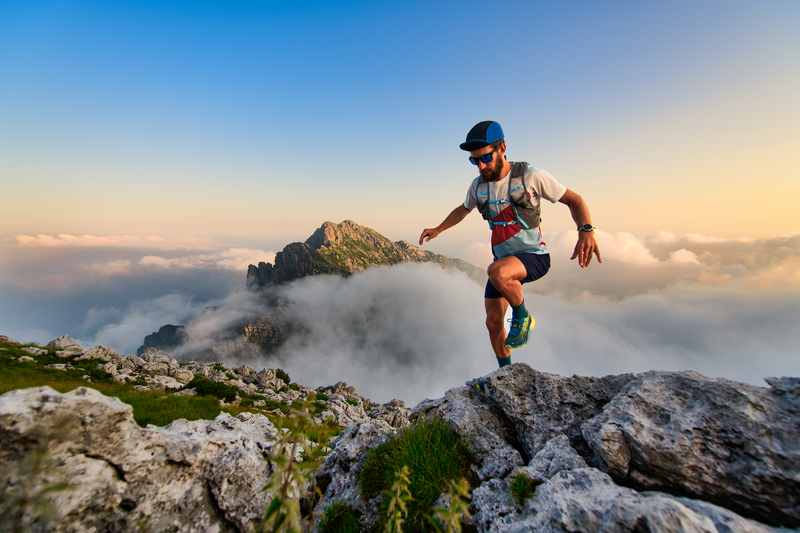
95% of researchers rate our articles as excellent or good
Learn more about the work of our research integrity team to safeguard the quality of each article we publish.
Find out more
ORIGINAL RESEARCH article
Front. Plant Sci. , 28 November 2023
Sec. Plant Development and EvoDevo
Volume 14 - 2023 | https://doi.org/10.3389/fpls.2023.1260596
This article is part of the Research Topic Model Organisms in Plant Developmental Biology — their effectiveness and limitations View all 14 articles
Liverworts represent one of six embryophyte lineages that have a Devonian, or earlier, origin, and are, at present, represented by only Marchantia polymorpha as an established model. Ricciocarpos natans is a secondarily monoicous aquatic liverwort with a worldwide distribution, being found on all continents except Antarctica. Ricciocarpos, a monotypic genus, forms a sister relationship with Riccia, the largest genus of the Marchantiopsida (~250 species), diverging from their common ancestor in the mid-Cretaceous. R. natans is typically found on small stagnant ponds and billabongs (seasonal pools), where it assumes a typical ‘aquatic’ form with long scale keels for stabilization on the water surface. But, as water bodies dry, plants may become stranded and subsequently shift their development to assume a ‘terrestrial’ form with rhizoids anchoring the plants to the substrate. We developed R. natans as a model to address a specific biological question — what are the genomic consequences when monoicy evolves from ancestral dioicy where sex is chromosomally determined? However, R. natans possesses other attributes that makes it a model to investigate a variety of biological processes. For example, it provides a foundation to explore the evolution of sexual systems within Riccia, where it appears monoicy may have evolved many times independently. Furthermore, the worldwide distribution of R. natans postdates plate tectonic driven continent separation, and thus, provides an intriguing model for population genomics. Finally, the transition from an aquatic growth form to a terrestrial growth form is mediated by the phytohormone abscisic acid, and represents convergent evolution with a number of other aquatic embryophytes, a concept we explore further here.
Why does one choose a particular species as a model system? For most of the 20th century plant biologists chose species based on attributes that facilitate experimental approaches to answer a specific biological question (Sussex, 1998). This approach led to a plethora of different species being investigated, each suited the questions being investigated. At one point, it became so parochial that each researcher worked on a different species, and as Ian Sussex once related, he was thinking about working on a particular species of plant, but was told by others that he should not, as that was professor X’s species. Despite the parochialism, during that time a few species, namely maize, petunia and snapdragon, gained some traction as models, especially among geneticists (Stubbe, 1966; Rhoades, 1984; Coe, 2001; Schwarz-Sommer et al., 2003; Candela and Hake, 2008; Vandenbussche et al., 2016). It was not until the 1980’s that the plant science community converged upon a dominant model system, Arabidopsis thaliana (Meyerowitz and Pruitt, 1985; Page and Grossniklaus, 2002; Somerville and Koornneef, 2002; Provart et al., 2015; Prunet and Meyerowitz, 2016). Subsequently, research with a small number of species amenable to genetic and molecular approaches facilitated rapid advances in our understanding of plant development, physiology, and even ecology. However, this canalization also led to other aspects of plant biology for which the model species were not appropriate, such as mycorrhizal fungal interactions and broader questions in evolution and ecology being neglected. With the advances of genomic sequencing and development of genome editing in the past decade, we are now in a position to return to the broader plant biology perspective of last century, where a wide spectrum of species could be developed as models given the specific biological question at hand. It is in this vein that we began research on the liverwort Ricciocarpos natans.
To determine optimal growth conditions in the laboratory (i.e. to mimic morphologies observed in nature), Ricciocarpos was growth in axenic aquatic culture, with liquid media containing various concentrations of Gamborg B-5 basal medium [PhytoTech Labs; www.phytotechlab.com; (Gamborg et al., 1968)], pH 6.0, with concentrations of 1x, 1/2, 1/4, 1/6, 1/8, 1/10, and 1/12 tested. Growth was under a 16-hour photoperiod at 20˚C. Light intensity was varied by growing plants at different distances from the light source. Conditions of 1/8 B-5 media and a light intensity of 80 µmol. m-2s-1 resulted a typical aquatic morphology with purple scale production. Addition of ABA at a concentration of 0.1µM to the above media successfully induced terrestrial characteristics of Ricciocarpos growing in liquid media; for the differential gene experiment, the addition of ABA was a single event at the initiation of the growth period.
The phylogenetic tree of Ricciocarpos was constructed using nucleotide sequences of 6 genes (nuclear: LOX1; chloroplast: rbcL, rps4, cpITS, trnL-F, 26S); while the Jerrybomberra Creek and Butner NC accessions were represented by most genes, the other accessions were represented by as few as one gene. Each sequence alignment was manually trimmed to exclude ambiguously aligned regions. The best substitution models for each of the six alignments obtained using the “optimize” function in raxmlGui2.0 (Edler et al., 2020). The six alignments were concatemerized producing a matrix consisting of 12,465 nucleotides representing 11 species/isolates. A maximum likelihood phylogeny was constructed, with nodal support calculated after 1000 replications, using raxmlGui2.0 (Edler et al., 2020).
Repeat Annotation: RepeatModeler (Smit and Hubley, 2008-2015) (version 1.0.8_RM4.0.7) was used for de novo repeat family identification. The output was used as a repeat library for RepeatMasker version 4.0.9 (Smit et al., 2013-2015).
RNA Extraction and Sequencing: RNA for sequencing was extracted from R. natans by submerging whole plants in liquid nitrogen and using a mortar and pestle to grind the tissue into a powder. For each line, 100mg of tissue was processed with the RNeasy mini kit (Qiagen), as per the manufacturer’s instructions for purification of total RNA from plant tissues. The total RNA for each sample was quantified with the NanoDrop 2000 (Thermo Scientific). Library preparation used polyA mRNA selection and MGIEasy stranded mRNA chemistry. Sequencing used MGI Tech MGISEQ-2000RS hardware (400 million raw reads per lane, 100-pb paired-end reads).
Gene Prediction: A total of 106,736 transcript assemblies were made from ∼57M pairs of paired-end Illumina RNA-seq reads with Trinity software-v2.12.0 described in Chapter 3. Ab-initio gene predictions were generated by AUGUSTUS-3.3.3 (Stanke et al., 2008). 37,626 transcript assemblies were constructed with RNA-seq-assisted prediction by PASA software (Haas et al., 2003) using RNA-seq transcript assemblies. Homology based gene prediction was done with EXONERATE alignments with the Marchantia v6.1 protein dataset to a repeat-soft-masked Ricciocarpos genome using RepeatMasker (Smit et al., 2013-2015). The Repeat library was generated using RepeatModeler (Smit and Hubley, 2008-2015).
The EVidenceModeler software, which combines ab-initio gene predictions, protein and transcript alignments into weighted consensus gene structures, was used to obtain consensus gene structures (Haas et al., 2008) in R. natans. Resultant gene structure annotations were updated by PASA. As Marchantia is used as model liverwort for comparison with R. natans, and is functionally well annotated, PASA-improved gene model proteins were subject to protein homology analysis to Marchantia to retrieve functional annotation of genes.
RNA-seq filtered libraries, in triplicate, were used for each sample. Scaffold level assembly of Ricciocarpos (https://genomevolution.org/coge/; Genome id65508) was used as the reference for mapping filtered transcripts to R. natans genome assembly using samtools (Li and Durbin, 2009). Transcript abundance was estimated using HT-Seq count with -no strand specific parameter (-s = no) and other parameters were kept as default. Differential gene expression (DGE) analysis of ABA treated plants (terrestrial) in contrast to no ABA (aquatic) was performed with DESeq2 (Love et al., 2014).
Liverworts are one of three bryophyte lineages (liverworts, mosses, hornworts) and comprise one of six land plant lineages that diverged from one another in the Devonian or earlier (Bowman, 2022). Ricciocarpos natans, hereafter Ricciocarpos, is a monoicous, largely aquatic, complex thalloid liverwort (Marchantiopsida) with a cosmopolitan distribution (Figures 1A–C). The genus is monotypic, being comprised of a single described species. Due to its resemblance to species of the genus Riccia, Ricciocarpos has traditionally been placed as one of the two genera of the Ricciaceae (Schuster, 1992), however, phylogenetic analyses using sequence data suggest it is more closely related to another genus, Oxymitra, and that they together are sister to Riccia [Figure 1A, (Villarreal et al., 2016)]. As liverworts were ancestrally dioicous and terrestrial, both monoicy and its aquatic habit are derived characters. However, development of Ricciocarpos as a model system could serve as a model for species in the genus Riccia, the largest genus by species number within the Marchantiopsida.
