- Center for Plant Cell Biology, Institute for Integrative Genome Biology, Department of Botany & Plant Sciences, University of California at Riverside, Riverside, CA, United States
Mentioned for the first time in an article 1971, the occurrence of the term “Macrophomina phaseolina” has experienced a steep increase in the scientific literature over the past 15 years. Concurrently, incidences of M. phaseolina-caused crop diseases have been getting more frequent. The high levels of diversity and plasticity observed for M. phasolina genomes along with a rich equipment of plant cell wall degrading enzymes, secondary metabolites and putative virulence effectors as well as the unusual longevity of microsclerotia, their asexual reproduction structures, make this pathogen very difficult to control and crop protection against it very challenging. During the past years several studies have emerged reporting on host defense measures against M. phaseolina, as well as mechanisms of pathogenicity employed by this fungal pathogen. While most of these studies have been performed in crop systems, such as soybean or sesame, recently interactions of M. phaseolina with the model plant Arabidopsis thaliana have been described. Collectively, results from various studies are hinting at a complex infection cycle of M. phaseolina, which exhibits an early biotrophic phase and switches to necrotrophy at later time points during the infection process. Consequently, responses of the hosts are complex and seem coordinated by multiple defense-associated phytohormones. However, at this point no robust and strong host defense mechanism against M. phaseolina has been described.
Introduction
Macrophomina phaseolina (Tassi) Goid is a soilborne fungal plant pathogen of the ascomycetes family Botryosphaeriaceae that causes charcoal rot, a root and stem disease characterized by black specs in the host tissue. It is distributed across the globe and is particularly problematic in arid climates (Kaur et al., 2012a). Charcoal rot symptoms are known to be more severe under heat and drought stress (Mughogho and Pande, 1983; Fang et al., 2011). More than 500 plant species have been reported to be susceptible to this pathogen, including many economically important crops such as canola, maize, sesame and soybean (Su et al., 2001; Gaetán et al., 2006; Saleh et al., 2010). Moreover, M. phaseolina is capable of human infection in immunosuppressed individuals, which highlights the range of host environments where it can thrive (Tan et al., 2008; Arora et al., 2012). Pathogens like M. phaseolina with a wide host range are described as “generalists”. M. phaseolina is difficult to eliminate from infected fields due to its production of microsclerotia, which are dark-colored asexual propagation structures that can survive for many years in soil without a host. Originally M. phaseolina was considered to be a necrotrophic pathogen, but detailed analysis of the early stages of infection in soybean revealed the presence of a distinct biotrophic phase of up to 36 hours preceding the onset of necrotic plant tissue collapse (Chowdhury et al., 2017). M. phaseolina is therefore often characterized as a hemibiotroph along the likes of Phytophthora infestans and Fusarium oxysporum. The asymptomatic biotrophic infection phase makes early identification of disease in the field difficult. Several factors seem to contribute to a growing number of M. phaseolina-related crop disease incidents. For example, strawberry collapse due to charcoal rot in the U.S. began in 2005, and by 2014 this disease was confirmed in most strawberry-growing counties (Koike et al., 2016). This rise of strawberry field infestation is correlated with high temperatures and drought, as well as the ban on methylbromide fumigation (Muchero et al., 2011). Along with its increased impact on crop production studies on mechanisms of M. phaseolina pathogenicity and host immunity against this root pathogen are emerging. However, our understanding of these processes is still fragmentary.
M. phaseolina: a global “crop destroyer”
Agricultural impact
Due to its wide host range, ubiquitous global distribution, and increasing impact on agriculture, M. phaseolina has been dubbed a “global crop destroyer”. Disease caused by M. phaseolina is most often referred to as charcoal rot, but this pathogen is also known to cause wilting, stem canker, seedling blight, rot, and damping-off (Singh et al., 1990; Kaur et al., 2012b). These contribute to yield loss, reduction in seed quality, and viability of infected plants (Mughogho and Pande, 1983). Moreover, the severity of symptoms is correlated with high temperatures and low soil water potential conditions. Thus, plants growing in (sub)-tropical arid regions are at higher risk of succumbing to disease caused by this pathogen (Mayek-Pérez et al., 2002; Chamorro et al., 2015; Lodha and Mawar, 2020). Pandey and Basandrai reviewed the current literature on factors related to climate change that could potentially worsen M. phaseolina infestation (Pandey and Basandrai, 2021). Both the visible traits and genetic architecture of this pathogen, which are further described below, show that M. phaseolina as a species is well adapted to diverse climates and equipped to withstand environmental change.
Many important crops including grains and legumes are included among the wide host range of this pathogen (Pandey and Basandrai, 2021). Known hosts of M. phaseolina such as alfalfa, maize, canola, soybean, and sunflower have vast global economic implications, while others like cassava, chickpea, and mungbean are critical for regional food security (Ammon et al., 1974; Bashir and Malik, 1988; Kaiser and Das, 1988; Msikita et al., 1998; Pratt et al., 1998; Gaetán et al., 2006; Weems et al., 2011; Lakhran et al., 2018). The combined estimated loss of soybean yields in ten countries due to charcoal rot exceeded 2 million metric tons during 1998, ranking third among the most common soybean diseases (Wrather et al., 2001). Furthermore, charcoal rot ranked second for economic losses per hectare in the U.S. across two decades and combined with soybean cyst nematode accounted for 33% of the total economic losses (Bandara et al., 2020).
M. phaseolina also poses a great threat to specialty crops, such as strawberry, pistachio, and sunflower (Mertely et al., 2005; Bokor, 2007; de los Santos et al., 2019; Nouri et al., 2020). Research on specialty crops is often underfunded and disproportionately lacking in comparison to the steady rise in value of production, despite the inherently high risk involved in marketing fresh produce (Alston and Pardey, 2008; Neill and Morgan, 2021). For example, strawberries ranked fourth among the top California crops produced in 2017, with a value of $3.10 billion (California Department of Food and Agriculture; Koike et al., 2016). Strawberries are highly susceptible to M. phaseolina. Fumigation with methylbromide and chloropicrin served as a common method of pathogen control. In 2005, the agricultural use of methylbromide was phased out. Since then, the relationship between yield and area of strawberry production reversed, as yields stagnated and new incidences of charcoal rot increased (Holmes et al., 2020).
Disease cycle
M. phaseolina is most often characterized by its production of microsclerotia, or small sclerotial bodies 200–600 μm in diameter, which appear as dark specs in infected plant tissue (Jackson and Jaronski, 2009). Microsclerotia are spherical clusters of hyphal cells, each with the ability to germinate and propagate when appropriate environmental conditions are met. They can remain dormant in dead host tissue or soil for an extended period of time, becoming the primary inoculum for the next growing season (Short et al., 1980). On potato dextrose agar (PDA), microsclerotium formation can be identified in hosts as early as 24–30 hours post-inoculation (Wyllie and Brown, 1970). Pycnidia, which are asexual reproductive structures that form spores, have also been documented for this fungus, but are rarely observed in vitro (Ma et al., 2010).
Microsclerotia germinate at a temperature range of 28–35°C, as the emerging mycelium extends radially until it identifies host tissue (Ammon et al., 1974; Mihail and Taylor, 1995). Appressoria-like structures, or hyphopodia, form when hyphal tips recognize host root cells, often penetrating the plant tissue between epidermal cells, before colonization is established in the middle lamella and intercellular space (Ammon et al., 1974). Early stages of infection remain mostly intercellular, but during host cell penetration the hyphae can invaginate the host plasma membrane creating an interface similar to that of haustoria of biotrophic pathogens (Figure 1A). Moreover, formation of intracellular vesicles, which is a characteristic of early hemibiotrophic infection, has been observed in the cortical tissue of infected soybean (Chowdhury et al., 2017). Production of pectolytic and cellulolytic enzymes by the pathogen has been observed both in vivo and in vitro, but it is not certain during which phases of the infection process this occurs (Radha, 1953; Chan and Sackston, 1969; Chan and Sackston, 1970). Development of thin, filamentous hyphae is thought to be an indicator of a biotrophy to necrotrophy switch (BNS), as subsequently, tissue begins to necrose (Chowdhury et al., 2017). M. phaseolina mycelia eventually colonize the host’s vascular tissue, and the formation of microsclerotia in the xylem vessels leads to blockage and consequently wilting of the host (Abawi and Corrales, 1990; Mayek-Pérez et al., 2002; Figure 1B). A deeper understanding of the initial stages of M. phaseolina infection may provide us with clues as to which modes of plant defense are effective against this pathogen.
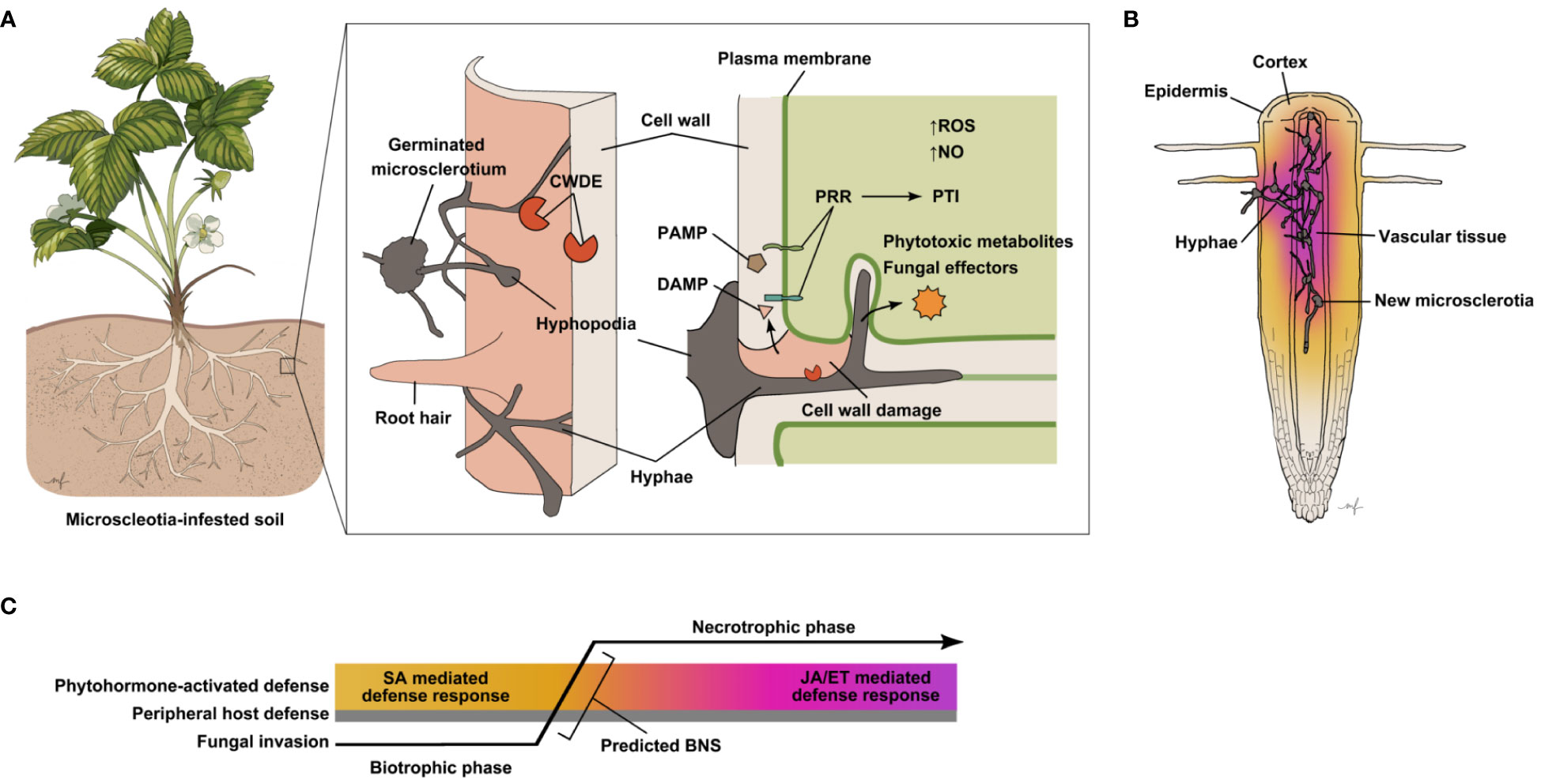
Figure 1 Presumed events and molecular interactions determining the outcome of host infection by M. phaseolina. (A) Schematic diagram of presumed M. phaseolina pathogenesis process and host defense responses. Microcsclerotia (asexual propagation structures) in the soil germinate under suitable conditions emitting hyphae that extend until a host plant, typically its root, is detected. The first line of defense a host plant possesses is its cell wall, which mainly serves as a passive physical barrier. M. phaseolina hyphopodia have been observed at sites of hyphal penetration of the host epidermis (Ammon et al., 1974; Bressano et al., 2010; Chowdhury et al., 2014). The M. phaseolina genome encodes a large number of cell wall degrading enzymes (CWDEs), predicted to aid in breaching host cell walls. Plant cell wall damage leads to the release of cell wall-derived molecules, some of which may be detected by host pattern recognition receptors (PRRs) as damage-associated molecular patterns (DAMPs) triggering pattern-triggered immunity (PTI). Host PRRs may also trigger PTI upon recognition of pathogen-associated molecular patterns (PAMPs), molecules directly derived from the pathogen. In the initial stages of infection, hyphae generally stay intercellular. At later stages hyphae can also be seen invaginating host cells, while remaining enveloped in host plasma membranes (Chowdhury et al., 2017). The M. phaseolina genome also encodes predicted effector proteins and has been shown to produce several phytotoxic compounds, which may further disrupt host cell function and defense processes. Whether such molecules are secreted into the host tissue to aid pathogenicity remains to be confirmed. Increased accumulation of reactive oxygen species (ROS), as well as nitric oxide (NO) have been observed in many plant hosts infected with M. phaseolina. While both ROS and NO accumulation are known defense responses in plants, it has also been suggested that M. phaseolina is capable of NO production (Sarkar et al., 2014). (B) M. phaseolina continues to invade host root tissue, spreading profusely through various tissue types and forming new microsclerotia. Common causes of plant collapse for M. phaseolina-infected plants are blockage and necrosis of vascular tissue. Activation of host defense by M. phaseolina described in the literature thus far represents that of whole roots, which includes different cell types at various stages of infection. The color gradient represents that photohormone-mediated defense responses are likely to vary and differ between cell types and infection stages. (C) Predicted sequence of events during M. phaseolina invasion of host plant tissue, spatially and temporally depicted. Defense activation in the host and fungal transition from biotrophy to necrotrophy, as it has been observed through current methods of detection, is averaged across space from cell to cell and time relative to the infection progress. Hence, phytohormone-mediated defense response is depicted as a gradient. As M. phaseolina penetrates peripheral host tissues it is presumably in its biotrophic phase eliciting SA-mediated host immune responses. A biotrophic to necrotrophic switch (BNS) likely occurs after hyphae further progress into host tissues leading to activation of JA and ET-mediated defense signaling.
