- 1College of Horticulture and Landscape Architecture, Zhongkai University of Agriculture and Engineering, Guangzhou, Guangdong, China
- 2Key Laboratory for New Technology Research of Vegetable, Vegetable Research Institute, Guangdong Academy of Agricultural Science, Guangzhou, China
- 3Department of Crop Physiology, Faculty of Crop Production, Sindh Agriculture University, Tandojam, Pakistan
- 4College of Agriculture and Biology, Zhongkai University of Agriculture and Engineering, Guangzhou, Guangdong, China
Magnesium (Mg2+) is pivotal for the vitality, yield, and quality of horticultural crops. Central to plant physiology, Mg2+ powers photosynthesis as an integral component of chlorophyll, bolstering growth and biomass accumulation. Beyond basic growth, it critically affects crop quality factors, from chlorophyll synthesis to taste, texture, and shelf life. However, Mg2 + deficiency can cripple yields and impede plant development. Magnesium Transporters (MGTs) orchestrate Mg2+ dynamics, with notable variations observed in horticultural species such as Cucumis sativus, Citrullus lanatus, and Citrus sinensis. Furthermore, Mg2+ is key in fortifying plants against environmental stressors and diseases by reinforcing cell walls and spurring the synthesis of defense substances. A burgeoning area of research is the application of magnesium oxide nanoparticles (MgO-NPs), which, owing to their nanoscale size and high reactivity, optimize nutrient uptake, and enhance plant growth and stress resilience. Concurrently, modern breeding techniques provide insights into Mg2+ dynamics to develop crops with improved Mg2+ efficiency and resilience to deficiency. Effective Mg2+ management through soil tests, balanced fertilization, and pH adjustments holds promise for maximizing crop health, productivity, and sustainability. This review unravels the nuanced intricacies of Mg2+ in plant physiology and genetics, and its interplay with external factors, serving as a cornerstone for those keen on harnessing its potential for horticultural excellence.
1 Introduction
Magnesium (Mg2+) is not only a quintessential macronutrient but also a cornerstone for the vitality and quality of horticultural crops. Its importance stretches across a broad spectrum of plant physiology, governing photosynthesis, nutrient metabolism, cell membrane stability, enzyme activation, and, notably, its resilience against various environmental stresses (Chen et al., 2018; Tian et al., 2021; Kumari et al., 2022). While its influence on agronomic plants has been meticulously explored in previous research, the depth of knowledge regarding horticultural crops remains comparatively shallow (Crusciol et al., 2019; Qin et al., 2020; Heidari et al., 2021; Sharma et al., 2022). This gap is surprising given the pronounced impact of Mg2+ on pivotal horticultural crop attributes, from enhancing flavor to influencing texture and extending shelf life (Zhang H, et al., 2020; Adnan et al., 2021). It is becoming apparent that the intricate interplay of Mg2+ with flavor nuances, texture modulation, and postharvest durability in horticultural crops is a fertile ground for exploration. In today’s context, where consumers exhibit an escalating appetite for premium-quality produce, unraveling the depth of Mg2+’s role is not just a scientific endeavor but also an economic imperative (Gelli et al., 2015; Adaskaveg and Blanco-Ulate, 2023). Addressing this will not merely align with market aspirations, but will also carve a competitive edge for growers. Moreover, although the qualitative advantages of Mg2+ are evident, its quantitative contribution to crop yields cannot be understood. Proper management of Mg2+ is not a luxury but a necessity for the economic viability and sustainability of horticultural enterprises (Adnan et al., 2021; He et al., 2022). The tapestry of horticultural crops, with their unique characteristics and demands, presents a compelling case for a more exhaustive examination of the role of Mg2+. By illuminating the myriad ways in which Mg2+ shapes the growth, development, and quality of these crops, we can equip growers with a refined toolkit for nutrient management, aligning scientific insight with on-ground farming practices.
2 The critical role of magnesium in horticultural crop physiology and productivity
Magnesium although present in plant tissues at relatively modest concentrations, is an indispensable macronutrient. It plays a multitude of pivotal roles in plant physiology, growth, development, productivity, and resilience to environmental stresses (Tang and Luan, 2020; Hamedeh et al., 2022; Bin et al., 2023). Despite its seemingly small presence, the myriad functions that Mg2+ undertakes within plant physiology affirms its critical importance for maintaining plant health and securing high yields. Mg2+ plays an indispensable role in plant physiology; from its key role as the central atom in the chlorophyll molecule, crucial for photosynthesis (Tang et al., 2023), to its function as an activator of myriad enzymes. This dual capacity not only underscores its importance in fundamental photosynthetic processes but also highlights its pervasive influence across a broad spectrum of plant metabolic activities, including nutrient uptake, energy transfer, and regulation of cellular processes (Morozova, 2022; Kleczkowski and Igamberdiev, 2023). Moreover, Mg2+ plays a significant role in transporting carbohydrates from leaves to other developing tissues (Jiao et al., 2023). In addition to its metabolic role, Mg2+ contributes to the maintenance of structural stability in plant cells. It aids in stabilizing cell structures, particularly cell membranes, ribosomes, mitochondria, chloroplasts, and nucleic acids, ensuring both the structural integrity and functional efficiency of plant cells (Rengel et al., 2015; Kleczkowski and Igamberdiev, 2023). Furthermore, an emerging body of research has underscored the importance of Mg2+ in bolstering plant resilience against diverse environmental stressors (Rengel et al., 2015; Silva et al., 2017).
2.1 Role in photosynthesis and energy metabolism
Mg2+ is a paramount macronutrient that serves as a linchpin for numerous aspects of plant growth, development, and function (Figure 1). Its role in photosynthesis is multifaceted and its presence can significantly influence this process, directly affecting plant productivity (Li et al., 2023b). The efficacy of photosynthetic processes depends heavily on Mg2+ availability (Peng et al., 2019). Its interaction with the chlorophyll absorption spectrum aligns with specific light wavelengths (Ma et al., 2023), primarily in red and blue ranges. Within the chlorophyll molecule, Mg2+ ions play a vital role in photon capture and the subsequent transfer of energy to the photosynthetic machinery of plants (Igamberdiev and Kleczkowski, 2011; Sun T. et al., 2023). Specifically, within the thylakoid membranes of chloroplasts, Mg2+-containing chlorophyll molecules assemble into photosystems I and II (Rankelytė et al., 2023). These structures orchestrate the light-dependent photosynthetic reactions. When chlorophyll absorbs light energy, Mg2+ ions are pivotal in initiating electron transfer and the formation of energy-rich molecules such as adenosine triphosphate (ATP) and nicotinamide adenine dinucleotide phosphate (NADPH) (Tang and Luan, 2017; Kleczkowski and Igamberdiev, 2023). This electron flow is vital for synthesizing ATP and fueling a myriad of cellular processes, including the conversion of carbon dioxide into organic compounds (Figure 2) (Jiao et al., 2023). Furthermore, Mg2+’s significance in photosynthesis permeates dark reactions, most notably in its role with the enzyme RuBisCO (ribulose-1,5-bisphosphate carboxylase/oxygenase), which catalyzes the fixation of carbon dioxide (CO2) and the subsequent formation of carbohydrates (Douglas-Gallardo et al., 2022). As an essential cofactor, Mg2+ empowers RuBisCO activation and optimization, facilitating the transformation of CO2 into organic molecules (Douglas-Gallardo et al., 2022). Conclusively, Mg2+ central position within the chlorophyll molecule is indispensable for light energy absorption and the onset of photosynthesis (Tang et al., 2023). Through electron transfer, ATP synthesis, CO2 fixation, and carbohydrate synthesis, Mg2+ acts as an underlying catalyst, nurturing plant growth, development, and productivity. Its strategic and multifunctional roles underscore its importance, reinforcing the necessity of recognizing and harnessing its potential within horticulture.
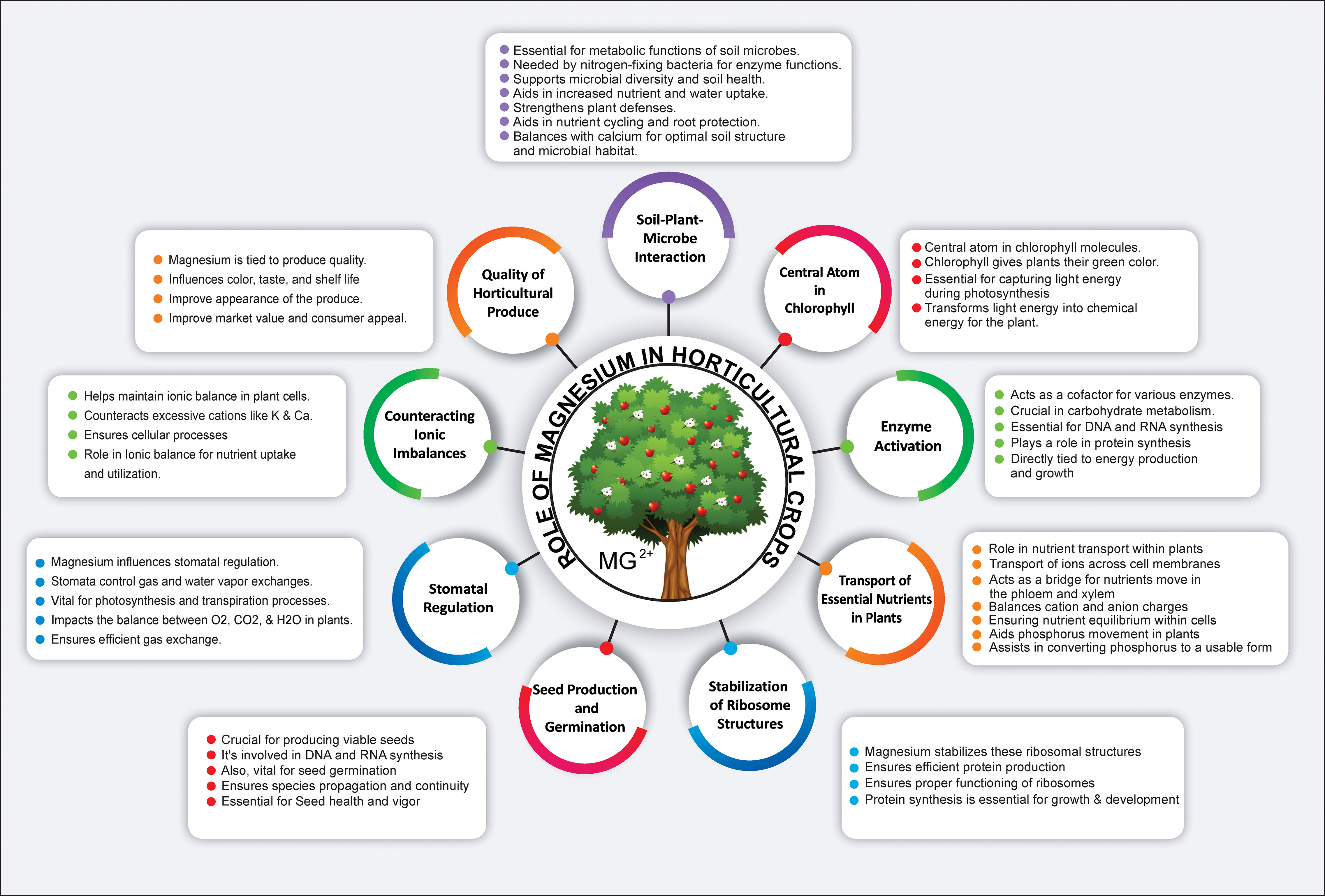
Figure 1 Role of magnesium in plant physiology: Central atom in chlorophyll, Enzymatic cofactor, Nutrient transporter, Ribosomal stabilizer, Seed production enhancer, Stomatal regulator, Ionic balance maintainer, and Influencer of horticultural quality and soil-microbe interactions.
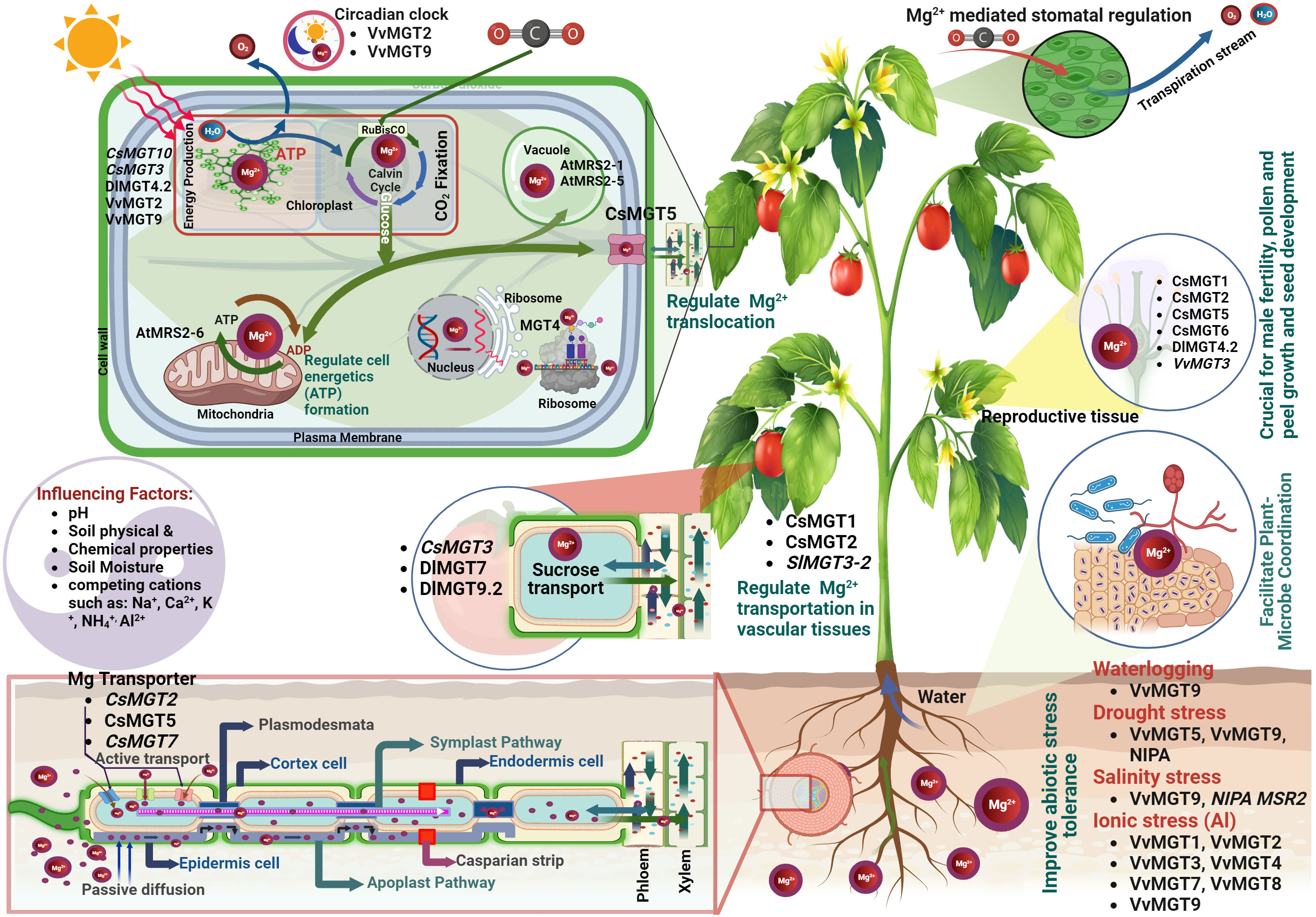
Figure 2 Roles of Mg2+ and Magnesium Transporters (MGTs) in plants: (Upon entry into the root stele, Mg2+ ions are shuttled into xylem vessels by transport proteins, aiding their distribution to the aerial parts. Mg2+ is central to photosynthesis because of its position in the chlorophyll. It catalyses ATP synthesis, CO2 fixation, and carbohydrate production, thereby fostering plant growth. Inside the cells, Mg2+ supports protein synthesis, DNA replication, and ATP utilization. These ions are essential for chlorophyll synthesis, enzyme activation and nucleic acid production. MGTs are also vital for Mg2+ absorption, transport, and utilization in reproductive organs, influencing pollen development, fruit quality, and stress tolerance).
Mg2+ serves as a multifunctional catalyst in plant physiology, playing a critical role in various aspects of enzyme activation and metabolism (Figure 1). Its function as a cofactor in numerous enzymatic reactions emphasizes its significance in the synthesis and metabolism of carbohydrates, proteins, and nucleic acids (Farhat et al., 2016; Douglas-Gallardo et al., 2022; Tang et al., 2022a). The influence of Mg2+ extends to essential processes such as protein synthesis, cell division, and DNA replication, reflecting its pervasive impact on plant metabolism (Gerendás and Führs, 2013; Wang et al., 2020; Zhang B, et al., 2020). Specifically, Mg2+ is fundamental in activating key enzymes such as pyruvate kinase, which catalyzes the conversion of phosphoenolpyruvate (PEP) to pyruvate during glycolysis (Schormann et al., 2019). Moreover, Mg2+ affects the activity of enzymes in the tricarboxylic acid (TCA) cycle, such as isocitrate dehydrogenase and α-ketoglutarate dehydrogenase (Yang et al., 2013; Jin et al., 2023), which are both crucial for energy production and the synthesis of metabolic intermediates. Moreover, Mg2+ is indispensable for protein synthesis in plants as it contributes to the structure and function of ribosomes (Figure 2). By interacting with ribosomal subunits, Mg2+ adds stability and aids in binding messenger RNA (mRNA) and transfer RNA (tRNA), ensuring the accurate translation of genetic information into functional proteins (Yu et al., 2023). In terms of nucleic acid metabolism, Mg2+ is actively involved in DNA replication and repair. It influences the function of enzymes, such as DNA polymerase and DNA ligase (Adhikari et al., 2006; Romani, 2011), as well as RNA synthesis and processing, affecting the activity of RNA polymerases and ribonucleases in transcription and RNA maturation (Yu et al., 2023).
Furthermore, Mg2+ plays a role in phosphate transport and metabolism by activating ATPases and phosphatases (Cakmak and Yazici, 2010). This helps to regulate the transition of phosphate ions across membranes and the conversion of organic phosphates into accessible inorganic forms for cellular use. In energy metabolism, Mg2+ is involved in the synthesis and utilization of adenosine triphosphate (ATP). Acting as a cofactor for ATP synthase, ATP synthesis is observed during oxidative phosphorylation in mitochondria (Cakmak and Kirkby, 2008; Igamberdiev and Kleczkowski, 2011). Additionally, Mg2+ affects the activity of enzymes that break down ATP, such as ATPases, thereby releasing energy for various cellular functions (Ma et al., 2023). Mg2+ serves as an essential cofactor in plant enzymatic reactions and plays a pivotal role in various metabolic pathways, which in turn influences plant physiology, growth, and overall development.
2.2 Protein synthesis
Mg2+ serves as a cornerstone in the intricate process of protein synthesis, which is a critical aspect of plant growth and development. Their involvement spans several stages of protein formation, all of which collectively contribute to the synthesis of functional proteins (Petrov et al., 2012). Mg2+ is integral to the structural formation and function of ribosomes, which are the cell organelles responsible for protein synthesis. Mg2+ aids in the stability of ribosomal subunits and promotes the binding of messenger RNA (mRNA) and transfer RNA (tRNA), thereby ensuring accurate translation of genetic information into proteins (Petrov et al., 2012; Verbruggen and Hermans, 2013; Yu et al., 2023). Furthermore, Mg2+ influences gene expression by modulating multiple phases of transcription and RNA processing. It activates and regulates enzymes and proteins essential for DNA replication, transcription, and RNA maturation, such as DNA and RNA polymerases (Adhikari et al., 2006; Andreadelli et al., 2021; El-Sharkawy et al., 2022). By interacting with various enzymes involved in DNA repair, Mg2+ enhances their activity and ensures the effective transcription of genetic information. Mg2+ also contributes to the stability and integrity of RNA by preventing its degradation by ribonucleases (RNases). Its involvement in pre-mRNA splicing is vital for the formation of mature and functional mRNA molecules (Adhikari et al., 2006). This precise splicing process, facilitated by Mg2+, ensures the proper removal of introns and retention of exons, leading to the synthesis of functional proteins (Johansson and Jacobson, 2010). The influence of Mg2+ extends to the compaction and packaging of DNA, where it plays a role in shaping the structure and organization of chromatin (Hartwig, 2001). Mg2+ interacts with histone proteins that bind to DNA, thereby facilitating the formation of nucleosomes and higher-order chromatin structures (Ohyama, 2019). These interactions help maintain chromatin stability and integrity, influencing gene accessibility and the regulation of transcription and translation. Mg2+ is essential for protein synthesis, weaving its way through various processes to ensure the accurate and effective production of proteins. From influencing ribosome structure to regulating gene expression and RNA splicing, the role of Mg2+ is multifaceted and crucial. It even extends to the structural components of DNA, maintaining its stability and integrity.
