- 1Department of Biotechnology, Graphic Era Deemed to be University, Dehradun, India
- 2Forest Pathology Discipline, Forest Protection Division, ICFRE-Forest Research Institute, Dehradun, India
- 3Genetics and Tree Improvement Division, ICFRE-Forest Research Institute, Dehradun, India
- 4Forest Ecology and Climate Change Division, ICFRE-Forest Research Institute, Dehradun, India
- 5Department of Zoology, Ram Chandra Uniyal Government Post Graduate College College, Uttarkashi, India
- 6Department of Microbiology, Graphic Era Deemed to be University, Dehradun, India
Turmeric (Curcuma longa L.), a significant commercial crop of the Indian subcontinent is widely used as a condiment, natural dye, and as a cure for different ailments. Various bioactive compounds such as turmerones and curcuminoids have been isolated from C. longa that have shown remarkable medicinal activity against various ailments. However, reduced soil fertility, climatic variations, rapid urbanization, and enhanced food demand, pose a multifaceted challenge to the current agricultural practices of C. longa. Plant growth-promoting microbes play a vital role in plant growth and development by regulating primary and secondary metabolite production. Rhizospheric associations are complex species-specific interconnections of different microbiota with a plant that sustain soil health and promote plant growth through nutrient acquisition, nitrogen fixation, phosphate availability, phytohormone production, and antimicrobial activities. An elaborative study of microbiota associated with the roots of C. longa is essential for rhizospheric engineering as there is a huge potential to develop novel products based on microbial consortium formulations and elicitors to improve plant health, stress tolerance, and the production of secondary metabolites such as curcumin. Primarily, the purpose of this review is to implicate the rhizospheric microbial flora as probiotics influencing overall C. longa health, development, and survival for an increase in biomass, enhanced yield of secondary metabolites, and sustainable crop production.
Introduction
According to United Nations, Department of Economic and Social Affairs (DESA), the world population is projected to reach 9 billion by November 2022 (World Population Prospects, 2022). India ranks among one of the most populous countries in the world, with its current population estimated to be 1.429 billion (United Nations Population Fund, 2022). However, in the future, it is expected that the Indian population would increase to 1.67 billion by the year 2050 (World Population Prospects, 2022).
Consequently, the demand for resources such as energy, water, and food will increase in the near future. To meet these growing demands sustainable practices of food production need to be adopted to minimize the impacts of current agricultural practices. Conventional agricultural practices in India, characterized by intensive crop cultivation, excessive usage of synthetic fertilizers, pesticides, and water, have led to the depletion of natural resources, environment, and land (Schlaeppi and Bulgarelli, 2015). Sustainable agriculture, which envisages the use of organic fertilizers, biofertilizers, and biopesticides for crop production and a reduction in the residual impact of harmful agrochemicals on the environment and food chain, is a fundamental necessity for food security (Kumar et al., 2017). Plants possess a diverse array of microorganisms on their surface (phylloplane and rhizoplane) as well as inner tissues (endosphere). These plant-microbe interactions are a complex interplay of harmful, neutral, as well as beneficial relations for the plant. Such interactions between plants and their environment have been an intriguing research area for the scientific community. Deciphering the potential use of such rhizospheric microbiota is pitched to be the long-term answer to the deleterious effects caused by agricultural practices following the “green revolution” and transition towards organic farming and sustainable agricultural practices for food security, human health, and reducing environmental pollution (Suman et al., 2022).
India a country renowned for its rich diversity of spices (Ramkumar and Karuppsamy, 2021) cultivates approximately 60 diverse varieties of spices, a fact that has been well-documented since ancient times (Mathew, 2013). Curcuma longa L. is one of the most widely used and economically important spice crops in India. C. longa, also known as ‘Indian saffron’ or ‘Golden spice’, is a rhizomatous herb of the Zingiberaceae family and is popularly known as Turmeric, haldi, and haridra. It is reported to have originated in south-eastern countries like India, Bangladesh, and China. India is the world’s largest consumer, producer as well as exporter of C. longa and hence, it is economically a significant crop for the Indian farmers. It serves as a spice, condiment, organic colour, and is also used in traditional medicine systems to treat various diseases (Nair, 2013). It has been utilised in Ayurvedic, Unani, and Siddha traditional medical practices since 4500 BCE. Numerous bioactive compounds such as turmerones and curcuminoids have been isolated from C. longa that have shown remarkable medicinal activity against diabetes, cancer, sinusitis, anaemia, skin diseases, intoxication, wounds, and ulcers. Studies have shown that C. longa has anti-oxidative, chemopreventive, anti-microbial, anti-diabetic, anti-carcinogenic, anti-mutagenic, anti-angiogenic, hepatoprotective, anti-thrombotic, wound healing properties, anti-inflammatory activity and therapeutic properties against Alzheimer’s disease (Nair, 2019).
C. longa rhizosphere hosts a myriad of microorganisms in its rhizosphere, which have been reported for their plant growth-promoting properties. Associations between the beneficial microbiota and roots are favoured by the release of carbon compounds or rhizodeposition, which facilitates the colonization of rhizospheric soil by species-specific microbes as compared to bulk soil (Kumar et al., 2017). Plant growth-promoting rhizobacteria (PGPR) such as Azospirillum (Dutta and Neog, 2015), Azotobacter (Kumar et al., 2014), Bacillus (Vinayarani and Prakash, 2018), Rhizobium (Chandran, 2019), and Pseudomonas (Kumar et al., 2016a) have been widely reported from the rhizosphere of C. longa. Klebsiella (Anisha et al., 2013), Penibacillus (Aswathy et al., 2013), Bacillus (Kumar et al., 2017), and Pseudomonas (Vinayarani and Prakash, 2018) are some of the important bacterial endophytes reported from the rhizome of C. longa. Rhizospheric associations are complex species-specific interconnections of different microbiota with a plant that not only help to sustain the soil environment but also promote the growth of plants. Beneficial microbes enhance plant growth by improving nutrition uptake by solubilizing minerals necessary for optimum plant growth such as Phosphorus (P), Potassium (K), Zinc (Zn), and Nitrogen fixation and production of certain plant growth regulators (PGR) such as Auxin viz. Indole-3-acetic acid (IAA), Cytokines, and Gibberellic acids (Hakim et al., 2021; Suman et al., 2022). PGPRs also produce antibacterial, antibiotic, and antifungal compounds, which help in the biocontrol of phytopathogens; Iron (Fe) chelating agents; stimulate induced systemic resistance; and help in biotic and abiotic stress amelioration. These PGPRs have also been reported to enrich the accumulation of important secondary metabolites such as curcuminoids in the rhizome of C. longa (Kumar et al., 2016a).
To date, studies have indicated that the rhizospheric microbiome in C. longa can significantly impact plant growth and enhance crop productivity. However, a comprehensive synthesis of the existing literature on this topic is lacking. This systematic review aims to bridge this knowledge gap by analyzing and synthesizing the current body of literature on rhizospheric microbiome interactions in C. longa. By systematically examining the available studies, we seek to unravel rhizosphere engineering (RE), including specific microbial communities associated with C. longa, their functions, and the mechanisms through which they influence plant growth and crop productivity.
The findings of this review hold significant implications for agricultural practices and the cultivation of C. longa. Understanding the intricate relationships between the rhizospheric microbiome and C. longa can aid in the development of targeted strategies to enhance plant growth, improve nutrient uptake efficiency, and mitigate diseases. Moreover, unravelling these interactions can potentially lead to the optimization of crop management practices, contributing to sustainable agriculture and increased crop yields. Therefore, this systematic compilation could be a valuable resource for researchers, agronomists, and farmers interested in harnessing the potential of the rhizospheric microbiome to enhance plant growth and maximize crop productivity.
Rhizosperic microbes of C. longa
The preferential enrichment of microbial populations in the rhizosphere zone partly relies on the rhizodeposition of carbon compounds excreted by plants (Hassan et al., 2019; Zhou et al., 2020). A comprehensive investigation conducted by Kumar et al. observed the prevalence of Proteobacteria (α, β, and γ), particularly Pseudomonas, Klebsiella, Agrobacterium, Azotobacter, and Burkholderia, accounting for two-thirds of the total bacterial population isolated from the rhizosphere of C. longa (Kumar et al., 2017). Similarly, in another study by the same research group focusing on endophytic bacteria of C. longa, various bacteria were identified and characterized, including Bacillus thuringiensis, Bacillus sp., Bacillus pumilis, Pseudomonas putida, and Clavibacter michiganensis (Kumar et al., 2016a). All the isolated rhizospheric and endophytic bacteria demonstrated indole-3-acetic acid (IAA) production, a key characteristic of rhizospheric bacteria (Sokolova et al., 2011). These rhizospheric and endophytic microbes (Table 1) play a crucial role in influencing crop productivity by promoting enhanced plant growth, evidenced by increased leaf count, heightened plant height, and other desirable traits (Subrahmanyam et al., 2020). Moreover, they actively contribute to the synthesis of secondary metabolites, equipping the plant with mechanisms to combat both abiotic and biotic stresses (Patel et al., 2020; Chandra et al., 2022). In a similar line of research, Kumar et al. isolated a free-living nitrogen-fixing bacterium, Azobacter chroococcum CL13, from the rhizosphere of C. longa and investigated its impact on plant growth and curcumin content (Kumar et al., 2014).
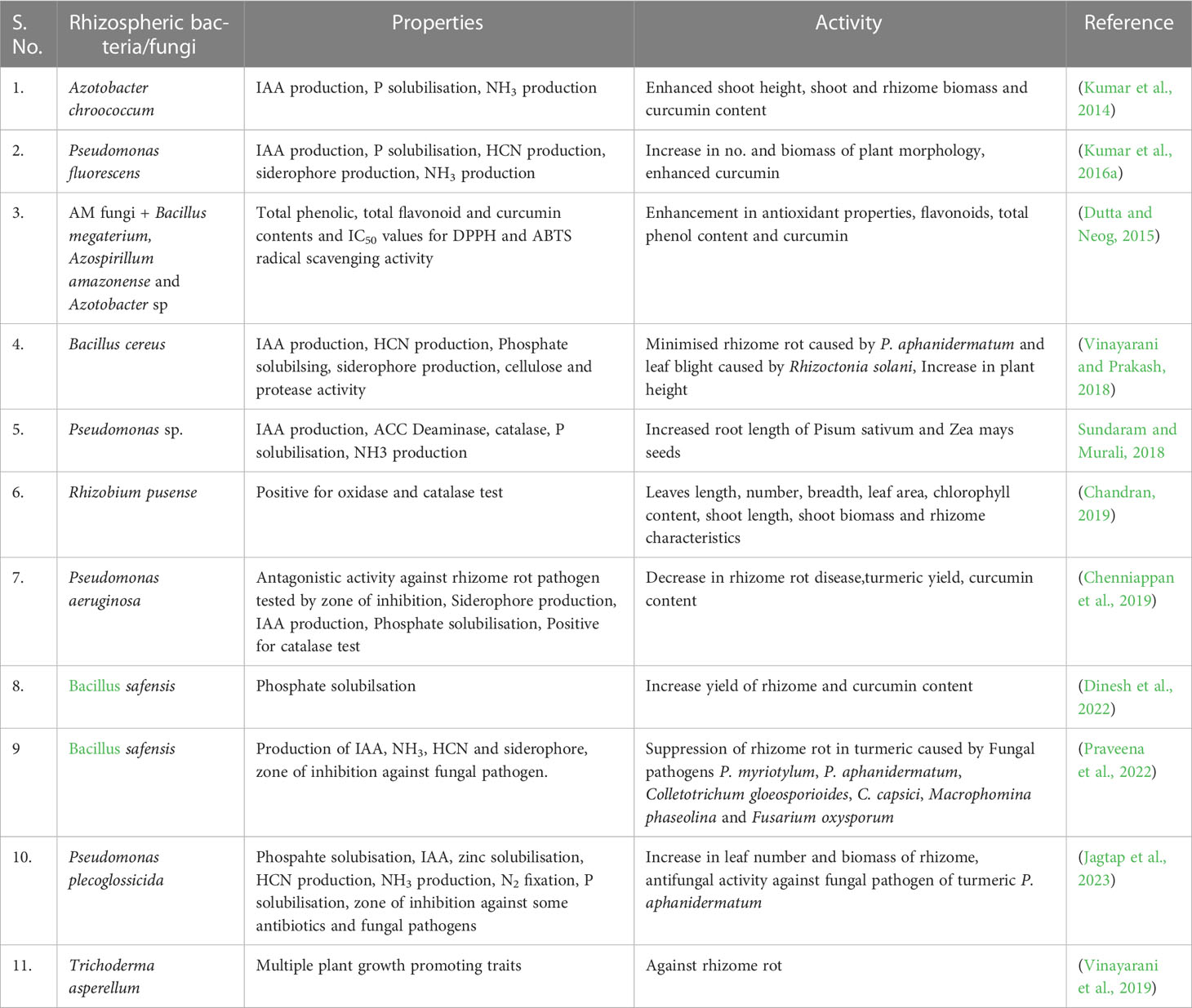
Table 1 Microbial isolates from the rhizosphere of C. longa and their role in plant growth promotion.
In addition, the bacteria residing in the rhizosphere zone also play a significant role in the production of secondary metabolites, providing the plant with defense mechanisms against various abiotic and biotic stresses (Patel et al., 2020; Chandra et al., 2022). Similarly, Kumar et al. conducted a similar study where they isolated a nitrogen-fixing bacterium, Azobacter chroococcum CL13, from the rhizosphere of C. longa. They examined the impact of this bacterium on plant growth and the content of curcumin, a bioactive compound present in turmeric (Kumar et al., 2014). Chenniappan et al. conducted a study demonstrating the biocontrol effectiveness of rhizobacteria derived from turmeric fields against fungal pathogens responsible for rhizome rot in turmeric. Out of the 157 isolated rhizobacteria, a total of 16 bacterial species showed notable inhibitory effects on the growth of various fungal pathogens, namely Rhizoctonia solani MML4001, Fusarium solani MML4002, Schizophyllum commune MML4003, Macrophomina phaseolina MML4004, Fusarium graminearum MML4005, Fusarium solani MML4006 and Fusarium solani MML4007. These bacterial species included Pseudomonas aeruginosa MML2424, P. aeruginosa MML2515, P. aeruginosa MML2519, Bacillus amyloliquefaciens MML2522, B. amyloliquefaciens MML2547, Bacillus tequilensis MML2476, Bacillus cereus MML2533, Bacillus subtilis MML2406, B. subtilis MML2411, B. subtilis MML2415, B. subtilis MML2451, B. subtilis MML2458, B. subtilis MML2473, B. subtilis MML2483, B. subtilis MML2490 and B. subtilis MML2518 (Chenniappan et al., 2019)
In order to investigate the role of rhizospheric bacteria in correlation to plant health, Kumar et al. (2019) has isolated nine bacterial species. These species included Bacillus subtilis CL1, Bacillus sp. CL3, Burkholderia thailandensis CL4, Agrobacterium tumefaciens CL5, Klebsiella sp. CL6, Bacillus cereus CL7, P. putida CL9, Pseudomonas fluorescens CLI2 and Azotobacter chroococcum CL13 from the rhizosphere of C. longa. All of these isolates exhibited activities related to phosphate solubilization, and IAA, and ammonia production, which are key characteristics for the identification of PGPR. Furthermore, they were characterized for salt tolerance, antimicrobial activities. Bacillus subtilis, P. putida, and demonstrated tolerance to 6% NaCl, whereas compared to Agrobacterium tumefaciens could only tolerate 1% NaCl. Additionally, almost all strains exhibited antifungal effects against Aspergillus niger, and Alterneria alternate. Based on their superior performance, a P. fluorescens strain was selected for the development of an inoculum (Kumar et al., 2016b).