Strategies for control
M. phaseolina is adapted to survive in diverse environmental conditions, including sodium salt concentrations of 1 to 8%, and pH ranges of 3.5 to 10 (Islam et al., 2012). Its microsclerotia can persist in soil for up to 15 years. There is no universal, economically, and environmentally reasonable protocol for eradicating this pathogen from an infected field. What is known is that there is a clear relationship between inoculum concentration, disease occurrence, and yield loss. Numerous strategies to suppress charcoal rot have been tested, and the results have been thoroughly reviewed by authors such as Lodha and Mawar (2020) and Marquez et al. (2021). Current approaches to controlling this disease can be grouped into four categories: (1) Agronomic practices, (2) Chemical control by using fungicides or herbicides, (3) Host genetic resistance, and (4) Biological control (Marquez et al., 2021).
While certain combinations of agronomic practices showed some promise, none of these measures proved to be fully sufficient. The situation seems to be similar for fungicides against charcoal rot. Although highly effective in controlling M. phaseolina, methylbromide has been phased out throughout the world per the “Montreal Protocol Guidelines on Substances that Deplete the Ozone Layer”, released in 1991 (Chamorro et al., 2016; Guthman, 2017). Fumigation of strawberry fields with other chemicals continues in California, and in fact, chloropicrin use has increased significantly in correlation to the drop in methylbromide use, as it is less effective alone (Guthman, 2017). Although alternatives to methylbromide, including chloropicrin, have been shown to reduce M. phaseolina density and plant mortality in the field, crop production cost and chemical use have only increased (Chamorro et al., 2016; Guthman, 2017).
Unlike resistance against many foliar pathogens, host resistance against M. phaseolina seems not to be mediated by individual disease resistance (R)-genes, and multiple genetic loci seem to make combined quantitative contributions in protecting plants against this root pathogen (Marquez et al., 2021). Only gradual differences in quantitative resistance against M. phaseolina have been observed among different varieties of certain crop species, such as cowpea, soybean, or strawberry (Muchero et al., 2011; Reznikov et al., 2019; Gomez et al., 2020). While the underlying quantitative trait loci can be useful for breeding, genetic engineering can also result in crop varieties with enhanced protection against M. phaseolina. For example, transgenic jute lines expressing the rice chitinase (chi11) gene showed significantly smaller lesion sizes and improved fiber yield in comparison to the wild type when infected with M. phaseolina (Majumder et al., 2018). However, efforts to combat M. phaseolina by transgenic crop lines have been scarce. This approach has been used more so to identify genes and pathways important for host resistance (Lygin et al., 2013; Dabi et al., 2020).
Biological control strategies have shown some promise in protecting crops against M. phaseolina. Dhingra et al. (1976) found that increasing organic matter in soil reduces M. phaseolina colonization of soybean and maize stems, likely due to the increased presence of other microbes. The implication that M. phaseolina has low competitive saprophytic ability has been tested and confirmed with multiple fungal and bacterial biocontrol agents (BCAs). For example, several species of Bacillus have been reported to antagonize M. phaseolina growth both in vitro and in vivo and to suppress disease symptoms in soybean, common bean and cowpea (Yasmin et al., 2020; Bojórquez-Armenta et al., 2021; Rangel-Montoya et al., 2022). Other rhizospheric bacteria such as fluorescent Pseudomonas spp. have also been identified as M. phaseolina BCAs (Gupta et al., 2002; Das et al., 2008). Many of these rhizobacterial species also have plant growth-promoting properties.
The genus Trichoderma is a well-known fungal BCA (Lorito et al., 2010). Indeed, examples like T. viride and T. harzianum successfully inhibit the growth of M. phaseolina and further have positive effects on plant growth (Sankar and Sharma, 2001; Khaledi and Taheri, 2016; Khalili et al., 2016). Interestingly, it has been shown that the combined application of T. harzianum and arbuscular mycorrhizal fungi (AMF) allows for AMF colonization in non-host Brassica plants, including Arabidopsis thaliana, and increases silique numbers (Poveda et al., 2019). Additionally, AMF co-treatment with M. phaseolina resulted in a much lower number of up-regulated genes in comparison to the application of the pathogen alone. Pre-mycorrhized plants also exhibited greater expression of serine carboxipeptidase-like (SCPL) and lectin genes, which have been suggested to prime the plant for pathogen attack (Marquez et al., 2018; Marquez et al., 2021).
Despite the growing interest in reducing agrochemical inputs, the biopesticide market constitutes only a small fraction (6.4% in 2018) of the total pesticide market (Pal and McSpadden Gardener, 2006; Regnault-Roger, 2020). Obstacles that remain to be addressed, such as storage, lack of commercialized products, narrow target application, and limited user awareness have hindered widespread adoption of biological control strategies.
M. phaseolina’s toolkit for pathogenicity
Pathogen variability
Comparisons between M. phaseolina isolates across host species and geographical distances have revealed high levels of morphological, physiological, pathogenic, and genetic variation. Despite this, the genus Macrophomina is monospecific, meaning that it constitutes a single species. Classification of M. phaseolina isolates based on chlorate sensitivity was previously proposed by Pearson et al. (1986). Chlorate is an analogue of nitrate and can be toxic to plants and fungi when reduced to chlorite via the nitrate reductase pathway. Since M. phaseolina isolates from corn were chlorate resistant and those from soybean were chlorate sensitive, it was suggested that the presence or absence of the nitrate reductase pathway could be a marker for host-specific strains (Pearson et al., 1986; Pearson et al., 1987). However, subsequent reports using this approach have had mixed results. Mihail and Taylor (1995) were unable to confirm relationships between the chlorate phenotype and origin host species when comparing 114 isolates. Su et al. (2001) found that both host species and location (root or soil) of a given isolate had effects on chlorate sensitivity, albeit at varying levels.
Random amplified polymorphic DNA (RAPD) analysis can also be used to genetically differentiate M. phaseolina isolates by host species (Su et al., 2001). Jana et al. (2005) also successfully used two different kinds of markers derived from repetitive sequences to fingerprint and differentiate isolates from soybean, cotton, and chickpea. Baird et al. (2010) surveyed 109 isolates across the U.S. using 12 simple sequence repeats and found them to group into clusters based on both the location and host from which these isolates were found, but exceptions were also not uncommon. There is no evidence of sexual reproduction in M. phaseolina (Marquez et al., 2021). In a study that examined isolates across host plant families and geographical distances, hyphal fusions were observed frequently enough (64.3%) for the authors to conclude that there are no genetic barriers to nonsexual genetic exchange amongst M. phaseolina isolates (Mihail and Taylor, 1995). The potential for gene flow across populations and the movement of agricultural products including soil likely contribute to the variability seen in this species. As nearly 4% of the annotated M. phaseolina genome encodes transposable elements, genetic transposition is another potential avenue for diversity due to the introduction of novel mutations, gene duplications, and horizontal gene transfers (Islam et al., 2012). Understanding M. phaseolina variance is important for developing resistant crop genotypes and highlights the importance of experimental design in studying this pathogen.
Host specialization
There are many conflicting reports regarding whether this heterogenous pathogen is adapted to infecting particular host species. To what degree the variability described above contributes to host specialization or preference is still unclear. For some examples of generalist necrotrophic fungi such as Botrytis cinerea, there is evidence suggesting that selective pressure maintains moderate virulence against a broad spectrum of host species (Caseys et al., 2021). In a study by Koike et al. (2016), M. phaseolina isolates from melon, thyme, and apple were tested against strawberry and failed to cause disease. Moreover, five cover crop species tested against strawberry-derived isolates also did not develop symptoms. However, results from Su et al. (2001) indicate that no specialization occurs for isolates from sorghum, cotton, or soybean, and while corn isolates seemed to colonize corn roots better than other isolates, it also was the most virulent against soybean. Similar cross-host species pathogenicity experiments have been carried out testing hosts against isolates collected across species and geographic distances, but no isolate had restricted virulence to a single host (Mayék-Pérez et al., 2001; Reyes-Franco et al., 2006). Although there is some evidence to suggest that host preference exists for certain isolates, it is not certain whether this is the result of evolutionary specialization or an artifact of environmental factors, which may alter the virulence of the pathogen against specific hosts. Recently, a large-scale analysis of three M. phaseolina isolates from three different hosts revealed structural chromosomal rearrangements and SNPs, an indicator of genomic flexibility, although no pattern was necessarily associated with a particular host (Burkhardt et al., 2019). However, ten genes were identified to be exclusive to strawberry pathogenic isolates (Burkhardt et al., 2019). Whether these genes (alleles)? are the causal factor for the host preference of these isolates has not been reported.
M. phaseolina -omics studies
In 2012, Islam et al. reported on the first draft M. phaseolina genome, from an isolate of infected jute. Cross-species homology analysis revealed its close synteny with another soil-borne generalist fungus, Fusarium oxysporum, which shares 54.10% of its predicted genes with M. phaseolina. Additionally, the authors identified 537 predicted proteins in the M. phaseolina genome that are included in the pathogen–host interaction database (PHI-base), a collection of experimentally verified virulence effectors from bacterial, fungal, and oomycete pathogens. So far, genomes of M. phaseolina isolates from a variety of hosts including jute, strawberry, alfalfa, sorghum, and castor have been sequenced (Islam et al., 2012; Verma et al., 2018; Burkhardt et al., 2019; Hossen et al., 2019; Purushotham et al., 2020; Marquez et al., 2021). Sequencing and assembly M. phaseolina genomes has led to comparative studies across isolates and geographic locations, and the development of new methods to study plant–M. phaseolina interactions to elucidate biological processes involved in pathogenicity.
Liu et al. (2022) used transcriptomic analysis to study the microsclerotia formation stages of M. phaseolina, revealing an enrichment of reactive oxygen species (ROS)-related genes among up-regulated differentially expressed genes.
The first documented proteomic analysis on M. phaseolina published by Zaman et al. (2020) compared the expression of proteins with and without challenge of the fungus by the jute endophytic bacterium Burkholderia contaminans NZ. A large number of hydrolyzing enzymes predicted in the M. phaseolina genome were confirmed to be expressed through this study. M. phaseolina co-cultured with this bacterial antagonist showed signs of decreased virulence, morphological changes, and altered metabolic processes switching to a more dormant state that prioritizes survival over growth. Characterization of the M. phaseolina mycelial proteome by Arafat et al. (2022) revealed a network of proteins that could be classified by their function including growth and reproduction, stress tolerance and virulence, nutrient synthesis, transcription and translation regulation, and more. Sinha et al. (2022) identified 117 proteins in the M. phaseolina secretome isolated from wheat bran samples, including cell wall degrading enzymes (CWDEs) and proteases which are vital for breaching plant defense mechanisms. Recently, Pineda-Fretez et al. (2023) identified 250 proteins secreted by M. phaseolina grown in liquid media supplemented with a soybean leaf infusion. Among these proteins were numerous CWDEs, and peptidases, as well as 54 putative effectors (Pineda-Fretez et al., 2023). These proteomic studies provide the groundwork for potential functional studies of specific genes or gene groups involved in virulence.