2.3 Magnesium in the transport of essential nutrients in plants
Mg2+ plays a pivotal role in the uptake and transport of vital nutrients within plants, ensuring proper growth, development, and metabolic function. This multifaceted role can be understood by exploring its influence on various nutrient transport systems. Mg2+ is pivotal in the regulation of phosphorus uptake and transport by modulating the activity of P transporters and channels. Mg2+ ensures the efficient movement of phosphate ions from the soil into plant roots, thus maintaining optimal intracellular P levels (Verbruggen and Hermans, 2013; Assunção et al., 2022). Mg2+ also plays a vital role in the regulation of K + transporters and channels, orchestrating the proper movement of K+ ions across cell membranes (Hermans et al., 2013). A deficiency in Mg2+ can disrupt K+ uptake, leading to an imbalance that affects physiological processes, such as osmoregulation, enzymatic activity, and stomatal function (Verbruggen and Hermans, 2013). Similarly, Mg2+ is involved in the regulation of Ca2+ transporters and channels, thereby controlling the influx of Ca2+ across membranes (Cakmak and Yazici, 2010). Imbalances in Ca2+, often stemming from Mg2+ deficiency, can affect essential functions, such as cell division, cell wall synthesis, and signal transduction. Moreover, Mg2+ has broad effects on the uptake and distribution of other essential nutrients including nitrogen (N), iron (Fe2+), and zinc (Zn2+). The interactions of Mg2+ with other nutrients are summarized in Table 1. Deficiencies in Mg2+ can lead to inadequate uptake and utilization of Fe2+ and Zn2+, resulting in micronutrient imbalances that can hinder plant growth and development (Cakmak and Yazici, 2010). Mg2+ establishes a delicate balance within the plant by interacting with and regulating various nutrient transport systems. This balance ensures proper nutrient distribution and supports various physiological processes (Cakmak and Yazici, 2010; Ye et al., 2019). Delving deeper into the intricate relationship between Mg2+ and nutrient transport, as explored in studies like those by Assunção et al. (2022), unveils promising avenues to bolster plant health and productivity. Tailoring fertilization strategies to include optimal levels of Mg2+ could mitigate nutrient deficiencies and enhance overall crop yields.
2.4 Magnesium in maintaining cellular functions
Mg2+ is a critical element that permeates various cellular functions within plants, acts as a catalyst in enzymatic reactions, stabilizes cell membranes, and contributes to ion homeostasis (Farhat et al., 2016; Tian et al., 2022). Its diverse roles are essential for the optimal growth, development, and productivity of horticultural crops (Andreadelli et al., 2021). Recognizing the paramount importance of Mg2+ in plants, the following sections will delve into the multifaceted roles of Mg2+, exploring how it orchestrates and supports essential cellular functions, from energy metabolism to structural stability and resilience.
In plants, Mg2+ plays an important role in maintaining cell membrane stability and in regulating ion homeostasis. Their presence influences the structure and function of cell membranes, thereby ensuring proper cellular processes and overall plant health (Li et al., 2023b). Mg2+ interacts with phospholipids, which are the main components of cell membranes, helping to maintain their fluidity and stability (Cakmak and Yazici, 2010; Li et al., 2023b). Mg2+ also plays a crucial role in preventing the destruction of lipid bilayers and maintaining the structural integrity of cell membranes under various environmental conditions (Kobayashi and Tanoi, 2015). It interacts with ion channels, modulates their activity, and controls the movement of ions, including potassium (K+), calcium (Ca2+), and other cations, across membranes (Hermans et al., 2013; Verma et al., 2021). Hence, it helps regulate the balance of important ions such as K+, Ca2+, and Na+ (Verma et al., 2021). Mg2+ ions interact with ion transporters and pumps, influencing the movement and distribution of ions across cell membranes, which is crucial for physiological processes, such as nutrient uptake, osmoregulation, and cellular signaling (Yang et al., 2012; Hermans et al., 2013; Ye et al., 2019). Mg2+ also contributes to the regulation of the cytoplasmic pH in plants. It influences the activity of proton pumps and ion channels involved in pH regulation, maintains an optimal pH environment within plant cells, and is essential for the proper functioning of enzymes and metabolic processes (Bose et al., 2011). The stability of the cell membrane is pivotal in protecting cells from various environmental stresses, such as drought, heat, cold, photooxidative damage, salinity, and heavy metal stress (Cakmak and Kirkby, 2008; Rengel et al., 2015; Silva et al., 2017; Adnan et al., 2021; Hamedeh et al., 2022; Li et al., 2023b). The role of Mg2+ in maintaining membrane stability helps preserve the viability and functionality of cells under adverse conditions (Rehman et al., 2018).
Mg2+ interacts with various negatively charged molecules, such as nucleotides and chlorophyll, thereby stabilizing their structure (Tang and Luan, 2017; Tränkner et al., 2018). This stabilization helps in maintaining the electrochemical gradient across cell membranes, which is essential for cellular activities (Bose et al., 2011). Mg2+ participates in controlling the activity of ionic channels in many tissues. Its mechanism of action relies on direct interaction with the channel, indirect modification of channel function through other proteins (e.g., enzymes or G proteins), or via membrane surface charges and phospholipids (Marschner, 2011). It also acts as a cofactor for several transport proteins and channels responsible for the movement of ions across membranes (Allen, 2013; Chen and Ma, 2013). It regulates the activity of these channels, thereby controlling the passage of vital ions such as K+ and Ca2+, which are crucial in various cellular signaling pathways (Hermans et al., 2013; Tang and Luan, 2017; Ge H. et al., 2022). A deficiency or imbalance in Mg2+ affects ion homeostasis, leading to dysfunctions in various cellular processes. The symptoms include poor plant growth, chlorosis, and increased susceptibility to stress (Yang et al., 2013; Peng et al., 2019; Ye et al., 2019). Continued research in this area can provide insights into maximizing crop yield and quality through proper Mg management (Yang et al., 2012; He et al., 2022). Therefore, Mg2+ is pivotal in regulating plant cellular ion balance, influencing overall health and development. Its connection with ion homeostasis provides insights into plant nutrition and potential agricultural advancements.
2.5 Role of magnesium in soil-plant-microbe interaction
The role of Mg2+ in soil-plant-microbe interactions is multifaceted and essential for sustainable horticultural crop production. Adequate Mg2+ levels in the soil promote the growth of beneficial microorganisms, improve nutrient availability, and enhance the overall health and resilience of horticultural crops (Yang et al., 2021; Nikolaou et al., 2023; Yang et al., 2023). Mg2+ availability in the soil not only affects plant growth and development but also has profound implications for the composition and activity of soil microbial communities. Mg2+ availability in the soil can modulate the abundance and diversity of soil microbial communities (Yang et al., 2023). Studies have shown that sufficient Mg2+ levels promote the growth of beneficial microorganisms such as mycorrhizal fungi (Hou et al., 2021; Yang et al., 2023). These mycorrhizal associations form symbiotic relationships with plant roots, facilitating nutrient uptake and promoting plant growth and stress tolerance (Qin et al., 2020; Liu et al., 2023). Mg2+ availability in the rhizosphere can also influence soil pH, nutrient solubility, and availability to plants. Adequate Mg2+ levels help to maintain optimal rhizosphere pH and enhance nutrient availability for plant uptake (Wang et al., 2020). It also influences the activity of plant growth-promoting rhizobacteria (PGPR) (Nikolaou et al., 2023). These beneficial bacteria colonize the rhizosphere and promote plant growth by facilitating nutrient acquisition, disease resistance, and stress tolerance (Kumar et al., 2021; Tahiri et al., 2022). Research has shown that Mg2+ availability can modulate the production of certain plant growth-promoting substances by PGPR, leading to beneficial effects on horticultural crop growth and yield (Yang et al., 2021; Tahiri et al., 2022; Yang et al., 2023). Mg2+ also plays a role in nutrient cycling within the soil, affecting the decomposition of organic matter, mineralization of nutrients, and nutrient availability to plants. Proper Mg2+ management helps to maintain soil fertility and nutrient balance, ensuring that essential nutrients are readily available for plant uptake (Ye et al., 2019; Nikolaou et al., 2023). Understanding these intricate interactions between Mg, soil, plants, and microbes can guide effective Mg2+ management practices, ultimately leading to optimized crop productivity and improved agricultural sustainability.
2.6 Magnesium in reproductive development
Magnesium, an essential macronutrient, plays a significant role in the reproductive development of plants, directly affecting yield quality and quantity. The role of Mg2+ has been underscored in numerous studies, particularly during the flowering and fruit development phases. These studies illustrate that Mg2+ deficiency can lead to suboptimal fruit and seed sets, thereby contributing to a significant reduction in crop yields (Gransee and Führs, 2013; Setiawati et al., 2020; Wang et al., 2020; Zhang H. et al., 2020). A crucial aspect of plant reproduction is the development of pollen, the primary vehicle for fertilization in plants. A recent study demonstrated that an insufficient Mg2+ supply adversely affects pollen development, thereby decreasing overall plant fertility (Xu X.F. et al., 2021). Consequently, poor fruit set is observed, which directly affects crop yield by reducing the number of fruits produced (Ceylan et al., 2016; Kanjana, 2020; Zhang et al., 2020; Zhang S. et al., 2021). Mg2+ deficiency can impede seed formation and maturation, resulting in fewer and poor-quality seeds (Ceylan et al., 2016; Kanjana, 2020). Such subpar seed formation contributes to yield reduction and can compromise the viability of future plant generations (Zhang et al., 2020). Importantly, the role of Mg2+ extends beyond immediate crop yield. Research suggests that Mg2+ deficiency can also lead to a long-term decline in plant fertility, thereby affecting the plant’s yield potential in successive growing seasons (Tang and Luan, 2017; Zhang B, et al., 2020). Furthermore, Mg2+ plays a pivotal role in both chlorophyll synthesis and photosynthesis, which directly influence plant growth and reproduction (Shaul, 2002; Hamedeh et al., 2022). An inadequate Mg2+ supply can impede these processes, thus indirectly affecting plant fertility and yield. Understanding the vital role of Mg2+ in plant reproductive development provides insights into maximizing crop yield and quality. Careful management of Mg2+ can enhance fertility and fruit and seed development, thereby supporting the sustainable growth of horticultural crops.
3 Impact of magnesium on crop development
3.1 Effects of magnesium on crop quality
Magnesium is an essential macronutrient vital for various physiological processes in plants, including growth, development, reproduction, and quality enhancement (Xu J. et al., 2021; Li et al., 2023c; Tian et al., 2023b). Its importance extends to influencing the taste, texture, shelf life, and nutritional content of fruits and vegetables, thereby driving consumer preferences and market value (Petrescu et al., 2020; Yousaf et al., 2021; Zhang S. et al., 2021; Mullins and Wolt, 2022). In recent years, extensive research has illuminated the complex roles of Mg2+, contributing to a nuanced understanding of its effects on horticultural products (Bashir et al., 2019; Zhang H, et al., 2020; Quddus et al., 2022). This study synthesizes these findings and offers a comprehensive examination of the multifaceted effects of Mg2+. Mg2+ has a profound effect on the taste and aroma of horticultural crops. Liu et al. (2022) demonstrated a positive correlation between Mg2+ content and sugar accumulation in navel oranges, substantiating the role of Mg2+ in sugar synthesis. Furthermore, Li et al. (2021) investigated Camellia sinensis and revealed that adequate Mg2+ levels enhance the production of volatile compounds, thus improving the quality constituents of Hydroponic-Cultivated Tea. In a study on oolong tea gardens, He et al. (2023) found that application of Mg2+ fertilizer significantly enhanced tea yield, improved tea quality, and boosted nitrogen use efficiency. In addition, Du et al. (2021) investigated the impact of Mg2+ stress on lemon varieties with a special focus on taste and aroma. Under Mg2+ stress, all varieties showed a decline in Mg2+ content, particularly in leaves, roots, fruits, and stems. Although the external appearance of the fruit remained largely unaffected, there were discernible reductions in juice yield, total soluble solids, total acid, and vitamin C content. Such changes, intimately tied to the lemon’s taste and aroma, are correlated with the fruit’s Mg2+ levels. Additionally, the taste profile was further influenced by an increase in nutrients, such as K, Ca, and Mn. These findings underscore the essential role of Mg2+ in preserving the taste and aroma characteristics of lemons. Texture is a significant determinant of crop quality, and Mg2+ plays an indispensable role in shaping it. Quddus et al. (2022) conducted research on tomato and found that Mg2+-supplemented fruits retained firmness for a more extended period, indicating Mg2+’s influence on cell wall composition. Preciado-Mongui et al. (2023) delved into lettuce and observed that Mg2+ levels enhanced the crispness and appeal, thereby boosting market value. A related study by Zheng and Moreira (2020) demonstrated Mg2+ ion impregnation in potato slices to improve cell integrity, reduce oil absorption during frying, and increase crispness. Extensive research underscores the multifaceted benefits of Mg2+ fertilizers in agriculture. Tan et al. (2000) highlighted its positive effects on a range of crops. Building on this foundation, recent studies have delved deeper into specific advantages. For instance, Djabou et al. (2018) found that Mg2+ delayed post-harvest deterioration in cassava. Similarly, kenaf plants exhibited enhanced growth when exposed to Mg2+, as documented by Salih et al. (2023). Watermelon genetics were also affected, with a distinct gene expression pattern in response to Mg2+, according to Heidari et al. (2022). Morales-Payan (2022) pinpointed optimal pineapple yields when magnesium sulfate was employed as the Mg2+ source. Zhang et al. (2022) identified variations in Soybean and Pomelo yields depending on the type of Mg2+ fertilizer used. The importance of Mg2+ is further emphasized by Mengutay et al. (2013), who reported that a deficiency in maize plants increased their susceptibility to heat stress. Moreira et al. (2015) established that a higher concentration of Mg2+ results in reduced disease severity and improved photosynthesis. Rajitha et al. (2019) documented Mg2+ positive influence on groundnuts, while Tan et al. (2000) acknowledged its role in optimizing the yields of sweet potatoes, sugarcane, and various vegetables.
The effects of Mg2+ go beyond immediate sensory qualities, extending the postharvest shelf life and quality. Aghofack and Yambou (2006) reported that Ca2+ and Mg2+ may play a role in stabilizing cell membranes, maintaining cell wall firmness, and inhibiting chlorophyll-degrading enzymes in climacteric banana fruits and non-climacteric pineapple fruits, as well as in inhibiting respiration and the breakdown of membrane lipids in climacteric banana fruits. Kumar et al. (2020) demonstrated that passive modified atmosphere packaging (PMAP) using a pectin-based bionanocomposite film reinforced with Mg2+ hydroxide nanoparticles effectively preserved the quality of cherry tomatoes at low temperatures (10°C), prolonging shelf life up to 24 days, offering a sustainable, biodegradable alternative to traditional synthetic packaging materials like low density polyethylene and polypropylene. A study by Aghofack-Nguemezi et al. (2014) demonstrated that the use of calcium- and magnesium-based fertilizers significantly reduced the incidence and severity of fungal diseases in tomato plants, promoted growth and yield, and extended the shelf life of fruits in the three most cultivated varieties of tomatoes in the Western Highlands of Cameroon. Mg2+ has consistently emerged as a pivotal element in determining nutrient content across a diverse range of crops, signifying its vital role in agricultural practices. Ye et al. (2019) highlighted this in their research on Citrus sinensis seedlings, where it was discovered that Mg2+ application considerably augmented the nutrient content of the leaf blades. Specifically, while Mg2 + deficiency resulted in a decrease in the concentrations of N, P, and Mg2+, it influenced the levels of other nutrients such as K, Ca, Mn, Fe, Cu, and Zn in different ways depending on the leaf’s position and age. This emphasizes the complex yet crucial interplay between Mg2+ and other nutrients in plant growth. Highlighting the broad influence of Mg2+, Nikolaou et al. (2023) reported that organic fertilization rich in Ca2+ and Mg2+ significantly boosted the plant’s bioactive compounds, notably acemannan and total phenolic content in Aloe vera. This not only underscores the role of Mg2+ in nutrient enhancement but also hints at its potential influence on the therapeutic properties of medicinal plants. Yousaf et al. (2021) broadened this perspective with their work on radishes, demonstrating that specific nitrogen and Mg2+ treatments distinctly impacted soluble protein, sugar, and ascorbic acid contents. This further supports the idea that the role of Mg2+ in nutrient enhancement is not limited to fruits or medicinal plants but extends across the vegetable spectrum. In a recent study on tomatoes, Quddus et al. (2022) highlighted the significance of precise application of Mg2+. A dose of 12 kg·ha−1 Mg2+ yielded not only the most abundant tomatoes but also tomatoes of superior nutritional quality, enriched with vitamin C, β-carotene, and protein. Collectively, these studies underscore Mg2+ pivotal role in enriching the nutrient profile of various crops, ranging from fruits to vegetables and medicinal plants, highlighting its significant impact on agriculture.
3.2 Magnesium and crop yield
Mg2+ is a fundamental macronutrient pivotal to various physiological and metabolic processes in horticultural crops, profoundly influencing the yield and quality of fruits, vegetables, and root crops. For instance, Tian et al. (2023b) revealed that Mg2+ supplementation in ‘Red Fuji’ apple trees bolstered nitrogen utilization, enhanced photosynthesis, and encouraged anthocyanin biosynthesis, thereby elevating fruit size, weight, and yield. Quddus et al. (2022) demonstrated that tomatoes benefited substantially from Mg2+ application. Specifically, 12 kg·ha−1 of Mg2+ notably improved photosynthetic efficiency, carbohydrate translocation, and overall yield, resulting in heavier, more nutritious fruits. Similarly, El-Sharkawy et al. (2022) found that spraying grapevines with 3% magnesium carbonate (MgCO3) amplified crop yield by 20% and enhanced berry biochemical properties, suggesting its role in strengthening plant defense mechanisms and optimizing grape growth and yield. Root crops such as potatoes also benefit from Mg2+ supplementation. A two-season study by El-Metwaly and Mansour (2019) showed that using MgSO4 combined with calcium chloride foliar application optimized growth characteristics, leading to significant improvements in tuber yield, dry matter, and starch content. The combined treatment resulted in yield spikes of approximately 42%. Leafy vegetables such as spinach and lettuce are also responsive to Mg2+. Jamali Jaghdani et al. (2021) found that Mg2+ deficiency in spinach reduces CO2 assimilation. Preciado-Mongui et al. (2023) highlighted an ideal fertilization combo for lettuce, optimizing yield while emphasizing the significance of balanced nutrient application. Zhang et al. (2022) further emphasized that slow-release Mg2+ fertilizers, predominantly MgO, outperform their fast-release counterparts in boosting soybean and pomelo yields in acidic soils. In studies on peppers and cucumbers, researchers observed that while Mg2+ supplementation significantly improved yields, its influence on nutrient composition and interactions with factors like irrigation and nitrogen was equally critical. A study by Li et al. (2023a) on cucumbers pinpointed the optimal fertilization combination, leveraging factors like yield, quality, and efficiency. Researchers have also explored the complex interplay between Mg2+ and other nutrients in horticultural crops. Findings by Li et al. (2023b), El-Metwaly and Mansour (2019) underscore the intricate relationship between these nutrients, highlighting the importance of a comprehensive nutrient-management strategy. Finally, reinforcing the necessity of Mg2+, Chen et al. (2023) discovered that while China’s soils are replete with N, P, and K, there is a glaring deficiency of Mg2+ in 73% of them, prompting the recommendation to curtail NPK usage and elevate Mg2+ supplementation to further sustainable agriculture. The interrelationship between Mg2+ and other nutrients underscores the importance of a holistic nutrient management strategy.
