- Laboratorio de Biología Molecular de Bacterias y Levaduras, Departamento de Microbiología, Escuela Nacional de Ciencias Biológicas, Instituto Politécnico Nacional, Ciudad de México, Mexico
The bacterial component of plant holobiont maintains valuable interactions that contribute to plants’ growth, adaptation, stress tolerance, and antagonism to some phytopathogens. Teosinte is the grass plant recognized as the progenitor of modern maize, domesticated by pre-Hispanic civilizations around 9,000 years ago. Three teosinte species are recognized: Zea diploperennis, Zea perennis, and Zea mays. In this work, the bacterial diversity of three species of Mexican teosinte seeds was explored by massive sequencing of 16S rRNA amplicons. Streptomyces, Acinetobacter, Olivibacter, Erwinia, Bacillus, Pseudomonas, Cellvibrio, Achromobacter, Devosia, Lysobacter, Sphingopyxis, Stenotrophomonas, Ochrobactrum, Delftia, Lactobacillus, among others, were the bacterial genera mainly represented. The bacterial alpha diversity in the seeds of Z. diploperennis was the highest, while the alpha diversity in Z. mays subsp. mexicana race was the lowest observed among the species and races. The Mexican teosintes analyzed had a core bacteriome of 38 bacterial genera, including several recognized plant growth promoters or fungal biocontrol agents such as Agrobacterium, Burkholderia, Erwinia, Lactobacillus, Ochrobactrum, Paenibacillus, Pseudomonas, Sphingomonas, Streptomyces, among other. Metabolic inference analysis by PICRUSt2 of bacterial genera showed several pathways related to plant growth promotion (PGP), biological control, and environmental adaptation. The implications of these findings are far-reaching, as they highlight the existence of an exceptional bacterial germplasm reservoir teeming with potential plant growth promotion bacteria (PGPB). This reserve holds the key to cultivating innovative bioinoculants and formidable fungal antagonistic strains, thereby paving the way for a more sustainable and eco-friendly approach to agriculture. Embracing these novel NGS-based techniques and understanding the profound impact of the vertical transference of microorganisms from seeds could revolutionize the future of agriculture and develop a new era of symbiotic harmony between plants and microbes.
1 Introduction
The domestication of plants has played a crucial role in the cultural and economic advancement of societies across the globe. Through domestication, humanity has cultivated plants that provide several benefits, including food, beverages, medicine, raw materials for industry, and even elements that have cultural or social significance (Milla et al., 2015; Purugganan, 2019).
The biological origin, diversification, and domestication of maize occurred in Mesoamerica, located in the center of Mexico. This grass of the Poaceae family had a seminal role in the origin, extension of agriculture, and culture of pre-Hispanic civilizations (Smith et al., 1981). One of the species of actual teosintes, Zea mays subsp. parviglumis, is the progenitor of all derivative Zea mays subsp. mays modern races. The human-driven domestication that started around 9,000 years ago is one of the most critical events in the history of agriculture (Doebley, 2004; Piperno et al., 2009; Sahoo et al., 2021).
Numerous groups of bacteria and fungi establish interactions with plants. It has been discovered that the overall health of plants is closely associated with the specific composition of microorganisms present both in the soil and the plants themselves (Gherbi et al., 2008; Miyambo et al., 2016; van der Heijden and Hartmann, 2016; Dastogeer et al., 2020). Plants maintain associations with microorganisms both outside and within their tissues. Endophytic microorganisms within the root, stem, leaves, flowers, and seeds maintain mutualistic symbiosis with the plant host (Frey-Klett et al., 2011; Mishra et al., 2015; De Mandal et al., 2021). Seed endophyte microorganisms can be transferred vertically to plant offspring, ensuring their permanence in favorable environments (Johnston-Monje & Raizada, 2011).
Few studies of culturable fractions of teosinte bacteria have been performed. Nitrogen-fixing Paraburkholderia tropica (formerly Burkholderia tropica) was isolated from the rhizosphere and stem of teosinte (Caballero-Mellado et al., 2004; Reis et al., 2004). Although this species has not been reported again associated with teosinte, other species and strains isolated from maize and sugarcane express plant growth promotion (PGP) and antifungal phenotypic features (Tenorio-Salgado et al., 2013; Bernabeu et al., 2018; Schlemper et al., 2018; Kuramae et al., 2020; Vio et al., 2022). Also, endophytic Bacillus, Enterobacter, Methylobacterium, and Pantoea, with variable PGP features, were repeatedly isolated from three different teosinte species (Johnston-Monje & Raizada, 2011). Paenibacillus polymyxa and Citrobacter sp. obtained from the same teosinte seeds inhibited fungal growth and mycotoxin production and maintained a potential to combat phytopathogens (Mousa et al., 2015). Currently, an important research topic is to elucidate how much of a plant’s phenotype, adaptive capacities, evolution, and productivity are due to its endospheric and rhizospheric microbiome (Santoyo et al., 2017; Kaur et al., 2021).
In that sense, next-generation sequencing (NGS) technologies have revolutionized the field of microbiology and have become an essential tool for studying the plant holobiont, which encompasses the plant and all its associated microorganisms. Identifying microbial species or microbiomes present in the plant holobiont is the first step to studying the complexity of the existing symbiosis (Simon et al., 2019; Marco et al., 2022).
In this work, the bacteriome of seeds of three teosinte species was explored by NGS of 16S rRNA gene. The alpha and beta diversities of bacterial genera, the core bacteriome of the teosinte species, and metabolic prediction of the main bacteria were documented. Many previously potential PGPB associated with maize were detected in teosintes. This work may lead efforts to isolate the cultivable fraction of these plant species that may be a reservoir of PGPB for use as biofertilizers and for biocontrol.
2 Materials and methods
2.1 Biological samples
Seeds of 6 different species, subspecies, and races of Mexican teosintes were used in this work: Zea perennis, Zea diploperennis, Zea mays subsp. mexicana race Nobogame, Zea mays subsp. mexicana race Mesa Central, Zea mays subsp. mexicana race Chalco and Zea mays subsp. parviglumis race Balsas. Teosinte seeds were provided by the International Maize and Wheat Improvement Center (CIMMYT) (Texcoco, Mexico). Information and access numbers for CIMMYT collections are presented in Figure 1; Table 1.
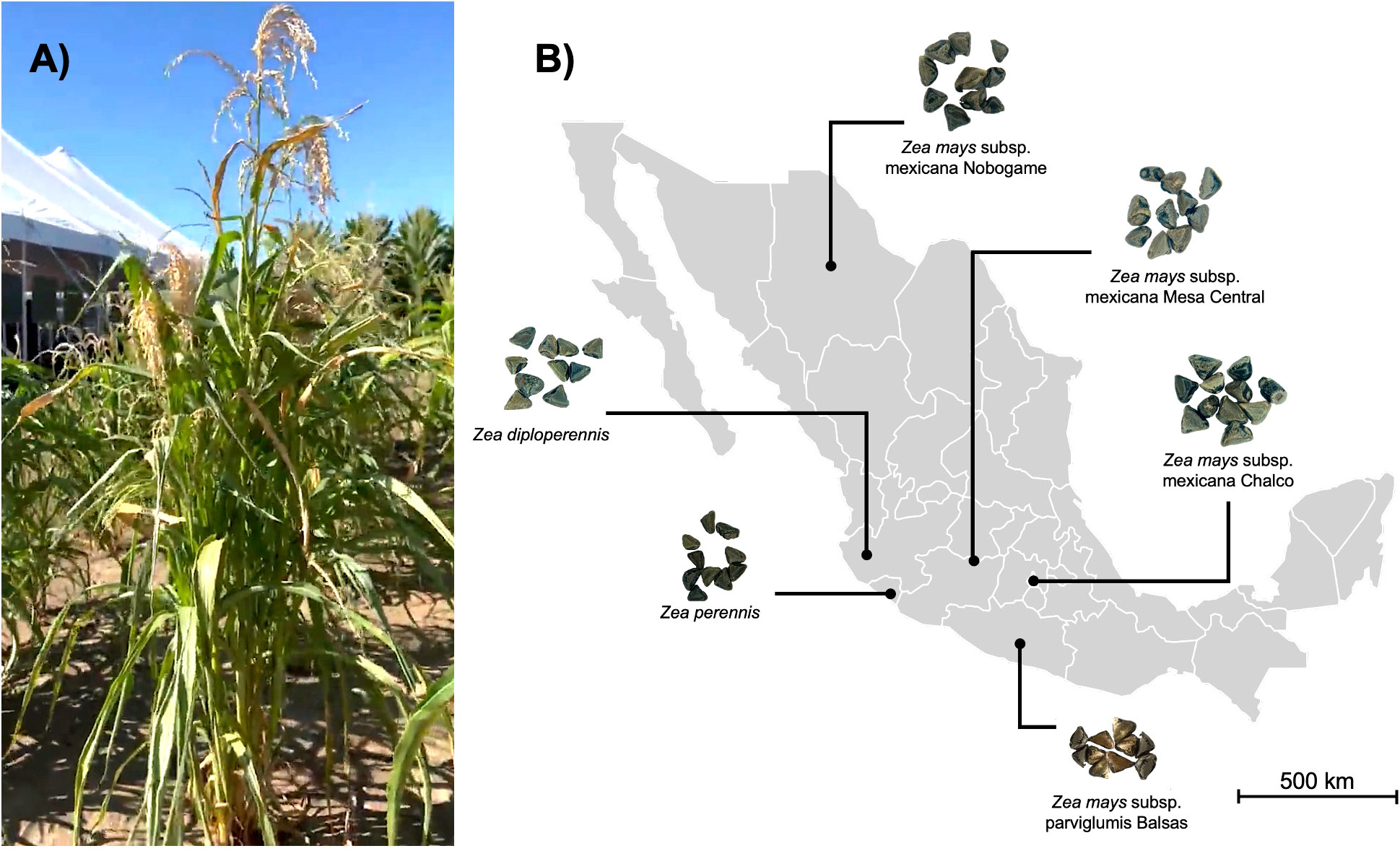
Figure 1 Teosinte in Mexico. (A) Mature teosinte plant, growing wild in maize fields. (B) Map of the distribution of the different races of teosintes in Mexico from which the samples were obtained.
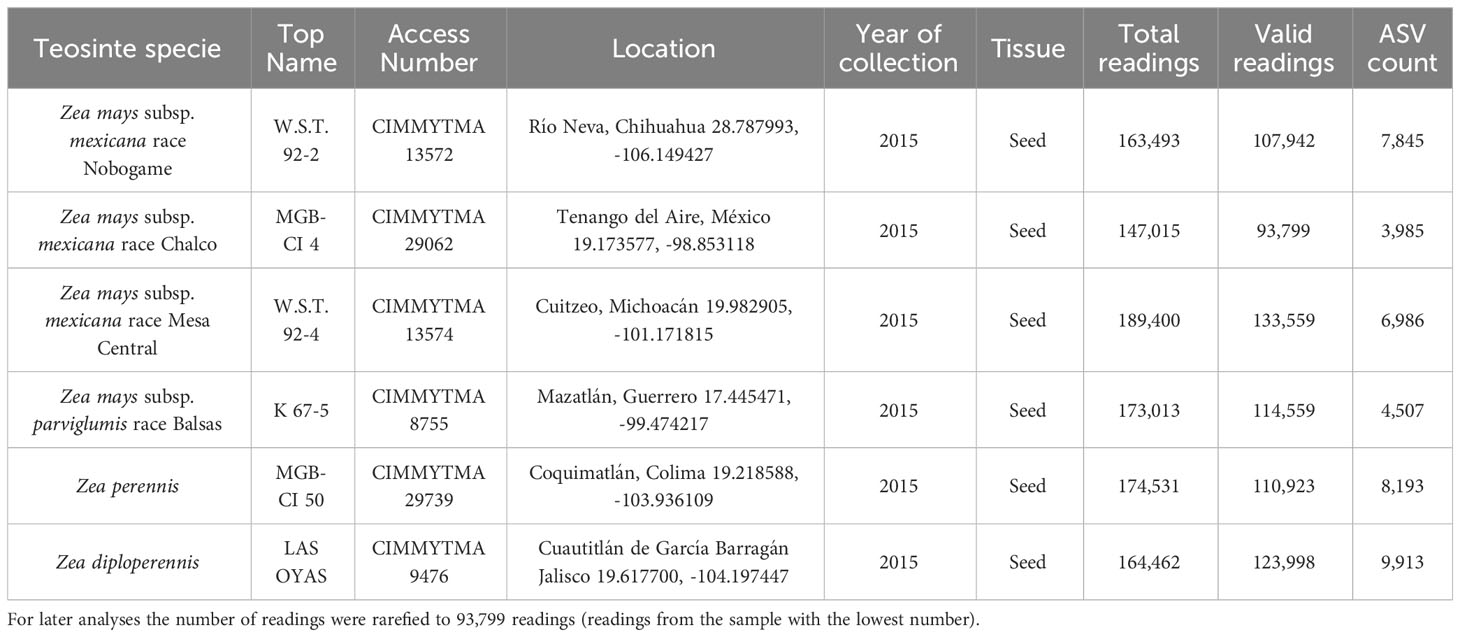
Table 1 Readings obtained and quality filtration from the massive sequencing of the 16S rRNA gene of teosinte seeds.
