- 1Department of Biotechnology, Yeungnam University, Gyeongsan, Gyeongbuk, Republic of Korea
- 2Department of Biotechnology, Central University of Kashmir, Ganderbal, India
- 3Department of Biological Sciences, Faculty of Science, King Abdulaziz University (KAU), Jeddah, Saudi Arabia
- 4Directorate of Programs, International Center for Biosaline Agriculture, Dubai, United Arab Emirates
Beneficial microbes or their products have been key drivers for improving adaptive and growth features in plants under biotic and abiotic stress conditions. However, the majority of these studies so far have been utilized against individual stressors. In comparison to individual stressors, the combination of many environmental stresses that plants experience has a greater detrimental effect on them and poses a threat to their existence. Therefore, there is a need to explore the beneficial microbiota against combined stressors or multiple stressors, as this will offer new possibilities for improving plant growth and multiple adaptive traits. However, recognition of the multifaceted core beneficial microbiota from plant microbiome under stress combinations will require a thorough understanding of the functional and mechanistic facets of plant microbiome interactions under different environmental conditions in addition to agronomic management practices. Also, the development of tailored beneficial multiple stress tolerant microbiota in sustainable agriculture necessitates new model systems and prioritizes agricultural microbiome research. In this review, we provided an update on the effect of combined stressors on plants and their microbiome structure. Next, we discussed the role of beneficial microbes in plant growth promotion and stress adaptation. We also discussed how plant-beneficial microbes can be utilized for mitigating multiple stresses in plants. Finally, we have highlighted some key points that warrant future investigation for exploring plant microbiome interactions under multiple stressors.
Introduction
Plants are very often confronted by different biotic and abiotic stressors that can occur individually or in combination, impeding their growth and output (Eckardt et al., 2023; Tyagi et al., 2023). Global climate change has significantly increased the prevalence of multiple stressors in plants, which has a negative impact on agricultural growth and productivity (Eckardt et al., 2023). The unprecedented droughts, heat waves, fires, and floods across the world are some of the major consequences of global warming that have threatened agricultural productivity and the earth’s biodiversity (Zandalinas et al., 2021). Abiotic stressors such as drought, salinity, heat, cold, waterlogging, and heavy metals are known to cause 51–82% production losses thereby posing a serious threat to global food security for growing human populations (Oshunsanya et al., 2019). During their lifespan, plants are frequently subjected to recurrent combinations of abiotic stressors that occur simultaneously or in sequence. For instance, the occurrence of heat and drought stress is very often confronted by plants which lead to severe crop damage and yield losses (Mittler et al., 2012; Li et al., 2013; Sánchez-Bermúdez et al., 2022). According to Ahmed et al. (2013) the combination of drought and salinity stress causes more harm to barley plants than stress alone, mostly via impairing physiological and biochemical properties. Similarly in cotton plants, it was found that a combination of drought and heat stress causes more detrimental effects than individual stress by altering not only photosynthesis and stomatal activities but also increasing leaf temperature (Carmo-Silva et al., 2012; Melandri et al., 2021). Numerous studies have suggested that an increase in ambient temperature over time can endanger plant viability by increasing the frequency of droughts as well as the harmful effects of heat stress on plants (Mittler et al., 2012; Li et al., 2013; Matiu et al., 2017; Aubert and Quinet, 2022). According to Su et al. (2015), a combination of drought and cold stressors has a more detrimental impact on grape development and yield than individual stress. Another recognized effect of abiotic stress combination is ozone and cold stress on wheat plants which impairs their ability to withstand frost (Barnes et al., 1988). It was reported that salinity and ozone stress significantly lower the yields of Oryza sativa and Cicer arietinum (Welfare et al., 2002). Further, we have summarized the impact of combined abiotic stressors on different crops in Table 1. As it is difficult to predict how crops will respond to too many stress combinations and the ensuing damage from them, therefore, it is crucial to comprehend the intricate interactions between plants and multiple abiotic stress combinations (Anderegg et al., 2015). This is especially important given the urgency of global climate change that could make relationships more complicated by raising both the severity and frequency of adverse weather occurrences (Ju-Qi et al., 2022).
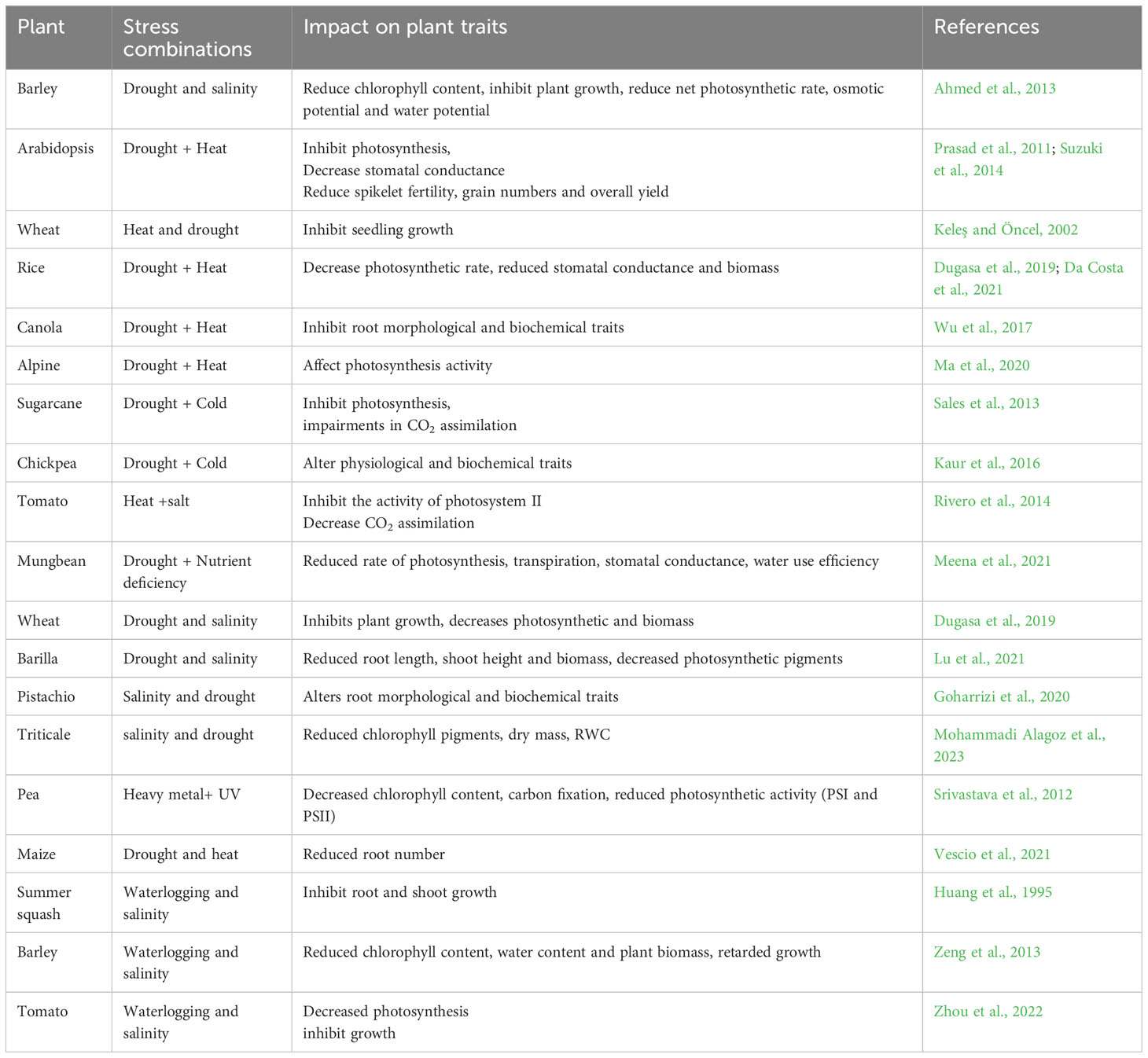
Table 1 Effect of combined abiotic stressors on plant morphological, physiological and biochemical traits.
