- 1The National Institute of Horticultural Research, Skierniewice, Poland
- 2University of Belgrade, Faculty of Agriculture, Belgrade, Serbia
- 3Valent BioSciences, Libertyville, IL, United States
- 4United States Department of Agriculture, Agricultural Research Service, Horticultural Crops Disease and Pest Management Research Unit, Corvallis, OR, United States
- 5Environmental Genomics and Systems Biology Research Group, Institute for Natural Resource Sciences, Zurich University of Applied Sciences (ZHAW), Wädenswil, Switzerland
Xanthomonas arboricola pv. corylina (Xac; formerly Xanthomonas campestris pv. corylina) is the causal agent of the bacterial blight of hazelnuts, a devastating disease of trees in plant nurseries and young orchards. Currently, there are no PCR assays to distinguish Xac from all other pathovars of X. arboricola. A comparative genomics approach with publicly available genomes of Xac was used to identify unique sequences, conserved across the genomes of the pathogen. We identified a 2,440 bp genomic region that was unique to Xac and designed identification and detection systems for conventional PCR, qPCR (SYBR® Green and TaqMan™), and loop-mediated isothermal amplification (LAMP). All PCR assays performed on genomic DNA isolated from eight X. arboricola pathovars and closely related bacterial species confirmed the specificity of designed primers. These new multi-platform molecular diagnostic tools may be used by plant clinics and researchers to detect and identify Xac in pure cultures and hazelnut tissues rapidly and accurately.
1 Introduction
Xanthomonas arboricola pv. corylina (Xac; formerly Xanthomonas campestris pv. corylina; Vauterin et al., 1995) is a Gram-negative plant pathogenic bacterium of the Lysobacteraceae family (earlier synonym of Xanthomonadaceae) (Saddler and Bradbury, 2005; Tindall, 2014). Xac is the causal agent of the bacterial blight of hazelnut (Corylus avellana L.). Other Corylus spp., including C. pontica, C. maxima and C. colurna, also can be infected by Xac (OEPP/EPPO, 1986; OEPP/EPPO, 2004). Bacterial blight of hazelnut is a devastating disease that is commonly observed in plant nurseries and young orchards, causing significant plant mortality (Miller et al., 1949; Moore, 2002; OEPP/EPPO, 2004; Lamichhane and Varvaro, 2014; Webber et al., 2021). The disease also can be seen in established production orchards, especially on susceptible cultivars. The main disease symptoms include angular necrotic lesions on leaves and the involucres of shells, as well as shoot necrosis and cankers. Lesions on the stalk and top of nuts results in reduced nut quality. Dieback of nut-bearing branches causes measurable yield reduction. Over time, bacterial blight of hazelnut reduces tree health and results in poor tree structure and continued yield losses (Obradović et al., 2010; Kałużna et al., 2021).
Xac has been a regulated pathogen and placed on the European and Mediterranean Plant Protection Organization (EPPO) list A2 of quarantine pathogens, but it was recently reclassified as a Regulated Non-Quarantine Pest (RNQP) (European Union, 2016; European Union, 2019). Currently, bacterial blight caused by Xac has been reported in nearly every hazelnut-producing country (OEPP/EPPO, 2004; Kałużna et al., 2021; Osdaghi, 2022). Identification of Xac is currently a tedious, multistep process, which is described below and can take several days to return a diagnostic result. Difficulty in identification arises largely because it is closely related to seven other pathovars of X. arboricola, including pv. pruni (Xap), pv. juglandis (Xaj), pv. fragariae, pv. celebensis, pv. arracaciae, pv. poinsettiicola and pv. zantedeschiae (Vauterin et al., 1995; Janse et al., 2001; Fischer-Le Saux et al., 2015; Kałużna et al., 2021). Two former X. arboricola pathovars were recently elevated to the species rank as X. guizotiae and X. populina (Zarei et al., 2022).
The diagnostic procedures for Xac as recommended by EPPO rely on the observation of disease symptoms, microscopic examination of the symptomatic tissues, isolation of the pathogen from the plant material on common microbiological media for xanthomonads (Schaad et al., 2001), observation of colony morphology, biochemical, phenotypic, and pathogenicity assays (Lelliott and Stead, 1987; OEPP/EPPO, 2004). Xac also can be identified with serological methods following the procedures described in EPPO protocols (OEPP/EPPO, 2010b).
Molecular tools for rapid diagnosis of Xac colonies currently include methods specific for the genus Xanthomonas (Maes, 1993) and for the species X. arboricola (Pothier et al., 2011a). To identify X. arboricola isolates to the pathovar level, rep-PCR and partial sequence alignments are generally used (Tuang et al., 1999; Schaad et al., 2001; Scortichini et al., 2002; Parkinson et al., 2007; Young et al., 2008; Calić et al., 2009; OEPP/EPPO, 2010a; Puławska et al., 2010; Webber et al., 2020). Moreover, it was reported that primers designed for identification of X. arboricola pv. pruni (XapY17-F/XapY17-R) can also generate amplicons of some Xac strains (Pothier et al., 2011a; Webber et al., 2020).
Currently, there are no rapid and sensitive diagnostic tools for Xac (Prokić et al., 2012; Kałużna et al., 2021). The conventional methods are too labor-intensive and slow for routine detection and diagnosis, as complete diagnostic protocols can take several days. Additionally, the symptoms of bacterial blight of hazelnuts may be confused with anthracnose, a fungal disease caused by Piggotia coryli (Roberge ex Desm.) B. Sutton (Syn. Gloeosporium coryli (Roberge ex Desm.) Sacc.). Disease misidentification can lead to applying ineffective management methods and use of unwarranted chemical applications.
Recently, next-generation sequencing (NGS) and comparative genomics have developed as effective methods to provide information on pathogen population structures, create species specific markers, and characterize virulence or antibiotic resistance genes. The genomes and/or plasmids of several pathovars of X. arboricola, including Xac, have been sequenced (Pothier et al., 2011b; Pothier et al., 2011c; Ibarra Caballero et al., 2013; Garita-Cambronero et al., 2014; Cesbron et al., 2015; Higuera et al., 2015; Ignatov et al., 2015; Garita-Cambronero et al., 2016a; Garita-Cambronero et al., 2016b; Garita-Cambronero et al., 2016c; Harrison et al., 2016; López-Soriano et al., 2016; Garita-Cambronero et al., 2017; Retamales et al., 2017; Fernandes et al., 2018; Fu et al., 2018; Gétaz et al., 2018; Nuñez Cerda et al., 2021; Teixeira et al., 2021; Cuesta-Morrondo et al., 2022; D’Amico-Willman et al., 2022; Herbert et al., 2022; Kałużna and Pothier, 2022; Pothier et al., 2022). The available sequence data and the needs of the grower community and diagnostic laboratories prompted us to develop rapid, accurate and sensitive tools for the bacterial blight of hazelnut causal agent. We developed molecular tools for identification of Xac that could be used with several platforms, including conventional PCR, qPCR, and Loop-mediated isothermal AMPlification (LAMP), to facilitate adoption based on available laboratory equipment. We validated each of the tools using genomic DNA isolated from pure cultures of Xac and DNA isolated from artificially inoculated and field-infected plant material. These fast and accurate identification and detection methods will aid in the diagnosis and management of bacterial blight of hazelnut in nursery stock tissues, nurseries, and in both young and established orchards.
2 Materials and methods
2.1 Bacterial strains
Xac isolates and strains collected from different geographical regions (n = 60) were tested to validate all diagnostic assays. Additionally, a collection of type and non-type strains of all pathovars of X. arboricola species, other closely related Xanthomonas species (n = 30), and microorganisms (bacteria and fungi) isolated from symptomatic hazelnut and walnut tissues, i.e. Pseudomonas spp., Pseudomonas avellanae, Sphingomonas spp. and Xanthomonas campestris (n = 46) were included in assays (Table 1, Supplementary Table S1).
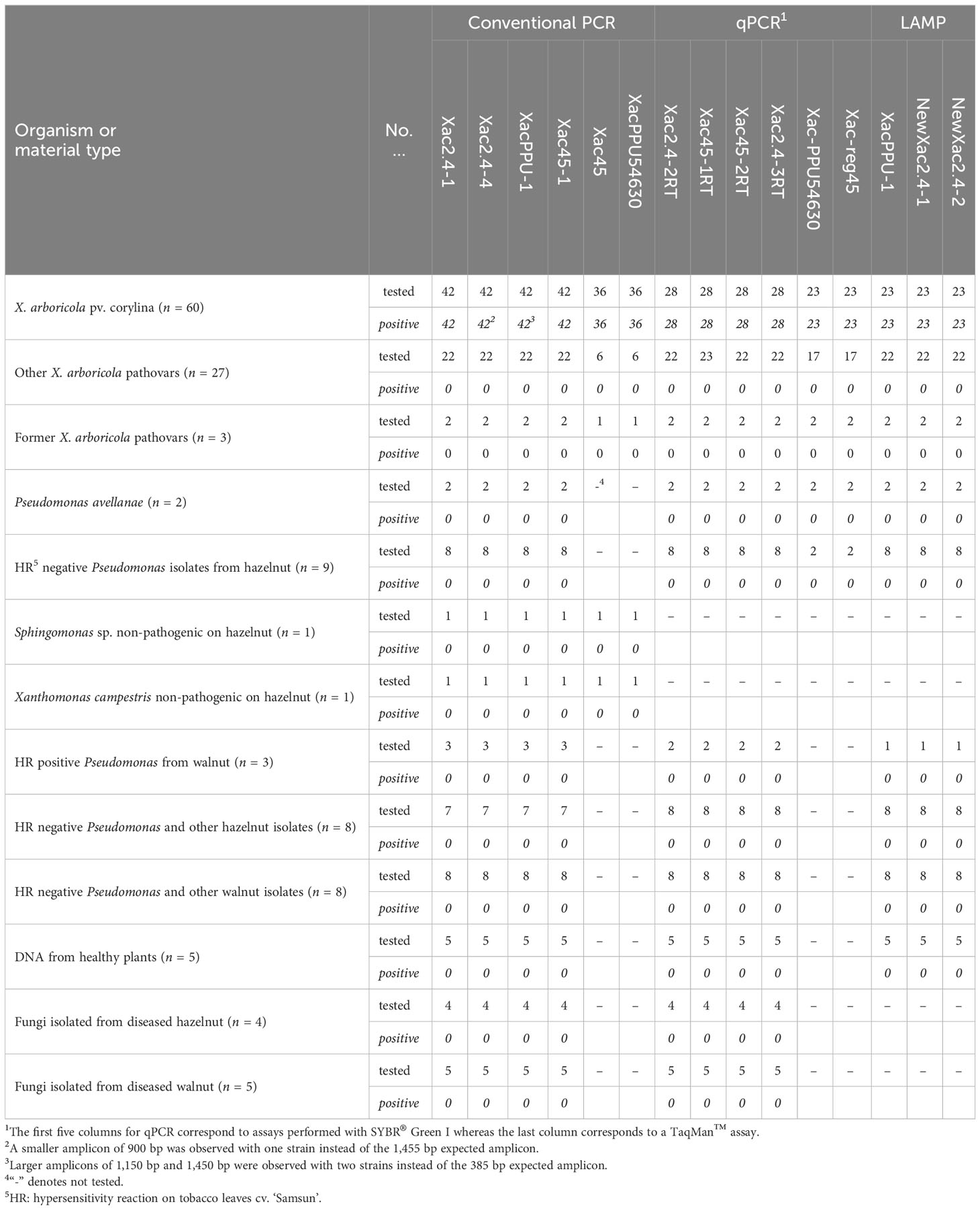
Table 1 Summary of in vitro primers specificity with the different Xanthomonas arboricola pv. corylina detection tools developed in this study.
