Prunus avium; Yang H. et al., 2021), kiwifruit (Brian et al., 2021), and Vitis spp. (Toups et al., 2020; Tan et al., 2023). However, as co-expression network inference is an in silico inference method to identify genes of interest, these predictions need to be validated via experimental approaches (Haque et al., 2019) such as chromatin immunoprecipitation sequencing (ChIP-seq) (Robertson et al., 2007) and DNA affinity purification sequencing (DAP-seq) (O’Malley et al., 2016).
ChIP-seq is an in vivo technique first implemented on mammalian HeLa cells which utilises antibody-driven chromatin immunoprecipitation and DNA sequencing to identify direct genetic targets of transcription factors via read mapping against a reference genome (Robertson et al., 2007). The potential of ChIP-seq was further expanded to identify histone modifications in the genome landscape which are correlated with gene expression changes (Pillai et al., 2009). ChIP-seq has since been used for studying both the targets of transcription factors as well as the genome-wide patterns of histone modifications underlying traits such as fruit ripening, dormancy, and stress-response in various horticulture crops including peach (de la Fuente et al., 2015; Canton et al., 2022; Jin et al., 2022; Zhao et al., 2023), sweet cherry (Vimont et al., 2019), kiwifruit (Wu et al., 2018), and apple (Chen et al., 2020; Hu et al., 2020; Chen et al., 2022; Hu et al., 2022a; Hu et al., 2022b).
In contrast to ChIP-seq which requires costly antibodies and is not easily upscaled, an alternative in vitro method called DAP-seq was created by O’Malley et al. (2016). The technique uses an affinity tagged transcription factor to bind genomic DNA and identify downstream target genes via DNA sequence read mapping. The number of regulators can be easily upscaled in this method, with O’Malley et al. (2016) using DAP-seq on 1812 Arabidopsis transcription factors and the maize ZmARF29 transcription factor. DAP-seq has since been used in numerous horticultural perennial crops including apple (Chen et al., 2020; Falavigna et al., 2021), sweet orange (Wang W. et al., 2023), grapevine (Vitis vinifera; Orduña et al., 2022) and avocado (Núñez-Lillo et al., 2023) to study traits like flowering, fruit softening, starch synthesis, disease resistance, and stress-induced responses.
Network inference is a promising tool for molecular breeding as it allows the use of transcriptomic data to confirm the association of genes previously identified through functional genomics experiments with traits of interest, and associate new genes with these traits. Furthermore, it can allow us to identify potential interactions between previously identified and newly identified genes for validation via techniques like ChIP-seq and DAP-seq. Finally, network inference can assist with selecting the best candidates for manipulation of single traits.
In planta bioassays – leveraging knowledge from trees in the field for selecting seedlings with desirable traits
Plants have evolved to synchronise flowering with favourable environmental conditions to ensure successful reproduction (Kim et al., 2009). The ability of plants to flower in response to the correct environmental stimuli is gained during either the vegetative or reproductive phases of organ development. The prolonged juvenile phase of perennials makes it difficult to unravel molecular mechanisms controlling reproductive organ development and floral initiation in the early years of perennial growth, and to assess horticultural traits such as fruit quality can take years.
There is a need for more creative approaches by comparing gene regulatory processes in different tissues from trees growing in the field with those growing in an environmentally controlled growth environment. For example, by examining transcriptomic profiles in field grown tree tissues responding to a decline in photoperiod, cold snaps, and progressive chilling period, it would be possible to untangle the gene regulatory networks sensing, responding, and modulating phases of floral bud dormancy and the hence the onset of flowering. An integrated approach that evaluates gene regulatory processes in source tissues such as the leaves and sink tissues such as floral buds could enable predictions of the key events modulating early to late cultivar floral initiation. By simulating these climate events within a controlled environment, it might be possible to replicate the transcriptomic networks of gene regulations within seedling tissues thereby enabling the fast-forwarding screening of individuals in a segregating population that perform according to the tree growing in the field.
This approach could be routinely established by also combining external chemical treatments to the seedlings growing in a controlled environment to further simulate the use of plant growth regulators used within field conditions to alter gene regulatory networks that facilitate flowering. The role of phytohormones and their crosstalk with environmental signals during flowering in annuals is well studied (Gerashchenkov and Rozhnova, 2013), but less so in perennials due to the long juvenile period. Mølmann et al. (2005) experimented with juvenile hybrid aspen by treating with paclobutrazol combined with low night temperature and short-day conditions. As expected, paclobutrazol reduced GA and caused growth cessation. It also promoted bud set and improved cold hardiness, a trait that is important for perennials to survive harsh environmental conditions during endodormancy. However, it did not promote dormancy suggesting that the ABA pathway can maintain dormancy independent of GA. The application of omics approaches to quantify hormones, metabolites, and gene expression in tissues from trees and seedlings grown in controlled environments, could fast-track knowledge of how the environment modulates dormancy and flowering. Ultimately, devising a tree to seedling bioassay could improve the development of new molecular markers to screen for important tree crop traits and reduce the number of trees from segregating populations to be planted in the field.
Functional genetics for trait characterisation and improvement
The development of the first transgenic plant in 1983 (Herrera-Estrella et al., 1983) gave scientists and plant breeders high hopes that transgenic technology would revolutionise our understanding of plant molecular biology and fast-track crop breeding. However, the negative public perception and intense biosecurity regulation did not allow transgenic technology to be used to its full potential. A prime example of this was the development of transgenic American chestnut (Castanea dentata) which is resistant to fungal blight disease (Powell et al., 2019). Blight disease has destroyed billions of wild-type American chestnuts, yet transgenic plants with resistance to this disease have not yet been deregulated for public use. Nevertheless, it must also be noted that the regulations restricting genetically modified organisms (GMOs) are slowly being relaxed in different countries. This has resulted in a steady increase in the total acreage under GM crop cultivation in the last decade (Lobato-Gómez et al., 2021). In Australia, commercial cultivation of four genetically-modified crops: canola (Brassica napus), mustard (Brassica juncea), cotton (Gossypium hirsutum) and safflower (Carthamus tinctorius) has been approved (OGTR, 2023). Globally, different transgenic crop cultivars including several horticultural crops such as apple, plum, papaya (Carica papaya), eggplant (Solanum melongena), sweet pepper (Capsicum frutescens) and watermelon (Citrullus lanatus) have been approved for commercial cultivation (Baranski et al., 2019). These GM crops have improved agronomic traits such as fruit browning in apple (Waltz, 2015), resistance to insects in eggplant (Ahmed et al., 2021), and resistance to viral disease in plum (Singh et al., 2021), papaya (Gonsalves et al., 2007), watermelon (Lin et al., 2012) and sweet pepper (Chen et al., 2003). The future of GM crops is encouraging since many crop cultivars are in the pipeline for deregulation, and public perception and legalities are changing around the world with many countries slowly beginning to embrace GM crops.
Transgenic technologies have provided alternatives to employing forward genetics in horticultural trees. Predicting gene functions using forward genetics requires developing mapping populations which is time-consuming and resource-intensive due to the long juvenile phase of trees and resources needed for maintaining a large number of trees. Transgenic technologies in addition to the public availability of genome databases of different horticultural trees and along with the progress in the development of different tools for phylogenetic analysis have simplified gene function studies in trees. These techniques have been used to identify homologs of genes from model plants in tree crops and transgenically overexpress those genes either in Arabidopsis or in the trees themselves. These techniques have been used in several tree crops for studying genes related to different biological processes, including those already described in this review such as tree architecture, bud dormancy and flowering time. In addition to these processes, transgenic approaches have also been used to study fruit quality, disease resistance and other traits of economic significance. For example, in Carrizo citrange, overexpression of Thionin increased resistance to citrus canker and Huanglongbing disease in transgenic citrus plants (Hao et al., 2016). While transgenic overexpression of peach NAC25 in Nanlin895 poplar (Populus x euramericana) shoots increased anthocyanin biosynthesis and transport resulting in redder shoot tips, indicating that NAC25 could play an important role in red fruit colour development in peach (Geng et al., 2022). Results from these and other studies have shown that gene function knowledge from model plants can be exploited using transgenic technologies to bridge the gap in functional genomic studies between model plants and tree crops.
In contrast to transgenic overexpression where the gain-of-function strategy is utilised to characterise gene functions, another transgenic tool called RNAi utilises a loss-of-function strategy to characterise gene functions. RNAi induced post-transcriptional gene silencing has been used to characterise genes related to different biological pathways in transgenic plants (Small, 2007). For example, in apple three DAM and two SVP genes were silenced simultaneously resulting in the development of an evergrowing phenotype (Wu et al., 2021) similar to that observed in the natural evergrowing peach mutant (Bielenberg et al., 2008). While in transgenic strawberries (Fragaria x ananassa), an RNAi construct designed using a jasmonic acid biosynthetic gene ALLENE OXIDE SYNTHASE (AOS) from grapevine triggered silencing of FaAOS and caused an un-colouring phenotype in strawberry fruit (Jia et al., 2016b). Apart from gene function studies, RNAi-based gene silencing has also been used for improving cultivars and rootstocks. One of the first RNAi-based transgenic commercial cultivars was developed in papaya to protect against Papaya ringspot virus (PRV; Ferreira et al., 2002). A similar strategy was used in developing the plum ‘Honeysweet’ cultivar, which contains an RNAi construct with plum pox virus coat protein-derived hairpin and is resistant to plum pox virus (Scorza et al., 1994). Apple cultivar Arctic®, grown by Okanagan Specialty Fruit Inc., is another RNAi-based cultivar with a browning resistance phenotype (https://osfruits.com/science/biotech-information/). Meanwhile, RNAi-induced knockdown of six apple GH3 genes increased drought tolerance in apple rootstock and increased scion growth and photosynthesis (Jiang et al., 2022). And in a sour cherry (Prunus cerasus x P. canescens) rootstock, expression of an RNAi construct containing a Prunus necrotic ringspot virus (PNRSV) RNA3 hairpin caused resistance to PNRSV in wild-type scion grafted on transgenic rootstock (Zhao and Song, 2014). These findings highlight the potential of RNAi in both gene-function studies and crop improvement.
Transgenic technologies have inherent limitations in fruit trees. For example, developing transgenic plants requires a long time and many fruit tree species are recalcitrant to tissue culture required for development of transgenic plants (Lobato-Gómez et al., 2021). These limitations can be overcome by using a virus-induced gene regulation (VIGR) system. VIGR technology exploits the ability of viruses to multiply in plants and the plant antiviral defence system to overexpress (virus-induced gene overexpression (VIGO)) and silence (VIGS) target genes in plants (Paudel et al., 2022). Tobacco rattle virus (TRV) and apple latent spherical virus (ALSV) based VIGR systems are two of the most common systems used for VIGS and VIGO in fruit trees such as peach, apple, European pear, Japanese pear, almond and mango (Paudel et al., 2022; Pathak et al., 2023). In fruit trees, VIGR systems have been mostly used for functional genomics to characterise genes regulating different biological pathways such as carotenoid accumulation and fruit ripening in peach (Li et al., 2017) and mango (Pathak et al., 2023), pathogen resistance in peach (Cui and Wang, 2017), and flowering in apple, citrus, Japanese pear and Vitis spp. (Velázquez et al., 2016; Yamagishi et al., 2016; Maeda et al., 2020). Apart from functional genomics studies, VIGR can also be used to reduce the juvenile phase in fruit trees for fast-track tree breeding (Figure 4). This approach was used in apple to select a fire blight-resistant apple genotype in fifth generation plants within seven years (Schlathölter et al., 2018). Considering that the juvenile phase of apple lasts for 5-12 years, this process would have taken several decades using traditional breeding methods.
However, there are also limitations to VIGR, including a limited host range of the viruses used (Silva et al., 2010), non-uniform gene silencing (Burch-Smith et al., 2004), and immunogenicity and unintended mutations in the host (Li et al., 2018). The use of nanoparticles to deliver RNA for gene silencing or plasmid DNA for gene expression may overcome some of these issues. One advantage of nanoparticles is their small size ranging from 1-100 nm in at least one dimension (Cunningham et al., 2018). As such nanoparticles can be designed to fit within the size exclusion limit of cell wall pores, usually ~20 nm (Kumar et al., 2020) allowing for the application of nanoparticles to potentially any plant species. Larger nanoparticles can also be modified to cause structural changes to the cell wall to allow their entry (Kumar et al., 2020), which is beneficial for delivering larger cargo such as plasmid DNA. Modifications to nanoparticles can also allow for targeted subcellular localisation such as to the chloroplast or mitochondria (Yoshizumi et al., 2018; Thagun et al., 2022). Furthermore, nanoparticles can deliver a wide range of cargo to the cell, including DNA, RNA, protein, plant hormones and chemicals such as pesticides and fertilisers (Kumar et al., 2020; Hu and Xianyu, 2021). While nanoparticles have been tested often in model species e.g. to deliver dsRNA or siRNA for gene silencing (Jiang et al., 2014; Demirer et al., 2019; Schwartz et al., 2020; Yong et al., 2022), plasmid DNA for transient overexpression (Chang et al., 2013; Demirer et al., 2019) or stable transformation (Burlaka et al., 2015; Zhao et al., 2017), or protein for stable transformation (Martin-Ortigosa et al., 2014), limited studies have been done in tree crops. Lipid-based nanovectors were used to deliver the phytohormones indole-3-butyric acid (IBA) and 1-Naphthaleneacetic acid (NAA) to olive trees to improve the rooting process both in vitro and in vivo (Clemente et al., 2018). And a recent study by Qiao et al. (2023) used nanovesicles for dsRNA delivery to induce gene silencing for crop protection against a fungal pathogen in grapevine, fox grape (Vitis labrusca), rose (Rosa hybrida), lettuce (Lactuca sativa) and tomato, demonstrating the applicability of this technology in tree crops and other perennial herbaceous crops.