The occurrence of numerous stress combinations, such as biotic and abiotic stressors (pathogens, drought, salinity, and heat), or abiotic stressors (drought, salinity, and heat), could further reduce agricultural productivity. For instance, it has been shown that drought significantly worsens the damage caused by pathogens to aerial (leaf and stem) and underground (root) structures, ultimately leading to a higher plant mortality rate (Jactel et al., 2012). The incidence and severity of necrotrophic pathogens increased dramatically under drought conditions (Barradas et al., 2018). Similarly, salt stress in tomato plants increases the occurrence of Fusarium wilt fungal disease which leads to more detrimental impact on them (Daami-Remadi et al., 2009). Increasing temperature also leads plants more susceptible to diseases and also to the development of more virulent pathovars (Ladányi and Horváth, 2010). Global warming will also change the pathogen shift from cold to warm or vice versa which may pose a serious threat to agriculturally important crops (Burgess et al., 2017). Many reports have found that heat stress decreases the host’s immunity against different pathogens resulting in severe yield losses (Jactel et al., 2012). In the future unprecedented abiotic stressors will make plants more susceptible to diseases by promoting their reproduction, spread, and evolution into more dangerous pathogens (Ali et al., 2017; Matsuhashi et al., 2020).
Our understanding of plants’ response to individual stressors is well understood but we are for a way to understand how the plant responds to combined stressors. Given the multiple external pressures plants face pose not only a serious threat to their survival but also to food security and the agricultural economy. Hence it is important to decipher how plants respond to multiple stressors and also develop new tools that will provide multiple stress tolerance in plants. In the past, a number of tools were used to boost crop yield and stress tolerance in various crop systems, but all had drawbacks. For instance, the use of chemical fertilizers is currently an essential part of agriculture, but they ultimately cause soil pollution, degradation, nutrient pollution, eutrophication, and greenhouse gas emissions (Arif et al., 2020). Additionally, only 0.1% of chemical pesticides reach their intended target; the remainder seeps into the soil and water thereby damaging the ecosystem (Fukamachi, 2020). Although genetically engineered (GM) crops continue to be a viable choice as a low-input, sustainable agriculture technique, the research and regulation required to generate new cultivars are time-consuming and expensive and can take decades to achieve market and regulatory clearance (Ab Rahman et al., 2018). One way to meet these challenges is to harness and integrate beneficial microbiomes i.e., those that promote plant growth, nutrient uptake, soil fertility, and host resistance to biotic and abiotic stressors (Busby et al., 2017; Ali et al., 2022; Ali et al., 2023). In the natural world, plants respond and adapt to abiotic and biotic challenges in the presence of microbial communities that live inside and outside of the host plants. Therefore, it is important to comprehend how plants undergo microbiome reprogramming amid combined abiotic stressors and how they enriched distinctive microbial communities that will help them to cope with stress. Indeed, in the last two decades, there have been significant reports on exploring plant microbiome diversity and their functions under different growth and environmental conditions in both crop and model plants viz., rice (Knief et al., 2012; Edwards et al., 2015); barley (Bulgarelli et al., 2015); corn (Aira et al., 2010); soybean (Rascovan et al., 2016); wheat (Donn et al., 2015); Arabidopsis thaliana (Bulgarelli et al., 2012). However, their expansion to the field has been limited when compared to classical PGPR microbes. There is still a long way to go before this knowledge can be effectively used in agricultural fields or in the development of tailored microbial consortia. Also, the successful incorporation of beneficial microbiomes into plant breeding and agronomical management practices is practically hampered due to the limited knowledge of plant microbiome interactions under different environmental stressors, host-microbe interactions, plant genotype variability, and microbe-microbe interactions in addition to lack of coordination between the academic scientific community and industrial researches. In this mini-review, we highlight the impact of combined stressors on sustainable agriculture and also highlight the importance of beneficial microbiota for combating multiple stressors. In addition, this review provides possible directions for future research for the development of tailored microbiota for tackling multiple stressors in sustainable agriculture.
Revisiting the role of beneficial microbes in plants
In nature, plants are associated with dynamic microbial communities of structurally and taxonomically diverse that offer a variety of beneficial services to them in terms of growth and adaptive response. For instance, beneficial microbes provide nutrients and modulate growth hormones by secreting auxins, gibberellins, and cytokinins. They also activate different defense hormonal pathways such as salicylic acid, jasmonic acid, ethylene, and abscisic acid which are the key regulators of biotic and abiotic adaptive responses (Ali et al., 2023). In order to combat abiotic stressors in plants, beneficial microbes perform a variety of functions, including activating the antioxidant system, osmobalancing, stomatal conductance, improving photosynthesis, water retention, improving root and shoot growth, and maintaining membrane stability, all of which are essential for plants’ survival in stressful situations Figure 1. Interestingly, some of the well-known microbial-assisted functions in plants are nitrogen fixation, phosphate solubilization, potassium solubilization, zinc solubilization, and iron sequestering. These processes not only benefit plant growth but also increase soil fertility and soil biota (Kamran et al., 2017; Mahmud et al., 2020). For instance, Rhizobium, Azotobacter, Azospirillum, Gluconaceotobacter, and Burkholderia are the most widely used nitrogen-fixing bacteria in sustainable agriculture (Aloo et al., 2022). The most important function of plant growth-promoting bacteria is secreting 1-aminocyclopropane-1-carboxylate (ACC) deaminase which breaks down ACC, an ethylene (ET) precursor and reduces the impacts of abiotic stresses such as salinity, drought, and waterlogging (Danish and Zafar-ul-Hye, 2019; Liu et al., 2019). Despite numerous reports of microorganisms having a variety of advantageous impacts we still know very little about the underlying molecular mechanisms. Customized microbial consortia can be developed for agricultural solutions if we have a mechanistic understanding of the interactions between plants and microbiota in heterogeneous abiotic stress environments. In nature, plants and their microbiome can very often face different combinations of multiple stressors such as drought and heat, heavy metal and cold, mechanical and other abiotic stresses, or a combination of biotic and abiotic stressors. It will be interesting to find out how these factors affect plant microbiome interactions and how will it affect the overall plant traits. We are still in the early stage of how combined stress affects plant microbiomes as most of the studies have focused on individual stress.
