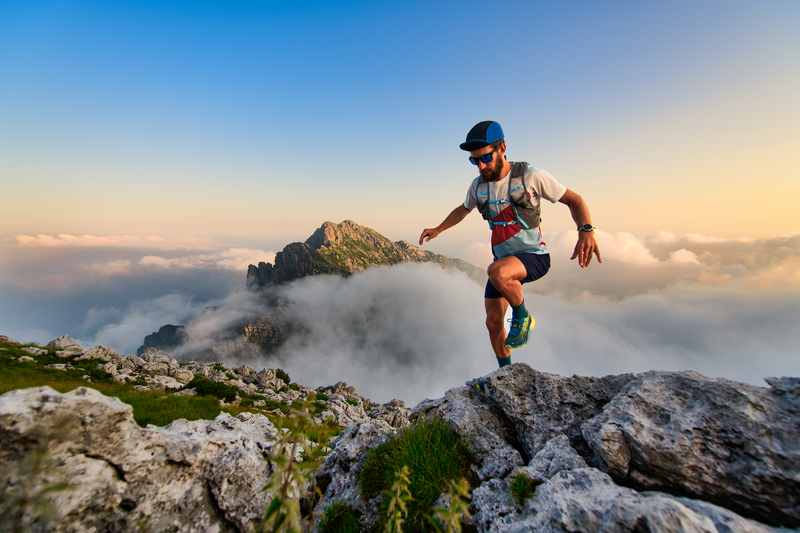
95% of researchers rate our articles as excellent or good
Learn more about the work of our research integrity team to safeguard the quality of each article we publish.
Find out more
ORIGINAL RESEARCH article
Front. Plant Sci. , 04 December 2023
Sec. Plant Abiotic Stress
Volume 14 - 2023 | https://doi.org/10.3389/fpls.2023.1278954
This article is part of the Research Topic Plant Responses to Salt Stress View all 12 articles
Using the halotolerant green microalgae Dunaliella salina as a model organism has special merits, such as a wide range of salt tolerance, unicellular organism, and simple life cycle and growth conditions. These unique characteristics make it suitable for salt stress study. In order to provide an overview of the response of Dunaliella salina to salt stress and hopefully to reveal evolutionarily conserved mechanisms of photosynthetic organisms in response to salt stress, the transcriptomes and the genome of the algae were sequenced by the second and the third-generation sequencing technologies, then the transcriptomes under salt stress were compared to the transcriptomes under non-salt stress with the newly sequenced genome as the reference genome. The major cellular biological processes that being regulated in response to salt stress, include transcription, protein synthesis, protein degradation, protein folding, protein modification, protein transport, cellular component organization, cell redox homeostasis, DNA repair, glycerol synthesis, energy metabolism, lipid metabolism, and ion homeostasis. This study gives a comprehensive overview of how Dunaliella salina responses to salt stress at transcriptomic level, especially characterized by the nearly ubiquitous up-regulation of the genes involving in protein folding, DNA repair, and cell redox homeostasis, which may confer the algae important mechanisms to survive under salt stress. The three fundamental biological processes, which face huge challenges under salt stress, are ignored by most scientists and are worth further deep study to provide useful information for breeding economic important plants competent in tolerating salt stress, other than only depending on the commonly acknowledged osmotic balance and ion homeostasis.
Salt stress of plants is an active area of research. A lots of researches have been carried out using the model plant Arabidopsis thaliana (Møller and Tester, 2007), partially because that Arabidopsis thaliana has a clear genetic background and is from the same family Brassicaceae as some economic important crops and vegetables, such as rapeseed, cabbage, radish et al., and the response mechanisms of Arabidopsis thaliana under salt stress may better reflect those of related crops (Kowalski et al., 1994).
Dunaliella salina is an extremely halotolerant, unicellular, eukaryotic, photosynthetic green microalgae, which is unique in its remarkable ability to survive in media containing NaCl at a wide range of concentrations, from about 0.05 M to 5.5 M (Ben-Amotz and Avron, 1973). Dunaliella salina can grow easily in aqueous media in a flask, which makes it very convenient to apply salt stress and other abiotic stresses, such as heavy metal, nutrition, temperature and light stress, on it for study. Compared with Arabidopsis thaliana, Dunaliella salina is unicellular, cells in log phase are highly homogeneous. For this reason, it is hopefully to find some of the fundamental and conserved mechanisms that remain uncovered due to cellular heterogeneity of study materials. These characteristics make Dunaliella salina a very good model organism for studying salt stress response and tolerance (Ben-Amotz and Avron, 1973).
Omics methods, such as methods of genomics, transcriptomics and proteomics, are powerful tools to reveal the mechanisms of salt tolerance and can give an overview of the response of plants to salt stress because omics aims at the collective characterization and quantification of pools of biological molecules (Mangelsen et al., 2011; Marco et al., 2011; Kang et al., 2020). With the development of high throughput sequencing technology, comparative transcriptomic analysis becomes an efficient and powerful method to reveal the response of plants to all kinds of stress at a global level, and some papers reported using this method to study the response of Dunaliella salina to salt stress (He et al., 2020a; Gao et al., 2021; Lv et al., 2021; Panahi and Hejazi, 2021). Considering that morphological change and glycerol synthesis in Dunaliella salina are almost accomplished in about 2 hours after salt stress (Chitlaru and Pick, 1989; Oren, 2005), it is universally acknowledged that most of the adaptive changes of Dunaliella salina should occur within about 2 hours under salt stress. Lv et al. (Lv et al., 2021) reported the transcriptomes at 15, 30, 60, and 120-min under salt stress of 4.5 M NaCl, finding that GO (Gene Ontology) terms of “chromosome and associated proteins”, “transporters”, and “cytoskeleton proteins” were enriched by differentially expressed genes (DEGs). They also analyzed the expression of enzymes in the core carbon metabolism pathway, such as starch catabolism, glycolysis, Calvin cycle and glycerol metabolic pathways. However, except the core carbon metabolism pathway, other biological processes and pathways weren’t analyzed in detail. He et al. (He et al., 2020a) reported the transcriptomes at 30, 60, and 120-min under salt stress of 2.5M NaCl, found that some biological processes, such as photosynthesis, lipid metabolism, amino acids and protein metabolism, starch and sucrose metabolism and glycerol synthesis, were enriched by the DEGs. They also constructed for the first time the carbon metabolism pathway from starch to glycerol in Dunaliella salina. In that study, the transcripts from the third-generation sequencing (PacBio sequencing) was used as the reference genes for gene expression quantification analysis due to there was no genome data of Dunaliella salina strain CCAP 19/30 available at the moment. Although this method has the advantage to detect alternatively spliced transcripts, there exist obvious drawbacks, since artificially spliced transcripts generated during incomplete reverse transcription could interfere the expressional quantification of the genes and the potential loss of low-abundance mRNAs could lower genome coverage (Van Dijk et al., 2018). Recently we sequenced the whole genome of Dunaliella salina strain CCAP 19/30 by PacBio sequencing, which gave a total of 13509 genes by annotation. Using this high-quality genome sequence as the reference, we redo in this study the comparative transcriptomic analysis for Dunaliella salina strain CCAP 19/30 under salt stress of 2.5 M NaCl within 2 hours duration. In addition to helping to focus on identifying and analyzing the major biological processes being regulated to reflect the responses of Dunaliella salina to salt stress at a global level, the high-quality reference genome also enables us to analyze the specific pathways, the glycerol synthesis, the fatty acid synthesis, and the TAG synthesis pathway in detail, especially the key enzymes that regulate a specific pathway. This work gives an overview of the response of Dunaliella salina to salt stress at transcriptomic level. The nearly ubiquitous up-regulation of the genes involved in protein folding, DNA repair, and cell redox homeostasis characterizes the expression profile of Dunaliella salina when confronting salt stress, which may be important mechanisms to enable the algae to survive under salt stress.
Dunaliella salina strain CCAP 19/30 was obtained from Mariela A. González and Thomas Pröschold. The algae grew in a controlled-environment chamber with 16 h lighting (17500 lx) and 8 h darkness at 20 °C. The growth medium contains 1 M NaCl, 5.0 mM NaNO3, 5.0 mM MgSO4 · 7H2O, 0.1 mM NaH2PO4 · 2H2O, 1.0 mM KCl, 10.0 mM NaHCO3, 0.3 mM CaCl2 · 2H2O, 4.6 uM H3BO3, 0.9 uM MnCl2.4H2O, 0.08 uM ZnSO4.7H2O, 0.03 uM CuSO4.5H2O, and 0.02 uM Na2MoO4.2H2O. Briefly, the algae grew in growth medium containing 1 M NaCl. Salt stress was applied by adding equal volume of high-salt medium (containing 4M NaCl) to the growth medium containing algae in log phase (1 106 cells/ml), which resulted in an increasing of salt concentration from 1 M to 2.5 M. Then the algae were collected at the time points of 0.5 h, 1 h and 2 h after the applying of salt stress, respectively, for RNA extraction. The algae were also collected before stress for RNA extraction to serve as the control. The salt stress and RNA extraction experiments were performed in triplicate.
The genomic DNA was extracted using plant genomic DNA extraction kit (DP305, TIANGEN, China) by omitting the step of tissue homogenization by liquid nitrogen. Following genomic DNA extraction, libraries were generated and sequenced by using either the Illumina (insert size 350 bp) or the PacBio platforms. Totally, 102.65 Gbp were produced including 25.44 Gbp Illumina data (sequencing depth is 92.7 and 77.21 Gbp PacBio data (sequencing depth is 281.4 The sequencing coverage is 99.38%. The genome was assembled using overlap-layout-consensus algorithm (Lieberman-Aiden et al., 2009). The main genome assembly is about 223.7 Mbp with contig N50 = 2.93 Mbp, scaffold N50 = 13.13 Mbp. Using the RNA-Seq data of Dunaliella salina strain CCAP 19/30 and the published genomic data of closely related algae, 13509 protein-coding genes were found in the genome and used for comparative transcriptomic analysis.