3.3 Magnesium and abiotic stress tolerance
Many environmental and intracellular factors affect Mg2+ homeostasis in plants, including Mg2+ deficiency and toxicity, high temperature, drought, high light irradiance, low or high pH, and antagonistic ions (Guo, 2017; Tariq Aftab, 2020). However, recent research has highlighted another critical aspect of Mg2+; its role in bolstering the resilience of horticultural crops to various abiotic stresses such as drought, heat, cold, high irradiance, salinity, and heavy metal toxicity (Boaretto et al., 2020; Jamali Jaghdani et al., 2021; Li et al., 2023b). Understanding the influence of Mg2+ on stress tolerance in horticultural crops provides key insights for the development of effective agricultural practices and supports sustainable horticulture in the face of a changing climate (Hamedeh et al., 2022; Li et al., 2023b). Drought is a significant abiotic stress factor that affects the growth and yield of horticultural crops. In a study by Hamedeh et al. (2022) The biostimulant EnNuVi® ALPAN®, rich in magnesium, was found to enhance drought tolerance in tomato plants by modulating molecular pathways associated with carbohydrate metabolism, stomatal regulation, and cellular homeostasis. This Mg2+-rich formulation mitigated the adverse effects of drought by preserving the photosynthetic pigment levels and reducing cellular oxidative damage. Heat stress is another principal concern in horticultural crop production, particularly given ongoing global warming. Recent studies on lemon trees and tea have demonstrated the beneficial effects of Mg2+ in mitigating the adverse impacts of heat stress (Du et al., 2021; Zhang Q. et al., 2021). In a study by Boaretto et al. (2020), young lemon trees supplemented with extra Mg2+ and/or nitrogen (N) displayed increased resilience to elevated irradiance and air temperature commonly associated with heatwaves. The trees maintained optimal photosynthetic and transpiration rates and Mg2+ enhanced the activity of the antioxidant enzyme system, thereby decreasing oxidative stress. Similarly, Silva et al. (2017) highlighted the importance of Mg2+ nutrition in mitigating the adverse effects of heat stress in coffee seedlings. Adequate Mg2+ nutrition is essential for maintaining lower hydrogen peroxide production and preventing lipid peroxidation and protein degradation under heat stress, emphasizing its indispensable role in preserving cellular integrity and enhancing antioxidant responses during temperature extremes.
Cold stress, particularly in temperate regions, can severely affect the yield of horticultural crops. A study by Li et al. (2023b) on tobacco (Nicotiana tabacum L.) demonstrated that under cold stress conditions, Mg2+ supplementation led to notable improvements in plant morphology, nutrient uptake, and photosynthetic attributes. Notably, tobacco plants treated with Mg2+ under cold stress exhibited significant increases in biomass, nutrient uptake, photosynthetic activity, and chlorophyll content. Furthermore, Mg2+ application positively influenced tobacco quality, including increased starch and sucrose content. These findings highlight that Mg2+ application can mitigate the adverse effects of cold stress and promote better growth and quality of tobacco plants. Mg2+ supplementation has been identified as a vital factor for improving the resilience of pepper plants to salinity stress. Zirek and Uzal (2020) investigated the effects of varying Mg2+ doses on pepper plants under salt-induced stress and discovered that higher Mg2+ doses led to increased plant growth, as demonstrated by greater total plant weights, especially in the exogenous application of Mg2+ @98.56 ppm + salt treatments. Additionally, elevated Mg2+ levels resulted in augmented chlorophyll content and heightened antioxidant enzyme activities, which play a role in combating oxidative stress. Simultaneously, there was a reduction in malondialdehyde (MDA) levels, an indicator of cellular damage. These findings suggest that increasing Mg2+ doses can mitigate the detrimental effects of salinity stress on pepper plants, thereby enhancing their growth and physiological health.
The growing concern over heavy metal toxicity in soils has sparked research into Mg2 the potential role in alleviating these toxic effects in horticultural crops. Lu et al. (2021a) explored the impact of Mg2+ on field-grown Chinese cabbage and discovered that it significantly enhanced the cabbage’s nutritional profile, with noticeable increases in vitamin C and water-soluble protein content. Most notably, Mg2+ reduced the uptake and accumulation of heavy metals, such as cadmium and nickel, in plant tissues, thereby minimizing their adverse health effects. Cr uptake increased, but the health risks posed by Cr were substantially lower than those posed by Cd and Ni. Therefore, soil-applied Mg2+, particularly in the range of 22.5–45 kg ha−1, can notably enhance the nutritional qualities of Chinese cabbage, while alleviating the potential health risks from heavy metal consumption. Luo et al. (2020) investigated the effects of nanometer magnesium hydroxide on the growth of Chinese cabbage and its ability to uptake cadmium (Cd) from polluted soil. These findings revealed that low doses of nanometer-sized magnesium hydroxide boosted the biomass of Chinese cabbage, whereas higher doses had toxic effects. Crucially, the presence of magnesium hydroxide, especially in its nanometer form, effectively decreased the concentration of Cd in various parts of the cabbage, especially under low Cd stress. This Mg2+ treatment also transformed the soil Cd into less bioavailable forms, reducing the exchangeable Cd content and increasing other bound Cd forms. This means that magnesium hydroxide, particularly in its nanometer form, can act as an effective agent in reducing harmful Cd concentrations in crops grown in contaminated soils, offering a potential avenue for mitigating heavy metal stress in agricultural settings. In a separate investigation by Faizan et al. (2022), it was reported that increasing the accumulation of metalloids, such as arsenic, poses significant threats to crop growth and yield. This study revealed that MgO-NPs could bolster plant growth and fortify plant resistance to metal/metalloid toxicity. When soybean plants were treated with MgO-NPs under arsenic-containing conditions, there was a marked improvement in various growth parameters, photosynthetic functions, and nutrient uptake. The nanoparticles also reduced arsenic uptake and associated oxidative damage. These findings highlight that Mg2+ supplementation could reduce the uptake and accumulation of these heavy metals, thus protecting plants from oxidative damage and preserving their growth and productivity. These findings underscore the crucial role of Mg2+ in bolstering the resilience of horticultural crops to various abiotic stresses and maintaining their productivity and quality. Nonetheless, further research is necessary to elucidate the precise mechanisms of Mg2+-mediated stress tolerance and to develop tailored Mg2+-based strategies for mitigating different types of abiotic stress in horticulture. In order to provide a comprehensive overview of the effects of magnesium supplementation on the tolerance of crops to environmental stresses, we have summarized recent research findings in Table 2.
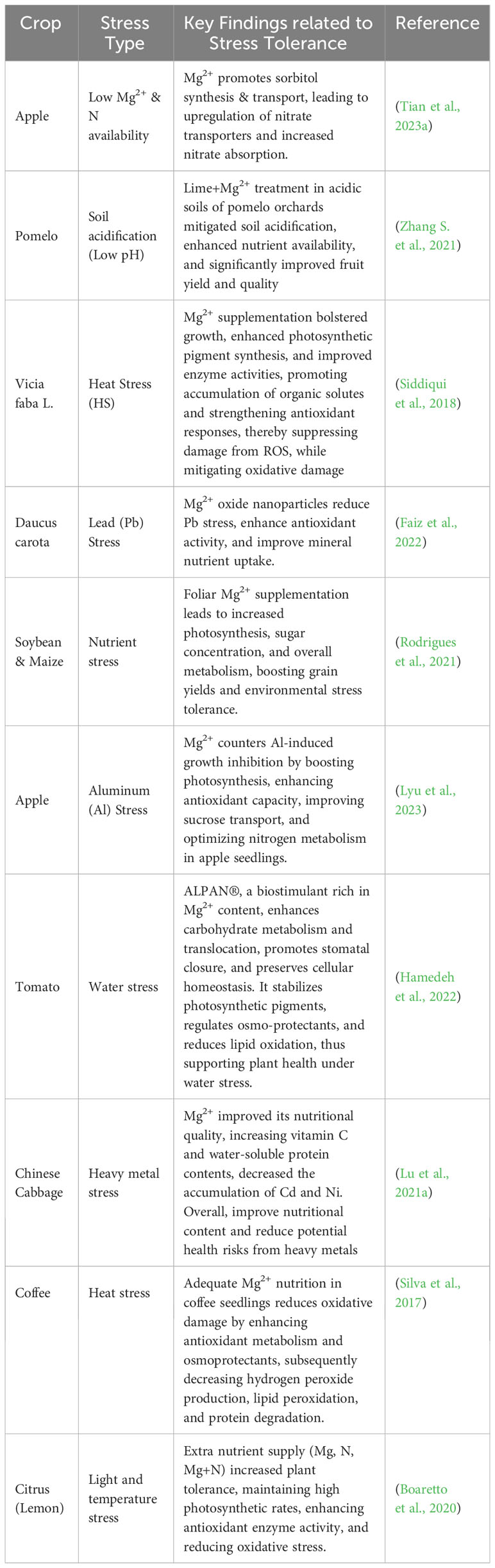
Table 2 Influence of magnesium supplementation on crop tolerance to various environmental stresses: a summary of recent studies.
3.4 Magnesium and its multifaceted role in biotic stress tolerance
Magnesium stands out as more than just an essential macronutrient for plants; its impact permeates deep into the physiological and biochemical frameworks of plants, steering their resistance against biotic stresses, and affirming its crucial role in modern agriculture (Huber and Jones, 2013). Central to the effect of Mg2+ on plant physiology is its role in photosynthesis. Their integral position in chlorophyll molecules resonates with the vitality of plants and their combined resistance to biotic threats (Tang and Luan, 2020). Mg2+ is a linchpin in the biosynthesis of salicylic acid (SA), which is a cornerstone of plant defense. In its nanoparticle form, Mg2+ amplifies the function of phenylalanine ammonia-lyase (PAL), a significant driver of SA production (Setiawati et al., 2020). As SA is fundamental to pathogen defense (Lefevere et al., 2020; Zhong et al., 2021), the interplay between Mg2+ and SA assumes colossal significance. This SA-mediated defense is multifaceted; it orchestrates immediate counteractions against biotrophic pathogens, while simultaneously harmonizing defense and growth via interactions with other hormones, particularly auxins (Zhong et al., 2021). Some plants, such as Fritillaria unibracteata, have demonstrated a role for Mg2+ influenced PAL in mediating drought tolerance by enhancing SA accumulation (Qin et al., 2022). However, SA is not a lone warrior in plant defenses. Other hormones, such as jasmonic acid (JA) and ethylene (ET), have distinctive roles in countering different threats, from (hemo) biotrophic pathogens to herbivorous pests (Huang et al., 2020; Wu and Ye, 2020). The versatility of Mg2+ has been highlighted by the mediation of these diverse pathways. For instance, when Arabidopsis plants were treated with MgO, SA content increased, boosting resistance against Ralstonia solanacearum, a pattern mediated by both SA biosynthesis and ROS production (Ota et al., 2019). However, in another instance, Mg2+’s influence pivoted towards JA signaling, which is critical for tomato plants to ward off Fusarium wilt (Fujikawa et al., 2021). In addition to hormones, Mg2+ tendrils influence various biochemical aspects of plant defence. It modulates metabolic pathways and shapes the production of secondary metabolites that are vital for defense (Guo et al., 2016). However, the influence of Mg2+ spans even further. It has a symbiotic relationship with the cell wall, fortifying plant cells against pathogen infiltration, while ensuring the optimal function of defense-signaling proteins (Ye et al., 2021; Hamedeh et al., 2022). Within this cellular symphony, Mg2+ guarantees the stability of RNA and the timely synthesis of defense-related proteins in the face of pathogenic threats, a response greatly amplified by its availability (Liang et al., 2017; Andreadelli et al., 2021). Additionally, as a co-factor for an array of enzymes, Mg2+ oversees reactive oxygen species detoxification and the synthesis of defence compounds, forming an immediate shield against biotic stressors (Faizan et al., 2022). Mg2+ also intricately modulates metabolic pathways governing secondary metabolite production, many of which underpin plant defense mechanisms (Guo et al., 2016). Another dimension of the Mg2+ defense strategy has emerged from studies pointing to the antimicrobial properties of MgO nanoparticles. These nanoparticles have shown efficacy against a spectrum of bacterial and fungal plant diseases (Liao et al., 2019; Liao et al., 2021). Copper (Cu) bactericides, which are extensively used in agriculture, pose environmental risks and foster Cu-tolerant pathogens such as Xanthomonas perforins. Recent research has found that MgO-NPs effectively reduced tomato bacterial spot disease severity without negative yield impacts or significant soil accumulation of metals, suggesting it as a potential environmentally friendly alternative to Cu bactericides (Liao et al., 2021). However, determining whether antimicrobial efficacy is attributed to the inherent properties of MgO or the unique characteristics of nanoparticles remains a compelling question. However, although the contribution of Mg2+ to plant defense is monumental, there is a balancing act at play. Extreme Mg2+ deprivation can induce stress in plants, leading to chlorophyll degradation and increased ROS generation, thus underscoring the nuanced role of Mg2+ in plant health (Peng et al., 2019).These insights present revolutionary prospects for enhancing plant defense and yield in contemporary agricultural landscapes, emphasizing the pivotal role of Mg2+ in the narrative of plant resilience.
4 Mechanisms and dynamics of magnesium in plants
4.1 Mechanisms of magnesium absorption
Plants absorb Mg2+ from their surrounding environment primarily through their roots via intricate and highly regulated mechanisms. These absorption processes are governed by various environmental factors and operate passively, following a concentration gradient, and actively against it (Hermans et al., 2013). When the Mg2+ content in the soil solution is high, passive diffusion primarily drives uptake (Figure 2). Mg2+ ions permeate the root epidermis and cortex from areas of higher concentration in the soil solution to areas of lower concentration within the roots. However, when soil Mg2+ levels are low, active transport mechanisms become crucial. This involves specialized proteins, known as Mg2+ transporters or channels, which are present in the membranes of root cells. Among these, the CorA, MRS2, and MGT family members have been identified as key players in this process (Li et al., 2016). These transporters facilitate the movement of Mg2+ ions against the concentration gradient into the root cells, an energy-dependent process that requires ATP. The root epidermis, which is the outermost cell layer of the root, acts as the primary site for Mg2+ uptake, with epidermal cells orchestrating the initial contact and uptake of Mg2+ ions from the soil solution (Figure 2). Once these ions infiltrate the root cortex, they traverse the plasma membrane of the root cells, a process assisted by membrane transport proteins including Mg2+ transporters or channels. Upon entering the cytoplasm of root cells, Mg2+ ions can either opt for a symplastic or apoplastic pathway. In the symplastic pathway, Mg2+ ions are transferred from one cell to another through plasmodesmata, the cytoplasmic connections between neighboring cells. Conversely, the apoplastic pathway enables Mg2+ ions to traverse through the cell walls and extracellular spaces between cells (Ishfaq et al., 2022). Following this, Mg2+ ions are transported towards the central root stele housing the xylem vessels, which serve as the primary highway for long-distance nutrient transport. Both the symplastic and apoplastic pathways participate in this movement. Once within the root stele, Mg2+ ions are actively loaded into the xylem vessels and transported upward to the aerial parts of the plant, a process facilitated by specific transport proteins in the plasma membrane of the xylem parenchyma cells (Ishfaq et al., 2022). This upward movement or xylem loading is heavily influenced by transpiration rates and can also be affected by the plant’s Mg2+ nutritional status. The phloem also plays a significant role in redistributing Mg2+ to areas of higher demand, particularly younger leaves and reproductive tissues (Koch et al., 2020). Once in the leaves, Mg2+ ions are vital for several physiological processes, including chlorophyll synthesis and operation of the photosystem II complex. Mg2+ also plays a pivotal role in the activation of numerous enzymes, assisting in carbohydrate partitioning and the synthesis of nucleic acids and proteins (Chen et al., 2018). Therefore, the journey of Mg2+ within a plant from root absorption to leaf utilization is an intricate, multifaceted process, as shown in Figure 2. Understanding these mechanisms offers profound insights into how to optimize the Mg2+ nutritional status of plants, which has implications for improving crop yield and overall plant health.
4.2 Factors affecting magnesium availability and intake
The availability of Mg2+ in the soil and its subsequent intake by plants depends on a variety of factors, such as soil pH, cation exchange capacity (CEC), soil organic matter, soil texture and structure, moisture and drainage, presence of other nutrients, and ambient temperature (Chen and Ma, 2013; Gransee and Führs, 2013; Mengutay et al., 2013; Sun et al., 2013). The availability of Mg2+ in the soil is significantly influenced by the pH level. When the soil is acidic (pH < 6.0), the solubility of Mg2+ decreases because it binds more readily to the soil particles, leading to less accessibility for plant uptake. Conversely, in alkaline soils (pH > 7.0), Mg2+ can become limited owing to the excessive precipitation of Ca and Mg carbonates (Koyama et al., 2001; Kobayashi et al., 2013). The CEC of a soil refers to its capacity to bind and exchange cations, including Mg2+. High CEC soils are advantageous because they have greater Mg2+ retention, thus promoting their availability for plant uptake (Mayland and Wilkinson, 1989). The organic matter content of soil can affect Mg2+ availability. This is because organic matter enhances the soil structure, facilitates cation exchange, and aids the release of Mg2+ from organic matter as it decomposes (Mayland and Wilkinson, 1989). Soil texture and structure also play crucial roles in determining Mg2+ availability. For instance, sandy soils, which have larger particles and low water-holding capacity, are susceptible to Mg2+ leaching, thereby reducing their accessibility to plants. In contrast, clay soils can retain Mg2+ better but may limit their accessibility due to poor drainage and limited root penetration (Senbayram et al., 2015).
The moisture content and drainage of soil can also affect the availability of Mg2+. If the soil is excessively wet or waterlogged, roots may be deprived of oxygen, leading to reduced Mg2+ uptake (Grzebisz, 2011; Gransee and Führs, 2013). The presence and concentration of other nutrients can also influence Mg2+ uptake. For instance, excessive Ca2+ can compete with Mg2+ for uptake by plant roots, potentially reducing Mg2+ availability. Similarly, an imbalance or excessive levels of other nutrients such as K+ or NH4+ can also affect Mg2+ uptake (Fageria, 2001; Römheld and Kirkby, 2009; Cakmak and Yazici, 2010). Mg2+ absorption rates are typically higher in warmer soil temperatures, which facilitates root activity and nutrient uptake. Conversely, cold temperatures can limit root activity and nutrient uptake, potentially affecting Mg2+ uptake during colder seasons (Jin et al., 2022). In conclusion, the availability and absorption of Mg2+ in plants are complex processes that are affected by various environmental and soil factors. Effective management of these factors through appropriate soil amendments, irrigation practices, and nutrient management strategies is critical to ensure optimal Mg2+ uptake and to meet the physiological needs of plants.
5 Magnesium transporters and their importance
5.1 Molecular insights into magnesium transporters: their pivotal roles in plant development and stress responses
Navigating these Mg2+ dynamics are Mg2+ transporter (MGTs) proteins, whose functionalities are garnering increasing research attention. It meticulously orchestrates the absorption, transportation, and storage of Mg2+ within plant cells, ensuring optimal growth and stress responses (Heidari et al., 2022; Bin et al., 2023; Tang et al., 2023). In the horticulture domain, the pertinence of these transporters is evident. Cucumis sativus and Citrullus lanatus, members of the Cucurbitaceae family, house 19 and 20 MGT genes, respectively, predominantly stationed in their root tissues (Heidari et al., 2022). In contrast, Citrus sinensis responds to Mg2+ deficiency with altered CO2 assimilation patterns and heightened expression of specific MGT genes, notably CsMGT3 (Yang et al., 2019; Ye et al., 2019). Furthermore, the intracellular Mg2+ ion dynamics of grapes can be mapped through the structure and function of their MGTs (Ge M. et al., 2022). Remarkably, despite its undeniable importance, Mg2+’s prominence in academia and agriculture often falls into shadow. Historically, cereal seeds have witnessed a decline in their Mg2+ content (Guo et al., 2016). However, with evolving research, especially concerning horticultural crops, the imperative to uncover and understand the molecular transport mechanisms of Mg2+ has surged to the forefront. This chapter ventures into this molecular journey, embarking from the roots, coursing through the xylem, navigating the leaves, and arriving at the blossoms and fruits, shedding light on the nuances of Mg2+ dynamics in these pivotal plant structures.
