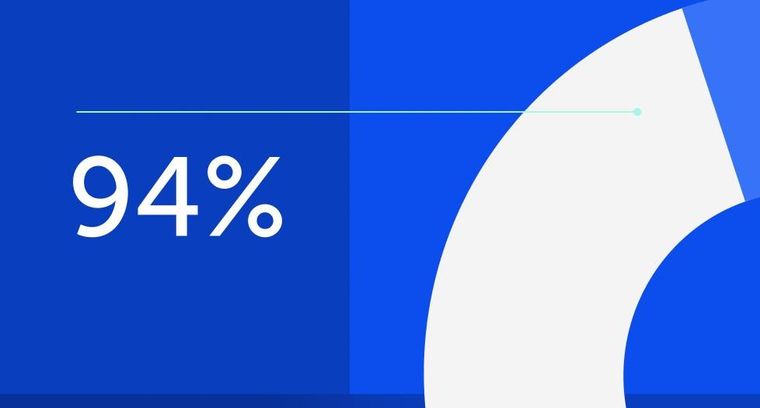
94% of researchers rate our articles as excellent or good
Learn more about the work of our research integrity team to safeguard the quality of each article we publish.
Find out more
ORIGINAL RESEARCH article
Front. Plant Sci., 15 September 2023
Sec. Plant Biotechnology
Volume 14 - 2023 | https://doi.org/10.3389/fpls.2023.1251046
This article is part of the Research TopicThe Utilization of Plants in Vaccine ResearchView all 9 articles
Bovine respiratory disease (BRD) affects feedlot cattle across North America, resulting in economic losses due to animal treatment and reduced performance. In an effort to develop a vaccine candidate targeting a primary bacterial agent contributing to BRD, we produced a tripartite antigen consisting of segments of the virulence factor Leukotoxin A (LktA) and lipoprotein PlpE from Mannheimia haemolytica, fused to a cholera toxin mucosal adjuvant (CTB). This recombinant subunit vaccine candidate was expressed in the leaves of Nicotiana benthamiana plants, with accumulation tested in five subcellular compartments. The recombinant protein was found to accumulate highest in the endoplasmic reticulum, but targeting to the chloroplast was employed for scaling up production due the absence of post-translational modification while still producing feasible levels. Leaves were freeze dried, then orally administered to mice to determine its immunogenicity. Sera from mice immunized with leaf tissue expressing the recombinant antigen contained IgG antibodies, specifically recognizing both LktA and PlpE. These mice also had a mucosal immune response to the CTB+LktA+PlpE protein as measured by the presence of LktA- and PlpE-specific IgA antibodies in lung and fecal material. Moreover, the antigen remained stable at room temperature with limited deterioration for up to one year when stored as lyophilized plant material. This study demonstrated that a recombinant antigen expressed in plant tissue elicited both humoral and mucosal immune responses when fed to mice, and warrants evaluation in cattle.
Bovine respiratory disease (BRD) is the most common and costly disease in North American feedlot cattle (Blakebrough-Hall et al., 2022). A 2010 survey of the United States cattle industry found that 26.5% of all non-predator-related deaths, a total of 460 000 cattle, resulted from BRD (USDA, 2011). As a result, economic losses in North American feedlots have been approximated to be greater than $500 million per year (Miles, 2009). Although multifactorial in nature, BRD is mainly caused by opportunistic bacterial pathogens which colonize the upper respiratory tract and is more likely to occur shortly after feedlot placement (Snyder and Credille, 2020). Stressors occurring from feedlot placement, including transportation, comingling, and primary viral infections (Rice et al., 2007; Timsit et al., 2016) contribute to innate immunity suppression by altering calf mucosal secretions, anionic peptides and β-defensins (Brogden et al., 1998). This contributes to bacterial pathogen proliferation in the upper respiratory tract, and translocation and infection in the lungs (Timsit et al., 2020). Current BRD mitigation strategies include antimicrobial treatment upon feedlot arrival (metaphylaxis) and vaccination against BRD pathogens. However, antimicrobial resistance has increased in BRD-associated bacterial pathogens as a result of broad antimicrobial use, and vaccines have afforded variable protection (Lemon and Mcmenamy, 2021; Andres-Lasheras et al., 2022). Therefore, novel methods to mitigate BRD pathogens, including new vaccines, are needed.
While several bacterial pathogens are implicated in BRD, Mannheimia haemolytica is a principal one, often detected in acute pneumonia cases (Booker et al., 2008). M. haemolytica are non-motile, gram-negative, coccobacilli that primarily inhabit the nasopharynx and tonsils of ruminants (Frank et al., 1995). Of the 12 M. haemolytica serotypes, the majority of BRD cases are associated with serotype A1 affecting approximately 75% of feedlot cattle in North America (Snyder and Credille, 2020). Consequently, efforts to understand and mitigate this pathogen have been strongly directed towards this serotype. The pathogenicity of M. haemolytica is in part due to the virulence factor leukotoxin (Lkt), a member of the repeats-in-toxin (RTX) family of cytotoxins. Lkt has detrimental effects on macrophages and other leukocytes, and more severe effects on neutrophils (Snyder and Credille, 2020).
There are currently several commercial vaccines available against M. haemolytica. These vaccines come in the form of cell culture supernatants, bacterin-toxoid, and extracted antigens, while they are administered via intramuscular, subcutaneous and more recently intranasal routes (Rice et al., 2007; Confer and Ayalew, 2018). However, the results of these vaccines in controlled trials have been inconsistent and yielded limited efficacy in production settings (Rice et al., 2007; Larson and Step, 2012). The relative success of protein-based, cell-free vaccines led to the development of M. haemolytica protein subunit vaccines which are comprised of partial antigens, intended to elicit specific antibody responses. Potential targets for vaccine development include virulence factors such as Lkt, as well as surface lipoproteins such as PlpE. An intranasal vaccination of cattle with a PlpE-LKT-cholera toxin subunit B chimeric protein (SAC102) produced in E. coli and purified by immobilized metal affinity chromatography (IMAC) stimulated serum and nasal antibodies in vaccinated calves, and intrabronchial challenge in these calves produced fewer clinical symptoms than unvaccinated animals (Ayalew et al., 2009). Nevertheless, microbial-produced antigens for cattle require administration to individual animals and thus have high labor demands associated with their use.