Total RNA was extracted with Trizol (Invitrogen, USA) by following the user manual for suspension cell culture. Briefly, the algae cells from salt stress treatment group and control group were collected immediately by centrifugation at room temperature for 5 min at , and quickly lysed by adding 1 ml Trizol. The time from centrifugation to lysing should be as short as possible to avoid additional stress. The remaining steps are the same as the manual recommends. The RNA pellets were kept in 75% ethanol and stored at -80°C before library construction. For library construction, mRNA was purified from total RNA and fragmented to perform cDNA synthesis. The cDNA was used to construct library with insert size of 350 bp. The libraries were sequenced by HiSeq 2000 platform to generate paired-end reads of 150 bp. The raw reads were processed to generate clean reads by removing adapter sequences, excluding reads contain >10% ambiguous bases N and > 50% bases with Qphred ≤20. The clean reads were used to quantify the values of expression of the reference genes from genome annotation of Dunaliella salina CCAP 19/30. Briefly, read count of each gene was calculated by mapping the clean reads from each RNA sample to the full-length coding sequence of the reference gene using HTSeq v0.6.1 (Anders et al., 2015). And then FPKM (expected number of Fragments per Kilobase of transcript sequence per Millions base pairs sequenced) of the genes was calculated by involving parameters of gene length, reads count and sequencing depth (Trapnell et al., 2010).
Differential expression analysis of the genes from two groups was performed by using the DESeq R package with the read counts of the genes (Anders and Huber, 2010). The resulting P values from DESeq were adjusted using the Benjamini and Hochberg’s approach for controlling the false discovery rate. Those with an adjusted P-value below 0.05 were considered as differentially expressed genes (DEGs). Heatmaps were made using online tool Chiplot (https://www.chiplot.online).
Functional categorization of the DEGs was performed by using Gene Ontology (GO) database and Kyoto Encyclopedia of Genes and Genomes (KEGG) database. GO enrichment analysis of DEGs was performed by using the BLAST2GO platform (Conesa et al., 2005) and the GOseq R package (Young et al., 2010). GO terms with corrected P value below 0.05 were considered significantly enriched. KEGG enrichment analysis of DEGs was performed by using the KEGG Automatic Annotation Server (Moriya et al., 2007) and KOBAS software (Mao et al., 2005). KEGG terms with false discovery rate (FDR) below 0.05 were considered significantly enriched.
In order to confirm the genes expression profiles resulting from high throughput RNA-seq analysis, we performed real-time PCR to analyze the expressions of the key genes from each pathway or functional group. The real-time PCR kit was from QIAGEN (208054, Germany). The primers used in real-time PCR were in the Supplementary Materials (Supplementary File 2). The reference genes used in real-time PCR were elongation factor 1-alpha. The relative expression quantification was calculated by the 2-ΔΔCt method (Livak and Schmittgen, 2001).
There were 13509 genes annotated in the genome of Dunaliella salina. Among the 9537 genes annotated by the GO database, the number of differently regulated genes (DEGs) increased from 4142 at 0.5-hour under stress, to 4568 at 1-hour, and to 6577 at 2-hour, accounting for about 31%, 34%, and 49% of the total genes. The number of DEGs increased with the increasing of stress time. By using GO enrichment analysis, most of these differently regulated genes (DEGs) can be clustered into some biological processes. These biological processes reflect the responses during the first two hours of Dunaliella salina when confronting salt stress at a more global level, include transcription, protein synthesis, protein degradation, protein folding, protein modification, protein transport, cellular component organization, cell redox homeostasis, DNA repair, glycerol synthesis, energy metabolism, lipid metabolism, and ion homeostasis. Among them, protein folding, DNA repair, and cell redox homeostasis are the three eye-catching ones characterized by the nearly ubiquitous up-regulation of the genes involved in them. All the biological processes are analyzed and discussed in the following sections.
There were 33 genes encoding subunits of DNA-directed RNA polymerases (RNAP) annotated in the genome of Dunaliella salina. Twenty-two of them were differentially expressed, including the largest and catalytic core components of RNAP I, II and III (subunit rpa1, subunit 1, and subunit rpc1 respectively), the second largest components of RNAP II and III (subunit RPB2 and subunit RPC2), components of RNAP II, IV and V (subunit 11 and 12), and other subunits of RNAPs. The chloroplastic/mitochondrial specific RNA polymerase 2 was also included (Supplementary File 1: Figure S1).
Lots of transcription factors (TFs) were differentially expressed, including general transcription factors and gene specific transcription factors. The general TFs included subunits of RNA polymerase II transcription factor B, transcription factor IIH subunit 2, transcription termination factor MTERF9, mediator of RNA polymerase II transcription subunit 6, etc. The gene specific TFs, which regulate specific genes, included ethylene-responsive transcription factor AIL1, transcription factor VIP1, heat stress transcription factor A-1d, leucine zipper transcription factor-like protein 1, etc. (Supplementary File 1: Figure S2). Previous studies show that these TFs participated in variety of biologic processes including response to abiotic stresses (salt, osmotic, and heat stress), DNA repair, cell cycle, lipid accumulation, protein transport, etc. (Table 1).
Besides the TFs involved in regulations of transcription, some genes involved in chromatin remodeling and histone modification were also differentially expressed, including histone-lysine N-methyltransferases, histone acetyltransferases, CHD3-type chromatin-remodeling factor PICKLE, protein chromatin remodeling 20, bromodomain-containing protein 7, etc. The regulation of these genes reflects pre-initiation regulations of transcription which affect the binding of the core transcriptional machinery proteins to the core promoter sequence on the coding region of the DNA.
Some genes involved in post-transcriptional regulations (RNA processing) were also differentially expressed, including pre-mRNA splicing factors, mRNA decapping enzymes, tRNA & rRNA methyltransferases, Zinc phosphodiesterases, Component of the SSU processome, RAP domain-containing protein, etc. These DEGs can be classified by function into groups of pre-mRNA splicing, mRNA decay, and tRNA and rRNA processing.
The GO term of peptide biosynthetic process was significantly enriched by DEGs. Lots of genes encoding ribosomal proteins were up-regulated, including chloroplast, mitochondrial, and cytosolic ribosomal protein genes (Supplementary File 1: Figure S3). Most of the 40S and 60S ribosomal proteins showed up-regulation at 0.5-hour and 1-hour under stress, and return to normal level at 2-hour under stress, on the other side, most of the 30S and 50S ribosomal proteins showed up-regulation at 2-hour of stress (Supplementary File 1: Figure S3). Lots of aminoacyl-tRNA ligases, translation initiation factors, and translation elongation factors were also up-regulated (Supplementary File 1: Figure S4). The up-regulation of these genes possibly reflect the accelerating of protein synthesis. The child term glycoprotein biosynthetic process was also enriched, subunits of dolichyldiphosphooligosaccharide-protein glycosyltransferase, Exostosin-like 2 & 3, and UDP-glucose:glycoprotein glucosyltransferase were up-regulated, which may reflect the regulation of glycoprotein synthesis.
On the other side, the GO term of ubiquitin-dependent protein catabolic process was enriched. Lots of ubiquitin-protein ligases, ubiquitin-conjugating enzymes (Supplementary File 1: Figure S5), and subunits of 26S proteasome were up-regulated (Supplementary File 1: Figure S6). The up-regulation of the genes possibly suggest the acceleration of protein degradation by ubiquitin proteasome system (UPS).
The accelerating of both protein synthesis and ubiquitin-dependent protein degradation possibly reflect the accelerating of protein dynamic change under salt stress.
In cytosol, protein folding starts with the help of a first tier of ribosome-associated chaperones (mainly Hsp70 and Hsp40 which stabilize the nascent polypeptides) which form complex with ribosome called ribosome-associated complex (RAC), then a second tier of components including chaperonins and Hsp90 act downstream in completing the folding process (Vabulas et al., 2010). The ubiquitous up-regulation of Hsp40s (also called chaperone protein DnaJ), Hsp70s, and Hsp90s were observed (Supplementary File 1: Figure S7). The subunits of T-complex protein 1 (the group II chaperonin) and prefoldin were also up-regulated (Supplementary File 1: Figure S7).
In endoplasmic reticulum, protein folding participants include luminal binding proteins (BiPs) which bind to nascent polypeptides to prevent their aggregation, ER-localized DNAJ domain-containing proteins (ERdj proteins) which are BiP cochaperones that assist protein folding, calreticulin (CRT, folding apparatus) which sequester nascent glycoproteins to facilitate folding, UDP-glucose: glycoprotein glucosyltransferase (UGGT) which tells CRT if the glycoprotein needs another round of folding by adding a terminal glucose to the glycoprotein, and protein disulfide isomerases (PDIs) which catalyzes the breaking and reforming of disulfide bonds (Liu and Howell, 2016). These genes were all up-regulated except one ERdj gene (the other ERdj was up-regulated) (Supplementary File 1: Figure S7). Besides the genes mentioned above, there were as much as 37 genes encoding peptidyl-prolyl cis-trans isomoerases, only 3 of them do not showed up-regulation (Supplementary File 1: Figure S8). We speculate that the non-up-regulated genes possibly been up-regulated before 0.5-hour of stress. The expressional profiles of these genes suggest that protein folding was strongly enhanced under salt stress.