2.2 DNA extraction and 16S rRNA metabarcoding sequencing
Teosinte seeds (3 groups of 20 seeds per species) were washed with sterile distilled water for 48 h. The wash water was decanted, and the seeds were soaked in 5% sodium hypochlorite for 10 min and washed five times with sterile distilled water for 1 min. Finally, the seeds were disinfected with 95% ethyl alcohol for 10 min and washed five times with sterile distilled water for 1 min.
Three groups of 20 seeds for each variety were used for DNA extraction; later, these extractions per variety were pulled and sequenced. The extraction of metagenomic DNA was performed using the cetyltrimethylammonium bromide (CTAB) technique (Aboul-Maaty and Oraby, 2019). Primers 341F (5-Clamp 1-CCTACGGGAGGCAGCAG-3)/806R (5-ATTACCGCGGCTGCTGG-3) were used to amplify the V3-V4 regions of the 16S rRNA gene of the pulled metagenomic DNA obtained (Yang et al., 2017). A single 6-nucleotide label was added to the 5′ end of the initiators to distinguish PCR products. All PCR amplifications were performed in 30 μL reaction volumes containing 15 μL of 2 Phusion Master Mix (New England Biolabs, Ipswich, MA, USA), 0.2 μM of each forward and reverse primers, and 10 ng of metagenomic DNA. The thermal cycle conditions were: initial denaturation at 98°C for 1 minute followed by 30 cycles of 95°C for 10 sec, 50°C for 30 sec, and 72°C for 30 sec, with a final extension at 72°C for 5 min.
The amplification products were separated by 2% agarose gel electrophoresis (p/v) and purified with a GeneJET Gel extraction kit (Thermo Fisher Scientific, Waltham, MA, USA). Purified PCR products were sequenced on the Illumina HiSeq 2000 platform at Novogene Bioinformatics Technology Co. Ltd. (Beijing, China).
2.3 NGS analyses
FastQC performed the quality control for high throughput sequence data was performed by FastQC Version 0.12.0 (Andrews et al., 2010). The low-quality reads (Phred quality score < 25) and sequences <200 or > 500 bp long, containing ambiguous characters, homopolymers >6 bp, and mismatches in primers > 14 were removed from subsequent analyses (Lawley and Tannock, 2017).
Sequencing data were analyzed using the QIIME2™ software package (Bolyen et al., 2019). Sequences were quality-filtered, trimmed, denoised, and merged using DADA2 plugin (Callahan et al., 2016). Chimeric sequences, singletons, and doubletons were detected and removed by the DADA2 workflow. Representative ASVs were aligned with MAFFT and used for phylogenetic reconstruction in FastTree using plugin alignment and phylogeny (Faith and Baker, 2006). A trained Naïve Bayes classifier-based SILVA database (https://www.arb-silva.de/documentation/release-132/) was applied to assign the taxonomy (Agnihortry et al., 2020; Kõljalg et al., 2020). ASVs that could not be taxonomically identified were manually checked by performing BLAST searches in RDP (http://rdp.cme.msu.edu/) (Bacci et al., 2015) based on similarity thresholds for family, genus, and species at >90, >95, and >97%, respectively (Rosselló-Móra et al., 2017).
2.4 Alpha diversity analysis
The microbial diversity and microbial communities’ composition analyses were estimated with a series of scripts from QIIME2, including generating rarefied amplicon sequence variant (ASV) tables. To calculate α-diversity within these communities in all samples, the species richness was estimated using the observed ASV number and Chao1 (Chao, 1984), species diversity with Shannon (Shannon, 1948), and the dominance with Simpson index (Simpson, 1949) in QIIME2. The diversity indices of the samples were compared using the Mann-Whitney U test to evaluate the statistical significance between the samples (P < 0.05). Good’s coverage estimator was used to calculate the sequence coverage obtained for the 16S rRNA region datasets (Good, 1953).
2.5 Beta diversity analysis
The β-diversity comparison of seed bacteria among teosinte species was performed using UniFrac distances (Lozupone et al., 2011), both unweighted (phylogenetic richness) and weighted (relative abundance and phylogenetic richness) in MEGAN 6.21 software (Bağcı et al., 2019). Also, the Bray-Curtis dissimilarity was estimated using PAST 4.03 software (Hammer et al., 2001). Significant differences among bacterial communities of teosinte species were tested with the Monte Carlo method and Adonis test for UniFrac distances and the Bray-Curtis index, respectively. A Principal Coordinates Analysis (PCoA) to explore multidimensional patterns of diversity variation of bacterial communities among teosinte species was performed using unweighted and weighted UniFrac distances in PAST 4.03 (Hammer et al., 2001).
2.6 Visualization of diversity and abundance of samples and core bacteriome
The visualization, analysis, comparison, and contrast of the information of the ASV tables, heat-map graphs of relative abundance, and taxonomic co-occurrence analysis were made with MEGAN 6.21 (Bağcı et al., 2019) and TBtools v1.108 tools (Chen et al., 2020). The cut-off to define the core bacteriome of ASV in teosinte samples was a strict core of 100% (Bağcı et al., 2019).
2.7 Prediction of functional profiling of teosinte seed endophytic bacteria
The predictive functional profile of the endophytic bacterial communities of different teosinte seeds was inferred using the Phylogenetic Investigation of Communities by Reconstruction of Unobserved States 2 (PICRUSt2) software (Langille et al., 2013; Douglas et al., 2020) through the web application Galaxy7 and employing KEGG database (Afgan et al., 2016). The accuracy of metagenome predictions was determined with the nearest sequence-weighted taxon index (NSTI) that summarizes the extent to which microorganisms in a sample are related to sequence genomes, and they represent the average branch length that separates each ASV in a sample from a reference bacterial genome, weighting their relative abundance in each sample. Low values of this index indicate a closer mean relationship.
3 Results
3.1 Data quality analysis
The DNA sequence quality trimming was performed. Table 1 summarizes the sample data and the number of trimmed DNA sequence data, showcasing only the high-quality, validated readings that met the predetermined quality criteria. The valid readings that oscillate between 93,799 and 133,559 are shown. The number of readings was reduced to the lowest value for subsequent analysis.
3.2 Bacterial communities’ analysis
The analysis of diversity to estimate richness and abundance in individual samples was carried out using multiple methods, as shown in Table 2. The samples of Z. diploperennis had the highest number of observed bacterial ASV (1822), while Z. perennis y Z. mays subsp. parviglumis teosinte harbored the greatest bacterial diversity estimated with the Simpson (0.0024) and reciprocal Simpson (3.8025) and Shannon (4.0142) indexes, respectively.
The α-diversity indices showed that Z. diploperennis, Z. perennis, and Z. mays subsp. parviglumis harbored higher diverse bacterial communities than Z. mays subsp. mexicana races Chalco, Nobogame, and Mesa Central. Moreover, the utilization of weighted UniFrac in β-diversity analysis unveiled that the estimated species turnover demonstrates the grouping of Z. diploperennis, Z. perennis, and Z. mays subsp. parviglumis within a single clade, while the races of Z. mays subsp. mexicana exhibit distribution in a separate clade.
The PCoA was performed using unweighted and weighted UniFrac distances and explained 78.6% (PCoA- 45.0%; PCoB-20.0%; PCoC- 13.6%) (Figure 2A) and 95.7% (PCoA: 73.6%; PCoB-18.5%; PCoC-3.6%) (Figure 2C) of the total bacterial genus-level variation, respectively. The unweighted PCoA showed that the bacterial diversity was different (P<0.05) among communities of teosinte races. However, in a weighted PCoA analysis, a rearrangement arose in the relationship among the different teosinte races according to bacterial communities’ diversity and abundance. Z. mays subsp. mexicana races Nobogame and Mesa Central were the most similar between them, followed by Z. perennis and Z. diploperennis pair, with his analysis does not show a clear grouping between the races of the species Zea mays (Figures 2B–D).
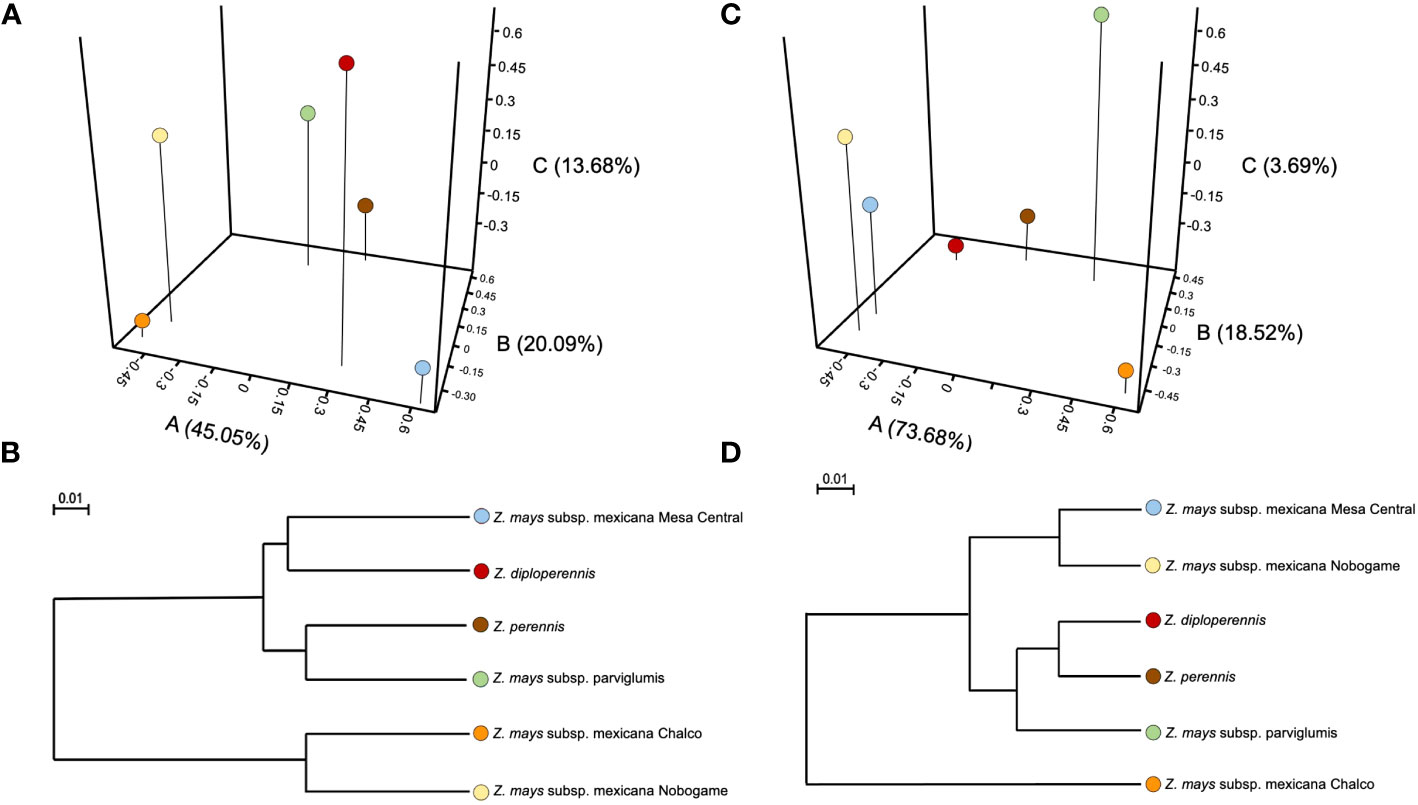
Figure 2 Principal coordinate analysis (PCoA) of β−bacterial diversity across all samples using unweighted (A, B) and weighted (C, D) UniFrac distances. Unweighted PCoA and UniFrac were performed to compare taxonomic groups assigned from massive sequencing of the 16S rRNA gene of seeds of different teosinte species and races.