Engineering new varieties by gene editing
Since the discovery of the Nobel prize winning CRISPR-Cas9 system by Jinek et al. (2012), the use of CRISPR-Cas9 and other Cas proteins to perform directed changes to the genome has dominated the field of genetic engineering. The benefits of this system are the precise manner in which these changes can be made, the potential for the technology to be applied to theoretically any organism, and the potential for engineering non-GM gene edited varieties. In fact, some CRISPR-edited crops have already been released such as ɣ-aminobutyric acid (GABA)-enriched tomatoes with improved nutritional profiles (Ezura, 2022) and mustard salad greens with loss-of-function myrosinase genes to improve the taste profile by eliminating pungency (Karlson et al., 2022). Several examples of CRISPR-edited tree and woody crops also exist (e.g. apple (Charrier et al., 2019), kiwifruit (Herath et al., 2022), Hongkong kumquat (Citrus hindsii; Zhu et al., 2019), sweet orange (Jia and Wang, 2014) and avocado (https://www.prnewswire.com/news-releases/ag-biotech-innovator-greenvenus-achieves-breakthrough-in-non-browning-avocado-through-gene-editing-301842939.html), although none have been commercially released yet, and many tree crops like macadamia and mango have no published examples of CRISPR-editing. Despite the successful use of CRISPR technologies in several tree crops, many challenges remain.
One challenge faced with CRISPR technologies is the transfection step. Agrobacterium is commonly used as the transfection vector for the CRISPR-Cas transgene, however, transfection rates are lower for differentiated tissue (e.g. epicotyl) compared to cell-based cultures (Dutt et al., 2018) and many species are not amenable to transfection by Agrobacterium (Song et al., 2019). The use of ribonucleoproteins (RNPs) (Mout et al., 2017b; Zhang et al., 2022) and/or nanoparticles (Mout et al., 2017a) to deliver CRISPR-Cas products may be one solution for species not amenable to Agrobacterium-mediated transformation. The use of cell-based cultures can also reduce the risk of chimerism, an issue often encountered when tissues such as epicotyls are transfected, however, this does not eliminate the risk completely (Sahijram and Bahadur, 2015). Chimerism may also be reduced through multiple rounds of shoot regeneration, as shown by Ding et al. (2020) in ‘Shanxin’ poplar (Populus davidiana × P. bolleana) trees. These methods also all require tissue culture to regenerate gene edited plants, which itself is a challenge in many tree crops. Recent innovative methods have successfully bypassed the tissue culture process by overexpressing developmental regulators to stimulate organogenesis in somatic cells generating gene edited plantlets within 2-4 weeks in maize (Lowe et al., 2018), Arabidopsis, Nicotiana benthamiana (Maher et al., 2020), and sorghum (Sorghum bicolor; Che et al., 2022). Another issue with many of these methods is the requirement for the insertion of transgenes expressing the CRISPR components. This means that the T1 generation is transgenic, and conventional breeding is required to select out the transgene. Yang L. et al. (2023) demonstrated a method to create a transgene-free T1 generation using rootstocks expressing mobile Cas9 mRNA and gRNA to create gene edited Arabidopsis and Brassica rapa. Mendelian genetics potentially allows for a transgene free T1 generation that carries a gene edit, even with a transgenic T0 generation, providing the transgene and gene edited allele are not linked. Cross-pollination allows for the T1 generation to be added to the conventional breeding pipeline, thus complementing, and not replacing known plant breeding techniques. However, the use of many of these advances in CRISPR have not yet been demonstrated in tree crops.
Another remaining challenge is the issue of chromatin accessibility influencing gene editing efficiency as chromatin can impede the Cas9-gRNA complex accessing the target sequence. Weiss et al. (2022) showed that methods to study chromatin accessibility, like ChIP-seq (by targeting histones associated with closed chromatin), Formaldehyde-Assisted Isolation of Regulatory Elements (FAIRE)-seq, and Assay for Transposase-Accessible Chromatin (ATAC)-seq, may also provide directions in improving editing efficiency of certain genes. Since gene expression also varies over time and per tissue type, gene expression data can also be leveraged to understand what tissues to target and when. Combining gene expression data with chromatin accessibility could also provide more defined targeting strategies.
The CRISPR system itself also has potential for improvement through methods like base-editing (Komor et al., 2016) and prime-editing (Anzalone et al., 2019). Both methods improve upon the standard CRISPR-Cas9 mechanism of inducing double-strand DNA breaks (DSBs) and relying on endogenous DNA repair pathway machinery to introduce errors, by inducing specific base pair edits without requiring DSBs. Base-editing utilises a Cas9 fused with a deaminase allowing for transition and some transversion mutations to occur without DSBs, while prime-editing utilises a Cas9 nickase fused with a reverse transcriptase variant to create a single-strand DNA break which, along with a guide RNA containing an edited RNA template, can introduce both transition and transversion mutations, as well as small insertion or deletion mutations (Chen and Liu, 2023). Although both base-editing and prime-editing have been demonstrated in several plant species (Azameti and Dauda, 2021), its use in plants is still in early stages and requires improvement to be viable for commercial breeding. Other improvements include the use of different Cas proteins, like Cpf1/Cas12a (Zetsche et al., 2015; Zhang et al., 2022) with different recognition sites.
Even if the science of CRISPR-Cas shows potential, challenges remain on the regulatory end. CRISPR-Cas9 is a patented system with Corteva and MIT, Broad Institute holding the licensing rights (Corteva Agriscience, n.d.), which means royalties from products using the technology must be considered. Whether CRISPR-edited crops are considered a GMO differs around the world. Jurisdictions like New Zealand (Kershen, 2015) and the European Union (EU; Court of Justice of the European Union (CJEU), 2018) regulate all CRISPR gene edited plants as GMOs. While in Australia, a CRISPR gene edited organism is not considered as a GMO if no foreign genetic material is present (Mallapaty, 2019), though this must be proven to regulators using techniques like genome sequencing. The legal landscape is also constantly evolving with the EU recently requesting for comment changes to the regulations surrounding gene editing (Laaninen, 2021; Mehta, 2023). Additionally, most CRISPR gene edited plants involve the insertion of a transgene in the T1 generation, making them GMO in many jurisdictions, and requiring multiple rounds of breeding to select out the transgene. The European Patent Office also decided a case in 1999 where a key decision stated that ‘plant varieties containing genes introduced into an ancestral plant by recombinant gene technology are excluded from patentability’ (European Patent Office, 1999, p.35). By extension, this decision likely applies to CRISPR-edited plants which are seen as GMOs in the EU further reducing incentives for using gene editing in the EU agricultural sector. Despite these legal challenges, CRISPR has opened multiple possibilities for improving tree breeding. However, the many limitations associated with the use of CRISPR in trees, along with regulatory hurdles mean that CRISPR-edited tree products will likely not get to the market immediately.
Concluding remarks
The rich history of traditional horticultural tree breeding has domesticated a diverse variety of fruits, refined through generations of crossing and selection. This process has generated high-yielding citrus, apple, almond and avocado cultivars, among others, that have become the staple fruits produced in orchards around the world. Yet, in the face of evolving challenges and opportunities posed by shifting climatic patterns, the growing demand for food and the emergence of automation, the limitations of conventional breeding methods are becoming increasingly apparent. To effectively address these threats to horticultural production and to rapidly develop resilient varieties, there is a need for greater integration of genome enabled technologies into horticultural tree breeding programs. Apple breeding is one of several industries that has led the way for integration, showcasing remarkable success in expediting the development of novel cultivars boasting enhanced disease resistance, drought resilience, and superior fruit quality. The advent of low-cost, large-scale genome sequencing and the expanding repertoire of genomic approaches, such as those outlined in this review, are shifting the research and development landscape in tree crop species enabling greater integration of genome-enabled technologies across the horticultural tree crop breeding sector. By embracing genome enabled technologies in traditional breeding programs, we not only increase our capacity to develop new varieties, but also produce tree crops better suited to existing and emerging challenges, safeguarding the future of next generation orchards.
Author contributions
SK: Conceptualization, Writing – original draft, Writing – review & editing, Visualization. SS: Writing – original draft, Writing – review & editing. LP: Writing – original draft, Writing – review & editing, Visualization. MM: Writing – original draft, Writing – review & editing. LS: Writing – original draft, Writing – review & editing. AJ: Writing – original draft. JV: Writing – original draft, Writing – review & editing. MT: Writing – review & editing. CC: Conceptualization, Writing – review & editing, Writing – original draft. EV-G: Conceptualization, Writing – original draft, Writing – review & editing, Visualization. PP: Writing – original draft, Writing – review & editing.
Funding
The author(s) declare financial support was received for the research, authorship, and/or publication of this article. This work was supported by the Hort Innovation National Tree Genomics Program (AS17000) and the Australian Government DAWE 2022 Science and Innovation Award.
Acknowledgments
We acknowledge Associate Professor Cassandra Collins from the University of Adelaide for providing the almond photos in Figures 2A, E, Ian Bally from the Queensland Department of Agriculture and Fisheries the Queensland for providing the mango photos in Figures 2B, D, and the New Zealand Institute for Plant and Food Research for providing the apple fruit photo in Figure 2C.
Conflict of interest
The authors declare that the research was conducted in the absence of any commercial or financial relationships that could be construed as a potential conflict of interest.
The author(s) declared that they were an editorial board member of Frontiers, at the time of submission. This had no impact on the peer review process and the final decision.
Publisher’s note
All claims expressed in this article are solely those of the authors and do not necessarily represent those of their affiliated organizations, or those of the publisher, the editors and the reviewers. Any product that may be evaluated in this article, or claim that may be made by its manufacturer, is not guaranteed or endorsed by the publisher.
References
Adami, M., De Franceschi, P., Brandi, F., Liverani, A., Giovannini, D., Rosati, C., et al. (2013). Identifying a carotenoid cleavage dioxygenase (ccd4) gene gontrolling yellow/white fruit flesh color of peach. Plant Mol. Biol. Rep. 31, 1166–1175. doi: 10.1007/s11105-013-0628-6
Agüero, J., Vives, M., del, C., Velázquez, K., Pina, J. A., Navarro, L., et al. (2014). Effectiveness of gene silencing induced by viral vectors based on Citrus leaf blotch virus is different in Nicotiana benthamiana and citrus plants. Virology 460–461, 154–164. doi: 10.1016/j.virol.2014.04.017
Agustí, M., Mesejo, C., Muñoz-Fambuena, N., Vera-Sirera, F., de Lucas, M., Martínez-Fuentes, A., et al. (2020). Fruit-dependent epigenetic regulation of flowering in Citrus. New Phytol. 225, 376–384. doi: 10.1111/nph.16044
Ahmed, A. U., Hoddinott, J., Abedin, N., Hossain, N. (2021). The impacts of GM foods: Results from a randomized controlled trial of Bt eggplant in Bangladesh. Am. J. Agric. Econ. 103, 1186–1206. doi: 10.1111/ajae.12162
Alburquerque, N., García-Montiel, F., Carrillo, A., Burgos, L. (2008). Chilling and heat requirements of sweet cherry cultivars and the relationship between altitude and the probability of satisfying the chill requirements. Environ. Exp. Bot. 64, 162–170. doi: 10.1016/j.envexpbot.2008.01.003
Allard, A., Bink, M. C. A. M., Martinez, S., Kelner, J.-J., Legave, J.-M., di Guardo, M., et al. (2016). Detecting QTLs and putative candidate genes involved in budbreak and flowering time in an apple multiparental population. J. Exp. Bot. 67, 2875–2888. doi: 10.1093/jxb/erw130
Amasino, R. (2009). Floral induction and monocarpic versus polycarpic life histories. Genome Biol. 10, 228. doi: 10.1186/gb-2009-10-7-228
An, L., Lei, H., Shen, X., Li, T. (2012). Identification and characterization of PpLFL, a homolog of FLORICAULA/LEAFY in peach (Prunus persica). Plant Mol. Biol. Rep. 30, 1488–1495. doi: 10.1007/s11105-012-0459-x
André, D., Marcon, A., Lee, K. C., Goretti, D., Zhang, B., Delhomme, N., et al. (2022). FLOWERING LOCUS T paralogs control the annual growth cycle in Populus trees. Curr. Biol. 32, 2988–2996.e4. doi: 10.1016/j.cub.2022.05.023
Anzalone, A. V., Randolph, P. B., Davis, J. R., Sousa, A. A., Koblan, L. W., Levy, J. M., et al. (2019). Search-and-replace genome editing without double-strand breaks or donor DNA. Nature 576, 149–157. doi: 10.1038/s41586-019-1711-4
Atkinson, C. J., Brennan, R. M., Jones, H. G. (2013). Declining chilling and its impact on temperate perennial crops. Environ. Exp. Bot. 91, 48–62. doi: 10.1016/j.envexpbot.2013.02.004
Aydinalp, C., Cresser, M. S. (2008). The effects of global climate change on agriculture. Am.-Eurasian J. Agric. Environ. Sci. 3, 672–676.
