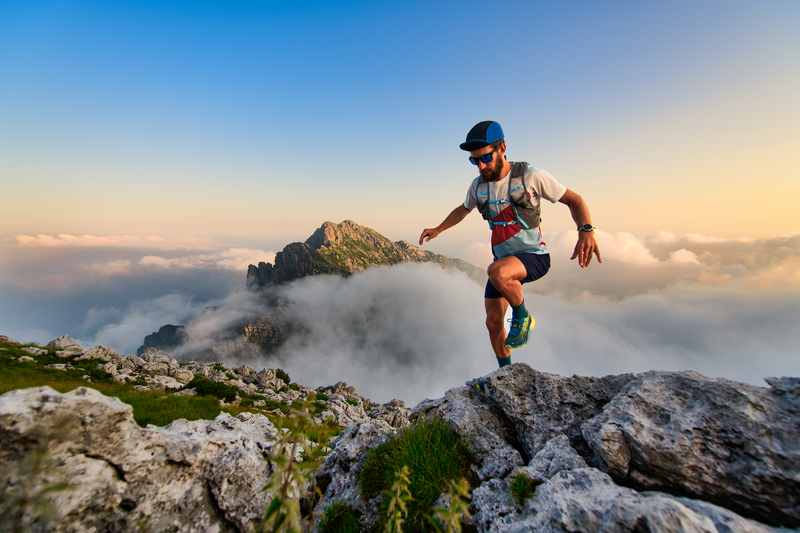
95% of researchers rate our articles as excellent or good
Learn more about the work of our research integrity team to safeguard the quality of each article we publish.
Find out more
ORIGINAL RESEARCH article
Front. Plant Sci. , 05 January 2024
Sec. Plant Abiotic Stress
Volume 14 - 2023 | https://doi.org/10.3389/fpls.2023.1289801
Iron deficiency is a major nutritional problem causing iron deficiency chlorosis (IDC) and yield reduction in soybean, one of the most important crops. The ATP-binding cassette G subfamily plays a crucial role in substance transportation in plants. In this study, we cloned the GmABCG5 gene from soybean and verified its role in Fe homeostasis. Analysis showed that GmABCG5 belongs to the ABCG subfamily and is subcellularly localized at the cell membrane. From high to low, GmABCG5 expression was found in the stem, root, and leaf of young soybean seedlings, and the order of expression was flower, pod, seed stem, root, and leaf in mature soybean plants. The GUS assay and qRT-PCR results showed that the GmABCG5 expression was significantly induced by iron deficiency in the leaf. We obtained the GmABCG5 overexpressed and inhibitory expressed soybean hairy root complexes. Overexpression of GmABCG5 promoted, and inhibition of GmABCG5 retarded the growth of soybean hairy roots, independent of nutrient iron conditions, confirming the growth-promotion function of GmABCG5. Iron deficiency has a negative effect on the growth of soybean complexes, which was more obvious in the GmABCG5 inhibition complexes. The chlorophyll content was increased in the GmABCG5 overexpression complexes and decreased in the GmABCG5 inhibition complexes. Iron deficiency treatment widened the gap in the chlorophyll contents. FCR activity was induced by iron deficiency and showed an extraordinary increase in the GmABCG5 overexpression complexes, accompanied by the greatest Fe accumulation. Antioxidant capacity was enhanced when GmABCG5 was overexpressed and reduced when GmABCG5 was inhibited under iron deficiency. These results showed that the response mechanism to iron deficiency is more actively mobilized in GmABCG5 overexpression seedlings. Our results indicated that GmABCG5 could improve the plant’s tolerance to iron deficiency, suggesting that GmABCG5 might have the function of Fe mobilization, redistribution, and/or secretion of Fe substances in plants. The findings provide new insights into the ABCG subfamily genes in the regulation of iron homeostasis in plants.
Iron (Fe) is an indispensable trace element for plant growth. Iron often forms as insoluble Fe(III) oxyhydroxide in calcite soils, meaning that plants often suffer from iron deficiency (Ishimaru et al., 2006; Hindt and Guerinot, 2012). Because iron plays a crucial role in electron transport and chlorophyll formation, an adequate supply of iron in the chloroplast is required to perform the photosynthetic process. Iron deficiency alters photosynthesis and reduces chlorosis, or the yellowing of plant leaves (Pandey et al., 2021; Prity et al., 2021; Therby-Vale et al., 2022). Iron starvation is a major nutritional problem that causes severe visual symptoms and yield reductions in plants. The calcareous soils favor the development of iron deficiency chlorosis (IDC) in soybean (Glycine max L. Merr.) and result in soybean yield losses (Atencio et al., 2021; Merry et al., 2022).
Higher plants have developed effective strategies to enhance iron uptake in soils with low Fe availability, such as the reduction-based strategy (Strategy I) in nongraminaceous plants and the chelation-based strategy (Strategy II) in graminaceous species. When dicotyledonous plants are subjected to Fe-limited conditions, a proton pump, H+-ATPase2 (AHA2), contributes to the acidification of the rhizosphere to facilitate the solubilization of Fe(III); the ferric-chelate reductase reduces Fe(III) to Fe(II), and Fe(II) is then transported into root epidermal cells by Iron Regulated Transporter1 (IRT1) (Kobayashi et al., 2019). Plant iron transporters such as yellow stripe-like proteins (YSL), natural resistance-associated macrophage proteins (NRAMP), and vacuolar iron transporters (VIT) are involved in the iron uptake, sequestration, translocation, and remobilization processes (Kurt and Filiz, 2020). The ability to improve the availability and efficiency of Fe strongly affects both crop yield and quality. Understanding the mechanisms of Fe uptake, transport, and storage is essential for breeding crops that are more tolerant to Fe-limited conditions. The iron transporter genes can be used as genetic improvement target genes to increase plant tolerance to iron deficiency (Curie et al., 2001).
Multiple phytohormones, such as auxin, abscisic acid (ABA), ethylene, and brassinosteroids, regulate the Fe deficiency response (Briat et al., 2012). Previous studies have shown that ABA participates in Fe reutilization and transport under Fe-deficient conditions (Zhang et al., 2019). The involvement of auxin in the regulation of the Fe deficiency response is also well known. Recently, it was demonstrated that Arabidopsis roots enhance the elongation of cells leaving the root meristem under Fe deficiency depend on the auxin-mediated response, indicating that auxin acts downstream in the transmission of the Fe deficiency signal (Giehl et al., 2012; Wu et al., 2012; Lin et al., 2016). Furthermore, the Fe deficiency-caused alterations in IRT1 and citrate synthase2 (PbCS2) expression are modulated by auxin (Blum et al., 2014; Li et al., 2016). These results strongly suggest that the iron deficiency-induced physiological responses are mediated by systemic auxin signaling.
ATPase-binding cassette (ABC) transporters are membrane transport proteins that use the energy released by the hydrolysis of ATP (Lefevre et al., 2018a). ABCs mediate the transport of hormones, pigments, toxic chemicals, secondary metabolites, lipid molecules, reactive oxygen species-related compounds, and heavy metal ions (Linton and Higgins, 2007; Kang et al., 2015; Hwang et al., 2016). In addition to transport, ABCs also act as ion channels and molecular switches to regulate a variety of cellular functions and physiological processes (Hinrichs et al., 2017; Zaynab et al., 2022). From microorganisms to mammals, members of the ATP-binding cassette G subfamily (ABCG subfamily) play a crucial role (Mcfarlane et al., 2010; Borghi et al., 2015; Dhara and Raichaudhuri, 2021; Niu et al., 2021). Genome studies have shown that the ABCG subfamily members are particularly abundant in plants, surpassing other eukaryotes such as human beings and yeast (Andolfo et al., 2015; Gupta et al., 2019). Transport substrates of ABCG transporters include terpenoids, flavonoids, plant hormones, and heavy metals in plants.
The ABCG subfamily includes two categories: White-brown Complex Homolog (WBC) and Pleiotropic Drug Resistance (PDR), both of which are involved in metal homeostasis. The full-length ABC transporter AtABCG37 (alias PDR9) mediates the secretion of the phenolic compound coumarin from Arabidopsis roots into the medium in response to iron deficiency. Expression of AtABCG37 was up-regulated by iron deficiency in Arabidopsis roots (Fourcroy et al., 2014; Ziegler et al., 2017). The Atabcg19 mutant had reduced iron uptake, accompanied by upregulation of the gene encoding iron reduction oxidase 6 (Mentewab et al., 2014). Expression of NtABCG3 (NtPDR3) in the tobacco root epidermis was strongly induced by iron deficiency. Silencing and overexpression experiments showed that NtABCG3 mediates the secretion of O-methylated coumarin into the rhizosphere in response to iron deficiency (Lefèvre et al., 2018b).