Bacterial communities in the seeds of three teosinte species were remarkably diverse and consisted of 39 phyla and about 342 families with at least 1% abundance in samples (Figure 3A). The teosinte seed endophytes exhibited a dominant presence of Proteobacteria (8-40%) across all samples, highlighting its prominence as the most abundant phylum. Notably, the relative abundance analysis revealed several prominent bacterial families, including Enterobacteriaceae (0.6-3.9%), Vibrionaceae (0.2-1.7%), Xanthomonadaceae (3.2-8.5%), Aeromonadaceae (0.1-2.2%), Comamonadaceae (0.1-1.7%), Moraxellaceae (0.2-28.7%), Pseudomonadaceae (0.5-10.1%), Cyclobacteriaceae (0.4-2.4%), Cytophagaceae (0.2-3.9%), Sphingobacteriaceae (0.6-19.6%), Hyphomicrobiaceae (0.1-5.1%), Rhizobiaceae (0.1-3.6%), Rhosdospirilaceae (0.3-1.4%), Alcaligeneaceae (0.2-15.2%), Bacillaceae (0.1-3.8%), Lactobacillaceae (0.1-3.6%), Clostridiaceae (0.3-5.1%), Heliobacteriaceae (0.1-5.7%), Rhodobiaceae (0.3-6.1%), Rhodobacteriaceae (0.2-3.7%), and Ruminococcaceae (0.1-1.2%). A total of 572 genera were assigned, and the most abundant were Streptomyces, Acinetobacter, Olivibacter, Erwinia, Bacillus, Pseudomonas, Cellvibrio, Achromobacter, Devosia, Lysobacter, Agrobacterium, Sphingopyxis, Stenotrophomonas, Ochrobactrum, Delftia, and Lactobacillus. Streptomyces was the most abundant genera associated with Z. mays subsp. mexicana Mesa Central (17.7%) and Z. diploperennis (21.8%), for Z. mays subsp. mexicana Nobogame (19.5%) and Z. mays subsp. parviglumis (40.3%) was Erwinia, for Z. perennis was Olivibacter (17.9%), and for Z. mays subsp. mexicana Chalco was Acinetobacter (64.2%) (Figure 3B).
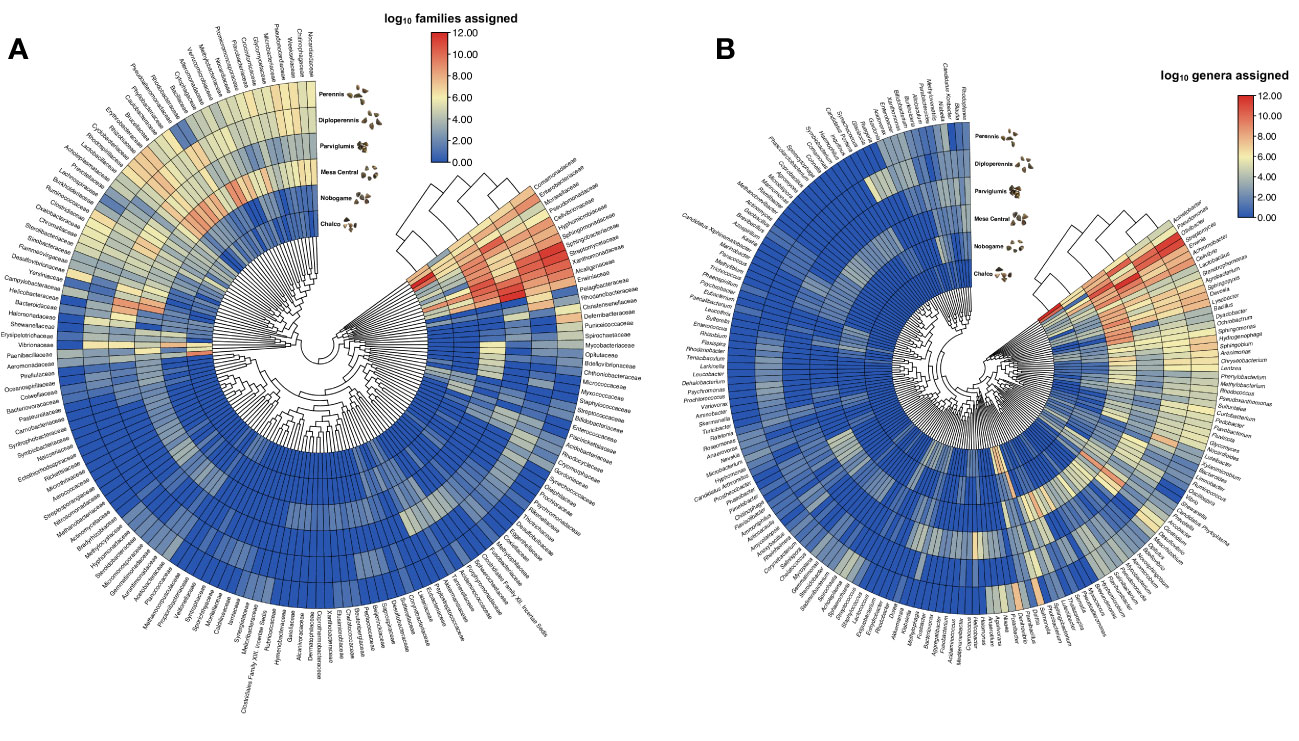
Figure 3 Endophytic bacterial diversity in Mexican teosinte seeds. (A) the relative abundance of families in teosinte seeds is expressed as the log10 of the total assigned readings, and (B) the relative abundance of bacterial genera is shown as the log10 of the total readings assigned. The bar color gradient represents high (red) and low (blue) readings.
In the initial analysis, the distribution patterns of bacterial genera were examined within each teosinte race. The results indicated that varying numbers of bacterial genera exclusively associated with each race. Z. mays subsp. mexicana Chalco and Z. mays subsp. mexicana Nobogame exhibited three exclusive bacterial genera, while Z. perennis, Z. mays subsp. mexicana parviglumis, Z. mays subsp. mexicana Mesa Central, and Z. diploperennis showed seven, nine, thirteen, and fourteen exclusive bacterial genera, respectively (Figure 4). Although these findings could suggest the presence of bacterial genus-specific relationships within each teosinte race, the experimental design does not allow reaching that conclusion. Further investigations, such as metagenomic sequencing or functional profiling of the associated bacterial communities, and an extensive sampling would provide a more comprehensive understanding of the specific bacteriome and its potential implications for teosinte races.
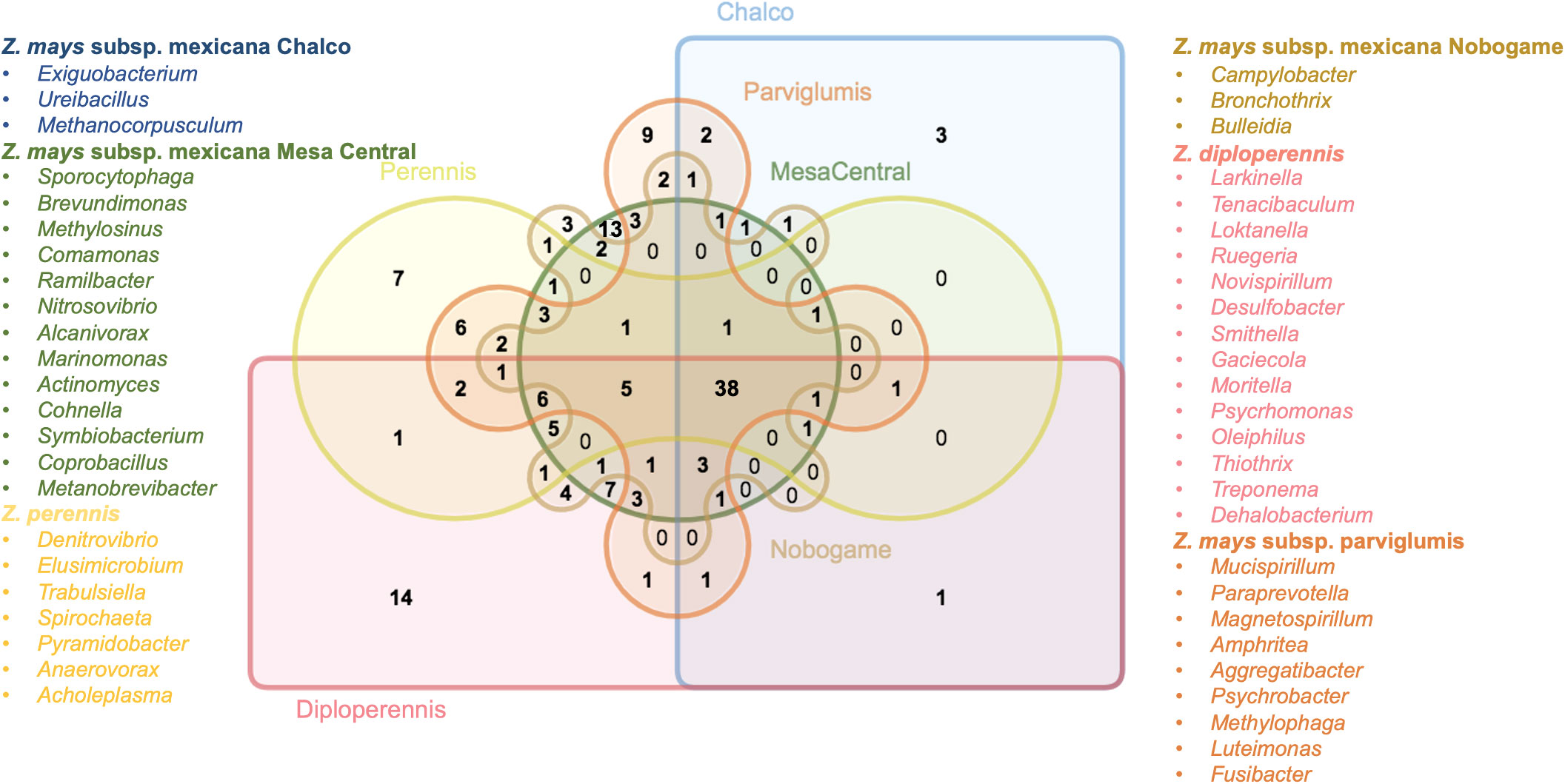
Figure 4 Comparison of bacterial genera in teosinte samples. Venn diagram showing the grouping relationships of microbial genera in the different species of teosinte (central bacteriome and accessory bacteriomes).
The analysis of the strict core bacteriome in three teosinte species and six races revealed a total of 38 genera that were present at 100% of presence with a high relative abundance (0.010% of detection) across all samples. These genera include Acinetobacter, Aeromonas, Agrobacterium, Arenimonas, Bacteroides, Blautia, Burkholderia, Cellvibrio, Chryseobacterium, Clostridium, Delftia, Devosia, Erwinia, Fibrobacteria, Glycomyces, Hydrogenophaga, Lactobacillus, Lentzea, Limnobacter, Luteibacter, Lysobacter, Methylobacterium, Ochrobactrum, Olivibacter, Oscillospira, Paenibacillus, Parabacteroides, Phenylobacterium, Phytoplasma, Prevotella, Pseudomonas, Pseudoxanthomonas, Ruminococcus, Salmonella, Sphingomonas, Sphingopyxis, Stenotrophomonas, and Streptomyces. In this same analysis, we noticed that within the negative interrelationships, at least six subgroups of between 5-15 genera are formed that share more than 80% co-occurrence, which could suggest that these genera probably also play a significant role in the specificity of each genotype in particular (Figure 5). Some of these genera have been found and studied in different maize samples under different techniques, which suggests the close relationship of these bacteria with maize and teosinte plants (Table 3).