5.2 Mg2+ absorption in roots: unraveling the transporters and mechanisms
Magnesium, a pivotal nutrient in plant physiology, relies on specialized proteins (MGTs) for its uptake and transportation across plant tissues. Recent research on MGTs in horticultural crops is summarized in Table 3. The role of MGTs is particularly pronounced in the root system, the primary gateway for nutrients from the soil to the plant. Roots serve as linchpins in plant nutrient acquisition, with Mg2+ absorption being central to a myriad of biological processes. As the primary interface between the plant and its soil environment, roots are equipped with an array of transporters to facilitate Mg2+ uptake (Tian et al., 2023a). The morphology of the roots is directly influenced by the Mg2+ levels they encounter. Studies such as those by Regon et al. (2019) highlight how low Mg2+ concentrations result in slender roots sprouting numerous hairs, whereas an abundance of Mg2+ produces thicker, nearly hairless roots. Diving deeper into molecular intricacies, MGTs in roots exhibit an array of functionalities. For instance, Arabidopsis leans on its MGT6 transporter under Mg2+-limited conditions, thereby ensuring high-affinity Mg2+ uptake (Yan et al., 2018). However, the narrative is more nuanced in varied crops. In Citrus sinensis, a host of MGTs, such as CsMGT2 and CsMGT7, modulates their activities according to soil Mg2+ levels. They ramp up expression during scarcity, working overtime to ensure the plant does not starve of this crucial nutrient (Yang et al., 2019; Bin et al., 2023). The tea plant story Camellia sinensis L. adds another chapter to the tale. Here, CsMGT5 stands out, especially during Mg2+ crunch times, to ensure sustained uptake (Bin et al., 2023). Similar to Arabidopsis MGT6, CsMGT5 exhibits evolutionary convergence of function across plant species (Li et al., 2023a). The subcellular localization of these transporters further unravels the depths of their intricacies. For instance, CsMGT5 localizes to the plasma membrane, positioning it perfectly for high-affinity Mg2+ transportation (Li et al., 2023a). When faced with challenging environments, CsMGT5 manifests resilience, emphasizing the transporter’s prowess (Li et al., 2023a; Tang et al., 2021). Moreover, its heterologous expression in Arabidopsis led to enhanced Mg2+ retention not only in the roots but also in the above-ground parts, underscoring its comprehensive role in Mg2+ dynamics (Li et al., 2023a). In conclusion, the multifaceted world of Mg2+ transporters in roots provides a picture of evolutionary brilliance. Their diverse functionalities, adaptive expression patterns, and strategic subcellular positioning collectively ensure that plants thrive, even when faced with fluctuating soil Mg2+ levels. Their role is a testament to the intricate the regulation of plant nutrition, adaptation, and survival.
5.3 Magnesium transport in shoots and leaves
Upon absorption from the soil, Mg2+ is transported via the xylem from the roots to the shoots and is particularly mobile within the phloem. This mobility facilitates the redistribution from mature to younger tissues, ensuring that newer tissues receive adequate Mg2+ levels (Tang and Luan, 2017). Specific transporters such as MGT-1 and MGT-2 in Arabidopsis thaliana play a pivotal role, especially under Mg2+ stress, aiding in the mobilization of Mg2+ from vacuoles (Meng et al., 2022; Tang et al., 2022b). This action ensures a balanced Mg2+ equilibrium within the plant, even when the external Mg2+ supply is limited (Tang et al., 2022b). Transporter expression is a dynamic process. During Mg2+ deficiency, there is an increase in the expression of specific MGT genes in various crops, such as CsMGT3 and CsMGT7, to maintain adequate internal Mg2+ levels (Tang et al., 2021). Furthermore, diverse plants, such as Dimocarpus longan and tomato (Solanum lycopersicum), display unique patterns. In Dimocarpus longan, MGT genes like DlMGT4.2 are expressed across various organs, suggesting a universal role in Mg2+ management (Lv et al., 2023). In contrast, tomato plants under Mg2+ stress exhibit heightened expression of genes such as SlMGT1-1 and SlMGT3-2 across roots and shoots (Regon et al., 2019). Delving into the subcellular localization of these transporters offers profound insights into their respective functionalities. Specifically, AtMGT2 and MGT3 are pivotal in channeling Mg2+ into mesophyll vacuoles (Tang and Luan, 2017). The suspected involvement of MGT10 in shuttling Mg2+ into the chloroplast envelope remains a riveting research topic (Tang and Luan, 2017).
Further highlighting the myriad roles of these transporters, the overexpression of AtMRS2-10 in tobacco was observed to enhance Mg2+ concentration within the plants, thereby conferring tolerance to low-Mg2+ and even aluminum stress (Deng et al., 2006). Functionality assays utilizing a Mg2+-deficient strain of Salmonella typhimurium (MM281) have indicated distinct transport capacities among different transporters (Tang et al., 2021). Notably, while CsMGT1 demonstrated a robust Mg2+ transport capacity, surpassing that of CsMGT2, both CsMGT2.1 and CsMGT3 exhibited negligible Mg2+ transport functions (Tang et al., 2021). These findings shed light on the diverse functionalities of the CsMGT family in Camellia sinensis, thereby providing a foundation for future endeavors aimed at optimizing Mg2+ utilization in these plants (Tang et al., 2021). In addition to their functions in cellular homeostasis, Mg2+ transporters also facilitate critical biochemical processes. ATP synthesis, a product of oxidative and photosynthetic phosphorylation occurring in mitochondria and chloroplasts, respectively, underscores the role of Mg2+ in maintaining electron transport and generating membrane potential (Tang and Luan, 2017; Kleczkowski and Igamberdiev, 2021). Intriguingly, Mg2+ transporters manifest in diverse cellular locations, including the plasma membrane, tonoplast, plastids, mitochondria, and ER (Tang and Luan, 2017; Chen et al., 2018). Some transporters, such as the Arabidopsis mitochondrial MGT5, display tissue specificity; it is expressed exclusively in anthers during the early stages of flower development, highlighting its potential involvement in pollen development and male fertility (Li et al., 2008). Conclusively, the Mg2+ transporter/mitochondrial RNA splicing 2 (MGT/MRS2) family stands out as a linchpin in maintaining Mg2+ homeostasis, thereby underpinning the plant’s growth and developmental trajectories (Li et al., 2023a; Yang et al., 2022; Lv et al., 2023). Beyond its role in photosynthesis, Mg2+ is also pivotal for enzymes such as Mg2+ chelatase (MgCh) in chlorophyll biosynthesis. Disruptions in this process, as observed in the strawberry species Fragaria pentaphylla, manifest as phenotypic changes, such as yellow-green leaves, underlining the importance of Mg2+ in plant health (Ma et al., 2023). In summary, the sophisticated interplay between Mg2+ transporters and their modulated expression in different plants highlights the critical role of Mg2+ in plant physiology and health (Figure 2).
5.4 Magnesium dynamics in reproductive parts
Magnesium transporters play crucial roles across plants, with significant functions detected in the reproductive parts, ensuring successful pollen development, male fertility, and fruit quality (Li et al., 2015; Xu X. F. et al., 2021; Tian et al., 2023b). Several members of the MGT family, including MGT4, MGT5, and MGT9, are vital for pollen development and male fertility. MGT4, which is situated in the endoplasmic reticulum, and MGT9, which is localized in the plasma membrane, are integral to pollen maturation processes (Tang and Luan, 2017; Chen et al., 2018). A comprehensive study of tea plants (Camellia sinensis L.) sheds light on four key MGT genes: CsMGT1, CsMGT2, CsMGT2.1, and CsMGT3. These genes were expressed across roots, stems, leaves, and flowers, with varying responses to Mg2+ levels. Intriguingly, while CsMGT1 and CsMGT2 have evident Mg2+ transport functionality (Figure 3), CsMGT2.1 and CsMGT3 showed negligible transport action. This discovery underscores the varied roles of these transporters in Mg2+ management within tea plants, with potential ramifications for agricultural utilization and Mg2+ enhancement in such crops (Bin et al., 2023; Tang et al., 2023).
Diverse expression patterns have also been observed in tomatoes (Solanum lycopersicum). Liu et al. (2023) pinpointed differential tissue-specific activity among SlMGT genes. SlMGT1-1, for instance, was more dynamically expressed in stems, leaves, and flowers, while genes like SlMGT3-1 and SlMGT5-1 showed minuscule transcript levels in stems and flowers. Such variations hint at the unique roles that each transporter plays in plant growth and physiological metabolism. Viticulture is another arena in which Mg2+ plays a vital role. Recent observations have highlighted increasing Mg2+ deficiencies in vineyards in southern China, which have adverse consequences for grape growth and fruit quality (Ge M. et al., 2022). In grapevines, VvMGT3 was prominently expressed only in pollen and rachis PFS, elucidating its potential role in the growth and development of these specific tissues (Ge M. et al., 2022). Additionally, the application of Mg2+ was found to promote fruit coloring in apples, with a marked upregulation in genes associated with anthocyanin synthesis in the fruit peel, underscoring the intertwined nature of Mg2+ with fruit quality (Tian et al., 2023b). Citrus is another domain in which Mg2+ transporters have unique roles. In citrus fruit pulp and peels, the expression of CsMGT3 outshone others, while CsMGT5’s presence was almost negligible (Bin et al., 2023; Tang et al., 2023). Such tissue-specific expression of CsMGT genes suggests their diverse functions during citrus plant growth and development phases. In summary, Mg2+ transporters, particularly the MGT family, demonstrate a plethora of roles across plant reproductive parts. These transporters not only ensure successful reproduction but also influence fruit development and quality, with implications for agricultural productivity and crop enhancement.
5.5 Magnesium transporters in plants: deciphering roles in biotic and abiotic stress responses and adaptation
Recent studies have significantly expanded our understanding of the role of MGTs in abiotic stress tolerance in plants. In grapes (Vitis vinifera), the VvMGT9 gene is notably upregulated under waterlogging, suggesting a key role in waterlogging resistance (Ge M. et al., 2022). The same gene, along with VvMGT5, also showed marked upregulation during drought stress, emphasizing its potential in drought tolerance (Ge M. et al., 2022). This was further corroborated by findings in watermelon (Citrullus lanatus), where two NIPA genes were found to be responsive to drought stress (Heidari et al., 2022). The role of MGTs in the salinity response has been highlighted in cucumber (Cucumis sativus). Here, NIPA and MSR2 genes exhibited upregulated expression when exposed to NaCl stress (Heidari et al., 2022). Metal stress, particularly from copper, magnesium, and aluminum, presents another dimension of the MGTs function. In grapes, several VvMGT genes show varied expression patterns under these stresses. Intriguingly, while magnesium treatment led to a peak in the expression of numerous VvMGT genes at 24 h, aluminum exposure mostly resulted in up-regulation, except for VvMGT2. This divergence in the response to aluminum hints at the unique mechanisms at play (Ge M. et al., 2022).
Furthermore, the potential of magnesium to mitigate aluminum toxicity has been recognized. Specifically, certain concentrations of Mg2+ alleviate the toxic effects of aluminum, coinciding with increased Al-induced citrate exudation and enhanced plasma membrane H+ ATPase activity (Chen et al., 2014). The regulatory role of Mg2+ is not limited to stress responses. Evidence from a study on potatoes suggests that the “plant hormone signal transduction pathway” may be influenced by Mg-ONPs without any detrimental effects (Wang et al., 2022). Additionally, watermelons show a particular response to melatonin treatment and low leaf nitrogen content, with two NIPA genes being more highly induced (Heidari et al., 2022). In terms of protection, MgONPs were identified as potential agents to shield potatoes against the detrimental effects of Phytophthora infestans at specific dosages (Wang et al., 2022). In Citrus sinensis, an intricate response is observed under Mg2+ deficiency, which is characterized by decreased CO2 assimilation and fluctuations in multiple compounds. RNA-Seq analyses from various studies have revealed the differential expression of a plethora of genes. Remarkably, among the seven identified CsMGTs, CsMGT3 showed the highest expression across diverse tissues (Bin et al., 2023; Tang et al., 2023). Collectively, these studies underscore the multifunctional role of MGTs in abiotic stress responses. Their varied and sometimes tissue-specific expression patterns in different plant species, from grapes to citrus, highlight their potential as prime targets for enhancing stress tolerance in crop plants.Highlighting the journey of Mg2+ within horticultural crops, this chapter traces its path from soil uptake to its integral functions within the fruit. Key transporters, particularly MGTs, ensure its optimal distribution, which is crucial not only for basic cellular operations but also for the plant’s critical reproductive stages. The adaptability of these transporters against both biotic and abiotic stresses emphasize their evolutionary importance and suggests potential pathways for crop enhancement. As global agriculture grapples with increasing challenges, a deep understanding of Mg2+ roles and its transport systems can guide us towards more sustainable and robust cropping paradigms.
6 Adverse effects of magnesium deficiency and toxicity
6.1 The consequences of magnesium deficiency
Mg2+ deficiency in plants can lead to a range of negative effects on growth, productivity, and general plant health. Recent research findings are summarized in Table 4 (Cakmak and Yazici, 2010; Yang et al., 2012; Verbruggen and Hermans, 2013). A key symptom of Mg2+ deficiency in plants is interveinal chlorosis, where the tissues between the leaf veins turn yellow, while the veins remain green. This typically starts in older leaves before progressing to younger ones (Figure 3). Severe deficiency can lead to necrosis or tissue death, particularly at the leaf margins and tips (Hermans and Verbruggen, 2005; Hermans et al., 2005; Hermans et al., 2010a). Plants lacking Mg2+ often exhibit reduced growth and development. They might appear smaller, with shorter internodes and poorer branching. This is because Mg2+ is a critical component of the chlorophyll molecule, and without sufficient Mg2+, plants cannot perform photosynthesis effectively, leading to reduced energy production and subsequently stunted growth (Hermans and Verbruggen, 2005; Cakmak and Yazici, 2010; Yang et al., 2012). Mg2+ deficiency can disrupt the uptake of other essential nutrients such as Ca2+, K+, and N. This can lead to an imbalance of these nutrients within the plant, which can exacerbate symptoms of Mg2+ deficiency and further hinder plant growth (Hermans and Verbruggen, 2005; Cakmak and Yazici, 2010; Malvi, 2011). As a cofactor for numerous enzymatic reactions, Mg2+ plays a key role in many plant metabolic processes. Therefore, Mg2+ deficiency can disrupt these functions and impede essential biochemical processes, such as carbohydrate metabolism and protein synthesis (Hermans and Verbruggen, 2005; Hermans et al., 2005; Farhat et al., 2014). Mg2+ deficiency can also weaken plants, making them more susceptible to various stress factors, such as drought, high temperatures, and diseases. This compromises the ability of plants to withstand and recover from adverse environmental conditions, leading to potential yield losses (Hermans and Verbruggen, 2005; Hermans et al., 2005; Ceppi et al., 2012). In addition to affecting yield, Mg2+ deficiency can negatively affect crop quality. With reduced chlorophyll content, the color, taste, and nutritional value of crops can be adversely affected (Hermans and Verbruggen, 2005; Nedim and Damla, 2015; Wang et al., 2020). Furthermore, visible symptoms such as chlorosis and necrosis can negatively impact the marketability and consumer acceptance of the produce (Hermans and Verbruggen, 2005; Cakmak and Yazici, 2010). To minimize these negative effects, it is critical to detect and rectify Mg2+ deficiencies in a timely manner. Soil and plant tissue analyses can be used to identify deficiencies, and appropriate fertilization strategies can be implemented to correct them. By addressing Mg2+ deficiency, farmers can not only improve plant health and maximize productivity, but also enhance the quality of their crops.
6.2 The consequences of magnesium toxicity
Mg2+ is integral to the plant physiology. Adequate levels are vital for numerous metabolic processes, particularly in horticultural crops. However, excessive levels can lead to toxicity and multiple adverse effects (Marschner, 2011; Römheld, 2012). Numerous studies have investigated the nuances of the role of Mg2+ and its impacts when present in excess. Older leaves can show interveinal chlorosis, a distinct yellowing between the veins (Figure 3). As toxicity progresses, leaf tissues, especially along the margins, can undergo necrosis or death (Römheld, 2012). High Mg2+ concentrations in the soil can compete with other cations, such as Ca2+ and K+, at the root uptake sites (Figure 2), potentially leading to deficiencies, even if the soil has ample amounts of these nutrients (Fageria, 2001; Römheld and Kirkby, 2009). An overabundance of Mg2+ can alter the physical properties of soil, making it sticky when wet, leading to compaction. These conditions hinder water movement and can lead to waterlogging. Additionally, compacted soil restricts aeration, thereby affecting root health and function (Kopittke and Menzies, 2007; Damm et al., 2013). Elevated Mg2+ levels can be toxic to root tips, stifling root growth, which in turn diminishes the plant’s ability to effectively absorb essential nutrients and water (Sun L. et al., 2023). Crops such as tomatoes exposed to excessive Mg2+ can experience compromised fruit quality, including blossom end rot (Kwon et al., 2019). Increased Mg2+ concentrations in peppers were correlated with a notable reduction in essential nutrients, such as calcium, zinc, and vitamin C. This decrease in nutritional value is believed to elevate health risks for Chinese adults consuming these peppers, especially due to lowered calcium and vitamin C levels (Lu et al., 2021b). The amalgamation of effects on root health, nutrient uptake, and general plant vitality can culminate in the reduced yield of horticultural crops (Marschner, 2011). Magnesium, particularly magnesium carbonate, can alter soil pH levels, indirectly influencing the availability and uptake of other essential nutrients (Machin and Navas, 2000). To manage Mg2+ toxicity, a multi-pronged approach involving reduced Mg input, improved soil drainage, and the use of soil amendments is recommended. Continuous research in this domain is vital to understand the evolving challenges and mitigation strategies related to Mg2+ toxicity in horticulture.
6.3 Factors influencing MGTs functionality in plants
Mg2+ is a fundamental nutrient that plays a critical role in many plants physiological processes, including chlorophyll biosynthesis, enzyme activation, and energy metabolism. However, the uptake and homeostasis of Mg2+ are not isolated events; they are influenced by a multitude of internal and external factors. Transcriptional regulators, hormonal cues, inter-nutrient dynamics, and environmental stresses converge to determine the function of MGTs in plants. These MGTs, in turn, dictate how well plants adapt to fluctuating soil Mg2+ levels and optimize their growth and development (Hermans et al., 2010b; Chaudhry et al., 2021; Ishfaq et al., 2022).
6.3.1 Hormonal interactions influencing MGTs expression in plants
Ethylene, a key phytohormone, plays a crucial role in modulating numerous growth and developmental processes in plants, including responses to Mg2+ starvation. This is exemplified by the induction of specific isoforms of the 1-aminocyclopropane-1-carboxylic acid synthase (ACS) family under Mg-deficient conditions. Notably, the ACS11 gene becomes active in both roots and leaves, whereas ACS2, ACS7, and ACS8 are primarily expressed in leaves, underscoring the profound role of ethylene in the Mg2+ starvation response (Hermans et al., 2010b). In addition to ethylene, other hormones also intersect with Mg2+ regulation. For instance, MGT has been identified as being under the control of JA (Chen et al., 2010). There is also evidence that hormones such as ABA and GA are responsive to Mg2+ toxicity. In particular, signaling factors, such as DELLA and ABI1, associated with ABA and GA, have been implicated in reactions to Mg2+ toxicity (Guo et al., 2014). Delving into specific genes, the AtMHX gene in Arabidopsis thaliana is noteworthy. This gene encodes a vacuolar transporter that is crucial for maintaining the metal and proton balance within cells, an essential process for photosynthesis. While both auxin and ABA influence AtMHX’s expression, it is especially active in tissues with photosynthetic potential. However, Mg toxicity can suppress its expression, although the exact upstream regulators in response to Mg levels have yet to be identified (David-Assael et al., 2006; Guo et al., 2014).
6.3.2 Interactions between Mg2+ and other nutrients in plant physiology
Mg2+ uptake and MGT expression are not only influenced by Mg2+ availability but also by interactions with other nutrients. The balance between Mg2+ and other elements, particularly calcium, is pivotal for maintaining nutrient homeostasis in plants (Lenz et al., 2013). Soil factors, such as type, pH, and temperature, play instrumental roles in nutrient uptake. For instance, high availability of K+ in the soil can potentially limit Mg2+ uptake because some MGTs also transport K+. In such cases, K+ may compete for transporter-binding sites, thereby reducing Mg2+ uptake. However, this interference is not reciprocal; elevated levels of Mg2+ do not typically hinder K+ uptake owing to the specificity of K+ transporters (Gransee and Führs, 2013). The importance of these interactions is further highlighted under specific environmental stresses. In rice, the Mg2+ transporter OsMGT1 not only aids in Mg2+ uptake but also plays a pivotal role in salt stress tolerance. It is believed to modulate the transport activity of the OsHKT1;5 sodium transporter, thus preventing excessive sodium accumulation in the plant shoots, which could otherwise be detrimental (Chen et al., 2017). Moreover, the influence of other nutrients on Mg uptake and MGT expression extends beyond that of K+. For example, the AtMGT1 transporter, while primarily responsible for Mg2+ transport, also has the capacity to transport other divalent cations, albeit at non-typical physiological concentrations (Li et al., 2001).