Transmembrane transport of ABA by ABCG transporters is one of the important methods in plants (Kuromori et al., 2010; Hwang et al., 2016; Kuromori et al., 2017; Pawela et al., 2019; Zhang et al., 2021; Kim et al., 2022). Hyperaccumulation of radioactive indole-3-butyric acid was observed in the root tips of AtABCG36 (atpdr8) seedlings (Strader and Bartel, 2009). Analyses of single and double-mutant phenotypes suggest that both ABCG36 and ABCG37 function cooperatively in auxin-controlled plant development. The effect of ABCG1 and ABCG16 promoted rapid pollen tube growth through their effects on auxin distribution and auxin flow in the pistil (Liu et al., 2020). The ABC transporter AtPGP4 is involved in auxin-mediated lateral root and root hair development (Santelia et al., 2005). In the ABCB subfamily, OsABCB14 was supported as an auxin transporter involved in Fe homeostasis (Xu et al., 2014).
Human ABCG5 functions in the intestinal absorption and biliary elimination of plant sterols and cholesterol, defects that result in sitosterolemia (Yu et al., 2005; Moitra and Dean, 2011; Lee et al., 2016). In Arabidopsis, ABCG5-mediated formation of a dense cuticle layer was required for early seedling establishment (Do et al., 2018). The atabcg5 seedlings failed to develop true leaves when grown under waterlogged conditions (Lee et al., 2021). The OsABCG5 from Oryza sativa is involved in the hypodermal suberization of roots and positively controls shoot branching by promoting the outgrowth of lateral shoots, known as reduced culm number1 (rcn1) (Yasuno et al., 2009; Shiono et al., 2014). OsABCG5 positively affects shoot branching independently of auxin signaling, while it is proposed to act on ABA translocation in guard cells (Yasuno et al., 2009; Matsuda et al., 2016). The orthologous of the OsABCG5 gene, LpABCG5, was identified in perennial ryegrass as a candidate for the plant type determinant (Shinozuka et al., 2011). NtABCG5 was reported to be involved in short- and long-term defense against insect/herbivore attacks by secreting toxic substrates (Bienert et al., 2012). The results indicate that ABCG5 plays an important role in the growth and development of monocotyledons.
Soybean is one of the most important crops in the world and is sensitive to iron deficiency. Iron deficiency chlorosis (IDC) in calcareous soils seriously affects the economic and nutritional value of soybean (Waters et al., 2018; Merry et al., 2022). Among soybean ABC families, the ABCG subfamily has the largest number of GmABCs with 117, or 44.8% (Verrier et al., 2008; Mishra et al., 2019; Huang et al., 2021). At present, there are few studies on the function and identification of the ABCG genes in soybean plants. Nevertheless, the function of the ABCG5 gene in dicotyledonous plants has been poorly investigated.
ABC transporter functions have been proposed using homologous expression and RNAi-silenced plant systems (Kim et al., 2007; Song et al., 2010). We have obtained several soybean iron deficiency response genes by screening the iron deficiency transcriptome database, and one of these genes, GmABCG5 (LOC100789722), was chosen for further study (Li et al., 2023). In order to verify the role of GmABCG5 in Fe homeostasis, we characterized the GmABCG5 gene using homologous expression and RNAi-silenced plant systems. The results will clarify the function of the GmABCG5 gene and provide new insights into the regulation of iron homeostasis in soybean.
Suinong37 (SN37) and William82 soybeans were donated by the Institute of Crops Tillage and Cultivation, Heilongjiang Academy of Agricultural Sciences. SN37 seeds were hydroponically grown in 1/2 Hoagland, and roots, stems, and leaves at the three-leaf stage were sampled. the roots, stems, leaves, flowers, 1-2 cm young pods, and mature seeds of SN37 soybean planted in the experimental field were sampled. William82 soybean was used for physiological index detection and GUS histochemical staining. All plants were grown in a greenhouse at 16/8 h (light/dark, 22/20°).
Total RNA was extracted from SN37 soybean seedlings using Total RNA Kit II (Omega BioTek, Norcross, GA, USA). The cDNA was synthesized using ReverTra Ace™ qPCR RT Master MIX (TOYOBO, Japan). The coding sequence of GmABCG5 was amplified from the cDNA. The total DNA of SN37 was extracted using the E.Z.N.A.® SP Plant DNA Kit (OMGAE, USA). After purification, the PCR products were sequenced (Sangon Biotechnology Co. Ltd., Shanghai, China) and analyzed (https://www.ncbi.nlm.nih.gov/orffinder/). From NCBI (https://www.ncbi.nlm.nih.gov) and Phytozome (https://phytozome-next.jgi.doe.gov/info/Gmax_Wm82_a2_v1) the GmABCG5 protein sequence was GmABCG5 promoter analysis by Plant CARE (http://bioinformatics.psb.ugent.be/webtools/plantcare/html/). The ABC protein sequence in the evolutionary tree was downloaded from NCBI and Phytozome and analyzed with MEGA7.0. Chromosome localization was analyzed with the TBtools software. Introns were analyzed with GSDS: http://GSDS.cbi.pku.edu.cn.
SN37 soybeans were grown in a 1/2 Hoagland nutrient solution to the three-leaf stage and then treated with iron deficiency for 7 d. The isolated RNA was used as a template, and qRT-PCR analysis was performed using SYBR®TremixExTaqTMII (TAKARA, Otsu, Japan) on CFX96 real-time PCR detection systems (Bio-Rad, Hercules, CA, USA). The GmACTIN gene was selected as an internal reference gene and was used as an internal control to normalize the data (Jian et al., 2008). The relative expression of genes was calculated by ▵▵Ct. The primers used are listed in Supplementary Table 1.
The GmABCG5 gene fragment without a stop codon was connected to GFP and expressed under the CaMV 35S promoter to construct the pBI121-GmABCG5-GFP recombinant vector. The 35S::GmABCG5-GFP fusion vector and the 35S::GFP vector (the control) were transformed into Agrobacterium GV3101 by the freeze-thaw method and then separately infiltrated into the leaves of a 3-week-old Nicotiana benthamiana leaf using 1-mL needless syringes. After 48 h, the GFP fluorescence was detected at 488 nm using a Zeiss LSM 510 Meta confocal laser scanning microscope with a 500-530 nm emission filter (Zeiss, Oberkochen, Germany).
The pCAMBIA1301-GmABCG5pro::GUS recombinant vector was constructed and transformed into Agrobacterium K599 by the freeze-thaw method, and soybean hairy roots were induced by needle insertion. The soybean complex was transferred to 1/2 Hoagland nutrient solution and 1/2 Hoagland nutrient solution without iron supply for 7 d. Tissue staining was conducted following the method described previously (Li et al., 2023). The stained tissues were photographed using a stereomicroscope (EZ4-HD LEICA, Germany) coupled with a color charge-coupled device (CCD) camera (Zeiss, Germany). The GUS enzyme activity was detected using a plant β-glucuronidase (GUS) ELISA kit. The absorbance (OD value) was measured at 450nm wavelength, with the standard concentration as the horizontal coordinate and the corresponding OD value as the ordinate. The standard linear regression line was drawn, and the GUS enzyme activity value was calculated according to the linear regression equation.
The pROKII-GmABCG5 overexpressed recombinant vector and the pZH01-GmABCG5 RNAi recombinant vector driven by the CaMV 35S promoter were constructed. Healthy soybean seeds with full particles were selected, disinfected with a 15% sodium hypochlorite solution for 5 min, and sown in sterile soil (nutrient soil: vermiculite = 1:1). After 5-7 d, soybean seedlings with good growth status were selected, and the surrounding tissues were punctured with the head of a syringe 2-3 mm below the cotyledon node. The recombinant plasmid pROKII empty vector, pROKII-GmABCG5, and pZH01-GmABCG5 were transformed into Agrobacterium K599 by the freeze-thaw method, and single bacterial populations were screened and smeared on the wound site. After inoculation, it was cultured in a warm and humid environment, and hairy roots grew after 9-12 d. The obtained hairy root complex of soybean is called empty vector (Ev), overexpression GmABCG5 soybean complex (OEGmABCG5), and inhibition GmABCG5 soybean complex (iGmABCG5). When the hairy root grew to 2-3 cm, it was buried with sterilized vermiculite and then cultured for 20-30 d. Hairy roots were detected by qRT-PCR.