Dutta and Neog (2015) formulated a consortium inoculum by utilizing microorganisms obtained from the rhizosphere of C. longa and investigated their impact on soil enzyme activities and microbial biomass. The inoculum was specifically composed of arbuscular mycorrhizal fungi (Glomus, Gigaspora and Acaulospora sp.) along with phosphate solubilizers (Bacillus megaterium), and nitrogen fixers (Azospirillum amazonense and Azotobacter sp.). The designed inoculum demonstrated a preferential ability to enhance the soil enzyme activities, including phosphatase, dehydrogenase, and urease as well as microbial biomass in the soil (Dutta and Neog, 2015). This research indicated that such inoculums, combining mycorrhizal fungi along with bacteria could serve as a viable alternative for the sustainable cultivation of C. longa. Antagonistic effect of PGPR and endophytes (isolated from C. longa) against Pythium aphanidermatum (Edson) Fitzp., and Rhizoctonia solani Kuhn., causing rhizome rot and leaf blight diseases respectively, in turmeric, investigated using dual culture and liquid culture methods (Vinayarani and Prakash, 2018). The results revealed that five PGPR isolates and four endophyte isolates were capable of suppressing 70% of the growth of both pathogens.
In a distinct approach, the effect of N2 fixer Rhizobium pusense isolated from the rhizosphere of C.longa, on the growth characteristics of C. longa was studied by Chandran (2019). In this approach rhizome was inoculated with Rhizobium pusense (108 CFU/mL) along with 1% CMC. The results showed significant improvements in various growth parameters, including the number and length of leaves, chlorophyll content, and rhizome biomass, indicating enhanced growth in the treated plants compared to the control group (Chandran, 2019). In a recent study, the impact of phosphate solubilizing bacteria; Bacillus safensis (NCBI-MT192800), B. marisflavi (NCBI-MT 192801), B. cereus (NCBI- MT192803), and P. aeruginosa (NCBI-MZ 540872) isolated from the rhizosphere of C. longa, on the yield, and quality of turmeric was studied in green house and as well as in field conditions (Dinesh et al., 2022). Among all the phosphate solubilizing bacteria, Bacillus safensis has shown promising results in phosphate solubilisation and consequently the yield of rhizome. Further in continuation of the work, Bacillus safensis was also tested for antifungal activity against Pythium myriotylum, P. aphanidermatum, Colletotrichum gloeosporioides, C. capsici, Macrophomina phaseolina and Fusarium oxysporum under in vitro conditions (Praveena et al., 2022). Bacillus safensis was identified with the presence of antimicrobial peptide (AMP) genes for bacillomycin, surfactin, and iturin. In conclusion, the research group highlighted that Bacillus safensis has the potential to serve as a valuable isolate for reducing the reliance on fungicide chemicals. Similarly, plant growth promoting, antifungal, primary (curcumin), and secondary metabolite production activities of Serratia nematodiphila RGK and P. plecoglossicida RGK isolated from the rhizosphere of the turmeric plant, were studied (Jagtap et al., 2023). These isolates were subjected to a pot experiment to assess their effects, and the results revealed that P. plecoglossicida exhibited superior outcomes compared to S. nematodiphila in terms of leaf count and rhizome biomass.
The role of rhizospheric fungus in promoting plant growth and providing resistance to rhizome rot disease was initially investigated by (Vinayarani et al., 2019). Out of the thirty fungal isolates obtained from the C. longa field, only five demonstrated significant inhibition (>70%) in the growth of P. aphanidermatum. These five potential isolates were identified as Trichoderma viride PGPFDOB-V6, Chaetomium sp. PGPFDOB-V13, T. viride PGPFDOB-V11, Trichoderma harzianum PGPFDOB-V22, and T. asperellum PGPFDOB-V36. Table 1 presents a compilation of PGPR isolates obtained from the rhizosphere of C. longa, along with their respective properties and roles in promoting growth and enhancing rhizome or curcumin yield.
Endophytes of C. longa
Many endophytic microorganisms have been discovered to be associated with C. longa L. (Table 2). These endophytic microbes play a crucial role in promoting plant growth and offering protection against plant pathogens through the production of various secondary metabolites (Ludwig-Müller, 2015; Pandey et al., 2020). Paenibacillus sp. is frequently encountered as an endophyte in woody plants like pine, coffee, and poplar. However, the research group led by Aswathy has also documented its presence as an endophyte in the rhizome of C. longa. It showed IAA production and may help in fighting stress conditions (Aswathy et al., 2013). P. aeruginosa, an endophytic bacterium obtained from the rhizome of C. longa, exhibits traits of PGPR. It has been demonstrated to be effective in reducing the incidence of rhizome rot and leaf blight by inhibiting the growth of the respective causative agents, P. aphanidermatum and R. solani (Vinayarani and Prakash, 2018). In a different application, (Singh et al., 2014) isolated an endophytic fungi viz., Penicillium sp. from the leaves of turmeric and were found to inhibit E.coli and S. aureus when applied with silver nanoparticles. An endophytic fungi; Eurotium Sp. isolated from the rhizome of C. longa is responsible for the production of asparaginase enzyme (Jalgaonwala and Mahajan, 2014). Similarly, an endophytic fungus of rhizome, namely Phoma herbarum exhibited a strong growth inhibition of fungal pathogen Colletotrichum gloeosporioides the causal organism of leaf spot of turmeric (Gupta et al., 2016). Endophytic fungi; A. foliicola and F. verticillioides of rhizome of C. longa were also reported for inhibiting the growth of Morganella morganii, a common histamine-producing bacteria in fish (Septiana et al., 2017).
Twenty different types of endophytic bacteria were isolated from C. longa (Vinayarani and Prakash, 2018) and characterized for antagonistic effect against two major pathogens, namely Pythium aphanidermatum (Edson) Fitzp., and Rhizoctonia solani Kuhn., causing rhizome rot and leaf blight diseases, respectively. Among all the endophytes, P. aeruginosa has shown most promising results by inhibiting the growth of both pathogens. Moreover, P. aeruginosa contributed in plant growth promotion as plant height, and rhizome yield significantly increased compared to untreated control.
PGPR: a compelling tool for rhizosphere engineering
Rhizosphere is a thin layer of soil which is in close proximity to the roots and is a hotspot of numerous microorganisms. The three major components of rhizosphere are the plant (root), soil and microbes (Figure 1). Each of the three components can be engineered to enhance plant productivity (Dessaux et al., 2016). Root architecture and rhizodepositions of a plant are important traits that shape the microbial activity in the rhizosphere (Kumar et al., 2017). Numerous studies of plant improvement either by genetic engineering or traditional breeding have been published for increased uptake of zinc, phosphorus, and iron (Clemens, 2014, disease resistance (Zhang et al., 2019) and photoremediation of heavy metals (Gunarathne et al., 2019). Despite the advantages of plant engineering their large scale application remains few and far between due to social acceptance, regulatory issues, environmental sustainability and human health concerns. Various methods, such as applying livestock manure, sewage waste, fly ash, zeolite, silicon, and biochar, have been used to enrich the soil (Dessaux et al., 2016; Villegas-Cornelio and Laines Canepa, 2017; Jakkula and Wani, 2018). Advancements in analytical instrumentation, microbial ecology, and plant genetics have also contributed significantly to soil fertility and crop productivity. However, the application of rhizospheric microbes as a microbial inoculum, known as rhizosphere engineering, has emerged as an easy and cost-effective approach to enhance soil fertility and crop productivity. As a result, rhizosphere engineering has become a preferred technique for manipulating the microbiome to promote better plant growth (Dessaux et al., 2016). PGPR inhabiting the rhizosphere play a vital role in plant growth and development by regulating primary and secondary metabolite production. Rhizospheric associations are complex species-specific interconnections of different microbiota with a plant that sustain soil health and promote plant growth through nutrient acquisition, nitrogen fixation, phosphate availability, phytohormone production, and antimicrobial activities (Suman et al., 2022; Figure 2). Kumar et al. (2014); Kumar et al. (2016b) isolated Azotobacter chroococcum and P. fluorescens, respectively, from C. longa rhizosphere and reported their role in increasing plant biomass and enhanced curcumin accumulation in the rhizome. Similarly, the use of Azospirillum amazonense and Azotobacter sp. and P. aeruginosa increased the curcumin accumulation in the rhizome and decreased the occurrence of rhizome rot disease in C. longa, respectively (Dutta and Neog, 2015; Chenniappan et al., 2019). Vinayarani et al. (2019) also reported T. asperellum from C. longa and showed that its exogenous use significantly reduced the chances of rhizome rot. Detailed description of rhizospheric and endophytic microbes associated with C. longa and their plant growth promoting activity is described in Tables 1, 2, respectively. Yanti et al. (2021) developed a rhizobacterial consortium between Bacillus thuringiensis strain RBI 2AB1.1, Cyanobacteria RZ2AB2.1., B. subtilis BSn5 RBI IPBL 2.3 and B. cereus strain APSB03 RBI 2AB 2.2 and reported enhanced growth in tomato plants along with protection from phytopathogens after its application. Similarly, Burkholderia gladioli, Pseudomonas sp. and B. subtilis were used as a consortium to improve the growth of fenugreek plants (Kumar et al., 2020). Use of Enterobacter hormaechei (AM122) and Lysinibacillus xylanilyticus (DB25) in a consortium enhanced growth, yield and aroma in basmati and nonbasmati rice varieties (Dhondge et al., 2021). A rhizobacterial consortium of helpful microorganisms such as the ones described above which promote the growth of plant by facilitating nutrient acquisition, fixing atmospheric nitrogen, help in accumulation of secondary metabolites and reduce the population of phytopathogens should be developed and promoted for sustainable growth of C. longa.
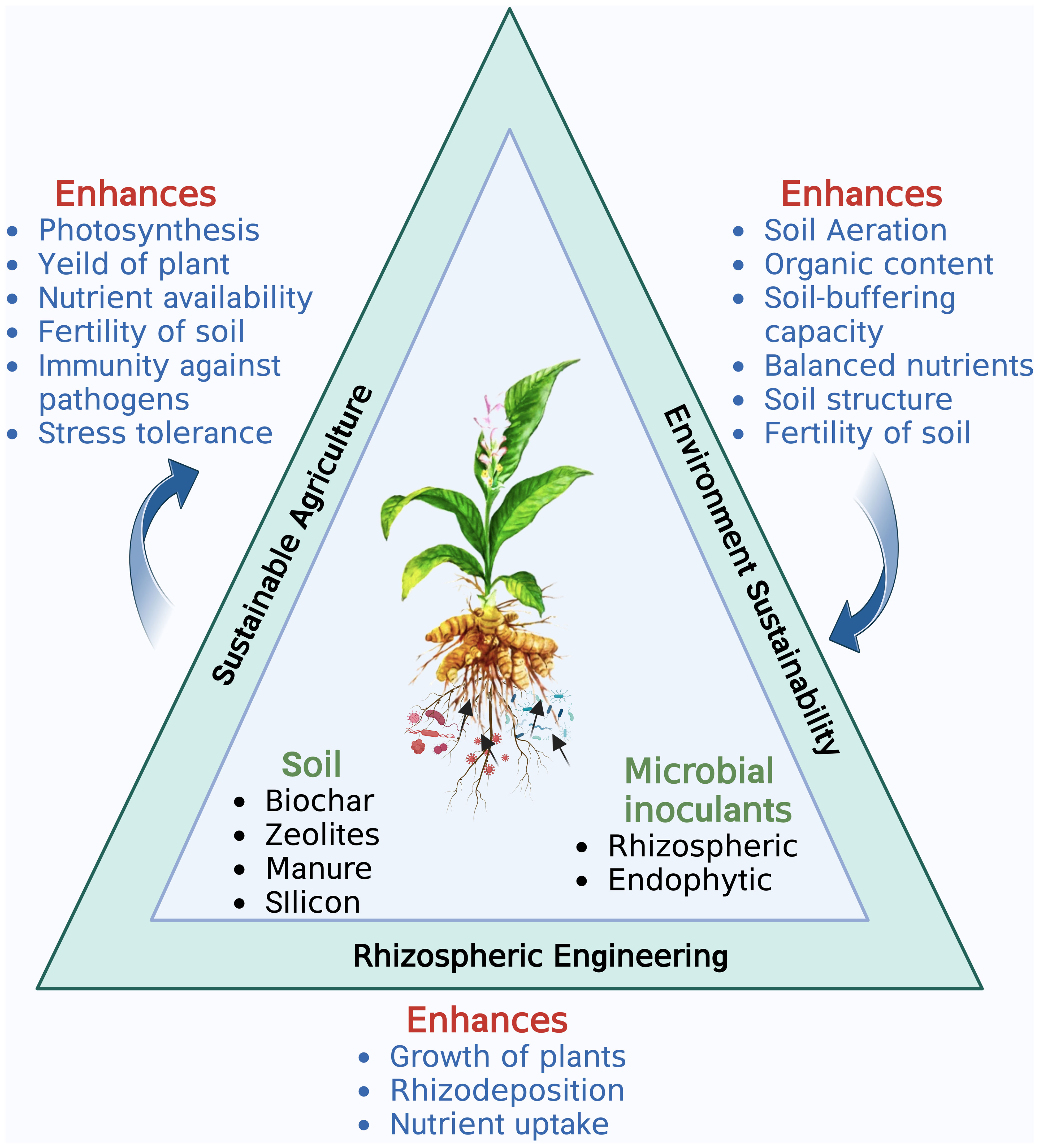
Figure 1 The major components of Rhizosphere engineering and their interconnections. Created with BioRender.com.