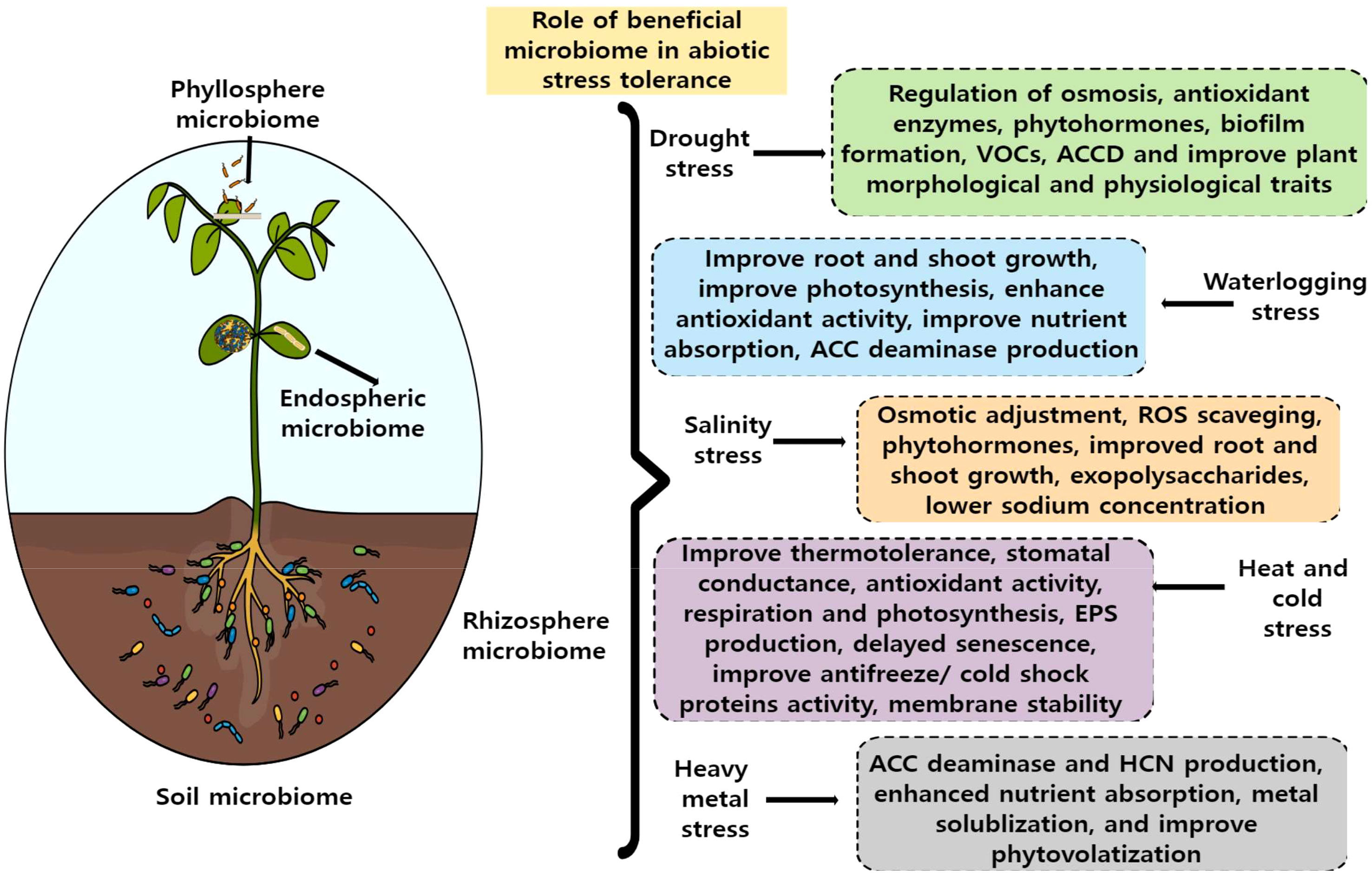
Figure 1 Role of plant beneficial microbiota in plant abiotic stress tolerance. Plant-beneficial microbial communities improve various morphological, physiological, biochemical, and nutritional traits in plants during abiotic stress conditions like drought, salt, heat, waterlogging, cold, and heavy metal stress. Some of the key functions that microbes involve to mitigate abiotic stressors are highlighted in the figure.
Plant-microbe interactions during abiotic stressors
Environmental stressors not only affect plant growth and adaptive traits but also have a huge impact on plant microbiota and their interactions. Therefore, plants, microbes, and environmental stressors are highly interconnected hence their interactions are dynamic and complex. Understanding the effects of environmental stressors (biotic and abiotic) stress on plants, microbes, and their interactions is essential for developing more robust microbial consortia in sustainable agriculture. Also, the identification of beneficial microbes and antagonistic microbes during multiple stressors in plants will provide new frontiers for crop improvement (Busby et al., 2017). Abiotic stressors are known to affect plant microbiome structure and assembly as well as plant exudate composition. Root exudates are a crucial factors that influence the microbiome of plants when they are under abiotic stress. Root exudation plays a role in improving plant resource utilization and promoting communication between soil bacteria and plants in order to reduce stress. Plant root exudates consists both primary (sugars, amino acids, carboxylates) and secondary metabolites (coumarin sorgoleone, flavonoids) (Ali et al., 2022). Abiotic stresses have been demonstrated to trigger key root exudation components like abscisic acid, proline, trehalose, betaine, and pinitol (ABA), which either directly increase plant tolerance or indirectly do so by shaping beneficial microbiota (Gargallo-Garriga et al., 2018; Chai and Schachtman, 2022). Soil microbes, which frequently have a carbon shortage under stress conditions can obtain a significant energy source from exudates. As a result, a process known as “soil priming” occurs, in which the microbial community becomes more active and may release nutrients crucial for plants due to its function in nutrient cycle (Yan et al., 2023). Previous studies have shown that plants exposed to drought display major shifts in their root microbiome. For example, enrichment of actinobacteria (Streptomyces spps) was found in different plants after drought stress. These findings suggest that Streptomyces spp. may be actively recruited by plants and have favorable effects on them (Fitzpatrick et al. (2018). However, it remains unknown at the molecular level how plants shape Actinobacteria during drought stress. Recently, it was discovered that rehydrating sorghum plants following drought stress results in bacterial community rearrangement that resembles that of control plants (Xu et al., 2018). In contrast, after rewatering in rice plants there was no restructuring of microbial communities when compared to non-stressed plants. Hence, investigating how much the difference between sorghum and rice’s capacities to reconstruct the microbiota is a result of the host’s genetics, the local soil, or microbial variation would be intriguing. There are numerous studies that have utilized different microbes for mitigating drought stress. For instance, inoculation of wheat plants by Burkholderia phytofirmans (Naveed et al., 2014)., Bacillus safensis and Ochrobactrum pseudogregnonense (Chakraborty et al., 2013) enhance drought tolerance by improving photosynthetic activity, soil moisture, and nutrient and water absorption, antioxidant activity. On the other hand inoculation of Azospirillum brasilense in Arabidopsis increased drought resilience enhancing ABA levels which is hallmark of drough signaling in plants Cohen et al. (2015). Plants also enriched particular microbiota during salinity stress to mitigate salt stress. For example, in the rhizosphere of groundnuts, salt stress increased the relative abundance of cyanobacteria and Acidobacteria while decreasing the number of Actinobacteria and Chloroflexi (Xu et al., 2020). According to Yaish et al., 2016, salt stress induces significant changes in microbiome structure with more abundance of Enterobacter spp. A previous study has revealed that salt stress changes microbial communities in both below and above-ground plant parts (Hou et al., 2021b). Many salt stress-related hormones such ABA and salicylic acid (SA) have been found to drive the microbial community changes during salt stress in Arabidopsis. These studies provide evidence that plants recruit special beneficial microbiota that promotes growth traits under salinity. However, more studies are required in different crop systems using both salt tolerant and sensitive to further unravel how plants recruit specific microbial communities under salt stress. Temperature (heat and cold stress) also triggers microbial community changes in plants (Wipf et al., 2021; Liu et al., 2022). These studies have highlighted that plants recruit specific microbial taxa or genre during abiotic