- Instituto de Bioingeniería, Universidad Miguel Hernández, Elche, Spain
The paralogous genes INCURVATA11 (ICU11) and CUPULIFORMIS2 (CP2) encode components of the epigenetic machinery in Arabidopsis and belong to the 2-oxoglutarate and Fe (II)-dependent dioxygenase superfamily. We previously inferred unequal functional redundancy between ICU11 and CP2 from a study of the synergistic phenotypes of the double mutant and sesquimutant combinations of icu11 and cp2 mutations, although they represented mixed genetic backgrounds. To avoid potential confounding effects arising from different genetic backgrounds, we generated the icu11-5 and icu11-6 mutants via CRISPR/Cas genome editing in the Col-0 background and crossed them to cp2 mutants in Col-0. The resulting mutants exhibited a postembryonic-lethal phenotype reminiscent of strong embryonic flower (emf) mutants. Double mutants involving icu11-5 and mutations affecting epigenetic machinery components displayed synergistic phenotypes, whereas cp2-3 did not besides icu11-5. Our results confirmed the unequal functional redundancy between ICU11 and CP2 and demonstrated that it is not allele or genetic background specific. An increase in sucrose content in the culture medium partially rescued the post-germinative lethality of icu11 cp2 double mutants and sesquimutants, facilitating the study of their morphological phenotypes throughout their life cycle, which include floral organ homeotic transformations. We thus established that the ICU11-CP2 module is required for proper flower organ identity.
Introduction
In Arabidopsis thaliana (hereafter, Arabidopsis), as in many other model species, the genetic dissection of biological phenomena typically involves the isolation and genetic analysis of mutants (Nüsslein-Volhard and Wieschaus, 1980; Jürgens et al., 1991; Koornneef et al., 1991; Wilkins, 1992; Haffter et al., 1996; Berná et al., 1999). The choice of the wild-type strain to be mutagenized is a key step in this endeavor, as mutant phenotypes clearly distinguishable from the wild type will not be produced for some genes in some genetic backgrounds (Lee et al., 1994; Koornneef et al., 2004; Chandler et al., 2013; Leng et al., 2022).
In addition, comparative analysis of the morphological, physiological, and molecular phenotypes of double or higher-order mutant combinations obtained by crossing single mutants is not always straightforward, given that different genetic backgrounds sometimes need to be mixed because of mutation availability in distinct strains (Huq and Quail, 2002; Scortecci et al., 2003; Clerkx et al., 2004). Indeed, phenotypes may be strongly influenced by modifiers present in the genomes of wild-type strains subjected to mutagenesis (Fernando et al., 2018). One strategy to partially overcome this problem is to first introgress each mutation of interest into an adequate genetic background, but this approach is time consuming and often leaves traces of the donor background (Rustérucci et al., 2001; Mouchel et al., 2004; Yoo et al., 2007; Zikherman et al., 2009; Kradolfer et al., 2013). An alternative approach is now accessible via clustered regularly interspaced short palindromic repeats (CRISPR)/CRISPR-associated nuclease (Cas)-mediated genome editing, which allows the relatively rapid isolation of single or multiple mutants in the same genetic background. CRISPR/Cas9 is now the preferred choice for directed mutagenesis due to its high specificity, efficiency, and simplicity (Jinek et al., 2012; Cong et al., 2013; Gaj et al., 2013; Jia et al., 2016; Zhao et al., 2016; Wang et al., 2022).
The Arabidopsis paralogous epigenetic factors INCURVATA11 (ICU11) and CUPULIFORMIS2 (CP2; Mateo-Bonmatí et al., 2018) belong to one of the largest known protein superfamilies, the 2-oxoglutarate and Fe (II)-dependent dioxygenases (2OGDs), which is represented by about 150 members in plants (Kawai et al., 2014; Martinez and Hausinger, 2015; Nadi et al., 2018). These proteins catalyze oxidation reactions using 2-oxoglutarate (also called α-ketoglutarate) and molecular oxygen as cosubstrates, and ferrous iron (Fe2+) as a cofactor (Islam et al., 2018). ICU11 is a POLYCOMB REPRESSIVE COMPLEX 2 (PRC2) accessory protein likely involved in removing the active histone mark H3K36me3 (trimethylation of lysine 36 of histone H3; Bloomer et al., 2020).
We previously described unequal functional redundancy between ICU11 and CP2 (Mateo-Bonmatí et al., 2018). The icu11-1 and icu11-2 mutant alleles in the S96 and Wassilewskija-2 (Ws-2) genetic backgrounds, respectively, showed mild but pleiotropic phenotypic defects, such as early flowering and curled (hyponastic) rosette leaves, while the cp2-1, cp2-2, and cp2-3 alleles in Columbia-0 (Col-0) were indistinguishable from their wild type. Notably, double mutant combinations between the icu11 null alleles and the hypomorphic cp2 alleles cp2-1 and cp2-2 skipped the vegetative phase and flowered immediately after germination, producing aberrant and sterile embryonic flowers. Double mutants with the null cp2-3 allele were not obtained. The reciprocal sesquimutants ICU11/icu11-1;cp2-3/cp2-3 and icu11-1/icu11-1;CP2/cp2-3, each only harboring one functional gene copy out of four, were not equivalent: while one copy of ICU11 was sufficient to obtain plants that were phenotypically wild type, a single copy of CP2 was not, with this sesquimutant producing lethal embryonic flowers (Mateo-Bonmatí et al., 2018).
It is not clear whether genetic background may influence redundancy in general or unequal redundancy in particular. An example of background-specific unequal redundancy has been described for the brassinosteroid receptor BRASSINOSTEROID INSENSITIVE 1 (BRI1) and its paralog BRI-LIKE1 (BRL1): in the Col-0 background, while no mutant phenotype is caused by brl1-2, bri1 mutants have altered vasculature and are dwarf, only the latter trait being enhanced in the bri1 brl1 double mutants. In contrast, the brl1-1 mutant in Ws-2 background is altered in vasculature development (Caño-Delgado et al., 2004; Briggs et al., 2006). An example of unequal redundancy not dependent on genetic background is provided by the SULFATE TRANSPORTER 1;1 (SULTR1;1) and SULTR1;2 paralogs, encoding high-affinity sulfate uptake transporters. Whereas the sultr1;1 mutant is similar to the wild type in root length, shoot biomass and sulfate uptake, the phenotype of the sultr1;1 sultr1;2 double mutant is extreme, and that of sultr1;2 is intermediate. These single and double mutants were studied both in Col-0 and Ws-2 and no effect of the genetic background was observed (Yoshimoto et al., 2007; Barberon et al., 2008).
Here, we obtained by CRISPR/Cas9-mediated gene editing alleles of ICU11 in the Col-0 and S96 backgrounds. They had differing phenotypes as single mutants but apparently identical genetic interactions with cp2 alleles in double mutant combinations. We therefore provide evidence that the lethal postembryonic phenotype of the icu11 cp2 double mutants and sesquimutants is not specific to the allele or the genetic background. We also discovered that this seedling lethality can be circumvented by increasing the sucrose content of the growth medium, which in turn allowed us to obtain evidence of the requirement of the ICU11-CP2 module for proper flower organ identity.
Materials and methods
Plant material, culture conditions, and crosses
Unless otherwise stated, all Arabidopsis thaliana (L.) Heynh. plants studied in this work were homozygous for the mutations indicated. The Nottingham Arabidopsis Stock Centre (NASC) provided seeds for the wild-type accessions Columbia-0 (Col-0; N1092), S96 (N914), and Wassilewskija-2 (Ws-2; N1601), as well as the following mutants: icu11-1 (N242) in the S96 background; curly leaf-2 (clf-2; N8853) in the Landsberg erecta (Ler) background; arabidopsis trithorax1-2 (atx1-2; N649002), arabidopsis trithorax-related protein 5 (atxr5; N630607), atxr6 (N866134), atxr7-1 (N667600), cp2-1 (N861581), cp2-2 (N828642), cp2-3 (N826626), demeter-like 2-3 (dml2-3; N631712), dml3-1 (N556440), dna methyltransferase-2-2 (dnmt2-2; N836854), domains rearranged methylase 1-2 (drm1-2; N521316), drm2-2 (N650863), histone acetyltransferase of the cbp family 1-3 (hac1-3; N580380), histone acetyltransferase of the myst family 1-1 (ham1-1; N655396), methyltransferase 1-4 (met1-4; N836155), repressor of silencing 1-4 (ros1-4; N682295), ros3-2 (N522363), terminal flower2-2 (tfl2-2; N3797), and embryonic flower2-3 (emf2-3; N16240) in the Col-0 background; icu2-1 (N329) and fasciata1-1 (fas1-1; N265) in the Enkheim2 (En-2) background; methyl-cpg-binding domain10-1 (mbd10-1; N872244) and variant in methylation 3-2 (vim3-2; N804664) in the Col-3 background; and histone deacetylase 6-6 (hda6-6; N66153) and hda6-7 (N66154) in the Col background. Seeds for early bolting in short days-1 (ebs-1, in the Ler background; Piñeiro et al., 2003) were provided by Manuel Piñeiro (CBGP, UPM-INIA-CSIC, Madrid, Spain), those of gigantea supressor5 (gis5, in the Col-0 background; Iglesias et al., 2015) by Pablo D. Cerdán (Fundación Instituto Leloir, IIBBA-CONICET, Buenos Aires, Argentina), and those of icu11-2 (in the Ws-2 background) by the Versailles Arabidopsis Stock Center (Brunaud et al., 2002). The presence and positions of all T-DNA insertions were confirmed by PCR amplification using gene-specific primers, with the LbB1.3 and LB1 primers used for the SALK and SAIL T-DNA insertions, respectively (Supplementary Table S1).
Unless otherwise stated, all seeds were surface sterilized, plated onto 140-mm (diameter) Petri dishes containing 100 ml half-strength Murashige and Skoog (MS) plant agar medium with 1% (w/v) sucrose at 20 ± 1°C, 60-70% relative humidity, and continuous illumination at ~75 µmol m–2 s–1, as previously described (Ponce et al., 1998). Crosses were performed as previously described (Quesada et al., 2000).
Plant morphology and pollen staining
Photographs showing morphology were taken with a Nikon SMZ1500 stereomicroscope equipped with a Nikon DXM1200F digital camera and the ACT-1 software (Nikon). Pollen grains were stained with Alexander red solution for 5–10 min before observation and photographed using a Leica DMRB microscope equipped with a Nikon DXM1200 digital camera.