Lots of DEGs were enriched in protein modification processes including phosphorylation, glycosylation, ubiquitination, lipidation, dephosphorylation, methylation, alkylation, acetylation, and mannosylation. Among these modifications, protein phosphorylation was the most eye-catching one with the largest number of DEGs involved, accounting for about 60% of the total DEGs of protein modification. A lot of protein kinases enriched in protein phosphorylation, included serine/threonine-protein kinases, cyclin-dependent kinases, calcium-dependent protein kinases, mitogen-activated protein kinases, subunits of cAMP-dependent protein kinases, etc. By GO enrichment analysis, these kinases participate in a variety of cellular biological processes including response to abiotic stress (including salt, osmotic, drought, oxidative, and cold stress), response to DNA damage and lead to cell cycle control, response to unfolded protein, RNA processing, carbohydrate metabolism, photosystem II regulating, microtubule organization, and chloroplast protein import (Table 2). These biological processes, except the response to abiotic stress which is a general expression, represent most of the aspects of this paper that are discussed or will be discussed in the following sections. Upon the whole, the regulation of these kinases suggests that most of the biological processes of Dunaliella salina in response to salt stress are carried out through the way of protein phosphorylation by kinases.
There are two kinds of protein transport, co-translational transport (vesicle-mediated transport) and post-translational transport (non-vesicular transport) (Jungnickel et al., 1994). Compared to post-translational transport, co-translational transport is reported to involve in abiotic stress response (Wang et al., 2020), so we analyze only co-translational transport here. The co-translational transport pathway utilizes the signal recognition particle (SRP) to deliver proteins to the ER membrane while they are still being synthesized by ribosomes (Nyathi et al., 2013). On ER membrane with the help of Sec translocon (Sec61 complex), the nascent polypeptides enter the lumen of ER where they fold to their final conformation (Denks et al., 2014). Then the protein cargos can be transported from ER to Golgi, subsequentially from Golgi to Lysosome, to Plasma membrane, or to out of cell by vesicles. Protein cargos can also be transported from Golgi back to ER, or from Plasma membrane to Lysosome by vesicles (Paez Valencia et al., 2016). During the process, there are proteins and protein machineries work together to create vesicles from donor membranes, to target the vesicles to different destinations, and to fuse vesicles to target membranes (Paez Valencia et al., 2016). In Dunaliella salina, the genes encoding the proteins and the subunits of the machineries that involved in vesicle-mediate transport are summarized in Table 3. The SRP proteins including the SRP54 protein which carries the core function, the alpha and gamma subunits of sec61 translocon, and the subunits of coat proteins including COPI (required for vesicles formation from cis Golgi membrane), COPII (required for vesicles formation from ER membrane), and Clathrin (required for vesicles formation from trans Golgi membrane and plasma membrane), were up-regulated. The adaptor proteins and syntaxins were also up-regulated. The up-regulation of these genes suggests the enhancing of vesicle-mediated protein transport under salt stress. Lots of vacuolar protein sorting-associated proteins (VPS), including components of ESCRT-I, ESCRT-II, and ESCRT-III complex which involved in ubiquitin tagged proteins sorting and delivery to the endosome for degradation, were differently regulated. The SNARE proteins, which drive membrane fusion, were also differently regulated. Studies report that Sec31 (coat protein) (Chung et al., 2016), Sar1a (small GTPase, mediating COPII vesicle formation) (Zeng et al., 2021), Vps23 (component of ESCRT-I) (Yu et al., 2016), LIP5 (accessory protein mediating endosomal sorting) (Wang et al., 2015), and SKD1 (AAA-type ATPase mediating endosomal sorting) (Ho et al., 2010) participated in abiotic stress including drought and salt stress. The homologs of the five genes in Dunaliella salina were also up-regulated, which reflects the conservation of the response mechanism between the algae and Arabidopsis thaliana (Table 3).
When confronting salt stress, the Dunaliella cells decrease their volume quickly due to high extracellular osmotic pressure, and then gradually increase their cell volume by increasing the intracellular glycerol content to balance the osmotic pressure across plasma membrane. The whole process accomplishes in about 2 hours (Ben-Amotz and Avron, 1973). During the process, cellular component organization is a must. GO terms, such as membrane organization, endomembrane system organization, cytoskeleton organization, chromosome organization, protein-containing complex organization, etc., were enriched (Supplementary File 1: Figure S9). Here we only discuss membrane organization, endomembrane system organization, and cytoskeleton organization. The rest GO terms will not be discussed due to the limits of paper length. For membrane organization, it is well-known that autophagy, which was enriched in our study, involves organization of plasma membrane and reported to be induced by salt stress (Liu and Bassham, 2012). For endomembrane system organization, it is known that the vesicle-mediated protein trafficking is carried out by the way of endomembrane system organization as described in the section of protein transport. Besides membrane and endomembrane system organization, the other eye-catching GO term is cytoskeleton organization containing the most DEGs among the child terms of organelle organization ((Supplementary File 1: Figure S9).
When confronting salt stress, Dunaliella cells rapidly shrink, then gradually recover its original volume by intracellular glycerol synthesis to balance the extracellular osmotic pressure (Ben-Amotz and Avron, 1973; Baek et al., 2011). The whole process finished in about 2 hours. Studies report that Dunaliella cells grown in 4 M NaCl contain about 8 M glycerol (Chitlaru and Pick, 1989; Oren, 2005). These studies indicate that rapid high-yield biosynthesis of glycerol is a special mechanism of Dunaliella to tolerate salt stress. In the last century, scientists suggested that the reserve starch pool is a carbon source of glycerol synthesis (Ben-Amotz and Avron, 1973), and proposed the glycerol cycle pathway which suggested that glycerol can be synthesized from and converted to DHAP (dihydroxyacetone phosphate or glycerone-phosphate) which is an intermediate metabolite of glycolysis (Haus and Wegmann, 1984) However a complete synthesis pathway from starch to glycerol was absent, here we propose a pathway from starch to glycerol based on our genomic data and the published papers (He et al., 2020b), the input of energy and reducing equivalents are also indicated (Figure 1A). The expressions of the enzymes were all up-regulated, the key enzymes are PYG, PFK, and the di-domain GPDH, which catalyze the three irreversible reactions.
Figure 1 Glycerol synthesis & energy metabolism. (A) the glycerol synthesis pathway from starch to glycerol, the enzymes on the pathway are shown by rectangles, the up-regulated enzymes are indicated by light red background, the two red dotted boxes indicate the two key genes of pentose phosphate pathway confirmed by real-time PCR; (B) the possible sources (rounded rectangles) of ATP and reducing equivalents (NADH or NADPH) for glycerol synthesis, the dotted arrows indicate the ATP and reducing equivalents could be used for glycerol synthesis, the red dotted box indicates the gene confirmed by real-time PCR.
Study also showed that photosynthesis is also a carbon source of glycerol synthesis (Goyal, 2007). In our study, the up-regulated genes enriched in the term photosynthesis included photosystem I reaction center subunits, photosystem II reaction center proteins, chlorophyII a-b binding proteins, oxygen-evolving enhancer proteins, PsbP domain-containing proteins, thylakoid luminal proteins, etc. We can see most of the genes showed a quick up-regulation of expression at 0.5 and 1-hour under stress followed by a down-regulation of expression at 2-hour under stress (Supplementary File 1: Figure S10). The expressional profiles of these genes suggest that photosynthesis was transiently up-regulated by salt stress.
Study report that photosynthesis and reserved starch are the carbon source of glycerol synthesis, and reserved starch is the main source (Goyal, 2007). From the glycerol synthesis pathway that we proposed, synthesis of one molecule of glycerol needs 0.5 molecule of ATP and one molecule of NADH or NADPH (reducing equivalents) (Figure 1A). So, synthesis of large amount of glycerol needs large amount of ATP and reducing equivalents. Where do these ATP and reducing equivalents come from the GO enrichment analysis, glycolysis, TCA cycle and oxidative phosphorylation, which can generate ATP or NADH or both of them, were enriched. It is worth noting that pentose phosphate pathway (PPP) was also enriched. Glucose-6-phosphate dehydrogenase, the rate-controlling enzyme of PPP, was significantly up-regulated (Stryer and Co, 1995). 6-phosphogluconate dehydrogenase, the second NADPH producing enzyme in PPP, was also significantly up-regulated. Since generation of NADPH is one of the outcomes of PPP, the up-regulation of PPP could be a source of reducing equivalents for glycerol synthesis. From the starch catabolism pathway (Figure 1A), the carbon flux branches at the point of fructose 1, 6-biphosphate, some carbon goes to glycerol synthesis, the other goes to TCA cycle and oxidative phosphorylation to generate NADH and ATP which can be used for glycerol synthesis. Taking together, it seems that the large amount of ATP and reducing equivalents needed for glycerol synthesis could be provided by starch catabolism through glycolysis, pentose phosphate pathway, TCA cycle and oxidative phosphorylation (Figure 1B).
Efficient flux of electrons is vital for photosynthesis and respiration of plant cells. Efficient flux of electrons also means that the oxidized and reduced forms of electron carriers in electron transport chains must be balanced, which is called redox homeostasis (Foyer and Noctor, 2005). However, salt stress can disrupt the balance and induce the rise of reactive oxygen species (ROS) and lead to oxidative stress. To survive, organisms need to regain cell redox homeostasis by getting the excessive ROS reduced by small molecule antioxidants, such as ascorbate, glutathione (GSH), and tocopherol. The genes involved in regeneration of these antioxidants, such as monodehydroascorbate reductase and glutathione reductase, were mostly up-regulated at 0.5-hour under stress (Supplementary File 1: Figure S11). Another type of antioxidants is antioxidative proteins, such as thioredoxins, glutaredoxins, and peroxiredoxins. Most of the genes encoding antioxidative proteins were up-regulated at 0.5-hour under stress (Supplementary File 1: Figure S11). The genes involved in regeneration of these antioxidative proteins, such as thioredoxin reductase and methionine sulfoxide reductase, were also up-regulated at 0.5-hour under stress. Taken together, the genes involved in cell redox homeostasis responded quickly and strongly to salt stress by up-regulation of their expressions.