stressors which might help them to survive under such conditions. However, these studies were carried out under single stress. Therefore, future studies are required to examine how plants shape unique microbial communities during multiple stress combinations in both model and crop systems which can be further explored for mitigating combined stressors (drought +heat, drought +salinity, heat +salt stress etc) in sustainable agriculture. As a result, it is necessary to use new methods and technologies like machine learning that can be used to underpin how plants modify their microbiomes under multiple stress conditions and how stress microbiomes aid plant survival in such circumstances. Also, the identification of beneficial microbes and antagonistic microbes during multiple stressors in plants will provide new frontiers in microbiome ecology. Given that plant responses to microorganisms are typically highly species-specific, one crucial question is how compatible the plant microbiota implicated in plant stress tolerance will be across plant species and conditions (Marasco et al., 2013). For instance, some plants are more dependent on beneficial fungi and some on free living microbes therefore it will be interesting to identify microbes that confer greater stress tolerance to a variety of plants rather than individual plants.
Development of tailored microbiota for plant growth promotion and multiple stress tolerance
Although the green revolution has dramatically increased crop production using chemical fertilizers, pesticides, and genetically improved crop cultivars but this achievement has been marred by numerous unsustainable practices, such as soil pollution, development of resistant pathovars, environmental damage, etc. Considering the future food demand under changing environmental conditions there is a need for a second green revolution but it should be achieved in a sustainable way. One way is to harness plant-beneficial microbiome or their products which provide eco-friendly services in terms of plant growth promotion and stress resilience (Arif et al., 2020; Ali et al., 2022; Ali et al., 2023). Microbes are known to flourish in extreme environments like high salinity, drought, heavy metal, high temperature, etc., and develop resistance to a variety of environmental insults and improper soil conditions. Therefore, it is obvious that microbes can assist plants under unfavorable environmental conditions and can be a more sustainable way to boost crop production and soil health. However, it will be intriguing to investigate if microorganisms can resist numerous stressors when they occur simultaneously or whether they act differently in response to individual stress.
For the development of tailored multiple tolerant microbiota, we need to first identify the core microbiome and its interactions with the host, microbes, and environments. These studies require the integration of cutting-edge technologies like metagenomics, metatranscriptomics, and temporal-spatial mapping of plant responses and plant-colonizing bacteria and machine learning (Doni et al., 2022). Further, the development of beneficial multiple stress-tolerant microbiota in sustainable agriculture necessitates new model systems and prioritizes agricultural microbiome research. For instance, 1) we have to develop plant microbiome systems in both model and crop systems where we can study different host-microbiome interaction traits under combined stress conditions. 2) Selection of core microbiome under combined stress conditions using both culture and non-culture-dependent methods. 3) An essential frontier of plant microbiome interactions under stress combinations will be evaluating how plants undergo transcriptional, metabolic, and posttranslational alterations associated with root exudate chemistry, a crucial driver for core microbiome construction 4) Plant-based microbiome engineering involves plant breeding and genetic engineering for developing tailored microbiome is another aspect of harnessing beneficial core microbiome 5) Finally, microbiome engineering and enivroomics will also play key role in developing SynComs for multiple stressors in sustainable agriculture Figure 2. Some studies have also reported metaorganism-based microbiome engineering which involves the co-engineering of both plants and their associated microbes for particular metabolic traits. To address the above points there is a need to integrate different molecular (multi-omics) and system biology tools along with plant phenotype screening that will provide novel insights into the complexity of plant microbiome and improve models for the predictive traits necessary for a successful SynCom design.
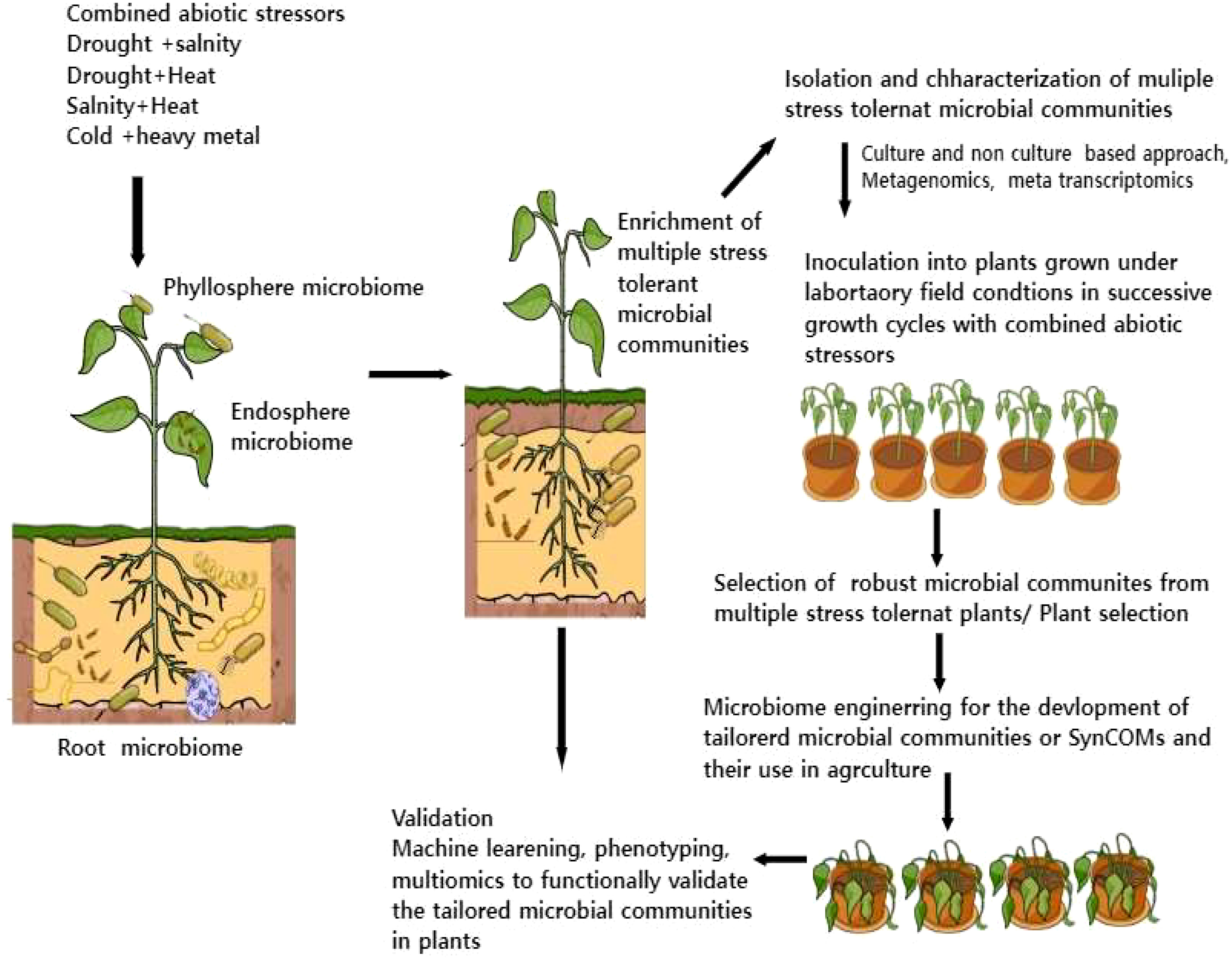
Figure 2 A systematic approach for the development of multiple stress-tolerant microbial communities in sustainable agriculture. This illustration highlights how plants could enrich selective microbial communities during combined stressors which can be further used for the development of tailored microbiota or SynComs using microbiome engineering. The role of omics tools, plant phenotyping, and machine learning for functional validation of tailored microbiota is also shown in the figure.