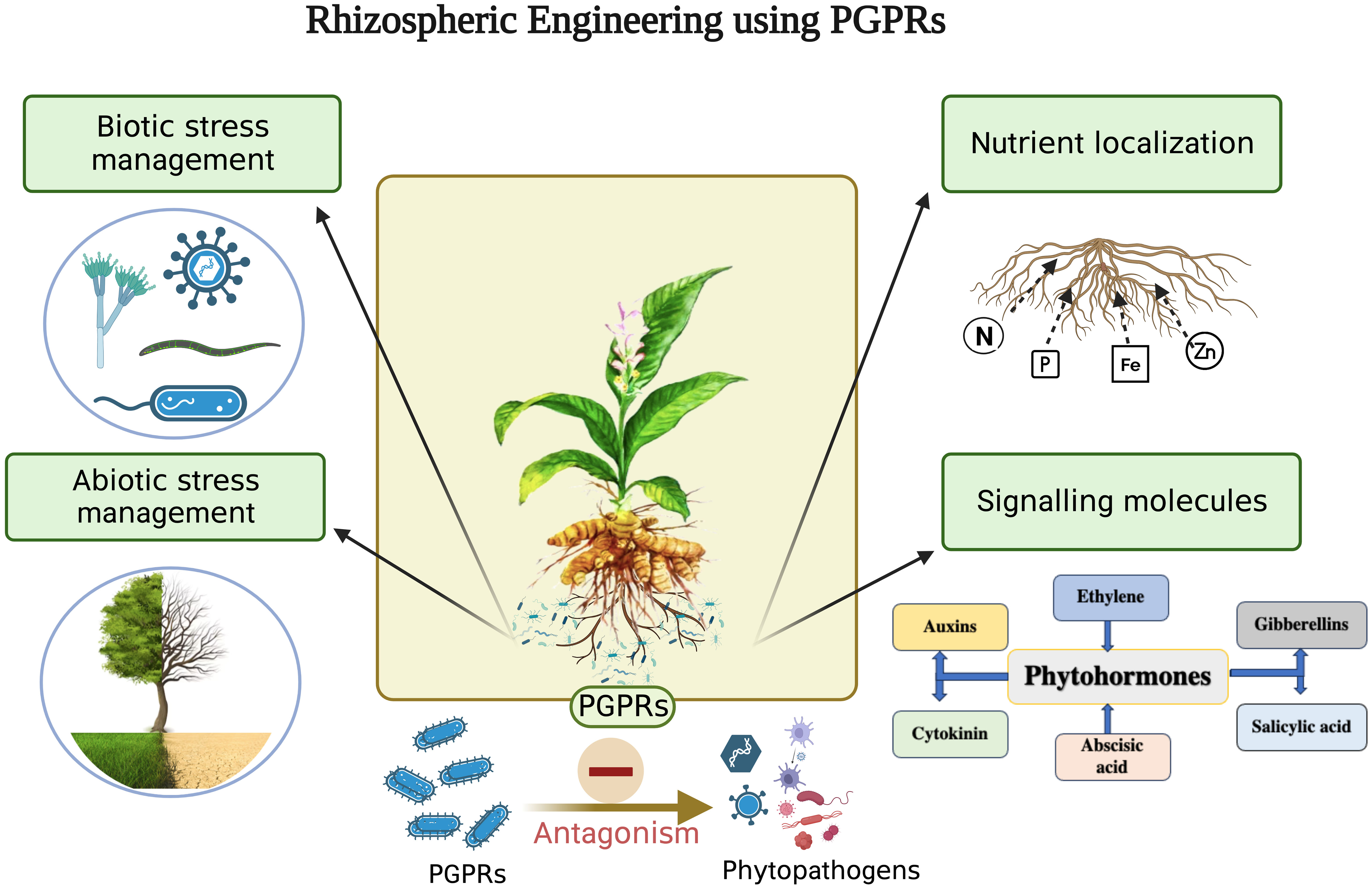
Figure 2 Role of PGPRs in sustainable agriculture (Legend: N - Nitrogen, P - Phosphorus, Fe - Iron, Zn – Zinc, PGPR – Plant growth promoting rhizobacteria). Created with BioRender.com.
Mechanism of PGPR in rhizosphere engineering
Rhizosphere engineering, an increasingly popular field of research, strives to comprehend and regulate plant and microbial interactions in order to boost plant growth, productivity in agriculture, and sustainability concerns (Ahkami et al., 2017). New approaches are being developed to solve the worldwide food crisis and promote sustainable agricultural practices by leveraging the power of the rhizosphere (Sharma et al., 2021) (Figure 1). Plant-microbe interactions in the rhizosphere are relatively complex and mutually beneficial (Schenk et al., 2012). PGPR are beneficial soil microbes that form symbiotic relationships with plants (Singh, 2023). They boost nutrient uptake in plants, increase resistance to pests and diseases, and contribute to overall plant health and vigour (Abdelaziz et al., 2023; Gul et al., 2023; Hyder et al., 2023). PGPR actively influences the microbial community composition and modulates plant-microbe interactions (Gul et al., 2023). There are various ways in which PGPRs engineer the rhizosphere environment viz. by solubilizing the nutrients, fixing atmospheric nitrogen, producing and accumulating phytohormones, production of biocontrol agents to fight against phytopathogens and providing resistance to plants against biotic and abiotic stresses (Borah et al., 2023; Gupta and Pandey, 2023) (Figure 2).
Rhizospheric nutrient mobilization
Rhizosphere is a dynamic and active ecosystem, which supports an eclectic mix of microorganisms such as bacteria, fungi, and protozoa that are essential to the cycling of nutrients (Murphy et al., 2015; Garcia and Kao-Kniffin, 2018). In order to make nutrients more readily available for plant absorption, microorganisms, and plants work together in the rhizosphere, which serves as a hotspot for nutrient mobilization and enhances the nutritional composition of soil by fixing nitrogen (Richardson et al., 2009; Figure 3).
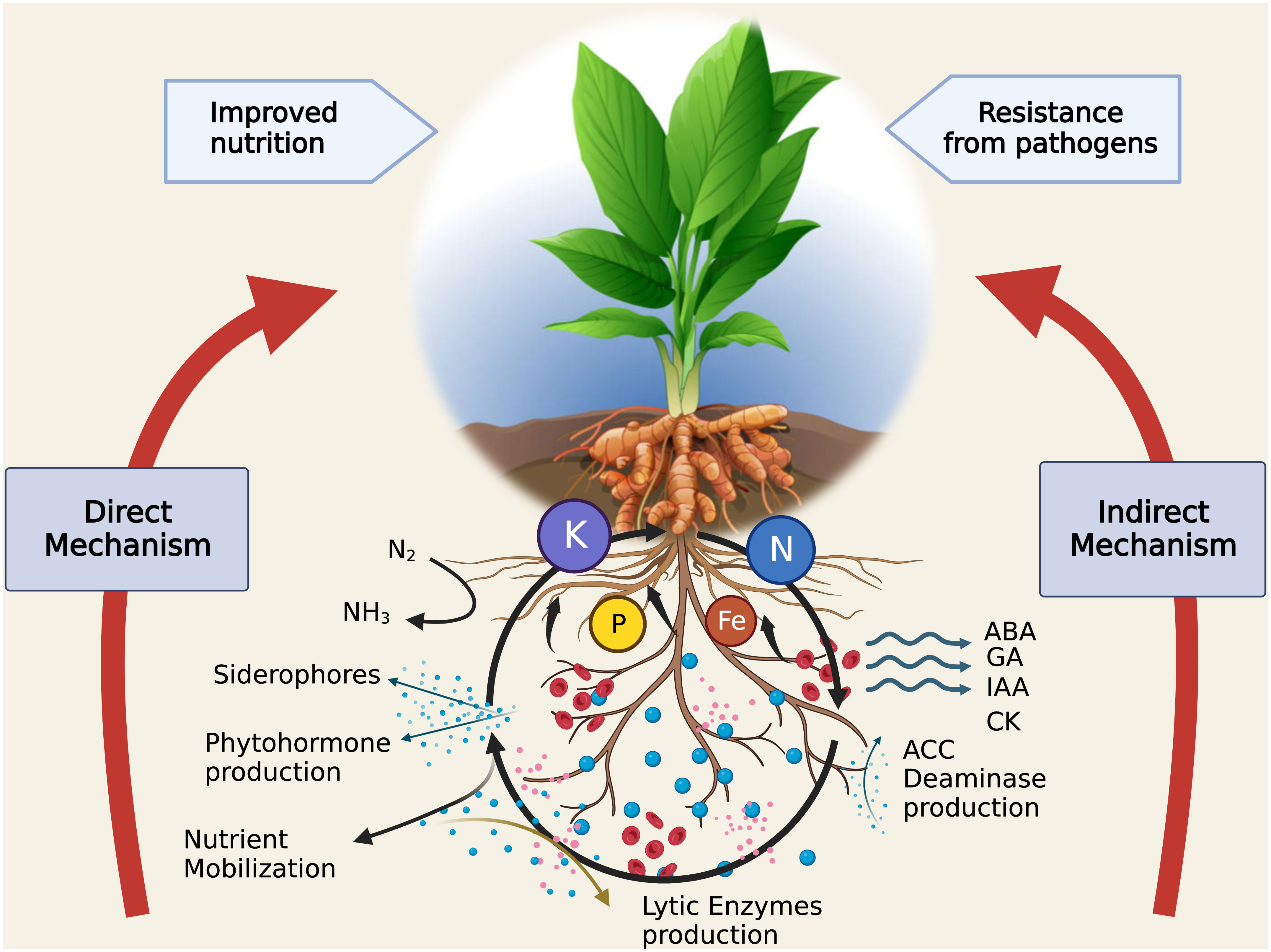
Figure 3 Intricate relationships between soil, rhizosphere, and plant for nutrient mobilization. (Legend: N/N2 - Nitrogen, NH3 – Ammonia, P - Phosphorus, Fe - Iron, K - Potassium, ABA - Abscisic acid, GA - Gibberellic acid, IAA - Indole-3-acetic acid, CK – Cytokines, ACC - 1-aminocyclopropane-1-carboxylic acid). Created with BioRender.com.
Phosphate solubilization
Phosphate (PO4-3) is an essential macronutrient for plant growth and its deficiency can hamper several crucial biological processes. It is present in both inorganic and organic forms in the soil, inorganic being an easily absorbable form by plants. However, a certain fraction remains bounded to soil rendering it unavailable to plants. Here comes the role of PGPRs, which can solubilize the phosphate by using several mechanisms and making it available to plants. These PGPRs in the form of biofertilizers and bioinoculants when applied to plant systems enhance their capabilities to solubilise the phosphorus. These biofertilizers are a group of bacteria, fungi, and archaea that works singly or as a consortium of microbes. They create acidic conditions around the rhizosphere by secreting organic acids like citric acid, oxalic acid, malic acid, succinic acid, acetic acid, fumaric acid, indoleacetic acid, ketogluconic acid (Paredes and Victoria, 2010) and acidic environment solubilize the phosphate from its inorganic state. Another mechanism through which PGPRs enhance the accessibility of phosphorus is by producing phosphatase enzymes; these enzymes liberate phosphorus from organic form to inorganic form. Studies carried out on different crop species highlight the role of PGPRs in phosphate solubilization viz. phosphorus uptake and nitrogen fixation in chickpeas (Wani et al., 2007), growth promotion in maize (Hameeda et al., 2008), improving plant health of tomato (Hariprasad and Niranjana, 2009) and in peanuts (Jiang et al., 2019) where PGPRs enhanced phosphorus solubilization in saline soil.
Zinc mobilization
Another crucial micronutrient that plays an important role in the biological processes of plants is zinc; it is mostly present in soluble form and insoluble complexes. Zinc deficiency is one of the most prevalent micronutrient deficits, especially in soils with high pH. PGPRs utilize two main approaches to enhance the availability of zinc for plants in the rhizosphere, where zinc often exists in insoluble complexes. The first approach involves the release of organic acids (OAs) to solubilize these complexes, while the second approach involves the secretion of chelating agents to accomplish the same goal. The role of zinc solubilizing bacteria has been studied widely in many crops like soybean (Srithaworn et al., 2023), capsicum (Bhatt and Maheshwari, 2020), wheat (Kamran et al., 2017), rice (Vaid et al., 2014) and for nutrient uptake in maize (Goteti et al., 2013). Organic acid production is the most widely used mechanism by PGPRs to solubilize the complex insoluble forms of nutrients. These organic acids have the ability to dissolve insoluble forms of zinc. Eshaghi et al. (2019) explain the role of proton transporters which pump protons (H+) into the rhizosphere and make the rhizospheric environment acidic. This acidic environment aids the solubilization of nutrients by converting them to soluble forms. In another mechanism, PGPRs employ alternative strategy i.e. secretion of chelating agents, such as siderophores, which are considered crucial in the solubilization of iron (Fe), zinc (Zn), and other essential micronutrients (Saravanan et al., 2011).
Iron sequestration
Iron plays a pivotal role in electron chains, contributing to energy production and serving as a cofactor for numerous essential enzymes. Insufficient iron levels can lead to chlorosis, characterized by yellowing of leaves with green veins. Several factors hinder iron availability to plants, including high pH levels, iron-bound compounds, and the low solubility of iron (Zhang et al., 2019). Enhancement of iron uptake and utilization by plants is achieved by the addition of PGPRs and they act through several mechanisms like acidification of root zone by forming organic acids and through proton pumps, as Iron solubilization requires low pH. Formation of siderophores is also done to solubilize the Iron. Siderophores are low molecular weight chelating molecules with a strong affinity for Iron, although they are also used in zinc and phosphorus mobilization. Siderophores bind to Fe ions, generating soluble complexes that plants can readily absorb (Shameer and Prasad, 2018). The formation of siderophores by PGPRs aids in the sequestration of Iron and the availability of Iron to plants in soil deficient in Iron. Certain phytohormones are produced by PGPRs like Auxins, they alter the root morphology by root hair development and expansion thereby increasing the surface area of roots for better uptake of Iron by plants. PGPRs increase the expression of genes involved in the Fe absorption mechanism in plants (Morrissey and Guerinot, 2009) like ferric chelate reductase, which converts insoluble Fe3+ to soluble Fe2+ forms. Furthermore, PGPRs can increase the activity of iron transporters in root cells, boosting Iron uptake (Singh et al., 2020), Azospirillum, Azotobacter, Bacillus, Enterobacter, Mycobacterium, and Pseudomonas are some of the bacteria reported for iron uptake in plants by siderophore production (Ghosh et al., 2020; Singh et al., 2020).