Xanthomonads were grown on yeast extract nutrient agar (YNA) or yeast extract dextrose calcium carbonate (YDC; Schaad et al., 2001) and pseudomonads were cultured on King’s B medium (King et al., 1954) at 28°C for 24 to 48 h. The nine fungal isolates were grown on PDA (potato dextrose agar; Becton Dickinson, Sparks, MD, USA) at 24°C with an 8 h light and 16 h dark photoperiod.
2.2 DNA isolation from bacterial and fungal cultures
Genomic bacterial DNA was isolated using the Genomic Mini bacterial DNA Purification Kit (A&A Biotechnology, Poland), the DNeasy Mericon Food Kit (Qiagen, Hilden, Germany) or Whole Blood and Tissue kit (Qiagen, Germantown, MD, USA), according to the manufacturer’s instructions. The total fungal DNA was extracted from 100 mg of mycelia scraped from 10-day-old PDA cultures with the GeneMatrix Plant & Fungi DNA Purification Kit (EURx, Gdańsk, Poland) according to the manufacturer’s instructions. The quality and total DNA concentration was estimated with a NanoDrop ND-100 or NanoDrop 2000c (ThermoFisherScientific, Waltham, MA, USA).
2.3 Genome-informed target identification
DNA sequences from three Xac whole genome shotgun sequencing projects (WGS) (CFBP 1159PT, CFBP 2565 and NCCB 100457; GenBank WGS prefixes MDEA01, MDSJ01 and APMC02, respectively) was used for comparative genomic analysis. A ‘dual-BLASTn’ comparative genomics pipeline was applied to select 300-bp regions shared among these three target WGS (Schneeberger et al., 2017). After segmentation into 300 bp length fragments, duplicates were removed and Xac unique sequences were selected using BLASTn+ v.2.8.1 (Altschul et al., 1990; Camacho et al., 2009) analysis against the database derived from the three genomes. Regions obtained from this workflow were further checked for Xac specificity using online BLASTn searches against the nr/nt and X. arboricola and Xanthomonas WGS NCBI databases (accessed in July 2019). Finally, Xac-specific DNA markers were also confirmed in three recently released Xac complete genomes (CFBP 1159PT, CFBP 6600 and Xac 301; GenBank assemblies GCA_905220785.1, GCA_905220805.1, and GCA_905220715.1, respectively; Pothier et al., 2022).
2.4 Primer design and synthesis
Three Xac-specific regions and their associated primers were given ‘in-house’ names during analyses, the genome context of the regions is illustrated in Figure 1. These regions were used to design primers for: 1) conventional PCR, 2) qPCR (SYBR® Green and TaqMan™), and 3) LAMP. The primers for conventional PCR and qPCR were designed using the PrimerSelect program of the LASERGENE package v.9 (DNASTAR, Madison, WI, USA) and Primer3Web v.4.1.0. (Untergasser et al., 2012). LAMP primers were designed using the online platform PrimerExplorer v.5 (Eiken Chemical Co., Ltd, Tokyo, Japan, http://primerexplorer.jp/lampv5e/index.html) also including loop primers (i.e. in total six primers) to speed up the LAMP reaction (Nagamine et al., 2002). Based on the regions selected (Figure 1), ten candidate primer sets were designed for conventional PCR (5, 2 and 3 primer sets based on the “region 2.4”, “PPU54630”, and “target 45” Xac-specific DNA markers, respectively), six for SYBR® Green I qPCR (3, 1 and 2 primer sets based on the “region 2.4”, “PPU54630”, and “target 45” Xac-specific DNA markers, respectively), two for TaqMan™ qPCR (one primer pair based on the “region 2.4” and one based on the “target 45” Xac-specific DNA markers), and three for LAMP (two primer pairs based on the “region 2.4” and one based on the “PPU54630” Xac-specific DNA markers). The primers for the TaqMan™ qPCR were purchased HPLC purified since this effectively increases the melting temperature (Tm) for shorter sequences, allowing for an overall shorter amplicon while remaining within temperature requirements. The TaqMan™ probes were designed with a 5′ FAM reporter dye and a 3’ BHQ-1 non-fluorescent quencher. Initially, the specificity of the primers, the TaqMan™ probe, and predicted amplicons to Xac were tested in silico with BLASTn searches against the nr/nt and WGS NCBI databases (accessed in July 2019). All these primer sets were then tested in vitro for specificity, sensitivity, and reproducibility during screening. Depending on the research institutions, primers were synthesized at Genomed S.A. (Warszawa, Poland), Invitrogen (ThermoFisherScientific, Waltham, MA, USA) and MilliporeSigma (Burlington, MA., USA).
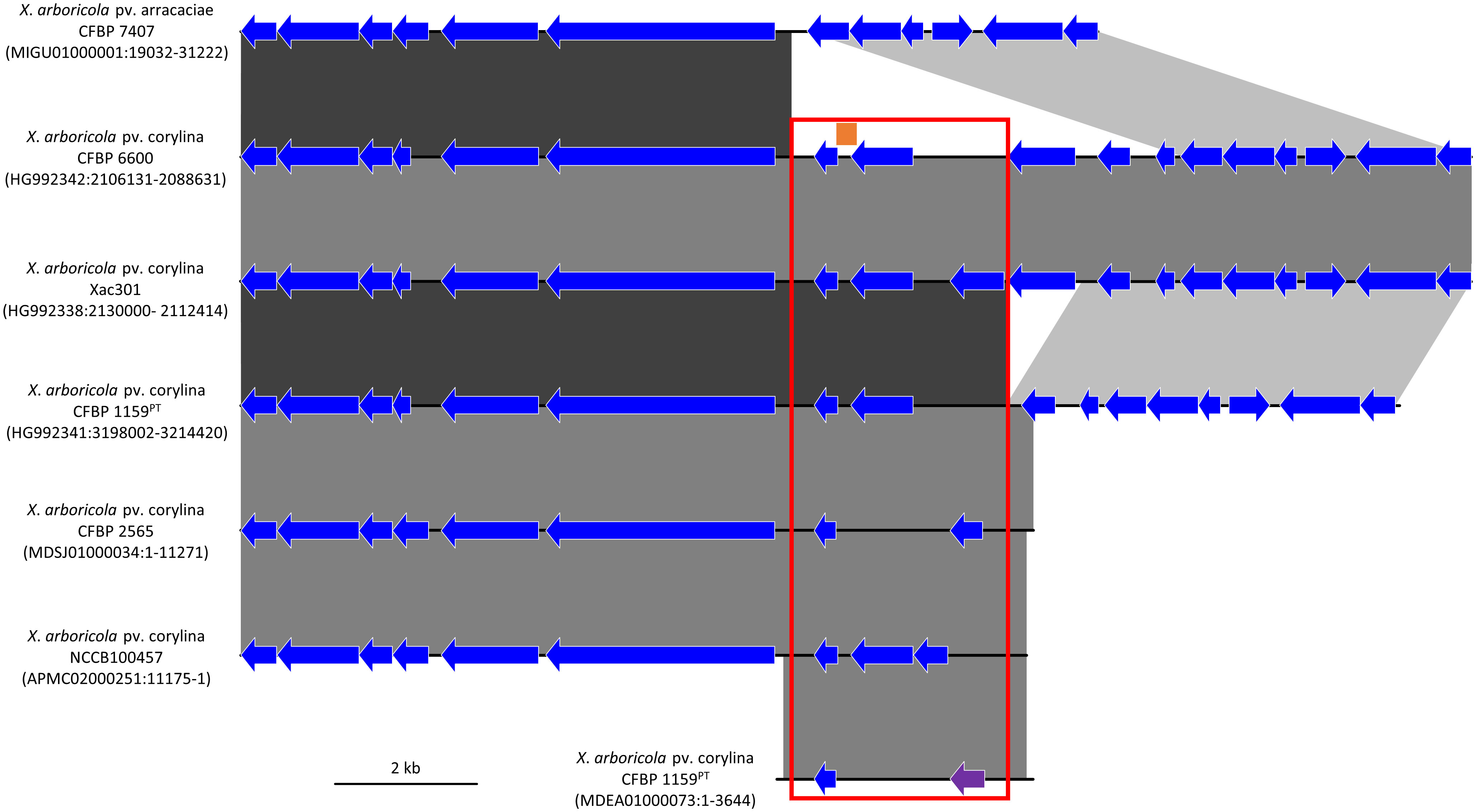
Figure 1 Comparison of the genetic environment of the Xanthomonas arboricola pv. corylina (Xac) specific DNA targets in six Xac draft and complete genomes and one draft X. arboricola pv. arracaciae draft genome. The 300 bp Xac-specific region called “target 45” is represented by an orange bar, the XaxcyCFBP1159_22010 singleton encoding the hypothetical protein “PPU54630” is displayed by a purple arrow, and the 2.4 kb region called “region 2.4” identified by comparative genomics is indicated by the red frame. Other CoDing Sequence (CDS) are shown with blue arrows, which do not denote any shared identity among the genomes. Regions with high DNA sequence identity between the genomes are represented with blocks using a black to grey scale with black representing the highest identity. The strain name is followed by the GenBank accession number and the location of the genomic region displayed.