6.3.3 Genetic influences on MGT expression and function in plants
MGTs expression in plants is intricately regulated by a complex network of genetic factors. Key transcription factors such as the MYB and WRKY families have been identified as significant players in this regulatory matrix. For instance, distinct genes, such as MYB108 and WRKY75, which display differential expression under Mg2+ deficiency (MD) in banana seedlings, have revealed an essential role in root hair development under MD conditions. MYB108’s function appears multifaceted, as it is also implicated in processes such as wound healing, and potentially in the regulation of genes linked to chlorophyll catabolism (Cui et al., 2013; Xu X. F. et al., 2021). The differential MGT activities observed across plant genotypes could be rooted in genetic polymorphisms within the regulatory regions of these genes. Highlighting the importance of individual MGTs, overexpression of PtrMGT5 in Arabidopsis demonstrated enhanced tolerance to MD, as evidenced by increased plant weight and Mg2+ content (Liu et al., 2019). Further emphasizing the importance of proper MGT regulation, combined knockouts of specific Arabidopsis genes, AtMRS2-1 and AtMRS2-5, led to pronounced developmental delays when exposed to low Mg2+ conditions (Lenz et al., 2013). The intricate connection between Mg2+ and other elements, particularly Ca2+, indicates a delicate balance in plant nutrient homeostasis. Under Mg2+ scarcity conditions, reducing calcium concentrations can ameliorate adverse growth effects, underscoring the tight link between these divalent cations (Lenz et al., 2013).
6.3.4 Internal Mg2+ status and nutrient homeostasis
A balanced mineral supply is vital for plant growth. Plants have evolved mechanisms to manage ion concentrations either by preventing excess ion entry or by sequestering them in vacuoles. MRS2 transporters are central to maintaining Mg2+ homeostasis under various conditions. Some MRS2 transporters, such as MRS2-2 and MRS2-7, have been identified with innate polymorphisms; MGTs functionality in plants is influenced by a web of interconnected factors, spanning from environmental to genetic scales (Turner et al., 2010; Oda et al., 2016). Given that Mg2 + plays a central role in plant physiology, understanding these influencing factors offers a roadmap for improving plant health and productivity in various and often challenging environments.
7 Strategies for magnesium management in horticulture
Effective management of Mg2+ is pivotal for optimal plant nutrition and prevention of deficiency symptoms in horticultural crops. Accordingly, strategies such as regular soil testing, appropriate fertilization, and vigilant plant monitoring are essential to ensure sufficient Mg2+ levels for crop growth and productivity. Effective management of Mg2+ is paramount for ensuring the optimal nutrition of plants and preventing deficiency symptoms in horticultural crops. Some of the strategies to manage Mg2+ levels in the soil include conducting regular soil tests, which can provide insights into the Mg2+ content of the soil as well as the soil pH, both of which can guide decisions about nutrient management. Regular testing of plant tissues can provide an accurate representation of the Mg2+ status within plants, allowing for the early detection of deficiencies or imbalances (Cakmak and Yazici, 2010; Gransee and Führs, 2013). Adjusting the soil pH is vital for optimal Mg2+ availability. In acidic soils (pH below 6.0), liming with materials such as dolomitic lime can help increase pH and Mg2+ availability. In alkaline soils, pH must be reduced using acidifiers to increase Mg2+ availability. Furthermore, chelated Mg can be used effectively in alkaline soils, as it is more soluble and available to plants than inorganic sources at higher pH levels (Gransee and Führs, 2013). Incorporating Mg2+-containing fertilizers, such as magnesium sulfate (Epsom salt) or magnesium oxide, into a balanced fertilization program can help provide Mg2+ to plants. While Epsom salt is highly soluble and provides an immediate source of Mg2+, magnesium oxide is a slow-release fertilizer that provides Mg2+ over an extended period (Cakmak and Yazici, 2010; Gransee and Führs, 2013).
In cases of severe Mg2+ deficiency, the foliar application of Mg2+ may be an effective strategy. Spraying soluble sources of Mg2+, such as magnesium sulfate, directly onto the leaves can quickly correct this deficiency (He et al., 2022). In a study on pomelo orchards in South China, adding Mg2+ to optimized fertilization significantly boosted yield, fruit quality, and economic benefits by up to 15% compared to traditional practices, while reducing greenhouse gas emissions and energy inputs by over 20%. This suggests that optimized fertilization can enhance productivity and sustainability under acidic soil conditions (Chen et al., 2022). Incorporating organic matter, such as compost or well-rotted manure, into the soil can help improve soil structure, cation exchange capacity, and nutrient storage, thereby improving the availability of Mg2+ in the soil (Gransee and Führs, 2013). Moreover, proper irrigation management is essential to ensure Mg2+ availability. Maintaining optimal soil moisture levels aids the efficient uptake of Mg2+ by plants (Marschner, 2011). Crop rotation and use of cover crops can also contribute to the management of Mg2+ in the soil. Different crops have different Mg2+ requirements, which can help to avoid excessive nutrient depletion or imbalances (Mauro et al., 2015). Regular review and refinement of management practices are also necessary to ensure the optimization of Mg2+ availability and utilization (Yeh et al., 2000; Verbruggen and Hermans, 2013). Implementing these strategies, while considering the specific requirements of the crop and soil conditions, can effectively manage Mg2+ levels in horticultural crops and support optimal growth, nutrition, and productivity.
7.1 Breeding and genetic engineering strategies to improve magnesium uptake and utilization in crop plants
Mg2+ is a pivotal nutrient that is integral to many physiological processes in plants, including photosynthesis and various cellular functions. Proper absorption and utilization in horticultural crops are vital for achieving desirable yield and quality (Adnan et al., 2021). Recently, the interplay of molecular and genetic studies has opened avenues for the development of robust strategies to enhance magnesium uptake in crops. Traditional breeding methods have capitalized on vast natural genetic variations associated with Mg2+ absorption and utilization. Diverse germplasm collections serve as a rich reservoir, where researchers have identified genotypes exhibiting superior nutrient uptake or resilience under nutrient-deficient scenarios (Kudapa et al., 2023). Complementing this approach is Quantitative Trait Locus (QTL) Mapping. This powerful technique identifies genomic regions intrinsically linked to magnesium-related traits, paving the way for the rapid marker-assisted breeding of magnesium-optimized plants (Zhi et al., 2023). Delving deeper, studies centered on the Malvaceae family, particularly Theobroma cacao and Gossypium hirsutum, have been revelatory. They have uncovered key MGT genes that regulate Mg2+ transport, offering invaluable insights for breeding not only within Malvaceae but extending to other families such as Cucurbitaceae (Heidari et al., 2021; Heidari et al., 2022). Concurrently, the realm of genetic engineering has seen remarkable advancements, presenting precise mechanisms for optimizing Mg2+ in crops. One such strategy involves the introduction and silencing of specific genes. For instance, the introduction of genes like AtMGT1 into Nicotiana benthamiana has showcased enhanced magnesium absorption and resilience against adversities like aluminum toxicity (Deng et al., 2006). In contrast, silencing genes, such as MGT6 in Arabidopsis thaliana, have been shown to have indispensable roles in magnesium uptake (Yan et al., 2018). Further magnification of the genetic precision was performed using the CRISPR-Cas9 system. Its application in editing the MgCh gene in sugarcane, pivotal for chlorophyll synthesis, is a testament to its transformative potential (Eid et al., 2021). In addition, the fascinating symbiotic relationship between plants and mycorrhizal fungi can be harnessed to enhance Mg2+ uptake. Beneficial fungi, in particular, have demonstrated their capability to amplify magnesium absorption in specific crops under stress, such as the salt affected Sesbania species (Giri and Mukerji, 2004).
Merging traditional breeding with advanced genomic tools can capture selection precision. In-depth genomic analyses of crops such as Vitis vinifera have unearthed SNPs intricately tied to Mg2+ uptake, offering refined markers for breeding programs (Eid et al., 2021). In addition, discoveries in Camellia sinensis and Vitis vinifera have highlighted pivotal Mg2+ transporter genes, enriching our understanding of Mg2+ uptake and adaptive stress responses (Tang et al., 2021; Ge M. et al., 2022). The combined efforts of breeding and genetic engineering present a bright future for enhancing Mg2+ utilization in crops. As our understanding of the crucial role of Mg2+ in plant health deepens, it is essential to weave these insights into practical agricultural techniques. As we venture further into this pioneering domain, thorough assessments of altered crops remain imperative for maintaining both environmental balance and food safety. Together, grounded in comprehensive research, these methods promise a sustainable agricultural future, particularly for areas with Mg2+ deficiencies, reinforcing the pillars of global food security.
7.2 Nano-Mg and integrated approaches: pioneering advances in horticultural crops
At the crossroads of food security and sustainable agriculture, nanotechnology has become an innovative solution. The application of Mg2+ nanoparticles stands out in this field, especially when addressing plant nutrition challenges (Singh and Shukla, 2020; Hayat et al., 2023). Although Mg2+ is a vital macronutrient, its occasional scarcity poses challenges. Nanofertilizers are lauded for their exceptional physicochemical traits, such as heightened reactivity and extensive surface area, and are a promising alternative to their traditional counterparts. By offering increased agricultural yields and acting as a protective shield against both biotic and abiotic stresses, they pave the way for a sustainable agricultural future by minimizing ecological impacts. In particular, magnesium oxide nanoparticles (MgO NPs) have garnered significant attention. Their biodegradability and non-toxicity coupled with their diverse applications make them indispensable. Fernandes et al. (2020) spotlighted their versatility, highlighting their role in areas ranging from environmental waste treatment to advanced medical applications, like anti-cancer treatments. Further accentuating the role of nanotechnology in agriculture is its potential to revolutionize plant breeding and genetic transformation processes. As Singh and Shukla (2020) posited, this cutting-edge technology is set to anchor future agricultural advancements. One of its most prominent manifestations is the rise of nanofertilizers. These advanced fertilizers, characterized by their vast surface areas and consistent nutrient release, have been championed by researchers, such as Yaseen Aljanabi (2021), as the next step in optimizing crop yields while mitigating environmental concerns associated with excessive agrochemical usage. Furthermore, bean plants showed optimized biomass and photosynthetic pigment production when treated with precise dosages of Mg NPs, as documented by Salcido-Martinez et al. (2020).
Luo et al. (2020) added another dimension to this burgeoning field by demonstrating the positive effects of nanometer MgOH on Chinese cabbage, especially under the stress of cadmium pollution, a common agricultural concern. Addressing another critical environmental concern in agriculture, the contamination by metalloids like arsenic, Faizan et al. (2022) explored the potential of MgOH. Their study highlighted the dual role of NPs in promoting plant growth in contaminated environments and simultaneously mitigating the oxidative stress induced by such pollutants. As for disease control, the versatility of Mg-NPs are noteworthy. Liao et al., in a series of studies from 2019 and 2021, brought to light the significant bactericidal activity of nano-sized MgO particles, suggesting them as potent, eco-friendly alternatives to traditional copper-based bactericides. Their findings are particularly timely given the increasing global challenges posed by Cu-tolerant pathogens in agriculture. Andreadelli et al. (2021) highlighted that hydrated MgO NPs sprays resulted in a profound alteration of the tomato phylloplane microbiota. This modification strengthens their immune systems, leading to a 70% reduction in fungal infestation. However, tomatoes are not the only beneficiaries. In a groundbreaking study by Wang et al. (2022), it was revealed that potatoes treated with MgO NPs garnered a protective layer against the notorious pathogen Phytophthora infestans. Beyond crop health, the sustainability wave driven by nanotechnology is reshaping agricultural packaging. Kumar et al. (2020) introduced a sustainable solution by developing a biodegradable pectin-based bionanocomposite film integrated with MgOH NPs. These films are not just environmentally friendly, but also compare favorably with conventional packaging materials in terms of performance. In essence, the confluence of nanotechnology and Mg-NPs holds the promise to usher in a new era in horticulture. From fortifying crops against pests and diseases to optimizing growth and even addressing broader environmental concerns, this innovative approach paves the way for sustainable, efficient, and resilient future practices in horticulture.
8 Future directions and research needs
Although significant progress has been made in understanding the role of Mg2+ in horticulture, certain knowledge gaps and areas require further exploration. Addressing these gaps could guide future research and enhance our understanding of the role of Mg2+ in crop production. Some potential research directions and needs include the following.
Comprehensive genomic and transcriptomic studies to identify Mg2+-responsive genes and transcription factors in horticultural plants. Better knowledge of the genetic regulation of Mg2+ uptake, transport, and utilization can pave the way for targeted breeding programs and genetic engineering strategies. Employing omics approaches such as metabolomics and proteomics to decipher the metabolic and biochemical pathways influenced by Mg2+ deficiency or excess (Yang et al., 2019; Cao et al., 2021). By monitoring changes in associated metabolites and proteins, researchers can unearth the underlying mechanisms and metabolic adaptations of plants to Mg2+ stress. Extensive research into the molecular mechanisms of Mg2+ transport in plants, from root uptake to distribution in various tissues and organs, is required to understand the role of transporters, channels, and regulatory factors involved in Mg2+ uptake, long-distance transport, and compartmentalization, which will further enhance our understanding of Mg2+ homeostasis in plants. Investigating the interactions between Mg2+ and other vital nutrients, such as calcium, potassium, and phosphorus, is essential to comprehend their synergistic and antagonistic effects on plant growth and development. Developing precise nutrient management strategies that affect spatial and temporal variations in Mg2+ availability and crop nutrient requirements (Chen et al., 2009). Remote sensing technologies, soil sensors, and predictive models can also be used to optimize the rates and timing of Mg2+ application to ensure that a precise nutrient supply meets crop needs. Probing the genetic regulation of Mg2+ homeostasis in horticultural crops is critical for the evolution of plant varieties with improved Mg2+ utilization (Hermans et al., 2013). Further studies are needed to identify and characterize the crucial genes, transcription factors, and signaling pathways involved in Mg2+ recognition, transport, and distribution. Studying Mg2+ dynamics in different soil types, including their interactions with soil pH, organic matter, and nutrient availability, is crucial for effective nutrient management (Mikkelsen, 2010; Neina, 2019). Further research should focus on Mg2+ availability, mobility, and transformation in diverse soil environments under various management practices. Designing sustainable Mg2+ management strategies that balance nutrient availability with minimal environmental impacts is crucial (Tully and Ryals, 2017). Future research should explore innovative approaches, such as precise nutrient management, using Mg2+-efficient plant genotypes, and optimizing fertilization techniques to enhance Mg2+ utilization and minimize nutrient losses. Examining the role of rhizosphere microbiome in regulating Mg2+ availability and uptake by plants is a promising research direction (Lazcano et al., 2021). Understanding the interactions between plant roots, soil microorganisms, and the Mg2+ cycle can provide insights into potential microbial strategies to improve Mg2+ uptake and nutrient acquisition. Furthermore, studies focusing on Mg2+ management under specific growing conditions, such as hydroponics, greenhouse cultivation, or controlled environments, would help optimize nutrient supply and crop performance. Integrating Mg2+ management practices with other sustainable cropping strategies, such as conservation agriculture, organic farming, and cover cropping, could provide holistic solutions (Altieri et al., 2017). The combined effects of these practices on Mg2+ availability, soil health, and crop performance should be evaluated to develop comprehensive approaches for improving nutrient use efficiency and sustainability. By addressing these research needs, we aim to refine our understanding of the role of Mg2+ in horticultural crops, improve Mg2+ management practices, develop nutrient-efficient crop varieties, and promote sustainable and resilient agriculture. This could fuel innovation in crop management, breeding programs, and nutrient optimization strategies, ultimately enhancing the efficiency, sustainability, and nutritional value of horticultural production.
9 Conclusion
In summary, this study emphasized the significant role of Mg2+ in the production and quality of horticultural crops. As a vital macronutrient, it is implicated in several physiological functions such as chlorophyll synthesis, enzyme activation, nutrient uptake, protein synthesis, and gene expression. Ensuring appropriate levels of Mg2+ contributes to higher quality plants by enhancing chlorophyll production, leading to vibrant leaf coloration and more efficient photosynthesis. Beyond mere aesthetics, Mg2+ also affects the nutrient content, flavor, texture, and shelf life of horticultural produce, increasing their consumer appeal. Conversely, Mg2+ deficiency can restrict plant growth, impair reproductive development, and lead to yield losses, underlining the importance of nutrients in crop yield potential. Another crucial aspect of Mg2+ is its role in bolstering stress tolerance, disease resistance, and pest defence mechanisms in plants. These factors can significantly affect the resilience and overall health of horticultural crops, thereby affecting their productivity and profitability. Interactions among Mg2+, plants, and soil microbiomes also require mention. The role of MGTs from various cellular levels to different organ levels and compartmentalization, and their role in Mg2+ homeostasis are also highlighted. Mg2+ availability can influence the rhizosphere microbiome composition, which in turn can affect plant health and nutrient acquisition. Microbial activities in the rhizosphere can also affect Mg2+ availability and form, which can affect plant uptake and utilization. Efficient Mg2+ management strategies, including soil testing, balanced fertilization, and precise nutrient management, are integral to ensuring optimal Mg2+ availability and utilization in horticultural cropping systems. Emphasizing the importance of Mg2+ and implementing effective management practices can enhance crop quality, yield, stress tolerance, and disease resistance. Ultimately, this can optimize horticultural productivity and profitability, underscoring the need for continued research and innovation in Mg2+ management in horticulture.
Author contributions
NA: Conceptualization, Formal Analysis, Investigation, Methodology, Software, Visualization, Writing – original draft, Writing – review & editing. BZ: Funding acquisition, Supervision, Writing – review & editing. BB: Investigation, Writing – review & editing. SC: Investigation, Visualization, Writing – review & editing. MR: Investigation, Writing – review & editing. JL: Conceptualization, Writing – review & editing. YL: Project administration, Writing – review & editing. FH: Methodology, Writing – review & editing. ZC: Formal Analysis, Writing – review & editing. PT: Funding acquisition, Project administration, Resources, Supervision, Writing – review & editing.
Funding
The author(s) declare financial support was received for the research, authorship, and/or publication of this article. Funding was jointly provided by the GuangDong Basic and Applied Basic Research Foundation (2023A1515030283), Agricultural Competitive Industry Discipline Team Building Project of Guangdong Academy of Agricultural Sciences (202103TD), Scarce and Quality Economic Forest Engineering Technology Research Center (2022GCZX002), and Meizhou Science and Technology Project (2021A0304001).
Conflict of interest
The authors declare that the research was conducted in the absence of any commercial or financial relationships that could be construed as a potential conflict of interest.
Publisher’s note
All claims expressed in this article are solely those of the authors and do not necessarily represent those of their affiliated organizations, or those of the publisher, the editors and the reviewers. Any product that may be evaluated in this article, or claim that may be made by its manufacturer, is not guaranteed or endorsed by the publisher.
References
Adaskaveg, J. A., Blanco-Ulate, B. (2023). Targeting ripening regulators to develop fruit with high quality and extended shelf life. Curr. Opin. Biotechnol. 79, 102872. doi: 10.1016/j.copbio.2022.102872
Adhikari, S., Toretsky, J. A., Yuan, L., Roy, R. (2006). Magnesium, essential for base excision repair enzymes, inhibits substrate binding of N-methylpurine-DNA glycosylase. J. Biol. Chem. 281, 29525–29532. doi: 10.1074/jbc.M602673200
Adnan, M., Tampubolon, K., Ur Rehman, F., Saeed, M. S., Hayyat, M. S., Imran, M., et al. (2021). Influence of foliar application of magnesium on horticultural crops: A review. Agrinula: Jurnal Agroteknologi dan Perkebunan 4, 13–21. doi: 10.36490/agri.v4i1.109
Aghofack, J. N., Yambou, T. N. (2006). Effects of Calcium chloride and magnesium sulphate treatments on the shelf-life of climacteric banana and non-climacteric pineapple fruits. Cameroon J. Exp. Biol. 1, 34–38. doi: 10.4314/cajeb.v1i1.37923
Aghofack-Nguemezi, J., Noumbo, G., Nkumbe, C. (2014). Influence of calcium and magnesium based fertilizers on fungal diseases, plant growth parameters and fruit quality of three varieties of tomato (Solanum lycopersicum). J. Sci. Technol. (Ghana) 34, 9–20. doi: 10.4314/just.v34i1.2
Allen, L. H. (2013). Magnesium. Encyclopedia Hum. Nutr. 3–4, 131–135. doi: 10.1016/B978-0-12-375083-9.00177-X
Almeida, H. J. d., Carmona, V. M. V., Cavalcante, V. S., Filho, A. B. C., Prado, R.d. M., Flores, R. A., et al. (2020). Nutritional and visual diagnosis in broccoli (Brassica oleracea var. italica L.) Plants: disorders in physiological activity, nutritional efficiency and metabolism of carbohydrates. Agronomy 10, 1572.