Salt stress induces the rise of ROS. ROS have strong oxidizing potential and lead to DNA damage. When DNA damage happens, cells stop cell cycle and activate DNA repair mechanisms to repair DNA. If DNA repairs are successful, cell cycle is reactivated. On the contrary, if the repairs fail and DNA lesions accumulate, cells undergo apoptosis (Hu et al., 2016) From this perspective, the strength of ability of DNA repair influences the degree of salt tolerance of an organism. Although we know that Dunaliella salina is extremely salt tolerant, it was still astonishing to see that so many genes involved in DNA repair were up-regulated by salt stress. The DEGs included DNA repair proteins, DNA repair ligases, DNA repair helicases, endonucleases, glycosylases, DNA polymerases, replication factor C subunits, recombinases, and DNA methyltransferases, etc. Most of these genes were up-regulated at 0.5-hour and 1-hour under stress (Supplementary File 1: Figure S12).
In general, the up-regulation of the key genes in the biosynthetic pathways indicates the acceleration of de novo fatty acid synthesis and TAG synthesis under salt stress. The up-regulation of the desaturases indicates that the fatty acids and lipids undergo desaturation.
The chloroplastic acetyl-CoA carboxylase (heteromeric ACC) catalyzing the irreversible carboxylation of acetyl-CoA to produce malonyl-CoA, which is involved in de novo fatty acid synthesis in plants (Konishi et al., 1996), is composed of four independent polypeptides: biotin carboxyl carrier protein (BCCP), biotin carboxylase (BC), α carboxyl transferase, and β carboxyl transferase. The four subunits were all significantly up-regulated at 0.5-hour and 1-hour under salt stress. The cytosolic acetyl-CoA carboxylase (homomeric ACC) was also significantly up-regulated. The malonyl-CoA-ACP transacylase (FabD) and the other subunits of type II fatty acid synthase (FAS) including 3-ketoacyl-ACP synthase II and III (KAS II or FabF and KAS III or FabH), 3-oxoacyl-ACP reductase (FabG), 3-hydroxyacyl-ACP dehydratase (FabZ), and enoyl-ACP reductase (FabI), were all significantly up-regulated at 0.5-hour and 1-hour under salt stress (Figure 2). The expression of the biotin carboxylase and cytosolic acetyl-CoA carboxylase were confirmed by real-time PCR (Figure 3; Supplementary File 2). The up-regulation of these genes reflects the acceleration of de novo fatty acid synthesis under salt stress. Acyl-protein thioesterases and long chain acyl-CoA synthetases were also up-regulated, which possibly suggest the synthesized acyl-CoA could be used for lipid synthesis (Ohlrogge and Browse, 1995).
Figure 2 Fatty acid biosynthesis. The pathway of fatty acid biosynthesis from acetyl-CoA to fatty acids are shown in the figure. Enzymes catalyzing the reactions on the pathway are shown by rectangles. The enzymes were all up-regulated as indicated by light red background. The two red dotted boxes indicate the two key genes (biotin carboxylase and acetyl-CoA carboxylase) confirmed by real-time PCR. The full name of the enzymes are acetyl-CoA carboxylase (ACC), malonyl-CoA-ACP transacylase (FabD), 3-ketoacyl-ACP synthase II and III (FabF and FabH), 3-oxoacyl-ACP reductase (FabG), 3-hydroxyacyl-ACP dehydratase (FabZ), and enoyl-ACP reductase (FabI), acyl-protein thioesterase, and long chain acyl-CoA synthetase (LACS).
Figure 3 Heatmap of the expressions from real-time PCR of the selected key genes in the biological processes. The colors from blue to red represent the gene expression values from low to high. Values of log2 (Fold change) are used to generate the heatmap. The significance is indicated by asterisks in the heatmap (* P<0.05,** P< 0.01, *** P< 0.001,**** P < 0.0001).
Glycerol-3-phosphate acyltransferase (EC: 2.3.1.15) and 1-acylglycerol-3-phosphate O-acyltransferase (EC: 2.3.1.51) were up-regulated at 0.5-hour under stress, which possibly suggest the acceleration of phosphatidic acid (PA) synthesis. The up-regulation of phosphatidate phosphatase (EC: 3.1.3.4) was detected at 10-min under stress by qPCR (Figure 3; Supplementary File 2). The up-regulation of the chloroplastic lipid phosphate phosphatase 3, which may exhibit phosphatidate phosphatase activity (Pierrugues et al., 2001; Nakamura et al., 2007), was also observed at 2-hour under stress. The expression of diacylglycerol O-acyltransferases (EC: 2.3.1.20) and phospholipid:diacylglycerol acyltransferase (EC: 2.3.1.158) were up-regulated at 10-min and 30-min under stress (Figures 3, 4). Taken together, the expression profiles of these genes possibly suggest the acceleration of triacylglycerol (TAG) synthesis under stress.
Figure 4 Simplified triacylglycerol biosynthesis pathway. The up-regulated enzymes catalyzing the reactions on the pathway are indicated by rectangles with light red background, the red dotted boxes indicate the genes confirmed by real-time PCR. The enzymes are glycerol-3-phosphate acyltransferase [2.3.1.15], 1-acylglycerol-3-phosphate O-acyltransferase [2.3.1.51], phosphatidate phosphatase [3.1.3.4], diacylglycerol O-acyltransferases [2.3.1.20], phospholipid:diacylglycerol acyltransferase [2.3.1.158], and phosphatidate cytidylyltransferases [2.7.7.41].
Many desaturases were up-regulated under salt stress, including stearoyl-[acyl-carrier-protein] 9-desaturase (EC: 1.14.19.2), acyl-lipid omega-3 desaturase (chloroplastic, EC: 1.14.19.25), Delta12 fatty acid desaturase (EC: 1.14.19.6), Delta7-sterol 5(6)-desaturase (EC: 1.14.19.20), Palmitoyl-monogalactosyldiacylglycerol delta-7 desaturase (chloroplastic, EC: 1.14.19.42), Acyl-lipid (7-3)-desaturase (chloroplastic, EC: 1.14.19.31), and acyl-lipid omega-3 desaturase (chloroplastic, EC: 1.14.19.25) (Supplementary File 1: Figure S13). Upon the whole, the up-regulation of these desaturases suggest the desaturation of lipids under salt stress.
Studies report that Na+/H+ antiporters (or exchangers) located on plasma membrane are responsible for sodium ion extrusion, while Na+/H+ exchangers located on vacuolar membrane are for sodium ion sequestration in vacuole (Zhu, 2003). There is one gene, named as DsaChr110059 and showing sequence similarity with Na+/H+ antiporter 7 of Arabidopsis thaliana (SOS1) which was reported to be responsible for Na+ and Li+ extrusion across plasma membrane (Wu et al., 1996; Shi et al., 2000), was significantly up-regulated at 0.5-hour and 1-hour under stress (Figure 3; Supplementary File 2). There are two genes, named as DsaChr121177 and DsaChr160120, that show sequence similarities with Na+/H+ exchanger 2 and 6 of Arabidopsis thaliana, respectively, which are reported to be involved in vacuolar sodium ion compartmentalization (Yokoi et al., 2002; Bassil et al., 2011). Real-time PCR show that DsaChr160120 was up-regulated at 10-min under stress, but DsaChr121177 was not up-regulated (Figure 3; Supplementary File 2).
Studies also report that plasma membrane-type and vacuolar-type proton ATPase are responsible for creating proton gradient across plasma membrane and vacuolar membrane respectively, which could benefit Na+ efflux and sequestration by Na+/H+ antiporters and Na+/H+ exchangers (Yang and Guo, 2018). There are two genes, named as DsaChr060031 and DsaChr080167, which show sequence similarities with plasma membrane ATPase 1 of Arabidopsis thaliana and plasma membrane ATPase of Dunaliella bioculata, respectively. DsaChr060031 showed up-regulation at 10-min and 30-min under stress, but DsaChr080167 didn’t show up-regulation (Figure 3; Supplementary File 2). There are 13 genes encoding subunits of vacuolar-type proton ATPase, 5 of them showed significant up-regulation. Besides the vacuolar-type proton ATPases, a gene encoding pyrophosphate-energized vacuolar membrane proton pump which also contributes to the proton gradient across vacuolar membrane showed up-regulation (Segami et al., 2018). The up-regulation of the vacuolar-type proton ATPases and the pyrophosphate-energized vacuolar membrane proton pump would lead to acidation of the vacuoles and benefit for Na+ sequestration.
Taken together, We did saw the up-regulation of a plasma membrane-type Na+/H+ antiporter (DsaChr110059), which shows sequence similarity with the well-studied SOS1 of Arabidopsis thaliana which was the main player of Na+ extrusion under salt stress (Zhu, 2003). We also saw the up-regulation of a vacuolar-type Na+/H+ exchanger (DsaChr160120) together with the up-regulation of some of the subunits of vacuolar-type proton ATPase and the up-regulation of a pyrophosphate-energized vacuolar membrane proton pump.
This research gives a more comprehensive overview of how Dunaliella cells response to salt stress than the previous published papers do (Li et al., 2019; Lv et al., 2021; Panahi and Hejazi, 2021) due to high quality genomic data of this algae and appropriated stress time course setting. From the point of evolution, all plant cells, including higher plants, lower plants, and green algae, have similar cellular structure and respond to environmental stress similarly (Chapin, 1991), so this is also an overview of how photosynthetic cells response to salt stress. Although this overview is only at transcriptomic level, there are published papers to support most of the biological processes being discussed in this research, which also suggests that the regulations of the biological processes are conserved mechanisms of plants in response to salt stress.