Effects on host cell wall and membrane permeability
A notable characteristic of the M. phaseolina genome is the abundance of genes involved in cell wall degradation. Numerous carbohydrate-active enzymes (CAZymes) including glycoside hydrolases (GH), glycosyltransferases (GT), carbohydrate esterases (CE), carbohydrate-binding modules (CBM), and polysaccharide lyases (PL), were predicted within the genome. The M. phaseolina genome possesses high levels of GHs (four times more than GTs), and CEs (Islam et al., 2012), which is consistent with previous observations that this pathogen has greater cellulolytic activity than most other fungi (Kaur et al., 2012a). A β-1,4-endoglucanase produced by this pathogen was previously characterized and shown to be similar to plant-derived endoglucanases (Wang and Jones, 1995). While the significance of this is unclear, use of a CWDE that is similar to a plant-derived enzyme may allow for surreptitious modification of the host cell wall without eliciting plant defense responses. A repertoire of genes associated with lignin depolymerization, including laccases, lignin peroxidases, galactose oxidases, chloroperoxidase, haloperoxidases, and heme peroxidases were also identified. The M. phaseolina genome encodes the highest number of laccases in comparison to seven other fungal species (Islam et al., 2012). Production of these CWDEs has also been observed in M. phaseolina grown in vitro (Ahmad et al., 2006; Ramos et al., 2016). The activity of phosphatidases, which hydrolyze phosphatide lipids and alter the permeability of cellular membranes, was also observed during Brassica juncea infection with M. phaseolina isolates of various levels of virulence. A strong correlation between host susceptibility, pathogen virulence, and phosphatidase activity was described, which peaked at 18 days post-inoculation (Srivastava and Dhawan, 1982). The extensive collection of genes associated with cell wall and membrane disruption suggests that this pathogen is well equipped to breach diverse wall polysaccharide and lipid bilayer compositions, which differ between plant lineages (Kubicek et al., 2014). This perhaps explains, at least in part, M. phaseolina’s wide host range.
An unusually high number of amino acid transporters, which are likely useful for accessing host protein degradation products, were predicted in the M. phaseolina genome. In addition, enzymes involved in amino acid metabolism are enriched in the mycelial proteome (Arafat et al., 2022). Uptake of host-derived metabolites, such as amino acids, is likely to occur via hyphopodia of M. phaseolina formed during its biotrophic infection phase (Figure 1A) as well as from leaking host cells with degraded cell walls and plasma membranes during its necrotrophic phase.
Signal transduction and host crosstalk
The M. phaseolina genome encodes a multitude of protein kinases and G-protein coupled receptors, some of which are known to facilitate host recognition in other pathogens (Islam et al., 2012). The abundance of such receptors and signal transduction components may contribute to the ability of M. phaseolina to recognize and utilize a great variety of plant species as hosts.
In fungi, redox signaling is essential for the regulation of developmental processes, crosstalk with plant hosts, and degradation of host lignocellulose (Breitenbach et al., 2015). M. phaseolina infection causes nitric oxide (NO) accumulation in jute (Corchorus capsularis), but the fungus itself is also capable of NO production in vitro (Sarkar et al., 2014). NO is a key signaling factor in the early stages of the host hypersensitive response (HR). In plants pathogen-induced NO production is accompanied by a burst of reactive oxygen species (ROS), such as superoxide (O2−) and hydrogen peroxide (H2O2) (Van Baarlen et al., 2004; Asai and Yoshioka, 2009). Balanced accumulation of both NO and H2O2 seems to be required to trigger HR-associated cell death (Delledonne et al., 1998; Delledonne et al., 2001), although there are somewhat conflicting reports as to whether NO and ROS act synergistically (Delledonne et al., 2001) or antagonistically (Asai and Yoshioka, 2009; Asai et al., 2010) in this process.
Interestingly, genes involved in ROS-related functions were found to be differentially expressed in M. phaseolina during microsclerotia formation, suggesting that ROS, specifically O2−, stimulates microsclerotia formation (Liu et al., 2022). Moreover, studies across multiple filamentous fungi have shown that ROS have functions in cell differentiation and development in fungi (Breitenbach et al., 2015). In vitro treatment of cultures with ROS further supported this hypothesis. A fascinating possibility is that ROS produced by M. phaseolina may interfere with ROS/NO-controlled processes in its hosts and vice versa. Pathogen-derived ROS and NO may contribute to HR in the host, which may increase its virulence during necrotrophy, while the defense-linked oxidative burst in the host may contribute to M. phaseolina microsclerotia formation.
Secondary metabolites
Fungi produce diverse bioactive natural products, some of which enhance their virulence, such as mycotoxins (Chooi and Tang, 2012). A total of 75 putative secondary metabolite-related genes were identified in the M. phaseolina genome, which is more than double of what can be found in each of the plant pathogenic fungi Magnaporthe grisea, B. cinerea, Sclerotinia sclerotiorum, and Fusarium graminearum. These include genes encoding polyketide synthases (PKS), non-ribosomal peptide synthases (NPRS) and PKS-NPRS hybrids, which synthesize precursors to various secondary metabolites including those involved in virulence (Islam et al., 2012). Additional expression analyses and functional studies are required to confirm the role of these genes. Examples of secondary metabolites produced by M. phaseolina include phaseolinone (Siddiqui et al., 1979; Dhar et al., 1982), phaseolinic acid (Mahato et al., 1987), (−)-botryodiplodin (Ramezani et al., 2007; Abbas et al., 2019), succinic acid, tyrosol, (R)-mellein, cis-(3R,4R)-4-hydroxymellein, azelaic acid (Salvatore et al., 2020), kojic acid, moniliformin, orsellinic acid (Khambhati et al., 2020), macrollins A–C (Gao et al., 2021), phaseocyclopentenones A and B, and guignardone A (Masi et al., 2021). However production of such metabolites seems to be dependent on a number of factors including isolate variability and the presence of host substrates. To what extent virulence is affected by these metabolites is largely unknown.
Phaseolinone was described to be a novel phytotoxin when it was first identified in M. phaseolina and has been documented to affect plant seed germination, wilting, and leaf necrosis (Dhar et al., 1982; Bhattacharya et al., 1992; Bhattacharya et al., 1994). The compound (−)-botryodiplodin is a well-documented toxic metabolite prevalent in ascomycetes such as M. phaseolina. When soybean plants were infected with M. phaseolina, this compound was isolated exclusively from root tissues displaying charcoal rot symptoms (Ramezani et al., 2007; Abbas et al., 2019), suggesting that it is produced during pathogenesis. In these assays, however, phaseolinone could not be detected in any soybean tissue examined despite previous reports describing it as a metabolite unique to M. phaseolina. Analysis by Shier et al. (2007) provides several possible reasons for this discrepancy, one being that Dhar et al. mistakenly characterized (−)-botryodiplodin as phaseolinone. Another possible explanation is that different isolates of M. phaseolina produce different “cocktails” of toxins. This is supported by the fact that mellein was identified in some, but not all, isolates of this pathogen recovered from symptomatic soybean plants collected in Mississippi, U.S. (Khambhati et al., 2020). The abundance of phytotoxic secondary metabolites potentially contributing to M. phaseolina pathogenicity is mirrored by the induced expression of numerous detoxification-related genes in A. thaliana after infection with this pathogen (Schroeder et al., 2019).
Host immunity and defense mechanisms
Plants have innate defense mechanisms that are widely conserved across species, and which allow for protection against a wide range of pathogens. The fact that within given host species varieties or cultivars with different levels of resistance against M. phaseolina have been described, strongly suggests the existence of plant immune mechanisms against this pathogen. Multiple efforts have been made to uncover the genetic basis of M. phaseolina resistance in crop species. Despite these recent advancements, molecular mechanisms that determine host resistance against M. phaseolina are still poorly understood.
One of the first lines of defense that plants possess is a physical barrier that simultaneously functions as a sensory organ – the cell wall. It can efficiently deter many pathogens, contributing to non-host resistance, an unspecific type of immunity protecting the plant against a wide-range of pathogens. Polysaccharides of diverse compositions form a network that supports the structural integrity of the cell wall, alongside proteins, which have roles in the remodeling and turnover of cell wall components. For pathogens that can breach and decompose this barrier, the cell wall may be a valuable nutrient source. However, disruptions in the integrity of the cell wall, as well as the activity of enzymes that cause its degradation, including those that originate from the host itself, are known to trigger signaling cascades eliciting biotic stress responses. Evidence for the role of cell wall maintenance in host defense is thoroughly reviewed by (Hamann, 2012). Molecular signals released as a result of cell wall damage can be perceived as damage-associated molecular patterns (DAMPs) by pattern recognition receptors (PRRs) residing on the plant plasma membrane (Figure 1A) leading to a robust local defense response called pattern triggered immunity (PTI) and possible systemic defense signaling (Hou et al., 2019).
PRRs can also detect conserved pathogen-associated molecular patterns (PAMPs) directly derived from invading pathogens and activate PTI (Figure 1A) (Bigeard et al., 2015). For example, the A. thaliana PRR FLS2, a receptor-like protein kinase (RLK), responds to flagellin, a ubiquitous bacterial PAMP conferring PTI (Gómez-Gómez and Boller, 2000). Responses to PAMP recognition include ionic influxes in the cytosol, increased ROS and NO production, activation of mitogen-activated protein kinase cascades, and transcriptional reprogramming that entails induction of pathogenesis related (PR) genes (Nürnberger et al., 2004). In turn, some pathogens have adapted to counter host defenses through proteins and secondary metabolites collectively called effectors that can interfere with PTI. Effectors can serve as toxic agents themselves or as a means to evade and suppress host defense mechanisms (Lo Presti et al., 2015). Although there are several characteristics common to many effector proteins, such as size, species exclusivity, and expression profile, exceptions are also common. In terms of total secreted proteins, hemibiotrophs on average have the greatest number of effectors than any other fungal lifestyle group (Lo Presti et al., 2015).
The perpetual coevolution of plant hosts and microbial pathogens, collectively described as the “zigzag model”, has contributed greatly to the repertoire of attack/defense mechanisms encoded by the genomes of both sides involved (Chisholm et al., 2006; Jones and Dangl, 2006). Pathogen effectors can be directly or indirectly identified by specific receptors encoded by host resistance (R) genes which then trigger an acute immune response that often results in the “hypersensitive response” (HR) a defense mechanism involving localized program cell death of plant cells. Many fungal avirulence (Avr) genes encoding Avr proteins have gene-for-gene relationships with plant host R genes and typically act as effectors. This tertiary layer of defense is called effector-triggered immunity (ETI) (Macho and Zipfel, 2014; Lo Presti et al., 2015). The A. thaliana ecotype Col-0 possesses approximately 165 NB-LRR genes, which is the largest class of R genes characterized by their nucleotide binding (NB) and leucine rich repeat (LRR) domains (Dangl and Jones, 2001; Wei et al., 2020). PTI and ETI make up the plant’s inducible defense core.
PAMP candidates of M. phaseolina
Eight orthologs of the Phytophthora parasitica cellulose-binding elicitor lectin (CBEL) gene, which has a role in the perception of host cell wall components and is a known PAMP, were found in the M. phaseolina genome (Gaulin et al., 2002; Islam et al., 2012). The 13 amino acid comprising peptide Pep-13 is a known PAMP motif in cell wall transglutaminases from Phytophthora sojae (Brunner et al., 2002). Three homologs containing the Pep-13 motif are found in M. phaseolina (Islam et al., 2012). As several M. phaseolina-derived PAMPs have been identified thus far, it is likely that plants recognize the presence of this pathogen through mechanisms similar to those of other pathosystems.
Phytohormones
Three phytohormones are crucial for the orchestration of plant defense responses: salicylic acid (SA), jasmonic acid (JA), and ethylene (ET) (Glazebrook, 2005; Bari and Jones, 2009). SA accumulates in host tissues, can trigger HR and comprehensive transcriptional reprogramming as a response to infection, and mediate efficient defense against biotrophic and hemibiotrophic pathogens. It is also known to induce systemic acquired resistance (SAR), in which distal tissues acquire heightened resistance to secondary infection due to elevated expression of pathogenesis related (PR) genes (Ross, 1961; Durrant and Dong, 2004). JA and ET are associated with comprehensive defense responses against necrotrophic pathogens (Bari and Jones, 2009). Similar to, but independent from SA, the phytohormones JA and ET are also known to trigger a systemic defense response called induced systemic resistance (ISR) (Pieterse et al., 1996). Although signaling pathways controlled by these plant hormones are tuned to defend against pathogens of distinct lifestyles, they are known to affect each other both synergistically and antagonistically (Clarke et al., 2000; Thomma et al., 2001; Mur et al., 2006; Koornneef and Pieterse, 2008). Cross-talk between phytohormone-signaling pathways allows the host plant to balance the energy cost of pathogen defense to that of its own growth and propagation (Pieterse et al., 2012).
Transcriptomic analysis in A. thaliana roots revealed that M. phaseolina induces JA-, ET-, and SA-responsive genes, which is consistent with the observation that this pathogen leads a hemibiotrophic lifestyle. Mutants compromised in signaling mediated by each of these three hormones exhibited increased susceptibility to M. phaseolina (Schroeder et al., 2019). M. phaseolina induced upregulation of JA- and ET-responsive genes has been reported in several species of hosts susceptible to this pathogen, both in the shoot and the root (Gaige et al., 2010). In Sesamum indicum, widely known as sesame, SA responsive differential gene expression in the root was in correlation with the early biotrophic phase of M. phaseolina infection both in resistant and susceptible varieties, although overall transcript levels were greater in the resistant variety (Chowdhury et al., 2017). Accumulation of JA- and ET-signaling gene transcripts was seen during the BNS and through the necrotrophic phase in these studies (Figure 1C). Moreover, the authors conducted a priming assay with each of these phytohormones and found that pre-treatment of sesame with JA and ET significantly reduced M. phaseolina-associated disease symptoms, while JA- and ET-inhibitors significantly increased susceptibility to this pathogen. Taken together, the evidence suggests that the timing and amplitude of SA, JA and ET signaling are critical in determining the susceptibility of the host. While all three of these defense-associated phytohormones seem to cooperate in protecting host roots against M. phaseolina, it is important to consider that results based on gene expression studies in whole organs reflect responses that are averaged across different cell types. Cell-type specific regulation of phytohormone-mediated defense has not been explored in M. phaseolina pathosystems.