Altieri, M. A., Nicholls, C. I., Montalba, R. (2017). Technological approaches to sustainable agriculture at a crossroads: An agroecological perspective. Sustainability 9, 349. doi: 10.3390/su9030349
Andreadelli, A., Petrakis, S., Tsoureki, A., Tsiolas, G., Michailidou, S., Baltzopoulou, P., et al. (2021). Effects of magnesium oxide and magnesium hydroxide microparticle foliar treatment on tomato pr gene expression and leaf microbiome. Microorganisms 9, 1217. doi: 10.3390/microorganisms9061217
Aparna, C. A. K., Singh, P. K. (2018). Estimation of toxic, trace and essential metals (Pb, cd, Fe, Zn, Mn, Cu, Mg, K) in fruit and vegetable product (jam, ketchup, pickles) by atomic absorption spectrophotometer. Pharm. Innov. 7, 313–317.
Assunção, A. G. L., Cakmak, I., Clemens, S., González-Guerrero, M., Nawrocki, A., Thomine, S. (2022). Micronutrient homeostasis in plants for more sustainable agriculture and healthier human nutrition. J. Exp. Bot. 73, 1789–1799. doi: 10.1093/jxb/erac014
Bashir, M. A., Noreen, A., Ikhlaq, M., Shabir, K., Altaf, F., Akhtar, N. (2019). Effect of Boric acid, Potassium nitrate and Magnesium sulphate on Managing Fruit Cracking and Improving Fruit Yield and Quality of Pomegranate. J. Hortic. Sci. Technol. 2, 49–53. doi: 10.46653/jhst190202049
Bin, M., Yi, G., Zhang, X. (2023). Discovery and characterization of magnesium transporter (MGT) gene family in Citrus sinensis and their role in magnesium deficiency stress. Plant Growth Regul. 100, 733–746. doi: 10.1007/s10725-023-00973-7
Boaretto, R. M., Hippler, F. W. R., Ferreira, G. A., Azevedo, R. A., Quaggio, J. A., Mattos, D. (2020). The possible role of extra magnesium and nitrogen supply to alleviate stress caused by high irradiation and temperature in lemon trees. Plant Soil 457, 57–70. doi: 10.1007/s11104-020-04597-y
Borowski, E., Michalek, S. (2010). The effect of foliar nutrition of spinach (Spinacia oleracea L.) with magnesium salts and urea on gas exchange, leaf yield and quality. Acta Agrobot 63.
Bose, J., Babourina, O., Rengel, Z. (2011). Role of magnesium in alleviation of aluminium toxicity in plants. J. Exp. Bot. 62, 2251–2264. doi: 10.1093/jxb/erq456
Cakmak, I., Kirkby, E. A. (2008). Role of magnesium in carbon partitioning and alleviating photooxidative damage. Physiol. Plant 133, 692–704. doi: 10.1111/J.1399-3054.2007.01042.X
Cakmak, I., Yazici, A. M. (2010). Magnesium: A forgotten element in crop production. Better Crops 94, 23–25.
Cao, Q., Li, L., Yang, Z., Wang, Y., Li, J., Chen, W., et al. (2021). Transcriptome analysis of genes in response to magnesium nitrate stress on cucumber leaf. Sci. Hortic. 288, 110391. doi: 10.1016/j.scienta.2021.110391
Ceppi, M. G., Oukarroum, A., Çiçek, N., Strasser, R. J., Schansker, G. (2012). The IP amplitude of the fluorescence rise OJIP is sensitive to changes in the photosystem I content of leaves: a study on plants exposed to magnesium and sulfate deficiencies, drought stress and salt stress. Physiol. Plant 144, 277–288. doi: 10.1111/j.1399-3054.2011.01549.x
Ceylan, Y., Kutman, U. B., Mengutay, M., Cakmak, I. (2016). Magnesium applications to growth medium and foliage affect the starch distribution, increase the grain size and improve the seed germination in wheat. Plant Soil 406, 145–156. doi: 10.1007/s11104-016-2871-8
Chaudhry, A. H., Nayab, S., Hussain, S. B., Ali, M., Pan, Z. (2021). Current understandings on magnesium deficiency and future outlooks for sustainable agriculture. Int. J. Mol. Sci. doi: 10.3390/ijms22041819
Chen, W., Bell, R. W., Brennan, R. F., Bowden, J. W., Dobermann, A., Rengel, Z., et al. (2009). Key crop nutrient management issues in the Western Australia grains industry: a review. Soil Res. 47, 1–18. doi: 10.1071/SR08097
Chen, Y., Hoehenwarter, W., Weckwerth, W. (2010). Comparative analysis of phytohormone-responsive phosphoproteins in Arabidopsis thaliana using TiO2-phosphopeptide enrichment and mass accuracy precursor alignment. Plant J. 63, 1–17. doi: 10.1111/j.1365-313X.2010.04218.x
Chen, Q., Kan, Q., Wang, P., Yu, W., Yu, Y., Zhao, Y., et al. (2014). Phosphorylation and interaction with the 14-3-3 protein of the plasma membrane H+-ATPase are involved in the regulation of magnesium-mediated increases in aluminum-induced citrate exudation in broad bean (Vicia faba. L). Plant Cell Physiol. 56, 1144–1153. doi: 10.1093/pcp/pcv038
Chen, Z. C., Ma, J. F. (2013). Magnesium transporters and their role in Al tolerance in plants. Plant Soil 368, 51–56. doi: 10.1007/s11104-012-1433-y
Chen, Z. C., Peng, W. T., Li, J., Liao, H. (2018). Functional dissection and transport mechanism of magnesium in plants. Semin. Cell Dev. Biol. 74, 142–152. doi: 10.1016/j.semcdb.2017.08.005
Chen, X., Wang, Z., Muneer, M. A., Ma, C., He, D., White, P. J., et al. (2023). Short planks in the crop nutrient barrel theory of China are changing: Evidence from 15 crops in 13 provinces. Food Energy Secur 12, e389. doi: 10.1002/fes3.389
Chen, Z. C., Yamaji, N., Horie, T., Che, J., Li, J., An, G., et al. (2017). A magnesium transporter OsMGT1 plays a critical role in salt tolerance in rice. Plant Physiol. 174, 1837–1849. doi: 10.1104/pp.17.00532
Chen, X., Yan, X., Muneer, M. A., Weng, X., Cai, Y., Ma, C., et al. (2022). Pomelo green production on acidic soil: reduce traditional fertilizers, but do not ignore magnesium. Front. Sustain Food Syst. 6. doi: 10.3389/fsufs.2022.948810
Crusciol, C. A. C., Moretti, L. G., Bossolani, J. W., Moreira, A., Micheri, P. H., Rossi, R. (2019). Can dunite promote physiological changes, magnesium nutrition and increased corn grain yield? Commun. Soil Sci. Plant Anal. 50, 2343–2353. doi: 10.1080/00103624.2019.1659304
Cui, F., Brosché, M., Sipari, N., Tang, S., Overmyer, K. (2013). Regulation of ABA dependent wound induced spreading cell death by MYB108. New Phytol. 200, 634–640. doi: 10.1111/nph.12456
Damm, S., Hofmann, B., Gransee, A., Christen, O. (2013). Zur Wirkung von Kalium auf ausgewählte bodenphysikalische Eigenschaften und den Wurzeltiefgang landwirtschaftlicher Kulturpflanzen. Arch. Agron. Soil Sci. 59, 1–19. doi: 10.1080/03650340.2011.596827
David-Assael, O., Berezin, I., Shoshani-Knaani, N., Saul, H., Mizrachy-Dagri, T., Chen, J., et al. (2006). AtMHX is an auxin and ABA-regulated transporter whose expression pattern suggests a role in metal homeostasis in tissues with photosynthetic potential. Funct. Plant Biol. 33, 661–672. doi: 10.1071/FP05295
Deng, W., Luo, K., Li, D., Zheng, X., Wei, X., Smith, W., et al. (2006). Overexpression of an Arabidopsis magnesium transport gene, AtMGT1, in Nicotiana benthamiana confers Al tolerance. J. Exp. Bot. 57, 4235–4243. doi: 10.1093/jxb/erl201
Djabou, A. S. M., Qin, Y., Thaddee, B., Figueiredo, P. G., Feifei, A., Carvalho, L. J. C. B., et al. (2018). Effects of calcium and magnesium fertilization on antioxidant activities during cassava postharvest physiological deterioration. Crop Sci. 58, 1385–1392. doi: 10.2135/cropsci2017.09.0526
Douglas-Gallardo, O. A., Murillo-López, J. A., Oller, J., Mulholland, A. J., Vöhringer-Martinez, E. (2022). Carbon dioxide fixation in ruBisCO is protonation-state-dependent and irreversible. ACS Catal 12, 9418–9429. doi: 10.1021/acscatal.2c01677
Du, Y., Li, J., Cun, D., Zhu, C., Liu, H., Li, D., et al. (2021). Effect of low magnesium stress on mineral element contents in various organs, yield and fruit quality in different lemon varieties. J. Fruit Sci. 38, 1330–1339. doi: 10.13925/j.cnki.gsxb.20200213
Eid, A., Mohan, C., Sanchez, S., Wang, D., Altpeter, F. (2021). Multiallelic, targeted mutagenesis of magnesium chelatase with CRISPR/cas9 provides a rapidly scorable phenotype in highly polyploid sugarcane. Front. Genome Ed 3. doi: 10.3389/fgeed.2021.654996
El-Metwaly, H. M. B., Mansour, F. Y. O. (2019). Effect of addition methods of magnesium and calcium foliar application on productivity and quality of potato crop in winter plantation. Fayoum J. Agric. Res. Dev. 33, 148–158. doi: 10.21608/fjard.2019.190242
El-Sharkawy, H. H. A., Rashad, Y. M., El-kenawy, M. A., Galilah, D. A. (2022). Magnesium carbonate elicits defense-related genes in King Ruby grapevines against downy mildew and improves its growth, yield, and berries quality. Pestic Biochem. Physiol. 184. doi: 10.1016/j.pestbp.2022.105075
Fageria, V. D. (2001). Nutrient interactions in crop plants. J. Plant Nutr. 24, 1269–1290. doi: 10.1081/PLN-100106981
Fageria, N. K., Oliveira, J. P. (2014). Nitrogen, phosphorus and potassium interactions in upland rice. J. Plant Nutr. 37, 1586–1600. doi: 10.1080/01904167.2014.920362
Faiz, S., Yasin, N. A., Khan, W. U., Shah, A. A., Akram, W., Ahmad, A., et al. (2022). Role of magnesium oxide nanoparticles in the mitigation of lead-induced stress in Daucus carota: modulation in polyamines and antioxidant enzymes. Int. J. Phytoremediation 24, 364–372. doi: 10.1080/15226514.2021.1949263
Faizan, M., Bhat, J. A., El-Serehy, H. A., Moustakas, M., Ahmad, P. (2022). Magnesium oxide nanoparticles (MgO-NPs) alleviate arsenic toxicity in soybean by modulating photosynthetic function, nutrient uptake and antioxidant potential. Metals (Basel) 12. doi: 10.3390/met12122030
Farhat, N., Elkhouni, A., Zorrig, W., Smaoui, A., Abdelly, C., Rabhi, M. (2016). Effects of magnesium deficiency on photosynthesis and carbohydrate partitioning. Acta Physiol. Plant 38, 145. doi: 10.1007/S11738-016-2165-Z
Farhat, N., Rabhi, M., Krol, M., Barhoumi, Z., Ivanov, A. G., McCarthy, A., et al. (2014). Starch and sugar accumulation in Sulla carnosa leaves upon Mg 2+ starvation. Acta Physiol. Plant 36, 2157–2165. doi: 10.1007/s11738-014-1592-y
Fernandes, M., Singh, R. B., Sarkar, T., Singh, P., Pratap Singh, R. (2020). Recent Applications of Magnesium Oxide (MgO) Nanoparticles in various domains. Adv. Mater Lett. 11, 1–10. doi: 10.5185/amlett.2020.081543
Fujikawa, I., Takehara, Y., Ota, M., Imada, K., Sasaki, K., Kajihara, H., et al. (2021). Magnesium oxide induces immunity against Fusarium wilt by triggering the jasmonic acid signaling pathway in tomato. J. Biotechnol. 325, 100–108. doi: 10.1016/j.jbiotec.2020.11.012
Ge, H., Shao, Q., Chen, J., Chen, J., Li, X., Tan, Y., et al. (2022). A metal tolerance protein, MTP10, is required for the calcium and magnesium homeostasis in Arabidopsis. Plant Signal Behav. 17, 2025322. doi: 10.1080/15592324.2021.2025322
Ge, M., Zhong, R., Sadeghnezhad, E., Hakeem, A., Xiao, X., Wang, P., et al. (2022). Genome-wide identification and expression analysis of magnesium transporter gene family in grape (Vitis vinifera). BMC Plant Biol. 22, 680. doi: 10.1186/s12870-022-03599-5
Gelli, A., Hawkes, C., Donovan, J., Harris, J., Allen, S. L., de Brauw, A., et al. (2015). Value chains and nutrition: A framework to support the identification, design, and evaluation of interventions. SSRN Electronic J. doi: 10.2139/ssrn.2564541
Gerendás, J., Führs, H. (2013). The significance of magnesium for crop quality. Plant Soil 368, 101–128. doi: 10.1007/s11104-012-1555-2
Giri, B., Mukerji, K. G. (2004). Mycorrhizal inoculant alleviates salt stress in Sesbania aEgyptiaca and Sesbania grandiflora under field conditions: Evidence for reduced sodium and improved magnesium uptake. Mycorrhiza 14, 307–312. doi: 10.1007/s00572-003-0274-1
Gransee, A., Führs, H. (2013). Magnesium mobility in soils as a challenge for soil and plant analysis, magnesium fertilization and root uptake under adverse growth conditions. Plant Soil 368, 5–21. doi: 10.1007/S11104-012-1567-Y/FIGURES/5
Guo, W. (2017). Magnesium homeostasis mechanisms and magnesium use efficiency in plants, in: Hossain, Mohammad Anwar, Kamiya, Takehiro, B., D.J., Tran, Lam-Son Phan, Fujiwara, Toru (Eds.), Plant Macronutrient Use Efficiency: Molecular and Genomic Perspectives in Crop Plants. Academic Press 197–213. doi: 10.1016/B978-0-12-811308-0.00011-9
Guo, W., Cong, Y., Hussain, N., Wang, Y., Liu, Z., Jiang, L., et al. (2014). The remodeling of seedling development in response to long-term magnesium toxicity and regulation by ABA-DELLA signaling in Arabidopsis. Plant Cell Physiol. 55, 1713–1726. doi: 10.1093/pcp/pcu102
Guo, W., Nazim, H., Liang, Z., Yang, D. (2016). Magnesium deficiency in plants: An urgent problem. Crop J. 4, 83–91. doi: 10.1016/j.cj.2015.11.003
Hamedeh, H., Antoni, S., Cocciaglia, L., Ciccolini, V. (2022). Molecular and physiological effects of magnesium–polyphenolic compound as biostimulant in drought stress mitigation in tomato. Plants 11, 586. doi: 10.3390/plants11050586
Hartwig, A. (2001). Role of magnesium in genomic stability. Mutat. Res. - Fundam. Mol. Mech. Mutagenesis. doi: 10.1016/S0027-5107(01)00074-4
Hayat, F., Khanum, F., Li, J., Iqbal, S., Khan, U., Javed, H. U., et al. (2023). Nanoparticles and their potential role in plant adaptation to abiotic stress in horticultural crops: A review. Sci. Hortic. 321, 112285. doi: 10.1016/j.scienta.2023.112285
He, D., Chen, X., Zhang, Y., Huang, Z., Yin, J., Weng, X., et al. (2023). Magnesium is a nutritional tool for the yield and quality of oolong tea (Camellia sinensis L.) and reduces reactive nitrogen loss. Sci. Hortic. 308, 111590. doi: 10.1016/j.scienta.2022.111590
He, H., Khan, S., Deng, Y., Hu, H., Yin, L., Huang, J. (2022). Supplemental foliar-applied magnesium reverted photosynthetic inhibition and improved biomass partitioning in magnesium-deficient banana. Horticulturae 8, 1050. doi: 10.3390/horticulturae8111050
Heidari, P., Abdullah, F., Poczai, P. (2021). Magnesium transporter gene family: Genome-wide identification and characterization in theobroma cacao, corchorus capsularis, and gossypium hirsutum of family malvaceae. Agronomy 11, 1651. doi: 10.3390/agronomy11081651
Heidari, P., Puresmaeli, F., Mora-Poblete, F. (2022). Genome-Wide Identification and Molecular Evolution of the Magnesium Transporter (MGT) Gene Family in Citrullus lanatus and Cucumis sativus. Agronomy 12, 2253. doi: 10.3390/agronomy12102253
Hermans, C., Bourgis, F., Faucher, M., Strasser, R. J., Delrot, S., Verbruggen, N. (2005). Magnesium deficiency in sugar beets alters sugar partitioning and phloem loading in young mature leaves. Planta 220, 541–549. doi: 10.1007/s00425-004-1376-5
Hermans, C., Conn, S. J., Chen, J., Xiao, Q., Verbruggen, N. (2013). An update on magnesium homeostasis mechanisms in plants. Metallomics. 5, 1170–1183. doi: 10.1039/c3mt20223b
Hermans, C., Verbruggen, N. (2005). Physiological characterization of Mg deficiency in Arabidopsis thaliana. J. Exp. Bot. 56, 2153–2161. doi: 10.1093/jxb/eri215
Hermans, C., Vuylsteke, M., Coppens, F., Craciun, A., Inzé, D., Verbruggen, N. (2010a). Early transcriptomic changes induced by magnesium deficiency in Arabidopsis thaliana reveal the alteration of circadian clock gene expression in roots and the triggering of abscisic acid-responsive genes. New Phytol. 187, 119–131. doi: 10.1111/j.1469-8137.2010.03258.x
Hermans, C., Vuylsteke, M., Coppens, F., Cristescu, S. M., Harren, F. J. M., Inzé, D., et al. (2010b). Systems analysis of the responses to long-term magnesium deficiency and restoration in Arabidopsis thaliana. New Phytol. 187, 132–144. doi: 10.1111/j.1469-8137.2010.03257.x
Hou, J., Cheng, X., Li, J., Dong, Y. (2021). Magnesium and nitrogen drive soil bacterial community structure under long-term apple orchard cultivation systems. Appl. Soil Ecol. 167, 104103. doi: 10.1016/J.APSOIL.2021.104103
Huang, S., Zhang, X., Fernando, W. G. D. (2020). Directing trophic divergence in plant-pathogen interactions: antagonistic phytohormones with NO doubt? Front. Plant Sci 11, 600063. doi: 10.3389/fpls.2020.600063
Huber, D. M., Jones, J. B. (2013). The role of magnesium in plant disease. Plant Soil 368, 73–85. doi: 10.1007/s11104-012-1476-0
Igamberdiev, A. U., Kleczkowski, L. A. (2011). Magnesium and cell energetics in plants under anoxia. Biochem. J. doi: 10.1042/BJ20110213
Ishfaq, M., Wang, Y., Yan, M., Wang, Z., Wu, L., Li, C., et al. (2022). Physiological essence of magnesium in plants and its widespread deficiency in the farming system of China. Front. Plant Sci. 13. doi: 10.3389/fpls.2022.802274
Jamali Jaghdani, S., Jahns, P., Tränkner, M. (2021). The impact of magnesium deficiency on photosynthesis and photoprotection in Spinacia oleracea. Plant Stress 2, 100040. doi: 10.1016/j.stress.2021.100040
Jiao, J., Li, J., Chang, J., Li, J., Chen, X., Li, Z., et al. (2023). Magnesium effects on carbohydrate characters in leaves, phloem sap and mesocarp in wax gourd (Benincasa hispida (Thunb.) cogn.). Agronomy 13, 455. doi: 10.3390/agronomy13020455
Jin, X., Ackah, M., Wang, L., Amoako, F. K., Shi, Y., Essoh, L. G., et al. (2023). Magnesium nutrient application induces metabolomics and physiological responses in mulberry (Morus alba) plants. Int. J. Mol. Sci. 24, 9650. doi: 10.3390/IJMS24119650/S1
Jin, S., Zhou, W., Meng, L., Chen, Q., Li, J. (2022). Magnesium fertilizer application and soil warming increases tomato yield by increasing magnesium uptake under PE-film covered greenhouse. Agronomy 12, 940. doi: 10.3390/agronomy12040940
Johansson, M. J. O., Jacobson, A. (2010). Nonsense-mediated mRNA decay maintains translational fidelity by limiting magnesium uptake. Genes Dev. 24, 1491–1495. doi: 10.1101/gad.1930710
Kanjana, D. (2020). Foliar application of magnesium oxide nanoparticles on nutrient element concentrations, growth, physiological, and yield parameters of cotton. J. Plant Nutr. 43, 3035–3049. doi: 10.1080/01904167.2020.1799001
Kashinath, B. L., Murthy, A. N. G., Senthivel, T., Pitchai, G. J., Sadashiva, A. T. (2013). Effect of applied magnesium on yield and quality of tomato in Alfisols of Karnataka. J. Hortic. Sci. 8, 55–59. doi: 10.24154/jhs.v8i1.335
Kleczkowski, L. A., Igamberdiev, A. U. (2021). Magnesium signaling in plants. Int. J. Mol. Sci. 22, 1159.22. doi: 10.3390/IJMS22031159
Kleczkowski, L. A., Igamberdiev, A. U. (2023). Magnesium and cell energetics: At the junction of metabolism of adenylate and non-adenylate nucleotides. J. Plant Physiol. 280, 53901. doi: 10.1016/J.JPLPH.2022.153901
Kobayashi, N. I., Iwata, N., Saito, T., Suzuki, H., Iwata, R., Tanoi, K., et al. (2013). Application of 28 Mg for characterization of Mg uptake in rice seedling under different pH conditions. J. Radioanal Nucl. Chem. 296, 531–534. doi: 10.1007/s10967-012-2010-9
Kobayashi, N. I., Tanoi, K. (2015). Critical issues in the study of magnesium transport systems and magnesium deficiency symptoms in plants. Int. J. Mol. Sci. 16, 23076–23093. doi: 10.3390/ijms160923076
Koch, M., Winkelmann, M. K., Hasler, M., Pawelzik, E., Naumann, M. (2020). Root growth in light of changing magnesium distribution and transport between source and sink tissues in potato (Solanum tuberosum L.). Sci. Rep. 10, 8796. doi: 10.1038/s41598-020-65896-z
Kopittke, P. M., Menzies, N. W. (2007). A review of the use of the basic cation saturation ratio and the “ideal”. soil. Soil Sci. Soc. America J. 71, 259–265. doi: 10.2136/sssaj2006.0186
Koyama, H., Toda, T., Hara, T. (2001). Brief exposure to low-pH stress causes irreversible damage to the growing root in Arabidopsis thaliana: pectin–Ca interaction may play an important role in proton rhizotoxicity. J. Exp. Bot. 52, 361–368.