Gene constructs and plant transformation
The pKI1.1R-ICU11_sgRNA1 plasmid was constructed as described by Tsutsui and Higashiyama (2017). In brief, the pKI1.1R plasmid (85808; Addgene) was linearized by restriction digest with AarI (Thermo Fisher Scientific), and treated with FastAP alkaline phosphatase (Thermo Fisher Scientific). The ICU11_sgRNA1_F/R oligonucleotides were phosphorylated using T4 polynucleotide kinase (New England Biolabs) and hybridized in a thermal cycler (Bio-Rad Laboratories T100). The ligation reaction was performed with T4 DNA ligase (Thermo Fisher Scientific), and the ligation product was transformed into chemically competent Escherichia coli DH5α cells using the heat-shock method. Plasmid and insert integrity were verified by Sanger sequencing using an Applied Biosystems 3500 Genetic Analyzer (Thermo Fisher Scientific). Putative off-targets were identified using the default parameters of the ChopChop tool (Labun et al., 2019). The pKI1.1R-ICU11_sgRNA1 plasmid was mobilized into Agrobacterium tumefaciens GV3101 (C58C1 RifR) cells, which were used to transform Arabidopsis S96 and Col-0 plants via the floral dip method (Clough and Bent, 1998). T1 Arabidopsis transgenic plants were selected on plates with half-strength MS medium containing 15 mg l–1 hygromycin B (Thermo Fisher Scientific). Absence of the transgene in T2 plants was verified by selecting DsRED negative seeds and confirmed by negative PCR amplifications using Cas9 specific primers (Supplementary Table S1).
Flowering time analysis
Flowering time was determined based on the total number of rosette leaves (counted when internode elongation was visible) and the number of days to bolting (Bouveret et al., 2006). To determine flowering time, all plants were grown on half-strength MS medium for five days and transferred to soil (a 2:2:1 mixture of perlite, vermiculite and sphagnum peat moss) in individual pots in a TC30 growth chamber (Conviron).
Accession numbers
Sequence data from this article can be found at The Arabidopsis Information Resource (http://www.arabidopsis.org) under the following accession numbers: ICU11 (At1g22950), CP2 (At3g18210), EBS (At4g22140), FAS1 (At1g65470), GIS5 (At5g63960), ICU2 (At5g67100), CLF (At2g23380), TFL2 (At5g17690), EMF2 (At5g51230), DML2 (At3g10010), DML3 (At4g34060), DNMT2 (At5g25480), DRM1 (At5g15380), DRM2 (At5g14620), MBD10 (At1g15340), MET1 (At5g49160), ROS1 (At2g36490), ROS3 (At5g58130), VIM3 (At5g39550), ATX1 (At2g31650), ATXR5 (At5g09790), ATXR6 (At5g24330), ATXR7 (At5g42400), HAC1 (At1g79000), HDA6 (At5g63110), and HAM1 (At5g64610).
Results
Isolation of novel icu11 mutants in a Col-0 background after CRISPR/Cas9 mutagenesis
We previously characterized two loss-of-function icu11 alleles: icu11-1 and icu11-2 (Mateo-Bonmatí et al., 2018). A third allele, icu11-3, was identified in an Ac/Ds transposon-tagging mutagenesis screen (Bancroft et al., 1993; Bloomer et al., 2020). Although these three mutants appear to carry null alleles (Figure 1), the hyponasty of icu11-1 leaves is stronger than that caused by the icu11-2 and icu11-3 alleles, which is likely due to their different genetic backgrounds (S96, Ws-2, and Ler, respectively). Different or hybrid genetic backgrounds may not facilitate proper comparisons of the morphological and molecular phenotypes of single and multiple mutants (Page and Grossniklaus, 2002; Chandler et al., 2013; Taylor and Ehrenreich, 2015). Therefore, as all cp2 alleles were in the Col-0 background but there were no available icu11 alleles in this background, we subjected Col-0 plants to CRISPR/Cas9 mutagenesis targeting ICU11. We also mutagenized S96 plants as a control. Accordingly, we designed a 20-nt single guide RNA (sgRNA) that targets the first exon of the ICU11 gene, with a predicted targeting efficiency of 49.7% (Figures 1A, B; Supplementary Figure S1A). We obtained seven chimeric T1 plants, from which we isolated four T2 independent homozygous lines: the icu11-4 and icu11-7 mutants in the S96 background, carrying deletions of 4 bp and 1 bp, respectively, and icu11-5 and icu11-6 in the Col-0 background, carrying a 1-bp insertion and a 1-bp deletion, respectively (Figure 1C; Supplementary Figure S1B). All these mutations are predicted to cause frameshifts that introduce premature stop codons, producing truncated proteins of only 86 (icu11-4), 44 (icu11-5), or 87 (icu11-6 and icu11-7) amino acids, instead of the 397 amino acids of wild-type ICU11 (Supplementary Figure S1C). We sequenced Cas9-free lines by Sanger sequencing to examine the two most likely off-targets, both of which presented four mismatches with at least one mismatch located in the 5 bp adjacent to the protospacer adjacent motif (PAM) of our sgRNA (Supplementary Figure S2A); neither off-target was mutated in subsequent generations of our mutant lines (Supplementary Figure S2B).
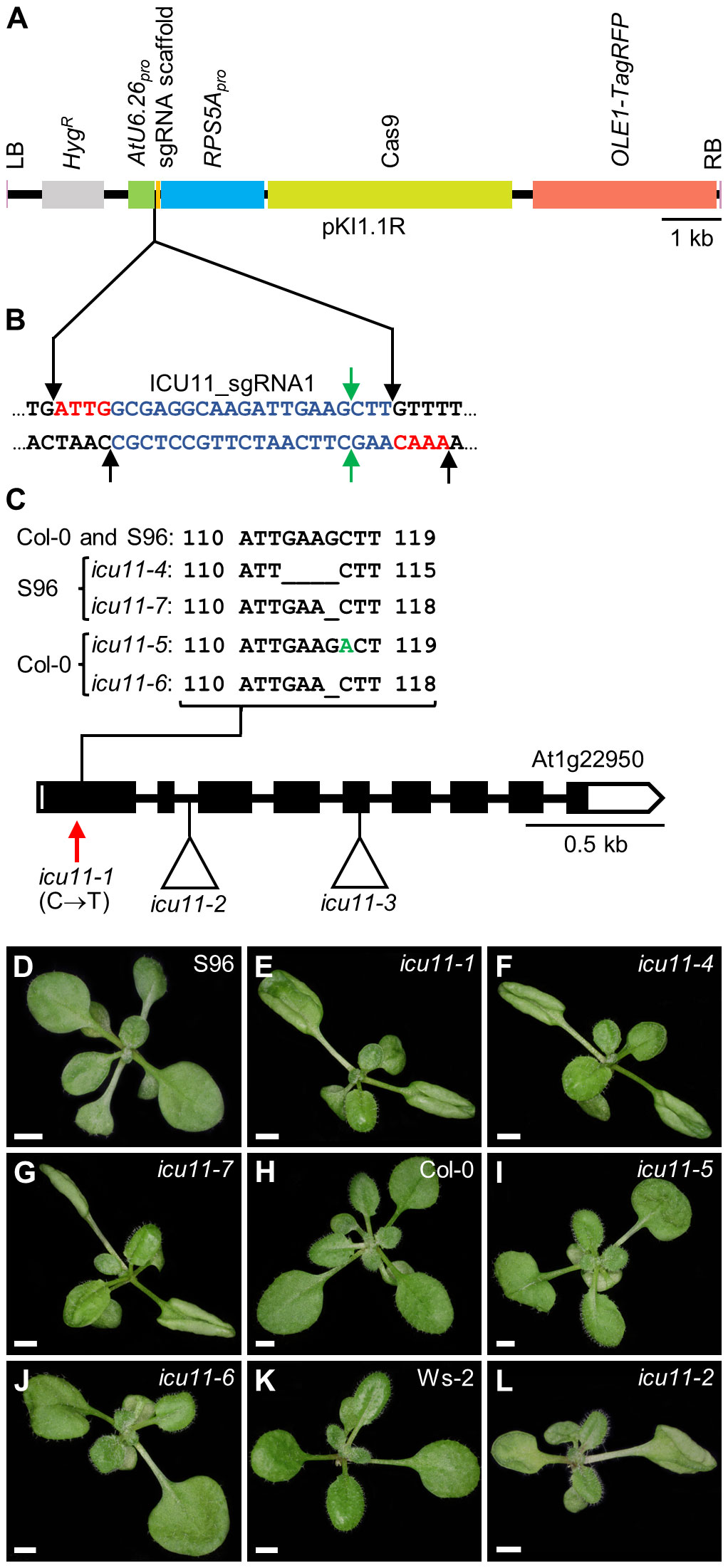
Figure 1 Molecular nature and morphological phenotypes of the icu11 mutations obtained in this work. (A) Diagram of the T-DNA fragment of the pKIR1.1R vector that is integrated into the plant genome. Structural features are represented as boxes: LB and RB, left and right T-DNA borders (pink); HygR, hygromycin resistance gene (gray); AtU6.26pro, promoter of U6 SMALL NUCLEOLAR RNA26 (green); sgRNA scaffold, the sequence that serves as a binding site for Streptococcus pyogenes Cas9 protein (orange); RPS5Apro, promoter of RIBOSOMAL PROTEIN 5A (blue); Cas9, CRISPR-associated protein 9 (pale green); OLE1-TagRFP, a translational fusion of the gene encoding OLEOSIN 1, the most abundant oleosin in Arabidopsis seeds, and that of the red fluorescent protein (RFP; red). Between the AtU6.26 promoter and the sgRNA scaffold, there are two restriction sites for the type IIS AarI restriction enzyme. (B) Nucleotide sequence of the sgRNA scaffold (black) and the ICU11 sgRNA1 (blue) with four-nucleotide overhangs used for cloning (red). Black and green arrows indicate the AarI restriction sites and predicted Cas9 cleavage sites, respectively. (C) Schematic representation of the structure of the ICU11 gene with indication of the nature and positions of icu11 mutations. White and black boxes represent untranslated and coding regions of exons, respectively; lines represent introns. A red vertical arrow indicates the icu11-1 point mutation, and triangles indicate the icu11-2 T-DNA and icu11-3 Ds insertions (not studied in this work). The sequences of the icu11-4, icu11-6, and icu11-7 deletions and the icu11-5 insertion (+1 bp, in green) are also shown. (D–L) Rosettes of the (D) S96, (H) Col-0, and (K) Ws-2 wild-type accessions, and the (E) icu11-1, (F) icu11-4, (G) icu11-7, (I) icu11-5, (J) icu11-6, and (L) icu11-2 single mutants. Photographs were taken 15 days after stratification (das). Scale bars, 2 mm.
The seedling lethal phenotype of the icu11 cp2 double mutants is independent of genetic background
As expected from their S96 background, the icu11-4 and icu11-7 mutants exhibited a morphological phenotype indistinguishable from that of icu11-1 (Figures 1D–G). The icu11-5 and icu11-6 mutants showed wavy leaves that did not reach hyponasty (Figures 1H–J), different to that of icu11-2 (Figures 1K, L) and icu11-3 (Bloomer et al., 2020). In addition, icu11-5 and icu11-6 shared other phenotypic traits with the other icu11 mutants, such as cotyledon epinasty (Figures 1D–L) and early flowering (Figures 2A, B). We observed significantly more unfertilized ovules per half silique in the icu11-4, icu11-5, icu11-6 and icu11-7 mutants compared to their respective wild types (Figures 2C–L). Taken together, these observations indicate that our new icu11 mutants are phenotypically similar to previously reported mutants, with only minor differences caused by their genetic background, which are particularly visible in their rosette leaf morphology.