ROS
As introduced earlier, plants produce ROS, primarily superoxide (O2−) and hydrogen peroxide (H2O2), in response to pathogen invasion. H2O2 resulting from such an oxidative burst can act as a diffusible signal in plants that activates defense-related genes and other defense reactions, ultimately triggering local HRs (Levine et al., 1994). The level of ROS in plant tissue is enzymatically controlled by superoxide dismutases (SOD), which catalyze the conversion of O2− to H2O2 and oxygen (Wang et al., 2018). When interactions of sesame plants with M. phaseolina were studied, the susceptible cultivar VRI-1 showed consistently higher accumulation of H2O2 in comparison to the resistant Nirmala variety, following the BNS (Chowdhury et al., 2017). Consistently, less lipid peroxidation and higher accumulation of SiSOD was observed during the necrotrophic phase in the resistant variety (Chowdhury et al., 2017).
Asai and Yoshioka (2009) found that necrotic lesions caused by Botrytis cinerea on Nicotiana benthamiana were reduced when the oxidative burst was suppressed, while disease lesions expanded when NO production was inhibited. HR induction in the host plant is favorable for pathogens during their necrotrophic phase, when they feed on dead tissue, especially when the HR is uncontrolled, as is expected if the pathogen produces additional ROS during infection (Sarkar et al., 2014; Liu et al., 2022). It has also been shown that changes in NO levels caused by M. phaseolina infection in sesame were dependent on the susceptibility of the cultivar, with resistant varieties exhibiting a rapid but moderate increase in NO, while susceptible varieties showed a delayed and robust decrease in NO (Acharya and Acharya, 2007). Similarly, in interactions of the necrotrophic fungus Sclerotinia sclerotiorum with A. thaliana, rapid and transient accumulation of NO was observed in resistant ecotypes, while NO accumulation was also observed in susceptible ecotypes at later time points (Perchepied et al., 2010). M. phaseolina-triggered accumulation of H2O2, however, was found between 24 and 72 hpi to be consistently higher in susceptible sesame genotypes compared to resistant ones (Chowdhury et al., 2017). At later time points when the pathogen has presumably entered its necrotrophic phase, resistant genotypes showed signs of ROS detoxification. Taken together these observations suggest that the timing and amplitude of NO and ROS production, interactions of these molecules and their turn-over are likely factors contributing to host susceptibility during necrotrophic phases of pathogen infections. While the oxidative burst is known to contribute to PTI and ETI against biotrophs, the degree of host resistance seems counter-correlated with ROS levels during necrotrophic infection phases.
Secondary metabolites
Like M. phaseolina, plants produce a diverse array of secondary metabolites with roles in biotic interactions. Antimicrobial secondary plant metabolites synthesized in response to biotic stress are collectively called phytoalexins (Ahuja et al., 2012), while antimicrobial compounds constitutively present in plants are referred to as phytoanticipins (Tiku, 2020). Potential phytoalexins and phytoanticipins found in A. thaliana include phenylpropanoids, glucosinolates, terpenoids, and camalexin (Kliebenstein, 2004). Consistent with the likely roles of host secondary metabolites as toxins against M. phaseolina, the genome of this fungus contains a large set of detoxification genes, including genes encoding dehydrogenases, acyl-coA N-acetyltransferases, monooxygenases, and cytochrome P450s (Islam et al., 2012).
One of the major plant metabolic pathways often discussed in relation to pathogen defense is the phenylpropanoid pathway, which is required for the synthesis of diverse phenolic metabolites including lignols, flavonoids, isoflavonoids, and tannins (Fraser and Chapple, 2011; Rehman et al., 2012). Soybean produces a repertoire of phytoalexins, including the isoflavonoid derivatives glyceollins, during both symbiotic and pathogenic microbial entry. Work by Lygin et al. (2013) showed that the downregulation of isoflavonoid biosynthesis, and consequently glyceollins, significantly increases charcoal rot disease severity in soybean. In Medicago truncatula, genes involved in the phenylpropanoid pathway, as well as flavonoid and isoflavonoid biosynthesis were up-regulated dramatically in the shoot but not in the root in response to M. phaseolina infection at 24 and 48 hpi (Gaige et al., 2010). As the authors pointed out, lack of induction of these genes in roots could be the result of pathogen suppression or tissue-specific gene regulation. Nonetheless this shows that M. phaseolina, despite being a soil-borne pathogen, strongly induces systemic upregulation of secondary metabolite synthesis genes. Accumulation of phenolic compounds synchronized with the induced activity of phenylalanine ammonia lyase (PAL), a key player phenylpropanoid pathway, was also seen in the root tissue of plate-grown sesame after infection (Chowdhury et al., 2017). Notably, this induction was stronger in the resistant Nirmala variety and it only occurred after the BNS. It is unknown according to this paper whether similar induction occurred in the shoot tissue. Radadiya et al. (2021) also observed upregulation of genes encoding key phenylpropanoid pathway enzymes in sesame roots post inoculation with M. phaseolina in soil through transcriptome analysis. Both Chowdhury et al. (2017) and Radadiya et al. (2021) reported earlier upregulation of these genes in different resistant varieties, and a delayed response in the susceptible varieties, suggesting that there is a correlation between timing of defense responses and susceptibility to this pathogen.
Glucosinolates, another major class of secondary metabolites, are known for their abundance in plants of the Brassicaceae family, which includes the model plant A. thaliana and several economically important crops like oilseed rape (Brassica napa). The glucosinolate-myrosinase system is a defense mechanism that releases toxic glucosinolate derivatives upon activation by physical damage to plant tissue (Wittstock and Halkier, 2002). This is commonly perceived as a primary defense against herbivores, but it is also known that some isothiocyanates, which are products of the glucosinolate-myrosinase reaction, have fungitoxic properties (Drobnica et al., 1967; Tierens et al., 2001; Smolinska et al., 2003). Cruciferous soil amendments have been used to successfully suppress generalist hemibiotrophic pathogens like F. oxysporum growth and protect crops from disease (Rosa and Rodrigues, 1999), however, it is still unclear whether this effect is the product of chemically active inputs or that of overall changes in the soil microbiome. Brassica seed meal amendment trials with strawberry have had limited success in protecting plants from M. phaseolina (Agostini, 2011; Mazzola et al., 2017). It remains to be demonstrated whether glucosinolate activity in known brassicaceae hosts like A. thaliana, Brassica napa, and Brassica juncea play a role in resistance against M. phaseolina.
Root-specific defense
M. phaseolina is a soil-borne pathogen, which means that the most accessible point of entry for hyphae will be in the root of host plants. While it is widely accepted that the two major plant defense hormones SA and JA act largely mutuality antagonistic, with each pathway corresponding to a particular pathogen life cycle (Glazebrook, 2005), several studies specifically focusing on root responses paint a more complex picture. Inoculation of Phytophthora parasitica on A. thaliana caused a simultaneous upregulation of both SA and JA pathway genes in the root (Attard et al., 2010). Fusarium oxysporum infection repressed many known defense-associated genes in roots, and only three genes overlapped with previously reported microarrays with inoculated leaves (Chen et al., 2014).
It is therefore necessary to mention that generalizations on plant immune responses should be made with caution if the data is derived from whole plants or even whole organs. For example, when JA is applied to Brassica oleracea to mimic wounding, the expression and regulation of known defense mechanisms like secondary metabolite synthesis and phytohormone responses varied depending on tissue type examined and the site of treatment (Tytgat et al., 2013). Root-specific pathogen-induced responses have been reviewed thoroughly by Chuberre et al. (2018), but it has yet to be confirmed whether defense responses to M. phaseolina are also tissue specific. In sorghum, M. phaseolina triggered a greater induction of chitinase genes in the aerial tissue in comparison to roots (Sharma et al., 2014).
Research on M. phaseolina–host relationships and future perspectives
Despite the long history of growers losing crop yield to disease caused by M. phaseolina, sustainable and effective protection strategies against this pathogen are still lacking. Several factors contribute to the obstacles prohibiting the advancement of research in this field, including the high diversity of this pathogen, and the susceptibility of field-generated data to environmental variability. Recently, a high-throughput pathosystem using the model plant A. thaliana has been established, which will likely accelerate research in this field (Schroeder et al., 2019). In addressing pathogen variability, it seems unusual that a monotypic genus can represent the level of genetic and morphological diversity seen in M. phaseolina without evidence of sexual reproduction. F. oxysporum also has not been documented to undergo sexual reproduction but is suspected to preserve its diversity through horizontal gene transfer (Gordon, 2017). Research has led to the following hypothetical scenario: virulent genotypes emerged through significant selection pressures such as agricultural activity while recent relatives that are capable of outcrossing, possibly within populations existing today, sustain a “reservoir” gene pool (Gordon, 2017). Although there is no direct evidence that M. phaseolina fit this model, this analysis may provide critical insights to the evolution of M. phaseolina, considering its striking similarity to F. oxysporum (Islam et al., 2012).
There are decades of records documenting M. phaseolina pathogenicity on a wide range of crop species, including soybean (Smith and Carvil, 1997; Coser et al., 2017; da Silva et al., 2019a), sesame (Chowdhury et al., 2017; Bedawy and Moharm, 2019; Radadiya et al., 2021; Yan et al., 2021), strawberries (Koike, 2008; Burkhardt et al., 2019; Nelson et al., 2021), and more. The advancement of high-throughput sequencing and genome-wide analysis technologies have allowed for the identification of many resistance-associated loci in cultivars of various host species, sampled from infected fields across the globe. Previously, Muchero et al. (2011) identified 9 QTL regions tied to M. phaseolina resistance in cowpea (Vigna unguiculata) through QTL mapping with a recombinant inbred line population. These regions include markers and genes associated with disease resistance homologous to genes in other species including soybean and medicago. Within the candidate gene sets identified, there is a noticeable presence of genes involved in pectin metabolism. A bimodal distribution of disease incidence was observed in castor (Ricinus communis), which suggests that a genetic variant with major effects on the disease resistance trait is present in the population (Tomar et al., 2017). The authors discovered three QTLs through linkage mapping with the most significant region predicted to explain 71.2% of the phenotypic variation, which combined with the phenotypic distribution supports the existence of major effect genes.
More recently, genome-wide association has been used to identify resistance loci in species with available reference genomes. Coser et al. (2017) conducted a disease screen using 459 soybean lines to identify M. phaseolina resistance loci through genome-wide association. In field and greenhouse experiments, a total of 19 SNPs were identified to have a significant association with the resistant phenotype. Orthologous gene regions to A. thaliana were also identified, and these included genes involved in biotic and abiotic stress response, cell wall composition, and ethylene signaling. Surprisingly, there were no overlaps between these SNP regions and QTL regions identified by da Silva et al. (2019b) using QTL-seq, which combines bulk segregant analysis with next-generation sequencing to statistically link loci to a quantitative trait. This could be because the segregating population used for QTL-seq was derived from parental lines (PI567562A and PI567437) that were not included in the set tested by Coser et al. Nonetheless, it should be taken into consideration that factors such as treatment conditions, sequencing methods, and genotypes of both host and pathogen could yield variable QTL. Nelson et al. (2021) identified three loci linked to M. phaseolina resistance from a breeding population obtained from controlled crosses of commercial strawberry. Many gene regions that exhibit strong associations with disease phenotype have not been narrowed down to candidate genes nor have they been assessed for their function. Consequently, evidence for a strong causal relationship between specific genes or molecular mechanisms and M. phaseolina resistance is still lacking. Cross-species synteny analysis may be a useful tool in identifying conserved trait loci.
Experimentation with M. phaseolina has primarily been focused on the field. However recently greenhouse studies have allowed for observation of disease progression in more controlled environments. Young sesame seedlings were inoculated in mycelium mixed soil and kept in artificial climate boxes in a study by Yan et al. (2021) and root tissue was collected for RNA-seq. Schroeder et al. (2019) used agar plates for assessing M. phaseolina interaction with small hosts like A. thaliana seedlings. While many studies have used pre-infected plots to study the relationship between native M. phaseolina and host crops (Miklas et al., 1998; Tomar et al., 2017), various methods of pathogen inoculation have been employed, including mixing mycelium in soil (Bedawy & Moharm, n.d.; Mahmoud et al., 2018), dipping roots in mycelium (Nelson et al., 2021), cut-stem inoculation (Lygin et al., 2013; Pawlowski et al., 2015), and incubating seeds with mycelium (Coser et al., 2017). It has not been evaluated whether the method of pathogen introduction to the host has an effect on the outcome of the infection. Commonly used metrics of disease susceptibility include necrotic lesion size, percent mortality, or overall disease symptom severity quantified by a numeric scoring scale. In order to evaluate the most suitable screening method for M. phaseolina resistance, Mengistu et al. (2007) tested five different disease metrics in soybean for their consistency across experimental years and environments. Colony-forming unit index (CFUI), calculated by counting colony forming units (CFU) derived from a set amount of ground tissue and dividing by the CFU count of the most susceptible genotype (highest CFU) within the experiment, was deemed to be the most consistent across all experimental conditions. This was followed by root and stem symptom severity (RSS), determined by discoloration of stem and taproot tissue, which the authors noted was a less time and resource consuming alternative disease assessment method (Mengistu et al., 2007).