Figure 1 Overview of Ricciocarpos. (A) Phylogenetic relationship of Ricciocarpos to its closest extant relatives and to Marchantia, with approximate divergence times indicated (MYA, million years ago). Tree topology and estimated times are based on an analysis of 12 genes of mixed organellar and nuclear origin and calibrated with the known fossil record, with 95% posterior density intervals indicated [from (Villarreal et al., 2016)]. Numbers in parentheses indicate number of species in genera. (B) Present day distribution (red squares) of Ricciocarpos displayed at inaturalist.org (19 August 2022). The eight major flyways for migrating birds are outlined in different colours (Boere and Stroud, 2006). (C) Relationship between accessions of R. natans: the genome of the present paper is from Jerrabombera Creek, ACT (Australia), while the 1kp transcriptome (Wickett et al., 2014) was from plants isolated near Butner, NC (USA), and other accessions are from data in Genbank. Names are colour-coded based on geographical location. (D) Clonal population of Ricciocarpos growing in water amongst algae. (E) Dichotomous branching and separation of thallus fragments. The numbers indicate the number of apical meristems in the thalli, with the asterisks demarking them in two thalli. the arrow indicated where this thallus with eight apices will separate. (F) The life cycle of Ricciocarpos; sexual reproduction in brown and vegetative reproduction in green. Sporophyte tissues are shown in brown shades, whereas gametophyte tissues are green, except scales (purple), egg cells (red), antheridia (blue). Plants most often undergo vegetative reproduction via successive cycles of dichotomous branching and subsequent abscision of plants along the midline. If plants become stranded on a terrestrial substrate, production of long scales ceases and rhizoid are produced; in addition, abscision following branching no longer occurs, with plants forming a rosette. Growth in inductive conditions (aquatic growth and likely longer warmer days in spring) leads to production of first antheridia and then archegonia along the dorsal midline. Self- or cross-fertilization leads to production of sporophytes, again along the dorsal midline and essentially enclosed by the maternal gametophyte. As sporophytes lack both foot and seta, maternal nutrient contributions must be transferred via the calyptra. Following meiosis and release of spores from tetrads, the unistratose capsule wall (dark brown) breaks down, releasing the spores into a cavity in the maternal gametophyte to be dispersed as the gametophyte senesces. Adapted from (Bischoff, 1835; Garber, 1904).
The morphology, anatomy and development of Ricciocarpos is similar to that of other complex thalloid liverworts. Growth occurs from a single apical cell in the shoot meristem, with the thallus undergoing periodic dichotomous branching [Figures 1D, E (Leitgeb, 1879; Garber, 1904)]. The dorsal surface is occupied by air chambers separated by unistratose (single cell layer) walls and forming an elaborate aerenchyma, with those at the dorsal surface having a complex air pore (Leitgeb, 1879; Kronestedt, 1981; Kronestedt, 1982a; Kronestedt, 1982b). The air chambers form schizogenously, i.e. via localized cell separation (Barnes and Land, 1907; Hirsh, 1910). The air in these chambers provides buoyancy such that the plants float on the water surface. As is typical of the Marchantiopsida, oil body cells are found as idioblasts (isolated cells differing from their neighbours) containing a single oil body (Kronestedt, 1983), and these likely function to deter herbivory as has been described for Marchantia (Kanazawa et al., 2020; Romani et al., 2020).
When Ricciocarpos is growing on an aquatic medium, ventral rhizoid production is suppressed, and large sword-like scales are produced that act as keels to stabilise the thallus on the water surface and prevent their overturning during windy periods [(Bischoff, 1835; Lindenberg, 1836; Kronestedt, 1981); e.g. Figure 2, Figures 3A, B]. The scales are unistratose, several cells wide (0.2-0.6 mm), and often with their length (10 mm) exceeding the width of the thallus (Kronestedt, 1981). The scales are often deeply pigmented, with a reddish-purple pigment that can almost appear black (Dillenius, 1741; Lindenberg, 1836; Schmidel, 1793). The pigment has been named riccionidin and its biosynthesis is related to that of the anthocyanin pathway (Kunz et al., 1994; Kunz and Becker, 1995; Albert et al., 2018; Kubo et al., 2018), and recent work on Marchantia has shown it to be an auronidin (Berland et al., 2019). This pigment appears to be polymerized in the cell wall, and thus provides both a possible sunscreen and also contributes mechanically to the stiffness of the scales (Kunz et al., 1994; Berland et al., 2019). Another attribute of the aquatic form is the periodic separation of thalli (Bischoff, 1835; Garber, 1904; Lewis, 1906; Pickett, 1925; Schmidel, 1793), via abscision and presumably involves programmed cell death (Figures 1D–F). Typical thalli have four shoot apices, and when each of these apices branch a short-lived thallus with eight shoot apices is formed that then undergoes abscission to produce two thalli with four apices once again [Figures 1D–F, (Ferreyra, 1990)]. This mode of vegetative reproduction allows for rapid proliferation of thalli on the water surface, with each ‘individual’ being able to float independently of the others. Consistent with its largely aquatic ecology, Ricciocarpos has lost the ability to form mycorrhizal fungal interactions (Stahl, 1949; Ligrone et al., 2007).
Figure 2 (A) A. dorsal surface; B. ventral surface; Plate 78, fig. 18 from Dillenius (Dillenius, 1741). (B) Riccia natans (Fringed Riccia), plate 77 in Smith’s English Botany (Smith, 1804). (C) Riccia natans, Plate LXXI, fig. V of (Bischoff, 1835). (1) Mature frond; (2-3) fronds split into two halves along the intermediate groove, each half consisting of two unequal parts; (4) the sporangium; (5) developing spores; (6) mature spores; (7, 10) scale; (8) sporangium within thallus; (9) thallus cross section within antheridium (A). (D) Riccia natans (plant juvenile), Plate LXX, fig. VI of (Bischoff, 1835). (1-3) Young fronds with quadricrenate apex, convex on both sides, attenuated on the outer edge, with an intermediate groove running out into two lateral grooves from the notches; (4) ventral side of 3; (5) frond cross section with young scales (A). (E, F) Riccia natans, from Plate XXXI of (Lindenberg, 1836). (E) 6. cross section of mature frond. (F) 2. Mature frond; (9) sporangium; (left) mature spores. (G) Riccia lutescens, from Plate XXVI of (Lindenberg, 1836). (1, 3) Mature frond; (4) ventral view; (5) enlarged view of 4; (6) thallus cross section.
Figure 3 Growth of Ricciocarpos in culture. (A) Growth curve with varying Gamborg B-5 concentration. (B) Variation in induction of purple scales in Ricciocarpos with light intensity. The plate with ⅛ Gamborg B-5 media and higher light intensity produced growth with prominent purple scales compared to the plate with the same nutrient concentration and less intense light.
When Ricciocarpos is growing on a terrestrial medium, scale production is suppressed and instead unicellular thin-walled rhizoids as long as 15 mm are produced and these anchor the plant to the substrate (Kronestedt, 1981). In addition, the terrestrial form does not undergo fragmentation via abscission, but rather stay together forming a tight circular rosette, similar to many Riccia species (Bischoff, 1835; Garber, 1904; Lewis, 1906; Pickett, 1925).
Ricciocarpos is monoicous, producing first antheridia and then archegonia along the dorsal furrow [Figure 1F; (Garber, 1904)]. As the sex organs are produced in a temporally distinct manner, there is some scope for outcrossing. The heterochronic production of sex organs is likely what led to some early researchers to suggest dioicy (Campbell, 1895; Leitgeb, 1879). It is this derived feature, the evolution of monoicy from ancestral dioicy, without a major karyotype change [n = 9 (Siler, 1934), from the ancestral state of liverworts (Berrie, 1960)], that induced us to develop Ricciocarpos as a model to investigate the genomic consequences of a shift in sexual systems (Singh et al., 2023). The antheridia and archegonia are produced along the dorsal furrow in a single row (Leitgeb, 1879; Garber, 1904; Lewis, 1906; Rieth, 1959), and their development are typical of the Marchantiopsida (Leitgeb, 1879; Garber, 1904; Lewis, 1906). Following fertilization the sporophyte develops essentially embedded within the maternal thallus [Figure 1F; (Garber, 1904; Lewis, 1906; Rieth, 1959)]. The sporophytes consist of a capsule whose wall is unistratose, and largely lack both a foot and seta (Figure 1F). All spore mother cells under meiosis producing haploid spores [about 500 per sporangium; (Rieth, 1959)], with no evidence of elaters (Figure 1F). Following spore formation, the capsule wall breaks down releasing the spores into a cavity in maternal gametophyte, with dispersal either due to degeneration of the maternal gametophyte or via bird consumption (see below).
The discovery and description of Ricciocarpos was given by Buddle in 1699 under the name ‘Lichen parvus vernus cordiformis, ima parte fimbriatus, Lentis palustris modo aquae innatans’ in his Hortus Siccus, a herbarium that he assembled. A description was first published by Jacob Petiver (Petiver, 1695-1703), “Lens palustris Roris Solis foliis cordatis, observed by my Reverend friend Mr. Adam Buddle in some ponds about Henley in Suffolk”, later corrected to ‘Hadley’ in Suffolk (Dillenius, 1741). It was later published under Buddle’s original description in John Ray’s Synopsis methodica stirpium Britannicarum, 3rd edition, and who noted that “It was suspected that it is being eaten by insects or ducks” (Ray, 1724). The first published image (Figure 2A) was in Dillenius’s Historia Muscorum (Dillenius, 1741). With respect to the cryptogams, Linneaus was a great lumper and placed Ricciocarpos with Riccia under the name Riccia natans (Linné, 1770); the species epithet ‘natans’ is derived from the Latin word for ‘swimming’. As is often the case, Schmidel’s description (of what he called Riccia capillata) added much detail, where he noted the dorsal air chambers and the serrated and coloured (black) nature of the ventral scales, and further suggested that it multiplied via dissociation along ‘nerves’ separating the lobes (Schmidel, 1793). A much less detailed drawing is presented in Smith’s English Botany [Figure 2B; (Smith, 1804)]. Hooker was the first to describe the position of the sporophyte (Hooker, 1830), with Bischoff subsequently describing the structures of the sunken antheridia and sporophytes in some detail (Figure 2C), and also detailing the vegetative propagation via repeated division along the central channel of older plants into two plants (Bischoff, 1835). As with the case of many liverworts, new techniques for fixation and sectioning allowed Leitgeb to provide a detailed anatomical description of Ricciocarpos, with foci on development from the apical cell and the formation of air chambers (Leitgeb, 1879). Anatomical details of antheridia, archegonia and sporophyte development were clarified some years later (Garber, 1904; Lewis, 1906).