Azameti, M. K., Dauda, W. P. (2021). Base editing in plants: applications, challenges, and future prospects. Front. Plant Sci. 12. doi: 10.3389/fpls.2021.664997
Azeez, A., Miskolczi, P., Tylewicz, S., Bhalerao, R. P. (2014). A tree ortholog of APETALA1 mediates photoperiodic control of seasonal growth. Curr. Biol. 24, 717–724. doi: 10.1016/j.cub.2014.02.037
Bally, I. S. E., Bombarely, A., Chambers, A. H., Cohen, Y., Dillon, N. L., Innes, D. J., et al. (2021). The ‘Tommy Atkins’ mango genome reveals candidate genes for fruit quality. BMC Plant Biol. 21, 108. doi: 10.1186/s12870-021-02858-1
Baranski, R., Klimek-Chodacka, M., Lukasiewicz, A. (2019). Approved genetically modified (GM) horticultural plants: A 25-year perspective. Folia Hortic. 31, 3–49. doi: 10.2478/fhort-2019-0001
Beauvieux, R., Wenden, B., Dirlewanger, E. (2018). Bud dormancy in perennial fruit tree species: a pivotal role for oxidative cues. Front. Plant Sci. 9. doi: 10.3389/fpls.2018.00657
Bielenberg, D. G., Wang, Y. E., Li, Z., Zhebentyayeva, T., Fan, S., Reighard, G. L., et al. (2008). Sequencing and annotation of the evergrowing locus in peach [Prunus persica (L.) Batsch] reveals a cluster of six MADS-box transcription factors as candidate genes for regulation of terminal bud formation. Tree Genet. Genomes 4, 495–507. doi: 10.1007/s11295-007-0126-9
Brian, L., Warren, B., McAtee, P., Rodrigues, J., Nieuwenhuizen, N., Pasha, A., et al. (2021). A gene expression atlas for kiwifruit (Actinidia chinensis) and network analysis of transcription factors. BMC Plant Biol. 21, 121. doi: 10.1186/s12870-021-02894-x
Brunner, A. M., Varkonyi-Gasic, E., Jones, R. C. (2017). “Phase change and phenology in trees,” in Comparative and evolutionary genomics of angiosperm trees. Eds. Groover, A., Cronk, Q. (Cham: Springer International Publishing), 227–274. doi: 10.1007/7397_2016_30
Bulley, S. M., Wilson, F. M., Hedden, P., Phillips, A. L., Croker, S. J., James, D. J. (2005). Modification of gibberellin biosynthesis in the grafted apple scion allows control of tree height independent of the rootstock. Plant Biotechnol. J. 3, 215–223. doi: 10.1111/j.1467-7652.2005.00119.x
Burch-Smith, T. M., Anderson, J. C., Martin, G. B., Dinesh-Kumar, S. P. (2004). Applications and advantages of virus-induced gene silencing for gene function studies in plants. Plant J. 39, 734–746. doi: 10.1111/j.1365-313X.2004.02158.x
Burlaka, O. M., Pirko, Y. V., Yemets, A. I., Blume, Y. B. (2015). Plant genetic transformation using carbon nanotubes for DNA delivery. Cytol. Genet. 49, 349–357. doi: 10.3103/S009545271506002X
Campoy, J. A., Ruiz, D., Egea, J. (2011). Dormancy in temperate fruit trees in a global warming context: a review. Sci. Hortic. 130, 357–372. doi: 10.1016/j.scienta.2011.07.011
Canton, M., Farinati, S., Forestan, C., Joseph, J., Bonghi, C., Varotto, S. (2022). An efficient chromatin immunoprecipitation (ChIP) protocol for studying histone modifications in peach reproductive tissues. Plant Methods 18, 43. doi: 10.1186/s13007-022-00876-0
Chagné, D., Vanderzande, S., Kirk, C., Profitt, N., Weskett, R., Gardiner, S. E., et al. (2019). Validation of SNP markers for fruit quality and disease resistance loci in apple (Malus × domestica Borkh.) using the OpenArray® platform. Hortic. Res. 6, 30. doi: 10.1038/s41438-018-0114-2
Chang, F.-P., Kuang, L.-Y., Huang, C.-A., Jane, W.-N., Hung, Y., Hsing, Y. C., et al. (2013). A simple plant gene delivery system using mesoporous silica nanoparticles as carriers. J. Mater. Chem. B 1, 5279–5287. doi: 10.1039/C3TB20529K
Charrier, A., Vergne, E., Dousset, N., Richer, A., Petiteau, A., Chevreau, E. (2019). Efficient targeted mutagenesis in apple and first time edition of pear using the CRISPR-Cas9 system. Front. Plant Sci. 10. doi: 10.3389/fpls.2019.00040
Che, P., Wu, E., Simon, M. K., Anand, A., Lowe, K., Gao, H., et al. (2022). Wuschel2 enables highly efficient CRISPR/Cas-targeted genome editing during rapid de novo shoot regeneration in sorghum. Commun. Biol. 5, 344 doi: 10.1038/s42003-022-03308-w
Chen, Z.-L., Gu, H., Li, Y., Su, Y., Wu, P., Jiang, Z., et al. (2003). Safety assessment for genetically modified sweet pepper and tomato. Toxicology 188, 297–307. doi: 10.1016/S0300-483X(03)00111-2
Chen, P. J., Liu, D. R. (2023). Prime editing for precise and highly versatile genome manipulation. Nat. Rev. Genet. 24, 161–177. doi: 10.1038/s41576-022-00541-1
Chen, T., Niu, J., Sun, Z., Chen, J., Wang, Y., Chen, J., et al. (2023). Transcriptome analysis and VIGS identification of key genes regulating citric acid metabolism in citrus. Curr. Issues Mol. Biol. 45, 4647–4664. doi: 10.3390/cimb45060295
Chen, W., Tamada, Y., Yamane, H., Matsushita, M., Osako, Y., Gao-Takai, M., et al. (2022). H3K4me3 plays a key role in establishing permissive chromatin states during bud dormancy and bud break in apple. Plant J. 111, 1015–1031. doi: 10.1111/tpj.15868
Chen, J., Xie, J., Duan, Y., Hu, H., Hu, Y., Li, W. (2016). Genome-wide identification and expression profiling reveal tissue-specific expression and differentially-regulated genes involved in gibberellin metabolism between Williams banana and its dwarf mutant. BMC Plant Biol. 16, 123. doi: 10.1186/s12870-016-0809-1
Chen, P., Yan, M., Li, L., He, J., Zhou, S., Li, Z., et al. (2020). The apple DNA-binding one zinc-finger protein MdDof54 promotes drought resistance. Hortic. Res. 7, 195. doi: 10.1038/s41438-020-00419-5
Chu, L. L., Yan, Z., Sheng, X. X., Liu, H. Q., Wang, Q. Y., Zeng, R. F., et al. (2023). Citrus ACC synthase CiACS4 regulates plant height by inhibiting gibberellin biosynthesis. Plant Physiol. 192, 1947–1968. doi: 10.1093/plphys/kiad159
Clemente, I., Menicucci, F., Colzi, I., Sbraci, L., Benelli, C., Giordano, C., et al. (2018). Unconventional and sustainable nanovectors for phytohormone delivery: insights on Olea europaea. ACS Sustain. Chem. Eng. 6, 15022–15031. doi: 10.1021/acssuschemeng.8b03489
Cooke, J. E. K., Eriksson, M. E., Junttila, O. (2012). The dynamic nature of bud dormancy in trees: environmental control and molecular mechanisms. Plant Cell Environ. 35, 1707–1728. doi: 10.1111/j.1365-3040.2012.02552.x
Corteva Agriscience CRISPR-Cas: growing more, sustainably. Available at: https://www.openinnovation.corteva.com/what-we-offer/crispr-cas-growing-more-sustainably.html.
Court of Justice of the European Union (CJEU). (2018). Case number = C-528/16. Available at: https://curia.europa.eu/juris/documents.jsf?num=C-528/16.
Cui, H., Wang, A. (2017). An efficient viral vector for functional genomic studies of Prunus fruit trees and its induced resistance to Plum pox virus via silencing of a host factor gene. Plant Biotechnol. J. 15, 344–356. doi: 10.1111/pbi.12629
Cunningham, F. J., Goh, N. S., Demirer, G. S., Matos, J. L., Landry, M. P. (2018). Nanoparticle-mediated delivery towards advancing plant genetic engineering. Trends Biotechnol. 36, 882–897. doi: 10.1016/j.tibtech.2018.03.009
Dardick, C., Callahan, A., Horn, R., Ruiz, K. B., Zhebentyayeva, T., Hollender, C., et al. (2013). PpeTAC1 promotes the horizontal growth of branches in peach trees and is a member of a functionally conserved gene family found in diverse plants species. Plant J. 75, 618–630. doi: 10.1111/tpj.12234
Das, A., Geetha, G. A., Ravishankar, K. V., Shivashankara, K. S., Roy, T. K., Dinesh, M. R. (2019). Interrelations of growth regulators, carbohydrates and expression of flowering genes (FT, LFY, AP1) in leaf and shoot apex of regular and alternate bearing mango (Mangifera indica L.) cultivars during flowering. Sci. Hortic. 253, 263–269. doi: 10.1016/j.scienta.2019.04.027
de Jong, M., Mariani, C., Vriezen, W. H. (2009). The role of auxin and gibberellin in tomato fruit set. J. Exp. Bot. 60, 1523–1532. doi: 10.1093/jxb/erp094
de la Fuente, L., Conesa, A., Lloret, A., Badenes, M. L., Ríos, G. (2015). Genome-wide changes in histone H3 lysine 27 trimethylation associated with bud dormancy release in peach. Tree Genet. Genomes 11, 45. doi: 10.1007/s11295-015-0869-7
Demirer, G. S., Zhang, H., Matos, J. L., Goh, N. S., Cunningham, F. J., Sung, Y., et al. (2019). High aspect ratio nanomaterials enable delivery of functional genetic material without DNA integration in mature plants. Nat. Nanotechnol. 14, 456–464. doi: 10.1038/s41565-019-0382-5
Dinesh, M. R., Reddy, B. M. C. (2012). “Physiological basis of growth and fruit yield characteristics of tropical and sub-tropical fruits to temperature,” in Tropical fruit tree species and climate change. Eds. Sthapit, B., Rao, V. R., Sthapit, S. (New Delhi: Bioversity International), 97–125.
Ding, L., Chen, Y., Ma, Y., Wang, H., Wei, J. (2020). Effective reduction in chimeric mutants of poplar trees produced by CRISPR/Cas9 through a second round of shoot regeneration. Plant Biotechnol. Rep. 14, 549–558. doi: 10.1007/s11816-020-00629-2
Ding, J., Nilsson, O. (2016). Molecular regulation of phenology in trees — because the seasons they are a-changin’. Curr. Opin. Plant Biol. 29, 73–79. doi: 10.1016/j.pbi.2015.11.007
Ding, F., Zhang, S., Chen, H., Peng, H., Lu, J., He, X., et al. (2018). Functional analysis of a homologue of the FLORICAULA/LEAFY gene in litchi (Litchi chinensis Sonn.) revealing its significance in early flowering process. Genes Genomics 40, 1259–1267. doi: 10.1007/s13258-018-0739-4
Ding, F., Zhang, S., Chen, H., Su, Z., Zhang, R., Xiao, Q., et al. (2015). Promoter difference of LcFT1 is a leading cause of natural variation of flowering timing in different litchi cultivars (Litchi chinensis Sonn.). Plant Sci. 241, 128–137. doi: 10.1016/j.plantsci.2015.10.004
Ding, T., Zhang, R., Zhang, H., Zhou, Z., Liu, C., Wu, M., et al. (2021). Identification of gene co-expression networks and key genes regulating flavonoid accumulation in apple (Malus × domestica) fruit skin. Plant Sci. 304, 110747. doi: 10.1016/j.plantsci.2020.110747
Doebley, J., Stec, A., Gustus, C. (1995). teosinte branched1 and the origin of maize: evidence for epistasis and the evolution of dominance. Genetics 141, 333–346. doi: 10.1093/genetics/141.1.333
Doebley, J., Stec, A., Hubbard, L. (1997). The evolution of apical dominance in maize. Nature 386, 485–488. doi: 10.1038/386485a0
Dougherty, L., Borejsza-Wysocka, E., Miaule, A., Wang, P., Zheng, D., Jansen, M., et al. (2023). A single amino acid substitution in MdLAZY1A dominantly impairs shoot gravitropism in Malus. Plant Physiol. 193, 1142–1160. doi: 10.1093/plphys/kiad373
Dutt, M., Erpen, L., Grosser, J. W. (2018). Genetic transformation of the ‘W Murcott’ tangor: comparison between different techniques. Sci. Hortic. 242, 90–94. doi: 10.1016/j.scienta.2018.07.026
Dutt, M., Mahmoud, L. M., Nehela, Y., Grosser, J. W., Killiny, N. (2022). The Citrus sinensis TILLER ANGLE CONTROL 1 (CsTAC1) gene regulates tree architecture in sweet oranges by modulating the endogenous hormone content. Plant Sci. 323, 111401. doi: 10.1016/j.plantsci.2022.111401
Duval, H., Van Ghelder, C., Callot, C., Esmenjaud, D. (2018). Characterization of the RMja resistance gene to root-knot nematodes from the ‘Alnem’ almond rootstock. Acta Hortic. (1219), 325–330. doi: 10.17660/ActaHortic.2018.1219.49
Elo, A., Lemmetyinen, J., Novak, A., Keinonen, K., Porali, I., Hassinen, M., et al. (2007). BpMADS4 has a central role in inflorescence initiation in silver birch (Betula pendula). Physiol. Plant 131, 149–158. doi: 10.1111/j.1399-3054.2007.00947.x
Elsysy, M., Hirst, P. M. (2019). Molecular basis of flower formation in apple caused by defoliation and gibberellins. J. Amer. Soc Hortic. Sci. 144, 414–419. doi: 10.21273/jashs04760-19
Endo, T., Shimada, T., Fujii, H., Kobayashi, Y., Araki, T., Omura, M. (2005). Ectopic expression of an FT homolog from Citrus confers an early flowering phenotype on trifoliate orange (Poncirus trifoliata L. Raf.). Transgenic Res. 14, 703–712. doi: 10.1007/s11248-005-6632-3
European Patent Office. (1999). G 0001/98 (Transgenic plant/NOVARTIS II) 20-12-1999. Available at: https://www.epo.org/en/case-law-appeals/decisions/recent/g980001ex1.
Ezura, H. (2022). Letter to the editor: the world’s first CRISPR tomato launched to a Japanese market: the social-economic impact of its implementation on crop genome editing. Plant Cell Physiol. 63, 731–733. doi: 10.1093/pcp/pcac048
Fadón, E., Herrera, S., Guerrero, B., Guerra, M., Rodrigo, J. (2020). Chilling and heat requirements of temperate stone fruit trees (Prunus sp.). Agronomy 10, 409. doi: 10.3390/agronomy10030409
Falavigna, V. D. S., Severing, E., Lai, X., Estevan, J., Farrera, I., Hugouvieux, V., et al. (2021). Unraveling the role of MADS transcription factor complexes in apple tree dormancy. New Phytol. 232, 2071–2088. doi: 10.1111/nph.17710
Fan, Z.-Y., He, X.-H., Fan, Y., Yu, H.-X., Wang, Y.-H., Xie, X.-J., et al. (2020). Isolation and functional characterization of three MiFTs genes from mango. Plant Physiol. Biochem. 155, 169–176. doi: 10.1016/j.plaphy.2020.07.009
Felipe, J. E. L., Lachica, J. A. P., Dela Cueva, F. M., Laurel, N. R., Alcasid, C. E., Sison, M. L. J., et al. (2022). Validation and molecular analysis of β-1,3-GLU2 SNP marker associated with resistance to Colletotrichum gloeosporioides in mango (Mangifera indica L.). Physiol. Mol. Plant Pathol. 118, 101804. doi: 10.1016/j.pmpp.2022.101804
Feng, C., Zhang, X., Du, B., Xiao, Y., Wang, Y., Sun, Y., et al. (2023). MicroRNA156ab regulates apple plant growth and drought tolerance by targeting transcription factor MsSPL13. Plant Physiol. 192, 1836–1857. doi: 10.1093/plphys/kiad099
Ferreira, S. A., Pitz, K. Y., Manshardt, R., Zee, F., Fitch, M., Gonsalves, D. (2002). Virus coat protein transgenic papaya provides practical control of Papaya ringspot virus in Hawaii. Plant Dis. 86, 101–105. doi: 10.1094/PDIS.2002.86.2.101
Fichtner, F., Lunn, J. E. (2021). The role of trehalose 6-phosphate (Tre6P) in plant metabolism and development. Annu. Rev. Plant Biol. 72, 737–760. doi: 10.1146/annurev-arplant-050718-095929
Fisher, D. V. (1969). Spur-type strains of McIntosh for high density plantings. Br. Columbia Fruit Growers’ Assoc. Q. Rep. 14, 3–10.