Nitrogen fixation
Nitrogen is an indispensable element used in the synthesis of proteins, nucleic acids, and photosynthetic pigment and is a major driver in plant growth and development. PGPRs help plants fix nitrogen in the soil through a symbiotic relationship. While rhizobia are predominantly responsible for nitrogen fixation in legumes, certain associative or endophytic Nitrogen-fixing PGPRs are also isolated which can also fix atmospheric nitrogen in non-legume species (Lugtenberg and Kamilova, 2009). The following steps are involved in the nitrogen fixation process by PGPRs: they colonize the rhizosphere and by using chemotaxis move towards roots and then penetrate the root cells through wounds or any opening. They use a similar strategy to fix nitrogen in legumes as rhizobium, through nodule formation and by nitrogenase enzyme but in non-legume species, nitrogen is fixed by associative or endophytic nitrogen-fixing PGPRs which do not form nodules but reside inside the intercellular spaces of the root cells. There are several PGPRs that have been reported for their nitrogen-fixing abilities in plants such as Pseudomonas spp. in maize (Piromyou et al., 2011) and sugarcane (Li et al., 2017; Singh et el., 2020), Rhizobium in common beans (Yadegari et al., 2010), kallar grass and rice (Malik et al., 1997) and soybean (Dashti et al., 1998)
Phytohormone production
Plant hormones or phyto-hormones are molecules present within plant system, which act as chemical messengers and regulate physiological processes (Davies, 2004). The classical phytohormones include auxins, cytokinins, gibberellins (GAs), ethylene (ETH), and abscisic acid (ABA). The former three are known as growth promoters whereas ETH and ABA are growth inhibitory. Besides these, jasmonates, salicylates, strigolactones and brassinosteroids are relatively novice additions to the group. Hakim et al. (2021) classified plant-rhizosphere interactions into direct and indirect mechanism to decipher the role of PGPR activities. PGPR-stimulated growth promotion via phyto-hormone induction is one of the direct-action mechanisms, which as suggested, aids plant growth by induction of plant hormones (auxins, cytokinins and GA) or regulation of higher levels (ethylene, Figure 3).
Auxins
Auxins are crucial phyto-hormones involved in development and growth of cells (Asgher et al., 2015). Apical dominance, phyllotaxis, root formation, embryogenesis, vascular differentiation, shoot architecture are a few of processes regulated by this class of phytohormones (Quint and Gray, 2006; Iqbal et al., 2022). Indole-3-acetic acid (IAA) is one of the most prominently explored auxins, found in plants. IAA synthesis involves multiple pathways amongst which indole-3-acetamide (IAM) synthesis (tryptophan as the precursor) is most common in bacteria (Tahir and Sarwar, 2013; Maheshwari et al., 2015). Auxin synthesising PGPRs have been identified amongst bacterial genera like Agrobacterium, Pantoea, Bacillus, Moraxella, Pseudomonas, Gluconobacter and Micrococcus (Barazani and Friedman, 1999; Liu et al., 2016). Inoculation with PGPRs increased IAA production (Liu et al., 2016), root biomass in wheat (Kudoyarova et al., 2017), yield in maize, cotton, canola (Iqbal et al., 2015, Iqbal et al., 2023; Qureshi et al., 2019) as well as certain active components viz essential oils in medicinal and aromatic plants (Santoro et al., 2011; Darzi et al., 2015; Santoro et al., 2015; Mishra et al., 2017). P. fluorescens and Bacillus spp. when inoculated to Curcumin longa (turmeric) reported an increase in curcumin content by 13 to 18 percent in comparison to control counterparts (Kumar et al., 2016a; Chauhan et al., 2017). Escalated level of secondary metabolites might be a reflection of enhanced metabolic functioning and nutrient availability (Çakmakçı et al., 2020).
Cytokinins
Cytokinins (CK) are another class of phyto-hormone family. These are growth regulating compounds present usually in low concentrations in plants all through the life cycle (Osugi and Sakkibara, 2015). Both natural and artificial CKs have been employed for plant growth regulations in different studies. Functions of CKs include promoting cell division and proliferation, embryogenesis; shoot differentiation, modulation of apical dominance, chlorophyll biosynthesis regulation and chloroplast genesis (Cortleven and Schmülling, 2015); root formation and architecture in combination with Auxin (Schaller et al., 2015) etc. PGPR induced CKs and their ability to reorient plant CK concentration have been studied to affect plant growth in a beneficial manner (Tsukanova et al., 2017). A plethora of bacterial genera is known to induce CKs in rhizosphere include Agrobacterium, Pseudomonas, Bacillus, Escherichia, Methylobacterium, Proteus, Klebsiella, Xanthomonas (Akiyoshi et al., 1987; García de Salamone et al., 2001; Karadeniz et al., 2006; Madhaiyan et al., 2006; Liu et al., 2013). CK producing PGPRs inoculation resulted in formation of fruit and shoot growth (Tsukanova et al., 2017); lateral root development in cucumber (Sokolova et al., 2011); resistance to infection with P. syringae in Arabidopsis thaliana (Grossinsky et al., 2016) and drought stress (Liu et al., 2013)
Gibberellins
Gibberellins (GAs) are well known plant growth regulators. The “bakane” disease of rice resulted in discovery of GAs, which were reported from and named after the fungus Gibberellin fujikuroi, better known as Fusarium fujikuroi now. Gibberellic acid (GA3), a diterpinoid acid is the most common and initially discovered member of gibberellins (Rodrigues et al., 2012). GAs are known to promote growth by targeting the growth inhibiting DELLA proteins and their subsequent degradation (Hedden and Sponsel, 2015). Cell elongation, division, overcoming dwarfism, flowering etc. are some of the primary function of this group. GAs have been known positively regulate leaf root meristem and leaf size (Martínez et al., 2016). Activation of stress-responsive genes via GA mediated phytohormone crosstalk has been reported to develop stress endurance against abiotic stresses (Jogawat et al., 2021). Other than plants, microbes are capable of synthesising GAs as well. Atzorn et al. (1988) were the first to demonstrate the ability of Rhizobium melitoli to produce bacterial gibberellins. Other genera of bacteria include Pseudomonas, Bacilus, Herbaspirillum, Azospirillum, Promicro, Leifsoniaxyli, Leifsonia, Acetobacter, (Bastián et al., 1998; Gutiérrez-Mañero et al., 2001; Probanza et al., 2002; Lenin and Jayanthi, 2010; Sharma et al., 2018). Fungal species reported to produce gibberellins include Fusarium fujikuroi, Fusarium moniliforme, Fusarium proliferatum (Machado et al., 2000; Camara et al., 2018) Fusarium oxysporum (Ben Rhouma et al., 2020), Penicillium variable (Isa and Mat Don, 2014) Paecilomyces spp (El-Sheikh et al., 2020).
Biocontrol of plant pathogens
Mitigation of stress is an important effect of beneficial microbes to host plants. These organisms could confer resistance directly by demonstrating antagonism to the phyto-pathogens. The indirect mechanisms include production of compounds which either check the growth of such pathogens or create competition for space, nutrients etc. Moreover, PGPRs released compounds also reduce the deteriorating effects of pathogen induced stress. ACC-deaminase synthesis, siderophore production, induced systemic resistance etc. are few of the indirect methods of plant-resistance induced by PGPRs (Figure 3). These have been discussed ahead.
ACC-deaminase synthesis
Ethylene production is one the most common effects of pathogen-induced stress in plants. Extreme conditions lead to enhanced ethylene release and its over-accumulation often has depreciating effects on plant health. Thus a high susceptibility is indicated by enhanced ethylene levels in plants (Glick, 2014; Müller and Munné-Bosch, 2015). 1-aminocyclopropane-1-carboxylate or ACC is the precursor for ethylene. ACC oxidase (ACO) oxidises ACC to ethylene and is a rate limiting step in Yang cycle (Wang et al., 2002). Rhizospheric micro biome inhabiting organisms produce ACC-deaminase, causing hydrolysis of ACC to α-ketobutyrate and ammonia (Saraf et al., 2010). Hence, reduction in ACC concentrations in rhizosphere furthers controls ethylene levels and promotes plant growth. The usual presence of ACC-deaminase producing PGPRs in the proximity of roots highlights the importance of these associations for plants (Orozco-Mosqueda et al., 2020). Studies across different plant-pathogen systems have revealed the ability of PGPRs to reduce susceptibility of host against pathogens. Fusarium oxysporum, Rhizoctonia solani, Phytopathora, Pythium spp, Xanthomonas oryzae are some pathogens which were controlled via ACC-deaminase activity (Pandey and Gupta, 2020; Hakim et al., 2021). Increasing productivity by mitigation of abiotic stress such as limited water availability in maize (Zarei et al., 2020), drought stress in wheat and chickpea (Timmusk et al., 2014; Tiwari et al., 2016), salinity stress (Saravanakumar and Samiyappan, 2007), phytoremediation of contaminated soil (Khan, 2005) are some of the other effects reported by ACC-deaminase producing microbes. Thus, manipulation of plant rhizosphere using ACC-deaminase producing PGPRs is a sustainable approach for bio-control of phyto-pathogens and promotion of plant growth for future.
Formation of siderophore
Siderophores are low molecular mass iron-chelating moieties, which increase the bioavailability of iron in the form of ferric/soluble Fe (III)-complexes, produced by microbes when iron is unavailable for nutrient uptake by plants (Sureshbabu et al., 2016). Buysens et al. (1996) discovered the anti-pathogenic ability of siderophores against soil borne microbes. Reduced availability of free iron in soil due to siderophores, increases competition in the soil and thus inhibits the growth of pathogens (Sahu and Sindhu, 2011; Shen et al., 2013; Reed et al., 2015). Rhodococcus, Enterobacter, Pseudomonas, Klebsiella, Bacillus, Bradyrhizobium, Streptomyces, Serratia, and Rhizobium are some genera of bacteria producing siderophores (Tian et al., 2009; Mustafa et al., 2019). Fungi, both freely living and symbionts, are also known to produce siderophores including T. harzianum, Trichoderma longibrachiatum, T. viride (Hussein and Joo, 2014; Ghosh et al., 2017), Ceratobasidium, Rhizoctonia (Haselwandter et al., 2006) and Aspergillus spp. (Schrettl et al., 2010). PGPRs producing siderophores could be used as green factories for Fe sequestration as well as green or biofertilizers. Reduced soil degradation and pollution, biological fixation of Fe, controlled leaching, reduced dependence on inorganic fertilizers and pesticides are some of the many benefits, which could be derived for sustainable approach to agriculture by employing PGPRs.
Antibiosis
Synthesis of allelopathic compounds in the rhizosphere, which check the growth of phytopathogens, is one reliable method to confer resistance in plants. Antibiotics are toxins produced by PGPRs which act antagonistically to check pathogens which are detrimental for host plant’s health and productivity. Antibiotics inhibit pathogen growth by impeding the processes of cell wall formation and structural membranes (Maksimov et al., 2011). The synthesis of antibiotics is stimulated with change in pH, temperature, available oxygen and even presence of other antibiotic molecules (Raaijmakers et al., 2002). PGPRs produced antibiotics have been a subject of keen interest over past few decades. 2,4-diacetylphloroglucinol (DAPG), pyoluteorin, phenazine, mycosubtilin, cyclic lipopeptides are a few to be named. Redox activity of phenazine, from Pseudomonas strains, was reported to inhibit Gaeumannomyces graminis and Fusarium oxysporum (Loper and Gross, 2007; Hakim et al., 2021). DAPG ceases zoospore formation and renders immunity to Phythium (Bhattacharyya and Jha, 2012). Bacillus cereus produced zwittermicin and kanosamine show anti-pathogen behaviour in-vitro (Milner et al., 1995; Milner et al., 1996). Stenotrophomonas sp., Serratia spp., Streptomyces spp., Agrobacterium radiobacter, Lysobacter spp., and Paenibacillus spp. are examples of genera studied for antibiotic production potential (Fridlender et al., 1993; Singh et al., 1999; Islam et al., 2005).
Secretion of lytic enzymes
Lytic enzymes are proteins which cause hydrolysis of specific organic components which constitute the cell walls and membranes. Dehrydrogenases, chitinases, phosphatases, lipases, β-1,3 glucanase are some of the enzymes produced by PGPRs naturally for protection against fungal and other bacterial pathogens. These PGPRs when inhabiting the rhizosphere of hosts provide a new line of defence against its natural pathogens (Lanteigne et al., 2012). The enzymes cause hydrolysis of the pathogen cell walls and membranes by changing structural composition and stability. Numerous PGPRs have been employed for their beneficial behaviour against plant pathogens. For example, chitinolytic activity of Serratia marcescens against Fusarium oxysporum and Rhizoctonia solani (Karthika et al., 2020), secretion of cellulase and β-1,3-glucanase by Streptomyces spp. and Paenibacillus conferred resistance to F. solani and Sclerotinia sclerotiorum (Mun et al., 2020). Similarly P. fluorescens is another important bio-control agent against root rots in Nicotiana tabacum and Brassica juncea (Arora et al., 2008; Gupta et al., 2015).
Induced systemic resistance
Plants, like other living organisms, have a congenital resistance to pathogenic microbes. However, resistance to such detrimental agents can also be induced in plants via application of certain chemicals or using beneficial microbes. Kloepper et al., 1992, delineated the former as systemic acquired resistance (SAR) whereas microbes-induced resistance is known as induced systemic resistance (ISR). ISR mediated resistance has been established and demonstrated across various species through inoculation with PGPRs. Plants recruit selective microbes in response to alteration of root exudation by phytopathogens (Berendsen et al., 2018; Chialva et al., 2018). Pseudomonas genus in itself has displayed its efficiency as a good resistance inducer in numerous plant species like tomato, sugarcane, rice, cucumber, tea, soyabean etc. (Nandakumar et al., 2001; Viswanathan and Ramasamy, 2001; Ramamoorthy et al., 2002; Saravanakumar and Samiyappan, 2007; Khalimi and Suprapta, 2011). The other genera involved in conferring resistance in hosts include Aeromonas, Azoarcus, Azospirillum, Azotobacter, Arthrobacter, Bacillus, Clostridium, Enterobacter, Gluconobacter, Klebsiella, Pseudomonas and Serratia (Annapurna et al., 2013). PGPR mediated ISR functions either by synthesising anti-microbial compounds (viz. coumarin) and furthering release of similar compound (Hu et al., 2018; Stringlis et al., 2018), modulating signalling of ethylene and jasmonic acid (Bukhat et al., 2020) or biofortification of cell wall in host resulting in enhanced resistance against pathogen like Fusarium oxysporum (Cha et al., 2016). Certain microbes also enhance adaptation and resistance by increasing nutrient solubilisation and uptake to the host (Kirankumar et al., 2008). The efficiency against a pathogen depends not only upon the inoculated strain but also the spatial distribution as well as number of PGPRs employed (Annapurna et al., 2013). Thus, inoculation with beneficial strains of microbes shows potential as a sustainable approach for biocontrol of phytopathogens and a good alternative for conventional pesticides.