The genus name Ricciocarpos was coined by Corda (Corda, 1828) to separate Ricciocarpos from other Riccia species, but the first illustrations under the moniker Ricciocarpos are very poor [see Tab. 32. in (Sturm, 1832)]. Furthermore, Corda’s distinguishing characters were shown not to be diagnostic (Bischoff, 1835), and thus it was subsequently often placed back into the genus Riccia. However, likely due to its similarity with other water plants and a lack of attention to detail, Ricciocarpos was described under other genera, e.g. Salviniella (natans) (Hübener, 1834). C. S. Rafinesque described Ricciocarpos growing on ponds on Long island, NY as Lemna dimidiata, perhaps ironically as he was didactic in correcting other botanist’s nomenclature (Rafinesque, 1817). However, to his credit, Rafinesque also formulated a prescient early view of evolution: “The truth is that Species and perhaps Genera also, are forming in organized beings by gradual deviations of shapes, forms and organs, taking place in the lapse of time. There is a tendency to deviations and mutations through plants and animals by gradual steps at remote irregular periods. This is a part of the great universal law of perpetual mutability in everything.” (Rafinesque, 1833), which was later acknowledged by Darwin. Bischoff disparaged this proliferation of genus names, saying of some of his contemporaries, “they could not resist the addiction to see their names behind a synonym, even if they were born as an invalid”, and in his description of Ricciocarpos he wished “to protect us and our descendants from Babylonian confusion” (Bischoff, 1835). Furthermore, “In order to finally prevent such a polyonomatomania, which might threaten to infiltrate our Riccia even further”, Bischoff also drew “attention to a plant which was discovered in February in the ditch of Lille near the Pont-de-France by Gay and (in the year 1834) to Prof. Al. Braun was notified” (Figure 2D). Bischoff interpreted “this plant, which at first glance could be taken to be a separate species, is in all probability only the Riccia natans in its youngest condition” (Bischoff, 1835). Bischoff noted that while the dorsal surfaces were nearly identical, the ventral surfaces were different, which we now interpret to be due to the differences in the production of rhizoids rather than scales. The terrestrial form was actually described some years earlier as a distinct species, Riccia lutescens, that was “found in abundance in an exsiccated swamp on the ground” in western North Carolina (Schweinitz, 1821), consistent with the habitat of terrestrial Ricciocarpos. Bischoff’s observations appear the first to equate the aquatic and terrestrial forms of Ricciocarpos to the same species. Lindenberg, who produced stunning drawings of Ricciocarpos (Figures 2E-G), also stated that when floating in the water large purple scales were produced, but “if the plant floats completely and consistently on the water, it is absolutely rootless, like R. fluitans in the same case; but as soon as it approaches the bank, or rests on the mud, it drives thin, delicate, rounded, hair-shaped, often articulated root fibers” [Figure 2E, (Lindenberg, 1836)]. Remarkably, despite these observations, Lindenberg also listed R. lutescens as a distinct species, with no cross reference to Ricciocarpos [Figure 2G, (Lindenberg, 1836)]. While some equated the two forms as a single species (Lindberg, 1882), R. lutescens was often listed as a separate species in other publications into the mid-20th century, despite Lewis conclusively demonstrating that the terrestrial form could be converted into the aquatic form (Lewis, 1906). An additional distinct species name for the terrestrial form, Riccia velutina, was also proposed in the mid-19th century (Hooker, 1840). The original R. lutescens specimen of Schweinitz was typified as an isolectotype of Ricciocarpos natans (Stotler and Crandall-Stotler, 2017). A full account of the historical nomenclature of Ricciocarpos, including its orthographical variant (Ricciocarpus natans), has been previously described (Duthie and Garside, 1936; Perold, 1995).
Ricciocarpos has a nearly cosmopolitan distribution, being found throughout temperate habitats on six continents in both hemispheres, absent from extreme alpine habitats, the Arctic and Antarctica [Figure 1B; (Scott, 1985; Schuster, 1992)]. While not widely reported from the tropics, it can be found in both the neotropics of the Americas and tropics of the old world in Africa (Jones, 1957; Bischler-Causse et al., 2005). Notably, not long after Ricciocarpos was being described across western Europe, e.g. Germany (Schmidel, 1793) and in Provence and Montmorency in France (Candolle and Lamarck, 1805), it was also described in eastern North America (Muhlenberg, 1813), and noted by Robert Brown in Australia [(Brown and Bauer, 1814); Brown was botanist on the Investigator captained by Flinders and which circumnavigated Australia and he was the discoverer of both the nucleus and ‘Brownian’ motion], described by Joseph Dalton Hooker in the North Island of New Zealand (Hooker, 1855), collected as early as 1840 in Omgeni, Durban, South Africa (Drège, 1843) and as early as 1839 at Porto Alegre in southern Brazil (Montagne, 1839), and identified in Japan in the early 1850’s (Perry et al., 1856). These early observations suggest its presence in these locales likely predated most human mediated dispersal. However, it was noted as early as the mid-19th century that Ricciocarpos was a suitable aquarium plant (Collier and Hooper, 1866) and this may have contributed to its dissemination in some local contexts. In regional floras, Ricciocarpos has been reported to be widely dispersed across the Americas, Eurasia, Africa, Australia and New Zealand [e.g. (Kashyap, 1929; Hassel de Menendez, 1962; Campbell, 1975; Scott, 1985; Piippo, 1990; Schuster, 1992; Fischer, 1995; Perold, 1995; Bischler-Causse et al., 2005; Frey et al., 2006; Lee and Gradstein, 2021; Acuña-Castillo et al., 2023)], with recent iNaturalist observations consistent with the published distribution (Figure 1B).
Ricciocarpos is thought to have diverged from its nearest extant relatives (the genera Oxymitra and Riccia) in the mid-Cretaceous (Villarreal et al., 2016), postdating the breakup of Pangea and indicating its present distribution has involved trans-oceanic dispersal. The evolution of both an aquatic lifestyle and monoicy likely evolved after the divergence of Ricciocarpos from Oxymitra and Riccia, and evolution of the two characters could be linked. The obvious vector for dispersal of aquatic plants over trans-oceanic distances, and shorter ones as well, is migratory waterbirds (Buch, 1954). For example, when Ricciocarpos was noted to be newly present at Lake Gjølsjø in eastern Norway, it was suspected that it was due to transport of plants from Swedish wetlands where Ricciocarpos is common, with the swan (Cygnus olor) the likely culprit (Skulberg, 1978). While it has not been demonstrated directly for Ricciocarpos, ectozoochory, including transequatorial dispersal of bryophyte diaspores in bird plumage has been documented (Lewis et al., 2014a). Endozoochory of moss spores or plant fragments via a number of bird species [e.g. upland goose (Chloephaga picta), white-bellied seedsnipe (Attagis malouinus), mallard (Anas platyrhnchos), skua (Stercororius sp.)] and even a flying fox (Pteropus conspicillatus), has been shown to be feasible (Parsons et al., 2007; Wilkinson et al., 2017; Lázaro et al., 2021; Maggio et al., 2022), and Ricciocarpos has been noted to be present in the faeces of mallards (Hartman, 1985). Thus, long distance dispersal via bird vectors (Viana et al., 2016) is a plausible mechanism to explain bryophyte species with disjunct, sometimes bipolar, geographic distributions (Schuster, 1983; Piñeiro et al., 2012; Lewis et al., 2014b). As Ricciocarpos is monoicous, only a single spore or thallus fragment is sufficient for dispersal to a new habitat, with evolution of monoicy an adaptation to its aquatic habit. Given the worldwide distribution of Ricciocarpos and its monoicous nature, it would be of interest to investigate the regional and global phylogenetic structure of the species and whether local adaptation can precede faster than dispersal.
In nature, the habitat of Ricciocarpos is limited to stagnant ponds and billabongs and their margins. Growth is most conspicuous during the summer months when plants may cover a significant fraction of the surface area. As temporary pools dry, the plants may become stranded on the margins, shifting to the terrestrial form with rhizoids anchoring the plants to the soil. If the waterholes refill while these stranded plants are still alive, pieces of plants originally stuck to the substrate will break free, possibly due to further growth being of the aquatic form, and the free thallus fragments can float to the pond surface once again. A similar scenario seems to occur in ponds that do not dry out, but freeze over. In this case plants growing in late autumn often sink to the bottom of the pond and over-winter there. With the coming of spring, as photosynthesis resumes, the plants then float back to the pond surface (Pickett, 1925). After some vegetative growth, first antheridia and then archegonia are produced in the late spring, with sporophytes maturing in the early summer (Garber, 1904; Pickett, 1925). If the population is undergoing the sexual life cycle, the spores can also act as over-wintering or desiccation tolerant propagules. Spores can apparently remain in the ‘seed’ bank for several years, with germination of Ricciocarpos observed following a ten year drought at Lake Ita, an ephemeral floodplain lake of the Lachlan River in the outback of southwestern New South Wales (Kelleway et al., 2021). Most reports suggest that Ricciocarpos primarily progresses through the sexual life cycle only in the aquatic form (Garber, 1904; Pickett, 1925; Maeda et al., 2016), but others have reported sexual reproduction in the terrestrial form (Lewis, 1906). One possible explanation is that sexual organs develop on the aquatic form, with sporophytes sometimes maturing after plants become stranded on the bank.
Ricciocarpos is often found in conjunction with a number of aquatic plants (often invasive weeds) including the angiosperms Lemna, Wolffia, Spirodela (i.e. the duckweeds), and Utricularia (the bladderworts), the fern Azolla, and in the northern hemisphere, another aquatic liverwort, Riccia fluitans (Scott, 1985; Schuster, 1992; Aoki et al., 2017). The specific community accompanying Ricciocarpos has been termed ‘Ricciocarpetum natantis’ (Scoppola et al., 1988), but to co-occurrence of different combination of free-floating, or pleustonic, plants appears to be random (Wolek, 1997; Wolek and Walanus, 2000). Competition among pleustonic plants is driven at least in part by nutrient supply (Peeters et al., 2016), with some evidence that increased eutrophication of Finnish lakes has facilitated the establishment and spread of Ricciocarpos where it had not been reported until after the 1930’s (Toivonen, 1985).
A number of studies have registered the effects of water contaminants on Ricciocarpos growth. Pollution of waterways by factories producing the auxin analogues 2,4-D and dikamba led to the loss of severe reduction in local populations of both Ricciocarpos and R. fluitans in Lower Silesia, Poland (Kolon and Sarosiek, 1995). In transplantation experiments from fresh to water polluted with detergents or high nitrogen concentrations, Ricciocarpos was less tolerant than vascular aquatic plants and succumbed in the polluted water (Agami et al., 1976). However, that Ricciocarpos might tolerate moderate levels of certain water contaminants was suggested by its growth in a coal strip mine impoundment in Illinois (Chimney, 1984). Growth of Ricciocarpos in different concentrations of heavy metals (zinc, copper, lead, cobalt, chromium, nickel and vanadium) induced specific phenotypic responses suggesting the plant might be used as a bioindicator of chemical water pollution (Sarosiek et al., 1987a; Sarosiek et al., 1987b), as did subsequent experiments with cadmium (Oh and Koh, 2013). All these heavy metals, along with manganese and aluminium (Gimenes et al., 2020), affect growth when at higher concentrations. An open question is whether Ricciocarpos can accumulate any to provide a tool for phytoremediation. In a similar vein, experiments demonstrate that Ricciocarpos exhibits high ciprofloxacin (an antibiotic) tolerance, with a capacity for uptake and accumulation despite ciprofloxacin negatively affecting photosynthetic capacity (Gomes et al., 2018). Ricciocarpos, and aquatic plants in general, likely evolved mechanisms to cope with water contaminants, either internally, or alternatively involving active secretion of chemicals to modify their immediate environment, both chemically and altering the microbiome composition. Ricciocarpos may be a comparable model for such studies along with duckweeds (Fourounjian et al., 2020) and Azolla (Li et al., 2018).