2.5 Primer selection based on in vitro specificity analysis
The in vitro specificity of all primers was tested with purified genomic DNA of the bacteria and fungi listed in Table 1 (detailed in Supplementary Table S1).
To exclude potential non-specific amplification of plant genomic DNA with the primers, total plant DNA was isolated from clean asymptomatic leaves of five hazelnut cultivars (cv. ‘Cosford’, cv. ‘Merveille de Bollwiller’, cv. ‘Garibaldi’, cv. ‘Webb’s Prize Cob’ and cv. ‘Hall’s Giant’) grown in a greenhouse. Total plant DNA was isolated from leaves using the GeneMATRIX Plant & Fungi DNA Purification Kit (EURx, Gdańsk, Poland), as well as the Genomic Mini DNA Extraction Kit (A&A Biotechnology, Gdynia, Poland) to isolate bacterial DNA. Both kits were used according to the manufacturer’s instructions with the following specifications concerning the starting material. To isolate plant DNA: 100 mg from hazelnut leaves were homogenized in liquid nitrogen in a cooled mortar and pestle and transferred to a 2 ml tube before addition of 400 μl lysis buffer L. For bacterial DNA isolation: 100 mg of crushed or cut leaf tissue was placed in 20 ml of PBS buffer, incubated for 1 h at 26°C with shaking (150 rpm), pelleted by centrifugation (5 min at 12,000 × g), and then re-suspended in 100 μl Tris EDTA (TE) buffer.
Three labs participated in the specificity validation of the assays: two assay development laboratories (Poland and Serbia) and one assay testing laboratory (USA).
The reactions were conducted according to the protocols established based on the optimization of all reagents and temperature gradient analysis performed separately for each primer pair. The amplification conditions for all the primers pairs/sets are listed in Table 2.
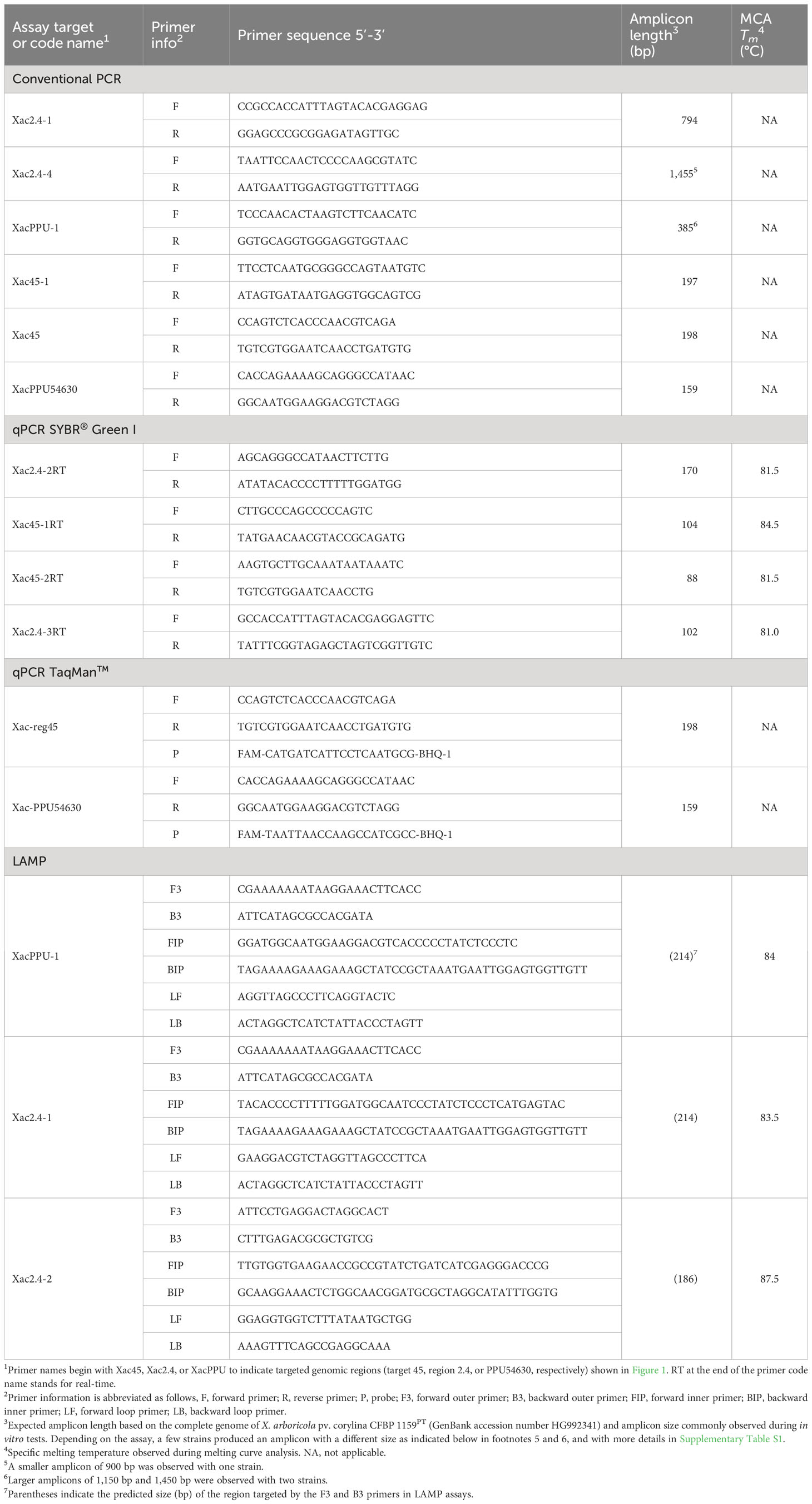
Table 2 Nucleotide sequences of specific primers developed in this study for the detection of Xanthomonas arboricola pv. corylina.
Amplification reactions with the four selected primer pairs for conventional PCR were conducted in a Biometra T3000 thermocycler (Biometra, Göttingen, Germany) in Poland, in a Thermo Cycler 2720 (Applied Biosystems, USA) in Serbia, and a Veriti 96-well Thermal Cycler 9902 (Applied Biosystems, USA) in the USA. The total amplification reaction mixtures for primers in 15 μl of volume included: 10 to 15 ng of DNA, 0.4 U of DreamTaq DNA Polymerase (ThermoFisherScientific, Waltham, MA, USA), 1× reaction DreamTaq Green buffer, 0.15 mM each dNTPs and 0.7 mM of each primer. The amplicons obtained in individual reactions for each primer pair were separated in 1.5% agarose gels in 0.5× TBE buffer (0.045 M Tris-boric acid, 0.001 M EDTA, pH 8.0) (Sambrook et al., 1989). To confirm the size of the obtained product O’GeneRuler100-bp DNA Ladder Plus (ThermoFisherScientific, Waltham, MA, USA) was used. Gels were stained in an ethidium bromide solution (0.5 μg ml-1) and obtained products were visualized under UV irradiation.
SYBR® Green I qPCRs were conducted in a Bio-Rad CFX96 (Bio-Rad, Hercules, CA, USA) with SsoAdvanced™ Universal SYBR® Green Supermix (Bio-Rad, Hercules, CA, USA) in Poland or a Mic qPCR Cycler (Bio Molecular Systems, Australia) in Serbia. The reaction mixture in 20 μl of total volume included 1× reaction SYBR® Green Supermix and 0.5 mM of each primer from the following primer sets: Xac2.4-2RT, Xac45-1RT, Xac45-2RT, Xac2.43RT, and 10 ng of DNA. The PCR programs for all above-listed primers are given in Table 3. The specificity of amplification products was verified by a melting curve analysis using a progressive denaturation of products at a rising temperature (Table 3). Specific melting temperatures observed are indicated in Table 2.
The validation of the TaqMan™ qPCR was also done in Poland. The sequence of probes and primers are indicated in Table 2. Reactions were conducted in a Bio-Rad CFX96 (Bio-Rad, Hercules, CA, USA) using the amplification conditions in Table 3. The TaqMan™ qPCR assays were carried out in a 10 μl total reaction mixture containing 1 μl of template DNA, 0.25 μl of primers Xac-PPU54630-F and Xac-PPU54630-R (0.25 μM final concentration of each), 0.15 μl of probe Xac-PPU54630-P (0.15 μM final concentration), 1× TaqMan™ Fast Universal PCR Master Mix (Applied Biosystems, USA).
Loop‐mediated isothermal amplifications were performed on a Bio-Rad CFX96 (Bio-Rad, Hercules, CA, USA) in Poland. The reactions mixture carried out in a total volume of 20 μl contained 1× Isothermal Mastermix (OptiGene, Horsham, UK) and primers at the final concentrations as follows: outer primers F3/B3 0.2 μM each, inner primers FIB/BIP 0.8 μM each and loop primers 0.4 μM each. Fluorescence was detected on the FAM channel. The LAMP reaction mixtures were run according to conditions detailed in Table 3.
2.6 Limits of detection of DNA- and crude bacterial cell-based assays
The limits of detection (LoD) of all the DNA-template based assays were tested with 10-fold dilutions series prepared in TE buffer using bacterial genomic DNA isolated from pure cultures of CFBP 1159PT and Xac 301. The dilution series ranged from ~10 ng μl-1 to 0.1 fg μl-1 based on the initial concentrations determined with a NanoDrop ND-100 (ThermoFisherScientific, Waltham, MA, USA). Additionally, bacterial genomic DNA was independently extracted from pure bacterial cultures of these two same strains using known bacterial concentrations ranging from ~108 to 100 CFU ml-1 as described in Kałużna et al. (2016).
For the crude bacterial cell-template based assays, 100 μl of different concentrations of aqueous suspensions of strain Xac 301 were added to 100 mg of crushed/cut fragments of leaves or stems. Then DNA was isolated from these ‘heterogeneous suspensions’ according to the methodology described by Kałużna et al. (2016).
For the PCR-based assays, the efficiency (E) was calculated from the slope (S) of the standard curve generated for each run using the following equation E = 10(−1/S) with E = 2 corresponding to 100% efficiency (Ramakers et al., 2003).