The basic way of a plant cell to response to environmental changes is the regulation of gene expression. Besides the expressional regulations of the core components of transcription machineries, lots of the TFs were regulated in response to salt stress, many of them were reported to participate in abiotic stress response (Table 1). Moreover, the regulations at pre-initiation of transcription and post-transcriptional levels were also indicated by the DEGs, some of the DEGs were reported to be involved in salt tolerance of Arabidopsis thaliana, such as histone-lysine N-methyltransferase, histone acetyltransferase GCN5, and pre-mRNA splicing factors (Baek et al., 2011; Zheng et al., 2019).
Gene expression includes not only the transcription process, but also the translation process. The functional enrichment analysis of the DEGs indicates that the protein synthesis process was enhanced. This is reasonable because cells need to synthesize new proteins to adapt to salt stress. There are studies reported the association of glycoprotein with salt tolerance in Arabidopsis (Frank et al., 2008; Kang et al., 2008; Nagashima et al., 2018). What is interesting is that protein degradation was also enriched. In the last century, scientists found that mammal cells exhibit increased rates of proteolysis following exposure to oxidative stress (Pacifici et al., 1989; Grune et al., 1997)and proposed that ubiquitin proteasome system (UPS) is a major way for protein degradation (Hershko and Ciechanover, 1992). In plants, scientists found many homologs of mammal UPS, and suggested that UPS is also a major way for protein degradation (Smalle and Vierstra, 2004), however published works that report protein degradation induced by abiotic stress are rare (Ferguson et al., 1990; Neelam and Subramanyam, 2013). Here our data show that lots of ubiquitin-protein ligases, ubiquitin-conjugating enzymes, and subunits of 26S proteasome were up-regulated under salt stress, which indicate that UPS plays an important role in protein degradation under salt stress.
The protein folding process accompanies the translation process. Salt stress disrupts protein folding in ER and lead to ER stress. ER stress activates signal transduction pathway to up-regulate the expression of genes that aid in protein folding, such as chaperones, peptidyl prolyl isomerases, prefoldins, etc. (Liu and Howell, 2016). We saw the nearly ubiquitous up-regulation of the genes involved in protein folding, which indicates the process was strongly enhanced. However, reports of genetic engineering of protein folding to increase salt tolerance are rare (Wang et al., 2004). There are lots of molecular chaperones and enzymes that aid in protein folding, it is not likely to randomly choose a target that will lead to significant improvement of salt tolerance.
In eukaryotic cells, many newly synthesized proteins go to the post-translational modification process to become functional forms. There are two kinds of protein modifications that obviously related to salt stress response, one is ubiquitination, as mentioned above, the misfolded or damaged proteins must be attached the tag of ubiquitin before degradation. The other is protein phosphorylation, protein phosphorylation usually plays important role in signal transduction of stress sensing and response. To modulating signal transduction of salt stress response is a hopeful way to increase salt tolerance by changing phosphorylation state of key component of signal cascade. For example, the activity of Na+/H+ antiporter (SOS1) in salt overly sensitive (SOS) pathway is control by phosphorylation (Shi et al., 2000; Dittmore et al., 2016). Phosphorylation can activates SOS1 and might increase Na+ exclusion and lead to improvement of salt tolerance. Besides ubiquitination and phosphorylation, reactive oxygen species (ROS) and reactive nitrogen species (RNS) were reported to regulate ion transporters by protein modifications. These modifications include cysteine oxidation, methionine oxidation, cysteine S-nitrosylation, and tyrosine nitration (Nieves-Cordones et al., 2019; Sandalio et al., 2023). Salt stress can induce the rise of ROS and probably RNS, which could lead to oxidation or nitration of the residues of ion transporters and regulate their activities. Garcia-Mata et al. (Garcia-Mata et al., 2010) demonstrated that the residue Cys168 of SKOR K+ Channel was essential for sensitivity to H2O2. However more experiments on other transporters at protein molecular or structural level are still needed to show how ROS and RNS regulate the activities of transporters through residues oxidation or nitration in response to salt stress.
Since proteins are synthesized far away from their functional sites, protein transport is a fundamental cellular process that can be regulated in response to environmental changes. The vesicle-mediated protein transport involves ER as the very important departure station of protein cargos. Salt stress disrupt protein folding in ER, leading to accumulation of unfolded or misfolded proteins in ER which is also called ER stress (Liu et al., 2010). ER needs to activate signal transduction pathway to up-regulate the expression of genes that aid in protein folding, such as chaperones, and to send the unfolded proteins cargos to degradation. This is how salt stress intersects protein transport. By loss of function mutation techniques, researchers identified some participators of vesicle-mediated protein transport involved in salt stress response. Loss of function mutants were sensitive to salt stress compare to wild type plants (Wang et al., 2020). Although we know that protein transport is tightly linked to salt stress response, the underlying mechanisms are largely unknown.
Cellular component organization plays an essential role in a variety of biological processes, such as vesicle-mediated protein transport, cytoskeleton dynamics, autophagy, etc. Autophagy is a bulk degradation pathway that is essential for cell survival under nutrient-limiting conditions (Levine and Klionsky, 2004). Later scientists found that autophagy helps maintain cellular homeostasis under oxidative stress by degradation and recycling of the oxidized proteins and other cellular components (Bassham, 2007). Studies showed directly that salt stress induced autophagy of Arabidopsis within 30 min and mutants defective in autophagy were sensitive to salt stress (Leshem et al., 2007; Luo et al., 2017). These studies indicate that autophagy plays an important role in plant response to salt stress. Leshem et al. also reported that phosphoinositide signaling pathway is involved in autophagy under salt stress (Leshem et al., 2007). Considering that phosphoinositide signaling is also involved in the organization of cytoskeleton (Yin and Janmey, 2003) and the regulation of vesicle trafficking (Roth, 2004), we speculate that these biological processes might be coordinated by phosphoinositide signaling pathway in response to salt stress. However more work needed to elucidate how they can be regulated.
Salt stress induces rise of ROS and leads to disruption of redox homeostasis which is vital for proper cellular function. To survive, cells need to regain redox homeostasis by removing the excessive ROS. We saw most of the genes involved in cellular redox homeostasis were up-regulated at 0.5-hour under stress and last to 2-hour under stress, which indicates that the process was quickly and strongly enhanced. The quick and strong type of response of these genes also reflects the strong ability of Dunaliella salina to maintain cell redox homeostasis under salt stress. Overexpression of the genes encoding antioxidative enzymes led to improved salt tolerance (Agarwal et al., 2013; Wang et al., 2020), which indicates the importance of redox homeostasis in response to salt stress.
The rise in ROS also brings another serious consequence that is DNA damage. When DNA damage happens, cells activate DNA repair mechanisms to repair DNA (Hu et al., 2016). We saw that most of the genes involved in DNA repair were up-regulated at 0.5-hour and 1-hour under stress, which reflects the quick and strong response of Dunaliella salina to maintain DNA correctness under salt stress. Dunaliella salina can survive in medium containing NaCl as high as 5.5 M, so it is unexpected that salt stress of 2.5 M NaCl would cause such a strong response. On the other side, the strong response of DNA repair possibly reflects the high efficiency of DNA repair, which may be a major reason for the algae to survive in hypersaline environment.
There are studies reported that salt stress induced synthesis of fatty acid and total lipids in some microalgae (Takagi et al., 2006; El Arroussi et al., 2015; Pandit et al., 2017), and desaturation of fatty acids were reported in a few species(López-Pérez et al., 2009; Harrathi et al., 2011; Sui and Han, 2014). In our study, we see the up-regulation of the key enzymes ACCs (both the chloroplastic form and the cytosolic form) of the de novo fatty acid synthesis pathway and the enzymes in TAG synthesis pathway, which indicates the synthesis of fatty acids and TAG. The TAG contents were indeed increased (our unpublished data) under salt stress. We also see the up-regulation of the fatty acids desaturases, which indicates the lipids undergo desaturation under salt stress. Our data are in consistent with the previous studies. Moreover, Overexpression of desA encoding Δ12 acyl-lipid desaturase in Synechococcus led to improved salt tolerance(Allakhverdiev et al., 2001). Although these studies indicate that lipid metabolism is relevant to salt stress response, the underlying mechanism is unclear. Scientists speculate that unsaturated fatty acids can act as modulators of cellular membrane to increasing the fluidity of membrane under salt stress (He and Ding, 2020), and they also suggested that glycerolipids can work as carbon and energy reservoir under stress (He and Ding, 2020).
Besides the above discussed aspects, salt stress has two direct impacts on plant cells, one is that high salt content can alter the osmotic potential across plasma membrane and results in dehydration of plasma. The rapid high-yield biosynthesis of glycerol is a special mechanism of Dunaliella to cope with osmotic stress (Chitlaru and Pick, 1989; Oren, 2005). Based on our data, we propose a pathway from starch to glycerol, and suggest that PYG, PFK, and the di-domain GPDH are the three rate-limiting enzymes of the glycerol synthesis pathway. Our published paper showed that the di-domain GPDH can convert DHAP directly to glycerol whereas a separate phosphatase protein is required for this conversion process in most organisms, and the homotetramer structure of di-domain GPDH likely contributes to the rapid biosynthesis of glycerol (He et al., 2020b).
The other impact is that under high salt content, Na+ enters cell more easily, which will disrupt the intrinsic homeostasis of high concentration of K+ and low concentration of Na+, which is vital for diverse cellular processes. We find in Dunaliella the up-regulation of the homolog of SOS1 of Arabidopsis which is the main player of Na+ extrusion under salt stress (Zhu, 2003). We also find the up-regulation of a vacuolar-type Na+/H+ exchanger, some of the subunits of vacuolar-type proton ATPase, and a pyrophosphate-energized vacuolar membrane proton pump. The up-regulation of these genes is consistent with the expressions of their homologs in Arabidopsis thaliana under salt stress (Wu et al., 1996; Shi et al., 2000; Yokoi et al., 2002), which might suggest that these genes are responsible for ion homeostasis.