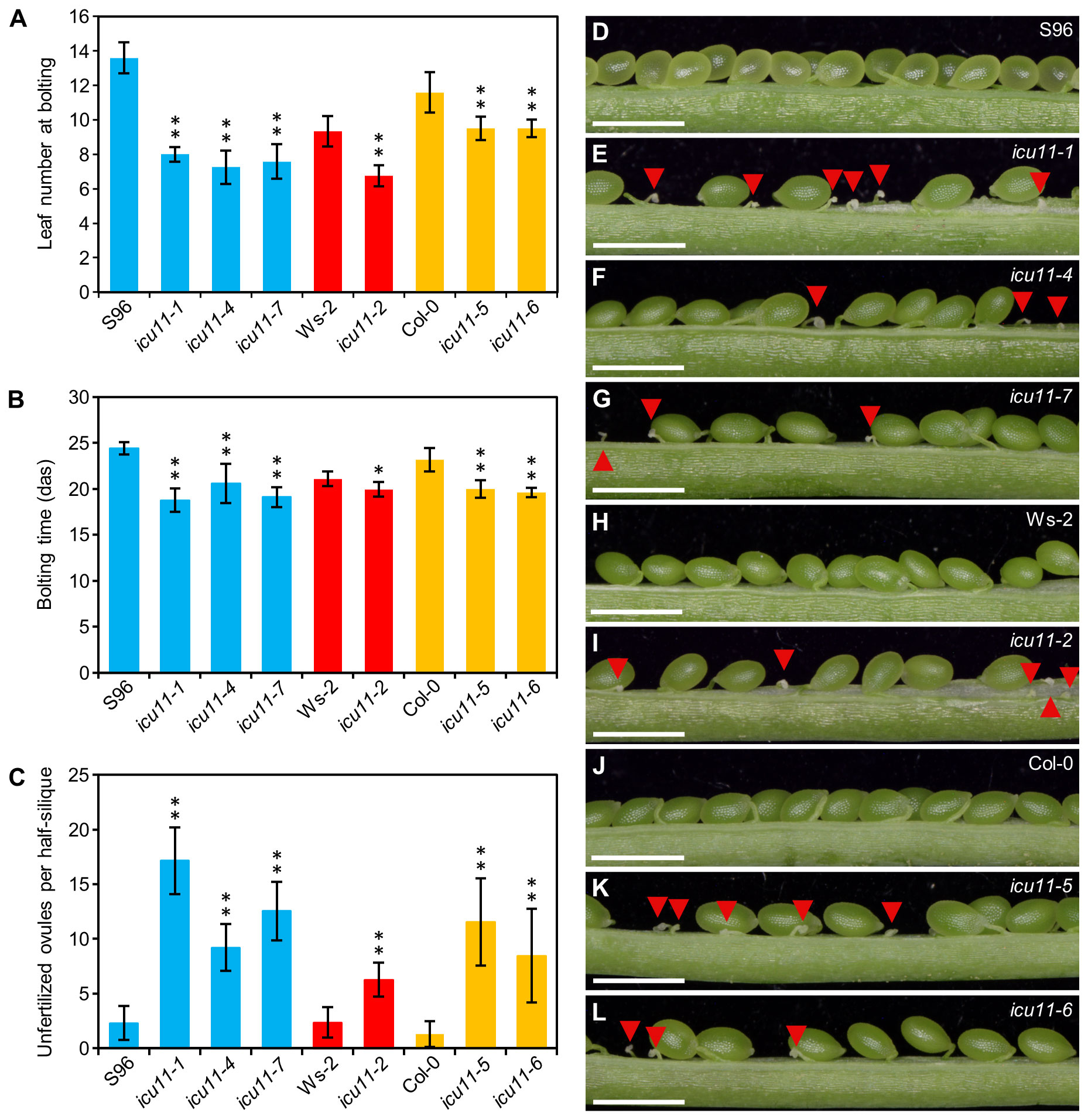
Figure 2 Flowering and reproductive phenotypes of the icu11-5 and icu11-6 mutants. (A, B) Flowering time of S96, icu11-1, icu11-4, icu11-7, Ws-2, icu11-2, Col-0, icu11-5, and icu11-6 plants, expressed as (A) leaf number at bolting and (B) number of days to bolting. (C) Number of unfertilized ovules per half silique for the indicated genotypes. Data are means ± standard deviation. Asterisks indicate values significantly different from the corresponding wild type in a Mann-Whitney U test (*P < 0.01 and **P < 0.001). Blue, red, and yellow bars indicate that the plants of the genotypes shown are in the S96, Ws-2, and Col-0 backgrounds, respectively. (D–L) Dissected fully elongated siliques with the indicated genotypes. Red arrowheads indicate unfertilized ovules. Photographs were taken 45 das. Scale bars, 1 mm. All siliques in (C–L) were collected from plants grown simultaneously within the same growth chamber. Pictures in (D, E, H, I, J) are similar to those that we published in the Supplemental Figure 2 (A–D, G) of Mateo-Bonmatí et al. (2018), respectively. Siliques of S96, icu11-1, Ws-2, icu11-2 and Col-0 are included here to allow comparison with icu11-4, icu11-7, icu11-5 and icu11-6. The numbers shown in (C) for S96, icu11-1, Ws-2, icu11-2 and Col-0 have been obtained independently of those of Supplemental Figure 2L of Mateo-Bonmatí et al. (2018).
Although the morphological phenotypes caused by the icu11 alleles are relatively mild, and the cp2 null mutants are indistinguishable from wild type, the phenotypes of icu11 cp2 double mutants are synergistic: they are seedling lethal, as might be expected for the genetic combination of mutations in two close paralogs with a high degree of functional redundancy (Ohno, 1970; Nowak et al., 1997; Cusack et al., 2021). Our previously obtained icu11 cp2 double mutants had hybrid genetic backgrounds, which prevented a clear conclusion as to the seedling lethality presented by these double mutants. Here, we thus crossed icu11-5 and icu11-6 with the cp2-1 and cp2-2 hypomorphic alleles of CP2, and with the cp2-3 null allele, all of which are in the Col-0 genetic background. The double homozygous mutant combinations between icu11-5 or icu11-6 and cp2-1 or cp2-2 exhibited an embryonic-flowering seedling-lethal phenotype, as did the icu11/icu11;CP2/cp2-3 sesquimutants (Figure 3). We obtained no icu11-5 cp2-3 or icu11-6 cp2-3 double mutants, as was previously published for icu11-1 cp2-3 (Mateo-Bonmatí et al., 2018). The ICU11/icu11;cp2-3/cp2-3 sesquimutants were indistinguishable from the wild type (Figures 3I, N), as were those we previously published in hybrid genetic backgrounds.
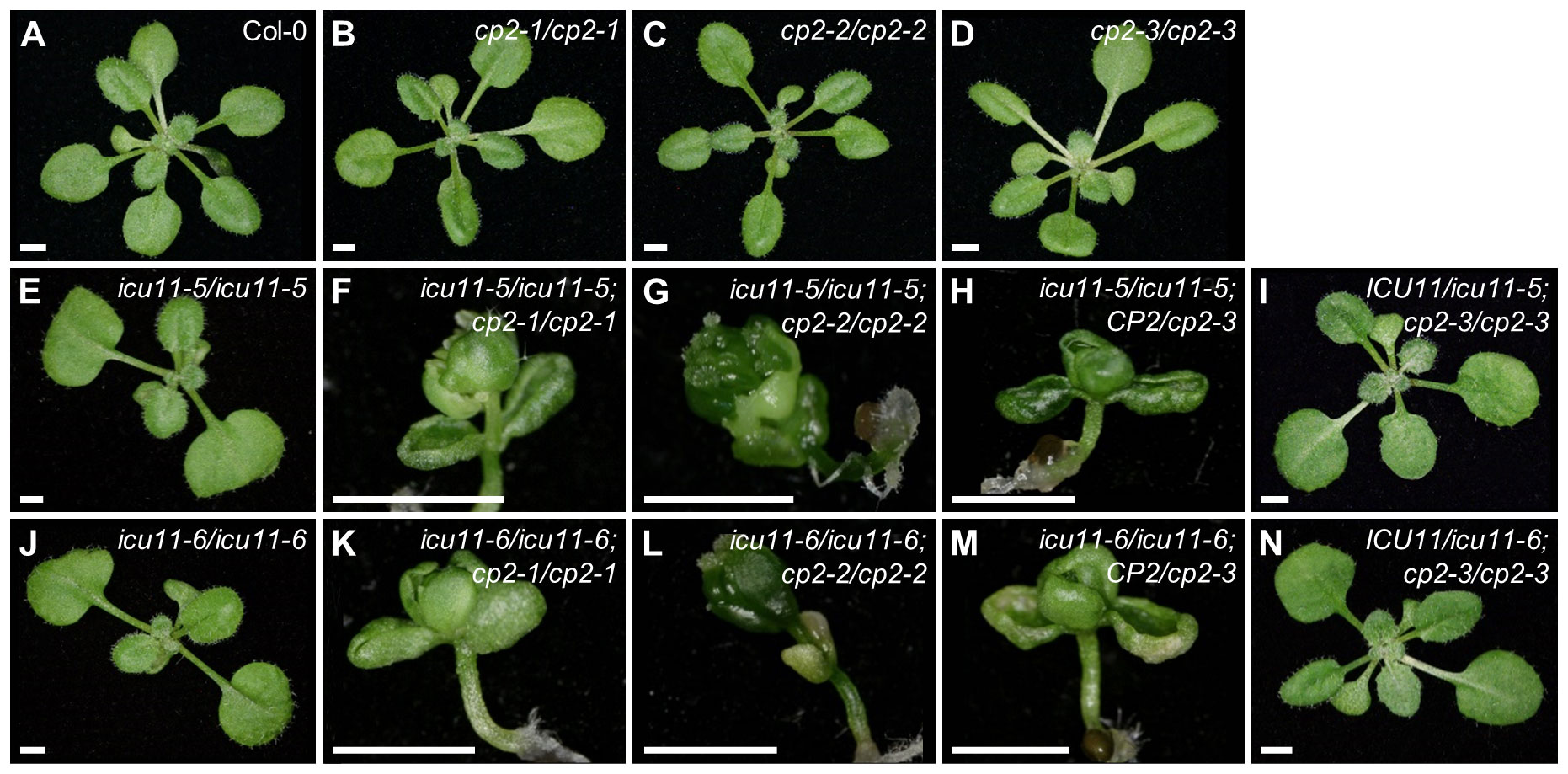
Figure 3 Genetic interactions between the loss-of-function icu11 and cp2 alleles in the Col-0 genetic background. icu11-5, icu11-6, and cp2-3 are null alleles, while cp2-1 and cp2-2 are hypomorphic. Rosettes of (A) the wild-type Col-0; the homozygous single mutants (B) cp2-1, (C) cp2-2, (D) cp2-3, (E) icu11-5, and (J) icu11-6; the double mutants (F) icu11-5 cp2-1, (G) icu11-5 cp2-2, (K) icu11-6 cp2-1, and (L) icu11-6 cp2-2; and the sesquimutants (H) icu11-5/icu11-5;CP2/cp2-3, (I) ICU11/icu11-5;cp2-3/cp2-3, (M) icu11-6/icu11-6;CP2/cp2-3 and (N) ICU11/icu11-6;cp2-3/cp2-3. Photographs were taken 16 das. Scale bars, 2 mm.
icu11-5, but not cp2-3, genetically interacts with loss-of-function alleles of genes encoding PRC2 core components and accessory proteins
Synergistic phenotypes visualized in double mutants shed light on functional relationships between genes (Pérez-Pérez et al., 2009), including those encoding components of the epigenetic machinery of Arabidopsis. For example, PWWP-DOMAIN INTERACTOR OF POLYCOMB1 (PWO1) is a histone reader that recruits PcG proteins (Hohenstatt et al., 2018), and BLISTER (BLI) is a PRC2 interactor and a regulator of stress-responsive genes (Kleinmanns et al., 2017). Both pwo1 and bli loss-of-function mutations display synergistic phenotypes when combined with strong mutant alleles of CURLY LEAF (CLF), which encodes a PRC2 core component responsible for the deposition of H3K27me3 repressive marks (Goodrich et al., 1997; Schatlowski et al., 2010).