2.7 Validation of assays on artificially and naturally infected hazelnuts
To test the usefulness of designed primers, positive controls for in planta detection were obtained from artificially inoculated hazelnut cvs. ‘Cosford’ and ‘Merveille de Bollwiller’ (two samples from each cultivar) maintained in a greenhouse, as well as from naturally infected material obtained from orchards (two samples). For artificial inoculation of the hazelnut cultivars, a 48-h culture of Xac 301 grown on YNA medium was suspended in sterile water (108 and 107 CFU ml-1) and infiltrated into hazelnut leaves with a needleless syringe and/or injected into green shoots using a hypodermic needle (0.7×30mm) attached to a syringe. Four to six weeks post-inoculation, symptomatic plant tissue was harvested. Leaf samples were rubbed for 10 s on both sides with a cotton-swab soaked in 70% ethanol. A sample consisting of three 1-cm2 segments including the lesion border was collected, crushed, and suspended in 1 to 2 ml sterile PBS for 15 min. We then tested two DNA extraction methods on the tissue macerate. In the first one, 10 μl of the plant macerate was added to 190 μl of TE buffer, boiled for 10 min at 100°C, and then centrifuged for 5 min at 9,500 × g. In the second approach, 10 μl of the plant macerate was added to 90 μl of TE buffer and total DNA was isolated using the Genomic Mini DNA Extraction Kit (A&A Biotechnology Gdynia, Poland) according to the manufacturer’s instructions. The boiled extract and purified DNA extract were used as templates in molecular assays. To confirm the infection by Xac, especially from naturally infected plant material, bacterial colony isolation was done simultaneously by plating on YNA medium.
3 Results
3.1 Genome-informed Xac-specific targets
The in silico analysis resulted in the detection of a highly conserved, Xac-specific sequence of 300 bp called “target 45” that had no hit with other bacteria in the database. A 2,440 bp genomic region called “region 2.4” encompassing “target 45” was identified after performing the comparative genomic analysis of target 45 in the six Xac whole genomes available (Figure 1). Region 2.4 located on the chromosome corresponds to an insertion in Xac that was not present in other X. arboricola pathovars, such as pv. arracaciae (Figure 1). The annotations for this region varied slightly between the different Xac genomes, but the region contains between two and three singletons that encode hypothetical proteins. A 494 bp singleton located within “region 2.4” in Xac CFBP 1159PT (locus_tag XaxcyCFBP1159_22010) and annotated as encoding the hypothetical protein PPU54630 was used for the further development of Xac-specific assays.
3.2 Candidate primer sets and Xac assays development
Out of the candidate primer sets designed for all three detection techniques, a few sets were discarded from further analysis due to the presence of non-specific products that persisted, even after adjusting annealing temperatures. After initial laboratory testing, we focused on validation and testing of six primer pairs for conventional PCR, four primer pairs for qPCR with SYBR® Green I, two primer pairs for TaqMan™ qPCR, and three primer pairs for LAMP. The sequences of these primer sets are reported in Table 2. A primer BLASTn analysis of selected primers showed no full similarity to any sequences of bacterial plant pathogens in GenBank in July 2019. This in silico result was also confirmed on 15 May 2023 with a final primer check performed in the course of writing this article.
3.3 Primers specificity for Xac in conventional, qPCR and LAMP in vitro and in planta
The genomic DNA of the 60 Xac strains was selectively amplified with all the primers developed for the different assays. No amplification was observed for the bacterial and fungal genomic DNA not belonging to the Xac pathovar (Table 1). Similarly, no amplification was observed with DNA templates obtained from clean, asymptomatic leaves of five hazelnut cultivars using two DNA extraction kits.
The PCR assays using primers designed for conventional PCR gave amplicons ranging from 197 bp to 1,455 bp depending on the primer pairs used (Table 2, Supplementary Table S1). The six primer sets designed for conventional PCR generated a single amplicon of the size predicted by genome analyses for nearly all the 60 strains of Xac evaluated (Table 1). Although, during validation of conventional PCR reactions on the JL26xx strains of Xac collected in Oregon, amplicons with an unexpected size were observed with Xac strain JL2600 with primers Xac2.4-4 and XacPPU-1. For Xac strain JL2600, the amplicon observed for primer pair for Xac2.4-4 was 1,166 bp instead of 1,455 bp and the amplicon for the primer pair for XacPPU-1 was 1,450 bp instead of 390 bp. The other conventional PCR primer pairs generated the predicted amplicon size for Xac strain JL2600. Conventional PCR reactions for all the other Xac strains in the JL26xx series (Supplementary Table S1) returned the expected amplicon size for each of the primer pairs.
In the SYBR® Green I qPCR assays, DNA from the Xac strains resulted in a positive reaction. However, non-specific, false-positive results after 28 cycles for a few bacteria not belonging to the Xanthomonas genus were observed when using the primers Xac45-1. Nonetheless, these non-specific amplicons were excluded based on the results of melting curve analysis i.e., having different melting temperature than the target product. The amplicons ranged from 88 bp to 170 bp and melting curve analysis performed on these specific products revealed a single peak characteristic of their already introduced line 492 Tm as reported in Table 2.
In the TaqMan™ qPCR, two primer sets designed resulted in a positive reaction for the tested DNA from the Xac strains tested (Table 1, Supplementary Table S1) and no product were observed in case of testing of bacterial and fungal genomic DNA not belonging to the Xac pathovar nor DNA templates obtained from clean, asymptomatic leaves of five hazelnut cultivars.
In the LAMP assays, DNA of the Xac strains gave a positive reaction as expected and no amplification was observed with DNA of other isolates. The Tm of products amplified using the LAMP primers are provided in Table 2.
Specificity, sensitivity, and efficiency of the Xanthomonas arboricola pv. corylina specific assays based on the organisms evaluated in this study are reported in Table 4.
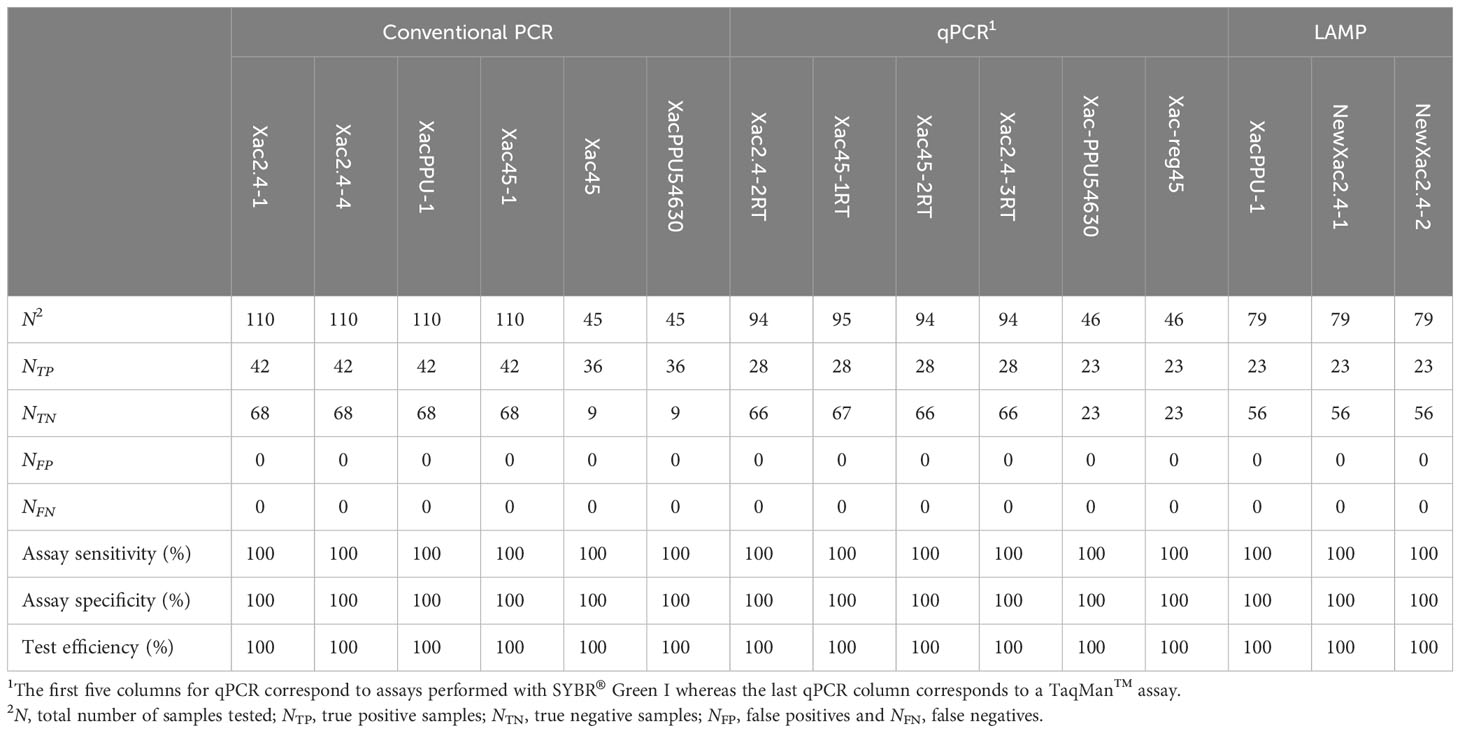
Table 4 Specificity, sensitivity, and efficiency of the Xanthomonas arboricola pv. corylina specific assays based on the organisms evaluated in this study.
The LAMP and both qPCR assays confirmed identity of verified Xac strains. Results for LAMP and qPCR platforms were obtained in less than 1 h.
3.4 Limits of detection of DNA- and crude bacterial cell-based assays
The sensitivity and detection limit of the Xac target DNA varies not only between the detection systems developed but also depending on the primer sets used. For four primer pairs designed for conventional PCR, 100 fg of genomic DNA generated a visible amplicon with primer pairs Xac2.4-4, and XacPPU-1; ~1 pg genomic DNA for primer pair Xac2.4-1; and 10 pg was detected with primer set Xac45-1. When crude, boiled bacterial cell templates were tested, the LoD was 1.8 × 101 CFU per reaction for Xac2.4-1 and XacPPU-1 primer sets, 1.8 × 100 CFU per reaction for Xac2.4-4 primer sets, 1.8 × 102 CFU per reaction for XacPPU54630 primer sets, and 1.8 × 103 CFU per reaction for Xac45-1 primer sets.
The LoD was lowered by 101 when using the primer pairs Xac2.4-1, Xac2.4-4, and XacPPU-1 to detect Xac in plant tissue macerates that contained the pathogen; for the primer pairs XacPPU54630 and Xac45-1 the LoD remained the same with or without plant tissues.
Among qPCR primers designed for SYBR® Green I, two primer sets (Xac2.4-3RT and Xac45-1RT) detected 1 fg of Xac genomic DNA (Figure 2), however the two other primer sets (Xac2.4-2RT and Xac45-2RT) detected about 10 fg of Xac genomic DNA. When crude boiled bacterial templates were tested, the limit of detection was 1 × 100 CFU per reaction. The same decrease of sensitivity as noticed for conventional PCR (lowered by 101) for boiled bacterial preparations and in combinations of plant tissues and bacteria. Parameters of the four qPCR SYBR® Green I assays are reported in Table 5.