Finally, what light on the expression profile is the nearly ubiquitous up-regulation of the genes involved in protein folding, DNA repair, and cell redox homeostasis, which suggests that the three biological processes were strongly enhanced under salt stress, in reverse, it also implicates that we can improve plants salt tolerance by enhancing the activities of the three biological processes. Modulating the signal transduction pathways that control the three biological processes might be a promising way to improve salt tolerance. Considering that salt tolerance is a high energy and resource costing process, fine tuning is very important to achieve the balance between growth rate and degree of salt tolerance.
The major cellular biological processes that being regulated in response to salt stress, are shown in Figure 5. The GO term of transcription was enriched, the regulation of transcription occurred at pre-initiation, initiation, and post transcription level; protein synthesis and protein degradation were enhanced at the same time, which implicates the acceleration of protein turnover; protein modification was enriched included a lot of protein kinases, which implicates protein phosphorylation plays an important role in response to salt stress; protein transport was enriched, the vesicle-mediated protein transport was enhanced; cellular component organization, which plays an essential role in vesicle-mediated protein transport, cytoskeleton dynamics, autophagy, was enriched; glycerol synthesis was enhanced, the energy for glycerol synthesis might be provided by starch catabolism through glycolysis, pentose phosphate pathway, TCA cycle and oxidative phosphorylation; the synthesis of fatty acids and TAG were enhanced, while desaturation of lipids was also enhanced; the up-regulation of the plasma membrane-type Na+/H+ antiporter and the vacuolar-type Na+/H+ exchanger together with the vacuolar-type proton ATPase and the pyrophosphate-energized vacuolar membrane proton pump might aid in ion homeostasis; protein folding, cell redox homeostasis, and DNA repair were greatly enhanced as indicated by the nearly ubiquitous up-regulation of the genes involved in the three biological processes, which may confer the algae important mechanisms to survive under salt stress, also implicates that enhancing the activities of the three biological processes are promising strategies to improve crop salt tolerance.
Figure 5 The major biological processes being regulated under salt stress. The biological processes include transcription, protein synthesis, protein degradation, protein folding, protein modification, protein transport, cellular component organization, cell redox homeostasis, DNA repair, glycerol synthesis, energy metabolism, lipid metabolism, and ion homeostasis.
The datasets presented in this study can be found in online repositories. The names of the repository/repositories and accession number(s) can be found below: Sequence Read Archive under accession number SRR8552788, and SRR8543799 to SRR8543810. The genome data is deposited in the Genbank under accession number JAVFKU000000000.
BZ: Data curation, Formal Analysis, Validation, Writing – review & editing. CD: Data curation, Formal Analysis, Validation, Writing – review & editing. SW: Formal Analysis, Investigation, Writing – review & editing. QD: Formal Analysis, Investigation, Writing – review & editing. YC: Formal Analysis, Validation, Writing – review & editing. ZB: Formal Analysis, Validation, Writing – review & editing. AH: Data curation, Formal Analysis, Validation, Writing – review & editing. QZ: Conceptualization, Supervision, Writing – review & editing, Writing – original draft. QH: Conceptualization, Funding acquisition, Methodology, Resources, Supervision, Writing – original draft, Writing – review & editing, Project administration.
The author(s) declare financial support was received for the research, authorship, and/or publication of this article. Funds was provided by Natural Science Foundation of Sichuan province (2022NSFSC0244).
The authors are grateful to Southwest Minzu University for providing the necessary facilities and support. The authors also would like to express their gratitude for the funding provided by Natural Science Foundation of Sichuan province and Innovation funds for postgraduates, Southwest Minzu University.
The authors declare that the research was conducted in the absence of any commercial or financial relationships that could be construed as a potential conflict of interest.
All claims expressed in this article are solely those of the authors and do not necessarily represent those of their affiliated organizations, or those of the publisher, the editors and the reviewers. Any product that may be evaluated in this article, or claim that may be made by its manufacturer, is not guaranteed or endorsed by the publisher.
The Supplementary Material for this article can be found online at: https://www.frontiersin.org/articles/10.3389/fpls.2023.1278954/full#supplementary-material
Agarwal, P. K., Shukla, P. S., Gupta, K., Jha, B. (2013). Bioengineering for salinity tolerance in plants: state of the art. Mol. Biotechnol. 54 (1), 102–123. doi: 10.1007/s12033-012-9538-3
Allakhverdiev, S. I., Kinoshita, M., Inaba, M., Suzuki, I., Murata, N. (2001). Unsaturated fatty acids in membrane lipids protect the photosynthetic machinery against salt-induced damage in Synechococcus. Plant Physiol. 125 (4), 1842–1853. doi: 10.1104/pp.125.4.1842
Anders, S., Huber, W. (2010). Differential expression analysis for sequence count data. Genome Biol. 11 (10), R106. doi: 10.1186/gb-2010-11-10-r106
Anders, S., Pyl, P. T., Huber, W. (2015). HTSeq–a Python framework to work with high-throughput sequencing data. Bioinformatics 31 (2), 166–169. doi: 10.1093/bioinformatics/btu638
Baek, D., Jiang, J., Chung, J. S., Wang, B., Chen, J., Xin, Z., et al. (2011). Regulated AtHKT1 gene expression by a distal enhancer element and DNA methylation in the promoter plays an important role in salt tolerance. Plant Cell Physiol. 52 (1), 149–161. doi: 10.1093/pcp/pcq182
Bassham, D. C. (2007). Plant autophagy–more than a starvation response. Curr. Opin. Plant Biol. 10 (6), 587–593. doi: 10.1016/j.pbi.2007.06.006
Bassil, E., Ohto, M. A., Esumi, T., Tajima, H., Zhu, Z., Cagnac, O., et al. (2011). The Arabidopsis intracellular Na+/H+ antiporters NHX5 and NHX6 are endosome associated and necessary for plant growth and development. Plant Cell 23 (1), 224–239. doi: 10.1105/tpc.110.079426
Ben-Amotz, A., Avron, M. (1973). The role of glycerol in the osmotic regulation of the halophilic alga dunaliella parva. Plant Physiol. 51 (5), 875–878. doi: 10.1104/pp.51.5.875
Chapin, F. S., III (1991). Integrated Responses of Plants to Stress: A centralized system of physiological responses. BioScience 41 (1), 29–36. doi: 10.2307/1311538
Chitlaru, E., Pick, U. (1989). Selection and characterization of dunaliella salina mutants defective in haloadaptation. Plant Physiol. 91 (2), 788–794. doi: 10.1104/pp.91.2.788
Chung, K. P., Zeng, Y., Jiang, L. (2016). COPII paralogs in plants: functional redundancy or diversity? Trends Plant Sci. 21 (9), 758–769. doi: 10.1016/j.tplants.2016.05.010
Conesa, A., Götz, S., García-Gómez, J. M., Terol, J., Talón, M., Robles, M. (2005). Blast2GO: a universal tool for annotation, visualization and analysis in functional genomics research. Bioinformatics 21 (18), 3674–3676. doi: 10.1093/bioinformatics/bti610
Denks, K., Vogt, A., Sachelaru, I., Petriman, N. A., Kudva, R., Koch, H. G. (2014). The Sec translocon mediated protein transport in prokaryotes and eukaryotes. Mol. Membr Biol. 31 (2-3), 58–84. doi: 10.3109/09687688.2014.907455
Dittmore, A., Silver, J., Sarkar, S. K., Marmer, B., Neuman, K. C. (2016). Internal strain drives spontaneous periodic buckling in collagen and regulates remodeling. Proc. Natl. Acad. Sci. U.S.A. 113 (30), 8436–8441. doi: 10.1073/pnas.1523228113
El Arroussi, H., Benhima, R., Bennis, I., El Mernissi, N., Wahby, I. (2015). Improvement of the potential of Dunaliella tertiolecta as a source of biodiesel by auxin treatment coupled to salt stress. Renewable Energy 77, 15–19. doi: 10.1016/j.renene.2014.12.010
Ferguson, D. L., Guikema, J. A., Paulsen, G. M. (1990). Ubiquitin pool modulation and protein degradation in wheat roots during high temperature stress 1. Plant Physiol. 92 (3), 740–746. doi: 10.1104/pp.92.3.740