Abiotic stress resistance
PGPRs aid plant in combating against both biotic and abiotic stress through various mechanisms. Drought, salinity and temperature extremities are the most common abiotic stresses which plants experience on a regular basis. Numerous studies have studied the effect of these abiotic stress on plants. Production of siderophores, phosphate solubilisation and increaseing reactive oxygen species (ROS) are some ways to alleviate heavy metal, draught and salinity stress in potato crop Gururani et al. (2013), phytohormone production and phosphate solubilization in tomato (Damodaran et al., 2013). Two PGPR strains P. aeruginosa and Burkholderia gladioli are reported in mitigating Cadmium toxicity in tomato seedlings by producing various phenolic compounds (Khanna et al., 2019). Another study reported that supplementing salicylic acid with PGPRs can increase the yields significantly and alleviate salinity stress in chickpea (Aneela et al., 2019). Bacillus velezensis provides resistance to wheat against various abiotic stresses viz. cold, frost, drought and heat by expressing genes and phenolic compounds which counteracts the stresses (Abd El-Daim et al., 2019). Heat stress is alleviated by Bacillus cereus by producing ACC-deaminase and polysaccharides in tomato (Mukhtar et al., 2020). ACC producing Pseudomonas sp. of bacteria previously isolated from turmeric (C. longa) were assessed in tomato against salt stress and it was found significant in alleviating both biotic and abiotic stresses (Pandey and Gupta, 2020). A transcriptomic and metabolomic study in tomato reveals that Pseudomonas oryzihabitans expresses ACC deaminase enzyme which is responsible for phosphate solubilization and siderophores production that further aids in providing salt tolerance to tomatoes (Mellidou et al., 2021, Figure 3).
Current challenges and future prospects
Sustainable cultivation of C. longa is a major challenge in agriculture today. Synthetic fertilizers and pesticides are commonly used to boost yield and enhance the contents of curcuminoids and sesquiterpenoids for the pharmaceutical industry. However, these practices harm the soil microbiome and disrupt plant-soil interactions. The application of PGPR can mitigate the negative effects of synthetic agrochemicals, improve soil fertility, and restore plant-rhizosphere-soil dynamics. Although microbial inoculants show promise for sustainable agriculture, their widespread adoption is hindered by inconsistent results in field trials, environmental and soil variations, farmer psychology, specificity of PGPR strains, formulation stability, re-inoculation, and regulatory concerns (Tabassum et al., 2017). The growing demand for safe organic food and interest in sustainable agriculture, and PGPR call for further research to understand climate change impacts on the soil microbiome. Identifying beneficial microorganisms from the rhizosphere of C. longa in different regions of India will help develop region-specific microbial consortia and enhance knowledge of plant-microbe interactions for secondary metabolite production. Additionally, advanced techniques like metagenomics, metatranscriptomics, and next-generation sequencing platforms are needed to study the dynamics of non-culturable microbes in the rhizosphere and the effects of PGPR on the accumulation of bioactive secondary metabolites, such as curcuminoids.
Conclusion
The current review highlights the advantageous contribution of C. longa rhizospheric microbiota in growth, yield, and secondary metabolite production. Current conventional agricultural practices, which include extensive use of chemical fertilizers and pesticides for plant growth, have resulted in the deterioration of soil health and fertility. In contrast, the application of PGPRs as biofertilizers and biocontrol has shown promising results for healthy and superior crop productivity with increased production of secondary metabolites such as curcuminoids. Leveraging microbial interventions for augmenting agricultural productivity through rhizospheric engineering presents an alluring approach to practicing sustainable agriculture, restoring soil health and productivity, and facilitating the synthesis of secondary metabolites. A comprehensive understanding of the diverse factors influencing plant-microbe interactions, particularly the ramifications of climate change, necessitates further investigation to devise future strategies for sustainable agriculture.
Author contributions
SK, A, SSh, KR, and AK prepared the first draft and made tables and figures. YS, MP, PR, MT, and AThap supervised the overall data collection and review of literature. SSi helped in revision of the manuscript. YS, MP, AThak, and SP edited the manuscript. YS and MP finalized the review. All authors contributed to the article and approved the submitted version.
Acknowledgments
The authors acknowledge the President and Vice Chancellor, Graphic Era Deemed to be University and Head, Department of Biotechnology, Graphic Era Deemed to be University for providing facility to conduct the research. The authors also acknowledge Miss. Poonam Pal, Dev Bhoomi Uttarakhand University, Navgaon, Dehradun for drawing a colour portrait of the turmeric plant for the manuscript.
Conflict of interest
The authors declare that the research was conducted in the absence of any commercial or financial relationships that could be construed as a potential conflict of interest.
Publisher’s note
All claims expressed in this article are solely those of the authors and do not necessarily represent those of their affiliated organizations, or those of the publisher, the editors and the reviewers. Any product that may be evaluated in this article, or claim that may be made by its manufacturer, is not guaranteed or endorsed by the publisher.
References
Abdelaziz, A. M., Hashem, A. H., El-Sayyad, G. S., El-Wakil, D. A., Selim, S., Alkhalifah, D. H., et al. (2023). Biocontrol of soil borne diseases by plant growth promoting rhizobacteria. Trop. Plant Pathol. 48 (2), 105–127. doi: 10.1007/s40858-022-00544-7
Abd El-Daim, I. A., Bejai, S., Meijer, J. (2019). Bacillus velezensis 5113 induced metabolic and molecular reprogramming during abiotic stress tolerance in wheat. Sci. Rep. 9 (1), 16282. doi: 10.1038/s41598-019-52567-x
Ahkami, A. H., White, R. A., III, Handakumbura, P. P., Jansson, C. (2017). Rhizosphere engineering: enhancing sustainable plant ecosystem productivity. Rhizosphere 3, 233–243. doi: 10.1016/j.rhisph.2017.04.012
Akiyoshi, D. E., Regier, D. A., Gordon, M. P. (1987). Cytokinin production by Agrobacterium and Pseudomonas spp. J Bacteriol. 169 (9), 4242–4248. doi: 10.1128/jb.169.9.4242-4248.1987
Aneela, R.İ.A.Z., Rafique, M., Aftab, M., Qureshi, M. A., Javed, H., Mujeeb, F., et al. (2019). Mitigation of salinity in chickpea by Plant Growth Promoting Rhizobacteria and salicylic acid. Eurasian J. @ Soil Sci. 8 (3), 221–228. doi: 10.18393/ejss.560745
Anisha, C., Mathew., J., Radhakrishnan, E. K. (2013). Plant growth promoting properties of endophytic Klebsiella sp. isolated from Curcuma longa. Int. J. Biol. Pharm. Allied Sci. 2 (3), 593–601.
Annapurna, K., Kumar, A., Kumar, L. V., Govindasamy, V., Bose, P., Ramadoss, D. (2013). “PGPR-Induced Systemic Resistance (ISR) in Plant Disease Management,” in Bacteria in Agrobiology: Disease Management. Ed. Maheshwari, D. K.(Berlin, Heidelberg: Springer), 405–425. doi: 10.1007/978-3-642-33639-3_15
Arora, N., Khare, E., Verma, A., Sahu, R. (2008). In vivo control of Macrophomina phaseolina by a chitinase and β-1,3-glucanase- producing Pseudomonad NDN1. Symbiosis 46, 129–135.
Asgher, M., Khan, M. I. R., Anjum, N. A., Khan, N. A. (2015). Minimising toxicity of cadmium in plants—role of plant growth regulators. Protoplasma 252, 399–413. doi: 10.1007/s00709-014-0710-4
Aswathy, A. J., Jasim, B., Jyothis, M., Radhakrishnan, E. K. (2013). Identification of two strains of Paenibacillus sp. as indole 3 acetic acid-producing rhizome-associated endophytic bacteria from Curcuma longa. 3 Biotech. 3, 219–224. doi: 10.1007/s13205-012-0086-0
Atzorn, R., Crozier, A., Wheeler, C. T., Sandberg, G. (1988). Production of gibberellins and indole-3-acetic acid by Rhizobium phaseoli in relation to nodulation of Phaseolus vulgaris roots. Planta 175, 532–538. doi: 10.1007/BF00393076
Barazani, O., Friedman, J. (1999). Is IAA the major root growth factor secreted from plant-growth-mediating bacteria? J. Chem. Ecol. 25, 2397–2406. doi: 10.1023/A:1020890311499
Bastián, F., Cohen, A., Piccoli, P., Luna, V., Bottini, R., Baraldi, R., et al. (1998). Production of indole-3-acetic acid and gibberellins A1 and A3 by Acetobacter diazotrophicus and Herbaspirillum seropedicae in chemically-defined culture media. Plant Growth Regul. 24, 7–11. doi: 10.1023/A:1005964031159
Ben Rhouma, M., Kriaa, M., Ben Nasr, Y., Mellouli, L., Kammoun, R. (2020). A new endophytic fusarium oxysporum gibberellic acid: optimization of production using combined strategies of experimental designs and potency on tomato growth under stress condition. BioMed. Res. Int. 2020, 1–14. doi: 10.1155/2020/4587148
Berendsen, R. L., Vismans, G., Yu, K., Song, Y., De Jonge, R., Burgman, W. P., et al. (2018). Disease-induced assemblage of a plant-beneficial bacterial consortium. ISME J. 12, 1496–1507. doi: 10.1038/s41396-018-0093-1
Bhatt, K., Maheshwari, D. K. (2020). Zinc solubilizing bacteria (Bacillus megaterium) with multifarious plant growth promoting activities alleviates growth in Capsicum annuum L. 3 Biotech. 10 (2), 36. doi: 10.1007/s13205-019-2033-9
Bhattacharyya, P. N., Jha, D. K. (2012). Plant growth-promoting rhizobacteria (PGPR): emergence in agriculture. World J. Microbiol. Biotechnol. 28, 1327–1350. doi: 10.1007/s11274-011-0979-9
Borah, P., Gogoi, N., Asad, S. A., Rabha, A. J., Farooq, M. (2023). An insight into plant growth-promoting rhizobacteria-mediated mitigation of stresses in plant. J. Plant Growth Regul. 42 (5), 3229–3256. doi: 10.1007/s00344-022-10787-y
Bukhat, S., Imran, A., Javaid, S., Shahid, M., Majeed, A., Naqqash, T. (2020). Communication of plants with microbial world: Exploring the regulatory networks for PGPR mediated defense signaling. Microbiol. Res. 238, 126486. doi: 10.1016/j.micres.2020.126486
Buysens, S., Heungens, K., Poppe, J., Hofte, M. (1996). Involvement of pyochelin and pyoverdin in suppression of Pythium-induced damping-off of tomato by Pseudomonas aeruginosa 7NSK2. Appl. Environ. Microbiol. 62 (3), 865–871. doi: 10.1128/aem.62.3.865-871.1996
Çakmakçı, R., Mosber, G., Milton, A. H., Alatürk, F., Ali, B. (2020). The effect of auxin and auxin-producing bacteria on the growth, essential oil yield, and composition in medicinal and aromatic plants. Curr. Microbiol. 77, 564–577. doi: 10.1007/s00284-020-01917-4
Camara, M. C., Vandenberghe, L. P. S., Rodrigues, C., de Oliveira, J., Faulds, C., Bertrand, E., et al. (2018). Current advances in gibberellic acid (GA3) production, patented technologies and potential applications. Planta 248, 1049–1062. doi: 10.1007/s00425-018-2959-x
Cha, J.-Y., Han, S., Hong, H.-J., Cho, H., Kim, D., Kwon, Y., et al. (2016). Microbial and biochemical basis of a Fusarium wilt-suppressive soil. ISME J. 10, 119–129. doi: 10.1038/ismej.2015.95
Chandra, P., Rai, A. K., Sundha, P., Basak, N., Kaur, H. (2022). “Rhizospheric Soil–Plant-Microbial Interactions for Abiotic Stress Mitigation and Enhancing Crop Performance,” in Environmental Science and Engineering. Eds. Shit, P. K., Adhikary, P. P., Bhunia, G. S., Sengupta, D. (Cham: Springer International Publishing), 593–614. doi: 10.1007/978-3-031-09270-1_26
Chandran, S. J. G. (2019). Isolation and characterization of N2 fixing bacteria from curcuma rhizosphere soil of western ghats. Int. J. Res. Anal. Rev. 6, 487–491.
Chauhan, A. K., Maheshwari, D. K., Dheeman, S., Bajpai, V. K. (2017). Termitarium-Inhabiting Bacillus spp. Enhanced Plant Growth and Bioactive Component in Turmeric (Curcuma longa L.). Curr. Microbiol. 74, 184–192. doi: 10.1007/s00284-016-1172-z
Chenniappan, C., Narayanasamy, M., Daniel, G. M., Ramaraj, G. B., Ponnusamy, P., Sekar, J., et al. (2019). Biocontrol efficiency of native plant growth promoting rhizobacteria against rhizome rot disease of turmeric. Biol. Control 129, 55–64. doi: 10.1016/j.biocontrol.2018.07.002
Chialva, M., Salvioli Di Fossalunga, A., Daghino, S., Ghignone, S., Bagnaresi, P., Chiapello, M., et al. (2018). Native soils with their microbiotas elicit a state of alert in tomato plants. New Phytol. 220, 1296–1308. doi: 10.1111/nph.15014
Clemens, S. (2014). Zn and fe biofortification: The right chemical environment for human bioavailability. Plant Sci. 225, 52–57. doi: 10.1016/j.plantsci.2014.05.014
Cortleven, A., Schmülling, T. (2015). Regulation of chloroplast development and function by cytokinin. J. Exp. Bot. 66, 4999–5013. doi: 10.1093/jxb/erv132
Damodaran, T., Sharma, D. K., Mishra, V. K., Jha, S. K., Kannan, R., Sah, V., et al. (2013). Isolation of Salt Tolerant Endophyticand Rhizospheric Bacteria by Natural Selection and screening for promising plant growth promoting Rhizobacteria [PGPR] and growth vigour in Tomato under sodic soil. Afr. J. Microbiol. Res. 7 (44), 5082–5089. doi: 10.5897/AJMR2013.6003
Darzi, M. T., Shirkhodaei, M., Haj SeyedHadi, M. R. (2015). Effects of vermicompost and nitrogen fixing bacteria on seed yield, yield components of seed and essential oil content of coriander (Coriandrum sativum). J. Med. Plants By-products 4 (1), 103–109. doi: 10.22092/jmpb.2015.108897
Dashti, N., Zhang, F., Hynes, R., Smith, D. L. (1998). Plant growth promoting rhizobacteria accelerate nodulation and increase nitrogen fixation activity by field grown soybean [Glycine max (L.) Merr.] under short season conditions. Plant Soil 200, 205–213. doi: 10.1023/A:1004358100856
Davies, P. J. (2004). Plant hormones: biosynthesis, signal transduction, action, 3rd ed (Dordrecht Boston: Kluwer Academic).