The soybean complex was transferred to 1/2 Hoagland nutrient solution and 1/2 Hoagland nutrient solution without iron supply for 7 d. The ferric chelate reductase assay was conducted as described previously (Yi and Guerinot, 1996). A Perls staining solution was mixed with 4% hydrochloric acid [v/v] and 4% [w/v] potassium ferricyanide in equal proportions (Roschzttardtz et al., 2009). The content of chlorophyll was determined by the 80% acetone and the light absorption values of the solution was tested at 663 nm and 645 nm (Manzoor et al., 2022). The content of superoxide anion (O2-) and malondialdehyde (MDA) was determined according to Dhindsa and other experimental methods with some modifications (Rajinder et al., 1982). Peroxidase (POD) and catalase (CAT) activities in plants were analyzed according to the published protocol with minor modifications (Chen and Zhang, 2016). Plant parts were blotted dry, and the fresh weight (g) of leaves and roots was measured using a digital scale. Root length (cm) was measured using a ruler.
For each experiment, at least three plants were sampled. Statistical analyses were performed using the SPSS software, version 18.0. Data are shown as mean ± SD. Differences in means between two groups were compared using a two-tailed Student’s t-test and among three or more groups by one-way ANOVA followed by Duncan’s multiple-range test. Data analysis is shown in Supplementary Tables 4-6.
As shown in the ABCG subfamily phylogenetic tree, eight ABCGs (GmABCG23, GmABCGSTR, GmABCG10, GmABCG6, GmABCG20, AtABCG5, and OsABCG5) were assigned to the same branch. Soybean GmABCG5 was closely related to the AtABCG5 of Arabidopsis thaliana and the GmABCG10 of Glycine max (Figure 1A; Supplementary Table 2). There were no introns in GmABCG5, AtABCG5, and OsABCG5 (Figure 1B). The 40 identified soybean GmABCG genes were unevenly distributed among the 14 chromosomes. The majority of the GmABCG genes weree located at the ends of the chromosomes. Some soybean chromosomes contained multiple GmABCG genes, for example, Ghr20 carried six GmABCG genes. GmABCG5 was located at the end of soybean Ghr5, with no other GmABCG genes nearby (Figure 1C; Supplementary Table 3).
Figure 1 Bioinformatic analysis of GmABCG5. (A) Phylogenetic tree of the ABCG subfamily in Arabidopsis thaliana, Glycine max, and Oryza sativa. A neighbor-joining tree showed the evolutionary relationships of the ABCG members of the three species, which are highlighted in different colors. (B) Structure of some ABCG genes in Arabidopsis thaliana, Glycine max, and Oryza sativa. (C) Chromosomal mapping of 40 GmABCG genes in soybean. The scale on the left represents the chromosome length in megabases (Mb). The chromosome number is indicated to the left of each chromosome.
The qRT-PCR results showed that the GmABCG5 expression ranged from high to low in the stem, root, and leaf of young soybean seedlings. There was not much difference in the expression of GmABCG5 in stem and root, while the expression of GmABCG5 in leaves was significantly lower than that in stem and root (p<0.05) (Figure 2A). The expression level of GmABCG5 from high to low was flower, pod, seed, root, stem, and leaf in mature soybean plants. The GmABCG5 expression was significantly higher in the flower than that in the pod and seed, and was significantly higher in the pod than that in the seed (p<0.05) (Figure 2B).
Figure 2 Tissue expression of GmABCG5. (A) Relative expression of GmABCG5 in vegetative organs of Suinong37 soybean young seedlings. The roots, stems, and leaves of three-leaf stage soybean in hydroponic soybean were detected. (B) Relative expression of GmABCG5 in the reproductive organs of Suinong37 soybean. The roots, stems and leaves, full-flowering flowers, 1-2 cm young pods, and mature seeds of field-grown Suinong37 soybean plants were detected. (C) GmABCG5 gene expression in hydroponically grown soybean under iron deficiency for 7 d. Experiments were repeated three times, and each treatment group contained five samples. Data represent the mean of the replicates. Lowercase letters indicate p<0.05.
GmABCG5 was fused to the green fluorescent protein (GFP) and driven by a 35S promoter. The 35S::GmABCG5-GFP fluorescence signal was observed exclusively at the plasma membrane of the epidermal cells of the Nicotiana benthamiana leaves, while the 35S::GFP fluorescence signal was scattered in the epidermal cells of the Nicotiana benthamiana leaves (Figure 3). The results showed that the GmABCG5 protein was located at the cell plasma membrane.
Figure 3 Subcellular localization of GmABCG5. The fusion protein 35S::GmABCG5-GFP was transiently transformed into epidermal cells of Nicotiana benthamiana leaves. 35S::GFP was used for comparison. These images were obtained using a confocal microscope. Bar = 100 µm.
In addition to the core cis-elements, the GmABCG5 promoter (GmABCG5pro) contains a large number of light-responsive elements, MYB binding sites, and ABA response elements (Figure 4A). The expression vector pCAMBIA1301-GmABCG5pro::GUS was constructed (Figure 4B), and the hairy root was induced by Agrobacterium-mediated acupuncture infection on soybean. The hairy roots of soybean showed blue staining under non-stress and iron deficiency treatments. The staining was deeper in soybean hairy roots under iron deficiency than under non-stress (Figure 4C). The results showed that GmABCG5pro could be induced by iron deficiency. The GUS enzyme activity of transgenic GmABCG5pro::GUS soybean hairy root was determined. The results showed that the GUS enzyme activity in GmABCG5pro::GUS soybean hairy roots was significantly higher than that of the control under iron deficiency stress (p<0.05), which was consistent with the results of GUS histochemical staining (Figure 4D; Supplementary Figure 1). The qRT-PCR results showed that GmABCG5 expression was significantly increased in stem and leaf under iron deficiency (p<0.05) compared with non-stress treatment (Figure 2C). The qRT-PCR result was consistent with the GUS assay, indicating that the GmABCG5 gene expression was induced by iron deficiency in the roots, the stem, and the leaf of soybean, especially in the leaf.
Figure 4 Response of the GmABCG5 promoter and GmABCG5 expression to iron deficiency. (A) Several predicted cis-elements in the GmABCG5 promoter. (B) The pCAMBIA1301-GmABCG5pro::GUS recombinant expression vector. (C) GUS staining of GmABCG5pro::GUS-transformed hairy root of soybean under iron deficiency for 7 d, with non-stress treatment as the CK. (a) The hairy root of soybean. (b) Staining of a soybean hairy root tip under CK. (c) Staining of a soybean hairy root tip under iron deficiency stress. (d) Transverse cutting view of a hairy root under non-stress treatment. (e) Transverse cutting view of hairy root under iron deficiency treatment. Bar = 10 µm. (D) GUS enzyme activity of GmABCG5pro::GUS transformed soybean hairy root under iron deficiency for 7 d, with non-stress treatment as the CK. The experiments were repeated three times, and each treatment group contained five samples. The data represent the mean of the replicates. * indicates p<0.05, ** indicates p< 0.01.
The pROKII-GmABCG5 was constructed and transformed into soybean. The 350-bp RNA interference target fragment (352-702) of the ABC2 membrane domain in the GmABCG5 gene was identified, and the RNAi vector pZH01-GmABCG5 was constructed (Figures 5A, B). Compared with Ev, OEGmABCG5 displayed longer hairy roots and greener leaves, and iGmABCG5 displayed shorter hairy roots and yellower leaves under iron deficiency (Figure 5C). The OEGmABCG5, iGmABCG5, and Ev soybean complexes were obtained by Agrobacterium-mediated acupuncture infection. The qRT-PCR results showed that GmABCG5 expression was significantly increased in OEGmABCG5 hairy roots and was significantly decreased in iGmABCG5 hairy roots, compared with Ev (p<0.01) (Figure 5D).
Figure 5 The phenotypes of OEGmABCG5, iGmABCG5, and Ev under iron deficiency. (A) The construction of the pROKII-GmABCG5 recombinant vector. (B) Construction of the pZH01-GmABCG5 recombinant vector. (C) The OEGmABCG5, iGmABCG5, and Ev were hydroponically cultivated in 1/2 Hoagland solution and treated with iron deficiency (0 mM Fe) for 7 d, the control was hydroponically grown in 1/2 Hoagland solution for equal time. (D) GmABCG5 expression in the hairy roots of OEGmABCG5, iGmABCG5, and Ev. ** indicates p <0.01. (E) The root length, the fresh root weight, and the fresh leaf weight of OEGmABCG5, iGmABCG5, and Ev complexes.
The root length, fresh root weight, and fresh leaf weight of OEGmABCG5, iGmABCG5, and Ev in the control were all higher than those under iron deficiency. The results proved that iron deficiency has a negative effect on the growth of soybean. The root length, the fresh root weight, and the fresh leaf weight in OEGmABCG5 were higher than those in Ev, while these indices in iGmABCG5 were lower than those in Ev. The results indicated that overexpression of GmABCG5 improved the plant growth of soybean complexes, while inhibition of GmABCG5 was stunted (Figure 5E).