Alternatively, plants have been explored in the past 30 years as bioreactors for the production of recombinant proteins. Plants are an excellent vehicle for the production of veterinary subunit vaccines targeted for oral mucosal delivery since farm animals consume plants as part of their diet and mass medication could be achieved through their use (Rybicki, 2018). There are several reports of oral vaccines produced in plants with demonstrated efficacy (Miletic et al., 2015; Shahid and Daniell, 2016; Sanchez-Lopez et al., 2021). It has been argued that the plant cell walls bioencapsulate antigens and protect them from degradation in the stomach (Kwon and Daniell, 2016; Daniell et al., 2019). In fact, it has been reported that plant tissue might prolong the residence time on the mucosa, thereby increasing antigen uptake and enhancing the immune response (Merlin et al., 2017; Sanchez-Lopez et al., 2021). Furthermore, oral delivery of subunit vaccines is less expensive than parenteral immunization as it obviates the need for purification which can constitute 70-80% of the cost of producing the vaccine (Tuse et al., 2014; Tschofen et al., 2016). This also reduces stress on the animals, reducing hands-on management, and promoting broad vaccination of farm animal populations (Kolotilin et al., 2014).
In this study, we fused segments of Lkt and PlpE to the B-subunit of the cholera toxin (CTB) to enhance mucosal immunity. This antigen was produced in the leaves of Nicotiana benthamiana plants, which were subsequently freeze-dried and orally administered to mice for evaluation of the immune response.
The structural gene of the cytolytic leukotoxin operon, LktA, and the outer membrane Pasteurella lipoprotein E (PlpE) had been previously shown to be part of effective subunit vaccines against M. haemolytica when produced in E. coli (Ayalew et al., 2008; Ayalew et al., 2009; Confer et al., 2009a; Confer et al., 2009b). Using a similar recombinant protein approach, we created a fusion protein beginning with the B subunit of cholera toxin (CTB) from Vibrio cholerae at the N-terminus (Figure 1). CTB acts as a strong mucosal adjuvant and is thought to facilitate protein uptake into epithelial cells, resulting in its immune-recognition and response, leading to potentially improved efficacy of vaccines (Sheoran et al., 2002; Holmgren et al., 2005). Indeed, a similar CTB-LktA protein was shown to bind the cholera toxin receptor GM1-ganglioside, highlighting the binding activity of the CTB component (Ayalew et al., 2009). We used a larger region of the LktA gene in our construct than the previously reported sequence (Ayalew et al., 2008), and fused it to the C terminus of CTB with a GPGP amino acid linker to separate the 2 domains. The LktA domain consisted of the nucleotides encoding amino acids 705 through to the C-terminus, amino acid 953, of the M. haemolytica LktA gene (GenBank: M20730.1). This region was selected based on previous work that used amino acids 719-939, which encompassed the neutralizing and non-neutralizing antibody epitopes (Rajeev et al., 2001). We included more amino acids on both sides of this segment, which were highly conserved among multiple serotypes of M. haemolytica, with the intent of providing further stability to the resultant protein and greater antigen recognition across multiple serotypes. The LktA segment was followed by a linker (GGGS)3 and the immunodominant surface epitope (R2) of the PlpE gene. Antibodies against R2 were previously shown to sufficiently stimulate complement-mediated bacteriolysis (Ayalew et al., 2004).
Figure 1 Schematic representation of the constructs used in the Agrobacterium-mediated transient expression of CTB+LktA+PlpE in N. benthamiana leaves. All five constructs are under the control of the double-enhanced cauliflower mosaic virus 35S promoter (2x35S), a translational enhancer from the tobacco cryptic upstream constitutive promoter (tCUP), and the nopaline synthase terminator (nosT). Recombinant protein accumulation was targeted to the (A) apoplast; (B) chloroplast; (C) cytoplasm; (D) endoplasmic reticulum; and (E) vacuole. Pr1b denotes the tobacco pathogenesis-related 1b signal peptide; RBC-S TP is the transit peptide from the small subunit of RuBisCo; thin blocks with diagonal lines, solid black or grey filling are Xpress, HA, and c-Myc tags, respectively. K represents the KDEL endoplasmic reticulum retrieval signal, while C is the barley lectin vacuole targeting signal. The CTB and LktA sequences are joined by a GPGP linker while the LktA and PlpE sequences are connected through a 3x(GGGS) linker. Construct segments are not drawn to scale.
The subcellular localization of a protein can affect its accumulation, and often proteins will not accumulate at all in some subcellular compartments (Pereira et al., 2014). For this reason, we explored targeting the CTB+LktA+PlpE fusion protein to five subcellular compartments: the apoplast (APO), chloroplast (CHL), cytoplasm (CYT), endoplasmic reticulum (ER), or vacuole (VAC) using expression vectors previously tested in our lab to direct the green fluorescent protein (GFP) to the correct subcellular compartment (Conley et al., 2009). The five expression constructs were transformed into Agrobacterium tumefaciens then co-infiltrated into N. benthamiana leaves with an Agrobacterium strain containing p19, a suppressor of post-transcriptional gene silencing from Cymbidium ringspot virus (Silhavy et al., 2002). To determine when the accumulation of the recombinant protein peaked after Agroinfiltration, a time course was conducted. Samples were collected from each of five infiltrated plants 4, 6, and 8 days post-infiltration (dpi) on three separate occasions (15 biological replicates). Each was extracted, then analyzed by SDS-PAGE and western blot to determine the accumulation levels of antigen in the subcellular targets. Data were expressed as averages of all the samples for each compartment.
Western blots of pooled extracts (Figure 2A) demonstrated the size variability in the products that were detected, but also showed that recombinant protein successfully accumulated in all five of the tested subcellular compartments of N. benthamiana leaves. However, we observed that the CTB+LktA+PlpE fusion protein accumulated to its highest level in all compartments on day 4 post-infiltration, and that its levels dropped with time (Figure 2B). Depending on the protein’s stability, conformation, and post-translational modifications, some proteins will become more or less abundant over the first week or two before they disappear completely. Some proteins remain stable over 3-8 days (Chin-Fatt et al., 2021; VanderBurgt et al., 2023), some increase their accumulation with time (Saberianfar et al., 2019), and others reach their highest accumulation at 4-6 days and then go down with time (Garabagi et al., 2012). Here, we found that accumulation levels are highest four days post-infiltration, and this is the time we subsequently harvested the infiltrated leaf material.
Figure 2 Accumulation of CTB+LktA+PlpE recombinant protein in various subcellular compartments following transient expression in the leaves of N. benthamiana. (A) Western blot hybridization of extracts pooled from 5 plants co-infiltrated with A tumefaciens containing constructs of p19 and of CTB+LktA+PlpE targeted to the apoplast (APO), chloroplast (CHL), cytoplasm (CYT), endoplasmic reticulum (ER), or vacuole (VAC). Blots were detected with an anti-c-Myc antibody which only recognizes a C-terminal tag on the CTB+LktA+PlpE protein. (B) Average recombinant protein accumulation levels in the five subcellular compartments as measured in 5 individual plants over three separate sets of infiltrations (n=15). Data are expressed as grams of recombinant protein per kilogram of fresh leaf weight at 4, 6, and 8 dpi. Error bars = ± standard error of the mean.