Flachowsky, H., Le Roux, P.-M., Peil, A., Patocchi, A., Richter, K., Hanke, M.-V. (2011). Application of a high-speed breeding technology to apple (Malus × domestica) based on transgenic early flowering plants and marker-assisted selection. New Phytol. 192, 364–377. doi: 10.1111/j.1469-8137.2011.03813.x
Flachowsky, H., Peil, A., Sopanen, T., Elo, A., Hanke, V. (2007). Overexpression of BpMADS4 from silver birch (Betula pendula Roth.) induces early-flowering in apple (Malus × domestica Borkh.). Plant Breed. 126, 137–145. doi: 10.1111/j.1439-0523.2007.01344.x
Flachowsky, H., Szankowski, I., Waidmann, S., Peil, A., Tränkner, C., Hanke, M.-V. (2012). The MdTFL1 gene of apple (Malus x domestica Borkh.) reduces vegetative growth and generation time. Tree Physiol. 32, 1288–1301. doi: 10.1093/treephys/tps080
Freiman, A., Golobovitch, S., Yablovitz, Z., Belausov, E., Dahan, Y., Peer, R., et al. (2015). Expression of flowering locus T2 transgene from Pyrus communis L. delays dormancy and leaf senescence in Malus × domestica Borkh, and causes early flowering in tobacco. Plant Sci. 241, 164–176. doi: 10.1016/j.plantsci.2015.09.012
Freiman, A., Shlizerman, L., Golobovitch, S., Yablovitz, Z., Korchinsky, R., Cohen, Y., et al. (2012). Development of a transgenic early flowering pear (Pyrus communis L.) genotype by RNAi silencing of PcTFL1-1 and PcTFL1-2. Planta 235, 1239–1251. doi: 10.1007/s00425-011-1571-0
Fu, X. Z., Khan, E. U., Hu, S. S., Fan, Q. J., Liu, J. H. (2011). Overexpression of the betaine aldehyde dehydrogenase gene from Atriplex hortensis enhances salt tolerance in the transgenic trifoliate orange (Poncirus trifoliata L. Raf.). Environ. Exp. Bot. 74, 106–113. doi: 10.1016/j.envexpbot.2011.05.006
Geng, M., Shao, Y., Zhang, M., Zheng, X., Tan, B., Wang, W., et al. (2022). Overexpression of peach NAC25 promotes anthocyanin biosynthesis in poplar shoots. Fruit Res. 2, 1–9. doi: 10.48130/FruRes-2022-0021
Gerashchenkov, G. A., Rozhnova, N. A. (2013). The involvement of phytohormones in the plant sex regulation. Russ. J. Plant Physiol. 60, 597–610. doi: 10.1134/S1021443713050063
Goldberg-Moeller, R., Shalom, L., Shlizerman, L., Samuels, S., Zur, N., Ophir, R., et al. (2013). Effects of gibberellin treatment during flowering induction period on global gene expression and the transcription of flowering-control genes in Citrus buds. Plant Sci. 198, 46–57. doi: 10.1016/j.plantsci.2012.09.012
Gonsalves, C., Lee, D. R., Gonsalves, D. (2007). The adoption of genetically modified papaya in Hawaii and its implications for developing countries. J. Dev. Stud. 43, 177–191. doi: 10.1080/00220380601055650
Goonetilleke, S. N., March, T. J., Wirthensohn, M. G., Arús, P., Walker, A. R., Mather, D. E. (2018). Genotyping by sequencing in almond: SNP discovery, linkage mapping, and marker design. G3: Genes Genomes Genet. 8, 161–172. doi: 10.1534/g3.117.300376
Guitton, B., Kelner, J. J., Celton, J. M., Sabau, X., Renou, J. P., Chagne, D., et al. (2016). Analysis of transcripts differentially expressed between fruited and deflowered “Gala” adult trees: a contribution to biennial bearing understanding in apple. BMC Plant Biol. 16, 22. doi: 10.1186/s12870-016-0739-y
Haberman, A., Ackerman, M., Crane, O., Kelner, J. J., Costes, E., Samach, A. (2016). Different flowering response to various fruit loads in apple cultivars correlates with degree of transcript reaccumulation of a TFL1-encoding gene. Plant J. 87, 161–173. doi: 10.1111/tpj.13190
Hackett, W. P. (1985). “Juvenility, maturation, and rejuvenation in woody plants,” in Horticultural reviews. Ed. Janick, J. (John Wiley & Sons, Ltd), 109–155. doi: 10.1002/9781118060735.ch3
Haim, D., Shalom, L., Simhon, Y., Shlizerman, L., Kamara, I., Morozov, M., et al. (2021). Alternate bearing in fruit trees: fruit presence induces polar auxin transport in citrus and olive stem and represses IAA release from the bud. J. Exp. Bot. 72, 2450–2462. doi: 10.1093/jxb/eraa590
Hao, G., Stover, E., Gupta, G. (2016). Overexpression of a modified plant thionin enhances disease resistance to citrus canker and huanglongbing (HLB). Front. Plant Sci. 7. doi: 10.3389/fpls.2016.01078
Haque, S., Ahmad, J. S., Clark, N. M., Williams, C. M., Sozzani, R. (2019). Computational prediction of gene regulatory networks in plant growth and development. Curr. Opin. Plant Biol. 47, 96–105. doi: 10.1016/j.pbi.2018.10.005
Haque, M. A., Sakimin, S. Z. (2022). Planting arrangement and effects of planting density on tropical fruit crops—a review. Horticulturae 8, 485. doi: 10.3390/horticulturae8060485
Herath, D., Voogd, C., Mayo-Smith, M., Yang, B., Allan, A. C., Putterill, J., et al. (2022). CRISPR-Cas9-mediated mutagenesis of kiwifruit BFT genes results in an evergrowing but not early flowering phenotype. Plant Biotechnol. J. 20, 2064–2076. doi: 10.1111/pbi.13888
Herath, D., Wang, T., Voogd, C., Peng, Y., Douglas, M., Putterill, J., et al. (2023). Strategies for fast breeding and improvement of Actinidia species. Hortic. Res. 10, uhad016. doi: 10.1093/hr/uhad016
Herrera-Estrella, L., Depicker, A., Van Montagu, M., Schell, J. (1983). Expression of chimaeric genes transferred into plant cells using a Ti-plasmid-derived vector. Nature 303, 209–213. doi: 10.1038/303209a0
Hollender, C. A., Dardick, C. (2015). Molecular basis of angiosperm tree architecture. New Phytol. 206, 541–556. doi: 10.1111/nph.13204
Hollender, C. A., Hadiarto, T., Srinivasan, C., Scorza, R., Dardick, C. (2016). A brachytic dwarfism trait (dw) in peach trees is caused by a nonsense mutation within the gibberellic acid receptor PpeGID1c. New Phytol. 210, 227–239. doi: 10.1111/nph.13772
Hollender, C. A., Pascal, T., Tabb, A., Hadiarto, T., Srinivasan, C., Wang, W., et al. (2018a). Loss of a highly conserved sterile alpha motif domain gene (WEEP) results in pendulous branch growth in peach trees. Proc. Natl. Acad. Sci. U.S.A. 115, E4690-E4699. doi: 10.1073/pnas.1704515115
Hollender, C. A., Waite, J. M., Tabb, A., Raines, D., Chinnithambi, S., Dardick, C. (2018b). Alteration of TAC1 expression in Prunus species leads to pleiotropic shoot phenotypes. Hortic. Res. 5, 1–9. doi: 10.1038/s41438-018-0034-1
Hsu, C.-Y., Adams, J. P., Kim, H., No, K., Ma, C., Strauss, S. H., et al. (2011). FLOWERING LOCUS T duplication coordinates reproductive and vegetative growth in perennial poplar. Proc. Natl. Acad. Sci. U.S.A. 108, 10756–10761. doi: 10.1073/pnas.1104713108
Hu, J., Gao, C. (2023). CRISPR-edited plants by grafting. Nat. Biotechnol. 41, 909–910. doi: 10.1038/s41587-022-01516-7
Hu, Y., Han, Z., Sun, Y., Wang, S., Wang, T., Wang, Y., et al. (2020). ERF4 affects fruit firmness through TPL4 by reducing ethylene production. Plant J. 103, 937–950. doi: 10.1111/tpj.14884
Hu, Y., Han, Z., Wang, T., Li, H., Li, Q., Wang, S., et al. (2022a). Ethylene response factor MdERF4 and histone deacetylase MdHDA19 suppress apple fruit ripening through histone deacetylation of ripening-related genes. Plant Physiol. 188, 2166–2181. doi: 10.1093/plphys/kiac016
Hu, J. H., Israeli, A., Ori, N., Sun, T. P. (2018). The interaction between DELLA and ARF/IAA mediates crosstalk between gibberellin and auxin signaling to control fruit initiation in tomato. Plant Cell 30, 1710–1728. doi: 10.1105/tpc.18.00363
Hu, Y., Sun, H., Han, Z., Wang, S., Wang, T., Li, Q., et al. (2022b). ERF4 affects fruit ripening by acting as a JAZ interactor between ethylene and jasmonic acid hormone signaling pathways. Hortic. Plant J. 8, 689–699. doi: 10.1016/j.hpj.2022.01.002
Hu, J., Xianyu, Y. (2021). When nano meets plants: a review on the interplay between nanoparticles and plants. Nano Today 38, 101143. doi: 10.1016/j.nantod.2021.101143
Huang, Y., He, J., Xu, Y., Zheng, W., Wang, S., Chen, P., et al. (2023). Pangenome analysis provides insight into the evolution of the orange subfamily and a key gene for citric acid accumulation in citrus fruits. Nat. Genet. 55, 1–12. doi: 10.1038/s41588-023-01516-6
Huang, M., Roose, M. L., Yu, Q., Stover, E., Hall, D. G., Deng, Z., et al. (2023). Mapping of QTLs and candidate genes associated with multiple phenotypic traits for Huanglongbing tolerance in citrus. Hortic. Plant J. 9, 705–719. doi: 10.1016/j.hpj.2022.10.008
Huang, X., Wang, Y., Wang, N. (2022). Highly efficient generation of canker-resistant sweet orange enabled by an improved CRISPR/Cas9 system. Front. Plant Sci. 12. doi: 10.3389/fpls.2021.769907
Imai, A., Kuniga, T., Yoshioka, T., Nonaka, K., Mitani, N., Fukamachi, H., et al. (2019). Single-step genomic prediction of fruit-quality traits using phenotypic records of non-genotyped relatives in citrus. PloS One 14, e0221880. doi: 10.1371/journal.pone.0221880
IPCC. (2023). AR6 synthesis report: climate change 2023. Available at: https://www.ipcc.ch/report/ar6/syr/ (Accessed September 22, 2023).