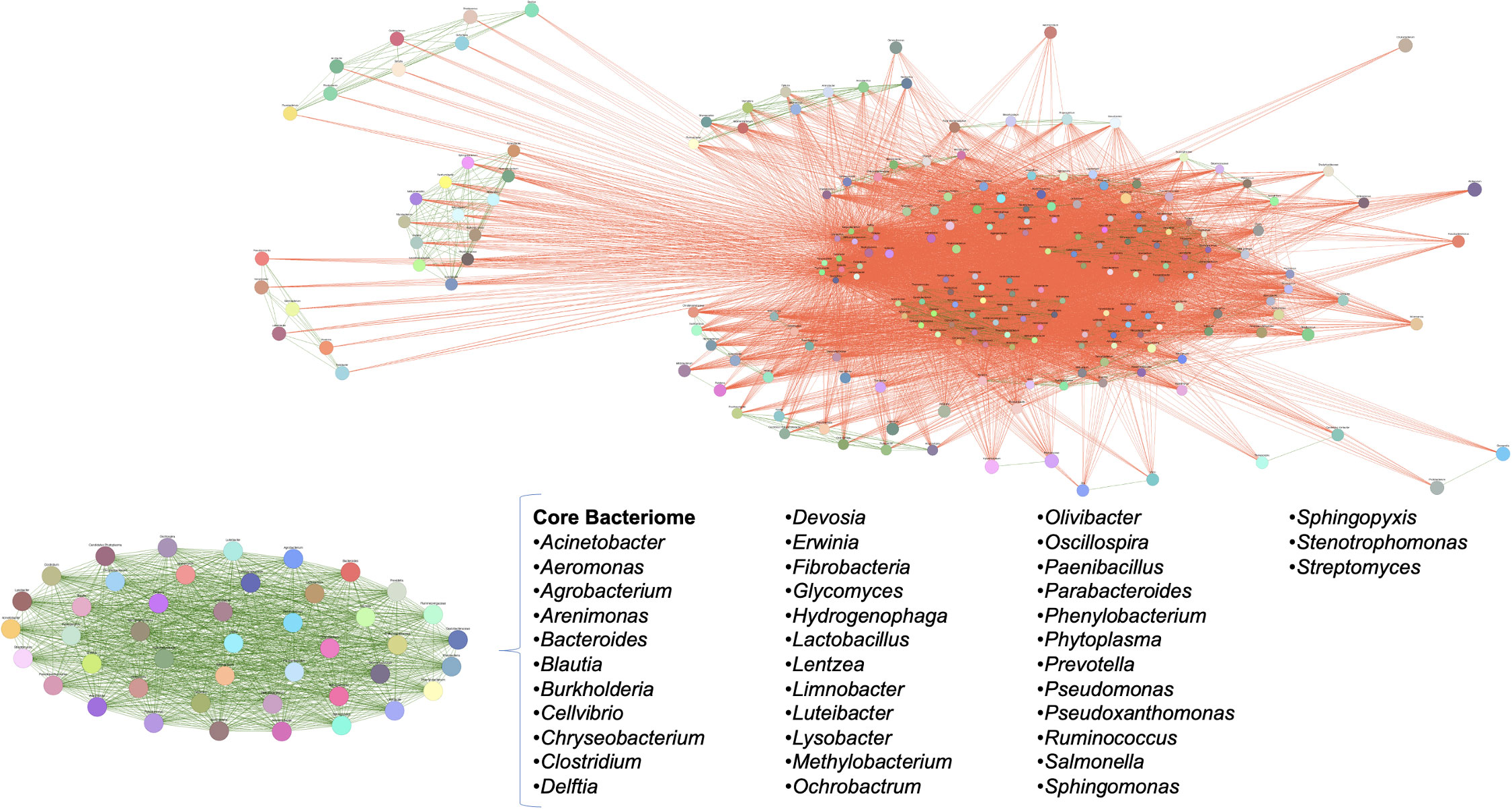
Figure 5 Taxonomic microbial interaction networks of representative individuals in teosinte samples. Co-occurrence diagram where green lines represent a positive correlation of bacterial genera in all analyzed samples, and red lines represent a negative correlation. The size of the circular area indicates the relative abundance of the genera. The core is shown as an oval of green lines, demonstrating that these organisms are present in all samples and can occur among them.
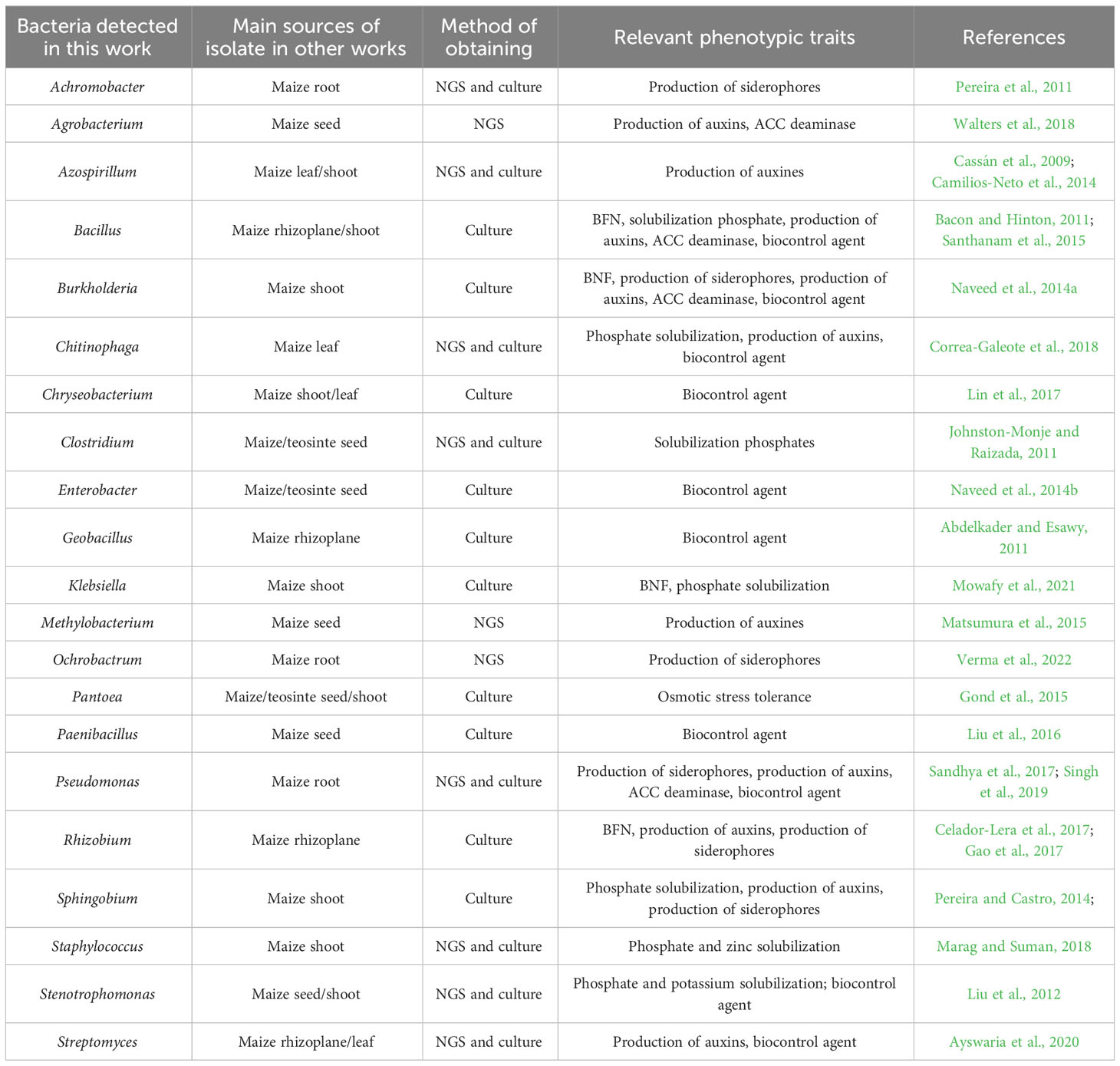
Table 3 Bacterial genera associated to teosinte and maize detected in this work and other previous papers.
3.3 Metabolic inference
The metabolic function profiles of microbial communities in teosinte seeds samples were analyzed using PICRUSt2 software and the TBtools-II v1.108 viewer. Notably, these findings are inferred through metabolic inference analysis; however, they provide valuable insights into the potential functional attributes of the microbial communities associated with different teosinte races focused on PGP and biocontrol traits. NSTI values are among 0.0011-0.0080, where Z. mays subsp. parviglumis (0.0076) and Z. perennis (0.0080) show a higher relative abundance of specific taxonomic groups than the other samples. The results show that Z. diploperennis harbors bacterial communities with a large number of genes related to plant growth promotion, including the carbohydrate phosphotransferase system, amino acids, sugar, and nitrogen metabolism, biosynthesis of plant hormones, proteins for enhancing seed germination and photosynthesis.
Additionally, genes responsible for the biosynthesis of biocontrol molecules, such as biosynthesis of antibiotics, antifungals, and siderophores, and genes related to adaptations to the host environment, such as chemotaxis, motility, protein export, transporters, peroxisomes, protein kinases, and degradation of recalcitrant compounds. In contrast, the Z. mays subsp. mexicana Chalco landrace exhibits the lowest number of genes associated with these traits, as shown in Figure 6.
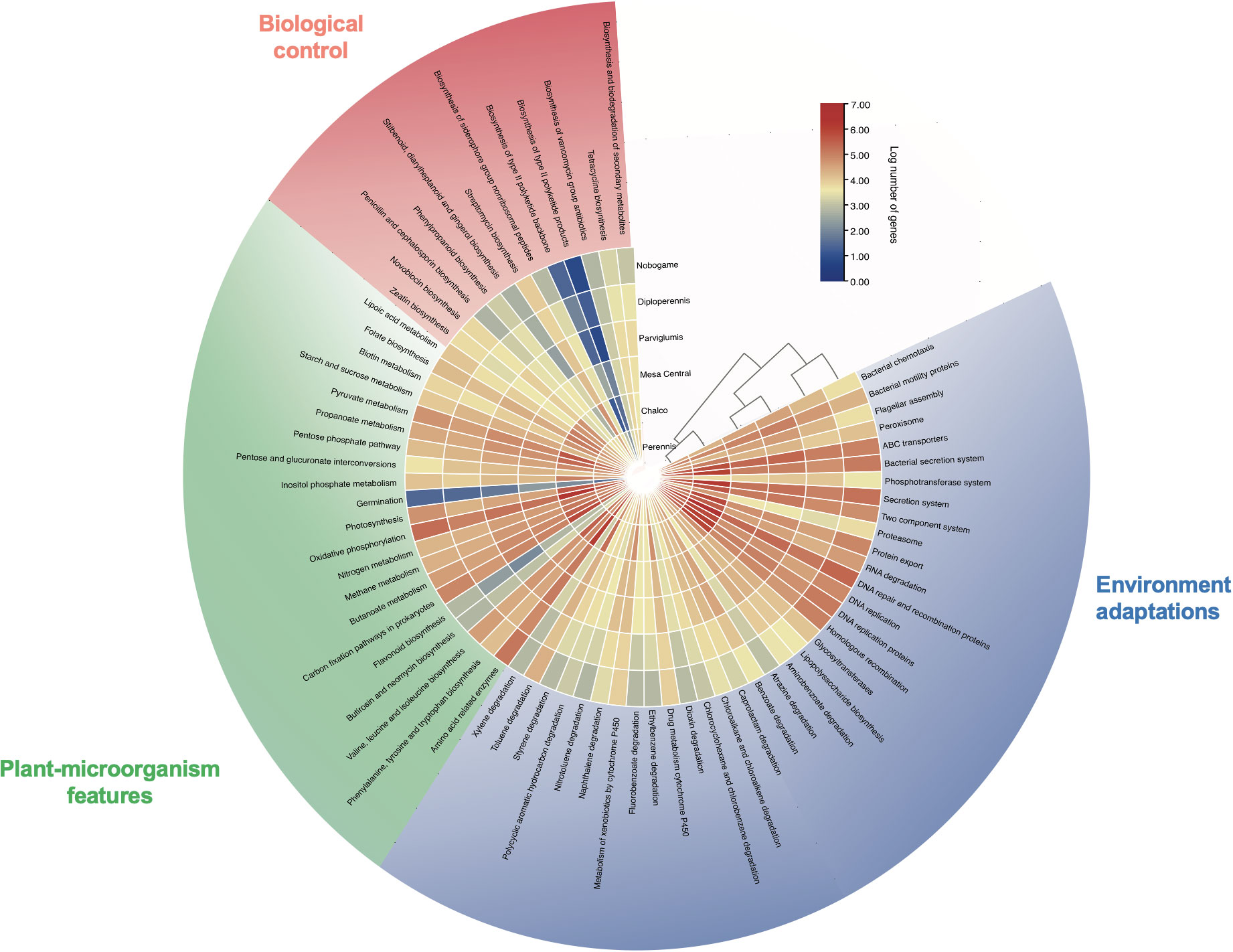
Figure 6 Visualization of relative gene abundance in predicted endophytic bacterial communities of teosinte seeds using a heatmap approach with PICRUSt-inferred genes. Outer semicircle, categories of environmental adaptations; inner semicircle log of the relative number of gene abundances. Some important holobiont features are biological control (pink), plant-microorganism interactions (green) and environmental adaptation (blue). The bar color gradient represents high gene abundances (red), and low (blue) abundances.