Dessaux, Y., Grandclément, C., Faure, D. (2016). Engineering the Rhizosphere. Trends in Plant Science 21 (3), 266–278. doi: 10.1016/j.tplants.2016.01.002
Dhondge, H. V., Pable, A. A., Barvkar, V. T., Dastager, S. G., Nadaf, A. B. (2021). Rhizobacterial consortium mediated aroma and yield enhancement in basmati and non-basmati rice (Oryza sativa l.). J. Biotechnol. 328, 47–58. doi: 10.1016/j.jbiotec.2021.01.012
Dinesh, R., Srinivasan, V., Praveena, R., Subila, K. P., George, P., Das, A., et al. (2022). Exploring the potential of P solubilizing rhizobacteria for enhanced yield and quality in turmeric (Curcuma longa L.). Ind. Crops Prod. 189, 115826. doi: 10.1016/j.indcrop.2022.115826
Dutta, S. C., Neog, B. (2015). Effects of AM fungi and plant growth-promoting Rhizobacteria on enzymatic activities of soil under turmeric (Curcuma longa L.) cultivation. J. Indian Soc Soil Sci. 63, 442–448. doi: 10.5958/0974-0228.2015.00059.6
El-Sheikh, M. A., Rajaselvam, J., Abdel-Salam, E. M., Vijayaraghavan, P., Alatar, A. A., Devadhasan Biji, G. (2020). Paecilomyces sp. ZB is a cell factory for the production of gibberellic acid using a cheap substrate in solid state fermentation. Saudi J. @ Biol. Sci. 27, 2431–2438. doi: 10.1016/j.sjbs.2020.06.040
Eshaghi, E., Nosrati, R., Owlia, P., Malboobi, M. A., Ghaseminejad, P., Ganjali, M. R. (2019). Zinc solubilization characteristics of efficient siderophore-producing soil bacteria. Iran. J. Microbiol. 11 (5), 419.
Fridlender, M., Inbar, J., Chet, I. (1993). Biological control of soilborne plant pathogens by a beta-1,3-glucanase-producing Pseudomonas cepacia. Soil Biol. Biochem. 5 (9), 1211–1221. doi: 10.1016/0038-0717(93)90217-Y
Garcia, J., Kao-Kniffin, J. (2018). Microbial group dynamics in plant rhizospheres and their implications on nutrient cycling. Front. Microbiol. 9. doi: 10.3389/fmicb.2018.01516
García de Salamone, I. E., Hynes, R. K., Nelson, L. M. (2001). Cytokinin production by plant growth promoting rhizobacteria and selected mutants. Can. J. Microbiol. 47 (5), 404–411. doi: 10.1139/w01-029
Ghosh, S. K., Banerjee, S., Sengupta, C. (2017). Bioassay, characterization and estimation of siderophores from some important antagonistic fungi. J. Biopestic 10 (2), 105–112. doi: 10.57182/jbiopestic.10.2.105-112
Ghosh, S. K., Bera, T., Chakrabarty, A. M. (2020). Microbial siderophore–A boon to agricultural sciences. Biol. Control 144, 104214. doi: 10.1016/j.biocontrol.2020.104214
Glick, B. R. (2014). Bacteria with ACC deaminase can promote plant growth and help to feed the world. Microbiol. Res. 169, 30–39. doi: 10.1016/j.micres.2013.09.009
Goteti, P. K., Emmanuel, L. D. A., Desai, S., Shaik, M. H. A. (2013). Prospective zinc solubilising bacteria for enhanced nutrient uptake and growth promotion in maize (Zea mays L.). Int. J. Microbiol. 2013, 869697. doi: 10.1155/2013/869697
Großkinsky, D. K., Tafner, R., Moreno, M. V., Stenglein, S. A., García de Salamone, I. E., Nelson, L. M., et al. (2016). Cytokinin production by Pseudomonas fluorescens G20-18 determines biocontrol activity against Pseudomonas syringae in Arabidopsis. Sci. Rep. 6 (1), 1–11. doi: 10.1038/srep23310
Gul, N., Wani, I. A., Mir, R. A., Nowshehri, J. A., Aslam, S., Gupta, R., et al. (2023). Plant growth promoting microorganisms mediated abiotic stress tolerance in crop plants: A critical appraisal. Plant Growth Regul. 100 (1), 7–24. doi: 10.1007/s10725-022-00951-5
Gunarathne, V., Mayakaduwa, S., Ashiq, A., Weerakoon, S. R., Biswas, J. K., Vithanage, M. (2019). “Transgenic plants In,” in Transgenic plant technology for remediation of toxic metals and metalloids, vol. pp. (Elsevier), 89–102. doi: 10.1016/B978-0-12-814389-6.00005-5
Gupta, S., Kaul, S., Singh, B., Vishwakarma, R. A., Dhar, M. K. (2016). Production of Gentisyl Alcohol from Phoma herbarum Endophytic in Curcuma longa L. and Its Antagonistic Activity Towards Leaf Spot Pathogen Colletotrichum gloeosporioides. Appl. Biochem. Biotechnol. 180, 1093–1109. doi: 10.1007/s12010-016-2154-0
Gupta, S., Pandey, S. (2023). “Plant Growth Promoting Rhizobacteria to Mitigate Biotic and Abiotic Stress in Plants,” in Sustainable Agriculture Reviews, vol. 60 . Eds. Singh, N., Chattopadhyay, A., Lichtfouse, E. (Cham: Springer). doi: 10.1007/978-3-031-24181-9_3
Gupta, G., Parihar, S. S., Ahirwar, N. K., Snehi, S. K., Singh, V. (2015). Plant growth promoting rhizobacteria (PGPR): current and future prospects for development of sustainable agriculture. J. Microb. Biochem. Technol. 7, 096–102. doi: 10.4172/1948-5948.1000188
Gururani, M. A., Upadhyaya, C. P., Baskar, V., Venkatesh, J., Nookaraju, A., Park, S. W. (2013). Plant growth-promoting rhizobacteria enhance abiotic stress tolerance in Solanum tuberosum through inducing changes in the expression of ROS-scavenging enzymes and improved photosynthetic performance. J. Plant Growth Regul. 32, 245–258. doi: 10.1007/s00344-012-9292-6
Gutiérrez-Mañero, F. J., Ramos-Solano, B., Probanza, A., Mehouachi, J., R. Tadeo, F., Talon, M. (2001). The plant-growth-promoting rhizobacteria Bacillus pumilus and Bacillus licheniformis produce high amounts of physiologically active gibberellins. Physiol. Plant 111, 206–211. doi: 10.1034/j.1399-3054.2001.1110211.x
Hakim, S., Naqqash, T., Nawaz, M. S., Laraib, I., Siddique, M. J., Zia, R., et al. (2021). Rhizosphere engineering with plant growth-promoting microorganisms for agriculture and ecological sustainability. Front. Sustain. Food Syst. 5. doi: 10.3389/fsufs.2021.617157
Hameeda, B., Harini, G., Rupela, O. P., Wani, S. P., Reddy, G. (2008). Growth promotion of maize by phosphate-solubilizing bacteria isolated from composts and macrofauna. Microbiol. Res. 163 (2), 234–242. doi: 10.1016/j.micres.2006.05.009
Hariprasad, P., NIranjana, S. R. (2009). Isolation and characterization of phosphate solubilizing rhizobacteria to improve plant health of tomato. Plant Soil 316, 13–24. doi: 10.1007/s11104-008-9754-6
Haselwandter, K., Passler, V., Reiter, S., Schmid, D. G., Nicholson, G., Hentschel, P., et al. (2006). Basidiochrome – A novel siderophore of the orchidaceous mycorrhizal fungi ceratobasidium and Rhizoctonia spp. Biometals 19, 335–343. doi: 10.1007/s10534-006-6986-x
Hassan, M. K., McInroy, J. A., Kloepper, J. W. (2019). The interactions of rhizodeposits with plant growth-promoting rhizobacteria in the rhizosphere: A review. Agriculture 9 (7), 142. doi: 10.3390/agriculture9070142
Hedden, P., Sponsel, V. (2015). A century of gibberellin research. J. Plant Growth Regul. 34, 740–760. doi: 10.1007/s00344-015-9546-1
Hu, L., Robert, C. A. M., Cadot, S., Zhang, X., Ye, M., Li, B., et al. (2018). Root exudate metabolites drive plant-soil feedbacks on growth and defense by shaping the rhizosphere microbiota. Nat. Commun. 9, 2738. doi: 10.1038/s41467-018-05122-7
Hussein, K. A., Joo, J. H. (2014). Potential of siderophore production by bacteria isolated from heavy metal: polluted and rhizosphere soils. Curr. Microbiol. 68, 717–723. doi: 10.1007/s00284-014-0530-y
Hyder, S., Rizvi, Z. F., Santos-Villalobos, S. de l., Santoyo, G., Gondal, A., Khalid, N., et al. (2023). Applications of plant growth-promoting rhizobacteria for increasing crop production and resilience. J. Plant Nutr. 46, 2551–2580. doi: 10.1080/01904167.2022.2160742
Iqbal, M. A., Khalid, M., Zahir, Z. A., Ahmad, R. (2015). Auxin producing plant growth promoting rhizobacteria improve growth, physiology and yield of maize under saline field conditions. Int. J. Agric. Biol. 18, 37–45. doi: 10.17957/IJAB/15.0059
Iqbal, M., Naveed, M., Sanaullah, M., Brtnicky, M., Hussain, M. I., Kucerik, J., et al. (2023). Plant microbe mediated enhancement in growth and yield of canola (Brassica napus L.) plant through auxin production and increased nutrient acquisition. J. Soils Sediments 23, 1233–1249. doi: 10.1007/s11368-022-03386-7
Iqbal, S., Wang, X., Mubeen, I., Kamran, M., Kanwal, I., Díaz, G. A., et al. (2022). Phytohormones trigger drought tolerance in crop plants: outlook and future perspectives. Front. Plant Sci. 12. doi: 10.3389/fpls.2021.799318
Isa, N. K. M., Mat Don, M. (2014). Investigation of the gibberellic acid optimization with a statistical tool from Penicillium variabile in batch reactor. Prep. Biochem. Biotechnol. 44, 572–585. doi: 10.1080/10826068.2013.844707
Islam, M., Hashidoko, Y., Deora, A., Ito, T., Tahara, S. (2005). Suppression of Damping-Off Disease in Host Plants by the Rhizoplane Bacterium Lysobacter sp. Strain SB-K88 Is Linked to Plant Colonization and Antibiosis against Soil borne Peronosporomycetes. Appl. Environ. Microbiol. 71, 3786–3796. doi: 10.1128/AEM.71.7.3786-3796.2005
Jagtap, R. R., Mali, G. V., Waghmare, S. R., Nadaf, N. H., Nimbalkar, M. S., Sonawane, K. D. (2023). Impact of plant growth promoting rhizobacteria Serratia nematodiphila RGK and Pseudomonas plecoglossicida RGK on secondary metabolites of turmeric rhizome. Biocatal. Agric. Biotechnol. 47, 102622. doi: 10.1016/j.bcab.2023.102622
Jakkula, V. S., Wani, S. P. (2018). Zeolites: Potential soil amendments for improving nutrient and water use efficiency and agriculture productivity. Sci. Rev. Chem. Commun. 8 (1), 1–15.
Jalgaonwala, R. E., Mahajan, R. T. (2014). Production of anticancer enzyme asparaginase from endophytic Eurotium Sp. isolated from rhizomes of Curcuma longa. Eur. J. Exp. Biol. 4, 36–43.
Jiang, H., Qi, P., Wang, T., Chi, X., Wang, M., Chen, M., et al. (2019). Role of halotolerant phosphate-solubilising bacteria on growth promotion of peanut (Arachis hypogaea) under saline soil. Ann. Appl. Biol. 174 (1), 20–30. doi: 10.1111/aab.12473
Jogawat, A., Yadav, B., Lakra, N., Singh, A. K., Narayan, O. P. (2021). Crosstalk between phytohormones and secondary metabolites in the drought stress tolerance of crop plants: A review. Physiol. Plant 172, 1106–1132. doi: 10.1111/ppl.13328
Kamran, S., Shahid, I., Baig, D. N., Rizwan, M., Malik, K. A., Mehnaz, S. (2017). Contribution of zinc solubilizing bacteria in growth promotion and zinc content of wheat. Front. Microbiol. 8. doi: 10.3389/fmicb.2017.02593
Karadeniz, A., Topcuoğlu, Ş, İnan, S. (2006). Auxin, gibberellin, cytokinin and abscisic acid production in some bacteria. World J. Microbiol. Biotechnol. 22, 1061–1064. doi: 10.1007/s11274-005-4561-1
Karthika, S., Midhun, S. J., Jisha, M. S. (2020). A potential antifungal and growth-promoting bacterium Bacillus sp. KTMA4 from tomato rhizosphere. Microb. Pathog. 142, 104049. doi: 10.1016/j.micpath.2020.104049
Khalimi, K., Suprapta, D. (2011). Induction of plant resistance against Soybean stunt virus using some formulations of Pseudomonas aeruginosa. Science 17 (1), 98–105.
Khan, A. G. (2005). Role of soil microbes in the rhizospheres of plants growing on trace metal contaminated soils in phytoremediation. J. Trace Elem. Med. Biol. 18, 355–364. doi: 10.1016/j.jtemb.2005.02.006
Khanna, K., Jamwal, V. L., Sharma, A., Gandhi, S. G., Ohri, P., Bhardwaj, R., et al. (2019). Supplementation with plant growth promoting rhizobacteria (PGPR) alleviates cadmium toxicity in Solanum lycopersicum by modulating the expression of secondary metabolites. Chemosphere 230, 628–639. doi: 10.1016/j.chemosphere.2019.05.072
KIrankumar, R., Jagadeesh, K. S., Krishnaraj, P. U., Patil, M. S. (2008). Enhanced growth promotion of tomato and nutrient uptake by plant growth promoting rhizobacterial isolates in presence of tobacco mosaic virus pathogen. Karnataka J. Agric. Sci. 21, 309–311.
Kloepper, J. W., Tuzun, S., Kuć, J. A. (1992). Proposed definitions related to induced disease resistance. Biocontrol Sci. Technol. 2 (4), 349–351. doi: 10.1080/09583159209355251
Kudoyarova, G. R., Vysotskaya, L. B., Arkhipova, T. N., Kuzmina, L., Galimsyanova, N. F., Sidorova, L. V., et al. (2017). Effect of auxin producing and phosphate solubilizing bacteria on mobility of soil phosphorus, growth rate, and P acquisition by wheat plants. Acta Physiol. Plant 39, 253. doi: 10.1007/s11738-017-2556-9
Kumar, A., Singh, R., Giri, D. D., Singh, P. K., Pandey, K. D. (2014). Effect of Azotobacter chroococcum CL13 inoculation on growth and curcumin content of turmeric (Curcuma long a L.). Int. J. Curr. Microbiol. Appl. Sci. 3, 275–283.