Conclusions
Movement towards reduced chemical use in agriculture is an imperative step towards sustainability. In order to develop resistant varieties of crops, it is necessary to first elucidate functions of specific genes/gene groups and quantify their contribution to host resistance and pathogen virulence. With modern sequencing technologies, high-throughput assay capabilities, and decades of research on M. phaseolina pathology, researchers are equipped to advance our understanding of generalist hemibiotrophic pathogens like F. oxysporum and M. phaseolina, which are notoriously difficult to control. Slowly, we are beginning to understand why M. phaseolina is a successful pathogen. First, its wide host range and prevalence throughout the globe indicate high genomic plasticity that has allowed this fungus to adapt to local conditions and host plant species. This is supported by the presence of repetitive sequences and transposable elements within its genome. Moreover, no evidence for sexual reproduction has been documented for M. phaseolina thus far. This is striking considering the level of genomic variability seen across isolates even from the same host species and geographic origins, and evidence for host specificity seen in multiple examples. Several possible explanations including horizontal gene transfer, sporadic mutations mediated by TEs and repeats, and epigenetics could be hypothesized, but nonetheless it is likely that the instability of the M. phaseolina genome contributes to its extensive virulence capabilities. It is also important to note that the sampling of M. phaseolina in published studies is overwhelmingly biased towards those collected from agricultural fields and may not be an accurate representation of naturally occurring populations. Recently, more and more studies describing M. phaseolina pathosystems, using various isolates and host species, from various levels of genetic regulation are becoming available.
Unfortunately, it seems that the likelihood of finding crop genotypes conferring complete immunity to this pathogen is very low. To date, no specific R-Avr gene interactions have been identified in any host interactions with M. phaseolina. Resistance, if seen at all, seems to be a quantitative trait. Several recurring themes can be identified in the literature, such as: cell wall metabolism, oxidative stress and detoxification. These known plant immune responses potentially contribute partially to the overall disease phenotype. Through what mechanisms, and to what extent these biological processes impact the phenotype remains to be answered. As M. phaseolina is a generalist pathogen, it may benefit researchers to view defense against this pathogen from a generalist perspective.
Assuming that host species of this pathogen possess similar basic forms of defense and that canonical plant defense-related signal transduction pathways are intact, temporal parameters are likely to determine the fate of the host plant: the speed at which host reacts to the pathogen, and the duration of the subsequent response. Moreover, it must be taken into account that each cell in the plant experiences pathogen invasion differently and that systemic defense activation is a gradient. Neither of these temporal parameters, to our knowledge, have been studied at the tissue nor cell type-specific level in M. phaseolina pathosystems. Spatio-temporal analysis of host defense gene activation, as well as an in-depth examination of the M. phaseolina secretome, are potential avenues of further research in this field.
Author contributions
TE: Conceptualization, Funding acquisition, Supervision, Writing – review & editing. MS: Conceptualization, Writing – original draft, Writing – review & editing.
Acknowledgments
Work on this article and work in TE’s lab on M. phaseolina was supported by National Science Foundation (NSF) grant IOS-2129302 to TE.
Conflict of interest
The authors declare that the research was conducted in the absence of any commercial or financial relationships that could be construed as a potential conflict of interest.
Publisher’s note
All claims expressed in this article are solely those of the authors and do not necessarily represent those of their affiliated organizations, or those of the publisher, the editors and the reviewers. Any product that may be evaluated in this article, or claim that may be made by its manufacturer, is not guaranteed or endorsed by the publisher.
References
Abawi, G. S., Corrales (1990). Root rots of beans in latin America and Africa: Diagnosis, research methodologies, and management strategies (Cali, Columbia: CIAT).
Abbas, H. K., Bellaloui, N., Accinelli, C., Smith, J. R., Shier, W. T. (2019). Toxin Production in Soybean (Glycine max L.) Plants with Charcoal Rot Disease and by Macrophomina phaseolina, the Fungus that Causes the Disease. Toxins 11 (11), 645. doi: 10.3390/toxins11110645
Acharya, R., Acharya, K. (2007). Evaluation of nitric oxide synthase status during disease progression in resistant and susceptible varieties of Sesamum indicum against Macrophomina phaseolina. Asian Australas. J. Plant Sci. Biotechnol. 1, 61–63.
Agostini, A. (2011) Effects of Brassicaceae Seed Meal Incorporation on Pre-plant Weed Emergence and Strawberry Productivity in Macrophomina Phaseolina infested and Non-infested Soil. Available at: http://sonoma-dspace.calstate.edu/handle/10211.1/1462.
Ahmad, Y., Hameed, A., Ghaffar, A. (2006). Enzymatic activity of fungal pathogens in corn. Pakistan J. Bot. 38 (4), 1305–1316.
Ahuja, I., Kissen, R., Bones, A. M. (2012). Phytoalexins in defense against pathogens. Trends Plant Sci. 17 (2), 73–90. doi: 10.1016/j.tplants.2011.11.002
Alston, J. M., Pardey, P. G. (2008). Public funding for research into specialty crops. HortScience: A Publ. Am. Soc. Hortic. Sci. 43 (5), 1461–1470. doi: 10.21273/HORTSCI.43.5.1461
Ammon, V., Wyllie, T. D., Brown, M. F. (1974). An ultrastructural investigation of pathological alterations induced by Macrophomina phaseolina (Tassi) Goid in seedlings of soybean, Glycine max (L.) Merrill. Physiol. Plant Pathol. 4 (1), 1–4. doi: 10.1016/0048-4059(74)90038-1
Arafat, M. Y., Narula, K., Nalwa, P., Sengupta, A., Chakraborty, N., Chakraborty, S. (2022). Proteomic analysis of phytopathogenic fungus Macrophomina phaseolina identify known and novel mycelial proteins with roles in growth and virulence. J. Proteins Proteomics 13 (3), 149–157. doi: 10.1007/s42485-022-00095-0
Arora, P., Dilbaghi, N., Chaudhury, A. (2012). Opportunistic invasive fungal pathogen Macrophomina phaseolina prognosis from immunocompromised humans to potential mitogenic RBL with an exceptional and novel antitumor and cytotoxic effect. Eur. J. Clin. Microbiol. Infect. Diseases: Off. Publ. Eur. Soc. Clin. Microbiol. 31 (2), 101–107. doi: 10.1007/s10096-011-1275-1
Asai, S., Mase, K., Yoshioka, H. (2010). Role of nitric oxide and reactive oxygen species in disease resistance to necrotrophic pathogens. Plant Signaling Behav. 5 (7), 872–874. doi: 10.4161/psb.5.7.11899
Asai, S., Yoshioka, H. (2009). Nitric Oxide as a Partner of Reactive Oxygen Species Participates in Disease Resistance to Necrotrophic Pathogen Botrytis cinerea in Nicotiana benthamiana. Mol. Plant-Microbe Interactions: MPMI 22 (6), 619–629. doi: 10.1094/MPMI-22-6-0619
Attard, A., Gourgues, M., Callemeyn-Torre, N., Keller, H. (2010). The immediate activation of defense responses in Arabidopsis roots is not sufficient to prevent Phytophthora parasitica infection. New Phytol. 187 (2), 449–460. doi: 10.1111/j.1469-8137.2010.03272.x
Baird, R. E., Wadl, P. A., Allen, T., McNeill, D., Wang, X., Moulton, J. K., et al. (2010). Variability of United States isolates of Macrophomina phaseolina based on simple sequence repeats and cross genus transferability to related genera within botryosphaeriaceae. Mycopathologia 170 (3), 169–180. doi: 10.1007/s11046-010-9308-3
Bandara, A. Y., Weerasooriya, D. K., Bradley, C. A., Allen, T. W., Esker, P. D. (2020). Dissecting the economic impact of soybean diseases in the United States over two decades. PLoS One 15 (4), e0231141. doi: 10.1371/journal.pone.0231141
Bari, R., Jones, J. D. G. (2009). Role of plant hormones in plant defense responses. Plant Mol. Biol. 69 (4), 473–488. doi: 10.1007/s11103-008-9435-0
Bashir, M., Malik, B. A. (1988). Diseases of major pulse crops in Pakistan—a review. Trop. Pest Manage. 34 (3), 309–314. doi: 10.1080/09670878809371262
Bedawy, I., Moharm, M. (2019). Reaction and performance of some sesame genotypes for resistance to Macrophomina phaseolina, the incitant of charcoal rot disease. Alexandria Sci. Exchange J. 40 (JANUARY-MARCH), 12–18. doi: 10.21608/asejaiqjsae.2019.26294
Bhattacharya, D., Dhar, T. K., Ali, E. (1992). An enzyme immunoassay of phaseolinone and its application in estimation of the amount of toxin in Macrophomina phaseolina-infected seeds. Appl. Environ. Microbiol. 58 (6), 1970–1974. doi: 10.1128/aem.58.6.1970-1974.1992
Bhattacharya, D., Dhar, T. K., Siddiqui, K. A. I., Ali, E. (1994). Inhibition of seed germination by Macrophomina phaseolina is related to phaseolinone production. J. Appl. Bacteriology 77 (2), 129–133. doi: 10.1111/j.1365-2672.1994.tb03055.x
Bigeard, J., Colcombet, J., Hirt, H. (2015). Signaling mechanisms in pattern-triggered immunity (PTI). Mol. Plant 8 (4), 521–539. doi: 10.1016/j.molp.2014.12.022
Bojórquez-Armenta, Y., de, J., Mora-Romero, G. A., López-Meyer, M., Maldonado-Mendoza, I. E., Castro-Martínez, C., et al. (2021). Evaluation of Bacillus spp. isolates as potential biocontrol agents against charcoal rot caused by Macrophomina phaseolina on common bean. J. Gen. Plant Pathology: JGPP 87 (6), 377–386. doi: 10.1007/s10327-021-01019-4
Bokor, P. (2007). Macrophomina phaseolina causing a charcoal rot of sunflower through Slovakia. Biologia 62 (2), 136–138. doi: 10.2478/s11756-007-0020-9
Breitenbach, M., Weber, M., Rinnerthaler, M., Karl, T., Breitenbach-Koller, L. (2015). Oxidative stress in fungi: its function in signal transduction, interaction with plant hosts, and lignocellulose degradation. Biomolecules 5 (2), 318–342. doi: 10.3390/biom5020318
Bressano, M., Lorena Giachero, M., Luna, C. M., Ducasse, D. A. (2010). An in vitro method for examining infection of soybean roots by Macrophomina phaseolina. Physiol. Mol. Plant Pathol. 74 (3), 201–204. doi: 10.1016/j.pmpp.2009.12.003
Brunner, F., Rosahl, S., Lee, J., Rudd, J. J., Geiler, C., Kauppinen, S., et al. (2002). Pep-13, a plant defense-inducing pathogen-associated pattern from Phytophthora transglutaminases. EMBO J. 21 (24), 6681–6688. doi: 10.1093/emboj/cdf667
Burkhardt, A. K., Childs, K. L., Wang, J., Ramon, M. L., Martin, F. N. (2019). Assembly, annotation, and comparison of Macrophomina phaseolina isolates from strawberry and other hosts. BMC Genomics 20 (1), 802. doi: 10.1186/s12864-019-6168-1
California Department of Food, & Agriculture (2018) CDFA > STATISTICS. Available at: https://www.cdfa.ca.gov/statistics/?xid=PS_smithsonian.
Caseys, C., Shi, G., Soltis, N., Gwinner, R., Corwin, J., Atwell, S., et al. (2021). Quantitative interactions: the disease outcome of Botrytis cinerea across the plant kingdom. G3 11 (8). doi: 10.1093/g3journal/jkab175
Chamorro, M., Domínguez, P., Medina, J. J., MIranda, L., Soria, C., Romero, F., et al. (2015). Assessment of chemical and biosolarization treatments for the control of Macrophomina phaseolina in strawberries. Scientia Hortic. 192, 361–368. doi: 10.1016/j.scienta.2015.03.029
Chamorro, M., Seijo, T. E., Noling, J. C., De los Santos, B., Peres, N. A. (2016). Efficacy of fumigant treatments and inoculum placement on control of Macrophomina phaseolina in strawberry beds. Crop Prot., 163–169. doi: 10.1016/j.cropro.2016.08.020
Chan, Y.-H., Sackston, W. E. (1969). Mechanisms of pathogenesis in Sclerotium bataticola on sunflowers. I. Production and translocation of a necrosis-inducing toxin. Can. J. Botany. J. Canadien Botanique 47 (7), 1147–1151. doi: 10.1139/b69-159
Chan, Y.-H., Sackston, W. E. (1970). Mechanisms of pathogenesis in Sclerotium bataticola on sunflowers. II. Pectolytic and cellulolytic enzyme production in vitro and in vivo. Can. J. Bot. 48 (6), 1073–1077. doi: 10.1139/b70-155
Chen, Y. C., Wong, C. L., Muzzi, F., Vlaardingerbroek, I., Kidd, B. N., Schenk, P. M. (2014). Root defense analysis against Fusarium oxysporum reveals new regulators to confer resistance. Sci. Rep. 4 (1), 1–10. doi: 10.1038/srep05584
Chisholm, S. T., Coaker, G., Day, B., Staskawicz, B. J. (2006). Host-microbe interactions: shaping the evolution of the plant immune response. Cell 124 (4), 803–814. doi: 10.1016/j.cell.2006.02.008
Chooi, Y.-H., Tang, Y. (2012). Navigating the fungal polyketide chemical space: from genes to molecules. J. Organic Chem. 77 (22), 9933–9953. doi: 10.1021/jo301592k
Chowdhury, S., Basu, A., Ray Chaudhuri, T., Kundu, S. (2014). In-vitro characterization of the behaviour of Macrophomina phaseolina (Tassi) Goid at the rhizosphere and during early infection of roots of resistant and susceptible varieties of sesame. European Journal of Plant Pathology / European Foundation for Plant Pathology 138 (2), 361–375.