With the aim to expand the spectrum of genes demonstrated to genetically interact with ICU11, we crossed icu11-1 to loss-of-function mutants of 17 genes encoding components of the epigenetic machinery that include proteins involved in DNA or histone methylation, acetylation, or deacetylation (Pikaard and Mittelsten Scheid, 2014). We identified 18 double mutants with additive phenotypes (Supplementary Table S2). Also we obtained double mutant combinations of icu11-5 with loss-of-function alleles of CLF, LIKE HETEROCHROMATIN PROTEIN 1 (LHP1; also named TFL2), FASCIATA1 (FAS1), EARLY BOLTING IN SHORT DAYS (EBS), GIGANTEA SUPRESSOR5 (GIS5), and ICU2, previously found to genetically interact with icu11-1 (Mateo-Bonmatí et al., 2018). FAS1 is a component of the Chromatin Assembly Factor 1 (CAF-1) complex that promotes the deposition of histone H3 and H4 at newly synthesized DNA during replication (Schönrock et al., 2006). EBS is an H3K27me3 and H3K4me3 reader that regulates the floral phase transition (Piñeiro et al., 2003; López-González et al., 2014; Yang et al., 2018). GIS5 and ICU2 are the catalytic subunits of DNA polymerase δ and α, respectively (Barrero et al., 2007; Iglesias et al., 2015). The double mutant combinations of icu11-5 with clf-2, ebs-1, gis5, icu2-1, tfl2-2, or fas1-1 exhibited strong synergistic phenotypes consisting of dwarf rosettes; extreme leaf hyponasty in the cases of gis5, icu2-1, and fas1-1; and some degree of anthocyanin accumulation in the icu11-5 tfl2-2 rosette center (Figure 4). The phenotypes of the double mutant combinations of icu11-5 with clf-2, gis5, and icu2-1 were similar to those previously reported using icu11-1; however, those involving tfl2-2, ebs-1, and fas1-1 were milder in combination with icu11-5 (Figures 4L–N) than with icu11-1 (Mateo-Bonmatí et al., 2018).
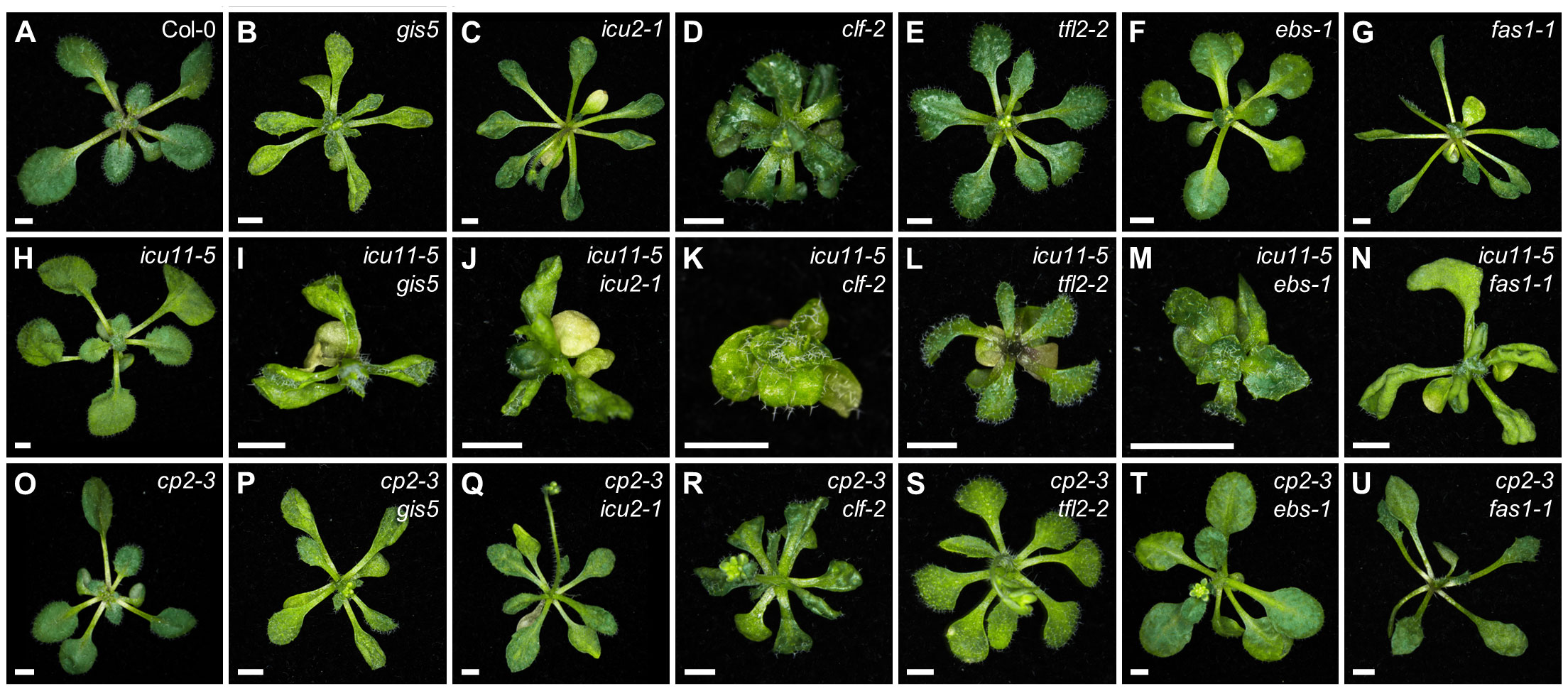
Figure 4 Phenotypes of double mutants involving icu11-5 and cp2-3 with gis5, icu2-1, clf-2, tfl2-2, ebs-1, and fas1-1. Rosettes of (A) the wild-type Col-0; the single mutants (B) gis5, (C) icu2-1, (D) clf-2, (E) tfl2-2, (F) ebs-1, (G) fas1-1, (H) icu11-5, and (O) cp2-3; and the double mutants (I) icu11-5 gis5, (J) icu11-5 icu2-1, (K) icu11-5 clf-2, (L) icu11-5 tfl2-2, (M) icu11-5 ebs-1, (N) icu11-5 fas1-1, (P) cp2-3 gis5, (Q) cp2-3 icu2-1, (R) cp2-3 clf-2, (S) cp2-3 tfl2-2, (T) cp2-3 ebs-1, and (U) cp2-3 fas1-1. Photographs were taken 15 das. Scale bars, 2 mm.
We also combined the mutations mentioned above with the cp2-3 mutation, which lacks a distinctive phenotype; the resulting F2 progeny showed rosette phenotypes that were indistinguishable from those of their corresponding phenotypically mutant parent (Figures 4P–U). We conclude that, unlike ICU11, the loss of CP2 function is not sufficient to modify the phenotypes caused by mutations in other genes involved in epigenetic modifications, confirming the previously proposed unequal functional redundancy between ICU11 and CP2 (Mateo-Bonmatí et al., 2018).
Sucrose partially rescues the lethality of the icu11 cp2 double mutants
There is an expanding list of Arabidopsis mutants exhibiting morphological phenotypes that are partially or fully rescued by the exogenous supplementation of sucrose, such as phosphatidylglycerol phosphate synthase 1 (pgp1) and cyclophilin 38 (cyp38), which are defective in the assembly and proper function of photosystem II protein complexes, respectively (Fu et al., 2007; Kobayashi et al., 2016; Duan et al., 2021). This phenomenon is true for other Arabidopsis genes that are functionally unrelated, but whose mutations directly or indirectly impair or abolish photosynthesis. We observed the same effect in icu11 cp2-1 and icu11 cp2-2 double mutant seedlings, in which photosynthesis is diminished because they do not form true leaves, instead developing embryonic flowers immediately after germination. These mutants had a very slow growth rate, did not develop further, and died 20–40 days after stratification (das; Figures 5A–C). When we raised the concentration of sucrose in the growth medium from 1% (w/v) to 3%, icu11-5 cp2-1 double mutant plants developed main and axillary shoots with long internodes, small cauline leaves, disorganized flowers, and short siliques. Most of the disorganized flowers showed homeotic transformations of sepals and petals into carpels and had few stamens (Figures 5D–H).