Kudapa, H., Barmukh, R., Vemuri, H., Gorthy, S., Pinnamaneni, R., Vetriventhan, M., et al. (2023). Genetic and genomic interventions in crop biofortification: Examples in millets. Front. Plant Sci 14, 1123655. doi: 10.3389/fpls.2023.1123655
Kumar, M., Giri, V. P., Pandey, S., Gupta, A., Patel, M. K., Bajpai, A. B., et al. (2021). Plant-growth-promoting rhizobacteria emerging as an effective bioinoculant to improve the growth, production and stress tolerance of vegetable crops. Int. J. Mol. Sci. 22, 12245. doi: 10.3390/ijms222212245
Kumar, N., Kaur, P., Devgan, K., Attkan, A. K. (2020). Shelf life prolongation of cherry tomato using magnesium hydroxide reinforced bio-nanocomposite and conventional plastic films. J. Food Process Preserv 44, e14379. doi: 10.1111/jfpp.14379
Kumari, V. V., Banerjee, P., Verma, V. C., Sukumaran, S., Chandran, M. A. S., Gopinath, K. A., et al. (2022). Plant nutrition: an effective way to alleviate abiotic stress in agricultural crops. Int. J. Mol. Sci. 23, 8519. doi: 10.3390/ijms23158519
Kwon, M. C., Kim, Y. X., Lee, S., Jung, E. S., Singh, D., Sung, J., et al. (2019). Comparative metabolomics unravel the effect of magnesium oversupply on tomato fruit quality and associated plant metabolism. Metabolites 9, 231. doi: 10.3390/metabo9100231
Lazcano, C., Boyd, E., Holmes, G., Hewavitharana, S., Pasulka, A., Ivors, K. (2021). The rhizosphere microbiome plays a role in the resistance to soil-borne pathogens and nutrient uptake of strawberry cultivars under field conditions. Sci. Rep. 11, 3188. doi: 10.1038/s41598-021-82768-2
Lefevere, H., Bauters, L., Gheysen, G. (2020). Salicylic acid biosynthesis in plants. Front. Plant Sci 11, 338. doi: 10.3389/fpls.2020.00338
Lenz, H., Dombinov, V., Dreistein, J., Reinhard, M. R., Gebert, M., Knoop, V. (2013). Magnesium deficiency phenotypes upon multiple knockout of arabidopsis thaliana MRS2 clade B genes can be ameliorated by concomitantly reduced calcium supply. Plant Cell Physiol. 54. doi: 10.1093/pcp/pct062
Li, H., Du, H., Huang, K., Chen, X., Liu, T., Gao, S., et al. (2016). Identification, and functional and expression analyses of the CorA/MRS2/MGT-type magnesium transporter family in maize. Plant Cell Physiol. 57, 1153–1168. doi: 10.1093/pcp/pcw064
Li, J., Hu, X., Zhang, R., Li, Q., Xu, W., Zhang, L., et al. (2023a). The plasma membrane magnesium transporter CsMGT5 mediates magnesium uptake and translocation under magnesium limitation in tea plants (Camellia sinensis L.). Sci. Hortic. 310. doi: 10.1016/j.scienta.2022.111711
Li, J., Huang, Y., Tan, H., Yang, X., Tian, L., Luan, S., et al. (2015). An endoplasmic reticulum magnesium transporter is essential for pollen development in Arabidopsis. Plant Sci. 231, 212–220. doi: 10.1016/j.plantsci.2014.12.008
Li, J., Li, Q. H., Zhang, X. Y., Zhang, L. Y., Zhao, P. L., Wen, T., et al. (2021). Exploring the effects of magnesium deficiency on the quality constituents of hydroponic-cultivated tea (Camellia sinensis L.) leaves. J. Agric. Food Chem. 69, 14278–14286. doi: 10.1021/ACS.JAFC.1C05141
Li, J., Muneer, M. A., Sun, A., Guo, Q., Wang, Y., Huang, Z., et al. (2023b). Magnesium application improves the morphology, nutrients uptake, photosynthetic traits, and quality of tobacco (Nicotiana tabacum L.) under cold stress. Front. Plant Sci. 14, 1078128. doi: 10.3389/FPLS.2023.1078128/BIBTEX
Li, L. G., Sokolov, L. N., Yang, Y. H., Li, D. P., Ting, J., Pandy, G. K., et al. (2008). A mitochondrial magnesium transporter functions in Arabidopsis pollen development. Mol. Plant 1, 675–685. doi: 10.1093/mp/ssn031
Li, L., Tutone, A. F., Drummond, R. S. M., Gardner, R. C., Luan, S. (2001). A novel family of magnesium transport genes in arabidopsis. Plant Cell 13, 2761–2775. doi: 10.1105/tpc.010352
Li, J., Yang, X., Zhang, M., Li, D., Jiang, Y., Yao, W., et al. (2023c). Yield, quality, and water and fertilizer partial productivity of cucumber as influenced by the interaction of water, nitrogen, and magnesium. Agronomy 13, 772. doi: 10.3390/agronomy13030772
Liang, W. W., Huang, J. H., Li, C. P., Yang, L. T., Ye, X., Lin, D., et al. (2017). MicroRNA-mediated responses to long-term magnesium-deficiency in Citrus sinensis roots revealed by Illumina sequencing. BMC Genomics 18, 1–16. doi: 10.1186/s12864-017-3999-5
Liao, Y. Y., Huang, Y., Carvalho, R., Choudhary, M., Da Silva, S., Colee, J., et al. (2021). Magnesium oxide nanomaterial, an alternative for commercial copper bactericides: field-scale tomato bacterial spot disease management and total and bioavailable metal accumulation in soil. Environ. Sci. Technol. 55, 13561–13570. doi: 10.1021/acs.est.1c00804
Liao, Y. Y., Strayer-Scherer, A., White, J. C., de la Torre-Roche, R., Ritchie, L., Colee, J., et al. (2019). Particle-size dependent bactericidal activity of magnesium oxide against Xanthomonas perforans and bacterial spot of tomato. Sci. Rep. 9, 18530. doi: 10.1038/s41598-019-54717-7
Liu, J., Fang, L., Pei, W., Li, F., Zhao, J. (2023). Effects of magnesium application on the arbuscular mycorrhizal symbiosis in tomato. Symbiosis 89, 73–82. doi: 10.1007/s13199-022-00862-z
Liu, X., Guo, L. X., Luo, L. J., Liu, Y. Z., Peng, S. A. (2019). Identification of the magnesium transport (MGT) family in Poncirus trifoliata and functional characterization of PtrMGT5 in magnesium deficiency stress. Plant Mol. Biol. 101, 551–560. doi: 10.1007/s11103-019-00924-9
Liu, X., Hu, C., Liu, X., Riaz, M., Liu, Y., Dong, Z., et al. (2022). Effect of magnesium application on the fruit coloration and sugar accumulation of navel orange (Citrus sinensis Osb.). Sci. Hortic. 304. doi: 10.1016/j.scienta.2022.111282
Lu, M., Liang, Y., Lakshmanan, P., Guan, X., Liu, D., Chen, X. (2021a). Magnesium application reduced heavy metal-associated health risks and improved nutritional quality of field-grown Chinese cabbage. Environ. pollut. 289, 117881. doi: 10.1016/j.envpol.2021.117881
Lu, M., Liu, D., Shi, Z., Gao, X., Liang, Y., Yao, Z., et al. (2021b). Nutritional quality and health risk of pepper fruit as affected by magnesium fertilization. J. Sci. Food Agric. 101, 582–592. doi: 10.1002/jsfa.10670
Luo, X., Zhang, C., Xu, W., Peng, Q., Jiao, L., Deng, J. (2020). Effects of nanometer magnesium hydroxide on growth, cadmium (Cd) uptake of Chinese cabbage (Brassica campestris) and soil cd form. Int. J. Agric. Biol. 24, 1006–1016. doi: 10.17957/IJAB/15.1527
Lv, X., Huang, S., Wang, J., Han, D., Li, J., Guo, D., et al. (2023). Genome-wide identification of Mg2+ transporters and functional characteristics of DlMGT1 in Dimocarpus longan. Front. Plant Sci. 14. doi: 10.3389/fpls.2023.1110005
Lyu, M., Liu, J., Xu, X., Liu, C., Qin, H., Zhang, X., et al. (2023). Magnesium alleviates aluminum-induced growth inhibition by enhancing antioxidant enzyme activity and carbon–nitrogen metabolism in apple seedlings. Ecotoxicol Environ. Saf. 249, 114421. doi: 10.1016/j.ecoenv.2022.114421
Ma, Y.-Y., Shi, J.-C., Wang, D.-J., Liang, X., Wei, F., Gong, C.-M., et al. (2023). A point mutation in the gene encoding magnesium chelatase I subunit influences strawberry leaf color and metabolism. Plant Physiol. 192, 2737–2755. doi: 10.1093/PLPHYS/KIAD247
Machin, J., Navas, A. (2000). Soil pH changes induced by contamination by magnesium oxides dust. Land Degrad Dev. 11, 37–50. doi: 10.1002/(SICI)1099-145X(200001/02)11:1<37::AID-LDR366>3.0.CO;2-8
Malvi, U. R. (2011). Interaction of micronutrients with major nutrients with special reference to potassium. Karnataka J. Agric. Sci. 24 (1), 106–109.
Marschner, P. (2011). Marschner’s Mineral Nutrition of Higher Plants, 3rd ed. Marschner’s Mineral Nutrition of Higher Plants: Third Edition. London: Academic press. doi: 10.1016/C2009-0-63043-9
Massimi, M., Radocz, L. (2021). The Action of Nutrients Deficiency on Growth Biometrics, Physiological Traits, Production Indicators, and Disease Development in Pepper (Capsicum annuum L.) Plant: A review. AMERICAN-EURASIAN J. OF Sustain. Agric. 15, 1–19.
Mauro, R. P., Anastasi, U., Lombardo, S., Pandino, G., Pesce, R., Alessia, R., et al. (2015). Cover crops for managing weeds, soil chemical fertility and nutritional status of organically grown orange orchard in Sicily. Ital. J. Agron. 10, 101–104. doi: 10.4081/ija.2015.641
Mayland, H. F., Wilkinson, S. R. (1989). Soil factors affecting magnesium availability in plant-animal systems: a review. J. Anim. Sci. 67, 3437–3444. doi: 10.2527/jas1989.67123437x
Meng, S. F., Zhang, B., Tang, R. J., Zheng, X. J., Chen, R., Liu, C. G., et al. (2022). Four plasma membrane-localized MGR transporters mediate xylem Mg2+ loading for root-to-shoot Mg2+ translocation in Arabidopsis. Mol. Plant 15, 805–819. doi: 10.1016/j.molp.2022.01.011
Mengutay, M., Ceylan, Y., Kutman, U. B., Cakmak, I. (2013). Adequate magnesium nutrition mitigates adverse effects of heat stress on maize and wheat. Plant Soil 368, 57–72. doi: 10.1007/s11104-013-1761-6
Morales-Payan, J. P. (2022). Performance of ‘MD-2’ pineapple as affected by magnesium source and rate. Acta Hortic., 367–372. doi: 10.17660/ActaHortic.2022.1333.49
Moreira, W. R., da Silva Bispo, W. M., Rios, J. A., Debona, D., Nascimento, C. W. A., Rodrigues, F.Á. (2015). Magnesium-induced alterations in the photosynthetic performance and resistance of rice plants infected with bipolaris oryzae. Sci. Agric. 72, 328–333. doi: 10.1590/0103-9016-2014-0312
Morozova, L. (2022). The role of magnesium ions for the growth and development of tomatoes when growing in protected soil conditions. Balanced Nat. using. 10, 112–118. doi: 10.33730/2310-4678.4.2022.275039
Mullins, C. A., Wolt, J. D. (2022). Effects of calcium and magnesium lime sources on yield, fruit quality, and elemental uptake of tomato. J. Am. Soc. Hortic. Sci. 108, 850–854. doi: 10.21273/jashs.108.5.850
Nedim, O., Damla, B. O. (2015). Effect of magnesium fertilization on some plant nutrient interactions and nut quality properties in Turkish hazelnut (Corylus avellana L.). Sci. Res. Essays 10, 465–470. doi: 10.5897/sre2014.5811
Neina, D. (2019). The role of soil pH in plant nutrition and soil remediation. Appl. Environ. Soil Sci. 2019, 1–9. doi: 10.1155/2019/5794869
Nikolaou, C. N., Chatziartemiou, A., Tsiknia, M., Karyda, A. G., Ehaliotis, C., Gasparatos, D. (2023). Calcium- and Magnesium-Enriched Organic Fertilizer and Plant Growth-Promoting Rhizobacteria Affect Soil Nutrient Availability, Plant Nutrient Uptake, and Secondary Metabolite Production in Aloe vera (Aloe barbadensis Miller) Grown under Field Conditions. Agronomy 13, 1–14. doi: 10.3390/agronomy13020482
Oda, K., Kamiya, T., Shikanai, Y., Shigenobu, S., Yamaguchi, K., Fujiwara, T. (2016). The arabidopsis Mg transporter, MRS2-4, is essential for Mg homeostasis under both low and high Mg conditions. Plant Cell Physiol. 57, 754–763. doi: 10.1093/pcp/pcv196
Ohyama, T. (2019). New aspects of magnesium function: A key regulator in nucleosome self-assembly, chromatin folding and phase separation. Int. J. Mol. Sci. 20, 4232. doi: 10.3390/ijms20174232
Ota, M., Imada, K., Sasaki, K., Kajihara, H., Sakai, S., Ito, S. (2019). MgO-induced defence against bacterial wilt disease in Arabidopsis thaliana. Plant Pathol. 68, 323–333. doi: 10.1111/ppa.12939
Pacumbaba, R. O., Jr., Beyl, C. A. (2011). Changes in hyperspectral reflectance signatures of lettuce leaves in response to macronutrient deficiencies. Adv. Space Res. 48, 32–42. doi: 10.1016/j.asr.2011.02.020
Peng, Y. Y., Liao, L. L., Liu, S., Nie, M. M., Li, J., Zhang, L. D., et al. (2019). Magnesium deficiency triggers sgr-mediated chlorophyll degradation for magnesium remobilization. Plant Physiol. 181, 262–275. doi: 10.1104/PP.19.00610
Peng, W. T., Qi, W. L., Nie, M. M., Xiao, Y. B., Liao, H., Chen, Z. C. (2020). Magnesium supports nitrogen uptake through regulating NRT2. 1/2.2 in soybean. Plant Soil 457, 97–111. doi: 10.1007/s11104-019-04157-z
Petrescu, D. C., Vermeir, I., Petrescu-Mag, R. M. (2020). Consumer understanding of food quality, healthiness, and environmental impact: A cross-national perspective. Int. J. Environ. Res. Public Health 17. doi: 10.3390/ijerph17010169
Petrov, A. S., Bernier, C. R., Hsiao, C., Okafor, C. D., Tannenbaum, E., Stern, J., et al. (2012). RNA-magnesium-protein interactions in large ribosomal subunit. J. Phys. Chem. 116. doi: 10.1021/jp304723w
Potarzycki, J., Grzebisz, W., Szczepaniak, W. (2022). Magnesium fertilization increases nitrogen use efficiency in winter wheat (Triticum aestivum L.). Plants 11, 2600. doi: 10.3390/plants11192600
Preciado-Mongui, I. M., Reyes-Medina, A. J., Romero-Barrera, Y., Álvarez-Herrera, J. G., Jaime-Guerrero, M. (2023). Growth and production of crisp lettuce (Lactuca sativa L.) using different doses of nitrogen and magnesium. Rev. Colombiana Cienc. Hortícolas 17, e15706. doi: 10.17584/RCCH.2023V17I1.15706
Qin, Y., Li, Q., An, Q., Li, D., Huang, S., Zhao, Y., et al. (2022). A phenylalanine ammonia lyase from Fritillaria unibracteata promotes drought tolerance by regulating lignin biosynthesis and SA signaling pathway. Int. J. Biol. Macromol 213, 574–588. doi: 10.1016/j.ijbiomac.2022.05.161
Qin, J., Wang, H., Cao, H., Chen, K., Wang, X. (2020). Combined effects of phosphorus and magnesium on mycorrhizal symbiosis through altering metabolism and transport of photosynthates in soybean. Mycorrhiza 30, 285–298. doi: 10.1007/s00572-020-00955-x
Quddus, M. A., Siddiky, M. A., Hussain, M. J., Rahman, M. A., Ali, M. R., Masud, M. A. T. (2022). Magnesium influences growth, yield, nutrient uptake, and fruit quality of tomato. Int. J. Vegetable Sci. 28, 441–464. doi: 10.1080/19315260.2021.2014614
Rajitha, G., Reddy, M. S., Ramesh Babu, P. V., Maheshwari, V. U. (2019). Influence of secondary and micronutrients on primary nutrient uptake by groundnut (Arachis hypogaea L.). Agric. Sci. Digest - A Res. J. 38, 285–288. doi: 10.18805/ag.d-4798
Rankelytė, G., Chmeliov, J., Gelžinis, A., Valkūnas, L. (2023). “Excited states of chlorophyll molecules in light-harvesting antenna of PSI,” in Chemistry and chemical technology: international conference CCT-2023, Vilnius: Conference Book. Vilnius university press. March 10, 2023. doi: 10.15388/CCT.2023
Regon, P., Chowra, U., Awasthi, J. P., Borgohain, P., Panda, S. K. (2019). Genome-wide analysis of magnesium transporter genes in Solanum lycopersicum. Comput. Biol. Chem. 80, 498–511. doi: 10.1016/j.compbiolchem.2019.05.014
Rehman, H., Alharby, H. F., Alzahrani, Y., Rady, M. M. (2018). Magnesium and organic biostimulant integrative application induces physiological and biochemical changes in sunflower plants and its harvested progeny on sandy soil. Plant Physiol. Biochem. 126, 97–105. doi: 10.1016/J.PLAPHY.2018.02.031
Rengel, Z., Bose, J., Chen, Q., Tripathi, B. N. (2015). Magnesium alleviates plant toxicity of aluminium and heavy metals. Crop Pasture Sci. 66, 1298–1307. doi: 10.1071/CP15284
Rodrigues, V. A., Crusciol, C. A. C., Bossolani, J. W., Moretti, L. G., Portugal, J. R., Mundt, T. T., et al. (2021). Magnesium foliar supplementation increases grain yield of soybean and maize by improving photosynthetic carbon metabolism and antioxidant metabolism. Plants 10, 797. doi: 10.3390/plants10040797
Romani, A. M. P. (2011). Cellular magnesium homeostasis. Arch. Biochem. Biophys. 512, 1–23. doi: 10.1016/j.abb.2011.05.010
Römheld, V. (2012). Diagnosis of deficiency and toxicity of nutrients, in: Marschner’s Mineral Nutrition of Higher Plants, 3rd edn. San Diego: Elsevier/Academic Press, 299–312.