Irenaeus, T., Mitra, S. K. (2014). Understanding the pollen and ovule characters and fruit set of fruit crops in relation to temperature and genotype – a review. J. Appl. Bot. Food Qual. 87, 157–167. doi: 10.5073/JABFQ.2014.087.023
Jia, H., Omar, A. A., Orbović, V., Wang, N. (2022). Biallelic editing of the LOB1 promoter via CRISPR/Cas9 creates canker-resistant ‘Duncan’ grapefruit. Phytopathology 112, 308–314. doi: 10.1094/PHYTO-04-21-0144-R
Jia, H., Orbovic, V., Jones, J. B., Wang, N. (2016a). Modification of the PthA4 effector binding elements in Type I CsLOB1 promoter using Cas9/sgRNA to produce transgenic Duncan grapefruit alleviating XccΔpthA4:dCsLOB1.3 infection. Plant Biotechnol. J. 14, 1291–1301. doi: 10.1111/pbi.12495
Jia, H., Wang, N. (2014). Targeted genome editing of sweet orange using Cas9/sgRNA. PloS One 9, e93806. doi: 10.1371/journal.pone.0093806
Jia, H., Zhang, Y., Orbović, V., Xu, J., White, F. F., Jones, J. B., et al. (2017). Genome editing of the disease susceptibility gene CsLOB1 in citrus confers resistance to citrus canker. Plant Biotechnol. J. 15, 817–823. doi: 10.1111/pbi.12677
Jia, H., Zhang, C., Pervaiz, T., Zhao, P., Liu, Z., Wang, B., et al. (2016b). Jasmonic acid involves in grape fruit ripening and resistant against Botrytis cinerea. Funct. Integr. Genomics 16, 79–94. doi: 10.1007/s10142-015-0468-6
Jiang, L., Ding, L., He, B., Shen, J., Xu, Z., Yin, M., et al. (2014). Systemic gene silencing in plants triggered by fluorescent nanoparticle-delivered double-stranded RNA. Nanoscale 6, 9965–9969. doi: 10.1039/C4NR03481C
Jiang, X., Liu, K., Peng, H., Fang, J., Zhang, A., Han, Y., et al. (2023). Comparative network analysis reveals the dynamics of organic acid diversity during fruit ripening in peach (Prunus persica L. Batsch). BMC Plant Biol. 23, 16. doi: 10.1186/s12870-023-04037-w
Jiang, Y., Liu, C., Yan, D., Wen, X., Liu, Y., Wang, H., et al. (2017). MdHB1 down-regulation activates anthocyanin biosynthesis in the white-fleshed apple cultivar ‘Granny Smith.’. J. Exp. Bot. 68, 1055–1069. doi: 10.1093/jxb/erx029
Jiang, L., Shen, W., Liu, C., Tahir, M. M., Li, X., Zhou, S., et al. (2022). Engineering drought-tolerant apple by knocking down six GH3 genes and potential application of transgenic apple as a rootstock. Hortic. Res. 9, uhac122. doi: 10.1093/hr/uhac122
Jiang, J., Tan, L., Zhu, Z., Fu, Y., Liu, F., Cai, H., et al. (2012). Molecular evolution of the TAC1 gene from rice (Oryza sativa L.). J. Genet. Genomics 39, 551–560. doi: 10.1016/j.jgg.2012.07.011
Jin, R., Klasfeld, S., Zhu, Y., Fernandez Garcia, M., Xiao, J., Han, S.-K., et al. (2021). LEAFY is a pioneer transcription factor and licenses cell reprogramming to floral fate. Nat. Commun. 12, 626. doi: 10.1038/s41467-020-20883-w
Jin, Z., Wang, J., Cao, X., Wei, C., Kuang, J., Chen, K., et al. (2022). Peach fruit PpNAC1 activates PpFAD3-1 transcription to provide ω-3 fatty acids for the synthesis of short-chain flavor volatiles. Hortic. Res. 9, uhac085. doi: 10.1093/hr/uhac085
Jinek, M., Chylinski, K., Fonfara, I., Hauer, M., Doudna, J. A., Charpentier, E. (2012). A programmable dual RNA–guided DNA endonuclease in adaptive bacterial immunity. Science 337, 816–822. doi: 10.1126/science.1225829
Jung, M., Keller, B., Roth, M., Aranzana, M. J., Auwerkerken, A., Guerra, W., et al. (2022). Genetic architecture and genomic predictive ability of apple quantitative traits across environments. Hortic. Res. 9, uhac028. doi: 10.1093/hr/uhac028
Karlgren, A., Gyllenstrand, N., Källman, T., Sundström, J. F., Moore, D., Lascoux, M., et al. (2011). Evolution of the PEBP gene family in plants: functional diversification in seed plant evolution. Plant Physiol. 156, 1967–1977. doi: 10.1104/pp.111.176206
Karlson, D., Mojica, J. P., Poorten, T. J., Lawit, S. J., Jali, S., Chauhan, R. D., et al. (2022). Targeted mutagenesis of the multicopy Myrosinase gene family in allotetraploid Brassica juncea reduces pungency in fresh leaves across environments. Plants 11, 2494. doi: 10.3390/plants11192494
Kawai, T., Gonoi, A., Nitta, M., Yamagishi, N., Yoshikawa, N., Tao, R. (2016). Virus-induced gene silencing in various Prunus species with the Apple latent spherical virus vector. Sci. Hortic. 199, 103–113. doi: 10.1016/j.scienta.2015.12.031
Kershen, D. L. (2015). Sustainability Council of New Zealand Trust v. The Environmental Protection Authority: Gene editing technologies and the law. GM Crops Food 6, 216–222. doi: 10.1080/21645698.2015.1122859
Khan, I. A., Cao, K., Guo, J., Li, Y., Wang, Q., Yang, X., et al. (2022). Identification of key gene networks controlling anthocyanin biosynthesis in peach flower. Plant Sci. 316, 111151. doi: 10.1016/j.plantsci.2021.111151
Khush, G. S. (1999). Green revolution: preparing for the 21st century. Genome 42, 646–655. doi: 10.1139/g99-044
Kim, D.-H., Doyle, M. R., Sung, S., Amasino, R. M. (2009). Vernalization: winter and the timing of flowering in plants. Annu. Rev. Cell Dev. Biol. 25, 277–299. doi: 10.1146/annurev.cellbio.042308.113411
Komor, A. C., Kim, Y. B., Packer, M. S., Zuris, J. A., Liu, D. R. (2016). Programmable editing of a target base in genomic DNA without double-stranded DNA cleavage. Nature 533, 420–424. doi: 10.1038/nature17946
Koshita, Y., Takahara, T., Ogata, T., Goto, A. (1999). Involvement of endogenous plant hormones (IAA, ABA, GAs) in leaves and flower bud formation of satsuma mandarin (Citrus unshiu Marc.). Sci. Hortic. 79, 185–194. doi: 10.1016/s0304-4238(98)00209-x
Kotoda, N., Hayashi, H., Suzuki, M., Igarashi, M., Hatsuyama, Y., Kidou, S., et al. (2010). Molecular characterization of FLOWERING LOCUS T-like genes of apple (Malus × domestica Borkh.). Plant Cell Physiol. 51, 561–575. doi: 10.1093/pcp/pcq021
Kotoda, N., Iwanami, H., Takahashi, S., Abe, K. (2006). Antisense expression of MdTFL1, a TFL1-like gene, reduces the juvenile phase in apple. J. Amer. Soc Hortic. Sci. 131, 74–81. doi: 10.21273/JASHS.131.1.74
Krishna, B., Vyavahare, S. N., Chaudhari, R. S., Subramaniam, Vr., Sane, P. V. (2017). Roles of Flowering Locus T (FT) and Terminal Flower 1 (TFL1) in flowering of mango. Acta Hortic. 1183, 125–132. doi: 10.17660/ActaHortic.2017.1183.17
Kumar, S., Chagné, D., Bink, M. C. A. M., Volz, R. K., Whitworth, C., Carlisle, C. (2012). Genomic selection for fruit quality traits in apple (Malus × domestica Borkh.). PloS One 7, e36674. doi: 10.1371/journal.pone.0036674
Kumar, S., Nehra, M., Dilbaghi, N., Marrazza, G., Tuteja, S. K., Kim, K.-H. (2020). Nanovehicles for plant modifications towards pest- and disease-resistance traits. Trends Plant Sci. 25, 198–212. doi: 10.1016/j.tplants.2019.10.007
Lai, X., Blanc-Mathieu, R., GrandVuillemin, L., Huang, Y., Stigliani, A., Lucas, J., et al. (2021). The LEAFY floral regulator displays pioneer transcription factor properties. Mol. Plant 14, 829–837. doi: 10.1016/j.molp.2021.03.004
Lang, G. A., Early, J. D., Arroyave, N. J., Darnell, R. L., Martin, G. C., Stutte, G. W. (1985). Dormancy: toward a reduced, universal terminology. HortScience 20, 809–812. doi: 10.21273/hortsci.20.5.809
Lapins, K. O. (1976). Inheritance of compact growth type in apple. J. Amer. Soc Hortic. Sci. 101, 133–135. doi: 10.21273/JASHS.101.2.133
Lee, T., Kim, H., Lee, I. (2015). Network-assisted crop systems genetics: network inference and integrative analysis. Curr. Opin. Plant Biol. 24, 61–70. doi: 10.1016/j.pbi.2015.02.001
Li, L., Hu, S., Chen, X. (2018). Non-viral delivery systems for CRISPR/Cas9-based genome editing: challenges and opportunities. Biomaterials 171, 207–218. doi: 10.1016/j.biomaterials.2018.04.031
Li, T., Jiang, Z., Zhang, L., Tan, D., Wei, Y., Yuan, H., et al. (2016). Apple (Malus domestica) MdERF2 negatively affects ethylene biosynthesis during fruit ripening by suppressing MdACS1 transcription. Plant J. 88, 735–748. doi: 10.1111/tpj.13289
Li, J., Li, F., Qian, M., Han, M., Liu, H., Zhang, D., et al. (2017). Characteristics and regulatory pathway of the PrupeSEP1 SEPALLATA gene during ripening and softening in peach fruits. Plant Sci. 257, 63–73. doi: 10.1016/j.plantsci.2017.01.004
Li, Y., Tan, X., Guo, J., Hu, E., Pan, Q., Zhao, Y., et al. (2022). Functional characterization of MdTAC1a gene related to branch angle in apple (Malus x domestica Borkh.). Int. J. Mol. Sci. 23, 1870. doi: 10.3390/ijms23031870
Li, P., Wang, Y., Qian, Q., Fu, Z., Wang, M., Zeng, D., et al. (2007). LAZY1 controls rice shoot gravitropism through regulating polar auxin transport. Cell Res. 17, 402–410. doi: 10.1038/cr.2007.38
Liao, X., Li, M. S., Liu, B., Yan, M. L., Yu, X. M., Zi, H. L., et al. (2018). Interlinked regulatory loops of ABA catabolism and biosynthesis coordinate fruit growth and ripening in woodland strawberry. Proc. Natl. Acad. Sci. U.S.A. 115, E11542–E11550. doi: 10.1073/pnas.1812575115
Lin, C.-Y., Ku, H.-M., Chiang, Y.-H., Ho, H.-Y., Yu, T.-A., Jan, F.-J. (2012). Development of transgenic watermelon resistant to Cucumber mosaic virus and Watermelon mosaic virus by using a single chimeric transgene construct. Transgenic Res. 21, 983–993. doi: 10.1007/s11248-011-9585-8
Liu, G., Li, H., Fu, D. (2021). Applications of virus-induced gene silencing for identification of gene function in fruit. Food Qual. Saf. 5, 1–9. doi: 10.1093/fqsafe/fyab018
Liu, J., Sherif, S. M. (2019). Hormonal orchestration of bud dormancy cycle in deciduous woody perennials. Front. Plant Sci. 10. doi: 10.3389/fpls.2019.01136
Lloret, A., Martínez-Fuentes, A., Agustí, M., Badenes, M. L., Ríos, G. (2017). Chromatin-associated regulation of sorbitol synthesis in flower buds of peach. Plant Mol. Biol. 95, 507–517. doi: 10.1007/s11103-017-0669-6
Lobato-Gómez, M., Hewitt, S., Capell, T., Christou, P., Dhingra, A., Girón-Calva, P. S. (2021). Transgenic and genome-edited fruits: background, constraints, benefits, and commercial opportunities. Hortic. Res. 8, 166. doi: 10.1038/s41438-021-00601-3
Lo Bianco, R., Proietti, P., Regni, L., Caruso, T. (2021). Planting systems for modern olive growing: strengths and weaknesses. Agriculture 11, 494. doi: 10.3390/agriculture11060494
Lotti, C., Minervini, A. P., Delvento, C., Losciale, P., Gaeta, L., Sánchez-Pérez, R., et al. (2023). Detection and distribution of two dominant alleles associated with the sweet kernel phenotype in almond cultivated germplasm. Front. Plant Sci. 14. doi: 10.3389/fpls.2023.1171195
Lowe, K., La Rota, M., Hoerster, G., Hastings, C., Wang, N., Chamberlin, M., et al. (2018). Rapid genotype “independent” Zea mays L. (maize) transformation via direct somatic embryogenesis. In Vitro Cell. Dev. Biol. Plant 54, 240–252. doi: 10.1007/s11627-018-9905-2
Luedeling, E. (2012). Climate change impacts on winter chill for temperate fruit and nut production: a review. Sci. Hortic. 144, 218–229. doi: 10.1016/j.scienta.2012.07.011
Maeda, K., Kikuchi, T., Kasajima, I., Li, C., Yamagishi, N., Yamashita, H., et al. (2020). Virus-induced flowering by apple latent spherical virus vector: effective use to accelerate breeding of grapevine. Viruses 12, 70. doi: 10.3390/v12010070
Maher, M. F., Nasti, R. A., Vollbrecht, M., Starker, C. G., Clark, M. D., Voytas, D. F. (2020). Plant gene editing through de novo induction of meristems. Nat. Biotechnol. 38, 84–89. doi: 10.1038/s41587-019-0337-2
Mallapaty, S. (2019). Australian gene-editing rules adopt ‘middle ground.’. Nature. doi: 10.1038/d41586-019-01282-8
Martín-Fontecha, E. S., Tarancón, C., Cubas, P. (2018). To grow or not to grow, a power-saving program induced in dormant buds. Curr. Opin. Plant Biol. 41, 102–109. doi: 10.1016/j.pbi.2017.10.001
Martin-Ortigosa, S., Peterson, D. J., Valenstein, J. S., Lin, V. S.-Y., Trewyn, B. G., Lyznik, L. A., et al. (2014). Mesoporous silica nanoparticle-mediated intracellular Cre protein delivery for maize genome editing via loxP site excision. Plant Physiol. 164, 537–547. doi: 10.1104/pp.113.233650
Mazzitelli, L., Hancock, R. D., Haupt, S., Walker, P. G., Pont, S. D. A., McNicol, J., et al. (2007). Co-ordinated gene expression during phases of dormancy release in raspberry (Rubus idaeus L.) buds. J. Exp. Bot. 58, 1035–1045. doi: 10.1093/jxb/erl266
McClure, K. A., Gardner, K. M., Douglas, G. M., Song, J., Forney, C. F., DeLong, J., et al. (2018). A genome-wide association study of apple quality and scab resistance. Plant Genome 11, 170075. doi: 10.3835/plantgenome2017.08.0075
Mehta, D. (2023). EU proposal on CRISPR-edited crops is welcome - but not enough. Nature 619, 437. doi: 10.1038/d41586-023-02328-8
Miller, A. J., Gross, B. L. (2011). From forest to field: perennial fruit crop domestication. Am. J. Bot. 98, 1389–1414. doi: 10.3732/ajb.1000522
Minamikawa, M. F., Nonaka, K., Kaminuma, E., Kajiya-Kanegae, H., Onogi, A., Goto, S., et al. (2017). Genome-wide association study and genomic prediction in citrus: potential of genomics-assisted breeding for fruit quality traits. Sci. Rep. 7, 4721. doi: 10.1038/s41598-017-05100-x