4 Discussion
The diversity of bacterial communities associated with the seed of three teosinte species: Z. diploperennis, Z. perennis, and Z. mays subsp. mexicana races Nobogame, Balsas, Mesa Central, and Chalco were explored in this work with culture-independent methods of NGS. Only some works have addressed the issue of bacterial diversity in the teosinte endosphere from the perspective of culture-independent methods. In this sense, previous efforts focused on using bacterial DNA fingerprinting (16S rDNA TRFLP) detected a core bacteriome composed of Clostridium, Paenibacillus, and two other unidentified genera in seeds and stems of three teosinte species (Johnston-Monje & Raizada, 2011). Additionally, 18 bacteria genera were isolated and cultured from the same samples, expanding the core bacteriome of teosinte with members of the Enterobacter, Methylobacterium, Pantoea, and Pseudomonas genres. In contrast, in this work, the core bacteriome detected with NGS includes 38 bacterial genera only in seeds, confirming the presence of all previously detected but adding 36 bacteria genera for the first time. However, although the investigation of bacterial diversity is just the beginning and the comparisons between works carried out with different experimental strategies should be taken carefully, previous research using TRFLP has raised crucial questions regarding maize domestication, evolution, ethnography, geographic migration, and ecology (Johnston-Monje & Raizada, 2011; Johnston-Monje et al., 2014), all legitimate questions that can now be reconsidered with the use of NGS.
Some of the bacterial genera found in this work have been previously described as culturable endophytes in maize and teosinte plants with relevant phenotypic traits for plant-microorganism interaction, plant growth promotion, biological control, and adaptation to the environment (Chowdhury et al., 2019; Mehta et al., 2021; Wallace, 2023). However, many non-cultured bacteria genera no previously associated with teosinte and maize endophytes were also detected in the seed endosphere of teosinte, such as Nitrospira, Scalindua, and Phytoplasma, among others. These bacteria expand the potential of the teosinte microbiome for developing PGPB and biocontrol agents. The work results may be the basis for renewing efforts for isolating bacterial genera and species in specific culture media and ambiental conditions designed for those bacteria that have yet to be isolated in pure cultures.
The dynamic symbiotic relationship of endophytes with the host has essential implications for adaptation, stress tolerance, evolution, and plant domestication (Hardoim et al., 2015). Most of 38 genera of central bacteriome (core) have been recognized as PGPB, and some are also among the most abundant microorganisms found in native landrace maize samples, such as the case of Burkholderia, Methylobacterium, Pseudomonas, Paenibacillus, Clostridium, Stenotrophomonas, Streptomyces, and Luteibacter (Johnston-Monje & Raizada, 2011). As has been suggested in previous works performed with the seeds of other plants, teosinte seeds are also a vast reservoir of microorganisms of evolutionary and biotechnological interest that remain in their host despite geographic and genetic differences (Chen et al., 2018; Hamonts et al., 2018; Koskella and Bergelson, 2020; Kuźniar et al., 2020; Noble et al., 2020; Rodríguez et al., 2020). The accessory bacteriome of teosintes is possibly related to the specialization of the bacteria with their particular host. Depending on the plant host’s sampling moment, geography, or ecology, it could also be a transitory event.
The UniFrac in β-diversity analysis unveiled two clades, one that included Z. mays subsp. mexicana and another to the rest of the species and races. The association between these bacteriomes, phylogenetic proximity, and geographical distribution highlight the significance of plant genotype in influencing microbiome selection and alterations, emphasizing the role of host genetics in shaping the microbial communities associated with these plant species (Yadav et al., 2023).
The phylogeny of Mexican annual teosintes performed with microsatellite analysis recognizes two clusters Zea mays subsp. mexicana and Z. mays subsp. parviglumis on one side; meanwhile, Z. diploperennis, and Z. perennis on the other share an earlier common ancestor (Matsuoka et al., 2002; Fukunaga et al., 2005). This phylogenetic scenario could be related to the greater bacterial diversity associated with earlier teosinte species since a longer available evolutionary time to establish a symbiosis and co-evolve with free-living bacteria than the other races. However, other geographic, ecological, or evolutive scenarios cannot be discarded.
The taxonomic microbial interaction network, constructed using representative bacterial genera from the core bacteriome in teosinte samples, exhibited a complex and extensive structure. These findings indicate that the seeds of teosinte act as a “Noah’s Ark,” which possibly facilitates the vertical transmission of essential symbiotic bacteria for the survival and growth of the subsequent plant generation in new and challenging environments. When considering the assumption that the teosinte seeds originate from diverse conditions, it becomes evident that the microbial interaction network within these seeds is crucial for the plants’ adaptation and resilience. This intricate network of interactions among microbial taxa suggests a cooperative and interdependent relationship between bacteria and their plant host (Li et al., 2019; Verma & White, 2019; Bomfim et al., 2020).
By implementing caution and considering the current state of knowledge, identifying bacteria at the genus level can provide valuable insights into their phenotypic characteristics and their ability to establish symbiotic relationships with plants (Fitzpatrick et al., 2020; Liu et al., 2020; Morella et al., 2020; Trivedi et al., 2020). The outstanding similarity in the diversity and relative abundance of bacterial genera among teosintes indicates the presence of a functional and stable microbiome despite variations in recognized bacterial taxonomy. By conducting a thorough analysis of bacterial diversity and their phenotypic traits, we can better understand the role and symbiotic interactions of these bacterial communities throughout the plants’ life cycle (Berg et al., 2010; Reinhold-Hurek and Hurek, 2011; Sessitsch et al., 2012; Belimov et al., 2015; Khatabi et al., 2019).
The prediction of the functional profiles of teosinte endophytes focuses on three critical components in the plant-microorganism symbiosis: adaptation to the host environment, specific symbiotic activities, and biological control of plant pathogens. The endophyte seed bacteria of teosinte participate in the potential establishment and development of the plant holobiont through the secretion of enzymes that break down complex organic matter, allowing adequate access to nutrients and bacterial motility to enable and facilitate colonization and establishing beneficial interactions and chemical communication systems such as quorum sensing to synchronize bacteria-bacteria interaction and production of phytohormones that lead plant-bacteria communication (Vandana et al., 2021). Also, bacterial genera with a potential capacity for degradation of xenobiotic compounds commonly present in contaminated soils, such as atrazine, xylene, chloroalkanes, and polycyclic aromatic compounds, were detected. These bacteria detoxify the soil, recirculate carbon from generally recalcitrant compounds, and offer the plant an adaptative advantage during colonization and initial growth of plants (Li et al., 2012; Pandey et al., 2013; Thelusmond et al., 2016; Regar et al., 2019; Huang X. et al., 2022).
Besides, the bacterial digestion of starch, sucrose metabolism, biosynthesis of amino acids, phytohormones, and intermediate compounds of vital biochemical cycles are metabolic activities that promote the development of plants from germination to advanced phenological stages (Hunting et al., 2015; Cui et al., 2019; Rehman et al., 2019; Yuan et al., 2021; Mishra et al., 2022). Finally, the biosynthesis of antibiotic compounds such as streptomycin, cephalosporin, tetracycline, polyketides, and non-ribosomal peptides such as siderophores can function as antifungals and protect the seed before and during germination and early growth (Abbas et al., 2022; Huang B. et al., 2022; Yadav et al., 2022), although they could also interfere with the establishment of mutualistic mycorrhizae (Schrey et al., 2012).
The microbiome of other plants highlights the importance of diversity, structure, composition, and core bacteriomes for the production of essential metabolites for ecology and plant-microorganism interaction, as is the example of Salvia miltiorrhiza (Chen et al., 2018), Hordeum vulgare L. (Rahman et al., 2018), Brassica napus (Rybakova et al., 2017), among others.
These efforts help lay the foundations for understanding the specific interactions between plants and microorganisms from an evolutionary and ecological point of view, complementing these studies with more precise tools such as holo-omics sciences (Xu et al., 2021).
The knowledge of the bacterial diversity in the progenitor plants of modern maize can allow us to propose lines of research that will explore the domestication, evolution, ecology, and biogeography of the different races of the plant to the symbiosis-plant microorganism that will allow us to recognize the bacteria that harbor a potential to improve agricultural productivity under more environmentally friendly conditions.
5 Conclusion
The endophytic bacterial diversity of seed teosintes, encompassing Z. diploperennis, Z. perennis, and Z. mays, displays a rich array of dozens of bacterial genera, forming a strict core. In contrast, many others reside in accessory bacteriomes specific to each plant species. Numerous PGB bacterial genera have been identified, alongside several previously unassociated with maize or teosinte. However, it is essential to acknowledge that further experiments are needed to demonstrate the reproducibility of these findings. The results also suggest that teosinte seeds are a reservoir of many important culturable and non-culturable bacteria, potentially microorganisms with exciting properties in plant-microorganism interaction as plant growth promoters or bio-control agents. These results lay the groundwork for future research on the functional role of members of the core bacteriome in symbiosis and their possible biotechnological applications in the intelligent design of bioinoculants. This work is the first step toward defining holobiont, holohabitat, and holoniche as previously defined (Malard and Guisan, 2023).
Data availability statement
The datasets presented in this study can be found in online repositories.The accesion number of the Bioproject in NCBI is PRJNA952205.
Author contributions
ED-L-V-C and JH-G performed the DNA extraction, data analysis, and bioinformatics performances; ED-L-V-C, JH-G, LV-T, and CH-R designed and coordinated the study; ED-L-V-C wrote the first draft manuscript; ED-L-V-C, JH-G, LV-T, and CH-R contributed to the manuscript editing. All authors read and approved the final manuscript.
Funding
This work was supported by the SIP-IPN (SIP-20211032 and SIP-20220795).
Acknowledgments
All authors would like to thank the review service for the English version. Special thanks to Bram Govaerts, Denise Costich, and Christian Zavala Espinosa from the International Maize and Wheat Improvement Center (CIMMyT) for providing the teosinte seeds. ED-L-V-C thanks the National Council of Science and Technology (CONACyT) and BEIFI-IPN for students’ fellowships. JH-G, LV-T, and CH-R are fellows of EDI-IPN, COFFA-IPN, and SNI-CONACyT.