Ricciocarpos is amenable to growth in axenic culture under a variety of growth conditions (Woodfin, 1976; Lorenzen et al., 1981; Kunz and Becker, 1995). A culture of Ricciocarpos natans was obtained from Dr. Christine Cargill, curator of cryptogam collections at the Australian National Botanic Gardens in Canberra. This culture was originally isolated from Jerrabomberra Creek, near a bridge over the creek in Jerrabomberra Wetlands in the Australian Capital Territory (35° 18’ S, 149° 9’ E). We established axenic cultures with conditions adapted to our growth rooms. The aquatic form of Ricciocarpos grows on the surface of stagnant water bodies, such as ponds and billabongs, conditions which are not necessarily nutrient rich. Thus, Ricciocarpos was grown with varying concentrations of Gamborg B-5 media, pH 6.0, (Gamborg et al., 1968) to identify a ‘wild-like’ morphology of aquatic form of Ricciocarpos, based on previous descriptions of the species growing in nature. Plants were grown for 4 weeks and plant morphology, as well as the quantity of growth produced, with each varying nutrient concentration was compared. A peak of growth, measured by surface area, was observed when plants were grown in 1/8 B-5 media (Figure 3A). Increasing light intensity from 45 µmol. m-2s-1 to 80 µmol. m-2s-1 was sufficient to induce the production of pigmented scales on the ventral surface (Figure 3B); this range is similar to light intensities used in some previous in vitro culture conditions (Lorenzen et al., 1981; Kunz and Becker, 1995).
We recently reported an assembly of the Ricciocarpos natans genome based on approximately 200x coverage of Illumina sequencing followed by scaffold assembly using Hi-C [available at https://genomevolution.org/; (Singh et al., 2023)]. The current version (v1.0) consists of 38 large scaffolds covering 185.50 Mb genome assembly. Structural annotation of Ricciocarpos genome revealed 18,813 protein coding genes in Ricciocarpos, which is similar to gene number with the reference species Marchantia polymorpha ruderalis, hereafter Marchantia, with 19,473 genes (Bowman et al., 2017; Montgomery et al., 2020). Marchantia has 23,399 proteins and Ricciocarpos has 21,958 proteins suggesting that Ricciocarpos has 3,145 additional isomers. Blast searches to identify how many Ricciocarpos proteins have orthologs in Marchantia were performed using Blast-P with the Ricciocarpos protein set against the Marchantia protein set with filter parameters of percentage identity >30%, bitscore of >50 and e-value <=1e-05. This revealed that out of total number of 21,958 Ricciocarpos proteins orthologs were identified for 13,910 proteins
The accession from which the genome presented is derived was isolated from Jerrybomberra Creek, ACT, Australia, while the1kp transcriptome data was derived from mRNA isolated from a plant from near Butner, NC, USA. Their geographically distinct origins afforded the opportunity to examine sequence divergence between the two accessions. Surprisingly, it was noted that the two accessions differ on average by approximately 4% in the coding regions analysed, which is more than is typically observed for individual comparisons within eukaryotic species (<1%), and is closer to upper values of combined divergence for populations (Leffler et al., 2012) and to that (5%) observed for bacterial ‘species’ (Jain et al., 2018). The diversification of subspecies of the Marchantia polymorpha complex are thought to date to the late Miocene, 5 Ma (highest posterior density 2-11 Ma) (Villarreal et al., 2016). The single nucleotide polymorphism frequency between Marchantia polymorpha subspecies is approximately 1.0-1.2% (Linde et al., 2020), while that between the Australian and North American Ricciocarpos isolates is approximately 4.1%. In the absence of fossil calibration, the nucleotide differences suggest divergence of the two Ricciocarpos populations perhaps in the early Miocene, 15-20 Ma.
We examined the phylogenetic relationships between Ricciocarpos natans accessions for which DNA sequence was available on Genbank, and found that sequences were distributed into at least two distinct clades (Figure 1C). One clade contained sequences from five accessions collected in Australia and Asia, including the Jerrybomberra Creek accession, while a second distinct clade was composed of sequences representing five accessions collected in North America. Ironically, a paucity of available DNA sequence from accessions collected in Europe, where Ricciocarpos was first described, prevented definitive placement of European accessions relative to the distinct two clades, with a single sequence with a long branch representing this continent. Given the sequence divergence between the Australasian and North American accessions, we suggest that these two clades might represent two distinct reproductively isolated Ricciocarpos populations and could be considered two separate species. Further work is required to ascertain whether there exist morphological or anatomical characters that define the two clades. Whether the European accessions might represent a third entity must await more sequence data from such accessions. Regardless of the phylogenetic position of the European accessions, it is of note that the divergence between the American and Australasian accessions could be related to distinct migratory bird flyways (Boere and Stroud, 2006). In this regard, it will be of interest whether genetic characterization of Ricciocarpos accessions from other geographic locations, such as South America, Africa, Central Asia and New Zealand also correlate with migratory patterns of birds.
Ricciocarpos is secondarily adapted to aquatic life and is found worldwide in stagnant water, e.g. ponds and billabongs. However, as water levels drop seasonally, Ricciocarpos plants may become stranded on the terrestrial margins. Ricciocarpos exhibits strong morphological differences based on the habitat in which it is growing, with a plant of the same genotype being able to transition from a free-floating aquatic form into a terrestrial form (Garber, 1904; Pickett, 1925). When growing in culture, the addition of abscisic acid (ABA) to the media of aquatically growing Ricciocarpos is sufficient to induce the transformation in growth habit from the aquatic to that of the terrestrial form (Hartung et al., 1994). This observation parallels similar experiments on another secondarily aquatic liverwort, Riccia fluitans, that usually grows submerged in water rather than floating on the surface. When Riccia fluitans is transferred from an aquatic medium to one exposed to the air, a transition from an aquatic growth form to a terrestrial form and during this transition process the concentration of ABA is increased 10-30 fold (Hellwege et al., 1992). Furthermore, treatment of Riccia fluitans with ABA can induce such characteristics even when the thallus is submerged in water (Hellwege et al., 1992), with concomitant changes in gene expression, including genes encoding Late Embryogenesis Abundant (LEA) proteins (Hellwege et al., 1996). The transition, which takes place over a couple weeks, includes changes to cell division patterns at the shoot apex such that the terrestrial form has larger air chambers and some air pores, in addition to the development of rhizoids (Althoff et al., 2022).
To further understand the aquatic to terrestrial transition in Ricciocarpos, we repeated earlier observations. In our growth conditions the morphological differences between aquatic and terrestrial forms of Ricciocarpos were successfully induced by addition of 0.1µM ABA (Figure 4A). The aquatic form is characterized by the development of long ventral scales that act as keels to keep the plants stable on the water surface (Figures 4B, C). The scales are usually heavily pigmented with riccionidin (Figure 4B), which is an auronidin localized to the cell wall and whose synthesis is biochemically related to that of anthocyanins that are common in other land plants (Kunz et al., 1994; Albert et al., 2018; Berland et al., 2019). In the aquatic form, the development of rhizoids is suppressed. Conversely, in the terrestrial form, the development of rhizoids is promoted, while that of scales is repressed. In addition, separation of the thallus via (presumably) programmed cell death, following dichotomous branching is a form of vegetative reproduction that allows dispersal of the plants across the water surface. Separation is suppressed in the terrestrial form such that plants form a rosette. When ABA is added to aquatically growing plants, a transition from production of scales to the production of rhizoids is observed and separation of thalli is suppressed (Figures 4A, C).
Figure 4 ABA induced formation of the terrestrial form. (A) When grown aquatically in ⅛ Gamborg B-5 media, plants produce copious ventral long scales, with their purple colour visible in the mocked plants (left); some of the thalli are flipped over to show the ventral scales. ABA treatment of leads to growth of the terrestrial form (right), lacking purple scales and. The diameter of petri plate is 9 cm. (B) The aquatic form of Ricciocarpos exhibits purple scales. The purple pigment of scales is cell wall bound, perhaps providing structural support. The image shows a thallus fixed in Formaldehyde Alcohol Acetic Acid - FAA and cleared in 100% Ethanol. (C) Both scales and rhizoids are observed in Ricciocarpos following a shift to ABA containing media. This plant was initially producing ventral scales, but began to produce rhizoids after ABA treatment; if treatment continues, eventually the scales will fall off and the plant will only have rhizoids; ventral thallus; s, scale; r, rhizoid.
In the aquatic form, the scales that develop from the ventral epidermis are deeply pigmented with riccionidin at maturity. However, immature scales in which cell division is still occurring lack riccionidin pigmentation, but do contain conspicuous chloroplasts (Figure 5A). As scales differentiate, riccionidin accumulation first appears proximally and then gradually extends to the distal tip (Figure 5B). As scales mature riccionidin accumulation continues and chloroplasts are no longer conspicuous (Figures 5C-E). Pigmentation is particularly intense in the marginal cells with protuberances giving the scale a fringed appearance (Figures 5C-E). As the riccionidin accumulation occurs as a polymerized derivative embedded in the cell wall (Kunz et al., 1994; Berland et al., 2019), it may act to stiffen the scales to aid their function as keels. The production of the cell wall pigment may also preclude subsequence cell division (Albert et al., 2018). In the terrestrial form the scales remain small and are restricted to the region of the apical meristem (Kronestedt, 1982a). The rhizoids that develop in the terrestrial form following ABA treatment are smooth rhizoids (Figure 5F)
Figure 5 Scales and rhizoids of Ricciocarpos. (A) An immature scale in which little riccionidin is visible. (a’) Magnification of (A) showing conspicuous chloroplasts. (B) In slightly older scales, the reddish-purple coloured riccionidin pigment initially appears at the proximal end of the scale and progresses towards the distal tip. (C, D) As scales differentiate, an increase in riccionidin pigmentation is distinctly visible. (c’ and d’) Magnifications of (C, D), respectively, showing the loss of chloroplasts as scales mature. (E) Mature scales retain a gradient of pigmentation. (F) Ricciocarpos rhizoids are similar to the smooth rhizoids of Marchantia (Shimamura, 2016; Bowman et al., 2022).