Chowdhury, S., Basu, A., Kundu, S. (2017). Biotrophy-necrotrophy switch in pathogen evoke differential response in resistant and susceptible sesame involving multiple signaling pathways at different phases. Sci. Rep. 7 (1), 17251. doi: 10.1038/s41598-017-17248-7
Chuberre, C., Plancot, B., Driouich, A., Moore, J. P., Bardor, M., Gügi, B., et al. (2018). Plant immunity is compartmentalized and specialized in roots. Front. Plant Sci. 9, 1692. doi: 10.3389/fpls.2018.01692
Clarke, J. D., Volko, S. M., Ledford, H., Ausubel, F. M., Dong, X. (2000). Roles of Salicylic acid, Jasmonic acid, and Ethylene in cpr-Induced Resistance in Arabidopsis. Plant Cell 12 (11), 2175–2190. doi: 10.1105/tpc.12.11.2175
Coser, S. M., Chowda Reddy, R. V., Zhang, J., Mueller, D. S., Mengistu, A., Wise, K. A., et al. (2017). Genetic architecture of charcoal rot (Macrophomina phaseolina) resistance in soybean revealed using a diverse panel. Front. Plant Sci. 8, 1626. doi: 10.3389/fpls.2017.01626
Dabi, M., Agarwal, P., Agarwal, P. K. (2020). Overexpression of JcWRKY2 confers increased resistance towards Macrophomina phaseolina in transgenic tobacco. 3 Biotech. 10 (11), 490. doi: 10.1007/s13205-020-02490-0
Dangl, J. L., Jones, J. D. (2001). Plant pathogens and integrated defence responses to infection. Nature 411 (6839), 826–833. doi: 10.1038/35081161
Das, I. K., Indira, S., Annapurna, A., Prabhakar, Seetharama, N. (2008). Biocontrol of charcoal rot in sorghum by fluorescent pseudomonads associated with the rhizosphere. Crop Prot. 27 (11), 1407–1414. doi: 10.1016/j.cropro.2008.07.001
da Silva, M. P., Klepadlo, M., Gbur, E. E., Pereira, A., Mason, R. E., Rupe, J. C., et al. (2019a). QTL mapping of charcoal rot resistance in PI 567562A soybean accession. Crop Sci. 59 (2), 474–479. doi: 10.2135/cropsci2018.02.0145
da Silva, M. P., Zaccaron, A. Z., Bluhm, B. H., Rupe, J. C., Wood, L., Mozzoni, L. A., et al. (2019b). Bulked segregant analysis using next-generation sequencing for identification of genetic loci for charcoal rot resistance in soybean. Physiol. Mol. Plant Pathol., 101440. doi: 10.1016/j.pmpp.2019.101440
Delledonne, M., Xia, Y., Dixon, R. A., Lamb, C. (1998). Nitric oxide functions as a signal in plant disease resistance. Nature 394 (6693), 585–588. doi: 10.1038/29087
Delledonne, M., Zeier, J., Marocco, A., Lamb, C. (2001). Signal interactions between nitric oxide and reactive oxygen intermediates in the plant hypersensitive disease resistance response. Proc. Natl. Acad. Sci. United States America 98 (23), 13454–13459. doi: 10.1073/pnas.231178298
de los Santos, B., Aguado, A., Borrero, C., Viejobueno, J., Avilés, M. (2019). First report of charcoal rot, caused by macrophomina phaseolina, on blueberry in southwestern Spain. Plant Dis. 03 (10), 2677–2677. doi: 10.1094/PDIS-04-19-0761-PDN
Dhar, T. K., Siddiqui, K. A. I., Ali, E. (1982). Structure of phaseolinone, a novel phytotoxin from macrophomina phaseolina. Tetrahedron Lett. 23 (51), 5459–5462. doi: 10.1016/0040-4039(82)80157-3
Dhingra, O. D., Tenne, F. D., Sinclair, J. B. (1976). Method for the determination of competitive saprophytic colonization of soil fungi. Trans. Br. Mycological Soc. 66 (3), 447–456. doi: 10.1016/S0007-1536(76)80215-X
Drobnica, L., Zemanová, M., Nemec, P., Antos, K., Kristián, P., Stullerová, A., et al. (1967). Antifungal activity of isothiocyanates and related compounds. I. Naturally occurring isothiocyanates and their analogues. Appl. Microbiol. 15 (4), 701–709. doi: 10.1128/am.15.4.701-709.1967
Durrant, W. E., Dong, X. (2004). Systemic acquired resistance. Annu. Rev. Phytopathol. 42, 185–209. doi: 10.1146/annurev.phyto.42.040803.140421
Fang, X., Phillips, D., Li, H., Sivasithamparam, K., Barbetti, M. J. (2011). Comparisons of virulence of pathogens associated with crown and root diseases of strawberry in Western Australia with special reference to the effect of temperature. Scientia Hortic. 131, 39–48. doi: 10.1016/j.scienta.2011.09.025
Fraser, C. M., Chapple, C. (2011). The phenylpropanoid pathway in Arabidopsis. The Arabidopsis Book / American Society of Plant Biologists, 9, e0152.
Gaetán, S. A., Fernandez, L., Madia, M. (2006). Occurrence of charcoal rot caused by macrophomina phaseolina on canola in Argentina. Plant Dis. 90 (4), 524. doi: 10.1094/PD-90-0524A
Gaige, A. R., Ayella, A., Shuai, B. (2010). Methyl jasmonate and ethylene induce partial resistance in Medicago truncatula against the charcoal rot pathogen Macrophomina phaseolina. Physiol. Mol. Plant Pathol. 74 (5), 412–418. doi: 10.1016/j.pmpp.2010.07.001
Gao, Y., Xie, M., Yu, C., Zhang, M., Huang, J., Li, Q., et al. (2021). Heterologous expression of macrollins from phytopathogenic Macrophomina phaseolina revealed a cytochrome P450 mono-oxygenase in the biosynthesis of β-hydroxyl tetramic acid. J. Agric. Food Chem. 69 (50), 15175–15183. doi: 10.1021/acs.jafc.1c05304
Gaulin, E., Jauneau, A., Villalba, F., Rickauer, M., Esquerré-Tugayé, M.-T., Bottin, A. (2002). The CBEL glycoprotein of Phytophthora parasitica var-nicotianae is involved in cell wall deposition and adhesion to cellulosic substrates. J. Cell Sci. 115 (Pt 23), 4565–4575. doi: 10.1242/jcs.00138
Glazebrook, J. (2005). Contrasting mechanisms of defense against biotrophic and necrotrophic pathogens. Annu. Rev. Phytopathol. 43, 205–227. doi: 10.1146/annurev.phyto.43.040204.135923
Gomez, A. O., De Faveri, J., Neal, J. M., Aitken, E. A. B., Herrington, M. E. (2020). Response of Strawberry Cultivars Inoculated with Macrophomina phaseolina in Australia. International Journal of Fruit Science 20 (sup2), 164–177.
Gómez-Gómez, L., Boller, T. (2000). FLS2: an LRR receptor-like kinase involved in the perception of the bacterial elicitor flagellin in Arabidopsis. Mol. Cell 5 (6), 1003–1011. doi: 10.1016/S1097-2765(00)80265-8
Gordon, T. R. (2017). Fusarium oxysporum and the fusarium wilt syndrome. Annu. Rev. Phytopathol. 55, 23–39. doi: 10.1146/annurev-phyto-080615-095919
Gupta, C., Dubey, R., Maheshwari, D. (2002). Plant growth enhancement and suppression of Macrophomina phaseolina causing charcoal rot of peanut by fluorescent. Pseudomonas. Biol. Fertility Soils 35 (6), 399–405. doi: 10.1007/s00374-002-0486-0
Guthman, J. (2017). Land access and costs may drive strawberry growers’ increased use of fumigation. California Agric. 71 (3), 184–191. doi: 10.3733/ca.2017a0017
Hamann, T. (2012). Plant cell wall integrity maintenance as an essential component of biotic stress response mechanisms. Front. Plant Sci. 3, 77. doi: 10.3389/fpls.2012.00077
Holmes, G. J., Mansouripour, S. M., Hewavitharana, S. S. (2020). Strawberries at the crossroads: management of soilborne diseases in california without methyl bromide. Phytopathology 110 (5), 956–968. doi: 10.1094/PHYTO-11-19-0406-IA
Hossen, Q. M. M., Islam, M. S., Emdadul, E. M., Haque, S., Alam, M., Alam, M. (2019). Whole-genome optical mapping: Improving assembly of Macrophomina phaseolina MS6 through spanning of twelve blunt end chromosomes by obviating all errors and misassembles. Afr. J. Biotechnol. 18 (31), 1031–1043. doi: 10.5897/AJB2019.16978
Hou, S., Liu, Z., Shen, H., Wu, D. (2019). Damage-associated molecular pattern-triggered immunity in plants. Front. Plant Sci. 10, 646. doi: 10.3389/fpls.2019.00646
Islam, M. S., Haque, M. S., Islam, M. M., Emdad, E. M., Halim, A., Hossen, Q. M. M., et al. (2012). Tools to kill: genome of one of the most destructive plant pathogenic fungi Macrophomina phaseolina. BMC Genomics 13, 493. doi: 10.1186/1471-2164-13-493
Jackson, M. A., Jaronski, S. T. (2009). Production of microsclerotia of the fungal entomopathogen Metarhizium anisopliae and their potential for use as a biocontrol agent for soil-inhabiting insects. Mycological Res. 113 (Pt 8), 842–850. doi: 10.1016/j.mycres.2009.03.004
Jana, T., Sharma, T. R., Singh, N. K. (2005). SSR-based detection of genetic variability in the charcoal root rot pathogen Macrophomina phaseolina. Mycological Res. 109 (Pt 1), 81–86. doi: 10.1017/S0953756204001364
Jones, J. D. G., Dangl, J. L. (2006). The plant immune system. Nature 444 (7117), 323–329. doi: 10.1038/nature05286
Kaiser, S. A. K. M., Das, S. N. (1988). Physical factors that influence the growth and spread of charcoal rot pathogen (Macrophomina phaseolina) infecting maize. Phytopathologische Zeitschrift. J. Phytopathol. 123 (1), 47–51. doi: 10.1111/j.1439-0434.1988.tb01035.x
Kaur, S., Dhillon, G. S., Brar, S. K., Chauhan, V. B. (2012a). Carbohydrate degrading enzyme production by plant pathogenic mycelia and microsclerotia isolates of Macrophomina phaseolina through koji fermentation. Ind. Crops Products 36 (1), 140–148. doi: 10.1016/j.indcrop.2011.08.020
Kaur, S., Dhillon, G. S., Brar, S. K., Vallad, G. E., Chand, R., Chauhan, V. B. (2012b). Emerging phytopathogen Macrophomina phaseolina: biology, economic importance and current diagnostic trends. Crit. Rev. Microbiol. 38 (2), 136–151. doi: 10.3109/1040841X.2011.640977
Khaledi, N., Taheri, P. (2016). Biocontrol mechanisms of Trichoderma harzianum against soybean charcoal rot caused by Macrophomina phaseolina. J. Plant Prot. Res. 56 (1), 21–31. doi: 10.1515/jppr-2016-0004
Khalili, E., Javed, M. A., Huyop, F., Rayatpanah, S., Jamshidi, S., Wahab, R. A. (2016). Evaluation of Trichoderma isolates as potential biological control agent against soybean charcoal rot disease caused by Macrophomina phaseolina. Biotechnology Biotechnol. Equip. 30 (3), 479–488. doi: 10.1080/13102818.2016.1147334
Khambhati, V. H., Abbas, H. K., Sulyok, M., Tomaso-Peterson, M., Shier, W. T. (2020). First report of the production of mycotoxins and other secondary metabolites by Macrophomina phaseolina (Tassi) Goid. Isolates from soybeans (Glycine max L.) symptomatic with charcoal rot disease. J. Fungi 6 (4), 332. doi: 10.3390/jof6040332
Kliebenstein, D. J. (2004). Secondary metabolites and plant/environment interactions: a view through Arabidopsis thaliana tinged glasses. Plant Cell Environ. 27 (6), 675–684. doi: 10.1111/j.1365-3040.2004.01180.x
Koike, S. T. (2008). Crown rot of strawberry caused by macrophomina phaseolina in california. Plant Dis. 92 (8), 1253–1253. doi: 10.1094/PDIS-92-8-1253B
Koike, S. T., Arias, R. S., Hogan, C. S., Martin, F. N., Gordon, T. R. (2016). Status of macrophomina phaseolina on strawberry in California and preliminary characterization of the pathogen. Int. J. Fruit Sci. 16 (sup1), 148–159. doi: 10.1080/15538362.2016.1195313
Koornneef, A., Pieterse, C. M. J. (2008). Cross talk in defense signaling. Plant Physiol. 146 (3), 839–844. doi: 10.1104/pp.107.112029
Kubicek, C. P., Starr, T. L., Glass, N. L. (2014). Plant cell wall–degrading enzymes and their secretion in plant-pathogenic fungi. Annu. Rev. Phytopathol. 52 (1), 427–451. doi: 10.1146/annurev-phyto-102313-045831
Lakhran, L., Ahir, R. R., Choudhary, M., Choudhary, S. (2018). Isolation, purification, identification and pathogenicity of Macrophomina phaseolina (Tassi) goid caused dry root rot of chickpea. J. Pharmacognosy Phytochem. 7 (3), 3314–3317.