Conclusion
Recent developments in biotechnological tools and culture-independent techniques have revealed that plants are profoundly colonized with diversified microbial communities which are essential to their survival under changing environmental conditions. Nevertheless, over the past few years, our understanding of how plants and microbes interact under abiotic stressors has significantly increased, we still lack a thorough mechanistic understanding of how the microbiome shifts occurs under combined or multiple stressors. Therefore, future studies are required to decipher how plants enrich their microbiome during combined stressors and how they vary under different stress combinations. Also, how plants modulate root exudates during combined stressors is another key frontier in plant microbiome research as they are the major drivers for microbiome assembly and hence warrant further attention. Future research is needed to understand how phytohormones like ABA, SA, JA, and ET affect microbiome structure and how they contribute to microbiome-mediated stress tolerance in plants under multiple stressors. In this regard, reductionist methods under controlled lab conditions or utilizing SynComs will provide more mechanistic and functional insights into how combined abiotic stressors affect plant microbiome structure and how microbes enhance a plant’s ability to withstand abiotic stressors. A combination of metagenomics, metatranscriptomics, and temporal-spatial mapping of plant responses and plant-colonizing bacteria as well as the use of machine learning will aid to underpin how plants modify their microbiomes under multiple stress conditions which will aid in identifying unique and robust microbiota. For long-term usage of beneficial microbiota, it is necessary to identify bonafide receptors, genes, or transcriptional factors in plants that regulate microbiome drivers (root exudates, hormones) under combined stresses which can be employed in plant breeding or plant genetic engineering to create a desired robust microbiome for improving multiple stress resilience in crops. Additionally, the use of traditional and biotechnological-based microbiome engineering could also play a key role in developing multiple stress-tolerant beneficial microbiota for sustainable agriculture.
Author contributions
SA: Conceptualization, Formal Analysis, Software, Supervision, Validation, Writing – original draft, Writing – review & editing. AT: Conceptualization, Software, Visualization, Writing – original draft, Writing – review & editing. IR: Conceptualization, Supervision, Writing – original draft, Writing – review & editing. YA: Writing – original draft, Writing – review & editing. RM: Writing – original draft, Writing – review & editing. HM: Conceptualization, Supervision, Writing – original draft, Writing – review & editing.
Funding
This research work was funded by Institutional Fund Projects, under grant number (IFPRP:119-130-1442). Therefore, authors gratefully acknowledge technical and financial support from the Ministry of Education and King Abdulaziz University, DSR, Jeddah, Saudi Arabia.
Acknowledgments
We gratefully acknowledge the support of the International Center for Biosaline Agriculture (ICBA) - Dubai, United Arab Emirates.
Conflict of interest
The authors declare that the research was conducted in the absence of any commercial or financial relationships that could be construed as a potential conflict of interest.
Publisher’s note
All claims expressed in this article are solely those of the authors and do not necessarily represent those of their affiliated organizations, or those of the publisher, the editors and the reviewers. Any product that may be evaluated in this article, or claim that may be made by its manufacturer, is not guaranteed or endorsed by the publisher.