Chlorophyll content was significantly higher in OEGmABCG5, iGmABCG5, and Ev s than those under iron deficiency (p<0.01). Compared with Ev, the chlorophyll content was significantly higher in OEGmABCG5 and was significantly lower in iGmABCG5 under the control and iron deficiency treatment (p<0.05) (Figure 6A). Combining the phenotypic changes, the results indicated that overexpression of GmABCG5 improved, while inhibition of GmABCG5 stunted chlorophyll synthesis regardless of iron deficiency (Figure 5C). FCR activity was significantly higher in OEGmABCG5, iGmABCG5, and Ev under iron deficiency than that under the control (p<0.01). There was no significant difference in FCR activity among the three soybean complexes in the control. After iron deficiency treatment, the FCR activity of OEGmABCG5 was significantly higher than that of iGmABCG5 and Ev (p<0.01). The increase in FCR activity of OEGmABCG5 caused by iron deficiency was significantly higher than that of iGmABCG5, and Ev (Figure 6B). The results indicated that when the plant was subjected to iron deficiency, overexpression of GmABCG5 activated FCR activity, while inhibition of GmABCG5 did not impact FCR activity. A Perls staining in the OEGmABCG5 hairy root showed the deepest, and iGmABCG5 showed the lightest color (Figure 6C). The results showed that OEGmABCG5 had the most Fe accumulation and iGmABCG5 had the least Fe accumulation.
Figure 6 Chlorophyll content, FCR activity, and Perls staining in OEGmABCG5, iGmABCG5, and Ev. (A) Chlorophyll content. (B) FCR activity. (C) Perls staining. The OEGmABCG5, iGmABCG5, and Ev were grown in 1/2 Hoagland solution for 14 d and transferred to 1/2 Hoagland solution with the CK (0.1 mM Fe) or iron deficiency (0 mM Fe) for 7 d. Experiments were repeated three times, and each treatment group contained five samples. Data represent the mean of the replicates. ** indicates p<0.01, the statistical difference between the normal condition and iron deficiency treatment groups. Lowercase letters indicate p<0.05, the statistical difference between OEGmABCG5, iGmABCG5, and Ev in the same treatment group.
Malondialdehyde (MDA) and Superoxide anion (O2-) contents were significantly higher in OEGmABCG5, iGmABCG5, and Ev under iron deficiency than in the control (p<0.01). MDA and O2- contents showed no significant difference in OEGmABCG5, iGmABCG5, and Ev in the control. Under iron deficiency, = MDA and O2- contents were significantly lower in OEGmABCG5 and significantly higher in iGmABCG5 than in Ev (p<0.05) (Figures 7A, B). The results indicated that overexpression of GmABCG5 reduced oxidative damage, while inhibition of GmABCG5 increased oxidative damage in plants under iron deficiency. In the control, the activities of peroxidase (POD) and catalase (CAT) showed no significant difference in OEGmABCG5, iGmABCG5, and Ev. After 7 d of iron deficiency, POD and CAT activities were significantly increased in the three soybean complexes compared with the control (p<0.01). The POD and CAT activities were significantly lower in OEGmABCG5 and significantly higher in iGmABCG5 than in Ev (p<0.05) (Figures 7C, D). The results indicated that overexpression of GmABCG5 enhanced, while inhibition of GmABCG5 reduced, the antioxidant capacity of plants under iron deficiency.
Figure 7 Physiological indices of OEGmABCG5, iGmABCG5, and Ev under iron deficiency. (A) Malondialdehyde content. (B) Superoxide anion content. (C) Catalase activity. (D) Peroxidase activity. The soybean complexes were grown in 1/2 Hoagland solution for 14 d and transferred to 1/2 Hoagland solution with the control (0.1 mM Fe) or iron deficiency (0 mM Fe) for 7 d. The experiments were repeated three times, and each treatment group contained five samples. Data represent the mean of the replicates. ** indicates p<0.01, the statistical difference between the normal condition and iron deficiency treatment groups. Lowercase letters indicate p<0.05, the statistical difference in OEGmABCG5, iGmABCG5, and Ev in the same treatment group.
Due to their ability to transport compounds in multiple biological processes, the applicability of ABC transporters in biotechnology is enormous. Evolutionary analysis showed that soybean GmABCG5 is closely related to AtABCG5 in Arabidopsis and OsABCG5 in Oryza sativa, with similar structures (Figures 1A, B), which indicates that ABCG5 genes are evolutionarily conserved. The characterization of GmABCG5 will also provide useful information about other function-unknown GmABCG genes.
We found that the expression of GmABCG5 in the reproductive organs was higher than that in the vegetative organs, which is consistent with the previous finding that GmABCG5 (Glyma.05G192700) was upregulated in the pod and seed of William82 soybean (Mishra et al., 2019). GmABCG5 may play an essential role in soybean reproduction, seed development, and maturation. Previous research also showed that ABCG5 expression is present at low levels in the roots of soybean (Mishra et al., 2019) and Arabidopsis (Lee et al., 2021), with no expression even in 6-week-old Medicago truncatula (Jasinski et al., 2009). On the contrary, in our study, the expression of GmABCG5 was higher in roots than in leaves. We speculated that the difference in tissue expression of these ABCG5 genes might be due to differences in species and functions. GmABCG5 may play a role in soybean roots.
In this study, GmABCG5 expression was significantly up-regulated in the roots and was dramatically up-regulated in the leaves of soybean under iron deficiency. GUS staining also showed that GmABCG5pro responded to iron deficiency (Figure 4C). Upregulation of iron transporter genes such as YSL (Senoura et al., 2017) and IRT (Huang and Dai, 2015) is an important strategy for plants to overcome iron deficiency because iron transporters could take up and redistribute iron and improve plant growth. Meanwhile, plants secrete organic substances that promote iron absorption. For example, members of the ABCG subfamily, AtABCG37, and NtABCG3, were strongly induced by iron deficiency in plant roots, secreting coumarins to promote iron absorption in the plant rhizosphere (Rodríguez-Celma et al., 2013; Fourcroy et al., 2014; Ziegler et al., 2017). The up-regulation of GmABCG5 by iron deficiency confirms that GmABCG5 is involved in the iron deficiency response, especially in the leaf. Our result is in contrast to the studies of Lefevre et al., which found that the GmABCG5 gene in stems and leaves was not induced by iron deficiency. GmABCG5 was located at the cell plasma membranes, which is consistent with the characteristics of transporters (Andolfo et al., 2015). When plants suffer from iron deficiency, iron transporters mobilize and redistribute Fe in the leaves to supply iron to organs that preferentially require Fe. Thus, we suggest that GmABCG5 may have the function of mobilizing and redistributing Fe and/or secreting Fe substances to maintain iron homeostasis in plants.
We found that the GmABCG5 promoter (GmABCG5pro) contains a large number of photoresponsive elements, MYB binding sites, and abscisic acid response elements, suggesting a role for GmABCG5’ in development and abiotic stress response (Mishra et al., 2019). The existence of several ABA-responsive elements in GmABCG5pro suggests that GmABCG5 could be induced by ABA. OsABCG5 has been shown to be associated with ABA in guard cells. Several reports have linked ABA to heavy metal tolerance responses, such as lead-related phenotypes, which are indirect effects of the ABA transport function of AtPDR12 (Atici et al., 2005; Fan et al., 2014). The relationship between auxin and ABCG5 has been discussed in previous works of literature. Although some ABCGs have been observed to be involved in the auxin pathway (Strader and Bartel, 2009), GmABCG5 is unlikely to be related to auxin as there is no auxin-responsive element in its promoter. GmABCG5 may directly affect root growth and development independently of auxin signaling, like OsABCG5, its homologous gene in rice. In this study, overexpressed GmABCG5 affects hairy root elongation, whether under iron deficiency treatment or not.