Foyer, C. H., Noctor, G. (2005). Redox homeostasis and antioxidant signaling: a metabolic interface between stress perception and physiological responses. Plant Cell 17 (7), 1866–1875. doi: 10.1105/tpc.105.033589
Frank, J., Kaulfürst-Soboll, H., Rips, S., Koiwa, H., von Schaewen, A. (2008). Comparative analyses of Arabidopsis complex glycan1 mutants and genetic interaction with staurosporin and temperature sensitive3a. Plant Physiol. 148 (3), 1354–1367. doi: 10.1104/pp.108.127027
Gao, F., Nan, F., Feng, J., Lü, J., Liu, Q., Liu, X., et al. (2021). Transcriptome profile of Dunaliella salina in Yuncheng Salt Lake reveals salt-stress-related genes under different salinity stresses. J. Oceanology Limnology 39 (6), 2336–2362. doi: 10.1007/s00343-021-0164-4
Garcia-Mata, C., Wang J Fau - Gajdanowicz, P., Gajdanowicz P Fau - Gonzalez, W., Gonzalez W Fau - Hills, A., Hills A Fau - Donald, N., Donald N Fau - Riedelsberger, J., et al. (2010). A minimal cysteine motif required to activate the SKOR K+ channel of Arabidopsis by the reactive oxygen species H2O2. J. Biol. Chem. 285 (38), 29286–29294. doi: 10.1074/jbc.M110.141176
Goyal, A. (2007). Osmoregulation in Dunaliella, Part I: Effects of osmotic stress on photosynthesis, dark respiration and glycerol metabolism in Dunaliella tertiolecta and its salt-sensitive mutant (HL 25/8). Plant Physiol. Biochem. 45 (9), 696–704. doi: 10.1016/j.plaphy.2007.05.008
Grune, T., Reinheckel, T., Davies, K. J. A. (1997). Degradation of oxidized proteins in mammalian cells. FASEB J. 11 (7), 526–534. doi: 10.1096/fasebj.11.7.9212076
Harrathi, J., Hosni, K., Karray-Bouraoui, N., Attia, H., Marzouk, B., Magné, C., et al. (2011). Effect of salt stress on growth, fatty acids and essential oils in safflower (Carthamus tinctorius L.). Acta Physiologiae Plantarum 34 (1), 129–137. doi: 10.1007/s11738-011-0811-z
Haus, M., Wegmann, K. (1984). Glycerol-3-phosphate dehydrogenase (EC 1.1.1.8) from Dunaliella tertiolecta. I. Purification and kinetic properties. Physiologia Plantarum 60 (3), 283–288. doi: 10.1111/j.1399-3054.1984.tb06063.x
He, M., Ding, N.-Z. (2020). Plant unsaturated fatty acids: multiple roles in stress response. Front. Plant Sci. 11. doi: 10.3389/fpls.2020.562785
He, Q., Lin, Y., Tan, H., Zhou, Y., Wen, Y., Gan, J., et al. (2020a). Transcriptomic profiles of Dunaliella salina in response to hypersaline stress. BMC Genomics 21 (1), 115. doi: 10.1186/s12864-020-6507-2
He, Q., Toh, J. D., Ero, R., Qiao, Z., Kumar, V., Serra, A., et al. (2020b). The unusual di-domain structure of Dunaliella salina glycerol-3-phosphate dehydrogenase enables direct conversion of dihydroxyacetone phosphate to glycerol. Plant J. 102 (1), 153–164. doi: 10.1111/tpj.14619
Hershko, A., Ciechanover, A. (1992). The ubiquitin system for protein degradation. 61 (1), 761–807. doi: 10.1146/annurev.bi.61.070192.003553
Ho, L.-W., Yang, T., Shieh, S.-S., Edwards, G. E., Yen, H. E. (2010). Reduced expression of a vesicle trafficking-related ATPase SKD1 decreases salt tolerance in Arabidopsis. Funct. Plant Biol. 37 (10), 962–973. doi: 10.1071/Fp10049
Hu, Z., Cools, T., De Veylder, L. (2016). Mechanisms used by plants to cope with DNA damage. Annu. Rev. Plant Biol. 67 (1), 439–462. doi: 10.1146/annurev-arplant-043015-111902
Jungnickel, B., Rapoport, T. A., Hartmann, E. (1994). Protein translocation: common themes from bacteria to man. FEBS Lett. 346 (1), 73–77. doi: 10.1016/0014-5793(94)00367-x
Kang, J. S., Frank, J., Kang, C. H., Kajiura, H., Vikram, M., Ueda, A., et al. (2008). Salt tolerance of Arabidopsis thaliana requires maturation of N-glycosylated proteins in the Golgi apparatus. Proc. Natl. Acad. Sci. U.S.A. 105 (15), 5933–5938. doi: 10.1073/pnas.0800237105
Kang, W. H., Sim, Y. M., Koo, N., Nam, J. Y., Lee, J., Kim, N., et al. (2020). Transcriptome profiling of abiotic responses to heat, cold, salt, and osmotic stress of Capsicum annuum L. Sci. Data 7 (1), 17. doi: 10.1038/s41597-020-0352-7
Konishi, T., Shinohara, K., Yamada, K., Sasaki, Y. (1996). Acetyl-CoA carboxylase in higher plants: most plants other than gramineae have both the prokaryotic and the eukaryotic forms of this enzyme. Plant Cell Physiol. 37 (2), 117–122. doi: 10.1093/oxfordjournals.pcp.a028920
Kowalski, S. P., Lan, T. H., Feldmann, K. A., Paterson, A. H. (1994). Comparative mapping of Arabidopsis thaliana and Brassica oleracea chromosomes reveals islands of conserved organization. Genetics 138 (2), 499–510. doi: 10.1093/genetics/138.2.499
Leshem, Y., Seri L Fau - Levine, A., Levine, A. (2007). Induction of phosphatidylinositol 3-kinase-mediated endocytosis by salt stress leads to intracellular production of reactive oxygen species and salt tolerance. Plant J. 51 (2), 185–197. doi: 10.1111/j.1365-313X.2007.03134.x
Levine, B., Klionsky, D. J. (2004). Development by self-digestion: molecular mechanisms and biological functions of autophagy. Dev. Cell 6 (4), 463–477. doi: 10.1016/s1534-5807(04)00099-1
Li, L., Zhang, X., He, N., Wang, X., Zhu, P., Ji, Z. (2019). Transcriptome profiling of the salt-stress response in the halophytic green alga dunaliella salina. Plant Mol. Biol. Rep. 37 (5), 421–435. doi: 10.1007/s11105-019-01168-z
Lieberman-Aiden, E., van Berkum, N. L., Williams, L., Imakaev, M., Ragoczy, T., Telling, A., et al. (2009). Comprehensive mapping of long-range interactions reveals folding principles of the human genome. Science 326 (5950), 289–293. doi: 10.1126/science.1181369
Liu, J. X., Howell, S. H. (2016). Managing the protein folding demands in the endoplasmic reticulum of plants. New Phytol. 211 (2), 418–428. doi: 10.1111/nph.13915
Liu, J. X., Srivastava, R., Che, P., Howell, S. H. (2010). Salt stress responses in Arabidopsis utilize a signal transduction pathway related to endoplasmic reticulum stress signaling. Plant J. 51 (5), 897–909. doi: 10.1111/j.1365-313X.2007.03195.x
Liu, Y., Bassham, D. C. (2012). Autophagy: pathways for self-eating in plant cells. Annu. Rev. Plant Biol. 63, 215–237. doi: 10.1146/annurev-arplant-042811-105441
Livak, K. J., Schmittgen, T. D. (2001). Analysis of relative gene expression data using real-time quantitative PCR and the 2(-Delta Delta C(T)) Method. Methods 25 (4), 402–408. doi: 10.1006/meth.2001.1262
López-Pérez, L., Martínez-Ballesta, M., Maurel, C., Carvajal, M. (2009). Changes in plasma membrane lipids, aquaporins and proton pump of broccoli roots, as an adaptation mechanism to salinity. Phytochemistry 70 (4), 492–500. doi: 10.1016/j.phytochem.2009.01.014
Luo, L., Zhang, P., Zhu, R., Fu, J., Su, J., Zheng, J., et al. (2017). Autophagy is rapidly induced by salt stress and is required for salt tolerance in arabidopsis. Front. Plant Sci. 8). doi: 10.3389/fpls.2017.01459
Lv, H., Kim, M., Park, S., Baek, K., Oh, H., Polle, J. E. W., et al. (2021). Comparative transcriptome analysis of short-term responses to salt and glycerol hyperosmotic stress in the green alga Dunaliella salina. Algal Res. 53, 102147. doi: 10.1016/j.algal.2020.102147
Mangelsen, E., Kilian, J., Harter, K., Jansson, C., Wanke, D., Sundberg, E. (2011). Transcriptome analysis of high-temperature stress in developing barley caryopses: early stress responses and effects on storage compound biosynthesis. Mol. Plant 4 (1), 97–115. doi: 10.1093/mp/ssq058
Mao, X., Cai, T., Olyarchuk, J. G., Wei, L. (2005). Automated genome annotation and pathway identification using the KEGG Orthology (KO) as a controlled vocabulary. Bioinformatics 21 (19), 3787–3793. doi: 10.1093/bioinformatics/bti430
Marco, F., Alcázar, R., Tiburcio, A. F., Carrasco, P. (2011). Interactions between polyamines and abiotic stress pathway responses unraveled by transcriptome analysis of polyamine overproducers. Omics 15 (11), 775–781. doi: 10.1089/omi.2011.0084
Møller, I. S., Tester, M. (2007). Salinity tolerance of Arabidopsis: a good model for cereals? Trends Plant Sci. 12 (12), 534–540. doi: 10.1016/j.tplants.2007.09.009
Moriya, Y., Itoh, M., Okuda, S., Yoshizawa, A. C., Kanehisa, M. (2007). KAAS: an automatic genome annotation and pathway reconstruction server. Nucleic Acids Res. 35 (Web Server issue), W182–W185. doi: 10.1093/nar/gkm321
Nagashima, Y., von Schaewen, A., Koiwa, H. (2018). Function of N-glycosylation in plants. Plant Sci. 274, 70–79. doi: 10.1016/j.plantsci.2018.05.007
Nakamura, Y., Tsuchiya, M., Ohta, H. (2007). Plastidic phosphatidic acid phosphatases identified in a distinct subfamily of lipid phosphate phosphatases with prokaryotic origin. J. Biol. Chem. 282 (39), 29013–29021. doi: 10.1074/jbc.M704385200