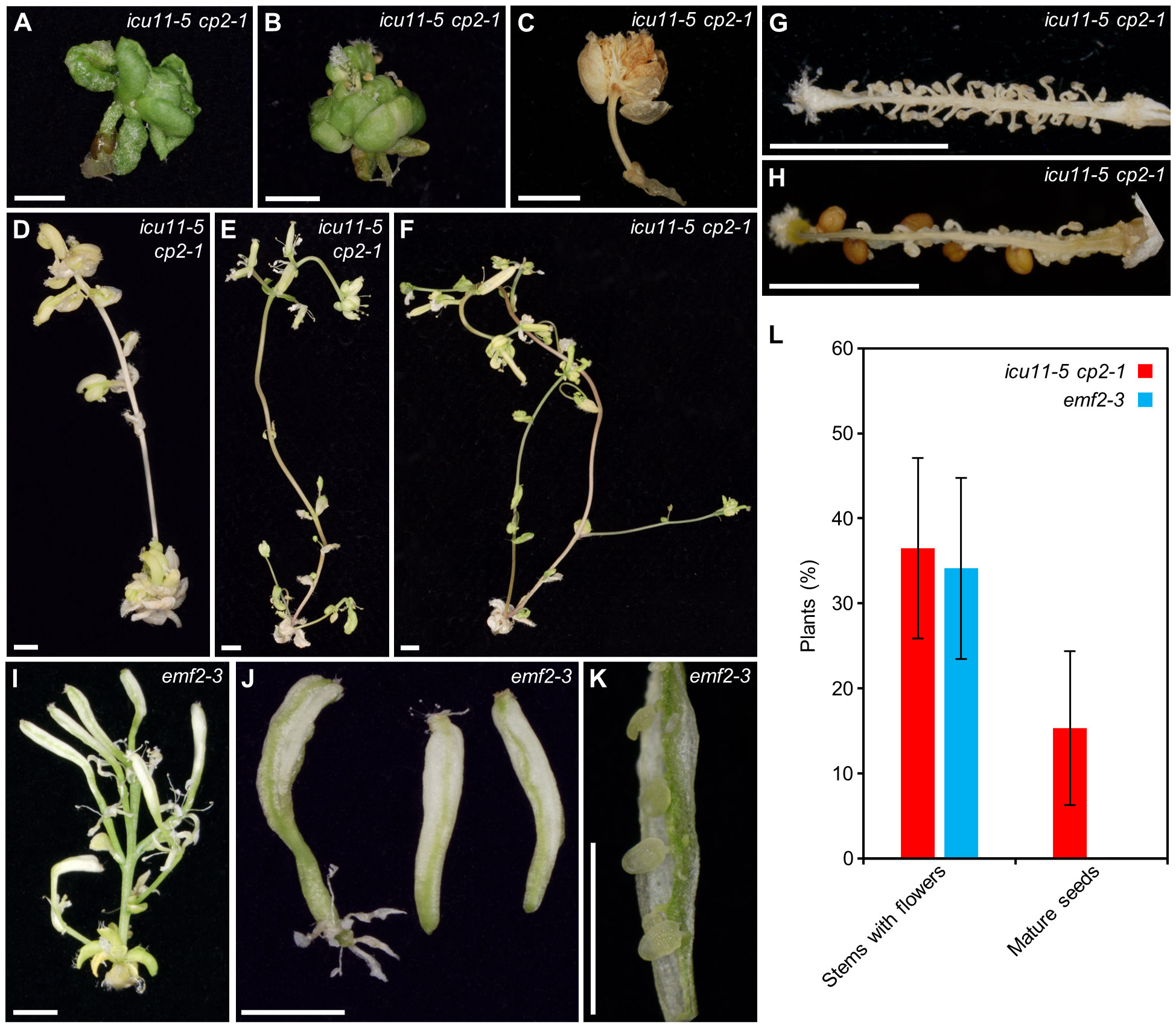
Figure 5 Partial rescue of the post-germinative lethality phenotype of icu11-5 cp2-1 and emf2-3 plants grown on culture medium supplemented with 3% sucrose. (A–K) Pictures of (A–H) the icu11-5 cp2-1 double mutant or (I–K) the emf2-3 single mutant, showing (A–C) embryonic flowers; seedlings developing (D–F) stems, (I) flowers, (J) siliques, and (G, H, K) dissected siliques. (L) Percentage of icu11-5 cp2-1 and emf2-3 plants with different phenotypes grown on culture medium supplemented with 3% sucrose. Error bars indicate standard deviation. A total of 136 icu11-5 cp2-1 and 110 emf2-3 plants were classified. Photographs were taken (A, B) 26, (D–F, I–K) 36, (G, H) 60, and (C) 84 das. Scale bars, 2 mm.
The emf2-3 single mutant (Yoshida et al., 2001), whose morphological phenotype is similar to that of icu11 cp2 double mutant plants, also exhibited a partial rescue under increased sucrose supplementation. These plants growing on 3% sucrose medium produced main and axillary shoots lacking apical dominance, as well as flowers with an altered structure that developed into short siliques with extended white sectors reminiscent of petal tissue (Figures 5I–K). When grown on growth medium supplemented with 3% sucrose, 36.5% of icu11-5 cp2-1 (n = 136) and 34.1% of emf2-3 (n = 110) plants developed stems with flowers, although even with hand pollination only 15.3% of icu11-5 cp2-1 plants and no emf2-3 plants produced mature seeds (Figure 5L). Only one out of the 23 seeds obtained in this manner from icu11-5 cp2-1 plants germinated on medium supplemented with 3% sucrose, and still developed embryonic flowers.
An alteration in pollen viability may explain why only some icu11-5 cp2-1 double mutant plants produced seeds. To assess pollen grain viability in the icu11-5, cp2-1, and icu11-5 cp2-1 mutants, we stained their anthers with Alexander solution. We detected no aberrations in anther shape or size or in pollen grain viability for the cp2-1 and icu11-5 anthers when compared to Col-0 (Figures 6A–F). However, 66% of the observed icu11-5 cp2-1 anthers were smaller and carried fewer but viable pollen grains (Figures 6G, H, K). The remaining 34% of anthers were also small and contained only non-viable pollen that turned blue/gray upon staining (Figures 6G–K).
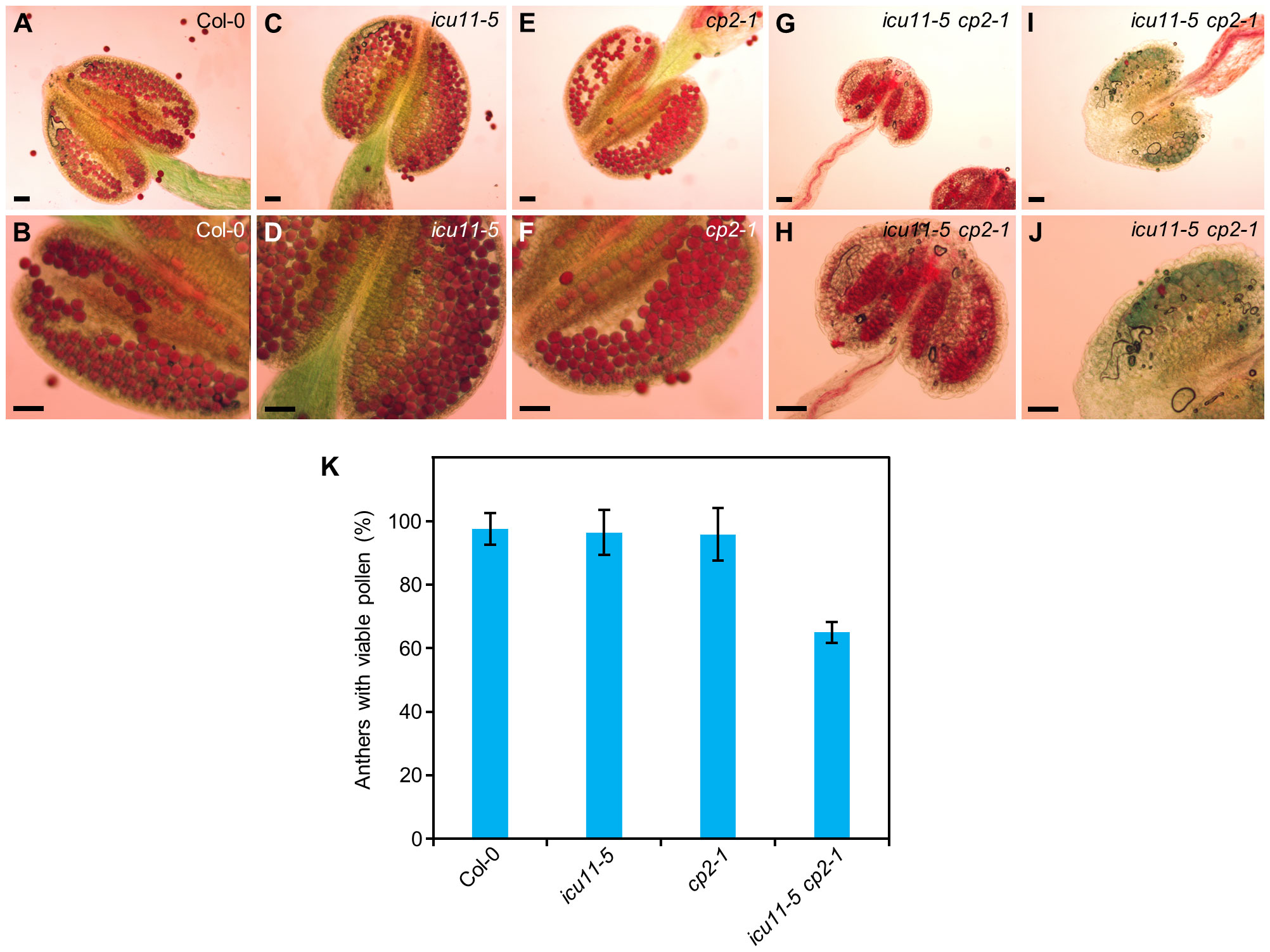
Figure 6 Pollen grain viability in icu11-5, cp2-1, and icu11-5 cp2-1. (A–J) Anthers of (A, B) Col-0, (C, D) icu11-5, (E, F) cp2-1, and (G–J) icu11-5 cp2-1 plants. Col-0, icu11-5, and cp2-1 plants were grown on standard culture medium (supplemented with 1% sucrose), while icu11-5 cp2-1 plants were grown on medium supplemented with 3% sucrose. Purple/red and blue/gray grains are viable and non-viable pollen, respectively. All samples were treated for 5 min with Alexander solution. Scale bars, 50 μm. (K) Percentage of anthers containing viable pollen after collecting and studying 28 anthers (three or four per plant). Error bars indicate standard deviation.
Discussion
The severity of leaf aberrations in the icu11 single mutants is dependent on genetic background
A common task in developmental genetics is the analysis of phenotypic differences between mutants carrying different alleles of a given gene, as well as the comparison of their corresponding double mutants. Many studies have shown that morphological phenotypes can vary depending on the genetic background; for example, the gibberellin biosynthesis (ga5) mutant in Ler displays a much more pronounced drop in shoot fresh weight than the ga20ox1-3 (another loss-of-function allele of the GA5 gene) mutant, in the Col-0 background (Barboza-Barquero et al., 2015). Such phenotypic difference can be due to allele specifity, the erecta mutation carried by Ler, or to any other of the many differences between the genetic backgrounds of Ler and Col-0. Another example is the transfer of transgenes carrying either the functional Ler allele or the loss-of-function allele from the Japanese accession Fuk of the MADS box transcription factor gene SHORT VEGETATIVE PHASE (SVP), which regulates flowering time, into five Arabidopsis accessions. In some of these accessions, the presence of SVP-Fuk accelerated flowering, whereas SVP-Ler delayed it; however, in other accessions, no noticeable differences were observed (Méndez-Vigo et al., 2013). The genetic modifiers partially or fully responsible for genetic background effects have been identified in a few cases. For example, the short stem and midrib (ssm) mutant in Col-0 is semi-dwarf and has wavy leaves; in the progeny of crosses of Ler to ssm, such phenotype was rescued by a gene apparently present in Ler but absent from Col-0. ssm was found to be a null allele of SYNTAXIN OF PLANTS 22 (SYP22), which encodes a syntaxin-related protein required for vacuolar assembly. The Ler allele of SYP23, a close paralog of SYP22, is functional and complements ssm, whereas the Col-0 allele of SYP23 is mutated (Ohtomo et al., 2005).