Miskolczi, P., Singh, R. K., Tylewicz, S., Azeez, A., Maurya, J. P., Tarkowská, D., et al. (2019). Long-range mobile signals mediate seasonal control of shoot growth. Proc. Natl. Acad. Sci. U.S.A. 116, 10852–10857. doi: 10.1073/pnas.1902199116
Mohamed, R., Wang, C.-T., Ma, C., Shevchenko, O., Dye, S. J., Puzey, J. R., et al. (2010). Populus CEN/TFL1 regulates first onset of flowering, axillary meristem identity and dormancy release in Populus. Plant J. 62, 674–688. doi: 10.1111/j.1365-313X.2010.04185.x
Mølmann, J. A., Asanta, D. K. A., Jensen, J. B., Krane, M. N., Ernstsen, A., Junttila, O., et al. (2005). Low night temperature and inhibition of gibberellin biosynthesis override phytochrome action and induce bud set and cold acclimation, but not dormancy in PHYA overexpressors and wild-type of hybrid aspen. Plant Cell Environ. 28, 1579–1588. doi: 10.1111/j.1365-3040.2005.01395.x
Monselise, S. P., Goldschmidt, E. E. (1982). Alternate bearing in fruit trees. Hortic. Rev. 4, 128–173. doi: 10.1002/9781118060773.ch5
Montalt, R., Cuenca, J., Vives, M. C., Mournet, P., Navarro, L., Ollitrault, P., et al. (2023). Genotyping by sequencing for SNP-based linkage analysis and the development of KASPar markers for male sterility and polyembryony in citrus. Plants 12, 1567. doi: 10.3390/plants12071567
Moser, M., Asquini, E., Miolli, G. V., Weigl, K., Hanke, M.-V., Flachowsky, H., et al. (2020). The MADS-box gene MdDAM1 controls growth cessation and bud dormancy in apple. Front. Plant Sci. 11. doi: 10.3389/fpls.2020.01003
Moss, S. M. A., Wang, T., Voogd, C., Brian, L. A., Wu, R., Hellens, R. P., et al. (2018). AcFT promotes kiwifruit in vitro flowering when overexpressed and Arabidopsis flowering when expressed in the vasculature under its own promoter. Plant Direct 2, 1–12. doi: 10.1002/pld3.68
Mout, R., Ray, M., Tay, T., Sasaki, K., Yesilbag Tonga, G., Rotello, V. M. (2017a). General strategy for direct cytosolic protein delivery via protein-nanoparticle Co-engineerinc. ACS Nano 11, 6416–6421. doi: 10.1021/acsnano.7b02884
Mout, R., Ray, M., Yesilbag Tonga, G., Lee, Y. W., Tay, T., Sasaki, K., et al. (2017b). Direct cytosolic delivery of CRISPR/Cas9-ribonucleoprotein for efficient gene editing. ACS Nano 11, 2452–2458. doi: 10.1021/acsnano.6b07600
Muñoz-Fambuena, N., Mesejo, C., Carmen González-Mas, M., Primo-Millo, E., Agustí, M., Iglesias, D. J. (2011). Fruit regulates seasonal expression of flowering genes in alternate-bearing ‘Moncada’ mandarin. Ann. Bot. 108, 511–519. doi: 10.1093/aob/mcr164
Muranty, H., Troggio, M., Sadok, I. B., Rifaï, M. A., Auwerkerken, A., Banchi, E., et al. (2015). Accuracy and responses of genomic selection on key traits in apple breeding. Hortic. Res. 2, 15060. doi: 10.1038/hortres.2015.60
Nakagawa, M., Honsho, C., Kanzaki, S., Shimizu, K., Utsunomiya, N. (2012). Isolation and expression analysis of FLOWERING LOCUS T-like and gibberellin metabolism genes in biennial-bearing mango trees. Sci. Hortic. 139, 108–117. doi: 10.1016/j.scienta.2012.03.005
Nilsson, O. (2022). Winter dormancy in trees. Curr. Biol. 32, R630–R634. doi: 10.1016/j.cub.2022.04.011
Niu, Q., Li, J., Cai, D., Qian, M., Jia, H., Bai, S., et al. (2016). Dormancy-associated MADS-box genes and microRNAs jointly control dormancy transition in pear (Pyrus pyrifolia white pear group) flower bud. J. Exp. Bot. 67, 239–257. doi: 10.1093/jxb/erv454
Núñez-Lillo, G., Ponce, E., Arancibia-Guerra, C., Carpentier, S., Carrasco-Pancorbo, A., Olmo-García, L., et al. (2023). A multiomics integrative analysis of color de-synchronization with softening of ‘Hass’ avocado fruit: a first insight into a complex physiological disorder. Food Chem. 408, 135215. doi: 10.1016/j.foodchem.2022.135215
O’Connor, K. M., Hayes, B. J., Hardner, C. M., Alam, M., Henry, R. J., Topp, B. L. (2021). Genomic selection and genetic gain for nut yield in an Australian macadamia breeding population. BMC Genom. 22, 370. doi: 10.1186/s12864-021-07694-z
O’Connor, K., Hayes, B., Hardner, C., Alam, M., Topp, B. (2019). Selecting for nut characteristics in macadamia using a genome-wide association study. HortScience 54, 629–632. doi: 10.21273/HORTSCI13297-18
OGTR. (2023). Genetically modified (GM) crops in Australia. Office of the Gene Technology Regulator, Department of Health, Australian Government. Available at: https://www.ogtr.gov.au/sites/default/files/files/2021-06/11_-_genetically_modified_gm_crops_in_Australia.pdf.
Okada, K., Wada, M., Moriya, S., Katayose, Y., Fujisawa, H., Wu, J., et al. (2016). Expression of a putative dioxygenase gene adjacent to an insertion mutation is involved in the short internodes of columnar apples (Malus × domestica). J. Plant Res. 129, 1109–1126. doi: 10.1007/s10265-016-0863-7
Okada, K., Wada, M., Takebayashi, Y., Kojima, M., Sakakibara, H., Nakayasu, M., et al. (2020). Columnar growth phenotype in apple results from gibberellin deficiency by ectopic expression of a dioxygenase gene. Tree Physiol. 40, 1205–1216. doi: 10.1093/treephys/tpaa049
Okemo, P., Wijesundra, U., Nakandala, U., Ananda, G. K. S., Vanambathina, P., Hasan, S., et al. (2022)The use of genome sequencing to improve crops for tropical agriculture. In: Next-generation sequencing and agriculture CABI Biotechnology Series (CAB International) (Accessed September 22, 2023).
O’Malley, R. C., Huang, S. C., Song, L., Lewsey, M. G., Bartlett, A., Nery, J. R., et al. (2016). Cistrome and epicistrome features shape the regulatory DNA landscape. Cell 165, 1280–1292. doi: 10.1016/j.cell.2016.04.038
Omori, M., Yamane, H., Osakabe, K., Osakabe, Y., Tao, R. (2021). Targeted mutagenesis of CENTRORADIALIS using CRISPR/Cas9 system through the improvement of genetic transformation efficiency of tetraploid highbush blueberry. J. Hortic. Sci. Biotechnol. 96, 153–161. doi: 10.1080/14620316.2020.1822760
Orduña, L., Li, M., Navarro-Payá, D., Zhang, C., Santiago, A., Romero, P., et al. (2022). Direct regulation of shikimate, early phenylpropanoid, and stilbenoid pathways by Subgroup 2 R2R3-MYBs in grapevine. Plant J. 110, 529–547. doi: 10.1111/tpj.15686
Pan, W., Liang, J., Sui, J., Li, J., Liu, C., Xin, Y., et al. (2021). ABA and bud dormancy in perennials: current knowledge and future perspective. Genes 12, 1635. doi: 10.3390/genes12101635
Pathak, G., Dudhagi, S. S., Raizada, S., Singh, R. K., Sane, A. P., Sane, V. A. (2023). Phosphomevalonate kinase regulates the MVA/MEP pathway in mango during ripening. Plant Physiol. Biochem. 196, 174–185. doi: 10.1016/j.plaphy.2023.01.030
Paudel, L., Kerr, S., Prentis, P., Tanurdžić, M., Papanicolaou, A., Plett, J. M., et al. (2022). Horticultural innovation by viral-induced gene regulation of carotenogenesis. Hortic. Res. 9, uhab008. doi: 10.1093/hr/uhab008
Peña, L., Martín-Trillo, M., Juárez, J., Pina, J. A., Navarro, L., Martínez-Zapater, J. M. (2001). Constitutive expression of Arabidopsis LEAFY or APETALA1 genes in citrus reduces their generation time. Nat. Biotechnol. 19, 263–267. doi: 10.1038/85719
Peng, Z., Bredeson, J. V., Wu, G. A., Shu, S., Rawat, N., Du, D., et al. (2020). A chromosome-scale reference genome of trifoliate orange (Poncirus trifoliata) provides insights into disease resistance, cold tolerance and genome evolution in Citrus. Plant J. 104, 1215–1232. doi: 10.1111/tpj.14993
Peng, A., Chen, S., Lei, T., Xu, L., He, Y., Wu, L., et al. (2017). Engineering canker-resistant plants through CRISPR/Cas9-targeted editing of the susceptibility gene CsLOB1 promoter in citrus. Plant Biotechnol. J. 15, 1509–1519. doi: 10.1111/pbi.12733
Petiteau, A., Denancé, C., Muranty, H., Durel, C.-E., García-Gómez, B. E., Aranzana, M. J., et al. (2023). Marker-assisted breeding (MAB) on apple and pear and new approaches for QTLs and major gene genotyping involved in disease resistance. Acta Hortic. 1362, 221–230. doi: 10.17660/ActaHortic.2023.1362.30
Pillai, S., Dasgupta, P., Chellappan, S. P. (2009). “Chromatin immunoprecipitation assays: analyzing transcription factor binding and histone modifications in vivo,” in Chromatin protocols methods in molecular biology. Ed. Chellappan, S. P. (Totowa, NJ: Humana Press), 323–339. doi: 10.1007/978-1-59745-190-1_22
Pin, P. A., Nilsson, O. (2012). The multifaceted roles of FLOWERING LOCUS T in plant development. Plant Cell Environ. 35, 1742–1755. doi: 10.1111/j.1365-3040.2012.02558.x
Polo-Oltra, Á., Romero, C., López, I., Badenes, M. L., Zuriaga, E. (2020). Cost-effective and time-efficient molecular assisted selection for PPV resistance in apricot based on ParPMC2 allele-specific PCR. Agronomy 10, 1292. doi: 10.3390/agronomy10091292
Pompili, V., Dalla Costa, L., Piazza, S., Pindo, M., Malnoy, M. (2020). Reduced fire blight susceptibility in apple cultivars using a high-efficiency CRISPR/Cas9-FLP/FRT-based gene editing system. Plant Biotechnol. J. 18, 845–858. doi: 10.1111/pbi.13253
Powell, W. A., Newhouse, A. E., Coffey, V. (2019). Developing blight-tolerant American chestnut trees. Cold Spring Harb. Perspect. Biol. 11, a034587. doi: 10.1101/cshperspect.a034587
Putterill, J., Varkonyi-Gasic, E. (2016). FT and florigen long-distance flowering control in plants. Curr. Opin. Plant Biol. 33, 77–82. doi: 10.1016/j.pbi.2016.06.008
Qiao, L., Niño-Sánchez, J., Hamby, R., Capriotti, L., Chen, A., Mezzetti, B., et al. (2023). Artificial nanovesicles for dsRNA delivery in spray-induced gene silencing for crop protection. Plant Biotechnol. J. 21, 854–865. doi: 10.1111/pbi.14001
Robertson, G., Hirst, M., Bainbridge, M., Bilenky, M., Zhao, Y., Zeng, T., et al. (2007). Genome-wide profiles of STAT1 DNA association using chromatin immunoprecipitation and massively parallel sequencing. Nat. Methods 4, 651–657. doi: 10.1038/nmeth1068
Rottmann, W. H., Meilan, R., Sheppard, L. A., Brunner, A. M., Skinner, J. S., Ma, C., et al. (2000). Diverse effects of overexpression of LEAFY and PTLF, a poplar (Populus) homolog of LEAFY/FLORICAULA, in transgenic poplar and Arabidopsis. Plant J. 22, 235–245. doi: 10.1046/j.1365-313x.2000.00734.x
Rutledge, Z., Taylor, J. E. (2023). “Economic and societal aspects,” in Advanced automation for tree fruit orchards and vineyards agriculture automation and control. Eds. Vougioukas, S. G., Zhang, Q. (Cham: Springer International Publishing), 219–241. doi: 10.1007/978-3-031-26941-7_10
Ruttink, T., Arend, M., Morreel, K., Storme, V., Rombauts, S., Fromm, J., et al. (2007). A molecular timetable for apical bud formation and dormancy induction in poplar. Plant Cell 19, 2370–2390. doi: 10.1105/tpc.107.052811
Sahijram, L., Bahadur, B. (2015). “Somatic embryogenesis,” in Plant biology and biotechnology plant genomics and biotechnology. Eds. Bahadur, B., Venkat Rajam, M., Sahijram, L., Krishnamurthy, K. V. (New Delhi: Springer), 315–327. doi: 10.1007/978-81-322-2283-5_15
Saito, T., Bai, S., Ito, A., Sakamoto, D., Saito, T., Ubi, B. E., et al. (2013). Expression and genomic structure of the dormancy-associated MADS box genes MADS13 in Japanese pears (Pyrus pyrifolia Nakai) that differ in their chilling requirement for endodormancy release. Tree Physiol. 33, 654–667. doi: 10.1093/treephys/tpt037
Salojärvi, J., Smolander, O.-P., Nieminen, K., Rajaraman, S., Safronov, O., Safdari, P., et al. (2017). Genome sequencing and population genomic analyses provide insights into the adaptive landscape of silver birch. Nat. Genet. 49, 904–912. doi: 10.1038/ng.3862
Sánchez-Pérez, R., Howad, W., Dicenta, F., Arús, P., Martínez-Gómez, P. (2007). Mapping major genes and quantitative trait loci controlling agronomic traits in almond. Plant Breed. 126, 310–318. doi: 10.1111/j.1439-0523.2007.01329.x
Sasaki, S., Yamagishi, N., Yoshikawa, N. (2011). Efficient virus-induced gene silencing in apple, pear and Japanese pear using Apple latent spherical virus vectors. Plant Methods 7, 15. doi: 10.1186/1746-4811-7-15
Schilling, S., Pan, S., Kennedy, A., Melzer, R. (2018). MADS-box genes and crop domestication: the jack of all traits. J. Exp. Bot. 69, 1447–1469. doi: 10.1093/jxb/erx479
Schlathölter, I., Jänsch, M., Flachowsky, H., Broggini, G. A. L., Hanke, M.-V., Patocchi, A. (2018). Generation of advanced fire blight-resistant apple (Malus × domestica) selections of the fifth generation within 7 years of applying the early flowering approach. Planta 247, 1475–1488. doi: 10.1007/s00425-018-2876-z
Schwartz, S. H., Hendrix, B., Hoffer, P., Sanders, R. A., Zheng, W. (2020). Carbon dots for efficient small interfering RNA delivery and gene silencing in plants. Plant Physiol. 184, 647–657. doi: 10.1104/pp.20.00733
Scorza, R. (1984). Characterization of four distinct peach tree growth types. J. Amer. Soc Hortic. Sci. 109, 455–457. doi: 10.21273/JASHS.109.4.455
Scorza, R. (2005). Theory and practice of genetically manipulating peach tree architecture. New York Fruit Q. 13, 27–31.