The conventional threshold of economic viability for recombinant protein production in plants is considered to begin at 0.1 g/kg of fresh weight (FW) of plant material (Schiefer et al., 1978; Rybicki, 2009; Saberianfar et al., 2019; Schillberg et al., 2019; Sanchez-Lopez et al., 2021; Schillberg and Finnern, 2021). The CTB+LktA+PlpE fusion protein achieved this benchmark in 4 of the 5 targeted subcellular compartments. Accumulation in each compartment peaked at 4 dpi, with the highest levels measured at 0.37 g/kg FW in the endoplasmic reticulum (ER), 0.22 g/kg FW in the apoplast (APO), 0.1 g/kg FW in chloroplasts (CHL), and 0.15 g/kg FW in the vacuole (VAC) (Figure 2B). The yield in the cytoplasm (CYT) was below the conventional threshold with a level of 0.06 g/kg FW, and was not considered going forward.
Since the accumulation levels of our fusion protein reached higher amounts in the secretory pathway, we considered the potential impact of glycosylation on this synthetic protein comprised exclusively of prokaryotic sequences. The heterogeneity in size of the recombinant protein in Figure 2A indicated that glycosylation was likely occurring in the secretory pathway. The predicted molecular weight of the fusion protein based on its amino acid sequence is 49.2 kDa, corresponding to the band observed in the CHL- or CYT-targeted proteins. Indeed the cytosol or chloroplasts do not have the machinery to glycosylate proteins. To test whether the recombinant protein targeted to the secretory pathway (ER, APO or VAC) is glycosylated, crude extracts were digested with PNGase F, separated by SDS-PAGE and detected by western blot. No shift in size was detected with the CHL- or CYT-targeted protein. However, a decrease in size was seen in the product when accumulation was targeted to the APO, ER and VAC (Figure 3). This demonstrates N-glycosylation of the recombinant fusion protein in the secretory pathway. The observed mass of the cleaved products from the APO, ER, and VAC, and the non-glycosylated protein in the CHL and CYT was approximately 50 kDa, close to the predicted 49.2 kDa molecular weight of the fusion protein. It is possible that the higher accumulation of the protein in the secretory pathway may be due to the presence of glycans that could shield the protein from proteolysis. However, the presence of those glycans may also shield the antigens from the immune system.
Figure 3 Glycosylation of transiently expressed CTB+LktA+PlpE. Extracts from N. benthamiana transiently expressing each of the five subcellularly-targeted constructs of CTB+LktA+PlpE were digested with PNGase F (+) or incubated without the enzyme (-), then analyzed by western blot.
Considering the prokaryotic origin of the three CTB+LktA+PlpE segments and the lack of glycosylation in their native forms, we scaled up production of the fusion protein targeted to the chloroplast to avoid potential interference of glycans with protein folding or masking immunogenic epitopes. Agrobacterium-infiltrated N. benthamiana leaves were harvested 4 days post-infiltration, frozen and lyophilized. Western blot analysis was performed on protein extracted from the lyophilized tissue to determine the amount of recombinant protein in the batch of dried leaves. On the blots, we observed a full-size band at about 50 kDa, and three smaller bands which may have resulted from protein cleavage during lyophilization. The full-size band alone was quantified against known amounts of a standard protein (Figure 4A). There was 6.1 mg CTB+LktA+PlpE in 19.5 g of lyophilized N. benthamiana leaves.
Figure 4 One year stability of transiently expressed CTB+LktA+PlpE recombinant protein in freeze dried N. benthamiana leaf tissue. (A) Western blot hybridization of extract from a pooled, freeze dried sample of N. benthamiana plants infiltrated with A tumefaciens containing constructs of p19 and of CTB+LktA+PlpE targeted to the chloroplast. The 4 µl loaded in the first lane represent the amount of soluble protein in 0.206 mg of lyophilized tissue. (B) Western blot hybridization of extract prepared from the same pool of infiltrated, freeze dried, N. benthamiana leaves that was milled following its storage in a sealed container at ambient temperature for one year. The 5 µl loaded in the first lane represent the amount of soluble protein in 0.254 mg of lyophilized tissue. Both blots were detected with an anti-HA antibody which only recognizes an N-terminal tag on the CTB+LktA+PlpE protein.
The stability of the CTB+LktA+PlpE antigen within the cells of the freeze dried N. benthamiana leaves was assessed again immediately before its use in the immunization of mice. The lyophilized leaf tissue had been stored in a sealed container at ambient temperature for 12 months prior to pulverization using a ball-grinding mill. Soluble protein from a sample of this milled material was extracted and analyzed by SDS-PAGE and western blot hybridization in the same fashion that was employed when the tissue was originally infiltrated and freeze dried (Figure 4B). There was little change in the amount of full-length product present when compared to the original quantification one year earlier. This high antigen stability of a plant-based vaccine has also been reported by Lee et al. (2008). In that study, antigen containing the plant material was oven-dried for a week at 50° C and was reported to be stable at ambient temperature for up to one year (Lee et al., 2008). The lack of need for refrigeration adds practicality to the administration of this potential vaccine to cattle.
To evaluate whether this plant expressed chimeric protein would elicit an immune response, mice were vaccinated by oral gavage of a slurry of leaf material containing 50 μg of the recombinant protein per dose (Table 1). Sera collected from mice 42 days post immunization did not have quantifiable levels of anti-PlpE or anti-LktA IgG (limit of quantification ≥ 7.8 ng/ml). However, on day 56, one animal from the leaf with antigen treatment group did exhibit an IgG response above the limit of quantification (data not shown). On day 70, all of the mice in the leaf with antigen treatment group displayed levels of both anti-PlpE and anti-LktA IgG (Figure 5). In contrast to this leaf with antigen treatment group, none of the mice administered only PBS had measurable IgG against either antigen. A single animal from the plant control leaf without antigen group (treatment 2) did show an antibody response on day 70, however it was below the limit of quantification, and likely due to background noise from the ELISA. Similar to IgG responses, anti-PlpE or anti-LktA IgA were only quantifiable in samples on day 70. An exception was the same animal in the leaf with antigen treatment group that had antigen-specific IgG on day 56, also had IgA against LktA and PlpE on day 56 in bronchoalveolar lavage (BAL) and fecal samples (data not shown). On day 70, mice from the leaf with antigen treatment had both anti-PlpE IgA and anti-LktA IgA in BAL and fecal samples, while the other groups did not have any detectable IgA antibodies against PlpE or LktA (P < 0.05; Figure 6). It is noteworthy that in our study, the antibody responses in BAL and feces were approximately a magnitude greater than sera IgG amounts. Combined, these data show that oral immunization of mice with lyophilized plant leaves expressing CTB+LktA+PlpE stimulated humoral and mucosal anti-PlpE and anti-LktA antibodies, indicating that the chimeric protein is immunogenic, and retained immunogenicity after oral administration. The combination of LktA and PlpE antigens were selected for use in our study because intranasal vaccination of calves with a similar chimera stimulated nasal antibodies after M. haemolytica challenge and enhanced protection against infection (Ayalew et al., 2009). In the study by Ayalew et al. (2009), the authors noted an increase in nasal anti-Lkt antibody responses, which was only significant after bacterial challenge, and not after the booster immunization alone. The next step will be determining whether the plant CTB+LktA+PlpE is similarly immunogenic in calves after in-feed administration.