Römheld, V., Kirkby, E. A. (2009). Magnesium functions in crop nutrition and yield. Nawozy i Nawożenie (Fertilisers Fertilization) 34, 163–182.
Sadeghi, F., Rezeizad, A., Rahimi, M. (2021). Effect of zinc and magnesium fertilizers on the yield and some characteristics of wheat (Triticum aestivum L.) seeds in two years. Int. J. Agron. 2021, 1–6. doi: 10.1155/2021/8857222
Salcido-Martinez, A., Sanchez, E., Licon-Trillo, L. P., Perez-Alvarez, S., Palacio-Marquez, A., Amaya-Olivas, N. I., et al. (2020). Impact of the foliar application of magnesium nanofertilizer on physiological and biochemical parameters and yield in green beans. Not Bot. Horti Agrobot Cluj Napoca 48, 2167–2181. doi: 10.15835/48412090
Salih, R. F., Ismail, T. N., Hamad, E. M. (2023). Foliar application of NPK improves growth, yield and fiber morphological properties of some kenaf (Hibiscus cannabunus L.) varieties. Passer J. Basic Appl. Sci. 5, 183–190. doi: 10.24271/PSR.2023.387988.1267
Schormann, N., Hayden, K. L., Lee, P., Banerjee, S., Chattopadhyay, D. (2019). An overview of structure, function, and regulation of pyruvate kinases. Protein Sci. 28, 1771–1784. doi: 10.1002/pro.3691
Senbayram, M., Gransee, A., Wahle, V., Thiel, H. (2015). Role of magnesium fertilisers in agriculture: plant–soil continuum. Crop Pasture Sci. 66, 1219–1229. doi: 10.1071/CP15104
Setiawati, W., Hasyim, A., Udiarto, B. K., Hudayya, A. (2020). Pengaruh Magnesium, Boron, dan Pupuk Hayati terhadap Produktivitas Cabai serta Serangan Hama dan Penyakit (Effect of Magnesium, Boron, and Biofertilizers on Chili Pepper Productivity and Impact of Pests and Diseases). Jurnal Hortikultura 30, 65–74. doi: 10.21082/jhort.v30n1.2020.p65-74
Sharma, P., Gautam, A., Kumar, V., Guleria, P. (2022). In vitro exposed magnesium oxide nanoparticles enhanced the growth of legume Macrotyloma uniflorum. Environ. Sci. pollut. Res. 29, 13635–13645. doi: 10.1007/s11356-021-16828-5
Shaul, O. (2002). Magnesium transport and function in plants: The tip of the iceberg. BioMetals. 15, 307–321. doi: 10.1023/A:1016091118585
Shehata, S. A., ZA, S., M Attia, M., Rageh, M. A. (2015). Effect of foliar application of micronutrients, magnesium and wrapping films on yield, quality and storability of green bean pods. Fayoum J. Agric. Res. Dev. 29, 121–138. doi: 10.21608/fjard.2015.192992
Siddiqui, M. H., Alamri, S. A., Al-Khaishany, M. Y. Y., Al-Qutami, M. A., Ali, H. M., Al-Whaibi, M. H., et al. (2018). Mitigation of adverse effects of heat stress on Vicia faba by exogenous application of magnesium. Saudi J. Biol. Sci. 25, 1393–1401. doi: 10.1016/j.sjbs.2016.09.022
Silva, D. M. d., Souza, K., Vilas Boas, L. V., Alves, Y. S., Alves, J. D. (2017). The effect of magnesium nutrition on the antioxidant response of coffee seedlings under heat stress. Sci. Hortic. 224, 115–125. doi: 10.1016/j.scienta.2017.04.029
Singh, N., Shukla, S. (2020). Nano technology for increasing productivity in agriculture. Vivechan Int. J. Res. 11, 2020.
Sotoodehnia-Korani, S., Iranbakhsh, A., Ebadi, M. (2020). Efficacy of magnesium nanoparticles in modifying growth, antioxidant activity, nitrogen status, and expression of wrky1 and bzip transcription factors in pepper (Capsicum annuum); an in vitro biological assessment. Russ J Plant Physiol 70, 39. doi: 10.1134/S1021443723600186
Sun, X., Kay, A. D., Kang, H., Small, G. E., Liu, G., Zhou, X., et al. (2013). Correlated biogeographic variation of magnesium across trophic levels in a terrestrial food chain. PloS One 8, e78444. doi: 10.1371/journal.pone.0078444
Sun, T., Wang, P., Rao, S., Zhou, X., Wrightstone, E., Lu, S., et al. (2023). Co-chaperoning of chlorophyll and carotenoid biosynthesis by ORANGE family proteins in plants. Mol. Plant 16, 1048–1065. doi: 10.1016/j.molp.2023.05.006
Sun, L., Zhang, P., Xing, M., Li, R., Yu, H., Ju, Q., et al. (2023). NAC32 alleviates magnesium toxicity-induced cell death through positive regulation of XIPOTL1 expression. Plant Physiol. 191, 849–853. doi: 10.1093/plphys/kiac562
Tahiri, A., Meddich, A., Raklami, A., Alahmad, A., Bechtaoui, N., Anli, M., et al. (2022). Assessing the potential role of compost, PGPR, and AMF in improving tomato plant growth, yield, fruit quality, and water stress tolerance. J. Soil Sci. Plant Nutr. 22, 1–22. doi: 10.1007/s42729-021-00684-w
Tan, H., Du, C., Zhou, L. (2000). Effect of magnesium fertilizer on sustaining upland agricultural development in Guangxi province. Better Crops Int. 14, 13–15.
Tang, R. J., Luan, S. (2017). Regulation of calcium and magnesium homeostasis in plants: from transporters to signaling network. Curr. Opin. Plant Biol. 39, 97–105. doi: 10.1016/j.pbi.2017.06.009
Tang, R. J., Luan, S. (2020). Rhythms of magnesium. Nat. Plants. 6, 742–743. doi: 10.1038/s41477-020-0706-3
Tang, R. J., Meng, S. F., Zheng, X. J., Zhang, B., Yang, Y., Wang, C., et al. (2022a). Conserved mechanism for vacuolar magnesium sequestration in yeast and plant cells. Nat. Plants 8, 181–190. doi: 10.1038/s41477-021-01087-6
Tang, L., Xiao, L., Chen, E., Lei, X., Ren, J., Yang, Y., et al. (2023). Magnesium transporter CsMGT10 of tea plants plays a key role in chlorosis leaf vein greening. Plant Physiol. Biochem. 201, 107842. doi: 10.1016/j.plaphy.2023.107842
Tang, L., Xiao, L., Huang, Y., Xiao, B., Gong, C. (2021). Cloning and magnesium transport function analysis of csMGTs genes in tea plants (Camellia sinensis). J. Tea Sci. 41, 761–776.
Tang, R. J., Yang, Y., Yan, Y. W., Mao, D. D., Yuan, H. M., Wang, C., et al. (2022b). Two transporters mobilize magnesium from vacuolar stores to enable plant acclimation to magnesium deficiency. Plant Physiol. 190, 1307–1320. doi: 10.1093/plphys/kiac330
Tariq Aftab, K. R. H. (2020). Plant Micronutrients: Deficiency and Toxicity Management (Springer: Springer Nature, Cham). doi: 10.1007/978-3-030-49856-6
Tian, X. Y., He, D. D., Bai, S., Zeng, W. Z., Wang, Z., Wang, M., et al. (2021). Physiological and molecular advances in magnesium nutrition of plants. Plant Soil. doi: 10.1007/s11104-021-05139-w
Tian, G., Liu, C., Xu, X., Xing, Y., Liu, J., Lyu, M., et al. (2022). Magnesium promotes nitrate uptake by increasing shoot-to-root translocation of sorbitol in apple seedlings. SSRN Electronic J. 19, 1–27. doi: 10.2139/ssrn.4290413
Tian, G., Liu, C., Xu, X., Xing, Y., Liu, J., Lyu, M., et al. (2023a). Effects of Magnesium on nitrate uptake and sorbitol synthesis and translocation in apple seedlings. Plant Physiol. Biochem. 196, 139–151. doi: 10.1016/j.plaphy.2023.01.033
Tian, G., Qin, H., Liu, C., Xing, Y., Feng, Z., Xu, X., et al. (2023b). Magnesium improved fruit quality by regulating photosynthetic nitrogen use efficiency, carbon–nitrogen metabolism, and anthocyanin biosynthesis in ‘Red Fuji’ apple. Front. Plant Sci. 14. doi: 10.3389/fpls.2023.1136179
Tränkner, M., Tavakol, E., Jákli, B. (2018). Functioning of potassium and magnesium in photosynthesis, photosynthate translocation and photoprotection. Physiol. Plant 163, 414–431. doi: 10.1111/ppl.12747
Trejo-Téllez, L. I., Gómez-Merino, F. C. (2014). Nutrient management in strawberry: Effects on yield, quality and plant health. Strawberries: Cultivation antioxidant properties Health benefits, 239–267.
Tully, K., Ryals, R. (2017). Nutrient cycling in agroecosystems: Balancing food and environmental objectives. Agroecol Sustain. Food Syst. 41, 761–798. doi: 10.1080/21683565.2017.1336149
Turner, T. L., Bourne, E. C., Von Wettberg, E. J., Hu, T. T., Nuzhdin, S. V. (2010). Population resequencing reveals local adaptation of Arabidopsis lyrata to serpentine soils. Nat. Genet. 42, 260–263. doi: 10.1038/ng.515
Verbruggen, N., Hermans, C. (2013). Physiological and molecular responses to magnesium nutritional imbalance in plants. Plant Soil 368, 87–99. doi: 10.1007/s11104-013-1589-0
Verma, P., Sanyal, S. K., Pandey, G. K. (2021). Ca2+–CBL–CIPK: a modulator system for efficient nutrient acquisition. Plant Cell Rep. 40, 2111–2122. doi: 10.1007/S00299-021-02772-8/FIGURES/3
Villeneuve, F., Geoffriau, E. (2020). “Carrot physiological disorders and crop adaptation to stress,” in Carrots and Related Apiaceae Crops Cabi, Wallingford, 156–170. doi: 10.1079/9781789240955.0156
Wang, Z., Hassan, M. U., Nadeem, F., Wu, L., Zhang, F., Li, X. (2020). Magnesium fertilization improves crop yield in most production systems: A meta-analysis. Front. Plant Sci 10, 1727. doi: 10.3389/fpls.2019.01727
Wang, Z., Zhang, X., Fan, G. J., Que, Y., Xue, F., Liu, Y. H. (2022). Toxicity Effects and Mechanisms of MgO Nanoparticles on the Oomycete Pathogen Phytophthora infestans and Its Host Solanum tuberosum. Toxics 10, 553. doi: 10.3390/toxics10100553
Wu, X., Ye, J. (2020). Manipulation of jasmonate signaling by plant viruses and their insect vectors. Viruses. 12, 1–16. doi: 10.3390/v12020148
Xie, K., Cakmak, I., Wang, S., Zhang, F., Guo, S. (2021). Synergistic and antagonistic interactions between potassium and magnesium in higher plants. Crop J. 9, 249–256. doi: 10.1016/j.cj.2020.10.005
Xu, X. F., Qian, X. X., Wang, K. Q., Yu, Y. H., Guo, Y. Y., Zhao, X., et al. (2021). Slowing development facilitates arabidopsis mgt mutants to accumulate enough magnesium for pollen formation and fertility restoration. Front. Plant Sci. 11. doi: 10.3389/FPLS.2020.621338/BIBTEX
Xu, J., Wu, L., Tong, B., Yin, J., Huang, Z., Li, W., et al. (2021). Magnesium supplementation alters leaf metabolic pathways for higher flavor quality of oolong tea. Agriculture (Switzerland) 11 (2), 1–12. doi: 10.3390/agriculture11020120
Yan, Y. W., Mao, D. D., Yang, L., Qi, J. L., Zhang, X. X., Tang, Q. L., et al. (2018). Magnesium transporter MGT6 plays an essential role in maintaining magnesium homeostasis and regulating high magnesium tolerance in Arabidopsis. Front. Plant Sci. 9. doi: 10.3389/fpls.2018.00274
Yang, W., Ji, Z., Wu, A., He, D., Rensing, C., Chen, Y., et al. (2023). Inconsistent responses of soil bacterial and fungal community’s diversity and network to magnesium fertilization in tea (Camellia sinensis) plantation soils. Appl. Soil Ecol. 191, 105055. doi: 10.1016/J.APSOIL.2023.105055
Yang, X., Kobayashi, N. I., Hayashi, Y., Ito, K., Moriwaki, Y., Terada, T., et al. (2022). Mutagenesis analysis of GMN motif in Arabidopsis thaliana Mg2+transporter MRS2-1. Biosci. Biotechnol. Biochem. 86, 870–874. doi: 10.1093/bbb/zbac064
Yang, G. H., Yang, L. T., Jiang, H. X., Li, Y., Wang, P., Chen, L. S. (2012). Physiological impacts of magnesium-deficiency in Citrus seedlings: Photosynthesis, antioxidant system and carbohydrates. Trees - Structure Funct. 26, 1237–1250. doi: 10.1007/S00468-012-0699-2
Yang, L. T., Yang, G. H., You, X., Zhou, C. P., Lu, Y. B., Chen, L. S. (2013). Magnesium deficiency-induced changes in organic acid metabolism of Citrus sinensis roots and leaves. Biol. Plant 57, 481–486. doi: 10.1007/s10535-013-0313-5
Yang, W., Zhang, X., Wu, L., Rensing, C., Xing, S. (2021). Short-term application of magnesium fertilizer affected soil microbial biomass, activity, and community structure. J. Soil Sci. Plant Nutr. 21, 675–689. doi: 10.1007/s42729-020-00392-x
Yang, L. T., Zhou, Y. F., Wang, Y. Y., Wu, Y. M., Ye, X., Guo, J. X., et al. (2019). Magnesium deficiency induced global transcriptome change in citrus sinensis leaves revealed by RNA-seq. Int. J. Mol. Sci. 20, 3129. doi: 10.3390/ijms20133129
Yaseen Aljanabi, H. A. (2021). Effects of nano fertilizers technology on agriculture production. Ann. Rom Soc. Cell Biol. 25, 6728–6739.
Ye, X., Chen, X. F., Deng, C. L., Yang, L. T., Lai, N. W., Guo, J. X., et al. (2019). Magnesium-deficiency effects on pigments, photosynthesis and photosynthetic electron transport of leaves, and nutrients of leaf blades and veins in citrus sinensis seedlings. Plants 8, 389. doi: 10.3390/plants8100389
Ye, X., Huang, H. Y., Wu, F. L., Cai, L. Y., Lai, N. W., Deng, C. L., et al. (2021). Molecular mechanisms for magnesium-deficiency-induced leaf vein lignification, enlargement and cracking in Citrus sinensis revealed by RNA-Seq. Tree Physiol. 41, 280–301. doi: 10.1093/treephys/tpaa128
Yeh, D.-M., Lin, L., Wright, C. J. (2000). Effects of mineral nutrient deficiencies on leaf development, visual symptoms and shoot–root ratio of Spathiphyllum. Sci. Hortic. 86, 223–233. doi: 10.1016/S0304-4238(00)00152-7
Yousaf, M., Bashir, S., Raza, H., Shah, A. N., Iqbal, J., Arif, M., et al. (2021). Role of nitrogen and magnesium for growth, yield and nutritional quality of radish. Saudi J. Biol. Sci. 28, 3021–3030. doi: 10.1016/j.sjbs.2021.02.043
Yu, T., Jiang, J., Yu, Q., Li, X., Zeng, F. (2023). Structural Insights into the Distortion of the Ribosomal Small Subunit at Different Magnesium Concentrations. Biomolecules 13, 566. doi: 10.3390/biom13030566
Zhang, B., Cakmak, I., Feng, J., Yu, C., Chen, X., Xie, D., et al. (2020). Magnesium deficiency reduced the yield and seed germination in wax gourd by affecting the carbohydrate translocation. Front. Plant Sci. 11, 797. doi: 10.3389/fpls.2020.00797
Zhang, W., Liu, Y., Muneer, M. A., Jin, D., Zhang, H., Cai, Y., et al. (2022). Characterization of different magnesium fertilizers and their effect on yield and quality of soybean and pomelo. Agronomy 12, 2693. doi: 10.3390/agronomy12112693
Zhang, Q., Ni, K., Yi, X., Liu, M., Ruan, J. (2021). Advances of magnesium nutrition in tea plant. J. Tea Sci. 41, 19–27.
Zhang, S., Yang, W., Muneer, M. A., Ji, Z., Tong, L., Zhang, X., et al. (2021). Integrated use of lime with Mg fertilizer significantly improves the pomelo yield, quality, economic returns and soil physicochemical properties under acidic soil of southern China. Sci. Hortic. 290, 110502. doi: 10.1016/j.scienta.2021.110502
Zhang, H., Zhao, D., Yan, S., Xu, K., Zhang, Y. (2020). Effect of potassium-magnesium ratio on “Zaosu” pear fruit quality. J. Fruit Sci. 37, 1667–1675. doi: 10.13925/j.cnki.gsxb.20200183
Zheng, T., Moreira, R. G. (2020). Magnesium ion impregnation in potato slices to improve cell integrity and reduce oil absorption in potato chips during frying. Heliyon 6, e05834. doi: 10.1016/j.heliyon.2020.e05834
Zhi, S., Zou, W., Li, J., Meng, L., Liu, J., Chen, J., et al. (2023). Mapping QTLs and gene validation studies for Mg2+ uptake and translocation using a MAGIC population in rice. Front. Plant Sci. 14. doi: 10.3389/fpls.2023.1131064
Zhong, Q., Hu, H., Fan, B., Zhu, C., Chen, Z. (2021). Biosynthesis and roles of salicylic acid in balancing stress response and growth in plants. Int. J. Mol. Sci. 22, 1–14. doi: 10.3390/ijms222111672
Keywords: biofortification, Mg2+ transporter, photosynthesis, absorption, stress tolerance, plant nutrition, deficiency and toxicity, nanocompoiste
Citation: Ahmed N, Zhang B, Bozdar B, Chachar S, Rai M, Li J, Li Y, Hayat F, Chachar Z and Tu P (2023) The power of magnesium: unlocking the potential for increased yield, quality, and stress tolerance of horticultural crops. Front. Plant Sci. 14:1285512. doi: 10.3389/fpls.2023.1285512
Received: 30 August 2023; Accepted: 09 October 2023;
Published: 24 October 2023.
Edited by:
Laichao Luo, Anhui Agricultural University, ChinaReviewed by:
Xiaohui Chen, Fujian Agriculture and Forestry University, ChinaViabhav Kumar Upadhayay, Dr. Rajendra Prasad Central Agricultural University, India
Copyright © 2023 Ahmed, Zhang, Bozdar, Chachar, Rai, Li, Li, Hayat, Chachar and Tu. This is an open-access article distributed under the terms of the Creative Commons Attribution License (CC BY). The use, distribution or reproduction in other forums is permitted, provided the original author(s) and the copyright owner(s) are credited and that the original publication in this journal is cited, in accordance with accepted academic practice. No use, distribution or reproduction is permitted which does not comply with these terms.
*Correspondence: Panfeng Tu, dHVwYW5mZW5nQDE2My5jb20=
†These authors have contributed equally to this work