Scorza, R., Ravelonandro, M., Callahan, A. M., Cordts, J. M., Fuchs, M., Dunez, J., et al. (1994). Transgenic plums (Prunus domestica L.) express the plum pox virus coat protein gene. Plant Cell Rep. 14, 18–22. doi: 10.1007/BF00233291
Shalit, A., Rozman, A., Goldschmidt, A., Alvarez, J. P., Bowman, J. L., Eshed, Y., et al. (2009). The flowering hormone florigen functions as a general systemic regulator of growth and termination. Proc. Natl. Acad. Sci. U.S.A. 106, 8392–8397. doi: 10.1073/pnas.0810810106
Shalom, L., Samuels, S., Zur, N., Shlizerman, L., Zemach, H., Weissberg, M., et al. (2012). Alternate bearing in citrus: changes in the expression of flowering control genes and in global gene expression in ON- versus OFF-crop trees. PloS One 7, 16. doi: 10.1371/journal.pone.0046930
Shao, X., Wu, S., Dou, T., Zhu, H., Hu, C., Huo, H., et al. (2020). Using CRISPR/Cas9 genome editing system to create MaGA20ox2 gene-modified semi-dwarf banana. Plant Biotechnol. J. 18, 17–19. doi: 10.1111/pbi.13216
Sharma, N., Singh, A. K., Singh, S. K., Mahato, A. K., Srivastav, M., Singh, N. K. (2020). Comparative RNA sequencing based transcriptome profiling of regular bearing and alternate bearing mango (Mangifera indica L.) varieties reveals novel insights into the regulatory mechanisms underlying alternate bearing. Biotechnol. Lett. 42, 1035–1050. doi: 10.1007/s10529-020-02863-8
Shen, F., Huang, Z., Zhang, B., Wang, Y., Zhang, X., Wu, T., et al. (2019). Mapping gene markers for apple fruit ring rot disease resistance using a multi-omics approach. G3: Genes Genomes Genet. 9, 1663–1678. doi: 10.1534/g3.119.400167
Sheng, X., Hsu, C.-Y., Ma, C., Brunner, A. M. (2022). Functional diversification of Populus FLOWERING LOCUS D-LIKE3 transcription factor and two paralogs in shoot ontogeny, flowering, and vegetative phenology. Front. Plant Sci. 13. doi: 10.3389/fpls.2022.805101
Sheng, X., Mahendra, R. A., Wang, C.-T., Brunner, A. M. (2023). CRISPR/Cas9 mutants delineate roles of Populus FT and TFL1/CEN/BFT family members in growth, dormancy release and flowering. Tree Physiol. 43, 1042–1054. doi: 10.1093/treephys/tpad027
Sideli, G. M., Mather, D., Wirthensohn, M., Dicenta, F., Goonetilleke, S. N., Martínez-García, P. J., et al. (2023). Genome-wide association analysis and validation with KASP markers for nut and shell traits in almond (Prunus dulcis [Mill.] D.A.Webb). Tree Genet. Genomes 19, 13. doi: 10.1007/s11295-023-01588-9
Silva, A. T., Nguyen, A., Ye, C., Verchot, J., Moon, J. H. (2010). Conjugated polymer nanoparticles for effective siRNA delivery to tobacco BY-2 protoplasts. BMC Plant Biol. 10, 291. doi: 10.1186/1471-2229-10-291
Singh, K., Callahan, A. M., Smith, B. J., Malinowski, T., Scorza, R., Jarošová, J., et al. (2021). Long-term efficacy and safety of RNAi-mediated virus resistance in ‘HoneySweet’plum. Front. Plant Sci. 12. doi: 10.3389/fpls.2021.726881
Singh, S., Chaudhary, C., Bharsakale, R. D., Gazal, S., Verma, L., Tarannum, Z., et al. (2023). PRpnp, a novel dual activity PNP family protein improves plant vigour and confers multiple stress tolerance in Citrus aurantifolia. Plant Biotechnol. J. 21, 726–741. doi: 10.1111/pbi.13989
Singh, R. K., Miskolczi, P., Maurya, J. P., Bhalerao, R. P. (2019). A tree ortholog of SHORT VEGETATIVE PHASE floral repressor mediates photoperiodic control of bud dormancy. Curr. Biol. 29, 128–133.e2. doi: 10.1016/j.cub.2018.11.006
Singh, R. K., Svystun, T., AlDahmash, B., Jönsson, A. M., Bhalerao, R. P. (2017). Photoperiod- and temperature-mediated control of phenology in trees – a molecular perspective. New Phytol. 213, 511–524. doi: 10.1111/nph.14346
Sinn, J. P., Held, J. B., Vosburg, C., Klee, S. M., Orbovic, V., Taylor, E. L., et al. (2021). Flowering Locus T chimeric protein induces floral precocity in edible citrus. Plant Biotechnol. J. 19, 215–217. doi: 10.1111/pbi.13463
Small, I. (2007). RNAi for revealing and engineering plant gene functions. Curr. Opin. Biotechnol. 18, 148–153. doi: 10.1016/j.copbio.2007.01.012
Soares, J. M., Weber, K. C., Qiu, W., Stanton, D., Mahmoud, L. M., Wu, H., et al. (2020). The vascular targeted citrus FLOWERING LOCUS T3 gene promotes non-inductive early flowering in transgenic Carrizo rootstocks and grafted juvenile scions. Sci. Rep. 10, 21404. doi: 10.1038/s41598-020-78417-9
Sobreiro, J., Patanita, M. I., Patanita, M., Tomaz, A. (2023). Sustainability of high-density olive orchards: hints for irrigation management and agroecological approaches. Water 15, 2486. doi: 10.3390/w15132486
Song, G. Q., Prieto, H., Orbovic, V. (2019). Agrobacterium-mediated transformation of tree fruit crops: methods, progress, and challenges. Front. Plant Sci. 10. doi: 10.3389/fpls.2019.00226
Spielmeyer, W., Ellis, M. H., Chandler, P. M. (2002). Semidwarf (sd-1), “green revolution” rice, contains a defective gibberellin 20-oxidase gene. Proc. Natl. Acad. Sci. U.S.A. 99, 9043–9048. doi: 10.1073/pnas.132266399
Srivastav, M., Radadiya, N., Ramachandra, S., Jayaswal, P. K., Singh, N., Singh, S., et al. (2023). High resolution mapping of QTLs for fruit color and firmness in Amrapali/Sensation mango hybrids. Front. Plant Sci. 14. doi: 10.3389/fpls.2023.1135285
Su, H., Wang, Y., Xu, J., Omar, A. A., Grosser, J. W., Calovic, M., et al. (2023). Generation of the transgene-free canker-resistant Citrus sinensis using Cas12a/crRNA ribonucleoprotein in the T0 generation. Nat. Commun. 14, 3957. doi: 10.1038/s41467-023-39714-9
Taagepera, R., Nemčok, M. (2023). World population growth over millennia: ancient and present phases with a temporary halt in-between. Anthr. Rev. 0. doi: 10.1177/20530196231172423
Tan, J. W., Shinde, H., Tesfamicael, K., Hu, Y., Fruzangohar, M., Tricker, P., et al. (2023). Global transcriptome and gene co-expression network analyses reveal regulatory and non-additive effects of drought and heat stress in grapevine. Front. Plant Sci. 14. doi: 10.3389/fpls.2023.1096225
Tang, M., Bai, X., Wang, J., Chen, T., Meng, X., Deng, H., et al. (2022). Efficiency of graft-transmitted JcFT for floral induction in woody perennial species of the Jatropha genus depends on transport distance. Tree Physiol. 42, 189–201. doi: 10.1093/treephys/tpab116
Tarancón, C., González-Grandío, E., Oliveros, J. C., Nicolas, M., Cubas, P. (2017). A conserved carbon starvation response underlies bud dormancy in woody and herbaceous species. Front. Plant Sci. 8. doi: 10.3389/fpls.2017.00788
Thagun, C., Horii, Y., Mori, M., Fujita, S., Ohtani, M., Tsuchiya, K., et al. (2022). Non-transgenic gene modulation via spray delivery of nucleic acid/peptide complexes into plant nuclei and chloroplasts. ACS Nano 16, 3506–3521. doi: 10.1021/acsnano.1c07723
Tomes, S., Gunaseelan, K., Dragulescu, M., Wang, Y.-Y., Guo, L., Schaffer, R. J., et al. (2023). A MADS-box gene-induced early flowering pear (Pyrus communis L.) for accelerated pear breeding. Front. Plant Sci. 14. doi: 10.3389/fpls.2023.1235963
Toups, H. S., Cochetel, N., Gray, D., Cramer, G. R. (2020). VviERF6Ls: an expanded clade in Vitis responds transcriptionally to abiotic and biotic stresses and berry development. BMC Genom. 21, 472. doi: 10.1186/s12864-020-06811-8
Tränkner, C., Lehmann, S., Hoenicka, H., Hanke, M.-V., Fladung, M., Lenhardt, D., et al. (2010). Over-expression of an FT-homologous gene of apple induces early flowering in annual and perennial plants. Planta 232, 1309–1324. doi: 10.1007/s00425-010-1254-2
Tuan, P. A., Bai, S., Saito, T., Ito, A., Moriguchi, T. (2017). Dormancy-associated MADS-box (DAM) and the abscisic acid pathway regulate pear endodormancy through a feedback mechanism. Plant Cell Physiol. 58, 1378–1390. doi: 10.1093/pcp/pcx074
van Dijken, A. J. H., Schluepmann, H., Smeekens, S. C. M. (2004). Arabidopsis trehalose-6-phosphate synthase 1 is essential for normal vegetative growth and transition to flowering. Plant Physiol. 135, 969–977. doi: 10.1104/pp.104.039743
Varkonyi-Gasic, E., Wang, T., Cooney, J., Jeon, S., Voogd, C., Douglas, M. J., et al. (2021). Shy Girl, a kiwifruit suppressor of feminization, restricts gynoecium development via regulation of cytokinin metabolism and signalling. New Phytol. 230, 1461–1475. doi: 10.1111/nph.17234
Varkonyi-Gasic, E., Wang, T., Voogd, C., Jeon, S., Drummond, R. S. M., Gleave, A. P., et al. (2019). Mutagenesis of kiwifruit CENTRORADIALIS-like genes transforms a climbing woody perennial with long juvenility and axillary flowering into a compact plant with rapid terminal flowering. Plant Biotechnol. J. 17, 869–880. doi: 10.1111/pbi.13021
Velázquez, K., Agüero, J., Vives, M. C., Aleza, P., Pina, J. A., Moreno, P., et al. (2016). Precocious flowering of juvenile citrus induced by a viral vector based on Citrus leaf blotch virus: a new tool for genetics and breeding. Plant Biotechnol. J. 14, 1976–1985. doi: 10.1111/pbi.12555
Vimont, N., Quah, F. X., Schöepfer, D. G., Roudier, F., Dirlewanger, E., Wigge, P. A., et al. (2019). ChIP-seq and RNA-seq for complex and low-abundance tree buds reveal chromatin and expression co-dynamics during sweet cherry bud dormancy. Tree Genet. Genomes 16, 9. doi: 10.1007/s11295-019-1395-9
Voogd, C., Brian, L. A., Wang, T., Allan, A. C., Varkonyi-Gasic, E. (2017). Three FT and multiple CEN and BFT genes regulate maturity, flowering, and vegetative phenology in kiwifruit. J. Exp. Bot. 68, 1539–1553. doi: 10.1093/jxb/erx044
Waltz, E. (2015). Nonbrowning GM apple cleared for market. Nat. Biotechnol. 33, 326–327. doi: 10.1038/nbt0415-326c
Wang, L., Chen, S., Peng, A., Xie, Z., He, Y., Zou, X. (2019). CRISPR/Cas9-mediated editing of CsWRKY22 reduces susceptibility to Xanthomonas citri subsp. citri in Wanjincheng orange (Citrus sinensis (L.) Osbeck). Plant Biotechnol. Rep. 13, 501–510. doi: 10.1007/s11816-019-00556-x
Wang, M., Le Moigne, M.-A., Bertheloot, J., Crespel, L., Perez-Garcia, M.-D., Ogé, L., et al. (2019). BRANCHED1: a key hub of shoot branching. Front. Plant Sci. 10. doi: 10.3389/fpls.2019.00076
Wang, W., Li, T., Chen, J., Zhang, X., Wei, L., Yao, S., et al. (2023). A self-regulated transcription factor CsWRKY33 enhances resistance of citrus fruit to Penicillium digitatum. Postharvest Biol. Technol. 198, 112267. doi: 10.1016/j.postharvbio.2023.112267
Wang, N., Liu, Y., Dong, C., Zhang, Y., Bai, S. (2022). MdMAPKKK1 regulates apple resistance to Botryosphaeria dothidea by interacting with MdBSK1. Int. J. Mol. Sci. 23, 4415. doi: 10.3390/ijms23084415
Wang, L., Pan, L., Niu, L., Cui, G., Wei, B., Zeng, W., et al. (2022). Fine mapping of the gene controlling the weeping trait of Prunus persica and its uses for MAS in progenies. BMC Plant Biol. 22, 459. doi: 10.1186/s12870-022-03840-1
Wang, F., Wang, M., Liu, X., Xu, Y., Zhu, S., Shen, W., et al. (2017). Identification of putative genes involved in limonoids biosynthesis in citrus by comparative transcriptomic analysis. Front. Plant Sci. 8. doi: 10.3389/fpls.2017.00782
Wang, X., Wang, Q., Yan, L., Hao, Y., Lian, X., Zhang, H., et al. (2023). PpTCP18 is upregulated by lncRNA5 and controls branch number in peach (Prunus persica) through positive feedback regulation of strigolactone biosynthesis. Hortic. Res. 10, uhac224. doi: 10.1093/hr/uhac224
Wang, F., Xu, Y., Liu, X., Shen, W., Zhu, S., Zhao, X. (2019). Use of TRV-mediated VIGS for functional genomics research in citrus. Plant Cell Tiss. Organ Cult. 139, 609–613. doi: 10.1007/s11240-019-01698-0