Kumar, A., Singh, A. K., Kaushik, M. S., Mishra, S. K., Raj, P., Singh, P. K., et al. (2017). Interaction of turmeric (Curcuma longa L.) with beneficial microbes: a review. 3 Biotech. 7, 357. doi: 10.1007/s13205-017-0971-7
Kumar, A., Singh, R., Yadav, A., Giri, D. D., Singh, P. K., Pandey, K. D. (2016a). Isolation and characterization of bacterial endophytes of Curcuma longa L. 3 Biotech. 6, 1–8. doi: 10.1007/s13205-016-0393-y
Kumar, S., Suyal, D. C., Yadav, A., Shouche, Y., Goel, R. (2019). Microbial diversity and soil physiochemical characteristic of higher altitude. PloS One 14 (3), e0213844. doi: 10.1371/journal.pone.0213844
Kumar, A., Vandana, Singh, M., Singh, P. P., Singh, S. K., Singh, P. K., et al. (2016b). Isolation of plant growth promoting rhizobacteria and their impact on growth and curcumin content in Curcuma longa L. Biocatal. Agric. Biotechnol. 8, 1–7. doi: 10.1016/j.bcab.2016.07.002
Kumar, P., Aeron, A., Shaw, N., Singh, A., Bajpai, V. K., Pant, S., et al. (2020). Seed bio-priming with tri-species consortia of phosphate solubilizing rhizobacteria (PSR) and its effect on plant growth promotion. Heliyon 6 (12). doi: 10.1016/j.heliyon.2020.e05701
Lanteigne, C., Gadkar, V. J., Wallon, T., Novinscak, A., Filion, M. (2012). Production of DAPG and HCN by pseudomonas sp. LBUM300 contributes to the biological control of bacterial canker of tomato. Phytopathology 102, 967–973. doi: 10.1094/PHYTO-11-11-0312
Lenin, G., Jayanthi, M. (2010). Indole acetic acid, gibberellic acid and siderophore production by PGPR isolates from rhizospheric soils of catharanthus roseus. Int. J. Pharm. Biol. Arch. 3 (4), 933–938.
Li, H. B., Singh, R. K., Singh, P., Song, Q. Q., Xing, Y. X., Yang, L. T., et al. (2017). Genetic diversity of nitrogen-fixing and plant growth promoting Pseudomonas species isolated from sugarcane rhizosphere. Front. Microbiol. 8. doi: 10.3389/fmicb.2017.01268
Liu, Y., Chen, L., Zhang, N., Li, Z., Zhang, G., Xu, Y., et al. (2016). Plant-microbe communication enhances auxin biosynthesis by a root-associated bacterium, bacillus amyloliquefaciens SQR9. Mol. Plant Mirobe. Interact. 29, 324–330. doi: 10.1094/MPMI-10-15-0239-R
Liu, F., Xing, S., Ma, H., Du, Z., Ma., B. (2013). Cytokinin-producing, plant growth-promoting rhizobacteria that confer resistance to drought stress in Platycladus orientalis container seedlings. Appl. Microbiol. Biotechnol. 97, 9155–9164. doi: 10.1007/s00253-013-5193-2
Loper, J. E., Gross, H. (2007). Genomic analysis of antifungal metabolite production by Pseudomonas fluorescens Pf-5. Eur. J. Plant Pathol. 119, 265–278. doi: 10.1007/s10658-007-9179-8
Ludwig-Müller, J. (2015). Plants and endophytes: equal partners in secondary metabolite production. Biotechnol. Lett. 37, 1325–1334. doi: 10.1007/s10529-015-1814-4
Lugtenberg, B., Kamilova, F. (2009). Plant-growth-promoting rhizobacteria. Annu. Rev. Microbiol. 63, 541–556. doi: 10.1146/annurev.micro.62.081307.162918
MaChado, C. M. M., Oliveira, B. H., Pandey, A., Soccol, C. R. (2000). “Coffee Husk as Substrate for the Production of Gibberellic Acid by Fermentation,” in Coffee Biotechnology and Quality. Eds. Sera, T., Soccol, Carlos, R., Pandey, A., Roussos, S. (Dordrecht: Springer Netherlands), 401–408. doi: 10.1007/978-94-017-1068-8_37
Madhaiyan, M., Poonguzhali, S., Ryu, J., Sa, T. (2006). Regulation of ethylene levels in canola (Brassica campestris) by 1-aminocyclopropane-1-carboxylate deaminase-containing Methylobacterium fujisawaense. Planta 224, 268–278. doi: 10.1007/s00425-005-0211-y
Maheshwari, D. K., Dheeman, S., Agarwal, M. (2015). “Phytohormone-Producing PGPR for Sustainable Agriculture,” in Bacterial Metabolites in Sustainable Agroecosystem, Sustainable Development and Biodiversity. Ed. Maheshwari, D. K. (Cham: Springer International Publishing), 159–182. doi: 10.1007/978-3-319-24654-3_7
Maksimov, I. V., Abizgil’dina, R. R., Pusenkova, L. I. (2011). Plant growth promoting microorganisms as alternative to chemical protection from pathogens. Prikl Biokhim Mikrobiol 47, 373–385. doi: 10.1134/S0003683811040090
Malik, K. A., Bilal, R., Mehnaz, S., Rasul, G., Mirza, M. S., Ali, S. (1997). “Association of nitrogen-fixing, plant-growth-promoting rhizobacteria (PGPR) with kallar grass and rice,” in Opportunities for Biological Nitrogen Fixation in Rice and Other Non-Legumes: Papers presented at the Second Working Group Meeting of the Frontier Project on Nitrogen Fixation in Rice held at the National Institute for Biotechnology and Genetic Engineering (NIBGE) (Faisalabad, Pakistan: Springer Netherlands), 37–44.
Martínez, C., Espinosa-Ruiz, A., Prat, S. (2016). “Gibberellins and plant vegetative growth,” in Annual Plant Reviews, vol. 49 . Eds. Hedden, P., Thomas, S. G. (United Kingdom: John Wiley & Sons, Ltd, Chichester, UK), 285–322. doi: 10.1002/9781119210436.ch10
Mathew, J. (2013). Indigenous aromatic and spice plants described in Van Rheed’s Hortus Indici Malabarici. Indian J. Appl. Res. 3, 30–33. doi: 10.15373/2249555X/NOV2013/10
Mellidou, I., Ainalidou, A., Papadopoulou, A., Leontidou, K., Genitsaris, S., Karagiannis, E., et al. (2021). Comparative transcriptomics and metabolomics reveal an intricate priming mechanism involved in PGPR-mediated salt tolerance in tomato. Front. Plant Sci. 12. doi: 10.3389/fpls.2021.713984
Milner, J. L., Raffel, S. J., Lethbridge, B. J., Handelsman, J. (1995). Culture conditions that influence accumulation of zwittermicin A by Bacillus cereus UW85. Appl. Microbiol. Biotechnol. 43, 685–691. doi: 10.1007/BF00164774
Milner, J. L., Silo-Suh, L., Lee, J. C., He, H., Clardy, J., Handelsman, J. (1996). Production of kanosamine by Bacillus cereus UW85. Appl. Environ. Microbiol. 62, 3061–3065. doi: 10.1128/aem.62.8.3061-3065.1996
Mishra, B. K., Dubey, P. N., Aishwath, O. P., Kant, K., Sharma, Y. K., Vishal, M. K. (2017). Effect of plant growth promoting rhizobacteria on coriander (Coriandrum sativum) growth and yield under semi-arid condition of India. Indian J. Agric. Sci. 87 (5), 607–612. doi: 10.56093/ijas.v87i5.70126
Morrissey, J., Guerinot, M. L. (2009). Iron uptake and transport in plants: the good, the bad, and the ionome. Chem. Rev. 109 (10), 4553–4567. doi: 10.1021/cr900112r
Mukhtar, T., Rehman, S. U., Smith, D., Sultan, T., Seleiman, M. F., Alsadon, A. A., et al. (2020). Mitigation of heat stress in Solanum lycopersicum L. by ACC-deaminase and exopolysaccharide producing Bacillus cereus: effects on biochemical profiling. Sustainability 12 (6), 2159. doi: 10.3390/su12062159
Müller, M., Munné-Bosch, S. (2015). Ethylene response factors: A key regulatory hub in hormone and stress signaling. Plant Physiol. 169, 32–41. doi: 10.1104/pp.15.00677
Mun, B. G., Lee, W. H., Kang, S. M., Lee, S. U., Lee, S. M., Lee, D. Y., et al. (2020). Streptomyces sp. LH 4 promotes plant growth and resistance against Sclerotinia sclerotiorum in cucumber via modulation of enzymatic and defense pathways. Plant Soil 448, 87–103. doi: 10.1007/s11104-019-04411-4
Murphy, C. J., Baggs, E. M., Morley, N., Wall, D. P., Paterson, E. (2015). Rhizosphere priming can promote mobilisation of N-rich compounds from soil organic matter. Soil Biol. Biochem. 81, 236–243. doi: 10.1016/j.soilbio.2014.11.027
Mustafa, S., Kabir, S., Shabbir, U., Batool, R. (2019). Plant growth promoting rhizobacteria in sustainable agriculture: from theoretical to pragmatic approach. Symbiosis 78, 115–123. doi: 10.1007/s13199-019-00602-w
Nair, K. P. (2013). The Agronomy and Economy of Turmeric and Ginger The Invaluable Medicinal Spice Crops (London: Elsevier). doi: 10.1016/C2011-0-07514-2
Nair, K. P. (2019). Turmeric (Curcuma longa L.) and Ginger (Zingiber officinale Rosc.) - World’s Invaluable Medicinal Spices (Switzerland: Springer Nature). doi: 10.1007/978-3-030-29189-1
Nandakumar, R., Babu, S., Viswanathan, R., Sheela, J., Raguchander, T., Samiyappan, R. (2001). A new bio-formulation containing plant growth promoting rhizobacterial mixture for the management of sheath blight and enhanced grain yield in rice. Bio Control 46, 493–510. doi: 10.1023/A:1014131131808
Orozco-Mosqueda, Ma. d. C., Glick, B. R., Santoyo, G. (2020). ACC deaminase in plant growth-promoting bacteria (PGPB): An efficient mechanism to counter salt stress in crops. Microbiol. Res. 235, 126439. doi: 10.1016/j.micres.2020.126439
Osugi, A., Sakakibara, H. (2015). Q&A: How do plants respond to cytokinins and what is their importance. BMC Biol. 13 (1), 1–10. doi: 10.1186/s12915-015-0214-5
Pandey, S., Gupta, S. (2020). Evaluation of Pseudomonas sp. for its multifarious plant growth promoting potential and its ability to alleviate biotic and abiotic stress in tomato (Solanum lycopersicum) plants. Sci. Rep. 10, 20951. doi: 10.1038/s41598-020-77850-0
Pandey, K. D., Patel, A. K., Singh, M., Vandana, Kumari, A., Jalaluddin (2020). “Secondary metabolites from bacteria and viruses,” in Natural Bioactive Compounds: Technological Advancements. Eds. Sinha, R. P., Häder, D.P.B.T.N.B.C (Academic Press), 19–40. doi: 10.1016/B978-0-12-820655-3.00002-1
Paredes-Mendoza, M., Espinosa-Victoria, D. (2010). Organic acids produced by phosphate solubilizing rhizobacteria: a critical review. Terra Latinoamericana 28 (1), 61–70.