The icu11-1 mutant exhibits a developmental phenotype previously observed in other mutants that carry alleles of genes encoding components of the epigenetic machinery (Mateo-Bonmatí et al., 2018). These characteristic aberrations, such as leaf hyponasty and early flowering, are in some cases associated with a reduced deposition of the epigenetic mark H3K27me3 across a large number of genes (Förderer et al., 2016). The gene-edited icu11-5 and icu11-6 null alleles of ICU11 that we obtained in the Col-0 genetic background in this study exhibited a leaf incurvature milder than that of icu11-1 in the S96 background. These differences are most likely due to differences in the genetic backgrounds, as we also obtained two CRISPR/Cas9 mutants in the S96 background, icu11-4 and icu11-7, which displayed identical leaf curvature as icu11-1. Furthermore, it is worth mentioning here that icu11-6 and icu11-7 carry independently obtained but identical mutations: a deletion of one nucleotide immediately downstream of the PAM; therefore, their differences in leaf phenotype can only be due to their different genetic backgrounds.
The unequal functional redundancy between the ICU11 and CP2 paralogs is not dependent on genetic background
CP2 and ICU11 are unequally redundant paralogs, as we inferred from the phenotype of the icu11-1/icu11-1;CP2/cp2-3 sesquimutant, which develops lethal embryonic flowers, while the reciprocal ICU11/icu11-1;cp2-3/cp2-3 sesquimutant is phenotypically wild type (Mateo-Bonmatí et al., 2018). The icu11-1 cp2-1 and icu11-1 cp2-2 double mutants also developed embryonic flowers, whereas the ICU11/icu11-1;cp2-1/cp2-1 and ICU11/icu11-1;cp2-2/cp2-2 sesquimutants were indistinguishable from wild type plants, and the icu11-1/icu11-1;CP2/cp2-1 and icu11-1/icu11-1;CP2/cp2-2 sesquimutants were indistinguishable from icu11-1 single mutant plants. While the genetic background of these double mutants and sesquimutants was hybrid (S96/Col-0), all combinations between the icu11-5, icu11-6, cp2-1, cp2-2, and cp2-3 mutations that we obtained here were in a single genetic background (Col-0). Furthermore, as previously shown for icu11-1/icu11-1;cp2-3/cp2-3, no icu11-5/icu11-5;cp2-3/cp2-3 or icu11-6/icu11-6;cp2-3/cp2-3 double mutants were obtained likely because of their gametic or early embryonic mortality. The genetic combination of our new icu11 alleles with cp2 alleles, all in the Col-0 background, confirmed that CP2 behaves as a haploinsufficient locus in a homozygous icu11 background (Pérez-Pérez et al., 2009; Meinke, 2013; Navarro-Quiles et al., 2023), and in particular, that the embryonic flower phenotype of the double mutant and some sesquimutant combinations of their alleles are not influenced by the S96, Ws-2, or Col-0 genetic backgrounds.
We also provided further evidence for the unequal functional redundancy between ICU11 and CP2 through the analysis of their genetic interactions with loss-of-function alleles of other epigenetic machinery components. We established that icu11-5 synergistically interacts with clf-2, ebs-1, gis5, icu2-1, tfl2-2, and fas1-1 in the presence of two wild-type CP2 copies, as previously reported for icu11-1. There were some minor differences between the synergistic phenotypes of the double mutant combinations of ebs-1 (in the Ler background), tfl2-2 (Col-0), and fas1-1 (En-2) with icu11-1 (S96) or icu11-5 (Col-0), which can be attributed to the genetic background. By contrast, the double mutant combinations of ebs-1, tfl2-2, or fas1-1 with the cp2-3 null allele, in the presence of two ICU11 wild-type copies, resulted in phenotypes indistinguishable from those of the ebs-1, tfl2-2, or fas1-1 single mutants. Apparently, the presence of a wild-type allele of ICU11 impedes the identification of CP2 genetic interactors, as they are redundant. Thus, the lethality of the icu11 cp2 double mutants is an obstacle for an independent characterization of CP2.
The viability of the double mutant and sesquimutant combinations of alleles of ICU11 and CP2 is dependent on exogenous carbon
The phenotype of a large number of mutants in genes that are primarily associated with photosynthesis or processes closely linked to it can be partially or completely alleviated by supplementation with an exogenous carbon source. Indeed, photosynthesis, primarily occurring in plant leaves, serves as the ultimate source of sugars, which are the primary carriers of both sunlight energy and carbon required for metabolism. Sugar availability is crucial for Arabidopsis development, leading to early developmental arrest in mutants with impaired photosynthesis, such as pgp1 (Kobayashi et al., 2016), and fructokinase-like 2-4 (fln2-4; Huang et al., 2013) or white cotyledons (wco; Yamamoto et al., 2000). Since WCO is only required for chloroplast biogenesis in cotyledons but not in true leaves, wco mutants can be supplied with 3% sucrose for a few days to enable them to survive and produce true leaves, after which the plants develop normally. Other mutations of genes involved in photosynthesis are not seedling or plant lethal but delay growth; for example, CYP38 participates in the assembly and maintenance of photosystem II, and loss-of-function cyp38 alleles show retarded growth and pale green leaves. A 1% sucrose supplementation was sufficient to rescue rosette growth, although cyp38 still displayed hypersensitivity to light, while higher concentrations of sucrose increased the length of the primary root (Fu et al., 2007; Duan et al., 2021).
Although several genes repress flowering in Arabidopsis, the emf single mutants are unique because they completely skip the vegetative phase after seed germination without generating true leaves (Sung et al., 1992; Yang et al., 1995). The icu11 cp2 double mutants resembled the emf single mutants, as they also lacked true leaves. Impaired carbon fixation results in the early-development arrest of these mutants, so they cannot produce reproductive structures beyond a disorganized embryonic flower. These mutants represent a class completely different from those mentioned above, as they are not mutated in genes primarily related to photosynthesis. Instead, they skip vegetative development and die because they cannot develop leaves, which does not allow them to photosynthesize properly.
ICU11 and CP2 appear to regulate flower organ identity genes
The increase from 1% to 3% sucrose in the growth medium was sufficient to allow the formation in our icu11 cp2 double mutants of axillary shoots, cauline leaves, and disorganized inflorescences exhibiting flowers with homeotic transformations. Analysis of these structures revealed that ICU11 and CP2 are not only required for vegetative development, as previously described (Mateo-Bonmatí et al., 2018), but also to ensure proper reproductive development. ICU11 and CP2 are expressed in both vegetative and reproductive organs, with CP2 showing higher expression levels than ICU11 in the flowers and siliques of wild-type plants (Mateo-Bonmatí et al., 2018).
Similar to the homeotic transformations that we observed in icu11-5 cp2-1 plants grown with 3% sucrose, the loss-of-function apetala2 (ap2) mutations and the overexpression of AGAMOUS (AG) and SEPALLATA3 (SEP3) result in the transformation of sepals and petals into carpelloid structures (Mizukami and Ma, 1992; Riechmann and Meyerowitz, 1997; Chen, 2004; Castillejo et al., 2005). Moreover, the weak emf2-10 allele causes the appearance of carpelloid sepals (Chanvivattana et al., 2004) reminiscent of those of the icu11-5 cp2-1 double mutant. Indeed, previous studies have described that ICU11 plays a role in repressing AG, SEP1, SEP2, and SEP3 expression during the vegetative phase (Mateo-Bonmatí et al., 2018; Bloomer et al., 2020). Therefore, based on our observations of icu11-5 cp2-1, we propose that ICU11 and CP2 are required to regulate the expression of floral identity genes during reproductive development.
The homeotic transformations of the flowers produced by icu11-5 cp2-1 and emf2-3 plants grown on medium supplemented with 3% sucrose impeded self-pollination. Hand pollination of the icu11-5 cp2-1 double mutant, but not the emf2-3 single mutant, resulted in a few seeds, only one of which germinated and developed an embryonic flower when grown on growth medium containing 3% sucrose. In this growth condition, icu11-5 cp2-1 anthers were smaller than those of Col-0, and only 64% contained small but viable pollen grains. When grown under short-day conditions, the mutant emf2-3 also develops a small shoot with two or three flowers and short siliques that did not produce mature seeds (Chen et al., 1997).
The moderate reduction in pollen viability that we observed by Alexander staining does not explain the extremely low production and viability of icu11-5 cp2-1 seeds. This clearly suggests the existence of additional problems with either ovule formation, fertilization or embryonic development. Further research would be needed to determine the causes of icu11-5 cp2-1 reduced fertility.
Data availability statement
The original contributions presented in the study are included in the article/Supplementary Material. Further inquiries can be directed to the corresponding author.
Author contributions
JLM conceived and supervised the study, provided resources, and obtained funding. RN, LJ-V, and JLM designed the methodology. RN, LJ-V, and EM-B performed the experiments. RN, LJ-V, and JLM wrote the original draft. All authors contributed to the article and approved the submitted version.
Funding
The author(s) declare financial support was received for the research, authorship, and/or publication of this article. This work was supported by the Ministerio de Ciencia e Innovación of Spain (PGC2018-093445-B-I00, EQC2018-005181-P and EQC2019-006592-P [MCI/AEI/FEDER, UE]) and the Generalitat Valenciana (PROMETEO/2019/117 and IDIFEDER/2020/019). RN, EM-B, and LJ-V held predoctoral fellowships from the Generalitat Valenciana (GRISOLIAP/2016/131) and the Ministerio de Universidades of Spain (FPU13/00371 and FPU16/03772), respectively.
Acknowledgments
The authors wish to thank JM Serrano and J Castelló for their excellent technical assistance. This manuscript was previously published as a preprint at: https://www.biorxiv.org/content/10.1101/2023.04.20.537354v1.
Conflict of interest
The authors declare that the research was conducted in the absence of any commercial or financial relationships that could be construed as a potential conflict of interest.
Publisher’s note
All claims expressed in this article are solely those of the authors and do not necessarily represent those of their affiliated organizations, or those of the publisher, the editors and the reviewers. Any product that may be evaluated in this article, or claim that may be made by its manufacturer, is not guaranteed or endorsed by the publisher.