Levine, A., Tenhaken, R., Dixon, R., Lamb, C. (1994). H2O2 from the oxidative burst orchestrates the plant hypersensitive disease resistance response. Cell 79 (4), 583–593. doi: 10.1016/0092-8674(94)90544-4
Liu, H.-H., Huang, C.-C., Lin, Y.-H., Tseng, M.-N., Chang, H.-X. (2022). Superoxide initiates the hyphal differentiation to microsclerotia formation of macrophomina phaseolina. Microbiol. Spectr. 10 (1), e0208421. doi: 10.1128/spectrum.02084-21
Lodha, S., Mawar, R. (2020). Population dynamics of Macrophomina phaseolina in relation to disease management: A review. Phytopathologische Zeitschrift. J. Phytopathol. 168 (1), 1–17. doi: 10.1111/jph.12854
Lo Presti, L., Lanver, D., Schweizer, G., Tanaka, S., Liang, L., Tollot, M., et al. (2015). Fungal effectors and plant susceptibility. Annu. Rev. Plant Biol. 66, 513–545. doi: 10.1146/annurev-arplant-043014-114623
Lorito, M., Woo, S. L., Harman, G. E., Monte, E. (2010). Translational research on Trichoderma: from ’omics to the field. Annu. Rev. Phytopathol. 48, 395–417. doi: 10.1146/annurev-phyto-073009-114314
Lygin, A. V., Zernova, O. V., Hill, C. B., Kholina, N. A., Widholm, J. M., Hartman, G. L., et al. (2013). Glyceollin is an important component of soybean plant defense against Phytophthora sojae and Macrophomina phaseolina. Phytopathology 103 (10), 984–994. doi: 10.1094/PHYTO-12-12-0328-R
Ma, J., Hill, C. B., Hartman, G. L. (2010). Production of macrophomina phaseolina conidia by multiple soybean isolates in culture. Plant Dis. 94 (9), 1088–1092. doi: 10.1094/PDIS-94-9-1088
Macho, A. P., Zipfel, C. (2014). Plant PRRs and the activation of innate immune signaling. Mol. Cell 54 (2), 263–272. doi: 10.1016/j.molcel.2014.03.028
Mahato, S. B., Siddiqui, K. A. I., Bhattacharya, G., Ghosal, T., Miyahara, K., Sholichin, M., et al. (1987). Structure and stereochemistry of phaseolinic acid: A new acid from macrophomina phaseolina. J. Natural Products 50 (2), 245–247. doi: 10.1021/np50050a024
Mahmoud, A. F., Abou-Elwafa, S. F., Shehzad, T. (2018). Identification of charcoal rot resistance QTLs in sorghum using association and in silico analyses. J. Appl. Genet. 59 (3), 243–251. doi: 10.1007/s13353-018-0446-5
Majumder, S., Datta, K., Sarkar, C., Saha, S. C., Datta, S. K. (2018). The development of macrophomina phaseolina (Fungus) resistant and glufosinate (Herbicide) tolerant transgenic jute. Front. Plant Sci. 9, 920. doi: 10.3389/fpls.2018.00920
Marquez, N., Giachero, M. L., Declerck, S., Ducasse, D. A. (2021). Macrophomina phaseolina: general characteristics of pathogenicity and methods of control. Front. Plant Sci. 12, 634397. doi: 10.3389/fpls.2021.634397
Marquez, N., Giachero, M. L., Gallou, A., Debat, H. J., Cranenbrouck, S., Di Rienzo, J. A., et al. (2018). Transcriptional Changes in Mycorrhizal and Nonmycorrhizal Soybean Plants upon Infection with the Fungal Pathogen Macrophomina phaseolina. Mol. Plant-Microbe Interactions: MPMI 31 (8), 842–855. doi: 10.1094/MPMI-11-17-0282-R
Masi, M., Sautua, F., Zatout, R., Castaldi, S., Arrico, L., Isticato, R., et al. (2021). Phaseocyclopentenones A and B, phytotoxic penta- and tetrasubstituted cyclopentenones produced by macrophomina phaseolina, the causal agent of charcoal rot of soybean in Argentina. J. Natural Products 84 (2), 459–465. doi: 10.1021/acs.jnatprod.0c01287
Mayek-Pérez, N., GarcÍa-Espinosa, R., LÓpez-CastaÑeda, C., Acosta-Gallegos, J. A., Simpson, J. (2002). Water relations, histopathology and growth of common bean (Phaseolus vulgaris L.) during pathogenesis of Macrophomina phaseolina under drought stress. Physiol. Mol. Plant Pathol. 60 (4), 185–195. doi: 10.1006/pmpp.2001.0388
Mayék-Pérez, N., López-Castañeda, C., González-Chavira, M., Garcia-Espinosa, R., Acosta-Gallegos, J., de la Vega, O. M., et al. (2001). Variability of Mexican isolates of Macrophomina phaseolina based on pathogenesis and AFLP genotype. Physiol. Mol. Plant Pathol. 59 (5), 257–264. doi: 10.1006/pmpp.2001.0361
Mazzola, M., Agostini, A., Cohen, M. F. (2017). Incorporation of Brassica seed meal soil amendment and wheat cultivation for control of Macrophomina phaseolina in strawberry. Eur. J. Plant Pathol. / Eur. Foundation Plant Pathol. 149 (1), 57–71. doi: 10.1007/s10658-017-1166-0
Mengistu, A., Ray, J. D., Smith, J. R., Paris, R. L. (2007). Charcoal rot disease assessment of soybean genotypes using a colony-forming unit index. Crop Sci. 47, 2453–2461. doi: 10.2135/cropsci2007.04.0186
Mertely, J., Seijo, T., Peres, N. (2005). First report of Macrophomina phaseolina causing a crown rot of strawberry in Florida. Plant Dis. 89 (4), 434–434. doi: 10.1094/PD-89-0434A
Mihail, J. D., Taylor, S. J. (1995). Interpreting variability among isolates of Macrophomina phaseolina in pathogenicity, pycnidium production, and chlorate utilization. Can. J. Botany. J. Canadien Botanique 73 (10), 1596–1603. doi: 10.1139/b95-172
Miklas, P. N., Stone, V., Urrea, C. A., Johnson, E., Beaver, J. S. (1998). Inheritance and QTL analysis of field resistance to ashy stem blight in common bean. Crop Sci. 38 (4), 916–921. doi: 10.2135/cropsci1998.0011183X003800040004x
Msikita, W., James, B., Wilkinson, H. T., Juba, J. H. (1998). First report of macrophomina phaseolina causing pre-harvest cassava root rot in Benin and Nigeria. Plant Dis. 82 (12), 1402–1402. doi: 10.1094/PDIS.1998.82.12.1402C
Muchero, W., Ehlers, J. D., Close, T. J., Roberts, P. A. (2011). Genic SNP markers and legume synteny reveal candidate genes underlying QTL for Macrophomina phaseolina resistance and maturity in cowpea [Vigna unguiculata (L) Walp.]. BMC Genomics 12, 8. doi: 10.1186/1471-2164-12-8
Mughogho, L. K., Pande, S. (1983). Charcoal Rot of Sorghum. Sorghum Root and Stalk Rots, a Critical Review, 11–24.
Mur, L. A. J., Kenton, P., Atzorn, R., Miersch, O., Wasternack, C. (2006). The outcomes of concentration-specific interactions between salicylate and jasmonate signaling include synergy, antagonism, and oxidative stress leading to cell death. Plant Physiol. 140 (1), 249–262. doi: 10.1104/pp.105.072348
Neill, C. L., Morgan, K. L. (2021). Beyond scale and scope: Exploring economic drivers of U.S. Specialty crop production with an application to edamame. Front. Sustain. Food Syst. 4. doi: 10.3389/fsufs.2020.582834
Nelson, J. R., Verma, S., Bassil, N. V., Finn, C. E., Hancock, J. F., Cole, G. S., et al. (2021). Discovery of three loci increasing resistance to charcoal rot caused by Macrophomina phaseolina in octoploid strawberry. G3. doi: 10.1093/g3journal/jkab037
Nouri, M. T., Lawrence, D. P., Kallsen, C. E., Trouillas, F. P. (2020). Macrophomina crown and root rot of pistachio in California. Plants 9 (2), 134. doi: 10.3390/plants9020134
Nürnberger, T., Brunner, F., Kemmerling, B., Piater, L. (2004). Innate immunity in plants and animals: striking similarities and obvious differences. Immunol. Rev. 198, 249–266. doi: 10.1111/j.0105-2896.2004.0119.x
Pal, K. K., McSpadden Gardener, B. (2006). Biological control of plant pathogens. Plant Health Instructor. doi: 10.1094/phi-a-2006-1117-02
Pandey, A. K., Basandrai, A. K. (2021). Will Macrophomina phaseolina spread in legumes due to climate change? A critical review of current knowledge. J. Plant Dis. Protection: Sci. J. German Phytomedical Soc. 128 (1), 9–18. doi: 10.1007/s41348-020-00374-2
Pawlowski, M. L., Hill, C. B., Hartman, G. L. (2015). Resistance to charcoal rot identified in ancestral soybean germplasm. Crop Sci. 55, 1230–1235. doi: 10.2135/cropsci2014.10.0687
Pearson, C. A. S., Leslie, J. F., Schwenk, F. W. (1986). Variable chlorate resistance in Macrophomina phaseolina from corn, soybean, and soil. Phytopathology 76 (6), 646–649. doi: 10.1094/Phyto-76-646
Pearson, C. A. S., Leslie, J. F., Schwenk, F. W. (1987). Nitrogen source utilization by chlorate-resistant and chlorate-sensitive isolates of Macrophomina phaseolina. Trans. Br. Mycological Soc. 88 (4), 497–502. doi: 10.1016/S0007-1536(87)80033-5
Perchepied, L., Balagué, C., Riou, C., Claudel-Renard, C., Rivière, N., Grezes-Besset, B., et al. (2010). Nitric oxide participates in the complex interplay of defense-related signaling pathways controlling disease resistance to Sclerotinia sclerotiorum in Arabidopsis thaliana. Mol. Plant-Microbe Interactions: MPMI 23 (7), 846–860. doi: 10.1094/MPMI-23-7-0846
Pieterse, C. M. J., van der Does, D., Zamioudis, C., Leon-Reyes, A., Van Wees, S. C. M. (2012). Hormonal modulation of plant immunity. Annu. Rev. Cell Dev. Biol. 28, 489–521. doi: 10.1146/annurev-cellbio-092910-154055
Pieterse, C. M., van Wees, S. C., Hoffland, E., van Pelt, J. A., van Loon, L. C. (1996). Systemic resistance in Arabidopsis induced by biocontrol bacteria is independent of salicylic acid accumulation and pathogenesis-related gene expression. Plant Cell 8 (8), 1225–1237. doi: 10.1105/tpc.8.8.1225
Pineda-Fretez, A., Orrego, A., Iehisa, J. C. M., Flores-Giubi, M. E., Barúa, J. E., Sánchez-Lucas, R., et al. (2023). Secretome analysis of the phytopathogen Macrophomina phaseolina cultivated in liquid medium supplemented with and without soybean leaf infusion. Fungal Biol. 127 (5), 1043–1052. doi: 10.1016/j.funbio.2023.04.001
Poveda, J., Hermosa, R., Monte, E., Nicolás, C. (2019). Trichoderma harzianum favours the access of arbuscular mycorrhizal fungi to non-host Brassicaceae roots and increases plant productivity. Sci. Rep. 9 (1), 11650. doi: 10.1038/s41598-019-48269-z
Pratt, R. G., McLaughlin, M. R., Pederson, G. A., Rowe, D. E. (1998). Pathogenicity of macrophomina phaseolina to mature plant tissues of alfalfa and white clover. Plant Dis. 82 (9), 1033–1038. doi: 10.1094/PDIS.1998.82.9.1033
Purushotham, N., Jones, A., Poudel, B., Nasim, J., Adorada, D., Sparks, A., et al. (2020). Draft genome resource for macrophomina phaseolina associated with charcoal rot in Sorghum. Mol. Plant-Microbe Interactions: MPMI 33 (5), 724–726. doi: 10.1094/MPMI-12-19-0356-A
Radadiya, N., Mangukia, N., Antala, V., Desai, H., Chaudhari, H., Dholaria, T. L., et al. (2021). Transcriptome analysis of sesame-Macrophomina phaseolina interactions revealing the distinct genetic components for early defense responses. Physiol. Mol. Biol. Plants: Int. J. Funct. Plant Biol. 27 (8), 1675–1693. doi: 10.1007/s12298-021-01039-6
Radha, K. (1953). The enzymic activity of Macrophomina phaseoli,(Maubl.), Ashby. Proceedings: Plant Sci. 38 (6), 231–234. doi: 10.1007/BF03050620
Ramezani, M., Shier, W. T., Abbas, H. K., Tonos, J. L., Baird, R. E., Sciumbato, G. L. (2007). Soybean Charcoal Rot Disease Fungus Macrophomina phaseolina in Mississippi Produces the Phytotoxin (–)-Botryodiplodin but No Detectable Phaseolinone. J. Natural Products 70 (1), 128–129. doi: 10.1021/np060480t
Ramos, A. M., Gally, M., Szapiro, G., Itzcovich, T., Carabajal, M., Levin, L. (2016). In vitro growth and cell wall degrading enzyme production by Argentinean isolates of Macrophomina phaseolina, the causative agent of charcoal rot in corn. Rev. Argent. Microbiologia 48 (4), 267–273. doi: 10.1016/j.ram.2016.06.002
Rangel-Montoya, E. A., Delgado-Ramírez, C. S., Sepulveda, E., Hernández-Martínez, R. (2022). Biocontrol of Macrophomina phaseolina Using Bacillus amyloliquefaciens Strains in Cowpea (Vigna unguiculata L.). Agronomy 12 (3), 676. doi: 10.3390/agronomy12030676
Regnault-Roger, C. (2020). “Trends for commercialization of biocontrol agents (Biopesticide),” in Plant defence: Biological control. Eds. Mérillon, J.-M., Ramawat, K. G. (Cham: Springer International Publishing), 445–471.