Table 1 Experimental design of mouse trial. Balb/c pathogen-free mice (n=18 mice per treatment) were immunized at 14-day intervals by oral gavage.
Figure 5 Systemic immune response in mice measured by ELISA on day 70. ELISA plates coated with either recombinant PlpE or LktA protein were used to measure (A) anti-PlpE and (B) anti-LktA antibodies in sera from immunized mice. Immune responses from three treatment groups were compared: 1) leaf with antigen (Intra-gastric inoculation of Agrobacterium-infiltrated, freeze dried, pulverized leaf tissue containing CTB+LktA+PlpE protein; mixed with PBS); 2) leaf without antigen (Intra-gastric inoculation of Agrobacterium-infiltrated, freeze dried, pulverized leaf tissue containing no protein; mixed with PBS); and 3) PBS only/control mice (Intra-gastric inoculation of PBS without any plant material or protein). The dashed line indicates the limit of detection (≥ 7.8 ng/ml). Results are expressed as mean ± standard error.
Figure 6 Mucosal immune response in mice measured by ELISA on day 70. ELISA plates coated with either recombinant PlpE or LktA protein were used to measure (A) anti-PlpE and (B) anti-LktA antibodies in bronchoalveolar lavage (BAL), and (C) anti-PlpE and (D) anti-LktA antibodies in feces from immunized mice. Fecal and BAL samples were collected and analyzed from three treatment groups of mice: 1) leaf with antigen (Intra-gastric inoculation of Agrobacterium-infiltrated, freeze dried, pulverized leaf tissue containing CTB+LktA+PlpE protein; mixed with PBS); 2) leaf without antigen (Intra-gastric inoculation of Agrobacterium-infiltrated, freeze dried, pulverized leaf tissue containing no protein; mixed with PBS); and 3) PBS only/control mice (Intra-gastric inoculation of PBS without any plant material or protein). Results are expressed as mean ± standard error.
While commercial vaccines exist for M. haemolytica, their subcutaneous or intranasal administration requires direct animal handling. An efficacious orally administered plant vaccine would be advantageous by allowing mass vaccination with reduced labor input, and lower production costs due to elimination of expenses associated with purification and formulation of the vaccine. Purification costs account for 70-80% of the cost of production of pharmaceutical proteins (Tuse et al., 2014; Tschofen et al., 2016). To date however, there are few reports of oral vaccines targeting BRD pathogens. Lee et al. (2008) evaluated transgenic alfalfa expressing the M. haemolytica GS60 outer membrane protein in rabbits. The authors reported that two oral immunizations with dried transgenic alfalfa resulted in seroconversion in one of six animals vaccinated. The low seroconversion may have been due to only two vaccinations being administered. Indeed, only one mouse showed seroconversion after four oral vaccinations in our study, and the number increased to all mice showing seroconversion after five immunizations. It is also possible that the varying immune responses reported in these studies stem from dissimilarities in the antigen design, the schedule of immunization, the adjuvant used, and the animal species being utilized (mice versus rabbits). Thus, it is important to determine the optimal number of immunizations for plant-based oral vaccines, especially concerning that differences in antigen structure and plant composition could affect digestion and antigen survival in the gastrointestinal tract.
In this study, we showed that when lyophilized leaf tissue expressing a putative subunit vaccine was orally administered to mice, the recombinant protein resisted degradation enough such that both LktA and PlpE antigenic components were taken up by M cells, allowing activation of immunocompetent cells in the mucosa-associated lymphoid tissue. Taghavian et al. (2013) have also found that Pichia-produced VP2 from inflammatory bursal disease virus survived digestion and induced an IgY immune response in chickens. The presence of antigen-specific secretory antibodies in lungs of mice indicated trafficking of stimulated immune cells from the gut occurred, while antigen-specific IgA in feces also showed a local response. Similarly, Shewen et al. (2009) found that feeding of transgenic alfalfa expressing Lkt50 led to an increase in Lkt-specific IgA in nasal secretions, though it could not be determined if immunity was stimulated in the lower gastrointestinal tract, or the upper respiratory tract through rumination and oropharyngeal exposure to antigen. Regardless, a plant-based vaccine could be targeted to confer protection against respiratory and gastrointestinal bovine pathogens. A vaccine delivering both respiratory and gastrointestinal protection against pathogens in calves would be highly valuable. Moreover, the antigen used in this plant-based vaccine remained stable for approximately one year while stored at room temperature. Thus the vaccine was shown to maintain long-term stability and elicit systemic and mucosal immunity in mice, and may have potential use as an in-feed vaccine in cattle to mitigate the BRD pathogen M. haemolytica.
The sequence of CTB used is from the Vibrio cholerae toxin B subunit gene (GenBank: AY804244.1). The signal peptide region spanning the first 63 nucleotides was not included. Immediately following the CTB sequence, 12 nucleotides encoding a GPGP linker connected to the LktA segment. The LktA fragment consisted of the nucleotides encoding amino acids 705-953 of the M. haemolytica LktA gene (GenBank: M20730.1). The segment used in this CTB+LktA+PlpE construct was taken from a previously reported sequence (Rajeev et al., 2001) and expanded to include 14 amino acids on either side. This was followed by twelve amino acids, three repeats of GGGS, joining the 3’ end of the LktA segment to the nucleotides encoding the immunodominant 55-amino acid R2 region of PlpE from M. haemolytica sequence (Ayalew et al., 2008). This gene fusion was capped by a hemagglutinin tag at the 5’ end and a c-Myc tag at the 3’ end.