References
Ab Rahman, S. F. S., Singh, E., Pieterse, C. M., Schenk, P. M. (2018). Emerging microbial biocontrol strategies for plant pathogens. Plant Sci. 267, 102–111. doi: 10.1016/j.plantsci.2017.11.012
Ahmed, I. M., Dai, H., Zheng, W., Cao, F., Zhang, G., Sun, D., et al. (2013). Genotypic differences in physiological characteristics in the tolerance to drought and salinity combined stress between Tibetan wild and cultivated barley. Plant Physiol. Biochem. 63, 49–60. doi: 10.1016/j.plaphy.2012.11.004
Aira, M., Gómez-Brandón, M., Lazcano, C., Bååth, E., Domínguez, J. (2010). Plant genotype strongly modifies the structure and growth of maize rhizosphere microbial communities. Soil Biol. Biochem. 42 (12), 2276–2281. doi: 10.1016/j.soilbio.2010.08.029
Ali, S., Mir, Z. A., Tyagi, A., Bhat, J. A., Chandrashekar, N., Papolu, P. K., et al. (2017). Identification and comparative analysis of Brassica juncea pathogenesis-related genes in response to hormonal, biotic and abiotic stresses. Acta physiologiae planta. 39, 1–15. doi: 10.1007/s11738-017-2565-8
Ali, S., Tyagi, A., Bae, H. (2023). Plant microbiome: an ocean of possibilities for improving disease resistance in plants. Microorganisms 11 (2), 392. doi: 10.3390/microorganisms11020392
Ali, S., Tyagi, A., Park, S., Mir, R. A., Mushtaq, M., Bhat, B., et al. (2022). Deciphering the plant microbiome to improve drought tolerance: Mechanisms and perspectives. Environ. Exp. Bot. 201, 104933. doi: 10.1016/j.envexpbot.2022.104933
Aloo, B. N., Tripathi, V., Makumba, B. A., Mbega, E. R. (2022). Plant growth-promoting rhizobacterial biofertilizers for crop production: The past, present, and future. Front. Plant Sci. 13. doi: 10.3389/fpls.2022.1002448
Anderegg, W. R., Hicke, J. A., Fisher, R. A., Allen, C. D., Aukema, J., Bentz, B., et al. (2015). Tree mortality from drought, insects, and their interactions in a changing climate. New Phytol. 208 (3), 674–683. doi: 10.1111/nph.13477
Arif, I., Batool, M., Schenk, P. M. (2020). Plant microbiome engineering: expected benefits for improved crop growth and resilience. Trends Biotechnol. 38 (12), 1385–1396. doi: 10.1016/j.tibtech.2020.04.015
Aubert, L., Quinet, M. (2022). Comparison of heat and drought stress responses among twelve Tartary buckwheat (Fagopyrum tataricum) varieties. Plants 11 (11), 1517. doi: 10.3390/plants11111517
Barnes, J. D., Reiling, K., Davison, A. W., Renner, C. J. (1988). Interaction between ozone and winter stress. Environ. pollut. 53 (1-4), 235–254. doi: 10.1016/0269-7491(88)90037-1
Barradas, C., Pinto, G., Correia, B., Castro, B. B., Phillips, A. J. L., Alves, A. (2018). Drought× disease interaction in Eucalyptus globulus under Neofusicoccum eucalyptorum infection. Plant Pathol. 67 (1), 87–96. doi: 10.1111/ppa.12703
Bulgarelli, D., Garrido-Oter, R., Münch, P. C., Weiman, A., Dröge, J., Pan, Y., et al. (2015). Structure and function of the bacterial root microbiota in wild and domesticated barley. Cell Host Microbe 17 (3), 392–403. doi: 10.1016/j.chom.2015.01.011
Bulgarelli, D., Rott, M., Schlaeppi, K., Ver Loren van Themaat, E., Ahmadinejad, N., Assenza, F., et al. (2012). Revealing structure and assembly cues for arabidopsis root-inhabiting bacterial microbiota. Nature 488 (7409), 91–95. doi: 10.1038/nature11336
Burgess, T. I., Scott, J. K., Mcdougall, K. L., Stukely, M. J., Crane, C., Dunstan, W. A., et al. (2017). Current and projected global distribution of Phytophthora cinnamomi, one of the world’s worst plant pathogens. Global Change Biol. 23 (4), 1661–1674. doi: 10.1111/gcb.13492
Busby, P. E., SOman, C., Wagner, M. R., Friesen, M. L., Kremer, J., Bennett, A., et al. (2017). Research priorities for harnessing plant microbiomes in sustainable agriculture. PloS Biol. 15 (3), e2001793. doi: 10.1371/journal.pbio.2001793
Carmo-Silva, A. E., Gore, M. A., Andrade-Sanchez, P., French, A. N., Hunsaker, D. J., Salvucci, M. E. (2012). Decreased CO2 availability and inactivation of Rubisco limit photosynthesis in cotton plants under heat and drought stress in the field. Environ. Exp. Bot. 83, 1–11. doi: 10.1016/j.envexpbot.2012.04.001
Chai, Y. N., Schachtman, D. P. (2022). Root exudates impact plant performance under abiotic stress. Trends Plant Sci. 27 (1), 80–91. doi: 10.1016/j.tplants.2021.08.003
Chakraborty, U., Chakraborty, B. N., Chakraborty, A. P., Dey, P. L. (2013). Water stress amelioration and plant growth promotion in wheat plants by osmotic stress tolerant bacteria. World J. Microbiol. Biotechnol. 29, 789–803. doi: 10.1007/s11274-012-1234-8
Cohen, A. C., Bottini, R., Pontin, M., Berli, F. J., Moreno, D., Boccanlandro, H., et al. (2015). Azospirillum brasilense ameliorates the response of Arabidopsis thaliana to drought mainly via enhancement of ABA levels. Physiologia Planta. 153 (1), 79–90. doi: 10.1111/ppl.12221
Daami-Remadi, M., Souissi, A., Oun, H. B., Mansour, M., Nasraoui, B. (2009). Salinity effects on Fusarium wilt severity and tomato growth. Dyn. Soil Dyn. Plant 3 (1), 61–69.
Da Costa, M. V. J., Ramegowda, Y., Ramegowda, V., Karaba, N. N., Sreeman, S. M., Udayakumar, M. (2021). Combined drought and heat stress in rice: responses, phenotyping and strategies to improve tolerance. Rice Sci. 28 (3), 233–242. doi: 10.1016/j.rsci.2021.04.003
Danish, S., Zafar-ul-Hye, M. (2019). Co-application of ACCdeaminase producing PGPR and timber-waste biochar improves pigments formation, growth and yield of wheat under drought stress. Sci. Rep. 9, 5999. doi: 10.1038/s41598-019-42374-9
Doni, F., Miranti, M., Mispan, M. S., Mohamed, Z., Uphoff, N. (2022). Multi-omics approaches for deciphering the microbial modulation of plants' genetic potentials: What's known and what's next? Rhizosphere 100613. doi: 10.1016/j.rhisph.2022.100613
Donn, S., Kirkegaard, J. A., Perera, G., Richardson, A. E., Watt, M. (2015). Evolution of bacterial communities in the wheat crop rhizosphere. Environ. Microbiol. 17 (3), 610–621. doi: 10.1111/1462-2920.12452
Dugasa, M. T., Cao, F., Ibrahim, W., Wu, F. (2019). Differences in physiological and biochemical characteristics in response to single and combined drought and salinity stresses between wheat genotypes differing in salt tolerance. Physiologia Planta. 165 (2), 134–143. doi: 10.1111/ppl.12743
Eckardt, N. A., Cutler, S., Juenger, T. E., Marshall-Colon, A., Udvardi, M., Verslues, P. E. (2023). Focus on climate change and plant abiotic stress biology. Plant Cell 35 (1), 1–3. doi: 10.1093/plcell/koac329
Edwards, J., Johnson, C., Santos-Medellín, C., Lurie, E., Podishetty, N. K., Bhatnagar, S., et al. (2015). Structure, variation, and assembly of the root-associated microbiomes of rice. Proc. Natl. Acad. Sci. 112 (8), E911–EE20. doi: 10.1073/pnas.1414592112