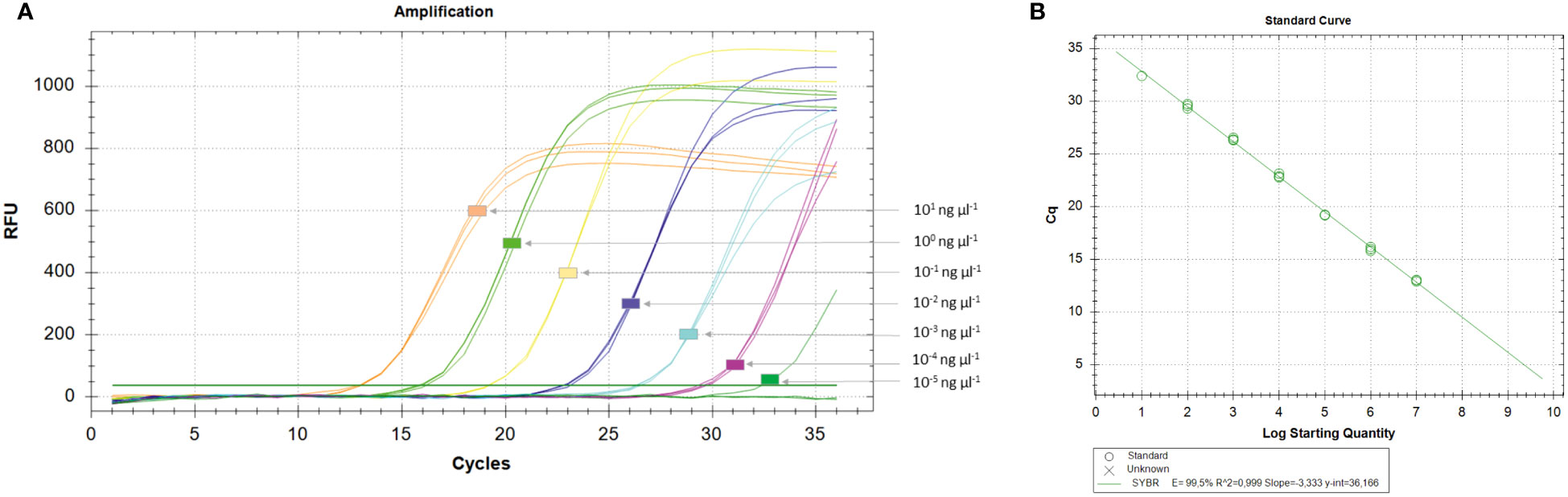
Figure 2 Determination of the limit of detection (LoD) of the Xanthomonas arboricola pv. corylina (Xac) qPCR SYBR® Green I assay (A) and standard curve (B). The representative amplifications were obtained with the Xac2.4-3RT SYBR® Green I assay using 10-fold dilutions (three technical replicates) of genomic DNA of known concentrations isolated from pure cultures of strain Xac 301. The efficacy reaction E, coefficient of determination (R2), slope and regression curve equations (y) were evaluated using the CFX Manager Software v.3.1 (Bio-Rad, Hercules, CA, USA).
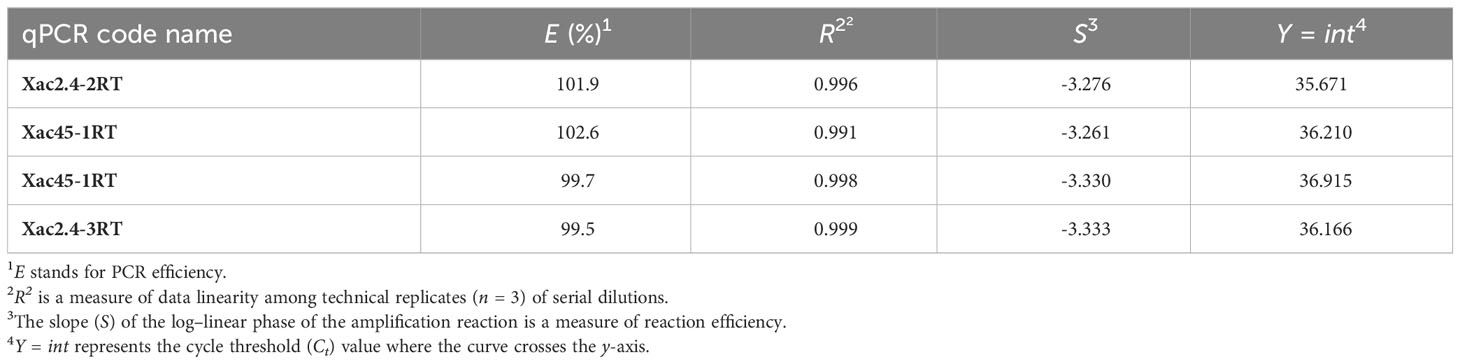
Table 5 Parameters of the four qPCR SYBR® Green I assays evaluated through the analysis of standard curves generated with serial dilutions of genomic DNA extracts from X. arboricola pv. corylina CFBP 1159PT and Xac 301 as templates.
For TaqMan™ qPCR, the primer sets Xac-reg45 and Xac-PPU54630 detected 80 and 8 pg of Xac genomic DNA, respectively. When crude boiled bacterial templates were tested, the limit of detection was 2 × 101 CFU per reaction for primer set Xac-PPU54630 and 2 × 103 CFU per reaction for primer set Xac-reg45.
When determining the sensitivity of LAMP primers, we detected 1 pg of purified genomic DNA from Xac isolates. When boiled bacterial cell templates were tested, the LoD was 1 × 100 CFU per reaction for the XacPPU-1 primer set and 1 × 101 CFU per reaction for the Xac2.4-1 and Xac2.4-2 primer sets. In purified DNA isolated from plant material combined with bacteria, the LoD was 1 × 103 CFU per reaction for all the primers tested.
3.5 Performance of the different detection tools on tissues from artificially inoculated and naturally infected hazelnuts
The detection of Xac in artificially inoculated plant material was done with four conventional primer sets (Xac2.4-1, Xac2.4-4, XacPPU-1 and Xac45-1), all SYBR® Green I qPCR (n = 4) and all LAMP primer sets (n = 3). All the primer sets used in the different platforms returned positive results for detection of Xac when the DNA was isolated using the kit procedure. Nonetheless, when the volume of the template of purified genomic DNA (µl or concentration per reaction) significantly increased, detection was decreased. Correspondingly, a one-tenth dilution of the purified genomic DNA template added to plant tissues allowed for consistent detection of Xac.
With all four conventional PCR primer sets, Xac was not detected when the assays were performed on DNA templates obtained via the boiling procedure of plant macerate (plants artificially or naturally infected). Because this was not the case with templates consisting of purified genomic DNA, we suspect that the boiling procedure did not eliminate possible plant inhibitors. The assays also remained negative when a tenfold dilution of the extracts was tested. For the SYBR® Green I qPCR primer sets, Xac was detected in DNA isolated with both procedures independent of the template DNA concentration. With the LAMP XacPPU-1, Xac2.4-1 and Xac2.4-2 primer sets, Xac was always detected with purified genomic DNA preparations. In case of DNA extracted by boiling, templates with only 0.5 and 1 µl of undiluted extract was detected.
4 Discussion
Based on a comparative genomics approach using five publicly available Xac genomes (Ibarra Caballero et al., 2013; Merda et al., 2017; Pothier et al., 2022) and several bacterial genomes from NCBI GenBank, we successfully identified unique DNA targets and designed highly specific tools capable of identifying Xac in pure culture and culture-independent in planta detection. We developed four different systems for conventional PCR and qPCR, as well as a LAMP protocol for the rapid and specific detection of Xac. This ensures a wide application of the developed detection methods, depending on the equipment or preferences of scientists, diagnosticians, inspectors, and producers. In addition, these methods offer an advantage over conventional testing as bacteria do not need to be cultured prior to detection (Palacio-Bielsa et al., 2009). This could prove especially useful in the context of screening nursery material for latent infections, which would otherwise go undetected and become a source of primary infection in the field. For regions where new hazelnut acreage is rapidly increasing, such as Serbia and Chile (Lamichhane et al., 2012; Obradović et al., 2010), disease-free planting material is a critical first step to keep Xac disease pressure low.
Historically, hazelnut bacterial blight diagnostics have relied upon a combination of classical microbiology, serology, and molecular techniques (Schaad et al., 2001; OEPP/EPPO, 2004; Pothier et al., 2011a; Prokić et al., 2012; Kałużna et al., 2021). While recommended by EPPO, these methods are time consuming and risk misdiagnosis (Prokić et al., 2012). Moreover, none of them provide a LoD. For example, the biochemical features of Polish strains differ from those described in the EPPO standard. As a result, the recommended phenotype testing methods are not applicable to strains from the Polish climatic zone (Puławska et al., 2010). Similar issues have emerged when conducting the recommended procedure of sequencing housekeeping genes to identify pathovars within X. arboricola. The multilocus sequence analysis within this species showed that using a restricted number of housekeeping gene loci did not have sufficient discriminatory power to differentiate isolates of Xaj and Xac into unique groupings. Moreover, the use of partial gyrB sequences alone cannot discriminate Xaj and Xac from Xap (Kałużna et al., 2014; Fischer-Le Saux et al., 2015; Webber et al., 2020). The molecular tools reported herein overcome these sub-species diagnostic shortcomings.