Supplementary material
The Supplementary Material for this article can be found online at: https://www.frontiersin.org/articles/10.3389/fpls.2023.1239093/full#supplementary-material
References
Bancroft, I., Jones, J. D., Dean, C. (1993). Heterologous transposon tagging of the DRL1 locus in Arabidopsis. Plant Cell 5, 631–368. doi: 10.1105/tpc.5.6.631
Barberon, M., Berthomieu, P., Clairotte, M., Shibagaki, N., Davidian, J. C., Gosti, F. (2008). Unequal functional redundancy between the two Arabidopsis thaliana high-affinity sulphate transporters SULTR1;1 and SULTR1;2. New Phytol. 180, 608–619. doi: 10.1111/j.1469-8137.2008.02604.x
Barboza-Barquero, L., Nagel, K. A., Jansen, M., Klasen, J. R., Kastenholz, B., Braun, S., et al. (2015). Phenotype of Arabidopsis thaliana semi-dwarfs with deep roots and high growth rates under water-limiting conditions is independent of the GA5 loss-of-function alleles. Ann. Bot. 116, 321–331. doi: 10.1093/aob/mcv099
Barrero, J. M., González-Bayón, R., Del Pozo, J. C., Ponce, M. R., Micol, J. L. (2007). INCURVATA2 encodes the catalytic subunit of DNA Polymerase α and interacts with genes involved in chromatin-mediated cellular memory in Arabidopsis thaliana. Plant Cell 19, 2822–2838. doi: 10.1105/tpc.107.054130
Berná, G., Robles, P., Micol, J. L. (1999). A mutational analysis of leaf morphogenesis in Arabidopsis thaliana. Genetics 152, 729–742. doi: 10.1093/genetics/152.2.729
Bloomer, R. H., Hutchison, C. E., Bäurle, I., Walker, J., Fang, X., Perera, P., et al. (2020). The Arabidopsis epigenetic regulator ICU11 as an accessory protein of Polycomb Repressive Complex 2. Proc. Natl. Acad. Sci. U. S. A. 117, 16660–16666. doi: 10.1073/pnas.1920621117
Bouveret, R., Schönrock, N., Gruissem, W., Hennig, L. (2006). Regulation of flowering time by Arabidopsis MSI1. Development 133, 1693–1702. doi: 10.1242/dev.02340
Briggs, G. C., Osmont, K. S., Shindo, C., Sibout, R., Hardtke, C. S. (2006). Unequal genetic redundancies in Arabidopsis – a neglected phenomenon? Trends Plant Sci. 11, 492–498. doi: 10.1016/j.tplants.2006.08.005
Brunaud, V., Balzergue, S., Dubreucq, B., Aubourg, S., Samson, F., Chauvin, S., et al. (2002). T-DNA integration into the Arabidopsis genome depends on sequences of pre-insertion sites. EMBO Rep. 3, 1152–1157. doi: 10.1093/embo-reports/kvf237
Caño-Delgado, A., Yin, Y., Yu, C., Vafeados, D., Mora-García, S., Cheng, J. C., et al. (2004). BRL1 and BRL3 are novel brassinosteroid receptors that function in vascular differentiation in Arabidopsis. Development 131, 5341–5351. doi: 10.1242/dev.01403
Castillejo, C., Romera-Branchat, M., Pelaz, S. (2005). A new role of the Arabidopsis SEPALLATA3 gene revealed by its constitutive expression. Plant J. 43, 586–596. doi: 10.1111/j.1365-313X.2005.02476.x
Chandler, C. H., Chari, S., Dworkin, I. (2013). Does your gene need a background check? How genetic background impacts the analysis of mutations, genes, and evolution. Trends Genet. 29, 358–366. doi: 10.1016/j.tig.2013.01.009
Chanvivattana, Y., Bishopp, A., Schubert, D., Stock, C., Moon, Y. H., Sung, Z. R., et al. (2004). Interaction of Polycomb-group proteins controlling flowering in Arabidopsis. Development 131, 5263–5276. doi: 10.1242/dev.01400
Chen, X. (2004). A microRNA as a translational repressor of APETALA2 in Arabidopsis flower development. Science 303, 2022–2025. doi: 10.1126/science.1088060
Chen, L., Cheng, J. C., Castle, L., Sung, Z. R. (1997). EMF genes regulate Arabidopsis inflorescence development. Plant Cell 9, 2011–2024. doi: 10.1105/tpc.9.11.2011
Clerkx, E. J., Blankestijn-De Vries, H., Ruys, G. J., Groot, S. P., Koornneef, M. (2004). Genetic differences in seed longevity of various Arabidopsis mutants. Physiol. Plant 121, 448–461. doi: 10.1111/j.0031-9317.2004.00339.x
Clough, S. J., Bent, A. F. (1998). Floral dip: a simplified method for Agrobacterium-mediated transformation of Arabidopsis thaliana. Plant J. 16, 735–743. doi: 10.1046/j.1365-313x.1998.00343.x
Cong, L., Ran, F. A., Cox, D., Lin, S., Barretto, R., Habib, N., et al. (2013). Multiplex genome engineering using CRISPR/Cas systems. Science 339, 819–823. doi: 10.1126/science.1231143
Cusack, S. A., Wang, P., Lotreck, S. G., Moore, B. M., Meng, F., Conner, J. K., et al. (2021). Predictive models of genetic redundancy in Arabidopsis thaliana. Mol. Biol. Evol. 38, 3397–3414. doi: 10.1093/molbev/msab111
Duan, L., Pérez-Ruiz, J. M., Cejudo, F. J., Dinneny, J. R. (2021). Characterization of CYCLOPHILLIN38 shows that a photosynthesis-derived systemic signal controls lateral root emergence. Plant Physiol. 185, 503–518. doi: 10.1093/plphys/kiaa032
Fernando, V. C. D., Al Khateeb, W., Belmonte, M. F., Schroeder, D. F. (2018). Role of Arabidopsis ABF1/3/4 during det1 germination in salt and osmotic stress conditions. Plant Mol. Biol. 97, 149–163. doi: 10.1007/s11103-018-0729-6
Förderer, A., Zhou, Y., Turck, F. (2016). The age of multiplexity: recruitment and interactions of Polycomb complexes in plants. Curr. Opin. Plant Biol. 29, 169–178. doi: 10.1016/j.pbi.2015.11.010
Fu, A., He, Z., Cho, H. S., Lima, A., Buchanan, B. B., Luan, S. (2007). A chloroplast cyclophilin functions in the assembly and maintenance of photosystem II in Arabidopsis thaliana. Proc. Natl. Acad. Sci. U. S. A. 104, 15947–15952. doi: 10.1073/pnas.0707851104
Gaj, T., Gersbach, C. A., Barbas, C. F. (2013). ZFN, TALEN, and CRISPR/Cas-based methods for genome engineering. Trends Biotechnol. 31, 397–405. doi: 10.1016/j.tibtech.2013.04.004
Goodrich, J., Puangsomlee, P., Martin, M., Long, D., Meyerowitz, E. M., Coupland, G. (1997). A Polycomb-group gene regulates homeotic gene expression in Arabidopsis. Nature 386, 44–51. doi: 10.1038/386044a0
Haffter, P., Granato, M., Brand, M., Mullins, M. C., Hammerschmidt, M., Kane, D. A., et al. (1996). The identification of genes with unique and essential functions in the development of the zebrafish, Danio rerio. Development 123, 1–36. doi: 10.1242/dev.123.1.1
Hohenstatt, M. L., Mikulski, P., Komarynets, O., Klose, C., Kycia, I., Jeltsch, A., et al. (2018). PWWP-DOMAIN INTERACTOR OF POLYCOMBS1 interacts with Polycomb-group proteins and histones and regulates Arabidopsis flowering and development. Plant Cell 30, 117–133. doi: 10.1105/tpc.17.00117
Huang, C., Yu, Q. B., Lv, R. H., Yin, Q. Q., Chen, G. Y., Xu, L., et al. (2013). The reduced plastid-encoded polymerase-dependent plastid gene expression leads to the delayed greening of the Arabidopsis fln2 mutant. PloS One 8, e73092. doi: 10.1371/journal.pone.0073092
Huq, E., Quail, P. H. (2002). PIF4, a phytochrome-interacting bHLH factor, functions as a negative regulator of phytochrome B signaling in Arabidopsis. EMBO J. 21, 2441–2450. doi: 10.1093/emboj/21.10.2441
Iglesias, F. M., Bruera, N. A., Dergan-Dylon, S., Marino-Buslje, C., Lorenzi, H., Mateos, J. L., et al. (2015). The Arabidopsis DNA polymerase δ has a role in the deposition of transcriptionally active epigenetic marks, development and flowering. PloS Genet. 11, e1004975. doi: 10.1371/journal.pgen.1004975
Islam, M. S., Leissing, T. M., Chowdhury, R., Hopkinson, R. J., Schofield, C. J. (2018). 2-oxoglutarate-dependent oxygenases. Annu. Rev. Biochem. 87, 585–620. doi: 10.1146/annurev-biochem-061516-044724
Jia, Y., Ding, Y., Shi, Y., Zhang, X., Gong, Z., Yang, S. (2016). The cbfs triple mutants reveal the essential functions of CBFs in cold acclimation and allow the definition of CBF regulons in Arabidopsis. New Phytol. 212, 345–353. doi: 10.1111/nph.14088
Jinek, M., Chylinski, K., Fonfara, I., Hauer, M., Doudna, J. A., Charpentier, E. (2012). A programmable dual RNA-guided DNA endonuclease in adaptive bacterial immunity. Science 337, 816–821. doi: 10.1126/science.1225829
Jürgens, G., Mayer, U., Torres Ruiz, R. A., Berleth, T., Miséra, S. (1991). Genetic analysis of pattern formation in the Arabidopsis embryo. Dev. Supplem. 113, 27–38. doi: 10.1242/dev.113.Supplement_1.27
Kawai, Y., Ono, E., Mizutani, M. (2014). Evolution and diversity of the 2-oxoglutarate-dependent dioxygenase superfamily in plants. Plant J. 78, 328–343. doi: 10.1111/tpj.12479
Kleinmanns, J. A., Schatlowski, N., Heckmann, D., Schubert, D. (2017). BLISTER regulates Polycomb-target genes, represses stress-regulated genes and promotes stress responses in Arabidopsis thaliana. Front. Plant Sci. 8. doi: 10.3389/fpls.2017.01530
Kobayashi, K., Endo, K., Wada, H. (2016). Multiple impacts of loss of plastidic phosphatidylglycerol biosynthesis on photosynthesis during seedling growth of Arabidopsis. Front. Plant Sci. 7. doi: 10.3389/fpls.2016.00336
Koornneef, M., Alonso-Blanco, C., Vreugdenhil, D. (2004). Naturally occurring genetic variation in Arabidopsis thaliana. Annu. Rev. Plant Biol. 55, 141–172. doi: 10.1146/annurev.arplant.55.031903.141605
Koornneef, M., Hanhart, C. J., van der Veen, J. H. (1991). A genetic and physiological analysis of late flowering mutants in Arabidopsis thaliana. Mol. Gen. Genet. 229, 57–66. doi: 10.1007/BF00264213
Kradolfer, D., Wolff, P., Jiang, H., Siretskiy, A., Köhler, C. (2013). An imprinted gene underlies postzygotic reproductive isolation in Arabidopsis thaliana. Dev. Cell 26, 525–535. doi: 10.1016/j.devcel.2013.08.006