Fitzpatrick, C. R., Copeland, J., Wang, P. W., Guttman, D. S., Kotanen, P. M., Johnson, M. T. (2018). Assembly and ecological function of the root microbiome across angiosperm plant species. Proc. Natl. Acad. Sci. 115 (6), E1157–E1165. doi: 10.1073/pnas.1717617115
Fukamachi, K. (2020). Building resilient socio-ecological systems in Japan: Satoyama examples from Shiga Prefecture. Ecosys. Serv. 46, 101187. doi: 10.1016/j.ecoser.2020.101187
Gargallo-Garriga, A., Preece, C., Sardans, J., Oravec, M., Urban, O., Peñuelas, J., et al. (2018). Root exudate metabolomes change under drought and show limited capacity for recovery. Sci. Rep. 8, 12696. doi: 10.1038/s41598-018-30150-0
Goharrizi, K. J., Baghizadeh, A., Kalantar, M., Fatehi, F. (2020). Combined effects of salinity and drought on physiological and biochemical characteristics of pistachio rootstocks. Scientia Horticul. 261, 108970. doi: 10.1016/j.scienta.2019.108970
Hou, S., Wolinska, K. W., Hacquard, S. (2021). Microbiota-root-shoot-environment axis and stress tolerance in plants. Curr. Opin. Plant Biol. 62, 102028. doi: 10.1016/j.pbi.2021.102028
Huang, B., Johnson, J. W., NeSmith, D. S., Bridges, D. C. (1995). Nutrient accumulation and distribution of wheat genotypes in response to waterlogging and nutrient supply. Plant Soil 173, 47–54. doi: 10.1007/BF00155517
Jactel, H., Petit, J., Desprez-Loustau, M. L., Delzon, S., Piou, D., Battisti, A., et al. (2012). Drought effects on damage by forest insects and pathogens: a meta-analysis. Global Change Biol. 18 (1), 267–276. doi: 10.1111/j.1365-2486.2011.02512.x
Ju-Qi, D. U. A. N., Jia-Shuang, Y. U. A. N., Xin-Wu, X. U., Hui, J. U. (2022). Interpretation of the IPCC AR6 report on agricultural systems. Adv. Climate Change Res. 18 (4), 422. doi: 10.12006/j.issn.1673-1719.2022.035
Kamran, S., Shahid, I., Baig, D. N., Rizwan, M., Malik, K. A., Mehnaz, S. (2017). Contribution of zinc solubilizing bacteria in growth promotion and zinc content of wheat. Front. Microbiol. 8, 2593. doi: 10.3389/fmicb.2017.02593
Kaur, S., Jairath, A., Singh, I., Nayyar, H., Kumar, S. (2016). Alternate mild drought stress (– 0.1 MPa PEG) immunizes sensitive chickpea cultivar against lethal chilling by accentuating the defense mechanisms. Acta Physiologiae Planta. 38, 1–11. doi: 10.1007/s11738-016-2212-9
Keleş, Y., Öncel, I. (2002). Response of antioxidative defence system to temperature and water stress combinations in wheat seedlings. Plant Sci. 163 (4), 783–790. doi: 10.1016/S0168-9452(02)00213-3
Knief, C., Delmotte, N., Chaffron, S., Stark, M., Innerebner, G., Wassmann, R., et al. (2012). Metaproteogenomic analysis of microbial communities in the phyllosphere and rhizosphere of rice. ISME J. 6 (7), 1378–1390. doi: 10.1038/ismej.2011.192
Ladányi, M., Horváth, L. (2010). a review of the potential climate change impact on insect populations- general and agricultural aspects. Appl. Ecol. Environ. Res. 8 (2), 143–152. doi: 10.15666/aeer/0802_143151
Li, J., Lin, X., Chen, A., Peterson, T., Ma, K., Bertzky, M., et al. (2013). Global priority conservation areas in the face of 21st century climate change. PloS One 8, e54839. doi: 10.1371/journal.pone.0054839
Liu, H., Khan, M. Y., Carvalhais, L. C., Delgado-Baquerizo, M., Yan, L., Crawford, M., et al. (2019). Soil amendments with ethylene precursor alleviate negative impacts of salinity on soil microbial properties and productivity. Sci. Rep. 9, 6892 41. doi: 10.1038/s41598-019-43305-4
Liu, X., Le Roux, X., Salles, J. F. (2022). The legacy of microbial inoculants in agroecosystems and potential for tackling climate change challenges. Iscience 25 (3). doi: 10.1016/j.isci.2022.103821
Lu, Y., Zhang, B., Li, L., Zeng, F., Li, X. (2021). Negative effects of long-term exposure to salinity, drought, and combined stresses on halophyte Halogeton glomeratus. Physiologia Planta. 173 (4), 2307–2322. doi: 10.1111/ppl.13581
Ma, L., Zhang, Z., Zhou, B., Xu, M., Zhou, H. (2020). Drought, heat, and their combined stress reduce the productivity and alter the photosynthetic characteristics of different alpine meadow plants. Authorea Preprints. doi: 10.22541/au.159985785.51409338
Mahmud, K., Makaju, S., Ibrahim, R., Missaoui, A. (2020). Current progress in nitrogen fixing plants and microbiome research. Plants 9 (1), 97. doi: 10.3390/plants9010097
Marasco, R., Rolli, E., Vigani, G., Borin, S., Sorlini, C., Ouzari, H., et al. (2013). Are drought-resistance promoting bacteria cross-compatible with different plant models? Plant Signaling Behav. 8 (10), e26741. doi: 10.4161/psb.26741
Matiu, M., Ankerst, D. P., Menzel, A. (2017). Interactions between temperature and drought in global and regional crop yield variability during 1961-2014. PloS One 12, e0178339. doi: 10.1371/journal.pone.0178339
Matsuhashi, S., Hirata, A., Akiba, M., Nakamura, K., Oguro, M., Takano, K. T., et al. (2020). Developing a point process model for ecological risk assessment of pine wilt disease at multiple scales. For. Ecol. Manage. 463, 118010. doi: 10.1016/j.foreco.2020.118010
Meena, S. K., Pandey, R., Sharma, S., Gayacharan, Kumar, T., Singh, M. P., et al. (2021). Physiological basis of combined stress tolerance to low phosphorus and drought in a diverse set of mungbean germplasm. Agronomy 11 (1), 99. doi: 10.3390/agronomy11010099
Melandri, G., Thorp, K. R., Broeckling, C., Thompson, A. L., Hinze, L., Pauli, D. (2021). Assessing drought and heat stress-induced changes in the cotton leaf metabolome and their relationship with hyperspectral reflectance. Front. Plant Sci. 12. doi: 10.3389/fpls.2021.751868
Mittler, R., Finka, A., Goloubinoff, P. (2012). How do plants feel the heat? Trends Biochem. Sci. 37, 118–125. doi: 10.1016/j.tibs.2011.11.007
Mohammadi Alagoz, S., Hadi, H., Toorchi, M., Pawłowski, T. A., Asgari Lajayer, B., Price, G. W., et al. (2023). Morpho-physiological responses and growth indices of triticale to drought and salt stresses. Sci. Rep. 13 (1), 8896. doi: 10.1038/s41598-023-36119-y
Naveed, M., Hussain, M. B., Zahir, Z. A., Mitter, B., Sessitsch, A. (2014). Drought stress amelioration in wheat through inoculation with Burkholderia phytofirmans strain PsJN. Plant Growth Regul. 73, 121–131. doi: 10.1007/s10725-013-9874-8
Oshunsanya, S. O., Nwosu, N. J., Li, Y. (2019). “Abiotic stress in agricultural crops under climatic conditions,” in Sustainable Agriculture, Forest and Environmental Management. Eds. Jhariya, M. K., Banerjee, A., Meena, R. S. (Singapore: Springer), 71–100.