The gene was commercially synthesized (Bio Basic Inc., CAN) following optimization of codon usage throughout the sequence for nuclear expression in N. benthamiana. Flanking sequences necessary for Golden Gate cloning with BsaI were added to both ends of the gene. It was subsequently cloned into five modified versions of the pCaMterX plant expression vector (Menassa et al., 2001), which had been altered to accommodate Golden Gate cloning, pCLGG. Each version of pCLGG targeted recombinant protein accumulation to a different subcellular compartment: the apoplast (APO), chloroplast (CHL), cytosol (CYT), endoplasmic reticulum (ER), and vacuole (VAC) (Figure 1). Cloning into these expression vectors was done using NEB Golden Gate Assembly Mix (New England Biolabs Inc., USA) according to the manufacturer’s instructions. Cloning of the CTB+LktA+PlpE gene fusion into the five expression vectors was verified by DNA sequencing.
The five vectors targeting the CTB+LktA+PlpE gene fusion to various subcellular compartments were transformed into Agrobacterium tumefaciens (EHA105). N. benthamiana plants were grown and infiltrated as previously described (Miletic et al., 2015; Saberianfar et al., 2019). For these experiments, four leaves of five plants were infiltrated on three separate occasions. From each infiltrated plant, a leaf disc (7 mm diameter) was taken from each of the four leaves and pooled as one sample. Samples were collected from the infiltrated plants 4, 6, and 8 days post-infiltration (dpi). Total soluble protein (TSP) was extracted from each sample in 200 μl of plant protein extraction buffer (PEB) [1xphosphate-buffered saline (PBS), 0.1% (v/v) Tween-20, 2% (w/v) polyvinylpolypyrrolidone (PVPP), 100mM ascorbic acid, 1mM ethylenediaminetetraacetic acid (EDTA), 1mM phenylmethanesulfonylfluoride (PMSF),1 μg/ml leupeptin] as detailed in (Pereira et al., 2014). To aid in cell lysis, crude extracts were also sonicated on ice for 30 seconds at a 30% amplitude. Following centrifugation at 20,000 x g for 10 minutes at 4°C to clarify the lysates, TSP was quantified.
Samples of extracts from each infiltration were prepared for SDS-PAGE by mixing with a reducing, denaturing loading buffer before heat-denaturation at 100°C for 10 minutes before separation on 4-20% acrylamide, continuous gradient gels (Genscript, Piscataway, USA). Gels were subsequently transferred to polyvinylidene difluoride (PVDF) membranes using the TransBlot Turbo system (Bio-Rad, Hercules, USA). Recombinant protein levels in extracts were determined by comparison to a known protein standard on western blots probed with an anti-c-Myc antibody (Genscript, USA). The protein standard used was a recombinant fusion of eGFP with multiple tags including HA, c-Myc, and 6xHis, expressed in N. benthamiana and purified by immobilized metal affinity chromatography. Accumulation levels of the recombinant antigen in each subcellular compartment were averaged for all individual plants across all three repetitions (Figure 2B).
Enzymatic deglycosylation of transiently produced CTB+LktA+PlpE targeted to the five subcellular compartments was done by digestion of the various extracts using PNGase F (New England Biolabs, USA) according to the manufacturer’s instructions. Digestion was allowed to proceed at 37°C overnight before analysis of the products via SDS-PAGE and western blot with the anti-c-Myc antibody.
To scale up the production of chloroplast-targeted CTB+LktA+PlpE, 6- to 7-week old N. benthamiana plants were infiltrated with a mixture of Agrobacteria containing the expression construct and a construct to express p19, prepared as described for the five targeting constructs, using vacuum. As a negative control, plants were infiltrated with Agrobacteria containing only the p19 expression construct. Whole plants were inverted into the Agrobacteria suspension, completely submerging the leaves. A vacuum of 85 kPa was applied for 1 min. The vacuum was slowly released over 30 seconds, then plants were removed from the Agrobacteria mixture. Any leaves that were not infiltrated were removed. All infiltrated leaf material was collected and pooled 4 days post infiltration, flash frozen in liquid nitrogen, then freeze dried, which reduced the weight of the leaf material by approximately 90%. The dried leaf material was pulverized into small particles using a ball-grinding mill, then analyzed for recombinant protein integrity and amount per dry weight as determined by SDS-PAGE and western blot. The dried N. benthamiana leaves were stored for 12 months, and the stability of the recombinant protein was reassessed prior to the mice study by SDS-PAGE and Western blotting.
The immunogenicity of recombinant CTB+LktA+PlpE protein was studied using pathogen-free Balb/c (Charles River) mice as the experimental animal model. Mice were housed at the vivarium of Lethbridge Research and Development Centre (LeRDC) and work described in this study was conducted in accordance with the Canadian Council on Animal Care guidelines (https://ccac.ca/Documents/Standards/Guidelines/Farm_Animals.pdf) under protocol # 1829 approved by the animal care committee of LeRDC. A total of 54 mice were divided into three experimental groups (n=18 mice per treatment): 1) Leaf with antigen: Agrobacterium-infiltrated, dried leaf material expressing both p19 and CTB+LktA+PlpE (50 μg recombinant protein/160 mg total dry weight/mouse, mixed in 0.5 ml PBS), 2) Leaf without antigen: Agrobacterium-infiltrated, dried leaf material expressing only p19 (160 mg total dry weight, mixed in 0.5 ml PBS), and 3) PBS only: PBS without any leaf material or any antigen (0.5 ml PBS). All treatments were delivered by oral gavage, twice a day, with half of the treatment dose being administered in the morning, and the second half prepared and administered 6 h later. The Leaf with antigen-treated group received 50 µg of CTB+LktA+PlpE protein per immunization day. Mice were vaccinated by oral gavage on days 0, 14, 28, 42 and 56. Samples (blood, bronchoalveolar lavage, and fecal pellets) were collected on days 42, 56 and 70. At each sampling point, 6 mice from each group were sacrificed by exsanguination under deep anesthesia. To determine immune response, each sample was analyzed quantitatively for antigen-specific IgG and IgA.
Antigen-specific antibody (IgG) responses in serum were quantified by enzyme-linked immunosorbent assay (ELISA) as described (Uddin et al., 2023). Sequences corresponding to individual constructs (i.e. LktA and PlpE) were commercially synthesized (BioBasic Inc., Markham, Canada), codon optimized for expression in Escherichia coli, and subsequently purified by immobilized metal affinity chromatography. The purified recombinant antigens LktA and PlpE were used as ligands to coat ELISA plates at concentrations of 50 ng/well and incubated at 4°C overnight. After blocking with 50 mM Tris, 0.14 M NaCl, 1% BSA, pH 8.0 for 1 h at 37°C, serum samples (100 µL) were dispensed into wells of microtiter plates in duplicates, and incubated for 1 h. After washing and adding HRP-conjugated anti-mouse secondary IgG (Cat. No. A90-131P; Bethyl Laboratories Inc.), plates were incubated for 1 h at room temperature. Color development was performed by addition of the TMB substrate (3,3´,5,5´-tetramentylbenzidine; ThermoFisher). Reactions were stopped with 0.2 M H2SO4, and plates were read at 450 nm on a Microtiter Plate Reader (Synergy HTX Microplate Reader, BioTek). Standard curves were generated using a Mouse IgG ELISA Quantitation kit (Cat. No. E90-131; Bethyl Laboratories Inc.) where Mouse Reference Serum (RS10-101-6; Bethyl Laboratories Inc.) was used as standard.