Conflict of interest
The authors declare that the research was conducted in the absence of any commercial or financial relationships that could be construed as a potential conflict of interest.
Publisher’s note
All claims expressed in this article are solely those of the authors and do not necessarily represent those of their affiliated organizations, or those of the publisher, the editors and the reviewers. Any product that may be evaluated in this article, or claim that may be made by its manufacturer, is not guaranteed or endorsed by the publisher.
References
Abbas, A., Duan, J., Abdoulaye, A. H., Fu, Y., Lin, Y., Xie, J., et al. (2022). Deciphering bacterial community of the fallow and paddy soil focusing on possible biocontrol agents. Agronomy 12, 431. doi: 10.3390/agronomy12020431
Abdelkader, A. F., Esawy, M. A. (2011). Case study of a biological control: Geobacillus caldoxylosilyticus (IRD) contributes to alleviate salt stress in maize (Zea mays L.) plants. Acta Physiol. Plant 33, 2289–2299. doi: 10.1007/s11738-011-0769-x
Aboul-Maaty, N., Oraby, H. (2019). Extraction of high-quality genomic DNA from different plant orders applying a modified CTAB-based method. Bull. Natl. Res. Cent. 43, 25. doi: 10.1186/s42269-019-0066-1
Afgan, E., Baker, D., van den Beek, M., Blankenberg, D., Bouvier, D., Čech, M., et al. (2016). The Galaxy platform for accessible, reproducible and collaborative biomedical analyses: 2016 update. Nucleic Acids Res. 44, W3–W10. doi: 10.1093/nar/gkw343
Agnihotry, S., Sarangi, A., Aggarwal, R. (2020). Construction and assessment of a unified curated reference database for improving the taxonomic classification of bacteria using 16S rRNA sequence data. Indian J. Med. Res. 151, 93. doi: 10.4103/ijmr.IJMR_220_18
Andrews, S., Krueger, F., Segonds-Pichon, A., Biggins, L., Krueger, C., Wingett, S. (2010). A quality control tool for high throughput sequence data (Cambridge: Babraham Institute). 370, FastQC.
Ayswaria, R., Vasu, V., Krishna, R. (2020). Diverse endophytic Streptomyces species with dynamic metabolites and their meritorious applications: a critical review. Crit. Rev. Microbiol. 46, 750–758. doi: 10.1080/1040841X.2020.1828816
Bacci, G., Bani, A., Bazzicalupo, M., Ceccherini, M. T., Galardini, M., Nannipieri, P., et al. (2015). Evaluation of the performances of Ribosomal Database Project (RDP) Classifier for taxonomic assignment of 16S rRNA metabarcoding sequences generated from Illumina-Solexa NGS. J. Genomics 3, 36–39. doi: 10.7150/jgen.9204
Bacon, C. W., Hinton, D. M. (2011). “Bacillus mojavensis: Its Endophytic Nature, the Surfactins, and Their Role in the Plant Response to Infection by Fusarium verticillioides,” in Bacteria in Agrobiology: Plant Growth Responses. Ed. Maheshwari, D. K. (Berlin, Heidelberg: Springer Berlin Heidelberg), 21–39. doi: 10.1007/978-3-642-20332-9_2
Bağcı, C., Beier, S., Górska, A., Huson, D. H. (2019). “Introduction to the analysis of environmental sequences: Metagenomics with MEGAN,” in Evolutionary Genomics Methods in Molecular Biology. Ed. Anisimova, M. (New York, NY: Springer New York), 591–604. doi: 10.1007/978-1-4939-9074-0_19
Belimov, A. A., Puhalsky, I. V., Safronova, V. I., Shaposhnikov, A. I., Vishnyakova, M. A., Semenova, E. V., et al. (2015). Role of plant genotype and soil conditions in symbiotic plant-microbe interactions for adaptation of plants to cadmium-polluted soils. Water Air Soil pollut. 226, 264. doi: 10.1007/s11270-015-2537-9
Berg, G., Egamberdieva, D., Lugtenberg, B., Hagemann, M. (2010). “Symbiotic plant–microbe interactions: stress protection, plant growth promotion, and biocontrol by stenotrophomonas,” in Symbioses and Stress Cellular Origin, Life in Extreme Habitats and Astrobiology. Eds. Seckbach, J., Grube, M. (Dordrecht: Springer Netherlands), 445–460. doi: 10.1007/978-90-481-9449-0_22
Bernabeu, P. R., García, S. S., López, A. C., Vio, S. A., Carrasco, N., Boiardi, J. L., et al. (2018). Assessment of bacterial inoculant formulated with Paraburkholderia tropica to enhance wheat productivity. World J. Microbiol. Biotechnol. 34, 81. doi: 10.1007/s11274-018-2461-4
Bolyen, E., Rideout, J. R., Dillon, M. R., Bokulich, N. A., Abnet, C. C., Al-Ghalith, G. A., et al. (2019). Reproducible, interactive, scalable and extensible microbiome data science using QIIME 2. Nat. Biotechnol. 37, 852–857. doi: 10.1038/s41587-019-0209-9
Bomfim, C. S. G., da Silva, V. B., Cursino, L. H. S., Mattos, W., da, S., Santos, J. C. S., et al. (2020). Endophytic bacteria naturally inhabiting commercial maize seeds occupy different niches and are efficient plant growth-promoting agents. Symbiosis 81, 255–269. doi: 10.1007/s13199-020-00701-z
Caballero-Mellado, J., Martínez-Aguilar, L., Paredes-Valdez, G., Santos, P.E.l. (2004). Burkholderia unamae sp. nov., an N2-fixing rhizospheric and endophytic species. Int. J. Syst. Evol. Microbiol. 54, 1165–1172. doi: 10.1099/ijs.0.02951-0
Callahan, B. J., Sankaran, K., Fukuyama, J. A., McMurdie, P. J., Holmes, S. P. (2016). Bioconductor workflow for microbiome data analysis: from raw reads to community analyses. F1000Res 5, 1492. doi: 10.12688/f1000research.8986.2
Camilios-Neto, D., Bonato, P., Wassem, R., Tadra-Sfeir, M. Z., Brusamarello-Santos, L. C., Valdameri, G., et al. (2014). Dual RNA-seq transcriptional analysis of wheat roots colonized by Azospirillum brasilense reveals up-regulation of nutrient acquisition and cell cycle genes. BMC Genomics 15, 378. doi: 10.1186/1471-2164-15-378
Cassán, F., Maiale, S., Masciarelli, O., Vidal, A., Luna, V., Ruiz, O. (2009). Cadaverine production by Azospirillum brasilense and its possible role in plant growth promotion and osmotic stress mitigation. Eur. J. Soil Biol. 45, 12–19. doi: 10.1016/j.ejsobi.2008.08.003
Celador-Lera, L., Menéndez, E., Peix, A., Igual, J. M., Velázquez, E., Rivas, R. (2017). Rhizobium zeae sp. nov., isolated from maize (Zea mays L.) roots. Int. J. Syst. Evol. Microbiol. 67, 2306–2311. doi: 10.1099/ijsem.0.001944
Chao, A. (1984). Nonparametric estimation of the number of classes in a population. Scand. J. Stat. 11, 265–270. doi: 10.4236/ojdm.2016.62006
Chen, C., Chen, H., Zhang, Y., Thomas, H. R., Frank, M. H., He, Y., et al. (2020). TBtools: An integrative toolkit developed for interactive analyses of big biological data. Mol. Plant 13, 1194–1202. doi: 10.1016/j.molp.2020.06.009
Chen, H., Wu, H., Yan, B., Zhao, H., Liu, F., Zhang, H., et al. (2018). Core microbiome of medicinal plant Salvia miltiorrhiza seed: A rich reservoir of beneficial microbes for secondary metabolism? IJMS 19, 672. doi: 10.3390/ijms19030672
Chowdhury, S., Lata, R., Kharwar, R. N., Gond, S. K. (2019). “Microbial Endophytes of maize seeds and their application in crop improvements,” in Seed Endophytes. Eds. Verma, S. K., White, J. F. (Cham: Springer International Publishing), 449–463. doi: 10.1007/978-3-030-10504-4_21
Correa-Galeote, D., Bedmar, E. J., Arone, G. J. (2018). Maize endophytic bacterial diversity as affected by soil cultivation history. Front. Microbiol. 9. doi: 10.3389/fmicb.2018.00484
Cui, E., Fan, X., Li, Z., Liu, Y., Neal, A. L., Hu, C., et al. (2019). Variations in soil and plant-microbiome composition with different quality irrigation waters and biochar supplementation. Appl. Soil Ecol. 142, 99–109. doi: 10.1016/j.apsoil.2019.04.026
Dastogeer, K. M. G., Tumpa, F. H., Sultana, A., Akter, M. A., Chakraborty, A. (2020). Plant microbiome–an account of the factors that shape community composition and diversity. Curr. Plant Biol. 23, 100161. doi: 10.1016/j.cpb.2020.100161
De Mandal, S., Sonali, Singh, S., Hussain, K., Hussain, T. (2021). “Plant–microbe association for mutual benefits for plant growth and soil health,” in Current Trends in Microbial Biotechnology for Sustainable Agriculture. Eds. Yadav, A. N., Singh, J., Singh, C., Yadav, N. (Singapore: Springer Singapore), 95–121. Book Series: Environmental and Microbial Biotechnology. doi: 10.1007/978-981-15-6949-4_5
Doebley, J. (2004). The genetics of maize evolution. Annu. Rev. Genet. 38, 37–59. doi: 10.1146/annurev.genet.38.072902.092425
Douglas, G. M., Maffei, V. J., Zaneveld, J. R., Yurgel, S. N., Brown, J. R., Taylor, C. M., et al. (2020). PICRUSt2 for prediction of metagenome functions. Nat. Biotechnol. 38, 685–688. doi: 10.1038/s41587-020-0548-6
Faith, D. P., Baker, A. M. (2006). Phylogenetic diversity (PD) and biodiversity conservation: some bioinformatics challenges. Evol. Bioinform. Online 2, 121–128. doi: 10.1177/117693430600200007
Fitzpatrick, C. R., Salas-González, I., Conway, J. M., Finkel, O. M., Gilbert, S., Russ, D., et al. (2020). The plant microbiome: From ecology to reductionism and beyond. Annu. Rev. Microbiol. 74, 81–100. doi: 10.1146/annurev-micro-022620-014327
Frey-Klett, P., Burlinson, P., Deveau, A., Barret, M., Tarkka, M., Sarniguet, A. (2011). Bacterial-fungal interactions: Hyphens between agricultural, clinical, environmental, and food microbiologists. Microbiol. Mol. Biol. Rev. 75, 583–609. doi: 10.1128/MMBR.00020-11
Fukunaga, K., Hill, J., Vigouroux, Y., Matsuoka, Y., Sanchez, G. J., Liu, K., et al. (2005). Genetic diversity and population structure of teosinte. Genetics 169, 2241–2254. doi: 10.1534/genetics.104.031393
Gao, J., Sun, P., Wang, X., Lv, F., Mao, X., Sun, J. (2017). Rhizobium wenxiniae sp. nov., an endophytic bacterium isolated from maize root. Int. J. Syst. Evol. Microbiol. 67, 2798–2803. doi: 10.1099/ijsem.0.002025
Gherbi, H., Markmann, K., Svistoonoff, S., Estevan, J., Autran, D., Giczey, G., et al. (2008). SymRK defines a common genetic basis for plant root endosymbioses with arbuscular mycorrhiza fungi, rhizobia, and Frankia bacteria. Proc. Natl. Acad. Sci. U.S.A. 105, 4928–4932. doi: 10.1073/pnas.0710618105
Gond, S. K., Torres, M. S., Bergen, M. S., Helsel, Z., White, J. F. (2015). Induction of salt tolerance and up-regulation of aquaporin genes in tropical corn by rhizobacterium Pantoea agglomerans. Lett. Appl. Microbiol. 60, 392–399. doi: 10.1111/lam.12385
Good, I. J. (1953). The population frequencies of species and the estimation of population parameters. Biometrika 40, 237–264. doi: 10.1093/biomet/40.3-4.237
Hammer, Ø., Harper, D. A., Paul, D. R. (2001). Past: Paleontological statistics software package for education and data analysis. Palaeontol. Electron. 4, 4.