Labun, K., Montague, T. G., Krause, M., Torres Cleuren, Y. N., Tjeldnes, H., Valen, E. (2019). CHOPCHOP v3: expanding the CRISPR web toolbox beyond genome editing. Nucleic Acids Res. 47, W171–W174. doi: 10.1093/nar/gkz365
Lee, I., Michaels, S. D., Masshardt, A. S., Amasino, R. M. (1994). The late-flowering phenotype of FRIGIDA and mutations in LUMINIDEPENDENS is suppressed in the Landsberg erecta strain of Arabidopsis. Plant J. 6, 903–909. doi: 10.1046/j.1365-313X.1994.6060903.x
Leng, Y. J., Yao, Y. S., Yang, K. Z., Wu, P. X., Xia, Y. X., Zuo, C. R., et al. (2022). Arabidopsis ERdj3B coordinates with ERECTA-family receptor kinases to regulate ovule development and the heat stress response. Plant Cell 34, 3665–3684. doi: 10.1093/plcell/koac226
López-González, L., Mouriz, A., Narro-Diego, L., Bustos, R., Martínez-Zapater, J. M., Jarillo, J. A., et al. (2014). Chromatin-dependent repression of the Arabidopsis floral integrator genes involves plant specific PHD-containing proteins. Plant Cell 26, 3922–3938. doi: 10.1105/tpc.114.130781
Martinez, S., Hausinger, R. P. (2015). Catalytic mechanisms of Fe(II)- and 2-oxoglutarate-dependent oxygenases. J. Biol. Chem. 290, 20702–20711. doi: 10.1074/jbc.R115.648691
Mateo-Bonmatí, E., Esteve-Bruna, D., Juan-Vicente, L., Nadi, R., Candela, H., Lozano, F. M., et al. (2018). INCURVATA11 and CUPULIFORMIS2 are redundant genes that encode epigenetic machinery components in Arabidopsis. Plant Cell 30, 1596–1616. doi: 10.1105/tpc.18.00300
Meinke, D. W. (2013). A survey of dominant mutations in Arabidopsis thaliana. Trends Plant Sci. 18, 84–91. doi: 10.1016/j.tplants.2012.08.006
Méndez-Vigo, B., Martínez-Zapater, J. M., Alonso-Blanco, C. (2013). The flowering repressor SVP underlies a novel Arabidopsis thaliana QTL interacting with the genetic background. PloS Genet. 9, e1003289. doi: 10.1371/journal.pgen.1003289
Mizukami, Y., Ma, H. (1992). Ectopic expression of the floral homeotic gene AGAMOUS in transgenic Arabidopsis plants alters floral organ identity. Cell 71, 119–131. doi: 10.1016/0092-8674(92)90271-D
Mouchel, C. F., Briggs, G. C., Hardtke, C. S. (2004). Natural genetic variation in Arabidopsis identifies BREVIS RADIX, a novel regulator of cell proliferation and elongation in the root. Genes Dev. 18, 700–714. doi: 10.1101/gad.1187704
Nadi, R., Mateo-Bonmatí, E., Juan-Vicente, L., Micol, J. L. (2018). The 2OGD superfamily: emerging functions in plant epigenetics and hormone metabolism. Mol. Plant 11, 1222–1224. doi: 10.1016/j.molp.2018.09.002
Navarro-Quiles, C., Lup, S. D., Muñoz-Nortes, T., Candela, H., Micol, J. L. (2023). The genetic and molecular basis of haploinsufficiency in flowering plants. Trends Plant Sci. doi: 10.1016/j.tplants.2023.07.009
Nowak, M. A., Boerlijst, M. C., Cooke, J., Smith, J. M. (1997). Evolution of genetic redundancy. Nature 388, 167–171. doi: 10.1038/40618
Nüsslein-Volhard, C., Wieschaus, E. (1980). Mutations affecting segment number and polarity in Drosophila. Nature 287, 795–801. doi: 10.1038/287795a0
Ohtomo, I., Ueda, H., Shimada, T., Nishiyama, C., Komoto, Y., Hara-Nishimura, I., et al. (2005). Identification of an allele of VAM3/SYP22 that confers a semi-dwarf phenotype in Arabidopsis thaliana. Plant Cell Physiol. 46, 1358–1365. doi: 10.1093/pcp/pci146
Page, D. R., Grossniklaus, U. (2002). The art and design of genetic screens: Arabidopsis thaliana. Nat. Rev. Genet. 3, 124–136. doi: 10.1038/nrg730
Pérez-Pérez, J. M., Candela, H., Micol, J. L. (2009). Understanding synergy in genetic interactions. Trends Genet. 25, 368–376. doi: 10.1016/j.tig.2009.06.004
Pikaard, C. S., Mittelsten Scheid, O. (2014). Epigenetic regulation in plants. Cold Spring Harb. Perspect. Biol. 6, a019315. doi: 10.1101/cshperspect.a019315
Piñeiro, M., Gómez-Mena, C., Schaffer, R., Martínez-Zapater, J. M., Coupland, G. (2003). EARLY BOLTING IN SHORT DAYS is related to chromatin remodeling factors and regulates flowering in Arabidopsis by repressing FT. Plant Cell 15, 1552–1562. doi: 10.1105/tpc.012153
Ponce, M. R., Quesada, V., Micol, J. L. (1998). Rapid discrimination of sequences flanking and within T-DNA insertions in the Arabidopsis genome. Plant J. 14, 497–501. doi: 10.1046/j.1365-313X.1998.00146.x
Quesada, V., Ponce, M. R., Micol, J. L. (2000). Genetic analysis of salt-tolerant mutants in Arabidopsis thaliana. Genetics 154, 421–436. doi: 10.1093/genetics/154.1.421
Riechmann, J. L., Meyerowitz, E. M. (1997). Determination of floral organ identity by Arabidopsis MADS domain homeotic proteins AP1, AP3, PI, and AG is independent of their DNA-binding specificity. Mol. Biol. Cell 8, 1243–1259. doi: 10.1091/mbc.8.7.1243
Rustérucci, C., Aviv, D. H., Holt, B. F., 3rd, Dangl, J. L., Parker, J. E. (2001). The disease resistance signaling components EDS1 and PAD4 are essential regulators of the cell death pathway controlled by LSD1 in Arabidopsis. Plant Cell 13, 2211–2224. doi: 10.1105/tpc.010085
Schatlowski, N., Stahl, Y., Hohenstatt, M. L., Goodrich, J., Schubert, D. (2010). The CURLY LEAF interacting protein BLISTER controls expression of Polycomb-group target genes and cellular differentiation of Arabidopsis thaliana. Plant Cell 22, 2291–2305. doi: 10.1105/tpc.109.073403
Schönrock, N., Exner, V., Probst, A., Gruissem, W., Hennig, L. (2006). Functional genomic analysis of CAF-1 mutants in Arabidopsis thaliana. J. Biol. Chem. 281, 9560–9568. doi: 10.1074/jbc.M513426200
Scortecci, K., Michaels, S. D., Amasino, R. M. (2003). Genetic interactions between FLM and other flowering-time genes in Arabidopsis thaliana. Plant Mol. Biol. 52, 915–922. doi: 10.1023/a:1025426920923
Sung, Z. R., Belachew, A., Shunong, B., Bertrand-Garcia, R. (1992). EMF, an Arabidopsis gene required for vegetative shoot development. Science 258, 1645–1647. doi: 10.1126/science.258.5088.1645
Taylor, M. B., Ehrenreich, I. M. (2015). Higher-order genetic interactions and their contribution to complex traits. Trends Genet. 31, 34–40. doi: 10.1016/j.tig.2014.09.001
Tsutsui, H., Higashiyama, T. (2017). pKAMA-ITACHI vectors for highly efficient CRISPR/Cas9-Mediated gene knockout in Arabidopsis thaliana. Plant Cell Physiol. 58, 46–56. doi: 10.1093/pcp/pcw191
Wang, L., Xu, D., Scharf, K., Frank, W., Leister, D., Kleine, T. (2022). The RNA-binding protein RBP45D of Arabidopsis promotes transgene silencing and flowering time. Plant J. 109, 1397–1415. doi: 10.1111/tpj.15637
Yamamoto, Y. Y., Puente, P., Deng, X. W. (2000). An Arabidopsis cotyledon-specific albino locus: a possible role in 16S rRNA maturation. Plant Cell Physiol. 41, 68–76. doi: 10.1093/pcp/41.1.68
Yang, C. H., Chen, L. J., Sung, Z. R. (1995). Genetic regulation of shoot development in Arabidopsis: role of the EMF genes. Dev. Biol. 169, 421–435. doi: 10.1006/dbio.1995.1158
Yang, Z., Qian, S., Scheid, R. N., Lu, L., Chen, X., Liu, R., et al. (2018). EBS is a bivalent histone reader that regulates floral phase transition in Arabidopsis. Nat. Genet. 50, 1247–1253. doi: 10.1038/s41588-018-0187-8
Yoo, S. Y., Kim, Y., Kim, S. Y., Lee, J. S., Ahn, J. H. (2007). Control of flowering time and cold response by a NAC-domain protein in Arabidopsis. PloS One 2, e642. doi: 10.1371/journal.pone.0000642
Yoshida, N., Yanai, Y., Chen, L., Kato, Y., Hiratsuka, J., Miwa, T., et al. (2001). EMBRYONIC FLOWER2, a novel Polycomb group protein homolog, mediates shoot development and flowering in Arabidopsis. Plant Cell 13, 2471–2481. doi: 10.1105/tpc.010227
Yoshimoto, N., Inoue, E., Watanabe-Takahashi, A., Saito, K., Takahashi, H. (2007). Posttranscriptional regulation of high-affinity sulfate transporters in Arabidopsis by sulfur nutrition. Plant Physiol. 145, 378–388. doi: 10.1104/pp.107.105742
Zhao, C., Zhang, Z., Xie, S., Si, T., Li, Y., Zhu, J. K. (2016). Mutational evidence for the critical role of CBF transcription factors in cold acclimation in Arabidopsis. Plant Physiol. 171, 2744–2759. doi: 10.1104/pp.16.00533
Keywords: epigenetic machinery, Arabidopsis thaliana, ICU11, 2OGD, CUPULIFORMIS2, CRISPR/Cas9
Citation: Nadi R, Juan-Vicente L, Mateo-Bonmatí E and Micol JL (2023) The unequal functional redundancy of Arabidopsis INCURVATA11 and CUPULIFORMIS2 is not dependent on genetic background. Front. Plant Sci. 14:1239093. doi: 10.3389/fpls.2023.1239093
Received: 12 June 2023; Accepted: 26 October 2023;
Published: 15 November 2023.
Edited by:
Baohua Wang, Nantong University, ChinaReviewed by:
Sang-Tae Kim, The Catholic University of Korea, Republic of KoreaCécile Raynaud, UMR9213 Institut des Sciences des Plantes de Paris Saclay (IPS2), France
Copyright © 2023 Nadi, Juan-Vicente, Mateo-Bonmatí and Micol. This is an open-access article distributed under the terms of the Creative Commons Attribution License (CC BY). The use, distribution or reproduction in other forums is permitted, provided the original author(s) and the copyright owner(s) are credited and that the original publication in this journal is cited, in accordance with accepted academic practice. No use, distribution or reproduction is permitted which does not comply with these terms.
*Correspondence: José Luis Micol, amxtaWNvbEB1bWguZXM=
†Present address: Eduardo Mateo-Bonmatí, Centro de Biotecnología y Genómica de Plantas (CBGP), Universidad Politécnica de Madrid (UPM) – Instituto Nacional de Investigación y Tecnología Agraria y Alimentaria (INIA)/CSIC, Pozuelo de Alarcón, Madrid, Spain