In this study, soybean hairy root growth is promoted by GmABCG5 and retarded by GmABCG5 inhibition, which further confirms the previously discovered growth-promoting function of ABCG5. The worse growth status of the soybean complex seedlings under iron deficiency confirms the importance of iron nutrients for plant growth (Yasuno et al., 2009). Iron deficiency has a very obvious inhibitory effect on hairy root elongation in soybean. At the same time, we also see that the contribution of GmABCG5 to hairy root elongation is closely related to iron nutrition. The rate of root length reduction caused by iron deficiency was the lowest in OEGmABCG5 and the highest in iGmABCG5. The result means that GmABCG5 could save the delayed hairy root elongation phenotype and that the knockdown of GmABCG5 has a synergistic effect with iron deficiency to delay hairy root elongation. The transport function of the transporter is conducive to substance synthesis and biological growth (Briat et al., 2015; Prity et al., 2021; Barnaby et al., 2022), which may explain the high biomass of OEGmABCG5. Here, the decrease in fresh weight of Ev, OEGmABCG5, and iGmABCG5 caused by iron deficiency may be due to the inhibition of iron-participating biological processes. It is very interesting that the decrease in plant root biomass caused by iron deficiency was alleviated in iGmABCG5. This may be a compensatory effect of iron deficiency, but the exact reason is not clear.
Chlorophyll content and FCR activity are both valuable parameters for the state of plant iron nutrition. Overexpression of GmABCG5 increased chlorophyll content, and inhibition of GmABCG5 decreased chlorophyll content, corresponding to the yellowed leaf phenotype. The higher chlorophyll content showed that the effect of iron deficiency on plants was reduced, indicating that GmABCG5 can increase a plant’s tolerance to iron deficiency (Wakabayashi et al., 2006; Frelet-Barrand et al., 2008; Mirzahossini et al., 2015; He et al., 2020). The increase in FCR activity is a typical response to iron deficiency because FCR promotes iron absorption in plants. The increase in FCR activity induced by iron deficiency was much higher in OEGmABCG5 than in the other two complexes. This result indicates that the response mechanism to iron deficiency in plants is more actively mobilized in OEGmABCG5, suggesting that GmABCG5 is related to the iron deficiency response. Accordingly, the iron content is high in OEGmABCG5 and low in iGmABCG5, which is consistent with previous studies (Sisó-Terraza et al., 2016; Dahuja et al., 2021). We also used Perls staining to visually demonstrate the iron content in plants, and the results showed that GmABCG5 overexpressed plants had high iron content. The results suggest that the GmABCG5 gene allows more iron to enter the plant, although the effects of other transporters cannot be excluded. Thus, we conclude that GmABCG5 may be involved in iron absorption and regulate iron homeostasis in plants.
Iron and ROS have attracted much interest because of their sophisticated (Reyt et al., 2015; Le et al., 2016; vonder Mark et al., 2021; Hornbergs et al., 2023). Furthermore, no difference was found in the three soybean complexes in the control, while under iron deficiency, less membrane damage and superoxide anion and higher antioxidant capacity were found in OEGmABCG5, and the opposite result was found in iGmABCG5. This difference indicates that GmABCG5 could counteract the damage caused by iron deficiency and improve the ability to protect against oxidative damage, which suggests a close relationship between GmABCG5 and iron (Dhara and Raichaudhuri, 2021). In plant tissues, severe iron deficiency stress catalyzes the production of reactive oxygen species (ROS), which cause irreversible damage to membrane lipids, proteins, and nucleic acids (Becana et al., 1998; Zingg and Jones, 1997). MDA, an indicator of oxidative stress, was found to be increased by iron deficiency stress in alfalfa (M’Sehli et al., 2014), similar to our results that MDA content increased significantly in soybean after iron deficiency. We also found that the MDA content increased less when GmABCG5L was overexpressed, indicating that oxidative damage was reduced in plants. Under iron deficiency, plants can reduce or remove the active oxygen in the cell to avoid the harm caused by the accumulation of ROS through the synergistic action of the SOD, CAT, and POD. It was found that iron deficiency inhibited soybean growth, plant growth slowed down, and the activities of antioxidant enzymes CAT and POD showed an increasing trend (Mahmoud et al., 2022), which is consistent with our results. The significantly higher antioxidant enzyme activity in OEGmABCG5 and lower in iGmABCG5 suggested that overexpression of GmABCG5L can produce more protective enzymes, effectively remove harmful substances, and resist oxidative damage induced by iron deficiency. However, Wang et al. found that iron deficiency culture had no obvious effect on the CAT activity of tobacco leaves but reduced their POD activity (Thoiron et al., 1997). The results of this study were inconsistent with theirs, which may be due to the inconsistent response of different species to iron deficiency.
In conclusion, the soybean GmABCG5 gene was cloned and characterized in this study. As an ABCG family transporter, soybean GmABCG5 is localized at the cell membrane. The GmABCG5 promoter (GmABCG5pro) contains a large number of stress response elements. The expression of GmABCG5 was high in the reproductive organs of soybean plants. Soybean GmABCG5 gene expression was induced by iron deficiency, especially in the leaf. Overexpression/inhibition of GmABCG5 in soybean hairy roots proved that GmABCG5 has a positive effect on plant growth. The results indicated that overexpression of GmABCG5 increased, while inhibition of GmABCG5 reduced, the tolerance capacity to iron deficiency in plants. The results elucidate the function of the GmABCG5 gene and provide new insights into the regulation of iron homeostasis in soybean.
The original contributions presented in the study are publicly available. This data can be found here: https://www.ncbi.nlm.nih.gov/gene/?term=LOC100789722.
YW: Writing – original draft. XZ: Writing – original draft. YY: Writing – original draft. TN: Writing – original draft. MZ: Writing – review & editing, Conceptualization, Project administration. CF: Writing – original draft. WL: Writing – original draft. YS: Writing – original draft. CG: Writing – review & editing. DG: Writing – original draft. YB: Writing – review & editing.
The author(s) declare financial support was received for the research, authorship, and/or publication of this article. This experiment was funded by the Key Research and Development Project of Heilongjiang Province (No.2022ZX02B05), the National Natural Science Foundation of China (No. U21a20182, 31972507), the National Key R&D Program of China (2022YFE0203300) the National Natural Science Foundation of China general project (No. 31771823), and the Graduate Venture Fund of Harbin Normal University (HSDSSCX2019-24).
The authors declare that the research was conducted in the absence of any commercial or financial relationships that could be construed as a potential conflict of interest.
All claims expressed in this article are solely those of the authors and do not necessarily represent those of their affiliated organizations, or those of the publisher, the editors and the reviewers. Any product that may be evaluated in this article, or claim that may be made by its manufacturer, is not guaranteed or endorsed by the publisher.