Patel, J. S., Yadav, S. K., Bajpai, R., Teli, B., Rashid, M. (2020). “PGPR secondary metabolites: an active syrup for improvement of plant health,” in Molecular Aspects of Plant Beneficial Microbes in Agriculture. Eds. Sharma, V., Salwan, R., Al-Ani, T. (Academic Press), 195–208. doi: 10.1016/b978-0-12-818469-1.00017-1
Piromyou, P., Buranabanyat, B., Tantasawat, P., Tittabutr, P., Boonkerd, N., Teaumroong, N. (2011). Effect of plant growth promoting rhizobacteria (PGPR) inoculation on microbial community structure in rhizosphere of forage corn cultivated in Thailand. Eur. J. Soil Biol. 47 (1), 44–54. doi: 10.1016/j.ejsobi.2010.11.004
Praveena, R., Srekha, K., Revathy, R., Srinivasan, V., Sarathambal, C., George, P., et al. (2022). New rhizobacteria strains with effective antimycotic compounds against rhizome rot pathogens and identification of genes encoding antimicrobial peptides. Rhizosphere 22, 100515. doi: 10.1016/j.rhisph.2022.100515
Probanza, A., Lucas Garcıía, J. A., Ruiz Palomino, M., Ramos, B., Gutiérrez Mañero, F. J. (2002). Pinus pinea L. seedling growth and bacterial rhizosphere structure after inoculation with PGPR Bacillus (B. licheniformis CECT 5106 and B. pumilus CECT 5105). Appl. Soil Ecol. 20, 75–84. doi: 10.1016/S0929-1393(02)00007-0
Quint, M., Gray, W. M. (2006). Auxin signaling. Curr. Opin. Plant Biol. 9, 448–453. doi: 10.1016/j.pbi.2006.07.006
Qureshi, M. A., Shahzad, H., Saeed, M. S., Ullah, S., Ali, M. A., Mujeeb, F., et al. (2019). Relative potential of rhizobium species to enhance the growth and yield attributes of cotton (Gossypium hirsutum L.). Eurasian J. Soil Sci. 8, 159–166. doi: 10.18393/ejss.544747
Raaijmakers, J. M., Vlami, M., de Souza, J. T. (2002). Antibiotic production by bacterial biocontrol agents. Antonie van Leeuwenhoek 81, 537–547. doi: 10.1023/A:1020501420831
Ramamoorthy, V., Raguchander, T., Samiyappan, R. (2002). Enhancing resistance of tomato and hot pepper to pythium diseases by seed treatment with fluorescent pseudomonads. Eur. J. Plant Pathol. 108, 429–441. doi: 10.1023/A:1016062702102
Ramkumar, T. R., Karuppusamy, S. (2021). Plant Diversity and Ethnobotanical Knowledge of Spices and Condiments. In Upadhyay, S. K., Singh, S. P. (Eds). Bioprospecting of Plant Biodiversity for Industrial Molecules (Wiley), 231–260. doi: 10.1002/9781119718017.ch12
Reed, S. C., Yang, X., Thornton, P. E. (2015). Incorporating phosphorus cycling into global modeling efforts: a worthwhile, tractable endeavor. New Phytol. 208, 324–329. doi: 10.1111/nph.13521
Richardson, A. E., Barea, J. M., McNeill, A. M., Prigent-Combaret, C. (2009). Acquisition of phosphorus and nitrogen in the rhizosphere and plant growth promotion by microorganisms. Plant Soil 321, 305–339. doi: 10.1007/s11104-009-9895-2
Rodrigues, C., Vandenberghe, L. P., de, S., de Oliveira, J., Soccol, C. R. (2012). New perspectives of gibberellic acid production: a review. Crit. Rev. Biotechnol. 32, 263–273. doi: 10.3109/07388551.2011.615297
Sahu, G. K., Sindhu, S. S. (2011). Disease control and plant growth promotion of green gram by siderophore producing pseudomonas sp. Res. J. @ Microbiol. 6, 735–749. doi: 10.3923/jm.2011.735.749
Santoro, M. V., Cappellari, L. R., Giordano, W., Banchio, E. (2015). Plant growth-promoting effects of native Pseudomonas strains on Mentha piperita (peppermint): an in vitro study. Plant Biol. J. 17, 1218–1226. doi: 10.1111/plb.12351
Santoro, M. V., Zygadlo, J., Giordano, W., Banchio, E. (2011). Volatile organic compounds from rhizobacteria increase biosynthesis of essential oils and growth parameters in peppermint (Mentha piperita). Plant Physiol. Biochem. 49, 1177–1182. doi: 10.1016/j.plaphy.2011.07.016
Saraf, M., Jha, C. K., Patel, D. (2010). “The Role of ACC Deaminase Producing PGPR in Sustainable Agriculture,” in Plant Growth and Health Promoting Bacteria, Microbiology Monographs. Ed. Maheshwari, D. K. (Berlin, Heidelberg: Springer Berlin Heidelberg), 365–385. doi: 10.1007/978-3-642-13612-2_16
Saravanakumar, D., Samiyappan, R. (2007). ACC deaminase from Pseudomonas fluorescens mediated saline resistance in groundnut (Arachis hypogea) plants. J. Appl. Microbiol. 102, 1283–1292. doi: 10.1111/j.1365-2672.2006.03179.x
Saravanan, V. S., Kumar, M. R., Sa, T. ,. M. (2011). “Microbial zinc solubilization and their role on plants,” in Bacteria in Agrobiology: Plant Nutrient Management. Ed. Maheshwari, D. K. (Berlin: Springer), 47–63. doi: 10.1007/978-3-642-21061-7_3
Schaller, G. E., Bishopp, A., Kieber, J. J. (2015). The yin-yang of hormones: cytokinin and auxin interactions in plant development. Plant Cell 27, 44–63. doi: 10.1105/tpc.114.133595
Schenk, P. M., Carvalhais, L. C., Kazan, K. (2012). Unraveling plant–microbe interactions: can multi-species transcriptomics help? Trends Biotechnol. 30 (3), 177–184. doi: 10.1016/j.tibtech.2011.11.002
Schlaeppi, K., Bulgarelli, D. (2015). The plant microbiome at work. Mol. Plant Microbe Interact. 212, 212–217. doi: 10.1094/MPMI-10-14-0334-FI
Schrettl, M., Ibrahim-Granet, O., Droin, S., Huerre, M., Latgé, J.-P., Haas, H. (2010). The crucial role of the Aspergillus fumigatus siderophore system in interaction with alveolar macrophages. Microbes Infect. 12, 1035–1041. doi: 10.1016/j.micinf.2010.07.005
Septiana, E., Sukarno, N., Simanjuntak, P. (2017). Endophytic fungi associated with turmeric (Curcuma longa L.) can inhibit histamine-forming bacteria in fish. HAYATI J. Biosci. 24, 46–52. doi: 10.1016/j.hjb.2017.05.004
Shameer, S., Prasad, T. (2018). Plant growth promoting rhizobacteria for sustainable agricultural practices with special reference to biotic and abiotic stresses. Plant Growth Regul. 84, 603–615. doi: 10.1007/s10725-017-0365-1
Sharma, S., Sharma, A., Kaur, M. (2018). Extraction and evaluation of gibberellic acid from Pseudomonas sp.: Plant growth promoting rhizobacteria. J. Pharmacogn. Phytochem. 7, 2790–2795.
Sharma, P., Sharma, M. M. M., Malik, A., Vashisth, M., Singh, D., Kumar, R., et al. (2021). “Rhizosphere, rhizosphere biology, and rhizospheric engineering,” in Plant growth-promoting microbes for sustainable biotic and abiotic stress management (Cham: Springer International Publishing), 577–624.
Shen, X., Hu, H., Peng, H., Wang, W., Zhang, X. (2013). Comparative genomic analysis of four representative plant growth-promoting rhizobacteria in Pseudomonas. BMC Genomics 14, 271. doi: 10.1186/1471-2164-14-271
Singh, P. (2023). Plant growth promoting-rhizobacteria (PGPR): their potential as biofertilizer and biopesticide agents: A review. Asian J. Adv. Agric. Res. 22 (1), 25–37. doi: 10.9734/ajaar/2023/v22i1431
Singh, D., Rathod, V., Ninganagouda, S., Hiremath, J., Singh, A. K., Mathew, J. (2014). Optimization and characterization of silver nanoparticle by endophytic fungi Penicillium sp. isolated from Curcuma longa (Turmeric) and application studies against MDR E. coli and S. aureus. Bioinorg. Chem. Appl. 2014, 408021. doi: 10.1155/2014/408021
Singh, P. P., Shin, Y. C., Park, C. S., Chung, Y. R. (1999). Biological control of fusarium wilt of cucumber by chitinolytic bacteria. Phytopathology 89, 92–99. doi: 10.1094/PHYTO.1999.89.1.92
Singh, R. K., Singh, P., Li, H. B., Song, Q. Q., Guo, D. J., Solanki, M. K., et al. (2020). Diversity of nitrogen-fixing rhizobacteria associated with sugarcane: a comprehensive study of plant-microbe interactions for growth enhancement in Saccharum spp. BMC Plant Biol. 20, 1–21. doi: 10.1186/s12870-020-02400-9
Sokolova, M. G., Akimova, G. P., Vaishlya, O. B. (2011). Effect of phytohormones synthesized by rhizosphere bacteria on plants. Appl. Biochem. Microbiol. 47, 274–278. doi: 10.1134/S0003683811030148
Srithaworn, M., Jaroenthanyakorn, J., Tangjitjaroenkun, J., SuriyaChadkun, C., Chunhachart, O. (2023). Zinc solubilizing bacteria and their potential as bioinoculant for growth promotion of green soybean (Glycine max L. Merr.). Peer J. 11, e15128. doi: 10.7717/peerj.15128
Stringlis, I. A., Yu, K., Feussner, K., De Jonge, R., Van Bentum, S., Van Verk, M. C., et al. (2018). MYB72-dependent coumarin exudation shapes root microbiome assembly to promote plant health. Proc. Natl. Acad. Sci. U.S.A 115 (22), E5213–E5222. doi: 10.1073/pnas.1722335115
Subrahmanyam, G., Kumar, A., Sandilya, S. P., Chutia, M., Yadav, A. N. (2020). “Diversity, Plant Growth Promoting Attributes, and Agricultural Applications of Rhizospheric Microbes,”. Eds. Yadav, A. N., Singh, J., Rastegari, A. A., Yadav, N. Plant Microbiomes for Sustainable Agriculture (Cham, Switzerland: Springer International Publishing) Vol. 25, 1–52. doi: 10.1007/978-3-030-38453-1_1
Suman, J., Rakshit, A., Ogireddy, S. D., Singh, S., Gupta, C., Chandrakala, J. (2022). Microbiome as a key player in sustainable agriculture and human health. Front. Soil Sci. 2. doi: 10.3389/fsoil.2022.821589
Sundaram, N. M., Murali, S. R. (2018). Isolation and characterization of bacteria from rhizospheric soils of Curcuma longa for different plant growth promotion (PGPR) activities, World J. Pharm. Res. 7, 692–700. doi: 10.20959/wjpr201813-12743
Sureshbabu, K., Amaresan, N., Kumar, K. (2016). Amazing multiple function properties of plant growth promoting rhizobacteria in the rhizosphere soil. Int. J. Curr. Microbiol. Appl. Sci. 5, 661–683. doi: 10.20546/ijcmas.2016.502.074
Tabassum, B., Khan, A., Tariq, M., Ramzan, M., Khan, M. S. I., Shahid, N., et al. (2017). Bottlenecks in commercialization and future prospects of PGPR. Appl. Soil Ecol. 121, 102–117. doi: 10.1016/j.apsoil.2017.09.030
Tahir, M., Sarwar, M. A. (2013). Plant Growth promoting rhizobacteria (PGPR): A budding complement of synthetic fertilizers for improving crop production. Pak. J. Life Soc Sci. 11, 1–7.
Tian, F., Ding, Y., Zhu, H., Yao, L., Du, B. (2009). Genetic diversity of siderophore-producing bacteria of tobacco rhizosphere. Braz. J. Microbiol. 40, 276–284. doi: 10.1590/S1517-838220090002000013
Timmusk, S., Abd El-Daim, I. A., Copolovici, L., Tanilas, T., Kännaste, A., Behers, L., et al. (2014). Drought-tolerance of wheat improved by rhizosphere bacteria from harsh environments: enhanced biomass production and reduced emissions of stress volatiles. PLoS One 9, e96086. doi: 10.1371/journal.pone.0096086
Tiwari, S., Lata, C., Chauhan, P. S., Nautiyal, C. S. (2016). Pseudomonas putida attunes morphophysiological, biochemical and molecular responses in Cicer arietinum L. during drought stress and recovery. Plant Physiol. Biochem. 99, 108–117. doi: 10.1016/j.plaphy.2015.11.001
Tsukanova, K. A., Сhеbоtаr, V.К., Meyer, J. J. M., Bibikova, T. N. (2017). Effect of plant growth-promoting Rhizobacteria on plant hormone homeostasis. S. Afr. J. Bot. 113, 91–102. doi: 10.1016/j.sajb.2017.07.007
United Nations Population Fund. (2022). Available at: https://www.unfpa.org/data/world-population-dashboard (Accessed May 1, 2023).
Vaid, S. K., Kumar, B., Sharma, A., Shukla, A. K., Srivastava, P. C. (2014). Effect of Zn solubilizing bacteria on growth promotion and Zn nutrition of rice. J. Soil Sci. Plant Nutr. 14 (4), 889–910. doi: 10.4067/S0718-95162014005000071
Villegas-Cornelio, V. M., Laines Canepa, J. R. (2017). Vermicompostaje: I avances y estrategias en el tratamiento de residuos sólidos orgánicos. Rev. Mexicana Cienc. Agrícolas 8 (2), 393–406. doi: 10.29312/remexca.v8i2.59
Vinayarani, G., Madhusudhan, K. N., Prakash, H. S. (2019). Induction of systemic resistance in turmeric by rhizospheric isolate Trichoderma asperellum against rhizome rot disease. J. Plant Pathol. 101, 965–980. doi: 10.1007/s42161-019-00303-9
Vinayarani, G., Prakash, H. S. (2018). Growth promoting rhizospheric and endophytic bacteria from Curcuma longa L. As biocontrol agents against rhizome rot and leaf blight diseases. Plant Pathol. J. 34, 218–235. doi: 10.5423/PPJ.OA.11.2017.0225
Viswanathan, R., Ramasamy, S. (2001). Role of chitinases in Pseudomonas spp. induced systemic resistance against Colletotrichum falcatum in sugarcane. Indian Phytopathol. 54, 418–423.
Wang, K. L.-C., Li, H., Ecker, J. R. (2002). Ethylene biosynthesis and signaling networks. Plant Cell 14, S131–S151. doi: 10.1105/tpc.001768
Wani, P. A., Khan, M. S., Zaidi, A. (2007). Synergistic effects of the inoculation with nitrogen-fixing and phosphate-solubilizing rhizobacteria on the performance of field-grown chickpea. J. Plant Nutr. Soil Sci. 170 (2), 283–287. doi: 10.1002/jpln.200620602
World Population Prospects. (2022). Available at: https://population.un.org/wpp/Graphs/DemographicProfiles/Line/356 (Accessed May 1, 2023).
Yadegari, M., Rahmani, H. A., Noormohammadi, G., Ayneband, A. (2010). Plant growth promoting rhizobacteria increase growth, yield and nitrogen fixation in Phaseolus vulgaris. J. Plant Nutr. 33 (12), 1733–1743. doi: 10.1080/01904167.2010.503776
Yanti, Y., Hamid, H., & reflin development of the PGPR and cyanobacteria consortium for growth promotion and control ralstonia syzigii subsp. indonesiensis of tomato. IOP Conf. Series: Earth Environ. Sci. 709 (1), 12085. doi: 10.1088/1755-1315/709/1/012085
Zarei, T., Moradi, A., Kazemeini, S. A., Akhgar, A., Rahi, A. A. (2020). The role of ACC deaminase producing bacteria in improving sweet corn (Zea mays L. varsaccharata) productivity under limited availability of irrigation water. Sci. Rep. 10, 20361. doi: 10.1038/s41598-020-77305-6
Zhang, X., Zhang, D., Sun, W., Wang, T. (2019). The adaptive mechanism of plants to iron deficiency via iron uptake, transport, and homeostasis. Int. J. Mol. Sci. 20, 2424. doi: 10.3390/ijms20102424
Keywords: plant-microbe interactions, plant growth-promotion, rhizospheirc association, microbiota, biotic and abiotic stress, secondary metabolites
Citation: Khan S, Ambika, Rani K, Sharma S, Kumar A, Singh S, Thapliyal M, Rawat P, Thakur A, Pandey S, Thapliyal A, Pal M and Singh Y (2023) Rhizobacterial mediated interactions in Curcuma longa for plant growth and enhanced crop productivity: a systematic review. Front. Plant Sci. 14:1231676. doi: 10.3389/fpls.2023.1231676
Received: 30 May 2023; Accepted: 21 July 2023;
Published: 24 August 2023.
Edited by:
Ziliang Zhang, University of Illinois at Urbana-Champaign, United StatesReviewed by:
Bhupinder Jatana, Clemson University, United StatesYonglei Jiang, Yunnan Academy of Tobacco Agricultural Sciences, China
Yongping Kou, Chinese Academy of Sciences (CAS), China
Copyright © 2023 Khan, Ambika, Rani, Sharma, Kumar, Singh, Thapliyal, Rawat, Thakur, Pandey, Thapliyal, Pal and Singh. This is an open-access article distributed under the terms of the Creative Commons Attribution License (CC BY). The use, distribution or reproduction in other forums is permitted, provided the original author(s) and the copyright owner(s) are credited and that the original publication in this journal is cited, in accordance with accepted academic practice. No use, distribution or reproduction is permitted which does not comply with these terms.
*Correspondence: Yashaswi Singh, eWFzaGFzd2kuc2luZ2hAZ21haWwuY29t; WWFzaGFzd2lzaW5naC5idEBnZXUuYWMuaW4=
†These authors have contributed equally to this work