To explore the role of ABA on gene expression in the Ricciocarpos thallus during the transition from an aquatic form to a terrestrial form, a differential expression analysis comparing plants growing aquatically to those grown for four weeks in the presence of ABA [0.1µM] was performed; given the time point the differentially expressed genes (DEG) will represent steady state levels following long-term ABA exposure. DEG were identified considering all data and observing the scaling at the gene level to identify differential expression (up or down). This analysis revealed that out of 15,440 genes with non-zero total read count with adjusted p-value < 0.05, a total of 2237 (15% of genes) are up-regulated (log2Foldchange > 0) and 2798 (18% of genes) are down-regulated (log2Foldchange < 0) (Figure 6A). A heatmap of the DEG facilitated hierarchical clustering to identify gene clusters displaying similar expression patterns amongst samples (Figure 6B). The heatmap was plotted with all significant genes with adjusted value < 0.05. The genes differentially expressed are grouped in 10 clusters.
Figure 6 Differential gene expression between aquatic and terrestrial forms. (A) Differentially expressed genes in Aquatic vs 4-week ABA treated samples: adjusted p-values of <0.05 are plotted in the above graph with log2Foldchange between -0.5 to 0.5 represented in grey, genes with log2Foldchange in range -0.5 to -1 and 0.5 to 1 shown in green. The down-regulated and up-regulated genes with log2Foldchange greater than 1 or smaller than -1 are shown in red and blue color, respectively. (B) Heatmap showing differential gene expression in ABA treated Ricciocarpos in contrast to the control sample with no ABA treatment. Orange color signifies up regulated genes and blue color signifies down-regulated genes, scaled to normalized read counts.
Response of ABA related Genes: To determine changes in ABA-related genes, transcriptomes were analysed to identify expression of each known ortholog of Marchantia ABA biosynthesis and response genes (Bowman et al., 2017). DEG analysis of ABA-related genes revealed Rn_08915.1, an ortholog of Marchantia MpNCED (9-cis-epoxycarotenoid dioxygenase1; Mp2g07800) and of NCED1 (At3g63520) of Arabidopsis (Bowman et al., 2017), is up-regulated upon ABA treatment (Table 1). In contrast, the PYRABACTIN RESISTANCE1-like (PYR1-like) ABA receptor, an ortholog of MpPYL1 and the fourteen PYR-related genes of Arabidopsis (Bowman et al., 2017; Jahan et al., 2019) was not found to be differentially expressed when comparing aquatic and ABA treated samples.
Table 1 ABA genes differentially expressed with ABA treated plants in comparison to aquatic R. natans.
ABA control expression of LEA-like genes: ABA reduces growth and enhances desiccation tolerance by increasing accumulation of intracellular sugars and various proteins such as those encoded by LEA-like genes (Akter et al., 2014; Hernández-Sánchez et al., 2022). Expression patterns of Ricciocarpos LEA genes are presented in Table 2. As expected, there is accumulation of several LEA genes upon ABA treatment, with Rn_05573.1 (LEA_1), Rn_18585.1 (LEA_1), and Rn_15939.1 (LEA_4) up-regulated in response to exogenous ABA application and Rn_16212.1 (LEA_2) and Rn_12186.1 (LEA_2) downregulated; LEA families as previously defined (Artur et al., 2018). In addition, several members of the NHL (NDR1, nonrace specific disease resistance 1; HIN1, hairpin-induced 1) gene family previously associated with disease resistance and ABA response (Bao et al., 2016) were differentially regulated, with Rn_11497.1, Rn_08770.1, and Rn_09383.1 downregulated in response to exogenous ABA.
Table 2 LEA genes are differentially expressed with ABA treated plants in comparison to aquatic R. natans.
Expression of the rhizoid gene, RSL1: The Marchantia gene MpRSL1 (Mp3g17930.1) is required for rhizoid initiation and is an ortholog of Arabidopsis ROOT HAIR DEFECTIVE6 that acts in root hair development (Proust et al., 2016). The orthologous Ricciocarpos gene is Rn_00421, and consistent with the observed morphology of ABA treated Ricciocarpos plants, this gene is up-regulated upon ABA treatment. An analysis examining gene expression at shorter intervals during the aquatic to terrestrial transition should identify additional gene regulatory networks associated with the developmental changes.
Several limnetic or riparian plants exhibit phenotypic plasticity wherein morphology is dependent upon environmental conditions, e.g. whether growing submerged or floating on the water versus aerial growth or on a terrestrial substrate. In many cases the shift from an aquatic morphology to a terrestrial morphology is correlated or mediated with increased endogenous ABA levels. For example, in two liverworts that evolved a secondarily aquatic habit independently, Riccia fluitans and Ricciocarpos natans, the terrestrial form can be induced by ABA (Hellwege et al., 1992; Hartung et al., 1994; Hellwege et al., 1996; Althoff et al., 2022). In each of these species, the terrestrial form develops rhizoids, while the aquatic form does not, but other morphological changes appear to be species specific, such as the loss of scale production and loss of thallus separation appear to be specific to Ricciocarpos. Similar aquatic-terrestrial transitions are also observed in vascular plants in the form of heterophylly, wherein leaf morphology is polymorphic depending upon environmental conditions (Nakayama et al., 2017; Li et al., 2019). Such heterophylly has evolved multiple times independently within angiosperms, and while in many instances ABA in implicated, its involvement is not universal. For example, abscisic acid induces formation of floating leaves in the heterophyllous aquatic angiosperm Potamogeton nodosus (Anderson, 1978) and abscisic acid induces land form characteristics in the fern Marsilea quadrifolia (Liu, 1984). In contrast, aquatic-terrestrial heterophylly in Rorippa aquatica another phytohormone, gibberellic acid, regulates leaf morphology in conjunction with KNOX1 genes known to influence leaf complexity (Nakayama et al., 2014) and in Ranunculus trichophyllus ABA works in conjunction with a third phytohormome, ethylene, to regulate heterophylly (Kim et al., 2018). Ethylene has also been implicated in heterophylly in other angiosperm species (Nakayama et al., 2017; Li et al., 2019). Both ethylene and ABA are implicated in adaptations to water stress, too much for the former and too little for the latter (Jackson, 2008; Sakata et al., 2013). Thus, these phytohormone signalling pathways are likely already active in the tissues that will develop heterophylly or other developmental modifications in aquatic plants, and can be integrated into gene regulatory networks controlling morphology as well as physiology.
While we developed Ricciocarpos natans as a ‘model’ system for examining genomic evolution during the transition for dioicy to monoicy (Singh et al., 2023), once some tools are developed for a species, other biological questions come calling. In today’s age, only a few prerequisites are required for establishing a model organism — e.g. ease of growth in culture, genomic resources, ability to introduce genome editing technologies. For Ricciocarpos, the former two have been established and it is likely that transformation using protocols for the related species, Riccia fluitans (Althoff and Zachgo, 2020), can be readily adapted to Ricciocarpos. Thus, Ricciocarpos is primed to be utilized to answer a spectrum of biological questions only limited by the imagination, and of course funding.
The data presented in the study are deposited at https://genomevolution.org/coge/, accession code: ‘Ricciocarpos’.
SS: Conceptualization, Formal Analysis, Investigation, Methodology, Writing – original draft. JB: Conceptualization, Formal Analysis, Funding acquisition, Supervision, Writing – original draft, Writing – review & editing.
The authors declare financial support was received for the research, authorship, and/or publication of this article. This work was supported by Monash University and funding from the Australian Research Council (CE200100015).
We thank Chris Cargill at the Australian National Botanic Gardens for the original Ricciocarpos natans isolate. We thank John Alvarez and Tom Fisher for helpful comments on the manuscript.
The authors declare that the research was conducted in the absence of any commercial or financial relationships that could be construed as a potential conflict of interest.
All claims expressed in this article are solely those of the authors and do not necessarily represent those of their affiliated organizations, or those of the publisher, the editors and the reviewers. Any product that may be evaluated in this article, or claim that may be made by its manufacturer, is not guaranteed or endorsed by the publisher.
Acuña-Castillo, R., Jiménez, J. E., Blanco Coto, M. A., Bezerra Silva, L. E. (2023). Ricciocarpos natans (Marchantiophyta, Ricciaceae), new to Costa Rica, with A survey of its presence in Latin America. Acta Botanica Mexicana 130, E2177. doi: 10.21829/abm130.2023.2177
Agami, M., Litav, M., Waisel, Y. (1976). The effects of various components of water pollution on the behavior of some aquatic macrophytes of the coastal rivers of Israel. Aquat. Bot. 2, 203–213. doi: 10.1016/0304-3770(76)90021-8
Akter, K., Kato, M., Sato, Y., Kaneko, Y., Takezawa, D. (2014). Abscisic acid-induced rearrangement of intracellular structures associated with freezing and desiccation stress tolerance in the liverwort marchantia polymorpha. J. Of Plant Physiol. 171, 1334–1343. doi: 10.1016/j.jplph.2014.05.004
Albert, N. W., Thrimawithana, A. H., Mcghie, T. K., Clayton, W. A., Deroles, S. C., Schwinn, K. E., et al. (2018). Genetic analysis of the liverwort Marchantia polymorpha reveals that R2r3myb activation of flavonoid production in response to abiotic stress is an ancient character in land plants. New Phytol. 218, 554–566. doi: 10.1111/nph.15002
Althoff, F., Wegner, L., Ehlers, K., Buschmann, H., Zachgo, S. (2022). Developmental plasticity of the amphibious liverwort Riccia fluitans. Front. In Plant Sci. 13, 909327. doi: 10.3389/fpls.2022.909327
Althoff, F., Zachgo, S. (2020). Transformation of riccia fluitans, an amphibious liverwort dynamically responding to environmental changes. Int. J. Of Mol. Biosci. 21, 5410. doi: 10.3390/ijms21155410
Anderson, L. W. (1978). Abscisic acid induces formation of floating leaves in the heterophyllous aquatic angiosperm Potamogeton nodosus. Science 201, 1135–1138. doi: 10.1126/science.201.4361.1135
Aoki, C., Teixeira-Gamarra, M. C., Gamarra, R. M., Medeiros, S. C. H. D., Pott, V. J., Junior, G. A. D., et al. (2017). Abiotic factors drive the structure of aquatic plant assemblages in riverine habitats of the Brazilian ‘‘Pantanal’’. Braz. J. Of Bot. 40, 405–415. doi: 10.1007/s40415-016-0345-0
Artur, M. A. S., Zhao, T., Ligterink, W., Schranz, E., Hilhorst, H. W. M. (2018). Dissecting thegenomicdiversificationof late embryogenesis abundant (Lea) protein gene families in plants. Genome Biol. And Evol. 11, 459–471. doi: 10.1093/gbe/evy248
Bao, Y., Song, W.-M., Zhang, H.-X. (2016). Role of arabidopsis Nhl family in aba and stress response. Plant Signaling Behav. 11, E1180493. doi: 10.1080/15592324.2016.1180493
Barnes, C. R., Land, W. J. G. (1907). Bryological papers I. The origin of air chambers - contributions from the hull botanical laboratory, 100. Botanical Gazette 44, 197–213. doi: 10.1086/329317
Berland, H., Albert, N. W., Stavland, A., Jordheim, M., Mcghie, T. K., Zhou, Y., et al. (2019). Auronidins are A previously unreported class of flavonoid pigments that challenges when anthocyanin biosynthesis evolved in plants. Proc. Of Natl. Acad. Of Sci. U.S.A. 116, 20232–20239. doi: 10.1073/pnas.1912741116
Berrie, G. K. (1960). The chromosome numbers of liverworts (Hepaticae and Anthocerotae). Trans. Of Br. Bryological Soc. 3, 688–705. doi: 10.1179/006813860804828963
Bischler-Causse, H., Gradstein, S. R., Jovet-Ast, S., Long, D. G., Allen, N. S. (2005). Marchantiidae. Flora Neotropica 97, 1–262.