The success of our work is based on comparing the genomes of all X. arboricola pathovars and related Xanthomonas species (Zarei et al., 2022), which allowed for the selection of a highly specific regions for Xac. The specificity of the region identified within the six genomes used for in silico development (three WGS and three complete genomes from five Xac strains) also was confirmed when tested with BLASTn analysis against three additional complete Xac genomes released after our assay development (namely: A7, assembly ASM1814170v1; IVIA 3978, assembly ASM2337497v1; CFBP 1846, assembly ASM2337499v1; data not shown). The success of our approach likely benefitted from the large number of genomes available for the X. arboricola species (about 100 genomes at the time of in silico development) thus allowing the development of assays at a sub-species level. The designed diagnostic tools allowed the detection of Xac genotypes from different worldwide geographical origins. A total of 60 Xac strains originating from eight countries in two continents and collected over 20 different years spanning the period 1939-2020 was tested successfully. The only exception was a result for the conventional PCR primer set Xac2.4-4 and XacPPU-1 when screening a set of Xac isolates from the United States. Xac isolate JL2600 amplified successfully, which indicates a Xac positive result, but the resulting amplicon was larger than expected. This result is particularly surprising because the dendrogram constructed using the concatenated partial sequences of rpoD and gyrB (Webber et al., 2020), had strain JL2600 clustered together with strain JL2606, an isolate for which the expected amplicon size was obtained. All other Xac strains (e.g., JL2610) belonging to the other Xac cluster described in the work by Webber et al. (2020) gave the expected amplicon size. This result reaffirms that validation testing of a comprehensive collection of strains, preferentially in different laboratories, is very important when developing novel identification and detection systems. Importantly, Xac specificity was confirmed by all detection assays and none of the non-X. arboricola pathovars tested returned a positive amplicon, which has happened in previous studies (Palacio-Bielsa et al., 2011; Pothier et al., 2011a; Fernandes et al., 2017). Also, none of the genomic DNA of Pseudomonas, other plant pathogenic and nonpathogenic bacteria, or fungi isolated from hazelnut and walnut gave a positive signal in the assays. In addition, no amplification was observed from DNA isolated from asymptomatic plants of different C. avellana cultivars, which means that the designed primers did not react with the hazelnut genome or its microbiota.
The methods and tools developed here can be applied for specific, reliable detection of Xac in infected plant material. Not having to first isolate and purify the pathogen significantly shortens the time required for diagnosis. All methods presented in this study allow for direct amplification of Xac DNA present in plant material. However, we observed that direct detection of DNA templates extracted by boiling can give false negative results, most likely due to the presence of inhibitory compounds. This phenomenon has already been observed with culture-independent detection of other pathogens in planta (De Boer et al., 1995; López et al., 2009; Palacio-Bielsa et al., 2009; Gétaz et al., 2017) and did not occur with the qPCR assays. The use of a DNA extraction kit eliminated putative DNA polymerase inhibitors and supports the finding of López et al. (2009) that the purification methods used should be evaluated for each combination of tested pathogen and plant before establishing and recommending the procedure for routine detection. Therefore, a DNA extraction kit is recommended for detection of Xac DNA in hazelnut tissues.
The LoD of the different assays was satisfactory for all the primer sets and allowed detection of between 1 pg to 10 fg per reaction or 1 × 100 to 1 × 103 CFU per reaction, with the highest sensitivity obtained for qPCRs. The qPCR procedure turned out to be the fastest of the protocols developed, with the whole reaction and melting curve analysis taking about 1 hour. The high sensitivity of these assays is especially important in the case of naturally infected plant material with low populations of the pathogen. LoD values, similar to the ones obtained in this study were observed previously during the development of detection methods for other X. arboricola pathovars (Palacio-Bielsa et al., 2011; Pothier et al., 2011a; Fernandes et al., 2017), as well as for diagnostics of other plant pathogenic bacteria from other species or genus, e.g., Pseudomonas morsprunorum race 1 and 2 (Kałużna et al., 2016), P. syringae pv. actinidiae (Gallelli et al., 2014), X. campestris pv. campestris (Eichmeier et al., 2019).
The Xac detection systems developed allow for quick and reliable determination of host plant infection without the requirement for isolation of the bacterial pathogen. These assays also can be used to improve our knowledge of this pathogen, such as exploration of other host plants and natural reservoir(s). Even in the presence of potential plant inhibitors, the sensitivity of the assays remained high and sample-to-result times ranged from 5 to 6 hours for conventional PCR down to 1 to 2 hours for qPCR and LAMP assays. So far, this group of molecular assays is the first such methods available for rapid detection of the Xac pathogen directly from plant material.
Data availability statement
Publicly available datasets were analyzed in this study. This data can be found here: https://www.ncbi.nlm.nih.gov/datasets/genome/GCF_002939845.1, https://www.ncbi.nlm.nih.gov/datasets/genome/GCA_002940125.1, https://www.ncbi.nlm.nih.gov/datasets/genome/GCA_000355635.2, https://www.ncbi.nlm.nih.gov/bioproject/PRJEB42844.
Author contributions
MK: conceptualization, funding-acquisition, investigation, methodology, visualization, writing-original-draft, writing-review-editing. AP: investigation, software, writing-review-editing. AO: funding-acquisition, writing-review-editing. WW: investigation, writing-review-editing. VS: investigation, writing-review-editing, funding-acquisition. JP: conceptualization, funding-acquisition, software, visualization, writing-original-draft, writing-review-editing.
Funding
This study was partly financed by the National Science Centre, Poland (Narodowe Centrum Nauki), grant UMO- 2017/26/M/NZ9/01024 granted to MK. AP was granted a Short-Term Scientific Mission by the European Cooperation in Science and Technology COST Action CA16107 EuroXanth to conduct some analysis in Wädenswil (Switzerland). AO and AP were supported by the Ministry of Science, Technological Development and Innovation, Republic of Serbia and the Faculty of Agriculture contract number 451-03-47/2023-01/200116. VS and WW were supported by base funds of USDA ARS Project 2072-22000-045-000D and a 2020 USDA ARS HQ Administrator-funded Postdoctoral Award. Support was also provided to JP by the Department of Life Sciences and Facility Management of the Zurich University of Applied Sciences (ZHAW) in Wädenswil. The open access article processing charges of this publication were funded by the National Science Centre, Poland (grant UMO- 2017/26/M/NZ9/01024).
Acknowledgments
The authors would like to thank the HPC team of the School for Life Sciences and Facility Management at ZHAW for providing computing resources and support. They also want to acknowledge Dr. Jan Nechwatal from Bavarian State Research Center for Agriculture (DE) for supplying Xac strains and Prof. Ewa Zalewska from University of Life Sciences in Lublin (PL) as well as all Polish producers for providing diseased plant material. This article is based upon work from COST Action CA16107 EuroXanth, supported by COST (European Cooperation in Science and Technology).
Conflict of interest
Author WW was employed by USDA-ARS as a Postdoctoral Fellow during this project. All authors declare that the research was conducted in the absence of any commercial or financial relationships that could be construed as a potential conflict of interest.
Mention of trade names or commercial products in this publication is solely for the purpose of providing specific information and does not imply recommendation or endorsement by the U.S. Department of Agriculture.
Publisher’s note
All claims expressed in this article are solely those of the authors and do not necessarily represent those of their affiliated organizations, or those of the publisher, the editors and the reviewers. Any product that may be evaluated in this article, or claim that may be made by its manufacturer, is not guaranteed or endorsed by the publisher.
Supplementary material
The Supplementary Material for this article can be found online at: https://www.frontiersin.org/articles/10.3389/fpls.2023.1254107/full#supplementary-material
References
Altschul, S. F., Gish, W., Miller, W., Myers, E. W., Lipman, D. J. (1990). Basic local alignment search tool. J. Mol. Biol. 215 (3), 403–410. doi: 10.1006/jmbi.1990.9999
Calić, A., Gašić, K., Ivanović, M., Obradović, A., Ferrante, P., Scortichini, M. (2009). “New occurence of Xanthomonas arboricola pv. corylina on European hazelnut in Serbia,” in Proceedings of the Annual COST 873 Meeting, Vol. 89, 89.
Camacho, C., Coulouris, G., Avagyan, V., Ma, N., Papadopoulos, J., Bealer, K., et al. (2009). BLAST+: architecture and applications. BMC Bioinf. 10 (1), 421. doi: 10.1186/1471-2105-10-421
Cesbron, S., Briand, M., Essakhi, S., Gironde, S., Boureau, T., Manceau, C., et al. (2015). Comparative genomics of pathogenic and nonpathogenic strains of Xanthomonas arboricola unveil molecular and evolutionary events linked to pathoadaptation. Front. Plant Sci. 6. doi: 10.3389/fpls.2015.01126
Cuesta-Morrondo, S., Redondo, C., Palacio-Bielsa, A., Garita-Cambronero, J., Cubero, J. (2022). Complete genome sequence resources of six strains of the most virulent pathovars of Xanthomonas arboricola using long- and short-read sequencing approaches. Phytopathology 112 (8), 1808–1813. doi: 10.1094/phyto-10-21-0436-a
D’Amico-Willman, K. M., Joglekar, P., Luna, E. K., Ritchie, D. F., Fagen, J., Huerta, A. I. (2022). Complete genome sequence of Xanthomonas arboricola pv. pruni strain Xcp1 isolated in 1984 from a bacterial spot spring canker on Prunus persica var. nucipersica cv. “Redgold”. Microbiol. Resour. Announc. 11 (12), e00209–e00222. doi: 10.1128/mra.00209-22
De Boer, S. H., Ward, L. J., Li, X., Chittaranjan, S. (1995). Attenuation of PCR inhibition in the presence of plant compounds by addition of BLOTTO. Nucleic Acids Res. 23 (13), 2567–2568. doi: 10.1093/nar/23.13.2567
Eichmeier, A., Peňázová, E., Pokluda, R., Vicente, J. G. (2019). Detection of Xanthomonas campestris pv. campestris through a real-time PCR assay targeting the Zur gene and comparison with detection targeting the hrpF gene. Eur. J. Plant Pathol. 155 (3), 891–902. doi: 10.1007/s10658-019-01820-0
European Union (2016). Regulation (EU) 2016/2031 of the European Parliament of the Council of 26 October 2016 on protective measures against pests of plants, amending Regulations (EU) No 228/2013, (EU) No 652/2014 and (EU) No 1143/2014 of the European Parliament and of the Council and repealing Council Directives 69/464/EEC, 74/647/EEC, 93/85/EEC, 98/57/EC 2000/29/EC 2006/91/EC and 2007/33/EC. Off. J. Eur. Union L317, 4–104.
European Union (2019). Commission implementing directive (EU) 2019/2072 of 28 november 2019 establishing uniform conditions for the implementation of regulation (EU) 2016/2031 of the European Parliament and the Council, as regards protective measures against pests of plants, and repealing commission regulation (EC) no 690/2008 and amending commission implementing regulation (EU) 2018/2019. Off. J. Eur. Union L319, 1–278.