Rehman, F., Khan, F. A., Badruddin, S. M. A. (2012). “Role of phenolics in plant defense against insect herbivory,” in Chemistry of phytopotentials: Health, energy and environmental perspectives. Eds. Khemani, L. D., Srivastava, M. M., Srivastava, S. (Berlin, Heidelberg: Springer), 309–313.
Reyes-Franco, M. C., Hernandez-Delgado, S., Beas-Fernandez, R., Medina-Fernandez, M., Simpson, J., Mayek-Perez, N. (2006). Pathogenic and Genetic Variability within Macrophomina phaseolina from Mexico and Other Countries. Phytopathologische Zeitschrift. J. Phytopathol. 154 (7-8), 447–453. doi: 10.1111/j.1439-0434.2006.01127.x
Reznikov, S., Chiesa, M. A., Pardo, E. M., De Lisi, V., Bogado, N., González, V., et al. (2019). Soybean-Macrophomina phaseolina-Specific Interactions and Identification of a Novel Source of Resistance. Phytopathology 109 (1), 63–73.
Rosa, E. A. S., Rodrigues, P. M. F. (1999). Towards a more sustainable agriculture system: The effect of glucosinolates on the control of soil-borne diseases. J. Hortic. Sci. Biotechnol. 74 (6), 667–674. doi: 10.1080/14620316.1999.11511170
Ross, A. F. (1961). Systemic acquired resistance induced by localized virus infections in plants. Virology 14 (3), 340–358. doi: 10.1016/0042-6822(61)90319-1
Saleh, A. A., Ahmed, H. U., Todd, T. C., Travers, S. E., Zeller, K. A., Leslie, J. F., et al. (2010). Relatedness of Macrophomina phaseolina isolates from tallgrass prairie, maize, soybean and sorghum. Mol. Ecol. 19 (1), 79–91. doi: 10.1111/j.1365-294X.2009.04433.x
Salvatore, M. M., Félix, C., Lima, F., Ferreira, V., Naviglio, D., Salvatore, F., et al. (2020). Secondary Metabolites Produced by Macrophomina phaseolina Isolated from Eucalyptus globulus. Collection FAO: Agric. 10 (3), 72. doi: 3390/agriculture10030072
Sankar, P., Sharma, R. C. (2001). Management of charcoal rot of maize with Trichoderma viride. Scientia Agricola 55, 1–7.
Sarkar, T. S., Biswas, P., Ghosh, S. K., Ghosh, S. (2014). Nitric oxide production by necrotrophic pathogen Macrophomina phaseolina and the host plant in charcoal rot disease of jute: complexity of the interplay between necrotroph-host plant interactions. PLoS One 9 (9), e107348. doi: 10.1371/journal.pone.0107348
Schroeder, M. M., Lai, Y., Shirai, M., Alsalek, N., Tsuchiya, T., Roberts, P., et al. (2019). A novel Arabidopsis pathosystem reveals cooperation of multiple hormonal response-pathways in host resistance against the global crop destroyer Macrophomina phaseolina. Sci. Rep. 9 (1), 20083. doi: 10.1038/s41598-019-56401-2
Sharma, I., Kumari, N., Sharma, V. (2014). Defense gene expression in Sorghum bicolor against Macrophomina phaseolina in leaves and roots of susceptible and resistant cultivars. J. Plant Interact. 9 (1), 315–323. doi: 10.1080/17429145.2013.832425
Shier, W. T., Abbas, H. K., Baird, R. E., Ramezani, M., Sciumbato, G. L. (2007). (-)-Botryodiplodin, a unique ribose-analog toxin. Toxin Rev. 26 (4), 343–386. doi: 10.1080/15569540701741866
Short, G. E., Wyllie, T. D., Bristow, P. R. (1980). Survival of Macrophomina phaseolina in soil and in residue of soybean. Survival 7 (13), 17.
Siddiqui, K. A., Gupta, A. K., Paul, A. K., Banerjee, A. K. (1979). Purification and properties of heat-resistant exotoxin produced by Macrophomina phaseolina (Tassi) Goid in culture. Experientia 35 (9), 1222–1223. doi: 10.1007/BF01963302
Singh, S. K., Nene, Y. L., Reddy, M. V. (1990). Influence of cropping systems on Macrophomina phaseolina population of Soil. Plant Dis. 74 (10), 812–814. doi: 10.1094/PD-74-0812
Sinha, N., Patra, S. K., Ghosh, S. (2022). Secretome analysis of macrophomina phaseolina identifies an array of putative virulence factors responsible for charcoal rot disease in plants. Front. Microbiol. 13, 847832. doi: 10.3389/fmicb.2022.847832
Smith, G. S., Carvil, O. N. (1997). Field screening of commercial and experimental soybean cultivars for their reaction to macrophomina phaseolina. Plant Dis. 81 (4), 363–368. doi: 10.1094/PDIS.1997.81.4.363
Smolinska, U., Morra, M. J., Knudsen, G. R., James, R. L. (2003). Isothiocyanates produced by brassicaceae species as inhibitors of fusarium oxysporum. Plant Dis. 87 (4), 407–412. doi: 10.1094/PDIS.2003.87.4.407
Srivastava, S. K., Dhawan, S. (1982). Phosphatidase Activity in Brassica juncea Plants Infected with Isolates of Macrophomina phaseolina and Its Role in Pathogenesis. Bull. Torrey Botanical Club 109 (4), 508–512. doi: 10.2307/2996491
Su, G., Suh, S. O., Schneider, R. W., Russin, J. S. (2001). Host specialization in the charcoal rot fungus. Macrophomina phaseolina. Phytopathol. 91 (2), 120–126. doi: 10.1094/PHYTO.2001.91.2.120
Tan, D. H. S., Sigler, L., Gibas, C. F. C., Fong, I. W. (2008). Disseminated fungal infection in a renal transplant recipient involving Macrophomina phaseolina and Scytalidium dimidiatum: case report and review of taxonomic changes among medically important members of the Botryosphaeriaceae. Med. Mycology: Off. Publ. Int. Soc. Hum. Anim. Mycology 46 (3), 285–292. doi: 10.1080/13693780701759658
Thomma, B. P., Penninckx, I. A., Broekaert, W. F., Cammue, B. P. (2001). The complexity of disease signaling in Arabidopsis. Curr. Opin. Immunol. 13 (1), 63–68. doi: 10.1016/S0952-7915(00)00183-7
Tierens, K. F., Thomma, B. P., Brouwer, M., Schmidt, J., Kistner, K., Porzel, A., et al. (2001). Study of the role of antimicrobial glucosinolate-derived isothiocyanates in resistance of Arabidopsis to microbial pathogens. Plant Physiol. 125 (4), 1688–1699. doi: 10.1104/pp.125.4.1688
Tiku, A. R. (2020). “Antimicrobial compounds (Phytoanticipins and phytoalexins) and their role in plant defense,” in Co-evolution of secondary metabolites. Eds. Mérillon, J.-M., Ramawat, K. G. (Cham: Springer International Publishing), 845–868.
Tomar, R. S., Parakhia, M. V., Rathod, V. M., Thakkar, J. R., Padhiyar, S. M., Thummar, V. D., et al. (2017). Molecular mapping and identification of QTLs responsible for charcoal rot resistance in Castor (Ricinus communis L.). Ind. Crops Products 95, 184–190. doi: 10.1016/j.indcrop.2016.10.026
Tytgat, T. O. G., Verhoeven, K. J. F., Jansen, J. J., Raaijmakers, C. E., Bakx-Schotman, T., McIntyre, L. M., et al. (2013). Plants know where it hurts: root and shoot jasmonic acid induction elicit differential responses in Brassica oleracea. PLoS One 8 (6), e65502. doi: 10.1371/annotation/4574edb2-15bd-4689-bf84-b947ebe81ed2
Van Baarlen, P., Staats, M., VAN Kan, J. A. L. (2004). Induction of programmed cell death in lily by the fungal pathogen Botrytis elliptica. Mol. Plant Pathol. 5 (6), 559–574. doi: 10.1111/j.1364-3703.2004.00253.x
Verma, S., Tomar, R. S., Rathode, V., Thakker, J. (2018). Genome sequencing analysis of Macrophomina phaseolina resistant and susceptible castor genotype. Biosci. Biotech. Res. Asia 15 (1). doi: 10.13005/bbra/2624
Wang, Y., Branicky, R., Noë, A., Hekimi, S. (2018). Superoxide dismutases: Dual roles in controlling ROS damage and regulating ROS signaling. J. Cell Biol. 217 (6), 1915–1928. doi: 10.1083/jcb.201708007
Wang, H., Jones, R. W. (1995). A unique endoglucanase-encoding gene cloned from the phytopathogenic fungus Macrophomina phaseolina. Appl. Environ. Microbiol. 61 (5), 2004–2006. doi: 10.1128/aem.61.5.2004-2006.1995
Weems, J. D., Ebelhar, S. A., Chapara, V., Pedersen, D. K., Zhang, G. R., Bradley, C. A. (2011). First report of charcoal rot caused by macrophomina phaseolina on sunflower in illinois. Plant Dis. 95 (10), 1318–1318. doi: 10.1094/PDIS-02-11-0102
Wei, H., Liu, J., Guo, Q., Pan, L., Chai, S., Cheng, Y., et al. (2020). Genomic Organization and Comparative Phylogenic Analysis of NBS-LRR Resistance Gene Family in Solanum pimpinellifolium and Arabidopsis thaliana. Evolutionary Bioinf. Online 16, 1176934320911055. doi: 10.1177/1176934320911055
Wittstock, U., Halkier, B. A. (2002). Glucosinolate research in the Arabidopsis era. Trends Plant Sci. 7 (6), 263–270. doi: 10.1016/S1360-1385(02)02273-2
Wrather, J. A., Anderson, T. R., Arsyad, D. M., Tan, Y., Ploper, L. D., Porta-Puglia, A., et al. (2001). Soybean disease loss estimates for the top ten soybean-producing countries in 1998. Can. J. Plant Pathology. Rev. Can. Phytopathologie 23 (2), 115–121. doi: 10.1080/07060660109506918
Wyllie, T. D., Brown, M. F. (1970). Ultrastructural formation of sclerotia of Macrophomina phaseoli. Phytopathology 60 (3), 524–528. doi: 10.1094/Phyto-60-524
Yan, W., Ni, Y., Liu, X., Zhao, H., Chen, Y., Jia, M., et al. (2021). The mechanism of sesame resistance against Macrophomina phaseolina was revealed via a comparison of transcriptomes of resistant and susceptible sesame genotypes. BMC Plant Biol. 21 (1), 159. doi: 10.1186/s12870-021-02927-5
Yasmin, H., Naz, R., Nosheen, A., Hassan, M. N., Ilyas, N., Sajjad, M., et al. (2020). Identification of New Biocontrol Agent against Charcoal Rot Disease Caused by Macrophomina phaseolina in Soybean (Glycine max L.). Sustainability: Sci. Pract. Policy 12 (17), 6856. doi: 10.3390/su12176856
Keywords: Macrophomina phaseolina, pathogenicity, crop destroyer, crop diseases, plant immunity, host defense, root-specific defense, plant defense signaling
Citation: Shirai M and Eulgem T (2023) Molecular interactions between the soilborne pathogenic fungus Macrophomina phaseolina and its host plants. Front. Plant Sci. 14:1264569. doi: 10.3389/fpls.2023.1264569
Received: 21 July 2023; Accepted: 28 August 2023;
Published: 14 September 2023.
Edited by:
Ramesh Raju Vetukuri, Swedish University of Agricultural Sciences, SwedenReviewed by:
Xiong Zhang, Chinese Academy of Agricultural Sciences, ChinaMaofeng Jing, Nanjing Agricultural University, China
Copyright © 2023 Shirai and Eulgem. This is an open-access article distributed under the terms of the Creative Commons Attribution License (CC BY). The use, distribution or reproduction in other forums is permitted, provided the original author(s) and the copyright owner(s) are credited and that the original publication in this journal is cited, in accordance with accepted academic practice. No use, distribution or reproduction is permitted which does not comply with these terms.
*Correspondence: Thomas Eulgem, dGhvbWFzLmV1bGdlbUB1Y3IuZWR1