Bischoff, G. W. (1835). Bemerkungen über die lebermoose vozüglich aus den gruppen der marchantieen und riccieen. Nova Acta Physico Med. A C L 17 (2), 909–1088. Tafeln Lxvii-Lxxi.
Boere, G. C., Stroud, D. A. (2006). “The flyway concept: what it is and what it isn’t,” in Waterbirds Around The World. Eds. Boere, G. C., Galbraith, C.A., Stroud, D. A. (Edinburgh, Uk: The Stationery Office).
Bowman, J. L. (2022). The origin of A land flora. Nat. Plants 8, 1352–1369. doi: 10.1038/s41477-022-01283-y
Bowman, J. L., Arteaga-Vazquez, M., Berger, F., Briginshaw, L. N., Carella, P., Aguilar-Cruz, A., et al. (2022). The renaissance and enlightenment of marchantia as A model system. Plant Cell 34, 3512–3542. doi: 10.1093/plcell/koac219
Bowman, J. L., Kohchi, T., Yamato, K. T., Jenkins, J., Shu, S., Ishizaki, K., et al. (2017). Insights into land plant evolution garnered from the Marchantia polymorpha genome. Cell 171, 287–304. doi: 10.1016/j.cell.2017.09.030
Brown, R., Bauer, F. (1814). General Remarks, Geographical And Systematical, On The Botany Of Terra Australis (W. Bulmer: London).
Buch, H. (1954). Om utbredning och spridningen av fissidens julianus, ricciocarpus natans och riccia fluitans I ostfennoskandia. Memoranda Societatis Pro Fauna Et Flora Fennica 29, 35–40.
Campbell, D. H. (1895). The structure and development of mosses and ferns (London: Macmillan And Co).
Campbell, E. O. (1975). Notes on the liverwort family ricciaceae in New Zealand. Tuatara 21, 121–129.
Candela, H., Hake, S. (2008). The art and design of genetic screens: maize. Nat. Rev. Genet. 9, 192–203. doi: 10.1038/nrg2291
Candolle, A. P. D., Lamarck, J.-B. P. A. (1805). Flore Française, Paris, Chez H. Agasse, Rue Des Poitevins, N°. 6 (De L'imprimerie De Stoupe). Paris.
Chimney, M. J. (1984). First report of Ricciocarpus natans (L.) corda (Marchantiales-ricciaceae) from A strip mine impoundment. Trans. Of Illinois Acad. Of Sci. 77, 43–44.
Coe, E. H. J. (2001). The origins of maize genetics. Nat. Rev. Genet. 2, 898–905. doi: 10.1038/35098524
Collier, J. H., Hooper, J. (1866). The American Parlor Aquarium, Or, Fluvial Aqua Vivarium : Being A Familiar Treatise On The Fresh Water Aquarium; The Best Mode Of Construction And Arrangement; Including A Brief Sketch Of All The Fresh Water Fishes And Aquatic Plants Adapted To The Same, Found In The United States New York. Collier, J. H.
Corda, A. J. C. (1828). Genera hepaticarum. Ph. M. Opiz Beiträgen Zur Naturgeschichte, 12, 643–655.
Dillenius, J. J. (1741). Historia Muscorum: A General History Of Land And Water, &C. Mosses And Corals, Containing All The Known Species (London: J. Millan).
Drège, J. F. (1843). Zwei Pflanzengeographische Documente, Stafleu & Cowan (Taxonomic Literature). doi: 10.5962/bhl.title.87612
Duthie, A. V., Garside, S. (1936). Studies in South African ricciaceae. Trans. Of R. Soc. Of South Afr. 2, 93–133. doi: 10.1080/00359193609520543
Edler, D., Klein, J., Antonelli, A., Silverstro, D. (2020). Raxmlgui 2.0: A graphical interface and toolkit for phylogenetic analyses using Raxml. Methods In Ecol. And Evol. 12, 373–377. doi: 10.1111/2041-210X.13512
Ferreyra, A. N. (1990). Notas sobre la morfologia vegetativa Y la reproducción asexual en Ricciocarpus natans L. Corda marchantiales hepaticae. Lilloa 37, 51–54.
Fischer, E. (1995). The genera ricciocarpos and riccia (Hepaticae, Ricciaceae) in Rwanda. Fragmenta Floristica Et Geobotanica 40, 93–111.
Fourounjian, P., Fakhoorian, T., Cao, X. H. (2020). “Importance of duckweeds in basic research and their industrial applications,” in The Duckweed Genomes. Eds. Cao, X. H., Fourounjian, P., Wang, W. (Springer Cham). doi: 10.1007/978-3-030-11045-1_1
Frey, W., Frahm, J.-P., Fischer, E., Lobin, W. (2006). The Liverworts, Mosses And Ferns Of Europe Martins, Greathorkesley, Colchester, Essexc06 4ah (England: Harley Books, B.H. & A. Harley Ltd).
Gamborg, O. L., Miller, R. A., Ojima, K. (1968). Nutrient requirements of suspension cultures of soybean root cells. Exp. Cell Res. 50, 151–158. doi: 10.1016/0014-4827(68)90403-5
Garber, J. F. (1904). The life history of Ricciocarpus natans. Botanical Gazette 37, 161–177. doi: 10.1086/328464
Gimenes, L. L. S., Freschi, G. P. G., Bianchini, I. J., Cunha Santino, M. B. D. (2020). Growth of the aquatic macrophyte Ricciocarpos natans (L.) corda in different temperatures and in distinct concentrations of aluminum and manganese. Aquat. Toxicol. 224, 105484. doi: 10.1016/j.aquatox.2020.105484
Gomes, M. P., Brito, J. C. E. M. D., Bicalho, E. M., Silva, J. G., Gomides, M. D. F. A., Garcia, Q. S., et al. (2018). Ciprofloxacin vs. Temperature- antibiotic toxicity in the free-floating liverwort Ricciocarpus natans from A climate change perspective. Chemosphere 202, 410–419. doi: 10.1016/j.chemosphere.2018.03.048
Haas, B. J., Delcher, A. L., Mount, S. M., Wortman, J. R., Smith, R. K., Hannick, L. I., et al. (2003). Improving the arabidopsis genome annotation using maximal transcript alignment assemblies. Nucleic Acids Res. 31, 5654–5666. C.OMMAJ.R.X.X.X. doi: 10.1093/nar/gkg770
Haas, B. J., Salzberg, S. L., Zhu, W., Pertea, M., Allen, J. E., Orvis, J., et al. (2008). Automated eukaryotic gene structure annotation using evidencemodeler and the program to assemble spliced alignments. Genome Biol. 9, R7. doi: 10.1186/gb-2008-9-1-r7
Hartman, G. (1985). Foods of male mallard, before and during moult, as determined by faecal analysis. Wildfowl J. 36, 65–71.
Hartung, W., Hellwege, E. M., Volk, O. H. (1994). The function of abscisic acid in bryophytes. J. Of Hattori Botanical Lab. 76, 59–65. 10.18968/jhbl.76.0_59
Hassel de Menendez, G. G. (1962). Estudio De Las Anthocerotales Y Marchantiales de la Argentina. Opera Lilloana 7, 1–97.
Hellwege, E. M., Dietz, K.-J., Hartung, W. (1996). Abscisic acid causes changes in gene expression involved in the induction of the landform of the liverwort Riccia fluitans L. Planta 198, 423–432. doi: 10.1007/BF00620059
Hellwege, E. M., Volk, O. H., Hartung, W. (1992). A physiological role of abscisic acid in the liverwort Riccia fluitans L. J. Plant Physiol. 140, 553–556. doi: 10.1016/S0176-1617(11)80788-1
Hernández-Sánchez, I. E., Maruri-López, I., Martinez-Martinez, C., Janis, B., Jiménez-Bremont, J. F., Covarrubias, A. A., et al. (2022). Leafing through literature: late embryogenesis abundant proteins coming of age—Achievements and perspectives. J. Of Exp. Bot. 73, 6525–6546. doi: 10.1093/jxb/erac293
Hirsh, P. E. (1910). The development of air chambers in the Ricciaceae. Bull. Of Torrey Botanical Club 37, 73–77. doi: 10.2307/2478961
Hooker, W. J. (1840). Icones Plantarum Or Figures, With Brief Descriptive Characters And Remarks, Of New Or Rare Plants, Selected From The Author's Herbarium, London: Longman, Rees, Orme, Brown, Green, & Longman.
Hooker, J. D. (1855). “The botany of the antarctic voyage of H. M. Discovery ships erebus and terror in the years 1839-1843 unde rthe command of captain sir james clark ross,” in Ii. Flora Novae-Zealandiae ii. Flowerless Plants (London: Lovell Reeve).
Hübener, J.W., P. (1834). Hepaticologia Germanica Oder Beschreibung Der Deutschen Lebermoose, Mannheim: Schwan - Götz'sche Hofbuchlandlung.