Wang, Y., Yu, H., He, X., Lu, T., Huang, X., Luo, C. (2022). Isolation and functional characterization of a LEAFY gene in mango (Mangifera indica L.). Int. J. Mol. Sci. 23, 3974. doi: 10.3390/ijms23073974
Watanabe, D., Takahashi, I., Jaroensanti-Tanaka, N., Miyazaki, S., Jiang, K., Nakayasu, M., et al. (2021). The apple gene responsible for columnar tree shape reduces the abundance of biologically active gibberellin. Plant J. 105, 1026–1034. doi: 10.1111/tpj.15084
Weigel, D., Nilsson, O. (1995). A developmental switch sufficient for flower initiation in diverse plants. Nature 377, 495–500. doi: 10.1038/377495a0
Weiss, T., Crisp, P. A., Rai, K. M., Song, M., Springer, N. M., Zhang, F. (2022). Epigenetic features drastically impact CRISPR–Cas9 efficacy in plants. Plant Physiol. 190, 1153–1164. doi: 10.1093/plphys/kiac285
Wenzel, S., Flachowsky, H., Hanke, M.-V. (2013). The fast-track breeding approach can be improved by heat-induced expression of the FLOWERING LOCUS T genes from poplar (Populus trichocarpa) in apple (Malus × domestica Borkh.). Plant Cell Tissue Organ Cult. 115, 127–137. doi: 10.1007/s11240-013-0346-7
Wickland, D. P., Hanzawa, Y. (2015). The FLOWERING LOCUS T/TERMINAL FLOWER gene family: functional evolution and molecular mechanisms. Mol. Plant 8, 983–997. doi: 10.1016/j.molp.2015.01.007
Wilkie, J. D., Sedgley, M., Olesen, T. (2008). Regulation of floral initiation in horticultural trees. J. Exp. Bot. 59, 3215–3228. doi: 10.1093/jxb/ern188
Wilkinson, M. J., Yamashita, R., James, M. E., Bally, I. S. E., Dillon, N. L., Ali, A., et al. (2022). The influence of genetic structure on phenotypic diversity in the Australian mango (Mangifera indica) gene pool. Sci. Rep. 12, 20614. doi: 10.1038/s41598-022-24800-7
Wisniewski, M., Norelli, J., Artlip, T. (2015). Overexpression of a peach CBF gene in apple: a model for understanding the integration of growth, dormancy, and cold hardiness in woody plants. Front. Plant Sci. 6. doi: 10.3389/fpls.2015.00085
Wu, R., Cooney, J., Tomes, S., Rebstock, R., Karunairetnam, S., Allan, A. C., et al. (2021). RNAi-mediated repression of dormancy-related genes results in evergrowing apple trees. Tree Physiol. 41, 1510–1523. doi: 10.1093/treephys/tpab007
Wu, Y.-M., Ma, Y.-J., Wang, M., Zhou, H., Gan, Z.-M., Zeng, R.-F., et al. (2022). Mobility of FLOWERING LOCUS T protein as a systemic signal in trifoliate orange and its low accumulation in grafted juvenile scions. Hortic. Res. 9, uhac056. doi: 10.1093/hr/uhac056
Wu, R., Tomes, S., Karunairetnam, S., Tustin, S. D., Hellens, R. P., Allan, A. C., et al. (2017). SVP-like MADS box genes control dormancy and budbreak in apple. Front. Plant Sci. 8. doi: 10.3389/fpls.2017.00477
Wu, R., Wang, T., Warren, B. A. W., Thomson, S. J., Allan, A. C., Macknight, R. C., et al. (2018). Kiwifruit SVP2 controls developmental and drought-stress pathways. Plant Mol. Biol. 96, 233–244. doi: 10.1007/s11103-017-0688-3
Xu, Y., Ma, K., Zhao, Y., Wang, X., Zhou, K., Yu, G., et al. (2021). Genomic selection: a breakthrough technology in rice breeding. Crop J. 9, 669–677. doi: 10.1016/j.cj.2021.03.008
Yamagishi, N., Kishigami, R., Yoshikawa, N. (2014). Reduced generation time of apple seedlings to within a year by means of a plant virus vector: a new plant-breeding technique with no transmission of genetic modification to the next generation. Plant Biotechnol. J. 12, 60–68. doi: 10.1111/pbi.12116
Yamagishi, N., Li, C., Yoshikawa, N. (2016). Promotion of flowering by Apple latent spherical virus vector and virus elimination at high temperature allow accelerated breeding of apple and pear. Front. Plant Sci. 7. doi: 10.3389/fpls.2016.00171
Yamagishi, N., Sasaki, S., Yamagata, K., Komori, S., Nagase, M., Wada, M., et al. (2011). Promotion of flowering and reduction of a generation time in apple seedlings by ectopical expression of the Arabidopsis thaliana FT gene using the Apple latent spherical virus vector. Plant Mol. Biol. 75, 193–204. doi: 10.1007/s11103-010-9718-0
Yamane, H., Singh, A. K., Cooke, J. E. K. (2021). Plant dormancy research: from environmental control to molecular regulatory networks. Tree Physiol. 41, 523–528. doi: 10.1093/treephys/tpab035
Yamane, H., Wada, M., Honda, C., Matsuura, T., Ikeda, Y., Hirayama, T., et al. (2019). Overexpression of Prunus DAM6 inhibits growth, represses bud break competency of dormant buds and delays bud outgrowth in apple plants. PloS One 14, e0214788. doi: 10.1371/journal.pone.0214788
Yang, Q., Gao, Y., Wu, X., Moriguchi, T., Bai, S., Teng, Y. (2021). Bud endodormancy in deciduous fruit trees: advances and prospects. Hortic. Res. 8, 139. doi: 10.1038/s41438-021-00575-2
Yang, L., Machin, F., Wang, S., Saplaoura, E., Kragler, F. (2023). Heritable transgene-free genome editing in plants by grafting of wild-type shoots to transgenic donor rootstocks. Nat. Biotechnol. 41, 958–967. doi: 10.1038/s41587-022-01585-8
Yang, H., Tian, C., Li, X., Gong, H., Zhang, A. (2021). Transcriptome co-expression network analysis identifies key genes and regulators of sweet cherry anthocyanin biosynthesis. Horticulturae 7, 123. doi: 10.3390/horticulturae7060123
Yang, Q., Wu, X., Gao, Y., Ni, J., Li, J., Pei, Z., et al. (2023). PpyABF3 recruits the COMPASS-like complex to regulate bud dormancy maintenance via integrating ABA signaling and GA catabolism. New Phytol. 237, 192–203. doi: 10.1111/nph.18508
Ye, J., Geng, Y., Zhang, B., Mao, H., Qu, J., Chua, N.-H. (2014). The Jatropha FT ortholog is a systemic signal regulating growth and flowering time. Biotechnol. Biofuels 7, 91. doi: 10.1186/1754-6834-7-91
Yong, J., Wu, M., Zhang, R., Bi, S., Mann, C. W. G., Mitter, N., et al. (2022). Clay nanoparticles efficiently deliver small interfering RNA to intact plant leaf cells. Plant Physiol. 190, 2187–2202. doi: 10.1093/plphys/kiac430
Yoshihara, T., Iino, M. (2007). Identification of the gravitropism-related rice gene LAZY1 and elucidation of LAZY1-dependent and -independent gravity signaling pathways. Plant Cell Physiol. 48, 678–688. doi: 10.1093/pcp/pcm042
Yoshizumi, T., Oikawa, K., Chuah, J.-A., Kodama, Y., Numata, K. (2018). Selective gene delivery for integrating exogenous DNA into plastid and mitochondrial genomes Using peptide–DNA complexes. Biomacromolecules 19, 1582–1591. doi: 10.1021/acs.biomac.8b00323
Yu, J., Conrad, A. O., Decroocq, V., Zhebentyayeva, T., Williams, D. E., Bennett, D., et al. (2020). Distinctive gene expression patterns define endodormancy to ecodormancy transition in apricot and peach. Front. Plant Sci. 11. doi: 10.3389/fpls.2020.00180
Yu, B., Lin, Z., Li, H., Li, X., Li, J., Wang, Y., et al. (2007). TAC1, a major quantitative trait locus controlling tiller angle in rice. Plant J. 52, 891–898. doi: 10.1111/j.1365-313X.2007.03284.x
Zeng, T., Liao, P., Zheng, C., Gao, H., Ye, X., Zhou, C., et al. (2023). The interaction between the lemon ribosomal protein ClRPS9-2 and citrus yellow vein clearing virus coat protein affects viral infection and gene silencing suppressor activity. Mol. Plant Pathol. 24, 1047–1062. doi: 10.1111/mpp.13347
Zetsche, B., Gootenberg, J. S., Abudayyeh, O. O., Slaymaker, I. M., Makarova, K. S., Essletzbichler, P., et al. (2015). Cpf1 is a single RNA-guided endonuclease of a class 2 CRISPR-Cas system. Cell 163, 759–771. doi: 10.1016/j.cell.2015.09.038
Zhang, Y., Cheng, Y., Fang, H., Roberts, N., Zhang, L., Vakulskas, C. A., et al. (2022). Highly efficient genome editing in plant protoplasts by ribonucleoprotein delivery of CRISPR-Cas12a nucleases. Front. Genome Ed. 4. doi: 10.3389/fgeed.2022.780238
Zhang, H., Harry, D. E., Ma, C., Yuceer, C., Hsu, C.-Y., Vikram, V., et al. (2010). Precocious flowering in trees: the FLOWERING LOCUS T gene as a research and breeding tool in Populus. J. Exp. Bot. 61, 2549–2560. doi: 10.1093/jxb/erq092
Zhang, F., Rossignol, P., Huang, T., Wang, Y., May, A., Dupont, C., et al. (2020). Reprogramming of stem cell activity to convert thorns into branches. Curr. Biol. 30, 2951–2961.e5. doi: 10.1016/j.cub.2020.05.068
Zhang, J., Tao, N., Xu, Q., Zhou, W., Cao, H., Xu, J., et al. (2009). Functional characterization of Citrus PSY gene in Hongkong kumquat (Fortunella hindsii Swingle). Plant Cell Rep. 28, 1737–1746. doi: 10.1007/s00299-009-0774-3
Zhang, F., Wang, Y., Irish, V. F. (2021). CENTRORADIALIS maintains shoot meristem indeterminacy by antagonizing THORN IDENTITY1 in Citrus. Curr. Biol. 31, 2237–2242.e4. doi: 10.1016/j.cub.2021.02.051
Zhao, Y.-L., Li, Y., Cao, K., Yao, J.-L., Bie, H.-L., Khan, I. A., et al. (2023). MADS-box protein PpDAM6 regulates chilling requirement-mediated dormancy and bud break in peach. Plant Physiol. 193, 448–465. doi: 10.1093/plphys/kiad291
Zhao, X., Meng, Z., Wang, Y., Chen, W., Sun, C., Cui, B., et al. (2017). Pollen magnetofection for genetic modification with magnetic nanoparticles as gene carriers. Nat. Plants 3, 956–964. doi: 10.1038/s41477-017-0063-z
Zhao, D., Song, G. (2014). Rootstock-to-scion transfer of transgene-derived small interfering RNA s and their effect on virus resistance in nontransgenic sweet cherry. Plant Biotechnol. J. 12, 1319–1328. doi: 10.1111/pbi.12243
Zhou, H., Bai, S., Wang, N., Sun, X., Zhang, Y., Zhu, J., et al. (2020). CRISPR/Cas9-mediated mutagenesis of MdCNGC2 in apple callus and VIGS-mediated silencing of MdCNGC2 in fruits improve resistance to Botryosphaeria dothidea. Front. Plant Sci. 11. doi: 10.3389/fpls.2020.575477
Zhu, C., Zheng, X., Huang, Y., Ye, J., Chen, P., Zhang, C., et al. (2019). Genome sequencing and CRISPR/Cas9 gene editing of an early flowering Mini-Citrus (Fortunella hindsii). Plant Biotechnol. J. 17, 2199–2210. doi: 10.1111/pbi.13132
Ziv, D., Zviran, T., Zezak, O., Samach, A., Irihimovitch, V. (2014). Expression profiling of FLOWERING LOCUS T-Like gene in alternate bearing ‘Hass’ avocado trees suggests a role for PaFT in avocado flower induction. PloS One 9, e110613. doi: 10.1371/journal.pone.0110613
Keywords: tree crops, architecture, precocity, flowering, dormancy, alternate bearing, genomics
Citation: Kerr SC, Shehnaz S, Paudel L, Manivannan MS, Shaw LM, Johnson A, Velasquez JTJ, Tanurdžić M, Cazzonelli CI, Varkonyi-Gasic E and Prentis PJ (2024) Advancing tree genomics to future proof next generation orchard production. Front. Plant Sci. 14:1321555. doi: 10.3389/fpls.2023.1321555
Received: 14 October 2023; Accepted: 26 December 2023;
Published: 19 January 2024.
Edited by:
Cristian Silvestri, University of Tuscia, ItalyReviewed by:
Concetta Licciardello, CREA Research Centre for Olive, Fruit and Citrus Crops, ItalyWen-Wu Guo, Huazhong Agricultural University, China
Copyright © 2024 Kerr, Shehnaz, Paudel, Manivannan, Shaw, Johnson, Velasquez, Tanurdžić, Cazzonelli, Varkonyi-Gasic and Prentis. This is an open-access article distributed under the terms of the Creative Commons Attribution License (CC BY). The use, distribution or reproduction in other forums is permitted, provided the original author(s) and the copyright owner(s) are credited and that the original publication in this journal is cited, in accordance with accepted academic practice. No use, distribution or reproduction is permitted which does not comply with these terms.
*Correspondence: Stephanie C. Kerr, c3RlcGhhbmllLmtlcnJAcXV0LmVkdS5hdQ==