The Supplementary Material for this article can be found online at: https://www.frontiersin.org/articles/10.3389/fpls.2023.1289801/full#supplementary-material
Andolfo, G., Ruocco, M., Donato, A., Frusciante, L., Lorito, M., Scala, F., et al. (2015). Genetic variability and evolutionary diversification of membrane ABC transporters in plants. BMC. Plant Biol. 15, 51. doi: 10.1186/s12870-014-0323-2
Atencio, L., Salazar, J., Lauter, A. N. M., Gonzales, M. D., Graham, M. A. (2021). Characterizing short and long term iron stress responses in iron deficiency tolerant and susceptible soybean (Glycine max L. Merr.). Plant Stress 2, 100012. doi: 10.1016/j.stress.2021.100012
Atici, O., Agar, G., Battal, P. (2005). Changes in phytohormone contents in chickpea seeds germinating under lead or zinc stress. Biol. Plant 49 (2), 215–222. doi: 10.1007/s10535-005-5222-9
Barnaby, J. Y., Mcclung, A. M., Edwards, J. D., Pinson, S. R. M. (2022). Identification of quantitative trait loci for tillering, root, and shoot biomass at the maximum tillering stage in rice. Sci. Rep. 12 (1), 13304. doi: 10.1038/s41598-022-17109-y
Becana, M., Moran, J. F., Iturbe-Ormaetxe, I. (1998). Iron-dependent oxygen free radical generation in plants subjected to environmental stress: toxicity and antioxidant protection. Plant Soil 201, 137–147. doi: 10.1023/A:1004375732137
Bienert, M. D., Siegmund, S. E., Drozak, A., Trombik, T., Bultreys, A., Baldwin, I. T., et al. (2012). A pleiotropic drug resistance transporter in Nicotiana tabacum is involved in defense against the herbivore Manduca sexta. Plant J. 72 (5), 745–757. doi: 10.1111/j.1365-313X.2012.05108.x
Blum, A., Brumbarova, T., Bauer, P., Ivanov, R. (2014). Hormone influence on the spatial regulation of IRT1 expression in iron-deficient Arabidopsis thaliana roots. Plant Signal. Behav. 9 (4), e28787. doi: 10.4161/psb.28787
Borghi, L., Kang, J., Ko, D., Lee, Y., Martinoia, E. (2015). The role of ABCG-type ABC transporters in phytohormone transport. Biochem. Soc Trans. 43 (5), 924–930. doi: 10.1042/bst20150106
Briat, J. F., Dubos, C., Gaymard, F. (2015). Iron nutrition, biomass production, and plant product quality. Trends Plant Sci. 20 (1), 33–40. doi: 10.1016/j.tplants.2014.07.005
Briat, J. F. O., Fobis-Loisy, I., Grignon, N., Lobréaux, S., Pascal, N., Savino, G., et al. (2012). Cellular and molecular aspects of iron metabolism in plants. Biol. Cell. 84 (1), 69–81. doi: 10.1016/0248-4900(96)81320-7
Chen, T., Zhang, B. (2016). Measurements of proline and malondialdehyde contents and antioxidant enzyme activities in leaves of drought stressed cotton. Bio. Protocol. 6, e1913. doi: 10.21769/BioProtoc.1913
Curie, C., Panaviene, Z., Loulergue, C., Dellaporta, S. L., Briat, J. F., Walker, E. L. (2001). Maize yellow stripe1 encodes a membrane protein directly involved in Fe(III) uptake. Nature 409 (6818), 346–349. doi: 10.1038/35053080
Dahuja, A., Kumar, R. R., Sakhare, A., Watts, A., Singh, B., Goswami, S., et al. (2021). Role of ATP-binding cassette transporters in maintaining plant homeostasis under abiotic and biotic stresses. Physiol. Plant 171 (4), 785–801. doi: 10.1111/ppl.13302
Dhara, A., Raichaudhuri, A. (2021). ABCG transporter proteins with beneficial activity on plants. Phytochemistr 184, 112663. doi: 10.1016/j.phytochem.2021.112663
Do, T. H. T., Martinoia, E., Lee, Y. (2018). Functions of ABC transporters in plant growth and development. Curr. Opin. Plant Biol. 41, 32–38. doi: 10.1016/j.pbi.2017.08.003
Fan, S. K., Fang, X. Z., Guan, M. Y., Ye, Y. Q., Lin, X. Y., Du, S. T., et al. (2014). Exogenous abscisic acid application decreases cadmium accumulation in Arabidopsis plants, which is associated with the inhibition of IRT1-mediated cadmium uptake. Front. Plant Sci. 5. doi: 10.3389/fpls.2014.00721
Fourcroy, P., Sisó-Terraza, P., Sudre, D., Savirón, M., Reyt, G., Gaymard, F., et al. (2014). Involvement of the ABCG37 transporter in secretion of scopoletin and derivatives by Arabidopsis roots in response to iron deficiency. New Phytol. 201 (1), 155–167. doi: 10.1111/nph.12471
Frelet-Barrand, A., Kolukisaoglu, H. U., Plaza, S., Rüffer, M., Azevedo, L., Hörtensteiner, S., et al. (2008). Comparative mutant analysis of Arabidopsis ABCC-type ABC transporters: AtMRP2 contributes to detoxification, vacuolar organic anion transport and chlorophyll degradation. Plant Cell Physiol. 49 (4), 557–569. doi: 10.1093/pcp/pcn034
Giehl, R. F., Lima, J. E., Wirén, N. (2012). Regulatory components involved in altering lateral root development in response to localized iron: evidence for natural genetic variation. Plant Signal. Behav. 7 (7), 711–713. doi: 10.4161/psb.20337
Gupta, B. B., Selter, L. L., Baranwal, V. K., Arora, D., Mishra, S. K., Sirohi, P., et al. (2019). Updated inventory, evolutionary and expression analyses of G (PDR) type ABC transporter genes of rice. Plant Physiol. Biochem. 142, 429–439. doi: 10.1016/j.plaphy.2019.08.004
He, Y., Shi, Y., Zhang, X., Xu, X., Wang, H., Li, L., et al. (2020). The osABCI7 transporter interacts with osHCF222 to stabilize the thylakoid membrane in rice. Plant Physiol. 184 (1), 283–299. doi: 10.1104/pp.20.00445
Hindt, M. N., Guerinot, M. L. (2012). Getting a sense for signals: regulation of the plant iron deficiency response. Biochim. Biophys. Acta 1823 (9), 1521–1530. doi: 10.1016/j.bbamcr.2012.03.010
Hinrichs, M., Fleck, A. T., Biedermann, E., Ngo, N. S., Schreiber, L., Schenk, M. K. (2017). An ABC transporter is involved in the silicon-induced formation of casparian bands in the exodermis of rice. Front. Plant Sci. 8. doi: 10.3389/fpls.2017.00671
Hornbergs, J., Montag, K., Loschwitz, J., Mohr, I., Poschmann, G., Schnake, A., et al. (2023). SEC14-GOLD protein PATELLIN2 binds IRON-REGULATED TRANSPORTER1 linking root iron uptake to vitamin E. Plant Physiol. 192 (1), 504–526. doi: 10.1093/plphys/kiac563
Huang, D., Dai, W. (2015). Two iron-regulated transporter (IRT) genes showed differential expression in poplar trees under iron or zinc deficiency. J. Plant Physiol. 186, 59–67. doi: 10.1016/j.jplph.2015.09.001
Huang, J., Li, X., Chen, X., Guo, Y., Liang, W., Wang, H. (2021). Genome-wide identification of soybean ABC transporters relate to aluminum toxicity. Int. J. Mol. Sci. 22 (12), 6556. doi: 10.3390/ijms22126556
Hwang, J. U., Song, W. Y., Hong, D., Ko, D., Yamaoka, Y., Jang, S., et al. (2016). Plant ABC transporters enable many unique aspects of a terrestrial plant's lifestyle. Mol. Plant 9 (3), 338–355. doi: 10.1016/j.molp.2016.02.003
Ishimaru, Y., Suzuki, M., Tsukamoto, T., Suzuki, K., Nakazono, M., Kobayashi, T., et al. (2006). Rice plants take up iron as an Fe3+-phytosiderophore and as Fe2+. Plant J. 45 (3), 335–346. doi: 10.1111/j.1365-313X.2005.02624.x
Jasinski, M., Banasiak, J., Radom, M., Kalitkiewicz, A., Figlerowicz, M. (2009). Full-size ABC transporters from the ABCG subfamily in medicago truncatula. Mol. Plant Microbe Interact. 22 (8), 921–931. doi: 10.1094/mpmi-22-8-0921
Jian, B., Liu, B., Bi, Y., Hou, W., Wu, C., Han, T. (2008). Validation of internal control for gene expression study in soybean by quantitative real-time PCR. BMC Mol. Biol. 9 (1), 1–14. doi: 10.1186/1471-2199-9-59
Kang, J., Yim, S., Choi, H., Kim, A., Lee, K. P., Lopez-Molina, L., et al. (2015). Abscisic acid transporters cooperate to control seed germination. Nat. Commun. 6, 8113. doi: 10.1038/ncomms9113
Kim, D. Y., Bovet, L., Maeshima, M., Martinoia, E., Lee, Y. (2007). The ABC transporter AtPDR8 is a cadmium extrusion pump conferring heavy metal resistance. Plant J. 50 (2), 207–218. doi: 10.1111/j.1365-313X.2007.03044.x
Kim, K., Choi, B. Y., Kang, J., Shim, D., Martinoia, E., Lee, Y. (2022). Arabidopsis ABCG27 plays an essential role in flower and leaf development by modulating abscisic acid content. Physiol. Plant 174 (3), e13734. doi: 10.1111/ppl.13734
Kobayashi, T., Nozoye, T., Nishizawa, N. K. (2019). Iron transport and its regulation in plants. Free Radic. Biol. Med. 133, 11–20. doi: 10.1016/j.freeradbiomed.2018.10.439
Kuromori, T., Miyaji, T., Yabuuchi, H., Shimizu, H., Sugimoto, E., Kamiya, A., et al. (2010). ABC transporter AtABCG25 is involved in abscisic acid transport and responses. Proc. Natl. Acad. Sci. U S A. 107 (5), 2361–2366. doi: 10.1073/pnas.0912516107
Kuromori, T., Sugimoto, E., Ohiraki, H., Yamaguchi-Shinozaki, K., Shinozaki, K. (2017). Functional relationship of AtABCG21 and AtABCG22 in stomatal regulation. Sci. Rep. 7 (1), 12501. doi: 10.1073/pnas.0912516107
Kurt, F., Filiz, E. (2020). Subcellular iron transport genes in Arabidopsis thaliana: insights into iron homeostasis. J. Bio. Sci. Biotech. 9 (1), 1–10.