Bronchoalveolar lavage (BAL), and fecal samples were processed for IgA quantification after pre-processing of the samples as described (Hoang et al., 2008). Briefly, BAL samples were washed with PBS containing 0.1% (w/v) BSA and 1 mM of PMSF, followed by centrifugation. Freshly voided feces were collected and stored frozen at -80°C until use. Approximately 100 mg of feces was incubated in 400 µL PBS containing 0.05% Tween 20, 1% BSA, 1 mM PMSF and 0.1% Triton X-100 with vortexing to disrupt solid material. Samples were centrifuged at 17,000 x g for 10 min and supernatants were used for analysis. Following pre-processing, ELISA was used to measure the concentrations of mucosal secretory IgA (sIgA) in accordance with the Mouse IgA ELISA Quantitation kit (Cat. No. E90-103; Bethyl Laboratories Inc.). Briefly, 96-well ELISA plates were coated with 50 ng/well of purified recombinant LktA or PlpE and incubated at 4°C overnight. After blocking in 50 mM Tris, 0.14 M NaCl, 1% BSA, pH 8.0 for 1 h at room temperature, samples were applied in duplicate, then plates were incubated for 1 h at room temperature. After washing and the addition of HRP-conjugated anti-Mouse IgA Detection Antibody (A90-103P; Bethyl Laboratories Inc.), plates were further incubated for 1 h at room temperature. TMB substrate was added for color development, and finally reactions were stopped by adding ELISA stop solution. The plates were read at 450 nm to determine optical density on a spectrophotometric plate reader.
Kruskal-Wallis test was performed to analyze the ELISA results. Significance was tested against the control by Kruskal-Wallis test with post-hoc Dunn’s multiple comparison test where p-value less than 0.05 was considered statistically significant. All analyses were performed using Microsoft Excel 2010 and GraphPad Prism version 9.4.1.
The original contributions presented in the study are included in the article/supplementary material. Further inquiries can be directed to the corresponding authors.
The animal study was approved by animal care committee of LeRDC. The study was conducted in accordance with the local legislation and institutional requirements.
AK designed the constructs, carried out the plant transformation, and expression experiments. MU conducted the animal experiments and analysis. RM and TA conceptualized the study. JO participated in developing the animal use protocol and in mouse sampling. CM participated in construct design. AK, MU, RM, and TA co-wrote the manuscript. All authors contributed to the article and approved the submitted version.
This work was funded by AAFC grant # J-001350.
We thank Alex Molnar for help with the figures, Dr. Igor Kolotilin for developing the Golden Gate compatible plant expression vectors, and Hong Zhu for technical support.
The authors declare that the research was conducted in the absence of any commercial or financial relationships that could be construed as a potential conflict of interest.
All claims expressed in this article are solely those of the authors and do not necessarily represent those of their affiliated organizations, or those of the publisher, the editors and the reviewers. Any product that may be evaluated in this article, or claim that may be made by its manufacturer, is not guaranteed or endorsed by the publisher.
Andres-Lasheras, S., Jelinski, M., Zaheer, R., Mcallister, T. A. (2022). Bovine respiratory disease: conventional to culture-independent approaches to studying antimicrobial resistance in north america. Antibiotics (Basel) 11, 4. doi: 10.3390/antibiotics11040487
Ayalew, S., Confer, A. W., Blackwood, E. R. (2004). Characterization of immunodominant and potentially protective epitopes of Mannheimia haemolytica serotype 1 outer membrane lipoprotein PlpE. Infect. Immun. 72 (12), 7265–7274. doi: 10.1128/IAI.72.12.7265-7274.2004
Ayalew, S., Confer, A. W., Payton, M. E., Garrels, K. D., Shrestha, B., Ingram, K. R., et al. (2008). Mannheimia haemolytica chimeric protein vaccine composed of the major surface-exposed epitope of outer membrane lipoprotein PlpE and the neutralizing epitope of leukotoxin. Vaccine 26 (38), 4955–4961. doi: 10.1016/j.vaccine.2008.07.023
Ayalew, S., Step, D. L., Montelongo, M., Confer, A. W. (2009). Intranasal vaccination of calves with Mannheimia haemolytica chimeric protein containing the major surface epitope of outer membrane lipoprotein PlpE, the neutralizing epitope of leukotoxin, and cholera toxin subunit B. Vet. Immunol. Immunopathol. 132 (2-4), 295–302. doi: 10.1016/j.vetimm.2009.06.005
Blakebrough-Hall, C., Hick, P., Mahony, T. J., Gonzalez, L. A. (2022). Factors associated with bovine respiratory disease case fatality in feedlot cattle. J. Anim. Sci. 100, 1. doi: 10.1093/jas/skab361
Booker, C. W., Abutarbush, S. M., Morley, P. S., Jim, G. K., Pittman, T. J., Schunicht, O. C., et al. (2008). Microbiological and histopathological findings in cases of fatal bovine respiratory disease of feedlot cattle in western Canada. Can. Vet. J. 49:5, 473–481.
Brogden, K. A., Lehmkuhl, H. D., Cutlip, R. C. (1998). Pasteurella haemolytica complicated respiratory infections in sheep and goats. Veterinary Res. 29:3-4, 233–254.