Jackson, M. B. (2008). Ethylene-promoted elongation: an adaptation to submergence stress. Ann. Of Bot. 101, 229–248. doi: 10.1093/aob/mcm237
Jahan, A., Komatsu, K., Wakida-Sekiya, M., Hiraide, M., Tanaka, K., Ohtake, R., et al. (2019). Archetypal roles of an abscisic acid receptor in drought and sugar responses in liverworts. Plant Physiol. 179, 317–328. doi: 10.1104/pp.18.00761
Jain, C., Rodriguez-R, L. M., Phillippy, A. M., Konstantinidis, K. T., Aluru, S. (2018). High throughput ani analysis of 90k prokaryotic genomes reveals clear species boundaries. Nat. Commun. 9, 5114. doi: 10.1038/s41467-018-07641-9
Jones, E. W. (1957). African hepatics: xiii. The ricciaceae in tropical Africa. Trans. Of Br. Bryological Soc. 3, 208–227. doi: 10.1179/006813857804829524
Kanazawa, T., Morinaka, H., Ebine, K., Shimada, T. L., Ishida, S., Minamino, N., et al. (2020). The liverwort oil body is formed by redirection of the secretory pathway. Nat. Commun. 11, 6152. doi: 10.1038/s41467-020-19978-1
Kashyap, S. R. (1929). Liverworts of The Western Himalayas and the Panjab Plain. Part I (Lahore: The University Of The Panjab).
Kelleway, J. J., Iles, J. A., Kobayashi, T., Ling, J. E. (2021). Resilience Of A native soil seed bank in A floodplain lake subjected to cropping, grazing and extended drought. Mar. And Freshw. Res. 72, 787–799. doi: 10.1071/MF19386
Kim, J., Joo, Y., Kyung, J., Jeon, M., Park, J. Y., Lee, H. G., et al. (2018). A molecular basis behind heterophylly in an amphibious plant, Ranunculus trichophyllus. PloS Genet. 14, E1007208. doi: 10.1371/journal.pgen.1007208
Kolon, K., Sarosiek, J. (1995). Das verschwinden der wasserlebermoose riccia fluitans und Ricciocarpos natans an irhen fundorten in niederschlesien und ihre empfindlichkeit gegen̈ber zwei herbiziden. Cryptogamica Helv. 18, 77–83.
Kronestedt, E. (1981). Anatomy of Ricciocarpus natans (L.) Corda, studied by scanning electron microscopy. Ann. Of Bot. 47, 817–827. doi: 10.1093/oxfordjournals.aob.a086081
Kronestedt, E. (1982a). Anatomy of Ricciocarpus natans, with emphasis on fine structure. Nordic J. Of Bot. 2, 353–367. doi: 10.1111/j.1756-1051.1982.tb01200.x
Kronestedt, E. (1982b). Structure and development of the air pores in Ricciocarpus natans. Nordic J. Of Bot. 2, 491–499. doi: 10.1111/j.1756-1051.1982.tb01214.x
Kronestedt, E. (1983). Cytology of oil–body cells in Ricciocarpus natans. Nordic J. Of Bot. 3, 547–558. doi: 10.1111/j.1756-1051.1983.tb01467.x
Kubo, H., Nozawa, S., Hiwatashi, T., Kondou, Y., Nakabayashi, R., Mori, T., et al. (2018). Biosynthesis of riccionidins and marchantins is regulated by R2r3- Myb transcription factors in marchantia polymorpha. J. Of Plant Res. 131, 849–864. doi: 10.1007/s10265-018-1044-7
Kunz, S., Becker, H. (1995). Cell wall pigment formation of in vitro cultures of the liverwort Ricciocarpos natans. Z. Naturforsch. 50, 235–240. doi: 10.1515/znc-1995-3-412
Kunz, S., Burkhardt, G., Becker, H. (1994). Riccionidins A and B, anthocyanidins from the cell walls of the liverwort Ricciocarpus natans. Phytochemistry 35, 233–235. doi: 10.1016/S0031-9422(00)90540-5
Lázaro, X. A., Mackenzie, R., Jiménez, J. E. (2021). Evidence of endozoochory in upland geese chloephaga picta and white-bellied seedsnipes attagis malouinus in sub-antarctic Chile. Ecol. And Evol. 11, 9191–9197. doi: 10.1002/ece3.7725
Lee, G. E., Gradstein, S. R. (2021). Guide to the Genera of Liverworts and Hornworts Of Malaysia (Tokyo, Japan: Hattori Botanical Laboratory).
Leffler, E. M., Bullaughey, K., Matute, D. R., Meyer, W. K., Ségurel, L., Venkat, A., et al. (2012). Revisiting an old riddle: what determines genetic diversity levels within species? PloS Biol. 10, E1001388. doi: 10.1371/journal.pbio.1001388
Leitgeb, H. (1879). Untersuchungen über die lebermoose. Heft iv. Die riccieen (Leuschner & Lubensky: Graz).
Lewis, C. E. (1906). The embryology and development of riccia lutescens and Riccia-crystallina. Botanical Gazette 41, 109–138. doi: 10.1086/328727
Lewis, L. R., Behling, E., Gousse, H., Qian, E., Elphick, C. S., Lamarre, J.-F., et al. (2014a). First evidence of bryophyte diaspores in the plumage of transequatorial migrant birds. Peerj 2, 424. doi: 10.7717/peerj.424
Lewis, L. R., Rozzi, R., Goffinet, B. (2014b). Direct long-distance dispersal shapes A new world amphitropical disjunction in the dispersal-limited dung moss tetraplodon (Bryopsida: Splachnaceae). J. Of Biogeography 41, 2385–2395. doi: 10.1111/jbi.12385
Li, F.-W., Brouwer, P., Carretero-Paulet, L., Cheng, S., Vries, J. D., Delaux, P.-M., et al. (2018). Fern genomes elucidate land plant evolution and cyanobacterial symbioses. Nat. Plants 4, 460–472. doi: 10.1038/s41477-018-0188-8
Li, H., Durbin, R. (2009). Fast and accurate short read alignment with burrows–wheeler transform. Bioinformatics 25, 1754–1760. doi: 10.1093/bioinformatics/btp324
Li, G., Hu, S., Hou, H., Kimura, S. (2019). Heterophylly: phenotypic plasticity of leaf shape in aquatic and amphibious plants. Plants 8, 420. doi: 10.3390/plants8100420
Ligrone, R., Carafa, A., Lumini, E., Bianciotto, V., Bonfante, P., Duckett, J. G. (2007). Glomeromycotean associations in liverworts- A molecular, cellular, and taxonomic analysis. Am. Jounal Of Bot. 94, 1756–1777. doi: 10.3732/ajb.94.11.1756
Lindberg, S. O. (1882). Bryological notes from the meetings of the society pro fauna et flora fennica. Rev. Bryologique 9, 81–85.
Linde, A.-M., Sawangproh, W., Cronberg, N., Szövényi, P., Lagercrantz, U. (2020). Evolutionary history of the Marchantia polymorpha complex. Front. In Plant Sci. 11, 829. doi: 10.3389/fpls.2020.00829
Lindenberg, J. B. W. (1836). Monographie der riccieen. Nova Acta Acad. Caes. Leopold.-Carol. Nat. Cur. 18, 360–504. Tafeln Xix-Xxxvii.
Linné, C. V. (1770). Systema Naturae Per Regna Tria Naturae, Secundum Classes, Ordines, Genera, Species, Cum Characteribus, Differentiis, Synonymis, Locis Vindobonae: Typis Ioannis Thomae Von Trattner.
Liu, B.-L. L. (1984). Abscisic acid induces land form characteristics in Marsilea quadrifolia L. Am. Jounal Of Bot. 71, 638–644. doi: 10.1002/j.1537-2197.1984.tb14170.x
Lorenzen, H., Kaiser, U., Foerster, M. (1981). Intensives wachstum von ricciocarpus natans (Lebermoos) in durchlüftungskultur. Berichte Der Deutschen Botanischen Gesellschaft 94, 719–725. doi: 10.1111/j.1438-8677.1981.tb03439.x
Love, M. I., Huber, W., Anders, S. (2014). Moderated estimation of fold change and dispersion for RNA-seq data with DESeq2. Genome biology 15 (12), 1–21 doi: 10.1186/s13059-014-0550-8
Maeda, M., Akiyama, H., Ashiya, M. (2016). Life history of Ricciocarpos natans L. 1. Development of sexual organs and sporophytes in rice field conditions. Humans And Nat. 27, 43–52. doi: 10.1590/0001-3765202220210436
Maggio, L. P., Schmitz, D., Putzke, J., Schaefer, C. E. G. R., Pereira, A. B. (2022). Pellets of Stercorarius spp. (Skua) as plant dispersers in the Antarctic peninsula. Anais Da Academia Bras. Cienc. 94, E20210436.
Meyerowitz, E. M., Pruitt, R. E. (1985). Arabidopsis thaliana and plant molecular genetics. Science 229, 1214–1218. doi: 10.1126/science.229.4719.1214
Montagne, C. (1839). Cryptogamae brasiliensis. Annales Des. Sci. Naturelles Botanique Ser. 2 T. 12, 42–55.
Montgomery, S. A., Tanizawa, Y., Galik, B., Wang, N., Ito, T., Mochizuki, T., et al. (2020). Chromatin organization in early land plants reveals an ancestral association between H3k27me3, transposons, and constitutive heterochromatin. Curr. Biol. 30, 573–588. doi: 10.1016/j.cub.2019.12.015
Nakayama, H., Nakayama, N., Seiki, S., Kojima, M., Sakakibara, H., Sinha, N., et al. (2014). Regulation of the Knox-ga gene module induces heterophyllic alteration in North American lake cress. Plant Cell 26, 4733–4748. doi: 10.1105/tpc.114.130229
Nakayama, H., Sinha, N. R., Kimura, S. (2017). How do plants and phytohormones accomplish heterophylly, leaf phenotypic plasticity, in response to environmental cues. Front. In Plant Sci. 8, 1717. doi: 10.3389/fpls.2017.01717
Oh, S., Koh, S. C. (2013). Chl A fluorescence characterization and biomarker selection from Ricciocarpos natans under cadmium stress. J. Of Environ. Sci. Int. 22, 1403–1413. doi: 10.5322/JESI.2013.22.11.1403
Page, D. R., Grossniklaus, U. (2002). The art and design of genetic screens: Arabidopsis thaliana. Nat. Rev. Genet. 3, 124–136. doi: 10.1038/nrg730
Parsons, J. G., Cairns, A., Johnson, C. N., Robson, S. K. A., Shilton, L. A., Westcott, D. A. (2007). Bryophyte dispersal by flying foxes: A novel discovery. Oecologia 152, 112–114. doi: 10.1007/s00442-006-0639-1
Peeters, E. T. H. M., Neefjes, R. E. M., Van Zuidam, B. G. (2016). Competition between free-floating plants is strongly driven by previously experienced phosphorus concentrations in thewater column. PloS One 11, E0162780. doi: 10.1371/journal.pone.0162780
Perold, S. M. (1995). The taxonomic history of the ricciaceae, (1937-1995) and A classification of sub-saharan ricciae. Bothalia 25, 211–231. doi: 10.4102/abc.v25i1.729
Perry, M. C., Lilly, L., Jones, G. (1856). Narrative of the expedition of an american squadron to the China seas and Japan : performed in the years 1852, 1853, and 1854, under the command of commodore M.C. Perry, United States navy, by order of the government of the United States (Wahsington: Beverley Tucker).