Hamonts, K., Trivedi, P., Garg, A., Janitz, C., Grinyer, J., Holford, P., et al. (2018). Field study reveals core plant microbiota and relative importance of their drivers. Environ. Microbiol. 20, 124–140. doi: 10.1111/1462-2920.14031
Hardoim, P. R., van Overbeek, L. S., Berg, G., Pirttilä, A. M., Compant, S., Campisano, A., et al. (2015). The hidden world within plants: Ecological and evolutionary considerations for defining functioning of microbial endophytes. Microbiol. Mol. Biol. Rev. 79, 293–320. doi: 10.1128/MMBR.00050-14
Huang, B., Wang, J., Han, X., Gou, J., Pei, Z., Lu, G., et al. (2022). The relationship between material transformation, microbial community and amino acids and alkaloid metabolites in the mushroom residue-prickly ash seed oil meal composting with biocontrol agent addition. Bioresour. Technol. 350, 126913. doi: 10.1016/j.biortech.2022.126913
Huang, X., Yang, X., Lin, J., Franks, A. E., Cheng, J., Zhu, Y., et al. (2022). Biochar alleviated the toxicity of atrazine to soybeans, as revealed by soil microbial community and the assembly process. Sci. Total Environ. 834, 155261. doi: 10.1016/j.scitotenv.2022.155261
Hunting, E. R., Vijver, M. G., van der Geest, H. G., Mulder, C., Kraak, M. H. S., Breure, A. M., et al. (2015). Resource niche overlap promotes stability of bacterial community metabolism in experimental microcosms. Front. Microbiol. 6. doi: 10.3389/fmicb.2015.00105
Johnston-Monje, D., Mousa, W. K., Lazarovits, G., Raizada, M. N. (2014). Impact of swapping soils on the endophytic bacterial communities of pre-domesticated, ancient and modern maize. BMC Plant Biol. 14, 233. doi: 10.1186/s12870-014-0233-3
Johnston-Monje, D., Raizada, M. N. (2011). Conservation and diversity of seed associated endophytes in Zea across boundaries of evolution, ethnography and ecology. PloS One 6, e20396. doi: 10.1371/journal.pone.0020396
Kaur, T., Devi, R., Kour, D., Yadav, A., Yadav, A. N., Dikilitas, M., et al. (2021). Plant growth promoting soil microbiomes and their potential implications for agricultural and environmental sustainability. Biologia 76, 2687–2709. doi: 10.1007/s11756-021-00806-w
Khatabi, B., Gharechahi, J., Ghaffari, M. R., Liu, D., Haynes, P. A., McKay, M. J., et al. (2019). Plant–microbe symbiosis: what has proteomics taught us? Proteomics 19, 1800105. doi: 10.1002/pmic.201800105
Kõljalg, U., Nilsson, H. R., Schigel, D., Tedersoo, L., Larsson, K.-H., May, T. W., et al. (2020). The taxon hypothesis paradigm—on the unambiguous detection and communication of taxa. Microorganisms 8, 1910. doi: 10.3390/microorganisms8121910
Koskella, B., Bergelson, J. (2020). The study of host–microbiome (co)evolution across levels of selection. Phil. Trans. R. Soc B 375, 20190604. doi: 10.1098/rstb.2019.0604
Kuramae, E. E., Derksen, S., Schlemper, T. R., Dimitrov, M. R., Costa, O. Y. A., Silveira, A. P. D. D. (2020). Sorghum growth promotion by Paraburkholderia tropica and Herbaspirillum frisingense: Putative mechanisms revealed by genomics and metagenomics. Microorganisms 8, 725. doi: 10.3390/microorganisms8050725
Kuźniar, A., Włodarczyk, K., Grządziel, J., Goraj, W., Gałązka, A., Wolińska, A. (2020). Culture-independent analysis of an endophytic core microbiome in two species of wheat: Triticum aestivum L. (cv. ‘Hondia’) and the first report of microbiota in Triticum spelta L. (cv. ‘Rokosz’). Syst. Appl. Microbiol. 43, 126025. doi: 10.1016/j.syapm.2019.126025
Langille, M. G. I., Zaneveld, J., Caporaso, J. G., McDonald, D., Knights, D., Reyes, J. A., et al. (2013). Predictive functional profiling of microbial communities using 16S rRNA marker gene sequences. Nat. Biotechnol. 31, 814–821. doi: 10.1038/nbt.2676
Lawley, B., Tannock, G. W. (2017). Analysis of 16S rRNA gene amplicon sequences using the QIIME software package. Oral. Biol. Methods Mol. Biol., 153–163. doi: 10.1007/978-1-4939-6685-1_9
Li, H., Parmar, S., Sharma, V. K., White, J. F. (2019). “Seed endophytes and their potential applications,” in Seed Endophytes. Eds. Verma, S. K., White, J. F. (Cham: Springer International Publishing), 35–54. doi: 10.1007/978-3-030-10504-4_3
Li, H. Y., Wei, D. Q., Shen, M., Zhou, Z. P. (2012). Endophytes and their role in phytoremediation. Fungal Divers. 54, 11–18. doi: 10.1007/s13225-012-0165-x
Lin, S. Y., Hameed, A., Liu, Y. C., Hsu, Y. H., Hsieh, Y. T., Lai, W. A., et al. (2017). Chryseobacterium endophyticum sp. nov., isolated from a maize leaf. Int. J. Syst. Evol. Microbiol. 67, 570–575. doi: 10.1099/ijsem.0.001656
Liu, H., Brettell, L. E., Singh, B. (2020). Linking the phyllosphere microbiome to plant health. Trends Plant Sci. 25, 841–844. doi: 10.1016/j.tplants.2020.06.003
Liu, Y., Wang, R., Cao, Y., Chen, C., Bai, F., Xu, T., et al. (2016). Identification and antagonistic activity of endophytic bacterial strain Paenibacillus sp. 5 L8 isolated from the seeds of maize (Zea mays L., Jingke 968). Ann. Microbiol. 66, 653–660. doi: 10.1007/s13213-015-1150-x
Liu, Y., Zuo, S., Xu, L., Zou, Y., Song, W. (2012). Study on diversity of endophytic bacterial communities in seeds of hybrid maize and their parental lines. Arch. Microbiol. 194, 1001–1012. doi: 10.1007/s00203-012-0836-8
Lozupone, C., Lladser, M. E., Knights, D., Stombaugh, J., Knight, R. (2011). UniFrac: an effective distance metric for microbial community comparison. ISME J. 5, 169–172. doi: 10.1038/ismej.2010.133
Malard, L. A., Guisan, A. (2023). Into the microbial niche. Trends Ecol. Evol. 38, 936–945. doi: 10.1016/j.tree.2023.04.015
Marag, P. S., Suman, A. (2018). Growth stage and tissue specific colonization of endophytic bacteria having plant growth promoting traits in hybrid and composite maize (Zea mays L.). Microbiol. Res. 214, 101–113. doi: 10.1016/j.micres.2018.05.016
Marco, S., Loredana, M., Riccardo, V., Raffaella, B., Walter, C., Luca, N. (2022). Microbe-assisted crop improvement: a sustainable weapon to restore holobiont functionality and resilience. Hortic. Res. 9, 160. doi: 10.1093/hr/uhac160
Matsumura, E. E., Secco, V. A., Moreira, R. S., dos Santos, O. J. A. P., Hungria, M., de Oliveira, A. L. M. (2015). Composition and activity of endophytic bacterial communities in field-grown maize plants inoculated with Azospirillum brasilense. Ann. Microbiol. 65, 2187–2200. doi: 10.1007/s13213-015-1059-4
Matsuoka, Y., Vigouroux, Y., Goodman, M. M., Sanchez G., J., Buckler, E., Doebley, J. (2002). A single domestication for maize shown by multilocus microsatellite genotyping. Proc. Natl. Acad. Sci. U.S.A. 99, 6080–6084. doi: 10.1073/pnas.052125199
Mehta, S., Singh, B., Patra, A., Tripathi, A., Easwaran, M., Choudhary, J. R., et al. (2021). “Maize microbiome: current insights for the sustainable agriculture,” in Microbiomes and Plant Health (Elsevier), 267–297. doi: 10.1016/B978-0-12-819715-8.00009-4. Available at: https://linkinghub.elsevier.com/retrieve/pii/B9780128197158000094.