Chin-Fatt, A. S., Saberianfar, R., Menassa, R. (2021). A rationally designed bovine IgA Fc scaffold enhances in planta accumulation of a VHH-Fc fusion without compromising binding to enterohemorrhagic E. coli. Front. Plant Sci. 12. doi: 10.3389/fpls.2021.651262
Confer, A. W., Ayalew, S. (2018). Mannheimia haemolytica in bovine respiratory disease: immunogens, potential immunogens, and vaccines. Anim. Health Res. Rev. 19 (2), 79–99. doi: 10.1017/S1466252318000142
Confer, A. W., Ayalew, S., Montelongo, M., Step, D. L., Wray, J. H., Hansen, R. D., et al. (2009a). Immunity of cattle following vaccination with a Mannheimia haemolytica chimeric PlpE-LKT (SAC89) protein. Vaccine 27:11, 1771–1776. doi: 10.1016/j.vaccine.2008.09.028
Confer, A. W., Ayalew, S., Step, D. L., Trojan, B., Montelongo, M. (2009b). Intranasal vaccination of young Holstein calves with Mannheimia haemolytica chimeric protein PlpE-LKT (SAC89) and cholera toxin. Vet. Immunol. Immunopathol. 132 (2-4), 232–236. doi: 10.1016/j.vetimm.2009.04.018
Conley, A. J., Joensuu, J. J., Menassa, R., Brandle, J. E. (2009). Induction of protein body formation in plant leaves by elastin-like polypeptide fusions. BMC Biol. 7, 48. doi: 10.1186/1741-7007-7-48
Daniell, H., Rai, V., Xiao, Y. (2019). Cold chain and virus-free oral polio booster vaccine made in lettuce chloroplasts confers protection against all three poliovirus serotypes. Plant Biotechnol. J. 17 (7), 1357–1368. doi: 10.1111/pbi.13060
Frank, G. H., Briggs, R., Zehr, E. (1995). Colonization of the tonsils and nasopharynx of calves by a rifampicin-resistant Pasteurella haemolytica and its inhibition by vaccination. Am. J. Vet. Res. 56 (7), 866–869.
Garabagi, F., Gilbert, E., Loos, A., McLean, M. D., Hall, J. C. (2012). Utility of the P19 suppressor of gene-silencing protein for production of therapeutic antibodies in Nicotiana expression hosts. Plant Biotechnol. J. 10, 1118–1128. doi: 10.1111/j.1467-7652.2012.00742.x
Hoang, T. H., Hong, H. A., Clark, G. C., Titball, R. W., Cutting, S. M. (2008). Recombinant Bacillus subtilis expressing the Clostridium perfringens alpha toxoid is a candidate orally delivered vaccine against necrotic enteritis. Infect. Immun. 76 (11), 5257–5265. doi: 10.1128/IAI.00686-08
Holmgren, J., Adamsson, J., Anjuere, F., Clemens, J., Czerkinsky, C., Eriksson, K., et al. (2005). Mucosal adjuvants and anti-infection and anti-immunopathology vaccines based on cholera toxin, cholera toxin B subunit and CpG DNA. Immunol. Lett. 97 (2), 181–188. doi: 10.1016/j.imlet.2004.11.009
Kolotilin, I., Topp, E., Cox, E., Devriendt, B., Conrad, U., Joensuu, J., et al. (2014). Plant-based solutions for veterinary immunotherapeutics and prophylactics. Vet. Res. 45, 375. doi: 10.1186/s13567-014-0117-4
Kwon, K. C., Daniell, H. (2016). Oral delivery of protein drugs bioencapsulated in plant cells. Mol. Ther. 24, 1342–50. doi: 10.1038/mt.2016.115
Larson, R. L., Step, D. L. (2012). Evidence-based effectiveness of vaccination against Mannheimia haemolytica, Pasteurella multocida, and Histophilus somni in feedlot cattle for mitigating the incidence and effect of bovine respiratory disease complex. Vet. Clin. North Am. Food Anim. Pract. 28 (97-106), 106e101–107, ix. doi: 10.1016/j.cvfa.2011.12.005
Lee, R. W., Cornelisse, M., Ziauddin, A., Slack, P. J., Hodgins, D. C., Strommer, J. N., et al. (2008). Expression of a modified Mannheimia haemolytica GS60 outer membrane lipoprotein in transgenic alfalfa for the development of an edible vaccine against bovine pneumonic pasteurellosis. J. Biotechnol. 135 (2), 224–231. doi: 10.1016/j.jbiotec.2008.03.006
Lemon, J. L., Mcmenamy, M. J. (2021). A review of UK-registered and candidate vaccines for bovine respiratory disease. Vaccines 9, 12. doi: 10.3390/vaccines9121403
Menassa, R., Nguyen, V., Jevnikar, A., Brandle, J. (2001). A self-contained system for the field production of plant recombinant interleukin-10. Mol. Breed 8 (2), 177–185. doi: 10.1023/A:1013376407362
Merlin, M., Pezzotti, M., Avesani, L. (2017). Edible plants for oral delivery of biopharmaceuticals. Br. J. Clin. Pharmacol. 83 (1), 71–81. doi: 10.1111/bcp.12949
Miles, D. G. (2009). Overview of the North American beef cattle industry and the incidence of bovine respiratory disease (BRD). Anim. Health Res. Rev. 10 (2), 101–103. doi: 10.1017/S1466252309990090
Miletic, S., Simpson, D. J., Szymanski, C. M., Deyholos, M. K., Menassa, R. (2015). A plant-produced bacteriophage tailspike protein for the control of salmonella. Front. Plant Sci. 6. doi: 10.3389/fpls.2015.01221
Pereira, E. O., Kolotilin, I., Conley, A. J., Menassa, R. (2014). Production and characterization of in planta transiently produced polygalacturanase from Aspergillus Niger and its fusions with hydrophobin or ELP tags. BMC Biotechnol. 14, 59. doi: 10.1186/1472-6750-14-59
Rajeev, S., Kania, S. A., Nair, R. V., Mcpherson, J. T., Moore, R. N., Bemis, D. A. (2001). Bordetella bronchiseptica fimbrial protein-enhanced immunogenicity of a Mannheimia haemolytica leukotoxin fragment. Vaccine 19 (32), 4842–4850. doi: 10.1016/s0264-410x(01)00226-2
Rice, J. A., Carrasco-Medina, L., Hodgins, D. C., Shewen, P. E. (2007). Mannheimia haemolytica and bovine respiratory disease. Anim. Health Res. Rev. 8 (2), 117–128. doi: 10.1017/S1466252307001375
Rybicki, E. P. (2009). Plant-produced vaccines: promise and reality. Drug Discovery Today 14 (1-2), 16–24. doi: 10.1016/j.drudis.2008.10.002
Rybicki, E. (2018). “History and promise of plant-made vaccines for animals,” in Prospects of Plant-Based Vaccines in Veterinary Medicine. Ed. MacDonald, J. (Cham: Springer). doi: 10.1007/978-3-319-90137-4_1
Saberianfar, R., Chin-Fatt, A., Scott, A., Henry, K. A., Topp, E., Menassa, R. (2019). Plant-produced chimeric VHH-sIgA against enterohemorrhagic E. coli intimin shows cross-serotype inhibition of bacterial adhesion to epithelial cells. Front. Plant Sci. 10. doi: 10.3389/fpls.2019.00270