Le, C. T., Brumbarova, T., Ivanov, R., Stoof, C., Weber, E., Mohrbacher, J., et al. (2016). Zinc finger of Arabidopsis thaliana12 (ZAT12) interacts with FER-like iron deficiency-induced transcription factor (FIT) linking iron deficiency and oxidative stress responses. Plant Physiol. 170 (1), 540–557. doi: 10.1104/pp.15.01589
Lee, E. J., Kim, K. Y., Zhang, J., Yamaoka, Y., Gao, P., Kim, H., et al. (2021). Arabidopsis seedling establishment under waterlogging requires ABCG5-mediated formation of a dense cuticle layer. New Phytol. 229 (1), 156–172. doi: 10.1111/nph.16816
Lee, J. Y., Kinch, L. N., Borek, D. M., Wang, J., Wang, J., Urbatsch, I. L., et al. (2016). Crystal structure of the human sterol transporter ABCG5/ABCG8. Nature 533 (7609), 561–564. doi: 10.1038/nature17666
Lefèvre, F., Boutry, M. (2018a). Towards identification of the substrates of ATP-binding cassette transporters. Plant Physiol. 178 (1), 18–39. doi: 10.1104/pp.18.00325
Lefèvre, F., Fourmeau, J., Pottier, M., Baijot, A., Cornet, T., Abadía, J., et al. (2018b). The Nicotiana tabacum ABC transporter NtPDR3 secretes O-methylated coumarins in response to iron deficiency. J. Exp. Bot. 69 (18), 4419–4431. doi: 10.1093/jxb/ery221
Li, X. G., Gao, Y., Li, Y. L., Y, S., Li, W. Z., Zhang, J. G. (2016). Isolation and characterization of PbCS2 gene regulated by iron deficiency and auxin-based systemic signals in Pyrus betulifolia. Sci. Hortic. 205, 25–31. doi: 10.1016/j.scienta.2016.04.017
Li, T., Zhang, X. M., Gao, J. L., Wang, L., Si, L., Shu, Y. J., et al. (2023). Soybean gmVIT1 gene confers plant tolerance to excess fe/mn stress. Agronomy 13 (2), 384. doi: 10.3390/agronomy13020384
Lin, X. Y., Ye, Y. Q., Fan, S. K., Jin, C. W., Zheng, S. J. (2016). Increased sucrose accumulation regulates iron-deficiency responses by promoting auxin signaling in arabidopsis plants. Plant Physiol. 170 (2), 907–920. doi: 10.1104/pp.15.01598
Linton, K. J., Higgins, C. F. (2007). Structure and function of ABC transporters: the ATP switch provides flexible control. Pflugers Arch. 453 (5), 555–567. doi: 10.1007/s00424-006-0126-x
Liu, L., Zhao, L., Chen, P., Cai, H., Hou, Z., Jin, X., et al. (2020). ATP binding cassette transporters ABCG1 and ABCG16 affect reproductive development via auxin signalling in Arabidopsis. Plant J. 102 (6), 1172–1186. doi: 10.1111/tpj.14690
Mahmoud, A. M., Ayad, A. A., Abdel-Aziz, H. M., Williams, L. L., El-Shazoly, R. M., et al. (2022). Foliar application of different iron sources improves morpho-physiological traits and nutritional quality of broad bean grown in sandy soil. Plants (Basel Switzerland) 11 (19), 2599. doi: 10.3390/plants11192599
Manzoor, H., Mehwish, Bukhat, S., Rasul, S., Rehmani, M., Noreen, S. (2022). Methyl jasmonate alleviated the adverse effects of cadmium stress in pea (Pisum sativum L.): A nexus of photosystem II activity and dynamics of redox balance. Front. Plant Sci. 24 (13). doi: 10.3389/fpls.2022.860664
Matsuda, S., Takano, S., Sato, M., Furukawa, K., Nagasawa, H., Yoshikawa, S., et al. (2016). Rice stomatal closure requires guard cell plasma membrane ATP-binding cassette transporter RCN1/osABCG5. Mol. Plant 9 (3), 417–427. doi: 10.1016/j.molp.2015.12.007
Mcfarlane, H. E., Shin, J. J., Bird, D. A., Samuels, A. L. (2010). Arabidopsis ABCG transporters, which are required for export of diverse cuticular lipids, dimerize in different combinations. Plant Cell 22 (9), 3066–3075. doi: 10.1105/tpc.110.077974
Mentewab, A., Matheson, K., Adebiyi, M., Robinson, S., Elston, B. (2014). RNA-seq analysis of the effect of kanamycin and the ABC transporter AtWBC19 on Arabidopsis thaliana seedlings reveals changes in metal content. PloS One 9 (10), e109310. doi: 10.1371/journal.pone.0109310
Merry, R., Espina, M. J., Lorenz, A. J., Stupar, R. M. (2022). Development of a controlled-environment assay to induce iron deficiency chlorosis in soybean by adjusting calcium carbonates, pH, and nodulation. Plant Methods 18 (1), 36. doi: 10.1186/s13007-022-00855-5
Mirzahossini, Z., Shabani, L., Sabzalian, M. R., Sharifi-Tehrani, M. (2015). ABC transporter and metallothionein expression affected by NI and Epichloe endophyte infection in tall fescue. Ecotoxicol. Environ. Saf. 120, 13–19. doi: 10.1016/j.ecoenv.2015.05.025
Mishra, A. K., Choi, J., Rabbee, M. F., Baek, K. H. (2019). In silico genome-wide analysis of the ATP-binding cassette transporter gene family in soybean (Glycine max L.) and their expression profiling. BioMed. Res. Int. 2019, 8150523. doi: 10.1155/2019/8150523
Moitra, K., Dean, M. (2011). Evolution of ABC transporters by gene duplication and their role in human disease. Biol. Chem. 392 (1), 29–37. doi: 10.1515/bc.2011.006
M’sehli, W., Houmani, H., Donnini, S., Zocchi, G., Abdelly, C., Gharsalli, M. (2014). Iron deficiency tolerance at leaf level in Medicago ciliaris plants. Am. J. Plant Sci. 5 (16), 2541–2553. doi: 10.4236/ajps.2014.516268
Niu, L., Li, H., Song, Z., Dong, B., Cao, H., Liu, T., et al. (2021). The functional analysis of ABCG transporters in the adaptation of pigeon pea (Cajanus cajan) to abiotic stresses. Peer J. 9, e10688. doi: 10.7717/peerj.10688
Pandey, R. N., Singh, B., Sharma, D. K., Lal, S. K., Anil, A. S. (2021). Adaptive strategies to minimise iron limiting stress under climate change scenario: A study pertinent to iron stress response of two soybean (Glycine max (L.) Merr.) Genotypes. Int. J. Chem. Stud. 9, 625–631. doi: 10.22271/chemi.2021.v9.i1i.11296
Pawela, A., Banasiak, J., Biała, W., Martinoia, E., Jasiński, M. (2019). MtABCG20 is an ABA exporter influencing root morphology and seed germination of Medicago truncatula. Plant J. 98 (3), 511–523. doi: 10.1111/tpj.14234
Prity, S. A., El-Shehawi, A. M., Elseehy, M. M., Tahura, S., Kabir, A. H. (2021). Early-stage iron deficiency alters physiological processes and iron transporter expression, along with photosynthetic and oxidative damage to sorghum. Saudi J. Biol. Sci. 28 (8), 4770–4777. doi: 10.1016/j.sjbs.2021.04.092
Rajinder, S., Dhindsa, Pamela, L., Plumb-Dhindsa, David, M. (1982). Leaf senescence and lipid peroxidation: effects of some phytohormones, and scavengers of free radicals and singlet oxygen. Physiol. Plantarum. 56 (4), 453–457. doi: 10.1111/j.1399-3054.1982.tb04539.x
Reyt, G., Boudouf, S., Boucherez, J., Gaymard, F., Briat, J. F. (2015). Iron- and ferritin-dependent reactive oxygen species distribution: impact on Arabidopsis root system architecture. Mol. Plant 8 (3), 439–453. doi: 10.1016/j.molp.2014.11.014
Rodríguez-Celma, J., Lin, W. D., Fu, G. M., Abadía, J., López-Millán, A. F., Schmidt, W. (2013). Mutually exclusive alterations in secondary metabolism are critical for the uptake of insoluble iron compounds by Arabidopsis and Medicago truncatula. Plant Physiol. 162 (3), 1473–1485. doi: 10.1104/pp.113.220426
Roschzttardtz, H., Conéjéro, G., Curie, C., Mari, S. (2009). Identification of the endodermal vacuole as the iron storage compartment in the Arabidopsis embryo. Plant Physiol. 151 (3), 1329–1338. doi: 10.1104/pp.109.144444
Santelia, D., Vincenzetti, V., Azzarello, E., Bovet, L., Fukao, Y., Düchtig, P., et al. (2005). MDR-like ABC transporter AtPGP4 is involved in auxin-mediated lateral root and root hair development. FEBS. Lett. 579 (24), 5399–5406. doi: 10.1016/j.febslet.2005.08.061