Milla, R., Osborne, C. P., Turcotte, M. M., Violle, C. (2015). Plant domestication through an ecological lens. Trends Ecol. Evol. 30, 463–469. doi: 10.1016/j.tree.2015.06.006
Mishra, S., Singh, A., Keswani, C., Saxena, A., Sarma, B. K., Singh, H. B. (2015). “Harnessing plant-microbe interactions for enhanced protection against phytopathogens,” in Plant Microbes Symbiosis: Applied Facets. Ed. Arora, N. K. (New Delhi: Springer India), 111–125. doi: 10.1007/978-81-322-2068-8_5
Mishra, A. K., Sudalaimuthuasari, N., Hazzouri, K. M., Saeed, E. E., Shah, I., Amiri, K. M. A. (2022). Tapping into plant–microbiome interactions through the lens of multi-omics techniques. Cells 11, 3254. doi: 10.3390/cells11203254
Miyambo, T., Makhalanyane, T. P., Cowan, D. A., Valverde, A. (2016). Plants of the fynbos biome harbour host species-specific bacterial communities. FEMS Microbiol. Lett. 363, 22. doi: 10.1093/femsle/fnw122
Morella, N. M., Weng, F. C. H., Joubert, P. M., Metcalf, C. J. E., Lindow, S., Koskella, B. (2020). Successive passaging of a plant-associated microbiome reveals robust habitat and host genotype-dependent selection. Proc. Natl. Acad. Sci. U.S.A. 117, 1148–1159. doi: 10.1073/pnas.1908600116
Mousa, W. K., Shearer, C. R., Limay-Rios, V., Zhou, T., Raizada, M. N. (2015). Bacterial endophytes from wild maize suppress Fusarium graminearum in modern maize and inhibit mycotoxin accumulation. Front. Plant Sci. 6. doi: 10.3389/fpls.2015.00805
Mowafy, A. M., Fawzy, M. M., Gebreil, A., Elsayed, A. (2021). Endophytic Bacillus, Enterobacter, and Klebsiella enhance the growth and yield of maize. Acta Agric. Scand. B Soil Plant Sci. 71, 237–246. doi: 10.1080/09064710.2021.1880621
Naveed, M., Mitter, B., Reichenauer, T. G., Wieczorek, K., Sessitsch, A. (2014a). Increased drought stress resilience of maize through endophytic colonization by Burkholderia phytofirmans PsJN and Enterobacter sp. FD17. Environ. Exp. Bot. 97, 30–39. doi: 10.1016/j.envexpbot.2013.09.014
Naveed, M., Mitter, B., Yousaf, S., Pastar, M., Afzal, M., Sessitsch, A. (2014b). The endophyte Enterobacter sp. FD17: a maize growth enhancer selected based on rigorous testing of plant beneficial traits and colonization characteristics. Biol. Fertil. Soils 50, 249–262. doi: 10.1007/s00374-013-0854-y
Noble, A. S., Noe, S., Clearwater, M. J., Lee, C. K. (2020). A core phyllosphere microbiome exists across distant populations of a tree species indigenous to New Zealand. PloS One 15, e0237079. doi: 10.1371/journal.pone.0237079
Pandey, S., Ghosh, P. K., Ghosh, S., De, T. K., Maiti, T. K. (2013). Role of heavy metal resistant Ochrobactrum sp. and Bacillus spp. strains in bioremediation of a rice cultivar and their PGPR like activities. J. Microbiol. 51, 11–17. doi: 10.1007/s12275-013-2330-7
Pereira, S. I. A., Castro, P. M. L. (2014). Diversity and characterization of culturable bacterial endophytes from Zea mays and their potential as plant growth-promoting agents in metal-degraded soils. Environ. Sci. pollut. Res. 21, 14110–14123. doi: 10.1007/s11356-014-3309-6
Pereira, P., Ibáñez, F., Rosenblueth, M., Etcheverry, M., Martínez-Romero, E. (2011). Analysis of the Bacterial Diversity Associated with the Roots of Maize (Zea mays L.) through Culture-Dependent and Culture-Independent Methods. ISRN Ecol. 2011, 1–10. doi: 10.5402/2011/938546
Piperno, D. R., Ranere, A. J., Holst, I., Iriarte, J., Dickau, R. (2009). Starch grain and phytolith evidence for early ninth millennium B.P. maize from the Central Balsas River Valley, Mexico. Proc. Natl. Acad. Sci. U.S.A. 106, 5019–5024. doi: 10.1073/pnas.0812525106
Purugganan, M. D. (2019). Evolutionary insights into the nature of plant domestication. Curr. Biol. 29, R705–R714. doi: 10.1016/j.cub.2019.05.053
Rahman, M. M., Flory, E., Koyro, H.-W., Abideen, Z., Schikora, A., Suarez, C., et al. (2018). Consistent associations with beneficial bacteria in the seed endosphere of barley (Hordeum vulgare L.). Syst. Appl. Microbiol. 41, 386–398. doi: 10.1016/j.syapm.2018.02.003
Regar, R. K., Gaur, V. K., Bajaj, A., Tambat, S., Manickam, N. (2019). Comparative microbiome analysis of two different long-term pesticide contaminated soils revealed the anthropogenic influence on functional potential of microbial communities. Sci. Total Environ. 681, 413–423. doi: 10.1016/j.scitotenv.2019.05.090
Rehman, A., Ijaz, M., Mazhar, K., Ul-Allah, S., Ali, Q. (2019). “Metagenomic approach in relation to microbe–microbe and plant–microbiome interactions,” in Microbiome in Plant Health and Disease. Eds. Kumar, V., Prasad, R., Kumar, M., Choudhary, D. K. (Singapore: Springer Singapore), 507–534. doi: 10.1007/978-981-13-8495-0_22
Reinhold-Hurek, B., Hurek, T. (2011). Living inside plants: Bacterial endophytes. Curr. Op. Plant Biol. 14, 435–443. doi: 10.1016/j.pbi.2011.04.004
Reis, V. M., Santos, P.E.l., Tenorio-Salgado, S., Vogel, J., Stoffels, M., Guyon, S., et al. (2004). Burkholderia tropica sp. nov., a novel nitrogen-fixing, plant-associated bacterium. Int. J. Syst. Evol. Microbiol. 54, 2155–2162. doi: 10.1099/ijs.0.02879-0
Rodríguez, C. E., Antonielli, L., Mitter, B., Trognitz, F., Sessitsch, A. (2020). Heritability and functional importance of the Setaria viridis bacterial seed microbiome. Phytobiomes J. 4, 40–52. doi: 10.1094/PBIOMES-04-19-0023-R
Rosselló-Móra, R., Trujillo, M. E., Sutcliffe, I. C. (2017). Introducing a digital protologue: A timely move towards a database-driven systematics of Archaea and bacteria. Syst. Appl. Microbiol. 40, 121–122. doi: 10.1016/j.syapm.2017.02.001
Rybakova, D., Mancinelli, R., Wikström, M., Birch-Jensen, A.-S., Postma, J., Ehlers, R.-U., et al. (2017). The structure of the Brassica napus seed microbiome is cultivar-dependent and affects the interactions of symbionts and pathogens. Microbiome 5, 104. doi: 10.1186/s40168-017-0310-6
Sahoo, S., Adhikari, S., Joshi, A., Singh, N. K. (2021). Use of wild progenitor teosinte in Maize (Zea mays subsp. mays) improvement: Present status and future prospects. Trop. Plant Biol. 14, 156–179. doi: 10.1007/s12042-021-09288-1
Sandhya, V., Shrivastava, M., Ali, S., Sai Shiva Krishna Prasad, V. (2017). Endophytes from maize with plant growth promotion and biocontrol activity under drought stress. Russ. Agricult. Sci. 43, 22–34. doi: 10.3103/S1068367417010165
Santhanam, R., Luu, V. T., Weinhold, A., Goldberg, J., Oh, Y., Baldwin, I. T. (2015). Native root-associated bacteria rescue a plant from a sudden-wilt disease that emerged during continuous cropping. Proc. Natl. Acad. Sci. U.S.A. 112, E5013–E5020. doi: 10.1073/pnas.1505765112
Santoyo, G., Hernández-Pacheco, C., Hernández-Salmerón, J., Hernández-León, R. (2017). The role of abiotic factors modulating the plant-microbe-soil interactions: toward sustainable agriculture. A review. Span. J. Agric. Res. 15, e03R01. doi: 10.5424/sjar/2017151-9990
Schlemper, T. R., Dimitrov, M. R., Silva Gutierrez, F. A. O., van Veen, J. A., Silveira, A. P. D., Kuramae, E. E. (2018). Effect of Burkholderia tropica and Herbaspirillum frisingense strains on sorghum growth is plant genotype dependent. Peer J 6, e5346. doi: 10.7717/peerj.5346
Schrey, S. D., Erkenbrack, E., Früh, E., Fengler, S., Hommel, K., Horlacher, N., et al. (2012). Production of fungal and bacterial growth modulating secondary metabolites is widespread among mycorrhiza-associated streptomycetes. BMC Microbiol. 12, 164. doi: 10.1186/1471-2180-12-164
Sessitsch, A., Hardoim, P., Döring, J., Weilharter, A., Krause, A., Woyke, T., et al. (2012). Functional characteristics of an endophyte community colonizing rice roots as revealed by metagenomic analysis. IS-MPMI 25, 28–36. doi: 10.1094/MPMI-08-11-0204
Shannon, C. E. (1948). A mathematical theory of communication. Bell Syst. Techn. J. 27, 379–423. doi: 10.1002/j.1538-7305.1948.tb01338.x
Simon, J. C., Marchesi, J. R., Mougel, C., Selosse, M. A. (2019). Host-microbiota interactions: from holobiont theory to analysis. Microbiome 7, 5. doi: 10.1186/s40168-019-0619-4
Singh, S., Singh, U. B., Trivedi, M., Sahu, P. K., Paul, S., Paul, D., et al. (2019). Seed Biopriming with Salt-Tolerant Endophytic Pseudomonas geniculata-Modulated Biochemical Responses Provide Ecological Fitness in Maize (Zea mays L.) Grown in Saline Sodic Soil. IJERPH 17, 253. doi: 10.3390/ijerph17010253
Smith, J. S. C., Goodman, M. M., Lester, R. N. (1981). Variation within teosinte. I. Numerical analysis of morphological data. Econ. Bot. 35, 187–203. doi: 10.1007/BF02858686
Tenorio-Salgado, S., Tinoco, R., Vazquez-Duhalt, R., Caballero-Mellado, J., Perez-Rueda, E. (2013). Identification of volatile compounds produced by the bacterium Burkholderia tropica that inhibit the growth of fungal pathogens. Bioengineered 4, 236–243. doi: 10.4161/bioe.23808
Thelusmond, J. R., Strathmann, T. J., Cupples, A. M. (2016). The identification of carbamazepine biodegrading phylotypes and phylotypes sensitive to carbamazepine exposure in two soil microbial communities. Sci. Total Environ. 571, 1241–1252. doi: 10.1016/j.scitotenv.2016.07.154
Trivedi, P., Leach, J. E., Tringe, S. G., Sa, T., Singh, B. K. (2020). Plant–microbiome interactions: from community assembly to plant health. Nat. Rev. Microbiol. 18, 607–621. doi: 10.1038/s41579-020-0412-1
Vandana, U. K., Rajkumari, J., Singha, L. P., Satish, L., Alavilli, H., Sudheer, P. D. V. N., et al. (2021). The endophytic microbiome as a hotspot of synergistic interactions, with prospects of plant growth promotion. Biology 10, 101. doi: 10.3390/biology10020101
van der Heijden, M. G. A., Hartmann, M. (2016). Networking in the plant microbiome. PloS Biol. 14. doi: 10.1371/journal.pbio.1002378
Verma, S., Kumar, M., Kumar, A., Das, S., Chakdar, H., Varma, A., et al. (2022). Diversity of bacterial endophytes of maize (Zea mays) and their functional potential for micronutrient biofortification. Curr. Microbiol. 79, 6. doi: 10.1007/s00284-021-02702-7
Verma, S. K., White, J. F., Jr (2019). Seed Endophytes: Biology and Biotechnology (Cham: Springer International Publishing). doi: 10.1007/978-3-030-10504-4
Vio, S. A., Bernabeu, P. R., García, S. S., Galar, M. L., Luna, M. F. (2022). Tracking and plant growth-promoting effect of Paraburkholderia tropica MTo-293 applied to Solanum lycopersicum. J. Basic Microbiol. 62, 875–886. doi: 10.1002/jobm.202100628
Wallace, J. G. (2023). Maize seed endophytes. Mol. Plant Pathol. 24, 801–810. doi: 10.1111/mpp.13278
Walters, W. A., Jin, Z., Youngblut, N., Wallace, J. G., Sutter, J., Zhang, W., et al. (2018). Large-scale replicated field study of maize rhizosphere identifies heritable microbes. Proc. Natl. Acad. Sci. U.S.A. 115, 7368–7373. doi: 10.1073/pnas.1800918115
Xu, L., Pierroz, G., Wipf, H. M.-L., Gao, C., Taylor, J. W., Lemaux, P. G., et al. (2021). Holo-omics for deciphering plant-microbiome interactions. Microbiome 9, 69. doi: 10.1186/s40168-021-01014-z
Yadav, U., Bano, N., Bag, S., Srivastava, S., Singh, P. C. (2022). An insight into the endophytic bacterial community of tomato after spray application of propiconazole and Bacillus subtilis strain NBRI-W9. Microbiol. Spectr. 10, e01186–e01122. doi: 10.1128/spectrum.01186-22
Yadav, P., Quattrone, A., Yang, Y., Owens, J., Kiat, R., Kuppusamy, T., et al. (2023). Zea mays genotype influences microbial and viral rhizobiome community structure. bioRxiv . doi: 10.1101/2023.06.09.544353
Yang, Y., Wang, N., Guo, X., Zhang, Y., Ye, B. (2017). Comparative analysis of bacterial community structure in the rhizosphere of maize by high-throughput pyrosequencing. PloS One 12, e0178425. doi: 10.1371/journal.pone.0178425
Keywords: teosinte, massive sequencing, next generation sequencing (NGS), maize, bacteriome
Citation: De-la-Vega-Camarillo E, Hernández-García JA, Villa-Tanaca L and Hernández-Rodríguez C (2023) Unlocking the hidden potential of Mexican teosinte seeds: revealing plant growth-promoting bacterial and fungal biocontrol agents. Front. Plant Sci. 14:1247814. doi: 10.3389/fpls.2023.1247814
Received: 26 June 2023; Accepted: 15 September 2023;
Published: 04 October 2023.
Edited by:
Domingo Martinez-Soto, Center for Scientific Research and Higher Education in Ensenada (CICESE), MexicoReviewed by:
Houlin Yu, University of Massachusetts Amherst, United StatesLuis Jesús Castillo Pérez, Autonomous University of San Luis Potosí, Mexico
Copyright © 2023 De-la-Vega-Camarillo, Hernández-García, Villa-Tanaca and Hernández-Rodríguez. This is an open-access article distributed under the terms of the Creative Commons Attribution License (CC BY). The use, distribution or reproduction in other forums is permitted, provided the original author(s) and the copyright owner(s) are credited and that the original publication in this journal is cited, in accordance with accepted academic practice. No use, distribution or reproduction is permitted which does not comply with these terms.
*Correspondence: César Hernández-Rodríguez, Y2hkZXozOEBob3RtYWlsLmNvbQ==