Prasad, P. V. V., Pisipati, S. R., Momčilović, I., Ristic, Z. (2011). Independent and combined effects of high temperature and drought stress during grain filling on plant yield and chloroplast EF-Tu expression in spring wheat. J. Agron. Crop Sci. 197 (6), 430–441. doi: 10.1111/j.1439-037X.2011.00477.x
Rascovan, N., Carbonetto, B., Perrig, D., Díaz, M., Canciani, W., Abalo, M., et al. (2016). Integrated analysis of root microbiomes of soybean and wheat from agricultural fields. Sci. Rep. 6, 28084. doi: 10.1038/srep28084
Rivero, R. M., Mestre, T. C., Mittler, R., Rubio, F., Garcia-Sanchez, F., Martinez, V. (2014). The combined effect of salinity and heat reveals a specific physiological, biochemical and molecular response in tomato plants. Plant Cell Environ. 37 (5), 1059–1073. doi: 10.1111/pce.12199
Sales, C. R., Ribeiro, R. V., Silveira, J. A., MaChado, E. C., Martins, M. O., Lagôa, A. M. M. (2013). Superoxide dismutase and ascorbate peroxidase improve the recovery of photosynthesis in sugarcane plants subjected to water deficit and low substrate temperature. Plant Physiol. Biochem. 73, 326–336. doi: 10.1016/j.plaphy.2013.10.012
Sánchez-Bermúdez, M., Del Pozo, J. C., Pernas, M. (2022). Effects of combined abiotic stresses related to climate change on root growth in crops. Front. Plant Sci. 13, 918537. doi: 10.3389/fpls.2022.918537
Srivastava, G., Kumar, S., Dubey, G., Mishra, V., Prasad, S. M. (2012). Nickel and ultraviolet-B stresses induce differential growth and photosynthetic responses in Pisum sativum L. seedlings. Biol. Trace Element Res. 149, 86–96. doi: 10.1007/s12011-012-9406-9
Su, L., Dai, Z., Li, S., Xin, H. (2015). A novel system for evaluating drought–cold tolerance of grapevines using chlorophyll fluorescence. BMC Plant Biol. 15, 1–12. doi: 10.1186/s12870-015-0459-8
Suzuki, N., Rivero, R. M., Shulaev, V., Blumwald, E., Mittler, R. (2014). Abiotic and biotic stress combinations. New Phytol. 203 (1), 32–43. doi: 10.1111/nph.12797
Tyagi, A., Sharma, S., Srivastava, H., Singh, A., Kaila, T., Ali, S., et al. (2023). Transcriptome profiling of two contrasting pigeon pea (Cajanus cajan) genotypes in response to waterlogging stress. Frontiers in Genetics 13, p.1048476.
Vescio, R., Malacrinò, A., Bennett, A. E., Sorgonà, A. (2021). Single and combined abiotic stressors affect maize rhizosphere bacterial microbiota. Rhizosphere 17, 100318. doi: 10.1016/j.rhisph.2021.100318
Welfare, K., Yeo, A. R., Flowers, T. J. (2002). Effects of salinity and ozone, individually and in combination, on the growth and ion contents of two chickpea (Cicer arietinum L.) varieties. Environ. pollut. 120 (2), 397–403. doi: 10.1016/S0269-7491(02)00109-4
Wipf, H. M. L., Bùi, T. N., Coleman-Derr, D. (2021). Distinguishing between the impacts of heat and drought stress on the root microbiome of Sorghum bicolor. Phytobiomes J. 5 (2), 166–176. doi: 10.1094/PBIOMES-07-20-0052-R
Wu, W., Duncan, R. W., Ma, B. L. (2017). Quantification of canola root morphological traits under heat and drought stresses with electrical measurements. Plant Soil 415, 229–244. doi: 10.1007/s11104-016-3155-z
Xu, L., Naylor, D., Dong, Z., Simmons, T., Pierroz, G., Hixson, K. K., et al. (2018). Drought delays development of the sorghum root microbiome and enriches for monoderm bacteria. Proc. Natl. Acad. Sci. 115 (18), E4284–E4293. doi: 10.1073/pnas.1717308115
Xu, Y., Zhang, G., Ding, H., Ci, D., Dai, L., Zhang, Z. (2020). Influence of salt stress on the rhizosphere soil bacterial community structure and growth performance of groundnut (Arachis hypogaea L.). Int. Microbiol. 23, 453–465. doi: 10.1007/s10123-020-00118-0
Yaish, M. W., Al-Lawati, A., Jana, G. A., Vishwas Patankar, H., Glick, B. R. (2016). Impact of soil salinity on the structure of the bacterial endophytic community identified from the roots of caliph medic (Medicago truncatula). PloS One 11 (7), e0159007. doi: 10.1371/journal.pone.0159007
Yan, S., Yin, L., Dijkstra, F. A., Wang, P., Cheng, W. (2023). Priming effect on soil carbon decomposition by root exudate surrogates: A meta-analysis. Soil Biol. Biochem. 178, 108955. doi: 10.1016/j.soilbio.2023.108955
Zandalinas, S. I., Fritschi, F. B., Mittler, R. (2021). Global warming, climate change, and environmental pollution: recipe for a multifactorial stress combination disaster. Trends Plant Sci. 26 (6), 588–599. doi: 10.1016/j.tplants.2021.02.011
Zeng, F., Shabala, L., Zhou, M., Zhang, G., Shabala, S. (2013). Barley responses to combined waterlogging and salinity stress: separating effects of oxygen deprivation and elemental toxicity. Front. Plant Sci. 4, 313. doi: 10.3389/fpls.2013.00313
Keywords: beneficial microbes, abiotic stressors, combined stress, climate change, tailored microbiota
Citation: Ali S, Tyagi A, Mir RA, Rather IA, Anwar Y and Mahmoudi H (2023) Plant beneficial microbiome a boon for improving multiple stress tolerance in plants. Front. Plant Sci. 14:1266182. doi: 10.3389/fpls.2023.1266182
Received: 24 July 2023; Accepted: 28 August 2023;
Published: 11 September 2023.
Edited by:
Kanika Khanna, Guru Nanak Dev University, IndiaReviewed by:
Javaid Akhter Bhat, Nanjing Agricultural University, ChinaMuhammad Shoib Nawaz, Clemson University, United States
Tariq Shah, North Carolina State University, United States
Copyright © 2023 Ali, Tyagi, Mir, Rather, Anwar and Mahmoudi. This is an open-access article distributed under the terms of the Creative Commons Attribution License (CC BY). The use, distribution or reproduction in other forums is permitted, provided the original author(s) and the copyright owner(s) are credited and that the original publication in this journal is cited, in accordance with accepted academic practice. No use, distribution or reproduction is permitted which does not comply with these terms.
*Correspondence: Sajad Ali, sajadmicro@yu.ac.kr; Irfan A. Rather, ammm@kau.edu.sa; Henda Mahmoudi, HMJ@biosaline.org.ae
†These authors have contributed equally to this work