Fernandes, C., Albuquerque, P., Sousa, R., Cruz, L., Tavares, F. (2017). Multiple DNA markers for identification of Xanthomonas arboricola pv. juglandis isolates and its direct detection in plant samples. Plant Dis. 101 (6), 858–865. doi: 10.1094/PDIS-10-16-1481-RE
Fernandes, C., Blom, J., Pothier, J. F., Tavares, F. (2018). High-quality draft genome sequence of Xanthomonas arboricola pv. juglandis CPBF 1521, isolated from leaves of a symptomatic walnut tree in Portugal without a past of phytosanitary treatment. Microbiol. Resour. Announc. 7 (16), e00887–e00818. doi: 10.1128/mra.00887-18
Fischer-Le Saux, M., Bonneau, S., Essakhi, S., Manceau, C., Jacques, M.-A. (2015). Aggressive emerging pathovars of Xanthomonas arboricola represent widespread epidemic clones that are distinct from poorly pathogenic strains, as revealed by multilocus sequence typing. Appl. Environ. Microbiol. 81 (14), 4651–4688. doi: 10.1128/aem.00050-15
Fu, B., Chen, Q., Wei, M., Zhu, J., Zou, L., Li, G., et al. (2018). Complete genome sequence of Xanthomonas arboricola pv. juglandis strain DW3F3, isolated from a Juglans regia L. bacterial blighted fruitlet. Genome Announc. 6 (8), e00023–e00018. doi: 10.1128/genomeA.00023-18
Gallelli, A., Talocci, S., Pilotti, M., Loreti, S. (2014). Real-time and qualitative PCR for detecting Pseudomonas syringae pv. actinidiae isolates causing recent outbreaks of kiwifruit bacterial canker. Plant Pathol. 63, 264–276. doi: 10.1111/ppa.12082
Garita-Cambronero, J., Palacio-Bielsa, A., López, M. M., Cubero, J. (2016a). Comparative genomic and phenotypic characterization of pathogenic and non-pathogenic strains of Xanthomonas arboricola reveals insights into the infection process of bacterial spot disease of stone fruits. PloS One 11 (8), e0161977. doi: 10.1371/journal.pone.0161977
Garita-Cambronero, J., Palacio-Bielsa, A., López, M. M., Cubero, J. (2016b). Draft genome sequence for virulent and avirulent strains of Xanthomonas arboricola isolated from Prunus spp. in Spain. Stand. Genomic Sci. 11 (1), 1–10. doi: 10.1186/s40793-016-0132-3
Garita-Cambronero, J., Palacio-Bielsa, A., López., M. M., Cubero, J. (2016c). Draft genome sequence of two strains of Xanthomonas arboricola isolated from Prunus persica which are dissimilar to strains that cause bacterial spot disease on Prunus spp. Genome Announc. 4 (5), e00974–e00916. doi: 10.1128/genomeA.00974-16
Garita-Cambronero, J., Palacio-Bielsa, A., López, M. M., Cubero, J. (2017). Pan-genomic analysis permits differentiation of virulent and non-virulent strains of Xanthomonas arboricola that cohabit Prunus spp. and elucidate bacterial virulence factors. Front. Microbiol. 8 (573). doi: 10.3389/fmicb.2017.00573
Garita-Cambronero, J., Sena-Vélez, M., Palacio-Bielsa, A., Cubero, J. (2014). Draft genome sequence of Xanthomonas arboricola pv. pruni strain Xap33, causal agent of bacterial spot disease on almond. Genome Announc. 2 (3), e00440–e00414. doi: 10.1128/genomeA.00440-14
Gétaz, M., Baeyen, S., Blom, J., Maes, M., Cottyn, B., Pothier, J. F. (2018). High-quality draft genome sequences of five Xanthomonas arboricola pv. fragariae isolates. Genome Announc. 6 (7), e01585–e01517. doi: 10.1128/genomeA.01585-17
Gétaz, M., Bühlmann, A., Schneeberger, P. H. H., Van Malderghem, C., Duffy, B., Maes, M., et al. (2017). A diagnostic tool for improved detection of Xanthomonas fragariae using a rapid and highly specific LAMP assay designed with comparative genomics. Plant Pathol. 66 (7), 1094–1102. doi: 10.1111/ppa.12665
Harrison, J., Grant, M. R., Studholme, D. J. (2016). Draft genome sequences of two strains of Xanthomonas arboricola pv. celebensis isolated from banana plants. Genome Announc. 4 (1), e01705–e01715. doi: 10.1128/genomeA.01705-15
Herbert, A., Hancock, C. N., Cox, B., Schnabel, G., Moreno, D., Carvalho, R., et al. (2022). Oxytetracycline and streptomycin resistance genes in Xanthomonas arboricola pv. pruni, the causal agent of bacterial spot in peach. Front. Microbiol. 13. doi: 10.3389/fmicb.2022.821808
Higuera, G., González-Escalona, N., Véliz, C., Vera, F., Romero, J. (2015). Draft genome sequences of four Xanthomonas arboricola pv. juglandis strains associated with walnut blight in Chile. Genome Announc. 3 (5), e01160–e01115. doi: 10.1128/genomeA.01160-15
Ibarra Caballero, J., Zerillo, M. M., Snelling, J., Boucher, C., Tisserat, N. (2013). Genome sequence of Xanthomonas arboricola pv. corylina, isolated from turkish filbert in Colorado. Genome Announc. 1 (3), e00246–e00213. doi: 10.1128/genomeA.00246-13
Ignatov, A. N., Kyrova, E. I., Vinogradova, S. V., Kamionskaya, A. M., Schaad, N. W., Luster, D. G. (2015). Draft genome sequence of Xanthomonas arboricola strain 3004, a causal agent of bacterial disease on barley. Genome Announc. 3 (1), e01572–e01514. doi: 10.1128/genomeA.01572-14
Janse, J. D., Rossi, M. P., Gorkink, R. F. J., Derks, J. H. J., Swings, J., Janssens, D., et al. (2001). Bacterial leaf blight of strawberry (Fragaria (×) ananassa) caused by a pathovar of Xanthomonas arboricola, not similar to Xanthomonas fragariae Kennedy & King. Description of the causal organism as Xanthomonas arboricola pv. fragariae (pv. nov., comb. nov.). Plant Pathol. 50 (6), 653–665. doi: 10.1046/j.1365-3059.2001.00644.x
Kałużna, M., Albuquerque, P., Tavares, F., Sobiczewski, P., Puławska, J. (2016). Development of SCAR markers for rapid and specific detection of Pseudomonas syringae pv. morsprunorum races 1 and 2, using conventional and real-time PCR. Appl. Microbiol. Biotechnol. 100 (8), 3693–3711. doi: 10.1007/s00253-016-7295-0
Kałużna, M., Fischer-Le Saux, M., Pothier, J. F., Jacques, M.-A., Obradović, A., Tavares, F., et al. (2021). Xanthomonas arboricola pv. juglandis and pv. corylina: brothers or distant relatives? Genetic clues, epidemiology, and insights for disease management. Mol. Plant Pathol. 22 (12), 1481–1499. doi: 10.1111/mpp.13073
Kałużna, M., Pothier, J. F. (2022). Complete genome sequence data of two Xanthomonas arboricola strains isolated from blueberry plants displaying bacterial leaf blight in Poland. Phytopathology 112 (8), 1814–1818. doi: 10.1094/phyto-11-21-0484-a
Kałużna, M., Pulawska, J., Waleron, M., Sobiczewski, P. (2014). The genetic characterisation of Xanthomonas arboricola pv. juglandis, the causal agent of walnut blight in Poland. Plant Pathol. 63 (6), 1404–1416. doi: 10.1111/ppa.12211
King, E. O., Ward, M. K., Raney, D. E. (1954). Two simple media for the demonstration of pyocyanin and fluorescin. J. Lab. Clin. Med. 44 (2), 301–307.
Lamichhane, J. R., Grau, P., Varvaro, L. (2012). Emerging hazelnut cultivation and the severe threat of bacterial blight in Chile. J. Phytopathol. 160, 752–754. doi: 10.1111/jph.12004
Lamichhane, J. R., Varvaro, L. (2014). Xanthomonas arboricola disease of hazelnut: current status and future perspectives for its management. Plant Pathol. 63, 243–254. doi: 10.1111/ppa.12152
Lelliott, R. A., Stead, D. E. (1987). Methods for the diagnosis of bacterial diseases of plants. (Oxford, UK: Blackwell Scientific Publications).
López, M. M., Llop, P., Olmos, A., Marco-Noales, E., Cambra, M., Bertolini, E. (2009). Are molecular tools solving the challenges posed by detection of plant pathogenic bacteria and viruses? Curr. Issues Mol. Biol. 11, 13–46. doi: 10.21775/cimb.011.013
López-Soriano, P., Boyer, K., Cesbron, S., Morente, M. C., Peñalver, J., Palacio-Bielsa, A., et al. (2016). Multilocus variable number of tandem repeat analysis reveals multiple introductions in Spain of Xanthomonas arboricola pv. pruni, the causal agent of bacterial spot disease of stone fruits and almond. PloS One 11 (9), e0163729. doi: 10.1371/journal.pone.0163729
Maes, M. (1993). Fast classification of plant-associated bacteria in the Xanthomonas genus. FEMS Microbiol. Lett. 113 (2), 161–165. doi: 10.1111/j.1574-6968.1993.tb06508.x
Merda, D., Briand, M., Bosis, E., Rousseau, C., Portier, P., Barret, M., et al. (2017). Ancestral acquisitions, gene flow and multiple evolutionary trajectories of the type three secretion system and effectors in Xanthomonas plant pathogens. Mol. Ecol. 26 (21), 5939–5952. doi: 10.1111/mec.14343
Miller, P., Bollen, W., Simmons, J. (1949). Filbert bacteriosis and its control. Technical bulletin 16. (Corvallis: Agricultural Experiment Station).
Moore, L. W. (2002). “Bacterial blight,” in Compendium of nut crop diseases in temperate zones. Eds. Teviotdale, B. L., Michailides, T. J., Pscheidt, J. W. (St Paul, MN: APS Press), 249–257.
Nagamine, K., Hase, T., Notomi, T. (2002). Accelerated reaction by loop-mediated isothermal amplification using loop primers. Mol. Cell. Probes 16 (3), 223–229. doi: 10.1006/mcpr.2002.0415
Nuñez Cerda, P. D., Muster, C., Lisperguer, M. J., Vargas, E., Bustos, S. (2021). Complete genome of Xanthomonas arboricola pv. corylina strain A7 isolated from southern Chile. Mol. Plant-Microbe Interact. 35 (1), 94–95. doi: 10.1094/mpmi-12-20-0363-a
Obradović, A., Ivanović, M., Ćalić, A. (2010). Bacterial diseases of hazelnut. Biljni Lekar (Plant Doctor) 38 (3), 192–201.