Petiver, J. (1695-1703). Musei petiveriani centuria prima-[Decima] rariora naturae; continens: viz. Animalia, fossilia, plantas, ex variis mundi plagis advecta, ordine digesta, et nominibus propriis signata,. Smith, S., Walford., B.: Londini (London).
Pickett, F. L. (1925). The life history of Ricciocarpus Natans. Bryologist 28, 1–3. doi: 10.1639/0007-2745(1925)28[1:TLHORN]2.0.CO;2
Piippo, S. (1990). Annotated Catalogue of Chinese hepaticae and anthocerotae. J. Of Hattori Botanical Lab. 68, 1–192. doi: 10.18968/jhbl.68.0_1
Piñeiro, R., Popp, M., Hassel, K., Listl, D., Westergaard, K. B., Flatberg, K. I., et al. (2012). Circumarctic dispersal and long-distance colonization of South America: the moss genus cinclidium. J. Of Biogeography 39, 2041–2051. doi: 10.1111/j.1365-2699.2012.02765.x
Proust, H., Honkanen, S., Jones, V. A. S., Morieri, G., Prescott, H., Kelly, S., et al. (2016). Rsl class I genes controlled the development of epidermal structures in the common ancestor of land plants. Curr. Biol. 26, 93–99. doi: 10.1016/j.cub.2015.11.042
Provart, N. J., Alonso, J., Assmann, S. M., Bergmann, D., Brady, S. M., Brkljacic, J., et al. (2015). 50 years of Arabidopsis research: highlights and future directions. New Phytol. 209, 921–944. doi: 10.1111/nph.13687
Prunet, N., Meyerowitz, E. M. (2016). Genetics and plant development. Comptes Rendus Biologies 339, 240–246. doi: 10.1016/j.crvi.2016.05.003
Rafinesque, C. S. (1817). First decade of undescribed American plants, or synopsis of new species, from the United States. Am. Monthly Magazine And Crit. Rev. 2, 43.
Rafinesque, C. S. (1833). Principles of the philosophy of New Genera and new species of plants and animals. Atlantic J. And Friend Of Knowledge 1, 163–164.
Ray, J. (1724). Synopsis methodica stirpium britannicarum. 3rd ed (Londini, Gulielmi & Joannis Innys).
Rhoades, M. M. (1984). The early years of maize genetics. Annu. Rev. Of Genet. 18, 1–29. doi: 10.1146/annurev.ge.18.120184.000245
Rieth, A. (1959). Bemerkungen über ricciocarpus natans (L.) corda. Kulturpflanze 7, 207–217. doi: 10.1007/BF02099389
Romani, F., Banic, E., Florent, S. N., Kanazawa, T., Goodger, J. Q. D., Mentink, R. A., et al. (2020). Oil body formation in marchantia polymorpha is controlled by Mpc1hdz and serves as A defense against arthropod herbivores. Curr. Biol. 30, 2815–2828. doi: 10.1016/j.cub.2020.05.081
Sakata, Y., Komatsu, K., Takezawa, D. (2013). ABA as a universal plant hormone. Prog. In Bot. 75, 57–96. doi: 10.1007/978-3-642-38797-5_2
Sarosiek, J., Wazakowska Natkaniec, H., Wiewiórka, Z. (1987a). The effect of heavy metals on the dynamics of A ricciocarpus natans (L.) corda population. Symp. Biologica Hungaria 35, 857–863.
Sarosiek, J., Wiewiórka, Z., Mróz, L. (1987b). Bioindication of heavy metal toxicity of water by the liverwort Ricciocarpus natans (L.) corda. Symp. Biologica Hungaria 35, 827–833.
Schmidel, C. C. (1793). Icones plantarvm et analyses partivm aeri incisae atqve vivis coloribvs insignatae (Joannen Jocobum Palm: Erlangae).
Schuster, R. M. (1983). “Phytogeography of the bryophytes,” in New manual of bryology vol. 1. Ed. Schuster, R.M. (Hattori Botanical Laboratory: NiChinan, Miyazaki, Japan).
Schuster, R. M. (1992). The hepaticae and anthocerotae of North America, vol. Vi (Columbia University Press: New York).
Schwarz-Sommer, Z., Davies, B., Hudson, A. (2003). An everlasting pioneer: the story of antirrhinum research. Nat. Rev. Genet. 4, 655–664. doi: 10.1038/nrg1127
Schweinitz, L. D. D. (1821). Specimen florae americae septentrionalis cryptogamicae; sistens: muscos hepaticos huc usque'in am. Sept. Observatos (Raleigh: J. Gales).
Scoppola, A., Spada, F., Blasi, C. (1988). Framework for A chronological and coenological characterization of A Ricciocarpus natna (L.) corda stand in the subcoastal district in central Italy. Documents Phytosociologiques 11, 423–432.
Scott, G. A. M. (1985). Southern Australian liverworts (Australian Government Publishing Service: Canberra).
Shimamura, M. (2016). Marchantia polymorpha; taxonomy, phylogeny and morphology of A model plant. Plant Cell Physiol. 57, 230–256. doi: 10.1093/pcp/pcv192
Siler, M. B. (1934). Chromosome numbers in certain ricciaceae. Proc. Of Natl. Acad. Of Sci. Of United States Of America 20, 603–607. doi: 10.1073/pnas.20.12.603
Singh, S., Davies, K. M., Chagné, D., Bowman, J. L. (2023). The fate of sex chromosomes during the evolution of monoicy from dioicy in liverworts. Curr. Biol. 33, 3597–3609. doi: 10.1016/J.Cub.2023.07.023
Skulberg, O. M. (1978). En ny lemnide I norsk flora - svanemat (Ricciocarpus natans) I gjølsjøen, halden’-vassdraget. Blyttia 36, 27–34.
Smith, J. E. (1804). English botany or coloured figures of british plants vol. Xvii (London: J. Taylor).
Somerville, C., Koornneef, M. (2002). A fortunate choice: the history of arabidopsis as A model plant. Nat. Rev. Genet. 3, 883–889. doi: 10.1038/nrg927
Stahl, M. (1949). Die mycorrhiza der lebermoose mit besonderer ber̈cksichtigung der thallosen formen. Planta 37, 103–148. doi: 10.1007/BF01929705
Stanke, M., Diekhans, M., Baertsch, R., Haussler, D. (2008). Using native and syntenically mapped cdna alignments to improve de novo gene finding. Bioinformatics 24, 637–644. doi: 10.1093/bioinformatics/btn013
Stotler, R. E., Crandall-Stotler, B. (2017). A synopsis of the liverwort flora of North America North of Mexico. Ann. Of Missouri Botanical Garden 102, 574–709. doi: 10.3417/2016027
Stubbe, H. (1966). Genetik und zytologie von antirrhinum L. Sect. Antirrhinum (Jena: Gustav Fischer).
Sturm, J. (1832). Deutschlands flora in abbildungen nach der natur (Nürnberg: Gedruckt Auf Kosten Des Verfassers).
Sussex, I. (1998). Themes in plant development. Annu. Rev. Plant Physiol. And Plant Mol. Biol. 49, Xiii–Xxii. doi: 10.1146/annurev.arplant.49.1.0
Toivonen, H. (1985). Changes in the pleustic macrophyte flora of 54 small finnish lakes in 30 years. Annales Botanici Fennici 22, 37–44.
Vandenbussche, M., Chambrier, P., Bentoand, S. R., Morel, P. (2016). Petunia,Your next supermodel? Front. In Plant Sci. 7, 72. doi: 10.3389/fpls.2016.00072
Viana, D. S., Santamaría, L., Figuerola, J. (2016). Migratory birds as global dispersal vectors. Trends In Ecol. Evol. 31, 763–775. doi: 10.1016/j.tree.2016.07.005
Villarreal, A. J. C., Crandall-Stotler, B. J., Hart, M. L., Long, D. G., Forrest, L. L. (2016). Divergence times and the evolution of morphological complexity in an early land plant lineage (Marchantiopsida) with A slow molecular rate. New Phytol. 209, 1734–1746. doi: 10.1111/nph.13716
Wickett, N. J., Mirarab, S., Nguyen, N., Warnow, T., Carpenter, E., Matasci, N., et al. (2014). Phylotranscriptomic analysis of the origin and early diversification of land plants. Proc. Of Natl. Acad. Of Sci. Of United States Of America 111, E4859–E4868. doi: 10.1073/pnas.1323926111
Wilkinson, D. M., Lovas-Kiss, A., Callaghan, D. A., Green, A. J. (2017). Endozoochory of large bryophyte fragments by waterbirds. Cryptogamie Bryologie 38, 223–228. doi: 10.7872/cryb/v38.iss2.2017.223
Wolek, J. (1997). Species co-occurrence patterns in pleustonic plant communities (Class lemnetea): are there assembly rules governing pleustonic community assembly? Fragmenta Floristica Et Geobotanica 0, 3–100.
Wolek, J., Walanus, A. (2000). Co-occurrence of lemnids in Argentina: A null model analysis. Fragmenta Floristica Et Geobotanica 45, 179–192.
Keywords: Ricciocarpos natans, liverwort, aquatic macrophytes, abscisic acid, evo devo
Citation: Singh S and Bowman JL (2023) The monoicous secondarily aquatic liverwort Ricciocarpos natans as a model within the radiation of derived Marchantiopsida. Front. Plant Sci. 14:1260596. doi: 10.3389/fpls.2023.1260596
Received: 18 July 2023; Accepted: 08 September 2023;
Published: 28 November 2023.
Edited by:
Verónica S. Di Stilio, University of Washington, United StatesReviewed by:
Eftychios Frangedakis, University of Cambridge, United KingdomCopyright © 2023 Singh and Bowman. This is an open-access article distributed under the terms of the Creative Commons Attribution License (CC BY). The use, distribution or reproduction in other forums is permitted, provided the original author(s) and the copyright owner(s) are credited and that the original publication in this journal is cited, in accordance with accepted academic practice. No use, distribution or reproduction is permitted which does not comply with these terms.
*Correspondence: John L. Bowman, am9obi5ib3dtYW5AbW9uYXNoLmVkdQ==
Disclaimer: All claims expressed in this article are solely those of the authors and do not necessarily represent those of their affiliated organizations, or those of the publisher, the editors and the reviewers. Any product that may be evaluated in this article or claim that may be made by its manufacturer is not guaranteed or endorsed by the publisher.
Research integrity at Frontiers
Learn more about the work of our research integrity team to safeguard the quality of each article we publish.