Senoura, T., Sakashita, E., Kobayashi, T., Takahashi, M., Aung, M. S., Masuda, H., et al. (2017). The iron-chelate transporter OsYSL9 plays a role in iron distribution in developing rice grains. Plant Mol. Biol. 95 (4), 375–387. doi: 10.1007/s11103-017-0656-y
Shinozuka, H., Cogan, N. O., Spangenberg, G. C., Forster, J. W. (2011). Comparative genomics in perennial ryegrass (Lolium perenne L.): identification and characterisation of an orthologue for the rice plant architecture-controlling gene osABCG5. Int. J. Plant Genomics 2011, 291563. doi: 10.1155/2011/291563
Shiono, K., Ando, M., Nishiuchi, S., Takahashi, H., Watanabe, K., Nakamura, M., et al. (2014). RCN1/OsABCG5, an ATP-binding cassette (ABC) transporter, is required for hypodermal suberization of roots in rice (Oryza sativa). Plant J. 80 (1), 40–51. doi: 10.1111/tpj.12614
Sisó-Terraza, P., Luis-Villarroya, A., Fourcroy, P., Briat, J. F., Abadía, A., Gaymard, F., et al. (2016). Accumulation and Secretion of Coumarinolignans and other Coumarins in Arabidopsis thaliana Roots in Response to Iron Deficiency at High pH. Front. Plant Sci. 7. doi: 10.3389/fpls.2016.01711
Song, W. Y., Park, J., Mendoza-Cózatl, D. G., Suter-Grotemeyer, M., Shim, D., Hörtensteiner, S., et al. (2010). Arsenic tolerance in Arabidopsis is mediated by two ABCC-type phytochelatin transporters. Proc. Natl. Acad. Sci. U.S.A. 107 (49), 21187–21192. doi: 10.1073/pnas.1013964107
Strader, L. C., Bartel, B. (2009). The Arabidopsis PLEIOTROPIC DRUG RESISTANCE8/ABCG36 ATP binding cassette transporter modulates sensitivity to the auxin precursor indole-3-butyric acid. Plant Cell 21 (7), 1992–2007. doi: 10.1105/tpc.109.065821
Therby-Vale, R., Lacombe, B., Rhee, S. Y., Nussaume, L., Rouached, H. (2022). Mineral nutrient signaling controls photosynthesis: focus on iron deficiency-induced chlorosis. Trends Plant Sci. 27 (5), 502–509. doi: 10.1016/j.tplants.2021.11.005
Thoiron, S., Pascal, N., Briat, J. F. (1997). Impact of iron deficiency and iron re-supply during the early stages of vegetative development in maize (Zea mays L.). Plant Cell &Environment 20 (8), 1051–1060. doi: 10.1111/j.1365-3040.1997.tb00681.x
Verrier, P. J., Bird, D., Burla, B., Dassa, E., Forestier, C., Geisler, M., et al. (2008). Plant ABC proteins–a unified nomenclature and updated inventory. Trends Plant Sci. 13 (4), 151–159. doi: 10.1016/j.tplants.2008.02.001
von der Mark, C., Ivanov, R., Eutebach, M., Maurino, V. G., Bauer, P., Brumbarova, T. (2021). Reactive oxygen species coordinate the transcriptional responses to iron availability in Arabidopsis. J. Exp. Bot. 72 (6), 2181–2195. doi: 10.1093/jxb/eraa522
Wakabayashi, K., Tamura, A., Saito, H., Onishi, Y., Ishikawa, T. (2006). Human ABC transporter ABCG2 in xenobiotic protection and redox biology. Drug Metab. Rev. 38 (3), 371–391. doi: 10.1080/03602530600727947
Waters, B. M., Amundsen, K., Graef, G. (2018). Gene expression profiling of iron deficiency chlorosis sensitive and tolerant soybean indicates key roles for phenylpropanoids under alkalinity stress. Front. Plant Sci. 9. doi: 10.3389/fpls.2018.00010
Wu, T., Zhang, H. T., Wang, Y., Jia, W. S., Xu, X. F., Zhang, X. Z., et al. (2012). Induction of root Fe(lll) reductase activity and proton extrusion by iron deficiency is mediated by auxin-based systemic signalling in Malus xiaojinensis. J. Exp. Bot. 63 (2), 859–870. doi: 10.1093/jxb/err314
Xu, Y., Zhang, S., Guo, H., Wang, S., Xu, L., Li, C., et al. (2014). OsABCB14 functions in auxin transport and iron homeostasis in rice (Oryza sativa L.). Plant J. 79 (1), 106–117. doi: 10.1111/tpj.12544
Yasuno, N., Takamure, I., Kidou, S. I., Tokuji, Y., Ureshi, A. N., Funabiki, A., et al. (2009). Rice shoot branching requires an ATP-binding cassette subfamily G protein. New Phytol. 182 (1), 91–101. doi: 10.1111/j.1469-8137.2008.02724.x
Yi, Y., Guerinot, M. L. (1996). Genetic evidence that induction of root Fe(III) chelate reductase activity is necessary for iron uptake under iron deficiency. Plant journal: Cell Mol. Biol. 10 (5), 835–844. doi: 10.1046/j.1365-313x.1996.10050835.x
Yu, L., Bergmann, K., Lütjohann, D., Hobbs, H. H., Cohen, J. C. (2005). Ezetimibe normalizes metabolic defects in mice lacking ABCG5 and ABCG8. J. Lipid Res. 46 (8), 1739–1744. doi: 10.1194/jlr.M500124-JLR200
Zaynab, M., Wang, Z., Hussain, A., Bahadar, K., Sajid, M., Sharif, Y., et al. (2022). ATP-binding cassette transporters expression profiling revealed its role in the development and regulating stress response in Solanum tuberosum. Mol. Biol. Rep. 49 (6), 5251–5264. doi: 10.1007/s11033-021-06697-z
Zhang, Y., Kilambi, H. V., Liu, J., Bar, H., Lazary, S., Egbaria, A., et al. (2021). ABA homeostasis and long-distance translocation are redundantly regulated by ABCG ABA importers. Sci. Adv. 7 (43), eabf6069. doi: 10.1126/sciadv.abf6069
Zhang, J. C., Wang, X. F., Wang, X. N., Wang, F. P., Ji, X. L., An, J. P., et al. (2019). Abscisic acid alleviates iron deficiency by regulating iron distribution in roots and shoots of apple. Scientia Horticultura 262, 109018. doi: 10.1016/j.scienta.2019.109018
Ziegler, J., Schmidt, S., Strehmel, N., Scheel, D., Abel, S. (2017). Arabidopsis Transporter ABCG37/PDR9 contributes primarily highly oxygenated Coumarins to Root Exudation. Sci. Rep. 7 (1), 3704. doi: 10.1038/s41598-017-03250-6
Keywords: ATP-binding cassette, GmABCG5, iron deficiency, soybean, RNAi
Citation: Wang Y, Zhang X, Yan Y, Niu T, Zhang M, Fan C, Liang W, Shu Y, Guo C, Guo D and Bi Y (2024) GmABCG5, an ATP-binding cassette G transporter gene, is involved in the iron deficiency response in soybean. Front. Plant Sci. 14:1289801. doi: 10.3389/fpls.2023.1289801
Received: 06 September 2023; Accepted: 24 November 2023;
Published: 05 January 2024.
Edited by:
Weiqiang Li, Chinese Academy of Sciences (CAS), ChinaReviewed by:
Rumen Ivanov, Heinrich Heine University of Düsseldorf, GermanyCopyright © 2024 Wang, Zhang, Yan, Niu, Zhang, Fan, Liang, Shu, Guo, Guo and Bi. This is an open-access article distributed under the terms of the Creative Commons Attribution License (CC BY). The use, distribution or reproduction in other forums is permitted, provided the original author(s) and the copyright owner(s) are credited and that the original publication in this journal is cited, in accordance with accepted academic practice. No use, distribution or reproduction is permitted which does not comply with these terms.
*Correspondence: Donglin Guo, Z3VvZG9uZ2xpbkBocmJudS5lZHUuY24=; Yingdong Bi, eWluZ2RvbmdiaUBoYWFzLmNu
†These authors have contributed equally to this work
Disclaimer: All claims expressed in this article are solely those of the authors and do not necessarily represent those of their affiliated organizations, or those of the publisher, the editors and the reviewers. Any product that may be evaluated in this article or claim that may be made by its manufacturer is not guaranteed or endorsed by the publisher.
Research integrity at Frontiers
Learn more about the work of our research integrity team to safeguard the quality of each article we publish.