OEPP/EPPO (1986). Xanthomonas campestris pv. corylina. EPPO Bull. 16 (1), 67–78. doi: 10.1111/j.1365-2338.1986.tb01139.x
OEPP/EPPO (2004). Xanthomonas arboricola pv. corylina. EPPO Bull. 34 (2), 179–181. doi: 10.1111/j.1365-2338.2004.00716.x
OEPP/EPPO (2010a). PM 7/100 (1) Rep-PCR tests for identification of bacteria. EPPO Bull. 40 (3), 365–368. doi: 10.1111/j.1365-2338.2010.02409.x
OEPP/EPPO (2010b). PM 7/101 (1) ELISA tests for plant pathogenic bacteria. EPPO Bull. 40 (3), 369–372. doi: 10.1111/j.1365-2338.2010.02420.x
Osdaghi, E. (2022). Xanthomonas arboricola pv. corylina (bacterial blight of hazelnut). PlantwisePlus Knowledge Bank. (CABI International). doi: 10.1079/pwkb.species.56930
Palacio-Bielsa, A., Cambra, M. A., López, M. M. (2009). PCR detection and identification of plant-pathogenic bacteria: updated review of protocols, (1989-2007). J. Plant Pathol. 91 (2), 249–297.
Palacio-Bielsa, A., Cubero, J., Cambra, M. A., Collados, R., Berruete, I. M., Lopez, M. M. (2011). Development of an efficient real-time quantitative PCR protocol for detection of Xanthomonas arboricola pv. pruni in Prunus species. Appl. Environ. Microbiol. 77 (1), 89–97. doi: 10.1128/aem.01593-10
Parkinson, N., Aritua, V., Heeney, J., Cowie, C., Bew, J., Stead, D. (2007). Phylogenetic analysis of Xanthomonas species by comparison of partial gyrase B gene sequences. Int. J. Syst. Evol. Microbiol. 57 (12), 2881–2887. doi: 10.1099/ijs.0.65220-0
Pothier, J. F., Kałużna, M., Prokić, A., Obradovic, A., Rezzonico, F. (2022). Complete genome and plasmid sequence data of three Xanthomonas arboricola pv. corylina strains, the bacterium responsible for bacterial blight of hazelnut. Phytopathology 12 (4), 956–960. doi: 10.1094/phyto-08-21-0356-a
Pothier, J. F., Pagani, M. C., Pelludat, C., Ritchie, D. F., Duffy, B. (2011a). A duplex-PCR method for species- and pathovar-level identification and detection of the quarantine plant pathogen Xanthomonas arboricola pv. pruni. J. Microbiol. Methods 86, 16–24. doi: 10.1016/j.mimet.2011.03.019
Pothier, J. F., Smits, T. H. M., Blom, J., Vorhölter, F., Goesmann, A., Pühler, A., et al. (2011b). Complete genome sequence of the stone fruit pathogen Xanthomonas arboricola pv. pruni. Phytopathology 101 (6S), S144–S145.
Pothier, J. F., Vorhölter, F.-J., Blom, J., Goesmann, A., Pühler, A., Smits, T. H. M., et al. (2011c). The ubiquitous plasmid pXap41 in the invasive phytopathogen Xanthomonas arboricola pv. pruni: complete sequence and comparative genomic analysis. FEMS Microbiol. Lett. 323 (1), 52–60. doi: 10.1111/j.1574-6968.2011.02352.x
Prokić, A., Gašić, K., Ivanović, M. M., Kuzmanović, N., Šević, M., Pulawska, J., et al. (2012). Detection and identification methods and new tests as developed and used in the framework of COST873 for bacteria pathogenic to stone fruits and nuts - Xanthomonas arboricola pv. corylina. J. Plant Pathol. 94 (S1), S127–S133. doi: 10.4454/jpp.v94i1sup.020
Puławska, J., Kałużna, M., Kołodziejska, A., Sobiczewski, P. (2010). Identification and characterization of Xanthomonas arboricola pv. corylina causing bacterial blight of hazelnut: a new disease in Poland. J. Plant Pathol. 92 (3), 803–806.
Ramakers, C., Ruijter, J. M., Deprez, R. H. L., Moorman, A. F. M. (2003). Assumption-free analysis of quantitative real-time polymerase chain reaction (PCR) data. Neurosci. Lett. 339 (1), 62–66. doi: 10.1016/S0304-3940(02)01423-4
Retamales, J., Segovia, C., Alvarado, R., Nuñez, P., Santander, J. (2017). Draft genome sequence of Xanthomonas arboricola pv. juglandis J303, isolated from infected walnut trees in Southern Chile. Genome Announc. 5 (39), e01085–e01017. doi: 10.1128/genomeA.01085-17
Saddler, G. S., Bradbury, J. F. (2005). “Xanthomonadales ord. nov,” in Bergey’s Manual of Systematic Bacteriology., 2nd ed (Boston, MA: Springer), 63–122.
Sambrook, J., Fritsch, E. F., Maniatis, T. (1989). Molecular cloning: a laboratory manual (New York: Cold Spring Harbour Laboratory).
Schaad, N. W., Jones, J. B., Chun, W. (2001). Laboratory guide for identification of plant pathogenic bacteria. (St. Paul, MN: APS Press).
Schneeberger, P. H. H., Pothier, J. F., Bühlmann, A., Duffy, B., Beuret, C., Utzinger, J., et al. (2017). Development and evaluation of a bioinformatics approach for designing molecular assays for viral detection. PLoS One 12 (5), e0178195. doi: 10.1371/journal.pone.0178195
Scortichini, M., Rossi, M. P., Marchesi, U. (2002). Genetic, phenotypic and pathogenic diversity of Xanthomonas arboricola pv. corylina strains question the representative nature of the type strain. Plant Pathol. 51 (3), 374–381. doi: 10.1046/j.1365-3059.2002.00691.x
Teixeira, M., Fernandes, C., Chaves, C., Pinto, J., Tavares, F., Fonseca, N. A. (2021). Complete genome sequence obtained by Nanopore and Illumina hybrid assembly of Xanthomonas arboricola pv. juglandis CPBF 427, isolated from buds of a walnut tree. Microbiol. Resour. Announc. 10 (10), e00085–e00021. doi: 10.1128/MRA.00085-21
Tindall, B. J. (2014). The family name Solimonadaceae Losey et al. 2013 is illegitimate, proposals to create the names ‘Sinobacter soli’ comb. nov. and ‘Sinobacter variicoloris’ contravene the Code, the family name Xanthomonadaceae Saddler and Bradbury 2005 and the order name Xanthomonadales Saddler and Bradbury 2005 are illegitimate and notes on the application of the family names Solibacteraceae Zhou et al. 2008, Nevskiaceae Henrici and Johnson 1935 (Approved Lists 1980) and Lysobacteraceae Christensen and Cook 1978 (Approved Lists 1980) and order name Lysobacteriales Christensen and Cook 1978 (Approved Lists 1980) with respect to the classification of the corresponding type genera Solibacter Zhou et al. 2008, Nevskia Famintzin 1892 (Approved Lists 1980) and Lysobacter Christensen and Cook 1978 (Approved Lists 1980) and importance of accurately expressing the link between a taxonomic name, its authors and the corresponding description/circumscription/emendation. Int. J. Syst. Evol. Microbiol. 64 (Pt_1), 293–297. doi: 10.1099/ijs.0.057158-0
Tuang, F. N., Rademaker, J. L. W., Alocilja, E. C., Louws, F. J., Bruijn, F. J. (1999). Identification of bacterial rep-PCR genomic fingerprints using a backpropagation neural network. FEMS Microbiol. Lett. 177 (2), 249–256. doi: 10.1111/j.1574-6968.1999.tb13740.x
Untergasser, A., Cutcutache, I., Koressaar, T., Ye, J., Faircloth, B. C., Remm, M., et al. (2012). Primer3—new capabilities and interfaces. Nucleic Acids Res. 40 (15), e115. doi: 10.1093/nar/gks596
Vauterin, L., Hoste, B., Kersters, K., Swings, J. (1995). Reclassification of Xanthomonas. Int. J. Syst. Bacteriol. 45 (3), 472–489. doi: 10.1099/00207713-45-3-472
Webber, J. B., Putnam, M., Serdani, M., Pscheidt, J. W., Wiman, N. G., Stockwell, V. O. (2020). Characterization of isolates of Xanthomonas arboricola pv. corylina, the causal agent of bacterial blight, from Oregon hazelnut orchards. J. Plant Pathol. 102, 799–812. doi: 10.1007/s42161-020-00505-6
Webber, J. B., Wada, S., Stockwell, V. O., Wiman, N. G. (2021). Susceptibility of some Corylus avellana L. cultivars to Xanthomonas arboricola pv. corylina. Front. Plant Sci. 12. doi: 10.3389/fpls.2021.800339
Young, J. M., Park, D.-C., Shearman, H. M., Fargier, E. (2008). A multilocus sequence analysis of the genus Xanthomonas. Syst. Appl. Microbiol. 31 (5), 366–377. doi: 10.1016/j.syapm.2008.06.004
Keywords: Corylus spp., Corylus avellana, diagnosis, PCR, LAMP, qPCR
Citation: Kałużna M, Prokić A, Obradović A, Weldon WA, Stockwell VO and Pothier JF (2023) Specific and sensitive detection tools for Xanthomonas arboricola pv. corylina, the causal agent of bacterial blight of hazelnut, developed with comparative genomics. Front. Plant Sci. 14:1254107. doi: 10.3389/fpls.2023.1254107
Received: 06 July 2023; Accepted: 18 August 2023;
Published: 13 September 2023.
Edited by:
Vittoria Catara, University of Catania, ItalyReviewed by:
Alessandro Passera, University of Milan, ItalyMassimiliano Morelli, National Research Council (CNR), Italy
Copyright © 2023 Kałużna, Prokić, Obradović, Weldon, Stockwell and Pothier. This is an open-access article distributed under the terms of the Creative Commons Attribution License (CC BY). The use, distribution or reproduction in other forums is permitted, provided the original author(s) and the copyright owner(s) are credited and that the original publication in this journal is cited, in accordance with accepted academic practice. No use, distribution or reproduction is permitted which does not comply with these terms.
*Correspondence: Monika Kałużna, bW9uaWthLmthbHV6bmFAaW5ob3J0LnBs; Joël F. Pothier, am9lbC5wb3RoaWVyQHpoYXcuY2g=