Sanchez-Lopez, E. F., Corigliano, M. G., Oliferuk, S., Ramos-Duarte, V. A., Rivera, M., Mendoza-Morales, L. F., et al. (2021). Oral immunization with a plant HSP90-SAG1 fusion protein produced in tobacco elicits strong immune responses and reduces cyst number and clinical signs of toxoplasmosis in mice. Front. Plant Sci. 12. doi: 10.3389/fpls.2021.726910
Schiefer, B., Ward, G., Moffatt, R. (1978). Correlation of microbiological and histological findings in bovine fibrinous pneumonia. Vet. Pathol. 15 (3), 313–321. doi: 10.1177/030098587801500305
Schillberg, S., Finnern, R. (2021). Plant molecular farming for the production of valuable proteins - Critical evaluation of achievements and future challenges. J. Plant Physiol. 258-259, 153359. doi: 10.1016/j.jplph.2020.153359
Schillberg, S., Raven, N., Spiegel, H., Rasche, S., Buntru, M. (2019). Critical analysis of the commercial potential of plants for the production of recombinant proteins. Front. Plant Sci. 10. doi: 10.3389/fpls.2019.00720
Shahid, N., Daniell, H. (2016). Plant based oral vaccines against zoonotic and non-zoonotic diseases. Plant Biotechnol. J. 14, 2079–2099. doi: 10.1111/pbi.12604
Sheoran, A. S., Artiushin, S., Timoney, J. F. (2002). Nasal mucosal immunogenicity for the horse of a SeM peptide of Streptococcus equi genetically coupled to cholera toxin. Vaccine 20 (11-12), 1653–1659. doi: 10.1016/s0264-410x(01)00488-1
Shewen, P. E., Carrasco-Medina, L., Mcbey, B. A., Hodgins, D. C. (2009). Challenges in mucosal vaccination of cattle. Vet. Immunol. Immunopathol. 128 (1-3), 192–198. doi: 10.1016/j.vetimm.2008.10.297
Silhavy, D., Molnar, A., Lucioli, A., Szittya, G., Hornyik, C., Tavazza, M., et al. (2002). A viral protein suppresses RNA silencing and binds silencing-generated, 21- to 25-nucleotide double-stranded RNAs. EMBO J. 21 (12), 3070–3080. doi: 10.1093/emboj/cdf312
Snyder, E., Credille, B. (2020). Mannheimia haemolytica and Pasteurella multocida in Bovine Respiratory Disease: How Are They Changing in Response to Efforts to Control Them? Vet. Clin. North Am. Food Anim. Pract. 36 (2), 253–268. doi: 10.1016/j.cvfa.2020.02.001
Taghavian, O., Spiegel, H., Hauck, R., Hafez, H. M., Fischer, R., Schillberg, S. (2013). Protective oral vaccination against infectious bursal disease virus using the major viral antigenic protein VP2 produced in Pichia pastoris. PloS One 8 (12), e83210. doi: 10.1371/journal.pone.0083210
Timsit, E., Mcmullen, C., Amat, S., Alexander, T. W. (2020). Respiratory bacterial microbiota in cattle: from development to modulation to enhance respiratory health. Vet. Clin. North Am. Food Anim. Pract. 36 (2), 297–320. doi: 10.1016/j.cvfa.2020.03.001
Timsit, E., Workentine, M., Schryvers, A. B., Holman, D. B., van der Meer, F., Alexander, T. W. (2016). Evolution of the nasopharyngeal microbiota of beef cattle from weaning to 40 days after arrival at a feedlot. Vet. Microbiol. 187, 75–81. doi: 10.1016/j.vetmic.2016.03.020
Tschofen, M., Knopp, D., Hood, E., Stoger, E. (2016). Plant molecular farming: much more than medicines. Annu. Rev. Anal. Chem. (Palo Alto Calif) 9 (1), 271–294. doi: 10.1146/annurev-anchem-071015-041706
Tuse, D., Tu, T., Mcdonald, K. A. (2014). Manufacturing economics of plant-made biologics: case studies in therapeutic and industrial enzymes. BioMed. Res. Int. 2014, 256135. doi: 10.1155/2014/256135
Uddin, M. S., Ortiz, J., Abbott, D. W., Inglis, G. D., Guan, L. L., Alexander, T. W. (2023). Development of a spore-based mucosal vaccine against the bovine respiratory pathogen Mannheimia haemolytica. Sci. Rep. 13, 1–11. doi: 10.1038/s41598-023-29732-4
USDA (2011) Cattle and calves nonpredator death loss in the U.S. 2010. Animal and Plant Health Inspection Service, United States Department of Agriculture. Available at: https://www.aphis.usda.gov/animal_health/nahms/general/downloads/cattle_calves_nonpred_2010.pdf.
Keywords: bovine respiratory disease, plant-made vaccine, oral vaccine, Mannheimia haemolytica, molecular farming, mucosal immunity, agroinfiltration
Citation: Kaldis A, Uddin MS, Guluarte JO, Martin C, Alexander TW and Menassa R (2023) Development of a plant-based oral vaccine candidate against the bovine respiratory pathogen Mannheimia haemolytica. Front. Plant Sci. 14:1251046. doi: 10.3389/fpls.2023.1251046
Received: 30 June 2023; Accepted: 07 August 2023;
Published: 15 September 2023.
Edited by:
Linda Avesani, University of Verona, ItalyReviewed by:
Hugh S. Mason, Arizona State University, United StatesCopyright © 2023 His Majesty the King in Right of Canada, as represented by the Minister of Agriculture and Agri-Food Canada for the contribution of Angelo Kaldis, Muhammed Salah Uddin, Jose Ortiz Guluarte, Coby Martin, Trevor W. Alexander, Rima Menassa. This is an open-access article distributed under the terms of the Creative Commons Attribution License (CC BY). The use, distribution or reproduction in other forums is permitted, provided the original author(s) and the copyright owner(s) are credited and that the original publication in this journal is cited, in accordance with accepted academic practice. No use, distribution or reproduction is permitted which does not comply with these terms.
*Correspondence: Rima Menassa, cmltYS5tZW5hc3NhQGFnci5nYy5jYQ==; Trevor W. Alexander, dHJldm9yLmFsZXhhbmRlckBhZ3IuZ2MuY2E=
†These authors have contributed equally to this work and share first authorship
Disclaimer: All claims expressed in this article are solely those of the authors and do not necessarily represent those of their affiliated organizations, or those of the publisher, the editors and the reviewers. Any product that may be evaluated in this article or claim that may be made by its manufacturer is not guaranteed or endorsed by the publisher.
Research integrity at Frontiers
Learn more about the work of our research integrity team to safeguard the quality of each article we publish.