Neelam, S., Subramanyam, R. (2013). Alteration of photochemistry and protein degradation of photosystem II from Chlamydomonas reinhardtii under high salt grown cells. J. Photochem. Photobiol. B: Biol. 124, 63–70. doi: 10.1016/j.jphotobiol.2013.04.007
Nieves-Cordones, M., López-Delacalle, M., Ródenas, R., Martínez, V., Rubio, F., Rivero, R. M. (2019). Critical responses to nutrient deprivation: A comprehensive review on the role of ROS and RNS. Environ. Exp. Bot. 161, 74–85. doi: 10.1016/j.envexpbot.2018.10.039
Nyathi, Y., Wilkinson, B. M., Pool, M. R. (2013). Co-translational targeting and translocation of proteins to the endoplasmic reticulum. Biochim. Biophys. Acta 1833 (11), 2392–2402. doi: 10.1016/j.bbamcr.2013.02.021
Ohlrogge, J., Browse, J. (1995). Lipid biosynthesis. Plant Cell 7 (7), 957–970. doi: 10.1105/tpc.7.7.957
Oren, A. (2005). A hundred years of Dunaliella research: 1905-2005. Saline Syst. 1, 2. doi: 10.1186/1746-1448-1-2
Pacifici, R. E., Salo, D. C., Davies, K. J. (1989). Macroxyproteinase (M.O.P.): A 670 kDa Proteinase complex that degrades oxidatively denatured proteins in red blood cells. Free Radic. Biol. Med. 7 (5), 521–536. doi: 10.1016/0891-5849(89)90028-2
Paez Valencia, J., Goodman, K., Otegui, M. S. (2016). Endocytosis and endosomal trafficking in plants. Annu. Rev. Plant Biol. 67, 309–335. doi: 10.1146/annurev-arplant-043015-112242
Panahi, B., Hejazi, M. A. (2021). Weighted gene co-expression network analysis of the salt-responsive transcriptomes reveals novel hub genes in green halophytic microalgae Dunaliella salina. Sci. Rep. 11 (1), 1607. doi: 10.1038/s41598-020-80945-3
Pandit, P. R., Fulekar, M. H., Karuna, M. S. L. (2017). Effect of salinity stress on growth, lipid productivity, fatty acid composition, and biodiesel properties in Acutodesmus obliquus and Chlorella vulgaris. Environ. Sci. Pollut. Res. 24 (15), 13437–13451. doi: 10.1007/s11356-017-8875-y
Pierrugues, O., Brutesco, C., Oshiro, J., Gouy, M., Deveaux, Y., Carman, G. M., et al. (2001). Lipid phosphate phosphatases in Arabidopsis. Regulation of the AtLPP1 gene in response to stress. J. Biol. Chem. 276 (23), 6896–6901. doi: 10.1074/jbc.M009726200
Roth, M. G. (2004). Phosphoinositides in constitutive membrane traffic. Physiol. Rev. 84 (3), 699–730. doi: 10.1152/physrev.00033.2003
Sandalio, L. M., Espinosa, J., Shabala, S. A.-O., León, J., Romero-Puertas, M. C. (2023). Reactive oxygen species- and nitric oxide-dependent regulation of ion and metal homeostasis in plants. J. Exp. Bot. 74 (19), 5970–5988. doi: 10.1093/jxb/erad349
Segami, S., Asaoka, M., Kinoshita, S., Fukuda, M., Nakanishi, Y., Maeshima, M. (2018). Biochemical, structural and physiological characteristics of vacuolar H+-pyrophosphatase. Plant Cell Physiol. 59 (7), 1300–1308. doi: 10.1093/pcp/pcy054
Shi, H., Ishitani, M., Kim, C., Zhu, J.-K. (2000). The Arabidopsis thaliana salt tolerance gene SOS1 encodes a putative Na+/H+ antiporter. Proc. Natl. Acad. Sci. U.S.A. 97 (12), 6896–6901. doi: 10.1073/pnas.120170197
Smalle, J., Vierstra, R. D. (2004). The ubiquitin 26s proteasome proteolytic pathway. Annu. Rev. Plant Biol. 55 (1), 555–590. doi: 10.1146/annurev.arplant.55.031903.141801
Sui, N., Han, G. (2014). Salt-induced photoinhibition of PSII is alleviated in halophyte Thellungiella halophila by increases of unsaturated fatty acids in membrane lipids. Acta Physiologiae Plantarum 36 (4), 983–992. doi: 10.1007/s11738-013-1477-5
Takagi, M., Karseno, Yoshida, T. (2006). Effect of salt concentration on intracellular accumulation of lipids and triacylglyceride in marine microalgae Dunaliella cells. J. Biosci. Bioeng. 101 (3), 223–226. doi: 10.1263/jbb.101.223
Trapnell, C., Williams, B. A., Pertea, G., Mortazavi, A., Kwan, G., van Baren, M. J., et al. (2010). Transcript assembly and quantification by RNA-Seq reveals unannotated transcripts and isoform switching during cell differentiation. Nat. Biotechnol. 28 (5), 511–515. doi: 10.1038/nbt.1621
Vabulas, R. M., Raychaudhuri, S., Hayer-Hartl, M., Hartl, F. U. (2010). Protein folding in the cytoplasm and the heat shock response. Cold Spring Harb. Perspect. Biol. 2 (12), a004390. doi: 10.1101/cshperspect.a004390
Van Dijk, E. L., Jaszczyszyn, Y., Naquin, D., Thermes, C. (2018). The third revolution in sequencing technology. Trends Genet. 34 (9), 666–681. doi: 10.1016/j.tig.2018.05.008
Wang, W., Vinocur, B., Shoseyov, O., Altman, A. (2004). Role of plant heat-shock proteins and molecular chaperones in the abiotic stress response. Trends Plant Sci. 9 (5), 244–252. doi: 10.1016/j.tplants.2004.03.006
Wang, X., Xu, M., Gao, C., Zeng, Y., Cui, Y., Shen, W., et al. (2020). The roles of endomembrane trafficking in plant abiotic stress responses. J. Integr. Plant Biol. 62 (1), 55–69. doi: 10.1111/jipb.12895
Wang, F., Yang, Y., Wang, Z., Zhou, J., Fan, B., Chen, Z. (2015). A critical role of lyst-interacting protein5, a positive regulator of multivesicular body biogenesis, in plant responses to heat and salt stresses. Plant Physiol. 169 (1), 497–511. doi: 10.1104/pp.15.00518
Wu, S. J., Ding, L., Zhu, J. K. (1996). SOS1, a genetic locus essential for salt tolerance and potassium acquisition. Plant Cell 8 (4), 617–627. doi: 10.1105/tpc.8.4.617
Yang, Y., Guo, Y. (2018). Elucidating the molecular mechanisms mediating plant salt-stress responses. New Phytol. 217 (2), 523–539. doi: 10.1111/nph.14920
Yin, H. L., Janmey, P. A. (2003). Phosphoinositide regulation of the actin cytoskeleton. Annu. Rev. Physiol. 65, 761–789. doi: 10.1146/annurev.physiol.65.092101.142517
Yokoi, S., Quintero, F. J., Cubero, B., Ruiz, M. T., Bressan, R. A., Hasegawa, P. M., et al. (2002). Differential expression and function of Arabidopsis thaliana NHX Na+/H+ antiporters in the salt stress response. Plant J. 30 (5), 529–539. doi: 10.1046/j.1365-313x.2002.01309.x
Young, M. D., Wakefield, M. J., Smyth, G. K., Oshlack, A. (2010). Gene ontology analysis for RNA-seq: accounting for selection bias. Genome Biol. 11 (2), R14. doi: 10.1186/gb-2010-11-2-r14
Yu, F., Lou, L., Tian, M., Li, Q., Ding, Y., Cao, X., et al. (2016). ESCRT-I component VPS23A affects ABA signaling by recognizing ABA receptors for endosomal degradation. Mol. Plant 9 (12), 1570–1582. doi: 10.1016/j.molp.2016.11.002
Zeng, Y., Li, B., Ji, C., Feng, L., Niu, F., Deng, C., et al. (2021). A unique AtSar1D-AtRabD2a nexus modulates autophagosome biogenesis in Arabidopsis thaliana. Proc. Natl. Acad. Sci. U.S.A. 118 (17), e2021293118. doi: 10.1073/pnas.2021293118
Zheng, M., Liu, X., Lin, J., Liu, X., Wang, Z., Xin, M., et al. (2019). Histone acetyltransferase GCN5 contributes to cell wall integrity and salt stress tolerance by altering the expression of cellulose synthesis genes. Plant J. 97 (3), 587–602. doi: 10.1111/tpj.14144
Keywords: salt stress, Dunaliella salina, comparative transcriptomic analysis, protein Folding, DNA repair, cellular redox homeostasis
Citation: Zhang B, Deng C, Wang S, Deng Q, Chu Y, Bai Z, Huang A, Zhang Q and He Q (2023) The RNA landscape of Dunaliella salina in response to short-term salt stress. Front. Plant Sci. 14:1278954. doi: 10.3389/fpls.2023.1278954
Received: 17 August 2023; Accepted: 10 November 2023;
Published: 04 December 2023.
Edited by:
Keni Cota-Ruiz, Utica University, United StatesReviewed by:
Manoj Kumar Solanki, University of Silesia in Katowice, PolandCopyright © 2023 Zhang, Deng, Wang, Deng, Chu, Bai, Huang, Zhang and He. This is an open-access article distributed under the terms of the Creative Commons Attribution License (CC BY). The use, distribution or reproduction in other forums is permitted, provided the original author(s) and the copyright owner(s) are credited and that the original publication in this journal is cited, in accordance with accepted academic practice. No use, distribution or reproduction is permitted which does not comply with these terms.
*Correspondence: Qinghua He, ZGVtZWF0cnlAZ21haWwuY29t; Qinglian Zhang, cWx6aGFuZzgwQDE2My5jb20=
†These authors have contributed equally to this work
Disclaimer: All claims expressed in this article are solely those of the authors and do not necessarily represent those of their affiliated organizations, or those of the publisher, the editors and the reviewers. Any product that may be evaluated in this article or claim that may be made by its manufacturer is not guaranteed or endorsed by the publisher.
Research integrity at Frontiers
Learn more about the work of our research integrity team to safeguard the quality of each article we publish.